the Creative Commons Attribution 4.0 License.
the Creative Commons Attribution 4.0 License.
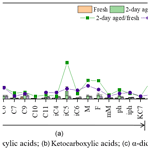
Distribution and stable carbon isotopic composition of dicarboxylic acids, ketocarboxylic acids and α-dicarbonyls in fresh and aged biomass burning aerosols
Minxia Shen
Kin Fai Ho
Wenting Dai
Suixin Liu
Ting Zhang
Qiyuan Wang
Jingjing Meng
Judith C. Chow
John G. Watson
Junji Cao
Biomass burning (BB) is a significant source of dicarboxylic acids (diacids) and related compounds that play important roles in atmospheric chemistry and climate change. In this study, a combustion chamber and oxidation flow reactor were used to generate fresh and aged aerosols from burned rice, maize and wheat straw to investigate atmospheric aging and the stable carbon isotopic (δ13C) composition of these emissions. Succinic acid (C4) was the most abundant species in fresh samples, while oxalic acid (C2) became dominant after atmospheric aging. Of all diacids, C2 had the highest aged to fresh emission ratios (), suggesting that C2 is largely produced through secondary photochemical processes. Compared with fresh samples, the emission factors of ketocarboxylic acids and α-dicarbonyls increased after 2 d but decreased after 7 d aging, indicating a short residence time and further atmospheric degradation from 2 to 7 d. The δ13C values of C2 for aged biomass samples were higher than those of urban aerosols but lower than marine or mountain aerosols, and the δ13C values of C2 became isotopically heavier during aging. Relationships between the reduction in volatile organic compounds (VOCs), such as toluene, benzene and isoprene, and increase in diacids after 2 d aging indicate that these VOCs led to the formation of diacids. However, no significant correlation was found between decreases in VOCs and increases in 7 d aged diacids. In addition, the of C2 was 50.8 at 2 d and 64.5 at 7 d, indicating that the conversion of VOCs to C2 was almost completed within 2 d. For the longer aging times, the particulate-phase compounds may undergo further degradation in the oxidation processes.
- Article
(877 KB) - Full-text XML
-
Supplement
(485 KB) - BibTeX
- EndNote
Dicarboxylic acids (diacids), ketocarboxylic acids and α-dicarbonyls are common components of the atmospheric organic aerosol, accounting for 1 %–3 % of the total organic carbon in urban areas and > 10 % of the carbon mass in remote regions (Kawamura and Usukura, 1993; Kawamura and Sakaguchi, 1999; Kerminen et al., 2000; Zhao et al., 2018). Due to their high water solubility and other physicochemical properties, diacids affect the hygroscopic growth of particulate matter (PM), and these compounds are involved in the activation of cloud condensation nuclei and formation of ice nuclei (Kawamura and Bikkina, 2016). Diacids and related compounds have been found in a wide variety of environments including urban settings (Ho et al., 2006; Kawamura and Ikushima, 1993; Meng et al., 2020; Sorathia et al., 2018; Wang et al., 2002, 2006, 2012), mountains ranges (Kawamura et al., 2013; Kunwar et al., 2019) and remote marine atmospheres (Hoque et al., 2020; Kawamura and Usukura, 1993). They also have been reported in both the Arctic and Antarctic aerosols (Kawamura et al., 1996a, b; Narukawa et al., 2002, 2003) as well as polar ice cores (Legrand and De Angelis, 1996; Kawamura et al., 2001). Various studies have assessed the molecular distributions, temporal variability and sources of diacids in different airsheds.
There are both primary and secondary sources of diacids (Mkoma and Kawamura, 2013). Primary sources include emissions from fossil fuel combustion (Kawamura and Kaplan, 1987; Rogge et al., 1993), cigarette burning (Rogge et al., 1994), cooking (Rogge et al., 1991) and biomass burning (BB) (Narukawa et al., 1999; Schauer et al., 2001). Of these, BB was found to be an important source of diacids and related compounds over regional and global scales (Kundu et al., 2010). Emissions from BB not only compose a major source of primary particles, but also introduce aerosol precursors to the atmosphere (Akagi et al., 2011; Gilman et al., 2015; Reid et al., 2005). Secondary sources include particles produced by chemical/photochemical oxidation reactions of volatile organic compounds (VOCs), especially those emitted from primary sources (Lim et al., 2013; Carlton et al., 2006, 2007).
Being one of the major contributors to the global budget of aerosols, BB emissions are of particular concern because they impact air quality, visibility, climate and human health (Hodshire et al., 2019). As the largest developing country and one that burns large quantities of biomass, China has long suffered from severe air pollution from BB (Chen et al., 2016; Fullerton et al., 2008). Domestic crop residues (e.g., rice, maize and wheat straw) and firewood are the most significant energy sources in most rural areas, and these are commonly used for cooking and heating (Li et al., 2021; Tao et al., 2018).
Diacids, ketocarboxylic acids and α-dicarbonyls are products of BB (Agarwal et al., 2010). Although these acids have been measured in ambient air in some areas dominated by BB sources (Falkovich et al., 2005; Kundu et al., 2010; Kawamura et al., 2013), there have been few BB source emission (e.g., chamber) measurements. It was reported that BB smoke was found to contain large amounts of gaseous pollutants, such as VOCs, nitrogen oxides (NOx), sulfur dioxide (SO2), and ammonia (NH3) (Akagi et al., 2011; Andreae and Merlet, 2001). Gas-phase compounds, especially VOCs, can be partitioned to the particle phase through nucleation, condensation and heterogeneous chemical reactions, creating secondary organic aerosol (SOA) and adding to aerosol mass (Hodshire et al., 2019; Lim et al., 2019). Oxalic acid (C2), the most abundant species of diacids (Kawamura and Sakaguchi, 1999), is formed by various VOCs in cloud droplets through photochemical oxidation and liquid-phase reactions. It is of interest to quantify emission factors (EFs) of diacids and related compounds during the combustion of different biomass fuels in the laboratory. Kalogridis et al. (2018) performed small-scale fire experiments using the Large Aerosol Chamber (LAC; 1800 m3) with a focus on BB from Siberian boreal coniferous forests and presented experimental data on EFs of diacids. However, this study only focused on the EFs of diacids of fresh pollutants that were directly emitted from BB, so it is necessary to further investigate the molecular composition of aged BB aerosols. In addition, limited data are available on the specific diacids emitted from the burning of agricultural residues. Therefore, it is important to investigate the molecular composition of diacids in both fresh and aged BB aerosols to advance current understanding of the potential environmental and climatic effects.
In this study, rice, maize and wheat straw were selected for laboratory simulations of fresh and aged BB aerosols. The study was conducted with the use of a combustion chamber and oxidation flow reactor (OFR). Fresh and aged BB aerosols were chemically analyzed for molecular characteristics and the stable carbon isotopic composition (δ13C) of selected diacids, ketocarboxylic acids, α-dicarbonyls and benzoic acid. The objectives of this study were to (1) investigate the emissions of diacids, ketocarboxylic acids and α-dicarbonyls from crop residue burning; (2) evaluate the effects of atmospheric aging processes on diacids and related compounds; and (3) investigate the relationship between VOCs with C2 and intermediates that form in the aging process to explore potential formation mechanisms of selected organic acids.
2.1 Preparation and collection of fresh and aged BB aerosols
The experimental setup is illustrated in the Supplement Fig. S1. Detailed procedures for sample preparation and collection may be found in previous studies (Li et al., 2020, 2021; Niu et al., 2020). Briefly, fresh smoke was generated by burning dry biomass fuels (i.e., rice, maize and wheat straw) in a combustion chamber, and the smoke was then passed through a potential aerosol mass oxidation flow reactor (PAM-OFR) (Aerodyne Research, LLC, Billerica, MA, USA) to simulate aging processes on the timescale of hours to days. The biomass combustion chamber had a volume of ∼ 8 m3 (1.8 m (W) × 1.8 m (L) × 2.2 m (H)) and was made of 3 mm thick aluminum to withstand high-temperature heating. The combustion chamber was equipped with a thermo anemometer, an air purification system, a heated sampling line, a dilution sampler and so on. More detailed information about the design and evaluation of the combustion chamber was described in Tian et al. (2015).
In order to get sufficient aerosol samples for measurements of chemical composition, around 1 kg biomass fuels were burned inside the chamber in 10 burning cycles. The entire burning cycle, including ignition, flaming, smoldering and extinction, intends to simulate real-world source characterization. Each burning cycle, containing ∼ 100 g biomass fuels, lasts around 12–18 min. The fresh smoke was diluted by 4.6 times using clean air controlled by the flow balance. A portion of the diluted smoke by a dilution sampler (Model 18, Baldwin Environmental Inc., Reno, NV, USA) was drawn through a quartz fiber filter (47 mm diameter, Whatman QM/A, Maidstone, UK) at 5 L min−1 using a miniVol PM2.5 sampler (Airmetrics, OR, USA) to capture fresh emission.
