the Creative Commons Attribution 4.0 License.
the Creative Commons Attribution 4.0 License.
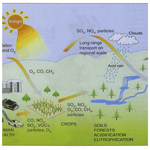
Opinion: Establishing a science-into-policy process for tropospheric ozone assessment
Richard G. Derwent
David D. Parrish
Ian C. Faloona
Elevated tropospheric ozone concentrations driven by anthropogenic precursor emissions are an environmental hazard scientifically similar to the depletion of the stratospheric ozone layer and global climate change; however, the tropospheric ozone issue lacks the generally accepted, international assessment efforts that have greatly informed our understanding of the other two. Here, we briefly review those successful science-into-policy approaches and outline the elements required to conduct a similar process for tropospheric ozone. Particular emphasis is placed on the need to establish a conceptual model to fully understand the underpinning science, useful policy metrics, and motivating international policy forums for regulating anthropogenic ozone production over the hemispheric and global scales, thereby expanding beyond the traditional regional, air basin approach that has dominated air quality regulatory philosophy to date.
- Article
(4281 KB) - Full-text XML
- BibTeX
- EndNote
The global environmental policy problems involving the depletion of the stratospheric ozone layer and global climate change have been identified, fully researched and moved into their respective policy arenas over the last 50 years or so. A number of features of the ozone depletion and climate change problems that have brought them to the forefront of environmental policy can be detailed and then compared with the corresponding features of the tropospheric ozone problem. The science-into-policy process is much less developed for tropospheric ozone, as it seems to have fallen between the two stools of ozone depletion and climate change.
While not presenting an existential crisis of the same magnitude as depletion of the stratospheric ozone layer or global climate change, tropospheric ozone is widely recognized as an important air pollutant (Monks et al., 2015). Beyond fundamentally controlling the oxidation potential of Earth's inhabited atmosphere, tropospheric ozone damages human health (Fleming et al., 2018), contributes to the global burden of disease (Cohen et al., 2017), impacts crops and vegetation (Mills et al., 2018; Feng et al., 2022), and is an anthropogenic greenhouse gas that is third in importance behind carbon dioxide and methane (IPCC, 2013; Skeie et al., 2020).
Urban and regional ozone has been subject to policy actions for several decades to reduce air pollution and ozone episodes; these measures have been largely successful in North America (Parrish et al., 2022) and Europe (Derwent and Parrish, 2022) and are making progress in other continents. An important counterexample to note here is the growth of tropospheric ozone over East Asia, where (in spite of large recent reductions in air pollutant emissions) regional ozone has generally risen (Wang et al., 2020). Regardless of the policy actions, exceedances of air quality standards and guidelines set to protect human health still occur and will do so for the foreseeable future. This is because ozone episodes sit on top of a baseline that is hemispheric and even global in scale. Furthermore, as the manifold, deleterious effects of ozone continue to be revealed by ongoing research, the policy targets of exposure are likely to be reduced even further or different exposure metrics developed. In either case, the relative importance of the background is going to become the dominant effect on future compliance or noncompliance.
Here, we outline a process by which understanding of the science underpinning tropospheric ozone could lead to robust international policy action with a view to simultaneously reducing the global-scale climate impacts of tropospheric ozone, reaching healthy air quality, and ameliorating damage to crops and vegetation.
Concerns about the possible impacts of anthropogenic chlorofluorocarbons (CFCs) on stratospheric ozone were first raised in the 1970s by Molina and Rowland (1974). These concerns were put into sharp focus by the discovery of the stratospheric ozone hole in Antarctica by Farman et al. (1985) in the 1980s. Policy action followed swiftly, not simply because of the importance of these discoveries but also because a number of potential policy hurdles or stumbling blocks had already been surmounted.
A “model” had been developed describing the mechanism by which the stratospheric ozone hole formed that was widely accepted by the atmospheric science community (Solomon et al., 1986; Crutzen and Arnold, 1986; Cox and Hayman, 1988). It was proposed that anthropogenic CFCs are photolyzed in the stratosphere to form active chlorine atoms and radicals which catalyze the ozone destruction. Armed with this model, the process of review and assessment began in earnest under the aegis of the World Meteorological Organization (WMO). A policy metric was developed, the ozone depletion potential (ODP), for the CFCs and, ultimately, for all ozone-depleting substances. It should be noted that ODPs cannot be “observed”. These policy metrics required the model of stratospheric ozone to be well understood so that they could be faithfully derived.
Policy actions were formulated within the Vienna Convention for the Protection of the Ozone Layer under the auspices of the United Nations Environment Programme (UNEP). The Vienna Convention used the WMO reviews and assessments to build a set of protocols identifying each ozone depleting substance in turn and moved toward their phase-out, in order of importance, as determined by the products of the ODPs and the abundances.
It has been postulated over the last 2 centuries by Eunice Foote, Joseph Fourier and John Tyndall that carbon dioxide would act as a greenhouse gas (Royal Institution, 2019), and Arrhenius (1896) quantified the global temperature increase that would result from increased CO2 levels. Charles Keeling identified the global-scale rise in atmospheric carbon dioxide levels from his observations on Mauna Loa, Hawaii, and at the South Pole in late 1950s (Keeling et al., 1989). Policymakers were first made aware of the emerging issue of global climate change in the 1980s. Scientific review and assessment began around this time under the Intergovernmental Panel on Climate Change (IPCC), spearheaded by Bert Bolin, who placed the greatest emphasis on assessment with a clear focus on science-into-policy process. The IPCC was formed under the aegis of the WMO, and its first scientific assessment was published in 1990 with subsequent major scientific assessment reports on the physical science basis in 1995, 2001, 2007, 2014, and 2022.
Daniel Albritton (Birks et al., 1992) conceptualized a model describing the basic scientific framework for the IPCC. It describes how atmospheric composition change drives radiative forcing, which in turns drives atmospheric responses in terms of changes in temperature, winds and rainfall (physical responses). These atmospheric responses drive changes in the climate (climate responses) which impact on the biosphere (biological responses), ultimately leading to ecosystem responses, as illustrated in Fig. 1a. Feedbacks occur when atmospheric responses, such as melting ice, modify radiative forcing or when ecosystem responses change atmospheric composition through, for example, changes in wetland methane emissions. In this model, global climate change is seen as a system of forcings and feedbacks, with anthropogenic composition change as the ultimate driving force. This system has been represented with increasing sophistication through the development of increasingly complex Earth system models (ESMs).
The series of IPCC reports has been presented to the United Nations Framework Convention on Climate Change (UN FCCC). In response to requests from the UN FCCC, the IPCC developed a policy metric, the global warming potential (GWP), so that the different propensities of a range of different trace gases to influence climate change could be represented on a common basis in policy contexts. Using GWPs the UN FCCC put together a basket of six trace gases and began developing strategies with the aim of reducing dangerous anthropogenic climate change. The basket, however, does not address tropospheric ozone, although it is the third most important anthropogenic greenhouse gas after CO2 and methane (Stevenson et al., 2013). Indeed, tropospheric ozone is one of the most important short-lived climate forcers (SLCFs).
