the Creative Commons Attribution 4.0 License.
the Creative Commons Attribution 4.0 License.
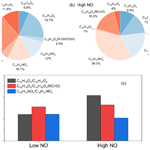
Formation of highly oxygenated organic molecules from the oxidation of limonene by OH radical: significant contribution of H-abstraction pathway
Hao Luo
Luc Vereecken
Hongru Shen
Sungah Kang
Iida Pullinen
Mattias Hallquist
Hendrik Fuchs
Andreas Wahner
Astrid Kiendler-Scharr
Highly oxygenated organic molecules (HOMs) play a pivotal role in the formation of secondary organic aerosol (SOA). Therefore, the distribution and yields of HOMs are fundamental to understand their fate and chemical evolution in the atmosphere, and it is conducive to ultimately assess the impact of SOA on air quality and climate change. In this study, gas-phase HOMs formed from the reaction of limonene with OH radicals in photooxidation were investigated with SAPHIR (Simulation of Atmospheric PHotochemistry In a large Reaction chamber), using a time-of-flight chemical ionization mass spectrometer with nitrate reagent ion (NO-CIMS). A large number of HOMs, including monomers (C9–10) and dimers (C17–20), were detected and classified into various families. Both closed-shell products and open-shell peroxy radicals (RO2) were identified under low NO (0.06–0.1 ppb) and high NO conditions (17 ppb). C10 monomers are the most abundant HOM products and account for over 80 % total HOMs. Closed-shell C10 monomers were formed from a two peroxy radical family, C10H15Ox• (x=6–15) and C10H17Ox• (x=6–15), and their respective termination reactions with NO, RO2, and HO2. While C10H17Ox• is likely formed by OH addition to C10H16, the dominant initial step of limonene plus OH, C10H15Ox•, is likely formed via H abstraction by OH. C10H15Ox• and related products contributed 41 % and 42 % of C10 HOMs at low and high NO, demonstrating that the H-abstraction pathways play a significant role in HOM formation in the reaction of limonene plus OH. Combining theoretical kinetic calculations, structure–activity relationships (SARs), data from the literature, and the observed RO2 intensities, we proposed tentative mechanisms of HOM formation from both pathways. We further estimated the molar yields of HOMs to be % and % at low and high NO, respectively. Our study highlights the importance of H abstraction by OH and provides the yield and tentative pathways in the OH oxidation of limonene to simulate the HOM formation and assess the role of HOMs in SOA formation.
- Article
(2618 KB) - Full-text XML
-
Supplement
(1606 KB) - BibTeX
- EndNote
Biogenic volatile organic compounds (BVOCs) are important precursors of atmospheric secondary organic aerosol particles (SOAs; Griffin et al., 1999; Kanakidou et al., 2005; Hallquist et al., 2009). In the Earth's atmosphere, monoterpenes (C10H16) are abundant BVOCs, with the second-largest emission rate of approximately 150 Tg yr−1 (Guenther et al., 2012). Among them, limonene accounts for 20 % of the total monoterpene emissions, ranking as the fourth largest (Guenther et al., 2012). In addition, as an important ingredient of the essential oil and common volatile chemical product (VCP), limonene is also widely used in house cleaning and personal care products due to its pleasant fragrance and antimicrobial property. Hence, limonene can play an important role in indoor SOA formation and indoor air quality (Waring, 2016; Rossignol et al., 2013; Nazaroff and Weschler, 2004). Although its concentration in the atmosphere is not the most abundant among monoterpenes, limonene has a high potential to form SOAs due to its high SOA yield and the high reactivity provided by the presence of an endocyclic and an exocyclic double bond (Koch et al., 2000; Chen and Hopke, 2010; Gong et al., 2018; Saathoff et al., 2009).
Limonene can undergo gas-phase reactions rapidly with atmospheric oxidants such as the hydroxyl radical (OH) and ozone (O3) in the daytime and the nitrate radical (NO3) and O3 in the nighttime, forming peroxy radicals (RO2; Lee et al., 2006b; Eddingsaas et al., 2012; Vereecken and Peeters, 2000, 2012; Zhang et al., 2018; Yu et al., 1999). In the reaction of limonene with OH, which is the main atmospheric daytime loss process of limonene, OH addition to one of the olefinic carbon atoms is the dominant channel, although the abstraction of an allylic hydrogen atom also occurs (Rio et al., 2010; Jokinen et al., 2014). Via OH addition, the reaction forms C10H17O3• as a first-generation RO2, which, under oxidative conditions, further reacts with RO2, HO2, and NO, forming oxidation products including limonaldehyde (C10H16O2), limonaketone (C9H14O), limonic acid (C10H16O3), ketolimononic acid (C9H14O4), peroxo-pinic acid or its isomer (C10H14O5), nitric acid ester (C9H13NO7), some organic acids with low molecular weight (formic acid, acetic acid, butyric acid, and methacrylic), and formaldehyde (HCHO; Pang et al., 2022; Friedman and Farmer, 2018; Romonosky et al., 2015; Jaoui et al., 2006; Lee et al., 2006a; Hakola et al., 1994). Although earlier studies have gained valuable insights into the products and mechanism of the reaction with OH, many products and their formation mechanisms are not well elucidated. For example, highly oxygenated organic molecule (HOM) products and their formation mechanism remain elusive.
HOMs were first discovered to be produced in forests and are defined as compounds containing six or more oxygen atoms formed in the gas phase via autoxidation (Bianchi et al., 2019; Ehn et al., 2012; Ehn et al., 2014). Among the myriad of oxygenated products, HOMs were found to be of great importance for the mass of SOA, new particle formation, and particle growth (Jokinen et al., 2014, 2015; Ehn et al., 2014, 2017; Mentel et al., 2015; Molteni et al., 2019; Kirkby et al., 2016; McFiggans et al., 2019; Crounse et al., 2013; Kenseth et al., 2018; Krechmer et al., 2015; Quéléver et al., 2019; Tröstl et al., 2016). Typically, HOMs are generated from the autoxidation of peroxy radicals (RO2), where a new, more oxygenated RO2 can be formed after an intramolecular H shift, followed by O2 addition in the resulting alkyl radical (Bianchi et al., 2019; Vereecken and Nozière, 2020; Crounse et al., 2013; Møller et al., 2019; Vereecken et al., 2007; Ehn et al., 2017; Nozière and Vereecken, 2019; Ehn et al., 2014). Bimolecular termination of the autoxidation chain occurs when an RO2 intermediate reacts with HO2, RO2, and NO to form a series of products (see below), which can be classified as organic hydroperoxide (ROOH; Reaction R1a), alcohol (ROH; Reaction R2), and carbonyl (R = O; Reaction R2), alkoxy radicals (RO; Reactions 3 and 4), or organic nitrates (ON; Reaction R5). The molecular masses of the termination products are M+1, M−15, M−17, and M+30, respectively, for an RO2 with a molecular mass of M (Mentel et al., 2015; Ehn et al., 2014). Meanwhile, unimolecular termination pathways generally lead to the formation of carbonyls (R = O; Reaction R6). In addition, two RO2 can also undergo an accretion reaction to form HOM dimers (Reaction R7), as seen in the reactions shown below (Berndt et al., 2018a; Valiev et al., 2019; Y. Zhao et al., 2018). Note that the RO2 reaction in Reaction R2 is considered for primary and secondary RO2. For tertiary RO2s, carbonyls cannot be formed. In addition, the unimolecular isomerization of RO (from Reactions R1b and R3–4) could produce RO2 in the same RO2 family.
HOM formation in the OH-initiated oxidation of monoterpenes, such as α-pinene and β-pinene, has been studied in the laboratory (Berndt, 2021; Xu et al., 2019; Berndt et al., 2016; Kirkby et al., 2016; Ehn et al., 2014; Shen et al., 2022) and is assumed to mainly start with the OH addition to the C = C double bond. The alkyl radical adduct can rapidly add O2 to the radical site to form a peroxy radical (Finlayson-Pitts and Pitts, 2000; Ziemann and Atkinson, 2012). A number of monomer and dimer products from several monoterpenes that followed the reaction with the hydroxyl radical (OH) were observed, such as C10H14–18O7–11 and C19–20H28–32O10–18 (Berndt et al., 2018a; Shen et al., 2022; Xu et al., 2019). Despite the molecular similarities of the HOM monomers or dimer products from different monoterpenes (Jokinen et al., 2015; Ehn et al., 2012, 2014), the HOM yields are quite different between different monoterpenes in a given oxidation reaction (e.g., with O3 or OH radical; Jokinen et al., 2015). It follows that the HOM formation mechanisms for other monoterpenes may not necessarily be applicable to limonene. Although HOM formation from limonene by ozonolysis and NO3 has been reported (Jokinen et al., 2015; Guo et al., 2022; Mayorga et al., 2022), to the best of our knowledge, no studies have systematically reported the HOM distribution in limonene plus OH. In the ozonolysis of limonene, Jokinen et al. (2014) attributed some HOMs (C10H17Ox• () (RO2)) to the reaction of limonene with OH produced in ozonolysis by comparing the mass spectra in the presence or absence of an OH scavenger. The composition and the formation mechanism of HOMs in limonene photooxidation by OH remains unclear. Moreover, although the HOM yield of limonene plus OH was determined, this yield was estimated based on the difference in the HOM yield in the ozonolysis both with and without the OH scavenger (HOM yields are 5.3 % (limonene plus O3) and 0.93 % (limonene plus OH); Jokinen et al., 2015). It is necessary to confirm the yield with its direct determination in the reaction of limonene plus OH. Interestingly, in our previous study, we found that hydrogen abstraction is important for HOM formation in the reaction of α-pinene plus OH (Shen et al., 2022), where intermediate alkoxy radical oxidation steps aid autoxidation by breaking the six-membered and four-membered rings. As limonene plus OH is known to have a high H-abstraction contribution of ∼34 % (Rio et al., 2010; Dash and Rajakumar, 2015; Braure et al., 2014), it is interesting to compare these two systems due to the similar but subtly different structures of α-pinene and limonene, with both containing a six-membered ring, whereas limonene does not contain a four-membered ring.
In this study, we investigated HOMs from the photooxidation of limonene with OH at low NO (0.06–0.1 ppb or parts per billion) and high NO (17 ppb) with SAPHIR (Simulation of Atmospheric PHotochemistry In a large Reaction chamber) at Forschungszentrum Jülich. HOMs were classified into monomers and dimers, and the product distribution is reported. The yields of HOMs from limonene with OH were estimated. The formation mechanism of HOMs in the OH reaction is proposed based on molecular formula of HOMs and quantum chemical calculation, in addition to the structure–activity relationships (SARs) of the RO2 autoxidation rates. The relative importance of the OH addition to C = C double bond and hydrogen abstraction by OH is discussed. We further investigated the effects of NOx, which changes the HOM composition via altering the RO2 fate.
2.1 Experiment design and setup
The experiments were conducted with SAPHIR at Forschungszentrum Jülich, Germany. Details of the chamber have been described in the previous studies (D. Zhao et al., 2018; Zhao et al., 2015a, b, 2021; Rohrer et al., 2005; Shen et al., 2021; Guo et al., 2022). In brief, SAPHIR is a 270 m3 Teflon chamber equipped with a louvre system to switch between natural sunlight for illumination and dark conditions. In this study, the experiments were conducted in sunlight, with the louvres opened. To avoid possible interference due to the long reaction time, the subsequent discussion focuses on the early stage (15 min) of the experiment. The initial experimental conditions are shown in Table S1 in the Supplement.
Gas- and particle-phase species were characterized by a comprehensive set of instruments, with the details described previously (Zhao et al., 2015b). A proton transfer reaction time-of-flight mass spectrometer (PTR-ToF-MS; Ionicon Analytik GmbH, Austria) was used for measuring volatile organic compounds (VOCs). A NOx analyzer (Eco Physics; TR480) and a UV photometer O3 analyzer (Ansyco; model O341M) were used to measure the concentrations of NO2, NO, and O3, respectively. A laser-induced fluorescence system (LIF) was used to measure the concentrations of OH, HO2, and RO2 (Fuchs et al., 2012). Note that the potential artifact in HO2 measurements from the concurrent chemical conversion of RO2 in the instrument that is making use of chemical conversion of HO2 through the reaction with NO can be avoided in this study through NO used so that no corrections of HO2 concentration measurements are required. The detection of RO2 radicals relies on the conversion of RO2 to HO2 in their reactions with NO. We applied a correction to the RO2 concentrations (Fuchs et al., 2011). We would like to note that only a few nitrated RO2 were observed to not form HO2 in the reaction with NO. In this study, we do not expect that there was large contribution of nitrate RO2 to the sum of all RO2, as seen in photochemistry experiments, as there is no significant fraction of nitrate RO2 formed. A scanning mobility particle sizer spectrometer (SMPS; TSI Incorporated; DMA 3081/CPC 3785) was used to obtain particle number distributions. Temperature and relative humidity were continuously measured.
Before an experiment was conducted, the chamber was flushed with high-purity synthetic air (purity > 99.9999 % N2 and O2). Experiments were conducted at ∼75 % relative humidity (RH) initially. In low NO conditions, no further NO was added, and the background concentration of NO, which mainly stems from HONO photolysis produced via a photolytic process from a Teflon wall, is 0.06–0.1 ppb (Fig. S1 in the Supplement). OH radicals were generated from the photolysis of HONO in both low and high NO experiments, and the HONO was formed from the Teflon chamber wall via a photolytic process. The details have been described by Rohrer et al. (2005). HO2 was produced from the reaction of O2 with RO, which can be formed in the reaction of RO2 plus NO in photooxidation during the experiments. The concentration of limonene was 7 ppb. The reaction time after the roof opened was 8 h. At high NO conditions, 17 ppb NO was added into the chamber first, and limonene was sequentially added after half an hour. The louvres were opened ∼40 min after adding limonene. To estimate the impact of ozone oxidation during photooxidation, we calculated the reaction rates of VOC plus OH and VOC plus O3 in the experiments of this study (Fig. S2). During the first 15 min, VOC plus OH accounted for >99 % limonene loss in both low and high NO conditions. We would like to note that the low NO does not refer to the case in which the RO2 loss is dominated by the reaction with HO2, e.g., in a remote ocean environment. At low NO, the RO2 loss was estimated to be dominated by its reactions with NO in the early period (within ∼15 min, RO2 plus HO2 contributed ∼15 % to the RO2 loss), and in later periods, a significant fraction of the RO2 loss was also contributed by the reaction of RO2 with HO2 (Fig. S3), based on the measured NOx, RO2, and HO2 concentrations and their rate constants for the reactions with RO2 (Jenkin et al., 1997, 2019). At high NO, the RO2 fate was by far dominated by the RO2 plus NO reaction.
