the Creative Commons Attribution 4.0 License.
the Creative Commons Attribution 4.0 License.
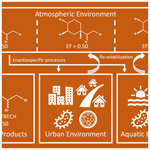
The atmospheric fate of 1,2-dibromo-4-(1,2-dibromoethyl)cyclohexane (TBECH): spatial patterns, seasonal variability, and deposition to Canadian coastal regions
Jenny Oh
Chubashini Shunthirasingham
Ying Duan Lei
Faqiang Zhan
Yuening Li
Abigaëlle Dalpé Castilloux
Amina Ben Chaaben
Kelsey Lee
Frank A. P. C. Gobas
Sabine Eckhardt
Nick Alexandrou
Hayley Hung
Brominated flame retardants (BFRs) that are gradually being phased out are being replaced by emerging BFRs. Here, we report the concentration of the α- and β-isomers of 1,2-dibromo-4-(1,2-dibromoethyl)cyclohexane (TBECH; also known as DBE-DBCH) in over 300 air, water, and precipitation samples collected between 2019 and 2022 using active air and deposition sampling as well as networks of passive air and water samplers. The sampling region includes Canada's most populated cities and areas along the St. Lawrence River and Estuary, Quebec, as well as around the Salish Sea, British Columbia. TBECH was detected in over 60 % of air samples at levels comparable to those of 2,2′,4,4′-tetrabromodiphenyl ether (BDE-47). Concentrations of TBECH and BDE-47 were typically higher in urban areas, with stronger correlations with population density during warmer deployments. Uniform α- β-TBECH ratios across space, time, and environmental media indicate the highly similar atmospheric fate of the two isomers. Although TBECH air concentrations were strongly related to temperature in urban Toronto and a remote site on the east coast, the lack of such dependence at a remote site on the west coast can be explained by the small seasonal temperature range and summertime air mass transport from the Pacific Ocean. Despite there being no evidence that TBECH has been produced, or imported for use, in Canada, it is now one of the most abundant gaseous BFRs in the Canadian atmosphere. The recorded spatial and temporal variability of TBECH suggest that its emissions are not constrained to specific locations but are generally tied to the presence of humans. The most likely explanation for its environmental occurrence in Canada is the release from imported consumer products containing TBECH. Chiral analysis suggests that despite its urban origin, at least some fraction of TBECH has experienced enantioselective processing, i.e., has volatilized from reservoirs where it has undergone microbial transformations. Microbial processes in urban soils and in marine waters may have divergent enantioselectivity.
- Article
(4108 KB) - Full-text XML
-
Supplement
(2709 KB) - BibTeX
- EndNote
Starting from the 1970s, brominated flame retardants (BFRs) have been used extensively in commercial products to reduce ignition and increase their resistance to fire. To date, at least 75 different kinds of BFRs have been commercially produced, with polybrominated diphenyl ethers (PBDEs) being historically the most used class (Alaee et al., 2003). However, their toxicity, potential for bioaccumulation, and persistence in the environment soon became apparent (Christensen et al., 2005; De Wit et al., 2006; Palm et al., 2002; Ruan et al., 2019), and PBDEs, along with other traditional BFRs, were eventually classified as persistent organic pollutants (POPs) (POPRC, 2009). As usage and production of these BFRs were restricted internationally in 2009 under the United Nations Environment Programme Stockholm Convention on POPs (UNEP-SC-POPs) (UNECE, 2018), emerging brominated flame retardants (EBFRs) entered into the market (Betts, 2008), which are touted to be less persistent than their traditional BFR counterparts. However, studies on their environmental behaviours have been limited.
1,2-Dibromo-4-(1,2-dibromoethyl)cyclohexane (TBECH) is an EBFR that is widely used in plastics, fabric adhesives, and building insulation materials (Alaee et al., 2003). TBECH exists as four diastereomers (α-, β-, γ-, and δ-TBECH). Furthermore, TBECH exhibits chirality, and thus each diastereomer consists of a pair of enantiomers. While commercial TBECH comprises mostly the α- and β-isomers (57.3 % and 42.5 %, respectively) (Ruan et al., 2018), TBECH has been reported to thermally isomerize to the γ- and δ-isomers when heated to 123 ∘C (Arsenault et al., 2008). Although global production volumes or emissions of TBECH are difficult to establish, reports on its detection in the environment have been increasing over the years, attributed to the rising demand for EBFRs (Bohlin et al., 2014; Cequier et al., 2014; Drage et al., 2016; Genisoglu et al., 2019; Gentes et al., 2012; Newton et al., 2015; Pasecnaja et al., 2021; Tao et al., 2017; Zacs et al., 2021). TBECH was first detected in sediment samples collected in 1996 near a discharge pipe of the Frutarom plastics plant near Haifa, Israel (Santillo et al., 1997). Since then, TBECH was found in various environmental media worldwide, such as biota and water, with α- and β-TBECH being the dominant isomers. TBECH concentrations appear to be particularly high in indoor settings, such as offices and residences (Melymuk et al., 2016; Newton et al., 2015; Wong et al., 2018).
There is evidence that TBECH displays toxic effects at low concentrations both in vitro and in vivo. All TBECH isomers have shown to be strong androgen agonists, where TBECH competitively binds to the androgen receptor active site in varying degrees, first determined in vitro in human cell lines (Khalaf et al., 2009; Larsson et al., 2006). Moreover, several studies suggest that all isomers are capable of multimodal endocrine disruption, which involves estrogenic (Asnake et al., 2014), anti-androgenic (Wong et al., 2016), and thyroidal processes (Porter et al., 2014). This disrupting potential of TBECH is also seen in the in vivo studies reporting changes in circulating hormones (Curran et al., 2017; Gemmill et al., 2011), organ structure (Park et al., 2011), and reproduction (Marteinson et al., 2012a, b) after exposure to low concentrations of TBECH. More details on the reported toxicological effects of TBECH from both in vivo and in vitro studies are discussed in Marteinson et al. (2021).
Presumably because no Canadian company reported the manufacture and importation of TBECH on a commercial scale (ECCC, 2017), it has been deemed a non-priority for assessment under Canada's Chemicals Management Plan (ECCC, 2019). As a result, TBECH remains unregulated at both national and international levels, despite being judged to be persistent and bioaccumulative. There is evidence, however, of its presence in the Canadian environment, as TBECH has been detected in different environmental media, ranging from urban outdoor air in Toronto (Shoeib et al., 2014) to whale blubber in the Arctic (Tomy et al., 2008). Only three studies have been published on the enantiomeric composition of TBECH in the environment (Ruan et al., 2018, 2019), with one study on Canadian urban soils (Wong et al., 2012). Therefore, concerted efforts to understand the sources, atmospheric behaviour, and enantiomeric profile of TBECH have been limited, not only in Canada but also worldwide.
With the objective to gain insight into the atmospheric fate of α- and β-TBECH in general and in southern Canada specifically, we sought to characterize the spatial and seasonal variability in their air concentrations and to explore their potential for atmospheric deposition. We also compare the atmospheric fate of TBECH with that of the PBDEs, in particular 2,2′,4,4′-tetrabromodiphenyl ether (BDE-47), one of the traditional BFRs that has been internationally banned under the UNEP-SC-POPs but can still be detected in the environment. Spatial patterns were investigated with two passive air sampler (PAS) networks in the coastal regions of Quebec (QC) and British Columbia (BC). Seasonal trends in air concentrations were studied using active air samplers (AASs) at an urban site in Toronto and two remote sites on either coast. The input to aquatic systems was studied by measuring year-round wet deposition and by deploying passive water samplers (PWSs) at selected coastal sites.
2.1 Sampling
2.1.1 Passive air sampling network
Networks of XAD-2-resin-based PASs (Wania et al., 2003) were installed in QC along the ca. 1000 km stretch of the St. Lawrence River and Estuary between Montreal and the Gulf of St. Lawrence, as well as in BC on the coast of the Central Salish Sea. PAS housings, containing two XAD-resin-filled mesh cylinders each, were deployed at a height of ca. 3 to 5 m above ground on trees or other structures at 118 unique sites between 2019 and 2022. Because PASs were deployed at some sites more than once, there was a total of 169 PASs. Maps of the networks are shown in Fig. S1 in the Supplement, while coordinates of the sampling sites, dates of deployment and retrieval, and deployment duration are provided in Table S1 in the Supplement. By revealing the spatial variability in the air concentration of TBECH and other BFRs across a wide region, the networks can identify the location of emissions to the atmosphere. The networks included sites in highly urbanized and industrialized area but also rural and remote sites. Additional details are provided in Text S1 in the Supplement. After retrieval, the PASs were stored in metal shipping containers sealed with Teflon-tape-coated stoppers at −20 ∘C until analysis.
