the Creative Commons Attribution 4.0 License.
the Creative Commons Attribution 4.0 License.
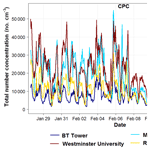
Interpretation of particle number size distributions measured across an urban area during the FASTER campaign
David C. S. Beddows
Mohammed S. Alam
Ajit Singh
James Brean
Ruixin Xu
Simone Kotthaus
Sue Grimmond
Particle number size distributions have been measured simultaneously by scanning mobility particle sizers (SMPSs) at five sites in central London for a 1 month campaign in January–February 2017. These measurements were accompanied by condensation particle counters (CPCs) to measure total particle number count at four of the sites and Aethalometers measuring black carbon (BC) at five sites. The spatial distribution and inter-relationships of the particle size distribution and SMPS total number counts with CPC total number counts and black carbon measurements have been analysed in detail as well as variations in the size distributions. One site (Marylebone Road) was in a street canyon with heavy traffic, one site (Westminster University) was on a rooftop adjacent to the Marylebone Road sampler, and a further sampler was located at Regent's University within a major park to the north of Marylebone Road. A fourth sampler was located nearby at 160 m above ground level on the BT tower and a fifth sampler was located 4 km to the west of the main sampling region at North Kensington. Consistent with earlier studies it was found that the mode in the size distribution had shifted to smaller sizes at the Regent's University (park) site, the mean particle shrinkage rate being 0.04 nm s−1 with slightly lower values at low wind speeds and some larger values at higher wind speeds. There was evidence of complete evaporation of the semi-volatile nucleation mode under certain conditions at the elevated BT Tower site. While the SMPS total count and black carbon showed typical traffic-dominated diurnal profiles, the CPC count data typically peaked during night-time as did CPC∕SMPS and CPC∕BC ratios. This is thought to be due to the presence of high concentrations of small particles (2.5–15 nm diameter) probably arising from condensational growth from traffic emissions during the cooler night-time conditions. Such behaviour was most marked at the Regent's University and Westminster University sites and less so at Marylebone Road, while at the elevated BT Tower site the ratio of particle number (CPC) to black carbon peaked during the morning rush hour and not at night-time, unlike the other sites. An elevation in nucleation mode particles associated with winds from the west and WSW sector was concluded to result from emissions from London Heathrow Airport, despite a distance of 22 km from the central London sites.
- Article
(7069 KB) - Full-text XML
-
Supplement
(1327 KB) - BibTeX
- EndNote
The adverse health consequences of air polluted by particulate matter are now well recognised (WHO, 2006). While the main focus has been on the public health impact of exposure to fine particulate matter measured by mass (PM2.5), there has also been concern over the possible contribution of ultrafine particles of less than 100 nm diameter to adverse health outcomes. While such particles contribute little to the total mass of particles in the atmosphere, they dominate particle number (Harrison et al., 2000) and authoritative reviews have concluded that although evidence is currently highly incomplete, they may contribute to the toxic hazard associated with ambient particulate matter (HEI, 2013; WHO, 2013). There have also been suggestions that particle surface area plays a major role in health impacts and this resides largely in the accumulation mode which is typically centred around 100–200 nm diameter (Harrison et al., 2000). Consequently, there is a strong interest from a health perspective in sub-micrometre particles and there are many reports of their concentrations and size distributions within the atmosphere (Asmi et al., 2011; Kumar et al., 2010, 2014).
In addition to concerns over human health, there are other reasons for the study of the size distribution of airborne particles. Not only does this strongly influence their location and efficiency of deposition in the human lung, the particle size distribution can also be a strong indicator of particle source, with there being some clear differences between the modal diameter of particles arising from different sources (Vu et al., 2015a). The clearest distinction is between particles arising from combustion and other high-temperature sources, which tend to be predominantly very small, and particles generated by attrition processes which are typically far more coarse. However, even within the particles generated from combustion and other high temperature sources, there may well be different modal diameters associated with different sources or even multiple modes associated with an individual source (Vu et al., 2015a). For example, exhaust emissions from diesel engines typically comprise both a nucleation mode and an overlapping Aitken mode, reflecting in the former case particles comprised mainly of condensed lubricating oil formed after the combustion process, and in the latter case solid carbonaceous particles formed within the combustion process (Shi and Harrison, 1999; Alam et al., 2016).
After their emission, particle size distributions are also liable to change through dynamic processes. These include evaporation, which causes particles to shrink without changing the overall number; condensation, which causes particles to grow without a change in total number; coagulation, which also causes growth but reduces the total particle number; and deposition, which causes a reduction in number and is a strong function of the particle size.
There are detailed assessments of the concentrations and size distributions of nanoparticles in the rural atmosphere (Van Dingenen et al., 2004; Asmi et al., 2011), and of their dynamics during atmospheric transport (Beddows et al., 2014), but urban studies have been limited. There has been much research on emissions from road transport (Zhu et al., 2002a, b; Kumar et al., 2011), with some attention given to shipping (Gonzalez et al., 2011) and to general modelling of sources (Posser and Pandis, 2015). However, most urban measurement studies have been limited to a single site (Morawska et al., 1998; Wang et al., 2011; Brines et al., 2015), although in a few instances more sites have been considered (Karl et al., 2016) but not as part of a concerted campaign.
Within this study, particle number size distributions were measured simultaneously by electrical mobility spectrometers at five separate sites across London and the size distributions are compared with a view to gaining a better understanding of the sources and processes affecting particles in the urban atmosphere.
Data were collected from 27 January 2017 to 16 February 2017 as part of the second campaign of the FASTER project. Data recovery was high (100 %, or close) at all sites except Westminster University, where good SMPS (scanning mobility particle sizer) data were collected on only 3 days, 30 and 31 January and 1 February 2017.
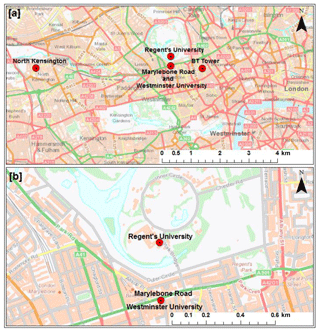
Figure 1Study area locations (a) in central London (UK) and (b) more detail of the Marylebone Road (MR), Westminster University (WU) and Regent's University (RU) sites.
2.1 Sampling sites
Data were collected at five sampling sites in total, three of which were established specifically for the FASTER campaign, Westminster University, Regent's University and BT Tower. The other two sites (London Marylebone Road and London North Kensington) collect data as part of the national Automatic Urban and Rural Network. The site locations (seen in Fig. 1) and characteristics are as follows:
-
Marylebone Road. Air sampling equipment is housed in a large kerbside cabin on the sidewalk of a busy central London street canyon with an inlet approximately 4 m above ground-level (a.g.l.). The adjacent six-lane highway carries around 80 000 vehicles per day. The highway is relatively straight and runs almost due east–west (angle 80∘ from north). The buildings on either side of the highway are around six storeys in height, giving a street canyon aspect ratio of approximately 1:1.
-
Westminster University. Air sampling instruments were located on the roof of the Westminster University building, almost directly above the Marylebone Road air sampling site on the southern side of the street. The instruments were housed in a temporary enclosure located approximately 26 m above street level and 4.5 m from the front edge of the roof where it overlooks the road, and with an inlet 1.5 m above the roof.
-
Regent's University. A temporary enclosure for the instruments was located on the roof of Regent's University, which is an isolated building within Regent's Park due north (i.e. 360∘) of the Marylebone Road and Westminster University sites. The only highway lying between Marylebone Road and the Regent's College site is a road with light traffic within Regent's Park. The distance between the Westminster University and Regent's University sites is estimated at 380 m. The instruments were located 17 m a.g.l. and 1 m from the edge of the roof.
-
London North Kensington. Instruments were sited in a permanent cabin located within the grounds of a high school in a suburban area with light traffic in central London, with an inlet approximately 2.5 m a.g.l. The air pollution climate at this site, often taken as representative of the background air quality within central London, has been characterised in detail by Bigi and Harrison (2010).
-
BT Tower. Instruments were sited on level T35 at approximately 160 m a.g.l. on a narrow tower which rises well above the surrounding buildings on a street with light traffic approximately 380 m to the south of Marylebone Road. The site was used extensively in the REPARTEE experiment (Harrison et al., 2012a).
2.2 Sampling instruments
The instruments (Table 1) were operated according to Wiedensohler et al. (2012) guidelines, with the omission of a dryer at three sites (discussed later), and calibrated and intercompared both before and after the sampling campaign. Small correction factors (<5 %) were applied to CPC (condensation particle counter) data as a result of the intercomparison. SMPS data were analysed using the AIM9 and AIM10 software provided by TSI as appropriate to the instrument. The national network sites (Marylebone Road and North Kensington) are fitted with diffusion dryers according to EUSAAR/ACTRIS protocols (Wiedensohler et al., 2012), but the other sites were not. The particle size ranges measured were 14.9–615.3 nm at Westminster University, Regent's University and BT Tower, 16.55–604.3 nm at Marylebone Road and North Kensington, and a further system with a short DMA (differential mobility analyser) gave 4.96–145.9 nm at Regent's University.
Table 1Location sites of instruments during the campaign. Mean sea level (m.s.l.), above ground level (a.g.l.), condensation particle counter (CPC), scanning mobility particle sizer (SMPS).
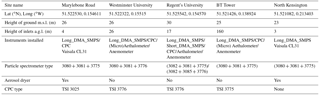
Note: The SMPS size ranges are given in Sect. 2.2. The lower size cuts (D50) of the CPCs are 3 nm (3025), 2.5 nm (3776) and 4 nm (3775).
