the Creative Commons Attribution 4.0 License.
the Creative Commons Attribution 4.0 License.
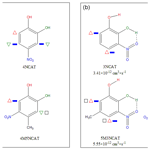
Investigations into the gas-phase photolysis and OH radical kinetics of nitrocatechols: implications of intramolecular interactions on their atmospheric behaviour
Claudiu Roman
Cecilia Arsene
Iustinian Gabriel Bejan
Romeo Iulian Olariu
The Environmental Simulation Chamber made of Quartz from the University “Alexandru Ioan Cuza” (ESC-Q-UAIC), at Iasi, Romania, was used to investigate the gas-phase reaction rate coefficients for four nitrocatechols toward OH radicals under simulated atmospheric conditions. Employing relative rate techniques at a temperature of 298 ± 2 K and a total air pressure of 1 atm, the obtained rate coefficients (in 10−12 cm3 s−1) were as follows: k3NCAT = (3.41 ± 0.37) for 3-nitrocatechol and k5M3NCAT = (5.55 ± 0.45) for 5-methyl-3-nitrocatechol at 365 nm, using CH3ONO photolysis as OH radicals source and dimethyl ether and cyclohexane as reference compounds, and k4NCAT = (1.27 ± 0.19) for 4-nitrocatechol and k4M5NCAT = (0.92 ± 0.14) for 4-methyl-5-nitrocatechol at 254 nm using H2O2 as OH radicals source and dimethyl ether and methanol as reference compounds. The photolysis rates in the actinic region, scaled to atmospheric relevant conditions by NO2 photolysis, were evaluated for 3-nitrocatechol and 5-methyl-3-nitrocatechol: J3NCAT = (3.06 ± 0.16) × 10−4 s−1 and J5M3NCAT = (2.14 ± 0.18) × 10−4 s−1, respectively. The photolysis rate constants at 254 nm were measured for 4-nitrocatechol and 4-methyl-5-nitrocatechol and the obtained values are J4NCAT = (6.7 ± 0.1) × 10−5 s−1 and J4M5NCAT = (3.2 ± 0.3) × 10−5 s−1. Considering the obtained results, our study suggests that photolysis may be the main degradation process for 3-nitrocatechol and 5-methyl-3-nitrocatechol in the atmosphere, with a photolytic lifetime in the atmosphere of up to 2 h. Results are discussed in terms of the reactivity of the four nitrocatechols under investigation toward OH-radical-initiated oxidation and their structural features. The rate coefficient values of the nitrocatechols are also compared with those estimated from the structure-activity relationship for monocyclic aromatic hydrocarbons and assessed in relation to their gas-phase IR spectra. Additional comparison with similar compounds is also presented, underlining the implications toward possible degradation pathways and atmospheric behaviour.
- Article
(2497 KB) - Full-text XML
-
Supplement
(1007 KB) - BibTeX
- EndNote
Aromatic hydrocarbons (AHs) are a class of volatile organic compounds (VOCs) present as primary pollutants in the atmosphere mainly because of anthropogenic activities. In urban areas, AHs are present owing to the use of solvents, incomplete combustion of fossil fuels, car-engine emissions and industrial processes (Piccot et al., 1992). Various monocyclic aromatics occur from biomass burning in rural and remote areas (Schauer et al., 2001). Atmospheric removal of these compounds occurs via reactions with different oxidants (Finlayson-Pitts and Pitts, 2000) and by wet and dry deposition (Warneck, 2000; Calvert et al., 2002). The gas-phase oxidation initiated by the OH radicals is the main reaction responsible for the atmospheric sink of aromatic hydrocarbons. The chemical degradation of AHs forms oxidation products that could further be aromatic in nature or that could lead to ring opening and fragmentation. AHs are also known to be important contributors to photo-oxidant and secondary organic aerosol (SOA) formation in the atmosphere (Calvert et al., 2002; Jenkin et al., 2017).
Hydroxylated aromatic compounds, such as phenol (Atkinson et al., 1992; Sørensen et al., 2002; Berndt and Böge, 2003), cresols (Atkinson et al., 1992; Coeur-Tourneur et al., 2006), dimethylphenols (Thüner et al., 2004), trimethylphenols (Bejan et al., 2012), methoxyphenols (Lauraguais et al., 2015), and catechols (Olariu et al., 2000) are the most reactive species toward OH radicals. According to the Master Chemical Mechanism (MCM), version 3.3.1 (Bloss et al., 2005), which describes the detailed gas-phase chemical processes involved in the tropospheric degradation of a series of primary emitted VOCs, the major oxidation pathway for monocyclic aromatic compounds is the addition of the OH radical to the aromatic ring. This may lead to ring-retaining products. The distribution of the hydroxylated isomers varies for each monocyclic aromatic compound depending on the stability of its OH adduct. Thus, the OH-addition reactivity channel leads to the formation of phenol and cresols with a range of yields from 5 % to 50 % in the case of benzene and toluene. These formation yields are very much affected by the levels of NOx (NOx = NO + NO2) in the reaction mixture, and the formation yields of the mono-hydroxylated aromatic compounds is decreasing in the presence of high NOx concentration (Atkinson and Aschmann, 1994; Klotz et al., 1998, 2002; Volkamer et al., 2002). The hydroxylated products, when photo-oxidized by the OH radicals under atmospheric conditions, in turn form catechols with formation yields of about 65 % to 90 % (Olariu et al., 2002). Formation of nitrophenols and nitrocatechols from the oxidation of phenols and catechols initiated by the OH radicals is assigned to the H-atom abstraction pathway from the phenolic hydroxyl group, accounting for about 10 % in the mono-hydroxylated aromatic compounds and for about 30 % in the di-hydroxylated aromatic compounds (Atkinson et al., 1992; Olariu et al., 2002; Finewax et al., 2018). High formation yields of nitrophenols (more than 50 %) and nitrocatechols (close to 100 %) were reported from the gas-phase reactions of the NO3 radical with the phenols and catechols, which may support the fact that the NO3 chemistry may play an important role in the formation of such nitroaromatic compounds (Olariu et al., 2004, 2013; Finewax et al., 2018).
Nitroaromatic hydrocarbon compounds (NAHs) have been found in particulate matter (Herterich and Herrmann, 1990; Delhomme et al., 2010), rainwater (Grosjean, 1991; Belloli et al., 2006), clouds (Lüttke et al., 1997), water (Herterich, 1991; Rubio et al., 2012), snow (Leuenberger et al., 1988), soil (Vozňáková et al., 1996), and fog (Richartz et al., 1990; Harrison et al., 2005), and even in remote areas such as in Terra Nova Bay, Antarctica (Vanni et al., 2001). More recently, nitroaromatics have been reported in aerosol composition (Vidović et al., 2018) from urban area samples from Nagoya, Japan (Ikemori et al., 2019), Paris, France (Lanzafame et al., 2021), and Beijing, China (Wang et al., 2021). Nitrocatechols have been found as tracer compounds in particulate matter emitted during biomass burning, correlating well with the levoglucosan and NOx levels (Kitanovski et al., 2012b, a; Salvador et al., 2021). In organic aerosols, the 4-nitrocatechol concentration exceeds in some cases 150 ng m−3, whereas for methylated nitrocatechols the overall concentration can be as high as 821 ng m−3 (Iinuma et al., 2010; Kitanovski et al., 2021). These high concentrations of nitrated monoaromatic hydrocarbons were determined in the winter season and have been correlated with household wood-burning use. However, in these studies, all identified nitrocatechols may cover up to 96 % of the nitrated monoaromatic hydrocarbons found in PM10 samples (Kitanovski et al., 2021). 4-nitrocatechol was found in the reaction of guaiacol initiated by OH radicals in the presence of NOx (Lauraguais et al., 2014). Besides 4-nitro-, 3-nitro-, and 6-nitroguaiacol formed as oxidation products, the occurrence of 4-nitrocatechol was explained via the -ipso addition channel of OH radical to the methoxy group. Schwantes et al. (2017) report the formation of highly oxygenated, low-volatile products from the chamber studies of o-cresol oxidation under high NOx conditions. The signal with detected by mass spectrometric techniques has been attributed to methyl-dihydroxy-nitrobenzene. Finewax et al. (2018) have reported 4-nitrocatechol in secondary organic aerosol (SOA) composition from the gas-phase oxidation of catechol initiated by both OH (30 %) and NO3 (91 %) radicals, respectively. Atmospheric production of nitroaromatic compounds, including nitrophenols, dinitrophenols, and nitrocatechols, is of great interest as they play a significant role in the formation of brown carbon and aerosols (Lin et al., 2016). Their low atmospheric reactivity and great ability to absorb large amounts of near-UV, visible and infrared radiation could lead to positive radiative forcing (Bejan et al., 2007; Zhang et al., 2017).
The present study is aimed at determining and discussing, to our knowledge for the first time, the OH kinetic rate coefficients for four nitrocatechols: 3-nitrocatechol (3NCAT), 4-nitrocatechol (4-NCAT), 5-methyl-3-nitrocatechol (5M3NCAT), and 4-methyl-5-nitrocatechol (4M5NCAT) by using the Environmental Simulation Chamber made of Quartz from University “Alexandru Ioan Cuza” at Iasi, Romania (ESC-Q-UAIC). Based on the ESC-Q-UAIC facilities, the photolysis frequencies of the investigated compounds were evaluated and scaled to the relevant atmospheric conditions. Detailed investigations with impact on the reactivity of highly substituted nitro-aromatic compounds are also depicted in this work.
