the Creative Commons Attribution 4.0 License.
the Creative Commons Attribution 4.0 License.
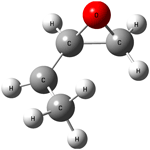
Kinetic study of the atmospheric oxidation of a series of epoxy compounds by OH radicals
Carmen Maria Tovar
Ian Barnes
Iustinian Gabriel Bejan
Peter Wiesen
In this work, we study the kinetics of the gas-phase reactions of hydroxyl radicals with cyclohexene oxide (CHO), 1,2-epoxyhexane (EHX), 1,2-epoxybutane (12EB), trans-2,3-epoxybutane (tEB) and cis-2,3-epoxybutane (cEB) using the relative rate technique. The experiments were conducted at (298 ± 3) K and (760 ± 10) Torr ((1.01 ± 0.01) × 105 Pa) total pressure of synthetic air using different reference compounds in a 1080 L Quartz Reactor (QUAREC) and a 480 L Duran glass chamber. The following room temperature rate coefficients (cm3 molecule−1 s−1) were obtained: , , , and . Except for previous studies on 1,2-epoxybutane and cyclohexene oxide, this is, to the best of our knowledge, the first kinetic study of the reaction of these compounds with OH radicals. We discuss the discrepancies found between the values obtained from the present study with values estimated from the structure–activity relationship method (SAR). Our findings indicate that pseudo-ethylenic character in the epoxy ring is an important factor to be included in the improvement of the SAR estimation method. Atmospheric lifetimes, reactivity trends and atmospheric implications are discussed considering the epoxy compound rate coefficients obtained in the present study.
- Article
(1225 KB) - Full-text XML
- BibTeX
- EndNote
Oxygenated volatile organic compounds (OVOCs) play an important role in atmospheric chemistry and have an impact on climate and human health (Calvert et al., 2011). From those OVOCs emitted from either biogenic or anthropogenic sources, cyclic ethers, with the exception of furans (Villanueva et al., 2009; Li et al., 2018), have received very little attention. Epoxides, as the simplest cyclic ethers, are an important and valuable class of raw materials and intermediates for chemical industry. They can polymerize for the production of homo- and copolymers as polyethers, polyols and polycarbonates (Hereijgers et al., 2012).
Epoxides are considered a key element in “click” chemistry (Kolb et al., 2001; Fokin and Wu, 2006). They are also relevant in the field of pharmaceutical applications because of their potential as protease inhibitors against several diseases like cancer, stroke, and parasitic or viral diseases (Powers et al., 2002; Otto and Schirmeister, 1997; Schirmeister and Klockow, 2003). A relatively new utilization of epoxides, which could have huge atmospheric implications and an impact on climate change, is their potential use for carbon capture and storage, i.e. the chemical fixation of CO2 in the form of cyclic carbonates in the presence of various catalysts (Zou and Hu, 2017; Zhang et al., 2020; Guo et al., 2021; Andrea and Kerton, 2021; Appaturi et al., 2021).
Epoxides are known to be formed from the reaction of O(3P) with isoprene at nearly 80 % yield (Atkinson et al., 1994b; Paulson et al., 1992). In the atmospheric ozonolysis of isoprene, epoxide yields of about 2 %–3 % were observed (Atkinson et al., 1994a). A few percent of epoxide formation have been reported from α-pinene ozonolysis as well as from the reaction of 1,2-dimethyl-1-cyclohexane with ozone (Alvarado et al., 1998). Atkinson et al. (1994a) concluded that epoxide formation is a process occurring in the ozonolysis of most terpenes and cycloalkenes. Low yields of isoprene-derived epoxides have also been observed from the gas-phase nitration of isoprene (Skov et al., 1994).
Furthermore, in indoor pollution studies the formation of epoxides from the heterogeneous oxidation of volatile organic compounds (VOCs) with gas-phase ozone has been reported (Zhou et al., 2017). Other compounds could also form epoxides during their gas-phase chemical degradation by OH radicals and ozone. More recent theoretical and mechanistic studies report epoxide formation during the gas-phase ozonolysis of methylbutenol and sabinene via the Criegee degradation mechanism (Calvert et al., 2000; Almatarneh et al., 2019a, b).
Epoxides are responsible for up to 10 % of the open-ring products from the degradation of aromatic hydrocarbons in the presence of OH radicals according to the MCM (Master Chemical Mechanism) model (Jenkin et al., 2015). In the past, benzene oxide has been reported as an epoxide-type product in the reaction of benzene with OH radicals (Klotz et al., 1997, 2000). In addition, a theoretical study has reported epoxide formation in the OH-radical-initiated oxidation of dimethylphenol isomers (Sandhiya et al., 2013).
Secondary organic aerosols (SOAs) constitute a substantial portion of the total ambient aerosol particles, which mainly originate from biomass burning and atmospheric reactions of volatile organic compounds (VOCs) (Kanakidou et al., 2005). Epoxides can polymerize easily, leading to the growth of SOA (Gao et al., 2004). A recent study shows that the yield of epoxides from the reaction of aromatic compounds with OH radicals is probably dependent on the abundance of NO, HO2 and RO2 in the reaction system (Vereecken, 2018). This aspect represents an uncertainty in atmospheric models to reliably estimate the expected SOA formation from reactive epoxide uptake by aerosols (Paulot et al., 2009). The presence of epoxides in SOA is highly probable for conditions prevailing in the atmosphere, leading to higher hygroscopicity of particles with potential cloud condensation nuclei activity by conversion to organosulfates through an acid-catalysed mechanism (Fuzzi et al., 2015).
Isoprene-derived epoxides are very important intermediates, which could explain at least in part the composition of ambient SOA both in urban areas (Lin et al., 2013) and remote regions (Jacobs et al., 2013; Stropoli et al., 2019; Paulot et al., 2009; Jacobs et al., 2013; Shrivastava et al., 2019).
The toxicity of ultrafine particles such as SOA is not only related to their atmospheric concentration but also to the nature and chemical properties of both the precursors and the formed SOA components (Jiang et al., 2019). In this sense, epoxides are of great concern because the epoxy functional group can act as an electrophile in its interaction with DNA and nucleosides, producing carcinogenic and mutagenic damage (Ehrenberg and Hussain, 1981).
The kinetic and mechanistic data available on the gas-phase reactions of epoxy compounds with the atmospheric oxidants OH and NO3 radicals, O3, and chlorine atoms are very scarce. For the compounds investigated in the present study, there is only one previous relevant study towards chlorine atoms at 298 K (Tovar et al., 2021). Two studies have reported the rate coefficients of the reaction of 1,2-epoxybutane towards OH radicals (Wallington et al., 1988a; El Othmani et al., 2021a), and more recently cyclohexene oxide with OH radicals have been measured as a function of temperature (El Othmani et al., 2021a).
In the present study we have performed, for the first time, a kinetic study of the reaction of OH radicals with 1,2-epoxyhexane, trans-2,3-epoxybutane and cis-2,3-epoxybutane at 298 K using the relative rate method.
Since several of the rate coefficients for the reactions of epoxides with OH radicals have been measured in the present study for the first time and cannot be compared with literature values accordingly, we have applied different approaches for the estimation of structure–activity relationship (SARs) for the compounds studied in this work.
The experiments were performed in two different environmental chambers, namely a 1080 L quartz–glass photoreactor and a 480 L Duran glass chamber. These two photoreactors are briefly described below.
2.1 480 L reactor
This reaction chamber consists of a cylindrical Duran glass vessel (3 m length, 45 cm diameter) closed at both ends by Teflon-coated aluminium end flanges. Integrated on the metal flanges are ports for the inlet of reactants into the chamber and for the collection of samples from the reaction mixtures for further analyses. Other accessories, like a mixing fan to ensure homogeneity of the reaction mixtures and a capacitance manometer, are also located on the flanges. Arranged concentrically around the outside of the reactor are 32 super actinic fluorescent lamps (Philips TLA 40 W, 300 460 nm, λmax = 360 nm). The vacuum (ca. 10−3 mbar) is maintained by means of a Leybold turbo-molecular pump, model RUVAC WZ 151 (500 m3 h−1), backed by a Leybold double-stage rotary fore pump, model D40B (200 m3 h−1). A White-type mirror system mounted inside the reactor is set to an overall optical path length of 51.6 m. The analysis of the reactants was done during the experiments by in situ FTIR (Fourier transform infrared) long-path spectroscopy using a resolution of 1 cm−1. The FTIR spectrometer (Nicolet Magna 520) and the transfer mirror system are covered with a protective box and are permanently purged with dry air to remove water vapour. The spectrometer is directly controlled by the OMNIC software, which is provided by Nicolet.
2.2 1080 L reactor
A detailed description of the reactor can be found in the literature (Barnes et al., 1994). Briefly, the reactor consists of the two quartz–glass tubes with an inner diameter of 47 cm and a wall thickness of 5 mm. The reactor has a total length of 6.2 m. Silicone rubber rings are used for all the glass–metal connections as well as for metal–metal connections. The reactor is connected to a turbo molecular pump system by which an end vacuum of 10−3 mbar can be achieved. A total of three fans are used for homogeneous mixing of compounds within the reactor. Different types of inlets are mounted on the end flanges for the introduction of chemicals and for pressure measurements.
