the Creative Commons Attribution 4.0 License.
the Creative Commons Attribution 4.0 License.
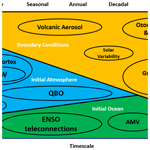
Long-range prediction and the stratosphere
Adam A. Scaife
Mark P. Baldwin
Amy H. Butler
Andrew J. Charlton-Perez
Daniela I. V. Domeisen
Chaim I. Garfinkel
Steven C. Hardiman
Peter Haynes
Alexey Yu Karpechko
Eun-Pa Lim
Shunsuke Noguchi
Judith Perlwitz
Lorenzo Polvani
Jadwiga H. Richter
John Scinocca
Michael Sigmond
Theodore G. Shepherd
Seok-Woo Son
David W. J. Thompson
Over recent years there have been concomitant advances in the development of stratosphere-resolving numerical models, our understanding of stratosphere–troposphere interaction, and the extension of long-range forecasts to explicitly include the stratosphere. These advances are now allowing for new and improved capability in long-range prediction. We present an overview of this development and show how the inclusion of the stratosphere in forecast systems aids monthly, seasonal, and annual-to-decadal climate predictions and multidecadal projections. We end with an outlook towards the future and identify areas of improvement that could further benefit these rapidly evolving predictions.
Daily weather fluctuations are thought to have a deterministic predictability horizon of around 2 weeks due to the sensitivity of the evolution of the atmospheric state to small errors in initial conditions (Lorenz, 1969) – the so-called “butterfly effect”. Recent estimates (Leung et al., 2020; Domeisen et al., 2018) as well as tests of the predictability of midlatitude daily weather using the latest global prediction models (Zhang et al., 2019; Son et al., 2020) produce similar estimates for this predictability limit. However, this does not preclude skilful forecasts of the statistics (most notably the average) of conditions at long range beyond this timescale (e.g. Shukla, 1981). This predictability owes its existence to slowly varying predictable components of the climate system in the ocean and in some cases the atmosphere, as well as externally forced changes such as volcanic or solar variability effects (e.g. Kushnir et al., 2019). Some of the more prominent examples of stratospheric variability such as sudden stratospheric warmings and their subsequent impact on the stratosphere and the troposphere (Baldwin et al., 2021) or the quasi-biennial oscillation and its associated teleconnections (Scaife et al., 2014a) have been shown to be predictable out to timescales well beyond the traditional 2-week predictability horizon from initial tropospheric conditions alone. Other examples involve stratospheric pathways for teleconnections originating in the troposphere or ocean (e.g. Schwartz and Garfinkel, 2017; Byrne et al., 2019) and are shown in Fig. 1. On longer timescales, boundary forcing, for example from composition changes such as ozone depletion and recovery, allows the stratosphere to provide relatively slowly varying conditions to guide the turbulent troposphere and hence provide long-range predictability (e.g. Thompson et al., 2011). The relative importance of stratospheric initial conditions to boundary conditions decreases with lead time as shown in the schematic in Fig. 1.
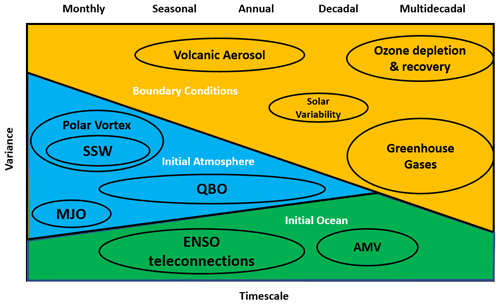
Figure 1Schematic representation of the role of the stratosphere in long-range prediction showing the transition from initial-condition predictability in the atmosphere (blue) and the ocean (green) to boundary-condition predictability at longer timescales (orange). Individual mechanisms involving the stratosphere are labelled in black. The width of the ellipses in the timescale direction shows the approximate range over which each phenomenon provides predictability. The width of the ellipses in the variance direction shows their relative contributions to forecast variance.
The extension of long-range prediction systems to explicitly include the representation of the stratosphere follows many years of development of stratosphere-resolving general circulation models (GCMs). By the late 20th century many leading centres for climate research had started to include the stratosphere in versions of their GCMs (Pawson et al., 2000; Gerber et al., 2012). Much of the early model development was motivated by the discovery of the ozone hole in the 1980s (Farman et al., 1985) and the need for simulations of ozone depletion and potential recovery of the ozone hole following the 1987 Montreal Protocol, which required atmospheric models that represented both the atmospheric dynamics and chemistry of stratospheric ozone depletion (Molina and Rowland, 1974; Crutzen, 1974). In most cases this was achieved by adding further quasi-horizontal layers to the domain of existing climate models to extend their representation of the atmosphere to the stratopause or beyond (e.g. Rind et al., 1988; Beagley et al., 1997; Swinbank et al., 1998; Sassi et al., 2002) while also incorporating key radiative (e.g. Fels et al., 1985), chemical (e.g. Steil et al., 1998), and dynamical (e.g. Scaife et al., 2000) processes.
The early development of so-called “high-top” climate models, which represent the whole depth of the stratosphere, in general preceded the discovery of the main body of evidence that the variability of the stratosphere is not only affected by but also interacts with the lower atmosphere and surface climate. Pioneering early studies suggested that the stratosphere might have direct effects on the troposphere and surface climate (e.g. Labitzke, 1965; Boville, 1984; Kodera et al., 1990, 1995; Haynes et al., 1991; Perlwitz and Graf, 1995). In subsequent years, as reliable observational records lengthened and large enough samples of stratospheric variability were amassed, it was unequivocally demonstrated that stratospheric variability precedes important tropospheric changes in the extratropics (Baldwin and Dunkerton, 1999, 2001). There was debate about causality and whether the stratosphere really does affect the atmosphere below (e.g. Plumb and Semeniuk, 2003). However, experiments where the stratosphere is perturbed in numerical models show changes in surface climate and reproduce similar patterns of response at the surface to those found in real-world observations (e.g. Polvani and Kushner, 2002; Norton et al., 2003; Scaife et al., 2005; Joshi et al., 2006; Scaife and Knight, 2008; Douville, 2009; Hitchcock and Haynes, 2016; White et al., 2020). These involve changes to planetary-scale waves and also baroclinic eddies in the troposphere that are consistent with changes in baroclinicity near the tropopause (Kushner and Polvani, 2004; Song and Robinson, 2004; Wittman et al., 2004, 2007; Scaife et al., 2012; Domeisen et al., 2013; Hitchcock and Simpson, 2014; White et al., 2020). Importantly, as we discuss below, the same mechanisms also appear to be at work across a broad range of timescales (Kidston et al., 2015).
In recent years, motivated by the evidence of surface effects of stratospheric variability in the midlatitudes, the high-top model configurations used for stratospheric research were incorporated into leading prediction systems. Improved vertical resolution was already known to improve the atmospheric data assimilation of satellite instrument observations whose sensitivity was often heavily weighted towards stratospheric altitudes. This also provided initial stratospheric conditions for sets of retrospective forecasts, some of which were internationally coordinated (e.g. Butler et al., 2016; Tompkins et al., 2017). A growing number of operational systems are now producing regular ensembles of predictions at lead times of months or years with coupled ocean–atmosphere models that extend to the stratopause or beyond, for example at Environment Canada (Merryfield et al., 2013), the Met Office in the UK (MacLachlan et al., 2015), the German Weather Service (DWD; Baehr et al., 2015), the Japan Meteorological Agency (Takaya et al., 2017), and the European Centre for Medium-Range Weather Forecasts (Johnson et al., 2019). In the following sections we document the emerging impacts and benefits of this new capability for surface climate predictions at monthly, seasonal, and annual-to-decadal lead times starting with the shorter-range initial-condition cases and ending with the longer-range boundary-condition cases.
The best-established phenomenon that gives rise to the predictability of surface climate from the stratosphere is the tropospheric circulation changes that follow strong and weak conditions in the stratospheric polar vortex (Baldwin and Dunkerton, 1999, 2001). For example, weak vortex conditions such as those found in a sudden stratospheric warming (SSW; Baldwin et al., 2021) are typically followed by a weakening and southward shift of the tropospheric midlatitude jet stream (see e.g. Kidston et al., 2015, and references therein) and thus the negative polarity of the North Atlantic Oscillation (NAO), Arctic Oscillation (AO), and Northern Annular Mode (NAM). These fluctuations also show a tendency to vacillate between strong westerly and weak (SSW) states on subseasonal timescales (Kuroda and Kodera, 2001; Hardiman et al., 2020a). The changes in the troposphere persist roughly as long as those in the lower stratosphere and last for around 2 months (Baldwin and Dunkerton, 2001; Baldwin et al., 2003; Hitchcock et al., 2013; Son et al., 2020; Domeisen, 2019). The impacts on surface climate also include changes in the frequency of extremes of temperature and rainfall (Scaife et al., 2008; King et al., 2019; Cai et al., 2016; Domeisen et al., 2020b).
Although major SSW events, involving a complete reversal of the zonal flow in the mid stratosphere, are rare in the Southern Hemisphere (Wang et al., 2020; Jucker et al., 2021), variations of the Antarctic polar vortex are likewise followed by similar signatures in the underlying tropospheric flow, in this case via the Southern Annular Mode (SAM). Weakening of the vortex is typically followed by a negative shift in the SAM and associated changes in rainfall and near-surface temperature (Thompson et al., 2005; Lim et al., 2018, 2019a, 2021; Rao et al., 2020d). These changes in Southern Hemisphere circulation typically take longer to reach the surface than their Northern Hemisphere counterparts (Graverson and Christiansen, 2003), perhaps due to the stronger stratospheric polar vortex and weaker wave driving in the Southern Hemisphere, but they are nonetheless better predicted by improving stratospheric resolution of forecast models (Roff et al., 2011). The timescale of weeks for the predictability of sudden warmings is limited by the predictability of weather patterns in the troposphere which might trigger SSW events (e.g. Mukougawa et al., 2005; Taguchi, 2016; Garfinkel and Schwarz, 2017; Jucker and Reichler, 2018; Lee et al., 2020a). However, if we add this timescale to the timescale of a month or more for the persistence of lower-stratospheric anomalies and their surface effects (e.g. Baldwin et al., 2003; Butler et al., 2019a), we arrive at the conclusion that on these occasions at least, initial conditions in the atmosphere can provide predictability well beyond the usual 2-week horizon for daily weather in either hemisphere.
Predictability of the atmosphere at monthly lead times is also known to originate in part from the Madden–Julian oscillation (MJO) in the troposphere and its teleconnection to the extratropics (e.g. Vitart, 2017). The circulation pattern associated with the MJO resembles a poleward- and eastward-propagating Rossby wave with centres of action over the Pacific and extending into the Atlantic sector where it also maps strongly onto the North Atlantic Oscillation. The lead time of around 10 d for the impact of a change in the MJO to appear in the extratropical flow (e.g. Cassou, 2008; Lin et al., 2009) is also consistent with the timescale for the poleward propagation of Rossby waves (e.g. Scaife et al., 2017). It turns out that this tropospheric MJO teleconnection on monthly timescales also interacts with the stratosphere (Garfinkel and Schwartz, 2017). The MJO teleconnection to the North Pacific affects the region most strongly associated with tropospheric precursors to SSW events, and consistent with this, SSWs in the observational record have tended to follow certain MJO phases. The subsequent weak vortex anomaly then propagates down to the troposphere (Garfinkel et al., 2012b), where it may strengthen and prolong any existing negative NAO signal that is directly linked to the MJO via the troposphere (Schwartz and Garfinkel, 2017, 2020; Barnes et al., 2019).
In addition to the interaction of the MJO with the extratropical stratosphere, a further, completely different link between the stratosphere and the MJO has recently been uncovered which modulates MJO amplitude and persistence in the troposphere via the phase of the quasi-biennial oscillation (QBO) in the tropical lower stratosphere (Liu et al., 2014; Yoo and Son, 2016; Martin et al., 2021). In this case, easterly phases of the QBO appear to energize the MJO compared to westerly QBO phases, likely due to changes in temperature and hence static stability close to the tropopause (Hendon and Abhik, 2018; Martin et al., 2019) with a potential contribution of cloud–radiation feedbacks (Son et al., 2017; see Martin et al., 2021, for a review). This modulation of the MJO is in turn important for predictability, as it gives rise to higher monthly prediction skill of the MJO and its surface teleconnections during the easterly phase of the QBO (Marshall et al., 2017; Abhik and Hendon, 2019; Lim et al., 2019b).
The traditional view of stratosphere–troposphere interaction involves upward propagation of planetary-scale Rossby waves (Charney and Drazin, 1961), but this linear theory applies equally well to downward propagation. Harnik and Lindzen (2001) and Perlwitz and Harnik (2003) identified a possible source of downward-propagating planetary waves in the form of reflecting surfaces in the winter stratosphere. Examples of specific reflection events, showing upward and then downward propagation have since been observed (e.g. Kodera et al., 2008; Harnik, 2009; Kodera and Mukougawa, 2017; Mukougawa et al., 2017; Matthias and Kretschmer, 2020). These results suggest that the details of the stratospheric circulation such as regions of negative vertical wind shear could be important for the formation of reflecting conditions (Shaw and Perlwitz, 2013) and may yet provide a further mechanism by which the stratosphere can affect the troposphere (Domeisen et al., 2019; Butler et al., 2019b).
Following studies demonstrating enhanced tropospheric predictability after SSW events in individual climate models (e.g. Kuroda, 2008; Mukougawa et al., 2009; Marshall and Scaife, 2010; Sigmond et al., 2013), subseasonal-forecast systems which explicitly represent the stratosphere in the climate system were developed and implemented at operational-prediction centres worldwide. It is often difficult to demonstrate significant increases in overall skill (e.g. Richter et al., 2020a), but routinely produced ensembles of subseasonal predictions show that both stratospheric variability and its subsequent tropospheric signature are predictable at monthly lead times (Domeisen et al., 2020a, b). The strongest surface impacts occur if the polar vortex in the lower stratosphere is in a weakened state at the time of the SSW (Karpechko et al., 2017), and there appears to be a roughly linear relationship between the strength of these lower-stratospheric anomalies and the tropospheric response (e.g. Runde et al., 2016; White et al., 2020; see Baldwin et al., 2019, for a review). We should note however that there is no one-to-one correspondence between stratospheric variability and tropospheric events, and some prominent examples of sudden stratospheric warmings are followed by differing tropospheric anomalies (e.g. Charlton-Perez et al., 2018; Knight et al., 2020; Butler et al., 2020; Rao et al., 2020a). Nevertheless, the canonical response is seen in the majority (∼ 70 %) of cases, and periods of intense wintertime stratospheric variability are important windows of opportunity to provide skilful monthly forecasts (Mariotti et al., 2020; Tripathi et al., 2015a).
These forecast systems are now important tools for national meteorological and hydrological services to monitor impending stratospheric variability and associated surface impacts in real time. Recent extreme examples illustrate the importance of this activity. In February 2018 a major SSW occurred and was followed by a strong negative NAO-like pattern at the surface with easterly wind anomalies over Europe and multiple cold-air outbreaks over the following weeks, including extreme snowfall across northern Europe (Fig. 2; Karpechko et al., 2018; Knight et al., 2020; Rao et al., 2020a) and an abrupt end to Iberian drought in southern Europe (Ayarzagueña et al., 2018b). Studies of monthly ensemble predictions of this event with operational stratosphere-resolving systems showed that the stratospheric event was predictable at least 2 weeks in advance (Fig. 2) and that the ensemble forecasts indicated an increased likelihood of cold surface conditions for several weeks after the event (Karpechko, 2018; Butler et al., 2020; Statnaia et al., 2020; Rao et al., 2020a). Again, as in the analysis of previous events, there was also a strong association with the MJO entering phase 7 with increased convection in the West Pacific (cf. Garfinkel and Schwartz, 2017) in the 2018 event. Finally, we should also note that cases of monthly forecasts where the stratosphere plays an important role are not restricted to winters with sudden stratospheric warmings; periods when the stratospheric polar vortex is above normal strength also provide opportunities for skilful monthly forecasts (Tripathi et al., 2015b; Scaife et al., 2016). In this case an opposite but symmetric surface response results, with a strong positive NAO. A very recent example occurred in February 2020, when, following an extremely strong polar vortex (Hardiman et al., 2020b; Lee et al., 2020b; Lawrence et al., 2020; Rao and Garfinkel, 2021), the tropospheric jet in the Atlantic sector strengthened, and the associated increased storminess and rainfall in this case resulted in UK monthly rainfall reaching a new record high (Davies et al., 2021).
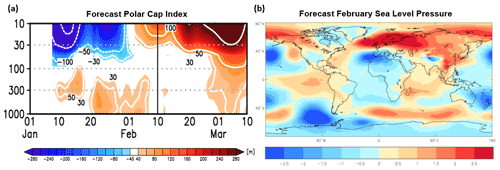
Figure 2Monthly forecasts prior to the 2018 sudden stratospheric warming and severe cold event over northern Europe. Forecast polar cap index (a) and February sea level pressure anomalies (b). Ensemble mean anomalies are shown for the average of forecasts initialized between 8 and 22 January 2018 relative to hindcasts over the 1993–2016 period using the Met Office Hadley Centre GloSea (global seasonal) prediction system (MacLachlan et al., 2015). Sea level pressure is measured in hectopascals (hPa), and the polar cap index is the geopotential height anomaly (m) averaged over 65∘ N to the North Pole.
