the Creative Commons Attribution 4.0 License.
the Creative Commons Attribution 4.0 License.
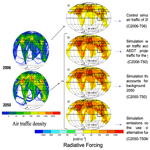
Understanding the role of contrails and contrail cirrus in climate change: a global perspective
Dharmendra Kumar Singh
Swarnali Sanyal
Donald J. Wuebbles
Globally, emissions from aviation affect Earth's climate via complex processes. Contrail cirrus and carbon dioxide emissions are the largest factors contributing to aviation's radiative forcing on climate. Contrail cirrus, like natural cirrus clouds, impacts Earth's climate. Even with the extensive ongoing research, the relative importance of the climate effects of contrails compared to other aviation effects on climate still has major uncertainties requiring further research. Contrail cirrus encompasses linear contrails and the associated cirrus clouds; these are characterized by ice particle properties, e.g., size, concentration, mixing, extinction, ice water content, optical depth, geometrical depth, and cloud coverage. The climate impact of contrails may intensify due to projected increases in air traffic. The radiative forcing from global contrail cirrus has the potential to triple and could reach as much as 160 mW m−2 by 2050. This projection is based on anticipated growth in air traffic and a potential shift to higher altitudes. The future climate impact of contrail cirrus is influenced by factors like the magnitude and geographical spread in air traffic, advancements in fuel efficiency, the effects of the use of alternative fuels, and the effects of the changing climate on the background atmosphere. This study reviews the microphysical processes affecting contrail formation and the aging of contrails and contrail cirrus. Furthermore, the study explores global observational datasets for contrails, current analyses, and future projections and will aid in evaluating the effectiveness and tradeoffs associated with various mitigation strategies. The research highlights gaps in knowledge and uncertainties while outlining research priorities for the future.
- Article
(4250 KB) - Full-text XML
- BibTeX
- EndNote
The increase in the atmospheric carbon dioxide (CO2) concentration has had the most substantial impact on human-induced climate change over recent decades (IPCC, 2021). Emissions from aviation are a minor but significant contributor to these changes in climate, especially because of emissions of CO2 and the effects of contrail formation from emitted water vapor (H2O). Aviation expansion is outpacing economic growth, with projections indicating that over the next 2 decades the demand for aviation could grow to about 3 times its present level (Wuebbles et al., 2007), with the International Civil Aviation Organization having previously projected a 4.3 % annual growth to 2050 (ICAO, 2012). Recent analyses by Boeing and Airbus suggest slightly lower rates of increase of 3.6 %–3.8 % (Boeing, 2022; Airbus, 2023). These analyses suggest that aviation could become an even more important contributor to climate change in the future. Taking both CO2 and non-CO2 effects into account. The global aviation industry comprises about 3.5 %–4 % of the current human-made (anthropogenic) activities forcing on climate (Lee et al., 2021; Klöwer et al., 2021; Grewe et al., 2021).
Aviation-induced cirrus is one of the most significant radiative forcing contributors from the aviation sector. (IPCC, 1999; Lee et al., 2009, 2021; Brasseur et al., 2016). However, considerable ambiguities persist, stemming from various origins, such as our incomplete understanding of cirrus cloud characteristics, their geographical distribution, and their life span. (Kärcher, 2018; Burkhardt et al., 2018; Schumann and Heymsfield, 2017). With current aircraft largely burning fossil fuel, CO2 emissions are the other major forcing. Additionally, NOx (oxides of nitrogen) emission also has a notable impact through the production of tropospheric ozone, but this is partially counteracted by chemical feedback effects on concentrations of atmospheric methane (CH4). Figure 1 (Lee et al., 2021) provides the most current assessment of the climate-forcing effects resulting from different aviation emissions on a global scale, spanning from 1940 to 2018; these effects are shown in terms of radiative forcing (RF) and effective radiative forcing (ERF). Radiative forcing in the context of contrails is due to the net radiative energy flux at the top of the atmosphere (TOA) caused by the presence of contrails in the atmosphere (Fuglestvedt et al., 2010). A positive radiative forcing (RF) signifies an increase in atmospheric warming, as depicted by the red bars in Fig. 1. ERF, on the other hand, adjusts the RF by accounting for rapid responses occurring within the Earth's climate system. While RF and ERF provide valuable insights, it is important to acknowledge that these are global metrics. Contrails primarily affect the upper troposphere and are short-lived, leading to potentially larger regional effects, particularly in densely populated areas with high air traffic.
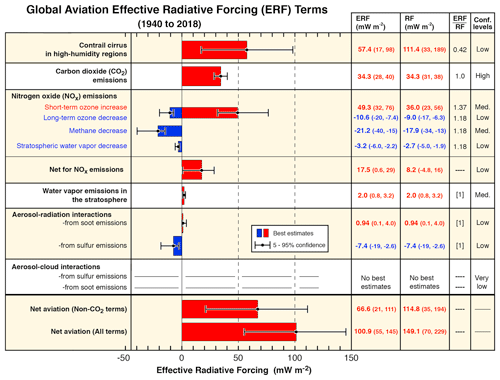
Figure 1Current best evaluation of the climate forcing between 1940 and 2018 from commercial aviation for different types of emissions. ERF accounts for short-term feedback in the climate system not accounted for in the traditional RF evaluation; adapted with permission from Lee et al. (2021).
In the scientific literature, contrail cirrus is defined as encompassing both linear contrails and the resulting formation of cirrus clouds. Figure 2 provides an overview of aviation exhaust plume emissions and factors influencing contrail formation. The characteristics of contrail cirrus include average ice particle sizes and concentrations, extinction, ice water content, optical depth, geometrical depth, and contrail coverage. Integral contrail properties involve parameters such as contrail cirrus volume, total number of ice particles, overall ice water content, and total extinction (area integral of extinction) per contrail length.
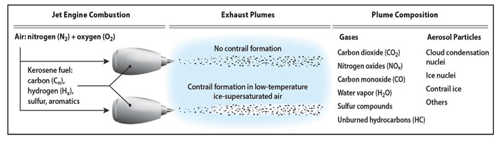
Figure 2Schematic overview of exhaust plumes (contrail formation in low temperature) and their composition; adapted with permission from Lee et al. (2021).
While significant progress has been made in recent years to incorporate contrail effects into global climate models, as evidenced by the pioneering work of Burkhardt and Kärcher (2011) and the continued advancements by others, like Bock and Burkhardt (2016a), Bier and Burkhardt (2022), Chen and Gettelman (2013), and Schumann et al. (2015), uncertainties remain in how well these models capture the long-term influence of contrails on cirrus clouds. These uncertainties arise from limitations in our understanding of how contrails age and spread (Bickel et al., 2020), the properties of the tiny ice crystals that make them up, and the behavior of contrails in regions with high ice content. Further research is crucial to address these uncertainties and improve the accuracy and reliability of contrail modeling for future climate projections.
The generation of persistent contrails depends on coming across ice-supersaturated conditions along a flight path, and these conditions show variability in both space and time within the troposphere and tropopause region (Irvine et al., 2014; Lamquin et al., 2012; Bier et al., 2017). Estimating the RF from contrail cirrus requires knowledge of complex microphysical processes, radiative transfer, and the interaction of contrails with background cloudiness (Burkhardt et al., 2010).
This review article aims to provide a comprehensive overview of our understanding of contrails, encompassing their formation, progression, and the consequent repercussions on Earth's climate system. In the process, the microphysical processes underlying contrail formation are explored along with their representation in the simulation of contrails in current climate-chemistry models of the global atmosphere. Furthermore, we address the uncertainties surrounding this topic and identify areas requiring further research in the future.
Microphysical processes play a pivotal role in the formation of contrails and their evolution in the atmosphere. This section is aimed at discussing these processes, their effects on the lifetime of contrails, and the possible transition of contrails to contrail cirrus in the upper troposphere.
2.1 The physics of contrail formation and aging
The fundamental principles for determining the formation of contrails were independently developed by E. Schmidt in 1941 and H. Appleman in 1953. Contrails appear when the hot and moist exhaust from aircraft quickly mixes with the cold and humid surrounding air, causing the humidity in the exhaust gases to exceed the saturation point for liquid water (Appleman, 1953; Schmidt, 1941; Schumann, 1996). A schematic representation of the Schmidt–Appleman criterion for contrail formation is shown in Fig. 3. In this diagram, the two solid curves depict saturation levels concerning liquid water (upper curve) and ice (lower curve). The phase trajectory of the mixture, consisting of exhaust gases and ambient air, follows a straight line from the upper right to the lower left in the e–T diagram, where e represents the partial pressure of water vapor in the mixture, and T represents its absolute temperature (as denoted by the dashed lines). The path that lines up with the water-saturation curve (dotted) specifies the highest temperatures at which contrail formation can take place. When the trajectory terminates in an ice-supersaturated state, the persisting contrails have the potential to disperse and transform into contrail cirrus.
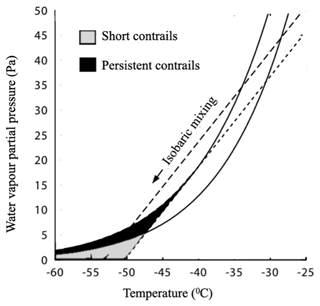
Figure 3Phase diagram with mixing lines for aircraft exhaust; adapted from Gierens et al. (2008) (licensed under CC BY 4.0).
In cases where the conditions do not meet the criteria, the contrail formed will have a brief lifespan, lasting only a few minutes. The mixing process is presumed to occur isobarically, resulting in a straight-line mixing (phase) trajectory on an e–T diagram. The Schmidt–Appleman criterion (SAC), which is primarily based on thermodynamics, establishes the threshold temperature for contrail formation. This threshold is determined by several factors, such as ambient air pressure, humidity, the amount of water and heat released by the aircraft per unit of fuel, and the overall efficiency of the aircraft engine's propulsion system. (Jensen et al., 1998; Schumann, 1996). Accurate assessments of contrails necessitate precise data on ambient meteorological conditions. The persistence of contrails is contingent on meeting a specific humidity threshold, as defined below.
The persistence of contrails hinges on the atmospheric-specific humidity. Temperature and vertical motions within the atmosphere play key roles in determining this humidity. Additionally, the optical characteristics and lifespan of contrails are key aspects of contrail formation and are influenced by various meteorological factors. These factors include turbulence, vertical motions, wind shear, and the presence of ambient cirrus clouds. The size and duration of the ice-supersaturated region; the availability of water vapor for ice crystal growth; and, consequently, the sedimentation of these ice crystals within the contrail are also essential considerations (Bock and Burkhardt, 2016). Crucially, the radiative forcing (RF) and the ensuing climate impacts depend on a multitude of meteorological parameters. Additionally, factors such as the albedo and the brightness temperature of the atmosphere in the absence of contrails play a significant role, as outlined by Schumann et al. (2012).
Upon reaching the ambient temperature through the process of mixing with the surrounding air, contrails undergo changes in size depending on the ambient humidity. When the ambient relative humidity remains supersaturated concerning ice (RHice>100 %), contrails experience growth in ice water content and can remain detectable for up to 5 h or even longer, as highlighted in various studies (Gierens and Vázquez-Navarro, 2018; Schumann and Heymsfield, 2017; Bier et al., 2017). This extended persistence contrasts with the mean lifetime of contrails, typically noted to be around 2–3 h (Schumann et al., 2015; Vázquez-Navarro et al., 2015; Tesche et al., 2016; Sausen et al., 1998). Contrails, under such conditions, have the potential to disperse and transform into thin cirrus layers. On the other hand, when the ambient relative humidity is not supersaturated concerning ice, contrail ice particles sublimate and dissipate. The timescale of this sublimation and dissipation process is contingent on the sizes of the contrail ice particles and the ambient air RHice (Schumann, 2012).
Combinations of satellite information and ground-based lidar measurements, such as in the Atlas et al. (2006) case study, showed a lifetime of more than 2 h and a mean optical thickness (integrated extinction coefficient over a vertical column of unit cross-section) of 0.35. Duda et al. (2004) studied the development of contrail clusters over the Great Lakes and derived optical thickness (optical depth, τ) from 0.1 to 0.6 for contrails that lasted several hours (Kärcher et al., 2009). Graf et al. (2012) studied the cirrus cover cycle and observed timescales between 2.3 to 4.1 h for contrail cirrus and 1.4 to 2.4 h for linear contrails.
The critical factor influencing this contrasting behavior lies in the concept of ice supersaturation. Ice supersaturation refers to conditions where the ambient humidity exceeds the saturation point for ice, even at temperatures below freezing. These conditions are essential for the persistence and growth of contrails. While the initial formation process might occur at varying humidity levels, studies like Ovarlez et al. (2002) highlight the presence of ice supersaturation within cirrus clouds, which can significantly impact contrail persistence. Jensen et al. (2001) revealed a surprisingly high frequency of ice supersaturation in the upper troposphere, even in the absence of visible ice clouds. This finding highlights the importance of considering ice supersaturation for accurate assessments of contrail persistence and its potential transformation into cirrus clouds.
Upon reaching the ambient temperature through the process of mixing with the surrounding air, contrails undergo changes in size depending on the ambient humidity. When the ambient relative humidity remains supersaturated concerning ice (RHice>100 %), contrails experience growth in ice water content and can remain detectable for up to 5 h or even longer. The microphysical properties of contrails, including ice crystal size distribution and habit, significantly influence their radiative effects and persistence. These properties are determined by the initial conditions during contrail formation and subsequent processes. Ice crystal shapes in contrails are primarily randomly oriented, small ice crystals, with a possibility of some horizontally oriented plates existing as well. This finding is supported by Iwabuchi et al. (2012), who analyzed contrails using a combination of MODIS and CALIPSO satellite data. Their study showed that contrails have larger backscattering coefficients than neighboring cirrus clouds, indicative of smaller ice crystals. The analysis also suggested a tendency for stronger depolarization when ice crystals are small, with a mean linear depolarization ratio (LDR) of approximately 0.4–0.45 for young contrails and contrail cores (Iwabuchi et al., 2012).
There has been ongoing uncertainty regarding whether persistent contrails exclusively form in cloud-free supersaturated areas or if they can also develop within existing cirrus clouds (Burkhardt et al., 2008). Subsequent modeling work (Tesche et al., 2016) suggested that persistent contrails indeed have the potential to form within cirrus clouds. While the formation of contrails is commonly observed in clear skies, they also emerge in conditions where the sky is covered with thin or even subvisible cirrus clouds (Immler et al., 2008).
The temperature threshold for contrail formation, known as the SAC threshold, is slightly higher in cirrus clouds compared to clear air (Gierens, 2012). This difference is attributed to the additional humidity introduced by the ice water content (IWC) from cirrus clouds (Verma and Burkhardt, 2022). In situ measurements have indicated high ice supersaturation both inside and outside cirrus clouds (Comstock et al., 2004). Moreover, observational evidence suggests that contrails embedded within cirrus clouds are not significantly thinner than contrails forming in clear air (Poellot et al., 1999).
A reliable estimation of the radiative effects stemming from contrail cirrus relies heavily on their optical properties, which are intricately linked to their microphysical properties and age, as well as their geographical distribution. The microphysical characteristics of contrail cirrus at various plume ages, as observed in diverse airborne campaigns, have been systematically compiled and detailed (Schröder et al., 2000; Schumann et al., 2017; Chauvigné et al., 2018). Fresh contrails, typically observed in plumes around 2 min old, exhibit distinct features, including an ice crystal number concentration reaching thousands per cubic centimeter and ice crystals measuring up to a few micrometers in diameter. This characterization is consistent with observations (Märkl et al., 2024; Bräuer et al., 2021a; Petzold et al., 1997). Contrails in their initial 2–5 min of existence have been frequently measured (Voigt et al., 2011; Gayet et al., 2012). The distinctive feature of these young contrails lies in their notably high ice crystal number concentration of small ice particles, making them easily discernible from natural cirrus formations. However, contrails often coexist with natural cirrus and may be incorporated into thin or subvisible cirrus (Kübbeler et al., 2011; Gierens, 2012; Unterstrasser et al., 2017). This coexistence poses a challenge in distinguishing between aged contrails and natural cirrus, complicating efforts to clarify the contribution of contrail cirrus to the radiative balance. Contrail cirrus is characterized by a low ice water content (IWC) ranging from 0.1 to about 10 mg m−3, a feature it shares with natural cirrus of in situ origin, as observed by Schumann et al. (2017). Ice crystals within these clouds have formed and enlarged in an ice-cloud environment (Luebke et al., 2016; Krämer et al., 2020). In contrast to contrail cirrus and in situ-origin cirrus, cirrus clouds initiating from liquid often yield a higher IWC since their ice crystals originally form as liquid drops (Krämer et al., 2016, 2020) in a warmer atmosphere with an ambient temperature exceeding 235 K (−38 °C), and particles undergo freezing as they are lifted into the cirrus temperature region of the atmosphere.
Chauvigné et al. (2018) applied a method based on principal component analysis to differentiate between particles in contrail cirrus at various stages and those observed in natural cirrus during the CONCERT 2008 campaign (Voigt et al., 2010). The success of the campaign stemmed from the fact that contrails sampled during the CONCERT initiative were relatively young and exhibited greater distinctiveness compared to natural cirrus formations. Despite this success, the comprehensive acquisition of all necessary optical and microphysical parameters proved challenging during single aircraft campaigns, limiting the widespread application of this method. The CONCERT dataset is relatively small, encompassing approximately 4.0 h of sampling time in total (Kübbeler et al., 2011). A commonly held assumption regarding the formation and evolution conditions of contrail cirrus is that it tends to persist particularly in ice-supersaturated regions (ISSRs) (Kärcher, 2018).
The collective presence of commercial, military, and other aircraft contributes to a global increase in cloudiness, primarily facilitated by the formation of persistent contrails when the surrounding atmosphere reaches supersaturation. Contrail cirrus exhibits both cooling and warming effects, with the nighttime impact being predominantly warming. Previous assessments of aviation's influence on climate (IPCC, 1999; Lee et al., 2009; Brasseur et al., 2016) were limited by the challenge of accurately quantifying the role of cloudiness arising from aging and spreading contrails (Minnis et al., 2013). The formation of a persistent contrail necessitates ice-supersaturated conditions along the aircraft's flight path. The life cycles of contrail cirrus are contingent upon the temporal and spatial scales of ice-supersaturated regions, which exhibit high variability in the troposphere and tropopause region (e.g., Lamquin et al., 2012; Irvine et al., 2013; Bier et al., 2017). Estimating the impact of contrail cirrus on upper tropospheric cloudiness requires the simulation of complex microphysical processes, contrail spreading, overlap with natural clouds, radiative transfer, and interaction with background cloudiness (Burkhardt et al., 2010).
Petzold et al. (2020) conducted a study investigating the frequency distribution of ice-supersaturated regions (ISSRs) through regular in situ observations made by passenger aircraft across northern midlatitude areas. Their research underscores the seasonal and geographical variability of ISSRs, indicating a higher likelihood of occurrence during winter and in specific geographic regions. This variability underscores the significance of considering the spatial and temporal distributions of ISSRs when assessing the potential for contrail formation.
