the Creative Commons Attribution 4.0 License.
the Creative Commons Attribution 4.0 License.
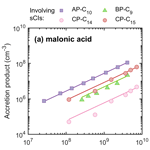
Gas-phase observations of accretion products from stabilized Criegee intermediates in terpene ozonolysis with two dicarboxylic acids
Lauri Franzon
Jiangyi Zhang
Nina Sarnela
Neil M. Donahue
Theo Kurtén
Criegee intermediates (CIs), formed from the ozonolysis of alkenes, are highly reactive species with diverse reaction pathways, with important roles in atmospheric chemistry. This study focuses on the formation of accretion products through reactions of thermally stabilized CIs (sCIs) from the ozonolysis of three different terpenes (α-pinene, β-pinene, and β-caryophyllene) with malonic and oxalic acids. Our experimental results demonstrate that these reactions efficiently produce the expected accretion products, though with apparent variations in yields depending on the specific terpene and acid involved. To our knowledge, this is the first study to report direct gas-phase observations of expected adducts from several different terpene-derived sCIs and carboxylic acids, paving the way for a better understanding of the importance and atmospheric implications of these reactions, especially in terms of the aerosol-forming capabilities of these large product molecules.
- Article
(903 KB) - Full-text XML
-
Supplement
(3087 KB) - BibTeX
- EndNote
Stabilized Criegee intermediates (sCIs), which are formed from the stabilization of excited Criegee intermediates (eCIs) during the ozonolysis of alkenes, play diverse roles in tropospheric chemistry. Depending on their structure, sCIs can have fast unimolecular reaction rates (Long et al., 2016; Chhantyal-Pun et al., 2015; Lester and Klippenstein, 2018; Drozd and Donahue, 2011) but also react with various trace atmospheric species, including water vapor, sulfur dioxide (SO2), nitrogen oxides (NOx), carboxylic acids, and carbonyl compounds (Cox et al., 2020; Osborn and Taatjes, 2015; Khan et al., 2018; Lin and Chao, 2017; Chhantyal-Pun et al., 2020; Gong and Chen, 2021; Taatjes et al., 2013). These reactions can produce low-volatility species that significantly contribute to the formation of both inorganic and organic aerosol components, consequently impacting air quality, climate, and human health.
Carboxylic acids are emitted directly into the atmosphere from both biogenic and anthropogenic sources and can also be produced in situ through various photochemical reactions. Observations have shown that carboxylic acids can maintain steady-state mixing ratios of up to a few parts per billion by volume (ppbv) at different locations globally (Kawamura et al., 1985; Chebbi and Carlier, 1996; Khan et al., 2015). Organic acids can react with stabilized sCIs to form adducts (Welz et al., 2014), facilitating a pathway through which alkenes are transformed into low-volatility compounds, thus contributing to the formation of secondary organic aerosol (SOA) (Chhantyal-Pun et al., 2018; Khan et al., 2018). Calculations of the reaction of formic acid with sCIs indicate that sCIs can target both the hydroxyl group and the carbonyl bond, forming stable adducts like peroxyesters, with the hydroxyl group attack being more favorable due to its higher exothermicity (Vereecken, 2017; Vereecken and Francisco, 2012). Recent direct kinetic investigations have measured reaction rate coefficients between sCIs and organic acids, finding values that greatly exceed those predicted by earlier theoretical computations or analyses of reaction end products (Chhantyal-Pun et al., 2017; Elsamra et al., 2016; Chhantyal-Pun et al., 2018). These studies have demonstrated that reactions of sCIs with carboxylic acids are typically at the gas-kinetic collision limit (Vereecken et al., 2014; Chhantyal-Pun et al., 2018).
While the significance of reactions between sCIs and organic acids has long been recognized, only recently has it become possible to measure some fundamental sCIs, enabling further experimental investigations into their properties and roles in the atmosphere (Sheps et al., 2014; Taatjes et al., 2013; Welz et al., 2014; Welz et al., 2012). Despite these advancements, the wide variety of carboxylic acids in the atmosphere, coupled with the diverse sCIs generated through the ozonolysis of alkenes, results in numerous potential reaction products. Identifying specific reaction pathways and products is especially challenging for sCIs from complex molecules like terpenes, which are a major source of atmospheric SOA (Lee et al., 2006; Yang et al., 2021; Faiola et al., 2018). Although many studies have posited that sCIs from such complex molecules reacting with organic acids could significantly contribute to the formation of SOA (Gong and Chen, 2021; Gong et al., 2024; Vansco et al., 2020; Yao et al., 2014; Wang et al., 2016), direct gas-phase observations of products from reactions involving larger sCIs – particularly those derived from monoterpenes and sesquiterpenes – are still extremely limited. Several papers have reported on the use of acids to scavenge sCIs (Berndt et al., 2017; Yao et al., 2014; Gong and Chen, 2021). However, these studies have primarily focused on the kinetics of sCIs and/or their role in aerosol formation and therefore have not tried to look into the formed products. Berndt et al. (2018) reported the formation of an abundant product from monoterpene sCI + acetic acid, but the data were not explicitly shown in that study. Even for smaller sCIs, very few direct observations of the expected products have been presented. Vansco et al. (2020) demonstrated the formation of functionalized hydroperoxide adducts from reactions between isoprene-derived sCIs and formic acid using multiplexed photoionization mass spectrometry.
In our previous work on the ozonolysis of the diterpene kaurene (Luo et al., 2022), we identified a series of oxidation products containing 21–29 carbon atoms, as depicted in Fig. 1a. Initially, we hypothesized that these products might have formed from cross-reactions of peroxy radicals (RO2) from kaurene with smaller RO2 formed from OH oxidation of various C2–9 organic contaminants within the chamber (Fig. 1c). However, we have later come to hypothesize that these might in fact have been products of sCIs with organic acid contaminants. Given that prior studies suggest a higher fraction of sCIs in larger molecules (Hakala and Donahue, 2023; Vereecken, 2017), we decided that these data are worth re-analyzing. To investigate sCI accretion products further, we conducted experiments in a flow tube reactor focusing on terpene-derived sCIs reacting with carboxylic acids. We used three different terpenes – α-pinene, β-pinene, and β-caryophyllene – and two commonly measured ambient dicarboxylic acids, malonic and oxalic acids, to explore sCIs' accretion pathways with acids across these chemical systems.
