the Creative Commons Attribution 4.0 License.
the Creative Commons Attribution 4.0 License.
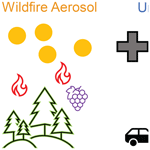
Gas–particle partitioning of semivolatile organic compounds when wildfire smoke comes to town
Rebecca A. Wernis
Kasper Kristensen
Nathan M. Kreisberg
Philip L. Croteau
Scott C. Herndon
Arthur W. H. Chan
Nga L. Ng
Wildfires have become an increasingly important source of organic gases and particulate matter in the western USA. A large fraction of organic particulate matter emitted in wildfires is semivolatile, and the oxidation of organic gases in smoke can form lower-volatility products that then condense on smoke particulates. In this research, we measured the gas- and particle-phase concentrations of semivolatile organic compounds (SVOCs) during the 2017 northern California wildfires in a downwind urban area, using semivolatile thermal desorption aerosol gas chromatography (SV-TAG), and measured SVOCs in a rural site affected by biomass burning using cTAG (comprehensive thermal desorption aerosol gas chromatography mass spectrometry) in Idaho in 2018. Commonly used biomass burning markers such as levoglucosan, mannosan, and nitrocatechols were found to stay predominantly in the particle phase, even when the ambient organic aerosol (OA) was relatively low. The phase partitioning of SVOCs is observed to be dependent on their saturation vapor pressure, while the equilibrium absorption model underpredicts the particle-phase fraction of most of the compounds measured. Wildfire organic aerosol enhanced the condensation of polar compounds into the particle phase but not some nonpolar compounds, such as polycyclic aromatic hydrocarbons.
- Article
(2306 KB) - Full-text XML
-
Supplement
(7338 KB) - BibTeX
- EndNote
In the western USA, wildfires have become an increasingly important source of organic gases and particulate matter in the atmosphere (Dennison et al., 2014; McClure and Jaffe, 2018; Iglesias et al., 2022). Biomass burning (BB) in wildfires emits thousands of organic compounds with vastly different properties (such as volatility and reactivity; Jen et al., 2019; Hatch et al., 2015; Liang et al., 2022a). Many of these compounds have known direct health impacts, and the oxidation of organic compounds can also produce secondary organic aerosols (SOAs), which are also hazardous (Kim et al., 2018; Tuet et al., 2017, 2019; Wong et al., 2019).
Among the wildfire emissions, the semivolatile and intermediate-volatility organic compounds (SVOCs and IVOCs), with effective saturation concentrations (C∗) between 10−1 and 106 µg m−3 are of particular interest because of their partitioning between the gas and particle phases, which can either decrease or increase the mass concentration of organic aerosol (OA, which only refers to the particles hereafter; Hodshire et al., 2019). May et al. (2013) found that the majority of primary organic aerosol (POA) emitted from BB is semivolatile. In a source apportionment analysis, an ideal tracer for a source-specific factor should be a stable compound with a relatively low volatility compared with the bulk BBOA (biomass burning organic aerosol; Donahue et al., 2012). However, levoglucosan, the most commonly used BB marker, was also found to evaporate and photochemically decay in the atmosphere (Hennigan et al., 2010; Xie et al., 2014). Through in situ measurement, Palm et al. (2020) found that up to a third of the BB POA evaporated as a wildfire smoke plume diluted by a factor of 5–10, and the majority of SOA formed was from the oxidation of evaporated BB POA. However, the molecular identities of these evaporated compounds are not known. The BB SOA compounds formed by atmospheric oxidation of primary emissions may also be semivolatile. For instance, Palm et al. (2020) also found that the nitrophenolic compounds, which were predicted to be in the particle-phase only from thermal desorption analysis (Finewax et al., 2018), may also exist in the gas phase. These knowledge gaps motivated our study of the gas–particle-partitioning behaviors of BB POA and SOA compounds in the atmosphere.
When wildfire smoke enters the urban area, the gases and particles in the smoke plumes interact with urban air pollutants. In absorptive partitioning theory that is assumed in most atmospheric models, the fraction of organic compounds in the particle phase increases with the mass of absorbing aerosol (Pankow, 1994; Donahue et al., 2006). Therefore, the massive amount of BBOA (which can exceed 100 µg m−3 of fine particulate matter) entering the urban atmosphere is expected to absorb SVOCs into the condensed phase to form SOA. The BBOA may also affect the downwind gas–particle partitioning of compounds such as polycyclic aromatic hydrocarbons (PAHs) and phthalates in the urban area, thereby altering the mechanisms and magnitude of their deposition in the respiratory tract (Pankow, 2001). However, whether preexisting organic aerosol can alter the partitioning behavior of SVOCs also depends on the aerosol composition (Asa-Awuku et al., 2009; Ye et al., 2016, 2018; Liu et al., 2021). Differences in the composition between urban aerosol and BBOA may make them (or some components of them) not miscible. Mahrt et al. (2022a) recently reported that two types of organic aerosol with difference under 0.265 can probably mix with each other, while two phases will remain if the difference in is above this threshold. BBOA can have ratios above 0.6, while cooking OA and hydrocarbon-like OA, which accounts for nearly half of the urban OA in Oakland, CA (a city adjacent to Berkeley), have ratios between 0.01 and 0.11 (Zhou et al., 2017; Shah et al., 2018). It is possible that the polar BBOA compounds can increase the activity coefficients of the relatively nonpolar organic compounds and expel them into the gas phase. In addition, BBOA was found to be semisolid, even under high relative humidity (RH ∼75 %), which can limit the gas–particle partitioning of organic compounds (Bateman et al., 2017). Measurements are therefore needed to find out whether wildfire aerosol can shift the gas–particle partitioning of SVOCs.
In this study, we measured the hourly concentrations of organic compounds using the semivolatile thermal desorption aerosol gas chromatography (SV-TAG) in Berkeley, California, during the October 2017 northern California wildfires. Concentrations of organic compounds were also measured in McCall, Idaho, in August 2018, as a part of the Fire Influence on Regional to Global Environments and Air Quality (FIREX-AQ) study, using the comprehensive thermal desorption aerosol gas chromatography (cTAG). The TAG instruments can achieve molecular speciation at an hourly time resolution, which enables us to better explore the impact of environmental factors on the gas–particle partitioning of individual compounds. Our main aims here are to examine the gas–particle partitioning behaviors of organic compounds, especially BBOA marker compounds when BB smoke is diluted and enters the urban atmosphere, and to evaluate the impact of the massive amount of wildfire aerosol on the partitioning behaviors of SVOCs (including IVOCs, hereafter).
2.1 Field sites, measurement periods, and the wildfires
The 2017 northern California wildfires started on 8 October in Napa and Sonoma counties and lasted for more than 8 weeks. The fuels and perimeters of these wildfires can be found in our previous publications (Liang et al., 2021, 2022b). These wildfires caused multiple air quality measurement stations in the San Francisco Bay Area to record PM2.5 (particulate matter with diameter less than 2.5 µm) exceeding 100 µg m−3 (Liang et al., 2021). These air masses contained fire emissions from mainly oak woodland landscapes, combined with suburban housing developments, and agricultural areas. Our SV-TAG measurements were conducted on the University of California (UC) Berkeley campus ( N, W), located in the urban San Francisco Bay Area, 55–65 km downwind of the major wildfires. SV-TAG data are reported for 10 to 19 October.
Data were also collected by the cTAG in rural Idaho between 12 and 27 August 2018. The McCall, Idaho, field site ( N, W) was a rural location approximately 4 km south of the town of McCall (population <3500), which is located 180 km north of Boise and surrounded by national forest. This site experienced smoke from the following four fires: Rattlesnake Creek (∼3300 ha; ∼45 km northwest of McCall), Mesa (∼14 000 ha; 38 km southwest of McCall), Rabbit Foot (∼15 000 ha; ∼145 km east of McCall), and a fire 60 km southwest of McCall and fires from central Oregon. More detailed descriptions of these fires can be found in Lindsay et al. (2022). Backward trajectory analysis by Lindsay et al. (2022) suggests that the smoke plumes traveled at least 3–5 h and up to 18–30 h prior to arriving at this site.
2.2 Speciated measurements by SV-TAG and cTAG
During the 2017 California wildfires, individual compounds were measured by the SV-TAG with in situ derivatization at hourly time resolution (Zhao et al., 2013b; Isaacman et al., 2014). Ambient air was pulled to the SV-TAG at a flow rate >1000 L min−1 through a 2 m×6 in. (15.2 cm) outer diameter duct to reduce condensation of gas-phase SVOCs in the tubing. The SV-TAG subsampled 20 L min−1 of air from the center of the airstream through passivated tubing and a 1.0 µm cutoff (PM1) cyclone. Then, 10 L min−1 of air was delivered to each of the two identical coated metal mesh filter cells, with one of the air samples passing through a carbon denuder that removes gases. This approach enables us to determine the particle-phase fraction (Fp) of each measured compound.
