the Creative Commons Attribution 4.0 License.
the Creative Commons Attribution 4.0 License.
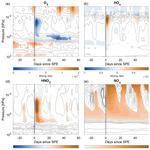
Statistical response of middle atmosphere composition to solar proton events in WACCM-D simulations: the importance of lower ionospheric chemistry
Pekka T. Verronen
Annika Seppälä
Monika E. Szeląg
Antti Kero
Daniel R. Marsh
Atmospheric effects of solar proton events (SPEs) have been studied for decades, because their drastic impact can be used to test our understanding of upper stratospheric and mesospheric chemistry in the polar cap regions. For example, odd hydrogen and odd nitrogen are produced during SPEs, which leads to depletion of ozone in catalytic reactions, such that the effects are easily observed from satellites during the strongest events. Until recently, the complexity of the ion chemistry in the lower ionosphere (i.e., in the D region) has restricted global models to simplified parameterizations of chemical impacts induced by energetic particle precipitation (EPP). Because of this restriction, global models have been unable to correctly reproduce some important effects, such as the increase in mesospheric HNO3 or the changes in chlorine species. Here we use simulations from the WACCM-D model, a variant of the Whole Atmosphere Community Climate Model, to study the statistical response of the atmosphere to the 66 strongest SPEs which occurred in the years 1989–2012. Our model includes a set of D-region ion chemistry, designed for a detailed representation of the atmospheric effects of SPEs and EPP in general. We use superposed epoch analysis to study changes in O3, HOx (OH + HO2), Clx (Cl + ClO), HNO3, NOx (NO + NO2) and H2O. Compared to the standard WACCM which uses an ion chemistry parameterization, WACCM-D produces a larger response in O3 and NOx and a weaker response in HOx and introduces changes in HNO3 and Clx. These differences between WACCM and WACCM-D highlight the importance of including ion chemistry reactions in models used to study EPP.
- Article
(6889 KB) - Full-text XML
- BibTeX
- EndNote
Solar proton events (SPEs) are observed on Earth when high-energy protons accelerated in the sun’s magnetic field during a solar coronal mass ejection strike Earth. These protons generally have energies in the range of tens or hundreds of megaelectronvolts per nucleon and due to their high energies are able to penetrate deep into the atmosphere at geomagnetic latitudes polewards of . This causes ionization and dissociation (mainly of the most abundant species N2 and O2) in the altitude range of roughly 30–90 km. Solar proton events typically last from a few hours to few days and can occur anytime during the 11-year solar cycle, although they are more common during solar maximum.
Ionization, excitation and dissociation caused by the SPEs, and energetic particle precipitation (EPP) in general, have a significant influence on neutral composition through ion-neutral chemistry (e.g., Verronen and Lehmann, 2013). Several studies have investigated the depletion of ozone in the polar mesosphere and upper stratosphere resulting from the increased production of odd hydrogen and odd nitrogen (e.g., Jackman et al., 2001; Seppälä et al., 2004; López-Puertas et al., 2005; Verronen et al., 2006). Ozone depletion can result in changes in temperatures during the largest SPEs (Jackman et al., 2007), and there is evidence that SPEs, as part of EPP, can modulate polar winter dynamics on decadal timescales (Seppälä et al., 2009; Semeniuk et al., 2011; Rozanov et al., 2012; Calisto et al., 2013). Recent results have also shown that SPEs play a role in the upper stratospheric variability of ozone and have to be considered when studying the expected recovery of the ozone layer (Stone et al., 2018). The state of the art of the impact of energetic particle precipitation, in particular SPE and geomagnetic forcing, has also been summarized in the recent WMO assessment (WMO, 2018, chap. 4.3.5.1, 4–25, and references therein).
Simulation results have suggested that the decrease in the polar total ozone column would be of the order of 1 %–2 % a few months after large SPEs (Jackman et al., 2014). However, local depletion has been reported to reach ≈10 % below the ozone layer peak at 50–100 hPa, based on a statistical analysis of almost 200 SPEs using ozone soundings (Denton et al., 2018). As the contribution of >300 MeV protons to direct ozone loss in the lower stratosphere would likely be negligible due to the relatively small fluxes at such high energies (Jackman et al., 2011), any observed ozone loss would likely be the result of indirect effects. Ozone may also increase in the lower stratosphere due to the enhanced NOx interfering with chlorine-driven catalytic ozone loss (Jackman et al., 2008).
The lower ionospheric, the D-region, chemistry, which connects SPE ionization to changes in neutral species, is rather complex compared to the five-ion chemistry that is adequate in the thermosphere. A special feature of the D-region is the presence of negative ions which are formed when electrons attach to neutral species. Another feature is the large number of different cluster ions, both positive and negative, some of which are among the most abundant ions in the region. Due to the large number of D-region ions and ionic reactions, atmospheric models have typically included the SPE, or EPP, effects using simple parameterizations of HOx and NOx production.
Funke et al. (2011) discussed shortcomings related to these parameterization schemes in a comparison study between observations and simulations of the October–November 2003 SPE. Among the outstanding issues in simulations have been the lack of nitric acid HNO3 increase (see also Jackman et al., 2008) and the lack of chlorine activation. The HNO3 production is driven through reactive nitrogen redistribution by negative ion chemistry (Verronen and Lehmann, 2013), and simulations could be improved either by improved parameterization (Päivärinta et al., 2016), or by considering the relevant ion chemistry explicitly (Verronen et al., 2008, 2011; Andersson et al., 2016). The differences between modeled and observed response of chlorine species to SPE reported by Jackman et al. (2008) can be explained by the conversion of HCl to active chlorine species (Cl, ClO, HOCl) by the ion chemical reactions (Winkler et al., 2009, 2011). In the lower ionosphere, the ion chemistry reactions are expected to lead to the depletion of water vapor during SPEs. For moderate-sized SPEs, this effect is small compared to the icy particle sublimation governed by the changes in temperature (von Savigny et al., 2007; Winkler et al., 2012).
The Whole Atmosphere Community Climate Model (WACCM) is a global chemistry–climate model and forms the atmospheric part of the Community Earth System Model (CESM). Recently, a variant called WACCM-D was developed for detailed simulations of D-region ion chemistry and EPP atmospheric effects (Verronen et al., 2016). In a case study of the January 2005 SPE, the consideration of ion chemistry in WACCM-D led to an improved SPE response in HNO3, Clx, NOx and HOx (Andersson et al., 2016). Since then, WACCM-D has been used to study mesospheric nitric acid and cluster ion composition during electron precipitation events (Orsolini et al., 2018) and magnetic latitude dependency of SPE ionospheric impact (Heino et al., 2019).
