the Creative Commons Attribution 4.0 License.
the Creative Commons Attribution 4.0 License.
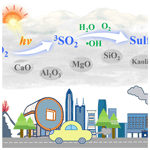
Photoenhanced sulfate formation by the heterogeneous uptake of SO2 on non-photoactive mineral dust
Wangjin Yang
Jiawei Ma
Hongxing Yang
Fu Li
Chong Han
Heterogeneous uptake of SO2 on mineral dust is a predominant formation pathway of sulfates, whereas the contribution of photo-induced SO2 oxidation to sulfates on the dust interfaces still remains unclear. Here, we investigated heterogeneous photochemical reactions of SO2 on five mineral oxides (SiO2, kaolinite, Al2O3, MgO, and CaO) without photocatalytic activity. Light enhanced the uptake of SO2, and its enhancement effects negatively depended on the basicity of mineral oxides. The initial uptake coefficient (γ0,BET) and the steady-state uptake coefficient (γs,BET) of SO2 positively relied on light intensity, relative humidity (RH), and O2 content, while they exhibited a negative relationship with the initial SO2 concentration. Rapid sulfate formation during photo-induced heterogeneous reactions of SO2 with all mineral oxides was confirmed to be ubiquitous, and H2O and O2 played key roles in the conversion of SO2 to sulfates. In particular, triplet states of SO2 (3SO2) were suggested to be the trigger for photochemical sulfate formation. Atmospheric implications supported a potential contribution of interfacial SO2 photochemistry on non-photoactive mineral dust to atmospheric sulfate sources.
- Article
(1976 KB) - Full-text XML
-
Supplement
(1819 KB) - BibTeX
- EndNote
As an important trace gas in the atmosphere, SO2 is mainly emitted by volcanic eruption and fuel combustion. There is an uneven distribution of atmospheric SO2 concentrations that show a distinctive seasonal and regional differentiation. Typical mixing ratios of SO2 in the troposphere are below 0.5 ppb for clean-weather days, rising to several hundred parts per billion during polluted days in urban regions (Ma et al., 2020). About half of SO2 is oxidized to sulfates (He et al., 2012), which are key components of fine particulates in the atmosphere. High sulfate loading in PM2.5 was observed (Shao et al., 2019), especially in polluted regions where high-sulfur fuels are usually used (Olson et al., 2021). They significantly alter physicochemical properties of aerosols in terms of hygroscopicity, acidity, and light absorption (Chan and Chan, 2003; Cao et al., 2013; Lim et al., 2018). Sulfates may lead to negative health outcomes, such as respiratory illnesses and cardiovascular diseases (Shiraiwa et al., 2017). In addition, the deposition of sulfates leads to adverse effects on ecosystems via the acidification of soils and lakes (Golobokova et al., 2020). Therefore, the oxidation of SO2 to form sulfates has attracted widespread attention in the past decades.
The conversion of SO2 to sulfates in the atmosphere usually occurs in different phases: gas-phase oxidation of SO2 by hydroxyl radicals (•OH) or Criegee intermediate radicals (Mauldin et al., 2012; Davis et al., 1979); aqueous-phase reactions of SO2 with O3, peroxides, or transition metal ions dissolved in cloud and fog droplets (Alexander et al., 2009; Herrmann et al., 2000; Harris et al., 2013; Liu et al., 2020; Li et al., 2020); and heterogeneous SO2 uptake on aerosols, including authentic mineral dust, soot, inorganic ion, and organic compounds (Adams et al., 2005; G. He et al., 2018; Ye et al., 2018; S. Wang et al., 2019; Yao et al., 2019; R. Zhang et al., 2020; Liu et al., 2020, 2021). However, the oxidation of SO2 in gas and aqueous phases fails to explain high sulfate concentrations in polluted areas. Model simulation suggests that the rapid sulfate formation can be attributed to heterogeneous SO2 uptake (Li et al., 2017). A positive relationship between the fraction of sulfates and mineral dust in haze days has been reported, implying that mineral dust may account for the formation of sulfates (J. Wang et al., 2020). Moreover, large numbers of sulfates were observed on the surface of mineral dust after long-distance transport (Prospero, 1999). Thus, investigating the heterogeneous oxidation of SO2 on mineral dust can provide basic data for the model calculation to evaluate atmospheric sulfates.
Mineral dust, as the dominant component of particulate matters in the atmosphere, accounts for about 30 %–60 % mass fractions of global aerosols (Dentener et al., 1996; Peng et al., 2012). It primarily contains SiO2 (40 %–80 %), followed by Al2O3 (10 %–15 %), Fe2O3 (6 %–13 %), CaO (3 %–10 %), MgO (1 %–7 %), and TiO2 (0.1 %–5 %) (Urupina et al., 2019, 2021; Usher et al., 2003). Mineral dust can provide active sites for the adsorption and reaction of gases. Up to now, the heterogeneous SO2 uptake on authentic mineral aerosols and model mineral oxides has been widely reported (Ma et al., 2019; Goodman et al., 2001; T. Wang et al., 2018, 2020), with the uptake coefficient (γ) of SO2 varying from 10−9 to 10−4 (Urupina et al., 2019; Usher et al., 2002).
It was recognized that light could significantly enhance the heterogeneous conversion of SO2 to sulfates on the surface of photocatalytic mineral dust (Chen et al., 2021; Li et al., 2019; T. Wang et al., 2020). Electron–hole pairs are produced via photo-induced electrons from the valence band to the conduction band of photocatalytic metal oxides and then react with H2O and O2 to generate reactive oxygen species (ROS) such as •OH and (Chu et al., 2019). Sulfates are produced by the heterogeneous reactions of SO2 with ROS (Park and Jang, 2016; Park et al., 2017; Langhammer et al., 2020; Bounechada et al., 2017). In particular, due to the large abundance of non-photoactive mineral dust (more than 85 % mass of total mineral dust in the atmosphere) (Usher et al., 2003; Liu et al., 2022), revealing the photooxidation processes of SO2 on these mineral dust is of great importance to better reevaluate the sulfate formation on aerosols at a global scale.
Photochemical SO2 uptake and sulfate formation on non-photoactive mineral oxides were investigated using a flow reactor and in situ diffuse reflectance infrared Fourier transform spectroscopy (DRIFTS). The conversion of SO2 to sulfates was examined under various conditions, and the roles of light intensity, SO2 concentration, H2O, O2, and basicity of mineral oxides were determined. Reaction mechanisms and atmospheric implications were proposed, highlighting an important pathway accounting for the photochemical uptake of SO2 to form sulfates on the non-photoactive surfaces.
2.1 Materials
Analytical-grade SiO2 (Sinopharm Chemical Reagent Co., Ltd.), kaolinite (Sinopharm Chemical Reagent Co., Ltd.), Al2O3 (Alfa Aesar), MgO (Sigma-Aldrich), and CaO (Sigma-Aldrich) were used in the experiments. Through the nitrogen Brunauer–Emmett–Teller (BET) physisorption analysis, their specific surface areas were detected to be 0.419, 6.407, 8.137, 10.948, and 6.944 m2 g−1, respectively. With BaSO4 used as the reference, the ultraviolet–visible (UV-vis) light absorption spectra of samples (Fig. S1 in the Supplement) in the wavelength range of 300–800 nm were obtained by the Shimadzu UV-2550 spectrophotometer, which was equipped with a diffuse-reflection integrating sphere attachment. The solid powder (0.2–5 g) was uniformly dispersed into 10.0 mL ethanol solution. The mixed liquid was poured into a rectangular quartz sample dish (14.0 cm × 7.0 cm) and dried to form a solid coating in an oven at 353 K for 10 h. SO2 standard gas (50 ppm in N2, Shenyang Air Liquide Co., Ltd.) and high-purity N2 and O2 (99.999 vol.%, Shenyang Air Liquide Co., Ltd.) were used as received. The solid sample powder (0.2 g) was immersed in 10 mL deionized water (20 mg mL−1), and then the suspension was vigorously stirred for 10 min. The pH of SiO2, kaolinite, Al2O3, MgO, and CaO suspension was measured to be 6.27, 6.58, 9.33, 10.61, and 12.72, respectively, using a pH meter.
