the Creative Commons Attribution 4.0 License.
the Creative Commons Attribution 4.0 License.
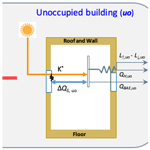
Revising the definition of anthropogenic heat flux from buildings: role of human activities and building storage heat flux
Yiqing Liu
Buildings are a major source of anthropogenic heat emissions, impacting energy use and human health in cities. The difference in magnitude and time lag between building energy consumption and building anthropogenic heat emission is poorly quantified. Energy consumption (QEC) is a widely used proxy for the anthropogenic heat flux from buildings (QF,B). Here we revisit the latter's definition. If QF,B is the heat emission to the outdoor environment from human activities within buildings, we can derive it from the changes in energy balance fluxes between occupied and unoccupied buildings. Our derivation shows that the difference between QEC and QF,B is attributable to a change in the storage heat flux induced by human activities (ΔSo-uo) (i.e. ). Using building energy simulations (EnergyPlus) we calculate the energy balance fluxes for a simplified isolated building (obtaining QF,B, QEC, ΔSo-uo) with different occupancy states. The non-negligible differences in diurnal patterns between QF,B and QEC are caused by thermal storage (e.g. hourly QF,B to QEC ratios vary between −2.72 and 5.13 within a year in Beijing, China). Negative QF,B can occur as human activities can reduce heat emission from a building but this is associated with a large storage heat flux. Building operations (e.g. opening windows, use of space heating and cooling system) modify the QF,B by affecting not only QEC but also the ΔSo-uo diurnal profile. Air temperature and solar radiation are critical meteorological factors explaining day-to-day variability of QF,B. Our new approach could be used to provide data for future parameterisations of both anthropogenic heat flux and storage heat fluxes from buildings. It is evident that storage heat fluxes in cities could also be impacted by occupant behaviour.
- Article
(5385 KB) - Full-text XML
-
Supplement
(4393 KB) - BibTeX
- EndNote
Human activities that influence energy exchanges are critical to a wide variety of disciplines (e.g. meteorology, building design, geography, climatology, hydrology, engineering). As disciplines often have interests in different scales, purposes and/or boundary conditions, the terminology and acceptable assumptions differ. However, disciplines may provide data to each other or help improve assumptions used. In this study we are concerned with the interface between meteorology, climatology and building design in urban areas.
To model the weather and climate in urban areas, an important additional source of energy to the environment is the anthropogenic heat flux (QF). This is defined as the heat converted from consumption of biological, chemical and electrical energy and released to the atmosphere due to human activities (Oke et al., 2017). The QF has three major sources, including metabolic (people and animals) activities (QF,M), transport (QF,T) and buildings (QF,B) (Grimmond, 1992). It can be large relative to incoming solar radiation in summer (e.g. 43 % in an area of Beijing, Nie et al. (2014)) and increases air temperature in cities (e.g. Ichinose et al., 1999; Fan and Sailor, 2005), subsequently contributing to higher cooling demand for buildings (Santamouris et al., 2001; Takane et al., 2019). In winter QF can contribute to the intensity of the urban heat island (Biggart et al., 2021). Not all heat generated within the building volume is directly ejected into the outdoor environment immediately but subject to change in magnitude and time lag. For example, the heat generated from human activities inside buildings is released initially indoors (via heating or cooling application), then transported through the building fabric by conduction, allowing it to be transported into the atmosphere by turbulent sensible heat flux and outgoing longwave radiation. In this process the net storage heat flux (ΔQS) of a building is modified since the building fabric temperature is changed by absorbing more heat from the internal heat generation.
In urban areas, ΔQS is the net uptake or release of energy from urban volume. This term is an important determinant of urban climate and is regarded as a key process in the genesis of urban heat island (Goward, 1981). The change in building ΔQS is modified when heat is released by human activities but the timing of the external emissions are impacted by the building fabric characteristics and the conduction process. As prior studies often used energy consumption (QEC) as a proxy for QF,B, derived from inventory-related approaches (e.g. Sailor and Lu, 2004; Iamarino et al., 2012) and building energy modelling (e.g. Heiple and Sailor, 2008; Nie et al., 2014), the impact on ΔQS is not addressed. To qualify the “real” QF,B and change of ΔQS, we revisit the definition of QF,B and attempt to understand how human activities affect the energy balance fluxes of buildings.
If QF,B is the heat released from buildings into the atmosphere as a result of human activities inside the building (including human metabolism), when the building is completely unoccupied (e.g. no operational appliances, no people: such as “ghost cities” in China (Shepard, 2015) or vacant in Dublin, (Kelly and Scott, 2018)), then QF,B is zero. However, heat released from the unoccupied building is non-zero as there is still heat exchange between the building and the ambient environment (see Eq. 1 and 2), as occurs in other environments with large mass, such as forests (e.g. Oliphant et al., 2004), and rocks (e.g. Wang et al., 2018). The QF,B differs from building heat emission (BHE) (e.g. Hong et al., 2020; Ferrando et al., 2021) as the latter is the total heat flux released from buildings to the ambient air () not due to human activities alone. Shortwave and longwave radiation can enter the unoccupied internal building space through windows and conduction through walls. It modifies the heat stored within the building volume and the temperature of the building envelope and indoor air, subsequently influencing the emission of heat via sensible heat flux, outgoing longwave radiation and air exchange. However, this energy leaving the unoccupied building is not anthropogenic heat flux. For an occupied building, the internal heat gain arises from
-
the equivalent sources and sinks as the unoccupied buildings; but also
-
the energy linked to the indoor human activities (metabolism, powered appliances and energy inputs to heating or cooling).
These will modify each of the energy balance fluxes. Some of this additional energy is transported out of buildings through indoor-outdoor ventilation exchange and/or heating ventilation and air conditioning (HVAC) systems, immediately contributes to QF,B, while some is stored in the building fabric, and later is released outdoors through various pathways (convection, radiation, conduction) to become QF,B with a time lag. Here, we derive QF,B by looking at the difference of heat fluxes between occupied and unoccupied buildings.
If the energy balance for the building system (including the indoor air and building envelope) for an unoccupied dry building (assuming latent heat is not important in this case) is
The radiation balance for an isolated unoccupied (uo) building can be expressed as:
where Q∗ is the net all-wave radiation, K is the shortwave radiation incoming (↓) and outgoing (↑) to the external surfaces. The longwave (L) radiation exchanges depend on the view factors (F) between the building of interest (boi), the surrounding facets of other surfaces or buildings (other b) and the sky:
In Eq. (1), QH is the turbulent sensible heat flux (convection) from external surfaces to the external ambient air and QBAE is the net energy exchange from the buildings through air exchange (e.g. ventilation). When the building is sealed QBAE is 0 W m−2, otherwise (e.g. open windows, cracks) it can be a source or sink of energy (environment ← building, or inverse). The ΔQS is the net storage heat flux of the building volume (i.e. fabric, contents, including the air). The left-hand side (LHS) of Eq. (1) is the inputs or source of energy to the building, whereas the right-hand side (RHS) is the sink or energy dissipation outputs. With no human activities within the building, the internal heat generation from human and infrastructure activities is zero.
