the Creative Commons Attribution 4.0 License.
the Creative Commons Attribution 4.0 License.
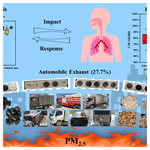
Source differences in the components and cytotoxicity of PM2.5 from automobile exhaust, coal combustion, and biomass burning contributing to urban aerosol toxicity
Weijie Huang
Guofeng Shen
Yuting Pang
Mingwei Tang
Weijun Li
Zhen Zhao
Hanhan Li
Yaqian Wei
Longjiao Xie
Tariq Mehmood
Although air quality guidelines generally use the atmospheric concentration of fine particulate matter (PM2.5) as a metric for air pollution evaluation and management, the fact cannot be ignored that different particle toxicities are unequal and significantly related to their sources and chemical compositions. Therefore, judging the most harmful source and identifying the toxic component would be helpful for optimizing air quality standards and prioritizing targeted PM2.5 control strategies to protect public health more effectively. Since the combustions of fuels, including oil, coal, and biomass, are the main anthropogenic sources of environmental PM2.5, their discrepant contributions to health risks of mixed ambient aerosol pollution dominated by the respective emission intensity and unequal toxicity of chemical components need to be identified. In order to quantify the differences between these combustion primary emissions, 10 types of PM2.5 from each typical source group, i.e., vehicle exhaust, coal combustion, and plant biomass (domestic biofuel) burning, were collected for comparative study with toxicological mechanisms. In total, 30 types of individual combustion samples were intercompared with representative urban ambient air PM2.5 samples, whose chemical characteristics and biological effects were investigated by component analysis (carbon, metals, soluble ions) and in vitro toxicity assays (cell viability, oxidative stress, inflammatory response) of human lung adenocarcinoma epithelial cells (A549). Carbonaceous fractions were plenteous in automobile exhaust and biomass burning, while heavy metals were more plentiful in PM2.5 from coal combustion and automobile exhaust. The overall ranking of mass-normalized cytotoxicity for source-specific PM2.5 was automobile exhaust > coal combustion > domestic plant biomass burning > ambient urban air, possibly with differential toxicity triggers, and showed that the carbonaceous fractions (organic carbon, OC; elemental carbon, EC) and redox-active transition metals (V, Ni, Cr) assisted by water-soluble ions (Ca2+, Mg2+, F−, Cl−) might play important roles in inducing cellular reactive organic species (ROS) production, causing oxidative stress and inflammation, resulting in cell injury and apoptosis, and thus damaging human health. Coupled with the source apportionment results of typical urban ambient air PM2.5 in eastern China, reducing toxic PM2.5 from these anthropogenic combustions will be greatly beneficial to public health. In addition to the air pollution control measures that have been implemented, like strengthening the vehicle emission standards, switching energy from coal to gas and electricity, and controlling the open incineration of agricultural straws, further methods could be considered, especially by preferentially reducing the diesel exhaust, lessening the coal combustion by replacement with low-ash clean coals, and depressing the rural crop straw biomass burning emissions.
- Article
(1118 KB) - Full-text XML
-
Supplement
(1995 KB) - BibTeX
- EndNote
As a mixture of multiple sources, ambient particulate matter (PM) arising from anthropogenic activities is continuously deteriorating urban air quality, particularly in developing countries. Among these, fine PM with an aerodynamic diameter of less than 2.5 µm (PM2.5) is recognized as a serious public health concern due to its long persistence in air, carcinogenicity, and acute toxicity to humans (Al-Kindi et al., 2020). There was extensive epidemiological evidence that airborne PM can cause serious negative effects on human health, such as respiratory and cardiovascular diseases, genetic mutations, and developmental disorders (Chowdhury et al., 2022; Clemens et al., 2017; Lelieveld et al., 2021; Smith, 2021). Currently, either the world air quality guidelines or the national air quality standards use the mass concentration of PM2.5 as a metric for PM2.5 pollution evaluation and management. However, the particle toxicities are unequal and significantly related to their sources and chemical compositions varying with space and time (Shiraiwa et al., 2017). Therefore, identifying which component(s) and source(s) of ambient PM are most harmful to health will be helpful for evaluating air quality and prioritizing targeted PM control strategies for protecting public health more effectively.
In addition to natural sources, most aerosols come from anthropogenic activities, especially energy consumption including the combustion of fossil fuels causing industrial emissions and automobile exhaust, together with biomass burning (McDuffie et al., 2021; Wu et al., 2022). These diverse sources make the ambient air PM2.5 a complex mixture with multiple chemical components, such as salts, organic carbon (OC), elemental carbon (EC), minerals, and trace metals (Bari and Kindzierski, 2016). The physiological mechanisms of PM-induced cell toxicity in respiratory systems have been continuously investigated with some progress (T. Li et al., 2022; Kelly and Fussell, 2012, 2020; Shiraiwa et al., 2017; Mack et al., 2019), such as metabolic activation, oxidative stress, inflammatory response, and apoptosis, which are focused on by the current study. Briefly, after inhalation and deposition onto the epithelium, redox-active materials in PM2.5 can induce the release of reactive organic species (ROS), which causes oxidative stress (an imbalance between ROS and antioxidants, i.e., disequilibrium of the redox state of a cell), followed by inflammation and cell death. The ROS can mediate subsequent signaling pathways, leading to biomolecular damage (e.g., DNA, lipids, and proteins) and cellular injury by mediating inflammatory response, including the release of proinflammatory cytokines like IL-6 and TNF-α by epithelial cells (Ahmed et al., 2020; Landwehr et al., 2021). For instance, oxidative stress could trigger the induction of proinflammatory transcription factors, such as nuclear factor (NF)-κB, via the mitogen-activated protein kinase (MAPK) signaling pathway. Components adsorbed on particle surfaces, such as redox-active metals (transition metals, Fe, Ni, V, Cr, Cu), organic compounds (polycyclic aromatic hydrocarbons, PAHs; quinones), or even carbonaceous cores of particles, are responsible for oxidative stress (Ahmed et al., 2020; Cachon et al., 2014). The non-redox-active metals (Zn, Pb, Al) can also influence the toxic effects of transition metals by exacerbating or lessening the production of free radicals. The EC may not be a directly toxic component of PM2.5 but may rather operate as a universal carrier of combustion-derived chemicals (semivolatile organic fractions, transition metals) of varying toxicities (Kelly and Fussell, 2020). Inorganic soluble sulfates and nitrates are acidic and can interact with and influence the solubility of other compositions like metal bioavailability (Fang et al., 2017; Weber et al., 2016). However, well-known toxic pollutants in the environment like heavy metals and PAHs, whose specific components and particular sources are the most critical factors dominating ambient aerosol health risks, still need be explored.
Past studies performed in various countries have focused on physicochemical characterization or biological effects of ambient air PM2.5, respectively (Weagle et al., 2018; Jia et al., 2017; Wang et al., 2020). For example, the source analysis of PM2.5 by photochemical modeling (Bao et al., 2018), the chemical composition of regional PM2.5 (Chi et al., 2022), and the mechanism of PM2.5 toxicity were independently reported recently (Jia et al., 2020). Because differences in particle compositions, sources, and toxicities appear in different urban environments (Zhao et al., 2019; Borlaza et al., 2018), source profiles of different emission inventories are needed to elucidate the local aerosol pollution characteristics for control strategies. For instance, it was reported that increased hospital admission risks were significantly associated with sources of vehicle exhaust, coal combustion, and secondary inorganic aerosols. In particular, coal combustion was positively correlated with increases in mortality risks (Du et al., 2021). Coal combustion and vehicle exhaust contributed more significantly to cancer risks of respiratory exposure to atmospheric heavy metals in Tianjin in northern China during the cold seasons (31 % and 11 %) than during the warm seasons (11 % and 4 %) (Tian et al., 2021), while in Nanjing in eastern China, traffic emissions and non-traffic combustion (coal, waste, and biomass) contributed 35 % and 31 % to carcinogenic risks of urban PM2.5-associated metals, respectively (Xie et al., 2020). Traffic was suggested to play the most crucial role in enhancing the toxicities of fine particles (Park et al., 2018). The particle composition of motor vehicle exhaust was related to automobile types with various fuels, engines, and loads (Lin et al., 2020). Strong catalytic reactivity of metals in PM emitted from diesel vehicles was observed by dithiothreitol (DTT) assay (Jesus et al., 2018). It was found that straw burning during the harvest season is a major trigger of severe air pollution in many regions (Sahu et al., 2021). Aerosols from open biomass burning in the Amazon had a stronger ability to induce ROS than laboratory-generated secondary organic aerosols (Tuet et al., 2019). Although there are emerging studies on particle emissions from single sources, quantitatively comparative studies on multisource pollutants as well as the differential composition and unequal toxicity of various sources are still limited.
The main objective of the current study was to compare the chemical components and corresponding mass-normalized toxicological effects of individual PM2.5 from various combustion sources and their unequal contributions to ambient aerosol health risks. The aim is to provide experimental evidence supporting the targeted control of specific anthropogenic sources with prominent risks based on their pivotal toxic components. Therefore, we collected both representative ambient PM2.5 samples (n=16) from urban air and typical source PM2.5 samples (n=30) from automobile exhaust, coal combustion, and plant biomass burning. Their independent profiles of chemical compositions and in vitro cytotoxicity (cell viability, oxidative stress, and inflammatory response) were investigated and intercompared to assess the differences in source-to-receptor toxicity and to infer the core toxic components and respective harmful contributions. The pivotal toxic components were identified based on the source–sink bidirectional composition-effect results, which were further used to assess the health toxicity contributions of various emission sources to ambient air PM2.5, supported by its source apportionment through positive matrix factorization (PMF) and chemical mass balance (CMB) models.
2.1 Collection of PM2.5 samples from primary emissions of 30 typical combustion sources and from representative ambient urban air
In total, 30 types of primary PM2.5 samples emitted directly from automobile exhaust, coal combustion, and plant biomass (domestic biofuel) burning were respectively collected as follows for both chemical and toxicological analyses.
A total of 10 types of vehicles were chosen for exhaust investigation. They were further categorized into seven subgroups, including small-duty gasoline coaches (SDGCs), small-duty diesel coaches (SDDCs), middle-duty diesel coaches (MDDCs), heavy-duty diesel coaches (HDDCs), light-duty diesel vans (LDDVs), middle-duty diesel vans (MDDVs), and heavy-duty diesel vans (HDDVs). The detailed information on these representative local automobiles is shown in Table S1.
