the Creative Commons Attribution 4.0 License.
the Creative Commons Attribution 4.0 License.
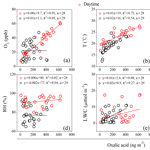
Molecular distribution and stable carbon isotopic compositions of dicarboxylic acids and related SOA from biogenic sources in the summertime atmosphere of Mt. Tai in the North China Plain
Jingjing Meng
Gehui Wang
Zhanfang Hou
Xiaodi Liu
Benjie Wei
Can Wu
Cong Cao
Jiayuan Wang
Jianjun Li
Junji Cao
Erxun Zhang
Jie Dong
Jiazhen Liu
Shuangshuang Ge
Yuning Xie
Molecular distributions and stable carbon isotopic (δ13C values) compositions of dicarboxylic acids and related secondary organic aerosols (SOA) in PM2.5 aerosols collected on a day/night basis at the summit of Mt. Tai (1534 m a.s.l.) in the summer of 2016 were analyzed to investigate the sources and photochemical aging process of organic aerosols in the forested highland region of the North China Plain. The molecular distributions of dicarboxylic acids and related SOA are characterized by the dominance of oxalic acid (C2), followed by malonic (C3), succinic (C4) and azelaic (C9) acids. The concentration ratios of C2 ∕ C4, diacid-C ∕ OC and C2 ∕ total diacids are larger in the daytime than in the nighttime, suggesting that the daytime aerosols are more photochemically aged than those in the nighttime due to the higher temperature and stronger solar radiation. Both ratios of C2 ∕ C4 (R2>0.5) and C3 ∕ C4 (R2>0.5) correlated strongly with the ambient temperatures, indicating that SOA in the mountaintop atmosphere are mainly derived from the photochemical oxidation of local emissions rather than long-range transport. The mass ratios of azelaic acid to adipic acid (C9 ∕ C6), azelaic acid to phthalic aid (C9 ∕ Ph) and glyoxal to methylglyoxal (Gly ∕ mGly) and the strong linear correlations of major dicarboxylic acids and related SOA (i.e., C2, C3, C4, ωC2, Pyr, Gly and mGly) with biogenic precursors (SOA tracers derived from isoprene, α/β-pinene and β-caryophyllene) further suggest that aerosols in this region are mainly originated from biogenic sources (i.e., tree emissions).
C2 concentrations correlated well with aerosol pH, indicating that particle acidity favors the organic acid formation. The stable carbon isotopic compositions (δ13C) of the dicarboxylic acids are higher in the daytime than in the nighttime, with the highest value ( ‰) found for C2 and the lowest value ( ‰) found for C9. An increase in δ13C values of C2 along with increases in C2 ∕ Gly and C2 ∕ mGly ratios was observed, largely due to the isotopic fractionation effect during the precursor oxidation process.
- Article
(2025 KB) - Full-text XML
-
Supplement
(124 KB) - BibTeX
- EndNote
Secondary organic aerosols (SOA) account for a substantial fraction (20 %–90 %) of the total PM2.5 mass in the troposphere, of which up to 80 % are water-soluble (Hallquist et al., 2009; Kroll and Seinfeld, 2008). Due to the low vapor pressures and high hygroscopicity (approximately less than 10−7 Pa) (Bilde et al., 2015; Ehn et al., 2014), dicarboxylic acids and related compounds are ubiquitously found in atmospheric water and particles (Kawamura and Bikkina, 2016; Sorooshian et al., 2007a). Because of the water-soluble and hygroscopic properties, dicarboxylic acids and related compounds play important roles in atmospheric aqueous chemistry and influence radiative forcing of aerosols by acting as cloud condensation nuclei (CCN) (Hoque et al., 2017; Wang et al., 2012, 2016; Zhang et al., 2016).
Although they can be emitted directly from sources such as incomplete combustion of fossil fuels (Kawamura and Kaplan, 1987) and biomass burning (Kawamura et al., 2013a, b; Narukawa et al., 1999), atmospheric dicarboxylic acids and related compounds are largely produced by photochemical oxidation of unsaturated fatty acids, PAHs (Kawamura et al., 1996), cyclic alkanes and other compounds (Kawamura and Usukura, 1993). Oxalic acid (C2) is the smallest and the most abundant dicarboxylic acid (Wang et al., 2009, 2015). Modeling studies and cloud measurements have suggested that C2 is largely produced from aqueous-phase oxidation of less oxygenated organic precursors such as glyoxal (Gly), methyglyoxal (mGly) and pyruvic acid (Pyr) in clouds or wet aerosols and the photochemical breakdown of longer chain dicarboxylic acids (Wang et al., 2012, 2015).
There is a growing consensus on highlighting the significance of oxalic acid and related SOA formation from the photochemical oxidation of anthropogenic/biogenic volatile organic compounds (VOCs) via the aqueous phase in clouds or aqueous aerosol from many field observations and laboratory experiments as well as modeling studies (Bikkina et al., 2017; Cheng et al., 2017; Ervens et al., 2014; Fu et al., 2008; Lim et al., 2005; Miyazaki et al., 2009; Mochizuki et al., 2017). A ubiquitous layer of dicarboxylic acids was found above the clouds by aircraft measurements in the USA, indicating that organic acids are important CCN in the free troposphere (Sorooshian et al., 2007a, b). Compared to the aerosols in lowland areas, alpine aerosols have a more significant influence on cloud formation because they are more accessible to clouds due to the higher elevation. Mt. Tai is an isolated peak located in the center of the North China Plain, one of the regions with the worst air pollution in the world (Wang et al., 2009; Yang et al., 2017). A few studies have been conducted to investigate the molecular compositions, sources and formation mechanisms of SOA including dicarboxylic acids at Mt. Tai, but most of them were performed in May and June and mainly focused on the impact of anthropogenic activities such as field burning of wheat straw (Kawamura et al., 2013a, b; Zhu et al., 2018), while very little information on dicarboxylic acids and related SOA at Mt. Tai during the typical summertime season (i.e., July and August) is available when the emission of biogenic volatile organic compounds (BVOCs) is dominant. A 3-D model simulation shows that about 79 % of oxalic acid in the global atmosphere is originated from the oxidation of natural vegetation emissions (Myriokefalitakis et al., 2011), suggesting the dominant contribution of BVOCs to the global SOA loading. Therefore, it is necessary to investigate the abundances, compositions and formation mechanisms of oxalic acid and related SOA when vegetation emission is dominant, especially in the forested highland region where aerosols are more accessible to clouds due to higher elevation.
Compound-specific stable carbon isotope analysis is a powerful tool to provide important information of the sources and atmospheric processing of organic aerosols because the isotopic fractionation of carbon occurs upon chemical reactions or phase transfer (Pavuluri and Kawamura, 2016; Zhang et al., 2016). Analyses of stable carbon isotope ratios (δ13C) of dicarboxylic acids and related SOA can be effectively applied to assess the photochemical aging level and relative contributions of primary emissions to aerosols in the atmosphere (Zhao et al., 2018). To the best of our knowledge, characteristics of the stable carbon isotopic compositions of dicarboxylic acids and related SOA in mountainous regions have not been investigated before. The current work will, for the first time, report the stable carbon isotopic compositions of dicarboxylic acids and related compounds in a mountainous area, which are very helpful for improving our understanding on the sources, formation mechanisms and atmospheric behavior of SOA. In this study, we first investigated the diurnal variations in molecular distributions and stable carbon isotopic compositions of dicarboxylic acids and related compounds. Then we discussed the impact of temperature (T), relative humidity (RH), particle acidity (pHIS), liquid water content (LWC) and O3 concentration on oxalic acid and related SOA to explore their sources and formation mechanisms in the forested highland region of the North China Plain.
2.1 Aerosol sampling
PM2.5 samples were collected at the Meteorological Observation Station of Mt. Tai, which is located at the summit of Mt. Tai (36.25∘ N, 117.10∘ E; 1534 m a.s.l.) in the North China Plain (Fig. 1). The sampling site was located about 1 km to the north of the peak, with an altitude of 1465 m a.s.l., and there is little anthropogenic emission nearby. About 80 % of the mountainous land is covered by vegetation known to comprise 989 species, which is densely wooded in summer (Fu et al., 2010). PM2.5 samples were collected from 20 July to 20 August 2016, each lasting 12 h on a day/night basis, using a mid-volume air sampler (KC-120H, Qingdao Laoshan Company, China) equipped with prebaked (450 ∘C, 8 h) quartz fiber filters (Whatman, USA) at an airflow rate of 100 L min−1. The daytime samples were collected from 08:00 to 20:00, while nighttime samples were collected from 20:00 to 08:00 of the next day. Field blank samples were also collected by mounting the blank filter onto the sampler for 15 min without sucking any air before and after the campaign. A total of 57 samples (daytime: 28; nighttime: 29) were collected during the campaign. After sampling, each filter was sealed in an aluminum foil bag and stored at −20 ∘C prior to laboratory analyses. Moreover, the concentration of ozone was simultaneously monitored at the site by a UV absorption analyzer (Model 49C, Thermo Electron Corporation).