From the two sections above, we can identify the essential elements of the science-into-policy process addressing ozone layer depletion and global climate change, as illustrated in Fig. 1b. These are, firstly, review and assessment of the underpinning science with strict and open peer review and encouragement of research with a clear focus on the science-into-policy process; secondly, development of a hierarchy of models of the underpinning science; and thirdly, development of a policy metric with full buy-in from the atmospheric science community. However, fourthly, and most importantly, we can identify the importance of having an international convention that brings together the policy-making and atmospheric science communities and provides a framework for taking account of both scientific and policy developments.
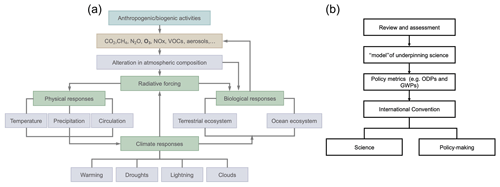
Figure 1Science-into-policy processes of global climate change. (a) A model of underpinned science representing the key processes involved with climate change, revised from Daniel Albritton (Birks et al., 1992). Forcings are represented by downward arrows. Feedbacks are processes by which responses lower down in the diagram drive changes further up. (b) Essential elements of the science-into-policy processes.
There are open and accessible data repositories covering many country-wide or regional monitoring networks, for example, those operated by the United States Environmental Protection Agency (US EPA), the United Nations Economic Commission for Europe (UN ECE) and the European Monitoring and Evaluation Programme (EMEP). In addition, there are some data portals that provide access to network data, including those operated by the Norwegian Institute for Air Research, the International Global Atmospheric Chemistry (IGAC) Tropospheric Ozone Assessment Report (TOAR) and the WMO World Data Centre for Greenhouse Gases. Some illustrative examples of long-term changes in both baseline and urban ozone concentrations are included in Fig. 2. Importantly, the interpretation of baseline ozone and its long-term changes remains an open scientific question.
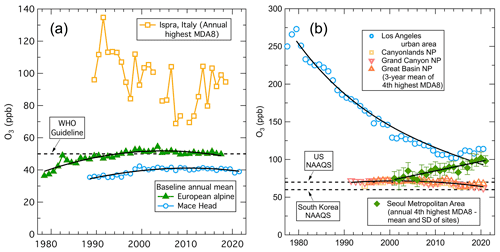
Figure 2Example long-term records of measured ozone. Baseline (Europe and US) and urban (Europe, US and Asia) ozone concentrations are included. Note that differing statistical metrics are used between the records. The data sources are as follows: Ispra, Italy, and the European alpine sites (the latter described in detail by Parrish et al., 2020) are from the TOAR database (https://join.fz-juelich.de, last access: 28 December 2022); Mace Head data are from Derwent et al. (2023); US data sets are from the US EPA Air Quality System (AQS) data archive (https://www.epa.gov/aqs, last access: 19 February 2023); and South Korea data are from Kim et al. (2023). The trend lines are quadratic polynomial fits to the baseline ozone data sets, a linear fit to the South Korean data and an exponential decrease above a baseline trend for the Los Angeles data (Parrish et al., 2022).
There are reviews of urban and regional ozone compiled by several organizations, including the US EPA, the Air Quality Expert Group (AQEG), the European Union (EU) and the EMEP, but they are generally restricted to single jurisdictions or single networks. Hemispheric and global-scale reviews are compiled by the UN ECE Task Force on Hemispheric Transport of Air Pollution (HTAP; Dentener et al., 2010) and TOAR. All of these reviews, whilst providing excellent coverage, suffer distinctly from a dichotomous consideration of urban and regional scales, on the one hand, and the hemispheric and global scales, on the other hand; they also lack a strategic focus on science-into-policy processes for tropospheric ozone mitigation issues.
In response to the human health impacts of elevated ozone levels, policymakers have taken extensive measures on local and regional scales to control the emissions of the main ozone precursors: oxides of nitrogen (NOx) and reactive organic compounds (Sillman, 1999; Ehlers et al., 2016; Lu et al., 2010). Control of motor vehicle exhaust emissions through the mandatory fitting of exhaust gas catalysts and evaporative cannisters has largely been a complete success, enabling large reductions to be achieved in ozone precursor emissions from road transport. Elevated ozone levels have declined in almost all legislative and administrative regions where ozone precursor emissions have been controlled. However, these declines have not gone far enough. Air quality targets, guidelines or standards set to protect human health have not always been achieved and, with the identification of adverse health impacts at lower ozone concentrations, there is growing pressure to further tighten standards; with some exceptions, non-attainment of air quality targets remains an important policy issue. Whilst assessment of long-term changes in urban and regional ozone levels point to the huge impact of the measures taken to reduce ozone precursor emissions, they also demonstrate that reductions in exceedances have slowed during the past decade (Parrish et al., 2022; Derwent and Parrish, 2022). The situation is also reflected by the atmospheric chemistry literature which is characterized by dichotomous views separated by scales: papers on urban ozone (Ehlers et al., 2016; Nelson et al., 2021; Tan et al., 2019; Cardelino and Chameides, 1995; Brune et al., 2016; Pusede et al., 2015) often ignore or oversimplify the impact of the background troposphere on the urban environment and papers on the background troposphere (Parrish et al., 2009; Wang and Jacob, 1998; Gaudel et al., 2018) often ignore or oversimplify the impact of urban and regional pollution.
A hierarchy of models of the underlying science is required to provide detailed advice for the science-into-policy process. There are excellent reviews and intercomparisons of complex ozone models on all scales from urban to regional to global, addressing issues of atmospheric chemistry, boundary layer processes and atmospheric transport (Simon et al., 2012; Turnock et al., 2020; Young et al., 2013). However, there is also a requirement for conceptual models that aim to advance our understanding of tropospheric ozone by simplifying and capturing the essence of the most salient physical and chemical processes that control observed ozone abundances. These refined models must be consistent with findings from observations and capture the overall behaviour of more complex models. Such simplified presentations are required to facilitate joint communication between different scientific communities and policymakers, and they can be instrumental in the continued development of the most complex chemical transport models.
Development of a widely accepted, simple, conceptual model that intuitively explains the broad features of how ozone sources, sinks and transport processes all interact to establish the observed local-, regional- and large-scale spatial distributions, seasonal cycles and long-term temporal changes in ozone is urgently required. Such a model would form the core of a robust assessment, would be invaluable to researchers in their efforts to understand the beautifully detailed observational data and chemistry transport model results that are presently available to the atmospheric community. Such a conceptual model would provide a firm foundation upon which to conceive and organize present and future research efforts into a more comprehensive understanding of all aspects of the spatial and temporal distribution of tropospheric ozone. Such an intuitive model would be an essential component of a modelling hierarchy, similar to those employed by the geophysical fluid dynamics community (Held, 2005), serving to complement the comprehensive numerical models that aim to simulate in full detail as much of the atmospheric chemistry, dynamics and coupling thereof as possible.
