the Creative Commons Attribution 4.0 License.
the Creative Commons Attribution 4.0 License.
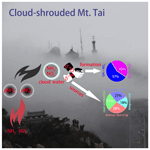
Isotopic constraints on the atmospheric sources and formation of nitrogenous species in clouds influenced by biomass burning
Yunhua Chang
Jiarong Li
Chongguo Tian
Linlin Song
Xiaoyao Zhai
Wenqi Zhang
Tong Huang
Yu-Chi Lin
Chao Zhu
Yunting Fang
Moritz F. Lehmann
Predicting tropospheric cloud formation and subsequent nutrient deposition relies on understanding the sources and processes affecting aerosol constituents of the atmosphere that are preserved in cloud water. However, this challenge is difficult to address quantitatively based on the sole use of bulk chemical properties. Nitrogenous aerosols, mainly ammonium () and nitrate (), play a particularly important role in tropospheric cloud formation. While dry and wet (mainly rainfall) deposition of and are regularly assessed, cloud water deposition is often underappreciated. Here we collected cloud water samples at the summit of Mt. Tai (1545 m above sea level) in eastern China during a long-lasting biomass burning (BB) event and simultaneously measured for the first time the isotopic compositions (mean ±1σ) of cloud water nitrogen species ( = −6.53 ‰ ± 4.96 ‰, = −2.35 ‰ ± 2.00 ‰, = 57.80 ‰ ± 4.23 ‰), allowing insights into their sources and potential transformation mechanism within the clouds. Large contributions of BB to the cloud water (32.9 % ± 4.6 %) and (28.2 % ± 2.7 %) inventories were confirmed through a Bayesian isotopic mixing model, coupled with our newly developed computational quantum chemistry module. Despite an overall reduction in total anthropogenic NOx emission due to effective emission control actions and stricter emission standards for vehicles, the observed cloud values suggest that NOx emissions from transportation may have exceeded emissions from coal combustion. values imply that the reaction of OH with NO2 is the dominant pathway of formation (57 % ± 11 %), yet the contribution of heterogeneous hydrolysis of dinitrogen pentoxide was almost as important (43 % ± 11 %). Although the limited sample set used here results in a relatively large uncertainty with regards to the origin of cloud-associated nitrogen deposition, the high concentrations of inorganic nitrogen imply that clouds represent an important source of nitrogen, especially for nitrogen-limited ecosystems in remote areas. Further simultaneous and long-term sampling of aerosol, rainfall, and cloud water is vital for understanding the anthropogenic influence on nitrogen deposition in the study region.
- Article
(2984 KB) - Full-text XML
- BibTeX
- EndNote
Nitrogenous aerosols, mainly nitrate () and ammonium (), formed from the emissions of nitrogen oxides (NOx = NO + NO2) and ammonia (NH3) and are major chemical components of aerosols, which serve as cloud condensation nuclei (CCN) and thus play an important role during cloud formation in the troposphere (Gioda et al., 2011; van Pinxteren et al., 2016). Cloud water nitrogenous compounds also represent a vital contributor to nitrogen (N) budgets of terrestrial (Li et al., 2016b; Liu et al., 2013; Weathers and Likens, 1997; Vega et al., 2019) and marine ecosystems (Kim et al., 2014; Okin et al., 2011). However, the sources and formation processes of cloud water N species are only poorly understood.
NOx can be emitted from both anthropogenic and natural sources. Globally, over 50 % of the NOx emissions derive from combustion of fossil fuels (∼25 Tg N yr−1; Jaegle et al., 2005; Richter et al., 2005; Duncan et al., 2016) with the remainder being primarily soil-related emissions (∼9 Tg N yr−1; Lamsal et al., 2011; Price et al., 1997; Yienger and Levy, 1995; Miyazaki et al., 2017) or deriving from biomass burning (∼6 Tg N yr−1) and lightning (2–6 Tg N yr−1) (Anenberg et al., 2017; Levy et al., 1996). The atmospheric sinks of NOx include the production of HNO3(g) and the formation of aerosol (Seinfeld and Pandis, 2012), the partitioning of which can vary with time (Morino et al., 2006). As for NH3, over 90 % of the NH3 emissions in terrestrial ecosystems originate from agricultural production, such as livestock breeding and NH3-based fertilizer application (Paulot and Jacobs, 2014; Kang et al., 2016; Reis et al., 2009; Bouwman et al., 1997; Heald et al., 2012; Zhang et al., 2018; Balasubramanian et al., 2015; Huang et al., 2011). In the urban atmosphere, recent studies suggest that nonagricultural activities like wastewater discharge (Zhang et al., 2017), coal burning (Li et al., 2016a), solid waste (Reche et al., 2012), on-road traffic (Suarez-Bertoa et al., 2014), and green spaces (Teng et al., 2017) also contribute to NH3 emissions. In reactions with H2SO4 and HNO3, NH3 contributes to the formation of salts, which typically make up from 20 % to 80 % of fine particles (PM2.5) in the atmosphere (Seinfeld and Pandis, 2012).
Biomass burning (BB) is an important source of N in the atmosphere (Lobert et al., 1990; Souri et al., 2017). During the harvest or hot season of eastern China, agricultural BB frequently occurs and modifies the concentration and composition of aerosols in the atmosphere (Chen et al., 2017; Zhang and Cao, 2015). For example, about 50 % of the N derived from biomass combustion can be released as NH3 and NOx to form particulate and , which then account for over 80 % of total nitrogenous species in BB smoke particles (Crutzen and Andreae, 1990). BB-induced aerosols have not only been associated with poor air quality and the detrimental effects on human health but have also shown to exert manifold effects on tropospheric clouds, altering regional or even global radiation budgets (Chen et al., 2014; Norris et al., 2016; Voigt and Shaw, 2015).
The optical and chemical properties of clouds (and thus their radiative forcing) are directly related to the aerosol and precipitation chemistry (Seinfeld et al., 2016). Moreover, clouds represent reactors of multiphase chemistry, contributing to many chemical transformations that would otherwise not take place or would proceed at much slower rates (Herrmann et al., 2015; Lance et al., 2017; Ravishankara, 1997; Schurman et al., 2018; Slade et al., 2017). Understanding the sources and fate of nitrogenous species in BB-influenced clouds is particularly important to comprehensively assess the environmental impacts of BB. But this challenge is difficult to address based on the sole use of bulk chemical properties (as most often done in previous studies).
Given that 15N can be preserved between the sources and sinks of NOx and NH3, the N isotopic composition of () and () can be related to different sources of NOx and NH3, and thus delivers useful information regarding the partitioning of the origins of atmospheric cloud water NOx and NH3, respectively (Hastings et al., 2013; Michalski et al., 2005; Morin et al., 2008; Chang et al., 2018). This is different for the O isotopes. production involves the oxidation of NO. The first step in the overall process is the conversion of NO into NO2, e.g., through the oxidation by either ozone (O3) or peroxy radicals (Michalski et al., 2011). Significant 18O enrichments and excess 17O (i.e., clear evidence for mass-independent fractionation) are observed in atmospheric collected across the globe (e.g., Michalski et al., 2005; Hastings et al., 2003). Such diagnostic isotope signatures, as well as their variability in space and time, have been linked to the extent of O3 oxidation (Michalski et al., 2011). Put another way, the oxygen isotope composition of () is largely determined by chemical reactions rather than the source, and it is primarily modulated by the O-atom exchange (Michalski et al., 2011) in the atmosphere. Therefore, has the potential to indicate the relative importance of various formation pathways (i.e., oxidation pathways during conversion of nitrogen oxides to NO3) (Alexander et al., 2009; Elliott et al., 2009).
The O isotope fractionation during the conversion of NOx to ( involves two oxidation pathways (Hastings et al., 2003)
where γ/(1-γ) represents the contribution ratio of the isotope fractionation associated with the formation of through the “OH+NO2” pathway ( and the hydrolysis of dinitrogen pentoxide (N2O5) (, respectively. The δ18O value of HNO3 produced by the former process reflects the O atom partitioning of two-thirds O3 and one-third OH (Chang et al., 2018):
As for the δ18O value of HNO3 formed during hydrolysis of N2O5, five-sixths of the O atoms are derived from O3 and one-sixth from OH (Hastings et al., 2003):
where refers to the fraction of NO2 in the total NOx pool. Values for vary between 0.2 and 0.95 (Walters and Michalski, 2015). δ18O-X is the O isotopic composition of X. The range of δ18O-H2O can be approximated using an estimated tropospheric water vapor δ18O range of −25 ‰ to 0 ‰ (Zong et al., 2017). The δ18O of NO2 and N2O5 varies between 90 ‰ and 122 ‰ (Zong et al., 2017). and represent the equilibrium O isotope fractionation factors between NO2 and NO and OH and H2O, respectively, which is temperature dependent:
where A, B, C, and D are experimental constants over the temperature range of 150–450 K. Based on Eqs. (1)–(4) and measured values for of cloud water, a Monte Carlo simulation was performed to generate 10 000 feasible solutions. The error between predicted and measured δ18O was less than 0.5 ‰.
