the Creative Commons Attribution 4.0 License.
the Creative Commons Attribution 4.0 License.
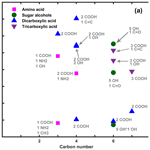
Hygroscopicity of organic compounds as a function of organic functionality, water solubility, molecular weight, and oxidation level
Shuang Han
Juan Hong
Qingwei Luo
Hanbing Xu
Haobo Tan
Qiaoqiao Wang
Jiangchuan Tao
Yaqing Zhou
Long Peng
Yao He
Jingnan Shi
Nan Ma
Yafang Cheng
Aerosol hygroscopicity strongly influences the number size distribution, phase state, optical properties, and multiphase chemistry of aerosol particles. Due to the large number of organic species in atmospheric aerosols, the determination of the hygroscopicity of ambient aerosols remains challenging. In this study, we measured the hygroscopic properties of 23 organics, including carboxylic acids, amino acids, sugars, and alcohols, using a hygroscopicity tandem differential mobility analyzer (HTDMA). Earlier studies have characterized the hygroscopicity either for a limited number of organic compounds using similar techniques or for particles at sizes beyond the microscale range or even bulk samples using other methodologies. Here, we validate these studies and extend the data by measuring the hygroscopicity of a broader suite of organics for particles with sizes under the submicrometer range that are more atmospherically relevant. Moreover, we systematically evaluate the roles of that related physicochemical properties play in organic hygroscopicity. We show that the hygroscopicity of organics varies widely with functional groups and organics with the same carbon number but that more functional groups show higher hygroscopicity. However, some isomers that are very similar in molecular structure show quite different hygroscopicity, demonstrating that other physicochemical properties, such as water solubility, may contribute to their hygroscopicity as well. If the organics are fully dissolved in water (solubility g mL−1), we found that their hygroscopicity is mainly controlled by their molecular weight. For the organics that are not fully dissolved in water (slightly soluble: g mL−1 < solubility < g mL−1), we observed that some of them show no obvious water uptake, which is probably due to the fact that they may not deliquesce under our studied conditions up to 90 % relative humidity (RH). The other type of slightly soluble organic material is moderately hygroscopic, and the larger its solubility is, the higher its hygroscopicity will be. Moreover, the hygroscopicity of organics generally increased with O:C ratios, although this relationship is not linear.
- Article
(2698 KB) - Full-text XML
-
Supplement
(2032 KB) - BibTeX
- EndNote
Atmospheric aerosol particles consist of numerous organic species with both anthropogenic and biogenic origins (Zhang et al., 2007, 2015; Jimenez et al., 2009; Wang et al., 2018). These organic species often contribute a significant fraction to the mass of submicrometer aerosols and have vital effects on air quality and climate (McFiggans et al., 2006; Randall et al., 2007; Zheng et al., 2015). To obtain a systematic understanding of their effects, it is necessary to acquire correct information on the chemical composition and physicochemical properties of these organics (Seinfeld and Pandis, 2016). Hygroscopicity is one of the most important physicochemical properties and describes the ability of particles to take up water and grow in size under subsaturated and supersaturated conditions (Petters and Kreidenweis, 2007). Thus, it strongly influences the number size distribution, phase state, optical properties, and multiphase chemistry of aerosol particles (Cheng et al., 2008; Su et al., 2010; Hong et al., 2018; Tang et al., 2019).
Given the large number of organic species in atmospheric aerosols, the determination of their hygroscopicity is quite experimentally difficult. Current models normally use aggregate quantities, such as the atomic oxygen-to-carbon (O:C) ratio or the average oxidation state of organics, to simply parameterize the hygroscopicity of organic species in ambient aerosols. However, recent studies show that the hygroscopicity of organic aerosols cannot be fully explained by their oxidation level and that the empirical relationship between hygroscopicity and O:C might not be linear (Lambe et al., 2011; Kuwata et al., 2013; Rickards et al., 2013; Marsh et al., 2017). This suggests that this simplified approach to quantify organic hygroscopicity might be problematic, and a more mechanistic understanding of the complex link between hygroscopicity and other physicochemical properties such as molecular functionality, molecular weight, and water solubility of organics should be examined.
Due to these challenges, prediction of the hygroscopicity of organic compounds sometimes relies on thermodynamic models that explicitly include these properties, for instance molecular functionality and molecular weight, into simulations. These thermodynamic models, including the Aerosol Inorganic-Organic Mixtures Functional groups Activity Coefficients (AIOMFAC) (Zuend et al., 2008, 2011), the Extended Aerosol Inorganic Model (E-AIM), and the University of Manchester System Properties (UManSysProp) (Clegg et al., 1998; Topping et al., 2016), use group contribution methods to calculate water activity for organic species of atmospheric relevance. However, involving these thermodynamic simulations in transport or climate models to predict the hygroscopicity for such a large number of organic compounds in ambient aerosols is computationally expensive. Moreover, these models, which are based on parameterizations from measurements, are semi-empirical and thus need more experimental data to constrain their predictions (Suda and Petters, 2013). These models may perform even worse and cannot capture the non-ideality of the solutions accurately, particularly when it comes to very dry conditions (Ohm et al., 2015). Therefore, quantifying the hygroscopicity of atmospherically relevant organic species through laboratory measurements by systematically varying the type of studied organics is an intrinsic necessity. Chan et al. (2008) studied the hygroscopic properties and cloud condensation nuclei (CCN) activities of a series of dicarboxylic acids and saccharides using an electrodynamic balance (EDB) and found that the CCN activities of highly water-soluble organic compounds can be predicted well by the Köhler theory. Suda et al. (2014) examined the hygroscopicity of a few synthetic organic compounds that are atmospherically relevant but not commercially available by using a CCN counter (CCNc). They found that the compounds with hydroxyl or carboxyl groups are the most hygroscopic, while the ones with nitrate or methylene are the least hygroscopic. Jing et al. (2016) investigated the hygroscopic properties of a series of dicarboxylic acids with levoglucosan using a hygroscopicity tandem differential mobility analyzer (HTDMA), but they mainly focused on the multicomponent interactions between organic compounds. Marsh et al. (2017) collected experimental hygroscopicity data for 23 organic compounds using a comparative kinetics EDB (CK-EDB) to compare with thermodynamic predictions and discussed the idea that the hygroscopicity of organic compounds with increasing branching and chain length is poorly represented by models.
All of the cases discussed above show that there is already some experimental hygroscopicity data for organics with high atmospheric abundance and relevance (Peng et al., 2001; Prenni et al., 2007; Chan et al., 2008; Lambe et al., 2011; Kuwata et al., 2013; Marsh et al., 2017; Lei et al., 2018). However, some of these measurements were conducted using different techniques, all of which have different limitations. Measurements using a CCNc could only probe the hygroscopic properties or CCN activities at supersaturated conditions, where many compounds may already fully dissolve in water droplets. The EDB or CK-EDB approaches normally analyze the droplets in the micrometer size range, far beyond the size range of atmospherically relevant aerosols. In contrast, the HTDMA system allows direct measurement of particle hygroscopicity at subsaturated conditions and for particles at the size from tens to hundreds of nanometers, which is a good method for closing the gaps that is beyond the reaches of other techniques. Furthermore, some of the aforementioned studies using similar HTDMA systems focused on quite a small number of organics, discussing only one or two properties potentially influencing the hygroscopicity, leading to a limited coverage of the experimental datasets. Thus, the general picture needed for understanding the observed hygroscopicity among different organic species still remains unclear.
Therefore, in this work we extend the compositional complexity and diversity of the studied organic compounds with varying functional groups, molecular structures and other relevant physicochemical properties. We try to form a systematic matrix of experimentally determined HTDMA data synthesizing a large suite of organics that provides unambiguous measurements of particles at atmospherically relevant size ranges. Combined with these experimental data, we aim to evaluate the roles of different physicochemical properties that play a role in organic hygroscopicity and gain new insight into their limitations and applicability.
Submicrometer aerosol particles were generated by nebulizing aqueous solutions (0.1 g L−1) of each compound using a constant output atomizer (TSI, 3076). The solutions were prepared by using ultrapure water (Millipore, resistivity ≥18.2 MΩ). The physicochemical properties of the studied 23 compounds are summarized in Table 1.
Table 1Substances and their relevant properties investigated in this study.
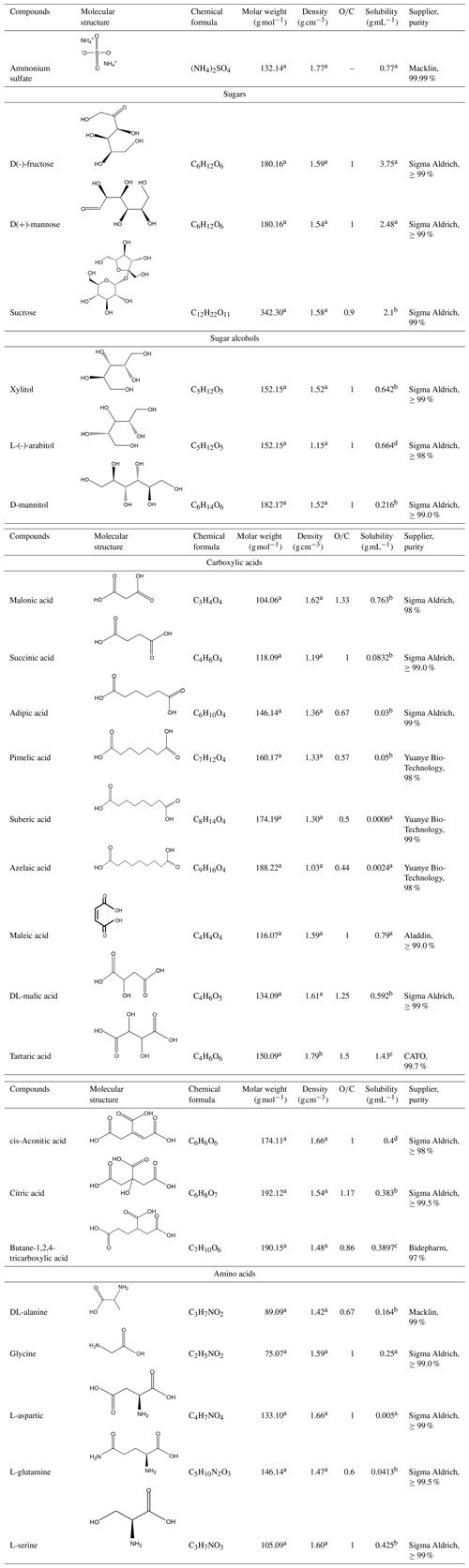
a https://www.chemicalbook.com/ (last access: 16 March 2022). b https://pubchem.ncbi.nlm.nih.gov/ (last access: 16 March 2022). c https://www.chemspider.com/ (last access: 16 March 2022). d https://hmdb.ca/ (last access: 16 March 2022). e Peng et al. (2001).
