the Creative Commons Attribution 4.0 License.
the Creative Commons Attribution 4.0 License.
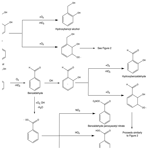
Quantifying primary oxidation products in the OH-initiated reaction of benzyl alcohol
Reina S. Buenconsejo
Sophia M. Charan
John H. Seinfeld
Benzyl alcohol is found in many volatile chemical products (VCPs) including a number of personal care products and industrial solvents. We report here on the products of the gas-phase oxidation of benzyl alcohol by OH and its dependence on nitric oxide (NO) levels. Using a gas chromatography in tandem with a chemical ionization mass spectrometer (CIMS) and gas chromatographer with a flame ionization detector (GC-FID), we measure the branching fractions to the major gas-phase oxidation products: hydroxybenzyl alcohol (HBA) and benzaldehyde. Later-generation oxidation products from both HBA and benzaldehyde pathways are also observed. In particular, catechol is a major gas-phase product of HBA. The fraction of H abstraction from benzyl alcohol leading to benzaldehyde formation is unaffected by [NO], with an average branching fraction of (21±10) %. The fraction of OH addition leading to HBA formation (36±18) % also does not appear to vary with [NO]. Consistent with the known high SOA yields of catechol, we find that HBA has a very high secondary organic aerosol (SOA) yield. Thus, benzyl alcohol and its oxidation products efficiently produce secondary organic aerosol – under some conditions approaching unity. Insights from the present study can help elucidate the chemistry of other atmospherically relevant aromatic compounds, especially those found in VCPs.
- Article
(1446 KB) - Full-text XML
- BibTeX
- EndNote
Emissions of volatile chemical products (VCPs) have been recently identified as being critical in driving air pollution chemistry, particularly as regulations drive a decrease in the contribution of vehicular-based emissions (McDonald et al., 2018; Coggon et al., 2021). VCPs play an important role in air quality because of their high potential to form secondary organic aerosol (SOA); an analysis of VCPs in the Los Angeles air basin indicates that VCPs could contribute up to 70 % of SOA formation in the Los Angeles air basin despite accounting for only approximately 4 % of total petrochemical product use (McDonald et al., 2018). The inclusion of VCP emission inventories has improved the agreement of regional-scale modeling compared to previous models that did not consider VCP emissions (Seltzer et al., 2021; Pennington et al., 2021). However, because many of the chemicals comprising VCP emissions have not been studied in laboratory settings, their contribution to SOA formation remains uncertain. Additional experimental work on VCPs is still needed.
Here, we evaluate the photochemistry of benzyl alcohol, a compound found prominently in VCPs. Benzyl alcohol is used in soaps and perfumes and is also used as a solvent in the manufacture of paints, inks, lacquers, and epoxies (Wang, 2015). In a previous study, the aerosol mass yield of benzyl alcohol was found to have a high mass yield under a variety of conditions – even approaching unity (Charan et al., 2020; Jaoui et al., 2023).
Experimental studies, including kinetic experiments on benzyl alcohol, have determined that the primary atmospheric oxidative pathway of benzyl alcohol proceeds via reaction with the hydroxyl (OH) radical (Bernard et al., 2013; Harrison and Wells, 2009). Harrison and Wells (2009) calculated that benzyl alcohol reacts with OH at a rate of cm3 per molecule per second. The reaction rate with O3 is too slow to significantly affect to benzyl alcohol mixing ratios in most environments (Harrison and Wells, 2009).
Previous experimental studies of benzyl alcohol identified several oxidation products including hydroxybenzyl alcohol (HBA) and benzaldehyde (Burkholder et al., 2017; Harrison and Wells, 2009; Bernard et al., 2013). A theoretical study on the mechanism of benzyl alcohol oxidation initiated by OH also predicted the major oxidation pathways form benzaldehyde, i-hydroxybenzyl alcohol, and o-hydroxybenzyl alcohol (Wang, 2015). Other products observed in past experimental studies include ring-opening products, such as 2-butenedial and 6-hydroxy-5-oxohex-2-enal (Harrison and Wells, 2009), as well as C6 compounds such as dihydroxybenzene (Bernard et al., 2013). These past experiments were conducted under high-NO conditions. Yet, understanding chemical mechanisms and branching fractions as a function of NO is important for understanding the impact of its oxidation on urban air quality as NOx-to-volatile-organic-compound (VOC) ratios have been shown to affect OH oxidation of VOCs and as NOx regimes continue to change in urban settings (Seinfeld and Pandis, 2016; Parker et al., 2020). This is particularly relevant as NOx continues to decline in many US urban areas (Parker et al., 2020).
In this study, we draw on past work which suggests HBA forms via addition of OH to the benzyl alcohol aromatic ring. We experimentally confirm products identified in past work, including products predicted in a theoretical study (Wang, 2015). We also quantify the branching to HBA, benzaldehyde, phenol, and 5-hydroxy-4-oxo-2-pentenal. We find the branching fraction for HBA, the primary oxidation product, is ∼36 %. Benzaldehyde has a branching fraction of ∼21 %.
Chamber experiments were conducted to elucidate the chemical mechanism of benzyl alcohol oxidation via OH. First-generation oxidation products were used to identify important pathways to SOA formation. Two setups were used to investigate this chemistry: (1) gas-phase experiments to determine the branching fractions and gas-phase oxidation chemistry and (2) particle-phase experiments to measure the SOA yields of the primary benzyl alcohol oxidation products.
2.1 Experimental design
Gas-phase experiments were conducted in a ∼0.8 m3 FEP Teflon-walled environmental chamber, henceforth referred to as Chamber G. Chamber G was filled and evacuated multiple times with purified air prior to experiments. Particle-phase experiments were conducted in a 19 m3 FEP Teflon-walled environmental chamber (henceforth referred to as Chamber P) which was continuously flushed for > 24 h prior to experiments. All experiments were run at room temperature (∼22 °C), low relative humidity (<10 % RH), and ambient pressure (∼1 atm). Benzyl alcohol (Sigma Millipore, ReagentPlus ≥99 %) and benzaldehyde (Sigma Millipore, ReagentPlus ≥99 %) were injected into the chamber by flowing warm air over a measured amount of precursor deposited on a Pall Teflon filter. For gas-phase experiments, o-hydroxybenzyl alcohol (o-HBA) (Sigma Millipore 99 %) was injected similarly. Because o-HBA is a relatively low-volatility compound, in order to achieve sufficiently high concentrations in Chamber P for particle-phase experiments, o-HBA was dissolved in Milli-Q water and injected by bubbling purified air through the solution.
For the high-NO gas-phase experiments G2 and G4, methyl nitrite was used as the oxidant precursor. Methyl nitrite was added to the reactor by measuring the pressure of methyl nitrite in an evacuated round-bottom bulb and back-filling the remainder of the bulb volume with nitrogen. NO (1993±20 ppm NO in N2; Matheson) was prepared in a similar manner. For high-NO particle-phase experiments, NO (506.9±10 ppm NO in N2) was injected using a mass flow controller (Sierra Instruments).