Figure 3 presents a schematic diagram illustrating some of the basic principles of the tropospheric ozone issue updated from Derwent et al. (1998b). The diagram envisages background air containing O3, CO and CH4 entering an urban area or rural region on the right-hand side. Urban and regional biogenic precursor emissions drive local- and regional-scale photochemical ozone production which elevates ozone concentrations above the global baseline levels, leading to human health effects and crop and vegetation damage. After 1 to several days travel downwind, the regionally polluted air with elevated levels of O3, CO, NOx and unreacted organic compounds is lofted from the continental boundary layer and rejoins the global circulation. One aspect of such a model requires particular attention: it should aim to bridge the dichotomous views of urban pollution ignoring or oversimplifying the impact of the background troposphere on the urban environment and the background troposphere ignoring or oversimplifying the impact of urban pollution.
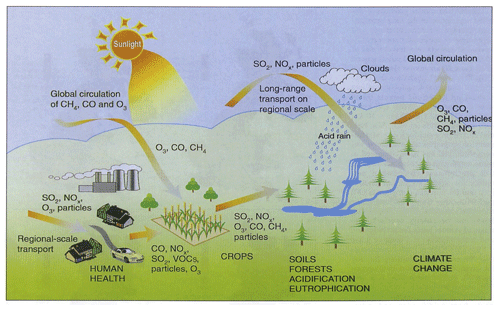
Figure 3Schematic illustration of the basic principles underpinning the tropospheric ozone issue. Note that tropospheric ozone sources include production in background, urban and rural areas as well as injection of ozone from the stratosphere. The ozone produced in the three distinctly different source areas is mixed within the troposphere, which depicts the difficulties of tropospheric ozone control.
As the manifold of processes determining the tropospheric ozone distribution is considerably simpler than that driving the climate system, this required model will be simpler than that developed by the IPCC. As examples of its utility, the model should be able to provide a clear description of northern midlatitude baseline ozone, including answering (1) why ozone maximizes in late spring in the free troposphere but peaks earlier in the spring with a summer minimum in the marine boundary layer, (2) why mean concentrations approximately doubled during the first half of the 20th century but have since decreased and (3) to what extent is ozone homogeneously mixed within the prevailing zonal flow. Moreover, the model should explain how ozone compares between the Northern and Southern hemispheres, both at present and in the pre-industrial atmosphere. Of great utility would be an interactive atlas, such as provided by the IPCC AR6 Synthesis Report, that quantifies background and anthropogenic contributions to ozone concentrations at any specified location on the globe, particularly if those quantities could be illustrated as a function of variable local or hemispheric precursor emissions.
A further important aspect of the model of tropospheric ozone is a close association with the model of climate change. For example, future changes in stratosphere–troposphere exchange due to an accelerated Brewer–Dobson circulation (Abalos et al., 2020) along with a changing Northern Hemisphere stratospheric ozone abundance (Woltmann et al., 2020) may raise background ozone concentrations over the next century. The observed increase in background NOx (Qu et al., 2021) could be the result of rising soil temperatures, increasing wildfire impacts and increasing lightning (Murray, 2018) production in a warming climate.
With the science-into-policy processes for stratospheric ozone and climate change, the scientific community developed the ODP and GWP metrics. These metrics provided a scientific focus for the actions of policymakers, which necessarily focused attention on the emissions of ozone-depleting substances and greenhouse gases, decreasing the emissions sooner and more strongly for species with the largest metric values.
Developing a policy metric like ODPs and GWPs for tropospheric ozone is more complex. The previously developed parameters such as OFPs (ozone formation potentials) (Carter et al., 1995) and POCPs (photochemical ozone creation potentials) (Derwent et al., 1998a) are not considered to be quite comparable with ODPs and GWPs, as they are highly state-dependent and so their efficacy as accurate metrics depends on local conditions, which vary over time and space.
A large variety of metrics have been proposed for tropospheric ozone, addressing its impacts on urban and regional air quality and impacts on human health and crops and vegetation (e.g. Monks et al., 2015). Indeed, Lefohn et al. (2018) propose 25 metrics in all, (4 for model–measurement intercomparison, 5 for characterization of ozone in the free troposphere, 11 for human health impacts and 5 for vegetation impacts). The choice of metric for our proposed science-into-policy process for tropospheric ozone will be an important discussion between the scientific and policy communities, and will likely include discussion of complicating issues, as has been the case for global climate change (e.g. Lynch et al., 2020) and stratospheric ozone depletion (e.g. Pyle et al., 2022).
However, as with the stratospheric ozone and climate change issues, policymakers will necessarily focus their attention on controlling the emissions of ozone precursor gases, decreasing the emissions sooner and more strongly for those ozone precursor emissions which more readily affect tropospheric ozone. Ozone precursor emission inventories have been established over at least 5 decades and have driven policy focus throughout the world. They can provide the essential policy focus for the tropospheric ozone issue.
Regional air quality and global models all require emission inventories (e.g. Fig. 4) and chemical mechanisms, along with observations to evaluate their performance. These models effectively convert ozone precursor emission inventory data into predictions of ozone concentrations over specific spatial and temporal scales in response to policy needs. By changing the ozone precursor emissions, whilst keeping all other input data the same, modellers can visualize the impact of particular ozone precursor emission control strategies on the tropospheric ozone distribution, for the benefit of policymakers.
Almost all urban areas and regions have been inventoried in greater or lesser detail for each of the major ozone precursors. Examples include the CHIEF (Clearinghouse for Inventories and Emissions Factors by US EPA), EMEP (by UN ECE), MEIC (Multi-resolution Emission Inventory for China), SAFAR (System of Air Quality and Weather Forecasting and Research), EDGAR (Emission Database for Global Atmospheric Research, from IGAC) and CEDS (Community Emission Data System) inventories (Hoesly et al., 2018).
Whilst the emission factor approach is well-defined for anthropogenic emissions, a different approach is required for biogenic emissions. Accurate estimation of the biogenic emissions of isoprene, terpenes and NOx requires information on plant and tree species, on ecosystem composition, and on the local meteorological conditions of temperature, radiation and soil moisture. Biomass burning is another source difficult to treat rigorously in ozone policy models; it is important to treat both agricultural biomass burning and wild fires separately for policy purposes.
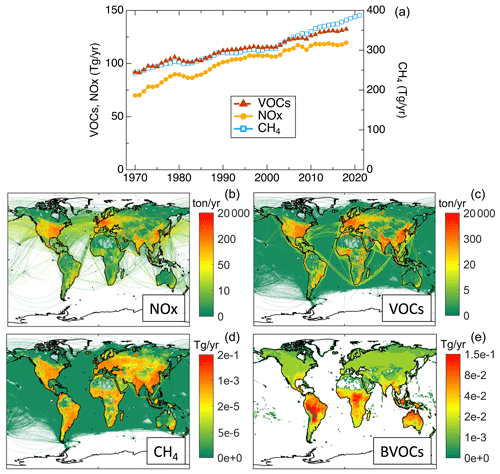
Figure 4Emission rates of key ozone precursors. (a) Time series of annual emission rates of anthropogenic NOx and VOCs on the left axis and total CH4 on the right axis. (b, c) Spatial distributions of mean annual emissions of NOx and VOCs (metric tons per year per unit area) over 1984–2015. (d, e) Spatial distributions of mean annual emissions of CH4 and biogenic volatile organic compounds (BVOCs) (teragrams per year per unit area) over 1984–2020. Underlying emission data are from EDGARv6.1, EDGARv7.0, and the Model of Emissions of Gases and Aerosols from Nature coupled with the Monitoring Atmospheric Composition and Climate reanalysis (MEGAN-MACC). Total annual mean BVOCs emissions are ∼ 200 Tg yr−1, with no significant trend.
