the Creative Commons Attribution 4.0 License.
the Creative Commons Attribution 4.0 License.
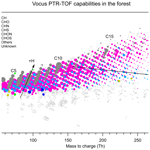
Terpenes and their oxidation products in the French Landes forest: insights from Vocus PTR-TOF measurements
Matthieu Riva
Pekka Rantala
Liine Heikkinen
Kaspar Daellenbach
Jordan E. Krechmer
Pierre-Marie Flaud
Douglas Worsnop
Markku Kulmala
Eric Villenave
Emilie Perraudin
Mikael Ehn
Federico Bianchi
The capabilities of the recently developed Vocus proton-transfer-reaction time-of-flight mass spectrometer (PTR-TOF) are reported for the first time based on ambient measurements. With the deployment of the Vocus PTR-TOF, we present an overview of the observed gas-phase (oxygenated) molecules in the French Landes forest during summertime 2018 and gain insights into the atmospheric oxidation of terpenes, which are emitted in large quantities in the atmosphere and play important roles in secondary organic aerosol production. Due to the greatly improved detection efficiency compared to conventional PTR instruments, the Vocus PTR-TOF identifies a large number of gas-phase signals with elemental composition categories including CH, CHO, CHN, CHS, CHON, CHOS, and others. Multiple hydrocarbons are detected, with carbon numbers up to 20. Particularly, we report the first direct observations of low-volatility diterpenes in the ambient air. The diurnal cycle of diterpenes is similar to that of monoterpenes and sesquiterpenes but contrary to that of isoprene. Various types of terpene reaction products and intermediates are also characterized. Generally, the more oxidized products from terpene oxidations show a broad peak in the day due to the strong photochemical effects, while the less oxygenated products peak in the early morning and/or in the evening. To evaluate the importance of different formation pathways in terpene chemistry, the reaction rates of terpenes with main oxidants (i.e., hydroxyl radical, OH; ozone, O3; and nitrate radical, NO3) are calculated. For the less oxidized non-nitrate monoterpene oxidation products, their morning and evening peaks have contributions from both O3- and OH-initiated monoterpene oxidation. For the monoterpene-derived organic nitrates, oxidations by O3, OH, and NO3 radicals all contribute to their formation, with their relative roles varying considerably over the course of the day. Through a detailed analysis of terpene chemistry, this study demonstrates the capability of the Vocus PTR-TOF in the detection of a wide range of oxidized reaction products in ambient and remote conditions, which highlights its importance in investigating atmospheric oxidation processes.
- Article
(4261 KB) - Full-text XML
-
Supplement
(2030 KB) - BibTeX
- EndNote
Organic aerosol (OA) constitutes a large fraction of atmospheric particles, having significant impacts on climate change, air quality, and human health (Maria et al., 2004; IPCC, 2013; Mauderly and Chow, 2008). On a global scale, secondary OA (SOA) is the largest source of OA, formed through the oxidation of volatile organic compounds (VOCs) (Jimenez et al., 2009). Biogenic VOCs (BVOCs) are released into the atmosphere in high amounts, with an annual global budget being 760 Tg C (Sindelarova et al., 2014). On average, SOA production from biogenic precursors ranges from 2.5 to 44.5 Tg C annually, which is much larger than that from anthropogenic sources (Tsigaridis and Kanakidou, 2003). Over the past decades, a considerable number of studies have been conducted to investigate the atmospheric chemistry of BVOCs (Kanakidou et al., 2005; Henze and Seinfeld, 2006; Hatfield and Huff Hartz, 2011; Calfapietra et al., 2013; Jokinen et al., 2015; Ng et al., 2017). However, an incomplete understanding of BVOC characteristics and their oxidation processes in the atmosphere remains and yields large uncertainties in quantitative estimates of air quality and climate effects of atmospheric aerosols (Carslaw et al., 2013; Zhu et al., 2019).
Terpenes make up the main fraction of BVOCs (Guenther et al., 1995), encompassing isoprene (C5H8), monoterpenes (C10H16), sesquiterpenes (C15H24), diterpenes (C20H32), and even larger compounds. With one or more C = C double bonds in their molecular structures, terpenes are highly reactive. After entering the atmosphere, terpenes can undergo oxidative chemistry with the common atmospheric oxidants including hydroxyl radical (OH), ozone (O3), and nitrate radical (NO3). These oxidation processes generate a large variety of organic species, with volatilities covering gas-phase volatile species (VOC), semivolatile and low volatility organic compounds (SVOC and LVOC), extremely low volatility organic compounds (ELVOC), and even ultra-low volatility organic compounds (ULVOC), which irreversibly contribute to SOA formation (Donahue et al., 2012). Due to the chemical complexity and low concentrations of BVOC oxidation products, it remains extremely challenging to provide a comprehensive understanding of terpene chemistry in the atmosphere.
With a high time response and sensitivity, proton-transfer-reaction mass spectrometry (PTR-MS) has been widely used to study the emissions and chemical evolution of VOCs in the atmosphere (Yuan et al., 2017). However, due to the relatively low sensitivity, previous PTR-MS instruments were not optimized to detect low-volatility compounds. For example, only a few ambient PTR-MS observations of sesquiterpenes are available (Kim et al., 2009; Jardine et al., 2011). Correspondingly, it is not surprising that ambient observations of diterpenes, which are generally considered to be non-volatile compounds, have never been reported. In addition, the existing PTR-MS is often not sensitive enough to quantify terpene oxidation products at atmospherically relevant concentrations (Yuan et al., 2017). To address these instrumental limitations, two new versions of PTR spectrometers were recently developed: the PTR3 (Breitenlechner et al., 2017) and the Vocus PTR-TOF (Krechmer et al., 2018), both coupled with a time-of-flight (TOF) mass analyzer. With the enhanced sensitivities by a factor of ∼10 (Holzinger et al., 2019), these instruments are capable of detecting a broader spectrum of VOCs, where the detection of low-volatility VOCs is significantly improved compared to conventional PTR-MS. Based on the laboratory evaluation by Riva et al. (2019a), the Vocus PTR-TOF is able to measure both monoterpenes and lots of monoterpene oxidation products containing up to six oxygen atoms.
Known for strong monoterpene emitters (Simon et al., 1994), the Landes forest in southwestern France is a suitable place to investigate atmospheric terpene chemistry. A previous study at this site reported a high nocturnal monoterpene loading and suggested that monoterpene oxidations play an important role in the formation of new particles and the consequent growth of atmospheric particles (Kammer et al., 2018). To better assess the roles of BVOCs in aerosol formation, the Characterization of Emissions and Reactivity of Volatile Organic Compounds in the Landes forest (CERVOLAND) campaign took place in July 2018. The recently developed Vocus PTR-TOF was deployed during the CERVOLAND campaign to characterize terpenes and their gas-phase oxidation products, which provides the first Vocus PTR-TOF measurements in a forested environment. In this work, we present a comprehensive summary of the identified gas-phase molecules and gain insights into terpene chemistry to demonstrate the Vocus PTR-TOF capabilities and the importance of its applications in atmospheric sciences. Characterizations of isoprene, monoterpenes, sesquiterpenes, and particularly the rarely detected diterpenes are reported. By comparing the reaction rates of different formation pathways, we explore the formation mechanisms of terpene oxidation products, including both non-nitrate and organic nitrate compounds.
2.1 Measurement site
The Vocus PTR-TOF measurements were performed from 8 to 20 July 2018 in the Landes forest (44∘ N, 0∘ W), as part of the CERVOLAND field campaign. The sampling site is situated at the European Integrated Carbon Observation System (ICOS) station at Bilos in southwestern France along the Atlantic coast, ∼40 km southwest from the nearest urban area of the Bordeaux metropole. Both population density and industrial emissions are low in this area. Due to the proximity of the Atlantic Ocean, the site has a strong maritime influence. The forest is largely composed of maritime pines (Pinus pinaster Aiton) and has an average height of ∼10 m. Monoterpenes are known to be strongly emitted in the forest (Simon et al., 1994), which provides a good place for BVOC characterization. More detailed descriptions of the site have been provided in earlier studies (Moreaux et al., 2011; Kammer et al., 2018; Bsaibes et al., 2019).
2.2 Instrumentation
Compared to conventional PTR instrument, the Vocus PTR-TOF used in this study is mainly differentiated in the following aspects:
-
a new chemical ionization source with a low-pressure reagent-ion source and focusing ion–molecule reactor (FIMR);
-
no dependence of the sensitivity on ambient sample humidity due to the high water mixing ratio (10 % v/v–20 % v/v) in the FIMR;
-
employment of a TOF mass analyzer with a longer flight tube and faster sampling data acquisition card (mass resolving power up to 15 000 m dm−1);
-
an enhanced inlet and source design that minimizes contact between analyte molecules and inlet or source walls, enabling detection of semivolatile and low-volatility compounds in a similar manner as chemical ionization mass spectrometer (CIMS) instruments (Liu et al., 2019).
Details about the Vocus PTR-TOF are well described by Krechmer et al. (2018). Compared to the ionization in a conventional PTR-MS at 2.0–4.0 mbar, a nitrate CIMS at ambient pressure or an iodide CIMS at around 100 mbar, the Vocus ionization source is generally operated at a low pressure (Krechmer et al., 2018). In this work, we operated the Vocus ionization source at a pressure of 1.5 mbar. During the campaign, the Vocus PTR-TOF measurements were performed at around 2 m above ground level (a.g.l.), thus within the canopy. Sample air was drawn in through a 1 m long PTFE tubing (10 mm o.d., 8 mm i.d.) with a flow rate of 4.5 L min−1, which helped to reduce inlet wall losses and sampling delay. Of the total sample flow, only 150 sccm went into the Vocus, while the remainder was directed to the exhaust. The design of the FIMR consists of a glass tube with a resistive coating on the inside surface and four quadrupole rods mounted radially on the outside. With an RF (radio frequency) field, ions are collimated to the central axis, improving the detection efficiency of product ions. The mass resolving power of the 1.2 m long TOF mass analyzer was 12 000–13 000 m dm−1 during the whole campaign. Data were recorded with a time resolution of 5 s. Background measurements using ultra-high-purity nitrogen (UHP N2) were automatically performed every hour.
The temperature, relative humidity (RH), wind speed, and ambient pressure were continuously monitored at 3.4 m a.g.l., whereas the solar radiation was measured at 15.6 m a.g.l. from a mast located at the site. The mixing ratios of nitrogen oxides (NOx) and ozone (O3) were measured at 4 m a.g.l. with UV absorption and chemiluminescence analyzers, respectively. All data are reported in Coordinated Universal Time (UTC).
