the Creative Commons Attribution 4.0 License.
the Creative Commons Attribution 4.0 License.
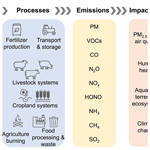
Opinion: Understanding the impacts of agriculture and food systems on atmospheric chemistry is instrumental to achieving multiple Sustainable Development Goals
Lina Luo
Biao Luo
Agriculture and food systems play important roles in shaping atmospheric chemistry and air quality, most dominantly via the release of reactive nitrogen (Nr) compounds but also via agricultural burning, energy use, and cropland and pastureland expansion. In this opinion article, we first succinctly review our current understanding of agricultural and food-system emissions of Nr and other atmospherically relevant compounds; their fates and impacts on air quality, human health, and terrestrial ecosystems; and how such emissions can be potentially mitigated through better cropland management, livestock management, and whole-food-system transformation. In doing so, we highlight important knowledge gaps that warrant more extensive research and argue that we scientists need to provide a more detailed, process-based understanding of the impacts of agriculture and food systems on atmospheric chemistry, including both chemical composition and processes, especially as the importance of emissions from other fossil-fuel-intensive sectors is fading in the face of regulatory measures worldwide. Such knowledge is necessary to guide food-system transformation in technologically feasible, economically viable, socially inclusive, and environmentally responsible ways and is essential to help society achieve multiple Sustainable Development Goals (SDGs), especially to ensure food security for people, protect human and ecosystem health, improve farmers' livelihoods, and ultimately help communities achieve socioeconomic and environmental sustainability.
- Article
(947 KB) - Full-text XML
- BibTeX
- EndNote
During and after the 2023 United Nations Climate Change Conference in Dubai, United Arab Emirates (UAE), commonly known as COP28, more than 150 nations signed the “UAE Declaration on Sustainable Agriculture, Resilient Food Systems, and Climate Action”, emphasizing the desperate need to integrate agriculture and food systems into their climate action to reach the climate goals set forth in the Paris Agreement. For the first time, agriculture has come under the spotlight of international climate negotiation, showcasing the important roles food systems play in shaping climate via their contribution to a third of global anthropogenic greenhouse gas (GHG) emissions (Crippa et al., 2021). Such momentum is arguably also a promising development for air quality managers and policymakers worldwide because agriculture and food systems are major sources of various short-lived chemical species that shape chemical composition and processes in the atmosphere, which in turn contribute to air pollution.
“The food we eat, the air we breathe”, a recent review article (Balasubramanian et al., 2021), highlights succinctly the deep interconnection between these two things everyone needs for survival but often thinks too little about. We all need a minimum amount of nutrition from food to survive and often a lot more for a thriving, productive, and good-quality life. Due to population growth, rising incomes, and shifting dietary habits across the world, the global food demand increased roughly 3-fold from 1960 to 2010 and is projected to rise further by 40 %–50 % by the year 2050 depending on the scenario (FAO, 2018). Despite substantial gains in agricultural production to meet the rising demand due to the advancement of “Green Revolution” technologies and intensified agricultural inputs as well as cropland and pastureland expansion, undernourishment remains prevalent with a global rate of 11 % in 2012; in low- and middle-income countries, the undernourishment rate can be as high as 20 % in sub-Saharan Africa and was 16 % in South Asia in 2012 (FAO, 2018). Even though global food systems can indeed produce enough food for everybody, persistent poverty, inequality, uneven distribution, conflicts, and socio-political instability cause people in many parts of the world to still go hungry on a daily basis. The challenge of satisfying the continuously rising food demand is further aggravated by environmental problems such as climate change and air pollution, which can severely threaten crop production and food security worldwide (Tai and Martin, 2017; Tai et al., 2014). Therefore, 193 member states of the United Nations (UN) came together in 2015 to endorse SDG 2, “Zero Hunger”, as 1 of the 17 Sustainable Development Goals (SDGs) for the 2030 Agenda for Sustainable Development, aiming primarily to end poverty, hunger, and malnutrition by the year 2030 and to make the food systems more sustainable and resilient to climate change. The UAE declaration mentioned above reinforced the importance of these food-centered goals for global sustainable development.
The tremendous gains in agricultural production in the past half-century have also posed severe threats to the environment, including the air we breathe. In addition to contributing to more than 30 % of global GHG emissions, agricultural expansion and intensification have constituted a major driver of deforestation, land and water degradation, and biodiversity loss (Foley et al., 2011). Global food systems, including all the stages of pre-production, production, post-production, consumption, and waste management, are estimated to account for 58 % of global anthropogenic emissions of primary fine particulate matter (PM2.5, i.e., particulate matter with a diameter of 2.5 µm or smaller), 72 % of ammonia (NH3), 13 % of nitrogen oxides (NOx = NO + NO2), 9 % of sulfur dioxide (SO2), and 19 % of non-methane volatile organic compounds (VOCs) (Balasubramanian et al., 2021). Such emissions were estimated to be responsible for 22 % of global mortality arising from poor air quality and 1.4 % of global crop production losses in the year 2018 (Crippa et al., 2022b). Moreover, reactive nitrogen (Nr) compounds of agricultural origins including NH3, NOx, nitrous acid (HONO), and their reaction products can readily be deposited back onto the land surface and waterbodies, bringing about various effects on terrestrial and aquatic ecosystems, including more serious nutrient leaching, soil acidification (Guo et al., 2010; Lu et al., 2011), and eutrophication (Deng et al., 2023, 2024a; Jickells et al., 2017; L. Liu et al., 2023). They may also enhance plant growth and soil carbon storage, especially where nitrogen is a limiting nutrient (Thomas et al., 2010; Zhao et al., 2017b; Liu et al., 2021b), but such enhancements generally favor the more competitive plant species and may ultimately reduce species diversity of plant communities (Bobbink et al., 2010). All these findings highlight the importance of agriculture and food systems in shaping atmospheric composition and chemical processes, as well as air pollution and the associated public health and ecosystem impacts.
The nitrogen load released by anthropogenic activities has also exceeded the so-called planetary boundary, meaning that human disturbances of the nitrogen cycle are destabilizing natural ecosystems to a possibly irreversible extent (Richardson et al., 2023). In a recent Nature Portfolio journals' collection called “Air pollution and global solutions”, out of 34 featured articles, only 5 directly addressed food-system emissions or food security issues, and all of them emphasized substantial knowledge gaps in understanding agricultural and food-system impacts on the atmospheric environment. Mitigating agricultural emissions will be even more important in the future as global air quality control efforts mostly targeting sources from the energy and transportation sectors have already substantially reduced NOx and SO2 emissions in many parts of the world. But how can we mitigate these emissions without compromising the needs of people to be food-secure and nourished? How can we achieve these multiple goals under the concurrent threat of climate change, which can both impair crop production and elevate agricultural emissions? Here we argue that, to protect people and ecosystems from the harmful effects of air pollution worldwide but especially in developing regions, society needs to lay more emphasis than it currently does on reforming food systems and mitigating their emissions of various pollutants while ensuring food security for people and the livelihoods of farmers. To support this, scientists need to provide a more solid understanding of how different parts and stages of the food systems emit different compounds; how these compounds are transported, transformed, and deposited back onto the surface; how all these processes are sensitive to climate change; and how the food systems can be modified in technologically feasible, economically viable, and socially equitable ways to abate emissions.
Agriculture and food systems profoundly impact the atmosphere, most dominantly through the substantial emissions of reactive nitrogen (Nr) compounds from cropland and livestock systems but also through other atmospheric pollutants such as primary particulate matter (PM), carbon monoxide (CO), methane (CH4), SO2, and VOCs via agricultural burning, energy use of whole food systems, and deforestation to clear lands for agriculture. Among these compounds, NH3, NOx, and HONO are inherently chemically active and play significant roles in atmospheric processes, leading to the formation of air pollutants such as PM2.5 and tropospheric ozone (O3), which subsequently harm human health. Previous studies have enhanced our understanding of the mechanisms and driving factors behind agricultural emissions, allowing for improved evaluation of their impacts on air quality, human health, and ecosystems (Butterbach-Bahl et al., 2013; Crippa et al., 2022a; Gu et al., 2023; Pilegaard, 2013). However, substantial uncertainties remain in these studies. The following text is intended not to be a comprehensive review but to highlight our understanding, as well as the gaps in our understanding, of agriculture and food systems on atmospheric composition and chemical processes. Figure 1 summarizes the important stages and impacts of agriculture and food systems in terms of shaping atmospheric chemistry.
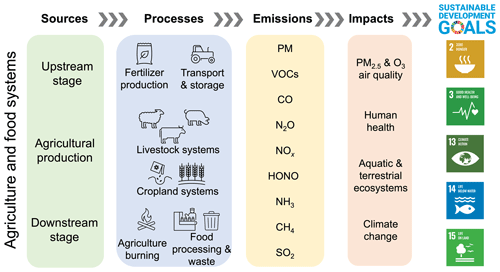
Figure 1Effects of agriculture and food systems on atmospheric chemistry and downstream impacts on human and ecosystem health, with direct linkages to various Sustainable Development Goals.
2.1 Emissions of reactive nitrogen
2.1.1 Sources, processes, and characteristics
Nitrogen is predominantly found in its inert form, dinitrogen (N2), in nature (Galloway et al., 2013). Only a small fraction of nitrogen is reactive as Nr and readily available to organisms. The advent of the Haber–Bosch process has revolutionized the way humans utilize nitrogen, allowing for the conversion of N2 into NH3 for fertilizer and other uses. Since then, the use of nitrogen-based fertilizers in agriculture has substantially increased, rising from 11.4 Tg N in 1961 to 108 Tg N in 2021 (FAO, 2024). This intensive and excessive use of fertilizers often surpasses crop nutrient demands, whereby only about half of the applied nitrogen is harvested in crops (Zhang et al., 2015). In livestock systems, nitrogen use efficiency (NUE) is even lower, with only 10 % of the nitrogen in feed being converted to livestock products (Uwizeye et al., 2020). Consequently, in both cropland and livestock systems, a significant portion of the added nitrogen is lost to the environment after undergoing various biogeochemical processes primarily mediated by microbes, leading to the emissions of many Nr compounds. Globally, around 60 % of agricultural NH3 emissions are from livestock production, with the rest coming from cropland systems (Y. Yang et al., 2023).
Specifically, NH3 is released through multi-stage volatilization processes. In cropland systems, NH3 production follows the deprotonation of the ammonium component in fertilizers, involving urea hydrolysis, NH3–ammonium equilibrium, and NH3 exchange between soil and air, with higher volatilization in regions with high temperatures and alkaline soils (Freney et al., 1981). In livestock systems, NH3 is primarily released during the housing, storage, and spreading of manure (Webb et al., 2005). Other Nr gases such as NOx and HONO, along with the potent greenhouse gas nitrous oxide (N2O), are predominantly produced from agricultural soils through microbially mediated nitrification (i.e., ammonium being oxidized to nitrate under aerobic conditions) and denitrification (i.e., nitrate being transformed into dinitrogen gas under anaerobic conditions) processes that release these gases into the atmosphere (Butterbach-Bahl et al., 2013; Pilegaard, 2013). When considering entire food systems beyond agricultural production, Nr emissions can be even higher. Food-system energy use, encompassing activities such as fertilizer production, transportation, and processing along with land use change driven by agricultural expansion, also contributes to substantial NH3 and NOx emissions (Balasubramanian et al., 2021).
Agricultural and food-system Nr emissions exhibit high spatiotemporal variations, responding nonlinearly to meteorological conditions, soil properties, and farming practices, influenced by microbial activities. Typically, regions with intensive fertilizer use and low NUE (i.e., the ratio of nitrogen removed with the harvest to nitrogen input) tend to have the highest emission levels. High temperature and precipitation also contribute to increased emissions and modulate their interannual variability (Griffis et al., 2017; Shen et al., 2020). NUE and Nr emission changes can be further driven by socioeconomic factors, with divergent patterns in different countries depending on the population level, economic growth, farm size, urbanization level, and international trade and their interactions. A series of global-scale, long-term analyses have suggested that developed regions with well-managed urban–rural development tend to have lower agricultural Nr emissions as their large-scale farming along with advanced agricultural technology and coupled cropland–livestock systems can enhance NUE and maintain agricultural productivity to support international trade (Deng et al., 2024b; Gu et al., 2020; Ren et al., 2022; Liu, 2023). In the future, fertilizer input is expected to further increase to feed the growing global population, potentially further elevating Nr emissions if not efficiently managed. Meanwhile, it has been estimated that climate change will increase Nr emissions by ∼ 80 % between 2011 and 2100, and the resulting more frequent extreme weather events may induce extensive dry–wet and freeze–thaw cycles that could further exacerbate such increases (Griffis et al., 2017; Shen et al., 2020; Wagner-Riddle et al., 2017).
