the Creative Commons Attribution 4.0 License.
the Creative Commons Attribution 4.0 License.
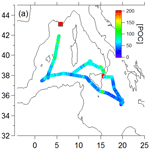
Mediterranean nascent sea spray organic aerosol and relationships with seawater biogeochemistry
Karine Sellegri
Alessia Nicosia
Leah R. Williams
Matteo Rinaldi
Jonathan T. Trueblood
André S. H. Prévôt
Melilotus Thyssen
Gérald Grégori
Nils Haëntjens
Julie Dinasquet
Ingrid Obernosterer
France Van Wambeke
Anja Engel
Birthe Zäncker
Karine Desboeufs
Eija Asmi
Hilkka Timonen
Cécile Guieu
The organic mass fraction from sea spray aerosol (SSA) is currently a subject of intense research. The majority of this research is dedicated to measurements in ambient air. However a number of studies have recently started to focus on nascent sea spray aerosol. This work presents measurements collected during a 5-week cruise in May and June 2017 in the central and western Mediterranean Sea, an oligotrophic marine region with low phytoplankton biomass. Surface seawater was continuously pumped into a bubble-bursting apparatus to generate nascent sea spray aerosol. Size distributions were measured with a differential mobility particle sizer (DMPS). Chemical characterization of the submicron aerosol was performed with a time-of-flight aerosol chemical speciation monitor (ToF-ACSM) operating with 10 min time resolution and with filter-based chemical analysis on a daily basis. Using positive matrix factorization analysis, the ToF-ACSM non-refractory organic matter (OMNR) was separated into four different organic aerosol types, identified as primary OA (POANR), oxidized OA (OOANR), methanesulfonic acid type OA (MSA-OANR), and mixed OA (MOANR). In parallel, surface seawater biogeochemical properties were monitored providing information on phytoplankton cell abundance and seawater particulate organic carbon (1 h time resolution) and seawater surface microlayer (SML) dissolved organic carbon (DOC) (on a daily basis). Statistically robust correlations (for n>500) were found between MOANR and nanophytoplankton cell abundance, as well as between POANR, OOANR, and particulate organic carbon (POC). Parameterizations of the contributions of different types of organics to the submicron nascent sea spray aerosol are proposed as a function of the seawater biogeochemical properties for use in models.
- Article
(1564 KB) - Full-text XML
-
Supplement
(1797 KB) - BibTeX
- EndNote
Oceans cover approximately 70 % of the Earth's surface, and sea spray emissions contribute up to 6 kt yr−1 of particulate matter, making them a major source of primary aerosol in the atmosphere (Lewis and Schwartz, 2004). The majority of the mass associated with sea spray emissions is in the form of coarse-mode sea salt particles. However, it is now well known that the submicron fraction of marine emissions is also important and contains a significant portion of organic compounds (Facchini et al., 2008). This organic fraction tends to be highest during phytoplankton bloom events (O'Dowd et al., 2004). Although the organic fraction of the aerosol population represents little mass, the high number concentration of these aerosol particles makes them a significant contributor to the potential cloud condensation nuclei concentration (Burrows et al., 2014). Organics in sea spray have also been shown to contribute to potential marine ice nuclei (McCluskey et al., 2017). Understanding how the organic fraction of marine aerosol particles transfer to the atmosphere is essential to help identify the contribution of the marine aerosols to the Earth's radiative budget.
In general, sea spray aerosol is generated through bubble bursting after wave breaking at the ocean surface, a process that has been described in early publications (Blanchard and Woodcock, 1980). Once bubbles burst, film and jet droplets are ejected into the atmosphere and dry to leave aerosol particles as residuals containing the different constituents of the bulk seawater and surface microlayer. The size distribution of this sea spray aerosol (SSA) extends from the fine particle range, with diameters < 100 nm, to the super-micron range (up to 3 µm). The super-micron range is known to be mainly composed of refractory NaCl and different MgCO3 or (Mg)2SO4 species, while the submicron fraction tends to be enriched with organic compounds (O'Dowd et al., 2004).
Traditionally, measurements in and around the marine boundary layer were made using offline filter measurements followed by laboratory-based analysis using either ion chromatography or organic carbon and elemental carbon analysis. However, over the last decade, there has been a significant increase in the number of studies deploying online aerosol mass spectrometry methods, including laser ablation mass spectrometry (Dall'Osto et al., 2019) and thermal vaporization followed by electron impact ionization methods (Giordano et al., 2017; Ovadnevaite et al., 2011; Schmale et al., 2013). The aerosol mass spectrometer (AMS, Aerodyne Research, Inc.) and aerosol chemical speciation monitor (ACSM, Aerodyne Research, Inc.) are examples of the latter type and are widely used instruments to monitor the chemical composition of submicron particulate matter in the atmosphere. The design of this instrument is optimized to investigate the non-refractory fraction (defined as material that vaporizes at 600 ∘C) of aerosol mass in the atmosphere. In the majority of atmospheric environments, the submicron fraction of the aerosol is dominated by non-refractory organic and inorganic species. In marine environments, aerosol mass spectrometry has been used to better characterize the chemical properties of ambient marine aerosol particles, from the Atlantic (Ovadnevaite et al., 2011) to Antarctic coastal environments (Schmale et al., 2013; Giordano et al., 2017). Using a combination of high-resolution aerosol mass spectrometry and positive matrix factorization analysis, different marine organic aerosols have been identified including secondary marine organic aerosols, organic aerosols containing methanesulfonic acid (MSA), and organic aerosols associated with amino acid (AA). The latter were thought to be primarily linked to local sea life emissions at the measurement site (Schmale et al., 2013).
Most of these studies measured ambient aerosol already modified through atmospheric chemical and physical processes. Current knowledge on the source and evolution of nascent sea spray organic emissions is still limited. This is attributed to the natural variability of marine organic aerosol and to the lack of high-temporal-resolution studies at the ocean–atmospheric interface. A limited number of studies have focused directly on the composition of nascent sea spray aerosol particles emitted from wave action in controlled simulation chambers (Wang et al., 2015; Collins et al., 2016) or through dedicated bubble-bursting experiments in ambient environments (Bates et al., 2012, 2020; Dall'Osto et al., 2019; Park et al., 2019). These studies in controlled environments identified the presence of aliphatic-rich and amino-acid-rich organic aerosols related to different phases of phytoplankton blooms (Bates et al., 2012; Wang et al., 2015). Dall'Osto et al. (2019) identified an amino acid contribution in both nascent sea spray aerosol and ambient aerosols. Park et al. (2019) observed that sea salt aerosol production was positively correlated with organic compounds in the water, notably dissolved organic carbon, marine microgels, and chlorophyll a (chl a). However, in all of these studies, samples were collected at point intervals and were not able to provide information on the evolution of aerosol physical and chemical properties over a large spatial area. In addition to this, previous studies do not provide measurements of seawater composition, making it impossible to provide the quantitative link between seawater biogeochemistry and the SSA organic composition.
In this work, we characterized the chemical composition of the nascent sea spray submicron aerosol continuously generated from an underway seawater system on the R/V Pourquoi Pas? over a 5-week campaign in the Mediterranean Sea. The Mediterranean Sea is a low-nutrient low-chlorophyll (LNLC) environment and was characterized by oligotrophic conditions along the whole field campaign (Guieu et al., 2020a). Understanding the formation of nascent sea spray aerosols in such an LNLC system can provide valuable information and be extrapolated to other oligotrophic environments.
2.1 The PEACETIME oceanographic campaign
The French research vessel, the R/V Pourquoi Pas?, was deployed for a 5-week-long period from 10 May to 10 June 2017 on the Mediterranean Sea, as part of the project PEACETIME (ProcEss studies at the Air-sEa Interface after dust deposition in The Mediterranean sea). The ship track (Fig. 1) started and ended in La Seyne-sur-Mer, France. The ship traveled clockwise, covering latitudes in the Mediterranean Sea from 35 to 42∘ and longitudes from 0 to 21∘.
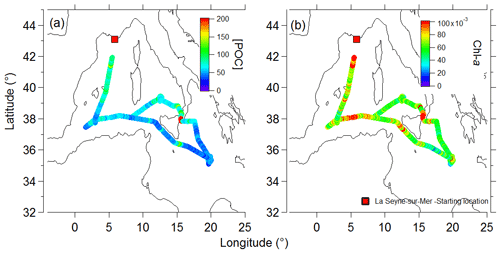
Figure 1The ship track in the Mediterranean Sea during the PEACETIME expedition. The trajectory is colored by (a) POC and (b) chl a.
Results from a suite of hydrology and biogeochemistry measurements performed on board are given in Guieu et al. (2020a). In addition to standard seawater temperature (T) and salinity (S) measurements, the concentrations of a wide range of chemical and microbiological parameters were monitored hourly. Several plankton functional groups were identified, including Synechococcus, Prochlorococcus, nanoeukaryotes, coccolithophore-like plankton, cryptophyte-like plankton, and microphytoplankton. The sea surface temperature (T) showed a gradual increase from the start to the end of the campaign from 19 to 23 ∘C. Sea surface salinity (S) varied from 36 to 39 PSU (practical salinity units), increasing from east to west. The sampling region was characteristic of open sea (average depth 2750 m ± 770 m along the transect). The sea was calm to moderately rough throughout the sampling period, with conditions always remaining below Beaufort 4. Wind speed varied between 10 and 20 m s−1.
Total chlorophyll a (chl a) and particulate organic carbon (POC) were also measured. In line with the oligotrophic state of the Mediterranean Sea during this period, the POC concentrations were highest at the most northern latitudes and gradually decreased along the ship transect (Fig. 1a), while the chl a concentration remained stable and low (0.07±0.013 mg m−3) throughout the sampling period (Fig. 1b).