Prior to the advent of dynamical forecast systems which explicitly represent the stratosphere, seasonal forecasts using empirical relationships and statistical methods were proposed. These relied on the prior state of the polar vortex and other predictable factors such as the QBO that are known to have links to surface climate (Thompson et al., 2002; Charlton et al., 2003; Christiansen, 2005; Boer and Hamilton, 2008). In some cases they indicated additional predictability that was absent in existing operational forecast systems, providing further evidence of predictability involving the stratosphere and further motivating the extension of dynamical forecast systems to properly represent the stratosphere. Similar empirical forecast studies continue, and although they cannot provide evidence of predictability that is as strong as from GCM experiments based on fundamental physical principles, they do continue to be useful to indicate sources of predictability that need to be properly represented in comprehensive forecast systems (e.g. Folland et al., 2012; Wang et al., 2017; Hall et al., 2017; Byrne and Shepherd, 2018).
Following the introduction of dynamical seasonal-forecast systems with a good representation of the stratosphere, clear links between successful seasonal prediction of the North Atlantic Oscillation, the closely related Arctic Oscillation, and the state of the stratospheric polar vortex have been identified in forecast output (e.g. Scaife et al., 2014b; Stockdale et al., 2015; Jia et al., 2017). Similar signals are also seen in the Southern Hemisphere in relation to predictability of the Southern Annular Mode (Seviour et al., 2014; Byrne et al., 2019; Lim et al., 2021). Statistically significant increases in overall skill directly attributable to the inclusion of the stratosphere in prediction systems is sometimes difficult to demonstrate (e.g. Butler et al., 2016), especially given that other factors such as horizontal resolution and physical parametrizations are often simultaneously changed. Nevertheless, the body of evidence now weighs heavily in favour of predictability of the NAO and SAM from the stratospheric polar vortex and from analyses showing reduced surface prediction skill in the absence of stratospheric variability (e.g. Hardiman et al., 2011; Sigmond et al., 2013; Scaife et al., 2016).
A second clear example of seasonal predictability originating in the stratosphere is the quasi-biennial oscillation (QBO). The QBO has such inherently long timescales that it persists for several months in seasonal forecasts from initial atmospheric conditions alone, and its regularity means that it can be predicted from simple composites of earlier cycles. Nevertheless, a growing number of numerical models used in seasonal-forecast systems can now simulate and predict the oscillation within climate forecasts (Garfinkel et al., 2018; Richter et al., 2020b; Stockdale et al., 2021) with the aid of forcing from parametrized non-orographic gravity waves, and there is skill in predicting QBO phase changes at lead times of a few months (e.g. Pohlman et al., 2013; Scaife et al., 2014a). The surface impact of the QBO is also well established and has stood the test of time since it was first identified in the 1970s (Ebdon, 1975; Thompson et al., 2002; Anstey and Shepherd, 2014; Gray et al., 2018). Yet again this response projects closely onto the North Atlantic Oscillation (and hence the Arctic Oscillation–Northern Annular Mode) and the Southern Annular Mode. The favoured mechanism involves refraction of vertically propagating Rossby waves in the lower stratosphere (Holton and Tan, 1980), although other pathways may also be involved (e.g. Inoue et al., 2011; Yamazaki et al., 2020; Rao et al., 2020b, 2021). The observed magnitude of the QBO teleconnection is also large enough to provide seasonal predictability of surface climate (Boer and Hamilton, 2008), but its modelled amplitude at the surface appears to be underrepresented in current operational-prediction systems and models (Scaife et al., 2014b; Garfinkel et al., 2018; O'Reilly et al., 2019; Rao et al., 2020b; Anstey et al., 2021).
In addition to the stratosphere acting as a source of predictability, other mechanisms by which the stratosphere plays a role in seasonal predictions involve a pathway for global-scale teleconnections. These often originate in the tropics where the longer timescales of coupled ocean–atmosphere variability such as the El Niño–Southern Oscillation (ENSO; L'Heureux et al., 2020) provide a predictable source of low-frequency variability. Effects on the extratropics can occur by tropical excitation of anomalous Rossby waves which propagate not only poleward but also upward into the stratosphere, as in the case of ENSO (Manzini et al., 2006; Domeisen et al., 2019), giving two pathways for extratropical influence (Butler et al., 2014; Kretschmer et al., 2021). These highly predictable tropical sources of climate variability alter the strength and position of the stratospheric polar vortex in the extratropics as well as the frequency of SSWs (Polvani et al., 2017), and these are followed by changes in the seasonal westerly jets in the troposphere and surface climate via the North Atlantic Oscillation (Ineson and Scaife, 2009; Cagnazzo and Manzini, 2009) or the Southern Annular Mode (Byrne et al., 2019). As might be expected, both the QBO and ENSO teleconnections are best represented in seasonal-forecast systems which contain a well-resolved stratosphere (Butler et al., 2016). We note that new examples of the stratosphere acting as a conduit for seasonal teleconnections are still being uncovered (Hurwitz et al., 2012; Woo et al., 2015). For example, the Indian Ocean Dipole (IOD) received little attention in this context until the recent record event of late 2019, when it appears to have driven an extreme winter strengthening of the Northern Hemisphere stratospheric polar vortex. This strengthening took many weeks to decay, giving rise to extreme yet highly predictable conditions in the stratosphere and around the Atlantic sector in late boreal winter (Hardiman et al., 2020b; Lee et al., 2020b). The same event was also implicated in extreme changes in the polar vortex and the near SSW in the Southern Hemisphere (Rao et al., 2020d), an event that itself likely helped to drive the extreme summer conditions and wildfires over Australia that year (Lim et al., 2021).
Apparent links between Arctic sea ice and seasonal winter climate in the midlatitudes have also been suggested to be mediated by the stratosphere, with increased Rossby wave activity and a weakening of the stratospheric polar vortex in response to reduced sea ice, especially in the Barents–Kara Sea (Honda et al., 2009; Jaiser et al., 2013; Kim et al., 2014; King et al., 2016; Kretschmer et al., 2016). Some studies also reproduced surface signals in response to sea ice anomalies in seasonal forecasts of particular years that are in apparent agreement with observational estimates (e.g. Balmaseda et al., 2010; Orsolini et al., 2012). However, recent updates to observational records show a weakening of these apparent effects (Blackport and Screen, 2020) and significant non-stationarity (Kolstad and Screen, 2019). Subsequent modelling studies with larger samples of simulations have provided mixed results (Zhang et al., 2018; Dai and Song, 2020), and some have argued that the atmospheric response to sea ice is weak (Smith et al., 2022) and that while the sensitivity to Barents–Kara sea ice may be stronger, the stratospheric response in particular is highly variable (McKenna et al., 2018). While there may well be a longer-term effect via the stratosphere from sea ice decline (Sun et al., 2015; Screen and Blackport, 2019; Kretschmer et al., 2020), sensitivity of the response to the background state complicates the issue (Labe et al., 2019; Smith et al., 2017), as do possible confounding influences from the tropics (Warner et al., 2020), and to date there is no clear consensus for strong enough year-to-year effects to provide significant seasonal predictability.
Other proposed teleconnections acting via the stratosphere have been found in observations but remain to be confirmed with successful reproduction in physically based climate models. A prominent example involves a proposed link between Eurasian snow amounts and the stratosphere, followed by a return influence on the NAO and surface climate. In this case, enhanced snow cover or depth is associated with high pressure over northern Eurasia, an increase in the flux of Rossby wave activity into the stratosphere, and a subsequent weakening of the stratospheric polar vortex, followed by the expected negative shift in the NAO and AO (Cohen and Entekhabi, 1999; Cohen and Jones, 2011; Cohen et al., 2014; Furtado et al., 2015). However, the strength of this link in climate models and seasonal predictions is modest (Fletcher et al., 2009; Riddle et al., 2013; Tyrrell et al., 2018, 2019) and does not agree with apparent links to the AO in observations (Kretschmer et al., 2016; Garfinkel et al., 2020) even when model mean state biases are corrected (Tyrrell et al., 2020). It has also been suggested that teleconnections to snow are non-stationary or non-causal, and there is continued debate about its long-term robustness (Peings et al., 2013; Henderson et al., 2018).
In summary, a number of mechanisms by which the stratosphere acts to provide seasonal predictability by acting directly either as a source of predictable variability (e.g. the QBO and SSWs) or as a conduit for teleconnections (e.g. ENSO, MJO, and IOD) have now been established in observations and have been confirmed using climate model simulations based on first principles. These operate in seasonal-forecast systems, albeit with remaining errors such as the weakness of the QBO connection to surface climate. Meanwhile, other mechanisms involving the stratosphere (for example the response to snow cover variations) have been proposed based on apparent observed relationships, but until we have agreement between these observations and theory (model simulations), scientists remain sceptical of whether they represent actual sources of seasonal predictability, and these remain topics of current research.
In recent years, initialized predictions on longer timescales were developed on the premise of multiyear memory in the ocean (e.g. Smith et al., 2007), and following the development pathway mapped out by seasonal forecasts in the past, these are now being run operationally to produce real time multimodel forecasts (Smith et al., 2013). Kushnir et al. (2019) mapped out this operational development of annual-to-decadal predictions and highlighted a number of sources of predictability, some of which involve the stratosphere (Fig. 3) but not all of which are fully represented in climate prediction systems.
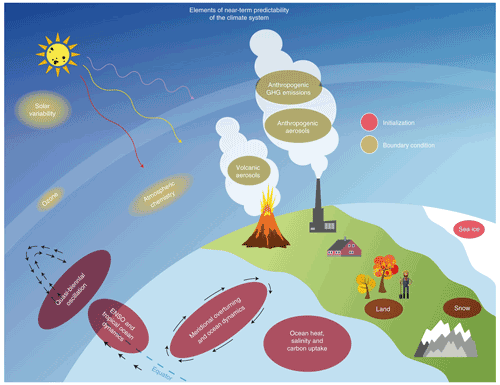
Figure 3Sources of annual-to-decadal predictability, some of which involve the stratosphere through the response to external forcing, internal atmospheric dynamics, or ozone chemistry changes. After Kushnir et al. (2019).
Despite common misconceptions, not all annual-to-decadal predictability stems from the ocean. Indeed, it has been clearly demonstrated that multiyear predictability of the QBO exists in current decadal predictions systems out to lead times of several years (Pohlman et al., 2013; Scaife et al., 2014a). This offers the prospect of a stratospheric contribution to multiyear predictability of the extratropics through the teleconnection with the Arctic Oscillation (Anstey and Shepherd 2014; Gray et al., 2018) and to tropical predictability through links to the MJO (e.g. Martin et al., 2021) and wider tropical climate variability (Haynes et al., 2021).
Although it is more important on multidecadal timescales (see below), external forcing of the stratosphere can also act as a source of decadal predictability. Forced climate signals from changes in greenhouse gases or stratospheric effects such as ozone depletion occur on a much longer timescale than the lead time of decadal forecasts, but their contribution to the skill of predictions is not trivial. For example, it is not immediately obvious whether the slow changes from multidecadal forced signals would simply be swamped by unpredictable internal variability on decadal timescales, rendering long-term external forcing changes useless for decadal predictions. However, this is not the case and long-term forcing is now known to be an important source of decadal prediction skill (Smith et al., 2019, 2020).
External forcing involving the stratosphere on shorter timescales is also important for annual-to-decadal predictions. The stratosphere has long been known to be influenced by volcanic eruptions, particularly in the case of tropical volcanic eruptions which are powerful enough to inject significant quantities of sulfur dioxide into the atmosphere. Here it reacts with water to form sulfuric acid and persists in aerosol form, leading to predictable multiyear global surface cooling, tropical stratospheric warming, and an intensification of the westerly stratospheric polar vortex in the extratropics (Robock and Mao, 1992). Although the sample of observed events is limited, modelling studies have reproduced an observed post-eruption intensification of the westerly winds in the stratosphere and some impacts on the surface Arctic Oscillation. However, generations of models have struggled to reproduce the 2-year persistence of volcanic effects seen in observations and the observed magnitude of the effect on the winter AO (e.g. Stenchikov et al., 2006; Marshall et al., 2009; Charlton-Perez et al., 2013; Bittner et al., 2016). In addition to these changes in the atmosphere, the intensification of stratospheric westerlies and hence Arctic Oscillation also combines with surface cooling of the ocean to generate predictable changes in the Atlantic meridional overturning circulation (Reichler et al., 2012) which can extend the volcanic influence to decadal timescales (Swingedouw et al., 2015). Finally, although the mechanism is debated, there is also evidence of a multiyear effect of tropical volcanic eruptions on ENSO, presumably requiring the persistent radiative forcing that arises through the long residence time of volcanic products, particularly sulfate aerosols, in the stratosphere. This reportedly increases the frequency of El Niño events by a factor of 2 in the years following volcanic eruptions (Adams et al., 2003), again suggesting an important source of multiannual predictability via the stratosphere.
A second source of multiannual predictability from external forcing originates from solar variability and in particular the 11-year solar activity cycle. Although a number of alternative mechanisms have been proposed (see Gray et al., 2010, for a review), the established mechanism for surface effects via the stratosphere is the change in the polar vortex that results from changes in upper-stratospheric heating over the course of each cycle between solar minimum and solar maximum. Interactions of atmospheric waves and mean flow amplify the initial radiatively driven change and drive its descent to the troposphere (Kodera and Kuroda, 2002; Marsh et al., 2007; Ineson et al., 2011; Givon et al., 2021), where changes in the extratropical jets result in a negative (positive) Arctic Oscillation pattern following solar minimum (maximum). There is also evidence that it contributes to interannual prediction skill (Dunstone et al., 2016), and an interesting aspect that has emerged in recent years is the integrating effect of the ocean on solar-induced changes in the NAO via interannual persistence of ocean heat content anomalies which lead to a lag of around 3 years ( cycles) in the peak response, as would be expected if the ocean is integrating the effects of a periodic solar forcing (Scaife et al., 2013; Gray et al., 2013; Andrews et al., 2015; Thiéblemont et al., 2015). However, debate continues as to whether the solar signal is indeed large enough to be detectable in observations in the presence of large internal tropospheric variability (Chiodo et al., 2019).
Perhaps the longest known timescale for predictability from initial conditions, which also involves the stratosphere, is the interaction of Atlantic multidecadal variability (AMV) with the stratospheric circulation. The Atlantic has followed pronounced multidecadal variations over the last century (Mann et al., 1995), and these variations are predictable out to years ahead (Hermanson et al., 2014). Some studies link these variations to the stratosphere and the NAO–AO (Reichler et al., 2012; Omrani et al., 2014). Indeed, the pronounced multidecadal increase in the surface NAO between the 1960s and 1990s is strongly coupled to changes in the strength of the stratospheric polar night jet (Scaife et al., 2005). Although current models simulate weak coupling between the AMV and the free atmosphere, this coupling appears to increase with model resolution (Lai et al., 2021), suggesting that the links between AMV, the stratosphere, and the NAO offer potential for improved decadal-scale prediction involving the stratosphere.
The currently recognized role of the stratosphere in decadal forecasts of surface climate again appears mainly via the impact on annular modes and, in the Northern Hemisphere, the North Atlantic Oscillation. Indeed, while current decadal prediction systems are now able to produce skilful predictions of variations in the NAO on multiyear lead times (Smith et al., 2019, 2020; Athanassiadis et al., 2020), much work is still needed to attribute these variations to external forcing or internal variability and to understand the interaction between boundary and initial conditions, which blurs the simple distinction between the two. These new results are important because they indicate newfound decadal predictability of events like the high NAO of the 1990s which yielded a run of mild but wet and stormy winters in northern Europe and the eastern USA. These winters are well known to have caused significant impact for example on the insurance sector (Leckebusch et al., 2007) and coincided with the longest observed absence of SSW events (Pawson and Naujokat, 1999; Domeisen, 2019). Given the indications of coupled stratosphere–troposphere variations on decadal timescales (Scaife et al., 2005; Omrani et al., 2014; Garfinkel et al., 2017; Woo et al., 2015), understanding the role of the stratosphere in extratropical decadal predictions needs further investigation.
The importance of the stratosphere for climate projections on multidecadal timescales was generally recognized before its role in predictions on shorter timescales. This is in part a legacy of the early development of stratosphere–troposphere models for ozone depletion studies described in the Introduction. On these longer timescales, coupling between stratospheric composition, thermal structure, and atmospheric circulation gives rise to improved climate projections.