Reutter et al. (2020) delved into the characteristics of ice-supersaturated regions using a combination of in situ water vapor measurements obtained from the IAGOS (In-service Aircraft for a Global Observing System) research program and data from the ERA-Interim reanalysis. Their findings showcase the potential for validating reanalysis data with high-resolution aircraft observations. This validation process is critical for enhancing the accuracy of global models utilized in evaluating contrail formation and their subsequent climatic impacts.
The ERF of contrail cirrus was estimated for 2011 (relative to an atmosphere without contrails) as 50 mW m−2 by Boucher et al. (2013), with uncertainty ranging from 20–150 mW m−2. Lee et al. (2021) presented a new estimate derived from the outcomes of global climate models implementing process-based contrail cirrus parameterizations. Recent analyses by Lee et al. (2021) of the current aviation fleet emissions evaluate the ERF through 2018 as 57 mW m−2, with an uncertainty range of 17–98 mW m−2. Given the limited availability of independent estimates, assessing uncertainty becomes crucial. This necessitates analyzing the sensitivities of relevant processes and incorporating the uncertainties associated with the underlying parameters and fields.
Contrails form in the early exhaust plumes of airplanes during flight (Kärcher et al., 2015; Aufm Kampe, 1943; Weickmann, 1945; Schumann and Wendling, 1990; Schumann, 1996). This happens when supercooled water droplets freeze into ice particles. At cruising altitudes, the atmospheric relative humidity (RH) is usually too low to sustain liquid water droplets but can support ice-phase particles (Gettelman et al., 2006; Lamquin et al., 2012). Consequently, freezing must occur soon after droplet formation within the moist exhaust plume to create persistent contrails. Appleman (1953) identified a key requirement for contrail formation, suggesting that many aerosol particles in the plume serve as centers for water condensation, known as the “water-saturation constraint.” Another essential factor is the visibility constraint, which requires at least 104 cm−3 within a plume age of 0.3 s for small-scale research aircraft (Appleman, 1953; Kärcher et al., 1996). These are the main constraints for contrail formation.
2.2 Transition of contrails into contrail cirrus and contrail-cirrus–soot interaction
Contrail cirrus consists of elongated contrails trailing high-altitude aircraft and thin cirrus patches formed by the dispersion of persistent contrails. The morphology of contrails evolves based on factors such as humidity, shear, stratification, waves, turbulence, and radiative heating. Contrails, individually and collectively, interact with other cirrus formations, giving rise to what is termed “contrail cirrus” (Schumann and Wendling, 1990). The total extinction is less pronounced when contrails overlap due to humidity competition. Consequently, the climate impact does not always exhibit a linear correlation with air traffic density (Unterstrasser and Sölch, 2013; Bickel et al., 2020). The transformation of a single contrail into a contrail cirrus is observable during aircraft spiral flights, as depicted in Fig. 4 (Haywood et al., 2009). “Contrail outbreaks” describe scenarios where numerous aged and young contrails coexist, often spanning expansive areas within the same airspace (Duda et al., 2001).
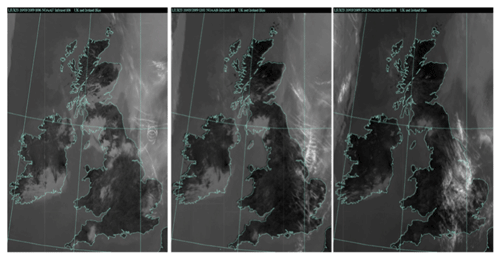
Figure 4Evolution of contrail cirrus over 1, 3, and 6.5 h. Contrail cirrus is identified by bright white areas with low infrared (10.8 mm) brightness temperature. The satellite scenes are from NOAA AVHRR (Advanced Very High Resolution Radiometer) for three UTC times: (left) 10:06 UTC (age after emission = 1 h), (middle) at 12:02 UTC (elapsed time: 3 h), and (on the right) 15:26 UTC (elapsed time: 6.5 h); adapted with permission from Haywood et al. (2009).
Soot emissions, composed of black carbon particles from aviation, have the potential to alter cirrus properties independently of contrail processing, leading to the formation of “soot cirrus” (Lee et al., 2010). Climate impact assessments of aviation-induced soot cirrus remain inconclusive due to uncertainties in soot abundance and their ice-nucleating properties (Gettelman and Chen, 2013; Zhou and Penner, 2014; Righi et al., 2021). Increased concentrations of small-sized cirrus particles resembling aviation soot emissions have been detected in cirrus regions with dense air traffic (Kristensson et al., 2000). It remains uncertain whether soot particles become incorporated into ice crystals during the initial nucleation process or through subsequent scavenging. The exact mechanism by which soot enters cirrus ice remains unclear.
A recent study by Testa et al. (2024) examines the processing of aviation soot within contrails and offered further insights into the potential influence of soot on ice nucleation. They specifically investigated the ice nucleation ability of contrail-processed soot particles at cirrus temperatures and found that these particles were generally poor ice-nucleating agents. This suggests that soot cirrus formed from processed aviation soot may have a weaker influence on cirrus cloud formation than previously thought.
Dischl et al. (2024) demonstrate a significant reduction in non-volatile particle emissions from aircraft engines using sustainable aviation fuel (SAF) compared to traditional jet fuel. This finding indirectly supports the notion that some soot particles might remain dry throughout contrail formation or that some ice particles might sublimate, as these processes could be influenced by particle properties.
The sublimation of cirrus particles containing soot and sulfate may lead to the formation of soot aggregates that can potentially act as efficient ice nuclei. A cirrus pattern observed near Munich, Germany, on 3 November 2012, displayed characteristics suggesting an association with aged soot plumes. The cirrus observed between 9.1 and 9.5 km in height, 8–10 km wide, and 35–50 km long, exhibited a distinctive pattern resembling parallel line clouds. Back trajectories indicated that soot was emitted upstream approximately 12 h before the event by aircraft. The air ascended, forming a cirrus about 4 h before the event, lasting for about 1–2 h before subsiding or sublimating. Analyses propose that this could be cirrus formed on pre-activated aircraft soot, but certainty is limited, and it cannot be ruled out that the same pattern might have formed without air traffic. (Schumann and Heymsfield, 2017).
A comprehensive understanding of the interplay between contrails, cirrus clouds, and aircraft emissions is essential for accurate assessments of their combined effect on Earth's climate. Recent investigations have illuminated this complex relationship, providing valuable scientific insights. Notably, Urbanek et al. (2018) documented ice clouds exhibiting atypical characteristics over Europe, suggesting potential deviations from standard ice crystal behavior. Additionally, these clouds displayed lower ice supersaturation, hinting at a possible modification in the usual ice formation processes (Urbanek et al., 2018). Intriguingly, the spatial distribution of these cloud formations appeared to correlate with regions experiencing high air traffic. While a definitive causal link between these observations and contrail cirrus formation remains elusive, they do suggest a potential indirect influence stemming from aircraft emissions (Urbanek et al., 2018).
In contrast, Kärcher and colleagues (2021) examine the impact of soot particles emitted by aircraft. Kärcher et al. (2021) used modeling to study how these soot particles influence the birth of new cirrus clouds. They found that only a tiny fraction of the soot particles played a role in cloud ice formation via the freezing of liquid aerosol droplets. Consequently, cirrus clouds affected by soot displayed negligible deviations in overall cloud ice content and optical depth compared to their naturally formed counterparts (Kärcher et al., 2021). These findings question the accuracy of current climate models, suggesting they might be overestimating the global radiative impact of interactions between aircraft soot and expansive cirrus clouds.
In a related study, Wolf et al. (2023) delve into the radiative effect (RE) of cirrus clouds, shedding light on the pivotal role played by ice crystal properties. Their investigations unveiled that ice crystal size and concentration are the linchpins dictating the overall RE of cirrus clouds. Intriguingly, smaller ice crystals, often associated with contrails, wield a dual-edged radiative effect, inducing both cooling and warming influences. This intricate interplay generally tips the scale towards an overall warming effect exerted by cirrus clouds (Wolf et al., 2023).
Beyond the realm of ice crystal properties, the study by Wolf et al. (2023) underscores the importance of other variables. Surface albedo emerges as a contributing factor, with cirrus clouds potentially inducing cooling effects over highly reflective surfaces, like ice, while warming effects over less reflective ones. Additionally, the presence of underlying liquid water clouds and the solar zenith angle also influence the radiative effect. Though their focus primarily centered on plane-parallel clouds, Wolf et al. (2023) acknowledge the potential significance of three-dimensional scattering effects, particularly under high sun angles.
2.3 Evolution of contrails and stages of aviation-induced cloud evolution
The review by Paoli and Shariff (2016) examined the primary physical processes and simulation efforts involved in four distinct phases of contrail evolution. These phases are commonly categorized as the jet, vortex, vortex dissipation, and diffusion phases. Contrail evolution is conveniently divided into these four regimes for clarity (Gerz et al., 1998) (see Fig. 5).
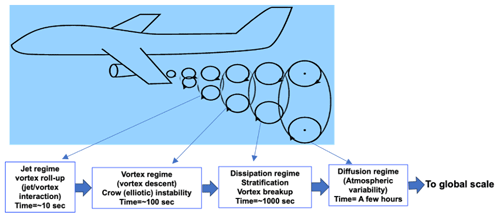
Figure 5Schematic classification of aircraft wakes evolution into four regimes; adapted from Paoli and Shariff (2016).
In the initial seconds after emission (the jet regime), the vortex sheet shed by the wings rolls up into a pair of counter-rotating vortices known as the primary wake. Simultaneously, newly formed ice crystals in the engine exhaust become entrapped around the cores of these vortices. Following this, in the minutes that follow (the vortex regime), the vortices descend into the atmosphere due to their mutually induced downward velocity.
The descent of vortices results in a contrast in density between the air contained within the vortices (within an oval-shaped region) and the ambient air. This process leads to the generation of vortices with opposite signs (in a stable stratified atmosphere) along the oval boundary. The vorticity shed in the upward direction gives birth to a “secondary wake”. Within this secondary wake, a portion of the exhaust gases and ice particles becomes incorporated. Within the regime of vortex dissipation, the primary vortex pair and secondary vorticity disintegrate and disperse, releasing both exhaust and ice crystals that eventually endure sublimation. Ice crystals released in the secondary wake can endure for a longer duration due to the lower temperature. In the fourth regime, known as the diffusion regime, the horizontal and vertical spreading of the contrail is influenced by atmospheric turbulence, particle sedimentation, radiative processes, and wind shear until complete mixing takes place, typically within a few hours.
Figure 6 illustrates the processes that influence the various stages of contrail formation. Exhaust plumes, generated by burning fuel-air mixtures at high temperatures and pressure within turbofan jet engines, contain both gaseous and particulate matter. In the freely expanding and cooling plumes (jet regime), particle types include emitted soot particles and aqueous aerosol particles formed within the exhaust, along with entrained ambient aerosol particles. In scenarios where turbulent mixing occurs, leading to cooling and generating plume supersaturation over liquid water, a significant number of plume particles transform water droplets (depicted as gray circles in Fig. 6).
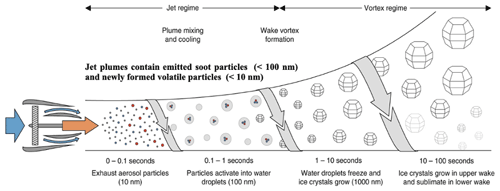
Figure 6Processes influencing the contrail formation stage. Figure adopted with permission from Kärcher (2018) (licensed under CC BY 4.0).
Water droplets rapidly freeze and increase in size by absorbing water vapor from their surroundings, eventually leading to the formation of a visible contrail. This occurs when the ambient temperature drops below the formation threshold. In exhaust rich in soot, most droplets contain soot inclusions. While droxtals, frozen water droplets with faceted surfaces, and hexagonal prisms and columns likely emerging from them may offer a realistic depiction of small ice crystals in fresh contrails, there is currently no direct observational evidence for their specific shapes.
Plumes from multiple aircraft engines combine with the two wing-tip vortices, creating an inhomogeneous wake. The subsequent evolution of ice crystals is contingent upon fluid-dynamical processes, particularly in the vortex regime. The downward movement of the vortex pair warms the surrounding air, resulting in the sublimation of ice crystals in the lower portion of the wake. A small fraction of contrail is amplified by the detrainment of air from the lower wake. Ice crystals present in the upper wake continue to grow by the uptake of entrained ice-supersaturated ambient water vapor. A few minutes past emission, the organized flow pattern collapses and mixes with ambient air (dissipation regime). Table 1 summarizes quantitative comparisons of contrail characteristics across different stages of evolution, including measurement techniques and modeling considerations.
Table 1Estimated changes in contrail properties during different stages with measurement techniques and modeling consideration. (The presented values for different stages of contrail properties and measurement techniques/modeling considerations are not based on specific references but represent a general understanding from Schumann et al., 1998, 2013, 2017, 2021; Schumann, 2005; Paoli and Shariff, 2016; Heymsfield et al., 2017; Bräuer et al., 2021a, b; Li et al., 2023a; Wang et al., 2023; Geraedts et al., 2024.)
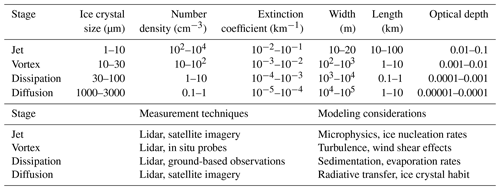
2.4 Contrail coverage
The radiative forcing (RF) for contrails, as determined by Lee et al. (2010), is defined by the product of contrail coverage and optical depth. For a single contrail segment, this product equates to total extinction. However, defining contrail coverage presents challenges for several reasons. Assessing contrail coverage separately from optical depth introduces difficulties. The identification of young contrails versus other cirrus clouds often relies on their distinctive line shape. Yet, using geometric characteristics for contrail coverage classification introduces uncertainty, particularly when contrails undergo deformation or shape changes (Mannstein et al., 1999). Only a small fraction of all linear contrails is detectable from satellites (Kärcher et al., 2009; Mannstein et al., 2010; Minnis et al., 2013).
Earlier studies examined global contrail coverage using regional satellite observations, estimating potential contrail coverage based on specific temperature and humidity data and traffic density (Sausen et al., 1998). The integration of observations to achieve hemispheric coverage has only recently become available (Duda et al., 2013). The coverage by contrail cirrus may be significantly higher than determined by line-shaped contrails, and uncertainty factors around an order of 10 have been reported (Burkhardt and Kärcher, 2011). However, this ratio is highly uncertain due to detection issues. Burkhardt and Kärcher (2011) conducted a quantification of contrail cirrus using the ECHAM contrail cirrus global climate model (CCMod), which incorporates a subgrid-scale cloud class representing young contrails. This model captures the life cycle of artificial clouds and simulates their global coverage, along with the changes in natural cloudiness they induce. Their calculations revealed contrail cirrus coverage to be approximately 0.23 %. Similar results were obtained from recent attempts to quantify contrail cirrus using MODIS data, accounting for more diffuse contrail contributions (Minnis et al., 2013).
Observations during the COVID-19 pandemic lockdowns provide valuable insights into the relationship between air traffic and contrail coverage. Duda et al. (2023) documented a significant decrease in contrail coverage (up to 41 %) over the conterminous United States during the lockdown period compared to pre-pandemic levels. This decrease highlights the direct impact of air traffic on contrail formation and underscores the potential for mitigating climate impact through air traffic management strategies. However, the study also emphasizes the importance of considering environmental factors alongside air traffic when assessing contrail coverage. While the reduction in air traffic was the primary driver of the observed decrease in contrail formation, changes in atmospheric conditions at cruise altitudes likely played a secondary, but significant, role.
In a modeling study, Huszar et al. (2013) adjusted the concentration of a tracer for contrails by multiplying it with a specific factor and then adding it to the large-scale cloud ice mixing ratio. They fine-tuned this factor through annual simulations spanning 2005 to achieve an appropriate global value of the top-of-the-atmosphere radiative forcing attributable to contrails and contrail-induced cirrus for that year, aiming for a target value of 31 mW m−2 as reported by Burkhardt and Kärcher (2011). The distribution of this forcing aligns with Burkhardt and Kärcher (2011) findings, showing peak values exceeding 300 mW m−2 over central Europe and the eastern USA, with significant parts of Europe and the USA experiencing a contrail-induced cirrus radiative forcing above 100 mW m−2. However, their estimates for eastern Asia surpass those of Burkhardt and Kärcher (2011), with maximum values exceeding 300 mW m−2, while ranging between 100 and 300 mW m−2 elsewhere. They suggested these differences could be attributed to how contrail cirrus is handled (a simpler parameterization in this study versus the more complex contrail-cirrus module used by Burkhardt and Kärcher), variations in simulated thermodynamic conditions over East Asia, and differences in emission inventories.
Schumann (2012) developed the Contrail Cirrus Prediction (CoCiP) tool based on a Lagrangian approach. In this model, global contrail coverage is computed by combining the optical depth (τ) contributions from individual contrails and ambient cirrus, counting fractions of areas where contrails cause the optical depth of the total cirrus to surpass a specific threshold (Schumann, 2012).
Contrails not only augment cloud coverage but also thicken existing cirrus, consequently influencing coverage (Minnis et al., 2013; Schumann and Graf, 2013). This thickening occurs by generating more ice particles with smaller effective radii at constant ice water content (Kristensson et al., 2000). While it is acknowledged that contrails consume humidity and may reduce natural cirrus (Burkhardt and Kärcher, 2011; Unterstrasser and Görsch, 2014; Schumann et al., 2015; Bickel et al., 2020), the prevailing evidence suggests that the thickening effect caused by numerous additional small ice crystals tends to dominate.
2.5 Contrail ice crystal nucleation
Kärcher and Yu (2009) systematically investigated the relationship between nucleated contrail ice crystal numbers and soot particle emissions. In exhaust rich in soot, there is a nearly proportional increase in both ice crystal and soot particle numbers. Close to the contrail threshold, the formation of ice crystals is limited as the low plume supersaturation hinders the activation of water on soot particles, resulting in only a few ice crystals forming. Under conditions rich in soot, the quantity of ice crystals reduces by about 100 times compared to soot-poor conditions, reaching constant low levels regulated by the number of particles present in the contrail environment. In soot-poor exhaust at low ambient temperatures, there is an increase in ice crystal numbers due to the activation of water and subsequent freezing of abundant aqueous plume particles. Figure 7 illustrates the ice crystal number emission index (per kilogram of fuel burned) in the jet regime, correlating with the number emission index of emitted soot particles as simulated by a parcel model (Kärcher and Yu, 2009). Two sets of results are presented: one for an ambient temperature (T) close to a contrail formation threshold temperature, Θ≈225 K (−48 °C), commonly encountered in extratropical cruise conditions, and another for a lower temperature of Θ (−60 °C). At intermediate ambient temperatures, nucleated ice crystal numbers increase due to enhanced water activation of either soot or ultrafine aqueous plume particles.