2.2 Chemical analyses
2.2.1 Sample extraction, derivatization, gas chromatograph–mass spectrometer (GC/MS) and gas chromatograph–flame ionization detector (GC/FID) quantification
Dicarboxylic acids, ketocarboxylic acids and α-dicarbonyls in PM2.5 were determined using the method described by previous studies (Kawamura et al., 1996; Meng et al., 2013, 2014). Briefly, one half of the filter was cut into pieces and extracted with pure Milli-Q water under ultrasonication three times each for 15 min. The water extracts were concentrated to near dryness and then reacted with 14 % BF3∕n-butanol at 100 ∘C for 1 h to form butyl esters/dibutoxy acetals. After derivatization, n-hexane was added and washed with pure water three times. Finally, the hexane layer was concentrated to 200 µL and determined using a capillary gas chromatograph (Agilent GC 7890A) coupled with a split/splitless injector. The GC was equipped with a flame ionization detector (FID) and a fused silica capillary column (HP-5, 0.2 mm × 25 m, film thickness 0.5 µm). The GC oven temperature was programmed from 50 (2 min) to 120 ∘C at 15 ∘C min−1, and then to 300 at 5 ∘C min−1 with a final isothermal hold at 300 ∘C for 16 min. Peak identification was performed by comparing the GC retention time with that of authentic standards and confirmed by mass spectrum of the sample using a GC–MS. Recoveries of the target compounds were 80 % for oxalic acid and 85 % to 110 % for other species. The target compounds in the field blank samples were lower than 4 % of those in the ambient samples. Data presented here were corrected for both field blanks and recoveries.
The analysis method of biogenic precursors has been reported elsewhere (Li et al., 2013). Briefly, one-quarter of the filter was cut and extracted with a mixture of dichloromethane and methanol (2 : 1, v∕v) under ultrasonication. The extracts were concentrated using a rotary evaporator under vacuum conditions and then dried using pure nitrogen. After reaction with a mixture of N,O-bis-(trimethylsilyl) trifluoroacetamide (BSTFA) and pyridine (5 : 1, v∕v) at 70 ∘C for 3 h, biogenic secondary organic aerosol (BSOA) tracers in the derivatized samples were determined using a GC–MS. These data were used in this study to explore the biogenic sources of dicarboxylic acids and related SOA.
2.2.2 Stable carbon isotope composition of dicarboxylic acids and related SOA
The stable carbon isotopic compositions (δ13C) of shorter chain dicarboxylic acids and related SOA were measured using the method developed by Kawamura and Watanabe (2004). Briefly, the δ13C values of the derivatized samples above were determined by gas chromatography–isotope ratio mass spectrometry (GC-IR-MS; Thermo Fisher, Delta V Advantage). The δ13C values were then calculated for free organic acids using an isotopic mass balance equation based on the measured δ13C values of derivatives and the derivatizing agent (BF3 ∕ n-butanol) (Kawamura and Watanabe, 2004). To ensure the analytical error of the δ13C values less than 0.2 ‰, each sample was measured three times. The δ13C data reported here are averaged values of the triplicate measurements.
2.2.3 Elemental carbon (EC), organic carbon (OC), inorganic ions, water-soluble organic carbon (WSOC), aerosol liquid water content (LWC) and in situ particle pH (pHIS)
Briefly, EC and OC in the PM2.5 samples were determined by using a DRI Model 2001 Carbon Analyzer following the Interagency Monitoring of Protected Visual Environments (IMPROVE) thermal/optical reflectance (TOR) protocol (Chow et al., 2004). As for the measurement of inorganic ions and WSOC, an aliquot of the sample filters was extracted with 30 mL Milli-Q water using an ultrasonic bath three times each for 15 min, and filtered through PTFE filters to remove insoluble particles and filter debris. The water extract was then separated into two parts. One part was analyzed for inorganic ions using an ion chromatograph (Dionex 600, Dionex, USA), and the other part of the water extract was used to determine WSOC using a Total Carbon Analyzer (TOC-L CPH, Shimadzu, Japan). As for the calculation of aerosol liquid water content (LWC) and in situ particle pH (pHIS), the Aerosol Inorganic Model (AIM), using a system (AIM-II), was employed (Li et al., 2013).
3.1 General description of chemical components at Mt. Tai
The concentrations of dicarboxylic acids and related SOA, EC, OC, WSOC and inorganic ions in PM2.5 samples from Mt. Tai are summarized in Table 1. During the campaign, the height of the boundary layer at Mt. Tai was frequently reduced to ∼600 m at night, which kept the sampling site in the free troposphere at night. In contrast, the boundary layer extended far above the mountaintop during the daytime (Zhu et al., 2018). However, as a tracer of the combustion source, EC concentration is very low and shows a similar level in the day and night periods, suggesting that the impact of anthropogenic emissions from the lowland region on the mountaintop atmosphere is insignificant. As seen in Tables 1 and 2, the day and night data presented a large variability. To verify if the day and night aerosol chemistry is of significant difference, Student's t test was performed for the day and night samples. As shown in Table S1, the concentrations and compositions of major species in PM2.5 between day and night show a p value less than 0.005, which clearly demonstrates that the abundances and compositions of the major species during the day and night are statistically different.
Table 2Concentrations (ng m−3) of dicarboxylic acids, ketocarboxylic acids and α-dicarbonyls of PM2.5 from Mt. Tai in the summer.
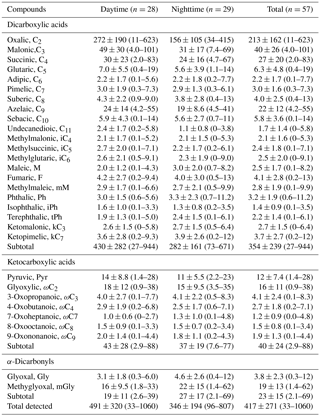
OC and WSOC in the PM2.5 samples in the daytime are similar to those in the nighttime (Table 1), but OC ∕ EC and WSOC ∕ OC ratios are around 1.4 times higher in the daytime than in the nighttime (Fig. 4), indicating an enhanced SOA production due to the stronger photochemical oxidation in the daytime rather than the changes in the planetary boundary layer heights (Hegde and Kawamura, 2012).
is mainly produced from aqueous-phase oxidation of SO2, which is favored by higher temperature and humid conditions (Kundu et al., 2010; Zhang et al., 2015; Wang et al., 2018). Thus, the concentration and relative abundance of are higher in the daytime than in the nighttime (Table 1 and Fig. S1). Conversely, the concentrations of and present higher values in the nighttime compared to those in the daytime (Table 1). Particulate is mainly formed via gas-phase oxidation of NO2 with OH radicals and subsequent partitioning into the aerosol phase with ammonia to form NH4NO3 (Pathak et al., 2009; Zhang et al., 2015). NH4NO3 is volatile and thus a lower temperature at night is favorable for NH4NO3 enriching in the aerosol phase (Bian et al., 2014), resulting in NH4NO3 being more abundant at night during the Mt. Tai observation period. As shown in Table 1, the remaining four kinds of cations (K+, Na+, Ca2+ and Mg2+), which can be regarded as the key markers of primary sources, did not exhibit significant diurnal variations, again suggesting that the effect of planetary boundary layer heights is minor. In this work, LWC and pHIS were calculated by using the AIM-II model because both species cannot be directly measured. LWC exhibits higher concentration (94±100 µg m−3) in the daytime than that (75±69 µg m−3) in the nighttime (Table 1). Aerosol LWC is controlled by the ambient relative humidity (Clegg et al., 1998) and the concentrations of inorganic salt (Fountoukis and Nenes, 2007). As shown in Table 1, the total concentration (21.7±11.5 µg m−3) of , and during the daytime was almost equivalent to that (20.4±8.2 µg m−3) during the nighttime, but the relative humidity (92±5.0 %) in the daytime was higher than that in the nighttime (77±8.2 %). Therefore, the concentration of LWC in the daytime was higher than that in the nighttime. In contrast, pHIS shows a lower value () in the daytime compared to that (0.4±0.6) in the nighttime (Table 1), indicating the daytime aerosols are more acidic.
3.2 Molecular distributions of dicarboxylic acids and related SOA
A homologous series of dicarboxylic acids (C2−C11), ketocarboxylic acids (ωC2–ωC9 and pyruvic acid) and α-dicarbonyls (glyoxal and methylglyoxal) in PM2.5 samples of Mt. Tai was determined (Table 2). The molecular compositions of these compounds are illustrated in Fig. 2.
Total dicarboxylic acids are 430±282 ng m−3 (27–944 ng m−3; Table 2) in the daytime, around 2 times higher than those in the nighttime (282±161 ng m−3, 73–671 ng m−3). The average concentration levels (354±239 ng m−3) are lower than those in Asian lowlands (e.g., 14 Chinese cities (892±457 ng m−3) (Ho et al., 2007), Chennai in India (502.9±117.9 ng m−3) (Pavuluri et al., 2010)) and the elevated regions (e.g., Mt. Hua in central China (744±340 ng m−3) (Meng et al., 2014) and the central Himalayas in Nainital, India (430 ng m−3) (Hegde and Kawamura, 2012)), but higher than those in the continental background areas such as Qinghai Lake in the Tibetan Plateau (231±119 ng m−3) (Meng et al., 2013) and marine regions such as the North Pacific (68 ng m−3) (Hoque et al., 2017) and the western North Pacific (99.2±86.4 ng m−3) (Boreddy et al., 2017).
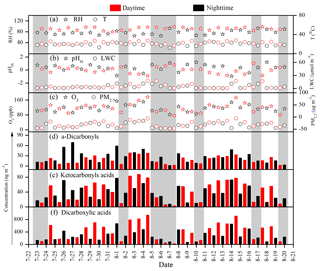
Figure 3Diurnal variations of relative humidity (RH), temperature (T), in situ acidity of particles (pHIS), liquid water content of particles (LWC), concentrations of O3, PM2.5, α-dicarbonyls, ketocarboxylic acids and dicarboxylic acids (rainy days are highlighted by the grey shading).
