the Creative Commons Attribution 4.0 License.
the Creative Commons Attribution 4.0 License.
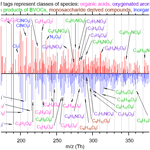
Chemical characterization of oxygenated organic compounds in the gas phase and particle phase using iodide CIMS with FIGAERO in urban air
Chenshuo Ye
Yi Lin
Zelong Wang
Weiwei Hu
Tiange Li
Wei Chen
Caihong Wu
Chaomin Wang
Shan Huang
Jipeng Qi
Baolin Wang
Chen Wang
Wei Song
Xinming Wang
E Zheng
Jordan E. Krechmer
Penglin Ye
Zhanyi Zhang
Xuemei Wang
Douglas R. Worsnop
Min Shao
The atmospheric processes under polluted environments involving interactions of anthropogenic pollutants and natural emissions lead to the formation of various and complex secondary products. Therefore, the characterization of oxygenated organic compounds in urban areas remains a pivotal issue in our understanding of the evolution of organic carbon. Here, we describe measurements of an iodide chemical ionization time-of-flight mass spectrometer installed with a Filter Inlet for Gases and AEROsols (FIGAERO-I-CIMS) in both the gas phase and the particle phase at an urban site in Guangzhou, a typical megacity in southern China, during the autumn of 2018. Abundant oxygenated organic compounds containing two to five oxygen atoms were observed, including organic acids, multi-functional organic compounds typically emitted from biomass burning, oxidation products of biogenic hydrocarbons and aromatics. Photochemistry played dominant roles in the formation of gaseous organic acids and isoprene-derived organic nitrates, while nighttime chemistry contributed significantly to the formation of monoterpene-derived organic nitrates and inorganics. Nitrogen-containing organic compounds occupied a significant fraction of the total signal in both the gas and particle phases, with elevated fractions at higher molecular weights. Measurements of organic compounds in the particle phase by FIGAERO-I-CIMS explained 24 ± 0.8 % of the total organic aerosol mass measured by aerosol mass spectrometer (AMS), and the fraction increased for more aged organic aerosol. The systematical interpretation of mass spectra of the FIGAERO-I-CIMS in the urban area of Guangzhou provides a holistic view of numerous oxygenated organic compounds in the urban atmosphere, which can serve as a reference for the future field measurements by FIGAERO-I-CIMS in polluted urban regions.
- Article
(1237 KB) - Full-text XML
-
Supplement
(1864 KB) - BibTeX
- EndNote
In urban air, atmospheric chemical processes are varied and complex, as the result of large emissions of both anthropogenic pollutants and biogenic volatile organic compounds, associated with strong interactions with each other (He et al., 2014; Karl et al., 2018; Shrivastava et al., 2019). Consequently, strong formation of secondary pollutants, e.g., ozone and secondary organic aerosol (SOA), are observed in urban and downwind regions (Huang et al., 2015; Zhang et al., 2014). Oxygenated organic compounds are not fully accounted for in some earlier studies, which may explain some of the discrepancies between observations and models for many unaddressed issues in atmospheric chemistry. Oxygenated organic compounds are supposed to be the top candidates for missing OH reactivity observed in various environments including pristine rainforests and urbanized areas (Noelscher et al., 2016; Yang et al., 2016, 2017). The photolysis of carbonyls serves as a critical radical source driving ozone formation in highly polluted industrialized areas (Edwards et al., 2014; Liu et al., 2012; Xue et al., 2016). Although it was discovered a long time ago that oxygenated organic compounds make up a substantial fraction of submicron aerosol mass (Kroll and Seinfeld, 2008), enormous difficulty still exists in accurately predicting the formation and evolution of SOA (de Gouw et al., 2005; Hodzic et al., 2010; Volkamer et al., 2006).
One of the biggest obstacles to understanding the role of oxygenated organic compounds is the characterization of these extremely complicated and diverse chemicals which encompass tens of thousands of individual species spanning a wide range of volatility. Chemical ionization mass spectrometry (CIMS) is a powerful technique for the molecular-level characterization of oxygenated organic compounds because of the following advantages (Zhao, 2018): direct measurements and fast time response to capture the rapid temporal change of short-lifetime intermediates, soft ionization providing chemical information on a molecular level, and selective ionization ensuring measurements for specific classes of species. The iodide anion ionizes species mainly through adduction (Iyer et al., 2016) and is used for the detection of oxygenated organic compounds, particularly organic compounds with two to five oxygen atoms (Lee et al., 2014; Lopez-Hilfiker et al., 2016; Riva et al., 2019). It has been shown that I-CIMS is an excellent technique to investigate the oxidation processes of volatile organic compounds (VOCs) and formation of SOA (Isaacman-VanWertz et al., 2018). Installed with a thermal desorption inlet that collects and heats aerosol to evaporate organic compounds, e.g., Filter Inlet for Gases and AEROsols (FIGAERO, Lopez-Hilfiker et al., 2014) and Micro-Orifice Volatilization Impactor (MOVI, Yatavelli et al., 2012), the CIMS instruments are capable of analyzing particle-phase species and gas-particle partitioning in a semi-continuous way (Stark et al., 2017; Stolzenburg et al., 2018).
Although FIGAERO-CIMS has gained recent popularity in atmospheric chemistry research, much of the published work was done in chambers or in the laboratory (D'Ambro et al., 2017, 2018; Hammes et al., 2019; Lopez-Hilfiker et al., 2015). As for the applications in field campaigns, most work has been mostly performed in forest or rural areas (Huang et al., 2019; Hunter et al., 2017; Lee et al., 2016, 2018b); measurements in the urban atmosphere by FIGAERO-CIMS are still limited (Le Breton et al., 2018b). Meanwhile, a systematic analysis on mass spectra of FIGAERO-CIMS in the ambient air is imperative, for a more holistic view in investigating emissions and chemistry of oxygenated organic compounds. In this study, we present the measurement results using FIGAERO-I-CIMS during a coordinated campaign in Guangzhou, a megacity in the Pearl River region of China. We describe the experimental design, instrumentation setup, calibration and data processing for this instrument in the campaign. This work will provide a detailed interpretation of the mass spectra of oxygenated species in both the gas phase and the particle phase. The bulk chemical properties will also be discussed to provide an overview of organic compounds.
2.1 Measurement site and supporting data
Measurements were conducted during the coordinated campaign “Particles, Radicals and Intermediates from oxiDation of primary Emissions over the Great Bay Area” (PRIDE-GBA) in October and November 2018. The Great Bay Area (GBA) refers to a highly industrialized and urbanized area in southern China, including the two special administrative regions of Hong Kong and Macau, and nine cities surrounding the Pearl River estuary. Affected by the subtropical monsoon climate, the weather in the region was characterized by high temperatures and relative humidity (RH) as well as sufficient sunshine (total solar radiation of the Pearl River Delta region in the fall of 2016 was ∼1200 MJ/m2, Liu et al., 2018). The city of Guangzhou lies in the north of the GBA and south of the mountains. Therefore, the city is extensively influenced by both anthropogenic and biogenic emissions. The urban site was located at Guangzhou Institute of Geochemistry, Chinese Academy of Sciences (23.14∘ N, 113.36∘ E). Online instruments sampled from inlets set up in laboratories on the eighth floor or ninth floor (about 25 m above the ground).
In addition to FIGAERO-I-CIMS discussed later, measurement data from a suite of other instruments were also used in this work. A high-resolution time-of-flight aerosol mass spectrometer (HR-ToF-AMS, Aerodyne Research, Inc.) was deployed to provide chemical composition and many other parameters of ambient aerosol including f60, liquid water content (LWC), particulate organic nitrate and elemental ratios (Hu et al., 2016, 2018). The parameter f60 is the ratio of the integrated signal at 60 to the total signal of organic components and is used as a tracer for biomass burning emissions (Cubison et al., 2011). LWC of aerosol was taken as the sum of water contributed by inorganic components predicted by the ISORROPIA II model and organic components calculated based on the organic hygroscopicity parameter (Fountoukis and Nenes, 2007; Guo et al., 2015). Based on AMS data, organic nitrate concentrations were determined by 2–3 times lower NO NO+ ratios for organic nitrate than inorganic nitrate (Fry et al., 2013). The calculation method of elemental ratios based on AMS data has been described elsewhere (Aiken et al., 2007; Canagaratna et al., 2015). Detailed information about AMS measurements from the PRIDE-GBA campaign is forthcoming in a separate paper. An online GC-MS/FID (Wuhan Tianhong Instrument Co., Ltd) and a proton transfer reaction time-of-flight mass spectrometer (PTR-ToF-MS, IONICON Analytik GmbH) (Yuan et al., 2017) served as the analytical techniques for measuring isoprene and other VOCs (e.g., monoterpenes, aromatics and a few oxygenated VOCs) (Wu et al., 2020), respectively. Trace gases (CO, O3, NO and NO2) were measured by commercial gas monitors (Thermo Fisher Scientific Inc.) (Z. Wang et al., 2020). Photolysis rates were measured by a PFS-100 photolysis spectrometer (Focused Photonics Inc.). Temperature and RH were measured by a Vantage Pro2 weather station (Davis Instruments Corp.). Time series and diurnal profiles of meteorological parameters, trace gases, the photolysis rate of NO2 () and several important VOCs (isoprene, monoterpenes, toluene and benzene) are shown in Fig. S1. The temperature during the campaign was between 17 and 33 ∘C, with an average of 24 ∘C, and RH was between 27 % and 97 %, with an average of 70 %.
2.2 FIGAERO-I-CIMS
2.2.1 Experimental setup
Our instrument consists of a Filter Inlet for Gases and AEROsols (FIGAERO) and a time-of-flight chemical ionization mass spectrometer coupled with an iodide ionization source (Bertram et al., 2011; Lee et al., 2014; Lopez-Hilfiker et al., 2014). The FIGAERO is a multi-port inlet assembly following a two-step procedure alternating between gas mode, in which online measurements of gases and semi-continuous sampling of particle-phase species are conducted, and particle mode, in which particulate composition is investigated via thermal desorption (Lopez-Hilfiker et al., 2014; Thornton et al., 2020). The iodide source is a “soft” ionization technique with little ionization-induced fragmentation and selective detection towards multi-functional organic compounds, providing elemental compositions for thousands of oxygenated compounds in the atmosphere (Hyttinen et al., 2018; Iyer et al., 2016; Lee et al., 2014; Riva et al., 2019).
The sample air was drawn into the ion molecule reaction (IMR) chamber where it intersected and reacted with primary ions generated by flowing 2 mL/min 1000 ppm methyl iodide in 2.4 L/min N2 through an X-ray source. The pressure in the IMR chamber was maintained at 370–390 mbar. Equipped with a long time-of-flight mass analyzer, our instrument was configured to measure singularly charged ions up to 603 Th with a mass resolving power of 10 000–11 000 (m/Δm at 50 % height) during the campaign (Fig. S2).
Ambient air was continuously sampled through two inlets protruding about 1.5 m out of a window on the ninth floor of a building. One was a 3 m PFA tubing ( in. o.d.) for gas-phase sampling, through which roughly 9 L/min air was drawn, and 2 L/min was directly taken into the instrument for gas measurements without removing particles, resulting in an inlet residence time of 0.24 s. The gas sampling line inside the room was covered by heat insulation associated with a heating cable to minimize condensation on the tubing surface. The other inlet for the particle phase was a 3.8 m metal tubing ( in. o.d.) fitted with a PM2.5 cyclone and a Nafion dryer (Perma Pure, model PD-07018T-12MSS) to reduce water content in the sampled air. The particle-phase inlet was drawn by a laminar flow at ∼ 8 L/min (Reynolds number of ∼ 1500), 3.8 L/min of which was collected on PTFE membrane filters (Zefluor®, Pall Inc., USA). The residence time was 1.3 s for the particle-phase sampling line. Semi-volatility and low-volatility compounds tend to interact with wall surfaces of both inlets and the IMR and thus extend response time (Krechmer et al., 2016). As accurate correction for wall losses remains impossible, no wall loss correction was performed in this study.
The FIGAERO worked in a cyclical 1 h pattern with two modes (Fig. S3): (i) measuring gas for the first 24 min while simultaneously collecting particles on the filter and (ii) then analyzing the particle-phase collection for another 36 min. In every 24 min gas mode, ambient air was measured for the first 21 min, followed by 3 min gas background by overflowing zero air at 5 L/min through a pinhole just in front of the IMR. The background measurements are inevitably influenced by wall interactions, especially for “sticky” species. Recently, Palm et al. (2019) proposed a new way to determine gas background (“fast background”) by fast switching between the ambient air and background, which greatly improves accurate determination of the CIMS background. In the remaining 36 min, the components of the collected particles were thermally desorbed and introduced into the CIMS with 2 L/min N2 carrier gas. The N2 flow was ramped from ambient temperature to 175 ∘C in 12 min and held for another 20 min. A schematic diagram of working modes and temperature profile of FIGAERO heating in a single cycle are shown in Fig. S4. Particle background was determined every sixth 1 h running cycle in which ambient air passed over a filter (Parker Balston, model 9922-11-CQ) in front of the FIGAERO filter.
2.2.2 Calibration experiments
Using various techniques, we calibrated dozens of chemical compounds in the laboratory. Table S1 summarizes the calibrated species and corresponding calibration methods. (1) Gas cylinders are commercially available for a few species (e.g., chlorine, hydrogen cyanide). The gaseous standards were diluted down to different concentrations and then introduced to the CIMS. (2) For those VOCs whose standards are liquid or solid, solutions with known concentrations are made and then vaporized using the liquid calibration unit (LCU, IONICON Analytik GmbH) to provide gaseous standards. (3) Commercial permeation tubes are available for some species (e.g., nitric acid). (4) Some gaseous chemicals were generated in the laboratory. For example, isocyanic acid was generated from thermal decomposition of cyanuric acid in a diffusion cell (Li et al., 2021; Z. Wang et al., 2020), and dinitrogen pentoxide was generated via the reaction of ozone with excess nitrogen dioxide in a flow reactor (Bertram et al., 2009). (5) Compounds of low vapor pressure were calibrated through the FIGAERO (Lopez-Hilfiker et al., 2014). Briefly, certain amounts of target species dissolved in organic solvents (e.g., isopropanol or acetone) were deposited onto the PTFE filter of the FIGAERO using a syringe, and the droplet was then subjected to a temperature-programmed thermal desorption by N2 gas. The sensitivity was determined as the integrated signals under thermogram profiles versus the amounts of deposited calibrant.
In addition to sensitivity calibration, the effect of humidity on the sensitivity for various species was investigated in the laboratory, some of which are shown in Fig. S5. Low-molecular-weight acids, e.g., formic acid and nitric acid, tend to be more sensitive to the humidity changes than multi-functional compounds. A similar tendency of multi-functional compounds associated with less humidity dependence was also reported in previous work (Lee et al., 2014). Considering water vapor pressure in the IMR, our humidity-dependent curves are generally consistent with those reported in Lee et al. (2014) (see detailed discussions in Sect. S3 in the Supplement).
