the Creative Commons Attribution 4.0 License.
the Creative Commons Attribution 4.0 License.
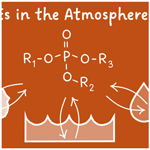
Seasonal air concentration variability, gas–particle partitioning, precipitation scavenging, and air–water equilibrium of organophosphate esters in southern Canada
Yuening Li
Faqiang Zhan
Chubashini Shunthirasingham
Ying Duan Lei
Jenny Oh
Amina Ben Chaaben
Kelsey Lee
Frank A. P. C. Gobas
Hayley Hung
In response to increasing production and application volumes, organophosphate esters (OPEs) have emerged as pervasively detected contaminants in various environmental media, with concentrations often exceeding those of traditional organic contaminants. Despite the recognition of the atmosphere's important role in dispersing OPEs and a substantial number of studies quantifying OPEs in air, investigations into atmospheric phase distribution processes are rare. Using measurements of OPEs in the atmospheric gas and particle phase, in precipitation, and in surface water collected in southern Canada, we explored the seasonal concentration variability, gas–particle partitioning behaviour, precipitation scavenging, and air–water equilibrium status of OPEs. Whereas consistent seasonal trends were not observed for OPE concentrations in precipitation or atmospheric particles, gas phase concentrations of several OPEs were elevated during the summer in suburban Toronto and at two remote sites on Canada's eastern and western coast. Apparent enthalpies of air–surface exchange fell mainly within or slightly above the range of air–water and air–octanol enthalpies of exchange, indicating the influence of local air–surface exchange processes and/or seasonally variable source strength. While many OPEs were present with a notable fraction in both the gas and particle phase, no clear relationship with compound volatility was apparent, although there was a tendency for higher particle-bound fractions at a lower temperature. High precipitation scavenging ratios for OPEs measured at the two coastal sites are consistent with low air–water partitioning ratios and the association with particles. Although beset by large uncertainties, air–water equilibrium calculations suggest net deposition of gaseous OPEs from the atmosphere to the Salish Sea and the St. Lawrence River and St. Lawrence Estuary. The measured seasonal concentration variability is likely less a reflection of temperature-driven air–surface exchange and instead indicates that more OPEs enter or are formed in the atmosphere in summer. More research is needed to better understand the atmospheric gas–particle partitioning behaviour of the OPEs and how it may be influenced by transformation reactions.
- Article
(2885 KB) - Full-text XML
-
Supplement
(3001 KB) - BibTeX
- EndNote
Organophosphate esters (OPEs) are synthetic organophosphorus compounds consisting of a central phosphate molecule substituted with nonhalogenated and halogenated alkyl or aryl groups. Widely used as flame retardants, plasticizers, stabilizers, and defoaming agents in various industries and consumer products (Environment and Climate Change Canada, 2023a, b, c, d; Salamova et al., 2016; van der Veen and de Boer, 2012), OPEs are typically physically incorporated into materials rather than chemically bonded (Wang et al., 2020b; Wong et al., 2018), facilitating their release into the environment. Following restrictions on many brominated flame retardants, e.g. through listing in the Stockholm Convention, OPE use has increased, reaching 620 kt globally in 2013, accounting for 30 % of total flame retardant usage (Sühring et al., 2016; Xie et al., 2022). The extensive application of OPEs, coupled with their potential for long-range atmospheric transport (Na et al., 2020; Sühring et al., 2016) and persistence (Möller et al., 2012; Salamova et al., 2014), has resulted in their ubiquitous presence in the environment (Han et al., 2020; Li et al., 2019a, b; Lu et al., 2017; Mi et al., 2023; Regnery and Püttmann, 2009; Stackelberg et al., 2007), often at concentrations exceeding those of traditional flame retardants and plasticizers (Salamova et al., 2014; Shoeib et al., 2014; Zhao et al., 2021b). Given their potential toxicity (Gu et al., 2019; Li et al., 2020; Rosenmai et al., 2021; Wang et al., 2022; Yan and Hales, 2019, 2020), understanding the fate, occurrence, and distribution of OPEs in the environment is critical for assessing their ecological and human health impacts.
The atmosphere plays a key role in the dispersion and transport of OPEs, with concentrations and spatial and temporal variability in air being influenced by emission sources, atmospheric transport, chemical transformation (Liu et al., 2023; Liu and Mabury, 2019), and deposition processes. The distribution of OPEs between different atmospheric phases (gas phase, particles, precipitation) affects these processes and is influenced by their partition properties. Most studies on OPEs in the atmosphere report concentrations in the particle phase, whereas studies on the presence in the gas phase are far more limited, which may be related to the relatively short half-lives of gas phase OPEs (Shi et al., 2024; Zhang et al., 2016). However, gaseous OPEs can constitute 15 % to 65 % of atmospheric OPEs (Möller et al., 2011), and diffusive air–water gas exchange of OPEs can be 2–3 orders of magnitude higher than dry particle deposition (Castro-Jiménez et al., 2016; Ma et al., 2021), highlighting the need for more research on OPE vapours.
Precipitation acts as a major pathway for the removal and redistribution of OPEs from the atmosphere to aquatic and terrestrial environments (Shi et al., 2024). It can scavenge and deposit both gas phase and particle-bound OPEs. Depending on regional emissions, temperature, precipitation type, and the physicochemical properties of the OPEs (Lei and Wania, 2004), the wet deposition flux of OPEs can be significantly larger than the dry deposition flux (Kim and Kannan, 2018). Despite its importance, fewer than 10 studies have reported OPE concentrations in precipitation (Bacaloni et al., 2008; Casas et al., 2021; Fries and Püttmann, 2003; Kim and Kannan, 2018; Marklund et al., 2005b; Mihajlović and Fries, 2012; Regnery and Püttmann, 2009; Zhang et al., 2020), and only one study has reported precipitation scavenging ratios for atmospheric OPEs (Casas et al., 2021).
OPEs can enter waterbodies through air–water gas exchange (Castro-Jiménez et al., 2016; Ma et al., 2021), wet and dry deposition (Castro-Jiménez et al., 2016; Kim and Kannan, 2018; Ma et al., 2021), wastewater effluent (Marklund et al., 2005a), industrial and municipal discharges (Bacaloni et al., 2008; Fries and Püttmann, 2003), and surface runoff (Awonaike et al., 2021; Regnery and Püttmann, 2010). Some OPEs, including tris (1-chloro-2-propyl) phosphate (TCPP) and tris (phenyl) phosphate (TPhP), have been detected in fish (Ma et al., 2013; Sundkvist et al., 2010). A comprehensive understanding of the environmental fate and occurrence of OPEs, in particular a better understanding of the contribution that the atmosphere makes for the delivery of OPEs to aquatic ecosystems, would benefit from investigations that quantify OPE concentrations in multiple environmental media sampled in the same area and at the same time. Despite the substantial number of studies on OPEs in the environment, those examining OPEs across three or more phases are very rare (He et al., 2019; Li et al., 2019b; Mi et al., 2023) and most studies focus on just one or two media, usually gas and/or particle phases (Li et al., 2018; Ma et al., 2022; Sühring et al., 2016; Zhao et al., 2021b) or the water phase (Choo and Oh, 2020; Ding et al., 2015; McDonough et al., 2018; Shi et al., 2020). No previous study has investigated OPEs in atmospheric gas and particle phases, precipitation, and surface water simultaneously.
To address this research gap and gather information on the contribution that the atmosphere makes to OPEs in coastal waters of southern Canada, we aimed to characterize the occurrence, behaviour, and fate of OPEs in different atmospheric phases. We measured OPEs in precipitation and the atmospheric gas and particle phase for 1 year at two remote sites on Canada's eastern and western coast, respectively, and complemented this dataset with the results of a year-long measurement campaign of OPEs in the gas and particle phase in Toronto. We further used passive samplers to gather data on the spatial variability in OPE concentrations in the atmospheric gas phase and in water in the two coastal regions. The data from passive air sampling have been presented previously (Li et al., 2025). This unique dataset allowed us to estimate the gas–particle distribution in the atmosphere, precipitation scavenging ratios, and the state of air–surface water equilibrium, often in their seasonal dependence or their variability between urban, rural, and remote locales. Finally, we used this dataset to explore the relative abundance of OPEs in the different types of samples.
