the Creative Commons Attribution 4.0 License.
the Creative Commons Attribution 4.0 License.
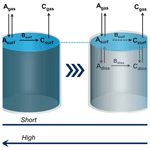
Opinion: Atmospheric multiphase chemistry – past, present, and future
Jonathan P. D. Abbatt
A. R. Ravishankara
Multiphase chemistry occurs between chemicals in different atmospheric phases, typically involving gas–solid and gas–liquid interactions. The importance of atmospheric multiphase chemistry has long been recognized. Its central role extends from acid precipitation and stratospheric ozone depletion to its impact on the oxidizing capacity of the troposphere and to the roles that aerosol particles play in driving chemistry–climate interactions and affecting human health. This opinion article briefly introduces the subject of multiphase chemistry and tracks its development before and after the start of Atmospheric Chemistry and Physics. Most of the article focuses on research opportunities and challenges in the field. Central themes are that a fundamental understanding of the chemistry at the molecular level underpins the ability of atmospheric chemistry to accurately predict environmental change and that the discipline of multiphase chemistry is strongest when tightly connected to atmospheric modeling and field observations.
- Article
(1362 KB) - Full-text XML
- BibTeX
- EndNote
When Atmospheric Chemistry and Physics was launched over 2 decades ago, Abbatt was invited to submit an article to the inaugural issue, which addressed the kinetics of the multiphase reaction between SO2 and H2O2 on ice surfaces (Clegg and Abbatt, 2001):
This contribution built upon concepts described in a review article published a few years earlier by Ravishankara (1997), who presented a conceptual view of tropospheric heterogeneous and multiphase chemistry. As part of the special issue entitled 20 Years of Atmospheric Chemistry and Physics, both authors value the current opportunity to contribute to the overall goal of the special issue “to reflect on the developments of the field of atmospheric chemistry and physics in the last 20 years and point to exciting directions for the future” by addressing the evolution of the field of atmospheric multiphase chemistry. Specifically, this paper will briefly describe multiphase chemistry, its origins, and the progress made in the past 20 years since the inauguration of Atmospheric Chemistry and Physics. It then focuses in depth on future research opportunities and associated challenges. For the sake of brevity, the citations in this paper are illustrative and not comprehensive. Therefore, the reader is directed to in-depth reviews on specific aspects of multiphase chemistry (Ravishankara, 1997; Jacob, 2000; Rudich, 2003; Usher et al., 2003; Finlayson-Pitts, 2003; Rudich et al., 2007; Kolb et al., 2010; George and Abbatt, 2010; Abbatt et al., 2012; McNeill et al., 2012; Pöschl and Shiraiwa, 2015; Herrmann et al., 2015; McNeill, 2015; Burkholder et al., 2017; Pye et al., 2020; Tilgner et al., 2021).
In the 1997 paper, Ravishankara distinguished between heterogeneous and multiphase chemistry based on the extent of diffusion into the bulk. At that time, the term “heterogeneous chemistry” was in vogue to describe ozone hole chemistry. Over the years, it has become clear that diffusion depths vary continuously from solid-like substrates to dilute water solutions. Therefore, in this article, we use the term “multiphase chemistry” to refer to all chemistry that involves more than one phase. Interfacial chemistry falls under this umbrella, with interfaces invariably present when more than one phase is present. We note that “heterogeneous chemistry” is a useful term to describe exclusively interfacial processes (Svehla, 1993), including the reactions of gas-phase molecules and atoms on solid material such as metallic or mineral catalysts. Similarly, “bulk chemistry” refers to chemistry that occurs mainly in only one phase. In this article, our focus is primarily on processes involving the gas phase interacting with atmospheric condensed phases, so we do not describe in-depth advances in the associated chemistry that takes place in the bulk phase.
One underlying theme in the paper is that understanding multiphase processes at the molecular level improves our ability to accurately predict atmospheric change, which, in turn, aids in developing sustainable environmental policy and practices. Positive impacts arise across multiple fields, from climate and air quality to human health and ecology. Another theme is that multiphase chemistry studies are most impactful when closely connected to the entire atmospheric science field, noting the interrelated nature of fundamental chemistry, field measurements, and atmospheric modeling that together constitute the “three-legged stool” model of our field (see Fig. 1) (Abbatt et al., 2014). Multiphase chemistry studies should be conducted to guide, interpret, and encourage field observations as well as to quantitatively inform atmospheric models.
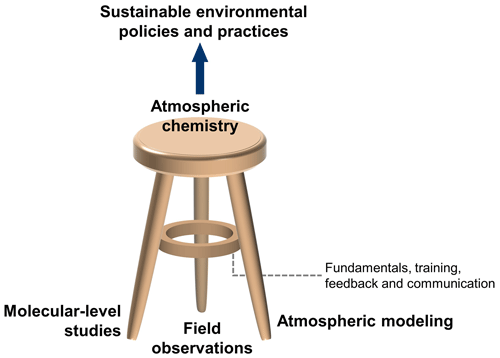
Figure 1The atmospheric chemistry three-legged stool, where the legs are as follows: (i) atmospheric modeling, (ii) field observations, and (iii) molecular-level studies via experimental and theoretical investigations of gas- and multiphase chemistry. The support ring represents the value arising from collaboration, training, and feedback that occurs across the field, along with the need to focus on fundamental science throughout. (Figure credit: Zilin Zhou.)
Multiphase chemistry involves interactions of chemical species present in two or more atmospheric phases, including gas–solid, gas–liquid, and liquid–solid processes. These interactions typically require both chemical reactions and mass transfer, i.e., the movement of a molecule within a phase or from one phase to another. Also important are processes in the interfacial regions, which are the thin transition zones from one phase to another.
To illustrate a multiphase process, consider Reaction (R1), a key reaction leading to acid precipitation. Gas-phase reactants must first partition to the condensed phase, such as an aerosol particle, cloud water droplet, or ice crystal. Once molecules collide with the interface, intermolecular forces promote adsorption for a short period, during which they can diffuse and react via an interfacial process (see Fig. 2). If diffusion into the bulk is sufficiently fast, they can also react in the bulk. In the case of Reaction (R1) in liquid water, dissolved SO2 forms HSO, which can be oxidized by dissolved H2O2 and O3 to form sulfate (Hoffmann and Edwards, 1975; Penkett et al., 1979). When the substrate is ice instead of liquid water, the reaction proceeds either at the gas–solid ice interface or within a thin, liquid-like layer prevalent on the ice's surface below its melting point (Girardet and Toubin, 2001; Abbatt, 2003).
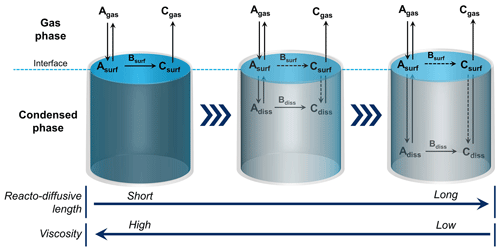
Figure 2Multiphase reactions occur at the interface and within the bulk of condensed-phase materials. Bare solids undergo heterogeneous chemical reactions at the interface. High-viscosity liquids may have sufficiently short reacto-diffusive lengths to restrict reactivity to the surface of the particle. As the viscosity lessens and the reacto-diffusive length deepens, multiphase chemistry can occur deeper into the bulk phase. For reactions that proceed in the bulk, some component of the reaction may also simultaneously occur at the interface (as indicated by the dashed reaction arrow). As noted in the text, the concept of the reacto-diffusive length, which is formally calculated from the bulk diffusion and rate constants, may break down in the interface region. (Figure credit: Zilin Zhou.)
The interface is involved in all multiphase processes, participating in mass transfer and reactivity. For a low-volatility material, such as a bare mineral or metal, there is a step function drop in the density moving from the condensed phase to the gas phase. A solid's interface, roughly one molecule (or atom) deep, can promote heterogeneous chemistry that occurs in a two-dimensional space (Tabazadeh and Turco, 1993). However, solid particles in the atmosphere, such as soot or mineral dust, frequently have mobile, semi-volatile molecules, such as water, sulfuric acid, and organics, deposited as multilayer-thick films or islands. In these cases, the multiphase chemistry is likely occurring in a three-dimensional space involving chemical interactions with not only the solid substrate but also the liquid coatings (Worsnop et al., 2002; Pöschl et al., 2007). For cloud droplets and liquid aerosol particles, which are very dynamic with large fluxes of substrate molecules being rapidly exchanged between the phases, the interfacial region is also thicker than it is for a pure solid (Gopalakrishnan et al., 2005). When the underlying substrate is a liquid, the depth to which a molecule diffuses from the interface into the bulk before reacting is called the reacto-diffusive length; this depth can be very short for species reacting close to the interface and extends to the size of an aerosol particle for reactions that occur throughout the volume of the particle (see Fig. 2) (Hanson et al., 1994). It is not easy to determine whether a reaction is occurring exclusively in a two-dimensional manner at an interface, where the concept of reacto-diffusive depth breaks down because of different energetics and solvation compared with the bulk phase. For particle chemistry, the reaction kinetics' dependence on particle size and reactant concentration can provide some information. Nevertheless, even in reactions that exhibit Langmuir–Hinshelwood kinetics (Pöschl et al., 2001), the depth of the reactive region at the surface of a particle is not clear for liquids or coated solids.
Although thermodynamically allowed, reactions between neutral closed-shell molecules are slow in the gas phase because of large reaction barriers. However, the rates of multiphase reactions involving the same reactants (or suitably altered versions in the condensed phase) can be larger than in the gas phase, either because the free energy barrier to reaction is lowered or because the concentrations of reactants are enhanced in the condensed phase. To illustrate, SO2 and H2O2 do not react efficiently in the gas phase, but oxidation with dissolved H2O2 can proceed once SO2 dissolves in water and forms HSO. Moreover, the gas-phase H2O2 is efficiently scavenged in clouds, enhancing its concentration for reaction.
Another example is the set of reactions that drive polar stratospheric ozone depletion (Solomon, 1999). Chlorine reservoir compounds such as ClONO2 and HCl do not react rapidly in the gas phase. However, HCl partitions strongly to polar stratospheric clouds by either adsorbing to their surfaces or dissolving within them. For example, it is likely that both adsorbed and dissolved HCl dissociates on/in ice to form chloride ions, which are reactive with ClONO2,
leading to the formation of Cl2, which is then released to the gas phase and is readily photolyzed, forming radicals that catalyze gas-phase ozone destruction in the Antarctic ozone hole. Moreover, ClONO2 can be protonated in strongly acidic cloud droplets or may dissociate to form Cl+ and NO (although there is no direct experimental evidence yet for forming Cl+ as an independently solvated species). Other possible mechanisms include a concerted reaction between Cl− and ClONO2 to produce Cl2 (Bianco and Hynes, 1999). It is unclear if such a reaction is truly an SN2-type process or if it can occur in a cage in the condensed phase.
Another distinguishing feature of multiphase chemistry is that it can lead to the formation of products that do not arise in the gas phase. Consider the acid-catalyzed nucleophilic reactions between sulfate and isoprene-derived epoxydiols that form organosulfate molecules and secondary organic aerosol (SOA) (Riva et al., 2019). Water molecules lower the transition state energies of such reactions. The solvent shell, which confines reactant partners via the so-called cage effect, can also promote novel products. For example, the formation of biologically active secondary ozonides is facile in the condensed-phase ozonolysis of unsaturated fatty esters and triglycerides, arising from reactions of Criegee and carbonyl intermediates that form in the same solvent shell after dissociation of a primary ozonide (Z. Zhou et al., 2019, 2022). In the gas phase, the solvent shell is essentially absent (except for some specific cases, such as cluster formation with H2O), so the carbonyl and Criegee intermediates fly apart, and secondary ozonides do not form so readily.
Lastly, some multiphase reactants, such as transition metal ions, are absent in the gas phase. More generally, ion–ion and ion–molecule regions play a much greater role in condensed-phase chemistry than in tropospheric and stratospheric gas-phase chemistry, leading to a wide variety of novel reaction pathways, with Reaction (R1) being an excellent example.
Studies of aerosol and cloud chemistry have proceeded in concert with the development of the wider atmospheric chemistry field, with many of the concepts of coupled reactivity and mass transfer initially developed by the process-oriented chemical engineering community (Dankwerts, 1970). Interest in multiphase reactions arose via the profound ways these sparse aerosol particles and cloud droplets can alter gas-phase composition. Moreover, as described in more detail below, it is now evident that the reverse is important, i.e., the gas phase alters the condensed media with important environmental consequences. Many aerosol and multiphase reaction studies were initially performed to develop parameterizations for atmospheric modeling. Even though this is still a major goal, much more effort is now given to understand the physicochemical processes, as this is essential for predictive capabilities.
The idea of reactive chemistry in hydrometeors goes back to the late 1960s and 1970s and possibly earlier. As in much of science, it is hard to pinpoint a specific paper that expounded this idea. The expectation that the SO2 pollutant can be oxidized to sulfuric acid in water droplets in the atmosphere spurred many studies, hypotheses, and definitive results. Early studies examined the potential oxidation of SO2 in the liquid phase via a variety of oxidants, including O3, H2O2, and NO2 (Hoffmann and Edwards, 1975; Erickson et al., 1977; Schroeder and Urone, 1978; Penkett et al., 1979). Furthermore, modeling studies showed the feasibility of such oxidation reactions occurring in the atmosphere (Jacob and Hoffmann, 1983, and references therein). The Great Dun Fell experiment observationally established that SO2 is indeed oxidized in the troposphere via reactions in cloud droplets (Choularton et al., 1997).
Similar multiphase reactions in the stratosphere were sometimes evoked, but they were never pursued with great vigor until the spectacular occurrence and subsequent explanation of the ozone hole by Solomon et al. (1986) and Solomon (1999) and a multitude of laboratory studies showing that there can indeed be chlorine activation (Molina et al., 1987; Tolbert et al., 1988; Leu, 1988; Hanson and Ravishankara, 1992; and many others).
Additional pioneering atmospheric multiphase chemistry studies arose from aerosol composition measurements conducted over a half-century ago. Specifically, continental aerosol particles always contain a measurable quantity of ammonium, indicating the uptake of gas-phase ammonia to acidic particles (Lee and Patterson, 1969; Kadowaki, 1976). Furthermore, particulate chloride levels in marine aerosol are depleted relative to their seawater abundance, due to replacement by sulfate or nitrate (Junge, 1956; Martens et al., 1973). This process was long thought to be the major source of gas-phase chlorine, whereby gaseous HCl is displaced from NaCl particles via the uptake of gas-phase strong acids:
Another early example of halogen chemistry demonstrated that volatile iodine species are released when dissolved oceanic iodide is exposed to either ultraviolet light or ozone (Miyake and Tsunogai, 1963; Garland et al., 1980). This multiphase chemistry is important for the dry deposition of ozone and the release of iodine into the atmosphere (Carpenter et al., 2013):
After these early studies, many additional important tropospheric multiphase chemical processes were identified prior to the launch of Atmospheric Chemistry and Physics. Some examples, which all address gas–particle and cloud droplet interactions, include the following: (i) the formation of reactive halogen species in the boundary layer (Finlayson-Pitts, 2003; Simpson et al., 2015); (ii) the uptake of tropospheric gases by mineral dust, especially nitric acid (Hanisch and Crowley, 2001; Usher et al., 2003); (iii) the scavenging of trace gases, such as nitric acid and small oxygenated volatile organic compounds (VOCs), by snow and ice crystals in the free and upper troposphere (Abbatt, 2003); (iv) the impact of aqueous cloud and aerosol chemistry on gas-phase HOx levels (Chameides and Davis, 1982; Calvert et al., 1985; Jacob, 1986; Lelieveld and Crutzen, 1991); (v) conversion of N2O5 to HNO3 on tropospheric aerosol, with impacts on the NOx budget (Dentener and Crutzen, 1993); (vi) uptake of HO2 to aerosol (Mozurkewich et al., 1987; Martin et al., 2003); and (vii) multiphase conversion of NO2 to HONO (Finlayson-Pitts et al., 2003). A critical point is that each of these multiphase processes affects the oxidizing capacity of the troposphere, frequently through modification of radical budgets and occurring via gas–aerosol or gas–droplet interactions. For example, these processes initiate oxidation in urban atmospheres through HONO photolysis, drive Arctic boundary layer ozone and mercury depletion via gas-phase halogen chemistry, and modulate the global oxidizing capacity via N2O5 or HO2 loss on aerosol particles. Additional work in the multiphase world at this time involved a wide variety of condensed-phase photochemistry studies, for example involving the interactions of light with nitrate (Zepp et al., 1987), which can lead to the release of NOx to the gas phase, and with transition metal ion complexes (Faust and Zepp, 1993).