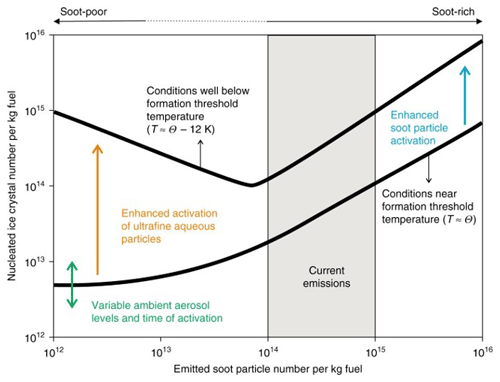
Figure 7Nucleation of ice crystals in jet aircraft exhaust plumes. Figure adapted with permission from Kärcher (2018) (licensed under CC BY 4.0).
The hatched area in Fig. 7 represents the approximate range of current in-flight soot emission indices. In exhaust rich in soot, the number of soot particles capable of water activation and freezing increases with decreasing ambient temperature. This, in turn, raises plume cooling rates and levels of plume supersaturation over liquid water. It is important to note that the primary driver of the increased cooling and supersaturation is the decreasing ambient temperature, with soot particles acting as additional ice nuclei under these cooler conditions. As the ambient temperature (T) approaches the contrail formation threshold (Θ) in soot-poor exhaust, ambient aerosol particles mixed into exhaust plumes become the sole source of contrail ice crystals. This is because fewer plume particles can be activated due to declining plume supersaturation. The number concentrations of ambient particles in contrails are significantly lower than current levels of soot emissions.
The plume cooling rate plays a crucial role in determining the timing of water activation of entrained ambient particles and, consequently, the number of ice crystals derived from them (Kärcher et al., 2015). In soot-poor exhaust, a substantial number of ultrafine aqueous plume particles are formed in the fresh exhaust, especially if the fuel contains condensable vapors besides water vapor. These small particles are anticipated to contribute significantly to ice crystal formation at low ambient temperatures well below average values at cruise levels (T≈218 K in the extratropics and T≈228 K in the tropics). If the formation of ultrafine particles cannot be mitigated, ice crystal numbers are expected to be lowest in an intermediate range of soot emissions, specifically between 1013 and 1014 kg−1 of fuel.
Ice crystal number (Ni), which quantifies the concentration of ice crystals in the surrounding atmosphere, plays a significant role in contrail properties (Kärcher, 2015). Studies have shown that Ni can influence factors such as contrail persistence, spreading, and optical characteristics (e.g., Lewellen, 2014). A higher Ni value typically indicates a greater concentration of ice particles within the contrail, which can affect its behavior in several ways.
The signs of variation in the number of ice crystals refer to the alteration in the ice particle concentration within a contrail, such as increased ice particle concentration in the contrail, greater persistence, and spreading of the contrail. Increased optical effects, such as increased brightness and reflectivity. The decreased ice crystal particle concentration in the contrail leads to reduced persistence and spreading of the contrail, and weaker optical effects. These variations in Ni may occur due to several factors, comprising changes in atmospheric conditions, aircraft engine emissions, and the presence of ice nuclei or other particles that impact ice particle formation.
Lewellen (2014) elucidates the pivotal role of initial ice crystal concentration (Ni) in contrail dynamics through extensive simulations and the proposition of a simplified model. Their simulations elucidate a discernible correlation between elevated Ni values and several significant effects on contrails. First, contrails characterized by higher Ni values demonstrate prolonged lifetimes, persisting for considerably extended durations, with simulations even indicating lifetimes surpassing 40 h (Lewellen, 2014). This protracted residence within the atmosphere translates to amplified influence on radiative forcing, as contrails with higher Ni values possess increased temporal opportunities for interaction with incident solar radiation. Second, heightened ice particle concentration within the contrail leads to augmented contrail spreading. As Ni escalates, interactions with atmospheric conditions become more conspicuous, potentially precipitating the formation of contrail cirrus, a prevalent cirrus cloud type intimately associated with contrail genesis (Lewellen, 2014). Finally, higher Ni values correlate with intensified optical effects. A greater abundance of ice crystals within the contrail facilitates more efficient scattering of incident sunlight, engendering brighter and more reflective contrails that perturb the radiative equilibrium in the atmosphere (Lewellen, 2014).
Lewellen (2014) delineates a valuable framework for understanding the influence of Ni on contrail significance through their simplified model: , where S∑ represents the lifetime-integrated ice crystal surface area, a metric for contrail impact, N is the total crystal number per length of flight path (i.e., ice crystal concentration), and D represents the number-averaged fall distance of ice crystals before sublimation. The coefficient α is approximately constant in the regime of interest. This equation underscores the critical role of Ni (N) in determining the overall radiative forcing exerted by contrails. A higher Ni value translates to a larger S∑, indicating a more significant impact on the atmosphere due to increased light scattering and longer contrail persistence.
2.6 Stages of aviation-induced cloud evolution and spreading stage
The microphysical and optical characteristics of ice crystals within contrails undergo alterations as they disperse or transform into contrail cirrus, contingent upon the prevailing meteorological conditions and microphysical processes (Fig. 8). Over time, persistent contrails undertake a transformation from their initial linear form to become contrail cirrus. In regions of heavy air traffic, these contrail cirrus formations overlap and merge, giving rise to extended layers of ice clouds characterized by alterations in shape, depth, and longevity. These patterns differ from natural cirrus clouds in terms of microphysical and optical properties; additionally, geometric properties also play a role. The ice-supersaturated layers that sustain these properties exhibit variations in both vertical structure and horizontal extent, impacting the exchange of water molecules between vapor and ice phases within them. Collectively, these factors contribute to the radiative forcing (RF) potential of aviation-induced cirrus (AIC). Figure 8 illustrates the augmentation in cloud coverage area attributed to the vertical shear of horizontal wind components.
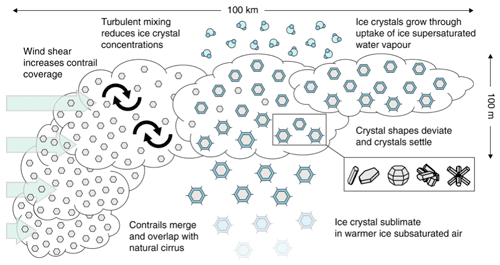
Figure 8Influential factors in aircraft-induced cloud formation. Figure adopted with permission from Kärcher (2018) (licensed under CC BY 4.0).
The turbulent mixing or entrainment processes result in a gradual reduction (dilution) of ice crystal concentrations over time. The sizes of ice crystals grow through the absorption (deposition) of water vapor from layers that are supersaturated with ice. Continuous deposition growth causes deviations in the shapes (habits) of ice crystals from their initial isometric forms.
The shapes and sizes of ice crystals in cirrus clouds significantly impact their growth rates, fall speeds, and optical properties. Larger ice crystals (>30 µm) tend to settle due to gravity with fall speeds exceeding 100 m h−1 and are more likely to sublimate in warmer or drier environments. Smaller ice crystals remain suspended around the flight levels due to their negligible fall speeds, provided that some degree of supersaturation can be maintained, which depends on the prevailing meteorological conditions.
The efficiency of sedimentation, the process by which ice crystals fall from clouds, is influenced by the depositional growth rate, which in turn is affected by ice supersaturation, the rate of air cooling, and the characteristics of the ice crystals themselves, including their size, shape, and number concentration. Sedimentation increases the vertical extent of cirrus clouds, enhancing their spreading rate and coverage under sheared flow conditions.
The settling of ice crystals, known as sedimentation, is a critical factor in determining the lifespan of contrails. As the contrail ages, larger ice particles, due to their increased mass, fall out of the plume more rapidly. This phenomenon can be explained by the concept of terminal velocity (Spichtinger and Gierens, 2009). Researchers have explored methods to calculate this velocity, considering the distribution of ice crystal sizes within the plume (Spichtinger and Gierens, 2009). This approach provides a more comprehensive understanding of the sedimentation process.
The size of an individual ice crystal directly influences its falling speed. Larger crystals, typically associated with persistent contrails, descend from the plume quicker than their smaller counterparts found in ephemeral contrails. This sedimentation process significantly impacts the vertical distribution of ice crystals within the contrail and affects its overall persistence. Ice crystals lofted higher, potentially due to weaker updrafts, may experience slower sedimentation and persist for longer durations, especially in colder atmospheric regions.
2.7 Lessons learned from observations of contrails and their properties
Contrails, as well as cirrus clouds, are frequently observed in air that lacks saturation (Kübbeler et al., 2011; Krämer et al., 2009). The prevailing explanation suggests that these clouds consist of large ice particles formed under supersaturated conditions, which then descend to lower and drier levels, undergoing slow sublimation. Notably, instances of contrails and contrail cirrus have been documented in ice-subsaturated air across various scenarios, extending beyond dedicated contrail research flights (Schumann et al., 2017; Voigt et al., 2011; Kübbeler et al., 2011; Gayet et al., 2012; Chauvigné et al., 2018). Such observations have been derived not only from contrail-specific research endeavors but also from IAGOS commercial aircraft data collected in the North Atlantic region (Petzold et al., 2017).
Apart from a high number of small contrail ice particles, large particles (ice particle diameter greater than 100 µm) were also detected but at relatively low concentrations (Voigt et al., 2010). Such large ice crystals were also observed in contrail cirrus during the ML-CIRRUS (midlatitude cirrus experiment) campaign (Voigt et al., 2017). However, attention to contrail cirrus in ice-subsaturated environments and the role that large ice particles play in contrail cirrus was raised by Kübbeler et al. (2011) and Schumann (2012).
Kübbeler et al. (2011) suggested that the subsaturation observed in contrail cirrus during the CONCERT campaign is caused by the sublimation of large ice particles that may have fallen from higher altitudes after forming under ice-supersaturated regions (ISSRs). However, due to the limited contrail cirrus data available, it is difficult to confirm whether contrail cirrus commonly occurs in ice-subsaturated environments.
Li et al. (2023b) explored an extended dataset derived from 14.7 h of cirrus cloud sampling conducted at a frequency of 1 Hz, with a maximum speed of approximately 290 m s−1, obtained during the ML-CIRRUS 2014 campaign. Utilizing readily available parameters that characterize cirrus microphysical properties – including ice number concentration, ice crystal sizes, and ice water content (IWC) – they employed a more straightforward statistical method to distinguish aviation-induced cirrus from natural cirrus, in contrast to the approach adopted by Chauvigné et al. (2018). It consists of the SAC, covering the most common aircraft cruising altitude range, and a recently developed algorithm for detecting aircraft exhaust plumes to identify matured contrail cirrus (>0.5 h lifetime; Voigt et al., 2017; Schumann et al., 2017) and natural cirrus. Contrail cirrus showed sharp differences from natural cirrus during the stages of formation and in the corresponding microphysical properties and occurrence conditions.
Mahnke et al. (2024) investigated the impact of aviation-induced aerosols on cirrus clouds and climate, which remains uncertain. Understanding these properties is vital for assessing aviation's climate effects. From July 2018 to March 2020, the IAGOS-CARIBIC Flying Laboratory identified over 1100 aircraft plume encounters. Findings showed consistent aerosol properties across various altitudes (upper troposphere, tropopause region, and lowermost stratosphere). The exhaust aerosols were primarily externally mixed, even after 1 to 3 h, with no increase in larger particles (diameter >250 nm) inside the plumes. The particle number emission indices (EIs) from aged plumes matched engine certification values, indicating that aviation aerosols maintain their emission state during plume expansion and that global models use accurate EI values.
Observational constraints
While there is growing evidence regarding contrail observations in ice-subsaturated environments, it is imperative to acknowledge the inherent limitations associated with measuring contrail properties. These limitations stem from various factors, including the following.
Spatial and temporal resolution of instrumentation. The comprehensive depiction of contrail phenomena may be impeded by the restricted range or sampling frequency of instruments employed for in situ or remote sensing observations. For instance, satellite-based observations might fail to capture minute-scale contrail features, while in situ measurements conducted via research aircraft may not fully encompass the spatial extent of a contrail layer.
Discriminating contrails from natural cirrus formations. The differentiation between contrails and natural cirrus clouds, particularly in ice-subsaturated conditions, presents a significant challenge and often necessitates sophisticated analytical techniques. Both varieties of ice clouds can exhibit comparable characteristics concerning size and ice crystal properties, rendering the definitive identification of their origin based on limited observations a challenging endeavor.
Restricted data availability. Observational datasets, particularly those focusing on fully developed contrail cirrus, may be scarce. This scarcity impedes the ability to draw definitive conclusions regarding the prevalence of contrails in subsaturated environments and their enduring influence on the climate system.
Understanding the multifaceted impacts of contrails necessitates employing models at various scales. Local-scale process and fluid-dynamic models offer valuable insights into how plume turbulence and wake dynamics influence the nucleation and properties of contrail ice crystals. These simulations can then be validated against in situ aircraft measurements of nucleated ice crystal concentrations and sublimation losses (Kärcher, 2018). For broader-scale analysis, cloud-resolving and regional models are utilized. These models incorporate meteorological boundary conditions and leverage optical parameterizations to capture the shortwave radiative response of micrometer-sized ice crystals. By co-locating these simulations with data from aircraft and satellite observations, scientists can gain a more comprehensive understanding of the radiative forcing (RF) exerted by contrail cirrus and its potential influence on pre-existing cirrus clouds (Kärcher, 2018).
The limitations of current instrumentation in measuring the microphysical and optical properties of contrail cirrus elevate the importance of models (Table 2) for estimating their RF. To provide robust predictions of climate change driven by aircraft emissions, models simulating ice nucleation and sublimation must effectively translate the current understanding of contrail formation and evolution processes into frameworks that lack the spatial or temporal resolution to explicitly capture the formation stage. This objective can be achieved by synergistically integrating findings from observations with local-scale, process-oriented models.
Table 2Modeling the complexities of aircraft-induced cloud radiative effects (adapted from Kärcher, 2018).
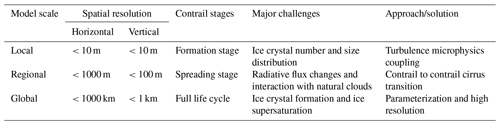
Improvements in a hierarchy of local- to global-scale models to be realized in conjunction with observations providing data for cloud and radiation parameterization development and overall model validation.
3.1 Modeling of individual contrails
Individual contrails have been studied by various authors through approaches ranging from simplified parameterizations to more complex numerical models. Parameterization approaches normally depend on simplified assumptions to estimate contrail properties based on simplified atmospheric and emission conditions. On the contrary, more sophisticated numerical models, such as the Contrail Cirrus Prediction (CoCiP) model, utilize detailed representations of atmospheric processes, ice particle growth, and radiative transfer to simulate the formation and evolution of contrails.
3.1.1 Contrail Cirrus Prediction (CoCiP) tool for individual contrails
Schumann et al. (2017) compiled a dataset of contrail properties from various sources, including previous publications, additional information gathered from experimenters, reanalysis of existing data, and comparisons with the CoCiP database. The dataset expands upon the work of Schumann and Heymsfield (2017) by incorporating data from the ML-CIRRUS campaign (Voigt et al., 2016). The data include both in situ measurements of contrails, such as ice particle size spectra obtained using optical particle spectrometers (Baumgardner et al., 2011; Wendisch and Brenguier, 2013), and remote sensing data from ground-based and airborne lidar, spectroradiometers, satellites, cameras, and visual observations. Remote sensing data provide information on contrail properties such as width and optical depth (Spinhirne et al., 1998; Duda et al., 2004).
3.1.2 MIT Aircraft Plume Chemistry, Emissions, and Microphysics Model (APCEMM)
APCEMM is applied to assess the impact of nonlinear plume chemistry and to deliver an initial estimate of the effects of contrails on atmospheric chemistry. Accurately determining the intricate relationship between contrail microphysics and chemistry often necessitates the use of costly large-eddy simulations (LESs). APCEMM, through simplified assumptions about plume dynamics, strives to close the disparity between Gaussian plume models and LESs, as outlined by Fritz et al. (2020). APCEMM simulates the growth and chemical progression of an individual aircraft plume. It computes chemical concentrations and aerosol characteristics for a two-dimensional cross-section of the plume, angled perpendicular to the flight path. Dynamics, chemistry, and microphysics are explicitly modeled within the plume, using two different approaches depending on the age of the plume.
3.1.3 NASA's global model for evaluation of individual linear contrails
The NASA Ames Research Center developed a computationally efficient aircraft contrail model designed to simulate aircraft-induced contrail formation. This model relies on the Appleman criterion and operates under static atmospheric conditions (Sridhar et al., 2011; Neil et al., 2010). Subsequently, researchers from NASA Ames extended this model to simulate the dynamic transport of contrails by incorporating a Lagrangian dispersion model and a cloud microphysics model (Li et al., 2013). The computational methods employed are grounded in well-established approaches utilized in other aircraft contrail models (Pruppacher and Klett, 2000; Schumann et al., 1995).
In comparison with models from Stanford (Naiman et al., 2009, 2011a; Jacobsen et al., 2011) and DLR (Deutsches Zentrum für Luft- und Raumfahrt; Burkhardt and Kärcher, 2009, 2011; Bier and Burkhardt, 2022), this dynamic contrail model diverges primarily in two aspects. (1) It excludes the initial contrail ice particle downwash process caused by airplane wake vortex turbulence. This process, typically lasting less than a minute, is crucial in determining contrail ice nucleation, initial contrail ice particle sizes, and displacements through a complex fluid dynamic process dependent on atmospheric, aircraft, and fuel parameters. In this model, the average initial ice particle size is predefined, and the initial contrail location is set based on the cell where contrail formation conditions are met. (2) The results from this model do not yet include additional radiative forcing caused by aircraft contrails. The researchers are in the process of adding a contrail radiative forcing module capable of computing the total aircraft contrail radiative forcing using inputs from the model, such as ice particle size and linear contrail cloud cover area.
3.1.4 DLR's ICON-LEM regional model
Verma and Burkhardt (2022) developed and implemented a model for contrail formation into the ICON-LEM (ICOsahedral Non-hydrostatic Large-Eddy Model; Zängl et al., 2014; Dipankar et al., 2015). This model includes parameterizations for ice nucleation in the jet phase and ice crystal loss during the contrail's vortex phase. It facilitates the investigation of modifications to cirrus clouds resulting from contrail formation. ICON is the new German numerical weather prediction/climate model co-developed by DWD (Deutscher Wetterdienst) and MPI (Max Planck Institute). It solves a set of equations on an unstructured triangular grid based on successive refinement of a spherical icosahedron (Zängl et al., 2014).
The model asserts high horizontal resolution coupled with a vertical resolution of approximately 150 m in the upper troposphere, enabling the resolution of pertinent cloud processes. Within ICON-LEM, a contrail scheme has been developed and implemented, incorporating the parameterization of contrail ice nucleation as proposed by Kärcher et al. (2015) and accounting for the survival of ice crystals within the vortex phase. (Unterstrasser, 2016), to study changes in cloud variables due to contrail formation within cirrus. Contrail formation, dependent on atmospheric as well as aircraft and fuel parameters, is calculated, and contrail ice nucleation and ice crystal loss in the contrail's vortex phase are estimated.