2.2 Characterization of HOMs
HOMs were characterized by a chemical ionization time-of-flight mass spectrometer (CIMS; Aerodyne Research Inc., USA) with nitrate (15NO) as the reagent ion, which has a mass resolution of ∼4000 (m Δm−1). The details of the instrument are described in previous publications (Pullinen et al., 2020; Mentel et al., 2015; Ehn et al., 2014). Briefly, 15NO, which was produced from 15N nitric acid, was used as the reagent ion to distinguish the complexation with the reagent ion from the NO3 groups in target molecules. NO CIMS is suitable for detecting oxygenated organic compounds with a high oxygen number. The mass spectra were analyzed by Tofware (version 2.5.7, TOFWERK/Aerodyne Research Inc.) in Igor Pro (version 6.37; WaveMetrics, Inc.). In this study, the mass spectra of HOM products during the first 15 min after the louvres were opened were analyzed because the particle number concentration (<30 # cm−1, where # refers to the particle numbers) remained low in the initial phase of the reaction. After attributing the molecular formulas of HOMs to different (mass-to-charge ratio), their concentrations were calculated using the calibration coefficient of H2SO4 (C is 2.5×1010 molec. cm−3 nc−1), as described before (Zhao et al., 2021; Pullinen et al., 2020), where the charge efficiency of HOMs and H2SO4 was assumed to be close to the collision limit (Pullinen et al., 2020; Ehn et al., 2014). The details of the calibration with H2SO4 are described in Sect. S1 in the Supplement. The loss of HOMs was corrected by using a wall loss rate of s−1 in fan-on conditions and s−1 in fan-off conditions, as quantified previously (Guo et al., 2022; D. Zhao et al., 2018), and a dilution loss rate s−1 (Zhao et al., 2015b). For details, we refer the reader to the aforementioned publications; briefly, the wall loss rate of HOMs in our chamber was estimated as being that of the decay of organic vapor (such as C10H15NO9–12 (nitrated compounds) and C10H14O8–11 (non-nitrated compounds) in the reaction of limonene with OH in the presence of NO) concentrations in the dark (Guo et al., 2022). Overall, the wall loss correction and dilution correction only affect the HOM yield by ∼5.8 % and <1 %, respectively.
2.3 Data analysis
The HOM yield was obtained using the concentration of the HOMs, divided by the concentration of limonene consumed by OH, which is the dominant oxidant of limonene and accounts for over 99 % of the limonene loss rate. HOM yield was calculated over the first 15 min after the opening of the louvres as follows:
where [HOM] means the concentrations of total HOMs corrected for wall loss and dilution loss, [VOC]r is the consumption concentrations of limonene corrected for wall loss and dilution loss, IHOM is the total signal intensity of HOMs normalized to the total signal, and C is the calibration coefficient of H2SO4. The calibration and wall loss and dilution loss correction are described in detail in the Supplement (Sect. S1).
Based on the C10 closed-shell products from unimolecular and bimolecular reactions of the C10 peroxy radical families C10H15Ox• and C10H17Ox•, C10H16Ox can be divided into carbonyls (R = O) and epoxides from C10H17Ox•, in addition to alcohols (ROH) and hydroperoxides (ROOH) from C10H15Ox•. The contribution of C10H17Ox•-related products to C10H16Ox was quantified as follows. For HOM-RO2, the production rate of alcohols (ROH) can be obtained according to Reaction R2.
The production rate of hydroperoxides (ROOH) can be obtained according to Reaction R1a, which forms ROOH with a yield β, where β is close to 1 for most RO2 (Jenkin et al., 2019).
The production rate of carbonyls (R = O) can be obtained according to Reactions R2 and R6.
By combining Eqs. (2)–(4), one can obtain Eq. (5), as follows:
where [RO2]T and [HO2] are the total concentrations of RO2 and the concentration of HO2 in the reaction system, respectively. kuni, , and represent the rate coefficient of the unimolecular termination of RO2 and the bimolecular reactions of RO2 with RO2 and HO2. α and 1−α are the carbonyl yield and the alcohol yield in reactions of RO2 plus RO2, respectively. Wall loss was neglected, due to its minor effects on the concentrations of the products (∼5.8 %).
For the C10H15Ox• family, Eq. (5) is equivalent to Eq. (6), as follows:
For the C10H17Ox• family, Eq. (5) is equivalent to Eq. (7), as follows:
As shown in Eq. (2), we assume the same kuni, , , α, and β for a given C10H15Ox• and C10H17Ox• family. Consequently, the ratios of for C10H15Ox• and C10H17Ox• are the same. Equation (8) can then be derived from Eqs. (6) and (7) to yield [R = O] %, which represents the contribution of the carbonyls produced from C10H17Ox• to C10H16Ox.
Based on this, about 90.1 % and 98.8 % of C10H16Ox were estimated to be carbonyls from C10H17Ox• at low and high NO, respectively. As the HOMs in this study are likely formed via a six-membered carbon ring opening, as discussed below, most HOM-RO2 are likely primary or secondary RO2, as shown in Schemes 1 and 2 and the RO2 distribution in Fig. S9. For primary and secondary HOM-RO2, although the carbonyl yield and alcohol yield does not necessarily equal to 1, they are most likely to be 1, according to Jenkin et al. (2019). With these equations, we can estimate the carbonyl fractions formed via C10H17Ox• under a reasonable assumption. We did a sensitivity analysis to test the influence of varying the kuni, , , and α using the ranges of these parameters reported in the literature on the fraction of carbonyl in C10H16Ox and on the importance of the H-abstraction channel in HOM formation. When kuni, , , and α were varied in the range of (0.01–1) × 10−12, (0.001–1) × 10−10, and (0.5–2) × 10−11 cm3 molec.−1 s−1, based on the values in the literature (Crounse et al., 2013; Berndt et al., 2018b; Ziemann and Atkinson, 2012), and 0.5, respectively, one can obtain the yield of carbonyl, according to Eqs. (6) and (7), which ranged from 90 %–96 % at low NO and 97 %–100 % at high NO. This indicated that the yields of carbonyl are not sensitive to these assumptions of k and α.
2.4 Theoretical kinetic study of C10H15O alkoxy and C10H15O2 peroxy radicals
The formation mechanism of C10H15O alkoxy and C10H15O2 peroxy radicals were considered in the theoretical kinetic study. The geometries of the intermediates and transition states for the first steps in the mechanism were first optimized using the M06-2X/cc-pVDZ methodology (Zhao and Truhlar, 2008; Dunning, 1989), with an exhaustive characterization of all conformers for each reactant and transition state. All geometries obtained were thus further optimized at the M06-2X-D3/aug-cc-pVTZ level of theory, which includes D3 diffusion corrections (Goerigk et al., 2017; Grimme et al., 2011). Moments of inertia for molecular rotation and wavenumbers for vibration were obtained at the same level of theory, with a vibrational scaling factor of 0.971 (Alecu et al., 2010; Dunning, 1989). The barrier heights were further improved by single-point calculations at the CCSD(T)/aug-cc-pVTZ level of theory (Dunning et al., 2001; Bartlett and Purvis, 1978; all T1 diagnostics ≤ 0.029). The expected uncertainty of the reaction barrier heights at this level of theory is ±0.5 kcal mol−1. All quantum chemical calculations were performed using the Gaussian 16 software suite (Frisch et al., 2016). The quantum chemical data underlying the theoretical kinetic calculations are provided in the Supplement.
The rate coefficients for the individual reactions at the high-pressure limit were then calculated using a multi-conformer transition state theory (MC-TST; Vereecken and Peeters, 2003), incorporating the data for all conformers obtained as described previously. Tunneling is accounted for by using an asymmetric Eckart barrier correction (Johnston and Heicklen, 1962; Eckart, 1930). Based on earlier work at a similar level of theory in comparison with experimental data on H migration in RO2• radicals with no or only one oxygenated functionality (Vereecken and Nozière, 2020; Nozière and Vereecken, 2019) and the available theoretical literature data on ring closure reactions (Vereecken et al., 2021), we estimate the thermal rates to be accurate to a factor 2 to 3.
2.5 SAR-based mechanism development for autoxidation
The kinetics of the chemistry following the ring breaking is expected to be fairly well described based on structure–activity relationships (SARs), and no explicit theoretical kinetic calculations were performed. We employ the same approach for deducing the mechanism as described in our recent work (Shen et al., 2022). Briefly, we only take into account a limited oxidation network by considering all possible reaction channels and select only the dominant channels, based on the rate predicted by SARs. For the rate coefficients for most H migrations in RO2• radicals, we base our findings on the SAR by Vereecken and Nozière (2020); this SAR was reported to reproduce scarce experimental data to within a factor of 2, but for multi-functionalized species, such as those studied in this work, the scatter on the data within each SAR category reaches an order of magnitude. For H migrations in cycloperoxides, we additionally rely on the systematic study by Vereecken et al. (2021), who explicitly calculated the rate coefficients for peroxy radicals formed after RO2• ring closure reactions. For those reaction classes that are not covered by either SAR, we estimate a rate by extrapolating the reactivity trends in the SARs, albeit with a large uncertainty. For ring closure reactions in unsaturated RO2•, we employ the SAR by Vereecken et al. (2021), where it is assumed that the presence of another cycloperoxide ring does not influence the rate. In assessing the fate of a RO2• radical with one or more −OOH groups, we account for the possibility of H atom scrambling, as described extensively in the literature (Praske et al., 2019; Møller et al., 2019; Jørgensen et al., 2016; Nozière and Vereecken, 2019; Knap and Jørgensen, 2017). These fast H migrations between the −OO• and −OOH groups are typically much faster than other unimolecular channels, leading to a fast equilibration among all accessible OOH-substituted RO2• radicals, and the dominant reaction is chosen among those available to the pool of RO2• radicals. We refer to Vereecken and Nozière (2020) for a more detailed description of this feature. In the early stages of the oxidation, we also use the recent work by Piletic and Kleindienst (2022). Finally, for alkoxy radical chemistry, we employ the SARs for decomposition and H migration by Novelli et al. (2021) and Vereecken and Peeters (2009, 2010). For hydroperoxyl-substituted alkoxy radicals, we note explicitly that the H migration of the hydroperoxide H atom, forming an alcohol and a RO2 radical, is typically very fast, k (298 K) ≥ 1010 s−1 (Vereecken and Nozière, 2020), and is often the most likely loss process in later alkoxy stages in the autoxidation chain. This fast H migration supports competitive autoxidation, even under high NO conditions, despite the formation of alkoxy intermediates that threaten to fragment the molecule.
3.1 Overview of HOM spectra
The mass spectra of gas-phase product HOMs formed in the oxidation of limonene by OH at two different NOx levels are demonstrated in Fig. 1. The HOM products can be classified according their mass-to-charge ratio () as either monomers (200–400 Th; including C6–C9 monomers and C10 monomers at 320–400 Th) or dimers (480–600 Th; C17–C20 dimers). We did not observe any trimer products (Fig. S4). The signal intensity of the monomers is higher than dimers at both low and high NO (Figs. 1 and 2), where monomers accounted for over 80 % of total HOMs. Both the signal intensity and the fraction of the observed dimers at high NO were much lower than that at low NO (Figs. 1 and 2). In the following (Sect. 3.2), we discuss the product distribution and formation mechanism of monomers and dimers in detail.
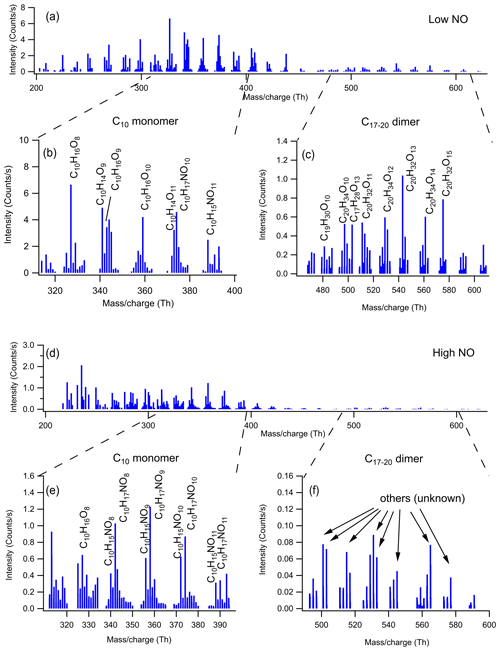
Figure 1Mass spectrum of the HOMs formed in the oxidation of limonene by OH in (a) low and (d) high NO conditions. The mass spectra were obtained within 15 min after opening the louvres and were averaged over 15 min. (b, c, e, f) The expanded mass spectra are presented, where major peaks are labeled with their molecular formulas.
3.2 Identification and formation mechanism of HOMs
3.2.1 Overview of HOM monomers
Closed-shell HOM monomers are characterized by the repeating pattern of molecular formulas with an increasing number of oxygen atoms in the mass spectrum. The repeating pattern in the mass spectrum at every 16 Th forms a series of HOM monomer families, such as C6HOx, C6HNOx, C7HOx, C7HNOx, C8HOx, C8HNOx, C9HOx, C9HNOx, C10HOx, and C10HNOx (Fig. S5). It is worth noting that some open-shell radicals, such as C10H15Ox• (x=6–15) and C10H17Ox• (x=6–15), were also observed; these are a significant fraction of HOM monomers and will be discussed in detail in Sect. 3.2.2. These open-shell molecules were likely RO2 radicals, as we are not able to detect the very short-lived alkoxy radicals (RO) and alkyl radicals (R). The closed-shell products (C≤10HyOx and C≤10HyNOx) will be discussed in the following from the perspective of RO2 chemistry (i.e., as termination products of RO2). It should be noted that each molecular mass can represent several isomers and that there may be more than one pathway to form a given product; our experiments do not allow us to discriminate these, and an in-depth discussion is beyond the scope of this work.
HOM monomers were classified into C10 and C6–9 monomers according to the number of carbon atoms. The abundance of C6–9 monomers (∼27.0 % combined) was lower than the C10 compounds (∼46.4 %) at low NO. In addition to C10 and C6–9 monomers, other monomers accounted for ∼18.9 % of all monomers, and unspecified monomers accounted for ∼7.7 %. As shown in Fig. S5, C6–10 monomers contain either no or one nitrogen atoms, and a higher fraction of organic nitrates was observed in high NO conditions (nitrate HOMs are 31 % at low NO and 41 % at high NO). This is as expected, given that the fraction of RO2 terminated by NO forming organic nitrate was higher at high NO. Higher fractions of organic nitrate have also been observed by the previous studies in the photooxidation of other monoterpenes at high NO (Pullinen et al., 2020; Shen et al., 2022).
