the Creative Commons Attribution 4.0 License.
the Creative Commons Attribution 4.0 License.
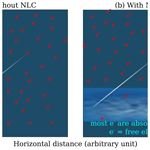
Mesospheric anomalous diffusion during noctilucent cloud scenarios
Gunter Stober
Jens Fiedler
Meers M. Oppenheim
Jorge L. Chau
Duggirala Pallamraju
Nicholas M. Pedatella
Masaki Tsutsumi
Toralf Renkwitz
The Andenes specular meteor radar shows meteor trail diffusion rates increasing on average by about 10 % at times and locations where a lidar observes noctilucent clouds (NLCs). This high-latitude effect has been attributed to the presence of charged NLC after exploring possible contributions from thermal tides. To make this claim, the current study evaluates data from three stations at high, middle, and low latitudes for the years 2012 to 2016 to show that NLC influence on the meteor trail diffusion is independent of thermal tides. The observations also show that the meteor trail diffusion enhancement during NLC cover exists only at high latitudes and near the peaks of NLC layers. This paper discusses a number of possible explanations for changes in the regions with NLCs and leans towards the hypothesis that the relative abundance of background electron density plays the leading role. A more accurate model of the meteor trail diffusion around NLC particles would help researchers determine mesospheric temperature and neutral density profiles from meteor radars at high latitudes.
- Article
(4163 KB) - Full-text XML
-
Supplement
(237 KB) - BibTeX
- EndNote
The motion and diffusion of meteor trails depend sensitively upon the properties of the neutral atmosphere where they ablate. Measuring meteor properties with radars enables researchers and weather modelers to estimate the state of the lower thermosphere and upper mesosphere. Meteor radars most often observe underdense meteors in which the radar frequency exceeds the plasma frequency set by the peak meteor plasma density. Typically they have lifetimes that vary from 0.01 to 0.3 s at altitudes below 110 km. Studies of the meteor trail decay time and effort to derive ambient temperatures from them have a long history (e.g., Greenhow and Neufeld, 1955; Murray, 1959), but even today there exist several subtle difficulties.
Theoretically the meteor trail diffusion (hereafter we refer it as MTD or Da) should increase exponentially with altitude. However, the MTD derived from echo fading times measured by meteor radars deviate away from exponential behavior at altitudes below about 85 km. Using chemistry based numerical simulation, Younger et al. (2014) reported that the deionization of the meteor trail by three-body attachment (a chemical process) at altitudes below 90 km could be responsible for the deviation. But they were open to contributions from background dusts, such as meteor smoke particles and noctilucent cloud (NLC). Moreover, in a recent study Hocking et al. (2016) argued that the chemical processes are more important for the long-lived (non-underdense) meteors, where the importance of ozone chemistry has been discussed. A study by Singer et al. (2008) showed different behavior of the MTD coefficient profiles during NLC and non-NLC cases. They also noted that the strong and weak meteor-based separation does show a partly similar behavior, so they could not conclude clearly the contributions from NLC. Also, the NLC occurrence has a local time or tidal dependence (Fiedler et al., 2011; Fiedler and Baumgarten, 2018; Gerding et al., 2013), which could bias the MTD segregation based on it. Here we investigate multiple years of NLC and MTD from different latitudes to investigate the lack of understanding in identifying the role of NLC and atmospheric dynamics.
Altitudinal profiles of temperature are essential for improved modeling of upper atmosphere dynamics at mesospheric heights. However, uninterrupted measurement of this parameter is not possible using traditional optical techniques due to cloud cover. If it were possible to derive temperature from MTD estimates, continuous temperature measurement could become a reality. Currently there are several difficulties in deriving temperatures from meteor diffusion measurements as there are several unknown and anomalous variabilities. Nevertheless, there are couple of techniques in use (e.g., Hocking et al., 1997; Hocking, 1999; Holdsworth et al., 2006; Stober et al., 2012; Holmen et al., 2016) which provide temperature estimates roughly at a cadence of about a day, but with their own merits and demerits.