The beam from an FT-IR spectrometer is coupled via a transfer mirror system into the reactor through KBr windows located in one of the end flanges. A White-type mirror system (base path length (5.91 ± 0.01) m), mounted inside the reactor, is used for multiple reflection of the infrared beam within the reactor before it reaches the detector. Reactants were monitored in situ in the reactor in the infrared using 82 traverses of the beam, which is equivalent to a total optical path length of (484.7 ± 0.8) m. All spectra in this work were recorded with a spectral resolution of 1 cm−1. The FTIR spectrometer NICOLET NEXUS was used, which is equipped with a liquid-nitrogen-cooled (77 K) mercury–cadmium–tellurium (MCT) detector. A Globar was used as IR light source. All mirrors are gold-coated to achieve optimum reflectivity.
Two different types of lamps (32 each) are installed around the reactor. They are mounted alternatively around the reactor to ensure homogeneity of the light intensity within the reactor. The first type, 32 superactinic fluorescent lamps (Philips TL05 40 W: 300–460 nm, max. intensity at ca. 360 nm) and 32 low-pressure mercury vapour lamps (Philips TUV 40 W: max. intensity at 254 nm), can be used to irradiate the reaction mixture.
Typically, 64 interferograms were co-added per spectrum over a period of approximately 1 min, and 15–20 such spectra were recorded per experiment.
2.3 Relative rate method
The relative rate method was used to determine the rate constant of the OH-radical-induced oxidation of the epoxy compounds.
The photolysis of CH3ONO in the presence of NO was used to produce OH radicals:
The concentrations or mixing ratios were measured from the exact amount of the compound added to the chamber. The liquids and gases were injected into the chamber via a septum using a gas-tight syringe in a flow of air with the reactor being under reduced pressure. The glass–steel inlet system was heated up to 60 ∘C to ensure the addition of the entire amount of epoxides and reference compounds into the reactor. After addition and filling the chamber to (760 ± 10) Torr ((1.01 ± 0.01) × 105 Pa) pressure of synthetic air, up to 15 infrared spectra (15 min) were recorded to prove the constant concentration of the compounds in the chamber. These tests were performed not only to check if there is a diffusion of the injected compounds from the inlet into the chamber, but also to evaluate the potential wall deposition of the added compounds to correct the rate coefficients for additional loss processes. Any diffusion from the inlet would affect the linearity of the kinetic plots, which otherwise would not have been obtained. The different epoxides and reference compounds employed in the present study did not show wall loss during the time of the experiment.
In the presence of the OH radical, the corresponding epoxide and reference compound are consumed by the following reactions:
Provided that the reference compound and the epoxide are lost only by Reactions (R1) and (R2), then it can be shown that
where [Epoxide]0, [Reference]0, [Epoxide]t and [Reference]t are the concentrations of the corresponding epoxy compound and reference compound at times t=0 and t, respectively, and kEpoxide and kReference. are the rate coefficients of Reactions (R1) and (R2), respectively.
To test for a possible loss of the reactants through photolysis, mixtures of the reactants in air in the absence of methyl nitrite were irradiated for 30 min, and photolysis was found to be negligible for both the epoxide and the reference compounds. Additionally, various tests were performed to assess possible loss of the reactants via deposition on the chamber walls, and no significant wall loss of the epoxy compounds and references was observed, leaving the compounds in the dark in the reactor.
A minimum of two experiments for each epoxide compound were performed in this study, and up to three reference compounds were used. Reference compounds were chosen based on similar reactivity as epoxides and suitability for FTIR subtraction procedures. Conversion of epoxides and reference compounds through the reaction with the OH radical was achieved up to 50 %. Initial mixing ratios used in the 1080 L reactor for the epoxides and reference compounds were as follows (in ppmv with 1 ppmv = 2.46 × 1013 molecule cm−3 at (298 ± 3) K and (760 ± 10) Torr ((1.01 ± 0.01) × 105 Pa) of total pressure of synthetic air): epoxides between 3–6 ppmv and reference compounds between 1 and 4 ppmv, as shown in Table 1. Concentrations used for the reactions performed in the 480 L reactor were up to 8 times higher. Around 8 ppmv CH3ONO was added in the 1080 L reactor and up to 10 times more in 480 L reactor. All epoxy and reference compounds used in this study were obtained from Sigma Aldrich and used without further purification. The stated purities were as follows: cyclohexene oxide, 98 %; 1,2-epoxyhexane, 97 %; 1,2-epoxybutane, 99 %; trans-2,3-epoxybutane, 97 %; cis-2,3-epoxybutane, 97 %; propylene, 99 %; butane, 99 %; iso-propyl acetate, 99.6 %; sec-butyl acetate, 99 %; and ethylene, 99.5 % and 99.985 % for synthetic air, which was from Messer.
Table 1Initial mixing ratios used in the 1080 L reactor and 480 L reactor for the epoxide and reference compounds in parts per million by volume (ppmv; 1 ppm = 2.46 × 1013 molecule cm−3) at (298 ± 3) K and (760 ± 10) Torr ((1.01 ± 0.01) × 105 Pa) of total pressure of air. The mixing ratios have been estimated from injections of the compounds into the reaction chambers and provide information about the amount of compounds used in this study.
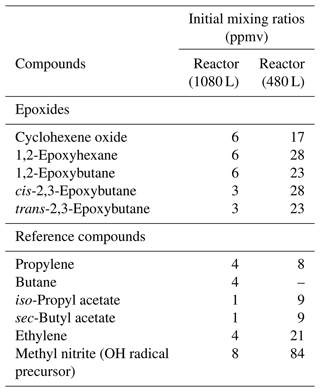
The experimental data from the kinetic experiments are plotted according to Eq. (1). Figure 1 shows the results obtained for the rate coefficient determinations from the OH-radical-initiated oxidation of cyclohexene oxide using ethylene, propylene and isobutene as reference compounds; 1,2-epoxyhexane using ethylene and propylene as reference compounds; 1,2-epoxybutane using ethylene and iso-propyl acetate as reference compounds; trans-2,3-epoxybutane using sec-butyl acetate, ethylene and propylene as reference compounds; and cis-2,3-epoxybutane using sec-butyl acetate, iso-propyl acetate and butane as reference compounds. The selection of the reference compounds employed in the kinetic study was constrained by the experimental technique since it is required to identify the infrared absorption bands which do not overlap with other infrared absorptions features from reaction mixture. All plots showed very good linearity despite the difficulties in handling the epoxides and subtracting the IR spectra.
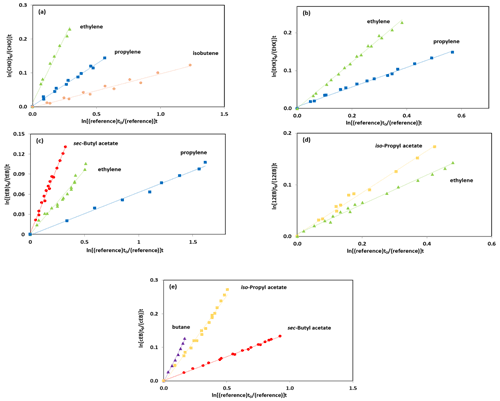
Figure 1Kinetic data for the reaction of OH radicals with (a) CHO, (b) EHX, (c) tEB, (d) 12EB and (e) cEB using ethylene, butane, sec-butyl acetate, iso-propyl acetate and propylene as reference compounds.
Table 2Measured rate coefficient ratios, , and the rate coefficients for the reactions of OH radical with epoxides at (298 ± 3) K derived from these ratios.
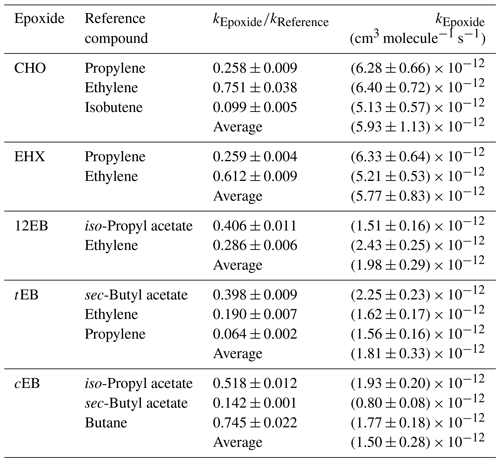
The rate coefficients kEpoxide given in Table 2 were put on an absolute basis using the following values for the reactions of the reference compounds (cm3 molecule−1 s−1): (IUPAC Task Group on Atmospheric Chemical Kinetic Data Evaluation, 2021), (Calvert et al., 2015), (IUPAC Task Group on Atmospheric Chemical Kinetic Data Evaluation, 2021), (Wallington et al., 1988c), (Wallington et al., 1988c) and (McGillen et al., 2020).