2.1 Laboratory experiments
We conducted experiments in a flow reactor at room temperature under dry conditions. The total flow was set at 16 L min−1, generated using a zero-air generator (AADCO, Series 737-14, Ohio, USA), resulting in a residence time of approximately 3 s. We utilized three different terpenes in each experiment: two monoterpenes, α-pinene and β-pinene (both C10H16), and one sesquiterpene, β-caryophyllene (C15H24), of which the structures are shown in Fig. S1. These were introduced into the flow reactor using a syringe pump setup, while ozone was added to the reactor from a Dasibi 1008-PC ozone generator. Once the terpene oxidation product signals had stabilized, we added either malonic acid (C3H4O4) or oxalic acid (C2H2O4) to titrate the sCIs. These dicarboxylic acids were chosen to enhance the detection of accretion products using our nitrate-ion-based chemical ionization mass spectrometer (hereafter NO3-CIMS). Dicarboxylic acids were chosen because they were expected to produce accretion products with functionalities that were detectable by the NO3-CIMS. The acids were vaporized into the reactor by flushing N2 through a vial containing the solid acid, which was heated (∼ 60 °C) using a water bath to promote evaporation.
Ozone concentrations were monitored using a UV photometric analyzer (model 49P, Thermo Environmental). Terpene concentrations were measured by a Vocus proton-transfer-reaction time-of-flight mass spectrometer (Vocus PTR, Tofwerk AG) (Krechmer et al., 2018), which was calibrated daily with a gas-phase standard (Apel-Riemer Environmental, Inc) containing 19 components, including α-pinene. The sensitivity for α-pinene was determined as 1370 ± 280 cps ppb−1. Since β-pinene and β-caryophyllene were not included in the standard, their sensitivities were estimated based on their proton affinity, fragmentation patterns, and transmission efficiencies, yielding sensitivities of 1400 ± 289 cps ppb−1 for β-pinene and 1780 ± 368 cps ppb−1 for β-caryophyllene, respectively. Highly oxygenated organic molecules (HOMs), accretion products, and carboxylic acids were detected using the NO3-CIMS (Tofwerk AG/Aerodyne Research Inc.), which has been shown to be very sensitive towards highly oxygenated species, typically with two or more H-bond donors (Junninen et al., 2010; Hyttinen et al., 2015). For such species, the charging is typically assumed to be collision-limited. The NO3-CIMS was not directly calibrated in this study, owing to various challenges related to HOM quantification (Riva et al., 2019), but a calibration factor of 1 × 1010 cm−3 was used to provide a rough estimate of product concentrations. This naturally introduces a large uncertainty, estimated as at least a factor of 3, but our focus will be on species identification and on comparing relative concentration changes, meaning that the lack of detailed quantification will not hamper our conclusions. Data from all instruments were collected at a frequency of 1 Hz. Data from both the Vocus PTR-TOF and NO3-CIMS were analyzed using the MATLAB tofTools package (version 612) (Junninen, 2014).
2.2 Quantum calculation
The simplified mechanism of the formation of accretion product from CIs + carboxylic acid reaction is shown in Scheme 1. Quantum chemical calculations were performed to help explain the experimental observations. Reaction rate coefficients with both dicarboxylic acids were estimated for all the α-pinene- and β-pinene-derived sCIs, and Gibbs free energies of clustering with the nitrate ion were calculated for the accretion products derived from these. β-caryophyllene-derived sCIs were not considered due to the power law dependence of computational cost on molecular size.
In a previous computational study, Vereecken (2017) found that gas-phase reactions between sCI and simple carboxylic acids are collision-limited using microvariational transition state theory. We considered such calculations to be beyond the scope of this work due to having a high computational cost for the C9 and C10 sCIs and simply performed a few test calculations to confirm that the sCI + dicarboxylic acid mechanism proceeds similarly to Vereecken's sCI + HCOOH mechanism and used the dipole-moment-based structure–activity relationship (SAR) by Chhantyal-Pun et al. (2018) to estimate reaction rate coefficients. Detailed results and method descriptions on these calculations are found in the Supplement.
The Gibbs free energies of clustering with the NO ion for the α-pinene- and β-pinene-derived accretion products were calculated similarly as in Hyttinen et al. (2015). The final Gibbs free energies of clustering were compared to the corresponding value for HNO3 to determine the detectability of each accretion product in our experimental conditions. The results are presented in Table S3.
In the computations, conformer sampling of all species was performed using the xTB-GFN2 level of theory with the CREST software (Pracht et al., 2024). For the quantum chemical calculations, geometry optimizations were performed using various density functionals (see Supplement for more details) and final single-point electronic energy corrections with DLPNO/CCSD(T). These quantum chemical calculations were performed using the ORCA program, versions 5.0.4 and 6.0 (Neese, 2022, 2023). Full details of all performed computations can be found in the Supplement.
The different sCIs expected to form from the ozonolysis of the selected terpenes are summarized in Table 1. When endocyclic C=C double bonds are cleaved, the sCI will retain all the atoms from the terpene and the O3; thus, e.g., α-pinene produces sCIs with the formula C10H16O3. β-pinene only features an exocyclic double bond, and the cleavage will form a C1 or C9 sCI. According to MCMv3.3.1 (Saunders et al., 2003), the branching ratios for the formation of the C1 and C9 CIs are 0.4 and 0.6, respectively, while Ahrens et al. (2014) reports a branching ratio of 0.1 for C1 and 0.45 each for both C9 isomers based on infrared spectroscopy experiments. β-caryophyllene has both endo- and exocyclic double bonds, and ozone may react with either of these bonds to form either C15H24O3 (endocyclic case) or (exocyclic case). A previous study indicated that ozone predominantly reacts with the endocyclic bond, with the exocyclic reaction being approximately 2 orders of magnitude slower (Winterhalter et al., 2009).
The ozonolysis of α-pinene forms four isomeric C10 sCIs, called syn-pinonaldehyde oxide, anti-pinonaldehyde oxide, syn-isopinonaldehyde oxide, and anti-isopinonaldehyde oxide (Molecular structures shown in the Supplement). The syn- and anti-conformers are not interconvertable, but crucially for these experiments, they form the same accretion product, as the non-rotatable carbonyl oxide C=O bond is converted into a rotatable hydroperoxide C–O bond. Similarly, the β-pinene C9 sCI has two non-convertible isomers, syn-nopinene oxide and anti-nopinene oxide, which also form the same accretion products. The latter two form the same accretion product. The β-caryophyllene-derived C14 and C15 sCIs have not been given systematic names as far as we are aware, but the same syn- and anti-isomerism applies to these as well (Winterhalter et al., 2009; Nguyen et al., 2009).