After particle generation, the particles were introduced into a custom-made HTDMA system where their hygroscopic growth factor (GF) can be measured. The GF of relative humidity (RH) is defined in Eq. (1) as follows:
where D(RH) and D0 are the equilibrium mobility diameter of the particles at a given RH and under dry conditions (<10 % RH), respectively. Figure S1 in the Supplement shows the schematic of the HTDMA system. A detailed schematic of the HTDMA system can be found in Tan et al. (2013). Residence time for humidification of the generated aerosols is around 2.7 s. Calibration of the system was performed using ammonium sulfate (AS) and the results given in Fig. S2 in the Supplement show that the measured hygroscopic behavior of AS agreed well with previous studies, with a deliquescence RH around 78 %.
Swietlicki et al. (2008) summarized the potential sources of error in HTDMA measurements and concluded that the reliability of the measured data is strongly associated with the stability and accuracy of DMA2 RH as well as the accurate measurement of particle diameter by DMAs. According to Mochida and Kawamura (2004), the uncertainty in the measured GF can be calculated by Eq. (2):
where GF is the measured growth factor with respect to any measured RH and and εRH are the errors in the measured Dp and RH. In our system, the accuracy of DMA2 RH was maintained to be ±1 %, and the uncertainty for the mobility diameter was ±1 % according to PSL (polystyrene latex particle) calibration. Hence, for our system, εRH and are 1 % and 0.01, respectively. The calculated uncertainty according to the abovementioned method is added in the measured GF in the following section.
According to κ-Köhler theory, we converted the measured hygroscopic growth factor to the single hygroscopicity parameter κ (Eqs. 3–6) to facilitate the comparison of the hygroscopic properties among different compounds (Petters and Kreidenweis, 2007):
where aw is the water activity, Mw and ρw are the molar mass and the density of pure water at temperature T, respectively, σsol is the solution droplet surface tension, which was assumed to be the surface tension of water (0.072 J m−2), and R is the ideal gas constant.
3.1 Hygroscopicity of individual organics
In this section, we summarized the measured hygroscopic properties of the 23 organic species, which are classified into three groups based on their functionality. Particles at the dry size of 200 nm were selected for analysis. Comparison with previous literature data for those compounds will be systematically conducted in the following section. The available information with respect to the deliquescence RH (DRH), efflorescence RH (ERH), phase transition, measured κ at 90 % RH for each compound, and the used instrument in different works is summarized in Table 2.
Table 2Summary of the measured properties with respect to their DRH, ERH, phase transition, and hygroscopicity parameter (90 % RH) obtained in this work and previous literature.
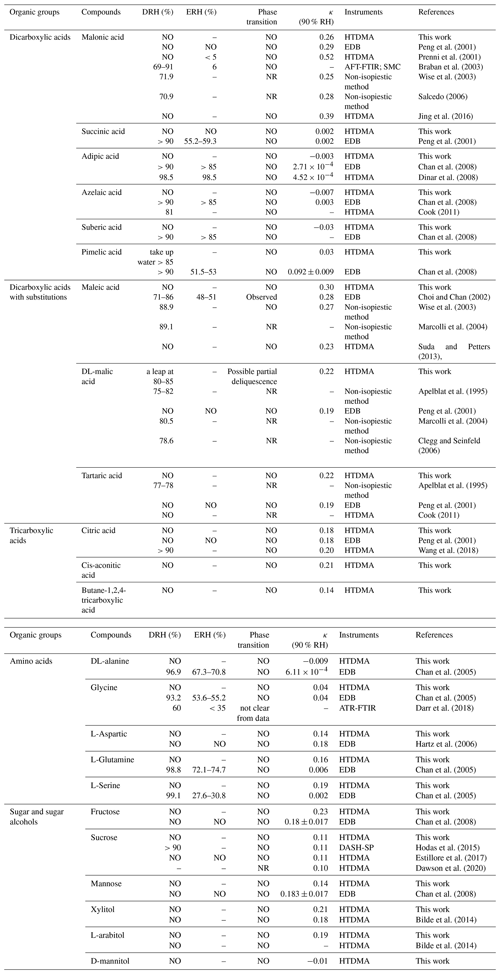
κ values were derived from hydration data at around of 90 % RH. NO indicates that no crystallization was observed. NR indicates that the value is not reported in the work. Dashes indicate that the value is not measured in the work.
3.1.1 Carboxylic acids
Carboxylic acids are the most abundant water-soluble components identified in atmospheric aerosols (Chebbi and Carlier, 1996; Mochida et al., 2003; Kundu et al., 2010). Hygroscopic properties of straight-chain dicarboxylic acids have been extensively investigated in previous studies (Chan et al., 2008; Kuwata et al., 2013; Rickards et al., 2013); however, HTDMA data for dicarboxylic acids with additional substitutions and tricarboxylic acids are limited. To achieve an overview of the hygroscopicity of carboxylic acids, we measured the water uptake of several common straight-chain dicarboxylic acids in the atmosphere and further extended the hygroscopic measurements for dicarboxylic acids with substitutions and tricarboxylic acids. Figures 1 and 2 show the measured humidograms of straight-chain dicarboxylic acids (Fig. 1a–f), dicarboxylic acids with substitutions (Fig. 2a–c), and three tricarboxylic acids (Fig. 2d–f).
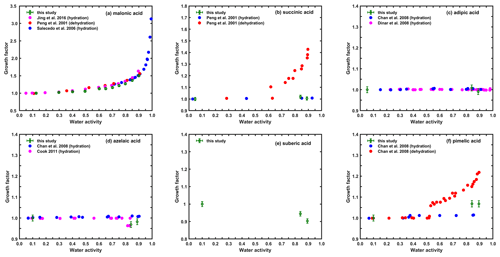
Figure 1The hygroscopic growth values of straight-chain dicarboxylic acid particles (200 nm) measured in this study and their individual comparison with previous studies.
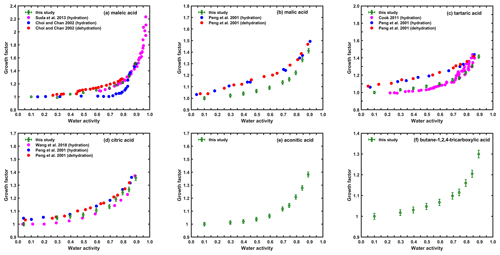
Figure 2The hygroscopic growth of straight-chain dicarboxylic acid particles with substitutions (a–c) and tricarboxylic acid (d–f) particles (200 nm) measured in this study and previous studies.
Among the studied straight-chain dicarboxylic acids, only malonic acid showed continuous hygroscopic growth with increasing RH, and the measured GF at 90 % RH was 1.47, as shown in Fig. 1a. This agrees quite well with previous studies using other HTDMA systems or other techniques with longer residence times for humidification, for instance EDB (Peng et al., 2001; Prenni et al., 2001; Wise et al., 2003; Salcedo, 2006; Jing et al., 2016). Previous studies (Swietlicki et al., 2008; Duplissy et al., 2009; Wu et al., 2011; Suda and Petters, 2013) suggested that the residence time for humidification may also potentially influence the observed water uptake of particles, as the measured particles, especially some organic compounds, may not reach their humidified equilibrium sizes during a short wetting period. However, current results show good consistency with other studies that have a much longer residence time, which suggests that malonic acid may already reach equilibrium during the short period of humidification in our system. On the other hand, Braban et al. (2003) reported that the DRH for malonic acid ranges between 69 % and 91 % RH. However, within this RH range we did not observe any clear evidence for phase transition. Braban et al. (2003) also reported that the ERH for malonic acid is around 6 %±3 % RH, which is lower than the condition that our system was able to reach. Thus, the generated malonic acid particles after drying in our study may not crystallize and thus exist in a liquid state, which is further confirmed by the good agreement between our results and the ones obtained from the dehydration process by Peng et al. (2001).
The other straight-chain dicarboxylic acids (i.e., succinic, adipic, suberic, and azelaic acids) did not show any water uptake at RH≤90 %, as shown in Fig. 1b–e. This non-hygroscopicity was also found for succinic acid in Peng et al. (2001), for adipic acid in Chan et al. (2008) and Dinar et al. (2008), and for azelaic acid in Chan et al. (2008) and Cook (2011), all of which either used a different HTDMA or an EDB. It has to be noted that no previous data were available for suberic acid. Although Chan et al. (2008) performed their hygroscopicity measurements, they failed to reported any results due to the trapping difficulties of this compound in their system. Thus, other measurements using different methods should be considered for future comparison. For all these dicarboxylic acids with no water uptake, Chan et al. (2008) explained that they have quite low solubility in water, and once crystallized they would not deliquesce even under high-RH conditions (e.g., RH<90 %). Moreover, we found that the measured GFs of azelaic and suberic acids were less than 1, which could be attributed to the adsorption of a small amount of water at the particle surface, leading to the rearrangement of the microstructure and compaction of the particle (Mikhailov et al., 2004, 2009). For pimelic acid, we observed a slightly weak hygroscopicity (Fig. 1f), which is significantly different from the result by Chan et al. (2008), where no water uptake was observed even at 90 % RH for this compound. Based on current comparison with limited data available, we were not be able to confirm which data are more accurate, especially as Chan et al. (2008) measured the particles in the micrometer size range using a different technique with a longer residence time. Therefore, the usage of the current dataset for this compound should be more careful, and thus repeated hygroscopicity measurements using different methods should be conducted, particularly when dealing with size-dependent hygroscopicity and the influence from residence time.
