the Creative Commons Attribution 4.0 License.
the Creative Commons Attribution 4.0 License.
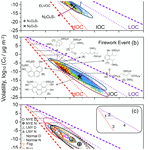
Increase of nitrooxy organosulfates in firework-related urban aerosols during Chinese New Year's Eve
Qiaorong Xie
Sihui Su
Jing Chen
Yuqing Dai
Siyao Yue
Haijie Tong
Wanyu Zhao
Lujie Ren
Yisheng Xu
Dong Cao
Zifa Wang
Cong-Qiang Liu
Kimitaka Kawamura
Guibin Jiang
Yafang Cheng
Little is known about the formation processes of nitrooxy organosulfates (OSs) by nighttime chemistry. Here we characterize nitrooxy OSs at a molecular level in firework-related aerosols in urban Beijing during Chinese New Year. High-molecular-weight nitrooxy OSs with relatively low H C and O C ratios and high unsaturation are potentially aromatic-like nitrooxy OSs. They considerably increased during New Year's Eve, affected by the firework emissions. We find that large quantities of carboxylic-rich alicyclic molecules possibly formed by nighttime reactions. The sufficient abundance of aliphatic-like and aromatic-like nitrooxy OSs in firework-related aerosols demonstrates that anthropogenic volatile organic compounds are important precursors of urban secondary organic aerosols (SOAs). In addition, more than 98 % of those nitrooxy OSs are extremely low-volatility organic compounds that can easily partition into and consist in the particle phase and affect the volatility, hygroscopicity, and even toxicity of urban aerosols. Our study provides new insights into the formation of nitrooxy organosulfates from anthropogenic emissions through nighttime chemistry in the urban atmosphere.
- Article
(3647 KB) - Full-text XML
-
Supplement
(3780 KB) - BibTeX
- EndNote
Secondary organic aerosols (SOAs) are essential components in atmospheric aerosols that are related to climate change, air quality, and human health. They are generated through not only daytime photooxidation, but also nighttime chemical oxidation from both biogenic and anthropogenic volatile organic compounds (VOCs) (Hallquist et al., 2009; Rollins et al., 2012; Nozière et al., 2015; Huang et al., 2019). Nitrooxy organosulfates (OSs) with nitrooxy (–ONO2) and sulfate ester groups (–OSO3H) (Surratt et al., 2008; Lin et al., 2012) substantially participate in the formation of SOA (Tolocka and Turpin, 2012; Ng et al., 2017; Bruggemann et al., 2020). Moreover, nitrooxy OSs can alter the surface hygroscopicity of aerosol particles because of their water-soluble and fat-soluble properties, promoting the production of cloud condensation nuclei (Schindelka et al., 2013) and also increasing the light absorption of organic aerosols (Nguyen et al., 2012).
Nitrooxy OSs can be generated from both biogenic (Iinuma et al., 2007b; Surratt et al., 2007; Gómez-González et al., 2008; Surratt et al., 2008) and anthropogenic VOCs (Tao et al., 2014; Riva et al., 2015). The main precursors of biogenic nitrooxy OSs were isoprene, monoterpenes, sesquiterpenes, and aldehyde, as previous studies observed biogenic nitrooxy OSs in isoprene chamber experiments (Gómez-González et al., 2008; Surratt et al., 2008), a forest (Iinuma et al., 2007b), and urban aerosols (Lin et al., 2012). Compared to biogenic nitrooxy OSs studies, few research activities have been carried out focusing on anthropogenic nitrooxy OSs. Tao et al. (2014) found that long-chain alkenes from traffic emissions are possible precursors of long-chain alkyl nitrooxy OSs in urban aerosols in Shanghai. A recent study reported the presence of nitrooxy OSs in the polar regions (Ye et al., 2021).
The formation of nitrooxy OSs due to nighttime chemistry has been less understood so far. Surratt et al. (2008) suggested that nitrooxy OSs can be formed from the combination of organonitrates and sulfates under acidification, while organonitrates are preferably produced by nighttime NO3 radical oxidation than daytime photooxidation (Rollins et al., 2012; Kiendler-Scharr et al., 2016; Huang et al., 2019). Iinuma et al. (2007a) reported that some monoterpene nitrooxy OSs (e.g., C10H17NO7S, C10H18N2O7S, and C5H10N2O11S) were only detected in nighttime aerosols, indicating the importance of NO3 radicals in nighttime chemistry. Firework displays are frequently conducted as a traditional activity to celebrate popular festivals, in particular New Year's Eve, emitting large quantities of pollutants into the atmosphere (Vecchi et al., 2008; Huang et al., 2012). It is found that lots of semi-volatile to volatile organic compounds, such as n-alkanes and polycyclic aromatic hydrocarbons (PAHs), released during firework-related events (Sarkar et al., 2010; Feng et al., 2012), can be essential precursors of anthropogenic nitrooxy OSs in aerosols (Tao et al., 2014; Riva et al., 2015). Even though the knowledge of chemical and physical behaviors of nitrooxy OSs in firework-related urban aerosols is very sparse, particularly for high-molecular-weight (HMW, molecular weight more than 500 Da) compounds because of their molecular complexity.
To fill this research gap, the molecular characterization of HMW nitrooxy OSs in firework-related aerosols during nighttime is reported in this study based on the measurements from Fourier transform ion cyclotron resonance mass spectrometry (FT-ICR MS) with ultrahigh resolution and mass accuracy. FT-ICR MS has been proven to be a powerful tool to reveal the complicated organic matter in environmental samples at a molecular level (Koch et al., 2007; Dzepina et al., 2015; Bao et al., 2018; Qi et al., 2020, 2021; Su et al., 2021). Our study presents elemental compositions and classifies the organic mixtures into different categories to identify potential origins of nitrooxy OSs and to investigate their possible chemical structures and precursors. The volatility of different nitrooxy OSs is predicted and discussed as well.
2.1 Aerosol sampling
Daytime and nighttime aerosol samples (n = 6) were sampled from 21 to 23 January 2012 in an urban site at the Institute of Atmospheric Physics, Chinese Academy of Sciences (39∘58′28′′ N, 116∘22′13′′ E), Beijing. The samples include NYE D (New Year's Eve daytime before the fireworks), NYE N (New Year's Eve nighttime during the fireworks), LNY D (lunar New Year's Day daytime after the fireworks), LNY N (lunar New Year's Day nighttime), normal D (normal day daytime), and normal N (normal day nighttime) (Xie et al., 2020b). Detailed sample information can be found in Table S1 in the Supplement. The total suspended particle (TSP) samples were collected on prebaked quartz filters (20 cm × 25 cm, Pallflex) using a high-volume air sampler (Kimoto, Japan) and then were refrigerated at −20 ∘C until analysis. Field blank filters were collected following the same procedure. Further, 2 d air mass backward trajectories show that the air was primarily originated from the clean northwest region during the sampling period.
2.2 FT-ICR MS analysis
The method to extract water-soluble organic carbon (WSOC) fractions from each aerosol sample was taken from our previous studies (Xie et al., 2020a, b). After being extracted with ultrapure Milli-Q water, WSOC fractions were eluted from a solid-phase extraction cartridge (Oasis HLB, Waters, USA) using methanol and were analyzed using a 15.0 T Bruker Solarix FT-ICR MS (Bruker Daltonik, GmbH, Bremen, Germany) with the negative electrospray ionization (ESI) mode. An average resolving power ( %) of over 400 000 at ∼ 400 was achieved (Cao et al., 2015). The detection mass ranges were 180–1000 Da. The mass spectra were internally calibrated via data analysis software. The mass accuracy was within 1 ppm, and peaks of the signal-to-noise ratio higher than 6 were assigned for further analysis. A molecular formula calculator was used to calculate formulas with elemental compositions up to 50 12C, 100 1H, 50 16O, 2 14N, and 1 32S atom. Several conservative rules were used as further restrictions for the formula calculation (i.e., the elemental ratios of H C < 2.5, O C < 1.2, and S C < 0.2, and the N rule for even electron ions) (Wozniak et al., 2008; Zhang et al., 2016). Unambiguous molecular formula assignment was determined with the help of the homologous series approach for multiple formula assignments (Koch et al., 2007; Herzsprung et al., 2014). The isotopic peaks were removed in this study.