2.2 Rectangular flow reactor
The uptake experiments of SO2 on mineral dust were performed in a horizontal rectangular flow reactor (26.0 cm length × 7.5 cm width × 2.0 cm height), which is depicted in Fig. S2. In a previous study, a similar rectangular flow reactor was designed, and the feasibility of the reactor has been verified (Knopf et al., 2007). The reactor was made of quartz to allow the transmission of light. The temperature was maintained at 298 K by circulating temperature-controlled water through the outer jacket of the reactor. Synthetic air with a volume ratio of 4:1 was introduced into the flow reactor, and its total flow rate was 1000 mL min−1. The Reynolds number (Re) was calculated to be 28.2 (Re < 200), as described in the Supplement, indicating a laminar flow state. SO2, together with high-purity N2 (100 mL min−1), was introduced into the reactor through a movable T-shaped injector equipped with six exit holes (0.2 mm diameter) so that the gas could be uniformly distributed over the width of the reactor. The SO2 concentration was 40–200 ppb and measured with a SO2 analyzer (Thermo 43i). Wet N2 generated with a bubbler containing deionized water was introduced by two parallel inlets on the side of a T-shaped injector. Relative humidity (RH, 10 %–75 %) was controlled by regulating the ratio of dry N2 to wet N2 and measured via a hygrometer (Center 314). The equivalent layer numbers of water on the surface were 0.9–4.0, according to the Brunauer–Emmett–Teller (BET) model (Sumner et al., 2004), and the thickness of the film of adsorbed water varied between 2.7–12 nm at RH = 10 %–75 %. There were three equally spaced exhaust ports to mitigate the outlet turbulence. A xenon lamp (CEL-LAX500, China Education Au-light Co., Ltd.) was used to simulate sunlight and vertically located above the reactor. A filter was placed on the reactor to remove the light with wavelengths shorter than 300 nm. The spectrum irradiance of the xenon lamp is displayed in Fig. S3 and was measured using a calibrated spectroradiometer (ULS2048CL-EVO, Avantes). The spectral irradiance was measured inside the reactor after passing the water cooling and in the absence of a sample. The total irradiance (0–7.93 × 1016 ) on the coating can be adjusted by varying the distance of the xenon lamp to the reactor.
2.3 Uptake coefficient of SO2
The heterogeneous reaction kinetics of SO2 with mineral dust can be described by a pseudo-first-order reaction. Figure S4 shows a linear relationship between the natural logarithm of the SO2 concentration and the reaction time. The apparent rate constant () of SO2 with SiO2 can be calculated using Eq. (1),
where C0 and Ct (ppb) are the initial SO2 concentration and the SO2 concentration at the exposure time t, respectively; t was calculated by diving the length of the reactive surface by the average flow velocity. The loss of SO2 on the internal wall of the reactor in blank experiments was carried out under various conditions (Fig. S5 as an example), and it has been deducted for the γ calculation. Assuming that the wall loss was constant in the experiments with and without samples, the geometric uptake coefficient (γgeo) was determined by Eq. (2) (Knopf et al., 2007),
where k (s−1), V (4 × 10−4 m3), S (9.8 × 10−3 m2), and ω (314.05 m s−1) are the reaction rate constant, the volume of the rectangular reactor, the surface area of the sample dish, and the mean molecular speed of SO2, respectively.
The uptake process of SO2 on SiO2 depended on the reaction of SO2 with SiO2 and the mass transport of SO2 to the surface. It can be expressed by Eq. (3),
where and kobs,wall (s−1) are the apparent rate constants measured with and without SiO2 samples, respectively. is the reaction rate constant of SO2 accounting for the diffusion effect; D (0.1337 cm2 s−1) is the diffusion coefficient of SO2 in air; a (1 cm) is one-half the height of the flow reactor; and Nu represents the Nusselt numbers obtained with a calculation method from Solbrig and Gidaspow (1967), which represents the mass transport. The corrected γ can be calculated by Eq. (2), where k was replaced by . In our experiments, the correction for γ was estimated to be 10 %. Initial uptake coefficients (γ0) and steady-state uptake coefficients (γs) were calculated by averaging the signals within the 1.0 and 40–60 min reaction time, respectively.
To understand the diffusion depth of SO2 and determine the interaction of SO2 with the underlying layers of SiO2, the uptake of SO2 as a function of the SiO2 mass under irradiation is shown in Fig. S6. The γ exhibited a linear increase in the SiO2 mass range of 0.05–2.0 g, while it remained unchanged at the SiO2 mass > 3.0 g. Therefore, the uptake coefficient of SO2 in the linear regions was normalized using the BET surface area of SiO2 by Eq. (4) (Brunauer et al., 1938),
where γBET is the SO2 uptake coefficient normalized to the BET surface area, Sgeo (9.8 × 10−3 m2) is the geometric area of the sample dish, SBET (0.419 m2 g−1) is the BET surface area of SiO2, and (0.05–2.0 g) is the SiO2 mass. The same method was also used to calculate the uptake coefficients of SO2 on other mineral oxides.
2.4 In situ DRIFTS analysis
The changes in the chemical compositions on mineral oxides in the SO2 uptake process were investigated by a Fourier transform infrared (FTIR) spectrometer (Thermo Nicolet iS50) equipped with an in situ diffuse reflectance accessory and a mercury cadmium telluride (MCT) detector. About 14 mg of mineral oxides was placed into a stainless-steel cup inside the reaction cell. To remove adsorbed impurities, SiO2 was purged with a 150 mL min−1 airflow ( volume ratio = 4:1) at RH = 40 % for 1 h. Then, a background spectrum of unreacted samples was collected. SO2 (2 ppm) was introduced into the reaction cell, and the IR spectra were recorded as a function of time at a resolution of 4 cm−1 by averaging 100 scans. The light from the xenon lamp (500 W) was transmitted into the DRIFTS reaction cell via a fiber. To verify the role of intermediates, Ru(bpy)3(Cl)2 and NaHCO3, acting as 3SO2 and •OH scavengers (Bulgakov and Safonova, 1996; Gen et al., 2019a), respectively, were mixed with SiO2 powder in an agate mortar, and the mixture was put in the reaction cell of DRIFTS.
.
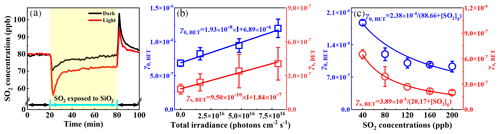
Figure 1(a) The temporal variation in the SO2 concentration on SiO2 in the dark and under irradiation (7.93 × 1016 ); the background changes in the SO2 concentration in the blank reactor have been deducted. (b) The γ0,BET and γs,BET of SO2 on SiO2 as a function of the light intensity. (c) The γ0,BET and γs,BET of SO2 on SiO2 at different SO2 concentrations under irradiation (7.93 × 1016 ). The fitting lines for γ0,BET and γs,BET were based on the Langmuir–Hinshelwood mechanism using Eq. (6). Reaction conditions: SiO2 mass of 0.2 g, temperature of 298 K, RH of 40 %, and O2 content of 20 %.