3.2.2 C10 monomer product distribution
At low and high NO conditions, C10 compounds (C10H14Ox, C10H15NOx, C10H16Ox, C10H17NOx, and C10H18Ox) were the abundant monomers, with a fractional contribution of ∼27 %–∼41 % (see Fig. 3). In this study, we observed two major RO2 radical families, C10H15Ox• (x=6–15) and C10H17Ox• (x=6–15), and their corresponding termination products, C10H14Ox−1, C10H16Ox−1, C10H16Ox, and C10H15NOx+1 and C10H16Ox−1, C10H18Ox−1, C10H18Ox, and C10H17NOx+1, respectively, which contained carbonyl, hydroxyl, hydroperoxyl, and nitrate, respectively (see Figs. 3 and S5; Tables S2, S3, S6, and S7). C10H14Ox and C10H16Ox were likely carbonyl compounds formed via the unimolecular termination of C10H15Ox• and C10H17Ox•, respectively. Overall, at high NO, organic nitrates were the most abundant among all classes of HOM monomers, and their relative proportion was much higher than at low NO (Fig. 3). Under low NO conditions, the C10H14Ox and C10H16Ox family are the two most abundant families among C10 monomers. This is similar to the C10 monomer products from the photooxidation of α-pinene by OH the radical (Shen et al., 2022).
3.2.3 Monomers with C<10 monomers and dimers
A number of monomers with a carbon number less than 10 (C<10) was observed in this study. The monomers (C<10) account for about 24.1 %–45.5 % of the total monomers. The two radicals C7H9Ox• and C7H11Ox• were likely produced from C10H15Ox• radicals and C10H17Ox• radicals, respectively (see below). The ratios of the total termination products from C7H9Ox• to those from C7H11Ox• are 0.7 at both low and high NO, which is generally consistent with the ratio of C10H15Ox• radicals and C10H17Ox• radicals for C10-related products. This suggests that the chemistry related to C10H15Ox• radicals plays a significant role in the formation of C<10 monomers. Although the concentrations of monomers (C<10) are smaller than those of C10 monomers, they may also contribute to the formation of dimers, which further condense onto particles.
We observed a number of dimer families, including C20H30Ox (x=10, 12–18), C20H32Ox (x=9–18), C20H34Ox (x=10–17), C19H32Ox (x=10–15), C19H31NOx (x=11–18), C19H30Ox (x=10–17), C18H30Ox (x=10–14), C19H28Ox (x=11–15), C17H26Ox (x=11–15), and C17H24Ox (x=12–17) in low NO conditions and C20H30Ox (x=12–16), C20H32Ox (x=11–18), C20H34Ox (x=10–15), C19H28Ox (x=11–17), and C17H26Ox (x=12–15) in high NO conditions (see Tables S4 and S5). Compared to the monomers, the peak intensity of dimers at both low and high NO conditions is much lower (Figs. S7 and S8). Furthermore, the peak intensity of dimers in low NO conditions is higher than that in high NO conditions. This inhibitory effect of NOx in dimer formation is consistent with the phenomenon described in previous studies (Pullinen et al., 2020; Yan et al., 2016; Shen et al., 2022). In the following discussion, we will focus on C10 monomers. However, more information on C<10 monomers and dimers can be found in Sect. S2.
3.3 Formation pathways of HOM monomers
In this section, we discuss the potential formation pathways for C10 monomers. These pathways are tentative only and are based on the current knowledge of autoxidation, as represented in various SARs, where we examine only those paths estimated as being the fastest. Our observations do not distinguish between different isomers, and we cannot validate the reaction scheme. We also observed HOMs with only 7, 8, or 9 carbon atoms, and their possible formation pathways, which involve a fragmentation step in an intermediate alkoxy radical, are inspected in the Supplement (see Schemes S1 and S2).
3.3.1 HOMs from OH addition
The C10H17Ox• radicals are formed from the OH addition reaction of limonene and subsequent autoxidation (see Table S3). Scheme 1 shows the possible oxidation routes for the OH addition to both double bonds, considering the particular channels leading to tertiary alkyl radicals and depicting the most likely reactions, as estimated based on theoretical work and SARs (Peeters et al., 1999; Vereecken et al., 2007; Jenkin et al., 2018). The C10H17O3• RO2 radical formed after O2 addition to the OH adducts has access to a wide range of reactions, but in most cases, the six-membered ring is retained in the first RO2 steps, leading to slow autoxidation steps (Vereecken et al., 2021). This allows the reaction with NO to become important, whereafter the decomposition of a β-OH or β-OOH alkoxy radical leads to carbonyl formation and thus chain termination. This analysis suggests that HOM formation after OH addition either proceeds through less favorable pathways or involves secondary chemistry. The complete explicit mechanism is highly branched and complex, and missing pathways in Scheme 1 could together contribute to sizable HOM formation. Furthermore, the rate estimates are tentative, with uncertainties that are an order of magnitude or more, so we cannot exclude that primary HOM formation is possible and important. The primary products predicted in Scheme 1 (or formed through similar termination reactions in other channels) can react again with OH, initiating secondary autoxidation. Most of these products have the six-membered ring broken and should be more amenable to further autoxidation steps and thus readily yield HOMs. However, this extra bimolecular OH reaction takes time and can delay the formation of HOMs. An analysis of the secondary chemistry is beyond the scope of this work, and at this time, we do not propose a reaction scheme that covers the full range of C10H17Ox• radicals and the related products observed.
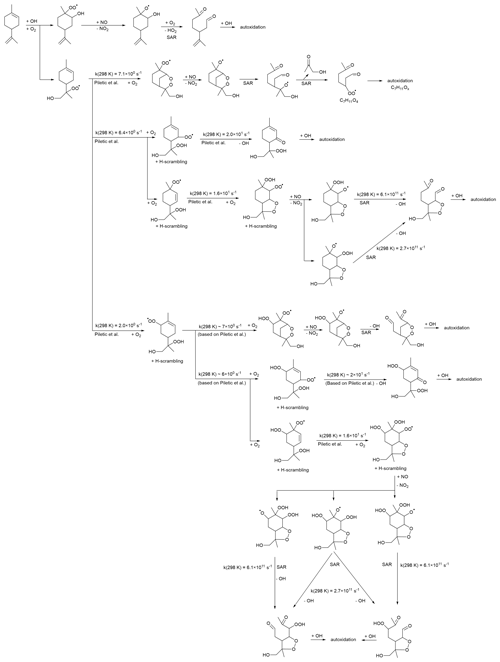
Scheme 1Example reaction scheme for C10H17Ox•RO2 formation after OH addition in limonene, based on the most likely reactions predicted in theoretical work and SAR predictions. All RO2 intermediates have competing reactions (not shown) under the current conditions with HO2 (forming hydroperoxides) and NO (forming alkoxy and nitrates).
In our previous study, the average bimolecular RO2 loss rate was estimated at ∼0.02 s−1 (low NO) and ∼3.5 s−1 (high NO; D. Zhao et al., 2018). In both cases, RO2 loss is due predominantly to the reaction with NO (see Fig. S3), which leads to the organic nitrate termination products, as illustrated in Fig. 3. Even at low NO with ∼0.2 ppb, organic nitrates contribute a large portion of HOMs (33 %). The relevant termination products from C10H17Ox• (x=8–13) in reactions with HO2 and RO2 are identified as C10H18Ox, C10H18Ox−1, and C10H16Ox−1. However, C10H18Ox accounted for only 5.2 % and 1 % in C10 monomers at low and high NO, respectively, as in high NO conditions these loss processes are overwhelmed by the RO2 plus NO reaction.
Table 1H migration, ring closure, and C−C bond scission reactions in RO2• and RO• radicals formed from limonene H abstraction, listing barrier heights Eb (kcal mol−1), rate coefficient k at 298 K (s−1), and parameters for a Kooij equation fit for the temperature range 200–450 K, calculated using the CCSD(T)//M06-2X-D3 level of theory.
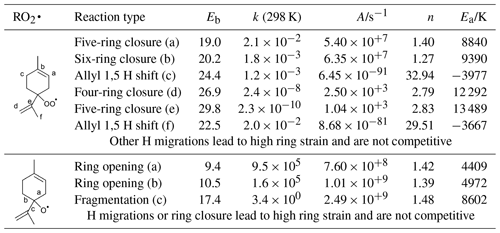
3.3.2 HOMs from H abstraction by OH
The observed C10H15Ox• radicals mainly originate from an H-abstraction reaction by OH, removing one of the limonene H atoms. In principle, C10H15Ox• peroxy radicals might also form through the secondary chemistry of first-generation C10 oxidation products of the limonene plus OH reaction. The limonaldehyde (C10H16O2) is the most abundant (99 %) first-generation C10 product reported in limonene plus OH reaction (Hakola et al., 1994; Larsen et al., 2001), which can form C10H15O4• and the C10H15Ox• family by further autoxidation through H abstraction and subsequent O2 addition. Therefore, we take into account limonaldehyde that is the most competitive candidate. For the early stages of our experiments (first 15 min), however, we find that secondary chemistry is not important (Sect. S2 and Fig. S9). An example pathway for C10H15Ox• RO2 radical formation is shown in Scheme 2, based on the most facile H-abstraction channel from limonene. Direct autoxidation of the nascent RO2 is slow, s−1, and formation of an alkoxy radical is to be expected immediately or after very few autoxidation steps, especially in high NO conditions. Once the ring structure is broken, fast autoxidation steps are accessible. All RO2 intermediates have competing reactions (not shown) under current conditions with HO2 (forming hydroperoxides) and NO (forming alkoxy radicals and nitrates). The alkoxy radicals formed thus can fragment, or continue autoxidation after ring breaking or fast migration of an hydroperoxide H atom, forming a wider variety of HOMs. The nascent peroxy radical formed after the H abstraction of the allylic tertiary H atom in limonene is not amenable to efficient autoxidation and HOM formation, due to the geometric constraints in the ring. Theoretical kinetic calculations (see Table 1) show that the fastest autoxidation steps occur at rates of s−1 (i.e., of a similar magnitude to the RO2 loss with NO in the low NO experiments but negligible against the RO2 plus NO rate at high NO). Our rate predictions agree with the recent theoretical study by Piletic and Kleindienst (2022). Further autoxidation steps with the ring structure intact are expected to be unfavorable, as in the case with autoxidation initiated through other H-abstraction sites (Vereecken et al., 2021; Piletic and Kleindienst, 2022). The reaction of the primary RO2 with NO, however, leads to an alkoxy radical that is most likely to break the six-membered ring, with a total rate coefficient exceeding ∼106 s−1 (see Table 1). This alkoxy-peroxy autoxidation step thus removes the geometric constraint, and fast autoxidation reactions become accessible, with rates enhanced by the presence of the double bonds (Vereecken and Nozière, 2020; Møller et al., 2019). Though further alkoxy-peroxy steps are not necessary for HOM formation from limonene, subsequent autoxidation steps will compete against bimolecular reactions with NO, suggesting that more alkoxy-peroxy steps may occur, even when the unimolecular lifetime of these later non-cyclic RO2 radicals is typically shorter than that of the early-stage cyclic RO2. Some of these alkoxy intermediates will preferentially break the carbon backbone, leading to fragmentation, while other alkoxy radicals will proceed by H migration and continue the autoxidation chain. In the latter case, autoxidation then no longer follows the systematic series of adding two O atoms per autoxidation step, and the C10H15Ox• formation mechanism can proceed with both even-numbered and odd-numbered oxygen numbers x. At high NO concentrations, formation of alkoxy radicals becomes more likely, and thus fragmentation can become more prominent, reducing the yield of C10H15Ox•-related HOMs. This is in agreement with the current observations, where more fragment C<10 monomers (47.2 % in total monomers) are observed at high NO than at low NO (27.0 %). Further autoxidation of the fragments is also possible, leading to C<10 monomers and dimer formation, as discussed in the Supplement. The need for a ring-breaking alkoxy-peroxy step in the proposed HOM formation mechanism does suggest that the highest yields of limonene HOM formation may occur at slightly higher NO concentrations than for non-cyclic VOCs, which autoxidize, even without alkoxy steps.
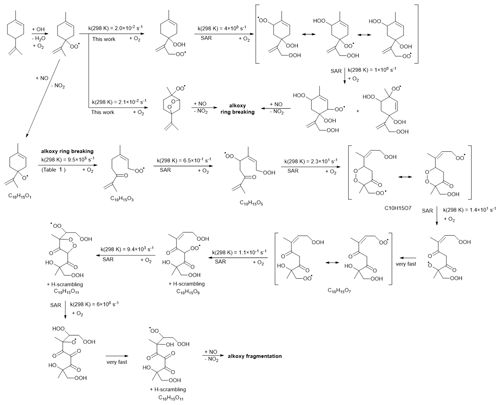
Scheme 2Example reaction scheme for HOM formation after H abstraction in limonene, based mostly on SAR prediction and starting at the fastest of the five allylic H-abstraction sites.
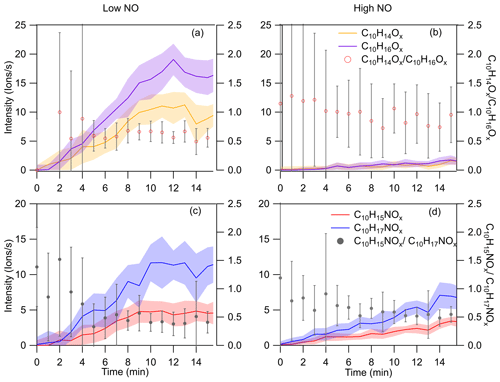
Figure 4Time series of C10H15Ox• and C10H17Ox•-related families of carbonyls and organic nitrates during the first 15 min of the experiments. The carbonyl families C10H14Ox (yellow), C10H16Ox (purple), and their concentration ratio () are shown for (a) low and (b) high NO experiments. Time series for organic nitrate families C10H15NOx (red) and C10H17NOx (blue) and the concentration ratio of , are similarly demonstrated in panel (c) for low NO experiments and panel (d) for high NO experiments. All data are averaged to 1 min, and the error bar represents 1 standard deviation.