Two major developments profoundly influenced multiphase chemistry: the first was the recognition of the importance of aerosol particles in changing the radiative balance of the Earth system, with impacts on climate, and the second was the continued recognition of the deleterious effects of particulate matter on human, animal, and ecosystem health. These two fields, climate change and air quality, have provided the impetus (and resources) for the development of the field. As a result, at the turn of the 21st century, additional research emphasis in the multiphase chemistry community was given to assess the impacts that arise on the composition of the particles.
Once inhaled, particles harm human health (Landrigan et al., 2018; GBD 2019 Risk Factors Collaborators, 2020), with recent studies implicating the secondary component of the particles in negative health outcomes (Pye et al., 2021). Research in the past 2 decades has focused strongly on the formation of SOA (Kroll and Seinfeld, 2008; Hallquist et al., 2009; Ziemann and Atkinson, 2012; Shrivastava et al., 2017b). SOA formation has required better knowledge of the kinetics and mechanisms of the gas-phase oxidation of SOA precursors (Crounse et al., 2013; Ehn et al., 2014). It has also needed a more complete understanding of gas–particle nucleation processes (Kulmala et al., 2014; Trostl et al., 2016; Xiao et al., 2021), volatility (Pankow, 1994; Donahue et al., 2011), and condensed-phase reactions that occur within aerosol particles. Specifically, volatility and multiphase reactivity can be coupled, as illustrated by the formation of high-molecular-weight, low-volatility species within particles (Kalberer et al., 2004). While such oligomers and highly oxygenated species may also form in the gas phase (Bianchi et al., 2019), they arise via a variety of reactions involving pairs of organic reactants, frequently forming esters and acetals/hemiacetals in the condensed phase (Tobias and Ziemann, 2000; Surratt et al., 2006; DeVault and Ziemann, 2021). These reactions may be acid-catalyzed (Jang et al., 2002). Moreover, multiphase oxidation by gas-phase oxidants can increase the average oxidation state of organic aerosol particles (Kroll et al., 2011) via a series of reactions that initially functionalize and eventually fragment the component molecules (Moise and Rudich, 2000; Molina et al., 2004; George et al., 2007; Kroll et al., 2009). Oxidation leads to a more soluble particle that increases its rate of wet deposition. In addition to forming organic aerosol via gas-to-particle conversion, they are produced from the evaporation of cloud droplets. Oxidation processes occur within cloud droplets (Herrmann et al., 2015), producing more oxidized organics that yield oxygenated aerosol upon evaporation. Similar reactions, proceeding at much higher organic reactant concentrations, can also occur within the aqueous component of tropospheric aerosol (Blando and Turpin, 2000).
Tightly connected to SOA formation and modification processes are the condensed-phase viscosity and phase state, which set mixing times within particles and are dependent on relative humidity and temperature (Virtanen et al., 2010; Koop et al., 2011; Renbaum-Wolff et al., 2013). Organic particles are likely glasses in the cold free troposphere (Shiraiwa et al., 2017b), which may affect SOA formation and growth, and restrict the degree to which heterogeneous oxidation can affect the aerosol composition. The particles are liquids in warm, wet boundary layers, with the full particle volume involved in partitioning with gas-phase molecules. The large variation in molecular diffusion coefficients and associated mobility determines where chemical reactions are important in the particles, from two-dimensional processes that occur solely at the gas–particle interface to three-dimensional chemistry with reactivity at the interface and deeper in the bulk (see Fig. 2). Overall, diffusion is a key parameter for determining whether a reaction is surface-area-limited or volume-limited (Hanson et al., 1994).
Multiphase chemistry also leads to the formation of secondary inorganic aerosol. For example, the hydrolysis of N2O5 converts NOx to HNO3; the gas–particle partitioning of HNO3 is then controlled by temperature, relative humidity, and ammonia levels. Furthermore, particulate sulfate is rapidly formed in polluted environments through multiphase aqueous chemistry, acting as the major formation mechanism in cloud-free settings (Cheng et al., 2016; Wang et al., 2016). Potential routes for fast sulfate formation in deliquesced particles include the following: the role of ionic strength in accelerating the rates of specific processes (Liu et al., 2020); fast interfacial chemistry (Liu and Abbatt, 2021); formation of condensed-phase oxidants through the photolysis of particulate nitrate (Zheng et al., 2020; see also Sect. 6.5); and the role of specific particle-phase reactants, such as organic hydroperoxides (Wang et al., 2019), hydroxymethanesulfonate (Song et al., 2019), and dissolved transition metal ions (J. Li et al., 2020). An accurate quantitative assessment of these and other reaction pathways is still developing but far from complete (T. Liu et al., 2021).
As noted earlier, the need to better understand aerosol–climate interactions has also motivated multiphase chemistry research in the past 20 years. Atmospheric processing leads to the formation of water-soluble condensed-phase products, such as sulfate or highly oxygenated organic molecules (Jimenez et al., 2009), enhancing the abilities of tropospheric aerosol particles to act as cloud condensation nuclei (CCN) and affecting their ability to scatter light (Cappa et al., 2011; Moise et al., 2015). Furthermore, the optical properties of the fraction of organic aerosol that absorbs near-ultraviolet (near-UV) and visible light (i.e., atmospheric “brown carbon” particles) are subject to change via multiphase oxidation and condensed-phase photochemistry (Laskin et al., 2015; C. Li et al., 2020; Hems et al., 2021; Schnitzler et al., 2022), potentially involving transition metals (Al-Abadleh and Nizkorodov, 2021). Although the rates of optical property changes remain uncertain, primary brown carbon particles, as formed in wildfires, tend to become less absorbing in the near-UV and visible parts of the spectrum on the timescale of days via a variety of multiphase aging mechanisms (Laskin et al., 2015; Hems et al., 2021), i.e., they are “bleached”. The diminution of light absorption is in accord with field observations (Forrister et al., 2015).
Multiphase chemistry can also affect the properties of ice-nucleating particles (INPs) by both gas–solid and liquid–solid interactions, noting that INPs can act in the deposition mode, where water vapor forms ice directly on solid substrates, and in the immersion mode, where a solid particle immersed in supercooled water leads to nucleation (Kanji et al., 2017). For example, mineral dust can have its IN activity decreased by the condensation of involatile materials, such as sulfate or by cloud processing (Sullivan et al., 2010b; Kilchhofer et al., 2021), and strong acids can react with carbonate-containing minerals, leading to particles that are less IN-active in the deposition mode but more active in the immersion mode (Sullivan et al., 2010a). Such effects can also arise when different gas and liquid species are exposed to volcanic ash (Maters et al., 2020; Fahy et al., 2022). Oxidation reactions can also occur so that efficient biological INPs, such as pollen fragments, lose activity upon oxidation by OH radicals, probably by morphological changes in surface proteins and carbohydrates (Gute and Abbatt, 2018). The mechanisms involving all of these interactions are very complex. In the case of mineral dusts, immersion INP activity can be changed by surface modification, ion exchange, adsorption of solutes such as ammonium, and acid dissolution (Sihvonen et al., 2014; Kumar et al., 2019; Yun et al., 2021).
6.1 Multiphase chemistry at the interfaces of the atmosphere
There are exciting opportunities to apply the conceptual, instrumental, and modeling tools developed for multiphase chemistry to understand chemistry occurring at the interfaces of the atmosphere with other environmental domains.
Consider the interface of the atmosphere and the ocean, where the sea-surface microlayer (SML) is a thin layer of ocean water that has enhanced concentrations of biological detritus, surface-active compounds, and gel-like substances (Cunliffe et al., 2013). Recognizing that individual molecule surrogates of the SML only capture specific aspects of the chemistry, experimental designs now involve either genuine seawater or water samples with significant biological components (Prather et al., 2013; Schneider et al., 2019). While we know that the SML can affect the composition of primary marine aerosol, an open question is the degree of chemical processing by photosensitization in the SML or by gas–surface heterogeneous oxidation, yielding volatile species that contribute to marine SOA formation (Donaldson and George, 2012; Rossignol et al., 2016; Mungall et al., 2017; Croft et al., 2019).
Another key role of multiphase reactions is in dry deposition to the ocean (e.g., see Reactions R4 and R5), vegetation, the built environment, and land surfaces (Garland et al., 1980; Fowler et al., 2009; Kavassalis and Murphy, 2017; Tuite et al., 2021). Deposition is a critical step that controls removing chemicals from the atmosphere. However, this process is a parameterization in models. Deposition in many environments needs to be predictive, which demands molecular-level understanding and quantification. This process is essentially a multiphase process that should be broken down into physicochemical steps, which can be independently measured and understood.
Indoor environments, with their vast surface-area-to-volume ratios, are another example of poorly explored multiphase processes (Morrison, 2008; Abbatt and Wang, 2020; Ault et al., 2020). Contrary to the outdoor environment, where aerosol particles may remain suspended for days to weeks, the indoor air-exchange timescale is on the order of 1 or 2 h. While this lessens the potential for gas–particle chemistry, multiphase chemistry occurs over much longer timescales on fixed indoor surfaces. For example, O3 is efficiently lost via dry deposition so that its mixing ratios are considerably lower indoors than outdoors (Weschler, 2000). This produces VOCs (Wisthaler and Weschler, 2009) and modifies the composition of sorbed molecules, in some cases forming species more toxic than their precursors (Pitts et al., 1978, 1980; Zhou et al., 2017). It can also lead to the formation of gas-phase OH radicals (Zannoni et al., 2022). This source of OH from alkene ozonolysis is in addition to the generation of OH from photolysis of HONO (Gomez Alvarez et al., 2013), which is partly formed by multiphase chemistry on indoor surfaces. Indoor surfaces are a chemically complex, poorly understood environment, with input from building materials, commercial products, humans, and cooking and cleaning activities. This chemistry is important because humans obtain most of their chemical exposure indoors, not only via inhalation but also through direct dermal uptake and by ingesting dust and contaminated foodstuffs (L. Li et al., 2019). Lastly, the light environment indoors can be substantially different than outside, bringing a new twist to multiphase photochemistry (Young et al., 2019).
6.2 Multiphase chemistry and human health
Epidemiological studies have conclusively shown that aerosol particle inhalation harms human health (Pope et al., 2009; Landrigan et al., 2018). For example, it is well recognized that inflammation occurs upon particle exposure (Brook et al., 2003). The current epidemiology (empirical evidence) does not readily distinguish the specific molecules in the particles and their formation pathways that lead to negative health outcomes nor the toxicity mechanism at the molecular level. Studies are currently examining oxidative stress, e.g., the reactive oxygen species (ROS) and reactive nitrogen species (RNS), as a mechanism for negative impacts (Shiraiwa et al., 2017a). Although there is debate over whether oxidants are largely endogenous or exogenous (Fang et al., 2022), one hypothesis is that the biochemical balance between oxidants and antioxidants is upset by inhaling harmful species (Miller, 2020). To contribute to this debate, the multiphase chemistry community needs to better describe the chemistry that occurs at the lung–air interface and the composition of respirable aerosol particles, especially the biologically active components that contain reactive functional groups (e.g., epoxides and hydroperoxides), redox-active materials (e.g., quinones), and reactive oxygen species (e.g., peroxides and HO2 / O). Many of these species are formed by multiphase oxidation processes.
An associated issue is how ultrafine particles influence health. These particles have been shown to be taken directly to the bloodstream and even move to the brain (Oberdorster et al., 2004; Maher et al., 2016). Although the chemistry involved is not the multiphase chemistry discussed here, the interactions of the particle in the liquid phase (i.e., impacting biological systems) are likely important. Many of the lessons learned from studies of multiphase processes are applicable to understanding such issues.
Largely unexplored until the recent COVID-19 pandemic is the impact of the atmosphere on airborne and surface-deposited biological pathogens, including bacteria and viruses. Early work in this area included the multiphase chemistry between NO2 and proteinaceous material, motivated by its potential to drive an allergenic response (Franze et al., 2005; Shiraiwa et al., 2012). Gas-phase O3 has also been examined with respect to its ability to affect the viability of bacteriophages, i.e., microorganisms with a lipid envelope and RNA core similar to the structure of SARS-CoV-2, deposited on surfaces (Tseng and Li, 2008). With the pandemic, research has accelerated into the impact of hygroscopic growth and water content on viral viability within respiratory particle surrogates that consist of viruses embedded in saline droplets containing surfactants, proteins, and carbohydrates. It is important to understand the changes in the acidity of these particles, the mass transfer within them, and the precipitation of salts as the particle water content changes (Lin et al., 2020; Oswin et al., 2022; Huynh et al., 2022).
The recent pandemic led to an emphasis on cleaning surfaces to reduce the potential for infection by fomites, i.e., via contact with contaminated surfaces. While cleaning agents such as chlorine bleach have well-established antimicrobial activity, their multiphase chemistry can release gases and particles that are deleterious to human health (Wong et al., 2017; Mattila et al., 2020). Understanding the multiphase chemistry associated with these cleaning activities and the outcomes of using air cleaners (Collins and Farmer, 2021) is essential for establishing healthy indoor environments.
Lastly, the pandemic prompted a re-examination of an overlooked aspect of our atmosphere: that it has an as yet unidentified germicidal component referred to as the open-air factor (Cox et al., 2021). In particular, it was shown many decades ago that fresh air led to better outcomes for tuberculosis patients and injured World War I soldiers than indoor air. Historically, sending sick people to pristine environments (e.g., the seaside) was a common medical recommendation. It is crucial to determine whether these effects are in some way related to multiphase chemistry occurring between reactive species in the gas phase interacting with biological molecules at the surface of the wounds and lungs.
Each of the above topics provides exciting opportunities for atmospheric chemists to collaborate with the environmental health, medical, and toxicological communities.
6.3 Chemistry of the free troposphere and lower stratosphere
Although the upper troposphere–lower stratosphere region was the focus of much attention in the 1980s and 1990s to understand the changes in ozone levels in these regions, most multiphase chemistry studies are currently conducted at room temperature. There is considerable motivation to re-explore chemistry at colder temperatures, given past work that illustrated the atmospheric impacts of a strongly nonlinear dependence of multiphase reactions rates on temperature (Murphy and Ravishankara, 1994) and extensive new observations from the Atom campaigns (Thompson, 2022) that sampled from the boundary layer to the upper troposphere over many latitudes and seasons. Moreover, there is emerging evidence for organic aerosol in the lower stratosphere, likely arising from wildfire injection, with potential influence on stratospheric ozone (Solomon et al., 2022; Strahan et al., 2022).
Organic aerosol viscosity and phase state depend on the environmental conditions (Koop et al., 2011), with semisolid and glassy organic particles predicted throughout much of the free troposphere (Shiraiwa et al., 2017b). Aside from those at the gas–particle interface, molecules in highly viscous organic particles are protected from heterogeneous oxidation (Shiraiwa et al., 2011; Zhou et al., 2012; Shrivastava et al., 2017a). Such protection increases the lifetimes of pollutants, e.g., brown carbon chromophores (Schnitzler et al., 2022), and lengthens particles' oxidation timescale and wet deposition lifetime.
In addition to continuing to address the fundamentals of cloud chemistry oxidation processes, the associated chemistry of transition metals, and the production of oxidants within cloud water and via uptake from the gas phase (Herrmann et al., 2015), there is a particular need to also study such processes at cold temperatures, including under supercooled water conditions. When supercooled water is frozen, solutes are excluded from the ice crystals and become highly concentrated at grain boundaries and in liquid and liquid-like layers at the surface, potentially leading to enhanced rates of aqueous-phase chemistry. Moreover, the Reynolds–Workman potential (Workman and Reynolds, 1950), arising at the ice–water interface, can drive chemistry.
A key factor affected by temperature is the solubility of various atmospheric constituents. Simple Henry's law constants and further equilibration steps that determine the overall solubilities are poorly known, especially below room temperature. Most of the published data on the solubilities in organics go back to chemical engineering literature that is more than half a century old. Furthermore, as solubilities vary according to Henry's law equilibria that vary exponentially with temperature, the accurate temperature dependence of solubilities is essential. Acid dissociation constants in organic acids and organic substrates are poorly known, and they determine the overall solubility of a chemical.