The PAM-OFR can be used to simulate an environment with extremely high oxidant concentrations with short residence times (Kang et al., 2007). Another portion of the exhaust (∼ 9 L min−1) was directed through a 19 L cylinder PAM-OFR (with a diameter of 20 cm and length of 60 cm) to simulate atmospheric aging. The residence time of PAM-OFR is estimated to be 90 ± 1 s at flow rate of 9 L min−1 (Li et al., 2021). Three oxidants (O3, ⋅OH and ⋅HO2) were generated in the PAM chamber using irradiation from ultraviolet (UV) lamps. The OH exposure values (OHexp) can be calculated by the concentration of SO2 and CO at the OFR inlet and outlet in a laboratory setting. Relative humidity (RH) inside the OFR was varied by passing different amounts of carrier gas through the OFR humidifier (MH-110). Additional details on smoke generation condition, test study and evaluation of the PAM-OFR were described by Cao et al. (2020).
In this study, the UV lamps operated at a voltage of 2 and 3.5 V, and OHexp in the chamber was estimated at 2.6 × 1011 and 8.8 × 1011 molecules s m−3, respectively. These levels corresponded to ∼ 2 and 7 d of aging (Chow et al., 2019; Watson et al., 2019), assuming a representative atmospheric ⋅OH level of 1.5 × 106 molecules m−3 (Mao et al., 2009). The aged aerosols were sampled by another miniVol PM2.5 sampler (5 L min−1) following the reactions in the PAM-OFR chamber. Each test was conducted in triplicate to account for experimental errors and to provide a measure of variability, which was calculated as standard deviations. A total of 36 samples were collected and analyzed for chemical composition.
2.2 Sample extraction, derivatization and quantification
For diacids, ketocarboxylic acids and α-dicarbonyls analysis, one-quarter of each filter sample was extracted three times (15 min each) with purified (18.2 MΩ) water (Milli-Q, Merch, France) and ultrasonication. The pH of the aerosol extracts was adjusted to 8.5 to 9.0 using a 0.1 M potassium hydroxide solution prior to drying that converts carboxylic acids into their salts (Bikkina et al., 2021). This drying step improves the recovery of smaller diacids, such as C2 (Hegde and Kawamura, 2012). Water extracts were concentrated to near-dryness with a rotary evaporator under vacuum and then reacted with 14 % BF3/n-butanol at 100 ∘C for 1 h to derivatize carboxyl groups to dibutyl esters and oxo groups to dibutoxyacetals.
After derivatization, n-hexane was added and washed with pure water three times to remove the water-soluble inorganics such as hydrogen fluoride and boric acid. The hexane layer was concentrated to near-dryness using a rotary evaporator under vacuum and a N2 blow-down technique, and then the esters and acetals of target analytes were dissolved in known amounts of n-hexane. Finally, the hexane layers were concentrated to 100 µL and analyzed using a capillary gas chromatograph (GC; HP 6890, Agilent Technology, Santa Clara, CA, USA) equipped with a split/splitless injector and a flame ionization detector (FID). Peak identification was performed by comparing the GC retention times with those of authentic standards and confirmed by a thermal desorption (TD) unit coupled with a gas chromatograph/mass spectrometric detector (TD-GC/MS, Models 7890A/5975C, Agilent Technology, Santa Clara, CA, USA). The detection limits for those organic compounds were 0.1 ng m−3, and the analytical errors, based on the replicate analyses, were less than 15 %. Recoveries of the target compounds were 83 % for C2 and 87 % to 110 % for the other species.
2.3 Emission factor calculations
Concentrations of the various species in the aged samples were affected by their initial emission and also undergo degradation and production through secondary chemical processes. Fresh and aged fuel-based EFs for each measured chemical compound were calculated by dividing its filter mass by the mass of combusted dry biomass fuel (Andreae and Merlet, 2001; Li et al., 2020; Tian et al., 2015); that is
where EFi (mg kg−1) is the EF of chemical compound i for the specific crop, mi (mg) is the mass of chemical compound i collected on the filter, vStk is the average stack flow velocity (m s−1) at standard conditions, D is the stack cross section (m2), tsample is the sampling duration (s), Qp is the sampling volume through the filter (m3) at standard temperature and pressure, and mfuel is the mass of burned biomass fuel (kg, dry weight).
The dilution ratio (DR) was determined from the CO2 concentrations measured at the stack, diluted stack and background, where
where CO2,Stk is the CO2 concentration in the stack, CO2,Bkg the background CO2 concentration in the atmosphere and CO2,Dil the CO2 concentration in the diluted smoke.
2.4 Stable carbon isotope composition of diacids
Stable carbon isotopic determinations (δ13C) of diacids, ketocarboxylic acids and α-dicarbonyls followed the techniques of Kawamura and Watanabe (2004). The isotope values of the derivatized samples were determined using a gas chromatography–isotope ratio mass spectrometer (GCIR-MS; Thermo Fisher, Delta V Advantage, Franklin, MA, USA). The δ13C values were then calculated for free organic acids using an isotope mass balance equation based on the measured δ13C values of derivatives and BF3/n-butanol (Kawamura and Watanabe, 2004). To ensure the analytical error of the δ13C values less than 0.2 ‰, each aerosol sample was analyzed in triplicate to obtain the average values.
3.1 Emission factors for diacids, ketocarboxylic acids and α-dicarbonyls
Fresh and aged PM2.5 EFs for a homologous series of diacids, ketocarboxylic acids (glyoxylic acid, ωC2, and pyruvic acid, Pyr), α-dicarbonyls (glyoxal, Gly, and methylglyoxal, mGly) and benzoic acid are presented in Table 1. The EFs for most fresh and aged diacids varied by several orders of magnitude, with higher EFs after atmospheric aging. The highest fresh EF (i.e. EFfresh) was found for wheat straw ranging 44–122 mg kg−1 for succinic acid (C4) and 67–102 mg kg−1 for Gly, higher than EFs found in maize and rice. The arithmetic means and standard deviations for the EFfresh of total diacids from burning of rice, maize and wheat straws were 63 ± 24, 117 ± 39 and 285 ± 135 mg kg−1, respectively.
Table 1Emission factors (EFs; mg kg−1) of fresh and aged dicarboxylic acids and related compounds from rice, maize and wheat straw burning.
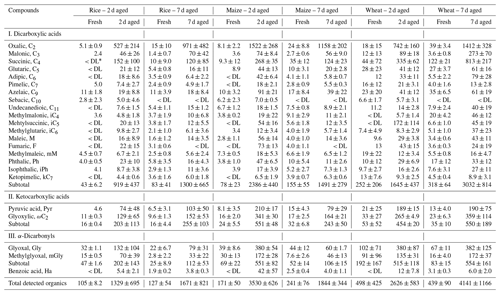
* < DL denotes emissions below method detection limit (MDL).
As is shown Fig. 1, distributions of diacids in fresh emissions varied by crop type and species. Of the saturated n-dicarboxylic acids, C4 acid was the most abundant species in the maize and wheat straw, with average EFfresh of 22 ± 12 and 83 ± 46 mg kg−1, respectively. Azelaic acid (C9) and C4 were the most abundant species from rice burning, with EFfresh of 11 ± 2.9 and 10 ± 9.0 mg kg−1, respectively. These findings are consistent with the fresh smoke aerosols in Siberian BB plumes (Kalogridis et al., 2018), in which C4 and C9 were more abundant than C2. Previous studies also showed C9 to be an oxidation product of unsaturated fatty acids in biomass smoke (Kawamura and Gagosian, 1987; Kawamura et al., 2013; Agarwal et al., 2010; Cao et al., 2017). C2 is the most abundant species of diacids and is one of the final products of the SOA reaction chain. In the fresh BB sample, C2 emissions were lower due to the short aging time.
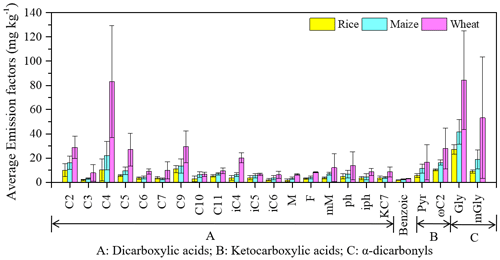
Figure 1Average emission factors of dicarboxylic acids and related compounds in fresh PM2.5 aerosols from biomass burning.
Similar to the diacids, the highest EFfresh for ketocarboxylic acids and α-dicarbonyls was also found in wheat straw samples, with 44 ± 31 and 138 ± 91 mg kg−1, respectively. Gly was the highest α-dicarbonyl, with an average EFfresh of 27 ± 3.9, 42 ± 10 and 84 ± 41 mg kg−1 for rice, maize and wheat straw, respectively. This is consistent with previous studies which showed that Gly is often more abundant than mGly in polluted aerosols collected from China (Pavuluri et al., 2010; Ho et al., 2007). Benzoic acid also was determined, and its EFfresh for rice, maize and wheat aerosols was 1.9 ± 0.2, 2.5 ± 0.4 and 3.1 ± 0.3 mg kg−1 (Table 1).