It was not possible to use identical SMPS systems at each site. The variants used are shown in Table 1. We expect little difference between the long column classifiers (TSI 3081) used at all sites but with different platforms (TSI 3080 and TSI 3082) and CPCs (TSI 3775 and 3776). Differences are expected to be minimal as platform-specific software was used to invert the data and both the CPCs are butanol-based, with only slightly different lower cut-points which were well outside of the range of measured particles. At the Regent's University site, both a long DMA (3081) and short column DMA (3085) were utilised and the data were merged to give a single continuous size distribution from 6 to 650 nm. A possible cause of divergence is the fact that two of the sites (Marylebone Road and North Kensington) used diffusion dryers according to the EUSAAR/ACTRIS Protocol. The dryers were tested when installed and showed very low particle losses (less than 5 %) and no significant change to particle size distributions (NPL, 2010). The dryer may, however, affect the particle size distribution due to the hygroscopicity of certain kinds of particles. Vu et al. (2015b) reviewed hygroscopic growth factors for submicron aerosols from different sources. Their data are difficult to extrapolate to this study as measurements of hygroscopic growth are typically made at very high relative humidities, normally around 90 %. Even at 99.5 % relative humidity, the growth of particles of less than 100 nm sampled from the atmosphere is relatively low (Vu et al., 2015b). Consequently, a reduction in humidity from 88 % typical of the campaign to the values of 30–40 % achieved in the dryer would be expected to have only a small effect on particle sizes, especially as fresh traffic-generated particles which comprise a large proportion of the sub-micrometre particulate matter in the urban atmosphere are hydrophobic and therefore undergo zero or very limited growth in humid atmospheres.
2.3 Weather conditions during the campaign
Wind speed and direction data were taken from Heathrow Airport to the west of London to reflect the synoptic flow minimally affected by local building effects. At the start of the campaign (27 January 2017) the wind direction was easterly and moved to southerly by 29 January, briefly passing through northerly before returning to a southerly circulation between 31 January and 3 February. During this time, wind speeds were typically around 4 m s−1 and temperatures mild for the time of the year (mostly 6–10 ∘C). From 4 to 8 February there was a period of lower wind speeds (1–4 m s−1) with variable wind directions and low nocturnal minima temperatures (down to 1 ∘C). From 8 to 12 Feburary, a period of northerly winds (speeds of 3–5 m s−1) and lower temperatures (1–3 ∘C) without appreciable diurnal variation occurred. After 12 February, the winds came from the east, moving to south-westerly by 17 February, with wind speeds variable (between 0 and 6 m s−1) and temperatures steadily rising to daily maxima of 12 ∘C.
The mixed layer heights (MLHs) were determined from Vaisala CL31 ceilometer data collected at the Marylebone Road site (Fig. 1, Table 1). The observed 15 s (10 m gates) aerosol attenuated backscatter profiles were pre-processed (Kotthaus et al., 2016) prior to using the CABAM algorithm (Kotthaus and Grimmond, 2018) to determine 15 min intervals of MLH. The multiple aerosol layers (e.g. nocturnal residual layers) in the atmosphere are detected (Kotthaus and Grimmond, 2018; Kotthaus et al., 2018). Here the lowest detected layer is analysed. At times the MLH cannot be detected (e.g. during rain or very weak gradients in attenuated backscatter), but a residual layer might still be indicated. The ceilometer detects periods of precipitation, including events that may not be recorded by ground-based stations (e.g. insufficient to trigger a tipping bucket rain gauge).
During the campaign the observed MLH varied from a daily minimum of 45 m a.g.l. to a daily maximum of 1312 m a.g.l. with an overall 15 min average (median) of 421 (382) m a.g.l. The daily average (median) maximum MLH was 777 (695) and minimum was 194 (197) m a.g.l. The daily range and the amount of data available per day are shown in Fig. S1 in the Supplement.
2.4 Modal analysis of size distributions
Modes were fitted to the 15 min data obtained at Marylebone Road and Regent's and Westminster Universities using curve fitting and data analysis software “Fityk (version 1.3.1)” developed by Wojdyr (2010). In the present analysis, a standard peak function (Eq. 1) was used to disaggregate the size distributions into lognormal modes:
By fitting a combination of n peaks () linearly to the number size distributions, the following information was calculated: (1) amplitude Ai and location of dN ∕ dlogD at the mode of the distribution ci, (2) area under the curve (nm cm−3), and (3) width of the lognormal curve Wi.
3.1 Particle size distributions
A time series of total particle number concentrations from the SMPS instruments appears in Fig. 2. A strong diurnal variation is seen at all sites and is exemplified by the average daily variation shown in Fig. 3.
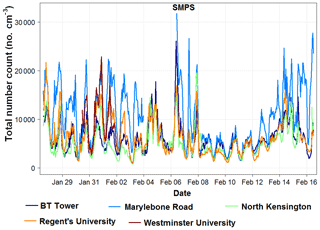
Figure 2Time series of total particle number count from the SMPS instruments at the five sites (Fig. 1, Table 1) over the campaign period.
The data stratified by the wind direction measured at London Heathrow airport (LHR) (Fig. 4) were used to perform the modal analysis. The log-normal modes fit to the size distribution were used to provide insights into the separate modes contributing to a measured size distribution. Although most measurements could be fit with three separate modes some distributions were best fit with only two modes. An example of a three-mode fit of a size distribution from North Kensington appears in the data for the 270∘ wind sector at this site (Fig. 5). It may be seen that using three modes gives a very good overall fit to the data. The details of the modes fitted and their relative magnitude and breadth appear in Table S1.
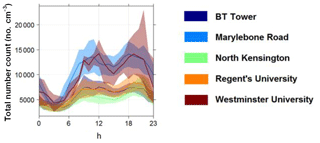
Figure 3Campaign-average diurnal variation of particle number counts derived from the SMPS instruments with median (line) and inter-quartile range (shading) shown.
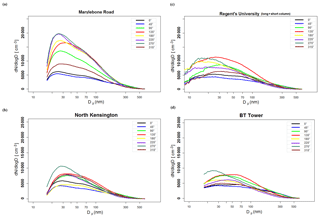
Figure 4Average particle number size distributions stratified by 45∘ wind directions sectors (∘, measured at LHR, value indicates mid-point of sector) for (a) Marylebone Road, (b) North Kensington (c) Regent's University, (d) BT Tower.
The Marylebone Road sampling site is located in a street canyon with heavy traffic (approx. 80 000 vehicles per day). The canyon is aligned almost east–west and the sampling site is at kerbside on the southern side of the street. The canyon has a height-to-width ratio of ∼1; consequently we expect skimming flow when flow is perpendicular, with one or more vortices established in the canyon (Oke et al., 2017). When there is one vortex, the sampler is exposed to freshly emitted traffic contaminants when the wind above the canyon is from the south (Fig. 6). Particle number concentration on Marylebone Road is highest for the 225 and 270∘ wind sectors (Fig. 4a) when traffic-generated pollutants are carried efficiently to the sampler. When winds have a northerly component such as those for 0 and 45∘ in Fig. 4a, the air reaching the sampler is typical of background air from north London and peak concentrations fall by a substantial margin. The particle size data from Marylebone Road (Table S1) show no strong effect of wind direction on the modal diameter for the first fitted mode in the distribution. The average diameter for the 180 and 225∘ wind sectors are 21.4 nm while for the 0 and 45∘ sectors they are 22.9 nm. The second and third mode in the distribution are far more sensitive to wind direction, with the southerly traffic-dominated wind directions showing modes at around 32 and 76 nm as opposed to 56 and 263 nm for the northerly mode data. The former values compare well with modes in the number distribution of around 20 and 50 nm, previously attributed to the nucleation mode and Aitken mode particles respectively, from engine exhaust when sampled at Marylebone Road, with data analysed by positive matrix factorization (Harrison et al., 2011).
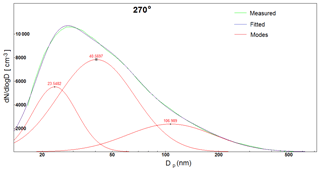
Figure 5Lognormal modes fitted to the average particle size spectrum at North Kensington for wind direction sector 270∘.
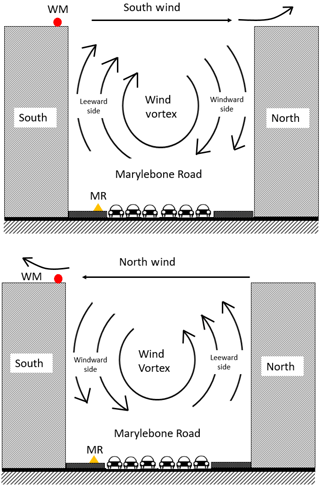
Figure 6A schematic diagram of the wind flows in the street canyon of Marylebone Road (six traffic lanes) during southerly and northerly winds. The orange marker represents the MR sampling site and red marker represents the WM sampling site.
The Westminster University sampling site is 22 m higher and slightly displaced (∼8 m) horizontally from the Marylebone Road air sampling station. The observations at roof level are influenced by the flow separation over the roof, if the air is entering or exiting the canyon, and the background concentrations. The particle size data (Table S1) indicate a nucleation mode very similar in size to that observed within the street canyon at the Marylebone Road site. Concentrations are elevated for the 135 and 180∘ wind bearings suggesting that enhanced concentrations occurring within the canyon on southerly winds are also elevated at the Westminster University sampler but the dataset is very small and hence not included in Fig. 4. The second mode appears to be broadly similar in size to that at Marylebone Road and falls within the range of modal diameters measured at Marylebone Road. Similarly, the third mode falls within the rather variable range also seen at Marylebone Road.