6.4 Reactive transformations of organic chemical contaminants
Over 40 years ago, it was recognized that multiphase oxidation of chemical contaminants leads to the rapid loss of surface-bound polycyclic aromatic hydrocarbons (PAHs) and the formation of more toxic and potentially carcinogenic products such as nitro-PAHs and oxygenated PAHs (Pitts et al., 1978, 1980). These reactions occur on a variety of surfaces with light, ozone, and NO2 reactants, some via Langmuir–Hinshelwood mechanisms (Pöschl et al., 2001; Mmereki and Donaldson, 2003; Kwamena et al., 2004). Buried PAHs are protected from heterogeneous loss by a crust of unreactive products that accumulate upon them and, when present, within viscous organic aerosol (S. Zhou et al., 2013, 2019), enabling the potential for long-range atmospheric transport (Mu et al., 2018). The chemistry of other organic contaminants, including smoking products such as nicotine (Destaillats et al., 2006) and tetrahydrocannabinol (Yeh et al., 2022), a few pesticides (Segal-Rosenheimer and Dubowski, 2007; Finlayson-Pitts et al., 2023), and organophosphate esters (Q. Liu et al., 2021), has also recently been explored.
However, these are largely exceptions, and the multiphase fate of most chemical contaminants, especially thousands of commercial products, has not been examined. Indeed, the atmospheric chemistry and chemical contaminant communities have traditionally not strongly interacted. Although assessment of the gas-phase OH reactivity is customarily performed in environmental fate analyses (L. Li et al., 2019), many commercial products have sufficiently low volatility such that they reside primarily on surfaces or within particles. It is important to establish whether organic contaminants traditionally viewed as persistent are indeed unreactive with respect to multiphase transformation.
6.5 Understanding the role of light
Many condensed-phase photochemical reactions proceed via indirect mechanisms where a photosensitizing molecule absorbs light, forming reactive species such as HO2 / O or 1O2 (George et al., 2015). Such chemistry, first identified for natural waters (Canonica et al., 1995), has been implicated in the daytime formation of HONO (George et al., 2005), the photoreactions of brown carbon aerosol (Laskin et al., 2015; Hems et al., 2021), the formation of active halogens (Reeser et al., 2009), and the reactivity of black carbon (Monge et al., 2010; M. Li et al., 2019). This chemistry has been illustrated using efficient photosensitizing agents, but quantitative assessments of atmospheric importance remain uncertain, largely because the character and quantity of atmospheric photosensitizers are not well established. Developing a tighter quantitative connection to the atmosphere will require using more representative photosensitizers, as is now being done using marine aerosol components (Ciuraru et al., 2015; Garcia et al., 2021). The wavelengths of interest for the troposphere are in the near-UV and visible part of the solar flux.
In addition to indirect sensitization, light can also lead to direct photochemistry. An important finding was that photolysis on ice and snow surfaces was demonstrated to form NOx in the midlatitude and polar regions (Honrath et al., 1999; Wolff et al., 2002; Domine and Shepson, 2002). This process, which likely proceeds in a wide range of environments, is now referred to as “re-noxification”, as it releases NOx from HNO3 that has deposited from the atmosphere. Other condensed-phase chemical processes of importance include the formation of oxidants from nitrate and nitrite photolysis (Zepp et al., 1987), photolysis of condensed-phase organic hydroperoxides and other highly oxygenated organics, and the photochemical activity of many transition metal ion complexes (Faust and Zepp, 1993; Weller et al., 2013). It is important to recognize that the absorption spectra and product quantum yields of dissolved species can be different than those in the gas phase (George et al., 2015), with aqueous nitrate being a prime example (Benedict et al., 2017). The variable viscosity of organic aerosol matrices can affect photolysis rates, products, and their temperature dependence (Lignell et al., 2014).
6.6 Developments in field observational capabilities
Our ability to characterize atmospheric composition continues to push the field of atmospheric chemistry forward. For multiphase chemistry, advances in analytical mass spectrometry have been transformative. Within the last 20 years, online characterization of aerosol composition has become commonplace (Canagaratna et al., 2007), studies of single-particle composition allow us to observe the variability in mixing state and chemical diversity (Zelenyuk and Imre, 2005; Murphy et al., 2006; Prather et al., 2008), and offline filter sampling has progressed from the characterization of a few targeted species to non-targeted analyses using a range of mass spectral ionization methods (Papazian et al., 2022; Ditto et al., 2022). Identifying specific molecular “markers” for organics and functional groups is still somewhat uncertain; developing such identification would be very helpful.
The continued development of analytical techniques will enable increasingly sophisticated characterization of aerosol particles and environmental surfaces, with the opportunity to deploy the same tools in both laboratory and field settings. However, challenges are arising as well. Despite the rapid development of low-cost sensors, affordable instrumentation for the long-term characterization of aerosol composition in many locations is still lacking. The increasing sophistication of analytical instrumentation also continues to unveil the high degree of chemical complexity present. Whereas high-resolution mass spectrometry yields chemical formulae in real time, there is often the need to identify chemical structures. This suggests that we should increasingly deploy separation techniques (e.g., chromatography and ion mobility) as front ends to our increasingly sophisticated mass spectrometric techniques (Krechmer et al., 2016; Claflin et al., 2021). There is also value to the expanded use of other classical chemical speciation methods, such as infrared (Russell, 2003) and nuclear magnetic resonance (Decesari et al., 2007) characterization of aerosol composition collected by filters. While these techniques have a low time resolution, they provide complementary quantitative and functional group information and can be inexpensively deployed for long-term analyses in a wide range of environments. Such analyses will also help with the source apportionment of aerosols.
Aerosol characteristics related to multiphase chemistry can be studied with increasingly sophisticated remote-sensing techniques. These approaches have been applied for many years to polar stratospheric clouds, whose composition and phase (via the degree of depolarization of a lidar probe) have been studied (Tritscher et al., 2021). Another example comes from satellite measurements of solid ammonium nitrate particles in the upper troposphere, driven by the Asian monsoon that uplifts ammonia-rich continental air (Hopfner et al., 2019). It is important to determine the role of these particles in ice nucleation and multiphase chemistry.
6.7 Developments in laboratory and molecular modeling techniques
In addition to our ability to conduct field observations, a revolution in laboratory analytical methods has occurred. This is most widely apparent with respect to applying sophisticated mass spectrometric techniques, increasingly involving a high mass resolution and a range of ionization schemes (Laskin et al., 2013). When coupled with other analytical methods, we can now determine the physicochemical properties of individual molecules and their mixtures in extreme detail. For example, this approach has been taken to characterize the optical properties of brown carbon aerosol materials (Fleming et al., 2020), the viscosity of organic aerosol (DeRieux et al., 2018), and the structural isomers of complex organic mixtures (Krechmer et al., 2016). There are significant opportunities for additional adoption of techniques from neighboring disciplines. Furthermore, the use of a number of these analytical techniques in both the laboratory and the field will enhance our ability to connect the laboratory to the field.
Molecular-level chemical models increasingly provide valuable insights into complex multiphase processes. For example, important insights into the nature of the chemistry occurring on polar stratospheric cloud materials were obtained from molecular dynamics modeling (Wang and Clary, 1996; Bianco and Hynes, 2006), and more recent studies have addressed gas–surface interactions and the roles of solvent molecules in small molecular clusters (Gerber et al., 2015; Fang et al., 2019; Yang et al., 2019). Whereas past computational methods only included a few solvent molecules, current dynamics models using state-of-the-art force fields can realistically simulate partitioning, surface adsorption constants, diffusion constants, and vapor pressures, representing an important point of contact to the physical chemistry and chemical physics communities (Tobias et al., 2013). We also note that machine learning techniques have very recently been applied to molecular dynamics simulations, for example, to describe the interactions of N2O5 with liquid water (Galib and Limmer, 2021) and the dissociation of strong acids at aqueous interfaces (de la Puente et al., 2022). To establish fundamental parameters that are experimentally challenging to measure, such as the likelihood that the collision of a molecule with a particle leads to uptake by the condensed phase (i.e., a mass accommodation coefficient), theoretical methods may be preferable to experiments in some situations.
6.8 Grappling with chemical complexity
Atmospheric aerosol particles and surfaces are morphologically and compositionally complex. This complexity can be enticing from a fundamental chemistry perspective, as we disentangle mass transfer, phase separation, and reactivity. However, it can impede the development of an accurate, quantitative description required to inform an atmospheric model. It can also be constraining if we study the detailed chemistry and lose sight of its overall impact on climate, air quality, or ecosystem health.
With enough care, the rate constant for a gas-phase, radical–molecule reaction can be measured with 10 %–20 % accuracy (Cox, 2012). Atmospheric modelers rely upon this confidence level as they assess their predictions. It is humbling to consider the accuracy of the available multiphase kinetics data for the modeling community. Take, for example, the reaction of N2O5 with tropospheric aerosol, which has been long known to impact the NOx fraction of NOy and active chlorine levels, with a secondary influence on OH, O3, and CH4 (Dentener and Crutzen, 1993). Although studies started in the 1980s, new mechanistic insights into N2O5 heterogeneous reactivity are still arising (Sobyra et al., 2019; Karimova et al., 2020). Laboratory reactive uptake coefficients for the hydrolysis of N2O5 vary over 1–2 orders of magnitude, with larger values reported for aqueous particles composed of sulfate or soluble organics and lower values for particles with less soluble organics and nitrate (Burkholder et al., 2020). Likewise, uptake coefficients inferred from field measurements or with genuine ambient particles vary by roughly 1 order of magnitude compared with those measured with laboratory surrogates (Brown et al., 2006; Bertram et al., 2009; Abbatt et al., 2012; Phillips et al., 2016; Tham et al., 2018). The discrepancies between field and laboratory studies are undoubtedly due to complex and variable particle composition and phase state. Simply put, unlike the case with gas-phase reactions, one of the “reactants” in this gas–particle reaction is highly variable. This complexity is exacerbated by the changes in the composition (including acidity), mixing state, and water content of the particle as it resides in the atmosphere. Added complexity arises from the differences in composition that occur in the bulk of particles and droplets compared with their interfacial composition (Wingen and Finlayson-Pitts, 2019).
Likewise, HOx loss on tropospheric aerosol may significantly impact ozone in high-NOx atmospheric regimes, as in East Asia. As particulate levels drop in such regions, HOx abundance and ozone mixing ratios will both rise (Martin et al., 2003; K. Li et al., 2019; Ivatt et al., 2022). However, reported HO2 uptake coefficients vary widely, from research group to research group and from the laboratory to the field (Burkholder et al., 2020), making modeling predictions highly uncertain.
Both bottom-up and top-down approaches can address chemical complexity. In the traditional bottom-up approach, the effects on the reaction system of step-by-step additions of chemical complexity are evaluated. This leads to a better understanding of the fundamental chemistry needed to develop our predictive abilities. Top-down approaches involve studying chemistry on ambient aerosol particles. This has been done for N2O5 and HO2 aerosol uptake (Bertram et al., 2009; Zhou et al., 2021), for heterogeneous OH oxidation (George et al., 2008), and to characterize SOA formation by using mobile reaction chambers (Jorga et al., 2021). Another top-down method constrains the rates of multiphase chemistry using detailed, simultaneous measurements of gas-phase composition under a range of environmental conditions (Brown et al., 2006). Combining top-down and bottom-up approaches enhances our understanding of the fundamental science while ensuring that parameterizations for atmospheric modeling are accurate.
Models working over a wide range of spatial and temporal scales can help address issues in chemical complexity. As mentioned in the previous section, molecular dynamics calculations are becoming increasingly sophisticated. Likewise, multiphase kinetics models that can incorporate insights gained at the molecular level into modeling frameworks that aim to couple the gas phase and condensed phases, including bulk reaction kinetics, mass transfer and interfacial processes, are also increasing in complexity (Pöschl et al., 2007; Tilgner et al., 2013; Woo and McNeill, 2015). A challenge is to couple bulk and interfacial processes correctly. As computing capabilities grow, the complexity of the multiphase and detailed molecular mechanism models that can be incorporated into chemical transport models will also increase. Moreover, Lagrangian-type models can increasingly model specific field observations (Zaveri et al., 2010). We note that a successful hierarchical approach has arisen in the indoor chemistry community where modeling groups using a wide range of tools, from molecular dynamics to large-scale computational fluid dynamics, interact closely with each other and with experimental scientists (Shiraiwa et al., 2019).
Multiphase chemistry has evolved alongside the wider field of atmospheric chemistry. While initial studies focused on its impacts on the gas phase, the field now addresses how chemistry affects particles. Although modification of aerosol composition has direct relevance to climate and human health, we should not lose sight of the connection of multiphase chemistry to the gas-phase composition of the atmosphere.
We need to understand chemical processes at the molecular level to improve our ability to interpret field observations and predict the nature of a changing atmosphere. Reinforcing an approach based on physicochemical understanding is necessary for detailed predictions of environmental change.
There are significant research opportunities for the characterization of the chemistry that occurs at the interface of the atmosphere with the rest of the environment, such as studies of ocean–atmosphere interactions, indoor air, aerosol health effects, atmosphere–cryosphere chemistry from the stratosphere all the way to the snowpack, and pathogen–air interactions.
With increasingly sophisticated experimental and theoretical tools, atmospheric chemical complexity becomes more apparent. While exciting, this presents challenges and constraints. We should emphasize not only highly detailed, molecular-level measurements but also more widespread and more prolonged aerosol characterization that has less chemical specificity but, nevertheless, provides valuable insights; there is also a role for both remote-sensing measurements and classical analytical techniques in this regard. This is akin to a need to understand and quantify thermal gas-phase reactions while also understanding and quantifying microcanonical reactivity.
Measurements of many fundamental physicochemical parameters such as solubility, diffusion coefficients, and liquid- or solid-phase reactivities are sorely needed.
Multiphase chemistry studies conducted under conditions that match those in the atmosphere, including those of the free troposphere and lower stratosphere, are needed.
Using the atmosphere as a laboratory to quantify rates of multiphase processes holds promise, with simultaneous measurements of many chemicals and other external parameters becoming more feasible through coordinated field measurements. Designing field studies with an eye toward quantification of the multiphase reactions is beneficial.
The field of atmospheric chemistry is healthiest when there is extensive communication and feedback between the fundamental chemistry, modeling, and field observation communities (see Fig. 1). To keep the three-legged stool balanced and strong, multiphase chemists should interact widely with not only other atmospheric scientists but also scientists in related fields such as meteorology, climate dynamics, ecology, and human health. This can be accomplished by participation in conferences and seminars that involve science from different legs of the stool; students exploring short-term training opportunities in diverse research groups; and collaborative grants that bring together laboratory and theory, modeling, and field measurement scientists.
No data sets were used in this article.
The two authors formulated the main concepts presented in this paper, and both contributed to writing and editing it.
The contact author has declared that neither of the authors has any competing interests.
Publisher's note: Copernicus Publications remains neutral with regard to jurisdictional claims in published maps and institutional affiliations.
This article is part of the special issue “20 years of Atmospheric Chemistry and Physics”. It is not associated with a conference.
The authors are grateful to Hind Al-Abadleh, Len Barrie, and Will Fahy for their comments on the manuscript and to Zilin Zhou for crafting the figures.
This paper was edited by Barbara Ervens and James Allan and reviewed by Hartmut Herrmann and two anonymous referees.
Abbatt, J. and Wang, C.: The atmospheric chemistry of indoor environments, Environ. Sci. Proc. Imp., 22, 25–48, https://doi.org/10.1039/c9em00386j, 2020.
Abbatt, J., George, C., Melamed, M., Monks, P., Pandis, S., and Rudich, Y.: New Directions: Fundamentals of atmospheric chemistry: Keeping a three-legged stool balanced, Atmos. Environ., 84, 390–391, https://doi.org/10.1016/j.atmosenv.2013.10.025, 2014.
Abbatt, J. P. D.: Interactions of atmospheric trace gases with ice surfaces: Adsorption and reaction, Chem. Rev., 103, 4783–4800, https://doi.org/10.1021/cr0206418, 2003.
Abbatt, J. P. D., Lee, A. K. Y., and Thornton, J. A.: Quantifying trace gas uptake to tropospheric aerosol: recent advances and remaining challenges, Chem. Soc. Rev., 41, 6555–6581, https://doi.org/10.1039/c2cs35052a, 2012.
Al-Abadleh, H. and Nizkorodov, S.: Open questions on transition metals driving secondary thermal processes in atmospheric aerosols, Commun. Chem., 4, 176, https://doi.org/10.1038/s42004-021-00616-w, 2021.
Ault, A., Grassian, V., Carslaw, N., Collins, D., Destaillats, H., Donaldson, D., Farmer, D., Jimenez, J., McNeill, V., Morrison, G., O'Brien, R., Shiraiwa, M., Vance, M., Wells, J., and Xiong, W.: Indoor Surface Chemistry: Developing a Molecular Picture of Reactions on Indoor Interfaces, Chem, 6, 3203–3218, https://doi.org/10.1016/j.chempr.2020.08.023, 2020.