2.1.2 Active air sampling and precipitation collection
To quantify the seasonal variability in the air concentration of TBECH and other BFRs in an urban source region, 48 consecutive week-long AASs were taken on the campus of the University of Toronto Scarborough in the eastern suburbs of Toronto, Ontario, between June 2020 and May 2021 (Li et al., 2023). These measurements were complemented by 24 h AASs taken once a month for 1 year at two remote coastal sites far from large urban centres and, therefore, less likely to be influenced by local emission sources. These were on Saturna Island, BC (L43 on Fig. S1; ca. 42 km NNE of Victoria; pop. ∼ 300; December 2019–November 2020; n=11), and Tadoussac, QC (near S57 on Fig. S1; ca. 190 km NE of Québec City; pop. ∼ 800; December 2020 and November 2021; n=12). Contaminants in the particle and gas phase were collected on a glass-fiber filter (GFF) and a polyurethane foam (PUF)–XAD-2–PUF sandwich, respectively. The sampling periods are listed in Table S2.
At Saturna Island and Tadoussac, monthly-integrated wet deposition samples were taken during the same 12-month period as the AAS. Precipitation samples were collected for 1 month each in sample bottles containing 0.2 L dichloromethane, which were connected to overflow bottles to capture any overflowing precipitation from the sample bottles. The sampling periods are listed in Table S3.
2.1.3 Passive water sampling network
Passive water samplers consisting of low-density polyethylene (LDPE) sheets in a metal mesh cage were deployed in 20 unique locations in BC and QC during the late spring and summer of 2021 (Table S9). Figure S2 provides maps with the sampling sites. Before deployment, the LPDE sheets were infused with several performance reference compounds (PRCs) to determine sampling rates. Details are provided in Text S2.
2.2 Sample analysis
The gas-phase sorbent from the AAS at Saturna Island and Tadoussac was Soxhlet extracted. All other air samples underwent pressurized liquid extraction using an accelerated solvent extractor. The precipitation samples were filtered through 0.7 µm GFFs, and the filtered samples were then subjected to liquid–liquid extraction. The LDPE sheets were simply soaked in solvent overnight. A total of 14 13C-labelled surrogates were spiked into all samples prior to extraction. All extracts were reduced in volume and dried with Na2SO4. Injection standards (13C-PCB-105 and 180) were added to the final extracts. Details on the extraction solvents, conditions, and standards are provided in Text S3.
All samples were analyzed for a suite of 28 BFRs, including 15 PBDE congeners (BDE-17, 28, 47, 49, 66, 71, 85, 99, 100, 138, 153, 154, 183, 190, and 209), α-TBECH, β-TBECH, allyl 2,4,6-tribromophenyl ether (ATE), 2-bromoallyl 2,4,6-tribromophenyl ether (BATE), pentabromobenzene (PBBz), hexabromobenzene (HBBz), pentabromotoluene (PBT), pentabromoethyl benzene (PBEB), 2,3-dibromopropyl-2,4,6-tribromophenyl ether (DBTE), 2-ethylhexyl-2,3,4,5-tetrabromobenzoate (EHTBB), 1,2-bis(2,4,6-tribromophenoxy)ethane (BTBPE), bis(2-ethylhexyl)-3,4,5,6-tetrabromo-phthalate (BEHTBP), and decabromodiphenyl ethane (DBDPE). Four additional chlorinated flame retardants (Dec-602, Dec-604, syn-DP, anti-DP) were also analyzed. Details of the instrumental analysis are provided in Text S4. This is also where information on the QA/QC procedures can be found, which includes many procedural and field blanks. Limit of detection (LOD) and limit of quantitation (LOQ) for different types of samples are provided in Table S12.
Both α- and β-TBECH are chiral molecules but can be expected to enter the environment as a racemate, i.e., with their two enantiomers being equally abundant (mean enantiomeric fraction EF). The unequal abundance of two enantiomers in environmental samples has been used to identify the occurrence of enantioselective processing, such as microbial transformation reactions. The enantiomeric composition of α- and β-TBECH was determined in samples with TBECH concentrations > LOQ from the PAS network in BC and QC, from the AAS in Toronto, and in a few PWSs. The amounts of β-TBECH in the extract were generally too low for reliable chiral analysis; therefore, only results on the enantiomeric analysis of α-TBECH are presented. A description of the analytical method for enantiomeric analysis is provided in Text S4.
2.3 Air and water concentration calculations
Volumetric air concentrations were derived from the amounts quantified in the PAS sorbent using sampling rates reported by Li et al. (2023). Volumetric water concentrations were calculated from the amount quantified in the LDPE sheets using the dissipation of PRCs and the approach by Booij and Smedes (2010). Details are again in Text S5. For statistical purposes, the measurements below the LOD are represented by the value of the compound specific LOD (Table S12).
2.4 Partitioning property calculations using COSMO-RS and other prediction tools
Equilibrium partition ratios between octanol and water (KOW), octanol and air (KOA), and air and water (KAW) of TBECH were estimated using quantitative structure–activity relationship (QSAR) models integrated in several chemical property prediction tools (EASE-Suite, EPI Suite, OPERA). Because these QSARs cannot distinguish between the properties of α- and β-TBECH, we also applied COSMOtherm, which is based on quantum chemistry and statistical thermodynamics. Details of these approaches have been described previously (Baskaran et al., 2021). More details on COSMOtherm are also given in Text S6. In general, the predicted partition ratios of TBECH from the different models are in good agreement; i.e., they are typically within 0.30 log units of each other (Table 1). COSMOtherm tends to give slightly lower log KOW and log KAW values, suggesting a higher solvation in the aqueous phase than the QSARs. COSMOtherm-predicted properties for the two isomers are also very similar, with α-TBECH being slightly more volatile than β-TBECH.
3.1 Air concentrations
3.1.1 Absolute concentration ranges, isomer composition, and comparison with previous measurements
α- and β-TBECH and BDE-47 were the most consistently detected BFRs in air samples taken across Canada, detected in 69 %, 59 %, and 80 % of samples from the PAS network, respectively. Table 2 summarizes the results, whereas data for each individual sample are documented in Tables S1 and S2. To a lesser extent, several other EBFRs and PBDE congeners were also detected in the samples of this study, with their detection frequencies or concentrations summarized in Tables S4 to S8. Several legacy BFRs (BDE-190 and 85) and EBFRs (HBBz and PBBz) were occasionally detected in the PAS network samples, with detection frequencies ranging between 7 % and 26 %. These compounds, however, were not detected in the active air samples. Moreover, other BFRs that were detected frequently in water samples (BDE-17, 28, 99, and 100) were rarely detected in the other sample types. Because of their much higher detection frequencies in all samples, the remainder of the paper is focused on TBECH and BDE-47.
Table 2Detection frequency, median, mean, and maximum of the concentrations and wet deposition fluxes of the three most frequently detected BFRs in air samples (gas phase) and water samples (dissolved) in Canada. For statistical purposes, measurements below the LOD were represented by the value of the compound specific LOD.
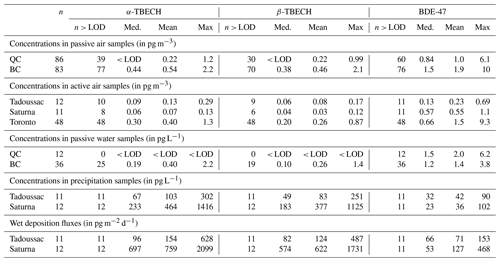
To place these concentrations into context, Table S13 summarizes all atmospheric concentrations of TBECH previously reported in the literature. A review of the occurrences of TBECH in other environmental media (e.g., soil, water, sediment) can be found in Marteinson et al. (2021). The concentrations of TBECH recorded here are at the lower end of the range of levels detected in urban outdoor air elsewhere. In particular, levels in Canadian urban locations are similar to those reported for outdoor air in Stockholm, Sweden (Newton et al., 2015; Wong et al., 2018), but somewhat lower than those measured in Birmingham, UK (Drage et al., 2016); Brno, Czech Republic (Bohlin et al., 2014; Melymuk et al., 2016); and previous measurements taken in Toronto (Shoeib et al., 2014). Much higher values had been reported for indoor air (Cequier et al., 2014; Genisoglu et al., 2019; Melymuk et al., 2016; Newton et al., 2015, 2016; Tao et al., 2016; Wong et al., 2018), an electronic waste facility in China (Hong et al., 2018), and – somewhat incongruously – in Longyearbyen, Svalbard (Carlsson et al., 2018). PAS deployed in remote regions (pop. < 10 000 in a 20 km radius) tended to have levels of TBECH below the LOD or LOQ. The levels above LOD measured in non-urban locations in this study are among the lowest ever reported, comparable to what has been reported for Tibet and Antarctica (Ma et al., 2017; Zhao et al., 2020). However, previous measurements of TBECH in air from non-urban locations are rare. Overall, the alignment of the air concentration data with those reported previously supports the validity of the results of this study.