Table 1Main sCIs derived from the ozonolysis of the studied terpenes and their corresponding potential accretion products with malonic (MA) and oxalic (OA) acids.
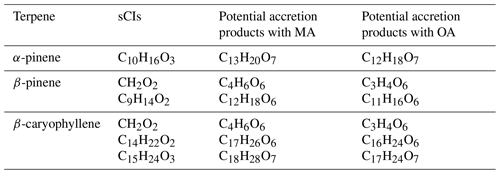
3.1 Accretion product formation in the flow reactor
From our previous work on kaurene ozonolysis, we identified C21–29 products (Fig. 1a). The primary sCI from kaurene is expected to be C19H30O2, while we also measured C2–9 contaminants in the chamber. If these contaminants were acids and they reacted with the C19H30O2 sCI, several accretion products could be expected to form. To test this, we took the part of the mass spectrum with the contaminants and shifted it by 290 Th (the mass of C19H30O2). As depicted in Fig. 1b, the spectrum aligns almost perfectly with the observed C21–29 products. This supports our hypothesis that the observed species were indeed sCI + acid accretion products. In the following sections, we explore this reaction with better controlled experiments.
Figure 2 presents the mass spectra of oxidation products resulting from the ozonolysis of α-pinene, β-pinene, and β-caryophyllene, both with and without the presence of malonic acid. The concentrations of the terpenes and ozone were maintained at similar levels before and after acid injection, ensuring comparable formation of sCIs. Prior to acid injection, we observed many of the typical HOMs associated with the different systems (Ehn et al., 2014; Jokinen et al., 2015; Richters et al., 2016a). For α-pinene, the predominant HOM monomers were C10H14,16O7,9, and dimers included C19H28O9, C20H30O12, and C20H32O9,11. In β-pinene ozonolysis, the major HOM monomers were C10H16O and C9H16O6, with dominant dimers such as C19H28O9,11. For β-caryophyllene, the most prevalent HOM monomers were C15H22,24O7,9, with dimers including C29H46O12 and C30H46O.
Following the injection of malonic acid, new peaks appeared at the masses corresponding to all the expected accretion products from Table 1 (Fig. 2). Notably, no other peaks appeared, strongly indicating that sCI + acid reactions were the driver for the observed changes. For β-caryophyllene, C18H28O7 was clearly the most abundant of the three new products, as expected, but in the β-pinene system, the signal intensity of C4H6O6 was more than twice that of C12H18O6. This may indicate that the branching towards the C1 CI was larger than expected or that there are differences in the sCI + acid reaction rate coefficients or yields. It is also possible that there is difference in the sensitivity of the NO3-CIMS towards the different products. For the corresponding oxalic acid experiments, we also detected all expected species at the expected masses for sCIs plus oxalic acid (Fig. S2). The largest difference compared to the malonic acid system was that the signal intensities for C3H4O6 in β-pinene and C17H26O7 in β-caryophyllene ozonolysis were substantially higher than those of other accretion products. These differences between the two acids were surprising, and for a more detailed look at the dependencies, we study the temporal behavior of the different species.
Figures S3–S8 display examples of time series for the dominant RO2, HOM monomers and dimers, acids, and sCI-derived accretion products in each of the six terpene + acid systems. Comparable observations were made for the systems, and we describe below the β-caryophyllene + malonic acid system (Fig. S5b). Without malonic acid, upon introducing ozone into the flow reactor with a stable β-caryophyllene concentration of approximately 30 ppb, the O3-initiated RO2 (e.g., C15H23O8) appears immediately, followed by the formation of HOM monomers and dimers. The trends in RO2, HOM monomers, and HOM dimers correlate well with the ozonolysis rate of β-caryophyllene. Background malonic acid was detected in the flow reactor prior to acid injection, leading to a minor signal of C18H28O7, the accretion product from sCI C15H24O3 and malonic acid. Upon injecting malonic acid at around 13:55 LT, the signal intensity of all the expected accretion products increased around 10-fold, while RO2, HOM monomers, and HOM dimers remained nearly constant, in line with earlier findings that sCIs are not intermediates in HOM formation (Richters et al., 2016b). The accretion products also responded as expected to the change of β-caryophyllene and O3 when the acid level was kept constant. The signal of C4H6O6 accumulated throughout the experiment and only decreased very slowly after the removal of β-caryophyllene and O3, while RO2, HOM, C18H28O7, and C17H26O6 all fell below detection limits. A similar pattern was observed for C3H4O6 in the oxalic acid system. We interpret this behavior as these species being semi-volatile; thus they accumulate on, and later desorb from, the wall of the reactor or sampling lines. Similar slow responses were observed also for malonic acid itself. In summary, the temporal trends also are in line with the proposed sCI + acid formation mechanism.
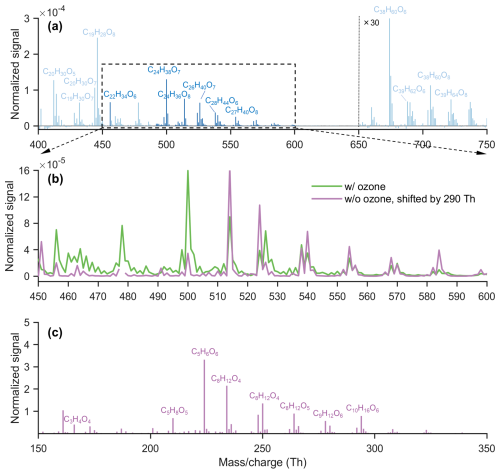
Figure 1(a) Mass spectrum of HOMs produced by kaurene ozonolysis, with the normalized signal amplified 30-fold in the HOM dimer region ( Th). All peaks were detected as clusters with NO. (b) Mass spectrum in the range that includes various species with 21–29 C atoms. The green spectrum represents measurements after ozone injection, while the purple spectrum, indicating chamber background, is the spectrum before ozone injected (panel c) was shifted by 290 Th (corresponding to the mass of C19H30O2, the main sCI from kaurene ozonolysis) after the signal had been reduced by a factor of 200. (c) Mass spectrum of chamber background before ozone injection.
