the Creative Commons Attribution 4.0 License.
the Creative Commons Attribution 4.0 License.
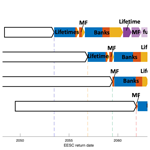
The return to 1980 stratospheric halogen levels: a moving target in ozone assessments from 2006 to 2022
Megan J. Lickley
John S. Daniel
Laura A. McBride
Ross J. Salawitch
Guus J. M. Velders
The international scientific assessment of ozone depletion is prepared every 4 years to support decisions made by the parties to the Montreal Protocol. In each assessment an outlook of ozone recovery time is provided. The year when equivalent effective stratospheric chlorine (EESC) returns to the level found in 1980 is an important metric for the recovery of the ozone layer. Over the past five assessments, the expected date for the return of EESC to the 1980 level, for mid-latitudes, was delayed, from the year 2049 in the 2006 assessment to 2066 in the 2022 assessment, which represents a delay of 17 years over a 16-year assessment period. Here, we quantify the primary drivers that have delayed the expected EESC recovery date between each of these assessments. We find that by using identical EESC formulations, the delay between the 2006 and 2022 assessments' expected return of EESC to 1980 levels is shortened to 12.6 years. Of this delay, bank calculation methods account for ∼ 4 years, changes in the assumed atmospheric lifetime for certain ozone-depleting substances (ODSs) account for ∼ 3.5 years, an underestimate of the emission of carbon tetrachloride accounts for ∼ 3 years, and updated historical mole fraction estimates of ODSs account for ∼ 1 year. Since some of the underlying causes of these delays are amenable to future controls (e.g., capture of ODSs from banks and limitations on future feedstock emissions), it is important to understand the reasons for the delays in the expected recovery date of stratospheric halogens.
- Article
(1649 KB) - Full-text XML
-
Supplement
(579 KB) - BibTeX
- EndNote
The Montreal Protocol is often lauded as the signature global environmental success story. Since its entry into force in 1989, it has led to large reductions in the production of ozone-depleting substances (ODSs) globally and has avoided a world with substantial ozone loss (Newman et al., 2009; Morgenstern et al., 2008). To inform potential policy decisions of the parties to the protocol, every 4 years a scientific assessment of ozone depletion (SAOD) report is prepared by leading international experts in atmospheric science and related fields under the auspices of the World Meteorological Organization (WMO) and the United Nations Environment Programme (UNEP). A key component of each SAOD report is an outlook of the timeline for recovery of the ozone layer. In addition to calculating the return of ozone itself, return dates to 1980 levels of equivalent effective stratospheric chlorine (EESC) are estimated and provided, given the best scientific understanding of atmospheric processes and the assumption of global compliance with the controls of the protocol at that time. EESC is a measure of the abundance of stratospheric inorganic chlorine and bromine and is a proxy for the chemical depletion of stratospheric ozone by halogens (discussed further in Sect. 2.1). While ozone depletion began before 1980, the return of EESC to the amount that had existed in 1980 is an adopted benchmark for the path to recovery. Over the past 16 years, the expected return of EESC to the 1980 level has been pushed back from 2049 in the 2006 SAOD (Daniel et al., 2007b) to 2066 in the 2022 SAOD (Daniel et al., 2022), a delay in the expected recovery of stratospheric chlorine and bromine of 17 years over a 16-year assessment period (Fig. 1a). The reasons for this expected delay in the return of stratospheric halogens to the 1980 level have not been fully elucidated, and changes to the Montreal Protocol do not explain this discrepancy, as the 2007 Amendment to the Montreal Protocol was the last major amendment with appreciable effects on EESC (e.g., see Fig. Q14-1 of Salawitch et al., 2018). However, the newer EESC formulation (Engel et al., 2018) first used in the 2018 SAOD may play an important role, as the return to 1980 levels was delayed by more than a decade simply from using this newer approach (WMO, 2018).
When projections of future mole fractions of ODSs and EESC recovery are updated, the underlying causes of any changes are important to understand. Changes can relate, for example, to an updated estimate of the global atmospheric lifetime of an ODS, new estimates of the amount of an ODS in banks, more extensive controls on the future production of an ODS, or the detection of an unexpected emission of an ODS. Here, the term “bank” refers to the quantity of ODSs stored in existing equipment, chemical stockpiles, foams, and other products with the potential to release ODSs into the atmosphere. An example of the unexpected emission was described by Montzka et al. (2018), who reported a slowdown in the rate of decline in atmospheric CFC-11 that they attributed to new unreported production in eastern Asia. This study brought into question the extent to which illicit production of CFC-11, inaccuracies in the assessed emission of CFC-11 from banks, variability in atmospheric transport (Ray et al., 2020), or possibly even inaccuracies in the atmospheric lifetime of CFC-11 were contributing to the delay in the expected decline in the global mean mole fraction of CFC-11 between the projection given in the 2006 SAOD and data presented in the 2014 SAOD. Subsequent studies confirmed the Montzka et al. (2018) findings by identifying emissions of CFC-11 originating from eastern Asia, likely resulting from new production in breach of the Montreal Protocol (Rigby et al., 2019; Benish et al., 2021; Montzka et al., 2021; Park et al., 2021). Furthermore, an upward revision in the magnitude of the bank of ODSs (Lickley et al., 2020, 2022) led to the recent realization that the return of EESC to the 1980 level would not occur as fast as once expected (Daniel et al., 2022). These studies underscore the role that SAOD reports have played in setting expectations for future mole fractions of ODSs. Considering that SAODs are assembled, in part, to provide the parties with our best knowledge of the effectiveness of current control measures and also to set an expectation of the future recovery of the ozone layer, a careful analysis of the drivers changing EESC projections between these past SAODs is warranted.
Here, we quantify the reasons for the delay in the projected recovery of EESC to the 1980 level given by the five most recent SAOD reports (Daniel et al., 2007b, 2011; Harris et al., 2014; Carpenter et al., 2018; Daniel et al., 2022). We henceforth refer to these five reports as the 2006, 2010, 2014, 2018, and 2022 SAODs. Specifically, we consider how the delay has been affected by the consistent underestimate of the global emissions of ODSs (Montzka et al., 2018; Rigby et al., 2019; Park et al., 2018; Lickley et al., 2022; Gamlen et al., 1986) as modeled through production, banks, and feedstocks and observed in part by measured mole fractions following the publication of each SAOD. In addition, we consider the role of changes in ODS atmospheric lifetime assumptions that affect future atmospheric abundances (SPARC, 2013), as well as variations in the scientific understanding of the best underlying approach used to compute EESC (Newman et al., 2007; Engel et al., 2018). We begin with the 2006 SAOD because knowledge of the release of ODSs from banks has evolved considerably since the publication of SAOD reports prior to 2006 (IPCC/TEAP, 2005). In addition, the 2006 SAOD report is the first to distinguish between mid-latitude and polar air for the return to 1980 levels of EESC, even though identical fractional release factors for ODSs were used for both regions. For completeness, the return-to-1980 dates for EESC provided in the past eight SAOD reports are given in the Supplement, Table S1.
We quantify the contributions from each of these primary drivers that have delayed the expected return to the 1980 date of EESC between each consecutive SAOD report from 2006 to 2022. To do so, we first re-evaluate each SAOD's historical and future EESC calculations using a common formulation for EESC (Engel et al., 2018). We are then able to compare the differences in projections of EESC due to changes in historical and projected atmospheric mole fractions alone, rather than confounding the issue with different formulations for the computation of EESC. Next, we identify the primary gases driving each change in the EESC return date between consecutive SAOD reports. We then isolate the effects of four primary gases (CFC-11, CFC-12, halon 1301, and carbon tetrachloride (CTC)) on EESC, as changing projections of these four compounds explain ∼ 90 % of the delay in expected EESC recovery from the 2006 SAOD to the 2022 SAOD. While the other 12 gases have led to both positive and negative changes in EESC between SAODs, their overall net contribution to the return of EESC to 1980 has been substantially smaller than the contribution of the primary four gases. Therefore, our focus is on quantifying the impact of changes in the mole fraction projections of CFC-11, CFC-12, halon 1301, and CTC on EESC. In Sect. 2, we detail the calculation of EESC given in each SAOD report, including various modeling assumptions. In Sect. 3, we present our methods for ODS selection and for quantifying each modeling component's contribution to delaying EESC return dates. We present the results of our analysis in Sect. 4. Finally, we discuss the implications for future assessments in Sect. 5.
The modeling approach used in the SAODs from 2006 to 2022 estimates the return of EESC to the 1980 level as a multi-step process. First, this estimate requires knowledge of pre-1980 atmospheric mole fractions of the 16 most abundant ODSs (see Table 2 for the complete list of gases) to establish the 1980 baseline level of EESC. Next, projections of future atmospheric mole fractions require assumptions about expected emissions from future production as well as about emissions from existing and future equipment (termed banks), along with an estimate of the atmospheric lifetime for each gas. Further, 4 additional years of observations between each assessment has required updating various assumptions such that the modeled atmospheric mole fractions of ODSs are consistent with new observations. Finally, once a historical and future time series of mole fractions has been constructed for each of the 16 primary ODSs, they are aggregated together with a calculation of EESC. Each step in this modeling process has been updated between various SAODs, reflecting the best scientific knowledge at the time of publication. A summary of the different modeling assumptions is provided in Table 1, along with the lifetimes of the four most important ODSs with regards to the variations in the return of EESC to the 1980 level across the assessments. In Table 1, the term FRF refers to the fractional release factor, a quantity that (as explained below) represents the conversion from organic to inorganic chlorine of each ODS.
Table 1Summary of key assumptions by scientific assessment of ozone depletion reports.
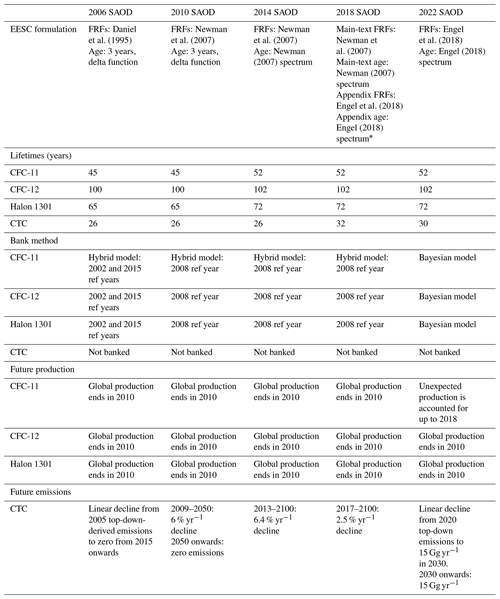
* The width of the age distribution was taken from Newman et al. (2007).