2.2 Emission estimates and associated uncertainties
Emission estimation plays a crucial role in investigating the impact of agriculture and food systems on atmospheric chemistry. Agricultural Nr emissions are typically estimated using two primary approaches: bottom-up and top-down methods. The bottom-up approach can be further categorized into multiplicative schemes based on emission factors (EFs) and mechanistic process-based models. The EF approach estimates agricultural emissions as multiplicative functions of agricultural activities (e.g., fertilizer use, livestock population) and their corresponding EFs under “standard” conditions (Misselbrook et al., 2000; Bouwman et al., 1997). Since agricultural emissions are influenced by multiple factors, including meteorological conditions, soil properties, and farming practices, the most advanced EF methods refine their EFs by localizing these factors as much as possible. For livestock systems, refined EFs can be developed for each stage along the manure management chain (e.g., housing, storage, spreading) to achieve more accurate estimation (Huang et al., 2012). Process-based methods that rely on agroecosystem models comprise the most advanced bottom-up approach to estimating emissions from croplands. Agroecosystem models, such as the DayCent and DeNitrification-DeComposition (DNDC) models, explicitly track the transport, biogeochemistry, and fates of Nr in the soil in a mechanistic manner (Vira et al., 2020; Li et al., 2000; Del Grosso et al., 2009) and can reflect the nonlinear responses of emissions to their major drivers.
Top-down inversion methods have also been developed to refine emission estimates. These use a priori bottom-up estimates (e.g., from EFs) and then assimilate observational data via air quality modeling to create a posteriori estimates, aiming to minimize discrepancies between observations and estimates. Satellite-derived observations of atmospheric NH3 and NOx column concentrations have widely been used to improve agricultural emission estimates, especially in regions lacking field measurements. The recent launch of geostationary satellites with high spatiotemporal resolutions (e.g., TEMPO, GEMS) is expected to further enhance the accuracy of agricultural emission estimates over North America and Asia (Zoogman et al., 2017; Kim et al., 2020).
Within the air quality research community, the bottom-up approach is the most commonly used for estimating agricultural emissions. However, the derived emission estimates often exhibit high uncertainties, with variations of up to a factor of 2 to 3 and, in some cases, even an order of magnitude difference from observations (Table 1). The refinement and localization of EFs rely on extensive field measurements, which render EF estimates more accurate in heavily researched regions such as the USA, Europe, and China (Ma et al., 2021; Vigan et al., 2019). However, for developing regions such as Latin America, Africa, and South Asia, sporadic field measurements are not sufficient for EF refinement and localization, where emission estimates often rely on general EFs obtained from more developed regions, lack accurate activity data (e.g., fertilizer input, manure use), and thus suffer significant uncertainties. This also contributes to the substantial differences between different global inventories. Another limitation is the relatively coarse temporal resolution (often on a monthly scale), which makes it difficult to capture the influence of abrupt increases in agricultural NH3 emissions on atmospheric composition, as NH3 typically peaks within several days of fertilizer application (Nelson et al., 2019).
Process-based models, often favored by the agroecosystem research community for analysis on field scales (∼ 100 ha) and daily timescales, face challenges in regional and larger-scale applications due to their high demand for input data. Nevertheless, recent studies have successfully integrated process-based models with air quality models to estimate Nr emissions and their impacts on the atmosphere (L. Luo et al., 2022; Balasubramanian et al., 2020), leading to enhanced spatiotemporal accuracy. Despite these improvements, most agroecosystem models are parameter-intensive, requiring field measurements to constrain the default parameter values. It remains questionable whether agroecosystem models can be effectively applied on larger, pan-regional scales, as even in the USA and China, field measurements are insufficient to cover all types of cropping systems. Furthermore, some recent studies have indicated that agricultural emissions may be substantial during non-growing seasons (Yang et al., 2022), may stem from some neglected nitrogen-cycle processes (Wrage-Mönnig et al., 2018), and may be stimulated by drying–wetting and freezing–thawing events (Del Grosso et al., 2022). Further refinement of the nitrogen-cycle representation within agroecosystem models is much warranted.
Along the entire food supply chain, emission estimation beyond the on-farm stage generally employs a similar EF method. Uncertainties associated with the estimates thus derived primarily stem from the activities themselves, as well as from the corresponding EFs, due to the paucity of activity data. This issue is particularly profound in emissions originating from food transportation, which involves aspects such as transportation distances, means (e.g., road, rail, or ship), and refrigeration technology. International trade further complicates such estimation. Additional uncertainties arise from how the boundaries of the food systems are defined; e.g., some studies have considered the transportation of fertilizers, machinery, and pesticides, while others have not (Li et al., 2022). A series of comprehensive assessment and life-cycle frameworks have recently been proposed to estimate global emissions from entire agriculture and food systems (Crippa et al., 2022a; Li et al., 2022, 2023). However, these frameworks still bear uncertainties in collecting activity data and assuming different food trade policies, underscoring the need for further refinement in their methodologies for emission estimation.
2.3 Emissions of other air pollutants: characteristics and uncertainties
In addition to Nr, agriculture and food systems are also major sources of a range of atmospherically relevant compounds, including PM, CO, CH4, SO2, and VOCs (Crippa et al., 2022a), much of which can be closely linked to agricultural burning practices (e.g., for managing crop residues, land clearance). About 11 % (83±14 Mha yr−1) of total burned area globally is attributed to crop residual management, primarily occurring in South and Southeast Asia, and sub-Saharan Africa (Chen et al., 2023). In developed countries such as the USA and European nations, agricultural burning is heavily regulated, with a focus on promoting alternative methods for managing crop residues and allowing controlled burning under specific meteorological conditions to minimize environmental impacts (Hall et al., 2021; Nematian et al., 2023). In contrast, agricultural burning remains widespread in developing regions, often due to the limited time between cropping seasons and high costs of alternative management methods (Lin and Begho, 2022). Additionally, deforestation accounts for 3.8±1.2 Mha yr−1 of the global burned area, which is frequently observed in South America and sub-Saharan Africa (Chen et al., 2023) and is largely driven by the expansion of pasturelands and croplands (e.g., soybean and palm tree cultivation).
Agricultural burning causes significant emissions of air pollutants, e.g., representing the largest source of primary PM from agriculture and food systems (Balasubramanian et al., 2021). The estimation of agricultural fire emissions often relies on satellite-derived datasets, such as the Global Fire Emissions Database (GFED) based on the 500 m MODIS Burned Area product (Van Der Werf et al., 2017), which poses challenges for detecting small fires such as those of crop residual burning. Although the “small fire boost” method has been applied in GFED v4.1s to enhance the identification of small fires, the improvement of accuracy is limited (Zhang et al., 2018). It is thus important to devote more attention to the characterization of air pollutants from agricultural burning, not only because of their large emissions, but also because they are important considerations in formulating equitable emission reduction policy in developing regions, where the poorer agricultural populations are disproportionately affected. Finally, other practices in food systems, including manure management and use of machinery and vehicles, contribute to the release of VOCs and SO2, responsible for 16 % and 12 % of the total global emissions of VOCs and SO2, respectively (Crippa et al., 2023). However, food-system energy use is rarely accounted for, which also limits the assessment of the impacts of entire food systems on the atmosphere.
2.4 Effects on atmospheric chemistry and ecosystems
Once released into the atmosphere, agricultural and food-system emissions are actively involved in atmospheric processes and contribute to the formation of health-damaging air pollutants including PM2.5 and O3 (Fig. 1). In particular, for PM2.5, agricultural NH3 and NOx can contribute to secondary inorganic aerosols, key components of PM2.5, which presents a major health risk worldwide, responsible for millions of premature deaths annually (Lelieveld et al., 2015). As the most abundant alkaline gas in the atmosphere, NH3 neutralizes sulfuric acid (H2SO4) to form ammonium sulfate and, when in excess, reacts with nitric acid (HNO3) produced from the oxidation of NOx to form ammonium nitrate. Agricultural burning also contributes to PM2.5 both as a component of primary PM and via secondary formation from emitted SO2, NOx, and VOCs. For O3, surface O3 is predominantly formed through the photochemical oxidation of CO and VOCs in the presence of NOx. Agriculture influences O3 formation mostly via its contribution to NO emissions. O3 is sensitive to either NOx or VOC emissions depending on whether the atmospheric chemical regime is NOx-limited (i.e., low-NOx environment) or VOC-limited (i.e., high-NOx environment). Agricultural emissions ultimately influence ecosystem health as primary and secondary Nr compounds of agricultural origins can finally be deposited back onto the surface, thus disrupting the nutrient content and cycling in the underlying ecosystems.
The significant roles that agriculture and food systems play in shaping chemical processes in the atmosphere are increasingly being realized. It is estimated that they contribute to 22 %–53 % of PM2.5 and 5 %–25 % of O3 pollution, which are contributions comparable to those of other well-regulated sources driven by fossil fuel combustion such as the energy and transportation sectors (Crippa et al., 2022a). However, there is still a lack of thorough investigation of these roles, particularly in underdeveloped regions such as Africa and South Asia. In this section, we highlight the latest findings, along with the uncertainties and limitations, associated with the impacts of agriculture and food systems on the atmospheric environment.
2.4.1 Impacts of agricultural NH3 emissions on PM2.5 pollution and human health
Agricultural NH3 emissions significantly contribute to PM2.5 pollution. Traditional PM2.5 control policies have targeted mainly combustion-related emissions of SO2 and NOx, which have already led to significant improvements in PM2.5 air quality in regions such as the USA; Europe; and, more recently, China, but ongoing efforts are still essential for further air quality improvements, especially in developing countries but even in cleaner regions such as the USA that still witness thousands of deaths every year (Thakrar et al., 2020; Tschofen et al., 2019). More importantly, NH3, another important precursor of PM2.5, has historically received much less attention, and its primary source, agriculture, has always been less regulated than other sectors. However, agricultural NH3 is an increasingly important contributor to PM2.5 globally, accounting for approximately 34 % of annual PM2.5 concentrations in Europe, 23 % in the western USA, 36 % in the eastern USA, and 31 %–33 % in China (Bauer et al., 2016; Han et al., 2020; Pozzer et al., 2017). The dominant influence of NH3 on PM2.5 is via affecting ammonium nitrate formation, especially during winter (Han et al., 2020; Pozzer et al., 2017).
Due to the strong nonlinearity of inorganic aerosol chemistry, the sensitivity of PM2.5 to NH3 emissions varies widely across different regions, mostly depending on the regional atmospheric conditions, seasonal meteorological conditions, and intensity of mitigation efforts (Thunis et al., 2021). The PM2.5 burden in China shows a higher sensitivity to agricultural NH3 emissions compared to combustion-related NOx emissions (Bauer et al., 2016). For the Beijing–Tianjin–Hebei region in particular, a joint control of NH3, NOx, and SO2 is essential, especially as NOx and SO2 levels remain high (Fu et al., 2017; Liu et al., 2021b). In the western USA, PM2.5 sensitivity to NH3 reductions is pronounced, with reduction intensities of 40 % to 60 % (Bauer et al., 2016). In the eastern USA and India, PM2.5 shows similar sensitivities to both combustion NOx and agricultural NH3, while Europe demonstrates greater sensitivity to NOx than to NH3 emissions, particularly in western Europe, but a joint control strategy is preferred in eastern Europe (Bauer et al., 2016; Liu et al., 2023a).
Furthermore, some studies have directly examined the health damage related to air quality associated with crop production processes, highlighting that animal-based foods contribute to higher PM pollution and subsequent health damage compared to plant-based foods, as livestock management results in greater NH3 emissions compared to fertilizer applications on croplands (Domingo et al., 2021). Health effects induced by fertilizer use are more significant in densely populated regions close to the farms (Hill et al., 2019).
In general, despite lower NH3 emissions at lower temperatures, the effects of mitigating agricultural NH3 are stronger in winter, when lower temperatures favor the formation of ammonium nitrate (Pozzer et al., 2017). PM2.5 formation is sensitive to reductions in NOx emissions in NH3-rich environments and becomes more sensitive to NH3 in environments with lower NH3 levels (Liu et al., 2023a; Holt et al., 2015; Ansari and Pandis, 1998). The relative effectiveness of controlling agricultural NH3 emissions may diminish when substantial amounts of NOx and SO2 are under control, as NH3 is more likely to remain in the gas phase rather than contributing to PM2.5 formation (Fu et al., 2017). Controlling agricultural emissions benefits not only rural areas but also downwind urban regions, especially for poorer populations near the farms (Hill et al., 2019). From a policymaking perspective, NH3 abatement may be even more cost-effective than NOx abatement for controlling PM2.5 pollution (Gu et al., 2021; Pinder et al., 2007).
Uncertainties and limitations still abound in our understanding of the impacts of agricultural NH3 on PM2.5 formation. A major source of uncertainty stems from the nonlinearity and high sensitivity of PM2.5 to the nitrate ammonium ratio, which may be prone to large errors due to uncertainties in both NOx and NH3 emission estimates. Consequently, even within the same region, the response of PM2.5 to agricultural NH3 emissions can vary between studies. For instance, one study suggested that reducing agricultural NH3 emissions by 40 % could decrease secondary inorganic PM in winter haze events by 21 % (Han et al., 2020), while another found that a reduction of over 50 % was needed to have similar effects in the same region (Guo et al., 2018; Song et al., 2019). Another source of uncertainty lies in the source apportionment methods used to estimate the contribution of agricultural emissions to PM2.5. Source apportionment studies relying on air quality models use either the brute-force method (BFM, also known as the zero-out method) or the tagged-species-based approach. A recent study applying both methods to estimate the impact of agricultural NH3 emissions found that the tagged-species-based method attributes a 16 % contribution to PM2.5, whereas estimates from BFM reach up to 33 % (Han et al., 2020). While BFM is effective for sensitivity analysis in examining the responses of PM2.5 to reductions in precursor emissions, the tagged-species-based method is more suitable for source contribution studies owing to the nonlinear nature of PM2.5 in relation to its precursors.
