the Creative Commons Attribution 4.0 License.
the Creative Commons Attribution 4.0 License.
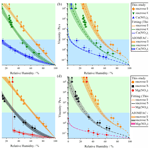
Viscosity and phase state of aerosol particles consisting of sucrose mixed with inorganic salts
Young-Chul Song
Joseph Lilek
Jae Bong Lee
Man Nin Chan
Zhijun Wu
Andreas Zuend
Mijung Song
Research on the viscosity and phase state of aerosol particles is essential because of their significant influence on the particle growth rate, equilibration times, and related evolution of mass concentration as well as heterogeneous reactions. So far, most studies of viscosity and phase state have been focused on organic aerosol particles, yet data on how viscosity can vary when the organic materials are mixed with inorganic salts remain scarce. Herein, using bead-mobility and poke-and-flow techniques, we quantified viscosities at 293 ± 1 K for binary mixtures of organic material H2O and inorganic salts H2O, as well as ternary mixtures of organic material inorganic salts H2O over the atmospheric relative humidity (RH) range. Sucrose as the organic species and calcium nitrate (Ca(NO3)2) or magnesium nitrate (Mg(NO3)2) as the inorganic salts were examined. For binary sucrose H2O particles, the viscosities gradually increased from ∼ 3 × 10−2 to ≳1 × 108 Pa s as RH decreased from ∼ 75 % to ∼ 25 %. Compared with the results for the sucrose H2O particles, binary and particles showed drastic enhancements to ≳1 × 108 Pa s at low RH close to the efflorescence RH. For ternary mixtures of sucrose Ca(NO3)2 H2O or sucrose Mg(NO3)2 H2O, with organic-to-inorganic mass ratios of 1:1, the viscosities of the particles gradually increased from ∼ 3 × 10−2 to greater than ∼ 1 × 108 Pa s for RH values from ∼ 75 % to ∼ 5 %. Compared to the viscosities of the particles, higher viscosities were observed for the ternary sucrose Ca(NO3)2 H2O particles, with values increased by about 1 order of magnitude at 50 % RH and about 6 orders of magnitude at 35 % RH. Moreover, we applied a thermodynamics-based group-contribution model (AIOMFAC-VISC, Aerosol Inorganic–Organic Mixtures Functional groups Activity Coefficients Viscosity) to predict aerosol viscosities for the studied systems. The model predictions and viscosity measurements show good agreement within ∼ 1 order of magnitude in viscosity. The viscosity measurements indicate that the studied mixed organic–inorganic particles range in phase state from liquid to semi-solid or even solid across the atmospheric RH range at a temperature of 293 K. These results support our understanding that organic inorganic H2O particles can exist in a liquid, semisolid, or even a solid state in the troposphere.
- Article
(5151 KB) - Full-text XML
-
Supplement
(598 KB) - BibTeX
- EndNote
Aerosol particles are emitted from various natural (e.g., the ocean and plants) and anthropogenic (e.g., transportation and fuel combustion) sources, as well as being produced by gas-to-particle conversion and equilibration processes due to chemical processing of gaseous species in the atmosphere (e.g., sulfur dioxide, nitrogen oxides, ammonia, and volatile organic compounds). Submicron-sized aerosol particles mainly consist of organic materials and inorganic salts (Jimenez et al., 2009; Huang et al., 2015; Cheng et al., 2016). Depending on the location and the season, the organic mass fraction of submicron particles ranges from ∼ 20 % up to ∼ 90 % (Zhang et al., 2007; R. Zhang et al., 2015; Jimenez et al., 2009; Hao et al., 2014; Huang et al., 2015; Cheng et al., 2016; Wang et al., 2016). Field measurements have shown that organic materials and inorganic salts are often internally mixed in an aerosol particle (Murphy et al., 2006; Y. C. Song et al., 2010, 2013). They can significantly affect the local air quality (Kulmala et al., 2011; Wang et al., 2015a; R. Zhang et al., 2015; Schmedding et al., 2020), regional climate (Russell et al., 1997; Kaufman et al., 2002; Kanakidou et al., 2005; Kulmala et al., 2011), and human health (Bhattarai et al., 2020). However, the physicochemical properties of internally mixed particles such as the relative humidity (RH)-dependent viscosities and morphologies remain poorly characterized.
The viscosity of aerosols can vary based on the RH and related water uptake, chemical composition, and temperature, with effects on the size distribution (Shiraiwa et al., 2013; Zaveri et al., 2014, 2018), mass concentration of aerosol particles (Shiraiwa and Seinfeld, 2012; Yli-Juuti et al., 2017; Kim et al., 2019), ice nucleation efficiency (Murray et al., 2010; Ladino et al., 2014; Knopf et al., 2018), and crystallinity of salts (Murray, 2008; Song et al., 2013; Ji et al., 2017; Wang et al., 2017). Depending on the viscosity, the phase state of aerosol particles can be determined; commonly the dynamic viscosity of a liquid is characterized as being less than 102 Pa s, that of a semi-solid is between 102 and 1012 Pa s, and that of a glassy or crystalline solid is typically greater than 1012 Pa s (Zobrist et al., 2008; Koop et al., 2011; Reid et al., 2018).
Most studies on this topic have focused on the determination of the viscosities and phase state of secondary organic aerosols (SOAs) (Mikhailov et al., 2009; Virtanen et al., 2010; Koop et al., 2011; Vaden et al., 2011; Saukko et al., 2012; Renbaum-Wolff et al., 2013; Hao et al., 2014; Kidd et al., 2014; Bateman et al., 2015; M. Song et al., 2015, 2016, 2019; Athanasiadis et al., 2016; Grayson et al., 2016; Hinks et al., 2016; Hosny et al., 2016; Yli-Juuti et al., 2017; Petters et al., 2019; Wallace and Preston, 2019; Gervasi et al., 2020; Schmedding et al., 2020). Different types of SOAs generated in environmental chambers and flow reactors have been examined. The dynamic viscosities of some types of SOA particles (e.g., generated from toluene and diesel vapors) ranged from ≲ 10−3 to ≳ 108 Pa s, which is approximately equal to the range of viscosities between that of water and tar pitch, depending on the RH, oxidation state, and chemical composition (Mikhailov et al., 2009; Virtanen et al., 2010; Vaden et al., 2011; Koop et al., 2011; Saukko et al., 2012; Renbaum-Wolff et al., 2013a; Hao et al., 2014; Kidd et al., 2014; Bateman et al., 2015; Y. Zhang et al., 2015; Athanasiadis et al., 2016; Grayson et al., 2016, 2017; Hinks et al., 2016; Hosny et al., 2016; Rothfuss and Petters, 2017; Yli-Juuti et al., 2017; Petters et al., 2019; M. Song et al., 2015, 2016, 2019; Gervasi et al., 2020). In addition to the viscosity of SOA, the viscosity effects of inorganic salts should also be investigated to better understand the viscosities and phase states of atmospherically relevant mixed particles.
Only a few studies have investigated the viscosity and phase state of aerosol particles consisting of organic materials and inorganic salts. Power et al. (2013) showed using holographic optical tweezers that the viscosity of sucrose H2O particles is significantly greater than that of NaCl H2O particles at any RH. They also showed that the viscosity of mixed sucrose NaCl H2O particles decreased as the NaCl concentration increased at a certain RH. Rovelli et al. (2019) used a different inorganic salt and observed that the viscosity of sucrose NaNO3 H2O particles decreased as the NaNO3 mass fraction increased at a certain RH. Richards et al. (2020a) reported that inorganic salt in organic and inorganic mixtures increased viscosity by the ion–molecule effect. Based on field measurements using a particle rebound technique, Bateman et al. (2017) showed that the phase state of atmospheric submicron aerosol particles consisting of biogenic organic compounds, sulfate, and black carbon in central Amazonia assumed a non-liquid state (i.e., viscosity > 102 Pa s) even at relatively high RH values during periods of anthropogenic influence. They reported that the aerosol particles influenced by a plume of urban pollution and biomass burning existed in a non-liquid state (viscosity > 102 Pa s). Liu et al. (2019) observed atmospheric submicron aerosol particles in a liquid phase state at RH > 60 % upon the enhancement of the aerosol nitrate fraction in Shenzhen, which is a subtropical coastal urban city in China. Considering the wide variety of complex atmospheric aerosol compositions, more information on the viscosity of mixed organic–inorganic particles is needed.
Using the bead-mobility and poke-and-flow techniques, at 293 ± 1 K, we measured the RH-dependent aerosol viscosities of binary mixtures of organic material H2O and inorganic salts H2O, as well as ternary mixtures of organic material inorganic salts H2O under dehydration conditions. Sucrose was selected as the model organic substance, because previous studies have frequently applied it as a surrogate species for SOA (Zobrist et al., 2011; Power et al., 2013; Grayson et al., 2017; Y. C. Song et al., 2016, 2020; Rothfuss and Petters, 2017; Rovelli et al., 2019). Ca(NO3)2 and Mg(NO3)2 were used as model inorganic salts, because they have been commonly observed in mineral dust particles (Usher et al., 2003; Laskin et al., 2005; Sullivan et al., 2007), and sea-salts (Gupta et al., 2015; Zieger et al., 2017) in the atmosphere (Usher et al., 2003; Laskin et al., 2005; Sullivan et al., 2007; Shi et al., 2008; Song et al., 2010, 2013; Pan et al., 2017). Moreover, both of these nitrate salts have a relatively low efflorescence RH in aqueous solutions, enabling viscosity measurements of crystal-free solutions from high RH down to at least 30 % RH. Using these binary and ternary mixtures, we explore how the viscosities vary as a function of RH and associated aerosol compositions. Such viscosity studies can provide a better knowledge of the physicochemical properties of atmospherically relevant aerosol particles consisting of organic material and inorganic salts.