Here, we take a statistical approach to look at the response of middle atmosphere chemistry to a number of SPEs of various intensities. As the SPE effects are largely known from previous work, we focus on the improvement provided by additional ion chemistry reactions included in the WACCM-D chemistry. In addition to the traditionally analyzed species, such as O3, HOx, NOx and HNO3, we also investigate water vapor and active chlorine species which have been less studied, especially with global models. While several of the largest SPEs included in the analysis have previously been studied individually, the statistical approach used here allows for inclusion of a number of moderate-sized events under various background atmosphere and illumination conditions. This approach also allows for the identification of climatological effects above natural variability. As the analysis includes SPEs of different sizes occurring during different seasons, a statistical approach is most useful for the study of temporal and spatial extent, rather than magnitude of the response.
2.1 WACCM-D simulations
WACCM is a global circulation model with fully coupled chemistry and dynamics extending from the surface to hPa (≈140 km). The horizontal resolution used is 1.9∘ latitude by 2.5∘ longitude. A description of the model physics in the MLT (mesosphere–lower thermosphere) as well as the simulations of dynamical and chemical response to radiative and geomagnetic forcing during solar maximum and minimum are described by Marsh et al. (2007). Marsh et al. (2013) present an overview of the model climate and describes the climate and the variability in a long-term simulation using version 4 of the WACCM. The standard chemistry package of WACCM includes photochemistry of 59 neutral species for the whole altitude range, and five ion species O+, NO+, , and N+ for the lower thermosphere, as well as excited species N(2D), O(1D), O2(1Δ) and O2(1Σ). For SPE effects, and energetic particle precipitation in general, HOx and NOx production is parameterized. For the SPE NOx effect, it is assumed that 1.25 N atoms are produced per ion pair with branching ratio of 0.55∕0.7 for N(4S)∕N(2D) (Jackman et al., 2005; Porter et al., 1976). This parameterization depends on a fundamental assumption of a fixed N2∕O2 ratio, and it has been shown to underestimate NOx production above about 65 km (Nieder et al., 2014; Andersson et al., 2016). For the SPE HOx effect, based on the work of Solomon et al. (1981), a maximum of two molecules are produced per ion pair in the lower mesosphere and below while in the upper mesosphere the production gradually decreases to zero with increasing altitude.
WACCM-D is a variant of WACCM in which the standard parameterizations of HOx and NOx production are replaced by a set of lower ionospheric photochemistry, with the aim of reproducing better the observed effects of EPP on the mesosphere and upper stratosphere neutral composition. The ion chemistry set was selected based on analysis of the latest knowledge of chemical reactions in the ionospheric D-region and their effects on the neutral atmosphere (Verronen and Lehmann, 2013). The set consists of 307 reactions of 20 positive ions and 21 negative ions, including cluster ions such as H+(H2O) and , which are important for HOx and HNO3 production, for example. The details on WACCM-D ion chemistry as well as its lower ionospheric evaluation were presented by Verronen et al. (2016), while the improvement in the atmospheric response during the January 2005 SPE was demonstrated in comparisons with satellite observations (Andersson et al., 2016). WACCM-D is now included as an official predefined component set (compset) since the CESM 2.0 release in June 2018.
In this study, we use WACCM version 4 simulations with the specified dynamics configuration (SD-WACCM) which is forced with meteorological fields (temperature, horizontal winds and surface pressure) from NASA’s Modern-Era Retrospective Analysis for Research and Applications (MERRA) (Rienecker et al., 2011; Lamarque et al., 2012) at every dynamics time step below about 50 km; above this, the model is free running (88 levels in total). It should be noted that even above 50 km model dynamics are strongly modulated by winds and wave fluxes from lower levels. This means that internal variability of dynamics in SD-WACCM is small.
For energetic particle precipitation, the simulations include forcing from auroral electrons (E<10 keV), solar protons and galactic cosmic rays. Medium-energy and high-energy electrons (E>10 keV) were excluded from the simulations. Two model simulations were made: (1) a reference run using a standard SD-WACCM compset (referred to as REF) and (2) a run with D-region ion chemistry (referred to as WD). Both simulations covered the years 1989 to 2012. We use daily mean SPE ionization rates based on GOES (Geostationary Operational Environmental Satellite) observations and described, for example, in Jackman et al. (2011). The energy range for protons is 1–300 MeV, and thus the direct atmospheric impact takes place at altitudes above ≈10 hPa (e,g, Turunen et al., 2009, Fig. 3).
Proton fluxes were only included for energies up to 300 MeV. However, Jackman et al. (2011) show that higher energies contribute very little to the total ozone or NOy impact. We only consider protons, and not the X-ray flares that are associated with some SPEs – in the polar regions we can assume that the proton effect is the dominant one, at least for large SPEs (Pettit et al., 2018).
2.2 Analysis methods
For our statistical analysis, SPEs were selected using proton flux data from satellite-based GOES observations (available from https://www.ngdc.noaa.gov/stp/satellite/goes/index.html, last access: 9 July 2020). An event was selected if the peak proton flux exceeded 100 particle flux units (pfu), with pfu defined as the 5 min average flux in units of particles cm−2 s−1 sr−1 for protons with energy larger than 10 MeV. To avoid obscuring the signal in composite means by introducing duplicates of major events in days preceding the zero epoch date, any event following a larger event onset within 7 d was excluded from the analysis. In total, 11 such events were removed. Following these criteria, 66 events given in Table A1 were identified in the simulation period. The seasonal distribution of the selected SPEs is shown in Fig. 1. As SPEs are sporadic, the distribution is somewhat uneven, with the Northern Hemisphere winter months (December–February) underrepresented. Of the 8 largest SPEs (above 10 000 pfu) in the analysis, five occurred in October or November, compared to only one each in March, July and September.
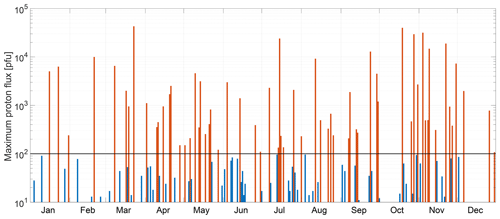
Figure 1Maximum proton flux of solar proton events used in the analysis as a function of the day of the year of the SPE onset. Events with maximum flux over threshold value (100 pfu) are shown as red bars and smaller events as blue bars.
For each of the 66 events, zero epoch day (D0) was defined as the first day when the proton flux exceeded 10 pfu. A 90 d epoch period around the zero epoch ([D0−29, D0+60]) was selected from the daily mean time series for the following constituents: O3, HOx (OH + HO2), Clx (Cl + ClO), HNO3, NOx (NO + NO2) and H2O. Definitions of abbreviations used in the following text for the statistical quantities from REF and WD simulations are shown in Table 1.
