the Creative Commons Attribution 4.0 License.
the Creative Commons Attribution 4.0 License.
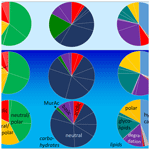
Amino acids, carbohydrates, and lipids in the tropical oligotrophic Atlantic Ocean: sea-to-air transfer and atmospheric in situ formation
Manuela van Pinxteren
Sebastian Zeppenfeld
Khanneh Wadinga Fomba
Nadja Triesch
Sanja Frka
This study examines carbohydrates, amino acids, and lipids as important contributors to organic carbon (OC) in the tropical Atlantic Ocean at the Cape Verde Atmospheric Observatory (CVAO). The above compounds were measured in both surface seawater and in ambient sub-micron aerosol particles to investigate their sea-to-air transfer, including their enrichment in the sea surface microlayer (SML), potential atmospheric in situ formation or degradation, and their oceanic contribution to the ambient marine aerosol particles.
In bulk seawater and the SML, similar distributions among species were found for the lipids and carbohydrates with moderate SML enrichments (enrichment factors EFSML = 1.3 ± 0.2 and 1.1 ± 0.5 respectively). In contrast, the amino acids exhibited a higher enrichment in the SML with an average EFSML of 2.3 ± 0.4, although they are less surface-active than lipids. The same compounds studied in the seawater were found on the ambient sub-micron aerosol particles, whereas the lipids' enrichment was more pronounced (EF) compared to the amino acids and carbohydrates (EF and 1.3×103 respectively), likely due to their high surface activity and/or the lipophilic character. Detailed molecular analysis of the seawater and aerosol particles revealed changes in the relative abundance of the individual organic compounds. They were most pronounced for the amino acids and are likely related to an in situ atmospheric processing by biotic and/or abiotic reactions.
On average, 49 % of the OC on the aerosol particles ( ng m−3) could be attributed to the specific components or component groups investigated in this study. The majority (43 %) was composed of lipids. Carbohydrates and amino acids made up less than 1 % of the OC. This shows that carbohydrates, at least when resolved via molecular measurements of single sugars, do not comprise a very large fraction of OC on marine aerosol particles, in contrast to other studies. However, carbohydrate-like compounds are also present in the high lipid fraction (e.g. as glycolipids), but their chemical composition could not be revealed by the measurements performed here.
Previously determined OC components at the CVAO, specifically amines, oxalic acid, and carbonyls, comprised an OC fraction of around 6 %.
Since the identified compounds constituted about 50 % of the OC and belong to the rather short-lived biogenic material probably originating from the surface ocean, a pronounced coupling between ocean and atmosphere was indicated for this oligotrophic region. The remaining, non-identified OC fraction might in part contain recalcitrant OC; however, this fraction does not constitute the vast majority of OC in the aerosol particles investigated here.
- Article
(1862 KB) - Full-text XML
-
Supplement
(1238 KB) - BibTeX
- EndNote
Marine aerosol particles and their composition, sources, and connection to the upper ocean are not yet fully understood but are important, as they impact the carbon cycle and radiative properties of aerosol particles (Abbatt et al., 2019; Brooks and Thornton, 2018; Burrows et al., 2013; Gantt and Meskhidze, 2013; Pagnone et al., 2019; Patel and Rastogi, 2020). Furthermore, the function of (marine) aerosol particles as cloud condensation nuclei (CCN) and ice-nucleating particles (INPs), i.e. marine aerosol–cloud interaction, is still elusive. Important information about marine CCN is still missing (e.g. Bertram et al., 2018). Ocean-derived INPs have been suggested as playing a dominant role in determining INP concentrations in near-surface air over remote areas such as the Southern Ocean, but their source strength in other oceanic regions and knowledge of which physicochemical properties determine INP efficiency remain largely unknown (Burrows et al., 2013; McCluskey et al., 2018a, b). Marine aerosol particles, notably in the sub-micrometre range, have been shown to contain a large part of organic carbon (OC) in field experiments and in laboratory studies, where nascent aerosol particles are generated by artificial bubble-bursting mechanisms (Facchini et al., 2008; Keene et al., 2007; O'Dowd et al., 2004). Notably, the laboratory experiments, where sources other than the ocean (such as long-range transport) can be excluded, suggest that a certain part of the OC on the aerosol particles is transferred directly from the ocean via bubble bursting (Facchini et al., 2008; Keene et al., 2007). The mechanisms of the OC enrichment finally observed in aerosol particles are not yet fully understood but are likely due to complex interactions at the ocean surface when air bubbles rise and break. Air bubbles collect (organic) matter at their surface (the gas–water interface) when they ascend through the water column and, when bursting, they produce film and jet droplets that transfer the OC to the atmosphere and form aerosol particles. At the ocean surface, the air bubbles enter the uppermost layer and the direct interface between the ocean and the atmosphere called the sea surface microlayer (SML) (Engel et al., 2017). The SML is described as a gel-like matrix that accumulates various organic and inorganic material (Cunliffe et al., 2013). The influence of the SML on the bubble bursting and the emission of OC into the atmosphere is difficult to determine and is still controversial (Engel et al., 2017).
Based on the OC-to-sodium ratios in the ocean and the atmosphere, the OC in marine aerosol particles has been shown to be strongly enriched compared to seawater concentrations. OC aerosol enrichment factors (EFaer.) of the order of 102 in super-micron aerosol particles and of the orders of 103 to 105 in sub-micron aerosol particles have been reported (Quinn et al., 2015, and references therein). However, individual chemical groups, such as amino acids, can be even more enriched, and EFaer. as high as 107 for these particular compounds have been measured in sub-micron particles resulting from bubble-bursting experiments within a tank study (Triesch et al., 2021c). The OC transfer from the ocean to the atmosphere is likely highly chemo-selective, and a hydrophobic nature as well as surface-active properties of organic compounds probably favour their transfer from the sea to the air (Rastelli et al., 2017; Schmitt-Kopplin et al., 2012).
An important component of understanding the OC on marine aerosol particles is the connection to oceanic bio-productivity. Several studies suggested that the marine aerosol composition is directly coupled to the productivity in the ocean, showing that at elevated chlorophyll-a (chl-a) concentrations in the seawater the OC on the aerosol particles is significantly higher when compared to the low oceanic productivity (O'Dowd et al., 2004; Facchini et al., 2008). A coupling between oceanic bio-productivity and aerosol composition is probably not straightforward. Wang et al. (2015) showed that two successive phytoplankton blooms in the tank seawater resulted in sea spray aerosols (SSAs) with vastly different compositions and properties. Other studies, however, propose that the OC transfer from the ocean to aerosol particles is non-correlated with oceanic bio-productivity. Quinn et al. (2014) suggested that the high reservoir of dissolved organic carbon (DOC) in the ocean is responsible for the organic enrichment in freshly emitted sea spray aerosol, thus dominating over any influence of recent local biological activity based on chlorophyll concentrations. Following this, Kieber et al. (2016) proposed that the major component in sub-micron sea spray particles is of a recalcitrant nature with a stability of months to millennia. They suggested that this persistent form of OC can very efficiently be transferred to the atmosphere via bubble bursting. Although they did not perform a detailed chemical analysis, they concluded that the recalcitrant organic matter exhibits surface-active properties. Applying natural-abundance radiocarbon (14C) measurements, it was recently suggested that 19 % to 40 % of the OC associated with freshly produced marine aerosol particles was refractory dissolved organic carbon (rDOC) (Beaupre et al., 2019).
In addition to the direct, or primary, transfer of organic compounds from the ocean to the atmosphere, atmospheric processing changes the composition. Once released from the ocean into the atmosphere, organic matter can be acidified within seconds due to a pH change in the atmospheric particles or undergo fast photochemical oxidation (Kieber et al., 2016). Moreover, biogenic in situ formation and degradation can change the OC composition in marine aerosol particles and marine cloud water (Bianco et al., 2019; Malfatti et al., 2019; Matulova et al., 2014). Ervens and Amato (2020) provided a framework for estimating the production of secondary biological aerosol mass in clouds through microbial cell growth and multiplication. This pathway could be a significant source of biological aerosol material (Ervens and Amato, 2020; Khaled et al., 2021; Zhang et al., 2021). In other recent studies, the in situ formation of amino acids by biotic and abiotic processes in cloud water was measured and modelled (Jaber et al., 2021), and gel-like, organic particles, originally present in the ocean, were suggested to form in situ in the marine atmosphere via biotic and/or abiotic pathways (Haddrell and Thomas, 2017; Klein et al., 2016; van Pinxteren et al., 2022). Nevertheless, despite some studies, the atmospheric in situ transformation of marine organic compounds and its significance have not yet been extensively studied.
To understand the transfer processes of OC from the ocean to the aerosol particles, potential atmospheric OC in situ formation, and the coupling of the OC on the aerosol particles to processes in the ocean, it is crucial to unravel the chemical composition of the aerosol OC content. In the present study, we investigated samples from the tropical Atlantic Ocean at the Cape Verde Atmospheric Observatory (CVAO). The focus of this study was on the analysis of amino acids, carbohydrates, and lipid components, as these OC groups are reported as the major marine organic matter groups in the seawater and are therefore likely transferred to the aerosol particles via bubble bursting (Burrows et al., 2014). We investigated these compounds on marine aerosol particles and in the ocean SML and bulk water. Specifically, we followed the concentrations and speciation of OC from the sea to the atmospheric particles, which helps to evaluate the enrichment factors of the various targeted compounds between the compartments. The results will help to gain a better understanding of the chemical composition of marine aerosol particles in this tropical location and its transfer from the ocean and in situ formation and, finally, help to elucidate the coupling of marine aerosol particles to the surface ocean in an oligotrophic region.
2.1 Aerosol and seawater sampling during the campaign
A field campaign, Marine biological production, organic aerosol particles and marine clouds: a Process chain (MarParCloud), was carried out at the CVAO (16∘51′49′′ N, 24∘52′02′′ E) in autumn 2017 (13 September–13 October 2017), and the sampling sites are illustrated and explained in detail in van Pinxteren et al. (2020). The CVAO is a remote marine station in the tropical Atlantic Ocean located on the north-eastern coast of the island of São Vicente and is described in Carpenter et al. (2010) and Fomba et al. (2014). The ocean around Cabo Verde has the lowest surface chlorophyll in the North Atlantic Ocean, with values below 0.2 µg L−1 for the majority of each year, with periodic events of slightly elevated concentrations of up to 0.7 µg L−1 (van Pinxteren et al., 2020, and references therein).
Sub-micron aerosol particles were sampled on pre-heated 150 mm quartz fibre filters (Munktell, MK 360) at a flow rate of about 700 L min−1 with a high-volume PM1 aerosol sampler (Digitel, Riemer, Germany) installed on the 30 m-high tower at the coastline. The sampling times were usually set to 24 h and are listed in Tables S4 and S5 in the Supplement and in Triesch et al. (2021b) and van Pinxteren et al. (2020). Regarding the aerosol sampling system, it needs to be underlined that artefact problems and overestimations due to gas-phase absorption and underestimations due to re-volatilizations of the analytes from the filters may represent a certain level of uncertainty. To this end, great care was taken to apply field blanks and correct for blank values as pointed out in detail in Sect. 2.2.3.
The seawater samples were taken at Bahia das Gatas, a coastal site that is upwind and about 4 km north-west of the CVAO (Fig. S1 in the Supplement). Fishing boats were rented to go out on the open ocean and the SML was sampled with a typical glass plate SML sampling strategy (Cunliffe and Wurl, 2014). To this end, a glass plate with a sampling area of 2000 cm2 was vertically immersed into the water and then slowly drawn upwards with a withdrawal rate between 5 and 10 cm s−1. The surface film adheres to the surface of the glass and is removed using framed Teflon wipers (Stolle et al., 2010; van Pinxteren et al., 2012). Bulk seawater was collected with a specially designed device consisting of a plastic bottle mounted on a telescopic rod. The bottle was opened underwater at a depth of 1 m with a specifically conceived seal opener.
For the sampling of the oceanic water samples, great care was taken that all parts that were in contact with the sample (glass plate, bottles) underwent an intense cleaning with 10 % HCl and rinsing with ultra-pure water (resistivity = 18.2 MΩ cm) prior to the campaign and in between sampling to avoid contamination and carry-over problems.
After the sampling, all seawater and aerosol samples were stored frozen at −20 ∘C and transported in a reefer at −20 ∘C to the TROPOS laboratories, where they were stored frozen until analysis.
2.2 Chemical analysis
2.2.1 Seawater and aerosol analysis: general considerations
Within the seawater analysis, we measured the dissolved amino acids (DAAs) and dissolved carbohydrates (DCHO) in the DOC fraction, as DOC represents by far the largest pool of organic material in the ocean (Riebesell et al., 2011). DOC is the fraction of OC that passes through a GF/F filter of 0.7 µm pore size (Wurl, 2009); however, filter pore sizes between 0.2 and 1.2 µm are often applied e.g. due to requirements of the analytical system (e.g. Romankevich, 1984; Zäncker et al., 2017). The lipid measurements (from the same samples) were taken from Triesch et al. (2021b), are included in the DOC fraction as well, being filtered via a 0.7 µm pore-sized filter (GF/F, Whatmann) and extracted in an organic solvent, and refer to dissolved lipids (DLs).