When the building is occupied (o) (e.g. appliances operating, people present), additional terms are needed in Eq. (1) to account for the supply of energy into the building for these activities and the release of energy:
The two additional sources of energy (LHS) are
-
QInternal, o: energy released within the building from lighting, powered appliances and metabolism (e.g. people, pets).
-
QHVAC, o: energy consumption in the building from heating, ventilation and air conditioning (HVAC) systems.
As the building may emit exhaust or waste heat (e.g. via HVAC systems), there is an additional sink (RHS) referred to here as QWaste, o. The cooling system, QWaste, o will remove energy from both anthropogenic (e.g. metabolism, lighting, electrical appliance and QHVAC, o) and natural sources (e.g. solar radiation through windows, heat diffusion through building envelope). Thus, only the natural sources occur in both the occupied and unoccupied states. In a “simple” occupied state, with HVAC operated only (i.e. no people or other appliances) there is a difference in the building storage heat flux because of the alternative route to transport this natural heat of the building out from additional source of energy.
Here QH only represents the convection heat transfer at building external surface (i.e. wall, roof and windows). Both QWaste, and QBAE will be incorporated into the turbulent sensible heat flux by the time they reach the inertial sub-layer (ISL) or constant flux layer (CFL). Hence, sensors (e.g. eddy covariance or large aperture scintillometry) located in the ISL would observe this as QH. The separation of these three terms is necessary for a better understanding of how human activities (e.g. open/closed windows, HVAC operation) influence each heat flux. Urban canopy parameterisation (UCP) can use this information about the separate sources and their roles in the urban energy balance to account for the modified fluxes by the time they reach the ISL. Additionally, it is clearer for multi-layer UCP where the energy should enter vertically.
To determine the impact of the occupancy (i.e. not just the physical building form) we can consider the difference between Eqs. (5) and (1). If the radiation balance for the occupied case is
we assume that the incoming and outgoing shortwave radiation remain unchanged because the reflectivity, transmissivity and absorptivity do not change by occupancy activities then:
The incoming longwave radiation is dependent on the surroundings, which are independent of the building state, so
Thus, the difference in radiative fluxes between occupied and unoccupied buildings () is
Similarly, the difference of the heat transfer through air exchange is
With the additional terms in Eq. (5) and the air exchange rate differences from the activities within the buildings, gives
As the change in surface temperature influences the sensible heat fluxes and storage heat fluxes:
By combining the Eqs. (1) and (5), we obtain
where the LHS accounts for the net available energy as a result of human activities in indoor environments and the RHS shows that these impact the longwave radiation, turbulent sensible and storage heat fluxes (in this dry case). With rearrangement:
The additional energy generation associated with human activities to the whole building system (LHS) is apparent, as traditionally defined as QF,B previously (Heiple and Sailor, 2008). Here, because the heat release from human metabolism indoors is considerably smaller than other sources, for simplicity of analysis we assume metabolic heat is also part of energy consumption (). In addition, some of additional energy is associated with the extra gain or release of stored heat within the building volume (ΔSo−uo). The rest is the heat released to the outdoor environment from the building due to human activities, which is the QF,B based on its definition:
Eq. (14) demonstrates that the QF,B is the relative heat emission at the exterior building boundary between unoccupied and occupied buildings through longwave radiation, convection, air exchange and waste heat from any mechanical heating/cooling system. The source of QF,B within the building volume gives (by combining Eqs. (13) and (14)
The sources of QF,B are from both energy consumption (QEC) and differences of storage heat flux (ΔSo-uo) between unoccupied and occupied buildings (QF,B) in this study includes part of QF,M from human metabolism). In most prior studies, the second term of Eq. (15) is ignored. Although the storage heat flux over a year should tend to zero, over short periods (e.g. sub-daily) ΔSo-uo is not zero causing time lag and magnitude difference between QF,B and QEC. Therefore, estimation of QF,B by differences in heat emission between occupied and unoccupied buildings can capture the impact of dynamic changes in the building storage heat flux especially in a sub-annual temporal cycle.
In this study, the objective is to understand the temporal profile of QF,B and how and why it differs from QEC at diurnal and seasonal time scales, by examining differences in energy balance fluxes between the same occupied and unoccupied building. A building energy simulation tool (EnergyPlus) is used to obtain the various energy balance fluxes from the building system.
2.1 Unoccupied (uo) and occupied (o) building energy simulation (BES)
Building energy simulation (BES) is widely used to estimate energy consumption, heat emission and heat storage within a building, while allowing changes in heat fluxes due to human activities to be estimated. Here we use EnergyPlus version 9.4 (DOE, 2020a) to study an isolated building (i.e. without a surrounding neighbourhood). The ASNI/ASHRAE standard 140 Case 900 test model (ASHRAE, 2017) is used, which is developed in software-to-software comparative tests for validating building thermal load. It is a 48 m2 one-story heavyweight rectangular prism with high mass fabrics (Fig. S1 in the Supplement), whose simple geometry is ideal to understand the process of how human activities change the building energy balance fluxes in a theoretical study.
Modifications of the original building model for this study, include: windows are reduced to one (6 m2 south-facing) for more appropriate EnergyPlus single-sided ventilation calculations (Daish et al., 2016) and internal heat gain, ventilation control strategy and HVAC system operation are varied with different scenarios considered (Table 1). For the simulations, the building is assumed to be located in Beijing as the climate has both hot summer and cold winter conditions. Chinese standard weather data (CSWD) selected to create a typical meteorological year (TMY) (China Meteorological Bureau et al., 2005) are used as the meteorological forcing, as these data were developed for simulating building thermal load and energy use.
Table 1Cases simulated differ based on building occupation state, internal heat gain (QInternal, o) and presence of natural ventilation and HVAC. Notation is defined in the text and nomenclature.
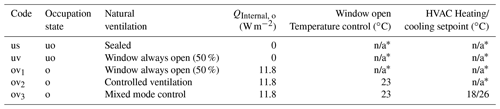
* n/a stands for not applicable.
The modelling scenarios (Table 1) vary with building occupation state. Two types of unoccupied (uo) buildings are considered. Neither have internal heat gains or HVAC systems, but they differ based on air exchange between (1) unoccupied sealed (us) with no infiltration nor ventilation, and (2) unoccupied ventilated (uv) with 50 % of window area kept open. The single-sided natural ventilation rate is estimated by including both the wind-driven ventilation rate (VW, m3 s−1) (Warren 1977):
and the stack buoyancy-driven ventilation rate (V, m3 s−1) (Warren 1977):
where Aeff is the effective opening area (m2), UW is the reference wind speed at the height of opening (m s−1). Cd is the discharge coefficient (usually taken as 0.6, Wang and Chen (2012)), ΔT is the indoor and outdoor air temperature difference (∘C), H is the height of opening (m), g the gravitational acceleration (m s−2) and Tave is the average indoor and outdoor air temperature (∘C). The combined ventilation rate is (Fan et al., 2021)
The three occupied (o) building simulations assume that occupant behaviour modifies internal heat generation, natural ventilation and HVAC systems (ov). First, ov1 has internal heat gains (QInternal, o) from human metabolism, lighting and other appliances based on local building code (MOHURD, 2018), with window always open (50 %, as uv). The internal heat gains are held constant allowing the fraction of heat in QF,B and ΔQS to be impacted by building and climate conditions but not the diurnal variability of human heat generation.
