the Creative Commons Attribution 4.0 License.
the Creative Commons Attribution 4.0 License.
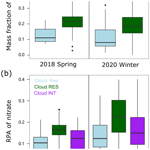
A comprehensive study about the in-cloud processing of nitrate through coupled measurements of individual cloud residuals and cloud water
Guohua Zhang
Xiaodong Hu
Wei Sun
Yuxiang Yang
Ziyong Guo
Yuzhen Fu
Haichao Wang
Shengzhen Zhou
Mingjin Tang
Zongbo Shi
Duohong Chen
Xinming Wang
While the formation and evolution of nitrate in airborne particles are extensively investigated, little is known about the processing of nitrate in clouds. Here we present a detailed investigation on the in-cloud formation of nitrate, based on the size-resolved mixing state of nitrate in the individual cloud residual and cloud-free particles obtained by single particle mass spectrometry, and also the mass concentrations of nitrate in the cloud water and PM2.5 at a mountain site (1690 m a.s.l. – above sea level) in southern China. The results show a significant enhancement of nitrate mass fraction and relative intensity of nitrate in the cloud water and the cloud residual particles, respectively, reflecting a critical role of in-cloud processing in the formation of nitrate. We first exclude the gas-phase scavenging of HNO3 and the facilitated activation of nitrate-containing particles as the major contribution for the enhanced nitrate, according to the size distribution of nitrate in individual particles. Based on regression analysis and theoretical calculations, we then highlight the role of N2O5 hydrolysis in the in-cloud formation of nitrate, even during the daytime, attributed to the diminished light in clouds. Nitrate is highly related (R2= ∼ 0.6) to the variations in [NOx][O3], temperature, and droplet surface area in clouds. Accounting for droplet surface area greatly enhances the predictability of the observed nitrate, compared with using [NOx][O3] and temperature. The substantial contribution of N2O5 hydrolysis to nitrate in clouds with diminished light during the daytime can be reproduced by a multiphase chemical box model. Assuming a photolysis rate at 30 % of the default setting, the overall contribution of N2O5 hydrolysis pathway to nitrate formation increases by ∼ 20 % in clouds. Given that N2O5 hydrolysis acts as a major sink of NOx in the atmosphere, further model updates would improve our understanding about the processes contributing to nitrate production in cloud and the cycling of odd nitrogen.
- Article
(2161 KB) - Full-text XML
- Companion paper
-
Supplement
(1208 KB) - BibTeX
- EndNote
Aerosol nitrate is an increasingly important component of PM2.5 that, in particular, contributes to haze formation in China (P. Liu et al., 2020; Xu et al., 2019; Zheng et al., 2020; Fu et al., 2020; Guo et al., 2014; Tian et al., 2019; Wen et al., 2018; Lu et al., 2019). As a key inorganic component in cloud water, nitrate can also modify microphysical properties of cloud, influence aqueous-phase processes in droplets, and affect the ecosystem after wet deposition (Schneider et al., 2017). Notably, aerosol nitrate is an important product in the cycling of odd nitrogen (Chang et al., 2011; Zheng et al., 2020; Zhang et al., 2021; Huang et al., 2018), playing a significant role in tropospheric ozone and OH production (Scharko et al., 2014; Kaur and Anastasio, 2017; Ye et al., 2017a, b), and contributing to net aerosol composition and radiative forcing (Bauer et al., 2007; Hauglustaine et al., 2014; Xu and Penner, 2012).
Aerosol nitrate originates from the oxidation of NOx, which refers to the gas-phase oxidation of NO2 by the hydroxyl radical (OH) followed by condensation (daytime chemistry) and the hydrolysis of N2O5 (nighttime chemistry) to nitrate in aqueous particles, initiated by the oxidation of NO2 by ozone (O3) to produce the NO3 radical (Seinfeld and Pandis, 2006). In contrast to aerosol sulfate formation, which is dominated by aqueous phase reactions, both gas-phase oxidation and the hydrolysis of N2O5 represent the major processes forming aerosol nitrate (Hayden et al., 2008; Sellegri et al., 2003; Fahey et al., 2005; Chen et al., 2020; Xiao et al., 2020). Extensive studies have shown that the formation and evolution of nitrate depend on various factors, such as the availability of ammonia (NH3), temperature (T), relative humidity (RH), and the presence of other ionic species in particulate phase (Chen et al., 2018; Shi et al., 2019; Chen et al., 2020; Lin et al., 2021; Fan et al., 2021).
Comparatively, detailed observational investigations and the possible mechanisms governing nitrate behavior upon in-cloud processes are scarce and poorly understood, although it is well-known that clouds play an important role in the transport and transformation of tropospheric pollutants (T. Li et al., 2020; Ervens, 2015; McNeill, 2017). Global model studies still disagree on the relative importance of in-cloud process contributing to the production of HNO3. While most have neglected N2O5 and NO3 uptake in clouds (Alexander et al., 2009; Hauglustaine et al., 2014; Xu and Penner, 2012), there is also research suggesting the significance of the in-cloud process (Holmes et al., 2019). Likewise, despite limited research, the role of clouds in nitrate formation from field observations remains controversial. Drewnick et al. (2007) and Prabhakar et al. (2014) reported that the relatively enhanced nitrate in clouds was associated with the composition of the activated cloud condensation nuclei (CCN) rather than the preferential scavenging of nitric acid (HNO3) in clouds. Contrastingly, there are also studies highlighting the predominant role of nitric acid partitioning in nitrate formation in clouds, which is contrary to the nucleation scavenging of sulfate (Schneider et al., 2017; Hayden et al., 2008; Leaitch et al., 1988). Hayden et al. (2008) also noted that potential contributions from gas-phase N2O5 cannot be ruled out. Therefore, more detailed information on the pathways of nitrate and controlling factors in clouds are still required for models to further integrate the role of clouds in the formation of nitrate in the troposphere (Zhu et al., 2020; Wu et al., 2021).
The aim of this study is to illustrate the in-cloud formation mechanisms of nitrate and evaluate the relative contribution of each pathway to nitrate in cloud water for daytime and nighttime. To this end, the mixing state of individual cloud residual, interstitial, and cloud-free particles were measured in a high time resolution with a single particle aerosol mass spectrometer (SPAMS). The combination of a counterflow virtual impactor (CVI) and aerosol mass spectrometry (including SPAMS) allows for the high time-resolved observations of the size and chemical compositions of submicron cloud residual particles (Boone et al., 2015; Hao et al., 2013; Zhang et al., 2017; Lin et al., 2017). In addition, cloud water and PM2.5 samples were collected, and the chemical compositions were measured to provide additional quantitative evidence.
2.1 Aerosol and cloud measurements
Aerosol and cloud measurements were performed at the Tianjing Mountain site (24∘41′56′′ N, 112∘53′56′′ E; 1690 m a.s.l.) in southern China, as described in detail by Lin et al. (2017), during 9 May–4 June 2018 and 13 November–9 December 2020. Cloud events can be distinguished by a sudden drop in visibility (to < ∼ 1 km) and a sharp increase of RH to > 95 %, as record by sensors equipped with a ground-based counterflow virtual impactor (GCVI; model 1205, Brechtel Manufacturing Inc., USA; Lin et al., 2017). Overall, 19 cloud events (lasting more than 6 h) were identified for spring 2018 and 10 for winter 2020, as also marked in Fig. S1. The visibility was generally lower than 0.1 km during the cloud events, compared to being as high as 80 km during the cloud-free periods. Besides a relatively long cloud event throughout 9–12 May, the cloud events were typically observed during nighttime for spring 2018 and associated with a prominently diurnal variation of RH and visibility. The RH during the daytime ranged between 70 %–80 % and increased to > 95 % during nighttime. The duration of cloud events was in a range of 6–24 h for 2020 winter. Air masses from the southern continental and marine areas dominated over the spring 2018 and winter 2020 periods, with air masses from the western continental areas being unique for winter 2020 (Fig. S2; obtained by HYSPLIT 4.9; http://ready.arl.noaa.gov/HYSPLIT.php, last access: 22 July 2022; Draxler and Rolph, 2012).