The rate coefficients obtained using two simulation chambers are in perfect agreement as shown in Fig. 2, which is a comparison of the data for EHX using propylene as reference compounds. The linearity observed in Fig. 2 was the same for all epoxides where we had the opportunity to perform the kinetic studies in both reactors with OH radicals and chlorine atoms. Therefore, considering they are the same family of compounds, we concluded the results are reproducible in both reactors. Accordingly, we plotted the data together for all the epoxides, regardless of the reactor where the experiment was performed.
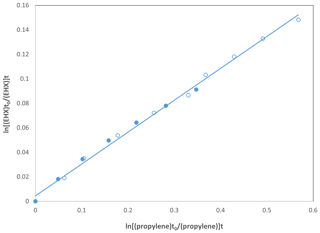
Figure 2Kinetic data for the reaction of OH radicals with EHX using propylene as reference compound performed in the 480 L reactor (•) and the 1080 L reactor (∘).
The rate coefficient ratios obtained from linear regression analyses of these plots are summarized in Table 2. The rate coefficients are averages from the experiments, with each reference compound from the experiments performed in both reactors (cm3 molecule−1 s−1): , , , and .
All the rate coefficients obtained in this study, except those for 12EB and CHO, were measured for the first time. The errors presented in Table 2 for the individual value of the slope represent 2σ of the least-squares fit of the data. The epoxides rate constants are placed on an absolute basis using different reference rate constants for which a uniform error of 10 % was applied, considering that the corrections for possible secondary processes (e.g., dilution, wall losses) were not necessary in the present study (Atkinson, 1986b). Thus, the errors quoted for individual rate constants of epoxides comprise the 2σ statistical errors resulted from lineal regression analysis of the plots and an additional 10 % contribution from the errors attributed to the recommended values of the rate constants for the reference compounds. The final rate constant has been calculated as an average of the individual rate constant, with the error calculated using the arithmetic calculation of the error propagation.
3.1 Correlation between the rate coefficients of the reaction of epoxides with OH radicals and chlorine atoms
The abstraction route defines the main reaction pathway of the epoxides with OH radicals. For epoxide series, it is likely to define a correlation of the rate coefficients from their reaction with OH radicals and chlorine atoms. Similar correlations were observed for the series of alkanes, saturated alcohols and acyclic ethers (Calvert et al., 2011). Figure 3 presents a log–log correlation plot between the Cl atoms and OH radical rate coefficients with epoxides for the series of propylene oxide, 1,2-epoxyhexane, 1,2-epoxybutane and both isomers of 2,3-epoxybutane. A very clear correlation (R2=0.9935) described by the relation was obtained. The compounds ethylene oxide and cyclohexene oxide are represented in Fig. 3 but are not included into the correlation plot, although including the cyclohexene oxide, the log–log correlation would change to with (R2=0.978). The reactivities of the epoxides with OH radicals and Cl atoms are clearly correlated for the series of epoxides. However, the log–log correlation for epoxides is different to the one presented by Calvert et al. (2011) and described by the relation with (R2=0.85) for the series of alkanes with those two atmospheric oxidants. In addition, the log–log correlation for the series of ethers and alcohols with these two oxidants presented by Calvert et al. (2011) described by the relation with (R2=0.72) is in better agreement with the log–log correlations obtained in this study for epoxides.
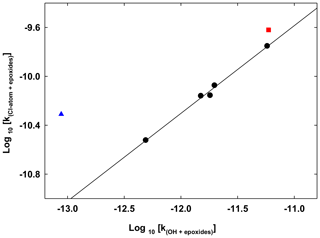
Figure 3Double logarithmic plot (log 10) of the rate coefficients for the reaction of Cl-atoms versus the reaction of OH radicals with the epoxides (propylene oxide, 1,2-epoxybutane, trans-2,3-epoxybutane, cis-2,3-epoxybutane and 1,2-epoxyhexane). The solid line represents the unweighted least-squares fit to the data. Cyclohexene oxide is represented by the red square, while ethylene oxide is represented by a blue triangle. Both were not included in the least-squares fit.
The log–log correlation plot can be used to predict rate coefficients. For example, the rate coefficient for the reaction of propylene oxide with chlorine atoms which has not been measured to date can be derived based on the proposed correlation, cm3 molecule−1 s−1. However, the knowledge of rate coefficients for the reactions of epoxides with atmospheric oxidants is still very limited.
3.2 Structure–activity relationship (SAR) calculations for epoxides
To date, the rate coefficients of hundreds of new compounds have been studied under laboratory conditions with different atmospheric oxidants. However, there are still many compounds for which the data are still very scarce, such as for cyclic ethers.
There are several methodologies to estimate the rate coefficients towards OH radicals. One of them is quantum theoretical calculations, useful for reactions which are very difficult to study directly in the laboratory. Another approach is the structure–activity relationship (SAR) method, which relies solely on the structure of the organic compounds and the effect of this structure on the reactivity. Both methodologies are critical to predict the chemical reactivity and physical behaviour of compounds where direct data are not available. The SAR estimation method applied for the epoxide type compounds studied in the present work treats the rate of abstraction from a group within an epoxide molecule. In the case of H atom abstraction from C–H bonds, the calculation of the overall H atom abstraction rate constants is based upon the estimation of –CH3, –CH2– and > CH– group rate constants.
The –CH3, –CH2– and > CH– group rate constants depend on the substituents influence around those groups.
For example, in the case of an α position, k(CH3-X)=kprimF(X); k(X-CH2-Y)=ksecF(X)F(Y); and k(X-CH(Y)(Z))=ktertF(X)F(Y)F(Z), where kprim, ksec and ktert are the rate constants per –CH3, –CH2– and > CH– group for a “standard” substituent; X, Y and Z are the substituent groups; and F(X), F(Y) and F(Z) are the corresponding substituent factors (Atkinson, 1987, 1986a, b). The validity and usefulness of a SAR depend on many factors, such as the amount and type of input data, reliability of prediction, scope of applicability and ease of implementation (Vereecken et al., 2018).
The rate constants of the reactions with OH radicals obtained in this work were used to derive reactivity trends towards the OH radical. Also, five different approaches have been used in the SAR estimations to evaluate the most suitable method to predict the reactivity of this series of epoxides towards OH radicals. The results obtained are described in more detail below.
Table 3Comparison of the rate coefficients for the reaction of OH radicals with the epoxides investigated in this study with the literature data and SAR predictions .
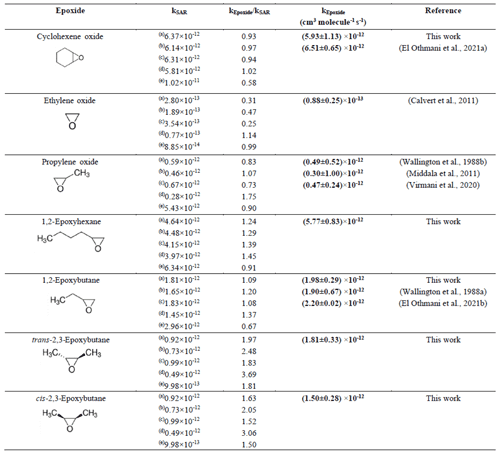
For isomers it has been observed that within the experimental error limits, the rate coefficients for the reaction of trans-2,3-epoxy butane with OH radicals are slightly different than for cis-2,3-epoxybutane. Table 3 shows the result of the SAR calculations for the epoxide reactions by using different factors from the literature for the total rate constant estimation. Method (a), Kwok and Atkinson (1995), used the substituent factor F(–OR) = 6 to describe the effect of one or two α–ether linkages, with the influence of a β–ether linkage. Method (b), Calvert et al. (2011), also took into consideration the influence of a β-ether linkage and a factor F(–OR) = 4.13. Method (c), Calvert et al. (2015), is similar to method (a) and (b) but assumes a factor F(–OR) = 8.4. Comparing these approaches with the experimental results, for 1,2-epoxybutane, the estimated value using method (a), Kwok and Atkinson (1995), is the closest to the experimental value. However, it is not the case for cis and trans isomers, where a small deviation is observed.
The SAR estimation using (d), Middala et al. (2011), proposed a corrected factor for ring strain, assuming that the effect of the presence of oxygen on the ring might have been underestimated. However, as shown in Table 3, the experimental data obtained in this work differ in most of the cases from the values predicted by SAR using this approach.
The SAR estimation using (e), Jenkin et al. (2018), proposes an improved new set of three rate coefficients for H atom abstraction for C atoms adjacent to ether linkages. These new sets of the rate coefficients are applied independently of neighbouring group substituent factors. Our experimental results show that the rate coefficient of 1, 2-epoxybutane is lower than the rate coefficient calculated with this approach. The rate coefficient calculated for cyclohexene oxide with this approach is the lowest with respect to all experimental values of epoxides and by other SAR estimation methods.
The influence of the ether group seems not to be the same for epoxides of the same number of carbon atoms with differences in the symmetry. Factors like geometrical disposition and neighbour groups could explain the H atom abstraction in places far from the ether linkage.