2.2 Surface seawater analysis
2.2.1 Flow cytometry
Phytoplankton cells were counted with 1 h time resolution using an automated Cytosense flow cytometer (Cytobuoy, NL) connected to a continuous-clean-pumping underway seawater system, as described in Thyssen et al. (2010) and Leroux et al. (2017). Particles were brought within a laminar-flow-filtered seawater sheath fluid and detected with forward scatter (FWS) and side scatter (SWS) as well as fluorescence in the red (FLR > 652 nm) and orange (FLO 552–652 nm) ranges in the size range of 1 to 800 µm. Two trigger levels were applied for the distinction between highly concentrated picophytoplankton and cyanobacteria groups (trigger level FLR 7.34 mV, sampling at a speed of 4 mm3 s−1 and analyzing 0.65±0.18 cm3) and less concentrated nano- and microphytoplankton (trigger level FLR 14.87 mV, at a speed of 8 mm3 s−1 and analyzing 3.57±0.97 cm3). Different sets of 2D projections were plotted in Cytoclus® software to manually gate phytoplankton groups. To follow stability of the flow cytometer, 2 µm red fluorescing polystyrene beads (Polyscience) were regularly analyzed. The use of silica beads (1, 2, 3, 5, and 7 µm in diameter, Bangs Laboratory) for size-retrieving estimates from FWS were used to separate picoplankton from nanoplankton clusters.
2.2.2 Chlorophyll a and POC
From the underway seawater system, chl a was derived from the particulate absorption spectrum line height at 676 nm (Boss et al., 2013) after the relationship was adjusted to PEACETIME chl a derived from HPLC (chl a = 194.41 × line_height1.131). POC was estimated from particulate attenuation at 660 nm using an empirical relationship specific to PEACETIME (POC = ), which was slightly higher than the literature value likely due to the small dynamic range (1.27 higher on average for the range observed; Cetinic et al., 2012). Particulate attenuation and absorption of surface water were measured continuously with a WET Labs spectral absorption and attenuation meter using a flow-through system similar to the setup described in Slade et al. (2010). Both the chl a and the POC were obtained with a time resolution of 1 min.
2.3 Surface microlayer (SML) sampling and analysis
2.3.1 Sampling
Surface microlayer SML sampling was conducted twice a day from a zodiac using a 50 cm × 26 cm silicate glass plate sampler (Harvey, 1966; Cunliffe and Wurl, 2014) with an effective sampling surface area of 2600 cm2 considering both sides. For sampling, the zodiac was positioned 0.5 nmi away from the research vessel and into the wind direction to avoid contamination. The glass plate was immersed perpendicular to the sea surface and withdrawn at ∼17 cm s−1. SML samples were removed from the plate using a Teflon wiper (Cunliffe and Wurl, 2014) and collected in an acid-cleaned and rinsed bottle. Prior to sampling, all equipment was cleaned with acid (10 % HCl), rinsed in Milli-Q water, and copiously rinsed with seawater directly before samples were taken.
2.3.2 DOC analysis
The concentration of dissolved organic carbon (DOC) was determined in samples filtered online (Sartoban © 300; 0.2 µm filters). Subsamples of 10 mL (in duplicate) were transferred to pre-combusted glass ampoules and acidified with H3PO4 (final pH = 2). The sealed glass ampoules were stored in the dark at room temperature until analysis. DOC measurements were performed on a Shimadzu TOC-V-CSH (Benner and Strom, 1993). Prior to injection, DOC samples were exposed to CO2-free air for 6 min to remove inorganic carbon. A 100 µL aliquot of sample was injected in triplicate, and the analytical precision was ±2 %. Standards were prepared with acetanilide. Analysis of DOC was performed on both SML and the underlying seawater sampled from the zodiac.
2.4 Sea spray generation and analysis
2.4.1 General setup
The sea spray generator has been characterized and deployed in a number of previous studies, and full details are reported in Schwier et al. (2015). Briefly, it consists of a 10 L glass tank, fitted with a plunging jet system for the water. A particle-free air-flushing system is placed perpendicular to the water surface at a distance of 1 cm to send a constant airflow across the surface of the water to replicate the effects of wind on the surface (13 m s−l). The sea spray generator was supplied with a continuous flow of seawater collected at a depth of 5 m by an underway seawater circulating system operated with a large peristaltic pump (Verder® VF40 with EPDM hose). The wastewater was evacuated downstream of the sampling location to avoid any contamination.
The aerosol instrumentation included a time-of-flight aerosol chemical speciation monitor (ToF-ACSM, Aerodyne Research, Inc.), a custom-made differential mobility particle sizer (DMPS) coupled with a condensation particle counter (CPC, model 3010, TSI), and an impactor (Dekati, PM1) for collecting submicron particulate matter for offline ion chromatography and chemical analysis. All three sampled from the headspace above the seawater in the tank. A silica gel dryer was connected to the output of the chamber, which was subsequently connected to a flow dispatcher having three outputs of equal length (<50 cm), one to the ToF-ACSM, one to the DMPS, and one to the impactor. The aerosol relative humidity was measured continuously and varied from 20 % to 40 % (Fig. S1 in the Supplement). The total sampling line length after the sea spray generator was approximately 2 m with a sampling flow of 5 L min−1 giving a residence time of less than 30 s. A schematic of the sampling setup is shown in Fig. S2. Regular tests were performed to ensure that the system was airtight and free from external aerosol influences.
2.4.2 Aerosol physical and chemical properties
Size distribution measurements
Particle size distribution and number concentration measurements were obtained using the DMPS. Measurements were provided approximately every 10 min for 25 different size bins ranging from 10 nm up to 500 nm. The size distribution was relatively constant throughout the measurement period, giving a principal size mode at 110 nm and a second mode at 300 nm. This size distribution is characteristic of the bubbler seawater generation method (Schwier et al., 2015) and is similar to that from other nascent seawater aerosol generators (Bates et al., 2012) and to that observed in the clean marine boundary layer (Yoon et al., 2007). Although the size distribution remained constant, the absolute number concentration varied by a factor of 3 over the sampling period. Details of these changes in aerosol number concentration as well as the associated cloud condensation nuclei activity are detailed in Sellegri et al. (2021).
Offline PM1 filter analysis
In parallel to the online aerosol physical and chemical measurements, the generated nascent sea spray aerosol particles were also sampled onto PM1 quartz filters in the impactor. Aerosol samples were stored in airtight containers and were transported to the laboratory for analysis following the field campaign. In the laboratory, aerosol samples were extracted in Milli-Q water by sonication (30 min) for analysis of the water-soluble components. Extracts were analyzed by ion chromatography for the quantification of the main inorganic ions (Sandrini et al., 2016). An IonPac CS16 3×250 mm Dionex separation column with gradient MSA elution and an IonPac AS11 2×250 mm Dionex separation column with gradient KOH elution were deployed for cations and anions, respectively. The contributions of the estimated ssSO4 (sea salt SO4), ssK, ssMg, ssCa, and ssCl were calculated based on the seawater theoretical ratio (Seinfeld and Pandis, 2016). These ratios are 0.25 for SO4, 0.06 for K, 0.12 for Mg, and 0.04 for Ca. The remaining non-sea-salt (nss) fraction of the inorganic aerosol was within the measurement error of the instrument.
The water-soluble organic carbon (WSOC) content of the extracts was quantified using a total organic carbon (TOC) thermal combustion analyzer (Shimadzu TOC-5000A) (detection limit (DL) = 1.9 µgC/filter). Measurements of the total carbon (TC) content were performed on a filter punch cut before water extraction by a thermal combustion analyzer equipped with a furnace for solid samples (Analytik Jena, multi NC2100S; Rinaldi et al., 2007) (DL = 37.9 µgC/filter). For the organic carbon (OC) analysis, the punch was acidified before analysis to remove inorganic carbon from TC and obtain OC. This process included positioning the punch in the instrument sample container, covered with 40 µL of H3PO4 (20 % w/w) and left under oxygen flow, at room temperature, for ca. 5 min to allow the volatilization of carbonates as carbon dioxide (CO2). The process was monitored online by nondispersive infrared (NDIR) spectroscopy CO2 detector: when the CO2 level went back to baseline conditions, the vessel was placed into the furnace (950 ∘C) for the OC analysis.
The water-insoluble organic carbon (WIOC) is not measured directly but is derived from the difference between WSOC and OC. As mentioned, the measurements of OC and WSOC are made with two different instruments with that for WSOC having a much lower limit of detection (DL). Although the quantification of WSOC was always possible, some samples had OC concentrations < DL, assuming that OC = WSOC (and WIOC = 0) would be incorrect and would result in significant error in the estimate of total OC. There could be a significant amount of WIOC that we cannot quantify because of the lower sensitivity of the OC analysis. For this reason, we presented WIOC and OC data only for samples that have both quantifiable OC and WSOC.
The amount of inorganic carbon varies between 12 % and 71 % of TC, and thus the acidification process for sea spray is an important step to follow for OC measurements. Organic carbon was converted to organic mass using conversion factors of 1.4 for the conversion of WIOC to WIOM and 1.8 for the conversion of WSOC and WSOM (Facchini et al., 2008). Although filters were only collected on a daily basis, they provided valuable information on the refractory component of the aerosol population. In order to compare filter measurements with volume concentrations measured by the DMPS, filter volume concentrations were calculated from the measured mass concentrations using a density value of 1.2 g cm−3 for organic matter and a density of 2.165 g cm−3 for the other remaining components, SO4, chl, Na, and Cl assuming a density for sea salt (Seinfeld and Pandis, 2016). These concentrations compared well with those from the DMPS (Fig. S3).