The same strategy was used for cTAG sampling in McCall, Idaho. The cTAG subsampled 10 L min−1 of air from the middle of a 25 cm diameter duct through a PM1 cyclone. The details of the cTAG can be found in Wernis et al. (2021). The cTAG measured volatile organic compounds (VOCs) and SVOCs concurrently, and this research focuses on the SVOCs. The main difference between the cTAG and the SV-TAG is that the cTAG alternated between gas plus particle and particle-only sampling by either having the sampled air passing through the denuder (same as the denuder for the SV-TAG) or bypassing the denuder. Since gas plus particle and particle-only organics were not collected simultaneously, we calculated the Fp value (2 h time resolution) of each compound using the interpolation method as follows:
where CP,n is the particle-phase concentration (proportional to the internal-standard-normalized signal) for sample n, and the denominator is the sum of gas plus particle concentrations in the previous hour and the subsequent hour (Zhao et al., 2013a). More materials and methods about SV-TAG and cTAG can be found in the Supplement.
2.3 Uncertainty in the measurements
The uncertainty in the SV-TAG measurements has been studied in Isaacman et al. (2014). Uncertainty in quantification of compounds was estimated to be 20 %–25 %. However, because Fp is the ratio of the signals from the two cells, the uncertainty of Fp only depends on how well samples from the two cells can be intercompared. We collected 11 bypass samples simultaneously on two cells as a direct comparison during this campaign to equalize the signal of the two cells. This equalization helps to reduce the cell-to-cell variabilities caused by differences in the collection efficiency, derivatization efficiency, etc. The uncertainty of Fp is estimated to be 15 %–25 % when this correction is applied, so a value of Fp greater than 1 is possible (Isaacman et al., 2014). For a particle-phase-dominant compound, we expect its Fp value to follow the normal distribution, with a mean value close to 1 (Isaacman et al., 2014). Another source of uncertainty comes from carryover from previous sample, which is found to be 2.6 % for a total ion chromatogram and 2.4 % for a median single ion chromatogram and independent of volatility (Kreisberg et al., 2014). We evaluated the bias caused by carryover in the Supplement. This level of carryover can create a noteworthy positive bias to the Fp value of compounds almost entirely in the gas phase (Fp less than 0.05). Those compounds are not considered in the activity coefficient analysis. The measurement uncertainty in the campaign average Fp value can be estimated from the error of the individual Fp value by the central limit theorem, which yields 1.4 %–2.4 % of uncertainty to the campaign average Fp value (in Fig. 1).
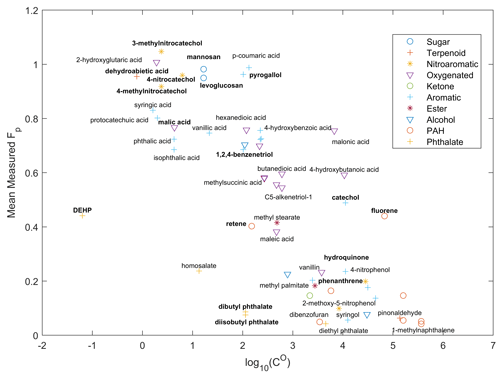
Figure 1Campaign average Fp value during the October 2017 northern California wildfires measured by the SV-TAG, classified by functional groups, and plotted against their logarithmic saturation concentrations CO (in µg m−3) at 298 K. Only a subset of the measured compounds are labeled. Compounds discussed in the text are in bold. Alkanes and mono-carboxylic acids are shown separately in Fig. S3.
2.4 Supporting measurements and models
During the 2017 northern California wildfires, PM2.5 filter samples (N=74) with 3 to 4 h time resolution were collected concurrently with SV-TAG measurements. The chemical composition of the filter samples were determined using two-dimensional gas chromatography coupled with high-resolution time-of-flight mass spectrometry (GC×GC HR-ToF-MS; Liang et al., 2021). Compounds identified from SV-TAG measurements were validated by matching with authentic standards and comparison with GC×GC measurements. More details can be found in the Supplement. In addition, the particle mass concentration was measured by a scanning mobility particle sizer (SMPS; TSI 1080 electrostatic classifier coupled with a TSI 3788 condensation particle counter; range = 10–600 nm, sampling time = 300 s; aerosol flow rate = 0.6 L min−1; sheath flow rate = 6 L min−1), assuming a density of PM1=1.1 µg m−3, according to Pokhrel et al. (2021), and averaged to the same sampling time base as SV-TAG measurements. The mass of PM1 was also measured by a Grimm 11 A OPC (GRIMM Aerosol Technik) for a shorter period. The linear fit of the SMPS measurement against the GRIMM measurement gives a slope of 0.98 and normalized root mean square error of 26 %, which suggests that the density assumed is reasonable. The mass of OA was not measured in Berkeley. In the biomass burning emission, OA was found to contribute 77 %–99 % to total aerosol mass (Lim et al., 2019). We therefore assume that 90 % of PM1 is organic in the following analysis. This assumption introduces some uncertainty but did not affect the conclusion. More discussion about the influence of this assumption is in Sect. 3.
In the FIREX-AQ 2018 campaign in McCall, the bulk properties of non-refractory PM2.5 were determined by an aerosol chemical speciation monitor (ACSM), using thermal vaporization and electron impact ionization mass spectrometry (Ng et al., 2011). The ACSM data were also averaged to the cTAG measurement sampling times. ACSM data and meteorological data in McCall can be accessed from Yacovitch et al. (2022).
Observed Fp values were compared with the values predicted based on the organic aerosol mass and vapor pressure of each compound by an absorption equilibrium partitioning model which assumes instantaneous equilibrium between the gas and particle phases (Pankow, 1994). With this assumption, the Fp value of compound i can be expressed as
where COA is the concentration of organic aerosol, γi is the unitless activity coefficient of compound i, is the saturation mass concentration of pure compound i, and is the effective saturation concentration of i (all concentrations are in µg m−3). The activity coefficient captures the non-ideal interactions between the compound with the aerosol mixture. A γ smaller than 1 means compound i is more likely to condense into the aerosol mixture than into pure compound i. A larger γ means that the compound is more likely to stay in the gas phase over this aerosol mixture, and very large γ (>10) may indicate phase separation (Liu et al., 2021; Donahue et al., 2011). The subcooled liquid vapor pressure of each compound, which is used to calculate , was calculated by the SIMPOL and EVAPORATION models (Pankow and Asher, 2008; Compernolle et al., 2011). We used the MPBPWIN component (modified Grain method) in the Estimation Program Interface (EPI) Suite of the U.S. Environment Protection Agency (US EPA, 2012) to estimate the vapor pressures of nitroaromatic compounds because the SIMPOL model substantially overestimates the vapor pressures of these compounds in general (Bannan et al., 2017; Wania et al., 2017). There is evidence that vapor pressures of multifunctional compounds may not be very accurate when by calculated SIMPOL and other group contribution methods (Barley and McFiggans, 2010; Dang et al., 2019; O'Meara et al., 2014). We used these methods to estimate CO, mainly because they are commonly used to describe the gas–particle partitioning of SVOCs. To better understand the influence of BBOA on the gas–particle partitioning of SVOCs, we modeled the activity coefficients of compounds above aerosol mixtures using the AIOMFAC model (Zuend et al., 2011). More details of these models are provided in the Supplement.