In the later part of the campaign (after 22 October), an isotopically labeled formic acid (DCOOH, Cambridge Isotope Laboratories, Inc.) permeation tube held at constant temperature (65∘) was mixed with 10 mL/min N2 and continuously delivered into the entrance of the sampling inlet in order to derive a humidity dependence function from the field measurements. DCOOH signals during the campaign exhibited a humidity-dependent curve consistent with formic acid obtained in the laboratory (Fig. S5). We applied humidity correction to the species with the humidity-dependent curves determined in the laboratory (underlined species in Table S1). For other compounds, humidity correction was not applied, as there is no universal pattern of humidity dependence for all detected species, and multi-functional compounds that comprise the majority of the species measured by FIGAERO-I-CIMS are usually less influenced by humidity.
The measured concentration of DCOOH was steady after humidity correction was applied (Fig. S6g), indicating the stability of our instrument. In addition, we also performed field calibrations throughout the campaign to check the instrument status by spotting a solution mixture of levoglucosan, heptaethylene glycol and octaethylene glycol onto the FIGAERO filter every 2–3 d (Fig. S6). Multiple-point calibrations for these organic species were performed in the beginning and the end of the campaign. The concentration of the solution used in the first two calibration experiments was too high, so we prepared a new solution for calibrations in November. The relative changes of the determined calibration factors in November were within 50 % for the calibrated species.
2.2.3 Data processing
The TofWare software (version 3.0.3; Tofwerk AG, Switzerland) was used to conduct the high-resolution peak fitting for the mass spectra data of ToF-CIMS, including mass calibration, instrumental parameter optimization (peak shape and peak width) and bunch fitting of high-resolution peaks (Stark et al., 2015). In this study, the signals of ions were normalized to the sum signals of I− and H2OI− at 106 cps. Hourly particle-phase data were obtained by integrating the signals of various ions during each FIGAERO desorption period. Background-corrected signals were obtained by subtracting linearly interpolated background signals from ambient signals (and integrated signals) for ions in the gas (and particle) phase.
In order to determine the sensitivities of uncalibrated species, a voltage scanning procedure was performed from time to time throughout the campaign covering different times of the day (Iyer et al., 2016; Lopez-Hilfiker et al., 2016). Here, we selected four representative periods including morning, afternoon, evening and night on polluted days. By performing sigmoidal fitting on the remaining signals as a function of voltages, a dV50 value of each ion from each period was determined, at which voltage half of one kind of ion dissociated (Lopez-Hilfiker et al., 2016). We observed a positive correlation between the sensitivities of the ions relative to maximum sensitivity and their average dV50 values (Fig. S7), consistent with previous studies (Isaacman-VanWertz et al., 2018; Lopez-Hilfiker et al., 2016). This relationship was used to calculate response factors for uncalibrated species, after taking into account the relative transmission efficiency for the ions (see Sect. S1 in the Supplement for detailed analysis).
3.1 Overview of detected species in the mass spectra
We identify 1334 ions adducted with iodide from the mass spectra, among which 427 are charged closed-shell organic compounds containing only C, H and O elements (CxHyOzI−) and 388 are charged closed-shell organic compounds containing C, H, O and N elements (CxHyN1,2OzI−). For species with the formula of CxHyOz, x ranges from 1 to 20, y is an even number and no more than 2x+2, and z is greater than or equal to 2. The range of carbon number x for the ions with CxHyN1,2Oz is the same as the ions with CxHyOz. For species containing one nitrogen (CxHyNOz), y is an odd number and less than 2x+2; z is larger than or equal to 2. For species containing two nitrogen atoms (CxHyN2Oz), y is an even number and less than 2x+1; z is larger than or equal to 4. Table 1 summarizes species discussed in the main text. Although iodide clusters with two nitrogen atoms and zero nitrogen atoms both lie on odd masses, they can be separated for certain ions with the current resolving power, as demonstrated by the peak fitting results of mass spectrum at 311 (Fig. S8).
The campaign-averaged mass spectra of detected ions in the both gas and particle phases are shown in Fig. 1. In general, molecules in the particle phase have larger molecular weights compared to those in the gas phase. Signals in the mass range of 150–300 Th comprise a large fraction of gas-phase compounds, and concentrations in the gas phase decrease quickly with higher than 250 Th. In contrast, the detected signals in the particle phase are mainly distributed within the range of 200–320 Th.
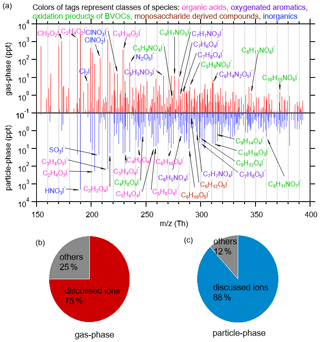
Figure 1(a) Mass spectra of the iodide charged ion within 150–400 Th in the gas phase (red) and the particle phase (blue), respectively. (b, c) The fractions of I-adduct ions discussed in the main text (Table 1) in the total ion signals for I-adduct ions measured in the gas phase (b) and the particle phase (c), respectively.
Average nighttime (22:00–06:00) and daytime (10:00–18:00) mixing ratios for various species were shown in Fig. 2. Most species have higher concentrations during the daytime, especially for relatively volatile compounds in the gas phase, despite the fact that lower boundary layer height at night should increase nighttime concentration, as many primary gases behaved, e.g., CO (Fig. S1) (Wu et al., 2020). The higher concentrations during the daytime for most species detected by FIGAERO-I-CIMS suggest the dominant role of photochemical-induced oxidation in forming these oxidized compounds. In addition to typical nocturnal species including nitryl chloride (ClNO2I−), chlorine nitrate (ClONO2I−) and dinitrogen pentoxide (N2O5I−), higher concentrations for the ions of C6H10O5I− and C6H12O5I− were also observed, which will be discussed in the next section. A large number of particulate N-containing organic compounds increase at night as well, as shown by mass defect diagrams of CxHyOz and CxHyN1,2Oz color-coded by the night-to-day ratios (Fig. S9).
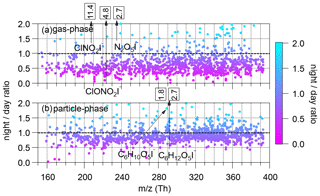
Figure 2The ratios of concentrations at night (22:00–06:00) to concentrations during the day (10:00–18:00) for ions ranging from 150 to 400 Th in the gas phase (a) and the particle phase (b). The range of the y axis is set between 0 and 2 for clarity, although the ratios of some compounds are larger than 2. The numbers in boxes indicate the night day ratios of tagged ions that exceed the y-axis ranges.
Based on the mass spectra shown in Fig. 1, we identify a number of ions associated with high concentrations in both the gas phase and the particle phase. In the following Sect. 3.2–3.7, we will perform interpretation of the mass spectra by analyzing variability and correlation of these important ions, including monosaccharide-derived compounds (with brown tags in Fig. 1), oxygenated aromatics (with purple tags), organic acids (with pink tags), oxidation products of biogenic volatile organic compounds (BVOCs, with green tags), sulfur-containing compounds and inorganics (with blue tags). After going through detailed analysis at the species level, Sect. 3.8 will provide an overall picture about bulk chemical characteristics of detected organic compounds in terms of the distributions of average carbon oxidation states, carbon number and oxygen number. Lastly, Sect. 3.9 will compare our measurement of organic aerosol (OA) with AMS data.
3.2 Monosaccharide-derived compounds
C6H10O5 and C6H12O5 are highly correlated with each other in aerosol (r= 0.92), and they are two of the few CxHyOz compounds with higher concentrations at night. Previous work attributed them to monosaccharide-derived compounds emitted from biomass burning (Bhattarai et al., 2019; Qi et al., 2019; Reyes-Villegas et al., 2018; Simoneit et al., 1999).
In this campaign, C6H10O5 was detected mostly in the particle phase (the fraction in the particle phase Fp= 0.81 ± 0.09) with an average concentration of 0.073 ± 0.076 µg/m3. Its diurnal profile started increasing at dusk, reaching a peak at about midnight, and then fell off, as shown in Fig. 3. The mass fraction of C6H10O5 in OA had a similar diurnal profile, and the ratios of C6H10O5 to CO increased at night (from 0.17 ± 0.02 to 0.5 ± 0.03 µg m−3/ppm, Fig. 3c), both suggesting enhanced emissions of this compound were related with combustion activities in the evening, e.g., residential biofuel burning for cooking as reported by some previous measurements in China (Q. Wang et al., 2020; Zhang et al., 2015). Furthermore, the time variations of particle-phase C6H10O5I− were very similar to those of the 60 fragment in AMS mass spectra (Fig. 3a), which is an identified tracer of biomass burning OA produced from the thermal decomposition of levoglucosan and similar compounds on the vaporizer of AMS (Brege et al., 2018; Cubison et al., 2011; Schneider et al., 2006). Therefore, C6H10O5 was probably levoglucosan and its isomers (mannosan and galactosan), and C6H12O5 was probably also a monosaccharide compound that had sources similar to C6H10O5.
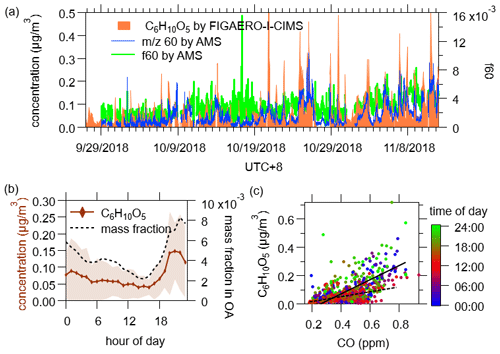
Figure 3(a) Time series of particulate C6H10O5 measured by FIGAERO-I-CIMS, 60 fragment and f60 measured by AMS. Background f60 = 0.3 % and background 60 = 0.3 % × OA were subtracted from f60 and 60 (Cubison et al., 2011; Hu et al., 2016). (b) Diurnal variations of particulate C6H10O5 and its mass fraction in OA. (c) Correlation between CO and particulate C6H10O5. The dash and solid lines indicate the ratios during daytime (10:00–18:00, 0.17 ± 0.02 µg m−3/ppm) and nighttime (22:00–06:00, 0.50 ± 0.03 µg m−3/ppm), respectively.
3.3 Oxygenated aromatic compounds
Combustion activities emit a great deal of compounds besides saccharides that the I-CIMS instrument can detect, including nitro-aromatics and guaiacol derivatives (Gaston et al., 2016; Kong et al., 2021). Nitro-benzenediols (C6H5NO4I−) as well as the highly correlated homologue methyl nitro-benzenediols (C7H7NO4I−) (r= 0.88 in the particle phase) exhibited double peaks in their diurnal profiles (Fig. 4). One was in the evening, similar to levoglucosan (C6H10O5). The other peak was at noon. The scatterplot of C6H5NO4 as the function of C6H10O5 exhibits two different slopes (Fig. 5): the lower slope at night (0.088 ± 0.005) indicates the contribution of biomass burning, while the higher slope during the daytime (0.26 ± 0.02) suggests there were other important sources for nitro-aromatics, potentially secondary formation from photooxidation of aromatics (Jenkin et al., 2003). Guaiacol derivatives may have similar sources with nitro-aromatics, as implied by the resemblance of the scatterplots of these two chemical classes versus levoglucosan (cf., Figs. S10 and 5).
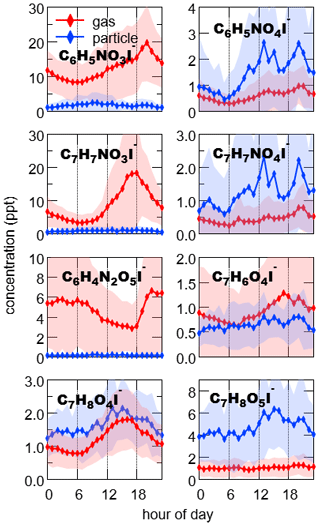
Figure 4Diurnal variations of oxidized aromatics including nitro-phenols (C6H5NO3I−), nitro-benzenediols (C6H5NO4I−), methyl nitro-phenols (C7H7NO3I−), methyl nitro-benzenediols (C7H7NO4I−), dinitro-phenols (C6H4N2O5I−), dihydroxy methyl benzoquinone (C7H6O4I−), tetrahydroxy toluene (C7H8O4I−), pentahydroxy toluene and fragments of C9 aromatics (C7H8O5I−). The shaded areas indicate 1 standard deviation.
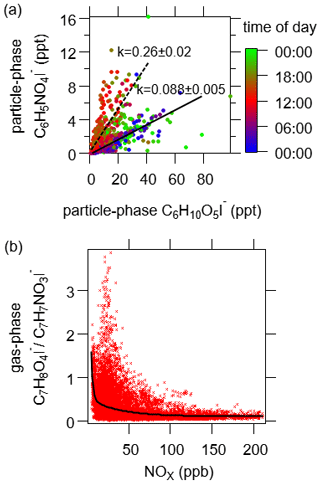
Figure 5(a) Correlation between particle-phase C6H5NO4I− and C6H10O5I−. The data points are color-coded using the time of the day. Solid and dash lines represent the slopes during the nighttime and daytime, respectively. (b) Relative concentration of C7H8O4I− and C7H7NO3I− in the gas phase as a function of NOx concentration. The black line is the fitted curve using a double exponential function.
Nitrophenols (C6H5NO3I−), methyl nitrophenols (C7H7NO3I−) and dinitrophenols (C6H4N2O5I−) were the most significant components of nitro-aromatics in the gas phase. Despite the fact that nitrated phenols could be formed by photochemical oxidation of their aromatic hydrocarbon precursors (Z. Wang et al., 2020; Yuan et al., 2016), none of them peaked in the daytime, consistent with a previous proposal on photolysis as the dominant loss pathway for these compounds (Chen et al., 2011; Yuan et al., 2016). C6H5NO3 and C7H7NO3 peaked in the evening, suggesting important contributions of NO3-induced reactions and/or primary emissions. The peak time of C6H4N2O5 was later than that of C6H5NO3, in agreement with dinitrophenols as the oxidation products from nitrophenols (Harrison et al., 2005).
We also detected non-N-containing compounds that were identified as oxidation products of aromatics in the literature, including C7H6O4I−, C7H8O4I− and C7H8O5I− (Mehra et al., 2020; Schwantes et al., 2017). C7H6O4 and C7H8O4 correlated well with each other (r= 0.72 in the gas phase and 0.91 in the particle phase). High concentrations of C7H6O4 and C7H8O4 were mainly observed during the periods with lower NOx concentration, which was a contrast to the variations of nitrophenols (Fig. S10). In addition, the concentration ratios of C7H8O4I− and C7H7NO3I− are lower for higher NOx concentration (Fig. 5), consistent with the literature stating that the formation of C7H6O4 and C7H8O4 is suppressed at high NOx concentration (Schwantes et al., 2017). C7H8O5 is reported as the ring-retaining oxidation product of C7H8O4, which is a typical oxidation product of toluene and cresol (Schwantes et al., 2017; M. Wang et al., 2020), as well as the ring-scission products of aromatic hydrocarbons with more carbon atoms, e.g., trimethyl benzenes (Mehra et al., 2020). Given that C7H8O5 closely followed with C7H8O4 (r= 0.93 in particles), toluene oxidation was probably the main contributor to this compound.
3.4 Organic acids and related compounds
Organic acids were one of the most abundant species classes detected by I-CIMS (Fig. 1). Low-molecular-weight organic acids (e.g., formic, acetic, glycolic and pyruvic acid) constituted a significant fraction of signals in the mass spectra of the gas phase. As shown in Fig. 6 (and also Fig. S11), they had very similar temporal trends with diurnal maxima in the afternoon, indicating photochemical oxidation played a dominant role in their formation (de Gouw et al., 2018; Yuan et al., 2015).