From the perspective of PM2.5 pollution control, region-specific investigation of the responses of PM2.5 to precursor reductions with higher spatial resolutions is strongly preferred to larger-scale (e.g., national) analysis, and such investigation should be updated periodically as emission inventories are revised. There is also a lack of studies with high temporal resolutions, such as weekly or daily, which is particularly important because NH3 emissions typically peak about 1 week after fertilizer application and such temporal details may influence episodic PM2.5 pollution but are lost if monthly emissions are used (Nelson et al., 2019). A more detailed spatiotemporal analysis would refine our understanding of the specific locations and periods most influenced by agricultural activities, possibly enabling more effective pollution mitigation strategies.
2.4.2 Impacts of agricultural burning on air quality and human health
Agricultural burning significantly shapes atmospheric chemistry, particularly in South Asia and Africa, leading to the formation of harmful air pollutants including PM2.5 and ozone (O3), mostly via substantial emissions of primary PM, CO, CH4, and VOCs. Once these pollutants are released into the atmosphere, they can not only affect local areas but also be transported to downwind regions. The pollution-related health burdens from agricultural burning disproportionately affect low-income individuals in rural areas or near the burning sites (Reddington et al., 2021). PM2.5 emissions from agricultural fires are often considered more harmful than those from other sources due to their composition and the potential for long-range transport (Lin and Begho, 2022). Specifically, in Delhi, India, agricultural burning is shown to be responsible for approximately 7 % to 78 % of the enhanced PM2.5 concentrations (Cusworth et al., 2018). In Southeast Asia, agricultural and deforestation fires are estimated to account for about 40 % to 70 % of annual PM2.5 concentrations in northern Thailand, Myanmar, Cambodia, and Laos, resulting in ∼ 59 000 annual premature deaths (Reddington et al., 2021). These fires also contribute to O3 pollution, accounting for 5 % of the average daily maximum 8 h O3 concentration and causing ∼ 3800 annual premature deaths (Reddington et al., 2021). Agricultural burning is a major source of PM2.5 pollution in South Asia, contributing to its status as one of the most polluted regions globally (Lan et al., 2022; Lin and Begho, 2022). In Africa, agricultural burning contributes to 22 % of the annual average PM2.5, leading to 106 000 premature deaths, though another study estimated a lower number of ∼ 43 000 deaths (Gordon et al., 2023).
Agricultural burning in South Asia, Southeast Asia, and Africa is challenging to detect and characterize quantitatively due to its small scale but large frequency, and estimates based on satellite observations suffer from inadequate resolutions for such detection, leading to significant uncertainties in emission estimates (Korontzi et al., 2006). In addition, the widely used bottom-up methods for emission inventories heavily rely on crop-type-specific EFs but often use fixed factors for different crops, further increasing uncertainties (T. Zhang et al., 2020). The lack of air monitoring networks in these regions further complicates the linkage between fire activities and pollution-related health damage. More field measurements to identify important emitted species and track their chemical transformation for different cropping systems or crop types, especially in developing regions, are very much warranted.
2.4.3 Impacts of agricultural NOx and HONO emissions on air quality
Agricultural emissions of NOx from fertilized soils, historically overlooked in O3 research, are now acknowledged for their impacts on O3 pollution in agriculturally intensive regions. Recent studies in rural areas with intensive agricultural activities have shown that NOx emissions from fertilized soils significantly enhance ozone formation (Romer et al., 2018). For example, in California, agricultural NO emissions account for approximately 40 % of the total NOx emissions and contribute to ∼ 23 % of O3 formation (Sha et al., 2021). In China, agricultural soil NOx emissions may also account for ∼ 40 % of O3 nonattainment in some regions (Huang et al., 2023). Similarly, a US study suggested that in low-NOx environments, controlling agricultural soil NOx emissions is more effective for O3 reduction than the same level of control on biogenic VOCs (Geddes et al., 2022). Beyond NOx-limited regions, agricultural NOx emissions are also influential in some NOx-saturated or transition-regime areas where agricultural NOx emissions are on a par with combustion-related NOx emissions; in these regions, controlling agricultural NOx emissions can be more effective than controlling other anthropogenic sources (Lu et al., 2021b).
As the mitigation of anthropogenic non-agricultural NOx emissions becomes more successful, many regions may eventually transition to being NOx-limited, suggesting that the importance of agricultural NOx to O3 control is expected to rise. A better understanding of the impacts of agricultural NOx on O3 chemistry requires more accurate emission estimation and more precise source apportionment analyses. Previous studies using air quality models have either neglected soil NOx emissions or relied on simplified EF-based methods that fail to capture spatiotemporal variability in emissions. Recent advancements in mechanistic parameterization schemes, such as the Berkeley Dalhousie Soil NOx Parameterization (BDSNP) scheme (Hudman et al., 2012), have improved our understanding of soil NOx and O3 chemistry, but more field measurements from poorly researched regions are much needed to enhance regionalized applicability.
Finally, beyond NH3 and NOx, agricultural emissions of HONO are also important for atmospheric chemistry by affecting chemical processes in the atmosphere, mostly because of HONO's photolysis product, the hydroxyl radical (OH), which is the primary oxidant in the troposphere and is heavily involved in PM2.5 and O3 chemistry (Oswald et al., 2013). A recent modeling study revealed that HONO emissions from fertilized agricultural soils could increase average daytime O3 and daily particulate nitrate concentrations across the North China Plain by 8 % and 47 %, respectively (Wang et al., 2021) and by 4.6 % and 14 %, respectively, even in non-growing seasons (Wang et al., 2023). However, more accurate parameterization for HONO emissions is needed to improve the estimates.
2.4.4 Impacts of nitrogen deposition on terrestrial and aquatic ecosystems
The Nr compounds of agricultural origins often undergo transport and chemical transformation and are eventually deposited back onto the surface of terrestrial and aquatic ecosystems, resulting in increased nitrification, nutrient leaching, soil acidification (Guo et al., 2010), eutrophication (L. Liu et al., 2023), and biodiversity loss (Simkin et al., 2016), while also possibly enhancing forest growth and carbon storage (Liu et al., 2022; Lu et al., 2021a; Quinn Thomas et al., 2010) as well as marine productivity (Jickells and Moore, 2015). Enhanced Nr deposition to the open ocean has been known to generate high-productivity, low-oxygen zones with disrupted ecosystem functions (Doney, 2010). Due to historically more stringent emission controls on combustion NOx than agricultural NH3 emissions, Nr deposition patterns are shifting from being nitrate-dominated to ammonium-dominated, a trend observed in the USA and China and expected in Europe (Chen et al., 2020; Li et al., 2016; Liu et al., 2020; Tan et al., 2020), not only over inland regions but also in coastal areas (L. Liu et al., 2023). Although the deposition of oxidized Nr compounds has decreased, increased deposition of reduced Nr compounds from agricultural NH3 emissions, particularly in regions with intensive fertilizer use or near animal feeding operations, may offset such reduction (Chen et al., 2020; Tan et al., 2020). Control measures for Nr deposition show varied effectiveness between oxidized and reduced forms. Each unit of NOx control can achieve 80 %–120 % reductions in oxidized deposition, whereas each unit of NH3 control can only achieve 60 %–80 % reductions in reduced-form Nr deposition (Tan et al., 2020).
A recent paper has systematically reviewed the quantification methods for nitrogen deposition and summarized the major uncertainties (C. H. Zhang et al., 2021). Global monitoring networks for nitrogen deposition have been established, especially in the USA, Europe, and East Asia, offering relatively accurate data for wet deposition. However, significant challenges remain in measuring dry deposition due to the need for highly advanced instruments and analysis methods. Further technological innovations in measurements are warranted. The spatial distribution of observation sites also needs to be optimized to cover more representative locations and reduce sampling time to prevent sample losses. In addition, integration of Earth system models and satellite retrievals has enhanced our understanding of the spatial distribution and temporal variations in Nr deposition and their ecosystem effects (Liu et al., 2017; Zhao et al., 2017a). Nevertheless, model estimation of Nr deposition still has substantial limitations that arise from poor representation of the bidirectional exchange of NH3, inaccurate dry-deposition velocities, poor representation of organic nitrogen compounds, and uncertainties in Nr emission estimates. The utility of satellite observations is also constrained by their spatiotemporal coverage and retrieval methods. To enhance our current understanding of Nr deposition, a comprehensive framework that integrates these methods, supported by international collaboration, is strongly encouraged.
3.1 Cropland systems
Nitrogen management in croplands is a crucial challenge of the 21st century, as we need to balance food production with pollution mitigation (Houlton et al., 2019; Davidson et al., 2015). To that end, NUE is a vital metric. The current global average NUE stands at ∼ 0.4, yet we need to increase it to ∼ 0.7 by 2050 to meet the growing global food demand while minimizing environmental degradation, in line with the UN SDGs (Alexandratos and Bruinsma, 2012; Zhang et al., 2015). NUE varies globally, with higher values in high-income countries such as the USA and Canada (0.68), as well as Europe (0.52), and lower values in middle-income countries such as China (0.25) and India (0.30). In sub-Saharan Africa (0.72), NUE is high due to low fertilizer use but is expected to decrease as fertilizer use increases (Zhang et al., 2015).
Agricultural Nr emissions are closely tied to farming practices aimed at boosting crop productivity. The goal of nitrogen management is to match nutrient supply with crop demands effectively. Therefore, choosing appropriate farming practices, particularly adhering to the principles of “4R nutrient stewardship” (i.e., applying fertilizer with the right source, at the right rate, at the right time, and in the right place) (Bruulsema et al., 2009), has shown potential in mitigating Nr emissions while maintaining or even enhancing crop productivity (Gu et al., 2023). Additionally, using enhanced-efficiency fertilizers (Akiyama et al., 2009; Qiao et al., 2015) such as slow-release and controlled-release fertilizers, fertilizers containing nitrification inhibitors (NIs), and/or urease inhibitors (UIs); adopting efficient irrigation practices (Holcomb et al., 2011); and incorporating biochar amendments (Luo et al., 2023) can also help reduce Nr emissions (summarized in Table 2).
Table 2Effects of different management strategies on agricultural nitrogen emissions, as adapted from Gu et al. (2023).
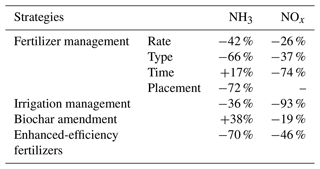
To mitigate agricultural burning emissions, eco-friendly crop residue management options have been explored. In situ methods such as reduced tillage hold much promise, yet they can also stimulate Nr emissions under certain conditions (Lin and Begho, 2022). Another approach involves converting crop residues into biochar or harnessing crop residues for renewable energy sources; however, these methods come with additional costs and technological requirements, making them less feasible in some developing countries (Lin and Begho, 2022). Effective crop residue management in South Asia and Africa remains a complex challenge that requires addressing various hurdles.
Cropland nitrogen management, while extensively researched, lacks a one-size-fits-all solution due to the diversity of cropping systems. The impact of various practices on Nr emissions varies significantly across regions and species. Managing Nr emissions often leads to trade-offs among different Nr species from fertilized soils (Gu et al., 2023; Pan et al., 2022; Qiao et al., 2015). Additionally, management strategies should account for other Nr losses, such as surface runoff, leaching, and potential changes in crop yield. Customized, region-specific, and even farm-specific evaluation is essential for harmonizing agricultural and environmental goals. Additionally, future climate change is likely to increase the occurrence of extreme weather events, imposing additional demands on cropland systems for resilience. This will further complicate nitrogen management, necessitating adaptive strategies to maintain agricultural productivity while managing Nr emissions effectively in the face of these evolving environmental challenges.
3.2 Livestock systems
Sustainable livestock management serves as another crucial pillar in achieving low-emission agriculture and food systems, with the pathway to this goal fundamentally rooted in the optimization of resource use efficiency. Guided by this principle, a series of measures regarding livestock management (e.g., sustainable intensification, animal health, and recoupling between cropping and livestock systems) and manure management (technological options at the feeding, housing, and storage stages) have been taken. Current estimates of emission reductions from these measures are limited, leaving great uncertainties in the outcomes of currently reported mitigation measures such as those listed in Table 3. Herd size can also help improve resource use efficiency (FAO, 2023). Industrial and intensive livestock farms can produce animal products more efficiently and have lower emissions compared to small farms (Herrero et al., 2013), but focusing solely on improving resource use efficiency may compromise other aspects, with issues such as local nutrient overload (Bai et al., 2022) and animal welfare harm (FAO, 2023). Furthermore, increasing use of industrial livestock farms disrupts nutrient recycling between livestock and croplands, inducing nutrient imbalances (Jin et al., 2020). It is important to note that a single measure may effectively control certain air pollutants while potentially increasing other air pollutants or greenhouse gas emissions. For example, anaerobic digestion, a biological process where bacteria degrade organic matter without oxygen, produces biogas for renewable energy, which can supply on-farm energy needs with lower emissions but may raise the NH3 emissions of the digestate (Yan et al., 2024). Overall, there are a number of abatement options, but more knowledge about their effectiveness, cost-effectiveness performance, and trade-offs is required to underpin the development of abatement measures or design of sustainable livestock systems.
