the Creative Commons Attribution 4.0 License.
the Creative Commons Attribution 4.0 License.
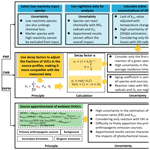
Review of source analyses of ambient volatile organic compounds considering reactive losses: methods of reducing loss effects, impacts of losses, and sources
Baoshuang Liu
Yao Gu
Yutong Wu
Shaojie Song
Philip K. Hopke
Chemical losses of ambient reactive volatile organic compounds (VOCs) is a long-term issue yet to be resolved in VOC source apportionments. These losses substantially reduce the concentrations of highly reactive species in the apportioned factor profiles and result in the underestimation of source contributions. This review assesses the common methods and existing issues in ways to reduce losses and loss impacts in source analyses and suggests research directions for improved VOC source apportionments. Positive matrix factorization (PMF) is now the main VOC source analysis method compared to other mathematical models. The issue in using any apportionment tool is the processing of the data to be analyzed to reduce the impacts of reactive losses. Estimating the initial concentrations of ambient VOCs based on photochemical age has become the primary approach to reduce reactive loss effects in PMF, except for selecting low-reactivity species or nighttime data into the analysis. Currently, the initial concentration method only considers daytime reactions with hydroxyl (•OH) radicals. However, the •OH rate constants vary with temperature, and that has not been considered. Losses from reactions with O3 and NO3 radicals, especially for alkene species, remain to be included. Thus, the accuracy of the photochemical age estimation is uncertain. Beyond developing accurate quantitative approaches for reactive losses, source analyses methods for the consumed VOCs and the accurate quantification of different source contributions to O3 and secondary organic aerosols are important additional directions for future research.
- Article
(3104 KB) - Full-text XML
-
Supplement
(862 KB) - BibTeX
- EndNote
Ambient volatile organic compounds (VOCs) are key precursors of ozone (O3) and secondary organic aerosol (SOA) formation (Li et al., 2016; J. Li et al., 2018; Wu and Xie, 2018). Accurate apportionment of their sources can be important in developing effective prevention and control measures for atmospheric O3 and SOA pollution (Carrillo-Torres et al., 2017; Meng et al., 1997; G. Wang et al., 2022). Current research on source analyses of atmospheric VOCs has been primarily conducted utilizing the species ratio method (Che et al., 2019; Zhang et al., 2021), the photochemical-age-based parameterization method (Huang et al., 2020; Zhu et al., 2021), and receptor models (e.g., positive matrix factorization (PMF) (Gu et al., 2020; Liu et al., 2016), chemical mass balance (CMB) (Song et al., 2019), and principal component analysis/multiple linear regression (PCA/MLR) (Jia et al., 2016; Sanchez et al., 2008)) based on the measured concentration data. Receptor models, especially PMF, are the most widely used source apportionment methods (Song et al., 2008; Vega et al., 2022; Y. Yang et al., 2022). However, compared to particulate matter, ambient VOC species can undergo rapid, complex chemical reactions with •OH radicals, NO3 radicals, O3, etc. (Atkinson and Arey, 2003), resulting in substantial chemical losses during their transport from their sources to the receptor site (Y. Yang et al., 2022; Yuan et al., 2012a). Therefore, the source-apportioned results based on the measured VOC data have difficulty in fully reflecting the actual impacts of emission sources on air quality (Y. T. Wu et al., 2023; T. Yang et al., 2022).
To reduce the impact of reactive losses, many studies have selected low-reactivity VOC species to conduct source analyses when using PMF analyses (Guan et al., 2020; T. Yang et al., 2022). However, this method cannot fully solve the issues related to reactive losses and provide complete source apportionments since some highly active marker species such as isoprene (Tan et al., 2020) cannot be excluded from the PMF input species without a substantial loss of information (B. S. Liu et al., 2023). Therefore, recent studies estimated the initial concentrations of ambient VOCs (i.e., the VOC concentrations in the fresh emissions before they can undergo chemical reactions) utilizing the photochemical-age-based parameterization method and then performed source analyses with PMF (He et al., 2019; Zou et al., 2023). However, there could still be high uncertainties in the estimated photochemical age of VOC species (Parrish et al., 2007; Yuan et al., 2012b).
There were studies that only used nighttime data for source analyses to reduce the loss impacts (Kim et al., 2005), but the representativeness of the apportioned results was likely limited since there could be daytime-only sources that would not be observed at night (Buzcu and Fraser, 2006). Some studies applied decay factors to correct the impact of reactive losses in using CMB for VOC source analyses (Friedlander, 1981; Lin and Milford, 1994; Na and Pyo Kim, 2007). However, there were relatively few studies, and the effectiveness of this method still needs to be assessed. In 2023, B. S. Liu et al. (2023) systematically investigated the impact of VOC photochemical losses on the PMF source-apportioned results and found that photochemical losses reduced the concentrations of highly reactive species in factor profiles, resulting in the contributions of biogenic emissions and polymer-production-related industrial sources being substantially underestimated. However, there has been little related research to assess the limitations of VOC apportionments.
With the substantial increase in O3 concentrations in many locations worldwide in recent years (K. Li et al., 2020; Zhang et al., 2018; Zhao et al., 2021), accurate source apportionment approaches of the key precursor VOCs have been acquired increasing attention (Gu et al., 2022). Thus, a related issue is the determination of the VOCs consumed in the formation of the observed O3 and SOA (Gu et al., 2023). Although some studies have investigated the underestimated contributions of emission sources by comparing the apportioned results based on the initial and measured data (He et al., 2019; Y. J. Wu et al., 2023), there are few publications that conducted source analyses for the consumed VOCs and apportioned the contributions of different sources to the formation of O3 and SOA. In 2023 and 2024, Gu et al. (2023) and Cui et al. (2024) attempted to develop a method for apportioning the primary and oxidative sources of the consumed VOCs, and Z. Y. Wang et al. (2022) conducted a similar study. Currently, although some studies have been conducted on these issues of VOC reactive losses and achieved some important results (Gu et al., 2023; Watson et al., 2001; Y. T. Wu et al., 2023), there remain unresolved issues. To better promote progress on the development of better VOCs source-apportioned methods, the present work systematically investigated the main methods and shortcomings of those methods that are currently applied to resolve these issues by reviewing relevant papers, with the aim of identifying directions for the future developments and improvement of VOCs source apportionment methods.
Relevant papers were collected by exhaustively searching Science Direct (Elsevier), the Web of Science, Scopus, Springer, Wiley, and China National Knowledge Infrastructure (CNKI) with the following keywords: volatile organic compounds (VOCs), oxygenated VOCs (OVOCs), initial ratios, chemical/photochemical losses, source apportionment/analysis, positive matrix factorization (PMF), chemical mass balance (CMB), receptor model, and photochemical-age-based parameterization method. The information extracted from each publication included methods for reducing the impacts of photochemical losses, the impacts of VOC photochemical losses on source analyses, the source-apportioned methods, and the results for the consumed VOCs in the atmosphere. This study identified 170 papers, of which 69 papers have been published since 2020, accounting for ∼ 41 % of the total publications; a total of 36 papers were published from 2015 to 2019, accounting for ∼ 21 %. There were 109 research papers reporting results in China, accounting for ∼ 64 % of the total papers. There were 40 papers located in the United States, accounting for ∼ 24 %. The number of papers located in India, South Korea, Canada, and Japan was only 4, 3, 3, and 3, respectively, and there were a few papers from other countries. Additionally, there were 19 research papers conducted in Beijing, 9 papers in Guangzhou, and 8 papers in Tianjin, accounting for ∼ 11 %, ∼ 5 %, and ∼ 5 % of the total papers, respectively. There were fewer reports from other cities.
3.1 Methods of reducing the impacts of reactive losses
Ambient VOCs can be substantially oxidized by O3, hydroxyl (•OH), and nitrate (NO3) radicals (Atkinson and Arey, 2003; Bey et al., 2001; Finlayson-Pitts and Pitts, 1997), especially oxidation by •OH radicals primarily during the daytime (Wang et al., 2013). Therefore, reducing the impacts of VOC reactive losses on source apportionment has long been an important but is not easy issue to resolve in VOC source apportionments (B. S. Liu et al., 2023). Ambient VOCs primarily include alkanes, alkenes, aromatic hydrocarbons, alkynes, OVOCs, and halogenated hydrocarbons. Studies suggested that the concentration of photochemical assessment monitoring station (PAMS) VOCs (i.e., alkanes, alkenes, aromatic hydrocarbons, and alkynes) accounted for ∼ 63 % of the total VOC (TVOC) concentration (i.e., average value of proportions from different literature), while OVOCs and halogenated hydrocarbons contributed ∼ 22 % and ∼ 14 % to the TVOCs, respectively (as shown in Table S1 in the Supplement). The reported number of measured species ranged from 13 to 124, including 0–32 alkanes, 2–16 alkenes, 1 alkyne (only acetylene), 3–19 aromatic hydrocarbons, 4–28 OVOCs, and 28–38 halogenated hydrocarbons. There were substantial differences in the identified emission sources for the different types of VOCs (Mo et al., 2016). For example, PAMS VOCs mainly originate from primary anthropogenic sources (Chen et al., 2010), while OVOCs can also be formed by the oxidation of PAMS VOCs, in addition to primary source emissions (Chen et al., 2014; Seinfeld and Pandis, 2006). The contributions of secondary formation to some OVOC species (e.g., acetaldehyde and propionaldehyde) can exceed 50 % (de Gouw et al., 2005). In addition to local emissions, the ambient concentrations of halogenated hydrocarbons can also be affected by the long-distance transport (Mintz and McWhinney, 2008). Therefore, utilizing only the same source analyses approach for multiple VOC species from different sources might produce results with high uncertainties in the apportionments.
Source analyses methods for ambient VOCs considering reactive losses mainly included PMF, CMB, and photochemical-age-based parameterization method (Table S2). PMF was the most common method used for source analyses (Y. Yang et al., 2022). Approximately 53 % of the reviewed publications focused on source analyses of PAMS species using PMF, while OVOCs primarily used the photochemical-age-based parameterization method for source apportionment but with only relatively few studies (Tables S2–S3). Additionally, the studies on data from simultaneous measurement of both PAMS and OVOC species utilized two methods to conduct source analyses. One method was to simultaneously input PAMS and some OVOC species into the receptor model (e.g., PMF) for source analyses and only separated primary and secondary source contributions of ambient VOCs (Han et al., 2023; Li et al., 2023; C. T. Liu et al., 2023; Tan et al., 2021) (Table S3). Another approach was to use the PMF and photochemical-age-based parameterization to obtain the source-apportioned results for ambient PAMS and OVOCs, respectively, and then combine the two apportioned results to obtain the final source-resolution results for the ambient VOCs (Zhu et al., 2021). However, the utilization of this method has been limited.
3.1.1 Methods for reducing reactive loss in PMF source analyses
Selecting low-activity species or incorporating night-only data into PMF
The methods of reducing the effects of reactive losses for source apportionments utilizing different models are shown in Fig. 1. At present, selecting the VOC species with lower reactivity to be input in PMF for apportionment is the most commonly used approach (Chen et al., 2019; Tan et al., 2020; Y. Yang et al., 2022). Many highly active alkene and aromatic hydrocarbon species were not included in the PMF calculations (Gu et al., 2023; B. S. Liu et al., 2023). For example, B. S. Liu et al. (2023) excluded highly active species such as 1-hexene, trans-2-butene, trans-2-pentene, cis-2-pentene, and cis-2-butene. In addition, the current approach of incorporating daytime and nighttime VOC data into PMF for source analyses (e.g., Gu et al., 2020; Z. Y. Li et al., 2020; Jain et al., 2022) assumes that the daytime and nighttime factor profiles are consistent. However, the daytime factor profiles can be substantially influenced by photochemistry (Liu et al., 2025). Therefore, the source contributions obtained by this method had relatively higher uncertainty. To reduce the impacts of reactive losses on the PMF-apportioned factor profiles and the corresponding contributions, some studies utilized only nighttime data when reactive losses would be lower for source analyses to obtain more accurate nighttime contributions of emission sources (Buzcu-Guven and Fraser, 2008; Buzcu and Fraser, 2006; Kim et al., 2005; Xie and Berkowitz, 2006).
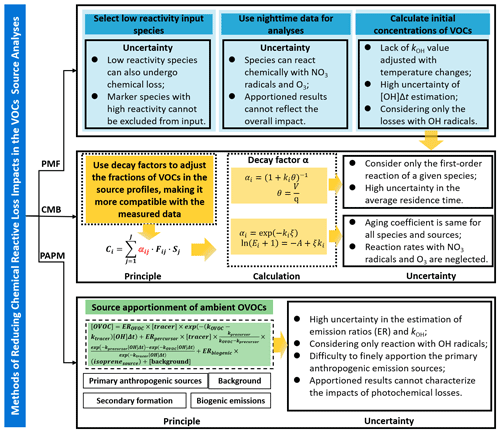
Figure 1Methods for reducing the impacts of reactive losses for different source analyses models. PMF represents the positive matrix factorization, CMB represents the chemical mass balance, and PAPM represents the photochemical-age-based parameterization method.
