the Creative Commons Attribution 4.0 License.
the Creative Commons Attribution 4.0 License.
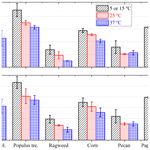
Water adsorption and hygroscopic growth of six anemophilous pollen species: the effect of temperature
Wenjun Gu
Yong Jie Li
Cheng Zhong
Sheng Li
Xin Yin
Ru-Jin Huang
Xinming Wang
Hygroscopicity largely affects environmental and climatic impacts of pollen grains, one important type of primary biological aerosol particles in the troposphere. However, our knowledge of pollen hygroscopicity is rather limited, and the effect of temperature in particular has rarely been explored before. In this work three different techniques, including a vapor sorption analyzer, diffusion reflectance infrared Fourier transform spectroscopy (DRIFTS) and transmission Fourier transform infrared spectroscopy (transmission FTIR) were employed to characterize six anemophilous pollen species and to investigate their hygroscopic properties as a function of relative humidity (RH, up to 95 %) and temperature (5 or 15, 25 and 37 ∘C). Substantial mass increase due to water uptake was observed for all the six pollen species, and at 25 ∘C the relative mass increase at 90 % RH, when compared to that at <1 % RH, ranged from ∼30 % to ∼50 %, varying with pollen species. It was found that the modified κ-Köhler equation can well approximate mass hygroscopic growth of all the six pollen species, and the single hygroscopicity parameter (κ) was determined to be in the range of 0.034±0.001 to 0.061±0.007 at 25 ∘C. In situ DRIFTS measurements suggested that water adsorption by pollen species was mainly contributed to by OH groups of organic compounds they contained, and good correlations were indeed found between hygroscopicity of pollen species and the number of OH groups, as determined using transmission FTIR. An increase in temperature would in general lead to a decrease in hygroscopicity, except for pecan pollen. For example, κ values decreased from 0.073±0.006 at 5 ∘C to 0.061±0.007 at 25 ∘C and to 0.057±0.004 at 37 ∘C for Populus tremuloides pollen, and decreased from 0.060±0.001 at 15 ∘C to 0.054±0.001 at 25 ∘C and 0.050±0.002 at 37 ∘C for paper mulberry pollen.
- Article
(3065 KB) - Full-text XML
-
Supplement
(542 KB) - BibTeX
- EndNote
Primary biological aerosol particles (PBAPs), an important type of aerosol particles in the troposphere, are directly emitted from the biosphere and include pollen, fungal spores, bacteria, viruses, algae and so on (Després et al., 2012; Fröhlich-Nowoisky et al., 2016). Emission and abundance of PBAPs are quite uncertain, and annual emission fluxes are estimated to be in the range of <10 to ∼1000 Tg for total PBAPs and 47–84 Tg for pollen (Després et al., 2012). Pollen, and PBAPs in general, are of great concern due to their various impacts on the Earth's system (Sun and Ariya, 2006; Ariya et al., 2009; Georgakopoulos et al., 2009; Morris et al., 2011, 2014; Fröhlich-Nowoisky et al., 2016). For example, they can be allergenic, infectious or even toxic, affecting the health of humans and other species in the ecological systems over different scales (Douwes et al., 2003; Reinmuth-Selzle et al., 2017; Shiraiwa et al., 2017). The geographical dispersion of anemophilous plants largely relies on pollen dispersal, which in turn depends on the emission, transport and deposition of pollen grains; therefore, pollen plays a key role in the evolution of many ecosystems (Womack et al., 2010; Fröhlich-Nowoisky et al., 2016). In addition, PBAPs can serve as giant cloud condensation nuclei (CCN) and ice-nucleating particles (INPs), significantly impacting the formation and properties of clouds and thus radiative balance and precipitation (Möhler et al., 2007; Ariya et al., 2009; Pratt et al., 2009; Pope, 2010; Pummer et al., 2012; Gute and Abbatt, 2018). It has also been proposed that PBAPs may have significant impacts on chemical composition of aerosol particles via heterogeneous and multiphase chemistry (Deguillaume et al., 2008; Estillore et al., 2016; Reinmuth-Selzle et al., 2017; Shiraiwa et al., 2017).
Hygroscopicity is one of the most important physicochemical properties of pollen (as well as aerosol particles in general). Hygroscopicity largely impacts the transport and deposition of pollen grains (Sofiev et al., 2006), therefore affecting their lifetimes, abundance and spatiotemporal distribution. In addition, hygroscopicity is closely linked to the ability of aerosol particles to serve as CCN and INPs (Petters and Kreidenweis, 2007; Kreidenweis and Asa-Awuku, 2014; Laaksonen et al., 2016; Tang et al., 2016). Several previous studies have measured the hygroscopicity and CCN activities of pollen (Diehl et al., 2001; Pope, 2010; Griffiths et al., 2012; Lin et al., 2015; Steiner et al., 2015; Prisle et al., 2018) and other PBAPs such as bacteria (Pasanen et al., 1991; Reponen et al., 1996; Franc and DeMott, 1998; Ko et al., 2000; Lee et al., 2002; Bauer et al., 2003). For example, water uptake of 11 pollen species was studied using an analytical balance (Diehl et al., 2001), and the mass of pollen was found to be increased by 3 %–16 % at 73 % RH and by ∼100 %–300 % at 95 % RH, compared to that at <1 % RH. An electrodynamic balance was employed to investigate hygroscopic growth of eight types of pollen (Pope, 2010; Griffiths et al., 2012), and it was found that their hygroscopic growth can be approximated by the modified κ-Köhler equation, with single hygroscopicity parameters being around 0.1 (depending on the assumed pollen density, as discussed in Sect. 3.2).
Previous measurements were mostly carried out at or close to room temperature, and the effects of temperature on hygroscopic properties of pollen and other types of PBAPs are yet to be elucidated. To our knowledge, only one previous study (Bunderson and Levetin, 2015) explored the effect of temperature (4, 15 and 20 ∘C) on water uptake by Juniperus ashei, Juniperus monosperma and Juniperus pinchotii pollen. It is important to account for the temperature effects, because ambient temperatures range from below −70 to >30 ∘C in the troposphere. In particular, the altitude of 0.5–2.0 km to which pollen can be easily transported (Noh et al., 2013) may have temperatures close to or lower than the chilling temperatures for vegetative species (up to 16.5 ∘C) (Melke, 2015). Moreover, the temperature in the respiratory tract can reach up to 37 ∘C (the physiological temperature). In the work presented here, a vapor sorption analyzer (VSA) was employed to investigate hygroscopic growth of pollen grains at different temperature (5 or 15, 25 and 37 ∘C), a range covering the chilling temperature to the physiological temperature. Water uptake by pollen was also examined using diffusion reflectance infrared Fourier transform spectroscopy at room temperature to complement the VSA results. Furthermore, transmission Fourier transformation infrared spectroscopy was used to characterize functional groups of dry pollen grains, in an attempt to seek potential links between chemical composition of pollen grains and their hygroscopic properties.
Six pollen species, all from anemophilous plants, were investigated in this work, including Populus tremuloides and Populus deltoides (provided by Sigma Aldrich) as well as ragweed, corn, pecan and paper mulberry (provided by Polysciences, Inc.). The six pollen species were chosen in our work primarily because they were commercially available. Furthermore, these plants are also widely distributed in the globe. For example, corn is the most produced grain in the world (International Grains Council, 2019), and up to 50 % of pollen-related allergic rhinitis cases in North America are caused by ragweed pollen (Taramarcaz et al., 2005).