In experiments G1, G3, G5, P1, and P2, hydrogen peroxide (H2O2) (Sigma Millipore, 50 wt % in H2O stabilized) was used as the OH precursor by injecting a known mass (G1, G3, and G5) or volume (P1 and P2) into a glass bulb and flowing purified air over the liquid droplets to add to the reactor. In particle-phase experiments, the H2O2 was heated in a water bath (∼42 °C) during injection.
All particle-phase experiments were seeded using a sonicated solution of 0.06 mol m−3 ammonium sulfate ((NH4)2SO4). During injection, the seed solution was run through a soft X-ray charge conditioner (TSI Model 3088). In all experiments, ∼1 h was allowed after all injections were completed and before the lights were turned on to allow for the mixing and collection of adequate background data. In particle-phase experiments, this time was also used to confirm previously calculated chamber wall loss parameters (Charan et al., 2018).
Ultraviolet (UV) broadband lights centered around ∼350 nm were used as the light source for photooxidation (Light Sources, Inc.); oxidation duration was determined so that ∼10 % of the precursor was reacted in gas-phase reactions. The and for Chamber G are s−1 and s−1, respectively. The and of Chamber P are measured to be s−1 and s−1, respectively, using methods described elsewhere (Zafonte et al., 1977). Additional information about the oxidants is found in Appendix A. Photooxidation was carried out for particle-phase experiments for >6 h. This oxidation time corresponds to about 50 %–100 % reaction of the initial VOC and is congruent with reaction times in the benzyl alcohol studies in Charan et al. (2020). A summary of experiments can be found in Table 1.
2.2 Instrumentation
In the particle-phase experiments, NO and NO2 were monitored using a commercially available Teledyne T200 NOx monitor. Temperature and RH were measured by a Vaisala HMM211 probe. In gas-phase experiments, NOx was monitored using a commercially available Teledyne M200EU NOx monitor. Additional instrumentation used in gas-phase and particle-phase experiments is described in the following sections.
2.2.1 Particle mass detection
Particle size distribution was monitored using a custom-built scanning mobility particle sizer (SMPS) which uses a commercially available TSI 3081 differential mobility analyzer (DMA) and a TSI 3010 condensation particle counter (CPC) (Mai, 2018). Prior to the DMA inlet, a soft X-ray charger provided a known charge distribution. Wall loss corrections and subsequent SOA yields were calculated based on work and model development explained in Charan et al. (2018) and Huang et al. (2018) and are detailed more specifically for these experiments in Charan et al. (2020). SOA yields were calculated based on a ratio of the mass of the VOC precursor that reacted and the mass of the SOA formed.
Here, ΔSOA is the change in the aerosol mass concentration while accounting for any aerosol loss to the chamber walls. Aerosol density was assumed to be 1.04 g cm−3 based on past work on benzyl alcohol (Charan et al., 2020; Li and Cocker, 2018).
2.2.2 Gas-phase detection
A chemical ionization triple quadrupole mass spectrometer (CIMS) with a CF3O− reagent ion was used to monitor gas-phase compounds in particle-phase experiments. This setup has been described in detail elsewhere (Schwantes et al., 2017). In brief, the CIMS operates by reacting with the gas-phase compounds in the sample that have an electron affinity sufficient to bind with the reagent ion cluster (CF3O−). Sampled compounds that are acidic may also transfer a fluoride ion (F−). The sample is then detected by a Varian 1200 triple quadrupole mass analyzer which measures masses from to 330. A custom-built inlet to the CIMS was set at a constant temperature, 25 °C.
Benzaldehyde, one of the primary products of benzyl alcohol OH oxidation, is not detectable using the CF3O− CIMS. Thus an HP 6890N gas chromatograph with a flame ionization detector (GC-FID) was used in experiments G1, G3, G5, P1, and P2 to detect benzaldehyde. Experiments were run with a DB-5 column. Information on the temperature profile and GC operation can be found in Appendix C.
In gas-phase experiments in Chamber G, the GC-CIMS was used to monitor the gas-phase precursors and subsequent photooxidation products. This experimental setup is described in detail elsewhere (Vasquez et al., 2018; Xu et al., 2020). Here, the GC-CIMS was operated in negative mode using CF3O− and for experiments G2 and G4 in positive mode using NO+. The chemistry involving CF3O− has been described previously and is detailed in Appendix B (Vasquez et al., 2018; Crounse et al., 2006). In positive mode, NO+ complexes with less acidic compounds and can be detected at [M + NO+] (also described further in Appendix B). A 2 m Restek Rtx-1701 column was used for all experiments for better chromatographic resolution of certain isomers. GC samples were cryogenically trapped at −20 °C on the column. Additional information on the GC-CIMS operation is in Appendix C.
2.2.3 Calibrations
The sensitivities for analytes in the GC-CIMS were generally determined from prepared standards and quantified using Fourier transform infrared (FTIR) spectroscopy. The GC-FID and the CIMS in experiments P1–P2 were calibrated by injecting the analyte of interest into a ∼100 L Teflon pillow bag using the same injection method as described for Chamber G. The sample was then measured via a FTIR spectrometer with a pathlength of 19 cm. Reference FTIR spectra from the Pacific Northwest National Laboratory (PNNL) database were used to tabulate cross sections and determine exact concentrations (Schwantes et al., 2017). The pillow bag was then diluted using dry N2 and sampled to determine the instrumental sensitivity (Xu et al., 2019).
Several substrates involved in this study are relatively non-volatile, leading to challenges with quantitative transfer into and out of the FTIR cell. Therefore some of the GC-CIMS sensitivities were determined using calculated polarizabilities and dipole moments to determine the ion–molecule collision rates of the analytes with the reagent ion relative to the collision rates with reference calibrants (Su and Chesnavich, 1982; Garden et al., 2009). Additional information on this procedure can be found in Appendix B.
2.3 Corrections
2.3.1 Vapor wall loss
Vapor wall loss was examined by sampling benzyl alcohol (and products) prior to oxidation and post-oxidation. Vapor wall loss periods were as long as or on timescales with similar orders of magnitude to the oxidation periods. During these sample periods, the signal of oxidation products remained stable. Thus, no vapor wall loss correction was applied. This is congruent with past work on quantifying benzyl alcohol products, as well as other work quantifying first-generation gas-phase products, which typically considered gas-phase wall loss to be negligible (Charan et al., 2020; Jaoui et al., 2023; Bernard et al., 2013; Harrison and Wells, 2009).
2.3.2 Secondary chemistry
Oxidation products of benzyl alcohol react as they are formed – for some of the oxidation products, their rate coefficient for reaction with OH is faster than benzyl alcohol. These secondary losses were accounted for in estimating the branching fractions of benzyl alcohol products, which are reported in Table 4. Branching fractions (BFs) were calculated as
The gas-phase, time-dependent yield (Y) is calculated as the amount of oxidation product formed divided by the amount of precursor reacted. To solve for the correction factor (CF), a constant [OH] is assumed for the time-dependent product concentration, which can be described as
The time-dependent concentration of benzyl alcohol is described as
Therefore, CF is defined as
The kinetic rate constants used to solve for Eq. (5) are found in Table 2. This correction factor is described elsewhere in greater detail (Atkinson et al., 1982). The correction factors are presented in Table 3.