The formulae containing C, H, N, O, and S atoms, namely CHONS compounds, in WSOC fractions of urban aerosols were assigned from the ESI FT-ICR MS. The number and the total intensity of CHONS compounds were attributed to 14 %–29 % and 10 %–28 % of the total assigned compounds, respectively (Fig. S1). As the predominant fraction occupying more than 85 %, CHONS compounds with O S ≥ 7 were tentatively deemed to be nitrooxy OSs in present work, supporting the assignment of a –OSO3H group and a –ONO2 group in molecules (Kuang et al., 2016; Wang et al., 2016). However, other sulfur-containing compounds (e.g., sulfonates) might also be introduced and impact the analysis due to lack of the structure information of ions from tandem MS experiments (El Haddad et al., 2013; Riva et al., 2015).
3.1 General molecular characterization of nitrooxy OSs
Table 1 shows that slightly higher compounds in number frequency were found in normal nighttime samples (1094 in normal N) than in normal daytime (885 in normal D), similar for the comparison between LNY N (1113) and LNY D (1097) samples, which is in agreement with previous studies (Pinxteren et al., 2009; O'Brien et al., 2014; Wang et al., 2016). However, 690 nitrooxy OSs were observed in NYE D before the firework period, while it considerably increased up to 2050 in NYE N during the firework period, indicating a pronounced increment at night during the firework event. This was consistent with the concentration trend of water-soluble organic nitrogen, which was significantly higher in the nighttime than that in the daytime, particularly in NYE N (Table S1). This can be explained by significant precursors emitted from fireworks (Kong et al., 2015) that produce nitrooxy OSs via nighttime NO3 radical chemistry (Riva et al., 2015). Meanwhile, the heavy emissions of nitrogen oxide during the firework event could elevate the production rate of NO3 radicals (Ljungström and Hallquist, 1996; Kiendler-Scharr et al., 2016), and a previous study showed a good correlation between NO3 and the total concentration of nitrooxy OSs at night (Nguyen et al., 2014).
Table 1The concentrations of chemical components and the number and elemental characteristics of nitrooxy OSs in the Beijing aerosol samples.
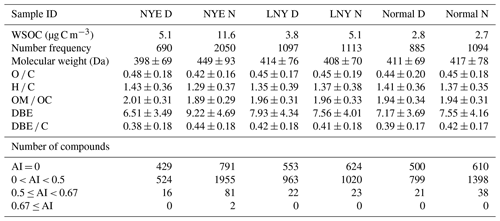
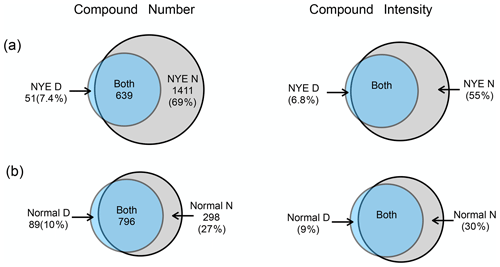
Figure 1The compound number and intensity of nitrooxy OSs in (a) samples NYE D and NYE N and (b) samples normal D and normal N. The common compounds in both daytime and nighttime samples are denoted by “Both”, along with a number. The percentage numbers represent the proportion of the unique ones in the total nitrooxy OSs of each sample.
Different from the detected compounds in NYE D, there were 1411 nitrooxy OSs that were only detected in NYE N, which contributed 69 % of the total number of nitrooxy OSs in the sample (Fig. 1). Moreover, their relative intensities also accounted for nearly half of the total intensities in NYE N. These results indicate that extensive burning of firecrackers offered many specific precursors for the formation of new nitrooxy OSs.
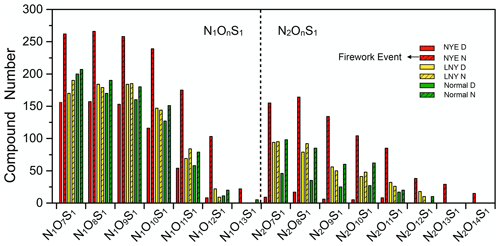
Figure 2Classification of nitrooxy OSs according to the numbers of N, S, and O atoms in their molecules.
Nitrooxy OSs were identified as N1O7S1–N1O13S1 and N2O7S1–N2O14S1 species (Figs. 2 and S2). Here, N1O7S1 compounds refer to formulae containing one nitrogen, seven oxygen, and one sulfur element, and this was similar for the other species. Their number concentrations decrease with the increase of oxygen content in molecules. N1OnS1 species are the predominant nitrooxy OSs; the number and the intensity of N1OnS1 species occupied 65 %–82 % and 62 %–80 % of the total detected nitrooxy OSs, respectively. During firework periods, up to 1300 species of N1OnS1 were detected in NYE N, twice as many as other samples. The effect of pyrotechnics on nitrooxy OSs becomes more substantial with the increased oxygen atom number (Fig. 2). Similarly, the intensity of N1OnS1 species doubled in NYE N compared to other samples. N2OnS1 species may have other nitrogenous functional groups (e.g., amino and nitro groups) in addition to the nitrate functional group. During non-firework periods, there were an average of 300 species of N2OnS1, but the number increased to 724 in NYE N. Moreover, the contribution of fireworks to N2OnS1 species was higher than that of N1OnS1 compounds, possibly because some released amino acids and their derivatives react to form nitrooxy OSs with two nitrogen-containing functional groups.
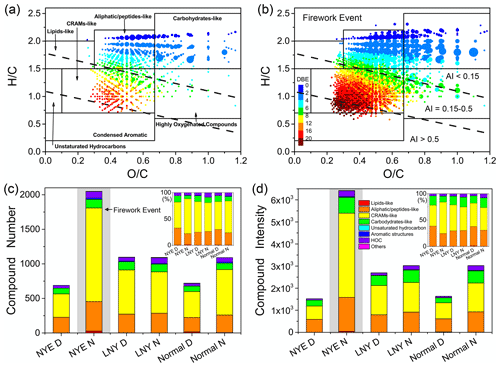
Figure 3Typical Van Krevelen symbols for (a) NYE D and (b) NYE N. Dotted black lines show various AI value ranges, and black lines denote class identification. The size of the plots represents the relative intensities of nitrooxy OSs on a logarithmic scale. The colored bars of (a) and (b) reflect the DBE values. Bar diagrams of (c) and (d) show the number and intensity contribution of major classes in different samples, respectively.