Second, ov2 considers natural ventilation based on passive cooling and thermal comfort. The window opening is controlled automatically. It is opened (50 % of window area) when the indoor air temperature is higher than both outdoor air temperature and the ventilation setting point (23∘C for “warm limit” in the bedroom (Oikonomou et al., 2012)). Otherwise, it is closed to reduce heat loss and keep the building warm. Third, since natural ventilation alone may not satisfy indoor thermal comfort, mixed mode ventilation with an auxiliary HVAC system (e.g. Wang and Chen, 2013; Wang and Greenberg, 2015; Chen et al., 2017) is considered in ov3. The mechanical heating and cooling systems are active when the indoor temperature reaches the threshold (18∘C for heating and 26∘C for cooling, MOHURD, 2018). The ventilation control strategy in ov3 is the same as ov2, but the EnergyPlus hybrid ventilation manager (DOE, 2020b) turns the HVAC off when natural ventilation is active to prevent simultaneous operation.
2.2 Determination of anthropogenic heat flux
The simulated hourly heat fluxes by radiation, convection, air exchange and waste heat generated from the HVAC system between the isolated building and the atmosphere (Table S3 in the Supplement) are analysed for each case (Table S2 in the Supplement). If cooling occurs, the waste heat consists of the cooling load and electrical energy consumed by the air conditioner (QHVAC). The QHVAC is predicted using a static coefficient of performance (COP) for the air conditioner, and the heat removed by an air conditioner (QAC) to the total amount of electricity consumed:
With a centralised heating system (as Beijing has), for simplicity we assume all energy associated with the heating system is released indoors, and waste heat due to boiler efficiency and pipe heat loss are not considered:
Combining mechanical heating and cooling, the energy consumption and corresponding waste heat from HVAC system gives
Each term in Eq. (14) is determined using an occupied (o) and unoccupied (uo) building result to determine QF,B and the other fluxes. The results are analysed by season as spring (March, April and May, MAM), summer (June, July, August, JJA), autumn (September, October, November, SON) and winter (December, January, February, DJF)) using the median (50 %) and interquartile range (IQR) between the 25th and 75th percentiles to assess the diurnal patterns.
2.3 Ratio of anthropogenic heat flux to energy consumption
If the energy consumed within the building is ejected immediately into the atmosphere (Heiple and Sailor, 2008), the change in ΔQS is not accounted for, and therefore QF,B is assumed to be only from energy consumption (QEC). The variation of ΔQS associated with human activities is considered when using the relative heat emissions in Eqs. (14) and (15). We use the ratio to determine the relative importance of building operation modes and choice of baselines on the discrepancy between QF,B and QEC.
Building energy balance fluxes vary through each day and season (Fig. 1) associated with when a building is occupied and people's activities inside the building. First, we consider one case in detail – an occupied building with both natural ventilation and HVAC (ov3, Table 1) relative to an unoccupied sealed building (us, Table 1) – their difference (ov3–us) allows us to obtain the fluxes needed (Sect. 1).
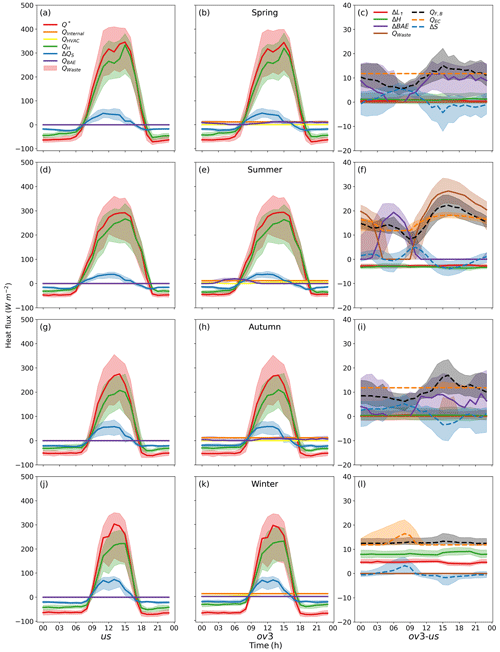
Figure 1Seasonal diurnal median (line) and interquartile range (IQR, shading) building heat fluxes for (a, d, g, j) unoccupied sealed (us), (b, e, h, k) occupied ventilated (ov3) building and their (c, f, i, l) difference (ov3–us) for (a–c) spring, (d–f) summer, (g–i) autumn and (j–l) winter. QF,B is estimated by either heat transfer difference (solid line components): in Eq. (14) or energy consumption and storage flux difference: (dashed line components) in Eq. (15)
As noted (Sect. 1), the shortwave and incoming longwave radiation fluxes for all cases (Table 1) are assumed to be identical, but all other terms of the building energy balance differ. Hence, the change in outgoing longwave radiation (ΔL↑o-uo, Fig. 1c) is equivalent to the net all-wave radiation difference (, Fig. 1a–b) for the occupied and unoccupied buildings. The positive sensible heat flux difference (Eq. 10, ΔHo-uo, Fig. 1c) and ΔL↑o-uo indicate the building is warmed up by internal heat gains (QInternal, o) with higher exterior surface temperatures. Their small magnitudes and flat patterns indicate small relative importance compared to the heat exchange from ventilation differences (Eq. 8, ΔBAEo-uo, Fig. 1c). The latter not only contributes the largest fraction of anthropogenic heat flux ( Fig. 1c), but also has a diurnal pattern consistent with QF,B, especially during spring and autumn (Fig. 1c, i). Rarely, heat (QWaste, o, Fig. 1i) is emitted by the air conditioner in the mid-afternoon (shading) at this time of year, but more importantly in summer (Fig. 1f) when cooling demand increases.
The QF,B (Eq. 14, Fig. 1c) has four components of emitted heat, whereas energy consumption (QEC, Fig. 1c) only has (in this case, constant) internal heat gains (QInternal, o=11.8 W m−2, Fig. 1b, Table 1) and energy use from HVAC systems (QHVAC, Fig. 1b). Their difference is the storage heat flux difference (Eq. 15 ΔSo-uo in Fig. 1c). If ΔSo-uo is positive, the building acts as a heat sink and stores the extra heat generated by human activities, or stored heat is released when ΔSo-uo is negative. Hence, we can identify the impacts of seasonal varying human activities and building operations on the diurnal variability in ΔSo-uo, QEC and QF,B.