BDE-47, despite its international ban under the UNEP-SC-POPS, can still be detected in the environment due to its persistence. Its presence in the atmosphere, along with other BDEs, has been documented over the years in Canada, such as in Ottawa, ON (Wilford et al., 2004); Alert, NU (Wong et al., 2021; Xiao et al., 2012); Yukon Territory (Yu et al., 2015); and the Great Lakes Basin (Shunthirasingham et al., 2018). In this study, BDE-47 was also detected in the air in all sampling regions, with comparable levels to TBECH. On Saturna Island, BDE-47 gas-phase concentrations have decreased by 1 order of magnitude relative to almost 2 decades prior (Noël et al., 2009). Levels in the atmospheric particle phase and in precipitation in the area have similarly been decreasing.
3.1.2 Spatial variability of air concentrations in Canadian coastal regions
The spatial patterns in the air concentrations of α- and β-TBECH in the coastal regions of BC and QC as obtained from the PAS networks (Fig. 1) show elevated levels in populated and urban areas. Specifically, in QC, higher levels are observed along the St. Lawrence River corridor between Montreal and Québec City contrasting with lower levels on the shores of the St. Lawrence Estuary. In BC, higher levels are apparent in the lower mainland and in Victoria. The overall higher levels observed in the PASs from BC when compared to those from QC are likely the result of more urbanized sampling locations in the former. Earlier studies also indicate that urban areas have higher TBECH air concentrations than remote regions (Table S13).
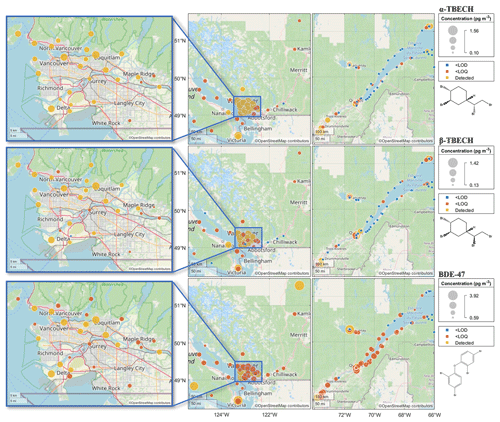
Figure 1The spatial distribution of the air concentrations of α-TBECH (top), β-TBECH (middle), and BDE-47 (bottom) in British Columbia and Quebec. Close-up maps (left) provide a detailed view of the Vancouver metropolitan area. Average air concentrations are displayed for sites with replicate deployments; therefore, the concentrations indicated on the figure may not align with concentrations listed in Tables 2 and S1. Air concentrations below the LOD are marked in blue and display the value of the LOD. Air concentrations above the LOD and below the LOQ are marked in dark orange. Air concentrations above LOQ are marked in yellow. Concentration values are listed in Table S1.
The α- β-TBECH ratio at sites where both isomers were present above their LODs (Fig. S3) is remarkably consistent in space on either coast; i.e., there is no indication that the ratio varies in space, e.g., by being correlated with the absolute concentration level. The ratio was typically close to or above one at sites with detectable amounts of both isomers in the air. An exception (α- β-TBECH = 0.29) was at a site located 10 km away from Québec City, QC (S26). However, because the air concentrations of both isomers measured at this site were below their LOQs, this value should be interpreted with caution.
Not only are the volumetric air concentrations of BDE-47 on the same order of magnitude as TBECH, but they also share a similar spatial distribution in the atmosphere (Fig. 1). This is also apparent from significant correlations between the concentration of both isomers of TBECH and those of BDE-47 in the PAS (Fig. S4, Table S14, R2>0.24, p<0.0001), which seem to be strongly influenced by the urban sites with elevated BFR concentrations. One difference is the notable presence of BDE-47 at one site in Alma, QC (S48), during the first deployment period, despite BDE-47 at other sites in Alma (S49–53) and in the wider Saguenay region (S54–56) being below the LOQ. PASs deployed at the same site (S48_2) and a site in the vicinity (S54_2) a year later had BDE-47 levels below the LOQ, suggesting that the first data point at S48 was an outlier and not an indication of a local point source.
In the BC PAS network, air concentrations are linearly correlated with population within a 20 km radius around a PAS deployment site (NASA, 2015) (Fig. S5, Table S15), more so for α- and β-TBECH (R2=0.27 and 0.23, respectively) than for BDE-47 (R2=0.09). The relationships were generally stronger when explored separately with concentration data obtained at different average deployment temperature (<10, 10–15, >15 ∘C; Fig. S5). Weaker or absent relationships at the warmest temperature (>15 ∘C) are likely caused by the relatively small number of summer deployments. Increasing slopes of these relationships at warmer temperatures indicate a higher seasonal concentration amplitude at sites in populated areas than in remote regions. This is also consistent with the expectation of a stronger temperature dependence of the atmospheric concentrations of semi-volatile chemicals in source areas than at sites without local sources (Wania et al., 1998). To further demonstrate this relationship with both temperature and population, multiple linear regression was used on the log-transformed partial pressure of TBECH against population and the inverse temperature, which resulted in higher correlations (Adjusted R2=0.56 and 0.42 for α- and β-TBECH, respectively). The seasonal variability in the air concentrations of TBECH and BDE-47 is discussed in more depth when discussing the AAS results in Sect. 3.1.3.
3.1.3 Seasonal variability of air concentrations in urban and remote regions of Canada
Seasonal variability in Toronto
The seasonal trends of AAS-derived air concentrations of TBECH and BDE-47 in Toronto, ON (Table S2, Fig. 2a and b) indicate a strong relationship with average air temperature (Fig. 2c), being higher in the summer (April to August) and lower in winter (September to March). Accordingly, log-transformed partial pressures of α- and β-TBECH and BDE-47 were significantly correlated with inverse absolute temperature (Fig. S6, Table S16, R2=0.61, 0.55, and 0.81, respectively, p<0.0001). Such a temperature dependence of the atmospheric concentration of semi-volatile organic compounds is often interpreted as temperature-driven air–surface exchange (Wania et al., 1998) or temperature-dependent rates of emission.
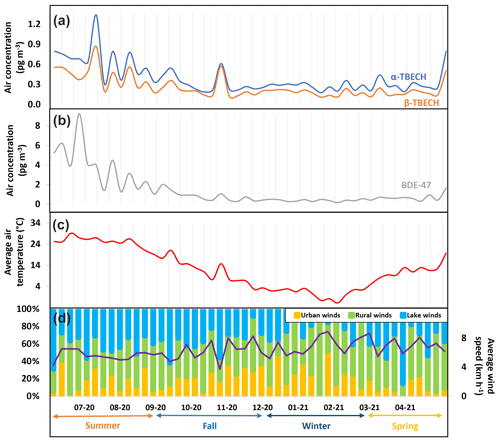
Figure 2The air concentration of α-TBECH, β-TBECH, and BDE-47 and meteorological parameters in Toronto, Ontario during 48 weekly active air sampling periods between June 2020 and May 2021.
With the sampling site located in an eastern suburb of a large urban conglomeration, wind also influenced the air concentrations of TBECH and BDE-47. Higher levels were recorded when weak winds were blowing from the urban core of Toronto, approximately 20 km to the SW of the sampling site. Text S7 and Fig. S7 provide detail on how an urban wind fraction was derived. The average wind speed and the fraction of wind originating over urban areas, rural regions, and Lake Ontario are shown in Fig. 2d. Multivariate regressions of the with both reciprocal temperature and the urban wind fraction are highly significant for all three BFRs and stronger than univariate regressions with reciprocal temperature only (Table S17).
Seasonal variability in Saturna Island and in Tadoussac
The AAS-derived air concentrations of TBECH and BDE-47 on Saturna Island were very low (Table S2). Whereas BDE-47 showed a clear seasonal pattern with higher levels in summer than in winter, any seasonal pattern of the TBECH isomers was obscured by levels below the LOD in air samples taken in December, June, and July (Fig. S8). Accordingly, whereas the of BDE-47 was significantly correlated with reciprocal temperature (R2=0.44 and p=0.027), for both TBECH isomers those relationships were generally weak and insignificant (Table S16). Weak or absent – relationships are typical for remote sites without local sources, where the concentration of a compound is controlled by advection from elsewhere (Wania et al., 1998).
In contrast to Saturna Island, AAS-derived air concentrations of TBECH and BDE-47 in Tadoussac (Table S2) showed a clear seasonal trend, with higher levels in summer and levels often below the LOD in winter (Fig. S9). – regressions (Fig. S6) were significant but only explained a relatively small fraction of the overall variability (R2>0.30; Table S16). One factor that may have contributed to the differences in the seasonal patterns of TBECH at the two coastal sites is the much larger seasonal temperature range in QC (−12 to +18 ∘C) than in BC (+1 to +18 ∘C).
We also sought to relate the temporal variabilities in the measured air concentrations with the history and origin of the sampled air masses using the Lagrangian atmospheric dispersion model FLEXPART (Pisso et al., 2019). TBECH levels below the LODs in June and July on Saturna Island despite the relatively warm temperatures may be related to air masses arriving at the sampling site directly from the Pacific Ocean, i.e., without encountering any potential urban source areas. Overall, TBECH levels on Saturna Island arise from competing influences of air mass origin and temperature. More detail is provided in Text S8.