At the end of July 2015, a large-scale BB event occurred over eastern and northern China. We took advantage of this special event to collect cloud water samples at a high-altitude mountaintop site in the North China Plain and to calibrate the isotopic signatures that BB events leave in the N pool of clouds. Integrating cloud water nitrogenous-species isotopic data (, , and ) into a Bayesian isotopic mixing model coupled with a newly developed computational quantum chemistry module (Chang et al., 2018), and using an isotopic mass balance approach, the sources and production pathways of inorganic nitrogen in cloud water were quantified. Although numerous studies have been conducted that involved the chemical characterization of fog water or cloud water, to our knowledge there are no reports on the N (and O) isotopic composition of both and in cloud water.
2.1 Cloud water sample collection
Mt. Tai (36∘18′ N, 117∘13′ E; 1545 m above sea level) is a world-recognized geopark of key natural, historical, and cultural significance, located in the eastern North China Plain (Fig. 1). It belongs to China's most important agricultural and industrial production areas, and the composition of the atmosphere near the mountain can be considered representative with regards to the quality and levels of atmospheric pollution in the region (Li et al., 2017; Liu et al., 2018). Given the opportunistic nature of this study, cloud water sampling commenced at the summit of Mt. Tai 3 d after the fire began (8 January 2015 19:12 LT (local time) to 8 March 2015 06:12 LT; Table 1). In total, six cloud water samples were collected during the long-lasting cloud event using a single-stage Caltech active strand cloud water collector (CASCC), as described by (Demoz et al., 1996). The cloud collector was cleaned prior to each sampling using high-purity deionized water. After sampling, cloud samples were filtered immediately using disposable syringe filters (0.45 µm) to remove any suspended particulate matter and then stored in a freezer at −80 ∘C until further analysis. More details on the monitoring site and sampling procedures can be found elsewhere (Li et al., 2017).
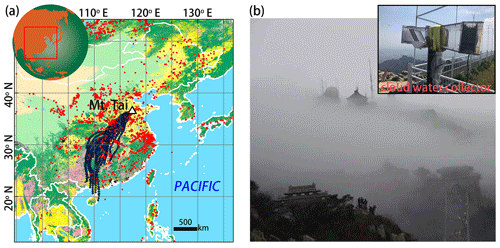
Figure 1(a) Location of Mt. Tai (triangle) and twenty-four 48 h back trajectories (black lines) of air masses simulated by the HYSPLIT model (http://ready.arl.noaa.gov/HYSPLIT.php, last access: 10 August 2019) based on the Global Data Assimilation System (GDAS) meteorological data set arriving at Mt. Tai on 1 August 2015 (04:00 UTC) at an altitude 1500 m a.s.l (close to the altitude of our sampling site). The base map of land use in China was modified from Chang et al. (2018). The red dots represent the positions of wildfires between 29 and 31 July 2015, based on the Moderate Resolution Imaging Spectroradiometer (MODIS) (http://modis-fire.umd.edu, last access: 10 August 2019). (b) Field photos of cloud-shrouded Mt. Tai and the cloud water collector.
2.2 Chemical and isotopic analysis
Inorganic ions (including , , Cl−, , K+, Ca2+, Mg2+, and Na+), as well as levoglucosan (a specific tracer of biomass burning), in cloud water samples were analyzed using a Dionex™ ICS-5000+ system (Thermo Fisher Scientific, Sunnyvale, USA). The IC (ion chromatograph) system was equipped with an automated sampler (AS-DV). Cloud water samples were measured using an IonPac CG12A guard column and a CS12A separation column with an aqueous methanesulfonic acid (MSA, 30 mM L−1) eluent at a flow rate of 1 mL min−1. Detailed information regarding sample processing, pretreatment, chemical analyses, and analytical protocol adaption can be found elsewhere (Cao et al., 2016, 2017). The detection limits for Na+, , K+, Mg2+, Ca2+, Cl−, , , , and levoglucosan are 0.06, 0.03, 0.12, 0.08, 0.13, 0.64, 1.11, 2.67, 1.41, and 1.29 ppb (parts per billion by weight in solution), respectively. The analytical errors from duplicate analyses were within 5 %.
Analysis of the isotopic compositions of () and ( and ) was based on the isotopic analysis of nitrous oxides (N2O) after chemical conversion of the respective target compound. More precisely, dissolved in cloud water samples was oxidized to by alkaline hypobromite (BrO−) and then reduced to N2O by hydroxylamine hydrochloride (NH2OH ⋅ HCl) (Liu et al., 2014). was initially transformed to by cadmium and then further reduced to N2O by sodium azide (NaN3) in an acetic acid buffer (McIlvin and Altabet, 2005; Tu et al., 2016). The produced N2O was analyzed using a purge and cryogenic trap system (Gilson GX-271, Isoprime Ltd., Cheadle Hulme, UK), coupled to an isotope ratio mass spectrometer (PT-IRMS) (Isoprime 100, Isoprime Ltd., Cheadle Hulme, UK). In order to correct for any machine drift and procedural blank contribution, international (IAEA N1, USGS 25, and USGS 26) and (IAEA N3, USGS 32, and USGS 34) standards were processed in the same way as samples. Standard regressions were made based on the known isotopic values of international standards and the measured standard δ15N values. For the NOderived NO analysis, the slope of the plot of the sample versus the standard δ15N (0.49) was very close to the expected slope (0.5), which can be predicted based on the fact that half of the N atoms were derived from the azide (McIlvin and Altabet, 2005). The r2 of the regression line was 0.999. The analytical precision for both multiple N and O isotopic analyses was better than 0.3 ‰ (n=5).
2.3 Bayesian mixing model analysis
By taking the uncertainty associated with the N isotopic signatures of multiple sources and associated isotope fractionation during (trans-)formations into account, the Bayesian method is more appropriate than simple linear mixing modeling to yield estimates on the source partitioning of a mixture like air pollutants (Chang et al., 2016, 2018). The relative contribution of each source in the Bayesian theorem is expressed as
where θ (data|fq) and P(fq) represent the likelihood of the given mixed isotope signature and the predetermined probability of the given state of nature, based on prior information, respectively. The denominator represents the numerical approximation of the marginal probability of the data. Here the Bayesian mixing model MixSIR (stable isotope mixing models using the sampling-importance-resampling method) was used to disentangle the various potential NH3 and NOx sources contributing to the cloud water and pools, respectively, by forming the true probability distributions through generating 10 000 solutions of source apportionment. Details on the model approach can be found in Appendix A.
The measured values of cloud water samples depend on the δ15N signatures of the original NOx sources (δ15N-NOx), the N isotope fractionation between nitrogen oxides (i.e., NO and NO2; Walters et al., 2016), and the N isotope enrichment factor (εN) associated with the kinetic transformation of NOx to HNO3 (Walters and Michalski, 2015). εN is considered a hybrid of two dominant processes: one is the reaction of NO2 and OH radicals to form and the other is the heterogeneous hydrolysis of dinitrogen pentoxide (N2O5) with water to form . We recently developed a quantum chemistry computation module to quantify the N fractionation during nitrate formation, which had been validated by field measurements (Chang et al., 2018). Here this module was adopted to calculate the N isotope fractionation during formation, and in turn to correct the raw values of cloud water samples.
While the N isotopic source signatures of NOx are relatively well constrained (Table B1 in Appendix B), this is not the case for NH3. We recently established a pool of isotopic source signatures of NH3 in eastern China, from which livestock breeding and fertilizer application were identified to produce NH3 with a δ15N of −29.1 ‰ ± 1.7 ‰ and −50.0 ‰ ± 1.8 ‰, respectively (Chang et al., 2016). Although fossil fuel combustion, urban waste, and natural soils also represent potential sources of NH3, their impacts are probably minor compared to those of agricultural and biomass burning emissions, at least on a regional (or greater) scale (Kang et al., 2016). For the N isotope signature of biomass-burning-derived NH3, we assumed 12 ‰ (Kawashima and Kurahashi, 2011), a value that has also been applied in other recent isotope-based source apportionment studies (e.g., Chellman et al., 2016; Wang et al., 2017a).