Table 2Kinetic rate constants used to determine the correction factors for branching fraction calculations.
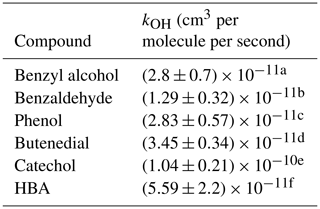
a Harrison and Wells (2009) and Bernard et al. (2013). b Calvert et al. (2002). c Rinke and Zetzsch (1984). d Martín et al. (2013). e Olariu et al. (2000). Note that for 5-hydroxy-4-oxo-2-pentenal, the kOH for butenedial is used to calculate the correction factor. f Calculated in the present work.
The time traces for HBA and catechol are consistent with the rapid rate of reaction of HBA with OH, which produces catechol in high yield. This was previously reported by Bernard et al. (2013). Because the kinetics of OH with HBA are unknown, we estimate kOH+HBA using the kinetic rate constant of catechol (Appendix H).
3.1 Gas-phase branching fractions
Gas-phase products were identified in experiments G1–G3 (Table 4). The secondary losses of first-generation products were minimized by limiting the reaction of benzyl alcohol to ∼10 %. The oxidation of benzyl alcohol forms C7 products, such as HBA and benzaldehyde. Additionally, we observed C6 (products such as phenol) and ring-opening products (such as 5-hydroxy-4-oxo-2-pentenal). A list of products and their corresponding CIMS chemistry can be found in Appendix D. Together, these products account for 65±32% of the loss of benzyl alcohol. The lack of mass closure may reflect the impact of the endo-cyclization route that is known to result in a large diversity of products (Xu et al., 2020), many of which may be unmeasurable with CF3O− CIMS or have very low vapor pressure, likely yielding aerosol (see “Particle-phase results” section).
3.1.1 Hydroxybenzyl alcohol and benzaldehyde
Here, we quantify the branching fractions of the primary benzyl alcohol oxidation products, HBA and benzaldehyde, under a range of NO concentrations. Previous studies of benzyl alcohol oxidation were generally performed under high-NO conditions. Thus, our interest is in understanding the extent to which NO affects benzyl alcohol chemistry.
While HBA is not currently included in the Master Chemical Mechanism (MCM) scheme (Jenkin et al., 2003; Bloss et al., 2005), its formation has been predicted and identified in past work on benzyl alcohol kinetics and mechanisms (Calvert et al., 2002; Bernard et al., 2013; Wang, 2015; Bloss et al., 2005; Jenkin et al., 2003). HBA forms via the addition of the OH radical to the aromatic ring. This leads to a radical intermediate which is stabilized by the electron delocalization of the remaining conjugated system. Subsequent abstraction of hydrogen by oxygen restores the aromaticity. We calculated the amount of HBA formed from benzyl alcohol oxidation from the gas-phase experiments conducted in Chamber G (experiments G1–G5 in Table 1). We find the branching fraction of HBA to be invariant with [NO]. By averaging over all experiments and accounting for systematic error, we estimate the averaged branching fraction of HBA to be (36±18) %. The primary oxidation product of HBA appears to be catechol (Appendix H). See Sect. 3.1.2 for a more detailed discussion of how C6 oxidation compounds are formed. Dihydroxybenzyl alcohol was observed in modest quantities, but given its low vapor pressure we are not able to accurately measure the yield. We also observed masses that corresponded to fragmentation products observed in past studies or believed to form via theoretical calculations such as hydroxyoxopropanal (Harrison and Wells, 2009; Wang, 2015; Jaoui et al., 2023). The mechanism for HBA formation and subsequent chemistry can be found in Fig. 1. This mechanism is based on observed compounds in the present study as well as our understanding of aromatic systems in general. An additional summary of compounds detected can be found in Appendix D.
Benzaldehyde forms via initial hydrogen abstraction from the CH2OH group followed by the addition of O2 and subsequent loss of HO2. Benzaldehyde was measured via NO+ CIMS in experiments G2 and G4 and via GC-FID in experiments G1, G3, and G5. The NO+ signal is highly water-dependent and therefore less stable than the CF3O− signal. However, the benzaldehyde branching fraction using NO+ and GC-FID are in reasonable agreement. We report an averaged branching fraction of (21±10) % under high- and low-NO conditions, consistent with the expectation that this channel should not have NO dependence when NO is less than several parts per million (Allen et al., 2018). Consistent with our findings, others have quantified the branching fraction to benzaldehyde to be 25 % (Bernard et al., 2013). Oxidation of benzaldehyde by OH can go on to form other closed-shell products such as benzyl hydroperoxy and benzaldehyde peroxyacetyl nitrate (Calvert et al., 2002). We observe that the oxidation of benzaldehyde by OH also produces hydroxybenzaldehyde following chemistry analogous to the formation of HBA from benzyl alcohol.
3.1.2 C6 compounds
Previous studies of the chemical composition of the SOA formed via OH oxidation of benzyl alcohol observed C6 compounds, such as nitrocatechol (Charan et al., 2020; Jaoui et al., 2023). In the present study, we observed nitrocatechol as well as other C6 compounds such as phenol and catechol. Past work has proposed one of two ways to form C6 products. Schwantes et al. (2017) found that C6 products, such as nitrocatechol, can form from the OH abstraction from the CH2O group. The second proposed mechanism via theoretical work done by Wang (2015) suggests that the formation of C6 compounds, such as phenol, can occur via OH addition to the ipso site of benzyl alcohol. The ipso radical intermediate can then decompose to form phenol. However, Wang (2015) estimated that the barrier to this decomposition was too high for the significant formation of phenol. Indeed, in the present study we observed initial branching fractions of phenol of <5 %. We also observed that the branching fraction of phenol decreases with decreasing NO, consistent with past studies showing the mechanism for phenol formation depends on the NO mixing ratio (Xu et al., 2020). Both mechanisms of C6 formation described in this section are illustrated in Fig. 1.
We also observed many C6 products in the aerosol phase. Phenol, catechol, and other C6 aromatic compounds react rapidly with OH to form other oxygenated aromatic compounds ( cm3 per molecule per second) (Calvert et al., 2002). We hypothesize many of the C6 compounds we observed in aerosol experiments are likely oxidation products of catechol.
3.2 Particle-phase results
We estimate the relative contributions of HBA and benzaldehyde to benzyl alcohol SOA formation by conducting individual SOA yield experiments using HBA and benzaldehyde as the VOC precursors. Conditions for SOA experiments were selected to match those of the benzyl alcohol SOA yield experiments in Charan et al. (2020). In brief, experiments were conducted with ∼80 ppb of initial NO, ∼80 ppb of VOC precursor, and cm−3 inorganic seed aerosol. SOA yield results were calculated using two treatments: one where the proportionality factor, ω, was set to unity and another in which ω=0. In the ω=0 case, oxidation products with a sufficiently low vapor pressure to condense were assumed to do so only on suspended particles and not on particles that had deposited on the chamber walls (Weitkamp et al., 2007). When ω=1, on the other hand, condensable oxidation products and particles deposited on the chamber walls during the experiment were assumed to be in equilibrium with one another (Weitkamp et al., 2007).