The North Kensington site is widely taken as representative of the background air pollution climate in central London (Bigi and Harrison, 2010; Bohnenstengel et al., 2015). At this site, the size of the first mode in the size distributions is remarkably constant at 22–26 nm, which is slightly larger than that observed at Marylebone Road. The second mode is also less variable than at most other sites and broadly within the range of the second mode sizes at Marylebone Road (see Table S1). The third mode is highly variable in size with wind direction but again broadly comparable to the data from Marylebone Road. The Beddows et al. (2015) positive matrix factorization of particle number size distributions data from this site identified four factors contributing to the particle number size distributions: a secondary component accounting for 4.4 % of particle number with a mode at around 250 nm, an urban background factor (43 % of particle number) peaking at around 50 nm, a traffic component (44.8 % of particle number) peaking at around 30 nm and a regional nucleation component (7.8 % of particle number) peaking at 20 nm. The regional nucleation component showed a strong seasonality with greatest prevalence in the summer months and is thought unlikely to have contributed significantly during the period of this campaign. This was a winter campaign without clear evidence of nucleation leading to new particle formation at any of the sites. A subsequent paper has investigated the factors influencing nucleation at three related sites, including North Kensington and Marylebone Road (Bousiotis et al., 2018). Consequently, the first mode observed in our current study is very comparable to the traffic mode observed by Beddows et al. (2015), and the second mode corresponds strongly to the urban background factor identified by Beddows et al. (2015) who associated this factor with aged traffic emissions and wood smoke, the latter of which is unlikely to have influenced the size distribution at Marylebone Road significantly.
3.2 Particle shrinkage
Previous London work has shown the tendency of nucleation mode traffic-generated particles sampled within Regent's Park to have shrunk by evaporation at rates of on average 0.13 nm s−1 (Harrison et al., 2016) while particles in the regional atmosphere typically undergo condensational growth at a rate of about 0.6–0.9 nm h−1 (Beddows et al., 2014). This reflects an initial local rapid loss of more volatile hydrocarbons, followed by a subsequent slower condensation of low-volatility species formed by atmospheric oxidation in the regional atmosphere.
Under southerly flows the Regent's University site is downwind of Marylebone Road (Fig. 1). The modal diameters measured at Regent's University in the nucleation mode (Table S1) are clearly indicative of a shrinkage of particle diameter for the wind sectors 180, 225 and 270∘, corresponding to air having passed over Marylebone Road. These data show that the nucleation mode is shrinking from a diameter in the range of 21–24 nm at Marylebone Road and 22–24 nm at Westminster University to a diameter of 14, 9 or 12 nm at the Regent's University site. In this case, particle shrinkage seems to be limited to those three wind sectors, with possibly some shrinkage in the 45∘ wind sector, but particles in other wind sectors retain broadly similar diameters to those measured at Marylebone Road and Westminster University. The second particle mode and third particle mode (where identifiable) at Regent's University are broadly similar and considerably larger than those measured at Marylebone Road or in the limited dataset at Westminster University.
In our earlier studies of the evolution of particle sizes between Marylebone Road and Regent's Park (Harrison et al., 2016), the nucleation mode in the Marylebone Road size distributions lay between 20 and 24 nm (i.e. very similar to this study). In Regent's Park this had reduced to within the range of 6–11 nm, with the largest sizes measured in the 0∘ wind sector and the smallest in the 180∘ wind sector. The current data show a similar general pattern, although the extent of size reduction is smaller. The travel distance to the Regent's University site is shorter, hence accounting in part for less shrinkage, but the overall shrinkage rate in the current study (0.04 nm s−1) was smaller than previously (0.13 nm s−1) (Harrison et al., 2016). This is probably explained by two factors. Firstly, with warmer mean air temperatures (12–18 ∘C) evaporation would be enhanced, and secondly, as the site used for collection of the data described in the Harrison et al. (2016) study was in the centre of the park and further from any major highways than the Regent's University site, it may have experienced lower vapour concentrations. Consequently, the two datasets appear highly consistent with one another.
Previous BT Tower site observations have reported loss of <20 nm particles (Dall'Osto et al., 2011). This loss was greatest when atmospheric turbulence levels were lowest and hence the time for ground to sampling height (160 m) transport was greatest. That analysis is not repeated in this study. However, the nucleation mode size (Table S1) has grown slightly from the sizes measured at Marylebone Road for the nucleation mode. It is notable that unlike the earlier results, the amplitude of this mode at the BT Tower was substantial and slightly larger than that observed at the ground-level background North Kensington site, suggesting that there was generally good coupling between ground level and the tower site. It is notable that the first mode diameter with greatest amplitude was for the 270∘ sector (Fig. 4d); this is discussed later. The particle size distribution associated with the 225∘ wind sector had only one mode at 40 nm, suggestive of the second solid particle mode with complete evaporation of the semi-volatile nucleation mode.
Earlier studies have shown that particle number concentrations (<100 nm) in a street canyon (Olivares et al., 2007) and urban air (Hussein et al., 2006) increase with reducing temperature. This is consistent with the semi-volatility of nucleation mode particles from road traffic (Harrison et al., 2016), and consequently it would be expected that the particle size distribution as well as the number concentration would be affected by ambient temperature. To investigate this, the size distributions collected in the lowest quartile of air temperatures (1.1 to 3.8 ∘C) were compared with those in the highest quartile of temperature (9.1 to 11.8 ∘C). This showed generally higher concentrations associated with the higher temperatures, and a clearer nucleation mode at higher temperatures, at all sites, and most notably at Marylebone Road. Such behaviour is contrary to expectations, as greater evaporative losses would be expected at higher temperatures, reducing the magnitude of the plot, or shifting the mode to smaller sizes. To understand this effect more clearly, wind directions with the coldest and hottest quartiles of temperature are analysed. The coldest periods all occurred during northerly flows (270 to 90∘) and >85 % of highest quartile of temperatures occur during southerlies (90 to 270∘). The behaviour, especially at Marylebone Road and Regent's University, therefore appears to be determined predominantly by synoptic wind conditions. For Marylebone Road, the street canyon flow (Fig. 6) is the dominant influence and at Regent's University the traffic sources are most proximate with southerly flows.
3.3 Particle number concentration (CPC) data
Average diurnal variations of total particle number count derived from the condensation particle counters produced using the OpenAir software package (Carslaw and Ropkins, 2012) appear in Fig. S2. At both Marylebone Road and Westminster University, these show a peak occurs between midnight and 06:00 LT (local time) before reducing and then rising to a second peak in the afternoon. CPC concentrations at these sites far exceed those at Regent's University and the BT Tower, whereas integrated counts from the SMPS instruments were considerably smaller and showed a diurnal variation broadly similar to that expected for road traffic emissions (Fig. 3). While it is quite normal for the CPC to give a higher count than the SMPS since it measures over a wider size range and may have lower internal losses (although the SMPS data analysis software corrects for internal losses), the ratio of CPC to SMPS is in our experience (e.g. Shi et al., 2001) typically around 2, but this value was significantly exceeded episodically, especially at Westminster University (Fig. S3). The overall pattern of CPC to SMPS ratios (Fig. 7) shows that some of the highest ratios were at Regent's University, with two individual occasions exceeding 13. Some high peak values were observed at Westminster University during the short SMPS time series. Wood burning is recognised as an influential source of particles in London (Harrison et al, 2012b; Crilley et al., 2015) and has a diurnal profile with higher concentrations typically at night. During the ClearfLo winter campaign the BT Tower was influenced substantially by wood smoke irrespective of boundary layer depth (Crilley et al., 2015). Since the BT Tower site was predominantly within the mixed layer during the 2017 campaign (Fig. S1) and the CPC∕SMPS average ratios at the tower show little nocturnal elevation, we consider it unlikely that wood smoke explains our observations. Furthermore, particle size distributions associated with biomass burning are typically larger than those from road traffic, and outside of the sub-15 nm size range (Vu et al., 2015a). The occurrence of the maximum in this behaviour at night-time (03:00–04:00 LT) suggests that other heating-related emissions (e.g. from natural gas combustion) are not the source.
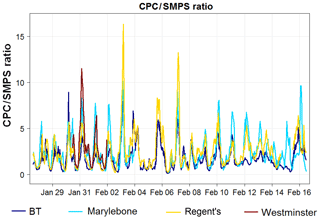
Figure 7Time series (15 min) of ratio of total particle number counts, CPC∕SMPS, for four sites over the campaign period.
To evaluate this phenomenon more closely, the black carbon data were examined. These are typically taken as a good tracer of diesel exhaust, which is expected to be the main source of the particle number count. The diurnal variation in black carbon (Fig. S4) conformed reasonably well to that expected for a traffic-generated pollutant, with Marylebone Road concentrations far exceeding those at the other sites and showing a typical traffic-associated pattern. The ratio of particle number (derived from the CPC) to black carbon (Fig. S5) shows huge diurnal variability similar to that seen in the ratio of particle number count from the CPC to that derived from the SMPS. We infer from this behaviour that a large number of particles smaller than the lower limit of the SMPS and above the lower limit of the CPC (i.e. 2.5–14.9 nm for the 3776 instrument at Westminster University and Regent's University; 4–14.9 nm for 3775 instrument at BT Tower; and 3–16.55 nm for 3025 instrument at Marylebone Road) were present in the atmosphere. Both the mean ratio of CPC to SMPS (Fig. S3) and CPC to black carbon (Fig. S6) have ratios that are greatest in the early morning (midnight to 06:00 LT). This is unexpected for the CPC∕SMPS ratio, as the contribution of traffic relative to regional aerosol is expected to be lowest and the coarser regional aerosol contains few particles in the size range below the lower limit of the SMPS instrument. Similarly, for the black carbon data, one would expect that if traffic is the main source of particles measured by the CPC, the latter would show a diurnal fluctuation like that of black carbon, which in London arises mostly from traffic emissions. Consequently, it seems likely that nucleation processes favoured by the cooler temperatures and lower condensation sink in the early hours of the morning are creating large numbers of particles in the range of 2.5–15 nm mobility diameter. These are forming as air moves away from the traffic source and hence are greatest at the rooftop Westminster University site and have diminished to some extent by coagulation or re-evaporation by the time they reach the Regent's University site, which still shows a marked elevation in the ratio of particle number to black carbon in the earlier hours of the morning compared to the Marylebone Road site.