2.1 Fourier transformation infrared spectroscopy
The adsorption of water by pollen species was studied using in situ diffusion reflectance infrared Fourier transform spectroscopy (DRIFTS) at room temperature (∼25 ∘C). This technique was described in detail in our previous work (Ma et al., 2010), and similar setups have also been used by other groups to investigate the adsorption of water by mineral dust (Joshi et al., 2017; Ibrahim et al., 2018). Infrared spectra were recorded using a Nicolet 6700 Fourier transformation infrared spectrometer (FTIR, Thermo Nicolet Instrument Corporation), equipped with an in situ diffuse reflection chamber and a high-sensitivity mercury cadmium telluride (MCT) detector cooled by liquid nitrogen. A pollen sample (about 10 mg for each sample) under investigation was placed into a ceramic crucible, which was located in the in situ chamber. A dry airflow and a humidified airflow were first mixed and then delivered into the chamber, and the total flow rate was set to 200 mL min−1 (standard condition). Relative humidity (RH) in the chamber could be adjusted by varying the flow rate ratio of the dry flow to the humidified flow and was monitored online using a moisture meter (CENTER 314) with an absolute uncertainty of ±2 %. Prior to each experiment, the sample was flushed with dry air for 3 h at 25 ∘C, and the reference spectrum was recorded after the pretreatment. Infrared spectra were collected and analyzed using OMNIC 6.0 software (Nicolet Corp.). All the spectra reported here were recorded with a wave number resolution of 4 cm−1, and 100 scans were averaged to produce a spectrum. Water adsorption was equilibrated for at least 30 min at each RH to ensure that the equilibrium between water vapor and adsorbed water was reached.
Pollen samples used in this work were also characterized using transmission FTIR equipped with a deuterated triglycine sulfate detector (DTGS) detector. Pollen grains and KBr were mixed with a mass ratio of approximately 1:100 and ground in an agate mortar, and the mixture was then pressed into a clear disc. Transmission FTIR was employed to examine these discs, and a pure KBr disc was used as the reference. All the spectra, each of which was the average of 100 scans, were also recorded with a wave number resolution of 4 cm−1.
2.2 Vapor sorption analyzer
Hygroscopic growth of pollen grains was further investigated using a vapor sorption analyzer (Q5000 SA, TA Instruments, New Castle, DE, USA) described in our previous work (Gu et al., 2017; Guo et al., 2019; Jia et al., 2018). In brief, this instrument measured the sample mass as a function of RH under isothermal conditions. The instrument can be operated in the temperature range of 5–85 ∘C with a temperature accuracy of ±0.1 ∘C and in the RH range of 0 %–98 % with an absolute accuracy of ±1 %. RH in the humidity chamber was regulated by using two mass flow controllers to control the dry and humidified nitrogen flows very precisely. The accuracy in RH control was routinely checked by measuring the DRH values for a series of standard compounds, e.g., NaCl, (NH4)2SO4 and KCl, and the difference between the measured and theoretical DRH was always <1 %. The mass measurement had a range of 0–100 mg and a sensitivity of ±0.01 µg. The initial mass of each sample used in this work was in the range of 0.5–1 mg. For each of the first three types of pollen species (Populus tremuloides, Populus deltoides and ragweed pollen), three samples in total were investigated, and each sample was studied under isothermal conditions at 5, 25 and 37 ∘C. For each of the other three types of pollen species (corn, pecan and paper mulberry pollen), experiments were carried out at 15 ∘C instead of 5 ∘C, because the instrument could only be cooled down to 15 ∘C due to a technical problem after we finished experiments for the first three pollen species.
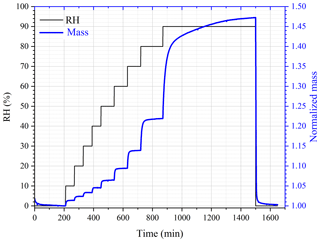
Figure 1Change in RH (black curve, left y axis) and normalized sample mass (blue curve, right y axis) with time for a typical experiment in which hygroscopic growth of pollen grains was measured. In this figure a data set for paper mulberry pollen at 25 ∘C is plotted as an example.
For the first sample, at each temperature the sample was first dried at 0 % RH (the actual RH was measured to be <1 %); after that, RH was increased stepwise to 95 % with an increment of 5 % per step and then switched back to <1 % to dry the sample again. At each RH, the sample was equilibrated with the environment (i.e., until the sample mass became stable) before RH was changed to the next value, and the sample mass was considered to be stabilized when the mass change was <0.05 % within 30 min. Such a measurement at one temperature could take several days. In order to reduce experimental time, the second and third samples were investigated in a similar way to the first sample, except that RH was increased stepwise to 90 % with an increment of 10 % per step. A typical experimental data set is displayed in Fig. 1, as an example to illustrate the change in RH and normalized sample mass with experimental time.
3.1 FTIR characterization of pollen samples
3.1.1 Infrared spectra of dry pollen samples
Figure 2 shows the transmission FTIR spectra of the six pollen species investigated in our work, and peak assignments can be found in Table 1. A broad band in the range of 3600–3000 cm−1, attributed to O-H stretching vibration (Stuart, 2004; Pummer et al., 2013), and two sharp peaks at 2920 and 2850 cm−1, attributed to C–H stretching (Eliason et al., 2003; Stuart, 2004; Pummer et al., 2013), were observed for all the pollen species. The two peaks at 1747 and 1658 cm−1 were assigned to alkyl ester carbonyls (Pappas et al., 2003; Najera et al., 2009; Pummer et al., 2013), and the two peaks at 1549 and 1458 cm−1 (1411 cm−1 for paper mulberry pollen) were assigned to C=C stretching and H–C–H deformation (Stuart, 2004; Pummer et al., 2013). In addition, the three peaks at 1053, 997 and 845 cm−1 were assigned to C–O stretching, C–C stretching and C–H out-of-plane bending, respectively (Stuart, 2004; Najera et al., 2009; Pummer et al., 2013).
OH groups and C–H groups in organic compounds are generally considered to be hydrophilic and hydrophobic, and one may expect that the number of OH groups (relative to that of C–H groups) that organic samples contain may affect their hygroscopicity. For example, it was found in many previous studies (Eliason et al., 2003; Asad et al., 2004; Hung et al., 2005; Najera et al., 2009) that heterogeneous reactions of organic materials with O3 and OH radicals would increase the IR absorption intensity for the O-H stretching mode and decrease the IR absorption intensity for the C–H stretching mode, meanwhile leading to the enhancement in their hygroscopicity. Therefore, in this work we use the intensity ratio of the O-H stretching vibration band (3000–3600 cm−1) to the C–H stretching mode (2920 cm−1) to qualitatively represent the number of OH groups pollen samples contain. As shown in Fig. 2, the six pollen species examined in our work can be roughly classified into two catalogues: (1) for Populus deltoides, Populus tremuloides and paper mulberry pollen, the O-H stretching vibration band is more intensive than the C–H stretching mode, indicating that they contain relatively high levels of OH groups; (2) for ragweed, pecan and corn pollen, the O-H stretching vibration band is less intensive than the C–H stretching mode, indicating that they contain relatively low levels of OH groups. The relation between the number of OH groups that pollen species contain and their hygroscopicity will be further discussed in Sect. 3.3.
