the Creative Commons Attribution 4.0 License.
the Creative Commons Attribution 4.0 License.
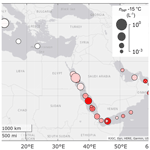
Ice-nucleating particles near two major dust source regions
Thomas C. J. Hill
Paul J. DeMott
Tobias Köneman
Michael Pikridas
Frank Drewnick
Hartwig Harder
Christopher Pöhlker
Jos Lelieveld
Bettina Weber
Minas Iakovides
Roman Prokeš
Jean Sciare
Meinrat O. Andreae
M. Dale Stokes
Kimberly A. Prather
Mineral dust and sea spray aerosol represent important sources of ice-nucleating particles (INPs), the minor fraction of aerosol particles able to trigger cloud ice crystal formation and, consequently, influence multiple climate-relevant cloud properties including lifetime, radiative properties and precipitation initiation efficiency. Mineral dust is considered the dominant INP source in many parts of the world due to its ice nucleation efficiency and its sheer abundance, with global emission rates of up to 4700 Tg a−1. However, INPs emitted from the ocean surface in sea spray aerosol frequently dominate INP populations in remote marine environments, including parts of the Southern Ocean where cloud-resolving model simulations have demonstrated that cloud radiative properties are likely strongly controlled by INPs. Here we report INP concentrations measured in aerosol and seawater samples during Air Quality and Climate Change in the Arabian Basin (AQABA), a shipborne campaign that spanned the Red Sea, Gulf of Aden, Arabian Sea, Arabian Gulf and part of the Mediterranean. In aerosol samples collected within a few hundred kilometers of the first and second ranked sources of dust globally, the Sahara and Arabian Peninsula, INP concentrations ranged from 0.2 to 11 L−1 at −20 ∘C with observed ice-active surface site densities (ns) 1–3 orders of magnitude below levels predicted by mineral dust INP parameterizations. Over half of the samples (at least 14 of 26) were collected during dust storms with average dust mass concentrations between 150 and 490 µg m−3 (PM10), as simulated by the Modern-Era Retrospective analysis for Research and Application, version 2 (MERRA-2). The impacts of heat and peroxide treatments indicate that organics dominated the observed ice nucleation (IN) activity at temperatures ≥ −15 ∘C with proteinaceous (heat-labile) INPs frequently observed at high freezing temperatures >−10 ∘C. INP concentrations in seawater samples ranged between 3 and 46 mL−1 at −19 ∘C, demonstrating the relatively low INP source potential of seawater in the region as compared to seawater from multiple other regions reported previously. Overall, our results demonstrate that despite proximity to the Sahara and the Arabian Peninsula and the dominance of mineral dust in the aerosol sampled, existing mineral dust parameterizations alone would not skillfully represent the near-surface ns in the observed temperature regime (−6 to −25 ∘C). Future efforts to develop or improve representations of dust INPs at modest supercooling ( ∘C) would benefit from a characterization of the specific organic species associated with dust INPs. More generally, an improved understanding of the organic species associated with increased IN activity and their variability across dust source regions would directly inform efforts to determine whether ns-based parameterizations are appropriate for faithful representation of dust INPs in this sensitive temperature regime, whether region-specific parameterizations are required, or whether an alternative to the ns approach is necessary.
- Article
(2209 KB) - Full-text XML
-
Supplement
(5881 KB) - BibTeX
- EndNote
Ice-nucleating particles (INPs) modulate the temperature and relative humidity at which ice particle formation occurs in the atmosphere and thus are a key factor that controls ice-phase partitioning in clouds. As initiators of ice formation and related phase-partitioning processes, INPs affect multiple cloud properties and exert a strong influence on cloud lifetime, radiative properties and precipitation initiation efficiency (e.g., Lohmann and Feichter, 2005; Vergara-Temprado et al., 2018; Brunner et al., 2021).
Globally, desert dust is likely the most abundant aerosol type by mass (Kinne et al., 2006; Kok et al., 2021). Furthermore, multiple studies have demonstrated that mineral dust is the dominant ice-nucleating species in many parts of the world based on observations (Ardon-Dryer and Levin, 2014; Boose et al., 2016; DeMott et al., 2015; Price et al., 2018a) and modeling of global INP distributions (Burrows et al., 2013; Hoose et al., 2010; Murray et al., 2012; Vergara-Temprado et al., 2017). Annual global dust emission rate estimates range between 400 and 4700 Tg a−1 (Huneeus et al., 2011; Kok et al., 2021). Of the average global dust loading in the atmosphere (20–29 Tg), North African source regions are estimated to contribute ∼ 50 % (11–15 Tg), and the Middle East and Central Asian source regions account for the bulk of the remainder, ∼ 30 % (7.7 Tg) (Kok et al., 2021). Analysis of satellite products indicates that dust emission rates are increasing over the Middle East at a rate of 15 % a−1 (Klingmüller et al., 2016; Yu et al., 2018).
While Hoose and Möhler (2012) showed that mineral dust INPs generally activate ice crystals at freezing temperatures < −15 ∘C, dust containing K-feldspar has been shown to nucleate ice at much warmer temperatures, up to −4 ∘C (Atkinson et al., 2013; Harrison et al., 2016; Niedermeier et al., 2015; Wex et al., 2014; Whale et al., 2015; Zolles et al., 2015). K-feldspars represent up to ∼ 24 % of Saharan and Asian dusts by mass (Nickovic et al., 2012). However, knowledge of the abundance and the available surface fraction of aerosolized K-feldspar would be necessary to evaluate the ice nucleation (IN) efficiency of dust at temperatures >−15 ∘C (Kanji et al., 2017).
Though mineral dust is considered to be the dominant INP source in many regions, multiple modeling and observational studies suggest that marine INPs are frequently dominant by number in remote ocean regions in air masses with low concentrations of terrestrial aerosol (McCluskey et al., 2018b, c; Vergara-Temprado et al., 2017; Wilson et al., 2015; DeMott et al., 2016). Using a global aerosol model to simulate marine organic and K-feldspar INP populations, Vergara-Temprado et al. (2017) showed that the relative contribution of marine organic versus dust INPs in remote regions varies seasonally and that marine organic INPs frequently outnumber K-feldspar INPs (up to 100 % of the simulated days in the Southern Ocean during summer). Results from a follow-up cloud-resolving model study showed that Southern Ocean cloud reflectivity is strongly modulated by INP concentrations, indicating that accurate estimates of the radiative energy budget in the Southern Ocean likely require improved and reliable representation of both dust and marine organic INPs (Vergara-Temprado et al., 2018). By generating isolated nascent sea spray aerosol over a range of biological conditions, mesocosm studies have shown that marine INPs are comprised of two classes: a dissolved organic carbon (DOC) type composed of IN-active molecules and a particulate organic carbon (POC) type linked to the death phase of phytoplankton blooms (McCluskey et al., 2017, 2018a).
Parameterizations for both marine and mineral dust populations are commonly implemented in atmospheric models to estimate dust and marine INP concentrations. There are multiple existing mineral dust INP parameterizations used to estimate their concentrations in aerosolized desert dust, some based exclusively on laboratory measurements (e.g., Niemand et al., 2012; Ullrich et al., 2017) and others derived from a combination of laboratory and field measurements (DeMott et al., 2015). There are, additionally, multiple mineral-specific INP parameterizations including illite (Broadley et al., 2012), kaolinite (Welti et al., 2012), quartz (Harrison et al., 2019) and K-feldspar (Atkinson et al., 2013; hereafter, “A13”). The parameterizations by Ullrich et al. (2017; hereafter, “U17”) and Niemand et al. (2012; hereafter, “N12”) were developed using dust samples from multiple deserts, and both found little variability in the IN activity between dusts from locations as disparate as the Sahara and Asia. DeMott et al. (2015; hereafter, “D15”) found agreement between their observation-based parameterization and N12, supporting the validity of laboratory-based parameterizations. Results in D15 also confirmed the conclusions of N12 and U17: that to first order, dusts from distinct regions can be parameterized as a single particle type. The D15 parameterization has been considered to be representative of dust that has undergone atmospheric photochemical and oxidative processes in transport (i.e., “aged” dust) because the parameterization was derived from observations made far (thousands of kilometers) from the dust emission sources (Boose et al., 2016).
By contrast, few studies report in situ INP measurements near (e.g., <1 d of transport) a major dust source, and the lack of observations near dust source regions inhibit the evaluation of the ability of existing dust INP parameterizations to represent nascent dust populations (Boose et al., 2016; Gong et al., 2020a; Price et al., 2018a). INP observations are particularly lacking for the sensitive temperature regime >−20 ∘C. Boose et al. (2016) found that D15 overpredicted INPs observed during Saharan dust events at a location within hundreds of kilometers west of the Sahara (Izaña, Tenerife, Spain) by 2–3 orders of magnitude, suggesting that aging may lead to increased IN efficiency in mineral dust and that D15 may be less representative of nascent dust. These conclusions were supported by Conen et al. (2015), who found that concentrations of INPs at −20 ∘C measured during Saharan dust events were 1 order of magnitude higher at Jungfraujoch in the Swiss Alps than in Izaña, where dust events had occurred 1–7 d prior to reaching Jungfraujoch. Gong et al. (2020a) measured INPs in a variety of atmospheric and seawater sample types at Cabo Verde and determined mineral dust to be the dominant source of INPs observed in the atmosphere but found that INPs with freezing temperatures >−10 ∘C were likely biological. At altitudes between 30 and 3500 m in the same region, Price et al. (2018a) found that measured concentrations of INPs ranged 2 orders of magnitude at a given temperature and that the observed concentrations related to the atmospheric dust loadings.
Recently, multiple studies have provided new, much-needed observations of ambient atmospheric INPs in marine environments (DeMott et al., 2016; Hartmann et al., 2020; McCluskey et al., 2018b; Yang et al., 2020) where data were historically lacking and, consequently, an impediment to achieving predictive understanding of global INP distributions (Burrows et al., 2013). There are now two parameterizations available for the estimation of atmospheric concentrations of marine INPs emitted from the ocean surface: Wilson et al. (2015), which estimates cumulative INPs from total organic carbon (TOC) concentrations in simulated sea spray aerosol (SSA), and McCluskey et al. (2018b), which estimates ice-active surface site density (ns) from aerosol surface area. Wilson et al. (2015) and McCluskey et al. (2018b; hereafter “M18”) derived marine INP parameterizations from field measurements of INPs in Atlantic and Arctic ocean sea surface microlayer samples and pristine SSA samples over the North Atlantic Ocean, respectively.
Here, we report observations of INPs measured in air masses influenced by both desert dust and marine aerosol (Edtbauer et al., 2020) in close proximity to the two greatest global dust aerosol sources: the Sahara (no. 1) and the Arabian Peninsula (no. 2) (Kok et al., 2021). INP concentrations were measured in 26 ambient aerosol samples collected during Air Quality and Climate Change in the Arabian Basin (AQABA), a shipborne campaign which took place during July–August 2017 on a transect that spanned the central and eastern parts of the Mediterranean, the Red Sea, the Gulf of Aden, the Arabian Sea and the Arabian Gulf. The rest of this study will be structured as follows. We present an overview of measurements and data sources in Sect. 2 Methods. In Results Sect. 3.1, an overview of INP concentrations observed is presented, followed by an assessment of subsurface seawater (SSW) source potential (Sect. 3.2). Observed ns values are compared to dust and marine INP parameterizations in Sect. 3.3, followed by an analysis of the contributions of heat-labile (e.g., proteinaceous) and heat-stable organic compounds to observed INP populations in aerosol (Sect. 3.4). The same analysis is applied to assess organic contributions to observed INPs in a soil dust sample from a likely source region in Sect. 3.5. We discuss the findings and potential INP sources and compare them with prior studies in the Discussion Sect. 5. Finally, in Sect. 5 we offer strategies to address the challenges of evaluating dust-specific INP parameterizations and recommend measurements needed to develop predictive understanding of dust INPs at modest supercooling (T≥ 15 ∘C).
2.1 Project overview
The AQABA campaign was conducted from 25 June to 3 September 2017 on board the RV Kommandor Iona. The research voyage was conducted in two transects: the first leg beginning in La-Seyne-sur-Mer, France, heading through the Suez Canal, around the Arabian Peninsula and ending in Kuwait, and the second leg was a return transect via the same route (Figs. S1–S2 in the Supplement). The campaign supported a large suite of on- and offline aerosol- and gas-phase measurements (Bourtsoukidis et al., 2019, 2020; Celik et al., 2020; Edtbauer et al., 2020; Eger et al., 2019; Friedrich et al., 2021; Pfannerstill et al., 2019; Tadic et al., 2020; Wang et al., 2020).
2.2 Aerosol and trace gas measurements
Aerosol size distributions were measured using an optical particle spectrometer (OPC, Grimm model 1.109) and a fast mobility particle spectrometer (FMPS, TSI model 3091). The OPC measures particles in the size range 0.25–32 µm, and the FMPS measures particles with sizes between 5.6 and 560 nm, with 6 and 1 s time resolution, respectively. The inlet for the aerosol instrumentation was located at the top of a measurement container at a horizontal distance of about 15 m from the INP filter sampling unit (Figs. S3–S4). To avoid condensation in inlet lines, aerosol samples were passed through a drying system, which reduced ambient relative humidity (RH) to an average value of ≈ 40 % in the measurement container. Ambient RH ranged between 67 % and 81 % during INP sampling periods. OPC and FMPS data were averaged over 1 min time intervals. A filter flag based on aerosol measurements was derived to identify and eliminate stack emissions and applied to all aerosol data. The filter flag was based on short-term variation in particle number concentration measured by a condensation particle counter (CPC, TSI model 3787), black carbon concentrations (aethalometer, Magee AE33), wind direction and wind speed. The flag was set when the apparent wind direction was from the direction of the stack (± 30∘) as seen from the aerosol inlet position (Fig. S3), and strong fluctuations of black carbon and/or particle number concentrations were observed relative to background levels. Particle losses were estimated using the particle loss calculator (PLC; von der Weiden et al., 2009). Losses were negligible (<1 %) up to 3.5 µm and increased to 40 % at 10 µm.
Particle surface area concentrations were derived from the 1 min time-averaged FMPS and OPC measurements as follows. Geometric diameters were estimated from the measured mobility diameters (FMPS) and optical particle diameters (OPC). Aerosols were assumed dry at sampling conditions following the drying system described above. To convert optical particle diameters into geometric diameters, it was assumed that all coarse particles (dp>3000 nm) were composed of sea salt and dust with a mass ratio of 25 % to 75 %, and using the respective refractive indices and shapes the measured optical particle diameters were converted into geometric diameters (Sect. S1.1). The sea salt : dust mass ratio was based on average dust and sea salt concentrations as measured in particles <10 µm (PM10; see Sect. S2 for details).