ToF-ACSM
The ToF-ACSM is based on the same operating principles as the aerosol mass spectrometer (Drewnick et al., 2009). The ToF-ACSM contains a critical orifice, a PM1 aerodynamic lens to focus submicron particles into a narrow beam that flows into a differentially pumped vacuum chamber, a standard vaporizer heated to 600 ∘C to vaporize particles, an electron-emitting tungsten filament (70 eV) to ionize the vapor, a compact time-of-flight mass analyzer (ETOF, Tofwerk AG, Thun, Switzerland), and a discrete dynode detector (Fröhlich et al., 2013). It does not have the ability to size aerosol particles but has the advantage of being more compact and more robust for continuous observations than the AMS (Fröhlich et al., 2013). The ToF-ACSM alternates between sampling ambient air and sampling through a filter in order to subtract the signal due to air.
During this experiment the ToF-ACSM was operated in a 2 min filter and 8 min sample mode with a measurement every 10 min. The aerodynamic lens transmits particles between 70 and 700 nm, making the ACSM approximately a PM1 measurement. The non-refractory particle material (NR-PM) is defined similarly as in DeCarlo et al. (2006) as aerosol particles that are vaporized using the 600 ∘C resistively heated vaporizer and detected during the instrument sampling interval. The relative ionization efficiency for NH4 was 3.12 and for SO4 was 0.8, determined from calibrations with ammonium nitrate and sulfate. However, considering that the measured SO4 concentrations represent sea salt (ss) SO4, we adjusted the relative ionization efficiency (RIE) of SO4 to 0.3 so that the mass concentration of from the ACSM agrees with that of the filters during the periods when filter and ACSM total mass concentrations were in agreement (before 30 May; see Fig. S3).
The temperature of the vaporizer and the size range do not permit efficient detection of sea salt particles with the ToF-ACSM. However, in situations of high sea salt concentrations, detection of sea salt ions and related halides have been reported (Bates et al., 2012; Giordano et al., 2017; Ovadnevaite et al., 2011; Schmale et al., 2013; Timonen et al., 2016). Likewise, in this study mass spectral signals associated with sea salt were observed. In addition, the contribution from chloride was very high (72 % of the total mass). In some quadrupole ACSM instruments, negative Cl peaks are sometimes observed (Tobler et al., 2020) due to slow evaporation of refractory material from the vaporizer relative to the 30 s switching time between filter and sample. This tends to overestimate the filter measurement and underestimate the sample measurement and can lead to negative values for the difference. However, during these measurements with the ToF-ACSM, negative Cl was not observed likely because of the long switching times.
The typical signature peaks for sea salt aerosol in our instrument were confirmed by atomizing pure aerosol particles generated from sea salt solution (Biokar, synthetic sea salt, lot: 0017475), passing the particles through a silica gel dryer and into the ToF-ACSM instrument. In the default fragmentation table used to assign the signals at individual 's to chemical species (Allan et al., 2004), peaks associated with sea salt were identified as organic aerosol fragments. In order to better represent the measured aerosol composition, we modified the standard fragmentation table by introducing a sea salt species that includes m/z fragments at 23 (Na+), 35 and 37 (35Cl−, 37Cl−), 58 and 60 (Na35Cl, Na37Cl), and 81 and 83 (NaCl2, NaCl37Cl). For 81, there is overlap with an SO4 fragment, and a correction suggested by Schmale et al. (2013) was applied (Eq. 1). This correction accounted for less than 10 % of the signal at 81 and 3 % of the total sulfate signal.
Quantification of sea salt is difficult in the ToF-ACSM as a result of inefficient vaporization and a nonlinear contribution to the Na+ signal from surface ionization on the vaporizer. Therefore, in this work we do not attempt to quantify the sea salt fraction, but instead we use the mass spectral information to separate it from the organic aerosols. A standard collection efficiency (CE) of 0.5 was applied to all data obtained from the ACSM (Middlebrook et al., 2012). Regular particle-free sampling periods were performed to ensure that there was no buildup of material on the vaporizer and that the sampling setup was leak-free.
Positive matrix factorization (PMF)
In order to identify the different organic aerosols present in the sea spray from primary seawater, unconstrained positive matrix factorization, using the SoFi interface (Canonaco et al., 2013), was performed on the ToF-ACSM organic mass spectra. The ToF-ACSM gives unit mass resolution (UMR) mass spectra, so it is not possible to distinguish between salt and non-salt ions at a given . A decision was made to remove all sea-salt-related ions from the organic mass spectral data matrix, giving a total of 116 from 0 up to 150. We are aware that removing the 's associated with NaCl (23, 35, 37, 58, 60, 81, and 83) will also remove contributions from organics at these 's. However, the organic contributions at these values are small relative to the rest of the organic MS and are typically a factor of 10 smaller than sea salt in the ACSM signal.
During sampling, the ToF-ACSM was run with a 2 min filter and 8 min sample cycle. When sampling with long times between filters, any drift in sensitivity can result in a difference signal that is an artifact. This is especially true for those signals with a high background (e.g., from air at 29 from 15NN), and when measuring low concentrations of organic mass (as is the case during this experiment). In PMF solutions including 29, one of the factors contained predominantly 29, and the time series was noisy and flat. Down-weighting 29 (by ×100) did not help distribute the signal at 29 into the other organic factors. Therefore, since 29 was only contributing to noise and not to chemical information, we removed it. The PMF solutions were explored up to six factors, as a function of f-peak values from −1 to +1 (Figs. S4 to S10). The four-factor solution was chosen, based on correlations with reference mass spectra. The correlations for the three- and five-factor solutions are illustrated in the Supplement (Figs. S5, S6, S9, and S10). The four identified factors, as well as their mass spectral fingerprints and time series, will be discussed in the following sections.
3.1 Time evolution of the chemical composition of nascent sea spray
Mass concentrations of aerosol chemical composition obtained from the submicron offline filter measurements are listed in Table 1. The soluble inorganic species concentrations were mostly found with proportions similar to the reference average seawater composition (Seinfeld and Pandis, 2016). However, enrichment in K+ (69 % of which was not explained by the average seawater composition) and a slight enrichment of Ca2+ were measured toward the end of the campaign (17 % of Ca2+ was not explained by reference seawater composition from 28 May onward). In contrast, the magnesium was slightly depleted (20 % less than expected in reference seawater composition) but less towards the end of the campaign. Filter-based organic matter (OM) was evenly split between WIOM (9 % ± 0.5 % of total mass) and WSOM (6 % ± 6 % of total mass), which contrasts with previous studies where organic matter in ambient marine aerosol was almost exclusively composed of WIOM (Facchini et al., 2008). However, these previous studies were conducted during phytoplankton bloom events of the North Atlantic Ocean, where POC is usually enhanced. Considering that the Mediterranean Sea is characterized by oligotrophic conditions during PEACETIME, it could explain the relatively low contributions of WIOM.
Table 1Concentrations of different chemical species in PM1 primary seawater aerosols measured using offline analysis of filters and online measurements from the ACSM. The cited uncertainty represents 1σ.
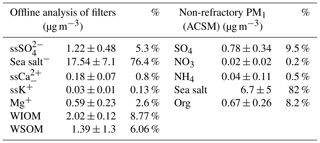
The chemical composition of SSA measured by the ACSM is shown in Fig. 2 and listed in Table 1 and was primarily composed of sea salt aerosol (determined from the signals at 23 (Na+), 35 and 37 (35Cl−, 37Cl−), 58 and 60 (Na35Cl, Na37Cl), and 81 and 83 (NaCl2, NaCl37Cl), representing 82 % ± 61 %), followed by SO4 at 9.5 % and organic matter at 8.2 %. The variability in the different chemical compositions throughout the sampling is thought to be a result of the differing associated contributions of refractory compounds (Ca2+, Mg+, K+, etc.) in the sea salt sample.
In order to determine how representative the ACSM PM1 measurements were of the total PM1 mass, the total ACSM PM1 mass concentration was converted into volume concentration (dividing organic mass concentrations by a density value of 1.2 g cm−3, and the other remaining components SO4, Cl, and NaCl by 2.165 g cm−3 (Seinfeld and Pandis, 2016). Contributions from NO3 and NH4 were less than 1 % of the mass and were not included in the volume calculation. The ACSM volume concentration was compared to the volume concentration measured by the DMPS, giving a correlation (r) of 0.49 and slope (b) of 1.21 (Fig. 3). The variation in the total sea spray mass concentration with time is a result of the variability in the number of sea spray particles generated from the sea spray generation device. The size distribution of the aerosol remained stable throughout the experiment, but the sea spray number emission flux is influenced by the variability in seawater biogeochemical properties. This is presented and discussed in detail in a companion paper (Sellegri et al., 2021), where we show a clear relationship between the sea spray number concentrations generated in the bubble-bursting system and the nanophytoplankton concentration of the seawater, not only in the PEACETIME experiment but also in other seawater types.
The agreement between the two instruments is relatively good in the first part of the campaign, but the difference between the ACSM-derived volume and the DMPS-derived volume increases during the later parts of the campaign from 1 to 10 June (Fig. 3). We ruled out the possibility that the high fraction of inorganics in the SSA led to an accumulation of refractory or semi-refractory material on the vaporizer and a corresponding decrease in the ability to measure non-refractory material by examining the background (filter) signals. Figure S11 shows the background signal at 58 (NaCl+) as a function of time; this signal varies as a function of total mass loading but does not progressively increase over time. Similarly, particle-free sampling periods at the start and the end of the field campaign show that 58 background levels dropped to comparably low values. Therefore, we conclude that overloading of the ACSM by refractory, or semi-refractory slowly vaporizing, material did not occur, and the missing mass is due to increased refractory content. The fraction of missing mass, FRefrac, is estimated as the difference between the DMPS volume and ACSM volume divided by the DMPS volume and is shown as the grey area in Fig. 2. The latter period of the field campaign corresponds to a change in seawater type with more inputs from the Atlantic Ocean and a corresponding decrease in practical seawater salinity (Fig. S13). It is possible that during this sampling period, the seawater contains higher fractions of refractory material that are less efficiently measured by the ACSM. An additional means to estimate the missing mass is to subtract the total ACSM mass loading from the total mass measured on the filters. Comparing this estimation of missing mass to the different species measured on the filters shows the best correlations with Mg2+, Ca2+, and (Fig. S14). This might suggest that the ability of the ACSM to measure NaCl particles depends on how NaCl is associated with other compounds in the sea spray.