Such behaviour is somewhat unexpected and a review of papers in which vertical gradients in particle number count have been measured above roadside sites showed no earlier evidence of such behaviour (Lingard et al., 2006; Agus et al., 2007; Nikolova et al., 2011; Ketzel et al., 2003; Longley et al., 2003; Kumar et al., 2008a, b, 2009; Li et al., 2007; Vakeva et al., 1999; Zhu et al., 2002b; Wehner et al., 2002). However, evidence is seen in some of Villa et al.'s (2017) observations that particle number count increased with height up to around 10 m above a multi-lane highway. The authors reported this unexpected pattern for some ascents and descents and attributed it to exhaust tubes of heavy-duty trucks tending to project vertically upwards and to be located at a height of several metres above ground. They suggest this is not the case in urban canyons.
Another possibility arises from the report of Rönkkö et al. (2017) that large numbers of sub-4 nm particles are observed in the exhaust of some diesel engines and the observation by Nosko et al. (2017) of substantial numbers of similarly sized particles amongst emissions from brake wear. Kontkanan et al. (2017) reported observations of sub-3 nm particles from many sites, the highest concentrations being in urban locations. The diurnal and regional variations did not relate clearly to photochemistry and it was concluded that sub-3 nm particle concentrations are affected by anthropogenic sources of precursor vapours. The correlation of sub-3 nm particle concentrations in Helsinki with nitrogen oxides suggested a link with traffic emissions. Shi et al. (2001) measured particles of >9.5 nm by SMPS, >7 nm by CPC and >3 nm by ultrafine CPC, finding large numbers of particles in urban air in the ranges 3–7 and 3–9.5 nm by differences of counts. Ratios of CPC ( (>9.5 nm) were highly variable, but typically around 4. Clear links to road traffic were seen, with drive-by experiments showing large numbers of particles in the 3–7 nm range in the exhausts of both diesel and gasoline vehicles (Shi et al., 2001). Nanoparticles were also produced in the plume downwind of a stationary combustion source (Shi et al., 2001). Herner et al. (2011) measured the size distribution of particles emitted from vehicles equipped with diesel particle filters, and with diesel filters and selective catalytic reduction. The dominant mode in the size distribution was at 10 nm diameter and comprised particles with a high fraction of sulfate. In highway and roadside measurements in Helsinki, Enroth et al. (2016) measured particle size distributions with a dominant mode at 10 nm diameter. Such particles would be largely below the lower threshold for counting by the SMPS but not the CPC. It is plausible that during the cooler hours of the night a tail of <2.5 nm particles might be subject to condensational growth if the co-emitted vapour were to be supersaturated in the atmosphere within the street canyon. The dominance of a 10 nm mode in the size distribution would appear to be the most plausible explanation for the high number concentration of particles observed at the Westminster University rooftop location and the apparent transport of a substantial proportion of such particles to the Regent's University measurement site. While this can explain the typically high CPC∕SMPS ratios observed, it does not explain their diurnal variation. This appears to require growth of sub-2.5 nm particles into the range measured by CPC in the cooler, more humid nocturnal conditions. Rönkkö et al. (2006) and Schneider et al. (2005) studied the formation of mechanisms and composition of diesel exhaust nucleation particles in the laboratory and during car chasing. They conclude that formation of nucleation mode particles depends upon formation of sulfate nuclei upon which hydrocarbons condense, consistent with earlier studies of Shi and Harrison (1999) and Shi et al. (2000) conducted in our laboratory. Factors favouring nucleation mode particle formation were found to be low temperature and high humidities, consistent with field measurements made on Marylebone Road (Charron and Harrison, 2003). Both factors prevail at night-time, probably contributing to the relative increase in 2.5–15 nm diameter particles seen most notably between midnight and 06:00 LT (Fig. S3). Salimi et al. (2017) reported nocturnal new particle formation events in Brisbane, Australia, finding that air masses associated with nocturnal events were typically transported over the ocean before reaching their sampling site, but the relevance to our study is unclear, although the maritime air might sometimes be expected to show lower temperature and higher humidity than that from the land.
Support for our observations also comes from the very detailed measurement and modelling study of Choi and Paulson (2016). Measuring particle number size distribution downwind of a major highway, they found a positive anomaly in particle number within the first 60 m of the plume peak, as the peak for the small particles appeared further downwind than the peak in accumulation mode particles. They attributed this to growth of unmeasured sub-5.6 nm particles into the smallest measurable size range and suggested condensational growth or self-coagulation as the mechanism (Choi and Paulson, 2016). Kerminen et al. (2007) measuring near a major road in Helsinki reported particle growth by condensation to be a dominant process during the road-to-ambient evolution stage at night-time in winter. They inferred that under such conditions (low wind speeds with a temperature inversion), traffic-generated particle numbers were enhanced and could affect submicron particle number concentrations over large areas around major roads. The distance scales for such processes in both studies (Choi and Paulson, 2016; Kerminen et al., 2007) were within 100 m of the source under the conditions of measurement but might conceivably extend over greater distance scales. Similar processes of particle evolution within an aircraft exhaust plume have been reported by Timko et al. (2013).
Pushpawela et al. (2018) report a phenomenon of hygroscopic particle growth at night-time, which can potentially be mistaken for new particle formation. This phenomenon was observed between 0.5 and 5.0 h after sunset, peaking at 3.5 h (Pushpawela et al., 2018). This would not appear to explain our observations, where the peak in N–SMPS and N–BC particle number plots (Figs. S2 and S5) is greatest at 03:00–04:00 LT, which in London in winter is some 10–11 h after sunset. Additionally, such a phenomenon would be expected to be unrelated to local traffic emissions, and hence more uniform across the various sites.
3.4 Spatial distribution of particles – horizontal and vertical
Figure 2 shows the time series of particle concentrations from the SMPS instruments throughout the campaign. Clearly, as expected, the Marylebone Road site shows the highest concentrations through the campaign period due to its proximity to the road traffic source. The other sites tend to track one another quite closely with no consistent ranking of concentrations. There are periods such as 1 to 3 February when Regent's University well exceeds North Kensington, but at other times, they are very similar (e.g. 10–12 February), or periods when North Kensington exceeds Regent's University (e.g. 7 February) but these are few. In the former period (1–3 February), winds were southerly and concentrations at Regent's University would be enhanced by passage of air across central London, including Marylebone Road. In the situation where concentrations were similar (10–12 February), winds were in the northerly sector, giving relatively low concentrations at all sites, and rather little spatial variation. The temporal pattern at all sites showed substantial similarity overall (Fig. 2), including diurnal patterns (Fig. 3), although the magnitude of concentrations varied.
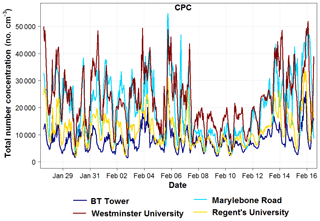
Figure 8Time series (15 min) of total particle number count from the CPC instruments located at four sites over the campaign period.
A time series of CPC particle number concentrations (Fig. 8) showed that under most conditions, the number count was lowest at the BT Tower site, and that the number count at Westminster University frequently exceeded that at Marylebone Road, with Regent's University lower, but above the concentration at the BT Tower (Fig. 8). During the period of northerly winds (8–12 February), all sites showed low concentrations in the SMPS data, with Regent's University and BT Tower similar for much of the time (Fig. 2). The highest CPC count concentrations during the latter were measured at Westminster University (Fig. 9), which was downwind of Marylebone Road at those times. The similarity seen between Westminster University and Marylebone Road for much of the campaign, with concentrations far in excess of those at BT Tower, is strongly suggestive of continuing particle growth into the size range 2.5–14.9 nm at Westminster University, with re-evaporation occurring before reaching the elevated BT Tower site, as previously observed by Dall'Osto et al. (2011). Elevations in N–BC data were seen at the BT Tower site (Figs. S4 and S6) but these occurred mainly during the morning rush hour period, presumably due to fresh traffic emissions, rather than overnight as at the other sites (Fig. S6).
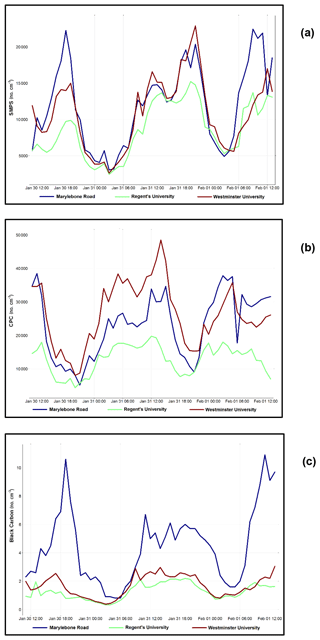
Figure 9Time series (15 min) of (a) SMPS-integrated counts, (b) particle number counts (CPC) and (c) black carbon from Marylebone Road, Westminster University and Regent's University for 30 January to 1 February 2017.