3.3 Whole food systems
Entire food systems include not only on-farm production but also upstream and downstream stages such as agricultural input (e.g., fertilizer, pesticide) production, food processing, distribution, storage, retail, and consumption. Emission estimation and mitigation strategies for these off-farm stages as well as along the whole food chain are further complicated by dietary changes and food loss and waste, which can affect emissions at any stage along the chain. The widespread dietary shifts from plant-based to meat-intensive diets are the key driver for the globally increasing food demand, and meat-intensive diets not only are linked to increased risks of cardiovascular diseases, cancers, and type-2 diabetes, but also pose severe environmental threats (GBD, 2019; Liu et al., 2021a). For instance, during 1980–2010 in China, dietary change alone could have increased NH3 emissions by 63 % and annual mean PM2.5 by up to ∼ 10 µg m−3 (Liu et al., 2021a). The cited study further suggested that adopting more sustainable, healthier, less meat-intensive diets could decrease annual mean PM2.5 by 2–6 µg m−3 in China. Likewise, a worldwide shift to plant-based diets could cut agricultural emissions significantly, by 44 %–86 %, especially in regions with extensive livestock production (Springmann et al., 2023). Such dietary changes are expected to lower PM2.5 and O3 pollution by 3 %–7 % and 2 %–4 %, respectively; reduce premature mortality by 3 %–6 %; and enhance economic output by 0.5 %–1.1 %. However, a recent detailed study on alternative dietary shifts argued that specific changes should be made cautiously, as some types of shifts aimed at improving health and nutrition may increase emissions (Guo et al., 2022). Dietary shifts toward a more plant-based diet, which drive more intake of fruits, vegetables, and dairy products, can sometimes increase Nr emissions if such shifts require higher fertilizer inputs in low-NUE croplands.
In addition, food loss typically occurs in the pre-production and production stages due to inadequate management and technology, whereas food waste happens during retail and consumption. About one-third of the total food production ( t) is discarded as food loss and waste (FLW) (Shafiee-Jood and Cai, 2016). Efforts to reduce FLW have shown promising results in mitigating NH3 emissions and PM2.5 pollution, with estimates suggesting a potential reduction of up to 11.5 Tg in NH3 emissions and a decrease of about 5 µg m−3 in PM2.5 levels worldwide (Guo et al., 2023). In relation to nutrition demand, populations with excessive calorie intake are recommended to shift their diets toward healthier nutritional patterns, which can also reduce FLW and emissions (Lopez Barrera and Hertel, 2023).
Overall, reducing FLW and dietary changes can have multiple benefits for people, prosperity, and the planet; specifically concerning atmospheric chemistry, they help mitigate pollutant emissions throughout the whole food supply chain by reducing the overall food demand. Currently, agricultural emission abatement is usually more focused on on-farm production and the food supply. Stronger emphasis on whole-food-system transformation and formulating integrated policies that target both the demand and the supply sides of food and agriculture are much warranted.
Sustainable development is development that aims to meet the needs of the present generation without compromising the ability of future generations to meet their own needs (Brundtland, 1987). It is a holistic approach that emphasizes that the “needs” of everyone, especially the poor and disadvantaged, should be prioritized and that there are “limitations” to the environment's ability to meet such needs. The goals of sustainable development are thus to seek economic prosperity for people in a way that is socially inclusive and environmentally responsible; that is, the economy, society, and the environment are equally important considerations when pursuing long-term human development. The 17 UN SDGs adopted in 2015 provide a framework for governments, businesses, and civil society to work toward sustainable development across all sectors, of which agriculture and food systems are among the most important, as most obviously indicated by SDG 2, “Zero Hunger”, which aims to end hunger, achieve food security, enhance nutrition, and promote sustainable and climate-resilient food systems. However, the SDGs are not meant to be standalone objectives; rather they are interconnected and need to be considered holistically to achieve various objectives, and here we argue that a better understanding of agricultural and food-system contributions to atmospheric chemistry, including both the composition and chemical processes of the atmosphere, is indeed crucial to help stakeholders achieve SDG 2 in synchrony with other SDGs, especially SDG 3, “Good Health and Well-being”; SDG 13, “Climate Action”; SDG 14, “Life Below Water”; and SDG 15, “Life on Land”, but also various other SDGs more indirectly.
The previous sections have highlighted how agriculture and food systems are important sources of Nr and other air-quality-relevant compounds and thus contribute substantially to PM2.5 pollution and, to a lesser but increasingly important extent, to O3 pollution. These pollutants are shown to cause some of the most fatal non-communicable diseases such as cardiovascular diseases, cancers, and respiratory diseases, taking a significant toll on human health and well-being worldwide. Therefore, better understanding and better quantification of these sources are crucial to achieving SDG 3, “Good Health and Well-being”, which aims to ensure healthy lives and promote well-being for all at all ages. This is particularly important now as so many emission control efforts have already been in place for decades to reduce non-agricultural sources of air pollutants, such as combustion-derived NOx and SO2 from the energy and transportation sectors, but relatively little has been done to mitigate agricultural emissions, and we foresee the increasing dominance of agricultural Nr as well as unmitigated agricultural burning in shaping future aerosol chemistry. To that end, as reviewed above (Sect. 2.1), a better understanding of the magnitudes and drivers of Nr emissions is much needed, and scientists need specifically to
-
conduct more field measurements in representative agricultural systems to better capture the responses of Nr emissions to driving factors and provide more comprehensive datasets for evaluating, calibrating, and refining emission models and estimates;
-
refine EFs within the EF approach by incorporating localized adjustments based on extensive field measurements for different crop types, cropping systems, and livestock systems across diverse regions;
-
incorporate process-based agroecosystem models with enhanced representation of the nitrogen cycle to improve emission estimates on fine spatiotemporal scales and to capture the episodic and dynamic responses of Nr emissions to fertilizer applications, extreme weather events, and changes in farming practices;
-
utilize geostationary satellite observations with more sophisticated retrieval methods, especially for agricultural burning detection and short-term soil responses to fertilizer applications.
These improvements would greatly help decrease uncertainties associated with Nr emission estimates and their adverse impacts on the atmospheric environment and thus help us devise better control policies. Currently, only the European Union has established NH3 emission control targets, aiming to reduce NH3 emissions by 19 % in 2030 compared to 2005 levels, as per the National Emission Ceilings Directive (EU, 2016). In China, in late 2023, a decade after the initial launch of the Action Plan for Fighting for a Blue Sky, new actions were announced to focus on controlling agricultural NH3 emissions (Council, 2023). These included specific targets for the Beijing–Tianjin–Hebei region, aiming for a 5 % reduction by 2025 compared to 2020 levels. Further improvements are still needed in Europe; China; and also the USA, where no specific mitigation targets have been planned, but relatively extensive research in these regions has already informed policy approaches elsewhere. Other countries and regions are expected to follow suit, and more research, especially for poorly researched developing regions such as those in South Asia, Southeast Asia, Africa, and Latin America, is necessary to guide their mitigation efforts.
To mitigate agricultural and food-system emissions of Nr and other pollutants, in light of the complex region- and species-specific responses of Nr emissions across multiple stages from whole food systems (Sect. 3), we also need to focus more research efforts on the various mitigation pathways, including
-
identifying strategies that can effectively mitigate multiple Nr species and benefit agricultural productivity without exacerbating other Nr losses, acknowledging the trade-offs commonly observed in mitigation strategies;
-
developing customized strategies tailored to the specific conditions of each region, farm, or facility, given the variability in the effectiveness of Nr emission control strategies;
-
enhancing our understanding of the costs and outcomes of various mitigation measures, which is crucial for developing socioeconomically sound strategies with minimal additional investment, particularly for low-income regions;
-
emphasizing integrated policies that consider the entire food supply chain and food demand to maximize the socioeconomic and environmental benefits of emission reduction measures.
Such efforts are recommended for both developing and developed regions.
Greater research efforts in the above can arguably help us address SDG 13, “Climate Action”, as well, which aims to take urgent action to combat climate change and its impacts, as many of the short-lived Nr species share common sources with N2O, the third most potent greenhouse gas. Furthermore, agriculture influences ecosystems not only via atmospheric Nr deposition but also via direct nutrient leaching and runoff to waterbodies. Nitrogen pollution can bring tremendous disruptions to terrestrial and aquatic ecosystems, often modifying both ecosystem productivity and biodiversity. Therefore, mitigating agricultural and food-system emissions also helps us strive toward SDG 14, “Life Below Water”, which aims to conserve marine and coastal ecosystems and sustainably use their resources for sustainable development, and SDG 15, “Life on Land”, which aims to protect, restore, and sustainably manage terrestrial ecosystems, promote biodiversity conservation, and combat desertification and land degradation. Although the fates of various agricultural Nr compounds in terrestrial and aquatic ecosystems may be more within the realm of biogeochemistry, atmospheric scientists are necessary to better quantify the ecosystem input of Nr via atmospheric deposition (Sect. 2.4.4), especially via (Q. Zhang et al., 2021)
-
enhancing the Nr deposition monitoring network with a focus on technological innovations for dry-deposition measurements and increased spatial resolutions by including more representative sites;
-
improving model-based analysis by better parameterizing both wet- and dry-deposition processes, as well as by providing more accurate Nr emission estimates to drive model simulations;
-
advancing satellite-based analysis with more refined retrieval methods;
-
developing a comprehensive framework that integrates monitoring, air quality modeling, and satellite observations.
It is essential to consider the mitigation strategies discussed above in synergy with other socioeconomic objectives. For instance, if top-down approaches are used to reform the food systems in ways that ignore the actual needs of the farmers or even deprive the farmers of their livelihood, cultural heritage, and social inclusion, such approaches do not adhere to the tenets of sustainability even if they are effective in abating food-system emissions. Indigenous knowledge, cultures, and traditions in the local food systems always have to be proactively considered. Often reducing food-system emissions would bring immediate health benefits to the farmers and people in agricultural regions in general due to their reduced exposure to airborne and waterborne (e.g., fertilizer, pesticide, and animal waste runoff) agricultural pollutants, which would in the long term improve their productivity and livelihoods. Furthermore, by promoting sustainable agricultural practices, supporting local food production, improving distribution networks, and reducing food waste, food-system transformation can help both rural and urban populations gain access to safe, nutritious, and affordable food, which is essential for fostering socially inclusive communities. Therefore, transforming the food systems in economically feasible, socially equitable, and environmentally responsible ways, facilitated by better understanding of the science of agriculture–environment interactions behind the systems, can also help us address SDG 1, “No Poverty”, which aims to end poverty by addressing its root causes, promoting social protection systems, and enhancing access to basic services and resources; SDG 6, “Clean Water and Sanitation”, which aims to ensure universal access to clean water and sanitation, improve water quality, and promote sustainable water management practices; and SDG 11, “Sustainable Cities and Communities”, which aims to make cities and human settlements inclusive, safe, resilient, and sustainable.
We therefore opine that, in consideration of the substantial impacts of agricultural and food-system emissions on atmospheric chemistry, on air pollution, and subsequently on terrestrial and aquatic ecosystems, we as a society need to take concrete actions to transform food systems so as to simultaneously ensure food security for the masses; lessen the human health and ecological impacts of agricultural pollutants; improve the livelihood of farmers and agricultural workers; and help cities and communities become economically, socially, and environmentally sustainable. That is, in essence, we need to take concrete actions to achieve multiple SDGs. To that end, scientists play vital roles in providing detailed, process-based understanding of agricultural and food-system emissions as well as of the fates and wider impacts of the emitted compounds. Above we have specifically highlighted several knowledge gaps and aspects that warrant much more research effort, which are necessary to guide food-system transformation along technologically and economically feasible as well as socially and environmentally responsible paths. This could be one of the key ways through which we scientists can fulfill not only our professional responsibility, but also our social responsibility.
No datasets were used in this article.
APKT conceived, wrote, and revised the opinion paper and diagnosed connections of atmospheric chemistry to sustainable development. LL and BL reviewed the current literature and drafted most parts on emissions, chemistry, and mitigation methods. LL was involved extensively in the revision of the manuscript.
At least one of the (co-)authors is a member of the editorial board of Atmospheric Chemistry and Physics. The peer-review process was guided by an independent editor, and the authors also have no other competing interests to declare.
Publisher's note: Copernicus Publications remains neutral with regard to jurisdictional claims made in the text, published maps, institutional affiliations, or any other geographical representation in this paper. While Copernicus Publications makes every effort to include appropriate place names, the final responsibility lies with the authors.
This article is part of the special issue “20 years of Atmospheric Chemistry and Physics”. It is not associated with a conference.