The PM1 mass concentrations of WSOM and WIOM calculated from the filters were additionally compared with the total OM measured from the ACSM (OMACSM) (Fig. S14). The OMACSM represented on average 27 % of the total filter OM. In the following section, we analyze in more detail the different organic aerosol species present in the SSA samples and determine to what extent they are related to seawater biogeochemical properties.
3.2 Marine organic aerosol speciation
As explained in Sect. 2 (Methodology), PMF was used to separate organic factors. Based on the correlations with reference mass spectra and on observations of the temporal variations, we chose a four-factor solution. These factors include an oxidized organic aerosol (OOANR), similar to ambient reference OOA (Ng et al., 2010), somewhat oxidized OA containing mixed amino acid and fatty acid signatures (mixed OA, MOANR), primary organics containing aliphatic signature peaks as well as several peaks corresponding to fatty acids signatures (POANR), and a methanesulfonic acid-like OA (MSA-OANR) (Phinney et al., 2006). The average composition for two distinct time periods, the mass concentrations as a function of time, and the mass spectra are shown in Fig. 4. The fractional contribution of each factor to the total mass as a function of time is shown in Fig. S7. The correlations of each of the identified factors with reference mass spectra are illustrated in Fig. S8. The OOANR contributed 51 % ± 2 % to OA and had signature peaks with high 44 and 28 and correlated with an OOA reference mass spectrum (r=0.98). It did not contain any other values that might suggest a contribution from other species. The ratio of the OOANR fraction was 1.6 (calculated using the method described in Canagaratna et al., 2015), which is significantly higher than the average ratio of LV-OOA found in terrestrial ambient aerosols (0.8) (Canagaratna et al., 2015). High ratios have been reported in ambient studies where carbonate species were thought to be measured by the ACSM (Bozzetti et al., 2017; Vlachou et al., 2019) and additionally have been associated with aerosols subjected to aqueous phase processing (Canagaratna et al., 2015). It is also possible that the PMF analysis wrongly attributed excess 44 to OOA at the expense of other species such as the MSA-OANR discussed below. This would impact the reported absolute concentrations of the OOANR vs. the MSA-OANR.
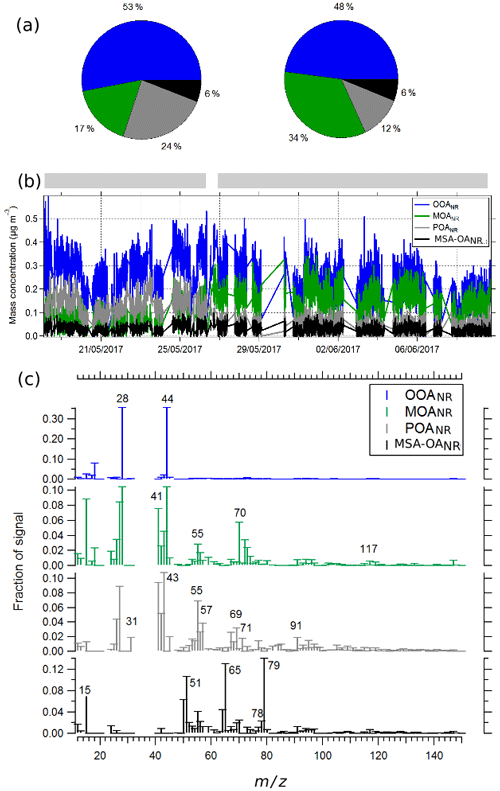
Figure 4(a) The contribution of the different organic factors during different periods of the PEACETIME ship campaign (identified by the grey bars at the top of b). (b) The mass concentrations of each factor (OOANR, MOANR, POANR, and MSA OANR) as a function of time. (c) The mass spectra of the factors.
The second most dominant species was defined as a mixed organic aerosol (MOANR). This factor contributed to 15 % of the total OANR at the start of the campaign and then increased to 28 % and 35 % later on in the campaign (Fig. 4a). This MOANR factor contained several mass peaks associated with amino acids (AAs) reported in reference mass spectral signatures of leucine and valine (Schneider et al., 2011). AA signature peaks were identified at 41, 70, 98, 112, 115, 117, 119, and 131 and were similar to signature peaks that had been identified in previous studies by Schmale et al. (2013) in ambient marine aerosols and by Schneider et al. (2011) during a series of laboratory studies on different AAs. Similar marker 's were present for fatty acid species such as palmitic and oleic acid (Alfarra et al., 2004). The MOANR factor had an of 0.53 and an of 1.39, hence much less oxidized than the OOANR type. These values are intermediate between those often calculated for low-volatility and semi-volatility OOANR in the ambient atmosphere (Canagaratna et al., 2015) and are similar to those identified by Schmale et al. (2013) for an amino-acid-type aerosol ( 0.35 and 1.65) detected in an ambient marine aerosol.
The third most prominent factor was identified as a primary organic aerosol (POANR) and contributed 26 % to the total organics at the start of the campaign, decreasing to 9 % near the end of the campaign (Fig. 4a). This factor contained typical aliphatic signatures and had little contribution from 44. The mass spectral signature of the POANR factor correlated well with reference mass spectra of leucine (r=0.56) and valine (r=0.51) but also with fatty acid mass spectra of oleic (r=0.69), palmitic acid (r=0.74), and hydrocarbon organic aerosol (HOA) (r=0.78). The ratio of this POANR was 0.1 and the was 1.64, which is typical for values of primary organic aerosol in the ambient atmosphere. The POANR factor identified in this work, as well as the ratio (1.64), was similar to the aliphatic-rich organic aerosol species measured in contained wave chamber experiments during a phytoplankton bloom (Wang et al., 2015). Once the bloom passed the of these aerosol particles decreased, and it was hypothesized that the primary organics were transformed through microbial activity in the water. During the PEACETIME campaign, the POANR factor had higher concentrations at the start of the field campaign and then later decreased. This decrease in POANR was accompanied by an increase in the more oxidized MOANR (Fig. 4b).
The last factor, MSA-OANR; contributed 6 % ± 1 %, contained typical signature peaks at 65, 79, and 96; and correlated with reference mass spectra of MSA (r=0.34) (Fig. S8). This mass spectrum is similar to that identified by Timonen et al. (2016) in Antarctica. However, unlike previously measured ambient MSA-like species (Schmale et al., 2013), it contained little or no oxygenated peaks at lower masses ( 43, 44, 45), making it impossible to calculate an ratio. As mentioned above, it is possible that, given the low temporal variability, the 44 was incorrectly attributed by the PMF analysis, resulting in an excess 44 in OOA and missing 44 in MSA-OANR. The ratio of 1.12 was similar to 1.2 measured by Ovadnevaite et al. (2011) but lower than the reported 1.6 by Schmale et al. (2013) for MSA-OANR, both detected in ambient aerosol. The presence of an MSA-OA in nascent sea spray generated in the present study suggests that this compound is already present in the seawater and not only produced from gas-phase DMS emissions and oxidation in the atmosphere. The Mediterranean Sea experiences a high level of radiation (MerMex Group, 2011) and could also explain the presence of MSA-like compounds from DMS oxidation within the seawater. Precursor species of MSA exist in seawater, such as dimethylsulfoniopropionate; however, the available reference mass spectra of this compound (determined using liquid chromatography MS) contain values at 63, 73, and 135, none of which are visible in our organic spectra (Swan et al., 2017; Spielmeyer and Pohnert, 2010). Therefore, we believe that the MSA-OANR species measured in these seawater samples resembles MSA more than one of its precursor species.
AAs containing OA have been measured at a number of coastal sites, and their formation in the ambient atmosphere is similar to that of MSA, where the AAs are formed from the gas-phase partitioning of amines such as trimethylamine or dimethylamine into the particulate phase (Facchini et al., 2008). These AA OA signatures were detected during measurements made on the east coast of America, in the Arctic (Dall'Osto et al., 2019), and also during controlled wave and bubble-bursting chamber experiments (Dall'Osto et al., 2019; Decesari et al., 2019; Kuznetsova et al., 2005; Wang et al., 2015).
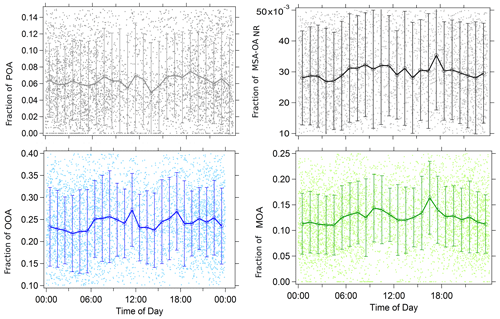
Figure 5Diurnal variation in the four PMF organic factors chosen to represent the measured non-refractory organic aerosol: average (circles) and standard deviation (vertical bars) of the measurements by hour during the whole PEACETIME cruise. The underlying raw data are shown with small dots.
In our experimental setup, the short time between particle generation and analysis (less than 30 s) does not allow for the formation of secondary aerosol through oxidation and partitioning of gas-phase species into the particle phase. Since these amino acid signatures are internally mixed with signatures for several different species, we assume that they are present in the organic matter of the seawater, similar to conclusions made by Dall'Osto et al. (2019).