Figure 3 shows results for SOA yield experiments using benzaldehyde and HBA. Note the OH exposures for benzaldehyde and HBA in these experiments were approximately 2.8×1010 cm−3 per molecule per second and 1.4×1010 cm−3 per molecule per second, respectively, which corresponds to ∼26 to 52 min of OH exposure on a typical Los Angeles summer day (Appendix F) (Griffith et al., 2016). Both experiments were allowed to react for the same amount of time, ∼350 min, to compare them to the SOA yield experiments conducted in Charan et al. (2020). Therefore, we report the SOA yields of HBA and benzaldehyde at t=350 min (SOA Y350 min) rather than at an equilibrium point. At the upper bound (ω=1), SOA Y350 min of HBA is (82±9) %, and at the lower bound (ω=0), SOA Y350 min of HBA is (69±9) %. For benzaldehyde, we report an upper bound of SOA Y350 min of (67±17) % and a lower bound of (46±17) %. While comparing SOA Y350 min is helpful in determining which pathways (addition versus abstraction) contribute to the high SOA yield of benzyl alcohol, this value does not necessarily inform atmospherically relevant SOA yields of benzaldehyde and HBA as inputs for models because modeled reaction time and conditions may not exactly match experimental ones. Therefore, we report the parameterized fit of the SOA yields as a function of absorbing organic mass concentration (M). In either case, it is clear that the subsequent oxidation of benzaldehyde and HBA contributes significantly to the total SOA yield observed in Charan et al. (2022).
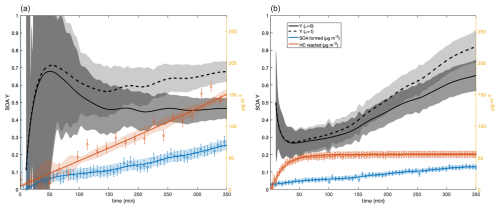
Figure 3Wall-loss-corrected SOA yields of benzaldehyde (a) and HBA (b). Solid yields are calculated assuming ω is zero. Dotted yields are calculated assuming ω equals unity. Red data are the amount of VOC precursor reacted in micrograms per cubic meter (µg m−3). Data displayed in blue are SOA formed in micrograms per cubic meter (µg m−3). Note that the first 10 min of SOA yield data is excluded because of the relatively low sensitivity and thus high errors in detecting the amount of hydrocarbon reacted and SOA formed at the start of an experiment.
We follow a one-product parameterization method that follows the multiple parameterization described in Odum et al. (1996), where
Here Kom is the partitioning coefficient and α is a constant relating the total concentration of products formed with the amount of organic gas-phase mass reacted. Two parameters were used to fit the present data. The parameters in Eq. (6) were chosen by minimizing the least square fit to the data. Results for benzaldehyde SOA yield are graphed in Fig. 4 for i=2 ( and ; and =0.73). For HBA, this approach becomes more complicated. The Odum et al. (1996) approach uses the steady state approximation (SSA) which states that the derivative of the concentration of an intermediate species appears to be zero. In other words, we assumed an approximate steady state of first-generation oxidation products which are reacting at roughly the same rate as their formation. The rates of reaction of HBA and subsequent oxidation products were likely unequal because the HBA reacts away early in the experiment; therefore, the SSA was not a sufficient approximation, and so we did not use the two-parameter Odum et al. (1996) fitting for HBA.
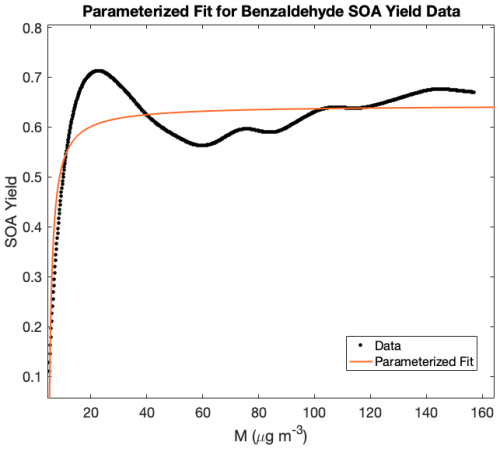
Figure 4Parameterization of SOA yield data as a function of organic mass reacted. Because SOA yields did not stabilize in these experiments, parameterization can be useful in contextualizing SOA yields under atmospherically relevant conditions.
At t=350 min, unreacted benzaldehyde remained in the chamber, whereas all of the HBA reacted within the first hour of the experiment. This may indicate that the SOA formed in the benzaldehyde experiments is from the very rapid chemistry of subsequent-generation oxidation products, as is the case with toluene. In the HBA experiments, most of the SOA is likely also generated from the oxidation of later-generation products, such as catechol.
HBA and benzaldehyde both exhibit potential for high SOA yields. HBA may have a slightly higher SOA yield than benzaldehyde and a large branching fraction from benzyl alcohol oxidation; therefore HBA contributes significantly to the large measured SOA yield of benzyl alcohol in our experiments. In aromatic oxidation chemistry, the addition of electron-donating groups (such as OH) lowers the barrier of reaction for additional OH chemistry (Calvert et al., 2002). In previously studied aromatic systems, adding electron-donating substituent groups can increase reactivity with OH (Calvert et al., 2002). In the benzyl alcohol system, after every subsequent reaction with the OH radical, we anticipate that the kinetics of HBA quickly lead to low-volatility, highly oxygenated products that readily partition into aerosol. The rapid reaction of HBA leads to catechol and other products that react quickly with OH.
3.3 Comparison with past work
Past studies have detected HBA and benzaldehyde from the oxidation of benzyl alcohol (Wang, 2015; Bernard et al., 2013; Harrison and Wells, 2009). We estimate the branching fraction to HBA to be (36±18) %. In comparison, Wang (2015) calculated o-HBA branching of 11 % using theoretical methods, while Bernard et al. (2013) estimated the branching fraction to be 21 %. The differences from Bernard et al. (2013) likely reflect the challenges of quantifying HBA and accounting for its very fast reactivity.
We observed only one isomer of HBA which we assume to be the ortho product. This assignment is based on past work that suggests the ortho position is a major product in aromatic oxidation chemistry by OH (Harrison and Wells, 2009; Finlayson-Pitts and Pitts, 1986; Baltaretu et al., 2009). Similarly, Wang (2015) predicted a single stable isomer of HBA: ortho. If additional HBA isomers existed in our system, it is likely they would have eluted at higher temperatures than were allowed by the current GC temperature profiles and were therefore undetected. However, secondary isomer formation is typically considered to be minor in other aromatic systems (Finlayson-Pitts and Pitts, 1986; Baltaretu et al., 2009).