For the aerosol particles (PM1), we focused on the water-soluble organic (WSOC) fraction of the amino acids (AAaer.) and carbohydrates (CHOaer.). The lipids, however, were extracted with an organic solvent and filtered (analogous to seawater), meaning they comprise the organic-soluble organic fraction of the aerosol particles (Lipidsaer.).
2.2.2 Analytical methods
For the analysis of the DCHO and CHOaer. as well as the DAAs and AAaer., molecular-resolved techniques were applied. DCHO in filtered (0.2 µm Millex syringe filters) and desalinated SML and bulk water samples and CHOaer. in the filtered PM1 extracts were quantified using high-performance anion-exchange chromatography coupled with pulsed amperometric detection (HPAEC-PAD) (Zeppenfeld et al., 2020, 2021). After an acid hydrolysis (0.8 M HCl, 100 ∘C, 20 h), the monosaccharides fucose (Fuc), rhamnose (Rha), arabinose (Ara), galactose (Gal), glucose (Glc), xylose (Xyl), mannose (Man), galactosamine (GalN), glucosamine (GlcN), muramic acid (MurAc), galacturonic acid (GalAc), and glucuronic acid (GlcAc) were measured.
For the DAA analysis, seawater samples (25.5 mL) were desalinated and concentrated to a few millilitres as described in Triesch et al. (2021a). For the AAaer. from PM1 particles, an aqueous extract of the amino acids was prepared by shaking a piece of the filter in 2 mL of water. After a filtration step (filter pore size: 0.2 µm), 25 µL of ascorbic acid (20 mg mL−1, purity 99 %, Sigma-Aldrich, St. Louis, Missouri, USA) was added to a 200 µL aliquot of the desalted seawater/aqueous filter extract to avoid the oxidation of the obtained amino acids as suggested in Mandalakis et al. (2010). Following the addition of 250 µL of HCl (Supra-quality, ROTIPURAN® Supra 35 %, Carl Roth, Karlsruhe, Germany), the hydrolysis was performed at 110 ∘C for 20 h. After cooling to room temperature, the hydrolysed filtrate was evaporated, resolved in 500 µL milliQ-water (Millipore Elix 3 and Element A10, Merck Millipore, Darmstadt, Germany), filtered, derivatized using the AccQ-Tag™ pre-column derivatization method (Waters, Eschborn, Germany), and measured by ultra-high-performance liquid chromatography with electrospray ionization and Orbitrap mass spectrometry (UHPLC/ESI-Orbitrap-MS), as described in Triesch et al. (2021a). The analytes include the amino acids glycine (Gly), alanine (Ala), serine (Ser), glutamic acid (Glu), threonine (Thr), proline (Pro), tyrosine (Tyr), valine (Val), phenylalanine (Phe), aspartic acid (Asp), isoleucine (Ile), leucine (Leu), methionine (Met), glutamine (Gln), and γ-aminobutyric acid (GABA) (purity ≥99 %, Sigma-Aldrich, St. Louis, Missouri, USA). The DL and Lipidsaer. measurements were taken from Triesch et al. (2021b), where the analysis was done with a semi-molecular technique. For a better understanding of the data, a short description is given in the following: the dissolved fraction of seawater samples (obtained after filtration through pre-combusted 0.7 µm GF/F filters) and aerosol filters were extracted with dichloromethane according to specific protocols after adding 2-hexadecanone as an internal standard, as described in more detail in Triesch et al. (2021b). The extract was analysed with thin-layer chromatography (TLC). Lipid classes were separated on Chromarods SIII and calibrated with an external calibration with a mixture of standard lipids by a chromatograph flame ionization detector (FID) Iatroscan MKVI (Iatron, Japan). The separation scheme included elution steps in the solvent systems with increasing polarity. The lipid classes included hydrocarbons (HC), fatty acid methyl esters (ME), free fatty acids (FFA), alcohols (ALC), 1,3-diacylglycerols (1,3 DG), 1,2-diacylglycerols (1,2 DG), monoacylglycerols (MG), wax esters (WE), triacylglycerols (TG), pigments (PIG), phospholipids (PP) including phosphatidylglycerols (PG), phosphatidylethanolamine (PE), phosphatidylcholines (PC), and glycolipids (GL), which cover sulfoquinovosyl diacylglycerols (SQDG), monogalactosyl diacylglycerols (MGDG), digalactosyl diacylglycerols (DGDG), and sterols (ST). It needs to be underlined that, as no single lipid compound but rather lipid groups (based on varying polarity in the TLC system) were measured, the lipid results can be classified as analysis on a semi-molecular level.
OC on the aerosol particles (PM1 samples) was measured by means of a thermal-optical method using the Sunset Laboratory Dual-Optical Carbonaceous Analyzer (Sunset Laboratory Inc., USA) from a filter piece with an area of 1.5 cm2. The EUSAAR 2 temperature protocol was utilized, and a charring correction was applied (Cavalli et al., 2010). The correction value for pyrolytic carbon was determined based on measurements of a sample transmission using a 678 nm laser. Samples were thermally desorbed from the filter medium under an inert He atmosphere followed by oxidizing an O2/He atmosphere while applying carefully controlled heating ramps. A flame ionization detector was used to quantify methane following a catalytic methanation of CO2.
Sodium was measured from filtered (0.45 µm syringe filter) aqueous extracts of the PM1 samples using ion chromatography (more details in Zeppenfeld et al., 2021; van Pinxteren et al., 2022). The details on sampling and the sample treatment are summarized in Table S1.
Atmospheric concentrations were calculated from the measured analyte concentrations on the filter or in the filter extract. The masses of the analytes on the filter were extrapolated to the total filter and related to the collected air volume that had flown through the filter.
2.2.3 Detection limits and blank handling
Detection limits were 0.5–2.5 µg L−1 (DAAs) and 0.1–0.2 pg m−3 (AAaer.) (Triesch et al., 2021a, c) and 0.13–0.7 µg L−1 (DCHO) and 0.5–5 pg m−3 (CHOaer.) (Zeppenfeld et al., 2021). Regarding the lipids, the method was carefully optimized for seawater analysis (Gašparović et al., 2015, 2017) and adopted for aerosol particle analytics as described in Triesch et al. (2021b). The detection limits were determined as the analyte concentrations corresponding to a signal-to-noise () ratio of 3 ranging from 0.06 to 0.33 µg as reported previously in Penezić et al. (2022).
For the seawater analysis, field blanks were taken by filling high-purity water in pre-cleaned plastic bottles and handled in the same way as the seawater samples. The seawater blanks were in general below 10 % and, for the lipids, always below 15 % (Triesch et al., 2021b). All data are blank-corrected by subtracting the field blank values from the samples.
Field blanks for aerosol particles were prepared using pre-baked quartz fibre filters without an active sampling and treated according to the same procedure as the field samples. The concentrations of the target analytes were calculated by external calibration. Each sample was measured twice with a relative standard deviation of typically <10 %, and field blanks, which were for most compounds negligible and for the lipid classes always below 20 % of the real aerosol particle sample, were subtracted. All presented values are corrected for the field blank.
2.3 Enrichment factors
The SML enrichment factor (EFSML) was calculated by dividing the concentration of the analyte in the SML by the concentration of the analyte in the bulk water after Eq. (1):
An enrichment in the SML is indicated with EFSML>1 and a depletion in the SML with EFSML<1.
The enrichment factor of aerosol (EFaer.) is a quantitative metric for comparing compounds in the ocean and in the atmosphere. The EFaer. concept is mainly applied to closed systems (Quinn et al., 2015, and references therein; Rastelli et al., 2017) as degradation and formation pathways on aerosol particles including photochemical and biotic atmospheric reactions and contributions from other (non-marine) sources are excluded from this parameter. Nevertheless, for comparison purposes, it is useful to apply the EFaer. to open systems as well, as shown in several studies (Russell et al., 2010; Triesch et al., 2021a, b; van Pinxteren et al., 2017; Zeppenfeld et al., 2021). To this end, the concentration of the analyte of interest in each compartment is related to the respective sodium concentration (Eq. 2), because sodium is regarded as a conservative sea salt tracer transferred to the atmosphere in the process of bubble bursting (Sander et al., 2003).
To account for the different timescales for ocean and atmospheric processes and to improve robustness, we applied the average concentration values of the respective compound groups in seawater and on aerosol particles as done by Zeppenfeld et al. (2021) and van Pinxteren et al. (2022). It should be noted that EFSML and EFaer. comparisons include samples from the same campaign but not necessarily from the same exact date (details in Tables 1 and S2–S5). However, as our focus was not on showing trends within the campaign (this was covered by Triesch et al., 2021a, b) but rather on comparing the three different OC groups with each other, the usage of average values is justified. To investigate the variability of the EFaer., the minimum ratio of versus the maximum ratio of was applied and vice versa. As seawater concentration, the bulk water or the SML concentration can be applied.
Statistical significance was evaluated using the analysis of variance (ANOVA).
Table 1Average concentrations of the organic groups and enrichment factors (EFs) in the SML (EFSML) and in the aerosol particles (EFaer.) after Eqs. (1) and (2). EFs were calculated from the average concentrations of the respective groups (values in Table 1). For EFaer. the average Na+ concentration in seawater (1.0×104 mg L−1) and the average Na+ concentrations in the PM1 particles from the MarParCloud campaign (100 ng m−3, values from Triesch et al., 2021b) were applied. To investigate the variability of the EFaer., the minimum ratio of versus the maximum ratio of was applied (and vice versa), and the range of EFaer. is given in parentheses. For comparison, the last column lists the EFaer. for PM1 from a chamber study (Rastelli et al., 2017).
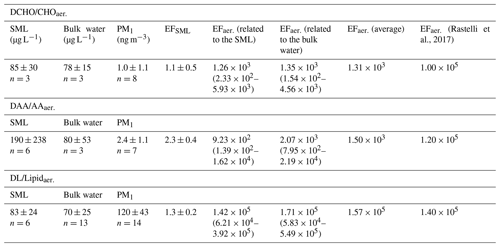
3.1 SML and bulk water: concentration and composition
Figure 1 shows the analyte concentrations in the bulk ocean water (DAA: 80 ± 53 µg L−1, DCHO: 78 ± 15 µg L−1, DL: 70 ± 25 µg L−1) and in the SML (DAA: 190 ± 238 µg L−1, DCHO: 85 ± 30 µg L−1, DL: 83 ± 24 µg L−1). Hence, the average concentrations for DCHO and DLs are similar in the bulk water and in the SML (detailed values are in Sect. 2.2.2 and Table 1, and DL concentrations can be found in Triesch et al., 2021b). For the DAAs, however, SML concentrations show a larger variability compared to the other compounds and to bulk water. However, despite the variability of the DAAs in the SML, they are not statistically different to the other two compound groups (DLs and DCHO) or the DAAs in the bulk water (ANOVA one way, p>0.5 at the 0.5 level) that are different in the case of free amino acids (FAAs). In total, no significant difference is present between the compound groups within the SML and the bulk water (ANOVA one way, p>0.5 at the 0.5 level). Resulting from the higher SML concentrations, the average SML enrichment factors of DAAs are 2.3 ± 0.4 (Table 1) and are therefore higher compared to the DCHO (EFSML = 1.1 ± 0.5) and DLs (EFSML = 1.3 ± 0.2). The high variability of the DAA concentrations agrees well with the FAAs that comprise the sum of unbound individual amino acids, i.e. not bound in a peptide or protein, and that were measured at this location during the MarParCloud campaign (Triesch et al., 2021a). However, the FAAs in the SML were significantly higher compared to the FAAs in the bulk water (Triesch et al., 2021a). Other studies have pointed out highly variable amino acid concentrations as well; for example, Zäncker et al. (2017) showed FAA concentrations between 32 and 1268 nmol L−1, and DAAs varied between 202 and 2007 nmol L−1 (for comparison, the DAA values presented here correspond on average to 1064 nmol L−1 in the bulk water and 2536 nmol L−1 in the SML). High enrichments of FAAs in the SML were reported (Kuznetsova and Lee, 2002; Kuznetsova et al., 2004; Reinthaler et al., 2008; van Pinxteren et al., 2012; Engel and Galgani, 2016), with FAA enrichments of up to 300 in the SML of the Cabo Verde seawaters (Triesch et al., 2021a). A preferential enrichment of FAAs over dissolved combined amino acids as a consistent microlayer feature was proposed (Kuznetsova et al., 2004).
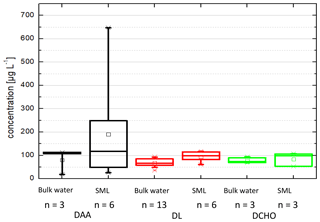
Figure 1Box-and-whisker plot of the concentrations in seawater (µg L−1), distinguished into SML and bulk water for the dissolved amino acids (DAAs) in the SML (n=6) and in bulk water (n=6), for the dissolved carbohydrates (DCHO) in the SML (n=3) and in bulk water (n=3), and for the dissolved lipids (DLs) in the SML (n=6) and in bulk water (n=13). Each box encloses 50 % of the data, with the mean value represented as an open square and the median value represented as a line. The bottom of the box marks the 25 % limit of the data, while the top marks the 75 % limit. The lines extending from the top and bottom of each box are the 5th and 95th percentiles within the data set, while the asterisks indicate the data points lying outside of this range (“outliers”).