2.1 Generation of particles
Sucrose (99.5 % purity, Sigma-Aldrich), Ca(NO3)2•4H2O (99.997 % purity, Sigma-Aldrich), and Mg(NO3)2•6H2O (99.99 % purity, Sigma-Aldrich) were purchased. Solutions containing each compound were prepared at 10 wt.% in pure water (resistivity ≥ 18.2 MΩ cm, Merck Millipore Synergy, Germany). For ternary mixtures, solutions were prepared at an organic-to-inorganic dry mass ratio (OIR) of 1:1. This dry mass ratio was chosen since it is expected to reveal well the effects of mixing of substantial amounts of both the organic and salt components on overall water uptake and viscosity. For the bead-mobility and poke-and-flow experiments, the aqueous solutions were nebulized on a hydrophobic substrate (Hampton Research, Canada). After nebulizing, the substrate with droplets was set in a flow cell which was placed below an objective mounted on an optical microscope (Olympus CKX53 with 40× objective) (Pant et al., 2006; Bertram et al., 2011; Song et al., 2012; Ham et al., 2019). The RH in the cell was adjusted by controlling the N2 mixing ratio of the dry and wet gas flows. The RH in the flow cell was continuously monitored by a digital humidity sensor (Sensirion, SHT C3, Switzerland). The RH reading from the flow cell was calibrated by measurement and comparison to the deliquescence RH (DRH) at 293 ± 1 K of saturated aqueous solutions of ammonium sulfate (80.5 %), sodium chloride (76.0 %), and potassium carbonate (44.0 %) (Winston and Bates, 1960). The uncertainty of the RH of the humidified N2 flow was ± 1.5 % RH based on the calibration tests. At the initiation of the bead-mobility and poke-and-flow experiments, the RH was maintained at ∼ 85 %, and the particles equilibrated for ∼ 20 min. Then, the RH was reduced to the target RH (∼ 80 % to ∼ 0 % RH) and equilibrated for ∼ 30 min for the bead-mobility experiments and for ≳ 2 h for the poke-and-flow experiments to give sufficient time for reaching equilibrium with the surrounding water vapor (Grayson et al., 2015, Song et al., 2019; Maclean et al., 2021). At a given RH, particles that were 20–100 µm in diameter were selected for the optical observation. All viscosity measurements were carried out at a temperature of 293 ± 1 K.
2.2 Bead-mobility technique
The bead-mobility technique was utilized, which can quantify a range of viscosities from ∼ 10−3 to ∼ 103 Pa s (Renbaum-Wolff et al., 2013b). At the beginning of the bead-mobility experiments, insoluble melamine beads (cat. no. 86296, Sigma-Aldrich), which were ∼ 1 µm in diameter and dispersed in pure water, were incorporated directly into the droplets deposited on the hydrophobic substrate. Aerosol particles were then placed into the flow cell, as described in Sect. 2.1. The total gas flow in the cell was 1200 sccm for the bead-mobility experiments. The continuous gas flow generates a shear stress on a particle's surface, which yields internal circulations of the beads in the particles during measurements. The movement of the beads at 293 ± 1 K was recorded every 1 s with a CMOS camera (MSC-M 3.0 UCMOS, China) and then quantified at a target RH. The viscosity was calculated from the bead speeds using a calibration line, which produced bead speeds for the sucrose particles at different RH values (see Fig. S1 in the Supplement). Figure S2 in the Supplement illustrates the mean bead speeds of each system as a function of the RH. When the bead speeds within a particle are too slow to be observed with this technique (i.e., below ∼ 10−6 µm m s−1 corresponding to ∼ 102 Pa s; see Figs. S1 and S2), we used the poke-and-flow technique (Sect. 2.3). For example, the movement of the beads within Mg(NO3)2 H2O particles at ∼ 35 % RH was too slow to be readily observed (Fig. S2). Further information on the calibration and the bead-mobility technique is given in Sect. S1 in the Supplement.
2.3 Poke-and-flow technique
Murray et al. (2012) developed the poke-and-flow technique for phase state determination, which was expanded for the quantification of viscosities ≳ 102 Pa s by Renbaum-Wolff et al. (2013a), Grayson et al. (2015), and Song et al. (2015). This technique uses a small hole on the top of the flow cell to allow us to poke the particles on the hydrophobic substrate using a sterilized sharp needle (Jung Rim Medical Industrial Co., South Korea). Using a micromanipulator (Narishige, model MO-152, Japan), the needle was controlled in the x, y, and z directions. The needle was passed through from the top to the bottom of a particle and then the needle was removed. After poking, the geometrical change of a deposited particle was observed and recorded by an optical microscope (Olympus CKX53 with 40× objective) with a CCD camera (MSC-M 3.0 UCMOS, China). All experiments were carried out at 293 ± 1 K.
Figure S3 in the Supplement shows an example of the geometrical changes in the sucrose H2O, sucrose Ca(NO3)2 H2O, and sucrose Mg(NO3)2 H2O particles during pre-poking, poking, and post-poking. Before poking by the needle, the particles had a geometry of a spherical cap. After poking by a needle, a half-torus geometry with an inner hole in the particle was observed. As time progressed, the particle recovered by adopting its original geometry by filling of the hole to minimize the surface energy. The experimental flow time (τ(exp, flow)) was obtained when the area of the inner hole just after poking (t = 0 s. Fig. S3) decreased to of the initial inner hole area (Renbaum-Wolff et al., 2013a; Grayson et al., 2015, 2016; Song et al., 2019). Figure S4 in the Supplement shows the τ(exp, flow) at different RH values for sucrose H2O, sucrose Mg(NO3)2 H2O, and sucrose Ca(NO3)2 H2O particles. The τ(exp, flow) of the particles was converted to the lower limit to the viscosity using the equation reported in Sellier et al. (2015). Using the poke-and-flow technique, the upper limit to viscosity is unknown in this work. For binary mixtures of inorganic salts H2O, we were unable to determine the viscosity between 102 to 108 Pa s because the poke-and-flow technique is not accessible for droplets that are supersaturated with respect to a crystalline state of involved inorganic salts. We also measured the RH value and corresponding viscosity at which the particles cracked. When the particles cracked upon poking, without any detectable flow after a duration of > 5 h at a given RH, we defined a lower limit to the viscosity of 1 × 108 Pa s based on the results of Renbaum-Wolff et al. (2013a), Grayson et al. (2015, 2016), and Song et al. (2019). Further details on these experiments can be found in Sect. S2 of the Supplement.
2.4 AIOMFAC-VISC model
The Aerosol Inorganic–Organic Mixtures Functional groups Activity Coefficients Viscosity model (AIOMFAC-VISC) is a thermodynamics-based group-contribution model for predicting the viscosity of aqueous organic mixtures (Gervasi et al., 2020). It is an extension module to the AIOMFAC model, which explicitly describes interactions among organic functional groups, inorganic ions, and water (Zuend et al., 2008, 2011). The AIOMFAC-VISC model offers predictive estimates of mixture viscosity covering the range from liquid-like low-viscosity aqueous solutions to highly viscous organic-rich amorphous solutions in the atmospherically relevant temperature range. An online version of AIOMFAC (AIOMFAC-web), which includes viscosity predictions for aqueous organic mixtures, can be run at https://aiomfac.lab.mcgill.ca (last access: 2 July 2021).
Aside from the focus on the viscosity effects of organic compounds, in recent work AIOMFAC-VISC has been further developed to enable the prediction of the viscosity of aqueous electrolyte solutions and aqueous inorganic–organic mixtures; with details provided elsewhere (Lilek and Zuend, 2021). Briefly, a semi-empirical approach was introduced to predict the mixture viscosity of aqueous electrolyte solutions as a function of temperature, ion activities, and ionic strength. Model parameters for the aqueous ion interaction approach were simultaneously fitted to room temperature viscosity measurements aggregated by Laliberté (2007, 2009) for many electrolyte solutions. The training dataset for AIOMFAC-VISC did not include measurements for the nitrate salts investigated in this study. For aqueous electrolyte solutions, AIOMFAC-VISC predictions currently achieve an excellent level of accuracy, comparable to that of the fitted expressions by Laliberté (2007). Furthermore, to more accurately capture the water uptake behavior of sucrose as a function of equilibrium RH (i.e., water activity of the solution), the version of AIOMFAC-VISC used to produce the viscosity predictions for the sucrose-containing systems in this work includes an improved treatment of the ether-group–water interactions of sucrose. Thus, viscosity predictions in this study for aqueous sucrose differ slightly from those of the AIOMFAC-web model version.
For the viscosities of ternary mixtures, a Zdanovskii–Stokes–Robinson (ZSR) type mixing rule is applied to predict the viscosity of the ternary mixtures of organic material inorganic salts H2O. This mixing rule is here adopted for viscosity applications since the AIOMFAC-VISC model does presently not include explicit ion–organic interaction effects on viscosity (only on activity coefficients). Therefore, a mixing rule is required to combine the predictions of the viscosity contributions from the electrolyte-free subsystem (sucrose H2O) and those from the organic-free aqueous electrolyte subsystem for the viscosity estimation of the full mixture. Generally, the ZSR approach involves combining some physical property of two or more (binary) mixtures at the same RH, often to determine the water content of the whole multicomponent mixture (Zdanovskii, 1936, 1948; Stokes and Robinson, 1966). Following the experimental conditions, the AIOMFAC-VISC predictions for the ternary systems use an OIR of 1:1, which constrains at each RH level the fractional contributions of mass (f1, f2) from each of the subsystems, sucrose H2O (1) or salt H2O (2). The viscosity of the overall mixture is then obtained as , with denoting unit viscosity (1 Pa s); see details in Sect. S5 in the Supplement.
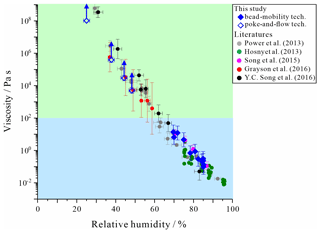
Figure 1Measured dynamic viscosities of sucrose H2O particles compared with previous studies at 293 K (Hosny et al., 2013, fluorescent lifetime imaging; Power et al., 2013, and Y. C. Song et al., 2016, aerosol optical tweezers; Grayson et al., 2015, and Song et al., 2015, bead-mobility and poke-and-flow techniques) and this study. The error bars in relative humidity (RH) were generated from the calibrated RH uncertainty and the standard deviation from grouped viscosity data (grouped by RH ranges), which included at least three data points. The error bars in viscosities were produced by 95 % prediction bands of viscosities (Fig. S1). Upward arrows represent lower limit to the viscosities of the particles that calculated by the experimental flow time and the equation reported in Sellier et al. (2015). Details are described in Fig. S2. Light blue and green regions indicate liquid and semi-solid regions, respectively.