Bernard et al. (2013) also reported a benzaldehyde yield of (25±4) % (which was used as input for the computations performed in Wang, 2015), while Harrison and Wells (2009) report a yield of 24 % (no error provided) (Bernard et al., 2013; Wang, 2015; Harrison and Wells, 2009). We report a benzaldehyde-averaged branching fraction of (21±10) %, in close agreement with these studies.
Benzyl alcohol oxidizes via OH to primarily form HBA and benzaldehyde. Significant additional chemistry occurred via endo cyclization following the addition of oxygen to fragmentation products such as 5-hydroxy-4-oxo-2-pentenal and butadiene. We found that [NO] does not affect product yields for HBA, benzaldehyde, or 5-hydroxy-4-oxo-2-pentenal. HBA was the dominant first-generation product with a branching fraction of ∼36 % over a range of NO conditions. The branching fraction of benzaldehyde is estimated to be ∼21 %.
Both HBA and benzaldehyde go on to form highly oxygenated gas-phase products. HBA oxidation leads to the formation of catechol and dihydroxybenzyl alcohol. Similarly, benzaldehyde oxidation forms products such as dihydroxybenzoic acid. These products indicate that subsequent OH addition to the aromatic ring occurs in both pathways. Both the addition and abstraction routes may also contribute to the formation of C6 products.
Aerosol yield studies using HBA and benzaldehyde as the precursors suggested that the HBA pathway is a very important contributor to the high SOA yields observed in benzyl alcohol oxidation. HBA is quickly oxidized by OH to form catechol and subsequently to low-volatility products which rapidly partition to the particle phase, thus contributing to the high SOA yield of benzyl alcohol. Though VCPs have been identified as increasingly important to SOA formation, key VOC components of VCPs remain uncharacterized. Products from benzyl alcohol oxidation via OH were identified here, elucidating its fast reactivity and high aerosol mass yields.
CH3ONO (synthesized following Taylor et al., 1980) and NO (1993±20 ppmv, Matheson) were injected into Chamber G (∼800 L) in a similar fashion. The analyte is introduced to an evacuated 0.5 L glass bulb and is serially diluted with N2 until the desired mixing ratio is achieved. CH3ONO was quantified via FTIR spectroscopy using a tabulated cross section prior to being injected into Chamber G. Ultraviolet lights (eight bulbs, Sylvania F40/350BL 40 W) centered around 350 nm were used. The measured and inferred from these lights are s−1 and s−1, respectively, for Chamber G.
CF3O− ions are produced by flowing the reagent ion source (CF3OOCF3) through a radioactive source (210Po). Similarly, is formed by flowing NO. In negative mode, CF3O− ions react with multifunctional organic compounds to form either clusters (Eq. B1) or F− transfer ions (Eq. B2). CF3O− CIMS chemistry has been documented extensively in past studies (Vasquez et al., 2018; St. Clair et al., 2010; Crounse et al., 2006).
In positive mode, is used (Eq. B3). The binds to less-oxygenated species than CF3O− including carbonyls such as benzaldehyde.
The CIMS and GC-FID in experiments in Chamber P were calibrated for benzyl alcohol and benzaldehyde, respectively. The GC-CIMS and GC-FID in experiments in Chamber G were calibrated for phenol and benzaldehyde, respectively. Individual calibrants were injected into a ∼100 L Teflon pillow bag using the same injection method as described previously. The sample was then measured via a Fourier transform infrared (FTIR) spectrometer with a path length of 19 cm. The reference FTIR spectrum from the Pacific Northwest National Laboratory (PNNL) database was used to tabulate cross sections to determine the exact concentration of the calibrant (Schwantes et al., 2017). The pillow bag was then diluted using dry N2 and sampled to determine the instrumental sensitivity.
Standards of catechol and decamethylcyclopentasiloxane (D5) phenol were prepared from temperature-controlled permeation tubes which were weighed periodically to quantify their emission rates. These standards were then used to calibrate the GC-CIMS for these compounds. The measured and calculated catechol sensitivities (determined relative to phenol) agree within 28 % of each other.
For other less-volatile analytes the calculated ion–molecule collision rate was used, relative to phenol (for G1–G4) or benzyl alcohol (for P1), to estimate the CIMS sensitivities. The sensitivities of o-HBA, p-HBA, 5-hydroxy-4-oxo-2-pentenal, catechol, and benzyl alcohol (G1–G4) were estimated in this way. Average dipole moments at 298 K and polarizabilities (at B3LYP/cc-pVTZ level) were calculated for all analytes of interest (Table B1). These were used to calculate the ion collision rate between analytes and reagent ions as described in Su and Chesnavich (1982). Assuming that the clusters are well bound, the sensitivity for a given analyte relative to a reference (here phenol) has been shown to be well represented by the ratio of their ion–molecule collision rates (Murphy et al., 2023).
For particle-phase experiments, HBA was calibrated on the CIMS relative to the CIMS sensitivity of benzyl alcohol by comparing the relative GC-CIMS sensitivities of HBA and benzyl alcohol in gas-phase experiments.
In gas-phase experiments, analyte samples were cryogenically trapped for 10 min on the head of the 20 m Restek Rtx-1701 at °C. The sample was then eluted through the GC using a ramp of 10 °C min−1 to 55 °C and then 2.5 °C min−1 to 130 °C. The slower ramp rate from 55–130 °C was used because most oxidation products eluted at this time and using a slower ramp ensured relevant products were sufficiently separated. When GC scans were not being taken, the CIMS sampled directly from the reaction chamber.
For the particle-phase experiments, the GC-FID was run from 40 to 250 °C with a ramp rate of 50 °C min−1. For gas-phase experiments, however, where benzyl alcohol was used as the precursor, we were interested in detecting and quantifying both benzaldehyde and benzyl alcohol. A DB-5 column in the GC-FID was used for all experiments.
Table D1Compound assignments from CIMS data. Note that several of the compounds listed have many isomeric structures though only one may be listed as an example.
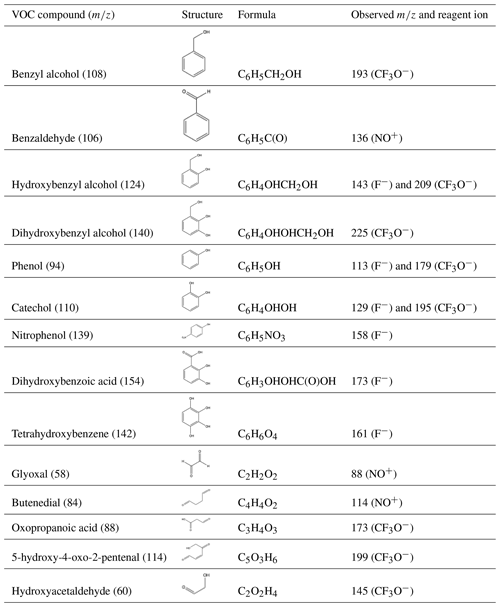
Table D1 summarizes masses detected by the GC-CIMS in gas-phase experiments (G1–G3). While some products were identified using authentic standards, other assignments are based on masses detected and our chemical understanding of this and other aromatic systems.