Regarding the composition of the individual DAAs measured here, clear differences between the SML and the bulk water characteristics were observed (Fig. 2, blue and orange bars, data in Tables S2–S5). Besides the higher concentrations in the SML, some DAAs were only present in the SML and not in the bulk water (below the detection limit). This was most pronounced for Glu but was also evident for Tyr and Iso (detailed values in Table S3).
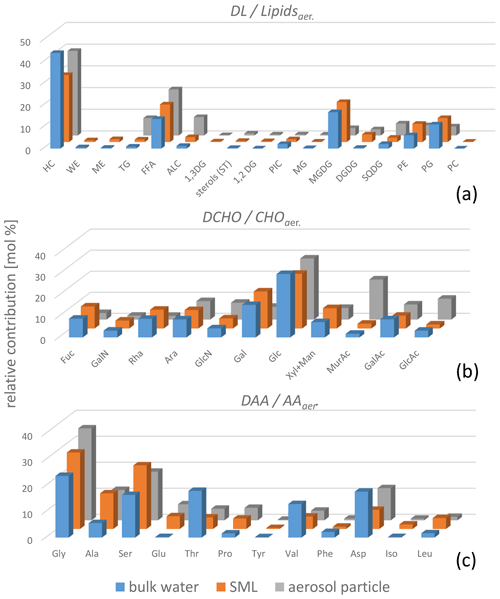
Figure 2Bar graph showing the average of the relative compositions (mol %) of DLs and Lipidsaer. (a), DCHO and CHOaer. (b), and DAAs and AAaer. (c) in the bulk water (blue bars), the SML (orange bars), and the PM1 aerosol particles (grey bars). Mol percentages were calculated from the molar masses of the respective analytes. For the lipid groups, the molar masses of the surrogate standard (Sect. 2.2.2 and Table S8) are applied. The relative mol fractions are relative to the total of each type (DAAs, DCHO, and DLs) analysed.
In contrast to the DAAs, the DCHO enrichment in the SML was less pronounced, with an average EFSML of 1.1 ± 0.5 (Table 1), similar to SML enrichment values obtained for DCHO close to the Peruvian upwelling regime (Zäncker et al., 2017) and the Antarctic Peninsula (Zeppenfeld et al., 2021). Regarding the relative composition, the DCHO showed a very homogeneous pattern and were similar in the SML and the bulk water (Fig. 2, blue and orange bars).
The enrichment of the DLs (EFSML = 1.3 ± 0.2, Table 1) was very similar to the DCHO enrichment and was discussed by Triesch et al. (2021b). From the individual lipid components and the lipolysis index, it was concluded that the lipids were degraded only to a small extent (Triesch et al., 2021b).
Altogether, the high and varying concentrations and enrichments of DAAs in the SML, in contrast to the DCHO and DL concentrations, underline that significant changes occur for the DAAs in the SML that are less pronounced for the other two compound groups. However, it is important to mention that the number of samples that have been analysed is different and limited (Table 1 and Fig. 1), and samples from partly different dates of the campaign have been analysed and compared.
3.2 Discussion of the SML enrichment
3.2.1 Surface versus bulk SML
The SML enrichment of DOC components is generally attributed to diffusion, turbulent mixing, scavenging, and transport of surface-active matter from rising gas bubbles in the water column (Liss and Duce, 1997). Within the groups investigated here, the DLs are the most hydrophobic compounds and are generally classified as highly surface-active compounds (Burrows et al., 2014). Although the surface-activity parameters (e.g. octanol–water partition coefficient, density, topological polar surface area) of the individual lipids differ among each other (values in Triesch et al., 2021b), the lipids are overall more non-polar and surface-active compared to the carbohydrates and amino acids (values in Triesch et al., 2021a). Nevertheless, the enrichment of the DLs in the SML was significantly lower compared to the carbohydrates and amino acids. This was obvious from a comparison of the averaged values and from single samples obtained on the same dates (3 and 7 October 2017, values in Tables S2 and S3 and Triesch et al., 2021b).
One explanation for the finding lies in the sampled SML thickness. With the glass plate technique, an SML thickness of about 100 µm has been sampled and reported for the CVAO area (van Pinxteren et al., 2017). Hence, the 100 µm-thick SML might be very well-mixed with regards to the soluble amino acids and carbohydrates, but the surface-active compounds, such as lipids, are potentially located at the very top and form a thin (nanometre-thick) monolayer. In the literature, the SML is described either as a series of sub-layers of wet and dry surfactants (Hardy, 1982) or as a gelatinous matrix (Sieburth, 1983). Independent of the model, it can be expected that a gradient along the surface likely forms with surfactants at the very top of the layer. The formation of a lipid-rich nanolayer at the very top agrees with surface-sensitive spectroscopy measurements that are able to tackle the uppermost layer and finds strong indications of a nanolayer dominated by soluble surfactants (Lass and Friedrichs, 2011) and hydrophobic low-molecular-weight lipids (Frka et al., 2012). The nanolayer, however, cannot be probed with currently applied SML sampling methods. Therefore, the measured SML concentrations may represent a very diluted (likely highly lipid-enriched) layer. Consequently, the SML structure is even more complex, which needs to be considered, particularly when discussing lipid enrichments in the SML. Here, a combination of bulk measurements with dedicated surface probing appears highly desirable.
3.2.2 Details of SML enrichment mechanisms
(i) Co-adsorption
Regarding the DAAs in detail, it is interesting to note that some compounds are exclusively present in the SML, as mentioned above. They belong to hydrophilic (Glu), hydrophobic (Iso), and neutral (Tyr) fractions of amino acids, underlining that their occurrence in the SML might not be related (solely) to their physicochemical properties. Besides an air-bubble-driven transfer to the surface, enrichment in the SML can be supported by co-adsorption mechanisms. Less surface-active compounds (e.g. amino acids and carbohydrates) can be attached due to ionic interactions/coulomb interactions to the head groups of the air-bubble-attached surfactants (e.g. lipids) that mediate their enrichment in the SML (Burrows et al., 2016; Hasenecz et al., 2019; Link et al., 2019; Schill et al., 2018). Co-adsorption can provide an explanation for the high occurrence of non-surface-active, very soluble compounds such as carbohydrates. A recent laboratory study showed different mechanisms for the co-adsorption of polysaccharides that form a second calcium-bridge sub-layer underneath the monolayer, whereas monosaccharides intercalate and induce reorganization within the nanolayer (Vazques de Vasquez et al., 2022). However, in the current study, only a small SML enrichment of the DCHO was observed and, hence, no indication of a strong co-adsorption.
(ii) In situ processing: abiotic versus biotic
Further explanations for the accumulation of dissolved compounds require in situ formation or degradation by SML-specific reactions that might be triggered by distinct environmental conditions in the SML. Biotic pathways and abiotic SML-specific (photo)chemical reactions may strongly impact OC cycling at the sea surface (Liss and Duce, 1997). The high abundance of the amino acid Glu in the SML observed here was also reported in the FAA fraction by Triesch et al. (2021a) and can likely be explained by in situ formation. In incubated cloud water, as another marine compartment, Glu has been shown to be produced via biotic and abiotic mechanisms, e.g. via the oxidation of proline (Jaber et al., 2021, and references therein), and such processes may be relevant in the ocean surface as well. Regarding biotic processes, it is well-known that microorganisms have complex and highly interconnected enzymatic networks and can biodegrade or biosynthesize organic compounds (Kyoto Encyclopedia of Genes and Genomes – KEEG – pathway). Kuznetsova and Lee (2002) suggested that stressed microorganisms, rich in dissolved combined amino acids, may be leached and release them, which in turn affects the pools of both these compounds in seawater. Although such formation mechanisms generally happen in the upper ocean, there are indications of SML-specific processes. Along a transect from upwelling regions toward oligotrophic gyres, it was found that while in the bulk water a clear trend toward degradation of amino acids was observed, the production and degradation patterns of amino acids in the SML were much more complex (Reinthaler et al., 2008). This is indicative of the role of the SML in the production of labile DOC driven by coupled microbial and photochemical processes. Similarly, Kuznetsova and Lee (2001) observed that peptide turnover was always faster in the SML than in sub-surface waters, likely due to the greater concentrations of DOC in the SML. The authors concluded that the accumulation of organic and inorganic compounds in the SML leads to a more nutritious medium for microbial growth and consequently enzymatic hydrolytic activity compared to the bulk water. Connecting this to the results presented here, this might suggest that changes induced by abiotic and biotic processing need to be considered when regarding the SML composition. Although such reactions likely also affect lipids and carbohydrates, they seem to be most pronounced for amino acids.
(iii) Microbial nitrogen fixation at the sea–air interface
A further mechanism contributing to the high and variable SML enrichment of the DAAs at the current location might be microbial nitrogen fixation at the sea–air interface. Measurements showed that cyanobacteria are a very pronounced phytoplankton group in this region (Franklin et al., 2009; Hepach et al., 2014; Zindler et al., 2012), which was dominant during the MarParCloud campaign (van Pinxteren et al., 2020). Cyanobacteria are able to take up nitrogen from the atmosphere (Zehr, 2011). Earlier studies showed that cyanobacteria-fixed nitrogen is incorporated into amino acids (specifically glutamine; Carpenter et al., 1992). The calculated net amino acid release from cyanobacterial colonies (Trichodesmium thiebautii) revealed that nitrogen fixation and the biogeochemical turnover of ambient amino acids are an important source of recently fixed (“new”) nitrogen within the oceanic surface water (Capone et al., 1994). These considerations are, however, highly speculative and demand further studies to investigate whether nitrogen fixation and biosynthesis via cyanobacteria, which is often observed in sub-tropical and tropical oceans (Montoya et al., 2007), might establish a considerable route for amino acid formation and enrichment in the SML from the atmospheric side.
(iv) Concluding remarks on the SML enrichment
Although SML enrichment factors for amino acids, carbohydrates, and lipids have been reported in the available literature, they have not previously been shown in such detailed analysis for samples collected from the same site as shown here. From this study it can be concluded that the amino acids are strongly enriched in the SML compared to carbohydrates and lipids, even under the same environmental conditions. In a recent study, we showed a strong enhancement of other nitrogen-containing species (aliphatic amines) in the SML at this location, while the amine concentration in the bulk water was often not detectable (van Pinxteren et al., 2019). This suggests that the pronounced SML enrichment specifically exists for nitrogen-containing organic species. In addition, the absence of a relation of the SML enrichment to physical compound parameters (e.g. hydrophobicity) suggests that enrichment processes based on physicochemical properties (e.g. surface activity) alone do not drive SML enrichment. Rather, an SML in situ formation mechanism impacts the abundance of amino acids and likely nitrogen-containing organic species in general.
3.3 Aerosol particles: concentration and composition
After evaluating the concentrations of the analytes in seawater and the SML, in the next step, their presence in the aerosol particles was investigated. The concentrations of AAaer. and CHOaer. were 2.4 ± 1.1 and 1.0 ± 1.1 ng m−3 respectively (Fig. 3, Table 1) and are not significantly different from bulk water (ANOVA one way, p>0.5 at the 0.5 level).
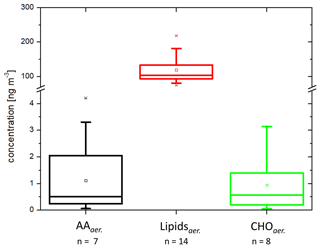
Figure 3Box-and-whisker plot of the concentrations in the PM1 aerosol particles (ng m−3): n=8 for CHOaer., n=7 for AAaer., n=14 for Lipidsaer. Each box encloses 50 % of the data, with the mean value represented as an open square and the median value represented as a line. The bottom of the box marks the 25 % limit of the data, while the top marks the 75 % limit. The lines extending from the top and bottom of each box are the 5th and 95th percentiles within the data set, while the asterisks indicate the data points lying outside of this range (“outliers”).
Compared to results from the polar regions, the CHOaer. concentrations in the tropical Atlantic Ocean analysed here are at the lower end. Leck et al. (2013) determined carbohydrates during the Arctic summer and found 0.7–20 ng m−3 in sub-micron particles. Zeppenfeld et al. (2021) found carbohydrate concentrations between 0.2 and 11.3 ng m−3 in PM10 atmospheric particles, in the Western Antarctic Peninsula, which contributed about 3 % to the OC. The same holds true for amino acids: the concentrations found here are slightly lower than those reported for other marine regions. For example, FAAs in Antarctic aerosol particles were on average 4.6 ng m−3 in Antarctic aerosol (Barbaro et al., 2015). Triesch et al. (2021a) found FAA concentrations between 1.5 and 3.0 ng m−3 in the aerosol particles from Cabo Verde. The AAaer. are generally of the same order of magnitude compared to other marine locations (Matos et al., 2016; Matsumoto and Uematsu, 2005; Renard et al., 2022; Wedyan and Preston, 2008). However, concentrations of amino acid and carbohydrates in urban, rural, and forest areas are significantly higher (2–3 orders of magnitude) compared to the values, and the spectrum of the compounds is strongly shifted (e.g. Dominutti et al., 2022; Samaké et al., 2019; Zhu et al., 2022).