2.1 Active air sampling and precipitation collection
Twelve 24 h air samples were collected monthly at a location on Saturna Island, British Columbia (BC) (48.7753° N, −123.1283° W), between December 2019 and November 2020 and in the vicinity of Tadoussac, Quebec (QC) (48.1415° N, −69.6991° W), between December 2020 and November 2021 using high-volume active air samplers (AASs) consisting of a Tisch sampling head (non-Teflon TE-1002 with a TE-1009 glass cartridge and a TE-1008-5 special silicone gasket, Pacwill Environmental, Ontario, Canada) and a high-volume pump (Gast R1102 regenerative blower, Cole-Parmer, Illinois, USA). Forty-eight consecutive week-long active air samples were taken with the same sampling head assembly and a mid-volume pump (AMETEK DFS 116643-03 centrifugal blower, RS, Texas, USA) in the eastern suburbs of Toronto (43.78371° N, −79.19027° W) (Li et al., 2023a, b, 2024) between June 2020 and May 2021. At all three sites, polyurethane foam (PUF)–XAD–PUF sandwiches and glass fiber filters (GFFs; CA28150-214, A/E, 102 mm diameter from VWR) were used to collect OPEs in the gas and particle phase, respectively. The XAD-2 was Supelpak™-2 polymeric adsorbent (21130-U, MilliporeSigma), and the PUF was a 7.62 cm TE-1010 (Pacwill Environmental, cut into a 2.54 cm top PUF and a 5.08 cm bottom PUF). Using the sampler described by Chan and Perkins (1989), precipitation (PCPN) samples were collected at the active air sampling locations in BC and QC during the same months as the air samples and the sampling length was ∼ 30 d (Oh et al., 2023; Zhan et al., 2023).
2.2 Passive air and water sampling
In QC, 86 passive air samplers (PASs) were deployed at 71 unique sampling sites on either shore of the St. Lawrence River and St. Lawrence Estuary, including in Montréal and Québec between 2019 and 2022. In BC, 83 PASs were deployed at 47 sites in the lower mainland around Vancouver and on the Canadian shore of the Salish Sea during different time periods between 2020 and 2022. More details are given in Table S5 in the Supplement of Li et al. (2025) and in previous publications (Oh et al., 2023; Zhan et al., 2023).
Forty-eight low-density-polyethylene-based (LDPE-based) passive water samplers (PWSs) were spiked with performance reference compounds, deployed at 10 sites in BC and 10 sites in QC, and collected after deployment lasting 20–35 d in BC and 27–70 d in QC. Detailed information on the passive water sampling is provided in the Supplement (Table S13) and previous publications (Oh et al., 2023; Zhan et al., 2023).
2.3 Sample analysis
Prior to extraction all samples were spiked with seven isotopically labelled OPEs (Table S1) as surrogates. XAD from the PASs and the PUF–XAD sandwiches and GFFs from the AASs were extracted using a Dionex Accelerated Solvent Extractor 350. The PCPN and PWSs were extracted using liquid–liquid extraction with dichloromethane and soaking in hexane, respectively. Extracts were concentrated to 0.5 mL using a rotary evaporator and nitrogen blow-down. Triamyl phosphate was added into the concentrated extracts as an injection standard. Gas chromatography–tandem mass spectrometry (GC-MS/MS) was used to detect and quantify 16 OPEs (Tables S1 and S2). Molecular structures of these 16 targeted OPEs are provided in Fig. S1.
2.4 Quality assurance and quality control
All extraction and concentration procedures were carried out in a trace analytical laboratory. The glassware was cleaned with detergents in a dishwasher, then rinsed with deionized water, and finally baked with GFFs at 450 °C in a muffle furnace for 24 h. Experimental materials that came into contact with samples or extracts were thoroughly cleaned and rinsed three times with solvents (acetone and hexane or dichloromethane) before use. Field blanks, procedure blanks, and solvent blanks were prepared with each batch of extractions and analyses (Oh et al., 2023; Zhan et al., 2023). OPEs were not found in procedure or solvent blanks. Only a few analytes were present in the field blanks, and for these, the average detected amount was subtracted from the amounts of target chemicals in the field samples. Method detection limits (MDLs) were calculated as 3 times the standard deviations of levels in field blanks when analytes were detected (signal-to-noise ratio () > 3); otherwise, MDLs were based on concentrations at which is 3 (Desimoni and Brunetti, 2015). MDLs are provided in the Supplement (Tables S5, S8, S10, S11, and S13). The average recoveries of five surrogates in AASs, PCPN samples, and PWSs ranged from 78 % to 232 % (Table S3). The concentrations reported have been corrected for recovery.
2.5 Data analysis
The water concentration of OPEs was calculated from the amounts quantified in PWS extracts following the method by Booij et al. (2003) and Booij and Smedes (2010), with details provided by Oh et al. (2023).
The fraction of an OPE in the particle phase (Φ, %) was obtained by dividing the particle phase concentration by the sum of concentrations in the gas and particle phase. Gas–particle partition ratios KPA (m3 air g−1 aerosol) were derived by dividing the measured concentrations of an OPE in the particle phase (pg m−3) by the product of the concentrations of particles less than 2.5 µm in diameter (PM2.5, g m−3) obtained from nearby national air pollution surveillance program (NAPS) stations (Table S8) and the measured concentrations of this OPE in the gas phase (pg m−3). More detail is given in previous publications (Li et al., 2023a; Oh et al., 2023; Zhan et al., 2023).
Measured scavenging ratios (SRs) were calculated as the ratios between the concentrations of an OPE in precipitation and air (sum of the gas and particle phase). We also estimated SRs by assuming OPE equilibrium between the atmospheric gas phase and water droplets (Oh et al., 2023) and that all OPEs are sorbed to the same particles, which are scavenged with a scavenging ratio WP of 200 000 (Kim et al., 2006). An estimated SR thus is , where KWA is the temperature-adjusted partition ratio between water and air (, Table S4).
The fugacities of OPEs in water fW, at average sea surface temperature TW (in K), were calculated using , and those in air (fA), at average air temperature TA (in K), were derived with , where CW and CA are the OPE concentrations (mol m−3) in water and air, respectively, and R is the gas constant.
3.1 OPEs in the atmospheric gas phase
The gas phase concentrations obtained during the three 1-year active air sampling campaigns in Tadoussac, on Saturna Island, and in Toronto are given in Table S5. The gas phase concentrations obtained by passive air sampling in QC and BC have been previously reported (Li et al., 2025), with tri-n-butyl phosphate (TBP), tris (2-chloroethyl) phosphate (TCEP), TCPP, and TPhP being reliably and ubiquitously detected. Due to the higher sampling volumes of the active air samples (∼ 520 m3) compared to the PASs (less than 200 m3), more OPEs could be detected above the MDLs in the active air samples. At all three locations, TBP, TCEP, TCPP, TPhP, and 2-ethylhexyl-diphenyl phosphate (EHDPP) were present above the MDLs. Additionally, triethyl phosphate (TEP) was detected on Saturna Island; TEP, tris (1,3-dichloro-2-propyl) phosphate (TDCPP), and tris (2-butoxyethyl) phosphate (TBEP) were detected in Tadoussac; and tripropyl phosphate (TPrP) and TDCPP were detected in Toronto. We are not comparing the gas phase concentrations recorded in our study with those reported previously here because that had already been done in Li et al. (2025).
For the four most frequently detected OPEs, it is possible to compare the levels obtained with the AASs on Saturna Island and in Tadoussac and by PASs at the nearby sites L43 and S57. On Saturna Island, the PAS deployment at site L43 overlapped with the time frame of the AASs (between May and October 2020) (Table S8). In Tadoussac, the deployment period of the PAS at S57 (November 2019–August 2020) preceded the active air sampling by about 1 year (December 2020–September 2021). Except for TBP and TPhP on Saturna Island, PAS levels generally trended lower than AAS levels at both locations, albeit within a factor of 5. One contributing factor to this difference could be the episodic 24 h active air sampling's inability to represent long-term concentration levels compared to PASs. For instance, AAS-measured concentrations of TBP in Tadoussac ranged from below the detection limit to approximately 200 pg m−3. Another factor could be the spatial-distribution variability in atmospheric OPEs. Despite our efforts to use PAS data from sites closest to AAS locations for comparison, the PAS and AAS sampling sites were not identical. To support the hypothesis that spatial and temporal variability in OPE concentrations contributes to the discrepancy, we also compared AAS and PAS results for hexachlorobutadiene and hexachlorobenzene, which exhibit uniform spatial distribution and consistent concentrations over time, using the same samples as for the OPEs. PAS levels for these two compounds closely aligned with AAS levels within a factor of 1.5. Similarly, halomethoxybenzene levels from PASs and AASs were within a factor of 3 (Zhan et al., 2023).
3.2 OPEs in atmospheric particle phase
The concentrations of five OPEs (TBP, TCEP, TCPP, TPhP, EHDPP) in the atmospheric particles from the three active air sampling locations are compiled in Table S9. Except for TPhP and EHDPP, which were not detected in particles from Saturna Island, all five OPEs most frequently detected in the gas phase could also be quantified in particle samples. Again, TCPP is the most abundant OPE at all three sites.