3.2 Effects of atmospheric aging processes
3.2.1 Diacids
The EFaged of 2 and 7 d diacids was 1650 ± 438 and 1957 ± 598 mg kg−1, respectively (Table S1), approximately 10 times greater than the EFfresh. High aged fresh emission ratios () imply that the bulk of the total diacids were secondarily produced through aging processes. Longer exposure time in the atmosphere increased the formation of diacids as ratios of average increased from 9.1 (2 d) to 10.8 (7 d) (Table S1). As shown in Fig. 2, C2 was the most abundant of all measured diacids among three crops, with the highest EFaged found in wheat (1412 ± 328 mg kg−1) after 7 d aging. These results provide further evidence that C2 is produced mainly through secondary photochemical processes rather than direct emission from BB. That is one possible reason that C2 is often the most abundant diacid in ambient samples, especially in the oceanic and other remote areas (Hoque et al., 2020; Kawamura and Usukura, 1993; Kawamura and Sakaguchi, 1999; Kunwar and Kawamura, 2014; Hegde and Kawamura, 2012; Kawamura and Bikkina, 2016; Wang et al., 2012). In addition, we found that the of C2 after 2 d aging was 50.8, and the change from 2 to 7 d was relatively small, only increasing by 13.7 (Table S1). These results meant that 2 d aging may be sufficient for most diacid formation. It can be inferred that although diacids are still generated at 7 d aging, a large number of VOCs may have been oxidized at 2 d aging and transferred to the particle phase by condensation, adsorption and other ways. Especially for maize straw, the EFaged of total detected organics at 7 d aging (1844 ± 344 mg kg−1) was lower than that of at 2 d aging (3530 ± 626 mg kg−1), which was mainly due to the predominant role of particulate diacid degradation in longer aging time. This phenomenon is consistent with the change of EFs of VOCs (precursors of C2) during maize straw combustion. The decreases in of ΣVOCEF after 2 d aging (1227 mg kg−1) were comparable with those of 7 d aging (884 mg kg−1) for maize straw (Niu et al., 2020).
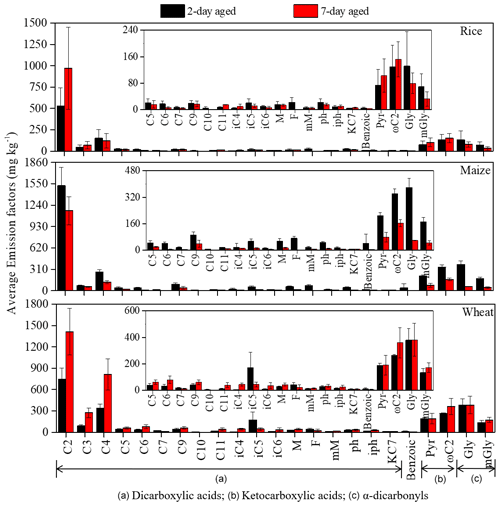
Figure 2Comparison between 2 and 7 d aged average PM2.5 emission factors of the sections marked as follows: (a) dicarboxylic acids, (b) ketocarboxylic acids and (c) α-carbonyls for laboratory combustion of rice, maize and wheat straw.
C4 ranked second in abundance after C2, with a 7–8-fold increase in EF after 2 and 7 d aging wheat. Although malonic acid (C3) is mainly produced by the photochemical oxidation of C4, it also can be formed through the incomplete combustion of fossil fuels and biomass (Kawamura and Ikushima, 1993). In the atmosphere, C4 is typically more abundant than C3 originated from BB, vehicular engine exhaust and biogenic emissions (Fu et al., 2013; Kawamura and Kaplan, 1987; Kundu et al., 2010). Figure 3 shows atmospheric aging increased the abundances of C3 and C4, with increasing from 16.2 to 31.1 for C3 and from 5.7 to 8.0 in C4 from 2 to 7 d of aging (Table S1). These findings add to the evidence that these diacids are produced by the photooxidation of primary pollutants emitted from combustion process. Higher in aged and fresh C3 acid than in C4 acid may be attributed to the rapid formation rate of C3 or decarboxylation processing of C4 diacid during aging (Zhao et al., 2018).
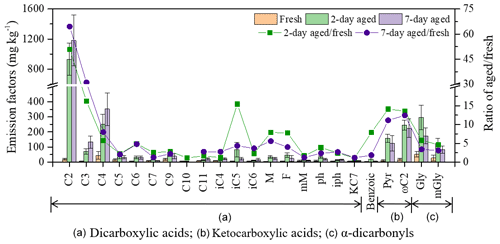
Figure 3Average emission factors of dicarboxylic acids and related compounds from biomass burning experiment for the fresh, 2 and 7 d aged PM2.5 aerosols. The squares and dots denote the ratios of aged to fresh () emission samples for the dicarboxylic acids and related compounds after 2 and 7 d aging.
As mentioned above, C9 is thought to be mainly formed through the photochemical oxidation of unsaturated fatty acids emitted by plants (Kawamura and Gagosian, 1987). Average EFs in C9 acid were low, ranging from 18 ± 7.3 mg kg−1 (fresh) to 51 ± 14 mg kg−1 (2 d), with an of C9 of 2.8 and 2.2 for the 2 and 7 d samples, respectively, suggesting that C9 is relatively stable with a short residence time. Figure 3 shows that of other long-chain diacids and branched diacids did not show apparent changes between the 2 and 7 d samples, which may be due to the degradation of long-chain diacids (Enami et al., 2015; Legrand et al., 2007; Miyazaki et al., 2010). It is also possible that the laboratory combustion experiment did not produce adequate quantities of certain diacids. For example, glutaric acid (C5) and adipic acid (C6) are commonly formed by reactions of cycloolefins emitted from anthropogenic sources with O3 (Hatakeyama et al., 1985) and phthalic acid as a product of the photochemical oxidation of aromatic hydrocarbon compounds (Kawamura and Ikushima, 1993). Additional laboratory experiments may be needed to reify different atmospheric process.
3.2.2 Ketocarboxylic acids and α-carbonyls
In contrast to the diacids, aging process were not apparent in ketocarboxylic acids as reduced by 16 % from 13.8 (2 d) to 11.9 (7 d). A similar phenomenon was found for α-carbonyls, with reduced by 64 % from 5.4 (2 d) to 3.3 (7 d). This suggests the possibility that the degradation of these intermediates to C2 is faster than their formation by oxidation after 2 d of aging. Figure 3 also show apparent reduction EF of 33 %–42 % from 2 to 7 d aging for Gly and mGly, which may be due to the fact both Gly and mGly initially can be oxidized to less volatile polar organic acids including Pyr and ωC2 and then further oxidized to C2 (Wang et al., 2012; Warneck, 2003).
3.3 Comparisons of diagnostic ratios of diacids in fresh and aged aerosols
Patterns in the relative abundances of diacids have been used to evaluate biogenic versus anthropogenic source strengths and the photochemical processing of organic aerosols (Kawamura et al., 2012). Previous studies have shown that C4 can be directly oxidized into C2 or via C3 into C2 (Jung et al., 2010; Sorooshian et al., 2007), with C2 being an end product of the photochemical oxidation (Wang et al., 2012). The ratios of C3 C4, C2 C4 and C2 total diacids can be regarded as indicators of aerosol aging (Cheng et al., 2013; Kunwar et al., 2019; Meng et al., 2018; Pavuluri et al., 2010), with higher ratios indicative of more aged aerosols (Kawamura and Sakaguchi, 1999). As shown in Table 2, the ratios in this study showed a clear atmospheric aging trend from fresh to 7 d aging, with ratios of 0.7 to 6.4 for C2 C4, 0.1 to 0.6 in C2 total diacids and 0.2 to 0.5 in C3 C4, indicating obvious photochemical oxidation.
Table 2Comparison of mass ratios of C3 C4, C2 C4, C2 total diacids, ωC2 C2 and Gly mGly in fresh and aged aerosols collected from biomass burning at different locations around the world.
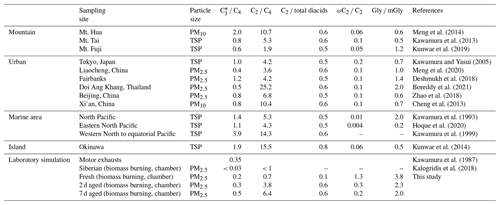
* See compound list in Table 1.
Ratios of ωC2 C2 and Gly mGly can also be used to evaluate the oxidation of organic aerosols (Cheng et al., 2013, 2015; Kawamura et al., 2013). In the study, apparent reduction of the ωC2 C2 ratios from 1.3 (fresh) to 0.2 (7 d) supports the potential oxidation pathways from precursor glyoxylic to oxalic acids. Aqueous-phase oxidation by OH is faster for Gly than for mGly, and the abundance of Gly relative to mGly is an indicator of aerosol aging (Cheng et al., 2013). The ratio of Gly mGly in Xi'an samples was lower on haze days than on clean days and lower in summer than in winter. Similarly, the Gly mGly ratios in the aged BB samples were higher in the fresh PM2.5 samples (3.8) compared to the 2 d (2.3) and 7 d (2.0) aging.