Gas-phase rate coefficients of the studied nitrocatechols toward OH radicals have been determined using the relative kinetic method. For this purpose, the ESC-Q-UAIC reactor, a 760-L and 4.2-m-long cylindrical photoreactor consisting of three quartz tubes surrounded by 32 Philips TL-DK 36W (with λmax = 365 nm) and 32 UV-C TUV 30W/G30 T8 (with λmax = 254 nm), has been employed. Experiments were performed at 1 atm of air and a temperature of 298 ± 2 K. Decay of the nitrocatechols and reference compounds in the reactor vessel was monitored by IR spectroscopy using a Bruker Vertex 80 FTIR coupled to a White type mirrors system mounted inside the chamber, providing a total optical path of 492 ± 1 m. Solid nitrocatechols were transferred into the reactor at low pressure, via a preheated glassware port. A stream of nitrogen flowing over the heated nitroaromatic compound was used to carry the reactants and reference compounds inside the reaction chamber. Two Teflon-blade fans were used to ensure homogeneous mixture. Dimethyl ether (C2H6O), cyclohexane (C6H12) and methanol (CH3OH) were chosen as reference compounds in the relative kinetic study owing to their IR spectral features and well-known rate coefficients for the reaction with OH radicals, rate coefficients close to those of the investigated compounds: = (2.80 ± 0.56) × 10−12 cm3 s−1 (Atkinson et al., 2004), = (6.38 ± 0.56) × 10−12 cm3 s−1 (Wilson et al., 2006) and = (0.90 ± 0.18) × 10−12 cm3 s−1 (Atkinson et al., 2004). Dimethyl ether (DME) was used as reference hydrocarbon for all the investigated nitrocatechols whereas cyclohexane was used for the kinetic studies involving 3-nitrocatechol and 5-methyl-3-nitrocatechol. Methanol was employed for the kinetic experiments of 4-nitrocatechol and 4-methyl-5-nitrocatechol. The OH radicals were generated in situ, (1) via photolysis of a mixture of CH3ONO NO at 365 nm as shown in Reactions (R1a) to (R1e), where NO has been added in excess to suppress ozone formation and potential interference processes in the reaction kinetics:
And (2) via photolysis of H2O2 at 254 nm for 4-nitrocatechol and 4-methyl-5-nitrocatechol as presented in Reaction (R2):
Preliminary tests showed that nitrocatechols photodissociate under experimental conditions. Corrections to reactants' concentration are needed to be made owing to the photolysis and wall deposition, as presented in the following reactions sequence:
By representing the total decay of both reference and reactant in time with respect to the correction mentioned above, the following kinetic expression shown in Eq. (1) could be used to determine the nitroaromatic rate coefficients:
where and are the initial concentrations of nitrocatechols and the reference compound at t0, and [reactant]t and [reference]t are the concentrations of nitrocatechols and the reference compounds during the reaction time t, k1, k2 are the gas-phase rate coefficients for the OH radical reactions with the reactants and reference compounds respectively, k3 is the photolysis frequency (J) of the reactants at 365/254 nm and k4 is the wall loss rate coefficient. The ratio of determined from the slope of the linear regression is used further to obtain the rate coefficient k1 by using well-determined k2 rate coefficients of the reference compounds. No wall loss or photolysis was observed for the reference hydrocarbons used in this study.
However, the photolysis of nitrocatechols at 254 and 365 nm has been measured using CO as OH-radical scavenger. Photolysis rate coefficients are corrected for wall loss and used to correct further rate coefficients from the reactions of nitrocatechols with OH radicals.
Initial concentrations of reactants in the reactor were: 6.5 × 1013 cm−3 for DME, 4.8 × 1013 cm−3 for cyclohexane, 12.5 × 1013 cm−3 for methanol, 12.9 × 1013 cm−3 for NO, 16.2 × 1013 cm−3 for CH3ONO, a maximum of 25.2 × 1013 cm−3 for H2O2. For the investigated nitrocatechols, their initial concentration varied between (2.5 and 7.7) × 1013 cm−3.
Chemical compounds used in the present study were: DME > 99.9 % (suitable for GC analysis, Sigma-Aldrich), cyclohexane > 99.5 % (anhydrous, Sigma-Aldrich), methanol (anhydrous, > 99.9 % suitable for HPLC analysis, Sigma-Aldrich), NO (> 99.5 % purity, Linde), H2O2 (40 % solution in water, Sigma-Aldrich), CO (> 99.997 % purity, Linde). CH3ONO was prepared in our laboratory using an adapted method from Taylor et al. (1980). All nitrocatechols have been synthesized using two methods of nitration according to available literature information proposed by Rosenblatt et al. (1953) and adapted by Iinuma et al. (2010) and Palumbo et al. (2002). Thus, both 3-nitrocatechols have been obtained by treating an anhydrous diethyl ether solution of the corresponding catechols with freshly prepared fuming nitric acid. 4-nitrocatechol and 4-methyl-5-nitrocatechol were prepared by treating a cooled aqueous solution of catechol and sodium nitrite with sulfuric acid. Extractions and purifications of the synthesized compounds were conducted by means of sublimation and recrystallization. The purity of the synthesized compounds from H-NMR analysis was found to be about 98 % (Roman et al., 2022).
Kinetic results for the reaction of nitrocatechols with OH radicals are presented in Figs. 1 to 4. For each compound, a minimum of four experiments have been performed. Despite difficulties in handling these low volatile compounds, their slow reactivity toward OH radicals, and the difficulties encountered in the IR spectra evaluation, the plots depicted in Figs. 1 to 4 show good linearity. Total conversion of nitrocatechols during the irradiation period was evaluated to range from about 25 % to 55 %. Up to 60 % of the decays of the nitrocatechols and the reference compounds have been attributed to the reaction with OH radicals during the kinetic experiments.
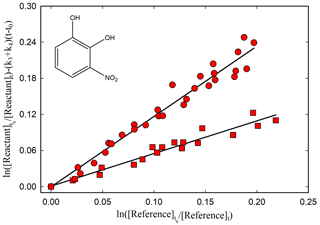
Figure 1Kinetic plot according to Eq. (1) for the reaction of 3-nitrocatechol with OH radicals relative to dimethyl ether (red circle) and cyclohexane (red square), using photolysis of CH3ONO NO mixture at 365 nm as OH-radical source, with (k3+k4) = (4.7 ± 0.41) × 10−4 s−1.
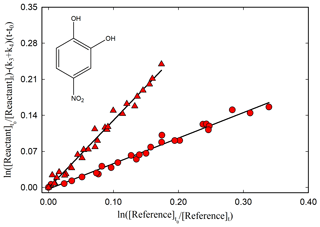
Figure 2Kinetic plot according to Eq. (1) for the reaction of 4-nitrocatechol with OH radicals relative to dimethyl ether (red circle), using photolysis of CH3ONO NO mixture at 365 nm as OH-radical source, and relative to methanol (red triangle), using photolysis of H2O2 at 254 nm as OH-radical source, with (k3+k4) = (1.37 ± 0.32) × 10−4 s−1.
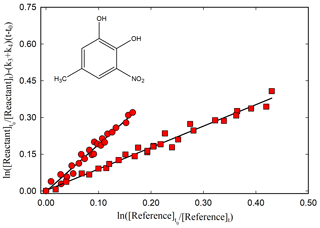
Figure 3Kinetic plot according to Eq. (1) for the reaction of 5-methyl-3-nitrocatechol with OH radicals relative to dimethyl ether (red circle) and cyclohexane (red square) using photolysis of CH3ONO NO mixture at 365 nm as OH radical source, with (k3+k4) = (4.27 ± 0.98) × 10−4 s−1.
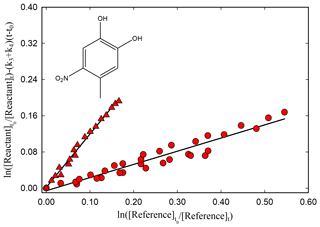
Figure 4Kinetic plot according to Eq. (1) for the reaction of 4-methyl-5-nitrocatechol with OH radicals relative to dimethyl ether (red circle), using photolysis of CH3ONO NO mixture at 365 nm as OH-radical source, and relative to methanol (red triangle), using photolysis of H2O2 at 254 nm as OH-radical source, with (k3+k4) = (1.02 ± 0.30) × 10−4 s−1.
Table 1 presents the kinetic data results from the present study. Relative ratios of determined from the kinetic plots (see Figs. 1 to 4), gas-phase rate coefficient (k1) calculated for the OH reactions with the investigated nitrocatechols, and the average kinetic coefficients (k1average) are also indicated in Table 1. Uncertainties for the represent 2σ from the linear regression analysis. The k1 rate coefficient errors are given from a combination of 2σ values from the linear regression and an additional error from the reference compound uncertainty. As for the k1 (average) and its uncertainty, the weighted average was used to estimate the best interval that accommodates the highest confidence of the obtained data. Based on the daytime average concentration of the OH radicals in the atmosphere (Prinn et al., 1995), of 1.6 × 106 cm−3, Table 1 lists also the atmospheric residence time of the studied nitrocatechols. No significant differences were observed for 4-nitrocatechol and 4-methyl-5-nitrocatechol rate coefficient values when NOx or NOx-free conditions have been employed.
Table 1Rate coefficient values of OH radical reactions with all investigated nitrocatechols in the present study.
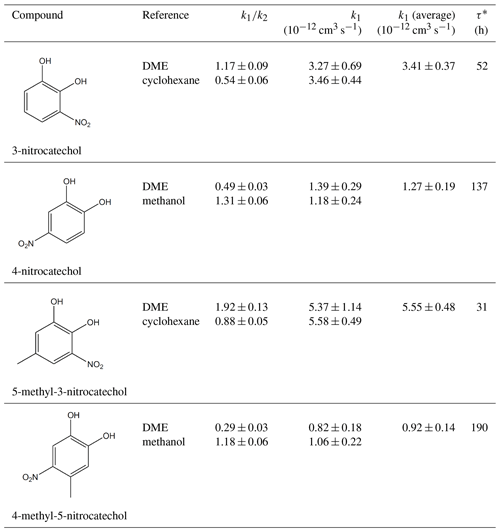
∗ Calculated by applying and considering the average daytime OH-radicals concentration () of 1.6 × 106 cm−3 (Prinn et al., 1995).