Interestingly, we found that the levels of dicarboxylic acids are equivalent to those at Mt. Fuji in Japan in the day and the night (day: 424 ng m−3; night: 266 ng m−3) (Mochizuki et al., 2017), which are dominantly derived from the oxidation of biogenic VOCs such as isoprene and α-pinene in summer (Mochizuki et al., 2017). Both mountains are located at the similar latitude in East Asia, and the altitudes of the sampling sites at Mt. Tai and Mt. Fuji are almost the same. In addition, both Mt. Tai and Mt. Fuji are dominated by broad-leaved forest. Thus, one may expect that the emissions of biogenic VOCs at both sites during the same season are similar. Moreover, the O3 level during the observation period at Mt. Tai is also similar to that at Mt. Fuji, Japan, ranging from a few ppb at night to about 60 ppb (Mochizuki et al., 2017) in the noontime, which means that photochemical activity at both sites during the campaigns is similar. Therefore, concentrations of dicarboxylic acids are comparable at both sites with a similar diurnal pattern.
At the Mt. Tai site, the concentrations of dicarboxylic acids in the daytime were about 2 times higher than in the nighttime, which can be ascribed to the stronger photochemical production of dicarboxylic acids and/or higher emissions of the precursors in the daytime. As shown in Fig. 2, oxalic acid (C2) is the dominant species at Mt. Tai, followed by malonic acid (C3), succinic acid (C4) and azelaic acid (C9) during the day and night, respectively. These four species account for 60, 12 %, 7.2 % and 6.9 % of the total dicarboxylic acids in the daytime and 53, 11 %, 8.5 % and 7.6 % of the total in the nighttime, respectively. The molecular compositions at Mt. Tai are similar to those in other remote areas such as Mt. Fuji, Japan, and Mt. Hua and Qinghai Lake, China, in the summer (Meng et al., 2013, 2014; Mochizuki et al., 2017), but different from that in Asian urban regions where phthalic and/or terephthalic acids are more abundant than C9 because of higher emissions of anthropogenic precursors (e.g., aromatics and plasticizers) (Cheng et al., 2015; He et al., 2014; Jung et al., 2010; Wang et al., 2002, 2017).
Ketocarboxylic acids in the atmosphere are the major intermediates of aqueous-phase photochemical oxidation producing dicarboxylic acids (Kawamura and Ikushima, 1993; Pavuluri and Kawamura, 2016). The concentrations of ketocarboxylic acids are 43±28 ng m−3 in the daytime and 37±19 ng m−3 in the nighttime, respectively, with glyoxylic acid (ωC2) being the dominant ω-oxoacid, followed by pyruvic acid (Pyr) and 3-oxobutanoic acid (ωC3) (Table 2 and Fig. 2). Previous studies have proposed that ωC2 can be initially formed from the photochemical oxidation of glyoxal with OH radicals and other oxidants in the aqueous phase and then further oxidized into oxalic acid (Wang et al., 2012; Rapf et al., 2017). In contrast to the diurnal variations of dicarboxylic and ketocarboxylic acids, the concentrations of α-dicarbonyls exhibit higher concentrations in the nighttime than in the daytime (Fig. 2). Because α-dicarbonyls in the aerosol phase are produced by the gas-phase photooxidation of isoprene and other VOCs and the subsequent partitioning into the aerosol aqueous phase (Carlton et al., 2006, 2007), the higher concentrations of α-dicarbonyls at night can in part be attributed to the nighttime lower temperatures, which are favorable for the partitioning of gaseous glyoxal and methylglyoxal into the aerosol phase. Since α-dicarbonyls are the major precursors of oxalic acid (Fu et al., 2008; Wang et al., 2012; Warneck, 2003), the opposite pattern suggests that the aerosol aqueous-phase oxidation in the daytime is more significant in comparison with that in the nighttime. The concentrations of glyoxal (Gly) are less than methylglyoxal (mGly), largely because of the stronger biogenic sources and the lower oxidation rate of mGly with OH radicals in the aerosol phase compared to Gly (Cheng et al., 2013; Meng et al., 2013) (see discussions in Sect. 3.3).
Temporal variations in concentrations of total dicarboxylic acids, ketocarboxylic acids and α-dicarbonyls are summarized in Fig. 3, along with the meteorological parameters. During the whole sampling periods, the concentrations of total dicarboxylic acids and related SOA fluctuated significantly, with a maximum (1060 ng m−3) on 4 August and a minimum (33 ng m−3) on 7 August. Our results showed that the levels of water-soluble organic compounds decreased by 30 %–80 % when it was rainy, suggesting that dicarboxylic acids and related SOA can be removed efficiently by wet deposition because these water-soluble compounds are not only easily washed out but also can be efficiently removed by serving as cloud condensation nuclei (CCN) during the wet deposition (Leaitch et al., 1996). Moreover, a reduced secondary formation due to weaker solar radiation and a reduced biogenic emission during the rainy days are also responsible for the lowest concentrations of dicarboxylic acid and related SOA.
3.3 Biogenic versus anthropogenic and local versus long-range transport sources
Previous studies have proposed that the hydroxylation of C4 can be further oxidized into C2 and C3, and C3 can also be oxidized into C2 through intermediate compounds such as hydroxymalonic acid or ketomalonic acid (Hoque et al., 2017; Kawamura and Usukura, 1993; Kunwar et al., 2017). Therefore, both ratios of C2 ∕ C4 and C3 ∕ C4 can be regarded as indicators of photochemical aging of organic aerosols. The C2 ∕ C4 and C3 ∕ C4 ratios in the mountainous atmosphere are 8.0±2.7 and 1.6±0.6, respectively, higher than those in aerosols freshly emitted from sources such as vehicle exhausts (C2 ∕ C4: 4.1; C3 ∕ C4: 0.35) (Kawamura and Kaplan, 1987) and biomass burning plumes (C2 ∕ C4: 5.0; C3 ∕ C4: 0.7) (Kundu et al., 2010), but lower than photochemically aged aerosols in remote regions such as a continental background site in the Tibetan Plateau (C2 ∕ C4: 11±7.2; C3 ∕ C4: 2.2±1.3) (Meng et al., 2013) and the North and South Pacific (C2 ∕ C4: 8.7; C3 ∕ C4: 3.0) (Hoque et al., 2017). Compared with those in the nighttime, the higher ratios of C2 ∕ C4 and C3 ∕ C4 (Fig. 4) in the daytime again indicated that the photochemical modification of aerosols is stronger. Decomposition of C4 and/or C3 into C2 is one of the major formation pathways of oxalic acid, which is favored by temperature (Kawamura and Ikushima, 1993). A few studies have reported that when local sources are dominant over long-range transport, both ratios of C2 ∕ C4 and C3 ∕ C4 correlate strongly with the ambient temperatures (Kawamura and Ikushima, 1993; Meng et al., 2013; Pavuluri et al., 2010). Temperature measured at the sampling site is a meteorological parameter, which only reflects the local meteorological conditions rather than the upwind conditions. Therefore, a significant correlation between C2 ∕ C4 and temperature can only be observed when SOA are largely derived from local precursor oxidation rather than from long-range transport. In the current work, the ratios of C2 ∕ C4 (R2>0.5) (Fig. 5a) and C3 ∕ C4 (R2≥0.5) (Fig. 5b) correlated well with the ambient temperatures in both the daytime and the nighttime, clearly suggesting that dicarboxylic acids and related SOA at Mt. Tai during the campaign are mostly derived from the local oxidation of BVOCs rather than long-range transport. Aggarwal et al. (2008) found that diacid-C ∕ OC and C2 ∕ total diacids should increase in the daytime when local emission and photooxidation are more significant than long-range transport. At the summit of Mt. Tai, the daytime ratios of diacid-C ∕ OC and C2 ∕ total diacids are 5.5 %±2.6 % and 60 %±7.7 %, which are about 1.2 and 1.3 times higher than those in the nighttime, respectively (Fig. 4), further indicating the stronger photochemical oxidation in the daytime and the dominance of local sources for SOA production in the atmosphere of Mt. Tai.
Table 3Concentrations of α-dicarbonyls in PM2.5 from Mt. Tai and Mt. Hua in China and the global budgets of atmospheric Gly and mGly.
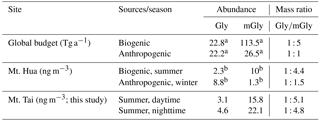
a Data are calculated from Fu et al. (2008). b Data are cited from Meng et al. (2014).
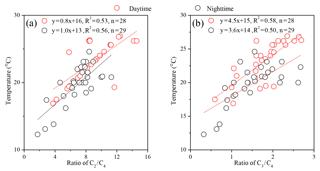
Figure 5Linear fit regression for temperature (T) with mass ratios of (a) C2 ∕ C4 and (b) C3 ∕ C4 (see the abbreviations in Table 1).