3.2 Concentrations in passive water samples
The water concentrations of the BFRs measured with PWSs in the coastal regions of BC and QC are summarized in Table 2, with all data provided in Table S9. Whereas the TBECH isomers were below the LODs in all water samples from QC, in BC, α- and β-TBECH ranged between < LOD to 1.75 pg L−1 and < LOD to 1.15 pg L−1, respectively, and were typically above the LODs at sites close to urban centres (Victoria, Vancouver) (Fig. S10). BDE-47 was detected at all sites of this study, with higher levels close to Victoria (Fig. S12). BDE-47 water concentrations were also generally slightly higher compared to those of TBECH, albeit on the same order of magnitude.
We compared the results from the PWS network in this study with water concentrations reported by the Federal Whales Initiative (FWI) of Canada (ECCC, 2022). Sampling campaigns conducted by the FWI occurred in the Fraser River, BC, including its main tributaries (Thompson River and Harrison River) between 2019 and 2021. The median water grab sampling concentrations of TBECH (sum of all isomers) and BDE-47 reported by the FWI were 22.9 pg L−1 (presumably incorrectly labelled as ng L−1 in the database) and 32 pg L−1, respectively, which are 1 to 2 orders of magnitude higher than the PWS measurements. However, the median calculated for TBECH only reflects the 3 out of 122 samples analyzed in total with concentrations above the LOD of 1.22 pg L−1. This may be attributed to the grab sampling technique used and the FWI sampling at inland freshwater sites, whereas the PWSs in this study were deployed in seawater, which is expected to be more diluted. BDE-47 concentrations measured in the water of the Juan de Fuca Strait, BC (Sun et al., 2023) were at comparable levels (0.6–2.0 pg L−1) to the ones in this study, giving credence to this theory.
3.3 Concentrations in precipitation samples
TBECH and BDE-47 could be quantified in almost all precipitation samples from Saturna Island and Tadoussac (Table S3), with levels generally being higher on the west coast. The concentrations of TBECH varied strongly between different months, without displaying a clear seasonal trend (Fig. S13). Both isomers exhibited similar fluctuations. The BDE-47 concentrations in precipitation were generally lower and did not fluctuate as strongly as TBECH.
3.4 Enantiomeric fractions of α-TBECH
The results of the chiral analysis are presented using enantiomeric fractions (EFs), where the chromatographic elution order of the enantiomers was used to calculate EF = . A racemate with equal amounts of the (+) and (−) enantiomers has an EF of 0.50. EFs that are significantly above or below 0.5 indicate the occurrence of enantioselective processes, typically biological pathways relying on enzymes or enantioselective membranes. The α-TBECH standard had an EF = 0.502 ± 0.001. The EFs of α-TBECH in the PAS extracts had values that were significantly above and below this value, ranging from 0.32 at L2 to 0.66 at L6 (Table S18). While PAS extracts from non-urban sites rarely had sufficient amounts of α-TBECH for reliable chiral analysis, samples from a few coastal sites on the St. Lawrence Estuary and in the Saguenay area in QC and on Vancouver Island in BC had EFs below 0.50 (Fig. 3). Conversely, samples from populated urban sites in Québec City and Vancouver generally had an EF greater than 0.50. Several sites in the Vancouver area and in Victoria with EF < 0.50 are located on the shore of Burrard Inlet and Vancouver Harbour, as well as Oak Bay, respectively.
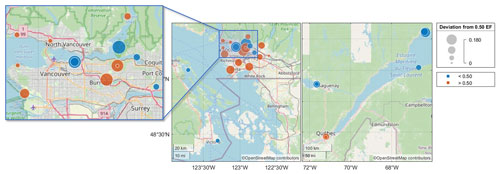
Figure 3The spatial distribution of the enantiomeric fraction (EF) of α-TBECH in selected BC and QC PASs. The absolute deviation from 0.50 (i.e., racemic mixture) of each site is plotted on the map, with values further from 0.00 indicating higher enantiomeric excess of one enantiomer. Sites with EF < 0.50 and EF > 0.50 are marked in blue and red, respectively.
Consistent with the PAS from urban deployments in BC and QC generally having EFs > 0.5, the EFs in the AAS from Toronto were also mostly above 0.502 ± 0.001 (only 1 out of 36 samples had an EF < 0.502) (Table S19). While there was no clear seasonal trend in the EF, α-TBECH tended to be close to racemic in summertime air (Fig. S14, Table S20; R2=0.14, p=0.023). Conversely, the EFs of α-TBECH in the PWS extracts (Table S21) were below 0.5, agreeing with the EF spatial trends observed in the PAS network.
Despite its urban origin, the TBECH in Canada has clearly experienced enantioselective processing; i.e., it has evaporated from reservoirs where it has undergone microbial transformations. This is in agreement with the observed enantiomeric excess for α-TBECH in soil experiments, with increasing excess seen after prolonged degradation (Wong et al., 2012). The consistent EF < 0.5 observed in water and marine air samples also suggests that at least some of the TBECH in the atmosphere evaporated from seawater. Moreover, the microbial processes occurring in urban soils and in marine waters seem to favour opposite enantiomers, leading to the divergent enantiomeric enrichment of TBECH.
4.1 Atmospheric deposition of TBECH
The log KOA values less than 9 (Table 1) imply that TBECH will not sorb appreciably to atmospheric particles. This was confirmed by the failure to detect either isomer in the GFFs of the AAS in Saturna and Tadoussac. Atmospheric deposition thus must occur by diffusive gas exchange or by precipitation scavenging from the gas phase. We paired the analytical results in different types of samples to study these deposition processes.
4.1.1 Diffusive air–water gas exchange
By having measured TBECH and BDE-47 in both air and water, we can investigate the water–air equilibrium status and the potential direction of diffusive air–water gas exchange in the Salish Sea. We estimated water–air fugacity ratios from the measured concentrations as described in Text S9. The ratios for both TBECH isomers and BDE-47 were below unity throughout BC, indicating net deposition of the BFRs from the atmosphere to the Salish Sea. This is also the case for Vancouver and Victoria Harbour (Figs. S15, S16). While this analysis could not be done in QC for TBECH, as it could not be detected in water, the ratios for BDE-47 in QC were found to also be below unity (Fig. S16). We caution that these air–water exchange calculations have considerable uncertainty, which stems from the uncertainty (i) of volumetric air and water concentrations derived from passive sampling techniques; (ii) in the KAW, which is apparent from differences in the predictions of different methods (Table 1); and (iii) arising from combining air and water data obtained during different time periods. While periods of deployment overlapped in this study, PWSs were deployed for approx. 1 month (Table S9), whereas PAS deployment periods were considerably longer (Table S1).
4.1.2 Scavenging ratios
The monthly measurements of BFRs in air and precipitation from Saturna Island and Tadoussac were used to estimate scavenging ratios, i.e., the concentration of TBECH and BDE-47 in precipitation divided by their concentration in air (Text S9). The scavenging ratios of the TBECH and BDE-47 ranged between 105 and 107 and between 104 and 105, respectively (Fig. S17). Exchange of vapours between the air and water droplets in the atmosphere is assumed to occur fast enough to achieve almost instant equilibrium. If equilibrium between the precipitation droplets and atmospheric gas phase was established, the scavenging ratio should equal the water–air partition ratio, KWA (). The measured scavenging ratios for TBECH in this study were 1 to 3 orders of magnitude higher than the predicted KWA values (Table 1). Accordingly, rainwater–air fugacity ratios of the BFRs in Saturna Island and Tadoussac (Fig. S18) were 1 to 3 orders of magnitude greater than the expected equilibrium value, i.e., 1.
Several reasons may contribute to this deviation from equilibrium. We have combined air concentration measurements for a 24 h period with precipitation samples collected over the course of an entire month. The AAS quantification is uncertain, due to the very low levels in the AAS extracts, as is the KAW and the temperature. Other potential reasons include (i) differences in air masses at different altitudes, where the warm continental air containing higher TBECH levels at the cloud level is scavenged by precipitation and has overridden the cooler clean ocean air at lower altitudes that was sampled by AASs; (ii) adsorption that occurs at the water–air interface (Hoff et al., 1993); or (iii) the BFRs experiencing sorption to dissolved organic matter and other sorption phases (Poster and Baker, 1996), all of which could lead to higher scavenging ratios. However, information on interface adsorption coefficients of the BFRs and other sorption phases at Saturna Island and Tadoussac is limited.
The month-to-month variability of the precipitation concentrations was also too large to reveal a clear seasonal pattern. This suggests that variability of TBECH levels in precipitation is controlled by factors that differ from those that control seasonal variability in air concentrations (e.g., temperature and air mass origin). Potential candidates for those factors are related to the nature of the precipitation events (e.g., frontal vs. convective storms, snow vs. rain, and precipitation rate). However, another phenomenon could also occur: higher temperatures in summer favour higher air concentrations but lower the precipitation scavenging efficiencies of vapours, due to the temperature dependence of KWA. This might explain why concentrations in precipitation do not peak in summer even if concentrations in air do.