3.3.3 Relative contribution of OH addition versus OH H abstraction
As C10H15Ox• (x=6–15) is formed via the OH H-abstraction pathway and C10H17Ox• is formed via OH addition, we can compare the relative importance of these two pathways via the ratios of C10H15Ox• to C10H17Ox• radicals and their termination products. At low NO, the ratio of the concentrations of C10H15Ox• to C10H17Ox• was 0.61, and the ratios of the concentrations of C10H15Ox•-related termination products carbonyls (C10H14Ox) and organic nitrates (C10H15NOx) to C10H17Ox•-related termination products carbonyls (C10H16Ox) and organic nitrates (C10H17NOx) are 0.78 and 0.61, respectively (Fig. 3c). At high NO, the ratio of the concentrations of C10H15Ox• to C10H17Ox• was ∼1.1 (Fig. 3), and the corresponding ratios of the concentrations of C10H15Ox•-related termination products to C10H17Ox•-related termination products are 0.82 and 0.53, respectively (Fig. 3c). Note that C10H16Ox can be termination products from either C10H15Ox• or C10H17Ox• radicals, depending on whether they contain hydroxyl or hydroperoxyl (ROH/ROOH) or carbonyl (RC = O) functionalities; the relative substituent contributions in C10H16Ox were quantified, using the method described in Sect. 2.2. The concentrations of C10H16Ox and C10H18Ox (alcohol and hydroperoxide products), formed from C10H15Ox• and C10H17Ox• radicals, respectively, were found to be negligible, and we can assign C10H16Ox products as carbonyls formed from the OH addition C10H17Ox• radicals. The NOx dependence on may be attributed to the differences in their reactivity. One explanation for the NOx dependence is that the autoxidation of C10H15Ox• RO2 radicals may be faster than that of C10H17Ox• RO2 radicals, which leads to the lower concentration of C10H15Ox• at high NO and thus higher sensitivity to NO concentrations. Ratios of can be derived with 0.47 and 0.02 at low and high NO, respectively. The decrease in at high NO compared to low NO was more evident than the decrease in . Theoretically, though, they should be similar. The difference may be attributed to the shift in C10H15Ox• distribution with a different number of O atoms, as evident in Fig. S6, and different isomers at high NO compared to low NO. At high NO, there might thus be more C10H15Ox• that reacts slower with HO2 or has a lower branching ratio that forms ROOH in RO2+HO2, which depends on the explicit RO2 structure, or has a lower yield that forms ROH in RO2 plus RO2. Overall, the C10H15Ox•-related products formed via the H-abstraction channel contribute 41 % and 42 % of C10 HOM monomer, respectively, at low and high NO. The ratio between the products of C10H15Ox• and C10H17Ox• radicals is stable at both low and high NO (Fig. 4), except for a small decrease at the early reaction times under low NO conditions (Fig. 4c), albeit with large error. At the same time, the nitrate ratio is lower than that of , which indicates that carbonyl production from C10H15Ox• is more efficient than from C10H17Ox•. The concentration of peroxy radicals C10H15Ox• and the related termination products C10H14Ox and C10H15NOx were comparable to C10H17Ox• and its corresponding products, illustrating the significant role of the H-abstraction pathway in the HOM formation from the limonene oxidation by OH at low and high NO. The much higher abundance of carbonyl than alcohol is unlikely to be explained by the RO plus O2 forming carbonyl because, for large CO (C10 in this study), the RO plus O2 is generally slower than unimolecular reactions, including H shift isomerization (i.e., alkoxy-peroxy pathway) and decomposition. The higher abundance of carbonyl products compared to alcohol products indicates that here a large fraction of the carbonyls is not formed from RO2 plus RO2 reactions (see also Fig. S3) but rather from termination reactions in HOOQOO• radicals eliminating an OH radical after an α-OOH H atom migration, thus forming O = QOOH. This observation is in agreement with recent findings for α-pinene (Shen et al., 2022) and previous studies (Miller et al., 2005; Taatjes, 2006; Rissanen et al., 2014; Bianchi et al., 2019).
The large contribution of C10H15Ox•-related products to HOM formation can be attributed to the significant contribution of H abstraction by OH in the initial step. While OH addition is believed to be the major initiation channel in the reaction of limonene plus OH, and H abstraction is often ignored in current chemical mechanisms (e.g., MCM v3.3.1), H abstraction by OH is clearly not negligible. Already previous studies have suggested that H abstraction can be a significant reaction pathway, with % branching ratio for the reactions of limonene with OH radical at 298 K (Rio et al., 2010; Dash and Rajakumar, 2015) and 33.6±4.8 % at 293 K (Braure et al., 2014). We found that the ratio of C10H15Ox• to C10H17Ox• at high NO conditions was higher than 2, emphasizing the importance of H abstraction. A similar abundance of carbonyls (C10H14Ox) and organic nitrates (C10H15NOx) stemming from C10H15Ox• radicals compared to their counterparts C10H16Ox and C10H17NOx from C10H17Ox• at both low and high NO levels (Fig. 3) likewise indicates that H abstraction in the first step of the limonene oxidation by OH is important for the subsequent oxidation. Moreover, the high relative yields of HOMs from the H-abstraction channel compared to its contribution in the limonene plus OH initiation reaction can be tentatively attributed to the difference in the autoxidation mechanisms (Schemes 1 and 2). Many of the autoxidation channels following the OH addition lead efficiently to termination products (Scheme 1) and require additional OH reactions to undergo further oxidation, whereas the RO2 formed from H abstraction readily lend themselves to a sequence of O2 additions once the ring structure is broken. The key difference is the presence of β-OH and β-OOH moieties in the alkoxy in the OH addition branch, which can form α-OH alkyl radicals and α-OOH alkyl radicals in fragmentation steps and further lead to HO2 formation from α-OH alkyl radicals plus O2 (Reaction R8) or by the regeneration of OH from α-OOH alkyl radicals (Reaction R9), thus preventing ring breaking without termination of the autoxidation chain, as follows:
In contrast, the alkoxy step after H abstraction breaks the six-membered ring to a new RO2, thereby enhancing autoxidation (Scheme 2). The propensity of the RO2 from OH adducts to terminate the autoxidation chain, especially at higher NO, where β-OH and β-OOH alkoxy decomposition is prevalent, then allows the H-abstraction RO2 to play a more dominant role. Contrary to our earlier studies on β-pinene or limonene with NO3 (Shen et al., 2021; Guo et al., 2022), where we were able to assign RO2 and products to first-, second-, or later-generation chemistry, no such clear nth-generation distinction can be made for the HOM mass spectrum traces in the limonene plus OH system. This suggests that the observed HOMs are a mixture formed in several generations of OH initiation reactions and lends further credence to our proposal that termination reactions requiring additional OH reactions are hampering the main OH addition channel from efficiently producing HOMs.
To our knowledge, no previous studies have observed C10H15Ox• radicals in limonene oxidation by OH, although in ozonolysis the formation of C10H15Ox• and their termination products, including C10H14–16O6–10 monomers and C18–20H28–34O6–16, was observed (Hammes et al., 2019; Tomaz et al., 2021), and field observations have shown the presence of C10H15Ox• radicals in the atmosphere (Yan et al., 2020; Massoli et al., 2018). Recent theoretical work from Piletic and Kleindienst (2022) concluded that H abstraction does not contribute to HOMs in limonene plus OH, but this study did not account for any alkoxy-peroxy autoxidation steps. Our study, then, shows for the first time that H abstraction significantly contributes to HOM formation in the reaction of limonene plus OH under reaction conditions that allow the formation of alkoxy radicals, such as by reaction with NO, RO2, or NO3, as commonly found in the atmosphere.
Currently, an absolute calibration using HOM standards is not possible, mainly due to the difficulty of synthesizing pure HOMs and the unclear chemical structures of many HOMs. However, we think that it is reasonable to expect a generally similar sensitivity for HOMs in this study for the following reasons. First, Hyttinen et al. (2017) found that the increase in binding energy with NO for molecules with an additional hydroxyperoxy group to two hydrogen bond donor functional groups is small for the HOMs formed in cyclohexene ozonolysis. As HOMs in this study generally contain more than two hydrogen bond donor functional groups, their sensitivity is expected to be similar. We used a unified H2SO4-based calibration coefficient for HOMs, which is commonly used to calibrate NO CIMS (Kirkby et al., 2016; Jokinen et al., 2015; Rissanen et al., 2014; Ehn et al., 2014). Second, although the underestimation of certain HOM-RO2 formed from the α-pinene plus OH reaction has been reported (Berndt et al., 2016), such an underestimation was mainly attributed to the steric hinderance in forming a HOM–nitrate cluster for HOMs with bicyclic structures (C10H17O7•; Berndt et al., 2016; see Sect. 3.2 of their Supplement), and thus, such underestimation is not common for all HOMs. In our study, we found the significance of the C10H15Ox•-related product at all oxygen contents, particularly for closed-shell products with the number of oxygen atoms being greater than eight, which is indicative of more H-donating functional groups (Fig. S6). This indicates that the significance of C10H15Ox•-related products is not affected by the detection sensitivity, which would mostly affect the sensitivity of less oxygenated compounds. And the presence of NO, particularly at high NO, leads to ring opening reactions, as shown in Scheme 1. Therefore, the HOM products from OH addition in this study are likely to form stable clusters with nitrate and thus have similar sensitivity with the HOMs formed via H abstraction in nitrate CIMS. Third, our previous study showed that using a unified sensitivity of H2SO4 only leads to a maximum uncertainty of a factor of 2 by comparing the condensation of HOMs and corresponding increase in aerosol mass (Pullinen et al., 2020). If for some currently unknown reason C10H17Ox•-related products had a higher sensitivity than the C10H15Ox•-related products, then this would lead us to underestimate of the significance of the OH H-abstraction pathway. This will not change our conclusion that the C10H15Ox•-related products contribute significantly to HOM formation.
We have further estimated the uncertainty in the fraction of C10H15Ox•-related products in C10-HOM that resulted from the allocation of carbonyls and alcohols in C10H16Ox in Eqs. (2)–(8). The contributions of C10H15Ox•-related products range from 39.5 % to 41.4 % at low NO and 42.2 % to 42.6 % at high NO, respectively. We found that fraction of C10H15Ox•-related products in C10-HOM was not affected much by how carbonyls and alcohols in C10H16Ox are allocated.
3.3.4 Comparison against α-pinene HOM formation
In our previous study, we observed the large contribution of H abstraction OH to HOM formation in the oxidation of α-pinene by OH (Shen et al., 2022). There, at low NO (0.03–0.1 ppb), the ratio of HOMs formed from H abstraction relative to OH addition increased at the beginning of the experiment and had a delay of 3–5 min before reaching 1:1. At high NO (∼17 ppb), an enhancement of the HOM yields formed through H abstraction was observed, which was both absolute and relative to the HOMs formed through OH addition. Alkoxy radical steps were found to be a prerequisite for the autoxidation and thus HOM formation in the hydrogen abstraction pathway. As discussed in Sect. 3.3.3, in limonene plus OH oxidation, the ratios of C10H14Ox to C10H16Ox and of C10H15NOx to C10H17NOx and the time series of the ratios were similar at low and high NO. These results indicate that the rate of autoxidation for both the limonene H abstraction and OH addition channels are affected to a similar extent by competing bimolecular reactions such as with NO. This contrasts with the enhancement of the HOM yield through H abstraction in the α-pinene system with increasing NO concentrations (Shen et al., 2022).
The different kinetic behavior relative to competing reactions can be attributed to the different molecular structures of α-pinene and limonene. α-pinene is a bicyclic rigid molecule, and the RO2 formed from H abstraction do not undergo autoxidation, as the rate coefficients are s−1 (Shen et al., 2022). Even after breaking the first ring in an alkoxy step, autoxidation rates remain fairly slow, s−1, requiring a second alkoxy step with ring breaking before fast autoxidation occurs. This makes HOM formation through H abstraction sensitive to the NO concentration in the first few steps. In contrast, limonene has only a single six ring, and only a single alkoxy ring breaking is needed to allow fast autoxidation in the resulting non-cyclic molecule. Moreover, the primary RO2 from the H abstraction of limonene can still undergo some slow autoxidation, with rates of s−1 (see Table 1), and possibly break the ring at a later stage (see Scheme 2). Overall, then, autoxidation in the limonene system after H abstraction is more competitive, even at lower NO than in the α-pinene system. We should note, though, that the low NO experiments of limonene oxidation were performed at NO concentrations that were higher by a factor of 2 to 7 compared to the low NO experiment for α-pinene (∼0.2 and 0.03–0.1 ppb, respectively); we speculate that an NO dependence on the ratio of HOMs that formed via H abstraction versus OH addition might even become apparent in the limonene system at strongly reduced NO levels. The higher absolute contribution of HOMs from the H-abstraction channel in limonene (H-abstraction-related HOM yields are 0.77 % low NO and 0.10 % at high NO, as shown in Sect. 3.4) is also affected by the higher branching ratio of H abstraction in limonene plus OH, ∼34 % (Rio et al., 2010; Dash and Rajakumar, 2015), compared to α-pinene plus OH, ∼11 % (Vereecken and Peeters, 2000).
3.4 HOM yield
The HOM molar yields during the first 60 min were estimated to be 1.97 % and 0.29 % at low and high NO, respectively. In brief, the uncertainty (−54 %/+128 %) was estimated from the HOM signal intensity, VOC concentration, and calibration coefficient of H2SO4, as discussed by Zhao et al. (2021; see Sect. S1 in the Supplement). It is noted that the HOM yield is not very sensitive to the vapor wall loss rate, since HOM yields only change 11 % to −6 % upon a 100 % increase and 50 % decrease in wall loss rate (Zhao et al., 2021). We further note that these HOM yields may be subject to uncertainties due to the assumption that HOMs have the same sensitivity as H2SO4, as we discussed in Sect. 3.3.3. As mentioned above, our previous study showed that using a unified sensitivity of H2SO4 only leads to a maximum uncertainty (that is a factor of 2) by comparing the condensation HOMs and corresponding increase in aerosol mass (Pullinen et al., 2020). The lower HOM yields at high NO can be partly attributed to the lower fractions of dimer products for the inhibitory effect of NOx, as discussed previously (Sect. 3.2.3). Within the uncertainty, the HOM yield at high NO in this study is comparable to the HOM yield (0.93±0.47) % from the photooxidation of limonene in a previous study (Jokinen et al., 2015). The difference in yield at low NO from Jokinen et al. (2015) may be attributed to different experimental methods and conditions. For example, Jokinen et al. (2015) derived the HOM yield from OH oxidation indirectly by comparing the HOM yield in ozonolysis with and without the OH scavenger, while in this study, we determined the HOM yield directly for the oxidation of limonene by OH. In ozonolysis, the cross-reactions of RO2 that formed from ozonolysis and OH oxidation may produce accretion products, thus possibly confounding the results. By directly measuring the HOM yield in the oxidation of limonene by OH, such an influence is avoided. The NO concentration might be also different between our study and that of Jokinen et al. (2015). Additionally, the HOM molar yields related to H abstraction were further determined to be 0.77 % at low NO and 0.10 % at high NO, when assuming that the ratio of all HOMs from H abstraction to those from OH addition is equal to the corresponding ratio for C10 monomers.