3.1 Chemical characterization of biomass-burning-influenced clouds
The Moderate Resolution Imaging Spectroradiometer (MODIS) wildfire map (Fig. 1) shows that there were intensive biomass burning events occurring over mainland China, end of July 2015, just before the study period. Moreover, analysis of the back trajectories of air masses at the study site revealed the strong influence of atmospheric transport from regions that also experienced intensive biomass burning events shortly before the sampling campaign. It can thus be assumed that large amounts of BB-related pollutants were transported from the southwest to the sampling site at Mt. Tai. Table 1 compiles sample information and results from the chemical and isotopic analysis of cloud water samples in this study. The concentrations of and ranged from 4.9 to 19.9 mg L−1 (10.1 mg L−1 on average) and from 4.9 to 18.0 mg L−1 (9.1 mg L−1 on average), respectively, much higher than during non-BB seasons (Chen et al., 2017; Desyaterik et al., 2013; Li et al., 2017, 2018; Lin et al., 2017). Similarly, levoglucosan in our cloud water samples varied between 12.1 and 35.1 µg L−1 (19.9 µg L−1 on average), and concentrations were thus 1 order of magnitude higher than those documented during non-BB seasons (Boone et al., 2015; Fomba et al., 2015). Although levoglucosan can be oxidized by OH radicals in the tropospheric aqueous phase (Sang et al., 2016), it is nevertheless a reliable marker compound for BB due to its high-emission factors and relatively high concentrations in the ambient aerosols (Hoffmann et al., 2010). In our study, the concentrations of (r2=0.55) and (r2=0.66) are strongly correlated with that of levoglucosan, suggesting that the pronounced increase in and levels observed here can at least be partly attributed to BB activities during the study period. Globally, BB accounts for around 10 % of NH3 and NOx emissions (Benkovitz et al., 1996; Bouwman et al., 1997; Olivier et al., 1998; Schlesinger and Hartley, 1992).
3.2 Isotopic characterization of biomass-burning-influenced clouds
The N (and O) isotopic composition of cloud water nitrogenous species was more () or less () variable (Table 1), with the average δ15N values of 6.53 ‰ and −2.35 ‰ for and , respectively. The average value was 57.80 ‰. These values are generally different from gas, rainwater, and aerosol values measured worldwide (Fig. 2). Various atmospheric processes can influence the isotopic composition of atmospheric nitrogenous species including the original emission source of NOx, seasonality of oxidation pathways, isotope fractionation during transport, partitioning between wet and dry components, and spatial gradients in atmospheric chemistry (Elliott et al., 2007; Hastings et al., 2003). These aspects may affect the δ15N and δ18O values differentially. For example, the δ15N of atmospheric retains spatial changes in the original NOx signature quite well, in contrast to the δ18O. On the other hand, the δ18O most strongly depends on the oxidation chemistry and formation pathway in the atmosphere (see Eqs. 1–4).
At present, there are no other reports on the isotope ratios of both and in cloud water, and a comparison is possible only with isotopic data from precipitation and aerosol N. Recently, Vega et al. (2019) reported the δ15N (−8 ‰ ± 2 ‰) and δ18O (71 ‰ ± 3 ‰) values of in fog water at a forest site in Sweden. The relatively high δ15N values in our study (−2.35 ‰) suggest more NOx was emitted from combustion processes. In contrast, the much higher δ18O values in Vega et al. (2019) indicate a much greater contribution from O3 in sub-Arctic environments. In Fig. 2a, N isotopic differences for NOx sources are greater (35 ‰) than for δ18O-NOx. In fact, the oxygen isotope signature of NOx is mainly driven by chemistry rather than determined by the source (see discussion below), and thus δ18O measurements cannot be used to address the uncertainty in the NOx sources that may remain when just looking at δ15N values alone. As shown in Fig. 2b, the δ15N values of aerosol are systematically higher than that of NH3. Significant εN during the conversion of gas to aerosol (up to 33 ‰) has been proposed to alter the δ15N values during the transformation of the source (NH3) to the sink (particulate ). Indeed, our compilation of previous results (Fig. 2b) reveals that particulate (particularly in the coarse aerosol fraction) is more enriched in 15N than NH3 (by >23 ‰ on average), as well as in precipitation (by 18 ‰ on average). This can most likely be attributed to the preferential absorption of 14N−NH3 associated with washout processes during precipitation (Zheng et al., 2018). We are aware of the fact that our sample and data used here are limited, resulting in a relatively large uncertainty with regards to the N isotope-based source apportionment. However, all values in cloud water samples fall within the observed range of values for fine particles (PM2.5), providing putative evidence that in cloud water is primarily derived from particulate rather than NH3 absorption.
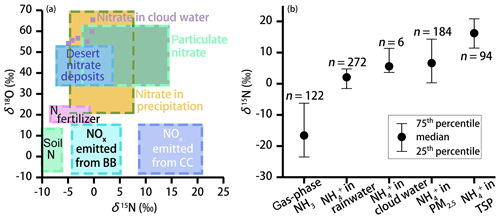
Figure 2(a) Observed range of typical δ18O and δ15N values of and NOx for different sources (adapted from Fenech et al., 2012). BB and CC represent biomass burning and coal combustion, respectively. The light purple squares represent nitrate isotope data in cloud water (this study). (b) The 25th percentiles, median, and 75th percentiles for the δ15N values of the ambient NH3 (Chang et al., 2016; Felix et al., 2013; Savard et al., 2018; Smirnoff et al., 2012) and in precipitation (Fang et al., 2011; Leng et al., 2018; Yang et al., 2014; Zhang et al., 2008), cloud water (this study), PM2.5 (particulate matter with aerodynamic diameter less than 2.5 µm; Lin et al., 2016; Park et al., 2018; Proemse et al., 2012; Smirnoff et al., 2012), and TSPs (total suspended particles with aerodynamic diameter less than 100 µm (Kundu et al., 2010; Savard et al., 2018; Yeatman et al., 2001) are shown.
3.3 Isotope-based assessment of the sources and formation of nitrogenous species in clouds
Using the MixSIR model, the relative contribution of four NH3 sources to can be calculated, based on the isotope data of ambient and considering the N fractionation and prior information on the site. As upper limit for the N isotope enrichment factor associated with the conversion of NH3 to (, we assumed 33 ‰ when using MixSIR but also considered lower values for (Fig. 3a) (given the conflicting evidence with regards to ; e.g., Deng et al., 2018; Li et al., 2012). Dependent of the choice for (between 0 ‰ and 33 ‰ proposed by Heaton et al., 1997) the relative contribution of biomass burning, fertilizer application, and livestock breeding to in cloud water ranges from 25.9 % to 85.4 %, 5.9 % to 37.0 %, and 8.7 % to 85.4 %, respectively. Irrespective of the uncertainty related to , the measurement of levoglucosan provides compelling evidence that biomass burning represents an important NH3 source, independently validating our isotope approach. Our sampling site is located in the North China Plain, also known as the granary of China. Although nonagricultural NH3 emissions like on-road traffic are important in the urban atmosphere (Chang et al., 2016), their contribution must be considered insignificant with respect to fertilizer application and livestock breeding in this region (Kang et al., 2016). Besides, coal-based heating in China is suspended during summertime, and coal combustion has been demonstrated to be a minor contributor of total NH3 emissions (Li et al., 2016a). Hence the partitioning between the three main NH3 sources appears plausible. Moreover, existing emission inventory data confirm that the ratio of NH3 emissions in the North China Plain from livestock breeding (1658 kt) and fertilizer application (1413 kt) was 1.17 (Zhang et al., 2010), which is very close to our estimate (between 0.98 and 1.14) when 25 ‰. For a range that we consider most plausible (i.e., between 25 ‰ and 33 ‰), the relative cloud water source partitioning between biomass burning, fertilizer application, and livestock is 32.9 % ± 4.6 %, 32.9 % ± 3.0 %, and 34.2 % ± 1.6 %, respectively (indicated as red square in Fig. 3a). It is important to note that a large uncertainty existed with regards to the assessment of biomass burning, which can partly be attributed to the lack of localized isotopic source signatures in China. In addition, the isotopic fractionation from the conversion of NH3 to was approximated in this study, and it was not possible to incorporate all of the possible equilibrium and kinetic fractionation scenarios.