An incorporation of a counterflow virtual impactor (CVI) or GCVI allows the separation of interstitial aerosols from cloud droplets that are evaporated to obtain the cloud residual particles (Bi et al., 2016; Roth et al., 2016; Pratt et al., 2009). Briefly, the GCVI was applied to collect the cloud droplets larger than the predefined sizes (i.e., 7.5–8.5 µm in the present study), with the cloud residual particles as output after being dried in the evaporation chamber (with an air flow temperature at 40 ∘C; Shingler et al., 2012). The influence of cloud-free air can be negligible as the number concentration of GCVI output particles was measured to be ∼ 1 cm−3, but at a magnitude of ∼ 103 cm−3 in the cloud-free air. In the present study, the average number concentration of the cloud residual particles sampled during the cloud events was at a level of ∼ 100 cm−3. In addition, a PM2.5 inlet was used to deliver cloud interstitial particles during the cloud events or the cloud-free particles.
2.2 SPAMS measurements and data processing
A SPAMS (Hexin Analytical Instrument Co., Ltd., Guangzhou, China), an Aethalometer (AE33; Magee Scientific, USA), and a scanning mobility particle sizer (SMPS; MSP Corporation, USA) were deployed to characterize the physical and chemical properties of the sampled particles. The instruments were connected downstream of the GCVI or PM2.5 inlets. Cloud residual and interstitial particles were alternately sampled with an interval of ∼ 1 h during some randomly selected cloud events. During the cloud-free period, these instruments were connected to the PM2.5 inlet in order to measure the cloud-free particles. In the present study, aerosol surface area (SA) for the cloud-free particles was directly calculated from the size distribution data obtained from the SMPS, whereas it can only be estimated based on the same data for the cloud residues by assuming a mean droplet size at 8 µm. We recognize the possible uncertainty, but the estimated SA should linearly correlate with real values and thus would not lead to ambiguous conclusions.
The vacuum aerodynamic diameter (dva) and mass spectral information for individual particles were measured by SPAMS (Li et al., 2011). A brief description on the performance of SPAMS can also be found in the Supplement. Over the sampling period for the spring 2018 and winter 2020 periods, a respective number of particles with mass spectral information were analyzed, using FATES (Flexible Analysis Toolkit for the Exploration of SPMS data), based on MATLAB (The MathWorks, Inc.; Sultana et al., 2017). The particles were classified by an adaptive resonance-theory-based neural network algorithm (Song et al., 1999), with the inputs of ion peak intensities. In total, seven types with distinct mass spectral characteristics (Fig. S3), accounting for > 95 % of all the detected particles, were obtained for further analysis. The presence of nitrate can be identified with ion peaks (defined as 5 times the noise signal) at [NO3]− or [NO2]−. Approximately 70 %–80 % of all the detected particles in the size range of 100–2000 nm contained nitrate ion signals for our measurements. Defined as fractional peak area of each relative to the sum of peak areas in a mass spectrum, a relative peak area (RPA) is applied to represent the relative amount of a species within a particle (Jeong et al., 2011; Healy et al., 2013).
2.3 Cloud water/PM2.5 collection and chemical analysis
A Caltech active strand cloud water collector (CASCC2) was applied to collect cloud water (with droplet size > 3.5 µm). The average cloud liquid water content (LWC) for each sampling period can be derived from LWC (Δt × η × Q), based on each sample mass (Δm), duration time (Δt), flow rate (Q= 5.8 m3 min−1), and collection efficiency (η= 86 %).
A total of 58/53 cloud water samples were collected over the 19/10 cloud events for the spring 2018 and winter 2020 periods, respectively, with the durations ranging between 2 and 10 h. The pH for collected samples were immediately measured using a pH meter (Mettler-Toledo, Switzerland) after being filtered through a 0.22 µm filter and then kept at −20 ∘C until the analysis.
PM2.5 samples were collected on quartz filters using a PM2.5 sampler (PM-PUF-300; Mingye Technology Co., Ltd., Guangzhou, China) at a flow rate of 300 L min−1. The filter were preconditioned in 450 ∘C for 6 h to eliminate the potential influence of organics. A total of PM2.5 samples were collected for the spring 2018 and winter 2020 periods, respectively. The samples were immediately kept at −20 ∘C until further analysis was conducted. These samples are representative for the cloud-free particles or cloud interstitial particles during the cloud events.
Cloud water and PM2.5 samples were analyzed with ion chromatograph (883 IC plus, Metrohm AG, Switzerland) for water-soluble inorganic ions (Na+, NH, K+, Ca2+, Mg2+, Cl−, NO, and SO) and total organic carbon analyzer (vario, Elementar, Germany, for the 2018 samples and TOC-V, Shimadzu, Japan, for the 2020 samples) for water-soluble organic carbon (WSOC). The overall uncertainty for the concentration of each species is calculated to be < 15 %, based on parallel analyses. The nitrate mass fractions in cloud water and PM2.5 were calculated by dividing the nitrate concentration by the sum of the measured water-soluble inorganic ions and water-soluble organic matter (estimated by 1.6 ⋅ WSOC).
2.4 Box modeling of nitrate formation in cloud
A multiphase chemical box model (Regional Atmospheric Chemistry Modeling–Chemical Aqueous Phase Radical Mechanism, RACM-CAPRAM) was used to simulate the production of nitrate in wet aerosols and cloud droplets. It couples the regional atmospheric chemistry mechanism version 2 (RACM2; including 363 chemical reactions) and the chemical aqueous-phase radical mechanism version 2.4 (CAPRAM2.4; including 438 chemical reactions) to account for gas- and aqueous-phase atmospheric chemistry (Ervens et al., 2003). As similarly performed in previous studies (Pathak et al., 2009; Wen et al., 2018), the following three major pathways for nitrate formation are considered: (1) the oxidation of NO2 by the OH radical produces HNO3 and partitioning of gaseous HNO3 into the aqueous phase, (2) the hydrolysis reactions of N2O5, and (3) the aqueous-phase reactions of NO3 radicals.
The average concentration of NO2 (∼ 25 ppb – parts per billion) and O3 (∼ 100 ppb) for gas-phase precursors and LWC (0.1 g m−3) for cloud droplets, obtained from the in situ measurements, were taken as representative parameters for the atmospheric condition at Tianjing Mountain and used as initial conditions for the model simulation. The detailed initial conditions for the model are listed in Table S1 in the Supplement. Several comparisons through varying the LWC and photolysis rate were considered in order to investigate the role of LWC and photolysis in the formation of nitrate in the cloud. It is also noted that only LWC and photolysis rate were reset in our scenario, with other factors (e.g., initial droplet composition and SO2) kept as the default setting in the model setup.
3.1 Enhanced in-cloud production of nitrate
Figure 1 shows the statistical results of the nitrate mass fractions in cloud water and PM2.5 and the hourly average relative intensity of nitrate (represented by the RPA) in the cloud-free, cloud residual, and cloud interstitial particles. The results clearly indicate the enhancement of nitrate in clouds. It can be seen that the mass fraction of nitrate in cloud water (∼ 20 % on average) is obviously higher than that in PM2.5 (< 15 % on average) during the cloud-free periods and the cloud events, for both the spring 2018 and winter 2020 periods. Moreover, the relative intensity of nitrate was substantially enhanced in the cloud interstitial particles and particularly cloud residues relative to the cloud-free particles. The influence of air mass on the enhanced nitrate can be ruled out for the 2018 spring period, as they, similarly, originated from southern areas over the whole campaign period (Fig. S2). While originating from different regions during the winter 2020 period, the air masses did not show a significant difference between the cloud-free periods and the cloud events (Figs. S1 and S2). Thus, the influence of the air mass on the enhanced nitrate in 2020 winter should also be limited.
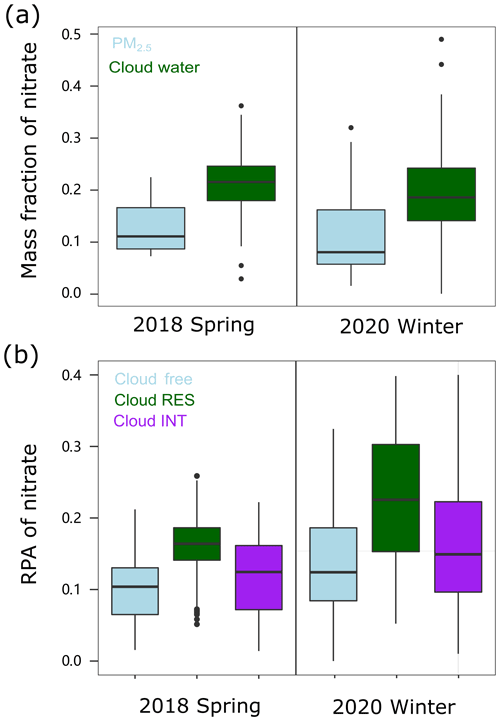
Figure 1Box-and-whisker plots of (a) the mass fraction of nitrate in PM2.5 and cloud water and (b) the RPA of nitrate separated for the cloud-free, cloud residual (RES), and cloud interstitial (INT) particles in spring 2018 and winter 2020, respectively. In a box-and-whisker plot, the lower, median, and upper line of the box denotes the 25th, 50th, and 75th percentiles, respectively. The lower and upper edges of the whisker denote the 10th and 90th percentiles, respectively.