In this study, the HOM products in the oxidation of limonene with OH were investigated by using a NO CIMS with SAPHIR at Forschungszentrum Jülich. A large number of HOM monomers (C6–10) and dimers (C17–20) was detected and classified according to the number of carbon atoms. Among HOM products, the proportion of HOM monomers is far higher than dimers in both low and high NO conditions. C10 HOMs were the most abundant monomers at both low and high NO. At low NO, the fraction of organic nitrates (31 %) was lower than that of non-nitrate HOMs (69 %). At high NO, a higher fraction of 41% was observed for organic nitrates. Two major RO2 radical families, C10H15Ox• and C10H17Ox•, were identified. While C10H17Ox• were formed via OH addition to a double bond, C10H15Ox• is proposed to be formed via H abstraction by OH on the basis of its molecular formula and the available literature. Although the concentrations of C10H15Ox•-related products were less than C10H17Ox•-related products, they remained at a comparable magnitude (38.9 % vs. 55.0 % at low NO and 33.7 % vs. 49.7 % at high NO). The formation pathways of C10H15Ox• and C10H17Ox• were proposed on the basis of observed signal intensity, theoretical kinetic calculations, and structure–activity relationships (SARs) for autoxidation. At both low and high NO, H abstraction by OH contributes a significant fraction of HOMs (41 % and 42 % of C10 HOM monomer, respectively), demonstrating that H abstraction is important to the formation of HOMs in the oxidation of limonene with OH radical and contributes more than expected, given the ∼34 % contribution in the initiation reaction. The key mechanistic difference between OH addition and H-abstraction pathways is that the former does not readily lead to breaking of the six-membered ring without chain termination, whereas H abstraction leads to fast first-generation autoxidation after an alkoxy-peroxy ring-breaking step. C6–C9 monomers are proposed to be formed via the fragmentation of alkoxy radicals, and C17–C20 dimers are proposed to be formed via accretion reactions.
The molar yields of total HOMs are estimated to be 1.97 % and 0.29 % in low and high NO conditions. Although the HOM yields in the oxidation of limonene with OH in this study are lower than that in the ozonolysis of limonene (Jokinen et al., 2015), the corresponding SOA mass yields (assuming the irreversible condensation of HOMs) can be as high as 4.3 % and 0.6 % at low NO and high NO, respectively. Similarly, while the gas-phase dimers observed in this study are clearly lower than the monomers in abundance, dimers may also be very efficient at condensing onto newly formed particles due to their large size and low volatility. These HOM dimer products are known to have an extremely low volatility due to their size and high degree of oxidation (Tröstl et al., 2016). At 17 ppb NO, the RO2 fate is exclusively a reaction with NO and is representative of all environments in which the RO2 loss is dominated by the reaction with NO. Such an environment includes urban regions and suburban regions, especially in developing countries such as East Asia and South Asia, where our HOM yield can be used to model these areas. In contrast, our HOM yield at low NO is representative of a rural and remote continental environment (Rohrer et al., 1998; Lelieveld et al., 2008; Whalley et al., 2011; Moiseenko et al., 2021; Wei et al., 2019). Combined, our results can thus be used directly in atmospheric chemical transport models to simulate the HOM concentrations in many atmospheric regimes (Pye et al., 2019; Xu et al., 2022) and help refine the simulations to assess its importance in new particle formation and particle growth (Zhao et al., 2020).
This study highlights the importance of the pathway of H abstraction by OH in competition with the OH addition to double bonds in the same molecule, at least for HOM formation, which has largely been neglected in current chemical mechanisms. The importance of the H-abstraction pathway to HOM formation also in the α-pinene plus OH oxidation has been shown in our recent study (Shen et al., 2022) and in recent experimental work by Williams et al. (2022). H abstraction in the case of limonene plus OH is more prominent than for α-pinene plus OH, but the OH abstraction pathway is important in both systems, suggesting its importance in other terpenes or even other unsaturated species. We propose that, in order to accurately simulate HOM formation from the oxidation of limonene by OH, H abstraction should be considered in the chemical mechanisms of atmospheric models. Considering the key role HOMs in SOA particle formation and growth, this study further enables a more accurate simulation of the chemical composition and concentrations of SOA, in addition to the growth of particles to the cloud condensation nuclei (CCN) size in order to assess the impact of SOA on climate.
The experiments in this study were conducted at ambient relevant conditions by using limonene and OH concentrations at ambient levels and using natural sunlight. The major RO2 loss rate in all experiments is via RO2 plus NO. Therefore, the HOM composition and formation pathway can represent a large part of the daytime continental environment. The low NO conditions are representative of forested regions with biogenic monoterpene emissions and are influenced by anthropogenic emissions and of rural regions. The experiments at high NO in this study are relevant for the reactions of limonene with OH in urban environment, as limonene is also a major component of VCP besides the biogenic sources (Nazaroff and Weschler, 2004; Rossignol et al., 2013; Waring, 2016; Gkatzelis et al., 2021).
The fraction of organic nitrate HOMs, such as C10H15NOx and C10H17NOx, was significant in the total HOM products in both the low and high NO conditions examined, highlighting the importance of organic nitrates (ONs). Even at ∼0.2 ppb NO, ONs account for a large part (31 %) of HOMs. ONs are important in the atmosphere, as they can serve as an NOx reservoir (Ng et al., 2017). Highly functionalized ONs have been inferred to be capable of strongly partitioning to the particle phase (Perraud et al., 2012; Ng et al., 2007). The significant amounts of monoterpene-derived ONs that were observed in field campaigns (Massoli et al., 2018; Huang et al., 2019; Lee et al., 2016) indicate that ONs make up a significant fraction of SOA. The abundant presence of ONs highlights the importance of NOx-driven chemistry in daytime. In most of the continental environment influenced by monoterpenes, ONs then likely contribute a large fraction of HOMs and SOA and contribute the organic nitrates and particle formation in the atmosphere.
The Supplement provides additional figures and tables and further information on the CIMS calibration and HOM monomers and dimers with reduced carbon number.
The quantum chemical data (geometries, vibrational wavenumbers, rotational constants, energies, and partition functions) can be found at https://doi.org/10.26165/JUELICH-DATA/9JVHEK (Vereecken, 2023).
The supplement related to this article is available online at: https://doi.org/10.5194/acp-23-7297-2023-supplement.
DZ and TFM designed the study. IP, HF, and DZ carried out instrument deployment and operation. HL analyzed the MS data and did the MCM simulation with the aid of DZ, HS. HL, DZ, TFM, and LV interpreted the compiled data set. LV performed the theoretical calculations and examined the reaction schemes. HL, DZ, TFM, and LV wrote the paper, and HS, IP, SK, LV, HF edited the paper. All the co-authors discussed the results and commented on the paper.
The contact author has declared that none of the authors has any competing interests.
Publisher's note: Copernicus Publications remains neutral with regard to jurisdictional claims in published maps and institutional affiliations.
Hao Luo, Hongru Shen, and Defeng Zhao would like to thank the funding support of the Science and Technology Commission of Shanghai Municipality (grant no. 20230711400), National Natural Science Foundation of China (grant no. 41875145), Shanghai International Science and Technology Partnership Project (grant no. 21230780200), and Tibet University–Fudan Joint Laboratory for Biodiversity and Global Change. Sungah Kang, Astrid Kiendler-Scharr, and Thomas F. Mentel acknowledge the support by the EU Project FORCeS (grant no. 821205).
This research has been supported by the Science and Technology Commission of Shanghai Municipality (grant no. 20230711400), National Natural Science Foundation of China (grant no. 41875145), Shanghai International Science and Technology Partnership Project (grant no. 21230780200), and the Horizon 2020 Framework Programme, H2020 European Institute of Innovation and Technology (grant no. 821205).
This paper was edited by Ivan Kourtchev and reviewed by two anonymous referees.
Alecu, I. M., Zheng, J., Zhao, Y., and Truhlar, D. G.: Computational thermochemistry: scale factor databases and scale factors for vibrational frequencies obtained from electronic model chemistries, J. Chem. Theor. Comput., 6, 2872–2887, https://doi.org/10.1021/ct100326h, 2010.
Bartlett, R. J. and Purvis, G. D.: Many-Body Perturbation-Theory, Coupled-Pair Many-Electron Theory, and Importance of Quadruple Excitations for Correlation Problem, Int. J. Quant. Chem., 14, 561–581, https://doi.org/10.1002/qua.560140504, 1978.
Berndt, T.: Peroxy Radical Processes and Product Formation in the OH Radical-Initiated Oxidation of α-Pinene for Near-Atmospheric Conditions, J. Phys. Chem. A, 125, 9151–9160, https://doi.org/10.1021/acs.jpca.1c05576, 2021.
Berndt, T., Richters, S., Jokinen, T., Hyttinen, N., Kurtén, T., Otkjær, R. V., Kjaergaard, H. G., Stratmann, F., Herrmann, H., Sipilä, M., Kulmala, M., and Ehn, M.: Hydroxyl radical-induced formation of highly oxidized organic compounds, Nat. Commun., 7, 13677, https://doi.org/10.1038/ncomms13677, 2016.
Berndt, T., Mentler, B., Scholz, W., Fischer, L., Herrmann, H., Kulmala, M., and Hansel, A.: Accretion Product Formation from Ozonolysis and OH Radical Reaction of alpha-Pinene: Mechanistic Insight and the Influence of Isoprene and Ethylene, Environ. Sci. Technol., 52, 11069–11077, https://doi.org/10.1021/acs.est.8b02210, 2018a.
Berndt, T., Scholz, W., Mentler, B., Fischer, L., Herrmann, H., Kulmala, M., and Hansel, A.: Accretion Product Formation from Self- and Cross-Reactions of RO2 Radicals in the Atmosphere, Angew. Chem. Int. Ed. Engl., 57, 3820–3824, https://doi.org/10.1002/anie.201710989, 2018b.
Bianchi, F., Kurtén, T., Riva, M., Mohr, C., Rissanen, M. P., Roldin, P., Berndt, T., Crounse, J. D., Wennberg, P. O., Mentel, T. F., Wildt, J., Junninen, H., Jokinen, T., Kulmala, M., Worsnop, D. R., Thornton, J. A., Donahue, N., Kjaergaard, H. G., and Ehn, M.: Highly Oxygenated Organic Molecules (HOM) from Gas-Phase Autoxidation Involving Peroxy Radicals: A Key Contributor to Atmospheric Aerosol, Chem. Rev., 119, 3472–3509, https://doi.org/10.1021/acs.chemrev.8b00395, 2019.
Braure, T., Bedjanian, Y., Romanias, M. N., Morin, J., Riffault, V., Tomas, A., and Coddeville, P.: Experimental study of the reactions of limonene with OH and OD radicals: kinetics and products, J. Phys. Chem. A, 118, 9482–9490, https://doi.org/10.1021/jp507180g, 2014.
Chen, X. and Hopke, P. K.: A chamber study of secondary organic aerosol formation by limonene ozonolysis, Indoor Air, 20, 320–328, https://doi.org/10.1111/j.1600-0668.2010.00656.x, 2010.
Crounse, J. D., Nielsen, L. B., Jørgensen, S., Kjaergaard, H. G., and Wennberg, P. O.: Autoxidation of Organic Compounds in the Atmosphere, J. Phys. Chem. Lett., 4, 3513–3520, https://doi.org/10.1021/jz4019207, 2013.
Dash, M. R. and Rajakumar, B.: Theoretical investigations of the gas phase reaction of limonene (C10H16) with OH radical, Mol. Phys., 113, 3202–3215, https://doi.org/10.1080/00268976.2015.1014002, 2015.
Dunning, T. H.: Gaussian basis sets for use in correlated molecular calculations. I. The atoms boron through neon and hydrogen, J. Chem. Phys., 90, 1007–1023, https://doi.org/10.1063/1.456153, 1989.
Dunning, T. H., Peterson, K. A., and Wilson, A. K.: Gaussian basis sets for use in correlated molecular calculations. X. The atoms aluminum through argon revisited, J. Chem. Phys., 114, 9244–9253, https://doi.org/10.1063/1.1367373, 2001.
Eckart, C.: The Penetration of a Potential Barrier by Electrons, Phys. Rev., 35, 1303–1309, https://doi.org/10.1103/PhysRev.35.1303, 1930.
Eddingsaas, N. C., Loza, C. L., Yee, L. D., Seinfeld, J. H., and Wennberg, P. O.: α-pinene photooxidation under controlled chemical conditions – Part 1: Gas-phase composition in low- and high-NOx environments, Atmos. Chem. Phys., 12, 6489–6504, https://doi.org/10.5194/acp-12-6489-2012, 2012.
Ehn, M., Kleist, E., Junninen, H., Petäjä, T., Lönn, G., Schobesberger, S., Dal Maso, M., Trimborn, A., Kulmala, M., Worsnop, D. R., Wahner, A., Wildt, J., and Mentel, T. F.: Gas phase formation of extremely oxidized pinene reaction products in chamber and ambient air, Atmos. Chem. Phys., 12, 5113–5127, https://doi.org/10.5194/acp-12-5113-2012, 2012.
Ehn, M., Thornton, J. A., Kleist, E., Sipilä, M., Junninen, H., Pullinen, I., Springer, M., Rubach, F., Tillmann, R., Lee, B., Lopez-Hilfiker, F., Andres, S., Acir, I. H., Rissanen, M., Jokinen, T., Schobesberger, S., Kangasluoma, J., Kontkanen, J., Nieminen, T., Kurtén, T., Nielsen, L. B., Jørgensen, S., Kjaergaard, H. G., Canagaratna, M., Maso, M. D., Berndt, T., Petäjä, T., Wahner, A., Kerminen, V. M., Kulmala, M., Worsnop, D. R., Wildt, J., and Mentel, T. F.: A large source of low-volatility secondary organic aerosol, Nature, 506, 476–479, https://doi.org/10.1038/nature13032, 2014.
Ehn, M., Berndt, T., Wildt, J., and Mentel, T.: Highly Oxygenated Molecules from Atmospheric Autoxidation of Hydrocarbons: A Prominent Challenge for Chemical Kinetics Studies, Int. J. Chem. Kinet., 49, 821–831, https://doi.org/10.1002/kin.21130, 2017.
Finlayson-Pitts, B. J. and Pitts, J. N. J.: Chemistry of the Upper and Lower Atmosphere: Theory, Experiments, and Applications, Academic Press, San Diego, San Francisco, New York, Boston, London, Sydney, Tokyo, ISBN 978-0-12-257060-5 https://doi.org/10.1016/B978-0-12-257060-5.X5000-X, 2000.
Friedman, B. and Farmer, D. K.: SOA and gas phase organic acid yields from the sequential photooxidation of seven monoterpenes, Atmos. Environ., 187, 335–345, https://doi.org/10.1016/j.atmosenv.2018.06.003, 2018.