3.1.5 Large-eddy simulations covering the entire life cycle, from initiation to termination
Lewellen et al. (2014) outlined the utilization of large-eddy simulations with size-resolved microphysics to model persistent aircraft contrails and the resulting contrail-induced cirrus clouds. These simulations aim to depict the dynamic evolution of contrails, spanning from a few wing spans behind the aircraft to their dissipation over an extended period. The emphasis of the study lies in the modeling approach, discussing the development of schemes for efficient numerical computation. The authors introduced dynamic local ice binning and updating, along with coupled radiation, to accurately capture microphysical processes and radiative properties within individual columns. The paper also addresses the challenge of maintaining realistic ambient turbulence over extended simulation times, proposing a quasi 3-D approach as a computationally feasible approximation of the full dynamics, allowing for exploration across a broader parameter space.
Lewellen (2014) provides an extensive analysis encompassing over 200 instances of long-lived contrails, spanning from their formation at several seconds old to their termination. The study investigated the complete lifespan of long-lived contrails originating from a single aircraft. Various factors were explored, including the effective ice crystal number emission index, temperature, relative humidity concerning ice, stratification, shear, supersaturated-layer depth, uplift/subsidence, and coupled radiation. The analysis delved into the scaling behaviors of contrail lifetime, width, ice mass, and surface area. The simulations unveiled contrail lifetimes exceeding 40 h, widths surpassing 100 km, and ice masses exceeding 50 kg m−1 of the flight path. The paper identified distinct behavioral regimes influenced by radiative forcing and proposed a simplified model to predict these regimes.
Key insights from the simulations highlight the notable impact of ice crystal number loss resulting from competition among different crystal sizes, influencing both young contrails and aging contrail cirrus. The sensitivity of contrail properties to the initial number of ice crystals decreases over time, highlighting the importance of uncertainties in ice crystal deposition coefficients and the Kelvin effect. The influence of atmospheric turbulence on contrail properties and lifetime is also emphasized. Additionally, the paper discusses the effects of ice crystal shape, coupled radiation, precipitation dynamics, and the role of water from fuel consumption in reducing ice crystal loss in colder contrails.
Lewellen's simulations provide valuable insights into contrail behavior, shedding light on the significance of crystal number loss mechanisms, the interaction between shear and ice sedimentation, the depth of the supersaturated layer, and the potential impact of cold subvisible contrails. The findings from these simulations contribute to estimating the effects of intricate contrail scenarios, formulating mitigation strategies, and enhancing our comprehension of the dynamics of natural cirrus clouds.
3.2 Treatment of contrails in global models
The consideration of contrail cirrus in global climate models has greatly improved in recent years. Despite these advancements, notable uncertainties persist, particularly in the depiction of contrail microphysics and the interaction between contrail cirrus and cirrus radiation. As mentioned earlier, the characteristics of young contrail cirrus diverge from those of natural cirrus primarily due to the elevated ice crystal number concentration typical in contrails. Consequently, microphysical process rates in contrail cirrus, influencing its lifespan, can exhibit significant deviations from those observed in natural cirrus. In this section, we examine the treatments of contrail and contrail cirrus in global models. Very few models account for contrails, so our focus is on those models that have been published extensively on contrail impacts, including modeling studies done by the Institute for Atmospheric Physics (DLR), the National Center for Atmospheric Research (NCAR), and Stanford University. The focus is on their modeling approaches and findings. We start with a description of the approach used to treat contrails in these models.
3.2.1 DLR Contrail Cirrus Prediction (CoCiP) model
CoCiP can be implemented within a global climate model (GCM) and used to study the global distributions of contrails and contrail cirrus (Schumann et al., 2015). The model is specifically crafted for the estimation of contrail cirrus coverage and the analysis of contrail climate impact, particularly in the context of aviation system optimization processes. It is engineered to simulate the entire life cycle of contrails. Contrail segments arise between waypoints along individual aircraft tracks in air masses that are cold and humid enough. The initial characteristics of contrails are contingent upon the specific aircraft involved. The advection and progression of contrails adhere to a Lagrangian Gaussian plume model. This model treats the contrail life cycle using bulk contrail ice physics, incorporating several simplifying assumptions. Notably, the model demonstrates efficiency in handling mixing and cloud processes in a quasi-analytical manner (Schumann, 2012). Contrails become extinct when the bulk ice content endures sublimation or precipitation.
The model takes into consideration the impact of both aircraft properties and ambient meteorological conditions. This encompasses established contrail formation thresholds, the effects of advection, turbulent mixing, and the formation of ice mass from both emitted and ambient humidity. The number of ice crystals is contingent upon the quantity of soot particles emitted. The model incorporates simplified approximations for the survival of ice particles in adiabatically sinking wake vortices and the loss of particles in aged contrails (Schumann, 2012).
CoCiP (Contrail Cirrus Prediction) depicted in Fig. 9 simulates the formation of contrails under specific meteorological conditions, either regionally or globally. Numerical weather prediction data are utilized to determine ambient meteorological conditions through linear interpolation at given positions and times. The model has been effectively employed to simulate contrails in both global and specific cases, and its results have been compared with outcomes from other models and in situ measurements (Schumann, 2012).
3.2.2 DLR version of the ECHAM5-HAM model
The global climate model ECHAM5-HAM (European Centre for Medium-Range Weather Forecasts (ECMWF) and Hamburg) was developed at the Max Planck Institute (MPI) for Meteorology in Hamburg, Germany (Roeckner et al., 2003; Stier et al., 2005), and adapted to the study of aviation effects on climate, including the modeling of the effects from contrails. The aerosol–climate modeling system ECHAM5-HAM is based on a flexible microphysical approach, and it predicts the evolution of an ensemble of microphysically interacting internally and externally mixed aerosol populations as well as their size distribution and composition. Bier and Burkhardt (2022) applied the ECHAM5-HAM model, incorporating a two-moment microphysical scheme that was expanded to introduce a novel cloud category – contrail cirrus. The contrail cirrus scheme encompasses a parameterization addressing contrail ice nucleation, the loss of ice crystals during the vortex phase, plume dilution, the spreading of contrails influenced by vertical wind shear, and inclusive microphysical and macrophysical processes aligned with the natural cloud scheme.
The contrail cirrus parameterization implemented in ECHAM5-HAM follows the framework established by Burkhardt and Kärcher (2009), who introduced contrail cirrus as a distinct cloud class alongside natural cirrus clouds within the model's natural cloud scheme. The water and heat budgets are balanced, with natural cirrus and contrails competing for available water vapor. Prognostic variables, including ice water content, contrail coverage, and the length of contrail cirrus, are computed based on factors such as persistence, advection, spreading, and deposition/sublimation.
Bock and Burkhardt (2016) extended this parameterization (CCMod) by incorporating a microphysical two-moment scheme (Lohmann et al., 2008). This enhancement significantly improved the representation of microphysical processes by introducing contrail cirrus ice crystal number concentration and volume as additional prognostic variables. This advancement is crucial for studies investigating the impact of aircraft particle number emissions on contrail cirrus properties and their overall climate influence.
Bier and Burkhardt (2022) describe the initialization of contrails within the model. However, the chosen initialization time of 450 s (7.5 min) warrants further discussion. This value represents half of a model time step, potentially suggesting computational efficiency as a factor in its selection. Nevertheless, a 450 s delay in contrail formation might be too long for accurate simulations, especially for studies focusing on the early stages of contrail evolution (Fig. 10). Future investigations need to explore the sensitivity of model results to this initialization time and potentially refine it for a more realistic representation of contrail formation processes.
In instances of contrail cirrus volumes exhibiting very low ice crystal number concentrations, as observed in aged contrails associated with heightened ice crystal sedimentation, the deposition of ice water is constrained, aligning with the depositional growth of ice crystals (Bock and Burkhardt, 2016). In more recent iterations of the model, specifically ECHAM5-CCMod, Bier and Burkhardt (2019) incorporated the contrail ice nucleation parameterization proposed by Kärcher et al. (2015). In their model approach, they suggest that the plume cools over time due to continuous mixing of the exhaust with ambient air, eventually becoming water-supersaturated when the SAC criterion is met. The number of activated aerosol particles, stemming from soot and ambient particles, is computed based on ambient conditions, fuel/engine characteristics, and exhaust particle properties. Additionally, Bier and Burkhardt (2022) introduced a parameterization for ice crystal loss during the vortex phase and conducted a thorough evaluation of their model.
3.2.3 NCAR CAM6 model with contrails
In the first NCAR study of the radiative forcing of linear contrails and contrail cirrus, Chen and Gettelman (2013) used the Community Atmosphere Model version 5 (CAM5), the atmospheric component of the National Center for Atmospheric Research (NCAR) Community Earth System Model (CESM). More recently, they have used the updated atmospheric component from the new version of CESM2 (Danabasoglu et al., 2020). The current atmospheric model implemented in CESM2 is CAM, version 6.2 (CAM6; Gettelman et al., 2020). CAM6 incorporates a detailed two-moment cloud microphysics scheme (Gettelman and Morrison, 2015) coupled with an aerosol microphysics and chemistry model (Liu et al., 2016; Gettelman et al., 2019). The latest version of the contrail parameterization (Chen et al., 2012) was utilized with CAM6 (Gettelman et al., 2021). While the ice-cloud microphysics and aerosols exhibit minimal differences between CAM5 and CAM6, the aerosol activation in CAM6 significantly differs, impacting natural cirrus clouds but not contrails. The assumed emission ice particle diameter was adjusted from the original parameterization (10 µm) to 7.5 µm to better align with observations (e.g., Lee et al., 2021).
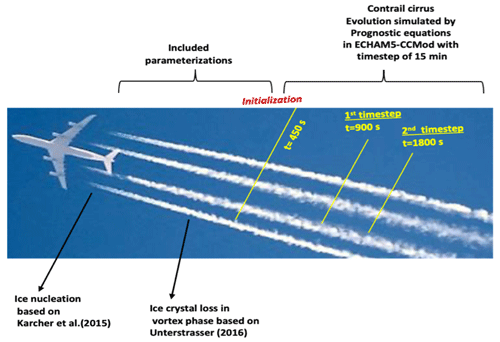
Figure 10Stages of contrails and their corresponding treatment into the global climate model ECHAM5-CCMod; adapted from Bier and Burkhardt (2022). Contrail image (credit: Adrian Pingstone (public domain)).
For contrail studies at NCAR, the standard version of CESM with 32 levels (to 3 hPa) vertical resolution and ∼1° horizontal resolution was employed. Winds and optional temperatures were relaxed to NASA's data assimilation analyses, specifically the Modern-Era Retrospective analysis for Research and Applications, version 2 (MERRA2), with wind nudging (Gettelman et al., 2020, 2021). CESM2 features a fully interactive land surface model (the Community Land Model, version 5). Sea surface temperatures (SSTs) are fixed to MERRA2 SST, and there is no interactive ocean.
These simulations allow for adjustments to atmospheric and surface temperatures, resulting in radiative flux perturbations representing an effective radiative forcing (ERF). Sensitivity tests were conducted, with temperatures nudged to MERRA2. The results were compared with previous contrail simulations using this model and others, as well as observational data. The pattern of contrail-induced changes to cloud fraction closely resembled the previous model documented in CAM5 (Chen and Gettelman, 2013), with peak effects observed in the Northern Hemisphere at midlatitudes. The radiative forcing in the CAM6 simulations exceeded that of the earlier Chen and Gettelman (2013) study due to a smaller initial contrail area (100 vs. 300 m) and smaller initial ice crystal sizes (7.5 vs. 10 µm diameter). The radiative forcing pattern and magnitude were qualitatively and quantitatively consistent with the analysis conducted by Lee et al. (2021), aligning with the intercomparison between contrail simulation models.
3.2.4 Stanford global model for contrail evaluation
Although it is no longer actively utilized in aviation studies, Stanford University developed a low-order contrail model and a large-eddy simulation (LES) model (Naiman et al., 2009, 2011b; Jacobsen et al., 2011). In their work, Jacobsen et al. (2011) assessed mass-conservative, positive-definite, unconditionally stable, and non-iterative numerical techniques for simulating the evolution of discrete, size, and composition-resolved aerosol and contrail particles within individual aircraft exhaust plumes. This simulation was conducted in a global or regional 3-D atmospheric model, incorporating the coupling of subgrid exhaust plume information to the grid scale (see Fig. 11). This approach represents a distinct method for simulating the impacts of aircraft on climate, contrails, and atmospheric composition.
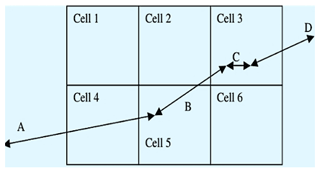
Figure 11Example of how flight segments cross grid cell boundaries in the Jacobsen et al., model. Segments A, B, C, and D are original segments. These are partitioned or aggregated into individual model grid cells 2, 3, 4, and 5 to form new segments; adapted with permission from Jacobson et al. (2011).
The microphysical processes addressed within each plume include size-resolved coagulation among and between aerosol and contrail particles, aerosol-to-hydrometeor particle ice and liquid nucleation, deposition/sublimation, and condensation/evaporation. Each plume is characterized by its own emission and supersaturation, and the spreading and shearing of each plume's cross-section are calculated over time. Aerosol and contrail-particle core compositions are tracked for each size and affect optical properties within each plume. When linear contrails sublimate or evaporate, their size- and composition-resolved aerosol cores and water vapor are introduced to the grid scale, where they influence large-scale clouds. The model's algorithmic properties were analyzed, and the final model was evaluated against in situ and satellite data. Table 3 summarizes the cross-model intercomparison of contrail simulation models covered in this study.
4.1 Contrail radiative forcing and efficacy
Contrails during the day can warm or cool depending on optical depth, zenith angle, and ice crystal shape (Meerkötter et al., 1999; Stuber et al., 2006; Newinger and Burkhardt, 2012). Stuber et al. (2006) suggested that moving all air traffic to the day decreases RF. Newinger and Burkhardt (2012) stated that we need to consider the lifetime of the contrails since daytime air traffic can cause a large contrail coverage at night. After all, it is the long-lived contrail cirrus outbreaks that are responsible for a large part of the climate impact (Burkhardt et al., 2018). As illustrated in Fig. 1, the overall effect reveals a positive net radiative forcing (RF), signifying a warming impact. The examination of this phenomenon is undertaken here in contrast to the comprehensive RF; the local RF (RF′) can be defined as the instantaneous change in net incoming radiation for 100 % contrail coverage in a specific location.
Contrail RF comprises both longwave (LW) and shortwave (SW) components, with each influenced by flight characteristics and the time of day. Unlike the systematic dependency on optical depth (τ), the relationship between RF and ice water path (IWP) is less consistent (De Leon et al., 2012; Schumann et al., 2012). The LW RF is positive both day and night, with the largest impact observed for a cold contrail (located near the tropopause) over a warm, cloud-free Earth surface. The shortwave radiative forcing (RF) is predominantly negative, with the greatest impact observed for contrails over darker surfaces, such as cloud-free oceans (Meerkötter et al., 1999). Studies conducted by Schumann et al. (2012), utilizing both their model and observational evaluations, suggest the existence of substantial regional RF′ values. The calculation of contrail RF within a global model is contingent on factors such as the representation of contrails, the atmospheric conditions, and the radiation transfer model employed (Myhre et al., 2009).
While radiative forcing (RF) provides a valuable measure of the impact of contrails on the radiative balance, it does not fully account for the efficacy of different forcing agents in driving surface temperature changes. Recent studies by Bickel et al. (2020) and Ponater et al. (2021) highlight the importance of effective radiative forcing (ERF) as a superior metric. ERF considers feedback mechanisms that can influence the ultimate climate impact of a forcing agent.
Contrail cirrus volumes often exhibit low ice crystal number concentrations due to ice crystal sedimentation. This aligns with the depositional growth of ice crystals, as described by Bock and Burkhardt (2016). Model simulations, such as those by Bier and Burkhardt (2019, 2022), incorporating the contrail ice nucleation parameterization from Kärcher et al. (2015), suggest that plume cooling through mixing leads to water supersaturation and subsequent ice crystal formation. The number of activated aerosol particles depends on ambient conditions, fuel/engine characteristics, and exhaust particle properties. These models also account for ice crystal loss during the vortex phase.
Bickel et al. (2020) analyzed the feedback processes and quantified the effective radiative forcing (ERF) of contrail cirrus, which refers to the cloud formations produced by aircraft engine exhaust. They highlighted that feedback analysis is a valuable tool for understanding climate sensitivity and the differences in efficacies between various climate-forcing agents. Previous climate model simulations have suggested a relatively low efficacy of contrails in forcing global mean surface temperature changes. They employed a climate model that incorporates a state-of-the-art representation of contrail cirrus and conducted the simulations with fixed sea surface temperatures to determine the ERF resulting from contrail cirrus.
Bickel et al. (2020) noticed that significant scaling up of aviation density is necessary to obtain statistically significant results from the simulations. Their study found that the ERF of contrail cirrus is less than 50 % of the respective instantaneous or stratosphere-adjusted radiative forcings. The best estimate of contrail cirrus ERF is approximately 35 %. In comparison, the reduction of ERF is more substantial for contrail cirrus than for a similar magnitude increase in CO2 concentrations. They identified the main factor contributing to the reduction in contrail cirrus ERF as a compensating effect of natural clouds that provide negative feedback. Additionally, they observed that the combined water vapor and lapse rate adjustment, which affects the distribution of water vapor in the atmosphere and the lapse rate (temperature decrease with altitude), has a less positive impact on contrail cirrus ERF compared to the reference case of CO2 forcing. Nevertheless, the negative feedback provided by natural clouds has a more pronounced effect in reducing contrail cirrus ERF compared to these adjustments.
Overall, Bickel et al. (2020) suggested that contrail cirrus has a lower climate impact than initially thought, with the reduction in ERF attributed to the compensating effect of natural clouds. Understanding the specific feedback processes and quantifying the ERF of different climate-forcing agents, such as contrail cirrus, is crucial for accurately assessing their contributions to surface temperature changes and overall climate dynamics.
While radiative forcing (RF) provides a measure of contrail impact on radiative balance, it does not fully account for the efficacy of different forcing agents in driving surface temperature changes. Recent studies by Ponater et al. (2021) emphasize the importance of effective radiative forcing (ERF) as a superior metric. ERF considers feedback mechanisms that influence a forcing agent's ultimate climate impact. Their findings suggest a potentially lower climate impact from contrail cirrus than previously assumed based on ERF calculations. However, as acknowledged by Ponater et al. (2021), further research is needed to confirm the efficacy of ERF in assessing the complete surface temperature response to contrail cirrus. Direct simulations of this response would be crucial for validating ERF as a reliable metric for contrail cirrus impact.
Similar to findings by Bickel (2023), this study highlights the potential limitations of using RF alone to estimate contrail cirrus impact. Here, climate model simulations revealed a significant reduction in the effective radiative forcing (ERF) of contrail cirrus compared to its conventional RF. This suggests a potentially lower climate impact from contrail cirrus than previously assumed based solely on RF calculations. The primary contributor to this reduced ERF is likely a negative cloud adjustment, where contrail cirrus formation leads to a decrease in natural cirrus cover. Further research is needed to confirm these findings across different climate models and refine our understanding of contrail cirrus efficacy in driving surface temperature changes.