The primary data used for this investigation are from the specular meteor radars (SMRs) at Andenes (69∘ N, 16∘ E) in northern Norway, Juliusruh (55∘ N, 13∘ E) in northern Germany, and Biak (1∘ S, 136∘ E) in Indonesia. All three radars are all-SKy interferometric METeor (SKiYMET) systems. Elaborate technical details and working principles of this type of systems can be found in Hocking et al. (2001). Specific technical details of the Biak system can be found in Batubara et al. (2018). In this study we use the decay time information estimated from underdense meteor trails as described in Hocking et al. (2001). Although the method is fairly robust, it does not account for meteor fragmentation, or other effects that might cause a deviation in the signal morphology deviating from a typical underdense trail. Further, we have to mention that the radars did undergo a change of the experiment settings for the Juliusruh and Andenes SMR. In the years 2014 and 2015 we changed the pulse repetition frequency from 2144 Hz (Juliusruh) and 2094 Hz (Andenes) to 625 Hz and the mono pulse was replaced by a 7-bit Barker code. The lower pulse repetition frequency together with an off-zenith filtering of angles larger than 65∘ eliminates a potential aliasing due to the range ambiguity of the meteor altitudes. The Biak SMR kept the experiment settings with a pulse repetition frequency of 2144 Hz and used a wide meteor layer, causing many ambiguous meteor positions. Thus, the data at the upper and lower edge of the meteor layer might be more prone to range aliasing issues. Other than these three radars, NLC data from a Rayleigh–Mie–Raman (RMR) lidar in Andenes are also used to study the characteristics of meteor radar diffusion during NLC presence and absence.
2.1 Specular meteor radar-based diffusion coefficients
The most commonly observed meteors using a 32 MHz meteor radar are of the underdense type, for which the amplitude profile A(t) decays approximately as per the following relation:
where t is time, Da is the ambipolar diffusion coefficient, λ is the wavelength of radar signal, and τ1∕2 is the decay time to reach half of maximum amplitude (A0).
Thus, knowing the decay rate τ1∕2 from the meteor echo received, the ambipolar diffusion coefficient can be estimated. As the number densities of the electrons in the meteor trail plasma are several orders of magnitude (at least 3 orders) greater than the background plasma, the trail diffusion could be assumed as an approximation of the mesospheric neutral diffusion. This is because the movement of the trail positive ions are governed by neutrals through collisions.
We have estimated diffusion coefficients from such meteor decay rates for all the available years of meteor detections. But for the current study, based on the availability of NLC data, four years (2012–2016, excluding 2014) are investigated in detail. Figure 1 shows the yearly composite (daily binned) Da values for all the available years of data obtained using the meteor radars located at low, middle, and high latitudes. It can be seen here that, in general, the diffusion decreases with altitude until about 85 km, above which it starts increasing again. In the current study, meteors qualifying the following selection criteria are considered: (i) zenith angle less than 65∘, (ii) those under auroral electrojet (AE) index conditions lower than 400 nT, and (iii) those with a signal-to-noise ratio (SNR) greater than 5 dB.
2.2 NLC data
The NLC data are obtained using the RMR lidar located at the Andoya (69∘ N, 16∘ E) island in northern Norway (Baumgarten, 2010), which is very close to the Andenes meteor radar site. Spectral and spatial filtering capability of this lidar enables continuous observations of NLC even during daylight conditions. Though the instrument existed for a long time, it had experienced several technical developments over the years. Since the year 2011 a pressure-controlled single Fabry–Pérot etalon is used to filter out the background, which increased the SNR of the system (Fiedler et al., 2017). So, the NLC data used here are from the years 2012 to 2016 during clear-sky hours of June–July–August over Andenes. The presence or absence of NLC are identified from integrated measurements, at roughly 15 min intervals, during all the clear-sky days.
Figure 2 shows diffusion coefficients estimated from Whole Atmosphere Community Climate Model Data Assimilation Research Testbed (WACCM+DART) (Pedatella et al., 2014) temperature profiles over the stations for the year 2007. Since WACCM+DART assimilates observations, temperatures are believed to provide not only close to realistic values as compared with satellite observations (e.g., Pedatella et al., 2014), but also a better local time coverage. The conversion from temperature to diffusion is done using the simple relation , where p, T, D, and K0 are respectively pressure, temperature, diffusion, and zero field mobility factor. The value of the factor K0 is debatable (e.g., Cervera and Reid, 2000; Hall et al., 2004) and we use m2 s−1 V−1 (e.g., Meek et al., 2013; Younger et al., 2014). Here it may be noted that the diffusion derived from model temperature follows the theoretically expected exponential law. But as mentioned above the observed diffusion from meteor radar-based fading time shows deviation away from exponential behavior. Some investigations attributed such deviation to be due to deionization of the trail by three-body chemistry (Younger et al., 2014; Lee et al., 2013). But it may also be possible that the assumption of the ambipolar diffusion and Gaussian profile of meter trail radial plasma distribution are too-simple approximations, which need further investigations.