Below, we review the calculation of EESC given in each SAOD from 2006 to 2022. We then present the method used for projecting future ODS atmospheric mole fractions and review each SAOD's input parameters for these calculations. While pre-1980 mole fractions of some of the 16 primary ODSs have been modified at times between assessments, this change represents a small fraction of total change in the EESC recovery time over the entire 16-year period and is therefore not the focus of the present analysis.
2.1 EESC calculations
Equivalent effective stratospheric chlorine (EESC) is a metric that has been developed to relate the surface level atmospheric abundance of ODSs to inorganic halogen loading in the stratosphere and thus to stratospheric ozone depletion. EESC was first introduced in Daniel et al. (1995), drawing in large part from the understanding gained in Solomon et al. (1992) and Solomon and Albritton (1992). EESC weighs surface mixing ratios of ODSs with their number of Cl (or Br) atoms and their factional release factors. It has since been refined with increasing specificity with regards to the timing of halogen releases and transport lag times, discussed further below. Methods for estimating EESC have generally followed the following functional form:
where ni is the number of chlorine or bromine atoms of an ODS, fi represents the value of the fractional release factor (FRF) of an ODS relative to CFC-11, and ρi is the mean stratospheric mole fraction that would be expected in the absence of chemical loss for the location of interest at time t. Values of ρi can be related to the surface level mole fraction for gas, i, by
where ρi,entry is the mixing ratio of the source gas at the time of entry into the stratosphere (Newman et al., 2007). The quantity α is the efficiency of ozone destruction by bromine radicals relative to the efficiency by chlorine radicals, which is commonly set to 60 for EESC of mid-latitude air and 65 for EESC in polar regions (Sinnhuber et al., 2009). The quantity a represents the fractional release of CFC-11 (Daniel et al., 1995; Newman et al., 2007). For the 2006 SAOD report, a single set of FRFs for the global stratosphere was used. From 2010 onwards, two sets of FRFs were used: one for the mid-latitude stratosphere and another for the polar stratosphere. G represents the distribution of times required to be transported from entry into the stratosphere to the region of interest and is referred to as the age spectrum. This transport time is referred to as the age of air of an air parcel and represents the amount of time since the parcel was last in the troposphere (Kida, 1983).
The 2006 and 2010 SAODs adopted the Daniel et al. (1995) EESC calculation approach, where G was assumed to be a delta function with a 3-year lag, so ρi represented a simple 3-year time lag from surface mole fractions (then adjusted by the factors described in the previous paragraph). The 2014 and 2018 SAODs adopted the Newman et al. (2007) formulation of EESC projections, which modified Eq. (1) such that both fi and ρi were time-weighted averages, reflecting the non-linear dependence of these terms on the age of air in the stratosphere. In the 2018 SAOD, the Engel et al. (2018) formulation (which employs slightly different fractional release values and a different age spectrum) is adopted in Chap. 1 on the historical estimates of EESC and in an appendix of Chap. 6, applied to future projections. The 2022 SAOD adopted the Engel et al. (2018) formulation for computation of EESC for both historical and future projections. The most significant difference introduced by the Engel et al. (2018) calculation of EESC is that it attempts to weight the age spectrum by the time when the source gas dissociates, rather than using the Newman et al. (2007) approach (and the delta function approach) in which the age spectrum is identical to the age spectrum of an inert tracer. For EESC, this change results in higher weighting of air with longer transit times through the stratosphere and lower weighting of air with shorter transit times (for which ODSs have been dissociated to a lesser degree, particularly in the mid-latitudes) compared to what was found using the approach of Newman et al. (2007). In summary, using the new formulation, EESC lags the troposphere more strongly than an inert tracer would.
2.2 Projecting ODS mole fractions
To calculate values of ρi in Eqs. (1) and (2), the SAODs each began with a time series from between 1951 and 1955 to 2100 of surface mole fractions for each ODS included in Table 2. The historical range of this period is developed using observed mole fractions when available. In recent decades, these have been highly precise and accurate atmospheric observations from the AGAGE (Prinn et al., 2018) and NOAA (https://gml.noaa.gov/dv/site/, last access: 1 February 2020) networks. For the period before routine and global atmospheric observations were available, there are observations from firn samples that can help constrain prior mole fractions for some of the compounds (Laube et al., 2014; Butler et al., 1999), particularly those with strong natural sources such as methyl bromide and methyl chloride. For the remaining ODSs, historical mole fractions between 1950 and 1980 were based on model calculations using the production data reported by the Alternative Fluorocarbons Environmental Acceptability Study (AFEAS, 2001), adjusting calculations to avoid discontinuities between modeled and observed mole fractions when observations first began for each compound. The projections of future mole fractions generally consider a range of future policy scenarios, where the baseline scenario reflects the current controls. We only consider the baseline scenarios here for comparison across SAOD reports. Baseline projections begin in the year prior to publication of the assessment, t0, where an initial mole fraction for ODS, i, is taken from observed surface mole fraction values. Each subsequent year, t, is then forward-simulated using a one-box model of the atmosphere following Eq. (3):
where τi is the atmospheric lifetime and Ai is a conversion factor relating emissions, Emiss, to surface mole fractions for gas, i, assuming all of the emissions are immediately deposited into the atmosphere. Values of Emissi,t are modeled as the sum of emissions from expected production and banks and are iteratively simulated using Eqs. (4) and (5):
where RFi is the yearly release fraction of the bank, Banki,t, and DEi is the fraction of production, Prodi,t for ODS, i, in year, t, that is emitted in the same year as the production. The size of the bank is then updated:
Therefore, future ODS mole fraction projections rely on assumptions about global lifetimes, bank sizes, bank release fractions, future production, and direct emissions from production. The different methods across assessments for the values used in Eqs. (1)–(4) are further summarized below.
2.3 Atmospheric lifetime assumptions
Atmospheric lifetimes of the ODSs are an important component of the projections of future mole fractions. For each SAOD report, an “assessed” best estimate for the lifetime of each compound is presented. Here, lifetime is defined as the global atmospheric mass, or burden, of a compound divided by the loss rate integrated over the entire atmosphere (SPARC, 2013). These lifetime estimates were calculated using numerous lifetime inference methods (see the Stratosphere-troposphere Processes and their Role in Climate (SPARC, 2013) report for more details). Lifetimes have been based on satellite-derived methods which convolve stratospheric distributions (as a function of altitude and pressure) of long-lived gases with photolysis rates of their destruction (Minschwaner et al., 1993); model inversion methods using ground-based measurements with prescribed emissions (Rigby et al., 2013); or tracer–tracer methods, which relate the slope of the mixing ratio of a particular species to the mixing ratio of another species with a well-established lifetime (Plumb and Ko, 1992). However, for many species, modeled lifetimes alone inform the atmospheric lifetimes used in the SAODs (as reported in SPARC, 2013). For CFCs and halons, atmospheric loss occurs primarily in the stratosphere through photolysis. For gases such as methyl chloroform that undergo removal in the troposphere due to processes such as reaction with OH, the lifetime may be revised due to better knowledge of the rate constant for reaction with OH or of the average OH concentration itself, as well as additional years of data from which the lifetime is inferred (Prinn et al., 2001; Montzka et al., 2011). A tabulation of the lifetime of the 16 major ODSs, from the five most recent SAOD reports, appears in the Supplement, Table S3. The lifetimes most central to our analysis are repeated in Table 1.
2.4 Bank modeling
An ODS bank refers to the quantity of gas contained in equipment or applications that is subject to later release. One approach to estimating the size of an ODS bank in a given year requires knowledge about how much of an ODS has been cumulatively produced and released prior to the year of interest. The difference is assumed to reside in the bank; this approach is referred to as a top-down approach (e.g., Montzka et al., 2003). An alternative method involves estimating the quantity of equipment and/or applications in a given year that contain a particular ODS and how much ODS resides in each piece of equipment/application; this approach is referred to as a bottom-up approach (Ashford et al., 2004; Campbell et al., 2005). Due to uncertainties in data and modeling assumptions, each method yields bank estimates with significant uncertainties. The various bank estimates for CFC-11, CFC-12, and halon 1301 from the 2006–2022 SAODs are shown in Fig. 1.
In earlier assessments (e.g., the 2002 SAOD; Montzka et al., 2003), projections of EESC were based on banks found using a top-down approach, where banks were estimated as
For the 2002 report, the production values, Prodi,t, came from AFEAS (2001) and UNEP's Ozone Secretariat and emissions, Emissi,t, were derived from observed mole fractions by rearranging Eq. (3). Banks were calculated by starting in the first year of production, with Bank equal to zero and iterating forwards.
Due to the inherent large uncertainties associated with this approach, since the bank is a small difference between two large numbers (cumulative production and cumulative emission), and due to the large discrepancies between bank estimates using this top-down approach and other bottom-up accounting methods (Daniel et al., 2007a), the 2006 SAOD used a hybrid modeling approach to estimate banks. Bank estimates for 2002 and 2015 were adopted from the Technology and Economic Assessment Panel (TEAP) bank estimate (IPCC/TEAP, 2006), which estimated banks using a careful bottom-up calculation of the inventory and expected release rates by application type. Such bottom-up estimates had not previously been available. Equation (5) was then used to solve for RFi, assuming DEi to be equal to RFi such that the 2002 and 2015 banks matched the prescribed 2002 and 2015 values while also accounting for the reported production during this period. After solving for RFi values, banks and emissions were simulated from 2015 onwards using Eqs. (4) and (5) and assuming RFi remained constant into the future.
This method was modified in the 2010, 2014, and 2018 SAODs, which started with a bottom-up estimate for the 2008 bank from a 2009 TEAP report (Kuijpers and Verdonik, 2009). Using Eq. (6), banks were calculated beginning in 2008 (forwards and backwards in time if necessary) for the 7 most recent years in which mole fraction observations were available. Emissions for Eq. (6) were calculated using Eq. (3), rearranged to solve for emissions. RFi was then estimated for each of these years by setting DEi to be equal to RFi in Eq. (4) and solving for RFi. For the 2014 and 2018 SAODs, the average RFi value over these 7 years was then used to project banks and emissions from 2008 onwards using Eqs. (3) and (4). For the 2010 SAOD, an RFi that was consistent with the values over the previous 5–10 years, depending on the compound, was used.
The 2022 SAOD bank estimates followed a Bayesian bank estimation method from Lickley et al. (2020, 2022). This method develops prior distributions of the input parameters for Eq. (4) to account for uncertainties in production, as well as uncertainties in RFi and DEi values. Equations (3)–(5) are then simulated starting in the first year of production for the respective ODS to the end of the observational record, which results in a joint prior distribution of banks, emissions, and atmospheric mole fractions. Joint posterior distributions are obtained by updating the prior with available globally averaged observed atmospheric mole fractions. This approach resulted in larger bank estimates than those used in previous assessments, largely due to allowing the possibility that ODS production was higher than reported; this higher posterior production primarily occurs because plausible RF values along with reported production values were inconsistent with atmospheric mole fractions, given the model assumptions. Projected ODS mole fractions were then estimated using posterior bank and RFi estimates in 2021 and forward-simulating Eqs. (4) and (5), with an assumed production time series in line with the controls set by the Montreal Protocol.