In less substituted epoxides, such as propylene oxide, the approaches presented by Kwok and Atkinson (1995) and Calvert et al. (2011, 2015) are the closest to the experimental values determined in this work.
There are discrepancies between the values calculated by different approaches of the SAR estimation method, as is shown in Table 3. In addition, there is no SAR estimation method that consistently generates a value close to the experimental value for all compounds which have been studied in the present work. These discrepancies had already been observed since the first SAR approximations for cyclic ethers, and they could be attributed to the limited database that still exists for this kind of compound (Kwok and Atkinson, 1995; Jenkin et al., 2018). In general, all these approaches were developed to fit the behaviour of cyclic alkanes, with one factor being attributed to ring strain and the presence of an ether group.
3.2.1 Pseudo-ethylenic character in the epoxy ring as the main factor affecting SAR estimation
The kinetics, reactivity trends and thermochemistry of cis- and trans-2,3-epoxybutane, 1,2-epoxybutane, 1,2-epoxyhexane and cyclohexene oxide initiated by Cl atoms were previously discussed (Tovar et al., 2021). The series of epoxides were theoretically investigated using density functional theory (DFT), applying the B3-LYP exchange–correlation function with Grimme's GD3-BJ empirical dispersion corrections. The calculation results showed that the abstraction channels of β-CH2 sites are dominant for all epoxides under study.
As we explained in Tovar et al. (2021), the sites in the molecule are more reactive in β position when the carbon atoms in ethylene oxide approach the sp2 state and its CH2 units are pseudo-ethylenic in character. That conjugation does not occur if the π orbitals of the unsaturated groups cannot orient themselves with their axes parallel to the plane of the epoxide ring. Such a geometric arrangement in the epoxy molecule would be necessary to allow for the abstraction of a hydrogen in the molecule in a preferential way, thus increasing the reactivity of the reaction.
In Sect. 3.2, we discuss the rate coefficients experimentally calculated for the same series of epoxides towards OH radical and the estimated rate constants of epoxides with a total of two to six carbon number using different SAR approaches. In these estimations, different substituent factors were applied. In the following discussion, we present the possible theoretical reasons these SAR estimations could differ between them and with respect to the experimental values based in novel theoretical and experimental studies, which confirms our previous findings.
Table 4Reactivity trends of the epoxy compounds towards OH radicals compared with their homologous alkanes, acyclic ethers and cycloalkanes with the same number of carbon atoms.
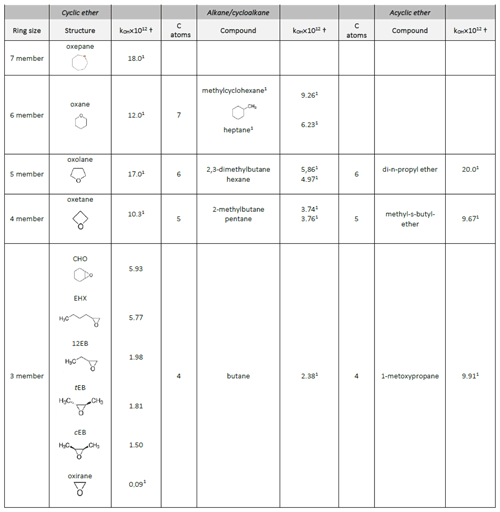
From Table 4, we can assess the influence of the ring size over a series of cyclic ethers. In this way, the cyclic ether reactivities towards OH radicals increase with ring size (k in 10−12 cm3 molecule−1 s−1) ; .
For previous studies, we know that the electron ability in hydrogen bonding can act as a measure of the relative electron density (Tamres et al., 1954). The very low electron donor ability of substituted ethylene oxides, compared with other cyclic ethers, in hydrogen bonding has already been shown in the past (Searles and Tamres, 1951; Searles et al., 1953). Thus, the oxygen in three-membered ring ethers is more electronegative than in larger ring ethers or in acyclic ethers. This observation can be explained from Walsh's proposal for the structure of ethylene oxide and cyclopropane (Walsh, 1949). Walsh's model implies a high electron density in the centre of the three-membered rings, which suggests that the exterior of such rings would have a lower electron density than normal for the atoms involved. This effect is explained for strained systems where the hybridized orbitals employed for a bonding attain maximum overlap in such a way that altered valence angles “bent bonds” are realized. Because the Walsh model does not correspond to the ground state of cyclopropane/epoxide, the description of bent bonds for cyclopropane by the Förster–Coulson–Moffitt model (Förster, 1939; Coulson and Moffitt, 1949, 1947) is the most frequently considered one (Wiberg, 1996). More recently, the topological analysis of the electron density method (ED) is applied to describe the electron distribution within a compound (Kutzelnigg, 1993; Bader et al., 1994; Coppens, 2005; Koritsanszky and Coppens, 2001). However, the ED method provides information on concentration and depletion of electrons but not on the pairing of electrons. In order to measure the electron pair localization, the electron ability indicator (ELI) is used (Kohout, 2004). A recent study presented by Grabowsky et al. (2010) has introduced the ELI based on an X-ray diffraction experiment by means of the X-ray constrained wave function fitting procedure. The method was applied on a series of epoxide derivatives and clearly indicated outwardly bent bonds according to the Förster–Coulson–Moffit model.
Also, this study shows that the maxima of deformation density and the valence shell charge concentrations (VSCCs) in the Laplacian maps are located clearly outside the bond axes for both the C–O and C–C bond, and there are no maxima inside the ring. The Förster–Coulson–Moffit model makes use of hybrid orbitals with a relation between s and p character like sp2 hybridization. This conducts to an orbital overlapping outside the direct bond axis forming three σ-type bonds. The orbitals used in these exterior bent bonds of the ring are in a favourable position for some overlap with p orbitals from adjacent atoms (Searles et al., 1953). The involvement of sp2-type orbitals in the epoxide ring instead of sp3 like in normal single bonds suggests that the bonds are not saturated and can interact with π–electron systems (Grabowsky et al., 2010). Our recent study on the reactivity of a series of epoxides towards chlorine atoms in gas phase using quantum mechanical calculations agrees with these findings. As we discussed in Tovar et al. (2021), if we consider the case of cis- and trans-epoxybutane, when the compound has lost one H of the CH3 group, it becomes trigonally (sp2) hybridized, facilitating the possible formation of a double bond with the singly occupied p-orbital after abstraction of H. This effect could explain the formation of acrolein from Cl-initiated oxidation of both cis-2,3-dimethyloxirane and trans-2,3-dimethyloxirane with yields of 36.49 ± 0.55 and 49.08 ± 1.29 at 650 and 800 K, respectively, in the work presented by Doner et al. (2021). The formation of the vinyl oxirane from β hydrogen abstraction and the subsequent formation of the keto hydro peroxy radical reported by Christianson et al. (2021) for the oxidation of 1,2-epoxybutane also confirm this theory. In addition, one of the most abundant products reported in the same work, 2,2-bioxirane (31.44 ± 6.49) at 640 K, would also respond to the same reactivity trend, with abstraction in the β position being the most predominant.
This structural effect has also been observed experimentally with cyclopropyl ketones (Rogers, 1947), α- and β-epoxyketones (Walsh, 1949; Cromwell and Graff, 1952), ethylenimine ketones (Cromwell and Graff, 1952) and more recently in the acid-catalysed ring opening of epoxides (Oshima et al., 2008). Moreover, there is some evidence of a conjugation of the epoxy ring with substituents using methods like UV spectroscopy, heat of combustion and molecular orbital (MO) calculations (Parker and Isaacs, 1959; Starit et al., 1964).
3.2.2 Ring strain in epoxides
From Table 4, we can see that the epoxides are less reactive towards OH radicals than the analogous alkanes with the same number of carbon atoms: (k in 10−12 cm3 molecule−1 s−1) , , . The effect is more pronounced for epoxides with four carbon atoms than for those with six carbon atoms: . The presence of a six-membered ring next to the epoxide group increases its reactivity .
Grabowsky et al. (2010) derived the experimental electron density of ethylene oxide from a multipole refinement of 100 K X-ray data and complemented it with density-functional calculations at experimental and optimized geometry. This study found that despite the high strain in the three-membered ring of ethylene oxide, most atomic and bond topological properties do not differ from comparable fragments in unstrained molecules. Grabowsky et al. (2010) concluded that the strained and unsaturated character of the epoxide ring is reflected in the populations of the bonds.
The three-membered ring strain is considered in the SAR estimations for its influence on the reactivity of epoxides. However, this factor itself does not play a dominant role in the rate coefficients of the epoxides. The presence of the epoxy ring would affect the reactivity of epoxides because the CH group attached favours the π overlap (Fig. 4). For this reason, the factors (F(O), F(three-member ring)) need to be treated specially in SAR and possibly as one single factor F (epoxy ring-β-CX) since their influence on the β carbon is very pronounced. Only then can one see the contributions of other groups, for example, linear, branched and rings on this basic structure. The reactivity trends observed in our previous (Tovar et al., 2021) and present work suggests that the rate constant of the β-channel could be the closest to the ktotal determining the overall reaction rate. These aspects had not been considered in SAR estimates to date; therefore new factors that include these complicated bond structures in epoxides need to be developed.