Direct photolysis of the hydroxylated nitroaromatics under similar conditions to those used in this study has been shown to produce HONO (Bejan et al., 2006), similar to the carboxylic acid formation mechanism in the aqueous phase proposed by Alif et al. (1991). Photolysis of HONO is a well-known source of OH radicals. To properly evaluate the photolysis rate coefficients at 365 and 254 nm for the investigated nitrocatechols by accounting for possible interference of the secondary chemistry owing to the OH radicals' reactions, a series of individual experiments were performed using carbon monoxide (CO) as OH scavenger. To override the low reactivity of the scavenger (kCO+OH = 2.41 × 10−13 cm3 s−1) (DeMore et al., 1997), a high amount of CO was introduced into the reactor vessel (of about 1.17 × 1017 cm−3) to ensure a scavenging efficiency of more than 98 %. Photolysis rates for all four nitrocatechols at 365 nm (J365) were evaluated. At this wavelength, no significant photolysis for 4-nitrocatechol and 4-methyl-5-nitrocatechol was observed, whereas for 3-nitrocatechol and 5-methyl-3-nitrocatechol photolysis frequencies of (1.53 ± 0.08) × 10−4 and (1.07 ± 0.09) × 10−4 s−1, respectively, have been measured (see Fig. S1 in the Supplement). Additionally, because for 4-nitrocatechol and 4-methyl-5-nitrocatechol the reaction rate coefficients were measured under NOx free condition by using H2O2 photolysis as OH-radical precursor, photolysis rate coefficients at 254 nm of these two compounds were evaluated. For 4-nitrocatechol the photolysis rate (J254) was (6.71 ± 0.99) × 10−5 s−1 and that for 4-methyl-5-nitrocatechol was (3.18 ± 0.32) × 10−5 s−1 (see Fig. S2). However, for 3-nitrocatechol and 5-methyl-3-nitrocatechol corrections have been also performed because of their high wall loss and their photolysis at 365 nm. Photolysis rate coefficients are presented in Table 2, in addition to the average lifetime with respect to photolysis under atmospheric conditions. Photolysis rate coefficients at 365 nm for 3-nitrophenols have been scaled to the atmospheric conditions, as Klotz et al. (1997) have described for 40∘ N latitude noontime and clear sky conditions, with a factor of 2 obtained from the NO2 photolysis frequency measured under atmospheric conditions versus the ESC-Q-UAIC reactor ( s−1 4.3 × 10−3 s). The wall loss (k4) measured for 3NCAT and 5M3NCAT was about (3.2 ± 0.4) × 10−4 s−1, whereas for 4NCAT and 4M5NCAT it was (0.7 ± 0.3) × 10−4 s−1. However, the wall loss was relatively constant over the different experiments employed in this study. Average quantum yields calculated for 3NCAT and 5M3NCAT over the 350- to 400-nm range were obtained using the theoretical approach described by Hofzumahaus et al. (1999), and the evaluated cross-sections of these two compounds in aqueous solutions are presented in Table S1 in the Supplement. The average quantum yields, calculated for the investigated range (φ350–400), are: (7.7 ± 0.7) × 10−3 for 3NCAT and (4.9 ± 0.5) × 10−3 for 5M3NCAT. The overall errors include contributions from the solution preparation, UV-Vis spectra recording (see Fig. S3a for 3NCAT and Fig. S3b for 5M3NCAT) and the photolysis values. A detailed description of the technique, data collection, evaluation and interpretation is presented in the Supplement. However, preliminary analysis of the residual spectra obtained after irradiation of 3NCAT and 5M3NCAT, presented in Fig. S4, reveals one signal at 2139 cm−1 for 3NCAT and 2135 cm−1 for 5M3NCAT that could be representative of a ketene-type product.
Table 2Rate coefficient values for the photolysis of all nitrocatechols investigated in this study scaled to atmospheric conditions and their average atmospheric lifetimes owing to photolysis (at 365 nm).
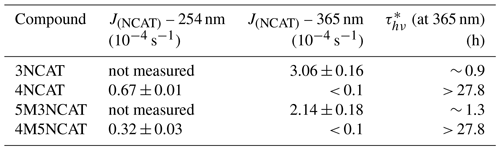
∗ Calculated by applying , where J(NCAT) at 365 nm has been estimated from the experimental determined J(NCAT ESC-Q-UAIC chamber) and using a scaled factor derived from the NO2 photolysis frequency for the atmospheric conditions described by Klotz et al. (1997) (see in text).
The rate coefficients obtained from the kinetic investigations of a series of nitrocatechols with OH radicals are reported for the first time in this study. No significant differences have been observed between the two reference compounds that have been employed to achieve the quality of the rate coefficient values. Based on collision theory and the standard gas kinetic theory, Sørensen et al. (2002) showed that the presence of inorganic aerosols does not influence the value of the OH radicals rate coefficients when relative kinetic technique is used. The OH radical deactivation from collision with surfaces, both with aerosols and chamber, were found to be negligible for all nitrocatechols. However, even if such a process occurs at a higher rate, using the relative kinetic technique, where the ratio is experimentally determined independent of the OH radical concentration, no systematic errors arising from the OH-radical concentration would influence the kinetic data.
A comparison with literature data is not possible. However, there are reported rate coefficient values for nitrophenol and methylated nitrophenols reaction with OH radicals (Atkinson et al., 1992; Bejan et al., 2007). Additionally, kinetic rate coefficients of the OH-radical-initiated oxidation of nitronaphthalene were studied by Atkinson et al. (1989). For the OH-radical reactions with the nitroaromatic compounds, however, all the reported values of the rate coefficients in the literature have the same order of magnitude of 10−12 cm3 s−1, as those presented in this work.
5.1 Electromeric effect of aromatic ring substituents
Prior to initiating discussions of the kinetic results, we should emphasize the influence of the substituent position on the aromatic ring for all the nitrocatechols and their effect on the reactivity. The electromeric effects influencing reactivity could be easily noticed on the structure of infrared spectra. Figure 5a presents the gas-phase infrared spectra of catechol (CAT), 3NCAT, and 4NCAT, and Fig. 5b the gas-phase infrared spectra of 4-methylcatechol (4MCAT), 5M3NCAT, and 4M5NCAT. Infrared spectra clearly show the presence of the H-bonds between OH (vibrations being marked with * and **) and NO2 group substituents attached to the aromatic ring in the nitrocatechols as follows: the compounds 3NCAT and 5M3NCAT exhibit an H-bond between the OH and one of the O atoms from the NO2 vicinal group; 4NCAT presents an H-bond between the H from the C1-linked OH and the O atom from the C2-linked OH; and 4M5NCAT reveals an H-bond between the H from the C2-linked OH and the O atom from the C1-linked OH in 4M5NCAT similar to 4NCAT. The existence of similar structure of vicinal OH groups could be observed in catechol and 4-methylcatechol. However, these H-bonds are not present in CAT and 4MCAT, as both OH groups are highlighted in the gas-phase IR spectra. H-bonds occurring in the nitrocatechols should be evaluated in light of the existing nitro group, which acts with its deactivating electromeric E− effect on the ring, withdrawing the electrons from its -ortho and -para positions. For the 3-nitrocatechols, the chemical structures reveal the presence of one OH group placed between the OH and NO2 groups. This hydroxyl group will be affected by the electron withdrawing effect of NO2, and as a consequence, the weakened O-H bond results in an H-atom that is more susceptible to forming an H-bond with the O from the NO2 group. This bond can also be observed in the IR spectra of 2-nitrophenols (Fig. S5) (Olariu et al., 2002, 2013; Bejan, 2006; Bejan et al., 2007) and is considered to be responsible for SOA and HONO formation via H-atom transfer during gas-phase photolytic processes (Bejan et al., 2006, 2020). As shown in the spectra from Fig. 5a and b, the structures of CAT and 4MCAT exhibit the presence of OH groups one next to the other and no visible H-bond between these substituents. This suggests that for 1,2-dihydroxybenzenes the E+ effect is less diminished through the presence of the vicinal OH groups.
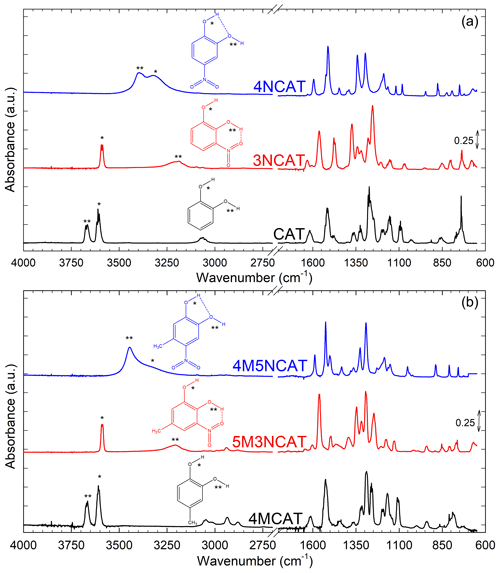
Figure 5(a) Gas-phase IR spectra of catechol (CAT), 3NCAT, 4NCAT; (b) Gas-phase IR spectra of 4-methylcatechol (4MCAT), 5M3NCAT, and 4M5NCAT. Evidence for the intramolecular H-bond occurrence between the substituents marked with */**: catechol and 4MCAT – both free OH present in the spectra; 3NCAT and 5M3NCAT – only one free OH; 4NCAT and 4M5NCAT – both OH involved in the H-bond.