2.3 Data analysis and quantification of multiple compounds
Data analysis was performed using the software package “Tofware” (https://www.tofwerk.com/software/tofware/, last access: 12 February 2020) that runs in the Igor Pro environment (WaveMetrics, OR, USA). Tofware enables time-dependent mass calibration, baseline subtraction, and assignment of a molecular formula to the identified ions by high-resolution analysis. Signals were averaged over 30 min before mass calibration. Due to the high resolving power of the LTOF (long time of flight) mass analyzer, isobaric ions were more clearly separated. Examples of peak identification are given in Fig. S1 in the Supplement.
The Vocus was calibrated twice a day during the campaign with a mixture (70 ppb each) of terpenes (m∕z 137: α-/β-pinene + limonene; m∕z 135: p-cymene) that was diluted using UHP N2. Similar to conventional PTR instruments, the sensitivities of different VOCs in the Vocus PTR-TOF are linearly related to their proton-transfer reaction rate constants (k) when ion transmission efficiency and fragmentation ions are considered (Sekimoto et al., 2017; Krechmer et al., 2018). Krechmer et al. (2018) have shown that within the Vocus PTR-TOF, the transmission efficiencies of ions 100 Th (thomson units) reach up to 99 %. Therefore, the influence of fragmentation correction should be included in this study. According to terpene calibrations, the residual fractions were on average 66 % and 55 % for protonated monoterpenes and p-cymene, respectively, after their fragmentation within the instrument. Based on the corrected sensitivities for fragmentation and the k values of monoterpenes and p-cymene, an empirical relationship between the sensitivity and k was built from the scatter plots using linear regression: sensitivity (cps ppb, where cps represents counts per second (Fig. S2). Once k is available, the sensitivity of a compound can be predicted. It should be noted that the established relationship in this study is not applicable to other conditions or instruments. Some studies found that isoprene may fragment significantly to m∕z 41 (Keck et al., 2008; Schwarz et al., 2009). However, with the ambient data in this work, isoprene seems not to fragment much to , and they correlate poorly with each other (Fig. S3). Therefore, the fragmentation of isoprene is not considered for its quantification. Sesquiterpenes and some terpene oxidation products were found to fragment to varying degrees (Kim et al., 2009; Kari et al., 2018). Due to the lack of calibrations using other terpenes or terpene oxidation products, their fragmentation patterns within the Vocus PTR-TOF are not known in this work. Therefore, all the other terpenes and terpene oxidation products were quantified without consideration of fragment ions, which should be regarded as the lower limit of their ambient concentrations.
Rate constants for the proton-transfer reactions have only been measured for a subset of compounds. To quantify terpenes and their oxidation products, we used the method proposed by Sekimoto et al. (2017) to calculate the rate constants of different compounds with the polarizability and permanent dipole moment of the molecule. According to Sekimoto et al. (2017), the polarizability and dipole moment of a molecule can be obtained based on the molecular mass, elemental composition, and functionality of the compound. For a class of VOCs with the same number of electronegative atoms, their polarizabilities can be well described using their molecular mass (Sekimoto et al., 2017). For VOCs containing a specific functional group, it is found that their dipole moments are relatively constant based on results in the CRC Handbook of Chemistry and Physics (Lide, 2005). Since no isomer information is provided by mass spectrometry alone, it is challenging to figure out the functionality of different compounds. Therefore, the polarizability and dipole moment of the compounds observed in this study were estimated only based on the molecular mass and elemental composition. In this work, based on the physical properties of various compounds in CRC Handbook of Chemistry and Physics (Lide, 2005) and the results in Sekimoto et al. (2017), we built the functions between polarizability (α) and molecular mass (MR) for different groups of VOCs and calculated the average dipole moment (μ) for each group. For example, the polarizabilities of hydrocarbons were approximated as α=0.142 MR−0.3, and the dipole moment was approximated to be zero. For the non-nitrate oxygenated compounds with one oxygen, α=0.133 MR−1.2, and the dipole moment was averaged to be 1.6.
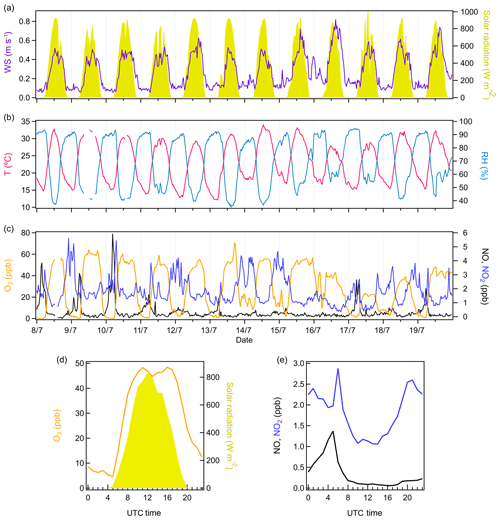
Figure 1Variations of meteorological conditions and trace gases. (a) Time series of wind speed and solar radiation. (b) Time series of temperature and relative humidity. (c) Time series of O3, NO, and NO2. (d) Diurnal cycles of O3 and solar radiation. (e) Diurnal cycles of NO and NO2.
It should be noted that uncertainties are introduced to the calculated sensitivities in the following factors. Firstly, the small difference between the rate coefficients of monoterpenes and p-cymene may lead to large uncertainty in the established linear regression function between sensitivity and k. Calibrations with more VOC compounds should be performed in future work to cover a larger range of k values. Secondly, as mentioned above, the theoretically calculated sensitivities of sesquiterpenes, diterpenes, and terpene oxidation products may be underestimated to varying extents without the consideration of their fragment ions. Further, some low-volatility compounds may experience wall losses inside the inlet tubing and the instrument and therefore have worse transmissions. The method in this work may overestimate the sensitivities of these low-volatility compounds. In addition to proton-transfer reactions, some VOCs can be ionized through ligand switching reactions with water cluster ((H2O)nH3O+) (Tani et al., 2004), thus increasing their sensitivity. However, with the calibration standards used in this study, it is hard to estimate the effect of ligand switching ionization. Lastly, uncertainties come from the estimation of polarizability and dipole moment of a molecule. With the method used in this study, the sensitivity is calculated to be within 50 % error when only the elemental composition of a compound is known (Sekimoto et al., 2017).
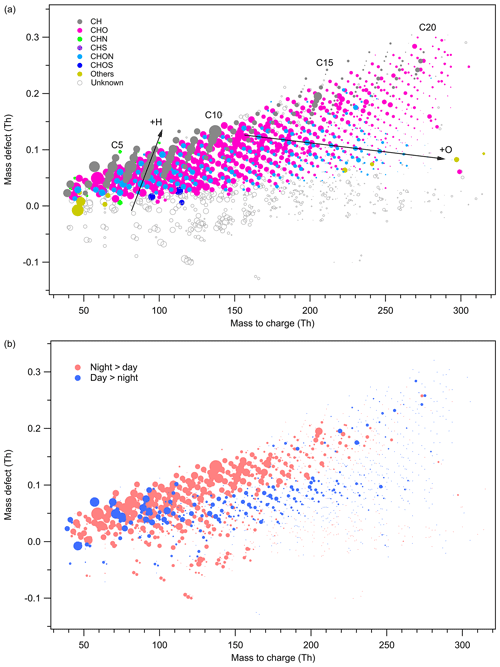
Figure 2Mass defect plot of the ions identified by high-resolution analysis of the Vocus PTR-TOF data set. The x axis shows the mass-to-charge ratio and the y axis shows the mass defect, which is the deviation of the exact mass from the nominal mass. Data points in (a) are color coded by ion family (CH, CHO, CHN, CHS, CHON, CHOS) and sized by the logarithm of peak area. Data points in (b) are shown in pink when signals are higher during nighttime and in blue when daytime signal is higher. The symbol size corresponds to the difference of daytime and nighttime signal for the molecule. It should be noted that ions <35 Th are detected at a much reduced efficiency due to a high-pass band filter in the BSQ.
3.1 Meteorology and trace gases
Figure 1 displays the time variations of meteorological conditions and trace gases during the observation period. The weather was mostly sunny, with solar radiation varying from 400 to 800 W m−2 during daytime, indicating strong photochemical activity. The ambient temperature and RH varied regularly every day. On average, the temperature was 22.8±5.9 ∘C (mean±SE), ranging from 12.1 to 35.0 ∘C, which is favorable for BVOC emissions in the forest. The average RH was 70.5 %±19.0 % during the campaign. Generally, the air masses were quite stable within the canopy. The wind speed never exceeded 1 m s−1, indicating the major influence of local sources on atmospheric processes in this study.
The O3 levels fluctuated dramatically between day and night during the campaign. The average O3 diurnal cycle showed that O3 concentration peaked up to ∼50 ppb in the daytime. However, during most of the nights, O3 concentration dropped below 2 ppb. Considering the high nighttime concentration of terpenes observed by the previous study at this site in the same season (Kammer et al., 2018), the low O3 level at night suggests the large consumption of O3 by terpenes. Such reactions of terpenes with O3 can produce low-volatility organic compounds, thus contributing to SOA formation (Presto et al., 2005; Jokinen et al., 2014). In addition, plant surface uptake is likely another important ozone sink in the canopy (Goldstein et al., 2004).
The NO concentration was generally low during the campaign, below detection limit (i.e., <0.5 ppb) most of the time. However, clear NO plumes were sometimes observed in the early morning (Fig. 1e). The NO concentration peak at 04:00 is probably the combination of local emission sources and a low boundary layer. With the increasing sunlight afterwards, the NO concentration started to decrease. A similar diel pattern of NO2 was observed by the previous study at this site (Kammer et al., 2018). The lower NO2 concentration during daytime is likely explained by dilution with increasing boundary layer height and NO2 photolysis.
3.2 Vocus PTR-TOF capabilities in the forest
While Krechmer et al. (2018) and Riva et al. (2019a) have described the novel setup and performance of the Vocus PTR-TOF and its application during a lab study, the instrument capability has not been fully explored in an ambient environment. Based on the CERVOLAND deployment, we provide here the first overview of gas-phase molecules measured by the Vocus PTR-TOF in the forest. For a better visualization of the complex data set from real atmosphere, mass defect plots (averaged over the whole campaign) are shown in Fig. 2 with the difference between the exact mass and the nominal mass of a compound plotted against its exact mass. With the addition of hydrogen atoms, the mass defect increases, while the addition of oxygen atoms decreases the mass defect. Therefore, changes in the mass defect plot help to provide information on chemical transformation such as oxidation.