The humidograms of the three dicarboxylic acids with substitutions (i.e., double bond or hydroxy groups) are illustrated in Fig. 2a–c. The continuous water uptake of maleic acid (Fig. 2a) indicates that these particles may be at liquid state under dry conditions, which agrees well with the results of Suda and Petters (2013) using another HTDMA at a humidification residence time of 6 s. However, the results of Choi and Chan (2002), using an EDB with humidification time of 40 min, show that maleic acid started to take up water at RH around 75 % and that this deliquescence process continued until the RH reached to 85 %, after which similar growth factor values to ours were observed. Using a non-isopiestic method, both Wise et al. (2003) and Marcolli et al. (2004) reported that the DRH of maleic acid would be around 89 % RH, which is different to Choi and Chan (2002), who used the EDB. Such a large variation in the phase state and the phase transition process for this compound provides a note of caution for other researchers when using different datasets. However, the good agreement in GF at 90 % RH among different studies suggests that further analysis in Sect. 3.2 using GF at 90 % RH should still be reliable.
Continuous water uptake was also found for malic acid and tartaric acid, similar to those measured by Peng et al. (2001) using an EDB with longer residence time or Cook et al. (2011) using a different HTDMA. However, for both compounds there was a clear and considerable difference in GF at RH lower than 80 % between our study and the ones by Peng et al. (2001) during the hydration process. This could be due to the short residence time of our HTDMA system, limiting the water uptake of aqueous malic acid. However, previous studies (Apelblat et al., 1995; Marcolli et al., 2004; Clegg and Seinfeld, 2006) suggested that both compounds may deliquesce during the wetting process, meaning that the DRH potentially ranges between 75 % and 82 % RH for malic acid and 77 % and 78 % RH for tartaric acid. Therefore, their conclusion was not consistent with the results of Peng et al. (2001), who reported no phase transition. In our study, a small leap in the GFs from 80 % to 85 % RH was observed for malic acid, implying that these particles were only partially deliquesced and that further dissolution occurred at elevated RH. However, this partly supports those studies by suggesting that the deliquescence of malic acid is during hydration. Taking both of these things into account, we are not sure which factor (the different residence time of humidification or the partial deliquescence) should be considered as responsible for the difference in GF at intermediate RHs, and thus further evidence from other measurements is essentially needed.
Gradual water uptake upon hydration was observed for citric acid (Fig. 2d), which is consistent with other studies (Peng et al., 2001; Wang et al., 2018). However, our measured GF at any specific RH was somewhat higher than those of Wang et al. (2018) using another HTDMA but lower than the ones of Peng et al. (2001) using an EDB. The residence time of wetting inside both instruments was longer than our system but caused the opposite effect on the measured GFs, indicating that the influence of residence time on the observed water uptake of particles is not conclusive based on current datasets. Continuous hygroscopic growth over the studied RH range was observed for the other two tricarboxylic acids (e.g., aconitic acid and butane-1,2,4-tricarboxylic acid; see Fig. 2e and f), indicating no obvious phase change for these particles upon hydration. Based on their GF values at 90 % RH, both compounds can be considered as more hygroscopic substances. It has to be noted this is, to our knowledge, the first time that their hygroscopicity has been reported within the aerosol community. These new datasets, despite needing to be further validated with other measurements, might benefit the elaboration of the current data pool with their extended coverage of atmospherically relevant compounds.
3.1.2 Amino acids
Figure 3 shows the measured humidograms of the five amino acids and their individual comparison with previous studies. Chan et al. (2005) reported that the DRH for alanine and glycine was 96.9 % and 93.2 % RH, respectively. Therefore, generally no water uptake at RH≤90 % was observed for both compounds in either our study or theirs. For glycine (see Fig. 3b) a continuous shrink in wet particle size was observed from 30 % to 80 % RH, and above 80 % RH the GFs increased slightly. Previous studies have reported that glycine particles started to absorb water above 60 % RH (Chan et al., 2005; Marsh et al., 2017; Darr et al., 2018) prior to deliquescence due to a capillary effect, which could be the potential reason for the shrinkage in their particle size in our study as a result of the microstructural rearrangement of particles upon humidification. The sizing of these structurally rearranged particles will be erroneous, especially at lower RH range, as the volume change of the particles upon wetting may be due not only to the water absorption but also the compaction of the original particles. This phenomena complicates the accurate estimation of the actual water amount absorbed by the particles due to their intrinsic hygroscopicity. In a recent study by Nakao et al. (2014), in order to avoid the influence from particle restructuring upon wetting, they sized wet particles without drying after generation and studied their droplet activation using a wet CCN. This approach might be easier to attempt, offering an unique solution for the current problems created by particle restructuring during the hydration processes.
Continuous water uptake was observed for particles of aspartic acid (Fig. 3c), indicating that no phase transition occurred during the hydration cycle. This pattern was consistent with the ones measured by Hartz et al. (2006) but with slightly lower GF values. As Hartz et al. (2006) used an EDB to measure the hygroscopicity of aspartic acid, the potential reason for the different GF would more likely be the different residence times of humidification between different techniques, although future data should be obtained to confirm this estimation.
Chan et al. (2005) reported that the DRH values for glutamine and serine were 98.8 % and 99.1 % RH, and thus those compounds were considered non-hygroscopic at RH below 90 %. Our observation deviated significantly from theirs, as gradual hygroscopic growth, indicating liquid state, was found for those two compounds. A previous study (Gregson et al., 2020) pointed out that particles without sufficient drying after generation would not crystallize; for instance, the sample RH after drying was higher than their ERH. However, Chan et al. (2005) also reported that the ERHs for glutamine and serine were 72.1 %–74.7 % and 27.6 %–30.8 % RH, respectively, much higher than that of our dried aerosols. Thus, this effect with respect to the drying process is not a plausible reason for the continuous water uptake of both compounds in our study. Ma et al. (2021) reviewed the ERH values for a series of inorganic and organic compounds and found that particles in the micrometer size range exhibited different ERH compared to those of submicrometer particles. On this basis, we speculated that the ERH values for glutamine and serine particles at nanometer range might be different (e.g., lower than the ones reported by Chan et al., 2005), and thus crystallization of these compounds did not occur in our setup. However, the conclusion from Ma et al. (2021) provides a hint that the DRH values for particles in the micrometer size range could also be different than those in the nanometer range. Based on this, the DRH of glutamine and serine and their phase states might also be dependent on size, potentially explaining the significant difference in the hygroscopic growth between our studies and the ones in Chan et al. (2005).
3.1.3 Sugars and sugar alcohols
The continuous hygroscopic growth and the measured GF values for particles of fructose and mannose (Fig. 4a and b) agree well with the results of Chan et al. (2008) during both hydration and dehydration processes. The only difference is that we observed a small leap in GF at RH between 70 % and 75 % for both compounds. However, considering the measurement uncertainties, this phenomena was not obvious, meaning that no further inference can be made. Based on the pattern of their continuous water uptake, we considered these particles to exist in liquid state at dry conditions, which is also confirmed by the fact that no DRH or ERH values were found for those compounds in previous studies. For sucrose (Fig. 4c) at intermediate RHs, our measured GFs were lower than the ones reported by Estillore et al. (2017), who used a different HTDMA with a much longer residence time (40 s), and the ones by Hodas et al. (2015), which were based on DASH-SP (differential aerosol sizing and hygroscopicity spectrometer probe) with a residence time of 4 s. The measured GFs among different studies show a strong dependence on the residence time of different instruments. As a viscous compound, the transport of water molecules within the particles and the particles to reach equilibrium with respect to their surrounding humid air might be quite slow. Thus, the short residence time inside the HTDMA may strongly limit the water uptake for aqueous sucrose. This conclusion was reinforced by the result of Rickards et al. (2015), who measured the water transport timescale in sucrose aerosols using aerosol optical tweezers. Water transport within such a viscous particle remained far from equilibrium even after more than 24 h. They also suggested that the diffusion of water or dissolution of sucrose was much faster at high RH levels. We note that our measured GF at high RH conditions, e.g., 90 % RH, was quite close to theirs, suggesting equilibrium at this point (i.e., 90 % RH) might be already reached or that the influence of residence time on the measured hygroscopicity is largely reduced. Therefore, the analysis in Sect. 3.2 using GF at 90 % RH should still be sound.
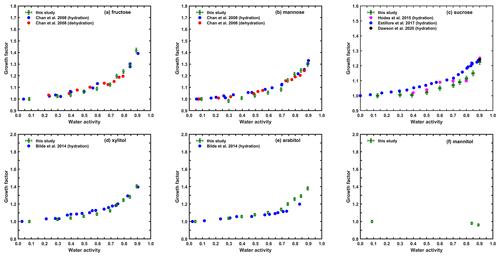
Figure 4Hygroscopic growth factors of sugars (a–c) and sugar alcohols (d–f) and comparison with previous studies.
The results for xylitol and arabitol are qualitatively consistent with the ones measured by Bilde et al. (2014), though they presented their results in an American Geophysical Union (AGU) abstract without detailed explanation or validation. According to the measured GF, both compounds were considered to be more hygroscopic, and they likely existed in liquid state under dry conditions. Mannitol, with a GF of less than unity at 90 % RH from the measured humidogram, can be considered as non-hygroscopic. This was also suggested by previous literature (Ohrem et al., 2014; Martău et al., 2020). However, no practical data were presented in these works, and thus only our measured data are given in Fig. 4f.