Both ratios of C9 ∕ C6 and C9 ∕ Ph can be used as indicators to qualitatively evaluate the source strength of anthropogenic versus biogenic precursors for producing dicarboxylic acids and related SOA (Jung et al., 2010) because C6 and Ph are largely produced by the oxidation of anthropogenic cyclohexene (Hatakeyama et al., 1987) and aromatic hydrocarbons such as naphthalene (Kawamura and Ikushima, 1993), respectively. In contrast, C9 is mainly produced by the oxidation of biogenic unsaturated oleic acid, which contains a double bond at the C-9 position (Wang et al., 2010). Therefore, both ratios of C9 ∕ C6 and C9 ∕ Ph are indicative of the source strengths of biogenic versus anthropogenic emissions. As shown in Fig. 4, both ratios of C9 ∕ C6 and C9 ∕ Ph are similar in the daytime to those in the nighttime. However, the average values of C9 ∕ C6 (14±9.0) and C9 ∕ Ph (7.2±2.2) at the mountaintop of Mt. Tai are higher than those in urban regions such as Xi'an, China (C9 ∕ C6: 3.1; C9 ∕ Ph: 5.6) (Cheng et al., 2013), and also higher than those in other mountainous areas during summer such as the central Himalayas, India (C9 ∕ C6: 2.1; C9 ∕ Ph: 0.2) (Hegde and Kawamura, 2012), and Mt. Fuji, Japan (C9 ∕ C6: 3.1) (Mochizuki et al., 2017), indicating the important contribution of biogenic sources to SOA in the Mt. Tai region. Model simulation (Fu et al., 2008) and field observations (Meng et al., 2014) have suggested that the concentration ratio of particulate Gly∕mGly is about 1 : 5 when biogenic sources are predominant and is about 1 : 1 when anthropogenic sources are predominant such as in urban areas. As shown in Table 3, the ratios of Gly∕mGly in the Mt. Tai atmosphere are 1 : 5.1 in the daytime and 1 : 4.8 in the nighttime, further suggesting that Gly and mGly in the Mt. Tai samples are mostly derived from biogenic sources. This result is also in agreement with the high abundance of C9 relative to the total dicarboxylic acids (7.2 %), which is about 2 times higher than that (3.5 %) in 14 Chinese megacities (Ho et al., 2007). Moreover, a trace amount of elemental carbon (EC) was found for most of the samples (Table 1), suggesting that the impact of pollutants derived from anthropogenic sources on the mountaintop atmosphere during the campaign is negligible. Consequently, it can be concluded that the summertime SOA of Mt. Tai are mainly derived from the local photochemical oxidation of biogenic precursors rather than the long-range transport of anthropogenic precursors during the sampling period.
3.4 Production of dicarboxylic acids and related SOA from biogenic sources
A 3-D modeling study has proposed that 79 % of oxalic acid is originated from the photochemical oxidation of isoprene and other biogenic hydrocarbons in cloud (Myriokefalitakis et al., 2011). Laboratory experiments and model simulations have demonstrated that the photooxidation of isoprene (Carlton et al., 2006, 2007; Huang et al., 2011) and monoterpenes (Fick et al., 2003; Lee et al., 2006) can produce Gly and mGly via reactions with OH radicals and/or O3 in the aerosol aqueous phase or the gas phase and subsequently partition into cloud droplets, where both carbonyls are oxidized further by OH radicals to form oxalic acid (Lim et al., 2005; Tan et al., 2010).
In order to further ascertain the contribution of BVOCs to dicarboxylic acids and related SOA during the high biological activity period at Mt. Tai, SOA tracers derived from isoprene, α-∕β-pinene and β-caryophyllene in the PM2.5 samples collected at the Mt. Tai site were determined. Their total concentrations (the sum of isoprene + α-∕β-pinene + β-caryophyllene-derived SOA tracers) are 1.3 times higher in the daytime (106±56 ng m−3) than those in the nighttime (79±38 ng m−3) (unpublished data), which is consistent with the diurnal variation patterns of dicarboxylic acids, ketocarboxylic acids and WSOC (Tables 1 and 2). Previous studies reported that 2-methylglyceric acid, which is an isoprene oxidation product, and 3-hydroxyglutaric acid, which is α-/β-pinene oxidation product, can serve as organic precursors for the production of dicarboxylic acids and ketocarboxylic acids (Fu et al., 2013). As shown in Table 4, major dicarboxylic acids and related SOA (e.g., C2, ωC2, Gly and mGly) correlated positively with the isoprene, α/β-pinene and β-caryophyllene oxidation products during the day and night (R>0.55, P<0.01) (Table 4), respectively, indicating that BVOCs' oxidation products can serve as precursors for the production of oxalic acid via α-dicarbonyls' oxidation (Myriokefalitakis et al., 2011). These strong correlations further highlight the important contribution of BSOA to dicarboxylic acids and related SOA at Mt. Tai in the summer season.
3.5 Effects of temperature, relative humidity and O3 concentrations on the formation of oxalic acid and related SOA
Because oxidants such as OH radicals were not measured at Mt. Tai, O3 is considered here as an indicator of the total oxidant concentrations in this study. A significant linear correlation of oxalic acid with O3 concentrations was observed for the daytime samples (R2=0.91), but no correlation (R2=0.05) was found for the nighttime samples (Fig. 6a). Such a phenomenon was also observed at Mt. Fuji, Japan (Mochizuki et al., 2017), and Beijing, China (He et al., 2014). Additionally, C2 ∕ Gly, C2 ∕ mGly and C2 ∕ total diacids ratios correlate positively with O3 concentrations in the daytime, but such correlations were not found in the nighttime (Fig. 8a–c). Mochizuki et al. (2017) have reported a robust correlation between concentration ratios of oxalic acid to isoprene plus α-pinene (oxalic acid ∕ (isoprene + α-pinene)) and O3 concentrations in a large forest region of Mt. Fuji, Japan, in the daytime. In the current work, BSOA tracers correlate strongly with O3 concentrations in the daytime (R>0.6, P<0.01), but no correlation was found at night (Table 4). These results suggest that the daytime oxalic acid and related SOA in the mountaintop of Mt. Tai are largely derived from O3 and OH radical oxidation of BVOCs such as isoprene and α-pinene, while the nighttime oxalic acid and related SOA might be mostly produced by NO3 radicals and other oxidizing agents such as H2O2 (Claeys et al., 2004; Herrmann et al., 1999). In addition, the titration of O3 by the residual NO in the nighttime atmosphere could also be responsible for the lack of correlation between BSOA tracers and O3 (Edwards et al., 2017).
As shown in Table 4, nearly all of the detected BSOA tracers including 2-methylglyceric acid, 3-hydroxyglutaric acid and β-caryophyllinic acid exhibit a strong correlation with the ambient temperature, largely due to the increased production of BSOA from enhanced emissions of BVOCs under higher temperature conditions. The BSOA tracer concentrations are higher in the daytime than in the nighttime. As seen in Table 1, O3 concentration during the sampling period is 50 % higher in the daytime than in the nighttime, clearly indicating that oxidation potential in the daytime at the Mt. Tai site is stronger. Moreover, isoprene is only emitted by trees during daytime. Thus, we think the higher loadings of BSOA tracers in the daytime are caused not only by stronger photochemical oxidation but also by enhanced emissions of BVOCs. In addition, oxalic acid and C2 ∕ total diacids ratios correlated strongly with temperatures (Figs. 6b and 8f) because higher temperature conditions can promote photochemical formation of oxalic acid. Such a temperature dependence is also observed in other regions such as Mt. Hua (Meng et al., 2014) and Beijing (Wang et al., 2017) in China.
Table 5Stable carbon isotopic compositions (δ13C, ‰) of major dicarboxylic acids and related SOA in PM2.5 of Mt. Tai in the North China Plain.
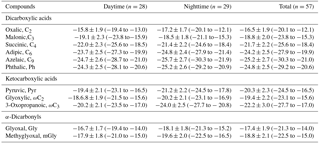
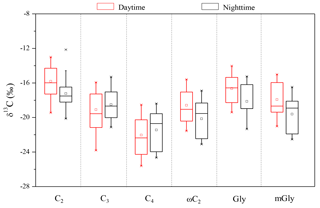
Figure 7Diurnal variations of stable carbon isotope composition of low molecular weight dicarboxylic acids (C2−C4), the smallest ketocarboxylic acids (ωC2) and α-dicarbonyls (Gly, mGly) in PM2.5 collected at the summit of Mt. Tai during the summer.
Online measurements, field observations and chamber studies (Cheng et al., 2017; Gao et al., 2004; McNeill, 2015; Meng et al., 2014; Wang et al., 2012, 2017) have suggested that oxalic acid is primarily derived from the acid-catalyzed heterogeneous oxidation of glyoxal and related precursors in the aqueous phase. Here we investigate the impact of LWC and pHIS on the formation of oxalic acid in Mt. Tai aerosols. Because the sampling site is far from agricultural sources, most of the sulfate is assumed to be acidic. As shown in Fig. 6c, a strong linear correlation between C2 and was found for the daytime (R2=0.89) and nighttime (R2=0.76) samples, respectively, which is consistent with the measurements observed in other mountainous regions (Meng et al., 2014) and Chinese cities (Wang et al., 2012, 2017; Yu et al., 2005), indicating that oxalic acid and sulfate are formed via a similar formation pathway such as in-cloud or aqueous-phase (Warneck, 2003). In this study, oxalic acid does not exhibit correlations with relative humidity (RH) and LWC (Fig. 6d and e), but presents a significant negative correlation with pHIS (R2>0.60) (Fig. 6f), largely due to the fact that acidic conditions can promote the formation of oxalic acid and their precursors. Therefore, a robust negative correlation was obtained for pHIS and the precursors of oxalic acid such as Gly, mGly and ωC2 (R2>0.50). A few studies have pointed out that aerosol acidity is favorable for the formation of biogenic SOA (BSOA) derived from isoprene oxidation such as 2-methylglyceric acid, which can be oxidized into Gly and mGly and then converted to oxalic acid (Meng et al., 2014; Surratt et al., 2007, 2010). Our previous studies have revealed that enhanced RH can reduce particle acidity (pHIS) and is thus unfavorable for oxalic acid formation by acid-catalyzed reactions occurring in the aerosol aqueous phase (Meng et al., 2014). Both RH and aerosol composition are key factors controlling the aerosol LWC (Bikkina et al., 2017). Deshmukh et al. (2017) and Bikkina et al. (2017) also found that RH and LWC correlated well with oxalic acid, indicating that humid conditions are favorable for the aqueous-phase formation of C2. Nevertheless, Zhang et al. (2011) pointed out that low RH conditions can promote SOA yields via the oxidation of isoprene. Higher RH and LWC can promote the partitioning of water-soluble semi-volatile organic precursors of oxalic acid (e.g., Gly and mGly) into the aerosol aqueous phase but can also suppress the acid-catalyzed formation of oxalic acid because of the lower aerosol acidity due to dilution. Therefore, C2 does not present any correlation with RH or LWC at Mt. Tai.