3.1 Impact of human activities on seasonal and diurnal variations of the fluxes
For the same ov3–us case (Table 1, Fig. 1), we consider the diurnal and seasonal variability of the fluxes. In spring and autumn (Fig. 1a–c, g–i), natural ventilation is the dominant factor contributing to diurnal variation in ΔSo-uo and QF,B, while QEC has minimal variability. The QEC is slightly larger than QInternal, o because of some short periods of HVAC use in the mid-afternoon (IQR shading in Fig. 1i). There is a clear diurnal cycle of QF,B (Fig. 1c) with the median varying between 8 W m−2 (07:00) and 15 W m−2 (15:00) relative to the constant internal heat gain (11.8 W m−2). The difference between QF,B and QEC (ΔSo-uo) is largely impacted by natural ventilation. During the night and early morning with closed windows, only part of the consumed energy is transferred externally to the atmosphere. The rest of the heat is stored in the building fabric (positive ΔSo-uo), hence QF,B is lower than QEC. However, when overheating may occur during the middle of the day, occupants keep the window opened (air conditioner is less frequently used) to cool the building down, with stored heat released (negative ΔSo-uo). This is consistent with the diurnal variability of ΔBAEo-uo, which has a minimum at night (window closed) and maximum in the mid-afternoon (window open).
In summer, the daytime natural ventilation is replaced by air conditioning as natural ventilation alone could not maintain thermal comfort indoors. Natural ventilation and waste heat from the air conditioner (QWaste, o) contribute to one peak QF,B at nighttime and daytime, respectively (Fig. 1f). QF,B is higher than QEC around these two peak periods (05:00–07:00 and 13:00–21:00). The peak QF,B at night reaches 14 W m−2 (median) at 05:00, which is mainly attributed to natural ventilation when outdoor air temperature is cooler than indoors. Conversely, in the afternoon when outdoor temperature is warmer, occupants “choose” mechanical cooling to achieve thermal comfort. The peak QF,B is 22 W m−2 at 16:00, approximately 22 % higher than QEC. It indicates that using QEC for the anthropogenic heat flux from buildings (e.g. Heiple and Sailor, 2008) may underestimate the effect of QF,B on urban atmospheric processes especially during the late afternoon/early evening. In addition, QF,B is always smaller than QWaste, o because of the negative ΔL↑o-uo and ΔHo-uo causing a cooler exterior surface. This suggests using QWaste, o as QF,B (e.g. Chow et al., 2014) may overestimate QF,B in summer.
However, in winter mechanical heating and thermal mass effect shape the temporal pattern of QF,B (Fig. 1i). The cool outdoor air temperature before sunrise results in a substantial heating supply and peak QEC (16.43 W m−2 for median line) at 08:00. This heat is stored in building fabric (positive ΔSo-uo) and has a relatively stable release through convection and longwave radiation. Therefore the diurnal profile QF,B is rather flatter and ΔSo-uo has a highly consistent temporal pattern to QEC.
Overall, this analysis recognises the crucial role of ΔSo-uoin distinguishing QF,B from QEC, which is highly dependent on HVAC operation and natural ventilation (i.e. human activity of window opening). These two factors can rapidly increase or decrease QF,B while convection and longwave radiation cannot. Whereas in winter, the larger IQR (shading) of QF,B than QEC indicates more day-to-day variation in QF,B diurnal profile than QEC. Estimates of QF,B using satellite remote sensing found heat storage plays an important role in moderating energy use within buildings (Yu et al., 2021). As the storage heat flux change modifies the diurnal sensible heat flux pattern it modifies the surface temperature increment (QF,B in remote sensing approach) and hence the apparent energy consumption.
The diurnal profiles of ΔSo-uo are not identical between seasons as people use different actions to achieve thermal comfort in different weather conditions. This suggests that the QF,B and QEC differences may vary between climates and with cultural practices. In inventory methods the diurnal profiles may be limited, e.g. large scale urban consumption of energy (LUCY, Allen et al. (2011), weekday/weekend by country, and ignore seasonal variations. However, ΔSo-uo behaviour type classes may benefit from distinguishing diurnal variation for different climates.
3.2 Impact of different building operation modes on seasonal and diurnal variations
Figure 2 illustrates the impact of different building operation modes (Table 1: ov1, ov2, ov3; cf. us) on the QF,B diurnal profiles. It suggests that the different ventilation strategies and HVAC systems do change QF,B in both temporal pattern and magnitude, but their impacts vary among seasons.
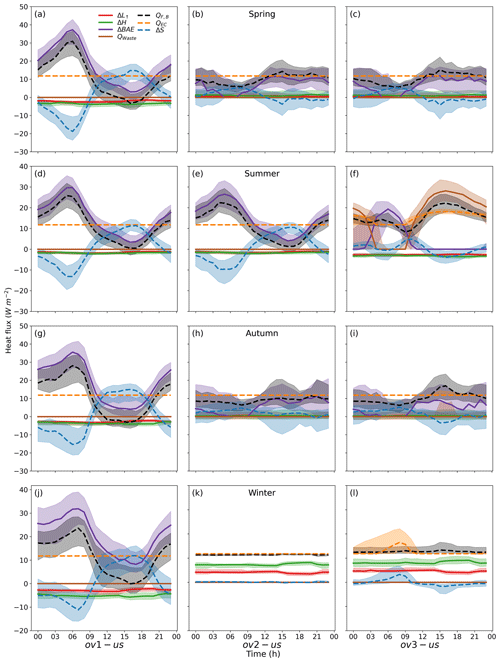
Figure 2As Fig. 1c, f, i, j , but comparing three different building operation types (a, d, g, j) ov1: window is always open without control, no HVAC; (b, e, h, k) ov2: controlled natural ventilation for indoor thermal comfort, no HVAC; (c, f, i, l) ov3: mixed mode ventilation.
In spring and autumn, different natural ventilation control strategies completely modify the QF,B diurnal profile, whereas a HVAC system only increases the peak QF,B slightly in autumn (Fig. 2i). The distinctly different (opposite) trend in diurnal QF,B pattern for ov1 cf. ov2 or ov3 (Fig. 2a–c, g–i) is largely explained by the diurnal change of ΔBAEo-uo in the three cases. In ov1 (window open, no control) the minimum outdoor air temperature before sunrise creates the maximum indoor and outdoor air temperature difference, therefore the highest ΔBAEo-uo and peak QF,B at 06:00 (30 W m−2 for the median in Fig. 2a). Whereas ov2 and ov3 have the window closed at night and early morning to avoid overcooling, therefore, the minimum QF,B is in the early morning (07:00). As outdoor air temperature increases through the day, QF,B follows the reduced ΔBAEo-uo in ov1, whereas natural ventilation is active in ov2 and ov3, leading to an increase in ΔBAEo-uo and QF,B. Unlike ov2, ov3 has a clear peak (16 W m−2 median, Fig. 2i) at 15:00, because natural ventilation alone cannot satisfy thermal comfort and ov3 air conditioning is activated. But their overall patterns (IQR) are very consistent, indicating afternoon use of air conditioning could increase QF,B magnitude but have a limited impact on other parts of the diurnal pattern. Surprisingly, negative QF,B occurs around 17:00 in spring (Fig. 2a), suggesting the occupied building has less heat emission than the unoccupied building, because the natural ventilation at night and morning cools the building down and reduced fabric exterior surface temperature leads to a larger reduction in longwave radiation and convection (ΔL↑o-uo and ΔHo-uo) than the increase in heat emission through natural ventilation (ΔBAEo-uo) in the afternoon. Also, the reduced overall emissions are converted into increase in storage heat flux (ΔSo-uo). Negative QF,B also occurs when the unoccupied building is always ventilated (uv) and the occupied building is ventilated with control (ov2 and ov3) in spring (e.g. Fig. S7b–c in the Supplement). The window is closed to avoid excessive cooling at night in ov2. With ΔBAEo-uo negative in this case, its magnitude is much larger than the increase in longwave radiation and convection ( and ΔHo-uo). The minimum QF,B frequently corresponds to the peak ΔSo-uo.