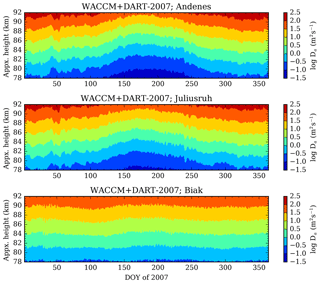
Figure 2Representative yearly diffusion values obtained by directly converting the WACCM+DART 2007 temperatures over the three stations. They show nearly similar seasonal variability, except the increased meteor trail diffusion at lower altitudes seen in Fig. 1. Also, the summer enhancement seen in Fig. 1 is not visible here. This implies that the enhanced diffusion is related to factors that are other than temperature variability.
From a comparison of Figs. 1 and 2, one can say that the broad seasonal features showing altitude shift of constant value surfaces are similar, but the increased values at lower altitudes in summer differ in the datasets. This suggests that additional physical processes are responsible for the MTD variability during summer.
In the high-latitude summer mesosphere upwelling occurs and the maximum of the upward motion lies close to the mesopause level (e.g., Smith, 2012; Laskar et al., 2017, and references therein). Due to such upward motion the summer mesosphere is the coldest region in the atmosphere. Under such cold conditions the saturated water vapor present and/or transported in the mesosphere freezes up and produces NLCs. NLCs are expected to remove free electrons and thus produce negatively charged ice particles. An earlier study by Singer et al. (2008) used 6 d of meteor trail diffusion data and reported that the diffusion profiles have different behavior if separated based on the NLC presence or absence. In order to systematically investigate the role of NLCs in larger datasets and for a greater number of years, we have used Andenes RMR-lidar-based NLC observation times to segregate the diffusion values. The leftmost column of Fig. 3 shows such a grouping based on NLC presence (yNLC) and absence (nNLC) for the measurements during clear-sky days of June–July–August of the years 2012–2016, excluding the year 2014 wherein we had many data gaps for the high-latitude station, Andenes. The horizontal histograms in the leftmost column represent the occurrences of NLC (total number of 15 min intervals with NLC presence) at a particular altitude for a particular summer. The middle and right columns in Fig. 3 are for the MTD data from Juliusruh (midlatitude) and Biak (low latitude) SMRs, but they were segregated and then grouped based on the NLC sampling at Andenes. As the meteor trail diffusion at a particular altitude is distributed log-normally, the solid (for yNLC) and dashed (for nNLC) lines here are the geometric mean, (e.g., Ballinger et al., 2008), profiles and the shaded regions represent their 95 % confidence intervals. As there are reports that neutral density and thus MTD are influenced by geomagnetic activity (e.g., Yi et al., 2018), we have considered only those meteors that occurred under relatively quiet geomagnetic conditions (AE index less than 400 nT).
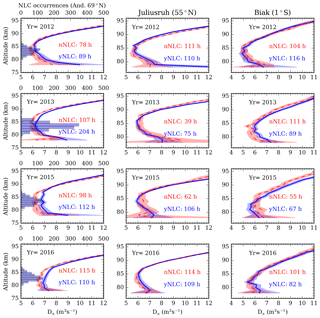
Figure 3Mean meteor trail diffusion coefficients after segregating them based on the presence of NLC (yNLC, blue) and non-NLC (nNLC, red) over Andenes station (leftmost column) are shown. Using the time sampling from NLC occurrences at Andenes, the Da measurements at midlatitude (Juliusruh; middle column) and low latitude (Biak; right column) are also grouped. The shaded regions around the averaged vertical profiles, dashed for nNLC and continuous for yNLC, are the 95 % confidence intervals. The histograms in the top axes of the leftmost column show the altitude variability of NLC occurrences measured using RMR lidar at Andenes during June–July–August. Notable features are that (i) Da under yNLC is enhanced compared to nNLC conditions, (ii) NLC-based grouping does not show separations or enhancements at middle and low latitudes, and (iii) the high-latitude enhancements are predominantly at NLC peak altitudes.
From the grouping based on NLC occurrence, as shown in Fig. 3, it can be seen clearly that there are differences between diffusion profiles in the presence and absence of NLC at high latitudes. Physical causes of such anomalous behavior are discussed below.
NLC particle sizes are of tens of nanometers and thus they are much heavier compared to ambient constituents. In the presence of such heavier particles, one may expect that a direct interaction with them, if any, would result in relatively smaller diffusion compared to their absence. Also, from the fact that NLCs are more probable during the cold phase of thermal tide one would expect lower values of MTD in the presence of NLC. But what we see from the leftmost column of Fig. 3 is the reverse, i.e., in the presence of NLC the SMR-radar-measured diffusion coefficient gets enhanced. Here we present a list of possible mechanisms through which NLC may influence or modulate MTD to give rise to the unexpected anomalous behavior. In the following paragraphs we discuss their role in explaining the anomaly. This includes (i) capturing trail electrons and thereby making the trail vanish faster in the eye of radar; (ii) radiative heating due to presence of semi-transparent NLC-layer; (iii) the possible introduction of a systematic artifact in our time sampling since NLC occurrence time shows a thermal tidal behavior; (iv) neutral turbulence possibly persisting longer during the relatively colder NLC occurrence durations, which could help to diffuse the trail faster; and (v) the NLC particles potentially absorbing background free electrons and thereby changing the electrodynamics of trail and background plasma.
