the Creative Commons Attribution 4.0 License.
the Creative Commons Attribution 4.0 License.
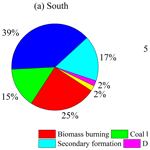
Measurement report: Oxidation potential of water-soluble aerosol components in the south and north of Beijing
Wei Yuan
Ru-Jin Huang
Chao Luo
Lu Yang
Wenjuan Cao
Jie Guo
Huinan Yang
Water-soluble components significantly contribute to the oxidative potential (OP) of atmospheric fine particles (PM2.5), but our understanding of the water-soluble PM2.5 OP and its sources, as well as its relationship with water-soluble components, is still limited. In this study, the water-soluble OP levels in wintertime PM2.5 in the south and north of Beijing, representing the difference in sources, were measured with the dithiothreitol (DTT) assay. The volume-normalized DTT (DTTv) in the north (3.5±1.2 nmol min−1 m−3) was comparable to that in the south (3.9±0.9 nmol min−1 m−3), while the mass-normalized DTT (DTTm) in the north (65±28 pmol min−1 µg−3) was almost twice that in the south (36±14 pmol min−1 µg−3). In both the south and north of Beijing, DTTv was better correlated with soluble elements instead of total elements. In the north, soluble elements (mainly Mn, Co, Ni, Zn, As, Cd and Pb) and water-soluble organic compounds, especially light-absorbing compounds (also known as brown carbon), had positive correlations with DTTv. However, in the south, DTTv was mainly related to soluble As, Fe and Pb. The sources of DTTv were further resolved using the positive matrix factorization (PMF) model. Traffic-related emissions (39 %) and biomass burning (25 %) were the main sources of DTTv in the south, and traffic-related emissions (>50 %) contributed the most to DTTv in the north. Our results indicate that vehicle emission was an important contributor to the OP in Beijing ambient PM2.5 and suggest that more study is needed to understand the intrinsic relationship between the OP and light-absorbing organic compounds.
- Article
(2048 KB) - Full-text XML
-
Supplement
(1439 KB) - BibTeX
- EndNote
Atmospheric fine particulate matter (PM2.5) pollution is one of the major global environmental issues, affecting air quality, climate and human health (Huang et al., 2014; Burnett et al., 2018; An et al., 2019; Zheng et al., 2020). The exposure to PM2.5 was estimated to be responsible for 8.9 million deaths worldwide in 2015, of which 28 % occurred in China (Burnett et al., 2018). Numerous studies have shown that oxidative stress is one of the possible mechanisms underlying the adverse effects of PM2.5 on human health (Chowdhury et al., 2019; Lelieveld et al., 2021; Yu et al., 2022a; Guascito et al., 2023). When entering the human body, PM2.5 can induce the production of excessive reactive oxygen species (ROS) (e.g., H2O2, ⋅ OH and ⋅ O), leading to cellular redox imbalance and generating oxidative stress effects. The ability of PM2.5 to cause oxidative stress is defined as oxidative potential (OP).
The methods to determine the OP of PM2.5 include cellular and acellular assays, and acellular methods are more widely used than cellular methods (Charrier and Anastasio, 2012; Xiong et al., 2017; Calas et al., 2018; Bates et al., 2019; Y. Wang et al., 2020; Campbell et al., 2021; Oh et al., 2023). Among acellular methods, the dithiothreitol (DTT) assay is extensively applied to determine the OP of ambient particles (Charrier and Anastasio, 2012; Xiong et al., 2017; Liu et al., 2018; Y. Wang et al., 2020; Puthussery et al., 2022; Wu et al., 2022a). DTT is a surrogate of cellular reductants, and the consumption rate of DTT was used to assess the OP of PM2.5. Previous studies have shown that organic matters (e.g., water-soluble organic species and polycyclic aromatic hydrocarbons (PAHs)) and some transition metals (e.g., Mn and Cu) are the important contributors to DTT consumption of PM2.5 (Charrier and Anastasio, 2012; Verma et al., 2015; Bates et al., 2019; Wu et al., 2022a, b). For example, Charrier and Anastasio (2012) measured the OP of PM2.5 in San Joaquin Valley, California, and reported that about 80 % of DTT consumption was contributed by transition metals. Verma et al. (2015) measured the OP of water-soluble PM2.5 in the southeastern United States and reported that about 60 % of DTT activity was contributed by water-soluble organics. The mixtures of metals and organics may produce synergistic or antagonistic effects; for example, ⋅ O produced from oxidation of DTT by quinones is more efficiently transformed to ⋅ OH in the presence of Fe, while the DTT consumption and ⋅ OH generation of quinones are reduced in the presence of Cu (Xiong et al., 2017; Yu et al., 2018; Bates et al., 2019).
A number of studies have investigated the OP of water-soluble components in PM2.5, which show that the average water-soluble OP values in urban areas ranged from 0.1 to 10 nmol min−1 m−3 (Fang et al., 2016; Liu et al., 2018; Chen et al., 2019; Wu et al., 2022a; Q. Yu et al., 2022; Xing et al., 2023). Due to the complexity in chemical composition and sources of PM2.5 that determine the OP levels, the sources of the OP are also diverse (Verma et al., 2015; Bates et al., 2019; Tuet et al., 2019; Yu et al., 2019; Cao et al., 2021). Several studies have investigated the emission sources and ambient samples to identify the sources of the OP (Tuet et al., 2019; Yu et al., 2019; Y. Wang et al., 2020; Cao et al., 2021), which include both primary and secondary sources. For example, Cao et al. (2021) measured the water-soluble OP of PM2.5 samples from six biomass and five coal burning emissions in China, with average values of 4.5–7.4 and 0.5–2.1 pmol min−1 µg−1, respectively. Tong et al. (2018) investigated the OPs of secondary organic aerosols (SOAs) from oxidation of naphthalene, isoprene and β-pinene with ⋅ OH or O3, which were 104±7.6, 48±7.9 and 36±3.1 pmol min−1 µg−1, respectively. Verma et al. (2014) identified the sources of the water-soluble OP of PM2.5 in Atlanta, United States, from June 2012 to September 2013 with positive matrix factorization (PMF) and chemical mass balance (CMB) methods, of which biomass burning was the largest contributor. Y. Wang et al. (2020) quantified the sources of the water-soluble OP of PM2.5 in Xi'an, China, in 2017 using PMF and multiple linear regression (MLR) methods, with significant contributions from secondary sulfates, vehicle emissions and coal combustion. Some studies have also measured the OP of particles with different particle sizes and reported that smaller size fractions typically have higher ROS activity compared to large PM size fractions (Saffari et al., 2014; Shafer et al., 2016; Besis et al., 2023). For example, Besis et al. (2023) measured the OP of the water-soluble fraction of size-segregated PM (<0.49, 0.49–0.95, 0.95–1.5, 1.5–3.0, 3.0–7.2 and >7.2 µm) collected during cold and warm periods at an urban site in Thessaloniki, northern Greece, and the results showed that the total DTT activity of the PM <3 µm size fraction was higher (2–5 times) than that of the PM >3 µm size fraction in both warm and cold periods. Despite these efforts, comparative studies on the differences in pollution levels and sources of the PM2.5 OP in different districts are still limited.
In this study, the DTT activity of water-soluble matter was measured in PM2.5 samples collected simultaneously in the southern and northern of Beijing in January 2018 were measured. The concentration and the light absorption of water-soluble organic carbon (WSOC), as well as the concentrations of 14 trace elements and 7 light-absorbing nitroaromatic compounds (NACs), were quantified. The sources of DTT activity were then identified with a PMF model. The results acquired in this study provide a comparison of the PM2.5 OP in different districts of Beijing and its connection with organic compounds, trace elements and sources, which could be helpful for further study of the regional differences in the effects of PM2.5 on human health.
