the Creative Commons Attribution 4.0 License.
the Creative Commons Attribution 4.0 License.
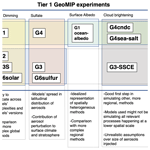
Opinion: The scientific and community-building roles of the Geoengineering Model Intercomparison Project (GeoMIP) – past, present, and future
Daniele Visioni
Ben Kravitz
Alan Robock
Simone Tilmes
Jim Haywood
Olivier Boucher
Mark Lawrence
Peter Irvine
Ulrike Niemeier
Gabriel Chiodo
Chris Lennard
Shingo Watanabe
John C. Moore
Helene Muri
The Geoengineering Model Intercomparison Project (GeoMIP) is a coordinating framework, started in 2010, that includes a series of standardized climate model experiments aimed at understanding the physical processes and projected impacts of solar geoengineering. Numerous experiments have been conducted, and numerous more have been proposed as “test-bed” experiments, spanning a variety of geoengineering techniques aimed at modifying the planetary radiation budget: stratospheric aerosol injection, marine cloud brightening, surface albedo modification, cirrus cloud thinning, and sunshade mirrors. To date, more than 100 studies have been published that used results from GeoMIP simulations. Here we provide a critical assessment of GeoMIP and its experiments.
We discuss its successes and missed opportunities, for instance in terms of which experiments elicited more interest from the scientific community and which did not, and the potential reasons why that happened. We also discuss the knowledge that GeoMIP has contributed to the field of geoengineering research and climate science as a whole: what have we learned in terms of intermodel differences, robustness of the projected outcomes for specific geoengineering methods, and future areas of model development that would be necessary in the future? We also offer multiple examples of cases where GeoMIP experiments were fundamental for international assessments of climate change.
Finally, we provide a series of recommendations, regarding both future experiments and more general activities, with the goal of continuously deepening our understanding of the effects of potential geoengineering approaches and reducing uncertainties in climate outcomes, important for assessing wider impacts on societies and ecosystems. In doing so, we refine the purpose of GeoMIP and outline a series of criteria whereby GeoMIP can best serve its participants, stakeholders, and the broader science community.
- Article
(1004 KB) - Full-text XML
- BibTeX
- EndNote
The comparison of results from nominally identical experiments in multiple, distinct climate models is useful for understanding models’ biases, for assessing robustness in the climate response to external forcings, and for partitioning sources of uncertainties in future climate projections (Lehner et al., 2020). There is a long history of such model intercomparison projects (MIPs) going back several decades (Cess et al., 1989). This process has become more formalized and rigorous and now falls under the auspices of the Coupled Model Intercomparison Project (CMIP; Meehl et al., 2005), which is one of the flagship efforts of the World Climate Research Programme. CMIP is key to our understanding of future climate change projections, and its results are prominently featured in the Intergovernmental Panel on Climate Change's assessment reports, among other numerous studies. With Phase 6 (CMIP6; Eyring et al., 2016), the decision was made to move from a centralized effort to a more distributed MIP approach, allowing different modeling groups to focus on different aspects of the Earth system. One of the most widely used MIPs is ScenarioMIP, which aims to produce “multi-model climate projections based on alternative scenarios of future emissions” (O'Neill et al., 2016), forming the basis for future projections of climate change. There are over 20 other MIPs spanning a wide variety of research topics, including the Chemistry-Climate Model Initiative (Morgenstern et al., 2017) aimed at evaluating model projections of the stratospheric ozone layer, tropospheric composition, and interactions with climate, the Volcanic Forcing Model Intercomparison Project (VolMIP; Zanchettin et al., 2016) aimed at assessing the robustness of the modeled response of the atmospheric–oceanic coupled system to a volcanic forcing, and the Land Use Model Intercomparison Project (LUMIP; Lawrence et al., 2016) aimed at understanding the climatic contribution of changes in land use activities.
Global mean surface air temperature in the decade 2011–2020 is around 1.2 ∘C higher than the preindustrial period (Chen et al., 2021), and most future climate projections suggest continued warming in the future, with only a very few ambitious scenarios managing to stabilize temperatures in the second half of the century. The rate of warming in recent decades is unprecedented in at least the last 2000 years. Mitigation efforts to reduce emissions of greenhouse gases, which are the root cause of global warming and the associated climate change, have been insufficient, with concentrations of atmospheric carbon dioxide over the last 30 years being accurately represented by the IS92a “business as usual” scenario that was developed over 30 years ago (Pedersen et al., 2020). Even though countries across the world agreed in the Paris Agreement (UNFCCC; The Paris Agreement, https://unfccc.int/documents/184656, last access: 3 May 2023) to keep “the increase in global average temperature to well below 2 ∘C above preindustrial levels and pursuing efforts to limit temperature increase to 1.5 ∘C”, their submitted Intended Nationally Determined Contributions (INDCs) of greenhouse gas emissions would be consistent with a projected median warming of between 2.6 and 3.1 ∘C by 2100 (Rogelj et al., 2016).
Because of the uncertainty of remaining below 1.5 or 2 ∘C of warming through emission cuts alone, around 10 years ago, an international group of researchers (Kravitz et al., 2011) proposed a new framework to coordinate climate modeling experiments to study proposals for solar geoengineering (also known as solar radiation modification – SRM – or solar climate intervention), aimed at understanding the impacts of proposed methods to offset the warming produced by an increase in greenhouse gases by directly intervening in the Earth's radiative balance. Fundamentally, these studies aim to produce a negative radiative forcing by increasing the planetary albedo to partly counteract the positive forcing of CO2 and other greenhouse gases. For comprehensive reviews of the scientific aspects raised by solar geoengineering techniques, see for instance Lawrence et al. (2018), Kravitz and MacMartin (2020), and international reports such as those from the National Academy of Science, Engineering and Medicine (National Academies of Sciences Engineering and Medicine, 2021) and EuTRACE (Schäfer et al., 2015). These summaries build upon the long history of discussion of solar geoengineering (see for instance Budyko, 1978; Keith, 2000; Govindasamy et al., 2003), but the 2006 editorial essay by Paul Crutzen, “Albedo Enhancement by Stratospheric Sulfur Injections: A Contribution to Resolve a Policy Dilemma?” (Crutzen, 2006), was a landmark in drawing the attention of the scientific community on the topic, as can be seen by the immediate responses it elicited (Bengtsson, 2006; Cicerone, 2006; Kiehl, 2006; MacCracken, 2006; Lawrence, 2006) and in the follow-up reflections a decade afterwards (Lawrence and Crutzen, 2017; Boettcher and Schäfer, 2017).
This international framework, the Geoengineering Model Intercomparison Project (GeoMIP), was initially coordinated in parallel with the European Union project “Implications and Risks of engineering solar radiation to limit climate change” (IMPLICC; Schmidt et al., 2012b), which included the intercomparison of simulations of four climate models for some of the same simulation setups as used in the first round of GeoMIP simulations (Schmidt et al., 2012a). The motivation for the initiation of this project was the lack of consistency between initial geoengineering studies, which resulted in very different climate outcomes, complicating the process of disentangling some of the observed differences (Rasch et al., 2008; Jones et al., 2010). A set of standardized experiments comprising a reduction in the solar constant and the injection of SO2 in the equatorial stratosphere was proposed and later expanded to encompass other geoengineering techniques (such as marine cloud brightening and cirrus cloud thinning) and following climate change scenarios described by CMIP6 (Kravitz et al., 2013, 2015). The GeoMIP community has produced over 100 papers (121 in November 2022 following the self-reported list tracked on the GeoMIP website, http://climate.envsci.rutgers.edu/GeoMIP/publications.html, last access: 3 November 2022) discussing or analyzing the impacts of these standardized experiments on the atmosphere, ocean, ecosystems, and human societies. As an officially endorsed part of CMIP, GeoMIP has enjoyed a collaborative relationship with other MIPs, exchanging findings and lessons learned and co-developing experiment protocols, moving our knowledge of climate geoengineering forward compared to 10 years ago.
The purpose of this paper is to take stock of GeoMIP as a project based on our collective experience in this field. What have been its successes in advancing knowledge around geoengineering or climate science as a whole? What are some shortcomings with its experiments, analysis, or coordination? What collaborations has it facilitated, and what are some missed opportunities? Perhaps most importantly, what are its next steps and the outstanding questions it needs to address? There are, of course, few objective answers to these questions; we have identified when we are making subjective judgments and upon what values those opinions are based. In the next three sections we provide overviews of past (CMIP5) and present GeoMIP (CMIP6) experiments, test beds for experiment development, and planned future experiments. Following that, in Sect. 5, we reflect on the role of GeoMIP and provide our conclusions and outlook in Sect. 6.
The CMIP protocol specifies that MIP experiments be divided into tiers. Tier-1 experiments are the most scientifically relevant to the broader community and hence are the highest-priority ones and are sometimes considered the minimum requirements for participation in that MIP. Subsequent tiers, while also scientifically relevant, are considered lower priority. The philosophy of GeoMIP has always been to keep the number of Tier-1 experiments small so as to reduce barriers to participation and increase the number of models conducting these core experiments. In Table 1, we provide a summary of all the formally adopted GeoMIP experiments to date, including the number of models that have participated in each experiment. Tier-1 experiments are summarized in Fig. 1.
Kravitz et al. (2011)Kravitz et al. (2015)Kravitz et al. (2013)Kravitz et al. (2011)Kravitz et al. (2011)Niemeier et al. (2013)Alterskjaer et al. (2013)Kravitz et al. (2011)Kravitz et al. (2013)Kravitz et al. (2013)Gabriel et al. (2017)Tilmes et al. (2015)Plummer et al. (2021)Kravitz et al. (2015)Kravitz et al. (2015)Kravitz et al. (2015)Tilmes et al. (2020)Weisenstein et al. (2022)Table 1Summary of all experiments in GeoMIP, with the specific reference of the paper in which they were described for further detail (last column). CDNC: cloud droplet number concentration, GHG: greenhouse gas, ODS: ozone-depleting substance, PI: preindustrial, SST: sea surface temperature, TOA: top of atmosphere.
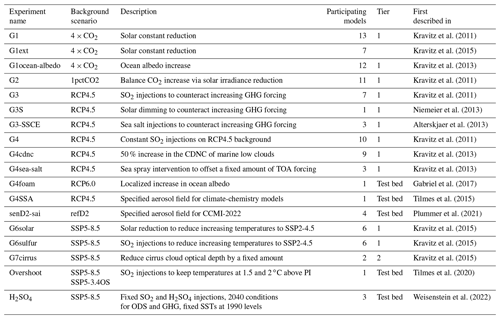
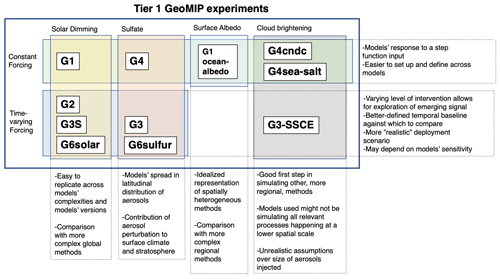
Figure 1Schematic summary of all Tier-1 GeoMIP experiments across different iterations (Kravitz et al., 2011, 2013, 2015). In rows, experiments are categorized based on how the geoengineering forcing is applied: constant or time-varying. In columns, experiments are categorized based on the method of applied forcing. Reference scenarios for each experiment are listed in Table 1.
2.1 Solar dimming: G1, G1ext, G2
Of all possible experiments, the simplest and easiest to replicate in different climate models aims to offset the radiative forcing from an increase in CO2 with a reduction in the model's solar constant. This method directly represents the idea of space sunshades (Angel, 2006): while potentially effective, technical feasibility and costs associated with deployment presently make such an approach prohibitive when compared to other proposed climate geoengineering methods (e.g., The Royal Society, 2009). More relevant for immediately practical geoengineering methods, solar dimming approximates the broad radiative effects of stratospheric aerosol injection.
Experiments G1 (CMIP5) and G1ext (CMIP6) involved offsetting the forcing from an instantaneous quadrupling of the CO2 concentration (abrupt4×CO2, a standard CMIP experiment) with solar constant reduction, so that the net top-of-atmosphere (TOA) radiative flux in each model was within ±0.1 W m−2 of the baseline value in the first decade of simulation (Kravitz et al., 2011). The high level of replicability means that results between CMIP5 and CMIP6 could be easily compared (Kravitz et al., 2021), even in some cases allowing for a comparison between different model versions; in Fig. 2 we show such a comparison across CMIP5 and CMIP6 models reproduced from Kravitz et al. (2021). For instance, such a comparison showed that across the 20 models that performed this experiment across two generations, the value of the solar reduction needed ranged from 3.8 % to 5.0 % (Kravitz et al., 2021). G1 has been extensively studied in terms of the hydrological response (Tilmes et al., 2013; Kravitz et al., 2013, 2014) and from an energetic and thermodynamics perspective (Russotto and Ackerman, 2018a, b; Virgin and Fletcher, 2022), highlighting both some commonalities in the response of the hydrological cycle to a reduction in incoming shortwave radiation and some large discrepancies in the cloud response. Similar experiments have also been performed outside of the GeoMIP framework: for instance, Irvine et al. (2019) used a higher-resolution model to understand changes in extremes and precipitation in a case where a doubling of preindustrial CO2 is partially offset by a reduction in the solar constant. Results from G1 have been used for more specific impact analyses (e.g., Bal et al., 2019; Harding et al., 2020), which poses two important issues. First, G1 is an extreme, idealized case, and thus a straightforward analysis of climate model output from G1 cannot be used as a prediction of what climate engineering would do under any practical deployment strategy. This is particularly true for variables such as regional precipitation for which solar dimming is a poor proxy for aerosol injections or other methods (see Niemeier et al., 2013; Visioni et al., 2021a) or for comparisons of tropical and high-latitude effects, considering that uniformly reducing the solar constant tends to produce a stronger cooling in the tropics (e.g., Govindasamy et al., 2003; Kravitz et al., 2013). Second, there is no single answer for what climate engineering “would do”, as the effects of climate engineering can, to some degree, be designed to mitigate any residual climate change impacts (e.g., Kravitz et al., 2016); this is discussed further in Sect. 3.5 below.
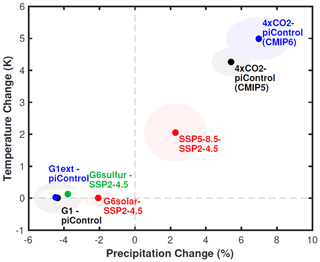
Figure 2A comparison of global temperature (K) and precipitation (%) changes for some Tier-1 GeoMIP experiments across CMIP5 and CMIP6. Points represent the multimodel averages for each experiment, and shaded areas represent 2 multimodel standard errors. Values for G1 and 4×CO2 (CMIP5, 13 models averaged) and G1ext and 4×CO2 (7 models) are from Kravitz et al. (2021), comparing against piControl values in the last 40 years of the experiment (years 11–50). Values for G6solar, G6sulfur, and SSP5-8.5 (six models) are from Visioni et al. (2021b), comparing against SSP2-4.5 values in the last 20 years of the experiment (2080–2099).
G2 similarly prescribes a solar reduction to offset an increase in the CO2 concentration, but in this case CO2 is increased by 1 % every year, and the solar constant is successively reduced each year, with similar intermodel differences to G1 (Jones et al., 2013). G2 has been more seldom used and usually as a way to test linearity assumptions in G1 (MacMartin and Kravitz, 2016). It was, however, featured in the first multimodel intercomparison of the termination effect (Jones et al., 2013); the gradual change in forcing allowed for a computation of rates of climate change under geoengineering with rates of change under termination.
G1 has substantial advantages that merit keeping it in future iterations of GeoMIP. First, while solar dimming does not capture some important features of climate response to stratospheric aerosol injection (Visioni et al., 2021a), it does capture some of the broad radiative effects, giving an indication of some of the radiative impacts. Also, G1 is easy to perform in all climate models, providing a low barrier to participation in GeoMIP, which is important for community-building and developing high confidence in results. Nevertheless, its limits as compared to more detailed representations of the effects of more practical geoengineering methods should always be well communicated (Reynolds, 2022).
2.2 Surface albedo modification: G1ocean-albedo
Marine cloud brightening (MCB; Latham, 1990) is also a commonly researched method of conducting geoengineering, but simulating MCB in a multimodel context has proven challenging for experimental design. Pre-GeoMIP simulations either injected sea salt aerosols directly into the marine boundary layer (e.g., Jones and Haywood, 2012) or increased the cloud droplet number concentration (CDNC) in marine low clouds (e.g., Jones et al., 2009; Rasch et al., 2009). However, because different models have different cloud cover amounts and locations and the key processes behind MCB need to be parameterized in global models, any multimodel comparison of these methods will necessarily impose different amounts and locations of forcing, and thus comparison and interpretation of the results may be challenging. While this can still be useful for a multimodel comparison (see Sect. 2.5 below), a more idealized experiment with a more controlled forcing could also be useful.
G1ocean-albedo involves offsetting the forcing from an abrupt quadrupling of the CO2 concentration with an increase in surface albedo over all ocean regions (Kravitz et al., 2013). While only loosely approximating the effects of MCB, it does capture differential forcing between land and ocean as well as a different perturbation to column absorption and vertical motion than would result from solar dimming. One study thus far has looked at a multimodel comparison of G1ocean-albedo results, finding that even though the models were in net top-of-atmosphere energy balance, global average temperature increased due to differential warming of the atmosphere and ocean, resulting in increased land–ocean energy transport (Kravitz et al., 2018). While perhaps this experiment has limited relevance for understanding potential geoengineering deployments, it does indicate the usefulness of geoengineering simulations for understanding fundamental climate responses to forcing.
2.3 Stratospheric aerosol injections: G3 and G4
One of the first proposed methods of conducting geoengineering is to mimic the cooling effects of a volcanic eruption by injecting sulfate aerosol precursors into the (tropical) stratosphere (Budyko, 1978). The first general circulation model simulations of this method of geoengineering (Robock et al., 2008) described their simulations in terms of or of a Pinatubo eruption every year (5 Tg SO2 and 10 Tg SO2 per year, respectively, based on Pinatubo estimates by Timmreck et al., 2018). The experiments G3 and G4 (Kravitz et al., 2011) aimed to reproduce this in multiple models: in G3, the injection of sulfate was aimed at maintaining net top-of-atmosphere radiative forcing at 2020 levels under an RCP4.5 scenario, and in G4, a fixed injection rate of 5 Tg of SO2 per year from 2020 to 2070. In both cases, the injection was at the Equator between 16 and 25 km in altitude, similar to how those same models would reproduce the Pinatubo eruption. As different models have varying assumptions in terms of the initial Pinatubo plume, this protocol led to some discrepancies between models: a more in-depth discussion of this intermodel discrepancy is given by Timmreck et al. (2018), which motivated the development of a modeling volcanic experiment named the Historical Eruption SO2 Emission Assessment (HErSEA) and whose results were recently described by Quaglia et al. (2023). Indeed, Pitari et al. (2014) reported large discrepancies in the amount of global aerosol optical depth necessary in G3 (0.025 in GISS by 2070 and 0.1 in ULAQ-CCM) and a general disagreement over the latitudinal distribution of the aerosols in the G4 experiment. The paucity of models that could reproduce the full cycle from SO2 oxidation to heterogeneous chemistry processes crucial for ozone chemistry was also highlighted. The termination of the implementation in 2070 has also produced interesting research on the impacts of a potential “termination effect”, were geoengineering to be stopped abruptly (Robock et al., 2008; Jones et al., 2013; Parker and Irvine, 2018; Trisos et al., 2018).