Table 1 and Fig. S3 present the arithmetic and weighted mean elemental ratios of total nitrooxy OSs for each sample, respectively. The average molecular weights rose from 411 ± 69 Da (normal D) to 417 ± 78 Da (normal N) and from 398 ± 69 Da (NYE D) to 449 ± 93 Da (NYE N). The average molecular formulae are C17H25O8.5N1.2S1.0 and C18H24O8.6N1.3S1.0 for normal D and normal N and C17H24O8.6N1.1S1.0 and C21H26O9.1N1.4S1.0 for NYE D and NYE N, respectively. Nitrooxy OSs in firework-related aerosols had relatively higher C and O contents, indicating that many HMW compounds had a higher extent of oxidation. Moreover, both O C and H C ratios of nitrooxy OSs lessened in NYE N, along with increases of unsaturation parameters of double-bone equivalent (DBE) values and DBE C ratios. Similar trends were found for the intensity-weighted average elemental ratios of compounds with high DBEw values but low O Cw and H Cw ratios (Fig. S3). Compared with other studies (Jiang et al., 2016; Lin et al., 2012), our results suggested that there were more aromatic compounds in aerosol samples. Additionally, lots of nitrooxy OSs with high DBE values (≥ 7) were only detected in NYE N (Fig. 3). They were mostly located in the region of aromatic index (AI) higher than 0.5, referring to their condensed aromatic ring structure. From Table 1, it is seen that 21 and 38 compounds with AI > 0.5 were observed in normal D and normal N and 22 and 23 compounds in LNY D and LNY N, respectively. Compared with 16 of these compounds in NYE D, there were up to 83 compounds in NYE N. These results possibly indicate that pyrotechnic emissions have substantial impacts on the formation of aromatic-like nitrooxy OSs.
3.2 Van Krevelen diagram division
The Van Krevelen (VK) diagram is widely applied to depict the evolution of organic mixtures and to identify possible origins of organic aerosols by differentiating major known categories of natural and anthropogenic organic matter (Nozière et al., 2015; Bianco et al., 2018). Here, we applied the VK to investigate nitrooxy OSs in firework-related aerosols. The seven specific classification areas are shown in Figs. 3 and S4, and their stoichiometric ranges are displayed in Table S2. The two most populated regions correspond to carboxylic-rich alicyclic molecules (CRAM-like)/lignin-like (49 %–66 % and 40 %–49 % of the total number and intensity) and aliphatic-/peptide-like (21 %–33 % and 24 %–38 % of the total number and intensity) classes, which are followed by carbohydrate-like (6 %–12 % and 11 %–19 % of total number and intensity) and tannin-like (4 %–7 % of total number and intensity) classes. It is found that more than 98 % of nitrooxy OSs belong to these four categories. Previous studies reported that the majority of WSOC fractions were lignin-, lipid-, and aliphatic-/peptide-like classes in aerosols and cloud water (Wozniak et al., 2008; Zhao et al., 2013; Bianco et al., 2018). However, nitrooxy OSs in the present work had relatively high O C ratios, potentially because they were consistent with covalently bound HSO (Romero and Oehme, 2005) in HMW sulfur-containing compounds (Wozniak et al., 2008). In addition, nitrooxy OSs also had high H C ratios, indicating that sulfation, nitration, or functionalization processes led to mostly saturated compounds.
Although the number of all seven types of nitrooxy OSs increased obviously in NYE N compared with other samples, the impacts of fireworks on nitrooxy OSs are different in categories. As for the most abundant CRAM-like nitrooxy OSs, they were more abundant during the nighttime (620 in normal N) than daytime (375 in normal D). However, NYE N contained about 1354 CRAM-like compounds, which was 3 times more than NYE D. The relative contribution of the number of these compounds was 60 % in NYE N, substantially higher than that in NYE D (45 %). The total intensity of such compounds in NYE N was about 8 times higher than of those in NYE D. These observations demonstrate that nighttime oxidation is important in the formation of CRAM-like nitrooxy OSs, especially in the presence of abundant firework-related precursors. CRAMs contain the structures of carboxylated alicyclic and large and fused nonaromatic rings with a high ratio of substituted carboxyl groups (Bianco et al., 2018). However, some CRAM-like nitrooxy OSs were located in the aromatic area (AI > 0.5). Thus, there may exist some aromatic-like compounds with some degree of alkylation that have been mistaken for a nonaromatic class (Kourtchev et al., 2016; Tong et al., 2016). Also, it was worth noting that nitrooxy OSs of aromatic region could be lignin-like compounds, which contains aromatic rings in their chemical structures. The results are consistent with the high unsaturation of compounds in the region as described above.
Aliphatic-/peptide-like nitrooxy OSs have low DBE values and H C ratios, indicating a high degree of saturation and long carbon lengths. Aliphatic-like nitrooxy OSs (e.g., acyclic compounds) are mainly derived from alkanes, alcohols, ethers, ketones, aldehydes, esters, etc., from anthropogenic and natural emissions. Peptide-like nitrooxy OSs are primarily derived from functionalized amino acids, peptides, and protein fragments. Unlike CRAM-like compounds, average relative contributions of both the number and the total intensity of aliphatic-/peptide-like nitrooxy OSs were more abundant during daytime (∼ 40 %) than nighttime (∼ 30 %). However, there are 427 species of aliphatic-/peptide-like nitrooxy OSs in NYE N, which is twice as many as other samples. The intensity of each compound was also higher in NYE N with bigger symbol sizes (Fig. 3b), and the total intensity was about 3 times higher than other samples. These results demonstrate the importance of anthropogenic precursors for the formation of aliphatic-/peptide-like nitrooxy OSs, though they could be more susceptible to photochemical reactions than nighttime chemistry from biogenic precursors.
Contrary to CRAM-like nitrooxy OSs, the carbohydrate-like nitrooxy OSs with high intensity have high H C and O C ratios and saturation, indicating they are highly oxidized and alkylated. The intensity of carbohydrate-like nitrooxy OSs was significantly higher than that of sulfur-free compounds reported in previous studies (Wozniak et al., 2008; Bianco et al., 2018). It is reasonable because carbohydrates and their derivatives are polyhydroxy aldehydes, polyhydroxy ketones, and organic compounds, which can be hydrolyzed to form polyhydroxy aldehydes or polyhydroxy ketones, tending to generate OSs and nitrooxy OSs (Passananti et al., 2016; Ogino, 2021). Moreover, N2OnS1 species were more abundant than N1OnS1 species (Fig. S5), indicating a trend toward easily functionalization. Although the number of carbohydrate-like compounds in NYE N was close to other samples, the intensity of them increased, potentially indicating an increase of the concentration of them. As for tannin-like classes, which are also highly oxygenated organic compounds (Bianco et al., 2018), their content in NYE N was also more abundant than other samples.
Considering the lipid-like nitrooxy OSs, unsaturated hydrocarbons, and aromatic structure classes, only less than 1.5 % compounds are in these regions. Lipid-like organics, containing monoglycerides, diglycerides, fats, fat-soluble vitamins, and sterols, primarily originate from biogenic materials and phospholipids (Gurganus et al., 2015; Bianco et al., 2018), but most nitrooxy OSs are of secondary origin. Unsaturated hydrocarbons compounds are mostly composed of carbon and hydrogen atoms, while nitrooxy OSs have lots of heteroatoms. As for aromatic compounds, they are mainly produced by combustion as the indicators of anthropogenic origin. The limited number of nitrooxy OSs detected in this region may be due to the tendency of some alkylated compounds to fall into other categories (e.g., CRAM-like). In summary, all nitrooxy OS categories were enhanced in NYE N, particularly for the lignin-like nitrooxy OSs. Moreover, the intensity of carbohydrate-like nitrooxy OSs increased due to the enhancement of nighttime chemistry.
3.3 Subgroups and potential precursors
Figure S6 showed that compared with NYE D, nitrooxy OSs (> 1500 compounds) were densely distributed with high DBE values (≥ 7) during the firework event, especially for those in the HMW region. Most of the nitrooxy OSs were aromatics with Xc higher than 2.5 (Yassine et al., 2014) and lower O C (≤ 0.5) and H C (≤ 1.5) ratios. Additionally, high intensities of these highly unsaturated compounds indicated their sufficient contents in aerosols. From Figs. 4 and 5, it is demonstrated that the DBE values and C numbers of N1OnS1 species of nitrooxy OSs in NYE N varied separately within the range of 0–23 and 6–35, which were higher than the average value of DBE (0–16) and C number (6–27) in other samples. Although the abundance of nitrooxy OSs of DBE (4–10) and C number (10–20) in the nighttime was higher than that during the daytime, the number of nitrooxy OSs with DBE and C number in the range of 4–18 and 10–20 was even higher in the NYE N. These highly unsaturated nitrooxy OSs are aromatics, which may be originated from firework-related aromatic VOCs or PAHs (Riva et al., 2015).