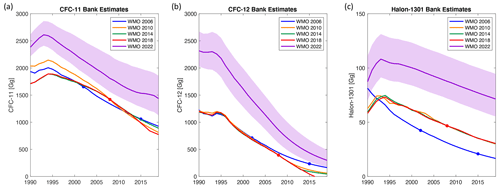
Figure 1Bank estimates of (a) CFC-11, (b) CFC-12, and (c) halon 1301 for each scientific assessment of ozone depletion report from 2006 to 2022, as indicated by the WMO date in the legend. The blue dots represent the bottom-up-derived banks from IPCC/TEAP (2006) that are adopted as an initial starting point for WMO 2006 (Daniel et al., 2007b) bank assessment. The red dot represents the starting point for WMO 2010, 2014, and 2018 assessments (Daniel et al., 2011; Harris et al., 2014; Carpenter et al., 2018), taken from the 2009 TEAP report (Kuijpers and Verdonik, 2009). The shaded region for WMO 2022 (Daniel et al., 2022) represents the 5th- and 95th-percentile confidence bounds around the median bank estimate.
2.5 Carbon tetrachloride modeling
We consider CTC separately from the banked ODSs because it is not thought to be a substantially banked chemical and because global emissions have been much less well understood. Therefore, CTC projections have been developed using an independent method compared to banked ODSs. The CTC budget remains an area of substantial uncertainty (SPARC, 2016), as noted in each SAOD report from 2010 to the present. Under the Montreal Protocol, CTC was scheduled to be phased out of production for dispersive uses by 2010, which was consistent with near-zero country-reported production values from that time onwards. Assuming CTC global production would follow the scheduled phaseout, the 2006 SAOD report adopted a future emissions pathway that began with a linear decrease from top-down-derived emissions values in 2005 of 65 Gg yr−1 to zero in 2015 and beyond. However, the expected rate of decline was not observed to be as quick as projected in the 2006 SAOD, which led to adjustments in CTC projections in subsequent SAODs. The emissions estimates for CTC used in the 2010 SAOD report and the subsequent three reports are shown in Fig. 2.
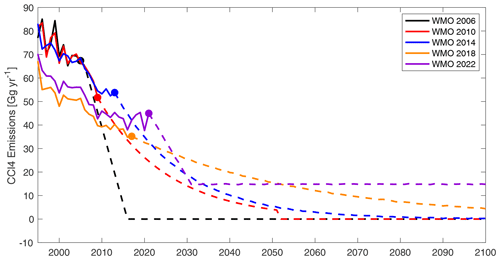
Figure 2Carbon tetrachloride emissions estimates from each scientific assessment of ozone depletion (SAOD) report from 2006 to 2022, as indicated by the WMO date in the legend. Solid lines represent observationally derived emissions using the assumed lifetime from the corresponding SAOD report. The dashed lines represent the emissions projection estimates from each SAOD, and the dots indicate the year of each publication, which separates the observationally derived emissions from projected emissions.
The 2010 and 2014 SAOD reports developed CTC emissions projections by extrapolating the top-down-derived CTC emissions trend from the previous 5 years, equivalent to a 6 % yr−1 and 6.4 % yr−1 decrease in emissions, respectively. The 2010 SAOD assumed zero emissions following 2050, whereas the 2014 SAOD assumed a continued 6.4 % yr−1 decrease in emissions (Fig. 2). However, observationally derived emissions values from 2000–2012, after accounting for updated atmospheric lifetime estimates, again did not reflect this assumed decline in emissions, but rather they were estimated to be relatively stable at 39 Gg yr−1 (Liang et al., 2014), highlighting the gap in understanding of emissions sources.
This projection assumption for CTC was again updated in the 2018 SAOD to better match top-down-derived emissions, which had been declining more slowly than expected at a rate of 2.5 % yr−1 over the previous 2 decades. This slower-than-expected decline was only partially explained by adjusted estimates in atmospheric lifetimes between the 2014 and 2018 SAODs (Table 1). Additional discrepancies were documented in the SPARC (2016) special report on the “mystery” of CTC, which pointed to previously unaccounted for by-product emissions during chloromethane and perchloroethylene (PCE) production; feedstock emissions for hydrofluorocarbons (HFCs) and PCE, where feedstocks refer to chemicals used in the process of manufacturing different chemicals; legacy emissions including from contaminated soils and landfills; and inadvertent emissions (Sherry et al., 2018; SPARC, 2016). Sherry et al. (2018) estimated bottom-up emissions in 2014 that included ∼ 15 Gg yr−1 from unreported non-feedstock and fugitive emissions and ∼ 10 Gg yr−1 of legacy emissions from chloro-alkali plants.
The 2022 SAOD thus adopted a hybrid CTC emissions projection that began with top-down-derived emissions equal to 45 Gg yr−1 in 2020 and assumed a linear decrease in emissions from 2020–2030, with constant emissions of 15 Gg yr−1 from 2030 onwards. The emissions pathway reflects an assumption that legacy emissions will decline linearly until their cessation in 2030 and an assumption of continued constant emission from, for example, feedstock sources. New knowledge of pathways of atmospheric emission of CTC continues to emerge (Li et al., 2024), which will likely result in further adjustments to future emissions in subsequent SAOD reports.
Here we explain the various steps that underlie our process for quantifying the contribution of updated modeling assumptions in delaying the return of EESC to the value found for January 1980. We use the beginning of 1980 as a marker for the return of EESC, since the return of EESC and atmospheric levels of ozone to the “pre-1980 value” is a commonly adopted metric for assessing the path to recovery of the ozone layer in the SAOD reports.
-
Step 1: update the EESC calculation method to that used in WMO (2022).
We recalculate the EESC time series for each assessment by applying the Engel et al. (2018) formulation, using each SAOD's original time series of atmospheric mole fractions for the 16 major ODSs given in Table 1. This calculation is performed first so that changes in the EESC computation method do not confound the interpretation of changes in ODS projections.
-
Step 2: identify the primary gases delaying EESC return dates.
We then identify the primary ODSs that have driven the delay in EESC return dates. This identification is performed by beginning with the original assessment's time series for all ODSs. We substitute in the subsequent assessment's time series and recalculate EESC and its expected 1980 return date one gas at a time with replacement. The ODSs that dominate the delay in EESC and are also subject to banking (that is, CFC-11, CFC-12, and halon 1301) are then considered in Steps 3–5. CTC, which is the most critical ODS in delaying expected EESC return dates between SAODs and is not assumed to be significantly banked, is then considered in Step 6.
-
Step 3: update lifetime assumptions to those of the 2022 SAOD for the most important banked gases.
For CFC-11, CFC-12, and halon 1301, we adopt the 2022 SAOD atmospheric lifetimes and recalculate emissions and banks following each assessment's original bank calculation method described earlier. We then use the updated bank and release fractions, along with the new atmospheric lifetimes, to project mole fractions to 2100. See Table S3 in the Supplement for a summary of the atmospheric lifetimes adopted for each SAOD.
-
Step 4: update mole fraction observations to those of WMO (2022) for the most important banked gases.
We recalculate atmospheric mole fractions by updating the bank and emissions calculations based on observed mole fractions up to 2021 while retaining the approaches of the respective SAODs. This step is taken to evaluate the extent to which differences in the projected mole fractions from 2006–2021 would have impacted the EESC return date using the original bank and emissions estimation methods.
-
Step 5: update banks to those of WMO (2022) for the most important banked gases.
After updating mole fractions and lifetimes, bank emissions are the only remaining discrepancy for CFC-11, CFC-12, and halon 1301. Therefore, we next update the entire projection time series using the 2022 SAOD 2020 bank values to account for the outstanding update, which comprises the updated bank values and approach. This step allows us to quantify the new estimated bank contributions to differences in the expected return of EESC to the 1980 level for these three gases.
-
Step 6: update CTC lifetimes and emissions projections.
CTC is treated separately from CFC-11, CFC-12 and halon 1301 because CTC is not a banked ODS; the sources of its emissions are also relatively poorly understood (Liang et al., 2014). We update the time series for CTC as follows. First, we update the lifetime of CTC to match the 2022 SAOD assumed lifetime of 30 years, and we then recalculate all future emissions based on the same approaches in each of the respective SAODs. This adjustment impacts future projected mole fractions of CTC due to the rate of atmospheric decline and also impacts observationally derived emissions that are used to inform the projected emissions. Next, we update the time series of observed mole fractions up to 2021 from the 2022 SAOD, as is done in Step 4, to reflect how the original projection methods from each SAOD would be impacted by the actual observed mole fractions. The final part of the modified CTC projection involves updating the future emissions projection method to what was used in the 2022 SAOD, which brings both historical and future CTC mole fraction time series to the time series used in the 2022 SAOD. We refer to this final part as accounting for future feedstock emissions, though the 15 Gg yr−1 of continued emission was meant to comprise all potential emissions, including those that might arise from unreported production.
-
Step 7: update all other gases to WMO (2022) values.
For the final step in quantifying contributions to expected delays in EESC, we update the time series for the abundance of the remaining 12 ODSs to the values given in the 2022 SAOD.
The various formulations of EESC used in the past five SAOD reports have resulted in substantial differences in the 1980 return date reported in these assessments (Fig. 3). There are relatively large differences in the magnitude of EESC given in the 2006 SAOD compared to all subsequent SAOD reports (Fig. 3a). The larger EESC magnitude in the 2006 report is due to the use of a single set of FRFs for each ODS representative of the global stratosphere given in Table 8-1 of the 2006 SAOD, rather than the adoption of separate sets of FRFs for the mid-latitude lower stratosphere (that is, 3-year-old air) and the polar stratosphere (5.5-year-old air), which commenced with the 2010 SAOD. Hence, the peak value of EESC given in the 2006 report falls in between the peaks of EESC for 3-year-old air and 5.5-year-old air given in the 2010 report. However, EESC return dates as reported in the assessments do not meaningfully change between the 2006 SAOD report and the subsequent three SAODs. Mid-latitude EESC values for the 2006–2018 SAOD reports yield a return-to-1980 date between 2046 and 2050. In contrast, the 2022 SAOD report provides an estimated return date of 2066. This disparity is due in part to the use of the Engel et al. (2018) formulation of EESC in the 2022 report, which effectively uses a new formulation that accounts for the interaction of tropospheric trends in the organic species with atmospheric loss of these compounds (Ostermöller et al., 2017; see also Sect. 2.1 and Box 1–4 of the 2018 SAOD report). Use of the Engel et al. (2018) approach in the 2022 SAOD results in a rightward shift in the EESC time series that reduces the value of EESC in 1980, consequently delaying the 1980 return date for EESC relative to all of the previous assessments (Fig. 3a). Various other counteracting changes in the formulation of EESC for the 2006–2018 SAOD reports resulted in near constancy of the return to the 1980 level for EESC. With each subsequent SAOD report, there was a tendency for the surface mixing ratios of ODSs, except for that of HCFC-22, to return to their 1980 levels at later dates, which, all else being equal, would have led to incremental delays in the return-to-1980 date of EESC. However, with each subsequent SAOD report, there were also incremental changes in the approach used to compute EESC that largely counteracted these incremental delays. It is therefore instructive to examine the return to 1980 EESC levels for the assessed time series of the 16 principal ODSs of each SAOD report using an identical formulation for EESC.