The General Research Fund supported this work (grant no. 14307722) granted by the Research Grants Council (RGC) to Amos P. K. Tai, as well as funding from the State Key Laboratory of Agrobiotechnology and the Innovation and Technology Commission (grant nos. 8300031, 8300036, 8300070) granted to Amos P. K. Tai.
This research has been supported by the Research Grants Council, University Grants Committee (grant no. 14307722), and the State Key Laboratory for Agrobiotechnology (grant nos. 8300031, 8300036, and 8300070).
This paper was edited by Barbara Ervens and James Allan and reviewed by two anonymous referees.
Akiyama, H., Yan, X., and Yagi, K.: Evaluation of effectiveness of enhanced-efficiency fertilizers as mitigation options for N2O and NO emissions from agricultural soils: meta-analysis: MITIGATION OPTIONS FOR N2O AND NO EMISSIONS, Global Change Biol., 16, 1837–1846, 10.1111/j.1365-2486.2009.02031.x, 2009.
Alexandratos, N. and Bruinsma, J.: World agriculture towards 2030/2050: the 2012 revision (No. 12-03), ESA Working Paper, World Food and Agricultural Organization, Rome, Italy, https://www.fao.org/4/ap106e/ap106e.pdf (last access: 19 December 2024), 2012.
Ansari, A. S. and Pandis, S. N.: Response of Inorganic PM to Precursor Concentrations, Environ. Sci. Technol., 32, 2706–2714, https://doi.org/10.1021/es971130j, 1998.
Bai, Z., Fan, X., Jin, X., Zhao, Z., Wu, Y., Oenema, O., Velthof, G., Hu, C., and Ma, L.: Relocate 10 billion livestock to reduce harmful nitrogen pollution exposure for 90 % of China's population, Nat. Food, 3, 152–160, https://doi.org/10.1038/s43016-021-00453-z, 2022.
Balasubramanian, S., McFarland, D. M., Koloutsou-Vakakis, S., Fu, K., Menon, R., Lehmann, C., and Rood, M. J.: Effect of grid resolution and spatial representation of NH3 emissions from fertilizer application on predictions of NH3 and PM2.5 concentrations in the United States Corn Belt, Environ. Res. Commun., 2, https://doi.org/10.1088/2515-7620/ab6c01, 2020.
Balasubramanian, S., Domingo, N. G. G., Hunt, N. D., Gittlin, M., Colgan, K. K., Marshall, J. D., Robinson, A. L., Azevedo, I. M. L., Thakrar, S. K., Clark, M. A., Tessum, C. W., Adams, P. J., Pandis, S. N., and Hill, J. D.: The food we eat, the air we breathe: a review of the fine particulate matter-induced air quality health impacts of the global food system, Environ. Res. Lett., 16, 103004, https://doi.org/10.1088/1748-9326/ac065f, 2021.
Bauer, S. E., Tsigaridis, K., and Miller, R.: Significant atmospheric aerosol pollution caused by world food cultivation, Geophys. Res. Lett., 43, 5394–5400, https://doi.org/10.1002/2016GL068354, 2016.
Bobbink, R., Hicks, K., Galloway, J., Spranger, T., Alkemade, R., Ashmore, M., Bustamante, M., Cinderby, S., Davidson, E., Dentener, F., Emmett, B., Erisman, J. W., Fenn, M., Gilliam, F., Nordin, A., Pardo, L., and De Vries, W.: Global assessment of nitrogen deposition effects on terrestrial plant diversity: a synthesis, Ecol. Appl., 20, 30–59, https://doi.org/10.1890/08-1140.1, 2010.
Bouwman, A. F., Lee, D. S., Asman, W. A. H., Dentener, F. J., Van Der Hoek, K. W., and Olivier, J. G. J.: A global high-resolution emission inventory for ammonia, Global Biogeochem. Cy., 11, 561–587, https://doi.org/10.1029/97gb02266, 1997.
Brundtland, G. H.: Our Common Future – Call for Action, Environ. Conserv., 14, 291–294, https://doi.org/10.1017/S0376892900016805, 1987.
Bruulsema, T., Lemunyon, J., and Herz, B.: Know your fertilizer rights, Crops and Soils, 42, 13–18, 2009.
Butterbach-Bahl, K., Baggs, E. M., Dannenmann, M., Kiese, R., and Zechmeister-Boltenstern, S.: Nitrous oxide emissions from soils: how well do we understand the processes and their controls?, Phil. Trans. R. Soc. B, 368, 20130122, https://doi.org/10.1098/rstb.2013.0122, 2013.
Chen, Y., Shen, H., Shih, J.-S., Russell, A. G., Shao, S., Hu, Y., Odman, M. T., Nenes, A., Pavur, G. K., Zou, Y., Chen, Z., Smith, R. A., Burtraw, D., and Driscoll, C. T.: Greater Contribution From Agricultural Sources to Future Reactive Nitrogen Deposition in the United States, Earth's Future, 8, e2019EF001453, https://doi.org/10.1029/2019EF001453, 2020.
Chen, Y., Hall, J., van Wees, D., Andela, N., Hantson, S., Giglio, L., van der Werf, G. R., Morton, D. C., and Randerson, J. T.: Multi-decadal trends and variability in burned area from the fifth version of the Global Fire Emissions Database (GFED5), Earth Syst. Sci. Data, 15, 5227–5259, https://doi.org/10.5194/essd-15-5227-2023, 2023.
Council, S.: Action Plan for Continuous Improvement of Air Quality, https://english.www.gov.cn/policies/latestreleases/202312/08/content_WS65724f25c6d0868f4e8e1fcb.html/ (last access: 19 December 2024), 2023.
Crippa, M., Guizzardi, D., Muntean, M., Schaaf, E., Dentener, F., van Aardenne, J. A., Monni, S., Doering, U., Olivier, J. G. J., Pagliari, V., and Janssens-Maenhout, G.: Gridded emissions of air pollutants for the period 1970–2012 within EDGAR v4.3.2, Earth Syst. Sci. Data, 10, 1987–2013, https://doi.org/10.5194/essd-10-1987-2018, 2018.
Crippa, M., Solazzo, E., Guizzardi, D., Monforti-Ferrario, F., Tubiello, F. N., and Leip, A.: Food systems are responsible for a third of global anthropogenic GHG emissions, Nat. Food, 2, 198–209, https://doi.org/10.1038/s43016-021-00225-9, 2021.
Crippa, M., Solazzo, E., Guizzardi, D., Van Dingenen, R., and Leip, A.: Air pollutant emissions from global food systems are responsible for environmental impacts, crop losses and mortality, Nat. Food, 3, 942–956, https://doi.org/10.1038/s43016-022-00615-7, 2022a.
Crippa, M., Solazzo, E., Guizzardi, D., Van Dingenen, R., and Leip, A.: Air pollutant emissions from global food systems are responsible for environmental impacts, crop losses and mortality, Nat. Food, 3, 942–956, https://doi.org/10.1038/s43016-022-00615-7, 2022b.
Crippa, M., Guizzardi, D., Butler, T., Keating, T., Wu, R., Kaminski, J., Kuenen, J., Kurokawa, J., Chatani, S., Morikawa, T., Pouliot, G., Racine, J., Moran, M. D., Klimont, Z., Manseau, P. M., Mashayekhi, R., Henderson, B. H., Smith, S. J., Suchyta, H., Muntean, M., Solazzo, E., Banja, M., Schaaf, E., Pagani, F., Woo, J.-H., Kim, J., Monforti-Ferrario, F., Pisoni, E., Zhang, J., Niemi, D., Sassi, M., Ansari, T., and Foley, K.: The HTAP_v3 emission mosaic: merging regional and global monthly emissions (2000–2018) to support air quality modelling and policies, Earth Syst. Sci. Data, 15, 2667–2694, https://doi.org/10.5194/essd-15-2667-2023, 2023.
Cusworth, D. H., Mickley, L. J., Sulprizio, M. P., Liu, T., Marlier, M. E., DeFries, R. S., Guttikunda, S. K., and Gupta, P.: Quantifying the influence of agricultural fires in northwest India on urban air pollution in Delhi, India, Environ. Res. Lett., 13, 044018, https://doi.org/10.1088/1748-9326/aab303, 2018.
Davidson, E. A., Suddick, E. C., Rice, C. W., and Prokopy, L. S.: More Food, Low Pollution (Mo Fo Lo Po): A Grand Challenge for the 21st Century, J. Environ. Qual., 44, 305–311, https://doi.org/10.2134/jeq2015.02.0078, 2015.
Del Grosso, S. J., Ojima, D. S., Parton, W. J., Stehfest, E., Heistemann, M., DeAngelo, B., and Rose, S.: Global scale DAYCENT model analysis of greenhouse gas emissions and mitigation strategies for cropped soils, Global Planet. Change, 67, 44–50, https://doi.org/10.1016/j.gloplacha.2008.12.006, 2009.
Del Grosso, S. J., Ogle, S. M., Nevison, C., Gurung, R., Parton, W. J., Wagner-Riddle, C., Smith, W., Winiwarter, W., Grant, B., Tenuta, M., Marx, E., Spencer, S., and Williams, S.: A gap in nitrous oxide emission reporting complicates long-term climate mitigation, P. Natl. Acad. Sci. USA, 119, e2200354119, https://doi.org/10.1073/pnas.2200354119, 2022.
Deng, J., Nie, W., Huang, X., Ding, A., Qin, B., and Fu, C.: Atmospheric Reactive Nitrogen Deposition from 2010 to 2021 in Lake Taihu and the Effects on Phytoplankton, Environ. Sci. Technol., 57, 8075–8084, https://doi.org/10.1021/acs.est.2c09434, 2023.
Deng, O., Huang, S., Wang, C., Wei, Y., Xia, Y., Liu, Z., Zhang, X., Xiao, W., He, T., Wu, X., Pradhan, M., and Gu, B.: Atmospheric Nitrogen Pollution Control Benefits the Coastal Environment, Environ. Sci. Technol., 58, 449–458, https://doi.org/10.1021/acs.est.3c07546, 2024a.
Deng, O., Wang, S., Ran, J., Huang, S., Zhang, X., Duan, J., Zhang, L., Xia, Y., Reis, S., Xu, J., Xu, J., de Vries, W., Sutton, M. A., and Gu, B.: Managing urban development could halve nitrogen pollution in China, Nat. Commun., 15, 401, https://doi.org/10.1038/s41467-023-44685-y, 2024b.
Domingo, N. G. G., Balasubramanian, S., Thakrar, S. K., Clark, M. A., Adams, P. J., Marshall, J. D., Muller, N. Z., Pandis, S. N., Polasky, S., Robinson, A. L., Tessum, C. W., Tilman, D., Tschofen, P., and Hill, J. D.: Air quality–related health damages of food, P. Natl. Acad. Sci. USA, 118, e2013637118, https://doi.org/10.1073/pnas.2013637118, 2021.
Doney, S. C.: The Growing Human Footprint on Coastal and Open-Ocean Biogeochemistry, Science, 328, 1512–1516, https://doi.org/10.1126/science.1185198, 2010.
EU: Directive (EU) 2016/2284 of the European Parliament and of the Council of 14 December 2016 on the reduction of national emissions of certain atmospheric pollutants, amending Directive 2003/35/EC and repealing Directive 2001/81/EC, https://www.legislation.gov.uk/eudr/2016/2284/introduction/ (last access: 19 December 2024), 2016.
FAO: The future of food and agriculture – Alternative pathways to 2050, Food and Agriculture Organization of the United Nations, Rome, Italy, 224, https://openknowledge.fao.org/server/api/core/bitstreams/2c6bd7b4-181e-4117-a90d-32a1bda8b27c/content/ (last access: 19 December 2024), 2018.
FAO: Pathways towards lower emissions A global assessment of the greenhouse gas emissions and mitigation options from livestock agrifood systems, Rome, https://doi.org/10.4060/cc9029en, 2023.
FAO: Fertilizers by Nutrient, https://www.fao.org/faostat/en/#data/ESB (last access: 19 December 2024), 2024.
Foley, J. A., Ramankutty, N., Brauman, K. A., Cassidy, E. S., Gerber, J. S., Johnston, M., Mueller, N. D., O'Connell, C., Ray, D. K., West, P. C., Balzer, C., Bennett, E. M., Carpenter, S. R., Hill, J., Monfreda, C., Polasky, S., Rockstrom, J., Sheehan, J., Siebert, S., Tilman, D., and Zaks, D. P. M.: Solutions for a cultivated planet, Nature, 478, 337–342, https://doi.org/10.1038/Nature10452, 2011.
Fowler, D., Coyle, M., Skiba, U., Sutton, M. A., Cape, J. N., Reis, S., Sheppard, L. J., Jenkins, A., Grizzetti, B., Galloway, J. N., Vitousek, P., Leach, A., Bouwman, A. F., Butterbach-Bahl, K., Dentener, F., Stevenson, D., Amann, M., and Voss, M.: The global nitrogen cycle in the twenty-first century, Philos. T. R. Soc. Lond. B, 368, 20130164, https://doi.org/10.1098/rstb.2013.0164, 2013.
Freney, J. R., Simpson, J. R., and Denmead, O. T.: AMMONIA VOLATILIZATION, Ecol. Bull., 291–302, 1981.