Ratios of C3 C4, C2 diacids, ωC2 C2 and Gly mGly are similar among studies, except for the higher C3 C4 ratio of 3.9 found in marine aerosols of over the Pacific region (Kawamura and Sakaguchi, 1999) and lower C3 C4 ratios in Siberian BB emissions in a large aerosol chamber (< 0.03) (Kalogridis et al., 2018). The largest difference was found for C2 C4, which varied from < 1 for fresh aerosol in Siberian BB (Kalogridis et al., 2018) to 25.2 from forest fire in Thailand (Boreddy et al., 2021). Elevated C2 C4 ratios exceeding 10 were found in the aged ambient atmosphere of Xi'an, China (10.4) (Cheng et al., 2013), Mt. Hua, China (10.7) (Meng et al., 2014), marine aerosol, Pacific Ocean (14.3) (Kawamura and Sakaguchi, 1999), and the ambient atmosphere of Okinawa Island, Japan (15.5) (Kunwar and Kawamura, 2014). These C2 C4 ratios are ∼ 63 % to 142 % higher than these reported in this study. Overall, these comparisons show the importance of photochemical aging; however, the atmospheric oxidation evidently was more extensive in aerosols from some remote mountain and marine environments.
3.4 Stable carbon isotopes of diacids
Stable carbon isotope ratios (δ13C) can provide insights into the sources of aerosols. Pavuluri and Kawamura (2016) reported that average δ13C values for C2 from biogenic aerosols (−15.8 ‰) were less negative; i.e., they contained more 13C and were isotopically more enriched than those from anthropogenic aerosols (−19.5 ‰). Data for δ13C can also provide information on the processing or aging of organic aerosols because isotopic fractionation results from chemical reactions or phase transfer (Pavuluri and Kawamura, 2016; Zhang et al., 2016). Mass loading of δ13C for diacids in the fresh BB samples was too low to be detected by the GCIR-MS, but the δ13C values for C2 ranged from −23.3 % to −21.0 ‰ (with an average of −21.9 ± 1.2 ‰) in 2 d and −19.1 ‰ to −15.5 ‰ (−17.3 ± 1.7 ‰) for 7 d aged samples (Table 3).
Table 3Stable carbon isotope ratios (δ13C, ‰) of C2 in atmospheric aerosols from selected locations.
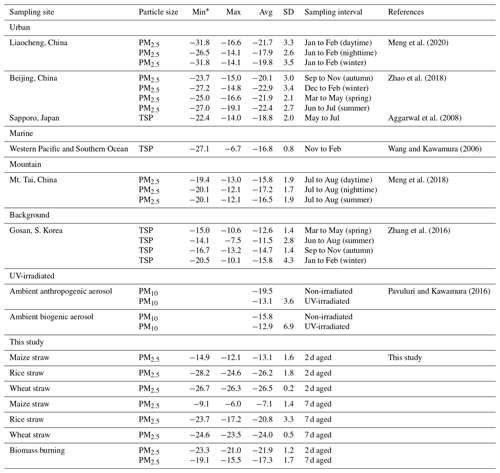
* Min, max, avg and SD stand for minimum, maximum, arithmetic mean and standard deviation.
Table 3 shows that the average δ13C values of C2 from aged maize samples were higher than those of rice and wheat. The reason for the isotope difference may be that maize is a C4 plant, while wheat and rice are both C3 plants. Song et al. (2018) showed that δ13CTC in C4 plants is isotopically heavier than in C3 plants. Moreover, the δ13C of C2 is more abundant in 7 than 2 d samples (Table 3), with −13.1 ± 1.6 ‰ (2 d) and −7.1 ± 1.4 ‰ (7 d) in maize; −26.2 ± 1.8 ‰ (2 d) and −20.8 ± 3.3 ‰ (7 d) in rice and −26.5 ± 0.2 ‰ (2 d) and −24.0 ± 0.5 ‰ (7 d) in wheat combustion. The δ13C data for C3, C4 and ωC2 (Table S2) showed similar trends, consistent with previous studies. For example, Zhao et al. (2018) found that the δ13C values of C2 were related to aging. Pavuluri and Kawamura (2016) analyzed diacids, ωC2 and Gly for δ13C in anthropogenic and biogenic aerosol samples by UV irradiation and reported more δ13C less negative with longer irradiation times. During atmospheric oxidation reactions, organic compounds react with OH radicals, causing the release of CO2 and CO which contain relatively the lighter 12C isotope and thus leaving the remaining substrate enriched in 13C (Hoefs, 1997; Sakugawa and Kaplan, 1995).
A comparison of δ13C values for C2 in the aerosols from selected environments is shown in Fig. 4. The average δ13C value (−21.9 ± 1.2 ‰) of 2 d aged biomass burning of C2 was comparable to the values reported for urban regions, such as Beijing (−21.8 ± 2.8 ‰) (Zhao et al., 2018) and Liaocheng (−19.8 ± 3.1 ‰) (Meng et al., 2020) (Table 3). With continued aging, the C2δ13C of the 7 d samples (−17.3 ± 1.7 ‰) was more similar in samples from Mt. Tai (−16.5 ± 1.8 ‰) (Meng et al., 2018) and western Pacific and Southern Ocean aerosol (−16.8 ± 0.8 ‰) (Wang and Kawamura, 2006), but it was significantly lighter than that of samples from the Gosan Climate Observatory at Gosan, South Korea (−13.7 ± 2.5 ‰), which is a mountain background site in East Asia (Zhang et al., 2016).
3.5 Relationships between decreases in VOCs and increases in diacids
The chamber experiment (Niu et al., 2020) measured VOC compounds. Table S3 presents the correlations between decreases in VOCs and increases in diacids from fresh to 2 d aged BB samples. Significant (0.01 < p < 0.05) correlations (R) were observed for toluene with Gly (R= 0.75), mGly (R= 0.81), Pyr (R= 0.78), ωC2 (R= 0.78) and C2 (R= 0.67) (Fig. 5), suggesting that toluene was converted to diacids during the aging process. Indeed, it has been reported that the photooxidation of toluene is a potential source of secondary organic aerosol (SOA) in urban air (Sato et al., 2007), and the major chemical components of SOA include hemiacetal, peroxy hemiacetal oligomers and diacids. It also can be seen that benzene had significant correlations with mGly and C2 (R > 0.59 in Fig. 5), implying that the oxidation of benzene led to diacid formation. Photooxidation of Gly and mGly is a major global and regional source of C2 diacid, and the two formation pathways are Gly–ωC2–C2 and mGly–Pyr–ωC2–C2, respectively (Yasmeen et al., 2010; Wang et al., 2012). As shown in Fig. 5, the slope (0.20–0.59) between the decrease of toluene and the increase of intermediates (Gly, mGly, Pyr and ωC2) is significantly higher than C2 (0.04). This was the same for benzene; the slope between the decrease of benzene and the increase of mGly is 0.55, while for C2 it is only 0.05.
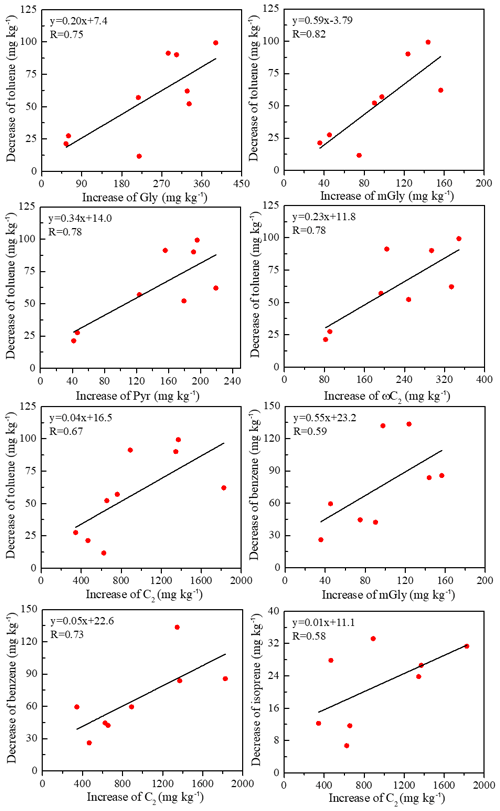
Figure 5Regressions between the decreases of specific VOCs (toluene, benzene and isoprene) and increases of C2 and its intermediates, methylglyoxal (mGly), glyoxal, (Gly), pyruvic acid (Pyr) and glyoxylic (ωC2).
On the global scale, isoprene is the most important precursor for C2, contributing 70 % to global C2, while anthropogenic VOCs contribute 21 % to C2 production (Myriokefalitakis et al., 2011). Thus, it is not surprising that isoprene correlated with C2 (R= 0.58) (Fig. 5). In addition, several alkenes and alkanes also had a significant correlation with C2 (Table S3), indicating that these species may react in secondary oxidation processes to generate C2. Previous studies have confirmed that diacids can be oxidation products of aromatic hydrocarbons (Borrás and Tortajada-Genaro, 2012) and cycloolefins (Hamilton et al., 2006) and may originate from diesel vehicle exhaust (Samy and Zielinska, 2010). However, no significant correlation was found between decreases in VOCs and increases in 7 d aged diacids. For the longer aging times, the particulate-phase compounds may be further oxidized to generate other compounds besides diacids. Such a correlation between decreases in VOCs and increases in diacids again suggests that 2 d aging may be sufficient to oxidize VOCs to diacids.