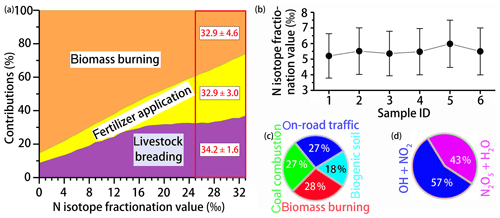
Figure 3(a) Source partitioning estimates for in cloud water as a function of . The red square highlights the best-guess estimates based on ‰. (b) Whisker plot of the N fractionation for the conversation of NOx to ( calculated by the computational quantum chemistry (CQC) module. The upper line, dot, and bottom line indicate the 25th percentile, median, and 75th percentile, respectively. Refer to Table 1 for sample ID. (c) Overall contribution of various NOx sources to in cloud water as estimated by the MixSIR model. (d) Overall contribution of the two dominant pathways to formation in cloud water, as estimated by the MixSIR model.
The computational quantum chemistry (CQC) module in MixSIR has proven to be a robust tool to quantify the N isotope enrichment factor during conversion ( (e.g., Zong et al., 2017; Chang et al., 2018). Cloud water sample-based data from this study reveal that values fall into a small range (5.21 ‰ to 5.98 ‰) (Fig. 3b), suggesting robust isotope effects during the N isotopic exchange reactions. Knowing , the overall contribution of various NOx sources to in cloud water can be estimated (Fig. 3c). As was expected, biomass burning was the largest contributor (28.2 % ± 2.7 %), followed by on-road traffic (27.1 % ± 2.2 %), coal combustion (26.8 % ± 3.4 %), and biogenic soil (17.9 % ± 3.9 %). The fundamental importance of biomass-burning-emitted NOx to in cloud water is supported by the observed correlation between the concentrations of levoglucosan and biomass-burning-derived (r2=0.66). The average contribution ratio of coal combustion and on-road transportation to NOx emissions in our study (0.99) is slightly lower than that calculated from regional emission inventories (9.0 Tg/7.4 Tg = 1.22) (Zhao et al., 2013). The apparent difference is likely real and reflects the fact that NOx emissions by anthropogenic activities changed significantly since 2010: a 17 % total emission decrease between 2010 and 2017 can primarily be attributed to upgraded emission standards and new “ultra-low emission” techniques in the coal-fired power plant sector, and given that NOx emitted from traffic likely increased as a consequence of the continuous expansion of the auto trade market during the last decade (Chang et al., 2018). In turn, our source partitioning estimate probably reflects the most updated status of NOx emissions in China, where transportation-related NOx emissions have reached levels that are comparable to NOx emissions by coal combustion. In this regard, our study demonstrates that Bayesian-based isotopic mixing modeling can be an effective and timely approach to track rapid emission changes in NOx in a fast-developing country like China.
Using the measured δ18O and Eqs. (1)–(4) (and the assumptions above), we can calculate γ and the relative importance of the two oxidation pathways of formation (Fig. 3d). On average, 57 % formation can be attributed to the “NO2 + OH” pathway and 43 % to the “N2O5 + H2O” pathway. In the low-latitude regions, where atmospheric OH concentrations are highest, particulate production via the NO2 + OH pathway predominates (up to 87 %) (Alexander et al., 2009). Sampling during summertime, oxidation of NO2 through OH was expected to be the dominant pathway of nitrate formation, in accordance with observations from the subtropics (Hastings et al., 2003). However, our results highlight that N2O5 hydrolysis can be an almost equally important process as the oxidization of NO2 with OH with regards to the formation in cloud water (Wang et al., 2017b).
In this study, we measured the isotopic composition of nitrogenous species in cloud water at the summit of Mt. Tai during a long-lasting biomass burning event in order to investigate the sources and processes involved in cloud water formation, and in turn to test our isotope-balance approach to constrain N source partitioning in cloud water. Using a Bayesian isotope mixing model, the δ15N-based estimates confirm that at least transiently biomass-burning-related NH3 and NOx emissions are a major source of cloud water N. Moreover, our data are in accordance with regional emission inventories for both NH3 and NOx, validating the Bayesian isotope mixing model approach. Based on cloud water nitrate δ18O measurements, the reaction of NO2 with OH turned out to be the dominant pathway to form cloud nitrate, yet the contribution from the heterogeneous hydrolysis of N2O5 to is almost equally important. Our study underscores the value of cloud-water-dissolved inorganic nitrogen isotopes as carriers of quantitative information on regional NOx emissions. It sheds light on the origin and production pathways of nitrogenous species in clouds and emphasizes the importance of BB-derived nitrogenous species as cloud condensation nuclei in China's troposphere. Moreover, it highlights the rapid evolution of NOx emissions in China. Despite an overall reduction in total anthropogenic NOx emissions due to effective emission control actions and stricter emission standards for vehicles, the relative contribution of transportation to total NOx emissions has increased over the last decade and may already have exceeded emissions from the power sector.
The Bayesian mixing model makes use of stable isotope data to determine the probability distribution of source contributions to a mixture, explicitly accounting for uncertainties associated with multiple sources, their isotopic signatures, and isotope fractionation during transformations. The model has been widely used in ecological studies, such as food-web analyses. In the Bayesian theorem, the contribution of each source is calculated based on mixed data and prior information, such that
where θ(data| fq) and p(fq) refer to the likelihood of the given mixed isotope signature and the predetermined probability of the given state of nature, based on prior information, respectively. The denominator represents the numerical approximation of the marginal probability of the data. In a Bayesian model (stable isotope in R, SIAR), isotope signatures from the mixed data pool are assumed to be normally distributed. Uncertainty in the distribution of isotope sources and associated isotope fractionation during transformations are factored into the model by defining respective mean (μ) and standard deviation (σ) parameters. Prior knowledge about proportional source contributions (fq) is parameterized using the Dirichlet distribution, with an interval of [0, 1]. To assess the likelihood of the given fq, the proposed proportional contribution is combined with a user-specified isotope distribution of sources and their associated isotope effects to develop a proposed isotope distribution for the mixture. The probability of fractional source contributions (fq) is calculated by the Hilborn sampling-importance-resampling method.
All data used to support the conclusion are presented in this paper. Additional data are available upon request; please contact the corresponding authors (Yanlin Zhang (dryanlinzhang@outlook.com) and Jianmin Chen (jmchen@fudan.edu.cn)).
YZ and JC designed the study. YC and YL conceived the study. YC, JL, LS, XZ, WZ, TH and CZ carried out the field experiments and laboratory analysis. YC wrote the paper with ML and YZ. All the authors contributed to the interpretation of the results obtained with the new approach here described and revised the paper content, giving final approval of the version to be submitted. YC and ML addressed the reviewers' comments.
The authors declare that they have no conflict of interest.
This article is part of the special issue “Regional transport and transformation of air pollution in eastern China”. It is not associated with a conference.
This study was supported by the National Key R&D Program of China (grant no. 2017YFC0212704), the National Natural Science Foundation of China (grant nos. 41975166, 41705100, 91644103, 41761144056, 91644103), the Provincial Natural Science Foundation of Jiangsu (grant nos. BK20180040, BK20170946), the University Science Research Project of Jiangsu Province (17KJB170011), the University of Basel research funds, the Joint Open Project of KLME & CIC-FEMD, NUIST (KLME201909), the opening project of Shanghai Key Laboratory of Atmospheric Particle Pollution and Prevention (LAP3), and the special fund of the State Key Joint Laboratory of Environment Simulation and Pollution Control (grant no. 19K01ESPCT).
This paper was edited by Sergey A. Nizkorodov and reviewed by three anonymous referees.
Alexander, B., Hastings, M. G., Allman, D. J., Dachs, J., Thornton, J. A., and Kunasek, S. A.: Quantifying atmospheric nitrate formation pathways based on a global model of the oxygen isotopic composition (Δ17O) of atmospheric nitrate, Atmos. Chem. Phys., 9, 5043–5056, https://doi.org/10.5194/acp-9-5043-2009, 2009.
Anenberg, S. C., Miller, J., Minjares, R., Du, L., Henze, D. K., Lacey, F., Malley, C. S., Emberson, L., Franco, V., Klimont, Z., and Heyes, C.: Impacts and mitigation of excess diesel-related NOx emissions in 11 major vehicle markets, Nature, 545, 467–471, 2017.
Balasubramanian, S., Koloutsou-Vakakis, S., McFarland, D. M., and Rood, M. J.: Reconsidering emissions of ammonia from chemical fertilizer usage in Midwest USA, J. Geophys. Res., 120, 6232–6246, 2015.