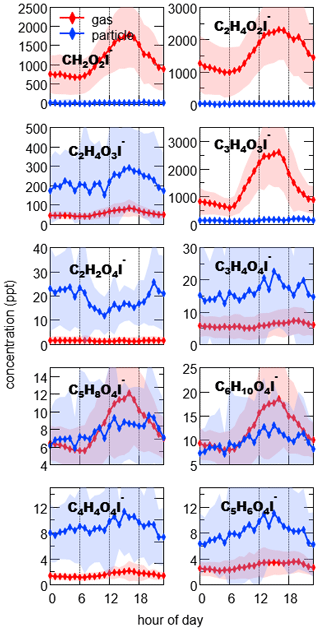
Figure 6Diurnal variations of organic acids in the gas phase (red) and particle phase (blue). The shaded area indicates 1 standard deviation.
In contrast to monocarboxylic acids, dicarboxylic acids partitioned mostly to the particle phase. As the dominant dicarboxylic acids in aerosol (Kawamura and Bikkina, 2016; Mellouki et al., 2015), 94 ± 5 % and 74 ± 13 % (mean ± 1 standard deviation of Fp) of C2H2O4 and C3H4O4, attributed to oxalic and malonic acid, were found in the particle phase, respectively. The concentrations of C4H6O4 were significantly lower compared to the C2 and C3 homologous series, but C5H8O4 and C6H10O4 had unexpected high abundance (Fig. 7). Additionally, C5H8O4 and C6H10O4 had considerable fractions in the gas phase (45 ± 13 % and 43± 11 %), which are significantly higher than their C2–C3 homologous series. These two compounds were correlated well with each other in temporal variations (r= 0.97 and 0.91 in the gas phase and the particle phase, respectively), and their diurnal variations were different from those of oxalic and malonic acid (Fig. 6). Therefore, dicarboxylic acids may not be the dominant contributing species for the two compounds. C5H8O4 and C6H10O4 have been observed from previous studies on isoprene oxidation (Berndt et al., 2018, 2019), attributing them to epoxy hydroperoxyl carbonyl and accretion product, respectively. However, the relative contributions from these possibilities remain unclear.
In addition to the series of CnH2n−2O4 (i.e., C2H2O4, C3H4O4), we also observed comparable concentrations of CnH2n−4O4 series, especially for carbon number of 4 and 5 (C4H4O4 and C5H6O4). Considering the double bonds in the molecules, CnH2n−4O4 should be more reactive than CnH2n−2O4, suggesting there were large sources for these compounds. Previous studies have reported photooxidation of aromatics can generate CnH2n−4O4, including C4H4O4 and C5H6O4 (Brege et al., 2018; Kawamura et al., 1996; Kawamura and Bikkina, 2016). Our measurements showed that temporal trends of C4H4O4 and C5H6O4 agreed well with those of aromatic hydrocarbons (Fig. S11b), and thus oxidation of aromatics could be an important contributor to CnH2n−4O4 in the urban air.
3.5 Oxidation products of biogenic VOCs
In addition to high anthropogenic emissions of aromatics, terrestrial vegetation nearby also released significant amounts of biogenic VOCs (BVOCs) (Wu et al., 2020). During the campaign, the concentrations of isoprene at noon were between 0.1 and 1.5 ppb, whereas the range of daily maxima of monoterpenes was 0.05–2.5 ppb. Hence, a number of oxidation products of BVOCs were detected (Figs. 8 and S12).
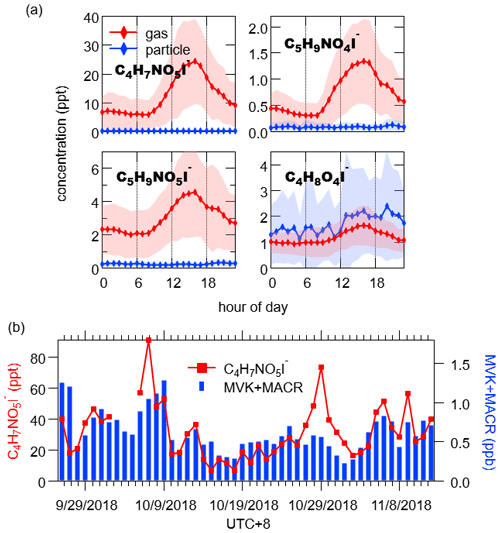
Figure 8(a) Diurnal variations of isoprene oxidation products in the gas phase (red) and particle phase (blue). The shaded area indicates 1 standard deviation. (b) Time series of daily maximum concentrations of gaseous C4H7NO5I− and MVK + MACR (C4H6OH+, 71.05) measured by PTR-ToF-MS.
The ion C4H7NO5I− was the most abundant N-containing C4 organic compounds that was detected in the gas phase. Its daily maxima occurred in the afternoon and correlated moderately with methyl vinyl ketone (MVK) + methacrolein (MACR) measured by PTR-ToF-MS (Fig. 8b, r= 0.58). We consequently attributed C4H7NO5 to MVK nitrates and MACR nitrates, which were reported as the second generation of organic nitrates formed from the oxidation of isoprene hydroxynitrates by OH in the presence of NOx (Fisher et al., 2016; Paulot et al., 2009). Strong correlations were observed between C5H9NO4I−, C5H9NO5I− and C4H7NO5I− (r= 0.93 and 0.80, respectively), which was in accordance with their similar formation pathways (Jacobs et al., 2014; Wennberg et al., 2018; Xiong et al., 2015). Hence, we expected these three compounds to be common oxidation products of isoprene in the polluted atmosphere. While in aerosol, 2-methylglyceric acid (C4H8O4) is a commonly reported oxidation product of isoprene formed in high-NOx conditions (Surratt et al., 2010). We observed the corresponding ion C4H8O4I− contributing to OA especially in dry conditions with strong sunlight (Fig. S13). This evidence indicates that isoprene oxidation may contribute to C4H8O4, but potential contribution from other sources cannot be ruled out in urban areas.
In terms of monoterpenes, a reasonable correlation (Fig. S14a, r= 0.63) was found between the ions C10H16O3I− and C10H16O2H+ measured by PTR-ToF-MS. C10H16O2H+ was attributed to pinonaldehyde formed from the oxidation of monoterpenes (Glasius et al., 2000; Larsen et al., 2001; Mutzel et al., 2016). Therefore, we tentatively attribute C10H16O3I− to pinonic acid and its oxocarboxylic acid isomers, which are formed via the oxidation of pinonaldehyde (Fang et al., 2017). C8H13NO6 also exhibited enhanced gas-phase formation during the day as pinonic acid did. The correlation coefficient of the two compounds was 0.71. In contrast to other monoterpene nitrates, particle-phase C8H11NO7 and C10H15NO6 peaked at night and decreased during the daytime (Fig. S12), indicative of the role of NO3 in producing organic nitrates as reported in the literature (Faxon et al., 2018). However, C10H15NO6 in the gas phase showed a distinct diurnal profile with peak before the noon. Two possible types of compounds were proposed for C10H15NO6 in previous studies: peroxyacetyl nitrate from pinonaldehyde (Faxon et al., 2018; Nah et al., 2016; Schwantes et al., 2020) or organic nitrates (Bean and Hildebrandt Ruiz, 2016; Boyd et al., 2015). Given the distinct diurnal profiles of C10H15NO6I− in the gas and particle phases and the fact that peroxyacetyl nitrate is supposed to dissociate during the FIGAERO heating (Slusher et al., 2004), we speculate that both compounds contributed to this ion. As shown in Fig. S15, C8H12O4 and C9H14O4 existed mostly in the particle phase (Fp= 0.63 ± 0.11 and 0.67 ± 0.10, respectively). We interpret them as products of monoterpenes via photochemical processes, consistent with the interpretations presented in previous work (Mohr et al., 2013; Mutzel et al., 2015).
3.6 S-containing compounds
Organosulfates are concerned as important components of SOA (Hallquist et al., 2009; Surratt et al., 2007), and they can be detected by iodide anion via proton abstraction (Le Breton et al., 2018b; Lee et al., 2014). We detected the ion C2H3SO with a diurnal peak in the afternoon (Fig. 9). This ion was attributed to glycolic acid sulfate, as suggested by previous work (Galloway et al., 2009; Liao et al., 2015).
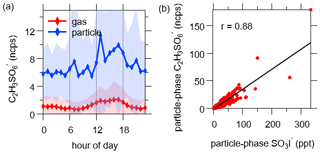
Figure 9(a) Diurnal variation of C2H3SO. The shaded areas indicate 1 standard deviation. (b) Correlation between particle-phase C2H3SO and SO3I−.
Abundant SO3I− was detected in particles, and it correlated well with the ion C2H3SO (Fig. 9b) and sulfates measured by AMS (Fig. S16). Previous work observed the sulfite ion radical (•SO) during the ionization of organosulfates in a liquid chromatography–electrospray ionization–tandem mass spectrometer (Huang et al., 2018). As a result, the ion SO3I− from FIGAERO-I-CIMS might be a potential indicator for the total organosulfates. However, more future work is needed for evaluating this possibility.
Other sulfate-related ions during gas-phase modes were also detected, including HSO (sulfuric acid) and CH3SO (methanesulfonic acid), which were enhanced in the gas phase during the daytime, in agreement with the notions of photochemistry-induced gas-phase oxidation (Brandt and van Eldik, 1995). However, these data were not available for quantification given that these low-volatility species would condense on our long gas sampling inlet. It should be noted that measuring sulfuric acid in the gas phase is difficult and generally requires a “wall-less” source design (Eisele and Tanner, 1993).
3.7 Inorganic compounds
There is a growing interest in N2O5 and its product nitryl chloride (ClNO2) because ClNO2 is found to serve as a nocturnal reservoir of Cl radical and reactive nitrogen, and hence enhance the ozone formation the next day (Osthoff et al., 2008; Wang et al., 2016). Time series of N2O5 and ClNO2 exhibited two patterns. During most of the nights, N2O5 started to increase quickly at sunset and lasted for only 2–3 h, and ClNO2 increased in the meantime and ultimately reached its maximum at night, indicative of local formation of ClNO2. However, sometimes a high level of N2O5 did not lead to an increase in ClNO2 (tinted background in Fig. 10a), probably due to the lack of chloride salts on the aerosol. Other nocturnal species including ClONO2 and Cl2 were highly correlated with ClNO2 as expected (r= 0.92 and 0.83, respectively), suggesting they had common formation mechanisms (Liu et al., 2017).
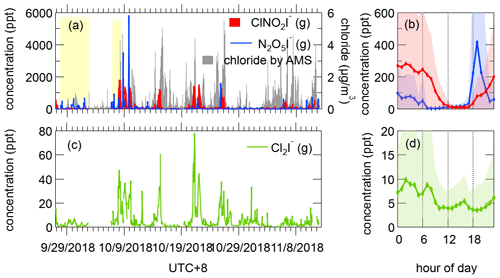
Figure 10Time series and diurnal variations of humidity-corrected concentrations of N2O5 and ClNO2 (a, b) and Cl2 (c, d). The tinted background indicates the days with high concentrations of N2O5 but low concentrations of ClNO2. The shaded areas indicate 1 standard deviation.
HNO3I− was observed as one of the most abundant species in the mass spectra of FIGAERO-I-CIMS both in the gas phase and the particle phase. In the gas phase, the ion HNO3I− from I-CIMS has been used to quantify nitric acid (Lee et al., 2018a). The concentrations of gas-phase nitric acid peaked in the afternoon, suggesting photochemistry in the daytime as the dominant source for gas-phase nitric acid.
Previous studies suggested that HNO3I− from particle-phase measurement by FIGAERO-I-CIMS can be indicative of nitrate in the particle phase (Lee et al., 2016). Here, the concentrations of HNO3I− in the particle phase were compared with particulate nitrate measured by AMS (Fig. 11c). A strong correlation was observed (r= 0.93), but the concentrations measured by FIGAERO-I-CIMS were higher (slope = 1.6), especially for higher concentrations of organic nitrates. Using a threshold of 1 µg/m3 for organic nitrates, the slopes and correlations were higher for the data points with particulate organic nitrates larger than 1 µg/m3 (slope = 1.8, r= 0.94) than those less than 1 µg/m3 (slope = 1.1, r= 0.90). In short, our measurements suggest that HNO3I− in the particle phase from FIGAERO-I-CIMS is formed from thermal decomposition of both inorganic nitrate (e.g., NH4NO3) and organic nitrates.
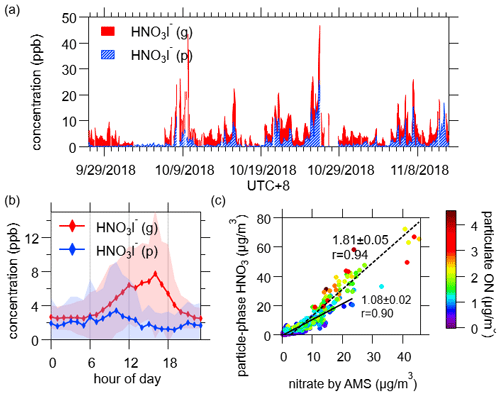
Figure 11(a) Time series of humidity-corrected HNO3I− in both phases. (b) Diurnal variation of humidity-corrected HNO3I−. The shaded areas indicate 1 standard deviation. (c) Comparison of particle-phase HNO3I− and nitrate measured by AMS. The color scale denotes particulate N-containing organic compounds measured by FIGAERO-I-CIMS (pON). The solid and dash lines show the fitted results for the dataset of pON less than 1 µg/m3 and more than 1 µg/m3, respectively. The concentration of gaseous HNO3I− shown here only included the last 5 min of every gas-phase working mode, as high level of HNO3 came out of aerosol, which then passed through the CIMS in a short time during particle analysis, and a substantial amount would subsequently accumulate on the inner surfaces, leading to a persistent carried over signal that was long enough to disturb the next gas measurement cycle (Palm et al., 2019).
3.8 Bulk chemical properties of detected organic compounds
The above discussions on individual chemical groups provide insights into the identification of the mass spectra from FIGAERO-I-CIMS, along with sources and chemistry of oxygenated organic compounds in the urban atmosphere. In this section and the following one, we will provide a bulk analysis of the detected organic compounds.
Organic compounds detected by FIGAERO-I-CIMS were comprehensively characterized with detailed elementary composition in space (Fig. 12), which depicts the average oxidation states of carbon for closed-shell CxHyOz and CxHyN1,2Oz compounds as a function of carbon number. The details in the calculation of can be found in Sect. S2 in the Supplement. S-containing compounds were omitted given their negligible variety and concentration compared to CxHyOz and CxHyN1,2Oz. The average in the particle phase was higher than that in the gas phase at the same carbon number, especially for carbon numbers between 2 and 10. This agrees with our expectation that more oxidized compounds would partition more strongly in aerosol, as indicated by larger fractions in particles (Fp) for higher . In addition, the average generally increased for lower carbon number, as a result of functionalization and fragmentation during VOCs aging. However, there was a notable exception in C5 which had a significantly reduced , probably as the result of emissions of isoprene. The analysis of the space indicates that the large number of organic compounds measured by FIGAERO-I-CIMS are useful to characterize the evolution of organic compounds in the atmosphere.