Perhaps the best-known case for the stratosphere affecting multidecadal projections of surface climate is the influence of ozone depletion on the Southern Annular Mode (SAM; Thompson and Solomon, 2002; Thompson et al., 2005; McLandress et al., 2011; Polvani et al., 2011; Son et al., 2008, 2018), where decreasing ozone in the late 20th century led to a strengthened pole-to-Equator temperature gradient, a stronger stratospheric polar vortex, and a shift to strong positive SAM phases at the surface. In this case, studies again show the importance of stratospheric resolution to generate the full response, consistent with a genuine downward influence (Karpechko et al., 2008). The associated poleward shift in the tropospheric jet is connected to a delay in the spring breakdown of the stratospheric polar vortex (Byrne et al., 2017) and delivered significant and prolonged changes in rainfall across many regions of the Southern Hemisphere (Kang et al., 2011b; Purich and Son, 2012). Implementation of the Montreal Protocol in 1987 and subsequent reductions in the rate of ozone depletion mean that recovery of the ozone layer is now expected over the coming decades, and the reversible effects of this on the surface climate form an important element of current multidecadal projections (Thompson et al., 2011; Previdi and Polvani, 2014; Solomon et al., 2016; Banarjee et al., 2020; Zambri et al., 2021), where they are expected to play an important role alongside other changes in the southern stratosphere due to continuing increases in greenhouse gases (Son et al., 2009; Barnes et al., 2014), some of which occur via the stratospheric polar vortex in a similar way to those from ozone depletion and recovery (Ceppi and Shepherd, 2019). The more limited effects of ozone depletion in the Northern Hemisphere meant that the role of the stratosphere in multidecadal projections took longer to become established. Some early studies found potential amplification of positive Arctic Oscillation trends under climate change when the stratosphere was included (Shindell et al., 2001). However, this was not borne out in later studies as simulations with other fully coupled ocean–troposphere–stratosphere models, suggesting weakening of the stratospheric polar vortex (e.g. Huebener et al., 2007). Subsequent studies with multiple models also indicated a southward shift in the polar night jet with weakening high-latitude winds and strengthening subtropical winds (Scaife et al., 2012; Manzini et al., 2014). These changes result from increased atmospheric wave driving of the winds which can overwhelm the cooling effect of greenhouse gases (Karpechko and Manzini, 2012) and can lead to important differences in future surface climate, for example in regional rainfall in areas typically affected by the stratosphere via the Arctic Oscillation and NAO (Scaife et al., 2012). There is still significant uncertainty due to the diversity of modelled stratospheric responses to greenhouse gas increases (Manzini et al., 2014; Simpson et al., 2018; Zappa and Shepherd, 2017), and it has proved difficult to identify any clear change in the frequency of sudden stratospheric warmings (Ayarzagüena et al., 2018a, 2020; Rao and Garfinkel, 2020). This is perhaps due to the competition between strengthening latitudinal temperature gradients near the tropopause and enhanced meridional overturning in the mid stratosphere. There is also strong inherent unpredictable variability from decade to decade in the frequency of SSW occurrence (Butchart et al., 2000; McLandress and Shepherd, 2009).
Other aspects of future climate change where the stratosphere plays a role have also been identified, for example, in the debate over the response to future levels of Arctic sea ice. In this case it seems that the response of the midlatitude circulation involves a negative shift in the Arctic Oscillation (Screen et al., 2018; Zappa et al., 2018; McKenna et al., 2018). This could again be amplified by interaction with the stratosphere, as some studies suggest that the stratospheric response is necessary for a large surface response (Kim et al., 2014), while others highlight that the stratospheric interaction is sensitive to the regional pattern of sea ice decline (McKenna et al., 2018), and still others show evidence of nonlinear stratospheric and stratosphere-mediated surface response (Manzini et al., 2018), coincident with the time when the Barents and Kara seas become ice-free (Kretschmer et al., 2020). Furthermore, studies also indicate that the surface climate response to sea ice decline depends systematically on the phase of the stratospheric QBO (Labe et al., 2019).
Although it is much less certain than anthropogenic climate change, there have also been suggestions of a multidecadal decline of external solar irradiance which can impact multidecadal climate projections via the stratosphere. Previous multidecadal solar minima, so-called “grand minima”, have occurred in sunspot records and have been connected to the Little Ice Age period around the end of the 17th century using proxy and other data (Owens et al., 2017). Given recent weak-amplitude 11-year solar cycles, there are now suggestions of a future solar “grand minimum”, where the 11-year cycle described above could become muted or even absent for a prolonged period (Lockwood et al., 2010). In this case, the upper-stratospheric cooling in the tropics and summer hemisphere can change the meridional temperature gradient in a similar fashion to the 11-year cycle (Maycock et al., 2015) and leads to a negative shift in the AO and the NAO and hence affects regional climate (Ineson et al., 2015). However, in this case it appears that while regional changes could be significant, they are generally much smaller than the surface warming due to anticipated levels of anthropogenic greenhouse gases (Anet et al., 2003; Ineson et al., 2015; Maycock et al., 2015).
Finally, we note that although low-frequency variability in teleconnections is observed (e.g. Garfinkel et al., 2019), it is often unclear whether this is a systematic variation or simply due to sampling variability of an underlying stationary process (Jain et al., 2019). Nevertheless, there is growing evidence for systematic climate change in some of the teleconnections by which the stratosphere enables surface predictability. Under future climate change it appears that some of the teleconnections discussed above may strengthen in amplitude. For example, the strengthening of ENSO-induced anomalies in the extratropical Atlantic–European sector increases in future climate projections (Müller and Roeckner, 2006; Fereday et al., 2020). Similarly, recent analyses suggest that the MJO teleconnection to the extratropics increases in amplitude under climate change (Samarasinghe et al., 2021). The same is also true of the extratropical effects of the stratospheric QBO, where in this case, the amplitude of the teleconnection in composite anomalies doubles under future climate change (Rao et al., 2020c) despite the QBO itself becoming weaker (Richter et al., 2020c).
Long-range prediction has evolved quickly in recent years (Merryfield et al., 2017, 2020; Butler et al., 2019b; Meehl et al., 2021), and this rapid development is due in part to the improved representation of stratospheric processes and stratospheric initial conditions in ensemble prediction systems. The long-range forecast community originally focused on predictability from initial ocean conditions, and this remains the primary source of long-range predictability, for example from ENSO, but some of these long-range prediction systems contained poor representations of the stratosphere. In the meantime, those working in parallel on climate modelling of the stratosphere were rarely involved in initialized long-range prediction, instead being driven primarily by the ozone depletion problem. Knowledge exchange across fields is important in science and precursors to a new paradigm often occur when a topic is investigated by researchers from outside the field (Kuhn, 1970). The crossover and collaboration between long-range prediction and stratospheric research communities is no exception, and the interaction between these communities has yielded rapid progress and new insights. Examples where initial atmospheric conditions can provide predictability beyond the usually assumed limit have been demonstrated, not only for the extratropics but also for the tropics, and we now know that in some situations, for example when sudden stratospheric warmings occur, the initial conditions in the stratosphere can have more impact than initial conditions in the ocean (Thompson et al., 2002; Scaife and Knight, 2008; Polvani et al., 2017). This suggests that initial atmospheric conditions in the stratosphere are likely to be more important for long-range forecasts than previously assumed (Mukougawa et al., 2005, 2009; Stockdale et al., 2015; Noguchi et al., 2016, 2020a; Choi and Son, 2019; O'Reilly et al., 2019; Nie et al., 2019), not least because the overturning and breaking of Rossby waves in the stratosphere is followed by long-lived atmospheric anomalies due to synoptic-scale eddy feedbacks that prolong the effects in the troposphere (Kunz and Greatbatch, 2013; Kang et al., 2011a; White et al., 2020). More research on the initial conditions in the stratosphere might therefore help to reveal potential for further improvements in prediction skill.
A notable simplification to understanding the role of the stratosphere, at least in extratropical long-range predictions, is its apparently seamless mechanism across different timescales and different phenomena. Following the early ground-breaking studies showing surface impacts of stratospheric variability (e.g. Labitzke, 1965; Boville, 1984) and a multitude of studies on individual teleconnections between the stratosphere and surface climate, the projection of stratospheric variability onto the Arctic Oscillation–North Atlantic Oscillation–Northern Annular Mode circulation patterns across timescales and hemispheres is now well established (see the review by Kidston et al., 2015). This suggests that similar coupling processes occur between the stratosphere and troposphere from months to decades, and these processes lead to some of the most intense extratropical climate extremes, in winter in the Northern Hemisphere and in late spring–early summer in the Southern Hemisphere (Karpechko et al., 2018; Fereday et al., 2012; Kautz et al., 2019; Domeisen and Butler, 2020). While studies point to changes in upper-tropospheric baroclinicity and tropospheric eddy feedbacks as crucial in these teleconnections, a full mechanistic understanding of how this occurs is still lacking.
Some, but not all, leading forecast systems now include a well-resolved stratosphere with a reasonable representation of relevant processes such as the body force from sub-grid orographic and non-orographic gravity waves. However, many outstanding problems remain. Although their number is increasing, only a subset of current GCMs have the ability to simulate a realistic QBO beyond its decay from initial conditions, and it seems that all GCMs have problems with the fidelity of modelled QBO teleconnections, which are either too weak or absent altogether (Scaife et al., 2014a; Kim et al., 2020; Anstey et al., 2021). Even the relatively well-studied ENSO teleconnection via the stratosphere to the extratropics still has outstanding questions, such as whether the Northern Hemisphere stratosphere exhibits more SSW events during the La Niña phase (Butler and Polvani, 2011; Song and Son, 2018). This is not generally reproduced in modelling systems (Garfinkel et al., 2012a) but occurred in the recent La Niña winter of 2020/21. Similarly, while the increased monthly predictability from the MJO during the easterly phase of the QBO has been detected in monthly forecast experiments, the QBO–MJO connection does not persist in longer predictions and simulations with current models (Kim et al., 2020). Research and model development on stratosphere–troposphere interaction, including tropical effects (Noguchi et al., 2020b), will no doubt lead to further progress in resolving this issue (Haynes et al., 2021).
Errors in the modelled climatological mean climate are inevitably present to varying degrees in even the latest climate models. The common protocol of running a set of retrospective predictions to allow these mean biases to be estimated and hence subtracted from real-time predictions may well correct for much of this error. However, the degree to which biases have a nonlinear, state-dependent impact on the predictions is not fully understood. In some contexts, the nonlinear impacts of biases may be minimal (Karpechko et al., 2021), while others show sensitivity (Sigmond et al., 2008, 2010) and increases of prediction skill occur under certain background conditions, for example during easterly QBO phases (Taguchi, 2018). Other processes generally omitted from long-range predictions include interactive variations of ozone and other trace gases. Although reports of impacts and benefits have varied, it is thought that surface signals on interannual timescales come mainly from dynamical rather than chemical changes (Seviour et al., 2014; Harari et al., 2019). Nevertheless, some studies suggest detectable effects from interannual variability of ozone, and it may be that ozone fluctuations could help to amplify surface signals (Karpechko et al., 2014; Son et al., 2013; Smith and Polvani, 2014; Oehrlein et al., 2020; Hendon et al., 2020), providing a further area for future development. Given that the cost of full atmospheric chemistry schemes remains computationally expensive, it seems likely that simple parametrizations of ozone chemistry (e.g. Monge-Sanz et al., 2021) would be valuable in this context.
We end with a pointer to an issue that has now been found to affect long-range predictions from monthly to seasonal to decadal and multidecadal timescales, particularly in the extratropics. So-called “perfect model studies”, which test the ability of models to predict their own ensemble members, are now known to underestimate the true predictability of climate in some regions, particularly around the Atlantic basin, and so models are better at predicting real-world variations than they are at predicting themselves. This so-called “signal-to-noise paradox” (Scaife and Smith, 2018) is at first surprising, because perfect model prediction scores are often assumed to represent an upper (rather than lower) limit for prediction skill of the real world. The problem can be understood in terms of unrealistically weak ensemble mean predictions (e.g. Eade et al., 2014), but whether the stratosphere is involved directly in the cause of this problem remains to be seen (Saito et al., 2017; Stockdale et al., 2015), as it initially appears in the troposphere rather than the stratosphere in long-range forecasts (Domeisen et al., 2020a). Nevertheless, the unrealistically weak amplitude of ensemble mean predictions may well have the same root cause as the weaker-than-observed amplitude of modelled teleconnections to the stratosphere discussed in this review, including, for example, the underrepresentation of the surface impact of the QBO. Resolving this problem will therefore likely amplify these signals, provide greater levels of prediction skill, and further strengthen the role of the stratosphere in long-range predictions of surface climate.
No data sets were used in this article.
AAS wrote the draft manuscript. All other co-authors contributed relevant references and input to revisions and edits of the manuscript. SWS helped produce Fig. 1.
The contact author has declared that neither they nor their co-authors have any competing interests.
Publisher’s note: Copernicus Publications remains neutral with regard to jurisdictional claims in published maps and institutional affiliations.
Adam A. Scaife and Steven C. Hardiman were supported by the Met Office Hadley Centre Climate Programme funded by BEIS (Department for Business, Energy & Industrial Strategy) and Defra (Department for Environment, Food & Rural Affairs). Mark P. Baldwin was supported by the Natural Environment Research Council (grant no. NE/M006123/1). Jadwiga H. Richter was supported by the Regional and Global Model Analysis (RGMA) component of the Earth and Environmental System Modeling programme of the U.S. Department of Energy's Office of Biological and Environmental Research (BER) via the National Science Foundation (NSF; interagency agreement no. 1844590). Shunsuke Noguchi was supported by the Japan Society for the Promotion of Science (KAKENHI; grant no. 19K14798). Eun-Pa Lim was supported by the Australian government's National Environmental Science Program phase 2 and the Victorian Water and Climate Initiative phase 2. Seok-Woo Son was supported by the National Research Foundation of Korea (NRF), funded by the government of the Republic of Korea (Ministry of Science and ICT; grant no. 2017R1E1A1A01074889). David W. J. Thompson is supported by the US National Science Foundation Climate and Large-Scale Dynamics programme. Daniella I. V. Domeisen is supported by the Swiss National Science Foundation (project nos. PP00P2_170523 and PP00P2_198896). Chaim I. Garfinkel was supported by a European Research Council starting grant under the European Union Horizon 2020 research and innovation programme (agreement no. 677756).
This paper was edited by Martin Dameris and reviewed by two anonymous referees.
Abhik, S. and Hendon, H. H.: Influence of the QBO on the MJO during coupled model multiweek forecasts, Geophys. Res. Lett., 46, 9213–9221, 2019.
Adams, B. J., Mann, M., and Ammann, C.: Proxy evidence for an El Niño-like response to volcanic forcing, Nature, 426, 274–278, 2003.
Andrews, M., Knight, J., and Gray, L.: A simulated lagged response of the North Atlantic Oscillation to the solar cycle over the period 1960–2009, Environ. Res. Lett., 10, L054022, https://doi.org/10.1088/1748-9326/10/5/054022, 2015.
Agnet, J. G., Rozanov, E. V., Muthers, S., Peter, T., Brönnimann, S., Arfeuille, F., Beer, J., Shapiro, A. I., Raible, C. C., Steinhilber, F., and Schmutz, W. K.: Impact of a potential 21st century “grand solar minimum” on surface temperatures and stratospheric ozone, Geophys. Res. Lett., 40, 4420–4425, https://doi.org/10.1002/grl.50806, 2003.
Anstey, J. A. and Shepherd, T. G.: High-latitude influence of the Quasi-Biennial Oscillation, Q. J. Roy. Meteor. Soc., 140, 1–21, 2014.
Anstey, J. A., Simpson, I. R., Richter, J. H., Naoe, H., Taguchi, M., Serva, F., Gray, L. J., Butchart, N., Hamilton, K., Osprey, S., Bellprat, O., Braesicke, P., Bushell, A. C., Cagnazzo, C., Chen, C.-C., Chun, H.-Y., Garcia, R. R., Holt, L., Kawatani, Y., Kerzenmacher, T., Kim, Y.-H., Lott, F., McLandress, C., Scinocca, J., Stockdale, T. N., Versick, S., Watanabe, S., Yoshida, K., and Yukimoto, S.: Teleconnections of the Quasi-Biennial Oscillation in a multi-model ensemble of QBO-resolving models, Q. J. Roy. Meteor. Soc., Special Section QBO Modelling Intercomparison, 1–26, 2021.
Athanasiadis, P. J., Yeager, S., Kwon, Y. O., Bellucci, A., Smith D. W., and Tibaldi S.: Decadal predictability of North Atlantic blocking and the NAO, npj Climate and Atmospheric Science, 3, 20, https://doi.org/10.1038/s41612-020-0120-6, 2020.
Ayarzagüena, B., Polvani, L. M., Langematz, U., Akiyoshi, H., Bekki, S., Butchart, N., Dameris, M., Deushi, M., Hardiman, S. C., Jöckel, P., Klekociuk, A., Marchand, M., Michou, M., Morgenstern, O., O'Connor, F. M., Oman, L. D., Plummer, D. A., Revell, L., Rozanov, E., Saint-Martin, D., Scinocca, J., Stenke, A., Stone, K., Yamashita, Y., Yoshida, K., and Zeng, G.: No robust evidence of future changes in major stratospheric sudden warmings: a multi-model assessment from CCMI, Atmos. Chem. Phys., 18, 11277–11287, https://doi.org/10.5194/acp-18-11277-2018, 2018a.
Ayarzagüena, B., Barriopedro, D., Garrido-Perez, J. M., Abalos, M., de la Cámara, A., García-Herrera, R., Calvo, N., and Ordóñez, C.: Stratospheric connection to the abrupt end of the 2016/2017 Iberian drought, Geophys. Res. Lett., 45, 12639–12646, 2018b.