3.3 Improving SAR estimation for epoxides
To provide a new set of “corrected” factors, especially for F (epoxy ring-β-CX) that can be used for an improved SAR estimation for epoxides, a new set of theoretical calculations with OH radicals are recommended. These kind of calculations are beyond of the scope of this work. However, a possible approach should be conducted to expand the kinetic database of epoxides (experimental and theoretical) in a wide temperature range. Experiment and theory are highly complementary in this regard, and their combined application allows for alleviation of some of the shortcomings in both approaches; for theoretical work specifically, even a single experimental datum point often allows for the optimization of the potential energy surface (PES) or energy transfer parameters to allow for temperature (T) and pressure (P) extrapolations with strongly enhanced reliability (Vereecken et al., 2015).
A recent work by Wang et al. (2022) proposed a concept of a combined experiment–SAR–DFT method to theoretically discuss the abstraction reaction of a series of alcohols and ketones. A similar approach could be used for epoxides with some considerations.
As has been shown in Tovar et al. (2021), the potential energy surface of chlorine atoms reacting with the series of epoxides possesses a barrierless reaction. For these cases, Piletic et al. (2017) proposed to use the Master Equation Solver for Multi-Energy Reactions (MESMER v.4.0) in order to compute the energy-dependent microcanonical rate constants for all forward and reverse reactions on the PES, with the option of computing the k(E)s using an inverse Laplace transform (ILT) technique. The k(E)s may be calculated using Arrhenius parameters from a fit of the temperature-dependent high-pressure rate coefficients.
A second method could include a semi-empirical method using the Arrhenius parameters in MESMER to fit phenomenological rate constants that are consistent with the experimentally measured rates. Our preliminary exploration of the PES of the series of epoxides towards chlorine atoms (Tovar et al. 2021) provides a hint of the complex structural analysis that must be developed in order to ensure more accurate rate coefficients and capture the reactivity trends of epoxides with the SAR estimation method.
Some studies have evaluated the temperature dependence for 1,2-epoxypropane and 1,2-epoxybutane towards OH radicals. Virmani et al. (2020) found for the reaction with 1,2-propylene oxide a very small (or possibly no) dependence on temperature within the temperature range between 261–355 K. El Othmani et al. (2021b) showed for the reaction with 1,2-epoxybutane a very weak negative temperature dependence at T ≤ 285 K and a transition toward a positive temperature dependence at T ≥ 295 K. El Othmani et al. (2021a) measured a stronger negative temperature dependence in the case of cyclohexene oxide. This behaviour could suggest the existence of van der Waals complexes that can play a role in the reaction mechanisms of epoxides at lower temperatures. More theoretical research is needed to further elucidate these findings.
All the electronic structure calculations will serve as input for the kinetic rate calculations using MESMER or another similar approach.
Even with the clear correlation between the rate coefficients of epoxides with OH radicals and chlorine atoms shown in Sect. 3.1, detailed structural optimizations and vibrational frequency calculations are also recommended for the reactions of the epoxides towards OH radicals.
Parallel to the present work, product studies of the reactions presented here have been undertaken, to delve a little deeper into the reaction mechanisms of these reactions.
Using the kinetic data obtained in the present work, in combination with daytime average radical concentrations, the estimation of the tropospheric lifetimes of the investigated compounds can be calculated using the following expression:
Therefore, for a globally averaged [OH] of 1.13 × 106 radicals cm−3 (Lelieveld et al., 2016) the atmospheric lifetimes for cyclohexene oxide, 1,2-epoxyhexane, 1,2-epoxybutane, trans-2,3-epoxybutane and cis-2,3-epoxybutane are estimated between 1 to 7 d, as shown in Table 5.
Table 5Comparison of the atmospheric lifetimes of epoxides towards OH radicals and chlorine atoms.
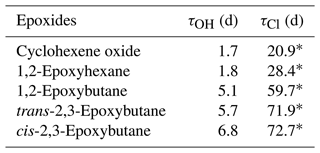
∗ Calculated values using the rate coefficients of epoxides towards Cl atoms (Tovar et al., 2021).
According to the rate coefficients presented in Tovar et al. (2021), and considering an estimated atmospheric concentration [Cl] of 2.3 × 103 atoms cm−3 (Young et al., 2014), an atmospheric lifetime between 21 and 73 d has been calculated for the epoxides. Accordingly, the reaction with OH radicals is the primary sink for epoxides in the atmosphere under these conditions.
However, in some cases, the atmospheric composition can be more than an order of magnitude more reactive with Cl than with OH (e.g Los Angeles during CalNex, where a maximum [Cl] of 8.7 × 103 atoms was measured; Young et al., 2014). In polluted continents during winter, ClNO2 chemistry drives increases in ozone of up to 8 ppb (Wang et al., 2019).
In the laboratory and ambient SOA, epoxides are considered as potential precursors for sulfate esters, polyols, hydroxy nitrates and halides. Epoxide concentrations in the aerosol composition will be limited by the rates of the gas uptake process, which will be largely determined by the gas-phase epoxide concentration levels (Minerath and Elrod, 2009; Minerath et al., 2009), the uptake process and particle acidity. Higher acidity of aerosol particles would produce more efficient heterogeneous reactions of epoxides. A recent study suggests that heterogeneous reaction of some epoxides on acidic aerosols is faster and more efficient than gaseous reactions with atmospheric oxidants, and thus these reactions could represent the major removal pathway for epoxides (Lal et al., 2012). Additionally, in urban environments, the particle acidity can be significantly high, with pH between 0–5. Under such conditions, epoxides can react rapidly, and the reaction products of the heterogeneous reactions could contribute to the growth of the SOA particles and to the possible modification of their physical and chemical properties (Zhang et al., 2007). Minerath and Elrod (2009) have shown that 1,2-epoxybutane, which possesses slower hydrolysis, has an increasing lifetime with increasing pH. It is expected that even at higher pH, most of the epoxides will have time to react on SOAs. The monitoring and evaluating of emissions of this kind of compound in different ambient conditions, such as pH and different regimens of NOx, are recommended in rural and urban locations.
The rate coefficients for the reaction of OH radicals with three different epoxides have been determined for the first time in this study. The rate coefficients for 1,2-epoxy butane and cyclohexene oxide are in very good agreement with previous studies (Wallington et al., 1988a; El Othmani et al., 2021b, a). A comparison of the reactivity trends from the gas-phase reaction of the epoxides obtained in this study showed a very good correlation with the reactivity trends of epoxides with chlorine atoms. Differences have been observed between the experimental OH rate coefficients and those obtained from SAR estimations. However, the values determined by the SAR method show some discrepancies dependent on the substituent factors, which were considered for the calculations. Such discrepancies could be explained by taking into consideration the structural effect of the ring acting in conjunction with the oxygen atom and the pseudo-ethylenic character of the epoxy ring. From the atmospheric chemistry viewpoint, the rate coefficients from the present study would help to extend the database for the reaction of cyclic ethers under atmospheric conditions.
Data can be provided upon request to the corresponding author, Carmen Maria Tovar (carmen.tovar_ramos@uni-wuppertal.de).
CMT designed and conducted the chamber experiments. CMT performed the data analysis. IB, IGB and PW made revisions at different stages of the study. CMT prepared the paper with contributions from all co-authors.
The contact author has declared that neither they nor their co-authors have any competing interests.
Publisher’s note: Copernicus Publications remains neutral with regard to jurisdictional claims in published maps and institutional affiliations.
The authors acknowledge the support provided by the European Union's Horizon 2020 Research and Innovation programme, through the EUROCHAMP-2020 Infrastructure Activity Grant (grant agreement no. 730997). Iustinian Gabriel Bejan acknowledges the PN-III-P4-ID-PCE-2016-4-0807 and PN-III-P2-2.1-PED-2019-4972 UEFISCDI projects. Carmen Maria Tovar is grateful for a PhD scholarship granted by FANTEL. We thank Alexander Haack for fruitful discussion.
This research has been supported by the Horizon 2020 (EUROCHAMP-2020 (grant no. 730997)), the Unitatea Executiva pentru Finantarea Invatamantului Superior, a Cercetarii, Dezvoltarii si Inovarii (grant nos. PN-III-P4-ID-PCE-2016-4-0807 and PN-III-P2-2.1-PED-2019-4972), and FANTEL (PhD scholarship).
This paper was edited by Thorsten Bartels-Rausch and reviewed by two anonymous referees.
Almatarneh, M. H., Elayan, I. A., Altarawneh, M., and Hollett, J. W.: A computational study of the ozonolysis of sabinene, Theor. Chem. Acc., 138, 30, https://doi.org/10.1007/s00214-019-2420-7, 2019a.
Almatarneh, M. H., Elayan, I. A., Abu-Saleh, A. A. A., Altarawneh, M., and Ariya, P. A.: The gas-phase ozonolysis reaction of methylbutenol: A mechanistic study, Int. J. Quantum Chem., 119, e25888, https://doi.org/10.1002/qua.25888, 2019b.