Benkovitz, C. M., Scholtz M. T., Pacyna J., Tarrasón L., Dignon J., Voldner E. C., Spiro P. A., Logan J. A., and Graedel T. E.: Global gridded inventories of anthropogenic emissions of sulfur and nitrogen, J. Geophys. Res., 101, 29239–29253, https://doi.org/10.1029/96JD00126, 1996.
Boone, E. J., Laskin, A., Laskin, J., Wirth, C., Shepson, P. B., Stirm, B. H., and Pratt, K. A.: Aqueous processing of atmospheric organic particles in cloud water collected via aircraft sampling, Environ. Sci. Technol., 49, 8523–8530, https://doi.org/10.1021/acs.est.5b01639, 2015.
Bouwman, A., Lee, D., Asman, W., Dentener, F., Van Der Hoek, K., and Olivier, J.: A global high-resolution emission inventory for ammonia, Global Biogeochem. Cy., 11, 561–587, https://doi.org/10.1029/97GB02266, 1997.
Cao, F., Zhang, S. C., Kawamura, K., and Zhang, Y. L.: Inorganic markers, carbonaceous components and stable carbon isotope from biomass burning aerosols in Northeast China, Sci. Total Environ., 572, 1244–1251, https://doi.org/10.1016/j.scitotenv.2015.09.099, 2016, 2016.
Cao, F., Zhang, S. C., Kawamura, K., Liu, X., Yang, C., Xu, Z., Fan, M., Zhang, W., Bao, M., Chang, Y., Song, W., Liu, S., Lee, X., Li, J., Zhang, G., and Zhang, Y. L.: Chemical characteristics of dicarboxylic acids and related organic compounds in PM2.5 during biomass-burning and non-biomass-burning seasons at a rural site of Northeast China, Environ. Pollut., 231, 654–662, https://doi.org/10.1016/j.envpol.2017.08.045, 2017.
Chang, Y. H., Liu, X., Deng, C., Dore, A. J., and Zhuang, G.: Source apportionment of atmospheric ammonia before, during, and after the 2014 APEC summit in Beijing using stable nitrogen isotope signatures, Atmos. Chem. Phys., 16, 11635–11647, https://doi.org/10.5194/acp-16-11635-2016, 2016.
Chang, Y. H., Zhang, Y., Tian, C., Zhang, S., Ma, X., Cao, F., Liu, X., Zhang, W., Kuhn, T., and Lehmann, M. F.: Nitrogen isotope fractionation during gas-to-particle conversion of NOx to in the atmosphere - implications for isotope-based NOx source apportionment, Atmos. Chem. Phys., 18, 11647–11661, https://doi.org/10.5194/acp-18-11647-2018, 2018.
Chellman, N. J., Hastings, M. G., and McConnell, J. R.: Increased nitrate and decreased in the Greenland Arctic after 1940 attributed to North American oil burning, The Cryosphere Discuss., https://doi.org/10.5194/tc-2016-163, 2016.
Chen, J. M., Li, C., Ristovski, Z., Milic, A., Gu, Y., Islam, M. S., Wang, S., Hao, J., Zhang, H., He, C., Guo, H., Fu, H., Miljevic, B., Morawska, L., Thai, P., Lam, Y. F., Pereira, G., Ding, A., Huang, X., and Dumka, U. C.: A review of biomass burning: Emissions and impacts on air quality, health and climate in China, Sci. Total Environ., 579, 1000–1034, https://doi.org/10.1016/j.scitotenv.2016.11.025, 2017.
Chen, Y. C., Christensen, M. W., Stephens, G. L., and Seinfeld, J. H.: Satellite-based estimate of global aerosol-cloud radiative forcing by marine warm clouds, Nat. Geosci., 7, 643, https://doi.org/10.1038/ngeo2214, 2014.
Crutzen, P. J. and Andreae, M. O.: Biomass burning in the tropics: Impact on atmospheric chemistry and biogeochemical cycles, Science, 250, 1669–1678, https://doi.org/10.1126/science.250.4988.1669, 1990.
Demoz, B. B., Collett, J. L., and Daube, B. C.: On the Caltech active strand cloudwater collectors, Atmos. Res., 41, 47–62, https://doi.org/10.1016/0169-8095(95)00044-5, 1996.
Deng, Y., Li, Y., and Li, L.: Experimental investigation of nitrogen isotopic effects associated with ammonia degassing at 0–70 ∘C, Geochim. Cosmochim. Ac., 226, 182–191, https://doi.org/10.1016/j.gca.2018.02.007, 2018.
Desyaterik, Y., Sun, Y., Shen, X., Lee, T., Wang, X., Wang, T., and Collett, J. L.: Speciation of “brown” carbon in cloud water impacted by agricultural biomass burning in eastern China, J. Geophys. Res., 118, 7389–7399, https://doi.org/10.1002/jgrd.50561, 2013.
Duncan, B. N., Lamsal, L. N., Thompson, A. M., Yoshida, Y., Lu, Z., Streets, D. G., Hurwitz, M. M., and Pickering, K. E.: A space-based, high-resolution view of notable changes in urban NOx pollution around the world (2005–2014), J. Geophys. Res., 121, 976–996, 2016.
Elliott, E., Kendall, C., Wankel, S. D., Burns, D., Boyer, E., Harlin, K., Bain, D., and Butler, T.: Nitrogen isotopes as indicators of NOx source contributions to atmospheric nitrate deposition across the Midwestern and northeastern United States, Environ. Sci. Technol., 41, 7661–7667, https://doi.org/10.1021/es070898t, 2007.
Elliott, E. M., Kendall, C., Boyer, E. W., Burns, D. A., Lear, G. G., Golden, H. E., Harlin, K., Bytnerowicz, A., Butler, T. J., and Glatz, R.: Dual nitrate isotopes in dry deposition: Utility for partitioning NOx source contributions to landscape nitrogen deposition, J. Geophys. Res., 114, G04020, https://doi.org/10.1029/2008jg000889, 2009.
Fang, Y. T., Koba, K., Wang, X. M., Wen, D. Z., Li, J., Takebayashi, Y., Liu, X. Y., and Yoh, M.: Anthropogenic imprints on nitrogen and oxygen isotopic composition of precipitation nitrate in a nitrogen-polluted city in southern China, Atmos. Chem. Phys., 11, 1313–1325, https://doi.org/10.5194/acp-11-1313-2011, 2011.
Felix, J. D. and Elliott, E. M.: The agricultural history of human-nitrogen interactions as recorded in ice core , Geophys. Res. Lett., 40, 1642–1646, https://doi.org/10.1002/grl.50209, 2013.
Felix, J. D., Elliott, E. M., and Shaw, S. L.: Nitrogen isotopic composition of coal-fired power plant NOx: Influence of emission controls and implications for global emission inventories, Environ. Sci. Technol., 46, 3528–3535, https://doi.org/10.1021/es203355v, 2012.
Felix, J. D., Elliott, E. M., Gish, T. J., McConnell, L. L., and Shaw, S. L.: Characterizing the isotopic composition of atmospheric ammonia emission sources using passive samplers and a combined oxidation-bacterial denitrifier approach, Rapid Commun. Mass Sp., 27, 2239–2246, https://doi.org/10.1002/rcm.6679, 2013.
Felix, J. D., Elliott, E. M., Avery, G. B., Kieber, R. J., Mead, R. N., Willey, J. D., and Mullaugh, K. M.: Isotopic composition of nitrate in sequential Hurricane Irene precipitation samples: Implications for changing NOx sources, Atmos. Environ., 106, 191–195, https://doi.org/10.1016/j.atmosenv.2015.01.075, 2015.
Fenech, C., Rock, L., Nolan, K., Tobin, J., and Morrissey, A.: The potential for a suite of isotope and chemical markers to differentiate sources of nitrate contamination: a review, Water Res., 46, 2023–2041, https://doi.org/10.1016/j.watres.2012.01.044, 2012.
Fibiger, D. L. and Hastings, M. G.: First measurements of the nitrogen isotopic composition of NOx from biomass burning, Environ. Sci. Technol., 50, 11569–11574, https://doi.org/10.1021/acs.est.6b03510, 2016.
Fomba, K. W., van Pinxteren, D., Müller, K., Iinuma, Y., Lee, T., Collett Jr., J. L., and Herrmann, H.: Trace metal characterization of aerosol particles and cloud water during HCCT 2010, Atmos. Chem. Phys., 15, 8751–8765, https://doi.org/10.5194/acp-15-8751-2015, 2015.