3.1 Photoenhanced uptake of SO2
Acting as the most abundant of the mineral oxides, SiO2 was used to investigate the uptake behaviors of SO2. As shown in Fig. 1a, when SO2 (80 ppb) was exposed to SiO2 in the dark, the SO2 concentration decreased to 70 ppb, and then it quickly increased and reached a steady state after 20 min. Upon exposure to SiO2 under irradiation, the SO2 concentration exhibited a greater drop than that in the dark. The deactivation of SO2 uptake on SiO2 was very slight after 20 min under irradiation. These results suggest that light can promote the heterogeneous reaction of SO2 on SiO2. Few studies have observed the photochemical uptake of SO2 on non-photoactive minerals (Xu et al., 2021; Zhang et al., 2022). When SO2 did not come into contact with SiO2, its concentration recovered rapidly. The desorption of SO2 was observed when SO2 was isolated from SiO2 in the dark and under irradiation, indicating that the physical adsorption partially contributed to the SO2 loss during the photochemical process. The proportion of the desorbed SO2 during the uptake process can be quantified by dividing the integral of reversible desorption of SO2 (t = 80–100 min) into the integral of the SO2 uptake (t = 20–80 min), which was calculated to be 95 % and 12 % in the dark and under irradiation, respectively. This implies that SO2 uptake in the dark was primarily ascribed to the physical adsorption of SO2, while SO2 uptake under irradiation was mainly attributed to chemical processes or irreversible adsorption.
The uptake coefficients of SO2 on SiO2 as a function of irradiation intensity are shown in Fig. 1b. The errors in all figures are the standard deviations of three repetitive experiments. Both γ0,BET and γs,BET displayed a strong linear relationship with the irradiation intensity. The γ0,BET and γs,BET under the irradiation of 7.93 × 1016 were 1.75 and 2.25 times those in the dark, respectively. This further confirms the photochemical nature of the reactions of SO2 on SiO2. In particular, γ0,BET and γs,BET on SiO2 under simulated solar irradiation were comparable with those (10−7–10−6) on Gobi Desert dust (GDD) and Arizona test dust (ATD) under UV irradiation, which contained photocatalytic metal oxides (Park et al., 2017). As for the SO2 uptake on TiO2, γ0,BET and γs,BET were measured to be 10−6 and 10−7, respectively, using the flow tube (Ma et al., 2019), which were similar to our results. It should be pointed out that the similar uptake coefficient did not mean the comparable ability of photoactive and non-photoactive mineral oxides to SO2 uptake, since the uptake coefficient was highly dependent on environmental conditions (SO2 concentration, relative humidity, mineral oxides mass, light source, and pressure) and reactor type (chamber and flow tube reactor). Table S1 in the Supplement shows that the percentage of SiO2 in the sample was 99.02 %, accompanied by a small amount of Al2O3, K2O, Fe2O3, and CaO. Photoactive substances (Fe2O3) were very few in the sample, and they should not be the main contributor to the photochemical uptake of SO2.
Figure 1c shows the evolution of γ0,BET and γs,BET at different SO2 concentrations under irradiation. An inverse dependence of γ0,BET and γs,BET on the SO2 concentration was observed, meaning that the efficiency of SO2 uptake decreased with increasing the SO2 concentration. The uptake of gases on the solid surfaces usually follows the Langmuir–Hinshelwood (L–H) mechanism (Ammann et al., 2003; T. Zhang et al., 2020), suggesting that gaseous molecules are quickly absorbed on the surfaces, and then the reactions occur among the absorbed species. Assuming that the adsorption of SO2 on SiO2 is in accordance with the Langmuir isotherm, the dependence of γ on the SO2 concentration can be described by Eq. (5) (T. Zhang et al., 2020):
where [SO2]g is the concentration of gaseous SO2, [SiO2]T is the total number of active sites on SiO2, k is the reaction rate constant of SO2 absorbed on SiO2, and represents the Langmuir adsorption constant of SO2. Because the SiO2 mass remained constant during the reaction, Eq. (5) can be written as Eq. (6):
where . As shown in Fig. 1c, Eq. (6) can well describe the correlation of the SO2 uptake coefficient with the SO2 concentration, suggesting that the L–H mechanism can explain the influence of the SO2 concentration on γ0,BET and γs,BET.
3.2 Photo-induced formation of sulfates by the SO2 uptake
To investigate the products formed on SiO2, in situ DRIFTS spectra were recorded, as shown in Fig. 2. The band at 1359 cm−1 was assigned to physically adsorbed SO2 on SiO2 (Urupina et al., 2019). The bidentate sulfates and bisulfates contributed to the bands at 1260 and 1229/1074 cm−1 (Urupina et al., 2019; Yang et al., 2020), respectively. The band at 1038 cm−1 may be related to the monodentate sulfites (Yang et al., 2019; Z. Wang et al., 2019). It was noted that the intensity of physically absorbed SO2 (1359 cm−1) under irradiation was lower than that in the dark (Fig. S7), which may be ascribed to further conversion of SO2 absorbed on SiO2 under irradiation. The sulfate bands in particular (1260, 1229, and 1074 cm−1) only appeared under irradiation, while the sulfites (1038 cm−1) were only detected in the dark. This suggests that light changed the SO2 conversion pathways on SiO2. As shown in Fig. S7, the bands at 1157/1055 cm−1 were assigned to the asymmetric stretching of Si–O (Hu et al., 2003). Sulfates generated on the surface may interact with SiO2, leading to a decrease in the intensity of peaks (1157/1055 cm−1).
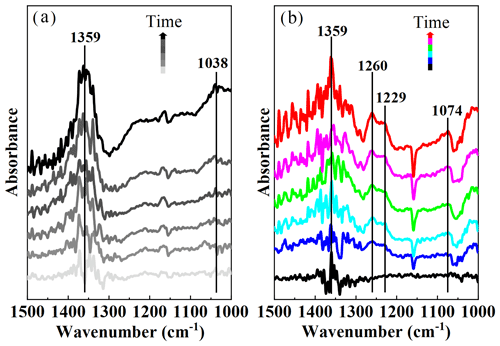
Figure 2In situ DRIFTS spectra of SiO2 during the uptake process of SO2 (2 ppm) in the dark (a) and under irradiation (b). Reaction conditions: RH of 40 %, temperature of 298 K, and O2 content of 20 %.
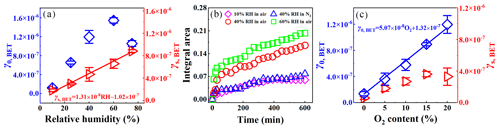
Figure 3(a) The dependence of γ0,BET and γs,BET on RH. (b) Integrated area of sulfates in DRIFTS spectra (1289–1202 cm−1) as a function of time. (c) The dependence of γ0,BET and γs,BET on O2. Reaction conditions: SiO2 mass of 0.2 g, irradiation intensity of 7.93 × 1016 , temperature of 298 K, O2 content of 20 % for (a), and RH of 40 % for (b).
3.3 Key roles of H2O and O2 in photochemical conversion of SO2 to sulfates
Figure S8a shows temporal variations in the SO2 concentration in the reaction with SiO2 at RH = 10 % and 60 % under irradiation. The uptake of SO2 was very weak at RH = 10 %, whereas it was obvious at RH = 60 %. Moreover, H2O prolonged the time to reach the steady-state uptake of SO2. This means that H2O plays an enhancement role in the photochemical uptake of SO2. As shown in Fig. 3a, γ0,BET had a continuous increase from (1.20 ± 0.04) × 10−7 to (1.54 ± 0.07) × 10−6 with increasing the RH in the 10 %–60 % range, but it decreased to (1.05 ± 0.09) × 10−6 at RH = 75 %. The γs,BET linearly depended on the RH, and linear fitting to γs,BET versus RH yielded an equation γs,BET = 1.31 × 10−8 × RH − 1.02 × 10−7. Adsorbed H2O promoted the hydration and dissociation of SO2 (Huang et al., 2015), and it may generate reactive oxygen species (ROS) such as •OH or HO2 radicals to oxidize SO2 under irradiation (Li et al., 2020; Ma et al., 2019), which would lead to positive effects of RH on the SO2 uptake. Adsorbed H2O also occupied adsorptive and active sites on the surface, leading to a decrease in SO2 adsorption. When this competitive role was dominant, the uptake of SO2 was hindered.