3.2 Relating the hygroscopicity of organic compounds to their physicochemical properties
In this section, we explore the effects from different physicochemical properties such as molecular functionality, water solubility, and organic oxidation level that potentially contribute to the observed hygroscopicity κ. According to the comparison with literature data available in Sect. 3.1, our measured growth factor at 90 % RH mostly agrees with or is close to the ones in previous works; therefore, the hygroscopicity parameter κ discussed in this section was converted by using growth factor data measured at 90 % RH.
3.2.1 κ vs. organic functionality
Figure 5a shows the measured hygroscopicity of the 23 organics as a function of carbon number. The functional groups with their corresponding numbers are indicated with colors and symbols. In order to facilitate the comparison of the compounds with the same carbons, the carbons with only one compound are not illustrated. For the studied organic compounds with the same carbon number, the hygroscopicity was increased via the addition of extra functional groups to the carbon backbone. For instance, maleic, malic, tartaric, and aspartic acid with extra functional groups (e.g., C=C, −OH and −NH2) with respect to succinic acid with only two −COOHs are more hygroscopic. For C7 compounds, adding an carboxylic acid group to the carbon backbone leads to an elevated hygroscopicity from pimelic to tricarboxylic acid. Moreover, organic compounds with the same carbon numbers but different molecular functionality presented quite distinct hygroscopicity. For example, for C3 compounds, when replacing the −CH3 with an −OH or replacing the −OH group with an −COOH in their parental molecules, the hygroscopicity was significantly increased. Taking another example from C4 compounds, the organics with a hydroxyl group (−OH) instead of an −NH2 or with a double bond (C=C) instead of the hydroxyl group in their carbon backbones were more hygroscopic. Similar differences in hygroscopicity were also observed between aconitic acid (C6) with a C=C and citric acid (C6) with an −OH. By summarizing the results in current study, κ increased with the functionality in the following order: (−CH3 or−NH2) < (−OH) < (−COOH or C=C or C=O). However, it has to be noted that this comparison is quite qualitative and might be ambiguous, and thus further evidence from other organic compounds is needed in order to drive a more general conclusion. Suda et al. (2012) and Chen et al. (2019) concluded that the hygroscopicity of organic compounds is closely related to their individual polarity and that highly polar compounds are usually more hygroscopic. Kier (1981) ranked the polarity of different functional groups in the sequence of −CH3 <−NH2 < −OH < −CHO < -NH2OH < −COOH, which could explain the difference in the hygroscopicity of organics with various functionalities in our study.
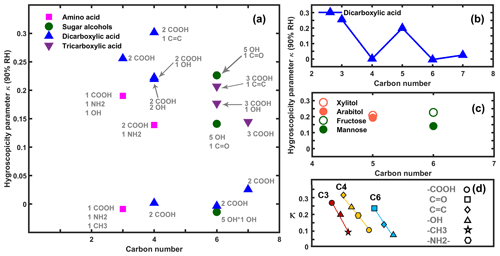
Figure 5Hygroscopicity of organics as a function of carbon number (a), hygroscopicity of dicarboxylic acids vs. carbon number (b), hygroscopicity of isomers (c), and organic hygroscopicity as a function of functionality with the same carbon number (d).
Figure 5b shows that the measured hygroscopicity of the straight-chain dicarboxylic acids alternates with the parity of the carbon numbers. It has to be noted that data of glutaric acid (C5) are quoted from Chan et al. (2008). Bilde et al. (2003) observed an alternation in the volatility of dicarboxylic acids with the number of carbon atoms similar to the ones we observed for their hygroscopicity. They attributed this to the alternation in the molar enthalpies of fusion of those compounds. Moreover, we observed that some compounds (xylitol vs. L-arabitol and fructose vs. mannose) share the same molecular formula or functionality but vary differently in regard to hygroscopicity, as shown in Fig. 5c. Both findings suggest that other physicochemical properties of organics besides molecular functionality may also contribute to the observed variation in their hygroscopicity. Previous studies (Marcolli and Peter, 2005; Petters et al., 2017) reported that the position of the functional groups could influence the hygroscopicity properties of organic compounds. For instance, Petters et al. (2017) suggested that organic molecules with the hydroperoxyl group close the end of carbon chain were more hygroscopic. Similarly, fructose observed in our study, with its hydroxyl group in the tail of the carbon chain and which is far away from the C=O group, is more hygroscopic than mannose (for which these two groups are much closer to each other).
3.2.2 κ vs. water solubility and molar volume
Previous studies suggested that for highly soluble compounds that are fully dissolved in the aqueous droplet, their hygroscopicity is mainly controlled by their molar volume (), while for slightly soluble compounds, their hygroscopicity is limited by their low water solubility (Petters et al., 2009; Kuwata et al., 2013; Nakao, 2017; Wang et al., 2019). Hence, we considered two regimes in our study: (A) compounds that fully dissolved (defined in this work as highly soluble with a solubility g mL−1, i.e., a regime that is not saturated) and (B) compounds that are not fully dissolved (defined in this work as slightly or sparingly soluble compounds with a solubility in the range between and g mL−1, i.e., a regime that is saturated) in the aqueous droplets under 90 % RH condition. In regime A, as shown in Fig. 6, the hygroscopicity decreases with increasing molar volume. Besides molar volume, the van't Hoff factor (i), which accounts for the degree of dissociation of a compound in water, could also contribute to the overall hygroscopicity for fully dissolved compounds. Sugars, as non-electrolytes with a van't Hoff factor of 1, do not dissociate in aqueous solutions (Giebl et al., 2002; Koehler et al., 2006; Rosenørn et al., 2006) and thus are less hygroscopic than the dicarboxylic acids that can dissociate in water and contribute to the reduction in water activity. Frosch et al. (2010) related the van't Hoff factor with the pKa values for a series of carboxylic acids and found that the stronger the acid with smaller values of pKa, the larger the van't Hoff factor. This could explain why maleic acid, even with a larger molar volume but smaller pKa value (1.8), is more hygroscopic than malonic acid (pKa=2.4).
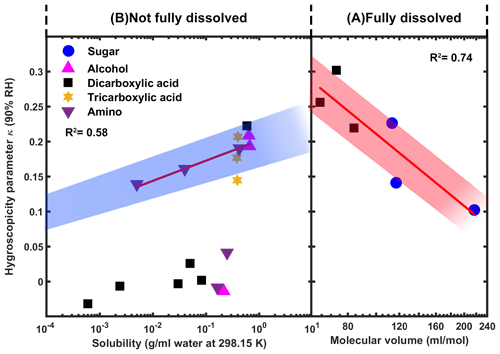
Figure 6Hygroscopicity of organic compounds as a function of molecular volume (A) and solubility (B).
Organic compounds with low water solubility (regime B) can clearly divided into two categories according to their hygroscopicity. One is non-hygroscopic or almost non-hygroscopic organics with κ close or equal to 0. These organics might present at solid or crystalline state and did not deliquesce at our measurement conditions during the whole RH range. Thus, their hygroscopicity is not only limited by their low water solubility but also their phase state and the energy that is needed for the phase transition. Compared to these non-hygroscopic slightly or sparingly soluble organic compounds, there are some other slightly or sparingly soluble organics that show moderate water uptake with κ values larger than 0.1. These organics with limited solubility may already partially deliquesce under our studied RH conditions (Hartz et al., 2006; Chan et al., 2008), and we found that their hygroscopicity increases with water solubility. Thus, it is physically reasonable that the aqueous droplet of these organics with limited solubility be considered as being composed of an effectively insoluble core with a saturated solution. The organic compound with the higher water solubility would dissolve more and have a higher molar concentration in the saturated solution. The higher molar concentration corresponds to a stronger reduction in water activity, which would lead the particles to become more hygroscopic.
3.2.3 κ vs. O:C ratio
Previous studies have suggested that the hygroscopicity parameter of organic species (κorg) is closely related to their O:C ratios (Jimenez et al., 2009; Chang et al., 2010; Massoli et al., 2010; Cappa et al., 2011; Lambe et al., 2011; Kuwata et al., 2013; Rickards et al., 2013). In this study, we plotted our measured κ of the 23 organic compounds with their O:C ratios in Fig. 7, and for a wider atmospheric implication we compared them against previous results obtained from different atmospheric environments (Mei et al., 2013; Wu et al., 2013, 2016; Hong et al., 2015, 2018; Deng et al., 2018; Kuang et al., 2020). Clearly, ambient organics show much lower O:C values, as seen in Fig. 7. Ng et al. (2010) compiled the measured O:C data from different environments and concluded that ambient organic aerosols mainly consist of oxygenated organic material (OOA) and hydrocarbon-like organic material (HOA) at most sites. HOA, which arises from vehicle emissions, is the least oxidized, with an average O:C value of less than 0.2 (Ng et al., 2010; Xu et al., 2015, 2016; Cao et al., 2019; Li et al., 2020). Hence, with the inclusion of HOA in ambient aerosols, the average O:C value of the bulk organic is less than 1, being generally lower than our laboratory-generated aerosols.
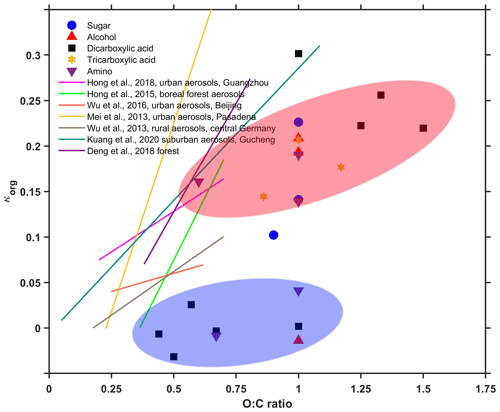
Figure 7Correlation between O:C ratio and κorg and comparison with previous literature results. Blue and red shades represent the fitting of results of non-hygroscopic and more hygroscopic organics, respectively.