3.6 Stable carbon isotopic composition of oxalic acid and related SOA
To further understand the formation mechanisms of C2 and related SOA, the stable isotopic composition of major dicarboxylic acids and related SOA in the Mt. Tai aerosols was investigated (Table 5). Generally, an increase in δ13C values was observed, with a decrease in carbon numbers of dicarboxylic acids. The averaged δ13C value (daytime: ‰; nighttime: ‰) of C2 is higher than other dicarboxylic acids and related SOA in the Mt. Tai atmosphere, and also higher than those observed in urban regions such as Xi'an, China (−22.7 ‰ to −22.0 ‰) (Wang et al., 2012), and Sapporo, Japan (18.8±2.0 ‰) (Aggarwal and Kawamura, 2008), and rural regions such as Morogoro, Tanzania (18.3 ‰±1.7 ‰) (Mkoma et al., 2014), but lower than those (11.5 ‰±2.8 ‰) (Zhang et al., 2016) at a background site (the Korea Climate Observatory at Gosan) in East Asia during the summer. Pavuluri et al. (2016) have reported that the average δ13C values of C2 from biogenic aerosols are higher than those from anthropogenic aerosols. The relatively higher δ13C values of C2 observed at Mt. Tai further demonstrate that the contribution of biogenic sources to C2 and related SOA is more significant than anthropogenic sources, which is consistent with our discussions above. The average δ13C values of C4 are more negative than C2 and C3 (Fig. 7). Such a phenomenon is also observed in other regions (Aggarwal and Kawamura, 2008; Wang et al., 2012; Zhang et al., 2016). Photochemical decomposition (or breakdown) of longer chain dicarboxylic acids (e.g., C3 or C4) in the aerosol aqueous phase can form C2 (Wang et al., 2017), during which C3 or C4 release CO2∕CO by reaction with OH radicals and other oxidants, resulting in C2 being more enriched in 13C due to kinetic isotope effects (KIEs) (Wang et al., 2012). The 13C enrichment in C2 is more distinguished in the daytime than in the nighttime (Table 5 and Fig. 7), largely due to the enhanced photochemical oxidation. However, such diurnal variation was not found for C3 and C4.
ωC2 is an important intermediate of the aqueous-phase photochemical oxidation of precursors such as Gly, mGly and Pyr during the C2 formation process (Carlton et al., 2006; Fu et al., 2008). Thus, the higher mass ratios of C2 ∕ ωC2, C2 ∕ Gly and C2 ∕ mGly indicate that organic aerosols are more aged (Wang et al., 2017). As shown in Fig. 8g–i, δ13C values of C2 correlate robustly with C2 ∕ Gly, C2 ∕ mGly and C2 ∕ total diacids, suggesting an enrichment of 13C during the organic aerosol ageing process. During the campaign, ωC2 is less enriched in 13C in comparison with Gly, mGly and Pyr because the lighter isotope (12C) is preferentially enriched in the products due to KIEs during the aqueous-phase irreversible chemical reactions (Wang et al., 2012). As one of the major precursors of Gly, isoprene emitted directly from vegetation is depleted in 13C, with a range from −32 ‰ to −27 ‰ (Affek and Yakir, 2003), but during the transport process, isoprene could gradually be enriched with 13C (δ13C value ‰) due to isotope fractionation associated with the reaction with OH radicals (Rudolph et al., 2003). Moreover, chamber experiments have shown that β-pinene is preferably enriched with 13C during its ozonolysis due to KIEs (Fisseha et al., 2009). Therefore, the δ13C values of Gly and mGly are relatively higher than fresh BVOCs such as isoprene, largely attributed to the secondary formation from the oxidation of isoprene and other biogenic precursors.
PM2.5 aerosols from the summit of Mt. Tai (1534 m a.s.l.) in the North China Plain during the summer of 2016 were analyzed for dicarboxylic acids, ketocarboxylic acids, α-dicarbonyls, EC, OC and WSOC. Molecular compositions of dicarboxylic acids and related compounds in the forested highland region are similar to those on the ground and other mountainous regions. The concentrations of total dicarboxylic acids and ketocarboxylic acids are higher in the daytime than those in the daytime, but α-dicarbonyls present lower values in the daytime, suggesting the mountainous atmospheric environment is more photochemically aged in the daytime than in the nighttime. The concentrations of oxalic acid and BSOA tracers and the mass ratios of C2 ∕ Gly, C2 ∕ mGly and C2 ∕ total diacids correlate positively with O3 concentrations in the daytime during the campaign, but such correlations were not found at night. Moreover, C2, C2 ∕ total diacids ratios and BSOA tracers correlate strongly with temperatures because higher temperature conditions can enhance the emissions of BVOCs and further promote the photochemical formation of C2. C2 has a robust correlation with pHIS and during the whole sampling period, indicating that acidic conditions can favor the formation of oxalic acid in the aqueous phase.
A significant enrichment of 13C in dicarboxylic acids was observed as a function of their carbon number. The observed larger δ13C values of lower carbon numbered dicarboxylic acids can be explained by isotopic fractionations resulting from the atmospheric decomposition of relatively longer chain diacids or their precursors. Increased δ13C values of C2 relative to C2 ∕ Gly and C2 ∕ mGly ratios also suggested an important effect of photochemical aging on the stable carbon isotopic composition of dicarboxylic acids.
The data for this paper are available upon request from the corresponding author (wanggh@ieecas.cn).
The supplement related to this article is available online at: https://doi.org/10.5194/acp-18-15069-2018-supplement.
GW designed and supervised the whole project. ZH collected the aerosol samples. JM and XL conducted the experiments. JM performed the data analyses and wrote the manuscript. All authors contributed to the paper with useful scientific discussions or comments.
The authors declare that they have no conflict of interest.
This article is part of the special issue “Regional transport and transformation of air pollution in eastern China”. It is not associated with a conference.
This work was supported by China National Science Funds (grant no. 41505112
and 41773117), the Natural Science Foundation of Shandong Province (grant no.
BS2015HZ002), the China National Natural Science Funds for Distinguished
Young Scholars (no. 41325014) and Open Funds of the State Key Laboratory of
Loess and Quaternary Geology, Institute of Earth Environment, Chinese Academy
of Sciences (grant nos. SKLLQG1509 and SKLLQG1504).
Edited by: Yuan Wang
Reviewed by: four anonymous referees
Affek, H. P. and Yakir, D.: Natural Abundance Carbon Isotope Composition of Isoprene Reflects Incomplete Coupling between Isoprene Synthesis and Photosynthetic Carbon Flow, Plant Physiol., 131, 1727–1736, 2003.
Aggarwal, S. G. and Kawamura, K.: Molecular distributions and stable carbon isotopic compositions of dicarboxylic acids and related compounds in aerosols from Sapporo, Japan: Implications for photochemical aging during long-range atmospheric transport, J. Geophys. Res., 113, D14301, https://doi.org/10.1029/2007jd009365, 2008.
Bian, Q., Huang, X. H. H., and Yu, J. Z.: One-year observations of size distribution characteristics of major aerosol constituents at a coastal receptor site in Hong Kong – Part 1: Inorganic ions and oxalate, Atmos. Chem. Phys., 14, 9013–9027, https://doi.org/10.5194/acp-14-9013-2014, 2014.
Bikkina, S., Kawamura, K., and Sarin, M.: Secondary Organic Aerosol Formation over Coastal Ocean: Inferences from Atmospheric Water-Soluble Low Molecular Weight Organic Compounds, Environ. Sci. Technol., 51, 4347-4357, https://doi.org/10.1021/acs.est.6b05986, 2017.
Bilde, M., Barsanti, K., Booth, M., Cappa, C. D., Donahue, N. M., Emanuelsson, E. U., McFiggans, G., Krieger, U. K., Marcolli, C., Topping, D., Ziemann, P., Barley, M., Clegg, S., Dennis-Smither, B., Hallquist, M., Hallquist, Å. M., Khlystov, A., Kulmala, M., Mogensen, D., Percival, C. J., Pope, F., Reid, J. P., Ribeiro da Silva, M. A. V., Rosenoern, T., Salo, K., Soonsin, V. P., Yli-Juuti, T., Prisle, N. L., Pagels, J., Rarey, J., Zardini, A. A., and Riipinen, I.: Saturation Vapor Pressures and Transition Enthalpies of Low-Volatility Organic Molecules of Atmospheric Relevance: From Dicarboxylic Acids to Complex Mixtures, Chem. Rev., 115, 4115–4156, https://doi.org/10.1021/cr5005502, 2015.
Boreddy, S. K. R., Kawamura, K., and Tachibana, E.: Long-term (2001–2013) observations of water-soluble dicarboxylic acids and related compounds over the western North Pacific: trends, seasonality and source apportionment, Sci. Rep.-UK, 7, 8518, https://doi.org/10.1038/s41598-017-08745-w, 2017.