Fu, X., Wang, S. X., Xing, J., Zhang, X. Y., Wang, T., and Hao, J. M.: Increasing Ammonia Concentrations Reduce the Effectiveness of Particle Pollution Control Achieved via SO2 and NOX Emissions Reduction in East China, Environ. Sci. Technol. Lett., 4, 221–227, https://doi.org/10.1021/acs.estlett.7b00143, 2017.
Galloway, J. N., Leach, A. M., Bleeker, A., and Erisman, J. W.: A chronology of human understanding of the nitrogen cycle, Phil. T. R. Soc. B, 368, 20130120, https://doi.org/10.1098/rstb.2013.0120, 2013.
GBD: Health effects of dietary risks in 195 countries, 1990–2017: a systematic analysis for the Global Burden of Disease Study 2017, Lancet, 393, 1958–1972, https://doi.org/10.1016/S0140-6736(19)30041-8, 2019.
Geddes, J. A., Pusede, S. E., and Wong, A. Y. H.: Changes in the Relative Importance of Biogenic Isoprene and Soil NOx Emissions on Ozone Concentrations in Nonattainment Areas of the United States, J. Geophys. Res.-Atmos., 127, e2021JD036361, https://doi.org/10.1029/2021JD036361, 2022.
Gordon, J. N. D., Bilsback, K. R., Fiddler, M. N., Pokhrel, R. P., Fischer, E. V., Pierce, J. R., and Bililign, S.: The Effects of Trash, Residential Biofuel, and Open Biomass Burning Emissions on Local and Transported PM(2.5) and Its Attributed Mortality in Africa, Geohealth, 7, e2022GH000673, https://doi.org/10.1029/2022GH000673, 2023.
Griffis, T. J., Chen, Z., Baker, J. M., Wood, J. D., Millet, D. B., Lee, X., Venterea, R. T., and Turner, P. A.: Nitrous oxide emissions are enhanced in a warmer and wetter world, P. Natl. Acad. Sci. USA, 114, 12081–12085, https://doi.org/10.1073/pnas.1704552114, 2017.
Gu, B., Song, Y., Yu, C., and Ju, X.: Overcoming socioeconomic barriers to reduce agricultural ammonia emission in China, Environ. Sci. Pollut. Res. Int., 27, 25813–25817, https://doi.org/10.1007/s11356-020-09154-9, 2020.
Gu, B., Zhang, L., Van Dingenen, R., Vieno, M., Van Grinsven, H. J. M., Zhang, X., Zhang, S., Chen, Y., Wang, S., Ren, C., Rao, S., Holland, M., Winiwarter, W., Chen, D., Xu, J., and Sutton, M. A.: Abating ammonia is more cost-effective than nitrogen oxides for mitigating PM2.5 air pollution, Science, 374, 758–762, https://doi.org/10.1126/science.abf8623, 2021.
Gu, B., Zhang, X., Lam, S. K., Yu, Y., Van Grinsven, H. J. M., Zhang, S., Wang, X., Bodirsky, B. L., Wang, S., Duan, J., Ren, C., Bouwman, L., De Vries, W., Xu, J., Sutton, M. A., and Chen, D.: Cost-effective mitigation of nitrogen pollution from global croplands, Nature, 613, 77–84, https://doi.org/10.1038/s41586-022-05481-8, 2023.
Guo, H., Otjes, R., Schlag, P., Kiendler-Scharr, A., Nenes, A., and Weber, R. J.: Effectiveness of ammonia reduction on control of fine particle nitrate, Atmos. Chem. Phys., 18, 12241–12256, https://doi.org/10.5194/acp-18-12241-2018, 2018.
Guo, J. H., Liu, X. J., Zhang, Y., Shen, J. L., Han, W. X., Zhang, W. F., Christie, P., Goulding, K. W. T., Vitousek, P. M., and Zhang, F. S.: Significant Acidification in Major Chinese Croplands, Science, 327, 1008–1010, https://doi.org/10.1126/science.1182570, 2010.
Guo, Y., He, P., Searchinger, T. D., Chen, Y., Springmann, M., Zhou, M., Zhang, X., Zhang, L., and Mauzerall, D. L.: Environmental and human health trade-offs in potential Chinese dietary shifts, One Earth, 5, 268–282, https://doi.org/10.1016/j.oneear.2022.02.002, 2022.
Hall, J. V., Zibtsev, S. V., Giglio, L., Skakun, S., Myroniuk, V., Zhuravel, O., Goldammer, J. G., and Kussul, N.: Environmental and political implications of underestimated cropland burning in Ukraine, Environ. Res. Lett., 16, 064019, https://doi.org/10.1088/1748-9326/abfc04, 2021.
Han, X., Zhu, L., Liu, M., Song, Y., and Zhang, M.: Numerical analysis of agricultural emissions impacts on PM2.5 in China using a high-resolution ammonia emission inventory, Atmos. Chem. Phys., 20, 9979–9996, https://doi.org/10.5194/acp-20-9979-2020, 2020.
Herrero, M., Havlik, P., Valin, H., Notenbaert, A., Rufino, M. C., Thornton, P. K., Blummel, M., Weiss, F., Grace, D., and Obersteiner, M.: Biomass use, production, feed efficiencies, and greenhouse gas emissions from global livestock systems, P. Natl. Acad. Sci. USA, 110, 20888–20893, https://doi.org/10.1073/pnas.1308149110, 2013.
Hill, J., Goodkind, A., Tessum, C., Thakrar, S., Tilman, D., Polasky, S., Smith, T., Hunt, N., Mullins, K., Clark, M., and Marshall, J.: Air-quality-related health damages of maize, Nat. Sustain., 2, 397–403, https://doi.org/10.1038/s41893-019-0261-y, 2019.
Holcomb, J. C., Sullivan, D. M., Horneck, D. A., and Clough, G. H.: Effect of Irrigation Rate on Ammonia Volatilization, Soil Sci. Soc. Am. J., 75, 2341–2347, https://doi.org/10.2136/sssaj2010.0446, 2011.
Holt, J., Selin, N. E., and Solomon, S.: Changes in Inorganic Fine Particulate Matter Sensitivities to Precursors Due to Large-Scale US Emissions Reductions, Environ. Sci. Technol., 49, 4834–4841, https://doi.org/10.1021/acs.est.5b00008, 2015.
Hou, Y., Velthof, G. L., and Oenema, O.: Mitigation of ammonia, nitrous oxide and methane emissions from manure management chains: a meta-analysis and integrated assessment, Glob. Change Biol., 21, 1293–1312, https://doi.org/10.1111/gcb.12767, 2015.
Houlton, B. Z., Almaraz, M., Aneja, V., Austin, A. T., Bai, E., Cassman, K. G., Compton, J. E., Davidson, E. A., Erisman, J. W., Galloway, J. N., Gu, B., Yao, G., Martinelli, L. A., Scow, K., Schlesinger, W. H., Tomich, T. P., Wang, C., and Zhang, X.: A World of Cobenefits: Solving the Global Nitrogen Challenge, Earth's Fut., 7, 865–872, https://doi.org/10.1029/2019EF001222, 2019.
Huang, L., Fang, J., Liao, J., Yarwood, G., Chen, H., Wang, Y., and Li, L.: Insights into soil NO emissions and the contribution to surface ozone formation in China, Atmos. Chem. Phys., 23, 14919–14932, https://doi.org/10.5194/acp-23-14919-2023, 2023.
Huang, T. B., Zhu, X., Zhong, Q. R., Yun, X., Meng, W. J., Li, B. G., Ma, J. M., Zeng, E. Y., and Tao, S.: Spatial and Temporal Trends in Global Emissions of Nitrogen Oxides from 1960 to 2014, Environ. Sci. Technol., 51, 7992–8000, https://doi.org/10.1021/acs.est.7b02235, 2017.
Huang, X., Song, Y., Li, M., Li, J., Huo, Q., Cai, X., Zhu, T., Hu, M., and Zhang, H.: A high-resolution ammonia emission inventory in China, Global Biogeochem. Cy., 26, 2011GB004161, https://doi.org/10.1029/2011gb004161, 2012.
Hudman, R. C., Moore, N. E., Mebust, A. K., Martin, R. V., Russell, A. R., Valin, L. C., and Cohen, R. C.: Steps towards a mechanistic model of global soil nitric oxide emissions: implementation and space based-constraints, Atmos. Chem. Phys., 12, 7779–7795, https://doi.org/10.5194/acp-12-7779-2012, 2012.
Jickells, T. and Moore, C. M.: The Importance of Atmospheric Deposition for Ocean Productivity, Ann. Rev. Ecol. Evolut. System., 46, 481–501, https://doi.org/10.1146/annurev-ecolsys-112414-054118, 2015.
Jickells, T. D., Buitenhuis, E., Altieri, K., Baker, A. R., Capone, D., Duce, R. A., Dentener, F., Fennel, K., Kanakidou, M., LaRoche, J., Lee, K., Liss, P., Middelburg, J. J., Moore, J. K., Okin, G., Oschlies, A., Sarin, M., Seitzinger, S., Sharples, J., Singh, A., Suntharalingam, P., Uematsu, M., and Zamora, L. M.: A reevaluation of the magnitude and impacts of anthropogenic atmospheric nitrogen inputs on the ocean, Global Biogeochem. Cy., 31, 289–305, https://doi.org/10.1002/2016gb005586, 2017.
Jin, S., Zhang, B., Wu, B., Han, D., Hu, Y., Ren, C., Zhang, C., Wei, X., Wu, Y., Mol, A. P. J., Reis, S., Gu, B., and Chen, J.: Decoupling livestock and crop production at the household level in China, Nat. Sustain., 4, 48–55, https://doi.org/10.1038/s41893-020-00596-0, 2020.
Kim, J., Jeong, U., Ahn, M. H., Kim, J. H., Park, R. J., Lee, H., Song, C. H., Choi, Y. S., Lee, K. H., Yoo, J. M., Jeong, M. J., Park, S. K., Lee, K. M., Song, C. K., Kim, S. W., Kim, Y. J., Kim, S. W., Kim, M., Go, S., Liu, X., Chance, K., Chan Miller, C., Al-Saadi, J., Veihelmann, B., Bhartia, P. K., Torres, O., Abad, G. G., Haffner, D. P., Ko, D. H., Lee, S. H., Woo, J. H., Chong, H., Park, S. S., Nicks, D., Choi, W. J., Moon, K. J., Cho, A., Yoon, J., Kim, S. K., Hong, H., Lee, K., Lee, H., Lee, S., Choi, M., Veefkind, P., Levelt, P. F., Edwards, D. P., Kang, M., Eo, M., Bak, J., Baek, K., Kwon, H. A., Yang, J., Park, J., Han, K. M., Kim, B. R., Shin, H. W., Choi, H., Lee, E., Chong, J., Cha, Y., Koo, J. H., Irie, H., Hayashida, S., Kasai, Y., Kanaya, Y., Liu, C., Lin, J., Crawford, J. H., Carmichael, G. R., Newchurch, M. J., Lefer, B. L., Herman, J. R., Swap, R. J., Lau, A. K. H., Kurosu, T. P., Jaross, G., Ahlers, B., Dobber, M., McElroy, C. T., and Choi, Y.: New Era of Air Quality Monitoring from Space: Geostationary Environment Monitoring Spectrometer (GEMS), B. Am. Meteorol. Soc., 101, E1–E22, https://doi.org/10.1175/Bams-D-18-0013.1, 2020.
Korontzi, S., McCarty, J., Loboda, T., Kumar, S., and Justice, C.: Global distribution of agricultural fires in croplands from 3 years of Moderate Resolution Imaging Spectroradiometer (MODIS) data, Global Biogeochem. Cy., 20, GB2021, https://doi.org/10.1029/2005gb002529, 2006.
Lan, R., Eastham, S. D., Liu, T., Norford, L. K., and Barrett, S. R. H.: Air quality impacts of crop residue burning in India and mitigation alternatives, Nat Commun, 13, 6537, https://doi.org/10.1038/s41467-022-34093-z, 2022.
Lelieveld, J., Evans, J. S., Fnais, M., Giannadaki, D., and Pozzer, A.: The contribution of outdoor air pollution sources to premature mortality on a global scale, Nature, 525, 367–371, https://doi.org/10.1038/nature15371, 2015.
Li, C., Aber, J., Stange, F., Butterbach-Bahl, K., and Papen, H.: A process-oriented model of N2O and NO emissions from forest soils: 1. Model development, J. Geophys. Res.-Atmos., 105, 4369–4384, https://doi.org/10.1029/1999JD900949, 2000.
Li, M., Jia, N., Lenzen, M., Malik, A., Wei, L., Jin, Y., and Raubenheimer, D.: Global food-miles account for nearly 20 % of total food-systems emissions, Nat. Food, 3, 445–453, https://doi.org/10.1038/s43016-022-00531-w, 2022.
Li, Y., Schichtel, B. A., Walker, J. T., Schwede, D. B., Chen, X., Lehmann, C. M. B., Puchalski, M. A., Gay, D. A., and Collett, J. L.: Increasing importance of deposition of reduced nitrogen in the United States, P. Natl. Acad. Sci. USA, 113, 5874–5879, https://doi.org/10.1073/pnas.1525736113, 2016.