Benedict, K., McFall, A., and Anastasio, C.: Quantum Yield of Nitrite from the Photolysis of Aqueous Nitrate above 300 nm, Environ. Sci. Technol., 51, 4387–4395, https://doi.org/10.1021/acs.est.6b06370, 2017.
Bertram, T. H., Thornton, J. A., Riedel, T. P., Middlebrook, A. M., Bahreini, R., Bates, T. S., Quinn, P. K., and Coffman, D. J.: Direct observations of N2O5 reactivity on ambient aerosol particles, Geophys. Res. Lett., 36, L19803, https://doi.org/10.1029/2009gl040248, 2009.
Bianchi, F., Kurten, T., Riva, M., Mohr, C., Rissanen, M., Roldin, P., Berndt, T., Crounse, J., Wennberg, P., Mentel, T., Wildt, J., Junninen, H., Jokinen, T., Kulmala, M., Worsnop, D., Thornton, J., Donahue, N., Kjaergaard, H., and Ehn, M.: Highly Oxygenated Organic Molecules (HOM) from Gas-Phase Autoxidation Involving Peroxy Radicals: A Key Contributor to Atmospheric Aerosol, Chem. Rev., 119, 3472–3509, https://doi.org/10.1021/acs.chemrev.8b00395, 2019.
Bianco, R. and Hynes, J.: A theoretical study of the reaction of ClONO2 with HCl on ice, J. Phys. Chem. A, 103, 3797–3801, https://doi.org/10.1021/jp990471b, 1999.
Bianco, R. and Hynes, J. T.: Heterogeneous reactions important in atmospheric ozone depletion: A theoretical perspective, Acc. Chem. Res., 39, 159–165, https://doi.org/10.1021/ar040197q, 2006.
Blando, J. D. and Turpin, B. J.: Secondary organic aerosol formation in cloud and fog droplets: a literature evaluation of plausibility, Atmos. Environ., 34, 1623–1632, https://doi.org/10.1016/S1352-2310(99)00392-1, 2000.
Brook, R., Brook, J., and Rajagopalan, S.: Air pollution: The “heart” of the problem, Curr. Hypertens. Rep., 5, 32–39, https://doi.org/10.1007/s11906-003-0008-y, 2003.
Brown, S. S., Ryerson, T. B., Wollny, A. G., Brock, C. A., Peltier, R., Sullivan, A. P., Weber, R. J., Dube, W. P., Trainer, M., Meagher, J. F., Fehsenfeld, F. C., and Ravishankara, A. R.: Variability in nocturnal nitrogen oxide processing and its role in regional air quality, Science, 311, 67–70, https://doi.org/10.1126/science.1120120, 2006.
Burkholder, J. B., Abbatt, J. P. D., Barnes, I., Roberts, J. M., Melamed, M. L., Ammann, M., Bertram, A. K., Cappa, C. D., Carlton, A. G., Carpenter, L. J., Crowley, J. N., Dubowski, Y., George, C., Heard, D. E., Herrmann, H., Keutsch, F. N., Kroll, J. H., McNeill, V. F., Ng, N. L., Nizkorodov, S. A., Orlando, J. J., Percival, C. J., Picquet-Varrault, B., Rudich, Y., Seakins, P. W., Surratt, J. D., Tanimoto, H., Thornton, J. A., Tong, Z., Tyndall, G. S., Wahner, A., Weschler, C. J., Wilson, K. R., and Ziemann, P. J.: The Essential Role for Laboratory Studies in Atmospheric Chemistry, Environ. Sci. Technol., 51, 2519–2528, https://doi.org/10.1021/acs.est.6b04947, 2017.
Burkholder, J. B., Abbatt, J. P. D., Cappa, C. D., Dibble, T. S., Kolb, C. E., Orkin, V. L., Wilmouth, D. M., Sander, S. P., Barker, J. R., Crounse, J. D., Huie, R. E., Kurylo, M. J., Percival, C. J., and Wine, P. H.: Chemical kinetics and photochemical data for use in atmospheric studies, NASA – JPL, Pasadena, CA, Evaluation No. 19, JPL Publication 19-5, https://jpldataeval.jpl.nasa.gov/ (last access: 29 August 2023), 2020.
Calvert, J., Lazrus, A., Kok, G., Heikes, B., Walega, J., Lind, J., and Cantrell, C.: Chemical mechanisms of acid generation in the troposphere, Nature, 317, 27–35, https://doi.org/10.1038/317027a0, 1985.
Canagaratna, M. R., Jayne, J. T., Jimenez, J. L., Allan, J. D., Alfarra, M. R., Zhang, Q., Onasch, T. B., Drewnick, F., Coe, H., Middlebrook, A., Delia, A., Williams, L. R., Trimborn, A. M., Northway, M. J., DeCarlo, P. F., Kolb, C. E., Davidovits, P., and Worsnop, D. R.: Chemical and microphysical characterization of ambient aerosols with the Aerodyne aerosol mass spectrometer, Mass Spectrom. Rev., 26, 185–222, 2007.
Canonica, S., Jans, U., Stemmler, K., and Hoigne, J.: Transformation kinetics of phenols in water – photosensitization by dissolved natural organic matter and aromatic ketones, Environ. Sci. Technol., 29, 1822–1831, https://doi.org/10.1021/es00007a020, 1995.
Cappa, C. D., Che, D. L., Kessler, S. H., Kroll, J. H., and Wilson, K. R.: Variations in organic aerosol optical and hygroscopic properties upon heterogeneous OH oxidation, J. Geophys. Res.-Atmos., 116, D15204, https://doi.org/10.1029/2011jd015918, 2011.
Carpenter, L., MacDonald, S., Shaw, M., Kumar, R., Saunders, R., Parthipan, R., Wilson, J., and Plane, J.: Atmospheric iodine levels influenced by sea surface emissions of inorganic iodine, Nat. Geosci., 6, 108–111, https://doi.org/10.1038/NGEO1687, 2013.
Chameides, W. and Davis, D.: The free-radical chemistry of cloud droplets and its impact upon the composition of rain, J. Geophys. Res., 87, 4863–4877, https://doi.org/10.1029/JC087iC07p04863, 1982.
Cheng, Y., Zheng, G., Wei, C., Mu, Q., Zheng, B., Wang, Z., Gao, M., Zhang, Q., He, K., Carmichael, G., Poschl, U., and Su, H.: Reactive nitrogen chemistry in aerosol water as a source of sulfate during haze events in China, Sci. Adv., 2, e1601530, https://doi.org/10.1126/sciadv.1601530, 2016.
Choularton, T., Colvile, R., Bower, K., Gallagher, M., Wells, M., Beswick, K., Arends, B., Mols, J., Kos, G., Fuzzi, S., Lind, J., Orsi, G., Facchini, M., Laj, P., Gieray, R., Wieser, P., Engelhardt, T., Berner, A., Kruisz, C., Moller, D., Acker, K., Wieprecht, W., Luttke, J., Levsen, K., Bizjak, M., Hansson, H., Cederfelt, S., Frank, G., Mentes, B., Martinsson, B., Orsini, D., Svenningsson, B., Swietlicki, E., Wiedensohler, A., Noone, K., Pahl, S., Winkler, P., Seyffer, E., Helas, G., Jaeschke, W., Georgii, H., Wobrock, W., Preiss, M., Maser, R., Schell, D., Dollard, G., Jones, B., Davies, T., Sedlak, D., David, M., Wendisch, M., Cape, J., Hargreaves, K., Sutton, M., StoretonWest, R., Fowler, D., Hallberg, A., Harrison, R., and Peak, J.: The Great Dun Fell Cloud Experiment 1993: An overview, Atmos. Environ., 31, 2393–2405, https://doi.org/10.1016/S1352-2310(96)00316-0, 1997.
Ciuraru, R., Fine, L., van Pinxteren, M., D'Anna, B., Herrmann, H., and George, C.: Photosensitized production of functionalized and unsaturated organic compounds at the air-sea interface, Sci. Rep., 5, 12741, https://doi.org/10.1038/srep12741, 2015.
Claflin, M., Pagonis, D., Finewax, Z., Handschy, A., Day, D., Brown, W., Jayne, J., Worsnop, D., Jimenez, J., Ziemann, P., de Gouw, J., and Lerner, B.: An in situ gas chromatograph with automatic detector switching between PTR- and EI-TOF-MS: isomer-resolved measurements of indoor air, Atmos. Meas. Tech., 14, 133–152, https://doi.org/10.5194/amt-14-133-2021, 2021.
Clegg, S. M. and Abbatt, J. P. D.: Oxidation of SO2 by H2O2 on ice surfaces at 228 K: a sink for SO2 in ice clouds, Atmos. Chem. Phys., 1, 73–78, https://doi.org/10.5194/acp-1-73-2001, 2001.
Collins, D. and Farmer, D.: Unintended Consequences of Air Cleaning Chemistry, Environ. Sci. Technol., 55, 12172–12179, https://doi.org/10.1021/acs.est.1c02582, 2021.
Cox, R., Ammann, M., Crowley, J., Griffiths, P., Herrmann, H., Hoffmann, E., Jenkin, M., McNeill, V., Mellouki, A., Penkett, C., Tilgner, A., and Wallington, T.: Opinion: The germicidal effect of ambient air (open-air factor) revisited, Atmos. Chem. Phys., 21, 13011–13018, https://doi.org/10.5194/acp-21-13011-2021, 2021.
Cox, R. A.: Evaluation of laboratory kinetics and photochemical data for atmospheric chemistry applications, Chem. Soc. Rev., 41, 6231–6246, https://doi.org/10.1039/c2cs35092k, 2012.
Croft, B., Martin, R., Leaitch, W., Burkart, J., Chang, R., Collins, D., Hayes, P., Hodshire, A., Huang, L., Kodros, J., Moravek, A., Mungall, E., Murphy, J., Sharma, S., Tremblay, S., Wentworth, G., Willis, M., Abbate, J., and Pierce, J.: Arctic marine secondary organic aerosol contributes significantly to summertime particle size distributions in the Canadian Arctic Archipelago, Atmos. Chem. Phys., 19, 2787–2812, https://doi.org/10.5194/acp-19-2787-2019, 2019.
Crounse, J. D., Nielsen, L. B., Jorgensen, S., Kjaergaard, H. G., and Wennberg, P. O.: Autoxidation of Organic Compounds in the Atmosphere, J. Phys. Chem. Lett., 4, 3513–3520, https://doi.org/10.1021/jz4019207, 2013.
Cunliffe, M., Engel, A., Frka, S., Gašparović, B., Guitart, C., Murrell, J. C., Salter, M., Stolle, C., Upstill-Goddard, R., and Wurl, O.: Sea surface microlayers: A unified physicochemical and biological perspective of the air–ocean interface, Prog. Oceanogr., 109, 104–116, https://doi.org/10.1016/j.pocean.2012.08.004, 2013.
Dankwerts, P. V.: Gas-Liquid Reactions, McGraw-Hill, ISBN 978-0070152878, 1970.
Decesari, S., Mircea, M., Cavalli, F., Fuzzi, S., Moretti, F., Tagliavini, E., and Facchini, M.: Source attribution of water-soluble organic aerosol by nuclear magnetic resonance spectroscopy, Environ. Sci. Technol., 41, 2479–2484, https://doi.org/10.1021/es061711l, 2007.
de la Puente, M., David, R., Gomez, A., and Laage, D.: Acids at the Edge: Why Nitric and Formic Acid Dissociations at Air-Water Interfaces Depend on Depth and on Interface Specific Area, J. Am. Chem. Soc., 144, 10524–10529, https://doi.org/10.1021/jacs.2c03099, 2022.
Dentener, F. J. and Crutzen, P. J.: Reaction of N2O5 on tropospheric aerosols – Impact on the global distributions of NOx, O3, and OH, J. Geophys. Res.-Atmos., 98, 7149–7163, https://doi.org/10.1029/92jd02979, 1993.
DeRieux, W.-S., Li, Y., Lin, P., Laskin, J., Laskin, A., Bertram, A. K., Nizkorodov, S. A., and Shiraiwa, M.: Predicting the glass transition temperature and viscosity of secondary organic material using molecular composition, Atmos. Chem. Phys., 18, 6331–6351, https://doi.org/10.5194/acp-18-6331-2018, 2018.
Destaillats, H., Singer, B. C., Lee, S. K., and Gundel, L. A.: Effect of ozone on nicotine desorption from model surfaces: Evidence for heterogeneous chemistry, Environ. Sci. Technol., 40, 1799–1805, https://doi.org/10.1021/es050914r, 2006.
DeVault, M. and Ziemann, P.: Gas- and Particle-Phase Products and Their Mechanisms of Formation from the Reaction of Delta-3-Carene with NO3 Radicals, J. Phys. Chem. A, 125, 10207–10222, https://doi.org/10.1021/acs.jpca.1c07763, 2021.
Ditto, J., Machesky, J., and Gentner, D.: Analysis of reduced and oxidized nitrogen-containing organic compounds at a coastal site in summer and winter, Atmos. Chem. Phys., 22, 3045–3065, https://doi.org/10.5194/acp-22-3045-2022, 2022.
Domine, F. and Shepson, P. B.: Air-snow interactions and atmospheric chemistry, Science, 297, 1506–1510, 2002.
Donahue, N. M., Epstein, S. A., Pandis, S. N., and Robinson, A. L.: A two-dimensional volatility basis set: 1. organic-aerosol mixing thermodynamics, Atmos. Chem. Phys., 11, 3303–3318, https://doi.org/10.5194/acp-11-3303-2011, 2011.
Donaldson, D. and George, C.: Sea-Surface Chemistry and Its Impact on the Marine Boundary Layer, Environ. Sci. Technol., 46, 10385–10389, https://doi.org/10.1021/es301651m, 2012.
Ehn, M., Thornton, J. A., Kleist, E., Sipila, M., Junninen, H., Pullinen, I., Springer, M., Rubach, F., Tillmann, R., Lee, B., Lopez-Hilfiker, F., Andres, S., Acir, I. H., Rissanen, M., Jokinen, T., Schobesberger, S., Kangasluoma, J., Kontkanen, J., Nieminen, T., Kurten, T., Nielsen, L. B., Jorgensen, S., Kjaergaard, H. G., Canagaratna, M., Dal Maso, M., Berndt, T., Petaja, T., Wahner, A., Kerminen, V. M., Kulmala, M., Worsnop, D. R., Wildt, J., and Mentel, T. F.: A large source of low-volatility secondary organic aerosol, Nature, 506, 476–480, https://doi.org/10.1038/nature13032, 2014.
Erickson, R., Yates, L., Clark, R., and McEwen, D.: Reaction of sulfur dioxde with ozone in water and its possible atmospheric significance, Atmos. Environ., 11, 813–817, https://doi.org/10.1016/0004-6981(77)90043-9, 1977.
Fahy, W., Maters, E., Miranda, R., Adams, M., Jahn, L., Sullivan, R., and Murray, B.: Volcanic ash ice nucleation activity is variably reduced by aging in water and sulfuric acid: the effects of leaching, dissolution, and precipitation, Environ. Sci.-Atmos., 2, 85–99, https://doi.org/10.1039/d1ea00071c, 2022.
Fang, T., Huang, Y., Wei, J., Mena, J., Lakey, P., Kleinman, M., Digman, M., and Shiraiwa, M.: Superoxide Release by Macrophages through NADPH Oxidase Activation Dominating Chemistry by Isoprene Secondary Organic Aerosols and Quinones to Cause Oxidative Damage on Membranes, Environ. Sci. Technol., 56, 17029–17038, https://doi.org/10.1021/acs.est.2c03987, 2022.
Fang, Y., Lakey, P. S. J., Riahi, S., McDonald, A. T., Shrestha, M., Tobias, D. J., Shiraiwa, M., and Grassian, V. H.: A molecular picture of surface interactions of organic compounds on prevalent indoor surfaces: limonene adsorption on SiO2, Chem. Sci., 10, 2906–2914, https://doi.org/10.1039/c8sc05560b, 2019.
Faust, B. and Zepp, R.: Photochemistry of aqueous iron(III) polycarboxylate complexes – roles in the chemistry of atmospheric and surface waters, Environ. Sci. Technol., 27, 2517–2522, https://doi.org/10.1021/es00048a032, 1993.
Finlayson-Pitts, B., Wingen, L., Sumner, A., Syomin, D., and Ramazan, K.: The heterogeneous hydrolysis of NO2 in laboratory systems and in outdoor and indoor atmospheres: An integrated mechanism, Phys. Chem. Chem. Phys., 5, 223–242, https://doi.org/10.1039/b208564j, 2003.