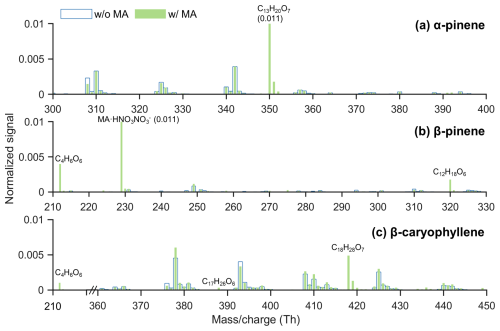
Figure 2Mass spectrum of products formed from the ozonolysis of (a) α-pinene, (b) β-pinene, and (c) β-caryophyllene, both with and without the presence of malonic acid. New peaks are labeled with their identified compositions, and numbers in parentheses denote signal strengths when peaks go off the scale.
Figure 3 illustrates the relationship between the concentration of accretion products and the ozonolysis rate of terpenes. It is evident that all accretion products, except those from β-caryophyllene ozonolysis sCI CH2O2 (Fig. S9), track the terpene reaction rate coefficients. The different behavior of the two accretion products from β-caryophyllene is attributed to their semi-volatile behavior, as previously mentioned.
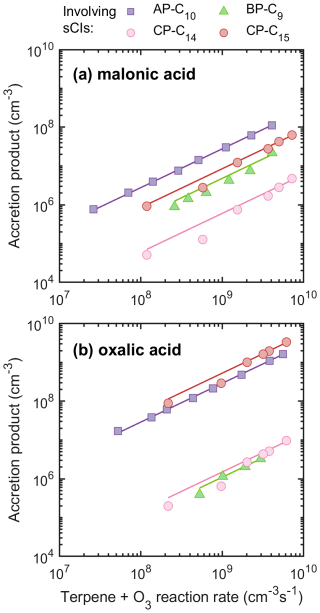
Figure 3Concentrations of accretion products from sCIs with (a) malonic acid and (b) oxalic acid plotted against the ozonolysis rate of terpenes. The lines represent linear fits. Legend labels indicate different accretion products formed from various sCIs in reaction with the acids. For instance, AP-C10 in panel (a) corresponds to the accretion product C13H20O7, formed from the sCI C10H16O3 with malonic acid; similarly, AP-C10 in panel (b) corresponds to the accretion product C12H18O7, formed from the same sCI reacting with oxalic acid.
In the case of malonic acid (Figs. 3a and S9a), α-pinene ozonolysis produced the highest concentration of accretion product at a given terpene reaction rate. Although incurring large uncertainties, we can estimate the molar yields of these accretion products, i.e. the fraction of reacted terpene molecules that result in sCI + acid accretion products. For the most abundant products from α-pinene, we estimate yields of ∼ 3 %, while yields of accretion products from β-pinene-derived sCI CH2O2 and β-caryophyllene-ozonolysis-derived sCI C15H24O3 were comparable at ∼ 1 %. Yields from β-pinene-derived sCI C9H14O2 and β-caryophyllene-derived sCI C14H22O2 were still lower, ∼ 0.5 % and ∼ 0.1 %, respectively. However, in the oxalic acid case (Figs. 3b and S9b), most accretion product yields increased dramatically compared to the malonic acid case, except for the β-pinene-derived sCI C9H14O2, which decreased by a factor of 4. The highest yield was from the β-caryophyllene-derived sCI C15H24O3 at 50 %, followed by the α-pinene-derived sCI at 30 %. The total yield of accretion products from β-pinene was approximately 5 %.
The yields of accretion products discussed above are determined by two key parameters: the stabilization yields of sCIs from the initial ozonolysis reaction and the rates of subsequent sCI + acid reactions. Clearly, the yield of the accretion products cannot exceed the yield of the sCIs from a given system. Earlier studies have reported total sCI yields from α-pinene, β-pinene, and β-caryophyllene ozonolysis to be approximately 15 %–34 % (Hakala and Donahue, 2023; Sipilä et al., 2014; Zhang and Zhang, 2005), 22 %–70 % (Ahrens et al., 2014; Yokouchi and Ambe, 1985; Zhang and Zhang, 2005; Hakala and Donahue, 2023), and ≥60 %–74 % (Nguyen et al., 2009; Winterhalter et al., 2009; Cox et al., 2020), respectively. This pattern (i.e., β-caryophyllene > β-pinene > α-pinene) diverges from our findings on the accretion product formation, but we also observed significant variations in the yields depending on which dicarboxylic acid we used, with more than a factor 50 difference in the case of β-caryophyllene-derived sCI C15H24O3. In addition, the yields of accretion products from β-pinene-derived sCI CH2O2 + acids are higher than products from β-pinene-derived sCI C9H14O2 + acids, although previous studies have reported higher yields of C9 sCI than CH2O2 sCI in β-pinene ozonolysis (Zhang and Zhang, 2005; Ahrens et al., 2014).
There are several potential explanations for the differences between our results and earlier studies. Firstly, as illustrated in Fig. S10, there was not always sufficient acid to fully terminate all the formed sCIs to sCI–acid accretion products, though a significant impact is only expected for the β-caryophyllene + OA case, which already showed a high yield. Another possible explanation is differing detection sensitivities in the NO3-CIMS. In our computations, the only sCI + acid accretion product (not including the C14–sCI products, for which calculations were not performed) with a lower detection sensitivity was one of the two isomers of the AP-C10 (C10 derived from β-pinene ozonolysis) + malonic acid, which could explain why the AP-C10 + malonic acid signal is 1 order of magnitude weaker than the AP-C10 + oxalic acid signal. However, if both accretion product isomers form in equal amounts, the signal should only decrease by a factor of 2. Thus, additional explanations are needed.
The above speculations concerned experimental artifacts, but we also looked into possible structural dependencies of sCIs + acid reaction rate coefficients. Previous studies suggest that the simplest sCI reactions with carboxylic acids are barrierless and largely driven by the long-range dipole–dipole interactions (Vereecken et al., 2014; Chhantyal-Pun et al., 2018). We were able to use the dipole-moment-based SAR model by Chhantyal-Pun et al. (2018) to estimate relative reaction rate coefficients for the sCI + malonic acid reactions (Sect. S2) and conclude that these do not explain the observed differences between the AP-C10, BP-C1, and BP-C9 signals (Figs. 3a and S9a), as the rate coefficients predict a formation rate ordering of BP-C9 > BP-C1 > AP-C10, which is the opposite of what we see, especially when accounting for the terpene-specific total sCI yields. Furthermore, the differences in rate coefficients all fit within a factor of 1.6, which is less than the spread in product formation we see. For the sCI + oxalic acid reactions we were not able to use this model due to the latter molecule's negligible dipole moment, and we instead used collision theory to estimate the rate coefficients (Sect. S2). While these results similarly imply minimal differences in rate coefficients, we note that the lack of dipole moment means that the relative orientation of the molecules is likely to play a larger role in determining the reaction rate coefficients. We speculate that the reduced signal of the BP-C9 accretion product in the oxalic acid experiments (Figs. 3b and S9b) compared to the malonic acid experiments (Figs. 3a and S9a) may be due to such orientation effects, as the bicyclic BP-C9 is more rigid in its internal motions than AP-C10 or CP-C14 and thus may be less likely to spontaneously rotate into the energy slope leading to the reaction. Lastly, differing decomposition of the products, either in the flow tube or inside the mass spectrometer, could result in fragments undetectable with these instruments.