3.1 Average partitioning behavior of BBOA marker compounds
After quality assurance and quality control (Sect. S1 in the Supplement), we selected 89 compounds from the 2017 study and 30 compounds from the 2018 study for detailed analysis. The campaign average Fp values for each compound measured during the 2017 northern California fires are shown in Figs. 1 and S3 (alkanes and aliphatic acids), with the distribution of Fp values shown in Fig. S4. The overall trend is as expected; the gas–particle partitioning behavior is related to the volatility of compounds. We focus our discussion on commonly used biomass burning markers. In contrast to Xie et al. (2014), primary BB tracers levoglucosan and mannosan (Eliasl et al., 2001; Simoneit, 2002) were found to be almost entirely in the particle phase. The same result is observed in the McCall data (Fig. S6). The discrepancy between our results and Xie et al. (2014) is likely due to the much higher mass of BBOA and levoglucosan and the lower temperature in our field campaigns. In Xie et al. (2014), gas-phase levoglucosan is comparable to particle-phase levoglucosan only in samples with less than 50 ng m−3 of levoglucosan at high ambient temperatures. These primary BB compounds have much higher Fp values compared with other compounds with similar saturation vapor pressures. The high particle-phase fraction of them makes them less likely to react with atmospheric oxidants and decay and therefore makes them very good BB marker compounds for source apportionment studies (Donahue et al., 2012). Surprisingly, dehydroabietic acid, although having a very low-saturation vapor pressure over pure compound, has a higher fraction in the gas phase compared with levoglucosan and mannosan in FIREX-AQ 2018 samples (Fig. S5). Nevertheless, it was predominantly in the particle phase in the Napa study. Retene (C18H18) is one of the most abundant PAHs emitted from BB (Jen et al., 2019; Liang et al., 2022a). In fresh BB emissions, where the level of OA is several hundreds of micrograms per cubic meter, retene is expected to be mainly in particle phase. In both campaigns, the average Fp value of retene was around 0.4–0.5, which suggests a substantial amount of retene was in the gas phase in the aged smoke plumes. This agrees with our results in the FIREX-AQ 2019 study, in which we found that the ratio of particle-phase PAHs to organic carbon decreased with increasing acetonitrilefuran ratio (with correlation coefficient ), which is an indicator of plume age (Liang et al., 2022a). The particle-phase PAHs, dominated by the diterpenoid-related ones such as retene, on average accounted for 7.6 % of quantified OA in the FIREX-AQ 2019 filter samples but only accounted for less than 0.3 % in the Berkeley filter samples collected simultaneously with the SV-TAG measurements (Liang et al., 2021, 2022a). Evaporation, in addition to the difference in biomass fuels, may help to explain this observed difference between fresh and aged BBOA. Given its low ratio and high molecular weight, the evaporated retene (and other diterpenoid-related PAHs) may react with oxidants and form SOA with a high yield (Gentner et al., 2012; Jimenez et al., 2009). Figure 1 also shows that isomers with very similar structures and therefore similar estimated saturation concentrations may have very different gas–particle partitioning behaviors. Pyrogallol (1,2,3-benzenetriol) has much higher fractions in the particle phase than 1,2,4-benzenetriol. It is either because the actual vapor pressures of these isomers are very different or because pyrogallol has specific interactions with the aerosol phase that their isomers do not have. Such interactions can reduce the activity coefficients of these SVOCs. A large variation in the vapor pressures among isomers has been reported before (Dang et al., 2019). Catechol also shows a much higher Fp value than hydroquinone. However, catechol could come from the decomposition of particle-phase-dominant compounds in GC. For example, protocatechuic acid (without catalyst; at 200 ∘C) is shown to produce catechol when heated in water (in CAS SciFinder, https://scifinder.cas.org/, last access: 9 February 2023). Further studies on the thermal stability of aromatic compounds in thermal desorption systems are needed to elucidate the partitioning behavior of such compounds.
Secondary BBOA markers, 4-nitrocatechol, 3-methylnitrocatechol, and 4-methylnitrocatechol, were predominantly in the particle phase. The sum of their concentrations reached 1.4 µg m−3 in the particle phase in the strongest wildfire plumes. The high Fp value and high concentration make them good BB SOA markers. Equilibrium absorption modeling using the vapor pressure estimated by the EPI Suite (US EPA, 2012) also predicted that these compounds stay mostly in the particle phase, while modeling using vapor pressure from the SIMPOL model predicted half of these compounds (by mass) would stay in the gas phase. The high Fp values of these compounds agree with the findings by Finewax et al. (2018) and Fredrickson et al. (2022), in which they found the group contribution methods overestimate the saturation vapor pressure of these compounds. Fredrickson et al. also reported that NO3-derived nitrocatechols are less volatile than the OH-derived nitrocatechols ( vs. 12 µg m−3) at 295 K, potentially due to the difference in the composition of the bulk SOA. However, there is no obvious diurnal trend in the Fp values of nitrocatechols in our study.
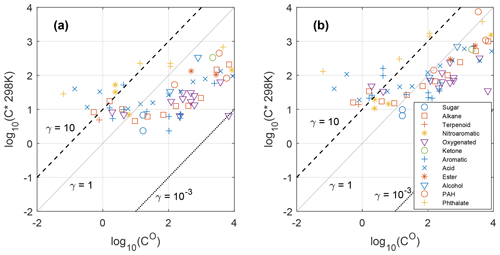
Figure 2The median effective saturation concentration C∗ (converted to 298 K) of each compound derived from Eq. (2) during the October 2017 northern California wildfires vs. the estimated saturation concentration over pure compound CO at 298 K on a logarithmic scale. Lines show the theoretical C∗ as a function of CO. (a) Weak BBOA scenario. (b) Strong BBOA scenario. C∗ and CO are in micrograms per cubic meter.
Table 1Mean and standard deviation of concentrations of levoglucosan, PM1, and levels of temperature and RH during different levels of BBOA influence in Berkeley during the 2017 northern California wildfires study.
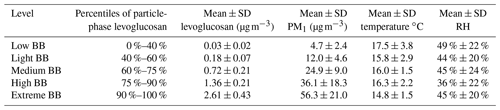
In many studies, such as Isaacman-VanWertz et al. (2016) and Nie et al. (2022), when predicting the gas–particle partitioning of organic compounds using Eq. (2), C∗ is assumed to be the same as CO(γ=1). To test how well the equilibrium absorptive partitioning model with the γ=1 assumption explains the partitioning behavior of individual compounds, we calculated the median C∗ of each compound from measured Fp values using Eq. (2), converted them to 298 K values (see details about the conversion in the Supplement), and plotted them against the CO over pure compound estimated by group contribution models (Fig. 2). Only compounds with CO between 10−2 and 104 µg m−3 were considered here because the partitioning method may not work for very volatile or nonvolatile compounds (Stark et al., 2017). It is worth noting that the results in Fig. 2 are not very sensitive to the assumed fraction of OA in PM1 because C∗ is proportional to COA. A 10 % change in COA will therefore only change log 10(C∗) by ∼0.04. As has been shown in our previous work, in October 2017, the level of organic carbon (OC) increased by ∼6 times during the wildfires, and the temporal variation in the levoglucosan correlated well with OC and thus BBOA (Liang et al., 2021). We therefore classified the data points into five concentration bins, based on the levels of particle-phase levoglucosan concentrations, which is a proxy for the BBOA influence (Table 1). The range of γ inferred from this study is similar to what Cappa et al. (2008) found for dicarboxylic acids in multicomponent mixtures. The γ inferred for adipic acid (∼0.3) is also within the range of γ inferred for adipic acid in adipic acid–ambient extract mixtures (Saleh and Khlystov, 2009). Under both strong BBOA (high to extreme BBOA in Table 1) and weak BBOA (low to light BBOA in Table 1) scenarios, most of the data points fall between the lines of γ=1 and . The result suggests these compounds have higher Fp values than what is predicted by the models (if γ=1 is assumed). A few exceptions include diethylhexyl phthalate (DEHP), dibutyl phthalate, diisobutyl phthalate, and homosalate, which were found to be more volatile than expected. The low Fp value of DEHP is likely related to DEHP in coarse particles (Ma et al., 2014), which is not measured in this study. However, this reason cannot fully explain the order of magnitudes deviation of measured C∗ from prediction. Many oxygenated compounds with at least two −OH groups were found to be BB SOA in these fire plumes in our previous study (Liang et al., 2021). In contrast to the GoAmazon wet season study in which they found that the Fp values of these compounds do not correlate with the vapor pressure (Isaacman-VanWertz et al., 2016), we saw that Fp and C∗ values of these compounds qualitatively follow the equilibrium absorptive partitioning models (Figs. 1 and 2), though the dependence on CO is not as strong as alkanes and carboxylic acids (Fig. S3). This is likely related to high concentration of organic aerosol (mainly from biomass burning; average estimated OA=20.1 µg m−3), the dominance of organic aerosol in biomass burning emissions (Lim et al., 2019; Fine et al., 2004), and relatively low average RH (47 %) in this study compared with the GoAmazon study (wet season average OA=1.2 µg m−3 with various sources; RH=90 %; De Sá et al., 2018; Isaacman-VanWertz et al., 2016). SOA formation catalyzed by inorganic compounds such as sulfate and water, which was observed in the GoAmazon study (Yee et al., 2020), was probably less important during the wildfire events in this study.
Comparing Fig. 2a and b, we found that the gas–particle partitioning behaviors of compounds are better described by the model in the strong BB case, especially for compounds with log 10CO between 1 and 3 (data points are closer to the γ=1 line; also shown in Fig. S7), which is probably due to the dominance of OA in the strong BB case. It is also worth noting that the deviation from the γ=1 line can be partly attributed to the inaccuracy in CO estimated by the group contribution models. When this area is not affected by wildfire smoke, inorganic aerosol accounts for a larger fraction of total aerosol mass (Shah et al., 2018). Inorganic compounds, liquid water in aerosol, and black carbon can therefore have stronger effects on the partitioning of the SVOCs in the low BB case. Also, the equilibration timescale of SVOCs between the gas and particle phases increases with decreasing mass of PM (Shiraiwa and Seinfeld, 2012). That can also limit the capability of the equilibrium absorption model in predicting the partitioning of many BB SVOCs under relatively clean scenarios. In addition, although log 10C∗ has a roughly linear relationship with log 10CO, the slope is only 0.35, even under the strong BB scenario. This nonideal behavior is in agreement with the trend observed in multicomponent temperature-programmed desorption experiments in which researchers found that larger dicarboxylic acids have higher γ than smaller ones (Cappa et al., 2008). Together, these results suggest that the inclusion of activity coefficients is needed to better predict the partitioning behaviors of individual organic compounds.