Frisch, M. J., Trucks, G. W., Schlegel, H. B., Scuseria, G. E., Robb, M. A., Cheeseman, J. R., Scalmani, G., Barone, V., Petersson, G. A., Nakatsuji, H., Li, X., Caricato, M., Marenich, A. V., Bloino, J., Janesko, B. G., Gomperts, R., Mennucci, B., Hratchian, H. P., Ortiz, J. V., Izmaylov, A. F., Sonnenberg, J. L., Williams-Young, D., Ding, F., Lipparini, F., Egidi, F., Goings, J., Peng, B., Petrone, A., Henderson, T., Ranasinghe, D., Zakrzewski, V. G., Gao, J., Rega, N., Zheng, G., Liang, W., Hada, M., Ehara, M., Toyota, K., Fukuda, R., Hasegawa, J., Ishida, M., Nakajima, T., Honda, Y., Kitao, O., Nakai, H., Vreven, T., Throssell, K., Montgomery, J. A., Jr., Peralta, J. E., Ogliaro, F., Bearpark, M. J., Heyd, J. J., Brothers, E. N., Kudin, K. N., Staroverov, V. N., Keith, T. A., Kobayashi, R., Normand, J., Raghavachari, K., Rendell, A. P., Burant, J. C., Iyengar, S. S., Tomasi, J., Cossi, M., Millam, J. M., Klene, M., Adamo, C., Cammi, R., Ochterski, J. W., Martin, R. L., Morokuma, K., Farkas, O., Foresman, J. B., and Fox, D. J.: Gaussian 09 Revision E.01, Gaussian, Inc., Wallingford CT, [code], https://gaussian.com/gaussian16/ (last access: 28 June 2023), 2016.
Fuchs, H., Bohn, B., Hofzumahaus, A., Holland, F., Lu, K. D., Nehr, S., Rohrer, F., and Wahner, A.: Detection of HO2 by laser-induced fluorescence: calibration and interferences from RO2 radicals, Atmos. Meas. Tech., 4, 1209–1225, https://doi.org/10.5194/amt-4-1209-2011, 2011.
Fuchs, H., Dorn, H.-P., Bachner, M., Bohn, B., Brauers, T., Gomm, S., Hofzumahaus, A., Holland, F., Nehr, S., Rohrer, F., Tillmann, R., and Wahner, A.: Comparison of OH concentration measurements by DOAS and LIF during SAPHIR chamber experiments at high OH reactivity and low NO concentration, Atmos. Meas. Tech., 5, 1611–1626, https://doi.org/10.5194/amt-5-1611-2012, 2012.
Gkatzelis, G. I., Coggon, M. M., McDonald, B. C., Peischl, J., Gilman, J. B., Aikin, K. C., Robinson, M. A., Canonaco, F., Prevot, A. S. H., Trainer, M., and Warneke, C.: Observations Confirm that Volatile Chemical Products Are a Major Source of Petrochemical Emissions in U.S. Cities, Environ. Sci. Technol., 55, 4332–4343, https://doi.org/10.1021/acs.est.0c05471, 2021.
Goerigk, L., Hansen, A., Bauer, C., Ehrlich, S., Najibi, A., and Grimme, S.: A look at the density functional theory zoo with the advanced GMTKN55 database for general main group thermochemistry, kinetics and noncovalent interactions, Phys. Chem. Chem. Phys., 19, 32184–32215, https://doi.org/10.1039/c7cp04913g, 2017.
Gong, Y., Chen, Z., and Li, H.: The oxidation regime and SOA composition in limonene ozonolysis: roles of different double bonds, radicals, and water, Atmos. Chem. Phys., 18, 15105–15123, https://doi.org/10.5194/acp-18-15105-2018, 2018.
Griffin, R. J., Cocker, D. R., Flagan, R. C., and Seinfeld, J. H.: Organic aerosol formation from the oxidation of biogenic hydrocarbons, J. Geophys. Res., 104, 3555–3567, https://doi.org/10.1029/1998jd100049, 1999.
Grimme, S., Ehrlich, S., and Goerigk, L.: Effect of the damping function in dispersion corrected density functional theory, J. Comput. Chem., 32, 1456–1465, https://doi.org/10.1002/jcc.21759, 2011.
Guenther, A. B., Jiang, X., Heald, C. L., Sakulyanontvittaya, T., Duhl, T., Emmons, L. K., and Wang, X.: The Model of Emissions of Gases and Aerosols from Nature version 2.1 (MEGAN2.1): an extended and updated framework for modeling biogenic emissions, Geosci. Model Dev., 5, 1471–1492, https://doi.org/10.5194/gmd-5-1471-2012, 2012.
Guo, Y., Shen, H., Pullinen, I., Luo, H., Kang, S., Vereecken, L., Fuchs, H., Hallquist, M., Acir, I.-H., Tillmann, R., Rohrer, F., Wildt, J., Kiendler-Scharr, A., Wahner, A., Zhao, D., and Mentel, T. F.: Identification of highly oxygenated organic molecules and their role in aerosol formation in the reaction of limonene with nitrate radical, Atmos. Chem. Phys., 22, 11323–11346, https://doi.org/10.5194/acp-22-11323-2022, 2022.
Hakola, H., Arey, J., Aschmann, S. M., and Atkinson, R.: Product Formation from the Gas-Phase Reactions of OH Radicals and O3 with a Series of Monoterpenes, J. Atmos. Chem., 18, 75–102, https://doi.org/10.1007/Bf00694375, 1994.
Hallquist, M., Wenger, J. C., Baltensperger, U., Rudich, Y., Simpson, D., Claeys, M., Dommen, J., Donahue, N. M., George, C., Goldstein, A. H., Hamilton, J. F., Herrmann, H., Hoffmann, T., Iinuma, Y., Jang, M., Jenkin, M. E., Jimenez, J. L., Kiendler-Scharr, A., Maenhaut, W., McFiggans, G., Mentel, Th. F., Monod, A., Prévôt, A. S. H., Seinfeld, J. H., Surratt, J. D., Szmigielski, R., and Wildt, J.: The formation, properties and impact of secondary organic aerosol: current and emerging issues, Atmos. Chem. Phys., 9, 5155–5236, https://doi.org/10.5194/acp-9-5155-2009, 2009.
Hammes, J., Lutz, A., Mentel, T., Faxon, C., and Hallquist, M.: Carboxylic acids from limonene oxidation by ozone and hydroxyl radicals: insights into mechanisms derived using a FIGAERO-CIMS, Atmos. Chem. Phys., 19, 13037–13052, https://doi.org/10.5194/acp-19-13037-2019, 2019.
Huang, W., Saathoff, H., Shen, X., Ramisetty, R., Leisner, T., and Mohr, C.: Chemical Characterization of Highly Functionalized Organonitrates Contributing to Night-Time Organic Aerosol Mass Loadings and Particle Growth, Environ. Sci. Technol., 53, 1165–1174, https://doi.org/10.1021/acs.est.8b05826, 2019.
Hyttinen, N., Rissanen, M. P., and Kurtén, T.: Computational Comparison of Acetate and Nitrate Chemical Ionization of Highly Oxidized Cyclohexene Ozonolysis Intermediates and Products, J. Phys. Chem. A, 121, 2172–2179, https://doi.org/10.1021/acs.jpca.6b12654, 2017.
Jaoui, M., Corse, E., Kleindienst, T. E., Offenberg, J. H., Lewandowski, M., and Edney, E. O.: Analysis of Secondary Organic Aerosol Compounds from the Photooxidation of d Limonene in the Presence of NOx and their Detection in Ambient PM2.5, Environ. Sci. Technol., 40, 3819–3828, 2006.
Jenkin, M. E., Saunders, S. M., and Pilling, M. J.: The tropospheric degradation of volatile organic compounds: a protocol for mechanism development, Atmos. Environ., 31, 81–104, 1997.
Jenkin, M. E., Valorso, R., Aumont, B., Rickard, A. R., and Wallington, T. J.: Estimation of rate coefficients and branching ratios for gas-phase reactions of OH with aliphatic organic compounds for use in automated mechanism construction, Atmos. Chem. Phys., 18, 9297–9328, https://doi.org/10.5194/acp-18-9297-2018, 2018.
Jenkin, M. E., Valorso, R., Aumont, B., and Rickard, A. R.: Estimation of rate coefficients and branching ratios for reactions of organic peroxy radicals for use in automated mechanism construction, Atmos. Chem. Phys., 19, 7691–7717, https://doi.org/10.5194/acp-19-7691-2019, 2019.
Johnston, H. S. and Heicklen, J.: Tunnelling Corrections for Unsymmetrical Eckart Potential Energy Barriers, J. Phys. Chem., 66, 532–533, 1962.
Jokinen, T., Sipilä, M., Richters, S., Kerminen, V. M., Paasonen, P., Stratmann, F., Worsnop, D., Kulmala, M., Ehn, M., Herrmann, H., and Berndt, T.: Rapid autoxidation forms highly oxidized RO2 radicals in the atmosphere, Angew. Chem. Int. Ed., 53, 14596–14600, https://doi.org/10.1002/anie.201408566, 2014.
Jokinen, T., Berndt, T., Makkonen, R., Kerminen, V. M., Junninen, H., Paasonen, P., Stratmann, F., Herrmann, H., Guenther, A. B., Worsnop, D. R., Kulmala, M., Ehn, M., and Sipilä, M.: Production of extremely low volatile organic compounds from biogenic emissions: Measured yields and atmospheric implications, P. Natl. Acad. Sci. USA, 112, 7123–7128, https://doi.org/10.1073/pnas.1423977112, 2015.
Jørgensen, S., Knap, H. C., Otkjær, R. V., Jensen, A. M., Kjeldsen, M. L., Wennberg, P. O., and Kjaergaard, H. G.: Rapid Hydrogen Shift Scrambling in Hydroperoxy-Substituted Organic Peroxy Radicals, J. Phys. Chem. A, 120, 266–275, https://doi.org/10.1021/acs.jpca.5b06768, 2016.
Kanakidou, M., Seinfeld, J. H., Pandis, S. N., Barnes, I., Dentener, F. J., Facchini, M. C., Van Dingenen, R., Ervens, B., Nenes, A., Nielsen, C. J., Swietlicki, E., Putaud, J. P., Balkanski, Y., Fuzzi, S., Horth, J., Moortgat, G. K., Winterhalter, R., Myhre, C. E. L., Tsigaridis, K., Vignati, E., Stephanou, E. G., and Wilson, J.: Organic aerosol and global climate modelling: a review, Atmos. Chem. Phys., 5, 1053–1123, https://doi.org/10.5194/acp-5-1053-2005, 2005.
Kenseth, C. M., Huang, Y., Zhao, R., Dalleska, N. F., Hethcox, J. C., Stoltz, B. M., and Seinfeld, J. H.: Synergistic O3+OH oxidation pathway to extremely low-volatility dimers revealed in beta-pinene secondary organic aerosol, P. Natl. Acad. Sci. USA, 115, 8301–8306, https://doi.org/10.1073/pnas.1804671115, 2018.
Kirkby, J., Duplissy, J., Sengupta, K., Frege, C., Gordon, H., Williamson, C., Heinritzi, M., Simon, M., Yan, C., Almeida, J., Tröstl, J., Nieminen, T., Ortega, I. K., Wagner, R., Adamov, A., Amorim, A., Bernhammer, A. K., Bianchi, F., Breitenlechner, M., Brilke, S., Chen, X., Craven, J., Dias, A., Ehrhart, S., Flagan, R. C., Franchin, A., Fuchs, C., Guida, R., Hakala, J., Hoyle, C. R., Jokinen, T., Junninen, H., Kangasluoma, J., Kim, J., Krapf, M., Kürten, A., Laaksonen, A., Lehtipalo, K., Makhmutov, V., Mathot, S., Molteni, U., Onnela, A., Peräkylä, O., Piel, F., Petäjä, T., Praplan, A. P., Pringle, K., Rap, A., Richards, N. A., Riipinen, I., Rissanen, M. P., Rondo, L., Sarnela, N., Schobesberger, S., Scott, C. E., Seinfeld, J. H., Sipilä, M., Steiner, G., Stozhkov, Y., Stratmann, F., Tomé, A., Virtanen, A., Vogel, A. L., Wagner, A. C., Wagner, P. E., Weingartner, E., Wimmer, D., Winkler, P. M., Ye, P., Zhang, X., Hansel, A., Dommen, J., Donahue, N. M., Worsnop, D. R., Baltensperger, U., Kulmala, M., Carslaw, K. S., and Curtius, J.: Ion-induced nucleation of pure biogenic particles, Nature, 533, 521–526, https://doi.org/10.1038/nature17953, 2016.
Knap, H. C. and Jørgensen, S.: Rapid Hydrogen Shift Reactions in Acyl Peroxy Radicals, J. Phys. Chem. A, 121, 1470–1479, https://doi.org/10.1021/acs.jpca.6b12787, 2017.
Koch, S., Winterhalter, R., Uherek, E., Kolloff, A., Neeb, P., and Moortgat, G. K.: Formation of new particles in the gas-phase ozonolysis of monoterpenes, Atmos. Environ., 34, 4031–4042, 2000.
Krechmer, J. E., Coggon, M. M., Massoli, P., Nguyen, T. B., Crounse, J. D., Hu, W., Day, D. A., Tyndall, G. S., Henze, D. K., Rivera-Rios, J. C., Nowak, J. B., Kimmel, J. R., Mauldin, R. L., Stark, H., Jayne, J. T., Sipilä, M., Junninen, H., Clair, J. M. S., Zhang, X., Feiner, P. A., Zhang, L., Miller, D. O., Brune, W. H., Keutsch, F. N., Wennberg, P. O., Seinfeld, J. H., Worsnop, D. R., Jimenez, J. L., and Canagaratna, M. R.: Formation of Low Volatility Organic Compounds and Secondary Organic Aerosol from Isoprene Hydroxyhydroperoxide Low-NO Oxidation, Environ. Sci. Technol., 49, 10330–10339, https://doi.org/10.1021/acs.est.5b02031, 2015.
Larsen, B. R., Di Bella, D., Glasius, M., Winterhalter, R., Jensen, N. R., and Hjorth, J.: Gas-phase OH oxidation of monoterpenes: Gaseous and particulate products, J. Atmos. Chem., 38, 231–276, https://doi.org/10.1023/A:1006487530903, 2001.
Lee, A., Goldstein, A. H., Kroll, J. H., Ng, N. L., Varutbangkul, V., Flagan, R. C., and Seinfeld, J. H.: Gas-phase products and secondary aerosol yields from the photooxidation of 16 different terpenes, J. Geophys. Res., 111, D17305, https://doi.org/10.1029/2006jd007050, 2006a.
Lee, A., Goldstein, A. H., Keywood, M. D., Gao, S., Varutbangkul, V., Bahreini, R., Ng, N. L., Flagan, R. C., and Seinfeld, J. H.: Gas-phase products and secondary aerosol yields from the ozonolysis of ten different terpenes, J. Geophys. Res., 111, D07302, https://doi.org/10.1029/2005jd006437, 2006b.