Finlayson-Pitts, B., Anderson, A., Lakey, P., Wang, W., Ezell, M., Wang, X., Wingen, L., Perraud, V., and Shiraiwa, M.: Oxidation of solid thin films of neonicotinoid pesticides by gas phase hydroxyl radicals, Environ. Sci.-Atmos., 3, 124–142, https://doi.org/10.1039/d2ea00134a, 2023.
Finlayson-Pitts, B. J.: The tropospheric chemistry of sea salt: A molecular-level view of the chemistry of NaCl and NaBr, Chem. Rev., 103, 4801–4822, 2003.
Fleming, L. T., Lin, P., Roberts, J. M., Selimovic, V., Yokelson, R., Laskin, J., Laskin, A., and Nizkorodov, S. A.: Molecular composition and photochemical lifetimes of brown carbon chromophores in biomass burning organic aerosol, Atmos. Chem. Phys., 20, 1105–1129, https://doi.org/10.5194/acp-20-1105-2020, 2020.
Forrister, H., Liu, J., Scheuer, E., Dibb, J., Ziemba, L., Thornhill, K. L., Anderson, B., Diskin, G., Perring, A. E., Schwarz, J. P., Campuzano-Jost, P., Day, D. A., Palm, B. B., Jimenez, J. L., Nenes, A., and Weber, R. J.: Evolution of brown carbon in wildfire plumes, Geophys. Res. Lett., 42, 4623–4630, https://doi.org/10.1002/2015gl063897, 2015.
Fowler, D., Pilegaard, K., Sutton, M., Ambus, P., Raivonen, M., Duyzer, J., Simpson, D., Fagerli, H., Fuzzi, S., Schjoerring, J., Granier, C., Neftel, A., Isaksen, I., Laj, P., Maione, M., Monks, P., Burkhardt, J., Daemmgen, U., Neirynck, J., Personne, E., Wichink-Kruit, R., Butterbach-Bahl, K., Flechard, C., Tuovinen, J., Coyle, M., Gerosa, G., Loubet, B., Altimir, N., Gruenhage, L., Ammann, C., Cieslik, S., Paoletti, E., Mikkelsen, T., Ro-Poulsen, H., Cellier, P., Cape, J., Horvath, L., Loreto, F., Niinemets, U., Palmer, P., Rinne, J., Misztal, P., Nemitz, E., Nilsson, D., Pryor, S., Gallagher, M., Vesala, T., Skiba, U., Brueggemann, N., Zechmeister-Boltenstern, S., Williams, J., O'Dowd, C., Facchini, M., de Leeuw, G., Flossman, A., Chaumerliac, N., and Erisman, J.: Atmospheric composition change: Ecosystems-Atmosphere interactions, Atmos. Environ., 43, 5193–5267, https://doi.org/10.1016/j.atmosenv.2009.07.068, 2009.
Franze, T., Weller, M., Niessner, R., and Poschl, U.: Protein nitration by polluted air, Environ. Sci. Technol., 39, 1673–1678, https://doi.org/10.1021/es0488737, 2005.
Galib, M. and Limmer, D.: Reactive uptake of N2O5 by atmospheric aerosol is dominated by interfacial processes, Science, 371, 921–924, https://doi.org/10.1126/science.abd7716, 2021.
Garcia, S., Pandit, S., Navea, J., and Grassian, V.: Nitrous Acid (HONO) Formation from the Irradiation of Aqueous Nitrate Solutions in the Presence of Marine Chromophoric Dissolved Organic Matter: Comparison to Other Organic Photosensitizers, ACS Earth Space Chem., 5, 3056–3064, https://doi.org/10.1021/acsearthspacechem.1c00292, 2021.
Garland, J., Elzerman, A., and Penkett, S.: The mechanism for dry deposition of ozoen to seawater surfaces, J. Geophys. Res.-Oceans, 85, 7488–7492, https://doi.org/10.1029/JC085iC12p07488, 1980.
GBD 2019 Risk Factors Collaborators: Global burden of 87 risk factors in 204 countries and territories, 1990–2019: a systematic analysis for the Global Burden of Disease Study 2019, Lancet, 396, 1223–1249, https://doi.org/10.1016/S0140-6736(20)30752-2, 2020.
George, C., Strekowski, R. S., Kleffmann, J., Stemmler, K., and Ammann, M.: Photoenhanced uptake of gaseous NO2 on solid-organic compounds: a photochemical source of HONO?, Faraday Discuss., 130, 195–210, https://doi.org/10.1039/b417888m, 2005.
George, C., Ammann, M., D'Anna, B., Donaldson, D. J., and Nizkorodov, S. A.: Heterogeneous Photochemistry in the Atmosphere, Chem. Rev., 115, 4218–4258, https://doi.org/10.1021/cr500648z, 2015.
George, I. J. and Abbatt, J. P. D.: Heterogeneous oxidation of atmospheric aerosol particles by gas-phase radicals, Nat. Chem., 2, 713–722, https://doi.org/10.1038/nchem.806, 2010.
George, I. J., Vlasenko, A., Slowik, J. G., Broekhuizen, K., and Abbatt, J. P. D.: Heterogeneous oxidation of saturated organic aerosols by hydroxyl radicals: uptake kinetics, condensed-phase products, and particle size change, Atmos. Chem. Phys., 7, 4187–4201, https://doi.org/10.5194/acp-7-4187-2007, 2007.
George, I. J., Slowik, J., and Abbatt, J. P. D.: Chemical aging of ambient organic aerosol from heterogeneous reaction with hydroxyl radicals, Geophys. Res. Lett., 35, L13811, https://doi.org/10.1029/2008GL033884, 2008.
Gerber, R., Varner, M., Hammerich, A., Riikonen, S., Murdachaew, G., Shemesh, D., and Finlayson-Pitts, B.: Computational Studies of Atmospherically-Relevant Chemical Reactions in Water Clusters and on Liquid Water and Ice Surfaces, Acc. Chem. Res., 48, 399–406, https://doi.org/10.1021/ar500431g, 2015.
Girardet, C. and Toubin, C.: Molecular atmospheric pollutant adsorption on ice: a theoretical survey, Surf. Sci. Rep., 44, 163–238, 2001.
Gomez Alvarez, E., Amedro, D., Afif, C., Gligorovski, S., Schoemaecker, C., Fittschen, C., Doussin, J.-F., and Wortham, H.: Unexpectedly high indoor hydroxyl radical concentrations associated with nitrous acid, P. Natl. Acad. Sci. USA, 110, 13294–13299, https://doi.org/10.1073/pnas.1308310110, 2013.
Gopalakrishnan, S., Jungwirth, P., Tobias, D., and Allen, H.: Air-liquid interfaces of aqueous solutions containing ammonium and sulfate: Spectroscopic and molecular dynamics studies, J. Phys. Chem. B, 109, 8861–8872, https://doi.org/10.1021/jp0500236, 2005.
Gute, E. and Abbatt, J. P. D.: Oxidative Processing Lowers the Ice Nucleation Activity of Birch and Alder Pollen, Geophys. Res. Lett., 45, 1647–1653, https://doi.org/10.1002/2017GL076357, 2018.
Hallquist, M., Wenger, J. C., Baltensperger, U., Rudich, Y., Simpson, D., Claeys, M., Dommen, J., Donahue, N. M., George, C., Goldstein, A. H., Hamilton, J. F., Herrmann, H., Hoffmann, T., Iinuma, Y., Jang, M., Jenkin, M. E., Jimenez, J. L., Kiendler-Scharr, A., Maenhaut, W., McFiggans, G., Mentel, Th. F., Monod, A., Prévôt, A. S. H., Seinfeld, J. H., Surratt, J. D., Szmigielski, R., and Wildt, J.: The formation, properties and impact of secondary organic aerosol: current and emerging issues, Atmos. Chem. Phys., 9, 5155–5236, https://doi.org/10.5194/acp-9-5155-2009, 2009.
Hanisch, F. and Crowley, J.: The heterogeneous reactivity of gaseous nitric acid on authentic mineral dust samples, and on individual mineral and clay mineral components, Phys. Chem. Chem. Phys., 3, 2474–2482, https://doi.org/10.1039/b101700o, 2001.
Hanson, D. R. and Ravishankara, A. R.: Investigation of the reactive and nonreactive processes involving ClONO2 and HCl on water and nitric acid doped ice, J. Phys. Chem., 96, 2682–2691, https://doi.org/10.1021/j100185a052, 1992.
Hanson, D. R., Ravishankara, A. R., and Solomon, S.: Heterogeneous reactions in sulfuric acid aerosols – A framework for model calculations, J. Geophys. Res.-Atmos., 99, 3615–3629, https://doi.org/10.1029/93jd02932, 1994.
Hems, R. F., Schnitzler, E. G., Liu-Kang, C., Cappa, C. D., and Abbatt, J. P. D.: Aging of Atmospheric Brown Carbon Aerosol, ACS Earth Space Chem., 5, 722–748, 2021.
Herrmann, H., Schaefer, T., Tilgner, A., Styler, S. A., Weller, C., Teich, M., and Otto, T.: Tropospheric Aqueous-Phase Chemistry: Kinetics, Mechanisms, and Its Coupling to a Changing Gas Phase, Chem. Rev., 115, 4259–4334, https://doi.org/10.1021/cr500447k, 2015.
Hoffmann, M. and Edwards, J.: Kinetics of oxidation of sulfite by hydrogen peroxide in acidic solution, J. Phys. Chem., 79, 2096–2098, https://doi.org/10.1021/j100587a005, 1975.
Honrath, R. E., Peterson, M. C., Guo, S., Dibb, J. E., Shepson, P. B., and Campbell, B.: Evidence of NOx production within or upon ice particles in the Greenland snowpack, Geophys. Res. Lett., 26, 695–698, https://doi.org/10.1029/1999gl900077, 1999.
Hopfner, M., Ungermann, J., Borrmann, S., Wagner, R., Spang, R., Riese, M., Stiller, G., Appel, O., Batenburg, A., Bucci, S., Cairo, F., Dragoneas, A., Friedl-Vallon, F., Hunig, A., Johansson, S., Krasauskas, L., Legras, B., Leisner, T., Mahnke, C., Mohler, O., Molleker, S., Muller, R., Neubert, T., Orphal, J., Preusse, P., Rex, M., Saathoff, H., Stroh, F., Weigel, R., and Wohltmann, I.: Ammonium nitrate particles formed in upper troposphere from ground ammonia sources during Asian monsoons, Nat. Geosci., 12, 608–613, https://doi.org/10.1038/s41561-019-0385-8, 2019.
Huynh, E., Olinger, A., Woolley, D., Kohli, R., Choczynski, J., Davies, J., Lin, K., Marr, L., and Davis, R.: Evidence for a semisolid phase state of aerosols and droplets relevant to the airborne and surface survival of pathogens, P. Natl. Acad. Sci. USA, 119, e2109750119, https://doi.org/10.1073/pnas.2109750119, 2022.
Ivatt, P., Evans, M., and Lewis, A.: Suppression of surface ozone by an aerosol-inhibited photochemical ozone regime, Nat. Geosci., 15, 536–540, https://doi.org/10.1038/s41561-022-00972-9, 2022.
Jacob, D.: Chemistry of OH in Remove Clouds and Its Role in the Production of Formic-Acid Peroxymonosulfate, J. Geophys. Res.-Atmos., 9807–9826, 1986.
Jacob, D.: Heterogeneous chemistry and tropospheric ozone, Atmos. Environ., 34, 2131–2159, https://doi.org/10.1016/S1352-2310(99)00462-8, 2000.
Jacob, D. J. and Hoffmann, M. R.: A dynamic model for the production of H+, NO, and SO in urban fog, J. Geophys. Res.-Oceans, 88, 6611–6621, https://doi.org/10.1029/JC088iC11p06611, 1983.
Jang, M. S., Czoschke, N. M., Lee, S., and Kamens, R. M.: Heterogeneous atmospheric aerosol production by acid-catalyzed particle-phase reactions, Science, 298, 814–817, https://doi.org/10.1126/science.1075798, 2002.
Jimenez, J. L., Canagaratna, M. R., Donahue, N. M., Prevot, A. S. H., Zhang, Q., Kroll, J. H., DeCarlo, P. F., Allan, J. D., Coe, H., Ng, N. L., Aiken, A. C., Docherty, K. S., Ulbrich, I. M., Grieshop, A. P., Robinson, A. L., Duplissy, J., Smith, J. D., Wilson, K. R., Lanz, V. A., Hueglin, C., Sun, Y. L., Tian, J., Laaksonen, A., Raatikainen, T., Rautiainen, J., Vaattovaara, P., Ehn, M., Kulmala, M., Tomlinson, J. M., Collins, D. R., Cubison, M. J., Dunlea, E. J., Huffman, J. A., Onasch, T. B., Alfarra, M. R., Williams, P. I., Bower, K., Kondo, Y., Schneider, J., Drewnick, F., Borrmann, S., Weimer, S., Demerjian, K., Salcedo, D., Cottrell, L., Griffin, R., Takami, A., Miyoshi, T., Hatakeyama, S., Shimono, A., Sun, J. Y., Zhang, Y. M., Dzepina, K., Kimmel, J. R., Sueper, D., Jayne, J. T., Herndon, S. C., Trimborn, A. M., Williams, L. R., Wood, E. C., Middlebrook, A. M., Kolb, C. E., Baltensperger, U., and Worsnop, D. R.: Evolution of Organic Aerosols in the Atmosphere, Science, 326, 1525–1529, https://doi.org/10.1126/science.1180353, 2009.
Jorga, S., Florou, K., Kaltsonoudis, C., Kodros, J., Vasilakopoulou, C., Cirtog, M., Fouqueau, A., Picquet-Varrault, B., Nenes, A., and Pandis, S.: Nighttime chemistry of biomass burning emissions in urban areas: A dual mobile chamber study, Atmos. Chem. Phys., 21, 15337–15349, https://doi.org/10.5194/acp-21-15337-2021, 2021.
Junge, C.: Recent investigations in air chemistry, Tellus, 8, 127–139, 1956.
Kadowaki, S.: Size distribution of atmospheric total aerosols, sulfate, ammonium and nitrate in Nagoya area, Atmos. Environ., 10, 39–43, https://doi.org/10.1016/0004-6981(76)90257-2, 1976.
Kalberer, M., Paulsen, D., Sax, M., Steinbacher, M., Dommen, J., Prevot, A. S. H., Fisseha, R., Weingartner, E., Frankevich, V., Zenobi, R., and Baltensperger, U.: Identification of polymers as major components of atmospheric organic aerosols, Science, 303, 1659–1662, https://doi.org/10.1126/science.1092185, 2004.
Kanji, Z., Ladino, L., Wex, H., Boose, Y., Burkert-Kohn, M., Cziczo, D., and Kramer, M.: Overview of Ice Nucleating Particles, in: Ice Formation and Evolution in Clouds and Precipitation: Measurement adn modeling challenges, vol. 58, edited by: Baumgardner, D., McFarquhar, G., and Heymsfield, A., American Meteorological Society, https://doi.org/10.1175/AMSMONOGRAPHS-D-16-0006.1, 2017.
Karimova, N., Chen, J., Gord, J., Staudt, S., Bertram, T., Nathanson, G., and Gerber, R.: SN2 Reactions of N2O5 with Ions in Water: Microscopic Mechanisms, Intermediates, and Products, J. Phys. Chem. A, 124, 711–720, https://doi.org/10.1021/acs.jpca.9b09095, 2020.
Kavassalis, S. and Murphy, J.: Understanding ozone-meteorology correlations: A role for dry deposition, Geophys. Res. Lett., 44, 2922–2931, https://doi.org/10.1002/2016GL071791, 2017.
Kilchhofer, K., Mahrt, F., and Kanji, Z.: The Role of Cloud Processing for the Ice Nucleating Ability of Organic Aerosol and Coal Fly Ash Particles, J. Geophys. Res.-Atmos., 126, e2020JD033338, https://doi.org/10.1029/2020JD033338, 2021.
Kolb, C. E., Cox, R. A., Abbatt, J. P. D., Ammann, M., Davis, E. J., Donaldson, D. J., Garrett, B. C., George, C., Griffiths, P. T., Hanson, D. R., Kulmala, M., McFiggans, G., Poschl, U., Riipinen, I., Rossi, M. J., Rudich, Y., Wagner, P. E., Winkler, P. M., Worsnop, D. R., and O'Dowd, C. D.: An overview of current issues in the uptake of atmospheric trace gases by aerosols and clouds, Atmos. Chem. Phys., 10, 10561–10605, https://doi.org/10.5194/acp-10-10561-2010, 2010.
Koop, T., Bookhold, J., Shiraiwa, M., and Poschl, U.: Glass transition and phase state of organic compounds: dependency on molecular properties and implications for secondary organic aerosols in the atmosphere, Phys. Chem. Chem. Phys., 13, 19238–19255, https://doi.org/10.1039/c1cp22617g, 2011.