For (i), in the presence of NLC it may be expected that ice particles absorb the trail electrons, which can lead to shorter lifetimes of the trail plasma. But the time constant of electron capture rate (order of seconds) (Rapp and Lübken, 2000) is longer than the typical lifetime of the underdense trails (order of milliseconds). Also, the abundance of NLC particles are at least 3 orders of magnitude less than trail electrons. Thus this process is very unlikely the cause for the enhanced diffusion. For (ii), the radiative influence on the background atmosphere due to changes in the optical properties in the presence of NLC could increase the NLC particle temperature by 1 to 2 K (e.g., Espy and Jutt, 2002). As the number of NLC particles are negligible compared to background neutral densities, such a rise in particle temperature would not contribute to the background temperature or diffusion.
To check if the anomaly during NLC scenarios could be occurring due to thermal tides, possibility (iii), we have used two additional stations: Juliusruh at midlatitude and Biak at low latitude. For the investigation of the tidal behavior in MTD, an hourly composite of the June–July 2012–2016 diffusion coefficient data for the high, middle, and low latitudes are shown in Fig. 4. Here it can be seen that the dominant variations are a semi-diurnal tide at high latitudes, a diurnal tide at low latitudes, and both at midlatitudes. A tidal behavior was also reported in the histogram of the local time occurrence of yNLC and nNLC during June–July–August (Fiedler et al., 2011; Fiedler and Baumgarten, 2018). Thus the tidal behavior in both MTD and NLC indicate that the difference between MTDs during yNLC and nNLC scenarios may arise from tides.
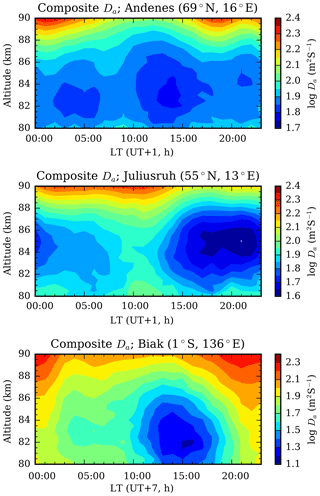
Figure 4Composite Da during June–July of the years 2012–2016, excluding the year 2014, over the three stations. It clearly shows that the semi-diurnal tide is the dominant tide at high latitudes and diurnal is dominant at low latitudes, while at midlatitudes it seems mixed. This signifies that MTD variation has a strong tidal variability.
In order to investigate the tidal variability of the NLC data used here, those NLC observation durations in which simultaneous MTD data over Andenes are present are used to make the histogram presented in Fig. 5. Since the MTD data at the other two stations have different durations of data availability, the histograms for them are different but the shape of the local variability is similar. So they are not shown here but are added in the Supplement Fig. S1. Because of the different availability of MTDs at different latitudes, the total intervals of yNLC or nNLC, as depicted in Fig. 3, are different for different stations. For example, for the year 2012 the yNLC durations are 89, 110, and 116 h for the Andenes, Juliusruh, and Biak stations, respectively. From Fig. 5 one can see that, except in the year 2013, there is no significant tide-like daily variability, and thus the sampling of MTDs based on NLC would have no tidal bias in those years. This, however, does not conflict with Fiedler et al. (2011) and Fiedler and Baumgarten (2018), where they showed a tidal dependence, as the time samplings here are different based on the common observations, though in 2013 there are some diurnal variations in sampling, which do not introduce any significant difference in diffusion even at low latitudes where the diurnal tide is dominant. Moreover, the separations between yNLC and nNLC diffusion profiles at high latitudes (as seen in Fig. 3) are of higher magnitude near the peak of the NLC layer.
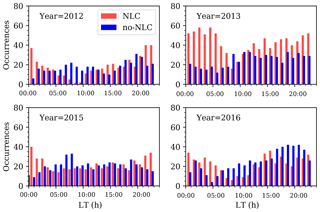
Figure 5NLC and non-NLC occurrences (number of 15 min intervals) over local time during the observation years from Andenes are shown. Similar figures for other stations are shown in Supplement Fig. S1. These samplings show that though the NLC and non-NLC occurrence and sampling are not uniform over local-time hours they do not vary significantly, except in the year 2013 where a diurnal-tide-like variability may be recognized.