To assess the anomalies and associated with the SPEs, daily climatological mean mixing ratios and were deducted from respective epoch mixing ratios REF and WD. Figure 2 shows the composite means of for the northern polar cap (60–90∘ N, area-weighted). Note that due to the seasonal distribution of the events some seasonal signals remain visible in the composite means, e.g., a slight increase in mesospheric O3 during the 90 d epoch period. It can also be seen that for some constituents, especially HNO3, mixing ratio enhancements following the events are large enough to visibly affect the daily climatology.
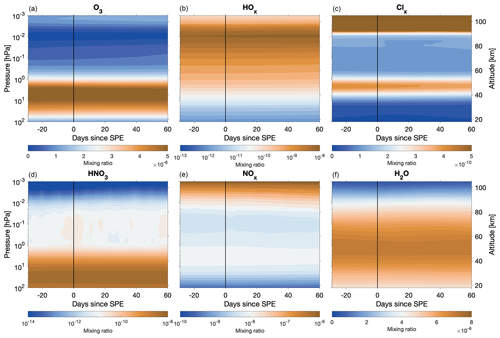
Figure 2Area-weighted northern polar cap (latitude N) average of composite mean of for O3, HOx, Clx, HNO3, NOx and H2O. The x axis shows the number of days before and after the event (day 0, solid black line) and the y axis pressure levels in the model (hPa, a, d) and approximate altitude (km, c, f).
For each constituent, we calculate the composite mean of difference μd between the epoch time series and the corresponding climatological time series
where r is the relevant mixing ratio epoch time series REF or WD, c is the epoch time series from daily climatology or , and d is the number of days before and after zero epoch . Subtracting the climatological values from the epoch time series helps to separate the SPE signal from the seasonal signals which can arise from the uneven monthly distribution of the SPEs (see Fig. 1). Variance of the composite means was approximated by bootstrapping, i.e., by re-sampling the random selection of dates 1000 times with replacement, with N=66 for each sample. Bootstrapping was chosen for variance estimation due to its independence from the shape of the distribution; i.e., it does not require a normal distribution. The standard deviation of the variance was then used to identify robust responses, i.e., SPE-driven changes that are clearly larger than the normal variability.
The SPE-driven anomalies are expected to occur following the SPE onset (zero epoch). However, in Sect. 3 we will show that the results sometimes have also anomalies that extend over the full epoch period. These anomalies are in most cases related to solar cycle variability, due to the SPE sampling over-representing the solar cycle maximum years when compared to climatology. Composite mean SPE ionization for the analyzed epochs is shown in Fig. 3. Daily composite mean peak ionization is 891 ion pairs cm−3 s−1, which is roughly similar in magnitude to peak ionization associated with January 2005 SPE. The largest individual events in the time series feature peak ionizations about 10 times higher, for example peak ionization of 8534 ion pairs cm−3 s−1 for the “Halloween” SPE. In addition to the main ionization peak within ca. 5 d following the onset of the SPE, secondary ionization peaks are evident in the epoch mean, due to closely separated SPEs. While these secondary peaks are much weaker (by roughly an order of magnitude) than the main peak, they can still cause noticeable anomalies in some of the analyzed constituents.
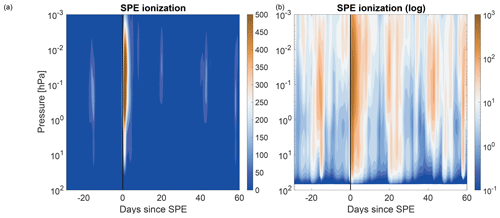
Figure 3Composite mean of epoch time series of SPE ionization rates in linear (a) and logarithmic (b) color scale. The x axis shows the number of days before and after the event (day 0, solid black line) and the y axis pressure levels in the model.
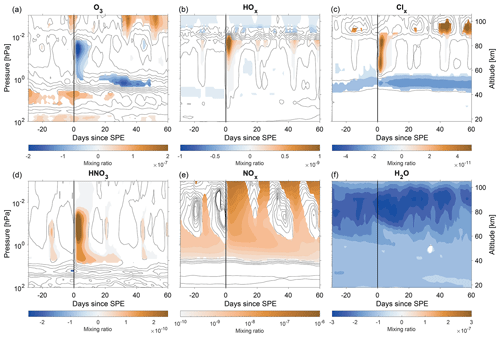
Figure 4Area-weighted northern polar cap averages (latitude N) of composite means of for O3, HOx, Clx, HNO3, NOx and H2O. The x axis shows the number of days before and after the event (day 0, solid black line) and the y axis pressure levels in the model (hPa, a, d) and approximate altitude (km, c, f). Note that the color scale for NOx panel is logarithmic, and all contours shown in that panel indicate positive difference.
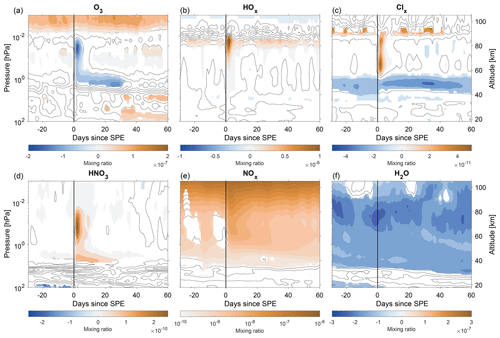
Figure 5Area-weighted southern polar cap averages (latitude S) of composite means of for O3, HOx, Clx, HNO3, NOx and H2O. The x axis shows the number of days before and after the event (day 0, solid black line) and the y axis pressure levels in the model (hPa, a, d) and approximate altitude (km, c, f). Note that the color scale for NOx panel is logarithmic, and all contours shown in that panel indicate positive difference.
In order to evaluate the contribution of the improved ion chemistry of WACCM-D, the same epoch analysis was made for both the REF and the WD simulations. The anomalies from the WACCM-D simulation () were then compared to anomalies from the standard WACCM () (Figs. 4 and 5). We considered the difference of composite means of differences in mixing ratio between the two simulations for the full altitude range (Figs. 6 and 7) as well as comparison of the SPE response at pressure levels chosen for maximum difference between two simulations (Figs. 8 and 9).
3.1 Statistical response from WACCM-D
Figures 4 (Northern Hemisphere, NH) and 5 (Southern Hemisphere, SH) show the mean anomaly of the super-imposed epochs for O3, HOx, Clx, HNO3, NOx and H2O. Statistically robust anomalies, i.e., those larger than 2 times the standard deviation of the bootstrap variance, are shown in color and discussed below.