Figure 2 suggests that vertical gradients between the proximate Regent's University and BT Tower sites were small in SMPS count (Fig. 2), but at certain times were substantial in the CPC count (Fig. 9). The particle size distributions measured at the BT Tower (Fig. 4d) differ from Marylebone Road and North Kensington (Fig. 4a and b) in having no obvious mode in the nucleation size range at 20–30 nm, a feature shared with Regent's University (Fig. 4c). Only during westerly winds (270∘) does the BT Tower show such a mode (Fig. 4d), while at Regent's University (Fig. 5) the 270∘ wind direction also shows differences from the others with a mode at below 20 nm. Anomalous behaviour in this wind sector is also observed at North Kensington (Fig. 4b), and at Marylebone Road. The most pronounced nucleation mode peak is associated with the 270 and 225∘ wind directions. In the Marylebone Road case, these wind directions are almost parallel to the highway, which might explain the high concentrations and pronounced nucleation mode, but this explanation does not work for the other sites. A more likely explanation is that all sites are affected by emissions from Heathrow Airport, which is to the west of London and has been recognised as a major source of nucleation mode particles associated with aircraft and road traffic emissions (Masiol et al., 2017). At a site 1 km from the northern boundary of Heathrow Airport, PMF factors attributed to aircraft (mode at <20 nm) and fresh road traffic emissions (mode at 18–35 nm) accounted respectively for 31.6 % and 27.9 % of particle number count in the warm season and 33.1 % and 35.2 % in the cold season (December 2014–January 2015) data (Masiol et al., 2017). Heathrow Airport is located approximately 22 km from our central London sites on a bearing of 255∘. Keuken et al. (2015) measured a large elevation in concentrations of particles of 10–20 nm diameter attributed to aircraft emissions (emission studies are reviewed by Masiol and Harrison, 2014) at a site 7 km east of Schiphol Airport (Netherlands) and have shown by modelling and measurement that concentrations are elevated to considerably greater downwind distances. Similarly, Hudda et al. (2014) reported PNC to have increased 4- to 5-fold at 8–10 km downwind of Los Angeles International Airport (USA).
The size distributions have also been analysed according to mixed layer height, determined by ceilometer (Kotthaus and Grimmond, 2018). Both Marylebone Road (Fig. S7) and Regent's University (Fig. S8) have the highest concentrations associated with the deepest MLH class (>1000 m). This seems likely to be due to an association with southerly winds and the street canyon circulation, whereas North Kensington (Fig. S9) has the highest concentrations during shallow MLH (<100 and 100–200 m) when dispersion is limited for the low-altitude emissions. The most interesting behaviour is seen at the elevated (160 m) BT Tower site, which is consistent with Harrison et al. (2012a) and Dall'Osto et al. (2011). During the shallowest MLH (<100 m) the measurement site is above the inversion and the size distribution lacks an obvious nucleation mode (Fig. S10). As the MLH deepens, a nucleation mode appears which dominates the size distribution for the deepest MLH categories (900–1000 and >1000 m) with a mode at 20–30 nm, similar to that seen at Marylebone Road for the same MLH depths (Fig. S7). The gradual transitioning of size distribution as the MLH deepens is consistent with the surface source (mainly road traffic) of nucleation mode particles and their evaporative loss, which increases with the timescale of vertical mixing to the height of the sampler, as reported by Dall'Osto et al. (2011), and the ultimate isolation of the sampler from ground-level emissions at the shallowest boundary layer heights, as observed by Harrison et al. (2012a).
3.5 Detailed comparison of Marylebone Road, Westminster University and Regent's University
Unfortunately, a full dataset for the Westminster University site was only collected over the period 30 January to 1 February due to a late set-up of the instrument and a malfunction after 1 February. This period, however, merits closer examination as it is the only period where SMPS data were available for all three sites. For much of the time the SMPS data for the Westminster University site looks surprisingly similar to that of the Marylebone Road site despite the former being on the rooftop and the latter being within the street canyon. A detailed analysis hour by hour showed that out of 51 hourly observations, in 23 the amplitude of the mode (dN ∕ dlogD) at Westminster University was within ±20 % of that at Marylebone Road while in 25 cases the amplitude was greater at Westminster University than at Marylebone Road, and in just two cases the amplitude was smaller at Westminster University. In an attempt to explain this observation, the meteorological data for the periods of similar magnitude and of different magnitudes were compared but no systematic difference was seen in wind direction, air temperature or relative humidity between any of the periods. Wind directions were generally in a south-easterly to easterly sector, mean temperatures around 8 ∘C and relative humidity high (85 and 99 %). The maximum MLH were low and there was a lot of rain (Fig. S1).
In order to gain further insight, the time series of observations were plotted for this period and appear in Fig. 9. The SMPS-integrated number counts shown in Fig. 9a show a remarkable similarity between Marylebone Road, Westminster University and Regent's University. For the first 2 days, Regent's University concentrations are lower than those from the other two sites, although on the third day they are very similar to those at Westminster University. On the first and last days, the peak concentrations at Marylebone Road exceed those at Westminster University but on the middle day (31 January) the differences between these two sites are very small. The CPC particle number counts shown in Fig. 9b are very similar to those at Marylebone Road on the first and last day but exceed those at Marylebone Road on 31 January. Concentrations at Regent's University are typically only around half or less of those measured at Westminster University. The magnitude of the CPC concentrations peaking at over 40 000 cm−3 is close to double the integrated SMPS counts, which peak at a little over 20 000 cm−3, indicating a large number of particles in the size range below 14.9 nm.
However, the black carbon data (Fig. 9c) have daytime concentrations at Marylebone Road that far exceed those at Westminster University and Regent's University, the latter sites tracking each other and having very similar concentrations. Since black carbon can be viewed as a conserved tracer of vehicle emissions over these small time and distance scales, the inference is that particle production must be continuing as the vehicle exhaust mixes upwards from the street canyon Marylebone Road site to the Westminster University rooftop site. The southerly wind directions likely associated with upward flow on the Westminster University canyon wall (Fig. 6) would carry vehicle exhaust past the Marylebone Road measurement station (south side of the road).
Air leaving the canyon and being entrained by the complex building roof flows could expose the Westminster University sampler to air exiting the street canyon and to the general flow towards Regent's University site (Figs. 6 and 1). Such behaviour is consistent with the observations of particle growth in the sub-SMPS size ranges reported in the previous section, extending into the SMPS size range. This is similar to behaviour observed by Kerminen et al. (2007) in Helsinki, who observed not only possible evaporation of some particles in the 7–30 nm range, but also an apparent growth of nucleation mode particles into the 30–63 nm size range between sampling points at 9 and 65 m downwind of a highway. The results in Fig. 9 are suggestive of a substantial growth of nuclei into the range of the CPC at Westminster University.
The measurement of particle number size distributions in the atmosphere is resource intensive and there have been rather few studies in which more than two samplers have been operated within a city. Typically if there are two sites, one is a traffic-influenced site and the other urban background. In this study, data have been collected at a total of five sites, although unfortunately the dataset from the Westminster University site is limited to only a few days. Nonetheless, the dataset allows some deep insights into the spatial distribution of particle sizes and number counts not only horizontally but also in the vertical dimension. Not unexpectedly, concentrations of particles at the street canyon Marylebone Road site considerably exceed concentrations at other sites, but there are nonetheless considerable similarities in diurnal profiles and the magnitude of concentrations at the other, background sites.
One of the main motivating factors for this study was to confirm earlier observations of shrinkage of the nucleation mode particles between traffic emissions on Marylebone Road and the downwind site at Regent's University within Regent's Park. Particle shrinkage was observed within the current study although at a slower mean rate (0.04 nm s−1) than in the earlier study (Harrison et al., 2016), in which the mean shrinkage rate was 0.13 nm s−1. However, temperatures in the current study all fell below those in the earlier work of Harrison et al. (2016). Other factors may also have been influential. There have been marked changes in the road vehicle fleet in London between the two measurement campaigns. The earlier dataset as reported by Dall'Osto et al. (2011) and Harrison et al. (2016) was collected in 2006, at which time the sulfur content of diesel fuel was regulated at below 50 ppm. Between the two campaigns, the sulfur content of both gasoline and diesel motor fuels was reduced to below 10 ppm sulfur in order to facilitate the introduction of diesel particle filters from 2011 onwards. The incorporation of a diesel particle filter on Euro 5 and Euro 6 vehicles leads to a substantial overall reduction in particulate matter emissions but also a change in the hydrocarbon content of the particles. Secondly, the Regent's Park sampling site used for the 2006 measurements was at about double the distance from Marylebone Road compared to the Regent's University used in the latest study. This would allow for greater dilution of the traffic plume from Marylebone Road and other adjacent highways, leading to a greater reduction in vapour-phase hydrocarbons at the more distant site, causing an accelerated evaporation process. The reduction in fuel sulfur content in 2007 was accompanied by a marked change in the size distribution of particles emitted from road traffic, including a reduction in the nucleation mode particles (Jones et al., 2012). The work of Dall'Osto et al. (2011) also analysed data from the BT Tower, showing increasing evaporative loss of nucleation mode particles as the travel time from ground level to the sampling site on the tower became longer with reduced atmospheric turbulence levels. Although that phenomenon has not been studied in detail in the latest dataset, the results are clearly consistent with such a process, and with an apparent total loss of the nucleation mode in particles associated with regional pollution sampled when the boundary layer top was below the sampling height on the tower.