3.1.2 Infrared spectra of pollen samples at different RHs
In situ DRIFTS was employed to explore the adsorption of water by pollen grains. Typical spectra of populous deltoides pollen as a function of RH up to 87 %, relative to that at <1 % RH, are displayed in Fig. 3. DRIFTS spectra of other pollen samples at different RHs can be found in Figs. S1–S5 in the Supplement and are very similar to those for populous deltoides pollen. As evident from Fig. 3, several IR peaks (e.g., 3593, 3205, 2135 and 1616 cm−1) appeared in the spectra at elevated RH, when compared with that at <1 % RH, and their intensities increased with increasing RH. The peaks at 3205, 2135 and 1616 cm−1 can be assigned to the stretching, association and bending modes of adsorbed water (Goodman et al., 2001; Schuttlefield et al., 2007a; Ma et al., 2010; Hatch et al., 2011; Song and Boily, 2013; Yeşilbaş and Boily, 2016; Joshi et al., 2017; Ibrahim et al., 2018).
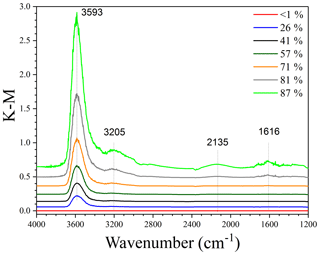
Figure 3In situ DRIFTS spectra of populous deltoides pollen as a function of RH (<1 %, 26 %, 41 %, 57 %, 71 %, 81 % and 87 %) at 25 ∘C.
Table 2Integrated areas of IR peaks (at ∼3600 cm−1) of adsorbed water as a function of RH for the six pollen species investigated in this work. Wave number ranges used for integration are 3750–3300 cm−1 for Populus deltoides pollen, 3750–3350 cm−1 for Populus tremuloides pollen, 3750–3400 cm−1 for ragweed pollen, 3750–3500 cm−1 for corn pollen, 3750–3450 cm−1 for pecan pollen and 3750–3300 cm−1 for paper mulberry pollen.
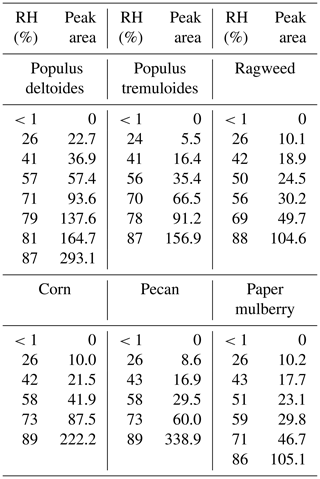
The peak at ∼3600 cm−1 was the most intensive one observed in the spectra, as shown in Fig. 3. For comparison, the IR peaks assigned to the stretching mode of adsorbed water on mineral dust and NaCl appeared at lower wave numbers, typically at around or lower than 3400 cm−1 (Schuttlefield et al., 2007a; Ma et al., 2010; Tang et al., 2016; Ibrahim et al., 2018). As a result, the peak at ∼3600 cm−1 may be assigned to the asymmetric stretching mode of water which interacted with OH groups in pollen samples (Iwamoto et al., 2003). These results imply that water adsorption by pollen samples could be mainly contributed to by OH groups of organic compounds they contained. Both C–OH and C(O)-OH groups can contribute to water adsorption by pollen samples, though their relative contribution cannot be resolved in our work. In addition, other factors, such as porosity and internal structure, may also be important for hygroscopic properties of pollen grains. The intensities of IR peaks at ∼3600 cm−1 were used to represent the amount of water adsorbed by pollen samples. Table 2 summarizes integrated areas of IR peaks at 3600 cm−1 as a function of RH for the six pollen species examined in our work, suggesting that the amount of adsorbed water by pollen samples increased with RH.
3.2 Mass hygroscopic growth
3.2.1 Hygroscopicity parameterizations
The single hygroscopicity parameter, κ, is widely used to describe the hygroscopicity of aerosol particles under both subsaturation and supersaturation (Petters and Kreidenweis, 2007). When the Kelvin effect is negligible (this is valid for pollen grains which are typically >1 µm), the dependence of diameter-based growth factor (GF) on RH can be linked to κ via Eq. (1) (Petters and Kreidenweis, 2007; Tang et al., 2016):
If we further assume that the particle is spherical, Eq. (1) can be transformed to Eq. (2):
where V, V0 and Vw are the volumes of the particle at the given RH, the dry particle and water associated with the particle at the given RH. In order for Eq. (2) to be valid, it is also assumed that at a given RH, V is equal to the sum of V0 and Vw. Equation (2) can be further transformed to Eqs. (3)–(4):
where ρw and ρp are the density of water and the dry particle, and m0 and mw are the mass of the dry particle and water associated with the particle at the given RH. Since the particle mass, m, is equal to the sum of m0 and mw, Eq. (5) can be derived from Eq. (4):
Using an electrodynamic balance, Pope (2010) and Griffiths et al. (2012) measured hygroscopic growth of eight types of pollen grains and found that their mass change with RH can be approximated by Eq. (5). It should be noted that the original equation derived by Pope (2010) and Griffiths et al. (2012) has a different format from but is essentially equivalent to Eq. (5). Equation (5) relates mass growth experimentally measured in our work to the single hygroscopicity parameter (κ), which has been widely used in atmospheric science to describe hygroscopic properties of aerosol particles under subsaturation as well as their CCN activities under supersaturation; nevertheless, a few assumptions are needed to derive Eq. (5), as discussed.
The Freundlich adsorption isotherm is another widely used equation that describes the change in sample mass with RH due to water uptake (Atkins, 1998; Skopp, 2009; Hatch et al., 2011; Tang et al., 2016):
where Af and Bf are empirical Freundlich constants related to the adsorption capacity and strength. One advantage of the Freundlich adsorption isotherm is that it provides a direct relationship between RH and mass growth, which was experimentally measured in our work, without any additional assumptions. In addition, the BET (Brunauer–Emmett–Teller) adsorption isotherm is also widely used to describe water adsorption by insoluble solid particles (Brunauer et al., 1938; Goodman et al., 2001; Henson, 2007; Ma et al., 2010; Tang et al., 2016; Joshi et al., 2017). While the BET adsorption isotherm typically works well for water adsorption of a few monolayers, the mass of adsorbed water, as shown in Sect. 3.2.2, can reach up to 50 % of the dry pollen mass at high RH; therefore, in this work we did not attempt to use the BET adsorption isotherm to describe water adsorption by pollen grains. Another reason that we did not attempt to use the BET adsorption isotherm is that the BET adsorption isotherm is mathematically more complex and requires the BET surface area to be known.
3.2.2 Mass hygroscopic growth at room temperature
Figure 4 displays the sample mass (normalized to that at 0 % RH) as a function of RH for pecan pollen at 25 ∘C. A significant increase in sample mass was observed at elevated RH due to uptake of water. Compared to that at <1 % RH, the sample mass increased by (2.3±0.3) % at 30 % RH, (6.4±0.2) % at 60 % RH, (30.3±0.4) % at 90 % RH, and up to ∼72 % at 95 % RH. As shown by the data compiled in Tables S1–S3 in the Supplement, substantial increases in sample mass were also observed for the other five types of pollen species at 25 ∘C (as well as 5 and 37 ∘C).