However, the volatile emission sources commonly contribute substantially to VOCs during the daytime (Gu et al., 2023). For example, Buzcu and Fraser (2006) used nighttime and all-day data to conduct VOC source apportionment in Houston, respectively. They found that the night-only data did not identify biogenic or evaporative gasoline sources due to minimal emissions at night. Thus, the contributions of the nighttime emission sources substantially increased compared to the all-day results because of the missing source types. Meanwhile, the all-day VOC source analysis conducted by Zhao et al. (2004) in Houston during the same period also showed substantial differences from the results reported by Buzcu and Fraser (2006), who utilized night-only data. An additional consideration is that VOCs can react with NO3 radicals and O3 (especially for highly reactive alkenes) at night, causing reactive losses (de Gouw et al., 2017). Thus, this approach does not eliminate the effects of all possible loss mechanisms. Although T. T. Wang et al. (2024) also considered reactions with O3 at night when estimating VOC chemical reactive losses, the number of related studies remains limited.
Incorporating VOC initial concentration estimated by photochemical-age-based parameterization method into PMF
The photochemical-age-based parameterization method is an approach to estimate the initial concentrations of ambient VOCs (Shao et al., 2011; Wang et al., 2013; Yuan et al., 2012b). This method assumes that the chemical loss of ambient VOCs mainly originates only from reactions of the VOCs with •OH radicals (Parrish et al., 1992; Sun et al., 2016; Wang et al., 2013). The photochemical ages (Δt) of VOC species are estimated and then combined with the rate constants for reactions of •OH radicals with the VOC species (k•OH) to calculate their initial concentrations and quantified the VOC photochemical losses (Shao et al., 2011; Wang et al., 2013; Zhan et al., 2021). The equation for the initial concentration calculation is shown in Eq. (1), where k•OH and Δt are the key parameters in this approach.
where [VOC]0 and [VOC]t are the initial and measured concentrations of VOC at time t, and [•OH] represents the concentration of the •OH radical. However, many studies estimated [•OH] Δt because of the lack of •OH radical concentration data and defined the product as the •OH exposure (Shao et al., 2011; Wei et al., 2022; Yuan et al., 2012b).
The k•OH of VOC species are substantially affected by atmospheric temperature (Atkinson and Arey, 2003), which could be commonly measured in laboratory (or chamber) experiments or through the use of detailed chemical computer models (Atkinson and Arey, 2003). According to the reviewed papers regarding the estimation of photochemical age, it was found that approximately 48 % of the publications used the Atkinson and Arey (2003) summary of k•OH values at 298° K, and approximately 8 % used the Carter (2010) summary of k•OH values at 300° K. The detailed k•OH values for different VOC species summarized in these two publications are provided in Table S4. However, other relevant studies cited k•OH values from other papers by Atkinson and/or Carter (Atkinson, 1991, 2007; Atkinson et al., 2006; Talukdar et al., 1994). The rate constant for a bimolecular reaction between a hydroxyl radical and a VOC molecule has a roughly exponential dependence on temperature in the Arrhenius equation (B. S. Liu et al., 2023). Therefore, these published papers only used k•OH values at specific temperatures, which made it difficult to characterize the actual reaction rates at the actual temperatures in the atmosphere. To address this issue, Z. Y. Wang et al. (2022) and Wang et al. (2023) utilized the Arrhenius expression to locally optimize the k•OH values of VOC species based on the actual temperature conditions to further improve the accuracy of estimating the VOC initial data. In addition, some studies used the k•OH of m,p-xylene instead of values of more reactive species to reduce high outliers when estimating the initial concentration (Wang et al., 2013). However, the validity of this method was difficult to assess.
There are two main methods (as shown in Table S5) for estimating Δt, namely the species ratio method (Roberts et al., 1984; Shao et al., 2011; Wan et al., 2022) and the sequential reaction model (Gong et al., 2018; Shao et al., 2011; Xie et al., 2008). The specific details are as follows.
(1) Estimation of Δt based on the species ratio method
where kA and kB are the reaction rate constants with •OH radicals for species A and B of a highly homologous species with substantial differences in reaction activity, respectively. is the initial concentration ratio of A to B in fresh emissions, and is the measured concentration ratio of A to B at time t. The determination of the initial concentration ratio of A to B was extremely critical for estimating the Δt. The Δt is used to then calculate the initial concentrations of the VOCs of interest using Eq. (1). The initial concentrations can then be the input variables to PMF (B. S. Liu et al., 2023).
This ratio method was first proposed by Roberts et al. (1984). The method has been commonly applied to PAMS species emitted by anthropogenic sources. There have been differences in the species used to calculate the initial ratio in different papers (Table S6). Generally, aromatic hydrocarbons (e.g., benzene, toluene, ethylbenzene, or m,p-xylene) were used as the selected species. The substantial differences in activity and high homology are generally the main basis for selecting species ratio types (Lin et al., 2011; Shao et al., 2009; Zou et al., 2021; Zou et al., 2023). Most publications used the initial ratios of () or () to calculate Δt (Table S6). These two species ratios ( and ) accounted for ∼ 34 % of the total reviewed publications of estimating initial ratios, respectively. Approximately 7 % of the papers used toluene/benzene (), while (), () or had limited use, accounting for ∼ 2 % and ∼ 11 % of the total reviewed publications of initial ratio estimation.
The methods for obtaining the initial species ratio in the reviewed papers mainly referred to prior similar studies (Wang et al., 2016), source emission inventory values (Wang et al., 2013), and estimations based on the observed concentration data at the receptor sites at times when low reactivity was expected (Borlaza-Lacoste et al., 2024; Fang et al., 2021; Han et al., 2019; Y. T. Wu et al., 2023). There were several papers using the first two methods to obtain the initial species ratios (Table S6). The initial species ratios obtained by these methods had difficulty in accurately characterizing the comprehensive impacts of multiple sources in the study area. Most studies were based on the observed data of low-photochemical-reaction periods (∼ 19:00 to 08:00 LT) to calculate the initial species ratios (Table S6). This method assumes that VOC species emitted by different sources during the certain periods of night would not undergo oxidative reaction, and the concentration ratios of two homologous VOC species remained unchanged during the transport process from source emissions to the receptor-measured sites (B. S. Liu et al., 2023; Sun et al., 2016; Yuan et al., 2012b). Since there could be residual reactions with nitrate radical and multiple sources of the indicator species, there remains uncertainty in the results. For example, McKeen and Liu (1993) and McKeen et al. (1996) found that the species ratios can be substantially influenced by the oxidative chemistry and atmospheric mixing. Comparing one species to another, both the model results and the observations were consistent with an average rate of dilution roughly equivalent to n-butane oxidation (McKeen et al., 1996). This result has negative implications for the use of hydrocarbon ratios as chemical reaction clocks (McKeen et al., 1996). Parrish et al. (2007) also suggested that there were uncertainties in the determination of Δt by the initial species ratios because of influence of fresh emissions along the transport path that perturb the results. However, this approach can still provide a useful measure of chemical processing in the atmosphere (Parrish et al., 2007).
There were differences in the estimation details of the initial species ratio in different reports (B. S. Liu et al., 2023; Shao et al., 2011; Yuan et al., 2012b). For example, Yuan et al. (2012b) utilized the m,p-xylene and benzene data measured between 00:00 and 05:00 LT to conduct a linear fit. The regression line was then extrapolated to the highest benzene concentration during this period so that the initial ratio could be estimated from the m,p-xylene to benzene concentration ratios at this point. Y. T. Wu et al. (2023) and T. Yang et al. (2022) used time periods (00:00–04:00 and 20:00–05:00 LT, respectively) with the lowest and the highest and ratios at night to calculate the average values of corresponding ratios less the various percentiles or the corresponding values above various percentiles and then determined the initial ratio by the minimum average ratio (i.e., ) or the maximum average ratio (i.e., ) of the two species with the highest homology (i.e., highest correlation) below different percentiles. Y. J. Wu et al. (2023) reported that the maximum value of at 01:00–06:00 LT was considered the initial species ratio. In addition, B. S. Liu et al. (2023) and Borlaza-Lacoste et al. (2024) utilized the slope of the linear fit of the scatterplots of corresponding ethylbenzene and m,p-xylene below the 10th percentile of ratios during 00:00–05:00 LT to determine the initial ratio. Overall, the in the reviewed papers ranged between 0.22 and 0.75, was between 3.14 and 4.48, was between 1.04 and 4.42, was between 1.30 and 1.32, was between 1.19 and 3.14, and was 2.2 (as shown in Table S6).
Zhang et al. (2020) attempted to confirm the feasibility and rationality of estimating Δt, based on utilizing the regressions of benzene and toluene versus CO and Δt. Zou et al. (2023) demonstrated the rationality of selecting the ratio of E and X to estimate the Δt through the high correlation of these two species having substantial activity differences. However, there were few studies to systematically assess how to choose the most suitable species ratios and the calculated method.
Multiple types of initial species ratios were used for estimating the Δt of VOCs (Table S6), and the results estimated by the different ratios could vary substantially. Shao et al. (2011) suggested that the differences between the initial concentrations calculated using the three pairs of compound ratios (i.e., , , and i-butene/propene ()) were generally within 50 %, and the and results were in good agreement. Zou et al. (2021) examined the sensitivity of estimating the initial concentration based on and initial ratios. They found that the relative variation range of the initial concentrations of PAMS species was between 0.41 % and 68.06 % for an initial of 1.3 when compared with an initial of 0.5.
Additionally, in the ratio method, the same Δt was calculated for different VOCs for each sample, and the paired species for estimating the ratio were aromatic hydrocarbons (Table S6), which are mainly emitted from anthropogenic emission sources such as solvent use and petrochemical enterprises (Mo et al., 2015; Na et al., 2004; Yuan et al., 2010; Zhang et al., 2016). Therefore, the Δt obtained based on these ratios may only reflect the chemical aging of VOC species emitted from these specific sources. It is difficult to accurately characterize the Δt for these species from other sources. In the future, different types of species ratios (not just aromatic hydrocarbons) need to be selected based on VOC species from different sources. The Δt derived from different types of VOC species should be calculated to improve traditional ratio methods.
An additional issue is that this method only considers the reactions between VOCs and •OH radicals during the day, while chemical reactions with NO3 radicals at night and/or O3 were commonly excluded. B. S. Liu et al. (2023) attempted to estimate the reactive losses between 19:00 and 23:00 LT, but they considered the gradual loss of •OH radicals after sunset and did not consider the losses caused by NO3 radical reactions that may be present at night since they are not photolyzed, as this occurs in the daylight. Additionally, alkenes can directly react with O3 in the atmosphere, causing losses. De Gouw et al. (2017) suggested that the nighttime removal of highly reactive alkenes by O3 and NO3 radicals was also substantial. However, there are currently no reports on the estimation methods for these reactive losses. In future studies, the ratio of two alkene species with substantial differences in reaction rates with O3 could be used as the base ratio as in Eq. (2) and combined with measured O3 reaction rate constants (Atkinson and Arey, 2003) to estimate the chemical reaction time (Δt) of alkene species with O3. Then, chemical losses of alkene species in the reaction with O3 could be estimated using Eqs. (1) and (9), and the combined effects of O3 and •OH could be estimated. De Gouw et al. (2017) proposed the calculated method of O3 exposure (i.e., [O3]Δt) at nighttime, which can be calculated from the measured ratio of benzene over cis-2-butene.
(2) Estimation of Δt based on the sequential reaction model
It has been found that using the ratio method to calculate the Δt of isoprene could result in overestimation (Y. J. Wu et al., 2023; Y. Yang et al., 2022). An alternative is the sequential reaction method. It is based on measurements of the reaction products of these reactions.
where MVK is methyl vinyl ketone, MACR is methacrolein; k1, k2, and k3 are the rate constants reacting with •OH of isoprene, MACR, and MVK, respectively; and [MVK]t, [MACR]t, and [isoprene]t are the measured ambient concentrations at time t.
This sequential reaction method was first proposed by Bertman et al. (1995) and was commonly used to estimate the Δt of isoprene emitted primarily from biogenic emissions (Gong et al., 2018; Roberts et al., 2006; Y. J. Wu et al., 2023). Assuming the •OH-driven isoprene oxidation mechanism (Eqs. 3–5) from the laboratory chamber studies of Carter and Atkinson (1996), Stroud et al. (2001) quantified the Δt of isoprene by the reaction relationship between isoprene and its oxidation products (Eqs. 6–7) (de Gouw et al., 2005; Y. J. Wu et al., 2023; Xie et al., 2008). The synchronous measurement of MVK and MACR is critical to obtain Δt when utilizing this method. Numerous studies failed to estimate the isoprene Δt due to the lack of MVK and MACR (Gu et al., 2023; B. S. Liu et al., 2023; Wang et al., 2023). Although some studies have used the average of Δt obtained from both MVK and MACR as the final Δt (Xie et al., 2008), there has been little assessment of which method was more suitable.