Long-lasting contrails contribute to global climate change. These contrails can form cirrus clouds, which are a type of high-altitude cloud that can trap heat in the atmosphere. This trapping of heat is known as radiative forcing. While the exact contrail contribution to radiative forcing remains uncertain, it is thought to be a significant factor in climate change (Brasseur et al., 2016). The RF metric is a backward-looking measure of the effect of emissions on the radiative flux balance and is commonly used to compare changes in climate forcings (Wuebbles et al., 2010). The RF linked to non-CO2 aviation emissions arises from processes occurring over different timescales. Contrails generally exist for a few hours after an aircraft's emissions occur, but other emissions can last much longer. Effects on the distribution of aerosols and on ozone produced from NOx emissions can remain for a few days to months, while changes in CH4 can be affected for longer than a decade. The limitations of using RF as a comprehensive metric for global non-CO2 aviation climate impacts are well-recognized due to such associated spatiotemporal variations (Wuebbles et al., 2007). The nonlinear interactions involved make it imprecise to represent the sum of RF for various non-CO2 components as a single value. Similarly, distinct climate responses are observed for different forcing mechanisms.
In 2016, Brasseur et al. investigated the radiative forcing (RF) through analyses involving seven global models (CAM4, CAM5, IGSM, GISS-E2, GEOSCCM, GATOR-GCMOM, and GEOS-CHEM) as part of the US Federal Aviation Administration's (FAA) Aviation Climate Change Research Initiative (ACCRI) program. The assessment covered climate impacts for 2006, and the initial five models projected impacts for 2050 scenarios, specifically focusing on selected aircraft emission components. In a separate study, Chen and Gettelman (2016) used the CAM5 model to estimate RF for contrails and contrail cirrus in the 2050 future scenario, employing the 2006 AEDT (Aviation Environmental Design Tool) dataset. Notably, the research highlighted the tendency to overestimate global linear contrail (LC) net RF when using the natural ice-cloud optical property parameterization as a stand-in for contrail counterparts in modeling studies. Furthermore, the distribution of regional RF indicated that in densely trafficked airspaces, such as the United States, RF could be up to 10 times higher than the global average (Brasseur et al., 2016).
Schumann and Graf (2013) determined a more significant AIC impact by using both observational data and the contrail cirrus prediction model (CoCiP; Schumann, 2012). They found an “aviation fingerprint” ascribed to a daily air traffic cycle within the diurnal cycle of cirrus properties in the North Atlantic region (NAR), associating with the annual mean diurnal patterns of cirrus cover and outgoing longwave radiation (OLR) derived from Meteosat data (Graf et al., 2012).
Figure 12 shows the global average annual radiative forcing (RF) values and uncertainty ranges for persistent contrails alone and together with contrail cirrus. The values are compiled from selected studies and assessments published since 1999 and include a recent development by Kärcher (2018). Over time, early assessments of contrail RF have been confirmed and the range of uncertainty has been narrowed down considerably.
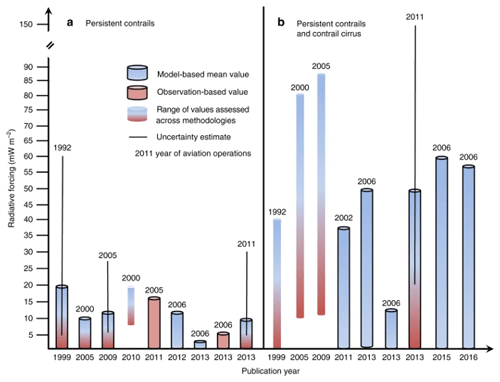
Figure 12Global annual average amount of radiative forcing caused by aircraft-induced clouds. Figure adapted with permission from Kärcher (2018) (licensed under CC BY 4.0).
The figure shows that the RF due to persistent contrails alone is −0.01 W m−2 (with an uncertainty range of 0.005–0.03 W m−2), and the RF due to persistent contrails together with contrail cirrus is ∼0.05 W m−2 (with an uncertainty range of 0.02–0.15 W m−2) (Kärcher, 2018). Figure 12 shows that analyses accounting only for linear contrails underestimate the total RF for contrails. More recent analyses tend to show an overall warming of around ∼45 mW m−2 (Bier and Burkhardt, 2022).
The models applied by DLR and NCAR's groups are summarized in Table 4 (updated from Bock and Burkhardt, 2016). Bock and Burkhardt (2016) evaluated the year 2002 using the AERO2k inventory and for year 2006 using the AEDT 2006 slant distance inventory. The corrected NCAR analyses and their more recent results are consistent with those from the DLR modeling studies (Lee et al., 2021). These findings further amplify the conclusions from Fig. 12.
Table 4Summary of existing contrail cirrus simulations and RF from the DLR and NCAR models; adapted from of Bock and Burkhardt (2016).
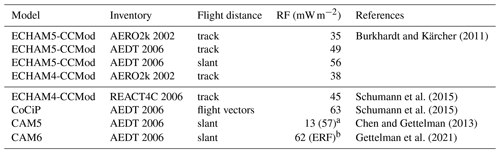
a The approximation of Chen and Gettelman (2013) was revised. b ERF (the scaling technique was derived from Lee et al. (2021), for 2006 to 2018 and then 9 % yr−1 scaled (2018 to 2019) by Gettelman et al. (2021). AERO2k – global aircraft emissions data project for climate impacts evaluation. AEDT – Aviation Environmental Design Tool. REACT4C – Reducing Emissions from Aviation by Changing Trajectories for the benefit of Climate.
4.2 Future RF projections
4.2.1 DLR's study for future RF projections
Bock and Burkhardt (2019) investigated how changes in air traffic between 2006 and 2050, including both volume increase and upward shift in flight altitudes (0.3–1.5 km), would impact contrail cirrus properties and radiative forcing. Table 5 shows their simulations considering this combined scenario. It projects a fourfold increase in air traffic and a maximum flight density at a lower altitude (200 hPa in 2050 compared to 240 hPa in 2006), which has climate implications.
Table 5Overview of the model simulations: Air traffic distance exists as ground-projected track distance. Coverage is furnished for all contrail cirrus, with coverage for visible contrail cirrus (visible optical depth >0.05) shown in brackets (Bock and Burkhardt, 2016). The radiative forcing is represented for both track distance and slant distance, with values enclosed in brackets (adapted from Bock and Burkhardt, 2019).

RCP6-Representative Concentration Pathway 6.0. * Asterisks denote extrapolated values resultant from the factor resolute by the radiative forcing in 2006, which is connected to air traffic volume and computed using slant distance and track distance (Bock and Burkhardt, 2016).
In Table 5, the air traffic distance is specified as the ground-projected track distance. The coverage is sketched out for all contrail cirrus, and the visibility of contrail cirrus (visible optical depth >0.05) is indicated in brackets (Bock and Burkhardt, 2016b). Radiative forcing estimates exist for both track distance and slant distance within brackets (Table 5). Remarkably, Bock and Burkhardt (2019) determined that the projected future increase in air traffic, coupled with a minor shift to higher altitudes, outcomes in a substantial rise in contrail cirrus coverage, optical depth, and radiative forcing. Particularly, air traffic contrail cirrus radiative forcing is estimated to increase threefold, from 49 to 159 mW m−2 (Fig. 13). The findings are a result of a future air traffic inventory, where the measurement of air traffic is signified in terms of track distance (ground projected) rather than slant distance (3-D). Declining the initial contrail ice particle number by 50 % results in a substantial drop in the climate impact of contrail cirrus, leading to a global decline in radiative forcing for the year 2050. The reduction is significant, amounting to a 14 % reduction from 160 to 137 mW m−2 (Fig. 13).
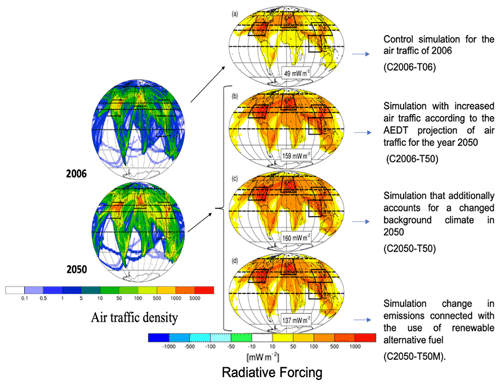
Figure 13The horizontal distribution depicts the vertically incorporated air traffic density () for the years 2006 and 2050, along with the radiative forcing in scenarios C2006-T06 (a), C2006-T50 (b), C2050-T50 (c), and C2050-T50M (d). Figure adapted with permission from Bock and Burkhardt (2019) (licensed under CC BY 4.0).
4.2.2 RF projections from the NCAR model
The study conducted by Chen and Gettelman (2016) investigated the radiative forcing curbing from aviation-induced cloudiness, using the Community Atmosphere Model version 5 (CAM5) for both present (2006) and future (up to 2050) scenarios. The researchers found a projected fourfold increase in global flight distance from 2006 to 2050. Despite this, the simulated radiative forcing from contrail cirrus in 2050 is predicted to reach 87 mW m−2, representing a sevenfold rise compared to 2006. This underlines a nonlinear correlation between radiative forcing and fuel emission mass, accredited to non-uniform regional escalations in air traffic and changes in contrail radiative forcing sensitivity through different regions.
The CAM5 simulations also indicate that the negative radiative forcing resulting from the indirect effect of aviation sulfate aerosols on liquid clouds in 2050 could be as large as −160 mW m−2, a fourfold increase from 2006. Consequently, when considering both aviation aerosols and contrail cirrus, the total radiative forcing in 2050 could possibly employ a cooling impact on the planet. Aerosols, particularly aviation sulfate aerosols distributed at cruise altitudes, may be transferred to the lower troposphere. This procedure raises aerosol concentrations and consequently enhances the cloud drop number concentration and persistence of low-level clouds. On the other hand, the study suggests that aviation black carbon aerosols have an insignificant net forcing affect globally, both in 2006 and 2050.
The researchers focus on specific regions, for instance, central Europe, eastern North America, and East Asia, and recommend quantitative evaluations of the forcing in each region for several scenarios. For example, in central Europe, the predicted contrail cirrus radiative forcing in 2050 is anticipated to locally peak at 2 W m−2, marking a twofold to threefold increase compared to 2006. In the eastern United States, the contrail cirrus radiative forcing could reach 800 mW m−2 in 2050, revealing a higher percentage increase compared to 2006. The most prominent rise in contrail cirrus radiative forcing is estimated in East Asia, where a sixfold increase is projected for 2050, associated with the region's anticipated significant rise in consumption of aviation fuel.
The inclusion of aviation aerosols in the simulations suggests a slight reduction in positive forcing over land, as compared to the forcing solely caused by contrail cirrus. On the other hand, over the ocean, a negative forcing rising from aviation emissions is measured. This can be accredited to the lower surface albedo and cleaner environment (fewer aerosols) in comparison to land areas. In the three regions with the highest projected air traffic in 2050 (eastern United States, central Europe, and East Asia), aviation aerosols reduce the regionally averaged positive radiative forcing induced by contrail cirrus by approximately 50 %, as indicated by the blue boxes in Fig. 14. The peak positive forcing within each of these regions is also reduced by 50 % due to aviation aerosols. The study provides detailed regional estimates of these effects (Table 6).
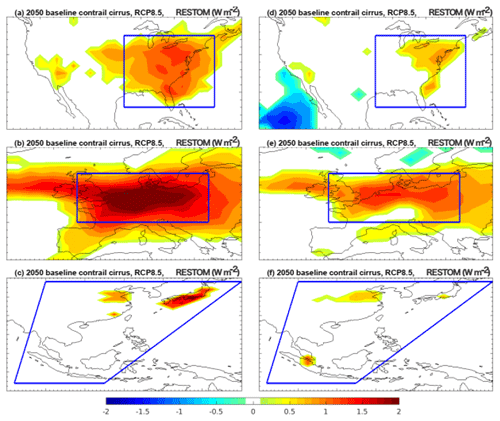
Figure 14Ensemble of the average regional radiative forcing (in W m−2), utilizing the baseline emission scenario for the year 2050 with RCP8.5 meteorology, focusing on contrail cirrus alone (a–c) and contrail cirrus combined with aviation aerosols (d–f). The term “RESTOM” represents the alteration in the net residual radiative flux at the top of the model. The plot includes only ensemble-mean perturbations that surpass 2 standard deviations of the averaged control simulations. Figure adopted with permission from Chen and Gettelman (2016) (licensed under CC BY 4.0).
Table 6Radiative forcing (mW m−2) attributed to aviation H2O emissions, with uncertainties derived from 2 standard deviations of the four-member ensemble (adapted from Chen and Gettelman, 2016).
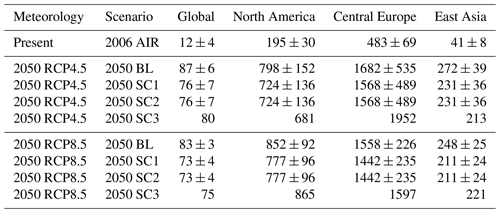
RCP4.5 – Representative Concentration Pathway 4.5. RCP8.5 – Representative Concentration Pathway 8.5. BL – base line. SC1, SC2, and SC3 – scenarios 1, 2, and 3.
The negative radiative forcing result from aviation aerosols, as detected in this study, supports the inferences represented by Righi et al. (2013). The extent of the cooling effect is estimated to be affected by the background cloud drop number concentration.
4.3 Issues representing cloud–contrail and contrail–contrail overlaps in modeling studies
A thorough examination of past methodologies for modeling cloud layer overlaps and several modeling studies recommends that overlapping with other cloud layers is prone to reduce both the shortwave (cooling) and longwave (warming) radiative forcing linked to contrails (Sanz-Morère et al., 2021). However, there is inadequate agreement on how the overlap between clouds and contrails might modify the net radiative forcing due to uncertainties regarding whether it would more strongly mitigate the shortwave or longwave components. Particularly, the precise impact of contrail–contrail overlap on global contrail radiative forcing has yet to be measured. In this section, we review previous literature referring to the treatment of multiple-layer overlap in the context of contrail radiative forcing calculations.
Schumann et al. (2012) determined that the net radiative forcing may augment if contrails overlap with low-level clouds but could go through significant variations when passing under natural cirrus clouds. This underscores the significance of precisely modeling natural clouds in contrail simulations. Nevertheless, when utilizing this method to simulate single contrails, accounting for the radiative interactions among multiple contrails turns into a challenging task. In prior examples of modeling contrail–contrail overlap, when simulating contrails in global climate models, various treatments have been utilized, as outlined in. Contrail parameterizations have been formulated for ECHAM4 (Ponater et al., 2002; Burkhardt and Kärcher, 2009), where maximum-random overlap is presumed between contrail and cloud layers, as well as among different contrails (Burkhardt and Kärcher, 2011; Marquart et al., 2003; Bock and Burkhardt, 2016; Frömming et al., 2011). Rädel and Shine (2008) and Rap et al. (2010) also use this parameterization, calibrating the outcomes by using satellite observations. Table 7 summarizes the existing methods for modeling contrail–contrail overlaps.
Table 7Existing methods for modeling contrail–contrail overlap when estimating global contrail RF. MRO: maximum-random overlap, defined by Geleyn and Hollingsworth (1978) as assuming that clouds in adjacent layers maximally overlap, while clouds separated by one or more clear layers randomly overlap (adapted from Sanz-Morère et al., 2021).
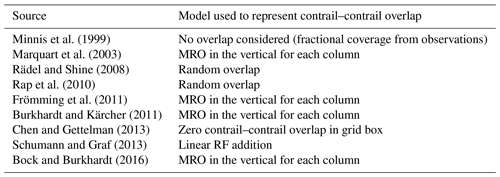
Chen and Gettelman (2013) included contrails in the CAM5 model by portraying them as an increase in the 3-D cloud fraction, where the model presumes maximum-random overlap. Nevertheless, in their method, they considered zero overlaps between newly formed contrails when placed at the same vertical level (approximately 1 km). Finally, the CoCiP Lagrangian contrail model (Schumann, 2012) reports contrail–contrail overlaps indirectly by linearly summing the radiative forcing of all contrails, taking into consideration any observed cirrus present above the simulated contrail. Nonetheless, this method does not clearly consider the overlap between simulated contrails. Inconsistencies rise in how contrail–contrail overlaps are modeled across various studies. The most efficient approach remains uncertain, and as of now, no analysis has measured the impact of contrail–contrail overlaps on global contrail RF. Since further expansion of the aviation sector is expected, it is anticipated that an increased number of instances of contrail overlap will occur. Hence, a more inclusive understanding of the degree and dynamics of contrail–contrail overlap is a necessity. Table 8 summarizes the agreement and disagreement between climate models on contrail cirrus radiative forcing.
Table 8Agreement and disagreement between climate models on contrail cirrus radiative forcing. (The models discussed include CoCiP, ECHAM5-HAM, CAM6, and the Stanford global model. Detailed descriptions of these models can be found in Sects. 3.2.1 through 3.2.4.)
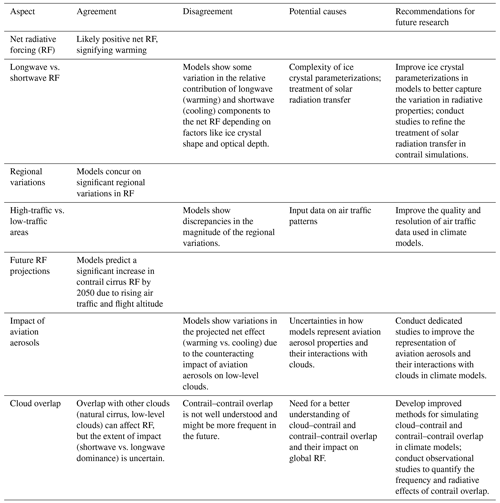
CoCiP: agreements on overall contrail effects but some discrepancies in initial ice crystal formation. ECHAM5-HAM: it captures basic contrail formation but has different ice crystal properties affecting long-term impacts. CAM6: aligns with others in radiative forcing but shows minor differences in contrail-induced cloud changes. Stanford global model: it agrees on initial formation but has contrasting plume mixing and microphysical schemes leading to varied impact predictions.
The vast majority of observations, comprised of airborne, satellite, and ground-based approaches, encompass jet aircraft exhaust contrails from 1972 onward. Nevertheless, it is remarkable that records of contrail observations date back to as early as 1915, as published by Ettenreich in 1919.
Early measurements performed behind a propeller-driven aircraft offered data supporting the concept that contrail ice formation requires liquid saturation to originate, associating with the Schmidt–Appleman criterion that is now well-established (Schmidt, 1941; Appleman, 1953; Schumann, 1996). These remarks, which encompassed the collection of ice particles on impactors and halo observations, also furnished initial insights into the size and shape of both contrail and cirrus ice particles (Weickmann, 1945).