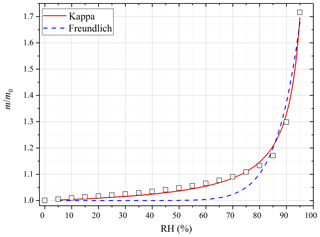
Figure 4Measured change in sample mass (normalized to that at dry conditions, i.e., m∕m0) of pecan pollen as a function of RH (0 %–95 %) at 25 ∘C. The experimental data are fitted with the modified κ-Köhler equation (solid red curve) and the Freundlich adsorption isotherm (dashed blue curve).
Hygroscopic properties exhibited considerable variations among different pollen species. Figure 5a compares the measured ratios of sample mass at 90 % RH to that at <1 % RH, m(90 %)∕m0, for the six pollen species investigated in this work. We specifically discuss mass changes of pollen grains at 90 % RH (relative to that at <1 % RH) because aerosol hygroscopic growth at 90 % RH was widely reported by laboratory and field studies (Kreidenweis and Asa-Awuku, 2014). As shown in Fig. 5a, m(90 %)∕m0 determined at 25 ∘C ranged from 1.293±0.028 (ragweed pollen) to 1.476±0.094 (Populus deltoides pollen); i.e., the amount of water adsorbed or absorbed by the six different pollen species at 90% RH varied between ∼30 % to ∼50 % of the dry mass.
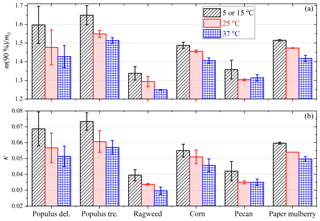
Figure 5Measured ratios of sample mass at 90 % RH to that at <1 % RH (a) and derived κ values (b) for six pollen species at different temperatures. The lowest temperatures were 5 ∘C for Populus deltoides (P. deltoides), Populus tremuloides (P. tremuloides) and ragweed pollen, and 15 ∘C for corn, pecan and paper mulberry pollen.
Table 3Single hygroscopicity parameters (κ) derived in this work for the six pollen species at different temperatures. All the errors given in this work are standard deviations.
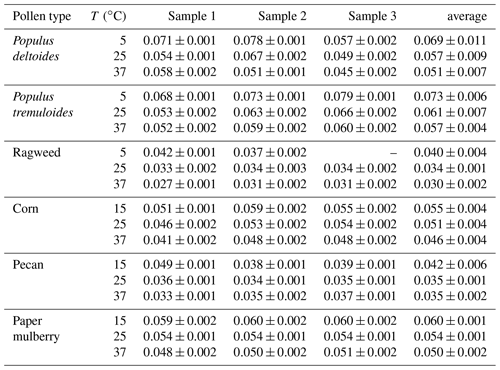
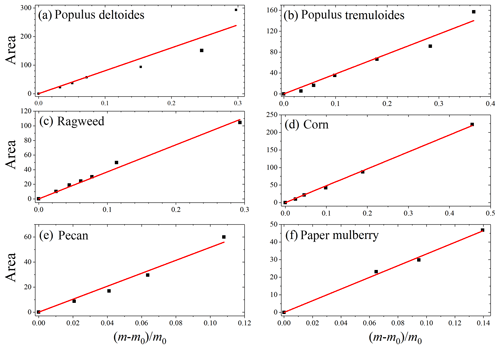
Figure 6Integrated areas of IR peaks at ∼3600 cm−1 vs. relative mass increase due to water uptake, (, for six pollen species: (a) Populus deltoides, (b) Populus tremuloides, (c) ragweed, (d) corn, (e) pecan and (f) paper mulberry.
As shown in Fig. 4, the increase in pecan pollen mass with RH at 25 ∘C could be satisfactorily described by the modified κ-Köhler equation for the entire RH range (up to 95 %). On the contrary, the Freundlich adsorption isotherm significantly underestimated the sample mass at low RH, although it represented the experimental data at high RH reasonably well. In addition, we found that the modified κ-Köhler equation could also approximate the dependence of sample mass on RH for all the six types of pollen species investigated in this work at different temperatures. If we use Eq. (5) to fit m∕m0 against RH, can be derived. The bulk densities of dry pollen grains were found to vary with species but typically fall into the range of 0.5–2 g cm−3 (Harrington and Metzger, 1963; Hirose and Osada, 2016), and for simplicity ρp was assumed to be 1 g cm−3 in this work (i.e., ρw∕ρp is equal to 1). With the assumptions on dry particle density and also particle sphericity, κ could then be derived from the measured RH-dependent sample mass at a given temperature.
Table 3 summarizes the average κ values at different temperatures for the six pollen species investigated in this work. At 25 ∘C, the κ values were found to increase from 0.034±0.001 for ragweed pollen to 0.061±0.007 for Populus tremuloides pollen, varied by almost a factor of 2. The κ values measured by Pope and co-workers (Pope, 2010; Griffiths et al., 2012) were approximately in the range of 0.05–0.11 (assuming that ρw∕ρp is equal to 1), in reasonably good agreement with these reported in our work. It should be noted that, in order to convert the measured mass growth to diameter growth and κ values, one key assumption is particle sphericity; nevertheless, pollen grains are known to be nonspherical and porous, and therefore our derived κ values might be smaller than the actual values. For example, although the mass increase was substantial (around 30 %–50 % at 90 % RH) for the six pollen species examined, their κ values at 25 ∘C were derived to be in the range of 0.034–0.061, significantly smaller than those (0.1–0.2) for typical secondary organic aerosols produced in smog chamber studies (Petters and Kreidenweis, 2007; Kreidenweis and Asa-Awuku, 2014).
3.3 Discussion
3.3.1 Reconciliation between IR and VSA results
Our in situ DRIFTS measurements, discussed in Sect. 3.1.2, suggested that water uptake by pollen samples was mainly contributed to by OH groups of organic compounds they contained; therefore, one may expect that pollen species which contain higher levels of OH groups would exhibit higher hygroscopicity. Transmission FTIR characterization of pollen species (Sect. 3.1.1) showed that Populus deltoides, Populus tremuloides and paper mulberry pollen contained relatively high levels of OH groups, and indeed their hygroscopicity (κ: 0.053–0.054 at 25 ∘C) was higher than the other three pollen species, as shown in Fig. 5 and Table 3. For comparison, ragweed and pecan pollen contained relatively low levels of OH groups and correspondingly exhibited lower hygroscopicity (κ: 0.033–0.036 at 25 ∘C). Corn pollen appeared to be an exception: it contained relatively low levels of OH groups but displayed medium hygroscopicity (κ: ∼0.046 at 25 ∘C). As a result, our results may imply that, in addition to chemical composition, other physicochemical properties, such as porosity and internal structure of pollen grains, could also play an important role in determining the hygroscopicity of pollen species. One clue came from environmental scanning electron microscopy observations (Pope, 2010), revealing that pollen grains started to swell internally before significant water uptake on the surface took place.