Additionally, there were studies using the isotopic hydrocarbon clock method (Kornilova et al., 2016; Rudolph and Czuba, 2000; Saito et al., 2009) to estimate the species Δt that was calculated by the decay of isotopes in the emissions to the ambient receptor site (Table S5). However, the studies only calculated the Δt without estimating the species's initial concentrations. Our study found that approximately 74 % of the publications that calculated the Δt used the species ratio method, approximately 11 % used both the ratio method and the sequential reaction model, while a few used other methods (Table S5).
3.1.2 Reducing the loss impacts in OVOC source analyses based on photochemical age
Ambient OVOCs are an important fraction of VOCs, which primarily included aldehydes, ketones, ethers, and alcohols (Mellouki et al., 2015) since OVOC photolysis is one of the main sources of •OH radicals (Z. Y. Li et al., 2018). The OVOCs can be directly emitted from biogenic and anthropogenic sources (Huang et al., 2019, 2020; Tanimoto et al., 2014) and can also be formed by oxidation of precursors (e.g., PAMS species) with •OH radicals, O3, and NO3 radicals (Legreid et al., 2008; Sahu et al., 2016; Tanimoto et al., 2014). OVOCs were commonly apportioned by the photochemical-age-based parameterization method, as shown in Eq. (8).
where [OVOC], [tracer], and [background] are the concentrations of measured ambient OVOCs, the tracer (e.g., benzene, acetylene (C2H2), or carbon monoxide (CO); Table S7) from primary anthropogenic sources, and background OVOC concentration, respectively; kOVOC, ktracer, and kprecursor are the •OH rate constants of the OVOCs, tracer, and precursor, respectively; and kOVOC and ktracer are commonly obtained from the related publications (Atkinson and Arey, 2003). EROVOC and ERprecursor are the emission ratios of OVOCs and precursors relative to the tracer, respectively; ERbiogenic is the emission ratio of OVOCs to the isoprene concentration emitted from biogenic sources (i.e., isoprenesource), and EROVOC, ERprecursor, ERbiogenic, kprecusor, and [background] can be determined from a linear least squares fit that minimizes the difference between the measured [OVOC] and those calculated from Eq. (8). ER values in the reviewed papers are listed in Table S8. [•OH]Δt represents the exposure of •OH radicals, which can be estimated by Eq. (2), and isoprenesource can be estimated by Eqs. (6)–(7) and (1), based on the measured concentrations of ambient isoprene and its photochemical products (i.e., MVK and MACR). The chemical removal of isoprene in the atmosphere is so rapid that it is impossible to differentiate between primary and secondary OVOC sources based on the measured data. Therefore, isoprenesource represents both primary and secondary biogenic sources (de Gouw et al., 2005).
However, this method remains highly uncertain because of the source complexities of OVOCs (Mo et al., 2016; Schlundt et al., 2017). This method assumes that (1) anthropogenic emissions of OVOCs and their precursors are proportional to the selected primary tracer, (2) the removal process of OVOCs is dominated by reactions with •OH radicals, (3) biogenic sources of OVOCs are proportional to the emission of isoprene, and (4) the photochemical age for a sampled air mass can be determined (de Gouw et al., 2005).
This method estimates the source contributions of primary and secondary anthropogenic sources, biogenic emissions, and background to different OVOC species, and then it further obtained the contributions of the four types of sources to the ambient OVOCs (de Gouw et al., 2005; Yuan et al., 2012b; Zhu et al., 2021). Using this approach made it difficult to finely apportion the contributions of primary anthropogenic sources and to obtain the contributions of primary and secondary biogenic emissions. Therefore, to obtain refined source-apportioned results for OVOCs, many studies incorporated both OVOC and PAMS species into the receptor model for source analyses (Guan et al., 2020; Yang et al., 2019; Zhou et al., 2022).
This method defines the measured concentrations of OVOCs as the sum of the concentrations after photochemical losses (i.e., losses caused by the formation of O3 and SOAs via photochemical reactions) of OVOCs directly emitted by anthropogenic sources (i.e., the first term in Eq. 8), the concentrations after the photochemical losses of OVOCs formed by the conversion of precursors emitted from anthropogenic sources (i.e., the second term in Eq. 8), the concentration from biogenic emissions (without considering losses because of small contributions of biogenic emissions) (i.e., the third term in Eq. 8), and the background concentration (i.e., the fourth term in Eq. 8). Therefore, although this approach considered the influences of photochemical losses in the calculation process, the final results only reflected the contributions of four types of sources to the measured OVOCs and could not characterize the impacts of photochemical losses. In addition, to compensate for the photolytic losses in OVOCs in Eq. (8), which was not considered by de Gouw et al. (2005), Wang et al. (2017) introduced a modification coefficient (m) before the kOVOC to modify it, assuming that the photolysis rate is proportional to the •OH reaction rate (de Gouw et al., 2018; Wang et al., 2017). Meanwhile, Huang et al. (2020) and Zhu et al. (2021) also conducted relevant studies using this coefficient. The m value depends on the relative rate of photolysis versus the •OH reaction for an OVOC species (Huang et al., 2020). However, related studies remain limited at present.
Additionally, some studies have attempted to estimate the initial concentrations of OVOCs using the traditional photochemical-age-based parameterization method (i.e., Eqs. 1–2) to correct their reactive losses and then incorporate initial data into PMF for source analyses (Cui et al., 2024; Li et al., 2023; Ren et al., 2024; L. L. Zhang et al., 2024). However, due to the complexity of the OVOC sources and the substantial differences in emission sources from PAMS species (de Gouw et al., 2018; Huang et al., 2020; Zhu et al., 2021), this method for correcting OVOC losses had high uncertainty. The rational estimation approaches remain to be studied.
3.1.3 Methods for reducing loss effects in CMB source apportionments
The chemical mass balance method uses known profiles measured at the source. Thus, any reactions in transit will result in a change in that source's profile and difficulty in fitting the data at the receptor site. Thus, corrections to the receptor site data are needed to make them comparable to the measured source profiles. As early as the 1980s and 1990s, studies had been conducted to reduce the impacts of different VOC species reactions on CMB estimation results, including the minimization of reaction effects by limiting source profiles to VOCs with similar reaction rates (Harley et al., 1992; Lewis et al., 1993; Nelson and Quigley, 1983; Wadden et al., 1986) and VOC samples obtained during winter (Aronian et al., 1989) or early in the morning (Scheff and Klevs, 1987). In 1983, Nelson and Quigley (1983) estimated the reaction extent by the changes in the ratios of xylene to ethylbenzene at the receptor and the release site and then obtained the decay factors of other VOC species. However, it was found that the concentrations adjusted by decay factors at the receptor site had little impact on their estimated source contributions (Nelson and Quigley, 1983). Since the emissions varied with time, especially during periods of intense photochemical reactions (Lin and Milford, 1994), some studies tried to use decay factors to adjust the fractions of VOC species in the source profiles at different times, making them more compatible with the data measured at the receptor site (Lin and Milford, 1994; Na and Pyo Kim, 2007). However, this approach has not been used other than in these two instances.
There are two methods to obtain the decay factors. One method was to consider an urban airshed as a continuous stirred-tank reactor and relating the decay factor for a given species to its first-order reaction rate constant (Friedlander, 1981). The other method was to conduct an estimation based on the reaction rate constants of specific VOC species and “aging coefficients” (Junninen et al., 2005; Lin and Milford, 1994). The details and limitations of the two methods are provided in Sect. S1. However, this method of adjusting source profiles cannot truly address the issue of reactive losses affecting the CMB-apportioned results, since the receptor-measured data and adjusted source profiles input to CMB were both data after reactive losses. With the progress of VOC source analyses studies in recent years, this method has not been widely applied and further developed in CMB source analyses. This change might be because CMB itself required input from VOC source profiles, but there were relatively few locally measured VOC source profiles due to high costs and difficulties in obtaining access to the source facilities (Y. Yang et al., 2022).
Alternatively, with the monitoring of highly time-resolved VOC data in recent years, it became infeasible to use CMB to conduct source analyses because CMB requires analysis of one sample (1 h) at a time resulting in needing thousands of separate analyses (Y. Yang et al., 2022). Additionally, the weights in the analyses are generally based only on the measurement uncertainties in the measurement samples from specific sources at a few specific times. Thus, CMB does not account for the variability in the source profiles that is included in the uncertainty values used to weight the data in PMF (Y. Yang et al., 2022). To reduce the impact of reactive losses, the initial concentration data estimated by the photochemical-age-based parameterization method has been used as input into CMB for source analyses (Shao et al., 2011). Additionally, the methods for reducing the impacts of reactive losses in PMF and CMB primarily focused on the PAMS species, and there were few considerations for OVOCs.
3.2 Effects of reactive losses on source analyses
According to the review of relevant publications, it was found that most of the current studies analyzed the impacts of VOC photochemical losses on the source analyses by comparing the PMF-apportioned results based on measured and initial concentrations estimated by a photochemical-age-based parameterization method (Gao et al., 2018; Gu et al., 2023; Kong et al., 2023; Li et al., 2023; Z. G. Liu et al., 2023; Zou et al., 2023) or comparing the apportioned results based on the daytime and nighttime VOC data (Liu et al., 2025). Reactive losses substantially reduced the concentrations (ppbv ppbv−1) of highly reactive VOC species in PMF-resolved factor profiles based on the measured data. For example, B. S. Liu et al. (2023) investigated the impacts of photochemical losses of ambient VOCs on the PMF-resolved profiles by comparing the initial and measured data results. They found that the concentrations of VOC species with relatively low reactivities (e.g., ethane, propane, n-butane, and i-butane) were higher in the factor profiles apportioned from the measured data, while those of VOC species with relatively high reactivities (e.g., m,p-xylene, isoprene, and propene) were lower in the measured data-resolved profiles. Gu et al. (2023) also reported the similar results. Meanwhile, Liu et al. (2025) also reported that reactive losses clearly reduced the concentrations of dominant VOC species with high reactivities in the profiles of solvent use, petrochemical industry emissions, and combustion sources by comparing the daytime- and nighttime-resolved profiles.
Additionally, VOC reactive losses can result in the substantial underestimation of the PMF-apportioned contributions of sources that emitted highly reactive species, and emission sources with substantially underestimated contributions varied in different cities. For example, Wang et al. (2013) found that the contributions of biogenic emissions and chemical industry in Shanghai were underestimated by 30 % and 10 %, respectively, due to photochemical reactive losses. He et al. (2024) found that the underestimations of the industrial source contributions in Guangzhou were markedly higher than those of other sources. B. S. Liu et al. (2023) suggested that biogenic emissions and polymer-production-related industrial sources in Tianjin were underestimated by 73 % and 50 %, respectively. In addition, Y. J. Wu et al. (2023), J. Q. Zhang et al. (2024), and Gu et al. (2023) also suggested that the underestimations of the contributions of biogenic emissions in Beijing, Langfang, and Qingdao were substantially higher than those of any other sources. However, T. T. Wang et al. (2024) found that the contributions of solvent usage and biomass burning in Zhengzhou were underestimated by 31.5 % and 15.4 %, thus higher than other sources. Cui et al. (2024) suggested that the contributions of petrochemical industries, diesel vehicle emissions, biogenic emissions, and oxidation formation in Shijiazhuang were underestimated by 72.0 %, 71.0 %, 64.5 %, and 44.0 %, respectively. However, due to the uncertainty in initial concentration estimation, the reliability of these results needs further validation and evaluation. Thus, further research is required.
3.3 Estimation methods of VOC reactive losses
There were two main methods for estimating the reactive losses of VOCs in the atmosphere. The first method was based on the differences between the initial and measured VOC concentrations (Table S9) (as shown in Eq. 9) (Wang et al., 2023; Y. T. Wu et al., 2023). The initial concentration was generally estimated using the photochemical-age-based parameterization method mentioned in Sect. 3.1.1 (as shown in Eq. 1) (B. S. Liu et al., 2023; Y. T. Wu et al., 2023). Due to the uncertainty in the initial concentration estimation, there were also uncertainties in the estimated photochemical losses.
where [VOC]C,t represents the photochemical loss of VOC at time t, kVOC represents the reaction rate constants with •OH radicals of VOC, and [•OH]Δt represents the exposure of •OH radicals.