In retrospect, these two experiments involved a learning process for GeoMIP. Experiment G3 was difficult to perform, as it involved regular radiative forcing calculations (often via a double-radiation call), and many groups reported having to redo periods of the experiment because they injected too much or too little SO2. Also, because the protocol specified that net top-of-atmosphere radiative forcing should remain at 2020 levels, temperature steadily increased in the simulations because the climate was already in imbalance in 2020. This exacerbated climate adjustments, increasing the spread and uncertainty in the multimodel ensemble. As a result, few models participated in G3, and most of the analysis of this experiment was used to supplement analysis of G4 (Berdahl et al., 2014; Yu et al., 2015), although the runs were used, for instance, to study the impacts on agriculture (Singh et al., 2020), Atlantic hurricanes (Moore et al., 2015), Arctic permafrost (Chen et al., 2020), and flood return frequency (Wei et al., 2018).
In turn, one of the intended purposes of G4 was to “do the experiment the same way that [you] would simulate Pinatubo”, and the groups would then look at the model spread in the climate outcomes. However, because the models had such different microphysics representations, aerosol distributions, and circulation patterns, it was difficult to attribute the spread to any particular processes or model features. However, these experiments did result in quite useful analysis and enabled some conclusions about tropical stratospheric aerosol geoengineering, amongst them the potential stratospheric ozone depletion at high latitudes (Berdahl et al., 2014) and its effect, its capability to reduce (but not halt altogether) ice melting (Berdahl et al., 2014; Zhao et al., 2017), and the dependency of the simulated precipitation reduction on the aerosol interaction with radiation (Ferraro and Griffiths, 2016). They were also used to study the impacts on ecosystems (Trisos et al., 2018). Nevertheless, they also provided important lessons about how to design a controlled experiment for GeoMIP and reinforced the community's notion that aerosol microphysics and circulation patterns are important contributors to model spread.
2.4 Compare and contrast: G6solar and G6sulfur
At the first GeoMIP meeting, there was a proposal (akin to what became the test bed) for G3solar, in which the protocol for G3 was followed up using solar reduction instead of stratospheric sulfate aerosol injection. Although this proposed experiment did not receive much participation, the idea of comparing solar dimming and sulfate aerosols in identical protocols emerged as a Tier-1 experiment in CMIP6 in the form of experiments G6solar and G6sulfur (Kravitz et al., 2015). Similar to G3, the amount of sulfate to be injected varies every year (or later modified to be every decade due to the difficulty in calculating forcing in transient runs) to obtain a certain target: in this case, the aim was to reduce global mean surface air temperatures from those under an SSP5-8.5 scenario to those under an SSP2-4.5 scenario, “mitigating” the warming produced by high greenhouse gas (GHG) concentrations. To do so, starting in 2020, models would reduce the solar constant (G6solar) or inject SO2 in a band between 10∘ N and 10∘ S and between 18 and 20 km in altitude (G6sulfur). The presence of two different experiments with similar targets allows for a broader assessment of the differences between solar dimming as a proxy and an actual sulfate injection (Niemeier et al., 2013; Visioni et al., 2021a, and Fig. 2) and has allowed us to assess the contribution of stratospheric uncertainties to overall uncertainties in the climate response to geoengineering (Jones et al., 2021; Visioni et al., 2021b; Bednarz et al., 2022). Of the six models that originally participated in G6, only two had comprehensive enough stratospheric chemistry to make them viable for assessing ozone changes. At the same time, three used a prescribed aerosol distribution and three used actual SO2 injections; the overlap between those with comprehensive chemistry and those with interactive aerosols was two (CESM2 and UKESM) (Tilmes et al., 2022). Experiment G4 had a similar mix of explicit and prescribed representations of these processes.
In G6, modeling centers have demonstrated that it is feasible to modify the amount of intervention in the models even once per decade to maintain a predetermined temperature target; the available comparison between temperatures under SSP2-4.5 (same global temperatures, different amount of CO2) allows for a contrast throughout the entire simulation period of multiple scenarios and can also be used to understand when the emerging signal of a geoengineering deployment would be distinguishable from natural variability.
The Max Planck Institute Earth System Model (MPI-ESM) performed two sets of G6 simulations with the same prescribed aerosol forcing but two different horizontal resolutions (roughly 200 and 300 km; Muller et al., 2018). A focused analysis of the differences in the climate response between these two versions could shed light on the impact of higher-resolution modeling on projected geoengineering impacts.
There is an interesting observation that can be made from the G6 experiments and that multiple users of the data have noticed. Six years passed from when the experiment was officially proposed in 2015 (Kravitz et al., 2015), when the modeling centers produced the simulations (early 2020 at the earliest), and when the first analyses came out in 2021 (Jones et al., 2021, with a subset of the models and Visioni et al., 2021b, with all six). With geoengineering studies being a novel, fast-paced field, in those 6 years multiple discussions and studies led many to question the relevance of both a high-emission scenario like SSP5-8.5 and the strategy aiming to “halve” warming to that of SSP2-4.5, as opposed to other, more moderate scenarios that try to discuss geoengineering in the light of the Paris Agreement targets (see Sect. 3.4 for instance). Furthermore, the injection strategy of injecting into the tropical pipe has also been found to be suboptimal (Kravitz et al., 2019; Visioni et al., 2021a). In this sense, some may feel that analyses of G6 results may already look outdated due to the relevance of the specific scenario selected. While this is not necessarily true, as there is a great deal of merit in analyzing results from the latest iteration of CMIP6 models, it is a valid concern. If there is a lesson to learn, it might be that there is a need for “future-proofing” the next generation of proposed GeoMIP experiments, so that they remain relevant for as long as possible. While a focus on “realistic” scenarios will produce results that are seen as more policy-relevant, there is a danger that they will not stand the test of time. Changes to baseline scenarios and assumptions about what is realistic may evolve, but idealized, high signal-to-noise experiments like G1 are timeless.
2.5 Sea spray geoengineering: G3-SSCE, G4sea-salt, and G4cdnc
As discussed in Sect. 2.2 above, GeoMIP developed several Tier-1 experiments to explore multimodel uncertainty in the climate response to marine cloud brightening. They were based on the G3 and G4 experiments described by Kravitz et al. (2013) but substituting sulfur injections with the addition of sea salt.
The first one, G3-SSCE, was described by Alterskjaer et al. (2013); three models involved with IMPLICC participated in that experiment, simulating the effect of an increase in sea salt aerosols in the lower atmosphere. Later, the experiment G4cdnc prescribed increasing the cloud droplet number concentration in all marine low clouds (lower in altitude than 680 hPa), which replicates the net microphysical effect of MCB but without relying on different parameterizations of aerosol–cloud interactions. G4sea-salt on the other hand involved direct aerosol injection into the marine boundary layer (between 30∘ S and 30∘ N), which is the most realistic representation of MCB in GeoMIP but also runs the risk of resulting in a large model spread due to dependence on aerosol–cloud interaction parameterizations as well as intermodel differences between cloud location and forcing strength. Both experiments are less controlled than G1ocean-albedo, in that in G4cdnc and G4sea-salt the MCB forcing will only be applied where there are clouds, which differs between models.
Nevertheless, G4cdnc and G4sea-salt revealed important insights about MCB, including the importance of cloud differences between models (Stjern et al., 2018) and that the aerosol direct forcing of sea salt aerosols can be quite important in MCB applications (Ahlm et al., 2017). Arguably, more of the model spread in these MCB experiments was attributable to specific processes than was the case for G3 and G4, which could reflect community investment in uncertainty: aerosol–cloud interactions have received vastly more attention than stratospheric processes. Results from G4cdnc and G4sea-salt have only been investigated in a few studies each (Xie et al., 2022), so it is presently difficult to draw conclusions about how one might design a more controlled MCB experiment for Earth system models.
2.6 Cirrus cloud thinning: G7cirrus
Due to increasing interest in cirrus cloud thinning (CCT; Mitchell and Finnegan, 2009; Storelvmo et al., 2013), GeoMIP proposed a Tier-1 experiment, G7cirrus, in which the fall speed of upper-tropospheric ice crystals was increased (Muri et al., 2014) to achieve a negative radiative forcing. However, due to large uncertainties in model representations of cirrus and upper-tropospheric ice water paths and a disconnect between the ice crystal distribution from increasing fall speed and the distribution that would result from CCT (Gasparini et al., 2020), the GeoMIP community chose to reclassify G7cirrus as a lower-tier experiment. This happened prior to most modeling groups conducting their simulations for Phase 6, which in part explains why only two models have performed G7cirrus simulations to date.
Nevertheless, the tier of the experiment has historically not been a huge barrier to participation, so we suspect there are other issues at play. Based on discussions with the community, there are several main reasons as to why G7cirrus has not received much attention. From a scientific standpoint, during the first Gordon Conference on Climate Engineering held in 2017, some members of the GeoMIP community pointed out that cirrus clouds are rather poorly represented in global circulation models (GCMs), and large challenges remain even in the observational record about the main sources of cirrus cloud formations and their properties (Gasparini et al., 2018; Sourdeval et al., 2018). Fundamentally, this would make the results of such an experiment rather untrustworthy.
From an operational point of view, G7cirrus is also not necessarily easy to perform, as it often requires code editing and testing. We hypothesize about potential ways to mitigate this in Sect. 5 below. Despite not necessarily being a realistic representation of CCT (as well as doubts about the effectiveness of CCT), a multimodel analysis of G7cirrus results would still represent a learning opportunity for the community. Indeed, GeoMIP has thrived on learning from experiments that lack realism, most prominently G1.
2.7 GeoMIP6 time-slice experiments
In addition to the Tier-1 experiments in GeoMIP6, there were several time-slice experiments proposed as Tier-2 simulations. These experiments involve 10-year simulations with fixed sea surface temperatures in which an external forcing is applied around a particular time, branched from the Tier-1 experiments. These were introduced to aid in separating the rapid adjustments from the feedback response, which was a major focus of GeoMIP analyses in previous iterations. As of the writing of this paper, few (if any) modeling groups have completed these experiments, and there does not appear to be widespread interest in conducting them. We suspect this is for a few reasons. First, the GeoMIP community seems to be uncertain about the value of these time-slice experiments, so they have been deprioritized. Also, many of the analysis directions in GeoMIP have moved away from fast–slow response diagnostics, due perhaps to a stronger focus on a “gradual” deployment, obviating the need to complete the time-slice experiments.
While these experiments would still be useful, they were introduced at a time when the analysis they would engender was not as popular. Perhaps the lesson learned is to design experiments that could serve multiple purposes rather than a narrow purpose (diagnosing fast–slow responses). However, one could argue that lower tiers are well suited for this sort of specificity, and even if only a few models conduct those simulations, it would still be effort well spent. Nevertheless, the time-slice experiments proposed could easily fit within the spirit of other MIPs that are aimed at diagnosing these processes, indicating that it would be prudent to more actively pursue coordination between GeoMIP and other MIPs.
Together with the experiments discussed in Sect. 2, numerous other experiments have been proposed and performed as test-bed experiments. Kravitz et al. (2015) initiated the GeoMIP test bed whereby groups could propose simulations and conduct them with a limited set of models, providing a pathway toward formal adoption by GeoMIP if those simulations go well. Here we discuss some of the proposed test-bed experiments and other relevant geoengineering experiments that have been or could be leveraged by the community and replicated.
3.1 Considering other sulfate precursors: SO2 and H2SO4 injections
Most model simulations of stratospheric aerosol injections have simulated the release of SO2, due to the volcanic analog. While this allows for some calibration based on observations of volcanic eruptions, modeling studies indicate that using SO2 injection results in large aerosols, which reduces the scattering efficiency, increases fall speed, and increases side-effects (like stratospheric heating). Pierce et al. (2010) and following works (Benduhn et al., 2016; Vattioni et al., 2019) have proposed direct injection of H2SO4 particles into the accumulation mode, which would avoid H2SO4 vapors formed from SO2 oxidation from coagulating on preexisting particles and having them grow too much. A test-bed experiment was carried out by Weisenstein et al. (2022), comparing and contrasting the injection of SO2 and H2SO4 with the aim of observing the response of a subset of GeoMIP models with interactive aerosol microphysics. The injection of 5, 10, and 25 Tg of S in either form was simulated in two different injection strategies, one uniformly spreading the aerosols between 30∘ N and 30∘ S at all longitudes and one injecting at 30∘ N and 30∘ S in only one grid box. As in G4, the injection of fixed amounts of materials highlighted models' differences in their formation of aerosols, forcing efficiency, and cascading impacts, such as the response of stratospheric dynamics (Franke et al., 2021), and confirmed the possibility of H2SO4 injections as a way to constrain aerosol sizes towards more efficient radii. The use of three models with different aerosol treatments also allowed for more in-depth analyses of models' differences in terms of simulated size distribution, possibly also highlighting the need for more detailed aerosol microphysics in climate models.
3.2 Isolating uncertainties through prescribed aerosol fields: G4SSA and Chemistry-Climate Model Initiative (CCMI) senD2-sai
The complex contribution of aerosol microphysics, chemistry, and dynamical changes in determining the overall stratospheric response has been highlighted in many of the general GeoMIP experiments (Visioni et al., 2021b). One way to constrain part of the response may be by prescribing an identical aerosol field in different models in order to obtain a similar perturbation and understand how and why models differ in their projection of stratospheric heating and chemical changes to an identical perturbation; such an experiment can also overcome the lack of a detailed interactive aerosol treatment in some ESMs. Tilmes et al. (2015) first proposed a similar experiment called G4 Specified Stratospheric Aerosols (G4SSA), offering the community a prescribed aerosol data set determined using the ECHAM5-HAM microphysical model. At least one model with detailed chemistry but lacking a high top and stratospheric aerosol treatment performed the simulations as they were prescribed (Xia et al., 2017), and one model scaled the field to perform G6sulfur simulations (Visioni et al., 2021b). However, in this latter case, while the model prescribed the aerosol distribution, it made its own assumption about the size distribution of the particles that form the optically thick cloud (Tilmes et al., 2022). This highlights the difficulties in properly performing such an experiment, making sure all models capture the same aerosol properties in order to reduce sources of uncertainty in projections.
Some insights into best practices for such an experiment could be gained by experiments with a similar philosophy described by Zanchettin et al. (2022) for VolMIP. After highlighting the intermodel disagreement in a Tambora-like simulation (Clyne et al., 2021) where injection rates of SO2 were prescribed, Zanchettin et al. (2022) discussed the prescription of a volcanic forcing input between models, so as to focus on intermodel differences in the surface climate response. They showed that, by combining the forcing prescription with a robust sampling of initial conditions between models (something that is fundamental in volcanic simulations but that would not be in long-term geoengineering ones), model disagreement could be reduced, and a further focus could be given to the climatic surface response.
CCMI has collaborated with some in the GeoMIP community to set up a new shared experiment in order to support the new phase of CCMI (CCMI-2022) meant to inform the 2026 World Meteorological Organization (WMO)/United Nation Environment Programme (UNEP) Scientific Assessments of Ozone Depletion. In particular, CCMI models expressed interest in an experiment similar to G4SSA in which chemistry-climate models could prescribe the same aerosol distribution and observe changes in key stratospheric quantities. This experiment, senD2-sai, described in the July 2021 Stratosphere-troposphere Processes And their Role in Climate (SPARC) newsletter (Plummer et al., 2021), will use a new aerosol field produced with CESM2-WACCM6, symmetrical around the Equator, maintaining sea surface temperatures fixed at present levels and running for 75 years from 2025 to 2100. The synergy between GeoMIP and CCMI, with all the expertise such a joint effort could leverage, is an exciting opportunity for GeoMIP.
3.3 Isolating the role of the stratospheric heating contribution to stratospheric aerosol injection (SAI) experiments
The absorption of longwave radiation by the sulfate aerosols and the enhanced absorption of shortwave radiation by ozone induced by aerosol scattering lead to a higher stratospheric warming that has long been known to produce numerous effects, both in the stratosphere and in the troposphere. Different sizes, spatial distributions, persistence, and chemical compositions of the injected aerosols would modify the specifics of this warming. Studies of past volcanic eruptions (Robock and Mao, 1995; Polvani et al., 2019; Coupe and Robock, 2021; DallaSanta and Polvani, 2022) have revealed little consensus about the dynamical effects of this stratospheric warming on surface climate. Furthermore, observing systems are either sparse or, in the case of satellite-based infrared sounders, influenced by the presence of the volcanic aerosols. Radiosonde and satellite-based microwave sounders do indicate some warming, but the amount remains uncertain. So, while experiments that prescribe the aerosol field can highlight how different models warm the lower stratosphere differently, experiments that directly prescribe a stratospheric heating perturbation (e.g., Simpson et al., 2019) may help isolate model spread in the dynamical and surface responses, such as the models' response to El Niño events (Liu et al., 2022). Nonetheless, these experiments could shed light on which processes contribute more to the overall intermodel spread in experiments such as G6sulfur.
Two shared protocols have been proposed for this purpose: one aimed at observing surface changes induced by the stratospheric heating and one aimed at observing stratospheric dynamics changes. The fact that two different sets of models have been used for this purpose highlights the possibility of exploring some of the processes involved in geoengineering through the use of multiple tools: for surface changes, lower-resolution models with prescribed ozone and preindustrial conditions that allow for the production of a larger ensemble of simulations in order to better quantify the significance of some of the observed changes; for stratospheric changes, models with high stratospheric vertical resolution used for the QBOi experiments (Butchart et al., 2018).
One of the issues with determining a shared protocol for this kind of experiment is the determination of the amount of stratospheric warming to simulate. A higher warming signal obviously allows for a larger signal-to-noise ratio, but there might be questions over the realism or the relevance of such warming signals, particularly if that large heat flux results in nonlinearities in the model response. For instance, in the G6sulfur experiments, for a global increase in optical depth of 0.1, models provide a range of warming in the lower tropical stratosphere between 1 and 5 K to offset a surface warming of roughly 0.5 K, whereas by the end of the century the stratospheric warming ranges between 5 and 14 K to offset a surface warming of (on average) 2 K. Simpson et al. (2019) and Visioni et al. (2021a) both imposed a stratospheric heating signal to isolate its impacts on the surface climate. They used an average value of 12 K based on results from GLENS simulations (described in Sect. 6) under an RCP8.5 scenario. Results found this would offset almost 5 K of surface warming.
On the other hand, the first proposed protocols mentioned in this section decided to use a stratospheric heating perturbation consistent with a forcing offset of 2 W m−2 derived from Dai et al. (2018), which would result in a much lower average stratospheric heating. This choice will thus produce results that would be expected for a “moderate” deployment and clearly a much lower signal for the surface perturbation obtained.
Both approaches have merits and shortcomings, depending on their specific intent. Nonetheless, both are fundamental pieces of the puzzle in trying to separate uncertainties related to the dynamical perturbations that stratospheric sulfate would produce. Such perturbations might be difficult to isolate in more comprehensive experiments such as G6sulfur. In this way, stratospheric heating experiments can be seen as complementary to experiments such as G1, isolating the contribution of changes in the incoming shortwave radiation (although solar dimming experiments might also modify stratospheric dynamics, as pointed out by Bednarz et al., 2022).