2.1 Sampling
Ambient 24 h integrated PM2.5 filter samples were collected from 1 to 31 January 2018 simultaneously in the south (the village of Dingfuzhuang (DFZ), Daxing District; 39.61° N, 116.28° E) and north (National Center for Nanoscience and Technology (NCNT), Haidian District; 39.99° N, 116.32° E) of Beijing (Fig. S1 in the Supplement). The distance between the two sampling sites is about 42 km. The south site is surrounded by agricultural, industrial and transportation areas, and the north site is surrounded by residential, transportation and commercial areas. PM2.5 samples were collected on pre-baked (780°, 3 h) quartz-fiber filters (20.3×25.4 cm; Whatman, QM-A, Clifton, NJ, USA) using high-volume PM2.5 samplers (1.13 m−3 min−1; Tisch Environmental, Cleveland, OH, USA) which were placed on the roof of buildings at heights of about 5 m (south) and 20 m (north) above the ground. A total of 31 samples were collected at each site. After collection, the samples were wrapped in baked aluminum foil and stored in a freezer (−20 °C) until further analysis.
2.2 Chemical analysis
The mass of PM2.5 on the filter was measured by a digital microbalance with a precision of 0.1 mg (LA130S-F, Sartorius, Germany) after 24 h equilibration in a chamber with constant temperature (20–23 °C) and humidity (35 %–45 %). Each filter was weighed at least two times, and the deviations for blank and sampled filters among the repetitions were less than 5 and 10 µg, respectively. The PM2.5 mass concentration was calculated by dividing the weight difference before and after sampling by the volume of sampled air.
For WSOC analysis, one punch (1.5 cm2 for concentration analysis and 0.526 cm2 for light absorption measurement) of filter was taken from each sample and extracted ultrasonically with ultrapure water (>18.2 MΩ cm) for 30 min. Afterwards, the extracts were filtered with a 0.45 µm PVDF pore syringe filter to remove insoluble substances. Finally, the concentration of WSOC was measured with a total organic carbon–total nitrogen analyzer (TOC-L, Shimadzu, Japan; (Ho et al., 2015)), and the light absorption of WSOC was measured by a UV–Vis spectrophotometer (300–700 nm; Ocean Optics, USA) equipped with a liquid waveguide capillary cell (LWCC-3100, World Precision Instruments, Sarasota, FL, USA; (Yuan et al., 2020)). The absorption coefficient (Abs) of WSOC was calculated according to formula S1 in the Supplement.
The total concentration and soluble fraction concentration of 14 trace elements (i.e., Ti, V, Cr, Mn, Fe, Co, Ni, Cu, Zn, As, Sr, Cd, Ba and Pb) were quantified by an inductively coupled plasma mass spectrometer (ICP-MS, 7700x, Agilent Technologies, USA), and details are shown in the Supplement. For soluble fraction concentration analysis, a punch of filter (47 mm diameter) was extracted with ultrapure water and then centrifuged from residues. For total concentration analysis, another 47 mm diameter filter of the same sample was used and digested with 10 mL HNO3 and 1 mL HF at 180° for 12 h. The extracts were then heated and concentrated to ∼0.1 mL and diluted to 5 mL with 2 % HNO3. Afterwards, the diluents were filtered with a 0.22 µm PTFE pore syringe filter and stored in a freezer (−4 °C) until further ICP-MS analysis.
The concentrations of organic markers (including levoglucosan, mannosan, galactosan, hopanes (including 17α(H)-22,29,30-trisnorhopane, 17α(H),21β(H)-30-norhopane, 17β(H),21α(H)-30-norhopane, 17β(H),21α(H)-hopane, 17β(H),21α(H)-hopane and 17β(H),21α(H)-hopane), picene, phthalic acid, isophthalic acid and terephthalic acid) and light-absorbing NACs (including 4-nitrophenol (4NP), 2-methyl-4-nitrophenol (2M4NP), 3-methyl-4-nitrophenol (3M4NP), 4-nitrocatechol (4NC), 3-methyl-5-nitrocatechol (3M5NC), 4-methyl-5-nitrocatechol (4M5NC) and 4-nitro-1-naphthol (4N1N)) were determined by a gas chromatograph–mass spectrometer (GC-MS; Agilent Technologies, Santa Clara, CA, USA) following the method described elsewhere (T. Wang et al., 2020), and more details about the analysis can be found in the Supplement. All of the results reported in this study were corrected for blanks.
2.3 Oxidative potential
The DTT assay was applied to determine the oxidative potential of water-soluble components in PM2.5 according to the method by Gao et al. (2017). In brief, a quarter of a 47 mm filter was ultrasonically extracted with 5 mL ultrapure water for 30 min and then filtered with a 0.45 µm PVDF pore syringe filter to remove insoluble substances. Several studies have shown that ultrasonic treatment of samples can lead to an increase in their OP values (Miljevic et al., 2014; Jiang et al., 2019); however, there was also a study that showed that the difference in OP values of water-soluble PM2.5 measured by the DTT assay was small for samples extracted by ultrasonic and shaking (Gao et al., 2017). Consistent with the extraction methods for organic markers and trace elements, an ultrasonic method was used to extract samples for DTT analysis. Afterwards, 0.5 mL of the extract was mixed with 1 mL of potassium phosphate buffer (pH =7.4) and 0.5 mL of 2 mM DTT in a brown vial and then placed in a water bath at 37 °C. Then, 20 µL of this mixture was taken at designated time intervals (2, 7, 13, 20 and 28 min) and mixed with 1 mL trichloroacetic acid (TCA; 1 % ) in another brown vial to terminate the reaction. Then, 0.5 mL of 5,5'-dithiobis-(2-nitrobenzoic acid) (DTNB; 2.5 µM) and 2 mL of tris buffer (pH =8.9) were added to form 2-nitro-5-thiobenzonic acid (TNB), which has a maximum light absorption at 412 nm. Finally, the absorption of TNB was measured by a UV–Vis spectrometer equipped with a LWCC. The DTT consumption rate was quantified by the remaining DTT concentration at different reaction times. Daily solution blanks and filter blanks were analyzed in parallel with samples to evaluate the consistency of the system performance. In addition, for every 10 samples, one sample was chosen to be measured three times to check the reproducibility, and the relative standard deviation was lower than 5 %. Ambient samples were corrected for filter blanks. The DTT activities were normalized by the volume of sampled air (DTTv; nmol min−1 m−3) and the mass concentration of PM2.5 (DTTm; pmol min−1 µg−1).
Considering that for samples containing a significant amount of substances whose DTT response is non-linear with PM2.5 concentration (e.g., Cu, Mn), the DTTm value depends on the concentration of PM2.5 added to the reaction solution (Charrier et al., 2016). The response of DTTm to PM2.5 concentration added to the reaction solution was analyzed using a sample containing high concentrations of soluble Cu and Mn (Fig. S2). When the PM2.5 concentration added to the reaction solution is less than 150 µg mL−1, the DTTm response is greatly affected by the difference in added PM2.5 concentration; however, when it is greater than 150 µg mL−1, the DTTm response is less affected by the difference in PM2.5 concentration (<12 %). In this study, the concentration of PM2.5 added to the reaction solution of most samples from the two sites was greater than 150 µg mL−1 (ranged from 79 to 749 µg mL−1, with an average of 409±164 and 207±95 µg mL−1 in the south and north, respectively); therefore, the difference in PM2.5 concentration added to the reaction solution of different samples should have had a relatively small impact on the difference in DTTm values of different samples. This study did not consider the impact of metal precipitation in the phosphate matrix on the measured DTT values, as there is not a straightforward method to correct the artifact caused by this phenomenon (Yalamanchili et al., 2023).
2.4 Source apportionment
The sources of DTT activities were identified and quantified using the PMF model implemented by the multilinear engine (ME-2; Paatero, 1997) following the method described in our previous studies (Huang et al., 2014; Yuan et al., 2020). For each site, 31 samples (a total of 62 samples) and 23 species were input into the PMF model. The number of samples is higher than the number of species. The input data include species concentration (including DTTv, 14 trace elements and 8 organic markers) and uncertainties. The species-specific uncertainties were calculated following Liu et al. (2017). For a clear separation of source profiles, the contribution of corresponding markers was set to 0 in the sources unrelated to the markers (see Table S1 in the Supplement). More details are described in the Supplement (PMF analysis).