To test whether the NLC-related differences could arise from some unknown systematic processes, say for example higher NLC occurring during the first part of the summer, we made two random samples using MTD during just the lidar observation durations. Such random samples do not show any difference between the average profiles between two groups (interested readers can see Supplement Fig. S2). A similar test by making two random samples using whole summer MTD data does not show such difference. From these results we hypothesize that the difference observed between MTD profiles during yNLC and nNLC scenarios at high latitudes are predominantly due to NLC influence.
With regard to the role of background turbulence, possibility (iv), Hall (2002) investigated the influence of neutral turbulence to explain the observed deviations of diffusion away from the exponential behavior. However, in a later report (Hall et al., 2005) they ruled out such a mechanism for radars with frequencies close to 30 MHz. They also estimated that the turbulence diffusion is in fact lower in magnitude during summer than in winter. Using 10 rocket flights that were capable of high-resolution measurements of neutral density Lübken et al. (2002) argued that neutral turbulence is very weak during summer and the adiabatic lapse rate condition to support persistent turbulence is hardly reached near the NLC layer. These earlier results imply that neutral turbulence is unlikely to be the cause for the enhanced diffusion during NLC scenarios.
For (v), in the absence of NLC the electrons in the trail could be short-circuited by the background free electrons and thus this would reduce the effective ambipolar diffusion as the lost electrons would no longer contribute to the diffusion. But when there is NLC they could absorb background electrons to reduce the density of the background free electrons, making a deficit to short-circuit the trail electrons. Under such conditions the ambipolar diffusion of the meteor trail would be higher due to additional pressure from the electrons that are not short-circuited as the background medium is less conductive. A schematic illustration for the background situation is depicted in Fig. 6, where the background electrons are less available in the NLC case (in right). This kind of explanation also suggests that the ambipolar diffusion assumption of the MTD is valid only when the background charges are very low compared to the trail electrons, similar to the situation as observed during the yNLC scenario. The possibility of such short-circuiting of the trail plasma by background free electrons was discussed both analytically and numerically by Dimant and Oppenheim (2006). This also suggest that for proper retrieval of the mesospheric diffusion we would need an estimate of background electron density.
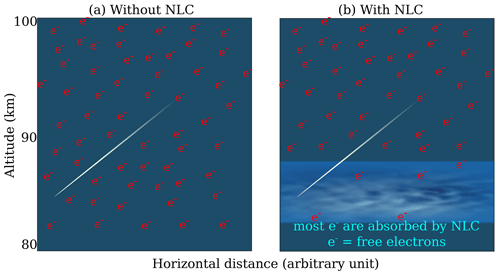
Figure 6A schematic illustration for the background state without NLC (a) and with NLC (b) is shown. In the with-NLC case background electrons at lower altitudes are mostly taken up by the NLC particles creating a deficiency of electrons, which therefore cannot take part in short-circuiting the trail electrons, and thus the meteor radar-measured diffusion appears to be enhanced.
Changes in the background chemistry could also have an influence but at lower altitudes where the reaction rates of the three-body reactions are comparable to the lifetime of the meteor trail. This kind of explanation was used earlier to explain the reversal or turnaround and then enhancement of the MTD coefficient at lower altitudes (e.g., Lee et al., 2013; Younger et al., 2014). But they did not rule out completely the importance of aerosols, such as NLC or meteor smoke.
For the high-latitude summertime data, Singer et al. (2008) used the assumption of the presence of neutral and charged dust, as was proposed by Havnes and Sigernes (2005), to explain the slower decay rate (i.e., higher diffusion as per Eq. 2) in the presence of NLC. They also expected that the strong and weak meteors would be affected differently by the presence or absence of NLC. With their limited data from only 6 d, they showed that yNLC and nNLC diffusion behavior is, to some extent, similar to diffusions during weak and strong meteor echoes. To investigate whether the enhancement during NLC is affected by strong and weak meteor bias, we also have carried out a test in which all those meteors with SNR greater than 12 dB (strong meteors) were used and it was found that the yNLC and nNLC difference scenario still persists as in Fig. 3, though they get narrower as the error limit increases due to the lower number of meteors. The test case figure is provided in the Supplement Fig. S3. This test also implies that the diffusion from weaker meteors could be more anomalous and it adds credence to our hypothesis presented in the previous paragraph.