Comparing the data obtained here to those from analytical techniques that use functional group information (Fourier-transform infrared spectroscopy – FT-IR) has shown that the latter techniques often attribute a large fraction of alcohol (hydroxyl) functional groups to the marine aerosol particles (Cravigan et al., 2020; Frossard et al., 2014; Russell et al., 2010). According to Russell et al. (2010), the primary marine signal in sub-micron marine aerosol over the North Atlantic and Arctic oceans is made on average for 88 % of hydroxyl groups corresponding to carbohydrate-like material. Such high fractions of carbohydrates were not found in the chromatographic analysis of CHOaer. presented here or in other studies using similar methodologies (e.g. Zeppenfeld et al., 2021). In a recent study, using thermal desorption mass spectrometry, it was suggested that carbohydrates only represented a minor fraction of the FT-IR alcohol group, and another thermally stable fraction, different to carbohydrates, was the main contributor to the alcohol group (Lawler et al., 2020). Hence, previous FT-IR measurements might have overpredicted the carbohydrate fraction of marine aerosol particles, and further (molecular-based) analysis should be conducted in comparison to resolve existing contradictions.
In contrast to the AAaer. and CHOaer., the Lipidaer. concentrations were 120 ± 43 ng m−3 and therefore 2 orders of magnitude higher than the other two organic groups (Fig. 3, Table 1). The Lipidaer. were significantly higher than the AAaer. () and the CHOaer. () (ANOVA one way, at the 0.5 level). One possible reason for the higher Lipidaer. concentration could lie in the difference in the extraction procedure (Sect. 2.2), as the AAaer. and CHOaer. were extracted in water, while the Lipidaer. were extracted in an organic solvent. Previous lipid analysis on a molecular level revealed concentrations between 0.19 and 23 ng m−3 for ALC and between 2.5 and 38 ng m−3 for free fatty acids on marine aerosol particles from the western North Pacific (Kawamura et al., 2003), and a recent study found marine fatty acid concentrations between 50 and 90 ng m−3 in coastal aerosol at Qingdao (Chen et al., 2021). Mochida et al. (2002) observed saturated fatty acids (C14–C19) on marine aerosol particles over the North Pacific in atmospheric concentrations between 0.8 and 24 ng m−3. Hence, these data are of the same order of magnitude as the lipid groups measured here (ALC: 6.3 ng m−3, free fatty acids: 18.5 ng m−3, values in Triesch et al., 2021b). Cochran et al. (2017) showed that lipid components (long-chain fatty acids) comprised a significant fraction of up to 75 % of the identified organic constituents in aerosol particles from a sea spray tank.
A recent study using Fourier-transform ion cyclotron resonance mass spectrometry (FT-ICR-MS) found that, among a large number of molecular formulas, large peak numbers were assigned to proteins and lignins (phenolic macro-molecules), followed by carbohydrates and lipids in aerosol originating from the Arctic Ocean (versus non-ocean aerosol) (Choi et al., 2019).
High lipid fractions in marine aerosol particles were also reported from nuclear magnetic resonance (NMR) measurements. Measurements of nascent aerosol particles produced from North Atlantic seawater showed that the water-soluble organic aerosol fraction was purely aliphatic, with hydroxylated moieties of sugars, esters, and polyols, aliphatic groups adjacent to carbonyls, amides, and acids, and aliphatic chains with terminal methyl groups typical of lipids (Facchini et al., 2008). The water-insoluble organic faction was dominated by lipopolysaccharides, known phytoplankton exudate components. A recent study applying NMR analysis to artificially produced aerosol particles after bubbling seawater from offshore areas also showed proof of polyols and lipids (Decesari et al., 2020). NMR measurements of lipids are mainly qualitative; however, the high fraction of lipid-like components from other regions agrees well with the high Lipidaer. concentrations presented here.
A high Lipidaer. concentration as observed in the present study agrees well with the modelling results of Burrows et al. (2014), where the ocean–atmosphere transfer was calculated according to the physicochemical properties of the distinct OC groups. Lipids, as the most surface-active OC group, comprise the largest fraction of the aerosol fraction, although their (modelled) concentration in the seawater is lower compared to carbohydrates and amino acids (Burrows et al., 2014). In the latter model modification, where additional co-adsorption processes were included in the calculations, a more pronounced saccharidic fraction was determined on the aerosol particles from the model results (Burrows et al., 2016), which is different from the findings here, at least regarding the CHOaer. measured on a molecular level. However, it needs to be considered that the lipids analysed here include glycolipids (MGDG, DGDG, SQDG), which are components that have the solubility properties of a lipid but that also contain one or more carbohydrate molecules. The glycolipids comprise a non-negligible portion of the OC on the aerosol particles (values in Tables S6 and S8). This underlines the complexity of attributing the OC to distinct organic groups and demonstrates that the applied analytical methods must be taken into account when comparing concentrations of substance groups. This is discussed in more detail in Sect. 3.7.1. Altogether, there seems to be a discrepancy between the measured concentrations and the modelled results, underlining that the transfer of the organic compounds from the ocean to the atmosphere based on their physicochemical properties might not be the only mechanism.
3.4 Aerosol enrichment
3.4.1 Aerosol enrichment factors
The finding that the Lipidsaer. were much more concentrated than the AAaer. and the CHOaer. resulted in a very different pattern compared to the similar seawater concentrations (Fig. 1 versus Fig. 3). To quantitatively compare the seawater and the aerosol concentrations, the EFaer. were calculated (values in Table 1).
For the amino acids, the EFaer. were between 9.2×102 (related to the SML) and 2.1×103 (related to the bulk water) and on average 1.5×103. For the carbohydrates, the EFaer. were between 1.3×103 (related to the SML) and 1.4×103 (related to the bulk water) and on average 1.3×103 and therefore similar to the EFaer. of the amino acids. For the lipids, however, the EFaer. were 2 orders of magnitude higher (EF, related to the SML; EF, related to the bulk water; EF on average, Table 1).
3.4.2 Oceanic transfer and atmospheric in situ formation
The overall high enrichment of OC in the aerosol particles is explained by complex, as-yet resolved interactions at the ocean surface where organic matter is enriched relative to sodium during the formation of film and jet droplets. Burrows et al. (2014) applied a conceptual model (“slab” model) where all organics partition to the surface of a “slab” of oceanic water or to both the outer and inner surfaces of a bubble film. The organic enrichment is therefore significantly higher for the thinner bubble films (bubble film thicknesses: 0.01 to 1 µm) than for the thicker SML (typically sampled SML thicknesses: 20 to 400 µm). This mechanism can explain EFaer. of OC in sub-micron aerosol particles of 102 to 103 compared to the SML (Burrows et al., 2014). However, EFaer. from ambient and laboratory-controlled observations show that, for some compounds, even higher EFaer. are obtained. In a controlled tank study, Rastelli et al. (2017) found strong enrichments for lipids (up to 1.4×105) as well as for proteins (up to 1.2×105) and carbohydrates (up to 1.0×105, Table 1). A recent controlled bubble-bursting laboratory study showed that amino acid enrichments can be up to 107 in sub-micron SSA between 0.029 and 0.060 µm (Triesch et al., 2021c). Similarly, Schmitt-Kopplin et al. (2012) showed that surface-active biomolecules are preferentially transferred from surface water into the atmosphere via bubble bursting. The ambient enrichment factor of the lipids (105) shown here and in Triesch et al. (2021b) agreed well with laboratory-derived ones (Rastelli et al., 2017), indicating that the transfer mechanisms simulated in laboratory experiments agree with observations performed here in the field. Hence, the high surface activity and/or lipophilic character of the lipid classes might explain their strong (chemo-selective) transfer to the aerosol particles. Even though the lipid composition on the aerosol particles varied slightly from the seawater concentration (Triesch et al., 2021b), their transfer is likely driven by their physicochemical properties (high surface activity and/or the lipophilic character). For the amino acids and carbohydrates, however, more complex mechanisms may determine their transfer to the atmosphere. Rastelli et al. (2017) suggested that diverse biological processes on the ocean drive the properties of proteins and carbohydrates on the ocean surface and in the atmosphere. Moreover, these compounds are known to be involved in marine gel-like particle formation, such as transparent polymer particles (TEPs) and Coomassie stained particles (CSPs), observed in the ocean and more recently in the atmosphere (Aller et al., 2017; Kuznetsova et al., 2005; van Pinxteren et al., 2022), adding more complexity to the system. Hence, not only a sea-to-air transfer but also atmospheric in situ formation and degradation might determine the concentration of the OC and notably of the amino acid and carbohydrates. This suggests that atmospheric processing plays an important role besides the physically driven bubble-bursting sea–air transfer of OC.
3.5 Limitations of the concept of an aerosol enrichment factor
When comparing OC in the ocean and the atmosphere, it needs to be considered that processes in the ocean and the atmosphere happen on different timescales. In addition, the seawater samples comprise spot samplings in the ocean, while the sampling period of PM1 aerosol particles at the CVAO covers a time span of 24 h. These issues make a comparison between the ocean and atmospheric data very challenging. However, the air masses arriving at the CVAO often followed the water current (Pena-Izquierdo et al., 2012; van Pinxteren et al., 2017) and suggest a strong link between the upper ocean and the aerosol particles, as mainly winds drive the ocean currents in the upper 100 m of the ocean. Besides the ocean, Saharan dust is a strong aerosol source at Cabo Verde, most pronounced in the months December to February (Fomba et al., 2014). The backward trajectories during the time of the campaign (Fig. S2) as well as the mass concentrations of inorganic ions and mineral dust tracers on the aerosol particles measured during the campaign suggested a predominant marine origin with low to medium dust influences (van Pinxteren et al., 2020). Moreover, dust generally influences the super-micron particles to a larger extent than the sub-micron particles analysed here (Fomba et al., 2013). Hence, although different factors certainly affect the aerosol composition, it is reasonable to assume a strong oceanic contribution.
3.6 Seawater and aerosol particles: comparison of the relative composition
Regarding the organic components on the aerosol particles, the same compounds that were present in the seawater were generally present on the aerosol particles (Fig. 2, grey bars, and values in Tables S2–S5). However, the relative composition of distinct compounds was, at least partly, different. Regarding the carbohydrate composition, the percentages of MurAc, GlcAc, and GlcN in the aerosol particles were higher compared to the seawater. MurAc and GlcN are important constituents in the cell walls of marine microorganisms and, notably, MurAc serves as a proxy for bacterial biomass (Mimura and Romano, 1985). Its high concentration might indicate an enrichment of bacteria on the aerosol particles. Zeppenfeld et al. (2021) detected similar (biogenic) carbohydrates in particles sampled in the Western Antarctic Peninsula and suggested that marine bacteria in atmospheric particles may metabolize a part of the oceanic carbohydrates in a selective enzymatic way analogous to the bacterial processes in seawater. Such processes might explain the changed carbohydrate composition and are likely not restricted to a specific oceanic regime, as they seem to happen in the Southern Ocean (Zeppenfeld et al., 2021) and in the tropical Atlantic Ocean, as observed here. The elevated relative occurrence of GlcAc found here agrees well with the recent finding of a high abundance of gel-like material in aerosol particles at the CVAO, strongly enriched towards sodium compared to seawater (van Pinxteren et al., 2022) as GlcAc is one main component of marine gelatinous exopolysaccharides (Casillo et al., 2018; Krembs et al., 2002). Regarding the lipids, surfactants, such as free fatty acids, and lipophilic compounds, such as hydrocarbons, had major contributions in the seawater and on the aerosol particles respectively. However, TG, an energy storage lipid, had a higher contribution to the aerosol particles versus the ocean water. In addition, some other, minorly contributing lipid classes were partly different within the ocean and atmosphere (Triesch et al., 2021b).
The most remarkable difference in relative composition in seawater and in aerosol particles was found for amino acids, as some DAAs were clearly present in the SML and in the aerosol particles but not in the bulk water (e.g. Iso and Glu, Fig. 4, individual values in Table S6). The amino acids generally differed a lot regarding their SML and bulk water composition. This was visible in the data set presented here for the DAAs and also reported for the FAAs measured from the same campaign (Triesch et al., 2021a). Recently it was reported that the acidic amino acid Glu (in the form of FAAs) is transferred to SSA to a large extent (Triesch et al., 2021c), and the results of the present study suggest that Glu might be transferred solely from the SML (and not from the bulk water) to the aerosol particles. However, besides the oceanic transfer, Glu can result from an in situ formation on the aerosol particles. Similarly to the seawater, Glu might form from biotic or abiotic reactions on the aerosol particles. From the measurements performed here, it is not possible to differentiate between a selective transfer of Glu from the SML and its biotic and abiotic in situ formation on aerosol particles. Recently, Jaber et al. (2021) and Renard et al. (2022) evaluated the atmospheric aging of the amino acids and considered biotic and abiotic (mainly oxidation) processing. Their calculations revealed different atmospheric lifetimes for the individual amino acids related to oxidation and biological processes respectively. For example, the amino acids Ser and Ala are degraded quickly by biological processes (lifetime of a few hours) but are more stable towards oxidation (Renard et al., 2022). Such studies can help to understand the patterns of the amino acids as observed here and relate them to sources and atmospheric processing. The presence of Ser and Ala in the aerosol particles investigated here could therefore indicate that biodegradation of these compounds was not pronounced. However, additional studies are needed to better understand atmospheric biotic and abiotic processing. In addition, the transfer of individual DAAs exclusively from the SML shall be investigated in further research, preferably within characterized and controlled bubbling systems.