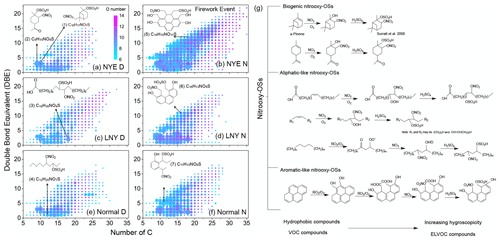
Figure 4(a–f) DBE vs. C number for N1OnS1 species. The color bar shows the number of O atoms. The size of the plots denotes the relative intensities of nitrooxy OSs on a logarithmic scale. (1)–(7) are proposed structures of some nitrooxy OSs, among which (1) and (2) have been reported previously (Surratt et al., 2008). Their relative intensities in each sample are shown in Table S3. (g) The proposed potential formation mechanisms of various groups of nitrooxy OSs.
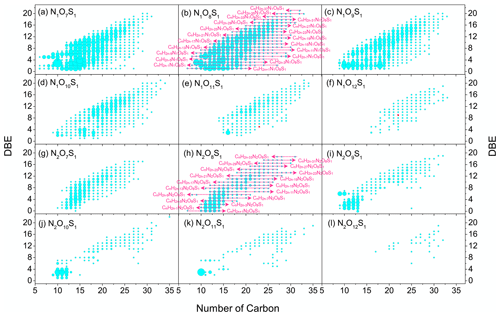
Figure 5Molecular formulae distributions of N1O7S1–N1O12S1 and N2O7S1–N2O12S1 class species. The C and DBE number distributions of N1OnS1 and N2OnS1 class species in the NYE N sample. The size of the symbols reflects the relative peak magnitudes of nitrooxy OSs on a logarithmic scale. The pink arrows and molecular formulae in N1O8S1 and N2O8S1 class species display the elemental composition of compounds as an example for all classes.
Nitrooxy OSs have an extensive range of unsaturation, with DBE values ranging from 0 to 23 (Fig. 4). Previous studies have reported that nitrooxy OSs (e.g., C10H17NO7S (1), C9H15NO8S (2)) can be formed from biogenic VOCs (e.g., α-pinene and limonene) (Iinuma et al., 2007a; Surratt et al., 2008; Cai et al., 2020). C10H17NO7S and C9H15NO8S (Fig. 4) have the same degree of unsaturation as their precursors and show the strongest intensity among all nitrooxy OSs, which demonstrate that α-pinene and limonene are the primary precursors of biogenic nitrooxy OSs. A continuous series of corresponding family series was also detected in firework-related aerosols, namely CnH2n−3NO7S (n = 9–22) and CnH2n−3NO8S (n = 9–24) (Fig. 5).
The nitrooxy OSs were divided into three main categories to illustrate the molecular difference. Group A comprises aliphatic-like nitrooxy OSs (DBE ≤ 2), which is featured by long alkyl carbon chains with high saturation; group B contains aromatic-like nitrooxy OSs (Xc > 2.5) with high unsaturation; group C represents the rest of the compounds similar to biogenic nitrooxy OSs with a moderate extent of saturation. As for the aliphatic-like nitrooxy OSs, such as C18H35NO9S (3) and C12H25NO7S (4) (Fig. 4), they have saturated and long carbon chains, which may source from precursors such as long-chain alkenes, alkanes, and fatty acids by photooxidation. Both had relatively high intensities and consecutive family series, i.e., CnH2n−1NO9S (n = 9–22) and CnH2n+1NO7S (n = 6–24), respectively (Fig. 5). These precursors may also produce nitrooxy OSs under high NOx conditions or break double bonds to form intermediate products through photooxidation and then form nitrooxy OSs with sulfates. Some possible formation mechanisms are proposed in Fig. 4g. It is noted that, except for compounds (1) and (2), the intensity of each aliphatic-like nitrooxy OS is higher than others in daytime samples, highlighting the importance of photooxidation to their generation.
The number of the aromatic-like nitrooxy OSs was the most abundant among all measured nitrooxy OSs. Figure S6d and h show that there were slight differences between NYE D and NYE N for aliphatic and biogenic nitrooxy OSs. Nonetheless, compared with the daytime, the number of aromatic-like nitrooxy OSs was considerably enhanced in NYE N with the HMW compounds. Riva et al. (2015), using side-by-side comparison experiments, proved that the generation of OSs and sulfonates from PAHs was enhanced with the existence of acidified sulfate seed aerosols. Their results implied that aromatic-like nitrooxy OSs might efficiently be generated through PAHs and sulfate ions released from fireworks at night without the participation of photochemistry. For instance, the aromatic-like nitrooxy OSs with multiple benzenes can be generated from carboxyl compounds, which is an oxidation product of pyrene (Juhasz and Naidu, 2000). Some possible structures, such as C18H15NO11S (5), C16H13NO9S (6), and C11H15NO8S (7), and proposed formation mechanisms are displayed in Fig. 4. Their corresponding family series (i.e., CnH2n−21NO11S (n = 17–28), CnH2n−19NO9S (n = 15–29), and CnH2n−7NO8S (n = 8–29)) have more carbon atoms than the biogenic and aliphatic nitrooxy OSs (Fig. 5), possibly because they are formed via the polymerization process or derived from aromatic compounds with high carbon content. In addition, it is noted that the intensity of each aromatic-like nitrooxy OS was lower than the other two groups and decreased with the increase of unsaturation (Fig. 4 and Table S3). This may be because the water solubility of aromatic-like nitrooxy OSs decreases with the increasing unsaturation. However, these nitrooxy OSs are possibly present in the non-WSOC fractions, which requires further investigation.
3.4 Volatility characteristics and molecular corridors
Molecular corridors that are constrained by two boundary lines of sugar alcohols CnH2n+2On with O C = 1 and linear n-alkanes CnH2n+2 with O C = 0 are used for a better understanding of the chemical and physical properties in SOA evolution (Shiraiwa et al., 2014; Li et al., 2016; Shiraiwa et al., 2017). From Fig. 6a–b, it is shown that more than 98 % of nitrooxy OSs detected in the present study were located in the region of extremely low-volatility organic compounds (ELVOCs) with saturation mass concentration (C0) < 3 × 10−4 µg m−3 (Donahue et al., 2011; Murphy et al., 2014) and a molar mass higher than 250 g mol−1. Moreover, the volatility varies among N1OnS1 and N2OnS1 species because of the differences in their molecular composition and structures. Compared with N1OnS1, many of the N2OnS1 species have lower volatility and a higher O C ratio, nearing the sugar alcohol CnH2n+2On line. Lots of these compounds with higher intensity were found in NYE N than other samples, which suggests that highly oxidized compounds are produced in large quantities via nighttime chemistry after firework emissions. Moreover, the volatility of nitrooxy OSs potentially decreased with the increase of molecular weight and unsaturation. Compared with compounds in NYE D, numbers of nitrooxy OSs with low volatility were only detected in NYE N, possibly because of the increase of HMW aromatic-like nitrooxy OSs affected by firework emissions. Further, it was worth noting that the volatility of nitrooxy OSs was lower than that of OSs (Xie et al., 2020a), possibly because they are highly functionalized compounds.