The DRIFTS spectra of SiO2 during the SO2 uptake at different RHs are shown in Fig. S9a. The band intensities of sulfates (1260 and 1229 cm−1) at RH = 60 % were stronger than those at RH = 10 %, suggesting that H2O promotes the sulfate formation. To further investigate the influence of H2O on the sulfate formation, the integrated area of sulfates in the DRIFTS spectra (1289–1202 cm−1) as a function of the time at different RHs is illustrated in Fig. 3b. Sulfates exhibited a fast formation in the initial 30 min, and then they were continuously generated at a relatively slow rate. SO2 absorption on the surface was blocked because of the accumulation of H2O and products (sulfites and sulfates), resulting in the gradual deactivation of the surface. It was noted that sulfate formation was more prominent at higher RHs, revealing that H2O can act as an important participator in the production of sulfates by the photochemical uptake of SO2 on SiO2.
Figure S8b displays effects of O2 on the photochemical uptake of SO2 on SiO2. Negligible SO2 uptake occurred in N2, while there was a significant decrease in the SO2 concentration in air. The γ0,BET greatly increased from (1.37 ± 0.45) × 10−7 under anaerobic conditions to (1.19 ± 0.13) × 10−6 under 20 % O2 content conditions (Fig. 3c), confirming that O2 was involved in the reaction of SO2 on SiO2. The γs,BET increased from (7.10 ± 2.85) × 10−8 under anaerobic conditions to (4.37 ± 0.58) × 10−7 under 15 % O2 content conditions, whereas it remained unchanged with O2 content further increasing.
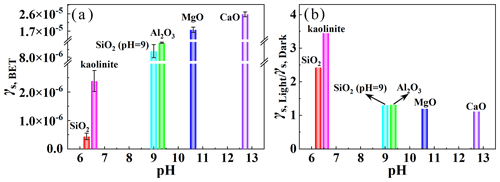
Figure 4(a) The dependence of γs,BET under irradiation on the basicity (pH) of mineral oxides. (b) The ratios of steady-state uptake coefficients under irradiation to those in the dark (). Reaction conditions: mineral oxides mass of 0.2 g, irradiation intensity of 7.93 × 1016 , temperature of 298 K, RH of 40 %, and O2 content of 20 %.
DRIFTS spectra of SiO2 during the SO2 uptake in N2 and air were compared in Fig. S9b. In both air and N2, the bands of absorbed SO2 were 1359 cm−1 and those of sulfates were 1260, 1229, and 1074 cm−1. Nevertheless, their intensities in N2 were weaker than those in air. According to the integrated area of sulfates in the DRIFTS spectra (1289–1202 cm−1), the formation trends of sulfates were similar in N2 and air (Fig. 3b), while the sulfate formation rate in N2 was obviously lower than that in air, meaning that O2 enhanced the sulfate production. It was reported that the production rate of sulfates from the SO2 uptake on TiO2 and by the photolysis of nitrates under UV irradiation in N2 was also smaller than that in air (Ma et al., 2019; Gen et al., 2019b). In addition, it was noted that sulfates can be generated in N2, meaning that O2 was not necessary and some pathways contributed to sulfates without O2.
3.4 Ubiquitously photoenhanced conversion of SO2 to sulfates
To better assess the potential for photochemical conversion of SO2 to sulfates, the SO2 uptake experiments were further performed on typical mineral oxides without photocatalytic activity. As displayed in Fig. S10, more obvious SO2 uptake on kaolinite, Al2O3, MgO, and CaO was observed under irradiation compared to that in the dark. Figure 4a shows that there was the largest γs,BET for CaO among five minerals, and γs,BET positively depended on the basicity (pH) of mineral oxides. Basic oxides generally contain more surface hydroxyls, which enhance the heterogeneous uptake of SO2 (Zhang et al., 2006). The ratios of steady-state uptake coefficients under irradiation to those in the dark () were larger than 1.0 for all mineral oxides (Fig. 4b). The experiments for the pH dependence on SiO2 have also been performed (Fig. S11). The pH of SiO2 suspension was adjusted to pH = 9, and γs,BET and were determined to be (8.79 ± 0.85) × 10−6 and 1.31, respectively (Fig. 4a and b). These results suggest that light can generally enhance the SO2 uptake on minerals at a wide pH range. However, the had smaller values with an increase in the basicity, suggesting that the promotion effect of the light was less remarkable for basic oxides.
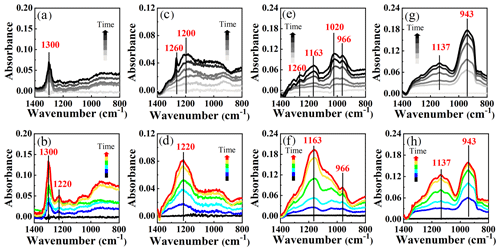
Figure 5In situ DRIFTS spectra of kaolinite (a, b), Al2O3 (c, d), MgO (e, f), and CaO (g, h) during the uptake process of SO2 (2 ppm) for 600 min in the dark (black lines) and under irradiation (colorful lines). Reaction conditions: RH of 40 %, temperature of 298 K, and O2 content of 20 %.
As shown in Fig. 5a and b, the band at 1300 cm−1 should be ascribed to the sulfates. The intensity of sulfates (1300 and 1220 cm−1) under irradiation was larger than those in the dark. Compared to weaker peaks of sulfates (1200 and 1260 cm−1) for Al2O3 in the dark (Fig. 5c), a stronger band of bisulfates appeared at 1220 cm−1 under irradiation (Fig. 5d). In contrast to the generation of sulfates for kaolinite and Al2O3, both sulfite and sulfate formations were observed for MgO and CaO (Fig. 5e–h). Sulfites were dominant in the dark, as shown by the peaks at 966 and 1020 cm−1 for MgO and 943 cm−1 for CaO, whereas the sulfate formation was significantly enhanced under irradiation according to peak intensities at 1163 cm−1 for MgO and 1137 cm−1 for CaO. It should be noted that these mineral oxides were non-photoactive because of their poor light absorption properties (Fig. S1). Nevertheless, the light can promote the formation of sulfates via the SO2 uptake process on mineral oxides without photocatalytic activity.
3.5 Conversion mechanisms of SO2 to sulfates
Heterogeneous photochemical reaction mechanisms of SO2 on non-photoactive mineral dust were proposed in light of experimental observations (Fig. 6). Gaseous SO2 was adsorbed on the surface (R1) and then reacted with H2O to form sulfites (R2). Under irradiation, adsorbed SO2 accepted photons to form its singlet states (1SO2) and 3SO2 (R3–R5) (Sidebottom et al., 1972; Martins-Costa et al., 2018). The reaction between 3SO2 and H2O resulted in the formation of HOSO• and •OH (R6), which can combine with SO2 to produce HOSO2• (R7). HOSO• and HOSO2• can be transformed into SO3, which reacted with H2O to drive the sulfate formation (R8 and R9). The interaction between 3SO2 and O2 may also generate SO3 directly, which would be subsequently converted to sulfates (R10). Theoretical calculations suggested that the multistep reactions between 3SO2 with H2O and O2 had small energy barriers or were barrier-free (Gong et al., 2022), which could enhance the generation of ROS and the transformation of S(IV) to S(VI). As displayed by R11 and R12, SO2 and H2SO3 adsorbed on the surface may be oxidized to form sulfates via the reactions with ROS, including •O, •OH, or HO2•, which were produced in R6 and R8–R10. In addition, gaseous SO2 could be dissolved into adsorbed H2O to generate bisulfites, which would be finally converted to sulfates by ROS (R13) (Urupina et al., 2019). As displayed in Fig. S12a, the IR peaks of sulfates were not observed when tris(2,2′-bipyridine)ruthenium dihydrochloride (Ru(bpy)3(Cl)2) was employed as the quencher of 3SO2. The peaks were assigned to the vibrations of excited Ru(bpy)3(Cl)2 (Mukuta et al., 2014). This definitely proves that 3SO2 is the key trigger for the sulfate formation. Figure S12b shows that the peaks of sulfates were weaker in the presence of NaHCO3, confirming the dominant contribution of •OH formed in R6 and R9 to the formation of sulfates.