Although the phenomenon of particle shrinkage had been seen in earlier work, there were two further major observations made in the current study which were not anticipated. The first was the clear influence of a major source to the west of London, almost certainly Heathrow Airport, upon concentrations of nucleation mode particles. The association of an enhanced nucleation mode in the 270 or 225∘ sector is indicative of a major source of very fine particles, and the work of Masiol et al. (2017) at a sampling site close to Heathrow Airport provides strong evidence for major emissions both from aircraft engines and the large volumes of road traffic attracted by the airport. Earlier research by Keuken et al. (2015) and Hudda et al. (2014) gives a clear precedent for measurement of strongly elevated concentrations of very fine particles several kilometres downwind of a major airport, but to our knowledge this is the first observation of concentrations above urban background at a distance of 22 km from the centre of the airport.
The other observation which was wholly unexpected was of the very poor relationship between total particle numbers measured by the scanning mobility particle sizers and the total particle numbers measured by co-located condensation particle counters. While both the SMPS counts and co-located black carbon measurements show a typical road traffic diurnal profile, the CPC data show a quite different diurnal profile peaking at night. This is most evident in the ratios of CPC∕SMPS and CPC∕BC seen at all sampling sites, with the exception of CPC∕BC at the elevated BT Tower site, which does not show a nocturnal maximum, but peaks during the morning rush hour period. Earlier studies such as that of Choi and Paulson (2016) and Kerminen et al. (2007) have reported data consistent with such a phenomenon, but with very modest elevations in particle count compared to those in the current data. The implication is of the presence of large numbers of particles within the range of 2.5–15 nm and hence observable with the CPC but below the lower cut of the SMPS. It seems likely that such particles grow at night from very small nuclei and it seems possible that the exceptional magnitude of this process within London results from the high density of diesel traffic, leading to substantial nocturnal concentrations of condensable vapours close to the traffic source. A common feature to such observations appears to be its association with still conditions on winter nights, which lead to poor dispersion of vehicle emissions and a pool of vapour co-emitted with traffic particles which becomes supersaturated as it cools in the ambient atmosphere, leading to condensation on small nuclei when the general particle concentrations and hence the condensation sink are relatively low in magnitude.
These very abundant particles within the 2.5–15 nm range are likely to prove ephemeral as they would be expected to re-evaporate as the air mass dilutes away from the source. However, the health effects of exposure to particles within this range are poorly known and no recommendation can be given as to whether health-related studies would be best to measure the particle size range covered by the SMPS as is most typically performed at present, or whether CPC data going down to smaller particles sizes would be more appropriate.
There are some additional general conclusions from the work. Firstly the results demonstrate the dynamic behaviour of traffic-generated (and other) particles within the urban atmosphere. Our earlier paper (Dall'Osto et al., 2011) referred to “remarkable dynamics”, and further remarkable dynamic processes have been observed in the current study. Secondly, as this work has revealed sources and processes that were not originally anticipated, although with the benefit of hindsight it might have been possible to predict them, there is clearly a need for further detailed observational studies of the behaviour of sub-100 nm particles within the urban atmosphere.
Data supporting this publication are openly available from the UBIRA eData repository at https://doi.org/10.25500/eData.bham.00000265.
The supplement related to this article is available online at: https://doi.org/10.5194/acp-19-39-2019-supplement.
DB, MA, JB and RX carried out the field measurements of particle size distributions, SK and SG collected and interpreted the ceilometer data, and DB and AS carried out data analyses. RH led the project and drafted the paper, with all co-authors contributing to subsequent enhancements.
The authors declare that they have no conflict of interest.
The authors are grateful to the management and staff of Westminster
University, Regent's University and British Telecom for access to their
buildings for air sampling. They also express gratitude to the National
Centre for Atmospheric Science (NCAS) for the loan of sampling instruments,
and to Paul Williams (NCAS) for facilitating the instrument
intercomparison. The operation of the ceilometers were supported by NERC
ClearfLo, NERC AirPro, Newton Fund/Met Office CSSP (SG, SK) and University
of Reading. We acknowledge the support of KCL LAQN for the instrument sites
and support and the Reading Urban Micromet group for maintaining the
instruments, notably Elliott Warren and Kjell zum Berge. The
work was funded by the European Research Council (ERC-2012-AdG, proposal
no. 320821) and the UK Natural Environment Research Council (R8/H12/83/011) and
a NCAS studentship (to JB).
Edited by: Veli-Matti Kerminen
Reviewed by: two anonymous referees
Agus, E. L., Young, D. T., Lingard, J. J. N., Smalley, R. J., Tate, J. E., Goodman, P. S., and Tomlin, A. S.: Factors influencing particle number concentrations, size distributions and modal parameters at a roof-level and roadside site in Leicester, UK, Sci. Total Environ., 386, 65–82, 2007.
Alam, M. S., Rezaei, S. Z., Stark, C. P., Liang, Z., Xu, H. M., and Harrison R. M.: The characterisation of diesel exhaust particles – composition, size distribution and partitioning, Faraday Discuss., 189, 69–84, 2016.
Asmi, A., Wiedensohler, A., Laj, P., Fjaeraa, A.-M., Sellegri, K., Birmili, W., Weingartner, E., Baltensperger, U., Zdimal, V., Zikova, N., Putaud, J.-P., Marinoni, A., Tunved, P., Hansson, H.-C., Fiebig, M., Kivekäs, N., Lihavainen, H., Asmi, E., Ulevicius, V., Aalto, P. P., Swietlicki, E., Kristensson, A., Mihalopoulos, N., Kalivitis, N., Kalapov, I., Kiss, G., de Leeuw, G., Henzing, B., Harrison, R. M., Beddows, D., O'Dowd, C., Jennings, S. G., Flentje, H., Weinhold, K., Meinhardt, F., Ries, L., and Kulmala, M.: Number size distributions and seasonality of submicron particles in Europe 2008–2009, Atmos. Chem. Phys., 11, 5505–5538, https://doi.org/10.5194/acp-11-5505-2011, 2011.
Beddows, D. C. S., Dall'Osto, M., Harrison, R. M., Kulmala, M., Asmi, A., Wiedensohler, A., Laj, P., Fjaeraa, A. M., Sellegri, K., Birmili, W., Bukowiecki, N., Weingartner, E., Baltensperger, U., Zdimal, V., Zikova, N., Putaud, J.-P., Marinoni, A., Tunved, P., Hansson, H.-C., Fiebig, M., Kivekäs, N., Swietlicki, E., Lihavainen, H., Asmi, E., Ulevicius, V., Aalto, P. P., Mihalopoulos, N., Kalivitis, N., Kalapov, I., Kiss, G., de Leeuw, G., Henzing, B., O'Dowd, C., Jennings, S. G., Flentje, H., Meinhardt, F., Ries, L., Denier van der Gon, H. A. C., and Visschedijk, A. J. H.: Variations in tropospheric submicron particle size distributions across the European continent 2008–2009, Atmos. Chem. Phys., 14, 4327–4348, https://doi.org/10.5194/acp-14-4327-2014, 2014.
Beddows, D. C. S., Harrison, R. M., Green, D. C., and Fuller, G. W.: Receptor modelling of both particle composition and size distribution from a background site in London, UK, Atmos. Chem. Phys., 15, 10107–10125, https://doi.org/10.5194/acp-15-10107-2015, 2015.
Bigi, A. and Harrison, R. M.: Analysis of the air pollution climate at a central urban background site, Atmos. Environ., 44, 2004–2012, 2010.
Bohnenstengel, S. I., Belcher, S. E., Aiken, A., Allan, J. D., Allen, G., Bacak, A., Bannan, T. J., Barlow, J. F., Beddows, D. C. S., Bloss, W. J., Booth, A. M., Chemel, C., Coceal, O., Di Marco, C. F., Dubey, M. K., Faloon, K. H., Fleming, Z. L., Furger, M., Geitl, J. K., Graves, R. R., Green, D. C., Grimmond, C. S. B., Halios, C. H., Hamilton, J. F., Harrison, R. M., Heal, M. R., Heard, D. E., Helfter, C., Herndon, S. C., Holmes, R. E., Hopkins, J. R., Jones, A. M., Kelly, F. J., Kotthaus, S., Langford, B., Lee, J. D., Leigh, R. J., Lewis, A. C., Lidster, R. T., Lopez-Hilfiker, F. D., McQuaid, J. B., Mohr, C., Monks, P. S., Nemitz, E., Ng, N. L., Percival, C. J., Prévôt, A. S. H., Ricketts, H. M. A., Sokhi, R., Stone, D., Thornton, J. A., Tremper, A. H., Valach, A. C., Visser, S., Whalley, L. K., Williams, L. R., Xu, L., Young, D. E., and Zotter, P.: Meteorology, air quality, and health in London: The ClearfLo project, Am. Meteorol. Soc., 779–804, https://doi.org/10.1175/BAMS-D-12-00245.1, 2015.
Bousiotis, D., Dall'Osto, M., Beddows, D. C. S., Pope, F. D., and Harrison, R. M.: Analysis of New Particle Formation (NPF) Events at Nearby Rural, Urban Background and Urban Roadside Sites, Atmos. Chem. Phys. Discuss., https://doi.org/10.5194/acp-2018-1057, in review, 2018.
Brines, M., Dall'Osto, M., Beddows, D. C. S., Harrison, R. M., Gómez-Moreno, F., Núñez, L., Artíñano, B., Costabile, F., Gobbi, G. P., Salimi, F., Morawska, L., Sioutas, C., and Querol, X.: Traffic and nucleation events as main sources of ultrafine particles in high-insolation developed world cities, Atmos. Chem. Phys., 15, 5929–5945, https://doi.org/10.5194/acp-15-5929-2015, 2015.