In summary, we consider that ozone precursor emission inventories can provide an up-to-the-task basis for driving policy formulation for tropospheric ozone. High annual emission rates (>100 t yr−1 per grid square) over densely populated regions (e.g. Asia, Europe and North America) dominate the total emission of global anthropogenic NOx and volatile organic compounds (VOCs) (Fig. 4), supporting the conclusions that intensive anthropogenic air pollutant emissions in these regions make great contributions to the non-attainment of ozone air quality standards. Moreover, CH4 emissions increased similarly to those of NOx and VOCs (Fig. 4), also indicating possible accelerated photochemical O3 production in background air masses. Importantly, these inventories require continual updating and extension to additional species (e.g. volatile chemical products, McDonald et al., 2018), emission sectors and global regions; top-down evaluation is essential as emission sources evolve (e.g. McDonald et al., 2018; Smith et al., 2022).
Whilst there are many international bodies that address issues relevant to improving scientific knowledge of the sources and distribution of tropospheric ozone, there is no international convention that could readily take up this issue and make policy progress globally. HTAP considers regions covering only Europe, USA and Canada. It specifically excludes Asia, Mexico and North Africa as well as with Southern Hemisphere countries.
The IPCC has identified tropospheric ozone as the third most important anthropogenic greenhouse gas after carbon dioxide and methane. The latter trace gas, methane, has been established as an important tropospheric ozone precursor (e.g. West et al., 2013). In its Working Group I, the Sixth Assessment Report from the IPCC addressed tropospheric ozone as a short-lived climate forcer and stressed the co-benefits of methane reductions to mitigate climate change and improve air quality. The Working Group II report underlines the effects of ozone on crops and warns of the health dangers of elevated ozone levels during heat waves. The Working Group III report dealt with decarbonizing strategies for tackling climate change and the co-benefits for ozone air quality.
The UN FCCC has pioneered the compilation of ozone precursor emission inventories from each country globally but does not include tropospheric ozone in its basket of trace gases; thus, there has been little focus on tropospheric ozone within the UN FCCC. If SLCFs could be moved up the UN FCCC agenda and policy actions focused on methane and tropospheric ozone, a rapid impact on climate forcing would result because of the relatively short global mean atmospheric lifetimes of both methane (12 years) and tropospheric ozone (∼1 month). There would also be a substantial improvement in urban and regional ozone air quality, bringing the possibility of achieving air quality standards and guidelines set to protect human health and crops and vegetation.
There are some crucial scientific issues that need resolution ahead of the proposed science-into-policy process and the development of policy advice leading to the regulation of tropospheric ozone. There is a conflict in the assessment of current ozone observations between two viewpoints concerning baseline ozone trends in the northern midlatitudes; namely, is baseline ozone continuing to rise (Gaudel et al., 2018; Tarasick et al., 2019) or has baseline ozone peaked during the 2000s and 2010s and is beginning to decline (Logan et al., 2012; Parrish et al., 2021). Because urban and regional ozone episodes sit on top of a hemispheric-scale ozone background, any trend in background ozone has a direct influence on the attainment of policy goals based on the achievement of air quality standards or guidelines set for the protection of human health.
The majority of these crucial scientific issues involve ozone modelling from the urban through to the global scale and, in particular, their uncertainties as quantified in international model intercomparison exercises. The magnitudes of model uncertainties imply that models should be regarded as indicative rather than prescriptive policy tools. As with climate science and climate models (Carslaw et al., 2018), there are areas where model uncertainties are apparent and where further scientific study aimed at reducing uncertainties should be encouraged. For tropospheric ozone, these include the following:
-
ozone trends since pre-industrial times (as these fix the radiative forcing from ozone; Stevenson et al., 2013), trends since the 1950s (covering the period of instrumental ozone observations; Parrish et al., 2014) and trends since the 1990s (covering the period of intense ozone monitoring);
-
ozone seasonal cycles and interhemispheric gradients (Derwent et al., 2016);
-
intercontinental ozone precursor source–receptor relationships for methane, carbon monoxide, NOx and VOCs linking to receptors in regional monitoring stations across the Northern Hemisphere continents (Fiore et al., 2009; HTAP, 2010);
-
biomass burning and wild fires as sources of tropospheric ozone (Jaffe et al., 2020) and air quality standard and guideline exceedance;
-
the impact of climate change on the strength of the Brewer–Dobson circulation in the stratosphere and the consequences for the stratosphere–troposphere exchange as a source of tropospheric ozone (Abalos et al., 2020).
We have every confidence that if a robust and cogent, peer-reviewed policy-oriented scientific review of the tropospheric ozone issue could be assembled by the atmospheric science community, then policy progress could be made under the auspices of the UNEP. Such a review could be built on the current TOAR and HTAP activities, with inclusion of participation by policy-associated scientists. Coordinated global action on tropospheric ozone holds the promise of delivering acceptable ozone air quality in all major population and industrial centres globally, a prospect that is unlikely to be achievable without such collective planning. Furthermore, future actions to reduce urban and regional ozone precursor emissions may be wasteful of resources if realistic account of the hemispheric or global baseline is not taken. In such a situation, the exceedance of air quality standards and guidelines will continue unchecked and the hands of local policy makers will be tied.
The highest annual daily maximum 8 h average concentration (MDA8) ozone data at Ispra, Italy, and the annual mean ozone data at European alpine sites (described in detail by Parrish et al., 2020) are available from the TOAR Surface Ozone Database via the JOIN web interface: https://join.fz-juelich.de (login required). Baseline annual mean ozone data from Mace Head are taken from Derwent et al. (2023). The 3-year mean of the fourth highest MDA8 data (i.e. the US ozone design value) from the four US data sets in Fig. 2 are available from the US EPA AQS data archive (https://www.epa.gov/aqs, login required). The surface monitoring data for South Korea are available from https://www.airkorea.or.kr/web/last_amb_hour_data?pMENU_NO=123 (NIER, 2022); the annual fourth highest MDA8 data for the Seoul Metropolitan Area are taken from Kim et al. (2023; https://doi.org/10.5194/acp-23-12867-2023). Globally averaged annual mean and grid maps of NOx and VOC data are obtained from the EDGARv6.1 and EDGARv7.0 Global Air Pollutant Emissions databases (https://edgar.jrc.ec.europa.eu/index.php/dataset_ap61, European Commission, 2023a; https://edgar.jrc.ec.europa.eu/dataset_ghg70, European Commission, 2023b). Global annual BVOC data are taken from the MEGAN-MACC biogenic emission inventory (Sindelarova et al., 2014) and are available from the Emissions of atmospheric Compounds and Compilation of Ancillary Data (ECCAD) database (https://eccad.aeris-data.fr/).