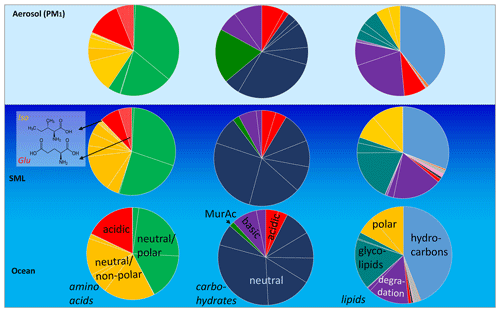
Figure 4Scheme underlining the seawater (SML and bulk water) and the PM1 relative compositions of DLs/Lipidsaer., DCHO/CHOaer., and DAA/AAaer. Assignment: amino acids: neutral/polar: Phe, Gly, Ser, Tyr, neutral/non-polar: Thr, Ala, Pro, Val, Leu, Iso, acidic: Aps, Glu; carbohydrates: basic: GlcN, GalN, neutral: Fuc, Rha, Ara, Gal, GlC, Xyl, Man, acidic: MurAc, GasAc, GlAc; lipids: hydrocarbons (HC), sterols (ST), pigments (PIC), fatty acid methyl ester (MW), membrane component: WE, metabolic reserve: TC, degradation lipids: FFA, ALC, 1,3 DG, 1,2 DG, MG, glycolipids: MGDG, DGDG, SQDG, polar lipids: PE, PG, PC.
3.7 Contribution to aerosol particle OC
3.7.1 Molecular and semi-molecular analysis
OC concentrations in marine aerosol particles during this campaign varied between 0.13 and 0.31 µg m−3 with an average value of 0.20 µg m−3 (values in Table S7). This agreed well with previous OC measurements from the CVAO that were on average 0.27 µg m−3 OC (van Pinxteren et al., 2017). To date, only a small percentage of OC on marine aerosol particles has been characterized on a molecular level, and organic biomarkers often comprise only a few percent of the OC (Chen et al., 2021). Fu et al. (2011) measured more than 140 different single organic species in marine aerosol from different oceanic areas; however, the identified species comprised less than 5.7 % of the OC. Taking together the OC components described here (Lipidsaer., AAaer., CHOaer.), the contribution of the identified components to the OC was calculated. Furthermore, the OC contribution of recently identified components from previous campaigns within the Cabo Verde region, in detail, aliphatic amines, methane-sulfonic acid (MSA), oxalic acid, and carbonyls (van Pinxteren et al., 2015), was included. The OC contribution of the single compounds and compound groups are shown in Fig. 5 (values in Table S8). Altogether, about 48 % of the average OC could be explained by the identified components. Regarding the maximum (0.31 µg m−3) and minimum (0.13 µg m−3) OC concentrations within the campaign, the OC contributions of the respective compounds are between 31 % (lower limit) and 74 % (upper limit). The major identified OC fractions (related to the average OC) were the Lipidsaer., with 43 %. They were followed by the aliphatic amines (4 %) that are in good agreement with a recent CVAO study, where they contributed on average 5 % to the (water-soluble) OC (van Pinxteren et al., 2019). MSA (0.9 %) and oxalic acid (0.3 %) were minor OC contributors. Similarly, the CHOaer. and the AAaer. made up minor percentages with 0.3 % and 0.4 % respectively. Regarding the lipids, it needs to be considered that the analysis performed here was not based on the detection of individual analytes but on an organic solvent extraction of the particle constituents and extract separation by solvents with different polarities applied in the TLC. The analytical method has been optimized for seawater analysis. Within atmospheric processing, additional organic compounds can form, which might contain a hydrophobic part and which are potentially included in the lipid analysis performed here. However, the large similarity of the lipid groups within the seawater and the aerosol particles as well as the agreeing concentrations of the single lipid groups (FFA, ALC) with measurements from other marine stations with molecular techniques (gas chromatography–mass spectrometry – GC-MS) suggests that the same compound classes were present in the particles. Future analysis of the lipid fraction with mass spectrometric techniques will help to better resolve this issue.
3.7.2 Non-identified, recalcitrant OC in aerosol particles
About 50 % of the aerosol OC remained uncharacterized on a molecular and semi-molecular level. The non-identified OC part may contain larger macro-molecules that might be composed of particulate or non-soluble forms in water (carbohydrates and proteins) or organic solvents (lipids) that were removed in the performed analysis during the sample preparation step. In addition, the unknown part might include component groups that belong to the soluble carbohydrates or amino acids but that are either too stable or too labile for the sample preparation procedure (e.g. within the hydrolysis step). Moreover, other complex molecules that cannot be captured with the methods applied here likely add to the unknown fraction including optical active parts summarized as chromophore dissolved organic matter (CDOM), humic-like substances (HULIS), brown carbon, and water-soluble pigments. As mentioned above, NMR analysis showed that SSA contains a large fraction of lipopolysaccharides comprising complex, macro-molecular groups of sugars, esters, carbonyls, acids, and lipids (Facchini et al., 2008). However, these components have not yet been analysed in aerosol particles using chromatographic techniques. The uncharacterized part may also contain particulate OC compounds, such as larger aggregates of marine gels or gel-like particles like TEPs. High TEP number concentrations in aerosol particles were recently identified in Cabo Verde (van Pinxteren et al., 2022), and high mass concentrations of TEPs (e.g. 1.2 µg m−3 for PM1) were found in the western North Atlantic atmosphere (Aller et al., 2017).
Kieber et al. (2016) suggested that the major OC component in sub-micron sea spray particles is recalcitrant, and recently Beaupre et al. (2019) proposed that 19 % to 40 % of the OC associated with freshly produced marine aerosol particles was refractory dissolved OC. This percentage agrees with the non-identified OC part from the present study. However, we cannot identify or classify the remaining OC fraction or attribute it with certainty to the recalcitrant OC. Further studies of the OC, in tropical and other areas of the world, are needed to continue resolving OC and related transfer and formation processes. Nevertheless, the potentially recalcitrant OC fraction in the oligotrophic region does not seem to constitute the majority of OC as reported by Kieber et al. (2016).
3.8 Sea-to-air fluxes of the individual OC groups
The CVAO is located in an oligotrophic region and should therefore be reasonably representative of most of the Earth's ocean surface. POA emission rates are strongly varying; however, modelling studies have estimated global sub-micron marine POA emission rates of 10 ± 5 Tg yr−1 (Gantt and Meskhidze, 2013). Based on this emission flux and the contribution of the compounds to the OC fraction, we estimated the fluxes of the DAAs, DLs, and DCHO. Accordingly, the annual rates of emission from the ocean to the atmosphere are 0.03 ± 0.01 Tg yr−1 for DCHO, 0.04 ± 0.02 Tg yr−1 for DAAs, and 4.2 ± 2.1 Tg yr−1 for DLs. The unknown OC that includes the potentially recalcitrant components has a sea-to-air flux of 4.8 ± 2.4 Tg yr−1. However, this approach only includes the bubble-bursting-mediated transfer of the respective compounds and neglects any potential seasonal changes, additional sources and formation processes. Ervens and Amato (2020) investigated the global impact of bacterial processes on carbon mass in cloud water and estimated formation rates of 3.7 Tg C yr−1 of secondary biological aerosol that are in the range of the POA emissions via sea spray (Gantt and Meskhidze, 2013). Hence, the emission fluxes presented here can change once such processes are quantified for these compounds.
A comprehensive chemical investigation of the OC in the tropical Atlantic Ocean and the atmosphere with a focus on its contribution to the OC on the marine aerosol particles in this particular region was performed.
Regarding seawater, a similar distribution of the DLs and DCHO was found with a small SML enrichment. However, the DAAs, and likely the N-containing compounds in general, exhibit a high and varying enrichment in the SML (although they are less surface-active than lipids). Although conclusions on the detailed processes that lead to the varying DAA concentrations and the high SML enrichments cannot be resolved here and the sample number is limited, the results suggest that processes leading to changes in the organic matter composition within the upper 100 µm oceanic layer are more pronounced for the group of amino acids (and possibly for nitrogen groups in general) compared to other organic compound groups such as lipids and carbohydrates. The SML is probably a very complex, heterogeneous, seasonality-dependent, and reactive matrix forming a lipid-rich nanolayer.
The same compounds studied in the seawater were found on the ambient sub-micron aerosol particles and were strongly enriched with respect to sea salt (EF for the carbohydrates and the amino acids). To this end, the lipids were even more enriched in the sub-micron aerosol particles (EF) compared to the other groups. This indicates a preferred transfer of the lipids (towards the carbohydrates and the amino acids) from the ocean to the atmosphere, likely driven by their physicochemical properties (high surface activity and/or the lipophilic character). Detailed molecular analysis of the seawater and aerosol particles revealed changes in the relative composition of the single compounds. They were most pronounced for the amino acids and are likely related to an in situ atmospheric processing by biotic and/or abiotic reactions that require further investigations. A high saccharide fraction, as described in other studies, could not be found on the aerosol particles, at least when regarding the molecular-resolved carbohydrate analysis. However, saccharidic-like components (e.g. glycolipids) are also included in the lipid fraction analysed here in non-negligible concentrations. This shows that, when comparing the concentrations of substance groups, the analytical methods used must be taken into account. Nevertheless, even small concentrations of carbohydrates and amino acids on marine aerosol particles can have a high impact in their microphysical properties, e.g. as ice-nucleating particles, and are worth studying further.
Altogether, the marine aerosol particle analysis applied here shows that half of the OC can be attributed to specific components or component groups. However, the molecular-level analysed fraction explains only a small part of the OC, and the CHOaer. and AAaer. made up less than 1 %. This shows that the typical representatives of carbohydrates and amino acids within the marine OC measured here can explain only a very small fraction of the organic composition of the aerosol particles on a molecular level. Amines, MSA, and oxalic acid carbonyls comprise a fraction of around 6 %. Lipid analysis reveals 43 % of the OC on the aerosol particles; however, the Lipidaer. composition on a molecular level cannot be obtained from the measurements performed here. Altogether, about 50 % of the OC remained uncharacterized on a molecular and semi-molecular level. Regarding further marine aerosol analysis, it will be important to resolve the large part of lipid compounds in more detail and to get molecular-level information on the remaining, unidentified OC. This shows the need for further detailed analytical OC studies in the marine environment to resolve formation and transfer mechanisms.
Nevertheless, the results obtained here show that, even in such an oligotrophic region, at least half of the OC on the aerosol particles consists of rather short-lived biogenic material, likely from the surface ocean, as (qualitatively) suggested by other studies (Choi et al., 2019; Schmitt-Kopplin et al., 2012). The non-resolved OC might be partly of a recalcitrant nature, as indicated in other studies (Beaupre et al., 2019; Kieber et al., 2016; Lawler et al., 2020). However, the (potentially) recalcitrant OC does not constitute the majority of the OC in the oligotrophic Atlantic Ocean. Future studies should complement the data presented here with investigations of the particulate OC fraction.
Finally, since large parts of the open oceans are oligotrophic, the findings of this study might be relevant to the majority of the world's oceans.
The amino acid and carbohydrate data are listed in the Supplement. The lipid data are available through the World Data Centre PANGAEA under the following link: https://doi.org/10.5194/acp-2022-832 (Triesch et al., 2023). Further data can be made available by the authors upon request.
The supplement related to this article is available online at: https://doi.org/10.5194/acp-23-6571-2023-supplement.
MvP led the MarParCloud campaign with support from KWF and HH. SZ performed the analytical measurements of the carbohydrates and supported the data analysis. SF was in charge of the lipid measurements. NT performed the measurements of the amino acids. MvP performed the data interpretation and wrote the manuscript with contributions from all the authors.
The contact author has declared that none of the authors has any competing interests.
Publisher’s note: Copernicus Publications remains neutral with regard to jurisdictional claims in published maps and institutional affiliations.
This article is part of the special issue “Marine organic matter: from biological production in the ocean to organic aerosol particles and marine clouds (ACP/OS inter-journal SI)”. It is not associated with a conference.
We thank the CVAO site manager Luis Neves, René Rabe, and Susanne Fuchs for technical and laboratory assistance. We were further professionally supported by the Ocean Science Centre Mindelo (OSCM) and the Instituto do Mar (IMar). Finally, the authors thank Simeon Schum for the review of the English language and two anonymous referees for their helpful input. The study contributes to the international SOLAS (Surface Ocean – Lower Atmosphere Study) programme.
We were funded by the Leibniz Association SAW in the project “Marine biological production, organic aerosol particles and marine clouds: a Process Chain (MarPar-Cloud)” (SAW-2016-TROPOS-2), the Research and Innovation Staff Exchange EU project MARSU (69089), and the Deutsche 15 Forschungsgemeinschaft (DFG, German Research Foundation) – Projektnummer 268020496 – TRR 172, within the Transregional Collaborative Research Center “ArctiC Amplification: Climate Relevant Atmospheric and SurfaCe Processes, and Feedback Mechanisms (AC)3” in the sub-project B04. Sanja Frka was supported by the Croatian Science Foundation under the IP-2018-01-3105 BiREADI project.
This paper was edited by Mario Hoppema and reviewed by two anonymous referees.