To cover all the coal types consumed in the city, 10 representative types of coal were gathered for investigation. They were further classified into four subgroups, including two types of honeycomb coal (HC), three types of anthracite coal (AC), two types of bituminous coal (BC) mainly for restaurant or household use, and three types of industrial coal (IC) for coal-fired power plants and the steel-smelting industry. The detailed characteristics of the physics and chemistry of these typical coals purchased from local markets are shown in Table S2.
Considering the plant biomass combustion in rural areas surrounding the megacity, 10 representative types of agricultural and forestry solid waste were gathered for investigation. Straws of rice, wheat, corn, soybean, peanut, rape, and sesame together with corncob and branches of peach and pine were selected as plant biomass fuels and further divided into two subgroups, including eight types of crop straw and two types of firewood. The detailed characteristic analysis of these typical plant biomass fuels collected from rural areas around Nanjing is shown in Table S3.
The PM2.5 samples directly emitted from these combustion sources were collected by the dilution channel sampling method (Fig. S1) using a four-channel particulate matter dilution sampler (HY-805, Hengyuan Technology Development Co., CN). Each sample included three parallel channels of a quartz microfiber filter (Fig. S2) and one channel of a Teflon membrane filter with diameters of 47 mm through a size selector for PM2.5 with a flow rate of 160 L min−1 (each channel is 40 L min−1). Clean air was pumped for 10 min before and after each sample was collected. Before use, the blank quartz filters were incinerated by a muffle furnace at 500 ∘C for 3 h to remove any possible organic matter, while the Teflon filters were baked at 60 ∘C for 4 h. After being equilibrated in a constant temperature and humidity chamber for 24 h, the filters were weighed both before and after sampling for gravimetric measurements, and then the mass of the collected PM2.5 could be calculated. The sampled filters were stored in a refrigerator at −20 ∘C before analysis. The quartz-filter-loaded PM2.5 samples were used for carbon and ion analysis and for toxicity tests, while the parallel Teflon-filter-loaded samples were used to determine metals.
As the actual mixture of various source particles in a real environment, a total of 16 ambient air PM2.5 samples (each time lasting 23 h) covering a year monthly were collected from December 2019 to October 2020 at an urban site surrounded by traffic, residential and commercial quarters of Nanjing, Yangtze River Delta of eastern China, using a high-volume air sampler (800 L min−1) with quartz microfiber filters (H. Li et al., 2022).
2.2 Chemical composition analysis
All collected source and ambient PM2.5 samples were conducted following component analysis (Li et al., 2023). For the concentrations of heavy metals in particulates, samples were digested by concentrated HNO3–HClO4 acids with a progressive heating program and determined by inductively coupled plasma optical emission spectrometry (ICP-OES; Optima8000, PerkinElmer, for Cr, Mn, Ni, and Pb), with elements (V, Co, As) at lower concentrations measured by ICP mass spectrometry (ICP-MS; NexIONTM300X, PerkinElmer). Blank filter, reagent blank, replicates, and standard reference material (NIST SRM 1648a, urban dust) were adopted for analytical quality control, with recoveries ranging from 90 % to 110 %. Carbonaceous species (OC and EC) in PM2.5 were determined using DRI-2001A (Atmoslytic Inc., Calabasas, CA, USA). For the concentrations of water-soluble ions (WSIs), the main cations (Na+, K+, Mg2+, Ca2+, NH) and anions (NO, SO, Cl−, F−) in PM2.5 were measured by ion chromatography (IC, Thermo Fisher Scientific, USA) using the Metrosep C6-150/4.0 column for cations and the Metrosep A Supp 5 150/4.0 column for anions, respectively.
2.3 Preparing mass-normalized PM2.5 suspension for cell exposure
In total, 30 source and 16 ambient PM2.5 samples also performed cytotoxicity tests. In order to elute the particles completely from the quartz membranes, a whole PM2.5-loaded sample filter was cut into small pieces, immerged in ultrapure water, and extracted six times (30 min for each) in an ultrasonic bath at 0 ∘C. Although the ultrasonication might impact the ROS (Miljevic et al., 2014), the inevitable systematic error was ignored in this study. The extract was then suction-filtered through a 2.6 µm pore-size nylon membrane to remove possible quartz fragments, and the bulk filtrate was freeze-dried back to pure PM2.5 powder. Ultimately, based on particle mass, the gathered PM2.5 was dispersed by sterile phosphate-buffered saline (PBS) to a concentration of 400 mg L−1 and then diluted to PM2.5 suspension of 80 mg L−1 with serum-free Dulbecco's modified eagle medium (DMEM) to follow in vitro cell exposure (H. Li et al., 2022).
2.4 Cell culture and cellular toxicity tests by in vitro PM2.5 exposure
Aerosol pollution can harm lung alveoli and epithelial cells, and the A549 adenocarcinoma epithelial cell has long been used as a suitable epithelial alveolar model (T. Li et al., 2022; Park et al., 2018). The A549 cells were cultured in RMPI-1640 medium (Gibco, USA) supplemented with 10 % fetal bovine serum (FBS, Hyclone, USA) and 1 % antibiotic penicillin-streptomycin (100 U mL−1) at 37 ∘C in a 5 % CO2 incubator. After PM2.5 exposure, cell viability and the indicators reflecting oxidative damage and inflammatory response, respectively, were determined. While the cell viability assay was helpful in determining PM2.5 doses to cells, the endogenous ROS measurements revealed the status of cellular oxidative potential after PM2.5 exposure followed by the relative effects of ROS on various stages of cellular toxicity like inflammatory response (Gali et al., 2019). The cell viability (metabolic activity) was evaluated by mitochondrial activity and determined by the methyl-thiazol-tetrazolium (MTT) assay (Chen et al., 2019). After trypsin action, the density of cells in the logarithmic growth phase was adjusted to 1×105 mL−1. Cell suspensions were inoculated into 96-well plates (Costar, USA) at 100 µL per well. The blank control well (without medium and PM2.5 suspension) and reagent control well (with medium but without PM2.5 suspension) were set together. After incubation for 24 h and removal of the cellular supernatant, various types of PM2.5 suspension (concentration of 80 mg L−1) were added to 96-well plates and incubated for 24 h. Based on pre-experiments, the oxidative stress and inflammation response are sensitive to this dose, while the cell viability can remain sufficient. Fresh medium and MTT reagent (Solarbio, Beijing, CN) were added to each well and the supernatant was discarded, and then 100 µL of formazan lysate was added to each well. The optical density (OD) values were measured at 490 nm using a microplate reader (Thermo MULTISKAN FC, USA). Cell viability was (%) = (ODtreatment − ODblank control) / (ODreagent control − ODblank control). The levels of cellular ROS production causing oxidative stress in cells, proinflammatory cytokines including tumor necrosis factor alpha (TNF-α), and interleukin-6 (IL-6) production for determining the expression of genes related to the inflammatory response in the supernatant were analyzed by enzyme-linked immunosorbent assay (ELISA) kits (Jiangsu Meibiao Biotechnology Co., Ltd., CN), and OD values were measured at 450 nm (Huang et al., 2020; Pang et al., 2020).
2.5 Data analysis
The statistical analysis was performed by IBM SPSS statistics 24 and was plotted by the Origin 2020b software. Spearman correlation coefficients were produced by the correlation analysis. The variance was statistically significant when the statistical test level was p<0.05 and extremely significant when p<0.01. Statistical analyses were performed using the Kruskal–Wallis test (Kruskal and Wallis, 1952).
The source apportionment of PM2.5 mass in urban ambient air was conducted by the receptor models PMF (EPA PMF version 5.0) and CMB (EPA CMB 8.0). All the measured constituents (OC, EC, Cu, Cr, Co, Ni, As, Pb, Mn, V, Na+, K+, Mg2+, Ca2+, NH, Cl−, F−, NO, and SO) were selected as PMF model input data, and a four-factor solution was chosen as the optimal solution based on an assessment of the interpretability of the source profiles and the seasonal variability of the source contributions. Due to the high concentrations of sulfate and nitrate in ambient PM2.5 and the lack of a specific actual source to emit sulfate and nitrate, we added the virtual source profiles of secondary sources in the CMB model (Table S4). The virtual source profiles of secondary sources are represented by the proportion of sulfate, nitrate, and ammonium in pure ammonium sulfate and ammonium nitrate.
3.1 Contributions of combustion primary sources to urban ambient air PM2.5
As shown in Fig. S3, although there have been significant improvements in national air quality in recent years, the estimated annual PM2.5 concentrations of the representative city Nanjing (59.1±20.5 µg m−3) were 1.7 times higher than the Chinese national standard (35 µg m−3) and 11.8 times higher than the WHO guidelines (5 µg m−3). Urban air PM2.5 pollution levels in the cold season were higher than the warm season. The similar source apportionment results from the PMF and CMB models are illustrated in Fig. 1. Four major sources of the ambient PM2.5 were produced by the PMF model (Fig. S4), including secondary aerosols and primary particles of automobile exhaust, coal combustion, and plant biomass burning, which account for 34.0 %, 27.7 %, 25.2 %, and 13.1 %, respectively, of the total PM2.5 mass concentration. The CMB model source profiles are shown in Table S4, and we normalized the contribution of secondary aerosols (32.4 %), automobile exhaust (32.2 %), coal combustion (25.1 %), and plant biomass burning (10.3 %). Therefore, although the contribution of secondary aerosols cannot be ignored, the main anthropogenic sources of urban air PM2.5 were primary emissions from the various fuel combustions.
3.2 Chemical compositions of different PM2.5 from 30 combustion sources and from representative urban ambient air
Typical chemical components, including carbonaceous fractions, heavy metals, and the WSIs of all the PM2.5 samples from both ambient air and combustion sources, were analyzed and compared with each other.