There are several pathways that might contribute to the enhanced nitrate in cloud droplets, including (1) the scavenging of gas-phase HNO3, (2) the preferential activation of nitrate-rich particles, and (3) in-cloud aqueous production of nitrate via reaction of NO3 radicals or hydrolysis of N2O5. The mechanism via the dissolution of NO2 and its aqueous phase oxidation is relatively slow and unlikely to be a significant source of cloud water nitrate (Seinfeld and Pandis, 2006).
We first exclude the scavenging of gas-phase HNO3 as a major pathway through the analysis of the size distribution of nitrate RPA and RPA ratio (nitrate/sulfate), although all the gas-phase HNO3 could be efficiently scavenged and presented in the aqueous phase in a typical cloud with LWC > 0.1 g m−3 (Seinfeld and Pandis, 2006). As can be seen in Fig. 2, the RPA of nitrate and the RPA ratios of nitrate to sulfate distributes relatively stable over the measured size range, which suggests that the gas-phase scavenging of HNO3 is not the dominant pathway in the present conditions. This is because gas-phase mass transfer would lead to enhanced nitrate in the smaller droplets with higher total surface area (Drewnick et al., 2007). Comparatively, the limited size dependence of nitrate for the cloud RES particles differs markedly from that observed by Hayden et al. (2008), showing a favorable presence of nitrate in the smaller size, rather than sulfate in the larger size. And their pattern could be well explained by the model calculation, assuming that all of the cloud nitrate comes from the uptake of HNO3. Therefore, our pattern at least indicates a limited contribution of gas-phase scavenging of HNO3 to the observed nitrate in the cloud RES particles. As also discussed in the following section, the formation of HNO3 would certainly be suppressed by the presence of clouds.
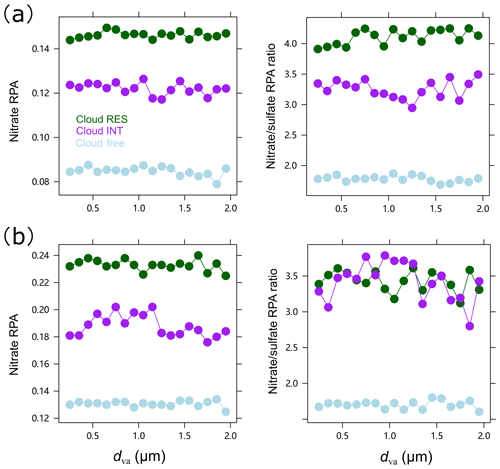
Figure 2Size-dependent RPA of nitrate and RPA ratio of nitrate/sulfate, separated for all the detected cloud-free, cloud residual (RES), and cloud interstitial (INT) particles, in (a) spring 2018 (May) and (b) winter 2020 (November–December), respectively.
We also indicate that the contribution of the preferential activation of the nitrate-rich particles should be limited, since such a process would lead to the depletion of nitrate in the cloud interstitial particles relative to the cloud-free particles. But this is not the case, as the RPA of nitrate and RPA ratios of nitrate to sulfate in the cloud interstitial particles are considerably higher than those in the cloud-free particles (Fig. 2). Both the enhanced nitrate in the cloud residual and interstitial particles suggest the in-cloud formation of nitrate, although the variation in nitrate RPA cannot provide a quantitative view. The enhancement of nitrate in the cloud interstitial particles may also indicate that the significant role of RH in the formation of nitrate, even in the inactivated particles. Similar results have also been observed in our previous study for oxalate (Zhang et al., 2017). In addition, the formation of nitrate in the cloud interstitial particles also extends their size towards the larger mode, as compared with the cloud-free particles (Fig. S4).
3.2 In-cloud nitrate formation
A theoretical estimation of nitrate production for winter 2020 is performed based on the well-established kinetic characteristic of reactions between NO2 and O3 and uptake of N2O5 onto aerosol/droplet surfaces that formed HNO3 (Sect. S1 in the Supplement), corresponding to the nighttime chemistry. It is reasonable since the heterogeneous hydrolysis of N2O5 within aerosol particles, fog, or cloud droplets has been shown to be much faster than homogeneous hydrolysis under typical tropospheric conditions (Chang et al., 2011; Wang et al., 2017). Through integrating the rate equations, as listed in Sect. S1, the solution for aqueous-phase production of HNO3 can be obtained as follows (Seinfeld and Pandis, 2006):
Thus, the conversion of NOx to HNO3 through the hydrolysis of N2O5 depends on the two lifetimes and , as defined by the reaction kinetics (Sect. S1). The key reaction that formed aqueous-phase nitrate is related to the effective reaction of N2O5 on the surface of wet aerosol or droplets (Holmes et al., 2019) and, therefore, depends on the concentration of NO2 and O3 ([NO2][O3]), the available SA for aerosol and droplets and temperature. Besides the reaction kinetics, temperature could also have influence on the hydrolysis of N2O5 (Chen et al., 2018; Chang et al., 2011).
As shown in Fig. 3, the theoretically calculated in-cloud nitrate production, assuming a typical uptake coefficient of N2O5γ= 0.06 (Seinfeld and Pandis, 2006) could match the measured nitrate concentrations well (with R2= 0.38 and 0.60 at p < 0.01, for daytime and nighttime, respectively), varying in a wide range from ∼ 1 to ∼ 60 mg L−1 for winter 2020. The correlation coefficients are obviously higher than those predicted using only [NOx][O3] (with R2= 0 and 0.54, for daytime and nighttime, respectively). This is consistent with previous results that the nighttime production of N2O5 and HNO3 would be proportional to the concentration of NO2 and O3 ([NO2][O3]) when assuming that N2O5 and the NO3 radical are both in a steady state when considering their short lifetimes (Li et al., 2018; Wang et al., 2017). The result also highlights the significance of SA in the in-cloud nitrate production through N2O5 hydrolysis, even during the daytime. A further comparison of [NOx][O3] and SA for the cloud events and the cloud-free periods, as shown in Fig. S5, also supports the above discussion that the higher fraction of nitrate cannot be well explained by the variations in [NOx][O3] but rather by the enhanced SA due to the presence of droplets (Fig. S5b), which is > 5 times on average that for aerosol particles during the cloud-free periods. In the present study, the average LWC of cloud droplets is at a level of ∼ 105 µg m−3, which is 3–4 levels of magnitude higher than those for urban haze conditions. As previously reported, high aerosol LWC (campaign average at ∼ 50 µg m−3) induced a fast heterogeneous uptake of N2O5 (γ= 0.048 on average) that is prevalent in urban haze (Wang et al., 2017), as compared with γ < 0.03 for normal periods, and thus results in enhanced nitrate in highly humid conditions (Neuman et al., 2003; Wang et al., 2009; Pathak et al., 2009).
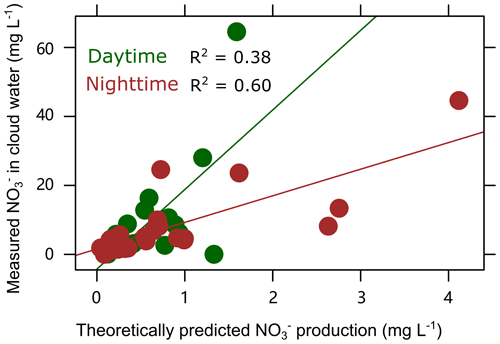
Figure 3Theoretical calculation of the trend of in-cloud-produced nitrate from the hydrolysis of N2O5 versus the temporal variations in NO concentrations in cloud water in winter 2020 (November–December).