In summer, in ov2 the window is open most of the time (as in ov1) for thermal comfort, therefore, the QF,B has no apparent difference to ov1. However for ov3, as the air conditioning runs from morning to late night there is a very different diurnal profile (cf. ov2 and ov1). Air conditioner use contributes to a much larger QF,B (cf. ov2) from 12:00 to 21:00. Not only is extra energy consumed, but it also removes heat from the building to the atmosphere in this period. In contrast, using natural ventilation as a cooling strategy (ov1 and ov2) contributes to a high QF,B at night and early morning but very low even negative extra heat emission in the afternoon. This implies that natural ventilation as a passive cooling strategy could not only improve the thermal conditions indoors but could also contribute to the improvement of outdoor climate by modifying the diurnal pattern of anthropogenic heat emissions (Duan et al., 2019).
Consistent with results in the other seasons, different ventilation control strategies in winter cause a large change in the QF,B profile between ov1 and ov2. However, the temporal pattern of QF,B (IQR) in ov2 is quite similar to ov3 because the supplied heat from the mechanical heating system does not immediately enhance QF,B with a closed window. Ov2 is the only scenario that has similar QF,B and QEC through the whole day. Comparison using an unoccupied ventilated (uv) baseline (Fig. S7) (cf. us Fig. 2) show that although QF,B profiles differ, the impacts of different building operation modes are consistent when the same occupied buildings are used. The impact of baselines with different air exchange on QF,B are analysed in Sect. 3.3.
3.3 Impact of unoccupied baseline chosen
Here two unoccupied baselines (us – unoccupied sealed building, uv – unoccupied ventilated building with uncontrolled open window) are used to assess the impact. A ratio between QF,B and QEC (R) is used (Fig. 3) to normalise the impact of baselines on their difference with different building operation modes. The largest difference in R occurs on 23 December at 11:00, with values of 5.13 (ov3–uv) and −2.72 (ov1–us), reflecting the considerable difference between QF,B and QEC.
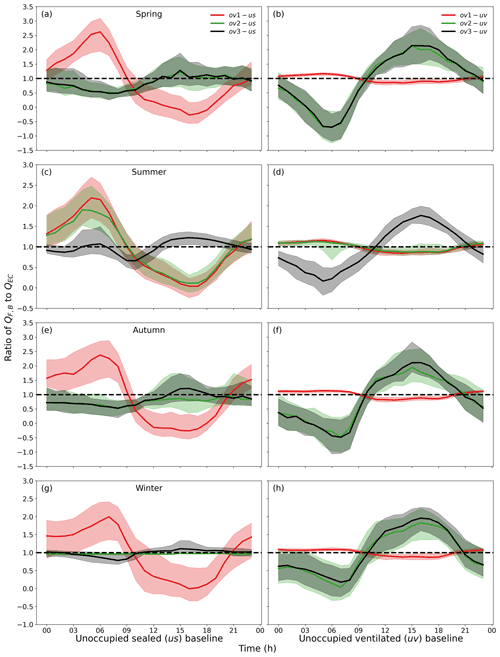
Figure 3QF,B to QEC ratio I median (line) and IQR (shading) for (a–b) spring, (c–d) summer, (e–f) autumn and (g–h) winter, using two unoccupied baselines: (a, c , e, g) sealed (us), and (b, d, f, h) ventilation (uv); each with three occupancy types (colour): ov1: only internal heat gains are applied and window is fully open; ov2: internal heat gains and natural ventilation control are applied. Ov3: internal heat gains, natural ventilation control and HVAC system are applied. Ratio R=1 (Black dotted line).
Two diurnal patterns of the R ratio are distinguished. When the window is always open (ov1 in all seasons, ov2 in summer), R>1 (QF,B>QEC) at night/early morning (22:00–08:00), reaching its maximum around 05:00–07:00 (near sunrise in all seasons). For the remaining periods, which are relatively warm R<1. Whereas, when window opening/closing is controlled and HVAC is used for thermal comfort an almost inverse temporal pattern of R occurs, with R>1 during the afternoon when either the window is open or the air conditioner is activated. The peak R occurs at 15:00 when both outdoor temperature and solar radiation are high.
When different unoccupied baselines are used, the temporal patterns of R are similar for all cases, but their magnitudes differ significantly. R is close to 1 when window states between unoccupied and occupied buildings are similar (e.g. ov1–uv in all seasons, ov2–uv in summer). Hence, a greater difference occurs in heat transfer from ventilation or mechanical heating/cooling between occupied and unoccupied buildings (i.e. larger R). Thus, the baseline chosen impacts the results and requires appropriate consideration for incorporating QF,B into atmospheric modelling.
3.4 Comparison between QF,B and building heat emission (BHE)
Comparison of building heat emissions (BHE), determined using the Hong et al. (2020) approach, to QF,B (this study) for one case (ov3–us) shows that the former is much larger than QF,B during the day but smaller at night and has different diurnal patterns (Fig. 4). Convection from the exterior envelope (QH, Fig. 1b, e, h, k) is the main contributor to BHE and therefore influences the BHE diurnal profile in each season. During the day, solar radiation is high and a major control whereas QF,B is relatively small and consistent but modified by building-human interactions (e.g. opening windows, activation of mechanical heating and cooling systems). In this scenario shown, natural ventilation and mechanical cooling dominate QF,B in summer and shoulder season (i.e. spring and autumn); while in winter in their absence, convection and longwave radiation are more important.