3.1 DTT activity and concentrations of water-soluble PM2.5 components
Figure 1 shows the daily variation of DTT activity, light absorption of WSOC at wavelength 365 nm (Abs365), together with the concentrations of PM2.5, WSOC, NACs and total elements in the south and north of Beijing. Their average values are shown in Table S2. Generally, the average values of PM2.5, WSOC, Abs365, NACs and total elements were higher in the south than in the north. Specifically, the concentrations of PM2.5 and WSOC in the south (122±49 µg m−3 and 8.1±5.0 µgC m−3, respectively) were both about 2 times higher than those in the north (62±28 µg m−3 and 4.0±2.0 µgC m−3, respectively), indicating that the proportion of WSOC in PM2.5 was similar in the south and north. However, the Abs365 in the south was about 3 times that in the north, indicating that the chemical composition of WSOC was different between the south and north. Previous studies have reported that NACs are the main water-soluble light-absorbing organic compounds (also known as brown carbon, BrC) of PM2.5 (Lin et al., 2017; Huang et al., 2020; Li et al., 2021). For the 7 NACs quantified in this study, the total concentration of nitrophenols (4NP, 2M4NP and 3M4NP), nitrocatechols (4NC, 3M5NC and 4M5NC) and 4N1N in the south (108±73, 118±91 and 12±8.2 ng m−3, respectively) was about 3, 5 and 4 times, respectively, that in the north (35±22, 24±30 and 3.1±3.0 ng m−3, respectively). These results indicate that the sources and emission strength of water-soluble organic compounds were different in the south and north of Beijing, suggesting the different contribution of water-soluble organic compounds to DTT activity. The concentration trends of total trace elements were also different between the south and north of Beijing, with Fe > Zn > Ti > Mn > Cu > Ba > Pb > Sr > Cr > As > V > Ni > Cd > Co in the south and Fe > Ti > Zn > Ba > Mn > Pb > Cu > Cr > Sr > As > Ni > V > Cd > Co in the north. It should be noted that although the contents of PM2.5, WSOC and total elements measured in this study were higher in the south than in the north, the average DTTv value in the south (3.9±0.9 nmol min−1 m−3) was comparable to that in the north (3.5±1.2 nmol min−1 m−3); meanwhile, the average DTTm value was much higher (1.8 times) in the north (65±28 pmol min−1 µg−1) than in the south (36±14 pmol min−1 µg−1). Ahmad et al. (2021) also reported that the concentrations of PM2.5, WSOC and most elements in Lahore, Pakistan, were higher than those in Peshawar, Pakistan, while the DTTv values of the two sites were similar, and the DTTm value in Peshawar was higher than that in Lahore. The lower DTTm in the south than in the north may be due to the increased PM2.5 in the south containing more substances with no or little contribution to DTT activity and indicates that the intrinsic OP of water-soluble components of PM2.5 was higher in the north than in the south. The similar DTTv values in the south and north indicate that the exposure-relevant OP of water-soluble components of PM2.5 was comparable at the two sites, and water-soluble DTTv was not consistent with the content of water-soluble substances. Due to the complex chemical composition of PM2.5, there may also be antagonistic and synergistic effects contributing to the inconsistent relationship between DTT activity and compounds' content (Xiong et al., 2017; Lionetto et al., 2021).
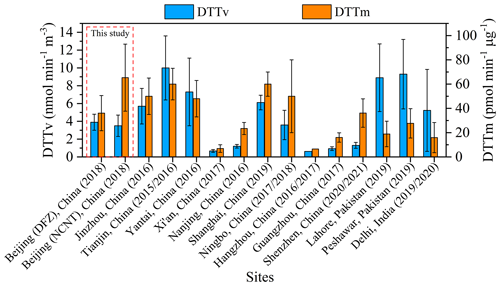
Figure 2Comparison of DTTv and DTTm values of water-soluble PM2.5 measured in this study with those measured in other areas of Asia during a similar period.
Figure 2 shows the comparison of water-soluble PM2.5 DTT activity measured in this study with that measured in other regions of Asia during similar periods. It can be seen that the DTTv values measured in Beijing in this study were lower than those in Jinzhou, Tianjin, Yantai, and Shanghai in China; Lahore and Peshawar in Pakistan; and Delhi in India (Liu et al., 2018; Ahmad et al., 2021; Puthussery et al., 2022; Wu et al., 2022a). They were higher than those in Xi'an, Nanjing, Hangzhou, Guangzhou and Shenzhen in China (Y. Wang et al., 2019, 2020; Ma et al., 2021; Yu et al., 2022b; Xing et al., 2023) and comparable with those in Ningbo, China (Chen et al., 2022). Different from DTTv, the DTTm value measured in NCNT in Beijing was similar to that in Jinzhou, Tianjin, Yantai, Shanghai and Ningbo in China (Liu et al., 2018; Chen et al., 2022; Wu et al., 2022a) and higher than that in other regions. The differences in water-soluble DTT activity of PM2.5 in different regions can be explained by the differences in chemical composition, sources and atmospheric formation processes (Tong et al., 2017; Wong et al., 2019; Daellenbach et al., 2020; Y. Wang et al., 2020; Cao et al., 2021). For example, Cao et al. (2021) reported the water-soluble DTT activity of PM2.5 from biomass and coal burning emissions in China, and the average value of biomass burning (4.5–7.4 pmol min−1 µg−1) was much higher than that of coal burning (0.5–2.1 pmol min−1 µg−1). Tuet et al. (2017) measured the water-soluble DTT activity of SOA generated under different precursors and reaction conditions, with SOA from naphthalene photooxidation under RO2+ NO-dominant dry reaction conditions having the highest DTT activity.
3.2 Correlation between DTT activity and water-soluble PM2.5 components
Figure 3 shows the correlations of DTTv with PM2.5, WSOC and Abs365 in the south and north of Beijing. It can be seen that the correlation coefficient between DTTv and PM2.5 was moderate in both the south (r=0.42) and north (r=0.45), indicating that the OP of particles cannot be evaluated solely by the total PM2.5 concentration. The correlations between DTTv and WSOC and Abs365 were strong in the north (r of 0.69 and 0.70, respectively), while they were relatively weak in the south (r of 0.41 and 0.40, respectively). The high correlations between DTTv and WSOC and Abs365 in the north of Beijing qualitatively agree with previous studies in Xi'an, China, and Atlanta, United States (Verma et al., 2012; Chen et al., 2019), and suggest that water-soluble organic matter, especially BrC, has a significant contribution to DTT consumption in the north. Light-absorbing BrC typically has conjugated electrons, making it more likely to transport electrons for catalytic reactions, thereby contributing to DTT activity (Chen et al., 2019; Wu et al., 2022). Further, in the north, DTTv was closely related to the concentrations of NACs (r of 0.57 to 0.79) (Fig. S3), suggesting that NACs may be important contributors to DTT consumption. Feng et al. (2022) reported positive correlations between NACs and biomarkers in saliva and urine (interleukin-6 and 8-hydrox-2'-deoxyguanosine). Zhang et al. (2023) also reported that NACs are major proinflammatory components in organic aerosols, contributing about 24 % of the interleukin-8 response of all compounds detected by Fourier transform ion cyclotron resonance mass spectrometry (FT-ICR-MS) in electrospray ionization negative mode (ESI−). Certainly, it may also be other substances related to NACs that contribute to the DTT activity, including those not detected in this study, driving the good correlation between NACs and DTTv in the north of Beijing, which is worth studying in the future.
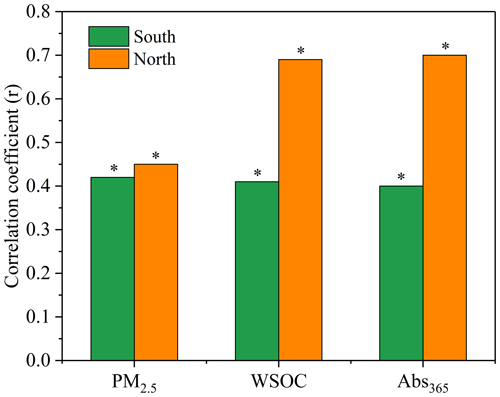
Figure 3Correlation coefficients between DTTv and PM2.5, WSOC and Abs365 in the south and north of Beijing (* indicates correlation is significant at the 0.05 level).