In our work two complementary techniques were employed to explore hygroscopic properties of pollen species. VSA measured the amount of water absorbed/adsorbed by pollen grains as a function of RH in a quantitative manner, whereas the intensities of IR peaks of adsorbed water at different RHs, as characterized by DRIFTS, can be used semiquantitatively to represent the amount of water associated with particles (Goodman et al., 2001; Schuttlefield et al., 2007b; Ma et al., 2010; Yeşilbaş and Boily, 2016; Joshi et al., 2017; Ibrahim et al., 2018). We compare our VSA results (i.e., the relative mass change due to water uptake) to the DRIFTS results (i.e., integrated area of IR peaks at ∼3600 cm−1). As shown in Fig. 6, good correlations between VSA and DRIFTS results are found for all the six pollen species, suggesting that DRIFTS can be used to represent the amount of adsorbed water, at least in a semiquantitative manner.
3.3.2 Effect of temperature
Figure 5a shows the comparison of the measured ratios of sample mass at 90 % RH to that at <1 % RH, m(90 %)∕m0, at different temperatures for the six pollen species. It can be concluded from Fig. 5a that, except for pecan pollen, for which a small increase in m(90 %)∕m0 occurred when temperature increased from 25 to 37 ∘C, an increase in temperature would lead to a small but nevertheless significant decrease in m(90 %)∕m0. For example, m(90 %)∕m0 decreased from 1.597±0.100 at 5 ∘C to 1.476±0.094 at 25 ∘C and to 1.427±0.060 at 37 ∘C for Populus deltoides pollen, and from 1.338±0.036 at 5 ∘C to 1.293±0.028 at 25 ∘C and to 1.249±0.002 at 37 ∘C for ragweed pollen.
We further derived κ values at different temperatures for the six pollen species, and the results are plotted in Fig. 5b and summarized in Table 3. An increase in temperature would lead to a decrease in κ values, except for pecan pollen. For example, κ decreased from 0.073±0.006 at 5 ∘C to 0.057±0.004 at 37 ∘C for Populus tremuloides pollen, and decreased from 0.060±0.001 at 15 ∘C to 0.050±0.002 at 37 ∘C for paper mulberry pollen.
Pollen grains are one of the most abundant types of primary biological aerosol particles in the troposphere and play important roles in many aspects of the Earth's system. Hygroscopicity is among the most important physicochemical properties of pollen grains and largely affects their environmental, health and climatic impacts. However, our knowledge of their hygroscopicity is still quite limited, and the temperature effect in particular has been rarely explored.
In this work we investigated hygroscopic properties of six types of pollen species as a function of RH (up to 95 %) at 5 (or 15), 25 and 37 ∘C. A substantial increase in pollen mass was observed at elevated RH due to water uptake for all the six pollen species. Therefore, a change in the mass of pollen grains and their aerodynamic properties at different RHs should be taken into account to better understand their transport and deposition in the troposphere. It was found that the mass hygroscopic growth of pollen grains can be well approximated by the modified κ-Köhler equation. The derived κ values at 25 ∘C ranged from 0.034±0.001 to 0.061±0.007, varying with pollen species. DRIFTS measurements indicated that water adsorption by pollen species were mainly contributed to by OH groups of organic compounds contained by pollen grains, and indeed pollen species that contained lower levels of OH groups (relative to C–H groups, as determined using transmission FTIR) showed lower hygroscopicity. One exception was corn pollen, which contained low levels of OH group but exhibited medium hygroscopicity, suggesting that, in addition to chemical composition, other physicochemical properties, such as porosity and internal structure, might also play an important role in determining the hygroscopicity of pollen grains. Due to their moderate hygroscopicity as well as large sizes, pollen grains can thus act as efficient giant CCN, which may have significant impacts on cloud and precipitation (Johnson, 1982; Feingold et al., 1999; Yin et al., 2000; Posselt and Lohmann, 2008). It is worth noting that only six different pollen species were examined in our work, and hygroscopic properties of other pollen species commonly found in the troposphere should be further investigated.
The effect of temperature on the hygroscopicity of pollen grains was systematically investigated in this work. An increase in temperature (from 5 or 15 to 25 and 37 ∘C), in a range from chilling temperatures to physiological temperatures, led to a small but detectable decrease in pollen hygroscopicity. For example, κ values were found to decrease from 0.073±0.006 at 5 ∘C to 0.061±0.007 at 25 ∘C and to 0.057±0.004 at 37 ∘C for Populus tremuloides pollen, and decrease from 0.060±0.001 at 15 ∘C to 0.054±0.001 at 25 ∘C to 0.050±0.002 at 37 ∘C for paper mulberry pollen. Our measurements at 37 ∘C (physiological temperature) provide very valuable parameters which can be used in numerical models to better understand the transport and deposition of pollen particles in the respiratory system and thus their impacts on human health (Yeh et al., 1996; Broday and Georgopoulos, 2001; Park and Wexler, 2008; Lambert et al., 2011; Longest and Holbrook, 2012; Tong et al., 2014). Nevertheless, it should be noted that, due to the short residence time in the respiratory system, pollen grains and other inhaled particles in general may not reach equilibrium with water vapor in the respiratory tract.
Due to technical challenges, the lowest temperature we could reach in this work was 5 ∘C, in the range of normal chilling temperatures for vegetative species, and also in the expected temperature range at the altitudes of 0.5–2.0 km to which pollen grains can be easily transported. Temperatures in the upper troposphere can be as low as below −70 ∘C, and it is yet to be explored whether a further decrease in temperature to far below 0 ∘C will lead to a large increase in pollen hygroscopicity. As a result, experimental measurements of pollen hygroscopicity at lower temperatures are warranted and would significantly help to better understand the transport of pollen grains in the troposphere. Since water vapor has to be adsorbed or condensed on ice-nucleating particles before heterogeneous ice nucleation can take place (Laaksonen et al., 2016), knowledge of hygroscopicity and water uptake at temperatures below 0 ∘C would provide fundamental insights into atmospheric ice nucleation, in which pollen grains may play an important role (Pratt et al., 2009; Prenni et al., 2009; Hoose et al., 2010; Pöschl et al., 2010; Murray et al., 2012; Creamean et al., 2013; Tang et al., 2018).
All the data are available from Mingjin Tang (mingjintang@gig.ac.cn) on request.
The supplement related to this article is available online at: https://doi.org/10.5194/acp-19-2247-2019-supplement.
MT, QM and YJL designed the research; WG, CZ, SL and XY took the measurements; MT, QM, YJL and RJH analyzed the results; MT, QM, YJL and RJH wrote the manuscript with contributions from all the co-authors.
The authors declare that they have no conflict of interest.
This article is part of the special issue “Multiphase chemistry of secondary aerosol formation under severe haze”. It is not associated with a conference.
This work was funded by National Natural Science Foundation of China
(91644106, 91744204 and 91644219), Chinese Academy of Sciences
(132744KYSB20160036), Science and Technology Development Fund of Macau
(016/2017/A1), and State Key Laboratory of Organic Geochemistry
(SKLOG2016-A05). Mingjin Tang would like to thank the CAS Pioneer Hundred
Talents program for providing a starting grant. This is contribution
no. IS-2661 from GIGCAS.
Edited by: Jingkun
Jiang
Reviewed by: two anonymous referees
Ariya, P. A., Sun, J., Eltouny, N. A., Hudson, E. D., Hayes, C. T., and Kos, G.: Physical and chemical characterization of bioaerosols – Implications for nucleation processes, Int. Rev. Phys. Chem., 28, 1–32, 2009.