While the ultimate reason for the differing yields estimated in our study and earlier ones remains an open question, we do stress that our main finding is that all the expected accretion products could be detected, and thus we can also look for them in the atmosphere.
3.2 Potential accretion product observations in the ambient air
A NO3-CIMS is permanently deployed at the Station for Measuring Ecosystem-Atmosphere Relations (SMEAR II) in Hyytiälä, southern Finland, to monitor low-volatility species. We analyzed data during late spring to summer (1 May to 31 August 2023) to investigate potential accretion products from monoterpene sCIs and organic acids, as monoterpene oxidation products have been shown to dominate the HOM spectrum at this site (Yan et al., 2016; Ehn et al., 2012; Lee et al., 2018). The time resolution of data in Hyytiälä is 30 min.
Measurements in Hyytiälä revealed a series of species whose chemical formulas corresponded to the mass of the α-pinene-ozonolysis-derived sCI C10H16O3 combined with malonic, oxalic, or formic acid. As demonstrated in Fig. S11, these potential accretion products correlated well with the respective acids during the whole observation period. The most abundant species was C11H18O5, which corresponds to the composition of the adduct of sCI C10H16O3 and formic acid. Given that formic acid was one of the most prevalent organic acids measured in the ambient environment (Stavrakou et al., 2012; Millet et al., 2015), its adduction with the sCI is possible, despite the NO3-CIMS's inability to detect formic acid directly. Malonic acid signals were significantly higher than those of oxalic acid, likely due to a higher detection efficiency of malonic acid in the NO3-CIMS (Ehn et al., 2012), but the levels of C13H20O7 and C12H18O7 were almost comparable. Despite these observations, a good correlation (Fig. S12) was also observed between these acids and various other C12 and C13 species. While it is possible that the discussed species are from sCI + acid reactions, it must be kept in mind that similar diurnal cycles can be caused by many different types of processes. Consequently, we cannot definitively conclude that these species are accretion products of sCIs with organic acids.
This study examined the reactions between sCIs and carboxylic acids to assess their potential to form low-volatility accretion products. Utilizing a flow reactor, we investigated the ozonolysis of three terpenes – α-pinene, β-pinene, and β-caryophyllene – combined with malonic and oxalic acids. We detected the expected accretion products from sCIs + acid in all systems. Our findings indicate that accretion products from sCIs + acids formed readily, with specific variances across different terpenes and acids. For instance, α-pinene ozonolysis yielded the most significant accretion product concentration with malonic acid. Conversely, β-caryophyllene ozonolysis with oxalic acid resulted in the highest yield among the tested combinations. These experimental results validate the hypothesis that sCIs originating from the ozonolysis of terpenes can significantly interact with organic acids forming accretion products but leave open questions regarding the yields between given sCI–acid pairs.
To our knowledge, this study is the first to show direct observations of gas-phase accretion products from terpene sCIs + acid reactions. The high efficiency of these reactions implies that sCIs could influence the dynamics and composition of organic aerosol formation in the troposphere, but future studies should look into the rate coefficients of suppression of the accretion products at different levels of humidity.
Data are available upon request by contacting the1 corresponding authors.
The supplement related to this article is available online at https://doi.org/10.5194/acp-25-4655-2025-supplement.
ME and YL conceptualized the study. The laboratory experiments and data analysis were conducted by YL. LF conducted the quantum chemical calculation. JZ performed the analysis of data from ambient air. YL prepared the original draft with large contributions from LF. All authors contributed to the discussion and commented on the manuscript.
The contact author has declared that none of the authors has any competing interests.
Publisher's note: Copernicus Publications remains neutral with regard to jurisdictional claims made in the text, published maps, institutional affiliations, or any other geographical representation in this paper. While Copernicus Publications makes every effort to include appropriate place names, the final responsibility lies with the authors.
This research has been supported by the Jane and Aatos Erkko Foundation (grant no. 220043); the Research Council of Finland, Luonnontieteiden ja Tekniikan Tutkimuksen Toimikunta (grant nos. 317380 and 320094); and the China Scholarship Council, University Postgraduate Program (grant no. 201906220191).
Open-access funding was provided by the Helsinki University Library.
This paper was edited by Carl Percival and reviewed by two anonymous referees.
Ahrens, J., Carlsson, P. T., Hertl, N., Olzmann, M., Pfeifle, M., Wolf, J. L., and Zeuch, T.: Infrared detection of Criegee intermediates formed during the ozonolysis of β-pinene and their reactivity towards sulfur dioxide, Angewandte Chemie International Edition, 53, 715–719, https://doi.org/10.1002/anie.201307327, 2014.
Berndt, T., Herrmann, H., and Kurtén, T.: Direct Probing of Criegee Intermediates from Gas-Phase Ozonolysis Using Chemical Ionization Mass Spectrometry, J. Am. Chem. Soc., 139, 13387–13392, https://doi.org/10.1021/jacs.7b05849, 2017.
Berndt, T., Mentler, B., Scholz, W., Fischer, L., Herrmann, H., Kulmala, M., and Hansel, A.: Accretion Product Formation from Ozonolysis and OH Radical Reaction of α-Pinene: Mechanistic Insight and the Influence of Isoprene and Ethylene, Environ. Sci. Technol., 52, 11069–11077, https://doi.org/10.1021/acs.est.8b02210, 2018.
Chebbi, A. and Carlier, P.: Carboxylic acids in the troposphere, occurrence, sources, and sinks: A review, Atmos. Environ., 30, 4233–4249, https://doi.org/10.1016/1352-2310(96)00102-1, 1996.
Chhantyal-Pun, R., Davey, A., Shallcross, D. E., Percival, C. J., and Orr-Ewing, A. J.: A kinetic study of the CH2OO Criegee intermediate self-reaction, reaction with SO2 and unimolecular reaction using cavity ring-down spectroscopy, Phys. Chem. Chem. Phys., 17, 3617–3626, https://doi.org/10.1039/C4CP04198D, 2015.