The theoretical estimate indicates that the hydrolysis of N2O5 may substantially contribute to the in-cloud production of nitrate even during the daytime, consistent with the observational results as discussed in Sect. 3.1. The theoretically predicted nitrate (NO) production from the hydrolysis of N2O5 represents ∼ 5 %–15 % of the measured nitrate (Fig. 3), based on our assumption. It could roughly explain up to 5 % increase of the nitrate mass fraction in clouds (Fig. 1). There are some factors that may contribute to the uncertainties in the estimation. One is that the assumed γ= 0.06 might not be representative for N2O5 uptake in cloud droplets, since the previously reported γ varies in a wide range, depending on various factors (e.g., droplet compositions, pH, and temperature; Bertram and Thornton, 2009; Holmes et al., 2019; Burkholder et al., 2015). Some higher γ (0.2–0.4) was also observed for deliquescent sodium sulfate particles (Burkholder et al., 2015). Another is that the SA estimated by the size distribution data of cloud residues obtained by the GCVI-SMPS only represents part (< 50 %) of the cloud droplets, as only droplets larger than 7.5 µm were collected in the present study. In addition, the scavenging of HNO3 may still contribute to the in-cloud nitrate production, as estimated in Sect. 3.3, although N2O5 hydrolysis still acts as the dominant pathway.
Furthermore, a simplified regression and a random forest analysis are also performed for the high time-resolved RPAs of nitrate obtained by the SPAMS, with [NOx][O3], SA, and temperature as inputs, separated for the cloud RES and the cloud-free particles, as detailed in Sect. S2. Note that the concentration of NOx is used here to represent that of NO2, since most of NO data were not available for spring 2018. The effect should be limited since NO could be negligible when the air masses were dominantly attributed to long range transport, which could also be supported by the data (NO, ∼ 0.1 µg m−3, and < 2 % of NO2 concentration) in winter 2020. As expected, the nitrate RPA in the cloud residual particles is highly correlated to the predicted ones (R2= 0.75 and 0.71, with p < 0.01, for daytime and nighttime, respectively), even during the daytime (Fig. 4). An inclusion of temperature and SA in the model substantially improves the correlation coefficient R2, which is originally 0.16 and 0.31 between the nitrate RPA and [NOx][O3] for the daytime and nighttime, respectively. Similarly, the correlation coefficients (R2= 0.45 and 0.66, for daytime and nighttime, respectively) are lower for spring 2018 than winter 2020, without the availability of SA data. The results are generally consistent with those obtained from random forest analysis, as shown in Fig. S6. Without the input of SA, [NOx][O3] and temperature only explain 52 %–61 % of the observed nitrate RPA for cloud residual particles in spring 2018, compared with 72 %–80 % in winter 2020. Compared with the cloud residual particles, the predictions for the nitrate RPA in the cloud-free particles are of lower coefficients. Such a difference between the cloud residual and the cloud-free particles also reflects the critical role of SA in the hydrolysis of N2O5 in cloud droplets.
3.3 Relative importance of N2O5 hydrolysis pathway to nitrate in clouds
The relative contribution of nitrate formation in the cloud droplets and the cloud-free particles is also assessed using the CAPRAM model, as shown in Fig. 5. The relative contribution difference between the cloud droplets and the cloud-free particles is primarily attributed to the different LWC setting, which is tightly linked to the cloud droplets' SA. Furthermore, the comparison between cloud scenarios with a different LWC setting (0.05 g m−3 versus 0.15 g m−3) also shows an enhanced contribution of N2O5 hydrolysis to nitrate with increasing LWC.
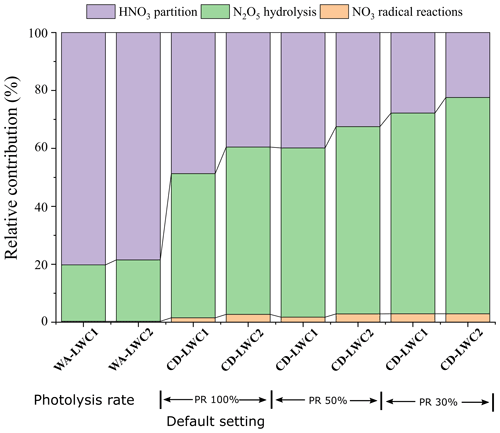
Figure 5Relative contribution of each pathway to the nitrate production in wet aerosols (WAs; 0.5 µm) and cloud droplets (CDs; 8 µm), respectively, simulated by the RACM-CAPRAM. The atmospheric conditions considered for comparison are LWC (10−5–10−4 g cm−3 for wet aerosols and 0.05–0.15 g cm−3) and photolysis rates (30 %, 50 %, 100 %).
Nitrate is known to form predominantly by the hydrolysis of N2O5 (> 80 %) for both the cloud droplets and the cloud-free particles for the nighttime. However, both Figs. 3 and 4 indicate the potential importance of the heterogeneous N2O5 hydrolysis to nitrate formation during the daytime. This is likely attributed to the substantial attenuation of the incident solar radiation by clouds in which the visibility was as low as < 0.1 km over this study. Previous studies have also indicated the effect of clouds in the vertical redistribution of the photochemical activity (Liu et al., 2006; Hall et al., 2018). Most comparatively, Brown et al. (2016) observed a discrepancy between the modeled and observed N2O5 during a daytime fog episode in Hong Kong and attributed to the uptake of N2O5 to fog droplets. Their calculation infers that the daytime production of soluble nitrate via N2O5 can be substantially faster than photochemical conversion through OH + NO2 in the polluted fog episodes (Brown et al., 2016). One may expect that the substantial attenuation of the incident solar radiation by clouds may inhibit the formation of O3, thereby affecting the formation of N2O5. However, the concentration of O3 showed relatively stable and limited variations throughout the cloud events (Fig. S1). Together with the similar [NOx][O3] observed during the cloud events and the cloud-free periods (Fig. S5), we indicate that the cloud events did not have much effect on the variation of O3 during our observation.
The model results in Fig. 5, with the consideration of photolysis rate, are, to some extent, consistent with our observations. The overall contribution of N2O5 hydrolysis pathways increases by ∼ 20 % (from ∼ 50 %–60 % to ∼ 70 %–80 %) when the photolysis rate is reduced to 30 % of the default setting. For daytime only, the contribution of this pathway also increases from nearly 0 % to ∼ 20 % during the noon hours and ∼ 40 % for the morning hours. A similar model study also indicates that N2O5 hydrolysis contributed to 30 % of daytime nitrate formation at Mount Tai (Zhu et al., 2020). Attributed to the substantial attenuation of incident solar radiation by clouds and the high loading of PM2.5, the daytime N2O5 hydrolysis has also been observed to be an important formation pathway for nitrate in the haze episodes in Xi'an (China), and the contribution increases from 8.2 % to 20.5 % of the total nitrate over 14:00–16:00 LT (local time) by model simulation (Wu et al., 2021). Similarly, L. Liu et al. (2020) showed that the daytime N2O5 hydrolysis contributed to ∼ 10 % of nitrate in the North China Plain in winter. Note that biogenic volatile organic compounds could also have a potentially important impact on nitrate formation through reacting with NO3 radical, which may lead to up to 35 % decrease of particulate nitrate (Fry et al., 2014; Aksoyoglu et al., 2017). However, the modeling results could still indicate the role of cloud in the hydrolysis of N2O5, which contributes to the enhanced nitrate.
The presented results provide direct evidence that in-cloud aqueous processing, in particular, the hydrolysis of N2O5, significantly contributes to the enhanced nitrate in cloud residues. We highlight that the hydrolysis of N2O5 serves as the critical route for the in-cloud formation of nitrate, even during the daytime. The dependence of in-cloud nitrate formation on the cloud droplets' SA extends the observation fact that higher RH facilities the formation of nitrate in wet aerosols (Neuman et al., 2003; Wang et al., 2009; Pathak et al., 2009). Given that N2O5 hydrolysis acts as a major sink of NOx in the atmosphere (Yan et al., 2019), further model updates may improve our understanding of the relative importance of nitrate production pathways (Chan et al., 2021; Alexander et al., 2020). In addition, significant hydrolysis of N2O5 in clouds may also pose a substantial effect on the tropospheric ozone budget (Riemer et al., 2003; Voulgarakis et al., 2009; Strode et al., 2017).
As sulfate would be reduced in the future through emission controls (S. Li et al., 2020; Chu et al., 2020), a higher nitrate fraction is expected in clouds (Herckes et al., 2007, 2015). However, the limited dependence of nitrate formation on the [NOx][O3] in the clouds suggests a possibility that controlling NOx and O3 might be offset in the cloudy regions. Given the significance of both emission and deposition on the variations in particulate nitrate (Zhai et al., 2021) and the contribution of the transported NOx and O3 to the notable effect and complex process of cross-regional nitrate formation (Qu et al., 2021), knowledge of the in-cloud formation of nitrate would also benefit PM2.5 pollution control target over a larger scale.