The mass defect plot in Fig. 2a is colored according to the retrieved elemental composition, with the black circle indicating unidentified molecules. The size of the markers is proportional to the logarithm of the peak area of the molecule. During the campaign, the Vocus PTR-TOF detected large amounts of (O)VOCs, with elemental composition categories of CH, CHO, CHN, CHS, CHON, CHOS, and others. For hydrocarbons, multiple series with different carbon numbers were measured, especially those compounds containing 5 (“C5”) to 10 carbon atoms (“C10”), 15 carbon atoms (“C15”), and 20 carbon atoms (“C20”). Some of the C5–C9 ions can be fragments of terpenes and their oxidation products (Tani et al., 2003; Tani, 2013; Kim et al., 2009; Kari et al., 2018). For ions <35 Th, the detection efficiency is much reduced due to a high-pass band filter of the big segmented quadrupole (BSQ; Krechmer et al., 2018). Compared to conventional PTR instruments, the observation of larger hydrocarbon molecules by the Vocus PTR-TOF is mainly caused by the much lower wall losses and increased detection efficiency. Hydrocarbon signals were largely contributed by monoterpene (C10H16H+) and its major fragment (C6H8H+), indicating the monoterpene-dominated environment in the Landes forest (Kammer et al., 2018). According to previous studies, monoterpene emissions in the Landes forest are dominated by α-pinene and β-pinene (Simon et al., 1994; Kammer et al., 2018). The identified compound with the elemental composition of ranked the third largest peak in hydrocarbons. A detailed discussion about ions can be found in the Supplement.
In addition to the emitted precursors, the Vocus PTR-TOF detected various VOC reaction products and intermediates. Similar to the PTR3 measurements in the CLOUD (Cosmics Leaving OUtdoor Droplets) chamber (Breitenlechner et al., 2017), many oxygenated compounds from terpene reactions with varying degrees of oxidation were observed in this study. However, as a potential limitation of the instrument, no dimers in the atmosphere were identified by the Vocus PTR-TOF, consistent with the results from a previous laboratory deployment (Riva et al., 2019a).
Figure 2b compares the daytime and nighttime variations of different molecules, with the marker sized by the signal difference between day and night. The daytime periods cover from 04:30 to 19:30, and the nighttime periods are from 19:30 to 04:30 of the next day (both are UTC time; local time equals UTC time + 2). The data points are colored in pink when the nighttime signal of the compound is larger than its daytime signal and in blue when the daytime signal is higher. Patterns in the figure clearly show the difference in the diurnal variations of gas molecules with different oxidation degrees. For example, most hydrocarbons are characterized with higher concentrations at night, which is largely caused by the stable nocturnal boundary layer. The more oxidized compounds with higher oxygen numbers are generally more abundant during the day due to enhanced photochemistry, whereas the concentrations of the less oxidized compounds are mostly higher at night. Details on the diurnal profiles of different oxidation products and their formation mechanisms are provided in Sect. 3.4.
3.3 Terpene characteristics
The characterizations of isoprene, monoterpenes, sesquiterpenes, and the rarely reported diterpenes are investigated in this study (Figs. 3, 4). On the global scale, isoprene is the most emitted BVOC species. It has been well established that photooxidation of isoprene in the atmosphere contributes to SOA formation through the multiphase reactions of isoprene-derived oxidation products (Claeys et al., 2004; Henze and Seinfeld, 2006; Surratt et al., 2010). However, recent advances in isoprene chemistry found that isoprene can impact both particle number and mass of monoterpene-derived SOA by scavenging hydroxyl and peroxy radicals (Kiendler-Scharr et al., 2009; Kanawade et al., 2011; McFiggans et al., 2019). During the CERVOLAND campaign, the average mixing ratio of isoprene was 0.6 ppb, consistent with the mean value of 0.4 ppb reported for the LANDEX campaign during summer 2017 at the same site (Mermet et al., 2019). These values are much lower than that in the southeastern United States (Xiong et al., 2015) and Amazon rainforest (Wei et al., 2018) but higher than observations in the boreal forest at the SMEAR II station in Finland (Hellén et al., 2018). Isoprene emissions are strongly light dependent (Monson and Fall, 1989; Kaser et al., 2013). Therefore, a pronounced diurnal pattern of isoprene was observed with maximum mixing ratios occurring during daytime and minima at night. It has been shown that the attribution of ions to isoprene with PTR instruments can be influenced by the fragmentation of many other compounds, i.e., cycloalkane and 2-methyl-3-buten-2-ol (MBO) (Karl et al., 2012; Gueneron et al., 2015). For example, using an E∕N ratio (the reduced electric field) of 106 Td (townsend units) in the PTR-MS with a quadrupole mass analyzer, 71 % of the parent MBO fragmented to ions (Warneke et al., 2003). However, in this study, the signal was around 10 times as high as the C5H11O+ signal, and both ions correlated poorly with each other (Fig. S4; r2=0.33). This information demonstrates that the fragmentation of MBO does not likely have a significant influence on the attribution of ions to isoprene in this work.
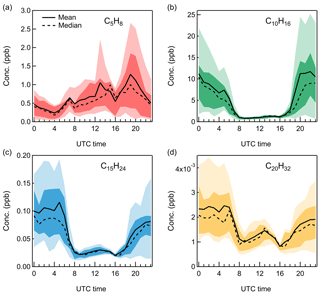
Figure 4Diurnal cycles of (a) C5H8, (b) C10H16, (c) C15H24, and (d) C20H32, with the 10th, 25th, 75th, and 90th percentiles shown in the shaded area.
As expected, monoterpenes showed the highest mixing ratios among all the terpenes, with an average value of 6.0 ppb. On 9 July, a heavy monoterpene episode occurred at night, with the monoterpene mixing ratio reaching as high as 41.2 ppb. Comparatively, the average monoterpene level observed in this work is similar to the measurements performed in 2015 and 2017 at the same site (Kammer et al., 2018; Mermet et al., 2019) and more than 10 times higher than that observed in the boreal forest at SMEAR II in summer (Hakola et al., 2012; Hellén et al., 2018). The high concentration of monoterpenes indicates the potential significance of monoterpene-related aerosol chemistry in the Landes forest. Unlike the light dependence of isoprene emissions, monoterpene emissions are found to be mainly controlled by temperature (Hakola et al., 2006; Kaser et al., 2013). At night, monoterpenes can be continuously emitted and accumulated within the boundary layer. Therefore, monoterpenes showed the opposite diel pattern to isoprene and peaked during nighttime. During daytime, the concentration of monoterpenes dropped to around 0.9 ppb, due to the increased atmospheric mixing after sunrise and the rapid photochemical consumptions.
A study in Hyytiälä concluded that sesquiterpenes, due to their higher reactivity, could play a more important role in O3 chemistry than monoterpenes, even though the concentration of sesquiterpenes was much lower (Hellén et al., 2018). However, the short lifetimes of sesquiterpenes also mean that their concentrations will be highly dependent on the sampling location at a given site. Some studies also proposed that sesquiterpene oxidation products are linked to atmospheric new particle formation (Bonn and Moortgat, 2003; Boy et al., 2007). Despite the potential importance of sesquiterpenes in aerosol chemistry, the available data on ambient sesquiterpene quantification remains still quite limited. In this work, the mixing ratios of sesquiterpenes were found to vary from 8.9 to 408.9 ppt in the Landes forest, with an average of 64.5 ppt during the observations. This sesquiterpene level is comparable to that reported by Mermet et al. (2019) in summer 2017 at the same site and observations by Jardine et al. (2011) in Amazonia but higher than previous measurements at the SMEAR II station (Hellén et al., 2018). Kim et al. (2009) show that different sesquiterpenes fragment on monoterpene parent and fragment ions to varying degrees inside PTR instruments. Without the consideration of sesquiterpene fragmentation, the quantification of sesquiterpenes in this work may be underestimated. As shown in Fig. 4, sesquiterpenes displayed a similar diurnal pattern with monoterpenes, consistent with observations in other areas (Jardine et al., 2011; Hellén et al., 2018).
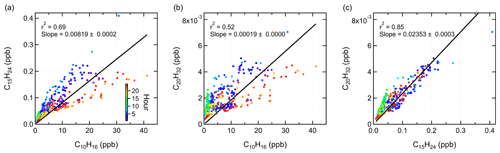
Figure 5Scatter plots of (a) C15H24 vs. C10H16, (b) C20H32 vs. C10H16, and (c) C20H32 vs. C15H24, colored by time of the day.
While diterpenes are present in all plants in the form of phytol, for a long time they have been thought to be not released by vegetation due to their low volatility (Keeling and Bohlmann, 2006). In 2004, von Schwartzenberg et al. (2004) reported for the first time the release of plant-derived diterpenes into the air. A recent study found that the emission rate of diterpenes by Mediterranean vegetation was in the same order of magnitude as monoterpenes and sesquiterpenes (Yáñez-Serrano et al., 2018). For the first time, this study reports the ambient concentration of diterpenes in a forest. According to the Vocus PTR-TOF measurements, the average mixing ratio of diterpenes was around 2 ppt in the Landes forest. Considering the low volatility of diterpenes and their potential wall losses inside the inlet tubing and the instrument, the diterpene concentration might be higher. Similar to monoterpenes and sesquiterpenes, diterpenes presented peak concentrations at night and lower levels during the day. Although the amounts of diterpenes in the atmosphere are hundreds to thousands of times lower than those of monoterpenes and sesquiterpenes, diterpenes potentially play a role in atmospheric chemistry due to their unsaturated structure and high molecular weight (Matsunaga et al., 2012). Up to now, there is no report on the possible atmospheric implications of diterpenes, which should deserve more attention in the future.
Considering the similar atmospheric behaviors of monoterpenes, sesquiterpenes, and diterpenes in this study, it is questioned if the observed sesquiterpenes and diterpenes are real signals in the atmosphere or generated by monoterpenes in the instrument. Bernhammer et al. (2018) have shown that secondary association reactions of protonated isoprene with isoprene can form monoterpenes within the PTR reaction chamber. Figure 5 illustrates the scatter plots among monoterpenes, sesquiterpenes, and diterpenes, colored by time of the day. At night, both sesquiterpenes and diterpenes correlated well with monoterpenes. However, their correlation with monoterpenes got weaker during daytime as the data points became more scattered. This suggests that the observations of sesquiterpenes and diterpenes are real emissions in the atmosphere. Comparatively, sesquiterpenes and diterpenes showed a strong correlation with each other through the whole day (r2=0.85).