The second method applies to other VOC species without isoprene in which the losses could be estimated utilizing isoprene conversion (Wiedinmyer et al., 2001). In this study, it was defined as the isoprene loss reference method (Table S9). Its principle is to first use the photochemical age parameter method based on the sequential reaction model, as shown in Eqs. (1) and (6)–(7), to estimate the photochemical loss of isoprene (i.e., Δ isoprene), and then the photochemical losses of other VOC species can be estimated by the proportional relationship ( between Δ isoprene and other species losses (Wiedinmyer et al., 2001; Xie et al., 2008) (as shown in Eq. 10). This method assumes that the relative source strengths of VOCs are constant in the immediate area surrounding the site and that atmospheric transport and dispersion are non-limiting factors when compared with chemistry (Xie et al., 2008). The photochemical losses of other VOC species can be calculated by Eq. (10).
where kisoprene represents the reaction rate constants with •OH radicals of isoprene, and [isoprene]t represents the measured concentration of isoprene at time t.
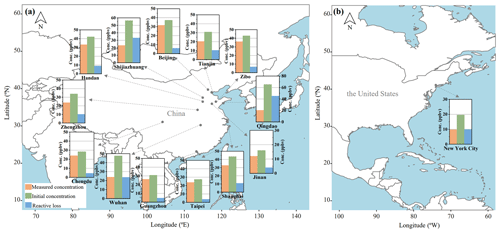
Figure 2The measured, initial, and reactive loss concentrations of ambient VOCs in Beijing (Gao et al., 2018; Ma et al., 2022; Zhan et al., 2021), Tianjin (B. S. Liu et al., 2023; Wang et al., 2023), Shanghai (Ren et al., 2024; Wang et al., 2013), Chengdu (Kong et al., 2023), Guangzhou (He et al., 2024; Wang et al., 2023), Qingdao (Gu et al., 2023), Shijiazhuang (Cui et al., 2024), Jinan (Z. G. Liu et al., 2023), Zhengzhou (T. T. Wang et al., 2024), Wuhan (Xu et al., 2023), Handan (Wei et al., 2022), Zibo (W. T. Wang et al., 2024), Taipei (Chen et al., 2023), and New York City (Borlaza-Lacoste et al., 2024). The data in Beijing, Tianjin, Shanghai, and Guangzhou were the average from all data in the published papers. The base map is from Natural Earth.
Isoprene mainly originates from natural sources (Fu et al., 2008; Kuhn et al., 2004; Lu et al., 2019), while many other VOC species are primarily emitted from anthropogenic sources (Li et al., 2021; Seinfeld and Pandis, 2006; Wei et al., 2011). Therefore, the assumption of this method itself has an obvious issue, which resulted in high uncertainties in the estimations. This defect substantially limits its application, only two publications have utilized this method to quantify chemical losses of VOCs in this study (Wiedinmyer et al., 2001; Xie et al., 2008), and most studies still used the first method (Table S9).
3.4 Spatiotemporal variation in VOC reactive losses
The VOC reactive losses in different cities are provided in Figs. 2–3 and S1 and Tables S10–S11. According to the papers reviewed in this study, we found that studies on the estimation of ambient VOC photochemical losses were primarily conducted in Chinese cities. To date, there are only two publications reporting quantitative research of VOC reactive losses conducted outside of China (i.e., Borlaza-Lacoste et al., 2024; Kalbande et al., 2022). The data of VOC reactive losses in the study conducted in New York City in the USA (Borlaza-Lacoste et al., 2024) have been included in Figs. 2–3. However, due to the fact that only nine VOC species were measured in the research conducted in Mumbai in India (Kalbande et al., 2022), the reactive loss data in their studies were difficult to compare and analyze with data from other cities; therefore, their estimated results were not shown in Figs. 2 and 3. Due to relatively limited number of studies, the representativeness of the analyses of the spatiotemporal distributions of photochemical losses of VOCs might also be limited. Meanwhile, due to the relative lack of the quantitative studies on the ambient VOC reactive losses in the cities outside of China, the comparative analyses of the differences in reactive losses of VOCs in different cities and the impacts of losses on air secondary pollution from a global perspective in this study could be insufficient. In addition, this study converted the unit of ppbC (parts per billion carbon) in some papers (Chen et al., 2023) to ppbv (parts per billion by volume) for better comparative analyses.
The photochemical reactive loss of ambient VOCs in Qingdao was the highest (45.1 ppbv), followed by Shijiazhuang (33.2 ppbv), Wuhan (23.7 ppbv), Shanghai (10.9 ppbv), Tianjin (10.4 ppbv), Zhengzhou (10.2 ppbv), New York City (9.84 ppbv), and Handan (8.90 ppbv) (Fig. 2). VOC reactive losses were relatively lower in Zibo (6.8 ppbv), Beijing (6.00 ppbv), Guangzhou (4.65 ppbv), Chengdu (4.48 ppbv), Jinan (4.00 ppbv), and Taipei (3.69 ppbv). The chemical loss rates (i.e., the proportion of chemical loss in the initial concentration; %) in Qingdao (69.1 %) and Shijiazhuang (58.9 %) were the highest, followed by New York City (50.2 %), Wuhan (49.8 %), and Tianjin (33.8 %). In contrast, chemical loss rates in Zhengzhou (29.9 %), Shanghai (25.1 %), Jinan (25.0 %), Handan (21.1 %), Beijing (16.1 %), Zibo (15.9 %), Chengdu (15.8 %), Guangzhou (15.1 %), and Taipei (13.4 %) were relatively lower. However, due to differences in observation periods and measured VOC species, the comparability of chemical reactive losses and loss rates between different cities is limited and the differences uncertain.
Compared to other VOC groups, alkenes had the highest reactive loss (Figs. 3 and S1), accounting for 36.7 %–93.3 % of the total losses, followed by aromatic hydrocarbons (3.8 %–24.3 %) and alkanes (2.3 %–13.6 %) (Fig. 3 and Table S11). There were substantial differences in VOC species with high losses in different cities (Fig. 3). The losses of ethene, propene, and isoprene in most cities were relatively higher than those of other species (Fig. 3), likely closely related to their high reactivities (Table S4). The isoprene losses in Beijing, Chengdu, Jinan, Taipei, and New York City were all the highest compared to other species (Fig. 3). However, the reactive losses of trans-2-butene and cis-2-butene in Qingdao and Zhengzhou were substantially higher than other VOC species. The reactive loss of 1-hexene in Tianjin was markedly higher compared to other species. Meanwhile, the trans-2-butene loss in Tianjin was also relatively higher (Fig. 3).
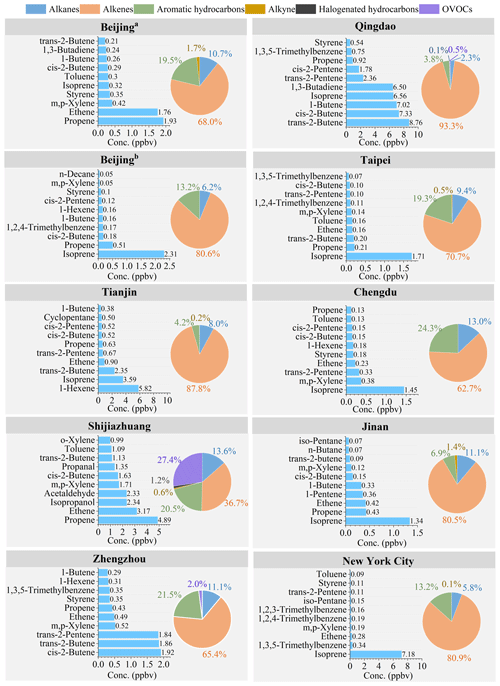
Figure 3The photochemical losses and percentages of the main VOCs in Beijing (a is cited from Gao et al., 2018, and b is cited from Zhan et al., 2021), Qingdao (Gu et al., 2023), Taipei (Chen et al., 2023), Tianjin (B. S. Liu et al., 2023), Chengdu (Kong et al., 2023), Shijiazhuang (Cui et al., 2024), Jinan (Z. G. Liu et al., 2023), Zhengzhou (T. T. Wang et al., 2024), and New York City (Borlaza-Lacoste et al., 2024).
In addition, the VOC reactive losses in spring and summer were substantially higher than those in autumn and winter. Atmospheric oxidation and meteorological factors can have impacts on the VOC losses. The trend of the reactive losses of VOCs was often consistent with the changes in the concentrations of Ox (O3+ NO2) in the atmosphere (Wang et al., 2013). In addition, studies also found that both solar radiation and temperature can have a substantial impact on the chemical reactions of VOCs (Yadav et al., 2016). However, compared to the solar radiation, temperature directly affects the reaction rates of VOC species (Atkinson and Arey, 2003). B. S. Liu et al. (2023) suggested that the reactive losses of ambient VOCs were highly correlated with temperature; when the temperature was above 25 °C, the losses of VOCs increased most substantially.
3.5 Source analyses of VOC reactive losses in the atmosphere
At present, source apportionments utilizing the measured and initial VOC concentrations do not consider the complementary issues of O3 and SOA formation (Cui et al., 2024; Gu et al., 2023; Z. Y. Wang et al., 2022). The chemical losses of VOCs by reaction (i.e., reactive VOCs forming SOA and O3) were real contributors to these pollutants (Ma et al., 2022; Wang et al., 2013). Thus, it is important to apportion the reactive losses of VOCs to provide the input needed to identify the responsible source types and thereby allow effective control of O3 and secondary aerosol pollution. In 2023, Gu et al. (2023) developed a source analyses method for consumed VOCs (i.e., the reactive losses of VOCs) in the atmosphere and conducted an applied study. This method required that the measured and initial data were first used to conduct source analyses by PMF, and then the difference in the contributions of the same factors from the paired analyses were considered to be the source contribution of consumed VOCs in the atmosphere. The ozone formation potential (OFP) and SOA formation potential (SOAFP) of the consumed VOCs from the different sources were estimated. To reduce the impacts of atmospheric dispersion on the apportioned results, dispersion-normalized PMF (DN-PMF) was applied for source analyses in this method, and its principle is provided by Dai et al. (2020) and Gu et al. (2022). The method flowchart is shown in Fig. 4. Similar studies were reported by Z. Y. Wang et al. (2022) and Wang et al. (2023). In 2024, based on the studies of Gu et al. (2023), Cui et al. (2024) developed a primary and oxidative source analyses method of consumed VOCs in the atmosphere. However, the current methods for apportioning the primary or oxidative sources of consumed VOCs in the atmosphere are still imperfect. The shortcomings included the uncertainty in estimating the initial concentrations of VOCs (as shown in Sect. 3.1.1) and the issue of factor identification, even if identified as the same type of factor; there were substantial differences in their factor profiles (B. S. Liu et al., 2023). The current studies primarily focused on the PAMS species, and reported apportionments of OVOC losses are still limited. There were numerous technical issues; for example, there is still a lack of rational methods for estimating the OVOC initial concentrations.
The source analyses of the consumed VOCs conducted by Gu et al. (2023) in Qingdao found that biogenic emissions (56.3 %), vehicle emissions (17.2 %), and gasoline evaporation (9.37 %) were the main sources of the consumed VOCs. However, the apportioned results from Wang et al. (2023) in Tianjin and Guangzhou suggested that biogenic emissions (43 % and 35 %, respectively), solvent usage (14 % and 18 %, respectively), and industrial sources (14 % and 22 %, respectively) were the major contributors of the consumed VOCs. Therefore, biogenic emissions might be an important source of reactive losses of VOCs. However, Cui et al. (2024) suggested that the petrochemical industries (36.9 % and 51.7 %) and oxidation formation (20.6 % and 35.6 %) were the largest contributions to the consumed VOCs and OVOCs in Shijiazhuang during the study period. The natural gas (5.0 % and 7.6 %) and the mixed source of liquefied petroleum gas and solvent use (3.1 % and 4.2 %) had relatively low contributions (Cui et al., 2024). However, due to the limited research currently available, the representativeness of the results is still insufficient at present.
This study systematically reviewed the major methods of reducing the impacts of reactive loss and the existing critical issues in the current VOC source analyses research. The purpose was to clarify the future research directions needed to improve the accuracy of VOC source apportionments and provide a potential supporting role in completely solving the issue of VOC chemical conversion. PMF is currently the most useful tool in treating highly time-resolved data compared to other receptor models. Estimating the initial concentrations of ambient VOCs based on photochemical age is the primary approach to reduce reactive loss effects in PMF. However, due to the shortcomings existing in the photochemical age estimation method, such as only considering the photochemical reactions with •OH radicals during the day and not considering the reactions with O3 (especially for alkene species) and NO3 radicals; difficulty in dynamically adjusting the required •OH-radical reaction rate constants with temperature changes; high uncertainty in the estimation of photochemical age; and difficulty in quantifying the initial concentrations of OVOCs, etc., resulting in substantial uncertainty in the PMF-apportioned results based on the initial concentration data. Furthermore, both the measured and initial VOC data do not match the ambient O3 or SOA measured synchronously because the VOCs consumed by reactions are real contributors to these pollutants. Source analyses of the consumed VOCs can effectively guide the prevention and control of O3 and SOA pollution in the atmosphere. Therefore, in addition to the research into more accurate quantitative approaches for ambient VOC reactive losses, the source analyses of consumed VOCs and the accurate quantification of their separate contributions to O3 and SOA should also be important directions for future research.