RGD and DDP were responsible for the overall design. RGD and DDP wrote the paper with input from ICF.
The contact author has declared that none of the authors has any competing interests.
Publisher’s note: Copernicus Publications remains neutral with regard to jurisdictional claims made in the text, published maps, institutional affiliations, or any other geographical representation in this paper. While Copernicus Publications makes every effort to include appropriate place names, the final responsibility lies with the authors.
Discussions with Keding Lu, Alex Archibald, David Stevenson, Daniel Jacob, Tao Wang, Ken Carslaw, Paul Monks, Jim Crawford and Martin Schultz are kindly acknowledged; they contributed to the discussions that led to the writing of the article, although they do not necessarily agree with the content.
This research has been supported by the National Institute of Food and Agriculture (Hatch project CA-D-LAW-2481-H).
This paper was edited by Andreas Hofzumahaus and Rolf Müller and reviewed by two anonymous referees.
Abalos, M., Orbe, C., Kinnison, D. E., Plummer, D., Oman, L. D., Jöckel, P., Morgenstern, O., Garcia, R. R., Zeng, G., Stone, K. A., and Dameris, M.: Future trends in stratosphere-to-troposphere transport in CCMI models, Atmos. Chem. Phys., 20, 6883–6901, https://doi.org/10.5194/acp-20-6883-2020, 2020.
Arrhenius, S.: On the influence of carbonic acid in the air upon the temperature of the ground, Philos. Mag., 41, 237–276, 1896.
Birks, J., Calvert, J. G., and Sievers, R.: CHEMRAWN VII, The Chemistry of the Atmosphere: Its Impact on Global Change, Perspectives and Recommendations, IUPAC, ISBN 978-084122 5336, 1992.
Brune, W. H., Baier, B. C., Thomas, J., Ren, X., Cohen, R. C., Pusede, S. E., Browne, E. C., Goldstein, A. H., Gentner, D. R., Keutsch, F. N., Thornton, J. A. Harrold, S. F., Lopez-Hilfiker, D., and Wennberg, P. O.: Ozone production chemistry in the presence of urban plumes, Faraday Discuss., 189, 169–189, https://doi.org/10.1039/c5fd00204d, 2016.
Cardelino, C. A. and Chameides, W. L.: An Observation-Based Model for Analyzing Ozone Precursor Relationships in the Urban Atmosphere, J. Air Waste Manage., 45, 161–180, 1995.
Carslaw, K. S., Lee, L. A., Regayre, L. A., and Johnson, J. S.: Climate models are uncertain, but we can do something about it, Eos, 99, https://doi.org/10.1029/2018EO093757, 26 February 2018.
Carter, W. P. L., Pierce, J. A., Luo, D. M., and Malkina, I. L.: Environmental Chamber Study of Maximum Incremental Reactivities of Volatile Organic-Compounds, Atmos. Environ., 29, 2499–2511, 1995.
Cohen, A. J., Brauer, M., Burnett, R., Anderson, H. R., Frostad, J., Estep, K., Balakrishnan, K., Brunekreef, B., Dandona, L., Dandona, R., Feigin, V., Freedman, G., Hubbell, B., Jobling, A., Kan, H., Knibbs, L., Liu, Y., Martin, R., Morawska, L., Pope III, C. A., Shin, H., Straif, K., Shaddick, G., Thomas, M., van Dingenen, R., van Donkelaar, A., Vos, T., Murray, C. J. L., and Forouzanfar, M. H.: Estimates and 25-year trends of the global burden of disease attributable to ambient air pollution: an analysis of data from the Global Burden of Diseases Study 2015, Lancet, 389, 1907–1918, https://doi.org/10.1016/S0140-6736(17)30505-6, 2017.
Cox, R. A. and Hayman, G. D.: The stability and photochemistry of dimers of the ClO radical and implications for Antarctic ozone depletion, Nature, 332, 796–800, 1988.
Crutzen, P. J. and Arnold, F.: Nitric acid cloud formation in the cold Antarctic stratosphere: a major cause for the springtime “ozone hole”, Nature, 324, 651–655, https://doi.org/10.1038/324651a0, 1986.
Dentener, F., Keating, T., and Akimoto, H.: Hemispheric Transport of Air Pollution (HTAP) 2010: Part A: Ozone and Particulate Matter, Air Pollution Studies No. 17, United Nations, Geneva, Switzerland, ISBN 978-92-1-117043-6, 2010.
Derwent, R. G. and Parrish, D. D.: Analysis and assessment of the observed long-term changes over three decades in ground-level ozone across north-west Europe from 1989–2018, Atmos. Environ., 286, 119222, https://doi.org/10.1016/j.atmosenv.2022.119222, 2022.
Derwent, R. G., Jenkin, M. E., Saunders, S. M., and Pilling, M. J.: Photochemical ozone creation potentials for organic compounds in northwest Europe calculated with a master chemical mechanism, Atmos. Environ., 32, 2429–2441, 1998a.
Derwent, R. G., Metcalfe, S. E., and Whyatt, J. D.: Environmental Benefits of NOx Control in Northwestern Europe, Ambio, 27, 518–527, 1998b.
Derwent, R. G., Parrish, D. D., Galbally, I. E., Stevenson, D. S., Doherty, R. M., Young, P. J., and Shallcross, D. E.: Interhemispheric differences in seasonal cycles of tropospheric ozone in the marine boundary layer: Observation-model comparisons, J. Geophys. Res.-Atmos., 121, 11075–11085, 2016.
Derwent, R. G., Parrish, D. D., Manning, A. J., Spain, T. G., Simmonds, P. G., and O’Doherty, S.: Ozone at Mace Head, Ireland from 1987 to 2021: Declining baselines, phase-out of European regional pollution, COVID-19 impacts, Atmos. Environ., in review, 2023.
Ehlers, C., Klemp, D., Rohrer, F., Mihelcic, D., Wegener, R., Kiendler-Scharr, A., and Wahner, A.: Twenty years of ambient observations of nitrogen oxides and specified hydrocarbons in air masses dominated by traffic emissions in Germany, Faraday Discuss., 189, 407–437, https://doi.org/10.1039/c5fd00180c, 2016.
European Commission: Global Air Pollutant Emissions, EDGAR – Emissions Database for Global Atmospheric Research [data set], https://edgar.jrc.ec.europa.eu/index.php/dataset_ap61, last access: 20 May 2023a.
European Commission: Global Greenhouse Gas Emissions, EDGAR – Emissions Database for Global Atmospheric Research [data set], https://edgar.jrc.ec.europa.eu/dataset_ghg70, last access: 20 May 2023b.