In Fig. 6, 3NCAT has been chosen as a master example to emphasize the electromeric effects manifested by NO2 and OH groups on the aromatic ring, in order to also identify their possible implications for molecule stability. For the 3NCAT structure, one could observe that the OH substituent next to the NO2 group would produce an H bond that is shielded to manifest its E+ effect on the ring. As reported by Bejan et al. (2006, 2020), photolysis of methylated 2-nitrophenols leads to HONO formation via H-transfer from the hydroxyl group to its vicinal nitro group. For 4NCAT and 4M5NCAT, where both OH are linked in the H-bonds, no significant photolysis at 365 nm and no E+ effect to enhance electron density on the aromatic ring occur.
5.2 Reactivity trends
It is well known that the presence of a nitro group has a high deactivating effect on the aromatic ring as the reactivities of nitroaromatic compounds are approximatively 5–20 times lower than their parent compounds. Some OH rate coefficients at 298 K for AHs and their corresponding NAHs are presented in Table 3. The reactivity of catechols against OH radicals drops even more than two orders of magnitude when a nitro group is attached to the ring (Olariu et al., 2000). Such a sudden loss in reactivity is due to the inhibition of the addition channel in aromatic hydrocarbons as discussed in the present study. Ring-retaining product studies from the gas-phase, OH-radical-initiated oxidation of phenol and cresols show that the addition pathway is the dominant oxidation mechanism, occurring in between 65 % and 90 % of the overall reactions, nitrophenols being formed via phenolic H-atom abstraction channel accounting for 5 % to 10 % (Olariu et al., 2002). Gas-phase reactions with NO3 radicals lead to a yield of ∼ 50 % of nitrocatechols and almost 100 % of nitric acid, suggesting that the H-atom abstraction channel represents at least half of the overall reactions (Olariu et al., 2013). In the gas-phase oxidation of catechol with OH radicals under high NOx conditions, Finewax et al. (2018) found 4NCAT in the SOA composition with a molar yield of 30 %. At least 90 % molar yield was obtained in their study in the oxidation initiated by NO3 radicals. These results suggest that the H-atom abstraction channel might make a greater contribution to catechol reactivity rather than the abstraction channel from phenol or cresol oxidation.
Table 3Rate coefficients at 298 K for aromatic hydrocarbons (AHs) and their correspondent nitroaromatic (NAHs) derivatives for the gas-phase reaction with the OH radical.
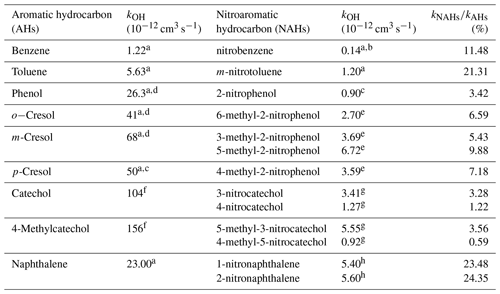
a Calvert et al. (2002). b Witte et al. (1986). c Atkinson et al. (1992). d Atkinson (1989). e Bejan et al. (2007). f Olariu et al. (2000). g This work. h Atkinson et al. (1989).
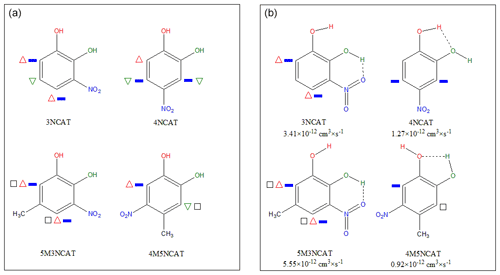
Figure 7Electromeric effect distribution on the aromatic ring for the all investigating nitrocatechols in the present study: (a) active sites toward electrophilic attack by hydroxyl (1st OH: red triangle/2nd OH: green triangle), nitro (blue bar), and methyl (black square) groups; (b) internal interactions of the substituents and consequences on the reactivity based on the gas phase FT-IR spectra.
The gas-phase rate coefficients in the investigated series of nitrocatechols vary as follows: . 4NCAT and 4M5NCAT could assume a small effect from the methyl group but shows little distinction from experimental determined rate coefficient values from this study. A distribution of possible free attack sites by OH radicals on the aromatic ring, accounted for also by the substituent's effects, is represented in Fig. 7a. The NO2 group deactivates the aromatic ring toward OH attack by the E− effect. By its I+ effect, the -CH3 substituent will probably influence the electrophilic addition, although its effect is diminished by the E− from the -NO2 substituent. According to Fig. 7a, the most stable adduct is the 4M5NCAT-OH, and one could propose an OH radical addition to the third position of the ring. However, this is not the case as the experimental data show that 4M5NCAT is the least reactive compound in this series, because of the substituent's interactions (see Fig. 7b). Differences between the reactivity of 3NCAT and 5M3NCAT in reaction with an electrophile suggest that two determining pathways might occur: addition to the ring in the NO2-deactivated fourth or sixth position oriented by the E+ electromeric effect of OH (C1) and the I+ effect from CH3, and H-atom abstraction from the C1-linked OH group. Although the difference between 3NCAT and 5M3NCAT is comparable with the difference between 2-nitrophenol (2NPh) and 4-methyl-2-nitrophenol (4M2NPh), we may consider that the E+ effect of OH (C1) is less present owing to the same orientation of the E− effect of NO2. Comparing the reactivity of 2NPh with those of methyl-2-nitrophenols, an increase in the reactivity of 2 to 7 times is observed, which is attributed to CH3 group presence and orientation. The most reactive compound is 5-methyl-2-nitrophenol (5M2NPh) with a k5M2NPh = 6.72 × 10−12 cm3 s−1, where the methyl I+ effect is manifested on both -ortho positions relative to itself and is not affected by the NO2 E− deactivating effect. In comparison, 4M2NPh, k4M2NPh = 3.59 × 10−12 cm3 s−1, both -ortho positions to methyl are likely deactivated by the NO2. In the methylated nitrophenol series, Bejan et al. (2007) found that the least reactive is 6-methyl-2-nitrophenol (6M2NPh), k6M2NPh = 2.70 × 10−12 cm3 s−1, with just one -ortho and one -para position that are both deactivated by NO2. Following the trend for 3-nitrocatechols with respect to the internal electromeric effects of the OH and NO2 groups and considering the reactivity trend in methyl-2-nitrophenols (Bejan et al., 2007) and the implication of the methyl group (Klotz et al., 1998; Bejan et al., 2012; Aschmann et al., 2013) on the addition of OH radicals to the (hydroxylated) aromatic hydrocarbons (Olariu et al., 2000), the rate coefficient for 6M3NCAT (not measured in this study) was estimated to fall between (5.0 and 6.5) × 10−12 cm3 s−1, similar to 5M3NCAT.
For 4NCAT and 4M5NCAT, the difference in the reactivity is not obvious as both OH groups involved in the H-bond have their E+ effect shielded by the H-bond. If the OH group could manifest its electromeric effect, the most reactive compound should be 4M5NCAT. However, the supplementary I+ effect of methyl group, -para oriented from the C1-linked OH in 4M5NCAT, seems to strengthen the C1 O-H bond, making the H abstraction harder. Even if H-abstraction from CH3 could be an option for 4M5NCAT, the overall reactivity of this compound is smaller or at least the same as that of 4NCAT, H-abstraction from the OH group C1-linked being the main degradation path. Seeking the trend in 4-nitrocatechols and 5-nitrocatechols based on these experimental observations, we could estimate that for 3M4NCAT the OH rate coefficient falls between (0.5 and 0.9) × 10−12 cm3 s−1 and for 3M5NCAT between (1.0 and 1.4) × 10−12 cm3 s−1. However, for all 4-nitrocatechols and 5-nitrocatechols, the rate coefficients could be considered to be around 1 × 10−12 cm3 s−1. These estimations should be treated carefully, as few experimental data are available at this time.