From this anomalous behavior of the meteor radar diffusion during NLC occurrence it is clear that some of the temperature profile estimation methods which use standard pressure levels will yield misleading results at lower altitudes in the presence of NLC. It also indicates that the use of MTD reversal altitude as the constant density surface would not be valid under NLC conditions, unless the NLC contribution has been deciphered. Further, for the derivation of temperature at NLC altitudes from SMR-diffusion measurements, a proper retrieval algorithm considering the NLC-related anomaly is very important. Such a retrieval would need information about background electron density, the size of NLC particles, and their charge state (Chau et al., 2014) and is a subject for future studies.
Meteor trail diffusion variations measured by SMRs at high- (Andenes), middle- (Juliusruh), and low- (Biak) latitude stations have been used to investigate the mesospheric diffusion variability during the summer season. The Andenes SMR-based diffusion coefficient during NLC scenarios has been found to be enhanced compared to over non-NLC periods. From a local time composite, overall, the SMR-based diffusion has been found to be dominated by the semidiurnal tide at high latitudes and the diurnal tide at low latitudes. Also, since the NLC occurrences have a well known tidal modulation, the meteor sampling based on this may be biased. However, applying the local time durations based on high-latitude NLC and non-NLC occurrence to sample the middle- and low-latitude SMR-based diffusions, we found no significant difference, which thus delineates tidal influence. Moreover, it has been observed that for the high-latitude station the enhancements in diffusion during NLC scenarios are of higher magnitude at NLC peak altitudes. This implies that the NLC influences SMR-based diffusion rates.
The NLC particles could absorb many of the background free electrons to create a background medium having less conductivity. Based on current results it is hypothesized that in such a background electron deficit situation, created by the presence of NLC particles, the trail diffusion would be enhanced as there are fewer free electrons to short-circuit the trail electrons. But in the absence of NLC the relatively higher number of background free electrons would help to short-circuit electrons from the trail, thereby reducing the ambipolar diffusion. From this statistical study of the anomalous behavior of SMR-based diffusion measurements we conclude that the temperature estimations from them would need a detailed retrieval algorithm to account for the influence of background electrons, ice particles, and other dusts or aerosols.
The meteor meteor position data of the Andenes and Juliusruh systems can be obtained from Gunter Stober (stober@iap-kborn.de). The Biak system data are available at IUGONET (http://www.iugonet.org, last access: 12 April 2019). The RMR-lidar-based NLC data can be obtained from Jens Fiedler. WACCM+DART-2007 data can be obtained from Nicholas M. Pedatella. The National Center for Atmospheric Research is sponsored by the US National Science Foundation.
The supplement related to this article is available online at: https://doi.org/10.5194/acp-19-5259-2019-supplement.
FIL and GS conceived the preliminary idea. FIL analyzed most of the presented data in coordination with GS, JLC, and JF. JLC also contributed by comparing the results with data from another independent analysis. MMO helped in the interpretation of the results and provided useful comments on presentation of the results. NMP provided the WACCM+DART data. DP, MT, and TR participated in discussions related to the interpretation and presentation of the results. All authors read and approved the final version of the paper.
The authors declare that they have no conflict of interest.
This article is part of the special issue “Layered phenomena in the mesopause region (ACP/AMT inter-journal SI)”. It is a result of the LPMR workshop 2017 (LPMR-2017), Kühlungsborn, Germany, 18–22 September 2017.
We acknowledge the support of the IAP staff for keeping the radars running.
This work is partially supported by the WaTiLa project (SAW-2015-IAP-1 383).
Data acquisition of meteor radar at Biak has been done by the Research
Institute for Sustainable Humanosphere (RISH), Kyoto University. Distribution
of the data has been partly supported by the IUGONET (Inter-university Upper
atmosphere Global Observation NETwork) project
(http://www.iugonet.org/, last access: 12 April 2019) funded by the
Ministry of Education, Culture, Sports, Science and Technology (MEXT), Japan.
The National Center for Atmospheric Research is sponsored by the US National
Science Foundation. We thank Cesar La-Hoz of the Arctic University of Norway
for his discussion and useful comments.
The
publication of this article was funded by the
Open Access
Fund of the Leibniz Association.
This paper was edited by Bernd Funke and reviewed by two anonymous referees.