Several photochemical mechanisms have been reported to explain the sulfate formation via the SO2 uptake on various surfaces. Photoactive mineral oxides (such as TiO2, F2O3, and ZnO) can accept photons to produce electron–hole pairs, which generated ROS for the conversion of SO2 to sulfates (Ma et al., 2019; Li et al., 2019; T. Wang et al., 2020). For example, •OH and HO2•, generated from the reaction of the hole with H2O and the electron with O2, respectively, can act as oxidizing agents for the reaction with SO2 (Ma et al., 2019). Similarly, the reaction of SO2 with photo-induced •OH obviously enhanced the formation of sulfates on diesel soot and actual PM2.5 (Zhang et al., 2022; Y. Zhang et al., 2020). NO2 and /HNO2 can be formed in the nitrates photolysis and primarily contribute to the oxidation of SO2 to sulfates on nitrates (Gen et al., 2019b, a). Theoretically, the mechanism proposed in this study should also occur on photo-excited substrates. Taking TiO2 as an example, SO2 competed with TiO2 for photons, and the production efficiency of 3SO2 and excited state of TiO2 () depended on their light absorption properties. Meanwhile, 3SO2 has competition with electron–hole pairs generated from for O2 and H2O. Thus, the dominant mechanism for the SO2 uptake on TiO2 should be related to light absorption properties of precursors and the reactivity for 3SO2 and to O2 and H2O. By contrast, all mineral oxides used here cannot be excited under irradiation according to their light absorption spectra (Fig. S1). Nevertheless, SO2 adsorbed on mineral oxides can absorb ultraviolet radiation (290–400 nm) to form the excited states of SO2 () (Kroll et al., 2018), which subsequently reacted with H2O and O2, finally converting SO2 to sulfates. The SO2 uptake experiment in the dark and the visible light (> 420 nm) was carried out (Fig. S13). An ignorable difference was observed for the SO2 concentration with or without visible light, suggesting that visible light had a minor contribution to the photoenhanced SO2 uptake.
According to the experimental results, some surfaces providing absorptive sites for SO2 can enhance the photooxidation of SO2 to sulfates. However, the promotion effect would vary with different substances. For example, the current experiments on some basic minerals indicate that light plays a minor enhancement role in the SO2 uptake (Fig. 4), but it could still enhance the sulfate formation (Fig. 5). The solubility and effective Henry's law constant of SO2 were positively dependent on pH. Thus, SO2 was more liable to be dissolved to form on a more alkaline surface, leading to a strong SO2 uptake in the dark (Fig. 4a and b) and abundant sulfites on surfaces (Fig. 5). Nevertheless, gaseous SO2 tends to be adsorbed on kaolinite and Al2O3 due to less solubility of SO2 on these surfaces, and then it is converted to sulfates under irradiation (Fig. 6). Accordingly, a promotion effect of light on SO2 uptake was observed on neutral and weakly alkaline surfaces (Fig. 4b).
The lifetime (τ) for photochemical loss of SO2 on mineral dust was given using Eq. (7),
where γ and ω are the uptake coefficient and the mean molecular speed of SO2, respectively. A is the surface area density of mineral dust, and it is estimated to be (1.4–4.8) × 10−5 cm2 cm−3 (Zhang et al., 2019; P. He et al., 2018). In this work, γs,BET of SO2 on several mineral oxides was measured to be 4.39 × 10−7–3.45 × 10−5 (reaction conditions: SO2 concentration of 40 ppb, irradiation intensity of 7.93 × 1016 , and RH of 40 %). Thus, the τ of SO2 with respect to the photooxidation on mineral dust was calculated to be 0.9–240 d, which was shorter than that (54 years) for the photochemical uptake of SO2 on TiO2 and the corresponding one (346 d) for the heterogeneous oxidation of SO2 on ATD in the presence of nitrates (Ma et al., 2019; Zhang et al., 2019). The reaction conditions in this study and those in the literature are different in some respects, and the previously reported SO2 uptake coefficient (10−7–10−6) had a lower value (Ma et al., 2019). The huge difference in the τ of SO2 was also ascribed to the variation in the surface area density. The content of TiO2 in mineral dust was only about 1 %. Thus, the surface area density of TiO2 was about 10−7 cm2 cm−3, leading to a longer τ (54 years) for SO2 on TiO2 (Ma et al., 2019). It was comparable to the lifetime (3.6–20 d) of SO2 for the gas-phase reaction with •OH at a concentration of ∼ 10−6 (Huang et al., 2015; Zhang et al., 2019). Therefore, the photochemical process with the excited state SO2 acting as a driver on mineral dust was an important pathway for the SO2 sink in the atmosphere.
Sulfates have a significant influence on the atmosphere, contributing to the haze formation, affecting the activity of aerosols acting as cloud condensation nuclei (CCN) and ice nuclei (IN), and modifying optical property and acidity of aerosols. A sulfate formation rate (R) can be obtained using γ in Eq. (8) (Cheng et al., 2016):
where RP (m) is the radius of mineral dust, which can be estimated using Eq. (9) (Li et al., 2020), which states
where [PM2.5] is average PM2.5 mass concentration and 300 µg m−3 is used for the polluted periods in typical China cities (Li et al., 2020; Guo et al., 2014). It was assumed that mineral dust accounted for 50 % of the mass of PM2.5 (Tohidi et al., 2022), and the mass fraction of SiO2, Al2O3, MgO, and CaO in mineral dust was 60 %, 12.5 %, 4 %, and 6.5 %, respectively (Urupina et al., 2019, 2021; Usher et al., 2003). Thus, R was determined to be 2.15 . This suggests that the SO2 uptake on non-photoactive surfaces may be an important sulfate formation pathway under irradiation in some dust-rich conditions.
The data used in this study are available from the corresponding author upon request (hanch@smm.neu.edu.cn).
The supplement related to this article is available online at: https://doi.org/10.5194/acp-24-6757-2024-supplement.
CH, WY, and JM designed and conducted experiments. CH, WY, and JM analyzed the data and prepared the paper with contributions from HY. FL conducted experiments. CH supervised the project.
The contact author has declared that none of the authors has any competing interests.
Publisher's note: Copernicus Publications remains neutral with regard to jurisdictional claims made in the text, published maps, institutional affiliations, or any other geographical representation in this paper. While Copernicus Publications makes every effort to include appropriate place names, the final responsibility lies with the authors.
This research has been supported by the National Natural Science Foundation of China (grant no. 42077198), the LiaoNing Revitalization Talents Program (grant no. XLYC1907185), and the Fundamental Research Funds for the Central Universities (grant nos. N2325034 and N2025011).
This paper was edited by Thorsten Bartels-Rausch and reviewed by Kangwei Li and two anonymous referees.
Adams, J. W., Rodriguez, D., and Cox, R. A.: The uptake of SO2 on Saharan dust: a flow tube study, Atmos. Chem. Phys., 5, 2679–2689, https://doi.org/10.5194/acp-5-2679-2005, 2005.
Alexander, B., Park, R. J., Jacob, D. J., and Gong, S.: Transition metal-catalyzed oxidation of atmospheric sulfur: Global implications for the sulfur budget, J. Geophy. Res., 114, 2309–2312, https://doi.org/10.1029/2008jd010486, 2009.
Ammann, M., Poschl, U., and Rudich, Y.: Effects of reversible adsorption and Langmuir–Hinshelwood surface reactions on gas uptake by atmospheric particles, Phys. Chem. Chem. Phys., 5, 351–356, https://doi.org/10.1039/b208708a, 2003.