5.3 Comparison with SAR estimated values
There are two Structure-Activity-Relationship (SAR) models for estimating gas-phase OH-kinetic rate coefficients of aromatic hydrocarbons, both based on substituent factor analysis, that influence the reactivity under atmospheric relevant conditions. The general model proposed by Kwok and Atkinson (1995) (used mainly for aliphatic hydrocarbons) considers four cumulative reaction pathways for the OH-initiated oxidations: H-atom abstraction from ring substituents, OH-radical addition to the unsaturated aliphatic (double or triple) bond of the substituents, OH-radical addition to the aromatic ring and OH-radical interactions with ring heteroatoms. The rate coefficient for the total reaction is assumed as a sum of rate coefficients from these four pathways. As for aromatics, this model proposes standard values for H-atom abstraction for the group rate coefficients and substituent factors. For addition to the aromatic ring, the Hammett constants for electrophilic addition, σ+, given by Brown and Okamoto (1958), were proposed by Zetzsch (1982) and updated by Kwok and Atkinson (1995) to fit to the equation log(kadd/cm3 s−1) = −11.71–1.34 × , where the most negative value of the sum has to be considered alone and any interaction among the substituents is neglected. The SAR estimation model from Jenkin et al. (2018a, b) updates the factors used in the calculation of rate coefficients and branching ratios for the gas-phase reactions of the OH radicals with monocyclic aromatic compounds. A scaling parameter for the OH-radical addition to each carbon atom in the aromatic cycle relative to positions of the ring substituents was introduced. Additionally, for the addition pathway, 17 possible ring substituent adjustment factors were introduced, including nitro and hydroxyl groups. In the H-atom abstraction pathway from aliphatic substituents, another scaling factor for group rate coefficients of is recommended to be used when alkyl substituents are present in -ortho or -para position relative to the attack site. Based on previous gas-phase kinetic and product studies on OH-radical- initiated oxidation of hydroxylated aromatic compounds, employed for cresols (Olariu et al., 2002; Coeur-Tourneur et al., 2006), methoxyphenols (Coeur-Tourneur et al., 2010b, a), catechols (Olariu et al., 2000; Finewax et al., 2018), and nitrophenols (Bejan et al., 2007, 2020), an abstraction rate coefficient from the phenolic OH of kabs (Ph-OH) = 2.6 × 10−12 cm3 s−1 was proposed by Jenkin et al. (2018b), a rate coefficient that is 20 times larger than those observed in aliphatic alcohols (Jenkin et al., 2018a). This has a great impact on the overall reactivity and the branching ratios of aromatic hydroxylated monocyclic compounds. Observations from the kinetic data of nitro-containing aromatic hydrocarbons result in re-evaluation of the H-atom abstraction channel from the OH substituent, and a more suitable value for the reactions occurring at 298 K is kabs (Ph-OH) = 1.4 × 10−13 cm3 s−1. EPI Suite – AOPWIN software 4.11 was developed based on Kwok and Atkinson's (1995) observations by the United States Environmental Protection Agency. Data in Table 4 show that each SAR tends to overestimate the OH gas-phase reactivity of hydroxylated nitrobenzenes. Along with nitrocatechols rate coefficient values obtained in the present study, Table 4 also lists the experimental kinetic rate coefficients for 2-nitrophenols determined by Bejan et al. (2007) and the estimated SAR values. The 2-nitrophenols estimated rate coefficient values of Jenkin et al. (2018b) fit better with the experimental data than other SARs, owing to the use of substituent adjustment factors R(Φ) for the substituent factors F(Φ) updated to the available literature and the rescaled abstraction rate coefficient previously assigned to -OH groups in aliphatic compounds of kabs (Ph-OH) = 1.28 × 10−12 × cm3 s−1 (1.4 × 10−13 cm3 s−1 at 298 K). In the case of nitrocatechols, Jenkin et al. (2018b) SAR values are a factor of 1.5 higher than the values of Kwok and Atkinson (1995). The difference between the estimated values is considered to appear because of the kadd estimation in Kwok and Atkinson's (1995) SAR, where the electrophilic substituent coefficient σ+ for the OH oriented in -meta position has no attributed contribution. For 1,2-dihydroxybenzenes molecules, the addition pathway might count only for one phenolic hydroxyl. This is in agreement with the experimental explanation given for 3-nitrocatechol's reactivity, where the E+ effect of the OH group from C2 has no electromeric effect on the aromatic ring as is visible in infrared spectra. Consistency between the rate coefficient values is given as both SARs assume that the addition pathway is at least 94 % of the overall reactivity. Based on the observations of Bejan et al. (2007) on methylated 2-nitrophenols, this assumption is reasonable.
Table 4Experimental and SAR-estimated rate coefficients of monoaromatic hydroxylated nitro compounds with the radicals. In brackets are the ratios of the estimated values versus experimental data ().
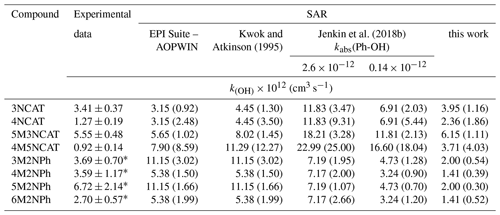
∗ Values from Bejan et al. (2007): 3M2NPh- 3-methyl-2-nitrophenol; 4M2NPh- 4-methyl-2-nitrophenol; 5M2NPh- 5-methyl-2-nitrophenol; 6M2NPh- 6-methyl-2-nitrophenol.
As the data in Table 4 show, no differences were observed between the rate coefficient values estimated by the EPI Suite – AOPWIN and Kwok and Atkinson's (1995) approach as the programme is based on the model calculation proposed study. However, for nitrocatechols there is a difference between the rate coefficients values. This difference is observed because the programme could not choose the correct parameters of the nitro group to properly calculate Σσ+. Instead of choosing the lowest value for NO2 substituent -meta oriented, it chooses the electrophilic substituent group constant from -ortho and -para, that gives the highest Σσ+, not the lowest, as is recommended. However, in further discussions we will no longer consider EPI Suite – AOPWIN software data.
In SAR approaches, the estimated rate coefficients values do not consider the interactions between the nitro and its vicinal OH group or between OH groups, as they all have a tendency to overestimate the gas-phase OH rate coefficients for hydroxylated nitro monocyclic automatics. However, Jenkin et al. (2018b) proposed a substituent adjustment factor for NO2 groups of 0.024 for the -ortho and -para relative to each of the addition sites on the ring and of 0.07 for the -ipso and -meta positions. In Kwok and Atkinson (1995), the SAR estimations assign the NO2 group substituent factor of Brown and Okamoto (1958) of +0.790 in -ortho or -para and of +0.674 for -meta or -ipso. All of these factors are calculated based on previous experimental data to fit alkyl substituted or low substituted aromatic compounds. Although the updated SAR of Jenkin et al. (2018b) is more complex, interactions between the substituents are not taken into consideration. These limitations lead in the case of 4NCAT or 4M5NCAT to different ratios of 5 and 18 times higher. For the 3NCAT and 5M3NCAT the estimated rate coefficient values are more consistent with the present experimental data, values being only ∼ 2 times larger. Differences between experimental values of 4NCAT and 4M5NCAT in comparison with those estimated by SAR models suggest that the addition pathway is of less importance than the H-atom abstraction from hydroxyl groups owing to the inhibition of the E+ effect of phenolic hydroxyls, and limited contribution from the presence of the CH3 group.
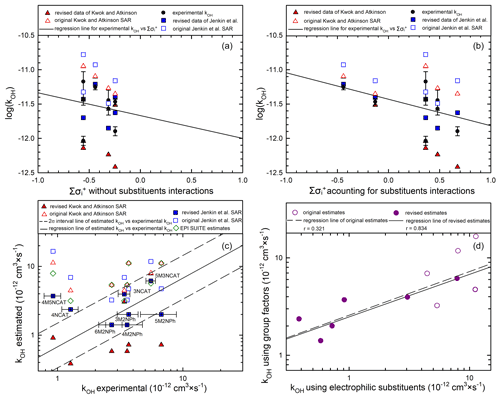
Figure 8Correlation analyses between the experimental, the original estimated, and the revised estimated OH gas-phase rate coefficients of nitrophenols and nitrocatechols.
If substituent interactions are taken into account by: (1) excluding the OH substituent effect on the addition channel (one substituent effect in 3NCAT, 5M3NCAT, and both substituents in 4NCAT and 4M5NCAT); (2) removing one H-atom abstraction channel in all the nitrocatechols; (3) considering the methyl group inhibiting the H-atom abstraction channel in 4M5NCAT; and (4) using a new neighbouring group factor for α-H-atom abstraction from the deactivated phenolic group F(-Ph deactivated) = 0.5, then the automated mechanism construction proposed by Jenkin et al. (2018b) would emphasize new estimated rate coefficient values approaching the experimental data obtained in this study as follows: for 3NCAT a rate of 3.95 × 10−12 cm3 s−1 with a = 0.67, for 5M3NCAT a rate of 6.15 × 10−12 cm3s−1 with a = 0.73, for 4NCAT a rate of 2.36 × 10−12 cm3 s−1 with a = 0.45 and for 4M5NCAT a rate of 3.71 × 10−12 cm3 s−1 with a = 0.69. However, based on the study of Jenkin et al. (2018b) and the behaviour of the investigated nitrocatechols it is probably better to use a value of 0.5 as a new parameter for the H-atom abstraction for the deactivated phenyl group F(-Ph deactivated) when kabs (Ph-OH) = 2.6 × 10−12 cm3 s−1 is considered. In this case, the H-atom abstraction from phenolic hydroxyl will gain significant importance and will be in agreement with previous kinetic studies of hydroxylated aromatic compounds. The new estimated values obtained within the present work are listed in Table 4 besides those of methylated nitrophenols, for which systematic lower values are reported. Table S2 in the Supplement presents the revised estimated kOH values for several nitroaromatic compounds using the Kwok and Atkinson (1995) SAR model updated with recent observations. Accounting for these experimental observations, revisions for the SAR model proposed by Jenkin et al. (2018b) were also performed. Figure 8 presents the correlation analyses between the experimental, the original estimated, and the revised estimated rate coefficient values. Thus, Fig. 8a presents such a correlation versus the variation of the Σσ+ calculated without substituents interactions, whereas Fig. 8b presents this correlation versus the variation of the Σσ+ calculated when substituent interactions are taken into account. Data presented in both figures show that the main data cluster would be shifted to positive Σσ+ when substituent interactions are considered in compliance with experimental values.
Figure 8c reflects the correlations between the estimated and experimental determined rate coefficient values for the nitrophenols and nitrocatechols. From Fig. 8c it is evident that the estimated revised values show reduced spread throughout the correlation lines of interest generated using the revised Jenkin et al. (2018b) SAR. Moreover, the correlation between estimated kOH values derived from using group factors Jenkin et al., 2018b) versus estimated kOH values derived using electrophilic substituents (Kwok and Atkinson, 1995) is presented in Fig. 8d. From Fig. 8d it is obvious that the original estimate values from both SAR methods present a poor correlation compared with the case when the same estimated kOH values were generated after the revision based on the newly obtained experimental data. The corrections introduced to account for the presence and influence of the H-bond on the rate coefficient values are reflected by the improvement in the Pearson coefficient value arising from r = 0.321 for the original estimates to r = 0.843 for the revised estimates. However, in the revised data the experimental rate constant values for nitrophenols seem to be underestimated and those for nitrocatechols overestimated. Under these circumstances, we believe that new experimental values for the rate coefficients of nitroaromatics with OH radicals would further help to better estimate the rate coefficient values for nitroaromatic compounds.