3.4 Insights into terpene chemistry
3.4.1 Comparison with chamber results
Due to the diverse precursors and changing environmental conditions in the ambient air, it is challenging to retrieve all the atmospheric chemical processes occurring within the Landes forest. To start with, we compare the ambient data with those from α-pinene ozonolysis in the presence of NOx conducted in the COALA chamber (named after the project for which it was constructed: Comprehensive molecular characterization of secondary Organic AerosoL formation in the Atmosphere) at the University of Helsinki. A detailed description of the laboratory experiment is provided elsewhere (Riva et al., 2019a, b). According to literature, monoterpenes undergo some degree of fragmentation within the PTR instrument, producing dominant ions of , , , etc. (Tani et al., 2003; Tani, 2013; Kari et al., 2018). As illustrated in Fig. 6, is the largest fragment produced by monoterpenes within the Vocus PTR-TOF. However, a clear difference in monoterpene fragmentation pattern is observed in the mass spectra of ambient observations and chamber experiments. While the signal of is lower than that of during the field deployment, the peak is higher than peak in the chamber study. Based on the monoterpene calibration data, the signal is around 40 % and 138 % of the protonated monoterpene signal in ambient deployment and chamber experiment, respectively. The larger presence of the peak in the chamber study can be likely explained by the much higher concentrations of oxygenated terpenoids during the chamber experiments. Indeed, previous studies have shown that oxygenated terpenoids, including linalool and pinonaldehyde, fragment inside the PTR instrument and produce a dominant ion at m∕z 81 (Maleknia et al., 2007; Tani, 2013). Different settings of the instrument can also contribute to different fragmentation patterns of monoterpenes (Tani et al., 2003; Tani, 2013; Kari et al., 2018). In our ambient and chamber studies, the E∕N values of the Vocus PTR-TOF are quite similar, 118 and 120 Td, respectively. In addition, the fragmentation patterns vary among individual monoterpene species due to their different physicochemical properties (Tani, 2013; Kari et al., 2018). Considering that α-pinene is the only monoterpene species injected in the chamber experiment, the combination of various monoterpenes in the atmosphere likely introduces additional differences in the fragmentation pattern.
Gas-phase ozonolysis of alkenes generates OH radicals in high yields (Rickard et al., 1999). Without an OH scavenger, both O3- and OH-initiated oxidations happened during α-pinene ozonolysis in the chamber. Using the Vocus PTR-TOF, various oxidation products were identified in the chamber study, with the dominant species being , C8H14O3−6, C9H14O1−5, and . In comparison, more oxygenated compounds which were directly emitted or from monoterpene reactions were observed in ambient air due to complex environmental conditions, with the oxygen number ranging from one to seven. Therefore, the Vocus PTR-TOF measurements provide the opportunity to characterize both the emitted precursors and the resulting oxidation products. During the chamber experiments, NO2 was injected and photolyzed using 400 nm LED lights to generate NO. In the presence of NOx, organic nitrates were formed from the reactions between NO and monoterpene-derived peroxy radicals (RO2). The major organic nitrates observed were and . Compared to the chamber study, more organic nitrates of C8, C9, and C10 from monoterpene reactions were identified in CERVOLAND data. It is worth pointing out that the combination of different monoterpene species in the ambient environment may result in various types of organic nitrates through different formation pathways.
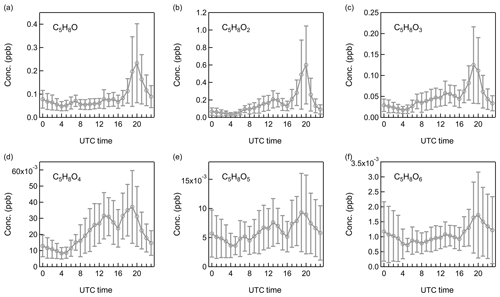
Figure 7Diurnal patterns of non-nitrate isoprene oxidation products: (a) C5H8O, (b) C5H8O2, (c) C5H8O3, (d) C5H8O4, (e) C5H8O5, and (f) C5H8O6.
3.4.2 Non-nitrate terpene oxidation products
Based on the ambient observations, the non-nitrate oxidation products from isoprene, monoterpenes, and sesquiterpenes are investigated in this study. Isoprene gas-phase products are mainly represented by C4 and C5 compounds (Wennberg et al., 2018). In this work, we consider C4H6,8On and n (n=1–6) as the dominant non-nitrate products from isoprene oxidations. The diurnal variations of C5H8On are displayed in Fig. 7 and the others in Figs. S5–S7. Generally, all these oxidation products displayed an evening peak at around 20:00, which may come from the O3- or OH-initiated isoprene oxidations. Reaction with OH represents the largest loss pathway for isoprene in the atmosphere and produces a population of isoprene peroxyl radicals (Wennberg et al., 2018). In the presence of NO, the major products are methyl vinyl ketone (MVK, C4H6O) and methacrolein (MACR, C4H6O). Globally, reactions with O3 contribute a small fraction of approximately 10 % to isoprene removal in the atmosphere (Wennberg et al., 2018). When isoprene reacts with O3, one carbon is always split off from the molecule (Criegee, 1975). Considering the peak concentration of isoprene at 20:00 and the relatively high O3 concentration at the moment (Figs. 1 and 4), isoprene ozonolysis is also likely contributing to the formation of C4 oxidation products. Because OH radicals can be efficiently produced from alkene ozonolysis (Pfeiffer et al., 2001), the OH-initiated oxidation of isoprene can also be an important formation pathway of these oxidation products in the evening. For example, as a predominant product from the reactions of isoprene with OH, C5H10O3 (corresponding to isoprene hydroxy hydroperoxide and/or isoprene epoxydiols) presented a clear single peak in the evening. To determine the relative importance of O3- and OH-initiated oxidations in isoprene chemistry at night, the reaction rates (R) of isoprene with O3 and OH radical were compared by Eqs. (1) and (2):
where k is the reaction rate coefficient of isoprene with OH or O3, and [ISO], [OH], and [O3] is the concentration of isoprene, OH radical, and O3, respectively.
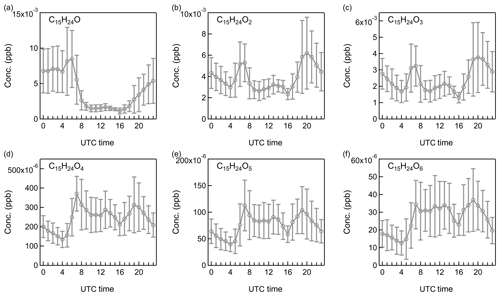
Figure 9Diurnal patterns of non-nitrate sesquiterpene oxidation products: (a) C15H24O, (b) C15H24O2, (c) C15H24O3, (d) C15H24O4, (e) C15H24O5, and (f) C15H24O6.
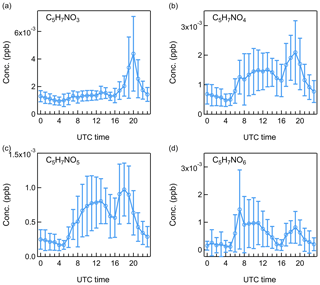
Figure 10Diurnal patterns of isoprene-derived organic nitrates: (a) C5H7NO3, (b) C5H7NO4, (c) C5H7NO5, and (d) C5H7NO6.
Taking the evening peak of isoprene oxidation products at 20:00 as an example, we compared the roles of O3 and OH radicals in their formation. Laboratory studies have shown that the reaction rate coefficient of isoprene with OH radical is generally 107 times larger than that of isoprene with O3 (Dreyfus et al., 2002; Karl et al., 2004). Based on the competition between OH production and removal processes at night (Dusanter et al., 2008), the steady-state OH concentration was estimated to be 0.012 ppt. Details can be found in the Supplement. With an O3 concentration of ∼20 ppb at 20:00, the reaction rate of isoprene with OH radical was around 6 times as high as that of isoprene with O3. For the more oxidized compounds from isoprene oxidations, their concentrations had a broad daytime presence from 10:00 to 20:00 due to strong photooxidation processes. Similar diurnal variations of and measured by nitrate CIMS have been observed in an isoprene-dominated environment at Centreville, Alabama (Massoli et al., 2018).
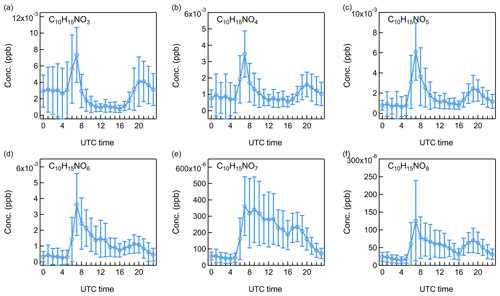
Figure 11Diurnal patterns of monoterpene-derived organic nitrates: (a) C10H15NO3, (b) C10H15NO4, (c) C10H15NO5, (d) C10H15NO6, (e) C10H15NO7, and (f) C10H15NO8.
The diurnal patterns of C8H12,14On, C9H14On, and n (n=1–6) were illustrated to characterize monoterpene oxidations in the Landes forest (Figs. 8, S8–S12). For the less oxidized compounds with oxygen numbers from one to four, most of them were observed with clear morning and evening peaks, which can be produced from O3- and OH-initiated monoterpene oxidations. For the morning peak at around 07:00, the relative roles of O3- and OH-initiated monoterpene oxidation were evaluated using a similar method as in Eqs. (1) and (2). The reaction rate coefficient of monoterpene + OH is approximately 106 times higher than that of monoterpene + O3 (Atkinson et al., 1990; Khamaganov and Hites, 2001; Gill and Hites, 2002; Hakola et al., 2012). In the morning, typical tropospheric OH concentrations have been observed to be around 1×105 to 1×106 molecule cm−3 (0.004–0.04 ppt) (Shirinzadeh et al., 1987; Ren et al., 2003; Khan et al., 2008; Petäjä et al., 2009; Stone et al., 2012). For an OH concentration of 1×105 molecule cm−3 (0.004 ppt), with the average O3 concentration of 15 ppb at 07:00, the reaction rate of monoterpene + OH was about 0.25 times as high as that of monoterpene + O3. If the OH concentration was up to 1×106 molecule cm−3 (0.04 ppt) at 07:00, the reaction rate of monoterpene with OH radical was 2.5 times higher than that of monoterpene with O3 according to the calculations. In other words, both oxidants are likely to be of importance at this time. For the evening peak of the less oxidized monoterpene oxidation products at 20:00, the relative importance of O3 and OH radical in monoterpene chemistry changed due to the lower OH concentration. With the average O3 concentration of ∼20 ppb and OH concentration of 0.012 ppt at 20:00, the reaction rates of monoterpenes with O3 and OH radical were at a similar level. Compared to other compounds, the evening peak of C9H14O, C10H16O, C10H18O, and C10H18O2 extended over midnight. C9H14O has been found to be one of the main products formed in the ozonolysis reactions of monoterpenes (Atkinson and Arey, 2003). O3-initiated oxidation with extremely high monoterpene levels might be responsible for the high concentration of C9H14O at night. Camphor (C10H16O), linalool (C10H18O), and linalool oxide (C10H18O2) can be emitted by leaves and flowers (Corchnoy et al., 1992; Lavy et al., 2002). Therefore, direct emissions from vegetation in the Landes forest may contribute to the high mixing ratios of these compounds during night. With strong photochemical oxidations during the day, the diurnal cycles of the more oxidized compounds were characterized with a broad daytime distribution peaking between 14:00 and 16:00 UTC.