A general trend showing an increase in κorg with increasing O:C has also been observed for laboratory results, but the correlation between κ and O:C falls into two categories. One is a non-hygroscopic organic group with a weak O:C dependence, which is shown in blue in Fig. 7. We have already suggested that these compounds with limited water solubility might not yet deliquesce under 90 % RH. The other slightly or sparingly soluble organics (shown in red in Fig. 7) are a moderately hygroscopic group with a slightly stronger O:C dependence. However, the correlation of both categories is not good, which may be affected by the other properties discussed above. Compared to those laboratory-generated pure organic compounds, ambient organics are more complex, with divergent O:C-dependent hygroscopicity in different environments. For instance, the hygroscopicity of urban aerosols in Beijing was almost constant, being less sensitive to the variations of the organic oxidation level, which is similar to our non-hygroscopic organics (Wu et al., 2016). In contrast, suburban aerosols in central Germany (Wu et al., 2013) and Guangzhou (Hong et al., 2018) exhibit a slightly stronger influence from their O:C ratio, being close to the behavior (slope≈0.12) of the moderate hygroscopic organics with relative higher water solubilities in our study. As discussed in previous works (Rickards et al., 2013), some of the laboratory-generated pure organics share identical O:C ratios but differ widely in hygroscopicity. However, no molecule-specific information could be concluded further for those ambient organics. This also indicates that large uncertainties may arise from the approximation of organic hygroscopicity based on their atomic O:C ratio for ambient aerosols. The use of a simplified average property (i.e., O:C ratio) to describe the hygroscopicity of ambient organics, whose makeup may be complex, is quite risky, as compounds with a similar O:C ratio may vary considerably in terms of hygroscopicity. Additional measurements of other properties (e.g., functionality or water solubility) may be difficult due to both the highly complex mixture of ambient aerosols and technique limitations. However, laboratory-generated surrogate mixtures representing the complexity of ambient aerosols should at least be examined to test the variety in the relationship between the O:C ratio and κ.
Our laboratory observations show some deviations with previous literature data measured using similar techniques with a different residence time or a completely different method. The residence time of humidification inside the instrument was suggested to be one of the reasons for this deviation. We noticed that some compounds with a longer time of humidification show higher GF values (e.g., sucrose at intermediate RHs). This is likely due to the kinetic limitations of water uptake, which is particularly important for viscous aerosols. This discrepancy further indicates the limitations of the current method, showing that extra care should be taken in the future and that viscosity information should always be taken into account when dealing with hygroscopicity data. However, for some other compounds, the techniques with a longer residence time are not always obtained the higher GF values. For instance, using STXM (scanning transmission x-ray microscopy) with a residence time that is much longer than our HTDMA, Piens et al. (2016) obtained a lower GF of fructose compared to our results, and the evaporation loss may not be caused by its low volatility. Moreover, for glutamine and serine particles, no deliquescence was observed by Chan et al. (2005) with a residence time longer than 40 min, while in our study a moderate water uptake for both compounds was observed. This evidence shows, for a large suite of different compounds with various complexities, that the influence of residence time on the observed water uptake of particles might not be conclusive. We may need to better understand the kinetics of water transport within particles and figure out which factors could introduce this variability (e.g., measurement timescale, the choice of compounds, and environmental conditions) before designing the measurement targeting the effect of residence time on aerosol hygroscopicity and eventually drawing conclusions.
The different phase transition observed between our study and the ones using the EDB (Chan et al., 2005), for instance for glutamine and serine, reveals other limitations of the current instrumentation in this study. One is that the drying process might not be sufficient. The inadequate drying may not be able to crystallize the generated aerosols if their ERH values are quite low. This will interfere with the generation of the correct phase state we require. The other limitation is that higher relative humidity conditions, e.g., >90 %, are difficult to access. We notice that many organic compounds have their DRH higher than 90 % RH, and thus measurements at those conditions are quite scarce. Therefore, different means, including both raising the drying capacity and making the elevated RH condition achievable, are all methods we should consider to break these limitations and improve the system.
On the other hand, these deviations also reveal that measurement of size-resolved particles, particularly relevant for atmospheric conditions, is still needed. As we notice, many of the data in the literature were obtained from bulk samples or particles in the micrometer size range. Previous studies pointed out that thermodynamic data of bulk samples or particles in the micrometer size range may not always represent the ones in the nanometer range, particularly in the size range of the atmospheric aerosol accumulation mode (e.g., ∼102 nm). For instance, Cheng et al. (2015) suggested that particle size can be an important factor influencing the solid–liquid equilibrium upon phase transition. Pöschl et al. (2015) and Reid et al. (2018) reported that thermodynamic properties of aerosols are strongly related to particle size (e.g., viscosity or diffusion coefficient). This could be another potential reason for the deviation between ours data and those of the literature. On the other hand, Rickards et al. (2015) concluded that the timescale of equilibrium for water transport inside particles is also size dependent. Larger particles need much more time to reach equilibrium than smaller particles. Thus, the aforementioned objective, i.e., investigating the effect of residence time on aerosol hygroscopicity, should also take the influence of particle size into account. Therefore, different approaches with capabilities accessing different particle size ranges, requiring different sample volumes, are still needed.
In the previous section discussing the roles that different physicochemical properties of organics play in aerosol hygroscopicity, the parameter κ was converted by the measured GF under 90 % RH. For those low-solubility or sparingly soluble organic compounds, the GF-derived κ (also known as apparent κ) is RH dependent (see Fig. S3 in the Supplement as an example) and cannot express their intrinsic κ (expressed by fully dissolved compounds) when compounds are sufficiently soluble in water. As the RH increases, further dissolution of these organic compounds with promoted hygroscopicity is expected. In the real atmosphere, different RH conditions, including both subsaturation and supersaturation can be reached. The measured GF or the apparent κ of ambient aerosols at a certain RH may not be able to reveal their real hygroscopicity under various atmospheric conditions. Further calculations of other variables such as the liquid water content (LWC), surface area of wet particles, and number concentration of CCN associated with the apparent κ will be significantly biased. If possible, hygroscopicity measurements over large saturation range up to supersaturation, especially with a combination of CCN measurements, provide an option to reduce these uncertainties. Therefore, a combination of different instruments, despite having different limitations to consider, should not be discarded but should instead be innovated upon.
The data used in this paper can be obtained from the corresponding author upon request.
A detailed description of the HTDMA implementation and the use of the hygroscopicity parameter κ as a function of RH for sparingly soluble organics can be found in the Supplement. The supplement related to this article is available online at: https://doi.org/10.5194/acp-22-3985-2022-supplement.
SH did the data analysis, plotted the figures, and wrote the original draft. JH collected the data, planned the study, and wrote and reviewed the manuscript. NM planned the study and reviewed the manuscript. HBX and HBT contributed to the instrumentation. JCT, YQZ, LP, and YH contributed to the discussion of the results. QQW, YFC, and HS contributed to fund acquisition. QWL and JNS contributed to data collection and data analysis.
At least one of the (co-)authors is a member of the editorial board of Atmospheric Chemistry and Physics. The peer-review process was guided by an independent editor, and the authors also have no other competing interests to declare.
Publisher's note: Copernicus Publications remains neutral with regard to jurisdictional claims in published maps and institutional affiliations.
This research has been supported by the National Natural Science Foundation of China (grant nos. 42175117 and 41877303), Special Fund Project for Science and Technology Innovation Strategy of Guangdong Province (grant no. 2019B121205004), and Guangdong Innovative and Entrepreneurial Research Team Program (grant no. 2016ZT06N263).
This paper was edited by Markus Ammann and reviewed by two anonymous referees.
Apelblat, A., Dov, M., Wisniak, J., and Zabicky, J.: The vapour pressure of water over saturated aqueous solutions of malic, tartaric, and citric acids, at temperatures from 288 K to 323 K, J. Chem. Thermodyn., 27, 35–41, https://doi.org/10.1006/jcht.1995.0004, 1995.
Bilde, M., Svenningsson, B., Mønster, J., and Rosenørn, T.: Even–odd alternation of evaporation rates and vapor pressures of C3–C9 dicarboxylic acid aerosols, Environ. Sci. Technol., 37, 1371–1378, https://doi.org/10.1021/es0201810, 2003.
Bilde, M., Zardini, A. A., Hong, J., Tschiskale, M., and Emanuelsson, E.: Atmospheric sugar alcohols: evaporation rates and saturation vapor pressures, AGU Fall Meeting Abstracts, A21K-3178, https://www.researchgate.net/publication/275890630 (last access: 16 March 2022), 2014.
Braban, C. F., Carroll, M. F., Styler, S. A., and Abbatt, J. P.: Phase transitions of malonic and oxalic acid aerosols, J. Phys. Chem. A, 107, 6594–6602, https://doi.org/10.1021/jp034483f, 2003.
Cao, L.-M., Huang, X.-F., Wang, C., Zhu, Q., and He, L.-Y.: Characterization of submicron aerosol volatility in the regional atmosphere in southern China, Chemosphere, 236, 124383, https://doi.org/10.1016/j.chemosphere.2019.124383, 2019.
Cappa, C. D., Che, D. L., Kessler, S. H., Kroll, J. H., and Wilson, K. R.: Variations in organic aerosol optical and hygroscopic properties upon heterogeneous OH oxidation, J. Geophys. Res.-Atmos., 116, D15204, https://doi.org/10.1029/2011JD015918, 2011.
Chan, M. N., Choi, M. Y., Ng, N. L., and Chan, C. K.: Hygroscopicity of water-soluble organic compounds in atmospheric aerosols: Amino acids and biomass burning derived organic species, Environ. Sci. Technol., 39, 1555–1562, https://doi.org/10.1021/es049584l, 2005.
Chan, M. N., Kreidenweis, S. M., and Chan, C. K.: Measurements of the hygroscopic and deliquescence properties of organic compounds of different solubilities in water and their relationship with cloud condensation nuclei activities, Environ. Sci. Technol., 42, 3602–3608, https://doi.org/10.1021/es7023252, 2008.