The precise testing of models necessitates reliable quantitative data, covering not only information on contrail and plume properties but also on contrail age, the generating aircraft, atmospheric conditions during contrail formation, and the observational methods employed (Schumann et al., 2017). Mean properties of individual contrails are characterized for a wide range of jet aircraft as a function of age during their life cycle from seconds to 11.5 h (7.4–18.7 km altitude, −88 to −31 °C ambient temperature), based on a compilation of about 230 in situ and remote sensing measurements (Schumann et al., 2017). Contrails from individual aircraft can remain visible for extended periods, as reported in analyses by Minnis et al., 1998, 2013) and Vázquez-Navarro et al. (2015). Schumann et al. (2017) furnished a brief description of individual datasets, integrating new analyses for their study, and combined them to create a “contrail library” (COLI). This dataset was then compared with the outcomes of the Contrail Cirrus Prediction (CoCiP) model. The observations corroborate that the quantity of ice particles in contrails is controlled by both the engine exhaust and the formation process in the jet phase. Some particle losses ensue in the wake vortex phase, with succeeding gradually diminishes over time.
Publicly available air traffic data are usually limited, but certain projects have gathered flight data from sources such as air traffic control or ground-based observations (Schumann et al., 2013, 2017). Distinguishable projects with air traffic subsets since 2005 are accredited. Garber et al. (2005) compiled traffic data for the United States and southern Canada for the years 2000–2005. In Germany, the Deutsche Flugsicherung (DFS) has been gathering and filing away traffic data from 2006 onwards for more new projects. The Aviation and Climate Change Research Initiative (ACCRI; Brasseur et al., 2016) furnished a global waypoint dataset for the year 2006 to researchers included in the ACCRI project.
The existing observation datasets on contrail properties and formation conditions are constantly expanding with the inclusion of innovative resources. The recently developed Global Aviation Emissions Inventory based on ADS-B (GAIA) (Teoh et al., 2024) leverages historical flight paths derived from Automatic Dependent Surveillance–Broadcast (ADS-B) technology, coupled with reanalyzed weather data from 2019 to 2021. GAIA paints a detailed picture of global aviation activity, encompassing the spatial and temporal variations in flight patterns; fuel consumption; and emissions of key pollutants like CO2, NOx, and particulate matter. This information proves particularly valuable for contrail research by offering insights into the connections between aircraft types, flight routes, and the potential for contrail formation in diverse regions. For example, by analyzing short-haul versus long-haul flight emissions within GAIA, researchers can glean insights into the contribution of various aircraft categories to contrail formation patterns across different geographical areas.
By seamlessly integrating GAIA with existing observation datasets like COLI (Schumann et al., 2017), researchers can cultivate a more nuanced understanding of the interplay between aviation activity, atmospheric conditions, and contrail occurrence. This enhanced knowledge ultimately empowers the improvement of contrail prediction models and informs strategies to mitigate their impact on climate.
Particulars about aircraft assets, including size, mass, speed, fuel consumption, and propulsion efficiency, were tracked from various references, including the base of aircraft data (BADA) (EUROCONTROL, 2009). Added engine properties, such as fuel consumption and emissions at surface pressure, can be attained from the ICAO Aircraft Engine Emissions Databank (EASA, 2023) and the 2006 AEDT emissions inventory (Barrett et al., 2010; Wilkerson et al., 2010).
Poll (2018) devised a straightforward model to estimate fuel burn for commercial aircraft, offering a transparent, publicly accessible option apart from the BADA and PIANO codes (Piano-X software, Lissys Ltd., 2008). Utilizing this method necessitates understanding the Mach number and flight level where an aircraft, under specific conditions, achieves its absolute minimum fuel burn rate. Yet, acquiring these data proves challenging, although an initial effort is outlined in Poll and Schumann (Poll, 2018), where input files are supplied for 53 aircraft types.
Two sets of observational-based capabilities exist that can provide the coverage needed to help evaluate the global modeling analyses for treating the effects of contrails, the contrail library (COLI), and the satellite-based record. The contrail library results are useful for evaluating limited areas of contrails but not for global analyses.
5.1 Contrail library (COLI)
The COLI database includes 236 entries describing properties of contrails with known ages, including mean data for 100 cases of in situ measurements, more than 70 cases from ground-based and airborne lidar observations, 50 cases from satellites, as well as a few camera observations. The data come from 33 observation projects during the last 45 years. Comparisons of data from various instruments revealed notable differences across the dataset. However, there was general agreement among all methods, with consistent results from both in situ and remote sensing studies, aligning with Schumann et al. (2017). Data included in situ contrail measurements and remote sensing observations from ground-based and airborne lidar and spectroradiometers, as well as satellite imagery and visual interpretations. Table 9 summarizes some of the projects that contributed to the COLI database, including the aircraft used and the primary location, the mode of reference, and the primary reference of the study.
Table 9Observations from the year 2000 to 2014 were incorporated into the COLI for the study of contrail (adapted from Schumann et al., 2017).
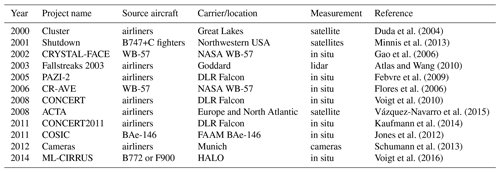
Accurate forecasting of contrail occurrence and persistence holds significant importance in addressing their potential climate impact. For this purpose, research conducted by Gierens et al. (2020) underscores the limitations of reanalysis data, primarily due to their coarse vertical resolution. This study shows the necessity for high-resolution observational data, especially concerning temperature and relative humidity in the upper troposphere and tropopause region, to understand contrail formation and behavior better. Initiatives such as the In-service Aircraft for a Global Observing System (IAGOS) (Gierens et al., 2020) are pivotal in providing such data by utilizing advanced instruments installed on passenger aircraft. The assessment of contrail formation models demands dependable data encompassing both contrail characteristics and atmospheric conditions during their formation. While the study by Gierens et al. (2020) evaluates the predictability of contrails using the Schmidt–Appleman criterion based on reanalysis data, it underscores the critical role of high-resolution data in assessing more advanced contrail prediction models. The availability of observational datasets containing parameters such as temperature, relative humidity, and air traffic data (as discussed in this section) are indispensable for such model assessments.
5.2 Satellite-based datasets
Over the past 2 decades, the detection of contrails in satellite imagery has primarily depended on the algorithm developed by Mannstein et al. (2019) (e.g., Palikonda et al., 2005; Vazquez-Navarro et al., 2010). This algorithm contains a series of convolution and thresholding operations related to brightness temperature images and, subsequently, the detection of linear associated components of fitting size. While the algorithm has been fine-tuned to attain either high precision or high remembrance in contrail detections, no single model has simultaneously surpassed in both aspects. Subsequently, empirical observations of contrail coverage often need broad lower-bound/upper-bound approaches (Duda et al., 2013). Minnis et al. (2013) reported the properties of contrail cirrus clouds formed during 11 different contrail outbreaks, in the context of objectively determined linear contrails and their properties. It was observed that the ratio of contrail cirrus to linear contrails is substantially affected by the satellite analysis algorithm utilized to allocate linear contrails. Furthermore, this ratio seems to be affected by the presence of overlapping contrails, which can obscure individual contrails.
The contrail cirrus optical depths were found to be 2–3 times greater than their linear contrail counterparts, and the associated ice crystal particle diameters were 20 % greater than the contrail particle sizes. The analyses of such satellite datasets are likely to be useful for evaluating global models of contrail radiative effects.
An exception to the application of the Mannstein et al. (2010) algorithm is prominent in Kulik (2019) and Meijer et al. (2021). These analyses employed a deep learning model for pixel-level contrail detection using GOES-16 satellite imagery. Upon detecting a contrail, these studies facilitate the assessment of its lifetime impact and the ascription of potentially relevant flights (Vazquez-Navarro et al., 2010, 2013, 2015). Mutually, these practices furnish a means to evaluate the efficacy of flight diversions in avoiding contrail formations. McCloskey et al. (2021) officially released the first large dataset of pixel-level contrail locations in Landsat-8 satellite imagery labeled by humans. Labelers should be able to accurately differentiate contrails from naturally occurring cirrus due to Landsat-8's high spatial resolution and the advected flight history information provided. This dataset will be useful in benchmarking contrail detection models and validating contrail research in colocated geostationary satellite imagery.
Satellite-based datasets offer valuable insights into global contrail occurrence and variability, although challenges arise when estimating contrail radiative forcing. Retrieving contrail optical thickness, a crucial parameter for such calculations, from current satellite observations, is particularly difficult. Moreover, distinguishing contrails from overlapping features and natural cirrus clouds remains challenging, especially with limited spatial resolution. Addressing these challenges is essential for enhancing the contribution of satellite observations to contrail radiative forcing estimations. Advancements in retrieval algorithms for contrail optical thickness and methods to differentiate contrails from overlapping features are necessary for more accurate assessments.
Despite these challenges, satellite-based contrail datasets have significant potential for monitoring contrail occurrence and coverage over time. This information is valuable for evaluating the effectiveness of flight diversion strategies aimed at minimizing contrail formation. Furthermore, the emergence of human-labeled contrail datasets like McCloskey et al. (2021) provides a valuable resource for training and validating future advancements in contrail detection using deep learning and machine learning algorithms. Continuous collaboration among satellite data providers, atmospheric scientists, and AI researchers is vital for realizing the full potential of satellite-based contrail datasets for contrail mitigation and climate impact assessments.
Ng et al. (2023) presented a human-labeled dataset named open contrails to train and evaluate contrail detection models based on GOES-16 Advanced Baseline Imager (ABI) data. Ng et al. (2023) proposed and evaluated a contrail detection model that incorporates temporal context for improved detection accuracy. The human-labeled dataset and the contrail detection outputs are publicly available on Google Cloud Storage at gs://goes_contrails_dataset.
Persuading policymakers and airlines about the climate benefits of flight diversions becomes more reasonable with the availability of satellite corroboration capabilities. Geostationary satellites like GOES-16 and low-Earth-orbit satellites such as Landsat-8 present both complementary strengths and weaknesses when employed for the task of contrail detection (i.e., coverage versus persistence of the observations). An automated contrail detection system is essential for developing and evaluating contrail avoidance systems. Deep neural networks can be employed as an automated contrail detection system, a form of artificial intelligence (AI) and machine learning (ML), that has been advanced to connect and categorize contrails in satellite imagery or other relevant data sources. This system can effectively analyze complex patterns and features associated with contrails, by leveraging the competencies of deep neural networks. By utilizing deep neural networks and machine learning techniques, the automated contrail detection system offers an efficient and reliable solution for detecting and monitoring contrails, facilitating further research and analysis of their impact on climate and aviation.
Siddiqui (2020) conducted a study where a neural network was used to distinguish contrail cirrus clouds from regular cirrus clouds from total sky imager (TSI) images. The study found that the neural network triumphed with a high accuracy of 98.5 % on the validation set, implying that the model was able to learn significant visual features of contrails that differentiate them from regular cirrus clouds. The success of this model opens various practical applications for studying contrails. For instance, the model can be used to analyze images from different months of data, agreeing for researchers to investigate the frequencies of contrail occurrences. This can impart valuable insights into air traffic trends, as well as how physical conditions like temperature and humidity vary seasonally and their potential impact on contrail formation. Researchers can better understand contrail behavior and their relationship with various environmental factors by leveraging the neural network's ability to detect and classify contrails accurately. This knowledge can be used to refine models and predictions related to contrail formation and persistence and contribute to a more comprehensive understanding of aviation's impact on the atmosphere.
Contrail studies have primarily focused on subsonic aviation due to the predominance of subsonic air traffic since the retirement of Concorde in 2003. Contrail formation and persistence for supersonic commercial aircraft pose challenges – persistent contrails are unlikely to form in the stratosphere when these aircraft are flying at supersonic speeds, but societal requirements for flying at subsonic speeds over land to avoid noise issues would still potentially produce persistent contrails. The importance of understanding the global climate effect of contrails linked to supersonic aviation is well-established (Stenke et al., 2008). Matthes et al. (2022) recently published a comprehensive review on the climate effects of supersonic aviation, encompassing non-CO2 effects like contrail formation. Their work highlights the need for a thorough understanding of existing research in this area and uncertainties persist regarding parameters controlling contrail ice crystal distribution evolution for these aircraft. Consequently, the presence or absence of contrails becomes a discernible marker of the aircraft's speed regime. Additionally, limited research, such as observations by Schumann et al. (2017), suggested the possibility of contrail formation even at high altitudes (18.7 km) under specific atmospheric conditions. These factors highlight the need for a comprehensive understanding of contrail formation and persistence for future supersonic aircraft.
7.1 Uncertainties
There are several crucial areas of uncertainty and research gaps that require focused investigation to achieve a more comprehensive understanding of contrail formation, evolution, and their effects:
-
Humidity observations and predictions. An important uncertainty lies in the accuracy and reliability of humidity observations and predictions at different altitudes. Improved measurements and modeling of humidity are critical for assessing the conditions conducive to persistent contrail formation and their subsequent behavior. Addressing this uncertainty will lead to more precise insights into contrail persistence and its associated radiative impacts.
-
Interaction with natural cirrus clouds. The interplay between natural cirrus clouds and contrails initiates an additional level of uncertainty, fostering obscuring the understanding of their individual and mutual effects on radiative forcing (RF).
-
Regional variations in atmospheric conditions. The sensitivity of contrail radiative impacts to regional variations in atmospheric conditions is not well understood and remains uncertain.
-
Uncertainties and soot particles. The role of soot particles in contrail formation remains subject to uncertainties, particularly regarding their ability to nucleate ice. Despite the presence of numerous ice particles in fresh contrails, ongoing debates, as highlighted in recent studies such as Righi et al. (2021), question whether soot particles directly facilitate ice nucleation. Clarifying this relationship is essential for comprehending the impact of soot emissions on contrail formation and subsequent evolution. To address this knowledge gap, further research efforts are imperative to explore the influence of soot particles on ice nucleation processes within contrails.
-
Radiative transfer scheme. Quantifying the radiative forcing exerted by contrails on Earth's climate remains an intricate task. Studies by Schumann and Graf (2013) identified a distinct signature, an “aviation fingerprint,” in cirrus cloud cover and outgoing longwave radiation (OLR) that mirrored air traffic patterns. This observation suggests a robust correlation between aviation activity and cirrus cloud formation. Their analysis even estimated a regional increase in heat-trapping (longwave radiative forcing) of 600–900 mW m−2, highlighting the potential influence of aviation on these high-altitude ice clouds. However, a crucial challenge lies in accurately modeling the net impact on Earth's energy balance. This difficulty stems from the fact that different climate models employ various approaches to simulate radiative transfer processes, which govern the movement of heat through the atmosphere. These variations in how models handle these radiative processes can significantly influence the estimated impact of contrails on our planet's energy equilibrium.
Significant uncertainties persist regarding the radiative forcing caused by aircraft contrails. These uncertainties arise from numerous factors, starting with uncertainties in the background meteorology and specific aircraft emissions. When assessing the climate impact of contrail cirrus, the primary uncertainties lie in (1) determining upper tropospheric water vapor concentrations and regions of supersaturation, (2) adequately representing contrail cirrus processes in global models, and (3) understanding the radiative response resulting from the contrail cirrus presence; typically, only approximate assessments of uncertainties associated with these processes are furnished.
Assessing the radiative response of contrail cirrus involves handling several crucial uncertainties, including the following.
Uncertainties in contrail cirrus radiative forcing. Estimating the radiative forcing (RF) of contrail cirrus clouds is inherently uncertain. Lee et al. (2021) categorizes these uncertainties into two main areas: (A) uncertainties related to the radiative response of contrail cirrus and (B) uncertainties associated with the upper-tropospheric water budget and the contrail cirrus scheme within climate models.
7.1.1 (A) Uncertainties in radiative response
Radiative transfer scheme (A1). Myhre et al. (2009) estimated an uncertainty of approximately 35 % arising from the radiative transfer scheme employed in climate models. This scheme simulates how different wavelengths of radiation interact with various atmospheric components, including contrail cirrus.
Cloud heterogeneity (A2). The inhomogeneity of ice crystals within a model grid box, vertical cloud overlap, and limitations of plane-parallel geometry contribute an estimated uncertainty of 35 % (Carlin et al., 2002; Pomroy and Illingworth, 2000; Gounou and Hogan, 2007).
Uncertainty in Radiative Transfer Estimation (A3). Uncertainty in estimating radiative transfer in a global climate model due to the presence of very small ice crystals within young contrails can reach approximately 10 % (Bock and Burkhardt, 2016a). This uncertainty is influenced by the ice water content in contrail cirrus.
Ice crystal habit (A4). Variations in the shape and form (habit) of ice crystals within contrail cirrus can significantly impact how they interact with radiation. Markowicz and Witek (2011) suggest an uncertainty of 20 % due to this factor.
Soot core impact (A5). The radiative transfer uncertainty associated with soot cores embedded within contrail cirrus ice crystals is significant, particularly for shortwave albedo (reflectivity), but remains unquantified (Liou et al., 2013). Soot cores can absorb radiation, potentially leading to a warming effect that is not currently accounted for in models.
7.1.2 (B) Uncertainties in water budget and contrail cirrus scheme
Ice supersaturation (B1). Uncertainties in the representation of upper-tropospheric ice supersaturation (Lamquin et al., 2012) contribute an estimated 20 % uncertainty.
Ice crystal number density (B2) and radiative transfer for young contrails (A3). Uncertainties in ice crystal number densities (Karcher et al., 2015; Kärcher, 2018) are linked to factors like water vapor saturation and soot emissions. Lee et al. (2021) recognized the dependence between A3 (radiative transfer for young contrails) and B2 (ice crystal number density). Consequently, they included the uncertainty from A3 within their overall category B uncertainty estimate.
Contrail cirrus lifetime (B3). The effect of contrail cirrus lifetime on estimated RF is relatively small, with an associated uncertainty estimated at 5 %–10 % (Chen and Gettelman, 2013; Newinger and Burkhardt, 2012). While Lewellen (2014) highlights the potential importance of lifetime through large-eddy simulations demonstrating contrail cirrus lifetimes exceeding 40 h and widths exceeding 100 km, several factors contribute to the seemingly counterintuitive finding in this context of estimated RF. Lewellen's (2014) simulations highlight the potential significance of contrail cirrus with extended lifetimes, particularly for their ice crystal surface area and overall radiative impact. However, the study focuses on the integrated effect over the entire lifetime, whereas the uncertainty estimate (5 %–10 %) pertains to the impact of variations in lifetime on the average RF. Further research is needed to better understand the complex interplay between contrail cirrus lifetime, ice crystal growth processes, atmospheric conditions, and their combined effect on radiative forcing.
Air traffic data resolution (B4). Sensitivity studies suggest an uncertainty of about 10 % arising from the temporal resolution of air traffic data used in climate models (Lee et al., 2021).
Natural cloud feedback (B5). The feedback mechanism by which contrail cirrus alters the upper tropospheric water budget, affecting natural clouds, introduces a significant uncertainty that remains unquantified (Burkhardt and Karcher, 2011; Schumann et al., 2015). Lee et al. (2021) assumed an uncertainty of 15 % for this factor.