3.4 Considering other scenarios: the Overshoot experiment
Tilmes et al. (2020) proposed a new GeoMIP test-bed experiment that aimed to examine the response to multiple stratospheric aerosol geoengineering scenarios aimed at keeping temperatures at two targets (2 and 1.5 ∘C above preindustrial), not only under a high-emission scenario (SSP5-8.5), but also under a scenario representing an “overshoot” (SSP5-3.4OS, Meinshausen et al., 2020), that is, a scenario with very large carbon dioxide removal after 2040 which results in only a brief exceedance of the 2 ∘C target. This idea of “peak shaving” has long been part of the discourse around geoengineering to represent temporary deployment to keep temperatures down while mitigation and negative-emission efforts are ramped up (Long and Shepherd, 2014; MacMartin et al., 2018). While this experiment could be part of a broader discussion around multiple scenarios involving high and low levels of intervention, SSP5-3.4OS is a Tier-2 experiment in ScenarioMIP, and thus modeling centers may not have the underlying emission scenario simulations. Furthermore, the geoengineering overshoot scenario uses the same feedback algorithm (Sect. 3.5 below) described by Kravitz et al. (2017), which not many modeling centers have implemented yet.
Lastly, some modelers might feel that when it comes to simulating “policy-relevant” scenarios, one should prioritize those that appear to be more plausible (although, while implausibility can be determined in some cases, plausibility is entirely subjective), and a scenario with very large amounts of greenhouse gas removal already by 2040 might very well be considered unrealistic. Meinshausen et al. (2020) describe SSP5-3.4OS as “geophysically interesting”, and perhaps the proposed geoengineering overshoot scenario should be viewed through the same lens. It also has the merit of fostering discussions about how to select policy-relevant scenarios that might be important for future CMIP choices; for example, considering a geoengineering scenario that includes carbon removal could lead to studies that quantify the interaction between solar geoengineering and carbon removal holistically (Xu et al., 2020). In particular, as SSP5-3.4OS relies heavily on bioenergy crops to reduce the CO2 concentration in the second half of the century (Melnikova et al., 2022), it will be interesting to investigate the interactions between stratospheric aerosols and the potential for mitigation through Bioenergy with Carbon Capture and Storage (BECCS).
3.5 Expanding the strategy space: single-point injection simulations and explicit feedback
The idea of using control theory to modify the annual SO2 injection amount (MacMartin et al., 2018; Kravitz et al., 2016, 2017) has been gaining traction in the geoengineering research community. In recent years, multiple experiments using CESM in various configurations have used a feedback algorithm to demonstrate that surface impacts of stratospheric aerosol geoengineering can be reduced if, instead of simulating equatorial or tropical injections of SO2, these injections are distributed over other latitudes (namely, 15∘ N, 15∘ S, 30∘ N, and 30∘ S) to control not just global mean temperatures, but also interhemispheric and Equator-to-pole temperature gradients (see Kravitz et al., 2017; Tilmes et al., 2018; Richter et al., 2022). The merits of this strategy have been discussed in depth by Kravitz et al. (2019) as they compare to equatorial injections, leading many to ask whether future GeoMIP experiments should incorporate an explicit strategy dimension (MacMartin et al., 2022); for example, future GeoMIP experiments could include off-equatorial injection strategies instead of the equatorial strategy used in G4 and G6sulfur.
Two obstacles limit this option: the working of the feedback algorithm that directly imposes in the model how much SO2 to inject every year may depend on exactly how each climate model imposes emissions in the model, requiring both an interactive SO2-to-sulfate aerosol treatment, which not all models have, and some software engineering applied to each model to make the algorithm work. Secondly, developing the control algorithm that manages more than one degree of freedom required previous sensitivity studies that determine the response of single-point injections at the required injection locations, as done by Tilmes et al. (2017) and MacMartin et al. (2017) for CESM1(WACCM). A GeoMIP test-bed experiment reproducing the single-point injection simulations and developing a similar algorithm for multiple models has been performed and described by Visioni et al. (2023) and Bednarz et al. (2023), but these studies were built upon the substantial body of work that had already been conducted. Any additional models wishing to engage with such a simulation would need to conduct their own single-location injection simulations, which is computationally expensive. The feasibility of expanding this idea to a larger host of GeoMIP models still needs to be discussed, and some groups may opt to study simpler strategies that still reduce impacts compared to equatorial injection but that are easier to implement.
The ARISE-SAI (Assessing Responses and Impacts of Solar climate intervention on the Earth system with stratospheric aerosol injection) protocols could offer an example of how such a feedback-driven experiment could work: the ideas behind the underlying scenario choices have been described by MacMartin et al. (2022), while Richter et al. (2022) clearly lays out the injection protocols, the overall setup for one model (CESM2-WACCM), and the required variables needed for analyses (that for things like aerosol fields might expand on what is recommended by CMIP in terms of required variables). A similar protocol (or the ARISE protocol itself) could be adopted as a future GeoMIP experiment.
3.6 The effects of surface brightening: G4foam
Experiment G4Foam (Gabriel et al., 2017) involves surface brightening, similar to G1ocean-albedo (Sect. 2.2), but only in selected oceanic regions where the forcing is expected to be amplified via cloud feedbacks. This idea is related to the idea of Green's function approaches to forcing that have been gaining traction recently in the study of climate feedbacks (Dong et al., 2019). Indeed, Harrop et al. (2018) showed that in CESM there are certain oceanic regions where, if one adds a positive heat flux, global mean temperature decreases. This raises the question as to whether there are high-leverage locations where small amounts of forcing could have robust, disproportionate effects. This area requires substantial further study, as it is not presently known whether these results are replicable in other models and, if so, what the physical mechanisms behind these effects may be. This experiment also reinforces the idea that issues central to geoengineering are also central to climate science in general – in this case, the relationships between forcing, rapid adjustments, feedbacks, and responses.
Based on the review of current and past experiments as well as community discussions, we have formulated opinions about which future experiments could be considered in geoengineering research. In the last two GeoMIP meetings, the GeoMIP community broadly agreed that the lack of person power, the computational expense, and the general uncertainty over future directions of CMIP indicated that the proposal of new Tier-1 experiments could be premature (Visioni and Robock, 2022); this of course might change in 2023, spurred by recent analyses, new community input, a notably increased interest in the theme by climate and impact scientists, and, perhaps, this piece. However, there was also a general agreement that we could use this time to better evaluate the models we currently have through process-based, more narrowly defined experiments to better constrain the intermodel spread observed in current GeoMIP iterations (Visioni et al., 2021b). These process-based experiments often do not need to be run with fully coupled Earth system models, relieving part of the computational cost and making these experiments interesting to a wider community. These efforts need to be run in parallel with efforts aimed at defining future policy-relevant scenarios that include geoengineering (Tilmes et al., 2020; MacMartin et al., 2022). In this section we describe some of these ideas and the reasons why we believe they are high priority for the research community.
Unlike other modeling experiments for which measurements are readily available to constrain model uncertainty (like volcanic eruptions or past stratospheric ozone evolution), there has been no implementation of geoengineering on a climate-relevant scale, and thus there are no observations of geoengineering. However, diagnosing the spread in the response of different models in a widening set of impacts on the natural and human world is always useful and may inform future plans for research (MacMartin and Kravitz, 2019) and, perhaps, contribute to the discussion around the need for future outdoor experiments (Golja et al., 2021).
4.1 Exploring Arctic-focused SAI: G6polar
In addition to equatorial injection, Robock et al. (2008) tested the climate effects of injecting SO2 into the polar stratosphere (67.5∘ N/S). Its inefficiency at reducing global mean surface temperatures and its impact on global precipitation, predominantly shifting the Intertropical Convergence Zone (ITCZ), was noted (Haywood et al., 2013). More recently, Lee et al. (2021) reevaluated polar injection by considering seasonal injections in the spring, which would result in less perturbation overall, and highlighted the efficacy of such a strategy in restoring sea ice and the benefit of having to loft material at lower altitudes given the lower height of the tropopause there (Smith et al., 2022). This illustrates that geoengineering may have important tradeoffs that need to be uncovered and discussed to inform future decisions around whether and how geoengineering might be deployed.
The need to address this question opens up the opportunity of devising a test-bed experiment aimed at analyzing the response to Arctic stratospheric injection in multiple models. However, because ITCZ shifts are a known consequence of single-hemisphere injection, care should be taken in considering the precipitation response, perhaps by devising an experiment that injects into both the North Pole and South Pole stratospheres in order to balance the forcing. If such an experiment is to be carried out, care should also be taken over the determination of a target: Lee et al. (2021) prescribed a fixed injection rate of 6 Tg-SO2 in various seasons and simply observed the resulting changes (like in a G4-like experiment), whereas Lee et al. (2023) and Jackson et al. (2015) devised a target based on restoration of sea ice. It is likely that a fixed injection rate would be easier to analyze in the beginning (and would ultimately inform development of a feedback algorithm to target specific objectives), leading to clearer differences between different models. A well-designed experiment such as G6polar could also serve as a useful tool to better understand the potential of SAI to provide an emergency brake on some high-latitudinal tipping elements of the Earth system (Lenton et al., 2008), which have been understudied up to now in the context of geoengineering.
4.2 Isolating uncertainties through specified dynamics simulations
The effects of intermodel differences in large-scale circulation have been highlighted in multiple venues, especially in CCMI (e.g., Eichinger et al., 2019). For stratospheric aerosol geoengineering, Niemeier et al. (2020) showed large differences in the baseline residual vertical velocities, aerosol confinement in the tropical pipe, and the surface response to an aerosol perturbation. Large-scale differences in the circulation result in different latitudinal distributions of the aerosols, especially if injections happen close to the tropical pipe (Laakso et al., 2017; Bednarz et al., 2023), but aerosol microphysical growth and the dynamical perturbation itself also play important roles (Visioni et al., 2023). It could therefore be posited that another way to separate specific differences between models can be through the nudging of stratospheric dynamics to common values, as was done in CCMI in the RefC1-SD experiments (Orbe et al., 2020). Nudging climate models to reanalysis meteorology fields has also been done for simulations of volcanic eruptions, for instance by Schmidt et al. (2018), resulting in better agreement with observations in terms of the global mean forcing produced. However, recent studies (Chrysanthou et al., 2019; Davis et al., 2020) observed that, in multiple climate models, nudging schemes may fail to better reproduce or constrain (compared to free-running simulations) long-term trends in the residual vertical upwelling.
So, while there could be merit in observing the response of GeoMIP models to nudged simulations in terms of the aerosol distribution, care should be taken in designing those simulations in multiple models. Another way could be the use of chemistry-transport models (CTMs) like GEOS-Chem that do not have interactive circulation at all, which allows for an actual prescription of circulation patterns. However, CTMs seldom have detailed aerosol microphysics in the stratosphere compared to some CCMs (Visioni et al., 2018).
In general, the use of such methods would prevent the simulation of the actual interactions between the stratospheric heating produced and the large-scale circulation: while this would not simulate the full response occurring in the atmosphere, it may help in separating the dynamical feedback from the uncertainties resulting from the aerosols.
4.3 Isolating uncertainties through analyses of model sensitivity to aerosol parameterization
While technically not a GeoMIP experiment, Laakso et al. (2022) explored using the same climate model (ECHAM-HAMMOZ) but with two different aerosol microphysical models. This highlighted the sensitivity of the baseline climate and the response to sulfate injections into the aerosol model, underscoring the need to pay more attention to aerosol parameterizations. Similarly, Visioni et al. (2023) compared two versions of GISS ModelE2.1, one with a bulk aerosol treatment and one with a quasi-modal microphysics, finding even larger discrepancies.
One possible way to more systematically understand differences between microphysical schemes could be a protocol analogous to Weisenstein et al. (2007), where an intercomparison was performed between a 0-D box model, a 2-D model (the Atmospheric and Environmental Research (AER) sectional model with multiple configurations), and a 3-D model (the Global Modeling Initiative (GMI) 3-D chemical-transport model). A similar comparison between models used for GeoMIP simulations with sulfur injections could highlight potential areas of needed improvements and perhaps even define a “minimal standard” of complexity (for some experiments) that climate models should have before they can be considered to produce robust results. This would represent an evolution of GeoMIP from its initial attitude of welcoming all 3-D models, regardless of complexity, to increase participation.
4.4 Isolating uncertainties through more in-depth analyses of the radiative transfer component
Recent studies have shown that some intermodel discrepancies in the response to CO2 can be traced to the shortwave radiation code (Chung and Soden, 2015; DeAngelis et al., 2015). Differences in radiative transfer have long been recognized, for example, in the Radiative Transfer Model Intercomparison Project (RTMIP; Collins et al., 2006). Boucher et al. (1998) performed a comparison of the shortwave response to sulfate aerosols for 15 radiative transfer codes produced by 12 different groups under a wide range of specified size distributions and aerosol and atmospheric optical properties. Unlike for the response to greenhouse gases, where the intermodel spread ranged up to 40 %, Boucher et al. (1998) found that the standard deviation of normalized forcing near the optimum size for maximum scattering was small (8 %). This highlights the importance of investigating fundamental model processes – we do not want to conclude erroneously that the response to geoengineering is uncertain if the actual uncertainty is in the radiative transfer code and thus somewhat independent of the forcing mechanism.
It would be extremely valuable to perform a similar intercomparison with current radiative transfer codes, especially those used or whose usage is planned for GeoMIP studies. This could be done for both shortwave and longwave forcing; in both cases, GeoMIP models show large spreads in efficiency of the forcing and in lower-stratospheric warmings (Visioni et al., 2021b; Tilmes et al., 2022). Understanding the behavior of the radiative codes separately could help understand which areas of model improvement require more focus. Recent proposed methods, such as by Jones et al. (2017) involving comparing a very specific set of diagnostics with a line-by-line radiative model, could suggest a way forward. This method has been adopted by the Radiative Forcing Model Intercomparison Project-Aerosol Instantaneous Radiative Forcing Component (RFMIP Aerosol-IRF) (Pincus et al., 2016), and a collaboration between the two MIPs could be extremely informative. Such focused experiments, including analyses of the radiative kernels, could help better understand and constrain the global response to solar geoengineering on the hydrological cycle (Kravitz et al., 2013, 2021; Tilmes et al., 2013; Kleidon et al., 2015), as has been done for a CO2 increase (DeAngelis et al., 2015).
4.5 How do we devise the next experiments for marine cloud brightening?
Questions regarding MCB can, to some degree, be separated into two categories. First, can clouds be brightened, and if so, by how much and under what conditions? Second, assuming clouds can be brightened, what are the climate effects of brightening clouds in specific areas? Experiment G4cdnc began addressing the second one, brightening all clouds where they existed. Indeed, this second category is well within the wheelhouse of Earth system models, as they are adept at understanding the climate response to imposed forcing. Specific experiments could be designed to test MCB in a multimodel capacity, such as choosing certain locations, different-sized regions, or seasons and performing Green's function approaches (analogous to those discussed in Sect. 2.2 above) in each model to exert a specified amount of forcing. One could envision either using the model's internal cloud field or prescribing a cloud field; each approach has its advantages and disadvantages.
The first category, as to whether clouds can be brightened, is somewhat more complicated. We argue that this category of studies is outside of the auspices of GeoMIP, although coordination with GeoMIP would certainly be valuable. Understanding this question deals with fundamental questions in aerosol–cloud interactions, namely, the susceptibility of cloud albedo to aerosol changes. This has been and continues to be the largest source of uncertainty in climate science (Chen et al., 2021). The answer varies depending upon location, atmospheric conditions, and the background aerosol state (Lee et al., 2016), among numerous other factors. Addressing these questions in a model context could be done through a series of large-eddy-simulation (LES) experiments. The advantage of LES is that because of the small grid size (sometimes on the order of meters), many cloud processes are resolved instead of parameterized, which relieves a substantial source of uncertainty. LES experiments could be conducted under a wide variety of atmospheric conditions and aerosol injection strategies to understand under what conditions marine low clouds can be brightened and by how much. This in turn could be used to constrain further GeoMIP experiments, in which regions to be brightened are selected dynamically rather than assuming that MCB automatically works. This would allow the research community to establish an upper bound on the effectiveness of MCB and to produce better assessments of the potential large-scale circulation response, which would be a necessary part of an overall assessment of MCB research (Diamond et al., 2022).
4.6 Impact assessment
A long-established need in geoengineering research is to understand downstream impacts, such as agriculture, ecosystems, and food/water security (Irvine et al., 2017). GeoMIP has been used in the past as driving information for impact models (e.g., Xia et al., 2014). Nevertheless, this process at present is woefully insufficient for quantifying benefits and risks of geoengineering. Any uncertainties or spread in the Earth system models (as discussed above) will propagate through impact models, which have their own uncertainties, leading to a wide range of results, even independent of different future scenarios of climate change and geoengineering. There is some effort to perform intercomparisons of impact models, such as in the InterSectoral Impacts Model Intercomparison Project (ISI-MIP; Rosenzweig et al., 2017) or the Agriculture Model Intercomparison Project (AgMIP; Rosenzweig et al., 2012), to understand and reduce uncertainties in impact assessments. Coordination between those MIPs and GeoMIP is in its initial stages, but developing a path forward, including specific GeoMIP experiments that are designed for impact assessment, would be of benefit to a wide variety of communities. A similar argument could be made for the ecological impacts of geoengineering, which are poorly understood (Zarnetske et al., 2021). It is essential to mention the DEGREES initiative in this respect (https://www.degrees.ngo, last access: 3 May 2023). One of the fundamental points of DEGREES is that researchers in the Global South, who are experts in climate impacts of most concern to their region, may also have access to high-frequency (at least daily) geoengineering model output in order to properly assess specific impacts that may be related to floods, droughts, freshwater availability, food security, human health, and other important impacts of their regions. The great advantage of bringing in these often poorly funded groups is that the research is community-driven from the grassroots upwards. Impacts that may interest the modeling community of GeoMIP may be quite different from those of most impacts locally. The scope for impact studies might be expanded in future to saline intrusion into ecosystems, fisheries, pests and diseases, human health, heat stress, and interactions with tropospheric pollutants.
From our perspective, and based on our experiences and feedback from the broader community of researchers, GeoMIP has been a resoundingly successful MIP. New analyses and publications are continually underway, and attendance at annual meetings continues to increase. GeoMIP has emerged as a flagship activity in the geoengineering research community and has served as a common venue where scientists interested in this topic can interact, and new partners are always welcome.
The experiments described in Sect. 2 have been instrumental in highlighting high-priority research areas for the community. While it has been pointed out that solar dimming has a limited value in representing stratospheric sulfate aerosol geoengineering (Visioni et al., 2021a), it is nevertheless a highly valuable experiment, since the straightforward setup allows us to be confident in the robust climate model responses to an experiment like G1 (Kravitz et al., 2021)). We are less confident in our understanding of stratospheric sulfate aerosols, and we are able to attribute those uncertainties largely to the complexities of aerosol microphysical growth, aerosol distribution, and stratospheric circulation. In doing so, we have provided an evidence base for more targeted approaches, such as the GeoMIP test-bed experiments described in Sect. 4.