Although very weak and within the measurement error, OOANR, MSA-OANR, and MOANR factors had a similar diurnal variation with increases during the early hours of the morning and again in the afternoon (Fig. 5). POANR showed similar variation to the other species in the morning but a second increase was not observed in the afternoon. A lack of strong diurnal variation in MSA-OANR and OOANR indicates that the secondary (oxygenated) nature of these compounds might be a result of biological processing rather than photochemical processes.
3.3 The sources and formation pathways of marine organic aerosol species
In several large-scale climate models chl a is used as a proxy of phytoplankton biomass to predict the organic fraction of sea spray. In this study, the measured chl a in the underway surface seawater was low and had little variability (0.07±0.013 mg m−3), therefore making it difficult to extract any significant relationship between our measured organic mass fractions and the measured chl a. No significant correlations were observed between the mass concentrations of the OM (measured by either the ACSM (OMACSM) or on filters (OMfilter = WIOM + WSOM) and chl a concentrations, or between the fraction of these two organic classes to the total sea spray mass and chl a (not shown). Therefore it is important to identify other marker species or processes that can be used to correctly link seawater chemical composition, biological activity, and the organic fraction in the seawater aerosol that can represent up to 15 % of the total submicron sea spray mass in oligotrophic waters.
In a companion paper, we illustrate that the total number of sea spray particles measured by the DMPS was correlated to the nanophytoplankton cell abundance (NanoPhyto) (r=0.33, n=501, p<0.001) (Sellegri et al., 2021). The hypothesis behind the dependence of the sea spray number concentration on NanoPhyto is that organic matter released by NanoPhyto influences the surface seawater (SSW) surface tension and therefore the bubble lifetime that drives the number of film drops ejected to the atmosphere.
3.3.1 High-time-resolution correlations
In this section we will investigate the dependence of each of the organic classes identified in sea spray on the SSW biogeochemical properties. The relationships of the total factor mass concentrations and the fractional factor contributions to the seawater biochemistry were investigated (Fig. 6).
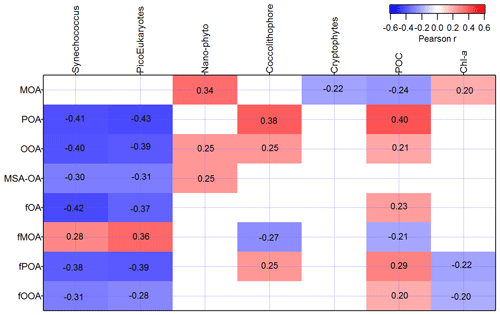
Figure 6Pearson correlation coefficients for the four different organic factors (MOANR, POANR, OOANR, and MSA-OANR) and their fraction of the total sea spray mass (fMOA, fPOA, fOOA, and fOA) with several phytoplankton functional group abundances (cells per cubic centimeter) (Synechococcus, picoeukaryotes, nanoeukaryotes (Nano-phyto), coccolithophore (coccolith), cryptophytes), total chl a (mg m−3), and POC (mg m−3) in the sampled seawater during the whole campaign. Sample number = 461. Correlations with absolute R values < 0.16 had Pearson's two-tailed significance values lower than 0.001 and were therefore left blank.
MOANR was strongly linked to NanoPhyto (r=0.34), as was MSA-OANR and OOANR but with less significance (r=0.25). Therefore, these organic classes follow the total sea spray mass and number behavior, as illustrated in Sellegri et al. (2021). The hypothesis of organic matter influencing the surface tension of seawater, bubble lifetime, and the number of film drops is therefore linked to this specific class of organic matter. Fatty acids, consistent with the MOANR and OOANR spectra, have been reported to be enriched in the SML (Cunliffe et al., 2013), which would explain their impact on the bubble-bursting process. POANR species are instead significantly correlated with particulate organic carbon concentrations, POC (r=0.40), and Coccolithophore-like abundance (r=0.38). A relationship between Coccolithophore-like cell abundance and [POC] is likely linked to the ability of the coccolithophores or similar groups of phytoplankton to secrete large amounts of sticky carbon which can result in the formation of gels and POC (Engel et al., 2004). As the time variation in POANR does not follow that of total sea spray mass, it is possible that POA is not linked to film drop formation and is ejected into the atmosphere via a separate mechanism, such as jet drops. It was recently shown by Wang et al. (2017) that jet drops can contribute significantly to the population of submicron SSA (up to 43 %). The jet-drop-originating SSA has a different chemical composition than the film-drop-originating SSA and is more influenced by the SML (Wang et al., 2017). The hypothesis of POANR being linked to jet drops is supported by its relationship to POC in SSW.
The time series of OOANR had positive relationships with NanoPhyto (r=0.25), but also with coccolithophore-like cell abundances (r=0.25) and POC (r=0.21), and hence OOANR seem to have an intermediate behavior between POANR and MOANR. All organic classes except MOANR are anticorrelated to the classes of small phytoplankton (picoeukaryotes and Synechococcus). This anticorrelation could be the result of the competition for nutrients between these small cells and the larger ones that rather drive the POC content.
Except for MOANR, all correlations for the absolute mass of these different types of organic matter are also observed when the fractional contribution of these species to the total mass of SSA (determined from the DMPS) are considered, although less significantly (lower part of Fig. 6). However, for MOANR species, correlations with NanoPhyto no longer hold if the fractional contribution of these species to the total mass of sea spray is considered. Instead, fMOA is correlated to picoeukaryotes and Synechococcus. This is likely due to the strong anticorrelation of the fraction of all other organic classes with these classes of small phytoplankton. At low picoeukaryotes and Synechococcus cell abundances, fPOA, fOOA, and fMSA-OA are higher, artificially decreasing the proportion of fMOA to the rest of the organic matter.
3.3.2 Filter-based resolution correlations
Since the non-refractory organic components analyzed using the ACSM technique are only a fraction of the marine organic mass, we investigate the relationships between off-line filter-based organic compounds and seawater biogeochemical properties. Filter-based organic fractions are also compared to seawater properties at the filter sampling time resolution. The organic mass concentration from filters is correlated to the coccolithophore cell abundance (r=0.88, n=13). The fraction of OM to total mass analyzed on filters (OMSS) was also correlated to coccolithophore cell abundance (r=0.72, n=13) and POC (r=0.6, n=13). This indicates that the total organic matter present in sea spray behaved similarly to the non-refractory POANR and OOANR analyzed by the ACSM. Previous studies observed a connection between seawater POC and SSA organic fraction. Facchini et al. (2008) found that WIOM in SSA was related to seawater POC derived from microgels. Furthermore, during mesocosm bubbling experiments using Emiliania huxleyi cultures and low heterotrophic prokaryote abundance counts, O'Dowd et al. (2015) suggested that the aggregation of dissolved organic carbon (DOC) into POC in the form of insoluble gel colloids was the driving force behind the enrichment of organic matter into submicron SSA. The authors hypothesized that the organic fraction of SSA can be controlled either by DOC or POC, depending on the biological state of the waters.
Measurements of DOC in the SML and underlying seawater were performed daily, as well as surface seawater bacterial total counts from daily surface water samples (5 m depth) (the description of these methods is provided in Sect. S2). DOC was slightly enriched in the SML compared to the underlying seawater, with enrichment factors varying between 1 and 1.2 (Fig. 7). The filter-based total organic content of sea spray aerosol was not correlated to DOC or total bacterial count in the SML and neither was OMSS. However we observe that MOANR was correlated to the enrichment of DOC in the SML (r=0.55, n=9), suggesting again that MOANR is likely linked to organic matter present in the SML. The correlation between MOA and DOC enrichment in the SML suggests that the fraction of DOC which is enriched in the SML contains lipids and amino acids found in the MOANR fraction. Although MOANR is an oxidized organic class, it does not seem to be the result of the bacterial production (BP) of organic matter in the seawater. Instead, we found a significant anticorrelation between MOANR and bacterial production (, n=10), indicating that MOANR could instead be consumed by this process.
3.3.3 Predicting organic matter in sea spray from seawater biogeochemical properties
By combining relationships between filter-based chemical analysis, ACSM organic source apportionment, and seawater properties, it is possible to propose a general relationship that can be used to predict the different fractions of organic matter in the nascent sea spray emitted from oligotrophic seawaters. These different organic fractions may have different atmospheric properties related to their climate impact, such as ice nuclei properties (Trueblood et al., 2021). We chose to parameterize the organic fractions of sea spray rather than computing organic mass fluxes, for an easier implementation in models that already have an inorganic sea spray source function. However, as shown in the preceding section, the total mass of sea spray is significantly influenced by SSW biology, and we recommend that the biology-dependent sea spray number flux modulation computed in Sellegri et al. (2021) is applied before biology-dependent organic fractions are calculated.
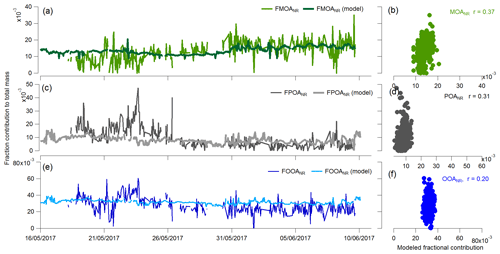
Figure 8Time series and correlation plots of each of the parameterizations (Eqs. 4 to 6) for the determination of the different organic fractions in nascent SSA.
We initially examined the relationship with offline filter organic measurements of water-insoluble and water-soluble organic matter fractions (FWIOM and FWSOM, respectively) with POC (Eqs. 2 and 3). These showed positive correlations of both WIOM and WSOM with POC measurements.
Using the higher-time-resolution data obtained from the ACSM, the non-refractory organic fraction of nascent sea spray can also be predicted using three different equations, with MSA-OANR being a negligible fraction of the total sea spray mass:
These relationships apply to the ranges of POC and picoeukaryotes measured during the PEACETIME cruise. Hence they may be applicable to other oligotrophic waters. If larger ranges of seawater biogeochemical properties are considered in the future, fractions of organic classes should be parameterized as logarithmic laws asymptotic to 1, in order to take into account the saturation of the organic fraction at 1 for the largest POC values.