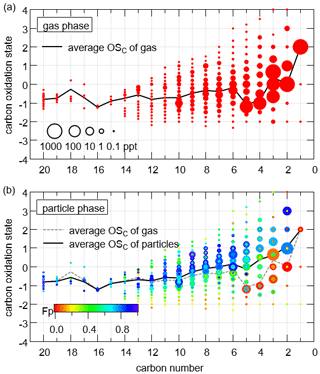
Figure 12 spaces for CxHyOz and CxHyN1,2Oz compounds in the gas phase (a) and the particle phase (b). The diameters of circles are proportional to the logarithmic average concentrations. The black lines are the average of each carbon number for compounds in the gas phase and the particle phase, respectively. The compounds in panel (b) are color-coded by their fractions in particles.
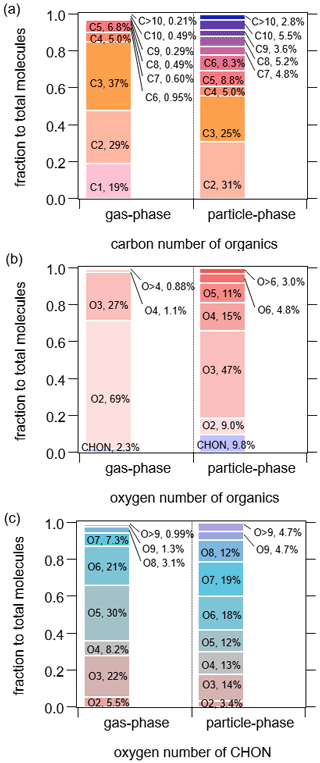
Figure 13Carbon number distribution (a) and oxygen number distribution of total CxHyOz and CxHyN1,2Oz compounds (b) and oxygen number distribution of CxHyN1,2Oz compounds (c).
The distributions of carbon and oxygen numbers of organic compounds were also investigated, as shown in Fig. 13. Most abundant organic compounds measured by FIGAERO-I-CIMS were C2–C3 compounds, which accounted for about 66 % in the gas phase and 56 % in the particle phase. It is unexpected that C2–C3 compounds made up such a significant portion in the particle phase, indicating a non-negligible role of thermal decomposition from low volatility compounds such as accretion products or extremely low volatility organic compounds which were reported from FIGAERO measurements on SOA (D'Ambro et al., 2018; Lopez-Hilfiker et al., 2014; Stark et al., 2017). Organic compounds with carbon numbers over five constituted only 3 % in the gas phase, while they accounted for 30 % in the particle phase. The oxygen numbers of the majority of gaseous organic compounds were no more than 3. Organic compounds containing two to three oxygen atoms had the largest contribution in both the gas phase (96 %) and the particle phase (56 %). CxHyN1,2Oz accounted for less than 10 % of the total oxygenated organic compounds. In the gas phase, compounds with five to six oxygen atoms accounted for 51 % of CxHyN1,2Oz, indicative of the high levels of organic nitrates in the urban atmosphere. Nitrophenols also contributed significantly to CxHyN1,2Oz compounds, as they accounted for 74 % of CxHyN1,2Oz containing three oxygen atoms, which in turn contributed to 22 % of CxHyN1,2Oz. In contrast, in the particle phase, the oxygen numbers of CxHyN1,2Oz distributed relatively evenly, as the fractions of compounds with three to eight oxygen atoms were similar (between 12 % and 19 %). Compared to measurements in a forest in the southeastern United States (cf., Table S1 from Lee et al., 2016), the fractions of N-containing organic compounds with less than five oxygen atoms were significantly larger in our measurements as a result of higher concentrations of nitro-aromatics.
We further determined the fractions of N-containing organic compounds in total organic compounds as a function of . It is clear that the observed fractions of N-containing organic compounds were higher for elevated (Fig. 14) and N-containing ions commonly dominated at even nominal masses (Fig. S17). The gas-phase CHON ions within the range of 250–350 Th accounted for about half of the organic compounds in this range. The fractions of CHON ions in the particle phase were somewhat smaller than those in the gas phase within the above range but were comparable for higher . A possible explanation for this is that functional groups of nitrate and nitro reduce less in vapor pressure for organic compounds than functional groups of carboxylic or oxygen-equivalent hydroxyl do (Capouet and Müller, 2006; Nannoolal et al., 2008; Pankow and Asher, 2008). Consequently, CHON compounds are generally more volatile than CHO compounds with similar molecular weights.
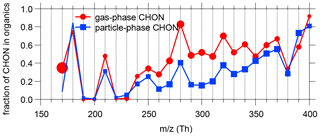
Figure 14The average fractions of CHON to total organic compounds (CHO + CHON + CHOS + CHONS) of every 10 Th in both phases. See Fig. S16 for the overall distribution of the contributions of species classes to the total concentrations. Marker sizes indicate the total concentration level in each bin. High ambient concentration of HNCO resulted in the large marker around 170 in the gas phase (Z. Wang et al., 2020).
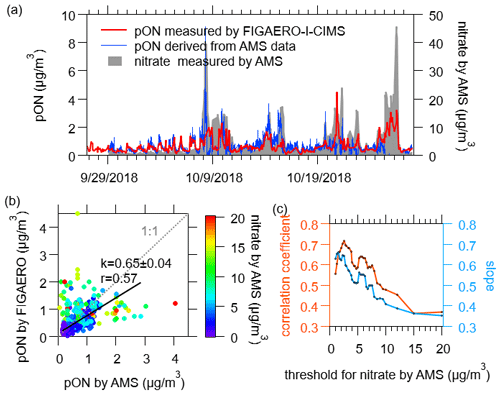
Figure 15(a) Time series of particulate N-containing organic compounds measured by FIGAERO-I-CIMS (pON by FIGAERO), particulate organic nitrates derived from AMS data (pON by AMS) as well as particulate inorganic nitrate. (b) Comparison of pON by FIGAERO and pON by AMS, color-coded by the concentrations of particulate inorganic nitrate measured by AMS. The black line presents the linear fit for nitrate by AMS below 8 µg/m3. (c) The determined slopes and correlation coefficients between pON by FIGAERO versus pON by AMS by filtering the data below different thresholds of particulate inorganic nitrate measured by AMS.
In the end, we determined the total concentration of N-containing organic compounds in the particle phase measured by FIGAERO-I-CIMS and compared it with the particulate organic nitrates derived from AMS (Fig. 15). Good agreement was achieved when the concentration of inorganic nitrate was relatively low, e.g., below 8 µg/m3. However, the discrepancies increased when inorganic nitrate concentration increased, which can affect the determination of organic nitrate from AMS. This encouraging result indicates that FIGAERO-I-CIMS is able to capture the variability of organic nitrates in the urban atmosphere, which can be helpful in understanding the sources and formation mechanism of these compounds.
3.9 Organic aerosol measurements
The total concentration of organic compounds in the particle phase measured by FIGAERO-I-CIMS was determined and compared with measurements of OA by AMS. The total organic compounds measured by FIGAERO-I-CIMS explained 24 ± 0.8 % (fitted slope ± 1 standard deviation) of the total OA on average (Fig. 16a), which is lower than the average fractions (∼ 50 %) reported previously in boreal and temperate forests (Lopez-Hilfiker et al., 2016; Stark et al., 2017). The lower fractions determined here might be the result of larger contributions to OA from primary emissions in the urban air, which are composed of a large number of compounds with little signals in I-CIMS (Zhao et al., 2016). As shown in Fig. 16a, organic compounds measured by FIGAERO-I-CIMS accounted for higher fractions in OA concentrations by AMS for more aged OA, consistent with the fact that I-CIMS is more sensitive to oxygenated organic compounds with multiple functional groups (Lee et al., 2014; Lopez-Hilfiker et al., 2016). Furthermore, we expect this fraction to change with the relative contributions of primary emissions and secondary formation for organic compounds in the atmosphere. Similar trends were found in Le Breton et al. (2019), in which an acetate source was used. Acetate ions have been reported to selectively ionize highly oxygenated organic compounds as an iodide source does (Aljawhary et al., 2013).
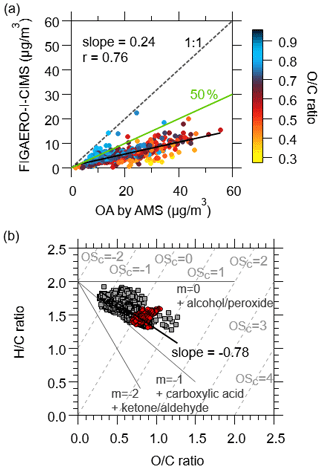
Figure 16(a) Comparison of particulate organic compounds measured by the FIGAERO-I-CIMS and AMS, color-coded by O C ratios measured by AMS. The black line is the slope which represents the fraction of OA explained by the measurements of FIGAERO-I-CIMS. The green line shows the results from previous work which were ∼ 50 % (Lopez-Hilfiker et al., 2016; Stark et al., 2017). (b) Van Krevelen diagrams for organic aerosol derived from AMS data (gray squares) and FIGAERO-I-CIMS data (red circles). The black line is the slope of AMS data. The gray dotted lines are estimated carbon oxidation state.
Comparison of the van Krevelen diagrams between FIGAERO-I-CIMS and AMS also provides useful insights on the measurement of organic compounds in OA. The van Krevelen diagram has been used as a tool for analyzing functional groups and OA aging by plotting H C ratios versus O C ratios (Heald et al., 2010; Lambe et al., 2012). As shown in Fig. 16b, the data points of the bulk OA from FIGAERO-I-CIMS followed the same trend as the data points from AMS. However, the bulk OA measured by FIGAERO-I-CIMS only occupied a much smaller region with O C ratios between 0.7 and 1.0. We further plotted all of the organic compounds in the H C versus O C space color-coded with their campaign-average concentrations (Fig. S18a). We observed that most data points from FIGAERO-I-CIMS distributed across the zone between the slope of 0 and −1.0. These observations provide additional evidence that FIGAERO-I-CIMS may only measure the more oxidized organic compounds in OA.
The correlation coefficients between the particle-phase concentrations at unit masses by FIGAERO-I-CIMS and OA mass concentration by AMS were calculated (Fig. S18b). The correlation coefficients were small for ions below 200, as these ions contributed little to organic aerosol. Moderate and strong correlations (r > 0.7) were observed for the ions of between 200 and 400 Th, implying that organic compounds with molecular weights of 100–300 g/mol may account for significant fractions in organic aerosol. The possible reason for the lower correlations for heavier compounds ( > 400 Th) with OA mass loadings is that these compounds might be related to specific sources or certain chemical processes, which might not contribute in large fractions to the total OA concentration.
We deployed a FIGAERO-I-CIMS instrument to measure oxygenated organic compounds in both the gas phase and the particle phase at a representative urban site in China. The experimental design and instrumentation setup were described in detail, which goes above and beyond typical studies, including (1) performing sensitivity calibrations in the laboratory using multiple methods for multiple species; (2) performing voltage scanning for unknown compounds detected in the ambient air; and (3) performing humidity calibrations for multiple types of species, which we have not seen anyone do after Lee et al. (2014).
From the mass spectra, a number of important compounds in the urban atmosphere were identified. We detected high concentrations of several monosaccharide species (e.g., levoglucosan). They were potentially emitted from biomass burning, which also contributed to the enhancement of many nitro-aromatic species. Photochemistry was also found to be a strong source of nitro-aromatics. Low-molecular-weight organic acids were mainly observed in the gas phase, and observations supported daytime photochemistry as the dominant source. Different diurnal profiles for various BVOC-derived organic nitrates were observed, reflecting their different formation pathways related to NOx chemistry (i.e., daytime photooxidation, nocturnal NO3 reactions). Local formation of nitryl chloride was observed, highlighting the potential importance of nighttime chemistry in the urban region. Our measurements show that oxygenated organic compounds dominated the majority of detected species by FIGAERO-I-CIMS, in which CHO and CHON compounds both accounted for significant fractions. Nitrogen-containing organic compounds occupied a significant fraction of the total signals in both the gas and particle phases, with elevated fractions at higher molecular weights. The most abundant organic compounds were formic acid and multifunctional organic compounds containing three to five oxygen atoms. Organic compounds containing two to three carbon atoms accounted for over half of the total organic compounds in both the gas phase and the particle phase measured by FIGAERO-I-CIMS. During the campaign, the FIGAERO-I-CIMS measurements explained 24 ± 0.8 % of OA mass measured by AMS, but the fractions were higher for more aged organic aerosol. This evidence, along with the analysis of the van Krevelen plot, indicates that FIGAERO-I-CIMS is measuring the more oxidized fraction of OA in the urban air.
Our observations suggest that oxygenated organic compounds in urban environments are complicated in both sources and chemistry. Oxygenated organic compounds can be both emitted from various emission sources (e.g., vehicular emissions and biomass burning) and also secondarily produced in the atmosphere. The chemistry in forming and removing these oxygenated organic compounds can be associated with daytime and nocturnal reactions initiated by both anthropogenic and biogenic precursors with strong influences from NOx chemistry. This work demonstrates that the rich information in both gas and particle phases provided by FIGAERO-I-CIMS can greatly promote the understanding of emission and chemistry of organic carbon in the atmosphere of urban regions.
The more detailed data can be provided by contacting the corresponding authors.
The supplement related to this article is available online at: https://doi.org/10.5194/acp-21-8455-2021-supplement.
BY and MS designed the research. CSY, YL, ZLW, TGL, WWH, WC, CHW, CMW, SH, JPQ, BLW, CW, WS, XMW, ZYZ and XMW contributed to data collection. CSY performed the data analysis with contributions from WWH and WC. CSY and BY prepared the manuscript with contributions from JEK and other authors. All the authors reviewed the manuscript.
The authors declare that they have no conflict of interest.
The authors would like to thank Felipe Lopez-Hilfiker for helping to tune the CIMS instrument. The authors appreciate valuable comments from four anonymous reviewers.
This research has been supported by the National Natural Science Foundation of China (grant no. 41877302), National Key R&D Plan of China (grant nos. 2019YFE0106300, 2018YFC0213904, 2016YFC0202206), Guangdong Natural Science Funds for Distinguished Young Scholar (grant no. 2018B030306037), Guangdong Provincial Key R&D Plan (grant no. 2019B110206001), Guangdong Soft Science Research Program (grant no. 2019B101001005), and Guangdong Innovative and Entrepreneurial Research Team Program (grant no. 2016ZT06N263). Weiwei Hu and Wei Chen were supported by the National Natural Science Foundation of China (grant no. 41875156), Natural Science Foundation of Guangdong (grant no. 2019A1515011153) and Guangdong Pearl River Talents Program (grant no. 2019QN01L948). This work was also supported by the Special Fund Project for Science and Technology Innovation Strategy of Guangdong Province (grant no. 2019B121205004).
This paper was edited by Arthur Chan and reviewed by four anonymous referees.
Aiken, A. C., DeCarlo, P. F., and Jimenez, J. L.: Elemental Analysis of Organic Species with Electron Ionization High-Resolution Mass Spectrometry, Anal. Chem., 79, 8350–8358, https://doi.org/10.1021/ac071150w, 2007.
Aljawhary, D., Lee, A. K. Y., and Abbatt, J. P. D.: High-resolution chemical ionization mass spectrometry (ToF-CIMS): application to study SOA composition and processing, Atmos. Meas. Tech., 6, 3211–3224, https://doi.org/10.5194/amt-6-3211-2013, 2013.