Chang, R. Y.-W., Slowik, J. G., Shantz, N. C., Vlasenko, A., Liggio, J., Sjostedt, S. J., Leaitch, W. R., and Abbatt, J. P. D.: The hygroscopicity parameter (κ) of ambient organic aerosol at a field site subject to biogenic and anthropogenic influences: relationship to degree of aerosol oxidation, Atmos. Chem. Phys., 10, 5047–5064, https://doi.org/10.5194/acp-10-5047-2010, 2010.
Chebbi, A. and Carlier, P.: Carboxylic acids in the troposphere, occurrence, sources, and sinks: A review, Atmos. Environ., 30, 4233–4249, https://doi.org/10.1016/1352-2310(96)00102-1, 1996.
Chen, J., Lee, W.-C., Itoh, M., and Kuwata, M.: A significant portion of water-soluble organic matter in fresh biomass burning particles does not contribute to hygroscopic growth: an application of polarity segregation by 1-Octanol–water partitioning method, Environ. Sci. Technol., 53, 10034–10042, https://doi.org/10.1021/acs.est.9b01696, 2019.
Cheng, Y., Wiedensohler, A., Eichler, H., Heintzenberg, J., Tesche, M., Ansmann, A., Wendisch, M., Su, H., Althausen, D., and Herrmann, H.: Relative humidity dependence of aerosol optical properties and direct radiative forcing in the surface boundary layer at Xinken in Pearl River Delta of China: An observation based numerical study, Atmos. Environ., 42, 6373–6397, https://doi.org/10.1016/j.atmosenv.2008.04.009, 2008.
Cheng, Y., Su, H., Koop, T., Mikhailov, E., and Pöschl, U.: Size dependence of phase transitions in aerosol nanoparticles, Nat. Commun., 6, 1–7, https://doi.org/10.1038/ncomms6923, 2015.
Choi, M. Y. and Chan, C. K.: Continuous measurements of the water activities of aqueous droplets of water-soluble organic compounds, J. Phys. Chem. A, 106, 4566–4572, https://doi.org/10.1021/jp013875o, 2002.
Clegg, S. L. and Seinfeld, J. H.: Thermodynamic models of aqueous solutions containing inorganic electrolytes and dicarboxylic acids at 298.15 K. 1. The acids as nondissociating components, J. Phys. Chem. A, 110, 5692–5717, https://doi.org/10.1021/jp056149k, 2006.
Clegg, S. L., Brimblecombe, P., and Wexler, A. S.: Thermodynamic model of the system H+––Na+–––Cl−–H2O at 298.15 K, J. Phys. Chem. A, 102, 2155–2171, https://doi.org/10.1021/jp973043j, 1998.
Cook, L.: Investigation of the hygroscopic and morphological properties of atmospheric aerosols, https://digitalcommons.bucknell.edu/masters_theses/13 (last access: 16 March 2022), 2011.
Darr, J. P., Gottuso, S., Alfarra, M., Birge, D., Ferris, K., Woods, D., Morales, P., Grove, M., Mitts, W. K., and Mendoza-Lopez, E.: The Hydropathy Scale as a Gauge of Hygroscopicity in Sub-Micron Sodium Chloride-Amino Acid Aerosols, J. Phys. Chem. A, 122, 8062–8070, https://doi.org/10.1021/acs.jpca.8b07119, 2018.
Dawson, J. N., Malek, K. A., Razafindrambinina, P. N., Raymond, T. M., Dutcher, D. D., Asa-Awuku, A. A., and Freedman, M. A.: Direct Comparison of the Submicron Aerosol Hygroscopicity of Water-Soluble Sugars, ACS Earth and Space Chemistry, 4, 2215–2226, https://doi.org/10.1021/acsearthspacechem.0c00159, 2020.
Deng, Y., Kagami, S., Ogawa, S., Kawana, K., Nakayama, T., Kubodera, R., Adachi, K., Hussein, T., Miyazaki, Y., and Mochida, M.: Hygroscopicity of Organic Aerosols and Their Contributions to CCN Concentrations Over a Midlatitude Forest in Japan, J. Geophys. Res.-Atmos., 123, 9703–9723, https://doi.org/10.1029/2017JD027292, 2018.
Dinar, E., Anttila, T., and Rudich, Y.: CCN activity and hygroscopic growth of organic aerosols following reactive uptake of ammonia, Environ. Sci. Technol., 42, 793–799, https://doi.org/10.1021/es071874p, 2008.
Duplissy, J., Gysel, M., Sjogren, S., Meyer, N., Good, N., Kammermann, L., Michaud, V., Weigel, R., Martins dos Santos, S., Gruening, C., Villani, P., Laj, P., Sellegri, K., Metzger, A., McFiggans, G. B., Wehrle, G., Richter, R., Dommen, J., Ristovski, Z., Baltensperger, U., and Weingartner, E.: Intercomparison study of six HTDMAs: results and recommendations, Atmos. Meas. Tech., 2, 363–378, https://doi.org/10.5194/amt-2-363-2009, 2009.
Estillore, A. D., Morris, H. S., Or, V. W., Lee, H. D., Alves, M. R., Marciano, M. A., Laskina, O., Qin, Z., Tivanski, A. V., and Grassian, V. H.: Linking hygroscopicity and the surface microstructure of model inorganic salts, simple and complex carbohydrates, and authentic sea spray aerosol particles, Phys. Chem. Chem. Phys., 19, 21101–21111, https://doi.org/10.1039/C7CP04051B, 2017.
Frosch, M., Zardini, A. A., Platt, S. M., Müller, L., Reinnig, M.-C., Hoffmann, T., and Bilde, M.: Thermodynamic properties and cloud droplet activation of a series of oxo-acids, Atmos. Chem. Phys., 10, 5873–5890, https://doi.org/10.5194/acp-10-5873-2010, 2010.
Giebl, H., Berner, A., Reischl, G., Puxbaum, H., Kasper-Giebl, A., and Hitzenberger, R.: CCN activation of oxalic and malonic acid test aerosols with the University of Vienna cloud condensation nuclei counter, J. Aerosol Sci., 33, 1623–1634, https://doi.org/10.1016/S0021-8502(02)00115-5, 2002.
Gregson, F., Robinson, J., Miles, R., Royall, C., and Reid, J.: Drying and Crystallization of Evaporating Sodium Nitrate Aerosol Droplets, J. Phys. Chem. B, 124, 6024–6036, https://doi.org/10.1021/acs.jpcb.0c04079, 2020.
Hartz, K. E. H., Tischuk, J. E., Chan, M. N., Chan, C. K., Donahue, N. M., and Pandis, S. N.: Cloud condensation nuclei activation of limited solubility organic aerosol, Atmos. Environ., 40, 605–617, https://doi.org/10.1016/j.atmosenv.2005.09.076, 2006.
Hodas, N., Zuend, A., Mui, W., Flagan, R. C., and Seinfeld, J. H.: Influence of particle-phase state on the hygroscopic behavior of mixed organic–inorganic aerosols, Atmos. Chem. Phys., 15, 5027–5045, https://doi.org/10.5194/acp-15-5027-2015, 2015.
Hong, J., Kim, J., Nieminen, T., Duplissy, J., Ehn, M., Äijälä, M., Hao, L. Q., Nie, W., Sarnela, N., Prisle, N. L., Kulmala, M., Virtanen, A., Petäjä, T., and Kerminen, V.-M.: Relating the hygroscopic properties of submicron aerosol to both gas- and particle-phase chemical composition in a boreal forest environment, Atmos. Chem. Phys., 15, 11999–12009, https://doi.org/10.5194/acp-15-11999-2015, 2015.
Hong, J., Xu, H., Tan, H., Yin, C., Hao, L., Li, F., Cai, M., Deng, X., Wang, N., Su, H., Cheng, Y., Wang, L., Petäjä, T., and Kerminen, V.-M.: Mixing state and particle hygroscopicity of organic-dominated aerosols over the Pearl River Delta region in China, Atmos. Chem. Phys., 18, 14079–14094, https://doi.org/10.5194/acp-18-14079-2018, 2018.
Jimenez, J. L., Canagaratna, M., Donahue, N., Prevot, A., Zhang, Q., Kroll, J. H., DeCarlo, P. F., Allan, J. D., Coe, H., and Ng, N.: Evolution of organic aerosols in the atmosphere, Science, 326, 1525–1529, https://doi.org/10.1126/science.1180353, 2009.
Jing, B., Tong, S., Liu, Q., Li, K., Wang, W., Zhang, Y., and Ge, M.: Hygroscopic behavior of multicomponent organic aerosols and their internal mixtures with ammonium sulfate, Atmos. Chem. Phys., 16, 4101–4118, https://doi.org/10.5194/acp-16-4101-2016, 2016.
Kier, L. B.: Quantitation of solvent polarity based on molecular structure, J. Pharm. Sci., 70, 930–933, https://doi.org/10.1002/jps.2600700825, 1981.
Koehler, K. A., Kreidenweis, S. M., DeMott, P. J., Prenni, A. J., Carrico, C. M., Ervens, B., and Feingold, G.: Water activity and activation diameters from hygroscopicity data – Part II: Application to organic species, Atmos. Chem. Phys., 6, 795–809, https://doi.org/10.5194/acp-6-795-2006, 2006.
Kuang, Y., Xu, W., Tao, J., Ma, N., Zhao, C., and Shao, M.: A Review on Laboratory Studies and Field Measurements of Atmospheric Organic Aerosol Hygroscopicity and Its Parameterization Based on Oxidation Levels, Curr. Pollut. Rep., 6, 410–424, https://doi.org/10.1007/s40726-020-00164-2, 2020.
Kundu, S., Kawamura, K., Andreae, T. W., Hoffer, A., and Andreae, M. O.: Molecular distributions of dicarboxylic acids, ketocarboxylic acids and α-dicarbonyls in biomass burning aerosols: implications for photochemical production and degradation in smoke layers, Atmos. Chem. Phys., 10, 2209–2225, https://doi.org/10.5194/acp-10-2209-2010, 2010.
Kuwata, M., Shao, W., Lebouteiller, R., and Martin, S. T.: Classifying organic materials by oxygen-to-carbon elemental ratio to predict the activation regime of Cloud Condensation Nuclei (CCN), Atmos. Chem. Phys., 13, 5309–5324, https://doi.org/10.5194/acp-13-5309-2013, 2013.