Several constituents show the most pronounced anomaly immediately following the event onset (zero epoch), which in the figures is marked with a black vertical line. For some constituents, most notably H2O and Clx, persistent anomalies extending throughout the epoch period are seen as well. These anomalies can be considered to represent the solar cycle signal because the distribution of SPEs concentrates on the years around the solar maximum. Some anomalies are clearly affected by the response to closely separated SPEs, i.e., when the separation is smaller than 60 d. These are most notable in NOx and HNO3 in NH around days −15, +22 and +45 with respect to zero epoch, corresponding to the secondary peaks of SPE ionization (see Fig. 3).
Ozone (Figs. 4 and 5, panel a) decreases between 1 and 0.01 hPa immediately following the SPE, lasting about 5–10 d. This is caused by catalytic destruction from enhanced HOx. Ozone loss also takes place around 1 hPa, driven by NOx and Clx. The depletion around 1 hPa lasts much longer, driven by the NOx increase, with maximum ozone decrease seen about 30 d after the event onset. In contrast, an increase in ozone is seen throughout the period near the secondary ozone maximum above 0.01 hPa, more robustly in the Southern Hemisphere. This increase is linked to enhanced atomic oxygen production by O2 photolysis in solar maximum conditions (see also Marsh et al., 2007). In the stratosphere below 5 hPa the ozone response is not consistently robust. However, an intermittent increase, likely caused by solar cycle effects, is seen throughout the epoch period in this region. Thus the decrease in the total ozone column, as reported by Denton et al. (2018), is not seen in our analysis. It should be noted that the highest energy protons (E>300 MeV), which can directly impact the lower stratosphere, are not included in the particle forcing used in our simulations. However, it is not clear whether inclusion of these protons would change the response because their fluxes should be too low to cause a significant extra response (Jackman et al., 2011).
A strong, short-lived increase in HOx is seen below 0.01 hPa (Figs. 4 and 5, panel b) immediately following the SPE, reaching down to the 10 hPa level, with the strongest and the most robust response in the mesosphere. Due to the short chemical lifetime of HOx, there is no longer-term anomaly beyond 10 d from the SPE onset. The later peaks just below 0.01 hPa are caused by SPEs occurring within 60 d of zero epoch of analyzed SPE. Above 0.01 hPa, a small negative anomaly is present throughout the period, related to solar maximum through less water vapor in the region and lowering of the HOx peak altitude. The persistent positive anomaly at just below 0.01 hPa, seen in the SH, is consistent with lowering of the HOx peak altitude. Factors affecting HOx peak altitude during solar maxima are the decrease in H2O and the increase in Lyman-α photolysis, balancing at the level of mesopause.
Clx mixing ratios (Figs. 4 and 5, panel c) are enhanced between 1 and 0.01 hPa, these SPE-driven increases last up to about a week. Below 1 hPa, at the altitude of Clx mixing ratio maximum, Clx is a reduced. This decrease is roughly consistent and continuous throughout the epoch period and so is likely, at least in part, connected to the solar cycle rather than individual events. However, the magnitude of the negative anomaly increases following the onset of the SPE, with maximum value in the SH reached after 30 d. A short-lived decrease in the NH polar upper stratosphere ClO during the January 2005 SPE has previously been reported both in satellite observations and models (Damiani et al., 2012; Andersson et al., 2016). In the short term decrease is likely caused by conversion of ClO to HOCl in the presence of enhanced HO2, while in the longer term, the decrease is probably connected to the descent of NOx and subsequent formation of ClONO2. Here we look at the polar average, but it should be noted that in the presence of SPE-generated HOx, the response of Clx in the polar atmosphere depends strongly on the sunlight conditions and varies across latitudes and altitudes (Funke et al., 2011). For example, in the polar night stratosphere ClO is converted to HOCl in reaction with HO2, while in the daytime HOCl is converted to ClO by OH.
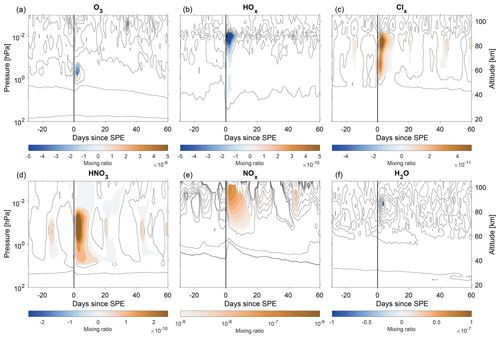
Figure 6Area-weighted northern polar cap averages (latitude N) of composite means of – (see Fig. 4). The x axis shows the number of days before and after the event (day 0, solid black line) and the y axis pressure levels in the model (hPa, a, d) and approximate altitude (km, c, f). Note that the color scale for NOx panel is logarithmic, and all contours shown in that panel indicate positive difference.
A large increase in HNO3 (Figs. 4 and 5, panel d) is seen up to 10 d after the SPE onset, with a maximum between 1 and 0.01 hPa. The absence of a longer-term effect is due to the fast photodissociation of HNO3 in sunlit conditions. Similar to HOx, the later peaks around days 20 and 45 in the NH are caused by nearly coincident SPEs. A longer-term enhancement persists for 20–30 d after SPEs below 1 hPa. The anomalies are weaker in the SH than in the NH, due to the strongest SPEs mostly occurring during NH winter (see Fig. 1) when there is less solar radiation and HNO3 photodissociation.
SPE-driven enhancements of NOx (Figs. 4 and 5, panel e) reach down to 1 hPa level and below. These enhancements start to diminish after about 10 d, but robust longer-term enhancements can be seen for several weeks afterwards. This is especially clear at lower altitudes (around 1 hPa), where transport from above contributes to persistence of enhancements. The response is different in the Northern and Southern Hemispheres due to the seasonal distribution of the events. In the NH, several strong, closely separated winter-time events show up as recurring anomalies, which are then transported to lower altitudes. The SH response is less variable, with small but robust enhancements seen through the two months following the SPE onset. In addition to the SPE-driven anomaly, there is a persistent, underlying anomaly seen above about 10 hPa from enhanced NOx production in the lower thermosphere by solar EUV and EPP during solar maximum times (see also Marsh et al., 2007).