As we discussed in depth in Sect. 4, GeoMIP should focus more on coordinating with other MIPs. While some may think that geoengineering itself does not overlap with the aims of other MIPs, the simulations and science objectives could serve dual purposes. As an example, during the design of CMIP6 experiments, we had conversations with the leads of the Cloud Forcing Model Intercomparison Project (CFMIP; Webb et al., 2017); their interest in comparing the effects of solar and CO2 forcing, as well as a delineation between fast and slow responses, aligns well with G1 and the time-slice experiments. Phase 6 of GeoMIP (Kravitz et al., 2015) had an overshoot scenario that was loosely coordinated with ScenarioMIP (O'Neill et al., 2016) and led to further studies of overshoot scenarios in the geoengineering research community (Tilmes et al., 2020, also see Section 3.4). The Scientific Assessment of Ozone Depletion: 2022 (Haywood et al., 2023), which required an assessment of possible changes due to SAI, has been an opportunity for renewed talks with the CCMI community, which resulted in the senD2-sai experiment (Table 1).
Through more focused coordination efforts with other MIPs, we could co-design new experiments that serve multiple communities. This could be a way to increase the likelihood that those simulations are conducted and analyzed. Due to the number of proposed simulations across all MIPS (there are 21 endorsed MIPs for CMIP6) and the budgetary and time constraints of the modeling centers, groups need to prioritize what experiments are worth their time. “MIP fatigue” is something we hear a lot in the climate modeling community: everyone thinks their experiments are relevant and interesting (and they are), and yet it is hard for modeling centers to keep up with all the new MIPs, with the requirements of the latest iteration of CMIP, and with improving the models, all of this while keeping an eye on what the funding agencies think is a priority for them.
Lastly, the analysis of output from geoengineering simulations has proven a viable and valuable means for involving researchers from countries that do not have the capacity for highly developed modeling programs, as has been demonstrated – albeit not to the extent of other modeling frameworks – through the DEGREES initiative (see Sect. 6.1.3 for further discussion of this initiative).
We also need to note that GeoMIP experiments have been critical for some recent high-level reports such as (i) the 2022 Quadrennial Ozone Report by the WMO (Haywood et al., 2023): without G6sulfur, there would have been no multimodel intercomparison of ESM results as related to the potential impacts of SAI on the ozone layer. (ii) The Intergovernmental Panel on Climate Change (IPCC) AR6 GeoMIP-based papers contribute to an assessment of SRM in a cross-chapter box in Working Group I (CCB10) and a cross-working group in Working Group II (chap. 6). (iii) The National Academy of Science, Technology and Medicine report (National Academies of Sciences Engineering and Medicine, 2021) also extensively references GeoMIP and its related works.
Based on this, and looking forward to the next decade, it is clear to us that modeling research into geoengineering will continue and most likely grow. As the topic expands and makes its way in international assessments, GeoMIP will have to adapt and change, and we explain how we think that should happen in Sect. 6.
5.1 Summary of outstanding scientific questions
Sections 3 and 4 offer many examples of questions that the GeoMIP community is interested in. This might feel like a scattershot of very different ideas, and in some ways it is so: the topic the GeoMIP community tries to tackle is a vast problem where the single pieces are always interconnected, and yet the community is (deliberately) diverse, with all participants bringing their own backgrounds and perspectives. Here, we highlight some of the key questions that continue to motivate our work.
What is the consensus of the most up-to-date models of the likely effects of solar geoengineering on the Earth system? This is certainly the one at the core of GeoMIP itself: we use highly advanced and, hopefully, independent climate models and try to discern commonalities in their response to various geoengineering techniques. If agreement is high for a certain aspect of the system, this is presumptively a likely response of the real Earth system. In general, the topic of “tipping points” (Lenton et al., 2008) and the potential of some form of solar geoengineering to delay or halt the emergence of dangerous instabilities in the planetary system are also ones that deserve far more attention in the future.
Highlighting disagreement, however, may be as important as highlighting consensus. Why do models disagree in their response to solar geoengineering? This may look similar to the previous question, but it shifts the focus from the projection itself to the tools used to derive it: is the multimodel average of the response “closer to the truth” than any single model or are there individual models that are “better” at capturing the effects of geoengineering than others (and how do we even characterize “closer” and “better” in a highly complex system)? If so, how can we constrain models with observations (past and future) to narrow down the uncertainty from multimodel ensemble simulations? In its latest assessment report, the IPCC highlights in multiple places the “large uncertainties” related to geoengineering. Together with a less vague quantification of such uncertainties, GeoMIP can also help identify areas of model agreement.
Moving to the various techniques tested, questions will differ based on the level of advancement in the field: for marine cloud brightening and cirrus cloud thinning, a major question that remains is the following. Could these geoengineering techniques measurably affect the global climate? For stratospheric aerosol injection, which has been studied more and for which more solid evidence behind its natural analog, volcanoes, exists, questions might be focused more on some of the details of the implementation. What are the impacts of different strategies of implementations of SAI? This is also a very broad question but one that focuses on the potential design aspect of geoengineering rather than on the uncertainty aspect of it, even if the two are connected (it is pointless to think about designing something of which we know very little).
GeoMIP is a framework for the intercomparison of geoengineering experiments using climate models. GeoMIP does not include all geoengineering research, and not all geoengineering research should go through GeoMIP: this is a fundamental point to put forward in order to understand the present and future roles of GeoMIP itself. There are clear examples of successful modeling efforts outside of GeoMIP, with the Geoengineering Large Ensemble (GLENS; Tilmes et al., 2018) being one of the most prominent. GLENS focused on producing a large ensemble (20 members) of simulations using one model (CESM1(WACCM)) and one, at the time, novel strategy (using an automated feedback loop capable of determining where and how much SO2 should be injected in the next year). This allowed for a more thorough exploration of, for instance, signal-to-noise emergence (MacMartin et al., 2019) and extreme events (Aswathy et al., 2015; Pinto et al., 2020; Tye et al., 2022). Both GeoMIP and GLENS have enabled numerous studies through the DEGREES initiative, enabling developing countries to assess changes they may experience under geoengineering.
With the urgency of climate change increasing impacts on societies and ecosystems, there is a great need to continue and accelerate geoengineering research (National Academies of Sciences Engineering and Medicine, 2021), and there remains much merit in scientific exploration through the use of coordinated multimodel experiments coupled with increased efforts to improve Earth observations in order to better assess the accuracy of current models. Overreliance of conclusions about geoengineering from just one climate model can be a potential pitfall and result in overconfidence in results that are model-specific. Multimodel comparisons can highlight outliers (not necessarily wrong, but different as compared to the mean), which can in turn spur further research behind the reasons why: this is for instance the case for the higher climate sensitivity of some CMIP6 models (Zelinka et al., 2020). This in turn can direct effort towards improving our understanding of model performance, with experiments such as those described in Sect. 4. In addition, knowledge-sharing and expertise-building are both at the core of GeoMIP and of fundamental importance in the field of geoengineering studies. In the previous sections we have tried to outline how much the process behind defining new experiments for GeoMIP relies on “learning by doing (and making mistakes)”. Yearly in-person GeoMIP meetings (except two online during the COVID pandemic) have been an opportunity for the community to grow and learn and share knowledge across different continents and levels of expertise: for each meeting, every year, a report is available (see the list at http://climate.envsci.rutgers.edu/GeoMIP/publications.html, last access: 4 May 2023) detailing both the discussions that took place and the novel science presented by researchers at every stage of their career.
Indeed, this opinion piece was strongly inspired by the discussions had during the Gordon Conference on Climate Engineering that took place in Newry, ME, in the summer of 2022, both in terms of the future plans and in terms of the level of enthusiasm we saw in the meeting, which involved over 50 early career researchers (while the first Gordon Conference, held in 2017, had fewer than 10). The yearly GeoMIP meeting was held during one of the afternoons of the Gordon Conference both times, and a very large portion of the people that were at the conference attended. This social aspect of GeoMIP is as valuable as the purely scientific one and, to some degree, those two aspects are inseparable.
With these reflections in mind, in this section we want to look even more broadly at the future of GeoMIP and discuss what direction we think the community should (and should not) move towards.
6.1 What should not be in GeoMIP's purview?
Here we want to summarize some of the most recent research or discussions around future geoengineering directions but which we think are either too premature to be considered in GeoMIP or which simply do not fall in its purview.
6.1.1 Is it necessary to simulate pathological scenarios in GeoMIP?
In the past, there have been studies aimed at understanding “pathological” SRM deployments or events: the effect of an abrupt termination (Jones et al., 2013; Trisos et al., 2018) or those of a single-hemispheric deployment (Haywood et al., 2013; Jones et al., 2017). The point of analyzing such scenarios is mostly to answer the question “what could go wrong?”, which is extremely important. However, it could also be misinterpreted in communication of the results as “this is what SRM would (always) do” (Reynolds, 2022), and therefore there can be a legitimate discussion over whether such scenarios are needed. In particular, because GeoMIP has served as a community-building and organizing effort, the project speaks with a loud voice; if GeoMIP explores pathological scenarios, this may send the signal that such scenarios are considered to potentially be realistic.
At the time the termination effect was proposed for inclusion in GeoMIP, few studies had explored it (e.g., Wigley, 2006; Robock et al., 2008). As such, there were numerous questions about the detailed effects of termination, including whether models would respond on similar timescales and with similar accelerations of climate change. The community has since learned a great deal from those simulations, and while there may remain open questions about termination, we presently see no need to conduct such investigations in numerous models. A similar discussion could be had for single-hemispheric deployment. This scenario is interesting and instructive from a basic-response standpoint and has resulted in fundamental knowledge that has informed geoengineering discussions. There is also some value in comparing the results of these experiments in multiple models (Haywood et al., 2016) to understand the degree of changes and whether there are robust signals. However, it is hard to envision a situation in which geoengineering would only be deployed in a single hemisphere for multiple decades. As such, these experiments may not be appropriate to adopt as formal GeoMIP experiments.
There has recently been increased discussion of uncoordinated geoengineering or “rogue actors” where there is no centralized decision-making about an optimal aerosol distribution or target, but rather multiple actors may be attempting to meet multiple, independent, perhaps conflicting targets (Rabitz, 2016). The lack of climate model simulations of this scenario in the geoengineering literature has been discussed before (McLaren and Corry, 2021). Nevertheless, we argue that this is not an experiment that should be adopted by GeoMIP. Policy experts or game-theory analysts could in principle devise a scenario involving multiple actors. However, the challenge would then be translating such a scenario into a specific experiment that could be run consistently in multiple models. Moreover, it is not obvious that there would be advantages to conducting such a specific simulation; for example, GLENS involved independent injections at four different locations, and it is unclear how different a simulation with decentralized geoengineering would be from GLENS. We do not rule out the possibility of this being explored in the future, especially as more concrete policy decisions emerge over the coming years, but we do not see a role for GeoMIP in these scenarios at this time.
6.1.2 Alternate materials for stratospheric aerosol injections
To date, all GeoMIP experiments involving solar geoengineering through the injection of aerosols into the stratosphere have consisted of injections of SO2 or H2SO4 (Weisenstein et al., 2022, Sect. 3.1). Global modeling of alternate aerosols is sparse, with some exceptions for black carbon or titania (Kravitz et al., 2012; Ferraro et al., 2015; Jones et al., 2016). Alternate, potentially less impactful materials such as calcium carbonate have been proposed to overcome the problem of ozone changes and lower-stratospheric temperature perturbations (see for instance Keith et al., 2016; Dykema et al., 2016), but since those materials are not naturally occurring in the atmosphere, information is still being sought on the chemical reaction rates (Dai et al., 2020). For this reason, it is clearly premature to consider such experiments for GeoMIP, but the potential for future intercomparisons would arise if the inclusion of these novel materials were to be tested in multiple climate models independently.
6.1.3 Subgrid-scale processes
One of the shortcomings of using climate models for SAI simulations is the inability to resolve fine-scale behaviors once the materials have been injected. In Sect. 2.5 we discussed some of the cloud processes that are unresolved at the scale of Earth system model grids, requiring parameterization. For stratospheric aerosol injection, there are additional uncertainties at the subgrid scale. For example, models often assume that once SO2 is injected into a grid box it becomes immediately uniformly distributed. This is uncharacteristic of diffusion processes or mixing forced by an aircraft jet. Additionally, many relevant weather and climate processes happen at the subgrid scale, which is particularly relevant for quantifying changes to extreme temperature and precipitation.
While many of these topics are certainly relevant for GeoMIP and may affect model capabilities and experiments in the future, as an Earth system model intercomparison, studying the importance of subgrid-scale processes is outside of the purview of GeoMIP and is better left to individual studies. There are numerous other MIPs and perturbed parameter ensembles that are focused on understanding parametric and structural uncertainty; through coordination and communication, such lessons can be conveyed to GeoMIP without requiring a specific GeoMIP experiment. New work involving a Lagrangian plume model that can be embedded in ESM grid boxes (Sun et al., 2022) is underway, but it needs further testing and has not been widely implemented, so using this capability in GeoMIP is premature.
Dynamical downscaling, either using a regional model or a regionally refined global model, is a standard technique for gaining finer-scale information. Downscaling has been shown to add value to GCM data over regions of topography, at land–water boundaries, and in better representing extremes (Ekström et al., 2015). Currently these methods are being tested for geoengineering applications (Wang et al., 2022), so adoption by GeoMIP is still premature. However, future efforts to coordinate between GeoMIP and the Coordinated Regional Climate Downscaling Experiment (CORDEX; Kotlarski et al., 2014) could prove to be fruitful in coming years, perhaps in concert with efforts to increase impact assessment.
6.2 What should GeoMIP do next?
As an official MIP under CMIP, the primary goal of GeoMIP for the coming years should be to prepare for CMIP7 and continue to harvest potentially valuable results from the efforts put into the CMIP6 simulations. This is especially important considering the potential for results from GeoMIP to be included in future international reports like the IPCC and WMO reports, as it has been in the past.
The experiments proposed in Sect. 4 would go a long way towards building more trust in the models used for future projections, in improving the modeling tools at our disposal in future iterations, and towards better understanding the underlying processes that comprise the overall response of the Earth system to geoengineering. However, in IPCC reports there is a strong push towards the use of complex emission scenarios, developed with the use of integrated assessment models (IAMs) that can represent a range of possible and plausible futures (Nakicenovic et al., 2000) spanning greenhouse gas emissions, land use, and population changes. The process that led to the development of the set of scenarios used in the Assessment Report 6 of the IPCC has been described in detail by O'Neill et al. (2016). The whole process was defined by the ScenarioMIP Scientific Steering Committee, which included extensive discussions with members of multiple scientific communities, numerous MIPs, and IPCC task forces. The main objectives that guided the decisions were (i) facilitating integrated research between climate analyses, scenario analyses, and feedbacks between climate and society, (ii) addressing targeted science questions regarding particular components of the overall forcing, and (iii) better quantifying projection uncertainties based on multimodel ensembles.
On the other hand, and with no surprise considering the smaller scope, previous GeoMIP scenarios have been defined by a much smaller (and narrower in terms of expertise) community, mostly taking into account the modelers’ needs for geoengineering scenarios that were straightforward to implement in different climate models. The amount of person time available to produce the GeoMIP simulations is perhaps 2 orders of magnitude lower than that made available by modeling centers for ScenarioMIP, and therefore it is unsurprising that GeoMIP has made use of available SSPs and added geoengineering on top. This is likely to continue to be the case for some time, but nonetheless the geoengineering research community should explore how geoengineering might be incorporated directly into the scenario development process. Learning from other MIPs and the history of geoengineering, we think it is important that future CMIP7 scenarios include geoengineering as well (in all its various forms). In our view, the scenarios that include them should address the following criteria.
-
Plausibility: in terms of possible start date, amount of cooling, characteristics of the deployment, and more, the scenarios should reflect to the best of our current knowledge realistic deployment options.
-
Policy relevance: the scenarios should be capable of informing policy-makers with regards to the possible outcomes of a geoengineering deployment by considering more than one possible scenario and strategy, as the analyses of just one case may lead to confusing a scenario-specific result with a result that is applicable to all geoengineering scenarios.
-
Scientific relevance: the analyses of the developed scenarios should aid in our scientific understanding of the outcomes of a geoengineering climate.
-
Reproducibility: the requests to the modeling teams should be as simple as possible in order to ensure the participation of as many models as possible, minimizing possible errors and ensuring reproducibility.
These four criteria present some tension between them: for instance, a scenario like G1 is very scientifically relevant, has a high signal-to-noise ratio, and is easily reproducible but not plausible or policy-relevant, whereas a scenario like G6sulfur might be scientifically relevant and policy-relevant but is not plausible (the start date has passed, and current emissions do not seem to track the SSP5-8.5 scenario), and future scenarios like those described by MacMartin et al. (2022) might not be easily reproducible in many climate models, which makes them not presently relevant for GeoMIP. In general, more plausible scenarios will have lower signal-to-noise ratios. However, there is the question of whether an effect (both direct and indirect) that is too small to be detected in a plausible simulation matters (“To whom? And for what?”) and needs to be investigated. We view this tension as a good thing, and indeed scenarios should not strive to meet all four criteria equally (although meeting the reproducibility criterion should be considered essential for inclusion as a Tier-1 GeoMIP experiment). Our purpose is not to prescribe at this stage what future GeoMIP experiments should be. Rather, we argue that proposed experiments should be interrogated to ensure that they are being true to purpose so that experiments are widely adopted by modeling groups and their results can be appropriately communicated and are useful for the scientific and policy-making community.
Moreover, the process for coming up with scenarios needs to be explicit about its intended audience. In particular, new scenarios should carefully consider (i) the need for an inclusive process that takes into account multiple lines of expertise across multiple fields (climate science, ecosystem sciences, social sciences), (ii) the needs of the community of modelers on which GeoMIP depends, and (iii) the needs of the community of scientists that want to use GeoMIP output. Balancing these three different sets of needs out will also result in some tensions (for instance, between the need for high-frequency output required by the impact modeling community and the difficulty in storing the large amount of data that would be produced), but it is a necessary discussion both in order to ensure that the scenarios are as representative as possible and in order to make sure that as many researchers as possible feel represented in GeoMIP.
To offer some specific examples of the needs of the users of GeoMIP, there is a growing community of ecologists interested in understanding the impacts of geoengineering on ecosystems (Zarnetske et al., 2021) that have already successfully used GeoMIP simulations. On the other hand, GeoMIP data have only been used in 3 out of the 12 papers published to date in the DEGREES project, supported by the DMF (DEGREES Modelling Fund), which aims to supports teams of researchers in developing countries (https://www.degrees.ngo/publications/, last access: 5 September 2022). In some cases this disparity makes sense – for example, DEGREES teams who want to study extreme events (e.g., Abiodun et al., 2021) would benefit from using a large ensemble like GLENS to obtain a good signal-to-noise ratio or be able to sample rare events. For some other groups, as the number of models increases, accessibility and usability of the data become harder, especially for teams with poor Internet connectivity or teams that require computing power to conduct their analyses. GLENS is a single model, and all data are available in one place, which can make it easier to use. There are important advances being undertaken for CMIP analyses, particularly by the Pangeo community (Odaka et al., 2020), who have enabled analysis of CMIP6 data in the cloud without requiring users to download terabytes of output. Nevertheless, widespread use of GeoMIP output, including data accessibility, is currently an unresolved issue.
Lastly, DEGREES teams have repeatedly reported their need to focus on small-scale, regional-impact assessments, which often require downscaling results from available climate models. Although several teams have conducted regional-impact modeling analyses (e.g., hydrology, health, and ocean modeling), only one study has employed dynamical downscaling of GeoMIP results, a study using WRF to downscale G4 output over Northeast China in comparison with statistical downscaling (Wang et al., 2022) and another to statistically downscale G4 output over the Indonesian maritime continent (Kuswanto et al., 2022). To statistically downscale climate data on a regional scale, however, the underlying data need to reliably replicate the climatological features of the baseline climate, which in some cases some models might fail to do. The issue of “which models are best to use” is therefore also a problem which DEGREES teams are faced with.