3.1 Viscosities of particles consisting of sucrose H2O
Figure 1 shows the RH-dependent viscosities of sucrose H2O particles obtained using the bead-mobility and poke-and-flow techniques. The viscosities of sucrose H2O particles were determined to be between ∼ 2 × 10−1 and ∼ 1 × 101 Pa s for RH values of ∼ 85 %–69 % and between ∼ 5 × 103 and ∼ 2 × 106 Pa s for RH values of ∼ 50 %–37 % (Fig. 1). The particles containing sucrose H2O cracked at ∼ 23 % RH when poked with a needle, and restorative flow did not occur over a time span of 5 h (Fig. 2a). Consequently, only a lower limit for the viscosity of ∼ 108 Pa s was obtained (Renbaum-Wolff et al., 2013a; Grayson et al., 2015, 2016; Song et al., 2015a, 2019). Figure 1 also includes results from previous studies for the viscosities of sucrose H2O particles using different techniques such as bead-mobility, poke-and-flow, holographic optical tweezers, and fluorescence lifetime imaging microscopy (Hosny et al., 2013, Power et al. 2013, Grayson et al., 2015, Song et al., 2015; Y. C. Song et al., 2016). As shown in Fig. 1, the viscosities for the sucrose H2O particles from this study and previous studies are consistent within ∼ 1 order of magnitude at given RH values. Using the entire dataset, it suggests that the sucrose H2O particle are in a liquid phase state for RH ≳65 %, in a semi-solid phase state for ∼ 25 % < RH ≲ 65 %, and in a semi-solid or solid phase state for RH ≲ 23 %.
3.2 Viscosities of particles consisting of Mg(NO3)2 H2O or Ca(NO3)2 H2O
Despite the abundant mass of inorganic species in the atmosphere (Jimenez et al., 2009; Cheng et al., 2016), few studies have reported the viscosity of single particles consisting of inorganic species covering the RH range to high salt concentrations (Power et al., 2013; Rovelli et al., 2019). Herein, the RH-dependent viscosity was quantified for particles consisting of Mg(NO3)2 H2O or Ca(NO3)2 H2O determined at 293 ± 1 K upon dehydration using the bead-mobility and poke-and-flow techniques.
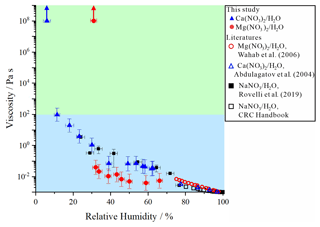
Figure 3Viscosities of inorganic salts containing Ca(NO3)2 H2O and Mg(NO3)2 H2O, as well as previous results (Abdulagatov et al., 2004; Wahab et al., 2006; Haynes, 2015; bulk measurements) and this study. Also, viscosities of NaNO3 H2O from previous studies are included (CRC Handbook; Rovelli et al., 2019, aerosol optical tweezers). Upward arrows represent a lower limit for the viscosities of the particles calculated by the experimental flow time and the equation reported in Sellier et al. (2015). The error bars in relative humidity (RH) were generated from combined RH sensor uncertainty and the standard deviation from the grouped viscosity data by RH, which included at least three data points. The error bars in viscosities were produced by 95 % prediction bands of viscosities (Fig. S1). Light blue region indicates a liquid phase, and light green region indicates a semi-solid phase.
Figure 3 illustrates the RH-dependent viscosities of Mg(NO3)2 H2O or Ca(NO3)2 H2O particles upon dehydration. The obtained viscosities of the Mg(NO3)2 H2O particles varied from ∼ 6 × 10−3 to ∼ 4 × 10−2 Pa s for RH values from ∼ 70 % to ∼ 35 %, whereas the values for Ca(NO3)2 H2O ranged from ∼ 3 × 10−2 to ∼ 9 × 101 Pa s for RH values from ∼ 65 % to ∼ 10 %. Previous studies have reported the viscosities of Ca(NO3)2 H2O and Mg(NO3)2 H2O solutions only at high RH values based on bulk solution measurements (Fig. 3) (Abdulagatov et al., 2004; Wahab et al., 2006). The values of the viscosities of the particles at high RH are consistent with our results within ∼ 1 order magnitude despite a limited number of data points due to the solubility limit restricting bulk solution measurements to the RH range above 70 %. In addition, Fig. 3 shows the viscosities of NaNO3 H2O particles measured by other groups (Haynes, 2015; Rovelli et al., 2019). The viscosities of the NaNO3 H2O particles were similar to those of the Ca(NO3)2 H2O particles for the RH range from ∼ 30 % to ∼ 100 %.
During the poke-and-flow experiments, the Mg(NO3)2 H2O or Ca(NO3)2 H2O particles cracked when poked with a needle at RH values of 30 % and 5 %, respectively. At these RH levels, noticeable restorative flow did not occur for over 5 h (Fig. 2b and c), which resulted in a lower estimated limit for the viscosity (∼ 108 Pa s) at the given RH values. The RH value where the particles shattered is similar to the efflorescence RH (ERH) of Mg(NO3)2 H2O. The ERH of Mg(NO3)2 H2O is known to be ∼ 30 % at 298 K (Li et al., 2008; Wang et al., 2015b) and RH of crystallization for Ca(NO3)2 H2O is reported to be ∼ 7 % at 298 K (Liu et al., 2008). In this study, we optically observed an ERH of 32.0 ± 2.5 % for Mg(NO3)2 H2O particles (i.e., Fig. S5b), but we did not optically observe the ERH of Ca(NO3)2 H2O at 293 ± 1 K with decreasing RH (Fig. S5c). Liu et al. (2008) also observed optically no efflorescence point for Ca(NO3)2 H2O particles down to 0 % RH using an optical microscope, but they confirmed the crystallization for Ca(NO3)2 H2O particles at RH of ∼ 7 % by Raman spectroscopy at 298 K. The RH values at which shattering of particles occurred for the binary inorganic salt particles of Mg(NO3)2 H2O or Ca(NO3)2 H2O were close to their reported ERH values and/or the RH of crystallization. When comparing the two inorganic salts, Ca(NO3)2 H2O particles showed slightly higher viscosities than the Mg(NO3)2 H2O particles, i.e., approximately 1 order of magnitude higher at equivalent RH for the RH range from ∼ 60 % to 30 % (Fig. 3). The difference in viscosities is likely due to the higher hygroscopicity of dissolved Mg(NO3)2 compared to Ca(NO3)2 (Guo et al., 2019). The Ca(NO3)2 H2O particles were a liquid phase state at RH ≳ 10 % and a semi-solid or solid phase state at RH ≲ 5 %. The Mg(NO3)2 H2O particles were a liquid phase state at RH ≳ 35 % and a semi-solid or solid phase state at RH ≲ 30 %. Based on the viscosity measurement, both inorganic particles underwent a phase change from liquid (< 102 Pa s) to semi-solid or solid (> 102 Pa s ) within a narrow RH range (Fig. 3) compared to the sucrose H2O particles (Fig. 1). Indeed, this discontinuity in viscosity with decreasing RH suggests a phase transition.
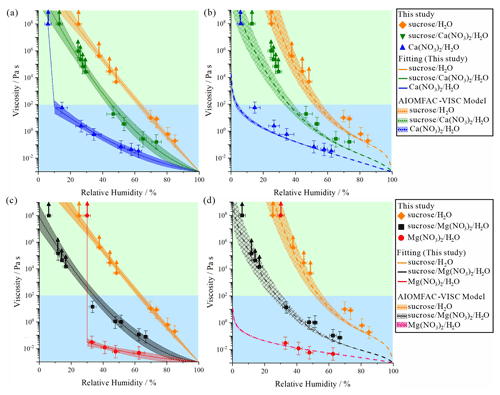
Figure 4Comparison of viscosities of binary and ternary systems obtained from the bead-mobility and poke-and-flow experiments. (a) Mean viscosities of sucrose H2O, Ca(NO3)2 H2O, and sucrose Ca(NO3)2 H2O particles with polynomial fits from measured viscosities for an organic-to-inorganic mass ratio (OIR) of 1:1. All data at a temperature of 293 ± 1 K, (b) Mean viscosities of sucrose H2O, Ca(NO3)2 H2O (grouped by relative humidity (RH) using data from Fig. 3), and sucrose Ca(NO3)2 H2O particles with AIOMFAC-VISC model predictions, (c) mean viscosities of sucrose H2O, Mg(NO3)2 H2O (grouped by RH using data from Fig. 3), and sucrose Mg(NO3)2 H2O particles with polynomial fits from measured viscosities for an OIR of 1:1, (d) mean viscosities of sucrose H2O, Mg(NO3)2 H2O, and sucrose Mg(NO3)2 H2O particles with AIOMFAC-VISC model predictions. The error bars in RH were generated from the RH uncertainty and the standard deviation of individual data grouped by RH. The error bars in viscosity represent grouped viscosity by RH including at least three data points. Symbols marked with upward arrows represent lower limits of the viscosities of the particles calculated based on the experimental flow time period and the equation reported in Sellier et al. (2015). Fitting (solid line curves and shaded areas) from measured viscosities are based on second-order polynomial curve fits with 95 % confidence bands. Predictions (dash line curves with checker shaded areas) are based on from AIOMFAC-VISC model. The parameterizations are described in Sect. S3 and Table S1 in the Supplement. Light blue and green regions represent liquid and semi-solid phase state ranges, respectively.
3.3 Viscosities of particles consisting of sucrose Ca(NO3)2 H2O or sucrose Mg(NO3)2 H2O
To explore the viscosity of more atmospherically relevant aerosol particle configurations, we investigated ternary systems containing sucrose mixed with either Ca(NO3)2 or Mg(NO3)2 for OIR of 1:1 at 293 ± 1 K. Shown in Fig. 4 are the viscosities for the sucrose Ca(NO3)2 H2O (Fig. 4a) and sucrose Mg(NO3)2 H2O particles (Fig. 4c) upon dehydration using the bead-mobility and the poke-and-flow measurement techniques.
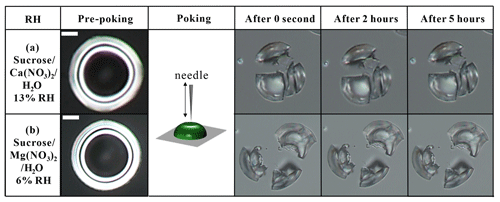
Figure 5Optical images during poke-and-flow experiments at the points of pre-poking, poking, and post-poking for particles consisting of (a) sucrose Ca(NO3)2 H2O particles and (b) sucrose Mg(NO3)2 H2O particles for relative humidity (RH) where the particles shattered. The scale bar indicates 5 µm.