Gioda, A., Reyes-Rodríguez, G. J., Santos-Figueroa, G., Collett, J. L., Decesari, S., Ramos, M. D. C. K. V., Bezerra Netto, H. J. C., de Aquino Neto, F. R., and Mayol-Bracero, O. L.: Speciation of water-soluble inorganic, organic, and total nitrogen in a background marine environment: Cloud water, rainwater, and aerosol particles, J. Geophys. Res., 116, D05203, https://doi.org/10.1029/2010JD015010, 2011.
Hastings, M., Sigman, D. M., and Lipschultz, F.: Isotopic evidence for source changes of nitrate in rain at Bermuda, J. Geophys. Res., 108, 4790, https://doi.org/10.1029/2003JD003789, 2003.
Hastings, M., Jarvis, J., and Steig, E.: Anthropogenic impacts on nitrogen isotopes of ice-core nitrate, Science, 324, 1288, https://doi.org/10.1126/science.1170510, 2009.
Hastings, M., Casciotti, K. L., and Elliott, E. M.: Stable isotopes as tracers of anthropogenic nitrogen sources, deposition, and impacts, Elements, 9, 339–344, https://doi.org/10.2113/gselements.9.5.339, 2013.
Heald, C. L., Collett Jr., J. L., Lee, T., Benedict, K. B., Schwandner, F. M., Li, Y., Clarisse, L., Hurtmans, D. R., Van Damme, M., Clerbaux, C., Coheur, P.-F., Philip, S., Martin, R. V., and Pye, H. O. T.: Atmospheric ammonia and particulate inorganic nitrogen over the United States, Atmos. Chem. Phys., 12, 10295–10312, https://doi.org/10.5194/acp-12-10295-2012, 2012.
Heaton, T. H. E., Spiro, B., and Robertson, S. M. C.: Potential canopy influences on the isotopic composition of nitrogen and sulphur in atmospheric deposition, Oecologia, 109, 600–607, https://doi.org/10.1007/s004420050122, 1997.
Herrmann, H., Schaefer, T., Tilgner, A., Styler, S. A., Weller, C., Teich, M., and Otto, T.: Tropospheric aqueous-phase chemistry: Kinetics, mechanisms, and its coupling to a changing gas phase, Chem. Rev., 115, 4259–4334, https://doi.org/10.1021/cr500447k, 2015.
Hoffmann, D., Tilgner, A., Iinuma, Y., and Herrmann, H.: Atmospheric stability of levoglucosan: A detailed laboratory and modeling study, Environ. Sci. Technol., 44, 694–699, https://doi.org/10.1021/es902476f, 2010.
Huang, C., Chen, C. H., Li, L., Cheng, Z., Wang, H. L., Huang, H. Y., Streets, D. G., Wang, Y. J., Zhang, G. F., and Chen, Y. R.: Emission inventory of anthropogenic air pollutants and VOC species in the Yangtze River Delta region, China, Atmos. Chem. Phys., 11, 4105–4120, https://doi.org/10.5194/acp-11-4105-2011, 2011.
Jaegle, L., Steinberger, L., Martin, R. V., and Chance, K.: Global partitioning of NOx sources using satellite observations: Relative roles of fossil fuel combustion, biomass burning and soil emissions, Faraday Discus., 130, 407–423, 2005.
Kang, Y., Liu, M., Song, Y., Huang, X., Yao, H., Cai, X., Zhang, H., Kang, L., Liu, X., Yan, X., He, H., Zhang, Q., Shao, M., and Zhu, T.: High-resolution ammonia emissions inventories in China from 1980 to 2012, Atmos. Chem. Phys., 16, 2043–2058, https://doi.org/10.5194/acp-16-2043-2016, 2016.
Kawashima, H. and Kurahashi, T.: Inorganic ion and nitrogen isotopic compositions of atmospheric aerosols at Yurihonjo, Japan: Implications for nitrogen sources, Atmos. Environ., 45, 6309–6316, https://doi.org/10.1016/j.atmosenv.2011.08.057, 2011.
Kim, I. N., Lee, K., Gruber, N., Karl, D. M., Bullister, J. L., Yang, S., and Kim, T. W.: Increasing anthropogenic nitrogen in the North Pacific Ocean, Science, 346, 1102–1106, https://doi.org/10.1126/science.1258396, 2014.
Kundu, S., Kawamura, K., and Lee, M.: Seasonal variation of the concentrations of nitrogenous species and their nitrogen isotopic ratios in aerosols at Gosan, Jeju Island: Implications for atmospheric processing and source changes of aerosols, J. Geophys. Res., 115, D20305, https://doi.org/10.1029/2009JD013323, 2010.
Lamsal, L. N., Martin, R. V., Padmanabhan, A., van Donkelaar, A., Zhang, Q., Sioris, C. E., Chance, K., Kurosu, T. P., and Newchurch, M. J.: Application of satellite observations for timely updates to global anthropogenic NOx emission inventories, Geophys. Res. Lett., 38, L05810, https://doi.org/10.1029/2010GL046476, 2011.
Lance, S., Barth, M., and Carlton, A.: Multiphase chemistry: Experimental design for coordinated measurement and modeling studies of cloud processing at a mountaintop, B. Am. Meteorol. Soc., 98, ES163–ES167, https://doi.org/10.1175/bams-d-17-0015.1, 2017.
Leng, Q., Cui, J., Zhou, F., Du, K., Zhang, L., Fu, C., Liu, Y., Wang, H., Shi, G., Gao, M., Yang, F., and He, D.: Wet-only deposition of atmospheric inorganic nitrogen and associated isotopic characteristics in a typical mountain area, southwestern China, Sci. Total Environ., 616, 55–63, https://doi.org/10.1016/j.scitotenv.2017.10.240, 2018.
Levy, H., Moxim, W. J., and Kasibhatla, P. S.: A global three-dimensional time-dependent lightning source of tropospheric NOx, J. Geophys. Res., 101, 22911–22922, 1996.
Li, J., Wang, X., Chen, J., Zhu, C., Li, W., Li, C., Liu, L., Xu, C., Wen, L., Xue, L., Wang, W., Ding, A., and Herrmann, H.: Chemical composition and droplet size distribution of cloud at the summit of Mount Tai, China, Atmos. Chem. Phys., 17, 9885–9896, https://doi.org/10.5194/acp-17-9885-2017, 2017.
Li, L., Lollar, B. S., Li, H., Wortmann, U. G., and Lacrampe-Couloume, G.: Ammonium stability and nitrogen isotope fractionations for NH3(aq)-NH3(gas) systems at 20–70 ∘C and pH of 2-13: Applications to habitability and nitrogen cycling in low-temperature hydrothermal systems, Geochim. Cosmochim. Ac., 84, 280–296, https://doi.org/10.1016/j.gca.2012.01.040, 2012.
Li, Q., Jiang, J., Cai, S., Zhou, W., Wang, S., Duan, L., and Hao, J.: Gaseous ammonia emissions from coal and biomass combustion in household stoves with different combustion efficiencies, Environ. Sci. Technol. Lett., 3, 98–103, https://doi.org/10.1021/acs.estlett.6b00013, 2016a.
Li, T., Wang, Y., Mao, H., Wang, S., Talbot, R. W., Zhou, Y., Wang, Z., Nie, X., and Qie, G.: Insights on chemistry of mercury species in clouds over Northern China: Complexation and adsorption, Environ. Sci. Technol., 52, 5125–5134, https://doi.org/10.1021/acs.est.7b06669, 2018.
Li, Y., Schichtel, B. A., Walker, J. T., Schwede, D. B., Chen, X., Lehmann, C. M. B., Puchalski, M. A., Gay, D. A., and Collett, J. L.: Increasing importance of deposition of reduced nitrogen in the United States, P. Natl. Acad. Sci. USA, 113, 5874–5879, https://doi.org/10.1073/pnas.1525736113, 2016b.
Lin, C. T., Jickells, T. D., Baker, A. R., Marca, A., and Johnson, M. T.: Aerosol isotopic ammonium signatures over the remote Atlantic Ocean, Atmos. Environ., 133, 165–169, https://doi.org/10.1016/j.atmosenv.2016.03.020, 2016.
Lin, Q., Zhang, G., Peng, L., Bi, X., Wang, X., Brechtel, F. J., Li, M., Chen, D., Peng, P., Sheng, G., and Zhou, Z.: In situ chemical composition measurement of individual cloud residue particles at a mountain site, southern China, Atmos. Chem. Phys., 17, 8473–8488, https://doi.org/10.5194/acp-17-8473-2017, 2017.