The Geoengineering Model Intercomparison Project community has been active for more than 10 years, and its participants span numerous countries on multiple continents (Visioni and Robock, 2022). Here we have reviewed past and present proposed GeoMIP experiments, including those determined by the community as high priority (Tier-1) and those proposed by specific members as “test-bed experiments”, and have reflected on the potential future development of GeoMIP.
While not a review of the state-of-the-art understanding of SRM, which exists elsewhere (e.g., Kravitz and MacMartin, 2020), critically assessing all available GeoMIP experiments is a useful exercise for understanding how the field of geoengineering modeling studies has evolved, as it gives an idea of the current areas where research has focused and how to move forward from there.
The inclusion of recent experiments and numerous new potential experiments or areas where experiments could be devised has multiple aims, many of which have been brought up repeatedly in publications and at various meetings of the research community, amongst them (i) offering the community a way to devise new, more specific analyses of current experiments that might have been underutilized and could still be leveraged, (ii) serving as a starting point for the community to more carefully devise new experiments, both more specific ones as we detailed and also for future CMIP experiments to be included in international assessments, and (iii) currently many calls are available for “more research” into solar geoengineering, such as the report from the National Academy of Science (National Academies of Sciences Engineering and Medicine, 2021). Nevertheless, those calls for “more experiments” or “more research” often lack detail, which is a barrier to action. Based on the discussions amongst the large GeoMIP community over the years, in this piece we have provided a critical examination of where GeoMIP's research has led and what gaps need to be filled, and we provide a number of conclusions that could provide needed specificity regarding future research directions and aligned programs.
One obvious gap, which has been repeatedly highlighted by the community, is a lack of people time more than simply computer time, both in defining shared protocols for experiments and in analyzing the available output. As a simple example, even just determining injections of SO2 in exactly the same grid box in multiple models requires more work than just specifying a certain height in the protocols: different models may have different kinds of vertical coordinates and different ways of specifying exogenous emissions. Coordinating between modeling centers and providing attention to these details are therefore crucial parts of a successful multimodel comparison. Related to this is limited funding to support geoengineering research, in terms of both people and computer time, and a lack of capacity in developing countries to operate in this space. As these countries are typically the most vulnerable to climate change, entraining local scientists into geoengineering research is especially important (Rahman et al., 2018).
We should underscore that multimodel comparisons are definitely not the only way to understand, constrain, and eventually reduce model uncertainty. As for climate change, uncertainty does not come from a single source. As explained, for instance, by Hawkins and Sutton (2009), it can come from internal variability, uncertainty in the climate response, scenario uncertainty, and parametric or structural uncertainty. Internal variability can be better studied in the context of large ensembles of simulations with single models, of which some are already available for geoengineering studies (Tilmes et al., 2018; Richter et al., 2022)): the use of multiple realizations from very similar initial conditions allows for a better separation of a given forcing signal from noise derived from the inherent chaotic nature of the atmospheric and oceanic system and makes exploring the timing of the emergence of such a signal easier (MacMartin et al., 2019; Tye et al., 2022). GeoMIP is suited to exploring uncertainties in the climate response as it allows an exploration of structural differences between models to a standardized forcing, but it does not directly address single-model uncertainty based on specific parameters in the physical representation of various aspects of the climate system: for such an endeavor, perturbed-physics ensembles within a single model may offer a much clearer answer. One example of such a perturbed-physics ensemble is the proposed Pinatubo Emulation in Multiple models (PoEMs) experiment in the Interactive Stratospheric Aerosols MIP (ISA-MIP) (Timmreck et al., 2018), in which modeling teams already simulating the 1991 Pinatubo eruption are asked to perform additional simulations modifying some of the possible parameters (in their case, such as aerosol nucleation, coagulation, or sedimentation rates) in order to span the possible space of parameters that most closely matches observations of the eruption. Some of the GeoMIP experiments, and especially some of the experiments we have proposed in Sect. 4, may help single-modeling teams better understand where to focus their efforts in terms of parameters to analyze and may even leverage existing protocols like PoEMs in order to longitudinally compare against other realizations with the same model and with other models. An initial effort along these lines was explored by Irvine et al. (2014) for G1 using HadCM3. Carefully designed protocols that can incorporate both model and parametric uncertainty would provide numerous advantages for quantifying and attributing uncertainty in processes.
Scenario uncertainty, which over multidecadal scales is larger than other sources in the CMIP context (Lehner et al., 2020), can also be very hard to sample in the GeoMIP context. Moreover, scenario exploration has historically required multiple decades of simulation for each scenario, which limits the number of scenarios that can be explored. Considering multiple, but shorter, simulations could perhaps free up the resources needed to span the scenario dimension but at the cost of perhaps undersampling the long-term climatic response: for future iterations of experiments that are part of CMIP, the community will have to make some choices on this aspect or perhaps distinctly consider short-term process details and long-term response characteristics in separate experiments. A possibility could be to also focus on studies that try to develop better emulators that are applicable in the geoengineering context: some currently exist and may work better for some variables than for others (MacMartin and Kravitz, 2016). These allow researchers to analyze multiple scenarios a posteriori after a lower number of possibly higher signal-to-noise scenarios has been simulated with the full host of climate models and to ultimately verify the emulator with a subset of available climate models afterwards.
There continues to be an important role for GeoMIP in geoengineering research, both scientifically and as a community effort. We recommend that, for activities in GeoMIP, participants carefully evaluate their purpose and participation to ensure that the objectives of GeoMIP continue to be met and support the ongoing development of GeoMIP. We also recommend active coordination with other MIPs, World Climate Research Program activities, core programs such as SPARC, the Earth System Modelling and Observations (ESMO), and the Safe Landings Lighthouse activity, and other geoengineering research efforts to identify synergies that will increase the use of GeoMIP efforts and encourage more participation in GeoMIP. Issues and uncertainties in geoengineering are rarely exclusive to that field – rather, they are often common to climate science research in general. Bringing geoengineering research into the mainstream, perhaps in part through efforts made by GeoMIP, will benefit both geoengineering research and broader climate research efforts.
CMIP5 and CMIP6 data discussed in this paper can be accessed from the following repositories: https://esgf-node.llnl.gov/search/cmip5/ (WCRP, 2023a) and https://esgf-node.llnl.gov/search/cmip6/ (WCRP, 2023b). They are described in the works discussed in the relevant sections.
DV and BK wrote the piece, with substantial contributions from all the other coauthors. DV: conceptualisation, writing of the original draft, visualization, review and editing. BK: writing – original draft preparation and editing. AR: supervision, review & editing. ST, JH, OB, ML, PI, UN, LX, GC, CL, SW, JM, HM: writing – review & editing.
At least one of the (co)authors is a member of the editorial board of Atmospheric Chemistry and Physics. The peer-review process was guided by an independent editor, and the authors also have no other competing interests to declare.
Publisher's note: Copernicus Publications remains neutral with regard to jurisdictional claims in published maps and institutional affiliations.
This article is part of the special issue “20 years of Atmospheric Chemistry and Physics”. It is not associated with a conference.
The authors would like to deeply thank the many scientists who, over the years, have contributed their time and effort to GeoMIP. Support for Ben Kravitz was provided in part by the Indiana University Environmental Resilience Institute, and the Prepared for Environmental Change Grand Challenge initiative. Pacific Northwest National Laboratory is operated for the US Department of Energy by Battelle Memorial Institute under contract DE-AC05-76RL01830. Ulrike Niemeier was supported by the Deutsche Forschungsgemeinschaft Research Unit VollImpact (FOR2820, grant no. 398006378) and used resources of the Deutsches Klimarechenzentrum (DKRZ) granted by its Scientific Steering Committee (WLA) under project no. bm0550.
This research has been supported by the National Science Foundation (grant no. SES-1754740), the Japan Society for the Promotion of Science (grant no. JP2103668), and the National Science Foundation (grant nos. ENG-2028541 and AGS-2017113).
This paper was edited by Rolf Müller and reviewed by K. Caldeira and one anonymous referee.
Abiodun, B. J., Odoulami, R. C., Sawadogo, W., Oloniyo, O. A., Abatan, A. A., New, M., Lennard, C., Izidine, P., Egbebiyi, T. S., and MacMartin, D. G.: Potential impacts of stratospheric aerosol injection on drought risk managements over major river basins in Africa, Climatic Change, 169, 31, https://doi.org/10.1007/s10584-021-03268-w, 2021. a
Ahlm, L., Jones, A., Stjern, C. W., Muri, H., Kravitz, B., and Kristjánsson, J. E.: Marine cloud brightening – as effective without clouds, Atmos. Chem. Phys., 17, 13071–13087, https://doi.org/10.5194/acp-17-13071-2017, 2017. a
Alterskjaer, K., Kristjánsson, J. E., Boucher, O., Muri, H., Niemeier, U., Schmidt, H., Schulz, M., and Timmreck, C.: Sea-salt injections into the low-latitude marine boundary layer: The transient response in three Earth system models, J. Geophys. Res.-Atmos., 118, 12195–12206, https://doi.org/10.1002/2013JD020432, 2013. a, b
Angel, R.: Feasibility of cooling the Earth with a cloud of small spacecraft near the inner Lagrange point (L1), P. Natl. Acad. Sci. USA, 103, 17184–17189, https://doi.org/10.1073/pnas.0608163103, 2006. a
Aswathy, V. N., Boucher, O., Quaas, M., Niemeier, U., Muri, H., Mülmenstädt, J., and Quaas, J.: Climate extremes in multi-model simulations of stratospheric aerosol and marine cloud brightening climate engineering, Atmos. Chem. Phys., 15, 9593–9610, https://doi.org/10.5194/acp-15-9593-2015, 2015. a
Bal, P. K., Pathak, R., Mishra, S. K., and Sahany, S.: Effects of global warming and solar geoengineering on precipitation seasonality, Environ. Res. Lett., 14, 034011, https://doi.org/10.1088/1748-9326/aafc7d, 2019. a
Bednarz, E. M., Visioni, D., Banerjee, A., Braesicke, P., Kravitz, B., and MacMartin, D. G.: The Overlooked Role of the Stratosphere Under a Solar Constant Reduction, Geophys. Res. Lett., 49, e2022GL098773, https://doi.org/10.1029/2022GL098773, 2022. a, b
Bednarz, E. M., Visioni, D., Kravitz, B., Jones, A., Haywood, J. M., Richter, J., MacMartin, D. G., and Braesicke, P.: Climate response to off-equatorial stratospheric sulfur injections in three Earth system models – Part 2: Stratospheric and free-tropospheric response, Atmos. Chem. Phys., 23, 687–709, https://doi.org/10.5194/acp-23-687-2023, 2023. a, b
Benduhn, F., Schallock, J., and Lawrence, M. G.: Early growth dynamical implications for the steerability of stratospheric solar radiation management via sulfur aerosol particles, Geophys. Res. Lett., 43, 9956–9963, https://doi.org/10.1002/2016GL070701, 2016. a
Bengtsson, L.: Geo-Engineering to Confine Climate Change: Is it at all Feasible?, Climatic Change, 77, 229, https://doi.org/10.1007/s10584-006-9133-3, 2006. a
Berdahl, M., Robock, A., Ji, D., Moore, J. C., Jones, A., Kravitz, B., and Watanabe, S.: Arctic cryosphere response in the Geoengineering Model Intercomparison Project G3 and G4 scenarios, J. Geophys. Res.- Atmos., 119, 1308–1321, https://doi.org/10.1002/2013JD020627, 2014. a, b, c
Boettcher, M. and Schäfer, S.: Reflecting upon 10 years of geoengineering research: Introduction to the Crutzen + 10 special issue, Earth's Future, 5, 266–277, https://doi.org/10.1002/2016EF000521, 2017. a
Boucher, O., Schwartz, S. E., Ackerman, T. P., Anderson, T. L., Bergstrom, B., Bonnel, B., Chýlek, P., Dahlback, A., Fouquart, Y., Fu, Q., Halthore, R. N., Haywood, J. M., Iversen, T., Kato, S., Kinne, S., Kirkevåg, A., Knapp, K. R., Lacis, A., Laszlo, I., Mishchenko, M. I., Nemesure, S., Ramaswamy, V., Roberts, D. L., Russell, P., Schlesinger, M. E., Stephens, G. L., Wagener, R., Wang, M., Wong, J., and Yang, F.: Intercomparison of models representing direct shortwave radiative forcing by sulfate aerosols, J. Geophys. Res.-Atmos., 103, 16979–16998, https://doi.org/10.1029/98JD00997, 1998. a, b
Budyko, M. I.: The Climate of the Future, American Geophysical Union, https://doi.org/10.1029/EO053i010p00868, 1978. a, b
Butchart, N., Anstey, J. A., Hamilton, K., Osprey, S., McLandress, C., Bushell, A. C., Kawatani, Y., Kim, Y.-H., Lott, F., Scinocca, J., Stockdale, T. N., Andrews, M., Bellprat, O., Braesicke, P., Cagnazzo, C., Chen, C.-C., Chun, H.-Y., Dobrynin, M., Garcia, R. R., Garcia-Serrano, J., Gray, L. J., Holt, L., Kerzenmacher, T., Naoe, H., Pohlmann, H., Richter, J. H., Scaife, A. A., Schenzinger, V., Serva, F., Versick, S., Watanabe, S., Yoshida, K., and Yukimoto, S.: Overview of experiment design and comparison of models participating in phase 1 of the SPARC Quasi-Biennial Oscillation initiative (QBOi), Geosci. Model Dev., 11, 1009–1032, https://doi.org/10.5194/gmd-11-1009-2018, 2018. a
Cess, R. D., Potter, G. L., Blanchet, J. P., Boer, G. J., Ghan, S. J., Kiehl, J. T., Treut, H. L., Li, Z.-X., Liang, X.-Z., Mitchell, J. F. B., Morcrette, J.-J., Randall, D. A., Riches, M. R., Roeckner, E., Schlese, U., Slingo, A., Taylor, K. E., Washington, W. M., Wetherald, R. T., and Yagai, I.: Interpretation of Cloud-Climate Feedback as Produced by 14 Atmospheric General Circulation Models, Science, 245, 513–516, https://doi.org/10.1126/science.245.4917.513, 1989. a
Chen, D., Rojas, M., Samset, B. H., Cobb, K., Diongue Niang, A., Edwards, P., Emori, S., Faria, S. H., Hawkins, E., Hope, P., Huybrechts, P., Meinshausen, M., Mustafa, S. K., Plattner, G. K., and Tréguier, A. M.: Framing, Context, and Methods, in: Climate Change 2021: The Physical Science Basis, Contribution of Working Group I to the Sixth Assessment Report of the Intergovernmental Panel on Climate Change, book section 1, edited by: Masson-Delmotte, V., Zhai, P., Pirani, A., Connors, S. L., Péan, C., Berger, S., Caud, N., Chen, Y., Goldfarb, L., Gomis, M. I., Huang, M., Leitzell, K., Lonnoy, E., Matthews, J. B. R., Maycock, T. K., Waterfield, T., Yelekçi, O., Yu, R., and Zhou, B., Cambridge University Press, Cambridge, UK and New York, NY, USA, https://www.ipcc.ch/report/ar6/wg1/downloads/report/IPCC_AR6_WGI_Chapter_01.pdf (last access: 3 May 2023), 2021. a, b
Chen, Y., Liu, A., and Moore, J. C.: Mitigation of Arctic permafrost carbon loss through stratospheric aerosol geoengineering, Nat. Commun., 11, 2430, https://doi.org/10.1038/s41467-020-16357-8, 2020. a