Ayarzagüena, B. , Charlton-Perez, A. J., Butler, A. H., Hitchcock, P., Simpson, I. R., Polvani, L. M., Butchart, N., Gerber, E. P., Gray, L., Hassler, B., Lin, P., Lott, F., Manzini, E., Mizuta, R., Orbe, C., Osprey, S., Saint-Martin, D., Sigmond, M., Taguchi, M., Volodin, E. M., and Watanabe, S.: Uncertainty in the response of stratospheric sudden warmings and stratosphere-troposphere coupling to quadrupled CO2 concentrations in CMIP6 models, J. Geophys. Res., 126, e2019JD032345, 2020.
Baehr, J., Fröhlich, K., Botzet, M., Domeisen, D. I. V., Kornblueh, L., Notz, D., Piontek, R., Pohlmann, H., Tietsche, S., and Müller, W. A.: The prediction of surface temperature in the new seasonal prediction system based on the MPI-ESM coupled climate model, Clim. Dynam., 44, 2723–2735, 2015.
Baldwin, M. P. and Dunkerton, T. J.: Propagation of the Arctic Oscillation from the stratosphere to the troposphere, J. Geophys. Res., 104, 30937–30946, 1999.
Baldwin, M. P. and Dunkerton, T. J.: Stratospheric Harbingers of Anomalous Weather Regimes, Science, 294, 581–584, 2001.
Baldwin M. P., Stephenson, D. B., Thompson, D. W. J., Dunkerton, T. J., Charlton, A. J., and O'Neill, A.: Stratospheric Memory and Skill of Extended-Range Weather Forecasts, Science, 301, 636–640, 2003.
Baldwin M. P., Birner, T., Brasseur, G., Burrows, J., Butchart, N., Garcia, R., Geller, M., Gray, L., Hamilton, K., Harnik, N., Hegglin, M. I., Langematz, U., Robock, A., Sato, K., and Scaife, A. A.: 100 Years of Progress in Understanding the Stratosphere and Mesosphere, Meteor. Mon., 59, 27.1–27.62, 2019.
Baldwin, M. P., Ayarzagüena, B., Birner, T., Butchart, N., Butler, A. H., Charlton-Perez, A. J., Domeisen, D. I. V., Garfinkel, C. I., Garny, H., Gerber, E. P., Hegglin, M. I., Langematz, U., and Pedatella, N. M.: Sudden stratospheric warmings, Rev. Geophys., 59, e2020RG000708, 2021.
Balmaseda, M. A., Ferranti, L., Molteni, F., and Palmer, T. N.: Impact of 2007 and 2008 Arctic ice anomalies on the atmospheric circulation: Implications for long-range predictions, Q. J. R. Meteor. Soc., 136, 1655–1664, 2010.
Banerjee, A., Fyfe, J. C., Polvani, L. M., Waugh, D., and Chang, K. L.: A pause in Southern Hemisphere circulation trends due to the Montreal Protocol, Nature, 579, 544–548, https://doi.org/10.1038/s41586-020-2120-4, 2020.
Barnes, E. A., Barnes, N. W., and Polvani, L. M.: Delayed Southern Hemisphere climate change induced by stratospheric ozone recovery, as projected by the CMIP5 models, 27, 852–867, 2014.
Barnes, E. A., Samarasinghe, S. M., Ebert-Uphoff, I., and Furtado, J. C.: Tropospheric and stratospheric causal pathways between the MJO and NAO, J. Geophys. Res.-Atmos., 124, 9356–9371, 2019.
Beagley, S. R., de Grandpre', J., Koshyk, J. N., McFarlane, N. A., and Shepherd, T. G.: Radiative-dynamical climatology of the first-generation Canadian middle atmosphere model, Atmos. Ocean, 35, 293–331, 1997.
Bittner, M., Timmreck, C., Schmidt, H., Toohey, M., and Krüger, K.: The impact of wave-mean flow interaction on the Northern Hemisphere polar vortex after tropical volcanic eruptions, J. Geophys. Res.-Atmos., 121, 5281–5297, 2016.
Blackport, R. and Screen, J. A.: Weakened evidence for mid-latitude impacts of Arctic warming, Nat. Clim. Change, 10, 1065–1666, 2020.
Boer, G. J. and Hamilton, K.: QBO influence on extratropical predictive skill, Clim. Dynam., 31, 987–1000, 2008.
Boville, B. A.: The Influence of the Polar Night Jet on the Tropospheric Circulation in a GCM, J. Atmos. Sci., 41, 1132–1142, 1984.
Butchart, N., Austin, J., Knight, J. R., Scaife, A. A., and Gallani, M. L.: The Response of the Stratospheric Climate to Projected Changes in the Concentrations of Well-Mixed Greenhouse Gases from 1992 to 2051, J. Climate, 13, 2142–2159, 2000.
Butler A. H. and Polvani, L. M.: El Niño, La Niña, and stratospheric sudden warmings: A re-evaluation in light of the observational record, Geophys. Res. Lett., 38, L13807, https://doi.org/10.1029/2011GL048084, 2011.
Butler, A. H., Polvani, L. M., and Deser, C.: Separating the stratospheric and tropospheric pathways of El Niño-Southern Oscillation teleconnections, Environ. Res. Lett., 9, 024014, https://doi.org/10.1088/1748-9326/9/2/024014, 2014.
Butler A. H., Arribas, A., Athanassiadou, M., Baehr, J., Calvo, N., Charlton-Perez, A., Deque, M., Domeisen, D. I. V., Fröhlich, K., Hendon, H., Imada, Y., Ishii, M., Iza, M., Karpechko, A. Y., Kumar, A., Maclachlan, C., Merryfield, W. J., Muller, W. A., O'Neill, A., Scaife, A. A., Scinocca, J., Sigmond, M., Stockdale, T. N., and Yasuda, T.: The Climate-system Historical Forecast Project: Do stratosphere-resolving models make better seasonal climate predictions in boreal winter?, Q. J. Roy. Meteor. Soc., 142, 1413–1427, https://doi.org/10.1002/qj.2743, 2016.
Butler, A. H., Charlton-Perez, A., Domeisen, D. I. V., Simpson, I. R., and Sjoberg J.: The predictability of Northern Hemisphere final stratospheric warmings and their surface impacts, Geophys. Res. Lett., 46, 10578–10588, 2019a.
Butler, A. H., Charlton-Perez, A., Domeisen, D. I. V., Garfinkel, C., Gerber, E. P., Hitchcock, P., Karpechko, A.-Y., Maycock, A. C., Sigmond, M., Simpson, I., and Son, S.-W.: Sub-seasonal Predictability and the Stratosphere, in: Chapter 11, The Gap Between Weather and Climate Forecasting, 223–241, 2019b.
Butler, A. H., Lawrence, Z. D., Lee, S. H., Lillo, S. P., and Long, C. S.: Differences between the 2018 and 2019 stratospheric polar vortex split events, Q. J. Roy. Meteor. Soc., 146, 3503–3521, https://doi.org/10.1002/qj.3858, 2020.
Byrne, N. J. and Shepherd, T. G.: Seasonal persistence of circulation anomalies in the Southern Hemisphere stratosphere and its implications for the troposphere, J. Climate, 31, 3467–3483, https://doi.org/10.1175/JCLI-D-17-0557.1, 2018.
Byrne, N. J., Shepherd, T. G., Woollings, T., and Plumb, R. A.: Non-stationarity in Southern Hemisphere climate variability associated with the seasonal breakdown of the stratospheric polar vortex, J. Climate, 30, 7125–7139, 2017.
Byrne, N. J., Shepherd, T. G., and Polichtchouk, I.: Subseasonal-to-seasonal predictability of the Southern Hemisphere eddy-driven jet during austral spring and early summer, J. Geophys. Res., 124, 6841–6855, 2019.
Cagnazzo, C. and Manzini, E.: Impact of the stratosphere on the winter tropospheric teleconnections between ENSO and the North Atlantic and European Region, J. Climate, 22, 1223–1238, 2009.
Cai, M., Yu, Y., Deng, Y., van den Dool, H. M., Ren, R., Saha, S., Wu, X., and Huang, J.: Feeling the pulse of the stratosphere: an emerging opportunity for predicting continental-scale cold-air outbreaks 1 month in advance, B. Am. Meteorol. Soc., 97, 1475–1489, 2016.
Cassou, C.: Intraseasonal interaction between the Madden–Julian Oscillation and the North Atlantic Oscillation, Nature, 455, 523–527, 2008.
Ceppi, P. and Shepherd, T. G.: The role of the stratospheric polar vortex for the austral jet response to greenhouse gas forcing, Geophys. Res. Lett., 46, 6972–6979, 2019.
Charlton, A. J., O'Neill, A., Stephenson, D. B., Lahoz, W. A., and Baldwin, M. P.: Can knowledge of the state of the stratosphere be used to improve statistical forecasts of the troposphere?, Q. J. R. Meteor. Soc., 129, 3205–3224, 2003.
Charlton-Perez, A. J., Baldwin, M. P., Birner, T., Black, R. X., Butler, A. H., Calvo, N., Davis, N. A., Gerber, E. P., Gillett, N., Hardiman, S., Kim, J., Krüger, K., Lee, Y.-Y., Manzini, E., McDaniel, B. A., Polvani, L., Reichler, T., Shaw, T. A., Sigmond, M., Son, S.-W., Toohey, M., Wilcox, L., Yoden, S., Christiansen, B., Lott, F., Shindell, D., Yukimoto, S., and Watanabe, S.: On the lack of stratospheric dynamical variability in low-top versions of the CMIP5 models, J. Geophys. Res.-Atmos., 118, 2494–2505, 2013.
Charlton-Perez, A. J., Ferranti, L., and Lee, R. W.: The influence of the stratospheric state on North Atlantic weather regimes, Q. J. Roy. Meteor. Soc., 144, 1140–1151, 2018.
Charney, J. G. and Drazin, P. G.: Propagation of planetary-scale disturbances from the lower into the upper atmosphere, J. Geophys. Res., 66, 83–109, https://doi.org/10.1029/JZ066i001p00083, 1961.
Chiodo, G., Oehrlein, J., Polvani, L. M., Fyfe, J. C., and Smith, A. K.: Insignificant influence of the 11-year solar cycle on the North Atlantic Oscillation, Nat. Geosci., 12, 94–99, 2019.
Choi, J. and Son, S.-W.: Stratospheric initial condition for skillful surface prediction in the ECMWF model, Geophys. Res. Lett., 21, 12556–12564, 2019.
Christiansen B.: Downward propagation and statistical forecast of the near-surface weather, J. Geophys. Res. Atmos., 110, D14104, https://doi.org/10.1029/2004JD005431, 2005.
Cohen, J. and Entekhabi, D.: Eurasian snow cover variability and northern hemisphere climate variability, Geophys. Res. Lett., 26, 345–348, 1999.
Cohen, J. and Jones, J.: A new index for more accurate winter predictions, Geophys. Res. Lett., 38, L21701, https://doi.org/10.1029/2011GL049626, 2011.
Cohen, J., Furtado, J. C., Jones, J., Barlow, M., Whittleston, D., and Entekhabi, D.: Linking Siberian snow cover to precursors of stratospheric variability, J. Climate, 27, 5422–5432, 2014.
Crutzen, P. J.: Estimates of Possible Variations in Total Ozone Due to Natural Causes and Human Activities, Ambio, 3, 201–210, 1974.
Dai, A. and Song, M.: Little influence of Arctic amplification on mid-latitude climate, Nat. Clim. Chang., 10, 231–237, 2020.
Davies, P. A., McCarthy, M., Christidis, N., Dunstone, N., Fereday, D., Kendon, M., Knight, J. R., Scaife, A. A., and Sexton, D.: The wet and stormy UK winter of 2019/2020, Weather, 76, 396–402, https://doi.org/10.1002/wea.3955, 2021.
Domeisen, D. I. V.: Estimating the Frequency of Sudden Stratospheric Warming Events From Surface Observations of the North Atlantic Oscillation, J. Geophys. Res.-Atmos., 124, 3180–3194, 2019.
Domeisen, D. I. V. and Butler, A. H.: Stratospheric drivers of extreme events at the Earth's surface, Commun. Earth Environ., 59, 1–8, 2020.
Domeisen, D. I. V., Sun, L., and Chen, G.: The role of synoptic eddies in the tropospheric response to stratospheric variability, Geophys. Res. Lett., 40, 1–5, 2013.
Domeisen, D. I. V., Badin, G., and Koszalka, I. M.: How Predictable Are the Arctic and North Atlantic Oscillations? Exploring the Variability and Predictability of the Northern Hemisphere, J. Climate, 31, 997–1014, 2018.
Domeisen, D. I. V., Garfinkel, C. I., and Butler, A. H.: The Teleconnection of El Niño Southern Oscillation to the Stratosphere, Rev. Geophys., 57, 5–47, 2019.
Domeisen, D. I. V., Butler, A. H., Charlton-Perez, A. J., Ayarzaguena, B., Baldwin, M. P., Dunn-Sigouin, E., Furtado, J. C., Garfinkel, C. I., Hitchcock, P., Karpechko, A. Y., Kim, H., Knight, J., Lang, A. L., Lim, E.-P., Marshall, A., Roff, G., Schwartz, C., Simpson, I. R., Son, S.-W., and Taguchi, M.: The role of stratosphere-troposphere coupling in sub-seasonal to seasonal prediction. 1. Predictability in the Stratosphere, J. Geophys. Res., 125, e2019JD030920, https://doi.org/10.1029/2019JD030923, 2020a.
Domeisen, D. I. V., Butler, A. H., Charlton-Perez, A. J., Ayarzaguena, B., Baldwin, M. P., Dunn-Sigouin, E., Furtado, J. C., Garfinkel, C. I., Hitchcock, P., Karpechko, A. Y., Kim, H., Knight, J., Lang, A. L., Lim, E.-P., Marshall, A., Roff, G., Schwartz, C., Simpson, I. R., Son, S.-W., and Taguchi, M.: The role of stratosphere-troposphere coupling in sub-seasonal to seasonal prediction. 2. Predictability arising from stratosphere-troposphere coupling, J. Geophys. Res., 125, e2019JD030923, https://doi.org/10.1029/2019JD030923, 2020b.
Douville, H.: Stratospheric polar vortex influence on Northern Hemisphere winter climate variability, Geophys. Res. Lett., 36, L18703, 2009.
Dunstone, N., Smith, D., Scaife, A., Hermanson, L., Eade, R., Robinson, N., Andrews, M., and Knight, J.: Skilful predictions of the winter North Atlantic Oscillation one year ahead, Nat. Geosci., 9, 809–814, https://doi.org/10.1038/ngeo2824, 2016.
Eade R., Smith, D., Scaife, A. A., and Wallace, E.: Do seasonal to decadal climate predictions underestimate the predictability of the real world?, Geophys. Res. Lett, 41, 5620–5628, https://doi.org/10.1002/2014GL061146, 2014.
Ebdon, R. A.: The quasi-biennial oscillation and its association with tropospheric circulation patterns, Meteor. Mag., 104, 282–297, 1975.
Farman, J., Gardiner, B., and Shanklin, J.: Large losses of total ozone in Antarctica reveal seasonal interaction, Nature, 315, 207–210, 1985.
Fels, S. B.: Radiative–Dynamical Interactions in the Middle Atmosphere, Adv. Geophys., 28, 277–300, 1985.
Fereday, D. R., Maidens, A., Arribas, A., Scaife, A. A., and Knight, J. R.: Seasonal forecasts of northern hemisphere winter 2009/10, Environ. Res. Lett., 7, 034031, https://doi.org/10.1088/1748-9326/7/3/034031, 2012.
Fereday, D. R., Chadwick, R., Knight, J. R., and Scaife, A. A.: Tropical rainfall drives stronger future ENSO-NAO teleconnection in CMIP5 models, Geophys. Res. Lett., 47, e2020GL088664, https://doi.org/10.1029/2020GL088664, 2020.
Fletcher, C. G., Hardiman, S. C., Kushner, P. J., and Cohen, J.: The dynamical response to snow cover perturbations in a large ensemble of atmospheric GCM integrations, J. Climate, 22, 1208–1222, https://doi.org/10.1175/2008JCLI2505.1, 2009.
Folland, C. K., Scaife, A. A., Lindesay, J., and Stephenson, D. B.: How potentially predictable is northern European winter climate a season ahead?, Int. J. Climatol., 32, 801–818, https://doi.org/10.1002/joc.2314, 2012.
Furtado, J. C., Cohen, J. L., Butler, A. H., Riddle, E. E., and Kumar, A.: Eurasian snow cover variability and links to winter climate in the CMIP5 models, Clim. Dynam., 45, 2591–2605, 2015.
Garfinkel, C. I. and Schwartz, C.: MJO-related tropical convection anomalies lead to more accurate stratospheric vortex variability in subseasonal forecast models, Geophys. Res. Lett., 44, 10054–10062, https://doi.org/10.1002/2017GL074470, 2017.
Garfinkel, C. I., Butler, A. H., Waugh, D. W., Hurwitz, M. M., and Polvani, L. M.: Why might stratospheric sudden warmings occur with similar frequency in El Nino and La Nina winters?, J. Geophys. Res. Atmos., 117, D19106, https://doi.org/10.1029/2012JD017777, 2012a.