Chhantyal-Pun, R., McGillen, M. R., Beames, J. M., Khan, M. A. H., Percival, C. J., Shallcross, D. E., and Orr-Ewing, A.: Temperature-Dependence of the Rates of Reaction of Trifluoroacetic Acid with Criegee Intermediates, Angewandte Chemie, 129, 9172–9175, https://doi.org/10.1002/ange.201703700, 2017.
Chhantyal-Pun, R., Rotavera, B., McGillen, M. R., Khan, M. A. H., Eskola, A. J., Caravan, R. L., Blacker, L., Tew, D. P., Osborn, D. L., Percival, C. J., Taatjes, C. A., Shallcross, D. E., and Orr-Ewing, A. J.: Criegee Intermediate Reactions with Carboxylic Acids: A Potential Source of Secondary Organic Aerosol in the Atmosphere, ACS Earth and Space Chemistry, 2, 833–842, https://doi.org/10.1021/acsearthspacechem.8b00069, 2018.
Chhantyal-Pun, R., Khan, M. A. H., Taatjes, C. A., Percival, C. J., Orr-Ewing, A. J., and Shallcross, D. E.: Criegee intermediates: production, detection and reactivity, Int. Rev. Phys. Chem., 39, 385–424, https://doi.org/10.1080/0144235X.2020.1792104, 2020.
Cox, R. A., Ammann, M., Crowley, J. N., Herrmann, H., Jenkin, M. E., McNeill, V. F., Mellouki, A., Troe, J., and Wallington, T. J.: Evaluated kinetic and photochemical data for atmospheric chemistry: Volume VII – Criegee intermediates, Atmos. Chem. Phys., 20, 13497–13519, https://doi.org/10.5194/acp-20-13497-2020, 2020.
Drozd, G. T. and Donahue, N. M.: Pressure Dependence of Stabilized Criegee Intermediate Formation from a Sequence of Alkenes, J. Phys. Chem. A, 115, 4381–4387, https://doi.org/10.1021/jp2001089, 2011.
Ehn, M., Kleist, E., Junninen, H., Petäjä, T., Lönn, G., Schobesberger, S., Dal Maso, M., Trimborn, A., Kulmala, M., Worsnop, D. R., Wahner, A., Wildt, J., and Mentel, Th. F.: Gas phase formation of extremely oxidized pinene reaction products in chamber and ambient air, Atmos. Chem. Phys., 12, 5113–5127, https://doi.org/10.5194/acp-12-5113-2012, 2012.
Ehn, M., Thornton, J. A., Kleist, E., Sipilä, M., Junninen, H., Pullinen, I., Springer, M., Rubach, F., Tillmann, R., and Lee, B.: A large source of low-volatility secondary organic aerosol, Nature, 506, 476–479, https://doi.org/10.1038/nature13032, 2014.
Elsamra, R. M., Jalan, A., Buras, Z. J., Middaugh, J. E., and Green, W. H.: Temperature-and pressure-dependent kinetics of CH2OO + CH3COCH3 and CH2OO + CH3CHO: direct measurements and theoretical analysis, Int. J. Chem. Kinet., 48, 474–488, https://doi.org/10.1002/kin.21007, 2016.
Faiola, C. L., Buchholz, A., Kari, E., Yli-Pirilä, P., Holopainen, J. K., Kivimäenpää, M., Miettinen, P., Worsnop, D. R., Lehtinen, K. E. J., Guenther, A. B., and Virtanen, A.: Terpene Composition Complexity Controls Secondary Organic Aerosol Yields from Scots Pine Volatile Emissions, Scientific Reports, 8, 3053, https://doi.org/10.1038/s41598-018-21045-1, 2018.
Gong, Y. and Chen, Z.: Quantification of the role of stabilized Criegee intermediates in the formation of aerosols in limonene ozonolysis, Atmos. Chem. Phys., 21, 813–829, https://doi.org/10.5194/acp-21-813-2021, 2021.
Gong, Y., Jiang, F., Li, Y., Leisner, T., and Saathoff, H.: Impact of temperature on the role of Criegee intermediates and peroxy radicals in dimer formation from β-pinene ozonolysis, Atmos. Chem. Phys., 24, 167–184, https://doi.org/10.5194/acp-24-167-2024, 2024.
Hakala, J. and Donahue, N. M.: Carbonyl Oxide Stabilization from Trans Alkene and Terpene Ozonolysis, J. Phys. Chem. A, 127, 8530–8543, https://doi.org/10.1021/acs.jpca.3c03650, 2023.
Hyttinen, N., Kupiainen-Määttä, O., Rissanen, M. P., Muuronen, M., Ehn, M., and Kurtén, T.: Modeling the Charging of Highly Oxidized Cyclohexene Ozonolysis Products Using Nitrate-Based Chemical Ionization, J. Phys. Chem. A, 119, 6339–6345, https://doi.org/10.1021/acs.jpca.5b01818, 2015.
Jokinen, T., Berndt, T., Makkonen, R., Kerminen, V. M., Junninen, H., Paasonen, P., Stratmann, F., Herrmann, H., Guenther, A. B., Worsnop, D. R., Kulmala, M., Ehn, M., and Sipila, M.: Production of extremely low volatile organic compounds from biogenic emissions: Measured yields and atmospheric implications, P. Natl. Acad. Sci. USA, 112, 7123–7128, https://doi.org/10.1073/pnas.1423977112, 2015.
Junninen, H.: Data cycle in atmospheric physics: From detected millivolts to understanding the atmosphere, PhD thesis, University of Helsinki, http://hdl.handle.net/10138/42512 (last access: 14 January 2014), 2014.
Junninen, H., Ehn, M., Petäjä, T., Luosujärvi, L., Kotiaho, T., Kostiainen, R., Rohner, U., Gonin, M., Fuhrer, K., Kulmala, M., and Worsnop, D. R.: A high-resolution mass spectrometer to measure atmospheric ion composition, Atmos. Meas. Tech., 3, 1039–1053, https://doi.org/10.5194/amt-3-1039-2010, 2010.
Kawamura, K., Ng, L. L., and Kaplan, I. R.: Determination of organic acids (C1–C10) in the atmosphere, motor exhausts, and engine oils, Environ. Sci. Technol., 19, 1082–1086, 1985.