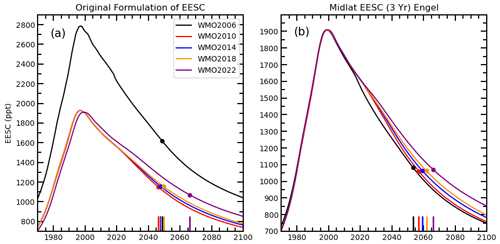
Figure 3EESC calculations applied to each WMO assessment atmospheric mole fraction table of the 16 primary ODSs. (a) EESC formulation as it appears in the original assessments. (b) Engel et al. (2018) EESC calculation applied to the atmospheric mole fractions given in each assessment. Dots and vertical lines on the x axis refer to the first month in which EESC returns to below 1980 levels for the respective WMO assessment.
Using the Engel et al. (2018) formulation for EESC applied to the time series of ODS mole fractions given in the past five SAOD reports, we see a near-consistent delay in the return to 1980 EESC levels between reports (Fig. 3b). For each consecutive SAOD, the return-to-1980 date lags that given in the prior report by about 2 to 4 years. Further, using the identical formulation for EESC (Engel et al., 2018) shortens the difference in the 1980 return date between the 2006 and 2022 SAOD reports from 17 to 12.6 years, which we investigate below. Note that the value of EESC in 1980 (that is, the return-to-1980 target) does not perfectly align, despite the use of an identical formulation for the calculation of EESC (Fig. 3b). These slight shifts are a result of updating historical atmospheric mole fractions of ODSs between assessments, with the most significant change arising from methyl bromide (Table 2).
The role of the choice of EESC formulation in delaying return dates is further explored in Fig. 4. The SAOD reports provide estimates of EESC for both mid-latitude (assumed to be 3-year-old air) and polar (5.5-year-old air) stratospheric regions, which are used in many papers (and the assessments) as proxies for the recovery of mid-latitude and polar ozone to perturbations caused by anthropogenic halogens. When normalizing the original formulations of EESC to the value in 1980 reported in those assessments, the 2022 SAOD report is a notable outlier for the EESC of mid-latitude regions (Fig. 4a).
Identical formulations of EESC, using the Engel et al. (2018), Newman et al. (2007), or Daniel et al. (1995) method, result in consistent delays in the return dates for mid-latitude EESC to 1980 levels with each subsequent SAOD report (Figs. 4b and c, S1). The return-to-1980 date for mid-latitude EESC is delayed by 12.6, 9.8, and 10.4 years when switching from the ODS mole fraction tables given in the 2006 SAOD report to the 2022 report upon use of the Engel et al. (2018), Newman et al. (2007), and Daniel et al. (1995) methods, respectively. Therefore, the mole fraction tables for ODSs given over the 16-year assessment period that are central to this study have played a key role in the delay in the return of stratospheric halogens to the 1980 level, regardless of which approach is used to compute EESC.
There are also large differences in the return-to-1980 dates between the various formulations for EESC. For example, return dates of mid-latitude EESC found using Engel et al. (2018) lag those of Newman et al. (2007) by 13.8 and 3.5 years for mid-latitude and polar air, respectively, when using the ODS mole fraction table given in the 2022 SAOD report. The later return date of the Engel et al. (2018) formulations is largely driven by the authors' use of a method that accounts for the relationship of tropospheric source gas trends and stratospheric chemical breakdown. Their EESC formulation takes into account the time needed to release the halogens from their source gases. The inorganic fraction, which EESC represents, is therefore weighted towards longer transit times and thus lags the troposphere more strongly than in the older formulation. The Engel et al. (2018) approach used in the 2022 SAOD report again leads to lower EESC values during the ascending phase of tropospheric halogen loading and higher EESC values during the descending (recovery) phase of tropospheric halogen loading and thus to a longer time frame needed to reach 1980 EESC values. Applying the Engel et al. (2018) formulation for EESC to the ODS mole fraction table of the 2010 SAOD delays the return to the 1980 value for mid-latitude air by about 3.5 years compared to the return date found using the mole fractions from the 2006 SAOD. Relative to the 2010 SAOD report, the 1980 return date is delayed by 2.4 years when using the mole fraction table of the 2014 report (Fig. 4b). The 2018 SAOD report exhibits another 2.5-year delay relative to the 2014 report. The 2022 SAOD report was the first report to adopt the Engel et al. (2018) formulation for EESC as the primary approach and showed an additional 4.1-year delay in the return date relative to the 2018 SAOD with the Engel et al. (2018) approach employed for both sets of ODS mole fractions. The top panels of Fig. 4 illustrate the important roles that ODS mole fraction changes and alternate formulations of EESC have played in delaying the return of EESC to the 1980 level for mid-latitude air over the past 16-year assessment period.
Table 2Independent incremental change (in years) between assessments by gas for mid-latitude EESC return date using the Engel et al. (2018) formulation. Gases are ordered by total contribution to change in the EESC return date between 2006 and 2022, where the 2022 SAOD's estimated EESC return date is 2066.0. For each gas' calculation, the subsequent SAOD's time series is adopted for that gas, while the 15 other gases maintain the time series from the original SAOD. The difference is then calculated relative to the original SAOD's time series for all 16 gases.
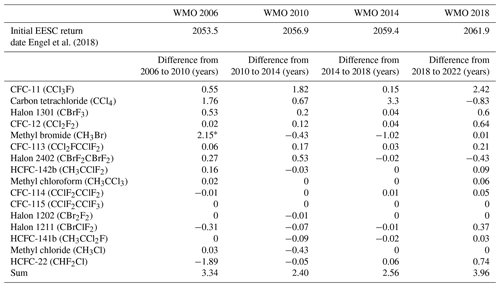
* Updating EESC values from changes in methyl bromide between WMO (2006) and WMO (2010) leads to a relatively large decrease in the value of EESC in 1980, from 1082 to 1060 ppt (parts per trillion).
While the Newman et al. (2007) and Engel et al. (2018) formulations result in large differences in EESC for mid-latitude regions, these two formulations result in similar EESC return dates for polar regions (Fig. 4e and f). This difference is due to the Engel et al. (2018) EESC formulation attempting to weight the age of air by the timing of source gas dissociation. This leads to differences in estimated mole fractions of active (inorganic) halogens in mid-latitudes, as photolysis largely occurs in the tropical stratosphere and affects some ODSs more than others. The formulations give similar estimates of EESC for polar regions because the transit through the stratosphere from injection (in the tropics) to polar descent includes the transit of air parcels through the upper branch of the Brewer–Dobson circulation. This results in nearly complete loss of most ODSs due to a longer residence time in the stratosphere and most importantly exposure to a more intense ultraviolet radiation environment than is seen for most 3-year-old mid-latitude air parcels. Thus, the age of air associated with dissociated ODSs is much more similar to the age of an inert tracer in polar regions than is the case for mid-latitude air.
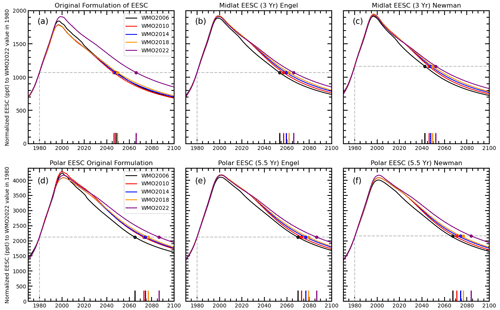
Figure 4EESC calculations applied to each WMO assessment's atmospheric mole fractions of the 16 primary ODSs, normalized to the value of EESC in 1980 reported by WMO (2022). (a) The original assessment's mid-latitude formulation of the EESC formulation. (b) Mid-latitude EESC estimates for each WMO assessment's atmospheric mole fractions using the Engel et al. (2018) formulation. (c) As in (b) but using the Newman et al. (2007) formulation. (d–f) As in (a)–(c) but for polar EESC calculations. Dots and vertical lines on the x axis refer to the first month in which EESC returns to below 1980 levels for the respective WMO assessment.
Table 2 provides the calculated contribution of each ODS to the delay in the return to 1980 EESC values. CFC-11 and CTC account for the largest delays in EESC recovery between the 2006 and 2022 SAOD reports, each delaying cumulative recovery over this period by ∼ 4.9 years (the ∼ 4.9-year delay is the sum of the four individual delays given for both CFC-11 and CTC in the table). Halon 1301 and CFC-12 contribute the third and fourth most substantial delays of 1.4 and 0.8 years, respectively. Note that the impacts on the 1980 return date shown in Table 2 are calculated for each ODS independently. The return-to-1980 date for EESC is affected by past and future changes in all ODSs in a small non-linear manner due to the non-linearity of the EESC time series, such that the sum of the independent impacts across all ODSs (Table 2), when all are changed simultaneously, is not precisely equal to the cumulative sum of the impacts on return date when all ODSs are changed individually (Table S2).
Of the four ODSs contributing to the largest delays in ozone recovery, CFC-11, CFC-12, and halon 1301 are subject to significant banking. We further investigate the role of each change in modeling assumptions for these three ODSs as well as the other key factors in the changing return dates in Fig. 5. Since 2006, projected mole fractions of HCFC-22 have decreased by a greater amount than had once been projected in the 2006 SAOD. However, the effect on EESC of a decline in the atmospheric abundance of HCFC-22 that is faster than had once been forecast has been offset by higher-than-expected atmospheric abundances of the other aggregated 11 ODSs (that is, the other 15 ODSs excluding the top 4 (CFC-11, CTC, halon 1301, and CFC-12)). Between the 2006 SAOD and 2010 SAOD reports, updates in methyl bromide delayed the EESC return date by ∼ 2 years. This change is a result of updates in pre-1980 methyl bromide mole fractions, which lowered the 1980 EESC baseline value between the 2006 and 2010 assessments (Fig. 3b).