The emission factors (EFs) of dicarboxylic acids (diacids) and related compounds in experimentally produced fresh and aged biomass burning (BB) aerosols were compared. For fresh emissions, succinic acid (C4) was the most abundant diacid species followed by azelaic acid (C9). After atmospheric aging, diacids were dominated by oxalic acid (C2), with elevated EFs. Ratios of aged to fresh () emissions for C2 increased from 50.8 (2 d) to 64.5 (7 d). These results suggest that the diacids in the atmosphere largely originated from secondary photochemical processes as opposed to primary emissions from BB. It is confirmed for the first time whether the contribution of BB source to diacids is formed by primary emission or secondary oxidation. In addition, by comparing the EFs and of 2 and 7 d aging, it was found that 2 d of aging is sufficient for many diacids. Moreover, the 2 d of azelaic acid (C9), 2.8, degraded by 27 % after 7 d, suggesting that this species is relatively stable with short residence time.
Decreasing trends in EFs were found for ketocarboxylic acids and α-dicarbonyls, from 2 to 7 d aging, with reducing from 13.8 to 11.9 and from 5.4 to 3.3, respectively. These results suggest that after 2 d aging, the net degradation of these intermediates was faster than their rates of formation. Compared with 2 d samples, the δ13C of C2, malonic acid (C3), C4 and glyoxylic acid (ωC2) in 7 d samples became more positive or isotopically heavier after the additional aging, likely due to kinetic isotope fractionation effects. Moreover, the δ13C values for the aged maize samples in both the 2 and 7 d samples were significantly more positive than those of rice and wheat. This may be due to their different plant types, with maize being a C4 plant, while wheat and rice are both C3 plants. The correlations between volatile organic compounds (VOCs) and C2 or intermediates indicated that the oxidation of VOCs led to the formation of diacids. This correlation exists only at 2 d aging but does not exist at 7 d aging, probably because the longer the aging time, the further the particle phase compounds may be oxidized to other compounds.
Diacids are highly water-soluble in nature, and thus their high abundances due to BB and intense photochemical aging would enhance the ability of aerosols to act as cloud condensation nuclei and modify the water-uptake properties of aerosol particles. Therefore, it is necessary to better understand the chemical and physical properties of the constituents of water-soluble organic smoke, as they may have a significant impact on climate forcings through indirect aerosol effects. The results provide in-depth understanding of secondary organic aerosol (SOA) formation in regions greatly affected by BB.
Data can be made available upon request from the corresponding authors.
The supplement related to this article is available online at: https://doi.org/10.5194/acp-22-7489-2022-supplement.
JC and JL conceived and designed the study. MS contributed to the literature search, sample and data analysis, and manuscript writing. JL, JC, JCC and JGW contributed to manuscript revision. KFH, WD, SL, TZ, QW and JM carried out the particulate samples and supervised the experiments. All authors commented on the manuscript and reviewed the manuscript.
The contact author has declared that neither they nor their co-authors have any competing interests.
Publisher’s note: Copernicus Publications remains neutral with regard to jurisdictional claims in published maps and institutional affiliations.
This work was jointly supported by a program from the National Nature Science Foundation of China (no. 41977332), the Strategic Priority Research Program of Chinese Academy of Sciences (no. XDB40000000), the Innovation Capability Support Program of Shaanxi (no. 2020KJXX-017) and the US National Science Foundation (AGS-1464501 and CHE 1214463). Jianjun Li also acknowledges the support of the Youth Innovation Promotion Association CAS (no. 2020407).
This research has been supported by the National Natural Science Foundation of China (grant no. 41977332), the Strategic Priority Research Program of Chinese Academy of Sciences (grant no. XDB40000000), the Innovation Capability Support Program of Shaanxi (grant no. 2020KJXX-017), the US National Science Foundation (grant nos. AGS-1464501 and CHE 1214463) and the Youth Innovation Promotion Association CAS (grant no. 2020407).
This paper was edited by James Allan and reviewed by two anonymous referees.
Agarwal, S., Aggarwal, S. G., Okuzawa, K., and Kawamura, K.: Size distributions of dicarboxylic acids, ketoacids, α-dicarbonyls, sugars, WSOC, OC, EC and inorganic ions in atmospheric particles over Northern Japan: implication for long-range transport of Siberian biomass burning and East Asian polluted aerosols, Atmos. Chem. Phys., 10, 5839–5858, https://doi.org/10.5194/acp-10-5839-2010, 2010.
Aggarwal, S. G. and Kawamura K.: Molecular distributions and stable carbon isotopic compositions of dicarboxylic acids and related compounds in aerosols from Sapporo, Japan: Implications for photochemical aging during long-range atmospheric transport, J. Geophys. Res., 113, D14301, https://doi.org/10.1029/2007JD009365, 2008.
Akagi, S. K., Yokelson, R. J., Wiedinmyer, C., Alvarado, M. J., Reid, J. S., Karl, T., Crounse, J. D., and Wennberg, P. O.: Emission factors for open and domestic biomass burning for use in atmospheric models, Atmos. Chem. Phys., 11, 4039–4072, https://doi.org/10.5194/acp-11-4039-2011, 2011.
Andreae, M. O. and Merlet, P.: Emission of trace gases and aerosols from biomass burning, Global Biogeochem. Cy., 15, 955–966, https://doi.org/10.1029/2000GB001382, 2001.
Bikkina, S., Kawamura, K., Sakamoto, Y., and Hirokawa, J.: Low molecular weight dicarboxylic acids, oxocarboxylic acids and α-dicarbonyls as ozonolysis products of isoprene: Implication for the gaseous-phase formation of secondary organic aerosols, Sci. Total Environ., 769, 14472, https://doi.org/10.1016/j.scitotenv.2020.144472, 2021.
Boreddy, S. K. R., Parvin, F., Kawamura, K., Zhu, C. M., and Lee, C. T.: Influence of forest fires on the formation processes of low molecular weight dicarboxylic acids, ω-oxocarboxylic acids, pyruvic acid and α-dicarbonyls in springtime fine (PM2.5) aerosols over Southeast Asia, Atmos. Environ., 246, 118065, https://doi.org/10.1016/j.atmosenv.2020.118065, 2021.
Borrás, E. and Tortajada-Genaro, L. A.: Secondary organic aerosol formation from the photo-oxidation of benzene, Atmos. Environ., 47, 154–163, https://doi.org/10.1016/j.atmosenv.2011.11.020, 2012.
Cao, F., Zhang, S. C., Kawamura, K., Liu, X. Y., Yang, C., Xu, Z. F., Fan, M. Y., Zhang, W. Q., Bao, M. Y., Chang, Y. H., Song, W. H., Liu, S. D., Lee, X. H., Li, J., Zhang, G., and Zhang, Y. L.: Chemical characteristics of dicarboxylic acids and related organic compounds in PM2.5 during biomass-burning and non-biomass-burning seasons at a rural site of Northeast China, Environ. Pollut., 231, 654–662, https://doi.org/10.1016/j.envpol.2017.08.045, 2017.
Cao, J. J., Wang, Q. Y., Li, L., Zhang, Y., Tian, J., Chen, L. W. A., Ho, S. S. H., Wang, X. L., Chow, J. C., and Watson, J. G.: Evaluation of the oxidation flow reactor for particulate matter emission limit certification, Atmos. Environ., 224, 117086, https://doi.org/10.1016/j.atmosenv.2019.117086, 2020.
Carlton, A. G., Turpin, B. J., Lim, H. J., Altieri, K. E., and Seitzinger, S.: Link between isoprene and secondary organic aerosol (SOA): Pyruvic acid oxidation yields low volatility organic acids in clouds, Geophy. Res. Lett., 33, L06822, https://doi.org/10.1029/2005GL025374, 2006.
Carlton, A. G., Turpin, B. J., Altieri, K. E., Seitzinger, S., Reff, A., Lim, H. J., and Ervens, B.: Atmospheric oxalic acid and SOA production from glyoxal: Results of aqueous photooxidation experiments, Atmos. Environ., 41, 7588–7602, https://doi.org/10.1016/j.atmosenv.2007.05.035, 2007.
Chen, J. M., Li, C. L., Ristovski, Z., Milic, A., Gu, Y. T., Islam, M. S., Wang, S. X., Hao, J. M., Zhang, H. F., He, C. R., Guo, H., Fu, H. B., Miljevic, B., Morawska, L., Thai, P., Fat LAM, Y., Pereira, G., Ding, A. J., Huang, X., and Dumka, U. C.: A review of biomass burning: Emissions and impacts on air quality, health and climate in China, Sci. Total Environ., 579, 1000–1034, https://doi.org/10.1016/j.scitotenv.2016.11.025, 2016.