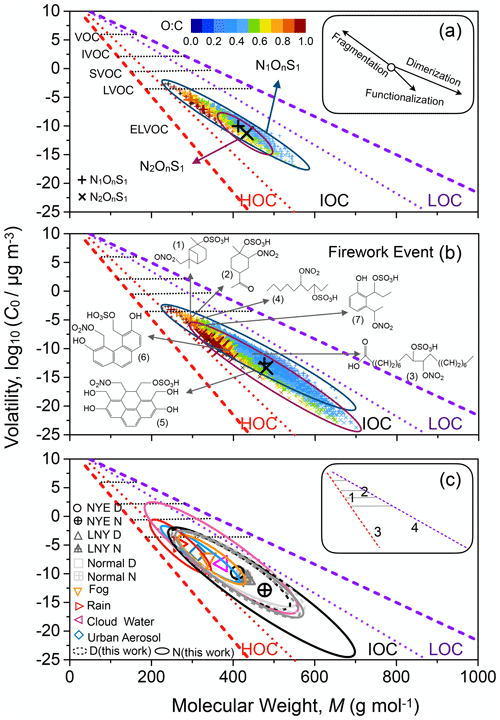
Figure 6Molecular corridors and volatility characteristics for nitrooxy OSs in (a) NYE D and (b) NYE N. (c) Comparison of nitrooxy OSs in the present work with those reported in urban aerosols (Lin et al., 2012; O'Brien et al., 2014), cloud water (Zhao et al., 2013), rain (Altieri et al., 2009), and fog (Mazzoleni et al., 2010). The top right of (c) shows the characteristic reaction pathways with most probable kinetic regimes (1. aqueous-phase reaction; 2. simple gas-phase oxidation; 3. gas- or particle-phase autoxidation; and 4. particle-phase dimerization) (Shiraiwa et al., 2014). The boundary lines denote sugar alcohols CnH2n+2On with O C = 1 (red) and linear n-alkanes CnH2n+2 with O C = 0 (purple). The small plots denote the individual nitrooxy OSs color-coded by O C ratio, and the larger ones show the surrogate nitrooxy OSs with the average values of M and C0.
The molecular corridor includes three primary parts, consisting of low, intermediate, and high O C ratio corridors (LOC, IOC, and HOC) (Shiraiwa et al., 2014). The plentiful gas-phase oxidation products of alkanes fall into LOC, which is near the alkane line. Conversely, the aqueous-phase reaction and autoxidation products were in HOC, close to the sugar alcohol line. The IOC corridor is the area connecting LOC and HOC. Nitrooxy OSs observed in this work are dominantly found in the IOC molecular corridors. N1OnS1 species are closer to the LOC corridors, while N2OnS1 species are closer to the HOC corridors. These results suggest that most firework-related nitrooxy OSs were possibly gas- and/or particle-phase autoxidation or dimerization products. For instance, nitrooxy OS can be formed through hydroxynitrate gas-phase products reactively uptaking onto acidified sulfate seed aerosols through the esterification of the hydroxyl group with sulfuric acid (Surratt et al., 2008). Jay and Stieglitz (1989) also found hydroxynitrates produced by the oxidation of α-pinene induced by NO3 at night. Also, several newly firework-related nitrooxy OSs with higher molecular weight were possibly generated from dimerization and oligomerization in the particle phase. These results demonstrate that the dimerization and functionalization of nitrooxy OSs can be substantially enhanced in the particle phase with rising pollutant concentrations and varying reaction scenarios.
Although nitrooxy OSs have been frequently reported within aerosols (Lin et al., 2012; O'Brien et al., 2014; Cai et al., 2020), deposited sediment (Zhang et al., 2016), and atmospheric water such as cloud water (Zhao et al., 2013), rain (Altieri et al., 2009), or fog (Mazzoleni et al., 2010), they were different from those in the present work because of the unique chemical reactions during the fireworks (Fig. 6c). Organics in atmospheric water with aqueous-phase reactions are highly oxidized and close to the sugar alcohol line. Although a fraction of cloud-water nitrooxy OSs overlap with those in aerosols, the firework-related nitrooxy OSs in our work showed a higher molar mass and lower volatility than urban aerosols reported previously (Lin et al., 2012; O'Brien et al., 2014), especially at NYE night, potentially because of increased dimerization and oligomerization reactions.
The present study provides unique information about the important contributions of anthropogenic precursors, as well as biogenic precursors, to the formation of nitrooxy OSs in ambient aerosols during firework events. Instead, numbers of nitrooxy OSs were potentially derived from alkene, fatty acids, and aromatics and their derivatives compared to biogenic-related nitrooxy OSs. The surfactant properties of ambient aerosol particles may be influenced after coupling with hydrophilic functional groups of nitrooxy and sulfate, which affect the formation of cloud condensation nuclei. Furthermore, influenced by the firework emission, a lot of organonitrates in the gas phase can partition into the particle phase by forming nitrooxy OSs with low volatility as ELVOCs, thus participating in the organic nitrogen cycle. In addition, they also affect the NOx cycle in the atmosphere. Our results highlight the fact that firework emission considerably contributes to the formation of nitrooxy OSs and will have an important influence on atmospheric physical and chemical processes. Nevertheless, nighttime chemistry of NO3 radicals is substantially involved in the generation of nitrooxy OSs, particularly for aromatic-like compounds. Such complex mechanisms warrant further investigation.
The dataset for this paper is available upon request from the corresponding author (fupingqing@tju.edu.cn).
The supplement related to this article is available online at: https://doi.org/10.5194/acp-21-11453-2021-supplement.
PF, YX, YC, and QX designed the research. QX, SS, JC, SY, and DC conducted the laboratory analysis. YD, SY, HS, HT, WZ, LR, YL, YS, ZW, CQL, KK, GJ, YC, and PF reviewed and commented on the paper. QX and PF wrote the paper.
The authors declare that they have no conflict of interest.
Publisher’s note: Copernicus Publications remains neutral with regard to jurisdictional claims in published maps and institutional affiliations.
The authors thank Lianfang Wei (Institute of Atmospheric Physics, Chinese Academy of Sciences, China) and Hong Ren (Tianjin University, China), for their helpful discussions.
This research has been supported by the National Natural Science Foundation of China (grant nos. 41625014 and 41961130384).
This paper was edited by Qiang Zhang and reviewed by two anonymous referees.
Altieri, K. E., Turpin, B. J., and Seitzinger, S. P.: Oligomers, organosulfates, and nitrooxy organosulfates in rainwater identified by ultra-high resolution electrospray ionization FT-ICR mass spectrometry, Atmos. Chem. Phys., 9, 2533–2542, https://doi.org/10.5194/acp-9-2533-2009, 2009.
Bao, H. Y., Niggemann, J., Luo, L., Dittmar, T., and Kao, S. J.: Molecular composition and origin of water-soluble organic matter in marine aerosols in the Pacific off China, Atmos. Environ., 191, 27–35, https://doi.org/10.1016/j.atmosenv.2018.07.059, 2018.
Bianco, A., Deguillaume, L., Vaïtilingom, M., Nicol, E., Baray, J. L., Chaumerliac, N., and Bridoux, M. C.: Molecular Characterization of Cloud Water Samples Collected at the puy de Dôme (France) by Fourier Transform Ion Cyclotron Resonance Mass Spectrometry, Environ. Sci. Technol., 52, 10275–10285, 2018.
Bruggemann, M., Xu, R. S., Tilgner, A., Kwong, K. C., Mutzel, A., Poon, H. Y., Otto, T., Schaefer, T., Poulain, L., Chan, M. N., and Herrmann, H.: Organosulfates in Ambient Aerosol: State of Knowledge and Future Research Directions on Formation, Abundance, Fate, and Importance, Environ. Sci. Technol., 54, 3767–3782, https://doi.org/10.1021/acs.est.9b06751, 2020.