To date the oxidation processes of sesquiterpenes have been rarely investigated despite its potential significance in new particle formation and SOA formation (Bonn and Moortgat, 2003; Winterhalter et al., 2009). In this study, various sesquiterpene oxidation products were observed, mainly including C14H22On, C15H22On, and C15H24On (n=1–6), providing the possibility to explore the oxidations of sesquiterpenes in the atmosphere. As shown in Fig. 9 and Figs. S13–S14, with the increase of oxygen numbers, sesquiterpene oxidation products displayed similar variations in their diurnal profiles with monoterpene oxidation products. The less oxidized products with one to three oxygens peaked both in the morning and in the evening, and the more oxidized compounds had a broad presence throughout the day. These results indicate a similar oxidation processes of sesquiterpenes with monoterpenes in the Landes forest.
3.4.3 Terpene-derived organic nitrates
Organic nitrates have been shown to represent a large fraction of submicron aerosol nitrate at both urban and rural sites in Europe (Kiendler-Scharr et al., 2016). During daytime, the reaction of peroxy radicals with NO can lead to the formation of organic nitrates. At night, NO3 radicals from the oxidation of NO2 by O3 can also react with unsaturated compounds mostly coming from BVOCs to generate organic nitrates (Ayres et al., 2015). In this study, the less oxidized organic nitrates from monoterpene oxidations presented a distinct morning peak at 07:00 (Figs. 11, S17–S18), which can come from O3- and OH-initiated monoterpene oxidations in the presence of NOx. In addition, both isoprene- and monoterpene-derived organic nitrates showed evening peaks at around 20:00 (Figs. 10, S15–S16). Using monoterpenes as an example, the relative roles of O3, OH radical, and NO3 radical in the nighttime formation of monoterpene-derived organic nitrates were evaluated by calculating the corresponding reaction rate (R):
where k is the reaction rate coefficient of monoterpenes with O3, OH radical, or NO3 radical, and [MT], [O3], [OH], and [NO3] represent the concentrations of monoterpenes, O3, OH radical, and NO3 radical, respectively.
Taking the peak concentration of monoterpene-derived organic nitrates at 20:00 as an example, the concentration of NO3 radical was calculated by assuming a steady state between its production from O3 and NO2 and its removal by oxidation reactions and losses. The details have been described by Allan et al. (2000) and Peräkylä et al. (2014). With the high O3 scavenging by monoterpenes in the evening, the estimated concentration of NO3 radical was 0.017 ppt. Using and cm3 molecule−1 s−1 taken from Peräkylä et al. (2014), the reaction rate of monoterpenes with O3 was ∼10 times higher than that of monoterpenes with NO3 radicals. However, while ozonolysis was likely to dominate the overall oxidation of monoterpenes, the organic nitrate formation from O3-initiated oxidation may still be much lower than those from NO3-initiated oxidations, depending on what fraction of RO2 radicals were reacting with NOx. The relative importance of O3 and OH radical in monoterpene chemistry at this time was the same as discussed in Sect. 3.4.2.
This work presented the deployment of the new state-of-the-art Vocus PTR-TOF in the French Landes forest during the CERVOLAND campaign. The Vocus PTR-TOF capabilities are evaluated for the first time in the actual ambient environment by the identification of the observed gas-phase molecules. With the improved detection efficiency and measurement precision compared to conventional PTR instruments, multiple hydrocarbons with carbon numbers varying from 3 to 20 were observed as well as various VOC oxidation products. Hydrocarbon signals were dominated by monoterpenes and their major fragment ions (e.g., C6H8H+) within the instrument, consistent with high monoterpene emissions in the Landes forest. In general, most hydrocarbon molecules and the less oxidized compounds were characterized with high signals at night, whereas the more oxidized compounds exhibited elevated intensity during the day.
To demonstrate the importance of the Vocus PTR-TOF application in atmospheric science study, the characteristics of terpenes and their oxidation products were investigated. In addition to the observation of isoprene, monoterpenes, and sesquiterpenes, this study presented the ambient characteristics of the rarely recorded diterpenes, which are traditionally considered non-volatile species in the atmosphere. On average, the concentration of diterpenes was 1.7 ppt in the Landes forest, which was 100 to 1000 times lower than that of monoterpenes (6.0 ppb) and sesquiterpenes (64.5 ppt). However, considering their low vapor pressure and high reactivity, diterpenes may potentially play an important part in atmospheric chemistry. The diurnal variations of diterpenes showed the maximum peak at night and low levels during the day, similar to those of monoterpenes and sesquiterpenes.
With strong photochemical oxidations of terpenes during the day, the more oxidized terpene reaction products were observed with a broad daytime peak, whereas the less oxidized terpene reaction products showed peak concentrations in the early morning and/or in the evening. By calculating the reaction rates of terpenes with the main oxidants, OH radical, O3, and NO3 radical, the contributions of different formation pathways to terpene oxidations were evaluated. The morning and evening peaks of non-nitrate terpene reaction products were contributed by both O3- and OH-induced terpene oxidations. For the formation of terpene-derived organic nitrates, the relative importance of O3-, OH-, and NO3-driven oxidation pathways was more difficult to evaluate. Overall, we have shown that the Vocus PTR-TOF is able to detect a very broad coverage of compounds from VOC precursors to various oxidation products. Therefore, the application of the Vocus PTR-TOF in atmospheric sciences will be fundamental to understand the chemical evolution of VOCs in the atmosphere and their roles in air quality and climate issues.
Data used in this study are available from the corresponding author upon request. Please contact Haiyan Li (haiyan.li@helsinki.fi).
The supplement related to this article is available online at: https://doi.org/10.5194/acp-20-1941-2020-supplement.
ME and MR conceived the study. MR, LH, PMF, EV, and EP conducted the field measurements. HL carried out the data analysis. MR, PR, KD, JEK, DW, MK, ME, and FB participated the data analysis. HL wrote the paper with inputs from all coauthors.
Jordan E. Krechmer and Douglas Worsnop both work for Aerodyne Research Inc.
The authors would like to thank the PRIMEQUAL program for financial support (ADEME, convention #1662C0024). This study has also been carried out with financial support from the French National Research Agency (ANR) in the frame of the “Investments for the Future” program, within the Cluster of Excellence COTE (ANR-10-LABX-45) of the University of Bordeaux. Special thanks to Elena Ormeño-Lafuente (IMBE) for the loan of the BVOC calibration gas cylinders and Christophe Chipeaux and Denis Loustau (ISPA-INRA) for their precious help in providing meteorological data and access to the ICOS station facility.
This research has been supported by the H2020 European Research Council (grant nos. ATM-GTP (742206), COALA (638703), and CHAPAs (850614)) and the Academy of Finland (grant nos. 317380, 320094).
Open access funding provided by Helsinki University Library.
This paper was edited by Alex B. Guenther and reviewed by two anonymous referees.
Allan, B. J., McFiggans, G., Plane, J. M. C., Coe, H., and McFadyen, G. G.: The nitrate radical in the remote marine boundary layer, J. Geophys. Res.-Atmos., 105, 24191–24204, https://doi.org/10.1029/2000JD900314, 2000.
Atkinson, R. and Arey, J.: Atmospheric Degradation of Volatile Organic Compounds, Chem. Rev., 103, 4605–4638, https://doi.org/10.1021/cr0206420, 2003.
Atkinson, R., Hasegawa, D., and Aschmann, S. M.: Rate constants for the gas-phase reactions of O3 with a series of monoterpenes and related compounds at 296±2 K, Int. J. Chem. Kinet., 22, 871–887, https://doi.org/10.1002/kin.550220807, 1990.
Ayres, B. R., Allen, H. M., Draper, D. C., Brown, S. S., Wild, R. J., Jimenez, J. L., Day, D. A., Campuzano-Jost, P., Hu, W., de Gouw, J., Koss, A., Cohen, R. C., Duffey, K. C., Romer, P., Baumann, K., Edgerton, E., Takahama, S., Thornton, J. A., Lee, B. H., Lopez-Hilfiker, F. D., Mohr, C., Wennberg, P. O., Nguyen, T. B., Teng, A., Goldstein, A. H., Olson, K., and Fry, J. L.: Organic nitrate aerosol formation via NO3 + biogenic volatile organic compounds in the southeastern United States, Atmos. Chem. Phys., 15, 13377–13392, https://doi.org/10.5194/acp-15-13377-2015, 2015.
Bernhammer, A.-K., Fischer, L., Mentler, B., Heinritzi, M., Simon, M., and Hansel, A.: Production of highly oxygenated organic molecules (HOMs) from trace contaminants during isoprene oxidation, Atmos. Meas. Tech., 11, 4763–4773, https://doi.org/10.5194/amt-11-4763-2018, 2018.
Bonn, B. and Moortgat, G. K.: Sesquiterpene ozonolysis: Origin of atmospheric new particle formation from biogenic hydrocarbons, Geophys. Res. Lett., 30, 1585, https://doi.org/10.1029/2003GL017000, 2003.
Boy, M., Bonn, B., Maso, M. D., Hakola, H., Hirsikko, A., Kulmala, M., Kurtén, T., Laakso, L., Mäkelä, J., Riipinen, I., Rannik, Ü., Sihto, S.-L., and Ruuskanen, T. M.: Biogenic Sesquiterpenes and Atmospheric New Particle Formation: A Boreal Forest Site Investigation, Nucleation and Atmospheric Aerosols, Dordrecht, 2007, 344–349, 2007.
Breitenlechner, M., Fischer, L., Hainer, M., Heinritzi, M., Curtius, J., and Hansel, A.: PTR3: An Instrument for Studying the Lifecycle of Reactive Organic Carbon in the Atmosphere, Anal. Chem., 89, 5824–5831, https://doi.org/10.1021/acs.analchem.6b05110, 2017.