Bean, J. K. and Hildebrandt Ruiz, L.: Gas–particle partitioning and hydrolysis of organic nitrates formed from the oxidation of α-pinene in environmental chamber experiments, Atmos. Chem. Phys., 16, 2175–2184, https://doi.org/10.5194/acp-16-2175-2016, 2016.
Berndt, T., Scholz, W., Mentler, B., Fischer, L., Herrmann, H., Kulmala, M., and Hansel, A.: Accretion Product Formation from Self- and Cross-Reactions of RO2 Radicals in the Atmosphere, Angew. Chem. Int. Edit., 57, 3820–3824, https://doi.org/10.1002/anie.201710989, 2018.
Berndt, T., Hyttinen, N., Herrmann, H., and Hansel, A.: First oxidation products from the reaction of hydroxyl radicals with isoprene for pristine environmental conditions, Commun. Chem., 2, 1–10, https://doi.org/10.1038/s42004-019-0120-9, 2019.
Bertram, T. H., Thornton, J. A., and Riedel, T. P.: An experimental technique for the direct measurement of N2O5 reactivity on ambient particles, Atmos. Meas. Tech., 2, 231–242, https://doi.org/10.5194/amt-2-231-2009, 2009.
Bertram, T. H., Kimmel, J. R., Crisp, T. A., Ryder, O. S., Yatavelli, R. L. N., Thornton, J. A., Cubison, M. J., Gonin, M., and Worsnop, D. R.: A field-deployable, chemical ionization time-of-flight mass spectrometer, Atmos. Meas. Tech., 4, 1471–1479, https://doi.org/10.5194/amt-4-1471-2011, 2011.
Bhattarai, H., Saikawa, E., Wan, X., Zhu, H., Ram, K., Gao, S., Kang, S., Zhang, Q., Zhang, Y., Wu, G., Wang, X., Kawamura, K., Fu, P., and Cong, Z.: Levoglucosan as a tracer of biomass burning: Recent progress and perspectives, Atmos. Res., 220, 20–33, https://doi.org/10.1016/j.atmosres.2019.01.004, 2019.
Boyd, C. M., Sanchez, J., Xu, L., Eugene, A. J., Nah, T., Tuet, W. Y., Guzman, M. I., and Ng, N. L.: Secondary organic aerosol formation from the β-pinene + NO3 system: effect of humidity and peroxy radical fate, Atmos. Chem. Phys., 15, 7497–7522, https://doi.org/10.5194/acp-15-7497-2015, 2015.
Brandt, C. and van Eldik, R.: Transition Metal-Catalyzed Oxidation of Sulfur(IV) Oxides. Atmospheric-Relevant Processes and Mechanisms, Chem. Rev., 95, 119–190, https://doi.org/10.1021/cr00033a006, 1995.
Brege, M., Paglione, M., Gilardoni, S., Decesari, S., Facchini, M. C., and Mazzoleni, L. R.: Molecular insights on aging and aqueous-phase processing from ambient biomass burning emissions-influenced Po Valley fog and aerosol, Atmos. Chem. Phys., 18, 13197–13214, https://doi.org/10.5194/acp-18-13197-2018, 2018.
Canagaratna, M. R., Jimenez, J. L., Kroll, J. H., Chen, Q., Kessler, S. H., Massoli, P., Hildebrandt Ruiz, L., Fortner, E., Williams, L. R., Wilson, K. R., Surratt, J. D., Donahue, N. M., Jayne, J. T., and Worsnop, D. R.: Elemental ratio measurements of organic compounds using aerosol mass spectrometry: characterization, improved calibration, and implications, Atmos. Chem. Phys., 15, 253–272, https://doi.org/10.5194/acp-15-253-2015, 2015.
Capouet, M. and Müller, J.-F.: A group contribution method for estimating the vapour pressures of α-pinene oxidation products, Atmos. Chem. Phys., 6, 1455–1467, https://doi.org/10.5194/acp-6-1455-2006, 2006.
Carlton, A. G., Turpin, B. J., Altieri, K. E., Seitzinger, S., Reff, A., Lim, H.-J., and Ervens, B.: Atmospheric oxalic acid and SOA production from glyoxal: Results of aqueous photooxidation experiments, Atmos. Environ., 41, 7588–7602, https://doi.org/10.1016/j.atmosenv.2007.05.035, 2007.
Carslaw, N.: A mechanistic study of limonene oxidation products and pathways following cleaning activities, Atmos. Environ., 80, 507–513, https://doi.org/10.1016/j.atmosenv.2013.08.034, 2013.
Chen, H. and Finlayson-Pitts, B. J.: New Particle Formation from Methanesulfonic Acid and Amines/Ammonia as a Function of Temperature, Environ. Sci. Technol., 51, 243–252, https://doi.org/10.1021/acs.est.6b04173, 2017.
Chen, J., Wenger, J. C., and Venables, D. S.: Near-Ultraviolet Absorption Cross Sections of Nitrophenols and Their Potential Influence on Tropospheric Oxidation Capacity, J. Phys. Chem. A, 115, 12235–12242, https://doi.org/10.1021/jp206929r, 2011.
Cubison, M. J., Ortega, A. M., Hayes, P. L., Farmer, D. K., Day, D., Lechner, M. J., Brune, W. H., Apel, E., Diskin, G. S., Fisher, J. A., Fuelberg, H. E., Hecobian, A., Knapp, D. J., Mikoviny, T., Riemer, D., Sachse, G. W., Sessions, W., Weber, R. J., Weinheimer, A. J., Wisthaler, A., and Jimenez, J. L.: Effects of aging on organic aerosol from open biomass burning smoke in aircraft and laboratory studies, Atmos. Chem. Phys., 11, 12049–12064, https://doi.org/10.5194/acp-11-12049-2011, 2011.
D'Ambro, E. L., Lee, B. H., Liu, J., Shilling, J. E., Gaston, C. J., Lopez-Hilfiker, F. D., Schobesberger, S., Zaveri, R. A., Mohr, C., Lutz, A., Zhang, Z., Gold, A., Surratt, J. D., Rivera-Rios, J. C., Keutsch, F. N., and Thornton, J. A.: Molecular composition and volatility of isoprene photochemical oxidation secondary organic aerosol under low- and high-NOx conditions, Atmos. Chem. Phys., 17, 159–174, https://doi.org/10.5194/acp-17-159-2017, 2017.
D'Ambro, E. L., Schobesberger, S., Zaveri, R. A., Shilling, J. E., Lee, B. H., Lopez-Hilfiker, F. D., Mohr, C., and Thornton, J.: Isothermal evaporation of α-pinene ozonolysis SOA: volatility, phase state, and oligomeric composition, ACS Earth Sp. Chem., 2, 1058–1067, https://doi.org/10.1021/acsearthspacechem.8b00084, 2018.
de Gouw, J. A., Middlebrook, A. M., Warneke, C., Goldan, P. D., Kuster, W. C., Roberts, J. M., Fehsenfeld, F. C., Worsnop, D. R., Canagaratna, M. R., Pszenny, A. A. P., Keene, W. C., Marchewka, M., Bertman, S. B., and Bates, T. S.: Budget of organic carbon in a polluted atmosphere: Results from the New England Air Quality Study in 2002, J. Geophys. Res.-Atmos., 110, 1–22, https://doi.org/10.1029/2004JD005623, 2005.
de Gouw, J. A., Gilman, J. B., Kim, S. W., Alvarez, S. L., Dusanter, S., Graus, M., Griffith, S. M., Isaacman-VanWertz, G., Kuster, W. C., Lefer, B. L., Lerner, B. M., McDonald, B. C., Rappenglück, B., Roberts, J. M., Stevens, P. S., Stutz, J., Thalman, R., Veres, P. R., Volkamer, R., Warneke, C., Washenfelder, R. A., and Young, C. J.: Chemistry of Volatile Organic Compounds in the Los Angeles Basin: Formation of Oxygenated Compounds and Determination of Emission Ratios, J. Geophys. Res.-Atmos., 123, 2298–2319, https://doi.org/10.1002/2017JD027976, 2018.
Edwards, P. M., Brown, S. S., Roberts, J. M., Ahmadov, R., Banta, R. M., DeGouw, J. A., Dubé, W. P., Field, R. A., Flynn, J. H., Gilman, J. B., Graus, M., Helmig, D., Koss, A., Langford, A. O., Lefer, B. L., Lerner, B. M., Li, R., Li, S. M., McKeen, S. A., Murphy, S. M., Parrish, D. D., Senff, C. J., Soltis, J., Stutz, J., Sweeney, C., Thompson, C. R., Trainer, M. K., Tsai, C., Veres, P. R., Washenfelder, R. A., Warneke, C., Wild, R. J., Young, C. J., Yuan, B., and Zamora, R.: High winter ozone pollution from carbonyl photolysis in an oil and gas basin, Nature, 514, 351–354, https://doi.org/10.1038/nature13767, 2014.
Eger, P. G., Schuladen, J., Sobanski, N., Fischer, H., Karu, E., Williams, J., Riva, M., Zha, Q., Ehn, M., Quéléver, L. L. J., Schallhart, S., Lelieveld, J., and Crowley, J. N.: Pyruvic acid in the boreal forest: gas-phase mixing ratios and impact on radical chemistry, Atmos. Chem. Phys., 20, 3697–3711, https://doi.org/10.5194/acp-20-3697-2020, 2020.
Eisele, F. L. and Tanner, D. J.: Measurement of the gas phase concentration of H2SO4 and methane sulfonic acid and estimates of H2SO4 production and loss in the atmosphere, J. Geophys. Res.-Atmos., 98, 9001–9010, https://doi.org/10.1029/93JD00031, 1993.
Fang, W., Gong, L., and Sheng, L.: Online analysis of secondary organic aerosols from OH-initiated photooxidation and ozonolysis of α-pinene, β-pinene, Δ3-carene and d-limonene by thermal desorption-photoionisation aerosol mass spectrometry, Environ. Chem., 14, 75–90, https://doi.org/10.1071/EN16128, 2017.
Faxon, C., Hammes, J., Le Breton, M., Pathak, R. K., and Hallquist, M.: Characterization of organic nitrate constituents of secondary organic aerosol (SOA) from nitrate-radical-initiated oxidation of limonene using high-resolution chemical ionization mass spectrometry, Atmos. Chem. Phys., 18, 5467–5481, https://doi.org/10.5194/acp-18-5467-2018, 2018.
Fisher, J. A., Jacob, D. J., Travis, K. R., Kim, P. S., Marais, E. A., Chan Miller, C., Yu, K., Zhu, L., Yantosca, R. M., Sulprizio, M. P., Mao, J., Wennberg, P. O., Crounse, J. D., Teng, A. P., Nguyen, T. B., St. Clair, J. M., Cohen, R. C., Romer, P., Nault, B. A., Wooldridge, P. J., Jimenez, J. L., Campuzano-Jost, P., Day, D. A., Hu, W., Shepson, P. B., Xiong, F., Blake, D. R., Goldstein, A. H., Misztal, P. K., Hanisco, T. F., Wolfe, G. M., Ryerson, T. B., Wisthaler, A., and Mikoviny, T.: Organic nitrate chemistry and its implications for nitrogen budgets in an isoprene- and monoterpene-rich atmosphere: constraints from aircraft (SEAC4RS) and ground-based (SOAS) observations in the Southeast US, Atmos. Chem. Phys., 16, 5969–5991, https://doi.org/10.5194/acp-16-5969-2016, 2016.
Fountoukis, C. and Nenes, A.: ISORROPIA II: a computationally efficient thermodynamic equilibrium model for K+–Ca2+–Mg2+–NH–Na+–SO–NO–Cl−–H2O aerosols, Atmos. Chem. Phys., 7, 4639–4659, https://doi.org/10.5194/acp-7-4639-2007, 2007.
Fry, J. L., Draper, D. C., Zarzana, K. J., Campuzano-Jost, P., Day, D. A., Jimenez, J. L., Brown, S. S., Cohen, R. C., Kaser, L., Hansel, A., Cappellin, L., Karl, T., Hodzic Roux, A., Turnipseed, A., Cantrell, C., Lefer, B. L., and Grossberg, N.: Observations of gas- and aerosol-phase organic nitrates at BEACHON-RoMBAS 2011, Atmos. Chem. Phys., 13, 8585–8605, https://doi.org/10.5194/acp-13-8585-2013, 2013.
Galloway, M. M., Chhabra, P. S., Chan, A. W. H., Surratt, J. D., Flagan, R. C., Seinfeld, J. H., and Keutsch, F. N.: Glyoxal uptake on ammonium sulphate seed aerosol: reaction products and reversibility of uptake under dark and irradiated conditions, Atmos. Chem. Phys., 9, 3331–3345, https://doi.org/10.5194/acp-9-3331-2009, 2009.
Gaston, C. J., Lopez-Hilfiker, F. D., Whybrew, L. E., Hadley, O., McNair, F., Gao, H., Jaffe, D. A., and Thornton, J. A.: Online molecular characterization of fine particulate matter in Port Angeles, WA: Evidence for a major impact from residential wood smoke, Atmos. Environ., 138, 99–107, https://doi.org/10.1016/j.atmosenv.2016.05.013, 2016.
Glasius, M., Lahaniati, M., Calogirou, A., Di Bella, D., Jensen, N. R., Hjorth, J., Kotzias, D., and Larsen, B. R.: Carboxylic acids in secondary aerosols from oxidation of cyclic monoterpenes by ozone, Environ. Sci. Technol., 34, 1001–1010, https://doi.org/10.1021/es990445r, 2000.
Gondwe, M., Krol, M., Gieskes, W., Klaassen, W., and de Baar, H.: The contribution of ocean-leaving DMS to the global atmospheric burdens of DMS, MSA, SO2, and NSS SO, Global Biogeochem. Cy., 17, 1056, https://doi.org/10.1029/2002GB001937, 2003.
Guo, H., Xu, L., Bougiatioti, A., Cerully, K. M., Capps, S. L., Hite Jr., J. R., Carlton, A. G., Lee, S.-H., Bergin, M. H., Ng, N. L., Nenes, A., and Weber, R. J.: Fine-particle water and pH in the southeastern United States, Atmos. Chem. Phys., 15, 5211–5228, https://doi.org/10.5194/acp-15-5211-2015, 2015.
Hallquist, M., Wenger, J. C., Baltensperger, U., Rudich, Y., Simpson, D., Claeys, M., Dommen, J., Donahue, N. M., George, C., Goldstein, A. H., Hamilton, J. F., Herrmann, H., Hoffmann, T., Iinuma, Y., Jang, M., Jenkin, M. E., Jimenez, J. L., Kiendler-Scharr, A., Maenhaut, W., McFiggans, G., Mentel, Th. F., Monod, A., Prévôt, A. S. H., Seinfeld, J. H., Surratt, J. D., Szmigielski, R., and Wildt, J.: The formation, properties and impact of secondary organic aerosol: current and emerging issues, Atmos. Chem. Phys., 9, 5155–5236, https://doi.org/10.5194/acp-9-5155-2009, 2009.
Hammes, J., Lutz, A., Mentel, T., Faxon, C., and Hallquist, M.: Carboxylic acids from limonene oxidation by ozone and hydroxyl radicals: insights into mechanisms derived using a FIGAERO-CIMS, Atmos. Chem. Phys., 19, 13037–13052, https://doi.org/10.5194/acp-19-13037-2019, 2019.