Furthermore, our results indicate that in-cloud formed nitrate remains in the particulate phase after cloud evaporation (Fig. S7), changing the mixing state of individual particles. Enhanced aerosol nitrate is expected to have higher hygroscopicity after cloud evaporation (Sun et al., 2018; Hodas et al., 2014) and, therefore, an increase in the particles' ability to act as CCN after their cloud's passage (Roth et al., 2016). This is different from the result observed along the Californian coast that the nitrate-to-sulfate mass ratio decreases rapidly with cloud height, due to the volatilization during drop evaporation that pushes NO3 to the gas phase (Prabhakar et al., 2014). In addition, vertical turbulent mixing of the residual aerosols from evaporating cloud droplets may contribute to the nitrate aerosol loading during the daytime at the ground level (Tao et al., 2018).
All the data can be obtained by contacting the corresponding author.
The supplement related to this article is available online at: https://doi.org/10.5194/acp-22-9571-2022-supplement.
GZ and XB designed the research (with input from LL, MT, and XW), analyzed the data (with input from XH and WS), and wrote the paper. YY, ZG, and YF performed the field measurements and analyzed the collected samples. DC, HW, SZ, and ZS provided constructive comments. All authors contributed to the refinement of the paper.
The contact author has declared that none of the authors has any competing interests.
Publisher’s note: Copernicus Publications remains neutral with regard to jurisdictional claims in published maps and institutional affiliations.
Thanks to Likun Xue (Shandong University) and Liang Wen (Leibniz Institute for Tropospheric Research), for their support of the box modeling of nitrate formation in cloud.
This work has been funded by the Natural Science Foundation of Guangdong Province (grant no. 2019B151502022), National Natural Science Foundation of China (grant nos. 42077322, 41775124, and 41877307), Youth Innovation Promotion Association CAS (grant no. 2021354), and Guangdong Foundation for Program of Science and Technology Research (grant no. 2020B1212060053).
This paper was edited by Katye Altieri and reviewed by two anonymous referees.
Aksoyoglu, S., Ciarelli, G., El-Haddad, I., Baltensperger, U., and Prévôt, A. S. H.: Secondary inorganic aerosols in Europe: sources and the significant influence of biogenic VOC emissions, especially on ammonium nitrate, Atmos. Chem. Phys., 17, 7757–7773, https://doi.org/10.5194/acp-17-7757-2017, 2017.
Alexander, B., Hastings, M. G., Allman, D. J., Dachs, J., Thornton, J. A., and Kunasek, S. A.: Quantifying atmospheric nitrate formation pathways based on a global model of the oxygen isotopic composition (Δ17O) of atmospheric nitrate, Atmos. Chem. Phys., 9, 5043–5056, https://doi.org/10.5194/acp-9-5043-2009, 2009.
Alexander, B., Sherwen, T., Holmes, C. D., Fisher, J. A., Chen, Q., Evans, M. J., and Kasibhatla, P.: Global inorganic nitrate production mechanisms: comparison of a global model with nitrate isotope observations, Atmos. Chem. Phys., 20, 3859–3877, https://doi.org/10.5194/acp-20-3859-2020, 2020.
Bauer, S. E., Koch, D., Unger, N., Metzger, S. M., Shindell, D. T., and Streets, D. G.: Nitrate aerosols today and in 2030: a global simulation including aerosols and tropospheric ozone, Atmos. Chem. Phys., 7, 5043–5059, https://doi.org/10.5194/acp-7-5043-2007, 2007.
Bertram, T. H. and Thornton, J. A.: Toward a general parameterization of N2O5 reactivity on aqueous particles: the competing effects of particle liquid water, nitrate and chloride, Atmos. Chem. Phys., 9, 8351–8363, https://doi.org/10.5194/acp-9-8351-2009, 2009.
Bi, X. H., Lin, Q. H., Peng, L., Zhang, G. H., Wang, X. M., Brechtel, F. J., Chen, D. H., Li, M., Peng, P. A., Sheng, G. Y., and Zhou, Z.: In situ detection of the chemistry of individual fog droplet residues in the Pearl River Delta region, China, J. Geophys. Res.-Atmos., 121, 9105–9116, https://doi.org/10.1002/2016JD024886, 2016.
Boone, E. J., Laskin, A., Laskin, J., Wirth, C., Shepson, P. B., Stirm, B. H., and Pratt, K. A.: Aqueous Processing of Atmospheric Organic Particles in Cloud Water Collected via Aircraft Sampling, Environ. Sci. Technol., 49, 8523–8530, https://doi.org/10.1021/acs.est.5b01639, 2015.
Brown, S. S., Dube, W. P., Tham, Y. J., Zha, Q. Z., Xue, L. K., Poon, S., Wang, Z., Blake, D. R., Tsui, W., Parrish, D. D., and Wang, T.: Nighttime chemistry at a high altitude site above Hong Kong, J. Geophys. Res.-Atmos., 121, 2457–2475, https://doi.org/10.1002/2015JD024566, 2016.
Burkholder, J. B., Sander, S. P., Abbatt, J., Barker, J. R., Huie, R. E., Kolb, C. E., Kurylo, M. J., Orkin, V. L., Wilmouth, D. M., and Wine, P. H.: Chemical kinetics and photochemical data for use in atmospheric studies C, edited by: Evaluation No. 18, JPL Publication, National Aeronautics and Space Administration, http://jpldataeval.jpl.nasa.gov (last access: 10 May 2022), 2015.
Chan, Y.-C., Evans, M. J., He, P., Holmes, C. D., Jaegle, L., Kasibhatla, P., Liu, X.-Y., Sherwen, T., Thornton, J. A., Wang, X., Xie, Z., Zhai, S., and Alexander, B.: Heterogeneous Nitrate Production Mechanisms in Intense Haze Events in the North China Plain, J. Geophys. Res.-Atmos., 126, e2021JD034688, https://doi.org/10.1029/2021jd034688, 2021.
Chang, W. L., Bhave, P. V., Brown, S. S., Riemer, N., Stutz, J., and Dabdub, D.: Heterogeneous Atmospheric Chemistry, Ambient Measurements, and Model Calculations of N2O5: A Review, Aerosol Sci. Tech., 45, 665–695, https://doi.org/10.1080/02786826.2010.551672, 2011.
Chen, X., Wang, H., Lu, K., Li, C., Zhai, T., Tan, Z., Ma, X., Yang, X., Liu, Y., Chen, S., Dong, H., Li, X., Wu, Z., Hu, M., Zeng, L., and Zhang, Y.: Field Determination of Nitrate Formation Pathway in Winter Beijing, Environ. Sci. Technol., 54, 9243–9253, https://doi.org/10.1021/acs.est.0c00972, 2020.
Chen, Y., Wolke, R., Ran, L., Birmili, W., Spindler, G., Schröder, W., Su, H., Cheng, Y., Tegen, I., and Wiedensohler, A.: A parameterization of the heterogeneous hydrolysis of N2O5 for mass-based aerosol models: improvement of particulate nitrate prediction, Atmos. Chem. Phys., 18, 673–689, https://doi.org/10.5194/acp-18-673-2018, 2018.
Chu, B., Ma, Q., Liu, J., Ma, J., Zhang, P., Chen, T., Feng, Q., Wang, C., Yang, N., Ma, H., Ma, J., Russell, A. G., and He, H.: Air Pollutant Correlations in China: Secondary Air Pollutant Responses to NOx and SO2 Control, Environ. Sci. Tech. Let., 7, 695–700, https://doi.org/10.1021/acs.estlett.0c00403, 2020.
Draxler, R. R. and Rolph, G. D.: HYSPLIT (HYbrid Single-Particle Lagrangian Integrated Trajectory), NOAA ARL READY, NOAA Air Resources Laboratory, MD, Silver Spring, http://ready.arl.noaa.gov/HYSPLIT.php (last access: 10 April 2022), 2012.
Drewnick, F., Schneider, J., Hings, S. S., Hock, N., Noone, K., Targino, A., Weimer, S., and Borrmann, S.: Measurement of ambient, interstitial, and residual aerosol particles on a mountaintop site in central Sweden using an aerosol mass spectrometer and a CVI, J. Atmos. Chem., 56, 1–20, https://doi.org/10.1007/s10874-006-9036-8, 2007.
Ervens, B., George, C., Williams, J. E., Buxton, G. V., Salmon, G. A., Bydder, M., Wilkinson, F., Dentener, F., Mirabel, P., Wolke, R., and Herrmann, H.: CAPRAM 2.4 (MODAC mechanism): An extended and condensed tropospheric aqueous phase mechanism and its application, J. Geophys. Res.-Atmos., 108, 4426, https://doi.org/10.1029/2002jd002202, 2003.