Garfinkel, C. I., Feldstein, S. B., Waugh, D. W., Yoo, C., and Lee, S.: Observed connection between stratospheric sudden warmings and the Madden-Julian Oscillation, Geophys. Res. Lett., 39, L18807, https://doi.org/10.1029/2012GL053144, 2012b.
Garfinkel, C. I., Son, S.-W., Song, K., Aquila, V., and Oman, L. D.: Stratospheric variability contributed to and sustained the recent hiatus in Eurasian winter warming, Geophys. Res. Lett., 44, 374–382, 2017.
Garfinkel, C. I., Schwartz, C., Domeisen, D. I. V., Son, S.-W., Butler, A. H., and White, I. P.: Extratropical atmospheric predictability from the Quasi-Biennial Oscillation in subseasonal forecast models, J. Geophys. Res., 123, 7855–7866, 2018.
Garfinkel, C. I., Schwartz, C., Butler, A. H., Domeisen, D. I. V., Son, S.-W., and White, I. P.: Weakening of the Teleconnection From El Niño–Southern Oscillation to the Arctic Stratosphere Over the Past Few Decades: What Can Be Learned From Subseasonal Forecast Models?, J. Geophys. Res.-Atmos., 124, 7683–7696, 2019.
Garfinkel, C. I., Schwartz, C., White, I. P., and Rao, J.: Predictability of the early winter Arctic oscillation from autumn Eurasian snowcover in subseasonal forecast models, Clim. Dynam., 55, 961–974, 2020.
Gerber, E. P., Butler, A., Calvo, N., Charlton-Perez, A., Giorgetta, M., Manzini, E., Perlwitz, J., Polvani, L. M., Sassi, F., Scaife, A. A., Shaw, T. A., Son, S.-W., and Watanabe, S.: Assessing and Understanding the Impact of Stratospheric Dynamics and Variability on the Earth System, B. Am. Meteorol. Soc., 93, 845–859, 2012.
Givon, Y., Garfinkel, C. I., and White, I.: Transient extratropical response to solar ultraviolet radiation in the Northern Hemisphere winter, J. Climate, 34, 3367–3383, 2021.
Graversen, R. G. and Christiansen, B.: Downward propagation from the stratosphere to the troposphere: A comparison of the two hemispheres, J. Geophys. Res. Atmos., 108, 4780, https://doi.org/10.1029/2003JD004077, 2003.
Gray, L. J., Beer, J., Geller, M., Haigh, J. D., Lockwood, M., Matthes, K., Cubasch, U., Fleitmann, D., Harrison, G., Hood, L., Luterbacher, J., Meehl, G. A., Shindell, D., van Geel, B., and White, W.: Solar influences on climate, Rev. Geophys., 48, RG4001, https://doi.org/10.1029/2009RG000282, 2010.
Gray, L. J., Scaife, A. A., Mitchell, D. M., Osprey, S., Ineson, S., Hardiman, S., Butchart, N., Knight, J., Sutton, R., and Kodera, K.: A lagged response to the 11 year solar cycle in observed winter Atlantic/European weather patterns, J. Geophys. Res. Atmos., 118, 13405–13420, 2013.
Gray, L. J., Anstey, J. A., Kawatani, Y., Lu, H., Osprey, S., and Schenzinger, V.: Surface impacts of the Quasi Biennial Oscillation, Atmos. Chem. Phys., 18, 8227–8247, https://doi.org/10.5194/acp-18-8227-2018, 2018.
Hall, R. J., Scaife, A. A., Hanna, E., Jones, J. M., and Erdélyi, R.: Simple statistical probabilistic forecasts of the winter NAO, Weather Forecast., 32, 1585–1601, 2017.
Harari, O., Garfinkel, C. I., Ziskin Ziv, S., Morgenstern, O., Zeng, G., Tilmes, S., Kinnison, D., Deushi, M., Jöckel, P., Pozzer, A., O'Connor, F. M., and Davis, S.: Influence of Arctic stratospheric ozone on surface climate in CCMI models, Atmos. Chem. Phys., 19, 9253–9268, https://doi.org/10.5194/acp-19-9253-2019, 2019.
Hardiman, S. C., Scaife, A. A., Dunstone, N. J., and Wang, L.: Subseasonal vacillations in the winter stratosphere, Geophys. Res. Lett., 47, e2020GL087766, https://doi.org/10.1029/2020GL087766, 2020a.
Hardiman, S. C., Dunstone, N. J., Scaife, A. A., Smith, D. M., Knight, J. R., Davies, P., Claus, M., and Greatbatch, R. J.: Predictability of European winter 2019/20: Indian Ocean dipole impacts on the NAO, Atmos. Sci. Lett., 2020, e1005, https://doi.org/10.1002/asl.1005, 2020b.
Hardiman, S. C., Butchart, N., Charlton-Perez, A. J., Shaw, T. A., Akiyoshi, H., Baumgaertner, A., Bekki, S., Braesicke, P., Chipperfield, M., Dameris, M., Garcia, R. R., Michou, M., Pawson, S., Rozanov, E., and Shibata, K.: Improved predictability of the troposphere using stratospheric final warmings, J. Geophys. Res., 116, D18113, https://doi.org/10.1029/2011JD015914, 2011.
Harnik N.: Observed stratospheric downward reflection and its relation to upward pulses of wave activity, J. Geophys. Res., 114, D08120, https://doi.org/2008JD010493, 2009.
Harnik, N. and Lindzen, R. S.: The effect of reflecting surfaces on the vertical structure and variability of stratospheric planetary waves, J. Atmos. Sci., 58, 2872–2894, 2001.
Haynes, P. H., McIntyre, M. E., Shepherd, T. G., Marks, C. J., and Shine, K. P.: On the “Downward Control” of Extratropical Diabatic Circulations by Eddy-Induced Mean Zonal Forces, J. Atmos. Sci., 48, 651–678, 1991.
Haynes, P., Hitchcock, P., Hitchman, M., Yoden, S., Hendon, H., Kiladis, G., Kodera, K., and Simpson, I.: The influence of the stratosphere on the tropical troposphere, J. Meteorol. Soc. Jpn., 99, 803–845, https://doi.org/10.2151/jmsj.2021-040, 2021.
Henderson, G. R., Peings, Y., Furtado, J. C., and Kushner, P. J.: Snow-atmosphere coupling in the northern hemisphere, Nat. Clim. Change, 8, 954–963, 2018.
Hendon, H. H. and Abhik, S.: Differences in vertical structure of the Madden-Julian oscillation associated with the quasi-biennial oscillation, Geophys. Res. Lett., 45, 4419–4428, 2018.
Hendon, H. H., Lim, E.-P., and Abhik, S.: Impact of Interannual Ozone Variations on the Downward Coupling of the 2002 Southern Hemisphere Stratospheric Warming, J. Geophys. Res.-Atmos., 125, 1–16, https://doi.org/10.1029/2020JD032952, 2020.
Hermanson L., Eade, R., Robinson, N. H., Andrews, M. B., Knight, J. R., Scaife, A. A., and Smith, D. M.: Forecast cooling of the Atlantic subpolar gyre and associated impacts, Geophys. Res. Lett., 41, 5167–5174, 2014.
Hitchcock, P. and Simpson, I. R.: The Downward Influence of Stratospheric Sudden Warmings, J. Atmos. Sci., 71, 3856–3876, 2014.
Hitchcock, P. and Haynes, P. H.: Stratospheric control of planetary waves, Geophys. Res. Lett., 43, 11884–11892, https://doi.org/10.1002/2016GL071372, 2016.
Hitchcock, P., Shepherd, T.G., Taguchi, M., Yoden, S., and Noguchi, S.: Lower-stratospheric radiative damping and Polar-night Jet Oscillation events, J. Atmos. Sci., 70, 1391–1408, 2013.
Holton, J. R. and Tan, H.-C.: The influence of the equatorial quasi-biennial oscillation on the global circulation at 50 mb, J. Atmos. Sci., 37, 2200–2208, 1980.
Honda, M., Inoue, J., and Yamane, S.: Influence of low Arctic sea-ice minima on anomalously cold Eurasian winters, Geophys. Res. Lett., 36, L08707, https://doi.org/10.1029/2008GL037079, 2009.
Huebener, H., Cubasch, U., Langematz, U., Spangehl, T., Niehörster, F., Fast, I., and Kunze, M.: Ensemble climate simulations using a fully coupled ocean–troposphere–stratosphere general circulation model, Phil. Trans. R. Soc. A., 365, 2089–2101, 2007.
Hurwitz, M. M., Newman, P. A., and Garfinkel, C. I.: On the influence of North Pacific sea surface temperature on the Arctic winter climate, J. Geophys. Res., 117, D19110, https://doi.org/10.1029/2012JD017819, 2012.
Ineson, S. and Scaife, A. A.: The role of the stratosphere in the European climate response to El Nino, Nat. Geosci., 2, 32–36, 2009.
Ineson S., Scaife, A. A., Knight, J. R., Manners, J. C., Dunstone, N. J., Gray, L. J., and Haigh, J. D.: Solar Forcing of Winter Climate Variability in the Northern Hemisphere, Nat. Geosci., 4, 753–757, 2011.
Ineson, S., Maycock, A., Gray, L. J., Scaife, A. A., Dunstone, N. J., Harder, J. W., Knight, J. R., Lockwood, M., Manners, J. C., and Wood, R. A.: Regional climate impacts of a possible future grand solar minimum, Nat. Commun., 6, 7535, https://doi.org/10.1038/ncomms8535, 2015.
Inoue, M., Takahashi, M., and Naoe, H.: Relationship between the stratospheric quasi-biennial oscillation and tropospheric circulation in northern autumn, J. Geophys. Res.-Atmos., 116, D24115, https://doi.org/10.1029/2011JD016040, 2011.
Jain, S., Scaife, A. A., and Mitra, A. K.: Skill of Indian summer monsoon rainfall prediction in multiple seasonal prediction systems, Clim. Dynam., 52, 5291–5301, 2019.
Jaiser, R., Dethloff, K., and Handorf, D: Stratospheric response to arctic sea ice retreat and associated planetary wave propagation changes, Tellus A., 65, 19375, https://doi.org/10.3402/tellusa.v65i0.19375, 2013.
Jia, L., Yang, X., Vecchi, G., Gudgel, R., Delworth, T., Fueglistaler, S., Lin, P., Scaife, A. A., Underwood, S., and Lin, S.: Seasonal Prediction Skill of Northern Extratropical Surface Temperature Driven by the Stratosphere, J. Climate, 30, 4463–4475, 2017.
Johnson, S. J., Stockdale, T. N., Ferranti, L., Balmaseda, M. A., Molteni, F., Magnusson, L., Tietsche, S., Decremer, D., Weisheimer, A., Balsamo, G., Keeley, S. P. E., Mogensen, K., Zuo, H., and Monge-Sanz, B. M.: SEAS5: the new ECMWF seasonal forecast system, Geosci. Model Dev., 12, 1087–1117, https://doi.org/10.5194/gmd-12-1087-2019, 2019.
Joshi, M. M., Charlton, A. J., and Scaife, A. A.: On the influence of stratospheric water vapor changes on the tropospheric circulation, Geophys. Res. Lett., 33, L09806, https://doi.org/10.1029/2006GL025983, 2006.
Jucker, M. and Reichler, T.: Dynamical precursors for statistical prediction of stratospheric sudden warming events, Geophys. Res. Lett., 45, 13124–13132, 2018.
Jucker, M., Reichler, T., and Waugh, D. W.: How frequent are Antarctic sudden stratospheric warmings in present and future climate?, Geophys. Res. Lett., 48, e2021GL093215, https://doi.org/10.1029/2021GL093215, 2021.
Kang, I. S., Kug, J. S., Lim, M. J., and Choi, D. H.: Impact of transient eddies on extratropical seasonal-mean predictability in DEMETER models, Clim. Dynam., 37, 509–519, 2011a.
Kang, S. M., Polvani, L. M., Fyfe, J. C., and Sigmond, M.: Impact of Polar Ozone Depletion on Subtropical Precipitation, Science, 332, 951–954, 2011b.
Karpechko, A. Y.: Predictability of Sudden Stratospheric Warmings in the ECMWF Extended-Range Forecast System, Mon. Wea. Rev., 146, 1063–1075, https://doi.org/10.1175/MWR-D-17-0317.1, 2018.
Karpechko, A. Y. and Manzini, E.: Stratospheric influence on tropospheric climate change in the Northern Hemisphere, J. Geophys. Res., 117, D05133, https://doi.org/10.1029/2011JD017036, 2012.
Karpechko, A., Gillett, N. P., Marshall, G. J., and Scaife, A. A.: Stratospheric influence on circulation changes in the Southern Hemisphere troposphere in coupled climate models, Geophys. Res. Lett., 35, L20806, https://doi.org/10.1029/2008GL035354, 2008.
Karpechko, A. Y., Perlwitz, J., and Manzini, E.: A model study of tropospheric impacts of the Arctic ozone depletion 2011, J. Geophys. Res.-Atmos., 119, 7999–8014, 2014.
Karpechko, A. Y., Hitchcock, P., Peters, D. H. W., and Schneidereit, A.: Predictability of downward propagation of sudden stratospheric warmings, Q. J. Roy. Meteor. Soc., 143, 1459–1470, 2017.
Karpechko, A. Y., Charlton-Perez, A., Balmaseda, M., Tyrrell, N., and Vitart, F.: Predicting sudden stratospheric warming 2018 and its climate impacts with a multimodel ensemble, Geophys. Res. Lett., 45, 13538–13546, 2018.
Karpechko, A. Y., Tyrrell, N. L., and Rast, S.: Sensitivity of QBO teleconnection to model circulation biases, Q. J. Roy. Meteor. Soc., 147, 2147–2159, 2021.
Kautz, L. A., Polichtchouk, I., Birner, T., Garny, H., and Pinto, J. G.: Enhanced extended-range predictability of the 2018 late-winter Eurasian cold spell due to the stratosphere, Q. J. Roy. Meteor. Soc., 146, 1040–1055, 2019.
Kidston, J., Scaife, A. A., Hardiman, S. C., Mitchell, D. M., Butchart, N., Baldwin, M. P., and Gray, L. J.: Stratospheric influence on tropospheric jet streams, storm tracks and surface weather, Nat. Geosci., 8, 433–440, 2015.
Kim, B. M., Son, S. W., Min, S. K., Jeong, J. H., Kim, S. J., Zhang, X., Shim, T., and Yoon, J. H.: Weakening of the stratospheric polar vortex by Arctic sea-ice loss, Nat. Comm., 5, 4646, https://doi.org/10.1038/ncomms5646, 2014.
Kim, H., Caron, J. M., Richter, J. H., and Simpson, I. R.: The lack of QBO-MJO connection in CMIP6 models, Geophys. Res. Lett., 47, e2020GL087295, https://doi.org/10.1029/2020GL087295, 2020.
King, M. P., Hell, M., and Keenlyside, N.: Investigation of the atmospheric mechanisms related to the autumn sea ice and winter circulation link in the Northern Hemisphere, Clim. Dynam., 46, 1185–1195, 2016.
King, A. D., Butler, A. H., Jucker, M., Earl, N. O., and Rudeva, I.: Observed relationships between sudden stratospheric warmings and European climate extremes, J. Geophys. Res., 124, 13943–13961, 2019.
Knight, J., Scaife, A., Bett, P. E., Collier, T., Dunstone, N., Gordon, M., Hardiman, S., Hermanson, L., Ineson, S., Kay, G., McLean, P., Pilling, C., Smith, D., Stringer, N., Thornton, H., and Walker B.: Predictability of European winters 2017/2018 and 2018/2019: Contrasting Influences from the Tropics and Stratosphere, Atmos. Sci. Lett., e1009, https://doi.org/10.1002/asl.1009, 2020.
Kodera, K.: On the origin and nature of the interannual variability of the winter stratospheric circulation in the northern hemisphere, J. Geophys. Res., 100, 14077–14087, 1995.
Kodera, K. and Kuroda, Y.: Dynamical response to the solar cycle, J. Geophys. Res., 107, 4749, https://doi.org/10.1029/2002JD002224, 2002.
Kodera K. and Mukougawa, H.: Eurasian Cold Surges Triggered by the Nonlinear Reflection of Stratospheric Planetary Waves in December 2012, SOLA, 13, 140–145, https://doi.org/10.2151/sola.2017-026, 2017.
Kodera, K., Yamazaki, K., Chiba, M., and Shibata, K.: Downward propagation of upper stratospheric mean zonal wind perturbation to the troposphere, Geophys. Res. Lett., 17, 1263–1266, 1990.
Kodera, K., Mukougawa, H., and Itoh, S.: Tropospheric impact of reflected planetary waves from the stratosphere, Geophys. Res. Lett., 35, L16806, https://doi.org/10.1029/2008GL034575, 2008.
Kolstad, E. W. and Screen, J. A.: Non-stationary relationship between autumn Arctic sea ice and the winter North Atlantic Oscillation, Geophys. Res. Lett., 46, 7583–7591, 2019.