3.5 Daily variation of fluxes in relation to meteorological conditions
Ambient air temperature is one of the most crucial factors controlling building energy consumption (Sailor and Vasireddy, 2006). Hence, it is often used to determine daily variability of QEC (e.g. Lindberg et al., 2013) and the resulting monthly variations (e.g. Allen et al., 2011). By accounting for ΔSo-uo in this study, the response of QF,B to ambient air temperature may differ to previous studies. To examine this we used the ov3–us case to consider the relations of daily mean (unless indicated) variables of air temperature (mean) , solar radiation (daily total) and simulated available energy to the building from human activities (ΔBo-uo) with anthropogenic heat flux (QF,B in Fig. 5a), energy consumption (QEC in Fig. 5b) and their difference (ΔSo-uo in Fig. 5c).The overall trends between QF,B and QEC to ambient air temperature are consistent, with QF,B and QEC smallest when temperatures are between 10–15 ∘C. This coincides with the Nicol and Humphreys (2002) monthly balance-point temperature of 12 ∘C, which has been regarded as the equivalent ambient air temperature with the minimum energy use within the building (e.g. Allen et al., 2011, Koralegedara et al., 2016). As the temperature increases (decreases), QEC increases proportionally with temperature due to mechanical cooling (heating). However, in contrast to QEC, QF,B has a much larger variability at the same temperature caused by a large range of ΔSo-uo (−7.7 to 9.0 W m−2), which is highly dependent on human activities on the diurnal scale (Sect. 3.1).
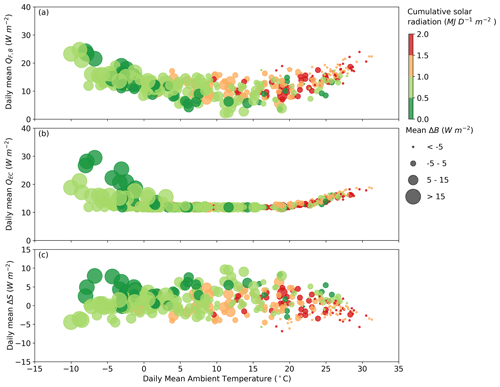
Figure 5Daily results for the ov3–us case stratified by daily cumulative solar radiation (colour) and daily mean available energy to the building (size) Eq. (9) associated with human activities, with mean external air (ambient) temperature and (a) mean anthropogenic heat flux, (b) energy consumption and (c) difference in storage heat flux.
To understand the large daily variability of ΔSo-uo, we use ΔBo-uo (net available energy from human activities in buildings in Eq. (9)) to indicate the effect of human activities (heat addition or removal) in one day. Higher ΔBo-uo (larger circles) are associated with higher ΔSo-uo at the same ambient air temperature, especially in winter (Fig. 5c). This is not unexpected as buildings will absorb more heat when extra internal energy is added into the building. Inversely, negative ΔBo-uo (small circles) contributes to much more heat release from heat storage (lower ΔSo-uo through either natural ventilation or mechanical cooling. The sign and magnitude of ΔBo-uo are linked to daily cumulative solar radiation. At the same ambient air temperature, more solar radiation enhances the need for larger heat removal or less heat addition to the building for thermal comfort, therefore leading to a smaller ΔBo-uo and lower ΔSo-uo. Consequently, we can conclude that both ambient air temperature and cumulative solar radiation are important meteorological factors to determine ΔSo-uo and QF,B.
Anthropogenic heat flux from buildings (QF,B) is defined as the additional heat released from the building into the atmosphere due to human activities. It is qualitatively and quantitatively different to building energy consumption (QEC) in temporal pattern and magnitude as a result of thermal inertia of the building (Iamarino et al., 2012). However, as there is no standard to quantify “real” QF,B most studies use QEC as a proxy via inventory and building energy modelling approaches. This paper proposes a new method to quantify a more appropriate QF,B by utilising the difference in heat fluxes between an occupied and unoccupied building (i.e. the built structure with absolutely no energy use and no human metabolism). We show that the difference between QEC and QF,B is attributable to a change in the storage heat flux induced by human activities (ΔSo-uo). QF,B has four components based on its dissipation pathways, including outgoing longwave radiation, turbulent sensible heat flux (convection), heat release due to air exchange and waste heat from HVAC systems. We use one simplified case study in Beijing to demonstrate the analysis using building energy simulations to quantify the temporal difference between QEC and QF,B and to understand the relative importance of building operations for thermal comfort and meteorological conditions on QF,B. The key conclusions are
-
Hourly ratios between QF,B and QEC can differ between −2.72 and 5.13 because of differences in occupancy use of the building (within a year, in Beijing's climate). Individual ratios frequently exceed 3 between 14:00 and 16:00 when controlled natural ventilation or mechanical cooling is activated in a shoulder season (i.e. spring and autumn). Thus, the differences in the definitions are large.
-
Natural ventilation (ΔBAEo-uo) or HVAC operation (QWaste, o for cooling and QHVAC for heating) are two predominant contributors to the storage heat flux. Hence, different building operations to control thermal comfort determine the diurnal profile of QF,B by affecting not only QEC but also ΔSo-uo.
-
The day-to-day variation of the QF,B diurnal profile is broader than that of QEC.
-
The diurnal profile of ΔSo-uo varies with season as occupants modify their behaviour and the interaction with buildings to achieve thermal comfort (e.g. cooling in summer and heating in winter), indicating that differences between QF,B and QEC will vary with both climate and cultural norms.
-
QF,B is sensitive to the unoccupied baseline chosen (here two are analysed unoccupied sealed vs unoccupied ventilated). An “unoccupied baseline” needs to be integrated into urban climate modelling in the future.
-
Daily mean temperature only accounts for the day-to-day variability in QEC rather than ΔSo-uo. Both ambient air temperature and cumulative solar radiation are important meteorological factors to determine ΔSo-uo and QF,B.
Our new approach should be used to provide data for future parameterisations of both anthropogenic heat flux from buildings and storage heat fluxes for urban weather and climate modelling. We conclude that storage heat fluxes in cities are also being modified by occupant behaviour, particularly by natural ventilation and mechanical cooling. It is expected that the diurnal variation of ΔSo-uo will vary with operation schedules for different building uses (e.g. residential vs. commercial buildings). Given that the release of stored heat has a critical influence on the nocturnal canopy layer urban heat island (CL-UHI), the impact of different HVAC operations on nocturnal UHI should be explored further. This is an important factor to determine diurnal pattern of QF,B in the shoulder season and can be expressed more accurately. However, in different climates and with different social cultural practices the periods most influenced will change. Further studies are being conducted to explore the impacts of these, while also addressing feedback at the neighbourhood scale.
For developers of urban canopy parameterisations (UCP) there are several considerations because of computational efficiencies which are essential for undertaking weather and climate modelling: (1) human activities within a building are modifying both the storage heat flux and the anthropogenic heat flux; (2) assuming within an UCP that a “simple” building energy model (BEM) (cf. a full building energy simulation scheme such as EnergyPlus) will require some human activities to be simplified, such as using a fixed ventilation rate, instead of dynamic natural ventilation depending on both outdoor weather conditions and thermal comfort requirements and (3) with a multi-layer UCP the appropriate levels for the impact of these energy exchanges can be accounted for. Our current research is extending this analysis to consider moisture and exploring the role of building materials, construction, other aspects of building design and external meteorology. The outcome of this work will also have implications for UCP development, as this can help identify what can be simplified and what are critical controls in different climates and urban settings.