The primary results of this analysis are shown in Fig. 5 and Table S2, which quantify the contribution of each modeling assumption to the delay in EESC return dates that is found for mid-latitude air for the past five SAOD reports. Updating the atmospheric lifetime for CFC-11 from the 45-year value used in the 2006 and 2010 SAOD reports to the 52-year value used in the 2014 to 2022 reports results in a 2.2-year delay in the EESC return date and is thus a key single factor. This delay is due to the projected atmospheric mole fractions declining more quickly in the earlier assessments with the shorter assumed CFC-11 lifetime and is also a result of the impact of lifetimes on earlier bank estimation methods. In the 2010 SAOD, for example, the shorter lifetime leads to higher inferred emissions during the time when atmospheric mole fraction observations were available, and because production was fixed, these higher emissions were modeled as emissions from banks. Therefore, banks were estimated to deplete more quickly when using the lower atmospheric lifetime.
Updates in observed atmospheric mole fractions do not substantially impact our EESC return date estimates for banked gases relative to the other factors. One initially counter-intuitive result is the impact of observed mole fractions of CFC-11 on the 1980 return date, for each SAOD report from 2006 to 2018 (Fig. 5). When observed mole fractions were higher than had been expected, this finding accelerated the estimated return date using each SAOD's respective projection method. The higher mole fractions were assumed to be due to higher-than-expected emissions from banks, which was achieved by increasing the release fractions from banks. This assumption led to estimated bank values decreasing more quickly as the date of each SAOD report advanced, thus being a smaller source of future emissions, which in turn moved up the return date for EESC. This result is in part due to the assumptions in SAODs from 2006 to 2018 that the global production of these banked gases was well known and that the uncertain parameters controlling bottom-up emissions were in bank release rates and not industrial production. This assumption regarding highly certain production values was relaxed in the bank modeling approach used in the 2022 SAOD report, which has led to bank release rates being less sensitive to observed mole fractions.
Updating the bank methodology to the 2022 SAOD report results in notable delays in EESC return dates for all three gases, though most substantially for CFC-11. The 2022 SAOD methodology results in significantly larger bank estimates compared to the prior assessments (Fig. 1), primarily driven by allowing for uncertainty in the values of the production of ODSs. By relaxing the assumption of completely accurate production reporting under the Montreal Protocol and even full compliance under the protocol, higher atmospheric mole fractions and inferred emissions of ODSs may result in higher posterior production estimates, which in turn accumulate into higher bank estimates. This was the case with unexpected emissions of CFC-11 after 2012 (Benish et al., 2021; Lickley et al., 2022; Montzka et al., 2018; Park et al., 2018; Rigby et al., 2019), where allowing for production uncertainty in the bank modeling framework over this time period resulted in posterior production estimates that were non-zero following 2010 and larger posterior bank values than what was inferred by assuming zero production uncertainty and full compliance with the protocol following 2010.
Changes in understanding of the processes impacting CTC have led to nearly consistent delays in expected EESC return dates (Fig. 5). Updating the atmospheric lifetime from 26 years, which was used in the 2006, 2010, and 2014 SAOD reports, to the 30-year value used in the 2022 SAOD had two competing effects on the EESC return date. The longer lifetime results in lower inferred emissions during the time atmospheric mole fraction observations were available; however, it also implies a slower decay of the gas in the atmosphere, resulting in a 0.9-year delay in the EESC return date relative to the date given in the 2006 SAOD. Likewise, the higher-than-projected observed mole fractions of CTC compared with earlier projections contributed an additional 1-year delay to the EESC return date. Because CTC is not a banked chemical, there is no compensating emission source to explain these higher-than-expected mole fractions in earlier SAODs, so the higher-than-expected inferred emissions would be modeled strictly as an emission source from new production of CTC. Finally, the additional assumption in the 2022 SAOD report of long-term, continuous feedstock emissions of 15 Gg yr−1, which was not accounted for in the projections used in any of the earlier SAODs (Fig. 2), contributes an addition 3.1 years to the delay in EESC compared to the 2006 SAOD report. Note, however, that this represents an acceleration in the EESC return date relative to the date in the 2018 SAOD, where the emissions decay function resulted in larger CTC emissions in the first half of the 21st century relative to the 2022 projections (Fig. 2). Overall, updated modeling assumptions for CTC between the 2006 and 2022 SAODs delay the expected EESC return date by a total of 4.9 years.
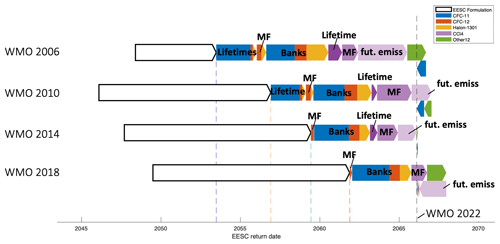
Figure 5EESC return dates to 1980 levels for each sequential update to the original assessment's methods. The vertical dashed lines correspond to the return date that is estimated after applying the identical Engel et al. (2018) EESC formulation to each of the original WMO atmospheric mole fraction time series for the 16 primary ODSs. The corresponding change resulting from the EESC formulation update is shown by the white arrows, followed by atmospheric lifetime assumptions for the three most prominent bank gases responsible for delaying the EESC return date (CFC-11, CFC-12, and halon 1301). The next update corresponds to the update in observed mole fractions for these three gases since publication of each assessment of those measurements used in the 2022 SAODs, indicated by MF, followed by the banks' update. The contribution of projections of carbon tetrachloride (CTC) to delaying EESC return dates is shown in purple for the lifetime updates (dark purple), the observed mole fraction updates (medium purple), and the future feedstock emissions projections (light purple). The remaining 12 gases are shown in green. If the update corresponds to a delay in the EESC return date, then the arrow points to the right. If updated assumptions accelerate the return date, the arrow points to the left.
Much of this work focuses on ODSs and their role in estimating EESC, which is an estimate of inorganic halogens in the stratosphere. Because stratospheric halogens originate from organic compounds that are accurately measured in the troposphere, the quantity of effective equivalent chlorine (EECl) (Montzka et al., 1996) has been considered in parallel with EESC when reporting trends in ODSs. Time series of EECl were highlighted in Fig. 1 of the “Executive Summary” of the 2018 and 2022 SAOD reports, though an unconventional definition of EECl was used, which did not account for FRFs in the calculation. Here, we term this alternative definition equivalent tropospheric chlorine (ETCl). Figure 6a shows historical and projected values of ETCl, found using the time series for 16 principal ODSs of each SAOD report considered in this analysis (Fig. 6a). The 1980 return dates of ETCl for SAODs from 2006 to 2018 are all clustered together around 2046 and 2047, whereas for the 2022 SAOD report, the 1980 return date of ETCl is around 2054. Repeating this calculation but weighting each ODS using FRFs, following the definition of Montzka et al. (1996), we find that the EECl return dates are more evenly delayed between various SAODs, more notably in the mid-latitudes than for polar regions (Fig. 6b, c). The reason for this difference is that ETCl assumes FRFs of unity for all species, resulting in a larger weighting for the HCFCs than is found for either EECl or EESC. Between the 2006 and 2010 SAODs, the projected emissions of HCFC-22 dropped by a factor of ∼ 2, reflecting the 2007 decision by the parties to accelerate the phaseout of HCFCs (Montzka et al., 2015). For ETCl, this earlier phaseout of HCFC-22 offsets the delay in the return to the 1980 values due to projected slower declines in other ODSs. While the drop in HCFC-22 makes a large contribution to ETCl, there is a substantially smaller effect on EECl (Fig. 6b, c) and EESC (Fig. 4) because of the use of FRFs for HCFC-22 of 0.15 and 0.44 for mid-latitude and polar air, respectively, compared to the use of FRFs equal to unity for HCFC-22 and all other species in the formulation of ETCl. Proper accounting of FRFs is needed, as is done in EECl and EESC but not in ETCl, should past and projected tropospheric abundances of ODSs be used as a proxy for how future ozone depletion will be affected by anthropogenic halogens. Finally, the much longer return-to-1980 dates of EESC (Fig. 4) compared to EECl (Fig. 6b, c) is caused by the time it takes for ODSs to reach various levels of the stratosphere as well as by the distribution of these times, as is included in the age-of-air spectrum inherent in the definition of EESC.
Between the 2006 and the 2022 SAOD reports, the expected year for which EESC of the mid-latitudes (3-year-old air) returns to the value of EESC at the start of 1980 was delayed by ∼ 17 years. This change suggests, all else being equal, an approximate 17-year delay in the recovery of mid-latitude ozone column with respect to the value that occurred in 1980. Reported EESC recovery dates were relatively consistent between the 2006 and 2018 SAOD reports, with the value given in the 2022 report appearing to be an outlier, though this was in part to be expected from the adoption of a new formulation for EESC (Engel et al., 2018). However, applying identical formulations for the computation of EESC to projections of the 16 principal ODSs of each SAOD report indicates, as shown above, that the EESC recovery time has been consistently delayed by 2 to 4 years between each successive SAOD report. Thus, the changing formulation of EESC between the 2006 and 2018 SAOD reports has obscured the fact that the assessed projections of the atmospheric abundances of ODSs as a whole have been consistently updated to higher values, on average, between consecutive reports. Applying an identical formulation for EESC (Engel et al., 2018) to the projections of ODSs from each SAOD report results in a delay of 12.6 years, between the 2006 and 2022 SAOD reports, for the recovery of the EESC of mid-latitude air to the value found for the start of 1980. Lifetime assumptions in the 2006 and 2010 assessments for CFC-11 and other key ODSs were lower than the current best estimates, contributing to an earlier expected return date for EESC than that found using lifetimes in the 2022 SAOD report. Since the 2006 SAOD report, changes in atmospheric lifetime estimates can explain approximately ∼ 3.5 years of the difference between the 2006 and 2022 SAOD-projected return dates. Higher-than-expected mole fractions of ODSs explain ∼ 1 year of the difference, largely due to observed mole fractions of CTC, which contributed a higher baseline and slower rate of decline in the future emissions projections. Changes in bank estimates account for another ∼ 4 years of the difference in EESC return date, and updated future emissions projections of CTC, largely due to assumed continued feedstock emissions, account for ∼ 3 years of the difference. The remaining 12 ODS mole fraction projection updates account for an additional net change of ∼ 1 year between SAODs.