Figure 8 shows the reconstruction of these organic factors using the parameterizations determined in Eqs. (4) to (6). The parameterizations correctly represent the absolute concentrations. However, the short-term variability is less well represented, with correlations varying from 0.20 to 0.37. For this sample size (n>450) the significance of this correlation is <0.001. These relatively low correlations suggest that there are parameters other than POC influencing the emission of these organic species, especially for OOA that is believed to be more linked to the SML properties than to the bulk seawater. However, these parameterizations are useful to provide a first approximation of the organic matter exported to the SSA and in this type of environment (LNLC) are a better choice than using chl a.
The primary objective of this experiment was to study the relationships between sea spray chemical properties and those of seawater. This work presents a unique dataset, which describes the first deployment of a ToF-ACSM to characterize, in a continuous way, the organic fraction present in sea spray aerosol generated from Mediterranean surface seawater. The non-refractory part of the organic content of sea spray was characterized by low concentrations and low variability along a 4300 km transect. Yet, using a positive matrix factorization on the ACSM organic mass spectra, it was possible to extract signatures for fatty acids, amino acids, and marine primary organic aerosols in non-refractory nascent sea spray. We identified four organic classes: two were composed of mixtures of amino acids and fatty acids (a primary aerosol POANR and a slightly oxidized MOANR factor), and two were identified as more oxidized organic aerosol (OOANR and MSA-OANR). The POANR factor was similar to that observed in wave chamber experiments and correlated well with POC concentrations in the seawater, as did the OOANR and MSA-OANR. The MOANR concentrations had a different behavior and correlated well with the nanophytoplankton cell abundance in the seawater, and also with the total sea spray number concentration and DOC enrichment in the surface microlayer. It is hypothesized that MOANR is related to surface tension properties that influence the bubble-bursting process and the resulting number of film drops ejected to the atmosphere. In contrast, the fraction of POANR, OOANR, and MSA-OANR classes is not connected to the sea spray number concentration but is linked to POC of the bulk surface seawater and more likely emitted with a different process such as through jet drops. Water-soluble and water-insoluble organic matter measured using offline filter analysis confirm this positive relationship between OM and POC measurements. This suggests that the refractory, as well as non-refractory, fractions of the particulate organic carbon in the seawater are transferred to the sea spray.
This work illustrates the value of continuous aerosol chemistry and physical characterization of the nascent sea spray aerosol in parallel with other biogeochemical measurements in surface seawater. It also illustrates that even under oligotrophic conditions, seawater biogeochemical properties influence the type and concentration of marine organic aerosols and therefore their ability to act as cloud condensation nuclei or ice nuclei. We provide a parameterization of the different marine organic components of nascent sea spray as a function of seawater biogeochemical properties typical for oligotrophic conditions in LNLC regions of the ocean that represent 60 % of the global ocean. Such parameterization used in models should allow a better prediction of the impact of living marine organisms on these properties in a future climate.
Underlying research data are available at https://doi.org/10.17882/75747 (Guieu et al., 2020b).
The supplement related to this article is available online at: https://doi.org/10.5194/acp-21-10625-2021-supplement.
CG and KD designed the PEACETIME project. KS designed the experiments specifically used in this paper. KS and AN performed the measurements aboard the ship. MT, GG, NH, JD, IO, FVW, AE, and BZ were responsible for collecting and analyzing the biogeochemical parameters in either the seawater or the surface microlayer. MR analyzed the offline filter measurements. EF analyzed the ACSM data with input from LRW and ASHP. EF and KS prepared the manuscript with contributions from all authors.
The authors declare that they have no conflict of interest.
This article is part of the special issue “Atmospheric deposition in the low-nutrient–low-chlorophyll (LNLC) ocean: effects on marine life today and in the future (ACP/BG inter-journal SI)”. It is not associated with a conference. This article is also part of the special issue “CHemistry and AeRosols Mediterranean EXperiments (ChArMEx) (ACP/AMT inter-journal SI)”. It does not belong to a conference.
The underway optical instrumentation was provided by Emmanuel Boss's group funded by NASA Ocean Biology and Biogeochemistry. We thank Oliver Grosso, Dominique Lefèvre, and Thibault Wagener for their contribution to the continuous surface pumping system and the follow-up of CytoSense during the cruise. More information on the PEACETIME cruise can be found at https://doi.org/10.17600/17000300. Sea2Cloud was endorsed by SOLAS. We thank the captain and the crew of R/V Pourquoi Pas? for their professionalism and their work at sea.
This study is a contribution to the PEACETIME project (http://peacetime-project.org, last access: 5 May 2021), a joint initiative of the MERMEX and ChArMEx components supported by CNRS-INSU, IFREMER, CEA, and Météo-France as part of the MISTRALS program coordinated by CNRS-INSU. PEACETIME was endorsed as a process study by GEOTRACES and is also a contribution to the IMBER and SOLAS international programs. This work has also received funding from the European Research Council (ERC) under the European Union's Horizon 2020 research and innovation program (Sea2Cloud grant agreement no. 771369).
This paper was edited by Maria Kanakidou and reviewed by two anonymous referees.
Alfarra, M. R., Coe, H., Allan, J. D., Bower, K. N., Boudries, H., Canagaratna, M. R., Jimenez, J. L., Jayne, J. T., Garforth, A., Li, S. M., and Worsnop, D. R.: Characterization of Urban and Rural Organic Aerosols In the Lower Fraser Valley Using Two Aerodyne Particulate Mass Spectrometers, Atmos. Environ., 38, 5745–5758, 2004.
Allan, J. D., Delia, A. E., Coe, H., Bower, K. N., Alfarra, M. R., Jimenez, J. L., Middlebrook, A. M., Drewnick, F., Onasch, T. B., and Canagaratna, M. R.: A generalised method for the extraction of chemically resolved mass spectra from Aerodyne aerosol mass spectrometer data, J. Aerosol Sci., 35, 909–922, https://doi.org/10.1016/j.jaerosci.2004.02.007, 2004.
Bates, T., Quinn, P., Frossard, A., Russell, L., Hakala, J., Petäjä, T., Kulmala, M., Covert, D., Cappa, C., and Li, S.: Measurements of ocean derived aerosol off the coast of California, J. Geophys. Res.-Atmos., 117, D00V15, https://doi.org/10.1029/2012JD017588, 2012.
Bates, T. S., Quinn, P. K., Coffman, D. J., Johnson, J. E., Upchurch, L., Saliba, G., Lewis, S., Graff, J., Russell, L. M., and Behrenfeld, M. J.: Variability in Marine Plankton Ecosystems Are Not Observed in Freshly Emitted Sea Spray Aerosol Over the North Atlantic Ocean, Geophys. Res. Lett., 47, e2019GL085938, https://doi.org/10.1029/2019GL085938, 2020.
Benner, R. and Strom, M.: A critical evaluation of the analytical blank associated with DOC measurements by high temperature catalytic oxidation, Mar. Chem., 41, 153–160, https://doi.org/10.1016/0304-4203(93)90113-3, 1993.
Blanchard, D. C. and Woodcock, A. H.: The production, concentration, and vertical distribution of the sea-salt aerosol, Ann. NY. Acad. Sci., 338, 330–347, https://doi.org/10.1111/j.1749-6632.1980.tb17130.x, 1980.
Boss, E., Picheral, M., Leeuw, T., Chase, A., Karsenti, E., Gorsky, G., Taylor, L., Slade, W., Ras, J., and Claustre, H.: The characteristics of particulate absorption, scattering and attenuation coefficients in the surface ocean, in: Contribution of the Tara Oceans expedition, Meth. Oceanogr., 7, 52–62, https://doi.org/10.1016/j.mio.2013.11.002, 2013.
Bozzetti, C., El Haddad, I., Salameh, D., Daellenbach, K. R., Fermo, P., Gonzalez, R., Minguillón, M. C., Iinuma, Y., Poulain, L., Elser, M., Müller, E., Slowik, J. G., Jaffrezo, J.-L., Baltensperger, U., Marchand, N., and Prévôt, A. S. H.: Organic aerosol source apportionment by offline-AMS over a full year in Marseille, Atmos. Chem. Phys., 17, 8247–8268, https://doi.org/10.5194/acp-17-8247-2017, 2017.
Burrows, S. M., Ogunro, O., Frossard, A. A., Russell, L. M., Rasch, P. J., and Elliott, S. M.: A physically based framework for modeling the organic fractionation of sea spray aerosol from bubble film Langmuir equilibria, Atmos. Chem. Phys., 14, 13601–13629, https://doi.org/10.5194/acp-14-13601-2014, 2014.
Canagaratna, M. R., Jimenez, J. L., Kroll, J. H., Chen, Q., Kessler, S. H., Massoli, P., Hildebrandt Ruiz, L., Fortner, E., Williams, L. R., Wilson, K. R., Surratt, J. D., Donahue, N. M., Jayne, J. T., and Worsnop, D. R.: Elemental ratio measurements of organic compounds using aerosol mass spectrometry: characterization, improved calibration, and implications, Atmos. Chem. Phys., 15, 253–272, https://doi.org/10.5194/acp-15-253-2015, 2015.
Canonaco, F., Crippa, M., Slowik, J. G., Baltensperger, U., and Prévôt, A. S. H.: SoFi, an IGOR-based interface for the efficient use of the generalized multilinear engine (ME-2) for the source apportionment: ME-2 application to aerosol mass spectrometer data, Atmos. Meas. Tech., 6, 3649–3661, https://doi.org/10.5194/amt-6-3649-2013, 2013.