5.4 Atmospheric implications
The estimated photolysis frequencies for the 3NCAT and 5M3NCAT extrapolated to atmospheric conditions (see Table 2) are comparable with those proposed by Bejan et al. (2007) for 2-nitrophenols. Photolysis of nitrocatechols with NO2 and OH groups in vicinal position would be the dominant atmospheric sink, being almost 10 times faster than the reaction with OH radicals. Similar values of photolysis frequencies have been also reported for nitronaphthalenes and methylnitronaphthalenes (Phousongphouang and Arey, 2003). Based on the obtained data, it may be suggested that all the nitroaromatic compounds that have the NO2 group in the vicinal position with a large electron density area might easily undergo photolysis, which may be the main sink pathway in the atmosphere. Similar to 4-nitro and 5-nitrocatechols, dry or wet deposition seems to be the most probable atmospheric removal process, with little or no photolysis in the boundary layer and with high chemical residence time regarding atmospheric oxidative degradation initiated by OH radicals.
Gas-phase reaction rate coefficients of OH radicals with four nitrocatechols have been investigated for the first time by using ESC-Q-UAIC chamber facilities. The reactivity of all investigated nitrocatechols is strongly influenced by the formation of intramolecular H-bonds that are directly influenced by the deactivating E− effect of the NO2 group. For the 3-nitrocatechol compounds, the electromeric effect of the “free” OH group is diminished by the deactivating E− effect of NO2 group. Thus, the rate coefficients of 3-nitrocatechol compounds are higher than the rate coefficient values of 4-nitrocatechol or 4-methyl-5-nitrocatechol, where no electromeric effect of the OH group seems to be active. As a consequence, in 4-nitro or 5-nitrocatechols, the H-abstraction pathway seems to play an important role for the entire OH-radical reaction, whereas in the 3-nitrocatechol case the OH-addition path remains dominant.
For 3-nitrocatechols the atmospheric average lifetime seems to be controlled by the photolysis processes, similar to 2-nitrophenols, with an average day-time lifetime of about 1 h. Reaction with OH radicals is the main atmospheric degradation process for 4-nitro and 5-nitrocatechols. Atmospheric day lifetimes of 4-nitro and 5-nitrocatechols are longer than 48 h because of OH-radical-initiated oxidation, being susceptible to long-range transport under atmospheric conditions.
As presented in the current study, the automated mechanism construction for OH rate coefficient SAR estimations is further recommended to consider the internal interactions between vicinal substituents. Moreover, all approaches tend to overestimate the gas-phase reactivity of highly substituted nitro-containing aromatic compounds. Use of an average rate coefficient for the H-atom abstraction from the OH group kabs(Ph-OH) = 2.6 × 10−12 cm3 s−1 is encouraged, along with a scaling parameter for the deactivated aromatic phenyl group, estimated to be 0.5 at 298 K. Also, the substituent adjustment factors R(Φ), relative to the F(Φ) values for nitro group should be re-evaluated, R(Φ) average for all possible addition sites being ∼ 0.009. However, more studies are required to support the proposed reactivity for nitrocatechols and to progress the knowledge of gas-phase aromatic chemistry further.
Additional relevant data and supporting information are given in the Supplement. All data used in this study are available upon request from the corresponding author.
The supplement related to this article is available online at: https://doi.org/10.5194/acp-22-2203-2022-supplement.
All authors defined the scope of the work. CR performed investigation, formal analysis, data curation, writing, reviewing and editing; CA was involved in data curation, formal analysis, funding acquisition, writing, reviewing and editing; IGB worked on formal analysis, conceptualization, funding acquisition, writing, reviewing and editing; RIO participated in conceptualization, formal analysis, data curation, funding acquisition, supervision, writing, reviewing, and editing. All authors have read and agreed to the final published version of the paper.
The contact author has declared that neither they nor their co-authors have any competing interests.
Publisher’s note: Copernicus Publications remains neutral with regard to jurisdictional claims in published maps and institutional affiliations.
This article is part of the special issue “Simulation chambers as tools in atmospheric research (AMT/ACP/GMD inter-journal SI)”. It is not associated with a conference.
All authors acknowledge the financial support from European Union's Horizon 2020 – Research and Innovation Framework Programme, through the EUROCHAMP-2020 Infrastructure Activity Grant (grant agreement No. 730997). Claudiu Roman and Iustinian Gabriel Bejan acknowledge the financial support offered by PN-III-P2-2.1-PED2019-4972 project from UEFISCDI. Claudiu Roman and Romeo Iulian Olariu are thankful to the support offer by UEFISCDI within the PN-III-P4-ID-PCE-2016-0270 Project (OLFA-ROA). Acknowledgement is given by Cecilia Arsene and Romeo Iulian Olariu to infrastructure support from the Operational Programme Competitiveness 2014–2020, Axis 1, under POC/448/1/1 Research infrastructure projects for public R&D institutions/Sections F 2018, through the Research Center with Integrated Techniques for Atmospheric Aerosol Investigation in Romania (RECENT AIR) project, under grant agreement MySMIS no. 127324.
This research has been supported by the Horizon 2020 (EUROCHAMP-2020 (grant no. 730997)), the Unitatea Executiva pentru Finantarea Invatamantului Superior, a Cercetarii, Dezvoltarii si Inovarii (grant nos. PN-III-P2-2.1-PED2019-4972 and PN-III-P4-ID-PCE-2016-0270), and the Operational Programme Competitiveness through the Research Center with Integrated Techniques for Atmospheric Aerosol Investigation in Romania (RECENT AIR) project (grant no. 127324).
This paper was edited by Andreas Hofzumahaus and reviewed by Cornelius Zetzsch and one anonymous referee.
Alif, A., Pilichowski, J. F., and Boule, P.: Photochemistry and environment XIII: Phototransformation of 2-nitrophenol in aqueous solution, J. Photochem. Photobiol. A Chem., 59, 209–219, https://doi.org/10.1016/1010-6030(91)87009-K, 1991.
Aschmann, S. M., Arey, J., and Atkinson, R.: Rate constants for the reactions of OH radicals with 1,2,4,5-tetramethylbenzene, pentamethylbenzene, 2,4,5-trimethylbenzaldehyde, 2,4,5-trimethylphenol, and 3-methyl-3-hexene-2,5-dione and products of OH + 1,2,4,5-tetramethylbenzene, J. Phys. Chem., 117, 2556–2568, https://doi.org/10.1021/jp8074018, 2013.
Atkinson, R.: Kinetics and mechanisms of the gas-phase reactions of the hydroxyl radical with organic compounds, J. Phys. Chem. Ref. Data, 1, 1–246, 1989.
Atkinson, R. and Aschmann, S. M.: Products of the gas-phase reactions of aromatic hydrocarbons: Effect of NO2 concentration, Int. J. Chem. Kinet., 26, 929–944, https://doi.org/10.1002/kin.550260907, 1994.
Atkinson, R., Aschmann, S. M., Arey, J., Barbara, Z., and Schuetzle, D.: Gas-phase atmospheric chemistry of 1- and 2-nitronaphthalene and 1,4-naphthoquinone, Atmos. Environ., 23, 2679–2690, https://doi.org/10.1016/0004-6981(89)90548-9, 1989.
Atkinson, R., Aschmann, S. M., and Arey, J.: Reactions of hydroxyl and nitrogen trioxide radicals with phenol, cresols, and 2-nitrophenol at 296 ± 2 K, Environ. Sci. Technol., 26, 1397–1403, https://doi.org/10.1021/es00031a018, 1992.
Atkinson, R., Baulch, D. L., Cox, R. A., Crowley, J. N., Hampson, R. F., Hynes, R. G., Jenkin, M. E., Rossi, M. J., and Troe, J.: Evaluated kinetic and photochemical data for atmospheric chemistry: Volume I – gas phase reactions of Ox, HOx, NOx and SOx species, Atmos. Chem. Phys., 4, 1461–1738, https://doi.org/10.5194/acp-4-1461-2004, 2004.
Bejan, I., Abd El Aal, Y., Barnes, I., Benter, T., Bohn, B., Wiesen, P., and Kleffmann, J.: The photolysis of ortho-nitrophenols: a new gas phase source of HONO, Phys. Chem. Chem. Phys., 8, 2028, https://doi.org/10.1039/b516590c, 2006.
Bejan, I., Barnes, I., Olariu, R., Zhou, S., Wiesen, P., and Benter, T.: Investigations on the gas-phase photolysis and OH radical kinetics of methyl-2-nitrophenols, Phys. Chem. Chem. Phys., 9, 5686, https://doi.org/10.1039/b709464g, 2007.
Bejan, I., Schurmann, A., Barnes, I., and Benter, T.: Kinetics of the gas-phase reactions of OH radicals with a series of trimethylphenols, Int. J. Chem. Kinet., 44, 117–124, https://doi.org/10.1002/kin.20618, 2012.
Bejan, I., Olariu, R., and Wiesen, P.: Secondary organic aerosol formation from nitrophenols photolysis under atmospheric conditions, Atmosphere, 11, 1346, https://doi.org/10.3390/atmos11121346, 2020.
Bejan, I. G.: Investigations on the gas phase atmospheric chemistry of nitrophenols and catechols, PhD thesis, Bergische Universität Wuppertal, http://d-nb.info/984997873/34 (last access: 1 February 2021), 2006.
Belloli, R., Bolzacchini, E., Clerici, L., Rindone, B., Sesana, G., and Librando, V.: Nitrophenols in air and rainwater, Environ. Eng. Sci., 23, 405–415, https://doi.org/10.1089/ees.2006.23.405, 2006.
Berndt, T. and Böge, O.: Gas-phase reaction of OH radicals with phenol, Phys. Chem. Chem. Phys., 5, 342–350, https://doi.org/10.1039/B208187C, 2003.
Bloss, C., Wagner, V., Jenkin, M. E., Volkamer, R., Bloss, W. J., Lee, J. D., Heard, D. E., Wirtz, K., Martin-Reviejo, M., Rea, G., Wenger, J. C., and Pilling, M. J.: Development of a detailed chemical mechanism (MCMv3.1) for the atmospheric oxidation of aromatic hydrocarbons, Atmos. Chem. Phys., 5, 641–664, https://doi.org/10.5194/acp-5-641-2005, 2005.