Seasonal differences in scavenging ratios for some organic contaminants have been observed previously, with higher values measured during colder seasons (Wania and Haugen, 1999). This is likely because KWA increases as the temperature lowers, resulting in more efficient scavenging. Another hypothesized reason is that snow at equivalent water content, having greater surface area, can act as a better scavenging medium than rain (Lei and Wania, 2004). The logarithm of the inverse scavenging ratio, −ln (SR) (i.e., ), was regressed with the reciprocal of the average air temperature. Whereas the scavenging ratios of TBECH did not significantly correlate with temperature (Table S22), the scavenging of BDE-47 at both sites increased with decreasing temperature (Fig. S19).
4.2 Do the two TBECH isomers show divergent atmospheric fate?
In this study, the relative abundance of α- and β-TBECH was very consistent, with the mean α- β-TBECH ratio generally ranging from 1.1 to 1.6, i.e., indicating a slightly higher abundance of the α-isomer relative to the β-isomer. In particular, this ratio was very similar in different parts of the country, with median α- β-TBECH in active air samples with concentrations of both isomers above the LOD being 1.56 (range 1.07 to 2.01) in Toronto, 1.72 (range 1.44 to 3.28) in Tadoussac, and 1.57 (range 1.08 to 2.28) on Saturna Island. Similarly, the median α- β-TBECH in PAS was the same in QC (1.12, range 0.29 to 1.99) and BC (1.16, range 0.51 to 3.20). This slightly higher abundance of the α-isomer relative to the β-isomer is consistent with earlier reports of TBECH elsewhere in the atmosphere (Table S13), as well as to the reported abundances in technical mixtures (Arsenault et al., 2008; Ruan et al., 2018).
The relative abundance of the two isomers is also generally very similar in different environmental media. The mean α- β-TBECH ratios in AASs (QC mean = 1.95; BC mean = 1.62; ON mean = 1.56) and PASs (QC mean = 1.03; BC mean = 1.21) bracket the values recorded in the PWS (BC mean = 1.52) and precipitation (QC mean = 1.21; BC mean = 1.22) (Fig. 4). Moreover, isomer abundance was very similar in different samples of the same type. The two isomers exhibited nearly identical spatial patterns in air (Fig. S3) and water (Fig. S11). The seasonal patterns in air (Figs. 2, S8, S9) and in precipitation (Fig. S13) were also highly consistent for α- and β-TBECH. Not surprisingly, α- and β-TBECH had similar water–air equilibrium status in the Salish Sea (Fig. S15) and essentially identical scavenging ratios (Fig. S16).
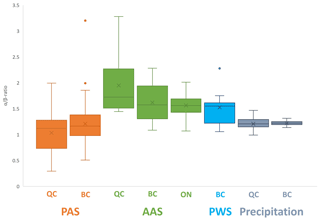
Figure 4Comparison of the α- β-TBECH ratio calculated in the sampling regions of this study, grouped into different environmental media/sampling methods. The mean α- β-TBECH ratio is indicated with an “×” for each method. For statistical analysis, outliers (α β > 2.0) were included but are explicitly marked on the plot as dots. Only measurements with both isomers above the LOD were used in this plot.
Information on the atmospheric degradation of TBECH has been extremely limited, with no experimental results in the literature to date. Using density functionals, Wang et al. (2021) predicted rate constants for the stereospecific hydroxyl radical-initiated transformation of and cm3 s−1 at 293 K for α- and β-TBECH, respectively, which, when applying 9.7 × 105 molec. cm−3 as the concentration of OH radicals in the atmosphere, corresponds to atmospheric lifetimes of 4.04 and 2.44 h. Such relatively short gas-phase lifetimes are difficult to reconcile with the relatively wide dispersal of TBECH recorded in the current study. Neither do our measurements support the idea that the atmospheric lifetime of the β-isomer is considerably short than that of α-TBECH, as this should have been apparent in an increasing relative abundance of α-TBECH with increasing distance from sources.
Rate constants predicted with COSMOtherm and the Atmospheric Oxidation Program for Microsoft Windows (AOPWIN) via EPI Suite (Table 1) are on the same order of magnitude and correspond to much longer atmospheric lifetimes (6.2 and 6.4 d for α- and β-TBECH, respectively, using COSMOtherm; 2.5 d for both isomers using AOPWIN) than those predicted by Wang et al. (2021). These longer lifetimes and the similar rates of reaction of the two main isomers are more consistent with the observed atmospheric dispersion of TBECH.
Taken together, this consistency in TBECH composition suggests that α- and β-TBECH exhibit similar atmospheric behaviours. This is also consistent with the very minor differences in partitioning behaviour predicted for the two congeners by COSMOtherm (Table 1). The slightly lower COSMOtherm-predicted air–water and slightly higher octanol–air partition ratios of the β-isomer compared to the α-isomer are not apparent in divergent atmospheric behaviour.
4.3 Sources of TBECH to the Canadian atmosphere
While literature on TBECH in the atmosphere has been limited, the few existing studies all point to TBECH levels generally being higher in populated urban areas. Using the spatial concentration patterns from this study, the first to compare urban and remote concentrations of TBECH within a single study, we were able to confirm that TBECH strongly correlates with population. Moreover, the temporal trends of TBECH indicate that the air concentrations increase during the warmer season with fixed isomeric composition, particularly in the urban centres of Canada. Its year-round presence in remote regions in this study also suggests that TBECH has the potential for long-range transport.
The inventory update phase 3 survey in 2017 under Canada's Chemical Management Plan (CMP) resulted in no reports of manufacturing or importing TBECH into the country by domestic companies, eliminating the possibility of point sources located in Canada for this flame retardant, such as factories that produce or import commercial TBECH. Similarly, the United States Environmental Protection Agency's (US EPA) Toxic Substances Control Act (TSCA) Chemical Substance Inventory contains no records of the production or importation of TBECH for commercial purposes in the United States (USEPA, 2023), indicating that there are also no point sources of TBECH located in the United States to contribute to the TBECH levels observed in Canada via atmospheric transport.
However, several recent international patents have included commercial TBECH as one of the flame retardants used mostly in electronics (Geng et al., 2018; Ke and Lv, 2020), pointing to the possibility that TBECH enters Canada almost exclusively as part of imported products. It is likely that most, if not all, of the TBECH detected in this study originates from the gradual release from these imported products. Populated urban areas, with typically greater overall usage of products containing flame retardants, e.g., electronics and plastics, have consistently shown higher TBECH levels with more pronounced temperature dependence compared to remote regions and therefore suggest greater leakage. This is also consistent with previous studies reporting higher TBECH levels in indoor air, compared to outdoor (Table S13).
Chiral compounds are typically commercially produced as a racemate, including technical mixtures of TBECH (Ruan et al., 2018). Abiotic processes occurring in the environment, such as hydrolysis, oxidation, and volatilization, are unable to distinguish between enantiomers, and therefore, the EF of the compound will remain unchanged through these forms of physical processes. Microbial degradation, however, can result in the preferential consumption of one enantiomer over another or, in other words, deviations in the EF from 0.5. The variations in the EF of a compound can therefore be used to observe the enantiomeric transformations of a molecule in the environment. Therefore, the EF results of this study also indicate that secondary emissions of TBECH may occur in Canada. Once TBECH is deposited, a portion of it may undergo enantioselective processes in the environment, e.g., uptake, translocation, and metabolism by microbes. TBECH that re-volatilizes from these reservoirs has an excess in one enantiomer, with different enantiomer preferences seen in different environments (urban soils vs. marine waters).
Environmental assessments of chemicals are currently prioritized by the Government of Canada based on their persistence; bioaccumulation potential; and the quantity of domestic industrial manufacture, import, and/or export of a chemical. With neither official records of importation nor manufacturing existing in Canada, TBECH is, for environmental assessment purposes, not considered to be present in Canada. Therefore, despite being classified as persistent and bioaccumulative, it has been deemed a nonpriority for evaluation by Environment and Climate Change Canada (ECCC) and other governmental programs. However, the results of this study reveal that TBECH is ubiquitous in the Canadian atmosphere and waters at comparable levels with a legacy flame retardant, BDE-47, with elevated levels in populated areas. This, along with the strong seasonal variability of TBECH observed in urban areas, suggests that TBECH is emitted primarily from imported products. At least some of the TBECH in the atmosphere has been subject to microbial processing; i.e., it entered the atmosphere after having been previously deposited to the surface (water/soil), resulting in enantiomeric enrichment.