The viscosities of the sucrose Ca(NO3)2 H2O particles ranged from ∼ 3 × 101 to ∼ 3 × 10−2 Pa s at ∼ 45 < RH ≲ 80 % and from ∼ 9 × 105 to ∼ 3 × 104 Pa s at ∼ 25 < RH ≲ 30 % (Fig. 4a). At ∼ 13 % RH, when poked with a needle, the sucrose Ca(NO3)2 H2O particles cracked. Thereafter, the particles did not exhibit any notable flow behavior for over 5 h (Fig. 5a), producing a lower limit for the viscosity of ∼ 108 Pa s. This result indicates that the sucrose Ca(NO3)2 H2O particles were in a liquid phase state at RH ≳ 45 %, in a semi-solid state at ∼ 25 % < RH ≲ 30 %, and in a semi-solid or solid state for RH ≲ 13 %.
For sucrose Mg(NO3)2 H2O particles, the viscosities ranged from ∼ 7 × 10−2 to ∼ 1 × 101 Pa s for RH values ranging from ∼ 70 % to ∼ 35 % and from ∼ 2 × 104 to ∼ 2 × 105 Pa s for the RH ranging from ∼ 17 % to ∼ 11 % (Fig. 4c). The sucrose Ca(NO3)2 H2O particles cracked at ∼ 6 % RH without flow over 5 h (Fig. 5b); and thus the lower limit of the viscosity was determined to be ∼ 108 Pa s. These results imply that the sucrose Ca(NO3)2 H2O particles are in a liquid phase state at RH ≳ 35 %, a semi-solid phase state for 17 % < RH < 11 %, and a semi-solid or solid phase state at RH ≲ 6 %. As shown in Fig. 4a and c, the viscosities of the sucrose Mg(NO3)2 H2O particles are approximately 1 order of magnitude lower at 50 % RH and ∼ 6 orders of magnitude lower at 35 % RH than the sucrose Ca(NO3)2 H2O particles over the same RH range. Both particles experienced a phase state change from a liquid phase state to a semi-solid or even solid phase state with decreasing RH. Finally, although not confirmed by our measurements, it is possible that in one or both of these ternary mixtures a gel phase transition may occur upon sufficient dehydration, as has been observed in mixtures of gluconic acid with CaCl2 (Richards et al., 2020a, b).
3.4 Model–measurement comparison of viscosity
Figure 4b and d show the model–measurement comparison of the RH-dependent viscosities of the binary and ternary systems. For the viscosities of sucrose H2O, the viscosities from the AIOMFAC-VISC model prediction agreed with the measurements within ∼ 1 order of magnitude. For the viscosities of Ca(NO3)2 H2O and Mg(NO3)2 H2O, the viscosity prediction from the AIOMFAC-VISC model showed a good agreement until the measured inorganic salt viscosities change steeply, indicating crystallization or another phase transition that the model ignores. When comparing AIOMFAC-VISC viscosity predictions to the measurements in this study, note that AIOMFAC-VISC assumes that mixtures remain in a metastable state to low water activity (or high solution concentration) and that solutes do not crystallize, which may explain the discrepancy among the model predictions and the measurements in the low RH region shown in Fig. 4b and d. Moreover, we note that the training of the AIOMFAC-VISC electrolyte solution model parameters did not include data from this study. The model predictions at RH levels below ∼ 20 % represent extrapolations of the model beyond the range of experimental data used in its training.
In AIOMFAC-VISC, the prediction of the temperature-dependent pure-component viscosity is based on the experimentally determined, yet uncertain, glass transition RH. As such, we include error thresholds of ± 5 % in the pure-component glass transition RH in Fig. 4 (Gervasi et al., 2020). Such uncertainty estimates are not necessary for aqueous electrolyte mixtures, so the AIOMFAC-VISC model sensitivity is shown instead, which is defined as the viscosity change due to a ± 2 % change in the mass fraction of water of the solution, while the ratio of the other components is preserved (Gervasi et al., 2020). The uncertainty in the aqueous sucrose viscosity dominates the modeled uncertainty of viscosity estimates for the ternary mixtures. For the viscosities of ternary sucrose Ca(NO3)2 H2O or sucrose Mg(NO3)2 H2O solutions, AIOMFAC-VISC viscosity predictions show agreement with the measurements within about 1 order of magnitude from high RH to about 30 % RH (considering measurement uncertainty). In the case of the ternary sucrose Ca(NO3)2 H2O system, the model–measurement deviation increases to about 1.5 orders of magnitude in viscosity at 30 % RH and lower, with AIOMFAC-VISC underestimating the measured viscosity. This result may be explained at least in part by the model predicting substantially lower viscosities for the binary aqueous Ca(NO3)2 system in this lower RH range, which affects the predictions for the ternary system via the deployed mixing rule. The good agreement between model and measurements for the ternary sucrose Mg(NO3)2 H2O system, even at low RH levels, may be interpreted as indicative of suppressed salt crystallization in the presence of sucrose, since no discontinuities in viscosity are observed (in contrast to the measurements for the binary salt particles).
Herein, we measured the RH-dependent viscosities at 293 ± 1 K for particles consisting of organic material H2O, inorganic salt H2O, and organic material inorganic salt H2O upon dehydration using the bead-mobility and poke-and-flow techniques. We selected sucrose as the organic species as previous studies have frequently applied it as a surrogate species of SOA, and this organic offers favorable properties for measurements. Ca(NO3)2 and Mg(NO3)2 were selected as the inorganic salts for viscosity measurements as these inorganic salts have been frequently observed from mineral dust and sea salt particles (Usher et al., 2003; Laskin et al., 2005; Sullivan et al., 2007). For the binary mixtures, the obtained viscosity of the sucrose H2O particles agreed well with those reported in previous studies, i.e., mixture viscosities < 102 Pa s at RH ≳ 65 %, which corresponds to a liquid phase state; mixture viscosities of ∼ 102 to 108 Pa s at RH values between ∼ 65 % and ∼ 25 %, which correspond to a semi-solid phase state; and mixture viscosities ≳108 Pa s at RH ≲ 25 %, which correspond to semi-solid or amorphous (or crystalline) solid phase states (Power et al., 2013; Grayson et al., 2017; Y. C. Song et al., 2016; Rothfuss and Petters, 2017; Rovelli et al., 2019). Upon dehydration, we also quantified the viscosities of the inorganic salts. The viscosities of the Mg(NO3)2 H2O particles were ≲ 4 × 10−2 Pa s for RH ≳ 35 % and ≳ 108 Pa s for RH ≲ 31 %, whereas those of the Ca(NO3)2 H2O particles were ≲ 9 × 100 Pa s for RH ≳ 10 % and ≳ 108 Pa s for RH ≲ 10 %. The particles containing either of these two inorganic salts cracked upon poking when the RH reached a value near the salt's ERH and/or the phase transition RH from a droplet to a solid phase state. These inorganic particles exhibited a sudden enhancement in the viscosity when the particle effloresced. In contrast, sucrose H2O particles showed a smooth enhancement in the viscosity with decreasing RH; this means that the viscosity of sucrose H2O particles gradually approached their glass transition RH. The AIOMFAC-VISC model prediction and viscosity measurements showed a good agreement within ∼ 1 order of magnitude, especially at RH levels above 30 %, where applicable. For sucrose Ca(NO3)2 H2O particles and sucrose Mg(NO3)2 H2O, predicted and measured viscosities showed good agreement over the whole RH range.
The phase states of aerosol particles have an impact on rate and potential for heterogeneous reactions as well as consequences for the resulting mass concentration of aerosol particles. The uptake coefficient of gas-phase oxidants depends on the phase states of aerosol particles (George and Abbatt, 2010; Xiao and Bertram, 2011; Kuwata and Martin, 2012; Slade and Knopf, 2014; Davies and Wilson, 2015; Berkemeier et al., 2016; Li et al., 2020; Xu et al., 2020). For example, ozone uptake coefficients (γO3) decreased by an order of magnitude in a semi-solid phase state compared to a liquid phase state (Steimer et al., 2015). Moreover, the effective mass concentration of aerosol particles can depend on the (assumed or modeled) phase state of aerosol particles (Shiraiwa and Seinfeld, 2012; Yli-Juuti et al., 2017; Kim et al., 2019). If a liquid aerosol phase state is assumed, the mass concentration may be overpredicted by up to 1 order of magnitude (Shiraiwa and Seinfeld, 2012). Based on the measurements and calculations, our results show that the studied aerosol particles consisting of organic material inorganic salt H2O range from liquid to semi-solid or solid phase states depending on the RH. A caveat is that a single OIR of 1:1 and relatively simple aerosol systems were used compared to the multicomponent, multiphase particles likely occurring in the real atmosphere (Murphy et al., 2006; Jimenez et al., 2009; Song et al., 2010, 2013; Huang et al., 2015; Cheng et al., 2016). Further studies are needed to confirm the equilibrating timescales of mixed organic–inorganic particles under ambient conditions, including the timescale for the equilibration of semivolatile organics and inorganics. Added complexity in particle morphology, such as phase separation, can also influence the equilibration timescale (e.g., Huang et al., 2021); however, our systems did not exhibit phase separation for the studied mixing ratios. Additional investigations are required to further explore and quantify how the viscosities and phase states of mixed organic–inorganic particles vary with the OIR, temperature, and functional group complexity.
Underlying material and related data for this paper are provided in the Supplement.
The supplement related to this article is available online at: https://doi.org/10.5194/acp-21-10215-2021-supplement.
MS and YCS designed this study. MS and JBL setup and calibrated the viscosity instrument. YCS and MS conducted viscosity experiments and analyzed the data. JL and AZ conducted AIOMFAC-VISC model predictions. YCS and MS prepared the article with contributions from JL, JBL, AZ, ZJ, and MNC.
The authors declare that they have no conflict of interest.
Publisher's note: Copernicus Publications remains neutral with regard to jurisdictional claims in published maps and institutional affiliations.
Mijung Song gives thanks to Dongwan Ham for the technical support.
This work was supported by the National Research Foundation of Korea (NRF) grant funded by the Korean government (MSIT) (grant no. NRF-2019R1A2C1086187) and by the Fine Particle Research Initiative in East Asia Considering National Differences (FRIEND) project (2020M3G1A1114548). This project was undertaken with the financial support of the government of Canada through the federal Department of Environment and Climate Change (grant no. GCXE20S049). This work was also supported by Alfred P. Sloan Foundation under Prime Award no. G-2020-13912 and the Regents of the University of California.
This paper was edited by Daniel Knopf and reviewed by two anonymous referees.
Abdulagatov, I. M., Zeinalova, A. A., and Azizov, N. D.: Viscosity of the aqueous Ca(NO3)2 solutions at temperatures from 298 to 573 K and at pressures up to 40 MPa, J. Chem. Eng. Data, 49, 1444–1450, https://doi.org/10.1021/je049853n, 2004.