Farman, J. C., Gardiner, B. G., and Shanklin, J. D.: Large losses of total ozone in Antarctica reveal seasonal ClOx/NOx interaction, Nature, 315, 207–210, 1985.
Feng, Z., Xu, Y., Kobayashi, K., Dai, L., Zhang, T., Agathokleous, E., Calatayud, V., Paoletti, E., Mukherjee, A., Agrawal, M., Park, R. J., Oak, Y. J., and Yue, X.: Ozone pollution threatens the production of major staple crops in East Asia, Nature Food, 3, 47–56, https://doi.org/10.1038/s43016-021-00422-6, 2022.
Fiore, A. M., Dentener, F., Wild, O., Cuvelier, C., Schultz, M., Hess, P., Textor, C., Schulz, M., Doherty, R., and Horowitz, L.: Multimodel estimates of intercontinental source-receptor relationships for ozone pollution, J. Geophys. Res.-Atmos., 114, D04301, https://doi.org/10.1029/2008JD010816, 2009.
Fleming, Z. L., Doherty, R. M., von Schneidemesser, E., Malley, C. S, Cooper, O. R., Pinto, J. P., Colette, A., Xu, X., Simpson, D., Schultz, M. G., Lefohn, A. S., Hamad, S., Moolla, R., Solberg, S., and Feng, Z.: Tropospheric Ozone Assessment Report: Present-day ozone distribution and trends relevant to human health, Elementa: Science of the Anthropocene, 6, 12, https://doi.org/10.1525/elementa.273, 2018.
Gaudel, A., Cooper, O. R., Ancellet, G., Barret, B., Boynard, A., Burrows, J. P., Clerbaux, C., Coheur, P.-F., Cuesta, J., Cuevas, E., Doniki, S., Dufour, G., Ebojie, F., Foret, G., Garcia, O., Granados Muños, M. J., Hannigan, J. W., Hase, F., Huang, G., Hassler, B., Hurtmans, D., Jaffe, D., Jones, N., Kalabokas, P., Kerridge, B., Kulawik, S. S., Latter, B., Leblanc, T., Le Flochmoën, E., Lin, W., Liu, J., Liu, X., Mahieu, E., McClure-Begley, A., Neu, J. L., Osman, M., Palm, M., Petetin, H., Petropavlovskikh, I., Querel, R., Rahpoe, N., Rozanov, A., Schultz, M. G., Schwab, J., Siddans, R., Smale, D., Steinbacher, M., Tanimoto, H., Tarasick, D. W., Thouret, V., Thompson, A. M., Trickl, T., Weatherhead, E., Wespes, C., Worden, H. M., Vigouroux, C., Xu, X., Zeng, G., and Ziemke, J.: Tropospheric Ozone Assessment Report: Present-day distribution and trends of tropospheric ozone relevant to climate and global atmospheric chemistry model evaluation, Elementa: Science of the Anthropocene, 6, 39, https://doi.org/10.1525/elementa.291, 2018.
Held, I. M.: The gap between simulation and understanding in climate modeling, B. Am. Meteorol. Soc., 86, 1609–1614, https://doi.org/10.1175/Bams-86-11-1609, 2005.
Hoesly, R. M., Smith, S. J., Feng, L., Klimont, Z., Janssens-Maenhout, G., Pitkanen, T., Seibert, J. J., Vu, L., Andres, R. J., Bolt, R. M., Bond, T. C., Dawidowski, L., Kholod, N., Kurokawa, J.-I., Li, M., Liu, L., Lu, Z., Moura, M. C. P., O'Rourke, P. R., and Zhang, Q.: Historical (1750–2014) anthropogenic emissions of reactive gases and aerosols from the Community Emissions Data System (CEDS), Geosci. Model Dev., 11, 369–408, https://doi.org/10.5194/gmd-11-369-2018, 2018.
IPCC: Climate Change 2013: The Physical Science Basis. Contribution of Working Group I to the Fifth Assessment Report of the Intergovernmental Panel on Climate Change, edited by: Stocker, T. F., Qin, D., Plattner, G.-K., Tignor, M., Allen, S. K., Boschung, J., Nauels, A., Xia, Y., Bex, V., and Midgley, P. M., Cambridge University Press, Cambridge, United Kingdom and New York, NY, USA, 1535 pp., 2013.
Jaffe, D. A., O'Neill, S. M., Larkin, N. K., Holder, A. L., Peterson, D. L., Halofsky, J. E., and Rappold, A. G.: Wildfire and prescribed burning impacts on air quality in the United States, J. Air Waste Manage., 70, 583–615, 2020.
Keeling, C. D., Bacastow, R. B., Carter, A. F., Piper, S. C., Whorf, T. P., Heimann, M., Mook, W. G., and Roeloffzen, H.: A three-dimensional model of atmospheric CO2 transport based on observed winds: 1. Analysis of observational data, Geophysical Monograph, 55, 305–363, 1989.
Kim, S.-W., Kim, K.-M., Jeong, Y., Seo, S., Park, Y., and Kim, J.: Changes in surface ozone in South Korea on diurnal to decadal timescales for the period of 2001–2021, Atmos. Chem. Phys., 23, 12867–12886, https://doi.org/10.5194/acp-23-12867-2023, 2023.
Lefohn, A. S., Malley, C. S., Smith, L., Wells, B., Hazucha, M., Simon, H., Naik, V., Mills, G., Schultz, M. G., Paoletti, E., De Marco, A., Xu, X., Zhang, L., Wang, T., Neufeld, H. S., Musselman, R. C., Tarasick, D., Brauer, M., Feng, Z., Tang, H., Kobayashi, K., Sicard, P., Solberg, S., and Gerosa, G.: Tropospheric ozone assessment report: Global ozone metrics for climate change, human health, and crop/ecosystem research, Elementa: Science of the Anthropocene, 6, 28, https://doi.org/10.1525/elementa.279, 2018.
Logan, J. A., Staehelin, J., Megretskaia, I. A., Cammas, J.-P., Thouret, V., Claude, H., De Backer, H., Steinbacher, M., Scheel, H. E., Stübi, R., Fröhlich, M., and Derwent, R.: Changes in ozone over Europe: Analysis of ozone measurements from sondes, regular aircraft (MOZAIC) and alpine surface sites, J. Geophys. Res., 117, D09301, https://doi.org/10.1029/2011JD016952, 2012.
Lu, K. D., Zhang, Y. H., Su, H., Brauers, T., Chou, C. C., Hofzumahaus, A., Liu, S. C., Kita, K., Kondo, Y., Shao, M., Wahner, A., Wang, J. L., Wang, X. S., and Zhu, T.: Oxidant (O3+NO2) production processes and formation regimes in Beijing, J. Geophys. Res.-Atmos., 115, D07303, https://doi.org/10.1029/2009jd012714, 2010.
Lynch, J., Cain, M., Pierrehumbert, R., and Allen, M.: Demonstrating GWP*: a means of reporting warming-equivalent emissions that captures the contrasting impacts of short- and long-lived climate pollutants, Environ. Res. Lett., 15, 044023, https://doi.org/10.1088/1748-9326/ab6d7e, 2020.