The data in this study are available from the corresponding authors upon request (lbsnankai@foxmail.com, fengyc@nankai.edu.cn).
The supplement related to this article is available online at: https://doi.org/10.5194/acp-24-12861-2024-supplement.
BL: writing (original draft and review and editing). YG: data curation and writing (original draft). YW: data curation. QD and SS: investigation and supervision. YF: supervision. PKH: supervision (review and editing).
The contact author has declared that none of the authors has any competing interests.
Publisher’s note: Copernicus Publications remains neutral with regard to jurisdictional claims made in the text, published maps, institutional affiliations, or any other geographical representation in this paper. While Copernicus Publications makes every effort to include appropriate place names, the final responsibility lies with the authors.
This research has been supported by the National Key R&D Program of China (grant no. 2023YFC3705801) and the National Natural Science Foundation of China (grant no. 42177085).
This paper was edited by Eva Y. Pfannerstill and reviewed by two anonymous referees.
Aronian, P. F., Scheff, P. A., and Wadden, R. A.: Wintertime source-reconciliation of ambient organics, Atmos. Environ., 23, 911–920, https://doi.org/10.1016/0004-6981(89)90295-3, 1989.
Atkinson, R.: Kinetics and mechanisms of the gas-phase reactions of the NO3 radical with organic compounds, J. Phys. Chem. Ref. Data, 20, 459–507, https://doi.org/10.1063/1.555887, 1991.
Atkinson, R. and Arey, J.: Atmospheric degradation of volatile organic compounds, Chem. Rev., 103, 4605–4638, https://doi.org/10.1002/chin.200410285, 2003.
Atkinson, R., Baulch, D. L., Cox, R. A., Crowley, J. N., Hampson, R. F., Hynes, R. G., Jenkin, M. E., Rossi, M. J., Troe, J., and IUPAC Subcommittee: Evaluated kinetic and photochemical data for atmospheric chemistry: Volume II – gas phase reactions of organic species, Atmos. Chem. Phys., 6, 3625–4055, https://doi.org/10.5194/acp-6-3625-2006, 2006.
Atkinson, R.: Gas-phase tropospheric chemistry of organic compounds: a review, Atmos. Environ., 41, 200–240, https://doi.org/10.1016/j.atmosenv.2007.10.068, 2007.
Bertman, S. B., Roberts, J. M., Parrish, D. D., Buhr, M. P., Goldan, P. D., Kuster, W. C., Fehsenfeld, F. C., Montzka, S. A., and Westberg, H.: Evolution of alkyl nitrates with air mass age, J. Geophys. Res., 100, 22805–22813, https://doi.org/10.1029/95JD02030, 1995.
Bey, I., Aumont, B., and Toupance, G.: A modeling study of the nighttime radical chemistry in the lower continental troposphere: 2. Origin and evolution of HOX, J. Geophys. Res., 106, 9991–10001, https://doi.org/10.1029/2000jd900348, 2001.
Borlaza-Lacoste, L., Bari, M. A., Lu, C. H., and Hopke, P. K.: Long-term contributions of VOC sources and their link to ozone pollution in Bronx, New York City, Environ. Int., 191, 108993, https://doi.org/10.1016/j.envint.2024.108993, 2024.
Buzcu-Guven, B. and Fraser, M. P.: Comparison of VOC emissions inventory data with source apportionment results for Houston, TX, Atmos. Environ., 42, 5032–5043, https://doi.org/10.1016/j.atmosenv.2008.02.025, 2008.
Buzcu, B. and Fraser, M. P.: Source identification and apportionment of volatile organic compounds in Houston, TX, Atmos. Environ., 40, 2385–2400, https://doi.org/10.1016/j.atmosenv.2005.12.020, 2006.
Carrillo-Torres, E. R., Hernández-Paniagua, I. Y., and Mendoza, A.: Use of combined observational- and model-derived photochemical indicators to assess the O3-NOx-VOC system sensitivity in urban areas, Atmosphere, 8, 22, https://doi.org/10.3390/atmos8020022, 2017.
Carter, W. P. L. and Atkinson, R.: Development and evaluation of a detailed mechanism for the atmospheric reactions of isoprene and NOx, Int. J. Chem. Kinet., 28, 497–530, https://doi.org/10.1002/(SICI)1097-4601(1996)28:7<497::AID-KIN4>3.0.CO;2-Q, 1996.
Carter, W. P. L.: Development of the SAPRC-07 chemical mechanism, Atmos. Environ., 44, 5324–5335, https://doi.org/10.1016/j.atmosenv.2010.01.026, 2010.
Che, H., Xia, X., Zhao, H., Dubovik, O., Holben, B. N., Goloub, P., Cuevas-Agulló, E., Estelles, V., Wang, Y., Zhu, J., Qi, B., Gong, W., Yang, H., Zhang, R., Yang, L., Chen, J., Wang, H., Zheng, Y., Gui, K., Zhang, X., and Zhang, X.: Spatial distribution of aerosol microphysical and optical properties and direct radiative effect from the China Aerosol Remote Sensing Network, Atmos. Chem. Phys., 19, 11843–11864, https://doi.org/10.5194/acp-19-11843-2019, 2019.
Chen, C.-H., Chuang, Y.-C., Hsieh, C.-C., and Lee, C.-S.: VOC characteristics and source apportionment at a PAMS site near an industrial complex in central Taiwan, Atmos. Pollut. Res., 10, 1060–1074, https://doi.org/10.1016/j.apr.2019.01.014, 2019.
Chen, S.-P., Liu, T.-H., Chen, T.-F., Yang, C.-F. O., Wang, J.-L., and Chang, J. S.: Diagnostic modeling of PAMS VOC observation, Environ. Sci. Technol., 44, 4635–4644, https://doi.org/10.1021/es903361r, 2010.
Chen, W. T., Shao, M., Lu, S. H., Wang, M., Zeng, L. M., Yuan, B., and Liu, Y.: Understanding primary and secondary sources of ambient carbonyl compounds in Beijing using the PMF model, Atmos. Chem. Phys., 14, 3047–3062, https://doi.org/10.5194/acp-14-3047-2014, 2014.
Chen, Z.-W., Ting, Y.-C., Huang, C.-H., and Ciou, Z.-J.: Sources-oriented contributions to ozone and secondary organic aerosol formation potential based on initial VOCs in an urban area of Eastern Asia, Sci. Total Environ., 892, 164392, https://doi.org/10.1016/j.scitotenv.2023.164392, 2023.
Cui, Y. Q., Liu, B. S., Yang, Y. F., Kang, S. C., Wang, F. Q., Xu, M., Wang, W., Feng, Y. C., and Hopke, P. K.: Primary and oxidative source analyses of consumed VOCs in the atmosphere, J. Hazard. Mater., 476, 134894, https://doi.org/10.1016/j.jhazmat.2024.134894, 2024.
Dai, Q. L., Liu, B. S., Bi, X. H., Wu, J. H., Liang, D. N., Zhang, Y. F., Feng, Y. C., and Hopke, P. K.: Dispersion normalized PMF provides insights into the significant changes in source contributions to PM2.5 after the COVID-19 outbreak, Environ. Sci. Technol., 54, 9917–9927, https://doi.org/10.1021/acs.est.0c02776, 2020.
de Gouw, J. A., Middlebrook, A. M., Warneke, C., Goldan, P. D., Kuster, W. C., Roberts, J. M., Fehsenfeld, F. C., Worsnop, D. R., Canagaratna, M. R., Pszenny, A. A. P., Keene, W. C., Marchewka, M., Bertman, S. B., and Bates, T. S.: Budget of organic carbon in a polluted atmosphere: Results from the New England Air Quality Study in 2002, J. Geophys. Res., 110, D16305, https://doi.org/10.1029/2004jd005623, 2005.
de Gouw, J. A., Gilman, J. B., Kim, S.-W., Lerner, B. M., Isaacman-VanWertz, G., McDonald, B. C., Warneke, C., Kuster, W. C., Lefer, B. L., Griffith, S. M., Dusanter, S., Stevens, P. S., and Stutz, J.: Chemistry of volatile organic compounds in the Los Angeles Basin: Nighttime removal of alkenes and determination of emission ratios, J. Geophys. Res., 122, 11843–11861, https://doi.org/10.1002/2017JD027459, 2017.
de Gouw, J. A., Gilman, J. B., Kim, S. W., Alvarez, S. L., Dusanter, S., Graus, M., Griffith, S. M., Isaacman-VanWertz, G., Kuster, W. C., Lefer, B. L., Lerner, B. M., McDonald, B. C., Rappenglück, B., Roberts, J. M., Stevens, P. S., Stutz, J., Thalman, R., Veres, P. R., Volkamer, R., Warneke, C., Washenfelder, R. A., and Young, C. J.: Chemistry of volatile organic compounds in the Los Angeles Basin: Formation of oxygenated compounds and determination of emission ratios, J. Geophys. Res.-Atmos., 123, 2298–2319, https://doi.org/10.1002/2017JD027976, 2018.
Fang, H., Luo, S. L., Huang, X. Q., Fu, X. W., Xiao, S. X., Zeng, J. Q., Wang, J., Zhang, Y. L., and Wang, X. M.: Ambient naphthalene and methylnaphthalenes observed at an urban site in the Pearl River Delta region: Sources and contributions to secondary organic aerosol, Atmos. Environ., 252, 118295, https://doi.org/10.1016/j.atmosenv.2021.118295, 2021.
Finlayson-Pitts, B. J. and Pitts, J. N.: Tropospheric air pollution: Ozone, airborne toxics, polycyclic aromatic hydrocarbons, and particles, Science, 276, 1045–1052, https://doi.org/10.1126/science.276.5315.1045, 1997.
Friedlander, S. K.: New Developments in Receptor Modeling Theory, in: Atmospheric Aerosol: Source/Air Quality Relationships, edited by: Macias, E. S. and Hopke, P. K., ACS Symposium Series No. 167, American Chemical Society: Washington, 19 pp., http://pubs.acs.org/doi/pdf/10.1021/bk-1981-0167.ch001 (last access: 12 November 2024), 1981.
Fu, T.-M., Jacob, D. J., Wittrock, F., Burrows, J. P., Vrekoussis, M., and Henze, D. K.: Global budgets of atmospheric glyoxal and methylglyoxal, and implications for formation of secondary organic aerosols, J. Geophys. Res., 113, D15303, https://doi.org/10.1029/2007jd009505, 2008.
Gao, J., Zhang, J., Li, H., Li, L., Xu, L. H., Zhang, Y. J., Wang, Z. S., Wang, X. Z., Zhang, W. Q., Chen, Y. Z., Cheng, X., Zhang, H., Peng, L., Chai, F. H., and Wei, Y. J.: Comparative study of volatile organic compounds in ambient air using observed mixing ratios and initial mixing ratios taking chemical loss into account – A case study in a typical urban area in Beijing, Sci. Total Environ., 628–629, 791–804, https://doi.org/10.1016/j.scitotenv.2018.01.175, 2018.
Gong, D., Wang, H., Zhang, S., Wang, Y., Liu, S. C., Guo, H., Shao, M., He, C., Chen, D., He, L., Zhou, L., Morawska, L., Zhang, Y., and Wang, B.: Low-level summertime isoprene observed at a forested mountaintop site in southern China: implications for strong regional atmospheric oxidative capacity, Atmos. Chem. Phys., 18, 14417–14432, https://doi.org/10.5194/acp-18-14417-2018, 2018.
Gu, Y., Liu, B. S., Li, Y. F., Zhang, Y. F., Bi, X. H., Wu, J. H., Song, C. B., Dai, Q. L., Han, Y., Ren, G., and Feng, Y. C.: Multi-scale volatile organic compound (VOC) source apportionment in Tianjin, China, using a receptor model coupled with 1-hr resolution data, Environ. Pollut., 265, 115023, https://doi.org/10.1016/j.envpol.2020.115023, 2020.
Gu, Y., Liu, B. S., Dai, Q. L., Zhang, Y. F., Zhou, M., Feng, Y. C., and Hopke, P. K.: Multiply improved positive matrix factorization for source apportionment of volatile organic compounds during the COVID-19 shutdown in Tianjin, China, Environ. Int., 158, 106979, https://doi.org/10.1016/j.envint.2021.106979, 2022.