Concentration levels on Saturna Island and in suburban Toronto are similar and higher by almost 1 order of magnitude than those in Tadoussac. The average TBP levels of 7, 3, and 9 pg m−3 on Saturna Island, in Tadoussac, and in Toronto are lower than those in Antarctica (23 pg m−3) (Wang et al., 2020a) and lower by 2 orders of magnitude than those detected in cities in the Great Lakes area (130 pg m−3) in 2012 (Salamova et al., 2013). Except in Tadoussac (1 pg m−3), the TCEP levels of 50 and 17 pg m−3 on Saturna Island and in Toronto are higher than those in Antarctica (5 pg m−3) (Wang et al., 2020a) and ca. 2–4 times lower than those detected in cities in the Great Lakes region (89 pg m−3) (Salamova et al., 2013) and lower by 2 orders of magnitude than the reported median concentration in Québec and near the St. Lawrence River (1903 pg m−3) (Sühring et al., 2016). TCPP in Tadoussac, at 3 pg m−3, is comparable to its level in Antarctica (6 pg m−3) (Wang et al., 2020a), and TCPP on Saturna Island (122 pg m−3) and in Toronto (90 pg m−3) is 3 and 4 times lower than the detected level in cities in the Great Lakes area (321 pg m−3) (Salamova et al., 2013) and lower by 1 order of magnitude than the detected level in Québec and near the St. Lawrence River (1557 pg m−3) (Sühring et al., 2016). TPhP and EHDPP levels in Antarctica (1 pg m−3) (Wang et al., 2020a) are close to those in Tadoussac (2 pg m−3) and lower by 1 order of magnitude than levels in Toronto (12 pg m−3) and those in Québec and near the St. Lawrence River (51 pg m−3) (Sühring et al., 2016). The relatively higher concentration levels of certain OPEs in Antarctica, such as TBP, may be due to preferential partitioning of TBP to particles at low temperatures. Compared to the sites in the Great Lakes region (Salamova et al., 2013) as well as Québec and near the St. Lawrence River region (Sühring et al., 2016), our sampling sites were more rural, which could explain lower OPE concentrations.
3.3 OPEs in precipitation
Eight OPEs, i.e. TEP, TBP, TCEP, TCPP, TDCPP, TPhP, TBEP, and EHDPP, were reliably detected in the PCPN samples from Saturna Island and Tadoussac (Tables 1 and S11). Concentrations are generally higher on Saturna Island than in Tadoussac. The OPE levels in Tadoussac were comparable to those in Antarctica (Casas et al., 2021). TDCPP detected on Saturna Island and in Tadoussac is higher by 2 times to 1 order of magnitude than the levels in Antarctica (Casas et al., 2021), Nanjing (Zhang et al., 2020), and New York (Kim and Kannan, 2018), and our measured EHDPP levels were higher than those detected in Antarctica. Overall, except for TDCPP and EHDPP, the average OPE concentrations detected in our study were comparable or lower by 1 order of magnitude than literature data (Table 1). Except for TBP in Tadoussac, OPE concentrations varied greatly between months, whereby no distinct and consistent seasonal trends were discernible (Table S11), which is consistent with previous observations (Regnery and Püttmann, 2009).
Table 1Summary of levels of OPEs in precipitation reported in the literature and our study. NA: not available.
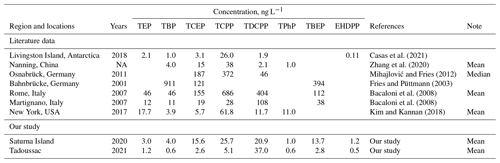
The concentrations of OPEs in snow and rainwater samples from five locations in Germany during 2007–2008 were reported (Regnery and Püttmann, 2009). However, as we could not calculate the average OPE concentrations in precipitation, we did not include these data in this table.
Marklund et al. (2005b) reported the concentrations of OPEs in combined dry and wet deposition samples, as there are no data for precipitation samples; therefore, these data were not included in this table either.
3.4 OPEs in water
The OPE concentrations in water, obtained with PWSs deployed in summer 2021, are reported in Table S13. Their spatial patterns are displayed in Figs. 1, S2, and S3. In BC, OPEs had elevated levels in the interior of Burrard Inlet close to Port Moody (V1 and V2), at the southern mouth of the Fraser River (V5), and at some sites around populated areas in Victoria, BC (V6–V8). TBP, TCPP, and TDCPP had higher concentrations close to an industrial area near Esquimalt (V10). In QC, the highest OPE water concentrations were usually detected at site W5, in the St. Lawrence River close to an industrial area in Sorel-Tracy, rather than at sites in Montréal (W1 and W2) or Québec (W8 and W9). W4 also had elevated concentrations for some OPEs such as TBP. Water concentrations at the one sampling site in the St. Lawrence Estuary were much lower than in the river. Overall, the spatial patterns suggest that the water concentrations of OPEs were related to both industrial activities and human populations in BC, whereas industrial activities might have a relatively higher impact on water concentrations of OPEs in QC.
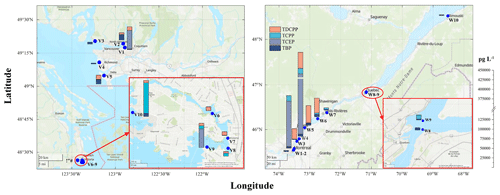
Figure 1Spatial patterns of OPEs in the water in British Columbia (left panel) and Quebec (right panel). The inserted maps at the bottom right of each panel show the sampling sites located within Victoria (left panel) and Québec (right panel). Concentrations in duplicate samples were averaged. The stacked bars indicate the total concentration levels of all detected OPEs and the individual OPE. Various colours are used for different OPEs. The dispersion plume of the Montréal wastewater treatment plant enters the river at 45°40′ N, 73°28′ W and stays on the north side of the river (Marcogliese et al., 2015); therefore, the OPEs in the dispersion plume might not be sampled at W3 and W4. The concentration scale is shown to the right of the maps, which were created using the basemap of MATLAB (copyright Esri, TomTom, Garmin, SafeGraph, GeoTechnologies, Inc, METI/NASA, USGS, Bureau of Land Management, EPA, NPS, US Census Bureau, USDA, USFWS, NRCan, and Parks Canada).
4.1 Seasonality and temperature dependence
Clear and consistent seasonal trends were not observed for particle-bound OPEs at any location during the 1 year of sampling (Table S9). However, OPE gas phase concentrations at all three active air sampling sites clearly varied seasonally, allowing us to investigate the influence of temperature on those concentrations (Fig. 2). Except for EHDPP and TDCPP, concentrations of frequently detected OPEs increased with increasing ambient temperature. The logarithm of the partial pressures of OPEs (ln p) were linearly regressed against the reciprocal of absolute temperatures () (Clausius–Clapeyron (CC) relationship), with the slopes, R2 values, and p values summarized in Table S7. Regressions for TBP were significant at the three AAS sites (p < 0.05), whereas EHDPP did not show significant relationships at any site. The CC relationships for other OPEs were only significant (p < 0.05) at some locations, i.e. TCEP and TCPP on Saturna Island; TEP and TPhP in Tadoussac; and TPrP, TCPP, TCEP, and TDCPP in Toronto. In cases with R2 > 0.10, the trends indicate higher partial pressures at higher temperatures.
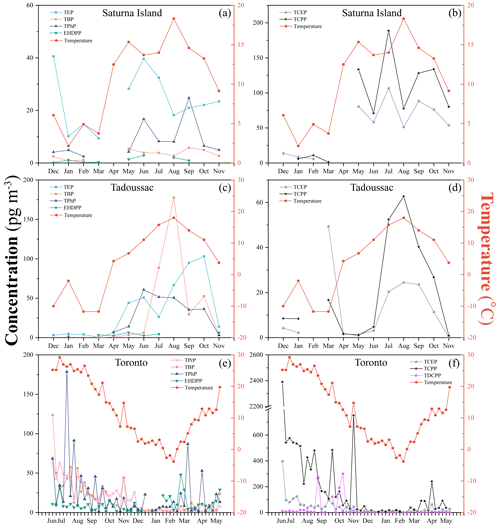
Figure 2Seasonal variability in ambient temperature (right y axis) and gas phase concentrations of OPEs (left y axis) in the atmosphere of (a, b) Saturna Island, British Columbia (2019–2020); (c, d) Tadoussac, Quebec (2020–2021); and (e, f) Toronto, Ontario (2020–2021). Only data for OPEs with a detection frequency higher than 50 % are shown.
Apparent enthalpies of air–surface exchange (ΔHAS-app) were obtained from the slopes of the CC relationships with R2 > 0.30 and p < 0.05 and compared with enthalpies of exchange between air and water (ΔHAW) and between air and octanol (ΔHAO), estimated using poly-parameter linear free energy relationships (UFZ-LSER database v 3.2.1, 2024) (Table S7). Values of ΔHAS-app that are similar to ΔHAW and ΔHAO have been interpreted as being indicative of a dominant contribution of temperature-driven local air–surface exchanges on the air concentration at a site (Bidleman et al., 2023; Wania et al., 1998; Zhan et al., 2023). If ΔHAS-app is much lower than ΔHAW and ΔHAO, advection from elsewhere is presumed to play a more important role. ΔHAS-app values of OPEs at the three sampling sites were mostly within the uncertainty range of ΔHAW and ΔHAO. In several instances the temperature dependence of air concentrations was even larger than might be expected from air–surface equilibrium; i.e. ΔHAS-app was larger than ΔHAW and ΔHAO. Examples are the ΔHAS-app values of TCPP on Saturna Island and in Toronto; TBP and TPhP in Tadoussac; and, to a lesser extent, TCEP on Saturna Island and in Toronto and TEP in Tadoussac.