Chrysanthou, A., Maycock, A. C., Chipperfield, M. P., Dhomse, S., Garny, H., Kinnison, D., Akiyoshi, H., Deushi, M., Garcia, R. R., Jöckel, P., Kirner, O., Pitari, G., Plummer, D. A., Revell, L., Rozanov, E., Stenke, A., Tanaka, T. Y., Visioni, D., and Yamashita, Y.: The effect of atmospheric nudging on the stratospheric residual circulation in chemistry–climate models, Atmos. Chem. Phys., 19, 11559–11586, https://doi.org/10.5194/acp-19-11559-2019, 2019. a
Chung, E.-S. and Soden, B. J.: An Assessment of Direct Radiative Forcing, Radiative Adjustments, and Radiative Feedbacks in Coupled Ocean–Atmosphere Models, J. Climate, 28, 4152–4170, https://doi.org/10.1175/JCLI-D-14-00436.1, 2015. a
Cicerone, R. J.: Geoengineering: Encouraging Research and Overseeing Implementation, Climatic Change, 77, 221–226, https://doi.org/10.1007/s10584-006-9102-x, 2006. a
Clyne, M., Lamarque, J.-F., Mills, M. J., Khodri, M., Ball, W., Bekki, S., Dhomse, S. S., Lebas, N., Mann, G., Marshall, L., Niemeier, U., Poulain, V., Robock, A., Rozanov, E., Schmidt, A., Stenke, A., Sukhodolov, T., Timmreck, C., Toohey, M., Tummon, F., Zanchettin, D., Zhu, Y., and Toon, O. B.: Model physics and chemistry causing intermodel disagreement within the VolMIP-Tambora Interactive Stratospheric Aerosol ensemble, Atmos. Chem. Phys., 21, 331–3343, https://doi.org/10.5194/acp-21-3317-2021, 2021. a
Collins, W. D., Ramaswamy, V., Schwarzkopf, M. D., Sun, Y., Portmann, R. W., Fu, Q., Casanova, S. E. B., Dufresne, J.-L., Fillmore, D. W., Forster, P. M. D., Galin, V. Y., Gohar, L. K., Ingram, W. J., Kratz, D. P., Lefebvre, M.-P., Li, J., Marquet, P., Oinas, V., Tsushima, Y., Uchiyama, T., and Zhong, W. Y.: Radiative forcing by well-mixed greenhouse gases: Estimates from climate models in the Intergovernmental Panel on Climate Change (IPCC) Fourth Assessment Report (AR4), J. Geophys. Res.-Atmos., 111, D14317, https://doi.org/10.1029/2005JD006713, 2006. a
Coupe, J. and Robock, A.: The Influence of Stratospheric Soot and Sulfate Aerosols on the Northern Hemisphere Wintertime Atmospheric Circulation, J. Geophys. Res.-Atmos., 126, e2020JD034513, https://doi.org/10.1029/2020JD034513, 2021. a
Crutzen, P. J.: Albedo Enhancement by Stratospheric Sulfur Injections: A Contribution to Resolve a Policy Dilemma?, Climatic Change, 77, 211–220, https://doi.org/10.1007/s10584-006-9101-y, 2006. a
Dai, Z., Weisenstein, D. K., and Keith, D. W.: Tailoring Meridional and Seasonal Radiative Forcing by Sulfate Aerosol Solar Geoengineering, Geophys. Res. Lett., 45, 1030–1039, https://doi.org/10.1002/2017GL076472, 2018. a
Dai, Z., Weisenstein, D. K., Keutsch, F. N., and Keith, D. W.: Experimental reaction rates constrain estimates of ozone response to calcium carbonate geoengineering, Commun. Earth Environ., 1, 63, https://doi.org/10.1038/s43247-020-00058-7, 2020. a
DallaSanta, K. and Polvani, L. M.: Volcanic stratospheric injections up to 160 Tg(S) yield a Eurasian winter warming indistinguishable from internal variability, Atmos. Chem. Phys., 22, 8843–8862, https://doi.org/10.5194/acp-22-8843-2022, 2022. a
Davis, N. A., Davis, S. M., Portmann, R. W., Ray, E., Rosenlof, K. H., and Yu, P.: A comprehensive assessment of tropical stratospheric upwelling in the specified dynamics Community Earth System Model 1.2.2 – Whole Atmosphere Community Climate Model (CESM (WACCM)), Geosci. Model Dev., 13, 717–734, https://doi.org/10.5194/gmd-13-717-2020, 2020. a
DeAngelis, A. M., Qu, X., Zelinka, M. D., and Hall, A.: An observational radiative constraint on hydrologic cycle intensification, Nature, 528, 249–253, https://doi.org/10.1038/nature15770, 2015. a, b
Diamond, M. S., Gettelman, A., Lebsock, M. D., McComiskey, A., Russell, L. M., Wood, R., and Feingold, G.: To assess marine cloud brightening's technical feasibility, we need to know what to study – and when to stop, P. Natl. Acad. Sci. USA, 119, e2118379119, https://doi.org/10.1073/pnas.2118379119, 2022. a
Dong, Y., Proistosescu, C., Armour, K. C., and Battisti, D. S.: Attributing Historical and Future Evolution of Radiative Feedbacks to Regional Warming Patterns using a Green's Function Approach: The Preeminence of the Western Pacific, J. Climate, 32, 5471–5491, https://doi.org/10.1175/JCLI-D-18-0843.1, 2019. a
Dykema, J. A., Keith, D. W., and Keutsch, F. N.: Improved aerosol radiative properties as a foundation for solar geoengineering risk assessment, Geophys. Res. Lett., 43, 7758–7766, https://doi.org/10.1002/2016GL069258, 2016. a
Eichinger, R., Dietmüller, S., Garny, H., Šácha, P., Birner, T., Bönisch, H., Pitari, G., Visioni, D., Stenke, A., Rozanov, E., Revell, L., Plummer, D. A., Jöckel, P., Oman, L., Deushi, M., Kinnison, D. E., Garcia, R., Morgenstern, O., Zeng, G., Stone, K. A., and Schofield, R.: The influence of mixing on the stratospheric age of air changes in the 21st century, Atmos. Chem. Phys., 19, 921–940, https://doi.org/10.5194/acp-19-921-2019, 2019. a
Ekström, M., Grose, M. R., and Whetton, P. H.: An appraisal of downscaling methods used in climate change research, WIREs Clim. Change, 6, 301–319, https://doi.org/10.1002/wcc.339, 2015. a
Eyring, V., Bony, S., Meehl, G. A., Senior, C. A., Stevens, B., Stouffer, R. J., and Taylor, K. E.: Overview of the Coupled Model Intercomparison Project Phase 6 (CMIP6) experimental design and organization, Geosci. Model Dev., 9, 1937–1958, https://doi.org/10.5194/gmd-9-1937-2016, 2016. a
Ferraro, A. J. and Griffiths, H. G.: Quantifying the temperature-independent effect of stratospheric aerosol geoengineering on global-mean precipitation in a multi-model ensemble, Environ. Res. Lett., 11, 034012, https://doi.org/10.1088/1748-9326/11/3/034012, 2016. a
Ferraro, A. J., Charlton-Perez, A. J., and Highwood, E. J.: Stratospheric dynamics and midlatitude jets under geoengineering with space mirrors and sulfate and titania aerosols, J. Geophys. Res.-Atmos., 120, 414–429, https://doi.org/10.1002/2014JD022734, 2015. a
Franke, H., Niemeier, U., and Visioni, D.: Differences in the quasi-biennial oscillation response to stratospheric aerosol modification depending on injection strategy and species, Atmos. Chem. Phys., 21, 8615–8635, https://doi.org/10.5194/acp-21-8615-2021, 2021. a
Gabriel, C. J., Robock, A., Xia, L., Zambri, B., and Kravitz, B.: The G4Foam Experiment: global climate impacts of regional ocean albedo modification, Atmos. Chem. Phys., 17, 595–613, https://doi.org/10.5194/acp-17-595-2017, 2017. a, b
Gasparini, B., Meyer, A., Neubauer, D., Münch, S., and Lohmann, U.: Cirrus Cloud Properties as Seen by the CALIPSO Satellite and ECHAM-HAM Global Climate Model, J. Climate, 31, 1983–2003, https://doi.org/10.1175/JCLI-D-16-0608.1, 2018. a
Gasparini, B., McGraw, Z., Storelvmo, T., and Lohmann, U.: To what extent can cirrus cloud seeding counteract global warming?, Environ. Res. Lett., 15, 054002, https://doi.org/10.1088/1748-9326/ab71a3, 2020. a
Golja, C. M., Chew, L. W., Dykema, J. A., and Keith, D. W.: Aerosol Dynamics in the Near Field of the SCoPEx Stratospheric Balloon Experiment, J. Geophys. Res.-Atmos., 126, e2020JD033438, https://doi.org/10.1029/2020JD033438, 2021. a
Govindasamy, B., Caldeira, K., and Duffy, P.: Geoengineering Earth's radiation balance to mitigate climate change from a quadrupling of CO2, Global Planet. Change, 37, 157–168, https://doi.org/10.1016/S0921-8181(02)00195-9, 2003. a, b
Harding, A. R., Ricke, K., Heyen, D., MacMartin, D. G., and Moreno-Cruz, J.: Climate econometric models indicate solar geoengineering would reduce inter-country income inequality, Nat. Commun., 11, 227, https://doi.org/10.1038/s41467-019-13957-x, 2020. a
Harrop, B. E., Lu, J., Liu, F., Garuba, O. A., and Leung, L. R.: Sensitivity of the ITCZ Location to Ocean Forcing Via Q-Flux Green's Function Experiments, Geophys. Res. Lett., 45, 13116–13123, https://doi.org/10.1029/2018GL080772, 2018. a
Hawkins, E. and Sutton, R.: The Potential to Narrow Uncertainty in Regional Climate Predictions, B. Am. Meteorol. Soc., 90, 1095–1108, https://doi.org/10.1175/2009BAMS2607.1, 2009. a
Haywood, J., Tilmes, S., Keutsch, F., Niemeier, U., Schmidt, A., Visioni, D.,, and Yu, P.: Stratospheric Aerosol Injection and its Potential Effect on the Stratospheric Ozone Layer, in: chap. 6 in Scientific Assessment of Ozone Depletion: 2022, Tech. Rep. GAW Report No. 278, World Meteorological Organization, https://csl.noaa.gov/assessments/ozone/2022/ (last access: 3 May 2023), 2023. a, b
Haywood, J. M., Jones, A., Bellouin, N., and Stephenson, D.: Asymmetric forcing from stratospheric aerosols impacts Sahelian rainfall, Nat. Clim. Change, 3, 660–665, https://doi.org/10.1038/nclimate1857, 2013. a, b
Haywood, J. M., Jones, A., Dunstone, N., Milton, S., Vellinga, M., Bodas-Salcedo, A., Hawcroft, M., Kravitz, B., Cole, J., Watanabe, S., and Stephens, G.: The impact of equilibrating hemispheric albedos on tropical performance in the HadGEM2-ES coupled climate model, Geophys. Res. Lett., 43, 395–403, https://doi.org/10.1002/2015GL066903, 2016. a
Irvine, P., Emanuel, K., He, J., Horowitz, L. W., Vecchi, G., and Keith, D.: Halving warming with idealized solar geoengineering moderates key climate hazards, Nat. Clim. Change, 9, 295–299, https://doi.org/10.1038/s41558-019-0398-8, 2019. a
Irvine, P. J., Boucher, O., Kravitz, B., Alterskjær, K., Cole, J. N. S., Ji, D., Jones, A., Lunt, D. J., Moore, J. C., Muri, H., Niemeier, U., Robock, A., Singh, B., Tilmes, S., Watanabe, S., Yang, S., and Yoon, J.-H.: Key factors governing uncertainty in the response to sunshade geoengineering from a comparison of the GeoMIP ensemble and a perturbed parameter ensemble, J. Geophys. Res.-Atmos., 119, 7946–7962, https://doi.org/10.1002/2013JD020716, 2014. a
Irvine, P. J., Kravitz, B., Lawrence, M. G., Gerten, D., Caminade, C., Gosling, S. N., Hendy, E. J., Kassie, B. T., Kissling, W. D., Muri, H., Oschlies, A., and Smith, S. J.: Towards a comprehensive climate impacts assessment of solar geoengineering, Earth's Future, 5, 93–106, https://doi.org/10.1002/2016EF000389, 2017. a
Jackson, L. S., Crook, J. A., Jarvis, A., Leedal, D., Ridgwell, A., Vaughan, N., and Forster, P. M.: Assessing the controllability of Arctic sea ice extent by sulfate aerosol geoengineering, Geophys. Res. Lett., 42, 1223–1231, https://doi.org/10.1002/2014GL062240, 2015. a
Jones, A. and Haywood, J. M.: Sea-spray geoengineering in the HadGEM2-ES earth-system model: radiative impact and climate response, Atmos. Chem. Phys., 12, 10887–10898, https://doi.org/10.5194/acp-12-10887-2012, 2012. a
Jones, A., Haywood, J., and Boucher, O.: Climate impacts of geoengineering marine stratocumulus clouds, J. Geophys. Res.-Atmos., 114, D10106, https://doi.org/10.1029/2008JD011450, 2009. a
Jones, A., Haywood, J., Boucher, O., Kravitz, B., and Robock, A.: Geoengineering by stratospheric SO2 injection: results from the Met Office HadGEM2 climate model and comparison with the Goddard Institute for Space Studies ModelE, Atmos. Chem. Phys., 10, 5999–6006, https://doi.org/10.5194/acp-10-5999-2010, 2010. a
Jones, A., Haywood, J. M., Alterskjær, K., Boucher, O., Cole, J. N. S., Curry, C. L., Irvine, P. J., Ji, D., Kravitz, B., Egill Kristjánsson, J., Moore, J. C., Niemeier, U., Robock, A., Schmidt, H., Singh, B., Tilmes, S., Watanabe, S., and Yoon, J.-H.: The impact of abrupt suspension of solar radiation management (termination effect) in experiment G2 of the Geoengineering Model Intercomparison Project (GeoMIP), J. Geophys. Res.-Atmos., 118, 9743–9752, https://doi.org/10.1002/jgrd.50762, 2013. a, b, c, d
Jones, A., Haywood, J. M., Jones, A. C., Tilmes, S., Kravitz, B., and Robock, A.: North Atlantic Oscillation response in GeoMIP experiments G6solar and G6sulfur: why detailed modelling is needed for understanding regional implications of solar radiation management, Atmos. Chem. Phys., 21, 1287–1304, https://doi.org/10.5194/acp-21-1287-2021, 2021. a, b
Jones, A. C., Haywood, J. M., and Jones, A.: Climatic impacts of stratospheric geoengineering with sulfate, black carbon and titania injection, Atmos. Chem. Phys., 16, 2843–2862, https://doi.org/10.5194/acp-16-2843-2016, 2016. a
Jones, A. L., Feldman, D. R., Freidenreich, S., Paynter, D., Ramaswamy, V., Collins, W. D., and Pincus, R.: A New Paradigm for Diagnosing Contributions to Model Aerosol Forcing Error, Geophys. Res. Lett., 44, 12004–12012, https://doi.org/10.1002/2017GL075933, 2017. a, b
Keith, D. W.: Geoengineering the climate: History and prospect, Ann. Rev. Energ. Environ., 25, 245–284, 2000. a
Keith, D. W., Weisenstein, D. K., Dykema, J. A., and Keutsch, F. N.: Stratospheric solar geoengineering without ozone loss, P. Natl. Acad. Sci. USA, 113, 14910–14914, https://doi.org/10.1073/pnas.1615572113, 2016. a
Kiehl, J. T.: Geoengineering climate Change: Treating the symptom over the cause?, Climatic Change, 77, 227–228, https://doi.org/10.1007/s10584-006-9132-4, 2006. a
Kleidon, A., Kravitz, B., and Renner, M.: The hydrological sensitivity to global warming and solar geoengineering derived from thermodynamic constraints, Geophys. Res. Lett., 42, 138–144, https://doi.org/10.1002/2014GL062589, 2015. a
Kotlarski, S., Keuler, K., Christensen, O. B., Colette, A., Déqué, M., Gobiet, A., Goergen, K., Jacob, D., Lüthi, D., van Meijgaard, E., Nikulin, G., Schär, C., Teichmann, C., Vautard, R., Warrach-Sagi, K., and Wulfmeyer, V.: Regional climate modeling on European scales: a joint standard evaluation of the EURO-CORDEX RCM ensemble, Geosci. Model Dev., 7, 1297–1333, https://doi.org/10.5194/gmd-7-1297-2014, 2014. a
Kravitz, B. and MacMartin, D. G.: Uncertainty and the basis for confidence in solar geoengineering research, Nat. Rev. Earth Environ., 1, 64–75, https://doi.org/10.1038/s43017-019-0004-7, 2020. a, b
Kravitz, B., Robock, A., Boucher, O., Schmidt, H., Taylor, K. E., Stenchikov, G., and Schulz, M.: The Geoengineering Model Intercomparison Project (GeoMIP), Atmos. Sci. Lett., 12, 162–167, https://doi.org/10.1002/asl.316, 2011. a, b, c, d, e, f, g, h
Kravitz, B., Robock, A., Shindell, D. T., and Miller, M. A.: Sensitivity of stratospheric geoengineering with black carbon to aerosol size and altitude of injection, J. Geophys. Res., 117, D09203, https://doi.org/10.1029/2011JD017341, 2012. a
Kravitz, B., Rasch, P. J., Forster, P. M., Andrews, T., Cole, J. N., Irvine, P. J., Ji, D., Kristjánsson, J. E., Moore, J. C., Muri, H., Niemeier, U., Robock, A., Singh, B., Tilmes, S., Watanabe, S., and Yoon, J. H.: An energetic perspective on hydrological cycle changes in the Geoengineering Model Intercomparison Project, J. Geophys. Res.-Atmos., 118, 13087–13102, https://doi.org/10.1002/2013JD020502, 2013. a, b, c, d, e, f, g, h, i, j
Kravitz, B., MacMartin, D. G., Robock, A., Rasch, P. J., Ricke, K. L., Cole, J. N. S., Curry, C. L., Irvine, P. J., Ji, D., Keith, D. W., Kristjánsson, J. E., Moore, J. C., Muri, H., Singh, B., Tilmes, S., Watanabe, S., Yang, S., and Yoon, J.-H.: A multi-model assessment of regional climate disparities caused by solar geoengineering, Environ. Res. Lett., 9, 074013, https://doi.org/10.1088/1748-9326/9/7/074013, 2014. a
Kravitz, B., Robock, A., Tilmes, S., Boucher, O., English, J. M., Irvine, P. J., Jones, A., Lawrence, M. G., MacCracken, M., Muri, H., Moore, J. C., Niemeier, U., Phipps, S. J., Sillmann, J., Storelvmo, T., Wang, H., and Watanabe, S.: The Geoengineering Model Intercomparison Project Phase 6 (GeoMIP6): simulation design and preliminary results, Geosci. Model Dev., 8, 3379–3392, https://doi.org/10.5194/gmd-8-3379-2015, 2015. a, b, c, d, e, f, g, h, i, j
Kravitz, B., MacMartin, D. G., Wang, H., and Rasch, P. J.: Geoengineering as a design problem, Earth Syst. Dynam., 7, 469–497, https://doi.org/10.5194/esd-7-469-2016, 2016. a, b
Kravitz, B., Lamarque, J.-F., Tribbia, J. J., Tilmes, S., Vitt, F., Richter, J. H., MacMartin, D. G., and Mills, M. J.: First Simulations of Designing Stratospheric Sulfate Aerosol Geoengineering to Meet Multiple Simultaneous Climate Objectives, J.Geophys. Res.-Atmos., 122, 12616–12634, https://doi.org/10.1002/2017jd026874, 2017. a, b, c
Kravitz, B., Rasch, P. J., Wang, H., Robock, A., Gabriel, C., Boucher, O., Cole, J. N. S., Haywood, J., Ji, D., Jones, A., Lenton, A., Moore, J. C., Muri, H., Niemeier, U., Phipps, S., Schmidt, H., Watanabe, S., Yang, S., and Yoon, J.-H.: The climate effects of increasing ocean albedo: an idealized representation of solar geoengineering, Atmos. Chem. Phys., 18, 13097–13113, https://doi.org/10.5194/acp-18-13097-2018, 2018. a
Kravitz, B., MacMartin, D. G., Tilmes, S., Richter, J. H., Mills, M. J., Cheng, W., Dagon, K., Glanville, A. S., Lamarque, J.-F., Simpson, I. R., Tribbia, J., and Vitt, F.: Comparing Surface and Stratospheric Impacts of Geoengineering With Different SO2 Injection Strategies, J. Geophys. Res.-Atmos., 124, 7900–7918, https://doi.org/10.1029/2019JD030329, 2019. a, b