Harrison, M. A. J., Barra, S., Borghesi, D., Vione, D., Arsene, C., and Iulian Olariu, R.: Nitrated phenols in the atmosphere: a review, Atmos. Environ., 39, 231–248, https://doi.org/10.1016/j.atmosenv.2004.09.044, 2005.
He, Q.-F., Ding, X., Wang, X.-M., Yu, J.-Z., Fu, X.-X., Liu, T.-Y., Zhang, Z., Xue, J., Chen, D.-H., Zhong, L.-J., and Donahue, N. M.: Organosulfates from Pinene and Isoprene over the Pearl River Delta, South China: Seasonal Variation and Implication in Formation Mechanisms, Environ. Sci. Technol., 48, 9236–9245, https://doi.org/10.1021/es501299v, 2014.
Heald, C. L., Kroll, J. H., Jimenez, J. L., Docherty, K. S., Decarlo, P. F., Aiken, A. C., Chen, Q., Martin, S. T., Farmer, D. K., and Artaxo, P.: A simplified description of the evolution of organic aerosol composition in the atmosphere, Geophys. Res. Lett., 37, L08803, https://doi.org/10.1029/2010GL042737, 2010.
Hodzic, A., Jimenez, J. L., Madronich, S., Canagaratna, M. R., DeCarlo, P. F., Kleinman, L., and Fast, J.: Modeling organic aerosols in a megacity: potential contribution of semi-volatile and intermediate volatility primary organic compounds to secondary organic aerosol formation, Atmos. Chem. Phys., 10, 5491–5514, https://doi.org/10.5194/acp-10-5491-2010, 2010.
Hu, W., Hu, M., Hu, W., Jimenez, J. L., Yuan, B., Chen, W., Wang, M., Wu, Y., Chen, C., Wang, Z., Peng, J., Zeng, L., and Shao, M.: Chemical composition, sources, and aging process of submicron aerosols in Beijing: Contrast between summer and winter, J. Geophys. Res.-Atmos., 121, 1955–1977, https://doi.org/10.1002/2015JD024020, 2016.
Hu, W., Day, D. A., Campuzano-Jost, P., Nault, B. A., Park, T., Lee, T., Croteau, P., Canagaratna, M. R., Jayne, J. T., Worsnop, D. R., and Jimenez, J. L.: Evaluation of the new capture vaporizer for aerosol mass spectrometers: Characterization of organic aerosol mass spectra, Aerosol Sci. Tech., 52, 725–739, https://doi.org/10.1080/02786826.2018.1454584, 2018.
Huang, R. J., Zhang, Y., Bozzetti, C., Ho, K. F., Cao, J. J., Han, Y., Daellenbach, K. R., Slowik, J. G., Platt, S. M., Canonaco, F., Zotter, P., Wolf, R., Pieber, S. M., Bruns, E. A., Crippa, M., Ciarelli, G., Piazzalunga, A., Schwikowski, M., Abbaszade, G., Schnelle-Kreis, J., Zimmermann, R., An, Z., Szidat, S., Baltensperger, U., El Haddad, I., and Prévôt, A. S. H.: High secondary aerosol contribution to particulate pollution during haze events in China, Nature, 514, 218–222, https://doi.org/10.1038/nature13774, 2015.
Huang, R.-J., Cao, J., Chen, Y., Yang, L., Shen, J., You, Q., Wang, K., Lin, C., Xu, W., Gao, B., Li, Y., Chen, Q., Hoffmann, T., O'Dowd, C. D., Bilde, M., and Glasius, M.: Organosulfates in atmospheric aerosol: synthesis and quantitative analysis of PM2.5 from Xi'an, northwestern China, Atmos. Meas. Tech., 11, 3447–3456, https://doi.org/10.5194/amt-11-3447-2018, 2018.
Huang, W., Saathoff, H., Shen, X., Ramisetty, R., Leisner, T., and Mohr, C.: Chemical Characterization of Highly Functionalized Organonitrates Contributing to Night-Time Organic Aerosol Mass Loadings and Particle Growth, Environ. Sci. Technol., 53, 1165–1174, https://doi.org/10.1021/acs.est.8b05826, 2019.
Hunter, J. F., Day, D. A., Palm, B. B., Yatavelli, R. L. N., Chan, A. W. H., Kaser, L., Cappellin, L., Hayes, P. L., Cross, E. S., Carrasquillo, A. J., Campuzano-Jost, P., Stark, H., Zhao, Y., Hohaus, T., Smith, J. N., Hansel, A., Karl, T., Goldstein, A. H., Guenther, A., Worsnop, D. R., Thornton, J. A., Heald, C. L., Jimenez, J. L., and Kroll, J. H.: Comprehensive characterization of atmospheric organic carbon at a forested site, Nat. Geosci., 10, 748–753, https://doi.org/10.1038/NGEO3018, 2017.
Hyttinen, N., Otkjær, R. V., Iyer, S., Kjaergaard, H. G., Rissanen, M. P., Wennberg, P. O., and Kurtén, T.: Computational Comparison of Different Reagent Ions in the Chemical Ionization of Oxidized Multifunctional Compounds, J. Phys. Chem. A, 122, 269–279, https://doi.org/10.1021/acs.jpca.7b10015, 2018.
Isaacman-VanWertz, G., Massoli, P., O'Brien, R., Lim, C., Franklin, J., Moss, J., Hunter, J., Nowak, J., Canagaratna, M., Misztal, P., Arata, C., Roscioli, J., Herndon, S., Onasch, T., Lambe, A., Jayne, J., Su, L., Knopf, D., Goldstein, A., Worsnop, D., and Kroll, J.: Chemical evolution of atmospheric organic carbon over multiple generations of oxidation, Nat. Chem., 10, 462–468, https://doi.org/10.1038/s41557-018-0002-2, 2018.
Iyer, S., Lopez-Hilfiker, F., Lee, B. H., Thornton, J. A., and Kurtén, T.: Modeling the Detection of Organic and Inorganic Compounds Using Iodide-Based Chemical Ionization, J. Phys. Chem. A, 120, 576–587, https://doi.org/10.1021/acs.jpca.5b09837, 2016.
Jacobs, M. I., Burke, W. J., and Elrod, M. J.: Kinetics of the reactions of isoprene-derived hydroxynitrates: gas phase epoxide formation and solution phase hydrolysis, Atmos. Chem. Phys., 14, 8933–8946, https://doi.org/10.5194/acp-14-8933-2014, 2014.
Jenkin, M. E., Saunders, S. M., Wagner, V., and Pilling, M. J.: Protocol for the development of the Master Chemical Mechanism, MCM v3 (Part B): tropospheric degradation of aromatic volatile organic compounds, Atmos. Chem. Phys., 3, 181–193, https://doi.org/10.5194/acp-3-181-2003, 2003.
Karl, T., Striednig, M., Graus, M., Hammerle, A., and Wohlfahrt, G.: Urban flux measurements reveal a large pool of oxygenated volatile organic compound emissions, P. Natl. Acad. Sci. USA, 115, 1186–1191, https://doi.org/10.1073/pnas.1714715115, 2018.
Kawamura, K. and Bikkina, S.: A review of dicarboxylic acids and related compounds in atmospheric aerosols: Molecular distributions, sources and transformation, Atmos. Res., 170, 140–160, https://doi.org/10.1016/j.atmosres.2015.11.018, 2016.
Kawamura, K., Kasukabe, H., and Barrie, L. A.: Source and reaction pathways of dicarboxylic acids, ketoacids and dicarbonyls in arctic aerosols: One year of observations, Atmos. Environ., 30, 1709–1722, https://doi.org/10.1016/1352-2310(95)00395-9, 1996.
Kong, X., Salvador, C. M., Carlsson, S., Pathak, R., Davidsson, K. O., Le Breton, M., Gaita, S. M., Mitra, K., Hallquist, Å. M., Hallquist, M., and Pettersson, J. B. C.: Molecular characterization and optical properties of primary emissions from a residential wood burning boiler, Sci. Total Environ., 754, 142143, https://doi.org/10.1016/j.scitotenv.2020.142143, 2021.
Krechmer, J. E., Pagonis, D., Ziemann, P. J., and Jimenez, J. L.: Quantification of Gas-Wall Partitioning in Teflon Environmental Chambers Using Rapid Bursts of Low-Volatility Oxidized Species Generated in Situ, Environ. Sci. Technol., 50, 5757–5765, https://doi.org/10.1021/acs.est.6b00606, 2016.
Kroll, J. H. and Seinfeld, J. H.: Chemistry of secondary organic aerosol: Formation and evolution of low-volatility organics in the atmosphere, Atmos. Environ., 42, 3593–3624, https://doi.org/10.1016/j.atmosenv.2008.01.003, 2008.
Lambe, A. T., Onasch, T. B., Croasdale, D. R., Wright, J. P., Martin, A. T., Franklin, J. P., Massoli, P., Kroll, J. H., Canagaratna, M. R., Brune, W. H., Worsnop, D. R., and Davidovits, P.: Transitions from functionalization to fragmentation reactions of laboratory Secondary Organic Aerosol (SOA) generated from the OH oxidation of alkane precursors, Environ. Sci. Technol., 46, 5430–5437, https://doi.org/10.1021/es300274t, 2012.
Larsen, B. R., Di Bella, D., Glasius, M., Winterhalter, R., Jensen, N. R., and Hjorth, J.: Gas-phase OH oxidation of monoterpenes: Gaseous and particulate products, J. Atmos. Chem., 38, 231–276, https://doi.org/10.1023/A:1006487530903, 2001.
Le Breton, M., Hallquist, Å. M., Pathak, R. K., Simpson, D., Wang, Y., Johansson, J., Zheng, J., Yang, Y., Shang, D., Wang, H., Liu, Q., Chan, C., Wang, T., Bannan, T. J., Priestley, M., Percival, C. J., Shallcross, D. E., Lu, K., Guo, S., Hu, M., and Hallquist, M.: Chlorine oxidation of VOCs at a semi-rural site in Beijing: significant chlorine liberation from ClNO2 and subsequent gas- and particle-phase Cl–VOC production, Atmos. Chem. Phys., 18, 13013–13030, https://doi.org/10.5194/acp-18-13013-2018, 2018a.
Le Breton, M., Wang, Y., Hallquist, Å. M., Pathak, R. K., Zheng, J., Yang, Y., Shang, D., Glasius, M., Bannan, T. J., Liu, Q., Chan, C. K., Percival, C. J., Zhu, W., Lou, S., Topping, D., Wang, Y., Yu, J., Lu, K., Guo, S., Hu, M., and Hallquist, M.: Online gas- and particle-phase measurements of organosulfates, organosulfonates and nitrooxy organosulfates in Beijing utilizing a FIGAERO ToF-CIMS, Atmos. Chem. Phys., 18, 10355–10371, https://doi.org/10.5194/acp-18-10355-2018, 2018b.
Le Breton, M., Psichoudaki, M., Hallquist, M., Watne, Å. K., Lutz, A., and Hallquist, Å. M.: Application of a FIGAERO ToF CIMS for on-line characterization of real-world fresh and aged particle emissions from buses, Aerosol Sci. Tech., 53, 244–259, https://doi.org/10.1080/02786826.2019.1566592, 2019.
Lee, B. H., Lopez-Hilfiker, F. D., Mohr, C., Kurtén, T., Worsnop, D. R., and Thornton, J. A.: An iodide-adduct high-resolution time-of-flight chemical-ionization mass spectrometer: Application to atmospheric inorganic and organic compounds, Environ. Sci. Technol., 48, 6309–6317, https://doi.org/10.1021/es500362a, 2014.
Lee, B. H., Mohr, C., Lopez-Hilfiker, F. D., Lutz, A., Hallquist, M., Lee, L., Romer, P., Cohen, R. C., Iyer, S., Kurtén, T., Hu, W., Day, D. A., Campuzano-Jost, P., Jimenez, J. L., Xu, L., Ng, N. L., Guo, H., Weber, R. J., Wild, R. J., Brown, S. S., Koss, A., de Gouw, J., Olson, K., Goldstein, A. H., Seco, R., Kim, S., McAvey, K., Shepson, P. B., Starn, T., Baumann, K., Edgerton, E. S., Liu, J., Shilling, J. E., Miller, D. O., Brune, W., Schobesberger, S., D'Ambro, E. L., and Thornton, J. A.: Highly functionalized organic nitrates in the southeast United States: Contribution to secondary organic aerosol and reactive nitrogen budgets, P. Natl. Acad. Sci. USA, 113, 1516–1521, https://doi.org/10.1073/pnas.1508108113, 2016.
Lee, B. H., Lopez-hilfiker, F. D., Veres, P. R., Mcduffie, E. E., Fibiger, D. L., Tamara, L., and Thornton, J. A.: Flight deployment of a high-resolution time-of-flight chemical ionization mass spectrometer: observations of reactive halogen and nitrogen oxide species, J. Geophys. Res.-Atmos., 123, 7670–7686, https://doi.org/10.1029/2017JD028082, 2018a.
Lee, B. H., Lopez-Hilfiker, F. D., D'Ambro, E. L., Zhou, P., Boy, M., Petäjä, T., Hao, L., Virtanen, A., and Thornton, J. A.: Semi-volatile and highly oxygenated gaseous and particulate organic compounds observed above a boreal forest canopy, Atmos. Chem. Phys., 18, 11547–11562, https://doi.org/10.5194/acp-18-11547-2018, 2018b.
Li, T., Wang, Z., Yuan, B., Ye, C., Lin, Y., Wang, S., Sha, Q., Yuan, Z., Zheng, J., and Shao, M.: Emissions of carboxylic acids, hydrogen cyanide (HCN) and isocyanic acid (HNCO) from vehicle exhaust, Atmos. Environ., 247, 118218, https://doi.org/10.1016/j.atmosenv.2021.118218, 2021.
Liao, J., Froyd, K. D., Murphy, D. M., Keutsch, F. N., Yu, G., Wennberg, P. O., St Clair, J. M., Crounse, J. D., Wisthaler, A., Mikoviny, T., Jimenez, J. L., Campuzano-Jost, P., Day, D. A., Hu, W., Ryerson, T. B., Pollack, I. B., Peischl, J., Anderson, B. E., Ziemba, L. D., Blake, D. R., Meinardi, S., and Diskin, G.: Airborne measurements of organosulfates over the continental U.S, J. Geophys. Res.-Atmos., 120, 2990–3005, https://doi.org/10.1002/2014JD022378, 2015.
Lim, H.-J., Carlton, A. G., and Turpin, B. J.: Isoprene Forms Secondary Organic Aerosol through Cloud Processing: Model Simulations, Environ. Sci. Technol., 39, 4441–4446, https://doi.org/10.1021/es048039h, 2005.
Liu, C., Deng, X., Zhu, B., and Yin, C.: Characteristics of GSR of China's three major economic regions in the past 10 years and its relationship with O3 and PM2.5, China Environ. Sci., 38, 2820–2829, https://doi.org/10.19674/j.cnki.issn1000-6923.2018.0295, 2018.
Liu, X., Qu, H., Huey, L. G., Wang, Y., Sjostedt, S., Zeng, L., Lu, K., Wu, Y., Hu, M., Shao, M., Zhu, T., and Zhang, Y.: High Levels of Daytime Molecular Chlorine and Nitryl Chloride at a Rural Site on the North China Plain, Environ. Sci. Technol., 51, 9588–9595, https://doi.org/10.1021/acs.est.7b03039, 2017.