Bounechada, D., Anderson, D., Skoglundh, M., and Carlsson, P.: SO2 adsorption on silica supported iridium, J. Chem. Phys., 146, 084701–084708, https://doi.org/10.1063/1.4976835, 2017.
Brunauer, B., Deming, L., Deming, W., and Teller, E.: Adsorption of gases in multimolecular layers, J. Am. Chem. Soc., 60, 309–319, https://doi.org/10.1021/ja01269a023, 1938.
Bulgakov, R. G. and Safonova, L. A.: Chemiluuminescence in the oxidation of Na2S by oxygen in water solutions, Russ. Chem. B.+, 45, 1775–1776, https://doi.org/10.1007/bf01431827, 1996.
Cao, J., Tie, X., Dabberdt, W. F., Jie, T., Zhao, Z., An, Z., Shen, Z., and Feng, Y.: On the potential high acid deposition in northeastern China, J. Geophy. Res.-Atmos., 118, 4834–4846, https://doi.org/10.1002/jgrd.50381, 2013.
Chan, M. and Chan, C.: Hygroscopic properties of two model humic-like substances and their mixtures with inorganics of atmospheric importance, Environ. Sci. Technol., 37, 5109–5115, https://doi.org/10.1021/es034272o, 2003.
Chen, Y., Tong, S., Li, W., Liu, Y., Tan, F., Ge, M., Xie, X., and Sun, J.: Photocatalytic oxidation of SO2 by TiO2: Aerosol formation and the key role of gaseous reactive oxygen species, Environ. Sci. Technol., 55, 9784–9793, https://doi.org/10.1021/acs.est.1c01608, 2021.
Cheng, Y., Zheng, G., Wei, C., Mu, Q., Zheng, B., Wang, Z., Gao, M., Zhang, Q., He, K., Carmichael, G., Pöschl, U., and Su, H.: Reactive nitrogen chemistry in aerosol water as a source of sulfate during haze events in China, Sci. Adv., 2, 1601530–1601540, https://doi.org/10.1126/sciadv.1601530, 2016.
Chu, B., Wang, Y., Yang, W., Ma, J., Ma, Q., Zhang, P., Liu, Y., and He, H.: Effects of NO2 and C3H6 on the heterogeneous oxidation of SO2 on TiO2 in the presence or absence of UV–Vis irradiation, Atmos. Chem. Phys., 19, 14777–14790, https://doi.org/10.5194/acp-19-14777-2019, 2019.
Davis, D. D., Ravishankara, A. R., and Fischer, S.: SO2 oxidation via the hydroxyl radical: Atmospheric fate of HSOx radicals, Geophys. Res. Lett., 6, 113–116, https://doi.org/10.1029/GL006i002p00113, 1979.
Dentener, F., Carmichael, G., Zhang, Y., Lelieveld, J., and Crutzen, P.: Role of mineral aerosol as a reactive surface in the global troposphere, J. Geophy. Res.-Atmos., 101, 22869–22889, https://doi.org/10.1029/96jd01818, 1996.
Gen, M., Zhang, R., Huang, D., Li, Y., and Chan, C.: Heterogeneous oxidation of SO2 in sulfate production during nitrate photolysis at 300 nm: Effect of pH, relative humidity, irradiation intensity, and the presence of organic compounds, Environ. Sci. Technol., 53, 8757–8766, https://doi.org/10.1021/acs.est.9b01623, 2019a.
Gen, M., Zhang, R., Huang, D., Li, Y., and Chan, C.: Heterogeneous SO2 oxidation in sulfate formation by photolysis of particulate nitrate, Environ. Sci. Tech. Let., 6, 86–91, https://doi.org/10.1021/acs.estlett.8b00681, 2019b.
Golobokova, L., Khodzher, T., Khuriganova, O., Marinayte, I., Onishchuk, N., Rusanova, P., and Potemkin, V.: Variability of chemical properties of the atmospheric aerosol above lake baikal during large wildfires in siberia, Atmosphere, 11, 1230–1250, https://doi.org/10.3390/atmos11111230, 2020.
Gong, C., Yuan, X., Xing, D., Zhang, D., Martins-Costa, M. T. C., Anglada, J. M., Ruiz-Lopez, M. F., Francisco, J. S., and Zhang, X.: Fast sulfate formation initiated by the spin-forbidden excitation of SO2 at the air-water interface, J. Am. Chem. Soc., 144, 22302–22308, https://doi.org/10.1021/jacs.2c10830, 2022.
Goodman, A., Li, P., Usher, C., and Grassian, V.: Heterogeneous uptake of sulfur dioxide on aluminum and magnesium oxide particles, J. Phys. Chem. A, 105, 6109–6120, https://doi.org/10.1021/jp004423z, 2001.
Guo, S., Hu, M., Zamora, M. L., Peng, J., Shang, D., Zheng, J., Du, Z., Wu, Z., Shao, M., Zeng, L., Molina, M. J., and Zhang, R.: Elucidating severe urban haze formation in China, P. Natl. Acad. Sci. USA, 111, 17373–17378, https://doi.org/10.1073/pnas.1419604111, 2014.
Harris, E., Sinha, B., van Pinxteren, D., Tilgner, A., Fomba, K. W., Schneider, J., Roth, A., Gnauk, T., Fahlbusch, B., Mertes, S., Lee, T., Collett, J., Foley, S., Borrmann, S., Hoppe, P., and Herrmann, H.: Enhanced role of transition metal ion catalysis during in-cloud oxidation of SO2, Science, 340, 727–730, https://doi.org/10.1126/science.1230911, 2013.
He, G., Ma, J., and He, H.: Role of carbonaceous aerosols in catalyzing sulfate formation, ACS Catal., 8, 3825–3832, https://doi.org/10.1021/acscatal.7b04195, 2018.
He, H., Li, C., Loughner, C. P., Li, Z., Krotkov, N. A., Yang, K., Wang, L., Zheng, Y., Bao, X., Zhao, G., and Dickerson, R. R.: SO2 over central China: Measurements, numerical simulations and the tropospheric sulfur budget, J. Geophy. Res.-Atmos., 117, 37–51, https://doi.org/10.1029/2011jd016473, 2012.
He, P., Alexander, B., Geng, L., Chi, X., Fan, S., Zhan, H., Kang, H., Zheng, G., Cheng, Y., Su, H., Liu, C., and Xie, Z.: Isotopic constraints on heterogeneous sulfate production in Beijing haze, Atmos. Chem. Phys., 18, 5515–5528, https://doi.org/10.5194/acp-18-5515-2018, 2018.
Herrmann, H., Ervens, B., Jacobi, H. W., Wolke, R., Nowacki, P., and Zellner, R.: CAPRAM2.3: A chemical aqueous phase radical mechanism for tropospheric chemistry, J. Atmos. Chem., 36, 231–284, https://doi.org/10.1023/A:1006318622743, 2000.
Hu, Q., Suzuki, H., Gao, H., Araki, H., Yang, W., and Noda, T.: High-frequency FTIR absorption of SiO2/Si nanowires, Chem. Phys. Lett., 378, 299–304, https://doi.org/10.1016/j.cplett.2003.07.015, 2003.
Huang, L., Zhao, Y., Li, H., and Chen, Z.: Kinetics of heterogeneous reaction of sulfur dioxide on authentic mineral dust: Effects of relative humidity and hydrogen peroxide, Environ. Sci. Technol., 49, 10797–10805, https://doi.org/10.1021/acs.est.5b03930, 2015.
Knopf, D., Cosman, L., Mousavi, P., Mokamati, S., and Bertram, A.: A novel flow reactor for studying reactions on liquid surfaces coated by organic monolayers: Methods, validation, and initial results, J. Phys. Chem. A, 111, 11021–11032, https://doi.org/10.1021/jp075724c, 2007.