An important update in the 2022 SAOD pertains to the assumptions that historical production of ODSs was in compliance with the Montreal Protocol and that reported production numbers were fully accurate. In the baseline scenarios of earlier SAODs, it was assumed that there was no unreported production, and therefore unexpected emissions were accounted for by higher release rates from banks. For the 2022 SAOD report, new production of controlled substances not in compliance with the Montreal Protocol was considered; this new production is included implicitly through increases to atmospheric mole fractions and explicitly through the bank size of the affected ODS. These updates have been made in light of evidence of unreported production of halocarbons in recent years (Benish et al., 2021; Lickley et al., 2022; Montzka et al., 2018; Park et al., 2018; Rigby et al., 2019; Montzka et al., 2021; Park et al., 2021; Sherry et al., 2018) as well as during the 1980s in the Soviet Union at an amount that accounted for ∼ 20 % of global production of CFC-11 (Gamlen et al., 1986), suggesting historical production may have been consistently underestimated in earlier SAOD reports. Production projections of CTC have similarly been consistently underestimated in the SAOD reports (SPARC, 2016). The CTC budget continues to be a source of uncertainty, as observationally derived emissions are consistently higher than bottom-up estimates (Daniel and Reimann, 2022). Recent studies have made progress on budget closure (Sherry et al., 2018; Liang et al., 2014; Park et al., 2018), though bottom-up sources of 15–25 Gg yr−1 (Sherry et al., 2018) are still not within the top-down global emissions range of 34–45 Gg yr−1 (Liang et al., 2014). Li et al. (2024) found current atmospheric emissions of CTC from numerous industrial sources such as the manufacture of general-purpose machinery, raw chemical materials, and chemical products. Currently, the use of CTC and other ODSs used as feedstock in the manufacturing process is exempt from control under the Montreal Protocol, likely due to an assumption that the associated atmospheric releases would remain small. The findings of Li et al. (2024), coupled with the continued tendency of atmospheric mole fractions of CTC to lie above prior projections, suggest a portion of the slower-than-expected decline in EESC since 2006 is caused by inadvertent atmospheric releases of CTC from a wide range of industrial activities.
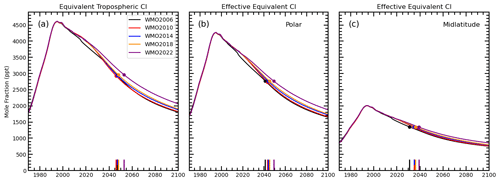
Figure 6Equivalent tropospheric chlorine (ETCl) and effective equivalent chlorine (EECl) estimates for each WMO assessment, using atmospheric mole fractions of the 16 primary ODSs. (a) ETCl, computed in the same unconventional manner as the quantity termed “Equivalent effective chlorine” that was shown in Fig. ES-1 of the “Executive Summary” of the 2018 and 2022 SAODs; (b) EECl calculated following Montzka et al. (1996) using polar time-independent fractional release factors from Table 2 of Engel et al. (2018); (c) EECl calculated following Montzka et al. (1996) using mid-latitude time-independent fractional release factors from Table 1 of Engel et al. (2018).
Following the publication of the 2022 SAOD, further evidence has emerged of increasing mole fractions of CFCs from 2010 to 2020 (Western et al., 2023), thought to be driven in part by feedstock-related emissions, and reports have emerged of unreported feedstock emissions at chemical plants (EIA, 2023). The apparent leakage from feedstock activity may warrant increasing controls on their production processes (Andersen et al., 2021; Lickley et al., 2021). Banks represent another opportunity for reducing future halocarbon emissions. While the CFC-11 bank resides largely in foams, which is difficult to recover, CFC-12 used in refrigeration and the use of halon 1301 as a fire suppressant may be more accessible for bank collection and subsequent destruction. Full recovery of CFC-12 and halon 1301 banks would accelerate estimated ozone recovery by ∼ 3 years, with total bank collection representing an opportunity for accelerating ozone recovery to ∼ 6 years of delay (Lickley et al., 2020, 2022). Unexpected emissions and additional controls on ODSs, such as the ones described above, would all impact estimates of the return-to-1980 date for EESC that will be given in future assessments.
In addition to changes in controls on feedstock and bank emissions, updates in the representation of atmospheric processes may also result in changes in the expected EESC recovery time in future SAOD reports. Future updates may include changes in estimated ODS atmospheric residence times resulting, for example, from changes driven by anthropogenic global warming in the Brewer–Dobson circulation (Prather et al., 2023; Fleming et al., 2011), ocean exchanges, and changes in the hydroxyl radical (Wang et al., 2023). There is emerging evidence that very short-lived (VSL) chlorine compounds, which are largely anthropogenic and are not controlled by the Montreal Protocol, might be responsible for the slower-than-expected decline in HCl in the lower stratosphere (Bednarz et al., 2022; Hossaini et al., 2015, 2019). If so, then VSL chlorine compounds might need to be considered in future formulations of EESC.
EESC baseline projections serve two important purposes for policymakers. First, they are designed to reflect how the current controls in place under the Montreal Protocol are expected to impact stratospheric halogens and hence the recovery of the ozone layer. Parties can use this information to identify which additional restrictions could potentially be considered for safeguarding the ozone layer and climate system. Second, the baseline mole fraction projections used to calculate EESC set expectations with regards to future abundances of ODSs. These projections have been proven valuable in identifying new and illicit production of banned substances in breach of the protocol (e.g., Montzka et al., 2018). While global compliance has not been absolute, the effectiveness of the protocol is clearly evidenced by the current declines in the value of EESC along with initial signs of the recovery of the ozone layer (Solomon et al., 2016; Dhomse et al., 2018; Weber et al., 2022). The present study shows that consistent delays in the estimated EESC return date to the 1980 level is partially due to unreported production of banned ODSs and partially driven by scientific uncertainty in atmospheric lifetimes and estimates of bank sizes. We would expect updates in the modeling of atmospheric processes to affect expected EESC return dates in the future, and it could be valuable for future SAOD reports to consider including uncertainty quantifications in their baseline projections to account for the uncertainty in current scientific understanding (for example the uncertainty in atmospheric lifetimes of ODSs). However, we would expect that each new update in the representation of atmospheric processes could lead to either accelerations of or delays in expected EESC return times. Changes in expected EESC return dates resulting mainly in delays are not expected to be a result of atmospheric uncertainties alone, but rather they may suggest the potential for either breaches in the protocol or significant emissions resulting from the use of ODSs as feedstock, which are not controlled by the protocol. A continued trend of delayed EESC return dates in future SAODs would suggest careful consideration is warranted regarding current reporting and monitoring procedures and regarding our understanding of ODS lifetimes and how to best characterize emissions over time.
All code and data used in this study are available through a public repository (https://doi.org/10.5281/zenodo.13952811, Lickley et al., 2024).
The supplement related to this article is available online at: https://doi.org/10.5194/acp-24-13081-2024-supplement.
All authors designed the methodology. MJL, JSD, and RJS conducted the analysis. MJL and LAM created the figures. All authors contributed to the writing and editing of the manuscript.
The contact author has declared that none of the authors has any competing interests.
Publisher’s note: Copernicus Publications remains neutral with regard to jurisdictional claims made in the text, published maps, institutional affiliations, or any other geographical representation in this paper. While Copernicus Publications makes every effort to include appropriate place names, the final responsibility lies with the authors.
We thank Andreas Engel and the anonymous referee for very constructive comments throughout the review process.
This research has been supported by the Atmospheric Chemistry Division of the National Science Foundation (grant no. 2128617), VoLo Foundation, and NASA Atmospheric Composition Modeling and Analysis Program (grant no. 80NSSC19K098).
This paper was edited by Marc von Hobe and reviewed by Andreas Engel and one anonymous referee.
AFEAS (Alternative Fluorocarbons Environmental Acceptability Study): Production, Sales and Calculated Emissions of Fluorocarbons Through 2000, AFEAS 2001 database, https://unfccc.int/files/methods/ (last access: 1 June 2021), 2001.
Andersen, S. O., Gao, S., Carvalho, S., Ferris, T., Gonzalez, M., Sherman, N. J., Wei, Y., and Zaelke, D.: Narrowing feedstock exemptions under the Montreal Protocol has multiple environmental benefits, P. Natl. Acad. Sci. USA, 118, e2022668118, https://doi.org/10.1073/pnas.2022668118, 2021.
Ashford, P., Clodic, D., McCulloch, A., and Kuijpers, L.: Emission profiles from the foam and refrigeration sectors comparison with atmospheric concentrations, Part 1: Methodology and data, Int. J. Refrig., 27, 687–700, https://doi.org/10.1016/j.ijrefrig.2004.07.025, 2004.
Bednarz, E. M., Hossaini, R., Chipperfield, M. P., Abraham, N. L., and Braesicke, P.: Atmospheric impacts of chlorinated very short-lived substances over the recent past – Part 1: Stratospheric chlorine budget and the role of transport, Atmos. Chem. Phys., 22, 10657–10676, https://doi.org/10.5194/acp-22-10657-2022, 2022.
Benish, S. E., Salawitch, R. J., Ren, X., He, H., and Dickerson, R. R.: Airborne Observations of CFCs Over Hebei Province, China in Spring 2016, J. Geophys. Res.-Atmos., 126, 1–18, https://doi.org/10.1029/2021JD035152, 2021.
Butler, J. H., Battle, M., Bender, M. L., Montzka, S. A., Clarke, A. D., Saltzman, E. S., Sucher, C. M., Severinghaus, J. P., and Elkins, J. W.: A record of atmospheric halocarbons during the twentieth century from polar firn air, Nature, 399, 749–755, https://doi.org/10.1038/21586, 1999.
Campbell, N., Shende, R., Bennett, M., Blinova, O., Derwent, R., McCulloch, A., Yamabe, M., Shevlin, J., and Vink, T.: HFCs and PFCs: Current and Future Supply, Demand and Emissions, plus Emissions of CFCs, HCFCs and Halons, in: IPCC/TEAP Special Report, Safeguarding the Ozone Layer and the Global Climate System: Issues Related to Hydrofluorocarbons and Perfluorocarbons, edited by: Metz, B., Kuijpers, L., Solomon, S., Andersen, S. O., Davidson, O., and Pons, J., WMO, 403–436, Cambridge University Press, ISBN 13: 9780521863360, 2005.
Carpenter, L. J., Daniel, J. S., Fleming, E., Hanaoka, T., Hu, J., Ravishankara, A. R., Ross, M. N., R., Tilmes, S., Wallington, T., and Wuebbles, D.: Scenarios and Information for Policymakers, Chap. 6, in: Scientific Assessment of Ozone Depletion: 2018, Global Ozone Research and Monitoring Project–Report No. 58, 588 pp., WMO, Geneva, Switzerland, ISBN: 978-1-7329317-1-8, 2018.
Daniel, J. S., Velders, G. J. M., Solomon, S., McFarland, M., and Montzka, S. A.: Present and future sources and emissions of halocarbons: Toward new constraints, J. Geophy. Res.-Atmos., 112, D02301, https://doi.org/10.1029/2006JD007275, 2007a.
Daniel, J. S., Velders, G. J. M., Douglass, A. R., Forster, P. M. D, Hauglustaine, D. A., Isaksen, I. S. A., Kuijpers, L. J. M, McCulloch, A., and Wallington, T. J.: Halocarbon Scenarios, Ozone Depletion Potentials, and Global Warming Potentials, Chap. 8, in: Scientific Assessment of Ozone Depletion: 2006, Global Ozone Research and Monitoring Project – Report No. 50, 572 pp., WMO, Geneva, ISBN: 9789280727579, 2007b.