Aeff | Effective area of window opening (m2) |
ΔBo-uo | Available energy to the building from human activities (W m−2) |
Δ BAEo-uo | Difference of heat transfer by air exchange between building and atmosphere between occupied (o) |
and unoccupied (uo) buildings (W m−2) | |
BHE | Building heat emission to ambient air (W m−2) |
ΔHo-uo | Difference in QH between occupied (o) and unoccupied (uo) buildings (W m−2) |
F[sky→boi] | View factor from sky to building of interest |
F[other b→boi] | View factor from other buildings to building of interest |
F[boi→sky] | View factor from building of interest to sky |
F[boi→other b] | View factor from building of interest to other buildings |
Cd | Discharge coefficient |
H | Height of window opening (m) |
K↑ | Outgoing shortwave radiation flux (W m−2) |
K↓ | Incoming shortwave radiation flux (W m−2) |
L↑ | Outgoing longwave radiation flux (W m−2) |
L↓ | Incoming longwave radiation flux (W m−2) |
ΔL↑o-uo | Difference in L↑ between occupied (o) and unoccupied (uo) buildings (W m−2) |
ΔQS | Net storage heat flux for the building volume (W m−2) |
Q∗ | Net all-wave radiation flux (W m−2) |
QAC | Sensible cooling load from air conditioning (W m−2) |
QBAE | Heat transfer by air exchange between building and atmosphere (W m−2) |
QF,B | Anthropogenic heat flux from building sector (W m−2) |
QF,M | Anthropogenic heat flux from metabolic activities (W m−2) |
QF,T | Anthropogenic heat flux from transport (W m−2) |
QH | Turbulent sensible heat flux (W m−2) |
QHS | Sensible heating load (W m−2) |
QHVAC | Energy consumption by heating ventilation and air conditioning (HVAC) system (W m−2) |
QInternal | Internal heat gain within the building (human metabolism, lighting and appliances) (W m−2) |
QWaste | Waste heat released to outdoor by HVAC system (W m−2) |
R | Ratio of anthropogenic heat flux from building (QF,B) to energy consumption (QEC) |
ΔSo-uo | Different in storage heat flux between occupied (o) and unoccupied (uo) buildings (W m−2) |
Tave | Average indoor and outdoor air temperatures (∘C) |
ΔT | Indoor and outdoor air temperature difference (∘C) |
UW | Reference wind speed at height of upstream airflow (m s−1) |
VStack | Buoyancy-driven ventilation rate (m3 s−1) |
VT | Total ventilation rate by combined wind and buoyancy effect |
VW | Wind-driven ventilation rate (m3 s−1) |
All data are deposited at https://doi.org/10.5281/zenodo.5903303 (Liu et al., 2022).
The supplement related to this article is available online at: https://doi.org/10.5194/acp-22-4721-2022-supplement.
Conceptualisation was done by SG and ZL, methods and analysis were performed by YL SG and ZL, the first draft and visualisation came from YL. YL, SG and ZL were responsible of writing and review submission. SG and ZL where in charge of the funding.
The contact author has declared that neither they nor their co-authors have any competing interests.
Publisher’s note: Copernicus Publications remains neutral with regard to jurisdictional claims in published maps and institutional affiliations.
This work has been funded as part of NERC-COSMA project (grant no. NE/S005889/1; ZL, SG), ERC urbisphere (grant no. 855005; SG) and Newton Fund/Met Office CSSP China Next Generation Cities (grant no. P107731; SG, ZL).
This work has been funded as part of NERC-COSMA project (grant no. NE/S005889/1; ZL, SG), ERC urbisphere (grant no. 855005; SG) and Newton Fund/Met Office CSSP China Next Generation Cities (grant no. P107731; SG, ZL).
This paper was edited by Stefano Galmarini and reviewed by Qi Li, Alberto Martilli and one anonymous referee.
Allen, L., Lindberg, F., and Grimmond, C. S. B.: Global to city scale urban anthropogenic heat flux: Model and variability, Int. J. Climatol., 31, 1990–2005, https://doi.org/10.1002/joc.2210, 2011.
ASHRAE: ANSI/ASHRAE Standard 140-2017 Standard method of test for the evaluation of building energy analysis computer programs, American Society of Heating, Refrigerating and Air-Conditioning Engineers, 2017.
Biggart, M., Stocker, J., Doherty, R. M., Wild, O., Carruthers, D., Grimmond, S., Han, Y., Fu, P., and Kotthaus, S.: Modelling spatiotemporal variations of the canopy layer urban heat island in Beijing at the neighbourhood scale, Atmos. Chem. Phys., 21, 13687–13711, https://doi.org/10.5194/acp-21-13687-2021, 2021.
Chen, X., Yang, H., and Wang, Y.: Parametric study of passive design strategies for high-rise residential buildings in hot and humid climates: miscellaneous impact factors, Renew. Sustain. Energy Rev., 69, 442–460, https://doi.org/10.1016/j.rser.2016.11.055, 2017.
China Meteorological Bureau, Climate Information Center, Climate Data Office and Tsinghua University, Department of Building Science and Technology.: China Standard Weather Data for Analyzing Building Thermal Conditions, Beijing: China Building Industry Publishing House, ISBN 7-112-07273-3, 2005.
Chow, W. T. L., Salamanca, F., Georgescu, M., Mahalov, A., Milne, J. M., and Ruddell, B. L.: A multi-method and multi-scale approach for estimating city-wide anthropogenic heat fluxes, Atmos. Environ., 99, 64–76, https://doi.org/10.1016/j.atmosenv.2014.09.053, 2014.
Daish, N. C., Carrilho da Graça, G., Linden, P. F., and Banks, D.: Impact of aperture separation on wind-driven single-sided natural ventilation, Build. Environ., 108, 122–134, https://doi.org/10.1016/j.buildenv.2016.08.015, 2016.
DOE: EnergyPlus™ Version 9.4.0, https://energyplus.net/ (last access: 13 October 2021), 2020a.
DOE: EnergyPlus™ Version 9.4.0, Input Output Reference, https://energyplus.net/documentation (last access: 13 October 2021), 2020b.
Duan, S., Luo, Z., Yang, X., and Li, Y.: The impact of building operations on urban heat/cool islands under urban densification: A comparison between naturally-ventilated and air-conditioned buildings, Appl. Energ., 235, 129–138, https://doi.org/10.1016/j.apenergy.2018.10.108, 2019.
Fan, H. and Sailor, D. J.: Modeling the impacts of anthropogenic heating on the urban climate of Philadelphia: A comparison of implementations in two PBL schemes, Atmos. Environ., 39, 73–84, https://doi.org/10.1016/j.atmosenv.2004.09.031, 2005.
Fan, S., Davies Wykes, M. S., Lin, W. E., Jones, R. L., Robins, A. G., and Linden, P. F.: A full-scale field study for evaluation of simple analytical models of cross ventilation and single-sided ventilation, Build. Environ., 187, 107386, https://doi.org/10.1016/j.buildenv.2020.107386, 2021.
Ferrando, M., Hong, T., and Causone, F.: A simulation-based assessment of technologies to reduce heat emissions from buildings, Build. Environ., 195, 107772, https://doi.org/10.1016/j.buildenv.2021.107772, 2021.