Brown, H. and Okamoto, Y.: Electrophilic substituent constants, J. Am. Chem. Soc., 80, 4979–4987, 1958.
Calvert, J. G., Atkinson, R., Becker, K. H., Kamens, R. M., Seinfeld, J. H., Wallington, T. J., and Yarwood, G.: The mechanisms of atmospheric oxidation of the aromatic hydrocarbons, Oxford University Press, 566 pp., ISBN 9780195146288, 2002.
Coeur-Tourneur, C., Henry, F., Janquin, M. A., and Brutier, L.: Gas-phase reaction of hydroxyl radicals with m-, o- and p-cresol, Int. J. Chem. Kinet., 38, 553–562, https://doi.org/10.1002/kin.20186, 2006.
Coeur-Tourneur, C., Foulon, V., and Laréal, M.: Determination of aerosol yields from 3-methylcatechol and 4-methylcatechol ozonolysis in a simulation chamber, Atmos. Environ., 44, 852–857, https://doi.org/10.1016/j.atmosenv.2009.11.027, 2010a.
Coeur-Tourneur, C., Cassez, A., and Wenger, J. C.: Rate coefficients for the gas-phase reaction of hydroxyl radicals with 2-methoxyphenol (guaiacol) and related compounds, J. Phys. Chem. A, 114, 11645–11650, https://doi.org/10.1021/jp1071023, 2010b.
Delhomme, O., Morville, S., and Millet, M.: Seasonal and diurnal variations of atmospheric concentrations of phenols and nitrophenols measured in the Strasbourg area, France, Atmos. Pollut. Res., 1, 16–22, https://doi.org/10.5094/APR.2010.003, 2010.
DeMore, W. B., Sander, S. P., Golden, D. M., Hampson, R. F., Kurylo, M. J., Howard, C. J., Ravishankara, A. R., Kolb, C. E., and Molina, M. J.: Chemical kinetics and photochemical data for use in stratospheric modeling, Jet Propulsion Laboratory, Pasadena, CA, NASA Evaluation No. 13, JPL Publication 97-4, 24–26, https://jpldataeval.jpl.nasa.gov/pdf/Atmos97_Anotated.pdf (last access: 10 March 2021), 1997.
Finewax, Z., De Gouw, J. A., and Ziemann, P. J.: Identification and quantification of 4-nitrocatechol formed from OH and NO3 radical-initiated reactions of catechol in air in the presence of NOx: implications for secondary organic aerosol formation from biomass burning, Environ. Sci. Technol., 52, 1981–1989, https://doi.org/10.1021/acs.est.7b05864, 2018.
Finlayson-Pitts, B. J. and Pitts, J. N.: Chemistry of the upper and lower atmosphere, Academic Press, https://doi.org/10.1016/B978-012257060-5/50007-1, 2000.
Grosjean, D.: Atmospheric fate of toxic aromatic compounds, Sci. Total Environ., 100, 367–414, https://doi.org/10.1016/0048-9697(91)90386-S, 1991.
Harrison, M. A. J., Barra, S., Borghesi, D., Vione, D., Arsene, C., and Iulian Olariu, R.: Nitrated phenols in the atmosphere: A review, Atmos. Environ., 39, 231–248, https://doi.org/10.1016/j.atmosenv.2004.09.044, 2005.
Herterich, R.: Gas chromatographic determination of nitrophenols in atmospheric liquid water and airborne particulates, J. Chromatogr. A, 549, 313–324, https://doi.org/10.1016/S0021-9673(00)91442-0, 1991.
Herterich, R. and Herrmann, R.: Comparing the distribution of nitrated phenols in the atmosphere of two German hill sites, Environ. Technol., 11, 961–972, https://doi.org/10.1080/09593339009384948, 1990.
Hofzumahaus, A., Kraus, A., and Müller, M.: Solar actinic flux spectroradiometry: a technique for measuring photolysis frequencies in the atmosphere, Appl. Opt., 38, 4443, https://doi.org/10.1364/ao.38.004443, 1999.
Iinuma, Y., Böge, O., and Herrmann, H.: Methyl-nitrocatechols: Atmospheric tracer compounds for biomass burning secondary organic aerosols, Environ. Sci. Technol., 44, 8453–8459, https://doi.org/10.1021/es102938a, 2010.
Ikemori, F., Nakayama, T., and Hasegawa, H.: Characterization and possible sources of nitrated mono- and di-aromatic hydrocarbons containing hydroxyl and/or carboxyl functional groups in ambient particles in Nagoya, Japan, Atmos. Environ., 211, 91–102, https://doi.org/10.1016/j.atmosenv.2019.05.009, 2019.
Jenkin, M. E., Derwent, R. G., and Wallington, T. J.: Photochemical ozone creation potentials for volatile organic compounds: Rationalization and estimation, Atmos. Environ., 163, 128–137, https://doi.org/10.1016/j.atmosenv.2017.05.024, 2017.
Jenkin, M. E., Valorso, R., Aumont, B., Rickard, A. R., and Wallington, T. J.: Estimation of rate coefficients and branching ratios for gas-phase reactions of OH with aliphatic organic compounds for use in automated mechanism construction, Atmos. Chem. Phys., 18, 9297–9328, https://doi.org/10.5194/acp-18-9297-2018, 2018a.
Jenkin, M. E., Valorso, R., Aumont, B., Rickard, A. R., and Wallington, T. J.: Estimation of rate coefficients and branching ratios for gas-phase reactions of OH with aromatic organic compounds for use in automated mechanism construction, Atmos. Chem. Phys., 18, 9329–9349, https://doi.org/10.5194/acp-18-9329-2018, 2018b.
Kitanovski, Z., Grgić, I., Yasmeen, F., Claeys, M., and Čusak, A.: Development of a liquid chromatographic method based on ultraviolet-visible and electrospray ionization mass spectrometric detection for the identification of nitrocatechols and related tracers in biomass burning atmospheric organic aerosol, Rapid Commun. Mass Spectrom., 26, 793–804, https://doi.org/10.1002/rcm.6170, 2012a.
Kitanovski, Z., Grgić, I., Vermeylen, R., Claeys, M., and Maenhaut, W.: Liquid chromatography tandem mass spectrometry method for characterization of monoaromatic nitro-compounds in atmospheric particulate matter, J. Chromatogr. A, 1268, 35–43, https://doi.org/10.1016/j.chroma.2012.10.021, 2012b.
Kitanovski, Z., Hovorka, J., Kuta, J., Leoni, C., Prokeš, R., Sáňka, O., Shahpoury, P., and Lammel, G.: Nitrated monoaromatic hydrocarbons (nitrophenols, nitrocatechols, nitrosalicylic acids) in ambient air: levels, mass size distributions and inhalation bioaccessibility, Environ. Sci. Pollut. Res., 28, 59131–59140, https://doi.org/10.1007/s11356-020-09540-3, 2021.
Klotz, B., Barnes, I., Becker, K. H., and Golding, B. T.: Atmospheric chemistry of benzene oxide/oxepin, J. Chem. Soc. Faraday Trans., 93, 1507–1516, https://doi.org/10.1039/a606152d, 1997.
Klotz, B., Sørensen, S., Barnes, I., Becker, K. H., Etzkorn, T., Volkamer, R., Platt, U., Wirtz, K., and Martın-Reviejo, M.: Atmospheric oxidation of toluene in a large-volume outdoor photoreactor: In situ determination of ring-retaining product yields, J. Phys. Chem. A, 102, 10289–10299, https://doi.org/10.1021/jp982719n, 1998.
Klotz, B., Volkamer, R., Hurley, M. D., Andersen, M. P. S., Nielsen, O. J., Barnes, I., Imamura, T., Wirtz, K., Becker, K. H., Platt, U., Wallington, T. J., and Washida, N.: OH-initiated oxidation of benzene part II. Influence of elevated NOx concentrations, Phys. Chem. Chem. Phys., 4, 4399–4411, https://doi.org/10.1039/b204398j, 2002.
Kwok, E. and Atkinson, R.: Estimation of hydroxyl radical reaction rate constants for gas-phase organic compounds using a structure-reactivity relationship: An update, Atmos. Environ., 29, 1685–1695, https://doi.org/10.1016/1352-2310(95)00069-B, 1995.
Lanzafame, G. M., Srivastava, D., Favez, O., Bandowe, B. A. M., Shahpoury, P., Lammel, G., Bonnaire, N., Alleman, L. Y., Couvidat, F., Bessagnet, B., and Albinet, A.: One-year measurements of secondary organic aerosol (SOA) markers in the Paris region (France): Concentrations, gas/particle partitioning and SOA source apportionment, Sci. Total Environ., 757, 143921, https://doi.org/10.1016/j.scitotenv.2020.143921, 2021.
Lauraguais, A., Coeur-Tourneur, C., Cassez, A., Deboudt, K., Fourmentin, M., and Choël, M.: Atmospheric reactivity of hydroxyl radicals with guaiacol (2-methoxyphenol), a biomass burning emitted compound: Secondary organic aerosol formation and gas-phase oxidation products, Atmos. Environ., 86, 155–163, https://doi.org/10.1016/j.atmosenv.2013.11.074, 2014.
Lauraguais, A., Bejan, I., Barnes, I., Wiesen, P., and Coeur, C.: Rate coefficients for the gas-phase reactions of hydroxyl radicals with a series of methoxylated aromatic compounds, J. Phys. Chem. A, 119, 6179–6187, https://doi.org/10.1021/acs.jpca.5b03232, 2015.