Cetinić, I., Perry, M. J., Briggs, N. T., Kallin, E., D'Asaro, E. A., and Lee, C. M.: Particulate organic carbon and inherent optical properties during 2008 North Atlantic Bloom Experiment, J. Geophys. Res.-Oceans, 117, C06028, https://doi.org/10.1029/2011JC007771, 2012.
Collins, D. B., Bertram, T. H., Sultana, C. M., Lee, C., Axson, J. L., and Prather, K. A.: Phytoplankton blooms weakly influence cloud forming ability of sea spray aerosol, Geophys. Res. Lett., 43, 9975–9983, https://doi.org/10.1002/2016GL069922, 2016.
Cunliffe, M. and Wurl, O.: Guide to best practices to study the ocean's surface? Occasional Publications of the Marine Biological Association of the United Kingdom serie, Marine Biological Association of the United Kingdom for SCOR, Plymouth, UK, 118 pp., http://hdl.handle.net/11329/261 (last access: 1 June 2021), 2014.
Cunliffe, M., Engel, A., Frka, S., Gasparovic, B., Guitart, C., Murrell, C., Salter, M., Stolle, C., Upstill-Goddard, R., and Wurl, O.: Sea surface microlayers: A unified physiochemical and biological perspective of the air-ocean interface, Prog. Oceanogr., 109, 104–116, https://doi.org/10.1016/j.pocean.2012.08.004, 2013.
Dall'Osto, M., Airs, R. L., Beale, R., Cree, C., Fitzsimons, M. F., Beddows, D., Harrison, R. M., Ceburnis, D., O'Dowd, C., and Rinaldi, M.: Simultaneous detection of alkylamines in the surface ocean and atmosphere of the Antarctic sympagic environment, ACS Earth Space Chem., 3, 854–862, https://doi.org/10.1021/acsearthspacechem.9b00028, 2019.
DeCarlo, P. F., Kimmel, J. R., Trimborn, A. M., Jayne, J. T., Aiken, A. C., Gonin, M., Fuhrer,K., Horvath, T., Docherty, K. S., Worsnop, D. R., and Jimenez, J. L.: A field-deployable high-resolution time-of-flight aerosol mass spectrometer, Anal. Chem., 78, 8281–8289, 2006.
Decesari, S., Paglione, M., Rinaldi, M., Dall'Osto, M., Simó, R., Zanca, N., Volpi, F., Facchini, M. C., Hoffmann, T., Götz, S., Kampf, C. J., O'Dowd, C., Ceburnis, D., Ovadnevaite, J., and Tagliavini, E.: Shipborne measurements of Antarctic submicron organic aerosols: an NMR perspective linking multiple sources and bioregions, Atmos. Chem. Phys., 20, 4193–4207, https://doi.org/10.5194/acp-20-4193-2020, 2020.
Drewnick, F., Hings, S. S., Alfarra, M. R., Prevot, A. S. H., and Borrmann, S.: Aerosol quantification with the Aerodyne Aerosol Mass Spectrometer: detection limits and ionizer background effects, Atmos. Meas. Tech., 2, 33–46, https://doi.org/10.5194/amt-2-33-2009, 2009.
Engel, A., Delille, B., Jacquet, S., Riebesell, U., Rochelle-Newall, E., Terbrüggen, A., and Zondervan, I.: Transparent exopolymer particles and dissolved organic carbon production by Emilianiahuxleyi exposed to different CO2 concentrations: a mesocosm experiment, Aquat. Microb. Ecol., 34, 93–104, https://doi.org/10.3354/ame034093, 2004.
Facchini, M. C., Rinaldi, M., Decesari, S., Carbone, C., Finessi, E., Mircea, M., Fuzzi, S., Ceburnis, D., Flanagan, R., and Nilsson, E. D.: Primary submicron marine aerosol dominated by insoluble organic colloids and aggregates, Geophys. Res. Lett., 35, L17814, https://doi.org/10.1029/2008GL034210, 2008.
Fröhlich, R., Cubison, M. J., Slowik, J. G., Bukowiecki, N., Prévôt, A. S. H., Baltensperger, U., Schneider, J., Kimmel, J. R., Gonin, M., Rohner, U., Worsnop, D. R., and Jayne, J. T.: The ToF-ACSM: a portable aerosol chemical speciation monitor with TOFMS detection, Atmos. Meas. Tech., 6, 3225–3241, https://doi.org/10.5194/amt-6-3225-2013, 2013.
Giordano, M. R., Kalnajs, L. E., Avery, A., Goetz, J. D., Davis, S. M., and DeCarlo, P. F.: A missing source of aerosols in Antarctica – beyond long-range transport, phytoplankton, and photochemistry, Atmos. Chem. Phys., 17, 1–20, https://doi.org/10.5194/acp-17-1-2017, 2017.
Guieu, C., D'Ortenzio, F., Dulac, F., Taillandier, V., Doglioli, A., Petrenko, A., Barrillon, S., Mallet, M., Nabat, P., and Desboeufs, K.: Introduction: Process studies at the air–sea interface after atmospheric deposition in the Mediterranean Sea – objectives and strategy of the PEACETIME oceanographic campaign (May–June 2017), Biogeosciences, 17, 5563–5585, https://doi.org/10.5194/bg-17-5563-2020, 2020a.
Guieu, C., Desboeufs, K., Albani, S., Alliouane, S., Aumont, O., Barbieux, M., Barrillon, S., Baudoux, A.-C., Berline, L., Bhairy, N., Bigeard, E., Bloss, M., Bressac, M., Brito, J., Carlotti, F., de Liege, G., Dinasquet, J., Djaoudi, K., Doglioli, A., D'Ortenzio, F., Doussin, J.-F. Duforet, L., Dulac, F., Dutay, J.-C., Engel, A., Feliu-Brito, G., Ferre, H., Formenti, P., Fu, F., Garcia, D., Garel, M., Gazeau, F., Giorio, C., Gregori, G., Grisoni, J.-M., Guasco, S., Guittonneau, J., Haëntjens, N., Heimburger, L.-E., Helias, S., Jacquet, S., Laurent, B., Leblond, N., Lefevre, D., Mallet, M., Marañón, E., Nabat, P., Nicosia, A., Obernosterer, I., Perez Lorenzo, M., Petrenko, A., Pulido-Villena, E., Raimbault, P., Ridame, C., Riffault, V., Rougier, G., Rousselet, L., Roy-Barman, M., Saiz-Lopez, A., Schmechtig, C., Sellegri, K., Siour, G., Taillandier, V., Tamburini, C., Thyssen, M., Tovar-Sanchez, A., Triquet, S., Uitz, J., Van Wambeke, F., Wagener, T., and Zaencker, B.: Biogeochemical dataset collected during the PEACETIME cruise, SEANOE, https://doi.org/10.17882/75747, 2020b.
Harvey, G.: Microlayer collection from the sea surface: a new method and intial results, Limnol. Oceanogr., 11, 608–613, https://doi.org/10.4319/lo.1966.11.4.0608, 1966.
Kuznetsova, M., Lee, C., and Aller, J.: Characterization of the proteinaceous matter in marine aerosols, Mar. Chem., 96, 359–377, https://doi.org/10.1016/j.marchem.2005.03.007, 2005.
Leroux, R., Grégori, G., Leblanc, K., Carlotti, F., Thyssen, M., Dugenne, M., Pujo-Pay, M., Conan, P.,Jouandet, M.-P., Bhairy, N., and Berline, L.: Combining laser diffraction, flow cytometry and optical microscopy to characterize a nanophytoplankton bloom in the northwestern Mediterranean, Prog. Oceanogr., 163, 248–259, https://doi.org/10.1016/j.pocean.2017.10.010, 2017.
Lewis, E. R. and Schwartz, S. E.: Sea Salt Aerosol Production: Mechanisms, Methods, Measurements and Models – a Critical Review, in: Geophys. Monogr. Ser., AGU, Washington, D.C., USA, 413 pp., 2004.
McCluskey, C. S., Hill, T. C., Malfatti, F., Sultana, C. M., Lee, C., Santander, M. V., Beall, C. M., Moore, K. A., Cornwell, G. C., and Collins, D. B.: A dynamic link between ice nucleating particles released in nascent sea spray aerosol and oceanic biological activity during two mesocosm experiments, J. Atmos. Sci., 74, 151–166, https://doi.org/10.1175/JAS-D-16-0087.1, 2017.
MerMex Group: Marine ecosystems' responses to climatic and anthropogenic forcings in the Mediterranean, Prog. Oceanogr., 91, 97–166, https://doi.org/10.1016/j.pocean.2011.02.003, 2011.
Middlebrook, A. M., Bahreini, R., Jimenez, J. L., and Canagaratna, M. R.: Evaluation of composition-dependent collection efficiencies for the aerodyne aerosol mass spectrometer using field data, Aerosol Sci. Tech., 46, 258–271, https://doi.org/10.1080/02786826.2011.620041, 2012.
Ng, N. L., Canagaratna, M. R., Zhang, Q., Jimenez, J. L., Tian, J., Ulbrich, I. M., Kroll, J. H., Docherty, K. S., Chhabra, P. S., Bahreini, R., Murphy, S. M., Seinfeld, J. H., Hildebrandt, L., Donahue, N. M., DeCarlo, P. F., Lanz, V. A., Prévôt, A. S. H., Dinar, E., Rudich, Y., and Worsnop, D. R.: Organic aerosol components observed in Northern Hemispheric datasets from Aerosol Mass Spectrometry, Atmos. Chem. Phys., 10, 4625–4641, https://doi.org/10.5194/acp-10-4625-2010, 2010.