This may simply be a result of high uncertainty, considering the relatively small number of samples available for deriving the CC relationships for Saturna Island and Tadoussac. It could also suggest that temperature influences not only the exchange between air and surface but also the OPE source strength to the atmosphere. This source strength could be correlated with temperature, e.g. because of enhanced release of OPEs from materials at higher temperatures or higher indoor–outdoor exchange rates in summer. Furthermore, the formation of TCPP, TCEP, and TPhP from precursor compounds, i.e. tris (2-chloroisopropyl) phosphite (TCPPi), tris (2-chloroethyl) phosphite (TCEPi), and triphenyl phosphite (TPhPi), by reaction with ozone could be higher in summer (Liu et al., 2023; Liu and Mabury, 2019; Turygin et al., 2018; Zhang et al., 2021), when photooxidant concentrations tend to be higher. Even though TCPP is widely used in large quantities, the spatial distribution and usage of its precursor TCPPi has not been reported. The high ΔHAS-app of TEP and TBP in Tadoussac may also be related to the conversion of their corresponding phosphite esters. The value of the measured ΔHAS-app may potentially contain information on the contribution of the transformation of organophosphite antioxidants to OPEs in the atmosphere; i.e. the extent to which ΔHAS-app exceeds ΔHAW and ΔHAO may indicate the extent of such a transformation. However, this would be beset by high uncertainties considering the complex set of factors influencing ΔHAS-app.
Incidentally, at 11 sites in BC, where PASs were deployed at least three times during different seasons with different average temperatures, higher OPE concentrations were generally also observed during warmer deployments (Tables S6 and S5 in the Supplement of Li et al., 2025). Of the 55 CC relationships using these PAS data 33 had R2 > 0.5, and 27 of these 33 were negative (Table S6). Considering the limited number of data points (three to four) for PAS sites with multiple deployments in different seasons, ΔHAS-app values may have high uncertainties (Table S6) and were therefore not compared with theoretical values.
4.2 Gas–particle partitioning
The fraction of the OPEs in the particle phase (Φ, %) is given in Table S9. As more than 50 % of Φ values for TPhP and EHDPP in Tadoussac were calculated using values below the MDLs, these data are not discussed further. Overall, Φ ranged between 32 % and 68 % and varied between OPEs and location. Among the five OPEs, the Φ of TCEP is the smallest at almost all three AAS sites. The Φ values for TBP and TCPP on Saturna Island (both ca. 66 %) are 12 % higher than that for TCEP (54 %). In Tadoussac, the Φ for TCPP (38 %) is comparable to that for TCEP (34 %), whereas the Φ for TBP (52 %) is the highest among the three OPEs. In Toronto, the Φ values of the five major OPEs were in the sequence of TCEP (50 %) = TBP (50 %) < TPhP (54 %) < TCPP (56 %) < EHDPP (68 %). This sequence is opposite to that found above the North Atlantic and in the Arctic (Wu et al., 2020).
Theoretically, TCEP, TCPP, and TBP have very similar volatility with logarithmic equilibrium partition ratios between octanol and air (log KOA) around 9 and a log (KPA, m3 g−1) of ∼ 1 at 15 °C estimated using the UFZ-LSER website (UFZ-LSER database v 3.2.1, 2024) (Table S9). These three chemicals are expected to be largely in the gas phase at ambient temperatures. TPhP and EHDPP have estimated values for log KOA of > 12 and log (KPA, m3 g−1) of ∼ 5 at 15 °C, which would indicate strong particle sorption in the atmosphere. However, the unexpectedly low fraction observed in the particle phase may suggest that TPhP and EHDPP are emitted at higher temperatures and are not in a state of equilibrium between the gas and particle phase (Zhao et al., 2021a). Alternatively, the fraction of TPhP and EHDPP in the gas phase may have been overestimated if very fine and ultrafine particles containing these OPEs passed through the glass fiber filters (Zhao et al., 2021b). While it has been suggested that the composition of the particles (Li et al., 2017), relative humidity (Storey et al., 1995; Li et al., 2017; Wu et al., 2020), and degradation of OPEs in the gas and particle phase may also influence the gas–particle partitioning of OPEs, we do not have the empirical data to explore the influence of these factors on our measurements.
The calculated Φ at the three AAS sites increases with decreasing ambient temperatures. This is consistent with lower temperatures favouring partitioning to particles (Table S9). This is also reflected in the positive linear relationships between ln KPA and the reciprocal temperature (in K) in Tadoussac, on Saturna Island, and in Toronto (Table S10).
4.3 Scavenging ratios
Measured SRs could be calculated for eight OPEs and ranged mainly from 104 to 107 (Table S12). These SRs are highly uncertain because of the uncertainty in the measured concentrations and because we combine a monthly precipitation sample with a 24 h air sample taken during the same month. The estimated SRs are also uncertain due to the possibly high uncertainty in the estimated KWA and the assumptions regarding equilibrium partitioning of OPE vapours between air and water droplets and the value and constancy of WP. Despite these uncertainties, estimated SRs for TBP and EHDPP are generally around 105 and therefore comparable to the measured ones, which indicates that equilibrium between precipitation and these chemicals in the atmosphere was achieved. The estimated SRs for other OPEs are mostly within the range of 2 × 106 to 109 and therefore higher by orders of magnitude than the measured SRs. At very high values, exceeding a threshold of ∼ 106, the SR concept loses its usefulness because the atmosphere will essentially be cleansed of such compounds at the onset of a precipitation event and subsequent precipitation will simply dilute the concentrations (Lei and Wania, 2004). As such, measured SRs that are smaller than these very high estimated ones are not too surprising.
4.4 Diffusive air–water gas exchange
The air–water equilibrium status was evaluated using fugacity ratios (), whereby values lower (higher) than 1 indicate a tendency for net deposition (volatilization). The estimated fugacity ratios for five OPEs (TBP, TCEP, TCPP, TPhP, and EHDPP) are given in Table S14. This estimation of incurs substantial uncertainty because of uncertainty in KAW and the passive sampling rates and because it involves combining air and water data obtained during different time periods (Oh et al., 2023; Zhan et al., 2023). Nevertheless, the values in BC and QC were so far below unity that one can confidently assert that all five OPEs were net-deposited from atmosphere to water. Ma et al. (2021) also reported that almost all five OPEs, except TBP, underwent net gas phase deposition in the lower Great Lakes region.
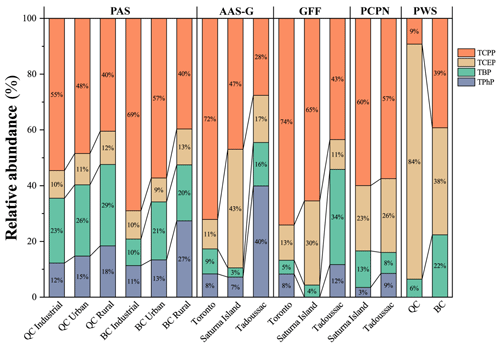
Figure 3The relative abundance of four frequently detected OPEs in the passive air samplers (PASs), gas phase active air samples (AAS-Gs), glass fiber filter (GFF) samples, precipitation (PCPN) samples, and passive water samplers (PWSs) in QC and BC. Due to the large concentration ranges, the geomean was used for calculating the relative abundance for PASs.
4.5 Relative abundance of OPEs in different environmental media
The frequent detection of TCPP, TCEP, TBP, and TPhP in PASs, the gas and particle phase of the AAS, PWSs, and PCPN samples in QC and BC, allows us to investigate the relative abundance of these OPEs in different environmental media (Fig. 3). Chlorinated compounds (TCPP and TCEP) were dominant in all environmental media regardless of sampling location, which is consistent with observations in gaseous and aqueous phases in the Great Lakes region (Ma et al., 2021). Specifically, TCPP was the most abundant of the four OPEs in all types of samples, except for the gas phase in Tadoussac and the PWS. By reporting the relative abundance of the OPEs in PASs separately for industrial, urban, and rural sites, we find a consistent pattern in both QC and BC, namely that the relative abundance of halogenated OPEs (TCPP and TCEP) decreased from industrial (65 % in QC and 79 % in BC) to urban (59 % in QC and 63 % in BC) to rural sites (52 % in QC and 53 % in BC) with a concomitant increase of two nonhalogenated OPEs (TBP and TPhP). This is consistent with previous studies (Kurt-Karakus et al., 2018; Zhang et al., 2019) but contrasts with the predominance of TCPP and TCEP reported for Antarctic air (Wang et al., 2020a). The higher abundance of TPhP at rural sites would be consistent with a relatively higher long-range transport potential (LRTP) estimated with the improved Organisation for Economic Co-operation and Development (OECD) Screening Tool for overall persistence (Pov) and long-range transport potential (LRTP) (OECD Tool) (Breivik et al., 2022) (Table S15). Even though the observed higher abundance of TBP in remote areas is inconsistent with its relatively low estimated LRTP, Sühring et al. (2020) indicated that non-chlorinated OPEs could be subject to LRTP.