Liu, Z., Wang, Y., Gu, D., Zhao, C., Huey, L. G., Stickel, R., Liao, J., Shao, M., Zhu, T., Zeng, L., Amoroso, A., Costabile, F., Chang, C.-C., and Liu, S.-C.: Summertime photochemistry during CAREBeijing-2007: ROx budgets and O3 formation, Atmos. Chem. Phys., 12, 7737–7752, https://doi.org/10.5194/acp-12-7737-2012, 2012.
Lopez-Hilfiker, F. D., Mohr, C., Ehn, M., Rubach, F., Kleist, E., Wildt, J., Mentel, Th. F., Lutz, A., Hallquist, M., Worsnop, D., and Thornton, J. A.: A novel method for online analysis of gas and particle composition: description and evaluation of a Filter Inlet for Gases and AEROsols (FIGAERO), Atmos. Meas. Tech., 7, 983–1001, https://doi.org/10.5194/amt-7-983-2014, 2014.
Lopez-Hilfiker, F. D., Mohr, C., Ehn, M., Rubach, F., Kleist, E., Wildt, J., Mentel, Th. F., Carrasquillo, A. J., Daumit, K. E., Hunter, J. F., Kroll, J. H., Worsnop, D. R., and Thornton, J. A.: Phase partitioning and volatility of secondary organic aerosol components formed from α-pinene ozonolysis and OH oxidation: the importance of accretion products and other low volatility compounds, Atmos. Chem. Phys., 15, 7765–7776, https://doi.org/10.5194/acp-15-7765-2015, 2015.
Lopez-Hilfiker, F. D., Iyer, S., Mohr, C., Lee, B. H., D'Ambro, E. L., Kurtén, T., and Thornton, J. A.: Constraining the sensitivity of iodide adduct chemical ionization mass spectrometry to multifunctional organic molecules using the collision limit and thermodynamic stability of iodide ion adducts, Atmos. Meas. Tech., 9, 1505–1512, https://doi.org/10.5194/amt-9-1505-2016, 2016.
Massoli, P., Stark, H., Canagaratna, M. R., Krechmer, J. E., Xu, L., Ng, N. L., Mauldin, R. L., Yan, C., Kimmel, J., Misztal, P. K., Jimenez, J. L., Jayne, J. T., and Worsnop, D. R.: Ambient Measurements of Highly Oxidized Gas-Phase Molecules during the Southern Oxidant and Aerosol Study (SOAS) 2013, ACS Earth Sp. Chem., 2, 653–672, https://doi.org/10.1021/acsearthspacechem.8b00028, 2018.
Mattila, J. M., Brophy, P., Kirkland, J., Hall, S., Ullmann, K., Fischer, E. V., Brown, S., McDuffie, E., Tevlin, A., and Farmer, D. K.: Tropospheric sources and sinks of gas-phase acids in the Colorado Front Range, Atmos. Chem. Phys., 18, 12315–12327, https://doi.org/10.5194/acp-18-12315-2018, 2018.
Mehra, A., Wang, Y., Krechmer, J. E., Lambe, A., Majluf, F., Morris, M. A., Priestley, M., Bannan, T. J., Bryant, D. J., Pereira, K. L., Hamilton, J. F., Rickard, A. R., Newland, M. J., Stark, H., Croteau, P., Jayne, J. T., Worsnop, D. R., Canagaratna, M. R., Wang, L., and Coe, H.: Evaluation of the chemical composition of gas- and particle-phase products of aromatic oxidation, Atmos. Chem. Phys., 20, 9783–9803, https://doi.org/10.5194/acp-20-9783-2020, 2020.
Mellouki, A., Wallington, T. J., and Chen, J.: Atmospheric Chemistry of Oxygenated Volatile Organic Compounds: Impacts on Air Quality and Climate, Chem. Rev., 115, 3984–4014, https://doi.org/10.1021/cr500549n, 2015.
Mohr, C., Lopez-Hilfiker, F. D., Zotter, P., Prévôt, A. S. H., Xu, L., Ng, N. L., Herndon, S. C., Williams, L. R., Franklin, J. P., Zahniser, M. S., Worsnop, D. R., Knighton, W. B., Aiken, A. C., Gorkowski, K. J., Dubey, M. K., Allan, J. D., and Thornton, J. A.: Contribution of Nitrated Phenols to Wood Burning Brown Carbon Light Absorption in Detling, United Kingdom during Winter Time, Environ. Sci. Technol., 47, 6316–6324, https://doi.org/10.1021/es400683v, 2013.
Mutzel, A., Poulain, L., Berndt, T., Iinuma, Y., Rodigast, M., Böge, O., Richters, S., Spindler, G., Sipilä, M., Jokinen, T., Kulmala, M., and Herrmann, H.: Highly Oxidized Multifunctional Organic Compounds Observed in Tropospheric Particles: A Field and Laboratory Study, Environ. Sci. Technol., 49, 7754–7761, https://doi.org/10.1021/acs.est.5b00885, 2015.
Mutzel, A., Rodigast, M., Iinuma, Y., Böge, O., and Herrmann, H.: Monoterpene SOA – Contribution of first-generation oxidation products to formation and chemical composition, Atmos. Environ., 130, 136–144, https://doi.org/10.1016/j.atmosenv.2015.10.080, 2016.
Nah, T., Sanchez, J., Boyd, C. M., and Ng, N. L.: Photochemical Aging of α-pinene and β-pinene Secondary Organic Aerosol formed from Nitrate Radical Oxidation, Environ. Sci. Technol., 50, 222–231, https://doi.org/10.1021/acs.est.5b04594, 2016.
Nannoolal, Y., Rarey, J., and Ramjugernath, D.: Estimation of pure component properties Part 3. Estimation of the vapor pressure of non-electrolyte organic compounds via group contributions and group interactions, Fluid Phase Equilib., 269, 117–133, https://doi.org/10.1016/j.fluid.2008.04.020, 2008.
Ng, N. L., Brown, S. S., Archibald, A. T., Atlas, E., Cohen, R. C., Crowley, J. N., Day, D. A., Donahue, N. M., Fry, J. L., Fuchs, H., Griffin, R. J., Guzman, M. I., Herrmann, H., Hodzic, A., Iinuma, Y., Jimenez, J. L., Kiendler-Scharr, A., Lee, B. H., Luecken, D. J., Mao, J., McLaren, R., Mutzel, A., Osthoff, H. D., Ouyang, B., Picquet-Varrault, B., Platt, U., Pye, H. O. T., Rudich, Y., Schwantes, R. H., Shiraiwa, M., Stutz, J., Thornton, J. A., Tilgner, A., Williams, B. J., and Zaveri, R. A.: Nitrate radicals and biogenic volatile organic compounds: oxidation, mechanisms, and organic aerosol, Atmos. Chem. Phys., 17, 2103–2162, https://doi.org/10.5194/acp-17-2103-2017, 2017.
Noelscher, A. C., Yanez-Serrano, A. M., Wolff, S., de Araujo, A. C., Lavric, J. V, Kesselmeier, J., and Williams, J.: Unexpected seasonality in quantity and composition of Amazon rainforest air reactivity, Nat. Commun., 7, , 10383, https://doi.org/10.1038/ncomms10383, 2016.
Osthoff, H. D., Roberts, J. M., Ravishankara, A. R., Williams, E. J., Lerner, B. M., Sommariva, R., Bates, T. S., Coffman, D., Quinn, P. K., Dibb, J. E., Stark, H., Burkholder, J. B., Talukdar, R. K., Meagher, J., Fehsenfeld, F. C., and Brown, S. S.: High levels of nitryl chloride in the polluted subtropical marine boundary layer, Nat. Geosci., 1, 324–328, https://doi.org/10.1038/ngeo177, 2008.
Palm, B. B., Liu, X., Jimenez, J. L., and Thornton, J. A.: Performance of a new coaxial ion–molecule reaction region for low-pressure chemical ionization mass spectrometry with reduced instrument wall interactions, Atmos. Meas. Tech., 12, 5829–5844, https://doi.org/10.5194/amt-12-5829-2019, 2019.
Pankow, J. F. and Asher, W. E.: SIMPOL.1: a simple group contribution method for predicting vapor pressures and enthalpies of vaporization of multifunctional organic compounds, Atmos. Chem. Phys., 8, 2773–2796, https://doi.org/10.5194/acp-8-2773-2008, 2008.
Paulot, F., Crounse, J. D., Kjaergaard, H. G., Kroll, J. H., Seinfeld, J. H., and Wennberg, P. O.: Isoprene photooxidation: new insights into the production of acids and organic nitrates, Atmos. Chem. Phys., 9, 1479–1501, https://doi.org/10.5194/acp-9-1479-2009, 2009.
Qi, L., Chen, M., Stefenelli, G., Pospisilova, V., Tong, Y., Bertrand, A., Hueglin, C., Ge, X., Baltensperger, U., Prévôt, A. S. H., and Slowik, J. G.: Organic aerosol source apportionment in Zurich using an extractive electrospray ionization time-of-flight mass spectrometer (EESI-TOF-MS) – Part 2: Biomass burning influences in winter, Atmos. Chem. Phys., 19, 8037–8062, https://doi.org/10.5194/acp-19-8037-2019, 2019.
Reyes-Villegas, E., Bannan, T., Le Breton, M., Mehra, A., Priestley, M., Percival, C., Coe, H., and Allan, J. D.: Online Chemical Characterization of Food-Cooking Organic Aerosols: Implications for Source Apportionment, Environ. Sci. Technol., 52, 5308–5318, https://doi.org/10.1021/acs.est.7b06278, 2018.
Riva, M., Rantala, P., Krechmer, J. E., Peräkylä, O., Zhang, Y., Heikkinen, L., Garmash, O., Yan, C., Kulmala, M., Worsnop, D., and Ehn, M.: Evaluating the performance of five different chemical ionization techniques for detecting gaseous oxygenated organic species, Atmos. Meas. Tech., 12, 2403–2421, https://doi.org/10.5194/amt-12-2403-2019, 2019.
Sander, R. and Crutzen, P. J.: Model study indicating halogen activation and ozone destruction in polluted air masses transported to the sea, J. Geophys. Res.-Atmos., 101, 9121–9138, https://doi.org/10.1029/95JD03793, 1996.
Schneider, J., Weimer, S., Drewnick, F., Borrmann, S., Helas, G., Gwaze, P., Schmid, O., Andreae, M. O., and Kirchner, U.: Mass spectrometric analysis and aerodynamic properties of various types of combustion-related aerosol particles, Int. J. Mass Spectrom., 258, 37–49, https://doi.org/10.1016/j.ijms.2006.07.008, 2006.
Schwantes, R. H., Teng, A. P., Nguyen, T. B., Coggon, M. M., Crounse, J. D., St Clair, J. M., Zhang, X., Schilling, K. A., Seinfeld, J. H., and Wennberg, P. O.: Isoprene NO3 Oxidation Products from the RO2 + HO2 Pathway, J. Phys. Chem. A, 119, 10158, https://doi.org/10.1021/acs.jpca.5b06355, 2015.
Schwantes, R. H., Schilling, K. A., McVay, R. C., Lignell, H., Coggon, M. M., Zhang, X., Wennberg, P. O., and Seinfeld, J. H.: Formation of highly oxygenated low-volatility products from cresol oxidation, Atmos. Chem. Phys., 17, 3453–3474, https://doi.org/10.5194/acp-17-3453-2017, 2017.
Schwantes, R. H., Emmons, L. K., Orlando, J. J., Barth, M. C., Tyndall, G. S., Hall, S. R., Ullmann, K., St. Clair, J. M., Blake, D. R., Wisthaler, A., and Bui, T. P. V.: Comprehensive isoprene and terpene gas-phase chemistry improves simulated surface ozone in the southeastern US, Atmos. Chem. Phys., 20, 3739–3776, https://doi.org/10.5194/acp-20-3739-2020, 2020.
Shrivastava, M., Andreae, M. O., Artaxo, P., Barbosa, H. M. J., Berg, L. K., Brito, J., Ching, J., Easter, R. C., Fan, J., Fast, J. D., Feng, Z., Fuentes, J. D., Glasius, M., Goldstein, A. H., Alves, E. G., Gomes, H., Gu, D., Guenther, A., Jathar, S. H., Kim, S., Liu, Y., Lou, S., Martin, S. T., McNeill, V. F., Medeiros, A., de Sá, S. S., Shilling, J. E., Springston, S. R., Souza, R. A. F., Thornton, J. A., Isaacman-VanWertz, G., Yee, L. D., Ynoue, R., Zaveri, R. A., Zelenyuk, A., and Zhao, C.: Urban pollution greatly enhances formation of natural aerosols over the Amazon rainforest, Nat. Commun., 10, 1046, https://doi.org/10.1038/s41467-019-08909-4, 2019.
Simoneit, B. R. T., Schauer, J. J., Nolte, C. G., Oros, D. R., Elias, V. O., Fraser, M. P., Rogge, W. F., and Cass, G. R.: Levoglucosan, a tracer for cellulose in biomass burning and atmospheric particles, Atmos. Environ., 33, 173–182, https://doi.org/10.1016/S1352-2310(98)00145-9, 1999.
Slusher, D. L., Huey, L. G., Tanner, D. J., Flocke, F. M., and Roberts, J. M.: A thermal dissociation–chemical ionization mass spectrometry (TD-CIMS) technique for the simultaneous measurement of peroxyacyl nitrates and dinitrogen pentoxide, J. Geophys. Res.-Atmos., 109, D19315, https://doi.org/10.1029/2004JD004670, 2004.
Stark, H., Yatavelli, R. L. N., Thompson, S. L., Kimmel, J. R., Cubison, M. J., Chhabra, P. S., Canagaratna, M. R., Jayne, J. T., Worsnop, D. R., and Jimenez, J. L.: Methods to extract molecular and bulk chemical information from series of complex mass spectra with limited mass resolution, Int. J. Mass Spectrom., 389, 26–38, https://doi.org/10.1016/j.ijms.2015.08.011, 2015.
Stark, H., Yatavelli, R. L. N., Thompson, S. L., Kang, H., Krechmer, J. E., Kimmel, J. R., Palm, B. B., Hu, W., Hayes, P. L., Day, D. A., Campuzano-Jost, P., Canagaratna, M. R., Jayne, J. T., Worsnop, D. R., and Jimenez, J. L.: Impact of Thermal Decomposition on Thermal Desorption Instruments: Advantage of Thermogram Analysis for Quantifying Volatility Distributions of Organic Species, Environ. Sci. Technol., 51, 8491–8500, https://doi.org/10.1021/acs.est.7b00160, 2017.