Fine particle (dp<700 nm) size was converted from optical diameter (dopt) into geometric diameter (dgeo) using the optical properties calculated from the chemical composition of particles <1 µm (PM1) as measured by a high-resolution time-of-flight aerosol mass spectrometer (Aerodyne HR-ToF-AMS), assuming spherical particles. For particles in the intermediate size range (700–3000 nm), log-linear interpolation of optical and spherical properties was applied for conversion of optical into geometric particle diameters (Sect. S1.2). The mobility diameters measured by the FMPS were considered equivalent to the geometric diameter, assuming spherical particles. From the resulting particle size distributions, particle surface area was calculated for each size bin. Total particle surface concentrations were determined by integrating the surface area distribution for particles up to 10 µm (dgeo). The overall uncertainty of derived particle surface area concentrations is estimated to be 30 %, including the uncertainty due to particle losses (see Sect. S3).
The water-soluble fraction of total suspended particles (TSPs) was monitored with hourly resolution using a Monitor for AeRosols and Gases in Ambient Air instrument (MARGA; Metrohm Applikon model S2, Herisau, Switzerland). Sea salt concentrations were estimated by scaling measured soluble Na+ concentrations by 3.27 following Manders et al. (2009) and were used as a proxy for SSA number concentrations. Size-resolved single particle chemical composition measurements have shown that sea salt represents 50 %–70 % of SSA particles by number (dp>0.5 µm) (Collins et al., 2014). Hourly composition data were linearly interpolated for four samples, for which 1–3 h (of 7–24 h total sampling time) was missing (Fig. S5). The MARGA sampling line was equipped with a PM10 cyclone, but the sample was not dried as the instrument is not prone to condensation. Particle transmission losses to the MARGA were estimated using the PLC and found to be consistent with the aerosol sizing instruments described above.
Nitric oxide (NO) concentrations were measured using a commercially available two-channel chemiluminescence monitor (CLD 790 SR, ECO Physics AG, Dürnten, Switzerland). During the AQABA campaign, the CLD 790 SR, MARGA, FMPS, OPC, HR-ToF-AMS, CPC and aethalometer were operated within laboratory containers on the main deck of the research vessel. The NO measurements were used to prevent stack sampling during INP collection (see Sect. 2.4).
2.3 Dust mass concentrations from MERRA-2
Since dust concentrations were not measured during the campaign, hourly dust surface mass concentrations along the cruise track were obtained from the (0.5×0.625∘) Modern-Era Retrospective analysis for Research and Application, version 2 (MERRA-2; Gelaro et al., 2017) and were averaged over the region covered during each sampling period. Buchard et al. (2017) showed a high degree of correlation between MERRA-2 and surface dust concentration observations (r≥0.69), particularly during dust storms (r≥0.92). MERRA-2 surface dust mass concentrations also correlated well with PM10 observed during AQABA (r≥0.71) (Fig. S6). MERRA-2 uses the GEOS-5 Earth system model (Molod et al., 2015; Rienecker et al., 2011) with 72 vertical layers between the surface and 0.01 hPa (∼ 80 km) and the three-dimensional variational data assimilation grid point statistical interpolation analysis system (Kleist et al., 2009; Wu et al., 2002; additional details in Sect. S4).
2.4 INP measurements in aerosol
Ambient aerosol sampling for offline measurement of INPs was conducted from 5 July to 31 August 2017 on the Kommandor Iona's wheelhouse top (platform above the bridge), ∼ 15 m horizontally from the online aerosol measurement inlet and ∼ 15 m from the ocean surface (Figs. S3–S4). Sampling locations along the cruise transect corresponding to each aerosol sample are shown in Figs. S1–S2.
Aerosol samples were collected over 3–28 h periods on polycarbonate filters (47 mm diameter, 0.2 µm pore size, Whatman® Nuclepore, Chicago, Illinois, USA) placed in open-face Nalgene® analytical filter units (Waltham, Massachusetts, USA). Sampling intervals and frequency were chosen with the aim of collecting >5000 L during dust events and >10 000 L when OPC particle counts were relatively low (e.g., during sampling periods f040–44), as conditions allowed. Aerosol sampling flow rates through the filter units were set to 10–13 L min−1 using a MassStream™ mass flow controller (Bethlehem, PA, USA) connected in line with a rotary vane pump (Thomas QR-0100, Gardner Denver ©, Monroe, LA, USA). To decrease exposure to stack emissions, the pump was automated to switch off when online measurements of NO exceeded 1 standard deviation above the average background concentration for over 1 min (∼ 0.4 ± 0.8 ppb). Comparing the stack contamination filter flag for aerosol measurements (Sect. 2.2) with INP sampling periods additionally indicates no influence of stack emissions on INP filter samples. Lacking a size-selective inlet for INP sampling, it is possible that aerosols >10 µm were present in INP samples during dust events. Surface area may be underestimated for these samples due to the PM10 cutoff for aerosol sizing (Sects. 2.2 and S3), but we do not expect this to affect our overall conclusions, as increased aerosol surface area would further reduce ns (see Results Sect. 3.3 and Discussion Sect. 4). Prior to sampling, filters were cleaned by soaking in 10 % peroxide (H2O2) for 10 min followed by rinsing three times with deionized water, the last rinse further “polished” by passage through a 0.1 µm pore-size syringe filter (Puradisc, Whatman®, Maidstone, UK). Filters were pre-loaded into filter units in a laminar flow hood to further minimize contamination from handling. After collection, each aerosol filter was placed in a 60 mm diameter sterile Petri dish (Life Science Products, Frederick, Colorado, USA) using pre-cleaned acetyl plastic forceps (Fine Science Tools, Foster City, California, USA), sealed with Parafilm and stored frozen (−20 ∘C). Samples were shipped in a dry shipper via Cryoport® High Volume Shipper at −180 ∘C and upon arrival at the laboratory were stored at −80 ∘C until processed, within 18 to 38 months of collection. To release collected particles, filters were immersed in 5–8 mL of ultrapure water (catalogue no. W4502, Sigma-Aldrich®, St. Louis, MO, USA) and shaken by hand for 20 min just prior to measurement.
INP concentrations were measured using the Scripps Institution of Oceanography automated ice spectrometer (SIO-AIS), an immersion freezing droplet assay instrument that is described in detail in Beall et al. (2017). Briefly, the aerosol sample suspensions and SSW samples were distributed in 30×50 µL aliquots into clean 96-well polypropylene sample trays (OPTIMUM® ULTRA Brand, Life Science Products). An equal number and volume of aliquots of ultrapure water accompanied each sample in the tray as a control. Trays were then inserted into an aluminum block that was cooled at −0.87 ∘C min−1 until the samples froze. Cumulative INP number concentrations per temperature per volume liquid are calculated using the fraction (f) of unfrozen wells per given temperature interval:
where Vd is the volume of the sample in each well (Vali, 1971). For aerosol filter samples, cumulative INP number concentrations are calculated using the ratio of the ultrapure water volume used for resuspension of the particles (Vre) to the volume of air sampled (VA):
Prior to calculating nINP, the fraction of unfrozen wells (f) was adjusted for contamination in the water used for suspension by subtracting the number of frozen ultrapure water wells per temperature interval from both the total number of unfrozen wells and total wells of the sample. The nINP was additionally adjusted for background INPs from filters and sampling handling processes. Background nINP were estimated using measured nINP in aerosol sample field blanks, which had been placed in the sampling apparatus ∼ 5 s (without actuating the pump) before removal and unloading and storage of the filter. Seven field blank samples were collected, one every ∼ 7 d of the cruise (Fig. S7). INP concentrations were measured in field blanks as described above, and the nINP was simulated using the mean air volume sampled (6680 L). Figure S7 shows the estimated nINP across the seven field blanks, which ranged between and L−1 at −20 ∘C. The freezing onset temperatures detected in the field blanks ranged between −6 and −27 ∘C. To correct nINP measured in aerosol samples for background INPs from sample handling, a linear regression based on the geometric mean nINP measured in field blank suspensions (mL−1 water) was used to estimate background concentrations of INPs in samples at all temperatures between −14.5 and −27 ∘C. The estimated background nINP was then subtracted from the INP concentration measured in filter sample suspension volumes in this temperature range prior to calculating nINP. The nINP measured in one aerosol sample (f033) fell within the estimated INP background levels.
For this study, the detection limit was 0.68 nINP mL−1 liquid or 0.001–0.0024 nINP L−1 air for the minimum and maximum air volume sampled, respectively. To extend the upper limit of detection (i.e., the point at which all droplets have frozen), dilutions of 1:10 and 1:100 were performed on eight samples (Fig. S8).
The ice-active surface site density, ns, is a metric used to define the ice-nucleating capabilities of an aerosol species (i.e., an aerosol sample of all the same particle type) (Kanji et al., 2017) as follows:
where Nice is the number of frozen droplets, Ntot is the total number of particles in a monodisperse aerosol population, and A is the surface area per particle.
The value of ns can also be approximated for polydisperse aerosol samples containing multiple aerosol types:
where Atot is the total surface area of the polydisperse aerosol. The difference between the ns approximation (Eq. 4) and ns (Eq. 3) is that many particle types are typically included in the ns approximation, and in an ambient aerosol measurement most of these are not IN active (see also Hiranuma et al., 2015, their Sect. 2.4). Furthermore, the subset of INPs in the sample are likely also of different types, which likely have different ns in the strict sense (Eq. 3). Nevertheless, the ns approximation is a useful metric for comparing the ice-nucleating ability of different air masses and source regions and is often used for comparing data across studies of INPs measured in ambient air. It is extremely challenging to separate measurements of INPs and surface area by each particle type and requires, for example, combining online measurements of single particle chemistry, size distributions and INPs Cornwell et al., 2019). All nINP and ns are reported normalized to a standard temperature of 273.15 K and pressure of 1013 hPa.
Heat and peroxide treatments were applied to a subset of samples (12 of 26) to test for heat-labile biological (e.g., proteinaceous) and organic INP composition, respectively, following the procedure described in McCluskey et al. (2018a) and Suski et al. (2018). The 12 samples were selected based on sampling location with the aim of getting a representative measurement from each region. For each heat-treated sample, a 2 mL aliquot of the original ultrapure water suspension was heated to 95 ∘C for 20 min in a water bath and re-tested for changes in nINP. For peroxide treatments, 1.6 mL of the original suspension was combined with 0.8 mL of 30 % peroxide (Sigma Aldrich®, St. Louis, Missouri, USA) to achieve a final concentration of 10 %, and then the mixture was heated to 95 ∘C for 20 min while being illuminated with two 26 W UVB fluorescent bulbs. To remove residual peroxide and prevent freezing point depression, the solution was cooled, and catalase (catalogue no. IC10042910, MP Biomedicals, Santa Ana, California, USA) was added. Figure S7 shows the estimated nINP in a heat- and peroxide-treated blank sample. Fisher's exact test was applied to frozen and unfrozen well fractions between each untreated sample and its corresponding treated sample to test for significant differences (p<0.05). Note that significant difference in frozen well fraction is insufficient as a sole indicator of sensitivity in peroxide-treated samples because samples are diluted 2 : 3 (by 33 %) compared to untreated samples. As nINP can be corrected for the dilution by scaling (as opposed to frozen well fractions), the overlap in 95 % binomial sampling confidence intervals (Agresti and Coull, 1998) between the untreated and peroxide-treated sample is an additional indicator of sensitivity for a given data point in the peroxide-treated sample spectrum within ± 0.2 ∘C, the uncertainty in the SIO-AIS temperature measurement (Beall et al., 2021). A lack of overlap in the 95 % binomial sampling confidence interval within ± 0.2 ∘C equates to a significance threshold of p<0.005 (Krzywinski and Altman, 2013).
INP concentrations were additionally measured in untreated, heat-treated and peroxide-treated subsamples from an archived suspension of the soil dust sample N12 SD for comparison with this study (DeMott et al., 2018; hereafter referred to as “N12-SD”). N12-SD (sample CD1 in Megahed 2007) is sedimented airborne dust that was carried in boundary layer winds north and then east from the desert in central Egypt during a sandstorm to a sampling point ∼ 50 km north of Cairo, collected on 18 February 2003. The sample was stored in a “clean bottle” (no temperature of storage given) for further analysis (Megahed, 2007). Briefly, the sample was generated during the recent laboratory intercomparison of INP measurements (collected on a 0.2 µm Nuclepore polycarbonate membrane filter; Whatman®, Chicago, Illinois, USA) and stored frozen at −20 ∘C until processed, as described in DeMott et al. (2018).
2.5 INP measurements in SSW
INP concentrations were additionally measured in 10 SSW samples. For seawater sampling, a water intake vertical steel pipe was positioned on the starboard side of the ship approximately 2 m below the sea surface level. The seawater was pumped into a 200 L stainless steel tank and continuously exchanged at a rate of 3000 L h−1. SSW samples for INP analysis were collected in 15 mL sterile centrifuge tubes (Falcon™, ThermoFisher Scientific, Waltham, Massachusetts, USA) and stored frozen at −20 ∘C until they could be shipped in a dry shipper via Cryoport® (−180 ∘C) and ultimately stored at −80 ∘C, as for aerosol samples until processed as described above (Sect. 2.4), within 18 to 38 months of collection. Storage duration was not correlated with INP concentration changes in frozen marine and coastal precipitation samples (Beall et al., 2020). Heat and peroxide treatments as described above were applied to 5 SSW samples from the Arabian Sea and the Gulf of Aden. The focus on these regions was motivated by the detection of marine aerosol originating from the upwelling region in Somalia reported in Edtbauer et al. (2020; see Sect. 3.3).To assess the contribution of submicron INPs to total measured INPs, 2 mL of SSW was filtered through a 0.2 µm sterile syringe-filter (Acrodisc® Pall®, Port Washington, New York, USA) and re-tested.
INP concentrations in SSW collected at the Ellen Browning Scripps Memorial Pier at Scripps Institution of Oceanography (SIO; 32.8662 N, 117.2544 W) were assessed in 17 samples for comparison with SSW collected during AQABA. Samples were collected between 31 January and 7 May 2016 in 15–30 mL sterile centrifuge tubes (Falcon™, ThermoFisher Scientific, Waltham, Massachusetts, USA) at depths of 1–3 m and processed immediately using the SIO-AIS as described above.