The relative abundance of OPEs in the gas and particle phase at the three AAS sites was similar. Mirroring the observation in the PASs, the relative contribution of chlorinated OPEs was higher in urban and industrial Toronto than on rural Saturna Island and in remote Tadoussac. Chen et al. (2019) and Salamova et al. (2013) reported a similar trend for OPEs in dust and atmospheric particles, respectively. Interestingly, in the atmospheric gas and particle phase, the higher abundance for TPhP in Tadoussac compared to Toronto suggests that long-range transport was dominant, despite Toronto being a highly populated city. This observation aligns with the above findings of a low ΔHAS-app; the low median concentration level in Toronto compared to that in Tadoussac (Table S5); and the spatial patterns, cluster analyses, and a weak linear relationship with population previously reported in Li et al. (2025). Collectively, these pieces of evidence indicate that TPhP is more closely related to industrial activities and subject to long-range transport.
The abundance of chlorinated OPEs in PCPN and PWS was much greater than that of the nonhalogenated OPEs, which is consistent with the dominance of TCPP and TCEP in the dissolved phase of water sampled from urban and rural watersheds in Toronto (Awonaike et al., 2021). Based on Sect. 4.4, net diffusion from the atmosphere to water occurred in BC and QC; therefore, the high abundance of chlorinated OPEs can be explained by their relatively high KWA (Tables S4 and S15).
Some observations made here conform with general expectations regarding the environmental behaviour of semi-volatile organic chemicals, such as higher gas phase concentrations and a decrease in the particle bound fraction at higher ambient temperatures. Also, the measured precipitation scavenging ratios, while high, can be reconciled with equilibrium partitioning ratios of gaseous OPEs that favour aqueous phases over the gas phase. Other observations are more puzzling, such as the general lack of a clear relationship between OPE volatility and the observed gas–particle partitioning behaviour. Furthermore, the strong temperature dependence of OPE gas phase concentrations that indicates the importance of temperature-driven local air–surface exchange processes is not entirely consistent with the low air–water fugacity ratios which suggest that gaseous air–water exchange is strongly depositional. One possible explanation is that the measured seasonal concentration variability is less a reflection of temperature-driven air–surface exchange and instead indicates that more OPEs enter or are formed in the atmosphere in summer. Potential mechanisms are (i) an increased release of OPEs at higher temperatures from outdoor materials to which they have been added (Kemmlein et al., 2003); (ii) faster ventilation of OPEs emitted indoors (Stamp et al., 2022; Han et al., 2024); (iii) more active industrial activities, such as construction, using products containing OPEs in the summer months; and (iv) the atmospheric oxidation of organophosphite precursors (Liu et al., 2023).
Our data also highlight that the understanding of the atmospheric dispersion potential of OPEs is still incomplete. While a relatively high LRTP for aryl-OPE (TPhP) is consistent with the results from the OECD Tool (Kung et al., 2022; Sühring et al., 2020), the higher or constant relative abundance of TBP at remote sites does not align with predictions, which indicate a limited LRTP for TBP. This, too, may be related to the unpredictable gas–particle partitioning behaviour of the OPEs and the role of gas and multi-phase transformation processes, e.g. the possibility that TBP originates in part from the transformation of precursors. More research is needed to better understand the atmospheric gas–particle partitioning behaviour of the OPEs and to elucidate the role that transformation reactions may play in this regard.
All data generated for this project are contained in the Supplement.
The supplement related to this article is available online at: https://doi.org/10.5194/acp-25-459-2025-supplement.
YL, FZ, and JO prepared and extracted the PASs and the Toronto AASs. YL and FZ also took the Toronto AASs. YDL prepared standards. CS prepared, obtained, and extracted samples from Saturna Island and Tadoussac as well as the PWSs and analyzed the particle samples. KL and FAPCG deployed and retrieved PASs and PWSs in British Columbia. ABC, ZL, HH, FZ, and FW deployed/retrieved PASs and PWSs in Quebec. YL compiled and interpreted data. YL wrote the manuscript under the guidance of FW with input from the other co-authors. HH coordinated the project. All authors reviewed the manuscript.
The contact author has declared that none of the authors has any competing interests.
Publisher's note: Copernicus Publications remains neutral with regard to jurisdictional claims made in the text, published maps, institutional affiliations, or any other geographical representation in this paper. While Copernicus Publications makes every effort to include appropriate place names, the final responsibility lies with the authors.
We thank Geri Crooks, Alexandre Costa, Yannick Lapointe, Louis-Georges Esquilat, Jocelyn Praud, Sandrine Vigneron, François Gagnon, Jonathan Pritchard, Alessia Colussi, Nicolas Alexandrou, Abigaëlle Dalpé Castilloux, Christian Boutot, Bruno Cayouette, Fella Moualek, Frédérik Bélanger, Claude Lapierre, Félix Ledoux, Samuel Turgeon, Sarah Duquette, and the Canadian Air and Precipitation Monitoring Network (CAPMoN) team for their assistance in deploying samplers and providing facilities and permission to access the sampling locations.
This research has been supported by Environment and Climate Change Canada under the Whales Initiative 1.0 (grants nos. GCXE20S008, GCXE20S010, and GCXE20S011) and a Connaught International Scholarship to Yuening Li.
This paper was edited by Jason Surratt and reviewed by two anonymous referees.
Awonaike, B., Lei, Y. D., and Wania, F.: Precipitation-induced transport and phase partitioning of organophosphate esters (OPEs) in urban and rural watersheds, Environ. Sci. Water Res. Technol., 7, 2106–2115, https://doi.org/10.1039/D1EW00329A, 2021.
Bacaloni, A., Cucci, F., Guarino, C., Nazzari, M., Samperi, R., and Laganà, A.: Occurrence of organophosphorus flame retardant and plasticizers in three volcanic lakes of Central Italy, Environ. Sci. Technol., 42, 1898–1903, https://doi.org/10.1021/es702549g, 2008.
Bidleman, T., Andersson, A., Brorström-Lundén, E., Brugel, S., Ericson, L., Hansson, K., and Tysklind, M.: Halomethoxybenzenes in air of the Nordic region, Environ. Sci. Ecotechnology, 13, 1–7, https://doi.org/10.1016/j.ese.2022.100209, 2023.
Booij, K. and Smedes, F.: An Improved Method for Estimating in Situ Sampling Rates of Nonpolar Passive Samplers, Environ. Sci. Technol., 44, 6789–6794, https://doi.org/10.1021/es101321v, 2010.
Booij, K., Hofmans, H. E., Fischer, C. V, and Van Weerlee, E. M.: Temperature-Dependent Uptake Rates of Nonpolar Organic Compounds by Semipermeable Membrane Devices and Low-Density Polyethylene Membranes, Environ. Sci. Technol., 37, 361–366, https://doi.org/10.1021/es025739i, 2003.
Breivik, K., McLachlan, M. S., and Wania, F.: The Emissions Fractions Approach to Assessing the Long-Range Transport Potential of Organic Chemicals, Environ. Sci. Technol., 56, 11983–11990, https://doi.org/10.1021/acs.est.2c03047, 2022.
Casas, G., Martinez-Varela, A., Vila-Costa, M., Jiménez, B., and Dachs, J.: Rain Amplification of Persistent Organic Pollutants, Environ. Sci. Technol., 55, 12961–12972, https://doi.org/10.1021/acs.est.1c03295, 2021.
Castro-Jiménez, J., González-Gaya, B., Pizarro, M., Casal, P., Pizarro-Álvarez, C., and Dachs, J.: Organophosphate ester flame retardants and plasticizers in the global oceanic atmosphere, Environ. Sci. Technol., 50, 12831–12839, https://doi.org/10.1021/acs.est.6b04344, 2016.
Chan, C. H. and Perkins, L. H.: Monitoring of trace organic contaminants in atmospheric precipitation, J. Great Lakes Res., 15, 465–475, https://doi.org/10.1016/S0380-1330(89)71502-1, 1989.
Chen, Y., Zhang, Q., Luo, T., Xing, L., and Xu, H.: Occurrence, distribution and health risk assessment of organophosphate esters in outdoor dust in Nanjing, China: Urban vs. rural areas, Chemosphere, 231, 41–50, https://doi.org/10.1016/j.chemosphere.2019.05.135, 2019.