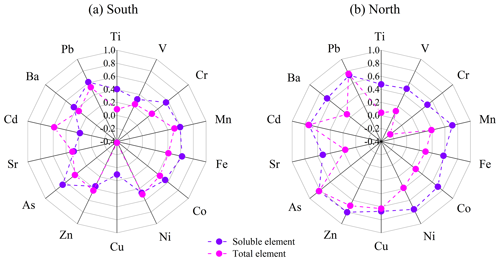
Figure 4Correlation coefficients between DTTv and elements in the (a) south and (b) north of Beijing.
The correlation coefficients between DTTv and 14 trace elements are shown in Fig. 4. Generally, the correlations between DTTv and soluble elements were higher than those between DTTv and total elements in both the south and north of Beijing. For soluble elements, in the south, DTTv showed positive correlations with Mn, Fe, Cr, Co, As and Pb (r>0.5), while in the north, it exhibited strong positive correlations with Mn, Co, Ni, Zn, As, Cd and Pb (r>0.7), indicating the different sources of DTTv in the south and north of Beijing. It is worth noting that the concentrations of all soluble elements were higher in the south than in the north (Fig. S4), while the correlation between DTTv and most soluble elements was lower in the south than in the north (Fig. 4). The high correlations between DTTv and soluble elements in the north of Beijing suggest that soluble elements also had a significant contribution to DTT consumption. The low correlations between DTTv and soluble elements in the south of Beijing may be due to the non-linear relationship between DTT consumption and element concentration (Charrier and Anastasio, 2012; Wu et al., 2022a). As shown in Fig. S5, the relationship between most soluble trace elements and DTTv was more non-linear than linear. As the concentration of soluble elements increases, the growth rate of DTTv obviously decreases.
In addition to being associated with individual water-soluble species, the interactions between metals and organic compounds also affect the consumption of DTT (Xiong et al., 2017; Wu et al., 2022b), with both synergistic and antagonistic effects. For example, Wu et al. (2022b) measured the DTT consumption of Fe(III) and Cu(II) interacting with 1,4-naphthoquinone, 9,10-phenanthraquinone, citric acid and 4-nitrocatechol, respectively. Their results showed that Cu(II) had antagonistic effects in interacting with most organics except for citric acid, and Fe(III) had an additive effect on DTT consumption of 1,4-naphthoquinone and citric acid, while it had an antagonistic effect on 1,4-naphthoquinone and 9,10-phenanthraquinone. Due to the complex composition of water-soluble organic aerosols, knowledge about the effects of organics and metal-organic interactions on DTT activity is still limited, especially the effects of BrC chromophores and their interactions with metals.
3.3 Sources of DTT activity
This study analyzed eight organic markers (including levoglucosan, mannosan and galactosan for biomass burning; hopanes for vehicle emissions; picene for coal combustion; and phthalic acid, isophthalic acid and terephthalic acid for secondary formation) to help identify the sources of DTT activity. The average concentrations of these organic markers are shown in Table S2. The correlation coefficients between DTTv and organic markers are shown in Fig. S6. In the south, levoglucosan, mannosan, galactosan and hopanes had moderate correlation with DTTv (r of 0.41 to 0.48); phthalic acid, isophthalic acid and terephthalic acid had low to moderate correlation with DTTv (r of 0.28 to 0.54); and picene had low correlation with DTTv (r of 0.21). These results suggest that biomass burning and vehicle emissions could have significant contribution to the water-soluble PM2.5 OP in the south. In the north, hopanes had the highest correlation with DTTv (r=0.70), indicating that vehicle emissions could have an important contribution. Levoglucosan, mannosan, galactosan, phthalic acid, isophthalic acid, terephthalic acid and picene had moderate to high correlations with DTTv in the north, suggesting that biomass and coal burning and secondary formation may also have a certain contribution to the water-soluble PM2.5 OP.
To further quantify the sources of DTT activity in the south and the north of Beijing, the PMF model, which had widely been used for the source apportionment of the PM2.5 OP (Liu et al., 2018; Shen et al., 2022; Cui et al., 2023), was applied. The input species include DTTv, soluble elements and organic markers, and five to seven factors were examined. Due to the oil factor being mixed with the vehicle emissions factor in the five-factor solution, there was no new reasonable factor when increasing the factor number to seven in the PMF analysis (Fig. S7). Finally, six factors were resolved and quantified using PMF model in the south and north of Beijing, including biomass burning, coal burning, traffic-related, dust, oil combustion and secondary formation, and the profiles of these sources are shown in Fig. S8. The uncertainties of PMF analysis for these sources were 2 %–14 %. Factor 1 is characterized by a high contribution of levoglucosan, mannosan and galactosan, mainly from biomass burning (Huang et al., 2014; Chow et al., 2022). The DTT activity of biomass burning organic aerosol was measured by Wong et al. (2019), which was 48±6 pmol min−1 µg−1 of WSOC. Liu et al. (2018) quantified the sources of DTTv in coastal cities (Jinzhou, Tianjin and Yantai) in China with the PMF model and multiple linear regression method, and the results showed that biomass burning contributed 28 % on average in winter. Factor 2 exhibits a large fraction of picene, Zn, Mn, Cd, As and Pb and is considered to be coal burning (Huang et al., 2014, 2018). Joo et al. (2018) measured the DTT activity of PM2.5 emitted from coal combustion at different temperatures, with the highest values of 26±21 pmol min−1 µg−1 and 0.10±0.06 nmol min−1 m−3 occurring at 550°. Factor 3 is identified as traffic-related emissions, which is characterized by the higher loading of hopanes, Ba, Sr, Cu and Ni (Huang et al., 2018; Chow et al., 2022). Vreeland et al. (2017) measured the DTT activity of PM2.5 emitted by side street and highway vehicles in Atlanta, with values of 0.78±0.60 and 1.1±0.60 nmol min−1 m−3, respectively. Ting et al. (2023) reported that the DTT activity of PM2.5 from vehicle emissions in Ziqing tunnel in Taiwan was 0.15–0.46 nmol min−1 m−3. Factor 4, secondary formation, is identified by high levels of phthalic acid, isophthalic acid and terephthalic acid (Al-Naiema and Stone, 2017; T. Wang et al., 2020). Verma et al. (2014) reported that secondary formation contributed about 30 % to the water-soluble DTT activity of PM2.5 in urban Atlanta. It is worth noting that the DTT activity of SOA generated from different precursors is different (Tuet et al., 2017; Tong et al., 2018). For example, the DTT activity of SOA from naphthalene was higher than that from isoprene (Tuet et al., 2017; Tong et al., 2018). Factor 5 is dominated by crustal elements Fe and Ti, mainly from dust (Huang et al., 2018). The DTT activity of atmospheric particulate matter during dust periods was reported in previous studies (Chirizzi et al., 2017; Khoshnamvand et al., 2023), and it has a low contribution in this study. Factor 6 is identified as oil combustion because of the high levels of V and Ni (Moreno et al., 2011; Minguillón et al., 2014; Huang et al., 2018).
The source contributions of DTTv in the south and north of Beijing are shown in Fig. 5, exhibiting obvious district differences. In the south, traffic-related emissions (39 %) and biomass burning (25 %) had the most contribution to DTTv, followed by secondary formation (17 %), coal burning (15 %), dust (2 %) and oil combustion (2 %). In the north, traffic-related emissions (52 %) had the highest contribution to DTTv, followed by coal burning (20 %), secondary formation (13 %), biomass burning (8 %), oil combustion (4 %) and dust (3 %). The absolute contribution of each source to DTTv varies by 1.2–3.4 times between the south and north of Beijing (Table S3). The large district differences in sources of DTTv of water-soluble PM2.5 call for more research on the relationship between sources, chemical composition, formation processes and the OP of PM2.5.