Carslaw, D. C. and Ropkins, K.: openair – An R package for air quality data analysis, Environ. Model. Softw., 27–28, 52–61, https://doi.org/10.1016/j.envsoft.2011.09.008, 2012.
Charron, A. and Harrison, R. M.: Primary particle formation from vehicle emissions during exhaust dilution in the roadside atmosphere, Atmos. Environ., 37, 4109–4119, 2003.
Choi, W. and Paulson, S. E.: Closing ultrafine particle number concentration budget at road-to-ambient scale: Implications for particle dynamics, Aerosol Sci. Technol., 50, 448–461, 2016.
Crilley, L. R., Bloss, W. J., Yin, J., Beddows, D. C. S., Harrison, R. M., Allan, J. D., Young, D. E., Flynn, M., Williams, P., Zotter, P., Prevot, A. S. H., Heal, M. R., Barlow, J. F., Halios, C. H., Lee, J. D., Szidat, S., and Mohr, C.: Sources and contributions of wood smoke during winter in London: assessing local and regional influences, Atmos. Chem. Phys., 15, 3149–3171, https://doi.org/10.5194/acp-15-3149-2015, 2015.
Dall'Osto, M., Thorpe, A., Beddows, D. C. S., Harrison, R. M., Barlow, J. F., Dunbar, T., Williams, P. I., and Coe, H.: Remarkable dynamics of nanoparticles in the urban atmosphere, Atmos. Chem. Phys., 11, 6623–6637, https://doi.org/10.5194/acp-11-6623-2011, 2011.
Enroth, J., Saarikoski, S., Niemi, J., Kousa, A., Ježek, I., Mocnik, G., Carbone, S., Kuuluvainen, H., Rönkkö, T., Hillamo, R., and Pirjola, L.: Chemical and physical characterization of traffic particles in four different highway environments in the Helsinki metropolitan area, Atmos. Chem. Phys., 16, 5497–5512, https://doi.org/10.5194/acp-16-5497-2016, 2016.
Gonzalez, Y., Rodriguez, S., Guerra Garcia, J. C., Trujillo, J. L., and Garcia, R.: Ultrafine particles pollution in urban coastal air due to ship emissions, Atmos. Environ., 45, 4907–4914, 2011.
Harrison, R. M., Shi, J. P., Xi, S., Khan, A., Mark, D., Kinnersley, R., and Yin, J.: Measurement of number, mass and size distribution of particles in the atmosphere, Phil. Trans. R. Soc. Lond., A, 358, 2567–2580, 2000.
Harrison, R. M., Beddows, D. C., and Dall'Osto, M.: PMF analysis of wide-range particle size spectra collected on a major highway, Environ. Sci. Technol., 45, 5522–5528, 2011.
Harrison, R. M., Dall'Osto, M., Beddows, D. C. S., Thorpe, A. J., Bloss, W. J., Allan, J. D., Coe, H., Dorsey, J. R., Gallagher, M., Martin, C., Whitehead, J., Williams, P. I., Jones, R. L., Langridge, J. M., Benton, A. K., Ball, S. M., Langford, B., Hewitt, C. N., Davison, B., Martin, D., Petersson, K. F., Henshaw, S. J., White, I. R., Shallcross, D. E., Barlow, J. F., Dunbar, T., Davies, F., Nemitz, E., Phillips, G. J., Helfter, C., Di Marco, C. F., and Smith, S.: Atmospheric chemistry and physics in the atmosphere of a developed megacity (London): an overview of the REPARTEE experiment and its conclusions, Atmos. Chem. Phys., 12, 3065–3114, https://doi.org/10.5194/acp-12-3065-2012, 2012a.
Harrison, R. M., Beddows, D. C. S., Hu, L., and Yin, J.: Comparison of methods for evaluation of wood smoke and estimation of UK ambient concentrations, Atmos. Chem. Phys., 12, 8271–8283, https://doi.org/10.5194/acp-12-8271-2012, 2012b.
Harrison, R. M., Jones, A. M., Beddows, D. C., and Dall'Osto, M.: Evaporation of traffic-generated nanoparticles during advection from source, Atmos. Environ., 125, 1–7, 2016.
HEI: Understanding the Health Effects of Ambient Ultrafine Particles, Health Effects Institute, HEI Perspectives 3, 2013.
Herner, D. H., Hu, S., Robertson, W. H., Huai, T., Chang, M. C. O., Riger, P., and Ayala, A.: Effect of Advanced Aftertreatment for PM and NOx Reduction on Heavy-Duty Diesel Engine Ultrafine Particle Emissions, Environ. Sci. Technol., 45, 2413–2419, 2011.
Hudda, N., Gould, T., Hartin, K., Larson, T. V., and Fruin, S. A.: Emissions from an international airport increase particle number concentrations 4-fold at 10 km downwind, Environ. Sci. Technol., 48, 6628–6635, 2014.
Hussein, T., Karppinen, A., Kukkonen, J., Harkonen, J., Aalto, P. P., Hameri, K., Kerminen, V.-M., and Kulmala, M.: Meteorological dependence of size-fractionated number concentrations of urban aerosol particles, Atmos. Environ., 40, 1427–1440, 2006.
Jones, A. M., Harrison, R. M., Barratt, B., and Fuller, G.: A large reduction in airborne particle number concentrations at the time of the introduction of “suphur free” diesel and the London Low Emission Zone, Atmos. Environ., 50, 129–138, 2012.
Karl, M., Kukkonen, J., Keuken, M. P., Lützenkirchen, S., Pirjola, L., and Hussein, T.: Modeling and measurements of urban aerosol processes on the neighborhood scale in Rotterdam, Oslo and Helsinki, Atmos. Chem. Phys., 16, 4817–4835, https://doi.org/10.5194/acp-16-4817-2016, 2016.
Kerminen, V. M., Pakkanen, T. A., Makela, T., Hillamo, R. E., Sillanpaa, M., Rönkkö, T., Virtanen, A., Keskinen, J., Pirjola, L., Hussein, T., and Hameri, K.: Development of particle number size distribution near a major road in Helsinki during an episodic inversion situation, Atmos. Environ., 41, 1759–1767, 2007.
Ketzel, M., Wahlin, P., Berkowicz, R., and Palmgren, F.: Particle and trace gas emission factors under urban driving conditions in Copenhagen based on street and roof-level observations, Atmos. Environ., 37, 2735–2749, 2003.
Keuken, M. P., Moerman, M., Zandveld, P., Henzing, J. S., and Hoek, G.: Total and size-resolved particle number and Black Carbon concentrations in urban areas near Schiphol airport (the Netherlands), Atmos. Environ., 104, 132–142, 2015.
Kontkanen, J., Lehtipalo, K., Ahonen, L., Kangasluoma, J., Manninen, H. E., Hakala, J., Rose, C., Sellegri, K., Xiao, S., Wang, L., Qi, X., Nie, W., Ding, A., Yu, H., Lee, S., Kerminen, V.-M., Petäjä, T., and Kulmala, M.: Measurements of sub-3 nm particles using a particle size magnifier in different environments: from clean mountain top to polluted megacities, Atmos. Chem. Phys., 17, 2163–2187, https://doi.org/10.5194/acp-17-2163-2017, 2017.
Kotthaus, S. and Grimmond, C. S. B.: Atmospheric boundary layer characteristics from ceilometer measurements Part 1: A new method to track mixed layer height and classify clouds, Q. J. Roy. Meteorol. Soc., 144, 1525–1538, https://doi.org/10.1002/qj.3299, 2018.
Kotthaus, S. and Grimmond, C. S. B.: Atmospheric boundary layer characteristics from ceilometer measurements, Part 2: Application to London's urban boundary layer, Q. J. Roy. Meteorol. Soc., 144, 1511–1524, https://doi.org/10.1002/qj.3298, 2018.
Kotthaus, S., O'Connor, E., Münkel, C., Charlton-Perez, C., Haeffelin, M., Gabey, A. M., and Grimmond, C. S. B.: Recommendations for processing atmospheric attenuated backscatter profiles from Vaisala CL31 ceilometers, Atmos. Meas. Tech., 9, 3769–3791, https://doi.org/10.5194/amt-9-3769-2016, 2016.
Kotthaus, S., Halios, C. H., Barlow, J. F., and Grimmond, C. S. B.: Volume for pollution dispersion: London's atmospheric boundary layer during ClearfLo observed with two ground-based lidar types, Atmos. Environ., 190, 401–414, 2018.
Kumar, P., Fennell, P., and Britter, R.: Measurements of particles in the 5–1000 nm range close to road level in an urban street canyon, Sci. Total Environ., 390, 437–447, 2008a.
Kumar, P., Fennell, P., Langley, D., and Britter, R.: Pseudo-simultaneous measurements for the vertical variation of coarse, fine and ultrafine particles in an urban street canyon, Atmos. Environ., 42, 4304–4319, 2008b.
Kumar, P., Garmory, A., Ketzel, M., Berkowicz, R., and Britter, R.: Comparative study of measured and modelled number concentrations of nanoparticles in an urban street canyon, Atmos. Environ., 43, 949–958, 2009.
Kumar, P., Robins, A., Vardoulakis, S., and Britter, R.: A review of the characteristics of nanoparticles in the urban atmosphere and the prospects for developing regulatory controls, Atmos. Environ., 44, 5035–5052, 2010.
Kumar, P., Ketzel, M., Vardoulakis, S., Pirjola, L., and Britter, R.: Dynamics and dispersion modelling of nanoparticles from road traffic in the urban atmospheric environment – a review, J. Aerosol Sci., 42, 580–602, 2011.
Kumar, P., Morawska, L., Birmili, W., Paasonen, P. H, M., Kulmala, M., Harriosn, R. M., Norford, L., and Britter, R.: Ultrafine particles in cites, Environ. Intl., 66, 1–10, 2014.