3.2 Temporal variations of Fp values and factors influencing Fp values of individual compounds
To explore the temporal variations in the individual compounds, we first plotted the correlation matrix of the Fp time series. The Fp values of lighter alkanes (C13–C20) correlate well with each other, while semivolatile alkanes (C22–C26) form a separate group (Fig. S8). This is probably because the lighter alkanes are so volatile that they do not respond to the levels of ambient aerosol like the semivolatile compounds do. As shown in Fig. 3, the Fp values of most PAHs have an anticorrelation with the Fp values of oxygenated compounds. Similar trends are observed in the FIREX-AQ 2018 study (Fig. S9), in which the partitioning behaviors of oxygenated compounds were not well-correlated with compounds without −OH groups. This is likely related to the varying ambient aerosol composition. Whether preexisting organic aerosol can alter the partitioning behavior of SVOCs depends on the aerosol composition because different components of organic aerosol are not always miscible (Ye et al., 2016; Mahrt et al., 2022a, b). Relatively nonpolar compounds in the ambient aerosol may change the OA matrix, thus enhancing the absorption of nonpolar into the particle phase, while they hardly enhance the condensation of polar compounds. In addition, the Fp values of methyl palmitate and methyl stearate correlated very well with each other, but their Fp values correlated poorly with the Fp values of other compounds (Fig. 3). Methyl palmitate and methyl stearate are emitted from cooking (Kristensen et al., 2019). Their Fp values were elevated (making their γ closer to 1) in the evening, and during breakfast or lunch hours (Fig. S10), which suggests the cooking aerosol enhanced the absorption of fatty acid esters into the particle phase.
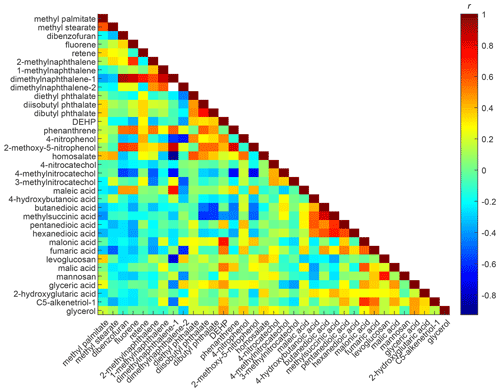
Figure 3Correlation matrix of Fp time series for selected compounds observed by SV-TAG in Berkeley, California, between 10 and 19 October 2017. Compounds are ordered by the number of −OH and −COOH groups. The white pixel indicates that the Fp values of those two compounds were both available in fewer than three samples.
We further explored the importance of environmental factors on the Fp values of each compound using a random forest model implemented in MATLAB R2022a. Details of the model can be found in the Supplement. The random forest algorithm is an ensemble approach that makes a prediction of the response (Fp in this study), with predictors (measured variables such as temperature) using the aggregation of multiple decision trees. In the 2017 northern California fires, constrained by the availability of data, we only used time of day, RH, temperature, PM1 and particle-phase levoglucosan concentration as predictors, and the Fp value of the compounds as the response. Possibly due to the lack of predictors, we were only able to achieve R2>0.45 for predicting the Fp value of out-of-bag data (data points not used in individual regression trees) of 4 compounds (fluorene, 4-nitrophenol, 1,2,4-benzenetriol, and pentanedioic acid) out of 89 compounds considered. The importance of predictors was estimated by permutation of out-of-bag predictor observations (Fig. S11). For fluorene, the levoglucosan concentration is the dominant predictor with a negative relationship. For other compounds, PM1 is the most important predictor (with a positive relationship). We also applied this model on the Fp value measured in the FIREX-AQ 2018 study in which more predictors are available. Out of 30 compounds considered, 5 of them were predicted with R2>0.45. As shown in Fig. 4, the level of OA is the dominant predictor of the Fp values for 2-methylglyceric acid and glyceric acid, while for the two C5 alkene triols, temperature and sulfate concentration become the most important factors. As temperature increases, their Fp value decreases ( between the Fp value of C5 alkene triol-1 and temperature and −0.67 for C5 alkene triol-2). The positive association of sulfate with the Fp value of C5 alkene triols and the positive association of the aerosol liquid water content (ALWC) with the Fp value of pinonaldehyde and C5 alkene triols are consistent with the catalytic roles of sulfate and water in the formation of these compounds (Yee et al., 2020; Jenkin et al., 2000). Nevertheless, the campaign-averaged particle-phase OA was 13 µg m−3, while the campaign-averaged ALWC was only 1.5 µg m−3, indicating that dissolution in aerosol liquid water was not the dominant gas–particle partitioning mechanism for most compounds considered in this study.
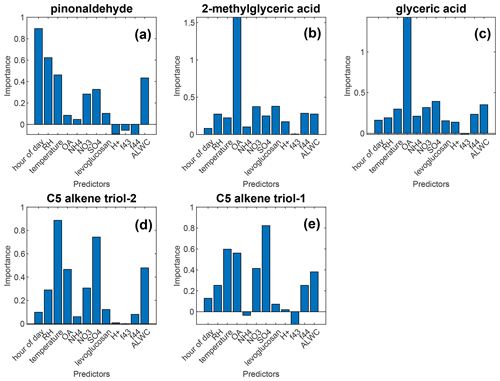
Figure 4The importance scores of factors on the Fp value of selected compounds, measured in the FIREX-AQ 2018 study. A high-importance score means that the factor is important. Importance scores close to zero and negative importance scores suggest low importance. Full descriptions of these predictors can be found in the Supplement.
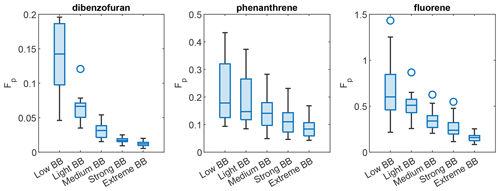
Figure 5Box plots showing the effect of BBOA on the particle-phase fraction on some nonpolar compounds measured in the 2017 northern California wildfire study. Each box plot shows the interquartile range, with whiskers extended to 1.5× the interquartile range. Central horizontal lines show the medians. Circles denote outliers.
3.3 Effect of BBOA on gas–particle partitioning of SVOCs
When BBOA dominated the ambient aerosol, the gas–particle partitioning of many compounds was different from clean periods. But such changes are not all in the same direction. Figure 5 shows the that Fp value of nonpolar compounds decreased with the level of wildfire smoke influence during the 2017 northern California wildfires. The Fp value of dimethylnapthalene (isomer 1) and 2-methylnaphthalene also decreases with increasing BBOA (p=0.021 and 0.026, respectively, for the linear fit between mean BBOA percentile in each bin and mean Fp value in each bin), although the changes are smaller. Conversely, levoglucosan, mannosan, 4-nitrocatechol, and hexanedioic acid (adipic acid), each with at least two −OH groups, did not follow this trend (Fig. S12). Environmental factors such as temperature and RH remained very similar at different BB influence levels in Berkeley (Table 1). They are therefore unlikely major causes for this trend. The high O:C ratios of the BBOA and the low O:C ratios of these nonpolar compounds, which makes them not miscible, is a more plausible cause of the decrease in their Fp value during the wildfires. We also compared the Fp value of these compounds in different ranges of PM concentrations, and a slightly weaker though still clear dependence of the Fp value on the PM concentration is observed for phenanthrene and fluorene (Fig. S13). The slightly weaker dependence of the Fp value on PM than on levoglucosan also suggests that BBOA is different from local PM and that it caused the decrease in the Fp value of these nonpolar compounds. Different effects of BBOA on gas–particle partitioning behaviors on relatively polar and relatively nonpolar compounds are also seen in the FIREX-AQ 2018 study. The median Fp value of the polar compounds increased almost monotonically from the low BB bin to the high BB bin, while the Fp value of the less polar compounds did not (Fig. S14). Nevertheless, we did not see the Fp value of these nonpolar compounds decrease monotonically when BB influence increased. It is probably because the aerosol in McCall, Idaho, did not have many contributions from urban sources such as traffic and cooking emissions, and the background aerosol (when BB influence was low) had similar compositions with the BBOA that impacted McCall. This is supported by the narrow distribution of f44 (the fraction of the OA mass spectrum signal at 44) measured by the ACSM, and therefore also the ratio (Aiken et al., 2008), and the lack of statistically significant difference of f44 under different BB influence levels (Fig. S15). Thus, the activity coefficients of these nonpolar compounds did not change as much as in the 2017 California study.
In the 2017 California study, from the low BB scenario to the high BB scenario, the level of PM1 and therefore COA increases by 10 times (COA might have increased by more than 10 times because a smaller fraction of local PM1 is OA). According to Eq. (2), to make the Fp value smaller, γ must increase by more than 10 times. We simulated the γ of these nonpolar compounds using the AIOMFAC model (in the Supplement). The model successfully predicted that γ of these compound increases was the fraction of the BBOA in the total ambient aerosol increases. However, the increase was predicted to be approximately a factor of 2 only (Fig. S16). It is worth noting that the simulations were based on the assumed composition of aerosol because many properties of the aerosol (e.g., bulk composition and liquid water content) were not measured in Berkeley, which introduced uncertainty to the simulations.