3.1 INP concentrations in aerosol
A total of 26 aerosol samples were collected in July–August 2017 during AQABA for offline measurements of INPs. The INP concentrations (nINP) measured in samples collected in the Mediterranean Sea, the Red Sea, the Gulf of Aden, Arabian Sea, Gulf of Oman and Arabian Gulf spanned up to 2 orders of magnitude at −15 ∘C (Fig. 1, Table 1), between and L−1. This range agrees within an order of magnitude with that of Prodi et al. (1983), who measured nINP in the Mediterranean, Red Sea, Gulf of Aden and Indian Ocean nearly 4 decades prior to the present study ( to 2 L−1 at −16 ∘C). Average ambient dust concentrations during each sampling period ranged from 2 to 490 µg m−3 (PM10 Table 1). There is no agreed-upon standard definition of extreme dust events in the literature, though the 24 h average WHO or US EPA health standards for average PM10 are commonly used (Gandham et al., 2020; Khaniabadi et al., 2017). Using the US EPA health standard for PM10 as a threshold for extreme events (150 µg m−3), 14 of the 26 samples were collected during dust events. This is conservative given the equivalent WHO guideline for PM10 is 50 µg m−3 (WHO, 2005), in which case 22 of the 26 sampling periods would be classified as dust events. Prior studies have reported comparable PM10 levels during dust events in the region (Gandham et al., 2020; Krasnov et al., 2016; Shahsavani et al., 2012).
Figures S9–S10 show the extent of k-means clustered FLEXPART (FLEXible PARTicle dispersion model) back trajectories below the altitude of 1500 m (see Sect. S5 for details). This threshold was applied to eliminate most of the free tropospheric parts of the back trajectories and was selected based on the MERRA-2 monthly average planetary boundary layer (PBL) heights during the campaign period, which were 200–700 m over the ocean and up to 1700 m over land. Air mass back trajectories show that source regions included the Mediterranean, Nile Delta, Sinai Peninsula (f006–f008, f038), northeast Egypt (f009–f010), Iran (f023–f024), and southern and eastern Europe (f040, f042, f044) (Figs. S9–S16). Many samples collected during extreme dust events (f013, f014, f016, f018 and f020) were influenced by emissions from North Africa and the Arabian Peninsula (Figs. S9–S10, S12–14). The ranges of aerosol surface area concentrations for all sampling periods were <320 µm2 cm−3, with the exception of f024, for which the aerosol surface area range was >600 µm2 cm−3 (Table 1).
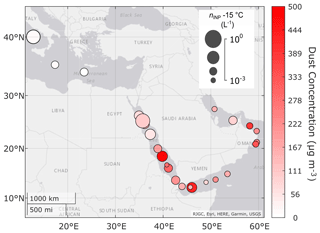
Figure 1Map of the sample locations for 26 aerosol samples collected on the RV Kommandor Iona during Air Quality and Climate Change in the Arabian Basin (AQABA; see also Figs. S1–S2 and S9–S10). Measured nINP spanned 2 orders of magnitude at −15 ∘C, from to L−1. Marker sizes indicate abundance of INPs. Marker colors indicate the average ambient dust mass concentration during the sampling period from hourly MERRA-2 reanalysis data.
3.2 Seawater source potential
The nINP values in 10 SSW samples collected during AQABA were used to characterize the INP source potential of SSA generated by bubble bursting (Wang et al., 2017). Results from prior studies have demonstrated that jet droplets are a more efficient transfer vehicle than film drops of INPs into SSA particles (Mitts et al., 2021; Wang et al., 2017). We measured the nINP in SSW to test whether the seawater source strength was comparable to that of prior studies or whether the SSW was possibly enriched with INPs due to biological activity or even dust deposition (Cornwell et al., 2020).
Figure S17 shows how the nINP measured at −19 ∘C in 10 seawater samples varied by the sample collection location. Concentrations ranged between 1 and 50 nINP mL−1 and were highest between the Gulf of Oman and the Gulf of Aden. This region exhibited relatively high chlorophyll a during the cruise, with levels between 1 and 30 mg m−3 (Fig. S18). In Fig. 2, nINP were compared with SSW from the Ellen Browning Scripps Memorial Pier in coastal southern California (SIO Pier), Cabo Verde in the Northeast Atlantic, the Southern Ocean (McCluskey et al., 2018c), and the Northwest Atlantic (Schnell, 1977). AQABA nINP were most comparable with Gong et al.'s (2020a) observations in Cabo Verde. The lack of any unusually high INP spectra suggests that INP enrichment due to dust deposition (Cornwell et al., 2020) was absent or infrequent. It is possible that storage of SSW samples (Sect. 2.5) could have decreased measured nINP, though we expect that nINP would be decreased by no more than 10× in untreated samples stored frozen (see discussion below).
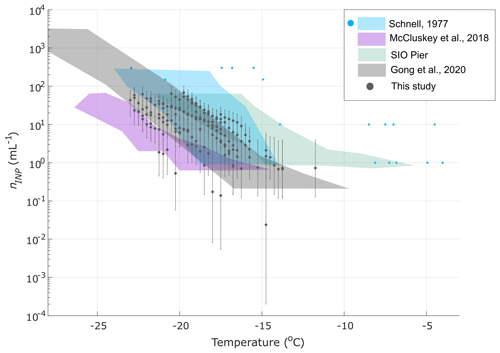
Figure 2Measured nINP in 10 SSW samples collected during AQABA. Also shown are the composite INP spectra of 14 coastal SSW samples collected on São Vicente Island, Cabo Verde (Gong et al., 2020b), 17 coastal SSW samples collected at the Ellen Browning Scripps Pier (green shading) and 12 SSW samples collected in the Southern Ocean (McCluskey et al., 2018d). Schnell's (1977) SSW measurements are represented as a composite spectrum of 24 samples (blue shaded region) and 5 additional spectra (blue markers) from samples that exhibited higher freezing temperatures. All spectra presented are uncorrected for freezing point depression.
Offline treatments for testing heat lability, organic composition and size were applied to 5 of the 10 seawater samples (Methods Sect. 2.5). Heat and 0.2 µm filtering treatments suggest that a large fraction of the seawater INPs were heat sensitive and larger than 0.2 µm (Fig. S19). These results are indicative of the POC type of marine INP defined in McCluskey et al. (2018a), though this result should be interpreted with caution as storage could potentially have increased sensitivity to filtering treatments.
Understanding of storage impacts on INPs measured in SSW is lacking. However, Beall et al. (2020) showed that average INP concentration changes for untreated coastal precipitation samples due to frozen storage were within 2× of nINP measured in fresh samples, with changes at the upper or lower end of the 95 % confidence interval exceeding 10× for some freezing temperatures. If SSW samples are similarly sensitive to storage, we would expect INP concentration changes to be within 2× on average but up to >10× for any particular untreated sample. Beall et al. (2020) also reported similar changes in INPs <0.45 µm with a greater tendency toward losses, which indicates that storage may have caused increased sensitivity to the filter treatments applied to stored samples.
3.3 Ice-active surface site densities in aerosol
In Fig. 3, approximated ice-active surface site densities (ns) in aerosol samples are compared with multiple population-specific observations and parameterizations for dust and marine INPs. The AQABA measurements are also compared with observations from dust-laden air over the Tropical Atlantic (Price et al., 2018a). Overall, observations nearly bridge the full regime between the M18 parameterization for marine INPs (McCluskey et al., 2018b) and multiple dust INP parameterizations based on laboratory studies of surface dust. At higher temperatures, between −5 and −12 ∘C, most observations show agreement with the composite spectrum of ns observed in a range of marine and coastal environments from DeMott et al. (2016) and Yang et al. (2020), and/or the A13 K-feldspar parameterization. Between −10 and −20 ∘C, several samples agree with the M18 marine INP parameterization within an order of magnitude, whereas two to three ns spectra approach the U17 and N12 laboratory-derived dust INP parameterizations within an order of magnitude (Niemand et al., 2012; Ullrich et al., 2017), depending on temperature. Multiple samples (∼ eight) additionally agreed with Price et al.'s (2018a) observations of INPs between 30 and 3500 m above the dust-laden Tropical Atlantic, and most agree with Gong et al.'s (2020a) surface-level observations, measured at Cabo Verde in the same region as Price et al. (2018a).
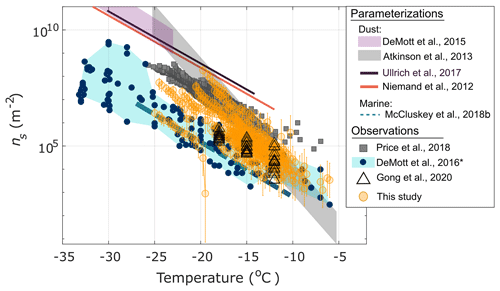
Figure 3Ice-active surface site densities (ns) as a function of temperature for 25 of 26 aerosol samples collected during AQABA. Gong et al. (2020a) and Price et al. (2018a) measured INPs in dust-dominant air masses in the tropical East Atlantic, with minor contributions from SSA, while the DeMott et al. (2016) measurements were collected across a range of locations and conditions within the marine boundary layer comprising air masses mostly dominated by relatively pristine marine SSA (Gong et al., 2020b; Price et al., 2018b). INP concentrations measured in sample f033 were below the detection limits imposed by field blanks (see Sect. 2.4, Fig. S7). Error bars represent 95 % binomial sampling confidence intervals (Agresti and Coull, 1998). Sample f020 is not shown due to missing aerosol surface area data during the sampling period. For the eight samples on which a dilution was performed (Fig. S8), ns for both the raw undiluted and diluted samples are shown. * DeMott et al. (2016) data shown have been updated with additional data from Yang et al. (2020).
Considering the frequency of dust events encountered (dust concentration >150 µg m−3, see Table 1) and the high probability that dust was the dominant aerosol source during most sampling periods, it is striking that most ns spectra observed are 1–3 orders of magnitude lower than the values predicted by dust parameterizations. As noted in Gong et al. (2020a), some deviations could be expected due to the difference between approximated ns based on total particle surface area in ambient measurements and true ns based on surface area of a homogeneous aerosol population (see Methods Sect. 2.4).
Given the marine environment in which sampling took place, a significant amount of sea spray aerosol (SSA) was also detected in many of the sampled air masses, using sea salt as a proxy (Table 1), and likely present in others for which no composition data were available. Edtbauer et al. (2020) reported the detection of high levels of dimethyl sulfide (DMS, up to 800 ppt; parts per trillion) in the Gulf of Aden associated with a local phytoplankton bloom during AQABA (as evidenced by visible bioluminescence around the ship at night), as well as high levels of dimethyl sulfone (DMSO2) and other marine biogenic volatile organic carbons (VOCs) from the Somalian upwelling region. As mentioned above, the ns for most samples between −6 and −18 ∘C agree with ns derived from observations across various locations within the marine boundary layer (Fig. 3). However, considering that SSA is associated with 1000× times fewer IN sites per unit surface area than dust (i.e., 1000× lower ns) (McCluskey et al., 2018b), the characteristically low IN activity of untreated SSW (even in light of the modest changes expected from storage, Sect. 3.2) and the frequency of dust events during AQABA, our findings suggest it is unlikely that the observed INPs originated from SSA. In general, detection of marine INPs in ambient aerosol is challenging due to their low relative abundance and decreased efficiency compared to dust (DeMott et al., 2016; McCluskey et al., 2018b). Thus, while SSA contributed to the measured aerosol surface area (Table 1), it is unlikely that the INPs observed in this study were marine in origin or at least that this is indiscernible in the present study or based on present parameterizations of these populations.
Heterogeneous aerosol composition in the sampled air masses likely contributed to some of the low ns spectra observed due to the contribution of non-INPs to the measured aerosol surface area (see description of ns approximation in Sect. 2.4). However, the difference between ns observed during the most extreme dust events, i.e., when the aerosol population was likely approaching homogeneity in composition, and the ns predicted from N12 and U17 was still greater than 2 orders of magnitude. Figure 4a and b show overlap in nINP and ns observed in samples collected in low dust and high dust conditions, indicating that the INP populations observed during AQABA exhibited similar IN activity despite variation in total aerosol composition and dust loading. No correlation was found between nINP and aerosol surface area (Fig. S20), PM10, or dust concentration. This result is in contrast to Price et al. (2018a), who found the variability in nINP to be largely determined by variability in dust loading or aerosol surface area. Price et al. (2018a) reported higher maximum aerosol surface area concentrations of ∼ 1500 µm cm−3 from three samples collected in an exceptionally optically thick layer, compared to the maximum of 965 µm cm−3 in the present study (Table 1). Yet overall, the aerosol surface area concentrations compare very well with those observed by Price et al. (2018a), indicative of comparable dustiness in the two studies. Excluding the case mentioned above the average aerosol surface area was 227 ± 68 µm2 cm−3 versus 226 ± 26 µm2 cm−3 for the present study. Furthermore, the sample with the highest ns at −15 ∘C (f044) was collected when dust concentrations were lowest (<10 µg m−3) (Fig. 4, Table 1). This is also in direct contrast to Price et al. (2018a), who found that the highest ns observed corresponded to the highest dust loading.
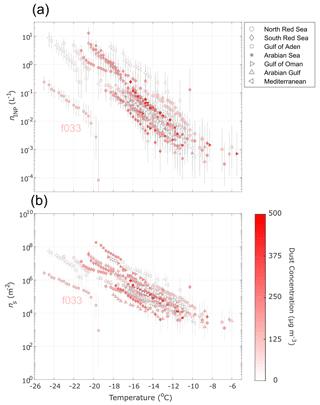
Figure 4INP concentrations (nINP) (a) and ice-active surface site densities (ns) (b) as a function of temperature for 26 aerosol samples collected during AQABA. Markers are colored by the average ambient dust concentration for the respective sampling period. Error bars represent 95 % binomial sampling confidence intervals (Agresti and Coull, 1998). The ns measured in samples collected during low dust conditions are equal to or greater than (up to 100×) the ns measured during dust events between −9 and −18 ∘C. INP concentrations measured in sample f033 were below the detection limits imposed by field blanks (see Sect. 2.4, Fig. S7). Sample f020 is not shown in (b) due to missing aerosol surface area data during the sampling period. For the eight samples on which a dilution was performed (Fig. S8), ns for both the raw undiluted and diluted samples are shown.