Kretschmer, M., Coumou, D., Donges, J. F., and Runge, J.: Using causal effect networks to analyze different Arctic drivers of midlatitude winter circulation, J. Climate, 29, 4069–4081, 2016.
Kretschmer, M., Zappa, G., and Shepherd, T. G.: The role of Barents–Kara sea ice loss in projected polar vortex changes, Weather Clim. Dynam., 1, 715–730, https://doi.org/10.5194/wcd-1-715-2020, 2020.
Kretschmer, M., Adams, S. V., Arribas, A., Prudden, R., Robinson, N., Saggioro, E., and Shepherd, T. G.: Quantifying causal pathways of teleconnections, B. Am. Meteorol. Soc., 102, E2247–E2263, https://doi.org/10.1175/BAMS-D-20-0117.1, 2021.
Kuhn, T. S.: The Structure of Scientific Revolutions, University of Chicago Press, Chicago, IL, USA, 1970.
Kunz, T. and Greatbatch, R. J.: On the Northern Annular Mode Surface Signal Associated with Stratospheric Variability, J. Atmos. Sci., 70, 2103–2118, 2013.
Kuroda, Y.: Role of the stratosphere on the predictability of medium-range weather forecast: A case study of winter 2003–2004, Geophys. Res. Lett., 35, L19701, https://doi.org/10.1029/2008GL034902, 2008.
Kuroda, Y. and Kodera, K.: Variability of the polar night jet in the northern and southern hemispheres, J. Geophys. Res., 106, 20703–20713, https://doi.org/10.1029/2001JD900226.2001, 2001.
Kushner, P. J. and Polvani, L. M.: Stratosphere-troposphere coupling in a relatively simple AGCM: The role of eddies, J. Climate, 17, 629–639, 2004.
Kushnir, Y., Scaife, A. A., Arritt, R., Balsamo, G., Boer, G., Doblas-Reyes, F., Hawkins, E., Kimoto, M., Kolli, R. K., Kumar, A., Matei, D., Matthes, K., Müller, W. A., O'Kane, T., Perlwitz, J., Power, S., Raphael, M., Shimpo, A., Smith, D., Tuma, M., and Wu, B.: Towards operational predictions of the near-term climate, Nat. Clim. Change, 9, 94–101, 2019.
Labe, Z., Peings, Y., and Magnusdottir, G.: The effect of QBO phase on the atmospheric response to projected Arctic sea ice loss in early winter, Geophys. Res. Lett., 46, 7663–7671, 2019.
Labitzke, K.: On the Mutual Relation between Stratosphere and Troposphere during Periods of Stratospheric Warmings in Winter, J. Appl. Meteorol. Clim., 4, 91–99, 1965.
Lai, W. K. M., Robson, J. I., Wilcox, L. J., and Dunstone, N.: Mechanisms of Internal Atlantic Multidecadal Variability in HadGEM3-GC3.1 at Two Different Resolutions, J. Climate, 35, 1365–1383, https://doi.org/10.1175/JCLI-D-21-0281.1, 2021.
Lawrence, Z. D., Perlwitz, J., Butler, A. H., Manney, G. L., Newman, P. A., Lee, S. H., and Nash, E. R.: The remarkably strong Arctic stratospheric polar vortex of winter 2020: Links to record-breaking Arctic Oscillation and ozone loss, J. Geophys. Res.-Atmos., 125, e2020JD033271, https://doi.org/10.1029/2020JD033271, 2020.
Leckebusch, G. C., Ulbrich, U., Fröhlich, L., and Pinto, J. G.: Property loss potentials for European midlatitude storms in a changing climate, Geophys. Res. Lett., 34, L05703, https://doi.org/10.1029/2006GL027663, 2007.
Lee, S. H., Charlton-Perez, A. J., Furtado, J. C., Woolnough, S. J.: Representation of the Scandinavia–Greenland pattern and its relationship with the polar vortex in S2S forecast models, Q. J. Roy. Meteor. Soc., 146, 4083–4098, 2020a.
Lee, S. H., Lawrence, Z. D., Butler, A. H., and Karpechko, A.: Seasonal forecasts of the exceptional Northern Hemisphere winter of 2020, Geophys. Res. Lett., 47, e2020GL090328, https://doi.org/10.1029/2020GL090328, 2020b.
Leung, T. Y., Leutbecher, M., Reich, S., and Shepherd, T. G.: Impact of the mesoscale range on error growth and the limits to atmospheric predictability, J. Atmos. Sci., 77, 3769–3779, 2020.
L'Heureux, M. L., Levine, A. F. Z., Newman, M., Ganter, C., Luo, J.-J., Tippett, M. K., and Stockdale, T. N.: ENSO Prediction, in: El Niño Southern Oscillation in a Changing Climate, edited by: McPhaden, M. J., Santoso, A., and Cai, W., AGU Monograph, Wiley, USA, 2020.
Lim, E.-P., Hendon, H. H., and Thompson, D. W. J.: Seasonal evolution of stratosphere-troposphere coupling in the Southern Hemisphere and implications for the predictability of surface climate, J. Geophys. Res.-Atmos., 123, 12002–12016, 2018.
Lim, E.-P., Hendon, H. H., Boschat, G., Hudson, D., Thompson, D. W. J., Dowdy, A. J., and Arblaster, J.: Australian hot and dry extremes induced by weakening of the stratospheric polar vortex, Nat. Geosci., 12, 896–901, 2019a.
Lim, Y., Son, S.-W., Marshall, A. G., Hendon, H. H., and Seo, K.-H.: Influence of the QBO on MJO prediction skill in the subseasonal-to-seasonal prediction models, Clim. Dynam., 53, 1681–1695, 2019b.
Lim, E.-P., Hendon, H. H., Butler, A. H., Thompson, D. W. J., Lawrence, Z. D., Scaife, A. A., Shepherd, T. G., Polichtchouk, I., Nakamura, H., Kobayashi, C., Comer, R., Coy, L., Dowdy, A., Garreaud, R. D., Newman, P. A., and Wang, G.: The 2019 Southern Hemisphere polar stratospheric warming and its impacts, B. Am. Meteorol. Soc., 102, E1150–E1171, 2021.
Lin, H., Brunet, G., and Derome, J.: An Observed Connection between the North Atlantic Oscillation and the Madden–Julian Oscillation, J. Climate, 22, 364–380, 2009.
Liu, C., Tian, B., Li, K.-F., Manney, G. L., Livesey, N. J., Yung, Y. L., and Waliser, D. E.: Northern Hemisphere mid-winter vortex-displacement and vortex-split stratospheric sudden warmings: influence of the Madden-Julian Oscillation and Quasi-Biennial Oscillation, J. Geophys. Res.-Atmos., 119, 12599–12620, 2014.
Lockwood, M.: Solar change and climate: An update in the light of the current exceptional solar minimum, Proc. R. Soc. A, 466, 303–329, 2010.
Lorenz, E. N.: The predictability of a flow which possesses many scales of motion, Tellus, 21, 289–307, 1969.
MacLachlan, C., Arribas, A., Peterson, K. A., Maidens, A., Fereday, D., Scaife, A. A., Gordon, M., Vellinga, M., Williams, A., Comer, R. E., Camp, J., Xavier, P., and Madec, G.: Global Seasonal forecast system version 5 (GloSea5): a high-resolution seasonal forecast system, Q. J. R. Meteorol. Soc., 141, 1072–1084, 2015.
Mann, M. E., Park, J., and Bradley, R. S.: Global interdecadal and century-scale climate oscillations during the past 5 centuries, Nature, 378, 266–270, 1995.
Manzini, E., Giorgetta, M. A., Esch, M., Kornblueh, L., and Roeckner, E.: The Influence of Sea Surface Temperatures on the Northern Winter Stratosphere: Ensemble Simulations with the MAECHAM5 Model, J. Climate, 19, 3863–3881, 2006.
Manzini, E., Karpechko, A. Y., Anstey, J., Baldwin, M. P., Black, R. X., Cagnazzo, C., Calvo, N., Charlton-Perez, A., Christiansen, B., Davini, P., Gerber, E., Giorgetta, M., Gray, L., Hardiman, S. C., Lee, Y.-Y., Marsh, D. R., McDaniel, B. A., Purich, A., Scaife, A. A., Shindell, D., Son, S.-W., Watanabe, S., and Zappa, G.: Northern winter climate change: Assessment of uncertainty in CMIP5 projections related to stratosphere-troposphere coupling, J. Geophys. Res. Atmos., 119, 7979–7998, https://doi.org/10.1002/2013JD021403, 2014.
Manzini, E., Karpechko, A. Y., and Kornblueh, L.: Nonlinear Response of the Stratosphere and the North Atlantic-European Climate to Global Warming, Geophys. Res. Lett., 45, 4255–4263, 2018.
Mariotti, A., Baggett, C., Barnes, E. A., Becker, E., Butler, A. H., Collins, D. C., Dirmeyer, P. A., Ferranti, L., Johnson, N. C., Jones, J., Kirtman, B. P., Lang, A. L., Molod, A., Newman, M., Robertson, A. W., Schubert, S., Waliser, D. E., and Albers J.: Windows of opportunity for skillful forecasts subseasonal to seasonal and beyond, B. Am. Meteorol. Soc., 101, E608–E625, 2020.
Marsh, D. R., Garcia, R. R., Kinnison, D. E., Boville, B. A., Sassi, F., Solomon, S. C., and Matthes, K.: Modeling the whole atmosphere response to solar cycle changes in radiative and geomagnetic forcing, J. Geophys. Res., 112, D23306, https://doi.org/10.1029/2006JD008306, 2007.
Marshall, A. and Scaife, A. A.: Improved predictability of stratospheric sudden warming events in an AGCM with enhanced stratospheric resolution, J. Geophys. Res., 115, D16114, https://doi.org/10.1029/2009JD012643, 2010.
Marshall, A. G., Scaife, A. A., and Ineson, S.: Enhanced Seasonal Prediction of European Winter Warming following Volcanic Eruptions, J. Climate, 22, 6168–6180, 2009.
Marshall, A. G., Hendon, H. H., Son, S. W., and Lim, Y.: Impact of the quasi-biennial oscillation on predictability of the Madden–Julian oscillation, Clim. Dynam., 49, 1365–1377, 2017.
Martin, Z., Wang, S., Nie, J., and Sobel, A.: The Impact of the QBO on MJO Convection in Cloud-Resolving Simulations, J. Atmos. Sci., 76, 669–688, 2019.
Martin, Z., Son, S. W., Butler, A., Hendon, H., Kim, H., Sobel, A., Yoden, S., and Zhang, C.: The influence of the quasi-biennial oscillation on the Madden–Julian oscillation, Nat. Rev. Earth Environ., 2, 477–489, 2021.
Matthias, V. and Kretschmer, M.: The Influence of Stratospheric Wave Reflection on North American Cold Spells, Mon. Weather Rev., 148, 1675–1690, 2020.
Maycock, A. C., Ineson, S., Gray, L. J., Scaife, A. A., Anstey, J. A., Lockwood, M., Butchart, N., Hardiman, S. C., Mitchell, D. M., and Osprey, S. M.: Possible impacts of a future grand solar minimum on climate: Stratospheric and global circulation changes, J. Geophys. Res.-Atmos., 120, 9043–9058, 2015.
McKenna, C. M., Bracegirdle, T. J., Shuckburgh, E. F., Haynes, P. H., and Joshi, M. M.: Arctic sea-ice loss in different regions leads to contrasting Northern Hemisphere impacts, Geophys. Res. Lett., 45, 945–954, 2018.
McLandress, C. and Shepherd, T. G.: Impact of climate change on stratospheric sudden warmings as simulated by the Canadian Middle Atmosphere Model, J. Climate, 22, 5449–5463, 2009.
McLandress, C., Shepherd, T. G., Scinocca, J. F., Plummer, D. A., Sigmond, M., Jonsson, A. I., and Reader, M. C.: Separating the dynamical effects of climate change and ozone depletion: Part 2. Southern Hemisphere Troposphere, J. Climate, 24, 1850–1868, 2011.
Meehl, G. A., Richter, J. H., Teng, H., Capotondi, A., Cobb, K., Doblas-Reyes, F., Donat, M. G., England, M. E., Fyfe, J. C., Han, W., Kim, H., Kirtman, B. P., Kushnir, Y., Lovenduski, N. S., Mann, M. E., Merryfield, W. J., Nieves, V., Pegion, K., Rosenbloom, N., Sanchez, S. C., Scaife, A. A., Smith, D., Subramanian, A. C., Sun, L., Thompson, D., Ummenhofer, C. C., and Xie, S. P. : Initialised Earth System Prediction from Subseasonal to Decadal Timescales, Nat. Rev. Earth. Environ., 2, 340–357, 2021.
Merryfield, W. J., Lee, W., Boer, G. J., Kharin, V. V., Scinocca, J. F., Flato, G. M., Ajayamohan, R. S., Fyfe, J. C., Tang, Y., and Polavarapu, S.: The Canadian Seasonal to Interannual Prediction System. Part I: Models and Initialization, Mon. Weather Rev., 141, 2910–2945, 2013.
Merryfield, W. J., Doblas-Reyes, F. J., Ferranti, L., Jeong, J.-H., Orsolini, Y. J., Saurral, R. I., Scaife, A. A., Tolstykh, M. A., and Rixen, M.: Advancing climate forecasting, Eos, 98, 17–21, 2017.
Merryfield, W. J., Baehr, J., Batté, L., Becker, E. J., Butler, A. H., Coelho, C. A. S., Danabasoglu, G., Dirmeyer, P. A., Doblas-Reyes, F. J., Domeisen, D. I. V., Ferranti, L., Ilynia, T., Kumar, A., Müller, W. A., Rixen, M., Robertson, A. W., Smith, D. M., Takaya, Y., Tuma, M., Vitart, F., White, C. J., Alvarez, M. S., Ardilouze, C., Attard, H., Baggett, C., Balmaseda, M. A., Beraki, A. F., Bhattacharjee, P. S., Bilbao, R., de Andrade, F. M., DeFlorio, M. J., Díaz, L. B., Ehsan, M. A., Fragkoulidis, G., Grainger, S., Green, B. W., Hell, M. C., Infanti, J. M., Isensee, K., Kataoka, T., Kirtman, B. P., Klingaman, N. P., Lee, J.-Y., Mayer, K., McKay, R., Mecking, J. V., Miller, D. E., Neddermann, N., Ng, C. H. J., Ossó, A., Pankatz, K., Peatman, S., Pegion, K., Perlwitz, J., Recalde-Coronel, G. C., Reintges, A., Renkl, C., Solaraju-Murali, B., Spring, A., Stan, C., Sun, Y. Q., Tozer, C. R., Vigaud, N., Woolnough, S., and Yeager, S.: Current and emerging developments in subseasonal to decadal prediction, B. Am. Meteorol. Soc., 101, E869–E896, 2020.
Molina, M. and Rowland, F.: Stratospheric sink for chlorofluoromethanes: chlorine atom-catalysed destruction of ozone, Nature, 249, 810–812, 1974.
Monge-Sanz, B. M., Bozzo, A., Byrne, N., Chipperfield, M. P., Diamantakis, M., Flemming, J., Gray, L. J., Hogan, R. J., Jones, L., Magnusson, L., Polichtchouk, I., Shepherd, T. G., Wedi, N., and Weisheimer, A.: A stratospheric prognostic ozone for seamless Earth System Models: performance, impacts and future, Atmos. Chem. Phys. Discuss. [preprint], https://doi.org/10.5194/acp-2020-1261, in review, 2021.
Müller, W. A. and Roeckner, E.: ENSO impact on midlatitude circulation patterns in future climate change projections, Geophys. Res. Lett., 33, L05711, https://doi.org/10.1029/2005GL025032, 2006.
Mukougawa, H., Sakai, H., and Hirooka, T.: High sensitivity to the initial condition for the prediction of stratospheric sudden warming, Geophys. Res. Lett., 32, L17806, https://doi.org/10.1029/2005GL022909, 2005.
Mukougawa, H., Hirooka, T., and Kuroda, Y.: Influence of stratospheric circulation on the predictability of the tropospheric Northern Annular Mode, Geophys. Res. Lett., 36, L08814, https://doi.org/10.1029/2008GL037127, 2009.
Mukougawa, H., Noguchi, S., Kuroda, Y., Mizuta, R., and Kodera, K.: Dynamics and predictability of downward-propagating stratospheric planetary waves observed in March 2007, J. Atmos. Sci., 74, 3533–3550, 2017.
Nie, Y., Scaife, A. A., Ren, H. L., Comer, R. E., Andrews, M. B., Davis, P., and Martin, N.: Stratospheric initial conditions provide seasonal predictability of the North Atlantic and Arctic Oscillations, Environ. Res. Lett., 14, 034006, https://doi.org/10.1088/1748-9326/ab0385, 2019.
Noguchi, S., Mukougawa, H., Kuroda, Y., Mizuta, R., Yabu, S., and Yoshimura, H.: Predictability of the stratospheric polar vortex breakdown: An ensemble reforecast experiment for the splitting event in January 2009, J. Geophys. Res.-Atmos., 121, 3388–3404, 2016.