Carlton, A. G., Turpin, B. J., Lim, H.-J., Altieri, K. E., and Seitzinger, S.: Link between isoprene and secondary organic aerosol (SOA): Pyruvic acid oxidation yields low volatility organic acids in clouds, Geophys. Res. Lett., 33, L06822, https://doi.org/10.1029/2005gl025374, 2006.
Carlton, A. G., Turpin, B. J., Altieri, K. E., Seitzinger, S., Reff, A., Lim, H.-J., and Ervens, B.: Atmospheric oxalic acid and SOA production from glyoxal: Results of aqueous photooxidation experiments, Atmos. Environ., 41, 7588–7602, https://doi.org/10.1016/j.atmosenv.2007.05.035, 2007.
Cheng, C., Wang, G., Zhou, B., Meng, J., Li, J., Cao, J., and Xiao, S.: Comparison of dicarboxylic acids and related compounds in aerosol samples collected in Xi'an, China during haze and clean periods, Atmos. Environ., 81, 443–449, https://doi.org/10.1016/j.atmosenv.2013.09.013, 2013.
Cheng, C., Wang, G., Meng, J., Wang, Q., Cao, J., Li, J., and Wang, J.: Size-resolved airborne particulate oxalic and related secondary organic aerosol species in the urban atmosphere of Chengdu, China, Atmos. Res., 161–162, 134–142, https://doi.org/10.1016/j.atmosres.2015.04.010, 2015.
Cheng, C., Li, M., Chan, C. K., Tong, H., Chen, C., Chen, D., Wu, D., Li, L., Wu, C., Cheng, P., Gao, W., Huang, Z., Li, X., Zhang, Z., Fu, Z., Bi, Y., and Zhou, Z.: Mixing state of oxalic acid containing particles in the rural area of Pearl River Delta, China: implications for the formation mechanism of oxalic acid, Atmos. Chem. Phys., 17, 9519–9533, https://doi.org/10.5194/acp-17-9519-2017, 2017.
Chow, J. C., Watson, J. G., Chen, L. W. A., Arnott, W. P., Moosmüller, H., and Fung, K.: Equivalence of Elemental Carbon by Thermal/Optical Reflectance and Transmittance with Different Temperature Protocols, Environ. Sci. Technol., 38, 4414–4422, https://doi.org/10.1021/es034936u, 2004.
Claeys, M., Graham, B., Vas, G., Wang, W., Vermeylen, R., Pashynska, V., Cafmeyer, J., Guyon, P., Andreae, M. O., Artaxo, P., and Maenhaut, W.: Formation of Secondary Organic Aerosols Through Photooxidation of Isoprene, Science, 303, 1173–1176, https://doi.org/10.1126/science.1092805, 2004.
Clegg, S. L., Brimblecombe, P., and Wexler, A. S.: Thermodynamic Model of the System at Tropospheric Temperatures, J. Phys. Chem. A, 102, 2137–2154, https://doi.org/10.1021/jp973042r, 1998.
Deshmukh, D. K., Kawamura, K., Deb, M. K., and Boreddy, S. K. R.: Sources and formation processes of water-soluble dicarboxylic acids, ω-oxocarboxylic acids, α-dicarbonyls, and major ions in summer aerosols from eastern central India, J. Geophys. Res.-Atmos., 122, 3630-3652, https://doi.org/10.1002/2016jd026246, 2017.
Edwards, P. M., Aikin, K. C., Dube, W. P., Fry, J. L., Gilman, J. B., de Gouw, J. A., Graus, M. G., Hanisco, T. F., Holloway, J., Huber, G., Kaiser, J., Keutsch, F. N., Lerner, B. M., Neuman, J. A., Parrish, D. D., Peischl, J., Pollack, I. B., Ravishankara, A. R., Roberts, J. M., Ryerson, T. B., Trainer, M., Veres, P. R., Wolfe, G. M., Warneke, C., and Brown, S. S.: Transition from high- to low-NOx control of night-time oxidation in the southeastern US, Nature Geoscience, 10, 490–496, 2017.
Ehn, M., Thornton, J. A., Kleist, E., Sipilä, M., Junninen, H., Pullinen, I., Springer, M., Rubach, F., Tillmann, R., Lee, B., Lopez-Hilfiker, F., Andres, S., Acir, I.-H., Rissanen, M., Jokinen, T., Schobesberger, S., Kangasluoma, J., Kontkanen, J., Nieminen, T., Kurtén, T., Nielsen, L. B., Jørgensen, S., Kjaergaard, H. G., Canagaratna, M., Maso, M. D., Berndt, T., Petäjä, T., Wahner, A., Kerminen, V.-M., Kulmala, M., Worsnop, D. R., Wildt, J., and Mentel, T. F.: A large source of low-volatility secondary organic aerosol, Nature, 506, 476–479, https://doi.org/10.1038/nature13032, 2014.
Ervens, B., Sorooshian, A., Lim, Y. B., and Turpin, B. J.: Key parameters controlling OH-initiated formation of secondary organic aerosol in the aqueous phase (aqSOA), J. Geophys. Res.-Atmos., 119, 3997–4016, https://doi.org/10.1002/2013jd021021, 2014.
Fick, J., Pommer, L., Nilsson, C., and Andersson, B.: Effect of OH radicals, relative humidity, and time on the composition of the products formed in the ozonolysis of α-pinene, Atmos. Environ., 37, 4087–4096, https://doi.org/10.1016/S1352-2310(03)00522-3, 2003.
Fisseha, R., Spahn, H., Wegener, R., Hohaus, T., Brasse, G., Wissel, H., Tillmann, R., Wahner, A., Koppmann, R., and Kiendler-Scharr, A.: Stable carbon isotope composition of secondary organic aerosol from β-pinene oxidation, J. Geophys. Res.-Atmos., 114, D02304, https://doi.org/10.1029/2008jd011326, 2009.
Fountoukis, C. and Nenes, A.: ISORROPIA II: a computationally efficient thermodynamic equilibrium model for aerosols, Atmos. Chem. Phys., 7, 4639–4659, https://doi.org/10.5194/acp-7-4639-2007, 2007.
Fu, P., Kawamura, K., Kanaya, Y., and Wang, Z.: Contributions of biogenic volatile organic compounds to the formation of secondary organic aerosols over Mt. Tai, Central East China, Atmos. Environ., 44, 4817–4826, https://doi.org/10.1016/j.atmosenv.2010.08.040, 2010.
Fu, P., Kawamura, K., Usukura, K., and Miura, K.: Dicarboxylic acids, ketocarboxylic acids and glyoxal in the marine aerosols collected during a round-the-world cruise, Mar. Chem., 148, 22–32, https://doi.org/10.1016/j.marchem.2012.11.002, 2013.
Fu, T.-M., Jacob, D. J., Wittrock, F., Burrows, J. P., Vrekoussis, M., and Henze, D. K.: Global budgets of atmospheric glyoxal and methylglyoxal, and implications for formation of secondary organic aerosols, J. Geophys. Res., 113, D15303, https://doi.org/10.1029/2007jd009505, 2008.
Gao, S., Ng, N. L., Keywood, M., Varutbangkul, V., Bahreini, R., Nenes, A., He, J., Yoo, K. Y., Beauchamp, J. L., Hodyss, R. P., Flagan, R. C., and Seinfeld, J. H.: Particle Phase Acidity and Oligomer Formation in Secondary Organic Aerosol, Environ. Sci. Technol., 38, 6582–6589, https://doi.org/10.1021/es049125k, 2004.
Hallquist, M., Wenger, J. C., Baltensperger, U., Rudich, Y., Simpson, D., Claeys, M., Dommen, J., Donahue, N. M., George, C., Goldstein, A. H., Hamilton, J. F., Herrmann, H., Hoffmann, T., Iinuma, Y., Jang, M., Jenkin, M. E., Jimenez, J. L., Kiendler-Scharr, A., Maenhaut, W., McFiggans, G., Mentel, Th. F., Monod, A., Prévôt, A. S. H., Seinfeld, J. H., Surratt, J. D., Szmigielski, R., and Wildt, J.: The formation, properties and impact of secondary organic aerosol: current and emerging issues, Atmos. Chem. Phys., 9, 5155–5236, https://doi.org/10.5194/acp-9-5155-2009, 2009.
Hatakeyama, S., Ohno, M., Weng, J., Takagi, H., and Akimoto, H.: Mechanism for the formation of gaseous and particulate products from ozone-cycloalkene reactions in air, Environ. Sci. Technol., 21, 52–57, https://doi.org/10.1021/es00155a005, 1987.
He, N., Kawamura, K., Okuzawa, K., Pochanart, P., Liu, Y., Kanaya, Y., and Wang, Z. F.: Diurnal and temporal variations of water-soluble dicarboxylic acids and related compounds in aerosols from the northern vicinity of Beijing: Implication for photochemical aging during atmospheric transport, Sci. Total Environ., 499, 154–165, https://doi.org/10.1016/j.scitotenv.2014.08.050, 2014.
Hegde, P. and Kawamura, K.: Seasonal variations of water-soluble organic carbon, dicarboxylic acids, ketocarboxylic acids, and a-dicarbonyls in Central Himalayan aerosols, Atmos. Chem. Phys., 12, 6645–6665, https://doi.org/10.5194/acp-12-6645-2012, 2012.
Herrmann, H., Ervens, B., Nowacki, P., Wolke, R., and Zellner, R.: A chemical aqueous phase radical mechanism for tropospheric chemistry, Chemosphere, 38, 1223–1232, https://doi.org/10.1016/S0045-6535(98)00520-7, 1999.