Liu, D., Fang, Y., Tu, Y., and Pan, Y.: Chemical method for nitrogen isotopic analysis of ammonium at natural abundance, Anal. Chem., 86, 3787–3792, https://doi.org/10.1021/ac403756u, 2014.
Liu, X., Zhang, Y., Han, W., Tang, A., Shen, J., Cui, Z., Vitousek, P., Erisman, J. W., Goulding, K., Christie, P., Fangmeier, A., and Zhang, F.: Enhanced nitrogen deposition over China, Nature, 494, 459–462, https://doi.org/10.1038/nature11917, 2013.
Liu, L., Zhang, J., Xu, L., Yuan, Q., Huang, D., Chen, J., Shi, Z., Sun, Y., Fu, P., Wang, Z., Zhang, D., and Li, W.: Cloud scavenging of anthropogenic refractory particles at a mountain site in North China, Atmos. Chem. Phys., 18, 14681–14693, https://doi.org/10.5194/acp-18-14681-2018, 2018.
Lobert, J. M., Scharffe, D. H., Hao, W. M., and Crutzen, P. J.: Importance of biomass burning in the atmospheric budgets of nitrogen-containing gases. Nature, 346, 552, https://doi.org/10.1038/346552a0, 1990.
McIlvin, M. R. and Altabet, M. A.: Chemical conversion of nitrate and nitrite to nitrous oxide for nitrogen and oxygen isotopic analysis in freshwater and seawater, Anal. Chem., 77, 5589–5595, https://doi.org/10.1021/ac050528s, 2005.
Michalski, G., Bockheim, J. G., Kendall, C., and Thiemens, M.: Isotopic composition of Antarctic Dry Valley nitrate: Implications for NOy sources and cycling in Antarctica, Geophys. Res. Lett., 32, L13817, https://doi.org/10.1029/2004GL022121, 2005.
Michalski, G., Bhattacharya, S., and Mase, F.: Oxygen isotope dynamics of atmospheric nitrate and its precursor molecules (Chapter 30), in: Handbook of Environmental Isotope Geochemistry, edited by: Baskaran, M., Advances in Isotope Geochemistry, https://doi.org/10.1007/978-3-642-10637-8_30, Springer-Verlag, Berlin Heidelberg, 2011.
Miyazaki, K., Eskes, H., Sudo, K., Boersma, K. F., Bowman, K., and Kanaya, Y.: Decadal changes in global surface NOx emissions from multi-constituent satellite data assimilation, Atmos. Chem. Phys., 17, 807–837, https://doi.org/10.5194/acp-17-807-2017, 2017.
Morin, S., Savarino, J., Frey, M. M., Yan, N., Bekki, S., Bottenheim, J. W., and Martins, J. M.: Tracing the origin and fate of NOx in the Arctic atmosphere using stable isotopes in nitrate. Science, 322, 730–732, https://doi.org/10.1126/science.1161910, 2008.
Morino, Y., Kondo, Y., Takegawa, N., Miyazaki, Y., Kita, K., Komazaki, Y., Fukuda, M., Miyakawa, T., Moteki, N., and Worsnop, D. R.: Partitioning of HNO3 and particulate nitrate over Tokyo: Effect of vertical mixing, J. Geophys. Res., 111, D15215, https://doi.org/10.1029/2005JD006887, 2006.
Norris, J. R., Allen, R. J., Evan, A. T., Zelinka, M. D., O'Dell, C. W., and Klein, S. A.: Evidence for climate change in the satellite cloud record. Nature, 536, 72, https://doi.org/10.1038/nature18273, 2016.
Okin, G. S., Baker, A. R., Tegen, I., Mahowald, N. M., Dentener, F. J., Duce, R. A., Galloway, J. N., Hunter, K., Kanakidou, M., Kubilay, N., Prospero, J. M., Sarin, M., Surapipith, V., Uematsu, M., and Zhu, T.: Impacts of atmospheric nutrient deposition on marine productivity: Roles of nitrogen, phosphorus, and iron. Global Biogeochem. Cy., 25, GB2022, https://doi.org/10.1029/2010GB003858, 2011.
Olivier, J., Bouwman, A., Van der Hoek, K., and Berdowski, J.: Global air emission inventories for anthropogenic sources of NOx, NH3 and N2O in 1990, Environ. Pollut., 102, 135–148, https://doi.org/10.1016/B978-0-08-043201-4.50024-1, 1998.
Park, Y., Park, K., Kim, H., Yu, S., Noh, S., Kim, M., Kim, J., Ahn, J., Lee, M., Seok, K., and Kim, Y.: Characterizing isotopic compositions of TC-C, -N, and -N in PM2.5 in South Korea: Impact of China's winter heating, Environ. Pollut., 233, 735–744, https://doi.org/10.1016/j.envpol.2017.10.072, 2018.
Paulot, F. and Jacob, D. J.: Hidden cost of US agricultural exports: particulate matter from ammonia emissions, Environ. Sci. Technol., 48, 903–908, 2014.
Price, C., Penner, J., and Prather, M.: NOx from lightning: 1. Global distribution based on lightning physics, J. Geophys. Res., 102, 5929–5941, 1997.
Proemse, B. C., Mayer, B., Chow, J. C., and Watson, J. G.: Isotopic characterization of nitrate, ammonium and sulfate in stack PM2.5 emissions in the Athabasca Oil Sands Region, Alberta, Canada, Atmos. Environ., 60, 555–563, https://doi.org/10.1016/j.atmosenv.2012.06.046, 2012.
Ravishankara, A. R.: Heterogeneous and multiphase chemistry in the troposphere, Science, 276, 1058–1065, https://doi.org/10.1126/science.276.5315.1058, 1997.
Reche, C., Viana, M., Pandolfi, M., Alastuey, A., Moreno, T., Amato, F., Ripoll, A., and Querol, X.: Urban NH3 levels and sources in a Mediterranean environment, Atmos. Environ., 57, 153–164, 2012.
Reis, S., Pinder, R. W., Zhang, M., Lijie, G., and Sutton, M. A.: Reactive nitrogen in atmospheric emission inventories, Atmos. Chem. Phys., 9, 7657–7677, https://doi.org/10.5194/acp-9-7657-2009, 2009.
Richter, A., Burrows, J. P., Nuss, H., Granier, C., and Niemeier, U.: Increase in tropospheric nitrogen dioxide over China observed from space, Nature, 437, 129–132, 2005.
Sang, X. F., Gensch, I., Kammer, B., Khan, A., Kleist, E., Laumer, W., Schlag, P., Schmitt, S. H., Wildt, J., Zhao, R., Mungall, E. L., Abbatt, J. P. D., and Kiendler-Scharr, A.: Chemical stability of levoglucosan: an isotopic perspective, Geophys. Res. Lett., 43, 5419–5424, https://doi.org/10.1002/2016GL069179, 2016.
Savard, M. M., Cole, A. S., Vet, R., and Smirnoff, A.: The Δ17O and δ18O values of atmospheric nitrates simultaneously collected downwind of anthropogenic sources – implications for polluted air masses, Atmos. Chem. Phys., 18, 10373–10389, https://doi.org/10.5194/acp-18-10373-2018, 2018.
Schlesinger, W. and Hartley, A.: A global budget for atmospheric NH3, Biogeochem., 15, 191–211, https://doi.org/10.1007/BF00002936, 1992.
Schurman, M. I., Boris, A., Desyaterik, Y., and Collett, J. L.: Aqueous secondary organic aerosol formation in ambient cloud water photo-oxidations, Aerosol Air Qual. Res., 18, 15–25, https://doi.org/10.4209/aaqr.2017.01.0029, 2018.
Seinfeld, J. and Pandis, S. N.: Atmospheric Chemistry and Physics: From air pollution to climate change, John Wiley and Sons, 2012.
Seinfeld, J. H., Bretherton, C., Carslaw, K. S., Coe, H., DeMott, P. J., Dunlea, E. J., Feingold, G., Ghan, S., Guenther, A. B., Kahn, R., Kraucunas, I., Kreidenweis, S. M., Molina, M. J., Nenes, A., Penner, J. E., Prather, K. A., Ramanathan, V., Ramaswamy, V., Rasch, P. J., Ravishankara, A. R., Rosenfeld, D., Stephens, G., and Wood, R.: Improving our fundamental understanding of the role of aerosol-cloud interactions in the climate system, P. Natl. Acad. Sci. USA, 113, 5781–5790, https://doi.org/10.1073/pnas.1514043113, 2016.