Ervens, B.: Modeling the Processing of Aerosol and Trace Gases in Clouds and Fogs, Chem. Rev., 115, 4157–4198, https://doi.org/10.1021/cr5005887, 2015.
Fahey, K. M., Pandis, S. N., Collett, J. L., and Herckes, P.: The influence of size-dependent droplet composition on pollutant processing by fogs, Atmos. Environ., 39, 4561–4574, https://doi.org/10.1016/j.atmosenv.2005.04.006, 2005.
Fan, M. Y., Zhang, Y. L., Lin, Y. C., Hong, Y., Zhao, Z. Y., Xie, F., Du, W., Cao, F., Sun, Y., and Fu, P.: Important Role of NO3 Radical to Nitrate Formation Aloft in Urban Beijing: Insights from Triple Oxygen Isotopes Measured at the Tower, Environ. Sci. Technol., 56, 6870–6879, https://doi.org/10.1021/acs.est.1c02843, 2021.
Fu, X., Wang, T., Gao, J., Wang, P., Liu, Y., Wang, S., Zhao, B., and Xue, L.: Persistent Heavy Winter Nitrate Pollution Driven by Increased Photochemical Oxidants in Northern China, Environ. Sci. Technol., 54, 3881–3889, https://doi.org/10.1021/acs.est.9b07248, 2020.
Fry, J. L., Draper, D. C., Barsanti, K. C., Smith, J. N., Ortega, J., Winkle, P. M., Lawler, M. J., Brown, S. S., Edwards, P. M., Cohen, R. C., and Lee, L.: Secondary Organic Aerosol Formation and Organic Nitrate Yield from NO3 Oxidation of Biogenic Hydrocarbons, Environ. Sci. Technol., 48, 11944–11953, https://doi.org/10.1021/es502204x, 2014.
Guo, S., Hu, M., Zamora, M. L., Peng, J., Shang, D., Zheng, J., Du, Z., Wu, Z., Shao, M., Zeng, L., Molina, M. J., and Zhang, R.: Elucidating severe urban haze formation in China, P. Natl. Acad. Sci. USA, 111, 17373, https://doi.org/10.1073/pnas.1419604111, 2014.
Hall, S. R., Ullmann, K., Prather, M. J., Flynn, C. M., Murray, L. T., Fiore, A. M., Correa, G., Strode, S. A., Steenrod, S. D., Lamarque, J.-F., Guth, J., Josse, B., Flemming, J., Huijnen, V., Abraham, N. L., and Archibald, A. T.: Cloud impacts on photochemistry: building a climatology of photolysis rates from the Atmospheric Tomography mission, Atmos. Chem. Phys., 18, 16809–16828, https://doi.org/10.5194/acp-18-16809-2018, 2018.
Hao, L., Romakkaniemi, S., Kortelainen, A., Jaatinen, A., Portin, H., Miettinen, P., Komppula, M., Leskinen, A., Virtanen, A., Smith, J. N., Sueper, D., Worsnop, D. R., Lehtinen, K. E. J., and Laaksonen, A.: Aerosol Chemical Composition in Cloud Events by High Resolution Time-of-Flight Aerosol Mass Spectrometry, Environ. Sci. Technol., 47, 2645–2653, https://doi.org/10.1021/es302889w, 2013.
Hauglustaine, D. A., Balkanski, Y., and Schulz, M.: A global model simulation of present and future nitrate aerosols and their direct radiative forcing of climate, Atmos. Chem. Phys., 14, 11031–11063, https://doi.org/10.5194/acp-14-11031-2014, 2014.
Hayden, K. L., Macdonald, A. M., Gong, W., Toom-Sauntry, D., Anlauf, K. G., Leithead, A., Li, S. M., Leaitch, W. R., and Noone, K.: Cloud processing of nitrate, J. Geophys. Res.-Atmos., 113, 1–18, https://doi.org/10.1029/2007jd009732, 2008.
Healy, R. M., Sciare, J., Poulain, L., Crippa, M., Wiedensohler, A., Prévôt, A. S. H., Baltensperger, U., Sarda-Estève, R., McGuire, M. L., Jeong, C.-H., McGillicuddy, E., O'Connor, I. P., Sodeau, J. R., Evans, G. J., and Wenger, J. C.: Quantitative determination of carbonaceous particle mixing state in Paris using single-particle mass spectrometer and aerosol mass spectrometer measurements, Atmos. Chem. Phys., 13, 9479–9496, https://doi.org/10.5194/acp-13-9479-2013, 2013.
Herckes, P., Chang, H., Lee, T., and Collett, J. L.: Air pollution processing by radiation fogs, Water Air Soil Poll., 181, 65–75, https://doi.org/10.1007/s11270-006-9276-x, 2007.
Herckes, P., Marcotte, A. R., Wang, Y., and Collett, J. L.: Fog composition in the Central Valley of California over three decades, Atmos. Res., 151, 20–30, https://doi.org/10.1016/j.atmosres.2014.01.025, 2015.
Hodas, N., Sullivan, A. P., Skog, K., Keutsch, F. N., Collett, J. L., Jr., Decesari, S., Facchini, M. C., Carlton, A. G., Laaksonen, A., and Turpin, B. J.: Aerosol liquid water driven by anthropogenic nitrate: implications for lifetimes of water-soluble organic gases and potential for secondary organic aerosol formation, Environ. Sci. Technol., 48, 11127–11136, https://doi.org/10.1021/es5025096, 2014.
Holmes, C. D., Bertram, T. H., Confer, K. L., Grahams, K. A., Ronan, A. C., Wirks, C. K., and Shah, V.: The Role of Clouds in the Tropospheeric NOx Cycle: A New Modeling Approach for Cloud Chemistry and Its Global Implications, Geophys. Res. Lett., 46, 4980–4990, https://doi.org/10.1029/2019gl081990, 2019.
Huang, D. D., Zhang, Q., Cheung, H. H. Y., Yu, L., Zhou, S., Anastasio, C., Smith, J. D., and Chan, C. K.: Formation and Evolution of aqSOA from Aqueous-Phase Reactions of Phenolic Carbonyls: Comparison between Ammonium Sulfate and Ammonium Nitrate Solutions, Environ. Sci. Technol., 52, 9215–9224, https://doi.org/10.1021/acs.est.8b03441, 2018.
Jeong, C.-H., McGuire, M. L., Godri, K. J., Slowik, J. G., Rehbein, P. J. G., and Evans, G. J.: Quantification of aerosol chemical composition using continuous single particle measurements, Atmos. Chem. Phys., 11, 7027–7044, https://doi.org/10.5194/acp-11-7027-2011, 2011.
Kaur, R. and Anastasio, C.: Light absorption and the photoformation of hydroxyl radical and singlet oxygen in fog waters, Atmos. Environ., 164, 387–397, https://doi.org/10.1016/j.atmosenv.2017.06.006, 2017.
Leaitch, W. R., Bottenheim, J. W., and Strapp, J. W.: Possible contribution of N2O5 scavenging to HNO3 observed in winter stratiform cloud, J. Geophys. Res.-Atmos., 93, 12569–12584, https://doi.org/10.1029/JD093iD10p12569, 1988.
Li, H., Zhang, Q., Zheng, B., Chen, C., Wu, N., Guo, H., Zhang, Y., Zheng, Y., Li, X., and He, K.: Nitrate-driven urban haze pollution during summertime over the North China Plain, Atmos. Chem. Phys., 18, 5293–5306, https://doi.org/10.5194/acp-18-5293-2018, 2018.
Li, L., Huang, Z. X., Dong, J. G., Li, M., Gao, W., Nian, H. Q., Fu, Z., Zhang, G. H., Bi, X. H., Cheng, P., and Zhou, Z.: Real time bipolar time-of-flight mass spectrometer for analyzing single aerosol particles, Int. J. Mass. Spectrom., 303, 118–124, https://doi.org/10.1016/j.ijms.2011.01.017, 2011.
Li, S., Zhang, F., Jin, X., Sun, Y., Wu, H., Xie, C., Chen, L., Liu, J., Wu, T., Jiang, S., Cribb, M., and Li, Z.: Characterizing the ratio of nitrate to sulfate in ambient fine particles of urban Beijing during 2018–2019, Atmos. Environ., 237, 117662, https://doi.org/10.1016/j.atmosenv.2020.117662, 2020.