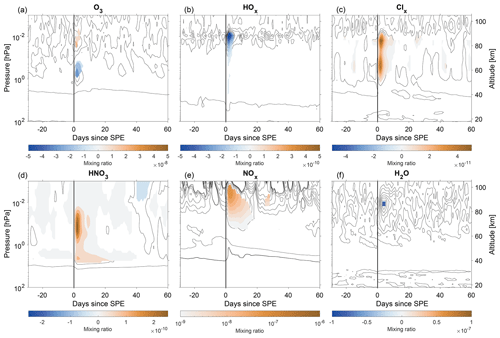
Figure 7Area-weighted southern polar cap averages (latitude S) of composite means of – (see Fig. 5). The x axis shows the number of days before and after the event (day 0, solid black line) and the y axis pressure levels in the model (hPa, a, d) and approximate altitude (km, c, f). Note that the color scale for NOx panel is logarithmic, and all contours shown in that panel indicate positive difference.
During an SPE, H2O would be expected to decrease when it is converted to HOx in ionic reactions. In our analysis, H2O (Figs. 4 and 5, panel f) shows a consistent and statistically robust negative anomaly throughout the period analyzed, except in the SH lower stratosphere. There is some indication of a stronger anomaly following the SPEs above 0.1 hPa, i.e., at altitudes where HOx increases. But in general the anomaly is mostly related to the solar cycle through the increased Lyman-α photodissociation during solar maximum decreasing H2O in the mesosphere–lower thermosphere (see also Schmidt et al., 2006; Marsh et al., 2007).
3.2 Effects of D-region ion chemistry
Figures 6 and 7 show the difference between epoch responses in the NH and SH, respectively, from WACCM-D and the standard WACCM. As the specified dynamics of the two simulations are identical, differences seen in these figures arise from the addition of the D-region ion chemistry. Note that the robustness threshold for these figures is set to 1 standard deviation rather than the 2 standard deviations used in Figs. 4 and 5. The differences shown in figures for constituents other than O3 and H2O also satisfy the threshold of 2 standard deviations. The effects on O3 and H2O, however, do not reach the threshold of 2 standard deviations. Further, Figs. 8 and 9 show line plots of epoch response from both WACCM-D () and standard WACCM () at pressure levels corresponding to maximum significant difference in Figs. 6 and 7.
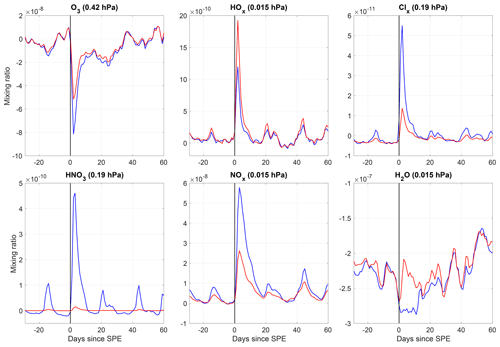
Figure 8Composite mean (blue) and (red) for the northern polar cap (area-weighted, latitude N) at selected pressure bands (three pressure levels, pressure of the center of the band shown in panel title). The x axis shows the number of days before and after the event (day 0, solid black line).
Ozone (Figs. 6 and 7, panel a) depletion is larger in WACCM-D for about 5 d after the onset at around 0.5–1 hPa. This effect is very similar in both hemispheres. Both the duration and altitude range of this extra ozone loss clearly correspond to enhanced Clx, while there is no clear connection to NOx or HOx differences. Less ozone depletion takes place in WACCM-D around the 0.01 hPa level, connected to the clearly lower HOx enhancement in WACCM-D. An anomaly of similar magnitude is observed in both hemispheres, although it is only robust in SH. The reduced HOx response in WACCM-D is a result of the lower water vapor amount, and thus lower HOx production, than what is assumed when HOx production is parameterized (Andersson et al., 2016).
Clx is enhanced in both hemispheres above 1 hPa in WACCM-D, while there is only a relatively weak increase in the standard WACCM. The reasons for the enhancement are the ion reactions included in WACCM-D that convert more HCl to active chlorine species Cl and ClO (Winkler et al., 2009) than the neutral gas-phase reactions which are included in both standard WACCM and WACCM-D. Enhancements relating to secondary ionization peaks can also be seen, most clearly around day 40. The negative anomaly of Clx below 1 hPa seen in Figs. 4 and 5 is not present here, again implying that this anomaly is not predominantly due to ion chemistry.
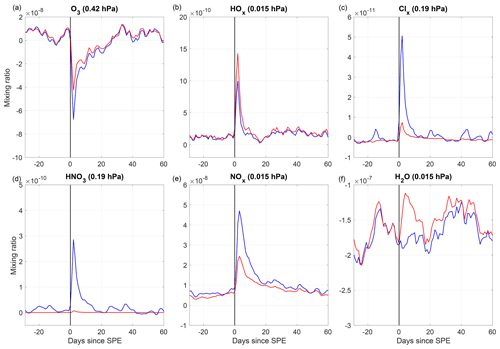
Figure 9Composite mean (blue) and (red) for the southern polar cap (area-weighted, latitude S) at selected pressure bands (three pressure levels, pressure of the center of the band shown in panel title). The x axis shows the number of days before and after the event (day 0, solid black line).
The HNO3 enhancement is clear and strong in WACCM-D, as ion chemistry produces it from other NOy species. Standard WACCM, like other models using an EPP lookup table parameterization, is known to underestimate the HNO3 mixing ratios when compared to observations (Jackman et al., 2008; Funke et al., 2011; Andersson et al., 2016). A discrepancy between WACCM-D and standard WACCM HNO3 enhancements is clearly seen in Figs. 8 and 9. Due to the dramatic increase in HNO3 following SPEs, peaks from other, close-by events are also clearly seen in Fig. 8.
In the mesosphere, the NOx enhancement is much larger in WACCM-D than in standard WACCM. Below 0.1 hPa there are some signs of an increase in transported NOx, although this effect is not statistically robust. Increased NOx is consistent between the hemispheres. Andersson et al. (2016) attribute the increased NOx to enhanced production above 80 km (ca. 0.01 hPa), mostly due to the reaction + N2 → , and consequent transport to lower altitudes.
Differences in H2O anomalies (Figs. 6–9, panel f) between WACCM-D and the standard WACCM are seen above 1.0 hPa but they are almost entirely overcome by the variance, which indicates that negative anomalies in Figs. 4 and 5 are not primarily connected to ion chemistry reactions but are a solar cycle signal. However, a short-lived negative difference, robust to a 1σ level, can be seen at 0.01 hPa immediately following the onset of SPE, even though the co-located HOx production in WACCM-D is smaller. This negative difference is more clearly seen in Figs. 8 and 9, where we see that mesospheric H2O anomalies are very consistent between the simulations, except within 10–15 d following the onset of SPE, where the WACCM-D negative anomalies are larger.