Choo, G. and Oh, J.-E.: Seasonal occurrence and removal of organophosphate esters in conventional and advanced drinking water treatment plants, Water Res., 186, 116359, https://doi.org/10.1016/j.watres.2020.116359, 2020.
Desimoni, E. and Brunetti, B.: About Estimating the Limit of Detection by the Signal to Noise Approach, Pharm. Anal. Acta, 6, 1–4, https://doi.org/10.4172/2153-2435.1000355, 2015.
Ding, J., Shen, X., Liu, W., Covaci, A., and Yang, F.: Occurrence and risk assessment of organophosphate esters in drinking water from Eastern China, Sci. Total Environ., 538, 959–965, https://doi.org/10.1016/j.scitotenv.2015.08.101, 2015.
Environment and Climate Change Canada: Draft screening assessment flame retardants group, Environment and Climate Change Canada, https://www.canada.ca/en/environment-climate-change/services/evaluating-existing-substances/draft-screening-assessment-flame-retardants-group.html#toc7, last access: 17 November 2023a.
Environment and Climate Change Canada: Risk management scope for TPHP, BPDP, BDMEPPP, IDDP, IPPP and TEP, Environment and Climate Change Canada, https://www.canada.ca/en/environment-climate-change/services/evaluating-existing-substances/risk-management-scope-tphp-bpdp-bdmeppp-iddp-ippp-tep.html, last access: 17 November 2023b.
Environment and Climate Change Canada: Screening assessment alkyl aryl phosphites, Environment and Climate Change Canada, https://www.canada.ca/en/environment-climate-change/services/evaluating-existing-substances/screening-assessment-alkyl-aryl-phosphites.html, last access: 17 November 2023c.
Environment and Climate Change Canada: Updated draft screening assessment – Certain organic flame retardants substance grouping – TCPP and TDCPP, Environment and Climate Change Canada, https://www.canada.ca/en/environment-climate-change/services/evaluating-existing-substances/updated-draft-screening-assessment-organic-flame-retardants-substance-grouping-tcpp-tdcpp.html, last access: 17 November 2023d.
Fries, E. and Püttmann, W.: Monitoring of the three organophosphate esters TBP, TCEP and TBEP in river water and ground water (Oder, Germany), J. Environ. Monit., 5, 346–352, https://doi.org/10.1039/b210342g, 2003.
Gu, J., Su, F., Hong, P., Zhang, Q., and Zhao, M.: 1H NMR-based metabolomic analysis of nine organophosphate flame retardants metabolic disturbance in Hep G2 cell line, Sci. Total Environ., 665, 162–170, https://doi.org/10.1016/j.scitotenv.2019.02.055, 2019.
Han, X., Hao, Y., Li, Y., Yang, R., Wang, P., Zhang, G., Zhang, Q., and Jiang, G.: Occurrence and distribution of organophosphate esters in the air and soils of Ny-Ålesund and London Island, Svalbard, Arctic, Environ. Pollut., 263, 114495, https://doi.org/10.1016/j.envpol.2020.114495, 2020.
Han, X., Li, W., Zhao, Y., Zhuang, Y., Jia, Q., Guan, H., Liu, J., and Wu, C.: Organophosphate Esters in Building Materials from China: Levels, Sources, Emissions, and Preliminary Assessment of Human Exposure, Environ. Sci. Technol., 58, 2434–2445, https://doi.org/10.1021/acs.est.3c08432, 2024.
He, M. J., Lu, J. F., and Wei, S. Q.: Organophosphate esters in biota, water, and air from an agricultural area of Chongqing, western China: Concentrations, composition profiles, partition and human exposure, Environ. Pollut., 244, 388–397, https://doi.org/10.1016/j.envpol.2018.10.085, 2019.
Kemmlein, S., Hahn, O., and Jann, O.: Emissions of Organophosphate and Brominated Flame Retardants from Selected Consumer Products and Building Materials, Atmos. Environ., 37, 5485–5493, https://doi.org/10.1016/j.atmosenv.2003.09.025, 2003.
Kim, H.-K., Shin, Y.-S., Lee, D.-S., Song, B.-J., and Kim, J.-G.: Estimation of Rain Scavenging Ratio for Particle Bound Polycyclic Aromatic Hydrocarbons and Polychlorinated Biphenyls, Environmental Engineering Research, 11, 33–44, https://doi.org/10.4491/eer.2006.11.1.033, 2006.
Kim, U. J. and Kannan, K.: Occurrence and Distribution of Organophosphate Flame Retardants/Plasticizers in Surface Waters, Tap Water, and Rainwater: Implications for Human Exposure, Environ. Sci. Technol., 52, 5625–5633, https://doi.org/10.1021/acs.est.8b00727, 2018.
Kung, H. C., Hsieh, Y. K., Huang, B. W., Cheruiyot, N. K., and Chang-Chien, G. P.: An Overview: Organophosphate Flame Retardants in the Atmosphere, Aerosol Air Qual. Res., 22, 220148, https://doi.org/10.4209/aaqr.220148, 2022.
Kurt-Karakus, P., Alegria, H., Birgul, A., Gungormus, E., and Jantunen, L.: Organophosphate ester (OPEs) flame retardants and plasticizers in air and soil from a highly industrialized city in Turkey, Sci. Total Environ., 625, 555–565, https://doi.org/10.1016/j.scitotenv.2017.12.307, 2018.
Lei, Y. D. and Wania, F.: Is rain or snow a more efficient scavenger of organic chemicals?, Atmos. Environ., 38, 3557–3571, https://doi.org/10.1016/j.atmosenv.2004.03.039, 2004.
Li, J., Xie, Z., Mi, W., Lai, S., Tian, C., Emeis, K. C., and Ebinghaus, R.: Organophosphate Esters in Air, Snow, and Seawater in the North Atlantic and the Arctic, Environ. Sci. Technol., 51, 6887–6896, https://doi.org/10.1021/acs.est.7b01289, 2017.
Li, J., Tang, J., Mi, W., Tian, C., Emeis, K. C., Ebinghaus, R., and Xie, Z.: Spatial Distribution and Seasonal Variation of Organophosphate Esters in Air above the Bohai and Yellow Seas, China, Environ. Sci. Technol., 52, 89–97, https://doi.org/10.1021/acs.est.7b03807, 2018.
Li, J., Zhao, L., Letcher, R. J., Zhang, Y., Jian, K., Zhang, J., and Su, G.: A review on organophosphate Ester (OPE) flame retardants and plasticizers in foodstuffs: Levels, distribution, human dietary exposure, and future directions, Environ. Int., 127, 35–51, https://doi.org/10.1016/j.envint.2019.03.009, 2019a.
Li, J., Cao, H., Mu, Y., Qu, G., Zhang, A., Fu, J., and Jiang, G.: Structure-Oriented Research on the Antiestrogenic Effect of Organophosphate Esters and the Potential Mechanism, Environ. Sci. Technol., 54, 14525–14534, https://doi.org/10.1021/acs.est.0c04376, 2020.
Li, W., Wang, Y., and Kannan, K.: Occurrence, distribution and human exposure to 20 organophosphate esters in air, soil, pine needles, river water, and dust samples collected around an airport in New York state, United States, Environ. Int., 131, 105054, https://doi.org/10.1016/j.envint.2019.105054, 2019b.
Li, Y., Zhan, F., Lei, Y. D., Shunthirasingham, C., Hung, H., and Wania, F.: Field calibration and PAS-SIM model evaluation of the XAD-based passive air samplers for semi-volatile organic compounds, Environ. Sci. Technol., 57, 9224–9233, https://doi.org/10.1021/acs.est.3c00809, 2023a.
Li, Y., Zhan, F., Shunthirasingham, C., Lei, Y. D., Hung, H., and Wania, F.: Unbiased Passive Sampling of All Polychlorinated Biphenyls Congeners from Air, Environ. Sci. Technol. Lett., 10, 565–572, https://doi.org/10.1021/acs.estlett.3c00271, 2023b.
Li, Y., Zhan, F., Su, Y., Lei, Y. D., Shunthirasingham, C., Zhou, Z., Abbatt, J. P. D., Hung, H., and Wania, F.: Uptake behavior of polycyclic aromatic compounds during field calibrations of the XAD-based passive air sampler across seasons and locations, Atmos. Meas. Tech., 17, 715–729, https://doi.org/10.5194/amt-17-715-2024, 2024.
Li, Y., Zhan, F., Shunthirasingham, C., Lei, Y. D., Oh, J., Weng, C., Chaaben, A. Ben, Lu, Z., Lee, K., Gobas, F. A. P. C., Hung, H., and Wania, F.: Inferring Atmospheric Sources of Gaseous Organophosphate Esters from Spatial Patterns, Environ. Toxicol. Chem., https://doi.org/10.1093/etojnl/vgae089, accepted, 2025.