Athanasiadis, A., Fitzgerald, C., Davidson, N. M., Giorio, C., Botchway, S. W., Ward, A. D., Kalberer, M., Pope, F. D., and Kuimova, M. K.: Dynamic viscosity mapping of the oxidation of squalene aerosol particles, Phys. Chem. Chem. Phys., 18, 30385–30393, https://doi.org/10.1039/C6CP05674A, 2016.
Bateman, A. P., Bertram, A. K., and Martin, S. T.: Hygroscopic influence on the semisolid-to-liquid transition of secondary organic materials, J. Phys. Chem. A, 119, 4386–4395, https://doi.org/10.1021/jp508521c, 2015.
Bateman, A. P., Gong, Z., Harder, T. H., de Sá, S. S., Wang, B., Castillo, P., China, S., Liu, Y., O'Brien, R. E., Palm, B. B., Shiu, H.-W., Cirino, G. G., Thalman, R., Adachi, K., Alexander, M. L., Artaxo, P., Bertram, A. K., Buseck, P. R., Gilles, M. K., Jimenez, J. L., Laskin, A., Manzi, A. O., Sedlacek, A., Souza, R. A. F., Wang, J., Zaveri, R., and Martin, S. T.: Anthropogenic influences on the physical state of submicron particulate matter over a tropical forest, Atmos. Chem. Phys., 17, 1759–1773, https://doi.org/10.5194/acp-17-1759-2017, 2017.
Berkemeier, T., Steimer, S. S., Krieger, U. K., Peter, T., Pöschl, U., Ammann, M., and Shiraiwa, M.: Ozone uptake on glassy, semi-solid and liquid organic matter and the role of reactive oxygen intermediates in atmospheric aerosol chemistry, Phys. Chem. Chem. Phys., 18, 12662–12674, 2016.
Bertram, A. K., Martin, S. T., Hanna, S. J., Smith, M. L., Bodsworth, A., Chen, Q., Kuwata, M., Liu, A., You, Y., and Zorn, S. R.: Predicting the relative humidities of liquid-liquid phase separation, efflorescence, and deliquescence of mixed particles of ammonium sulfate, organic material, and water using the organic-to-sulfate mass ratio of the particle and the oxygen-to-carbon elemental ratio of the organic component, Atmos. Chem. Phys., 11, 10995–11006, https://doi.org/10.5194/acp-11-10995-2011, 2011.
Bhattarai, G., Lee, J. B., Kim, M. H., Ham, S., So, H. S., Oh, S., Sim, H. J., Lee, J. C., Song, M., and Kook, S. H.: Maternal exposure to fine particulate matter during pregnancy induces progressive senescence of hematopoietic stem cells under preferential 30 impairment of the bone marrow microenvironment and aids development of myeloproliferative disease, Leukemia 34, 1481–1484, https://doi.org/10.1038/s41375-019-0665-8, 2020
Cheng, Z., Luo, L., Wang, S., Wang, Y., Sharma, S., Shimadera, H., Wang, X., Bressi, M., de Miranda, R. M., Jiang, J., Zhou, W., Fajardo, O., Yan, N., and Hao, J.: Status and characteristics of ambient PM2.5 pollution in global megacities, Environ. Int., 89–90, 212–221, https://doi.org/10.1016/j.envint.2016.02.003, 2016.
Davies, J. F. and Wilson, K. R.: Nanoscale interfacial gradients formed by the reactive uptake of OH radicals onto viscous aerosol surfaces, Chem. Sci., 6, 7020–7027, https://doi.org/10.1039/C5SC02326B, 2015.
George, I. J. and Abbatt, J. P. D.: Heterogeneous oxidation of atmospheric aerosol particles by gas-phase radicals, Nat. Chem., 2, 713–722, 2010.
Gervasi, N. R., Topping, D. O., and Zuend, A.: A predictive group-contribution model for the viscosity of aqueous organic aerosol, Atmos. Chem. Phys., 20, 2987–3008, https://doi.org/10.5194/acp-20-2987-2020, 2020.
Grayson, J. W., Song, M., Sellier, M., and Bertram, A. K.: Validation of the poke-flow technique combined with simulations of fluid flow for determining viscosities in samples with small volumes and high viscosities, Atmos. Meas. Tech., 8, 2463–2472, https://doi.org/10.5194/amt-8-2463-2015, 2015.
Grayson, J. W., Zhang, Y., Mutzel, A., Renbaum-Wolff, L., Böge, O., Kamal, S., Herrmann, H., Martin, S. T., and Bertram, A. K.: Effect of varying experimental conditions on the viscosity of α-pinene derived secondary organic material, Atmos. Chem. Phys., 16, 6027–6040, https://doi.org/10.5194/acp-16-6027-2016, 2016.
Grayson, J. W., Evoy, E., Song, M., Chu, Y., Maclean, A., Nguyen, A., Upshur, M. A., Ebrahimi, M., Chan, C. K., Geiger, F. M., Thomson, R. J., and Bertram, A. K.: The effect of hydroxyl functional groups and molar mass on the viscosity of non-crystalline organic and organic–water particles, Atmos. Chem. Phys., 17, 8509–8524, https://doi.org/10.5194/acp-17-8509-2017, 2017.
Guo, L., Gu, W., Peng, C., Wang, W., Li, Y. J., Zong, T., Tang, Y., Wu, Z., Lin, Q., Ge, M., Zhang, G., Hu, M., Bi, X., Wang, X., and Tang, M.: A comprehensive study of hygroscopic properties of calcium- and magnesium-containing salts: implication for hygroscopicity of mineral dust and sea salt aerosols, Atmos. Chem. Phys., 19, 2115–2133, https://doi.org/10.5194/acp-19-2115-2019, 2019.
Gupta, D., Eom, H.-J., Cho, H.-R., and Ro, C.-U.: Hygroscopic behavior of NaCl–MgCl2 mixture particles as nascent sea-spray aerosol surrogates and observation of efflorescence during humidification, Atmos. Chem. Phys., 15, 11273–11290, https://doi.org/10.5194/acp-15-11273-2015, 2015.
Ham, S., Babar, Z. B., Lee, J. B., Lim, H.-J., and Song, M.: Liquid–liquid phase separation in secondary organic aerosol particles produced from α-pinene ozonolysis and α-pinene photooxidation with/without ammonia, Atmos. Chem. Phys., 19, 9321–9331, https://doi.org/10.5194/acp-19-9321-2019, 2019.
Hao, L. Q., Kortelainen, A., Romakkaniemi, S., Portin, H., Jaatinen, A., Leskinen, A., Komppula, M., Miettinen, P., Sueper, D., Pajunoja, A., Smith, J. N., Lehtinen, K. E. J., Worsnop, D. R., Laaksonen, A., and Virtanen, A.: Atmospheric submicron aerosol composition and particulate organic nitrate formation in a boreal forestland–urban mixed region, Atmos. Chem. Phys., 14, 13483–13495, https://doi.org/10.5194/acp-14-13483-2014, 2014.
Haynes, W. M.: CRC handbook of chemistry and physics, 97rd edn., CRC Press, New York, 2015.
Hinks, M. L., Brady, M. V, Lignell, H., Song, M., Grayson, J. W., Bertram, A. K., Lin, P., Laskin, A., Laskin, J., and Nizkorodov, S. A.: Effect of viscosity on photodegradation rates in complex secondary organic aerosol materials, Phys. Chem. Chem. Phys., 18, 8785–8793, https://doi.org/10.1039/c5cp05226b, 2016.
Hosny, N. A., Fitzgerald, C., Tong, C., Kalberer, M., Kuimova, M. K., and Pope, F. D.: Fluorescent lifetime imaging of atmospheric aerosols: a direct probe of aerosol viscosity, Faraday Discuss., 165, 343–356, https://doi.org/10.1039/C3FD00041A, 2013.
Hosny, N. A., Fitzgerald, C., Vysniauskas, A., Athanasiadis, T., Berkemeier, T., Uygur, N., Pöschl, U., Shiraiwa, M., Kalberer, M., Pope, F. D., and Kuimova, M. K.: Direct imaging of changes in aerosol particle viscosity upon hydration and chemical aging, Chem. Sci., 7, 1357–1367, https://doi.org/10.1039/C5SC02959G, 2016.
Huang, R. J., Zhang, Y., Bozzetti, C., Ho, K. F., Cao, J. J., Han, Y., Daellenbach, K. R., Slowik, J. G., Platt, S. M., Canonaco, F., Zotter, P., Wolf, R., Pieber, S. M., Bruns, E. A., Crippa, M., Ciarelli, G., Piazzalunga, A., Schwikowski, M., Abbaszade, G., Schnelle-Kreis, J., Zimmermann, R., An, Z., Szidat, S., Baltensperger, U., El Haddad, I., and Prévôt, A. S. H.: High secondary aerosol contribution to particulate pollution during haze events in China, Nature, 514, 218–222, https://doi.org/10.1038/nature13774, 2015.
Huang, Y., Mahrt, F., Xu, S., Shiraiwa, M., Zuend, A., and Bertram, A. K.: Coexistence of three liquid phases in individual atmospheric aerosol particles, P. Natl. Acad. Sci. USA, 118, e2102512118, https://doi.org/10.1073/pnas.2102512118, 2021.
Ji, Z. R., Zhang, Y., Pang, S. F., and Zhang, Y. H.: Crystal nucleation and crystal growth and mass transfer in internally mixed sucrose/NaNO3 particles, J. Phys. Chem. A, 121, 7968–7975, https://doi.org/10.1021/acs.jpca.7b08004, 2017.