Asad, A., Mmereki, B. T., and Donaldson, D. J.: Enhanced uptake of water by oxidatively processed oleic acid, Atmos. Chem. Phys., 4, 2083–2089, https://doi.org/10.5194/acp-4-2083-2004, 2004.
Atkins, P. W.: Physical Chemistry, 6th Edn., Oxford University Press, Oxford, UK, 1998.
Bauer, H., Giebl, H., Hitzenberger, R., Kasper-Giebl, A., Reischl, G., Zibuschka, F., and Puxbaum, H.: Airborne bacteria as cloud condensation nuclei, J. Geophys. Res.-Atmos, 108, 4658, https://doi.org/10.1029/2003JD003545, 2003.
Broday, D. M. and Georgopoulos, P. G.: Growth and Deposition of Hygroscopic Particulate Matter in the Human Lungs, Aerosol Sci. Tech., 34, 144–159, 2001.
Brunauer, S., Emmett, P. H., and Teller, E.: Adsorption of Gases in Multimolecular Layers, J. Am. Chem. Soc., 60, 309–319, 1938.
Bunderson, L. D. and Levetin, E.: Hygroscopic weight gain of pollen grains from Juniperus species, Int. J. Biometeorol., 59, 533–540, 2015.
Creamean, J. M., Suski, K. J., Rosenfeld, D., Cazorla, A., DeMott, P. J., Sullivan, R. C., White, A. B., Ralph, F. M., Minnis, P., Comstock, J. M., Tomlinson, J. M., and Prather, K. A.: Dust and Biological Aerosols from the Sahara and Asia Influence Precipitation in the Western U.S., Science, 339, 1572–1578, 2013.
Deguillaume, L., Leriche, M., Amato, P., Ariya, P. A., Delort, A.-M., Pöschl, U., Chaumerliac, N., Bauer, H., Flossmann, A. I., and Morris, C. E.: Microbiology and atmospheric processes: chemical interactions of primary biological aerosols, Biogeosciences, 5, 1073–1084, https://doi.org/10.5194/bg-5-1073-2008, 2008.
Després, V. R., Huffman, J. A., Burrows, S. M., Hoose, C., Safatov, A. S., Buryak, G., Fröhlich-Nowoisky, J., Elbert, W., Andreae, M. O., Pöschl, U., and Jaenicke, R.: Primary biological aerosol particles in the atmosphere: a review, Tellus B, 64, 15598, https://doi.org/10.3402/tellusb.v64i0.15598, 2012.
Diehl, K., Quick, C., Matthias-Maser, S., Mitra, S. K., and Jaenicke, R.: The ice nucleating ability of pollen – Part I: Laboratory studies in deposition and condensation freezing modes, Atmos. Res., 58, 75–87, 2001.
Douwes, J., Thorne, P., Pearce, N., and Heederik, D.: Bioaerosol health effects and exposure assessment: Progress and prospects, Ann. Occup. Hyg., 47, 187–200, 2003.
Eliason, T. L., Aloisio, S., Donaldson, D. J., Cziczo, D. J., and Vaida, V.: Processing of unsaturated organic acid films and aerosols by ozone, Atmos. Environ., 37, 2207–2219, 2003.
Estillore, A. D., Trueblood, J. V., and Grassian, V. H.: Atmospheric chemistry of bioaerosols: heterogeneous and multiphase reactions with atmospheric oxidants and other trace gases, Chem. Sci., 7, 6604–6616, 2016.
Feingold, G., Cotton, W. R., Kreidenweis, S. M., and Davis, J. T.: The Impact of Giant Cloud Condensation Nuclei on Drizzle Formation in Stratocumulus: Implications for Cloud Radiative Properties, J. Atmos. Sci., 56, 4100–4117, 1999.
Fröhlich-Nowoisky, J., Kampf, C. J., Weber, B., Huffman, J. A., Pöhlker, C., Andreae, M. O., Lang-Yona, N., Burrows, S. M., Gunthe, S. S., Elbert, W., Su, H., Hoor, P., Thines, E., Hoffmann, T., Després, V. R., and Pöschl, U.: Bioaerosols in the Earth system: Climate, health, and ecosystem interactions, Atmos. Res., 182, 346–376, 2016.
Franc, G. D. and DeMott, P. J.: Cloud activation characteristics of airborne Erwinia carotovora cells, J. Appl. Meteorol., 37, 1293–1300, 1998.
Georgakopoulos, D. G., Després, V., Fröhlich-Nowoisky, J., Psenner, R., Ariya, P. A., Pósfai, M., Ahern, H. E., Moffett, B. F., and Hill, T. C. J.: Microbiology and atmospheric processes: biological, physical and chemical characterization of aerosol particles, Biogeosciences, 6, 721–737, https://doi.org/10.5194/bg-6-721-2009, 2009.
Goodman, A. L., Bernard, E. T., and Grassian, V. H.: Spectroscopic Study of Nitric Acid and Water Adsorption on Oxide Particles: Enhanced Nitric Acid Uptake Kinetics in the Presence of Adsorbed Water, J. Phys. Chem. A, 105, 6443–6457, 2001.
Griffiths, P. T., Borlace, J. S., Gallimore, P. J., Kalberer, M., Herzog, M., and Pope, F. D.: Hygroscopic growth and cloud activation of pollen: a laboratory and modelling study, Atmos. Sci. Lett., 13, 289–295, 2012.
Gu, W., Li, Y., Zhu, J., Jia, X., Lin, Q., Zhang, G., Ding, X., Song, W., Bi, X., Wang, X., and Tang, M.: Investigation of water adsorption and hygroscopicity of atmospherically relevant particles using a commercial vapor sorption analyzer, Atmos. Meas. Tech., 10, 3821–3832, https://doi.org/10.5194/amt-10-3821-2017, 2017.
Guo, L., Gu, W., Peng, C., Wang, W., Li, Y. J., Zong, T., Tang, Y., Wu, Z., Lin, Q., Ge, M., Zhang, G., Hu, M., Bi, X., Wang, X., and Tang, M.: A comprehensive study of hygroscopic properties of calcium- and magnesium-containing salts: implication for hygroscopicity of mineral dust and sea salt aerosols, Atmos. Chem. Phys., 19, 2115–2133, https://doi.org/10.5194/acp-19-2115-2019, 2019.
Gute, E. and Abbatt, J. P. D.: Oxidative Processing Lowers the Ice Nucleation Activity of Birch and Alder Pollen, Geophys. Res. Lett., 45, 1647–1653, 2018.
Harrington, J. B. and Metzger, K.: Ragweed Pollen Density, Am. J. Bot., 50, 532–539, 1963.
Hatch, C. D., Wiese, J. S., Crane, C. C., Harris, K. J., Kloss, H. G., and Baltrusaitis, J.: Water Adsorption on Clay Minerals As a Function of Relative Humidity: Application of BET and Freundlich Adsorption Models, Langmuir, 28, 1790–1803, 2011.
Henson, B. F.: An adsorption model of insoluble particle activation: Application to black carbon, J. Geophys. Res.-Atmos., 112, D24S16, https://doi.org/10.1029/2007JD008549, 2007.
Hirose, Y. and Osada, K.: Terminal settling velocity and physical properties of pollen grains in still air, Aerobiologia, 32, 385–394, 2016.