3.4 Characterization of INPs in aerosol
Offline treatments for testing heat lability and organic composition of INPs were performed on 12 samples via heat and peroxide treatments, respectively (Fig. 5). Prior studies have shown that the IN-active component of various types of mineral dusts are insensitive to heat treatments (Conen et al., 2011; Hara et al., 2016; Hill et al., 2016; O'Sullivan et al., 2014). The IN activity of K-feldspar, the dominant ice-nucleating component of mineral dust, was additionally found to be insensitive to digestion with peroxide (O'Sullivan et al., 2014). A small number of studies reported degradation of IN activity with peroxide treatment and/or heat treatment in Arizona Test Dust (ATD) that they attributed to organic material (Perkins et al., 2020; Yadav et al., 2019). Thus, we assume here that any degradation of IN activity due to heat and peroxide treatment corresponds to loss of heat-labile (e.g., proteinaceous) and heat-stable organic INPs, respectively.
Fisher's exact test was applied to frozen and unfrozen well fractions for each untreated sample and its corresponding treated sample to test for significant differences (p<0.05). Sensitivity to peroxide in most samples (i.e., INP degradation) demonstrate the consistent presence of stable organic INPs at temperatures ∘C. The lack of peroxide sensitivity at temperatures <−15 ∘C indicates dominance by mineral dust INPs at lower temperatures. Heat sensitivity in five samples suggests that biological INPs contributed to their warmest freezing INPs. Gong et al. (2020a) similarly found heat sensitivity in INPs at temperatures >−10 ∘C. A total of 4 of the 12 samples exhibited heat sensitivity at relatively moderate temperatures of −11 to −18 ∘C, including the two samples collected in the Mediterranean Sea. One sample (f010) exhibited increased nINP in freezing temperatures <−18 ∘C after heat and peroxide treatments. That the response to both heat and peroxide were nearly identical (Fig. 5) suggests that compounds may have been released from the surface during heating, uncovering a more active IN-active surface underneath (heating was common to both procedures). The increased nINP post heat and peroxide treatment is an unexpected result given previous studies on treated soil dust measurements (Conen et al., 2011; Hill et al., 2016; O'Sullivan et al., 2014; Tobo et al., 2014). However, increases in IN activity after heat treatment have been reported previously for airborne Saharan desert dust and aerosol collected during Saharan dust intrusions (Boose et al., 2019; Conen et al., 2022), as well as SSA and precipitation (Martin et al., 2019; McCluskey et al., 2018a), and should be further investigated in future studies. An increase in IN activity after peroxide treatment has also been reported in a Himalayan dust sample (Paramonov et al., 2018).
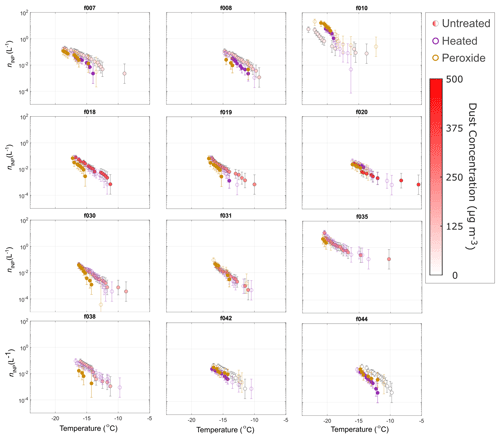
Figure 5INPs in aerosol samples treated with heat and peroxide (Methods Sect. 2.4) to test for INP heat lability and organic composition. Markers of untreated spectra are colored by the average dust concentration during the sampling period. Markers of heat-treated and peroxide-treated samples are filled to indicate significant INP concentration difference from untreated samples according to Fisher's exact test (p<0.05). Sensitivity to peroxide is evident for all samples ∘C, indicative of stable organic INPs. Heat lability is also evident at high to moderate temperatures in multiple samples, demonstrating that biological (e.g., proteinaceous) INPs also contributed to INPs observed during AQABA.
Given the frequency of dust events and generally high concentrations of dust during most sampling periods, it is surprising that most samples exhibit peroxide sensitivity. Aridisols and Entisols are the dominant soil types in North Africa and the Arabian Peninsula (Nortcliff, 2012). Both types are associated with the lowest levels of organic carbon, commonly used as a proxy for total soil organic matter, compared to other soil types (3 and 9 g kg−1, respectively) (Yost and Hartemink, 2019).
3.5 Characterization of INPs in a soil dust sample
INP measurements of soil dusts in this region are scarce and have only been reported for a single surface dust soil sample, sample “SD”, collected 50 km north of Cairo (Niemand et al., 2012). FLEXPART back trajectories indicate this source region for several samples (f006–10, f038), though it should be noted that dust sources cannot be confirmed in this study lacking aerosol and soil dust mineralogy. For comparison with this study, we measured INPs in untreated, heat-treated and peroxide-treated subsamples of an archived suspension of the N12-SD sample (Methods Sect. 2.4; DeMott et al., 2018). Sample N12-SD exhibits sensitivity to both heat and peroxide at temperatures >−16 ∘C, indicating biological composition of INPs at high freezing temperatures. Multiple AQABA samples influenced by desert air mass sources show similar sensitivities at higher temperatures: f006, f007, f019 and f020. Several others exhibit only peroxide sensitivity in this temperature range. Overall, the heat and peroxide sensitivities in the N12-SD sample indicate that desert dusts may contribute biological and/or organic INPs at moderate to high freezing temperatures, such as those observed in AQABA samples. Gong et al.'s (2020a) results showing heat sensitivity in INPs at temperatures >−10 ∘C further demonstrate the contribution of biological INPs at high temperatures in dust-laden air masses near North Africa.
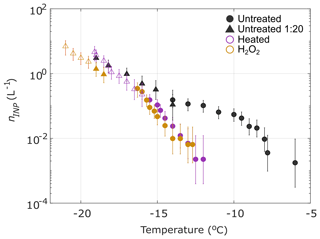
Figure 6Measured concentrations of INPs in an aerosolized soil dust sample “N12-SD”, collected 50 km north of Cairo, Egypt (Niemand et al., 2012), that was treated with heat and peroxide to test for INP heat lability and organic composition, same as in Fig. 5 above (Methods Sect. 2.4). A 1:20 dilution of the sample is shown (triangles), and markers of heat-treated and peroxide-treated samples are filled to indicate significant INP concentration differences from untreated samples according to Fisher's exact test (p<0.05). Sensitivity to peroxide and heat treatments indicates biological INPs between −6 and −16 ∘C.
Considering the high freezing temperatures observed, evidence of organic composition, and FLEXPART back trajectories showing that aerosol sources included populous regions and at least one agriculturally active region (the Nile River Delta; Figs. S9–S16), it is possible that agricultural soil dusts contributed to some of the relatively higher ns, nINP, and heat and peroxide sensitivity observed during AQABA. A range of ns values have been reported in studies of agricultural soil dusts, the lower end of which agrees with the ns observed in the present study between −8 and −25 ∘C (Fig. 3; Steinke et al., 2016; Tobo et al., 2014; O'Sullivan et al., 2014). Samples from air masses influenced by the Nile River Delta or southern Europe (f007–8, f010, f038, f042, f044) show a higher fraction of heat-sensitive INPs (Fig. 5). Heat sensitivity is indicative of biological INPs, which have been associated with agricultural soil dusts in prior studies (Hill et al., 2016; O'Sullivan et al., 2014). Hill et al. (2016) and O'Sullivan et al. (2014) showed peroxide sensitivity in agricultural soil dusts at temperatures >−18 to −15 ∘C, respectively, a range which aligns with the peroxide sensitivity exhibited in the present study.
Organic material can condense or adsorb onto aerosols during photochemical and oxidative processes, representing another potential source of organic INPs during AQABA (Dall'Osto et al., 2010; Hinz et al., 2005; Krueger et al., 2004). Could aging explain the organics and decreased ns observed? Though dust aerosol was collected within 1 d of transport from source regions throughout this study, we cannot rule out the possibility of aging impacts, lacking single particle chemistry measurements. In addition to field observations of nINP demonstrating that aging increased the IN efficiency of desert dust INPs (see Introduction; Boose et al., 2016; Conen et al., 2015), prior studies of the effects of aging on mineral dust INPs have yielded mixed and sometimes contradictory results, indicating that the impact of aging on IN properties likely depends on multiple factors including the ice nucleation pathway, the type of aging process, surface morphology and mineralogy (Perkins et al., 2020, and references therein). Multiple studies have investigated the effects of various aging processes on Arizona Test Dust (ATD) as a proxy for diverse natural dust samples. These included exposure to sulfuric acid, nitric acid vapor and solution-phase processes (Cziczo et al., 2009; Eastwood et al., 2009; Knopf and Koop, 2006; Salam et al., 2007; Sullivan et al., 2010a, b). Perkins et al. (2020) demonstrated the INP lability in ATD through multiple solution-phase aging processes (e.g., incubation in water, exposure to acid or salt), with up to 1000× reductions in INP abundance at freezing temperatures >10 ∘C. This result contrasts with the increase in IN activity attributed to aging reported in Boose et al. (2016) and Conen et al. (2015). Perkins et al. (2020) additionally reported that the lability of IN activity in ATD is temperature dependent, with large reductions evident at freezing temperature >10 ∘C, yet little to no change at temperatures <−15 ∘C. By contrast, most of the nsspectra in AQABA samples were 10–1000× lower than established dust parameterizations even at temperatures <−15 ∘C. In summary, it has proven difficult to determine any consistent impact of atmospheric processing on the IN activity of dust in model systems such as ATD (Perkins et al., 2020), and few studies have investigated impacts of aging on ambient desert dust, especially at modest supercooling (Boose et al., 2016). Furthermore, the use of ATD as a proxy for natural dust in INP studies has been questioned due to the complex ice-nucleating properties of natural dust, including mineral composition and defect sites at the particle surface, the latter of which is likely affected by the mechanical processing and milling involved in ATD production (e.g., Perkins et al., 2020, and references therein).
Gong et al. (2020a) also observed ns lower by more than 2 orders of magnitude compared to N12 and U17 despite the large fraction of supermicron INPs (77 %–83 % depending on temperature) and that the supermicron particles were mainly mineral dust. The cause of the decreased ns observed here and in Gong et al. (2020a) compared to dust ns parameterizations remains elusive. Both studies were conducted in air masses dominated by dust near major sources. In contrast, Price et al. (2018a) found agreement near the region of the Gong et al. (2020a) study. One obvious difference is that Price et al. (2018a) conducted measurements at higher altitudes, between 30 and 3500 m. A prior study that compared nINP in dust-laden air masses at the surface with nINP collected between 0.5 and 3 km above sea level found that median nINP increased by up to 10× above the surface and correlated to dust loading (Schrod et al., 2017). The differences between Price et al. (2018a) and the two surface-based studies draws attention to the need for vertical profiles of ns>−25 ∘C in dust-laden air masses.
The decreased ns compared to Price et al. (2018a) is also unlikely to be related to differences in INP measurement. In all three studies, cold stage or droplet assay measurements of immersion mode INPs were used in resuspensions of aerosol collected on filter samples. Recent studies that intercompared instruments designed for measurement of immersion mode INPs showed excellent agreement (i.e., within measurement uncertainty) in measurements of standardized dust and biological samples (DeMott et al., 2018) and when co-sampling ambient aerosol (DeMott et al., 2017). Moreover, the DeMott et al. (2018) intercomparison study demonstrated good agreement in multiple natural dust samples between the various measurement methods used to derive D15, N12 and U17 and the droplet assay methods applied in Gong et al. (2020a), Price et al. (2018a), and the present study.
Storage protocol represents another difference between Price et al. (2018a) and the two surface-based studies. Gong et al. (2020a) and the present study stored samples frozen prior to analysis, whereas Price et al. (2018a) processed samples immediately after collection. An understanding of storage impacts on INPs collected on filters is lacking (Wex et al., 2019), but we note that the discrepancies in ns between the two surface-based studies and Price et al. (2018a) exceed the range of INP concentration changes reported in untreated INP precipitation samples stored frozen (Beall et al., 2020).
Thus, the large differences between parameterized ns for dust and ns observed in both Gong et al. (2020a) and the present study between −12 and −25 ∘C indicate that existing ns-based parameterizations may not faithfully represent ns at moderate freezing temperatures despite proximity to major source regions. Whereas DeMott et al. (2015) found that for temperatures <−20 ∘C, mineral dust particles from Saharan and Asian deserts may be parameterized as a common particle type, our findings suggest that characteristic ns parameterizations for dust from different source regions may be needed for >−20 ∘C or, alternatively, that this temperature regime requires an alternative to an ns-based parameterizations. Gong et al. (2019) demonstrated that predicting nINP from surface area size distributions alone may not be feasible in environments where the aerosol and/or INP composition are unknown and proposed a probability density function (PDF)-based approach to predict INPs at a given freezing temperature.
In light of the evidence from this study that INPs were primarily influenced by organics associated with dust, especially at higher temperatures, and the lack of relationship between dust loading, ns, and nINP, we offer the following points for consideration. Prior studies of aerosolized dust demonstrated that it is frequently enriched in organic matter (6–20×) compared to soil dust and that wind erosion selectively removes the chemically enriched, fine portion of the soil higher in plant nutrients, organic matter and metals (Aryal et al., 2012; Delany and Zenchelsky, 1976; Van Pelt and Zobeck, 2007). Furthermore, a recent study that measured airborne concentrations of prokaryotic cells over the Red Sea characterized the region as a “global hot spot” with average concentrations of 155 000 (± 65 000) cells m−3, 19× higher than that over the subtropical and tropical open oceans (Mayol et al., 2014; Yahya et al., 2019). Yahya et al. (2019) demonstrated that the microbial loading was very likely related to the high concentrations of dust, as 99.9 % of the cells were attached to dust particles. Organic and biological species have been shown to dominate IN activity at temperatures ≳ −15 ∘C in many studies (e.g., Ladino et al., 2019; O'Sullivan et al., 2018; Kanji et al., 2017, and references therein). Thus, a faithful representation of dust INPs may require two parameterizations: one for the IN activity dominated by minerals ≲ −15 ∘C such as D15, U17 and N12 and another for the dust-associated organics ≳ −15 ∘C. As IN-active organics are limited compared to the IN-active mineral component of dust, we could expect an increase in ns slope between warm and cold regimes. The apparent decreased ns observed in this study between −18 and −12 ∘C could potentially be related to a plateau in ns through the transition between the mineral and organic “modes” (see untreated samples in Figs. 5–6). This study underscores the need to characterize the IN-active organic species associated with dust from major source regions and to investigate the extent to which biological and/or organic particles contribute to INP populations in dust-laden air masses at high to moderate freezing temperatures ∘C.