Leuenberger, C., Czuczwa, J., Heyerdahl, E., and Giger, W.: Aliphatic and polycyclic aromatic hydrocarbons in urban rain, snow and fog, Atmos. Environ., 22, 695–705, https://doi.org/10.1016/0004-6981(88)90007-8, 1988.
Lin, P., Aiona, P. K., Li, Y., Shiraiwa, M., Laskin, J., Nizkorodov, S. A., and Laskin, A.: Molecular characterization of brown carbon in biomass burning aerosol particles, Environ. Sci. Technol., 50, 11815–11824, https://doi.org/10.1021/acs.est.6b03024, 2016.
Lüttke, J., Scheer, V., Levsen, K., Wünsch, G., Cape, J. N., Hargreaves, K. J., Storeton-West, R. L., Acker, K., Wieprecht, W., and Jones, B.: Occurrence and formation of nitrated phenols in and out of cloud, Atmos. Environ., 31, 2637–2648, https://doi.org/10.1016/S1352-2310(96)00229-4, 1997.
Olariu, R. I., Barnes, I., Becker, K. H., and Klotz, B.: Rate coefficients for the gas-phase reaction of OH radicals with selected dihydroxybenzenes and benzoquinones, Int. J. Chem. Kinet., 32, 696–702, 2000.
Olariu, R. I., Klotz, B., Barnes, I., Becker, K. H., and Mocanu, R.: FT-IR study of the ring-retaining products from the reaction of OH radicals with phenol, o-, m-, and p-cresol, Atmos. Environ., 36, 3685–3697, https://doi.org/10.1016/S1352-2310(02)00202-9, 2002.
Olariu, R. I., Bejan, I., Barnes, I., Klotz, B., Becker, K. H., and Wirtz, K.: Rate coefficients for the gas-phase reaction of NO3 radicals with selected dihydroxybenzenes, Int. J. Chem. Kinet., 36, 577–583, https://doi.org/10.1002/kin.20029, 2004.
Olariu, R. I., Barnes, I., Bejan, I., Arsene, C., Vione, D., Klotz, B., and Becker, K. H.: FT-IR product study of the reactions of NO3 radicals with ortho-, meta-, and para-cresol, Environ. Sci. Technol., 47, 7729–7738, https://doi.org/10.1021/es401096w, 2013.
Palumbo, A., Napolitano, A., and D'Ischia, M.: Nitrocatechols versus nitrocatecholamines as novel competitive inhibitors of neuronal nitric oxide synthase: Lack of the aminoethyl side chain determines loss of tetrahydrobiopterin-antagonizing properties, Bioorganic Med. Chem. Lett., 12, 13–16, https://doi.org/10.1016/S0960-894X(01)00680-1, 2002.
Phousongphouang, P. T. and Arey, J.: Rate constants for the photolysis of the nitronaphthalenes and methylnitronaphthalenes, J. Photochem. Photobiol. A Chem., 157, 301–309, https://doi.org/10.1016/S1010-6030(03)00072-8, 2003.
Piccot, S. D., Watson, J. J., and Jones, J. W.: A global inventory of volatile organic compound emissions from anthropogenic sources, J. Geophys. Res., 97, 9897–9912, https://doi.org/10.1029/92JD00682, 1992.
Prinn, R. G., Weiss, R. F., Miller, B. R., Huang, J., Alyea, F. N., Cunnold, D. M., Fraser, P. J., Hartley, D. E., and Simmonds, P. G.: Atmospheric trends and lifetime of CH3CCl3 and global OH concentrations, Science, 269, 187–192, https://doi.org/10.1126/science.269.5221.187, 1995.
Richartz, H., Reischl, A., Trautner, F., and Hutzinger, O.: Nitrated phenols in fog, Atmos. Environ. Part A, Gen. Top., 24, 3067–3071, https://doi.org/10.1016/0960-1686(90)90485-6, 1990.
Roman, C., Roman, T., Arsene, C., Bejan, I. G., and Olariu, R. I.: Gas-phase IR cross-sections and single crystal structures data for atmospheric relevant nitrocatechols, Spectrochim. Acta-Part A Mol. Biomol. Spectrosc., 265, 120379, https://doi.org/10.1016/j.saa.2021.120379, 2022.
Rosenblatt, D. H., Epstein, T., and Levitch, M.: Some nuclearly substituted catechols and their acid dissociation constants, J. Am. Chem. Soc., 75, 3277–3278, https://doi.org/10.1021/ja01109a511, 1953.
Rubio, M. A., Lissi, E., Herrera, N., Pérez, V., and Fuentes, N.: Phenol and nitrophenols in the air and dew waters of Santiago de Chile, Chemosphere, 86, 1035–1039, https://doi.org/10.1016/j.chemosphere.2011.11.046, 2012.
Salvador, C. M. G., Tang, R., Priestley, M., Li, L., Tsiligiannis, E., Le Breton, M., Zhu, W., Zeng, L., Wang, H., Yu, Y., Hu, M., Guo, S., and Hallquist, M.: Ambient nitro-aromatic compounds – biomass burning versus secondary formation in rural China, Atmos. Chem. Phys., 21, 1389–1406, https://doi.org/10.5194/acp-21-1389-2021, 2021.
Schauer, J. J., Kleeman, M. J., Cass, G. R., and Simoneit, B. R. T.: Measurement of emissions from air pollution sources. 3. C1-C29 organic compounds from fireplace combustion of wood, Environ. Sci. Technol., 35, 1716–1728, https://doi.org/10.1021/es001331e, 2001.
Schwantes, R. H., Schilling, K. A., McVay, R. C., Lignell, H., Coggon, M. M., Zhang, X., Wennberg, P. O., and Seinfeld, J. H.: Formation of highly oxygenated low-volatility products from cresol oxidation, Atmos. Chem. Phys., 17, 3453–3474, https://doi.org/10.5194/acp-17-3453-2017, 2017.
Sørensen, M., Hurley, M. D., Wallington, T. J., Dibble, T. S., and Nielsen, O. J.: Do aerosols act as catalysts in the OH radical initiated atmospheric oxidation of volatile organic compounds?, Atmos. Environ., 36, 5947–5952, https://doi.org/10.1016/S1352-2310(02)00766-5, 2002.
Taylor, W. D., Allston, T. D., Moscato, M. J., Fazekas, G. B., Kozlowski, R., and Takacs, G. A.: Atmospheric photodissociation lifetimes for nitromethane, methyl nitrite, and methyl nitrate, Int. J. Chem. Kinet., 12, 231–240, https://doi.org/10.1002/kin.550120404, 1980.
Thüner, L. P., Bardini, P., Rea, G. J., and Wenger, J. C.: Kinetics of the gas-phase reactions of OH and NO3 radicals with dimethylphenols, J. Photochem. Photobiol. A Chem., 108, 11019–11025, https://doi.org/10.1021/jp046358p, 2004.
Vanni, A., Pellegrino, V., Gamberini, R., and Calabria, A.: An evidence for nitrophenols contamination in Antarctic fresh-water and snow. Simultaneous determination of nitrophenols and nitroarenes at ng/L levels, Int. J. Environ. Anal. Chem., 79, 349–365, https://doi.org/10.1080/03067310108044394, 2001.
Vidović, K., Lašič Jurković, D., Šala, M., Kroflič, A., and Grgić, I.: Nighttime aqueous-phase formation of nitrocatechols in the atmospheric condensed phase, Environ. Sci. Technol., 52, 9722–9730, https://doi.org/10.1021/acs.est.8b01161, 2018.
Volkamer, R., Klotz, B., Barnes, I., Imamura, T., Wirtz, K., Washida, N., Becker, K. H., and Platt, U.: OH-initiated oxidation of benzene, Phys. Chem. Chem. Phys., 4, 1598–1610, https://doi.org/10.1039/b108747a, 2002.
Vozňáková, Z., Podehradská, J., and Kohlíčková, M.: Determination of nitrophenols in soil, Chemosphere, 33, 285–291, https://doi.org/10.1016/0045-6535(96)00171-3, 1996.
Wang, Z., Zhang, J., Zhang, L., Liang, Y., and Shi, Q.: Characterization of nitroaromatic compounds in atmospheric particulate matter from Beijing, Atmos. Environ., 246, 118046, https://doi.org/10.1016/j.atmosenv.2020.118046, 2021.
Warneck, P. (Ed.): Chemistry of the natural atmosphere, second edition, Academic Press, https://doi.org/10.1016/S0074-6142(00)80028-5, 2000.
Wilson, E. W., Hamilton, W. A., Kennington, H. R., Evans, B., Scott, N. W., and Demore, W. B.: Measurement and estimation of rate constants for the reactions of hydroxyl radical with several alkanes and cycloalkanes, J. Phys. Chem. A, 110, 3593–3604, https://doi.org/10.1021/jp055841c, 2006.
Witte, F., Urbanik, E., and Zetzsch, C.: Temperature dependence of the rate constants for the addition of OH to benzene and to some monosubstituted aromatics (aniline, bromobenzene, and nitrobenzene) and the unimolecular decay of the adducts. Kinetics into a quasi-equilibrium, J. Phys. Chem., 90, 3251–3259, https://doi.org/10.1021/j100405a040, 1986.
Zetzsch, C.: Predicting the rate of OH-addition to aromatics using σ+-electrophilic substituents constants for mono- and polysubstituted benzene, in: XIth Informal Conference on Photochemistry, 27 June–1 July 1982, Stanford, California, USA, 1982.
Zhang, Y., Forrister, H., Liu, J., Dibb, J., Anderson, B., Schwarz, J. P., Perring, A. E., Jimenez, J. L., Campuzano-Jost, P., Wang, Y., Nenes, A., and Weber, R. J.: Top-of-atmosphere radiative forcing affected by brown carbon in the upper troposphere, Nat. Geosci., 10, 486–489, https://doi.org/10.1038/ngeo2960, 2017.
freeOH group is diminished by the deactivating E-effect of the NO2 group.