Liu, Q., Liu, R., Zhang, X., Li, W., Harner, T., Saini, A., Liu, H., Yue, F., Zeng, L., Zhu, Y., Xing, C., Li, L., Lee, P., Tong, S., Wang, W., Ge, M., Wang, J., Wu, X., Johannessen, C., Liggio, J., Li, S. M., Hung, H., Xie, Z., Mabury, S. A., and Abbatt, J. P. D.: Oxidation of commercial antioxidants is driving increasing atmospheric abundance of organophosphate esters: Implication for global regulation, One Earth, 6, 1202–1212, https://doi.org/10.1016/j.oneear.2023.08.004, 2023.
Liu, R. and Mabury, S. A.: Organophosphite Antioxidants in Indoor Dust Represent an Indirect Source of Organophosphate Esters, Environ. Sci. Technol., 53, 1805–1811, https://doi.org/10.1021/acs.est.8b05545, 2019.
Lu, Z., Martin, P. A., Burgess, N. M., Champoux, L., Elliott, J. E., Baressi, E., De Silva, A. O., de Solla, S. R., and Letcher, R. J.: Volatile Methylsiloxanes and Organophosphate Esters in the Eggs of European Starlings (Sturnus vulgaris) and Congeneric Gull Species from Locations across Canada, Environ. Sci. Technol., 51, 9836–9845, https://doi.org/10.1021/acs.est.7b03192, 2017.
Ma, Y., Cui, K., Zeng, F., Wen, J., Liu, H., Zhu, F., Ouyang, G., Luan, T., and Zeng, Z.: Microwave-assisted extraction combined with gel permeation chromatography and silica gel cleanup followed by gas chromatography-mass spectrometry for the determination of organophosphorus flame retardants and plasticizers in biological samples, Anal. Chim. Acta, 786, 47–53, https://doi.org/10.1016/j.aca.2013.04.062, 2013.
Ma, Y., Vojta, S., Becanova, J., Habtemichael, A. Z., Adelman, D. A., Muir, D., and Lohmann, R.: Spatial distribution and air-water exchange of organophosphate esters in the lower Great Lakes, Environ. Pollut., 286, 117349, https://doi.org/10.1016/j.envpol.2021.117349, 2021.
Ma, Y., Luo, Y., Zhu, J., Zhang, J., Gao, G., Mi, W., Xie, Z., and Lohmann, R.: Seasonal variation and deposition of atmospheric organophosphate esters in the coastal region of Shanghai, China, Environ. Pollut., 300, 118930, https://doi.org/10.1016/j.envpol.2022.118930, 2022.
Marcogliese, D. J., Blaise, C., Cyr, D., de Lafontaine, Y., Fournier, M., Gagné, F., Gagnon, C., and Hudon, C.: Effects of a major municipal effluent on the St. Lawrence River: A case study, Ambio, 44, 257–274, https://doi.org/10.1007/s13280-014-0577-9, 2015.
Marklund, A., Andersson, B., and Haglund, P.: Organophosphorus flame retardants and plasticizers in Swedish sewage treatment plants, Environ. Sci. Technol., 39, 7423–7429, https://doi.org/10.1021/es051013l, 2005a.
Marklund, A., Andersson, B., and Haglund, P.: Traffic as a source of organophosphorus flame retardants and plasticizers in snow, Environ. Sci. Technol., 39, 3555–3562, https://doi.org/10.1021/es0482177, 2005b.
McDonough, C. A., De Silva, A. O., Sun, C., Cabrerizo, A., Adelman, D., Soltwedel, T., Bauerfeind, E., Muir, D. C. G., and Lohmann, R.: Dissolved organophosphate esters and polybrominated diphenyl ethers in remote marine environments: Arctic surface water distributions and net transport through Fram Strait, Environ. Sci. Technol., 52, 6208–6216, https://doi.org/10.1021/acs.est.8b01127, 2018.
Mi, L., Xie, Z., Zhang, L., Waniek, J. J., Pohlmann, T., Mi, W., and Xu, W.: Organophosphate Esters in Air and Seawater of the South China Sea: Spatial Distribution, Transport, and Air–Sea Exchange, Environ. Heal., 1, 191–202, https://doi.org/10.1021/envhealth.3c00059, 2023.
Mihajlović, I. and Fries, E.: Atmospheric deposition of chlorinated organophosphate flame retardants (OFR) onto soils, Atmos. Environ., 56, 177–183, https://doi.org/10.1016/j.atmosenv.2012.03.054, 2012.
Möller, A., Xie, Z., Caba, A., Sturm, R., and Ebinghaus, R.: Organophosphorus flame retardants and plasticizers in the atmosphere of the North Sea, Environ. Pollut., 159, 3660–3665, https://doi.org/10.1016/j.envpol.2011.07.022, 2011.
Möller, A., Sturm, R., Xie, Z., Cai, M., He, J., and Ebinghaus, R.: Organophosphorus flame retardants and plasticizers in airborne particles over the Northern Pacific and Indian Ocean toward the polar regions: Evidence for global occurrence, Environ. Sci. Technol., 46, 3127–3134, https://doi.org/10.1021/es204272v, 2012.
Na, G., Hou, C., Li, R., Shi, Y., Gao, H., Jin, S., Gao, Y., Jiao, L., and Cai, Y.: Occurrence, distribution, air-seawater exchange and atmospheric deposition of organophosphate esters (OPEs) from the Northwestern Pacific to the Arctic Ocean, Mar. Pollut. Bull., 157, 111243, https://doi.org/10.1016/j.marpolbul.2020.111243, 2020.
Oh, J., Shunthirasingham, C., Lei, Y. D., Zhan, F., Li, Y., Dalpé Castilloux, A., Ben Chaaben, A., Lu, Z., Lee, K., Gobas, F. A. P. C., Eckhardt, S., Alexandrou, N., Hung, H., and Wania, F.: The atmospheric fate of 1,2-dibromo-4-(1,2-dibromoethyl)cyclohexane (TBECH): spatial patterns, seasonal variability, and deposition to Canadian coastal regions, Atmos. Chem. Phys., 23, 10191–10205, https://doi.org/10.5194/acp-23-10191-2023, 2023.
Regnery, J. and Püttmann, W.: Organophosphorus Flame Retardants and Plasticizers in Rain and Snow from Middle Germany, CLEAN – Soil, Air, Water, 37, 334–342, https://doi.org/10.1002/clen.200900050, 2009.
Regnery, J. and Püttmann, W.: Occurrence and fate of organophosphorus flame retardants and plasticizers in urban and remote surface waters in Germany, Water Res., 44, 4097–4104, https://doi.org/10.1016/j.watres.2010.05.024, 2010.
Rosenmai, A. K., Winge, S. B., Möller, M., Lundqvist, J., Wedebye, E. B., Nikolov, N. G., Lilith Johansson, H. K., and Vinggaard, A. M.: Organophosphate ester flame retardants have antiandrogenic potential and affect other endocrine related endpoints in vitro and in silico, Chemosphere, 263, 127703, https://doi.org/10.1016/j.chemosphere.2020.127703, 2021.
Salamova, A., Ma, Y., Venier, M., and Hites, R. A.: High Levels of Organophosphate Flame Retardants in the Great Lakes Atmosphere, Environ. Sci. Technol. Lett., 1, 8–14, https://doi.org/10.1021/ez400034n, 2013.
Salamova, A., Hermanson, M. H., and Hites, R. A.: Organophosphate and Halogenated Flame Retardants in Atmospheric Particles from a European Arctic Site, Environ. Sci. Technol., 48, 6133–6140, https://doi.org/10.1021/es500911d, 2014.
Salamova, A., Peverly, A. A., Venier, M., and Hites, R. A.: Spatial and temporal trends of particle phase organophosphate ester concentrations in the atmosphere of the great lakes, Environ. Sci. Technol., 50, 13249–13255, https://doi.org/10.1021/acs.est.6b04789, 2016.
Shi, T., Li, R., Fu, J., Hou, C., Gao, H., Cheng, G., Zhang, H., Jin, S., Kong, L., and Na, G.: Fate of organophosphate esters from the Northwestern Pacific to the Southern Ocean: Occurrence, distribution, and fugacity model simulation, J. Environ. Sci., 137, 347–357, https://doi.org/10.1016/j.jes.2023.03.001, 2024.
Shi, Y., Zhang, Y., Du, Y., Kong, D., Wu, Q., Hong, Y., Wang, Y., Tam, N. F. Y., and Leung, J. Y. S.: Occurrence, composition and biological risk of organophosphate esters (OPEs) in water of the Pearl River Estuary, South China, Environ. Sci. Pollut. Res., 27, 14852–14862, https://doi.org/10.1007/s11356-020-08001-1, 2020.