According to the comparisons of PM2.5-bound carbonaceous fractions (Fig. 2), automobile- and biomass-sourced PM2.5 contained a significantly higher total carbon (TC) content than coal combustion and ambient air, while the ratio trend was ambient air > coal combustion > biomass burning > automobile exhaust sources. This indicated that the carbon content of the ambient PM2.5 mixture was lower and more dominated by OC than that of combustion primary sources, implying that the OC in ambient air may be aged or cleaned. The OC undergoes various chemical reactions in the atmosphere, such as oxidization by ozone and hydroxyl radicals, resulting in degradation. Figures S4–7 show the detailed carbon fraction characteristics (contents and ratios) of PM2.5 from each specific source. Carbonaceous fractions in automobile exhaust PM2.5 were high, but the difference between the OC and EC contents was small. Depending on the diverse automobile fuels, loads, and tailpipe emission standards, the concentrations of carbon fractions in exhaust PM2.5 varied widely with vehicle categories. The carbonaceous portion of PM2.5 gradually declines as emission regulations rise, and EC likewise declines dramatically (Fig. S5). However, such differences between coal types were lower, except for the bituminous coal with extremely high OC (Fig. S6). The carbonaceous fraction of PM2.5 from domestic plant biomass burning differed from raw material species in that tree-branch-sourced PM2.5 generally contained higher carbon contents than those from crop straws (Fig. S7).
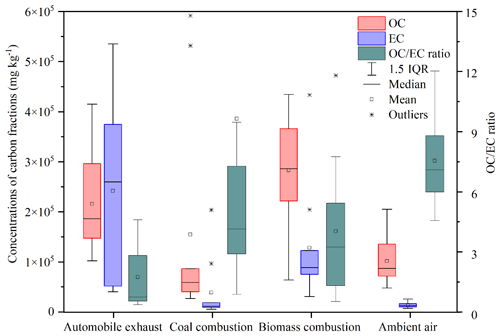
Figure 2Carbon contents (mg kg−1) and ratios in PM2.5 from various specific sources (n=10 for each combustion source and n=16 for urban ambient air).
Based on the grouped (Fig. 3) and individual (Figs. S9–12) distributions of the measured heavy metals in various PM2.5, the V concentrations of combustion sources were generally higher, while Co and Mn were lower than ambient urban air. Coal combustion emissions had the highest levels of Pb and were enriched in Cu and As (Fig. S10), while biomass burning was rich in Cr and Ni (Fig. S11). However, automobile exhausts were enriched in most heavy metals, especially Cu, Cr, Ni, V, and Mn (Fig. S9). Heavy metals from different types of automobile exhausts with the same emission standard vary greatly. Anthracite and industrial coal combustions contain similar heavy metals much more than bituminous coal. Generally, Pb, V, Mn, As, and Cu in branch-sourced PM2.5 were higher than straws, while Cr, Ni, and Co were dominant and higher in straw-burning emissions. A special discovery was that corncob-burning PM2.5 had more heavy metals than corn straw and was the biomass with the highest emission levels of heavy metals. Correspondingly, ambient air PM2.5 was also rich in most metals, especially Mn, Pb, Ni, Cu, and Cr. Therefore, coal combustion sources might contribute the most Pb to urban ambient air and contribute significant Cu and As with automobile exhaust emissions, while plant biomass burning and automobile sources contribute the Cr and Ni. In addition to natural dust, automobile exhaust should be the main anthropogenic source of airborne Mn. Considering the PMF source apportionments of ambient aerosols, automobile exhaust should be the main source of Cr in urban air PM2.5 and also the source of Cu together with coal combustion.
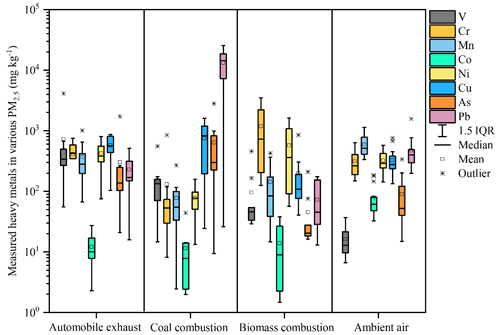
Figure 3Heavy-metal contents (mg kg−1) in PM2.5 from various specific sources (n=10 for each combustion source and n=16 for urban ambient air).
According to the comparisons of water-soluble cation and anion concentrations in various PM2.5 (Fig. 4), coal combustions contained the highest SO and NH, automobile exhausts had the highest contents of NO, Na+ and Ca2+, plant biomass burning sources contained the highest K+ and Cl−, but Mg2+ was the lowest for all the sources. However, the urban ambient air PM2.5 contained the highest NO and was also dominated by SO and NH, for which NO should be mainly contributed by secondary aerosols and automobile primary sources and SO and NH should be from coal combustion to a significant degree. In addition to NO, Na+ and Ca2+, automobile source PM2.5 also had higher F− and Mg2+ concentrations than other sources. The detailed concentration distributions of WSIs in PM2.5 from each specific source are provided in Figs. S12–14. The WSI levels vary widely with specific source categories. PM2.5 from LDDVs-2 had the lowest number of WSIs compared to the other automobile exhausts (Fig. S13). Similarly to the metal composition, bituminous coal also had the lowest number of WSIs among all the coals (Fig. S14). Compared to branches, PM2.5 from burning crop straws had much higher levels of K+, Cl− and SO and lower levels of F− and NO (Fig. S15).
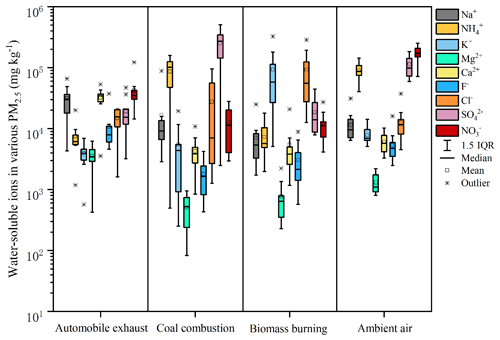
Figure 4Water-soluble ion (WSI) contents (mg kg−1) in PM2.5 from various specific sources (n=10 for each combustion source and n=16 for urban ambient air).
To summarize, the overall concentrations of measured TC, cumulated heavy metals and WSIs in PM2.5 from each source type are shown in Fig. 5. Among all the source emission and environmental receptor samples, the cumulated heavy metals from coal combustion were highest, and automobile exhaust was higher than ambient PM2.5, the overall carbon contents from automobile exhaust and biomass burning were both higher than ambient PM2.5, and only the cumulated soluble ions in PM2.5 from primary sources of coal combustion were equivalent to the ambient aerosols. In a word, chemical compositions of PM2.5 were distributed very diversely and varied significantly with the specific source types of combustion emissions.
3.3 Cell viability, oxidative stress, and inflammation levels exposed to various mass-normalized PM2.5
Multiple toxicological endpoints (cell viability, oxidative stress, and inflammation) that facilitate identification of the specific particle-triggering ROS and inflammatory response resulting in cell death were evaluated for source-specific PM2.5. After 24 h exposure to the same dose of different PM2.5 obtained from specific emission sources, the A549 lung cells also showed varying toxicological responses (Fig. 6). The survival rate of cells exposed to automobile exhaust PM2.5 was much lower than ambient air PM2.5 by 16.6 % (Fig. 6a). Automobile exhaust PM2.5 induced higher ROS production in cells than biomass burning, which was 26.4 % and 14.8 % higher than ambient PM2.5 (Fig. 6b). Coal combustion induced the highest cellular IL-6 production, followed by automobile exhaust, which was 13.1 % and 4.48 % higher than ambient air PM2.5, while the PM2.5 from automobile exhaust and biomass burning induced a similarly (10.4 %) higher cellular production of TNF-α than ambient PM2.5 (Fig. 6c, d). These results suggested that combustion primary emission PM2.5 had a stronger ability to induce oxidative stress and inflammatory injury in lung cells than ambient air PM2.5, thus resulting in the higher probability of apoptosis induction (Victor and Gottlieb, 2002; Wang et al., 2013). Generally, the mass-normalized PM2.5 from primary sources of automobile exhaust had the strongest overall toxicity. Therefore, to protect public health by controlling PM2.5 pollution, these anthropogenic combustions were key target sources, and the most toxic automobile PM2.5 especially should be reduced preferentially.
3.4 Correlations between various PM2.5 components and toxicity endpoints
Spearman correlation coefficients between chemical compositions and cellular toxicological response indicators were applied to screen the key components of all PM2.5 involved in cell injury (Fig. 7). It was found that the degrees of correlations varied with the toxicological mechanisms of different airborne chemicals. Based on the overall PM2.5 samples from various sources, the proinflammatory cytokine IL-6 showed significantly strong positive correlations with some heavy metals (As, Pb, V, Cu), while TNF-α and oxidative stress (ROS) had similar significantly positive correlations with aerosol components of carbon fractions (EC, OC) and transition metals (V, Cr, Ni). The TNF-α also showed a positive correlation with water-soluble Cl− and K+, and ROS correlated with F−, Ca2+, and Mg2+.
4.1 Chemical markers for source apportionments of ambient air PM2.5
Combustion emissions are key anthropogenic sources contributing to urban air PM2.5 through both primary and secondary aerosols, which were 66 % and 34 % as estimated by the PMF model and 67.6 % and 32.4 % as estimated by the CMB model, respectively (Fig. 1). Compared to the PMF results, the proportions of coal combustion and secondary sources in the CMB model results show minimal changes, while biomass contributions are slightly underestimated, and there is a slight increase in the proportion attributed to vehicular emissions. The high concentrations of chemical markers are usually used in source analysis, such as ammonium sulfate and nitrate for secondary aerosols which originated mainly from gaseous precursors (e.g., NH3, SO2, and NOX) (Mahilang et al., 2021), the EC, Cu, Mn, and Ni for vehicle exhaust (Srivastava et al., 2021), the As, Pb, OC, EC, SO, and relatively low ratios for coal combustion (Dai et al., 2020), and soluble K+ and Cl− for plant burning (Jain et al., 2020). The detailed chemical species of these specific source emission PM2.5 samples also supported the results. Moreover, low ratios of high TC content, high NO, F−, Na+, Ca2+ and Mg2+, V, and Mn of automobile exhaust; Pb and As, SO, and NH of coal combustion; soluble K+ and Cl−; and a high ratio of high TC for plant biomass burning found in the current study (Figs. 2–5) could also be corresponding potential aerosol source markers.