In this study, the water-soluble OPs of ambient PM2.5 collected in winter in the south and north of Beijing were quantified, together with the concentration and light absorption of WSOC and concentrations of 7 light-absorbing NACs and 14 trace elements. The average DTTv value was comparable in the south (3.9±0.9 nmol min−1 m−3) and north (3.5±1.2 nmol min−1 m−3), while the DTTm was higher in the north (65±28 pmol min−1 µg−1) than in the south (36±14 pmol min−1 µg−1), indicating that the exposure-relevant OP of water-soluble components of PM2.5 was similar at the two sites and that the intrinsic OP of water-soluble components of PM2.5 was higher in the north than in the south. The correlation between DTTv and soluble elements was higher than that between DTTv and total elements in both the south and north. In the north, DTTv was strongly correlated with soluble Mn, Co, Ni, Zn, As, Cd and Pb (r>0.7), and in the south it was positively correlated with Mn, Fe, Cr, Co, As and Pb (r>0.5). In addition, in the north DTTv was also positively correlated with WSOC, Abs365 and NACs (r of 0.56 to 0.79), while in the south it was weakly correlated (r≤0.4). These results indicate that in the north, trace elements and water-soluble organic compounds, especially BrC chromophores, both had significant contributions to DTT consumption, and in the south the consumption of DTT may mainly be from trace elements. Six sources of DTTv were resolved with the PMF model, including biomass burning, coal burning, traffic-related, dust, oil combustion and secondary formation. On average, traffic-related emissions (39 %) and biomass burning (25 %) were the major contributors of DTTv in the south, and traffic-related emissions (52 %) was the predominant source in the north. The differences in DTTv sources in the south and north of Beijing suggest that the relationship between source emissions and atmospheric processes and the PM2.5 OP deserves further exploration in order to better understand the regional differences of health impacts of PM2.5. In addition, in order to gain a more comprehensive understanding of the regional differences in the PM2.5 OP, sources and its relationship with chemical composition, longer periods and different seasonal datasets are also need to be studied in the future.
Raw data used in this study can be obtained from https://doi.org/10.5281/zenodo.10791126 (Yuan et al., 2024). They are also available on request by contacting the corresponding authors.
The supplement related to this article is available online at: https://doi.org/10.5194/acp-24-13219-2024-supplement.
RJH designed the study. Data analysis was done by WY, CL, LY, HY and RJH. WY, CL, LY, HY and RJH interpreted data, prepared the figures and wrote the manuscript. All authors commented on and discussed the manuscript.
The contact author has declared that none of the authors has any competing interests.
Publisher’s note: Copernicus Publications remains neutral with regard to jurisdictional claims made in the text, published maps, institutional affiliations, or any other geographical representation in this paper. While Copernicus Publications makes every effort to include appropriate place names, the final responsibility lies with the authors.
We are very grateful to the editor and the referees for the kind and valuable comments that have improved this paper.
This work was supported by the National Natural Science Foundation of China (NSFC) (grant no. 41925015), the Strategic Priority Research Program of Chinese Academy of Sciences (XDB40000000), the Key Research Program of Frontier Sciences from the Chinese Academy of Sciences (ZDBS-LY-DQC001), the New Cornerstone Science Foundation through the XPLORER PRIZE and the Postdoctoral Fellowship Program of CPSF (no. GZC20232628).
This paper was edited by Xavier Querol and reviewed by four anonymous referees.
Ahmad, M., Yu, Q., Chen, J., Cheng, S., Qin, W., and Zhang, Y.: Chemical characteristics, oxidative potential, and sources of PM2.5 in wintertime in Lahore and Peshawar, Pakistan, J. Environ. Sci., 102, 148–158, https://doi.org/10.1016/j.jes.2020.09.014, 2021.
Al-Naiema, I. M. and Stone, E. A.: Evaluation of anthropogenic secondary organic aerosol tracers from aromatic hydrocarbons, Atmos. Chem. Phys., 17, 2053–2065, https://doi.org/10.5194/acp-17-2053-2017, 2017.
An, Z., Huang, R. J., Zhang, R., Tie, X., Li, G., Cao, J., Zhou, W., Shi, Z., Han, Y., Gu, Z., and Ji, Y.: Severe haze in northern China: A synergy of anthropogenic emissions and atmospheric processes, P. Natl. Acad. Sci. USA, 116, 8657–8666, https://doi.org/10.1073/pnas.1900125116, 2019.
Bates, J. T., Fang, T., Verma, V., Zeng, L., Weber, R. J., Tolbert, P. E., Abrams, J. Y., Sarnat, S. E., Klein, M., Mulholland, J. A., and Russell, A. G.: Review of Acellular Assays of Ambient Particulate Matter Oxidative Potential: Methods and Relationships with Composition, Sources, and Health Effects, Environ. Sci. Technol., 53, 4003–4019, https://doi.org/10.1021/acs.est.8b03430, 2019.
Besis, A., Romano, M. P., Serafeim, E., Avgenikou, A., Kouras, A., Lionetto, M. G., Guascito, M. R., De Bartolomeo, A. R., Giordano, M. E., Mangone, A., Contini, D., and Samara, C.: Size-Resolved Redox Activity and Cytotoxicity of Water-Soluble Urban Atmospheric Particulate Matter: Assessing Contributions from Chemical Components, Toxics, 11, 59, https://doi.org/10.3390/toxics11010059, 2023.
Burnett, R., Chen, H., Szyszkowicz, M., Fann, N., Hubbell, B., Pope, C. A., 3rd, Apte, J. S., Brauer, M., Cohen, A., Weichenthal, S., Coggins, J., Di, Q., Brunekreef, B., Frostad, J., Lim, S. S., Kan, H., Walker, K. D., Thurston, G. D., Hayes, R. B., Lim, C. C., Turner, M. C., Jerrett, M., Krewski, D., Gapstur, S. M., Diver, W. R., Ostro, B., Goldberg, D., Crouse, D. L., Martin, R. V., Peters, P., Pinault, L., Tjepkema, M., van Donkelaar, A., Villeneuve, P. J., Miller, A. B., Yin, P., Zhou, M., Wang, L., Janssen, N. A. H., Marra, M., Atkinson, R. W., Tsang, H., Quoc Thach, T., Cannon, J. B., Allen, R. T., Hart, J. E., Laden, F., Cesaroni, G., Forastiere, F., Weinmayr, G., Jaensch, A., Nagel, G., Concin, H., and Spadaro, J. V.: Global estimates of mortality associated with long-term exposure to outdoor fine particulate matter, P. Natl. Acad. Sci. USA, 115, 9592–9597, https://doi.org/10.1073/pnas.1803222115, 2018.
Calas, A., Uzu, G., Kelly, F. J., Houdier, S., Martins, J. M. F., Thomas, F., Molton, F., Charron, A., Dunster, C., Oliete, A., Jacob, V., Besombes, J.-L., Chevrier, F., and Jaffrezo, J.-L.: Comparison between five acellular oxidative potential measurement assays performed with detailed chemistry on PM10 samples from the city of Chamonix (France), Atmos. Chem. Phys., 18, 7863–7875, https://doi.org/10.5194/acp-18-7863-2018, 2018.
Campbell, S. J., Wolfer, K., Utinger, B., Westwood, J., Zhang, Z.-H., Bukowiecki, N., Steimer, S. S., Vu, T. V., Xu, J., Straw, N., Thomson, S., Elzein, A., Sun, Y., Liu, D., Li, L., Fu, P., Lewis, A. C., Harrison, R. M., Bloss, W. J., Loh, M., Miller, M. R., Shi, Z., and Kalberer, M.: Atmospheric conditions and composition that influence PM2.5 oxidative potential in Beijing, China, Atmos. Chem. Phys., 21, 5549–5573, https://doi.org/10.5194/acp-21-5549-2021, 2021.
Cao, T., Li, M., Zou, C., Fan, X., Song, J., Jia, W., Yu, C., Yu, Z., and Peng, P.: Chemical composition, optical properties, and oxidative potential of water- and methanol-soluble organic compounds emitted from the combustion of biomass materials and coal, Atmos. Chem. Phys., 21, 13187–13205, https://doi.org/10.5194/acp-21-13187-2021, 2021.
Charrier, J. G. and Anastasio, C.: On dithiothreitol (DTT) as a measure of oxidative potential for ambient particles: evidence for the importance of soluble transition metals, Atmos. Chem. Phys., 12, 9321–9333, https://doi.org/10.5194/acp-12-9321-2012, 2012.
Charrier, J. G., McFall, A. S., Vu, K. K.-T., Baroi, J., Olea, C., Hasson, A., and Anastasio, C.: A Bias in the “Mass-Normalized” DTT Response-An Effect of Non-Linear Concentration Response Curves for Copper and Manganese, Atmos. Environ., 144, 325–334, https://doi.org/10.1016/j.atmosenv.2016.08.071, 2016.