With the current assessment requisites in place, TBECH and other chemicals of emerging concern (CECs) that are supposedly “nonexistent” in Canada would remain low priority for environmental assessment. This is particularly concerning for compounds that can enter in the country as part of imported products, potentially evading documentation. Such evasion has already been observed in Canada with short-chained chlorinated paraffins, which have been detected in imported products despite a ban on manufacture, usage, and import in 2013 (Kutarna et al., 2023). This raises the question of whether the current assessment criteria are sufficient to address the relevant CECs in Canada. The increasing development and production of novel chemicals aimed to replace legacy POPs in their functions will only further highlight the limitations of the assessment requisites in place. We emphasize, with the results of this study, the importance of the long-term environmental monitoring for EBFRs and other CECs as part of working towards proper screening and risk assessment.
All data generated in this study are provided in the Supplement.
The supplement related to this article is available online at: https://doi.org/10.5194/acp-23-10191-2023-supplement.
FZ, YL, and JO prepared and extracted the passive air samplers (PASs) and the Toronto active air samples (AASs). FZ and YL also took the Toronto AAS. YDL prepared standards and performed the instrumental analysis on all samples. CS prepared and obtained samples from Saturna Island and Tadoussac as well as the passive water samplers (PWSs) and performed the chiral analysis. KL and FAPCG deployed/retrieved PASs and PWSs in BC. ABC, ADC, ZL, NA, HH, FZ, and FW deployed/retrieved PASs and PWSs in Quebec. JO compiled and interpreted data, with input from FW. JO and FW wrote the manuscript with input by the other co-authors. HH coordinated the project. SE performed the FLEXPART simulations.
The contact author has declared that none of the authors has any competing interests.
Publisher's note: Copernicus Publications remains neutral with regard to jurisdictional claims in published maps and institutional affiliations.
We thank Geri Crooks, Alexandre Costa, Yannick Lapointe, Louis-Georges Esquilat, Jocelyn Praud, Sandrine Vigneron, François Gagnon, Jonathan Pritchard, Alessia Colussi, Christian Boutot, Bruno Cayouette, Fella Moualek, Frédérik Bélanger, Claude Lapierre, Félix Ledoux, Samuel Turgeon, Sarah Duquette, and the CAPMON team for their assistance in deploying samplers and providing facilities/permissions to the sampling locations.
We acknowledge OpenStreetMap for providing data to generate all maps, available under Open Database License (https://www.openstreetmap.org/copyright, last access: 23 May 2023).
This research has been supported by the Government of Canada, Environment Canada (grant nos. GCXE20S008, GCXE20S010, and GCXE20S011). Sabine Eckhardt was funded by COPE (Norwegian Research Council grant no. 287114).
This paper was edited by Ivan Kourtchev and reviewed by Terry Bidleman and one anonymous referee.
Alaee, M., Arias, P., Sjödin, A., and Bergman, Å.: An overview of commercially used brominated flame retardants, their applications, their use patterns in different countries/regions and possible modes of release, Environ. Int., 29, 683–689, https://doi.org/10.1016/S0160-4120(03)00121-1, 2003.
Arsenault, G., Lough, A., Marvin, C., McAlees, A., McCrindle, R., MacInnis, G., Pleskach, K., Potter, D., Riddell, N., Sverko, E., Tittlemier, S., and Tomy, G.: Structure characterization and thermal stabilities of the isomers of the brominated flame retardant 1,2-dibromo-4-(1,2-dibromoethyl)cyclohexane, Chemosphere, 72, 1163–1170, https://doi.org/10.1016/j.chemosphere.2008.03.044, 2008.
Asnake, S., Pradhan, A., Banjop-Kharlyngdoh, J., Modig, C., and Olsson, P.-E.: 1,2-dibromo-4-(1,2 dibromoethyl) cyclohexane (TBECH)–mediated steroid hormone receptor activation and gene regulation in chicken LMH cells, Environ. Toxicol. Chem., 33, 891–899, https://doi.org/10.1002/etc.2509, 2014.
Baskaran, S., Lei, Y. D., and Wania, F.: Reliable Prediction of the Octanol–Air Partition Ratio, Environ. Toxicol. Chem., 40, 3166–3180, https://doi.org/10.1002/etc.5201, 2021.
Betts, K.: New flame retardants detected in indoor and outdoor environments, Environ. Sci. Technol., 42, 6778–6778, https://doi.org/10.1021/es802145r, 2008.
Bohlin, P., Audy, O., Škrdlíková, L., Kukučka, P., Přibylová, P., Prokeš, R., Vojta, Š., and Klánová, J.: Outdoor passive air monitoring of semi volatile organic compounds (SVOCs): a critical evaluation of performance and limitations of polyurethane foam (PUF) disks, Environ. Sci.-Proc. Imp., 16, 433–444, https://doi.org/10.1039/C3EM00644A, 2014.
Booij, K. and Smedes, F.: An Improved Method for Estimating in Situ Sampling Rates of Nonpolar Passive Samplers, Environ. Sci. Technol., 44, 6789–6794, https://doi.org/10.1021/es101321v, 2010.
Carlsson, P., Vrana, B., Sobotka, J., Borgå, K., Bohlin Nizzetto, P., and Varpe, Ø.: New brominated flame retardants and dechlorane plus in the Arctic: Local sources and bioaccumulation potential in marine benthos, Chemosphere, 211, 1193–1202, https://doi.org/10.1016/j.chemosphere.2018.07.158, 2018.
Cequier, E., Ionas, A. C., Covaci, A., Marcé, R. M., Becher, G., and Thomsen, C.: Occurrence of a Broad Range of Legacy and Emerging Flame Retardants in Indoor Environments in Norway, Environ. Sci. Technol., 48, 6827–6835, https://doi.org/10.1021/es500516u, 2014.
Christensen, J. R., MacDuffee, M., Macdonald, R. W., Whiticar, M., and Ross, P. S.: Persistent Organic Pollutants in British Columbia Grizzly Bears: Consequence of Divergent Diets, Environ. Sci. Technol., 39, 6952–6960, https://doi.org/10.1021/es050749f, 2005.
Curran, I. H. A., Liston, V., Nunnikhoven, A., Caldwell, D., Scuby, M. J. S., Pantazopoulos, P., Rawn, D. F. K., Coady, L., Armstrong, C., Lefebvre, D. E., and Bondy, G. S.: Toxicologic effects of 28-day dietary exposure to the flame retardant 1,2-dibromo-4-(1,2-dibromoethyl)-cyclohexane (TBECH) in F344 rats, Toxicology, 377, 1–13, https://doi.org/10.1016/j.tox.2016.12.001, 2017.
de Wit, C. A., Alaee, M., and Muir, D. C. G.: Levels and trends of brominated flame retardants in the Arctic, Chemosphere, 64, 209–233, https://doi.org/10.1016/j.chemosphere.2005.12.029, 2006.
Drage, D. S., Newton, S., de Wit, C. A., and Harrad, S.: Concentrations of legacy and emerging flame retardants in air and soil on a transect in the UK West Midlands, Chemosphere, 148, 195–203, https://doi.org/10.1016/j.chemosphere.2016.01.034, 2016.
ECCC: Information received in response to the 2017 Inventory Update (chemicals and polymers), ECCC [data set], https://open.canada.ca/data/en/dataset/ec43e97c-4487-442e-ab2b-2b9eaf77ee28 (last access: 26 April 2023), 2017.
ECCC: Detailed categorization results of the Domestic Substances List, ECCC [data set], https://open.canada.ca/data/en/dataset/1d946396-cf9a-4fa1-8942-4541063bfba4 (last access: 26 April 2023), 2019.
ECCC: Federal Whales Initiative – Freshwater and sediment data (Pacific Region), ECCC [data set], https://open.canada.ca/data/en/dataset/04f5b200-bab1-4ecf-bd1a-0cd2f55cb34d (last access: 14 January 2023), 2022.
Gemmill, B., Pleskach, K., Peters, L., Palace, V., Wautier, K., Park, B., Darling, C., Rosenberg, B., McCrindle, R., and Tomy, G. T.: Toxicokinetics of tetrabromoethylcyclohexane (TBECH) in juvenile brown trout (Salmo trutta) and effects on plasma sex hormones, Aquat. Toxicol., 101, 309–317, https://doi.org/10.1016/j.aquatox.2010.11.003, 2011.
Geng, B., Tan, Y., Zhu, L., and Chen, F.: Stable polypropylene (PP) resin to be bonded with glass fiber, patent no. CN107815021A, https://worldwide.espacenet.com/patent/search/family/061609775/publication/CN107815021A?q=CN107815021A (last access: 8 September 2023), 2018.
Genisoglu, M., Sofuoglu, A., Kurt-Karakus, P. B., Birgul, A., and Sofuoglu, S. C.: Brominated flame retardants in a computer technical service: Indoor air gas phase, submicron (PM1) and coarse (PM10) particles, associated inhalation exposure, and settled dust, Chemosphere, 231, 216–224, https://doi.org/10.1016/j.chemosphere.2019.05.077, 2019.