Krechmer, J., Groessl, M., Zhang, X., Junninen, H., Massoli, P., Lambe, A., Kimmel, J., Cubison, M., Graf, S., Lin, Y., Budisulistiorini, S., Zhang, H., Surratt, J., Knochenmuss, R., Jayne, J., Worsnop, D., Jimenez, J., and Canagaratna, M.: Ion mobility spectrometry-mass spectrometry (IMS-MS) for on- and offline analysis of atmospheric gas and aerosol species, Atmos. Meas. Tech., 9, 3245–3262, https://doi.org/10.5194/amt-9-3245-2016, 2016.
Kroll, J. H. and Seinfeld, J. H.: Chemistry of secondary organic aerosol: Formation and evolution of low-volatility organics in the atmosphere, Atmos. Environ., 42, 3593–3624, 2008.
Kroll, J. H., Smith, J. D., Che, D. L., Kessler, S. H., Worsnop, D. R., and Wilson, K. R.: Measurement of fragmentation and functionalization pathways in the heterogeneous oxidation of oxidized organic aerosol, Phys. Chem. Chem. Phys., 11, 8005–8014, https://doi.org/10.1039/b905289e, 2009.
Kroll, J. H., Donahue, N. M., Jimenez, J. L., Kessler, S. H., Canagaratna, M. R., Wilson, K. R., Altieri, K. E., Mazzoleni, L. R., Wozniak, A. S., Bluhm, H., Mysak, E. R., Smith, J. D., Kolb, C. E., and Worsnop, D. R.: Carbon oxidation state as a metric for describing the chemistry of atmospheric organic aerosol, Nat. Chem., 3, 133–139, https://doi.org/10.1038/nchem.948, 2011.
Kulmala, M., Petaja, T., Ehn, M., Thornton, J., Sipila, M., Worsnop, D., and Kerminen, V.: Chemistry of Atmospheric Nucleation: On the Recent Advances on Precursor Characterization and Atmospheric Cluster Composition in Connection with Atmospheric New Particle Formation, Annu. Rev. Phys. Chem., 65, 21–37, https://doi.org/10.1146/annurev-physchem-040412-110014, 2014.
Kumar, A., Marcolli, C., and Peter, T.: Ice nucleation activity of silicates and aluminosilicates in pure water and aqueous solutions – Part 3: Aluminosilicates, Atmos. Chem. Phys., 19, 6059–6084, https://doi.org/10.5194/acp-19-6059-2019, 2019.
Kwamena, N., Thornton, J., and Abbatt, J.: Kinetics of surface-bound benzo[a]pyrene and ozone on solid organic and salt aerosols, J. Phys. Chem. A, 108, 11626–11634, https://doi.org/10.1021/jp046161x, 2004.
Landrigan, P., Fuller, R., Acosta, N., Adeyi, O., Arnold, R., Basu, N., Balde, A., Bertollini, R., Bose-O'Reilly, S., Boufford, J., Breysse, P., Chiles, T., Mahidol, C., Coll-Seck, A., Cropper, M., Fobil, J., Fuster, V., Greenstone, M., Haines, A., Hanrahan, D., Hunter, D., Khare, M., Krupnick, A., Lanphear, B., Lohani, B., Martin, K., Mathiasen, K., McTeer, M., Murray, C., Ndahimananjara, J., Perera, F., Potocnik, J., Preker, A., Ramesh, J., Rockstrom, J., Salinas, C., Samson, L., Sandilya, K., Sly, P., Smith, K., Steiner, A., Stewart, R., Suk, W., van Schayck, O., Yadama, G., Yumkella, K., and Zhong, M.: The Lancet Commission on pollution and health, Lancet, 391, 462–512, https://doi.org/10.1016/S0140-6736(17)32345-0, 2018.
Laskin, A., Laskin, J., and Nizkorodov, S. A.: Chemistry of Atmospheric Brown Carbon, Chem. Rev., 115, 4335–4382, https://doi.org/10.1021/cr5006167, 2015.
Laskin, J., Laskin, A., and Nizkorodov, S. A.: New mass spectrometry techniques for studying physical chemistry of atmospheric heterogeneous processes, Int. Rev. Phys. Chem., 32, 128–170, https://doi.org/10.1080/0144235x.2012.752904, 2013.
Lee, R. and Patterson, R.: Size determination of atmospheric phosphate, nitrate, chloride and ammonium particulate in several urban areas, Atmos. Environ., 3, 249–261, 1969.
Lelieveld, J. and Crutzen, P.: The Role of Clouds in Tropospheric Photochemistry, J. Atmos. Chem., 12, 229–267, https://doi.org/10.1007/BF00048075, 1991.
Leu, M. T.: Heterogeneous reactions of N2O5 with H2O and HCl on ice surfaces – Implications for Antarctic ozone depletion, Geophys. Res. Lett., 15, 851–854, https://doi.org/10.1029/GL015i008p00851, 1988.
Li, C., He, Q., Fang, Z., Brown, S. S., Laskin, A., Cohen, S. R., and Rudich, Y.: Laboratory Insights into the Diel Cycle of Optical and Chemical Transformations of Biomass Burning Brown Carbon Aerosols, Environ. Sci. Technol., 54, 11827–11837, https://doi.org/10.1021/acs.est.0c04310, 2020.
Li, J., Zhang, Y., Cao, F., Zhang, W., Fan, M., Lee, X., and Michalski, G.: Stable Sulfur Isotopes Revealed a Major Role of Transition-Metal Ion-Catalyzed SO2 Oxidation in Haze Episodes, Environ. Sci. Technol., 54, 2626–2634, https://doi.org/10.1021/acs.est.9b07150, 2020.
Li, K., Jacob, D., Liao, H., Zhu, J., Shah, V., Shen, L., Bates, K., Zhang, Q., and Zhai, S.: A two-pollutant strategy for improving ozone and particulate air quality in China, Nat. Geosci., 12, 906–911, https://doi.org/10.1038/s41561-019-0464-x, 2019.
Li, L., Arnot, J., and Wania, F.: How are Humans Exposed to Organic Chemicals Released to Indoor Air?, Environ. Sci. Technol., 53, 11276–11284, https://doi.org/10.1021/acs.est.9b02036, 2019.
Li, M., Bao, F., Zhang, Y., Sheng, H., Chen, C., and Zhao, J.: Photochemical Aging of Soot in the Aqueous Phase: Release of Dissolved Black Carbon and the Formation of 1O2, Environ. Sci. Technol., 53, 12311–12319, https://doi.org/10.1021/acs.est.9b02773, 2019.
Lignell, H., Hinks, M. L., and Nizkorodov, S. A.: Exploring matrix effects on photochemistry of organic aerosols, P. Natl. Acad. Sci. USA, 111, 13780–13785, https://doi.org/10.1073/pnas.1322106111, 2014.
Lin, K., Schulte, C., and Marr, L.: Survival of MS2 and Φ 6 viruses in droplets as a function of relative humidity, pH, and salt, protein, and surfactant concentrations, PLOS ONE, 15, e0243505, https://doi.org/10.1371/journal.pone.0243505, 2020.
Liu, Q., Li, L., Zhang, X., Saini, A., Li, W., Hung, H., Hao, C., Li, K., Lee, P., Wentzell, J., Huo, C., Li, S., Harner, T., and Liggio, J.: Uncovering global-scale risks from commercial chemicals in air, Nature, 600, 456–459, https://doi.org/10.1038/s41586-021-04134-6, 2021.
Liu, T. and Abbatt, J. P. D.: Oxidation of sulfur dioxide by nitrogen dioxide accelerated at the interface of deliquesced aerosol particles, Nat. Chem., 13, 1173–1177, 2021.
Liu, T., Clegg, S., and Abbatt, J.: Fast oxidation of sulfur dioxide by hydrogen peroxide in deliquesced aerosol particles, P. Natl. Acad. Sci. USA, 117, 1354–1359, https://doi.org/10.1073/pnas.1916401117, 2020.
Liu, T., Chan, A. W. H., and Abbatt, J. P. D.: Multiphase Oxidation of Sulfur Dioxide in Aerosol Particles: Implications for Sulfate Formation in Polluted Environments, Environ. Sci. Technol., 55, 4227–4242, 2021b.
Maher, B., Ahmed, I., Karloukovski, V., MacLaren, D., Foulds, P., Allsop, D., Mann, D., Torres-Jardon, R., and Calderon-Garciduenas, L.: Magnetite pollution nanoparticles in the human brain, P. Natl. Acad. Sci. USA, 113, 10797–10801, https://doi.org/10.1073/pnas.1605941113, 2016.
Martens, C., Wesolowski, J., Harriss, R., and Kaifer, R.: Chlorine loss from Puerto Rican and San Francisco Pay Area Marine Aerosols, J. Geophys. Res., 78, 8778–8792, https://doi.org/10.1029/JC078i036p08778, 1973.
Martin, R. V., Jacob, D. J., Yantosca, R. M., Chin, M., and Ginoux, P.: Global and regional decreases in tropospheric oxidants from photochemical effects of aerosols, J. Geophys. Res.-Atmos., 108, 4097, https://doi.org/10.1029/2002jd002622, 2003.
Maters, E., Cimarelli, C., Casas, A., Dingwell, D., and Murray, B.: Volcanic ash ice-nucleating activity can be enhanced or depressed by ash-gas interaction in the eruption plume, Earth Planet. Sc. Lett., 551, 116587, https://doi.org/10.1016/j.epsl.2020.116587, 2020.
Mattila, J., Lakey, P., Shiraiwa, M., Wang, C., Abbatt, J., Arata, C., Goldstein, A., Ampollini, L., Katz, E., DeCarlo, P., Zhou, S., Kahan, T., Cardoso-Saldana, F., Hildebrandt Ruiz, L., Abeleira, A., Boedicker, E., Vance, M., and Farmer, D.: Multiphase Chemistry Controls Inorganic Chlorinated and Nitrogenated Compounds in Indoor Air during Bleach Cleaning, Environ. Sci. Technol., 54, 1730–1739, https://doi.org/10.1021/acs.est.9b05767, 2020.
McNeill, V. F.: Aqueous Organic Chemistry in the Atmosphere: Sources and Chemical Processing of Organic Aerosols, Environ. Sci. Technol., 49, 1237–1244, https://doi.org/10.1021/es5043707, 2015.
McNeill, V. F., Grannas, A. M., Abbatt, J. P. D., Ammann, M., Ariya, P., Bartels-Rausch, T., Domine, F., Donaldson, D. J., Guzman, M. I., Heger, D., Kahan, T. F., Klán, P., Masclin, S., Toubin, C., and Voisin, D.: Organics in environmental ices: sources, chemistry, and impacts, Atmos. Chem. Phys., 12, 9653–9678, https://doi.org/10.5194/acp-12-9653-2012, 2012.
Miller, M.: Oxidative stress and the cardiovascular effects of air pollution, Free Radic. Biol. Med., 151, 69–87, https://doi.org/10.1016/j.freeradbiomed.2020.01.004, 2020.
Miyake, Y. and Tsunogai, S.: Evaporation of iodine from ocean, J. Geophys. Res., 68, 3989–3993, https://doi.org/10.1029/JZ068i013p03989, 1963.
Mmereki, B. T. and Donaldson, D. J.: Direct observation of the kinetics of an atmospherically important reaction at the air-aqueous interface, J. Phys. Chem. A, 107, 11038–11042, https://doi.org/10.1021/jp036119m, 2003.
Moise, T. and Rudich, Y.: Reactive uptake of ozone by proxies for organic aerosols: Surface versus bulk processes, J. Geophys. Res.-Atmos., 105, 14667–14676, https://doi.org/10.1029/2000jd900071, 2000.
Moise, T., Flores, J., and Rudich, Y.: Optical Properties of Secondary Organic Aerosols and Their Changes by Chemical Processes, Chem. Rev., 115, 4400–4439, https://doi.org/10.1021/cr5005259, 2015.
Molina, M., Tso, T., Molina, L., and Fang, F.: Antarctic stratospheric chemistry of chlorine nitrate, hydrogen chloride and ice – release of active chlorine, Science, 238, 1253–1257, https://doi.org/10.1126/science.238.4831.1253, 1987.
Molina, M. J., Ivanov, A. V., Trakhtenberg, S., and Molina, L. T.: Atmospheric evolution of organic aerosol, Geophys. Res. Lett., 31, L22104, https://doi.org/10.1029/2004GL020910, 2004.
Monge, M. E., D'Anna, B., Mazri, L., Giroir-Fendler, A., Ammann, M., Donaldson, D. J., and George, C.: Light changes the atmospheric reactivity of soot, P. Natl. Acad. Sci. USA, 107, 6605–6609, https://doi.org/10.1073/pnas.0908341107, 2010.
Morrison, G.: Interfacial chemistry in indoor environments, Environ. Sci. Technol., 42, 3494–3499, 2008.
Mozurkewich, M., McMurry, P. H., Gupta, A., and Calvert, J. G.: Mass Accommodation for HO2 Radicals on Aqueous Particles, J. Geophys. Res.-Atmos., 92, 4163–4170, 1987.
Mu, Q., Shiraiwa, M., Octaviani, M., Ma, N., Ding, A., Su, H., Lammel, G., Poschl, U., and Cheng, Y.: Temperature effect on phase state and reactivity controls atmospheric multiphase chemistry and transport of PAHs, Sci. Adv., 4, eaap731, https://doi.org/10.1126/sciadv.aap7314, 2018.
Mungall, E. L., Abbatt, J. P. D., Wentzell, J. J. B., Lee, A. K. Y., Thomas, J. L., Blais, M., Gosselin, M., Miller, L. A., Papakyriakou, T., Willis, M. D., and Liggio, J.: Microlayer source of oxygenated volatile organic compounds in the summertime marine Arctic boundary layer, P. Natl. Acad. Sci. USA, 114, 6203, https://doi.org/10.1073/pnas.1620571114, 2017.
Murphy, D. and Ravishankara, A.: Temperature averages and rates of stratospheric reactions, Geophys. Res. Lett., 21, 2471–2474, https://doi.org/10.1029/94GL02287, 1994.
Murphy, D., Cziczo, D., Froyd, K., Hudson, P., Matthew, B., Middlebrook, A., Peltier, R., Sullivan, A., Thomson, D., and Weber, R.: Single-particle mass spectrometry of tropospheric aerosol particles, J. Geophys. Res.-Atmos., 111, D23S32, https://doi.org/10.1029/2006JD007340, 2006.
Oberdorster, G., Sharp, Z., Atudorei, V., Elder, A., Gelein, R., Kreyling, W., and Cox, C.: Translocation of inhaled ultrafine particles to the brain, Inhal. Toxicol., 16, 437–445, https://doi.org/10.1080/08958370490439597, 2004.
Oswin, H., Haddrell, A., Otero-Fernandez, M., Mann, J., Cogan, T., Hilditch, T., Tian, J., Hardy, D., Hill, D., Finn, A., Davidson, A., and Reid, J.: The dynamics of SARS-CoV-2 infectivity with changes in aerosol microenvironment, P. Natl. Acad. Sci. USA, 119, e2200109119, https://doi.org/10.1073/pnas.2200109119, 2022.
Pankow, J. F.: An Absorption Model of the Gas/Aerosol Partitioning Involved in the Formation of Secondary Organic Aerosol, Atmos. Environ., 28, 189–193, 1994.
Papazian, S., D'Agostino, L., Sadiktsis, I., Froment, J., Bonnefille, B., Sdougkou, K., Xie, H., Athanassiadis, I., Budhavant, K., Dasari, S., Andersson, A., Gustafsson, O., and Martin, J.: Nontarget mass spectrometry and in silico molecular characterization of air pollution from the Indian subcontinent, Commun. Earth Environ., 3, 35, https://doi.org/10.1038/s43247-022-00365-1, 2022.
Penkett, S., Jones, B., Brice, K., and Eggleton, A.: Importance of atmospheric ozone and hydrogen peroxide in oxidizing sulfur dioxide in cloud and rainwater, Atmos. Environ., 13, 123–137, https://doi.org/10.1016/0004-6981(79)90251-8, 1979.
Phillips, G., Thieser, J., Tang, M., Sobanski, N., Schuster, G., Fachinger, J., Drewnick, F., Borrmann, S., Bingemer, H., Lelieveld, J., and Crowley, J.: Estimating N2O5 uptake coefficients using ambient measurements of NO3, N2O5, ClNO2 and particle-phase nitrate, Atmos. Chem. Phys., 16, 13231–13249, https://doi.org/10.5194/acp-16-13231-2016, 2016.