Observations from the 2-month AQABA campaign in the Mediterranean, Red Sea, Arabian Sea and Arabian Gulf are among the first INP measurements made in close proximity to the two largest dust sources globally: the Sahara and the Arabian Peninsula (Kok et al., 2021). Observed nINP measured in 26 aerosol samples spanned 2 orders of magnitude ( to L−1 at −15 ∘C).
In summary, INPs observed during AQABA were very likely dominated by mineral dust with some additional contributions possibly from densely populated and/or agricultural regions including the Nile River Delta region and southern or eastern Europe. Despite proximity to major dust sources and a high frequency of dust events with MERRA-2 simulated mass concentrations up to 490 µg m−3 (PM10), the observed ns for most samples was lower by 1–3 orders of magnitude compared to ns predicted by dust parametrizations N12 and U17 at T<−12 ∘C. Observed ns values in 48 % of the samples were equivalent within uncertainty to that of the A13 parameterization for K-feldspar (Atkinson et al., 2013), an ice-active component of desert dust. Observed ns agreed with many observations within the marine boundary layer (DeMott et al., 2016; Yang et al., 2020) and with Price et al.'s (2018a) measurements of nINP in dust-laden air masses over the Tropical Atlantic, within measurement uncertainty. Peroxide sensitivity was evident in all samples tested (12 of 26) at temperatures ∘C, demonstrating a consistent contribution of organic material to warm-temperature INPs. Heat sensitivity further suggested the presence of biological (e.g., proteinaceous) INPs in a subset of samples, particularly at high freezing temperatures >−10 ∘C. A soil dust sample from North Africa (originally from N12) exhibited heat and peroxide sensitivity between −5 and −16 ∘C, further demonstrating that the IN activity of mineral dust could be associated with organic and/or biological material. Contrary to Price et al. (2018a), who measured INP in the dust-laden Tropical Atlantic, no correlation was found between dust loading and nINP or ns. Results from this study and Gong et al. (2020a) indicate that the existing ns parameterizations alone do not skillfully represent mineral dust associated INPs at modest supercooling near major dust sources.
The source strengths of Red Sea, Mediterranean, Arabian Sea, and Arabian Gulf bulk seawater were also evaluated. The maximum source potential was observed in the Arabian Sea (50 INP mL−1 at −19 ∘C). The observed nINP values for SSW samples were equivalent to those of Gong et al. (2020a) at Cabo Verde within the 95 % binomial sampling confidence intervals (Agresti and Coull, 1998).
Considering that desert dust parameterizations overpredicted the ns values observed during AQABA despite proximity to major global emissions sources, this study demonstrates the need to evaluate the fidelity of dust INP parameterizations in nascent versus aged dust populations. The discrepancies underscore the challenges of evaluating dust-specific INP parameterizations: limited observations at modest supercooling, few assured methods for distinguishing between different INP sources in ambient aerosol, a dearth of characteristic soil dust samples from major dust sources, and limited knowledge of the specific composition and characteristics of dust INPs at temperatures >−15 ∘C. Vertical profiles of ns in dust-laden air masses are also needed to determine whether ns is consistently lower at the surface and examine the variability in ns with altitude. Potential storage impacts on INPs collected on filters are an additional factor worthy of future investigation, though storage alone does not likely explain the relatively decreased ns compared to parameterizations observed in this study, as U17 and N12 were both derived from stored dust samples.
In addition to providing observations at high to moderate freezing temperatures, future studies could apply the methods developed in Gong et al. (2020a) to estimate the contribution of marine INPs to the aerosol sampled by assuming equivalent distributions of sea salt and INPs between seawater and air. Furthermore, given the combination of marine, dust, and anthropogenically influenced air masses encountered, as well as the evidence of organic and biological INPs at modest supercooling in this study and Gong et al. (2020a), future studies could benefit from advances in on-line light-induced fluorescence (LIF) measurement techniques. Whereas the interpretation of fluorescence data from most LIF-based instruments has been limited by the lack of spectroscopic information, newer instruments support real-time spectrally resolved size and fluorescence measurement information for single particles (Fennelly et al., 2018; Huffman et al., 2020; Könemann et al., 2019). This information could be used to potentially “tag” different classes of organics and biological aerosols, enabling investigations of relationships between ns, nINP and organic signatures in, for example, mineral dusts and agricultural soil dusts. Finally, the decreased ns observed in this study further motivate comprehensive aerosol–ice nucleation studies, which aim to achieve closure between measured and predicted ambient nINP by simultaneously characterizing ambient INPs and relevant ice nucleation properties of the total aerosol population, such as composition and aerosol chemical mixing state (Sullivan et al., 2007).
The data set supporting this paper is hosted by the UCSD Library Digital Collections (https://doi.org/10.6075/J0X0676P, Beall et al., 2022).
The supplement related to this article is available online at: https://doi.org/10.5194/acp-22-12607-2022-supplement.
CMB, TCJH, PJD, MOA, CP, JL, JS, FD, BW, HH, MDS and KAP designed the study. CMB performed the INP measurements, FLEXPART modeling and analysis with support from TCH, PJD, MOA, MDS, MP and KAP. TCH, PJD and MOA contributed significantly to the writing, preparation of figures and analysis. TK, MI, RP and HH supported the field collection of aerosol for INP analysis, and TK additionally provided aerosol number concentration data. JS and MP provided aerosol water-soluble composition data. FD oversaw the aerosol sizing and AMS composition measurements and analysis. All authors contributed to the writing of the article.
At least one of the (co-)authors is a member of the editorial board of Atmospheric Chemistry and Physics. The peer-review process was guided by an independent editor, and the authors also have no other competing interests to declare.
Publisher's note: Copernicus Publications remains neutral with regard to jurisdictional claims in published maps and institutional affiliations.
The authors acknowledge collaborations with King Abdullah University of Science and Technology (KAUST), the Cyprus Institute (CyI) and the Kuwait Institute for Scientific Research (KISR). We additionally thank Marcel Dorf and Claus Koeppel for the organization of the campaign, as well as Horst Fischer, Ivan Tadic and Uwe Parchatka for provision of the NO data. Analyses and visualizations of dust mass concentrations and chlorophyll a used in this paper were produced with the Giovanni online data system, developed and maintained by the NASA GES DISC. Maps throughout this article were created using ArcGIS® software by Esri. We would also like to thank Hays Ships Ltd. and the Kommandor Iona's crew for their attention to the safety and well-being of the researchers. Finally, we thank the three anonymous reviewers whose insightful comments strengthened this paper.
Funding was provided by the Highly Cited Program at King Saud University and the Max Planck Society and the
University of California San Diego (UCSD) Understanding and Protecting the
Planet initiative.
The article processing charges for this open-access publication were covered by the Max Planck Society.
This paper was edited by Corinna Hoose and reviewed by three anonymous referees.
Agresti, A. and Coull, B. A.: Approximate Is Better than “Exact” for Interval Estimation of Binomial Proportions, Am. Stat., 52, 119, https://doi.org/10.2307/2685469, 1998.
Ardon-Dryer, K. and Levin, Z.: Ground-based measurements of immersion freezing in the eastern Mediterranean, Atmos. Chem. Phys., 14, 5217–5231, https://doi.org/10.5194/acp-14-5217-2014, 2014.
Aryal, R., Kandel, D., Acharya, D., Chong, M. N., and Beecham, S.: Unusual Sydney dust storm and its mineralogical and organic characteristics, Environ. Chem., 9, 537–546, https://doi.org/10.1071/EN12131, 2012.
Atkinson, J. D., Murray, B. J., Woodhouse, M. T., Whale, T. F., Baustian, K. J., Carslaw, K. S., Dobbie, S., O'Sullivan, D., and Malkin, T. L.: The importance of feldspar for ice nucleation by mineral dust in mixed-phase clouds, Nature, 498, 355–358, https://doi.org/10.1038/nature12278, 2013.
Beall, C. M., Stokes, M. D., Hill, T. C., DeMott, P. J., DeWald, J. T., and Prather, K. A.: Automation and heat transfer characterization of immersion mode spectroscopy for analysis of ice nucleating particles, Atmos. Meas. Tech., 10, 2613–2626, https://doi.org/10.5194/amt-10-2613-2017, 2017.
Beall, C. M., Lucero, D., Hill, T. C., DeMott, P. J., Stokes, M. D., and Prather, K. A.: Best practices for precipitation sample storage for offline studies of ice nucleation in marine and coastal environments, Atmos. Meas. Tech., 13, 6473–6486, https://doi.org/10.5194/amt-13-6473-2020, 2020.
Beall, C. M., Michaud, J. M., Fish, M. A., Dinasquet, J., Cornwell, G. C., Stokes, M. D., Burkart, M. D., Hill, T. C., Demott, P. J., and Prather, K. A.: Cultivable halotolerant ice-nucleating bacteria and fungi in coastal precipitation, Atmos. Chem. Phys., 21, 9031–9045, https://doi.org/10.5194/acp-21-9031-2021, 2021.
Beall, C. M., Hill, T. C. J., DeMott, P. J., Könemann, T., Pikridas, M., Drewnick, F., Harder, H., Pöhlker, C., Lelieveld, J., Weber, B., Iakovides, M., Prokeš, R., Sciare, J., Andreae, M. O., Stokes, M. D., and Prather, K. A.: Data from: Ice-nucleating Particles Near Two Major Dust Source Regions. In Center for Aerosol Impacts on Chemistry of the Environment (CAICE), UC San Diego Library Digital Collections [data set], https://doi.org/10.6075/J0X0676P, 2022.
Boose, Y., Sierau, B., Isabel García, M., Rodríguez, S., Alastuey, A., Linke, C., Schnaiter, M., Kupiszewski, P., Kanji, Z. A., and Lohmann, U.: Ice nucleating particles in the Saharan Air Layer, Atmos. Chem. Phys., 16, 9067–9087, https://doi.org/10.5194/acp-16-9067-2016, 2016.
Boose, Y., Baloh, P., Plötze, M., Ofner, J., Grothe, H., Sierau, B., Lohmann, U., and Kanji, Z. A.: Heterogeneous ice nucleation on dust particles sourced from nine deserts worldwide – Part 2: Deposition nucleation and condensation freezing, Atmos. Chem. Phys., 19, 1059–1076, https://doi.org/10.5194/acp-19-1059-2019, 2019.
Bourtsoukidis, E., Ernle, L., Crowley, J. N., Lelieveld, J., Paris, J.-D., Pozzer, A., Walter, D., and Williams, J.: Non-methane hydrocarbon (C2–C8) sources and sinks around the Arabian Peninsula, Atmos. Chem. Phys., 19, 7209–7232, https://doi.org/10.5194/acp-19-7209-2019, 2019.
Bourtsoukidis, E., Pozzer, A., Sattler, T., Matthaios, V. N., Ernle, L., Edtbauer, A., Fischer, H., Könemann, T., Osipov, S., Paris, J.-D., Pfannerstill, E. Y., Stönner, C., Tadic, I., Walter, D., Wang, N., Lelieveld, J., and Williams, J.: The Red Sea Deep Water is a potent source of atmospheric ethane and propane, Nat. Commun., 11, 447, https://doi.org/10.1038/s41467-020-14375-0, 2020.
Broadley, S. L., Murray, B. J., Herbert, R. J., Atkinson, J. D., Dobbie, S., Malkin, T. L., Condliffe, E., and Neve, L.: Immersion mode heterogeneous ice nucleation by an illite rich powder representative of atmospheric mineral dust, Atmos. Chem. Phys., 12, 287–307, https://doi.org/10.5194/acp-12-287-2012, 2012.
Brunner, C., Brem, B. T., Collaud Coen, M., Conen, F., Hervo, M., Henne, S., Steinbacher, M., Gysel-Beer, M., and Kanji, Z. A.: The contribution of Saharan dust to the ice-nucleating particle concentrations at the High Altitude Station Jungfraujoch (3580 m a.s.l.), Switzerland, Atmos. Chem. Phys., 21, 18029–18053, https://doi.org/10.5194/acp-21-18029-2021, 2021.
Buchard, V., Randles, C. A., da Silva, A. M., Darmenov, A., Colarco, P. R., Govindaraju, R., Ferrare, R., Hair, J., Beyersdorf, A. J., Ziemba, L. D., and Yu, H.: The MERRA-2 Aerosol Reanalysis, 1980 Onward, Part II: Evaluation and Case Studies, J. Clim., 30, 6851–6872, https://doi.org/10.1175/JCLI-D-16-0613.1, 2017.
Burrows, S. M., Hoose, C., Pöschl, U., and Lawrence, M. G.: Ice nuclei in marine air: biogenic particles or dust?, Atmos. Chem. Phys., 13, 245–267, https://doi.org/10.5194/acp-13-245-2013, 2013.
Celik, S., Drewnick, F., Fachinger, F., Brooks, J., Darbyshire, E., Coe, H., Paris, J.-D., Eger, P. G., Schuladen, J., Tadic, I., Friedrich, N., Dienhart, D., Hottmann, B., Fischer, H., Crowley, J. N., Harder, H., and Borrmann, S.: Influence of vessel characteristics and atmospheric processes on the gas and particle phase of ship emission plumes: in situ measurements in the Mediterranean Sea and around the Arabian Peninsula, Atmos. Chem. Phys., 20, 4713–4734, https://doi.org/10.5194/acp-20-4713-2020, 2020.
Collins, D. B., Zhao, D. F., Ruppel, M. J., Laskina, O., Grandquist, J. R., Modini, R. L., Stokes, M. D., Russell, L. M., Bertram, T. H., Grassian, V. H., Deane, G. B., and Prather, K. A.: Direct aerosol chemical composition measurements to evaluate the physicochemical differences between controlled sea spray aerosol generation schemes, Atmos. Meas. Tech., 7, 3667–3683, https://doi.org/10.5194/amt-7-3667-2014, 2014.
Conen, F., Morris, C. E., Leifeld, J., Yakutin, M. V., and Alewell, C.: Biological residues define the ice nucleation properties of soil dust, Atmos. Chem. Phys., 11, 9643–9648, https://doi.org/10.5194/acp-11-9643-2011, 2011.
Conen, F., Rodríguez, S., Hüglin, C., Henne, S., Herrmann, E., Bukowiecki, N., and Alewell, C.: Atmospheric ice nuclei at the high-altitude observatory Jungfraujoch, Switzerland, Tellus B, 67, 1–10, https://doi.org/10.3402/tellusb.v67.25014, 2015.