Abbatt, J. P. D., Leaitch, W. R., Aliabadi, A. A., Bertram, A. K., Blanchet, J.-P., Boivin-Rioux, A., Bozem, H., Burkart, J., Chang, R. Y. W., Charette, J., Chaubey, J. P., Christensen, R. J., Cirisan, A., Collins, D. B., Croft, B., Dionne, J., Evans, G. J., Fletcher, C. G., Galí, M., Ghahreman, R., Girard, E., Gong, W., Gosselin, M., Gourdal, M., Hanna, S. J., Hayashida, H., Herber, A. B., Hesaraki, S., Hoor, P., Huang, L., Hussherr, R., Irish, V. E., Keita, S. A., Kodros, J. K., Köllner, F., Kolonjari, F., Kunkel, D., Ladino, L. A., Law, K., Levasseur, M., Libois, Q., Liggio, J., Lizotte, M., Macdonald, K. M., Mahmood, R., Martin, R. V., Mason, R. H., Miller, L. A., Moravek, A., Mortenson, E., Mungall, E. L., Murphy, J. G., Namazi, M., Norman, A.-L., O'Neill, N. T., Pierce, J. R., Russell, L. M., Schneider, J., Schulz, H., Sharma, S., Si, M., Staebler, R. M., Steiner, N. S., Thomas, J. L., von Salzen, K., Wentzell, J. J. B., Willis, M. D., Wentworth, G. R., Xu, J.-W., and Yakobi-Hancock, J. D.: Overview paper: New insights into aerosol and climate in the Arctic, Atmos. Chem. Phys., 19, 2527–2560, https://doi.org/10.5194/acp-19-2527-2019, 2019.
Aller, J. Y., Radway, J. C., Kilthau, W. P., Bothe, D. W., Wilson, T. W., Vaillancourt, R. D., Quinn, P. K., Coffman, D. J., Murray, B. J., and Knopf, D. A.: Size-resolved characterization of the polysaccharidic and proteinaceous components of sea spray aerosol, Atmos. Environ., 154, 331–347, https://doi.org/10.1016/j.atmosenv.2017.01.053, 2017.
Barbaro, E., Zangrando, R., Vecchiato, M., Piazza, R., Cairns, W. R. L., Capodaglio, G., Barbante, C., and Gambaro, A.: Free amino acids in Antarctic aerosol: potential markers for the evolution and fate of marine aerosol, Atmos. Chem. Phys., 15, 5457–5469, https://doi.org/10.5194/acp-15-5457-2015, 2015.
Beaupre, S. R., Kieber, D. J., Keene, W. C., Long, M. S., Maben, J. R., Lu, X., Zhu, Y. T., Frossard, A. A., Section, J. D. K., Duplessis, P., Chang, R. Y. W., and Bisgrove, J.: Oceanic efflux of ancient marine dissolved organic carbon in primary marine aerosol, Sci. Adv., 5, eaax653, https://doi.org/10.1126/sciadv.aax6535, 2019.
Bertram, T. H., Cochran, R. E., Grassian, V. H., and Stone, E. A.: Sea spray aerosol chemical composition: elemental and molecular mimics for laboratory studies of heterogeneous and multiphase reactions, Chem. Soc. Rev., 47, 2374–2400, https://doi.org/10.1039/c7cs00008a, 2018.
Bianco, A., Deguillaume, L., Chaumerliac, N., Vaïtilingom, M., Wang, M., Delort, A.-M., and Bridoux, M. C.: Effect of endogenous microbiota on the molecular composition of cloud water: a study by Fourier-transform ion cyclotron resonance mass spectrometry (FT-ICR MS), Sci. Rep., 9, 7663, https://doi.org/10.1038/s41598-019-44149-8, 2019.
Brooks, S. D. and Thornton, D. C. O.: Marine Aerosols and Clouds, in: Annual Review of Marine Science, Vol. 10, edited by: Carlson, C. A. and Giovannoni, S. J., Annual Review of Marine Science, Annual Reviews, Palo Alto, 289–313, https://doi.org/10.1146/annurev-marine-121916-063148, 2018.
Burrows, S. M., Hoose, C., Pöschl, U., and Lawrence, M. G.: Ice nuclei in marine air: biogenic particles or dust?, Atmos. Chem. Phys., 13, 245–267, https://doi.org/10.5194/acp-13-245-2013, 2013.
Burrows, S. M., Ogunro, O., Frossard, A. A., Russell, L. M., Rasch, P. J., and Elliott, S. M.: A physically based framework for modeling the organic fractionation of sea spray aerosol from bubble film Langmuir equilibria, Atmos. Chem. Phys., 14, 13601–13629, https://doi.org/10.5194/acp-14-13601-2014, 2014.
Burrows, S. M., Gobrogge, E., Fu, L., Link, K., Elliott, S. M., Wang, H. F., and Walker, R.: OCEANFILMS-2: Representing coadsorption of saccharides in marine films and potential impacts on modeled marine aerosol chemistry, Geophys. Res. Lett., 43, 8306–8313, https://doi.org/10.1002/2016gl069070, 2016.
Capone, D. G., Ferrier, M. D., and Carpenter, E. J.: Amino Acid Cycling in Colonies of the Planktonic Marine Cyanobacterium TRICHODESMIUM-THIEBAUTII, Appl. Environ. Microb., 60, 3989–3995, https://doi.org/10.1128/aem.60.11.3989-3995.1994, 1994.
Carpenter, E. J., Bergman, B., Dawson, R., Siddiqui, P. J. A., Soderback, E., and Capone, D. G.: Glutamine Synthetase and Nitrogen Cycling in Colinies of the Marine Diazotrophic Cyanobacteria TRICHODESMIUM SPP, Appl. Environ. Microb., 58, 3122–3129, https://doi.org/10.1128/aem.58.9.3122-3129.1992, 1992.
Carpenter, L. J., Fleming, Z. L., Read, K. A., Lee, J. D., Moller, S. J., Hopkins, J. R., Purvis, R. M., Lewis, A. C., Müller, K., Heinold, B., Herrmann, H., Fomba, K. W., van Pinxteren, D., Müller, C., Tegen, I., Wiedensohler, A., Müller, T., Niedermeier, N., Achterberg, E. P., Patey, M. D., Kozlova, E. A., Heimann, M., Heard, D. E., Plane, J. M. C., Mahajan, A., Oetjen, H., Ingham, T., Stone, D., Whalley, L. K., Evans, M. J., Pilling, M. J., Leigh, R. J., Monks, P. S., Karunaharan, A., Vaughan, S., Arnold, S. R., Tschritter, J., Pohler, D., Friess, U., Holla, R., Mendes, L. M., Lopez, H., Faria, B., Manning, A. J., and Wallace, D. W. R.: Seasonal characteristics of tropical marine boundary layer air measured at the Cape Verde Atmospheric Observatory, J. Atmos. Chem., 67, 87–140, https://doi.org/10.1007/s10874-011-9206-1, 2010.
Casillo, A., Lanzetta, R., Parrilli, M., and Corsaro, M. M.: Exopolysaccharides from Marine and Marine Extremophilic Bacteria: Structures, Properties, Ecological Roles and Applications, Mar. Drugs, 16, 69, https://doi.org/10.3390/md16020069, 2018.
Cavalli, F., Viana, M., Yttri, K. E., Genberg, J., and Putaud, J.-P.: Toward a standardised thermal-optical protocol for measuring atmospheric organic and elemental carbon: the EUSAAR protocol, Atmos. Meas. Tech., 3, 79–89, https://doi.org/10.5194/amt-3-79-2010, 2010.
Chen, Q., Guo, Z. G., Yu, M., Sachs, J. P., Hou, P. F., Li, L., Jin, G. E., Liu, Y. Y., and Zhao, M. X.: Lipid biomarker estimates of seasonal variations of aerosol organic carbon sources in coastal Qingdao, China, Org. Geochem., 151, 104148, https://doi.org/10.1016/j.orggeochem.2020.104148, 2021.
Choi, J. H., Jang, E., Yoon, Y. J., Park, J. Y., Kim, T. W., Becagli, S., Caiazzo, L., Cappelletti, D., Krejci, R., Eleftheria, K., Park, K. T., and Jang, K. S.: Influence of Biogenic Organics on the Chemical Composition of Arctic Aerosols, Global Biogeochem. Cy., 33, 1238–1250, https://doi.org/10.1029/2019gb006226, 2019.
Cochran, R. E., Ryder, O. S., Grassian, V. H., and Prather, K. A.: Sea Spray Aerosol: The Chemical Link between the Oceans, Atmosphere, and Climate, Accounts Chem. Res., 50, 599–604, https://doi.org/10.1021/acs.accounts.6b00603, 2017.
Cravigan, L. T., Mallet, M. D., Vaattovaara, P., Harvey, M. J., Law, C. S., Modini, R. L., Russell, L. M., Stelcer, E., Cohen, D. D., Olsen, G., Safi, K., Burrell, T. J., and Ristovski, Z.: Sea spray aerosol organic enrichment, water uptake and surface tension effects, Atmos. Chem. Phys., 20, 7955–7977, https://doi.org/10.5194/acp-20-7955-2020, 2020.
Cunliffe, M. and Wurl, O.: Guide to best practices to study the ocean's surface, Occasional Publications of the Marine Biological Association of the United Kingdom, Plymouth, UK, 118 pp., https://plymsea.ac.uk/id/eprint/6523/ (last access: 24 May 2023), 2014.
Cunliffe, M., Engel, A., Frka, S., Gasparovic, B., Guitart, C., Murrell, J. C., Salter, M., Stolle, C., Upstill-Goddard, R., and Wurl, O.: Sea surface microlayers: A unified physicochemical and biological perspective of the air-ocean interface, Prog. Oceanogr., 109, 104–116, https://doi.org/10.1016/j.pocean.2012.08.004, 2013.
Decesari, S., Paglione, M., Rinaldi, M., Dall'Osto, M., Simó, R., Zanca, N., Volpi, F., Facchini, M. C., Hoffmann, T., Götz, S., Kampf, C. J., O'Dowd, C., Ceburnis, D., Ovadnevaite, J., and Tagliavini, E.: Shipborne measurements of Antarctic submicron organic aerosols: an NMR perspective linking multiple sources and bioregions, Atmos. Chem. Phys., 20, 4193–4207, https://doi.org/10.5194/acp-20-4193-2020, 2020.
Dominutti, P. A., Renard, P., Vaïtilingom, M., Bianco, A., Baray, J.-L., Borbon, A., Bourianne, T., Burnet, F., Colomb, A., Delort, A.-M., Duflot, V., Houdier, S., Jaffrezo, J.-L., Joly, M., Leremboure, M., Metzger, J.-M., Pichon, J.-M., Ribeiro, M., Rocco, M., Tulet, P., Vella, A., Leriche, M., and Deguillaume, L.: Insights into tropical cloud chemistry in Réunion (Indian Ocean): results from the BIO-MAÏDO campaign, Atmos. Chem. Phys., 22, 505–533, https://doi.org/10.5194/acp-22-505-2022, 2022.
Engel, A. and Galgani, L.: The organic sea-surface microlayer in the upwelling region off the coast of Peru and potential implications for air–sea exchange processes, Biogeosciences, 13, 989–1007, https://doi.org/10.5194/bg-13-989-2016, 2016.
Engel, A., Bange, H., Cunliffe, M., Burrows, S., Friedrichs, G., Galgani, L., Herrmann, H., Hertkorn, N., Johnson, M., Liss, P., Quinn, P., Schartau, M., Soloviev, A., Stolle, C., Upstill-Goddard, R., van Pinxteren, M., and Zäncker, B.: The Ocean's Vital Skin: Toward an Integrated Understanding of the Sea Surface Microlayer, Front. Mar. Sci., 4, 165, https://doi.org/10.3389/fmars.2017.00165, 2017.
Ervens, B. and Amato, P.: The global impact of bacterial processes on carbon mass, Atmos. Chem. Phys., 20, 1777–1794, https://doi.org/10.5194/acp-20-1777-2020, 2020.
Facchini, M. C., Rinaldi, M., Decesari, S., Carbone, C., Finessi, E., Mircea, M., Fuzzi, S., Ceburnis, D., Flanagan, R., Nilsson, E. D., de Leeuw, G., Martino, M., Woeltjen, J., and O'Dowd, C. D.: Primary submicron marine aerosol dominated by insoluble organic colloids and aggregates, Geophys. Res. Lett., 35, L17814, https://doi.org/10.1029/2008gl034210, 2008.
Fomba, K. W., Müller, K., van Pinxteren, D., and Herrmann, H.: Aerosol size-resolved trace metal composition in remote northern tropical Atlantic marine environment: case study Cape Verde islands, Atmos. Chem. Phys., 13, 4801–4814, https://doi.org/10.5194/acp-13-4801-2013, 2013.
Fomba, K. W., Müller, K., van Pinxteren, D., Poulain, L., van Pinxteren, M., and Herrmann, H.: Long-term chemical characterization of tropical and marine aerosols at the Cape Verde Atmospheric Observatory (CVAO) from 2007 to 2011, Atmos. Chem. Phys., 14, 8883–8904, https://doi.org/10.5194/acp-14-8883-2014, 2014.
Franklin, D., Poulton, J. A., Steinke, M., Young, J., Peeken, I., and Malin, G.: Dimethylsulphide, DMSP-lyase activity and microplankton community structure inside and outside of the Mauritanian upwelling, Prog. Oceanogr., 83, 134–142, 2009.