Lambe, A. T., Onasch, T. B., Massoli, P., Croasdale, D. R., Wright, J. P., Ahern, A. T., Williams, L. R., Worsnop, D. R., Brune, W. H., and Davidovits, P.: Laboratory studies of the chemical composition and cloud condensation nuclei (CCN) activity of secondary organic aerosol (SOA) and oxidized primary organic aerosol (OPOA), Atmos. Chem. Phys., 11, 8913–8928, https://doi.org/10.5194/acp-11-8913-2011, 2011.
Lei, T., Zuend, A., Cheng, Y., Su, H., Wang, W., and Ge, M.: Hygroscopicity of organic surrogate compounds from biomass burning and their effect on the efflorescence of ammonium sulfate in mixed aerosol particles, Atmos. Chem. Phys., 18, 1045–1064, https://doi.org/10.5194/acp-18-1045-2018, 2018.
Li, J., Liu, Z., Gao, W., Tang, G., Hu, B., Ma, Z., and Wang, Y.: Insight into the formation and evolution of secondary organic aerosol in the megacity of Beijing, China, Atmos. Environ., 220, 117070, https://doi.org/10.1016/j.atmosenv.2019.117070, 2020.
Ma, S., Pang, S., Li, J., and Zhang, Y.: A review of efflorescence kinetics studies on atmospherically relevant particles, Chemosphere, 130320, https://doi.org/10.1016/j.chemosphere.2021.130320, 2021.
Marcolli, C. and Peter, Th.: Water activity in polyol/water systems: new UNIFAC parameterization, Atmos. Chem. Phys., 5, 1545–1555, https://doi.org/10.5194/acp-5-1545-2005, 2005.
Marcolli, C., Luo, B., and Peter, T.: Mixing of the organic aerosol fractions: Liquids as the thermodynamically stable phases, J. Phys. Chem. A, 108, 2216–2224, https://doi.org/10.1021/jp036080l, 2004.
Marsh, A., Miles, R. E. H., Rovelli, G., Cowling, A. G., Nandy, L., Dutcher, C. S., and Reid, J. P.: Influence of organic compound functionality on aerosol hygroscopicity: dicarboxylic acids, alkyl-substituents, sugars and amino acids, Atmos. Chem. Phys., 17, 5583–5599, https://doi.org/10.5194/acp-17-5583-2017, 2017.
Martău, G. A., Coman, V., and Vodnar, D. C.: Recent advances in the biotechnological production of erythritol and mannitol, Crit. Rev. Biotechnol., 40, 608–622, https://doi.org/10.1080/07388551.2020.1751057, 2020.
Massoli, P., Lambe, A., Ahern, A., Williams, L., Ehn, M., Mikkilä, J., Canagaratna, M., Brune, W., Onasch, T., and Jayne, J.: Relationship between aerosol oxidation level and hygroscopic properties of laboratory generated secondary organic aerosol (SOA) particles, Geophys. Res. Lett., 37, L24801, https://doi.org/10.1029/2010GL045258, 2010.
McFiggans, G., Artaxo, P., Baltensperger, U., Coe, H., Facchini, M. C., Feingold, G., Fuzzi, S., Gysel, M., Laaksonen, A., Lohmann, U., Mentel, T. F., Murphy, D. M., O'Dowd, C. D., Snider, J. R., and Weingartner, E.: The effect of physical and chemical aerosol properties on warm cloud droplet activation, Atmos. Chem. Phys., 6, 2593–2649, https://doi.org/10.5194/acp-6-2593-2006, 2006.
Mei, F., Hayes, P. L., Ortega, A., Taylor, J. W., Allan, J. D., Gilman, J., Kuster, W., de Gouw, J., Jimenez, J. L., and Wang, J.: Droplet activation properties of organic aerosols observed at an urban site during CalNex-LA, J. Geophys. Res.-Atmos., 118, 2903–2917, https://doi.org/10.1002/jgrd.50285, 2013.
Mikhailov, E., Vlasenko, S., Niessner, R., and Pöschl, U.: Interaction of aerosol particles composed of protein and saltswith water vapor: hygroscopic growth and microstructural rearrangement, Atmos. Chem. Phys., 4, 323–350, https://doi.org/10.5194/acp-4-323-2004, 2004.
Mikhailov, E., Vlasenko, S., Martin, S. T., Koop, T., and Pöschl, U.: Amorphous and crystalline aerosol particles interacting with water vapor: conceptual framework and experimental evidence for restructuring, phase transitions and kinetic limitations, Atmos. Chem. Phys., 9, 9491–9522, https://doi.org/10.5194/acp-9-9491-2009, 2009.
Mochida, M. and Kawamura, K.: Hygroscopic properties of levoglucosan and related organic compounds characteristic to biomass burning aerosol particles, J. Geophys. Res.-Atmos., 109, D21202, https://doi.org/10.1029/2004jd004962, 2004.
Mochida, M., Kawabata, A., Kawamura, K., Hatsushika, H., and Yamazaki, K.: Seasonal variation and origins of dicarboxylic acids in the marine atmosphere over the western North Pacific, J. Geophys. Res.-Atmos., 108, 4193, https://doi.org/10.1029/2002JD002355, 2003.
Nakao, S.: Why would apparent κ linearly change with ? Assessing the role of volatility, solubility, and surface activity of organic aerosols, Aerosol Sci. Tech., 51, 1377–1388, https://doi.org/10.1080/02786826.2017.1352082, 2017.
Nakao, S., Suda, S. R., Camp, M., Petters, M. D., and Kreidenweis, S. M.: Droplet activation of wet particles: development of the Wet CCN approach, Atmos. Meas. Tech., 7, 2227–2241, https://doi.org/10.5194/amt-7-2227-2014, 2014.
Ng, N. L., Canagaratna, M. R., Zhang, Q., Jimenez, J. L., Tian, J., Ulbrich, I. M., Kroll, J. H., Docherty, K. S., Chhabra, P. S., Bahreini, R., Murphy, S. M., Seinfeld, J. H., Hildebrandt, L., Donahue, N. M., DeCarlo, P. F., Lanz, V. A., Prévôt, A. S. H., Dinar, E., Rudich, Y., and Worsnop, D. R.: Organic aerosol components observed in Northern Hemispheric datasets from Aerosol Mass Spectrometry, Atmos. Chem. Phys., 10, 4625–4641, https://doi.org/10.5194/acp-10-4625-2010, 2010.
Ohm, P. B., Asato, C., Wexler, A. S., and Dutcher, C. S.: Isotherm-Based Thermodynamic Model for Electrolyte and Nonelectrolyte Solutions Incorporating Long-and Short-Range Electrostatic Interactions, J. Phys. Chem. A, 119, 3244–3252, https://doi.org/10.1021/jp512646k, 2015.
Ohrem, H. L., Schornick, E., Kalivoda, A., and Ognibene, R.: Why is mannitol becoming more and more popular as a pharmaceutical excipient in solid dosage forms?, Pharm. Dev. Technol., 19, 257–262, https://doi.org/10.3109/10837450.2013.775154, 2014.
Peng, C., Chan, M. N., and Chan, C. K.: The hygroscopic properties of dicarboxylic and multifunctional acids: Measurements and UNIFAC predictions, Environ. Sci. Technol., 35, 4495–4501, https://doi.org/10.1021/es0107531, 2001.
Petters, M., Kreidenweis, S., Prenni, A., Sullivan, R., Carrico, C., Koehler, K. A., and Ziemann, P.: Role of molecular size in cloud droplet activation, Geophys. Res. Lett., 36, L22801, https://doi.org/10.1029/2009GL040131, 2009.
Petters, M. D. and Kreidenweis, S. M.: A single parameter representation of hygroscopic growth and cloud condensation nucleus activity, Atmos. Chem. Phys., 7, 1961–1971, https://doi.org/10.5194/acp-7-1961-2007, 2007.
Petters, S. S., Pagonis, D., Claflin, M. S., Levin, E. J., Petters, M. D., Ziemann, P. J., and Kreidenweis, S. M.: Hygroscopicity of organic compounds as a function of carbon chain length and carboxyl, hydroperoxy, and carbonyl functional groups, J. Phys. Chem. A, 121, 5164–5174, https://doi.org/10.1021/acs.jpca.7b04114, 2017.
Piens, D. S., Kelly, S. T., Harder, T. H., Petters, M. D., O'Brien, R. E., Wang, B., Teske, K., Dowell, P., Laskin, A., and Gilles, M. K.: Measuring mass-based hygroscopicity of atmospheric particles through in situ imaging, Environ. Sci. Technol., 50, 5172–5180, https://doi.org/10.1021/acs.est.6b00793, 2016.
Pöschl, U. and Shiraiwa, M.: Multiphase chemistry at the atmosphere–biosphere interface influencing climate and public health in the anthropocene, Chem. Rev., 115, 4440–4475, https://doi.org/10.1021/cr500487s, 2015.
Prenni, A. J., DeMott, P. J., Kreidenweis, S. M., Sherman, D. E., Russell, L. M., and Ming, Y.: The effects of low molecular weight dicarboxylic acids on cloud formation, J. Phys. Chem. A, 105, 11240–11248, https://doi.org/10.1021/jp012427d, 2001.
Prenni, A. J., Petters, M. D., Kreidenweis, S. M., DeMott, P. J., and Ziemann, P. J.: Cloud droplet activation of secondary organic aerosol, J. Geophys. Res.-Atmos., 112, D10223, https://doi.org/10.1029/2006JD007963, 2007.
Randall, D. A., Wood, R. A., Bony, S., Colman, R., Fichefet, T., Fyfe, J., Kattsov, V., Pitman, A., Shukla, J., and Srinivasan, J.: Climate models and their evaluation, in: Climate change 2007: The physical science basis. Contribution of Working Group I to the Fourth Assessment Report of the IPCC (FAR), Cambridge University Press, 589–662, ISBN 978052188009-1, 2007.