Conen, F., Einbock, A., Mignani, C., and Hüglin, C.: Measurement report: Ice-nucleating particles active ≥ −15 ∘C in free tropospheric air over western Europe, Atmos. Chem. Phys., 22, 3433–3444, https://doi.org/10.5194/acp-22-3433-2022, 2022.
Cornwell, G. C., McCluskey, C. S., Levin, E. J. T., Suski, K. J., DeMott, P. J., Kreidenweis, S. M., and Prather, K. A.: Direct Online Mass Spectrometry Measurements of Ice Nucleating Particles at a California Coastal Site, J. Geophys. Res.-Atmos., 124, 12157–12172, https://doi.org/10.1029/2019JD030466, 2019.
Cornwell, G. C., Sultana, C. M., Prank, M., Cochran, R. E., Hill, T. C. J., Schill, G. P., DeMott, P. J., Mahowald, N., and Prather, K. A.: Ejection of Dust From the Ocean as a Potential Source of Marine Ice Nucleating Particles, J. Geophys. Res.-Atmos., 125, e2020JD033073, https://doi.org/10.1029/2020JD033073, 2020.
Cziczo, D. J., Froyd, K. D., Gallavardin, S. J., Moehler, O., Benz, S., Saathoff, H., and Murphy, D. M.: Deactivation of ice nuclei due to atmospherically relevant surface coatings, Environ. Res. Lett., 4, 44013, https://doi.org/10.1088/1748-9326/4/4/044013, 2009.
Dall'Osto, M., Harrison, R. M., Highwood, E. J., O'Dowd, C., Ceburnis, D., Querol, X., and Achterberg, E. P.: Variation of the mixing state of Saharan dust particles with atmospheric transport, Atmos. Environ., 44, 3135–3146, https://doi.org/10.1016/j.atmosenv.2010.05.030, 2010.
Delany, A. C. and Zenchelsky, S.: The Organic Component Of Wind-Erosion-Generated Soil-Derived Aerosol, Soil Sci., 121, 146–155, 1976.
DeMott, P. J., Prenni, A. J., McMeeking, G. R., Sullivan, R. C., Petters, M. D., Tobo, Y., Niemand, M., Möhler, O., Snider, J. R., Wang, Z., and Kreidenweis, S. M.: Integrating laboratory and field data to quantify the immersion freezing ice nucleation activity of mineral dust particles, Atmos. Chem. Phys., 15, 393–409, https://doi.org/10.5194/acp-15-393-2015, 2015.
DeMott, P. J., Hill, T. C. J., McCluskey, C. S., Prather, K. A., Collins, D. B., Sullivan, R. C., Ruppel, M. J., Mason, R. H., Irish, V. E., Lee, T., Hwang, C. Y., Rhee, T. S., Snider, J. R., McMeeking, G. R., Dhaniyala, S., Lewis, E. R., Wentzell, J. J. B., Abbatt, J., Lee, C., Sultana, C. M., Ault, A. P., Axson, J. L., Diaz Martinez, M., Venero, I., Santos-Figueroa, G., Stokes, M. D., Deane, G. B., Mayol-Bracero, O. L., Grassian, V. H., Bertram, T. H., Bertram, A. K., Moffett, B. F., and Franc, G. D.: Sea spray aerosol as a unique source of ice nucleating particles, P. Natl. Acad. Sci. USA, 113, 5797–5803, https://doi.org/10.1073/pnas.1514034112, 2016.
DeMott, P. J., Hill, T. C. J., Petters, M. D., Bertram, A. K., Tobo, Y., Mason, R. H., Suski, K. J., McCluskey, C. S., Levin, E. J. T., Schill, G. P., Boose, Y., Rauker, A. M., Miller, A. J., Zaragoza, J., Rocci, K., Rothfuss, N. E., Taylor, H. P., Hader, J. D., Chou, C., Huffman, J. A., Pöschl, U., Prenni, A. J., and Kreidenweis, S. M.: Comparative measurements of ambient atmospheric concentrations of ice nucleating particles using multiple immersion freezing methods and a continuous flow diffusion chamber, Atmos. Chem. Phys., 17, 11227–11245, https://doi.org/10.5194/acp-17-11227-2017, 2017.
DeMott, P. J., Möhler, O., Cziczo, D. J., Hiranuma, N., Petters, M. D., Petters, S. S., Belosi, F., Bingemer, H. G., Brooks, S. D., Budke, C., Burkert-Kohn, M., Collier, K. N., Danielczok, A., Eppers, O., Felgitsch, L., Garimella, S., Grothe, H., Herenz, P., Hill, T. C. J., Höhler, K., Kanji, Z. A., Kiselev, A., Koop, T., Kristensen, T. B., Krüger, K., Kulkarni, G., Levin, E. J. T., Murray, B. J., Nicosia, A., O'Sullivan, D., Peckhaus, A., Polen, M. J., Price, H. C., Reicher, N., Rothenberg, D. A., Rudich, Y., Santachiara, G., Schiebel, T., Schrod, J., Seifried, T. M., Stratmann, F., Sullivan, R. C., Suski, K. J., Szakáll, M., Taylor, H. P., Ullrich, R., Vergara-Temprado, J., Wagner, R., Whale, T. F., Weber, D., Welti, A., Wilson, T. W., Wolf, M. J., and Zenker, J.: The Fifth International Workshop on Ice Nucleation phase 2 (FIN-02): laboratory intercomparison of ice nucleation measurements, Atmos. Meas. Tech., 11, 6231–6257, https://doi.org/10.5194/amt-11-6231-2018, 2018.
Eastwood, M. L., Cremel, S., Wheeler, M., Murray, B. J., Girard, E., and Bertram, A. K.: Effects of sulfuric acid and ammonium sulfate coatings on the ice nucleation properties of kaolinite particles, Geophys. Res. Lett., 36, L02811, https://doi.org/10.1029/2008GL035997, 2009.
Edtbauer, A., Stönner, C., Pfannerstill, E. Y., Berasategui, M., Walter, D., Crowley, J. N., Lelieveld, J., and Williams, J.: A new marine biogenic emission: methane sulfonamide (MSAM), dimethyl sulfide (DMS), and dimethyl sulfone (DMSO2) measured in air over the Arabian Sea, Atmos. Chem. Phys., 20, 6081–6094, https://doi.org/10.5194/acp-20-6081-2020, 2020.
Eger, P. G., Friedrich, N., Schuladen, J., Shenolikar, J., Fischer, H., Tadic, I., Harder, H., Martinez, M., Rohloff, R., Tauer, S., Drewnick, F., Fachinger, F., Brooks, J., Darbyshire, E., Sciare, J., Pikridas, M., Lelieveld, J., and Crowley, J. N.: Shipborne measurements of ClNO2 in the Mediterranean Sea and around the Arabian Peninsula during summer, Atmos. Chem. Phys., 19, 12121–12140, https://doi.org/10.5194/acp-19-12121-2019, 2019.
Fennelly, M. J., Sewell, G., Prentice, M. B., O'Connor, D. J., and Sodeau, J. R.: Review: The Use of Real-Time Fluorescence Instrumentation to Monitor Ambient Primary Biological Aerosol Particles (PBAP), Atmosphere, 9, 1, https://doi.org/10.3390/atmos9010001, 2018.
Friedrich, N., Eger, P., Shenolikar, J., Sobanski, N., Schuladen, J., Dienhart, D., Hottmann, B., Tadic, I., Fischer, H., Martinez, M., Rohloff, R., Tauer, S., Harder, H., Pfannerstill, E. Y., Wang, N., Williams, J., Brooks, J., Drewnick, F., Su, H., Li, G., Cheng, Y., Lelieveld, J., and Crowley, J. N.: Reactive nitrogen around the Arabian Peninsula and in the Mediterranean Sea during the 2017 AQABA ship campaign, Atmos. Chem. Phys., 21, 7473–7498, https://doi.org/10.5194/acp-21-7473-2021, 2021.
Gandham, H., Dasari, H. P., Langodan, S., Karumuri, R. K., and Hoteit, I.: Major Changes in Extreme Dust Events Dynamics Over the Arabian Peninsula During 2003–2017 Driven by Atmospheric Conditions, J. Geophys. Res.-Atmos., 125, e2020JD032931, https://doi.org/10.1029/2020JD032931, 2020.
Gelaro, R., McCarty, W., Suárez, M. J., Todling, R., Molod, A., Takacs, L., Randles, C. A., Darmenov, A., Bosilovich, M. G., Reichle, R., Wargan, K., Coy, L., Cullather, R., Draper, C., Akella, S., Buchard, V., Conaty, A., da Silva, A. M., Gu, W., Kim, G.-K., Koster, R., Lucchesi, R., Merkova, D., Nielsen, J. E., Partyka, G., Pawson, S., Putman, W., Rienecker, M., Schubert, S. D., Sienkiewicz, M., and Zhao, B.: The Modern-Era Retrospective Analysis for Research and Applications, Version 2 (MERRA-2), J. Clim., 30, 5419–5454, https://doi.org/10.1175/JCLI-D-16-0758.1, 2017.
Gong, X., Wex, H., Müller, T., Wiedensohler, A., Höhler, K., Kandler, K., Ma, N., Dietel, B., Schiebel, T., Möhler, O., and Stratmann, F.: Characterization of aerosol properties at Cyprus, focusing on cloud condensation nuclei and ice-nucleating particles, Atmos. Chem. Phys., 19, 10883–10900, https://doi.org/10.5194/acp-19-10883-2019, 2019.
Gong, X., Wex, H., van Pinxteren, M., Triesch, N., Fomba, K. W., Lubitz, J., Stolle, C., Robinson, T.-B., Müller, T., Herrmann, H., and Stratmann, F.: Characterization of aerosol particles at Cabo Verde close to sea level and at the cloud level – Part 2: Ice-nucleating particles in air, cloud and seawater, Atmos. Chem. Phys., 20, 1451–1468, https://doi.org/10.5194/acp-20-1451-2020, 2020a.
Gong, X., Wex, H., van Pinxteren, M., Triesch, N., Fomba, Khanneh, W., Lubitz, J., Stolle, C., Robinson, T.-B., Müller, T., Herrmann, H., and Stratmann, .F: Ice nucleating particles measured in air, cloud and seawater at the Cape Verde Atmospheric Observatory (CVAO), PANGAEA [data set], https://doi.org/10.1594/PANGAEA.906946, 2020b.
Hara, K., Maki, T., Kakikawa, M., Kobayashi, F., and Matsuki, A.: Effects of different temperature treatments on biological ice nuclei in snow samples, Atmos. Environ., 140, 415–419, https://doi.org/10.1016/j.atmosenv.2016.06.011, 2016.
Harrison, A. D., Whale, T. F., Carpenter, M. A., Holden, M. A., Neve, L., O'Sullivan, D., Vergara Temprado, J., and Murray, B. J.: Not all feldspars are equal: a survey of ice nucleating properties across the feldspar group of minerals, Atmos. Chem. Phys., 16, 10927–10940, https://doi.org/10.5194/acp-16-10927-2016, 2016.
Harrison, A. D., Lever, K., Sanchez-Marroquin, A., Holden, M. A., Whale, T. F., Tarn, M. D., McQuaid, J. B., and Murray, B. J.: The ice-nucleating ability of quartz immersed in water and its atmospheric importance compared to K-feldspar, Atmos. Chem. Phys., 19, 11343–11361, https://doi.org/10.5194/acp-19-11343-2019, 2019.
Hartmann, M., Adachi, K., Eppers, O., Haas, C., Herber, A., Holzinger, R., Hünerbein, A., Jäkel, E., Jentzsch, C., van Pinxteren, M., Wex, H., Willmes, S., and Stratmann, F.: Wintertime Airborne Measurements of Ice Nucleating Particles in the High Arctic: A Hint to a Marine, Biogenic Source for Ice Nucleating Particles, Geophys. Res. Lett., 47, e2020GL087770, https://doi.org/10.1029/2020GL087770, 2020.
Hill, T. C. J., DeMott, P. J., Tobo, Y., Fröhlich-Nowoisky, J., Moffett, B. F., Franc, G. D., and Kreidenweis, S. M.: Sources of organic ice nucleating particles in soils, Atmos. Chem. Phys., 16, 7195–7211, https://doi.org/10.5194/acp-16-7195-2016, 2016.
Hinz, K.-P., Trimborn, A., Weingartner, E., Henning, S., Baltensperger, U., and Spengler, B.: Aerosol single particle composition at the Jungfraujoch, J. Aerosol Sci., 36, 123–145, https://doi.org/10.1016/j.jaerosci.2004.08.001, 2005.
Hiranuma, N., Augustin-Bauditz, S., Bingemer, H., Budke, C., Curtius, J., Danielczok, A., Diehl, K., Dreischmeier, K., Ebert, M., Frank, F., Hoffmann, N., Kandler, K., Kiselev, A., Koop, T., Leisner, T., Möhler, O., Nillius, B., Peckhaus, A., Rose, D., Weinbruch, S., Wex, H., Boose, Y., DeMott, P. J., Hader, J. D., Hill, T. C. J., Kanji, Z. A., Kulkarni, G., Levin, E. J. T., McCluskey, C. S., Murakami, M., Murray, B. J., Niedermeier, D., Petters, M. D., O'Sullivan, D., Saito, A., Schill, G. P., Tajiri, T., Tolbert, M. A., Welti, A., Whale, T. F., Wright, T. P., and Yamashita, K.: A comprehensive laboratory study on the immersion freezing behavior of illite NX particles: a comparison of 17 ice nucleation measurement techniques, Atmos. Chem. Phys., 15, 2489–2518, https://doi.org/10.5194/acp-15-2489-2015, 2015.
Hoose, C. and Möhler, O.: Heterogeneous ice nucleation on atmospheric aerosols: a review of results from laboratory experiments, Atmos. Chem. Phys., 12, 9817–9854, https://doi.org/10.5194/acp-12-9817-2012, 2012.
Hoose, C., Kristjánsson, J. E., Chen, J.-P., and Hazra, A.: A Classical-Theory-Based Parameterization of Heterogeneous Ice Nucleation by Mineral Dust, Soot, and Biological Particles in a Global Climate Model, J. Atmos. Sci., 67, 2483–2503, https://doi.org/10.1175/2010JAS3425.1, 2010.