In gas-phase experiments, the major source of error in our calculation of branching ratios comes from the knowledge of the CIMS sensitivity to the analytes measured. A part of the uncertainty comes from propagating the error in phenol calibrated via the permeation tubes since several of the analytes were calibrated relative to phenol. Some uncertainty is also derived from the calculated ion–molecule collision rates which were based on the computationally derived dipole moments and polarizabilities. A smaller part of the reported error is also determined from the standard deviation of signals. This varies from compound to compound and is also dependent on the reagent gas used. As an example, catechol was calibrated using both the permutation tube method and the computational method. The two calibration factors varied by ∼28 %. Based on this, we estimate the error for the normalized branching fractions to be ∼45 %, congruent with past work using similar experimental setups (Praske et al., 2018; Yu et al., 2023). HBA branching ratios have a slightly higher uncertainty because of our treatment of its secondary chemistry (see Sect. 2.3.2).
[OH] was calculated for SOA yield experiments using the kinetic equation.
Here, was determined by finding the log of the slope of the starting reagent against time. For benzaldehyde, the log of the slope over the entire experiment was used, also assuming kOH = cm3 per molecule per second. For HBA, the log of the slope for the first 15 min was used to determine [OH] for the experiment, as well as assuming kOH of HBA = cm3 per molecule per second.
Particle wall loss was accounted for in SOA yield experiments using methods described in Charan et al. (2020) and Charan et al. (2018). In short, the SOA data were fit by parameterizing the eddy diffusivity coefficient (ke). The mean electric field experienced within the chamber (E) was assumed to be zero because the environmental chambers are enclosed and undisturbed prior to the experiments and therefore have no charge source. For experiment P1, ke=0.720 was used, and for experiment P2, ke=0.346 was used.
We use the kinetics of cresol to estimate kOH+HBA in order to make secondary chemistry corrections for the branching ratio of HBA. KOH+cresol is used because of its similar structure to HBA. We use a structure additivity correction to account for the abstractable hydrogens of HBA compared to cresol. We find that this rate is broadly consistent with the observed time dependence of HBA and catechol (Fig. H1).
One experiment was excluded from the data presented. This gas-phase experiment was run under similar conditions to those reported in the main text and with 0 ppb NO. This experiment was excluded because the relatively low amount of benzyl alcohol reacted resulted in very large uncertainty in the branching fractions of HBA and benzaldehyde.
Data from this study can be made available upon request. Data for experiments P1 and P2 can be found through the Integrated Chamber Atmospheric Data Repository for Unified Science (ICARUS) and upon request.
RSB: study conceptualization, data collection and analysis, result interpretation, and writing. SMC: analysis code and writing. JHS: supervision and writing. POW: supervision, result interpretation, and writing.
The contact author has declared that none of the authors has any competing interests.
Publisher’s note: Copernicus Publications remains neutral with regard to jurisdictional claims made in the text, published maps, institutional affiliations, or any other geographical representation in this paper. While Copernicus Publications makes every effort to include appropriate place names, the final responsibility lies with the authors.
The authors thank Yuanlong Huang for help in troubleshooting instrumentation in the lab, Nathan Dalleska for his insights into the GC-FID, and Katherine Ball for calibrations. We thank Henrik Kjaaragard, Copenhagen University, for providing the dipole moments and polarizabilities listed in Table B1.
This research has been supported by the National Science Foundation (grant no. CHE-2305204 and 1745301) and the Alfred P. Sloan Foundation (grant no. G-2019-12281).
This paper was edited by Frank Keutsch and reviewed by two anonymous referees.
Allen, H. M., Crounse, J. D., Bates, K. H., Teng, A. P., Krawiec-Thayer, M. P., Rivera-Rios, J. C., Keutsch, F. N., St. Clair, J. M., Hanisco, T. F., Møller, K. H., Kjaergaard, H. G., and Wennberg, P. O.: Kinetics and Product Yields of the OH Initiated Oxidation of Hydroxymethyl Hydroperoxide, J. Phys. Chem. A, 122, 6292–6302, https://doi.org/10.1021/acs.jpca.8b04577, 2018. a
Atkinson, R., Aschmann, S. M., Carter, W. P., Winer, A. M., and Pitts, J. N.: Alkyl nitrate formation from the NO-air photooxidations of C2-C8 n-alkanes, J. Phys. Chem., 86, 7, https://doi.org/10.1021/j100220a022, 1982. a
Baltaretu, C. O., Lichtman, E. I., Hadler, A. B., and Elrod, M. J.: Primary atmospheric oxidation mechanism for toluene, J. Phys. Chem. A, 113, 221–230, https://doi.org/10.1021/jp806841t, 2009. a, b
Bernard, F., Magneron, I., Eyglunent, G., Daële, V., Wallington, T. J., Hurley, M. D., and Mellouki, A.: Atmospheric chemistry of benzyl alcohol: kinetics and mechanism of reaction with OH radicals, Environ. Sci. Technol., 47, 3182–3189, https://doi.org/10.1021/es304600z, 2013. a, b, c, d, e, f, g, h, i, j, k, l, m
Bloss, C., Wagner, V., Jenkin, M. E., Volkamer, R., Bloss, W. J., Lee, J. D., Heard, D. E., Wirtz, K., Martin-Reviejo, M., Rea, G., Wenger, J. C., and Pilling, M. J.: Development of a detailed chemical mechanism (MCMv3.1) for the atmospheric oxidation of aromatic hydrocarbons, Atmos. Chem. Phys., 5, 641–664, https://doi.org/10.5194/acp-5-641-2005, 2005. a, b