4.2 Common PM2.5 components related to specific combustion sources
Generally, the automobile exhaust PM2.5 had a high TC content and a low value with a considerable EC content (Fig. 2), varying with specific vehicle types (Fig. S5–8). The contents of the carbon fractions from diesel vehicles were 2.39 times more than gasoline exhausts (Fig. S5), and the ratios of diesel exhausts were 37.3 % of gasoline vehicles, owing to both considerable contents of EC and OC from diesel vehicle emission PM2.5. Some diesel vehicles showed higher EC emissions with age, so exhaust-cleaning devices for them are suggested. In addition, the amounts of OC and EC in exhausts gradually decreased with the strengthened emission standards they met (Wong et al., 2020). In PM2.5 samples obtained from coal combustion (Fig. S6), the TC contents of bituminous coals were 3.97, 6.41, and 11.6 times higher than those of honeycomb coals, anthracite coals, and industrial coals, respectively, because bituminous coals contain higher volatile fractions. Emissions of non-methane VOCs increase with the volatile content of the coal (He et al., 2022). The vast majority of organic aerosols from bituminous coal are generated in the ignition and fierce combustion phases, which account for 99.9 % of the entire combustion process, while these two phases of anthracite coal generate only 77 % of the entire process (Zhou et al., 2016). Moreover, as the volatile matter in the coal decreases, the temperatures at which weight loss begins and ends shift to higher values, which may be due to the lower number of aliphatic chains present. It has been reported that bituminous maximum weight loss occurs in the range 490–600 ∘C, while in the case of anthracite coals it occurs between 750 and 870 ∘C (De la Puente et al., 1998). Therefore, in addition to the way of combustion and the use of combustion stoves, the coal quality related to different coal types and origins determines the carbonaceous fractions of the PM emitted by coal combustion (Zhang et al., 2022). In the PM2.5 samples from plant biomass combustion (Fig. S7), OC contents were 2.21 times higher than EC contents, except that pine branches contained higher EC and rapeseed straw had considerable contents of EC and OC. The OC in ambient PM2.5 dominated the carbonaceous component (Fig. S8), consistent with the North China Plain and Indo-Ganges Plain (Flores et al., 2020; Xu et al., 2019). Combining the TC contents and ratios, carbonaceous components in ambient PM2.5 mainly originate from semivolatile organic compounds (SVOCs) (Wang et al., 2018). Previous studies have reported that carbonaceous aerosols mainly originated from fossil fuel combustion in transportation, coal combustion in power plants and industries, and biomass combustion (Kang et al., 2018; Zhang et al., 2015). Thus, to control the ambient carbon aerosol pollution, in addition to reducing the precursor emissions of secondary organic aerosols (SOA), controlling primary aerosols, especially EC from diesel vehicles, might be an effective measure.
Airborne redox-active metals are usually linked to the oxidation stress of PM2.5. Different types of automobiles emitted diverse metal contents (Fig. S9). Metal elements in automobile exhaust are primarily contributed by fuels, lubricants, and engine component abrasion. Because Mn is a common anti-detonator that delays and prevents the oxidation of hydrocarbons and increases the octane number, which not only increases the thermal efficiency of the engine, but also improves the emission performance of the vehicle (Cheung et al., 2010), the Mn content was greater in gasoline vehicle exhausts than in diesel vehicles. Although there are multiple sources of traffic Pb emissions such as fuel combustion and brake wear (Wang et al., 2019; Panko et al., 2019), the automobile exhaust Pb content of gasoline vehicles were greater than diesel vehicles owing to oil combustion. Moreover, for the same vehicle type (LDDVs-1 and LDDVs-2; HDDVs-1 and HDDVs-2; SDGCs-1 and SDGCs-2), the stricter the emission standard required, the lower the exhaust metal contents. The metal contents in the PM2.5 of trucks was higher than that of passenger cars (Wu et al., 2016). In the combustion PM2.5 of 10 coal types (Fig. S10), Pb contents were the highest than other heavy metals, similar to available findings (Zhang et al., 2020). The PM2.5 metals from bituminous coal were significantly lower, as indicated by the coal quality analysis, bituminous coal has a low ash content which is mainly derived from non-combustible minerals in coal. These findings suggested that coal maturity might be an important factor influencing the metal composition of particulates emitted from coal combustion (Shen et al., 2021; Zhang et al., 2021). Heavy-metal contents in biomass-burned PM2.5 varied widely with raw plant types (Fig. S11) but were dominated by Cr and Ni. Different plant species and even different plant parts differ significantly in their ability to take up and accumulate metals from the soil (Zhao et al., 2020). Moreover, because of the high enrichment factors of some metals for crop straws (Zhang et al., 2016; Sun et al., 2019), they also released more Cr, Ni, and Co during burning than fuel woods. Total metal emissions were highest in corn cob but lowest in peanut straw burning PM2.5. The heavy metals enriched in urban ambient air PM2.5 showed a slightly seasonal pattern (Fig. S12), while contents of V, Co, and As were relatively low and less affected by seasonal changes. Accordingly, supported by the metal profiles of anthropogenic combustion sources and ambient aerosols, to control the environmental airborne heavy-metal pollution, key targets might be the Pb, Cu, and As from honeycomb, anthracite, and industrial coal combustion; Cu from vehicle exhausts and especially V from light-duty diesel vans with the CN.III emission standard and Mn from gasoline vehicles, Cr and Ni from biomass, especially crop straw burning.
Epidemiological studies have also shown mortality closely related to the WSIs such as sulfate and nitrate in aerosols (Ostro et al., 2009; Liang et al., 2022). Among the WSIs contents of various automobile exhaust PM2.5 (Fig. S13), NO and Ca2+ were the most abundant anion and cation, respectively. The high NO in the automobile PM2.5 may be due to NOx production during high-temperature combustion, while the high Ca2+ content should be related to additives in automobile fuels and calcium-based lubricants (Hao et al., 2019; Yang et al., 2019). Moreover, the exhaust WSIs decreased with the strengthened automobile emission standards required. Coal combustion PM2.5 contained relatively higher SO and NH concentrations followed by Cl− than other WSIs species (Fig. S14). Among various coal types, industrial coals emitted highest SO followed by honeycomb and industrial coal with also high NH, but bituminous coals emitted low WSIs which were mainly NO, F− and Na+, Ca2+. The WSIs emission factors of honeycomb coal were generally higher than those of lump coal (Yan et al., 2020). For biomass combustion emissions (Fig. S15), Cl− and K+ were dominant WSIs in PM2.5 from straw-type fuels (Tao et al., 2016; Sillapapiromsuk et al., 2013), but fuelwood-type combustion emitted high NO. Plant species absolutely determine the emissions (Liao et al., 2021). Finally, there were also high levels of NO, SO, and NH in ambient air PM2.5 (Zhang et al., 2019) (Fig. S16), even higher than the investigated combustion sources, so other sources like the secondary aerosols may also contribute. Consequently, target combustion primary aerosols WSIs might include the NO from vehicle exhausts and fuelwood burning; SO and NH from honeycomb, anthracite, and industrial coal combustion; and Cl− and K+ from biomass (especially crop straw) burning.
4.3 PM2.5 toxicity related to specific sources by pivotal chemical components
The complexity of the sources and compositions of atmospheric PM2.5 leads to different toxicological effects (Newman et al., 2020; Kelly, 2021). The toxicological effects of PM2.5 are not comparable among different studies, owing to distinct exposure concentrations, biological models, endpoints, and PM2.5-generation methods (Kelly and Fussell, 2020; Park et al., 2018). In this study, we employed same exposure conditions and biological endpoints in order to obtain comparable toxicity data for PM2.5 from different sources. Our mass-normalized results demonstrated that automobile exhaust PM2.5 induced the highest lethality and cellular ROS and TNF-α production, that coal combustion PM2.5 induced the highest cellular IL-6 production, and that plant biomass burning PM2.5 induced considerable cellular TNF-α and ROS production (Fig. 6). Generally, various toxicities of combustion emission primary PM2.5 were much greater than the urban ambient air PM2.5 (Fig. 6), owing to the higher concentrations of specific toxic components in PM2.5 from these sources. The Supplement included exhaustive cytotoxicity indicators from each individual source (Figs. S17–20). While the survival rate of cells exposed to the CN.III emission standard PM2.5 was the lowest and the capacity to induce cells to produce ROS was the highest for CN.IV, automobile exhaust had a similar potential to cause cells to produce inflammatory cytokines (Fig. S17). The capability to induce IL-6 production in cells was highest for industrial coal PM2.5, whereas bituminous coal had the highest survival rate of the cells and TNF-α induction capacity (Fig. S18). From Fig. S19, we can see that the PM2.5 cytotoxicity of straw and branch burning was analogous, but it should be noted that the cell viability of various straw PM2.5 differ significantly, which may be related to the raw fuel characteristics.
These possible mechanisms were implied by the overall relationships between the measured chemical components with cytotoxicity indicators of PM2.5 from various specific sources (Fig. 7). In general, both TNF-α and ROS were significantly positively correlated with carbonaceous fractions and redox-active transition metals (V, Cr, Ni), which were the main contributors of automobile exhausts and biomass burning. The IL-6 was significantly positively correlated with some heavy metals (As and Pb, V and Cu), which were the main contributors of coal combustion sources. Potential mechanisms include carbon fractions bound in PM2.5 possibly being transformed into reactive metabolites and then inducing ROS production in cells (Stevanovic et al., 2019), and the PM2.5-bound transition metals could also induce ROS production through the Fenton reaction and disrupt the function of enzymes in cells (Verma et al., 2010; Sørensen et al., 2005). Oxidative stress can lead to inflammatory infiltration of neutrophils and stimulate immune cells to produce inflammatory cytokines, among which TNF-α and IL-6 play important roles in inflammation development (Xu et al., 2020). Ultimately, excessive production of ROS leads to dysfunctional endoplasmic reticulum responses, and dysfunctional lipid metabolism in ROS bursts can result in cell membrane damage and even cell death (Piao et al., 2018; Zhao et al., 2004). There have been some related supporting reports. For instance, the OC and EC were significantly associated with biological responses of PM from vehicle emissions collected in tunnels (Niu et al., 2020). The polar or quinone fractions of PAHs in diesel engine exhaust particles significantly contributed to the heightened toxic response (Xia et al., 2004). The PM2.5 generated from biomass burning contained a substantial concentration of carbonaceous components. In addition, Cr and Ni in PM10 from straws were highly associated with ROS (Li et al., 2023). In the current study, cellular ROS were also correlated with water-soluble Ca2+, F−, and Mg2+, which were the main contributors of automobile exhaust PM2.5. The Ca2+ controls the membrane potential and regulates mitochondrial adenosine triphosphate (ATP) production, and excessive Ca2+ leads to energy loss and more ROS production (Madreiter-Sokolowski et al., 2020). Moreover, the TNF-α was also positively correlated with water-soluble Cl− and K+, which were the main contributors to plant burning PM2.5. Therefore, the accumulations of some organic matters with high carbonaceous content (OC, EC) in PM2.5, typically from automobile exhausts and plant biomass burning, redox-active metals (V, Cr, Ni) and water-soluble anions (Cl−, F−) and cations (Ca2+, Mg2+) contributed by various combustions, might induce ROS production in cells, cause cellular damage through oxidative stress and inflammatory response, impair cell viability, and finally harm human health.