McDonald, B. C., De Gouw, J. A., Gilman, J. B., Jathar, S. H., Akherati, A., Cappa, C. D., Jimenez, J. L., Lee-Taylor, J., Hayes, P. L., McKeen, S. A., Cui, Y. Y., Kim, S. W., Gentner, D. R., Isaacman-VanWertz, G., Goldstein, A. H., Harley, R. A., Frost, G. J., Roberts, J. M., Ryerson, T. B., and Trainer, M.: Volatile chemical products emerging as largest petrochemical source of urban organic emissions, Science, 359, 760–764, https://doi.org/10.1126/science.aaq0524, 2018.
Mills, G., Pleijel, H., Malley, C. S., Sinha, B., Cooper, O. R., Schultz, M. G., Neufeld, H. S., Simpson, D., Sharps, K., Feng, Z., Gerosa, G., Harmens, H., Kobayashi, K., Saxena, P., Paoletti, E., Sinha, V., and Xu, X.: Tropospheric Ozone Assessment Report: Present-day tropospheric ozone distribution and trends relevant to vegetation, Elementa: Science of the Anthropocene, 6, 47, https://doi.org/10.1525/elementa.302, 2018.
Molina, M. J. and Rowland, F. S.: Stratospheric sink for chlorofluoromethanes: chlorine atomc-atalysed destruction of ozone, Nature, 249, 810–812, https://doi.org/10.1038/249810a0, 1974.
Monks, P. S., Archibald, A. T., Colette, A., Cooper, O., Coyle, M., Derwent, R., Fowler, D., Granier, C., Law, K. S., Mills, G. E., Stevenson, D. S., Tarasova, O., Thouret, V., von Schneidemesser, E., Sommariva, R., Wild, O., and Williams, M. L.: Tropospheric ozone and its precursors from the urban to the global scale from air quality to short-lived climate forcer, Atmos. Chem. Phys., 15, 8889–8973, https://doi.org/10.5194/acp-15-8889-2015, 2015.
Murray, L. T.: An uncertain future for lightning, Nat. Clim. Change, 8, 191–192, https://doi.org/10.1038/s41558-018-0094-0, 2018.
National Institute of Environmental Research (NIER): Airkorea surface observation networks, NIER [data set], https://www.airkorea.or.kr/web/last_amb_hour_data?pMENU_NO=123 (last access: 28 July 2022), 2022.
Nelson, B. S., Stewart, G. J., Drysdale, W. S., Newland, M. J., Vaughan, A. R., Dunmore, R. E., Edwards, P. M., Lewis, A. C., Hamilton, J. F., Acton, W. J., Hewitt, C. N., Crilley, L. R., Alam, M. S., Şahin, Ü. A., Beddows, D. C. S., Bloss, W. J., Slater, E., Whalley, L. K., Heard, D. E., Cash, J. M., Langford, B., Nemitz, E., Sommariva, R., Cox, S., Shivani, Gadi, R., Gurjar, B. R., Hopkins, J. R., Rickard, A. R., and Lee, J. D.: In situ ozone production is highly sensitive to volatile organic compounds in Delhi, India, Atmos. Chem. Phys., 21, 13609–13630, https://doi.org/10.5194/acp-21-13609-2021, 2021.
Parrish, D. D., Millet, D. B., and Goldstein, A. H.: Increasing ozone in marine boundary layer inflow at the west coasts of North America and Europe, Atmos. Chem. Phys., 9, 1303–1323, https://doi.org/10.5194/acp-9-1303-2009, 2009.
Parrish, D. D., Lamarque, J.-F., Naik, V., Horowitz, L., Shindell, D. T., Staehelin, J., Derwent, R., Cooper, O. R., Tanimoto, H., Volz-Thomas, A., Gilge, S., Scheel, H.-E., Steinbacher, M., and Fröhlich, M.: Long-term changes in lower tropospheric baseline ozone concentrations: Comparing chemistry-climate models and observations at northern midlatitudes, J. Geophys. Res.-Atmos., 119, 5719–5736, 2014.
Parrish, D. D., Derwent, R. G., Steinbrecht, W., Stubi, R., Van Malderen, R., Steinbacher, M., Trickl, T., Ries, L., and Xu, X.: Zonal similarity of long-term changes and seasonal cycles of baseline ozone at northern mid-latitudes, J. Geophys. Res.-Atmos., 125, e2019JD031908, https://doi.org/10.1029/2019JD031908, 2020 (data available at: https://join.fz-juelich.de, last access: 28 December 2022).
Parrish, D. D., Derwent, R. G., and Staehelin, J.: Long-term changes in northern mid-latitude tropospheric ozone concentrations: Synthesis of two recent analyses, Atmos. Environ., 248, 118227, https://doi.org/10.1016/j.atmosenv.2021.118227, 2021.
Parrish, D. D., Faloona, I. C., and Derwent, R. G.: Observational-based assessment of contributions to maximum ozone concentrations in the western United States, J. Air Waste Manage., 72, 434–454, https://doi.org/10.1080/10962247.2022.2050962, 2022.
Pusede, S. E., Steiner, A. L., and Cohen, R. C.: Temperature and Recent Trends in the Chemistry of Continental Surface Ozone, Chem. Rev., 115, 3898–3918, https://doi.org/10.1021/cr5006815, 2015.
Pyle, J. A., Keeble, J., Abraham, N. L., Chipperfield, M. P., and Griffiths, P. T.: Integrated ozone depletion as a metric for ozone recovery, Nature, 608, 719–723, 2022.
Qu, Z., Jacob, D. J., Silvern, R. F., Shah, V., Campbell, P. C., Valin, L. C., and Murray, L. T.: US COVID-19 Shutdown Demonstrates Importance of Background NO2 in Inferring NOx Emissions From Satellite NO2 Observations, Geophys. Res. Lett., 48, e2021GL092783, https://doi.org/10.1029/2021GL092783, 2021.
Royal Institution: Who discovered the greenhouse effect?, https://www.rigb.org/explore-science/explore/blog/who-discovered-greenhouse-effect (last access: 18 May 2023), 2019.
Sillman, S.: The relation between ozone, NOx and hydrocarbons in urban and polluted rural environments, Atmos. Environ., 33, 1821–1845, 1999.
Simon, H., Baker, K. R., and Phillips, S.: Compilations and interpretations of photochemical model performance statistics published between 2006 and 2012, Atmos. Environ., 61, 124–139, 2012.
Sindelarova, K., Granier, C., Bouarar, I., Guenther, A., Tilmes, S., Stavrakou, T., Müller, J.-F., Kuhn, U., Stefani, P., and Knorr, W.: Global data set of biogenic VOC emissions calculated by the MEGAN model over the last 30 years, Atmos. Chem. Phys., 14, 9317–9341, https://doi.org/10.5194/acp-14-9317-2014, 2014 (data available at: https://eccad.aeris-data.fr/, last access: 20 May 2023).