Noguchi, S., Kuroda, Y., Mukougawa, H., Mizuta, R., and Kobayashi, C.: Impact of satellite observations on forecasting sudden stratospheric warmings, Geophys. Res. Lett., 47, e2019GL086233, https://doi.org/10.1029/2019GL086233, 2020a.
Noguchi, S., Kuroda, Y., Kodera, K., and Watanabe, S.: Robust enhancement of tropical convective activity by the 2019 Antarctic sudden stratospheric warming, Geophys. Res. Lett., 47, e2020GL088743, https://doi.org/10.1029/2020GL088743, 2020b.
Norton, W. A.: Sensitivity of northern hemisphere surface climate to simulation of the stratospheric polar vortex, Geophys. Res. Lett., 30, 1627, https://doi.org/10.1029/2003GL016958, 2003.
Oehrlein, J., Chiodo, G., and Polvani, L. M.: The effect of interactive ozone chemistry on weak and strong stratospheric polar vortex events, Atmos. Chem. Phys., 20, 10531–10544, https://doi.org/10.5194/acp-20-10531-2020, 2020.
Omrani, N. E., Keenlyside, N. S., Bader, J., and Manzini, E.: Stratosphere key for wintertime atmospheric response to warm Atlantic decadal conditions, Clim. Dynam., 42, 649–663, 2014.
O'Reilly, C. H., Weisheimer, A., Woollings, T., Gray, L. J., and MacLeod, D.: The importance of stratospheric initial conditions for winter North Atlantic Oscillation predictability and implications for the signal-to-noise paradox, Q. J. Roy. Meteor. Soc., 145, 131–146, 2019.
Orsolini, Y. J., Senan, R., Benestad, R. E., and Melsom, A.: Autumn atmospheric response to the 2007 low Arctic sea ice extent in coupled ocean–atmosphere hindcasts, Clim. Dynam., 38, 2437–2448, 2012.
Owens, M. J., Lockwood, M., Hawkins, E., Usoskin, I., Jones, G. S., Barnard, L., Schurer, A., and Fasullo, J.: The Maunder minimum and the Little Ice Age: an update from recent reconstructions and climate simulations, J. Space Weather Space Clim., 7, A33, https://doi.org/10.1051/swsc/2017034, 2017.
Pawson, S. and B. Naujokat: The cold winters of the middle 1990s in the northern lower stratosphere, J. Geophys. Res., 104, 14209–14222, 1999.
Pawson, S., Kodera, K., Hamilton, K., Shepherd, T. G., Beagley, S. R., Boville, B. A., Farrara, J. D., Fairlie, T. D. A., Kitoh, A., Lahoz, W. A., Langematz, U., Manzini, E., Rind, D. H., Scaife, A. A., Shibata, K., Simon, P., Swinbank, R., Takacs, L., Wilson, R. J., Al-Saadi, J. A., Amodei, M., Chiba, M., Coy, L., de Grandpré, J., Eckman, R. S., Fiorino, M., Grose, W. L., Koide, H., Koshyk, J. N., Li, D., Lerner, J., Mahlman, J. D., McFarlane, N. A., Mechoso, C. R., Molod, A., O'Neill, A., Pierce, R. B., Randel, W. J., Rood, R. B., and Wu, F.: The GCM-Reality Intercomparison Project for SPARC (GRIPS): Scientific Issues and Initial Results, B. Am. Meteorol. Soc., 81, 781–796, 2000.
Peings, Y., Brun, E., Mauvais, V., and Douville, H.: How stationary is the relationship between Siberian snow and Arctic Oscillation over the 20th century?, Geophys. Res. Lett., 40, 183–188, 2013.
Perlwitz, J. and Graf, H.: The Statistical Connection between Tropospheric and Stratospheric Circulation of the Northern Hemisphere in Winter, J. Climate, 8, 2281–2295, 1995.
Perlwitz, J. and Harnik, N.: Observational evidence of a stratospheric influence on the troposphere by planetary wave reflection, J. Climate, 16, 3011–3026, 2003.
Plumb, R. A. and Semeniuk, K.: Downward migration of extratropical zonal wind anomalies, J. Geophys. Res., 108, 4223, https://doi.org/10.1029/2002JD002773, 2003.
Pohlmann, H., Müller, W. A., Kulkarni, K., Kameswarrao, M., Matei, D., Vamborg, F. S. E., Kadow, C., Illing, S., and Marotzke, J.: Improved forecast skill in the tropics in the new MiKlip decadal climate predictions, Geophys. Res. Lett., 40, 5798–5802, https://doi.org/10.1002/2013GL058051, 2013.
Polvani, L. M. and Kushner, P. J.: Tropospheric response to stratospheric perturbations in a relatively simple general circulation model, Geophys. Res. Lett., 29, 18-1–18-4, https://doi.org/10.1029/2001GL014284, 2002.
Polvani, L. M., Waugh, D. W., Correa, G. J. P., and Son, S.-W.: Stratospheric ozone depletion: the main driver of 20th Century atmospheric circulation changes in the Southern Hemisphere, J. Climate, 24, 795–812, 2011.
Polvani, L. M., Sun, L., Butler, A. H., Richter, J. H., and Deser, C.: Distinguishing stratospheric sudden warmings from ENSO as key drivers of wintertime climate variability over the North Atlantic and Eurasia, J. Climate, 30, 1959–1969, 2017.
Previdi, M. and Polvani, L. M.: Climate System Response to Stratospheric Ozone Depletion and Recovery, Q. J. Roy. Meteor. Soc., 140, 2401–2419, 2014.
Purich, A. and Son, S.-W.: Impact of Antarctic ozone depletion and recovery on Southern Hemisphere precipitation, evaporation and extreme changes, J. Climate, 25, 3145–3154, 2012.
Rao, J. and Garfinkel, C. I.: CMIP5/6 Models Project Little Change in the Statistical Characteristics of Sudden Stratospheric Warmings in the 21st Century, Environ. Res. Lett., 16, 034024, https://doi.org/10.1088/1748-9326/abd4fe, 2020.
Rao, J. and Garfinkel, C. I.: The Strong Stratospheric Polar Vortex in March 2020 in Sub-Seasonal to Seasonal Models: Implications for Empirical Prediction of the Low Arctic Total Ozone Extreme, J. Geophys. Res.-Atmos., 126, e2020JD034190, https://doi.org/10.1029/2020JD034190, 2021.
Rao, J., Garfinkel, C. I., and White, I. P.: Predicting the downward and surface influence of the February 2018 and January 2019 sudden stratospheric warming events in subseasonal to seasonal (S2S) models, J. Geophys. Res.-Atmos., 125, e2019JD031919, https://doi.org/10.1029/2019JD031919, 2020a.
Rao, J., Garfinkel, C. I., White, I. P., and Schwartz, C.: How does the Quasi-Biennial Oscillation affect the boreal winter tropospheric circulation in CMIP5/6 models?, J. Climate, 33, 8975–8996, 2020b.
Rao, J., Garfinkel, C. I., and White, I. P.: Projected strengthening of the extratropical surface impacts of the stratospheric Quasi Biennial Oscillation, Geophys. Res. Lett., 47, e2020GL089149, 2020c.
Rao, J., Garfinkel, C. I., White, I. P., and Schwartz, C.: The Southern Hemisphere Minor Sudden Stratospheric Warming in September 2019 and its predictions in S2S Models, J. Geophys. Res.-Atmos., 125, e2020JD032723, https://doi.org/10.1029/2020JD032723, 2020d.
Rao, J., Garfinkel, C. I., and White, I. P.: Development of the Extratropical Response to the Stratospheric Quasi-Biennial Oscillation, J. Climate, 34, 7239–7255, 2021.
Reichler, T., Kim, J., Manzini, E., and Kröger, J.: A stratospheric connection to Atlantic climate variability, Nat. Geosci., 5, 783–787, 2012.
Richter, J. H., Pegion, K., Sun, L., Kim, H., Caron, J. M., Glanville, A., LaJoie, E., Yeager, S., Kim, W. M., Tawfik, A., and Collins, D.: Subseasonal Prediction with and without a Well-Represented Stratosphere in CESM1, Weather Forecast., 35, 2589–2602, 2020a.
Richter, J. H., Anstey, J. A., Butchart, N., Kawatani, Y., Meehl, G. A., Osprey, S., and Simpson, I. R.: Progress in simulating the quasi-biennial oscillation in CMIP models, J. Geophys. Res.-Atmos., 125, e2019JD032362, https://doi.org/10.1029/2019JD032362, 2020b.
Richter, J. H., Butchart, N., Kawatani, Y., Bushell, A. C., Holt, L., Serva, F., Anstey, J., Simpson, I. R., Osprey, S., Hamilton, K., Braesicke, P., Cagnazzo, C., Chen, C. C., Garcia, R. R., Gray, L. J., Kerzenmacher, T., Lott, F., McLandress, C., Naoe, H., Scinocca, J., Stockdale, T. N., Versick, S., Watanabe, S., Yoshida, K., and Yukimoto S.: Response of the Quasi-Biennial Oscillation to a warming climate in global climate models, Q. J. Roy. Meteor. Soc., Special Section QBO Modelling Intercomparison, 1–29, https://doi.org/10.1002/qj.3749, 2020c.
Riddle, E. E., Butler, A. H., Furtado, J. C., Cohen, J. L., and Kumar, A.: CFSv2 ensemble prediction of the wintertime Arctic Oscillation, Clim. Dynam., 41, 1099–1116, 2013.
Rind, D., Suozzo, R., Balachandran, N. K., Lacis, A., and Russell, G.: The GISS global climate-middle atmosphere model. Part I: Model structure and climatology, J. Atmos. Sci., 45, 329–370, 1988.
Robock, A. and Mao, J.: Winter warming from large volcanic eruptions, Geophys. Res. Lett., 19, 2405–2408, 1992.
Roff, G., Thompson, D. W. J., and Hendon, H.: Does increasing model stratospheric resolution improve extended-range forecast skill?, Geophys. Res. Lett., 38, L05809, https://doi.org/10.1029/2010GL046515, 2011.
Runde, T., Dameris, M., Garny, H., and Kinnison, D. E.: Classification of stratospheric extreme events according to their downward propagation to the troposphere, Geophys. Res. Lett., 43, 6665–6672, 2016.
Saito, N., Maeda, S., Nakaegawa, T., Takaya, Y., Imada, Y., and Matsukawa, C.: Seasonal predictability of the North Atlantic Oscillation and zonal mean fields associated with stratospheric influence in JMA/MRI-CPS2, Scientific Online Letters on the Atmosphere, 13, 209–213, 2017.
Samarasinghe, S. M., Connolly, C., Barnes, E. A., Ebert-Uphoff, I., and Sun, L.: Strengthened causal connections between the MJO and the North Atlantic with climate warming, Geophys. Res. Lett., 48, e2020GL091168, https://doi.org/10.1002/essoar.10504546.2, 2021.
Sassi, F., Garcia, R. R., Boville, B. A., and Liu, H.: On temperature inversions and the mesospheric surf zone, J. Geophys. Res., 107, 4380, https://doi.org/10.1029/2001JD001525, 2002.
Scaife, A. A. and Knight, J. R.: Ensemble simulations of the cold European winter of 2005/6, Q. J. R. Meteorol. Soc., 134, 1647–1659, 2008.
Scaife, A. A. and Smith, D.: A signal-to-noise paradox in climate science, npj Climate and Atmospheric Science, 1, 28, https://doi.org/10.1038/s41612-018-0038-4, 2018.
Scaife, A. A., Butchart, N., Warner, C. D., Stainforth, D., Norton, W. A., and Austin, J.: Realistic Quasi-Biennial Oscillations in a simulation of the global climate, Geophys. Res. Lett., 27, 3481–3484, 2000.
Scaife, A. A., Knight, J. R., Vallis, G. K., Folland, C. K.: A stratospheric influence on the winter NAO and North Atlantic surface climate, Geophys. Res. Lett., 32, L18715, https://doi.org/10.1029/2005GL023226, 2005.
Scaife, A. A., Folland, C. K., Alexander, L., Moberg, A., and Knight, J. R.: European climate extremes and the North Atlantic Oscillation, J. Climate, 21, 72–83, 2008.
Scaife, A. A., Spangehl, T., Fereday, D., Cubasch, U., Langematz, U., Akiyoshi, H., Bekki, S., Braesicke, P., Butchart, N., Chipperfield, M., Gettelman, A., Hardiman, S., Michou, M., Rozanov, E., and Shepherd, T. G.: Climate Change and Stratosphere-Troposphere Interaction, Clim. Dynam., 38, 2089–2097, 2012.
Scaife, A. A., Ineson, S., Knight, J. R., Gray, L., Kodera, K., and Smith, D. M.: A mechanism for lagged North Atlantic climate response to solar variability, Geophys. Res. Lett., 40, 434–439, https://doi.org/10.1002/grl.50099, 2013.
Scaife, A. A., Athanassiadou, M., Andrews, M., Arribas, A., Baldwin, M. P., Dunstone, N., Knight, J., MacLachlan, C., Manzini, E., Müller, W. A., Pohlmann, H., Smith, D., Stockdale, T., and Williams, A.: Predictability of the quasi-biennial oscillation and its northern winter teleconnection on seasonal to decadal timescales, Geophys. Res. Lett., 41, 1752–1758, 2014a.
Scaife, A. A., Arribas, A., Blockley, E., Brookshaw, A., Clark, R. T., Dunstone, N., Eade, R., Fereday, D., Folland, C. K., Gordon, M., Hermanson, L., Knight, J. R., Lea, D. J., MacLachlan, C., Maidens, A., Martin, M., Peterson, A. K., Smith, D., Vellinga, M., Wallace, E., Waters, J., and Williams, A.: Skillful long-range prediction of European and North American winters, Geophys. Res. Lett., 41, 2514–2519, 2014b.
Scaife, A. A., Karpechko, A.-Y., Baldwin, M. P., Brookshaw, A., Butler, A. H., Eade, R., Gordon, M., MacLachlan, C., Martin, N., Dunstone, N., and Smith, D.: Seasonal winter forecasts and the stratosphere, Atmos. Sci. Lett., 17, 51–56, https://doi.org/10.1002/asl.598, 2016.
Scaife, A. A., Comer, R. E., Dunstone, N. J., Knight, J. R., Smith, D. M., MacLachlan, C., Martin, N., Peterson, K. A., Rowlands, D., Carroll, E. B., Belcher, S., and Slingo, J.: Tropical rainfall, Rossby waves and regional winter climate predictions, Q. J. Roy. Meteor. Soc., 143, 1–11, https://doi.org/10.1002/qj.2910, 2017.
Schwartz, C. and Garfinkel, C. I.: Relative Roles of the MJO and Stratospheric Variability in North Atlantic and European Winter Climate, J. Geophys. Res., 122, 4184–4201, https://doi.org/10.1002/2016JD025829, 2017.
Schwartz, C. and Garfinkel, C. I.: Troposphere-stratosphere coupling in subseasonal-to-seasonal models and its importance for a realistic extratropical response to the Madden-Julian Oscillation, J. Geophys. Res.-Atmos., 125, e2019JD032043, https://doi.org/10.1029/2019JD032043, 2020.
Screen, J. A. and Blackport, R.: How robust is the atmospheric response to projected Arctic sea ice loss across climate models?, Geophys. Res. Lett., 46, 11406–11415, 2019.
Screen, J. A., Deser, C., Smith, D. M., Zhang, X., Blackport, R., Kushner, P. J., Oudar, T., McCusker, K. E., and Sun, L.: Consistency and discrepancy in the atmospheric response to Arctic sea-ice loss across climate models, Nat. Geosci., 11, 155–163, 2018.
Seviour, W. J. M., Hardiman, S. C., Gray, L. J., Butchart, N., MacLachlan, C., and Scaife, A. A.: Skillful seasonal prediction of the Southern Annular Mode and Antarctic ozone, J. Climate, 27, 7462–7474, https://doi.org/10.1175/JCLI-D-14-00264.1, 2014.
Shaw, T. A. and Perlwitz, J.: The life cycle of Northern Hemisphere downward wave coupling between the stratosphere and troposphere, J. Climate, 26 1745–1763, https://doi.org/10.1175/JCLI-D-12-00251.1, 2013.
Shindell, D. T., Schmidt, G. A., Miller, R. L., and Rind, D.: Northern hemisphere winter climate response to greenhouse gas, ozone, solar, and volcanic forcing, J. Geophys. Res., 106, 7193–7210, 2001.
Shukla, J.: Dynamical Predictability of Monthly Means, J. Atmos. Sci., 38, 2547–2572, 1981.
Sigmond, M. and Scinocca, J. F.: The influence of basic state on the Northern Hemisphere circulation response to climate change, J. Climate, 23, 1434–1446, 2010.
Sigmond, M., Scinocca, J. F., and Kushner, P. J.: The impact of the stratosphere on tropospheric climate change, Geophys. Res. Lett., 35, L12706, https://doi.org/10.1029/2008GL033573, 2008.
Sigmond, M., Scinocca, J. F., Kharin, V. V., and Shepherd, T. G.: Enhanced seasonal forecast skill following stratospheric sudden warmings, Nat. Geosci., 6, 98–102, 2013.
Simpson, I. R., Hitchcock, P., Seager, R., Wu, Y., and Callaghan, P.: The Downward Influence of Uncertainty in the Northern Hemisphere Stratospheric Polar Vortex Response to Climate Change, J. Climate, 31, 6371–6391, 2018.