Considering the multiple endpoints measured and the PM2.5 toxicity mechanisms mentioned above, based on the cell viability, the ROS, the inflammatory markers, and the significantly related toxic chemical composition contents (Park et al., 2018), we put forward a general sequence of overall mass-normalized toxicity for these combustion source PM2.5 to managers. To improve the urban environmental air quality for better public health benefits by controlling aerosol pollution, considering the differential toxicity intensity of each chemical component and its contributions from various sources to ambient aerosols, preferential targets of specific primary PM2.5 sources and bound pollutants from anthropogenic combustions are suggested as following this sequence: reducing the automobile exhaust PM2.5 containing high contents of EC, transition metals (V, Cu, Ni, Cr), and ions (Ca2+, Mg2+, F−, Na+) from diesel exhausts by strengthening the emission standards and accelerating the phasing out of highly polluting vehicles; lessening coal combustion rich in heavy metals (As, Pb, Cu) by replacement with low-ash clean coals; and decreasing biomass burning containing high OC, Ni, Cr, Cl−, and K+ from rural crop straw emissions and promoting domestic cleaner energy such as natural gas.
4.4 Limitations and perspectives
In the current study, we selected the A549 cell based on previous abundant experimental experiences and also because it has been used popularly in in vitro toxicology studies to elucidate the cellular and molecular mechanisms of PM involved in lungs for many decades (T. Li et al., 2022). However, recently, the human normal bronchial epithelial cell BEAS-2B was preferred over the human lung adenocarcinoma epithelial cell A549. For instance, both cells were used in an aerosol study (Bonetta et al., 2017), the results of which also highlighted the higher sensitivity of BEAS-2B cells with respect to A549 in samples with low levels of pollutants, because the PM0.5 samples from Italian towns can induce genotoxicity in normal cells, while cancer cells might be resistant to their adverse effects. Therefore, although our results are reasonable under the same exposure conditions, there were still potential limitations of A549 cells since they may be more resistant to exposure to external compounds, and the generally more sensitive BEAS-2B cells are suggested for future studies.
In toxicity assessments, cell vitality reflects the overall health of cells, encompassing factors such as cell membrane integrity, intracellular metabolic activity, and cell proliferation capacity. Decreased cellular vitality may be associated with cell damage, toxic effects, or cellular apoptosis. Inflammation markers are employed to assess the extent and nature of inflammatory reactions, including the production of cytokines and inflammatory mediators, as well as the activation status of inflammatory cells. Inflammation is a complex physiological response typically delineated by the immune and inflammatory reactions of the body to stimuli such as injury or infection. Alterations in inflammation markers can indicate the intensity and nature of the inflammatory response. In this study, multiple biological responses of epithelial cells to various PM2.5 were evaluated, including that cell viability evaluated the mitochondrial dehydrogenase activity of the living cells, excessive intracellular ROS formation induced by PM2.5 was responsible for oxidative stress on the cells, and cytokines IL-6 and TNF-α were determined for the effect of PM2.5 on proinflammatory response in cells. In general, in vitro data can be used to rank various types of particles in terms of the toxic potential, including possible carcinogenicity. Each marker will help one to understand the hazard and toxicity of PM2.5. However, the toxicity of PM2.5 may be the result of multiple components acting through disparate physiological mechanisms, with inconsistent relationships among endpoints (Park et al., 2018). For instance, in BEAS-2B cells, oxidative stress generated by H2O2 exposure rather than by stimulating cytokine or chemokine responses often results in cytotoxicity, sometimes with no correlation between oxidative damage and cytokine or chemokine responses. Moreover, the TNF-α gene was not detected in BEAS-2B cells exposed to atmospheric PM collected from Benin, but the gene expressions of other inflammatory cytokines (IL-1β, IL-6, and IL-8) were significantly induced, and decreasing cell viability was highly correlated with high secretions of all the studied cytokines (Cachon et al., 2014). Therefore, in the present study, it was impossible to analyze all the chemicals in PM2.5 and to determine all the related toxicological endpoints, so unmeasured chemicals and endpoints might also play roles in the incongruous or unexplained results, and we also cannot over-explain the mechanisms just based on statistical relations. To overcome these hurdles, standardization of toxicological studies (experimental methodologies) and reporting guidelines is necessary for tracking and comparing results.
This study ranked the unequal “toxic effects” based on the same mass concentration of PM2.5 exposure in body lung fluid systems, while the “health risks” usually related to the inhalation exposure concentration of PM2.5 in ambient air were not calculated and evaluated quantitatively. Moreover, nonlinear concentration–response functions for various endpoints and different exposure concentrations might also limit the straightforward use of toxicological data to predict health effects (morbidity, mortality) in human populations. Therefore, drawing precise conclusions quantifying or ranking the health risks of PM2.5 from specific sources or of individual PM2.5 components is still not an easy task (Kelly and Fussell, 2020). Coupled with source apportionment and exposure levels of ambient aerosol pollution, toxicology and epidemiology studies linking these factors and indicating scientific mechanisms would help one to reach conclusions.
Moreover, the exact effective measures to control these specific key toxic components from the emissions of various combustion sources are indeed a challenge but still need to be explored. The findings of this research provide a specific direction for better air pollution control and public health. In addition to the environmental technological methods of controlling toxic components targeting source materials, combustion processes, and final emissions, the environmental management policies are also beneficial to such aims, like the choice of fuel types, especially for the management of domestic biomass fuel burning. For example, potential solutions include promoting new green energy vehicles and low-ash clean coals and decreasing the diesel exhaust and rural crop straw burning emissions.
In the current study, we found that two-thirds of the mass of urban ambient air PM2.5 in a typical megacity of eastern China originated from primary sources of anthropogenic combustions, including coal, automobiles, and biomass. Because of the significant differences in the chemical compositions, the diverse PM2.5 from both mixed ambient air and directly from individual combustion sources showed very different mass-normalized in vitro toxicity to human lung epithelial cells, either for the environmental aerosol samples collected from different seasons or for the primary emissions of PM2.5 from various specific source types. According to the comparative study and correlation analysis, the carbonaceous fractions (OC, EC) and redox-active heavy metals (V, Ni, Cr) assisted by water-soluble ions (Ca2+, Mg2+, F−, Cl−) might play important roles in inducing cellular ROS production, causing oxidative stress and inflammation, resulting in cell injury and apoptosis, and damaging human health. These toxic pollutants accumulated in specific-source PM2.5 varied by the emission types and raw fuel properties. Combined with the chemical composition and general cytotoxicity rank, the preferential controlling targets of specific combustion sources might be automobile exhaust (diesel vehicles with emission standards inferior to CN.IV), coal combustion (high ash and high sulfur coals), and rural plant biomass burning (crop straws). While showing the synthetic effects of mixed compositions and complex sources, in addition to preventing the secondary aerosols from combustions, preferentially targeted reductions of toxic PM2.5 direct emissions from these primary sources would produce great benefits for public health with improved ambient air quality. Overall, the chemical findings of our toxicological research could help to support precise, oriented, effective, efficient, and economical composition-source-based strategies for urban aerosol pollution control. However, as a prospect, the detailed mechanisms for unequal toxicity of PM with complicated components from various sources and their quantitative contributions to the health effects of an ambient air PM2.5 mixture still need in-depth study.
All the raw data can be provided by the corresponding authors upon request.
The supplement related to this article is available online at: https://doi.org/10.5194/acp-24-1345-2024-supplement.
XSL conceived and supervised the study. WH, YP, MT, HL, and ZZ collected the samples. WH, YP, MT, WL, HL, ZZ, GS, and LX analyzed the chemical compositions. WH, YP, and MT performed the toxicity tests. WH, YP, MT, and XSL analyzed the data. WH and XSL wrote the manuscript draft. XSL, WH, GS, and TM reviewed and edited the manuscript.
The contact author has declared that none of the authors has any competing interests.
Publisher’s note: Copernicus Publications remains neutral with regard to jurisdictional claims made in the text, published maps, institutional affiliations, or any other geographical representation in this paper. While Copernicus Publications makes every effort to include appropriate place names, the final responsibility lies with the authors.
The authors are very grateful to the anonymous reviewers for their constructive comments.
This research has been supported by the National Natural Science Foundation of China (grant nos. 41977349 and 41471418).
This paper was edited by Hang Su and reviewed by three anonymous referees.
Ahmed, C. S., Yang, J., Chen, J. Y., Jiang, H., Cullen, C., Karavalakis, G., and Lin, Y.-H.: Toxicological responses in human airway epithelial cells (BEAS-2B) exposed to particulate matter emissions from gasoline fuels with varying aromatic and ethanol levels, Sci. Total Environ., 706, 135732, https://doi.org/10.1016/j.scitotenv.2019.135732, 2020.
Al-Kindi, S. G., Brook, R. D., Biswal, S., and Rajagopalan, S.: Environmental determinants of cardiovascular disease: lessons learned from air pollution, Nat. Rev. Cardiol., 17, 656–672, https://doi.org/10.1038/s41569-020-0371-2, 2020.
Bao, F., Li, M., Zhang, Y., Chen, C., and Zhao, J.: Photochemical aging of Beijing urban PM2.5: HONO production, Environ. Sci. Technol., 52, 6309–6316, https://doi.org/10.1021/acs.est.8b00538, 2018.
Bari, M. A. and Kindzierski, W. B.: Eight-year (2007–2014) trends in ambient fine particulate matter (PM2.5) and its chemical components in the Capital Region of Alberta, Canada, Environ. Int., 91, 122–132, https://doi.org/10.1016/j.envint.2016.02.033, 2016.