O'Dowd, C., Ceburnis, D., Ovadnevaite, J., Bialek, J., Stengel, D. B., Zacharias, M., Nitschke, U., Connan, S., Rinaldi, M., Fuzzi, S., Decesari, S., Facchini, M. C., Marullo, S., Santoleri, R., Dell'Anno, A., Corinaldesi, C., Tangherlini, M., and Danovaro, R.: Connecting marine productivity to sea-spray via nanoscale biological processes: Phytoplankton Dance or Death Disco?, Sci Rep.-UK, 5, 14883, https://doi.org/10.1038/srep14883, 2015.
O'Dowd, C. D., Facchini, M. C., Cavalli, F., Ceburnis, D., Mircea, M., Decesari, S., Fuzzi, S., Yoon, Y. J., and Putaud, J.-P.: Biogenically driven organic contribution to marine aerosol, Nature, 431, 676–680, https://doi.org/10.1038/nature02959, 2004.
Ovadnevaite, J., O'Dowd, C., Dall'Osto, M., Ceburnis, D., Worsnop, D. R., and Berresheim, H.: Detecting high contributions of primary organic matter to marine aerosol: A case study, Geophys. Res. Lett., 38, L02807, https://doi.org/10.1029/2010GL046083, 2011.
Park, J., Dall'Osto, M., Park, K., Kim, J.-H., Park, J., Park, K.-T., Hwang, C. Y., Jang, G. I., Gim, Y., Kang, S., Park, S., Keun, Y., Yong, J., Jin, K., Yum, S. S., Simó, R., and Yoon, Y. J.: Arctic Primary Aerosol Production Strongly Influenced by Riverine Organic Matter, Environ. Sci. Technol., 53, 8621–8630, https://doi.org/10.1021/acs.est.9b03399, 2019.
Phinney, L., Leaitch, W. R., Lohmann, U., Boudries, H., Worsnop, D. R., Jayne, J. T., Toom-Sauntry, D., Wadleigh, M., Sharma, S., and Shantz, N.: Characterization of the aerosol over the subarctic north east Pacific Ocean, Deep-Sea Res. Pt. II, 53, 2410–2433, https://doi.org/10.1016/j.dsr2.2006.05.044, 2006.
Rinaldi, M., Emblico, L., Decesari, S., Fuzzi, S., Facchini, M. C., and Librando, V.: Chemical characterization and source apportionment of size-segregated aerosol collected at an urban site in Sicily, Water Air Soil Poll., 185, 311–321, https://doi.org/10.1007/s11270-007-9455-4, 2007.
Sandrini, S., van Pinxteren, D., Giulianelli, L., Herrmann, H., Poulain, L., Facchini, M. C., Gilardoni, S., Rinaldi, M., Paglione, M., Turpin, B. J., Pollini, F., Bucci, S., Zanca, N., and Decesari, S.: Size-resolved aerosol composition at an urban and a rural site in the Po Valley in summertime: implications for secondary aerosol formation, Atmos. Chem. Phys., 16, 10879–10897, https://doi.org/10.5194/acp-16-10879-2016, 2016.
Schmale, J., Schneider, J., Nemitz, E., Tang, Y. S., Dragosits, U., Blackall, T. D., Trathan, P. N., Phillips, G. J., Sutton, M., and Braban, C. F.: Sub-Antarctic marine aerosol: dominant contributions from biogenic sources, Atmos. Chem. Phys., 13, 8669–8694, https://doi.org/10.5194/acp-13-8669-2013, 2013.
Schneider, J., Freutel, F., Zorn, S. R., Chen, Q., Farmer, D. K., Jimenez, J. L., Martin, S. T., Artaxo, P., Wiedensohler, A., and Borrmann, S.: Mass-spectrometric identification of primary biological particle markers and application to pristine submicron aerosol measurements in Amazonia, Atmos. Chem. Phys., 11, 11415–11429, https://doi.org/10.5194/acp-11-11415-2011, 2011.
Schwier, A. N., Rose, C., Asmi, E., Ebling, A. M., Landing, W. M., Marro, S., Pedrotti, M.-L., Sallon, A., Iuculano, F., Agusti, S., Tsiola, A., Pitta, P., Louis, J., Guieu, C., Gazeau, F., and Sellegri, K.: Primary marine aerosol emissions from the Mediterranean Sea during pre-bloom and oligotrophic conditions: correlations to seawater chlorophyll a from a mesocosm study, Atmos. Chem. Phys., 15, 7961–7976, https://doi.org/10.5194/acp-15-7961-2015, 2015.
Seinfeld, J. H. and Pandis, S. N.: Atmospheric Chemistry and Physics: From Air Pollution to Climate Change, 3rd Edn., John Wiley & Sons, Inc., Hoboken, New Jersey, USA, 2016.
Sellegri, K., Nicosia, A., Freney, E., Uitz, J., Thyssen, M., Grégori, G., Engel, A., Zäncker, B., Haëntjens, N., Mas, S., Picard, D., Saint-Macary, A., Peltola, M., Rose, C., Trueblood, J., Lefevre, D., D'Anna, B., Desboeufs, K., Meskhidze, N., Guieu, C., and Law, C. S.: Surface ocean microbiota determine cloud precursors, Sci. Rep., 11, 281, https://doi.org/10.1038/s41598-020-78097-5, 2021.
Slade, W. H., Boss, E., Dall'Olmo, G., Langner, M. R., Loftin, J., Behrenfeld, M. J., and Roesler, C.: Underway and moored methods for improving accuracy in measurement of spectral particulate absorption and attenuation, J. Atmos. Ocean. Tech., 27, 1733–1746, https://doi.org/10.1175/2010JTECHO755.1, 2010.
Spielmeyer, A. and Pohnert, G.: Direct quantification of dimethylsulfoniopropionate (DMSP) with hydrophilic interaction liquid chromatography/mass spectrometry, J. Chromatogr. B, 878, 3238–3242, 2010.
Swan, H. B., Deschaseaux, E. S. M., Jones, G. B., and Eyre, B. D.: Quantification of dimethylsulfoniopropionate (DMSP) in Acropora spp. of reef-building coral using mass spectrometry with deuterated internal standard, Anal. Bioanal. Chem., 409, 1929–1942, https://doi.org/10.1007/s00216-016-0141-5, 2017.
Thyssen, M., Grégori, G., Grisoni, J.-M., Pedrotti, M., Mousseau, L., Artigas, L. F., Marro, S., Garcia, N., Passafiume O., and Denis, M. J.: Onset of the spring bloom in the northwestern Mediterranean Sea: influence of environmental pulse events on the in situ hourly-scale dynamics of the phytoplankton community structure, Front. Microbiol., 5, 387, https://doi.org/10.3389/fmicb.2014.00387, 2010.
Timonen, H., Cubison, M., Aurela, M., Brus, D., Lihavainen, H., Hillamo, R., Canagaratna, M., Nekat, B., Weller, R., Worsnop, D., and Saarikoski, S.: Applications and limitations of constrained high-resolution peak fitting on low resolving power mass spectra from the ToF-ACSM, Atmos. Meas. Tech., 9, 3263–3281, https://doi.org/10.5194/amt-9-3263-2016, 2016.
Tobler, A. K., Skiba, A., Wang, D. S., Croteau, P., Styszko, K., Nęcki, J., Baltensperger, U., Slowik, J. G., and Prévôt, A. S. H.: Improved chloride quantification in quadrupole aerosol chemical speciation monitors (Q-ACSMs), Atmos. Meas. Tech., 13, 5293–5301, https://doi.org/10.5194/amt-13-5293-2020, 2020.
Trueblood, J. V., Nicosia, A., Engel, A., Zäncker, B., Rinaldi, M., Freney, E., Thyssen, M., Obernosterer, I., Dinasquet, J., Belosi, F., Tovar-Sánchez, A., Rodriguez-Romero, A., Santachiara, G., Guieu, C., and Sellegri, K.: A two-component parameterization of marine ice-nucleating particles based on seawater biology and sea spray aerosol measurements in the Mediterranean Sea, Atmos. Chem. Phys., 21, 4659–4676, https://doi.org/10.5194/acp-21-4659-2021, 2021.
Vlachou, A., Tobler, A., Lamkaddam, H., Canonaco, F., Daellenbach, K. R., Jaffrezo, J.-L., Minguillón, M. C., Maasikmets, M., Teinemaa, E., Baltensperger, U., El Haddad, I., and Prévôt, A. S. H.: Development of a versatile source apportionment analysis based on positive matrix factorization: a case study of the seasonal variation of organic aerosol sources in Estonia, Atmos. Chem. Phys., 19, 7279–7295, https://doi.org/10.5194/acp-19-7279-2019, 2019.
Wang, X., Sultana, C. M., Trueblood, J., Hill, T. C., Malfatti, F., Lee, C., Laskina, O., Moore, K. A., Beall, C. M., and McCluskey, C. S.: Microbial control of sea spray aerosol composition: a tale of two blooms, ACS Cent. Sci., 1, 124–131, https://doi.org/10.1021/acscentsci.5b00148, 2015.
Wang, X., Deane, G. B., Moore, K. A., Ryder, O. S., Stokes, M. D., Beall, C. M., Collins, D. B., Santander, M. V., Burrows, S. M., Sultana, C. M., and Prather, K. A.: The role of jet and film drops in controlling the mixing state of submicron sea spray aerosol particles, P. Natl. Acad. Sci. USA, 114, 6978–6983, https://doi.org/10.1073/pnas.1702420114, 2017.
Yoon, Y., Ceburnis, D., Cavalli, F., Jourdan, O., Putaud, J., Facchini, M., Decesari, S., Fuzzi, S., Sellegri, K., and Jennings, S.: Seasonal characteristics of the physicochemical properties of North Atlantic marine atmospheric aerosols, J. Geophys. Res.-Atmos., 112, D04206, https://doi.org/10.1029/2005JD007044, 2007.