Frka, S., Pogorzelski, S., Kozarac, Z., and Ćosović, B.: Physicochemical Signatures of Natural Sea Films from Middle Adriatic Stations, J. Phys. Chem. A, 116, 6552–6559, https://doi.org/10.1021/jp212430a, 2012.
Frossard, A. A., Russell, L. M., Burrows, S. M., Elliott, S. M., Bates, T. S., and Quinn, P. K.: Sources and composition of submicron organic mass in marine aerosol particles, J. Geophys. Res.-Atmos., 119, 12977–13003, https://doi.org/10.1002/2014jd021913, 2014.
Fu, P., Kawamura, K., and Miura, K.: Molecular characterization of marine organic aerosols collected during a round-the-world cruise, J. Geophys. Res.-Atmos., 116, D13302, https://doi.org/10.1029/2011jd015604, 2011.
Gantt, B. and Meskhidze, N.: The physical and chemical characteristics of marine primary organic aerosol: a review, Atmos. Chem. Phys., 13, 3979–3996, https://doi.org/10.5194/acp-13-3979-2013, 2013.
Gašparović, B., Kazazić, S. P., Cvitešić, A., Penezić, A., and Frka, S.: Improved separation and analysis of glycolipids by Iatroscan thin-layer chromatography-flame ionization detection, J. Chromatogr. A, 1409, 259–267, https://doi.org/10.1016/j.chroma.2015.07.047, 2015.
Gašparović, B., Kazazić, S. P., Cvitešić, A., Penezić, A., and Frka, S.: Improved separation and analysis of glycolipids by Iatroscan thin-layer chromatography-flame ionization detection (vol 1409, pg 259, 2015), J. Chromatogr. A, 1521, 168–169, https://doi.org/10.1016/j.chroma.2017.09.038, 2017.
Haddrell, A. E. and Thomas, R. J.: Aerobiology: Experimental Considerations, Observations, and Future Tools, Appl. Environ. Microb., 83, e00809-17, https://doi.org/10.1128/aem.00809-17, 2017.
Hardy, J. T.: The Sea-Surface Microlayer – Biology, Chemistry and Antrophogenic Enrichment, Prog. Oceanogr., 11, 307–328, https://doi.org/10.1016/0079-6611(82)90001-5, 1982.
Hasenecz, E. S., Kaluarachchi, C. P., Lee, H. D., Tivanski, A. V., and Stone, E. A.: Saccharide Transfer to Sea Spray Aerosol Enhanced by Surface Activity, Calcium, and Protein Interactions, ACS Earth Space Chem., 3, 2539–2548, https://doi.org/10.1021/acsearthspacechem.9b00197, 2019.
Hepach, H., Quack, B., Ziska, F., Fuhlbrügge, S., Atlas, E. L., Krüger, K., Peeken, I., and Wallace, D. W. R.: Drivers of diel and regional variations of halocarbon emissions from the tropical North East Atlantic, Atmos. Chem. Phys., 14, 1255–1275, https://doi.org/10.5194/acp-14-1255-2014, 2014.
Jaber, S., Joly, M., Brissy, M., Leremboure, M., Khaled, A., Ervens, B., and Delort, A.-M.: Biotic and abiotic transformation of amino acids in cloud water: experimental studies and atmospheric implications, Biogeosciences, 18, 1067–1080, https://doi.org/10.5194/bg-18-1067-2021, 2021.
Kawamura, K., Ishimura, Y., and Yamazaki, K.: Four years' observations of terrestrial lipid class compounds in marine aerosols from the western North Pacific, Global Biogeochem. Cy., 17, 3-1–3-19, https://doi.org/10.1029/2001gb001810, 2003.
Keene, W. C., Maring, H., Maben, J. R., Kieber, D. J., Pszenny, A. A. P., Dahl, E. E., Izaguirre, M. A., Davis, A. J., Long, M. S., Zhou, X., Smoydzin, L., and Sander, R.: Chemical and physical characteristics of nascent aerosols produced by bursting bubbles at a model air-sea interface, J. Geophys. Res.-Atmos., 112, D21202, https://doi.org/10.1029/2007jd008464, 2007.
Khaled, A., Zhang, M., Amato, P., Delort, A.-M., and Ervens, B.: Biodegradation by bacteria in clouds: an underestimated sink for some organics in the atmospheric multiphase system, Atmos. Chem. Phys., 21, 3123–3141, https://doi.org/10.5194/acp-21-3123-2021, 2021.
Kieber, D. J., Keene, W. C., Frossard, A. A., Long, M. S., Maben, J. R., Russell, L. M., Kinsey, J. D., Tyssebotn, I. M. B., Quinn, P. K., and Bates, T. S.: Coupled ocean-atmosphere loss of marine refractory dissolved organic carbon, Geophys. Res. Lett., 43, 2765–2772, https://doi.org/10.1002/2016gl068273, 2016.
Klein, A. M., Bohannan, B. J. M., Jaffe, D. A., Levin, D. A., and Green, J. L.: Molecular Evidence for Metabolically Active Bacteria in the Atmosphere, Front. Microbiol., 7, 772, https://doi.org/10.3389/fmicb.2016.00772, 2016.
Krembs, C., Eicken, H., Junge, K., and Deming, J. W.: High concentrations of exopolymeric substances in Arctic winter sea ice: implications for the polar ocean carbon cycle and cryoprotection of diatoms, Deep-Sea Res. Pt. I, 49, 2163–2181, https://doi.org/10.1016/s0967-0637(02)00122-x, 2002.
Kuznetsova, M. and Lee, C.: Enhanced extracellular enzymatic peptide hydrolysis in the sea-surface microlayer, Mar. Chem., 73, 319–332, https://doi.org/10.1016/s0304-4203(00)00116-x, 2001.
Kuznetsova, M. and Lee, C.: Dissolved free and combined amino acids in nearshore seawater, sea surface microlayers and foams: Influence of extracellular hydrolysis, Aquat. Sci., 64, 252–268, https://doi.org/10.1007/s00027-002-8070-0, 2002.
Kuznetsova, M., Lee, C., Aller, J., and Frew, N.: Enrichment of amino acids in the sea surface microlayer at coastal and open ocean sites in the North Atlantic Ocean, Limnol. Oceanogr., 49, 1605–1619, 2004.
Kuznetsova, M., Lee, C., and Aller, J.: Characterization of the proteinaceous matter in marine aerosols, Mar. Chem., 96, 359–377, https://doi.org/10.1016/j.marchem.2005.03.007, 2005.
Lass, K. and Friedrichs, G.: Revealing structural properties of the marine nanolayer from vibrational sum frequency generation spectra, J. Geophys. Res.-Oceans, 116, C08042, https://doi.org/10.1029/2010jc006609, 2011.
Lawler, M. J., Lewis, S. L., Russell, L. M., Quinn, P. K., Bates, T. S., Coffman, D. J., Upchurch, L. M., and Saltzman, E. S.: North Atlantic marine organic aerosol characterized by novel offline thermal desorption mass spectrometry: polysaccharides, recalcitrant material, and secondary organics, Atmos. Chem. Phys., 20, 16007–16022, https://doi.org/10.5194/acp-20-16007-2020, 2020.
Leck, C., Gao, Q., Mashayekhy Rad, F., and Nilsson, U.: Size-resolved atmospheric particulate polysaccharides in the high summer Arctic, Atmos. Chem. Phys., 13, 12573–12588, https://doi.org/10.5194/acp-13-12573-2013, 2013.
Link, K. A., Spurzem, G. N., Tuladhar, A., Chase, Z., Wang, Z. M., Wang, H. F., and Walker, R. A.: Organic Enrichment at Aqueous Interfaces: Cooperative Adsorption of Glucuronic Acid to DPPC Monolayers Studied with Vibrational Sum Frequency Generation, J. Phys. Chem. A, 123, 5621–5632, https://doi.org/10.1021/acs.jpca.9b02255, 2019.
Liss, P. S. and Duce, R. A.: The sea surface and global change, Cambridge University Press, Cambridge, https://doi.org/10.1017/CBO9780511525025, 1997.
Malfatti, F., Lee, C., Tinta, T., Pendergraft, M. A., Celussi, M., Zhou, Y. Y., Sultana, C. M., Rotter, A., Axson, J. L., Collins, D. B., Santander, M. V., Morales, A. L. A., Aluwihare, L. I., Riemer, N., Grassian, V. H., Azam, F., and Prather, K. A.: Detection of Active Microbial Enzymes in Nascent Sea Spray Aerosol: Implications for Atmospheric Chemistry and Climate, Environ. Sci. Tech. Let., 6, 171–177, https://doi.org/10.1021/acs.estlett.8b00699, 2019.
Mandalakis, M., Apostolaki, M., and Stephanou, E. G.: Trace analysis of free and combined amino acids in atmospheric aerosols by gas chromatography-mass spectrometry, J. Chromatogr. A, 1217, 143–150, https://doi.org/10.1016/j.chroma.2009.11.021, 2010.
Matos, J. T. V., Duarte, R., and Duarte, A. C.: Challenges in the identification and characterization of free amino acids and proteinaceous compounds in atmospheric aerosols: A critical review, Trac-Trends in Anal. Chem., 75, 97–107, https://doi.org/10.1016/j.trac.2015.08.004, 2016.
Matsumoto, K. and Uematsu, M.: Free amino acids in marine aerosols over the western North Pacific Ocean, Atmos. Environ., 39, 2163–2170, https://doi.org/10.1016/j.atmosenv.2004.12.022, 2005.
Matulova, M., Husarova, S., Capek, P., Sancelme, M., and Delort, A. M.: Biotransformation of Various Saccharides and Production of Exopolymeric Substances by Cloud-Borne Bacillus sp 3B6, Environ. Sci. Technol., 48, 14238–14247, https://doi.org/10.1021/es501350s, 2014.
McCluskey, C. S., Hill, T. C. J., Humphries, R. S., Rauker, A. M., Moreau, S., Strutton, P. G., Chambers, S. D., Williams, A. G., McRobert, I., Ward, J., Keywood, M. D., Harnwell, J., Ponsonby, W., Loh, Z. M., Krummel, P. B., Protat, A., Kreidenweis, S. M., and DeMott, P. J.: Observations of Ice Nucleating Particles Over Southern Ocean Waters, Geophys. Res. Lett., 45, 11989–11997, https://doi.org/10.1029/2018gl079981, 2018a.
McCluskey, C. S., Ovadnevaite, J., Rinaldi, M., Atkinson, J., Belosi, F., Ceburnis, D., Marullo, S., Hill, T. C. J., Lohmann, U., Kanji, Z. A., O'Dowd, C., Kreidenweis, S. M., and DeMott, P. J.: Marine and Terrestrial Organic Ice-Nucleating Particles in Pristine Marine to Continentally Influenced Northeast Atlantic Air Masses, J. Geophys. Res.-Atmos., 123, 6196–6212, https://doi.org/10.1029/2017jd028033, 2018b.
Mimura, T. and Romano, J. C.: Muramin acid measurements for bacterial investigations in marine environments by high-pressure-liquid-chromatography, Appl. Environ. Microb., 50, 229–237, https://doi.org/10.1128/aem.50.2.229-237.1985, 1985.
Mochida, M., Kitamori, Y., Kawamura, K., Nojiri, Y., and Suzuki, K.: Fatty acids in the marine atmosphere: Factors governing their concentrations and evaluation of organic films on sea-salt particles, J. Geophys. Res.-Atmos., 107, AAC 1-1–AAC 1-10, https://doi.org/10.1029/2001jd001278, 2002.
Montoya, J. P., Voss, M., and Capone, D. G.: Spatial variation in N2-fixation rate and diazotroph activity in the Tropical Atlantic, Biogeosciences, 4, 369–376, https://doi.org/10.5194/bg-4-369-2007, 2007.
O'Dowd, C. D., Facchini, M. C., Cavalli, F., Ceburnis, D., Mircea, M., Decesari, S., Fuzzi, S., Yoon, Y. J., and Putaud, J. P.: Biogenically driven organic contribution to marine aerosol, Nature, 431, 676–680, https://doi.org/10.1038/Nature02959, 2004.
Pagnone, A., Volker, C., and Ye, Y.: Processes affecting dissolved iron across the Subtropical North Atlantic: a model study, Ocean Dynam., 69, 989–1007, https://doi.org/10.1007/s10236-019-01288-w, 2019.
Patel, A. and Rastogi, N.: Chemical Composition and Oxidative Potential of Atmospheric PM10 over the Arabian Sea, ACS Earth Space Chem., 4, 112–121, https://doi.org/10.1021/acsearthspacechem.9b00285, 2020.
Pena-Izquierdo, J., Pelegri, J. L., Pastor, M. V., Castellanos, P., Emelianov, M., Gasser, M., Salvador, J., and Vazquez-Dominguez, E.: The continental slope current system between Cape Verde and the Canary Islands, Sci. Mar., 76, 65–78, https://doi.org/10.3989/scimar.03607.18C, 2012.
Penezić, A., Drozdowska, V., Novak, T., and Gasparovic, B.: Distribution and characterization of organic matter within the sea surface microlayer in the Gulf of Gdansk, Oceanologia, 64, 631–650, https://doi.org/10.1016/j.oceano.2022.05.003, 2022.
Quinn, P. K., Bates, T. S., Schulz, K. S., Coffman, D. J., Frossard, A. A., Russell, L. M., Keene, W. C., and Kieber, D. J.: Contribution of sea surface carbon pool to organic matter enrichment in sea spray aerosol, Nat. Geosci., 7, 228–232, https://doi.org/10.1038/ngeo2092, 2014.