Bonetta, S., Bonetta, S., Feretti, D., Moretti, M., Verani, M., De Donno, A., Schilirò, T., Carraro, E., and Gelatti, U.: DNA damage induced by PM0.5 samples in A549 and BEAS-2B human cell lines: Results of the MAPEC study, Toxicol. Lett., 280, 208–208, https://doi.org/10.1016/j.toxlet.2017.07.571, 2017.
Borlaza, L. J. S., Cosep, E. M. R., Kim, S., Lee, K., Joo, H., Park, M., Bate, D., Cayetano, M. G., and Park, K.: Oxidative potential of fine ambient particles in various environments, Environ. Pollut., 243, 1679–1688, https://doi.org/10.1016/j.envpol.2018.09.074, 2018.
Cachon, B. F., Firmin, S., Verdin, A., Ayi-Fanou, L., Billet, S., Cazier, F., Martin, P. J., Aissi, F., Courcot, D., and Sanni, A.: Proinflammatory effects and oxidative stress within human bronchial epithelial cells exposed to atmospheric particulate matter (PM2.5 and PM>2.5) collected from Cotonou, Benin, Environ. Pollut., 185, 340–351, https://doi.org/10.1016/j.envpol.2013.10.026, 2014.
Chen, Q., Luo, X.-S., Chen, Y., Zhao, Z., Hong, Y., Pang, Y., Huang, W., Wang, Y., and Jin, L.: Seasonally varied cytotoxicity of organic components in PM2.5 from urban and industrial areas of a Chinese megacity, Chemosphere, 230, 424–431, https://doi.org/10.1016/j.chemosphere.2019.04.226, 2019.
Cheung, K., Ntziachristos, L., Tzamkiozis, T., Schauer, J., Samaras, Z., Moore, K., and Sioutas, C.: Emissions of particulate trace elements, metals and organic species from gasoline, diesel, and biodiesel passenger vehicles and their relation to oxidative potential, Aerosol Sci. Technol., 44, 500–513, https://doi.org/10.1080/02786821003758294, 2010.
Chi, K. H., Huang, Y.-T., Nguyen, H. M., Tran, T. T.-H., Chantara, S., and Ngo, T. H.: Characteristics and health impacts of PM2.5-bound PCDD/Fs in three Asian countries, Environ. Int., 167, 107441, https://doi.org/10.1016/j.envint.2022.107441, 2022.
Chowdhury, S., Pozzer, A., Haines, A., Klingmuller, K., Munzel, T., Paasonen, P., Sharma, A., Venkataraman, C., and Lelieveld, J.: Global health burden of ambient PM2.5 and the contribution of anthropogenic black carbon and organic aerosols, Environ. Int., 159, 107020, https://doi.org/10.1016/j.envint.2021.107020, 2022.
Clemens, T., Turner, S., and Dibben, C.: Maternal exposure to ambient air pollution and fetal growth in North-East Scotland: A population-based study using routine ultrasound scans, Environ. Int., 107, 216–226, https://doi.org/10.1016/j.envint.2017.07.018, 2017.
Dai, Q., Liu, B., Bi, X., Wu, J., Liang, D., Zhang, Y., Feng, Y., and Hopke, P. K.: Dispersion normalized PMF provides insights into the significant changes in source contributions to PM2.5 after the COVID-19 outbreak, Environ. Sci. Technol., 54, 9917–9927, https://doi.org/10.1021/acs.est.0c02776, 2020.
De la Puente, G., Iglesias, M. J., Fuente, E., and Pis, J. J.: Changes in the structure of coals of different rank due to oxidation – effects on pyrolysis behaviour, J. Anal. Appl. Pyrol., 47, 33–42, https://doi.org/10.1016/S0165-2370(98)00087-4, 1998.
Du, H., Liu, Y., Shi, G., Wang, F., He, M. Z., and Li, T.: Associations between source-specific fine particulate matter and mortality and hospital admissions in Beijing, China, Environ. Sci. Technol., 56, 1174–1182, https://doi.org/10.1021/acs.est.1c07290, 2021.
Fang, T., Guo, H., Zeng, L., Verma, V., Nenes, A., and Weber, R. J.: Highly Acidic Ambient Particles, Soluble Metals, and Oxidative Potential: A Link between Sulfate and Aerosol Toxicity, Environ. Sci. Technol., 51, 2611–2620, https://10.1021/acs.est.6b06151, 2017.
Flores, R. M., Mertoğlu, E., Özdemir, H., Akkoyunlu, B. O., Demir, G., Ünal, A., and Tayanç, M.: A high-time resolution study of PM2.5, organic carbon, and elemental carbon at an urban traffic site in Istanbul, Atmos. Environ., 223, 117241, https://doi.org/10.1016/j.atmosenv.2019.117241, 2020.
Gali, N. K., Li, G., Ning, Z., and Brimblecombe, P.: Diurnal trends in redox characteristics of water-soluble and-insoluble PM components, Environ. Pollut., 254, 112841, https://doi.org/10.1016/j.envpol.2019.07.009, 2019.
Hao, Y., Gao, C., Deng, S., Yuan, M., Song, W., Lu, Z., and Qiu, Z.: Chemical characterisation of PM2.5 emitted from motor vehicles powered by diesel, gasoline, natural gas and methanol fuel, Sci. Total Environ., 674, 128–139, https://doi.org/10.1016/j.scitotenv.2019.03.410, 2019.
He, K., Shen, Z., Zhang, B., Sun, J., Zou, H., Zhou, M., Zhang, Z., Xu, H., Ho, S. S. H., and Cao, J.: Emission profiles of volatile organic compounds from various geological maturity coal and its clean coal briquetting in China, Atmos. Res., 274, 106200, https://doi.org/10.1016/j.atmosres.2022.106200, 2022.
Huang, W., Pang, Y., Luo, X.-S., Chen, Q., Wu, L., Tang, M., Hong, Y., Chen, J., and Jin, L.: The cytotoxicity and genotoxicity of PM2.5 during a snowfall event in different functional areas of a megacity, Sci. Total Environ., 741, 140267, https://doi.org/10.1016/j.scitotenv.2020.140267, 2020.
Jain, S., Sharma, S., Vijayan, N., and Mandal, T.: Seasonal characteristics of aerosols (PM2.5 and PM10) and their source apportionment using PMF: a four year study over Delhi, India, Environ. Pollut., 262, 114337, https://doi.org/10.1016/j.envpol.2020.114337, 2020.
Jesus, R. M. d., Mosca, A. C., Guarieiro, A. L., Rocha, G. O. d., and Andrade, J. B. d.: In vitro evaluation of oxidative stress caused by fine particles (PM2.5) exhausted from heavy-duty vehicles using diesel/biodiesel blends under real world conditions, J. Braz. Chem. Soc., 29, 1268–1277, https://doi.org/10.21577/0103-5053.20170223, 2018.
Jia, Y.-Y., Wang, Q., and Liu, T.: Toxicity research of PM2.5 compositions in vitro, Int. J. Environ. Res. Publ. Health, 14, 232, https://doi.org/10.3390/ijerph14030232, 2017.
Jia, Y., Li, X., Nan, A., Zhang, N., Chen, L., Zhou, H., Zhang, H., Qiu, M., Zhu, J., and Ling, Y.: Circular RNA 406961 interacts with ILF2 to regulate PM2.5-induced inflammatory responses in human bronchial epithelial cells via activation of STAT3/JNK pathways, Environ. Int., 141, 105755, https://doi.org/10.1016/j.envint.2020.105755, 2020.
Kang, M., Ren, L., Ren, H., Zhao, Y., Kawamura, K., Zhang, H., Wei, L., Sun, Y., Wang, Z., and Fu, P.: Primary biogenic and anthropogenic sources of organic aerosols in Beijing, China: Insights from saccharides and n-alkanes, Environ. Pollut., 243, 1579–1587, https://doi.org/10.1016/j.envpol.2018.09.118, 2018.
Kelly, F.: Air pollution and chronic bronchitis: the evidence firms up, Thorax, 76, 744–745, https://doi.org/10.1136/thoraxjnl-2021-216883, 2021.
Kelly, F. J. and Fussell, J. C.: Size, source and chemical composition as determinants of toxicity attributable to ambient particulate matter, Atmos. Environ., 60, 504–526, https://doi.org/10.1016/j.atmosenv.2012.06.039, 2012.
Kelly, F. J. and Fussell, J. C.: Toxicity of airborne particles – established evidence, knowledge gaps and emerging areas of importance, Philos. T. R. Soc. A, 378, 20190322, https://doi.org/10.1098/rsta.2019.0322, 2020.
Kruskal, W. H. and Wallis, W. A.: Use of ranks in one-criterion variance analysis, J. Am. Stat. Assoc., 47, 583–621, https://doi.org/10.2307/2280779, 1952.
Landwehr, K. R., Hillas, J., Mead-Hunter, R., Brooks, P., King, A., O'Leary, R. A., Kicic, A., Mullins, B. J., and Larcombe, A. N.: Fuel feedstock determines biodiesel exhaust toxicity in a human airway epithelial cell exposure model, J. Hazard. Mater., 420, 126637, https://doi.org/10.1016/j.jhazmat.2021.126637, 2021.
Lelieveld, S., Wilson, J., Dovrou, E., Mishra, A., Lakey, P. S. J., Shiraiwa, M., Poschl, U., and Berkemeier, T.: Hydroxyl Radical Production by Air Pollutants in Epithelial Lining Fluid Governed by Interconversion and Scavenging of Reactive Oxygen Species, Environ. Sci. Technol., 55, 14069–14079, https://doi.org/10.1021/acs.est.1c03875, 2021.
Li, H., Zhao, Z., Luo, X.-S., Fang, G., Zhang, D., Pang, Y., Huang, W., Mehmood, T., and Tang, M.: Insight into urban PM2.5 chemical composition and environmentally persistent free radicals attributed human lung epithelial cytotoxicity, Ecotoxicol. Environ. Saf., 234, 113356, https://doi.org/10.1016/j.ecoenv.2022.113356, 2022a.
Li, H., Tang, M., Luo, X., Li, W., Pang, Y., Huang, W., Zhao, Z., Wei, Y., Long, T., and Mehmood, T.: Compositional characteristics and toxicological responses of human lung epithelial cells to inhalable particles (PM10) from ten typical biomass fuel combustions, Particuology, 78, 16–22, https://doi.org/10.1016/j.partic.2022.09.006, 2023.