Li, Y., Zhong, H., Shan, Y., Hang, Y., Wang, D., Zhou, Y., and Hubacek, K.: Changes in global food consumption increase GHG emissions despite efficiency gains along global supply chains, Nat. Food, 4, 483–495, https://doi.org/10.1038/s43016-023-00768-z, 2023.
Lin, M. and Begho, T.: Crop residue burning in South Asia: A review of the scale, effect, and solutions with a focus on reducing reactive nitrogen losses, J. Environ. Manage., 314, 115104, https://doi.org/10.1016/j.jenvman.2022.115104, 2022.
Liu, L.: Urbanization is reshaping food production in China, Nature, 621, 42, https://doi.org/10.1038/d41586-023-02753-9, 2023.
Liu, L., Zhang, X., Xu, W., Liu, X., Li, Y., Lu, X., Zhang, Y., and Zhang, W.: Temporal characteristics of atmospheric ammonia and nitrogen dioxide over China based on emission data, satellite observations and atmospheric transport modeling since 1980, Atmos. Chem. Phys., 17, 9365–9378, https://doi.org/10.5194/acp-17-9365-2017, 2017.
Liu, L., Zhang, X., Xu, W., Liu, X., Zhang, Y., Li, Y., Wei, J., Lu, X., Wang, S., Zhang, W., Zhao, L., Wang, Z., and Wu, X.: Fall of oxidized while rise of reduced reactive nitrogen deposition in China, J. Clean. Produc., 272, 122875, https://doi.org/10.1016/j.jclepro.2020.122875, 2020.
Liu, L., Xu, W., Lu, X., Zhong, B., Guo, Y., Lu, X., Zhao, Y., He, W., Wang, S., Zhang, X., Liu, X., and Vitousek, P.: Exploring global changes in agricultural ammonia emissions and their contribution to nitrogen deposition since 1980, P. Natl. Acad. Sci. USA, 119, e2121998119, https://doi.org/10.1073/pnas.2121998119, 2022.
Liu, L., Xu, W., Wen, Z., Liu, P., Xu, H., Liu, S., Lu, X., Zhong, B., Guo, Y., Lu, X., Zhao, Y., Zhang, X., Wang, S., Vitousek, P. M., and Liu, X.: Modeling global oceanic nitrogen deposition from food systems and its mitigation potential by reducing overuse of fertilizers, P. Natl. Acad. Sci. USA, 120, e2221459120, https://doi.org/10.1073/pnas.2221459120, 2023.
Liu, X., Tai, A. P. K., Chen, Y., Zhang, L., Shaddick, G., Yan, X., and Lam, H. M.: Dietary shifts can reduce premature deaths related to particulate matter pollution in China, Nat. Food, 2, 997–1004, https://doi.org/10.1038/s43016-021-00430-6, 2021a.
Liu, X., Tai, A. P. K., and Fung, K. M.: Responses of surface ozone to future agricultural ammonia emissions and subsequent nitrogen deposition through terrestrial ecosystem changes, Atmos. Chem. Phys., 21, 17743–17758, https://doi.org/10.5194/acp-21-17743-2021, 2021b.
Liu, Z. H., Rieder, H. E., Schmidt, C., Mayer, M., Guo, Y. X., Winiwarter, W., and Zhang, L.: Optimal reactive nitrogen control pathways identified for cost-effective PM2.5 mitigation in Europe, Nat. Commun., 14, 4246, https://doi.org/10.1038/s41467-023-39900-9, 2023a.
Liu, Z. H., Zhou, M., Chen, Y. F., Chen, D., Pan, Y. P., Song, T., Ji, D. S., Chen, Q., and Zhang, L.: The nonlinear response of fine particulate matter pollution to ammonia emission reductions in North China, Environ. Res. Lett., 16, 034014, https://doi.org/10.1088/1748-9326/abdf86, 2021b.
Lopez Barrera, E. and Hertel, T.: Solutions to the double burden of malnutrition also generate health and environmental benefits, Nat. Food, 4, 616–624, https://doi.org/10.1038/s43016-023-00798-7, 2023.
Lu, M., Yang, Y. H., Luo, Y. Q., Fang, C. M., Zhou, X. H., Chen, J. K., Yang, X., and Li, B.: Responses of ecosystem nitrogen cycle to nitrogen addition: a meta-analysis, New Phytol., 189, 1040–1050, https://doi.org/10.1111/j.1469-8137.2010.03563.x, 2011.
Lu, X., Vitousek, P. M., Mao, Q., Gilliam, F. S., Luo, Y., Turner, B. L., Zhou, G., and Mo, J.: Nitrogen deposition accelerates soil carbon sequestration in tropical forests, P. Natl. Acad. Sci. USA, 118, e2020790118, https://doi.org/10.1073/pnas.2020790118, 2021a.
Lu, X., Ye, X., Zhou, M., Zhao, Y., Weng, H., Kong, H., Li, K., Gao, M., Zheng, B., Lin, J., Zhou, F., Zhang, Q., Wu, D., Zhang, L., and Zhang, Y.: The underappreciated role of agricultural soil nitrogen oxide emissions in ozone pollution regulation in North China, Nat. Commun., 12, 5021, https://doi.org/10.1038/s41467-021-25147-9, 2021b.
Luo, L., Ran, L., Rasool, Q. Z., and Cohan, D. S.: Integrated Modeling of U.S. Agricultural Soil Emissions of Reactive Nitrogen and Associated Impacts on Air Pollution, Health, and Climate, Environ. Sci. Technol., 56, 9265–9276, https://doi.org/10.1021/acs.est.1c08660, 2022.
Luo, L., Cohan, D. S., Masiello, C. A., Lychuk, T. E., and Gao, X.: Agroecosystem modeling of reactive nitrogen emissions from U.S. agricultural soils with carbon amendments, Biochar, 5, 72, https://doi.org/10.1007/s42773-023-00271-5, 2023.
Luo, Z., Zhang, Y., Chen, W., Van Damme, M., Coheur, P.-F., and Clarisse, L.: Estimating global ammonia (NH3) emissions based on IASI observations from 2008 to 2018, Atmos. Chem. Phys., 22, 10375–10388, https://doi.org/10.5194/acp-22-10375-2022, 2022.
Ma, R., Zou, J., Han, Z., Yu, K., Wu, S., Li, Z., Liu, S., Niu, S., Horwath, W. R., and Zhu-Barker, X.: Global soil-derived ammonia emissions from agricultural nitrogen fertilizer application: A refinement based on regional and crop-specific emission factors, Glob. Change Biol., 27, 855–867, https://doi.org/10.1111/gcb.15437, 2021.
McDuffie, E. E., Smith, S. J., O'Rourke, P., Tibrewal, K., Venkataraman, C., Marais, E. A., Zheng, B., Crippa, M., Brauer, M., and Martin, R. V.: A global anthropogenic emission inventory of atmospheric pollutants from sector- and fuel-specific sources (1970–2017): an application of the Community Emissions Data System (CEDS), Earth Syst. Sci. Data, 12, 3413–3442, https://doi.org/10.5194/essd-12-3413-2020, 2020.
Misselbrook, T. H., Van Der Weerden, T. J., Pain, B. F., Jarvis, S. C., Chambers, B. J., Smith, K. A., Phillips, V. R., and Demmers, T. G. M.: Ammonia emission factors for UK agriculture, Atmos. Environ., 34, 871–880, https://doi.org/10.1016/s1352-2310(99)00350-7, 2000.
Miyazaki, K., Eskes, H., Sudo, K., Boersma, K. F., Bowman, K., and Kanaya, Y.: Decadal changes in global surface NOx emissions from multi-constituent satellite data assimilation, Atmos. Chem. Phys., 17, 807–837, https://doi.org/10.5194/acp-17-807-2017, 2017.
Nelson, A. J., Lichiheb, N., Koloutsou-Vakakis, S., Rood, M. J., Heuer, M., Myles, L., Joo, E., Miller, J., and Bernacchi, C.: Ammonia flux measurements above a corn canopy using relaxed eddy accumulation and a flux gradient system, Agr. Forest Meteorol., 264, 104–113, https://doi.org/10.1016/j.agrformet.2018.10.003, 2019.
Nematian, M., Ng'ombe, J. N., and Keske, C.: Sustaining agricultural economies: regional economic impacts of biochar production from waste orchard biomass in California's Central Valley, Environ. Develop. Sustain., 26, 30701–30721, https://doi.org/10.1007/s10668-023-03984-6, 2023.
Oswald, R., Behrendt, T., Ermel, M., Wu, D., Su, H., Cheng, Y., Breuninger, C., Moravek, A., Mougin, E., Delon, C., Loubet, B., Pommerening-Röser, A., Sörgel, M., Pöschl, U., Hoffmann, T., Andreae, M. O., Meixner, F. X., and Trebs, I.: HONO Emissions from Soil Bacteria as a Major Source of Atmospheric Reactive Nitrogen, Science, 341, 1233–1235, https://doi.org/10.1126/science.1242266, 2013.
Pan, S.-Y., He, K.-H., Lin, K.-T., Fan, C., and Chang, C.-T.: Addressing nitrogenous gases from croplands toward low-emission agriculture, npj Clim. Atmos. Sci., 5, 43, https://doi.org/10.1038/s41612-022-00265-3, 2022.
Pilegaard, K.: Processes regulating nitric oxide emissions from soils, Phil. Trans. R. Soc. B, 368, 20130126, https://doi.org/10.1098/rstb.2013.0126, 2013.
Pinder, R. W., Adams, P. J., and Pandis, S. N.: Ammonia emission controls as a cost-effective strategy for reducing atmospheric particulate matter in the eastern United States, Environ. Sci. Technol., 41, 380–386, https://doi.org/10.1021/es060379a, 2007.
Pozzer, A., Tsimpidi, A. P., Karydis, V. A., de Meij, A., and Lelieveld, J.: Impact of agricultural emission reductions on fine-particulate matter and public health, Atmos. Chem. Phys., 17, 12813–12826, https://doi.org/10.5194/acp-17-12813-2017, 2017.
Qiao, C., Liu, L., Hu, S., Compton, J. E., Greaver, T. L., and Li, Q.: How inhibiting nitrification affects nitrogen cycle and reduces environmental impacts of anthropogenic nitrogen input, Global Change Biol., 21, 1249–1257, https://doi.org/10.1111/gcb.12802, 2015.
Quinn Thomas, R., Canham, C. D., Weathers, K. C., and Goodale, C. L.: Increased tree carbon storage in response to nitrogen deposition in the US, Nat. Geosci., 3, 13–17, https://doi.org/10.1038/ngeo721, 2010.
Reddington, C. L., Conibear, L., Robinson, S., Knote, C., Arnold, S. R., and Spracklen, D. V.: Air Pollution From Forest and Vegetation Fires in Southeast Asia Disproportionately Impacts the Poor, Geohealth, 5, e2021GH000418, https://doi.org/10.1029/2021gh000418, 2021.
Ren, C., Zhang, X., Reis, S., and Gu, B.: Socioeconomic barriers of nitrogen management for agricultural and environmental sustainability, Agr. Ecosyst. Environ., 333, 107950, https://doi.org/10.1016/j.agee.2022.107950, 2022.
Richardson, K., Steffen, W., Lucht, W., Bendtsen, J., Cornell, S. E., Donges, J. F., Drüke, M., Fetzer, I., Bala, G., von Bloh, W., Feulner, G., Fiedler, S., Gerten, D., Gleeson, T., Hofmann, M., Huiskamp, W., Kummu, M., Mohan, C., Nogués-Bravo, D., Petri, S., Porkka, M., Rahmstorf, S., Schaphoff, S., Thonicke, K., Tobian, A., Virkki, V., Wang-Erlandsson, L., Weber, L., and Rockström, J.: Earth beyond six of nine planetary boundaries, Sci. Adv., 9, eadh2458, https://doi.org/10.1126/sciadv.adh2458, 2023.
Romer, P. S., Duffey, K. C., Wooldridge, P. J., Edgerton, E., Baumann, K., Feiner, P. A., Miller, D. O., Brune, W. H., Koss, A. R., de Gouw, J. A., Misztal, P. K., Goldstein, A. H., and Cohen, R. C.: Effects of temperature-dependent NOx emissions on continental ozone production, Atmos. Chem. Phys., 18, 2601–2614, https://doi.org/10.5194/acp-18-2601-2018, 2018.
Sha, T., Ma, X., Zhang, H., Janechek, N., Wang, Y., Wang, Y., García, L. C., Jenerette, G. D., and Wang, J.: Impacts of Soil NOx Emission on O3 Air Quality in Rural California, Environ. Sci. Technol., 55, 7113–7122, https://doi.org/10.1021/acs.est.0c06834, 2021.
Shafiee-Jood, M. and Cai, X.: Reducing Food Loss and Waste to Enhance Food Security and Environmental Sustainability, Environ. Sci. Technol., 50, 8432–8443, https://doi.org/10.1021/acs.est.6b01993, 2016.