Bsaibes, S., Al Ajami, M., Mermet, K., Truong, F., Batut, S., Hecquet, C., Dusanter, S., Léornadis, T., Sauvage, S., Kammer, J., Flaud, P.-M., Perraudin, E., Villenave, E., Locoge, N., Gros, V., and Schoemaecker, C.: Variability of OH reactivity in the Landes maritime Pine forest: Results from the LANDEX campaign 2017, Atmos. Chem. Phys. Discuss., https://doi.org/10.5194/acp-2019-548, in review, 2019.
Calfapietra, C., Fares, S., Manes, F., Morani, A., Sgrigna, G., and Loreto, F.: Role of Biogenic Volatile Organic Compounds (BVOC) emitted by urban trees on ozone concentration in cities: A review, Environ. Pollut., 183, 71–80, https://doi.org/10.1016/j.envpol.2013.03.012, 2013.
Carslaw, K. S., Lee, L. A., Reddington, C. L., Pringle, K. J., Rap, A., Forster, P. M., Mann, G. W., Spracklen, D. V., Woodhouse, M. T., Regayre, L. A., and Pierce, J. R.: Large contribution of natural aerosols to uncertainty in indirect forcing, Nature, 503, 67, https://doi.org/10.1038/nature12674, 2013.
Claeys, M., Graham, B., Vas, G., Wang, W., Vermeylen, R., Pashynska, V., Cafmeyer, J., Guyon, P., Andreae, M. O., Artaxo, P., and Maenhaut, W.: Formation of Secondary Organic Aerosols Through Photooxidation of Isoprene, J. Sci., 303, 1173–1176, https://doi.org/10.1126/science.1092805, 2004.
Corchnoy, S. B., Arey, J., and Atkinson, R.: Hydrocarbon emissions from twelve urban shade trees of the Los Angeles, California, Air Basin, Atmos. Environ. B., 26, 339–348, https://doi.org/10.1016/0957-1272(92)90009-H, 1992.
Criegee, R.: Mechanism of Ozonolysis, Angewandte Chemie International Edition in English, 14, 745–752, https://doi.org/10.1002/anie.197507451, 1975.
Donahue, N. M., Kroll, J. H., Pandis, S. N., and Robinson, A. L.: A two-dimensional volatility basis set – Part 2: Diagnostics of organic-aerosol evolution, Atmos. Chem. Phys., 12, 615–634, https://doi.org/10.5194/acp-12-615-2012, 2012.
Dreyfus, G. B., Schade, G. W., and Goldstein, A. H.: Observational constraints on the contribution of isoprene oxidation to ozone production on the western slope of the Sierra Nevada, California, J. Geophys. Res.-Atmos., 107, ACH1-1–ACH1-17, https://doi.org/10.1029/2001JD001490, 2002.
Dusanter, S., Vimal, D., and Stevens, P. S.: Technical note: Measuring tropospheric OH and HO2 by laser-induced fluorescence at low pressure. A comparison of calibration techniques, Atmos. Chem. Phys., 8, 321–340, https://doi.org/10.5194/acp-8-321-2008, 2008.
Gill, K. J. and Hites, R. A.: Rate Constants for the Gas-Phase Reactions of the Hydroxyl Radical with Isoprene, α- and β-Pinene, and Limonene as a Function of Temperature, The J. Phys. Chem. A, 106, 2538–2544, https://doi.org/10.1021/jp013532q, 2002.
Goldstein, A. H., McKay, M., Kurpius, M. R., Schade, G. W., Lee, A., Holzinger, R., and Rasmussen, R. A.: Forest thinning experiment confirms ozone deposition to forest canopy is dominated by reaction with biogenic VOCs, Geophys. Res. Lett., 31, L22106, https://doi.org/10.1029/2004GL021259, 2004.
Gueneron, M., Erickson, M. H., VanderSchelden, G. S., and Jobson, B. T.: PTR-MS fragmentation patterns of gasoline hydrocarbons, Int. J. Mass Spectro., 379, 97–109, https://doi.org/10.1016/j.ijms.2015.01.001, 2015.
Guenther, A., Hewitt, C. N., Erickson, D., Fall, R., Geron, C., Graedel, T., Harley, P., Klinger, L., Lerdau, M., McKay, W. A., Pierce, T., Scholes, B., Steinbrecher, R., Tallamraju, R., Taylor, J., and Zimmerman, P.: A global model of natural volatile organic compound emissions, J. Geophys. Res.-Atmos., 100, 8873–8892, https://doi.org/10.1029/94JD02950, 1995.
Hakola, H., Tarvainen, V., Bäck, J., Ranta, H., Bonn, B., Rinne, J., and Kulmala, M.: Seasonal variation of mono- and sesquiterpene emission rates of Scots pine, Biogeosciences, 3, 93–101, https://doi.org/10.5194/bg-3-93-2006, 2006.
Hakola, H., Hellén, H., Hemmilä, M., Rinne, J., and Kulmala, M.: In situ measurements of volatile organic compounds in a boreal forest, Atmos. Chem. Phys., 12, 11665–11678, https://doi.org/10.5194/acp-12-11665-2012, 2012.
Hatfield, M. L. and Huff Hartz, K. E.: Secondary organic aerosol from biogenic volatile organic compound mixtures, Atmos. Environ., 45, 2211–2219, https://doi.org/10.1016/j.atmosenv.2011.01.065, 2011.
Hellén, H., Hakola, H., Pystynen, K.-H., Rinne, J., and Haapanala, S.: C2-C10 hydrocarbon emissions from a boreal wetland and forest floor, Biogeosciences, 3, 167–174, https://doi.org/10.5194/bg-3-167-2006, 2006.
Hellén, H., Praplan, A. P., Tykkä, T., Ylivinkka, I., Vakkari, V., Bäck, J., Petäjä, T., Kulmala, M., and Hakola, H.: Long-term measurements of volatile organic compounds highlight the importance of sesquiterpenes for the atmospheric chemistry of a boreal forest, Atmos. Chem. Phys., 18, 13839–13863, https://doi.org/10.5194/acp-18-13839-2018, 2018.
Henze, D. K. and Seinfeld, J. H.: Global secondary organic aerosol from isoprene oxidation, Geophys. Res. Lett., 33, L09812, https://doi.org/10.1029/2006GL025976, 2006.
Holzinger, R., Acton, W. J. F., Bloss, W. J., Breitenlechner, M., Crilley, L. R., Dusanter, S., Gonin, M., Gros, V., Keutsch, F. N., Kiendler-Scharr, A., Kramer, L. J., Krechmer, J. E., Languille, B., Locoge, N., Lopez-Hilfiker, F., Materić, D., Moreno, S., Nemitz, E., Quéléver, L. L. J., Sarda Esteve, R., Sauvage, S., Schallhart, S., Sommariva, R., Tillmann, R., Wedel, S., Worton, D. R., Xu, K., and Zaytsev, A.: Validity and limitations of simple reaction kinetics to calculate concentrations of organic compounds from ion counts in PTR-MS, Atmos. Meas. Tech., 12, 6193–6208, https://doi.org/10.5194/amt-12-6193-2019, 2019.
Intergovernmental Panel on Climate Change (IPCC), Climate Change 2013: The Physical Science Basis. Contribution of Working Group I to the Fifth Assessment Report of the Intergovernmental Panel on Climate Change, 1535 pp., Cambridge Univ. Press, Cambridge, UK, and New York, 2013.
Jardine, K., Yañez Serrano, A., Arneth, A., Abrell, L., Jardine, A., van Haren, J., Artaxo, P., Rizzo, L. V., Ishida, F. Y., Karl, T., Kesselmeier, J., Saleska, S., and Huxman, T.: Within-canopy sesquiterpene ozonolysis in Amazonia, J. Geophys. Res.Atmos., 116, D19301, https://doi.org/10.1029/2011JD016243, 2011.
Jimenez, J. L., Canagaratna, M. R., Donahue, N. M., Prevot, A. S. H., Zhang, Q., Kroll, J. H., DeCarlo, P. F., Allan, J. D., Coe, H., Ng, N. L., Aiken, A. C., Docherty, K. S., Ulbrich, I. M., Grieshop, A. P., Robinson, A. L., Duplissy, J., Smith, J. D., Wilson, K. R., Lanz, V. A., Hueglin, C., Sun, Y. L., Tian, J., Laaksonen, A., Raatikainen, T., Rautiainen, J., Vaattovaara, P., Ehn, M., Kulmala, M., Tomlinson, J. M., Collins, D. R., Cubison, M. J., Dunlea, J., Huffman, J. A., Onasch, T. B., Alfarra, M. R., Williams, P. I., Bower, K., Kondo, Y., Schneider, J., Drewnick, F., Borrmann, S., Weimer, S., Demerjian, K., Salcedo, D., Cottrell, L., Griffin, R., Takami, A., Miyoshi, T., Hatakeyama, S., Shimono, A., Sun, J. Y., Zhang, Y. M., Dzepina, K., Kimmel, J. R., Sueper, D., Jayne, J. T., Herndon, S. C., Trimborn, A. M., Williams, L. R., Wood, E. C., Middlebrook, A. M., Kolb, C. E., Baltensperger, U., and Worsnop, D. R.: Evolution of Organic Aerosols in the Atmosphere, Science, 326, 1525–1529, https://doi.org/10.1126/science.1180353, 2009.
Jokinen, T., Sipilä, M., Richters, S., Kerminen, V.-M., Paasonen, P., Stratmann, F., Worsnop, D., Kulmala, M., Ehn, M., Herrmann, H., and Berndt, T.: Rapid Autoxidation Forms Highly Oxidized RO2 Radicals in the Atmosphere, Angew. Chem. Int. Edit., 53, 14596–14600, https://doi.org/10.1002/anie.201408566, 2014.
Jokinen, T., Berndt, T., Makkonen, R., Kerminen, V.-M., Junninen, H., Paasonen, P., Stratmann, F., Herrmann, H., Guenther, A. B., Worsnop, D. R., Kulmala, M., Ehn, M., and Sipilä, M.: Production of extremely low volatile organic compounds from biogenic emissions: Measured yields and atmospheric implications, P. Natl. Acad. Sci. USA, 112, 7123–7128, https://doi.org/10.1073/pnas.1423977112, 2015.
Kammer, J., Perraudin, E., Flaud, P. M., Lamaud, E., Bonnefond, J. M., and Villenave, E.: Observation of nighttime new particle formation over the French Landes forest, Sci. Total Environ., 621, 1084–1092, https://doi.org/10.1016/j.scitotenv.2017.10.118, 2018.