3.3 Effect from individual events
The SPE effects are dependent on the background atmosphere and season of the year. Here we discuss this by looking at the effects of individual events at selected altitudes. This is also an additional check on the robustness of our analysis.
Figure 10 shows the individual time series of differences of epochs from the climatology around 0.05 hPa pressure level (calculated as the mean of three pressure levels centered at 0.04 hPa). SPEs used in this figure are screened for closely separated events, i.e., events which are followed by a larger event within 7 d are not shown. Individual events are sorted from top to bottom by the maximum observed proton flux, with strongest events on top of the figure. Dashed horizontal lines show the cut-offs for 1000 pfu (top line) and 100 pfu (bottom line) SPEs.
As expected, the largest SPEs cause a stronger response in O3, HOx and NOx. The response to events is more pronounced in the NH due to the seasonal distribution of SPEs; i.e., there are more events in NH winter. This is especially apparent for NOx, as its lifetime is heavily influenced by the amount of sunlight available. On visual inspection, the response is dominated by the largest SPEs, with anomalies following the onset of SPEs being visually indistinguishable for the weakest events. Below the 100 pfu threshold, any anomalies following the event onset are dominated by larger events occurring within the analysis period, rather than by the analyzed event itself. Thus, the decision to only analyze larger events seems reasonable because the events smaller than 100 pfu would only add a substantial amount of noise to the analysis.
We present an analysis of the chemical impacts of SPE on the middle atmosphere using simulations from WACCM-D, a variant of the Whole Atmosphere Community Climate Model including a set of D-region ion chemistry reactions. The aim of our analysis is to study the impact and improvement from a detailed ion chemistry scheme, which is done by comparing WACCM-D results to those from the standard WACCM scheme which used a simple parameterization of HOx and NOx production. Instead of analyzing individual SPE events, we present a statistical analysis of the 66 largest SPEs over the years 1989–2012 to allow for more general conclusions in a range of different background atmospheric conditions and with the observed seasonal distribution of SPEs. This statistical approach allowed for the incorporation of smaller events which would have been difficult to analyze separately. Analysis was performed by means of a superposed epoch method with bootstrapping to identify statistically robust responses.
Statistically robust SPE signals were present in WACCM-D for all analyzed species other than water vapor (O3, HOx, Clx, HNO3, NOx). These signals are qualitatively in line with previously published modeling results and satellite observations considering individual events. In addition, our analysis shows longer-term, solar-cycle-type signals for several species due to SPEs predominantly occurring during solar maximum years. In general, the responses are consistent between the two hemispheres.
Compared to the standard WACCM, WACCM-D provides a solution to some known shortcomings in chemistry–climate models, particularly in the case of HNO3 and Clx response to SPEs. Ozone loss following the SPEs was enhanced at 0.5 hPa in WACCM-D. As there is no corresponding increase in HOx or NOx at this altitude, this loss is connected to increased conversion of HCl to reactive Clx species by the ion reactions (Winkler et al., 2009), now accounted for in WACCM-D. Conversely, less ozone loss took place around 0.01 hPa in the SH, due to less production of HOx, in agreement with Andersson et al. (2016). NOx production in the mesosphere is enhanced by the inclusion of ion chemistry and subsequent downward transport, extending the effect to lower altitudes for up to 20 d following the SPE onset. Ion chemistry was found to be crucial for characterization of HNO3 production following the SPEs, which confirms the previous results from 1-D chemistry models (Verronen et al., 2008, 2011). Some effect was also found in H2O in an altitude region near the mesopause (around 0.01 hPa or 85 km). This, however, has a large 11-year solar cycle signal, partially masking the SPE impact.
In summary, incomplete representation of EPP-driven chemistry in simulations, for example regarding the HNO3, has been recognized as one of the outstanding questions in understanding the solar influence in the middle atmosphere and below (Jackman et al., 2008; Funke et al., 2011). Our results clearly show the importance of D-region ion chemistry in capturing the effects of energetic particle precipitation in the mesosphere–lower thermosphere simulations. Improved global modeling with ion chemistry, such as that in WACCM-D, provides an important tool for interpretation of a wider range of satellite-based observations of neutral species and allows for global studies of ionospheric D region.
All model data used are available from corresponding author by request (niilo.kalakoski@fmi.fi). CESM source code is distributed freely through a public subversion code repository (http://www.cesm.ucar.edu/models/cesm1.0/, UCAR, 2020).
NK, PTV and MES planned the research and simulation. MES carried out the simulations. NK carried out the analysis and led the writing of the paper. All authors contributed to the discussion and the writing of the final paper.
The authors declare that they have no conflict of interest.
This material is based upon work supported in part by the National Center for Atmospheric Research, which is a major facility sponsored by the National Science Foundation under cooperative agreement no. 1852977. Work was carried out as a part of International Space Science Institute (ISSI) project “Space Weather Induced Direct Ionisation Effects On The Ozone Layer”.
DRM has been supported by NSF (Award no. 1650918, “Collaborative Research: CEDAR – Quantifying the Impact of Radiation Belt Electron Precipitation on Atmospheric Reactive Nitrogen Oxides (NOx) and Ozone (O3)”). Antti Kero is funded by the Tenure Track Project in Radio Science at Sodankylä Geophysical Observatory.
This paper was edited by Gabriele Stiller and reviewed by three anonymous referees.