Ballinger, A. P., Chilson, P. B., Palmer, R. D., and Mitchell, N. J.: On the validity of the ambipolar diffusion assumption in the polar mesopause region, Ann. Geophys., 26, 3439–3443, https://doi.org/10.5194/angeo-26-3439-2008, 2008. a
Batubara, M., Yamamoto, M.-Y., Madkour, W., and Manik, T.: Long-Term Distribution of Meteors in a Solar Cycle Period Observed by VHF Meteor Radars at Near-Equatorial Latitudes, 123, 10403–10415, J. Geophys. Res.-Space, https://doi.org/10.1029/2018ja025906, 2018. a
Baumgarten, G.: Doppler Rayleigh/Mie/Raman lidar for wind and temperature measurements in the middle atmosphere up to 80 km, Atmos. Meas. Tech., 3, 1509–1518, https://doi.org/10.5194/amt-3-1509-2010, 2010. a
Cervera, M. A. and Reid, I. M.: Comparison of atmospheric parameters derived from meteor observations with CIRA, Radio Sci., 35, 833–843, https://doi.org/10.1029/1999RS002226, 2000. a
Chau, J. L., Strelnikova, I., Schult, C., Oppenheim, M. M., Kelley, M. C., Stober, G., and Singer, W.: Nonspecular meteor trails from non-field-aligned irregularities: Can they be explained by presence of charged meteor dust?, Geophys. Res. Lett., 41, 3336–3343, https://doi.org/10.1002/2014gl059922, 2014. a
Dimant, Y. S. and Oppenheim, M. M.: Meteor trail diffusion and fields: 2. Analytical theory, J. Geophys. Res.-Space, 111, A12313, https://doi.org/10.1029/2006JA011798, 2006. a
Espy, P. and Jutt, H.: Equilibrium temperature of water–ice aerosols in the high-latitude summer mesosphere, J. Atmos. Sol.-Terr. Phys., 64, 1823–1832, https://doi.org/10.1016/s1364-6826(02)00191-8, 2002. a
Fiedler, J. and Baumgarten, G.: Solar and lunar tides in noctilucent clouds as determined by ground-based lidar, Atmos. Chem. Phys., 18, 16051–16061, https://doi.org/10.5194/acp-18-16051-2018, 2018. a, b, c
Fiedler, J., Baumgarten, G., Berger, U., Hoffmann, P., Kaifler, N., and Lübken, F.-J.: NLC and the background atmosphere above ALOMAR, Atmos. Chem. Phys., 11, 5701–5717, https://doi.org/10.5194/acp-11-5701-2011, 2011. a, b, c
Fiedler, J., Baumgarten, G., Berger, U., and Lübken, F.-J.: Long-term variations of noctilucent clouds at ALOMAR, J. Atmos. Sol.-Terr. Phys., 162, 79–89, https://doi.org/10.1016/j.jastp.2016.08.006, 2017. a
Gerding, M., Kopp, M., Hoffmann, P., Höffner, J., and Lübken, F.-J.: Diurnal variations of midlatitude NLC parameters observed by daylight-capable lidar and their relation to ambient parameters, Geophys. Res. Lett., 40, 6390–6394, https://doi.org/10.1002/2013gl057955, 2013. a
Greenhow, J. and Neufeld, E.: The diffusion of ionized meteor trails in the upper atmosphere, J. Atmos. Sol.-Terr. Phys., 6, 133–140, https://doi.org/10.1016/0021-9169(55)90020-9, 1955. a
Hall, C. M.: On the influence of neutral turbulence on ambipolar diffusivities deduced from meteor trail expansion, Ann. Geophys., 20, 1857–1862, https://doi.org/10.5194/angeo-20-1857-2002, 2002. a
Hall, C. M., Aso, T., Tsutsumi, M., Höffner, J., and Sigernes, F.: Multi-instrument derivation of 90 km temperatures over Svalbard (78∘ N 16∘ E), Radio Sci., 39, https://doi.org/10.1029/2004rs003069, 2004. a
Hall, C. M., Aso, T., Tsutsumi, M., Nozawa, S., Manson, A. H., and Meek, C. E.: Letter to the Editior Testing the hypothesis of the influence of neutral turbulence on the deduction of ambipolar diffusivities from meteor trail expansion, Ann. Geophys., 23, 1071–1073, https://doi.org/10.5194/angeo-23-1071-2005, 2005. a
Havnes, O. and Sigernes, F.: On the influence of background dust on radar scattering from meteor trails, J. Atmos. Sol.-Terr. Phys., 67, 659–664, https://doi.org/10.1016/j.jastp.2004.12.009, 2005. a
Hocking, W., Fuller, B., and Vandepeer, B.: Real-time determination of meteor-related parameters utilizing modern digital technology, J. Atmos. Sol.-Terr. Phys., 63, 155–169, https://doi.org/10.1016/s1364-6826(00)00138-3, 2001. a, b