Burkholder, J. B., Abbatt, J. P. D., Barnes, I., Roberts, J. M., Melamed, M. L., Ammann, M., Bertram, A. K., Cappa, C. D., Carlton, A. G., Carpenter, L. J., Crowley, J. N., Dubowski, Y., George, C., Heard, D. E., Herrmann, H., Keutsch, F. N., Kroll, J. H., McNeill, V. F., Ng, N. L., Nizkorodov, S. A., Orlando, J. J., Percival, C. J., Picquet-Varrault, B., Rudich, Y., Seakins, P. W., Surratt, J. D., Tanimoto, H., Thornton, J. A., Tong, Z., Tyndall, G. S., Wahner, A., Weschler, C. J., Wilson, K. R., and Ziemann, P. J.: The essential role for laboratory studies in atmospheric chemistry, Environ. Sci. Technol., 51, 2519–2528, https://doi.org/10.1021/acs.est.6b04947, 2017. a
Calvert, J. G., Atkinson, R., Becker, K. H., Kamens, R. M., Seinfeld, J. H., Wallington, T. J., and Yarwood, G.: The mechanism of atmospheric oxidation of aromatic hydrocarbons, Oxford University Press, New York, New York, 2002. a, b, c, d, e, f
Charan, S. M., Kong, W., Flagan, R. C., and Seinfeld, J. H.: Effect of particle charge on aerosol dynamics in Teflon environmental chambers, Aerosol Sci. Technol., 52, 854–871, https://doi.org/10.1080/02786826.2018.1474167, 2018. a, b, c
Charan, S. M., Buenconsejo, R. S., and Seinfeld, J. H.: Secondary organic aerosol yields from the oxidation of benzyl alcohol, Atmos. Chem. Phys., 20, 13167–13190, https://doi.org/10.5194/acp-20-13167-2020, 2020. a, b, c, d, e, f, g, h, i
Charan, S. M., Huang, Y., Buenconsejo, R. S., Li, Q., Cocker III, D. R., and Seinfeld, J. H.: Secondary organic aerosol formation from the oxidation of decamethylcyclopentasiloxane at atmospherically relevant OH concentrations, Atmos. Chem. Phys., 22, 917–928, https://doi.org/10.5194/acp-22-917-2022, 2022. a
Coggon, M. M., Gkatzelis, G. I., McDonald, B. C., Gilman, J. B., Schwantes, R. H., Abuhassan, N., Aikin, K. C., Arend, M. F., Berkoff, T. A., Brown, S. S., Campos, T. L., Dickerson, R. R., Gronoff, G., Hurley, J. F., Isaacman-VanWertz, G., Koss, A. R., Li, M., McKeen, S. A., Moshary, F., Peischl, J., Pospisilova, V., Ren, X., Wilson, A., Wu, Y., Trainer, M., and Warneke, C.: Volatile chemical product emissions enhance ozone and modulate urban chemistry, P. Natl. Acad. Sci. USA, 118, e2026653118, https://doi.org/10.1073/pnas.2026653118, 2021. a
Crounse, J. D., McKinney, K. A., Kwan, A. J., and Wennberg, P. O.: Measurement of gas-phase hydroperoxides by chemical ionization mass spectrometry, Anal. Chem., 78, 6726–6732, https://doi.org/10.1021/ac0604235, 2006. a, b
Finlayson-Pitts, B. and Pitts, J. N. Jr.: Atmospheric chemistry: fundamentals and experimental techniques, Wiley-Interscience, United States, ISBN 0-471-88227-5, 1986. a, b
Garden, A. L., Paulot, F., Crounse, J. D., Maxwell-Cameron, I. J., Wennberg, P. O., and Kjaergaard, H. G.: Calculation of conformationally weighted dipole moments useful in ion–molecule collision rate estimates, Chem. Phys. Lett., 474, 45–50, https://doi.org/10.1016/j.cplett.2009.04.038, 2009. a
Griffith, S. M., Hansen, R. F., Dusanter, S., Michoud, V., Gilman, J. B., Kuster, W. C., Veres, P. R., Graus, M., Gouw, J. A., Roberts, J., Young, C., Washenfelder, R., Brown, S. S., Thalman, R., Waxman, E., Volkamer, R., Tsai, C., Stutz, J., Flynn, J. H., Grossberg, N., Lefer, B., Alvarez, S. L., Rappenglueck, B., Mielke, L. H., Osthoff, H. D., and Stevens, P. S.: Measurements of hydroxyl and hydroperoxy radicals during CalNex‐LA: Model comparisons and radical budgets, J. Geophys. Res.-Atmos., 121, 4211–4232, https://doi.org/10.1002/2015JD024358, 2016. a
Harrison, J. C. and Wells, J.: Gas-phase chemistry of benzyl alcohol: Reaction rate constants and products with OH radical and ozone, Atmos. Environ., 43, 798–804, https://doi.org/10.1016/j.atmosenv.2008.11.001, 2009. a, b, c, d, e, f, g, h, i, j, k, l
Huang, Y., Zhao, R., Charan, S. M., Kenseth, C. M., Zhang, X., and Seinfeld, J. H.: Unified Theory of Vapor–Wall Mass Transport in Teflon-Walled Environmental Chambers, Environ. Sci. Technol., 52, 2134–2142, https://doi.org/10.1021/acs.est.7b05575, 2018. a
Jaoui, M., Docherty, K. S., Lewandowski, M., and Kleindienst, T. E.: Yields and molecular composition of gas-phase and secondary organic aerosol from the photooxidation of the volatile consumer product benzyl alcohol: formation of highly oxygenated and hydroxy nitro-aromatic compounds, Atmos. Chem. Phys., 23, 4637–4661, https://doi.org/10.5194/acp-23-4637-2023, 2023. a, b, c, d
Jenkin, M. E., Saunders, S. M., Wagner, V., and Pilling, M. J.: Protocol for the development of the Master Chemical Mechanism, MCM v3 (Part B): tropospheric degradation of aromatic volatile organic compounds, Atmos. Chem. Phys., 3, 181–193, https://doi.org/10.5194/acp-3-181-2003, 2003. a, b
Li, L. and Cocker, D. R.: Molecular structure impacts on secondary organic aerosol formation from glycol ethers, Atmos. Environ., 180, 206–215, https://doi.org/10.1016/j.atmosenv.2017.12.025, 2018. a
Mai, H.: Scanning Electrical Mobility Methods for Aerosol Characterization, Ph.D. thesis, California Institute of Technology, https://doi.org/10.7907/9D4H-QS44, 2018. a
Martín, P., Cabañas, B., Colmenar, I., Salgado, M. S., Villanueva, F., and Tapia, A.: Reactivity of E-butenedial with the major atmospheric oxidants, Atmos. Environ., 70, 351–360, https://doi.org/10.1016/j.atmosenv.2013.01.041, 2013. a
McDonald, B. C., de Gouw, J. A., Gilman, J. B., Jathar, S. H., Akherati, A., Cappa, C. D., Jimenez, J. L., Lee-Taylor, J., Hayes, P. L., McKeen, S. A., Cui, Y. Y., Kim, S.-W., Gentner, D. R., Isaacman-VanWertz, G., Goldstein, A. H., Harley, R. A., Frost, G. J., Roberts, J. M., Ryerson, T. B., and Trainer, M.: Volatile chemical products emerging as largest petrochemical source of urban organic emissions, Science, 359, 760–764, https://doi.org/10.1126/science.aaq0524, 2018. a, b
Murphy, S. E., Crounse, J. D., Møller, K. H., Rezgui, S. P., Hafeman, N. J., Park, J., Kjaergaard, H. G., Stoltz, B. M., and Wennberg, P. O.: Accretion product formation in the self-reaction of ethene-derived hydroxy peroxy radicals, Environ. Sci.-Atmos., 3, 882–893, https://doi.org/10.1039/D3EA00020F, 2023. a