Cheng, C. L., Wang, G. H., Zhou, B. H., Meng, J. J., Li, J. J., and Cao, J. J.: Comparison of dicarboxylic acids and related compounds in aerosol samples collected in Xi'an, China during haze and clean periods, Atmos. Environ., 81, 443–449, https://doi.org/10.1016/j.atmosenv.2013.09.013, 2013.
Cheng, C. L., Wang, G. H., Meng, J. J., Wang, Q. Y., Cao, J. J., Li, J. J., and Wang, J. Y.: Size-resolved airborne particulate oxalic and related secondary organic aerosol species in the urban atmosphere of Chengdu, China, Atmos. Res., 161–162, 134–142, https://doi.org/10.1016/j.atmosres.2015.04.010, 2015.
Chow, J. C., Cao, J., Antony Chen, L.-W., Wang, X., Wang, Q., Tian, J., Ho, S. S. H., Watts, A. C., Carlson, T. B., Kohl, S. D., and Watson, J. G.: Changes in PM2.5 peat combustion source profiles with atmospheric aging in an oxidation flow reactor, Atmos. Meas. Tech., 12, 5475–5501, https://doi.org/10.5194/amt-12-5475-2019, 2019.
Deshmukh, D. K., Haque, M. M., Kawamura, K. and Kim, Y. W.: Dicarboxylic acids, oxocarboxylic acids and alpha-dicarbonyls in fine aerosols over central Alaska: Implications for sources and atmospheric processes, Atmos. Res., 202, 128–139, https://doi.org/10.1016/j.atmosres.2017.11.003, 2018.
Enami, S., Hoffmann, M. R., and Colussi, A. J.: Stepwise Oxidation of Aqueous Dicarboxylic Acids by Gas-Phase OH Radicals, J. Phys. Chem. Lett., 6, 527–534, https://doi.org/10.1021/jz502432j, 2015.
Falkovich, A. H., Graber, E. R., Schkolnik, G., Rudich, Y., Maenhaut, W., and Artaxo, P.: Low molecular weight organic acids in aerosol particles from Rondônia, Brazil, during the biomass-burning, transition and wet periods, Atmos. Chem. Phys., 5, 781–797, https://doi.org/10.5194/acp-5-781-2005, 2005.
Fu, P. Q., Kawamura, K., Usukura, K., and Miura, K.: Dicarboxylic acids, ketocarboxylic acids and glyoxal in the marine aerosols collected during a round-the-world cruise, Mar. Chem., 148, 22–32, https://doi.org/10.1016/j.marchem.2012.11.002, 2013.
Fullerton, D. G., Nigel, B., and Gordon, S. B.: Indoor air pollution from biomass fuel smoke is a major health concern in the developing world, T. Roy. Soc. Trop. Med. H., 102, 843–851, https://doi.org/10.1016/j.trstmh.2008.05.028, 2008.
Gilman, J. B., Lerner, B. M., Kuster, W. C., Goldan, P. D., Warneke, C., Veres, P. R., Roberts, J. M., de Gouw, J. A., Burling, I. R., and Yokelson, R. J.: Biomass burning emissions and potential air quality impacts of volatile organic compounds and other trace gases from fuels common in the US, Atmos. Chem. Phys., 15, 13915–13938, https://doi.org/10.5194/acp-15-13915-2015, 2015.
Hamilton, J. F., Lewis, A. C., Reynolds, J. C., Carpenter, L. J., and Lubben, A.: Investigating the composition of organic aerosol resulting from cyclohexene ozonolysis: low molecular weight and heterogeneous reaction products, Atmos. Chem. Phys., 6, 4973–4984, https://doi.org/10.5194/acp-6-4973-2006, 2006.
Hatakeyama, S., Tanonaka, T., Weng, J., Bandow, H., Takagi, H., and Akimoto, H.: Ozone-cyclohexene reaction in air: quantitative analysis of particulate products and the reaction mechanism, Environ. Sci. Technol., 19, 935–942, https://doi.org/10.1021/es00140a008, 1985.
Hegde, P. and Kawamura, K.: Seasonal variations of water-soluble organic carbon, dicarboxylic acids, ketocarboxylic acids, and α-dicarbonyls in Central Himalayan aerosols, Atmos. Chem. Phys., 12, 6645–6665, https://doi.org/10.5194/acp-12-6645-2012, 2012.
Ho, K. F., Lee, S. C., Cao, J. J., Kawamura, K., Watanabe, T., Cheng, Y., and Chow, J. C.: Dicarboxylic acids, ketocarboxylic acids and dicarbonyls in the urban roadside area of Hong Kong, Atmos. Environ., 40, 3030–3040, https://doi.org/10.1016/j.atmosenv.2005.11.069, 2006.
Ho, K. F., Cao, J. J., Lee, S. C., Kawamura, K., Zhang, R. J., Chow, J. C., and Watson, J. G.: Dicarboxylic acids, ketocarboxylic acids, and dicarbonyls in the urban atmosphere of China, J. Geophys. Res.-Atmos., 112, D22S27, https://doi.org/10.1029/2006JD008011, 2007.
Hodshire, A. L., Akherati, A., Alvarado, M. J., Brown-Steiner, B., Jathar, S. H., Jimenez, J. L., Kreidenweis, S. M., Lonsdale, C. R., Onasch, T. B., Ortega, A. M., and Pierce, J. R.: Aging effects on biomass burning aerosol mass and composition: a critical review of field and laboratory studies, Environ. Sci. Technol., 53, 10007–10022, https://doi.org/10.1021/acs.est.9b02588, 2019.
Hoefs, J.: Stable Isotope Geochemistry, Springer, New York, 1997.
Hoque, M., Kawamura, K., Nagayama, T., Kunwar, B., and Gagosian, R. B.: Molecular characteristics of water-soluble dicarboxylic acids, ω-oxocarboxylic acids, pyruvic acid and α-dicarbonyls in the aerosols from the eastern North Pacific, Mar. Chem., 224, 103812, https://doi.org/10.1016/j.marchem.2020.103812, 2020.
Jung, J. S., Tsatsral, B., Kim, Y. J., and Kawamura, K.: Organic and inorganic aerosol compositions in Ulaanbaatar, Mongolia, during the cold winter of 2007 to 2008 : Dicarboxylic acids, ketocarboxylic acids, and α-dicarbonyls, J. Geophys. Res.-Atmos., 115, D22203, https://doi.org/10.1029/2010JD014339, 2010.
Kalogridis, A. C., Popovicheva, O. B., Engling, G., Diapouli, E., Kawamura, K., Tachibana, E., Ono, K., Kozlov, V. S., and Eleftheriadis, K.: Smoke aerosol chemistry and aging of Siberian biomass burning emissions in a large aerosol chamber, Atmos. Environ., 185, 15–28, https://doi.org/10.1016/j.atmosenv.2018.04.033, 2018.
Kang, E., Root, M. J., Toohey, D. W., and Brune, W. H.: Introducing the concept of Potential Aerosol Mass (PAM), Atmos. Chem. Phys., 7, 5727–5744, https://doi.org/10.5194/acp-7-5727-2007, 2007.
Kawamura, K. and Usukura, K.: Distributions of low molecular weight dicarboxylic acids in the North Pacific aerosol samples, J. Oceanogr., 49, 271–283, https://doi.org/10.1007/BF02269565, 1993.
Kawamura, K. and Bikkina, S.: A review of dicarboxylic acids and related compounds in atmospheric aerosols: Molecular distributions, sources and transformation, Atmos. Res., 170, 140–160, https://doi.org/10.1016/j.atmosres.2015.11.018, 2016.
Kawamura, K. and Gagosian, R. B.: Implications of ω-oxocarboxylic acids in the remote marine atmosphere for photo-oxidation of unsaturated fatty acids, Nature, 325, 330–332, 1987.
Kawamura, K. and Ikushima, K.: Seasonal changes in the distribution of dicarboxylic acids in the urban atmosphere, Environ. Sci. Technol., 27, 2227–2235, https://doi.org/10.1021/es00047a033, 1993.
Kawamura, K. and Kaplan, I. R.: Motor exhaust emissions as a primary source for dicarboxylic acids in Los Angeles ambient air, Environ. Sci. Technol., 21, 105–110, https://doi.org/10.1021/es00155a014, 1987.
Kawamura, K. and Sakaguchi, F.: Molecular distributions of water soluble dicarboxylic acids in marine aerosols over the Pacific Ocean including tropics, J. Geophys. Res.-Atmos., 104, 3501–3509, https://doi.org/10.1029/1998JD100041, 1999.
Kawamura, K. and Watanabe, T.: Determination of stable carbon isotopic compositions of low molecular weight dicarboxylic acids and ketocarboxylic acids in atmospheric aerosol and snow samples, Anal. Chem., 76, 5762–5768, https://doi.org/10.1021/ac049491m, 2004.
Kawamura, K. and Yasui, O.: Diurnal changes in the distribution of dicarboxylic acids, ketocarboxylic acids and dicarbonyls in the urban Tokyo atmosphere, Atmos. Environ., 39, 1945–1960, https://doi.org/10.1016/j.atmosenv.2004.12.014, 2005.