The high viscosity of BBOA may contribute to the γ of these compounds increasing with fraction of BB influence. Under the RH and temperature in Berkeley during these fires, the BBOA is expected to be semisolid (Li et al., 2020). This can affect the evaporation and condensation of the SVOCs (Zelenyuk et al., 2012). However, the ages of the plumes are at least 2 h (Liang et al., 2022b), which are long enough for these nonpolar compounds to reach gas–particle partitioning, even when the particles are mainly in a semisolid state (Shiraiwa and Seinfeld, 2012). If the effect of viscosity is important here, then the compounds mainly emitted in the particle phase from wildfires would have Fp values higher than what is predicted from the equilibrium absorptive model, while compounds later condensed into the particle phase (such as SVOCs mainly emitted in the urban area, including phthalates and fatty acid esters) would have Fp values lower than prediction when BBOA dominates. However, we did not find such a trend, which may suggest the effect of viscosity on Fp value is overshadowed by other factors.
The observed partitioning phenomenon of BBOA has at least two interesting implications. First, the high gas-phase fraction of the nonpolar compounds can reduce their atmospheric lifetimes. For instance, the gas-phase lifetimes of fluorene and phenanthrene are 21 and 9 h under a typical atmospheric environment ([OH]=106 molecules cm−3, [O3]=50 ppb), respectively (Keyte et al., 2013). However, these compounds have much longer lifetimes in the particle phase because OA can shield them against heterogeneous oxidation and evaporation (Zelenyuk et al., 2012). The low-particle-phase fraction of these compounds in the BB plumes can shorten their lifetimes (May et al., 2012), thus reducing their long-range transport. Nonetheless, the gas-phase PAHs can react with oxidants to form oxygenated and nitrated PAHs, which may have higher toxicity than the parent PAHs (Idowu et al., 2019). Also, the result implies that PAHs, which have urban sources, do not necessarily partition more to the condensed phase when the urban area is affected by wildfire smoke. The phase distribution of compounds directly affects where they will deposit in the respiratory tract, what kind of cells will be exposed to these compounds, and, consequently, the health impacts (Liu et al., 2017). However, the PAHs observed in this research are not as toxic as heavy PAHs such as benzopyrenes. Future work on the gas–particle partitioning behaviors of more toxic PAHs is still needed.
In this work, we evaluated the measured phase partitioning behaviors of various BB markers. Levoglucosan, mannosan, and nitrocatechols were found to stay mainly in the particle phase, even when the ambient OA was relatively low, which suggests that they are good marker compounds for source apportionment. The phase partitioning of SVOCs is observed to be strongly dependent on the saturation vapor pressure, yet despite whether BBOA is abundant, the equilibrium absorption model underpredicts the particle-phase fraction of the majority of the compounds measured. BBOA enhanced the condensation of polar compounds into the particle phase but not some nonpolar compounds, such as PAHs.
Although we were able to qualitatively find out the factors influencing the gas–particle partitioning of compounds, with the current dataset, even using machine learning algorithms, we were not able to accurately predict the partitioning behavior of the majority of compounds. Future longer-term measurements of gas-phase and particle-phase SVOCs simultaneously with measurements of environmental factors and bulk aerosol composition may help to further address this problem.
Data used in this research are available from the corresponding authors upon request.
The supplement related to this article is available online at: https://doi.org/10.5194/acp-23-12441-2023-supplement.
YL, RAW, NMK, and AHG designed the research. YL, RAW, KK, NMK, PLC, and SCH performed the research. YL, RAW, AWHC, NLN, and AHG analyzed the data. YL and AHG wrote the paper, with suggestions from all coauthors.
At least one of the (co-)authors is a member of the editorial board of Atmospheric Chemistry and Physics. The peer-review process was guided by an independent editor, and the authors also have no other competing interests to declare.
Publisher's note: Copernicus Publications remains neutral with regard to jurisdictional claims in published maps and institutional affiliations.
The authors acknowledge Connor Daube, Francesca Majluf, Joseph Roscioli, Edward Fortner, Christoph Dyroff, Tim Onasch, Jordan Krechmer, and Tara Yacovitch of Aerodyne Research, Inc., and Andrew Lindsay from Drexel University for the planning, operation, support, and analysis of Aerodyne-operated instruments in the field. The authors also thank David Lunderberg from UC Berkeley for helpful discussions and for locating and retrieving the SV-TAG data.
This research has been supported by the National Science Foundation, Directorate for Geosciences (grant no. 1810641) and the National Oceanic and Atmospheric Administration, Climate Program Office (grant nos. NA16OAR4310107, NA17OAR4310102, and NA16OAR4310104).
This paper was edited by Alexander Laskin and reviewed by two anonymous referees.
Aiken, A. C., Decarlo, P. F., Kroll, J. H., Worsnop, D. R., Huffman, J. A., Docherty, K. S., Ulbrich, I. M., Mohr, C., Kimmel, J. R., Sueper, D., Sun, Y., Zhang, Q., Trimborn, A., Northway, M., Ziemann, P. J., Canagaratna, M. R., Onasch, T. B., Alfarra, M. R., Prevot, A. S. H., Dommen, J., Duplissy, J., Metzger, A., Baltensperger, U., and Jimenez, J. L.: and ratios of primary, secondary, and ambient organic aerosols with high-resolution time-of-flight aerosol mass spectrometry, Environ. Sci. Technol., 42, 4478–4485, https://doi.org/10.1021/es703009q, 2008.
Asa-Awuku, A., Miracolo, M. A., Kroll, J. H., Robinson, A. L., and Donahue, N. M.: Mixing and phase partitioning of primary and secondary organic aerosols, Geophys. Res. Lett., 36, L15827, https://doi.org/10.1029/2009GL039301, 2009.
Bannan, T. J., Booth, A. M., Jones, B. T., O'Meara, S., Barley, M. H., Riipinen, I., Percival, C. J., and Topping, D.: Measured Saturation Vapor Pressures of Phenolic and Nitro-aromatic Compounds, Environ. Sci. Technol., 51, 3922–3928, https://doi.org/10.1021/acs.est.6b06364, 2017.
Barley, M. H. and McFiggans, G.: The critical assessment of vapour pressure estimation methods for use in modelling the formation of atmospheric organic aerosol, Atmos. Chem. Phys., 10, 749–767, https://doi.org/10.5194/acp-10-749-2010, 2010.
Bateman, A. P., Gong, Z., Harder, T. H., de Sá, S. S., Wang, B., Castillo, P., China, S., Liu, Y., O'Brien, R. E., Palm, B. B., Shiu, H.-W., Cirino, G. G., Thalman, R., Adachi, K., Alexander, M. L., Artaxo, P., Bertram, A. K., Buseck, P. R., Gilles, M. K., Jimenez, J. L., Laskin, A., Manzi, A. O., Sedlacek, A., Souza, R. A. F., Wang, J., Zaveri, R., and Martin, S. T.: Anthropogenic influences on the physical state of submicron particulate matter over a tropical forest, Atmos. Chem. Phys., 17, 1759–1773, https://doi.org/10.5194/acp-17-1759-2017, 2017.
Cappa, C. D., Lovejoy, E. R., and Ravishankara, A. R.: Evidence for liquid-like and nonideal behavior of a mixture of organic aerosol components, P. Natl. Acad. Sci. USA, 105, 18687–18691, https://doi.org/10.1073/pnas.0802144105, 2008.
Compernolle, S., Ceulemans, K., and Müller, J.-F.: EVAPORATION: a new vapour pressure estimation method for organic molecules including non-additivity and intramolecular interactions, Atmos. Chem. Phys., 11, 9431–9450, https://doi.org/10.5194/acp-11-9431-2011, 2011.
Dang, C., Bannan, T., Shelley, P., Priestley, M., Worrall, S. D., Waters, J., Coe, H., Percival, C. J., and Topping, D.: The effect of structure and isomerism on the vapor pressures of organic molecules and its potential atmospheric relevance, Aerosol Sci. Tech., 53, 1040–1055, https://doi.org/10.1080/02786826.2019.1628177, 2019.
Dennison, P. E., Brewer, S. C., Arnold, J. D., and Moritz, M. A.: Large wildfire trends in the western United States, 1984–2011, Geophys. Res. Lett., 41, 2928–2933, https://doi.org/10.1002/2014GL059576, 2014.
de Sá, S. S., Palm, B. B., Campuzano-Jost, P., Day, D. A., Hu, W., Isaacman-VanWertz, G., Yee, L. D., Brito, J., Carbone, S., Ribeiro, I. O., Cirino, G. G., Liu, Y., Thalman, R., Sedlacek, A., Funk, A., Schumacher, C., Shilling, J. E., Schneider, J., Artaxo, P., Goldstein, A. H., Souza, R. A. F., Wang, J., McKinney, K. A., Barbosa, H., Alexander, M. L., Jimenez, J. L., and Martin, S. T.: Urban influence on the concentration and composition of submicron particulate matter in central Amazonia, Atmos. Chem. Phys., 18, 12185–12206, https://doi.org/10.5194/acp-18-12185-2018, 2018.