Kanakidou, M., Seinfeld, J. H., Pandis, S. N., Barnes, I., Dentener, F. J., Facchini, M. C., Van Dingenen, R., Ervens, B., Nenes, A., Nielsen, C. J., Swietlicki, E., Putaud, J. P., Balkanski, Y., Fuzzi, S., Horth, J., Moortgat, G. K., Winterhalter, R., Myhre, C. E. L., Tsigaridis, K., Vignati, E., Stephanou, E. G., and Wilson, J.: Organic aerosol and global climate modelling: a review, Atmos. Chem. Phys., 5, 1053–1123, https://doi.org/10.5194/acp-5-1053-2005, 2005.
Kanawade, V. P., Jobson, B. T., Guenther, A. B., Erupe, M. E., Pressley, S. N., Tripathi, S. N., and Lee, S.-H.: Isoprene suppression of new particle formation in a mixed deciduous forest, Atmos. Chem. Phys., 11, 6013–6027, https://doi.org/10.5194/acp-11-6013-2011, 2011.
Kari, E., Miettinen, P., Yli-Pirilä, P., Virtanen, A., and Faiola, C. L.: PTR-ToF-MS product ion distributions and humidity-dependence of biogenic volatile organic compounds, Int. J. Mass Spectro., 430, 87–97, https://doi.org/10.1016/j.ijms.2018.05.003, 2018.
Karl, M., Brauers, T., Dorn, H. P., Holland, F., Komenda, M., Poppe, D., Rohrer, F., Rupp, L., Schaub, A., and Wahner, A.: Kinetic Study of the OH-isoprene and O3-isoprene reaction in the atmosphere simulation chamber, SAPHIR, Geophys. Res. Lett., 31, L05117, https://doi.org/10.1029/2003GL019189, 2004.
Karl, T., Hansel, A., Cappellin, L., Kaser, L., Herdlinger-Blatt, I., and Jud, W.: Selective measurements of isoprene and 2-methyl-3-buten-2-ol based on NO+ ionization mass spectrometry, Atmos. Chem. Phys., 12, 11877–11884, https://doi.org/10.5194/acp-12-11877-2012, 2012.
Kaser, L., Karl, T., Guenther, A., Graus, M., Schnitzhofer, R., Turnipseed, A., Fischer, L., Harley, P., Madronich, M., Gochis, D., Keutsch, F. N., and Hansel, A.: Undisturbed and disturbed above canopy ponderosa pine emissions: PTR-TOF-MS measurements and MEGAN 2.1 model results, Atmos. Chem. Phys., 13, 11935–11947, https://doi.org/10.5194/acp-13-11935-2013, 2013.
Keck, L., Hoeschen, C., and Oeh, U.: Effects of carbon dioxide in breath gas on proton transfer reaction-mass spectrometry (PTR-MS) measurements, Int. J. Mass Spectro., 270, 156–165, https://doi.org/10.1016/j.ijms.2007.12.009, 2008.
Keeling, C. I. and Bohlmann, J.: Diterpene resin acids in conifers, Phytochemistry, 67, 2415–2423, https://doi.org/10.1016/j.phytochem.2006.08.019, 2006.
Khamaganov, V. G. and Hites, R. A.: Rate Constants for the Gas-Phase Reactions of Ozone with Isoprene, α- and β-Pinene, and Limonene as a Function of Temperature, The J. Phys. Chem. A, 105, 815–822, https://doi.org/10.1021/jp002730z, 2001.
Khan, M. A. H., Ashfold, M. J., Nickless, G., Martin, D., Watson, L. A., Hamer, P. D., Wayne, R. P., Canosa-Mas, C. E., and Shallcross, D. E.: Night-time NO3 and OH radical concentrations in the United Kingdom inferred from hydrocarbon measurements, Atmos. Sci. Lett., 9, 140–146, https://doi.org/10.1002/asl.175, 2008.
Kiendler-Scharr, A., Wildt, J., Maso, M. D., Hohaus, T., Kleist, E., Mentel, T. F., Tillmann, R., Uerlings, R., Schurr, U., and Wahner, A.: New particle formation in forests inhibited by isoprene emissions, Nature, 461, 381, https://doi.org/10.1038/nature08292, 2009.
Kiendler-Scharr, A., Mensah, A. A., Friese, E., Topping, D., Nemitz, E., Prevot, A. S. H., Äijälä, M., Allan, J., Canonaco, F., Canagaratna, M., Carbone, S., Crippa, M., Dall Osto, M., Day, D. A., De Carlo, P., Di Marco, C. F., Elbern, H., Eriksson, A., Freney, E., Hao, L., Herrmann, H., Hildebrandt, L., Hillamo, R., Jimenez, J. L., Laaksonen, A., McFiggans, G., Mohr, C., O'Dowd, C., Otjes, R., Ovadnevaite, J., Pandis, S. N., Poulain, L., Schlag, P., Sellegri, K., Swietlicki, E., Tiitta, P., Vermeulen, A., Wahner, A., Worsnop, D., and Wu, H. C.: Ubiquity of organic nitrates from nighttime chemistry in the European submicron aerosol, Geophys. Res. Lett., 43, 7735–7744, https://doi.org/10.1002/2016GL069239, 2016.
Kim, S., Karl, T., Helmig, D., Daly, R., Rasmussen, R., and Guenther, A.: Measurement of atmospheric sesquiterpenes by proton transfer reaction-mass spectrometry (PTR-MS), Atmos. Meas. Tech., 2, 99–112, https://doi.org/10.5194/amt-2-99-2009, 2009.
Krechmer, J., Lopez-Hilfiker, F., Koss, A., Hutterli, M., Stoermer, C., Deming, B., Kimmel, J., Warneke, C., Holzinger, R., Jayne, J., Worsnop, D., Fuhrer, K., Gonin, M., and de Gouw, J.: Evaluation of a New Reagent-Ion Source and Focusing Ion–Molecule Reactor for Use in Proton-Transfer-Reaction Mass Spectrometry, Anal. Chem., 90, 12011–12018, https://doi.org/10.1021/acs.analchem.8b02641, 2018.
Lavy, M., Zuker, A., Lewinsohn, E., Larkov, O., Ravid, U., Vainstein, A., and Weiss, D.: Linalool and linalool oxide production in transgenic carnation flowers expressing the Clarkia breweri linalool synthase gene, Mol. Breeding, 9, 103–111, https://doi.org/10.1023/A:1026755414773, 2002.
Lide, D. R.: CRC Handbook of Chemistry and Physics; Internet Version 2005, CRC Press, Boca Raton, FL, 2005.
Liu, X., Deming, B., Pagonis, D., Day, D. A., Palm, B. B., Talukdar, R., Roberts, J. M., Veres, P. R., Krechmer, J. E., Thornton, J. A., de Gouw, J. A., Ziemann, P. J., and Jimenez, J. L.: Effects of gas-wall interactions on measurements of semivolatile compounds and small polar molecules, Atmos. Meas. Tech., 12, 3137–3149, https://doi.org/10.5194/amt-12-3137-2019, 2019.
Maleknia, S. D., Bell, T. L., and Adams, M. A.: PTR-MS analysis of reference and plant-emitted volatile organic compounds, Int. J. Mass Spectro., 262, 203–210, https://doi.org/10.1016/j.ijms.2006.11.010, 2007.
Maria, S. F., Russell, L. M., Gilles, M. K., and Myneni, S. C. B.: Organic Aerosol Growth Mechanisms and Their Climate-Forcing Implications, Science, 306, 1921–1924, https://doi.org/10.1126/science.1103491 , 2004.
Massoli, P., Stark, H., Canagaratna, M. R., Krechmer, J. E., Xu, L., Ng, N. L., Mauldin, R. L., Yan, C., Kimmel, J., Misztal, P. K., Jimenez, J. L., Jayne, J. T., and Worsnop, D. R.: Ambient Measurements of Highly Oxidized Gas-Phase Molecules during the Southern Oxidant and Aerosol Study (SOAS) 2013, ACS Earth Space Chem., 2, 653–672, https://doi.org/10.1021/acsearthspacechem.8b00028, 2018.
Matsunaga, S. N., Chatani, S., Nakatsuka, S., Kusumoto, D., Kubota, K., Utsumi, Y., Enoki, T., Tani, A., and Hiura, T.: Determination and potential importance of diterpene (kaur-16-ene) emitted from dominant coniferous trees in Japan, Chemosphere, 87, 886–893, https://doi.org/10.1016/j.chemosphere.2012.01.040, 2012.
Mauderly, J. L. and Chow, J. C.: Health Effects of Organic Aerosols, Inhal. Toxicol., 20, 257–288, https://doi.org/10.1080/08958370701866008, 2008.
McFiggans, G., Mentel, T. F., Wildt, J., Pullinen, I., Kang, S., Kleist, E., Schmitt, S., Springer, M., Tillmann, R., Wu, C., Zhao, D., Hallquist, M., Faxon, C., Le Breton, M., Hallquist, Å. M., Simpson, D., Bergström, R., Jenkin, M. E., Ehn, M., Thornton, J. A., Alfarra, M. R., Bannan, T. J., Percival, C. J., Priestley, M., Topping, D., and Kiendler-Scharr, A.: Secondary organic aerosol reduced by mixture of atmospheric vapours, Nature, 565, 587–593, https://doi.org/10.1038/s41586-018-0871-y, 2019.
Mermet, K., Sauvage, S., Dusanter, S., Salameh, T., Léonardis, T., Flaud, P.-M., Perraudin, É., Villenave, É., and Locoge, N.: Optimization of a gas chromatographic unit for measuring biogenic volatile organic compounds in ambient air, Atmos. Meas. Tech., 12, 6153–6171, https://doi.org/10.5194/amt-12-6153-2019, 2019.
Monson, R. K. and Fall, R.: Isoprene Emission from Aspen Leaves, Influence of Environment and Relation to Photosynthesis and Photorespiration, J. Plant. Physiol., 90, 267–274, https://doi.org/10.1104/pp.90.1.267, 1989.
Moreaux, V., Lamaud, É., Bosc, A., Bonnefond, J.-M., Medlyn, B. E., and Loustau, D.: Paired comparison of water, energy and carbon exchanges over two young maritime pine stands (Pinus pinaster Ait.): effects of thinning and weeding in the early stage of tree growth, Tree Physiol., 31, 903–921, https://doi.org/10.1093/treephys/tpr048, 2011.
Ng, N. L., Brown, S. S., Archibald, A. T., Atlas, E., Cohen, R. C., Crowley, J. N., Day, D. A., Donahue, N. M., Fry, J. L., Fuchs, H., Griffin, R. J., Guzman, M. I., Herrmann, H., Hodzic, A., Iinuma, Y., Jimenez, J. L., Kiendler-Scharr, A., Lee, B. H., Luecken, D. J., Mao, J., McLaren, R., Mutzel, A., Osthoff, H. D., Ouyang, B., Picquet-Varrault, B., Platt, U., Pye, H. O. T., Rudich, Y., Schwantes, R. H., Shiraiwa, M., Stutz, J., Thornton, J. A., Tilgner, A., Williams, B. J., and Zaveri, R. A.: Nitrate radicals and biogenic volatile organic compounds: oxidation, mechanisms, and organic aerosol, Atmos. Chem. Phys., 17, 2103–2162, https://doi.org/10.5194/acp-17-2103-2017, 2017.