Chen, K., Xu, J., Famiyeh, L., Sun, Y., Ji, D., Xu, H., Wang, C., Metcalfe, S. E., Betha, R., Behera, S. N., Jia, C., Xiao, H., and He, J.: Chemical constituents, driving factors, and source apportionment of oxidative potential of ambient fine particulate matter in a Port City in East China, J. Hazard. Mater., 440, 129864, https://doi.org/10.1016/j.jhazmat.2022.129864, 2022.
Chen, Q., Wang, M., Wang, Y., Zhang, L., Li, Y., and Han, Y.: Oxidative Potential of Water-Soluble Matter Associated with Chromophoric Substances in PM2.5 over Xi'an, China, Environ. Sci. Technol., 53, 8574–8584, https://doi.org/10.1021/acs.est.9b01976, 2019.
Chirizzi, D., Cesari, D., Guascito, M. R., Dinoi, A., Giotta, L., Donateo, A., and Contini, D.: Influence of Saharan dust outbreaks and carbon content on oxidative potential of water-soluble fractions of PM2.5 and PM10, Atmos. Environ., 163, 1–8, https://doi.org/10.1016/j.atmosenv.2017.05.021, 2017.
Chow, W. S., Huang, X. H. H., Leung, K. F., Huang, L., Wu, X., and Yu, J. Z.: Molecular and elemental marker-based source apportionment of fine particulate matter at six sites in Hong Kong, China, Sci. Total Environ., 813, 152652, https://doi.org/10.1016/j.scitotenv.2021.152652, 2022.
Chowdhury, P. H., He, Q., Carmieli, R., Li, C., Rudich, Y., and Pardo, M.: Connecting the Oxidative Potential of Secondary Organic Aerosols with Reactive Oxygen Species in Exposed Lung Cells, Environ. Sci. Technol., 53, 13949–13958, https://doi.org/10.1021/acs.est.9b04449, 2019.
Cui, Y., Zhu, L., Wang, H., Zhao, Z., Ma, S., and Ye, Z.: Characteristics and Oxidative Potential of Ambient PM2.5 in the Yangtze River Delta Region: Pollution Level and Source Apportionment, Atmosphere, 14, 425, https://doi.org/10.3390/atmos14030425, 2023.
Daellenbach, K. R., Uzu, G., Jiang, J., Cassagnes, L. E., Leni, Z., Vlachou, A., Stefenelli, G., Canonaco, F., Weber, S., Segers, A., Kuenen, J. J. P., Schaap, M., Favez, O., Albinet, A., Aksoyoglu, S., Dommen, J., Baltensperger, U., Geiser, M., El Haddad, I., Jaffrezo, J. L., and Prevot, A. S. H.: Sources of particulate-matter air pollution and its oxidative potential in Europe, Nature, 587, 414–419, https://doi.org/10.1038/s41586-020-2902-8, 2020.
Fang, T., Verma, V., Bates, J. T., Abrams, J., Klein, M., Strickland, M. J., Sarnat, S. E., Chang, H. H., Mulholland, J. A., Tolbert, P. E., Russell, A. G., and Weber, R. J.: Oxidative potential of ambient water-soluble PM2.5 in the southeastern United States: contrasts in sources and health associations between ascorbic acid (AA) and dithiothreitol (DTT) assays, Atmos. Chem. Phys., 16, 3865–3879, https://doi.org/10.5194/acp-16-3865-2016, 2016.
Feng, R., Xu, H., Gu, Y., Wang, Z., Han, B., Sun, J., Liu, S., Lu, H., Ho, S. S. H., Shen, Z., and Cao, J.: Variations of Personal Exposure to Particulate Nitrated Phenols from Heating Energy Renovation in China: The First Assessment on Associated Toxicological Impacts with Particle Size Distributions, Environ. Sci. Technol., 56, 3974–3983, https://doi.org/10.1021/acs.est.1c07950, 2022.
Gao, D., Fang, T., Verma, V., Zeng, L., and Weber, R. J.: A method for measuring total aerosol oxidative potential (OP) with the dithiothreitol (DTT) assay and comparisons between an urban and roadside site of water-soluble and total OP, Atmos. Meas. Tech., 10, 2821–2835, https://doi.org/10.5194/amt-10-2821-2017, 2017.
Guascito, M. R., Lionetto, M. G., Mazzotta, F., Conte, M., Giordano, M. E., Caricato, R., De Bartolomeo, A. R., Dinoi, A., Cesari, D., Merico, E., Mazzotta, L., and Contini, D.: Characterisation of the correlations between oxidative potential and in vitro biological effects of PM10 at three sites in the central Mediterranean, J. Hazard. Mater., 448, 130872, https://doi.org/10.1016/j.jhazmat.2023.130872, 2023.
Ho, K. F., Ho, S. S. H., Huang, R.-J., Liu, S. X., Cao, J.-J., Zhang, T., Chuang, H.-C., Chan, C. S., Hu, D., and Tian, L.: Characteristics of water-soluble organic nitrogen in fine particulate matter in the continental area of China, Atmos. Environ., 106, 252–261, https://doi.org/10.1016/j.atmosenv.2015.02.010, 2015.
Huang, R. J., Zhang, Y., Bozzetti, C., Ho, K. F., Cao, J. J., Han, Y., Daellenbach, K. R., Slowik, J. G., Platt, S. M., Canonaco, F., Zotter, P., Wolf, R., Pieber, S. M., Bruns, E. A., Crippa, M., Ciarelli, G., Piazzalunga, A., Schwikowski, M., Abbaszade, G., Schnelle-Kreis, J., Zimmermann, R., An, Z., Szidat, S., Baltensperger, U., El Haddad, I., and Prevot, A. S.: High secondary aerosol contribution to particulate pollution during haze events in China, Nature, 514, 218–222, https://doi.org/10.1038/nature13774, 2014.
Huang, R. J., Cheng, R., Jing, M., Yang, L., Li, Y., Chen, Q., Chen, Y., Yan, J., Lin, C., Wu, Y., Zhang, R., El Haddad, I., Prevot, A. S. H., O'Dowd, C. D., and Cao, J.: Source-Specific Health Risk Analysis on Particulate Trace Elements: Coal Combustion and Traffic Emission As Major Contributors in Wintertime Beijing, Environ. Sci. Technol., 52, 10967–10974, https://doi.org/10.1021/acs.est.8b02091, 2018.
Huang, R. J., Yang, L., Shen, J., Yuan, W., Gong, Y., Guo, J., Cao, W., Duan, J., Ni, H., Zhu, C., Dai, W., Li, Y., Chen, Y., Chen, Q., Wu, Y., Zhang, R., Dusek, U., O'Dowd, C., and Hoffmann, T.: Water-Insoluble Organics Dominate Brown Carbon in Wintertime Urban Aerosol of China: Chemical Characteristics and Optical Properties, Environ. Sci. Technol., 54, 7836–7847, https://doi.org/10.1021/acs.est.0c01149, 2020.
Jiang, H., Xie, Y., Ge, Y., He, H., and Liu, Y.: Effects of ultrasonic treatment on dithiothreitol (DTT) assay measurements for carbon materials, J. Environ. Sci., 84, 51–58, https://doi.org/10.1016/j.jes.2019.04.019, 2019.
Joo, H. S., Batmunkh, T., Borlaza, L. J. S., Park, M., Lee, K. Y., Lee, J. Y., Chang, Y. W., and Park, K.: Physicochemical properties and oxidative potential of fine particles produced from coal combustion, Aerosol Sci. Technol., 52, 1134–1144, https://doi.org/10.1080/02786826.2018.1501152, 2018.
Khoshnamvand, N., Nodehi, R. N., Hassanvand, M. S., and Naddafi, K.: Comparison between oxidative potentials measured of water-soluble components in ambient air PM1 and PM2.5 of Tehran, Iran, Air Qual. Atmos. Hlth., 16, 1311–1320, https://doi.org/10.1007/s11869-023-01343-y, 2023.