Quinn, P. K., Collins, D. B., Grassian, V. H., Prather, K. A., and Bates, T. S.: Chemistry and Related Properties of Freshly Emitted Sea Spray Aerosol, Chem. Rev., 115, 4383–4399, https://doi.org/10.1021/cr500713g, 2015.
Rastelli, E., Corinaldesi, C., Dell'Anno, A., Lo Martire, M., Greco, S., Facchini, M. C., Rinaldi, M., O'Dowd, C., Ceburnis, D., and Danovaro, R.: Transfer of labile organic matter and microbes from the ocean surface to the marine aerosol: an experimental approach, Sci. Rep., 7, 11475, https://doi.org/10.1038/s41598-017-10563-z, 2017.
Reinthaler, T., Sintes, E., and Herndl, G. J.: Dissolved organic matter and bacterial production and respiration in the sea-surface microlayer of the open Atlantic and the western Mediterranean Sea, Limnol. Oceanogr., 53, 122–136, https://doi.org/10.4319/lo.2008.53.1.0122, 2008.
Renard, P., Brissy, M., Rossi, F., Leremboure, M., Jaber, S., Baray, J.-L., Bianco, A., Delort, A.-M., and Deguillaume, L.: Free amino acid quantification in cloud water at the Puy de Dôme station (France), Atmos. Chem. Phys., 22, 2467–2486, https://doi.org/10.5194/acp-22-2467-2022, 2022.
Riebesell, U., Fabry, V. J., Hansson L., and Gattuso J.-P. (Eds.): Guide to best practices for ocean acidification research and data reporting [reprinted edition including erratum], Luxembourg, Publications Office of the European Union, 258 pp., https://doi.org/10.2777/66906, 2011.
Romankevich, E. A.: Geochemistry of Organic Matter in the Ocean, Springer, https://doi.org/10.1002/aheh.19850130604, 1984.
Russell, L. M., Hawkins, L. N., Frossard, A. A., Quinn, P. K., and Bates, T. S.: Carbohydrate-like composition of submicron atmospheric particles and their production from ocean bubble bursting, P. Natl. Acad. Sci. USA, 107, 6652–6657, https://doi.org/10.1073/pnas.0908905107, 2010.
Samaké, A., Jaffrezo, J.-L., Favez, O., Weber, S., Jacob, V., Albinet, A., Riffault, V., Perdrix, E., Waked, A., Golly, B., Salameh, D., Chevrier, F., Oliveira, D. M., Bonnaire, N., Besombes, J.-L., Martins, J. M. F., Conil, S., Guillaud, G., Mesbah, B., Rocq, B., Robic, P.-Y., Hulin, A., Le Meur, S., Descheemaecker, M., Chretien, E., Marchand, N., and Uzu, G.: Polyols and glucose particulate species as tracers of primary biogenic organic aerosols at 28 French sites, Atmos. Chem. Phys., 19, 3357–3374, https://doi.org/10.5194/acp-19-3357-2019, 2019.
Sander, R., Keene, W. C., Pszenny, A. A. P., Arimoto, R., Ayers, G. P., Baboukas, E., Cainey, J. M., Crutzen, P. J., Duce, R. A., Hönninger, G., Huebert, B. J., Maenhaut, W., Mihalopoulos, N., Turekian, V. C., and Van Dingenen, R.: Inorganic bromine in the marine boundary layer: a critical review, Atmos. Chem. Phys., 3, 1301–1336, https://doi.org/10.5194/acp-3-1301-2003, 2003.
Schill, S. R., Burrows, S. M., Hasenecz, E. S., Stone, E. A., and Bertram, T. H.: The Impact of Divalent Cations on the Enrichment of Soluble Saccharides in Primary Sea Spray Aerosol, Atmosphere, 9, 476, https://doi.org/10.3390/atmos9120476, 2018.
Schmitt-Kopplin, P., Liger-Belair, G., Koch, B. P., Flerus, R., Kattner, G., Harir, M., Kanawati, B., Lucio, M., Tziotis, D., Hertkorn, N., and Gebefügi, I.: Dissolved organic matter in sea spray: a transfer study from marine surface water to aerosols, Biogeosciences, 9, 1571–1582, https://doi.org/10.5194/bg-9-1571-2012, 2012.
Sieburth, J. M.: Microbiological and organic-chemical processes in the surface and mixed layers, in: Air-Sea Exchange of Gases and Particles, edited by: Liss, P. S. and Slinn, W. G. N., Reidel Publishers Co, Hingham, MA, 121–172, 1983.
Stolle, C., Nagel, K., Labrenz, M., and Jürgens, K.: Succession of the sea-surface microlayer in the coastal Baltic Sea under natural and experimentally induced low-wind conditions, Biogeosciences, 7, 2975–2988, https://doi.org/10.5194/bg-7-2975-2010, 2010.
Triesch, N., van Pinxteren, M., Engel, A., and Herrmann, H.: Concerted measurements of free amino acids at the Cabo Verde islands: high enrichments in submicron sea spray aerosol particles and cloud droplets, Atmos. Chem. Phys., 21, 163–181, https://doi.org/10.5194/acp-21-163-2021, 2021a.
Triesch, N., van Pinxteren, M., Frka, S., Stolle, C., Spranger, T., Hoffmann, E. H., Gong, X., Wex, H., Schulz-Bull, D., Gašparović, B., and Herrmann, H.: Concerted measurements of lipids in seawater and on submicrometer aerosol particles at the Cabo Verde islands: biogenic sources, selective transfer and high enrichments, Atmos. Chem. Phys., 21, 4267–4283, https://doi.org/10.5194/acp-21-4267-2021, 2021b.
Triesch, N., van Pinxteren, M., Salter, M., Stolle, C., Pereira, R., Zieger, P., and Herrmann, H.: Sea Spray Aerosol Chamber Study on Selective Transfer and Enrichment of Free and Combined Amino Acids, ACS Earth Space Chem., 5, 1564–1574, https://doi.org/10.1021/acsearthspacechem.1c00080, 2021c.
Triesch, N., van Pinxteren, M., Frka, S., Stolle, C., Spranger, T., Hoffmann, E. H., Gong, X., Wex, H., Schulz-Bull, D., Gašparović, B., and Herrmann, H.: Measurements of lipids on submicron aerosol particles at the Cape Verde Islands, PANGAEA [data set], https://doi.org/10.1594/PANGAEA.921832, 2023.
van Pinxteren, M., Müller, C., Iinuma, Y., Stolle, C., and Herrmann, H.: Chemical Characterization of Dissolved Organic Compounds from Coastal Sea Surface Micro layers (Baltic Sea, Germany), Environ. Sci. Technol., 46, 10455–10462, https://doi.org/10.1021/es204492b, 2012.
van Pinxteren, M., Fiedler, B., van Pinxteren, D., Iinuma, Y., Koertzinger, A., and Herrmann, H.: Chemical characterization of sub-micrometer aerosol particles in the tropical Atlantic Ocean: marine and biomass burning influences, J. Atmos. Chem., 72, 105–125, https://doi.org/10.1007/s10874-015-9307-3, 2015.
van Pinxteren, M., Barthel, S., Fomba, K., Müller, K., von Tümpling, W., and Herrmann, H.: The influence of environmental drivers on the enrichment of organic carbon in the sea surface microlayer and in submicron aerosol particles – measurements from the Atlantic Ocean, Elementa 5, 35, https://doi.org/10.1525/elementa.225, 2017.
van Pinxteren, M., Fomba, K. W., van Pinxteren, D., Triesch, N., Hoffmann, E. H., Cree, C. H. L., Fitzsimons, M. F., von Tumpling, W., and Herrmann, H.: Aliphatic amines at the Cape Verde Atmospheric Observatory: Abundance, origins and sea-air fluxes, Atmos. Environ., 203, 183–195, https://doi.org/10.1016/j.atmosenv.2019.02.011, 2019.
van Pinxteren, M., Fomba, K. W., Triesch, N., Stolle, C., Wurl, O., Bahlmann, E., Gong, X., Voigtländer, J., Wex, H., Robinson, T.-B., Barthel, S., Zeppenfeld, S., Hoffmann, E. H., Roveretto, M., Li, C., Grosselin, B., Daële, V., Senf, F., van Pinxteren, D., Manzi, M., Zabalegui, N., Frka, S., Gašparović, B., Pereira, R., Li, T., Wen, L., Li, J., Zhu, C., Chen, H., Chen, J., Fiedler, B., von Tümpling, W., Read, K. A., Punjabi, S., Lewis, A. C., Hopkins, J. R., Carpenter, L. J., Peeken, I., Rixen, T., Schulz-Bull, D., Monge, M. E., Mellouki, A., George, C., Stratmann, F., and Herrmann, H.: Marine organic matter in the remote environment of the Cape Verde islands – an introduction and overview to the MarParCloud campaign, Atmos. Chem. Phys., 20, 6921–6951, https://doi.org/10.5194/acp-20-6921-2020, 2020.
van Pinxteren, M., Robinson, T.-B., Zeppenfeld, S., Gong, X., Bahlmann, E., Fomba, K. W., Triesch, N., Stratmann, F., Wurl, O., Engel, A., Wex, H., and Herrmann, H.: High number concentrations of transparent exopolymer particles in ambient aerosol particles and cloud water – a case study at the tropical Atlantic Ocean, Atmos. Chem. Phys., 22, 5725–5742, https://doi.org/10.5194/acp-22-5725-2022, 2022.
Vazques de Vasquez, M. G., Rogers, M. M., Carter-Fenk, K. A., and Allen, H. C.: Discerning Poly- and Monosaccharide Enrichment Mechanisms: Alginate and Glucuronate Adsorption to a Stearic Acid Sea Surface Microlayer, ACS Earth Space Chem., 6, 1581–1595, https://doi.org/10.1021/acsearthspacechem.2c00066, 2022.
Wang, X., Sultana, C. M., Trueblood, J., Hill, T. C. J., Malfatti, F., Lee, C., Laskina, O., Moore, K. A., Beall, C. M., McCluskey, C. S., Cornwell, G. C., Zhou, Y., Cox, J. L., Pendergraft, M. A., Santander, M. V., Bertram, T. H., Cappa, C. D., Azam, F., DeMott, P. J., Grassian, V. H., and Prather, K. A.: Microbial Control of Sea Spray Aerosol Composition: A Tale of Two Blooms, ACS Central Science, 1, 124–131, https://doi.org/10.1021/acscentsci.5b00148, 2015.
Wedyan, M. A. and Preston, M. R.: The coupling of surface seawater organic nitrogen and the marine aerosol as inferred from enantiomer-specific amino acid analysis, Atmos. Environ., 42, 8698–8705, https://doi.org/10.1016/j.atmosenv.2008.04.038, 2008.
Wurl, O.: Sampling and sample treatments, in: Practical Guidelines for the Analysis of Seawater, edited by: Wurl, O., CRC Press, Boca Raton, 329 pp., https://doi.org/10.1201/9781420073072, 2009.
Zäncker, B., Bracher, A., Röttgers, R., and Engel, A.: Variations of the Organic Matter Composition in the Sea Surface Microlayer: A Comparison between Open Ocean, Coastal, and Upwelling Sites Off the Peruvian Coast, Front. Microbiol., 8, 2369, https://doi.org/10.3389/fmicb.2017.02369, 2017.
Zehr, J. P.: Nitrogen fixation by marine cyanobacteria, Trend. Microbiol., 19, 162–173, https://doi.org/10.1016/j.tim.2010.12.004, 2011.
Zeppenfeld, S., van Pinxteren, M., Engel, A., and Herrmann, H.: A protocol for quantifying mono- and polysaccharides in seawater and related saline matrices by electro-dialysis (ED) – combined with HPAEC-PAD, Ocean Sci., 16, 817–830, https://doi.org/10.5194/os-16-817-2020, 2020.
Zeppenfeld, S., van Pinxteren, M., van Pinxteren, D., Wex, H., Berdalet, E., Vaqué, D., Dall'Osto, M., and Herrmann, H.: Aerosol Marine Primary Carbohydrates and Atmospheric Transformation in the Western Antarctic Peninsula, ACS Earth Space Chem., 5, 1032–1047, https://doi.org/10.1021/acsearthspacechem.0c00351, 2021.
Zhang, M., Khaled, A., Amato, P., Delort, A.-M., and Ervens, B.: Sensitivities to biological aerosol particle properties and ageing processes: potential implications for aerosol–cloud interactions and optical properties, Atmos. Chem. Phys., 21, 3699–3724, https://doi.org/10.5194/acp-21-3699-2021, 2021.
Zhu, R.-G., Xiao, H.-Y., Cheng, L., Zhu, H., Xiao, H., and Gong, Y.: Measurement report: Characterization of sugars and amino acids in atmospheric fine particulates and their relationship to local primary sources, Atmos. Chem. Phys., 22, 14019–14036, https://doi.org/10.5194/acp-22-14019-2022, 2022.
Zindler, C., Peeken, I., Marandino, C. A., and Bange, H. W.: Environmental control on the variability of DMS and DMSP in the Mauritanian upwelling region, Biogeosciences, 9, 1041–1051, https://doi.org/10.5194/bg-9-1041-2012, 2012.