Cai, D. M., Wang, X. K., Chen, J. M., and Li, X.: Molecular Characterization of Organosulfates in Highly Polluted Atmosphere Using Ultra-High-Resolution Mass Spectrometry, J. Geophys. Res.-Atmos., 125, e2019JD032253, https://doi.org/10.1029/2019jd032253, 2020.
Cao, D., Huang, H. G., Hu, M., Cui, L., Geng, F. L., Rao, Z. Y., Niu, H. Y., Cai, Y. Q., and Kang, Y. H.: Comprehensive characterization of natural organic matter by MALDI- and ESI-Fourier transform ion cyclotron resonance mass spectrometry, Anal. Chim. Acta, 866, 48–58, https://doi.org/10.1016/j.aca.2015.01.051, 2015.
Donahue, N. M., Epstein, S. A., Pandis, S. N., and Robinson, A. L.: A two-dimensional volatility basis set: 1. organic-aerosol mixing thermodynamics, Atmos. Chem. Phys., 11, 3303–3318, https://doi.org/10.5194/acp-11-3303-2011, 2011.
Dzepina, K., Mazzoleni, C., Fialho, P., China, S., Zhang, B., Owen, R. C., Helmig, D., Hueber, J., Kumar, S., Perlinger, J. A., Kramer, L. J., Dziobak, M. P., Ampadu, M. T., Olsen, S., Wuebbles, D. J., and Mazzoleni, L. R.: Molecular characterization of free tropospheric aerosol collected at the Pico Mountain Observatory: a case study with a long-range transported biomass burning plume, Atmos. Chem. Phys., 15, 5047–5068, https://doi.org/10.5194/acp-15-5047-2015, 2015.
El Haddad, I., Marchand, N., D'Anna, B., Jaffrezo, J. L., and Wortham, H.: Functional group composition of organic aerosol from combustion emissions and secondary processes at two contrasted urban environments, Atmos. Environ., 75, 308–320, https://doi.org/10.1016/j.atmosenv.2013.04.019, 2013.
Feng, J., Sun, P., Hu, X., Zhao, W., Wu, M., and Fu, J.: The chemical composition and sources of PM2.5 during the 2009 Chinese New Year's holiday in Shanghai, Atmos. Res., 118, 435–444, 2012.
Gómez-González, Y., Surratt, J. D., Cuyckens, F., Szmigielski, R., Vermeylen, R., Jaoui, M., Lewandowski, M., Offenberg, J. H., Kleindienst, T. E., and Edney, E. O.: Characterization of organosulfates from the photooxidation of isoprene and unsaturated fatty acids in ambient aerosol using liquid chromatography/(–) electrospray ionization mass spectrometry, J. Mass Spectrom., 43, 371–382, 2008.
Gurganus, S. C., Wozniak, A. S., and Hatcher, P. G.: Molecular characteristics of the water soluble organic matter in size-fractionated aerosols collected over the North Atlantic Ocean, Mar. Chem., 170, 37–48, 2015.
Hallquist, M., Wenger, J. C., Baltensperger, U., Rudich, Y., Simpson, D., Claeys, M., Dommen, J., Donahue, N. M., George, C., Goldstein, A. H., Hamilton, J. F., Herrmann, H., Hoffmann, T., Iinuma, Y., Jang, M., Jenkin, M. E., Jimenez, J. L., Kiendler-Scharr, A., Maenhaut, W., McFiggans, G., Mentel, Th. F., Monod, A., Prévôt, A. S. H., Seinfeld, J. H., Surratt, J. D., Szmigielski, R., and Wildt, J.: The formation, properties and impact of secondary organic aerosol: current and emerging issues, Atmos. Chem. Phys., 9, 5155–5236, https://doi.org/10.5194/acp-9-5155-2009, 2009.
Herzsprung, P., Hertkorn, N., von Tumpling, W., Harir, M., Friese, K., and Schmitt-Kopplin, P.: Understanding molecular formula assignment of Fourier transform ion cyclotron resonance mass spectrometry data of natural organic matter from a chemical point of view, Anal. Bioanal. Chem., 406, 7977–7987, https://doi.org/10.1007/s00216-014-8249-y, 2014.
Huang, K., Zhuang, G., Lin, Y., Wang, Q., Fu, J. S., Zhang, R., Li, J., Deng, C., and Fu, Q.: Impact of anthropogenic emission on air quality over a megacity – revealed from an intensive atmospheric campaign during the Chinese Spring Festival, Atmos. Chem. Phys., 12, 11631–11645, https://doi.org/10.5194/acp-12-11631-2012, 2012.
Huang, W., Saathoff, H., Shen, X., Ramisetty, R., Leisner, T., and Mohr, C.: Chemical Characterization of Highly Functionalized Organonitrates Contributing to Night-time Organic Aerosol Mass Loadings and Particle Growth, Environ. Sci. Technol., 53, 1165–1174, https://doi.org/10.1021/acs.est.8b05826, 2019.
Iinuma, Y., Muller, C., Berndt, T., Boge, O., Claeys, M., and Herrmann, H.: Evidence for the existence of organosulfates from beta-pinene ozonolysis in ambient secondary organic aerosol, Environ. Sci. Technol., 41, 6678–6683, https://doi.org/10.1021/es070938t, 2007a.
Iinuma, Y., Müller, C., Böge, O., Gnauk, T., and Herrmann, H.: The formation of organic sulfate esters in the limonene ozonolysis secondary organic aerosol (SOA) under acidic conditions, Atmos. Environ., 41, 5571–5583, https://doi.org/10.1016/j.atmosenv.2007.03.007, 2007b.
Jay, K. and Stieglitz, L.: The gas phase addition of NOx to olefins, Chemosphere, 19, 1939–1950, 1989.
Jiang, B., Kuang, B. Y., Liang, Y., Zhang, J., Huang, X. H., Xu, C., Yu, J. Z., and Shi, Q.: Molecular composition of urban organic aerosols on clear and hazy days in Beijing: a comparative study using FT-ICR MS, Environ. Chem., 13, 888–901, 2016.
Juhasz, A. L. and Naidu, R.: Bioremediation of high molecular weight polycyclic aromatic hydrocarbons: a review of the microbial degradation of benzo [a] pyrene, Int. Biodeter. Biodegr, 45, 57–88, 2000.
Kiendler-Scharr, A., Mensah, A. A., Friese, E., Topping, D., Nemitz, E., Prevot, A. S. H., Aijala, M., Allan, J., Canonaco, F., Canagaratna, M., Carbone, S., Crippa, M., Dall Osto, M., Day, D. A., De Carlo, P., Di Marco, C. F., Elbern, H., Eriksson, A., Freney, E., Hao, L., Herrmann, H., Hildebrandt, L., Hillamo, R., Jimenez, J. L., Laaksonen, A., McFiggans, G., Mohr, C., O'Dowd, C., Otjes, R., Ovadnevaite, J., Pandis, S. N., Poulain, L., Schlag, P., Sellegri, K., Swietlicki, E., Tiitta, P., Vermeulen, A., Wahner, A., Worsnop, D., and Wu, H. C.: Ubiquity of organic nitrates from nighttime chemistry in the European submicron aerosol, Geophys. Res. Lett., 43, 7735–7744, https://doi.org/10.1002/2016gl069239, 2016.
Koch, B. P., Dittmar, T., Witt, M., and Kattner, G.: Fundamentals of molecular formula assignment to ultrahigh resolution mass data of natural organic matter, Anal. Chem., 79, 1758–1763, https://doi.org/10.1021/ac061949s, 2007.