Lee, B. H., Mohr, C., Lopez-Hilfiker, F. D., Lutz, A., Hallquist, M., Lee, L., Romer, P., Cohen, R. C., Iyer, S., Kurtén, T., Hu, W., Day, D. A., Campuzano-Jost, P., Jimenez, J. L., Xu, L., Ng, N. L., Guo, H., Weber, R. J., Wild, R. J., Brown, S. S., Koss, A., de Gouw, J., Olson, K., Goldstein, A. H., Seco, R., Kim, S., McAvey, K., Shepson, P. B., Starn, T., Baumann, K., Edgerton, E. S., Liu, J., Shilling, J. E., Miller, D. O., Brune, W., Schobesberger, S., D'Ambro, E. L., and Thornton, J. A.: Highly functionalized organic nitrates in the southeast United States: Contribution to secondary organic aerosol and reactive nitrogen budgets, P. Natl. Acad. Sci. USA, 113, 1516–1521, https://doi.org/10.1073/pnas.1508108113, 2016.
Lelieveld, J., Butler, T. M., Crowley, J. N., Dillon, T. J., Fischer, H., Ganzeveld, L., Harder, H., Lawrence, M. G., Martinez, M., Taraborrelli, D., and Williams, J.: Atmospheric oxidation capacity sustained by a tropical forest, Nature, 452, 737–740, https://doi.org/10.1038/nature06870, 2008.
Massoli, P., Stark, H., Canagaratna, M. R., Krechmer, J. E., Xu, L., Ng, N. L., Mauldin, Ó., Roy L., Yan, C., Kimmel, J., Misztal, P. K., Jimenez, J. L., Jayne, J. T., and Worsnop, D. R.: Ambient Measurements of Highly Oxidized Gas-Phase Molecules during the Southern Oxidant and Aerosol Study (SOAS) 2013, ACS Earth Space Chem., 2, 653–672, https://doi.org/10.1021/acsearthspacechem.8b00028, 2018.
Mayorga, R., Xia, Y., Zhao, Z., Long, B., and Zhang, H.: Peroxy Radical Autoxidation and Sequential Oxidation in Organic Nitrate Formation during Limonene Nighttime Oxidation, Environ. Sci. Technol., 56, 15337–15346, https://doi.org/10.1021/acs.est.2c04030, 2022.
McFiggans, G., Mentel, T. F., Wildt, J., Pullinen, I., Kang, S., Kleist, E., Schmitt, S., Springer, M., Tillmann, R., Wu, C., Zhao, D., Hallquist, M., Faxon, C., Le Breton, M., Hallquist, Å. M., Simpson, D., Bergström, R., Jenkin, M. E., Ehn, M., Thornton, J. A., Alfarra, M. R., Bannan, T. J., Percival, C. J., Priestley, M., Topping, D., and Kiendler-Scharr, A.: Secondary organic aerosol reduced by mixture of atmospheric vapours, Nature, 565, 587–593, https://doi.org/10.1038/s41586-018-0871-y, 2019.
Mentel, T. F., Springer, M., Ehn, M., Kleist, E., Pullinen, I., Kurtén, T., Rissanen, M., Wahner, A., and Wildt, J.: Formation of highly oxidized multifunctional compounds: autoxidation of peroxy radicals formed in the ozonolysis of alkenes – deduced from structure–product relationships, Atmos. Chem. Phys., 15, 6745–6765, https://doi.org/10.5194/acp-15-6745-2015, 2015.
Miller, J. A., Pilling, M. J., and Troe, J.: Unravelling combustion mechanisms through a quantitative understanding of elementary reactions, P. Combust. Inst., 30, 43–88, https://doi.org/10.1016/j.proci.2004.08.281, 2005.
Moiseenko, K. B., Vasileva, A. V., Skorokhod, A. I., Belikov, I. B., Repin, A. Y., and Shtabkin, Y. A.: Regional Impact of Ozone Precursor Emissions on NOx and O3 Levels at ZOTTO Tall Tower in Central Siberia, Earth Space Sci., 8, e2021EA001762, https://doi.org/10.1029/2021ea001762, 2021.
Møller, K. H., Bates, K. H., and Kjaergaard, H. G.: The Importance of Peroxy Radical Hydrogen-Shift Reactions in Atmospheric Isoprene Oxidation, J. Phys. Chem. A, 123, 920–932, https://doi.org/10.1021/acs.jpca.8b10432, 2019.
Molteni, U., Simon, M., Heinritzi, M., Hoyle, C. R., Bernhammer, A.-K., Bianchi, F., Breitenlechner, M., Brilke, S., Dias, A., Duplissy, J., Frege, C., Gordon, H., Heyn, C., Jokinen, T., Kürten, A., Lehtipalo, K., Makhmutov, V., Petäjä, T., Pieber, S. M., Praplan, A. P., Schobesberger, S., Steiner, G., Stozhkov, Y., Tomé, A., Tröstl, J., Wagner, A. C., Wagner, R., Williamson, C., Yan, C., Baltensperger, U., Curtius, J., Donahue, N. M., Hansel, A., Kirkby, J., Kulmala, M., Worsnop, D. R., and Dommen, J.: Formation of Highly Oxygenated Organic Molecules from α-Pinene Ozonolysis: Chemical Characteristics, Mechanism, and Kinetic Model Development, ACS Earth Space Chem., 3, 873–883, https://doi.org/10.1021/acsearthspacechem.9b00035, 2019.
Nazaroff, W. W. and Weschler, C. J.: Cleaning products and air fresheners: exposure to primary and secondary air pollutants, Atmos. Environ., 38, 2841–2865, https://doi.org/10.1016/j.atmosenv.2004.02.040, 2004.
Ng, N. L., Chhabra, P. S., Chan, A. W. H., Surratt, J. D., Kroll, J. H., Kwan, A. J., McCabe, D. C., Wennberg, P. O., Sorooshian, A., Murphy, S. M., Dalleska, N. F., Flagan, R. C., and Seinfeld, J. H.: Effect of NOx level on secondary organic aerosol (SOA) formation from the photooxidation of terpenes, Atmos. Chem. Phys., 7, 5159–5174, https://doi.org/10.5194/acp-7-5159-2007, 2007.
Ng, N. L., Brown, S. S., Archibald, A. T., Atlas, E., Cohen, R. C., Crowley, J. N., Day, D. A., Donahue, N. M., Fry, J. L., Fuchs, H., Griffin, R. J., Guzman, M. I., Herrmann, H., Hodzic, A., Iinuma, Y., Jimenez, J. L., Kiendler-Scharr, A., Lee, B. H., Luecken, D. J., Mao, J., McLaren, R., Mutzel, A., Osthoff, H. D., Ouyang, B., Picquet-Varrault, B., Platt, U., Pye, H. O. T., Rudich, Y., Schwantes, R. H., Shiraiwa, M., Stutz, J., Thornton, J. A., Tilgner, A., Williams, B. J., and Zaveri, R. A.: Nitrate radicals and biogenic volatile organic compounds: oxidation, mechanisms, and organic aerosol, Atmos. Chem. Phys., 17, 2103–2162, https://doi.org/10.5194/acp-17-2103-2017, 2017.
Novelli, A., Cho, C., Fuchs, H., Hofzumahaus, A., Rohrer, F., Tillmann, R., Kiendler-Scharr, A., Wahner, A., and Vereecken, L.: Experimental and theoretical study on the impact of a nitrate group on the chemistry of alkoxy radicals, Phys. Chem. Chem. Phys., 23, 5474–5495, https://doi.org/10.1039/d0cp05555g, 2021.
Nozière, B. and Vereecken, L.: Direct Observation of Aliphatic Peroxy Radical Autoxidation and Water Effects: An Experimental and Theoretical Study, Angew. Chem. Int. Ed., 58, 13976–13982, https://doi.org/10.1002/anie.201907981, 2019.
Pang, J. Y. S., Novelli, A., Kaminski, M., Acir, I.-H., Bohn, B., Carlsson, P. T. M., Cho, C., Dorn, H.-P., Hofzumahaus, A., Li, X., Lutz, A., Nehr, S., Reimer, D., Rohrer, F., Tillmann, R., Wegener, R., Kiendler-Scharr, A., Wahner, A., and Fuchs, H.: Investigation of the limonene photooxidation by OH at different NO concentrations in the atmospheric simulation chamber SAPHIR (Simulation of Atmospheric PHotochemistry In a large Reaction Chamber), Atmos. Chem. Phys., 22, 8497–8527, https://doi.org/10.5194/acp-22-8497-2022, 2022.
Peeters, J., Vandenberk, S., Piessens, E., and Pultau, V.: H-atom abstraction in reactions of cyclic polyalkenes with OH, Chemosphere, 38, 1189–1195, 1999.
Perraud, V., Bruns, E. A., Ezell, M. J., Johnson, S. N., Yu, Y., Alexander, M. L., Zelenyuk, A., Imre, D., Chang, W. L., Dabdub, D., Pankow, J. F., and Finlayson-Pitts, B. J.: Nonequilibrium atmospheric secondary organic aerosol formation and growth, P. Natl. Acad. Sci. USA, 109, 2836–2841, https://doi.org/10.1073/pnas.1119909109, 2012.
Piletic, I. R. and Kleindienst, T. E.: Rates and Yields of Unimolecular Reactions Producing Highly Oxidized Peroxy Radicals in the OH-Induced Autoxidation of α-Pinene, β-Pinene, and Limonene, J. Phys. Chem. A, 126, 88–100, https://doi.org/10.1021/acs.jpca.1c07961, 2022.
Praske, E., Otkjær, R. V., Crounse, J. D., Hethcox, J. C., Stoltz, B. M., Kjaergaard, H. G., and Wennberg, P. O.: Intramolecular Hydrogen Shift Chemistry of Hydroperoxy-Substituted Peroxy Radicals, J. Phys. Chem. A, 123, 590–600, https://doi.org/10.1021/acs.jpca.8b09745, 2019.
Pullinen, I., Schmitt, S., Kang, S., Sarrafzadeh, M., Schlag, P., Andres, S., Kleist, E., Mentel, T. F., Rohrer, F., Springer, M., Tillmann, R., Wildt, J., Wu, C., Zhao, D., Wahner, A., and Kiendler-Scharr, A.: Impact of NOx on secondary organic aerosol (SOA) formation from α-pinene and β-pinene photooxidation: the role of highly oxygenated organic nitrates, Atmos. Chem. Phys., 20, 10125–10147, https://doi.org/10.5194/acp-20-10125-2020, 2020.
Pye, H. O. T., D'Ambro, E. L., Lee, B., Schobesberger, S., Takeuchi, M., Zhao, Y., Lopez-Hilfiker, F., Liu, J. M., Shilling, J. E., Xing, J., Mathur, R., Middlebrook, A. M., Liao, J., Welti, A., Graus, M., Warneke, C., de Gouw, J. A., Holloway, J. S., Ryerson, T. B., Pollack, I. B., and Thornton, J. A.: Anthropogenic enhancements to production of highly oxygenated molecules from autoxidation, P. Natl. Acad. Sci. USA, 116, 6641–6646, https://doi.org/10.1073/pnas.1810774116, 2019.
Quéléver, L. L. J., Kristensen, K., Normann Jensen, L., Rosati, B., Teiwes, R., Daellenbach, K. R., Peräkylä, O., Roldin, P., Bossi, R., Pedersen, H. B., Glasius, M., Bilde, M., and Ehn, M.: Effect of temperature on the formation of highly oxygenated organic molecules (HOMs) from alpha-pinene ozonolysis, Atmos. Chem. Phys., 19, 7609–7625, https://doi.org/10.5194/acp-19-7609-2019, 2019.
Rio, C., Flaud, P. M., Loison, J. C., and Villenave, E.: Experimental revaluation of the importance of the abstraction channel in the reactions of monoterpenes with OH radicals, Chem. Phys. Chem., 11, 3962–3970, https://doi.org/10.1002/cphc.201000518, 2010.
Rissanen, M. P., Kurtén, T., Sipilä, M., Thornton, J. A., Kangasluoma, J., Sarnela, N., Junninen, H., Jørgensen, S., Schallhart, S., Kajos, M. K., Taipale, R., Springer, M., Mentel, T. F., Ruuskanen, T., Petäjä, T., Worsnop, D. R., Kjaergaard, H. G., and Ehn, M.: The formation of highly oxidized multifunctional products in the ozonolysis of cyclohexene, J. Am. Chem. Soc., 136, 15596–15606, https://doi.org/10.1021/ja507146s, 2014.
Rohrer, F., Bruning, D., Grobler, E. S., Weber, M., Ehhalt, D. H., Neubert, R., Schussler, W., and Levin, I.: Mixing ratios and photostationary state of NO and NO2 observed during the POPCORN field campaign at a rural site in Germany, J. Atmos. Chem., 31, 119–137, https://doi.org/10.1023/A:1006166116242, 1998.
Rohrer, F., Bohn, B., Brauers, T., Brüning, D., Johnen, F.-J., Wahner, A., and Kleffmann, J.: Characterisation of the photolytic HONO-source in the atmosphere simulation chamber SAPHIR, Atmos. Chem. Phys., 5, 2189–2201, https://doi.org/10.5194/acp-5-2189-2005, 2005.
Romonosky, D. E., Laskin, A., Laskin, J., and Nizkorodov, S. A.: High-resolution mass spectrometry and molecular characterization of aqueous photochemistry products of common types of secondary organic aerosols, J. Phys. Chem. A, 119, 2594–2606, https://doi.org/10.1021/jp509476r, 2015.
Rossignol, S., Rio, C., Ustache, A., Fable, S., Nicolle, J., Même, A., D'Anna, B., Nicolas, M., Leoz, E., and Chiappini, L.: The use of a housecleaning product in an indoor environment leading to oxygenated polar compounds and SOA formation: Gas and particulate phase chemical characterization, Atmos. Environ., 75, 196–205, https://doi.org/10.1016/j.atmosenv.2013.03.045, 2013.
Saathoff, H., Naumann, K.-H., Möhler, O., Jonsson, Å. M., Hallquist, M., Kiendler-Scharr, A., Mentel, Th. F., Tillmann, R., and Schurath, U.: Temperature dependence of yields of secondary organic aerosols from the ozonolysis of α-pinene and limonene, Atmos. Chem. Phys., 9, 1551–1577, https://doi.org/10.5194/acp-9-1551-2009, 2009.
Shen, H., Zhao, D., Pullinen, I., Kang, S., Vereecken, L., Fuchs, H., Acir, I. H., Tillmann, R., Rohrer, F., Wildt, J., Kiendler-Scharr, A., Wahner, A., and Mentel, T. F.: Highly Oxygenated Organic Nitrates Formed from NO3 Radical-Initiated Oxidation of β-Pinene, Environ. Sci. Technol., 55, 15658–15671, https://doi.org/10.1021/acs.est.1c03978, 2021.