Kroll, J., Frandsen, B., Kjaergaard, H., and Vaida, V.: Atmospheric hydroxyl radical source: Reaction of triplet SO2 and water, J. Phys. Chem. A, 122, 4465–4469, https://doi.org/10.1021/acs.jpca.8b03524, 2018.
Langhammer, D., Kullgren, J., and Osterlund, L.: Photoinduced adsorption and oxidation of SO2 on anatase TiO2, J. Am. Chem. Soc., 142, 21767–21774, https://doi.org/10.1021/jacs.0c09683, 2020.
Li, G., Bei, N., Cao, J., Huang, R., Wu, J., Feng, T., Wang, Y., Liu, S., Zhang, Q., Tie, X., and Molina, L. T.: A possible pathway for rapid growth of sulfate during haze days in China, Atmos. Chem. Phys., 17, 3301–3316, https://doi.org/10.5194/acp-17-3301-2017, 2017.
Li, J., Zhang, Y. L., Cao, F., Zhang, W., Fan, M., Lee, X., and Michalski, G.: Stable sulfur isotopes revealed a major role of transition-metal ion-catalyzed SO2 oxidation in haze episodes, Environ. Sci. Technol., 54, 2626–2634, https://doi.org/10.1021/acs.est.9b07150, 2020.
Li, K., Kong, L., Zhanzakova, A., Tong, S., Shen, J., Wang, T., Chen, L., Li, Q., Fu, H., and Zhang, L.: Heterogeneous conversion of SO2 on nano á-Fe2O3: the effects of morphology, light illumination and relative humidity, Environ. Sci.: Nano, 6, 1838–1851, https://doi.org/10.1039/c9en00097f, 2019.
Lim, S., Lee, M., Kim, S., and Laj, P.: Sulfate alters aerosol absorption properties in East Asian outflow, Sci. Rep.-UK, 8, 5172–5178, https://doi.org/10.1038/s41598-018-23021-1, 2018.
Liu, T., Clegg, S., and Abbatt, J. P. D.: Fast oxidation of sulfur dioxide by hydrogen peroxide in deliquesced aerosol particles, P. Natl. Acad. Sci. USA, 117, 1354–1359, https://doi.org/10.1073/pnas.1916401117, 2020.
Liu, T., Chan, A. W. H., and Abbatt, J. P. D.: Multiphase oxidation of sulfur dioxide in aerosol particles: Implications for sulfate formation in polluted environments, Environ. Sci. Technol., 55, 4227–4242, https://doi.org/10.1021/acs.est.0c06496, 2021.
Liu, Y., Deng, Y., Liu, J., Fang, X., Wang, T., Li, K., Gong, K., Bacha, A. U., Nabi, I., Ge, Q., Zhang, X., George, C., and Zhang, L.: A novel pathway of atmospheric sulfate formation through carbonate radicals, Atmos. Chem. Phys., 22, 9175–9197, https://doi.org/10.5194/acp-22-9175-2022, 2022.
Ma, J., Dörner, S., Donner, S., Jin, J., Cheng, S., Guo, J., Zhang, Z., Wang, J., Liu, P., Zhang, G., Pukite, J., Lampel, J., and Wagner, T.: MAX-DOAS measurements of NO2, SO2, HCHO, and BrO at the Mt. Waliguan WMO GAW global baseline station in the Tibetan Plateau, Atmos. Chem. Phys., 20, 6973–6990, https://doi.org/10.5194/acp-20-6973-2020, 2020.
Ma, Q., Wang, L., Chu, B., Ma, J., and He, H.: Contrary role of H2O and O2 in the kinetics of heterogeneous photochemical reactions of SO2 on TiO2, J. Phys. Chem. A, 123, 1311–1318, https://doi.org/10.1021/acs.jpca.8b11433, 2019.
Martins-Costa, M., Anglada, J., Francisco, J., and Ruiz-Lopez, M.: Photochemistry of SO2 at the air-water interface: A source of OH and HOSO radicals, J. Am. Chem. Soc., 140, 12341–12344, https://doi.org/10.1021/jacs.8b07845, 2018.
Mauldin, R., Berndt, T., Sipila, M., Paasonen, P., Petaja, T., Kim, S., Kurten, T., Stratmann, F., Kerminen, V., and Kulmala, M.: A new atmospherically relevant oxidant of sulphur dioxide, Nature, 488, 193–196, https://doi.org/10.1038/nature11278, 2012.
Mukuta, T., Fukazawa, N., Murata, K., Inagaki, A., Akita, M., Tanaka, S., Koshihara, S. Y., and Onda, K.: Infrared vibrational spectroscopy of [Ru(bpy)2(bpm)]2+ and [Ru(bpy)3]2+ in the excited triplet state, Inorg. Chem., 53, 2481–2490, https://doi.org/10.1021/ic402474t, 2014.
Olson, E., Michalski, G., Welp, L., Valdivia, A., Larico, J., Pen, J., Fang, H., Gomez, K., and Li, J.: Mineral dust and fossil fuel combustion dominate sources of aerosol sulfate in urban Peru identified by sulfur stable isotopes and water-soluble ions, Atmos. Environ., 260, 118482–118495, https://doi.org/10.1016/j.atmosenv.2021.118482, 2021.
Park, J. and Jang, M.: Heterogeneous photooxidation of sulfur dioxide in the presence of airborne mineral dust particles, RSC Adv., 6, 58617–58627, https://doi.org/10.1039/c6ra09601h, 2016.
Park, J., Jang, M., and Yu, Z.: Heterogeneous photo-oxidation of SO2 in the presence of two different mineral dust particles: Gobi and arizona dust, Environ. Sci. Technol., 51, 9605–9613, https://doi.org/10.1021/acs.est.7b00588, 2017.
Peng, Y., von Salzen, K., and Li, J.: Simulation of mineral dust aerosol with Piecewise Log-normal Approximation (PLA) in CanAM4-PAM, Atmos. Chem. Phys., 12, 6891–6914, https://doi.org/10.5194/acp-12-6891-2012, 2012.
Prospero, J.: Long-range transport of mineral dust in the global atmosphere: Impact of African dust on the environment of the southeastern United States, P. Natl. Acad. Sci. USA, 96, 3396–3403, https://doi.org/10.1073/pnas.96.7.3396, 1999.
Shao, J., Chen, Q., Wang, Y., Lu, X., He, P., Sun, Y., Shah, V., Martin, R. V., Philip, S., Song, S., Zhao, Y., Xie, Z., Zhang, L., and Alexander, B.: Heterogeneous sulfate aerosol formation mechanisms during wintertime Chinese haze events: air quality model assessment using observations of sulfate oxygen isotopes in Beijing, Atmos. Chem. Phys., 19, 6107–6123, https://doi.org/10.5194/acp-19-6107-2019, 2019.
Shiraiwa, M., Ueda, K., Pozzer, A., Lammel, G., Kampf, C. J., Fushimi, A., Enami, S., Arangio, A. M., Frohlich-Nowoisky, J., Fujitani, Y., Furuyama, A., Lakey, P. S. J., Lelieveld, J., Lucas, K., Morino, Y., Poschl, U., Takahama, S., Takami, A., Tong, H., Weber, B., Yoshino, A., and Sato, K.: Aerosol health effects from molecular to global scales, Environ. Sci. Technol., 51, 13545–13567, https://doi.org/10.1021/acs.est.7b04417, 2017.
Sidebottom, H. W., Badcock, C. C., Jackson, G. E., Calvert, J. G., Reinhardt, G. W., and Damon, E. K.: Photooxidation of sulfur dioxide, Environ. Sci. Technol., 6, 72–79, https://doi.org/10.1080/00022470.1971.10469552, 1972.