Kong, S. F., Li, X. X., Li, L., Yin, Y., Chen, K., Yuan, L., Zhang, Y. J., Shan, Y. P., and Ji, Y. Q.: Variation of polycyclic aromatic hydrocarbons in atmospheric PM2.5 during winter haze period around 2014 Chinese Spring Festival at Nanjing: Insights of source changes, air mass direction and firework particle injection, Sci. Total Environ., 520, 59–72, https://doi.org/10.1016/j.scitotenv.2015.03.001, 2015.
Kourtchev, I., Godoi, R. H. M., Connors, S., Levine, J. G., Archibald, A. T., Godoi, A. F. L., Paralovo, S. L., Barbosa, C. G. G., Souza, R. A. F., Manzi, A. O., Seco, R., Sjostedt, S., Park, J.-H., Guenther, A., Kim, S., Smith, J., Martin, S. T., and Kalberer, M.: Molecular composition of organic aerosols in central Amazonia: an ultra-high-resolution mass spectrometry study, Atmos. Chem. Phys., 16, 11899–11913, https://doi.org/10.5194/acp-16-11899-2016, 2016.
Kuang, B. Y., Lin, P., Hu, M., and Yu, J. Z.: Aerosol size distribution characteristics of organosulfates in the Pearl River Delta region, China, Atmos. Environ., 130, 23–35, 2016.
Li, Y., Pöschl, U., and Shiraiwa, M.: Molecular corridors and parameterizations of volatility in the chemical evolution of organic aerosols, Atmos. Chem. Phys., 16, 3327–3344, https://doi.org/10.5194/acp-16-3327-2016, 2016.
Lin, P., Yu, J. Z., Engling, G., and Kalberer, M.: Organosulfates in humic-like substance fraction isolated from aerosols at seven locations in East Asia: A study by ultra-high-resolution mass spectrometry, Environ. Sci. Technol., 46, 13118–13127, 2012.
Ljungström, E. and Hallquist, M.: Nitrate radical formation rates in scandinavia, Atmos. Environ., 30, 2925–2932, 1996.
Mazzoleni, L. R., Ehrmann, B. M., Shen, X., Marshall, A. G., and Collett, J. L.: Water-Soluble Atmospheric Organic Matter in Fog: Exact Masses and Chemical Formula Identification by Ultrahigh-Resolution Fourier Transform Ion Cyclotron Resonance Mass Spectrometry, Environ. Sci. Technol., 44, 3690–3697, https://doi.org/10.1021/es903409k, 2010.
Murphy, B. N., Donahue, N. M., Robinson, A. L., and Pandis, S. N.: A naming convention for atmospheric organic aerosol, Atmos. Chem. Phys., 14, 5825–5839, https://doi.org/10.5194/acp-14-5825-2014, 2014.
Ng, N. L., Brown, S. S., Archibald, A. T., Atlas, E., Cohen, R. C., Crowley, J. N., Day, D. A., Donahue, N. M., Fry, J. L., Fuchs, H., Griffin, R. J., Guzman, M. I., Herrmann, H., Hodzic, A., Iinuma, Y., Jimenez, J. L., Kiendler-Scharr, A., Lee, B. H., Luecken, D. J., Mao, J., McLaren, R., Mutzel, A., Osthoff, H. D., Ouyang, B., Picquet-Varrault, B., Platt, U., Pye, H. O. T., Rudich, Y., Schwantes, R. H., Shiraiwa, M., Stutz, J., Thornton, J. A., Tilgner, A., Williams, B. J., and Zaveri, R. A.: Nitrate radicals and biogenic volatile organic compounds: oxidation, mechanisms, and organic aerosol, Atmos. Chem. Phys., 17, 2103–2162, https://doi.org/10.5194/acp-17-2103-2017, 2017.
Nguyen, Q. T., Christensen, M. K., Cozzi, F., Zare, A., Hansen, A. M. K., Kristensen, K., Tulinius, T. E., Madsen, H. H., Christensen, J. H., Brandt, J., Massling, A., Nøjgaard, J. K., and Glasius, M.: Understanding the anthropogenic influence on formation of biogenic secondary organic aerosols in Denmark via analysis of organosulfates and related oxidation products, Atmos. Chem. Phys., 14, 8961–8981, https://doi.org/10.5194/acp-14-8961-2014, 2014.
Nguyen, T. B., Lee, P. B., Updyke, K. M., Bones, D. L., Laskin, J., Laskin, A., and Nizkorodov, S. A.: Formation of nitrogen- and sulfur-containing light-absorbing compounds accelerated by evaporation of water from secondary organic aerosols, J. Geophys. Res.-Atmos., 117, D01207, https://doi.org/10.1029/2011JD016944, 2012.
Nozière, B., Kaberer, M., Claeys, M., Allan, J., D'Anna, B., Decesari, S., Finessi, E., Glasius, M., Grgic, I., Hamilton, J. F., Hoffmann, T., Iinuma, Y., Jaoui, M., Kahno, A., Kampf, C. J., Kourtchev, I., Maenhaut, W., Marsden, N., Saarikoski, S., Schnelle-Kreis, J., Surratt, J. D., Szidat, S., Szmigielski, R., and Wisthaler, A.: The Molecular Identification of Organic Compounds in the Atmosphere: State of the Art and Challenges, Chem. Rev., 115, 3919–3983, https://doi.org/10.1021/cr5003485, 2015.
O'Brien, R. E., Laskin, A., Laskin, J., Rubitschun, C. L., Surratt, J. D., and Goldstein, A. H.: Molecular characterization of S-and N-containing organic constituents in ambient aerosols by negative ion mode high-resolution Nanospray Desorption Electrospray Ionization Mass Spectrometry: CalNex 2010 field study, J. Geophys. Res.-Atmos., 119, 12706–12720, 2014.
Ogino, I.: Conversion of Carbohydrates to High Value Products, Heterogeneous Catalysts, 2, 617–632, https://doi.org/10.1002/9783527813599.ch34, 2021.
Passananti, M., Kong, L., Shang, J., Dupart, Y., Perrier, S., Chen, J., Donaldson, D. J., and George, C.: Organosulfate Formation through the Heterogeneous Reaction of Sulfur Dioxide with Unsaturated Fatty Acids and Long-Chain Alkenes, Angew. Chem. Int. Ed., 55, 10336–10339, 2016.
Pinxteren, D. V., Brueggemann, E., Gnauk, T., Iinuma, Y., Mueller, K., Nowak, A., Achtert, P., Wiedensohler, A., and Herrmann, H.: Size and time-resolved chemical particle characterization during CAREBeijing-2006: Different pollution regimes and diurnal profiles, J. Geophys. Res.-Atmos., 114, D00G09, https://doi.org/10.1029/2008JD010890, 2009.
Qi, Y. L., Fu, P. Q., Li, S. L., Ma, C., Liu, C. Q., and Volmer, D. A.: Assessment of molecular diversity of lignin products by various ionization techniques and high-resolution mass spectrometry, Sci. Total Environ., 713, 136573, https://doi.org/10.1016/j.scitotenv.2020.136573, 2020.
Qi, Y. L., Fu, P. Q., and Volmer, D. A.: Analysis of natural organic matter via fourier transform ion cyclotron resonance mass spectrometry: an overview of recent non-petroleum applications, Mass Spectrom. Rev., https://doi.org/10.1002/mas.21634, online first, 2021.
Riva, M., Tomaz, S., Cui, T., Lin, Y.-H., Perraudin, E., Gold, A., Stone, E. A., Villenave, E., and Surratt, J. D.: Evidence for an unrecognized secondary anthropogenic source of organosulfates and sulfonates: Gas-phase oxidation of polycyclic aromatic hydrocarbons in the presence of sulfate aerosol, Environ. Sci. Technol., 49, 6654–6664, 2015.