Donahue, N. M., Robinson, A. L., Stanier, C. O., and Pandis, S. N.: Coupled partitioning, dilution, and chemical aging of semivolatile organics, Environ. Sci. Technol., 40, 2635–2643, https://doi.org/10.1021/es052297c, 2006.
Donahue, N. M., Epstein, S. A., Pandis, S. N., and Robinson, A. L.: A two-dimensional volatility basis set: 1. organic-aerosol mixing thermodynamics, Atmos. Chem. Phys., 11, 3303–3318, https://doi.org/10.5194/acp-11-3303-2011, 2011.
Donahue, N. M., Kroll, J. H., Pandis, S. N., and Robinson, A. L.: A two-dimensional volatility basis set – Part 2: Diagnostics of organic-aerosol evolution, Atmos. Chem. Phys., 12, 615–634, https://doi.org/10.5194/acp-12-615-2012, 2012.
Eliasl, V. O., Simoneit, B. R. T., Cordeiro, R. C., and Turcq, B.: Evaluating levoglucosan as an indicator of biomass burning in Carajás, amazônia: A comparison to the charcoal record, Geochim. Cosmochim. Ac., 65, 267–272, https://doi.org/10.1016/S0016-7037(00)00522-6, 2001.
Fine, P. M., Cass, G. R., and Simoneit, B. R. T.: Chemical characterization of fine particle emissions from the wood stove combustion of prevalent united states tree species, Environ. Eng. Sci., 21, 705–721, https://doi.org/10.1089/ees.2004.21.705, 2004.
Finewax, Z., De Gouw, J. A., and Ziemann, P. J.: Identification and Quantification of 4-Nitrocatechol Formed from OH and NO3 Radical-Initiated Reactions of Catechol in Air in the Presence of NOx: Implications for Secondary Organic Aerosol Formation from Biomass Burning, Environ. Sci. Technol., 52, 1981–1989, https://doi.org/10.1021/acs.est.7b05864, 2018.
Fredrickson, C. D., Palm, B. B., Lee, B. H., Zhang, X., Orlando, J. J., Tyndall, G. S., Garofalo, L. A., Pothier, M. A., Farmer, D. K., Decker, Z. C. J., Robinson, M. A., Brown, S. S., Murphy, S. M., Shen, Y., Sullivan, A. P., Schobesberger, S., and Thornton, J. A.: Formation and Evolution of Catechol-Derived SOA Mass, Composition, Volatility, and Light Absorption, ACS Earth Sp. Chem., 6, 1067–1079, https://doi.org/10.1021/acsearthspacechem.2c00007, 2022.
Gentner, D. R., Isaacman, G., Worton, D. R., Chan, A. W. H., Dallmann, T. R., Davis, L., Liu, S., Day, D. A., Russell, L. M., Wilson, K. R., Weber, R., Guha, A., Harley, R. A., and Goldstein, A. H.: Elucidating secondary organic aerosol from diesel and gasoline vehicles through detailed characterization of organic carbon emissions, P. Natl. Acad. Sci. USA, 109, 18318–18323, https://doi.org/10.1073/pnas.1212272109, 2012.
Hatch, L. E., Luo, W., Pankow, J. F., Yokelson, R. J., Stockwell, C. E., and Barsanti, K. C.: Identification and quantification of gaseous organic compounds emitted from biomass burning using two-dimensional gas chromatography–time-of-flight mass spectrometry, Atmos. Chem. Phys., 15, 1865–1899, https://doi.org/10.5194/acp-15-1865-2015, 2015.
Hennigan, C. J., Sullivan, A. P., Collett, J. L., and Robinson, A. L.: Levoglucosan stability in biomass burning particles exposed to hydroxyl radicals, Geophys. Res. Lett., 37, L09806, https://doi.org/10.1029/2010GL043088, 2010.
Hodshire, A. L., Akherati, A., Alvarado, M. J., Brown-Steiner, B., Jathar, S. H., Jimenez, J. L., Kreidenweis, S. M., Lonsdale, C. R., Onasch, T. B., Ortega, A. M., and Pierce, J. R.: Aging Effects on Biomass Burning Aerosol Mass and Composition: A Critical Review of Field and Laboratory Studies, Environ. Sci. Technol., 53, 10007–10022, https://doi.org/10.1021/acs.est.9b02588, 2019.
Idowu, O., Semple, K. T., Ramadass, K., O'Connor, W., Hansbro, P., and Thavamani, P.: Beyond the obvious: Environmental health implications of polar polycyclic aromatic hydrocarbons, Environ. Int., 123, 543–557, https://doi.org/10.1016/J.ENVINT.2018.12.051, 2019.
Iglesias, V., Balch, J. K., and Travis, W. R.: U. S. fires became larger, more frequent, and more widespread in the 2000s, Sci. Adv., 8, eabc0020, https://doi.org/10.1126/sciadv.abc0020, 2022.
Isaacman, G., Kreisberg, N. M., Yee, L. D., Worton, D. R., Chan, A. W. H., Moss, J. A., Hering, S. V., and Goldstein, A. H.: Online derivatization for hourly measurements of gas- and particle-phase semi-volatile oxygenated organic compounds by thermal desorption aerosol gas chromatography (SV-TAG), Atmos. Meas. Tech., 7, 4417–4429, https://doi.org/10.5194/amt-7-4417-2014, 2014.
Isaacman-VanWertz, G., Yee, L. D., Kreisberg, N. M., Wernis, R., Moss, J. A., Hering, S. V, De Sá, S. S., Martin, S. T., Alexander, M. L., Palm, B. B., Hu, W., Campuzano-Jost, P., Day, D. A., Jimenez, J. L., Riva, M., Surratt, J. D., Viegas, J., Manzi, A., Edgerton, E., Baumann, K., Souza, R., Artaxo, P., and Goldstein, A. H.: Ambient Gas-Particle Partitioning of Tracers for Biogenic Oxidation, Environ. Sci. Technol., 50, 9952–9962, https://doi.org/10.1021/acs.est.6b01674, 2016.
Jen, C. N., Hatch, L. E., Selimovic, V., Yokelson, R. J., Weber, R., Fernandez, A. E., Kreisberg, N. M., Barsanti, K. C., and Goldstein, A. H.: Speciated and total emission factors of particulate organics from burning western US wildland fuels and their dependence on combustion efficiency, Atmos. Chem. Phys., 19, 1013–1026, https://doi.org/10.5194/acp-19-1013-2019, 2019.
Jenkin, M. E., Shallcross, D. E., and Harvey, J. N.: Development and application of a possible mechanism for the generation of cis-pinic acid from the ozonolysis of α- and β-pinene, Atmos. Environ., 34, 2837–2850, https://doi.org/10.1016/S1352-2310(00)00087-X, 2000.
Jimenez, J. L., Canagaratna, M. R., Donahue, N. M., Prevot, A. S. H., Zhang, Q., Kroll, J. H., DeCarlo, P. F., Allan, J. D., Coe, H., Ng, N. L., Aiken, A. C., Docherty, K. S., Ulbrich, I. M., Grieshop, A. P., Robinson, A. L., Duplissy, J., Smith, J. D., Wilson, K. R., Lanz, V. A., Hueglin, C., Sun, Y. L., Tian, J., Laaksonen, A., Raatikainen, T., Rautiainen, J., Vaattovaara, P., Ehn, M., Kulmala, M., Tomlinson, J. M., Collins, D. R., Cubison, M. J., Dunlea, J., Huffman, J. A., Onasch, T. B., Alfarra, M. R., Williams, P. I., Bower, K., Kondo, Y., Schneider, J., Drewnick, F., Borrmann, S., Weimer, S., Demerjian, K., Salcedo, D., Cottrell, L., Griffin, R., Takami, A., Miyoshi, T., Hatakeyama, S., Shimono, A., Sun, J. Y., Zhang, Y. M., Dzepina, K., Kimmel, J. R., Sueper, D., Jayne, J. T., Herndon, S. C., Trimborn, A. M., Williams, L. R., Wood, E. C., Middlebrook, A. M., Kolb, C. E., Baltensperger, U., and Worsnop, D. R.: Evolution of Organic Aerosols in the Atmosphere, Science, 326, 1525–1529, https://doi.org/10.1126/science.1180353, 2009.
Keyte, I. J., Harrison, R. M., and Lammel, G.: Chemical reactivity and long-range transport potential of polycyclic aromatic hydrocarbons – a review, Chem. Soc. Rev., 42, 9333–9391, https://doi.org/10.1039/c3cs60147a, 2013.