Li, T., Wang, Z., Wang, Y., Wu, C., Liang, Y., Xia, M., Yu, C., Yun, H., Wang, W., Wang, Y., Guo, J., Herrmann, H., and Wang, T.: Chemical characteristics of cloud water and the impacts on aerosol properties at a subtropical mountain site in Hong Kong SAR, Atmos. Chem. Phys., 20, 391–407, https://doi.org/10.5194/acp-20-391-2020, 2020.
Lin, Q., Zhang, G., Peng, L., Bi, X., Wang, X., Brechtel, F. J., Li, M., Chen, D., Peng, P., Sheng, G., and Zhou, Z.: In situ chemical composition measurement of individual cloud residue particles at a mountain site, southern China, Atmos. Chem. Phys., 17, 8473–8488, https://doi.org/10.5194/acp-17-8473-2017, 2017.
Lin, Y. C., Zhang, Y. L., Yu, M., Fan, M. Y., Xie, F., Zhang, W. Q., Wu, G., Cong, Z., and Michalski, G.: Formation Mechanisms and Source Apportionments of Airborne Nitrate Aerosols at a Himalayan-Tibetan Plateau Site: Insights from Nitrogen and Oxygen Isotopic Compositions, Environ. Sci. Technol., 55, 12261–12271, https://doi.org/10.1021/acs.est.1c03957, 2021.
Liu, H. Y., Crawford, J. H., Pierce, R. B., Norris, P., Platnick, S. E., Chen, G., Logan, J. A., Yantosca, R. M., Evans, M. J., Kittaka, C., Feng, Y., and Tie, X. X.: Radiative effect of clouds on tropospheric chemistry in a global three-dimensional chemical transport model, J. Geophys. Res.-Atmos., 111, D20303, https://doi.org/10.1029/2005jd006403, 2006.
Liu, L., Bei, N. F., Hu, B., Wu, J. R., Liu, S. X., Li, X., Wang, R. N., Liu, Z. R., Shen, Z. X., and Li, G. H.: Wintertime nitrate formation pathways in the north China plain: Importance of N2O5 heterogeneous hydrolysis, Environ. Pollut., 266, 10, https://doi.org/10.1016/j.envpol.2020.115287, 2020.
Liu, P., Ye, C., Xue, C., Zhang, C., Mu, Y., and Sun, X.: Formation mechanisms of atmospheric nitrate and sulfate during the winter haze pollution periods in Beijing: gas-phase, heterogeneous and aqueous-phase chemistry, Atmos. Chem. Phys., 20, 4153–4165, https://doi.org/10.5194/acp-20-4153-2020, 2020.
Lu, K., Fuchs, H., Hofzumahaus, A., Tan, Z., Wang, H., Zhang, L., Schmitt, S. H., Rohrer, F., Bohn, B., Broch, S., Dong, H., Gkatzelis, G. I., Hohaus, T., Holland, F., Li, X., Liu, Y., Liu, Y., Ma, X., Novelli, A., Schlag, P., Shao, M., Wu, Y., Wu, Z., Zeng, L., Hu, M., Kiendler-Scharr, A., Wahner, A., and Zhang, Y.: Fast Photochemistry in Wintertime Haze: Consequences for Pollution Mitigation Strategies, Environ. Sci. Technol., 53, 10676–10684, https://doi.org/10.1021/acs.est.9b02422, 2019.
McNeill, V. F.: Atmospheric Aerosols: Clouds, Chemistry, and Climate, Annu. Rev. Chem. Biomol., 8, 427–444, https://doi.org/10.1146/annurev-chembioeng-060816-101538, 2017.
Neuman, J. A., Nowak, J. B., Brock, C. A., Trainer, M., Fehsenfeld, F. C., Holloway, J. S., Hubler, G., Hudson, P. K., Murphy, D. M., Nicks, D. K., Orsini, D., Parrish, D. D., Ryerson, T. B., Sueper, D. T., Sullivan, A., and Weber, R.: Variability in ammonium nitrate formation and nitric acid depletion with altitude and location over California, J. Geophys. Res.-Atmos., 108, 4557, https://doi.org/10.1029/2003jd003616, 2003.
Pathak, R. K., Wu, W. S., and Wang, T.: Summertime PM2.5 ionic species in four major cities of China: nitrate formation in an ammonia-deficient atmosphere, Atmos. Chem. Phys., 9, 1711–1722, https://doi.org/10.5194/acp-9-1711-2009, 2009.
Prabhakar, G., Ervens, B., Wang, Z., Maudlin, L. C., Coggon, M. M., Jonsson, H. H., Seinfeld, J. H., and Sorooshian, A.: Sources of nitrate in stratocumulus cloud water: Airborne measurements during the 2011 E-PEACE and 2013 NiCE studies, Atmos. Environ., 97, 166–173, https://doi.org/10.1016/j.atmosenv.2014.08.019, 2014.
Pratt, K. A., DeMott, P. J., French, J. R., Wang, Z., Westphal, D. L., Heymsfield, A. J., Twohy, C. H., Prenni, A. J., and Prather, K. A.: In situ detection of biological particles in cloud ice-crystals, Nat. Geosci., 2, 397–400, 2009.
Qu, K., Wang, X., Xiao, T., Shen, J., Lin, T., Chen, D., He, L.-Y., Huang, X.-F., Zeng, L., Lu, K., Ou, Y., and Zhang, Y.: Cross-regional transport of PM2.5 nitrate in the Pearl River Delta, China: Contributions and mechanisms, Sci. Total. Environ., 753, 142439, https://doi.org/10.1016/j.scitotenv.2020.142439, 2021.
Riemer, N., Vogel, H., Vogel, B., Schell, B., Ackermann, I., Kessler, C., and Hass, H.: Impact of the heterogeneous hydrolysis of N2O5 on chemistry and nitrate aerosol formation in the lower troposphere under photosmog conditions, J. Geophys. Res.-Atmos., 108, 4144, https://doi.org/10.1029/2002jd002436, 2003.
Roth, A., Schneider, J., Klimach, T., Mertes, S., van Pinxteren, D., Herrmann, H., and Borrmann, S.: Aerosol properties, source identification, and cloud processing in orographic clouds measured by single particle mass spectrometry on a central European mountain site during HCCT-2010, Atmos. Chem. Phys., 16, 505–524, https://doi.org/10.5194/acp-16-505-2016, 2016.
Scharko, N. K., Berke, A. E., and Raff, J. D.: Release of Nitrous Acid and Nitrogen Dioxide from Nitrate Photolysis in Acidic Aqueous Solutions, Environ. Sci. Technol., 48, 11991–12001, https://doi.org/10.1021/es503088x, 2014.
Schneider, J., Mertes, S., van Pinxteren, D., Herrmann, H., and Borrmann, S.: Uptake of nitric acid, ammonia, and organics in orographic clouds: mass spectrometric analyses of droplet residual and interstitial aerosol particles, Atmos. Chem. Phys., 17, 1571–1593, https://doi.org/10.5194/acp-17-1571-2017, 2017.
Seinfeld, J. H., and Pandis, S. N.: Atmospheric Chemistry and Physics: From Air Pollution to Climate Change, John Wiley & Sons, Inc., New Jersey, ISBN 9780471720188, 2006.
Sellegri, K., Laj, P., Marinoni, A., Dupuy, R., Legrand, M., and Preunkert, S.: Contribution of gaseous and particulate species to droplet solute composition at the Puy de Dôme, France, Atmos. Chem. Phys., 3, 1509–1522, https://doi.org/10.5194/acp-3-1509-2003, 2003.
Shi, X., Nenes, A., Xiao, Z., Song, S., Yu, H., Shi, G., Zhao, Q., Chen, K., Feng, Y., and Russell, A. G.: High-Resolution Data Sets Unravel the Effects of Sources and Meteorological Conditions on Nitrate and Its Gas-Particle Partitioning, Environ. Sci. Technol., 53, 3048–3057, https://doi.org/10.1021/acs.est.8b06524, 2019.
Shingler, T., Dey, S., Sorooshian, A., Brechtel, F. J., Wang, Z., Metcalf, A., Coggon, M., Mülmenstädt, J., Russell, L. M., Jonsson, H. H., and Seinfeld, J. H.: Characterisation and airborne deployment of a new counterflow virtual impactor inlet, Atmos. Meas. Tech., 5, 1259–1269, https://doi.org/10.5194/amt-5-1259-2012, 2012.