Smith, D. M., Cusack, S., Colman, A. W., Folland, C. K., Harris, G. R., and Murphy, J. M.: Improved surface temperature prediction for the coming decade from a global climate model, Science, 317, 796–799, 2007.
Smith, D. M., Scaife, A. A., Boer, G. J., Caian, M., Doblas-Reyes, F. J., Guemas, V., Hawkins, E., Hazeleger, W., Hermanson, L., Ho, C. K., Ishii, M., Kharin, V., Kimoto, M., Kirtman, B., Lean, J., Matei, D., Merryfield, W. J., Müller, W. A., Pohlmann, H., Rosati, A., Wouters, B., and Wyser, K.: Real-time multi-model decadal climate predictions, Clim. Dynam., 41, 2875–2888, https://doi.org/10.1007/s00382-012-1600-0, 2013.
Smith, D. M., Eade, R., Scaife, A. A., Caron, L.-P., Danabasoglu, G., DelSole, T. M., Delworth, T., Doblas-Reyes, F. J., Dunstone, N. J., Hermanson, L., Kharin, V., Kimoto, M., Merryfield, W. J., Mochizuki, T., Müller, W. A., Pohlmann, H., Yeager, S., and Yang, X.: Robust skill of decadal climate predictions, npj Climate and Atmospheric Science, 2, 13, https://doi.org/10.1038/s41612-019-0071-y, 2019.
Smith, D. M., Dunstone, N. J., Scaife, A. A., Fiedler, E. K., Copsey, D., and Hardiman, S. C.: Atmospheric Response to Arctic and Antarctic Sea Ice: The Importance of Ocean–Atmosphere Coupling and the Background State, J. Climate, 30, 4547–4565, 2017.
Smith, D. M., Scaife, A. A., Eade, R., Athanasiadis, P., Bellucci, A., Bethke, I., Bilbao, R., Borchert, L. F., Caron, L.-P., Counillon, F., Danabasoglu, G., Delworth, T., Doblas-Reyes, F. J., Dunstone, N. J., Estella-Perez, V., Flavoni, S., Hermanson, L., Keenlyside, N., Kharin, V., Kimoto, M., Merryfield, W. J., Mignot, J., Mochizuki, T., Modali, K., Monerie, P.-A., Müller, W. A., Nicolí, D., Ortega, P., Pankatz, K., Pohlmann, H., Robson, J., Ruggieri, P., Sospedra-Alfonso, R., Swingedouw, D., Wang, Y., Wild, S., Yeager, S., Yang, X., and Zhang, L.: North Atlantic climate far more predictable than models imply, Nature, 583, 796–800, https://doi.org/10.1038/s41586-020-2525-0, 2020
Smith, D. M., Eade, R., Andrews, M. B., Ayres, H., Clark, A., Chripko, S., Deser, C., Dunstone, N. J., García-Serrano, J., Gastineau, G., Graff, L. S., Hardiman, S. C., He, B., Hermanson, L., Jung, T., Knight, J., Levine, X., Magnusdottir, G., Manzini, E., Matei, D., Mori, M., Msadek, R., Ortega, P., Peings, Y., Scaife, A. A., Screen, J. A., Seabrook, M., Semmler, T., Sigmond, M., Streffing, J., Sun, L., and Walsh, A.: Robust but weak winter atmospheric circulation response to future Arctic sea ice loss, Nat. Commun., 13, 727, 2022.
Smith, K. L. and Polvani, L. M.: The surface impacts of Arctic stratospheric ozone anomalies, Environ. Res. Lett., 9, 074015, https://doi.org/10.1088/1748-9326/9/7/074015, 2014.
Solomon, S., Ivy, D. J., Kinnison, D., Mills, M. J., Neely, R. R., and Schmidt, A.: Emergence of healing in the Antarctic ozone layer, Science, 353, 269–274, 2016.
Son, S. W., Polvani, L. M., Waugh, D. W., Akiyoshi, H., Garcia, R., Kinnison, D., Pawson, S., Rozanov, E., Shepherd, T. G., and Shibata, K.: The impact of stratospheric ozone recovery on the Southern Hemisphere westerly jet, Science, 320, 1486–1489, 2008.
Son, S.-W., Tandon, N. F., Polvani, L. M., and Waugh, D. W.: Ozone hole and Southern Hemisphere climate change, Geophys. Res. Lett., 36, L15705, https://doi.org/10.1029/2009GL038671, 2009.
Son, S.-W., Purich, A., Hendon, H. H., Kim, B.-M., and Polvani, L. M.: Improved seasonal forecast using ozone hole variability?, Geophys. Res. Lett., 40, 6231–6235, 2013.
Son, S.-W., Lim, Y., Yoo, C., Hendon, H. H., and Kim, J.: Stratospheric control of Madden-Julian Oscillation, J. Climate, 30, 1909–1922, 2017.
Son, S.-W., Han, B.-R., Garfinkel, C. I., Kim, S. Y., Park, R., Abraham, N. L., Akiyoshi, H., Archibald, A. T., Butchart, N., Chipperfield, M. P., Dameris, M., Deushi, M., Dhomse, S. S., Hardiman, S. C., Jöckel, P., Kinnison, D., Michou, M., Morgenstern, O., O'Connor, F. M., Oman, L. D., Plummer, D. A., Pozzer, A., Revell, L. E., Rozanov, E., Stenke, A., Stone, K., Tilmes, S., Yamashita, Y., and Zeng, G.: Tropospheric jet response to Antarctic ozone depletion: An update with Chemistry-Climate Model Initiative (CCMI) models, Environ. Res. Lett., 13, 054024, https://doi.org/10.1088/1748-9326/aabf21, 2018.
Son, S.-W., Kim, H., Song, K., Kim, S.-W., Martineau, P., Hyun, Y.-K., and Kim, Y.: Extratropical prediction skill of the subseasonal-to-seasonal (S2S) prediction models, J. Geophys. Res.-Atmos., 125, e2019JD031273, 2020.
Song, K. and Son, S.: Revisiting the ENSO–SSW Relationship, J. Climate, 31, 2133–2143, 2018.
Song, Y. and Robinson, W. A.: Dynamical Mechanisms for Stratospheric Influences on the Troposphere, J. Atmos. Sci., 61, 1711–1725, 2004.
Statnaia, I. A., Karpechko, A. Y., and Järvinen, H. J.: Mechanisms and predictability of sudden stratospheric warming in winter 2018, Weather Clim. Dynam., 1, 657–674, https://doi.org/10.5194/wcd-1-657-2020, 2020.
Steil, B., Dameris, M., Brühl, C., Crutzen, P. J., Grewe, V., Ponater, M., and Sausen, R.: Development of a chemistry module for GCMs: first results of a multiannual integration, Ann. Geophys., 16, 205–228, https://doi.org/10.1007/s00585-998-0205-8, 1998.
Stenchikov, G., Hamilton, K., Stouffer, R. J., Robock, A., Ramaswamy, V., Santer, B., and Graf, H.-F.: Arctic Oscillation response to volcanic eruptions in the IPCC AR4 climate models, J. Geophys. Res., 111, D07107, https://doi.org/10.1029/2005JD006286, 2006.
Stockdale, T. N., Molteni, F., and Ferranti, L.: Atmospheric initial conditions and the predictability of the Arctic Oscillation, Geophys. Res. Lett., 42, 1173–1179, https://doi.org/10.1002/2014GL062681, 2015.
Stockdale, T. N., Kim, Y.-H., Anstey, J. A., Palmeiro, F. M., Butchart, N., Scaife, A. A., Andrews, M., Bushell, A. C., Dobrynin. M., Garcia-Serrano, J., Hamilton, K., Kawatani, Y., Lott, F., McLandress, C., Naoe, H., Osprey, S., Pohlmann, H., Scinocca, J., Watanabe, S., Yoshida, K., and Yukimoto, S.: Multi-Model Predictions of the Quasi-Biennial Oscillation, Q. J. Roy. Meteor. Soc., Special Section QBO Modelling Intercomparison, 1–22, https://doi.org/10.1002/qj.3919, 2021.
Sun, L., Deser, C., and Tomas, R. A.: Mechanisms of Stratospheric and Tropospheric Circulation Response to Projected Arctic Sea Ice Loss, J. Climate, 28, 7824–7845, 2015.
Swinbank, R., Douglas, C. S., Lahoz, W. A., O'Neill, A., and Heaps, A.: Middle atmosphere variability in the UK Meteorological Office Unified Model, Q. J. R. Meteorol. Soc., 124, 1485–1525, 1998.
Swingedouw, D., Ortega, P., Mignot, J., Guilyardi, E., Masson-Delmotte, V., Butler, P. G., Khodri., M., and Séférian, R.: Bidecadal North Atlantic ocean circulation variability controlled by timing of volcanic eruptions, Nat. Commun., 6, 6545, https://doi.org/10.1038/ncomms7545, 2015.
Taguchi, M.: Predictability of Major Stratospheric Sudden Warmings: Analysis Results from JMA Operational 1-Month Ensemble Predictions from 2001/02 to 2012/13, J. Atmos. Sci., 73, 789–806, 2016.
Taguchi, M.: Seasonal Winter forecasts of the northern stratosphere and troposphere: Results from JMA seasonal hindcast experiments, J. Atmos. Sci., 75, 827–840, 2018.
Takaya, Y., Yasuda, T., Fujii, Y., Matsumoto, S., Soga, T., Mori, H., Hirai, M., Ishikawa, I., Sato, H., Shimpo, A., Kamachi, M., and Ose, T.: Japan Meteorological Agency/Meteorological Research Institute-Coupled Prediction System version 1 (JMA/MRI-CPS1) for operational seasonal forecasting, Clim. Dynam., 1–2, 313–333, 2017.
Thiéblemont, R., Matthes, K., Omrani, N. E., Kodera, K., and Hansen, F.: Solar forcing synchronizes decadal North Atlantic climate variability, Nat. Commun., 6, 8268, https://doi.org/10.1038/ncomms9268, 2015.
Thompson, D. W. J. and Solomon, S.: Interpretation of Recent Southern Hemisphere Climate Change, Science, 296, 895–899, 2002.
Thompson, D. W. J., Baldwin, M. P., and Wallace, J. M.: Stratospheric connection to Northern Hemisphere wintertime weather: Implications for prediction, J. Climate, 15, 1421–1428, 2002.
Thompson, D. W. J., Baldwin, M. P., and Solomon, S.: Stratosphere–troposphere coupling in the Southern Hemisphere, J. Atmos. Sci., 62, 708–715, 2005.
Thompson, D., Solomon, S., Kushner, P., England, M. H., Grise, K. M., and Karoly, D. J.: Signatures of the Antarctic ozone hole in Southern Hemisphere surface climate change, Nat. Geosci., 4, 741–749, 2011.
Tompkins, A. M., Ortiz De Zárate, M. I., Saurral, R. I., Vera, C., Saulo, C., Merryfield, W. J., Sigmond, M., Lee, W., Baehr, J., Braun, A., Butler, A., Déqué, M., Doblas-Reyes, F. J., Gordon, M., Scaife, A. A., Imada, Y., Ishii, M., Ose, T., Kirtman, B., Kumar, A., Müller, W. A., Pirani, A., Stockdale, T., Rixen, M., and Yasuda, T.: The Climate-System Historical Forecast Project: Providing Open Access to Seasonal Forecast Ensembles from Centers around the Globe, B. Am. Meteorol. Soc., 98, 2293–2301, 2017.
Tripathi, O. P., Baldwin, M., Charlton-Perez, A., Charron, M., Eckermann, S., Gerber, E., Harrison, G., Jackson, D., Kim, B., Kuroda, Y., Lang, A., Lee, C., Mahmood, S., Mizuta, R., Roff, G., Sigmond, M., and Son, S.-W.: The predictability of the extra-tropical stratosphere on monthly timescales and its impact on the skill of tropospheric forecasts, Q. J. R. Meteorol. Soc., 141, 987–1003, 2015a.
Tripathi, O., Charlton-Perez, A., Sigmond, M., and Vitart, F.: Enhanced long-range forecast skill in boreal winter following stratospheric strong vortex conditions, Environ. Res. Lett., 10, 104007, https://doi.org/10.1088/1748-9326/10/10/104007, 2015b.
Tyrrell, N. L., Karpechko, A. Y., and Raisanen, P.: The Influence of Eurasian Snow Extent on the Northern Extratropical Stratosphere in a QBO Resolving Model, J. Geophys. Res., 123, 315–328, 2018.
Tyrrell, N. L., Karpechko, A. Y., Uotila, P., and Vihma, T.: Atmospheric circulation response to anomalous Siberian forcing in October 2016 and its long-range predictability, Geophys. Res. Lett., 46, 2800–2810, 2019.
Tyrrell, N., Karpechko, A. Y., and Rast, S.: Siberian snow forcing in a dynamically bias-corrected model, J. Climate, 33, 10455–10467, 2020.
Vitart, F.: Madden–Julian Oscillation prediction and teleconnections in the S2S database, Q. J. R. Meteorol. Soc., 143, 2210–2220, 2017.
Wang, L., Ting, M., and Kushner, P. J.: A robust empirical seasonal prediction of winter NAO and surface climate, Sci. Rep., 7, 279, https://doi.org/10.1038/s41598-017-00353-y, 2017.
Wang, L., Hardiman, S. C., Bett, P. E., Comer, R. E., Kent, C., and Scaife, A. A.: What chance of a sudden stratospheric warming in the southern hemisphere?, Environ. Res. Lett., 15, 104038, https://doi.org/10.1088/1748-9326/aba8c1, 2020.
Warner, J. L., Screen, J. A., and Scaife, A. A.: Links between Barents-Kara sea ice and the extratropical atmospheric circulation explained by internal variability and tropical forcing, Geophys. Res. Lett., 47, e2019GL085679, https://doi.org/10.1029/2019GL085679, 2020.
White, I., Garfinkel, C. I., Gerber, E. P., Jucker, M., Hitchcock, P., and Rao, J.: The generic nature of the tropospheric response to sudden stratospheric warmings, J. Climate, 33, 5589–5610, 2020.
Wittman, L., Polvani, M., Scott, R. K., and Charlton, A. J.: Stratospheric influence on baroclinic lifecycles and its connection to the Arctic Oscillation, Geophys. Res. Lett., 31, L16113, https://doi.org/10.1029/2004GL020503, 2004.
Wittman, A., Charlton, J., and Polvani, L. M.: The effect of lower stratospheric shear on baroclinic instability, J. Atmos. Sci., 64, 479–496, 2007.
Woo, S. H., Sung, M. K., Son, S. W. and Kug, J. S.: Connection between weak stratospheric vortex events and the Pacific Decadal Oscillation, Clim. Dynam., 45, 3481–3492, 2015.
Yamazaki, K., Nakamura, T., Ukita, J., and Hoshi, K.: A tropospheric pathway of the stratospheric quasi-biennial oscillation (QBO) impact on the boreal winter polar vortex, Atmos. Chem. Phys., 20, 5111–5127, https://doi.org/10.5194/acp-20-5111-2020, 2020.
Yoo, C. and Son, S.-W.: Modulation of the boreal wintertime Madden-Julian oscillation by the stratospheric quasi-biennial oscillation, Geophys. Res. Lett., 43, 1392–1398, 2016.
Zambri, B., Solomon, S., Thompson, D. W. J., and Fu, Q.: Emergence of Southern Hemisphere stratospheric circulation changes in response to ozone recovery, Nat. Geosci. 14, 638–644, https://doi.org/10.1038/s41561-021-00803-3, 2021.
Zappa, G. and Shepherd, T. G.: Storylines of atmospheric circulation change for European regional climate impact assessment, J. Climate, 30, 6561–6577, 2017.
Zappa, G., Pithan, F., and Shepherd, T. G.: Multimodel evidence for an atmospheric circulation response to Arctic sea ice loss in the CMIP5 future projections, Geophys. Res. Lett., 45, 1011–1019, 2018.
Zhang, F., Sun, Y. Q., Magnusson, L., Buizza, R., Lin, S., Chen, J., and Emanuel, K.: What Is the Predictability Limit of Midlatitude Weather?, J. Atm. Sci., 76, 1077–1091, 2019.
Zhang, P., Wu, Y., Simpson, I. R., Smith, K. L., Zhang, X., De, B., and Callaghan, P.: A stratospheric pathway linking a colder Siberia to Barents-Kara Sea sea ice loss, Science Advances, 4, eaat6025, https://doi.org/10.1126/sciadv.aat6025, 2018.
- Abstract
- Introduction
- The stratosphere and monthly prediction
- The stratosphere and seasonal prediction
- The stratosphere and annual-to-decadal prediction
- The stratosphere and multidecadal projection
- Outlook
- Data availability
- Author contributions
- Competing interests
- Disclaimer
- Financial support
- Review statement
- References
- Abstract
- Introduction
- The stratosphere and monthly prediction
- The stratosphere and seasonal prediction
- The stratosphere and annual-to-decadal prediction
- The stratosphere and multidecadal projection
- Outlook
- Data availability
- Author contributions
- Competing interests
- Disclaimer
- Financial support
- Review statement
- References