Slade, J. H., Shiraiwa, M., Arangio, A., Su, H., Pöschl, U., Wang, J., and Knopf, D. A.: Cloud droplet activation through oxidation of organic aerosol influenced by temperature and particle phase state, Geophys. Res. Lett., 44, 1583–1591, https://doi.org/10.1002/2016GL072424, 2017.
Smirnoff, A., Savard, M. M., Vet, R., and Simard, M. C.: Nitrogen and triple oxygen isotopes in near-road air samples using chemical conversion and thermal decomposition, Rapid Commun. Mass Sp., 26, 2791–2804, https://doi.org/10.1002/rcm.6406, 2012.
Souri, A. H., Choi, Y., Jeon, W., Kochanski, A. K., Diao, L., Mandel, J., Bhave, P. V., and Pan, S.: Quantifying the impact of biomass burning emissions on major inorganic aerosols and their precursors in the U.S, J. Geophys. Res., 122, 12, https://doi.org/10.1002/2017JD026788, 2017.
Suarez-Bertoa, R., Zardini, A. A., and Astorga, C.: Ammonia exhaust emissions from spark ignition vehicles over the New European Driving Cycle, Atmos. Environ., 97, 43–53, 2014.
Teng, X., Hu, Q., Zhang, L., Qi, J., Shi, J., Xie, H., Gao, H., and Yao, X.: Identification of major sources of atmospheric NH3 in an urban environment in Northern China during wintertime, Environ. Sci. Technol., 51, 6839–6848, 2017.
Tu, Y., Fang, Y., Liu, D., and Pan, Y.: Modifications to the azide method for nitrate isotope analysis, Rapid Commun. Mass Sp., 30, 1213–1222, https://doi.org/10.1002/rcm.7551, 2016.
van Pinxteren, D., Fomba, K. W., Mertes, S., Müller, K., Spindler, G., Schneider, J., Lee, T., Collett, J. L., and Herrmann, H.: Cloud water composition during HCCT-2010: Scavenging efficiencies, solute concentrations, and droplet size dependence of inorganic ions and dissolved organic carbon, Atmos. Chem. Phys., 16, 3185–3205, https://doi.org/10.5194/acp-16-3185-2016, 2016.
Vega, C., Mårtensson, M., Wideqvist, U., Kaiser, J., Zieger, P., and StrÖm, J.: Composition, isotopic fingerprint and source attribution of nitrate deposition from rain and fog at a Sub-Arctic Mountain site in Central Sweden (Mt Åreskutan), Tellus B, 71, 1445379, https://doi.org/10.1080/16000889.2018.1559398, 2019.
Voigt, A. and Shaw, T. A.: Circulation response to warming shaped by radiative changes of clouds and water vapour, Nat. Geosci., 8, 102–106, https://doi.org/10.1038/ngeo2345, 2015.
Walters, W. W. and Michalski, G.: Theoretical calculation of nitrogen isotope equilibrium exchange fractionation factors for various NOy molecules, Geochim. Cosmochim. Ac., 164, 284–297, https://doi.org/10.1016/j.gca.2015.05.029, 2015.
Walters, W. W., Simonini, D. S., and Michalski, G.: Nitrogen isotope exchange between NO and NO2 and its implications for δ15N variations in tropospheric NOx and atmospheric nitrate, Geophys. Res. Lett., 43, 440–448, https://doi.org/10.1002/2015gl066438, 2016.
Wang, Y. L., Liu, X. Y., Song, W., Yang, W., Han, B., Dou, X. Y., Zhao, X. D., Song, Z. L., Liu, C. Q., and Bai, Z. P.: Source appointment of nitrogen in PM2.5 based on bulk δ15N signatures and a Bayesian isotope mixing model, Tellus B, 69, 1299672, https://doi.org/10.1080/16000889.2017.1299672, 2017a.
Wang, Z., Wang, W., Tham, Y. J., Li, Q., Wang, H., Wen, L., Wang, X., and Wang, T.: Fast heterogeneous N2O5 uptake and ClNO2 production in power plant and industrial plumes observed in the nocturnal residual layer over the North China Plain, Atmos. Chem. Phys., 17, 12361–12378, https://doi.org/10.5194/acp-17-12361-2017, 2017b.
Weathers, K. C. and Likens, G. E.: Clouds in Southern Chile: An important source of nitrogen to nitrogen-limited ecosystems?, Environ. Sci. Technol., 31, 210–213, https://doi.org/10.1021/es9603416, 1997.
Yang, J.-Y. T., Hsu, S.-C., Dai, M. H., Hsiao, S. S.-Y., and Kao, S.-J.: Isotopic composition of water-soluble nitrate in bulk atmospheric deposition at Dongsha Island: sources and implications of external N supply to the northern South China Sea, Biogeosciences, 11, 1833–1846, https://doi.org/10.5194/bg-11-1833-2014, 2014.
Yeatman, S., Spokes, L., Dennis, P., and Jickells, T.: Comparisons of aerosol nitrogen isotopic composition at two polluted coastal sites, Atmos. Environ., 35, 1307–1320, https://doi.org/10.1016/S1352-2310(00)00408-8, 2001.
Yienger, J. J. and Levy, H.: Empirical model of global soil-biogenic NOx emissions, J. Geophys. Res., 100, 11447–11464, 1995.
Zhang, C., Geng, X., Wang, H., Zhou, L., and Wang, B.: Emission factor for atmospheric ammonia from a typical municipal wastewater treatment plant in South China, Environ. Pollut., 220, 963–970, 2017.
Zhang, L., Chen, Y., Zhao, Y., Henze, D. K., Zhu, L., Song, Y., Paulot, F., Liu, X., Pan, Y., Lin, Y., and Huang, B.: Agricultural ammonia emissions in China: reconciling bottom-up and top-down estimates, Atmos. Chem. Phys., 18, 339–355, https://doi.org/10.5194/acp-18-339-2018, 2018.
Zhang, Y. L., and Cao, F.: Is it time to tackle PM2.5 air pollutions in China from biomass-burning emissions?, Environ. Pollut., 202, 217–219, https://doi.org/10.1016/j.envpol.2015.02.005, 2015.
Zhang, Y., Zheng, L. X., Liu, X. J., Jickells, T., Cape, J. N., Goulding, K., Fangmeier, A., and Zhang, F. S.: Evidence for organic N deposition and its anthropogenic sources in China, Atmos. Environ., 42, 1035–1041, https://doi.org/10.1016/j.atmosenv.2007.12.015, 2008.
Zhang, Y., Dore, A. J., Ma, L., Liu, X. J., Ma, W. Q., Cape, J. N., and Zhang, F. S.: Agricultural ammonia emissions inventory and spatial distribution in the North China Plain, Environ. Pollut., 158, 490–501, https://doi.org/10.1016/j.atmosenv.2007.12.015, 2010.
Zhao, Y., Zhang, J., and Nielsen, C. P.: The effects of recent control policies on trends in emissions of anthropogenic atmospheric pollutants and CO2 in China, Atmos. Chem. Phys., 13, 487–508, https://doi.org/10.5194/acp-13-487-2013, 2013.
Zheng, X. D., Liu, X. Y., Song, W., Sun, X. C., and Liu, C. Q.: Nitrogen isotope variations of ammonium across rain events: Implications for different scavenging between ammonia and particulate ammonium, Environ. Pollut., 239, 392–398, https://doi.org/10.1016/j.envpol.2018.04.015, 2018.
Zong, Z., Wang, X., Tian, C., Chen, Y., Fang, Y., Zhang, F., Li, C., Sun, J., Li, J., and Zhang, G.: First assessment of NOx sources at a regional background site in North China using isotopic analysis linked with modeling, Environ. Sci. Technol., 51, 5923–5931, https://doi.org/10.1021/acs.est.6b06316, 2017.
- Abstract
- Introduction
- Materials and methods
- Results and discussion
- Conclusions
- Appendix A: Bayesian isotopic mixing model
- Appendix B: Isotopic signatures of NOx emitted from various sources
- Data availability
- Author contributions
- Competing interests
- Special issue statement
- Financial support
- Review statement
- References
- Abstract
- Introduction
- Materials and methods
- Results and discussion
- Conclusions
- Appendix A: Bayesian isotopic mixing model
- Appendix B: Isotopic signatures of NOx emitted from various sources
- Data availability
- Author contributions
- Competing interests
- Special issue statement
- Financial support
- Review statement
- References