Shen, H., Vereecken, L., Kang, S., Pullinen, I., Fuchs, H., Zhao, D., and Mentel, T. F.: Unexpected significance of a minor reaction pathway in daytime formation of biogenic highly oxygenated organic compounds, Sci. Adv., 8, 1–11, 2022.
Taatjes, C. A.: Uncovering the Fundamental Chemistry of Alkyl + O2 Reactions via Measurements of Product Formation, J. Phys. Chem. A, 110, 4299–4312, 2006.
Tomaz, S., Wang, D., Zabalegui, N., Li, D., Lamkaddam, H., Bachmeier, F., Vogel, A., Monge, M. E., Perrier, S., Baltensperger, U., George, C., Rissanen, M., Ehn, M., El Haddad, I., and Riva, M.: Structures and reactivity of peroxy radicals and dimeric products revealed by online tandem mass spectrometry, Nat. Commun., 12, 300, https://doi.org/10.1038/s41467-020-20532-2, 2021.
Tröstl, J., Chuang, W. K., Gordon, H., Heinritzi, M., Yan, C., Molteni, U., Ahlm, L., Frege, C., Bianchi, F., Wagner, R., Simon, M., Lehtipalo, K., Williamson, C., Craven, J. S., Duplissy, J., Adamov, A., Almeida, J., Bernhammer, A. K., Breitenlechner, M., Brilke, S., Dias, A., Ehrhart, S., Flagan, R. C., Franchin, A., Fuchs, C., Guida, R., Gysel, M., Hansel, A., Hoyle, C. R., Jokinen, T., Junninen, H., Kangasluoma, J., Keskinen, H., Kim, J., Krapf, M., Kürten, A., Laaksonen, A., Lawler, M., Leiminger, M., Mathot, S., Möhler, O., Nieminen, T., Onnela, A., Petäjä, T., Piel, F. M., Miettinen, P., Rissanen, M. P., Rondo, L., Sarnela, N., Schobesberger, S., Sengupta, K., Sipilä, M., Smith, J. N., Steiner, G., Tomè, A., Virtanen, A., Wagner, A. C., Weingartner, E., Wimmer, D., Winkler, P. M., Ye, P., Carslaw, K. S., Curtius, J., Dommen, J., Kirkby, J., Kulmala, M., Riipinen, I., Worsnop, D. R., Donahue, N. M., and Baltensperger, U.: The role of low-volatility organic compounds in initial particle growth in the atmosphere, Nature, 533, 527–531, https://doi.org/10.1038/nature18271, 2016.
Valiev, R. R., Hasan, G., Salo, V. T., Kubeèka, J., and Kurten, T.: Intersystem Crossings Drive Atmospheric Gas-Phase Dimer Formation, J. Phys. Chem. A, 123, 6596–6604, https://doi.org/10.1021/acs.jpca.9b02559, 2019.
Vereecken, L.: Replication Data for: Formation of highly oxygenated organic molecules from the oxidation of limonene by OH radical: significant contribution of H-abstraction pathway, Jülich DATA [data set], https://doi.org/10.26165/JUELICH-DATA/9JVHEK, 2023.
Vereecken, L. and Nozière, B.: H migration in peroxy radicals under atmospheric conditions, Atmos. Chem. Phys., 20, 7429–7458, https://doi.org/10.5194/acp-20-7429-2020, 2020.
Vereecken, L. and Peeters, J.: Theoretical Study of the Formation of Acetone in the OH-Initiated Atmospheric Oxidation of α-Pinene, J. Phys. Chem. A, 104, 11140–11146, 2000.
Vereecken, L. and Peeters, J.: The 1,5-H-shift in 1-butoxy: A case study in the rigorous implementation of transition state theory for a multirotamer system, J. Chem. Phys., 119, 5159–5170, https://doi.org/10.1063/1.1597479, 2003.
Vereecken, L. and Peeters, J.: Decomposition of substituted alkoxy radicals – part I: a generalized structure-activity relationship for reaction barrier heights, Phys. Chem. Chem. Phys., 11, 9062–9074, https://doi.org/10.1039/b909712k, 2009.
Vereecken, L. and Peeters, J.: A structure-activity relationship for the rate coefficient of H-migration in substituted alkoxy radicals, Phys. Chem. Chem. Phys., 12, 12608–12620, https://doi.org/10.1039/c0cp00387e, 2010.
Vereecken, L. and Peeters, J.: A theoretical study of the OH-initiated gas-phase oxidation mechanism of β-pinene (C10H16): first generation products, Phys. Chem. Chem. Phys., 14, 3802–3815, https://doi.org/10.1039/c2cp23711c, 2012.
Vereecken, L., Müller, J. F., and Peeters, J.: Low-volatility poly-oxygenates in the OH-initiated atmospheric oxidation of á-pinene: impact of non-traditional peroxyl radical chemistry, Phys Chem Chem Phys, 9, 5241-5248, 10.1039/b708023a, 2007.
Vereecken, L., Vu, G., Wahner, A., Kiendler-Scharr, A., and Nguyen, H. M. T.: A structure activity relationship for ring closure reactions in unsaturated alkylperoxy radicals, Phys. Chem. Chem. Phys., 23, 16564–16576, https://doi.org/10.1039/d1cp02758a, 2021.
Waring, M. S.: Secondary organic aerosol formation by limonene ozonolysis: Parameterizing multi-generational chemistry in ozone- and residence time-limited indoor environments, Atmos. Environ., 144, 79–86, https://doi.org/10.1016/j.atmosenv.2016.08.051, 2016.
Wei, D., Fuentes, J. D., Gerken, T., Trowbridge, A. M., Stoy, P. C., and Chamecki, M.: Influences of nitrogen oxides and isoprene on ozone-temperature relationships in the Amazon rain forest, Atmos. Environ., 206, 280–292, https://doi.org/10.1016/j.atmosenv.2019.02.044, 2019.
Whalley, L. K., Edwards, P. M., Furneaux, K. L., Goddard, A., Ingham, T., Evans, M. J., Stone, D., Hopkins, J. R., Jones, C. E., Karunaharan, A., Lee, J. D., Lewis, A. C., Monks, P. S., Moller, S. J., and Heard, D. E.: Quantifying the magnitude of a missing hydroxyl radical source in a tropical rainforest, Atmos. Chem. Phys., 11, 7223–7233, https://doi.org/10.5194/acp-11-7223-2011, 2011.
Williams, P. J. H., Boustead, G. A., Heard, D. E., Seakins, P. W., Rickard, A. R., and Chechik, V.: New Approach to the Detection of Short-Lived Radical Intermediates, J. Am. Chem. Soc., 144, 15969–15976, https://doi.org/10.1021/jacs.2c03618, 2022.
Xu, L., Møller, K. H., Crounse, J. D., Otkjær, R. V., Kjaergaard, H. G., and Wennberg, P. O.: Unimolecular Reactions of Peroxy Radicals Formed in the Oxidation of α-Pinene and β-Pinene by Hydroxyl Radicals, J. Phys. Chem. A, 123, 1661–1674, https://doi.org/10.1021/acs.jpca.8b11726, 2019.
Xu, R., Thornton, J. A., Lee, B. H., Zhang, Y., Jaeglé, L., Lopez-Hilfiker, F. D., Rantala, P., and Petäjä, T.: Global simulations of monoterpene-derived peroxy radical fates and the distributions of highly oxygenated organic molecules (HOMs) and accretion products, Atmos. Chem. Phys., 22, 5477–5494, https://doi.org/10.5194/acp-22-5477-2022, 2022.
Yan, C., Nie, W., Äijälä, M., Rissanen, M. P., Canagaratna, M. R., Massoli, P., Junninen, H., Jokinen, T., Sarnela, N., Häme, S. A. K., Schobesberger, S., Canonaco, F., Yao, L., Prévôt, A. S. H., Petäjä, T., Kulmala, M., Sipilä, M., Worsnop, D. R., and Ehn, M.: Source characterization of highly oxidized multifunctional compounds in a boreal forest environment using positive matrix factorization, Atmos. Chem. Phys., 16, 12715–12731, https://doi.org/10.5194/acp-16-12715-2016, 2016.
Yan, C., Nie, W., Vogel, A. L., Dada, L., Lehtipalo, K., Stolzenburg, D., Wagner, R., Rissanen, M. P., Xiao, M., Ahonen, L., Fischer, L., Rose, C., Bianchi, F., Gordon, H., Simon, M., Heinritzi, M., Garmash, O., Roldin, P., Dias, A., Ye, P., Hofbauer, V., Amorim, A., Bauer, P. S., Bergen, A., Bernhammer, A. K., Breitenlechner, M., Brilke, S., Buchholz, A., Mazon, S. B., Canagaratna, M. R., Chen, X., Ding, A., Dommen, J., Draper, D. C., Duplissy, J., Frege, C., Heyn, C., Guida, R., Hakala, J., Heikkinen, L., Hoyle, C. R., Jokinen, T., Kangasluoma, J., Kirkby, J., Kontkanen, J., Kurten, A., Lawler, M. J., Mai, H., Mathot, S., Mauldin, R. L., Molteni, U., Nichman, L., Nieminen, T., Nowak, J., Ojdanic, A., Onnela, A., Pajunoja, A., Petaja, T., Piel, F., Quelever, L. L. J., Sarnela, N., Schallhart, S., Sengupta, K., Sipila, M., Tome, A., Trostl, J., Vaisanen, O., Wagner, A. C., Ylisirnio, A., Zha, Q., Baltensperger, U., Carslaw, K. S., Curtius, J., Flagan, R. C., Hansel, A., Riipinen, I., Smith, J. N., Virtanen, A., Winkler, P. M., Donahue, N. M., Kerminen, V. M., Kulmala, M., Ehn, M., and Worsnop, D. R.: Size-dependent influence of NOx on the growth rates of organic aerosol particles, Sci. Adv., 6, eaay4945, https://doi.org/10.1126/sciadv.aay4945, 2020.
Yu, J., Cocker, D. R., Griffin, R. J., Flagan, R. C., and Seinfeld, J. H.: Gas-phase ozone oxidation of monoterpenes: Gaseous and particulate products, J. Atmos. Chem., 34, 207–258, https://doi.org/10.1023/A:1006254930583, 1999.
Zhang, H., Yee, L. D., Lee, B. H., Curtis, M. P., Worton, D. R., Isaacman-VanWertz, G., Offenberg, J. H., Lewandowski, M., Kleindienst, T. E., Beaver, M. R., Holder, A. L., Lonneman, W. A., Docherty, K. S., Jaoui, M., Pye, H. O. T., Hu, W., Day, D. A., Campuzano-Jost, P., Jimenez, J. L., Guo, H., Weber, R. J., de Gouw, J., Koss, A. R., Edgerton, E. S., Brune, W., Mohr, C., Lopez-Hilfiker, F. D., Lutz, A., Kreisberg, N. M., Spielman, S. R., Hering, S. V., Wilson, K. R., Thornton, J. A., and Goldstein, A. H.: Monoterpenes are the largest source of summertime organic aerosol in the southeastern United States, P. Natl. Acad. Sci. USA, 115, 2038–2043, https://doi.org/10.1073/pnas.1717513115, 2018.
Zhao, B., Shrivastava, M., Donahue, N. M., Gordon, H., Schervish, M., Shilling, J. E., Zaveri, R. A., Wang, J., Andreae, M. O., Zhao, C., Gaudet, B., Liu, Y., Fan, J., and Fast, J. D.: High concentration of ultrafine particles in the Amazon free troposphere produced by organic new particle formation, P. Natl. Acad. Sci. USA, 117, 25344–25351, https://doi.org/10.1073/pnas.2006716117, 2020.
Zhao, D., Schmitt, S. H., Wang, M., Acir, I.-H., Tillmann, R., Tan, Z., Novelli, A., Fuchs, H., Pullinen, I., Wegener, R., Rohrer, F., Wildt, J., Kiendler-Scharr, A., Wahner, A., and Mentel, T. F.: Effects of NOx and SO2 on the secondary organic aerosol formation from photooxidation of α-pinene and limonene, Atmos. Chem. Phys., 18, 1611–1628, https://doi.org/10.5194/acp-18-1611-2018, 2018.
Zhao, D., Pullinen, I., Fuchs, H., Schrade, S., Wu, R., Acir, I.-H., Tillmann, R., Rohrer, F., Wildt, J., Guo, Y., Kiendler-Scharr, A., Wahner, A., Kang, S., Vereecken, L., and Mentel, T. F.: Highly oxygenated organic molecule (HOM) formation in the isoprene oxidation by NO3 radical, Atmos. Chem. Phys., 21, 9681–9704, https://doi.org/10.5194/acp-21-9681-2021, 2021.
Zhao, D. F., Buchholz, A., Kortner, B., Schlag, P., Rubach, F., Kiendler-Scharr, A., Tillmann, R., Wahner, A., Flores, J. M., Rudich, Y., Watne, Å. K., Hallquist, M., Wildt, J., and Mentel, T. F.: Size-dependent hygroscopicity parameter (κ) and chemical composition of secondary organic cloud condensation nuclei, Geophys. Res. Lett., 42, 10920–10928, https://doi.org/10.1002/2015gl066497, 2015a.
Zhao, D. F., Kaminski, M., Schlag, P., Fuchs, H., Acir, I. H., Bohn, B., Häseler, R., Kiendler-Scharr, A., Rohrer, F., Tillmann, R., Wang, M. J., Wegener, R., Wildt, J., Wahner, A., and Mentel, T. F.: Secondary organic aerosol formation from hydroxyl radical oxidation and ozonolysis of monoterpenes, Atmos. Chem. Phys., 15, 991–1012, https://doi.org/10.5194/acp-15-991-2015, 2015b.
Zhao, Y. and Truhlar, D. G.: The M06 suite of density functionals for main group thermochemistry, thermochemical kinetics, noncovalent interactions, excited states, and transition elements: two new functionals and systematic testing of four M06-class functionals and 12 other functionals, Theor. Chem. Account., 120, 215–241, https://doi.org/10.1007/s00214-007-0310-x, 2008.
Zhao, Y., Thornton, J. A., and Pye, H. O. T.: Quantitative constraints on autoxidation and dimer formation from direct probing of monoterpene-derived peroxy radical chemistry, P. Natl. Acad. Sci. USA, 115, 12142–12147, https://doi.org/10.1073/pnas.1812147115, 2018.
Ziemann, P. J. and Atkinson, R.: Kinetics, products, and mechanisms of secondary organic aerosol formation, Chem. Soc. Rev., 41, 6582–6605, https://doi.org/10.1039/c2cs35122f, 2012.