Kravitz, B., MacMartin, D. G., Visioni, D., Boucher, O., Cole, J. N. S., Haywood, J., Jones, A., Lurton, T., Nabat, P., Niemeier, U., Robock, A., Séférian, R., and Tilmes, S.: Comparing different generations of idealized solar geoengineering simulations in the Geoengineering Model Intercomparison Project (GeoMIP), Atmos. Chem. Phys., 21, 423–4247, https://doi.org/10.5194/acp-21-4231-2021, 2021. a, b, c, d, e, f
Kuswanto, H., Kravitz, B., Miftahurrohmah, B., Fauzi, F., Sopahaluwaken, A., and Moore, J.: Impact of solar geoengineering on temperatures over the Indonesian Maritime Continent, Int. J. Climatol., 42, 2795–2814, https://doi.org/10.1002/joc.7391, 2022. a
Laakso, A., Korhonen, H., Romakkaniemi, S., and Kokkola, H.: Radiative and climate effects of stratospheric sulfur geoengineering using seasonally varying injection areas, Atmos. Chem. Phys., 17, 6957–6974, https://doi.org/10.5194/acp-17-6957-2017, 2017. a
Laakso, A., Niemeier, U., Visioni, D., Tilmes, S., and Kokkola, H.: Dependency of the impacts of geoengineering on the stratospheric sulfur injection strategy – Part 1: Intercomparison of modal and sectional aerosol modules, Atmos. Chem. Phys., 22, 93–118, https://doi.org/10.5194/acp-22-93-2022, 2022. a
Latham, J.: Control of global warming?, Nature, 347, 339–340, https://doi.org/10.1038/347339b0, 1990. a
Lawrence, D. M., Hurtt, G. C., Arneth, A., Brovkin, V., Calvin, K. V., Jones, A. D., Jones, C. D., Lawrence, P. J., de Noblet-Ducoudré, N., Pongratz, J., Seneviratne, S. I., and Shevliakova, E.: The Land Use Model Intercomparison Project (LUMIP) contribution to CMIP6: rationale and experimental design, Geosci. Model Dev., 9, 2973–2998, https://doi.org/10.5194/gmd-9-2973-2016, 2016. a
Lawrence, M. G.: The Geoengineering Dilemma: To Speak or not to Speak, Climatic Change, 77, 245–248, https://doi.org/10.1007/s10584-006-9131-5, 2006. a
Lawrence, M. G. and Crutzen, P. J.: Was breaking the taboo on research on climate engineering via albedo modification a moral hazard, or a moral imperative?, Earth's Future, 5, 136–143, https://doi.org/10.1002/2016EF000463, 2017. a
Lawrence, M. G., Schäfer, S., Muri, H., Scott, V., Oschlies, A., Vaughan, N. E., Boucher, O., Schmidt, H., Haywood, J., and Scheffran, J.: Evaluating climate geoengineering proposals in the context of the Paris Agreement temperature goals, Nat. Commun., 9, 3734, https://doi.org/10.1038/s41467-018-05938-3, 2018. a
Lee, L. A., Reddington, C. L., and Carslaw, K. S.: On the relationship between aerosol model uncertainty and radiative forcing uncertainty, P. Natl. Acad. Sci. USA, 113, 5820–5827, https://doi.org/10.1073/pnas.1507050113, 2016. a
Lee, W. R., MacMartin, D. G., Visioni, D., and Kravitz, B.: High-Latitude Stratospheric Aerosol Geoengineering Can Be More Effective if Injection Is Limited to Spring, Geophys. Res. Lett., 48, e2021GL092696, https://doi.org/10.1029/2021GL092696, 2021. a, b
Lee, W. R., MacMartin, D. G., Visioni, D., Kravitz, B., Chen, Y., Moore, J. C., Leguy, G., Lawrence, D. M., and Bailey, D. A.: High-Latitude Stratospheric Aerosol Injection to Preserve the Arctic, Earth's Future, 11, e2022EF003052, https://doi.org/10.1029/2022EF003052, 2023. a
Lehner, F., Deser, C., Maher, N., Marotzke, J., Fischer, E. M., Brunner, L., Knutti, R., and Hawkins, E.: Partitioning climate projection uncertainty with multiple large ensembles and CMIP5/6, Earth Syst. Dynam., 11, 491–508, https://doi.org/10.5194/esd-11-491-2020, 2020. a, b
Lenton, T. M., Held, H., Kriegler, E., Hall, J. W., Lucht, W., Rahmstorf, S., and Schellnhuber, H. J.: Tipping elements in the Earth's climate system, P. Natl. Acad. Sci. USA, 105, 1786–1793, https://doi.org/10.1073/pnas.0705414105, 2008. a, b
Liu, F., Gao, C., Chai, J., Robock, A., Wang, B., Li, J., Zhang, X., Huang, G., and Dong, W.: Tropical volcanism enhanced the East Asian summer monsoon during the last millennium, Nat. Commun., 13, 3429, https://doi.org/10.1038/s41467-022-31108-7, 2022. a
Long, J. C. S. and Shepherd, J. G.: The Strategic Value of Geoengineering Research, Springer Netherlands, Dordrecht, 757–770, https://doi.org/10.1007/978-94-007-5784-4_24, 2014. a
MacCracken, M. C.: Geoengineering: Worthy of Cautious Evaluation?, Climatic Change, 77, 235–243, https://doi.org/10.1007/s10584-006-9130-6, 2006. a
MacMartin, D. G. and Kravitz, B.: Dynamic climate emulators for solar geoengineering, Atmos. Chem. Phys., 16, 15789–15799, https://doi.org/10.5194/acp-16-15789-2016, 2016. a, b
MacMartin, D. G. and Kravitz, B.: Mission-driven research for stratospheric aerosol geoengineering, P. Natl. Acad. Sci. USA, 116, 1089–1094, https://doi.org/10.1073/pnas.1811022116, 2019. a
MacMartin, D. G., Kravitz, B., Mills, M. J., Tribbia, J. J., Tilmes, S., Richter, J. H., Vitt, F., and Lamarque, J.-F.: The Climate Response to Stratospheric Aerosol Geoengineering Can Be Tailored Using Multiple Injection Locations, J. Geophys. Res.-Atmos., 122, 12574–12590, https://doi.org/10.1002/2017jd026868, 2017. a
MacMartin, D. G., Ricke, K. L., and Keith, D. W.: Solar geoengineering as part of an overall strategy for meeting the 1.5 ∘C Paris target, Philos. T. Roy. Soc. A, 376, 20160454, https://doi.org/10.1098/rsta.2016.0454, 2018. a, b
MacMartin, D. G., Wang, W., Richter, J. H., Mills, M. J., Kravitz, B., and Tilmes, S.: Timescale for Detecting the Climate Response to Stratospheric Aerosol Geoengineering, J. Geophys. Res.-Atmos., 124, 1233–1247, https://doi.org/10.1029/2018jd028906, 2019. a, b
MacMartin, D. G., Visioni, D., Kravitz, B., Richter, J., Felgenhauer, T., Lee, W. R., Morrow, D. R., Parson, E. A., and Sugiyama, M.: Scenarios for modeling solar radiation modification, P. Natl. Acad. Sci. USA, 119, e2202230119, https://doi.org/10.1073/pnas.2202230119, 2022. a, b, c, d
McLaren, D. and Corry, O.: Clash of Geofutures and the Remaking of Planetary Order: Faultlines underlying Conflicts over Geoengineering Governance, Global Policy, 12, 20–33, https://doi.org/10.1111/1758-5899.12863, 2021. a
Meehl, G. A., Covey, C., McAvaney, B., Latif, M., and Stouffer, R. J.: Overview Of The Coupled Model Intercomparison Project, B. Am. Meteorol. Soc., 86, 89–93, 2005. a
Meinshausen, M., Nicholls, Z. R. J., Lewis, J., Gidden, M. J., Vogel, E., Freund, M., Beyerle, U., Gessner, C., Nauels, A., Bauer, N., Canadell, J. G., Daniel, J. S., John, A., Krummel, P. B., Luderer, G., Meinshausen, N., Montzka, S. A., Rayner, P. J., Reimann, S., Smith, S. J., van den Berg, M., Velders, G. J. M., Vollmer, M. K., and Wang, R. H. J.: The shared socio-economic pathway (SSP) greenhouse gas concentrations and their extensions to 2500, Geosci. Model Dev., 13, 3571–3605, https://doi.org/10.5194/gmd-13-3571-2020, 2020. a, b
Melnikova, I., Boucher, O., Cadule, P., Tanaka, K., Gasser, T., Hajima, T., Quilcaille, Y., Shiogama, H., Séférian, R., Tachiiri, K., Vuichard, N., Yokohata, T., and Ciais, P.: Impact of bioenergy crop expansion on climate–carbon cycle feedbacks in overshoot scenarios, Earth Syst. Dynam., 13, 779–794, https://doi.org/10.5194/esd-13-779-2022, 2022. a
Mitchell, D. L. and Finnegan, W.: Modification of cirrus clouds to reduce global warming, Environ. Res. Lett., 4, 045102, https://doi.org/10.1088/1748-9326/4/4/045102, 2009. a
Moore, J. C., Grinsted, A., Guo, X., Yu, X., Jevrejeva, S., Rinke, A., Cui, X., Kravitz, B., Lenton, A., Watanabe, S., and Ji, D.: Atlantic hurricane surge response to geoengineering, P. Natl. Acad. Sci. USA, 112, 13794–13799, https://doi.org/10.1073/pnas.1510530112, 2015. a
Morgenstern, O., Hegglin, M. I., Rozanov, E., O'Connor, F. M., Abraham, N. L., Akiyoshi, H., Archibald, A. T., Bekki, S., Butchart, N., Chipperfield, M. P., Deushi, M., Dhomse, S. S., Garcia, R. R., Hardiman, S. C., Horowitz, L. W., Jöckel, P., Josse, B., Kinnison, D., Lin, M., Mancini, E., Manyin, M. E., Marchand, M., Marécal, V., Michou, M., Oman, L. D., Pitari, G., Plummer, D. A., Revell, L. E., Saint-Martin, D., Schofield, R., Stenke, A., Stone, K., Sudo, K., Tanaka, T. Y., Tilmes, S., Yamashita, Y., Yoshida, K., and Zeng, G.: Review of the global models used within phase 1 of the Chemistry–Climate Model Initiative (CCMI), Geosci. Model Dev., 10, 639–671, https://doi.org/10.5194/gmd-10-639-2017, 2017. a
Muller, W. A., Jungclaus, J. H., Mauritsen, T., Baehr, J., Bittner, M., Budich, R., Bunzel, F., Esch, M., Ghosh, R., Haak, H., Ilyina, T., Kleine, T., Kornblueh, L., Li, H., Modali, K., Notz, D., Pohlmann, H., Roeckner, E., Stemmler, I., Tian, F., and Marotzke, J.: A Higher-resolution Version of the Max Planck Institute Earth System Model (MPI-ESM1.2-HR), J. Adv. Model. Earth Syst., 10, 1383–1413, https://doi.org/10.1029/2017MS001217, 2018. a
Muri, H., Kristjánsson, J. E., Storelvmo, T., and Pfeffer, M. A.: The climatic effects of modifying cirrus clouds in a climate engineering framework, J. Geophys. Res.-Atmos., 119, 4174–4191, https://doi.org/10.1002/2013JD021063, 2014. a
Nakicenovic, N., Davidson, O., Davis, G., Grübler, Arnulf, Kram, T., La Lebre Rovere, E., Metz, B., Morita, T., Pepper, P. H., Sankovski, A., Shukla, P., Swart, R., Watson, R., and Dadi, Z.: IPCC Special Report: Emissions scenarios: Summary for policymakers, Intergovernmental Panel on Climate Change, Genf, https://www.ipcc.ch/site/assets/uploads/2018/03/sres-en.pdf (last access: 3 May 2023), 2000. a
National Academies of Sciences Engineering and Medicine: Reflecting Sunlight: Recommendations for Solar Geoengineering Research and Research Governance, The National Academies Press, Washington, DC, https://doi.org/10.17226/25762, 2021. a, b, c, d
Niemeier, U., Schmidt, H., Alterskjær, K., and Kristjánsson, J. E.: Solar irradiance reduction via climate engineering: Impact of different techniques on the energy balance and the hydrological cycle, J. Geophys. Res.-Atmos., 118, 11905–11917, https://doi.org/10.1002/2013JD020445, 2013. a, b, c
Niemeier, U., Richter, J. H., and Tilmes, S.: Differing responses of the quasi-biennial oscillation to artificial SO2 injections in two global models, Atmos. Chem. Phys., 20, 8975–8987, https://doi.org/10.5194/acp-20-8975-2020, 2020. a
Odaka, T. E., Banihirwe, A., Eynard-Bontemps, G., Ponte, A., Maze, G., Paul, K., Baker, J., and Abernathey, R.: The Pangeo Ecosystem: Interactive Computing Tools for the Geosciences: Benchmarking on HPC, in: Tools and Techniques for High Performance Computing, edited by: Juckeland, G. and Chandrasekaran, S., Springer International Publishing, Cham, 190–204, ISBN 9783030447274, 2020. a
O'Neill, B. C., Tebaldi, C., van Vuuren, D. P., Eyring, V., Friedlingstein, P., Hurtt, G., Knutti, R., Kriegler, E., Lamarque, J.-F., Lowe, J., Meehl, G. A., Moss, R., Riahi, K., and Sanderson, B. M.: The Scenario Model Intercomparison Project (ScenarioMIP) for CMIP6, Geosci. Model Dev., 9, 3461–3482, https://doi.org/10.5194/gmd-9-3461-2016, 2016. a, b, c
Orbe, C., Plummer, D. A., Waugh, D. W., Yang, H., Jöckel, P., Kinnison, D. E., Josse, B., Marecal, V., Deushi, M., Abraham, N. L., Archibald, A. T., Chipperfield, M. P., Dhomse, S., Feng, W., and Bekki, S.: Description and Evaluation of the specified-dynamics experiment in the Chemistry-Climate Model Initiative, Atmos. Chem. Phys., 20, 3809–3840, https://doi.org/10.5194/acp-20-3809-2020, 2020. a
Parker, A. and Irvine, P. J.: The Risk of Termination Shock From Solar Geoengineering, Earth's Future, 6, 456–467, https://doi.org/10.1002/2017EF000735, 2018. a
Pedersen, J. S. T., van Vuuren, D. P., Aparício, B. A., Swart, R., Gupta, J., and Santos, F. D.: Variability in historical emissions trends suggests a need for a wide range of global scenarios and regional analyses, Commun. Earth Environ., 1, 41, https://doi.org/10.1038/s43247-020-00045-y, 2020. a
Pierce, J. R., Weisenstein, D. K., Heckendorn, P., Peter, T., and Keith, D. W.: Efficient formation of stratospheric aerosol for climate engineering by emission of condensible vapor from aircraft, Geophys. Res. Lett., 37, L18805, https://doi.org/10.1029/2010GL043975, 2010. a
Pincus, R., Forster, P. M., and Stevens, B.: The Radiative Forcing Model Intercomparison Project (RFMIP): experimental protocol for CMIP6, Geosci. Model Dev., 9, 3447–3460, https://doi.org/10.5194/gmd-9-3447-2016, 2016. a
Pinto, I., Jack, C., Lennard, C., Tilmes, S., and Odoulami, R. C.: Africa's Climate Response to Solar Radiation Management With Stratospheric Aerosol, Geophys. Res. Lett., 47, e2019GL086047, https://doi.org/10.1029/2019GL086047, 2020. a
Pitari, G., Aquila, V., Kravitz, B., Robock, A., Watanabe, S., Cionni, I., Luca, N. D., Genova, G. D., Mancini, E., and Tilmes, S.: Stratospheric ozone response to sulfate geoengineering: Results from the Geoengineering Model Intercomparison Project (GeoMIP), J. Geophys. Res.-Atmos., 119, 2629–2653, https://doi.org/10.1002/2013JD020566, 2014. a
Plummer, D., Nagashima, T., Tilmes, S., Archibald, A., Chiodo, G., Fadnavis, S., Garny, H., Josse, B., Kim, J., Lamarque, J.-F., Morgenstern, O., Murray, L., Orbe, C., Tai, A., Chipperfield, M., Funke, B., Juckes, M., Kinnison, D., Kunze, M., Luo, B., Matthes, K., Newman, P. A., Pascoe, C., and Peter, T.: CCMI-2022: A new set of Chemistry-Climate Model Initiative (CCMI) Community Simulations to Update the Assessment of Models and Support Upcoming Ozone Assessment Activities, Newsletter no. 57, July 2021, p. 22, https://www.sparc-climate.org/publications/newsletter/ (last access: 3 May 2023), 2021. a, b
Polvani, L. M., Banerjee, A., and Schmidt, A.: Northern Hemisphere continental winter warming following the 1991 Mt. Pinatubo eruption: reconciling models and observations, Atmos. Chem. Phys., 19, 6351–6366, https://doi.org/10.5194/acp-19-6351-2019, 2019. a
Quaglia, I., Timmreck, C., Niemeier, U., Visioni, D., Pitari, G., Brodowsky, C., Brühl, C., Dhomse, S. S., Franke, H., Laakso, A., Mann, G. W., Rozanov, E., and Sukhodolov, T.: Interactive stratospheric aerosol models' response to different amounts and altitudes of SO2 injection during the 1991 Pinatubo eruption, Atmos. Chem. Phys., 23, 921–948, https://doi.org/10.5194/acp-23-921-2023, 2023. a
Rabitz, F.: Going rogue? Scenarios for unilateral geoengineering, Futures, 84, 98–107, https://doi.org/10.1016/j.futures.2016.11.001, 2016. a
Rahman, A. A., Artaxo, P., Asrat, A., and Parker, A.: Developing countries must lead on solar geoengineering research, Nature, 556, 22–24, https://doi.org/10.1038/d41586-018-03917-8, 2018. a
Rasch, P. J., Tilmes, S., Turco, R. P., Robock, A., Oman, L., Chen, C.-C., Stenchikov, G. L., and Garcia, R. R.: An overview of geoengineering of climate using stratospheric sulphate aerosols, Philos. T. Roy. Soc. A, 366, 4007–4037, https://doi.org/10.1098/rsta.2008.0131, 2008. a
Rasch, P. J., Latham, J., and Chen, C.-C. J.: Geoengineering by cloud seeding: influence on sea ice and climate system, Environ. Res. Lett., 4, 045112, https://doi.org/10.1088/1748-9326/4/4/045112, 2009. a
Reynolds, J. L.: Communication of solar geoengineering science: Forms, examples, and explanation of skewing, Anthropocene Rev., https://doi.org/10.1177/20530196221095569, in press, 2022. a, b
Richter, J. H., Visioni, D., MacMartin, D. G., Bailey, D. A., Rosenbloom, N., Dobbins, B., Lee, W. R., Tye, M., and Lamarque, J.-F.: Assessing Responses and Impacts of Solar climate intervention on the Earth system with stratospheric aerosol injection (ARISE-SAI): protocol and initial results from the first simulations, Geosci. Model Dev., 15, 8221–8243, https://doi.org/10.5194/gmd-15-8221-2022, 2022. a, b, c
Robock, A. and Mao, J.: The Volcanic Signal in Surface Temperature Observations, J. Climate, 8, 1086–1103, https://doi.org/10.1175/1520-0442(1995)008<1086:TVSIST>2.0.CO;2, 1995. a
Robock, A., Oman, L., and Stenchikov, G. L.: Regional climate responses to geoengineering with tropical and Arctic SO2 injections, J. Geophys. Res.-Atmos., 113, D16101, https://doi.org/10.1029/2008JD010050, 2008. a, b, c, d
Rogelj, J., den Elzen, M., Höhne, N., Fransen, T., Fekete, H., Winkler, H., Schaeffer, R., Sha, F., Riahi, K., and Meinshausen, M.: Paris Agreement climate proposals need a boost to keep warming well below 2 ∘C, Nature, 534, 631–639, https://doi.org/10.1038/nature18307, 2016. a
Rosenzweig, C., Jones, J. W., Hatfield, J. L., Mutter, C. Z., Adiku, S. G. K., Ahmad, A., Beletse, Y., Gangwar, B., Guntuku, D., Kihara, J., Masikati, P., Paramasivan, P., Rao, K. P. C., and Zubair, L.: The Agricultural Model Intercomparison and Improvement Project (AgMIP): Integrated regional assessment projects, in: ICP Series on Climate Change Impacts, Adaptation, and Mitigation, Vol. 2, Imperial College Press, London, 263–280, https://doi.org/10.1142/9781848169845_0014, 2012. a