Lelieveld, S., Wilson, J., Dovrou, E., Mishra, A., Lakey, P. S. J., Shiraiwa, M., Poschl, U., and Berkemeier, T.: Hydroxyl Radical Production by Air Pollutants in Epithelial Lining Fluid Governed by Interconversion and Scavenging of Reactive Oxygen Species, Environ. Sci. Technol., 55, 14069–14079, https://doi.org/10.1021/acs.est.1c03875, 2021.
Li, X., Hu, M., Wang, Y., Xu, N., Fan, H., Zong, T., Wu, Z., Guo, S., Zhu, W., Chen, S., Dong, H., Zeng, L., Yu, X., and Tang, X.: Links between the optical properties and chemical compositions of brown carbon chromophores in different environments: Contributions and formation of functionalized aromatic compounds, Sci. Total Environ., 786, 147418, https://doi.org/10.1016/j.scitotenv.2021.147418, 2021.
Lin, P., Bluvshtein, N., Rudich, Y., Nizkorodov, S. A., Laskin, J., and Laskin, A.: Molecular chemistry of atmospheric brown carbon inferred from a nationwide biomass burning event, Environ. Sci. Technol., 51, 11561–11570, https://doi.org/10.1021/acs.est.7b02276, 2017.
Lionetto, M., Guascito, M., Giordano, M., Caricato, R., De Bartolomeo, A., Romano, M., Conte, M., Dinoi, A., and Contini, D.: Oxidative Potential, Cytotoxicity, and Intracellular Oxidative Stress Generating Capacity of PM10: A Case Study in South of Italy, Atmosphere, 12, 464, https://doi.org/10.3390/atmos12040464, 2021.
Liu, W., Xu, Y., Liu, W., Liu, Q., Yu, S., Liu, Y., Wang, X., and Tao, S.: Oxidative potential of ambient PM2.5 in the coastal cities of the Bohai Sea, northern China: Seasonal variation and source apportionment, Environ. Pollut., 236, 514–528, https://doi.org/10.1016/j.envpol.2018.01.116, 2018.
Liu, Y., Yan, C. Q., Ding, X., Wang, X. M., Fu, Q. Y., Zhao, Q. B., Zhang, Y. H., Duan, Y. S., Qiu, X. H., and Zheng, M.: Sources and spatial distribution of particulate polycyclic aromatic hydrocarbons in Shanghai, China, Sci. Total Environ., 584–585, 307–317, https://doi.org/10.1016/j.scitotenv.2016.12.134, 2017.
Ma, X., Nie, D., Chen, M., Ge, P., Liu, Z., Ge, X., Li, Z., and Gu, R.: The Relative Contributions of Different Chemical Components to the Oxidative Potential of Ambient Fine Particles in Nanjing Area, Int. J. Environ. Res. Pub. He., 18, 2789, https://doi.org/10.3390/ijerph18062789, 2021.
Miljevic, B., Hedayat, F., Stevanovic, S., Fairfull-Smith, K. E., Bottle, S. E., and Ristovski, Z. D.: To sonicate or not to sonicate PM filters: reactive oxygen species generation upon ultrasonic irradiation, Aerosol. Sci. Technol., 48, 1276–1284, https://doi.org/10.1080/02786826.2014.981330, 2014.
Minguillón, M. C., Cirach, M., Hoek, G., Brunekreef, B., Tsai, M., de Hoogh, K., Jedynska, A., Kooter, I. M., Nieuwenhuijsen, M., and Querol, X.: Spatial variability of trace elements and sources for improved exposure assessment in Barcelona, Atmos. Environ., 89, 268–281, https://doi.org/10.1016/j.atmosenv.2014.02.047, 2014.
Moreno, T., Querol, X., Alastuey, A., Reche, C., Cusack, M., Amato, F., Pandolfi, M., Pey, J., Richard, A., Prévôt, A. S. H., Furger, M., and Gibbons, W.: Variations in time and space of trace metal aerosol concentrations in urban areas and their surroundings, Atmos. Chem. Phys., 11, 9415–9430, https://doi.org/10.5194/acp-11-9415-2011, 2011.
Oh, S. H., Park, K., Park, M., Song, M., Jang, K. S., Schauer, J. J., Bae, G. N., and Bae, M. S.: Comparison of the sources and oxidative potential of PM2.5 during winter time in large cities in China and South Korea, Sci. Total Environ., 859, 160369, https://doi.org/10.1016/j.scitotenv.2022.160369, 2023.
Paatero, P.: Least squares formulation of robust non negative factor analysis, Chemometr. Intell. Lab., 37, 23–35, https://doi.org/10.1016/S0169-7439(96)00044-5, 1997.
Puthussery, J. V., Dave, J., Shukla, A., Gaddamidi, S., Singh, A., Vats, P., Salana, S., Ganguly, D., Rastogi, N., Tripathi, S. N., and Verma, V.: Effect of Biomass Burning, Diwali Fireworks, and Polluted Fog Events on the Oxidative Potential of Fine Ambient Particulate Matter in Delhi, India, Environ. Sci. Technol., 56, 14605–14616, https://doi.org/10.1021/acs.est.2c02730, 2022.
Saffari, A., Daher, N., Shafer, M. M., Schauer, J. J., and Sioutas, C.: Global perspective on the oxidative potential of airborne particulate matter: a synthesis of research findings, Environ. Sci. Technol., 48, 7576–7583, https://doi.org/10.1021/es500937x, 2014.
Shafer, M. M., Hemming, J. D., Antkiewicz, D. S., and Schauer, J. J.: Oxidative potential of size-fractionated atmospheric aerosol in urban and rural sites across Europe, Faraday Discuss., 189, 381–405, https://doi.org/10.1039/c5fd00196j, 2016.
Shen, J., Taghvaee, S., La, C., Oroumiyeh, F., Liu, J., Jerrett, M., Weichenthal, S., Del Rosario, I., Shafer, M. M., Ritz, B., Zhu, Y., and Paulson, S. E.: Aerosol Oxidative Potential in the Greater Los Angeles Area: Source Apportionment and Associations with Socioeconomic Position, Environ. Sci. Technol., 56, 17795–17804, https://doi.org/10.1021/acs.est.2c02788, 2022.
Ting, Y. C., Chang, P. K., Hung, P. C., Chou, C. C., Chi, K. H., and Hsiao, T. C.: Characterizing emission factors and oxidative potential of motorcycle emissions in a real-world tunnel environment, Environ. Res., 234, 116601, https://doi.org/10.1016/j.envres.2023.116601, 2023.
Tong, H., Lakey, P. S. J., Arangio, A. M., Socorro, J., Kampf, C. J., Berkemeier, T., Brune, W. H., Poschl, U., and Shiraiwa, M.: Reactive oxygen species formed in aqueous mixtures of secondary organic aerosols and mineral dust influencing cloud chemistry and public health in the Anthropocene, Faraday Discuss., 200, 251–270, https://doi.org/10.1039/c7fd00023e, 2017.
Tong, H., Lakey, P. S. J., Arangio, A. M., Socorro, J., Shen, F., Lucas, K., Brune, W. H., Poschl, U., and Shiraiwa, M.: Reactive Oxygen Species Formed by Secondary Organic Aerosols in Water and Surrogate Lung Fluid, Environ. Sci. Technol., 52, 11642–11651, https://doi.org/10.1021/acs.est.8b03695, 2018.
Tuet, W. Y., Chen, Y., Xu, L., Fok, S., Gao, D., Weber, R. J., and Ng, N. L.: Chemical oxidative potential of secondary organic aerosol (SOA) generated from the photooxidation of biogenic and anthropogenic volatile organic compounds, Atmos. Chem. Phys., 17, 839–853, https://doi.org/10.5194/acp-17-839-2017, 2017.
Tuet, W. Y., Liu, F., de Oliveira Alves, N., Fok, S., Artaxo, P., Vasconcellos, P., Champion, J. A., and Ng, N. L.: Chemical Oxidative Potential and Cellular Oxidative Stress from Open Biomass Burning Aerosol, Environ. Sci. Technol. Lett., 6, 126–132, https://doi.org/10.1021/acs.estlett.9b00060, 2019.