Li, T., Yu, Y., Sun, Z., and Duan, J.: A comprehensive understanding of ambient particulate matter and its components on the adverse health effects based from epidemiological and laboratory evidence, Part. Fibre Toxicol., 19, 67, https://doi.org/10.1186/s12989-022-00507-5, 2022b.
Liang, R., Chen, R., Yin, P., van Donkelaar, A., Martin, R. V., Burnett, R., Cohen, A. J., Brauer, M., Liu, C., and Wang, W.: Associations of long-term exposure to fine particulate matter and its constituents with cardiovascular mortality: A prospective cohort study in China, Environ. Int., 162, 107156, https://doi.org/10.1016/j.envint.2022.107156, 2022.
Liao, X., Zhang, S., Wang, X., Shao, J., Zhang, X., Wang, X., Yang, H., and Chen, H.: Co-combustion of wheat straw and camphor wood with coal slime: Thermal behavior, kinetics, and gaseous pollutant emission characteristics, Energy, 234, 1–11, https://doi.org/10.1016/j.energy.2021.121292, 2021.
Lin, Y.-C., Li, Y.-C., Amesho, K. T., Shangdiar, S., Chou, F.-C., and Cheng, P.-C.: Chemical characterization of PM2.5 emissions and atmospheric metallic element concentrations in PM2.5 emitted from mobile source gasoline-fueled vehicles, Sci. Total Environ., 739, 139942, https://doi.org/10.1016/j.scitotenv.2020.139942, 2020.
Mack, S. M., Madl, A. K., and Pinkerton, K. E.: Respiratory health effects of exposure to ambient particulate matter and bioaerosols, Compr. Physiol., 10, 1, https://doi.org/10.1002/cphy.c180040, 2019.
Madreiter-Sokolowski, C. T., Thomas, C., and Ristow, M.: Interrelation between ROS and Ca2+ in aging and age-related diseases, Redox Biol., 36, 101678, https://doi.org/10.1016/j.redox.2020.101678, 2020.
Mahilang, M., Deb, M. K., and Pervez, S.: Biogenic secondary organic aerosols: A review on formation mechanism, analytical challenges and environmental impacts, Chemosphere, 262, 127771, https://doi.org/10.1016/j.chemosphere.2020.127771, 2021.
McDuffie, E. E., Martin, R. V., Spadaro, J. V., Burnett, R., Smith, S. J., O'Rourke, P., Hammer, M. S., van Donkelaar, A., Bindle, L., Shah, V., Jaegle, L., Luo, G., Yu, F., Adeniran, J. A., Lin, J., and Brauer, M.: Source sector and fuel contributions to ambient PM2.5 and attributable mortality across multiple spatial scales, Nat. Commun., 12, 3594, https://doi.org/10.1038/s41467-021-23853-y, 2021.
Miljevic, B., Hedayat, F., Stevanovic, S., Fairfull-Smith, K., Bottle, S., and Ristovski, Z.: To sonicate or not to sonicate PM filters: reactive oxygen species generation upon ultrasonic irradiation, Aerosol Sci. Technol., 48, 1276–1284, https://doi.org/10.1080/02786826.2014.981330, 2014.
Newman, J. D., Bhatt, D. L., Rajagopalan, S., Balmes, J. R., Brauer, M., Breysse, P. N., Brown, A. G. M., Carnethon, M. R., Cascio, W. E., Collman, G. W., Fine, L. J., Hansel, N. N., Hernandez, A., Hochman, J. S., Jerrett, M., Joubert, B. R., Kaufman, J. D., Malik, A. O., Mensah, G. A., Newby, D. E., Peel, J. L., Siegel, J., Siscovick, D., Thompson, B. L., Zhang, J., and Brook, R. D.: Cardiopulmonary Impact of Particulate Air Pollution in High-Risk Populations: JACC State-of-the-Art Review, J. Am. Coll. Cardiol., 76, 2878–2894, https://doi.org/10.1016/j.jacc.2020.10.020, 2020.
Niu, X., Chuang, H.-C., Wang, X., Ho, S. S. H., Li, L., Qu, L., Chow, J. C., Watson, J. G., Sun, J., Lee, S., Cao, J., and Ho, K. F.: Cytotoxicity of PM2.5 vehicular emissions in the Shing Mun Tunnel, Hong Kong, Environ. Pollut., 263, 114386, https://doi.org/10.1016/j.envpol.2020.114386, 2020.
Ostro, B., Roth, L., Malig, B., and Marty, M.: The effects of fine particle components on respiratory hospital admissions in children, Environ. Health Perspect., 117, 475–480, https://doi.org/10.1289/ehp.11848, 2009.
Pang, Y., Huang, W., Luo, X.-S., Chen, Q., Zhao, Z., Tang, M., Hong, Y., Chen, J., and Li, H.: In-vitro human lung cell injuries induced by urban PM2.5 during a severe air pollution episode: variations associated with particle components, Ecotoxicol. Environ. Saf., 206, 111406, https://doi.org/10.1016/j.ecoenv.2020.111406, 2020.
Panko, J. M., Hitchcock, K. M., Fuller, G. W., and Green, D.: Evaluation of Tire Wear Contribution to PM2.5 in Urban Environments, Atmosphere, 10, 99, https://doi.org/10.3390/atmos10020099, 2019.
Park, M., Joo, H. S., Lee, K., Jang, M., Kim, S. D., Kim, I., Borlaza, L. J. S., Lim, H., Shin, H., Chung, K. H., Choi, Y.-H., Park, S. G., Bae, M.-S., Lee, J., Song, H., and Park, K.: Differential toxicities of fine particulate matters from various sources, Sci. Rep., 8, 17007, https://10.1038/s41598-018-35398-0, 2018.
Piao, M. J., Ahn, M. J., Kang, K. A., Ryu, Y. S., Hyun, Y. J., Shilnikova, K., Zhen, A. X., Jeong, J. W., Choi, Y. H., Kang, H. K., Koh, Y. S., and Hyun, J. W.: Particulate matter 2.5 damages skin cells by inducing oxidative stress, subcellular organelle dysfunction, and apoptosis, Arch. Toxicol., 92, 2077–2091, https://doi.org/10.1007/s00204-018-2197-9, 2018.
Sahu, S. K., Mangaraj, P., Beig, G., Samal, A., Pradhan, C., Dash, S., and Tyagi, B.: Quantifying the high resolution seasonal emission of air pollutants from crop residue burning in India, Environ. Pollut., 286, 117165, https://doi.org/10.1016/j.envpol.2021.117165, 2021.
Shen, H., Luo, Z., Xiong, R., Liu, X., Zhang, L., Li, Y., Du, W., Chen, Y., Cheng, H., Shen, G., and Tao, S.: A critical review of pollutant emission factors from fuel combustion in home stoves, Environ. Int., 157, 106841, https://doi.org/10.1016/j.envint.2021.106841, 2021.
Shiraiwa, M., Ueda, K., Pozzer, A., Lammel, G., Kampf, C. J., Fushimi, A., Enami, S., Arangio, A. M., Fröhlich-Nowoisky, J., and Fujitani, Y.: Aerosol health effects from molecular to global scales, Environ. Sci. Technol., 51, 13545–13567, https://doi.org/10.1021/acs.est.7b04417, 2017.
Sillapapiromsuk, S., Chantara, S., Tengjaroenkul, U., Prasitwattanaseree, S., and Prapamontol, T.: Determination of PM10 and its ion composition emitted from biomass burning in the chamber for estimation of open burning emissions, Chemosphere, 93, 1912–1919, https://doi.org/10.1016/j.chemosphere.2013.06.071, 2013.
Smith, S. J.: Cleaning cars, grid and air, Nat. Energy, 6, 19–20, https://doi.org/10.1038/s41560-020-00769-3, 2021.
Sørensen, M., Schins, R. P. F., Hertel, O., and Loft, S.: Transition Metals in Personal Samples of PM2.5 and Oxidative Stress in Human Volunteers, Cancer Epidem. Biomar., 14, 1340–1343, https://doi.org/10.1158/1055-9965.Epi-04-0899, 2005.
Srivastava, D., Xu, J., Vu, T. V., Liu, D., Li, L., Fu, P., Hou, S., Moreno Palmerola, N., Shi, Z., and Harrison, R. M.: Insight into PM2.5 sources by applying positive matrix factorization (PMF) at urban and rural sites of Beijing, Atmos. Chem. Phys., 21, 14703–14724, https://doi.org/10.5194/acp-21-14703-2021, 2021.
Stevanovic, S., Gali, N. K., Salimi, F., Brown, R., Ning, Z., Cravigan, L., Brimblecombe, P., Bottle, S., and Ristovski, Z. D.: Diurnal profiles of particle-bound ROS of PM2.5 in urban environment of Hong Kong and their association with PM2.5, black carbon, ozone and PAHs, Atmos. Environ., 219, 117023, https://doi.org/10.1016/j.atmosenv.2019.117023, 2019.
Sun, J., Shen, Z., Zhang, Y., Zhang, Q., Lei, Y., Huang, Y., Niu, X., Xu, H., Cao, J., Ho, S. S. H., and Li, X.: Characterization of PM2.5 source profiles from typical biomass burning of maize straw, wheat straw, wood branch, and their processed products (briquette and charcoal) in China, Atmos. Environ., 205, 36–45, https://doi.org/10.1016/j.atmosenv.2019.02.038, 2019.
Tao, J., Zhang, L., Zhang, R., Wu, Y., Zhang, Z., Zhang, X., Tang, Y., Cao, J., and Zhang, Y.: Uncertainty assessment of source attribution of PM2.5 and its water-soluble organic carbon content using different biomass burning tracers in positive matrix factorization analysis – a case study in Beijing, China, Sci. Total Environ., 543, 326–335, https://doi.org/10.1016/j.scitotenv.2015.11.057, 2016.
Tian, Y., Li, Y., Liang, Y., Xue, Q., Feng, X., and Feng, Y.: Size distributions of source-specific risks of atmospheric heavy metals: An advanced method to quantify source contributions to size-segregated respiratory exposure, J. Hazard. Mater., 407, 124355, https://doi.org/10.1016/j.jhazmat.2020.124355, 2021.