Ho, K. F., Cao, J. J., Lee, S. C., Kawamura, K., Zhang, R. J., Chow, J. C., and Watson, J. G.: Dicarboxylic acids, ketocarboxylic acids, and dicarbonyls in the urban atmosphere of China, J. Geophys. Res.-Atmos., 112, D22S27, https://doi.org/10.1029/2006jd008011, 2007.
Hoque, M. M. M., Kawamura, K., and Uematsu, M.: Spatio-temporal distributions of dicarboxylic acids, ω-oxocarboxylic acids, pyruvic acid, α-dicarbonyls and fatty acids in the marine aerosols from the North and South Pacific, Atmos. Res., 185, 158–168, https://doi.org/10.1016/j.atmosres.2016.10.022, 2017.
Huang, D., Zhang, X., Chen, Z. M., Zhao, Y., and Shen, X. L.: The kinetics and mechanism of an aqueous phase isoprene reaction with hydroxyl radical, Atmos. Chem. Phys., 11, 7399–7415, https://doi.org/10.5194/acp-11-7399-2011, 2011.
Jung, J., Tsatsral, B., Kim, Y. J., and Kawamura, K.: Organic and inorganic aerosol compositions in Ulaanbaatar, Mongolia, during the cold winter of 2007 to 2008: Dicarboxylic acids, ketocarboxylic acids, and α-dicarbonyls, J. Geophys. Res.-Atmos., 115, D22203, https://doi.org/10.1029/2010jd014339, 2010.
Kawamura, K. and Bikkina, S.: A review of dicarboxylic acids and related compounds in atmospheric aerosols: Molecular distributions, sources and transformation, Atmos. Res., 170, 140–160, https://doi.org/10.1016/j.atmosres.2015.11.018, 2016.
Kawamura, K. and Ikushima, K.: Seasonal changes in the distribution of dicarboxylic acids in the urban atmosphere, Environ. Sci. Technol., 27, 2227–2235, https://doi.org/10.1021/es00047a033, 1993.
Kawamura, K. and Kaplan, I. R.: Motor exhaust emissions as a primary source for dicarboxylic acids in Los Angeles ambient air, Environ. Sci. Technol., 21, 105–110, https://doi.org/10.1021/es00155a014, 1987.
Kawamura, K. and Usukura, K.: Distributions of low molecular weight dicarboxylic acids in the North Pacific aerosol samples, J. Oceanogr., 49, 271–283, https://doi.org/10.1007/bf02269565, 1993.
Kawamura, K., Kasukabe, H., and Barrie, L. A.: Source and reaction pathways of dicarboxylic acids, ketoacids and dicarbonyls in arctic aerosols: One year of observations, Atmos. Environ., 30, 1709–1722, https://doi.org/10.1016/1352-2310(95)00395-9, 1996.
Kawamura, K. and Watanabe, T.: Determination of Stable Carbon Isotopic Compositions of Low Molecular Weight Dicarboxylic Acids and Ketocarboxylic Acids in Atmospheric Aerosol and Snow Samples, Anal. Chem., 76, 5762–5768, https://doi.org/10.1021/ac049491m, 2004.
Kawamura, K., Okuzawa, K., Aggarwal, S. G., Irie, H., Kanaya, Y., and Wang, Z.: Determination of gaseous and particulate carbonyls (glycolaldehyde, hydroxyacetone, glyoxal, methylglyoxal, nonanal and decanal) in the atmosphere at Mt. Tai, Atmos. Chem. Phys., 13, 5369–5380, https://doi.org/10.5194/acp-13-5369-2013, 2013a.
Kawamura, K., Tachibana, E., Okuzawa, K., Aggarwal, S. G., Kanaya, Y., and Wang, Z. F.: High abundances of water-soluble dicarboxylic acids, ketocarboxylic acids and a-dicarbonyls in the mountaintop aerosols over the North China Plain during wheat burning season, Atmos. Chem. Phys., 13, 8285–8302, https://doi.org/10.5194/acp-13-8285-2013, 2013b.
Kroll, J. H. and Seinfeld, J. H.: Chemistry of secondary organic aerosol: Formation and evolution of low-volatility organics in the atmosphere, Atmos. Environ., 42, 3593–3624, https://doi.org/10.1016/j.atmosenv.2008.01.003, 2008.
Kundu, S., Kawamura, K., Andreae, T. W., Hoffer, A., and Andreae, M. O.: Diurnal variation in the water-soluble inorganic ions, organic carbon and isotopic compositions of total carbon and nitrogen in biomass burning aerosols from the LBA-SMOCC campaign in Rondônia, Brazil, J. Aerosol Sci., 41, 118–133, https://doi.org/10.1016/j.jaerosci.2009.08.006, 2010.
Kunwar, B., Torii, K., and Kawamura, K.: Springtime influences of Asian outflow and photochemistry on the distributions of diacids, oxoacids and α-dicarbonyls in the aerosols from the western North Pacific Rim, Tellus B, 69, 1369341, https://doi.org/10.1080/16000889.2017.1369341, 2017.
Leaitch, W. R., Li, S. M., Liu, P. S. K., Banic, C. M., Macdonald, A. M., Isaac, G. A., Couture, M. D., and Strapp, J. W.: Relationships among ccn, aerosol size distribution and ion chemistry from airborne measurements over the bay of fundy in august-september, 1995 A2 – Kulmala, Markku, in: Nucleation and Atmospheric Aerosols 1996, edited by: Wagner, P. E., Pergamon, Amsterdam, 840–843, 1996.
Lee, A., Goldstein, A. H., Kroll, J. H., Ng, N. L., Varutbangkul, V., Flagan, R. C., and Seinfeld, J. H.: Gas-phase products and secondary aerosol yields from the photooxidation of 16 different terpenes, J. Geophys. Res.-Atmos., 111, D17305, https://doi.org/10.1029/2006jd007050, 2006.
Li, J. J., Wang, G. H., Cao, J. J., Wang, X. M., and Zhang, R. J.: Observation of biogenic secondary organic aerosols in the atmosphere of a mountain site in central China: temperature and relative humidity effects, Atmos. Chem. Phys., 13, 11535–11549, https://doi.org/10.5194/acp-13-11535-2013, 2013.
Lim, H.-J., Carlton, A. G., and Turpin, B. J.: Isoprene Forms Secondary Organic Aerosol through Cloud Processing:? Model Simulations, Environ. Sci. Technol., 39, 4441–4446, https://doi.org/10.1021/es048039h, 2005.
McNeill, V. F.: Aqueous Organic Chemistry in the Atmosphere: Sources and Chemical Processing of Organic Aerosols, Environ. Sci. Technol., 49, 1237–1244, https://doi.org/10.1021/es5043707, 2015.
Meng, J., Wang, G., Li, J., Cheng, C., and Cao, J.: Atmospheric oxalic acid and related secondary organic aerosols in Qinghai Lake, a continental background site in Tibet Plateau, Atmos. Environ., 79, 582–589, https://doi.org/10.1016/j.atmosenv.2013.07.024, 2013.
Meng, J., Wang, G., Li, J., Cheng, C., Ren, Y., Huang, Y., Cheng, Y., Cao, J., and Zhang, T.: Seasonal characteristics of oxalic acid and related SOA in the free troposphere of Mt. Hua, central China: Implications for sources and formation mechanisms, Sci. Total Environ., 493, 1088–1097, https://doi.org/10.1016/j.scitotenv.2014.04.086, 2014.
Miyazaki, Y., Aggarwal, S. G., Singh, K., Gupta, P. K., and Kawamura, K.: Dicarboxylic acids and water-soluble organic carbon in aerosols in New Delhi, India, in winter: Characteristics and formation processes, J. Geophys. Res.-Atmos., 114, D19206, https://doi.org/10.1029/2009jd011790, 2009.
Mkoma, S. L., Kawamura, K., and Tachibana, E.: Stable carbon isotopic compositions of low-molecular-weight dicarboxylic acids, glyoxylic acid and glyoxal in tropical aerosols: implications for photochemical processes of organic aerosols, Tellus B, 66, 23702, https://doi.org/10.3402/tellusb.v66.23702, 2014.
Mochizuki, T., Kawamura, K., Miyazaki, Y., Wada, R., Takahashi, Y., Saigusa, N., and Tani, A.: Secondary formation of oxalic acid and related organic species from biogenic sources in a larch forest at the northern slope of Mt. Fuji, Atmos. Environ., 166, 255–262, https://doi.org/10.1016/j.atmosenv.2017.07.028, 2017.
Myriokefalitakis, S., Tsigaridis, K., Mihalopoulos, N., Sciare, J., Nenes, A., Kawamura, K., Segers, A., and Kanakidou, M.: In-cloud oxalate formation in the global troposphere: a 3-D modeling study, Atmos. Chem. Phys., 11, 5761–5782, https://doi.org/10.5194/acp-11-5761-2011, 2011.
Narukawa, M., Kawamura, K., Takeuchi, N., and Nakajima, T.: Distribution of dicarboxylic acids and carbon isotopic compositions in aerosols from 1997 Indonesian forest fires, Geophys. Res. Lett., 26, 3101–3104, https://doi.org/10.1029/1999gl010810, 1999.
Pathak, R. K., Wu, W. S., and Wang, T.: Summertime PM2.5 ionic species in four major cities of China: nitrate formation in an ammonia-deficient atmosphere, Atmos. Chem. Phys., 9, 1711–1722, https://doi.org/10.5194/acp-9-1711-2009, 2009.
Pavuluri, C. M., Kawamura, K., and Swaminathan, T.: Water-soluble organic carbon, dicarboxylic acids, ketoacids, and α-dicarbonyls in the tropical Indian aerosols, J. Geophys. Res.-Atmos., 115, D11302, https://doi.org/10.1029/2009jd012661, 2010.