Andersson, M. E., Verronen, P. T., Marsh, D. R., Päivärinta, S.-M., and Plane, J. M. C.: WACCM-D – Improved modeling of nitric acid and active chlorine during energetic particle precipitation, J. Geophys. Res.-Atmos., 121, 10328–10341, https://doi.org/10.1002/2015JD024173, 2016. a, b, c, d, e, f, g, h, i
Calisto, M., Usoskin, I., and Rozanov, E.: Influence of a Carrington-like event on the atmospheric chemistry, temperature and dynamics: revised, Environ. Res. Lett., 8, 045010, https://doi.org/10.1088/1748-9326/8/4/045010, 2013. a
Damiani, A., Funke, B., Marsh, D. R., López-Puertas, M., Santee, M. L., Froidevaux, L., Wang, S., Jackman, C. H., von Clarmann, T., Gardini, A., Cordero, R. R., and Storini, M.: Impact of January 2005 solar proton events on chlorine species, Atmos. Chem. Phys., 12, 4159–4179, https://doi.org/10.5194/acp-12-4159-2012, 2012. a
Denton, M. H., Kivi, R., Ulich, T., Clilverd, M. A., Rodger, C. J., and von der Gathen, P.: Northern hemisphere stratospheric ozone depletion caused by solar proton events: the role of the polar vortex, Geophys. Res. Lett., 45, 2115–2124, 2018. a, b
Funke, B., Baumgaertner, A., Calisto, M., Egorova, T., Jackman, C. H., Kieser, J., Krivolutsky, A., López-Puertas, M., Marsh, D. R., Reddmann, T., Rozanov, E., Salmi, S.-M., Sinnhuber, M., Stiller, G. P., Verronen, P. T., Versick, S., von Clarmann, T., Vyushkova, T. Y., Wieters, N., and Wissing, J. M.: Composition changes after the ”Halloween” solar proton event: the High Energy Particle Precipitation in the Atmosphere (HEPPA) model versus MIPAS data intercomparison study, Atmos. Chem. Phys., 11, 9089–9139, https://doi.org/10.5194/acp-11-9089-2011, 2011. a, b, c, d
Heino, E., Verronen, P. T., Kero, A., Kalakoski, N., and Partamies, N.: Cosmic noise absorption during solar proton events in WACCM-D and riometer observations, J. Geophys. Res.-Space, 124, 1361–1376, https://doi.org/10.1029/2018JA026192, 2019. a
Jackman, C. H., McPeters, R. D., Labow, G. J., Fleming, E. L., Praderas, C. J., and Russel, J. M.: Northern hemisphere atmospheric effects due to the July 2000 solar proton events, Geophys. Res. Lett., 28, 2883–2886, 2001. a
Jackman, C. H., DeLand, M. T., Labow, G. J., Fleming, E. L., Weisenstein, D. K., Ko, M. K. W., Sinnhuber, M., and Russell, J. M.: Neutral atmospheric influences of the solar proton events in October–November 2003, J. Geophys. Res., 110, A09S27, https://doi.org/10.1029/2004JA010888, 2005. a
Jackman, C. H., Roble, R. G., and Fleming, E. L.: Mesospheric dynamical changes induced by the solar proton events in October–November 2003, Geophys. Res. Lett., 34, L04812, https://doi.org/10.1029/2006GL028328, 2007. a
Jackman, C. H., Marsh, D. R., Vitt, F. M., Garcia, R. R., Fleming, E. L., Labow, G. J., Randall, C. E., López-Puertas, M., Funke, B., von Clarmann, T., and Stiller, G. P.: Short- and medium-term atmospheric constituent effects of very large solar proton events, Atmos. Chem. Phys., 8, 765–785, https://doi.org/10.5194/acp-8-765-2008, 2008. a, b, c, d, e
Jackman, C. H., Marsh, D. R., Vitt, F. M., Roble, R. G., Randall, C. E., Bernath, P. F., Funke, B., López-Puertas, M., Versick, S., Stiller, G. P., Tylka, A. J., and Fleming, E. L.: Northern Hemisphere atmospheric influence of the solar proton events and ground level enhancement in January 2005, Atmos. Chem. Phys., 11, 6153–6166, https://doi.org/10.5194/acp-11-6153-2011, 2011. a, b, c, d
Jackman, C. H., Randall, C. E., Harvey, V. L., Wang, S., Fleming, E. L., López-Puertas, M., Funke, B., and Bernath, P. F.: Middle atmospheric changes caused by the January and March 2012 solar proton events, Atmos. Chem. Phys., 14, 1025–1038, https://doi.org/10.5194/acp-14-1025-2014, 2014. a
Lamarque, J.-F., Emmons, L. K., Hess, P. G., Kinnison, D. E., Tilmes, S., Vitt, F., Heald, C. L., Holland, E. A., Lauritzen, P. H., Neu, J., Orlando, J. J., Rasch, P. J., and Tyndall, G. K.: CAM-chem: description and evaluation of interactive atmospheric chemistry in the Community Earth System Model, Geosci. Model Dev., 5, 369–411, https://doi.org/10.5194/gmd-5-369-2012, 2012. a
López-Puertas, M., Funke, B., Gil-López, S., von Clarmann, T., Stiller, G. P., Höpfner, M., Kellmann, S., Fischer, H., and Jackman, C. H.: Observation of NOx enhancement and ozone depletion in the Northern and Southern Hemispheres after the October–November 2003 solar proton events, J. Geophys. Res., 110, A09S43, https://doi.org/10.1029/2005JA011050, 2005. a
Marsh, D. R., Garcia, R. R., Kinnison, D. E., Boville, B. A., Sassi, F., Solomon, S. C., and Matthes, K.: Modeling the whole atmosphere response to solar cycle changes in radiative and geomagnetic forcing, J. Geophys. Res.-Atmos., 112, D23306, https://doi.org/10.1029/2006JD008306, 2007. a, b, c, d
Marsh, D. R., Mills, M., Kinnison, D., Lamarque, J.-F., Calvo, N., and Polvani, L.: Climate change from 1850 to 2005 simulated in CESM1(WACCM), J. Climate, 26, 7372–7391, https://doi.org/10.1175/JCLI-D-12-00558.1, 2013. a
Nieder, H., Winkler, H., Marsh, D. R., and Sinnhuber, M.: NOx production due to energetic particle precipitation in the MLT region: Results from ion chemistry model studies, J. Geophys. Res.-Space, 119, 2137–2148, https://doi.org/10.1002/2013JA019044, 2014. a
Orsolini, Y. J., Smith-Johnsen, C., Marsh, D. R., Stordal, F., Rodger, C. J., Verronen, P. T., and Clilverd, M. A.: Mesospheric nitric acid enhancements during energetic electron precipitation events simulated by WACCM-D, J. Geophys. Res.-Atmos., 123, 6984–6998, https://doi.org/10.1029/2017JD028211, 2018. a
Päivärinta, S.-M., Verronen, P. T., Funke, B., Gardini, A., Seppälä, A., and Andersson, M. E.: Transport versus energetic particle precipitation: Northern polar stratospheric NOx and ozone in January–March 2012, J. Geophys. Res.-Atmos., 121, 6085–6100, https://doi.org/10.1002/2015JD024217, 2016. a