Daniel, J. S., Velders, G. J. M., Morgenstern, O., Toohey, D., Wallington, T., Wuebbles, D., Akiyoshi, H., Bais, A. F., Flemin, E. L., Jackman, C. H., Kuijpers, L. J. M., McFarland, M., Montzka, S. A., Ross, M. N., Tilmes, S., and Tully, M. B.: A focus on Information and Options for Policymakers, Chap. 5, in: Scientific Assessment of Ozone Depletion: 2010, Global Ozone Research and Monitoring Project-Report No. 52, 516 pp., WMO, Geneva, Switzerland, ISBN: 9966-7319-6-2, 2011.
Daniel, J. S., Reimann, S., Ashford, P., Fleming, E., Hossaini, R., Lickley, M., Schofield, R., and Walter-Terrinoni, H.: Scenarios and Information for Policymakers, Chap. 7, in: Scientific Assessment of Ozone Depletion: 2022, GAW Report No 278, 509 pp., WMO, Geneva, ISBN: 978-9914-733-97-6, 2022.
Daniel, J. S., Solomon, S., and Albritton, D. L: On the evaluation of halocarbon radiative forcing and global warming potentials, J. Geophys. Res.-Atmos., 100, 1271–1285, https://doi.org/10.1029/94JD02516, 1995.
Dhomse, S. S., Kinnison, D., Chipperfield, M. P., Salawitch, R. J., Cionni, I., Hegglin, M. I., Abraham, N. L., Akiyoshi, H., Archibald, A. T., Bednarz, E. M., Bekki, S., Braesicke, P., Butchart, N., Dameris, M., Deushi, M., Frith, S., Hardiman, S. C., Hassler, B., Horowitz, L. W., Hu, R.-M., Jöckel, P., Josse, B., Kirner, O., Kremser, S., Langematz, U., Lewis, J., Marchand, M., Lin, M., Mancini, E., Marécal, V., Michou, M., Morgenstern, O., O'Connor, F. M., Oman, L., Pitari, G., Plummer, D. A., Pyle, J. A., Revell, L. E., Rozanov, E., Schofield, R., Stenke, A., Stone, K., Sudo, K., Tilmes, S., Visioni, D., Yamashita, Y., and Zeng, G.: Estimates of ozone return dates from Chemistry-Climate Model Initiative simulations, Atmos. Chem. Phys., 18, 8409–8438, https://doi.org/10.5194/acp-18-8409-2018, 2018.
EIA: F-gases at the Fenceline: Exposing the Fluorochemical Production Sector's Undisclosed Emissions, Environmental Investigation Agency, https://eia.org/wp-content/uploads/2023/10/EIA-GasmetReport-FINAL-3sm.pdf (last access: 15 August 2024), 2023.
Engel, A., Bönisch, H., Ostermöller, J., Chipperfield, M. P., Dhomse, S., and Jöckel, P.: A refined method for calculating equivalent effective stratospheric chlorine, Atmos. Chem. Phys., 18, 601–619, https://doi.org/10.5194/acp-18-601-2018, 2018.
Fleming, E. L., Jackman, C. H., Stolarski, R. S., and Douglass, A. R.: A model study of the impact of source gas changes on the stratosphere for 1850–2100, Atmos. Chem. Phys., 11, 8515–8541, https://doi.org/10.5194/acp-11-8515-2011, 2011.
Gamlen, P. H., Lane, B. C., Midgley, P. M., and Steed, J. M.: The production and release to the atmosphere of CCl3F and CCl2F2 (chlorofluorocarbons CFC 11 and CFC 12), Atmos. Environ., 20, 1077–1085, https://doi.org/10.1016/0004-6981(86)90139-3, 1986.
Harris, N. R. P., Wuebbles, D. J., Daniel, J. S., Hu, J., Kuijpers, L. J. M., Law, K. S., Prather, M. J., and Schofield, R.: Scenarios and Information for Policymakers, Chap. 5, in: Scientific Assessment of Ozone Depletion: 2014, Global Ozone Research and Monitoring Project – Report No. 55, WMO, Geneva, Switzerland, ISBN: 978-9966-076-01-4, 2014.
Hossaini, R., Chipperfield, M. P., Montzka, S. A., Rap, A., Dhomse, S., and Feng, W.: Efficiency of short-lived halogens at influencing climate through depletion of stratospheric ozone, Nat. Geosci., 8, 186–190, https://doi.org/10.1038/ngeo2363, 2015.
Hossaini, R., Atlas, E., Dhomse, S. S., Chipperfield, M. P., Bernath, P. F., Fernando, A. M., Muhle, J., Leeson, A. A., Montzka, S. A., Feng, W., Harrison, J. J., Krummel, P., Vollmer, M. K., Reimann, S., O'Doherty, S., Young, D., Maione, M., Arduini, J., and Lunder, C. R.: Recent trends in stratospheric chlorine from very short-lived substances, J. Geophys. Res-Atmos., 124, 2318–2335, https://doi.org/10.1029/2018JD029400, 2019.
IPCC/TEAP: Task Force on Emissions Discrepancies Report, Technical Report. Nairobi, Kenya, UNEP, 2006.
Kida, H.: General circulation of air parcels and transport characteristics derived from a hemispheric GCM Part 2, Very long-term motions of air parcels in the troposphere and stratosphere, J. Meteorol. Soc. Jpn. Ser. II, 61, 510–523, https://doi.org/10.2151/jmsj1965.61.4_510, 1983.
Kuijpers, L. J. M. and Verdonik, D.: TEAP (Technology and Economic Assessment Panel), Task Force Decision XX/8 Report, Assessment of Alternatives to HCFCs and HFCs and Update of the TEAP 2005 Supplement Report Data. Nairobi, Kenya, UNEP, 2009.
Laube, J. C., Newland, M. J., Hogan, C., Brenninkmeijer, C. A., Fraser, P. J., Martinerie, P., Oram, D. E., Reeves, C. E., Rockmann, T., Schwander, J., Witrant, E., and Sturges, W. T.: Newly detected ozone-depleting substances in the atmosphere, Nat. Geosci., 7, 266–269, https://doi.org/10.1038/ngeo2109, 2014.
Li, B., Huang, J., Hu, X., Zhang, L., Ma, M., Hu, L., Chen, D., Du, W., Sun, Y., Cai, Z., Chen, A., Li, X., Feng, R., Prinn, R. G., and Fang, X.: CCl4 emissions in eastern China during 2021–2022 and exploration of potential new sources, Nat. Commun., 15, 1725, https://doi.org/10.1038/s41467-024-45981-x, 2024.
Liang, Q., Newman, P. A., Daniel, J. S., Reimann, S., Hall, B. D., Dutton, G., and Kuijpers, L. J.: Constraining the carbon tetrachloride (CCl4) budget using its global trend and inter-hemispheric gradient, Geophys. Res. Lett., 41, 5307–5315, https://doi.org/10.1002/2014GL060754, 2014.
Lickley, M., Solomon, S., Fletcher, S., Rigby, M., Velders, G. J. M., Daniel, J., Rigby, M., Montzka, S. A., Kuijpers, L. J. M., and Stone, K.: Quantifying contributions of chlorofluorocarbon banks to emissions and impacts on the ozone layer and climate, Nat. Commun., 11, 1380, https://doi.org/10.1038/s41467-020-15162-7, 2020.
Lickley, M., Fletcher, S., Rigby, M., and Solomon, S.: Joint inference of CFC lifetimes and banks suggests previously unidentified emissions, Nat. Commun., 12, 2920, https://doi.org/10.1038/s41467-021-23229-2, 2021.
Lickley, M. J., Daniel, J. S., Fleming, E. L., Reimann, S., and Solomon, S.: Bayesian assessment of chlorofluorocarbon (CFC), hydrochlorofluorocarbon (HCFC) and halon banks suggest large reservoirs still present in old equipment, Atm. Chem. Phys., 22, 11125–11136, https://doi.org/10.5194/acp-22-11125-2022, 2022.
Lickley, M. J., Salawitch, R., Daniel, J., and McBride, L.: meglickley/The-return-to-1980-stratospheric-halogen- levels-A-moving-target-in-ozone-assessments: v1: Code for Lickley et al. (2024), ACP (Version v1), Zenodo [code and data set], https://doi.org/10.5281/zenodo.13952812, 2024.
Minschwaner, K., Salawitch, R. J., and McElroy, M. B.: Absorption of solar radiation by O2: Implications for O3 and lifetimes of N2O, CFCl3, and CF2Cl2, J. Geophys. Res.-Atmos., 98, 10543–10561, https://doi.org/10.1029/93JD00223, 1993.
Montzka, S. A., Butler, J. H., Myers, R. C., Thompson, T. M., Swanson, T. H., Clarke, A. D., Lock, L. R., and Elkins, J. W.: Decline in the tropospheric abundance of halogen from halocarbons: Implications for stratospheric ozone depletion, Science, 272, 1318–1322, https://doi/10.1126/science.272.5266.1318, 1996.
Montzka, S. A. and Fraser, P. J., Butler, J. H., Connell, P. S., Cunnold, D. M., Daniel, J. S., Derwent, R. G., Lal, S., McCulloch, A., Oram, D. E., Reeves, C. E., Sanhueza, E., Steele, L. P., Velders, G. J. M., Weiss, R. F., and Zander, R. J.: Controlled Substances and Other Source Gases, in: Scientific Assessment of Ozone Depletion: 2002, Global Ozone Research and Monitoring Project – Report No. 47, Geneva, 498 pp., WMO, ISBN: 92-807-2261-1, 2002.
Montzka, S. A., Krol, M., Dlugokencky, E., Hall, B., Jöckel, P., and Lelieveld, J.: Small interannual variability of global atmospheric hydroxyl, Science, 331, 67–69, https://doi/10.1126/science.1197640, 2011.
Montzka, S. A., McFarland, M., Andersen, S. O., Miller, B. R., Fahey, D. W., Hall, B. D., Hu, L., Siso, C., and Elkins, J. W.: Recent trends in global emissions of hydrochlorofluorocarbons and hydrofluorocarbons: Reflecting on the 2007 adjustments to the Montreal Protocol, J. Phys. Chem. A, 119, 4439–4449, https://doi.org/10.1021/jp5097376, 2015.
Montzka, S. A., Dutton, G. S., Yu, P., Ray, E., Portmann, R. W., Daniel, J. S., Kuijpers, L., Hall, B. D., Mondeel, D., Siso, C., Nance, J. D., Rigby, M., Manning, A. J., Hu, L., Moore, F., Miller, B. R., and Elkinds, J. W.: An unexpected and persistent increase in global emissions of ozone-depleting CFC-11, Nature, 557, 413–417, https://doi.org/10.1038/s41586-018-0106-2, 2018.