Khan, M. A. H., Cooke, M. C., Utembe, S. R., Xiao, P., Morris, W. C., Derwent, R. G., Archibald, A. T., Jenkin, M. E., Percival, C. J., and Shallcross, D. E.: The global budgets of organic hydroperoxides for present and pre-industrial scenarios, Atmos. Environ., 110, 65–74, https://doi.org/10.1016/j.atmosenv.2015.03.045, 2015.
Khan, M. A. H., Percival, C. J., Caravan, R. L., Taatjes, C. A., and Shallcross, D. E.: Criegee intermediates and their impacts on the troposphere, Environmental Science: Processes Impacts, 20, 437–453, https://doi.org/10.1039/C7EM00585G, 2018.
Krechmer, J., Lopez-Hilfiker, F., Koss, A., Hutterli, M., Stoermer, C., Deming, B., Kimmel, J., Warneke, C., Holzinger, R., Jayne, J., Worsnop, D., Fuhrer, K., Gonin, M., and de Gouw, J.: Evaluation of a New Reagent-Ion Source and Focusing Ion-Molecule Reactor for Use in Proton-Transfer-Reaction Mass Spectrometry, Anal. Chem., 90, 12011–12018, https://doi.org/10.1021/acs.analchem.8b02641, 2018.
Lee, A., Goldstein, A. H., Kroll, J. H., Ng, N. L., Varutbangkul, V., Flagan, R. C., and Seinfeld, J. H.: Gas-phase products and secondary aerosol yields from the photooxidation of 16 different terpenes, J. Geophys. Res.-Atmos., 111, D17305, https://doi.org/10.1029/2006JD007050, 2006.
Lee, B. H., Lopez-Hilfiker, F. D., D'Ambro, E. L., Zhou, P., Boy, M., Petäjä, T., Hao, L., Virtanen, A., and Thornton, J. A.: Semi-volatile and highly oxygenated gaseous and particulate organic compounds observed above a boreal forest canopy, Atmos. Chem. Phys., 18, 11547–11562, https://doi.org/10.5194/acp-18-11547-2018, 2018.
Lester, M. I. and Klippenstein, S. J.: Unimolecular Decay of Criegee Intermediates to OH Radical Products: Prompt and Thermal Decay Processes, Accounts Chem. Res., 51, 978–985, https://doi.org/10.1021/acs.accounts.8b00077, 2018.
Lin, J. J.-M. and Chao, W.: Structure-dependent reactivity of Criegee intermediates studied with spectroscopic methods, Chem. Soc. Rev., 46, 7483–7497, https://doi.org/10.1039/c7cs00336f, 2017.
Long, B., Bao, J. L., and Truhlar, D. G.: Atmospheric Chemistry of Criegee Intermediates: Unimolecular Reactions and Reactions with Water, J. Am. Chem. Soc., 138, 14409–14422, https://doi.org/10.1021/jacs.6b08655, 2016.
Luo, Y., Garmash, O., Li, H., Graeffe, F., Praplan, A. P., Liikanen, A., Zhang, Y., Meder, M., Peräkylä, O., Peñuelas, J., Yáñez-Serrano, A. M., and Ehn, M.: Oxidation product characterization from ozonolysis of the diterpene ent-kaurene, Atmos. Chem. Phys., 22, 5619–5637, https://doi.org/10.5194/acp-22-5619-2022, 2022.
Millet, D. B., Baasandorj, M., Farmer, D. K., Thornton, J. A., Baumann, K., Brophy, P., Chaliyakunnel, S., de Gouw, J. A., Graus, M., Hu, L., Koss, A., Lee, B. H., Lopez-Hilfiker, F. D., Neuman, J. A., Paulot, F., Peischl, J., Pollack, I. B., Ryerson, T. B., Warneke, C., Williams, B. J., and Xu, J.: A large and ubiquitous source of atmospheric formic acid, Atmos. Chem. Phys., 15, 6283–6304, https://doi.org/10.5194/acp-15-6283-2015, 2015.
Neese, F.: Software update: The ORCA program system–Version 5.0, WIRES Comp. Mol. Sci., 12, e1606, https://doi.org/10.1002/wcms.1606, 2022.
Neese, F.: The SHARK integral generation and digestion system, J. Comput. Chem., 44, 381–396, https://doi.org/10.1002/jcc.26942, 2023.
Nguyen, T. L., Winterhalter, R., Moortgat, G., Kanawati, B., Peeters, J., and Vereecken, L.: The gas-phase ozonolysis of β-caryophyllene (C15H24). Part II: A theoretical study, Phys. Chem. Chem. Phys., 11, 4173–4183, https://doi.org/10.1039/b817913a, 2009.
Osborn, D. L. and Taatjes, C. A.: The physical chemistry of Criegee intermediates in the gas phase, Int. Rev. Phys. Chem., 34, 309–360, https://doi.org/10.1080/0144235X.2015.1055676, 2015.
Pracht, P., Grimme, S., Bannwarth, C., Bohle, F., Ehlert, S., Feldmann, G., Gorges, J., Müller, M., Neudecker, T., and Plett, C.: CREST – A program for the exploration of low-energy molecular chemical space, J. Chem. Phys., 160, 114110, https://doi.org/10.1063/5.0197592, 2024.
Richters, S., Herrmann, H., and Berndt, T.: Highly Oxidized RO2 Radicals and Consecutive Products from the Ozonolysis of Three Sesquiterpenes, Environ. Sci. Technol., 50, 2354–2362, https://doi.org/10.1021/acs.est.5b05321, 2016a.
Richters, S., Herrmann, H., and Berndt, T.: Different pathways of the formation of highly oxidized multifunctional organic compounds (HOMs) from the gas-phase ozonolysis of β-caryophyllene, Atmos. Chem. Phys., 16, 9831–9845, https://doi.org/10.5194/acp-16-9831-2016, 2016b.
Riva, M., Rantala, P., Krechmer, J. E., Peräkylä, O., Zhang, Y., Heikkinen, L., Garmash, O., Yan, C., Kulmala, M., Worsnop, D., and Ehn, M.: Evaluating the performance of five different chemical ionization techniques for detecting gaseous oxygenated organic species, Atmos. Meas. Tech., 12, 2403–2421, https://doi.org/10.5194/amt-12-2403-2019, 2019.
Saunders, S. M., Jenkin, M. E., Derwent, R. G., and Pilling, M. J.: Protocol for the development of the Master Chemical Mechanism, MCM v3 (Part A): tropospheric degradation of non-aromatic volatile organic compounds, Atmos. Chem. Phys., 3, 161–180, https://doi.org/10.5194/acp-3-161-2003, 2003.