Goward, S. N.: Thermal behavior of uban landscapes and the urban heat island, Phys. Geogr., 2, 19–33, https://doi.org/10.1080/02723646.1981.10642202, 1981.
Grimmond, C. S. B.: The suburban energy balance: Methodological considerations and results for a mid-latitude west coast city under winter and spring conditions, Int. J. Climatol., 12, 481–497, https://doi.org/10.1002/joc.3370120506, 1992.
Heiple, S. and Sailor, D. J.: Using building energy simulation and geospatial modeling techniques to determine high resolution building sector energy consumption profiles, Energ. Buildings, 40, 1426–1436, https://doi.org/10.1016/j.enbuild.2008.01.005, 2008.
Hong, T., Ferrando, M., Luo, X., and Causone, F.: Modeling and analysis of heat emissions from buildings to ambient air, Appl. Energ., 277, 115566, https://doi.org/10.1016/j.apenergy.2020.115566, 2020.
Iamarino, M., Beevers, S., and Grimmond, C. S. B.: High-resolution (space, time) anthropogenic heat emissions: London 1970–2025, Int. J. Climatol., 32, 1754–1767, https://doi.org/10.1002/joc.2390, 2012.
Ichinose, T., Shimodozono, K., and Hanaki, K.: Impact of anthropogenic heat on urban climate in Tokyo, Atmos. Environ., 33, 3897–3909, https://doi.org/10.1016/S1352-2310(99)00132-6, 1999.
Kelly, O. and Scott, P.: City vacant: Dublin's hundreds of multimillion-euro empty sites and properties, https://www.irishtimes.com/news/environment/city-vacant- dublin-s-hundreds-of-multimillion-euro-empty-sites-and-properties-1.3635595 (last access: 8 December 2021), 2018.
Koralegedara, S. B., Lin, C. Y., Sheng, Y. F., and Kuo, C. H.: Estimation of anthropogenic heat emissions in urban Taiwan and their spatial patterns, Environ. Pollut., 215, 84–95, https://doi.org/10.1016/j.envpol.2016.04.055, 2016.
Lindberg, F., Grimmond, C. S. B., Yogeswaran, N., Kotthaus, S., and Allen, L.: Impact of city changes and weather on anthropogenic heat flux in Europe 1995–2015, Urban Clim., 4, 1–15, https://doi.org/10.1016/j.uclim.2013.03.002, 2013.
Liu, Y., Luo, Z., and Grimmond, S.: Revising the definition of anthropogenic heat flux from buildings: role of human activities and building storage heat flux, Zenodo [data set], https://doi.org/10.5281/zenodo.5903303, 2021.
MOHURD: Design standard for energy efficiency of residential building in severe cold and cold zones, JGJ 26-2018, Ministry of Housing and Urban-Rural, ISBN 151133345, 2018 (in Chinese).
Nicol, J. F. and Humphreys, M. A.: Adaptive thermal comfort and sustainable thermal standards for buildings, Energ. Buildings, 34, 563–572, https://doi.org/10.1016/S0378-7788(02)00006-3, 2002.
Nie, W. S., Sun, T., and Ni, G. H.: Spatiotemporal characteristics of anthropogenic heat in an urban environment: A case study of Tsinghua Campus, Build. Environ., 82, 675–686, https://doi.org/10.1016/j.buildenv.2014.10.011, 2014.
Oikonomou, E., Davies, M., Mavrogianni, A., Biddulph, P., Wilkinson, P., and Kolokotroni, M.: Modelling the relative importance of the urban heat island and the thermal quality of dwellings for overheating in London, Build. Environ., 57, 223–238, https://doi.org/10.1016/j.buildenv.2012.04.002, 2012.
Oke, T. R., Mills, G., Christen, A., and Voogt, J. A.: Urban Climates, Cambridge University Press, https://doi.org/10.1017/9781139016476, 2017.
Oliphant, A. J., Grimmond, C. S. B., Zutter, H. N., Schmid, H. P., Su, H. B., Scott, S. L., Offerle, B., Randolph, J. C., and Ehman, J.: Heat storage and energy balance fluxes for a temperate deciduous forest, Agric. Forest Meteorol., 126, 185–201, https://doi.org/10.1016/j.agrformet.2004.07.003, 2004.
Sailor, D. J. and Lu, L.: A top-down methodology for developing diurnal and seasonal anthropogenic heating profiles for urban areas, Atmos. Environ., 38, 2737–2748, https://doi.org/10.1016/j.atmosenv.2004.01.034, 2004.
Sailor, D. J. and Vasireddy, C.: Correcting aggregate energy consumption data to account for variability in local weather, Environ. Modell. Softw., 21, 733–738, https://doi.org/10.1016/j.envsoft.2005.08.001, 2006.
Santamouris, M., Papanikolaou, N., Livada, I., Koronakis, I., Georgakis, C., Argiriou, A., and Assimakopoulos, D. N.: On the impact of urban climate on the energy consuption of building, Sol. Energy, 70, 201–216, https://doi.org/10.1016/S0038-092X(00)00095-5, 2001.
Shepard, W.: Ghost cities of China: The story of cities without people in the world's most populated country, Zed Books Ltd., ISBN-10: 178360218X, 2015.
Takane, Y., Kikegawa, Y., Hara, M., and Grimmond, C. S. B.: Urban warming and future air-conditioning use in an Asian megacity: importance of positive feedback, NPJ Clim. Atmos. Sci., 2, 1–11, https://doi.org/10.1038/s41612-019-0096-2, 2019.
Wang, H. and Chen, Q.: A new empirical model for predicting single-sided, wind-driven natural ventilation in buildings, Energ. Buildings, 54, 386–394, https://doi.org/10.1016/j.enbuild.2012.07.028, 2012.
Wang, H. and Chen, Q.: A semi-empirical model for studying the impact of thermal mass and cost-return analysis on mixed-mode ventilation in office buildings, Energ. Buildings, 67, 267–274, https://doi.org/10.1016/j.enbuild.2013.08.025, 2013.
Wang, K., Li, Y., Li, Y., and Lin, B.: Stone forest as a small-scale field model for the study of urban climate, Int. J. Climatol., 38, 3723–3731, https://doi.org/10.1002/joc.5536, 2018.
Wang, L. and Greenberg, S.: Window operation and impacts on building energy consumption, Energ. Buildings, 92, 313–321, https://doi.org/10.1016/j.enbuild.2015.01.060, 2015.
Warren, P.: Ventilation through openings on one wall only, in: Proceedings of International Centre for Heat and Mass Transfer Seminar “Energy Conservation in Heating, Cooling, and Ventilating Buildings, Washington, 29 August, 189–209, 1977.
Yu, Z., Hu, L., Sun, T., Albertson, J., and Li, Q.: Impact of heat storage on remote-sensing based quantification of anthropogenic heat in urban environments, Remote Sens. Environ., 262, 112520, https://doi.org/10.1016/j.rse.2021.112520, 2021.