Peräkylä, O., Vogt, M., Tikkanen, O.-P., Laurila, T., Kajos, M. K., Rantala, P. A., Patokoski, J., Aalto, J., Yli-Juuti, T., Ehn, M., Sipilä, M., Paasonen, P., Rissanen, M., Nieminen, T., Taipale, R., Keronen, P., Lappalainen, H. K., Ruuskanen, T. M., Rinne, J., Kerminen, V.-M., Kulmala, M., Bäck, J., and Petäjä, T.: Monoterpenes' oxidation capacity and rate over a boreal forest: temporal variation and connection to growth of newly formed particles, Boreal Environ. Res., 19, 293–310, 2014.
Petäjä, T., Mauldin, III, R. L., Kosciuch, E., McGrath, J., Nieminen, T., Paasonen, P., Boy, M., Adamov, A., Kotiaho, T., and Kulmala, M.: Sulfuric acid and OH concentrations in a boreal forest site, Atmos. Chem. Phys., 9, 7435–7448, https://doi.org/10.5194/acp-9-7435-2009, 2009.
Pfeiffer, T., Forberich, O., and Comes, F. J.: The contribution of the ozonolysis of terpenes to tropospheric OH concentrations, Can. J. Phys., 79, 131–142, https://doi.org/10.1139/p01-030, 2001.
Presto, A. A., Huff Hartz, K. E., and Donahue, N. M.: Secondary Organic Aerosol Production from Terpene Ozonolysis. 2. Effect of NOx Concentration, Environ. Sci. Technol., 39, 7046–7054, https://doi.org/10.1021/es050400s, 2005.
Ren, X., Harder, H., Martinez, M., Lesher, R. L., Oliger, A., Shirley, T., Adams, J., Simpas, J. B., and Brune, W. H.: HOx concentrations and OH reactivity observations in New York City during PMTACS-NY2001, Atmos. Environ., 37, 3627–3637, https://doi.org/10.1016/S1352-2310(03)00460-6, 2003.
Rickard, A. R., Johnson, D., McGill, C. D., and Marston, G.: OH yields in the gas-phase reactions of ozone with alkenes, J. Phys. Chem. A, 103, 7656–7664, 1999.
Riva, M., Rantala, P., Krechmer, J. E., Peräkylä, O., Zhang, Y., Heikkinen, L., Garmash, O., Yan, C., Kulmala, M., Worsnop, D., and Ehn, M.: Evaluating the performance of five different chemical ionization techniques for detecting gaseous oxygenated organic species, Atmos. Meas. Tech., 12, 2403–2421, https://doi.org/10.5194/amt-12-2403-2019, 2019a.
Riva, M., Heikkinen, L., Bell, D. M., Peräkylä, O., Zha, Q., Schallhart, S., Rissanen, M. P., Imre, D., Petäjä, T., Thornton, J. A., Zelenyuk, A., and Ehn, M.: Chemical transformations in monoterpene-derived organic aerosol enhanced by inorganic composition, Clim. Atmos. Sci., 2, 2, https://doi.org/10.1038/s41612-018-0058-0, 2019b.
Schwarz, K., Filipiak, W., and Amann, A.: Determining concentration patterns of volatile compounds in exhaled breath by PTR-MS, J. Breath Res., 3, 027002, https://doi.org/10.1088/1752-7155/3/2/027002, 2009.
Sekimoto, K., Li, S.-M., Yuan, B., Koss, A., Coggon, M., Warneke, C., and de Gouw, J.: Calculation of the sensitivity of proton-transfer-reaction mass spectrometry (PTR-MS) for organic trace gases using molecular properties, Int. J. Mass Spectro., 421, 71–94, https://doi.org/10.1016/j.ijms.2017.04.006, 2017.
Shirinzadeh, B., Wang, C. C., and Deng, D. Q.: Diurnal variation of the OH concentration in ambient air, Geophys. Res. Lett., 14, 123–126, https://doi.org/10.1029/GL014i002p00123, 1987.
Simon, V., Clement, B., Riba, M.-L., and Torres, L.: The Landes experiment: Monoterpenes emitted from the maritime pine, J. Geophys. Res., 99, 16501–16510, https://doi.org/10.1029/94JD00785, 1994.
Sindelarova, K., Granier, C., Bouarar, I., Guenther, A., Tilmes, S., Stavrakou, T., Müller, J.-F., Kuhn, U., Stefani, P., and Knorr, W.: Global data set of biogenic VOC emissions calculated by the MEGAN model over the last 30 years, Atmos. Chem. Phys., 14, 9317–9341, https://doi.org/10.5194/acp-14-9317-2014, 2014.
Stone, D., Whalley, L. K., and Heard, D. E.: Tropospheric OH and HO2 radicals: field measurements and model comparisons, Chem. Soc. Rev., 41, 6348–6404, https://doi.org/10.1039/C2CS35140D, 2012.
Surratt, J. D., Chan, A. W. H., Eddingsaas, N. C., Chan, M., Loza, C. L., Kwan, A. J., Hersey, S. P., Flagan, R. C., Wennberg, P. O., and Seinfeld, J. H.: Reactive intermediates revealed in secondary organic aerosol formation from isoprene, P. Natl. Acad. Sci. USA, 107, 6640–6645, https://doi.org/10.1073/pnas.0911114107, 2010.
Tani, A.: Fragmentation and Reaction Rate Constants of Terpenoids Determined by Proton Transfer Reaction-mass Spectrometry, Environ. Control Biol., 51, 23–29, https://doi.org/10.2525/ecb.51.23, 2013.
Tani, A., Hayward, S., and Hewitt, C. N.: Measurement of monoterpenes and related compounds by proton transfer reaction-mass spectrometry (PTR-MS), Int. J. Mass Spectro., 223–224, 561–578, https://doi.org/10.1016/S1387-3806(02)00880-1, 2003.
Tani, A., Hayward, S., Hansel, A., and Hewitt, C. N.: Effect of water vapour pressure on monoterpene measurements using proton transfer reaction-mass spectrometry (PTR-MS), Int. J. Mass Spectro., 239, 161–169, https://doi.org/10.1016/j.ijms.2004.07.020, 2004.
Tsigaridis, K. and Kanakidou, M.: Global modelling of secondary organic aerosol in the troposphere: a sensitivity analysis, Atmos. Chem. Phys., 3, 1849–1869, https://doi.org/10.5194/acp-3-1849-2003, 2003.
von Schwartzenberg, K., Schultze, W., and Kassner, H. J. P. C. R.: The moss Physcomitrella patens releases a tetracyclic diterpene, Plant Cell Rep., 22, 780–786, https://doi.org/10.1007/s00299-004-0754-6, 2004.
Warneke, C., de Gouw, J. A., Kuster, W. C., Goldan, P. D., and Fall, R.: Validation of Atmospheric VOC Measurements by Proton-Transfer- Reaction Mass Spectrometry Using a Gas-Chromatographic Preseparation Method, Environ. Sci. Technol., 37, 2494–2501, https://doi.org/10.1021/es026266i, 2003.
Wei, D., Fuentes, J. D., Gerken, T., Chamecki, M., Trowbridge, A. M., Stoy, P. C., Katul, G. G., Fisch, G., Acevedo, O., Manzi, A., von Randow, C., and dos Santos, R. M. N.: Environmental and biological controls on seasonal patterns of isoprene above a rain forest in central Amazonia, Agr. Forest Meteorol., 256–257, 391–406, https://doi.org/10.1016/j.agrformet.2018.03.024, 2018.
Wennberg, P. O., Bates, K. H., Crounse, J. D., Dodson, L. G., McVay, R. C., Mertens, L. A., Nguyen, T. B., Praske, E., Schwantes, R. H., Smarte, M. D., St Clair, J. M., Teng, A. P., Zhang, X., and Seinfeld, J. H.: Gas-Phase Reactions of Isoprene and Its Major Oxidation Products, Chem. Rev., 118, 3337–3390, 2018.
Winterhalter, R., Herrmann, F., Kanawati, B., Nguyen, T. L., Peeters, J., Vereecken, L., and Moortgat, G. K.: The gas-phase ozonolysis of β-caryophyllene (C15H24). Part I: an experimental study, Phys. Chem. Chem. Phys., 11, 4152–4172, https://doi.org/10.1039/B817824K, 2009.
Xiong, F., McAvey, K. M., Pratt, K. A., Groff, C. J., Hostetler, M. A., Lipton, M. A., Starn, T. K., Seeley, J. V., Bertman, S. B., Teng, A. P., Crounse, J. D., Nguyen, T. B., Wennberg, P. O., Misztal, P. K., Goldstein, A. H., Guenther, A. B., Koss, A. R., Olson, K. F., de Gouw, J. A., Baumann, K., Edgerton, E. S., Feiner, P. A., Zhang, L., Miller, D. O., Brune, W. H., and Shepson, P. B.: Observation of isoprene hydroxynitrates in the southeastern United States and implications for the fate of NOx, Atmos. Chem. Phys., 15, 11257–11272, https://doi.org/10.5194/acp-15-11257-2015, 2015.
Yáñez-Serrano, A. M., Nölscher, A. C., Bourtsoukidis, E., Gomes Alves, E., Ganzeveld, L., Bonn, B., Wolff, S., Sa, M., Yamasoe, M., Williams, J., Andreae, M. O., and Kesselmeier, J.: Monoterpene chemical speciation in a tropical rainforest:variation with season, height, and time of dayat the Amazon Tall Tower Observatory (ATTO), Atmos. Chem. Phys., 18, 3403–3418, https://doi.org/10.5194/acp-18-3403-2018, 2018.
Yuan, B., Koss, A. R., Warneke, C., Coggon, M., Sekimoto, K., and de Gouw, J. A.: Proton-Transfer-Reaction Mass Spectrometry: Applications in Atmospheric Sciences, Chem. Rev., 117, 13187–13229, 2017.
Zhu, J., Penner, J. E., Yu, F., Sillman, S., Andreae, M. O., and Coe, H.: Decrease in radiative forcing by organic aerosol nucleation, climate, and land use change, Nat. Commun., 10, 423, https://doi.org/10.1038/s41467-019-08407-7, 2019.