Sheps, L., Scully, A. M., and Au, K.: UV absorption probing of the conformer-dependent reactivity of a Criegee intermediate CH3CHOO, Phys. Chem. Chem. Phys., 16, 26701–26706, https://doi.org/10.1039/c4cp04408h, 2014.
Sipilä, M., Jokinen, T., Berndt, T., Richters, S., Makkonen, R., Donahue, N. M., Mauldin III, R. L., Kurtén, T., Paasonen, P., Sarnela, N., Ehn, M., Junninen, H., Rissanen, M. P., Thornton, J., Stratmann, F., Herrmann, H., Worsnop, D. R., Kulmala, M., Kerminen, V.-M., and Petäjä, T.: Reactivity of stabilized Criegee intermediates (sCIs) from isoprene and monoterpene ozonolysis toward SO2 and organic acids, Atmos. Chem. Phys., 14, 12143–12153, https://doi.org/10.5194/acp-14-12143-2014, 2014.
Stavrakou, T., Müller, J. F., Peeters, J., Razavi, A., Clarisse, L., Clerbaux, C., Coheur, P. F., Hurtmans, D., De Mazière, M., Vigouroux, C., Deutscher, N. M., Griffith, D. W. T., Jones, N., and Paton-Walsh, C.: Satellite evidence for a large source of formic acid from boreal and tropical forests, Nat. Geosci., 5, 26–30, https://doi.org/10.1038/ngeo1354, 2012.
Taatjes, C. A., Welz, O., Eskola, A. J., Savee, J. D., Scheer, A. M., Shallcross, D. E., Rotavera, B., Lee, E. P. F., Dyke, J. M., Mok, D. K. W., Osborn, D. L., and Percival, C. J.: Direct Measurements of Conformer-Dependent Reactivity of the Criegee Intermediate CH3CHOO, Science, 340, 177–180, https://doi.org/10.1126/science.1234689, 2013.
Vansco, M. F., Caravan, R. L., Pandit, S., Zuraski, K., Winiberg, F. A., Au, K., Bhagde, T., Trongsiriwat, N., Walsh, P. J., and Osborn, D. L.: Formic acid catalyzed isomerization and adduct formation of an isoprene-derived Criegee intermediate: experiment and theory, Phys. Chem. Chem. Phys., 22, 26796–26805, https://doi.org/10.1039/D0CP05018K, 2020.
Vereecken, L.: The reaction of Criegee intermediates with acids and enols, Phys. Chem. Chem. Phys., 19, 28630–28640, https://doi.org/10.1039/C7CP05132H, 2017.
Vereecken, L. and Francisco, J. S.: Theoretical studies of atmospheric reaction mechanisms in the troposphere, Chem. Soc. Rev., 41, 6259–6293, https://doi.org/10.1039/C2CS35070J, 2012.
Vereecken, L., Harder, H., and Novelli, A.: The reactions of Criegee intermediates with alkenes, ozone, and carbonyl oxides, Phys. Chem. Chem. Phys., 16, 4039–4049, https://doi.org/10.1039/C3CP54514H, 2014.
Wang, M., Yao, L., Zheng, J., Wang, X., Chen, J., Yang, X., Worsnop, D. R., Donahue, N. M., and Wang, L.: Reactions of Atmospheric Particulate Stabilized Criegee Intermediates Lead to High-Molecular-Weight Aerosol Components, Environ. Sci. Technol., 50, 5702–5710, https://doi.org/10.1021/acs.est.6b02114, 2016.
Welz, O., Savee, J. D., Osborn, D. L., Vasu, S. S., Percival, C. J., Shallcross, D. E., and Taatjes, C. A.: Direct Kinetic Measurements of Criegee Intermediate (CH2OO) Formed by Reaction of CH2I with O2, Science, 335, 204–207, https://doi.org/10.1126/science.1213229, 2012.
Welz, O., Eskola, A. J., Sheps, L., Rotavera, B., Savee, J. D., Scheer, A. M., Osborn, D. L., Lowe, D., Murray Booth, A., and Xiao, P.: Rate coefficients of C1 and C2 Criegee intermediate reactions with formic and acetic acid near the collision limit: direct kinetics measurements and atmospheric implications, Angewandte Chemie International Edition, 53, 4547–4550, https://doi.org/10.1002/anie.201400964, 2014.
Winterhalter, R., Herrmann, F., Kanawati, B., Nguyen, T. L., Peeters, J., Vereecken, L., and Moortgat, G. K.: The gas-phase ozonolysis of β-caryophyllene (C15H24). Part I: an experimental study, Phys. Chem. Chem. Phys., 11, 4152–4172, https://doi.org/10.1039/b817824k, 2009.
Yan, C., Nie, W., Äijälä, M., Rissanen, M. P., Canagaratna, M. R., Massoli, P., Junninen, H., Jokinen, T., Sarnela, N., Häme, S. A. K., Schobesberger, S., Canonaco, F., Yao, L., Prévôt, A. S. H., Petäjä, T., Kulmala, M., Sipilä, M., Worsnop, D. R., and Ehn, M.: Source characterization of highly oxidized multifunctional compounds in a boreal forest environment using positive matrix factorization, Atmos. Chem. Phys., 16, 12715–12731, https://doi.org/10.5194/acp-16-12715-2016, 2016.
Yang, W., Cao, J., Wu, Y., Kong, F., and Li, L.: Review on plant terpenoid emissions worldwide and in China, Sci. Total Environ., 787, 147454, https://doi.org/10.1016/j.scitotenv.2021.147454, 2021.
Yao, L., Ma, Y., Wang, L., Zheng, J., Khalizov, A., Chen, M., Zhou, Y., Qi, L., and Cui, F.: Role of stabilized Criegee Intermediate in secondary organic aerosol formation from the ozonolysis of α-cedrene, Atmos. Environ., 94, 448–457, https://doi.org/10.1016/j.atmosenv.2014.05.063, 2014.
Yokouchi, Y. and Ambe, Y.: Aerosols formed from the chemical reaction of monoterpenes and ozone, Atmos. Environ., 19, 1271–1276, https://doi.org/10.1016/0004-6981(85)90257-4, 1985.
Zhang, D. and Zhang, R.: Ozonolysis of α-pinene and β-pinene: kinetics and mechanism, J. Chem. Phys., 122, 114308, https://doi.org/10.1063/1.1862616, 2005.