Shen, H., Chen, Y., Hu, Y., Ran, L., Lam, S. K., Pavur, G. K., Zhou, F., Pleim, J. E., and Russell, A. G.: Intense Warming Will Significantly Increase Cropland Ammonia Volatilization Threatening Food Security and Ecosystem Health, One Earth, 3, 126–134, https://doi.org/10.1016/j.oneear.2020.06.015, 2020.
Simkin, S. M., Allen, E. B., Bowman, W. D., Clark, C. M., Belnap, J., Brooks, M. L., Cade, B. S., Collins, S. L., Geiser, L. H., Gilliam, F. S., Jovan, S. E., Pardo, L. H., Schulz, B. K., Stevens, C. J., Suding, K. N., Throop, H. L., and Waller, D. M.: Conditional vulnerability of plant diversity to atmospheric nitrogen deposition across the United States, P. Natl. Acad. Sci. USA, 113, 4086–4091, https://doi.org/10.1073/pnas.1515241113, 2016.
Song, S., Nenes, A., Gao, M., Zhang, Y., Liu, P., Shao, J., Ye, D., Xu, W., Lei, L., Sun, Y., Liu, B., Wang, S., and McElroy, M. B.: Thermodynamic Modeling Suggests Declines in Water Uptake and Acidity of Inorganic Aerosols in Beijing Winter Haze Events during 2014/2015–2018/2019, Environ. Sci. Technol. Lett., 6, 752–760, https://doi.org/10.1021/acs.estlett.9b00621, 2019.
Springmann, M., Van Dingenen, R., Vandyck, T., Latka, C., Witzke, P., and Leip, A.: The global and regional air quality impacts of dietary change, Nat. Commun., 14, 6227 https://doi.org/10.1038/s41467-023-41789-3, 2023.
Tai, A. P. K. and Martin, M. V.: Impacts of ozone air pollution and temperature extremes on crop yields: Spatial variability, adaptation and implications for future food security, Atmos. Environ., 169, 11–21, https://doi.org/10.1016/j.atmosenv.2017.09.002, 2017.
Tai, A. P. K., Martin, M. V., and Heald, C. L.: Threat to future global food security from climate change and ozone air pollution, Nat. Clim. Change, 4, 817–821, https://doi.org/10.1038/Nclimate2317, 2014.
Tan, J., Fu, J. S., and Seinfeld, J. H.: Ammonia emission abatement does not fully control reduced forms of nitrogen deposition, P. Natl. Acad. Sci. USA, 117, 9771–9775, https://doi.org/10.1073/pnas.1920068117, 2020.
Thakrar, S. K., Balasubramanian, S., Adams, P. J., Azevedo, I. M. L., Muller, N. Z., Pandis, S. N., Polasky, S., Pope, C. A., Robinson, A. L., Apte, J. S., Tessum, C. W., Marshall, J. D., and Hill, J. D.: Reducing Mortality from Air Pollution in the United States by Targeting Specific Emission Sources, Environ. Sci. Technol. Lett., 7, 639–645, https://doi.org/10.1021/acs.estlett.0c00424, 2020.
Thomas, R. Q., Canham, C. D., Weathers, K. C., and Goodale, C. L.: Increased tree carbon storage in response to nitrogen deposition in the US, Nat. Geosci., 3, 13–17, https://doi.org/10.1038/Ngeo721, 2010.
Thunis, P., Clappier, A., Beekmann, M., Putaud, J. P., Cuvelier, C., Madrazo, J., and de Meij, A.: Non-linear response of PM2.5 to changes in NOx and NH3 emissions in the Po basin (Italy): consequences for air quality plans, Atmos. Chem. Phys., 21, 9309–9327, https://doi.org/10.5194/acp-21-9309-2021, 2021.
Tschofen, P., Azevedo, I. L., and Muller, N. Z.: Fine particulate matter damages and value added in the US economy, P. Natl. Acad. Sci. USA, 116, 19857–19862, https://doi.org/10.1073/pnas.1905030116, 2019.
Uwizeye, A., de Boer, I. J. M., Opio, C. I., Schulte, R. P. O., Falcucci, A., Tempio, G., Teillard, F., Casu, F., Rulli, M., Galloway, J. N., Leip, A., Erisman, J. W., Robinson, T. P., Steinfeld, H., and Gerber, P. J.: Nitrogen emissions along global livestock supply chains, Nat. Food, 1, 437–446, https://doi.org/10.1038/s43016-020-0113-y, 2020.
van der Werf, G. R., Randerson, J. T., Giglio, L., van Leeuwen, T. T., Chen, Y., Rogers, B. M., Mu, M., van Marle, M. J. E., Morton, D. C., Collatz, G. J., Yokelson, R. J., and Kasibhatla, P. S.: Global fire emissions estimates during 1997–2016, Earth Syst. Sci. Data, 9, 697–720, https://doi.org/10.5194/essd-9-697-2017, 2017.
Vigan, A., Hassouna, M., Guingand, N., Brame, C., Edouard, N., Eglin, T., Espagnol, S., Eugène, M., Génermont, S., Lagadec, S., Lorinquer, E., Loyon, L., Ponchant, P., and Robin, P.: Development of a Database to Collect Emission Values for Livestock Systems, J. Environ. Qual., 48, 1899–1906, https://doi.org/10.2134/jeq2019.01.0007, 2019.
Vira, J., Hess, P., Melkonian, J., and Wieder, W. R.: An improved mechanistic model for ammonia volatilization in Earth system models: Flow of Agricultural Nitrogen version 2 (FANv2), Geosci. Model Dev., 13, 4459–4490, https://doi.org/10.5194/gmd-13-4459-2020, 2020.
Wagner-Riddle, C., Congreves, K. A., Abalos, D., Berg, A. A., Brown, S. E., Ambadan, J. T., Gao, X., and Tenuta, M.: Globally important nitrous oxide emissions from croplands induced by freeze–thaw cycles, Nat. Geosci., 10, 279–283, https://doi.org/10.1038/ngeo2907, 2017.
Wang, Y., Fu, X., Wu, D., Wang, M., Lu, K., Mu, Y., Liu, Z., Zhang, Y., and Wang, T.: Agricultural Fertilization Aggravates Air Pollution by Stimulating Soil Nitrous Acid Emissions at High Soil Moisture, Environ. Sci. Technol., 55, 14556–14566, https://doi.org/10.1021/acs.est.1c04134, 2021.
Wang, Y., Fu, X., Wang, T., Ma, J., Gao, H., Wang, X., and Pu, W.: Large Contribution of Nitrous Acid to Soil-Emitted Reactive Oxidized Nitrogen and Its Effect on Air Quality, Environ. Sci. Technol., 57, 3516–3526, https://doi.org/10.1021/acs.est.2c07793, 2023.
Webb, J., Menzi, H., Pain, B. F., Misselbrook, T. H., Dammgen, U., Hendriks, H., and Dohler, H.: Managing ammonia emissions from livestock production in Europe, Environ. Pollut., 135, 399–406, https://doi.org/10.1016/j.envpol.2004.11.013, 2005.
Wrage-Mönnig, N., Horn, M. A., Well, R., Müller, C., Velthof, G., and Oenema, O.: The role of nitrifier denitrification in the production of nitrous oxide revisited, Soil Biol. Biochem., 123, A3–A16, https://doi.org/10.1016/j.soilbio.2018.03.020, 2018.
Yan, X., Ying, Y., Li, K., Zhang, Q., and Wang, K.: A review of mitigation technologies and management strategies for greenhouse gas and air pollutant emissions in livestock production, J. Environ. Manage, 352, 120028, https://doi.org/10.1016/j.jenvman.2024.120028, 2024.
Yang, Y., Liu, L., Liu, P., Ding, J., Xu, H., and Liu, S.: Improved global agricultural crop- and animal-specific ammonia emissions during 1961–2018, Agr. Ecosyst. Environ., 344, 108289, https://doi.org/10.1016/j.agee.2022.108289, 2023.
Yang, Y., Liu, L., Zhou, W., Guan, K., Tang, J., Kim, T., Grant, R. F., Peng, B., Zhu, P., Li, Z., Griffis, T. J., and Jin, Z.: Distinct driving mechanisms of non-growing season N2O emissions call for spatial-specific mitigation strategies in the US Midwest, Agr. Forest Meteorol., 324, 109108, https://doi.org/10.1016/j.agrformet.2022.109108, 2022.
Yang, Y. Y., Liu, L., Liu, P., Ding, J., Xu, H., and Liu, S.: Improved global agricultural crop- and animal-specific ammonia emissions during 1961–2018, Agr. Ecosyst. Environ., 344, 108289, https://doi.org/10.1016/j.agee.2022.108289, 2023.
Zhang, C. H., Guo, H. R., Huang, H., Ma, T. Y., Song, W., Chen, C. J., and Liu, X. Y.: Atmospheric nitrogen deposition and its responses to anthropogenic emissions in a global hotspot region, Atmos. Res., 248, 105137, https://doi.org/10.1016/j.atmosres.2020.105137, 2021.
Zhang, Q., Li, Y. A., Wang, M. R., Wang, K., Meng, F. L., Liu, L., Zhao, Y. H., Ma, L., Zhu, Q. C., Xu, W., and Zhang, F. S.: Atmospheric nitrogen deposition: A review of quantification methods and its spatial pattern derived from the global monitoring networks, Ecotox Environ. Safe, 216, 112180, https://doi.org/10.1016/j.ecoenv.2021.112180, 2021.
Zhang, T., Wooster, M., de Jong, M., and Xu, W.: How Well Does the `Small Fire Boost' Methodology Used within the GFED4.1s Fire Emissions Database Represent the Timing, Location and Magnitude of Agricultural Burning?, Remote Sens., 10, 823, https://doi.org/10.3390/rs10060823, 2018.
Zhang, T., de Jong, M. C., Wooster, M. J., Xu, W., and Wang, L.: Trends in eastern China agricultural fire emissions derived from a combination of geostationary (Himawari) and polar (VIIRS) orbiter fire radiative power products, Atmos. Chem. Phys., 20, 10687–10705, https://doi.org/10.5194/acp-20-10687-2020, 2020.
Zhang, X., Davidson, E. A., Mauzerall, D. L., Searchinger, T. D., Dumas, P., and Shen, Y.: Managing nitrogen for sustainable development, Nature, 528, 51–59, https://doi.org/10.1038/nature15743, 2015.
Zhang, X., Gu, B., van Grinsven, H., Lam, S. K., Liang, X., Bai, M., and Chen, D.: Societal benefits of halving agricultural ammonia emissions in China far exceed the abatement costs, Nat. Commun., 11, 4357, https://doi.org/10.1038/s41467-020-18196-z, 2020.
Zhao, Y., Zhang, L., Chen, Y., Liu, X., Xu, W., Pan, Y., and Duan, L.: Atmospheric nitrogen deposition to China: A model analysis on nitrogen budget and critical load exceedance, Atmos. Environ., 153, 32–40, https://doi.org/10.1016/j.atmosenv.2017.01.018, 2017a.
Zhao, Y., Zhang, L., Tai, A. P. K., Chen, Y., and Pan, Y.: Responses of surface ozone air quality to anthropogenic nitrogen deposition in the Northern Hemisphere, Atmos. Chem. Phys., 17, 9781–9796, https://doi.org/10.5194/acp-17-9781-2017, 2017b.
Zoogman, P., Liu, X., Suleiman, R. M., Pennington, W. F., Flittner, D. E., Al-Saadi, J. A., Hilton, B. B., Nicks, D. K., Newchurch, M. J., Carr, J. L., Janz, S. J., Andraschko, M. R., Arola, A., Baker, B. D., Canova, B. P., Chan Miller, C., Cohen, R. C., Davis, J. E., Dussault, M. E., Edwards, D. P., Fishman, J., Ghulam, A., González Abad, G., Grutter, M., Herman, J. R., Houck, J., Jacob, D. J., Joiner, J., Kerridge, B. J., Kim, J., Krotkov, N. A., Lamsal, L., Li, C., Lindfors, A., Martin, R. V., McElroy, C. T., McLinden, C., Natraj, V., Neil, D. O., Nowlan, C. R., O'Sullivan, E. J., Palmer, P. I., Pierce, R. B., Pippin, M. R., Saiz-Lopez, A., Spurr, R. J. D., Szykman, J. J., Torres, O., Veefkind, J. P., Veihelmann, B., Wang, H., Wang, J., and Chance, K.: Tropospheric emissions: Monitoring of pollution (TEMPO), J. Quant. Spectrosc. Ra., 186, 17–39, https://doi.org/10.1016/j.jqsrt.2016.05.008, 2017.
- Abstract
- Introduction
- How agriculture and food systems shape atmospheric chemistry and air pollution
- How agriculture and food systems can be transformed to mitigate emissions
- How the science of agriculture–environment interactions contributes to sustainable development
- Data availability
- Author contributions
- Competing interests
- Disclaimer
- Special issue statement
- Acknowledgements
- Financial support
- Review statement
- References
- Abstract
- Introduction
- How agriculture and food systems shape atmospheric chemistry and air pollution
- How agriculture and food systems can be transformed to mitigate emissions
- How the science of agriculture–environment interactions contributes to sustainable development
- Data availability
- Author contributions
- Competing interests
- Disclaimer
- Special issue statement
- Acknowledgements
- Financial support
- Review statement
- References