Stolzenburg, D., Fischer, L., Vogel, A. L., Heinritzi, M., Schervish, M., Simon, M., Wagner, A. C., Dada, L., Ahonen, L. R., Amorim, A., Baccarini, A., Bauer, P. S., Baumgartner, B., Bergen, A., Bianchi, F., Breitenlechner, M., Brilke, S., Buenrostro Mazon, S., Chen, D., Dias, A., Draper, D. C., Duplissy, J., El Haddad, I., Finkenzeller, H., Frege, C., Fuchs, C., Garmash, O., Gordon, H., He, X., Helm, J., Hofbauer, V., Hoyle, C. R., Kim, C., Kirkby, J., Kontkanen, J., Kürten, A., Lampilahti, J., Lawler, M., Lehtipalo, K., Leiminger, M., Mai, H., Mathot, S., Mentler, B., Molteni, U., Nie, W., Nieminen, T., Nowak, J. B., Ojdanic, A., Onnela, A., Passananti, M., Petäjä, T., Quéléver, L. L. J., Rissanen, M. P., Sarnela, N., Schallhart, S., Tauber, C., Tomé, A., Wagner, R., Wang, M., Weitz, L., Wimmer, D., Xiao, M., Yan, C., Ye, P., Zha, Q., Baltensperger, U., Curtius, J., Dommen, J., Flagan, R. C., Kulmala, M., Smith, J. N., Worsnop, D. R., Hansel, A., Donahue, N. M., Winkler, P. M., Nie, W., Passananti, M., Leiminger, M., Stolzenburg, D., Yan, C., Wimmer, D., Buenrostro Mazon, S., Kontkanen, J., Wang, M., Garmash, O., Kulmala, M., Petäjä, T., Bianchi, F., Chen, D., Nieminen, T., Brilke, S., Nowak, J. B., Duplissy, J., El Haddad, I., Simon, M., Wagner, A. C., Kürten, A., Smith, J. N., Kim, C., et al.: Rapid growth of organic aerosol nanoparticles over a wide tropospheric temperature range, P. Natl. Acad. Sci., 115, 201807604, https://doi.org/10.1073/pnas.1807604115, 2018.
Surratt, J. D., Murphy, S. M., Kroll, J. H., Ng, N. L., Hildebrandt, L., Sorooshian, A., Szmigielski, R., Vermeylen, R., Maenhaut, W., Claeys, M., Flagan, R. C., and Seinfeld, J. H.: Chemical Composition of Secondary Organic Aerosol Formed from the Photooxidation of Isoprene, J. Phys. Chem. A, 110, 9665–9690, https://doi.org/10.1021/jp061734m, 2006.
Surratt, J. D., Kroll, J. H., Kleindienst, T. E., Edney, E. O., Claeys, M., Sorooshian, A., Ng, N. L., Offenberg, J. H., Lewandowski, M., Jaoui, M., Flagan, R. C., and Seinfeld, J. H.: Evidence for Organosulfates in Secondary Organic Aerosol, Environ. Sci. Technol., 41, 517–527, https://doi.org/10.1021/es062081q, 2007.
Surratt, J. D., Chan, A. W. H., Eddingsaas, N. C., Chan, M., Loza, C. L., Kwan, A. J., Hersey, S. P., Flagan, R. C., Wennberg, P. O., and Seinfeld, J. H.: Reactive intermediates revealed in secondary organic aerosol formation from isoprene, P. Natl. Acad. Sci. USA, 107, 6640–6645, https://doi.org/10.1073/pnas.0911114107, 2010.
Thornton, J. A., Mohr, C., Schobesberger, S., D'Ambro, E. L., Lee, B. H., and Lopez-Hilfiker, F. D.: Evaluating Organic Aerosol Sources and Evolution with a Combined Molecular Composition and Volatility Framework Using the Filter Inlet for Gases and Aerosols (FIGAERO), Acc. Chem. Res., 53, 1415–1426, https://doi.org/10.1021/acs.accounts.0c00259, 2020.
Volkamer, R., Jimenez, J. L., San Martini, F., Dzepina, K., Zhang, Q., Salcedo, D., Molina, L. T., Worsnop, D. R., and Molina, M. J.: Secondary organic aerosol formation from anthropogenic air pollution: Rapid and higher than expected, Geophys. Res. Lett., 33, L17811, https://doi.org/10.1029/2006GL026899, 2006.
Wang, H., Gao, Y., Wang, S., Wu, X., Liu, Y., Li, X., Huang, D., Lou, S., Wu, Z., Guo, S., Jing, S., Li, Y., Huang, C., Tyndall, G. S., Orlando, J. J., and Zhang, X.: Atmospheric Processing of Nitrophenols and Nitrocresols from Biomass Burning Emissions, J. Geophys. Res.-Atmos., 125, e2020JD033401, https://doi.org/10.1029/2020JD033401, 2020.
Wang, M., Chen, D., Xiao, M., Ye, Q., Stolzenburg, D., Hofbauer, V., Ye, P., Vogel, A. L., Mauldin, R. L., Amorim, A., Baccarini, A., Baumgartner, B., Brilke, S., Dada, L., Dias, A., Duplissy, J., Finkenzeller, H., Garmash, O., He, X.-C., Hoyle, C. R., Kim, C., Kvashnin, A., Lehtipalo, K., Fischer, L., Molteni, U., Petäjä, T., Pospisilova, V., Quéléver, L. L. J., Rissanen, M., Simon, M., Tauber, C., Tomé, A., Wagner, A. C., Weitz, L., Volkamer, R., Winkler, P. M., Kirkby, J., Worsnop, D. R., Kulmala, M., Baltensperger, U., Dommen, J., El-Haddad, I., and Donahue, N. M.: Photo-oxidation of Aromatic Hydrocarbons Produces Low-Volatility Organic Compounds, Environ. Sci. Technol., 54, 7911–7921, https://doi.org/10.1021/acs.est.0c02100, 2020.
Wang, Q., He, X., Zhou, M., Huang, D. D., Qiao, L., Zhu, S., Ma, Y., Wang, H., Li, L., Huang, C., Huang, X. H. H., Xu, W., Worsnop, D., Goldstein, A. H., Guo, H., and Yu, J. Z.: Hourly Measurements of Organic Molecular Markers in Urban Shanghai, China: Primary Organic Aerosol Source Identification and Observation of Cooking Aerosol Aging, ACS Earth Sp. Chem., 4, 1670–1685, https://doi.org/10.1021/acsearthspacechem.0c00205, 2020.
Wang, T., Tham, Y. J., Xue, L., Li, Q., Zha, Q., Wang, Z., Poon, S. C. N., Dube, W. P., Blake, D. R., Louie, P. K. K., Luk, C. W. Y., Tsui, W., Brown, S. S., Osthoff, H. D., Roberts, J. M., Ravishankara, A. R., Williams, E. J., Lerner, B. M., Sommariva, R., Bates, T. S., Coffman, D., Quinn, P. K., Dibb, J. E., Stark, H., Burkholder, J. B., Talukdar, R. K., Meagher, J., Fehsenfeld, F. C., and Brown, S. S.: Observations of nitryl chloride and modeling its source and effect on ozone in the planetary boundary layer of southern China, J. Geophys. Res., 121, 2476–2489, https://doi.org/10.1002/2015JD024556, 2016.
Wang, X., Jacob, D. J., Eastham, S. D., Sulprizio, M. P., Zhu, L., Chen, Q., Alexander, B., Sherwen, T., Evans, M. J., Lee, B. H., Haskins, J. D., Lopez-Hilfiker, F. D., Thornton, J. A., Huey, G. L., and Liao, H.: The role of chlorine in global tropospheric chemistry, Atmos. Chem. Phys., 19, 3981–4003, https://doi.org/10.5194/acp-19-3981-2019, 2019.
Wang, Z., Yuan, B., Ye, C., Roberts, J., Wisthaler, A., Lin, Y., Li, T., Wu, C., Peng, Y., Wang, C., Wang, S., Yang, S., Wang, B., Qi, J., Wang, C., Song, W., Hu, W., Wang, X., Xu, W., Ma, N., Kuang, Y., Tao, J., Zhang, Z., Su, H., Cheng, Y., Wang, X., and Shao, M.: High Concentrations of Atmospheric Isocyanic Acid (HNCO) Produced from Secondary Sources in China, Environ. Sci. Technol., 11818–11826, https://doi.org/10.1021/acs.est.0c02843, 2020.
Wennberg, P. O., Bates, K. H., Crounse, J. D., Dodson, L. G., McVay, R. C., Mertens, L. A., Nguyen, T. B., Praske, E., Schwantes, R. H., Smarte, M. D., St Clair, J. M., Teng, A. P., Zhang, X., and Seinfeld, J. H.: Gas-Phase Reactions of Isoprene and Its Major Oxidation Products, Chem. Rev., 118, 3337–3390, https://doi.org/10.1021/acs.chemrev.7b00439, 2018.
Wu, C., Wang, C., Wang, S., Wang, W., Yuan, B., Qi, J., Wang, B., Wang, H., Wang, C., Song, W., Wang, X., Hu, W., Lou, S., Ye, C., Peng, Y., Wang, Z., Huangfu, Y., Xie, Y., Zhu, M., Zheng, J., Wang, X., Jiang, B., Zhang, Z., and Shao, M.: Measurement report: Important contributions of oxygenated compounds to emissions and chemistry of volatile organic compounds in urban air, Atmos. Chem. Phys., 20, 14769–14785, https://doi.org/10.5194/acp-20-14769-2020, 2020.
Xiong, F., McAvey, K. M., Pratt, K. A., Groff, C. J., Hostetler, M. A., Lipton, M. A., Starn, T. K., Seeley, J. V., Bertman, S. B., Teng, A. P., Crounse, J. D., Nguyen, T. B., Wennberg, P. O., Misztal, P. K., Goldstein, A. H., Guenther, A. B., Koss, A. R., Olson, K. F., de Gouw, J. A., Baumann, K., Edgerton, E. S., Feiner, P. A., Zhang, L., Miller, D. O., Brune, W. H., and Shepson, P. B.: Observation of isoprene hydroxynitrates in the southeastern United States and implications for the fate of NOx, Atmos. Chem. Phys., 15, 11257–11272, https://doi.org/10.5194/acp-15-11257-2015, 2015.
Xue, L., Gu, R., Wang, T., Wang, X., Saunders, S., Blake, D., Louie, P. K. K., Luk, C. W. Y., Simpson, I., Xu, Z., Wang, Z., Gao, Y., Lee, S., Mellouki, A., and Wang, W.: Oxidative capacity and radical chemistry in the polluted atmosphere of Hong Kong and Pearl River Delta region: analysis of a severe photochemical smog episode, Atmos. Chem. Phys., 16, 9891–9903, https://doi.org/10.5194/acp-16-9891-2016, 2016.
Yang, Y., Shao, M., Wang, X., Nölscher, A. C., Kessel, S., Guenther, A., and Williams, J.: Towards a quantitative understanding of total OH reactivity: A review, Atmos. Environ., 134, 147–161, https://doi.org/10.1016/j.atmosenv.2016.03.010, 2016.
Yang, Y., Shao, M., Keßel, S., Li, Y., Lu, K., Lu, S., Williams, J., Zhang, Y., Zeng, L., Nölscher, A. C., Wu, Y., Wang, X., and Zheng, J.: How the OH reactivity affects the ozone production efficiency: case studies in Beijing and Heshan, China, Atmos. Chem. Phys., 17, 7127–7142, https://doi.org/10.5194/acp-17-7127-2017, 2017.
Yasmeen, F., Szmigielski, R., Vermeylen, R., Gomez-Gonzalez, Y., Surratt, J. D., Chan, A. W. H., Seinfeld, J. H., Maenhaut, W., and Claeys, M.: Mass spectrometric characterization of isomeric terpenoic acids from the oxidation of α-pinene, β-pinene, d-limonene, and Δ3-carene in fine forest aerosol, J. Mass Spectrom., 46, 425–442, https://doi.org/10.1002/jms.1911, 2011.
Yatavelli, R. L. N., Lopez-Hilfiker, F., Wargo, J. D., Kimmel, J. R., Cubison, M. J., Bertram, T. H., Jimenez, J. L., Gonin, M., Worsnop, D. R., and Thornton, J. A.: A Chemical Ionization High-Resolution Time-of-Flight Mass Spectrometer Coupled to a Micro Orifice Volatilization Impactor (MOVI-HRToF-CIMS) for Analysis of Gas and Particle-Phase Organic Species, Aerosol Sci. Tech., 46, 1313–1327, https://doi.org/10.1080/02786826.2012.712236, 2012.
Yuan, B., Veres, P. R., Warneke, C., Roberts, J. M., Gilman, J. B., Koss, A., Edwards, P. M., Graus, M., Kuster, W. C., Li, S.-M., Wild, R. J., Brown, S. S., Dubé, W. P., Lerner, B. M., Williams, E. J., Johnson, J. E., Quinn, P. K., Bates, T. S., Lefer, B., Hayes, P. L., Jimenez, J. L., Weber, R. J., Zamora, R., Ervens, B., Millet, D. B., Rappenglück, B., and de Gouw, J. A.: Investigation of secondary formation of formic acid: urban environment vs. oil and gas producing region, Atmos. Chem. Phys., 15, 1975–1993, https://doi.org/10.5194/acp-15-1975-2015, 2015.
Yuan, B., Liggio, J., Wentzell, J., Li, S.-M., Stark, H., Roberts, J. M., Gilman, J., Lerner, B., Warneke, C., Li, R., Leithead, A., Osthoff, H. D., Wild, R., Brown, S. S., and de Gouw, J. A.: Secondary formation of nitrated phenols: insights from observations during the Uintah Basin Winter Ozone Study (UBWOS) 2014, Atmos. Chem. Phys., 16, 2139–2153, https://doi.org/10.5194/acp-16-2139-2016, 2016.
Yuan, B., Koss, A. R., Warneke, C., Coggon, M., Sekimoto, K., and De Gouw, J. A.: Proton-Transfer-Reaction Mass Spectrometry: Applications in Atmospheric Sciences, Chem. Rev., 117, 13187–13229, https://doi.org/10.1021/acs.chemrev.7b00325, 2017.
Zhang, Q., Yuan, B., Shao, M., Wang, X., Lu, S., Lu, K., Wang, M., Chen, L., Chang, C.-C., and Liu, S. C.: Variations of ground-level O3 and its precursors in Beijing in summertime between 2005 and 2011, Atmos. Chem. Phys., 14, 6089–6101, https://doi.org/10.5194/acp-14-6089-2014, 2014.
Zhang, Y. J., Tang, L. L., Wang, Z., Yu, H. X., Sun, Y. L., Liu, D., Qin, W., Canonaco, F., Prévôt, A. S. H., Zhang, H. L., and Zhou, H. C.: Insights into characteristics, sources, and evolution of submicron aerosols during harvest seasons in the Yangtze River delta region, China, Atmos. Chem. Phys., 15, 1331–1349, https://doi.org/10.5194/acp-15-1331-2015, 2015.
Zhao, R.: The Recent Development and Application of Chemical Ionization Mass Spectrometry in Atmospheric Chemistry, in: Encyclopedia of Analytical Chemistry, American Cancer Society, John Wiley & Sons, Ltd., Chichester, UK, 1–33, 2018.
Zhao, Y., Nguyen, N. T., Presto, A. A., Hennigan, C. J., May, A. A., and Robinson, A. L.: Intermediate Volatility Organic Compound Emissions from On-Road Gasoline Vehicles and Small Off-Road Gasoline Engines, Environ. Sci. Technol., 50, 4554–4563, https://doi.org/10.1021/acs.est.5b06247, 2016.
Zhou, Y., Huang, X. H., Bian, Q., Griffith, S. M., Louie, P. K. K., and Yu, J. Z.: Sources and atmospheric processes impacting oxalate at a suburban coastal site in Hong Kong: Insights inferred from 1 year hourly measurements, J. Geophys. Res.-Atmos., 120, 9772–9788, https://doi.org/10.1002/2015JD023531, 2015.