Hoose, C., Kristjansson, J. E., and Burrows, S. M.: How important is biological ice nucleation in clouds on a global scale?, Environ. Res. Lett., 5, 024009, https://doi.org/10.1088/1748-9326/5/2/024009, 2010.
Hung, H. M., Katrib, Y., and Martin, S. T.: Products and mechanisms of the reaction of oleic acid with ozone and nitrate radical, J. Phys. Chem. A, 109, 4517–4530, 2005.
Ibrahim, S., Romanias, M. N., Alleman, L. Y., Zeineddine, M. N., Angeli, G. K., Trikalitis, P. N., and Thevenet, F.: Water Interaction with Mineral Dust Aerosol: Particle Size and Hygroscopic Properties of Dust, ACS Earth and Space Chem., 2, 376–386, 2018.
International Grains Council: Grain Market Report GMR 495, 2019.
Iwamoto, R., Matsuda, T., Sasaki, T., and Kusanagi, H.: Basic interactions of water with organic compounds, J. Phys. Chem. B, 107, 7976–7980, 2003.
Jia, X. H., Gu, W. J., Li, Y. J., Cheng, P., Tang, Y. J., Guo, L. Y., Wang, X. M., and Tang, M. J.: Phase transitions and hygroscopic growth of Mg(ClO4)2, NaClO4, and NaClO4•H2O: implications for the stability of aqueous water in hyperarid environments on Mars and on Earth, ACS Earth Space Chem., 2, 159–167, 2018.
Johnson, D. B.: The Role of Giant and Ultragiant Aerosol Particles in Warm Rain Initiation, J. Atmos. Sci., 39, 448–460, 1982.
Joshi, N., Romanias, M. N., Riffault, V., and Thevenet, F.: Investigating water adsorption onto natural mineral dust particles: Linking DRIFTS experiments and BET theory, Aeolian Res., 27, 35–45, 2017.
Ko, G., First, M. W., and Burge, H. A.: Influence of relative humidity on particle size and UV sensitivity of Serratia marcescens and Mycobacterium bovis BCG aerosols, Tubercle Lung Dis., 80, 217–228, 2000.
Kreidenweis, S. M. and Asa-Awuku, A.: 5.13 – Aerosol Hygroscopicity: Particle Water Content and Its Role in Atmospheric Processes, in: Treatise on Geochemistry, edited by: Turekian, K. K., 2nd Edn., Elsevier, Oxford, 331–361, 2014.
Laaksonen, A., Malila, J., Nenes, A., Hung, H. M., and Chen, J. P.: Surface fractal dimension, water adsorption efficiency, and cloud nucleation activity of insoluble aerosol, Sci. Rep.-UK, 6, 25504, https://doi.org/10.1038/srep25504, 2016.
Lambert, A. R., O'Shaughnessy, P. T., Tawhai, M. H., Hoffman, E. A., and Lin, C. L.: Regional Deposition of Particles in an Image-Based Airway Model: Large-Eddy Simulation and Left-Right Lung Ventilation Asymmetry, Aerosol Sci. Tech., 45, 11–25, 2011.
Lee, B. U., Kim, S. H., and Kim, S. S.: Hygroscopic growth of E-coli and B-subtilis bioaerosols, J. Aerosol. Sci., 33, 1721–1723, 2002.
Lin, H., Lizarraga, L., Bottomley, L. A., and Carson Meredith, J.: Effect of water absorption on pollen adhesion, J. Colloid Interf. Sci., 442, 133–139, 2015.
Longest, P. W. and Holbrook, L. T.: In silico models of aerosol delivery to the respiratory tract – Development and applications, Adv. Drug Deliver. Rev., 64, 296–311, 2012.
Ma, Q. X., He, H., and Liu, Y. C.: In Situ DRIFTS Study of Hygroscopic Behavior of Mineral Aerosol, J. Environ. Sci., 22, 555–560, 2010.
Melke, A.: The Physiology of Chilling Temperature Requirements for Dormancy Release and Bud-break in Temperate Fruit Trees Grown at Mild Winter Tropical Climate, Journal of Plant Studies, 4, 110–156, 2015.
Möhler, O., DeMott, P. J., Vali, G., and Levin, Z.: Microbiology and atmospheric processes: the role of biological particles in cloud physics, Biogeosciences, 4, 1059–1071, https://doi.org/10.5194/bg-4-1059-2007, 2007.
Morris, C. E., Sands, D. C., Bardin, M., Jaenicke, R., Vogel, B., Leyronas, C., Ariya, P. A., and Psenner, R.: Microbiology and atmospheric processes: research challenges concerning the impact of airborne micro-organisms on the atmosphere and climate, Biogeosciences, 8, 17–25, https://doi.org/10.5194/bg-8-17-2011, 2011.
Morris, C. E., Conen, F., Alex Huffman, J., Phillips, V., Pöschl, U., and Sands, D. C.: Bioprecipitation: a feedback cycle linking Earth history, ecosystem dynamics and land use through biological ice nucleators in the atmosphere, Glob. Chang. Biol., 20, 341–351, 2014.
Murray, B. J., O'Sullivan, D., Atkinson, J. D., and Webb, M. E.: Ice nucleation by particles immersed in supercooled cloud droplets, Chem. Soc. Rev., 41, 6519–6554, 2012.
Najera, J. J., Percival, C. J., and Horn, A. B.: Infrared spectroscopic studies of the heterogeneous reaction of ozone with dry maleic and fumaric acid aerosol particles, Phys. Chem. Chem. Phys., 11, 9093–9103, 2009.
Noh, Y. M., Lee, H., Mueller, D., Lee, K., Shin, D., Shin, S., Choi, T. J., Choi, Y. J., and Kim, K. R.: Investigation of the diurnal pattern of the vertical distribution of pollen in the lower troposphere using LIDAR, Atmos. Chem. Phys., 13, 7619–7629, https://doi.org/10.5194/acp-13-7619-2013, 2013.
Pappas, C. S., Tarantilis, P. A., Harizanis, P. C., and Polissiou, M. G.: New method for pollen identification by FT-IR spectroscopy, Appl. Spectrosc., 57, 23–27, 2003.
Park, S. S. and Wexler, A. S.: Size-dependent deposition of particles in the human lung at steady-state breathing, J. Aerosol. Sci., 39, 266–276, 2008.
Pasanen, A. L., Pasanen, P., Jantunen, M. J., and Kalliokoski, P.: Significance of air humidity and air velocity for fungal spore release into the air, Atmos. Environ., 25, 459–462, 1991.
Petters, M. D. and Kreidenweis, S. M.: A single parameter representation of hygroscopic growth and cloud condensation nucleus activity, Atmos. Chem. Phys., 7, 1961–1971, https://doi.org/10.5194/acp-7-1961-2007, 2007.
Pope, F. D.: Pollen grains are efficient cloud condensation nuclei, Environ. Res. Lett., 5, 044015, https://doi.org/10.1088/1748-9326/5/4/044015, 2010.
Pöschl, U., Martin, S. T., Sinha, B., Chen, Q., Gunthe, S. S., Huffman, J. A., Borrmann, S., Farmer, D. K., Garland, R. M., Helas, G., Jimenez, J. L., King, S. M., Manzi, A., Mikhailov, E., Pauliquevis, T., Petters, M. D., Prenni, A. J., Roldin, P., Rose, D., Schneider, J., Su, H., Zorn, S. R., Artaxo, P., and Andreae, M. O.: Rainforest Aerosols as Biogenic Nuclei of Clouds and Precipitation in the Amazon, Science, 329, 1513–1516, 2010.