Initial ice crystal assumptions (B6). The estimation of RF by Chen and Gettelman (2013) is subject to uncertainties associated with assumptions about initial ice crystal radii and contrail cross-sectional areas, leading to an estimated uncertainty of 33 %.
7.1.3 Overall uncertainty
Following Lee et al. (2021), the uncertainty in the radiative response to contrail cirrus (excluding soot cores) is estimated to be around 55 %, assuming independence of different uncertainties. This aligns with their findings and highlights the dominance of radiative response uncertainties (category A) in the overall uncertainty budget. Category B uncertainties (water budget and contrail cirrus scheme) remain significant and require further investigation, particularly the combined effect of ice crystal number density and radiative transfer for young contrails.
7.1.4 The uncertainty tightrope
While scientists continue to refine their understanding of how contrails form and influence our climate, the ever-present threat of climate change demands that we do not wait for complete certainty before taking action. The evidence of our warming planet is undeniable, and aviation's contribution to this issue necessitates exploring all possible solutions, even in the face of some lingering unknowns.
Fortunately, research like that conducted by Frias et al. (2024) offers a glimmer of hope. Their study suggests that practical strategies to avoid contrail formation can be implemented, with minimal disruption to flight operations, while significantly reducing the overall climate impact of these contrails. This finding underscores the potential for immediate action alongside ongoing scientific inquiry. By embracing a two-pronged approach that combines continued research with real-world mitigation efforts, we can work towards minimizing aviation's environmental footprint.
7.2 Research needs and gaps
In the pursuit of a comprehensive understanding of contrail impacts on climate, there is a critical need to establish clear research needs and requirements.
Improving weather models demands better predictions of humidity and clouds with enhanced resolution in time, space, and altitude. The models must also adapt to evolving weather conditions. Accurate water vapor data during cruise altitudes are crucial, emphasizing the need for small, cost-effective humidity sensors. To achieve this, there is a need for the production, testing, and evaluation of precise sensors. Lidar technology and weather model assessments play a key role in advancing observational capabilities for more accurate predictions. Enhancing cirrus cloud forecasts requires a dual-focus on data and artificial intelligence. Ensemble simulations are vital for estimating uncertainties in cirrus cloud predictions. Additionally, there is an urgent need for more climate and contrail models, ensuring a comprehensive understanding and evaluation of these complex atmospheric processes.
Research by Ovarlez et al. (2000) highlights discrepancies between measured water vapor content and data from weather forecasts. Their findings emphasize the importance of accurate humidity measurements, particularly for studies on contrail formation and persistence. Weather forecasts might underestimate actual humidity, potentially leading to inaccurate assessments of contrail behavior. This underlines the need for ongoing research to improve the accuracy of humidity data in weather models.
Furthermore, high-quality observations, effectively integrated into weather models, are crucial for setting accurate starting points for forecasts (Bauer et al., 2015). This highlights the need for robust data collection strategies that combine information from diverse sources. These sources can include in situ measurements taken directly within the atmosphere, radar systems that track precipitation patterns, and satellite sensors that provide a global view. Advanced data assimilation techniques then play a vital role by merging these observations with model outputs. This combined approach creates a more precise picture of the initial atmospheric state, ultimately leading to improved weather forecasts (Bauer et al., 2015).
Gierens et al. (2020) highlighted a significant issue with the ERA-5 reanalysis data, showing that they underestimate the frequency and degree of ice supersaturation. This underestimation impacts the reliability of forecasts for contrail persistence and the accuracy of estimates for contrail optical thickness and instantaneous radiative forcing. Their research, which involved comparing predictions from weather and climate models with actual atmospheric data from the IAGOS/MOZAIC project and the EMAC model, revealed that while temperature predictions are generally accurate, relative humidity predictions are often significantly off. This discrepancy undermines the effectiveness of using reanalysis data to predict real-world contrail formation along aircraft trajectories. The study underscores the necessity of improving ice-supersaturation predictions to enhance the reliability of contrail avoidance strategies and ensure they can be operationally viable (Gierens et al., 2020).
Sun and Roosenbrand (2023) reported the limitations in traditional computer vision approaches for contrail detection, particularly in handling the complexity of satellite images under varying conditions. Earlier machine learning methods relied on simpler convolutional neural network models, primarily demanding extensive labeled data for contrail presence determination. Notably, there is a gap in the existing literature, lacking research specifically tailored to machine learning approaches for contrail detection, setting it apart from other image segmentation or detection tasks. A significant challenge lies in the absence of adequate loss functions optimized for linear features like contrails during training, making detection notably difficult at lower resolutions, especially when multiple contrails are close. Addressing these gaps is crucial for advancing the efficacy of contrail detection methods (Sun and Roosenbrand, 2023).
While a complete understanding of contrail effects on climate may not be achievable, further research is crucial to reduce uncertainties and improve our ability to predict and mitigate their impact. Here are some of the key areas of research gaps that should be explored to address existing uncertainties:
-
Properties of contrail particles. Understanding contrail effects on climate requires reflection on key factors such as particle size, layer height, cloud overlap, and optical properties. Larger particles tend to have a different radiative impact than smaller ones. The altitude of contrail formation impacts their interaction with solar and terrestrial radiation. Precise climate modeling requires proper accounting for cloud overlap, impacting radiative calculations. Contrail optical properties, comprising albedo and emissivity, determine their reflective and trapping abilities for solar and terrestrial radiation. The concentration and size distribution of contrail particles affect their formation and radiative impact. Accurate estimation of contrail forcing, important for climate modeling and policy decisions, depends on understanding particle number, size, and layer height.
-
Assessment of effective radiative forcing. Effective radiative forcing (ERF) is a valuable tool for evaluating the global mean radiative impact of contrail cirrus on climate. However, it is important to recognize that ERF primarily focuses on this average effect and may not fully capture regional variations or complex feedback mechanisms associated with contrail formation and persistence.
-
Emissions and alternative fuels. Corroborating a precise connection between soot and contrail ice crystal numbers is important for understanding the environmental impact of sustainable aviation fuels, such as biofuels. Biofuels are being studied as a more sustainable alternative to conventional aviation fuels, and evaluating their impact on contrail formation is crucial for assessing environmental benefits. Scientific research, notifying regulatory decisions, relies on the interpretation of the correlation between soot and ice crystal numbers. This connection is integral for complete visions of the environmental implications of alternative aviation fuels. Precise data on contrail formation, containing the impact of biofuels, are crucial for climate models predicting the impact of aviation emissions on climate. The experimental establishment of the soot–ice-crystal connection gives this understanding.
-
Properties of soot particle. A study into the bimodal size distributions of soot particles in aircraft emissions is critical for focusing on uncertainties in contrail formation and properties, with inferences for climate science and aviation practices (Schumann et al., 2002). In-flight measurements typically show a single peak in the number size distribution of freshly emitted soot particles (Schumann et al., 2002). However, some cases reveal a second, larger particle mode in near-field contrails and dry plumes. The source of this larger mode remains uncertain, suggesting that the bimodal distribution might be present in unrefined jet engine emissions rather than being solely caused by contrail processing or coagulation during plume development (Schumann et al., 2002). Precise data on soot particle properties, including any potential bimodal size distribution, are essential for climate models incorporating contrail effects. Resolving the origin of this bimodal distribution is crucial to improving model representations of contrail properties. Policymakers and regulatory bodies rely on scientific research for progressing aviation-related environmental policies, making an understanding of soot particle size distributions significant for up-to-date decision-making.
-
Examining the microphysical interaction between soot particles and contrail ice crystals emerges as a critical research priority. Laboratory experiments simulating contrail formation conditions offer valuable insights into the ice-nucleating potential of soot particles under controlled environments. Moreover, field measurements focusing on the in situ characterization of soot particles and ice crystals within nascent contrails provide indispensable data for validating models. By integrating these methodologies, researchers can advance their understanding of the role of soot particles in contrail formation and refine climate models accordingly.
-
Remote sensing for contrail cirrus. Organized regional campaigns are important for estimating key variables in aging contrail cirrus and aircraft plumes. This involves in situ and remote sensing data to portray the growth, decay, and trajectories of contrail ice particles, delivering essential data for interpretation of transformation and radiative effects. Estimation of contrail particles, ambient aerosols, and gaseous aerosol precursors notifies climate modeling and environmental assessments, connecting emissions to contrail properties. Gathered data validate climate models, enhancing their accuracy and improving the representation of contrail cirrus effects. Understanding contrail cirrus development supports developing mitigation strategies, informing contrail avoidance, and reducing aviation's climate impact.
In addition to these research issues, there is a need to bridge the gaps with the assessment of the above-reported uncertainties and to investigate and improve the understanding of contrail avoidance and climate tradeoffs. Research should explore how to mitigate the climate impact of contrails without compromising aviation safety and efficiency.
7.3 Contrail avoidance and climate tradeoffs
While existing studies explore contrail avoidance strategies and their impact on fuel burn and climate, a comprehensive large-scale evaluation remains elusive. Ideally, such an evaluation would encompass a significant number of routes, account for weather variations, enable full flight-level optimization, quantify contrail impacts on individual flights, and compare results to a fuel-optimal baseline to isolate the specific effects of contrail avoidance. This comprehensive approach would provide a more definitive understanding of the fuel–climate tradeoffs associated with contrail avoidance strategies.
Gierens et al. (2008) proposed several potential ways to mitigate the impact of contrails, encompassing both technical and operational approaches. Regarding the technical aspect, they recognized the challenge of completely preventing contrail formation due to its primarily thermodynamic nature. However, they suggested the exploration of new engine cycles and technical infrastructures that might help suppress contrails. Additionally, reducing the number of emitted particles was considered to mitigate contrail formation. While such evaluations would not eliminate contrails completely, they would produce thinner contrails with rarer but larger crystals. These heavier ice crystals would incline more rapidly on average, causing shorter contrail lifetimes. The analysis also investigated operational mitigation options. Striking strict constraints, such as fixed maximum flight levels, was studied unreasonably due to the significant challenges it would pose for air traffic controllers and the resulting safety concerns. Their proposed strategies focused on addressing contrail formation both at the technical level through engine and infrastructure improvements and at the operational level by implementing flexible measures that consider real-time weather conditions. These approaches aimed to strike a balance between contrail reduction and the practical needs of aviation.
Recent studies investigating targeted contrail avoidance strategies offer promising results. Teoh et al. (2020) suggest that focusing on a small percentage of flights with the highest contrail impact could be an effective approach, minimizing the overall increase in fuel consumption and CO2 emissions. Additionally, advancements in engine design hold promise as a long-term solution for reducing contrail formation.
Molloy et al. (2022) address a critical step towards implementing effective contrail avoidance strategies in practice. Their work outlines the practical considerations for conducting large-scale trials, which are essential for evaluating the feasibility and effectiveness of these strategies in real-world conditions. The proposed deployable options based on existing air traffic management processes offer a promising path forward for initiating such trials.
Frias et al. (2024) present a compelling case for the practicality and economic viability of contrail avoidance. Their findings demonstrate that significant reductions in contrail climate impact can be achieved with minimal operational burdens. This study provides strong motivation for airlines and air traffic controllers to embrace contrail avoidance strategies as a means to reduce aviation's overall climate footprint.
In a recent study, Sausen et al. (2023) presented an experiment that aimed to avoid contrail formation during real-world operations. This experiment took place in the Maastricht Upper Area Control region, covering parts of Germany, the Benelux countries, and the North Sea, in the year 2021. The researchers highlighted that contrail avoidance could serve as an effective method for mitigating the climate impact of aviation. To conduct their trial experiment, air traffic was deliberately diverted every other day by adjusting the flight altitude, either increasing or decreasing it by up to 2000 ft (approximately 610 m), whenever potential persistent contrails were predicted. The effectiveness of these deviations was assessed by analyzing satellite images of high clouds and employing a contrail detection algorithm that utilized contrail properties. Despite the ongoing challenge of accurately forecasting persistent contrails, the trial achieved a significant level of success, reaching 97.5 %, suggesting that, on average, persistent contrails can be avoided during regular flights in the real world through minor adjustments to the vertical flight path. Contrail avoidance through minor adjustments to flight paths represents a practical approach to mitigate the formation of persistent contrails. It demonstrates the feasibility of implementing such measures within air traffic management to reduce the environmental impact of aviation. The study highlights the potential for real-world applications of contrail avoidance as a means of addressing the climate effects of contrails. The findings of this experiment mark an important milestone in implementing operational measures in air traffic management to decrease the impact of climate from aviation.
The limitations of simply avoiding all contrails become evident when we consider their diverse climate effects. While some contrails can have a net cooling effect, others contribute to warming. This distinction necessitates a more nuanced approach to contrail avoidance. We should focus on mitigating the formation of contrails that contribute to warming, while potentially allowing or even encouraging the formation of those with a net cooling effect. This distinction requires reliable methods for differentiating between the two contrail types.
7.3.1 Future considerations: optimizing contrail avoidance
The Sausen et al. (2023) study represents a significant step forward, demonstrating the feasibility of operational contrail avoidance through minor flight adjustments. To optimize this approach for maximum climate benefit, future research should focus on the following three key areas.
Distinguishing warming from cooling contrails. Developing robust methods to differentiate between these contrail types is crucial. Improved weather forecasting models and contrail prediction algorithms that consider factors like altitude, atmospheric conditions, and cirrus cloud cover can contribute to this goal.
Large-scale implementation. Evaluating the broader applicability of contrail avoidance strategies necessitates conducting large-scale trials across diverse airspace with varying weather patterns. This will provide a more comprehensive understanding of the effectiveness and potential tradeoffs associated with contrail avoidance in different operational contexts.
Integration with air traffic management. For widespread adoption, efficient protocols for integrating contrail avoidance measures within existing air traffic management systems are essential. This may involve developing streamlined decision-making tools and communication channels for air traffic controllers and pilots.
By addressing these considerations, contrail avoidance can evolve from a general strategy to a targeted approach that maximizes its potential for mitigating aviation's climate impact. This approach would focus on reducing the formation of warming contrails while allowing for or even encouraging the formation of cooling contrails.
In a recent Ph.D. thesis, Elmourad (2023) evaluated the fuel–climate tradeoffs arising from contrail avoidance strategies applied on a large scale. This study raises several key questions about contrail avoidance. What fraction of aviation's global total contrail length can be avoided using vertical re-routing exclusively? What is the fuel penalty of such an avoidance strategy? How does the fuel penalty or contrail reduction vary between flights or seasons? Can the fuel penalty be constrained? What effect does that have on the ability to do contrail avoidance? What net climate benefit can be achieved from contrail avoidance strategies? How does this differ from avoiding all contrails or only nighttime contrails? What are the relative orders of magnitude between the climate benefits from contrail avoidance and the climate damages from additional fuel burn? How does this difference compare with the uncertainties associated with contrail impacts?
As the global volume of air traffic continues to rise, the aviation industry grapples with a significant challenge in mitigating its environmental impact on climate change. Contrails, which contribute to global warming by trapping terrestrial radiation, have the potential to counteract the benefits of reduced emissions resulting from optimized flight paths. In a study by Roosenbrand et al. (2023), the authors conducted a global assessment of flights that contribute to contrail formation and assessed the altitude adjustments required to avoid these contrail-prone areas. This analysis utilized a combination of data from the Integrated Global Radiosonde Archive (IGRA), which provides measurements from weather balloons with global coverage and high vertical resolution, and flight data from OpenSky. The study identified midwestern Europe, the eastern United States of America, and Japan as regions characterized by both high air traffic volumes and a substantial percentage of flights forming contrails. Importantly, these regions offer opportunities for altitude adjustments of less than 1 km to minimize contrail formation. The research also pinpointed other regions where relatively minor operational interventions could yield significant climate benefits.
Fuel–climate tradeoffs. While there have been numerous studies on operational contrail avoidance, there are still some research gaps in the field when it comes to understanding the fuel–climate tradeoffs that would follow a large-scale global application of contrail avoidance strategies.
Sustainable aviation fuel (SAF). Much still needs to be understood about what SAF will look like as it becomes the dominant fuel of the future. Moore et al. (2017) examined the impact of biofuel blends compared to traditional jet fuel on aircraft emissions. They found that biofuel blends significantly reduced the quantity and weight of particles emitted, suggesting biofuels can reduce aviation's environmental impact. Their measurements showed a notable decrease in total and non-volatile particles with the biofuel blend and a shift to smaller particle sizes. This is due to a larger reduction in bigger soot particles acting as condensation nuclei. The drop in soot emissions enhances the formation of new particles. Mixing petroleum fuels with HEFA (hydroprocessed esters and fatty acids) jet biofuel cut volatile and non-volatile particle emissions by 50 %–70 % during cruise conditions. However, soot particle emissions remain high, indicating biofuels help but do not eliminate issues related to contrail formation. The emissions of soot and other particles from SAF are still not well understood and will have a significant effect on future contrail production and lifetimes. According to Bräuer et al. (2021b), sustainable aviation fuels have been identified as capable of reducing both contrail ice numbers and the radiative forcing caused by contrail cirrus. Their study involved the measurement of apparent ice emission indices across different fuels with varying aromatic content at altitudes ranging from 9.1 to 9.8 km and 11.4 to 11.6 km. The data were collected during the ECLIF II/NDMAX flight experiment in January 2018, encompassing a variety of fuels differing in aromatic quantity and type. A comparison between a sustainable aviation fuel blend and a reference fuel (Jet A-1) revealed a maximum reduction of 40 % in apparent ice emission indices, highlighting the potential impact of sustainable aviation fuels on mitigating contrail-related environmental effects.
Alternative aircraft and new fuels. There is a lot of ongoing discussion about the potential development of aircraft using hydrogen as fuel. Such an aircraft engine would still emit water vapor and therefore could produce contrails. However, the major reduction in particulates, e.g., no soot, could affect the lifetime of the contrail and whether it could result in contrail cirrus. Research is needed to evaluate the potential for contrail production and resulting lifetimes. Other alternative fuels would also need to be evaluated.
There is a necessity for government research organizations as well as industry partners to venture into the investigation and establishment of sensor prototypes. An example is a humidity sensor prototype with a range of great precision covering 20 parts per million by volume (ppmv) to more than 10 000 ppmv. The purpose is to improve the accuracy of measurements, especially on parameters like temperature and relative humidity, at altitudes preserved during flight to counteract contrail mitigation. Additionally, improving measurement at lower altitudes is vital for improving the precision of weather forecasting. These accumulated data will play a pivotal role in evaluating and integrating these insights into forecast models, enhancing the robustness of these calculations. An additional area of focus is the refinement of model calculations on the impact of contrails, necessitating improved handling of variables such as clouds, aerosols, and specific aircraft characteristics.
Tackling the pressing challenge of climate change requires the exploration and adoption of effective contrail mitigation strategies. Recent studies show promise in the utilization of alternative fuels, particularly sustainable aviation fuels (SAFs), which boast lower soot particle emissions compared to conventional jet fuel. Initial flight trials have shown a marked decrease in contrail formation when SAFs are used, potentially attributed to the reduced concentration of ice nucleation particles in SAF exhaust. Moreover, ongoing research focuses on optimizing flight path planning and air traffic management (ATM) to minimize contrail formation and persistence. Research conducted by Sausen et al. (2023) demonstrates the feasibility of contrail avoidance through minor adjustments to flight paths during real-world operations. Continued progress and application of these strategies, coupled with advancements in contrail observation technologies such as high-precision humidity sensors, offer significant potential to mitigate the climate impact of aviation.