Pitts, J., Van Cauwenbergh, K., Grosjean, D., SCHMID, J., Fitz, D., Belser, W., Knudson, G., and Hynds, P.: Atmospheric reactions fo polycyclic aromatic hydrocarbons – Facile formation of mutagenic nitro-derivatives, Science, 202, 515–519, https://doi.org/10.1126/science.705341, 1978.
Pitts, J., Lokensgard, D., Ripley, P., Van Cauwenberghe, K., Van Vaeck, L., Shaffer, S., Thill, A., and Belser, W.: Atmospheric epoxidation of benzo[a]pyrene by ozone – Formation of the metabolite benzo[a]pyrene-4,5-oxide, Science, 210, 1347–1349, https://doi.org/10.1126/science.210.4476.1347, 1980.
Pope, C. A., Ezzati, M., and Dockery, D. W.: Fine-Particulate Air Pollution and Life Expectancy in the United States, N. Engl. J. Med., 360, 376–386, 2009.
Pöschl, U. and Shiraiwa, M.: Multiphase Chemistry at the Atmosphere-Biosphere Interface Influencing Climate and Public Health in the Anthropocene, Chem. Rev., 115, 4440–4475, https://doi.org/10.1021/cr500487s, 2015.
Pöschl, U., Letzel, T., Schauer, C., and Niessner, R.: Interaction of ozone and water vapor with spark discharge soot aerosol particles coated with benzo[a]pyrene: O3 and H2O adsorption, benzo[a]pyrene degradation, and atmospheric implications, J. Phys. Chem. A, 105, 4029–4041, 2001.
Pöschl, U., Rudich, Y., and Ammann, M.: Kinetic model framework for aerosol and cloud surface chemistry and gas-particle interactions – Part 1: General equations, parameters, and terminology, Atmos. Chem. Phys., 7, 5989–6023, https://doi.org/10.5194/acp-7-5989-2007, 2007.
Prather, K., Bertram, T., Grassian, V., Deane, G., Stokes, M., DeMott, P., Aluwihare, L., Palenik, B., Azam, F., Seinfeld, J., Moffet, R., Molina, M., Cappa, C., Geiger, F., Roberts, G., Russell, L., Ault, A., Baltrusaitis, J., Collins, D., Corrigan, C., Cuadra-Rodriguez, L., Ebben, C., Forestieri, S., Guasco, T., Hersey, S., Kim, M., Lambert, W., Modini, R., Mui, W., Pedler, B., Ruppel, M., Ryder, O., Schoepp, N., Sullivan, R., and Zhao, D.: Bringing the ocean into the laboratory to probe the chemical complexity of sea spray aerosol, P. Natl. Acad. Sci. USA, 110, 7550–7555, https://doi.org/10.1073/pnas.1300262110, 2013.
Prather, K. A., Hatch, C. D., and Grassian, V. H.: Analysis of Atmospheric Aerosols, Annu. Rev. Anal. Chem., 1, 485–514, https://doi.org/10.1146/annurev.anchem.1.031207.113030, 2008.
Pye, H., Ward-Caviness, C., Murphy, B., Appel, K., and Seltzer, K.: Secondary organic aerosol association with cardiorespiratory disease mortality in the United States, Nat. Commun., 12, 7215, https://doi.org/10.1038/s41467-021-27484-1, 2021.
Pye, H. O. T., Nenes, A., Alexander, B., Ault, A. P., Barth, M. C., Clegg, S. L., Collett Jr., J. L., Fahey, K. M., Hennigan, C. J., Herrmann, H., Kanakidou, M., Kelly, J. T., Ku, I.-T., McNeill, V. F., Riemer, N., Schaefer, T., Shi, G., Tilgner, A., Walker, J. T., Wang, T., Weber, R., Xing, J., Zaveri, R. A., and Zuend, A.: The acidity of atmospheric particles and clouds, Atmos. Chem. Phys., 20, 4809–4888, https://doi.org/10.5194/acp-20-4809-2020, 2020.
Ravishankara, A. R.: Heterogeneous and multiphase chemistry in the troposphere, Science, 276, 1058–1065, https://doi.org/10.1126/science.276.5315.1058, 1997.
Reeser, D. I., George, C., and Donaldson, D. J.: Photooxidation of Halides by Chlorophyll at the Air-Salt Water Interface, J. Phys. Chem. A, 113, 8591–8595, https://doi.org/10.1021/jp903657j, 2009.
Renbaum-Wolff, L., Grayson, J. W., Bateman, A. P., Kuwata, M., Sellier, M., Murray, B. J., Shilling, J. E., Martin, S. T., and Bertram, A. K.: Viscosity of α-pinene secondary organic material and implications for particle growth and reactivity, P. Natl. Acad. Sci. USA, 110, 8014–8019, https://doi.org/10.1073/pnas.1219548110, 2013.
Riva, M., Chen, Y., Zhang, Y., Lei, Z., Olson, N., Boyer, H., Narayan, S., Yee, L., Green, H., Cui, T., Zhang, Z., Baumann, K., Fort, M., Edgerton, E., Budisulistiorini, S., Rose, C., Ribeiro, I., Oliveira, R., dos Santos, E., Machado, C., Szopa, S., Zhao, Y., Alves, E., de Sa, S., Hu, W., Knipping, E., Shaw, S., Duvoisin, S., de Souza, R., Palm, B., Jimenez, J., Glasius, M., Goldstein, A., Pye, H., Gold, A., Turpin, B., Vizuete, W., Martin, S., Thornton, J., Dutcher, C., Ault, A., and Surratt, J.: Increasing Isoprene Epoxydiol-to-Inorganic Sulfate Aerosol Ratio Results in Extensive Conversion of Inorganic Sulfate to Organosulfur Forms: Implications for Aerosol Physicochemical Properties, Environ. Sci. Technol., 53, 8682–8694, https://doi.org/10.1021/acs.est.9b01019, 2019.
Rossignol, S., Tinel, L., Bianco, A., Passananti, M., Brigante, M., Donaldson, D. J., and George, C.: Atmospheric photochemistry at a fatty acid–coated air-water interface, Science, 353, 699–702, https://doi.org/10.1126/science.aaf3617, 2016.
Rudich, Y.: Laboratory perspectives on the chemical transformations of organic matter in atmospheric particles, Chem. Rev., 103, 5097–5124, https://doi.org/10.1021/cr020508f, 2003.
Rudich, Y., Donahue, N. M., and Mentel, T. F.: Aging of Organic Aerosol: Bridging the Gap Between Laboratory and Field Studies, Annu. Rev. Phys. Chem., 58, 321–352, https://doi.org/10.1146/annurev.physchem.58.032806.104432, 2007.
Russell, L.: Aerosol organic-mass-to-organic-carbon ratio measurements, Environ. Sci. Technol., 37, 2982–2987, https://doi.org/10.1021/es026123w, 2003.
Schneider, S. R., Collins, D. B., Lim, C. Y., Zhu, L., and Abbatt, J. P. D.: Formation of Secondary Organic Aerosol from the Heterogeneous Oxidation by Ozone of a Phytoplankton Culture, ACS Earth Space Chem., 3, 2298–2306, https://doi.org/10.1021/acsearthspacechem.9b00201, 2019.
Schnitzler, E., Gerrebos, N., Carter, T., Huang, Y., Healdc, C., Bertram, A., and Abbatt, J.: Rate of atmospheric brown carbon whitening governed by environmental conditions, P. Natl. Acad. Sci. USA, 119, e2205610119, https://doi.org/10.1073/pnas.2205610119, 2022.
Schroeder, W. H. and Urone, P.: Isolation and identification of nitrosium hydrogen sulfate as a photochemical reaction product in air containing sulfur dioxide and nitrogen dioxide, Environ. Sci. Technol., 12, 545–550, https://doi.org/10.1021/es60141a016, 1978.
Segal-Rosenheimer, M. and Dubowski, Y.: Heterogeneous ozonolysis of cypermethrin using real-time monitoring FTIR techniques, J. Phys. Chem. C, 111, 11682–11691, 2007.
Shiraiwa, M., Ammann, M., Koop, T., and Poeschl, U.: Gas-uptake and chemical aging of semisolid organic aerosol particles, P. Nat. Acad. Sci. USA, 108, 11003–11008, 2011.
Shiraiwa, M., Selzle, K., Yang, H., Sosedova, Y., Ammann, M., and Poschl, U.: Multiphase Chemical Kinetics of the Nitration of Aerosolized Protein by Ozone and Nitrogen Dioxide, Environ. Sci. Technol., 46, 6672–6680, https://doi.org/10.1021/es300871b, 2012.
Shiraiwa, M., Ueda, K., Pozzer, A., Lammel, G., Kampf, C., Fushimi, A., Enami, S., Arangio, A., Frohlich-Nowoisky, J., Fujitani, Y., Furuyama, A., Lakey, P., Lelieveld, J., Lucas, K., Morino, Y., Poschl, U., Takaharna, S., Takami, A., Tong, H., Weber, B., Yoshino, A., and Sato, K.: Aerosol Health Effects from Molecular to Global Scales, Environ. Sci. Technol., 51, 13545–13567, https://doi.org/10.1021/acs.est.7b04417, 2017a.
Shiraiwa, M., Li, Y., Tsimpidi, A., Karydis, V., Berkemeier, T., Pandis, S., Lelieveld, J., Koop, T., and Poschl, U.: Global distribution of particle phase state in atmospheric secondary organic aerosols, Nat. Commun., 8, 15002, https://doi.org/10.1038/ncomms15002, 2017b.
Shiraiwa, M., Carslaw, N., Tobias, D. J., Waring, M. S., Rim, D., Morrison, G., Lakey, P. S. J., Kruza, M., von Domaros, M., Cummings, B. E., and Won, Y.: Modelling consortium for chemistry of indoor environments (MOCCIE): integrating chemical processes from molecular to room scales, Environ. Sci. Process. Imp., 21, 1240–1254, https://doi.org/10.1039/C9EM00123A, 2019.
Shrivastava, M., Lou, S., Zelenyuk, A., Easter, R., Corley, R., Thrall, B., Rasch, P., Fast, J., Simonich, S., Shen, H., and Tao, S.: Global long-range transport and lung cancer risk from polycyclic aromatic hydrocarbons shielded by coatings of organic aerosol, P. Natl. Acad. Sci. USA, 114, 1246–1251, https://doi.org/10.1073/pnas.1618475114, 2017a.
Shrivastava, M., Cappa, C., Fan, J., Goldstein, A., Guenther, A., Jimenez, J., Kuang, C., Laskin, A., Martin, S., Ng, N., Petaja, T., Pierce, J., Rasch, P., Roldin, P., Seinfeld, J., Shilling, J., Smith, J., Thornton, J., Volkamer, R., Wang, J., Worsnop, D., Zaveri, R., Zelenyuk, A., and Zhang, Q.: Recent advances in understanding secondary organic aerosol: Implications for global climate forcing, Rev. Geophys., 55, 509–559, https://doi.org/10.1002/2016RG000540, 2017b.
Sihvonen, S., Schill, G., Lyktey, N., Veghte, D., Tolbert, M., and Freedman, M.: Chemical and Physical Transformations of Aluminosilicate Clay Minerals Due to Acid Treatment and Consequences for Heterogeneous Ice Nucleation, J. Phys. Chem. A, 118, 8787–8796, https://doi.org/10.1021/jp504846g, 2014.
Simpson, W. R., Brown, S. S., Saiz-Lopez, A., Thornton, J. A., and von Glasow, R.: Tropospheric Halogen Chemistry: Sources, Cycling, and Impacts, Chem. Rev., 115, 4035–4062, https://doi.org/10.1021/cr5006638, 2015.
Sobyra, T., Pliszka, H., Bertram, T., and Nathanson, G.: Production of Br2 from N2O5 and Bromide in Salty and Surfactant-Coated Water Microjets, J. Phys. Chem. A, 123, 8942–8953, https://doi.org/10.1021/acs.jpca.9b04225, 2019.
Solomon, S.: Stratospheric ozone depletion: A review of concepts and history, Rev. Geophys., 37, 275–316, 1999.
Solomon, S., Garcia, R. R., Rowland, F. S., and Wuebbles, D. J.: On the depletion of Antarctic ozone, Nature, 321, 755–758, https://doi.org/10.1038/321755a0, 1986.
Solomon, S., Dube, K., Stone, K., Yu, P., Kinnison, D., Toon, O., Strahan, S., Rosenlof, K., Portmann, R., Davis, S., Randel, W., Bernath, P., Boone, C., Bardeen, C., Bourassa, A., Zawada, D., and Degenstein, D.: On the stratospheric chemistry of midlatitude wildfire smoke, P. Natl. Acad. Sci. USA, 119, e2117325119, https://doi.org/10.1073/pnas.2117325119, 2022.
Song, S., Gao, M., Xu, W., Sun, Y., Worsnop, D., Jayne, J., Zhang, Y., Zhu, L., Li, M., Zhou, Z., Cheng, C., Lv, Y., Wang, Y., Peng, W., Xu, X., Lin, N., Wang, Y., Wang, S., Munger, J., Jacob, D., and McElroy, M.: Possible heterogeneous chemistry of hydroxymethanesulfonate (HMS) in northern China winter haze, Atmos. Chem. Phys., 19, 1357–1371, https://doi.org/10.5194/acp-19-1357-2019, 2019.
Strahan, S., Smale, D., Solomon, S., Taha, G., Damon, M., Steenrod, S., Jones, N., Liley, B., Querel, R., and Robinson, J.: Unexpected Repartitioning of Stratospheric Inorganic Chlorine After the 2020 Australian Wildfires, Geophys. Res. Lett., 49, e2022GL098290, https://doi.org/10.1029/2022GL098290, 2022.
Sullivan, R., Minambres, L., DeMott, P., Prenni, A., Carrico, C., Levin, E., and Kreidenweis, S.: Chemical processing does not always impair heterogeneous ice nucleation of mineral dust particles, Geophys. Res. Lett., 37, L24805, https://doi.org/10.1029/2010GL045540, 2010a.
Sullivan, R., Petters, M., DeMott, P., Kreidenweis, S., Wex, H., Niedermeier, D., Hartmann, S., Clauss, T., Stratmann, F., Reitz, P., Schneider, J., and Sierau, B.: Irreversible loss of ice nucleation active sites in mineral dust particles caused by sulphuric acid condensation, Atmos. Chem. Phys., 10, 11471–11487, https://doi.org/10.5194/acp-10-11471-2010, 2010b.
Surratt, J. D., Murphy, S. M., Kroll, J. H., Ng, N. L., Hildebrandt, L., Sorooshian, A., Szmigielski, R., Vermeylen, R., Maenhaut, W., Claeys, M., Flagan, R. C., and Seinfeld, J. H.: Chemical Composition of Secondary Organic Aerosol Formed from the Photooxidation of Isoprene, J. Phys. Chem. A, 110, 9665–9690, https://doi.org/10.1021/jp061734m, 2006.
Svehla, G.: Nomenclature of kinetic methods of analysis, Pure Appl. Chem., 65, 2291–2298, https://doi.org/10.1351/pac199365102291, 1993.
Tabazadeh, A. and Turco, R.: A model for heterogeneous chemical processes on the surfaces of ice and nitric aid trihydrate particles, J. Geophys. Res.-Atmos., 98, 12727–12740, https://doi.org/10.1029/93JD00947, 1993.
Tham, Y., Wang, Z., Li, Q., Wang, W., Wang, X., Lu, K., Ma, N., Yan, C., Kecorius, S., Wiedensohler, A., Zhang, Y., and Wang, T.: Heterogeneous N2O5 uptake coefficient and production yield of ClNO2 in polluted northern China: roles of aerosol water content and chemical composition, Atmos. Chem. Phys., 18, 13155–13171, https://doi.org/10.5194/acp-18-13155-2018, 2018.
Thompson, C.: The NASA Atmospheric Tomography (ATom) Mission: Imaging the Chemistry of the Global Atmosphere, B. Am. Meteorol. Soc., 761–790, https://doi.org/10.1175/BAMS-D-20-0315.1, 2022.
Tilgner, A., Brauer, P., Wolke, R., and Herrmann, H.: Modelling multiphase chemistry in deliquescent aerosols and clouds using CAPRAM3.0i, J. Atmos. Chem., 70, 221–256, https://doi.org/10.1007/s10874-013-9267-4, 2013.
Tilgner, A., Schaefer, T., Alexander, B., Barth, M., Collett, J., Fahey, K., Nenes, A., Pye, H., Herrmann, H., and McNeill, V.: Acidity and the multiphase chemistry of atmospheric aqueous particles and clouds, Atmos. Chem. Phys., 21, 13483–13536, https://doi.org/10.5194/acp-21-13483-2021, 2021.