Alvarado, A., Tuazon, E. C., Aschmann, S. M., Atkinson, R., and Arey, J.: Products of the gas-phase reactions of O (3P) atoms and O3 with a-pinene and 1,2-dimethyl-1-cyclohexene, J. Geophys. Res.-Atmos., 103, 25541, https://doi.org/10.1029/98JD00524, 1998.
Andrea, K. A. and Kerton, F. M.: Iron-catalyzed reactions of CO2 and epoxides to yield cyclic and polycarbonates, Polym. J., 53, 29–46, https://doi.org/10.1038/s41428-020-00395-6, 2021.
Appaturi, J., Ramalingam, R., Gnanamani, M., Periyasami, G., Arunachalam, P., Adnan, R., Adam, F., Wasmiah, M., and Al-Lohedan, H.: Review on Carbon Dioxide Utilization for Cycloaddition of Epoxides by Ionic Liquid-Modified Hybrid Catalysts: Effect of Influential Parameters and Mechanisms Insight, Catalysts, 11, 4, https://doi.org/10.3390/catal11010004, 2021.
Atkinson, R.: Estimations of OH radical rate constants from H-atom abstraction from C-H and O-H bonds over the temperature range 250–1000 K, Int. J. Chem. Kinet., 18, 555–568, https://doi.org/10.1002/kin.550180506, 1986a.
Atkinson, R.: Kinetics and mechanisms of the gas-phase reactions of the hydroxyl radical with organic compounds under atmospheric conditions, Chem. Rev., 86, 69–201, https://doi.org/10.1021/cr00071a004, 1986b.
Atkinson, R.: A structure-activity relationship for the estimation of rate constants for the gas-phase reactions of OH radicals with organic compounds, Int. J. Chem. Kinet., 19, 799–828, https://doi.org/10.1002/kin.550190903, 1987.
Atkinson, R., Arey, J., Aschmann, S. M., and Tuazon, E. C.: Formation of O (3P) atoms and epoxides from the gas- phase reaction of O3 with isoprene, Res. Chem. Intermediat., 20, 385–394, https://doi.org/10.1163/156856794X00388, 1994a.
Atkinson, R., Aschmann, S. M., Arey, J., and Tuazon, E. C.: Formation yields of epoxides and O (3P) atoms from the gas-phase reactions of O3 with a series of alkenes, Int. J. Chem. Kinet., 26, 945–950, https://doi.org/10.1002/kin.550260908, 1994b.
Bader, R. F. W., Popelier, P. L. A., and Keith, T. A.: Theoretical Definition of a Functional Group and the Molecular Orbital Paradigm, Angew. Chem. Int. Ed., 33, 620–631, https://doi.org/10.1002/anie.199406201, 1994.
Barnes, I., Becker, K. H., and Mihalopoulos, N.: An FTIR product study of the photooxidation of dimethyl disulfide, J. Atmos. Chem., 18, 267–289, https://doi.org/10.1007/BF00696783, 1994.
Calvert, G., Atkinson, R., Kerr, A., Madronich, S., Moortgat, G., Wallington, J., and Yarwood, G.: The Mechanisms of Atmospheric Oxidation of the Alkenes, Oxford University Press, http://n2t.net/ark:/85065/d7cc11x7 (last access: 24 May 2022), 2000.
Calvert, J., Mellouki, A., Orlando, J., Pilling, M., and Wallington, T.: Mechanisms of Atmospheric Oxidation of the Oxygenates, Oxford University Press, https://doi.org/10.1093/oso/9780199767076.001.0001, 2011.
Calvert, J. G., Orlando, J. J., Stockwell, W. R., and Wallington, T. J.: The Mechanisms of Reactions Influencing Atmospheric Ozone, Oxford University Press, https://doi.org/10.1093/oso/9780190233020.001.0001, 2015.
Christianson, M. G., Doner, A. C., Davis, M. M., Koritzke, A. L., Turney, J. M., Schaefer III, H. F., Sheps, L., Osborn, D. L., Taatjes, C. A., and Rotavera, B.: Reaction mechanisms of a cyclic ether intermediate: Ethyloxirane, Int. J. Chem. Kinet., 53, 43–59, https://doi.org/10.1002/kin.21423, 2021.
Coppens, P.: Charge Densities Come of Age, Angew. Chem. Int. Ed., 44, 6810–6811, https://doi.org/10.1002/anie.200501734, 2005.
Coulson, C. A. and Moffitt, W. E.: Strain in Non-Tetrahedral Carbon Atoms, J. Chem. Phys., 15, 151, https://doi.org/10.1063/1.1746450, 1947.
Coulson, C. A. and Moffitt, W. E.: I. The properties of certain strained hydrocarbons, The London, Edinburgh, and Dublin Philosophical Magazine and Journal of Science, 40, 1–35, https://doi.org/10.1080/14786444908561208, 1949.
Cromwell, N. H. and Graff, M. A.: Three-Ring carbonyl hyperconjugation in cis and trans aryl-aroyl ethylene imines and related compounds, J. Org. Chem., 17, 414–425, https://doi.org/10.1021/jo01137a014, 1952.
Doner, A. C., Davis, M. M., Koritzke, A. L., Christianson, M. G., Turney, J. M., Schaefer III, H. F., Sheps, L., Osborn, D. L., Taatjes, C. A., and Rotavera, B.: Isomer-dependent reaction mechanisms of cyclic ether intermediates: cis-2,3-dimethyloxirane and trans-2,3-dimethyloxirane, Int. J. Chem. Kinet., 53, 127–145, https://doi.org/10.1002/kin.21429, 2021.
Ehrenberg, L. and Hussain, S.: Genetic toxicity of some important epoxides, Mutation Research/Reviews in Genetic Toxicology, Mutat. Res./Reviews in Genetic Toxicology, 86, 1–113, https://doi.org/10.1016/0165-1110(81)90034-8, 1981.
El Othmani, H., Ren, Y., Mellouki, A., Daële, V., and McGillen, M. R.: Gas-phase rate coefficient of OH + cyclohexene oxide measured from 251 to 373 K, Chem. Phys. Lett., 783, 139056, https://doi.org/10.1016/j.cplett.2021.139056, 2021a.
El Othmani, H., Ren, Y., Bedjanian, Y., El Hajjaji, S., Tovar, C., Wiesen, P., Mellouki, A., McGillen, M. R., and Daële, V.: Gas-Phase Rate Coefficient of OH + 1,2-Epoxybutane Determined between 220 and 950 K, ACS Earth and Space Chemistry, 5, 960–968, https://doi.org/10.1021/acsearthspacechem.1c00050, 2021b.
Fokin, V. V. and Wu, P.: Epoxides and Aziridines in Click Chemistry, in: Aziridines and Epoxides in Organic Synthesis, John Wiley & Sons, Ltd, 443–477, https://doi.org/10.1002/3527607862.ch12, 2006.
Förster, T.: Die gegenseitige Beeinflussung der Valenzen im Kohlenstoffatom, Z. Phys. Chem., 43B, 58–78, https://doi.org/10.1515/zpch-1939-4306, 1939.
Fuzzi, S., Baltensperger, U., Carslaw, K., Decesari, S., Denier van der Gon, H., Facchini, M. C., Fowler, D., Koren, I., Langford, B., Lohmann, U., Nemitz, E., Pandis, S., Riipinen, I., Rudich, Y., Schaap, M., Slowik, J. G., Spracklen, D. V., Vignati, E., Wild, M., Williams, M., and Gilardoni, S.: Particulate matter, air quality and climate: lessons learned and future needs, Atmos. Chem. Phys., 15, 8217–8299, https://doi.org/10.5194/acp-15-8217-2015, 2015.
Gao, S., Ng, N. L., Keywood, M., Varutbangkul, V., Bahreini, R., Nenes, A., He, J., Yoo, K. Y., Beauchamp, J. L., Hodyss, R. P., Flagan, R. C., and Seinfeld, J. H.: Particle Phase Acidity and Oligomer Formation in Secondary Organic Aerosol, Environ. Sci. Technol., 38, 6582–6589, https://doi.org/10.1021/es049125k, 2004.
Grabowsky, S., Jayatilaka, D., Mebs, S., and Luger, P.: The Electron Localizability Indicator from X-Ray Diffraction Data – A First Application to a Series of Epoxide Derivatives, Chem.-Eur. J., 16, 12818–12821, https://doi.org/10.1002/chem.201002061, 2010.
Guo, L., Lamb, K. J., and North, M.: Recent developments in organocatalysed transformations of epoxides and carbon dioxide into cyclic carbonates, Green Chem., 23, 77–118, https://doi.org/10.1039/D0GC03465G, 2021.
Hereijgers, B. P. C., Parton, R. F., and Weckhuysen, B. M.: Mechanistic insights in the olefin epoxidation with cyclohexyl hydroperoxide, Catal. Sci. Technol., 2, 951–960, https://doi.org/10.1039/C2CY00455K, 2012.