Gu, Y., Liu, B. S., Meng, H., Song, S. J., Dai, Q. L., Shi, L. Y., Feng, Y. C., and Hopke, P. K.: Source apportionment of consumed volatile organic compounds in the atmosphere, J. Hazard Mater., 459, 132138, https://doi.org/10.1016/j.jhazmat.2023.132138, 2023.
Guan, Y. N., Wang, L., Wang, S. J., Zhang, Y. H., Xiao, J. Y., Wang, X. L., Duan, E. H., and Hou, L. A.: Temporal variations and source apportionment of volatile organic compounds at an urban site in Shijiazhuang, China, J. Environ. Sci., 97, 25–34, https://doi.org/10.1016/j.jes.2020.04.022, 2020.
Han, Y., Huang, X. F., Wang, C., Zhu, B., and He, L. Y.: Characterizing oxygenated volatile organic compounds and their sources in rural atmospheres in China, J. Environ. Sci., 81, 148–155, https://doi.org/10.1016/j.jes.2019.01.017, 2019.
Han, S. W., Tan, Y., Gao, Y., Li, X. W., Ho, S. S. H., Wang, M., and Lee, S. C.: Volatile organic compounds at a roadside site in Hong Kong: Characteristics, chemical reactivity, and health risk assessment, Sci. Total Environ., 866, 161370, https://doi.org/10.1016/j.scitotenv.2022.161370, 2023.
Harley, R. A., Hannigan, M. P., and Cass, G. R.: Respeciation of organic gas emissions and the detection of excess unburned gasoline in the atmosphere, Environ. Sci. Technol., 26, 2395–2408, https://doi.org/10.1021/es00036a010, 1992.
He, Z., Wang, X., Ling, Z., Zhao, J., Guo, H., Shao, M., and Wang, Z.: Contributions of different anthropogenic volatile organic compound sources to ozone formation at a receptor site in the Pearl River Delta region and its policy implications, Atmos. Chem. Phys., 19, 8801–8816, https://doi.org/10.5194/acp-19-8801-2019, 2019.
He, C. Q., Zou, Y., Lv, S. J., Flores, R. M., Yan, X. L., Deng, T., and Deng, X. J.: The importance of photochemical loss to source analysis and ozone formation potential: Implications from in-situ observations of volatile organic compounds (VOCs) in Guangzhou, China, Atmos. Environ., 320, 120320, https://doi.org/10.1016/j.atmosenv.2023.120320, 2024.
Huang, X.-F., Wang, C., Zhu, B., Lin, L.-L., and He, L.-Y.: Exploration of sources of OVOCs in various atmospheres in southern China, Environ. Pollut., 249, 831–842, https://doi.org/10.1016/j.envpol.2019.03.106, 2019.
Huang, X.-F., Zhang, B., Xia, S.-Y., Han, Y., Wang, C., Yu, G.-H., and Feng, N.: Sources of oxygenated volatile organic compounds (OVOCs) in urban atmospheres in North and South China, Environ. Pollut., 261, 114152, https://doi.org/10.1016/j.envpol.2020.114152, 2020.
Jain, V., Tripathi, S. N., Tripathi, N., Sahu, L. K., Gaddamidi, S., Shukla, A. K., Bhattu, D., and Ganguly, D.: Seasonal variability and source apportionment of non-methane VOCs using PTR-TOF-MS measurements in Delhi, India, Atmos. Environ., 283, 119163, https://doi.org/10.1016/j.atmosenv.2022.119163, 2022.
Jia, C. H., Mao, X. X., Huang, T., Liang, X. X., Wang, Y. N., Shen, Y. J., Jiang, W. Y. H., Wang, H. Q., Bai, Z. L., Ma, M. Q., Yu, Z. S., Ma, J. M., and Gao, H.: Non-methane hydrocarbons (NMHCs) and their contribution to ozone formation potential in a petrochemical industrialized city, Northwest China, Atmos. Res., 169, 225–236, https://doi.org/10.1016/j.atmosres.2015.10.006, 2016.
Junninen, H., Borbon, A., Astorga, C., Locoge, N., and Larsen, B. R.: Source apportionment of Ozone precursor VOCs in urban atmospheres by receptor modelling, in 5th International Conference on Urban Air Quality, Valencia, Spain (CD-ROM), 29–31 March 2005, https://www.researchgate.net/publication/236972501 (last access: 12 November 2024), 2005.
Kalbande, R., Yadav, R., Maji, S., Rathore, D. S., and Beig, G.: Characteristics of VOCs and their contribution to O3 and SOA formation across seasons over a metropolitan region in India, Atmos. Pollut. Res., 13, 101515, https://doi.org/10.1016/j.apr.2022.101515, 2022.
Kim, E., Brown, S. G., Hafner, H. R., and Hopke, P. K.: Characterization of non-methane volatile organic compounds sources in Houston during 2001 using positive matrix factorization, Atmos. Environ., 39, 5934–5946, https://doi.org/10.1016/j.atmosenv.2005.06.045, 2005.
Kong, L., Zhou, L., Chen, D. Y., Luo, L., Xiao, K., Chen, Y., Liu, H. F., Tan, Q. W., and Yang, F. M.: Atmospheric oxidation capacity and secondary pollutant formation potentials based on photochemical loss of VOCs in a megacity of the Sichuan Basin, China, Sci. Total Environ., 901, 166259, https://doi.org/10.1016/j.scitotenv.2023.166259, 2023.
Kornilova, A., Huang, L., Saccon, M., and Rudolph, J.: Stable carbon isotope ratios of ambient aromatic volatile organic compounds, Atmos. Chem. Phys., 16, 11755–11772, https://doi.org/10.5194/acp-16-11755-2016, 2016.
Kuhn, U., Rottenberger, S., Biesenthal, T., Wolf, A., Schebeske, G., Ciccioli, P., Brancaleoni, E., Frattoni, M., Tavares, T. M., and Kesselmeier, J.: Seasonal differences in isoprene and light-dependent monoterpene emission by Amazonian tree species, Glob. Change Biol., 10, 663–682, https://doi.org/10.1111/j.1529-8817.2003.00771.x, 2004.
Legreid, G., Folini, D., Staehelin, J., Lööv, J. B., Steinbacher, M., and Reimann, S.: Measurements of organic trace gases including oxygenated volatile organic compounds at the high alpine site Jungfraujoch (Switzerland): Seasonal variation and source allocations, J. Geophys. Res., 113, D05307, https://doi.org/10.1029/2007jd008653, 2008.
Lewis, C. W., Conner, T. L., Stevens, R. K., Collins, J. F., and Henry, R. C.: Receptor modeling of volatile hydrocarbons measured in the 1990 Atlanta Ozone Precursor Study, paper No. 93-TP-58.04, 86th Annual Meeting, Denver, CO, Air and Waste Management Association, Pittsburgh, PA, 13–18 June 1993, https://www.zhangqiaokeyan.com/ntis-science-report_pb_thesis/02071928258.html (last access: 12 November 2024), 1993.
Li, B. W., Ho, S. S. H., Li, X. H., Guo, L. Y., Chen, A. O., Hu, L. T., Yang, Y., Chen, D., Lin, A. A., and Fang, X. K.: A comprehensive review on anthropogenic volatile organic compounds (VOCs) emission estimates in China: Comparison and outlook, Environ. Int., 156, 106710, https://doi.org/10.1016/j.envint.2021.106710, 2021.
Li, B. W., Yu, S. C., Shao, M., Li, X. H., Ho, S. S. H., Hu, X. Y., Wang, H. L., Feng, R., and Fang, X. K.: New insights into photochemical initial concentrations of VOCs and their source implications, Atmos. Environ., 298, 119616, https://doi.org/10.1016/j.atmosenv.2023.119616, 2023.
Li, J., Wu, R., Li, Y., Hao, Y., Xie, S., and Zeng, L.: Effects of rigorous emission controls on reducing ambient volatile organic compounds in Beijing, China, Sci. Total Environ., 557–558, 531–541, https://doi.org/10.1016/j.scitotenv.2016.03.140, 2016.
Li, J., Zhai, C. Z., Yu, J. Y., Liu, R. L., Li, Y. Q., Zeng, L. M., and Xie, S. D.: Spatiotemporal variations of ambient volatile organic compounds and their sources in Chongqing, a mountainous megacity in China, Sci. Total Environ., 627, 1442–1452, https://doi.org/10.1016/j.scitotenv.2018.02.010, 2018.
Li, K., Jacob, D. J., Shen, L., Lu, X., De Smedt, I., and Liao, H.: Increases in surface ozone pollution in China from 2013 to 2019: anthropogenic and meteorological influences, Atmos. Chem. Phys., 20, 11423–11433, https://doi.org/10.5194/acp-20-11423-2020, 2020.
Li, Z. Y., Xue, L. K., Yang, X., Zha, Q. Z., Tham, Y. J., Yan, C., Louie, P. K. K., Luk, C. W. Y., Wang, T., and Wang, W. X.: Oxidizing capacity of the rural atmosphere in Hong Kong, Southern China, Sci. Total Environ., 612, 1114–1122, https://doi.org/10.1016/j.scitotenv.2017.08.310, 2018.
Li, Z. Y., Ho, K. F., and Yim, S. H. L.: Source apportionment of hourly-resolved ambient volatile organic compounds: Influence of temporal resolution, Sci. Total Environ., 725, 138243, https://doi.org/10.1016/j.scitotenv.2020.138243, 2020.
Lin, C. and Milford, D. B.: Decay-adjusted chemical mass balance receptor modeling for volatile organic compounds, Atmos. Environ., 28, 3261–3276, https://doi.org/10.1016/1352-2310(94)00163-F, 1994.
Lin, C.-C., Lin, C., Hsieh, L.-T., Chen, C.-Y., and Wang, J.-P.: Vertical and diurnal characterization of volatile organic compounds in ambient air in urban areas, J. Air Waste Manage., 61, 714–720, https://doi.org/10.3155/1047-3289.61.7.714, 2011.
Liu, B. S., Liang, D. N., Yang, J. M., Dai, Q. L., Bi, X. H., Feng, Y. C., Yuan, J., Xiao, Z. M., Zhang, Y. F., and Xu, H.: Characterization and source apportionment of volatile organic compounds based on 1-year of observational data in Tianjin, China, Environ. Pollut., 218, 757–769, https://doi.org/10.1016/j.envpol.2016.07.072, 2016.
Liu, B. S., Yang, Y., Yang, T., Dai, Q. L., Zhang, Y. F., Feng, Y. C., and Hopke, P. K.: Effect of photochemical losses of ambient volatile organic compounds on their source apportionment, Environ. Int., 172, 107766, https://doi.org/10.1016/j.envint.2023.107766, 2023.
Liu, B. S., Yang, T., Kang, S. C., Wang, F. Q., Zhang, H. X., Xu, M., Wang, W., Bai, J. R., Song, S. J., Dai, Q. L., Feng, Y. C., and Hopke, P. K.: Changes in factor profiles deriving from photochemical losses of volatile organic compounds: Insight from daytime and nighttime positive matrix factorization analyses, J. Environ. Sci., 151, 627–639, https://doi.org/10.1016/j.jes.2024.04.032, 2025.
Liu, C. T., Xin, Y. Y., Zhang, C. L., Liu, J. F., Liu, P. F., He, X. W., and Mu, Y. J.: Ambient volatile organic compounds in urban and industrial regions in Beijing: Characteristics, source apportionment, secondary transformation and health risk assessment, Sci. Total Environ., 855, 158873, https://doi.org/10.1016/j.scitotenv.2022.158873, 2023.
Liu, Z. G., Wang, B. L., Wang, C., Sun, Y. C., Zhu, C. Y., Sun, L., Yang, N., Fan, G. L., Sun, X. Y., Xia, Z. Y., Pan, G., Zhu, C. T., Gai, Y. C., Wang, X. Y., Xiao, Y., Yan, G. H., and Xu, C. Q.: Characterization of photochemical losses of volatile organic compounds and their implications for ozone formation potential and source apportionment during summer in suburban Jinan, China, Environ. Res., 238, 117158, https://doi.org/10.1016/j.envres.2023.117158, 2023.
Lu, X., Zhang, L., and Shen, L.: Meteorology and climate influences on tropospheric ozone: a review of natural sources, chemistry, and transport patterns, Curr. Pollut. Rep., 5, 238–260, https://doi.org/10.1007/s40726-019-00118-3, 2019.
Ma, W., Feng, Z., Zhan, J., Liu, Y., Liu, P., Liu, C., Ma, Q., Yang, K., Wang, Y., He, H., Kulmala, M., Mu, Y., and Liu, J.: Influence of photochemical loss of volatile organic compounds on understanding ozone formation mechanism, Atmos. Chem. Phys., 22, 4841–4851, https://doi.org/10.5194/acp-22-4841-2022, 2022.