Montzka, S. A., Dutton, G. S., Portmann, R. W., Chipperfield, M. P., Davis, S., Feng, W., Manning, A. J., Ray, E., Rigby, M., Hall, B. D., Siso, C., Nance, J. D., Krummel, P. B., Muhle, J., Young, D., O'Doherty, S., Salameh, P. K., Harth, C. M., Prinn, R. G., Weiss, R. F., Elkins, J. W., Water-Terrinoni, H., and Theodoridi, C.: A decline in global CFC-11 emissions during 2018–2019, Nature, 590, 428–432, https://doi.org/10.1038/s41586-021-03260-5, 2021.
Morgenstern, O., Braesicke, P., Hurwitz, M. M., O'Connor, F. M., Bushell, A. C., Johnson, C. E., and Pyle, J. A.: The world avoided by the Montreal Protocol, Geophy. Res. Lett., 35, L16811, https://doi.org/10.1029/2008GL034590, 2008.
Newman, P. A., Daniel, J. S., Waugh, D. W., and Nash, E. R.: A new formulation of equivalent effective stratospheric chlorine (EESC), Atmos. Chem. Phys. 7, 4537–4552, https://doi.org/10.5194/acp-7-4537-2007, 2007.
Newman, P. A., Oman, L. D., Douglass, A. R., Fleming, E. L., Frith, S. M., Hurwitz, M. M., Kawa, S. R., Jackman, C. H., Krotkov, N. A., Nash, E. R., Nielsen, J. E., Pawson, S., Stolarski, R. S., and Velders, G. J. M.: What would have happened to the ozone layer if chlorofluorocarbons (CFCs) had not been regulated?, Atmos. Chem. Phys., 9, 2113–2128, https://doi.org/10.5194/acp-9-2113-2009, 2009.
Ostermöller, J., Bönisch, H., Jöckel, P., and Engel, A.: A new time-independent formulation of fractional release, Atmos. Chem. Phys., 17, 3785–3797, https://doi.org/10.5194/acp-17-3785-2017, 2017.
Park, S., Li, S., Mühle, J., O'Doherty, S., Weiss, R. F., Fang, X., Reimann, S., and Prinn, R. G.: Toward resolving the budget discrepancy of ozone-depleting carbon tetrachloride (CCl4): an analysis of top-down emissions from China, Atmos. Chem. Phys., 18, 11729–11738, https://doi.org/10.5194/acp-18-11729-2018, 2018.
Park, S., Western, L. M., Saito, T., Redington, A. L., Henne, S., Fang, X., Prinn, R. G., Manning, A. J., Montzka, S. A., Fraser, P. J., Ganesan, A. L., Harth, C. M., Kim, J., Krummel, P. B., Liang, Q., Muhle, J., O'Doherty, S., Park, H., Park, M., Reimann, S., Salameh, P. K., Weiss, R. F., and Rigby, M.: A decline in emissions of CFC-11 and related chemicals from eastern China, Nature, 590, 433–437, https://doi.org/10.1038/s41586-021-03277-w, 2021.
Plumb, R. A. and Ko, M. K.: Interrelationships between mixing ratios of long-lived stratospheric constituents, J Geophys. Res.-Atmos., 97, 10145–10156, https://doi/10.1029/92JD00450, 1992.
Prather, M. J., Froidevaux, L., and Livesey, N. J.: Observed changes in stratospheric circulation: decreasing lifetime of N2O, 2005–2021, Atmos. Chem. Phys., 23, 843–849, https://doi.org/10.5194/acp-23-843-2023, 2023.
Prinn, R. G., Huang, J., Weiss, R. F., Cunnold, D. M., Fraser, P. J., Simmonds, P. G., McCulloch, A., Harth, C., Salameh, P., O'doherty, S., and Wang, R. H. J.: Evidence for substantial variations of atmospheric hydroxyl radicals in the past two decades, Science, 292, 1882–1888, https://doi.org/10.1126/science.1058673, 2001.
Prinn, R. G., Weiss, R. F., Arduini, J., Arnold, T., DeWitt, H. L., Fraser, P. J., Ganesan, A. L., Gasore, J., Harth, C. M., Hermansen, O., Kim, J., Krummel, P. B., Li, S., Loh, Z. M., Lunder, C. R., Maione, M., Manning, A. J., Miller, B. R., Mitrevski, B., Muhle, J., O'Doherty, S., Park, S., Reimann, S., Rigby, M., Saito, T., Salameh, P. K., Schmidt, R., Simmonds, P. G., Steele, P., Vollmer, M. K., Wang, R. H., Yao, B., Yokouchi, Y., Young, D., and Zhou, L.: History of chemically and radiatively important atmospheric gases from the Advanced Global Atmospheric Gases Experiment (AGAGE), Carbon Dioxide Information Analysis Center (CDIAC), Oak Ridge National Laboratory (ORNL), Oak Ridge, TN (United States), ESS-DIVE repository [data set], https://doi.org/10.3334/CDIAC/ATG.DB1001, 2018.
Ray, E. A., Portmann, R. W., Yu, P., Daniel, J., Montzka, S. A., Dutton, G. S., Hall, B. D., Moore, F. L., and Rosenlof, K. H.: The influence of the stratospheric Quasi-Biennial Oscillation on trace gas levels at the Earth’s surface, Nat. Geosci., 13, 22–27, https://doi.org/10.1038/s41561-019-0507-3, 2020.
Rigby, M., Prinn, R. G., O'Doherty, S., Montzka, S. A., McCulloch, A., Harth, C. M., Mühle, J., Salameh, P. K., Weiss, R. F., Young, D., and Simmonds, P. G.: Re-evaluation of the lifetimes of the major CFCs and CH3CCl3 using atmospheric trends, Atmos. Chem. Phys, 13, 2691–2702, https://doi.org/10.5194/acp-13-2691-2013, 2013.
Rigby, M., Park, S., Saito, T., Western, L. M., Redington, A. L., Fang, X., Henne, S., Manning, A. J., Prinn, R. G., Dutton, G. S., Fraser, P. J., Gaensan, A. L., Hall, B. D., Harth, C. M., Kim, J., Kim, K.-R., Krummel, P. B., Lee, T., Li, S., Liang, Q., Lunt, M. F., Montzka, S. A., Muhle, J., O'Doherty, S., Park, M.-K., Reimann, S., Salameh, P. K., Simmonds, P., Tunnicliffe, R. L. M., Weiss, R. F., Yokouchi, Y., and Young, D.: Increase in CFC-11 emissions from eastern China based on atmospheric observations, Nature, 569, 546–550, https://doi.org/10.1038/s41586-019-1193-4, 2019.
Salawitch, R. J., Fahey, D. W., Hegglin, M. I., McBride, L. A., Tribett, W. R., and Doherty S. J.: Twenty Questions and Answers About the Ozone Layer: 2018 Update, Scientific Assessment of Ozone Depletion: 2018, 84 pp., WMO, Geneva, Switzerland, ISBN: 92-807-2261-1, 2019.
Sherry, D., McCulloch, A., Liang, Q., Reimann, S., and Newman, P. A.: Current sources of carbon tetrachloride (CCl4) in our atmosphere, Environ. Res. Lett., 13, 024004, https://doi.org/10.1088/1748-9326/aa9c87, 2018.
Sinnhuber, B. M., Sheode, N., Sinnhuber, M., Chipperfield, M. P., and Feng, W.: The contribution of anthropogenic bromine emissions to past stratospheric ozone trends: a modelling study, Atmos. Chem. Phys., 9, 2863–2871, https://doi.org/10.5194/acp-9-2863-2009, 2009.
Solomon, S. and Albritton, D. L.: Time-dependent ozone depletion potentials for short-and long-term forecasts, Nature, 357, 33–37, https://doi.org/10.1038/357033a0, 1992.
Solomon, S., Mills, M., Heidt, L. E., Pollock, W. H., and Tuck, A. F.: On the evaluation of ozone depletion potentials, J. Geophys. Res.-Atmos., 97, 825–842, https://doi.org/10.1029/91JD02613, 1992.
Solomon, S., Ivy, D. J., Kinnison, D., Mills, M. J., Neely III, R. R., and Schmidt, A.: Emergence of healing in the Antarctic ozone layer, Science, 353, 269–274, 2016.
SPARC: Report on Lifetimes of Stratospheric Ozone-Depleting Substances, Their Replacements, and Related Species, edited by: Ko, M., Newman, P., Reimann, S., and Strahan, S., SPARC Report No. 6, WCRP-15, WCRP, 2013.
SPARC: Report on the Mystery of Carbon Tetrachloride, edited by: Liang, Q., Newman, P. A., and Reimann, S., SPARC Report No. 7, WCRP-13, WCRP, 2016.
Wang, P., Solomon, S., Lickley, M., Scott, J. R., Weiss, R. F., and Prinn, R. G.: On the influence of hydroxyl radical changes and ocean sinks on estimated HCFC and HFC emissions and banks, Geophys. Res. Lett., 50, e2023GL105472, https://doi.org/10.1029/2023GL105472, 2023.
Weber, M., Arosio, C., Coldewey-Egbers, M., Fioletov, V. E., Frith, S. M., Wild, J. D., Tourpali, K., Burrows, J. P., and Loyola, D.: Global total ozone recovery trends attributed to ozone-depleting substance (ODS) changes derived from five merged ozone datasets, Atmos. Chem. Phys., 22, 6843–6859, https://doi.org/10.5194/acp-22-6843-2022, 2022.
Western, L. M., Vollmer, M. K., Krummel, P. B., Adcock, K. E., Crotwell, M., Fraser, P. J., Harth C. M., Langenfelds, R. L., Montzka, S. A., Muhle, J., O'Doherty, S., Oram, D. E., Reimann, S., Rigby, M., Vimont, I., Weiss, R. F., Young, D., and Laube, J. C.: Global increase of ozone-depleting chlorofluorocarbons from 2010 to 2020, Nat. Geosci., 16, 309–313, https://doi.org/10.1038/s41561-023-01147-w, 2023.
WMO: Scientific Assessment of Ozone Depletion: 2002, Global Ozone Research and Monitoring Project – Report No. 47, 498 pp., Geneva, WMO, ISBN: 92-807-2261-1, 2003.
- Abstract
- Introduction
- A review of SAOD calculations for estimating EESC return dates
- Quantifying drivers of delayed EESC return dates
- Results and discussion
- Conclusions
- Code and data availability
- Author contributions
- Competing interests
- Disclaimer
- Acknowledgements
- Financial support
- Review statement
- References
- Supplement
- Abstract
- Introduction
- A review of SAOD calculations for estimating EESC return dates
- Quantifying drivers of delayed EESC return dates
- Results and discussion
- Conclusions
- Code and data availability
- Author contributions
- Competing interests
- Disclaimer
- Acknowledgements
- Financial support
- Review statement
- References
- Supplement