IUPAC Task Group on Atmospheric Chemical Kinetic Data Evaluation: https://iupac-aeris.ipsl.fr/, last access: 26 October 2021.
Jacobs, M. I., Darer, A. I., and Elrod, M. J.: Rate Constants and Products of the OH Reaction with Isoprene-Derived Epoxides, Environ. Sci. Technol., 47, 12868–12876, https://doi.org/10.1021/es403340g, 2013.
Jenkin, M. E., Young, J. C., and Rickard, A. R.: The MCM v3.3.1 degradation scheme for isoprene, Atmos. Chem. Phys., 15, 11433–11459, https://doi.org/10.5194/acp-15-11433-2015, 2015.
Jenkin, M. E., Valorso, R., Aumont, B., Rickard, A. R., and Wallington, T. J.: Estimation of rate coefficients and branching ratios for gas-phase reactions of OH with aliphatic organic compounds for use in automated mechanism construction, Atmos. Chem. Phys., 18, 9297–9328, https://doi.org/10.5194/acp-18-9297-2018, 2018.
Jiang, X., Tsona, N. T., Jia, L., Liu, S., Zhang, H., Xu, Y., and Du, L.: Secondary organic aerosol formation from photooxidation of furan: effects of NOx and humidity, Atmos. Chem. Phys., 19, 13591–13609, https://doi.org/10.5194/acp-19-13591-2019, 2019.
Kanakidou, M., Seinfeld, J. H., Pandis, S. N., Barnes, I., Dentener, F. J., Facchini, M. C., Van Dingenen, R., Ervens, B., Nenes, A., Nielsen, C. J., Swietlicki, E., Putaud, J. P., Balkanski, Y., Fuzzi, S., Horth, J., Moortgat, G. K., Winterhalter, R., Myhre, C. E. L., Tsigaridis, K., Vignati, E., Stephanou, E. G., and Wilson, J.: Organic aerosol and global climate modelling: a review, Atmos. Chem. Phys., 5, 1053–1123, https://doi.org/10.5194/acp-5-1053-2005, 2005.
Klotz, B., Barnes, I., Becker, K. H., and Golding, B. T.: Atmospheric chemistry of benzeneoxide/oxepin, J. Chem. Soc. Faraday T., 93, 1507–1516, https://doi.org/10.1039/A606152D, 1997.
Klotz, B., Barnes, I., Golding, B. T., and Becker, K.-H.: Atmospheric chemistry of toluene-1,2-oxide/2-methyloxepin, Phys. Chem. Chem. Phys., 2, 227–235, https://doi.org/10.1039/A908365K, 2000.
Kohout, M.: A measure of electron localizability, Int. J. Quantum Chem., 97, 651–658, https://doi.org/10.1002/qua.10768, 2004.
Kolb, H. C., Finn, M. G., and Sharpless, K. B.: Click Chemistry: Diverse Chemical Function from a Few Good Reactions, Angew. Chem. Int. Edit., 40, 2004–2021, https://doi.org/10.1002/1521-3773(20010601)40:11<2004::AID-ANIE2004>3.0.CO;2-5, 2001.
Koritsanszky, T. S. and Coppens, P.: Chemical Applications of X-ray Charge-Density Analysis, Chem. Rev., 101, 1583–1628, https://doi.org/10.1021/cr990112c, 2001.
Kutzelnigg, W.: Book Review: Atoms in Molecules. A Quantum Theory (International Series Monographs on Chemistry, Vol. 22). By R. F. W. Bader, Angew. Chem. Int. Ed., 32, 128–129, https://doi.org/10.1002/anie.199301282, 1993.
Kwok, E. S. C. and Atkinson, R.: Estimation of hydroxyl radical reaction rate constants for gas-phase organic compounds using a structure-reactivity relationship: An update, Atmos. Environ., 29, 1685–1695, https://doi.org/10.1016/1352-2310(95)00069-B, 1995.
Lal, V., Khalizov, A. F., Lin, Y., Galvan, M. D., Connell, B. T., and Zhang, R.: Heterogeneous Reactions of Epoxides in Acidic Media, J. Phys. Chem. A, 116, 6078–6090, https://doi.org/10.1021/jp2112704, 2012.
Lelieveld, J., Gromov, S., Pozzer, A., and Taraborrelli, D.: Global tropospheric hydroxyl distribution, budget and reactivity, Atmos. Chem. Phys., 16, 12477–12493, https://doi.org/10.5194/acp-16-12477-2016, 2016.
Li, M., Liu, Y., and Wang, L.: Gas-phase ozonolysis of furans, methylfurans, and dimethylfurans in the atmosphere, Phys. Chem. Chem. Phys., 20, 24735–24743, https://doi.org/10.1039/C8CP04947E, 2018.
Lin, Y.-H., Zhang, H., Pye, H. O. T., Zhang, Z., Marth, W. J., Park, S., Arashiro, M., Cui, T., Budisulistiorini, S. H., Sexton, K. G., Vizuete, W., Xie, Y., Luecken, D. J., Piletic, I. R., Edney, E. O., Bartolotti, L. J., Gold, A., and Surratt, J. D.: Epoxide as a precursor to secondary organic aerosol formation from isoprene photooxidation in the presence of nitrogen oxides, P. Natl. Acad. Sci. USA, 110, 6718–6723, https://doi.org/10.1073/pnas.1221150110, 2013.
McGillen, M. R., Carter, W. P. L., Mellouki, A., Orlando, J. J., Picquet-Varrault, B., and Wallington, T. J.: Database for the kinetics of the gas-phase atmospheric reactions of organic compounds, Earth Syst. Sci. Data, 12, 1203–1216, https://doi.org/10.5194/essd-12-1203-2020, 2020.
Middala, S., Campbell, S., Olea, C., Scruggs, A., and Hasson, A. S.: Kinetics and mechanism of the reaction of propylene oxide with chlorine atoms and hydroxy radicals, Int. J. Chem. Kinet., 43, 507–521, https://doi.org/10.1002/kin.20580, 2011.
Minerath, E. C. and Elrod, M. J.: Assessing the Potential for Diol and Hydroxy Sulfate Ester Formation from the Reaction of Epoxides in Tropospheric Aerosols, Environ. Sci. Technol., 43, 1386–1392, https://doi.org/10.1021/es8029076, 2009.
Minerath, E. C., Schultz, M. P., and Elrod, M. J.: Kinetics of the Reactions of Isoprene-Derived Epoxides in Model Tropospheric Aerosol Solutions, Environ. Sci. Technol., 43, 8133–8139, https://doi.org/10.1021/es902304p, 2009.
Oshima, T., Asahara, H., Kubo, E., Miyamoto, S., and Togaya, K.: Conformational Effects in Acid-Mediated Ring Opening of Epoxides: A Prominent Role of the Oxirane Walsh Orbital, Org. Lett., 10, 2413–2416, https://doi.org/10.1021/ol800535c, 2008.
Otto, H.-H. and Schirmeister, T.: Cysteine Proteases and Their Inhibitors, Chem. Rev., 97, 133–172, https://doi.org/10.1021/cr950025u, 1997.
Parker, R. E. and Isaacs, N. S.: Mechanisms Of Epoxide Reactions, Chem. Rev., 59, 737–799, https://doi.org/10.1021/cr50028a006, 1959.
Paulot, F., Crounse, J. D., Kjaergaard, H. G., Kürten, A., St Clair, J. M., Seinfeld, J. H., and Wennberg, P. O.: Unexpected epoxide formation in the gas-phase photooxidation of isoprene, Science, 325, 730—733, https://doi.org/10.1126/science.1172910, 2009.
Paulson, S. E., Flagan, R. C., and Seinfeld, J. H.: Atmospheric photooxidation of isoprene part I: The hydroxyl radical and ground state atomic oxygen reactions, Int. J. Chem. Kinet., 24, 79–101, https://doi.org/10.1002/kin.550240109, 1992.
Piletic, I. R., Edney, E. O., and Bartolotti, L. J.: Barrierless Reactions with Loose Transition States Govern the Yields and Lifetimes of Organic Nitrates Derived from Isoprene, J. Phys. Chem. A, 121, 8306–8321, https://doi.org/10.1021/acs.jpca.7b08229, 2017.
Powers, J. C., Asgian, J. L., Ekici, Ö. D., and James, K. E.: Irreversible Inhibitors of Serine, Cysteine, and Threonine Proteases, Chem. Rev., 102, 4639–4750, https://doi.org/10.1021/cr010182v, 2002.
Rogers, M. T.: The Electric Moments and Ultraviolet Absorption Spectra of Some Derivatives of Cyclopropane and of Ethylene Oxide, J. Am. Chem. Soc., 69, 2544–2548, https://doi.org/10.1021/ja01202a081, 1947.
Sandhiya, L., Kolandaivel, P., and Senthilkumar, K.: Mechanism and Kinetics of the Atmospheric Oxidative Degradation of Dimethylphenol Isomers Initiated by OH Radical, J. Phys. Chem. A, 117, 4611–4626, https://doi.org/10.1021/jp3120868, 2013.