Kawamura, K., Kasukabe, H., and Barrie, L. A.: Source and reaction pathways of dicarboxylic acids, ketoacids and dicarbonyls in arctic aerosols: one year of observations, Atmos. Environ., 30, 1709–1722, https://doi.org/10.1016/1352-2310(95)00395-9, 1996a.
Kawamura, K., Sempéré, R., Imai, Y., Fujii, Y., and Hayashi, M.: Water soluble dicarboxylic acids and related compounds in Antarctic aerosols, J. Geophys. Res.-Atmos., 101, 18721–18728, https://doi.org/10.1029/96JD01541, 1996b.
Kawamura, K., Yokoyama, K., Fujii, Y., and Watanabe, O.: A Greenland ice core record of low molecular weight dicarboxylic acids, ketocarboxylic acids, and α-dicarbonyls: A trend from Little Ice Age to the present (1540 to 1989 A.D.), J. Geophys. Res.-Atmos., 106, 1331–1345, https://doi.org/10.1029/2000JD900465, 2001.
Kawamura, K., Ono, K., Tachibana, E., Charriére, B., and Sempéré, R.: Distributions of low molecular weight dicarboxylic acids, ketoacids and α-dicarbonyls in the marine aerosols collected over the Arctic Ocean during late summer, Biogeosciences, 9, 4725–4737, https://doi.org/10.5194/bg-9-4725-2012, 2012.
Kawamura, K., Tachibana, E., Okuzawa, K., Aggarwal, S. G., Kanaya, Y., and Wang, Z. F.: High abundances of water-soluble dicarboxylic acids, ketocarboxylic acids and α-dicarbonyls in the mountaintop aerosols over the North China Plain during wheat burning season, Atmos. Chem. Phys., 13, 8285–8302, https://doi.org/10.5194/acp-13-8285-2013, 2013.
Kerminen, V. M., Ojanen, C., Pakkanen, T., Hillamo, R., Aurela, M., and Meriläinen, J.: Low-molecular-weight dicarboxylic acids in an urban and rural atmosphere, J. Aerosol Sci., 31, 349–362, https://doi.org/10.1016/S0021-8502(99)00063-4, 2000.
Kundu, S., Kawamura, K., Andreae, T. W., Hoffer, A., and Andreae, M. O.: Molecular distributions of dicarboxylic acids, ketocarboxylic acids and α-dicarbonyls in biomass burning aerosols: implications for photochemical production and degradation in smoke layers, Atmos. Chem. Phys., 10, 2209–2225, https://doi.org/10.5194/acp-10-2209-2010, 2010.
Kunwar, B. and Kawamura, K.: Seasonal distributions and sources of low molecular weight dicarboxylic acids, v-oxocarboxylic acids, pyruvic acid, a-dicarbonyls and fatty acids in ambient aerosols from subtropical Okinawa in the western Pacific Rim, Environ. Chem., 11, 673–689, https://doi.org/10.1071/EN14097, 2014.
Kunwar, B., Kawamura, K., Fujiwara, S., Fu, P. Q., Miyazaki, Y., and Pokhrel, A.: Dicarboxylic acids, oxocarboxylic acids and α-dicarbonyls in atmospheric aerosols from Mt. Fuji, Japan: Implication for primary emission versus secondary formation, Atmos. Res., 221, 58–71, https://doi.org/10.1016/j.atmosres.2019.01.021, 2019.
Legrand, M. and De Angelis, M.: Light carboxylic acids in Greenland ice: A record of past forest fires and vegetation emissions from the boreal zone, J. Geophys. Res.-Atmos., 101, 4129–4145, https://doi.org/10.1029/95JD03296, 1996.
Legrand, M., Preunkert, S., Oliveira, T., Pio, C. A., Hammer, S., Gelencsér, A., And, K. G., and Laj, P.: Origin of C2–C5 dicarboxylic acids in the European atmosphere inferred from year-round aerosol study conducted at a west-east transect, J. Geophys. Res.-Atmos., 112, D23S07, https://doi.org/10.1029/2006JD008019, 2007.
Li, J. J., Li, J., Wang, G. H., Zhang, T., Dai, W. T., Ho, K. F., Wang, Q., Shao, Y., Wu, C., and Li, L.: Molecular characteristics of organic compositions in fresh and aged biomass burning aerosols, Sci. Total Environ., 741, 140247, https://doi.org/10.1016/j.scitotenv.2020.140247, 2020.
Li, J. J., Li, J., Wang, G. H., Ho, K. F., Dai, W. T., Zhang, T., Wang, Q., Wu, C., Li, L., Li, L., and Zhang, Q.: Effects of atmospheric aging processes on in vitro induced oxidative stress and chemical composition of biomass burning aerosols, J. Hazard. Mater., 401, 123750, https://doi.org/10.1016/j.jhazmat.2020.123750, 2021.
Lim, C. Y., Hagan, D. H., Coggon, M. M., Koss, A. R., Sekimoto, K., de Gouw, J., Warneke, C., Cappa, C. D., and Kroll, J. H.: Secondary organic aerosol formation from the laboratory oxidation of biomass burning emissions, Atmos. Chem. Phys., 19, 12797–12809, https://doi.org/10.5194/acp-19-12797-2019, 2019.
Lim, Y. B., Tan, Y., and Turpin, B. J.: Chemical insights, explicit chemistry, and yields of secondary organic aerosol from OH radical oxidation of methylglyoxal and glyoxal in the aqueous phase, Atmos. Chem. Phys., 13, 8651–8667, https://doi.org/10.5194/acp-13-8651-2013, 2013.
Mao, J., Ren, X., Brune, W. H., Olson, J. R., Crawford, J. H., Fried, A., Huey, L. G., Cohen, R. C., Heikes, B., Singh, H. B., Blake, D. R., Sachse, G. W., Diskin, G. S., Hall, S. R., and Shetter, R. E.: Airborne measurement of OH reactivity during INTEX-B, Atmos. Chem. Phys., 9, 163–173, https://doi.org/10.5194/acp-9-163-2009, 2009.
Meng, J., Wang, G., Hou, Z., Liu, X., Wei, B., Wu, C., Cao, C., Wang, J., Li, J., Cao, J., Zhang, E., Dong, J., Liu, J., Ge, S., and Xie, Y.: Molecular distribution and stable carbon isotopic compositions of dicarboxylic acids and related SOA from biogenic sources in the summertime atmosphere of Mt. Tai in the North China Plain, Atmos. Chem. Phys., 18, 15069–15086, https://doi.org/10.5194/acp-18-15069-2018, 2018.
Meng, J. J., Wang, G. H., Li, J. J., Cheng, C. L., Ren, Y. Q., Huang, Y., Cheng, Y. T., Cao, J. J., and Zhang, T.: Seasonal characteristics of oxalic acid and related SOA in the free troposphere of Mt. Hua, central China: Implications for sources and formation mechanisms, Sci. Total Environ., 493, 1088–1097, https://doi.org/10.1016/j.scitotenv.2014.04.086, 2014.
Meng, J. J., Liu, X. D., Hou, Z. F., Yi, Y. N., Yan, L., Li, Z., Cao, J. J., Li, J. J., and Wang, G. H.: Molecular characteristics and stable carbon isotope compositions of dicarboxylic acids and related compounds in the urban atmosphere of the North China Plain: Implications for aqueous phase formation of SOA during the haze periods, Sci. Total Environ., 705, 135256, https://doi.org/10.1016/j.scitotenv.2019.135256, 2020.
Miyazaki, Y., Kimitaka, K., and Sawano, M.: Size distributions and chemical characterization of water-soluble organic aerosols over the western North Pacific in summer, J. Geophys. Res.-Atmos., 115, D23210, https://doi.org/10.1029/2010JD014439, 2010.
Mkoma, S. L. and Kawamura, K.: Molecular composition of dicarboxylic acids, ketocarboxylic acids, α-dicarbonyls and fatty acids in atmospheric aerosols from Tanzania, East Africa during wet and dry seasons, Atmos. Chem. Phys., 13, 2235–2251, https://doi.org/10.5194/acp-13-2235-2013, 2013.
Myriokefalitakis, S., Tsigaridis, K., Mihalopoulos, N., Sciare, J., Nenes, A., Kawamura, K., Segers, A., and Kanakidou, M.: In-cloud oxalate formation in the global troposphere: a 3-D modeling study, Atmos. Chem. Phys., 11, 5761–5782, https://doi.org/10.5194/acp-11-5761-2011, 2011.
Narukawa, M., Kawamura, K., Takeuchi, N., and Nakajima, T.: Distribution of dicarboxylic acids and carbon isotopic compositions in aerosols from 1997 Indonesian forest fires, Geophys. Res. Lett., 26, 3101–3104, https://doi.org/10.1029/1999GL010810, 1999.
Narukawa, M., Kawamura, K., Li, S. M., and Bottenheim, J. W.: Dicarboxylic acids in the arctic aerosols and snowpacks collected during ALERT 2000, Atmos. Environ., 36, 2491–2499, https://doi.org/10.1016/S1352-2310(02)00126-7, 2002.