Pettit, J., Randall, C. E., Marsh, D. R., Bardeen, C. G., Qian, L., Jackman, C. H., Woods, T. N., Coster, A., and Harvey, V. L.: Effects of the September 2005 solar flares and solar proton events on the middle atmosphere in WACCM, J. Geophys. Res.-Space, 123, 5747–5763, https://doi.org/10.1029/2018JA025294, 2018. a
Porter, H. S., Jackman, C. H., and Green, A. E. S.: Efficiencies for production of atomic nitrogen and oxygen by relativistic proton impact in air, J. Chem. Phys., 65, 154–167, 1976. a
Rienecker, M. M., Suarez, M. J., Gelaro, R., Todling, R., Bacmeister, J., Liu, E., Bosilovich, M. G., Schubert, S. D., Takacs, L., Kim, G.-K., Bloom, S., Chen, J., Collins, D., Conaty, A., da Silva, A., Gu, W., Joiner, J., Koster, R. D., Lucchesi, R., Molod, A., Owens, T., Pawson, S., Pegion, P., Redder, C. R., Reichle, R., Robertson, F. R., Ruddick, A. G., Sienkiewicz, M., and Woollen, J.: MERRA: NASA's Modern-Era Retrospective Analysis for Research and Applications, J. Climate, 24, 3624–3648, https://doi.org/10.1175/JCLI-D-11-00015.1, 2011. a
Rozanov, E., Calisto, M., Egorova, T., Peter, T., and Schmutz, W.: The influence of precipitating energetic particles on atmospheric chemistry and climate, Surv. Geophys., 33, 483–501, https://doi.org/10.1007/s10712-012-9192-0, 2012. a
Schmidt, H., Brasseur, G. P., Charron, M., Manzini, E., Giorgetta, M. A., Diehl, T., Fomichev, V. I., Kinnison, D., Marsh, D., and Walters, S.: The HAMMONIA chemistry climate model: Sensitivity of the mesopause region to the 11-year solar cycle and CO2 doubling, J. Climate, 19, 3903–3931, 2006. a
Semeniuk, K., Fomichev, V. I., McConnell, J. C., Fu, C., Melo, S. M. L., and Usoskin, I. G.: Middle atmosphere response to the solar cycle in irradiance and ionizing particle precipitation, Atmos. Chem. Phys., 11, 5045–5077, https://doi.org/10.5194/acp-11-5045-2011, 2011. a
Seppälä, A., Verronen, P. T., Kyrölä, E., Hassinen, S., Backman, L., Hauchecorne, A., Bertaux, J. L., and Fussen, D.: Solar proton events of October–November 2003: Ozone depletion in the Northern Hemisphere polar winter as seen by GOMOS/Envisat, Geophys. Res. Lett., 31, L19107, https://doi.org/10.1029/2004GL021042, 2004. a
Seppälä, A., Randall, C. E., Clilverd, M. A., Rozanov, E., and Rodger, C. J.: Geomagnetic activity and polar surface air temperature variability, J. Geophys. Res., 114, A10312, https://doi.org/10.1029/2008JA014029, 2009. a
Solomon, S., Rusch, D. W., Gérard, J.-C., Reid, G. C., and Crutzen, P. J.: The effect of particle precipitation events on the neutral and ion chemistry of the middle atmosphere: II. Odd hydrogen, Planet. Space Sci., 8, 885–893, 1981. a
Stone, K. A., Solomon, S., and Kinnison, D. E.: On the identification of ozone recovery, Geophys. Res. Lett., 45, 5158–5165, https://doi.org/10.1029/2018GL077955, 2018. a
UCAR: CESM1.0 PUBLIC RELEASE, available at: http://www.cesm.ucar.edu/models/cesm1.0/, last access: 9 July 2020. a
Turunen, E., Verronen, P. T., Seppälä, A., Rodger, C. J., Clilverd, M. A., Tamminen, J., Enell, C.-F., and Ulich, T.: Impact of different precipitation energies on NOx generation during geomagnetic storms, J. Atmos. Sol.-Terr. Phys., 71, 1176–1189, https://doi.org/10.1016/j.jastp.2008.07.005, 2009. a
Verronen, P. T. and Lehmann, R.: Analysis and parameterisation of ionic reactions affecting middle atmospheric HOx and NOy during solar proton events, Ann. Geophys., 31, 909–956, https://doi.org/10.5194/angeo-31-909-2013, 2013. a, b, c
Verronen, P. T., Seppälä, A., Kyrölä, E., Tamminen, J., Pickett, H. M., and Turunen, E.: Production of odd hydrogen in the mesosphere during the January 2005 solar proton event, Geophys. Res. Lett., 33, L24811, https://doi.org/10.1029/2006GL028115, 2006. a
Verronen, P. T., Funke, B., López-Puertas, M., Stiller, G. P., von Clarmann, T., Glatthor, N., Enell, C.-F., Turunen, E., and Tamminen, J.: About the increase of HNO3 in the stratopause region during the Halloween 2003 solar proton event, Geophys. Res. Lett., 35, L20809, https://doi.org/10.1029/2008GL035312, 2008. a, b
Verronen, P. T., Santee, M. L., Manney, G. L., Lehmann, R., Salmi, S.-M., and Seppälä, A.: Nitric acid enhancements in the mesosphere during the January 2005 and December 2006 solar proton events, J. Geophys. Res., 116, D17301, https://doi.org/10.1029/2011JD016075, 2011. a, b
Verronen, P. T., Andersson, M. E., Marsh, D. R., Kovács, T., and Plane, J. M. C.: WACCM-D – Whole Atmosphere Community Climate Model with D-region ion chemistry, J. Adv. Model. Earth Sy., 8, 954–975, https://doi.org/10.1002/2015MS000592, 2016. a, b
von Savigny, C., Sinnhuber, M., Bovensmann, H., Burrows, J., Kallenrode, M.-B., and Schwartz, M.: On the disappearance of noctilucent clouds during the January 2005 solar proton events, Geophys. Res. Lett., 34, L02805, https://doi.org/10.1029/2006GL028106, 2007. a
Winkler, H., Kazeminejad, S., Sinnhuber, M., Kallenrode, M.-B., and Notholt, J.: Conversion of mesospheric HCl into active chlorine during the solar proton event in July 2000 in the northern polar region, J. Geophys. Res., 114, D00I03, https://doi.org/10.1029/2008JD011587, 2009. a, b, c
Winkler, H., Kazeminejad, S., Sinnhuber, M., Kallenrode, M.-B., and Notholt, J.: Correction to “Conversion of mesospheric HCl into active chlorine during the solar proton event in July 2000 in the northern polar region”, J. Geophys. Res., 116, D17303, https://doi.org/10.1029/2011JD016274, 2011. a
Winkler, H., von Savigny, C., Burrows, J. P., Wissing, J. M., Schwartz, M. J., Lambert, A., and García-Comas, M.: Impacts of the January 2005 solar particle event on noctilucent clouds and water at the polar summer mesopause, Atmos. Chem. Phys., 12, 5633–5646, https://doi.org/10.5194/acp-12-5633-2012, 2012. a
WMO: Scientific Assessment of Ozone Depletion: 2018, Global Ozone Research and Monitoring Project – Report No. 58, World Meteorological Organization, Geneva, Switzerland, 2018. a