McKeen, S. A. and Liu, S. C.: Hydrocarbon ratios and photochemical history of air masses, Geophys. Res. Lett., 20, 2363–2366, https://doi.org/10.1029/93GL02527, 1993.
McKeen, S. A., Liu, S. C., Hsie, E.-Y., Lin, X., Bradshaw, J. D., Smyth, S., Gregory, G. L., and Blake, D. R.: Hydrocarbon ratios during PEM-WEST A: A model perspective, J. Geophys. Res., 101, 2087–2109, https://doi.org/10.1029/95JD02733, 1996.
Mellouki, A., Wallington, T. J., and Chen, J.: Atmospheric chemistry of oxygenated volatile organic compounds: impacts on air quality and climate, Chem. Rev., 115, 3984–4014, https://doi.org/10.1021/cr500549n, 2015.
Meng, Z., Dabdub, D., and Seinfeld, J. H.: Chemical coupling between atmospheric ozone and particulate matter, Science, 277, 116–119, https://doi.org/10.1126/science.277.5322.116, 1997.
Mintz, R. and McWhinney, R. D.: Characterization of volatile organic compound emission sources in Fort Saskatchewan, Alberta using principal component analysis, J. Atmos. Chem., 60, 83–101, https://doi.org/10.1007/s10874-008-9110-5, 2008.
Mo, Z. W., Shao, M., Lu, S. H., Qu, H., Zhou, M. Y., Sun, J., and Gou, B.: Process-specific emission characteristics of volatile organic compounds (VOCs) from petrochemical facilities in the Yangtze River Delta, China, Sci. Total Environ., 533, 422–431, https://doi.org/10.1016/j.scitotenv.2015.06.089, 2015.
Mo, Z. W., Shao, M., and Lu, S. H.: Compilation of a source profile database for hydrocarbon and OVOC emissions in China, Atmos. Environ., 143, 209–217, https://doi.org/10.1016/j.atmosenv.2016.08.025, 2016.
Na, K., Kim, Y. P., Moon, I., and Moon, K.-C.: Chemical composition of major VOC emission sources in the Seoul atmosphere, Chemosphere, 55, 585–594, https://doi.org/10.1016/j.chemosphere.2004.01.010, 2004.
Na, K. and Pyo Kim, Y.: Chemical mass balance receptor model applied to ambient C2–C9 VOC concentration in Seoul, Korea: Effect of chemical reaction losses, Atmos. Environ., 41, 6715–6728, https://doi.org/10.1016/j.atmosenv.2007.04.054, 2007.
Nelson, P. F. and Quigley, S. M.: The m,p-xylenes: ethylbenzene ratio. A technique for estimating hydrocarbon age in ambient atmospheres, Atmos. Environ., 17, 659–662, https://doi.org/10.1016/0004-6981(83)90141-5, 1983.
Parrish, D. D., Hahn, C. J., Williams, E. J., Norton, R. B., Fehsenfeld, F. C., Singh, H. B., Shetter, J. D., Gandrud, B. W., and Ridley, B. A.: Indications of photochemical histories of Pacific air masses from measurements of atmospheric trace species at Point Arena, California, J. Geophys. Res., 97, 15883–15901, https://doi.org/10.1029/92JD01242, 1992.
Parrish, D. D., Stohl, A., Forster, C., Atlas, E. L., Blake, D. R., Goldan, P. D., Kuster, W. C., and de Gouw, J. A.: Effects of mixing on evolution of hydrocarbon ratios in the troposphere, J. Geophys. Res.-Atmos., 112, D10S34, https://doi.org/10.1029/2006jd007583, 2007.
Ren, H. R., Xia, Z. Y., Yao, L. B., Qin, G. M., Zhang, Y., Xu, H., Wang, Z., and Cheng, J. P.: Investigation on ozone formation mechanism and control strategy of VOCs in petrochemical region: insights from chemical reactivity and photochemical loss, Sci. Total Environ., 914, 169891, https://doi.org/10.1016/j.scitotenv.2024.169891, 2024.
Roberts, J. M., Fehsenfeld, F. C., Liu, S. C., Bollinger, M. J., Hahn, C., Albritton, D. L., and Sievers, R. E.: Measurements of aromatic hydrocarbon ratios and NOx concentrations in the rural troposphere: Observation of air mass photochemical aging and NOx removal, Atmos. Environ., 18, 2421–2432, https://doi.org/10.1016/0004-6981(84)90012-X, 1984.
Roberts, J. M., Marchewka, M., Bertman, S. B., Goldan, P., Kuster, W., de Gouw, J., Warneke, C., Williams, E., Lerner, B., Murphy, P., Apel, E., and Fehsenfeld, F. C.: Analysis of the isoprene chemistry observed during the New England Air Quality Study (NEAQS) 2002 intensive experiment, J. Geophys. Res., 111, D23S12, https://doi.org/10.1029/2006jd007570, 2006.
Rudolph, J. and Czuba, E.: On the use of isotopic composition measurements of volatile organic compounds to determine the “photochemical age” of an air mass, Geophys. Res. Lett., 27, 3865–3868, https://doi.org/10.1029/2000gl011385, 2000.
Sahu, L. K., Yadav, R., and Pal, D.: Source identification of VOCs at an urban site of western India: Effect of marathon events and anthropogenic emissions, J. Geophys. Res., 121, 2416–2433, https://doi.org/10.1002/2015jd024454, 2016.
Saito, T., Kawamura, K., Tsunogai, U., Chen, T. Y., Matsueda, H., Nakatsuka, T., Gamo, T., Uematsu, M., and Huebert, B. J.: Photochemical histories of nonmethane hydrocarbons inferred from their stable carbon isotope ratio measurements over east Asia, J. Geophys. Res., 114, D11303, https://doi.org/10.1029/2008jd011388, 2009.
Sanchez, M., Karnae, S., and John, K.: Source characterization of volatile organic compounds affecting the air quality in a coastal urban area of South Texas, Int. J. Env. Res. Pub. He., 5, 130–138, https://doi.org/10.3390/ijerph5030130, 2008.
Scheff, P. A. and Klevs, M.: Source-receptor analysis of volatile hydrocarbons, J. Environ. Eng., 113, 994–1005, https://doi.org/10.1061/(ASCE)0733-9372(1987)113:5(994), 1987.
Schlundt, C., Tegtmeier, S., Lennartz, S. T., Bracher, A., Cheah, W., Krüger, K., Quack, B., and Marandino, C. A.: Oxygenated volatile organic carbon in the western Pacific convective center: ocean cycling, air–sea gas exchange and atmospheric transport, Atmos. Chem. Phys., 17, 10837–10854, https://doi.org/10.5194/acp-17-10837-2017, 2017.
Seinfeld, J. H. and Pandis, S. N.: Atmospheric Chemistry and Physics: From Air Pollution to Climate Change, 2nd edn., John Wiley & Sons, Inc., New York, https://www.gbv.de/dms/goettingen/50408920X.pdf (last access: 12 November 2024), 2006.
Shao, M., Lu, S. H., Liu, Y., Xie, X., Chang, C. C., Huang, S., and Chen, Z. M.: Volatile organic compounds measured in summer in Beijing and their role in ground-level ozone formation, J. Geophys. Res., 114, D00G06, https://doi.org/10.1029/2008jd010863, 2009.
Shao, M., Wang, B., Lu, S. H., Yuan, B., and Wang, M.: Effects of Beijing Olympics control measures on reducing reactive hydrocarbon species, Environ. Sci. Technol., 45, 514–519, https://doi.org/10.1021/es102357t, 2011.
Song, S.-K., Shon, Z.-H., Kang, Y.-H., Kim, K.-H., Han, S.-B., Kang, M., Bang, J.-H., and Oh, I.: Source apportionment of VOCs and their impact on air quality and health in the megacity of Seoul, Environ. Pollut., 247, 763–774, https://doi.org/10.1016/j.envpol.2019.01.102, 2019.
Song, Y., Dai, W., Shao, M., Liu, Y., Lu, S. H., Kuster, W., and Goldan, P.: Comparison of receptor models for source apportionment of volatile organic compounds in Beijing, China, Environ. Pollut., 156, 174–183, https://doi.org/10.1016/j.envpol.2007.12.014, 2008.
Stroud, C. A., Roberts, J. M., Goldan, P. D., Kuster, W. C., Murphy, P. C., Williams, E. J., Hereid, D., Parrish, D., Sueper, D., Trainer, M., Fehsenfeld, F. C., Apel, E. C., Riemer, D., Wert, B., Henry, B., Fried, A., Martinez-Harder, M., Harder, H., Brune, W. H., Li, G., Xie, H., and Young, V. L.: Isoprene and its oxidation products, methacrolein and methylvinyl ketone, at an urban forested site during the 1999 Southern Oxidants Study, J. Geophys. Res., 106, 8035–8046, https://doi.org/10.1029/2000jd900628, 2001.
Sun, J., Wu, F. K., Hu, B., Tang, G. Q., Zhang, J. K., and Wang, Y. S.: VOC characteristics, emissions and contributions to SOA formation during hazy episodes, Atmos. Environ., 141, 560–570, https://doi.org/10.1016/j.atmosenv.2016.06.060, 2016.
Talukdar, R. K., Mellouki, A., Gierczak, T., Barone, S., Chiang, S. Y., and Ravishankara, A. R.: Kinetics of the reactions of OH with alkanes, Int. J. Chem. Kinet., 26, 973–990, https://doi.org/10.1002/kin.550261003, 1994.
Tan, Q. W., Zhou, L., Liu, H. F., Feng, M., Qiu, Y., Yang, F. M., Jiang, W. J., and Wei, F. S.: Observation-based summer O3 control effect evaluation: A Case study in Chengdu, a megacity in Sichuan Basin, China, Atmosphere, 11, 1278, https://doi.org/10.3390/atmos11121278, 2020.
Tan, Y., Han, S. W., Chen, Y., Zhang, Z. Z., Li, H. W., Li, W. Q., Yuan, Q., Li, X. W., Wang, T., and Lee, S. C.: Characteristics and source apportionment of volatile organic compounds (VOCs) at a coastal site in Hong Kong, Sci. Total Environ., 777, 146241, https://doi.org/10.1016/j.scitotenv.2021.146241, 2021.
Tanimoto, H., Kameyama, S., Iwata, T., Inomata, S., and Omori, Y.: Measurement of air-sea exchange of dimethyl sulfide and acetone by PTR-MS coupled with gradient flux technique, Environ. Sci. Technol., 48, 526–533, https://doi.org/10.1021/es4032562, 2014.
Vega, E., Ramírez, O., Sánchez-Reyna, G., Chow, J. C., Watson, J. G., López-Veneroni, D., and Jaimes-Palomera, M.: Volatile organic compounds and carbonyls pollution in Mexico City and an urban industrialized area of Central Mexico, Aerosol Air Qual. Res., 22, 210386, https://doi.org/10.4209/aaqr.210386, 2022.
Wadden, R. A., Uno, I., and Wakamatsu, S.: Source discrimination of short-term hydrocarbon samples measured aloft, Environ. Sci. Technol., 20, 473–483, https://doi.org/10.1021/es00147a006, 1986.
Wan, Z. C., Song, K., Zhu, W. F., Yu, Y., Wang, H., Shen, R. Z., Tan, R., Lv, D. Q., Gong, Y. Z., Yu, X. N., Chen, S. Y., Zeng, L. M., Lou, S. R., Yu, Y. J., and Guo, S.: A closure study of secondary organic aerosol estimation at an urban site of Yangtze River Delta, China, Atmosphere, 13, 1679, https://doi.org/10.3390/atmos13101679, 2022.
Wang, B. L., Liu, Y., Shao, M., Lu, S. H., Wang, M., Yuan, B., Gong, Z. H., He, L. Y., Zeng, L. M., Hu, M., and Zhang, Y. H.: The contributions of biomass burning to primary and secondary organics: A case study in Pearl River Delta (PRD), China, Sci. Total Environ., 569, 548–556, https://doi.org/10.1016/j.scitotenv.2016.06.153, 2016.
Wang, C., Huang, X. F., Han, Y., Zhu, B., and He, L. Y.: Sources and potential photochemical roles of formaldehyde in an urban atmosphere in South China, J. Geophys. Res., 122, 11934–11947, https://doi.org/10.1002/2017jd027266, 2017.
Wang, G., Zhao, N., Zhang, H. Y., Li, G. H., and Xin, G.: Spatiotemporal distributions of ambient volatile organic compounds in China: Characteristics and sources, Aerosol Air Qual. Res., 22, 210379, https://doi.org/10.4209/aaqr.210379, 2022.