Song, X. H., Hopke, P. K., Fergenson, D. P., and Prather, K. A.: Classification of single particles analyzed by ATOFMS using an artificial neural network, ART-2A, Anal. Chem., 71, 860–865, 1999.
Strode, S. A., Douglass, A. R., Ziemke, J. R., Manyin, M., Nielsen, J. E., and Oman, L. D.: A Model and Satellite-Based Analysis of the Tropospheric Ozone Distribution in Clear Versus Convectively Cloudy Conditions, J. Geophys. Res.-Atmos., 122, 11948–11960, https://doi.org/10.1002/2017jd027015, 2017.
Sultana, C. M., Cornwell, G. C., Rodriguez, P., and Prather, K. A.: FATES: a flexible analysis toolkit for the exploration of single-particle mass spectrometer data, Atmos. Meas. Tech., 10, 1323–1334, https://doi.org/10.5194/amt-10-1323-2017, 2017.
Sun, J. X., Liu, L., Xu, L., Wang, Y. Y., Wu, Z. J., Hu, M., Shi, Z. B., Li, Y. J., Zhang, X. Y., Chen, J. M., and Li, W. J.: Key Role of Nitrate in Phase Transitions of Urban Particles: Implications of Important Reactive Surfaces for Secondary Aerosol Formation, J. Geophys. Res.-Atmos., 123, 1234–1243, https://doi.org/10.1002/2017JD027264, 2018.
Tao, J., Zhang, Z., Tan, H., Zhang, L., Wu, Y., Sun, J., Che, H., Cao, J., Cheng, P., Chen, L., and Zhang, R.: Observational evidence of cloud processes contributing to daytime elevated nitrate in an urban atmosphere, Atmos. Environ., 186, 209–215, https://doi.org/10.1016/j.atmosenv.2018.05.040, 2018.
Tian, M., Liu, Y., Yang, F. M., Zhang, L. M., Peng, C., Chen, Y., Shi, G. M., Wang, H. B., Luo, B., Jiang, C. T., Li, B., Takeda, N., and Koizumi, K.: Increasing importance of nitrate formation for heavy aerosol pollution in two megacities in Sichuan Basin, southwest China, Environ. Pollut., 250, 898–905, https://doi.org/10.1016/j.envpol.2019.04.098, 2019.
Voulgarakis, A., Wild, O., Savage, N. H., Carver, G. D., and Pyle, J. A.: Clouds, photolysis and regional tropospheric ozone budgets, Atmos. Chem. Phys., 9, 8235–8246, https://doi.org/10.5194/acp-9-8235-2009, 2009.
Wang, H., Lu, K., Chen, X., Zhu, Q., Chen, Q., Guo, S., Jiang, M., Li, X., Shang, D., Tan, Z., Wu, Y., Wu, Z., Zou, Q., Zheng, Y., Zeng, L., Zhu, T., Hu, M., and Zhang, Y.: High N2O5 Concentrations Observed in Urban Beijing: Implications of a Large Nitrate Formation Pathway, Environ. Sci. Tech. Lett., 4, 416–420, https://doi.org/10.1021/acs.estlett.7b00341, 2017.
Wang, X. F., Zhang, Y. P., Chen, H., Yang, X., Chen, J. M., and Geng, F. H.: Particulate Nitrate Formation in a Highly Polluted Urban Area: A Case Study by Single-Particle Mass Spectrometry in Shanghai, Environ. Sci. Technol., 43, 3061–3066, https://doi.org/10.1021/es8020155, 2009.
Wen, L., Xue, L., Wang, X., Xu, C., Chen, T., Yang, L., Wang, T., Zhang, Q., and Wang, W.: Summertime fine particulate nitrate pollution in the North China Plain: increasing trends, formation mechanisms and implications for control policy, Atmos. Chem. Phys., 18, 11261–11275, https://doi.org/10.5194/acp-18-11261-2018, 2018.
Wu, C., Liu, L., Wang, G., Zhang, S., Li, G., Lv, S., Li, J., Wang, F., Meng, J., and Zens, Y.: Important contribution of N2O5 hydrolysis to the daytime nitrate in Xi'an, China during haze periods: Isotopic analysis and WRF-Chem model simulation, Environ. Pollut., 288, 117712, https://doi.org/10.1016/j.envpol.2021.117712, 2021.
Xiao, H.-W., Zhu, R.-G., Pan, Y.-Y., Guo, W., Zheng, N.-J., Liu, Y.-H., Liu, C., Zhang, Z.-Y., Wu, J.-F., Kang, C.-A., Luo, L., and Xiao, H.-Y.: Differentiation Between Nitrate Aerosol Formation Pathways in a Southeast Chinese City by Dual Isotope and Modeling Studies, J. Geophys. Res.-Atmos., 125, 13, https://doi.org/10.1029/2020jd032604, 2020.
Xu, L. and Penner, J. E.: Global simulations of nitrate and ammonium aerosols and their radiative effects, Atmos. Chem. Phys., 12, 9479–9504, https://doi.org/10.5194/acp-12-9479-2012, 2012.
Xu, Q., Wang, S., Jiang, J., Bhattarai, N., Li, X., Chang, X., Qiu, X., Zheng, M., Hua, Y., and Hao, J.: Nitrate dominates the chemical composition of PM2.5 during haze event in Beijing, China, Sci. Total. Environ., 689, 1293–1303, https://doi.org/10.1016/j.scitotenv.2019.06.294, 2019.
Yan, C., Tham, Y. J., Zha, Q. Z., Wang, X. F., Xue, L. K., Dai, J. N., Wang, Z., and Wang, T.: Fast heterogeneous loss of N2O5 leads to significant nighttime NOx removal and nitrate aerosol formation at a coastal background environment of southern China, Sci. Total. Environ., 677, 637–647, https://doi.org/10.1016/j.scitotenv.2019.04.389, 2019.
Ye, C., Heard, D. E., and Whalley, L. K.: Evaluation of Novel Routes for NOx Formation in Remote Regions, Environ. Sci. Technol., 51, 7442–7449, https://doi.org/10.1021/acs.est.6b06441, 2017a.
Ye, C., Zhang, N., Gao, H., and Zhou, X.: Photolysis of Particulate Nitrate as a Source of HONO and NOx, Environ. Sci. Technol., 51, 6849–6856, https://doi.org/10.1021/acs.est.7b00387, 2017b.
Zhai, S., Jacob, D. J., Wang, X., Liu, Z., Wen, T., Shah, V., Li, K., Moch, J. M., Bates, K. H., Song, S., Shen, L., Zhang, Y., Luo, G., Yu, F., Sun, Y., Wang, L., Qi, M., Tao, J., Gui, K., Xu, H., Zhang, Q., Zhao, T., Wang, Y., Lee, H. C., Choi, H., and Liao, H.: Control of particulate nitrate air pollution in China, Nat. Geosci., 14, 389–395, https://doi.org/10.1038/s41561-021-00726-z, 2021.
Zhang, G., Lin, Q., Peng, L., Yang, Y., Fu, Y., Bi, X., Li, M., Chen, D., Chen, J., Cai, Z., Wang, X., Peng, P., Sheng, G., and Zhou, Z.: Insight into the in-cloud formation of oxalate based on in situ measurement by single particle mass spectrometry, Atmos. Chem. Phys., 17, 13891–13901, https://doi.org/10.5194/acp-17-13891-2017, 2017.
Zhang, R., Gen, M., Fu, T. M., and Chan, C. K.: Production of Formate via Oxidation of Glyoxal Promoted by Particulate Nitrate Photolysis, Environ. Sci. Technol., 55, 5711–5720, https://doi.org/10.1021/acs.est.0c08199, 2021.
Zheng, H., Song, S., Sarwar, G., Gen, M., Wang, S., Ding, D., Chang, X., Zhang, S., Xing, J., Sun, Y., Ji, D., Chan, C. K., Gao, J., and McElroy, M. B.: Contribution of Particulate Nitrate Photolysis to Heterogeneous Sulfate Formation for Winter Haze in China, Environ. Sci. Tech. Let., 7, 632–638, https://doi.org/10.1021/acs.estlett.0c00368, 2020.
Zhu, Y., Tilgner, A., Hoffmann, E. H., Herrmann, H., Kawamura, K., Yang, L., Xue, L., and Wang, W.: Multiphase MCM–CAPRAM modeling of the formation and processing of secondary aerosol constituents observed during the Mt. Tai summer campaign in 2014, Atmos. Chem. Phys., 20, 6725–6747, https://doi.org/10.5194/acp-20-6725-2020, 2020.