Jimenez, J. L., Canagaratna, M. R., Donahue, N. M., Prevot, a. S. H., Zhang, Q., Kroll, J. H., DeCarlo, P. F., Allan, J. D., Coe, H., Ng, N. L., Aiken, a. C., Docherty, K. S., Ulbrich, I. M., Grieshop, A. P., Robinson, a. L., Duplissy, J., Smith, J. D., Wilson, K. R., Lanz, V. a., Hueglin, C., Sun, Y. L., Tian, J., Laaksonen, A., Raatikainen, T., Rautiainen, J., Vaattovaara, P., Ehn, M., Kulmala, M., Tomlinson, J. M., Collins, D. R., Cubison, M. J., Dunlea, J., Huffman, J. A., Onasch, T. B., Alfarra, M. R., Williams, P. I., Bower, K., Kondo, Y., Schneider, J., Drewnick, F., Borrmann, S., Weimer, S., Demerjian, K., Salcedo, D., Cottrell, L., Griffin, R., Takami, a., Miyoshi, T., Hatakeyama, S., Shimono, A., Sun, J. Y., Zhang, Y. M., Dzepina, K., Kimmel, J. R., Sueper, D., Jayne, J. T., Herndon, S. C., Trimborn, a. M., Williams, L. R., Wood, E. C., Middlebrook, A. M., Kolb, C. E., Baltensperger, U., Worsnop, D. R., Dunlea, E. J., Huffman, J. A., Onasch, T. B., Alfarra, M. R., Williams, P. I., Bower, K., Kondo, Y., Schneider, J., Drewnick, F., Borrmann, S., Weimer, S., Demerjian, K., Salcedo, D., Cottrell, L., Griffin, R., Takami, a., Miyoshi, T., Hatakeyama, S., Shimono, A., Sun, J. Y., Zhang, Y. M., Dzepina, K., Kimmel, J. R., Sueper, D., Jayne, J. T., Herndon, S. C., Trimborn, a. M., Williams, L. R., Wood, E. C., Middlebrook, A. M., Kolb, C. E., Baltensperger, U., Worsnop, D. R., Dunlea, J., Huffman, J. A., Onasch, T. B., Alfarra, M. R., Williams, P. I., Bower, K., Kondo, Y., Schneider, J., Drewnick, F., Borrmann, S., Weimer, S., Demerjian, K., Salcedo, D., Cottrell, L., Griffin, R., Takami, A., Miyoshi, T., Hatakeyama, S., Shimono, A., Sun, J. Y., Zhang, Y. M., Dzepina, K., Kimmel, J. R., Sueper, D., Jayne, J. T., Herndon, S. C., Trimborn, A. M., Williams, L. R., Wood, E. C., Middlebrook, A. M., Kolb, C. E., Baltensperger, U., and Worsnop, D. R.: Evolution of organic aerosols in the atmosphere, Science, 326, 1525–1529, https://doi.org/10.1126/science.1180353, 2009.
Kanakidou, M., Seinfeld, J. H., Pandis, S. N., Barnes, I., Dentener, F. J., Facchini, M. C., Van Dingenen, R., Ervens, B., Nenes, A., Nielsen, C. J., Swietlicki, E., Putaud, J. P., Balkanski, Y., Fuzzi, S., Horth, J., Moortgat, G. K., Winterhalter, R., Myhre, C. E. L., Tsigaridis, K., Vignati, E., Stephanou, E. G., and Wilson, J.: Organic aerosol and global climate modelling: a review, Atmos. Chem. Phys., 5, 1053–1123, https://doi.org/10.5194/acp-5-1053-2005, 2005.
Kaufman, Y. J., Tanré, D., and Boucher, O.: A satellite view of aerosols in the climate system, Nature, 419, 215–223, 2002.
Kidd, C., Perraud, V., Wingen, L. M., and Finlayson-Pitts, B. J.: Integrating phase and composition of secondary organic aerosol from the ozonolysis of α-pinene, P. Natl. Acad. Sci. USA, 111, 7552–7, https://doi.org/10.1073/pnas.1322558111, 2014.
Kim, Y., Sartelet, K., and Couvidat, F.: Modeling the effect of non-ideality, dynamic mass transfer and viscosity on SOA formation in a 3-D air quality model, Atmos. Chem. Phys., 19, 1241–1261, https://doi.org/10.5194/acp-19-1241-2019, 2019.
Knopf, D. A., Alpert, P., and Wang, B.: The role of organic aerosol in atmospheric ice nucleation – A Review, ACS Earth Sp. Chem., 2, 168–202, https://doi.org/10.1021/acsearthspacechem.7b00120, 2018.
Koop, T., Bookhold, J., Shiraiwa, M., and Pöschl, U.: Glass transition and phase state of organic compounds: dependency on molecular properties and implications for secondary organic aerosols in the atmosphere, Phys. Chem. Chem. Phys., 13, 19238–19255, https://doi.org/10.1039/c1cp22617g, 2011.
Kulmala, M., Asmi, A., Lappalainen, H. K., Baltensperger, U., Brenguier, J.-L., Facchini, M. C., Hansson, H.-C., Hov, Ø., O'Dowd, C. D., Pöschl, U., Wiedensohler, A., Boers, R., Boucher, O., de Leeuw, G., Denier van der Gon, H. A. C., Feichter, J., Krejci, R., Laj, P., Lihavainen, H., Lohmann, U., McFiggans, G., Mentel, T., Pilinis, C., Riipinen, I., Schulz, M., Stohl, A., Swietlicki, E., Vignati, E., Alves, C., Amann, M., Ammann, M., Arabas, S., Artaxo, P., Baars, H., Beddows, D. C. S., Bergström, R., Beukes, J. P., Bilde, M., Burkhart, J. F., Canonaco, F., Clegg, S. L., Coe, H., Crumeyrolle, S., D'Anna, B., Decesari, S., Gilardoni, S., Fischer, M., Fjaeraa, A. M., Fountoukis, C., George, C., Gomes, L., Halloran, P., Hamburger, T., Harrison, R. M., Herrmann, H., Hoffmann, T., Hoose, C., Hu, M., Hyvärinen, A., Hõrrak, U., Iinuma, Y., Iversen, T., Josipovic, M., Kanakidou, M., Kiendler-Scharr, A., Kirkevåg, A., Kiss, G., Klimont, Z., Kolmonen, P., Komppula, M., Kristjánsson, J.-E., Laakso, L., Laaksonen, A., Labonnote, L., Lanz, V. A., Lehtinen, K. E. J., Rizzo, L. V., Makkonen, R., Manninen, H. E., McMeeking, G., Merikanto, J., Minikin, A., Mirme, S., Morgan, W. T., Nemitz, E., O'Donnell, D., Panwar, T. S., Pawlowska, H., Petzold, A., Pienaar, J. J., Pio, C., Plass-Duelmer, C., Prévôt, A. S. H., Pryor, S., Reddington, C. L., Roberts, G., Rosenfeld, D., Schwarz, J., Seland, Ø., Sellegri, K., Shen, X. J., Shiraiwa, M., Siebert, H., Sierau, B., Simpson, D., Sun, J. Y., Topping, D., Tunved, P., Vaattovaara, P., Vakkari, V., Veefkind, J. P., Visschedijk, A., Vuollekoski, H., Vuolo, R., Wehner, B., Wildt, J., Woodward, S., Worsnop, D. R., van Zadelhoff, G.-J., Zardini, A. A., Zhang, K., van Zyl, P. G., Kerminen, V.-M., S Carslaw, K., and Pandis, S. N.: General overview: European Integrated project on Aerosol Cloud Climate and Air Quality interactions (EUCAARI) – integrating aerosol research from nano to global scales, Atmos. Chem. Phys., 11, 13061–13143, https://doi.org/10.5194/acp-11-13061-2011, 2011.
Kuwata, M. and Martin, S. T.: Phase of atmospheric secondary organic material affects its reactivity, P. Natl. Acad. Sci. USA, 109, 17354-17359, 2012.
Ladino, L. A., Zhou, S., Yakobi-Hancock, J. D., Aljawhary, D., and Abbatt, J. P. D.: Factors controlling the ice nucleating abilities of α-pinene SOA particles, J. Geophys. Res., 119, 9041–9051, https://doi.org/10.1002/2014JD021578, 2014.
Laliberté, M.: Model for calculating the viscosity of aqueous aolutions, J. Chem. Eng. Data, 52, 1507–1508, https://doi.org/10.1021/je700232s, 2007.
Laliberté, M.: A model for calculating the heat capacity of aqueous solutions, with updated density and viscosity data, J. Chem. Eng. Data, 54, 1725–1760, https://doi.org/10.1021/je8008123, 2009.
Laskin, A., Iedema, M. J., Ichkovich, A., Graber, E. R., Taraniuk, I., and Rudich, Y.: Direct observation of completely processed calcium carbonate dust particles, Faraday Discuss., 130, 453–468, https://doi.org/10.1039/b417366j, 2005.
Li, J., Forrester, S. M., and Knopf, D. A.: Heterogeneous oxidation of amorphous organic aerosol surrogates by O3, NO3, and OH at typical tropospheric temperatures, Atmos. Chem. Phys., 20, 6055–6080, https://doi.org/10.5194/acp-20-6055-2020, 2020.
Li, X. H., Zhao, L. J., Dong, J. L., Xiao, H. S., and Zhang, Y. H.: Confocal raman studies of Mg(NO3)2 aerosol particles deposited on a quartz substrate: supersaturated structures and complicated phase transitions, J. Phys. Chem. B, 112, 5032–5038, https://doi.org/10.1021/jp709938x, 2008.
Liu, Y., Wu, Z., Huang, X., Shen, H., Bai, Y., Qiao, K., Meng, X., Hu, W., Tang, M., and He, L.: Aerosol Phase State and Its Link to Chemical Composition and Liquid Water Content in a Subtropical Coastal Megacity, Environ. Sci. Technol., 53, 5027–5033, https://doi.org/10.1021/acs.est.9b01196, 2019.
Lilek, J. and Zuend, A.: A predictive viscosity model for aqueous electrolytes and mixed organic–inorganic aerosol phase, in preparation, 2021.
Liu, Y. J., Zhu, T., Zhao, D. F., and Zhang, Z. F.: Investigation of the hygroscopic properties of Ca(NO3)2 and internally mixed Ca(NO3)2 CaCO3 particles by micro-Raman spectrometry, Atmos. Chem. Phys., 8, 7205–7215, https://doi.org/10.5194/acp-8-7205-2008, 2008.
Maclean, A. M., Smith, N. R., Li, Y., Huang, Y., Hettiyadura, A. P. S., Crescenzo, G. V., Shiraiwa, M., Laskin, A., Nizkorodov, S. A., and Bertram, A. K.: Humidity-Dependent Viscosity of Secondary Organic Aerosol from Ozonolysis of β-Caryophyllene: Measurements, Predictions, and Implications, ACS Earth Sp. Chem., 5, 305–318, https://doi.org/10.1021/acsearthspacechem.0c00296, 2021.
Mikhailov, E., Vlasenko, S., Martin, S. T., Koop, T., and Pöschl, U.: Amorphous and crystalline aerosol particles interacting with water vapor: conceptual framework and experimental evidence for restructuring, phase transitions and kinetic limitations, Atmos. Chem. Phys., 9, 9491–9522, https://doi.org/10.5194/acp-9-9491-2009, 2009.