Hocking, W. K.: Temperatures Using radar-meteor decay times, Geophys. Res. Lett., 26, 3297–3300, https://doi.org/10.1029/1999GL003618, 1999. a
Hocking, W. K., Thayaparan, T., and Jones, J.: Meteor decay times and their use in determining a diagnostic mesospheric Temperature-pressure parameter: Methodology and one year of data, Geophys. Res. Lett., 24, 2977–2980, https://doi.org/10.1029/97GL03048, 1997. a
Hocking, W. K., Silber, R. E., Plane, J. M. C., Feng, W., and Garbanzo-Salas, M.: Decay times of transitionally dense specularly reflecting meteor trails and potential chemical impact on trail lifetimes, Ann. Geophys., 34, 1119–1144, https://doi.org/10.5194/angeo-34-1119-2016, 2016. a
Holdsworth, D. A., Morris, R. J., Murphy, D. J., Reid, I. M., Burns, G. B., and French, W. J. R.: Antarctic mesospheric temperature estimation using the Davis mesosphere-stratosphere-troposphere radar, J. Geophys. Res., 111, D05108, https://doi.org/10.1029/2005jd006589, 2006. a
Holmen, S. E., Hall, C. M., and Tsutsumi, M.: Neutral atmosphere temperature trends and variability at 90 km, 70∘ N, 19∘ E, 2003–2014, Atmos. Chem. Phys., 16, 7853–7866, https://doi.org/10.5194/acp-16-7853-2016, 2016. a
Laskar, F. I., Chau, J. L., St.-Maurice, J. P., Stober, G., Hall, C. M., Tsutsumi, M., Höffner, J., and Hoffmann, P.: Experimental Evidence of Arctic Summer Mesospheric Upwelling and Its Connection to Cold Summer Mesopause, Geophys. Res. Lett., 44, 9151–9158, https://doi.org/10.1002/2017gl074759, 2017. a
Lee, C. S., Younger, J. P., Reid, I. M., Kim, Y. H., and Kim, J.-H.: The effect of recombination and attachment on meteor radar diffusion coefficient profiles, J. Geophys. Res.-Atmos., 118, 3037–3043, https://doi.org/10.1002/jgrd.50315, 2013. a, b
Lübken, F.-J., Rapp, M., and Hoffmann, P.: Neutral air turbulence and temperatures in the vicinity of polar mesosphere summer echoes, J. Geophys. Res.-Atmos., 107, 4273, https://doi.org/10.1029/2001JD000915, 2002. a
Meek, C. E., Manson, A. H., Hocking, W. K., and Drummond, J. R.: Eureka, 80∘ N, SKiYMET meteor radar temperatures compared with Aura MLS values, Ann. Geophys., 31, 1267–1277, https://doi.org/10.5194/angeo-31-1267-2013, 2013. a
Murray, E.: Ambipolar diffusion of a meteor trail and its relation with height, Planet. Space Sci., 1, 125–129, https://doi.org/10.1016/0032-0633(59)90008-x, 1959. a
Pedatella, N. M., Raeder, K., Anderson, J. L., and Liu, H.-L.: Ensemble data assimilation in the Whole Atmosphere Community Climate Model, J. Geophys. Res.-Atmos., 119, 9793–9809, https://doi.org/10.1002/2014jd021776, 2014. a, b
Rapp, M. and Lübken, F.-J.: Electron temperature control of PMSE, Geophys. Res. Lett., 27, 3285–3288, https://doi.org/10.1029/2000gl011922, 2000. a
Singer, W., Latteck, R., Millan, L. F., Mitchell, N. J., and Fiedler, J.: Radar Backscatter from Underdense Meteors and Diffusion Rates, Earth Moon Planets, 102, 403–409, https://doi.org/10.1007/s11038-007-9220-0, 2008. a, b, c
Smith, A. K.: Global Dynamics of the MLT, Surv. Geophys., 33, 1177–1230, https://doi.org/10.1007/s10712-012-9196-9, 2012. a
Stober, G., Jacobi, C., Matthias, V., Hoffmann, P., and Gerding, M.: Neutral air density variations during strong planetary wave activity in the mesopause region derived from meteor radar observations, J. Atmos. Sol.-Terr. Phys., 74, 55–63, https://doi.org/10.1016/j.jastp.2011.10.007, 2012. a
Yi, W., Reid, I. M., Xue, X., Murphy, D. J., Hall, C. M., Tsutsumi, M., Ning, B., Li, G., Younger, J. P., Chen, T., and Dou, X.: High- and Middle-Latitude Neutral Mesospheric Density Response to Geomagnetic Storms, Geophys. Res. Lett., 45, 436–444, https://doi.org/10.1002/2017gl076282, 2018. a
Younger, J. P., Lee, C. S., Reid, I. M., Vincent, R. A., Kim, Y. H., and Murphy, D. J.: The effects of deionization processes on meteor radar diffusion coefficients below 90 km, J. Geophys. Res.-Atmos., 119, 10027–10043, https://doi.org/10.1002/2014JD021787, 2014. a, b, c, d