Gentes, M.-L., Letcher, R. J., Caron-Beaudoin, É., and Verreault, J.: Novel Flame Retardants in Urban-Feeding Ring-Billed Gulls from the St. Lawrence River, Canada, Environ. Sci. Technol., 46, 9735–9744, https://doi.org/10.1021/es302099f, 2012.
Hoff, J. T., Mackay, D., Gillham, R., and Shiu, W. Y.: Partitioning of organic chemicals at the air-water interface in environmental systems, Environ. Sci. Technol., 27, 2174–2180, https://doi.org/10.1021/es00047a026, 1993.
Hong, W.-J., Jia, H., Ding, Y., Li, W.-L., and Li, Y.-F.: Polychlorinated biphenyls (PCBs) and halogenated flame retardants (HFRs) in multi-matrices from an electronic waste (e-waste) recycling site in Northern China, J. Mater. Cycles Waste Manage., 20, 80–90, https://doi.org/10.1007/s10163-016-0550-8, 2018.
Ke, H. and Lv, X.: Material for high-glow wire wall switch panel, patent no. CN111607161A, https://worldwide.espacenet.com/patent/search/family/072198230/publication/CN111607161A?q=CN111607161A (last access: 8 September 2023), 2020.
Khalaf, H., Larsson, A., Berg, H., McCrindle, R., Arsenault, G., and Olsson, P.-E.: Diastereomers of the Brominated Flame Retardant 1,2-Dibromo-4-(1,2 dibromoethyl)cyclohexane Induce Androgen Receptor Activation in the HepG2 Hepatocellular Carcinoma Cell Line and the LNCaP Prostate Cancer Cell Line, Environ. Health Persp., 117, 1853–1859, https://doi.org/10.1289/ehp.0901065, 2009.
Kutarna, S., Du, X., Diamond, M. L., Blum, A., and Peng, H.: Widespread presence of chlorinated paraffins in consumer products, Environ. Sci.-Proc. Imp., 25, 893–900, https://doi.org/10.1039/D2EM00494A, 2023.
Larsson, A., Eriksson, L. A., Andersson, P. L., Ivarson, P., and Olsson, P.-E.: Identification of the Brominated Flame Retardant 1,2-Dibromo-4-(1,2-dibromoethyl)cyclohexane as an Androgen Agonist, J. Med. Chem., 49, 7366–7372, https://doi.org/10.1021/jm060713d, 2006.
Lei, Y. D. and Wania, F.: Is rain or snow a more efficient scavenger of organic chemicals?, Atmos. Environ., 38, 3557–3571, https://doi.org/10.1016/j.atmosenv.2004.03.039, 2004.
Li, Y., Zhan, F., Lei, Y. D., Shunthirasingham, C., Hung, H., and Wania, F.: Field Calibration and PAS-SIM Model Evaluation of the XAD-Based Passive Air Sampler for Semi-Volatile Organic Compounds, Environ. Sci. Technol., 57, 9224–9233, https://doi.org/10.1021/acs.est.3c00809, 2023.
Ma, W.-L., Li, W.-L., Zhang, Z.-F., Liu, L.-Y., Song, W.-W., Huo, C.-Y., Yuan, Y.-X., and Li, Y.-F.: Occurrence and source apportionment of atmospheric halogenated flame retardants in Lhasa City in the Tibetan Plateau, China, Sci. Total Environ., 607–608, 1109–1116, https://doi.org/10.1016/j.scitotenv.2017.07.112, 2017.
Marteinson, S. C., Bird, D. M., Letcher, R. J., Sullivan, K. M., Ritchie, I. J., and Fernie, K. J.: Dietary exposure to technical hexabromocyclododecane (HBCD) alters courtship, incubation and parental behaviors in American kestrels (Falco sparverius), Chemosphere, 89, 1077–1083, https://doi.org/10.1016/j.chemosphere.2012.05.073, 2012a.
Marteinson, S. C., Letcher, R. J., Graham, L., Kimmins, S., Tomy, G., Palace, V. P., Ritchie, I. J., Gauthier, L. T., Bird, D. M., and Fernie, K. J.: The Flame Retardant β-1,2-Dibromo-4-(1,2-dibromoethyl)cyclohexane: Fate, Fertility, and Reproductive Success in American Kestrels (Falco sparverius), Environ. Sci. Technol., 46, 8440–8447, https://doi.org/10.1021/es301032a, 2012b.
Marteinson, S. C., Bodnaryk, A., Fry, M., Riddell, N., Letcher, R. J., Marvin, C., Tomy, G. T., and Fernie, K. J.: A review of 1,2-dibromo-4-(1,2-dibromoethyl)cyclohexane in the environment and assessment of its persistence, bioaccumulation and toxicity, Environ. Res., 195, 110497, https://doi.org/10.1016/j.envres.2020.110497, 2021.
Melymuk, L., Bohlin-Nizzetto, P., Kukučka, P., Vojta, Š., Kalina, J., Čupr, P., and Klánová, J.: Seasonality and indoor/outdoor relationships of flame retardants and PCBs in residential air, Environ. Pollut., 218, 392–401, https://doi.org/10.1016/j.envpol.2016.07.018, 2016.
NASA: Earth Science Data Systems, NASA [data set], https://www.earthdata.nasa.gov/ (last access: 26 April 2023), 2015.
Newton, S., Sellström, U., and de Wit, C. A.: Emerging Flame Retardants, PBDEs, and HBCDDs in Indoor and Outdoor Media in Stockholm, Sweden, Environ. Sci. Technol., 49, 2912–2920, https://doi.org/10.1021/es505946e, 2015.
Newton, S., Sellström, U., Harrad, S., Yu, G., and de Wit, C. A.: Comparisons of indoor active and passive air sampling methods for emerging and legacy halogenated flame retardants in Beijing, China offices, Emerging Contam., 2, 80–88, https://doi.org/10.1016/j.emcon.2016.02.001, 2016.
Noël, M., Dangerfield, N., Hourston, R. A. S., Belzer, W., Shaw, P., Yunker, M. B., and Ross, P. S.: Do trans-Pacific air masses deliver PBDEs to coastal British Columbia, Canada?, Environ. Pollut., 157, 3404–3412, https://doi.org/10.1016/j.envpol.2009.06.025, 2009.
Palm, A., Cousins, I. T., Mackay, D., Tysklind, M., Metcalfe, C., and Alaee, M.: Assessing the environmental fate of chemicals of emerging concern: a case study of the polybrominated diphenyl ethers, Environ. Pollut., 117, 195–213, https://doi.org/10.1016/S0269-7491(01)00276-7, 2002.
Park, B. J., Palace, V., Wautier, K., Gemmill, B., and Tomy, G.: Thyroid Axis Disruption in Juvenile Brown Trout (Salmo trutta) Exposed to the Flame Retardant β-Tetrabromoethylcyclohexane (β-TBECH) via the Diet, Environ. Sci. Technol., 45, 7923–7927, https://doi.org/10.1021/es201530m, 2011.
Pasecnaja, E., Perkons, I., Bartkevics, V., and Zacs, D.: Legacy and alternative brominated, chlorinated, and organophosphorus flame retardants in indoor dust – levels, composition profiles, and human exposure in Latvia, Environ. Sci. Pollut. R., 28, 25493–25502, https://doi.org/10.1007/s11356-021-12374-2, 2021.
Pisso, I., Sollum, E., Grythe, H., Kristiansen, N. I., Cassiani, M., Eckhardt, S., Arnold, D., Morton, D., Thompson, R. L., Groot Zwaaftink, C. D., Evangeliou, N., Sodemann, H., Haimberger, L., Henne, S., Brunner, D., Burkhart, J. F., Fouilloux, A., Brioude, J., Philipp, A., Seibert, P., and Stohl, A.: The Lagrangian particle dispersion model FLEXPART version 10.4, Geosci. Model Dev., 12, 4955–4997, https://doi.org/10.5194/gmd-12-4955-2019, 2019.
POPRC: SC-4/10− SC-4/18: The new POPs under the Stockholm Convention, Stockholm Convention on Persistent Organic Pollutants, https://www.pops.int/TheConvention/ThePOPs/TheNewPOPs/tabid/2511/Default.aspx (last access: 8 September 2023), 2009.
Porter, E., Crump, D., Egloff, C., Chiu, S., and Kennedy, S. W.: Use of an avian hepatocyte assay and the avian toxchip polymerse chain reaction array for testing prioritization of 16 organic flame retardants, Environ. Sci. Technol., 33, 573–582, https://doi.org/10.1002/etc.2469, 2014.
Poster, D. L. and Baker, J. E.: Influence of Submicron Particles on Hydrophobic Organic Contaminants in Precipitation. 1. Concentrations and Distributions of Polycyclic Aromatic Hydrocarbons and Polychlorinated Biphenyls in Rainwater, Environ. Sci. Technol., 30, 341–348, https://doi.org/10.1021/es9406804, 1996.