Verma, V., Rico-Martinez, R., Kotra, N., King, L., Liu, J., Snell, T. W., and Weber, R. J.: Contribution of water-soluble and insoluble components and their hydrophobic/hydrophilic subfractions to the reactive oxygen species-generating potential of fine ambient aerosols, Environ. Sci. Technol., 46, 11384–11392, https://doi.org/10.1021/es302484r, 2012.
Verma, V., Fang, T., Guo, H., King, L., Bates, J. T., Peltier, R. E., Edgerton, E., Russell, A. G., and Weber, R. J.: Reactive oxygen species associated with water-soluble PM2.5 in the southeastern United States: spatiotemporal trends and source apportionment, Atmos. Chem. Phys., 14, 12915–12930, https://doi.org/10.5194/acp-14-12915-2014, 2014.
Verma, V., Fang, T., Xu, L., Peltier, R. E., Russell, A. G., Ng, N. L., and Weber, R. J.: Organic aerosols associated with the generation of reactive oxygen species (ROS) by water-soluble PM2.5, Environ. Sci. Technol., 49, 4646–4656, https://doi.org/10.1021/es505577w, 2015.
Vreeland, H., Weber, R., Bergin, M., Greenwald, R., Golan, R., Russell, A. G., Verma, V., and Sarnat, J. A.: Oxidative potential of PM2.5 during Atlanta rush hour: Measurements of in-vehicle dithiothreitol (DTT) activity, Atmos. Environ., 165, 169–178, https://doi.org/10.1016/j.atmosenv.2017.06.044, 2017.
Wang, J., Lin, X., Lu, L., Wu, Y., Zhang, H., Lv, Q., Liu, W., Zhang, Y., and Zhuang, S.: Temporal variation of oxidative potential of water soluble components of ambient PM2.5 measured by dithiothreitol (DTT) assay, Sci. Total Environ., 649, 969–978, https://doi.org/10.1016/j.scitotenv.2018.08.375, 2019.
Wang, T., Huang, R. J., Li, Y., Chen, Q., Chen, Y., Yang, L., Guo, J., Ni, H., Hoffmann, T., Wang, X., and Mai, B.: One-year characterization of organic aerosol markers in urban Beijing: Seasonal variation and spatiotemporal comparison, Sci. Total Environ., 743, 140689, https://doi.org/10.1016/j.scitotenv.2020.140689, 2020.
Wang, Y., Wang, M., Li, S., Sun, H., Mu, Z., Zhang, L., Li, Y., and Chen, Q.: Study on the oxidation potential of the water-soluble components of ambient PM2.5 over Xi'an, China: Pollution levels, source apportionment and transport pathways, Environ. Int., 136, 105515, https://doi.org/10.1016/j.envint.2020.105515, 2020.
Wong, J. P. S., Tsagkaraki, M., Tsiodra, I., Mihalopoulos, N., Violaki, K., Kanakidou, M., Sciare, J., Nenes, A., and Weber, R. J.: Effects of Atmospheric Processing on the Oxidative Potential of Biomass Burning Organic Aerosols, Environ. Sci. Technol., 53, 6747–6756, https://doi.org/10.1021/acs.est.9b01034, 2019.
Wu, N., Lu, B., Chen, Q., Chen, J., and Li, X.: Connecting the Oxidative Potential of Fractionated Particulate Matter With Chromophoric Substances, J. Geophys. Res.-Atmos., 127, e2021JD035503, https://doi.org/10.1029/2021jd035503, 2022a.
Wu, N., Lyu, Y., Lu, B., Cai, D., Meng, X., and Li, X.: Oxidative potential induced by metal-organic interaction from PM2.5 in simulated biological fluids, Sci. Total Environ., 848, 157768, https://doi.org/10.1016/j.scitotenv.2022.157768, 2022b.
Xing, C., Wang, Y., Yang, X., Zeng, Y., Zhai, J., Cai, B., Zhang, A., Fu, T. M., Zhu, L., Li, Y., Wang, X., and Zhang, Y.: Seasonal variation of driving factors of ambient PM2.5 oxidative potential in Shenzhen, China, Sci. Total Environ., 862, 160771, https://doi.org/10.1016/j.scitotenv.2022.160771, 2023.
Xiong, Q., Yu, H., Wang, R., Wei, J., and Verma, V.: Rethinking Dithiothreitol-Based Particulate Matter Oxidative Potential: Measuring Dithiothreitol Consumption versus Reactive Oxygen Species Generation, Environ. Sci. Technol., 51, 6507–6514, https://doi.org/10.1021/acs.est.7b01272, 2017.
Yalamanchili, J., Hennigan, C. J., and Reed, B. E.: Measurement artifacts in the dithiothreitol (DTT) oxidative potential assay caused by interactions between aqueous metals and phosphate buffer, J. Hazard. Mater., 456, 131693, https://doi.org/10.1016/j.jhazmat.2023.131693, 2023.
Yu, H., Wei, J., Cheng, Y., Subedi, K., and Verma, V.: Synergistic and Antagonistic Interactions among the Particulate Matter Components in Generating Reactive Oxygen Species Based on the Dithiothreitol Assay, Environ. Sci. Technol., 52, 2261–2270, https://doi.org/10.1021/acs.est.7b04261, 2018.
Yu, Q., Chen, J., Qin, W., Ahmad, M., Zhang, Y., Sun, Y., Xin, K., and Ai, J.: Oxidative potential associated with water-soluble components of PM2.5 in Beijing: The important role of anthropogenic organic aerosols, J. Hazard. Mater., 433, 128839, https://doi.org/10.1016/j.jhazmat.2022.128839, 2022.
Yu, S., Liu, W., Xu, Y., Yi, K., Zhou, M., Tao, S., and Liu, W.: Characteristics and oxidative potential of atmospheric PM2.5 in Beijing: Source apportionment and seasonal variation, Sci. Total Environ., 650, 277–287, https://doi.org/10.1016/j.scitotenv.2018.09.021, 2019.
Yu, Y., Sun, Q., Li, T., Ren, X., Lin, L., Sun, M., Duan, J., and Sun, Z.: Adverse outcome pathway of fine particulate matter leading to increased cardiovascular morbidity and mortality: An integrated perspective from toxicology and epidemiology, J. Hazard. Mater., 430, 128368, https://doi.org/10.1016/j.jhazmat.2022.128368, 2022a.
Yu, Y., Cheng, P., Li, Y., Gu, J., Gong, Y., Han, B., Yang, W., Sun, J., Wu, C., Song, W., and Li, M.: The association of chemical composition particularly the heavy metals with the oxidative potential of ambient PM2.5 in a megacity (Guangzhou) of southern China, Environ. Res., 213, 113489, https://doi.org/10.1016/j.envres.2022.113489, 2022b.
Yuan, W., Huang, R.-J., Yang, L., Guo, J., Chen, Z., Duan, J., Wang, T., Ni, H., Han, Y., Li, Y., Chen, Q., Chen, Y., Hoffmann, T., and O'Dowd, C.: Characterization of the light-absorbing properties, chromophore composition and sources of brown carbon aerosol in Xi'an, northwestern China, Atmos. Chem. Phys., 20, 5129–5144, https://doi.org/10.5194/acp-20-5129-2020, 2020.
Yuan, W., Huang, R.-J., Luo, C., Yang, L., Cao, W., Guo, J., and Yang, H.: Measurement report: Oxidation potential of water-soluble aerosol components in the southern and northern of Beijing, Zenodo [data set], https://doi.org/10.5281/zenodo.10791126, 2024.
Zhang, Q., Ma, H., Li, J., Jiang, H., Chen, W., Wan, C., Jiang, B., Dong, G., Zeng, X., Chen, D., Lu, S., You, J., Yu, Z., Wang, X., and Zhang, G.: Nitroaromatic Compounds from Secondary Nitrate Formation and Biomass Burning Are Major Proinflammatory Components in Organic Aerosols in Guangzhou: A Bioassay Combining High-Resolution Mass Spectrometry Analysis, Environ. Sci. Technol., 57, 21570–21580, https://doi.org/10.1021/acs.est.3c04983, 2023.
Zheng, Y., Davis, S. J., Persad, G. G., and Caldeira, K.: Climate effects of aerosols reduce economic inequality, Nat. Clim. Change, 10, 220–224, https://doi.org/10.1038/s41558-020-0699-y, 2020.