Murphy, D. M., Cziczo, D. J., Froyd, K. D., Hudson, P. K., Matthew, B. M., Middlebrook, A. M., Peltier, R. E., Sullivan, A., Thomson, D. S., and Weber, R. J.: Single-peptide mass spectrometry of tropospheric aerosol particles, J. Geophys. Res.-Atmos., 111, 1–15, https://doi.org/10.1029/2006JD007340, 2006.
Murray, B. J.: Inhibition of ice crystallisation in highly viscous aqueous organic acid droplets, Atmos. Chem. Phys., 8, 5423–5433, https://doi.org/10.5194/acp-8-5423-2008, 2008.
Murray, B. J., Wilson, T. W., Dobbie, S., Cui, Z., Al-Jumur, S. M. R. K., Möhler, O., Schnaiter, M., Wagner, R., Benz, S., Niemand, M., Saathoff, H., Ebert, V., Wagner, S., and Kärcher, B.: Heterogeneous nucleation of ice particles on glassy aerosols under cirrus conditions, Nat. Geosci., 3, 233–237, https://doi.org/10.1038/ngeo817, 2010.
Murray, B. J., Haddrell, A. E., Peppe, S., Davies, J. F., Reid, J. P., O'Sullivan, D., Price, H. C., Kumar, R., Saunders, R. W., Plane, J. M. C., Umo, N. S., and Wilson, T. W.: Glass formation and unusual hygroscopic growth of iodic acid solution droplets with relevance for iodine mediated particle formation in the marine boundary layer, Atmos. Chem. Phys., 12, 8575–8587, https://doi.org/10.5194/acp-12-8575-2012, 2012.
Pan, X., Uno, I., Wang, Z., Nishizawa, T., Sugimoto, N., Yamamoto, S., Kobayashi, H., Sun, Y., Fu, P., Tang, X., and Wang, Z.: Real-time observational evidence of changing Asian dust morphology with the mixing of heavy anthropogenic pollution, Sci. Rep.-UK, 7, 1–8, https://doi.org/10.1038/s41598-017-00444-w, 2017.
Pant, A., Parsons, M. T., and Bertram, A. K.: Crystallization of aqueous ammonium sulfate particles internally mixed with soot and kaolinite: Crystallization relative humidities and nucleation rates, J. Phys. Chem. A, 110, 8701–8709, https://doi.org/10.1021/Jp060985s, 2006.
Petters, S. S., Kreidenweis, S. M., Grieshop, A. P., Ziemann, P. J., and Petters, M. D.: Temperature- and humidity-dependent phase states of secondary organic aerosols, Geophys. Res. Lett., 46, 1005–1013, https://doi.org/10.1029/2018GL080563, 2019.
Power, R. M., Simpson, S. H., Reid, J. P., and Hudson, A. J.: The Transition from liquid to solid-like behaviour in ultrahigh viscosity aerosol particles, Chem. Sci., 4, 2597–2604, https://doi.org/10.1039/c3sc50682g, 2013.
Reid, J. P., Bertram, A. K., Topping, D. O., Laskin, A., Martin, S. T., Petters, M. D., Pope, F. D., and Rovelli, G.: The viscosity of atmospherically relevant organic particles, Nat. Commun., 9, 956, https://doi.org/10.1038/s41467-018-03027-z, 2018.
Renbaum-Wolff, L., Grayson, J. W., Bateman, A. P., Kuwata, M., Sellier, M., Murray, B. J., Shilling, J. E., Martin, S. T., and Bertram, A. K.: Viscosity of α-pinene secondary organic material and implications for particle growth and reactivity, P. Natl. Acad. Sci. USA, 110, 8014–9, https://doi.org/10.1073/pnas.1219548110, 2013.
Richards, D. S., Trobaugh, K. L., Hajek-Herrera, J., Price, C. L., Sheldon, C. S., Davies, J. F., and Davis, R. D.: Ion-molecule interactions enable unexpected phase transitions in organic-inorganic aerosol, Science Advances, 6, 1–12. https://doi.org/10.1126/sciadv.abb5643, 2020a.
Richards, D. S., Trobaugh, K. L., Hajek-Herrera, J., and Davis, R. D.: Dual-Balance Electrodynamic Trap as a Microanalytical Tool for Identifying Gel Transitions and Viscous Properties of Levitated Aerosol Particles, Anal. Chem., 92, 3086–3094, https://doi.org/10.1021/acs.analchem.9b04487, 2020b.
Rothfuss, N. E. and Petters, M. D.: Influence of functional groups on the viscosity of organic aerosol, Environ. Sci. Technol., 51, 271–279, https://doi.org/10.1021/acs.est.6b04478, 2017.
Rovelli, G., Song, Y. C., Maclean, A. M., Topping, D. O., Bertram, A. K., and Reid, J. P.: Comparison of approaches for measuring and predicting the viscosity of ternary component aerosol particles, Anal. Chem., 91, 5074–5082, https://doi.org/10.1021/acs.analchem.8b05353, 2019.
Russell, P. B., Kinne, S. A., and Bergstrom, R. W.: Aerosol climate effects: local radiative forcing and column closure experiments, J. Geophys. Res.-Atmos., 102, 9397–9407, https://doi.org/10.1029/97jd00112, 1997.
Saukko, E., Lambe, A. T., Massoli, P., Koop, T., Wright, J. P., Croasdale, D. R., Pedernera, D. A., Onasch, T. B., Laaksonen, A., Davidovits, P., Worsnop, D. R., and Virtanen, A.: Humidity-dependent phase state of SOA particles from biogenic and anthropogenic precursors, Atmos. Chem. Phys., 12, 7517–7529, https://doi.org/10.5194/acp-12-7517-2012, 2012.
Schmedding, R., Rasool, Q. Z., Zhang, Y., Pye, H. O. T., Zhang, H., Chen, Y., Surratt, J. D., Lopez-Hilfiker, F. D., Thornton, J. A., Goldstein, A. H., and Vizuete, W.: Predicting secondary organic aerosol phase state and viscosity and its effect on multiphase chemistry in a regional-scale air quality model, Atmos. Chem. Phys., 20, 8201–8225, https://doi.org/10.5194/acp-20-8201-2020, 2020.
Sellier, M., Grayson, J. W., Renbaum-Wolff, L., Song, M., and Bertram, A. K.: Estimating the viscosity of a highly viscous liquid droplet through the relaxation time of a dry spot, J. Rheol., 59, 733–750, https://doi.org/10.1122/1.4917240, 2015.
Shi, Z., Zhang, D., Hayashi, M., Ogata, H., Ji, H., and Fujiie, W.: Influences of sulfate and nitrate on the hygroscopic behaviour of coarse dust particles, Atmos. Environ., 42, 822–827, https://doi.org/10.1016/j.atmosenv.2007.10.037, 2008.
Shiraiwa, M. and Seinfeld, J. H.: Equilibration timescale of atmospheric secondary organic aerosol partitioning, Geophys. Res. Lett., 39, L24801, https://doi.org/10.1029/2012GL054008, 2012.
Shiraiwa, M., Yee, L. D., Schilling, K. A., Loza, C. L., Craven, J. S., Zuend, A., Ziemann, P. J., and Seinfeld, J. H.: Size distribution dynamics reveal particle-phase chemistry in organic aerosol formation, P. Natl. Acad. Sci. USA, 110, 11746–11750, https://doi.org/10.1073/pnas.1307501110, 2013.
Slade, J. H. and Knopf, D. A.: Multiphase OH oxidation kinetics of organic aerosol: The role of particle phase state and relative humidity, Geophys. Res. Lett., 41, 5297–5306. https://doi.org/10.1002/2014GL060582, 2014.
Song, M., Marcolli, C., Krieger, U. K., Zuend, A., and Peter, T.: Liquid-liquid phase separation and morphology of internally mixed dicarboxylic acids/ammonium sulfate/water particles, Atmos. Chem. Phys., 12, 2691–2712, https://doi.org/10.5194/acp-12-2691-2012, 2012.
Song, M., Liu, P. F., Hanna, S. J., Li, Y. J., Martin, S. T., and Bertram, A. K.: Relative humidity-dependent viscosities of isoprene-derived secondary organic material and atmospheric implications for isoprene-dominant forests, Atmos. Chem. Phys., 15, 5145–5159, https://doi.org/10.5194/acp-15-5145-2015, 2015.
Song, M., Liu, P. F., Hanna, S. J., Zaveri, R. A., Potter, K., You, Y., Martin, S. T., and Bertram, A. K.: Relative humidity-dependent viscosity of secondary organic material from toluene photo-oxidation and possible implications for organic particulate matter over megacities, Atmos. Chem. Phys., 16, 8817–8830, https://doi.org/10.5194/acp-16-8817-2016, 2016.
Song, M., Maclean, A. M., Huang, Y., Smith, N. R., Blair, S. L., Laskin, J., Laskin, A., DeRieux, W.-S. W., Li, Y., Shiraiwa, M., Nizkorodov, S. A., and Bertram, A. K.: Liquid–liquid phase separation and viscosity within secondary organic aerosol generated from diesel fuel vapors, Atmos. Chem. Phys., 19, 12515–12529, https://doi.org/10.5194/acp-19-12515-2019, 2019.
Song, Y. C., Ryu, J., Malek, M. A., Jung, H. J., and Ro, C. U.: Chemical speciation of individual airborne particles by the combined use of quantitative energy-dispersive electron probe X-ray microanalysis and attenuated total reflection Fourier transform-infrared imaging techniques, Anal. Chem., 82, 7987–7998, https://doi.org/10.1021/ac1014113, 2010.
Song, Y.-C., Eom, H.-J., Jung, H.-J., Malek, M. A., Kim, H. K., Geng, H., and Ro, C.-U.: Investigation of aged Asian dust particles by the combined use of quantitative ED-EPMA and ATR-FTIR imaging, Atmos. Chem. Phys., 13, 3463–3480, https://doi.org/10.5194/acp-13-3463-2013, 2013.
Song, Y. C., Haddrell, A. E., Bzdek, B. R., Reid, J. P., Bannan, T., Topping, D. O., Percival, C., and Cai, C.: Measurements and pedictions of binary component aerosol particle viscosity, J. Phys. Chem. A, 120, 8123–8137, https://doi.org/10.1021/acs.jpca.6b07835, 2016.
Song, Y. C., Ingram, S., Arbon, R. E., Topping, D. O., Glowacki, D. R., and Reid, J. P.: Transient Cavity Dynamics and Divergence from the Stokes-Einstein Equation in Organic Aerosol, Chem. Sci., 11, 2999–3006, https://doi.org/10.1039/C9SC06228A, 2020.