Rollins, A. W., Browne, E. C., Min, K.-E., Pusede, S. E., Wooldridge, P. J., Gentner, D. R., Goldstein, A. H., Liu, S., Day, D. A., and Russell, L. M.: Evidence for NOx control over nighttime SOA formation, Science, 337, 1210–1212, 2012.
Romero, F. and Oehme, M.: Organosulfates–a new component of humic-like substances in atmospheric aerosols?, J. Atmos. Chem., 52, 283–294, 2005.
Sarkar, S., Khillare, P. S., Jyethi, D. S., Hasan, A., and Parween, M.: Chemical speciation of respirable suspended particulate matter during a major firework festival in India, J. Hazard. Mater., 184, 321–330, https://doi.org/10.1016/j.jhazmat.2010.08.039, 2010.
Schindelka, J., Iinuma, Y., Hoffmann, D., and Herrmann, H.: Sulfate radical-initiated formation of isoprene-derived organosulfates in atmospheric aerosols, Faraday Discuss., 165, 237–259, 2013.
Shiraiwa, M., Berkemeier, T., Schilling-Fahnestock, K. A., Seinfeld, J. H., and Pöschl, U.: Molecular corridors and kinetic regimes in the multiphase chemical evolution of secondary organic aerosol, Atmos. Chem. Phys., 14, 8323–8341, https://doi.org/10.5194/acp-14-8323-2014, 2014.
Shiraiwa, M., Li, Y., Tsimpidi, A. P., Karydis, V. A., Berkemeier, T., Pandis, S. N., Lelieveld, J., Koop, T., and Pöschl, U.: Global distribution of particle phase state in atmospheric secondary organic aerosols, Nat. Commun., 8, 15002, https://doi.org/10.1038/ncomms15002, 2017.
Su, S. H., Xie, Q. R., Lang, Y. C., Cao, D., Xu, Y. S., Chen, J., Chen, S., Hu, W., Qi, Y. L., Pan, X. L., Sun, Y. L., Wang, Z. F., Liu, C. Q., Jiang, G. B., and Fu, P. Q.: High Molecular Diversity of Organic Nitrogen in Urban Snow in North China, Environ. Sci. Technol., 55, 4344–4356, https://doi.org/10.1021/acs.est.0c06851, 2021.
Surratt, J. D., Kroll, J. H., Kleinsienst, T. E., Edney, E. O., Claeys, M., Sorooshian, A., Ng, N. L., Offenberg, J. H., Lewandowski, M., Jaoui, M., Jaouri, R., Flagan, R. C., and Seinfield, J. H.: Evidence for organosulfates in secondary organic aerosol, Environ. Sci. Technol., 41, 517–527, 2007.
Surratt, J. D., Gómez-González, Y., Chan, A. W., Vermeylen, R., Shahgholi, M., Kleindienst, T. E., Edney, E. O., Offenberg, J. H., Lewandowski, M., and Jaoui, M.: Organosulfate formation in biogenic secondary organic aerosol, J. Phys. Chem. A, 112, 8345–8378, 2008.
Tao, S., Lu, X., Levac, N., Bateman, A. P., Nguyen, T. B., Bones, D. L., Nizkorodov, S. A., Laskin, J., Laskin, A., and Yang, X.: Molecular characterization of organosulfates in organic aerosols from Shanghai and Los Angeles urban areas by nanospray-desorption electrospray ionization high-resolution mass spectrometry, Environ. Sci. Technol., 48, 10993–11001, 2014.
Tolocka, M. P. and Turpin, B.: Contribution of organosulfur compounds to organic aerosol mass, Environ. Sci. Technol., 46, 7978–7983, 2012.
Tong, H., Kourtchev, I., Pant, P., Keyte, I. J., O'Connor, I. P., Wenger, J. C., Pope, F. D., Harrison, R. M., and Kalberer, M.: Molecular composition of organic aerosols at urban background and road tunnel sites using ultra-high resolution mass spectrometry, Faraday Discuss., 189, 51–68, 2016.
Vecchi, R., Bernardoni, V., Cricchio, D., D'Alessandro, A., Fermo, P., Lucarelli, F., Nava, S., Plazzalunga, A., and Valli, G.: The impact of fireworks on airborne particles, Atmos. Environ., 42, 1121–1132, https://doi.org/10.1016/j.atmosenv.2007.10.047, 2008.
Wang, X. K., Rossignol, S., Ma, Y., Yao, L., Wang, M. Y., Chen, J. M., George, C., and Wang, L.: Molecular characterization of atmospheric particulate organosulfates in three megacities at the middle and lower reaches of the Yangtze River, Atmos. Chem. Phys., 16, 2285–2298, https://doi.org/10.5194/acp-16-2285-2016, 2016.
Wozniak, A. S., Bauer, J. E., Sleighter, R. L., Dickhut, R. M., and Hatcher, P. G.: Technical Note: Molecular characterization of aerosol-derived water soluble organic carbon using ultrahigh resolution electrospray ionization Fourier transform ion cyclotron resonance mass spectrometry, Atmos. Chem. Phys., 8, 5099–5111, https://doi.org/10.5194/acp-8-5099-2008, 2008.
Xie, Q., Li, Y., Yue, S., Su, S., Cao, D., Xu, Y., Chen, J., Tong, H., Su, H., Cheng, Y., Zhao, W., Hu, W., Wang, Z., Yang, T., Pan, X., Sun, Y., Wang, Z., Liu, C.-Q., Kawamura, K., Jiang, G., Shiraiwa, M., and Fu, P.: Increase of High Molecular Weight Organosulfate with Intensifying Urban Air Pollution in the Megacity Beijing, J. Geophys. Res.-Atmos., 125, e2019JD032200, https://doi.org/10.1029/2019JD032200, 2020a.
Xie, Q., Su, S., Chen, S., Xu, Y., Cao, D., Chen, J., Ren, L., Yue, S., Zhao, W., Sun, Y., Wang, Z., Tong, H., Su, H., Cheng, Y., Kawamura, K., Jiang, G., Liu, C.-Q., and Fu, P.: Molecular characterization of firework-related urban aerosols using Fourier transform ion cyclotron resonance mass spectrometry, Atmos. Chem. Phys., 20, 6803–6820, https://doi.org/10.5194/acp-20-6803-2020, 2020b.
Yassine, M. M., Harir, M., Dabek-Zlotorzynska, E., and Schmitt-Kopplin, P.: Structural characterization of organic aerosol using Fourier transform ion cyclotron resonance mass spectrometry: aromaticity equivalent approach, Rapid Commun. Mass Spectrom., 28, 2445–2454, 2014.
Ye, Y. Q., Zhan, H., Yu, X., Li, J., Wang, X. M., and Xie, Z. Q.: Detection of organosulfates and nitrooxy-organosulfates in Arctic and Antarctic atmospheric aerosols, using ultra-high resolution FT-ICR mass spectrometry, Sci. Total Environ., 767, 144339, https://doi.org/10.1016/j.scitotenv.2020.144339, 2021.
Zhang, L., Wang, S., Xu, Y., Shi, Q., Zhao, H., Jiang, B., and Yang, J.: Molecular characterization of lake sediment WEON by Fourier transform ion cyclotron resonance mass spectrometry and its environmental implications, Water. Res., 106, 196–203, 2016.
Zhao, Y., Hallar, A. G., and Mazzoleni, L. R.: Atmospheric organic matter in clouds: exact masses and molecular formula identification using ultrahigh-resolution FT-ICR mass spectrometry, Atmos. Chem. Phys., 13, 12343–12362, https://doi.org/10.5194/acp-13-12343-2013, 2013.