Rosenzweig, C., Arnell, N. W., Ebi, K. L., Lotze-Campen, H., Raes, F., Rapley, C., Smith, M. S., Cramer, W., Frieler, K., Reyer, C. P. O., Schewe, J., van Vuuren, D., and Warszawski, L.: Assessing inter-sectoral climate change risks: the role of ISIMIP, Environ. Res. Lett., 12, 010301, https://doi.org/10.1088/1748-9326/12/1/010301, 2017. a
Russotto, R. D. and Ackerman, T. P.: Changes in clouds and thermodynamics under solar geoengineering and implications for required solar reduction, Atmos. Chem. Phys., 18, 11905–11925, https://doi.org/10.5194/acp-18-11905-2018, 2018a. a
Russotto, R. D. and Ackerman, T. P.: Energy transport, polar amplification, and ITCZ shifts in the GeoMIP G1 ensemble, Atmos. Chem. Phys., 18, 2287–2305, https://doi.org/10.5194/acp-18-2287-2018, 2018b. a
Schäfer, S., Lawrence, M., Stelzer, H., , Born, W., Low, S., Aaheim, A., Adriázola, P., Betz, G., Boucher, O., Carius, A., Devine-Right, P., Gullberg, A., Haszeldine, S., Haywood, J., Houghton, K., Ibarrola, R., Irvine, P. J., Kristjánsson, J. E., Lenton, T. M., Link, J. S. A., Maas, A., Meyer, L. H., Muri, H., Oschlies, A., Proelss, A., Rayner, T., Rickels, W., Ruthner, L., Scheffran, J., Schmidt, H., Schulz, M., Scott, V., Shackley, S., Tänzler, D., Watson, M., and Vaughan, N. E.: The European Transdisciplinary Assessment of Climate Engineering (EuTRACE): Removing Greenhouse Gases from the Atmosphere and Reflecting Sunlight away from Earth, Tech. rep., European Union, https://www.rifs-potsdam.de/sites/default/files/files/rz_150715_eutrace_digital_0.pdf (last access: 3 May 2023), 2015. a
Schmidt, A., Mills, M. J., Ghan, S., Gregory, J. M., Allan, R. P., Andrews, T., Bardeen, C. G., Conley, A., Forster, P. M., Gettelman, A., Portmann, R. W., Solomon, S., and Toon, O. B.: Volcanic Radiative Forcing From 1979 to 2015, J. Geophys. Res.-Atmos., 123, 12491–12508, https://doi.org/10.1029/2018JD028776, 2018. a
Schmidt, H., Alterskjær, K., Bou Karam, D., Boucher, O., Jones, A., Kristjánsson, J. E., Niemeier, U., Schulz, M., Aaheim, A., Benduhn, F., Lawrence, M., and Timmreck, C.: Solar irradiance reduction to counteract radiative forcing from a quadrupling of CO2: climate responses simulated by four earth system models, Earth Syst. Dynam., 3, 63–78, https://doi.org/10.5194/esd-3-63-2012, 2012a. a
Schmidt, H., Niemeier, U., Timmreck, C., Aaheim, A., Romstad, B., Wei, T., Kristjánsson, J. E., Alterskjær, K., Muri, H., Lawrence, M., Benduhn, F., Schulz, M., BouKaram, D., and Boucher, O.: The FP7 project IMPLICC: Implications and risks of engineering solar radiation to limit climate change, Tech. rep., European Union, https://implicc.mpimet.mpg.de/fileadmin/user_upload/implicc/other_documents/implicc_final_report_20121130_publishable_summary.pdf (last access: 3 May 2023), 2012b. a
Simpson, I., Tilmes, S., Richter, J., Kravitz, B., MacMartin, D., Mills, M., Fasullo, J., and Pendergrass, A.: The regional hydroclimate response to stratospheric sulfate geoengineering and the role of stratospheric heating, J. Geophys. Res.-Atmos., 124, 2019JD031093, https://doi.org/10.1029/2019JD031093, 2019. a, b
Singh, J., Sahany, S., and Robock, A.: Can stratospheric geoengineering alleviate global warming-induced changes in deciduous fruit cultivation? The case of Himachal Pradesh (India), Climatic Change, 162, 1323–1343, https://doi.org/10.1007/s10584-020-02786-3, 2020. a
Smith, W., Bhattarai, U., MacMartin, D. G., Lee, W. R., Visioni, D., Kravitz, B., and Rice, C. V.: A subpolar-focused stratospheric aerosol injection deployment scenario, Environ. Res. Commun., 4, 095009, https://doi.org/10.1088/2515-7620/ac8cd3, 2022. a
Sourdeval, O., Gryspeerdt, E., Krämer, M., Goren, T., Delanoë, J., Afchine, A., Hemmer, F., and Quaas, J.: Ice crystal number concentration estimates from lidar–radar satellite remote sensing – Part 1: Method and evaluation, Atmos. Chem. Phys., 18, 14327–14350, https://doi.org/10.5194/acp-18-14327-2018, 2018. a
Stjern, C. W., Muri, H., Ahlm, L., Boucher, O., Cole, J. N. S., Ji, D., Jones, A., Haywood, J., Kravitz, B., Lenton, A., Moore, J. C., Niemeier, U., Phipps, S. J., Schmidt, H., Watanabe, S., and Kristjánsson, J. E.: Response to marine cloud brightening in a multi-model ensemble, Atmos. Chem. Phys., 18, 621–634, https://doi.org/10.5194/acp-18-621-2018, 2018. a
Storelvmo, T., Kristjansson, J. E., Muri, H., Pfeffer, M., Barahona, D., and Nenes, A.: Cirrus cloud seeding has potential to cool climate, Geophys. Res. Lett., 40, 178–182, https://doi.org/10.1029/2012GL054201, 2013. a
Sun, H., Eastham, S., and Keith, D.: Developing a Plume-in-Grid Model for Plume Evolution in the Stratosphere, J. Adv. Model. Earth Syst., 14, e2021MS002816, https://doi.org/10.1029/2021MS002816, 2022. a
The Royal Society: Geoengineering the climate: science, governance and uncertainty, The Royal Society, London, https://royalsociety.org/~/media/Royal_Society_Content/policy/publications/2009/8693.pdf (last access: 3 May 2023), 2009. a
Tilmes, S., Fasullo, J., Lamarque, J.-F., Marsh, D. R., Mills, M., Alterskjær, K., Muri, H., Kristjánsson, J. E., Boucher, O., Schulz, M., Cole, J. N. S., Curry, C. L., Jones, A., Haywood, J., Irvine, P. J., Ji, D., Moore, J. C., Karam, D. B., Kravitz, B., Rasch, P. J., Singh, B., Yoon, J.-H., Niemeier, U., Schmidt, H., Robock, A., Yang, S., and Watanabe, S.: The hydrological impact of geoengineering in the Geoengineering Model Intercomparison Project (GeoMIP), J. Geophys. Res.-Atmos., 118, 11036–11058, https://doi.org/10.1002/jgrd.50868, 2013. a, b
Tilmes, S., Mills, M. J., Niemeier, U., Schmidt, H., Robock, A., Kravitz, B., Lamarque, J.-F., Pitari, G., and English, J. M.: A new Geoengineering Model Intercomparison Project (GeoMIP) experiment designed for climate and chemistry models, Geosci. Model Dev., 8, 43–49, https://doi.org/10.5194/gmd-8-43-2015, 2015. a, b
Tilmes, S., Richter, J. H., Mills, M. J., Kravitz, B., Macmartin, D. G., Vitt, F., Tribbia, J. J., and Lamarque, J. F.: Sensitivity of aerosol distribution and climate response to stratospheric SO2 injection locations, J. Geophys. Res.-Atmos., 122, 12591–12615, https://doi.org/10.1002/2017JD026888, 2017. a
Tilmes, S., Richter, J. H., Kravitz, B., Macmartin, D. G., Mills, M. J., Simpson, I. R., Glanville, A. S., Fasullo, J. T., Phillips, A. S., Lamarque, J. F., Tribbia, J., Edwards, J., Mickelson, S., and Ghosh, S.: CESM1(WACCM) stratospheric aerosol geoengineering large ensemble project, B. Am. Meteorol. Soc., 99, 2361–2371, https://doi.org/10.1175/BAMS-D-17-0267.1, 2018. a, b, c
Tilmes, S., MacMartin, D. G., Lenaerts, J. T. M., van Kampenhout, L., Muntjewerf, L., Xia, L., Harrison, C. S., Krumhardt, K. M., Mills, M. J., Kravitz, B., and Robock, A.: Reaching 1.5 and 2.0 ∘C global surface temperature targets using stratospheric aerosol geoengineering, Earth Syst. Dynam., 11, 579–601, https://doi.org/10.5194/esd-11-579-2020, 2020. a, b, c, d
Tilmes, S., Visioni, D., Jones, A., Haywood, J., Séférian, R., Nabat, P., Boucher, O., Bednarz, E. M., and Niemeier, U.: Stratospheric ozone response to sulfate aerosol and solar dimming climate interventions based on the G6 Geoengineering Model Intercomparison Project (GeoMIP) simulations, Atmos. Chem. Phys., 22, 4557–4579, https://doi.org/10.5194/acp-22-4557-2022, 2022. a, b, c
Timmreck, C., Mann, G. W., Aquila, V., Hommel, R., Lee, L. A., Schmidt, A., Brühl, C., Carn, S., Chin, M., Dhomse, S. S., Diehl, T., English, J. M., Mills, M. J., Neely, R., Sheng, J., Toohey, M., and Weisenstein, D.: The Interactive Stratospheric Aerosol Model Intercomparison Project (ISA-MIP): motivation and experimental design, Geosci. Model Dev., 11, 2581–2608, https://doi.org/10.5194/gmd-11-2581-2018, 2018. a, b, c
Trisos, C. H., Amatulli, G., Gurevitch, J., Robock, A., Xia, L., and Zambri, B.: Potentially dangerous consequences for biodiversity of solar geoengineering implementation and termination, Nat. Ecol. Evol., 2, 475–482, https://doi.org/10.1038/s41559-017-0431-0, 2018. a, b, c
Tye, M. R., Dagon, K., Molina, M. J., Richter, J. H., Visioni, D., Kravitz, B., and Tilmes, S.: Indices of extremes: geographic patterns of change in extremes and associated vegetation impacts under climate intervention, Earth Syst. Dynam., 13, 1233–1257, https://doi.org/10.5194/esd-13-1233-2022, 2022. a, b
Vattioni, S., Weisenstein, D., Keith, D., Feinberg, A., Peter, T., and Stenke, A.: Exploring accumulation-mode H2SO4 versus SO2 stratospheric sulfate geoengineering in a sectional aerosol-chemistry-climate model, Atmos. Chem. Phys., 19, 4877–4897, https://doi.org/10.5194/acp-19-4877-2019, 2019. a
Virgin, J. G. and Fletcher, C. G.: On the Linearity of External Forcing Response in Solar Geoengineering Experiments, Geophys. Res. Lett., 49, e2022GL100200, https://doi.org/10.1029/2022GL100200, 2022. a
Visioni, D. and Robock, A.: Future Geoengineering Scenarios: Balancing Policy Relevance and Scientific Significance, B. Am. Meteorol. Soc., 103, E817–E820, https://doi.org/10.1175/BAMS-D-21-0201.1, 2022. a, b
Visioni, D., Pitari, G., Tuccella, P., and Curci, G.: Sulfur deposition changes under sulfate geoengineering conditions: quasi-biennial oscillation effects on the transport and lifetime of stratospheric aerosols, Atmos. Chem. Phys., 18, 2787–2808, https://doi.org/10.5194/acp-18-2787-2018, 2018. a
Visioni, D., MacMartin, D. G., and Kravitz, B.: Is Turning Down the Sun a Good Proxy for Stratospheric Sulfate Geoengineering?, J. Geophys. Res.-Atmos., 126, e2020JD033952, https://doi.org/10.1029/2020JD033952, 2021a. a, b, c, d, e, f
Visioni, D., MacMartin, D. G., Kravitz, B., Boucher, O., Jones, A., Lurton, T., Martine, M., Mills, M. J., Nabat, P., Niemeier, U., Séférian, R., and Tilmes, S.: Identifying the sources of uncertainty in climate model simulations of solar radiation modification with the G6sulfur and G6solar Geoengineering Model Intercomparison Project (GeoMIP) simulations, Atmos. Chem. Phys., 21, 10039–10063, https://doi.org/10.5194/acp-21-10039-2021, 2021b. a, b, c, d, e, f, g
Visioni, D., Bednarz, E. M., Lee, W. R., Kravitz, B., Jones, A., Haywood, J. M., and MacMartin, D G.: Climate response to off-equatorial stratospheric sulfur injections in three Earth system models – Part 1: Experimental protocols and surface changes, Atmos. Chem. Phys., 23, 663–685, https://doi.org/10.5194/acp-23-663-2023, 2023. a, b, c
Wang, J., Moore, J. C., Zhao, L., Yue, C., and Di, Z.: Regional dynamical and statistical downscaling temperature, humidity and wind speed for the Beijing region under stratospheric aerosol injection geoengineering, Earth Syst. Dynam., 13, 1625–1640, https://doi.org/10.5194/esd-13-1625-2022, 2022. a, b
WCRP: CMIP5, https://esgf-node.llnl.gov/search/cmip5/ (last access: 3 May 2023), 2023a. a
WCRP: CMIP6, https://esgf-node.llnl.gov/search/cmip6/ (last access: 3 May 2023), 2023b. a
Webb, M. J., Andrews, T., Bodas-Salcedo, A., Bony, S., Bretherton, C. S., Chadwick, R., Chepfer, H., Douville, H., Good, P., Kay, J. E., Klein, S. A., Marchand, R., Medeiros, B., Siebesma, A. P., Skinner, C. B., Stevens, B., Tselioudis, G., Tsushima, Y., and Watanabe, M.: The Cloud Feedback Model Intercomparison Project (CFMIP) contribution to CMIP6, Geosci. Model Dev., 10, 359–384, https://doi.org/10.5194/gmd-10-359-2017, 2017. a
Wei, L., Ji, D., Miao, C., Muri, H., and Moore, J. C.: Global streamflow and flood response to stratospheric aerosol geoengineering, Atmos. Chem. Phys., 18, 16033–16050, https://doi.org/10.5194/acp-18-16033-2018, 2018. a
Weisenstein, D. K., Penner, J. E., Herzog, M., and Liu, X.: Global 2-D intercomparison of sectional and modal aerosol modules, Atmos. Chem. Phys., 7, 2339–2355, https://doi.org/10.5194/acp-7-2339-2007, 2007. a
Weisenstein, D. K., Visioni, D., Franke, H., Niemeier, U., Vattioni, S., Chiodo, G., Peter, T., and Keith, D. W.: An interactive stratospheric aerosol model intercomparison of solar geoengineering by stratospheric injection of SO2 or accumulation-mode sulfuric acid aerosols, Atmos. Chem. Phys., 22, 2955–2973, https://doi.org/10.5194/acp-22-2955-2022, 2022. a, b, c
Wigley, T. M. L.: A Combined Mitigation/Geoengineering Approach to Climate Stabilization, Science, 314, 452–454, https://doi.org/10.1126/science.1131728, 2006. a
Xia, L., Robock, A., Cole, J., Curry, C. L., Ji, D., Jones, A., Kravitz, B., Moore, J. C., Muri, H., Niemeier, U., Singh, B., Tilmes, S., Watanabe, S., and Yoon, J.-H.: Solar radiation management impacts on agriculture in China: A case study in the Geoengineering Model Intercomparison Project (GeoMIP), J. Geophys. Res.-Atmos., 119, 8695–8711, https://doi.org/10.1002/2013JD020630, 2014. a
Xia, L., Nowack, P. J., Tilmes, S., and Robock, A.: Impacts of stratospheric sulfate geoengineering on tropospheric ozone, Atmos. Chem. Phys., 17, 11913–11928, https://doi.org/10.5194/acp-17-11913-2017, 2017. a
Xie, M., Moore, J. C., Zhao, L., Wolovick, M., and Muri, H.: Impacts of three types of solar geoengineering on the Atlantic Meridional Overturning Circulation, Atmos. Chem. Phys., 22, 4581–4597, https://doi.org/10.5194/acp-22-4581-2022, 2022. a
Xu, Y., Lin, L., Tilmes, S., Dagon, K., Xia, L., Diao, C., Cheng, W., Wang, Z., Simpson, I., and Burnell, L.: Climate engineering to mitigate the projected 21st-century terrestrial drying of the Americas: a direct comparison of carbon capture and sulfur injection, Earth Syst. Dynam., 11, 673–695, https://doi.org/10.5194/esd-11-673-2020, 2020. a
Yu, X., Moore, J. C., Cui, X., Rinke, A., Ji, D., Kravitz, B., and Yoon, J.-H.: Impacts, effectiveness and regional inequalities of the GeoMIP G1 to G4 solar radiation management scenarios, Global Planet. Change, 129, 10–22, https://doi.org/10.1016/j.gloplacha.2015.02.010, 2015. a
Zanchettin, D., Khodri, M., Timmreck, C., Toohey, M., Schmidt, A., Gerber, E. P., Hegerl, G., Robock, A., Pausata, F. S. R., Ball, W. T., Bauer, S. E., Bekki, S., Dhomse, S. S., LeGrande, A. N., Mann, G. W., Marshall, L., Mills, M., Marchand, M., Niemeier, U., Poulain, V., Rozanov, E., Rubino, A., Stenke, A., Tsigaridis, K., and Tummon, F.: The Model Intercomparison Project on the climatic response to Volcanic forcing (VolMIP): experimental design and forcing input data for CMIP6, Geosci. Model Dev., 9, 270–2719, https://doi.org/10.5194/gmd-9-2701-2016, 2016. a
Zanchettin, D., Timmreck, C., Khodri, M., Schmidt, A., Toohey, M., Abe, M., Bekki, S., Cole, J., Fang, S.-W., Feng, W., Hegerl, G., Johnson, B., Lebas, N., LeGrande, A. N., Mann, G. W., Marshall, L., Rieger, L., Robock, A., Rubinetti, S., Tsigaridis, K., and Weierbach, H.: Effects of forcing differences and initial conditions on inter-model agreement in the VolMIP volc-pinatubo-full experiment, Geosci. Model Dev., 15, 2265–2292, https://doi.org/10.5194/gmd-15-2265-2022, 2022. a, b
Zarnetske, P. L., Gurevitch, J., Franklin, J., Groffman, P. M., Harrison, C. S., Hellmann, J. J., Hoffman, F. M., Kothari, S., Robock, A., Tilmes, S., Visioni, D., Wu, J., Xia, L., and Yang, C.-E.: Potential ecological impacts of climate intervention by reflecting sunlight to cool Earth, P. Natl. Acad. Sci. USA, 118, e1921854118, https://doi.org/10.1073/pnas.1921854118, 2021. a, b
Zelinka, M. D., Myers, T. A., McCoy, D. T., Po-Chedley, S., Caldwell, P. M., Ceppi, P., Klein, S. A., and Taylor, K. E.: Causes of Higher Climate Sensitivity in CMIP6 Models, Geophys. Res. Lett., 47, e2019GL085782, https://doi.org/10.1029/2019GL085782, 2020. a
Zhao, L., Yang, Y., Cheng, W., Ji, D., and Moore, J. C.: Glacier evolution in high-mountain Asia under stratospheric sulfate aerosol injection geoengineering, Atmos. Chem. Phys., 17, 6547–6564, https://doi.org/10.5194/acp-17-6547-2017, 2017. a
- Abstract
- Introduction
- An assessment of past and present GeoMIP experiments
- Test-bed experiments and other relevant experiments
- Future experiments
- Lessons learned
- The role of GeoMIP
- Conclusions
- Data availability
- Author contributions
- Competing interests
- Disclaimer
- Special issue statement
- Acknowledgements
- Financial support
- Review statement
- References
- Abstract
- Introduction
- An assessment of past and present GeoMIP experiments
- Test-bed experiments and other relevant experiments
- Future experiments
- Lessons learned
- The role of GeoMIP
- Conclusions
- Data availability
- Author contributions
- Competing interests
- Disclaimer
- Special issue statement
- Acknowledgements
- Financial support
- Review statement
- References