Posselt, R. and Lohmann, U.: Influence of Giant CCN on warm rain processes in the ECHAM5 GCM, Atmos. Chem. Phys., 8, 3769–3788, https://doi.org/10.5194/acp-8-3769-2008, 2008.
Pratt, K. A., DeMott, P. J., French, J. R., Wang, Z., Westphal, D. L., Heymsfield, A. J., Twohy, C. H., Prenni, A. J., and Prather, K. A.: In situ detection of biological particles in cloud ice-crystals, Nat. Geosci., 2, 397–400, 2009.
Prenni, A. J., Petters, M. D., Kreidenweis, S. M., Heald, C. L., Martin, S. T., Artaxo, P., Garland, R. M., Wollny, A. G., and Pöschl, U.: Relative roles of biogenic emissions and Saharan dust as ice nuclei in the Amazon basin, Nat. Geosci., 2, 401–404, 2009.
Prisle, N. L., Lin, J. J., Purdue, S. K., Lin, H., Meredith, J. C., and Nenes, A.: CCN activity of six pollenkitts and the influence of their surface activity, Atmos. Chem. Phys. Discuss., https://doi.org/10.5194/acp-2018-394, in review, 2018.
Pummer, B. G., Bauer, H., Bernardi, J., Bleicher, S., and Grothe, H.: Suspendable macromolecules are responsible for ice nucleation activity of birch and conifer pollen, Atmos. Chem. Phys., 12, 2541–2550, https://doi.org/10.5194/acp-12-2541-2012, 2012.
Pummer, B. G., Bauer, H., Bernardi, J., Chazallon, B., Facq, S., Lendl, B., Whitmore, K., and Grothe, H.: Chemistry and morphology of dried-up pollen suspension residues, J. Raman Spectrosc., 44, 1654–1658, 2013.
Reinmuth-Selzle, K., Kampf, C. J., Lucas, K., Lang-Yona, N., Frohlich-Nowoisky, J., Shiraiwa, M., Lakey, P. S. J., Lai, S. C., Liu, F. B., Kunert, A. T., Ziegler, K., Shen, F. X., Sgarbanti, R., Weber, B., Bellinghausen, I., Saloga, J., Weller, M. G., Duschl, A., Schuppan, D., and Poschl, U.: Air Pollution and Climate Change Effects on Allergies in the Anthropocene: Abundance, Interaction, and Modification of Allergens and Adjuvants, Environ. Sci. Technol., 51, 4119–4141, 2017.
Reponen, T., Willeke, K., Ulevicius, V., Reponen, A., and Grinshpun, S. A.: Effect of relative humidity on the aerodynamic diameter and respiratory deposition of fungal spores, Atmos. Environ., 30, 3967–3974, 1996.
Schuttlefield, J. D., Al-Hosney, H., Zachariah, A., and Grassian, V. H.: Attenuated Total Reflection Fourier Transform Infrared Spectroscopy to Investigate Water Uptake and Phase Transitions in Atmospherically Relevant Particles, Appl. Spectrosc., 61, 283–292, 2007a.
Schuttlefield, J. D., Cox, D., and Grassian, V. H.: An investigation of water uptake on clays minerals using ATR-FTIR spectroscopy coupled with quartz crystal microbalance measurements, J. Geophys. Res.-Atmos., 112, D21303, https://doi.org/10.1029/2007JD008973, 2007b.
Shiraiwa, M., Ueda, K., Pozzer, A., Lammel, G., Kampf, C. J., Fushimi, A., Enami, S., Arangio, A. M., Frohlich-Nowoisky, J., Fujitani, Y., Furuyama, A., Lakey, P. S. J., Lelieveld, J., Lucas, K., Morino, Y., Poschl, U., Takaharna, S., Takami, A., Tong, H. J., Weber, B., Yoshino, A., and Sato, K.: Aerosol Health Effects from Molecular to Global Scales, Environ. Sci. Technol., 51, 13545–13567, 2017.
Skopp, J.: Derivation of the Freundlich Adsorption Isotherm from Kinetics, J. Chem. Educ., 86, 1341–1343, 2009.
Sofiev, M., Siljamo, P., Ranta, H., and Rantio-Lehtimäki, A.: Towards numerical forecasting of long-range air transport of birch pollen: theoretical considerations and a feasibility study, Int. J. Biometeorol., 50, 392–402, 2006.
Song, X. W. and Boily, J. F.: Water Vapor Adsorption on Goethite, Environ. Sci. Technol., 47, 7171–7177, 2013.
Steiner, A. L., Brooks, S. D., Deng, C. H., Thornton, D. C. O., Pendleton, M. W., and Bryant, V.: Pollen as atmospheric cloud condensation nuclei, Geophys. Res. Lett., 42, 3596–3602, 2015.
Stuart, B.: Infrared Spectroscopy: Fundamentals and Applications, John Wiley & Sons, Ltd., New York, 2004.
Sun, J. M. and Ariya, P. A.: Atmospheric organic and bio-aerosols as cloud condensation nuclei (CCN): A review, Atmos. Environ., 40, 795–820, 2006.
Tang, M. J., Cziczo, D. J., and Grassian, V. H.: Interactions of Water with Mineral Dust Aerosol: Water Adsorption, Hygroscopicity, Cloud Condensation and Ice Nucleation, Chem. Rev., 116, 4205–4259, 2016.
Tang, M. J., Chen, J., and Wu, Z. J.: Ice nucleating particles in the troposphere: Progresses, challenges and opportunities, Atmos. Environ., 192, 206–208, 2018.
Taramarcaz, P., Lambelet, C., Clot, B., Keimer, C., and Hauser, C.: Ragweed (Ambrosia) progression and its health risks: will Switzerland resist this invasion?, Swiss Med. Wkly., 135, 538–548, 2005.
Tong, H. J., Fitzgerald, C., Gallimore, P. J., Kalberer, M., Kuimova, M. K., Seville, P. C., Ward, A. D., and Pope, F. D.: Rapid interrogation of the physical and chemical characteristics of salbutamol sulphate aerosol from a pressurised metered-dose inhaler (pMDI), Chem. Commun., 50, 15499–15502, 2014.
Womack, A. M., Bohannan, B. J. M., and Green, J. L.: Biodiversity and biogeography of the atmosphere, Philos. T. R. Soc. B, 365, 3645–3653, 2010.
Yeh, H. C., Cuddihy, R. G., Phalen, R. F., and Chang, I. Y.: Comparisons of calculated respiratory tract deposition of particles based on the proposed NCRP model and the new ICRP66 model, Aerosol Sci. Tech., 25, 134–140, 1996.
Yeşilbaş, M. and Boily, J.-F.: Particle Size Controls on Water Adsorption and Condensation Regimes at Mineral Surfaces, Sci. Rep.-UK, 6, 32136, https://doi.org/10.1038/srep32136, 2016.
Yin, Y., Levin, Z., Reisin, T. G., and Tzivion, S.: The effects of giant cloud condensation nuclei on the development of precipitation in convective clouds – a numerical study, Atmos. Res., 53, 91–116, 2000.