Steimer, S. S., Berkemeier, T., Gilgen, A., Krieger, K. U., Peter, T., Shiraiwa, M., and Ammann, M.: Shikimic acid ozonolysis kinetics in the transition from liquid aqueous solution to highly viscous glass, Phys. Chem. Chem. Phys., 17, 31101–31109, https://doi.org/10.1039/C5CP04544D, 2015.
Stokes, R. H. and Robinson, R. A.: Interactions in aqueous nonelectrolyte solutions .i. solute-solvent equilibria, J. Phys. Chem., 70, 2126–2130, 1966.
Sullivan, R. C., Guazzotti, S. A., Sodeman, D. A., and Prather, K. A.: Direct observations of the atmospheric processing of Asian mineral dust, Atmos. Chem. Phys., 7, 1213–1236, https://doi.org/10.5194/acp-7-1213-2007, 2007.
Usher, C. R., Michel, A. E., and Grassian, V. H.: Reactions on mineral dust, Chem. Rev., 103, 4883–4939, https://doi.org/10.1021/cr020657y, 2003.
Vaden, T. D., Imre, D., Beranek, J., Shrivastava, M., and Zelenyuk, A.: Evaporation kinetics and phase of laboratory and ambient secondary organic aerosol, P. Natl. Acad. Sci. USA, 108, 2190–2195, https://doi.org/10.1073/pnas.1013391108, 2011.
Virtanen, A., Joutsensaari, J., Koop, T., Kannosto, J., Yli-Pirilä, P., Leskinen, J., Mäkelä, J. M., Holopainen, J. K., Pöschl, U., Kulmala, M., Worsnop, D. R., and Laaksonen, A.: An amorphous solid state of biogenic secondary organic aerosol particles, Nature, 467, 824–827, https://doi.org/10.1038/nature09455, 2010.
Wahab, A., Mahiuddin, S., Hefter, G., and Kunz, W.: Densities, ultrasonic velocities, viscosities, and electrical conductivities of aqueous solutions of Mg(OAc)2 and Mg(NO3)2, J. Chem. Eng. Data, 51, 1609–1616, https://doi.org/10.1021/je060107n, 2006.
Wallace, B. J. and Preston, T. C.: Water Uptake and Loss in Viscous Aerosol Particles with Concentration-Dependent Diffusivities, J. Phys. Chem. A, 123, 3374–3382, https://doi.org/10.1021/acs.jpca.9b00907, 2019.
Wang, G., Zhang, R., Gomez, M. E., Yang, L., Levy Zamora, M., Hu, M., Lin, Y., Peng, J., Guo, S., Meng, J., Li, J., Cheng, C., Hu, T., Ren, Y., Wang, Y., Gao, J., Cao, J., An, Z., Zhou, W., Li, G., Wang, J., Tian, P., Marrero-Ortiz, W., Secrest, J., Du, Z., Zheng, J., Shang, D., Zeng, L., Shao, M., Wang, W., Huang, Y., Wang, Y., Zhu, Y., Li, Y., Hu, J., Pan, B., Cai, L., Cheng, Y., Ji, Y., Zhang, F., Rosenfeld, D., Liss, P. S., Duce, R. A., Kolb, C. E., and Molina, M. J.: Persistent sulfate formation from London fog to Chinese haze, P. Natl. Acad. Sci. USA, 113, 13630–13635, https://doi.org/10.1073/pnas.1616540113, 2016.
Wang, L. N., Cai, C., and Zhang, Y. H.: Kinetically determined hygroscopicity and efflorescence of sucrose-ammonium sulfate aerosol droplets under lower RH, J. Phys. Chem. B, 121, 8551–8557, https://doi.org/10.1021/acs.jpcb.7b05551, 2017.
Wang, S., Nan, J., Shi, C., Fu, Q., Gao, S., Wang, D., Cui, H., Saiz-Lopez, A., and Zhou, B.: Atmospheric ammonia and its impacts on regional air quality over the megacity of Shanghai, China, Sci. Rep.-UK, 5, 1–13, https://doi.org/10.1038/srep15842, 2015a.
Wang, Y., Ma, J. B., Zhou, Q., Pang, S. F., and Zhang, Y. H.: Hygroscopicity of mixed glycerol Mg(NO3)2 water droplets affected by the interaction between magnesium ions and glycerol molecules, J. Phys. Chem. B, 119, 5558–5566, https://doi.org/10.1021/acs.jpcb.5b00458, 2015b.
Winston, P. W. and Bates, D. H.: Saturated solutions for the control of humidity in biological research, Ecology, 41, 232–237, 1960.
Xiao, S. and Bertram, A. K.: Reactive uptake kinetics of NO3 on multicomponent and multiphase organic mixtures containing unsaturated and saturated organics, Phys. Chem. Chem. Phys, 13, 6628–6636, 2011.
Xu, R., Lam, H. K., Wilson, K. R., Davies, J. F., Song, M., Li, W., Tse, Y.-L. S., and Chan, M. N.: Effect of inorganic-to-organic mass ratio on the heterogeneous OH reaction rates of erythritol: implications for atmospheric chemical stability of 2-methyltetrols, Atmos. Chem. Phys., 20, 3879–3893, https://doi.org/10.5194/acp-20-3879-2020, 2020.
Yli-Juuti, T., Pajunoja, A., Tikkanen, O. P., Buchholz, A., Faiola, C., Väisänen, O., Hao, L., Kari, E., Peräkylä, O., Garmash, O., Shiraiwa, M., Ehn, M., Lehtinen, K., and Virtanen, A.: Factors controlling the evaporation of secondary organic aerosol from α-pinene ozonolysis, Geophys. Res. Lett., 44, 2562–2570, https://doi.org/10.1002/2016GL072364, 2017.
Zaveri, R. A., Easter, R. C., Shilling, J. E., and Seinfeld, J. H.: Modeling kinetic partitioning of secondary organic aerosol and size distribution dynamics: representing effects of volatility, phase state, and particle-phase reaction, Atmos. Chem. Phys., 14, 5153–5181, https://doi.org/10.5194/acp-14-5153-2014, 2014.
Zaveri, R. A., Shilling, J. E., Zelenyuk, A., Liu, J. M., Bell, D. M., D'Ambro, E. L., Gaston, C., Thornton, J. A., Laskin, A., Lin, P., Wilson, J., Easter, R. C., Wang, J., Bertram, A. K., Martin, S. T., Seinfeld, J. H., and Worsnop, D. R.: Growth kinetics and size distribution dynamics of viscous secondary organic aerosol, Environ. Sci. Technol., 52, 3, 1191–1199, https://doi.org/10.1021/acs.est.7b04623, 2018.
Zdanovskii, A. B.: Trudy Solyanoi Laboratorii (Transactions of the Salt Laboratory), Akad. Nauk SSSR, 6, 5–70, 1936.
Zdanovskii, A. B.: New methods of calculating solubilities of electrolytes in multicomponent systems, Zh. Fiz. Khim+., 22, 1478–1485, 1948.
Zhang, Q., Jimenez, J. L., Canagaratna, M. R., Allan, J. D., Coe, H., Ulbrich, I., Alfarra, M. R., Takami, A., Middlebrook, A. M., Sun, Y. L., Dzepina, K., Dunlea, E., Docherty, K., DeCarlo, P. F., Salcedo, D., Onasch, T., Jayne, J. T., Miyoshi, T., Shimono, A., Hatakeyama, S., Takegawa, N., Kondo, Y., Schneider, J., Drewnick, F., Borrmann, S., Weimer, S., Demerjian, K., Williams, P., Bower, K., Bahreini, R., Cottrell, L., Griffin, R. J., Rautiainen, J., Sun, J. Y., Zhang, Y. M., and Worsnop, D. R.: Ubiquity and dominance of oxygenated species in organic aerosols in anthropogenically-influenced Northern Hemisphere midlatitudes, Geophys. Res. Lett., 34, 1–6, https://doi.org/10.1029/2007GL029979, 2007.
Zhang, R., Wang, G., Guo, S., Zamora, M. L., Ying, Q., Lin, Y., Wang, W., Hu, M., and Wang, Y.: Formation of urban fine particulate matter, Chem. Rev., 115, 3803–3855, https://doi.org/10.1021/acs.chemrev.5b00067, 2015.
Zhang, Y., Sanchez, M. S., Douet, C., Wang, Y., Bateman, A. P., Gong, Z., Kuwata, M., Renbaum-Wolff, L., Sato, B. B., Liu, P. F., Bertram, A. K., Geiger, F. M., and Martin, S. T.: Changing shapes and implied viscosities of suspended submicron particles, Atmos. Chem. Phys., 15, 7819–7829, https://doi.org/10.5194/acp-15-7819-2015, 2015.
Zieger, P., Vaisanen, O., Corbin, J. C., Partridge, D. G., Bastelberger, S., Mousavi-Fard, M., Rosati, B., Gysel, M., Krieger, U. K., Leck, C., Nenes, A., Riipinen, I., Virtanen, A., and Salter, M. E.: Revising the hygroscopicity of inorganic sea salt particles, Nat. Commun., 8, 15883, https://doi.org/10.1038/ncomms15883, 2017.
Zobrist, B., Marcolli, C., Pedernera, D. A., and Koop, T.: Do atmospheric aerosols form glasses?, Atmos. Chem. Phys., 8, 5221–5244, https://doi.org/10.5194/acp-8-5221-2008, 2008.
Zobrist, B., Soonsin, V., Luo, B. P., Krieger, U. K., Marcolli, C., Peter, T., and Koop, T.: Ultra-slow water diffusion in aqueous sucrose glasses, Phys. Chem. Chem. Phys., 13, 3514–26, https://doi.org/10.1039/c0cp01273d, 2011.
Zuend, A., Marcolli, C., Luo, B. P., and Peter, T.: A thermodynamic model of mixed organic-inorganic aerosols to predict activity coefficients, Atmos. Chem. Phys., 8, 4559–4593, https://doi.org/10.5194/acp-8-4559-2008, 2008.
Zuend, A., Marcolli, C., Booth, A. M., Lienhard, D. M., Soonsin, V., Krieger, U. K., Topping, D. O., McFiggans, G., Peter, T., and Seinfeld, J. H.: New and extended parameterization of the thermodynamic model AIOMFAC: calculation of activity coefficients for organic-inorganic mixtures containing carboxyl, hydroxyl, carbonyl, ether, ester, alkenyl, alkyl, and aromatic functional groups, Atmos. Chem. Phys., 11, 9155–9206, https://doi.org/10.5194/acp-11-9155-2011, 2011.