Schirmeister, T. and Klockow, A.: Cysteine Protease Inhibitors Containing Small Rings, Mini-Rev. Med. Chem., 3, 585–596, 2003.
Searles, S. and Tamres, M.: Hydrogen Bond Formation with Saturated Cyclic Ethers1, J. Am. Chem. Soc., 73, 3704–3706, https://doi.org/10.1021/ja01152a041, 1951.
Searles, S., Tamres, M., and Lippincott, E. R.: Hydrogen Bonding Ability and Structure of Ethylene Oxides, J. Am. Chem. Soc., 75, 2775–2777, https://doi.org/10.1021/ja01107a517, 1953.
Shrivastava, M., Andreae, M. O., Artaxo, P., Barbosa, H. M. J., Berg, L. K., Brito, J., Ching, J., Easter, R. C., Fan, J., Fast, J. D., Feng, Z., Fuentes, J. D., Glasius, M., Goldstein, A. H., Alves, E. G., Gomes, H., Gu, D., Guenther, A., Jathar, S. H., Kim, S., Liu, Y., Lou, S., Martin, S. T., McNeill, V. F., Medeiros, A., de Sá, S. S., Shilling, J. E., Springston, S. R., Souza, R. a. F., Thornton, J. A., Isaacman-VanWertz, G., Yee, L. D., Ynoue, R., Zaveri, R. A., Zelenyuk, A., and Zhao, C.: Urban pollution greatly enhances formation of natural aerosols over the Amazon rainforest, Nat. Commun., 10, 1046, https://doi.org/10.1038/s41467-019-08909-4, 2019.
Skov, H., Benter, T., Schindler, R. N., Hjorth, J., and Restelli, G.: Epoxide formation in the reactions of the nitrate radical with 2,3-dimethyl-2-butene, cis- and trans-2-butene and isoprene, Atmos. Environ., 28, 1583–1592, https://doi.org/10.1016/1352-2310(94)90304-2, 1994.
Starit, L. A., Ketcham, Roger., Jambotkar, D., and Shah, V. P.: Three-Membered Rings. I. Conjugative Properties and Electrinic Spectra of Arylcyclopropanes, Oxiranes, and Thiiranes, J. Am. Chem. Soc., 86, 4628–4630, https://doi.org/10.1021/ja01075a022, 1964.
Stropoli, S. J., Miner, C. R., Hill, D. R., and Elrod, M. J.: Assessing Potential Oligomerization Reaction Mechanisms of Isoprene Epoxydiols on Secondary Organic Aerosol, Environ. Sci. Technol., 53, 176–184, https://doi.org/10.1021/acs.est.8b05247, 2019.
Tamres, M., Searles, S., Leighly, E. M., and Mohrman, D. W.: Hydrogen Bond Formation with Pyridines and Aliphatic Amines1, J. Am. Chem. Soc., 76, 3983–3985, https://doi.org/10.1021/ja01644a035, 1954.
Tovar, C. M., Haack, A., Barnes, I., Bejan, I. G., and Wiesen, P.: Experimental and theoretical study of the reactivity of a series of epoxides with chlorine atoms at 298 K, Phys. Chem. Chem. Phys., 23, 5176–5186, https://doi.org/10.1039/D0CP06033J, 2021.
Vereecken, L.: Reaction Mechanisms for the Atmospheric Oxidation of Monocyclic Aromatic Compounds, in: Advances in Atmospheric Chemistry, edited by: Barker, J. R., Steiner, A. L., and Wallington, T. J., World Scientific, 377–527, https://doi.org/10.1142/9789813271838_0006, 2018.
Vereecken, L., Glowacki, D. R., and Pilling, M. J.: Theoretical Chemical Kinetics in Tropospheric Chemistry: Methodologies and Applications, Chem. Rev., 115, 4063–4114, https://doi.org/10.1021/cr500488p, 2015.
Vereecken, L., Aumont, B., Barnes, I., Bozzelli, J. W., Goldman, M. J., Green, W. H., Madronich, S., Mcgillen, M. R., Mellouki, A., Orlando, J. J., Picquet-Varrault, B., Rickard, A. R., Stockwell, W. R., Wallington, T. J., and Carter, W. P. L.: Perspective on Mechanism Development and Structure-Activity Relationships for Gas-Phase Atmospheric Chemistry, Int. J. Chem. Kinet., 50, 435–469, https://doi.org/10.1002/kin.21172, 2018.
Villanueva, F., Cabañas, B., Monedero, E., Salgado, S., Bejan, I., and Martin, P.: Atmospheric degradation of alkylfurans with chlorine atoms: Product and mechanistic study, Atmos. Environ., 43, 2804–2813, https://doi.org/10.1016/j.atmosenv.2009.02.030, 2009.
Virmani, A., Walavalkar, M. P., Sharma, A., Sengupta, S., Saha, A., and Kumar, A.: Kinetic studies of the gas phase reaction of 1,2-propylene oxide with the OH radical over a temperature range of 261–335 K, Atmos. Environ., 237, 117709, https://doi.org/10.1016/j.atmosenv.2020.117709, 2020.
Wallington, T. J., Dagaut, P., and Kurylo, M. J.: Correlation between gas-phase and solution-phase reactivities of hydroxyl radicals towards saturated organic compounds, J. Phys. Chem., 92, 5024–5028, https://doi.org/10.1021/j100328a039, 1988a.
Wallington, T. J., Liu, R., Dagaut, P., and Kurylo, M. J.: The gas phase reactions of hydroxyl radicals with a series of aliphatic ethers over the temperature range 240–440 K, 20, 41–49, https://doi.org/10.1002/kin.550200106, 1988b.
Wallington, T. J., Dagaut, P., Liu, R., and Kurylo, M. J.: The gas phase reactions of hydroxyl radicals with a series of esters over the temperature range 240–440 K, Int. J. Chem. Kinet., 20, 177–186, https://doi.org/10.1002/kin.550200210, 1988c.
Walsh, A. D.: The structures of ethylene oxide, cyclopropane, and related molecules, T. Faraday Soc., 45, 179–190, https://doi.org/10.1039/TF9494500179, 1949.
Wang, Q., Ni, S., Bai, F., and Pan, X.: Theoretical investigation on atmospheric reaction mechanism, kinetics and SAR estimations of four-carbon ketones and alcohols, Atmos. Environ., 271, 118915, https://doi.org/10.1016/j.atmosenv.2021.118915, 2022.
Wang, X., Jacob, D. J., Eastham, S. D., Sulprizio, M. P., Zhu, L., Chen, Q., Alexander, B., Sherwen, T., Evans, M. J., Lee, B. H., Haskins, J. D., Lopez-Hilfiker, F. D., Thornton, J. A., Huey, G. L., and Liao, H.: The role of chlorine in global tropospheric chemistry, Atmos. Chem. Phys., 19, 3981–4003, https://doi.org/10.5194/acp-19-3981-2019, 2019.
Wiberg, K. B.: Bent Bonds in Organic Compounds, Acc. Chem. Res., 29, 229–234, https://doi.org/10.1021/ar950207a, 1996.
Young, C. J., Washenfelder, R. A., Edwards, P. M., Parrish, D. D., Gilman, J. B., Kuster, W. C., Mielke, L. H., Osthoff, H. D., Tsai, C., Pikelnaya, O., Stutz, J., Veres, P. R., Roberts, J. M., Griffith, S., Dusanter, S., Stevens, P. S., Flynn, J., Grossberg, N., Lefer, B., Holloway, J. S., Peischl, J., Ryerson, T. B., Atlas, E. L., Blake, D. R., and Brown, S. S.: Chlorine as a primary radical: evaluation of methods to understand its role in initiation of oxidative cycles, Atmos. Chem. Phys., 14, 3427–3440, https://doi.org/10.5194/acp-14-3427-2014, 2014.
Zhang, F., Wang, Y., Zhang, X., Zhang, X., Liu, H., and Han, B.: Recent advances in the coupling of CO2 and epoxides into cyclic carbonates under halogen-free condition, Green Chemical Engineering, 1, 82–93, https://doi.org/10.1016/j.gce.2020.09.008, 2020.
Zhang, Q., Jimenez, J. L., Worsnop, D. R., and Canagaratna, M.: A Case Study of Urban Particle Acidity and Its Influence on Secondary Organic Aerosol, Environ. Sci. Technol., 41, 3213–3219, https://doi.org/10.1021/es061812j, 2007.
Zhou, S., Yeung, L. W. Y., Forbes, M. W., Mabury, S., and Abbatt, J. P. D.: Epoxide formation from heterogeneous oxidation of benzo[a]pyrene with gas-phase ozone and indoor air, Environ. Sci.-Proc. Imp., 19, 1292–1299, https://doi.org/10.1039/C7EM00181A, 2017.
Zou, B. and Hu, C.: Halogen-free processes for organic carbonate synthesis from CO2, Current Opinion in Green and Sustainable Chemistry, 3, 11–16, https://doi.org/10.1016/j.cogsc.2016.10.007, 2017.