Kim, Y. H., Warren, S. H., Krantz, Q. T., King, C., Jaskot, R., Preston, W. T., George, B. J., Hays, M. D., Landis, M. S., Higuchi, M., Demarini, D. M., and Gilmour, M. I.: Mutagenicity and lung toxicity of smoldering vs. Flaming emissions from various biomass fuels: Implications for health effects from wildland fires, Environ. Health Persp., 126, 017011, https://doi.org/10.1289/EHP2200, 2018.
Kreisberg, N. M., Worton, D. R., Zhao, Y., Isaacman, G., Goldstein, A. H., and Hering, S. V.: Development of an automated high-temperature valveless injection system for online gas chromatography, Atmos. Meas. Tech., 7, 4431–4444, https://doi.org/10.5194/amt-7-4431-2014, 2014.
Kristensen, K., Lunderberg, D. M., Liu, Y., Misztal, P. K., Tian, Y., Arata, C., Nazaroff, W. W., and Goldstein, A. H.: Sources and dynamics of semivolatile organic compounds in a single-family residence in northern California, Indoor Air, 29, 645–655, https://doi.org/10.1111/ina.12561, 2019.
Li, Y., Day, D. A., Stark, H., Jimenez, J. L., and Shiraiwa, M.: Predictions of the glass transition temperature and viscosity of organic aerosols from volatility distributions, Atmos. Chem. Phys., 20, 8103–8122, https://doi.org/10.5194/acp-20-8103-2020, 2020.
Liang, Y., Jen, C. N., Weber, R. J., Misztal, P. K., and Goldstein, A. H.: Chemical composition of PM2.5 in October 2017 Northern California wildfire plumes, Atmos. Chem. Phys., 21, 5719–5737, https://doi.org/10.5194/acp-21-5719-2021, 2021.
Liang, Y., Stamatis, C., Fortner, E. C., Wernis, R. A., Van Rooy, P., Majluf, F., Yacovitch, T. I., Daube, C., Herndon, S. C., Kreisberg, N. M., Barsanti, K. C., and Goldstein, A. H.: Emissions of organic compounds from western US wildfires and their near-fire transformations, Atmos. Chem. Phys., 22, 9877–9893, https://doi.org/10.5194/acp-22-9877-2022, 2022a.
Liang, Y., Weber, R. J., Misztal, P. K., Jen, C. N., and Goldstein, A. H.: Aging of Volatile Organic Compounds in October 2017 Northern California Wildfire Plumes, Environ. Sci. Technol., 56, 1557–1567, https://doi.org/10.1021/acs.est.1c05684, 2022b.
Lim, C. Y., Hagan, D. H., Coggon, M. M., Koss, A. R., Sekimoto, K., de Gouw, J., Warneke, C., Cappa, C. D., and Kroll, J. H.: Secondary organic aerosol formation from the laboratory oxidation of biomass burning emissions, Atmos. Chem. Phys., 19, 12797–12809, https://doi.org/10.5194/acp-19-12797-2019, 2019.
Lindsay, A. J., Anderson, D. C., Wernis, R. A., Liang, Y., Goldstein, A. H., Herndon, S. C., Roscioli, J. R., Dyroff, C., Fortner, E. C., Croteau, P. L., Majluf, F., Krechmer, J. E., Yacovitch, T. I., Knighton, W. B., and Wood, E. C.: Ground-based investigation of HOx and ozone chemistry in biomass burning plumes in rural Idaho, Atmos. Chem. Phys., 22, 4909–4928, https://doi.org/10.5194/acp-22-4909-2022, 2022.
Liu, C., Zhang, Y., and Weschler, C. J.: Exposure to SVOCs from Inhaled Particles: Impact of Desorption, Environ. Sci. Technol., 51, 6220–6228, https://doi.org/10.1021/acs.est.6b05864, 2017.
Liu, X., Day, D. A., Krechmer, J. E., Ziemann, P. J., and Jimenez, J. L.: Determining Activity Coefficients of SOA from Isothermal Evaporation in a Laboratory Chamber, Environ. Sci. Tech. Let., 8, 212–217, https://doi.org/10.1021/acs.estlett.0c00888, 2021.
Ma, J., Chen, L., Guo, Y., Wu, Q., Yang, M., Wu, M., and Kannan, K.: Phthalate diesters in Airborne PM2.5 and PM10 in a suburban area of Shanghai: Seasonal distribution and risk assessment, Sci. Total Environ., 497–498, 467–474, https://doi.org/10.1016/j.scitotenv.2014.08.012, 2014.
Mahrt, F., Huang, Y., Zaks, J., Devi, A., Peng, L., Ohno, P. E., Qin, Y. M., Martin, S. T., Ammann, M., and Bertram, A. K.: Phase Behavior of Internal Mixtures of Hydrocarbon-like Primary Organic Aerosol and Secondary Aerosol Based on Their Differences in Oxygen-to-Carbon Ratios, Environ. Sci. Technol., 56, 3960–3973, https://doi.org/10.1021/acs.est.1c07691, 2022a.
Mahrt, F., Peng, L., Zaks, J., Huang, Y., Ohno, P. E., Smith, N. R., Gregson, F. K. A., Qin, Y., Faiola, C. L., Martin, S. T., Nizkorodov, S. A., Ammann, M., and Bertram, A. K.: Not all types of secondary organic aerosol mix: two phases observed when mixing different secondary organic aerosol types, Atmos. Chem. Phys., 22, 13783–13796, https://doi.org/10.5194/acp-22-13783-2022, 2022b.
May, A. A., Saleh, R., Hennigan, C. J., Donahue, N. M., and Robinson, A. L.: Volatility of organic molecular markers used for source apportionment analysis: Measurements and implications for atmospheric lifetime, Environ. Sci. Technol., 46, 12435–12444, https://doi.org/10.1021/es302276t, 2012.
May, A. A., Levin, E. J. T., Hennigan, C. J., Riipinen, I., Lee, T., Collett, J. L., Jimenez, J. L., Kreidenweis, S. M., and Robinson, A. L.: Gas-particle partitioning of primary organic aerosol emissions: 3. Biomass burning, J. Geophys. Res.-Atmos., 118, 11327–11338, https://doi.org/10.1002/jgrd.50828, 2013.
McClure, C. D. and Jaffe, D. A.: US particulate matter air quality improves except in wildfire-prone areas, P. Natl. Acad. Sci. USA, 115, 7901–7906, https://doi.org/10.1073/pnas.1804353115, 2018.
Ng, N. L., Herndon, S. C., Trimborn, A., Canagaratna, M. R., Croteau, P. L., Onasch, T. B., Sueper, D., Worsnop, D. R., Zhang, Q., Sun, Y. L., and Jayne, J. T.: An Aerosol Chemical Speciation Monitor (ACSM) for Routine Monitoring of the Composition and Mass Concentrations of Ambient Aerosol, Aerosol Sci. Tech., 45, 780–794, https://doi.org/10.1080/02786826.2011.560211, 2011.
Nie, W., Yan, C., Huang, D. D., Wang, Z., Liu, Y., Qiao, X., Guo, Y., Tian, L., Zheng, P., Xu, Z., Li, Y., Xu, Z., Qi, X., Sun, P., Wang, J., Zheng, F., Li, X., Yin, R., Dallenbach, K. R., Bianchi, F., Petäjä, T., Zhang, Y., Wang, M., Schervish, M., Wang, S., Qiao, L., Wang, Q., Zhou, M., Wang, H., Yu, C., Yao, D., Guo, H., Ye, P., Lee, S., Li, Y. J., Liu, Y., Chi, X., Kerminen, V. M., Ehn, M., Donahue, N. M., Wang, T., Huang, C., Kulmala, M., Worsnop, D., Jiang, J., and Ding, A.: Secondary organic aerosol formed by condensing anthropogenic vapours over China's megacities, Nat. Geosci., 15, 255–261, https://doi.org/10.1038/s41561-022-00922-5, 2022.
O'Meara, S., Booth, A. M., Barley, M. H., Topping, D., and McFiggans, G.: An assessment of vapour pressure estimation methods, Phys. Chem. Chem. Phys., 16, 19453–19469, https://doi.org/10.1039/c4cp00857j, 2014.
Palm, B. B., Peng, Q., Fredrickson, C. D., Lee, B. H., Garofalo, L. A., Pothier, M. A., Kreidenweis, S. M., Farmer, D. K., Pokhrel, R. P., Shen, Y., Murphy, S. M., Permar, W., Hu, L., Campos, T. L., Hall, S. R., Ullmann, K., Zhang, X., Flocke, F., Fischer, E. V., and Thornton, J. A.: Quantification of organic aerosol and brown carbon evolution in fresh wildfire plumes, P. Natl. Acad. Sci. USA, 117, 29469–29477, https://doi.org/10.1073/pnas.2012218117, 2020.