Shoeib, M., Ahrens, L., Jantunen, L., and Harner, T.: Concentrations in air of organobromine, organochlorine and organophosphate flame retardants in Toronto, Canada, Atmos. Environ., 99, 140–147, https://doi.org/10.1016/j.atmosenv.2014.09.040, 2014.
Stackelberg, P. E., Gibs, J., Furlong, E. T., Meyer, M. T., Zaugg, S. D., and Lippincott, R. L.: Efficiency of conventional drinking-water-treatment processes in removal of pharmaceuticals and other organic compounds, Sci. Total Environ., 377, 255–272, https://doi.org/10.1016/j.scitotenv.2007.01.095, 2007.
Stamp, S., Burman, E., Shrubsole, C., Chatzidiakou, L., Mumovic, D., and Davies, M.: Seasonal Variations and the Influence of Ventilation Rates on IAQ: A Case Study of Five Low-Energy London Apartments, Indoor Built Environ., 31, 607–623, https://doi.org/10.1177/1420326X211017175, 2022.
Storey, J. M. E., Luo, W., Isabelle, L. M., and Pankow, J. F.: Gas/Solid Partitioning of Semivolatile Organic Compounds to Model Atmospheric Solid Surfaces as a Function of Relative Humidity. 1. Clean Quartz, Environ. Sci. Technol., 29, 2420–2428, https://doi.org/10.1021/es00009a039, 1995.
Sühring, R., Diamond, M. L., Scheringer, M., Wong, F., Pućko, M., Stern, G., Burt, A., Hung, H., Fellin, P., Li, H., and Jantunen, L. M.: Organophosphate esters in Canadian Arctic air: Occurrence, levels and trends, Environ. Sci. Technol., 50, 7409–7415, https://doi.org/10.1021/acs.est.6b00365, 2016.
Sühring, R., Scheringer, M., Rodgers, T. F. M. M., Jantunen, L. M., and Diamond, M. L.: Evaluation of the OECD P OV and LRTP screening tool for estimating the long-range transport of organophosphate esters, Environ. Sci. Process. Impacts, 22, 207–216, https://doi.org/10.1039/c9em00410f, 2020.
Sundkvist, A. M., Olofsson, U., and Haglund, P.: Organophosphorus flame retardants and plasticizers in marine and fresh water biota and in human milk, J. Environ. Monit., 12, 943–951, https://doi.org/10.1039/B921910B, 2010.
Turygin, V. V., Sokhadze, L. A., Golubeva, Y. Y., Platonova, L. V., Afanas'eva, A. A., Nazarenko, D. I., and Shvetsova-Shilovskaya, T. N.: New Approach to Obtain Neutral Ester of Phosphoric Acid: Tris(2-chloroisopropyl) Phosphate, Theor. Found. Chem. Eng., 52, 643–647, https://doi.org/10.1134/S0040579518040280, 2018.
UFZ-LSER database v 3.2.1: http://www.ufz.de/lserd, last access: 13 January 2024.
van der Veen, I. and de Boer, J.: Phosphorus flame retardants: Properties, production, environmental occurrence, toxicity and analysis, Chemosphere, 88, 1119–1153, https://doi.org/10.1016/j.chemosphere.2012.03.067, 2012.
Wang, C., Wang, P., Zhao, J., Fu, M., Zhang, L., Li, Y., Yang, R., Zhu, Y., Fu, J., Zhang, Q., and Jiang, G.: Atmospheric organophosphate esters in the Western Antarctic Peninsula over 2014–2018: Occurrence, temporal trend and source implication, Environ. Pollut., 267, 115428, https://doi.org/10.1016/j.envpol.2020.115428, 2020a.
Wang, X., Zhu, Q., Yan, X., Wang, Y., Liao, C., and Jiang, G.: A review of organophosphate flame retardants and plasticizers in the environment: Analysis, occurrence and risk assessment, Sci. Total Environ., 731, 139071, https://doi.org/10.1016/j.scitotenv.2020.139071, 2020b.
Wang, X., Luu, T., Beal, M. A., Barton-Maclaren, T. S., Robaire, B., and Hales, B. F.: The Effects of Organophosphate Esters Used as Flame Retardants and Plasticizers on Granulosa, Leydig, and Spermatogonial Cells Analyzed Using High-Content Imaging, Toxicol. Sci., 186, 269–287, https://doi.org/10.1093/toxsci/kfac012, 2022.
Wania, F., Haugen, J. E., Lei, Y. D., and Mackay, D.: Temperature dependence of atmospheric concentrations of semivolatile organic compounds, Environ. Sci. Technol., 32, 1013–1021, https://doi.org/10.1021/es970856c, 1998.
Wong, F., de Wit, C. A., and Newton, S. R.: Concentrations and variability of organophosphate esters, halogenated flame retardants, and polybrominated diphenyl ethers in indoor and outdoor air in Stockholm, Sweden, Environ. Pollut., 240, 514–522, https://doi.org/10.1016/j.envpol.2018.04.086, 2018.
Wu, Y., Venier, M., and Salamova, A.: Spatioseasonal Variations and Partitioning Behavior of Organophosphate Esters in the Great Lakes Atmosphere, Environ. Sci. Technol., 54, 5400–5408, https://doi.org/10.1021/acs.est.9b07755, 2020.
Xie, Z., Wang, P., Wang, X., Castro-Jiménez, J., Kallenborn, R., Liao, C., Mi, W., Lohmann, R., Vila-Costa, M., and Dachs, J.: Organophosphate ester pollution in the oceans, Nat. Rev. Earth Environ., 3, 309–322, https://doi.org/10.1038/s43017-022-00277-w, 2022.
Yan, H. and Hales, B. F.: Effects of Organophosphate Ester Flame Retardants on Endochondral Ossification in Ex Vivo Murine Limb Bud Cultures, Toxicol. Sci., 168, 420–429, https://doi.org/10.1093/toxsci/kfy301, 2019.
Yan, H. and Hales, B. F.: Exposure to tert-Butylphenyl Diphenyl Phosphate, an Organophosphate Ester Flame Retardant and Plasticizer, Alters Hedgehog Signaling in Murine Limb Bud Cultures, Toxicol. Sci., 178, 251–263, https://doi.org/10.1093/toxsci/kfaa145, 2020.
Zhan, F., Shunthirasingham, C., Li, Y., Oh, J., Lei, Y. D., Ben Chaaben, A., Dalpé Castilloux, A., Lu, Z., Lee, K., Gobas, F. A. P. C., Alexandrou, N., Hung, H., and Wania, F.: Sources and environmental fate of halomethoxybenzenes, Sci. Adv., 9, eadi8082, https://doi.org/10.1126/sciadv.adi8082, 2023.
Zhang, Q., Li, X., Wang, Y., Zhang, C., Cheng, Z., Zhao, L., Li, X., Sun, Z., Zhang, J., Yao, Y., Wang, L., Li, W., and Sun, H.: Occurrence of novel organophosphate esters derived from organophosphite antioxidants in an e-waste dismantling area: Associations between hand wipes and dust, Environ. Int., 157, 106860, https://doi.org/10.1016/j.envint.2021.106860, 2021.
Zhang, W., Wang, P., Li, Y., Wang, D., Matsiko, J., Yang, R., Sun, H., Hao, Y., Zhang, Q., and Jiang, G.: Spatial and temporal distribution of organophosphate esters in the atmosphere of the Beijing-Tianjin-Hebei region, China, Environ. Pollut., 244, 182–189, https://doi.org/10.1016/j.envpol.2018.09.131, 2019.
Zhang, X., Sühring, R., Serodio, D., Bonnell, M., Sundin, N., and Diamond, M. L.: Novel flame retardants: Estimating the physical-chemical properties and environmental fate of 94 halogenated and organophosphate PBDE replacements, Chemosphere, 144, 2401–2407, https://doi.org/10.1016/j.chemosphere.2015.11.017, 2016.
Zhang, Z., Lin, G., Lin, T., Zhang, R., Jin, L., and Di, Y.: Occurrence, behavior, and fate of organophosphate esters (OPEs) in subtropical paddy field environment: A case study in Nanning City of South China, Environ. Pollut., 267, 115675, https://doi.org/10.1016/j.envpol.2020.115675, 2020.
Zhao, F., Riipinen, I., and MacLeod, M.: Steady-State Mass Balance Model for Predicting Particle–Gas Concentration Ratios of PBDEs, Environ. Sci. Technol., 55, 9425–9433, https://doi.org/10.1021/acs.est.0c04368, 2021a.
Zhao, S., Tian, L., Zou, Z., Liu, X., Zhong, G., Mo, Y., Wang, Y., Tian, Y., Li, J., Guo, H., and Zhang, G.: Probing Legacy and Alternative Flame Retardants in the Air of Chinese Cities, Environ. Sci. Technol., 55, 9450–9459, https://doi.org/10.1021/acs.est.0c07367, 2021b.