Skeie, R. B. Myhre, G., Hodnebrog, Ø., Cameron-Smith, P. J., Deushi, M., Hegglin, M. I., Horowitz, L. W., Kramer, R. J., Michou, M., Mills, M. J., Olivié, D. J. L., Connor, F. M. O., Paynter, D., Samset, B. H., Sellar, A., Shindell, D., Takemura, T., Tilmes, S., and Wu, T.: Historical total ozone radiative forcing derived from CMIP6 simulations, npj Clim. Atmos. Sci., 3, 32, https://doi.org/10.1038/s41612-020-00131-0, 2020.
Smith, S. J., McDuffie, E. E., and Charles, M.: Opinion: Coordinated development of emission inventories for climate forcers and air pollutants, Atmos. Chem. Phys., 22, 13201–13218, https://doi.org/10.5194/acp-22-13201-2022, 2022.
Solomon, S., Garcia, R. R., Rowland, F. S., and Wuebbles, D. J.: On the depletion of Antarctic ozone, Nature, 321, 755–758, 1986.
Stevenson, D. S., Young, P. J., Naik, V., Lamarque, J.-F., Shindell, D. T., Voulgarakis, A., Skeie, R. B., Dalsoren, S. B., Myhre, G., Berntsen, T. K., Folberth, G. A., Rumbold, S. T., Collins, W. J., MacKenzie, I. A., Doherty, R. M., Zeng, G., van Noije, T. P. C., Strunk, A., Bergmann, D., Cameron-Smith, P., Plummer, D. A., Strode, S. A., Horowitz, L., Lee, Y. H., Szopa, S., Sudo, K., Nagashima, T., Josse, B., Cionni, I., Righi, M., Eyring, V., Conley, A., Bowman, K. W., Wild, O., and Archibald, A.: Tropospheric ozone changes, radiative forcing and attribution to emissions in the Atmospheric Chemistry and Climate Model Intercomparison Project (ACCMIP), Atmos. Chem. Phys., 13, 3063–3085, https://doi.org/10.5194/acp-13-3063-2013, 2013.
Tan, Z., Lu, K., Jiang, M., Su, R., Wang, H., Lou, S., Fu, Q., Zhai, C., Tan, Q., Yue, D., Chen, D., Wang, Z., Xie, S., Zeng, L., and Zhang, Y.: Daytime atmospheric oxidation capacity in four Chinese megacities during the photochemically polluted season: a case study based on box model simulation, Atmos. Chem. Phys., 19, 3493–3513, https://doi.org/10.5194/acp-19-3493-2019, 2019.
Tarasick, D., Galbally, I. E., Cooper, O. R., Schultz, M. G., Ancellet, G., Leblanc, T., Wallington, T. J., Ziemke, J., Liu, X., Steinbacher, M., Staehelin, J., Vigouroux, C., Hannigan, J. W., García, O., Foret, G., Zanis, P., Weatherhead, E., Petropavlovskikh, I., Worden, H., Osman, M., Liu, J., Chang, K.-L., Gaudel, A., Lin, M., Granados-Muñoz, M., Thompson, A. M., Oltmans, S. J., Cuesta, J., Dufour, G., Thouret, V., Hassler, B., Trickl, T., and Neu, J. L.: Tropospheric Ozone Assessment Report: Tropospheric ozone from 1877 to 2016, observed levels, trends and uncertainties, Elementa: Science of the Anthropocene, 7, 39, https://doi.org/10.1525/elementa.376, 2019.
Turnock, S. T., Allen, R. J., Andrews, M., Bauer, S. E., Deushi, M., Emmons, L., Good, P., Horowitz, L., John, J. G., Michou, M., Nabat, P., Naik, V., Neubauer, D., O'Connor, F. M., Olivié, D., Oshima, N., Schulz, M., Sellar, A., Shim, S., Takemura, T., Tilmes, S., Tsigaridis, K., Wu, T., and Zhang, J.: Historical and future changes in air pollutants from CMIP6 models, Atmos. Chem. Phys., 20, 14547–14579, https://doi.org/10.5194/acp-20-14547-2020, 2020.
Wang, Y., Gao, W., Wang, S., Song, T., Gong, Z., Ji, D., Wang, L., Liu, Z., Tang, G., Huo, Y., Tian, S., Li, J., Li, M., Yang, Y., Chu, B., Petäjä, T., Kerminen, V. M., He, H., Hao, J., Kulmala, M., Wang, Y., and Zhang, Y.: Contrasting trends of PM2.5 and surface-ozone concentrations in China from 2013 to 2017, Natl. Sci. Rev., 7, 1331–1339, https://doi.org/10.1093/nsr/nwaa032, 2020.
Wang, Y. H. and Jacob, D. J. Anthropogenic forcing on tropospheric ozone and OH since preindustrial times, J. Geophys. Res.-Atmos., 103, 31123–31135, 1998.
West, J. J. Smith, S. J., Silva, R. A., Naik, V., Zhang, Y., Adelman, Z., Fry, M. M., Anenberg, S., Horowitz, L. W., and Lamarque, J. F.: Co-benefits of mitigating global greenhouse gas emissions for future air quality and human health, Nat. Clim. Change, 3, 885–889, https://doi.org/10.1038/Nclimate2009, 2013.
Young, P. J., Archibald, A. T., Bowman, K. W., Lamarque, J.-F., Naik, V., Stevenson, D. S., Tilmes, S., Voulgarakis, A., Wild, O., Bergmann, D., Cameron-Smith, P., Cionni, I., Collins, W. J., Dalsøren, S. B., Doherty, R. M., Eyring, V., Faluvegi, G., Horowitz, L. W., Josse, B., Lee, Y. H., MacKenzie, I. A., Nagashima, T., Plummer, D. A., Righi, M., Rumbold, S. T., Skeie, R. B., Shindell, D. T., Strode, S. A., Sudo, K., Szopa, S., and Zeng, G.: Pre-industrial to end 21st century projections of tropospheric ozone from the Atmospheric Chemistry and Climate Model Intercomparison Project (ACCMIP), Atmos. Chem. Phys., 13, 2063–2090, https://doi.org/10.5194/acp-13-2063-2013, 2013.
- Abstract
- Introduction
- Science-into-policy processes of stratospheric ozone layer depletion
- Science-into-policy processes of global climate change
- Essential elements of the science-into-policy process
- Review and assessment of tropospheric ozone
- Development of a hierarchy of models to understand the essential science of tropospheric ozone
- Policy-relevant metrics
- Implications for future policy and science
- Data availability
- Author contributions
- Competing interests
- Disclaimer
- Acknowledgements
- Financial support
- Review statement
- References
- Abstract
- Introduction
- Science-into-policy processes of stratospheric ozone layer depletion
- Science-into-policy processes of global climate change
- Essential elements of the science-into-policy process
- Review and assessment of tropospheric ozone
- Development of a hierarchy of models to understand the essential science of tropospheric ozone
- Policy-relevant metrics
- Implications for future policy and science
- Data availability
- Author contributions
- Competing interests
- Disclaimer
- Acknowledgements
- Financial support
- Review statement
- References