Pankow, J. F.: An absorption model of gas/particle partitioning of organic compounds in the atmosphere, Atmos. Environ., 28, 185–188, https://doi.org/10.1016/1352-2310(94)90093-0, 1994.
Pankow, J. F.: A consideration of the role of gas/particle partitioning in the deposition of nicotine and other tobacco smoke compounds in the respiratory tract, Chem. Res. Toxicol., 14, 1465–1481, https://doi.org/10.1021/tx0100901, 2001.
Pankow, J. F. and Asher, W. E.: SIMPOL.1: a simple group contribution method for predicting vapor pressures and enthalpies of vaporization of multifunctional organic compounds, Atmos. Chem. Phys., 8, 2773–2796, https://doi.org/10.5194/acp-8-2773-2008, 2008.
Pokhrel, R. P., Gordon, J., Fiddler, M. N., and Bililign, S.: Impact of combustion conditions on physical and morphological properties of biomass burning aerosol, Aerosol Sci. Tech., 55, 80–91, https://doi.org/10.1080/02786826.2020.1822512, 2021.
Saleh, R. and Khlystov, A.: Determination of activity coefficients of semi-volatile organic aerosols using the integrated volume method, Aerosol Sci. Tech., 43, 838–846, https://doi.org/10.1080/02786820902959474, 2009.
Shah, R. U., Robinson, E. S., Gu, P., Robinson, A. L., Apte, J. S., and Presto, A. A.: High-spatial-resolution mapping and source apportionment of aerosol composition in Oakland, California, using mobile aerosol mass spectrometry, Atmos. Chem. Phys., 18, 16325–16344, https://doi.org/10.5194/acp-18-16325-2018, 2018.
Shiraiwa, M. and Seinfeld, J. H.: Equilibration timescale of atmospheric secondary organic aerosol partitioning, Geophys. Res. Lett., 39, L24801, https://doi.org/10.1029/2012GL054008, 2012.
Simoneit, B. R. T.: Biomass burning – A review of organic tracers for smoke from incomplete combustion, Appl. Geochem., 17, 129–162, https://doi.org/10.1016/S0883-2927(01)00061-0, 2002.
Stark, H., Yatavelli, R. L. N., Thompson, S. L., Kang, H., Krechmer, J. E., Kimmel, J. R., Palm, B. B., Hu, W., Hayes, P. L., Day, D. A., Campuzano-Jost, P., Canagaratna, M. R., Jayne, J. T., Worsnop, D. R., and Jimenez, J. L.: Impact of Thermal Decomposition on Thermal Desorption Instruments: Advantage of Thermogram Analysis for Quantifying Volatility Distributions of Organic Species, Environ. Sci. Technol., 51, 8491–8500, https://doi.org/10.1021/acs.est.7b00160, 2017.
Tuet, W. Y., Chen, Y., Fok, S., Champion, J. A., and Ng, N. L.: Inflammatory responses to secondary organic aerosols (SOA) generated from biogenic and anthropogenic precursors, Atmos. Chem. Phys., 17, 11423–11440, https://doi.org/10.5194/acp-17-11423-2017, 2017.
Tuet, W. Y., Liu, F., De Oliveira Alves, N., Fok, S., Artaxo, P., Vasconcellos, P., Champion, J. A., and Ng, N. L.: Chemical Oxidative Potential and Cellular Oxidative Stress from Open Biomass Burning Aerosol, Environ. Sci. Tech. Let., 6, 126–132, https://doi.org/10.1021/acs.estlett.9b00060, 2019.
US EPA: Estimation Programs Interface SuiteTM for Microsoft® Windows, https://www.epa.gov/tsca-screening-tools/epi-suitetm-estimation-program-interface (last access: 28 September 2023), 2012.
Wania, F., Awonaike, B., and Goss, K. U.: Comment on “measured Saturation Vapor Pressures of Phenolic and Nitro-Aromatic Compounds,” Environ. Sci. Technol., 51, 7742–7743, https://doi.org/10.1021/acs.est.7b02079, 2017.
Wernis, R. A., Kreisberg, N. M., Weber, R. J., Liang, Y., Jayne, J., Hering, S., and Goldstein, A. H.: Development of an in situ dual-channel thermal desorption gas chromatography instrument for consistent quantification of volatile, intermediate-volatility and semivolatile organic compounds, Atmos. Meas. Tech., 14, 6533–6550, https://doi.org/10.5194/amt-14-6533-2021, 2021.
Wong, J. P. S., Tsagkaraki, M., Tsiodra, I., Mihalopoulos, N., Violaki, K., Kanakidou, M., Sciare, J., Nenes, A., and Weber, R. J.: Effects of Atmospheric Processing on the Oxidative Potential of Biomass Burning Organic Aerosols, Environ. Sci. Technol., 53, 6747–6756, https://doi.org/10.1021/acs.est.9b01034, 2019.
Xie, M., Hannigan, M. P., and Barsanti, K. C.: Gas/Particle Partitioning of 2-Methyltetrols and Levoglucosan at an Urban Site in Denver, Environ. Sci. Technol., 48, 2835–2842, https://doi.org/10.1021/es405356n, 2014.
Yacovitch, T. I., Onasch, T. B., Croteau, P. L., Lindsay, A. J., Wood, E. C., and Cross, E. S.: Aerodyne ARI-GROUND Data, https://doi.org/10.26023/TTD7-CXHA-DS0E, 2022.
Ye, J., Gordon, C. A., and Chan, A. W. H.: Enhancement in Secondary Organic Aerosol Formation in the Presence of Preexisting Organic Particle, Environ. Sci. Technol., 50, 3572–3579, https://doi.org/10.1021/acs.est.5b05512, 2016.
Ye, J., Van Rooy, P., Adam, C. H., Jeong, C. H., Urch, B., Cocker, D. R., Evans, G. J., and Chan, A. W. H.: Predicting Secondary Organic Aerosol Enhancement in the Presence of Atmospherically Relevant Organic Particles, ACS Earth Space Chem., 2, 1035–1046, https://doi.org/10.1021/acsearthspacechem.8b00093, 2018.
Yee, L. D., Isaacman-VanWertz, G., Wernis, R. A., Kreisberg, N. M., Glasius, M., Riva, M., Surratt, J. D., de Sá, S. S., Martin, S. T., Alexander, M. L., Palm, B. B., Hu, W., Campuzano-Jost, P., Day, D. A., Jimenez, J. L., Liu, Y., Misztal, P. K., Artaxo, P., Viegas, J., Manzi, A., de Souza, R. A. F., Edgerton, E. S., Baumann, K., and Goldstein, A. H.: Natural and Anthropogenically Influenced Isoprene Oxidation in Southeastern United States and Central Amazon, Environ. Sci. Technol., 54, 5980–5991, https://doi.org/10.1021/acs.est.0c00805, 2020.
Zelenyuk, A., Imre, D., Beránek, J., Abramson, E., Wilson, J., and Shrivastava, M.: Synergy between secondary organic aerosols and long-range transport of polycyclic aromatic hydrocarbons, Environ. Sci. Technol., 46, 12459–12466, https://doi.org/10.1021/es302743z, 2012.
Zhao, Y., Kreisberg, N. M., Worton, D. R., Isaacman, G., Weber, R. J., Liu, S., Day, D. A., Russell, L. M., Markovic, M. Z., Vandenboer, T. C., Murphy, J. G., Hering, S. V, and Goldstein, A. H.: Insights into secondary organic aerosol formation mechanisms from measured gas/particle partitioning of specific organic tracer compounds, Environ. Sci. Technol., 47, 3781–3787, https://doi.org/10.1021/es304587x, 2013a.
Zhao, Y., Kreisberg, N. M., Worton, D. R., Teng, A. P., Hering, S. V., and Goldstein, A. H.: Development of an in situ thermal desorption gas chromatography instrument for quantifying atmospheric semi-volatile organic compounds, Aerosol Sci. Tech., 47, 258–266, https://doi.org/10.1080/02786826.2012.747673, 2013b.
Zhou, S., Collier, S., Jaffe, D. A., Briggs, N. L., Hee, J., Sedlacek III, A. J., Kleinman, L., Onasch, T. B., and Zhang, Q.: Regional influence of wildfires on aerosol chemistry in the western US and insights into atmospheric aging of biomass burning organic aerosol, Atmos. Chem. Phys., 17, 2477–2493, https://doi.org/10.5194/acp-17-2477-2017, 2017.
Zuend, A., Marcolli, C., Booth, A. M., Lienhard, D. M., Soonsin, V., Krieger, U. K., Topping, D. O., McFiggans, G., Peter, T., and Seinfeld, J. H.: New and extended parameterization of the thermodynamic model AIOMFAC: calculation of activity coefficients for organic-inorganic mixtures containing carboxyl, hydroxyl, carbonyl, ether, ester, alkenyl, alkyl, and aromatic functional groups, Atmos. Chem. Phys., 11, 9155–9206, https://doi.org/10.5194/acp-11-9155-2011, 2011.