Li, X. L., Wang, J. S., Tu, X. D., Liu, W., and Huang, Z.: Vertical variations of particle number concentration and size distribution in a street canyon in Shanghai, China, Sci. Total Environ., 378, 306–316, 2007.
Lingard, J. J. N., Agus, E. L., Young, D. T., Andrew, G. E., and Tomlin, A. S.: Observations of urban airborne particle number concentrations during rush-hour conditions: analysis of the number based size distributions and modal parameters, J. Environ., Monitor., 8, 1203–1218, 2006.
Longley, I. D., Gallagher, M. W., Dorsey, J. R., Flynn, M., Allan, J. D., Alfarra, M. R., and Inglis, D.: A case study of aerosol (4.6 nm µm) number and mass size distribution measurements in a busy street canyon in Manchester, UK, Atmos. Environ., 37, 1563–1571, 2003.
Masiol, M. and Harrison, R. M.: Aircraft engine exhaust emissions and other airport-related contributions to ambient air pollution: A review, Atmos. Environ., 95, 409–455, 2014.
Masiol, M., Harrison, R. M., Vu, T. V., and Beddows, D. C. S.: Sources of sub-micrometre particles near a major international airport, Atmos. Chem. Phys., 17, 12379–12403, https://doi.org/10.5194/acp-17-12379-2017, 2017.
Morawska, L., Thomas, S., Bofinger, N., Wainwright, D., and Neale, D.: Comprehensive characterization of aerosols in a subtropical urban atmosphere: Particle size distribution and correlation with gaseous pollutants, Atmos. Environ., 32, 2467–2478, 1998.
Nikolova, I., Janssen, S., Vos, P., Vrancken, K., Mishra, V., and Berghmans, P.: Dispersion modelling of traffic induced ultrafine particles in a street canyon in Antwerp, Belgium and comparison with observations, Sci. Total Environ., 412, 336–343, 2011.
Nosko, O., Vanhanen, J., and Olofsson, U.: Emission of 1.3-10 nm airborne particles from brake materials, Aerosol Sci. Technol., 51, 91–96, 2017.
NPL: Design, construction and testing of a humidity management system for ultrafine particle field measurements, National Physical Laboratory, NPL Report AS 48, 2010.
Oke, T., Mills, G., Christen, A., and Voogt, J.: Urban Climates, Cambridge University Press, https://doi.org/10.1017/9781139016476, 2017.
Olivares, G., Johansson, C., Strom, J., and Hansson, H.-C.: The role of ambient temperature for particle number concentrations in a street canyon, Atmos. Environ., 41, 2145–2155, 2007.
Posser, L. N. and Pandis, S. N.: Sources of ultrafine particles in the Eastern United States, Atmos. Environ., 111, 103–112, 2015.
Pushpawela, B., Jayaratne, R., and Morawska, L.: Differentiating between particle formation and growth events in an urban environment, Atmos. Chem. Phys., 18, 11171–11183, https://doi.org/10.5194/acp-18-11171-2018, 2018.
Rönkkö, T., Virtanen, A., Vaaraslahti, K., Keskinen, J., Pirjola, L., and Lappi, M.: Effect of dilution conditions and driving parameters on nucleation mode particles in diesel exhaust: Laboratory and on-road study, Atmos. Environ., 40, 2893–2901, 2006.
Rönkkö, T., Kuuluvainen, H., Karjalainen, P., Keskinen, J., Hillamo, R., Niemi, J. V., Pirjola, L., Timonen, H. J., Saarikoski, S., Saukko, E., Järvinen, A., Silvennoinen, H., Rostedt, A., Olin, M., Yli-Ojanperä, J., Nousiainen, P., Kousa, A., and Dal Maso, M.: Traffic is a major source of atmospheric nanocluster aerosol, Proc. Natl. Acad. Sci., 114, 7549–7554, 2017.
Salimi, F., Rahman, Md. M., Clifford, S., Ristovski, Z., and Morawska, L.: Nocturnal new particle formation events in urban environments, Atmos. Chem. Phys., 17, 521–530, https://doi.org/10.5194/acp-17-521-2017, 2017.
Schneider, J., Hock, N., Weimer, S., Borrmann, S., Kirchner, U., Vogt, R., and Scheer, V.: Nucleation Particles in Diesel Exhaust: Composition Inferred from In Situ Mass Spectrometric Analysis, Environ. Sci. Technol., 39, 6153–6161, 2005.
Shi, J. P. and Harrison, R. M.: Investigation of ultrafine particle formation during diesel exhaust dilution, Environ. Sci. Technol., 33, 3730–3736, 1999.
Shi, J. P., Mark, D., and Harrison, R. M.: Characterization of Particles from a Current Technology Heavy-Duty Diesel Engine, Environ. Sci. Technol., 34, 748–755, 2000.
Shi, J. P., Evans, D. E., Khan, A. A., and Harrison, R. M.: Sources and concentration of nanoparticles (<10 nm diameter) in the urban atmosphere, Atmos. Environ., 35, 1193–1202, 2001.
Timko, M. T., Fortner, E., Franklin J., Yu, Z., Wong, W., Onasch, T. B., Miake-Lye, R. C., and Herndon, S. C.: Atmospheric Measurements of the Physical Evolution of Aircraft Exhaust Plumes, Environ. Sci. Technol., 47, 3513–3520, 2013.
Vakeva, M., Hameri, K., Kulmala, M., Lahdes, R., Ruuskanen, J., and Laitinen, T.: Street level versus rooftop concentrations of submicron aerosol particles and gaseous pollutants in an urban street canyon, Atmos. Environ., 33, 1385–1397, 1999.
Van Dingenen, R., Raes, F., Putaud, J.-P., Baltensperger, U., Charron, A., Facchini, M.-C., Decesari, S., Fuzzi, S., Gehrig, R., Hansson, H.-C., Harrison, R. M., Hüglin, C., Jones, A. M., Laj, P., Lorbeer, G., Maenhaut, W., Palmgren, F., Querol, X., Rodriguez, S., Schneider, J., ten Brink, H., Tunved, P., Tørseth, K., Wehner, B., Weingartner, E., Wiedensohler, A., and Wåhlin, P.: A European aerosol phenomenology – 1: Physical characteristics of particulate matter at kerbside, urban, rural and background sites in Europe, Atmos. Environ., 38, 2561–2577, 2004.
Villa, T. F., Jayaratne, E. R., Gonzalez, L. F., and Morawska, L.: Determination of the vertical profile of particle number concentration adjacent to a motorway using an unmanned aerial vehicle, Environ. Pollut., 230, 143–142, 2017.
Vu, T. V., Delgado-Saborit, J. M., and Harrison, R. M.: Review: Particle number size distributions from seven major sources and implications for source apportionment studies, Atmos. Environ., 122, 114–132, 2015a.
Vu, T. V., Delgado-Saborit, J. M., and Harrison, R. M.: A review of hygroscopic growth factors of submicron aerosols from different sources and its implication for calculation of lung deposition efficiency of ambient aerosol, Air Qual. Atmos. Health, 8, 429–440, https://doi.org/10.1007/s11869-015-0365-0, 2015b.
Wang, Y., Hopke, P. K., Chalupa, D. C., and Utell, M. J.: Long-term study of urban ultrafine particles and other pollutants, Atmos. Environ., 45, 7672–7680, 2011.
Wehner, B., Birmili, W., Gnauk, T., and Wiedensohler, A.: Particle number size distributions in a street canyon and their transformation into the urban-air background: measurements and a simple model study, Atmos. Environ., 36, 2215–2223, 2002.
WHO: Air Quality Guidelines – Global Update 2005, World Health Organization, Copenhagen, 2006.
WHO: Review of Evidence on Health Aspects of Air Pollution – REVIHAAP Project, World Health Organizatrion, Copenhagen, 2013.
Wiedensohler, A., Birmili, W., Nowak, A., Sonntag, A., Weinhold, K., Merkel, M., Wehner, B., Tuch, T., Pfeifer, S., Fiebig, M., Fjäraa, A. M., Asmi, E., Sellegri, K., Depuy, R., Venzac, H., Villani, P., Laj, P., Aalto, P., Ogren, J. A., Swietlicki, E., Williams, P., Roldin, P., Quincey, P., Hüglin, C., Fierz-Schmidhauser, R., Gysel, M., Weingartner, E., Riccobono, F., Santos, S., Grüning, C., Faloon, K., Beddows, D., Harrison, R., Monahan, C., Jennings, S. G., O'Dowd, C. D., Marinoni, A., Horn, H.-G., Keck, L., Jiang, J., Scheckman, J., McMurry, P. H., Deng, Z., Zhao, C. S., Moerman, M., Henzing, B., de Leeuw, G., Löschau, G., and Bastian, S.: Mobility particle size spectrometers: harmonization of technical standards and data structure to facilitate high quality long-term observations of atmospheric particle number size distributions, Atmos. Meas. Tech., 5, 657–685, https://doi.org/10.5194/amt-5-657-2012, 2012.
Wojdyr M.: Fityk: A General purpose peak fitting program, J. Appl. Cryst., 43, 1126–1128, 2010.
Zhu, Y., Hinds, W. C., Kim, S., and Sioutas, C.: Concentration and size distribution of ultrafine particles near a major highway, JAWMA, 52, 1032–1042, 2002a.
Zhu, Y., Hinds, W. C., Kim, S., Shen, S., and Sioutas, C.: Study of ultrafine particles near a major highway with heavy-duty diesel traffic, Atmos. Environ., 36, 4323–4335, 2002b.