Pavuluri, C. M. and Kawamura, K.: Enrichment of (13)C in diacids and related compounds during photochemical processing of aqueous aerosols: New proxy for organic aerosols aging, Sci. Rep.-UK, 6, 36467, https://doi.org/10.1038/srep36467, 2016.
Rapf, R. J., Dooley, M. R., Kappes, K., Perkins, R. J., and Vaida, V.: pH Dependence of the Aqueous Photochemistry of α-Keto Acids, J. Phys. Chem.A, 121, 8368–8379, https://doi.org/10.1021/acs.jpca.7b08192, 2017.
Rudolph, J., Anderson, R. S., Czapiewski, K. V., Czuba, E., Ernst, D., Gillespie, T., Huang, L., Rigby, C., and Thompson, A. E.: The Stable Carbon Isotope Ratio of Biogenic Emissions of Isoprene and the Potential Use of Stable Isotope Ratio Measurements to Study Photochemical Processing of Isoprene in the Atmosphere, J. Atmos. Chem., 44, 39–55, https://doi.org/10.1023/a:1022116304550, 2003.
Sorooshian, A., Lu, M.-L., Brechtel, F. J., Jonsson, H., Feingold, G., Flagan, R. C., and Seinfeld, J. H.: On the Source of Organic Acid Aerosol Layers above Clouds, Environ. Sci. Technol., 41, 4647–4654, https://doi.org/10.1021/es0630442, 2007a.
Sorooshian, A., Ng, N. L., Chan, A. W. H., Feingold, G., Flagan, R. C., and Seinfeld, J. H.: Particulate organic acids and overall water-soluble aerosol composition measurements from the 2006 Gulf of Mexico Atmospheric Composition and Climate Study (GoMACCS), J. Geophys. Res., 112, D13201, https://doi.org/10.1029/2007jd008537, 2007b.
Surratt, J. D., Lewandowski, M., Offenberg, J. H., Jaoui, M., Kleindienst, T. E., Edney, E. O., and Seinfeld, J. H.: Effect of Acidity on Secondary Organic Aerosol Formation from Isoprene, Environ. Sci. Technol., 41, 5363–5369, https://doi.org/10.1021/es0704176, 2007.
Surratt, J. D., Chan, A. W. H., Eddingsaas, N. C., Chan, M., Loza, C. L., Kwan, A. J., Hersey, S. P., Flagan, R. C., Wennberg, P. O., and Seinfeld, J. H.: Reactive intermediates revealed in secondary organic aerosol formation from isoprene, P. Natl. Acad. Sci. USA, 107, 6640–6645, https://doi.org/10.1073/pnas.0911114107, 2010.
Tan, Y., Carlton, A. G., Seitzinger, S. P., and Turpin, B. J.: SOA from methylglyoxal in clouds and wet aerosols: Measurement and prediction of key products, Atmos. Environ., 44, 5218–5226, https://doi.org/10.1016/j.atmosenv.2010.08.045, 2010.
Wang, G., Kawamura, K., Umemoto, N., Xie, M., Hu, S., and Wang, Z.: Water-soluble organic compounds in PM2.5 and size-segregated aerosols over Mount Tai in North China Plain, J. Geophys. Res.-Atmos., 114, D19208, https://doi.org/10.1029/2008jd011390, 2009.
Wang, G., Xie, M., Hu, S., Gao, S., Tachibana, E., and Kawamura, K.: Dicarboxylic acids, metals and isotopic compositions of C and N in atmospheric aerosols from inland China: implications for dust and coal burning emission and secondary aerosol formation, Atmos. Chem. Phys., 10, 6087–6096, https://doi.org/10.5194/acp-10-6087-2010, 2010.
Wang, G., Kawamura, K., Cheng, C., Li, J., Cao, J., Zhang, R., Zhang, T., Liu, S., and Zhao, Z.: Molecular Distribution and Stable Carbon Isotopic Composition of Dicarboxylic Acids, Ketocarboxylic Acids, and α-Dicarbonyls in Size-Resolved Atmospheric Particles From Xi'an City, China, Environ. Sci. Technol., 46, 4783–4791, https://doi.org/10.1021/es204322c, 2012.
Wang, G., Cheng, C., Meng, J., Huang, Y., Li, J., and Ren, Y.: Field observation on secondary organic aerosols during Asian dust storm periods: Formation mechanism of oxalic acid and related compounds on dust surface, Atmos. Environ., 113, 169–176, https://doi.org/10.1016/j.atmosenv.2015.05.013, 2015.
Wang, G., Zhang, F., Peng, J., Duan, L., Ji, Y., Marrero-Ortiz, W., Wang, J., Li, J., Wu, C., Cao, C., Wang, Y., Zheng, J., Secrest, J., Li, Y., Wang, Y., Li, H., Li, N., and Zhang, R.: Particle acidity and sulfate production during severe haze events in China cannot be reliably inferred by assuming a mixture of inorganic salts, Atmos. Chem. Phys., 18, 10123–10132, https://doi.org/10.5194/acp-18-10123-2018, 2018.
Wang, G., Zhang, R., Gomez, M. E., Yang, L., Levy Zamora, M., Hu, M., Lin, Y., Peng, J., Guo, S., Meng, J., Li, J., Cheng, C., Hu, T., Ren, Y., Wang, Y., Gao, J., Cao, J., An, Z., Zhou, W., Li, G., Wang, J., Tian, P., Marrero-Ortiz, W., Secrest, J., Du, Z., Zheng, J., Shang, D., Zeng, L., Shao, M., Wang, W., Huang, Y., Wang, Y., Zhu, Y., Li, Y., Hu, J., Pan, B., Cai, L., Cheng, Y., Ji, Y., Zhang, F., Rosenfeld, D., Liss, P. S., Duce, R. A., Kolb, C. E., and Molina, M. J.: Persistent sulfate formation from London Fog to Chinese haze, P. Natl. Acad. Sci. USA, 113, 13630–13635, https://doi.org/10.1073/pnas.1616540113, 2016.
Wang, J., Wang, G., Gao, J., Wang, H., Ren, Y., Li, J., Zhou, B., Wu, C., Zhang, L., Wang, S., and Chai, F.: Concentrations and stable carbon isotope compositions of oxalic acid and related SOA in Beijing before, during, and after the 2014 APEC, Atmos. Chem. Phys., 17, 981–992, https://doi.org/10.5194/acp-17-981-2017, 2017.
Warneck, P.: In-cloud chemistry opens pathway to the formation of oxalic acid in the marine atmosphere, Atmos. Environ., 37, 2423-2427, https://doi.org/10.1016/S1352-2310(03)00136-5, 2003.
Yang, X., Xue, L., Yao, L., Li, Q., Wen, L., Zhu, Y., Chen, T., Wang, X., Yang, L., Wang, T., Lee, S., Chen, J., and Wang, W.: Carbonyl compounds at Mount Tai in the North China Plain: Characteristics, sources, and effects on ozone formation, Atmos. Res., 196, 53–61, https://doi.org/10.1016/j.atmosres.2017.06.005, 2017.
Yu, J. Z., Huang, X.-F., Xu, J., and Hu, M.: When Aerosol Sulfate Goes Up, So Does Oxalate?: Implication for the Formation Mechanisms of Oxalate, Environ. Sci. Technol., 39, 128–133, https://doi.org/10.1021/es049559f, 2005.
Zhang, H., Surratt, J. D., Lin, Y. H., Bapat, J., and Kamens, R. M.: Effect of relative humidity on SOA formation from isoprene/NO photooxidation: enhancement of 2-methylglyceric acid and its corresponding oligoesters under dry conditions, Atmos. Chem. Phys., 11, 6411–6424, https://doi.org/10.5194/acp-11-6411-2011, 2011.
Zhang, R., Wang, G., Guo, S., Zamora, M. L., Ying, Q., Lin, Y., Wang, W., Hu, M., and Wang, Y.: Formation of urban fine particulate matter, Chem. Rev., 115, 3803–3855, https://doi.org/10.1021/acs.chemrev.3805b00067, 2015.
Zhang, Y.-L., Kawamura, K., Cao, F., and Lee, M.: Stable carbon isotopic compositions of low-molecular-weight dicarboxylic acids, oxocarboxylic acids, α-dicarbonyls, and fatty acids: Implications for atmospheric processing of organic aerosols, J. Geophys. Res.-Atmos., 121, 3707–3717, https://doi.org/10.1002/2015jd024081, 2016.
Zhao, W., Kawamura, K., Yue, S., Wei, L., Ren, H., Yan, Y., Kang, M., Li, L., Ren, L., Lai, S., Li, J., Sun, Y., Wang, Z., and Fu, P.: Molecular distribution and compound-specific stable carbon isotopic composition of dicarboxylic acids, oxocarboxylic acids and α-dicarbonyls in PM2.5 from Beijing, China, Atmos. Chem. Phys., 18, 2749–2767, https://doi.org/10.5194/acp-18-2749-2018, 2018.
Zhu, Y., Yang, L., Chen, J., Kawamura, K., Sato, M., Tilgner, A., van Pinxteren, D., Chen, Y., Xue, L., Wang, X., Simpson, I. J., Herrmann, H., Blake, D. R., and Wang, W.: Molecular distributions of dicarboxylic acids, oxocarboxylic acids and α-dicarbonyls in PM2.5 collected at the top of Mt. Tai, North China, during the wheat burning season of 2014, Atmos. Chem. Phys., 18, 10741–10758, https://doi.org/10.5194/acp-18-10741-2018, 2018.