Tuet, W. Y., Liu, F., de Oliveira Alves, N., Fok, S., Artaxo, P., Vasconcellos, P., Champion, J. A., and Ng, N. L.: Chemical oxidative potential and cellular oxidative stress from open biomass burning aerosol, Environ. Sci. Technol. Lett., 6, 126–132, https://doi.org/10.1021/acs.estlett.9b00060, 2019.
Verma, V., Shafer, M. M., Schauer, J. J., and Sioutas, C.: Contribution of transition metals in the reactive oxygen species activity of PM emissions from retrofitted heavy-duty vehicles, Atmos. Environ., 44, 5165–5173, https://doi.org/10.1016/j.atmosenv.2010.08.052, 2010.
Victor, F. C. and Gottlieb, A. B.: TNF-alpha and apoptosis: implications for the pathogenesis and treatment of psoriasis, J. Drugs Dermatol., 1, 264–275, 2002
Wang, S., Hu, G., Yan, Y., Wang, S., Yu, R., and Cui, J.: Source apportionment of metal elements in PM2.5 in a coastal city in Southeast China: Combined Pb-Sr-Nd isotopes with PMF method, Atmos. Environ., 198, 302–312, https://doi.org/10.1016/j.atmosenv.2018.10.056, 2019.
Wang, T., Tian, M., Ding, N., Yan, X., Chen, S.-J., Mo, Y.-Z., Yang, W.-Q., Bi, X.-H., Wang, X.-M., and Mai, B.-X.: Semivolatile Organic Compounds (SOCs) in Fine Particulate Matter (PM2.5) during Clear, Fog, and Haze Episodes in Winter in Beijing, China, Environ. Sci. Technol., 52, 5199–5207, https://doi.org/10.1021/acs.est.7b06650, 2018.
Wang, Y., Cao, M., Liu, A., Di, W., Zhao, F., Tian, Y., and Jia, J.: Changes of inflammatory cytokines and neurotrophins emphasized their roles in hypoxic–ischemic brain damage, Int. J. Neurosci., 123, 191–195, https://10.3109/00207454.2012.744755, 2013.
Wang, Y., Wang, M., Li, S., Sun, H., Mu, Z., Zhang, L., Li, Y., and Chen, Q.: Study on the oxidation potential of the water-soluble components of ambient PM2.5 over Xi'an, China: Pollution levels, source apportionment and transport pathways, Environ. Int., 136, 105515, https://doi.org/10.1016/j.envint.2020.105515, 2020.
Weagle, C. L., Snider, G., Li, C., van Donkelaar, A., Philip, S., Bissonnette, P., Burke, J., Jackson, J., Latimer, R., and Stone, E.: Global sources of fine particulate matter: interpretation of PM2.5 chemical composition observed by SPARTAN using a global chemical transport model, Environ. Sci. Technol., 52, 11670–11681, https://doi.org/10.1021/acs.est.8b01658, 2018.
Weber, R. J., Guo, H., Russell, A. G., and Nenes, A.: High aerosol acidity despite declining atmospheric sulfate concentrations over the past 15 years, Nat. Geosci., 9, 282–285, https://10.1038/ngeo2665, 2016.
Wong, Y. K., Huang, X., Louie, P. K., Yu, A. L., Chan, D. H., and Yu, J. Z.: Tracking separate contributions of diesel and gasoline vehicles to roadside PM2.5 through online monitoring of volatile organic compounds and PM2.5 organic and elemental carbon: a 6-year study in Hong Kong, Atmos. Chem. Phys., 20, 9871–9882, https://doi.org/10.5194/acp-20-9871-2020, 2020.
Wu, B., Shen, X., Cao, X., Yao, Z., and Wu, Y.: Characterization of the chemical composition of PM2.5 emitted from on-road China III and China IV diesel trucks in Beijing, China, Sci. Total Environ., 551, 579–589, https://doi.org/10.1016/j.scitotenv.2016.02.048, 2016.
Wu, D., Zheng, H., Li, Q., Jin, L., Lyu, R., Ding, X., Huo, Y., Zhao, B., Jiang, J., and Chen, J.: Toxic potency-adjusted control of air pollution for solid fuel combustion, Nat. Energy, 7, 194–202, https://doi.org/10.1038/s41560-021-00951-1, 2022.
Xia, T., Korge, P., Weiss, J. N., Li, N., Venkatesen, M. I., Sioutas, C., and Nel, A.: Quinones and aromatic chemical compounds in particulate matter induce mitochondrial dysfunction: implications for ultrafine particle toxicity, Environ. Health Perspect., 112, 1347–1358, https://doi.org/10.1289/ehp.7167, 2004.
Xie, J., Jin, L., Cui, J., Luo, X., Li, J., Zhang, G., and Li, X.: Health risk-oriented source apportionment of PM2.5-associated trace metals, Environ. Pollut., 262, 114655, https://doi.org/10.1016/j.envpol.2020.114655, 2020.
Xu, F., Shi, X., Qiu, X., Jiang, X., Fang, Y., Wang, J., Hu, D., and Zhu, T.: Investigation of the chemical components of ambient fine particulate matter (PM2.5) associated with in vitro cellular responses to oxidative stress and inflammation, Environ. Int., 136, 105475, https://doi.org/10.1016/j.envint.2020.105475, 2020.
Xu, W., Liu, X., Liu, L., Dore, A. J., Tang, A., Lu, L., Wu, Q., Zhang, Y., Hao, T., Pan, Y., Chen, J., and Zhang, F.: Impact of emission controls on air quality in Beijing during APEC 2014: Implications from water-soluble ions and carbonaceous aerosol in PM2.5 and their precursors, Atmos. Environ., 210, 241–252, https://doi.org/10.1016/j.atmosenv.2019.04.050, 2019.
Yan, Q., Kong, S., Yan, Y., Liu, H., Wang, W., Chen, K., Yin, Y., Zheng, H., Wu, J., Yao, L., Zeng, X., Cheng, Y., Zheng, S., Wu, F., Niu, Z., Zhang, Y., Zheng, M., Zhao, D., Liu, D., and Qi, S.: Emission and simulation of primary fine and submicron particles and water-soluble ions from domestic coal combustion in China, Atmos. Environ., 224, 117308, https://doi.org/10.1016/j.atmosenv.2020.117308, 2020.
Yang, H.-H., Dhital, N. B., Wang, L.-C., Hsieh, Y.-S., Lee, K.-T., Hsu, Y.-T., and Huang, S.-C.: Chemical Characterization of Fine Particulate Matter in Gasoline and Diesel Vehicle Exhaust, Aerosol Air Qual. Res., 19, 1439–1449, https://doi.org/10.4209/aaqr.2019.04.0191, 2019.
Zhang, J., Liu, L., Xu, L., Lin, Q., Zhao, H., Wang, Z., Guo, S., Hu, M., Liu, D., Shi, Z., Huang, D., and Li, W.: Exploring wintertime regional haze in northeast China: role of coal and biomass burning, Atmos. Chem. Phys., 20, 5355–5372, https://doi/org/10.5194/acp-20-5355-2020, 2020.
Zhang, L., Liu, Y., and Hao, L.: Contributions of open crop straw burning emissions to PM2.5 concentrations in China, Environ. Res. Lett., 11, 014014, https://doi.org/10.1088/1748-9326/11/1/014014, 2016.
Zhang, Q., Li, Z., Shen, Z., Zhang, T., Zhang, Y., Sun, J., Zeng, Y., Xu, H., Wang, Q., Hang Ho, S. S., and Cao, J.: Source profiles of molecular structure and light absorption of PM2.5 brown carbon from residential coal combustion emission in Northwestern China, Environ. Pollut., 299, 118866, https://doi.org/10.1016/j.envpol.2022.118866, 2022.
Zhang, X., Zhao, X., Ji, G., Ying, R., Shan, Y., and Lin, Y.: Seasonal variations and source apportionment of water-soluble inorganic ions in PM2.5 in Nanjing, a megacity in southeastern China, J. Atmos. Chem., 76, 73–88, https://doi.org/10.1007/s10874-019-09388-z, 2019.
Zhang, Y., Shen, Z., Sun, J., Zhang, L., Zhang, B., Zou, H., Zhang, T., Hang Ho, S. S., Chang, X., Xu, H., Wang, T., and Cao, J.: Parent, alkylated, oxygenated and nitrated polycyclic aromatic hydrocarbons in PM2.5 emitted from residential biomass burning and coal combustion: A novel database of 14 heating scenarios, Environ. Pollut., 268, 115881, https://doi.org/10.1016/j.envpol.2020.115881, 2021.
Zhang, Y. L., Huang, R. J., El Haddad, I., Ho, K. F., Cao, J. J., Han, Y., Zotter, P., Bozzetti, C., Daellenbach, K. R., Canonaco, F., Slowik, J. G., Salazar, G., Schwikowski, M., Schnelle-Kreis, J., Abbaszade, G., Zimmermann, R., Baltensperger, U., Prévôt, A. S. H., and Szidat, S.: Fossil vs. non-fossil sources of fine carbonaceous aerosols in four Chinese cities during the extreme winter haze episode of 2013, Atmos. Chem. Phys., 15, 1299–1312, https://10.5194/acp-15-1299-2015, 2015.
Zhao, K., Zhao, G. M., Wu, D., Soong, Y., Birk, A. V., Schiller, P. W., and Szeto, H. H.: Cell-permeable peptide antioxidants targeted to inner mitochondrial membrane inhibit mitochondrial swelling, oxidative cell death, and reperfusion injury, J. Biol. Chem., 279, 34682–34690, https://doi.org/10.1074/jbc.M402999200, 2004.
Zhao, M., Zeng, S., Liu, S., Li, Z., and Jing, L.: Metal accumulation by plants growing in China: Capacity, synergy, and moderator effects, Ecol. Eng., 148, 105790, https://doi.org/10.1016/j.ecoleng.2020.105790, 2020.
Zhao, X., Zhou, W., Han, L., and Locke, D.: Spatiotemporal variation in PM2.5 concentrations and their relationship with socioeconomic factors in China's major cities, Environ. Int., 133, 105145, https://doi.org/10.1016/j.envint.2019.105145, 2019.
Zhou, W., Jiang, J., Duan, L., and Hao, J.: Evolution of Submicrometer Organic Aerosols during a Complete Residential Coal Combustion Process, Environ. Sci. Technol., 50, 7861–7869, https://10.1021/acs.est.6b00075, 2016.