Huffman, J. A., Perring, A. E., Savage, N. J., Clot, B., Crouzy, B., Tummon, F., Shoshanim, O., Damit, B., Schneider, J., Sivaprakasam, V., Zawadowicz, M. A., Crawford, I., Gallagher, M., Topping, D., Doughty, D. C., Hill, S. C., and Pan, Y.: Real-time sensing of bioaerosols: Review and current perspectives, Aerosol Sci. Technol., 54, 465–495, https://doi.org/10.1080/02786826.2019.1664724, 2020.
Huneeus, N., Schulz, M., Balkanski, Y., Griesfeller, J., Prospero, J., Kinne, S., Bauer, S., Boucher, O., Chin, M., Dentener, F., Diehl, T., Easter, R., Fillmore, D., Ghan, S., Ginoux, P., Grini, A., Horowitz, L., Koch, D., Krol, M. C., Landing, W., Liu, X., Mahowald, N., Miller, R., Morcrette, J.-J., Myhre, G., Penner, J., Perlwitz, J., Stier, P., Takemura, T., and Zender, C. S.: Global dust model intercomparison in AeroCom phase I, Atmos. Chem. Phys., 11, 7781–7816, https://doi.org/10.5194/acp-11-7781-2011, 2011.
Kanji, Z. A., Ladino, L. A., Wex, H., Boose, Y., Burkert-Kohn, M., Cziczo, D. J., and Krämer, M.: Overview of Ice Nucleating Particles, Meteorol. Monogr., 58, 1.1–1.33, https://doi.org/10.1175/amsmonographs-d-16-0006.1, 2017.
Khaniabadi, Y. O., Daryanoosh, S. M., Amrane, A., Polosa, R., Hopke, P. K., Goudarzi, G., Mohammadi, M. J., Sicard, P., and Armin, H.: Impact of Middle Eastern Dust storms on human health, Atmos. Pollut. Res., 8, 606–613, https://doi.org/10.1016/j.apr.2016.11.005, 2017.
Kinne, S., Schulz, M., Textor, C., Guibert, S., Balkanski, Y., Bauer, S. E., Berntsen, T., Berglen, T. F., Boucher, O., Chin, M., Collins, W., Dentener, F., Diehl, T., Easter, R., Feichter, J., Fillmore, D., Ghan, S., Ginoux, P., Gong, S., Grini, A., Hendricks, J., Herzog, M., Horowitz, L., Isaksen, I., Iversen, T., Kirkevåg, A., Kloster, S., Koch, D., Kristjansson, J. E., Krol, M., Lauer, A., Lamarque, J. F., Lesins, G., Liu, X., Lohmann, U., Montanaro, V., Myhre, G., Penner, J., Pitari, G., Reddy, S., Seland, O., Stier, P., Takemura, T., and Tie, X.: An AeroCom initial assessment – optical properties in aerosol component modules of global models, Atmos. Chem. Phys., 6, 1815–1834, https://doi.org/10.5194/acp-6-1815-2006, 2006.
Kleist, D. T., Parrish, D. F., Derber, J. C., Treadon, R., Wu, W.-S., and Lord, S.: Introduction of the GSI into the NCEP Global Data Assimilation System, Weather Forecast., 24, 1691–1705, https://doi.org/10.1175/2009WAF2222201.1, 2009.
Klingmüller, K., Pozzer, A., Metzger, S., Stenchikov, G. L., and Lelieveld, J.: Aerosol optical depth trend over the Middle East, Atmos. Chem. Phys., 16, 5063–5073, https://doi.org/10.5194/acp-16-5063-2016, 2016.
Knopf, D. A. and Koop, T.: Heterogeneous nucleation of ice on surrogates of mineral dust, J. Geophys. Res.-Atmos., 111, D12201, https://doi.org/10.1029/2005JD006894, 2006.
Kok, J. F., Adebiyi, A. A., Albani, S., Balkanski, Y., Checa-Garcia, R., Chin, M., Colarco, P. R., Hamilton, D. S., Huang, Y., Ito, A., Klose, M., Li, L., Mahowald, N. M., Miller, R. L., Obiso, V., Pérez García-Pando, C., Rocha-Lima, A., and Wan, J. S.: Contribution of the world's main dust source regions to the global cycle of desert dust, Atmos. Chem. Phys., 21, 8169–8193, https://doi.org/10.5194/acp-21-8169-2021, 2021.
Könemann, T., Savage, N., Klimach, T., Walter, D., Fröhlich-Nowoisky, J., Su, H., Pöschl, U., Huffman, J. A., and Pöhlker, C.: Spectral Intensity Bioaerosol Sensor (SIBS): an instrument for spectrally resolved fluorescence detection of single particles in real time, Atmos. Meas. Tech., 12, 1337–1363, https://doi.org/10.5194/amt-12-1337-2019, 2019.
Krasnov, H., Katra, I., and Friger, M.: Increase in dust storm related PM10 concentrations: A time series analysis of 2001–2015, Environ. Pollut., 213, 36–42, https://doi.org/10.1016/j.envpol.2015.10.021, 2016.
Krueger, B. J., Grassian, V. H., Cowin, J. P., and Laskin, A.: Heterogeneous chemistry of individual mineral dust particles from different dust source regions: the importance of particle mineralogy, Atmos. Environ., 38, 6253–6261, https://doi.org/10.1016/j.atmosenv.2004.07.010, 2004.
Krzywinski, M. and Altman, N.: Error bars, Nat. Methods, 10, 921–922, https://doi.org/10.1038/nmeth.2659, 2013.
Ladino, L. A., Raga, G. B., Alvarez-Ospina, H., Andino-Enríquez, M. A., Rosas, I., Martínez, L., Salinas, E., Miranda, J., Ramírez-Díaz, Z., Figueroa, B., Chou, C., Bertram, A. K., Quintana, E. T., Maldonado, L. A., García-Reynoso, A., Si, M., and Irish, V. E.: Ice-nucleating particles in a coastal tropical site, Atmos. Chem. Phys., 19, 6147–6165, https://doi.org/10.5194/acp-19-6147-2019, 2019.
Lohmann, U. and Feichter, J.: Global indirect aerosol effects: a review, Atmos. Chem. Phys., 5, 715–737, https://doi.org/10.5194/acp-5-715-2005, 2005.
Manders, A. M., Schapp, M., Jozwicka, M., van Arkel, F., Weijers, E., and Matthijsen, J.: The contribution of sea salt to PM10 and PM2.5 in the Netherlands, http://www.pbl.nl/sites/default/files/cms/publicaties/500099004.pdf (last access: 20 June 2022), 2009.
Martin, A. C., Cornwell, G., Beall, C. M., Cannon, F., Reilly, S., Schaap, B., Lucero, D., Creamean, J., Ralph, F. M., Mix, H. T., and Prather, K.: Contrasting local and long-range-transported warm ice-nucleating particles during an atmospheric river in coastal California, USA, Atmos. Chem. Phys., 19, 4193–4210, https://doi.org/10.5194/acp-19-4193-2019, 2019.
Mayol, E., Jiménez, M. A., Herndl, G. J., Duarte, C. M., and Arrieta, J. M.: Resolving the abundance and air-sea fluxes of airborne microorganisms in the North Atlantic Ocean, Front. Microbiol., 5, 557, https://doi.org/10.3389/fmicb.2014.00557, 2014.
McCluskey, C. S., Hill, T. C. J., Malfatti, F., Sultana, C. M., Lee, C., Santander, M. V, Beall, C. M., Moore, K. A., Cornwell, G. C., Collins, D. B., Prather, K. A., Jayarathne, T., Stone, E. A., Azam, F., Kreidenweis, S. M., and DeMott, P. J.: A Dynamic Link between Ice Nucleating Particles Released in Nascent Sea Spray Aerosol and Oceanic Biological Activity during Two Mesocosm Experiments, J. Atmos. Sci., 74, 151–166, https://doi.org/10.1175/JAS-D-16-0087.1, 2017.
McCluskey, C. S., Hill, T. C. J., Sultana, C. M., Laskina, O., Trueblood, J., Santander, M. V, Beall, C. M., Michaud, J. M., Kreidenweis, S. M., Prather, K. A., Grassian, V., and DeMott, P. J.: A Mesocosm Double Feature: Insights into the Chemical Makeup of Marine Ice Nucleating Particles, J. Atmos. Sci., 75, 2405–2423, https://doi.org/10.1175/JAS-D-17-0155.1, 2018a.
McCluskey, C. S., Ovadnevaite, J., Rinaldi, M., Atkinson, J., Belosi, F., Ceburnis, D., Marullo, S., Hill, T. C. J., Lohmann, U., Kanji, Z. A., O'Dowd, C., Kreidenweis, S. M., and DeMott, P. J.: Marine and Terrestrial Organic Ice-Nucleating Particles in Pristine Marine to Continentally Influenced Northeast Atlantic Air Masses, J. Geophys. Res.-Atmos., 123, 6196–6212, https://doi.org/10.1029/2017JD028033, 2018b.
McCluskey, C. S., Hill, T. C. J., Humphries, R. S., Rauker, A. M., Moreau, S., Strutton, P. G., Chambers, S. D., Williams, A. G., McRobert, I., Ward, J., Keywood, M. D., Harnwell, J., Ponsonby, W., Loh, Z. M., Krummel, P. B., Protat, A., Kreidenweis, S. M., and DeMott, P. J.: Observations of Ice Nucleating Particles Over Southern Ocean Waters, Geophys. Res. Lett., 45, 11989–11997, https://doi.org/10.1029/2018GL079981, 2018c.
McCluskey, C. S., Hill, T. C. J., Humphries, R. S., Rauker, A. M., Moreau, S., Strutton, P. G., Chambers, S. D., Williams, A. G., McRobert, I., Ward, J., Keywood, M. D., Harnwell, J., Ponsonby, W., Loh, Z. M., Krummel, P. B., Protat, A., Kreidenweis, S. M., and DeMott, P. J.: Dataset associated with “Observations of ice nucleating particles over Southern Ocean waters”, Mountain Scholar [data set], https://doi.org/10.25675/10217/192127, 2018d.
Megahed, K.: The impact of mineral dust aerosol particles on cloud formation, Ph.D. dissertation, Rheinische Friedrich-Wilhelms-University Bonn, 175 pp., https://bonndoc.ulb.uni-bonn.de/xmlui/handle/20.500.11811/3083 (last access: 24 July 2022), 2007.
Mitts, B., Wang, X., Lucero, D., Beall, C., Deane, G., DeMott, P., and Prather, K.: Importance of Supermicron Ice Nucleating Particles in Nascent Sea Spray, Geophys. Res. Lett., 48, e2020GL089633, https://doi.org/10.1029/2020GL089633, 2021.
Molod, A., Takacs, L., Suarez, M., and Bacmeister, J.: Development of the GEOS-5 atmospheric general circulation model: evolution from MERRA to MERRA2, Geosci. Model Dev., 8, 1339–1356, https://doi.org/10.5194/gmd-8-1339-2015, 2015.
Murray, B. J., O'Sullivan, D., Atkinson, J. D., and Webb, M. E.: Ice nucleation by particles immersed in supercooled cloud droplets, Chem. Soc. Rev., 41, 6519–54, https://doi.org/10.1039/c2cs35200a, 2012.
Nickovic, S., Vukovic, A., Vujadinovic, M., Djurdjevic, V., and Pejanovic, G.: Technical Note: High-resolution mineralogical database of dust-productive soils for atmospheric dust modeling, Atmos. Chem. Phys., 12, 845–855, https://doi.org/10.5194/acp-12-845-2012, 2012.
Niedermeier, D., Augustin-Bauditz, S., Hartmann, S., Wex, H., Ignatius, K., and Stratmann, F.: Can we define an asymptotic value for the ice active surface site density for heterogeneous ice nucleation?, J. Geophys. Res.-Atmos., 120, 5036–5046, https://doi.org/10.1002/2014JD022814, 2015.
Niemand, M., Möhler, O., Vogel, B., Vogel, H., Hoose, C., Connolly, P., Klein, H., Bingemer, H., Demott, P., Skrotzki, J., and Leisner, T.: A particle-surface-area-based parameterization of immersion freezing on desert dust particles, J. Atmos. Sci., 69, 3077–3092, https://doi.org/10.1175/JAS-D-11-0249.1, 2012.
Nortcliff, S.: World Soil Resources and Food Security, edited by: Lal, R. and Stewart, B. A., Boca Raton, Fl, USA, CRC Press, 574 pp., ISBN-13 978-1439844502, Exp. Agric., 48, 305–306, 2012.
O'Sullivan, D., Murray, B. J., Malkin, T. L., Whale, T. F., Umo, N. S., Atkinson, J. D., Price, H. C., Baustian, K. J., Browse, J., and Webb, M. E.: Ice nucleation by fertile soil dusts: relative importance of mineral and biogenic components, Atmos. Chem. Phys., 14, 1853–1867, https://doi.org/10.5194/acp-14-1853-2014, 2014.
O'Sullivan, D., Adams, M. P., Tarn, M. D., Harrison, A. D., Vergara-Temprado, J., Porter, G. C. E., Holden, M. A., Sanchez-Marroquin, A., Carotenuto, F., Whale, T. F., McQuaid, J. B., Walshaw, R., Hedges, D. H. P., Burke, I. T., Cui, Z., and Murray, B. J.: Contributions of biogenic material to the atmospheric ice-nucleating particle population in North Western Europe, Sci. Rep., 8, 13821, https://doi.org/10.1038/s41598-018-31981-7, 2018.
Paramonov, M., David, R. O., Kretzschmar, R., and Kanji, Z. A.: A laboratory investigation of the ice nucleation efficiency of three types of mineral and soil dust, Atmos. Chem. Phys., 18, 16515–16536, https://doi.org/10.5194/acp-18-16515-2018, 2018.
Perkins, R. J., Gillette, S. M., Hill, T. C. J., and DeMott, P. J.: The Labile Nature of Ice Nucleation by Arizona Test Dust, ACS Earth Sp. Chem., 4, 133–141, https://doi.org/10.1021/acsearthspacechem.9b00304, 2020.
Pfannerstill, E. Y., Wang, N., Edtbauer, A., Bourtsoukidis, E., Crowley, J. N., Dienhart, D., Eger, P. G., Ernle, L., Fischer, H., Hottmann, B., Paris, J.-D., Stönner, C., Tadic, I., Walter, D., Lelieveld, J., and Williams, J.: Shipborne measurements of total OH reactivity around the Arabian Peninsula and its role in ozone chemistry, Atmos. Chem. Phys., 19, 11501–11523, https://doi.org/10.5194/acp-19-11501-2019, 2019.