Tobias, D., Stern, A., Baer, M., Levin, Y., and Mundy, C.: Simulation and Theory of Ions at Atmospherically Relevant Aqueous Liquid-Air Interfaces, Annu. Rev. Phys. Chem., 64, 339–359, https://doi.org/10.1146/annurev-physchem-040412-110049, 2013.
Tobias, H. and Ziemann, P.: Thermal desorption mass spectrometric analysis of organic aerosol formed from reactions of 1-tetradecene and O3 in the presence of alcohols and carboxylic acids, Environ. Sci. Technol., 34, 2105–2115, https://doi.org/10.1021/es9907156, 2000.
Tolbert, M. A., Rossi, M. J., and Golden, D. M.: Antarctic ozone depletion chemistry – Reactions of N2O5 with H2O and HCl on ice surfaces, Science, 240, 1018–1021, https://doi.org/10.1126/science.240.4855.1018, 1988.
Tritscher, I., Pitts, M., Poole, L., Alexander, S., Cairo, F., Chipperfield, M., Grooss, J., Hopfner, M., Lambert, A., Luo, B., Molleker, S., Orr, A., Salawitch, R., Snels, M., Spang, R., Woiwode, W., and Peter, T.: Polar Stratospheric Clouds: Satellite Observations, Processes, and Role in Ozone Depletion, Rev. Geophys., 59, e2020RG000702, https://doi.org/10.1029/2020RG000702, 2021.
Trostl, J., Chuang, W. K., Gordon, H., Heinritzi, M., Yan, C., Molteni, U., Ahlm, L., Frege, C., Bianchi, F., Wagner, R., Simon, M., Lehtipalo, K., Williamson, C., Craven, J. S., Duplissy, J., Adamov, A., Almeida, J., Bernhammer, A. K., Breitenlechner, M., Brilke, S., Dias, A., Ehrhart, S., Flagan, R. C., Franchin, A., Fuchs, C., Guida, R., Gysel, M., Hansel, A., Hoyle, C. R., Jokinen, T., Junninen, H., Kangasluoma, J., Keskinen, H., Kim, J., Krapf, M., Kurten, A., Laaksonen, A., Lawler, M., Leiminger, M., Mathot, S., Mohler, O., Nieminen, T., Onnela, A., Petaja, T., Piel, F. M., Miettinen, P., Rissanen, M. P., Rondo, L., Sarnela, N., Schobesberger, S., Sengupta, K., Sipilaa, M., Smith, J. N., Steiner, G., Tome, A., Virtanen, A., Wagner, A. C., Weingartner, E., Wimmer, D., Winkler, P. M., Ye, P. L., Carslaw, K. S., Curtius, J., Dommen, J., Kirkby, J., Kulmala, M., Riipinen, I., Worsnop, D. R., Donahue, N. M., and Baltensperger, U.: The role of low-volatility organic compounds in initial particle growth in the atmosphere, Nature, 533, 527–530, https://doi.org/10.1038/nature18271, 2016.
Tseng, C. and Li, C.: Inactivation of surface viruses by gaseous Ozone, J. Environ. Health, 70, 56–62, 2008.
Tuite, K., Thomas, J., Veres, P., Roberts, J., Stevens, P., Griffith, S., Dusanter, S., Flynn, J., Ahmed, S., Emmons, L., Kim, S., Washenfelder, R., Young, C., Tsai, C., Pikelnaya, O., and Stutz, J.: Quantifying Nitrous Acid Formation Mechanisms Using Measured Vertical Profiles During the CalNex 2010 Campaign and 1D Column Modeling, J. Geophys. Res.-Atmos., 126, e2021JD034689, https://doi.org/10.1029/2021JD034689, 2021.
Usher, C. R., Michel, A. E., and Grassian, V. H.: Reactions on mineral dust, Chem. Rev., 103, 4883–4939, https://doi.org/10.1021/cr020657y, 2003.
Virtanen, A., Joutsensaari, J., Koop, T., Kannosto, J., Yli-Pirila, P., Leskinen, J., Makela, J. M., Holopainen, J. K., Poschl, U., Kulmala, M., Worsnop, D. R., and Laaksonen, A.: An amorphous solid state of biogenic secondary organic aerosol particles, Nature, 467, 824–827, https://doi.org/10.1038/nature09455, 2010.
Wang, G., Zhang, R., Gomez, M., Yang, L., Zamora, M., Hu, M., Lin, Y., Peng, J., Guo, S., Meng, J., Li, J., Cheng, C., Hu, T., Ren, Y., Wang, Y., Gao, J., Cao, J., An, Z., Zhou, W., Li, G., Wang, J., Tian, P., Marrero-Ortiz, W., Secrest, J., Du, Z., Zheng, J., Shang, D., Zeng, L., Shao, M., Wang, W., Huang, Y., Wang, Y., Zhu, Y., Li, Y., Hu, J., Pan, B., Cai, L., Cheng, Y., Ji, Y., Zhang, F., Rosenfeld, D., Liss, P., Duce, R., Kolb, C., and Molina, M.: Persistent sulfate formation from London Fog to Chinese haze, P. Natl. Acad. Sci. USA, 113, 13630–13635, https://doi.org/10.1073/pnas.1616540113, 2016.
Wang, L. and Clary, D.: Time-dependent wave-packet studies on the sticking of HCl to an ice surface, J. Chem. Phys., 104, 5663–5673, https://doi.org/10.1063/1.471772, 1996.
Wang, S., Zhou, S., Tao, Y., Tsui, W. G., Ye, J., Yu, J. Z., Murphy, J. G., McNeill, V. F., Abbatt, J. P. D., and Chan, A. W. H.: Organic Peroxides and Sulfur Dioxide in Aerosol: Source of Particulate Sulfate, Environ. Sci. Technol., 53, 10695–10704, https://doi.org/10.1021/acs.est.9b02591, 2019.
Weller, C., Horn, S., and Herrmann, H.: Effects of Fe(III)-concentration, speciation, excitation-wavelength and light intensity on the quantum yield of iron(III)-oxalato complex photolysis, J. Photochem. Photobiol.-Chem., 255, 41–49, https://doi.org/10.1016/j.jphotochem.2013.01.014, 2013.
Weschler, C.: Ozone in indoor environments: Concentration and chemistry, Indoor Air, 10, 269–288, https://doi.org/10.1034/j.1600-0668.2000.010004269.x, 2000.
Wingen, L. and Finlayson-Pitts, B.: Probing surfaces of atmospherically relevant organic particles by easy ambient sonic-spray ionization mass spectrometry (EASI-MS), Chem. Sci., 10, 884–897, https://doi.org/10.1039/c8sc03851a, 2019.
Wisthaler, A. and Weschler, C. J.: Reactions of ozone with human skin lipids: Sources of carbonyls, dicarbonyls, and hydroxycarbonyls in indoor air, P. Natl. Acad. Sci. USA, 107, 6568–6575, https://doi.org/10.1073/pnas.0904498106, 2009.
Wolff, E., Jones, A., Martin, T., and Grenfell, T.: Modelling photochemical NOx production and nitrate loss in the upper snowpack of Antarctica, Geophys. Res. Lett., 29, 944, https://doi.org/10.1029/2002GL015823, 2002.
Wong, J. P. S., Carslaw, N., Zhao, R., Zhou, S., and Abbatt, J. P. D.: Observations and impacts of bleach washing on indoor chlorine chemistry, Indoor Air, 27, 1082–1090, https://doi.org/10.1111/ina.12402, 2017.
Woo, J. and McNeill, V.: simpleGAMMA v1.0 – a reduced model of secondary organic aerosol formation in the aqueous aerosol phase (aaSOA), Geosci. Model Dev., 8, 1821–1829, https://doi.org/10.5194/gmd-8-1821-2015, 2015.
Workman, E. J. and Reynolds, S. E.: Electrical Phenomena Occurring during the Freezing of Dilute Aqueous Solutions and Their Possible Relationship to Thundrstorm Electricity, Phys. Rev., 78, 254–259, 1950.
Worsnop, D. R., Morris, J. W., Shi, Q., Davidovits, P., and Kolb, C. E.: A chemical kinetic model for reactive transformations of aerosol particles, Geophys. Res. Lett., 29, 1996, https://doi.org/10.1029/2002gl015542, 2002.
Xiao, M., Hoyle, C. R., Dada, L., Stolzenburg, D., Kürten, A., Wang, M., Lamkaddam, H., Garmash, O., Mentler, B., Molteni, U., Baccarini, A., Simon, M., He, X.-C., Lehtipalo, K., Ahonen, L. R., Baalbaki, R., Bauer, P. S., Beck, L., Bell, D., Bianchi, F., Brilke, S., Chen, D., Chiu, R., Dias, A., Duplissy, J., Finkenzeller, H., Gordon, H., Hofbauer, V., Kim, C., Koenig, T. K., Lampilahti, J., Lee, C. P., Li, Z., Mai, H., Makhmutov, V., Manninen, H. E., Marten, R., Mathot, S., Mauldin, R. L., Nie, W., Onnela, A., Partoll, E., Petäjä, T., Pfeifer, J., Pospisilova, V., Quéléver, L. L. J., Rissanen, M., Schobesberger, S., Schuchmann, S., Stozhkov, Y., Tauber, C., Tham, Y. J., Tomé, A., Vazquez-Pufleau, M., Wagner, A. C., Wagner, R., Wang, Y., Weitz, L., Wimmer, D., Wu, Y., Yan, C., Ye, P., Ye, Q., Zha, Q., Zhou, X., Amorim, A., Carslaw, K., Curtius, J., Hansel, A., Volkamer, R., Winkler, P. M., Flagan, R. C., Kulmala, M., Worsnop, D. R., Kirkby, J., Donahue, N. M., Baltensperger, U., El Haddad, I., and Dommen, J.: The driving factors of new particle formation and growth in the polluted boundary layer, Atmos. Chem. Phys., 21, 14275–14291, https://doi.org/10.5194/acp-21-14275-2021, 2021.
Yang, J., Li, L., Wang, S., Li, H., Francisco, J., Zeng, X., and Gao, Y.: Unraveling a New Chemical Mechanism of Missing Sulfate Formation in Aerosol Haze: Gaseous NO2 with Aqueous HSO/SO, J. Am. Chem. Soc., 141, 19312–19320, https://doi.org/10.1021/jacs.9b08503, 2019.
Yeh, K., Ditto, J., and Abbatt, J.: Ozonolysis Lifetime of Tetrahydrocannabinol in Thirdhand Cannabis Smoke, Environ. Sci. Technol. Lett., 9, 599–603, https://doi.org/10.1021/acs.estlett.2c00311, 2022.
Young, C. J., Zhou, S., Siegel, J. A., and Kahan, T. F.: Illuminating the dark side of indoor oxidants, Environ. Sci Process. Imp., 21, 1229–1239, https://doi.org/10.1039/C9EM00111E, 2019.
Yun, J., Kumar, A., Removski, N., Shchukarev, A., Link, N., Boily, J., and Bertram, A.: Effects of Inorganic Acids and Organic Solutes on the Ice Nucleating Ability and Surface Properties of Potassium-Rich Feldspar, ACS Earth Space Chem., 5, 1212–1222, https://doi.org/10.1021/acsearthspacechem.1c00034, 2021.
Zannoni, N., Lakey, P., Won, Y., Shiraiwa, M., Rim, D., Weschler, C., Wang, N., Ernle, L., Li, M., Beko, G., Wargocki, P., and Williams, J.: The human oxidation field, Science, 377, 1071–1076, https://doi.org/10.1126/science.abn0340, 2022.
Zaveri, R., Barnard, J., Easter, R., Riemer, N., and West, M.: Particle-resolved simulation of aerosol size, composition, mixing state, and the associated optical and cloud condensation nuclei activation properties in an evolving urban plume, J. Geophys. Res.-Atmos., 115, D17210, https://doi.org/10.1029/2009JD013616, 2010.
Zelenyuk, A. and Imre, D.: Single particle laser ablation time-of-flight mass spectrometer: An introduction to SPLAT, Aerosol Sci. Tech., 39, 554–568, https://doi.org/10.1080/027868291009242, 2005.
Zepp, R. G., Hoigne, J., and Bader, H.: Nitrate-induced photooxidation of trace organic chemicals in water, Environ. Sci. Technol., 21, 443–450, https://doi.org/10.1021/es00159a004, 1987.
Zheng, H., Song, S., Sarwar, G., Gen, M., Wang, S., Ding, D., Chang, X., Zhang, S., Xing, J., Sun, Y., Ji, D., Chan, C., Gao, J., and McElroy, M.: Contribution of Particulate Nitrate Photolysis to Heterogeneous Sulfate Formation for Winter Haze in China, Environ. Sci. Technol. Lett., 7, 632–638, https://doi.org/10.1021/acs.estlett.0c00368, 2020.
Zhou, J., Sato, K., Bai, Y., Fukusaki, Y., Kousa, Y., Ramasamy, S., Takami, A., Yoshino, A., Nakayama, T., Sadanaga, Y., Nakashima, Y., Li, J., Murano, K., Kohno, N., Sakamoto, Y., and Kajii, Y.: Kinetics and impacting factors of HO2 uptake onto submicron atmospheric aerosols during the 2019 Air QUAlity Study (AQUAS) in Yokohama, Japan, Atmos. Chem. Phys., 21, 12243–12260, https://doi.org/10.5194/acp-21-12243-2021, 2021.
Zhou, S., Lee, A. K. Y., McWhinney, R. D., and Abbatt, J. P. D.: Burial Effects of Organic Coatings on the Heterogeneous Reactivity of Particle-Borne Benzo[a]pyrene (BaP) toward Ozone, J. Phys. Chem. A, 116, 7050–7056, https://doi.org/10.1021/jp3030705, 2012.
Zhou, S., Yeung, L. W. Y., Forbes, M. W., Mabury, S., and Abbatt, J. P. D.: Epoxide formation from heterogeneous oxidation of benzo[a]pyrene with gas-phase ozone and indoor air, Environ. Sci. Process. Imp., 19, 1292–1299, https://doi.org/10.1039/C7EM00181A, 2017.
Zhou, S., Hwang, B. C. H., Lakey, P. S. J., Zuend, A., Abbatt, J. P. D., and Shiraiwa, M.: Multiphase reactivity of polycyclic aromatic hydrocarbons is driven by phase separation and diffusion limitations, P. Natl. Acad. Sci. USA, 116, 11658–11663, https://doi.org/10.1073/pnas.1902517116, 2019.
Zhou, S. M., Shiraiwa, M., McWhinney, R. D., Poschl, U., and Abbatt, J. P. D.: Kinetic limitations in gas-particle reactions arising from slow diffusion in secondary organic aerosol, Faraday Discuss., 165, 391–406, https://doi.org/10.1039/c3fd00030c, 2013.
Zhou, Z., Zhou, S., and Abbatt, J. P. D.: Kinetics and Condensed-Phase Products in Multiphase Ozonolysis of an Unsaturated Triglyceride, Environ. Sci. Technol., 53, 12467–12475, https://doi.org/10.1021/acs.est.9b04460, 2019.
Zhou, Z., Lakey, P., von Domaros, M., Wise, N., Tobias, D., Shiraiwa, M., and Abbatt, J.: Multiphase Ozonolysis of Oleic Acid-Based Lipids: Quantitation of Major Products and Kinetic Multilayer Modeling, Environ. Sci. Technol., 56, 7716–7728, https://doi.org/10.1021/acs.est.2c01163, 2022.
Ziemann, P. J. and Atkinson, R.: Kinetics, products, and mechanisms of secondary organic aerosol formation, Chem. Soc. Rev., 41, 6582–6605, https://doi.org/10.1039/c2cs35122f, 2012.
- Abstract
- Introduction
- What is atmospheric multiphase chemistry?
- How does multiphase chemistry differ from gas-phase chemistry?
- Early studies of atmospheric multiphase chemistry
- Progress in the past 20 years
- The future of atmospheric multiphase chemistry studies
- Concluding thoughts
- Data availability
- Author contributions
- Competing interests
- Disclaimer
- Special issue statement
- Acknowledgements
- Review statement
- References
- Abstract
- Introduction
- What is atmospheric multiphase chemistry?
- How does multiphase chemistry differ from gas-phase chemistry?
- Early studies of atmospheric multiphase chemistry
- Progress in the past 20 years
- The future of atmospheric multiphase chemistry studies
- Concluding thoughts
- Data availability
- Author contributions
- Competing interests
- Disclaimer
- Special issue statement
- Acknowledgements
- Review statement
- References