Odum, J. R., Hoffmann, T., Bowman, F., Collins, D., Flagan, R. C., and Seinfeld, J. H.: Gas/Particle Partitioning and Secondary Organic Aerosol Yields, Environ. Sci. Technol., 30, 2580–2585, https://doi.org/10.1021/es950943+, 1996. a, b, c
Olariu, R. I., Barnes, I., Becker, K. H., and Klotz, B.: Rate coefficients for the gas-phase reaction of OH radicals with selected dihydroxybenzenes and benzoquinones, Int. J. Chem. Kinet., 32, 696–702, https://doi.org/10.1002/1097-4601(2000)32:11<696::AID-KIN5>3.0.CO;2-N, 2000. a
Parker, H. A., Hasheminassab, S., Crounse, J. D., Roehl, C. M., and Wennberg, P. O.: Impacts of Traffic Reductions Associated With COVID‐19 on Southern California Air Quality, Geophys. Res. Lett., 47, 23, https://doi.org/10.1029/2020GL090164, 2020. a, b
Pennington, E. A., Seltzer, K. M., Murphy, B. N., Qin, M., Seinfeld, J. H., and Pye, H. O. T.: Modeling secondary organic aerosol formation from volatile chemical products, Atmos. Chem. Phys., 21, 18247–18261, https://doi.org/10.5194/acp-21-18247-2021, 2021. a
Praske, E., Otkjær, R. V., Crounse, J. D., Hethcox, J. C., Stoltz, B. M., Kjaergaard, H. G., and Wennberg, P. O.: Atmospheric autoxidation is increasingly important in urban and suburban North America, P. Natl. Acad. Sci. USA, 115, 64–69, https://doi.org/10.1073/pnas.1715540115, 2018. a
Rinke, M. and Zetzsch, C.: Rate Constants for the Reactions of OH Radicals with Aromatics: Benzene, Phenol, Aniline, and 1,2,4-Trichlorobenzene, Berichte der Bunsengesellschaft für physikalische Chemie, 88, 55–62, https://doi.org/10.1002/bbpc.19840880114, 1984. a
Schwantes, R. H., Schilling, K. A., McVay, R. C., Lignell, H., Coggon, M. M., Zhang, X., Wennberg, P. O., and Seinfeld, J. H.: Formation of highly oxygenated low-volatility products from cresol oxidation, Atmos. Chem. Phys., 17, 3453–3474, https://doi.org/10.5194/acp-17-3453-2017, 2017. a, b, c, d
Seinfeld, J. H. and Pandis, S. N.: Atmospheric chemistry and physics: From air pollution to climate change, John Wiley & Sons, Hoboken, New Jersey, 3rd edn., ISBN 978-1-119-22116-6 978-1-119-22117-3, 2016. a
Seltzer, K. M., Pennington, E., Rao, V., Murphy, B. N., Strum, M., Isaacs, K. K., and Pye, H. O. T.: Reactive organic carbon emissions from volatile chemical products, Atmos. Chem. Phys., 21, 5079–5100, https://doi.org/10.5194/acp-21-5079-2021, 2021. a
St. Clair, J. M., McCabe, D. C., Crounse, J. D., Steiner, U., and Wennberg, P. O.: Chemical ionization tandem mass spectrometer for the in situ measurement of methyl hydrogen peroxide, Rev. Sci. Instrum., 81, 094102, https://doi.org/10.1063/1.3480552, 2010. a
Su, T. and Chesnavich, W. J.: Parametrization of the ion-polar molecule collision rate constant by trajectory calculations, J. Chem. Phys., 76, 5183–5185, https://doi.org/10.1063/1.442828, 1982. a, b
Taylor, W., Allston, T., Moscato, M., Fazekas, G., Kozlowski, R., and Takacs, G.: Atmospheric photodissociation lifetimes for nitromethane, methyl nitrite, and methyl nitrate, Int. J. Chem. Kinet., 12, 231–240, https://doi.org/10.1002/kin.550120404, 1980. a
Vasquez, K. T., Allen, H. M., Crounse, J. D., Praske, E., Xu, L., Noelscher, A. C., and Wennberg, P. O.: Low-pressure gas chromatography with chemical ionization mass spectrometry for quantification of multifunctional organic compounds in the atmosphere, Atmos. Meas. Tech., 11, 6815–6832, https://doi.org/10.5194/amt-11-6815-2018, 2018. a, b, c
Wang, L.: The Atmospheric Oxidation Mechanism of Benzyl Alcohol Initiated by OH Radicals: The Addition Channels, Chem. Phys. Chem., 16, 1542–1550, https://doi.org/10.1002/cphc.201500012, 2015. a, b, c, d, e, f, g, h, i, j, k
Weitkamp, E. A., Sage, A. M., Pierce, J. R., Donahue, N. M., and Robinson, A. L.: Organic Aerosol Formation from Photochemical Oxidation of Diesel Exhaust in a Smog Chamber, Environ. Sci. Technol., 41, 6969–6975, https://doi.org/10.1021/es070193r, 2007. a, b
Xu, L., Møller, K. H., Crounse, J. D., Otkjær, R. V., Kjaergaard, H. G., and Wennberg, P. O.: Unimolecular Reactions of Peroxy Radicals Formed in the Oxidation of alpha-Pinene and beta-Pinene by Hydroxyl Radicals, J. Phys. Chem. A, 123, 1661–1674, https://doi.org/10.1021/acs.jpca.8b11726, 2019. a
Xu, L., Møller, K. H., Crounse, J. D., Kjaergaard, H. G., and Wennberg, P. O.: New Insights into the Radical Chemistry and Product Distribution in the OH-Initiated Oxidation of Benzene, Environ. Sci. Technol., 54, 13467–13477, https://doi.org/10.1021/acs.est.0c04780, 2020. a, b, c
Yu, H., Møller, K. H., Buenconsejo, R. S., Crounse, J. D., Kjaergaard, H. G., and Wennberg, P. O.: Atmospheric photo-oxidation of 2-ethoxyethanol: autoxidation chemistry of glycol ethers, J. Phys. Chem. A, 127, 9564–9579, https://doi.org/10.1021/acs.jpca.3c04456, 2023. a
Zafonte, L., Rieger, P. L., and Holmes, J. R.: Nitrogen dioxide photolysis in the Los Angeles atmosphere, Environ. Sci. Technol., 11, 483–487, https://doi.org/10.1021/es60128a006, 1977. a
- Abstract
- Introduction
- Methods
- Results and discussion
- Conclusions
- Appendix A: Experimental conditions
- Appendix B: CIMS calibration and instrument sensitivity
- Appendix C: GC operation
- Appendix D: Oxidation products detected
- Appendix E: Estimation of uncertainty
- Appendix F: OH exposure
- Appendix G: Wall loss
- Appendix H: Secondary chemistry
- Appendix I: Excluded data
- Data availability
- Author contributions
- Competing interests
- Disclaimer
- Acknowledgements
- Financial support
- Review statement
- References
- Abstract
- Introduction
- Methods
- Results and discussion
- Conclusions
- Appendix A: Experimental conditions
- Appendix B: CIMS calibration and instrument sensitivity
- Appendix C: GC operation
- Appendix D: Oxidation products detected
- Appendix E: Estimation of uncertainty
- Appendix F: OH exposure
- Appendix G: Wall loss
- Appendix H: Secondary chemistry
- Appendix I: Excluded data
- Data availability
- Author contributions
- Competing interests
- Disclaimer
- Acknowledgements
- Financial support
- Review statement
- References