Price, H. C., Baustian, K. J., McQuaid, J. B., Blyth, A., Bower, K. N., Choularton, T., Cotton, R. J., Cui, Z., Field, P. R., Gallagher, M., Hawker, R., Merrington, A., Miltenberger, A., Neely III, R. R., Parker, S. T., Rosenberg, P. D., Taylor, J. W., Trembath, J., Vergara-Temprado, J., Whale, T. F., Wilson, T. W., Young, G., and Murray, B. J.: Atmospheric Ice-Nucleating Particles in the Dusty Tropical Atlantic, J. Geophys. Res.-Atmos., 123, 2175–2193, https://doi.org/10.1002/2017JD027560, 2018a.
Price, H. C., Baustian, K. J., McQuaid, J. B., Blyth, A., Bower, K. N., Choularton, T., Cotton, R. J., Cui, Z., Field, P. R., Gallagher, M., Hawker, R., Merrington, A., Miltenberger, A., Neely III, R. R., Parker, S. T., Rosenberg, P. D., Taylor, J. W., Trembath, J. Vergara-Temprado, J., Whale, T. F., Wilson, T.W., Young, G., and Murray, B. J.: Datasets associated with “Atmospheric ice-nucleating particles in the dusty tropical Atlantic”, University of Leeds [data set], https://doi.org/10.5518/302, 2018b.
Prodi, F., Santachiara, G., and Oliosi, F.: Characterization of aerosols in marine environments (Mediterranean, Red Sea, and Indian Ocean), J. Geophys. Res.-Ocean., 88, 10957–10968, https://doi.org/10.1029/JC088iC15p10957, 1983.
Rienecker, M. M., Suarez, M. J., Gelaro, R., Todling, R., Bacmeister, J., Liu, E., Bosilovich, M. G., Schubert, S. D., Takacs, L., Kim, G.-K., Bloom, S., Chen, J., Collins, D., Conaty, A., da Silva, A., Gu, W., Joiner, J., Koster, R. D., Lucchesi, R., Molod, A., Owens, T., Pawson, S., Pegion, P., Redder, C. R., Reichle, R., Robertson, F. R., Ruddick, A. G., Sienkiewicz, M., and Woollen, J.: MERRA: NASA's Modern-Era Retrospective Analysis for Research and Applications, J. Clim., 24, 3624–3648, https://doi.org/10.1175/JCLI-D-11-00015.1, 2011.
Salam, A., Lohmann, U., and Lesins, G.: Ice nucleation of ammonia gas exposed montmorillonite mineral dust particles, Atmos. Chem. Phys., 7, 3923–3931, https://doi.org/10.5194/acp-7-3923-2007, 2007.
Schnell, R. C.: Ice Nuclei in Seawater, Fog Water and Marine Air off the Coast of Nova Scotia: Summer 1975, J. Atmos. Sci., 34, 1299–1305, https://doi.org/10.1175/1520-0469(1977)034<1299:INISFW>2.0.CO;2, 1977.
Schrod, J., Weber, D., Drücke, J., Keleshis, C., Pikridas, M., Ebert, M., Cvetkoviæ, B., Nickovic, S., Marinou, E., Baars, H., Ansmann, A., Vrekoussis, M., Mihalopoulos, N., Sciare, J., Curtius, J., and Bingemer, H. G.: Ice nucleating particles over the Eastern Mediterranean measured by unmanned aircraft systems, Atmos. Chem. Phys., 17, 4817–4835, https://doi.org/10.5194/acp-17-4817-2017, 2017.
Shahsavani, A., Naddafi, K., Jafarzade Haghighifard, N., Mesdaghinia, A., Yunesian, M., Nabizadeh, R., Arahami, M., Sowlat, M. H., Yarahmadi, M., Saki, H., Alimohamadi, M., Nazmara, S., Motevalian, S. A., and Goudarzi, G.: The evaluation of PM10, PM2.5, and PM1 concentrations during the Middle Eastern Dust (MED) events in Ahvaz, Iran, from april through september 2010, J. Arid Environ., 77, 72–83, https://doi.org/10.1016/j.jaridenv.2011.09.007, 2012.
Steinke, I., Funk, R., Busse, J., Iturri, A., Kirchen, S., Leue, M., Möhler, O., Schwartz, T., Schnaiter, M., Sierau, B., Toprak, E., Ullrich, R., Ulrich, A., Hoose, C., and Leisner, T.: Ice nucleation activity of agricultural soil dust aerosols from Mongolia, Argentina, and Germany, J. Geophys. Res.-Atmos., 121, 13559–13576, https://doi.org/10.1002/2016JD025160, 2016.
Sullivan, R. C., Guazzotti, S. A., Sodeman, D. A., and Prather, K. A.: Direct observations of the atmospheric processing of Asian mineral dust, Atmos. Chem. Phys., 7, 1213–1236, https://doi.org/10.5194/acp-7-1213-2007, 2007.
Sullivan, R. C., Miñambres, L., DeMott, P. J., Prenni, A. J., Carrico, C. M., Levin, E. J. T., and Kreidenweis, S. M.: Chemical processing does not always impair heterogeneous ice nucleation of mineral dust particles, Geophys. Res. Lett., 37, L24805, https://doi.org/10.1029/2010GL045540, 2010a.
Sullivan, R. C., Petters, M. D., DeMott, P. J., Kreidenweis, S. M., Wex, H., Niedermeier, D., Hartmann, S., Clauss, T., Stratmann, F., Reitz, P., Schneider, J., and Sierau, B.: Irreversible loss of ice nucleation active sites in mineral dust particles caused by sulphuric acid condensation, Atmos. Chem. Phys., 10, 11471–11487, https://doi.org/10.5194/acp-10-11471-2010, 2010b.
Suski, K. J., Hill, T. C. J., Levin, E. J. T., Miller, A., DeMott, P. J., and Kreidenweis, S. M.: Agricultural harvesting emissions of ice-nucleating particles, Atmos. Chem. Phys., 18, 13755–13771, https://doi.org/10.5194/acp-18-13755-2018, 2018.
Tadic, I., Crowley, J. N., Dienhart, D., Eger, P., Harder, H., Hottmann, B., Martinez, M., Parchatka, U., Paris, J.-D., Pozzer, A., Rohloff, R., Schuladen, J., Shenolikar, J., Tauer, S., Lelieveld, J., and Fischer, H.: Net ozone production and its relationship to nitrogen oxides and volatile organic compounds in the marine boundary layer around the Arabian Peninsula, Atmos. Chem. Phys., 20, 6769–6787, https://doi.org/10.5194/acp-20-6769-2020, 2020.
Tobo, Y., DeMott, P. J., Hill, T. C. J., Prenni, A. J., Swoboda-Colberg, N. G., Franc, G. D., and Kreidenweis, S. M.: Organic matter matters for ice nuclei of agricultural soil origin, Atmos. Chem. Phys., 14, 8521–8531, https://doi.org/10.5194/acp-14-8521-2014, 2014.
Ullrich, R., Hoose, C., Möhler, O., Niemand, M., Wagner, R., Höhler, K., Hiranuma, N., Saathoff, H., and Leisner, T.: A new ice nucleation active site parameterization for desert dust and soot, J. Atmos. Sci., 74, 699–717, https://doi.org/10.1175/JAS-D-16-0074.1, 2017.
Vali, G.: Quantitative Evaluation of Experimental Results an the Heterogeneous Freezing Nucleation of Supercooled Liquids, J. Atmos. Sci., 28, 402–409, https://doi.org/10.1175/1520-0469(1971)028<0402:QEOERA>2.0.CO;2, 1971.
Van Pelt, R. S. and Zobeck, T. M.: Chemical Constituents of Fugitive Dust, Environ. Monit. Assess., 130, 3–16, https://doi.org/10.1007/s10661-006-9446-8, 2007.
Vergara-Temprado, J., Murray, B. J., Wilson, T. W., O'Sullivan, D., Browse, J., Pringle, K. J., Ardon-Dryer, K., Bertram, A. K., Burrows, S. M., Ceburnis, D., Demott, P. J., Mason, R. H., O'Dowd, C. D., Rinaldi, M., and Carslaw, K. S.: Contribution of feldspar and marine organic aerosols to global ice nucleating particle concentrations, Atmos. Chem. Phys., 17, 3637–3658, https://doi.org/10.5194/acp-17-3637-2017, 2017.
Vergara-Temprado, J., Miltenberger, A. K., Furtado, K., Grosvenor, D. P., Shipway, B. J., Hill, A. A., Wilkinson, J. M., Field, P. R., Murray, B. J., and Carslaw, K. S.: Strong control of Southern Ocean cloud reflectivity by ice-nucleating particles, P. Natl. Acad. Sci. USA, 115, 2687–2692, https://doi.org/10.1073/pnas.1721627115, 2018.
Wang, N., Edtbauer, A., Stönner, C., Pozzer, A., Bourtsoukidis, E., Ernle, L., Dienhart, D., Hottmann, B., Fischer, H., Schuladen, J., Crowley, J. N., Paris, J.-D., Lelieveld, J., and Williams, J.: Measurements of carbonyl compounds around the Arabian Peninsula: overview and model comparison, Atmos. Chem. Phys., 20, 10807–10829, https://doi.org/10.5194/acp-20-10807-2020, 2020.
Wang, X., Deane, G. B., Moore, K. A., Ryder, O. S., Stokes, M. D., Beall, C. M., Collins, D. B., Santander, M. V, Burrows, S. M., Sultana, C. M., and Prather, K. A.: The role of jet and film drops in controlling the mixing state of submicron sea spray aerosol particles, P. Natl. Acad. Sci. USA, 114, 6978–6983, https://doi.org/10.1073/pnas.1702420114, 2017.
von der Weiden, S.-L., Drewnick, F., and Borrmann, S.: Particle Loss Calculator – a new software tool for the assessment of the performance of aerosol inlet systems, Atmos. Meas. Tech., 2, 479–494, https://doi.org/10.5194/amt-2-479-2009, 2009.
Welti, A., Lüönd, F., Kanji, Z. A., Stetzer, O., and Lohmann, U.: Time dependence of immersion freezing: an experimental study on size selected kaolinite particles, Atmos. Chem. Phys., 12, 9893–9907, https://doi.org/10.5194/acp-12-9893-2012, 2012.
Wex, H., DeMott, P. J., Tobo, Y., Hartmann, S., Rösch, M., Clauss, T., Tomsche, L., Niedermeier, D., and Stratmann, F.: Kaolinite particles as ice nuclei: learning from the use of different kaolinite samples and different coatings, Atmos. Chem. Phys., 14, 5529–5546, https://doi.org/10.5194/acp-14-5529-2014, 2014.
Wex, H., Huang, L., Zhang, W., Hung, H., Traversi, R., Becagli, S., Sheesley, R. J., Moffett, C. E., Barrett, T. E., Bossi, R., Skov, H., Hünerbein, A., Lubitz, J., Löffler, M., Linke, O., Hartmann, M., Herenz, P., and Stratmann, F.: Annual variability of ice-nucleating particle concentrations at different Arctic locations, Atmos. Chem. Phys., 19, 5293–5311, https://doi.org/10.5194/acp-19-5293-2019, 2019.
Whale, T. F., Murray, B. J., O'Sullivan, D., Wilson, T. W., Umo, N. S., Baustian, K. J., Atkinson, J. D., Workneh, D. A., and Morris, G. J.: A technique for quantifying heterogeneous ice nucleation in microlitre supercooled water droplets, Atmos. Meas. Tech., 8, 2437–2447, https://doi.org/10.5194/amt-8-2437-2015, 2015.
Wilson, T. W., Ladino, L. a., Alpert, P. a., Breckels, M. N., Brooks, I. M., Browse, J., Burrows, S. M., Carslaw, K. S., Huffman, J. A., Judd, C., Kilthau, W. P., Mason, R. H., McFiggans, G., Miller, L. a., Nájera, J. J., Polishchuk, E., Rae, S., Schiller, C. L., Si, M., Temprado, J. V., Whale, T. F., Wong, J. P. S., Wurl, O., Yakobi-Hancock, J. D., Abbatt, J. P. D., Aller, J. Y., Bertram, A. K., Knopf, D. A., and Murray, B. J.: A marine biogenic source of atmospheric ice-nucleating particles, Nature, 525, 234–238, https://doi.org/10.1038/nature14986, 2015.
Wu, W.-S., Purser, R. J., and Parrish, D. F.: Three-Dimensional Variational Analysis with Spatially Inhomogeneous Covariances, Mon. Weather Rev., 130, 2905–2916, https://doi.org/10.1175/1520-0493(2002)130<2905:TDVAWS>2.0.CO;2, 2002.
Yadav, S., Venezia, R. E., Paerl, R. W., and Petters, M. D.: Characterization of Ice-Nucleating Particles Over Northern India, J. Geophys. Res.-Atmos., 124, 10467–10482, https://doi.org/10.1029/2019JD030702, 2019.
Yahya, R. Z., Arrieta, J. M., Cusack, M., and Duarte, C. M.: Airborne Prokaryote and Virus Abundance Over the Red Sea, Front. Microbiol., 10, 1112, https://doi.org/10.3389/fmicb.2019.01112, 2019.
Yang, J., Wang, Z., Heymsfield, A. J., DeMott, P. J., Twohy, C. H., Suski, K. J., and Toohey, D. W.: High ice concentration observed in tropical maritime stratiform mixed-phase clouds with top temperatures warmer than −8 ∘C, Atmos. Res., 233, 104719, https://doi.org/10.1016/j.atmosres.2019.104719, 2020.
Yost, J. L. and Hartemink, A. E.: Chapter Four – Soil organic carbon in sandy soils: A review, Vol. 158, edited by: Sparks, D. L., 217–310, Academic Press, ISBN: 9780128174128, https://doi.org/10.1016/bs.agron.2019.07.004 2019.
Yu, Y., Kalashnikova, O. V, Garay, M. J., Lee, H., and Notaro, M.: Identification and Characterization of Dust Source Regions Across North Africa and the Middle East Using MISR Satellite Observations, Geophys. Res. Lett., 45, 6690–6701, https://doi.org/10.1029/2018GL078324, 2018.
Zolles, T., Burkart, J., Häusler, T., Pummer, B., Hitzenberger, R., and Grothe, H.: Identification of Ice Nucleation Active Sites on Feldspar Dust Particles, J. Phys. Chem. A, 119, 2692–2700, https://doi.org/10.1021/jp509839x, 2015.