Reid, J. P., Bertram, A. K., Topping, D. O., Laskin, A., Martin, S. T., Petters, M. D., Pope, F. D., and Rovelli, G.: The viscosity of atmospherically relevant organic particles, Nat. Commun., 9, 1–14, https://doi.org/10.1038/s41467-018-03027-z, 2018.
Rickards, A. M., Miles, R. E., Davies, J. F., Marshall, F. H., and Reid, J. P.: Measurements of the sensitivity of aerosol hygroscopicity and the κ parameter to the O/C ratio, J. Phys. Chem. A, 117, 14120–14131, https://doi.org/10.1021/jp407991n, 2013.
Rickards, A. M., Song, Y.-C., Miles, R. E., Preston, T. C., and Reid, J. P.: Variabilities and uncertainties in characterising water transport kinetics in glassy and ultraviscous aerosol, Phys. Chem. Chem. Phys., 17, 10059–10073, https://doi.org/10.1039/C4CP05383D, 2015.
Rosenørn, T., Kiss, G., and Bilde, M.: Cloud droplet activation of saccharides and levoglucosan particles, Atmos. Environ., 40, 1794–1802, https://doi.org/10.1016/j.atmosenv.2005.11.024, 2006.
Salcedo, D.: Equilibrium phase diagrams of aqueous mixtures of malonic acid and sulfate/ammonium salts, J. Phys. Chem. A, 110, 12158–12165, https://doi.org/10.1021/jp063850v, 2006.
Seinfeld, J. H. and Pandis, S. N.: Atmospheric chemistry and physics: from air pollution to climate change, 3rd edition, John Wiley & Sons, Inc., New York, ISBN 1118947401, 2016.
Su, H., Rose, D., Cheng, Y. F., Gunthe, S. S., Massling, A., Stock, M., Wiedensohler, A., Andreae, M. O., and Pöschl, U.: Hygroscopicity distribution concept for measurement data analysis and modeling of aerosol particle mixing state with regard to hygroscopic growth and CCN activation, Atmos. Chem. Phys., 10, 7489–7503, https://doi.org/10.5194/acp-10-7489-2010, 2010.
Suda, S. R. and Petters, M. D.: Accurate determination of aerosol activity coefficients at relative humidities up to 99 % using the hygroscopicity tandem differential mobility analyzer technique, Aerosol Sci. Tech., 47, 991–1000, https://doi.org/10.1080/02786826.2013.807906, 2013.
Suda, S. R., Petters, M., Matsunaga, A., Sullivan, R., Ziemann, P., and Kreidenweis, S.: Hygroscopicity frequency distributions of secondary organic aerosols, J. Geophys. Res.-Atmos., 117, D04207, https://doi.org/10.1029/2011JD016823, 2012.
Suda, S. R., Petters, M. D., Yeh, G. K., Strollo, C., Matsunaga, A., Faulhaber, A., Ziemann, P. J., Prenni, A. J., Carrico, C. M., and Sullivan, R. C.: Influence of functional groups on organic aerosol cloud condensation nucleus activity, Environ. Sci. Technol., 48, 10182–10190, https://doi.org/10.1021/es502147y, 2014.
Swietlicki, E., Hansson, H. C., Hämeri, K., Svenningsson, B., Massling, A., McFiggans, G., McMurry, P. H., Petäjä, T., Tunved, P., Gysel, M., Topping, D., Weingartner, E., Baltensperger, U., Rissler, J., Wiedensohler, A., and Kulmala, M.: Hygroscopic properties of submicrometer atmospheric aerosol particles measured with H-TDMA instruments in various environments – a review, Tellus B, 60, 432–469, https://doi.org/10.1111/j.1600-0889.2008.00350.x, 2008.
Tan, H. B., Xu, H. B., Wan, Q. L., Li, F., Deng, X. J., Chan, P. W., Xia, D., and Yin, Y.: Design and Application of an Unattended Multifunctional H-TDMA System, J. Atmos. Ocean. Tech., 30, 1136–1148, https://doi.org/10.1175/Jtech-D-12-00129.1, https://doi.org/10.1175/JTECH-D-12-00129.1, 2013.
Tang, M., Chan, C. K., Li, Y. J., Su, H., Ma, Q., Wu, Z., Zhang, G., Wang, Z., Ge, M., Hu, M., He, H., and Wang, X.: A review of experimental techniques for aerosol hygroscopicity studies, Atmos. Chem. Phys., 19, 12631–12686, https://doi.org/10.5194/acp-19-12631-2019, 2019.
Topping, D., Barley, M., Bane, M. K., Higham, N., Aumont, B., Dingle, N., and McFiggans, G.: UManSysProp v1.0: an online and open-source facility for molecular property prediction and atmospheric aerosol calculations, Geosci. Model Dev., 9, 899–914, https://doi.org/10.5194/gmd-9-899-2016, 2016.
Wang, J., Shilling, J. E., Liu, J., Zelenyuk, A., Bell, D. M., Petters, M. D., Thalman, R., Mei, F., Zaveri, R. A., and Zheng, G.: Cloud droplet activation of secondary organic aerosol is mainly controlled by molecular weight, not water solubility, Atmos. Chem. Phys., 19, 941–954, https://doi.org/10.5194/acp-19-941-2019, 2019.
Wang, Z., Jing, B., Shi, X., Tong, S., Wang, W., and Ge, M.: Importance of water-soluble organic acid on the hygroscopicity of nitrate, Atmos. Environ., 190, 65–73, https://doi.org/10.1016/j.atmosenv.2018.07.010, 2018.
Wise, M. E., Surratt, J. D., Curtis, D. B., Shilling, J. E., and Tolbert, M. A.: Hygroscopic growth of ammonium sulfate/dicarboxylic acids, J. Geophys. Res.-Atmos., 108, 4638, https://doi.org/10.1029/2003JD003775, 2003.
Wu, Z. J., Nowak, A., Poulain, L., Herrmann, H., and Wiedensohler, A.: Hygroscopic behavior of atmospherically relevant water-soluble carboxylic salts and their influence on the water uptake of ammonium sulfate, Atmos. Chem. Phys., 11, 12617–12626, https://doi.org/10.5194/acp-11-12617-2011, 2011.
Wu, Z. J., Poulain, L., Henning, S., Dieckmann, K., Birmili, W., Merkel, M., van Pinxteren, D., Spindler, G., Müller, K., Stratmann, F., Herrmann, H., and Wiedensohler, A.: Relating particle hygroscopicity and CCN activity to chemical composition during the HCCT-2010 field campaign, Atmos. Chem. Phys., 13, 7983–7996, https://doi.org/10.5194/acp-13-7983-2013, 2013.
Wu, Z. J., Zheng, J., Shang, D. J., Du, Z. F., Wu, Y. S., Zeng, L. M., Wiedensohler, A., and Hu, M.: Particle hygroscopicity and its link to chemical composition in the urban atmosphere of Beijing, China, during summertime, Atmos. Chem. Phys., 16, 1123–1138, https://doi.org/10.5194/acp-16-1123-2016, 2016.
Xu, J., Shi, J., Zhang, Q., Ge, X., Canonaco, F., Prévôt, A. S. H., Vonwiller, M., Szidat, S., Ge, J., Ma, J., An, Y., Kang, S., and Qin, D.: Wintertime organic and inorganic aerosols in Lanzhou, China: sources, processes, and comparison with the results during summer, Atmos. Chem. Phys., 16, 14937–14957, https://doi.org/10.5194/acp-16-14937-2016, 2016.
Xu, L., Suresh, S., Guo, H., Weber, R. J., and Ng, N. L.: Aerosol characterization over the southeastern United States using high-resolution aerosol mass spectrometry: spatial and seasonal variation of aerosol composition and sources with a focus on organic nitrates, Atmos. Chem. Phys., 15, 7307–7336, https://doi.org/10.5194/acp-15-7307-2015, 2015.
Zhang, Q., Jimenez, J. L., Canagaratna, M., Allan, J. D., Coe, H., Ulbrich, I., Alfarra, M., Takami, A., Middlebrook, A., and Sun, Y.: Ubiquity and dominance of oxygenated species in organic aerosols in anthropogenically-influenced Northern Hemisphere midlatitudes, Geophys. Res. Lett., 34, L13801, https://doi.org/10.1029/2007GL029979, 2007.
Zhang, R., Wang, G., Guo, S., Zamora, M. L., Ying, Q., Lin, Y., Wang, W., Hu, M., and Wang, Y.: Formation of urban fine particulate matter, Chem. Rev., 115, 3803–3855, https://doi.org/10.1021/acs.chemrev.5b00067, 2015.
Zheng, G. J., Duan, F. K., Su, H., Ma, Y. L., Cheng, Y., Zheng, B., Zhang, Q., Huang, T., Kimoto, T., Chang, D., Pöschl, U., Cheng, Y. F., and He, K. B.: Exploring the severe winter haze in Beijing: the impact of synoptic weather, regional transport and heterogeneous reactions, Atmos. Chem. Phys., 15, 2969–2983, https://doi.org/10.5194/acp-15-2969-2015, 2015.
Zuend, A., Marcolli, C., Luo, B. P., and Peter, T.: A thermodynamic model of mixed organic-inorganic aerosols to predict activity coefficients, Atmos. Chem. Phys., 8, 4559–4593, https://doi.org/10.5194/acp-8-4559-2008, 2008.
Zuend, A., Marcolli, C., Booth, A. M., Lienhard, D. M., Soonsin, V., Krieger, U. K., Topping, D. O., McFiggans, G., Peter, T., and Seinfeld, J. H.: New and extended parameterization of the thermodynamic model AIOMFAC: calculation of activity coefficients for organic-inorganic mixtures containing carboxyl, hydroxyl, carbonyl, ether, ester, alkenyl, alkyl, and aromatic functional groups, Atmos. Chem. Phys., 11, 9155–9206, https://doi.org/10.5194/acp-11-9155-2011, 2011.