Narukawa, M., Kawamura, K., Anlauf, K. G., and Barrie, L. A.: Fine and coarse modes of dicarboxylic acids in the Arctic aerosols collected during the Polar Sunrise Experiment 1997, J. Geophy. Res.-Atomos., 108, 4575, https://doi.org/10.1029/2003JD003646, 2003.
Niu, X. Y., Li, J. J., Wang, Q. Y., Ho, S. S. H., Sun, J., Li, L., Cao, J. J., and Ho, K. F.: Characteristics of fresh and aged volatile organic compounds from open burning of crop residues, Sci. Total Environ., 726, 138545, https://doi.org/10.1016/j.scitotenv.2020.138545, 2020.
Pavuluri, C. M. and Kawamura, K.: Enrichment of 13C in diacids and related compounds during photochemical processing of aqueous aerosols: New proxy for organic aerosols aging, Sci. Rep.-UK, 6, 36467, https://doi.org/10.1038/srep36467, 2016.
Pavuluri, C. M., Kawamura, K., and Swaminathan, T.: Water-soluble organic carbon, dicarboxylic acids, ketoacids, and α-dicarbonyls in the tropical Indian aerosols, J. Geophy. Res.-Atomos., 115, D11302, https://doi.org/10.1029/2009JD012661, 2010.
Reid, J. S., Eck, T. F., Christopher, S. A., Koppmann, R., Dubovik, O., Eleuterio, D. P., Holben, B. N., Reid, E. A., and Zhang, J.: A review of biomass burning emissions part III: intensive optical properties of biomass burning particles, Atmos. Chem. Phys., 5, 827–849, https://doi.org/10.5194/acp-5-827-2005, 2005.
Rogge, W. F., Hildemann, L. M., Mazurek, M. A., Cass, G. R., and Simoneit, B. R.: Sources of fine organic aerosol. 1. Charbroilers and meat cooking operations, Environ. Sci. Technol., 25, 1112–1125, https://doi.org/10.1021/es00018a015, 1991.
Rogge, W. F., Hildemann, L. M., Mazurek, M. A., Cass, G. R., and Simoneit, B. R.: Sources of fine organic aerosol. 2. Noncatalyst and catalyst-equipped automobiles and heavy-duty diesel trucks, Environ. Sci. Technol., 27, 636–651, https://doi.org/10.1021/es00041a007, 1993.
Rogge, W. F., Hildemann, L. M., and Mazurek, M. A.: Sources of fine oganic aerosol. 6. Cigarette-smoke in the urban atmosphere, Environ. Sci. Technol., 28, 1375–1388, https://doi.org/10.1021/Es00056a030, 1994.
Sakugawa, H. and Kaplan, I. R.: Stable carbon isotope measurements of atmospheric organic acids in Los Angeles, California, Geophy. Res. Lett., 22, 1509–1512, https://doi.org/10.1029/95GL01359, 1995.
Samy, S. and Zielinska, B.: Secondary organic aerosol production from modern diesel engine emissions, Atmos. Chem. Phys., 10, 609–625, https://doi.org/10.5194/acp-10-609-2010, 2010.
Sato, K., Hatakeyama, S., and Imamura, T.: Secondary organic aerosol formation during the photooxidation of toluene: NOx dependence of chemical composition, J. Phys. Chem. A, 111, 9796–9808, https://doi.org/10.1021/jp071419f, 2007.
Schauer, J. J., Kleeman, M. J., Cass, G. R., and Simoneit, B. R. T.: Measurement of emissions from air pollution sources.3. C1–C29 organic compounds from fireplace combustion of wood, Environ. Sci. Technol., 35, 1716–1728, https://doi.org/10.1021/es001331e, 2001.
Song, J. W., Zhao, Y., Zhang, Y. Y., Fu, P. Q., Zheng, L. S., Yuan, Q., Wang, S., Huang, X. F., Xu, W. H., Cao, Z. X., Gromov, S., and Lai, S.: Influence of biomass burning on atmospheric aerosols over the western South China Sea: Insights from ions, carbonaceous fractions and stable carbon isotope ratios, Environ. Pollut., 242, 1800–1809, https://doi.org/10.1016/j.envpol.2018.07.088, 2018.
Sorathia, F., Rajput, P., and Gupta, T.: Dicarboxylic acids and levoglucosan in aerosols from Indo-Gangetic Plain: Inferences from day night variability during wintertime, Sci. Total Environ., 624, 451–460, https://doi.org/10.1016/j.scitotenv.2017.12.124, 2018.
Sorooshian, A., Ng, N. L., Chan, A. W. H., Feingold, G., Flagan, R. C., and Seinfeld, J. H.: Particulate organic acids and overall water-soluble aerosol composition measurements from the 2006 Gulf of Mexico Atmospheric Composition and Climate Study (GoMACCS), J Geophy. Res.-Atmos., 112, D13201, https://doi.org/10.1029/2007JD008537, 2007.
Tao, S., Ru, M. Y., Du, W., Zhu, X., Zhong, Q. R., Li, B. G., Shen, G. F., Pan, X. L., Meng, W. J., Chen, Y. L., Shen, H. Z., Lin, N., Su, S., Zhuo, S. J., Huang, T. B., Xu, Y., Yun, X., Liu, J. F., Wang, X. L., Liu, W. X., Cheng, H. F., and Zhu, D. Q.: Quantifying the rural residential energy transition in China from 1992 to 2012 through a representative national survey, Nat. Energy, 3, 567–573, https://doi.org/10.1038/s41560-018-0158-4, 2018.
Tian, J., Watson, J. G., Han, Y. M., Ni, H. Y., Chen, L. W. A., Wang, X. L., Huang, R. J., Moosmüller, H., Chow, J. C., and Cao, J. J.: A biomass combustion chamber: Design, evaluation, and a case study of wheat straw combustion emission tests, Aerosol Air Qual. Res., 15, 2104–2114, https://doi.org/10.4209/aaqr.2015.03.0167, 2015.
Wang, G. H., Niu, S. L., Liu, C., and Wang, L. S.: Identification of dicarboxylic acids and aldehydes of PM10 and PM2.5 aerosols in Nanjing, China, Atmos. Environ., 36, 1941–1950, https://doi.org/10.1016/S1352-2310(02)00180-2, 2002.
Wang, G. H., Kawamura, K., Watanabe, T., Lee, S. C., Ho, K. F., and Cao, J. J.: High loadings and source strengths of organic aerosols in China, Geophys. Res. Lett., 33, L22801, https://doi.org/10.1029/2006GL027624, 2006.
Wang, G. H., Kawamura, K., Cheng, C. L., Li, J. J., Cao, J. J., Zhang, R., Zhang, T., Liu, S. X., and Zhao, Z. Z.: Molecular distribution and stable carbon isotopic composition of dicarboxylic acids, ketocarboxylic acids, and alpha-dicarbonyls in size-resolved atmospheric particles from Xi'an City, China, Environ. Sci. Technol., 46, 4783–4791, https://doi.org/10.1021/es204322c, 2012.
Wang, H. B. and Kawamura, K.: Stable carbon isotopic composition of low-molecular-weight dicarboxylic acids and ketoacids in remote marine aerosols, J. Geophys. Res.-Atomos., 111, D07304, https://doi.org/10.1029/2005JD006466, 2006.
Warneck, P.: In-cloud chemistry opens pathway to the formation of oxalic acid in the marine atmosphere, Atmos. Environ., 37, 2423–2427, https://doi.org/10.1016/S1352-2310(03)00136-5, 2003.
Watson, J. G., Cao, J., Chen, L.-W. A., Wang, Q., Tian, J., Wang, X., Gronstal, S., Ho, S. S. H., Watts, A. C., and Chow, J. C.: Gaseous, PM2.5 mass, and speciated emission factors from laboratory chamber peat combustion, Atmos. Chem. Phys., 19, 14173–14193, https://doi.org/10.5194/acp-19-14173-2019, 2019.
Yasmeen, F., Sauret, N., Gal, J.-F., Maria, P.-C., Massi, L., Maenhaut, W., and Claeys, M.: Characterization of oligomers from methylglyoxal under dark conditions: a pathway to produce secondary organic aerosol through cloud processing during nighttime, Atmos. Chem. Phys., 10, 3803–3812, https://doi.org/10.5194/acp-10-3803-2010, 2010.
Zhang, Y. L., Kawamura, K., Cao, F., and Lee, M.: Stable carbon isotopic compositions of low-molecular-weight dicarboxylic acids, oxocarboxylic acids, α-dicarbonyls, and fatty acids, J. Geophys. Res.-Atmos., 3707–3717, https://doi.org/10.1002/2015JD024081, 2016.
Zhao, W., Kawamura, K., Yue, S., Wei, L., Ren, H., Yan, Y., Kang, M., Li, L., Ren, L., Lai, S., Li, J., Sun, Y., Wang, Z., and Fu, P.: Molecular distribution and compound-specific stable carbon isotopic composition of dicarboxylic acids, oxocarboxylic acids and α-dicarbonyls in PM2.5 from Beijing, China, Atmos. Chem. Phys., 18, 2749–2767, https://doi.org/10.5194/acp-18-2749-2018, 2018.