Ruan, Y., Zhang, X., Qiu, J.-W., Leung, K. M. Y., Lam, J. C. W., and Lam, P. K. S.: Stereoisomer-Specific Trophodynamics of the Chiral Brominated Flame Retardants HBCD and TBECH in a Marine Food Web, with Implications for Human Exposure, Environ. Sci. Technol., 52, 8183–8193, https://doi.org/10.1021/acs.est.8b02206, 2018.
Ruan, Y., Zhang, K., Lam, J. C. W., Wu, R., and Lam, P. K. S.: Stereoisomer-specific occurrence, distribution, and fate of chiral brominated flame retardants in different wastewater treatment systems in Hong Kong, J. Hazard. Mater., 374, 211–218, https://doi.org/10.1016/j.jhazmat.2019.04.041, 2019.
Santillo, D., Labounskaia, I., Stringer, R., and Johnston, P.: Report on the analysis of industrial wastewaters from the Frutarom VCM/PVC plant, near Haifa, Israel, and adjacent shoreline sediments for organic contaminants, Greenpeace Research Laboratories, 1–25, https://greenpeace.to/publications/TN_03_97.pdf (last access: 26 April 2023), 1997.
Shoeib, M., Ahrens, L., Jantunen, L., and Harner, T.: Concentrations in air of organobromine, organochlorine and organophosphate flame retardants in Toronto, Canada, Atmos. Environ., 99, 140–147, https://doi.org/10.1016/j.atmosenv.2014.09.040, 2014.
Shunthirasingham, C., Alexandrou, N., Brice, K. A., Dryfhout-Clark, H., Su, K., Shin, C., Park, R., Pajda, A., Noronha, R., and Hung, H.: Temporal trends of halogenated flame retardants in the atmosphere of the Canadian Great Lakes Basin (2005–2014), Environ. Sci.-Proc. Imp., 20, 469–479, https://doi.org/10.1039/C7EM00549K, 2018.
Sun, Y., Francois, R., Pawlowicz, R., Maldonado, M. T., Stevens, S. W., and Soon, M.: Distribution, sources and dispersion of polybrominated diphenyl ethers in the water column of the Strait of Georgia, British Columbia, Canada, Sci. Total Environ., 873, 162174, https://doi.org/10.1016/j.scitotenv.2023.162174, 2023.
Tao, F., Abdallah, M. A.-E., and Harrad, S.: Emerging and Legacy Flame Retardants in UK Indoor Air and Dust: Evidence for Replacement of PBDEs by Emerging Flame Retardants?, Environ. Sci. Technol., 50, 13052–13061, https://doi.org/10.1021/acs.est.6b02816, 2016.
Tao, F., Abou-Elwafa Abdallah, M., Ashworth, D. C., Douglas, P., Toledano, M. B., and Harrad, S.: Emerging and legacy flame retardants in UK human milk and food suggest slow response to restrictions on use of PBDEs and HBCDD, Environ. Int., 105, 95–104, https://doi.org/10.1016/j.envint.2017.05.010, 2017.
Tomy, G. T., Pleskach, K., Arsenault, G., Potter, D., McCrindle, R., Marvin, C. H., Sverko, E., and Tittlemier, S.: Identification of the Novel Cycloaliphatic Brominated Flame Retardant 1,2-Dibromo-4-(1,2-dibromoethyl)cyclohexane in Canadian Arctic Beluga (Delphinapterus leucas), Environ. Sci. Technol., 42, 543–549, https://doi.org/10.1021/es072043m, 2008.
UNECE: UNECE High-level Group for the Modernisation of Official Statistics, UNECE, https://statswiki.unece.org/display/hlgbas/High-Level+Group+for+the+Modernisation+of+Official+Statistics (last access: 26 April 2023), 2018.
USEPA: How to Access the TSCA Inventory, USEPA [data set], https://www.epa.gov/tsca-inventory/how-access-tsca-inventory (last access: 26 June 2023), 2023.
Wang, N., He, L., Lv, G., and Sun, X.: Potential environmental fate and risk based on the hydroxyl radical-initiated transformation of atmospheric 1,2-dibromo-4-(1,2dibromoethyl)cyclohexane stereoisomers, J. Hazard. Mater., 417, 126031, https://doi.org/10.1016/j.jhazmat.2021.126031, 2021.
Wania, F. and Haugen, J. E.: Long term measurements of wet deposition and precipitation scavenging of hexachlorocyclohexanes in Southern Norway, Environ. Pollut., 105, 381–386, https://doi.org/10.1016/S0269-7491(99)00038-X, 1999.
Wania, F., Haugen, J.-E., Lei, Y. D., and Mackay, D.: Temperature Dependence of Atmospheric Concentrations of Semivolatile Organic Compounds, Environ. Sci. Technol., 32, 1013–1021, https://doi.org/10.1021/es970856c, 1998.
Wania, F., Shen, L., Lei, Y. D., Teixeira, C., and Muir, D. C. G.: Development and Calibration of a Resin-Based Passive Sampling System for Monitoring Persistent Organic Pollutants in the Atmosphere, Environ. Sci. Technol., 37, 1352–1359, https://doi.org/10.1021/es026166c, 2003.
Wilford, B. H., Harner, T., Zhu, J., Shoeib, M., and Jones, K. C.: Passive Sampling Survey of Polybrominated Diphenyl Ether Flame Retardants in Indoor and Outdoor Air in Ottawa, Canada: Implications for Sources and Exposure, Environ. Sci. Technol., 38, 5312–5318, https://doi.org/10.1021/es049260x, 2004.
Wong, F., Kurt-Karakus, P., and Bidleman, T. F.: Fate of Brominated Flame Retardants and Organochlorine Pesticides in Urban Soil: Volatility and Degradation, Environ. Sci. Technol., 46, 2668–2674, https://doi.org/10.1021/es203287x, 2012.
Wong, F., de Wit, C. A., and Newton, S. R.: Concentrations and variability of organophosphate esters, halogenated flame retardants, and polybrominated diphenyl ethers in indoor and outdoor air in Stockholm, Sweden, Environ. Pollut., 240, 514–522, https://doi.org/10.1016/j.envpol.2018.04.086, 2018.
Wong, F., Hung, H., Dryfhout-Clark, H., Aas, W., Bohlin-Nizzetto, P., Breivik, K., Mastromonaco, M. N., Lundén, E. B., Ólafsdóttir, K., Sigurðsson, Á., Vorkamp, K., Bossi, R., Skov, H., Hakola, H., Barresi, E., Sverko, E., Fellin, P., Li, H., Vlasenko, A., Zapevalov, M., Samsonov, D., and Wilson, S.: Time trends of persistent organic pollutants (POPs) and Chemicals of Emerging Arctic Concern (CEAC) in Arctic air from 25 years of monitoring, Sci. Total Environ., 775, 145109, https://doi.org/10.1016/j.scitotenv.2021.145109, 2021.
Wong, L. I. L., Reers, A. R., Currier, H. A., Williams, T. D., Cox, M. E., Elliott, J. E., and Beischlag, T. V.: The Effects of the Organic Flame-Retardant 1,2-Dibromo-4-(1,2-dibromoethyl) Cyclohexane (TBECH) on Androgen Signaling in Human Prostate Cancer Cell Lines, J. Biochem. Mol. Toxicol., 30, 239–242, https://doi.org/10.1002/jbt.21784, 2016.
Xiao, H., Shen, L., Su, Y., Barresi, E., DeJong, M., Hung, H., Lei, Y.-D., Wania, F., Reiner, E. J., Sverko, E., and Kang, S.-C.: Atmospheric concentrations of halogenated flame retardants at two remote locations: The Canadian High Arctic and the Tibetan Plateau, Environ. Pollut., 161, 154–161, https://doi.org/10.1016/j.envpol.2011.09.041, 2012.
Yu, Y., Hung, H., Alexandrou, N., Roach, P., and Nordin, K.: Multiyear Measurements of Flame Retardants and Organochlorine Pesticides in Air in Canada's Western Sub-Arctic, Environ. Sci. Technol., 49, 8623–8630, https://doi.org/10.1021/acs.est.5b01996, 2015.
Zacs, D., Perkons, I., Abdulajeva, E., Pasecnaja, E., Bartkiene, E., and Bartkevics, V.: Polybrominated diphenyl ethers (PBDEs), hexabromocyclododecanes (HBCDD), dechlorane-related compounds (DRCs), and emerging brominated flame retardants (EBFRs) in foods: The levels, profiles, and dietary intake in Latvia, Sci. Total Environ., 752, 141996, https://doi.org/10.1016/j.scitotenv.2020.141996, 2021.
Zhao, J., Wang, P., Wang, C., Fu, M., Li, Y., Yang, R., Fu, J., Hao, Y., Matsiko, J., Zhang, Q., and Jiang, G.: Novel brominated flame retardants in West Antarctic atmosphere (2011–2018): Temporal trends, sources and chiral signature, Sci. Total Environ., 720, 137557, https://doi.org/10.1016/j.scitotenv.2020.137557, 2020.