Wang, H. L., Chen, C. H., Wang, Q., Huang, C., Su, L. Y., Huang, H. Y., Lou, S. R., Zhou, M., Li, L., Qiao, L. P., and Wang, Y. H.: Chemical loss of volatile organic compounds and its impact on the source analysis through a two-year continuous measurement, Atmos. Environ., 80, 488–498, https://doi.org/10.1016/j.atmosenv.2013.08.040, 2013.
Wang, T. T., Tao, J., Li, Z., Lu, X., Liu, Y. L., Zhang, X. R., Wang, B., Zhang, D., and Yin, S. S.: Characteristic, source apportionment and effect of photochemical loss of ambient VOCs in an emerging megacity of Central China, Atmos. Res., 305, 107429, https://doi.org/10.1016/j.atmosres.2024.107429, 2024.
Wang, W. T., Zheng, Z. S., Liu, Y. H., Xu, B., Yang, W., Wang, X. L., Geng, C. M., and Bai, Z. P.: Quantification for photochemical loss of volatile organic compounds upon ozone formation chemistry at an industrial city (Zibo) in North China Plain, Environ. Res., 256, 119088, https://doi.org/10.1016/j.envres.2024.119088, 2024.
Wang, Z. Y., Shi, Z. B., Wang, F., Liang, W. Q., Shi, G. L., Wang, W. C., Chen, D., Liang, D. N., Feng, Y. C., and Russell, A. G.: Implications for ozone control by understanding the survivor bias in observed ozone-volatile organic compounds system, npj Clim. Atmos. Sci., 5, 39, https://doi.org/10.1038/s41612-022-00261-7, 2022.
Wang, Z. Y., Tian, X., Li, J., Wang, F., Liang, W. Q., Zhao, H., Huang, B., Wang, Z. H., Feng, Y. C., and Shi, G. L.: Quantitative evidence from VOCs source apportionment reveals O3 control strategies in northern and southern China, Environ. Int., 172, 107786, https://doi.org/10.1016/j.envint.2023.107786, 2023.
Watson, J. G., Chow, J. C., and Fujita, E. M.: Review of volatile organic compound source apportionment by chemical mass balance, Atmos. Environ., 35, 1567–1584, https://doi.org/10.1016/s1352-2310(00)00461-1, 2001.
Wei, W., Wang, S. X., Hao, J. M., and Cheng, S. Y.: Projection of anthropogenic volatile organic compounds (VOCs) emissions in China for the period 2010–2020, Atmos. Environ., 45, 6863–6871, https://doi.org/10.1016/j.atmosenv.2011.01.013, 2011.
Wei, W., Chen, S. S., Wang, Y., Cheng, L., Wang, X. Q., and Cheng, S. Y.: The impacts of VOCs on PM2.5 increasing via their chemical losses estimates: A case study in a typical industrial city of China, Atmos. Environ., 273, 118978, https://doi.org/10.1016/j.atmosenv.2022.118978, 2022.
Wiedinmyer, C., Friedfeld, S., Baugh, W., Greenberg, J., Guenther, A., Fraser, M., and Allen, D.: Measurement and analysis of atmospheric concentrations of isoprene and its reaction products in central Texas, Atmos. Environ., 35, 1001–1013, https://doi.org/10.1016/s1352-2310(00)00406-4, 2001.
Wu, R. and Xie, S.: Spatial distribution of secondary organic aerosol formation potential in China derived from speciated anthropogenic volatile organic compound emissions, Environ. Sci. Technol., 52, 8146–8156, https://doi.org/10.1021/acs.est.8b01269, 2018.
Wu, Y. J., Fan, X. L., Liu, Y., Zhang, J. Q., Wang, H., Sun, L. A., Fang, T. E., Mao, H. J., Hu, J., Wu, L., Peng, J. F., and Wang, S. L.: Source apportionment of VOCs based on photochemical loss in summer at a suburban site in Beijing, Atmos. Environ., 293, 119459, https://doi.org/10.1016/j.atmosenv.2022.119459, 2023.
Wu, Y. T., Liu, B. S., Meng, H., Dai, Q. L., Shi, L. Y., Song, S. J., Feng, Y. C., and Hopke, P. K.: Changes in source apportioned VOCs during high O3 periods using initial VOC-concentration-dispersion normalized PMF, Sci. Total Environ., 896, 165182, https://doi.org/10.1016/j.scitotenv.2023.165182, 2023.
Xie, X., Shao, M., Liu, Y., Lu, S. H., Chang, C.-C., and Chen, Z.-M.: Estimate of initial isoprene contribution to ozone formation potential in Beijing, China, Atmos. Environ., 42, 6000–6010, https://doi.org/10.1016/j.atmosenv.2008.03.035, 2008.
Xie, Y. L. and Berkowitz, C. M.: The use of positive matrix factorization with conditional probability functions in air quality studies: An application to hydrocarbon emissions in Houston, Texas, Atmos. Environ., 40, 3070–3091, https://doi.org/10.1016/j.atmosenv.2005.12.065, 2006.
Xu, K., Liu, Y. F., Li, F., Li, C. L., Zhang, C., Zhang, H., Liu, X. G., Li, Q. J., and Xiong, M.: A retrospect of ozone formation mechanisms during the COVID-19 lockdown: The potential role of isoprene, Environ. Pollut., 317, 120728, https://doi.org/10.1016/j.envpol.2022.120728, 2023.
Yadav, R., Sahu, L. K., Beig, G., and Jaaffrey, S. N. A.: Role of long-range transport and local meteorology in seasonal variation of surface ozone and its precursors at an urban site in India, Atmos. Res., 176, 96–107, https://doi.org/10.1016/j.atmosres.2016.02.018, 2016.
Yang, T., Liu, B. S., Yang, Y., Dai, Q. L., Zhang, Y. F., Feng, Y. C., and Hopke, P. K.: Improved positive matrix factorization for source apportionment of volatile organic compounds in vehicular emissions during the Spring Festival in Tianjin, China, Environ. Pollut., 303, 119122, https://doi.org/10.1016/j.envpol.2022.119122, 2022.
Yang, Y., Ji, D. S., Sun, J., Wang, Y. H., Yao, D., Zhao, S., Yu, X. N., Zeng, L. M., Zhang, R. J., Zhang, H., Wang, Y. H., and Wang, Y. S.: Ambient volatile organic compounds in a suburban site between Beijing and Tianjin: Concentration levels, source apportionment and health risk assessment, Sci. Total Environ., 695, 133889, https://doi.org/10.1016/j.scitotenv.2019.133889, 2019.
Yang, Y., Liu, B. S., Hua, J., Yang, T., Dai, Q. L., Wu, J. H., Feng, Y. C., and Hopke, P. K.: Global review of source apportionment of volatile organic compounds based on highly time-resolved data from 2015 to 2021, Environ. Int., 165, 107330, https://doi.org/10.1016/j.envint.2022.107330, 2022.
Yuan, B., Shao, M., Lu, S. H., and Wang, B.: Source profiles of volatile organic compounds associated with solvent use in Beijing, China, Atmos. Environ., 44, 1919–1926, https://doi.org/10.1016/j.atmosenv.2010.02.014, 2010.
Yuan, B., Chen, W. T., Shao, M., Wang, M., Lu, S. H., Wang, B., Liu, Y., Chang, C. C., and Wang, B. G.: Measurements of ambient hydrocarbons and carbonyls in the Pearl River Delta (PRD), China, Atmos. Res., 116, 93–104, https://doi.org/10.1016/j.atmosres.2012.03.006, 2012a.
Yuan, B., Shao, M., de Gouw, J., Parrish, D. D., Lu, S. H., Wang, M., Zeng, L. M., Zhang, Q., Song, Y., Zhang, J. B., and Hu, M.: Volatile organic compounds (VOCs) in urban air: How chemistry affects the interpretation of positive matrix factorization (PMF) analysis, J. Geophys. Res.-Atmos., 117, D24302, https://doi.org/10.1029/2012jd018236, 2012b.
Zhan, J. L., Feng, Z. M., Liu, P. F., He, X. W., He, Z. M., Chen, T. Z., Wang, Y. F., He, H., Mu, Y. J., and Liu, Y. C.: Ozone and SOA formation potential based on photochemical loss of VOCs during the Beijing summer, Environ. Pollut., 285, 117444, https://doi.org/10.1016/j.envpol.2021.117444, 2021.
Zhang, C., Liu, X. G., Zhang, Y. Y., Tan, Q. W., Feng, M., Qu, Y., An, J. L., Deng, Y. J., Zhai, R. X., Wang, Z., Cheng, N. L., and Zha, S. P.: Characteristics, source apportionment and chemical conversions of VOCs based on a comprehensive summer observation experiment in Beijing, Atmos. Pollut. Res., 12, 183–194, https://doi.org/10.1016/j.apr.2020.12.010, 2021.
Zhang, F., Shang, X. N., Chen, H., Xie, G. Z., Fu, Y., Wu, D., Sun, W. W., Liu, P. F., Zhang, C. L., Mu, Y. J., Zeng, L. M., Wan, M., Wang, Y. S., Xiao, H., Wang, G. H., and Chen, J. M.: Significant impact of coal combustion on VOCs emissions in winter in a North China rural site, Sci. Total Environ., 720, 137617, https://doi.org/10.1016/j.scitotenv.2020.137617, 2020.
Zhang, J. Q., Liu, Z., Wu, Y. J., Zhu, Y., Cao, T., Ling, D. Y., Wang, H., and Wang, S. L.: The impacts of photochemical loss on the source apportionment of ambient volatile organic compounds: A case study in Northern China, Atmos. Environ., 333, 120671, https://doi.org/10.1016/j.atmosenv.2024.120671, 2024.
Zhang, L. L., Xu, T., Wu, G. C., Zhang, C. L., Li, Y., Wang, H., Gong, D. C., Li, Q. Q., and Wang, B. G.: Photochemical loss with consequential underestimation in active VOCs and corresponding secondary pollutions in a petrochemical refinery, China, Sci. Total Environ., 918, 170613, https://doi.org/10.1016/j.scitotenv.2024.170613, 2024.
Zhang, W. J., Lin, S., Hopke, P. K., Thurston, S. W., van Wijngaarden, E., Croft, D., Squizzato, S., Masiol, M., and Rich, D. Q.: Triggering of cardiovascular hospital admissions by fine particle concentrations in New York state: Before, during, and after implementation of multiple environmental policies and a recession, Environ. Pollut., 242, 1404–1416, https://doi.org/10.1016/j.envpol.2018.08.030, 2018.
Zhang, Z., Zhang, Y. L., Wang, X. M., Lü, S. J., Huang, Z. H., Huang, X. Y., Yang, W. Q., Wang, Y. S., and Zhang, Q.: Spatiotemporal patterns and source implications of aromatic hydrocarbons at six rural sites across China's developed coastal regions, J. Geophys. Res., 121, 6669–6687, https://doi.org/10.1002/2016jd025115, 2016.
Zhao, C. K., Sun, Y., Zhong, Y. P., Xu, S. H., Liang, Y., Liu, S., He, X. D., Zhu, J. H., Shibamoto, T., and He, M.: Spatio-temporal analysis of urban air pollutants throughout China during 2014–2019, Air Qual. Atmos. Hlth., 14, 1619–1632, https://doi.org/10.1007/s11869-021-01043-5, 2021.
Zhao, W., Hopke, P. K., and Karl, T.: Source identification of volatile organic compounds in Houston, Texas, Environ. Sci. Technol., 38, 1338–1347, https://doi.org/10.1021/es034999c, 2004.
Zhou, B. A., Zhao, T. Y., Ma, J., Zhang, Y. X., Zhang, L. J., Huo, P., and Zhang, Y.: Characterization of VOCs during nonheating and heating periods in the typical suburban area of Beijing, China: Sources and health assessment, Atmosphere, 13, 560, https://doi.org/10.3390/atmos13040560, 2022.
Zhu, B., Huang, X.-F., Xia, S.-Y., Lin, L.-L., Cheng, Y., and He, L.-Y.: Biomass-burning emissions could significantly enhance the atmospheric oxidizing capacity in continental air pollution, Environ. Pollut., 285, 117523, https://doi.org/10.1016/j.envpol.2021.117523, 2021.
Zou, Y., Charlesworth, E., Wang, N., Flores, R. M., Liu, Q. Q., Li, F., Deng, T., and Deng, X. J.: Characterization and ozone formation potential (OFP) of non-methane hydrocarbons under the condition of chemical loss in Guangzhou, China, Atmos. Environ., 262, 118630, https://doi.org/10.1016/j.atmosenv.2021.118630, 2021.
Zou, Y., Yan, X., Flores, R. M., Zhang, L. Y., Yang, S., Fan, L. Y., Deng, T., Deng, X., and Ye, D.: Source apportionment and ozone formation mechanism of VOCs considering photochemical loss in Guangzhou, China, Sci. Total Environ., 903, 166191, https://doi.org/10.1016/j.scitotenv.2023.166191, 2023.