Solbrig, C. W. and Gidaspow, D.: Convective diffusion in a parallel plate duct with one catalytic wall, laminar flow, first-order reaction-part one, Can. J. Chem. Eng., 45, 35–39, https://doi.org/10.1016/0304-5102(89)80197-X, 1967.
Sumner, A. L., Menke, E. J., Dubowski, Y., Newberg, J. T., Penner, R. M., Hemminger, J. C., Wingen, L. M., Brauers, T., and Finlayson-Pitts, B. J.: The nature of water on surfaces of laboratory systems and implications for heterogeneous chemistry in the troposphere, Phys. Chem. Chem. Phys., 6, 604–613, https://doi.org/10.1039/B308125G, 2004.
Tohidi, R., Farahani, V., and Sioutas, C.: Real-time measurements of mineral dust concentration in coarse particulate matter PM10–2.5 by employing a novel optical-based technique in Los Angeles, Sci. Total Environ., 838, 156215–156226, https://doi.org/10.1016/j.scitotenv.2022.156215, 2022.
Urupina, D., Lasne, J., Romanias, M. N., Thiery, V., Dagsson-Waldhauserova, P., and Thevenet, F.: Uptake and surface chemistry of SO2 on natural volcanic dusts, Atmos. Environ., 217, 116942–116959, https://doi.org/10.1016/j.atmosenv.2019.116942, 2019.
Urupina, D., Romanias, M. N., and Thevenet, F.: How relevant is it to use mineral proxies to mimic the atmospheric reactivity of natural dust samples? A reactivity study using SO2 as probe molecule, Minerals, 11, 282–299, https://doi.org/10.3390/min11030282, 2021.
Usher, C., Al-Hosney, H., Carlos-Cuellar, S., and Grassian, V.: A laboratory study of the heterogeneous uptake and oxidation of sulfur dioxide on mineral dust particles, J. Geophys. Res.-Atmos., 107, 4713–4729, https://doi.org/10.1029/2002jd002051, 2002.
Usher, C., Michel, A., and Grassian, V.: Reactions on mineral dust, Chem. Rev., 103, 4883–4939, https://doi.org/10.1021/cr020657y, 2003.
Wang, J., Li, J., Ye, J., Zhao, J., Wu, Y., Hu, J., Liu, D., Nie, D., Shen, F., Huang, X., Huang, D. D., Ji, D., Sun, X., Xu, W., Guo, J., Song, S., Qin, Y., Liu, P., Turner, J. R., Lee, H. C., Hwang, S., Liao, H., Martin, S. T., Zhang, Q., Chen, M., Sun, Y., Ge, X., and Jacob, D. J.: Fast sulfate formation from oxidation of SO2 by NO2 and HONO observed in Beijing haze, Nat. Commun., 11, 2844–2850, https://doi.org/10.1038/s41467-020-16683-x, 2020.
Wang, S., Zhou, S., Tao, Y., Tsui, W. G., Ye, J., Yu, J. Z., Murphy, J. G., McNeill, V. F., Abbatt, J. P. D., and Chan, A. W. H.: Organic peroxides and sulfur dioxide in aerosol: Source of particulate sulfate. Environ. Sci. Technol., 53, 10695–10704, https://doi.org/10.1021/acs.est.9b02591, 2019.
Wang, T., Liu, Y., Deng, Y., Fu, H., Zhang, L., and Chen, J.: The influence of temperature on the heterogeneous uptake of SO2 on hematite particles, Sci. Total Environ., 644, 1493–1502, https://doi.org/10.1016/j.scitotenv.2018.07.046, 2018.
Wang, T., Liu, Y. Y., Deng, Y., Cheng, H. Y., Yang, Y., Li, K. J., Fang, X. Z., and Zhang, L. W.: Irradiation intensity dependent heterogeneous formation of sulfate and dissolution of ZnO nanoparticles, Environ. Sci.: Nano, 7, 327–338, https://doi.org/10.1039/c9en01148j, 2020.
Wang, Z., Wang, T., Fu, H., Zhang, L., Tang, M., George, C., Grassian, V. H., and Chen, J.: Enhanced heterogeneous uptake of sulfur dioxide on mineral particles through modification of iron speciation during simulated cloud processing, Atmos. Chem. Phys., 19, 12569–12585, https://doi.org/10.5194/acp-19-12569-2019, 2019.
Xu, M., Qiu, P., He, Y., Guo, S., Bai, Y., Zhang, H., Zhao, S., Shen X., Zhu, B., Guo, Q., Guo, Z., Sulfur isotope composition during heterogeneous oxidation of SO2 on mineral dust: The effect of temperature, relative humidity, and light intensity, Atmos. Res., 254, 105513, https://doi.org/10.1016/j.atmosres.2021.105513, 2021.
Yang, N., Tsona, N. T., Cheng, S., Li, S., Xu, L., Wang, Y., Wu, L., and Du, L.: Competitive reactions of SO2 and acetic acid on á-Al2O3 and CaCO3 particles, Sci. Total Environ., 699, 134362–134370, https://doi.org/10.1016/j.scitotenv.2019.134362, 2020.
Yang, W., Ma, Q., Liu, Y., Ma, J., Chu, B., and He, H.: The effect of water on the heterogeneous reactions of SO2 and NH3 on the surfaces of á-Fe2O3 and ã-Al2O3, Environ. Sci.: Nano, 6, 2749–2758, https://doi.org/10.1039/c9en00574a, 2019.
Yao, M., Zhao, Y., Hu, M., Huang, D., Wang, Y., Yu, J. Z., and Yan, N.: Multiphase reactions between secondary organic aerosol and sulfur dioxide: Kinetics and contributions to sulfate formation and aerosol aging. Environ. Sci. Technol. Lett., 6, 768–774, https://doi.org/10.1021/acs.estlett.9b00657, 2019.
Ye, J., Abbatt, J. P. D., and Chan, A. W. H.: Novel pathway of SO2 oxidation in the atmosphere: reactions with monoterpene ozonolysis intermediates and secondary organic aerosol, Atmos. Chem. Phys., 18, 5549–5565, https://doi.org/10.5194/acp-18-5549-2018, 2018.
Zhang, P., Chen, T., Ma, Q., Chu, B., Wang, Y., Mu, Y., Yu, Y., and He, H.: Diesel soot photooxidation enhances the heterogeneous formation of H2SO4, Nat. Commun., 13, 5364–5372, https://doi.org/10.1038/s41467-022-33120-3, 2022.
Zhang, R., Gen, M., Huang, D., Li, Y., and Chan, C.: Enhanced sulfate production by nitrate photolysis in the presence of halide ions in atmospheric particles, Environ. Sci. Technol., 54, 3831–3839, https://doi.org/10.1021/acs.est.9b06445, 2020.
Zhang, T., Yang, W., Han, C., Yang, H., and Xue, X.: Heterogeneous reaction of ozone with syringic acid: Uptake of O3 and changes in the composition and optical property of syringic acid, Environ. Pollut., 257, 113632–113638, https://doi.org/10.1016/j.envpol.2019.113632, 2020.
Zhang, X., Zhuang, G., Chen, J., Wang, Y., Wang, X., An, Z., and Zhang, P.: Heterogeneous reactions of sulfur dioxide on typical mineral particles, J. Phys. Chem. B, 110, 12588–12596, https://doi.org/10.1021/jp0617773, 2006.
Zhang, Y., Bao, F., Li, M., Chen, C., and Zhao, J.: Nitrate-enhanced oxidation of SO2 on mineral dust: A vital role of a proton, Environ. Sci. Technol., 53, 10139–10145, https://doi.org/10.1021/acs.est.9b01921, 2019.
Zhang, Y., Bao, F., Li, M., Xia, H., Huang, D., Chen, C., and Zhao, J.: Photoinduced uptake and oxidation of SO2 on Beijing urban PM2.5, Environ. Sci. Technol., 54, 14868–14876, https://doi.org/10.1021/acs.est.0c01532, 2020.