This study reviews the current understanding of the impacts of contrail formation by aircraft on the Earth's climate system; it provides insights into the current state of contrail research, offering perspectives on contrail formation, characteristics, life cycle, and potential future impacts. The study underscores the importance of confronting uncertainties in climate models and emphasizes the need for further research to enhance our understanding of contrail effects.
Aviation emissions, encompassing both contrail cirrus clouds and carbon dioxide emissions, exert an important influence on the Earth's climate. Extensive research efforts have significantly improved our understanding of contrail formation and aging; however, key microphysical processes remain a subject of ongoing investigation. Our examination of global observational data and projections highlights the potential for a significant increase in contrail cirrus radiative forcing by the mid-21st century, driven by factors such as anticipated growth in air traffic, potential improvements in fuel efficiency, and evolving atmospheric conditions. While uncertainties regarding the precise magnitude of this increase exist, the potential consequences necessitate proactive mitigation strategies. Several promising approaches are being explored, including operational measures to optimize flight paths, the development of new contrail suppression technologies, and the adoption of sustainable aviation fuels. Implementing a combination of these strategies, while continuously refining our understanding of contrail effects, is crucial for minimizing the overall climate impact of aviation.
For readers interested in replicating specific aspects of our study, we encourage them to contact the corresponding authors of the cited papers for access to the underlying code and data.
DKS prepared the manuscript with partial support from SS and supervision from DJW.
The contact author has declared that none of the authors has any competing interests.
Publisher's note: Copernicus Publications remains neutral with regard to jurisdictional claims made in the text, published maps, institutional affiliations, or any other geographical representation in this paper. While Copernicus Publications makes every effort to include appropriate place names, the final responsibility lies with the authors.
This work was supported by the National Aeronautics and Space Administration (NASA) under award number NNA16BD14C for NASA Academic Mission Services (NAMS).
We thank Andrew Gettelman (Pacific Northwest National Laboratory, PNNL, USA), Ulrike Burkhardt (German Aerospace Center, DLR, Germany), Bernd Kärcher (DLR), Phillip J. Ansell (University of Illinois at Urbana-Champaign, USA), David Simon Lee (Manchester Metropolitan University, UK), and Steven L. Baughcum (The Boeing Company) for reviewing prior drafts of this report.
This research has been supported by the Universities Space Research Association (grant no. 08600-031).
This paper was edited by Martina Krämer and reviewed by two anonymous referees.
Airbus: Global Market Forecast 2023–2042, https://www.airbus.com/en/products-services/commercial-aircraft/market/global-market-forecast (last access: 28 December 2023), 2023.
Appleman, H.: The formation of exhaust condensation trails by jet aircraft, B. Am. Meteorol. Soc., 34, 14–20, https://doi.org/10.1175/1520-0477-34.1.14, 1953.
Atlas, D. and Wang, Z.: Contrails of small and very large optical depth, J. Atmos. Sci., 67, 3065–3073, https://doi.org/10.1175/2010JAS3403.1, 2010.
Atlas, D., Wang, Z., and Duda, D. P.: Contrails to cirrus: Morphology, microphysics, and radiative properties, J. Appl. Meteorol., 45, 5–19, https://doi.org/10.1175/JAM2335.1, 2006.
Aufm Kampe, H. J.: Die Physik der Auspuffwolken hinter Flugzeugen, Luftwissen, 10, 171–173, 1943.
Barrett, S., Prather, M., Penner, J., Selkirk, H., Balasubramanian, S., Dopelheuer, A., Fleming, G., Gupta, M., Halthore, R., Hileman, J., and Jacobson, M.: Guidance on the use of AEDT gridded aircraft emissions in atmospheric models. A technical note, 2010.
Bauer, H. S., Schwitalla, T., Wulfmeyer, V., Bakhshaii, A., Ehret, U., Neuper, M., and Caumont, O.: Quantitative precipitation estimation based on high-resolution numerical weather prediction and data assimilation with WRF – a performance test, Tellus A, 67, 25047, https://doi.org/10.3402/tellusa.v67.25047, 2015.
Baumgardner, D., Brenguier, J. L., Bucholtz, A., Coe, H., DeMott, P., Garrett, T. J., Gayet, J. F., Hermann, M., Heymsfield, A., Korolev, A., and Krämer, M.: Airborne instruments to measure atmospheric aerosol particles, clouds and radiation: A cook's tour of mature and emerging technology, Atmos. Res., 102, 10–29, https://doi.org/10.1016/j.atmosres.2011.08.010, 2011.
Bickel, M.: Climate Impact of Contrail Cirrus, PhD thesis, Ludwig-Maximilians-Universität München, 133 pp., https://doi.org/10.57676/mzmg-r403, 2023.
Bickel, M., Ponater, M., Bock, L., Burkhardt, U., and Reineke, S.: Estimating the effective radiative forcing of contrail cirrus, J. Climate, 33, 1991–2005, https://doi.org/10.1175/JCLI-D-19-0531.1, 2020.
Bier, A. and Burkhardt, U.: Variability in contrail ice nucleation and its dependence on soot number emissions, J. Geophys. Res.-Atmos., 124, 3384–3400, https://doi.org/10.1029/2018JD029155, 2019.
Bier, A. and Burkhardt, U.: Impact of parametrizing microphysical processes in the jet and vortex phase on contrail cirrus properties and radiative forcing, J. Geophys. Res.-Atmos., 127, e2022JD036677, https://doi.org/10.1029/2022JD036677, 2022.
Bier, A., Burkhardt, U., and Bock, L.: Synoptic control of contrail cirrus life cycles and their modification due to reduced soot number emissions, J. Geophys. Res.-Atmos., 122, 11584–11603, https://doi.org/10.1002/2017JD027011, 2017.
Bock, L. and Burkhardt, U.: Reassessing properties and radiative forcing of contrail cirrus using a global climate model, J. Geophys. Res.-Atmos., 121, 9717–9736, https://doi.org/10.1002/2015JD024688, 2016a.
Bock, L. and Burkhardt, U.: The temporal evolution of a long-lived contrail cirrus cluster: Simulations with a global climate model, J. Geophys. Res.-Atmos., 121, 3548–3565, https://doi.org/10.1002/2015JD024475, 2016b.
Bock, L. and Burkhardt, U.: Contrail cirrus radiative forcing for future air traffic, Atmos. Chem. Phys., 19, 8163–8174, https://doi.org/10.5194/acp-19-8163-2019, 2019.
Boeing: Commercial Market Outlook 2022–2041, https://www.boeing.com/commercial/market/commercial-market-outlook/index.page (last access: 16 August 2024), 2022.
Boucher, O., Randall, D., Artaxo, P., Bretherton, C., Feingold, G., Forster, P., Kerminen, V.-M., Kondo, Y., Liao, H., Lohmann, U., Rasch, P., Satheesh, S. K., Sherwood, S., Stevens, B., and Zhang, X. Y.: Clouds and aerosols, in: Climate Change 2013: The Physical Science Basis. Contribution of Working Group I to the Fifth Assessment Report of the Intergovernmental Panel on Climate Change, edited by: Stocker, T. F., Qin, D., Plattner, G.-K., Tignor, M., Allen, S. K., Doschung, J., Nauels, A., Xia, Y., Bex, V., and Midgley, P. M., Cambridge University Press, 571–657, https://doi.org/10.1017/CBO9781107415324.016, 2013.
Brasseur, G. P., Gupta, M., Anderson, B. E., Balasubramanian, S., Barrett, S., Duda, D., Fleming, G., Forster, P. M., Fuglestvedt, J., Gettelman, A., and Halthore, R. N.: Impact of aviation on climate: FAA’s aviation climate change research initiative (ACCRI) phase II, B. Am. Meteor. Soc., 97, 561–583, 2016.
Bräuer, T., Voigt, C., Sauer, D., Kaufmann, S., Hahn, V., Scheibe, M., Schlager, H., Diskin, G. S., Nowak, J. B., DiGangi, J. P., and Huber, F.: Airborne measurements of contrail ice properties-Dependence on temperature and humidity, Geophys. Res. Lett., 48, e2020GL092166, https://doi.org/10.1029/2020GL092166, 2021a.
Bräuer, T., Voigt, C., Sauer, D., Kaufmann, S., Hahn, V., Scheibe, M., Schlager, H., Huber, F., Le Clercq, P., Moore, R. H., and Anderson, B. E.: Reduced ice number concentrations in contrails from low-aromatic biofuel blends, Atmos. Chem. Phys., 21, 16817–16826, https://doi.org/10.5194/acp-21-16817-2021, 2021b.
Burkhardt, U. and Kärcher, B.: Process-based simulation of contrail cirrus in a global climate model, J. Geophys. Res.-Atmos., 114, D16201, https://doi.org/10.1029/2008JD011675, 2009.
Burkhardt, U. and Kärcher, B.: Global radiative forcing from contrail cirrus, Nat. Clim. Change, 1, 54–58, https://doi.org/10.1038/nclimate1068, 2011.
Burkhardt, U., Kärcher, B., Ponater, M., Gierens, K., and Gettelman, A.: Contrail cirrus supporting areas in model and observations, Geophys. Res. Lett., 35, L16808, https://doi.org/10.1029/2008GL034056, 2008.
Burkhardt, U., Kärcher, B., and Schumann, U.: Global modeling of the contrail and contrail cirrus climate impact, B. Am. Meteorol. Soc., 91, 479–484, 2010.
Burkhardt, U., Bock, L., and Bier, A.: Mitigating the contrail cirrus climate impact by reducing aircraft soot number emissions, npj Clim. Atmos. Sci., 1, 37, https://doi.org/10.1038/s41612-018-0046-4, 2018.
Carlin, B., Fu, Q., Lohmann, U., Mace, G., Sassen, K., and Comstock, J.: High-cloud horizontal inhomogeneity and solar albedo bias, J. Climate, 15, 2321–2339, https://doi.org/10.1175/1520-0442(2002)015<2321:HCHIAS>2.0.CO;2, 2002.
Chauvigné, A., Jourdan, O., Schwarzenboeck, A., Gourbeyre, C., Gayet, J. F., Voigt, C., Schlager, H., Kaufmann, S., Borrmann, S., Molleker, S., Minikin, A., Jurkat, T., and Schumann, U.: Statistical analysis of contrail to cirrus evolution during the Contrail and Cirrus Experiment (CONCERT), Atmos. Chem. Phys., 18, 9803–9822, https://doi.org/10.5194/acp-18-9803-2018, 2018.
Chen, C.-C. and Gettelman, A.: Simulated radiative forcing from contrails and contrail cirrus, Atmos. Chem. Phys., 13, 12525–12536, https://doi.org/10.5194/acp-13-12525-2013, 2013.
Chen, C.-C. and Gettelman, A.: Simulated 2050 aviation radiative forcing from contrails and aerosols, Atmos. Chem. Phys., 16, 7317–7333, https://doi.org/10.5194/acp-16-7317-2016, 2016.
Chen, C.-C., Gettelman, A., Craig, C., Minnis, P., and Duda, D.: Global contrail coverage simulated by CAM5 with the inventory of 2006 global aircraft emissions, J. Adv. Model. Earth Sy., 4, M04003, https://doi.org/10.1029/2011MS000105, 2012.
Chen, N., Sridhar, B., and Ng, H.: Prediction and use of contrail frequency index for contrail reduction strategies, in: AIAA Guidance, Navigation, and Control Conference, AIAA 2010-7849, https://doi.org/10.2514/6.2010-7849, 2010.
Comstock, J. M., Ackerman, T. P., and Turner, D. D.: Evidence of high ice supersaturation in cirrus clouds using ARM Raman lidar measurements, Geophys. Res. Lett., 31, L11106, https://doi.org/10.1029/2004GL019705, 2004.
Danabasoglu, G., Lamarque, J. F., Bacmeister, J., Bailey, D. A., DuVivier, A. K., Edwards, J., Emmons, L. K., Fasullo, J., Garcia, R., Gettelman, A., and Hannay, C.: The community earth system model version 2 (CESM2), J. Adv. Model. Earth Sy., 12, e2019MS001916, https://doi.org/10.1029/2019MS001916, 2020.
De León, R. R., Krämer, M., Lee, D. S., and Thelen, J. C.: Sensitivity of radiative properties of persistent contrails to the ice water path, Atmos. Chem. Phys., 12, 7893–7901, https://doi.org/10.5194/acp-12-7893-2012, 2012.
Dipankar, A., Stevens, B., Heinze, R., Moseley, C., Zängl, G., Giorgetta, M., and Brdar, S.: Large eddy simulation using the general circulation model ICON, J. Adv. Model. Earth Sy., 7, 963–986, https://doi.org/10.1002/2015MS000431, 2015.
Dischl, R. K., Sauer, D., Voigt, C., Harlaß, T., Sakellariou, F., Märkl, R. S., Schumann, U., Scheibe, M., Kaufmann, S., Roiger, A., Dörnbrack, A., Renard, C., Gauthier, M., Swann, P., Madden, P., Luff, D., Johnson, M., Ahrens, D., Sallinen, R., Schripp, T., Eckel, G., Bauder, U., and Le Clercq, P.: Measurements of particle emissions of an A350-941 burning 100 % sustainable aviation fuels in cruise, EGUsphere [preprint], https://doi.org/10.5194/egusphere-2024-1224, 2024.
Duda, D. P., Minnis, P., and Nguyen, L.: Estimates of cloud radiative forcing in contrail clusters using GOES imagery, J. Geophys. Res.-Atmos., 106, 4927–4937, 2001.
Duda, D. P., Minnis, P., Nguyen, L., and Palikonda, R.: A case study of the development of contrail clusters over the Great Lakes, J. Atmos. Sci., 61, 1132–1146, https://doi.org/10.1175/1520-0469(2004)061<1132:ACSOTD>2.0.CO;2, 2004.
Duda, D. P., Minnis, P., Khlopenkov, K., Chee, T. L., and Boeke, R.: Estimation of 2006 Northern Hemisphere contrail coverage using MODIS data, Geophys. Res. Lett., 40, 612–617, https://doi.org/10.1002/grl.50126, 2013.
EASA (European Union Aviation Safety Agency): ICAO Aircraft Engine Emissions Databank, http://www.easa.europa.eu/document-library/icao-aircraft-engine-emissions-databank (last access: 16 August 2024), 2023.
Elmourad, J. A.: Evaluating Fuel-Climate Tradeoffs in Contrail Avoidance, Doctoral dissertation, Massachusetts Institute of Technology, https://hdl.handle.net/1721.1/150282, 2023.
Ettenreich, R.: Wolkenbildung über einer Feuersbrunst und an Flugzeugabgasen, Meteorol. Z., 36, 355–356, 1919.
EUROCONTROL: Aircraft Performance Summary Tables for the Base of Aircraft Date (BADA), 3.7, European Organisation for the Safety of Air Navigation, Brétigny-sur-Orge, 103, 2009.
Febvre, G., Gayet, J. F., Minikin, A., Schlager, H., Shcherbakov, V., Jourdan, O., Busen, R., Fiebig, M., Kärcher, B., and Schumann, U.: On optical and microphysical characteristics of contrails and cirrus, J. Geophys. Res., 114, D02204, https://doi.org/10.1029/2008JD010184, 2009.
Flores, J. M., Baumgardner, D., Kok, G., Raga, G., and Hermann, R.: Tropical subvisual cirrus and contrails at −85 ◦C, 12th Conference on Cloud Physics, 10–14 July 2006, Madison, WI, https://ams.confex.com/ams/Madison2006/techprogram/paper_112323.htm (last access: 16 August 2024), 2006
Frias, A. M., Shapiro, M. L., Engberg, Z., Zopp, R., Soler, M., and Stettler, M. E. J.: Feasibility of contrail avoidance in a commercial flight planning system: an operational analysis, Environmental Research: Infrastructure and Sustainability, 4, 015013, https://doi.org/10.1088/2634-4505/ad310c, 2024.
Fritz, T. M., Eastham, S. D., Speth, R. L., and Barrett, S. R. H.: The role of plume-scale processes in long-term impacts of aircraft emissions, Atmos. Chem. Phys., 20, 5697–5727, https://doi.org/10.5194/acp-20-5697-2020, 2020.
Frömming, C., Ponater, M., Burkhardt, U., Stenke, A., Pechtl, S., and Sausen, R.: Sensitivity of contrail coverage and contrail radiative forcing to selected key parameters, Atmos. Environ., 45, 1483–1490, https://doi.org/10.1016/j.atmosenv.2010.12.039, 2011.
Fuglestvedt, J. S., Shine, K. P., Berntsen, T., Cook, J., Lee, D. S., Stenke, A., Skeie, R. B., Velders, G. J. M., and Waitz, I. A.: Transport impacts on atmosphere and climate: Metrics, Atmos. Environ., 44, 4648–4677, https://doi.org/10.1016/j.atmosenv.2009.04.044, 2010.
Gao, R. C., Fahey, D. W., Popp, P. J., Marcy, T. P., Herman, R. L., Weinstock, E. M., Smith, J. B., Sayres, D. S., Pittman, J. V., Rosenlof, K. H., Thompson, T. L., Bui, P. T., Baumgardner, D. G., Anderson, B. E., Kok, G., and Weinheimer, A. J.: Measurements of relative humidity in a persistent contrail, Atmos. Environ., 40, 1590–1600, https://doi.org/10.1016/j.atmosenv.2005.11.021, 2006.
Garber, D. P., Minnis, P., and Costulis, P. K.: A commercial flight track database for upper tropospheric aircraft emission studies over the USA and southern Canada, Meteorol. Z., 14, 445–452, https://doi.org/10.1127/0941-2948/2005/0040, 2005.
Gayet, J.-F., Shcherbakov, V., Voigt, C., Schumann, U., Schäuble, D., Jessberger, P., Petzold, A., Minikin, A., Schlager, H., Dubovik, O., and Lapyonok, T.: The evolution of microphysical and optical properties of an A380 contrail in the vortex phase, Atmos. Chem. Phys., 12, 6629–6643, https://doi.org/10.5194/acp-12-6629-2012, 2012.
Geleyn, J. F. and Hollingsworth, A.: An economical analytical method for the computation of the interaction between scattering and line absorption of radiation, Beiträge zur Physik der Atmosphäre, 52, 1–16, 1978.
Geraedts, S., Brand, E., Dean, T. R., Eastham, S., Elkin, C., Engberg, Z., Hager, U., Langmore, I., McCloskey, K., Ng, J. Y. H., and Platt, J. C.: A scalable system to measure contrail formation on a per-flight basis, Environ. Res. Commun., 6, 015008, https://doi.org/10.1088/2515-7620/ad11ab, 2024.
Gerz, T., Durbeck, T., and Konopka, P.: Transport and effective diffusion of aircraft emissions, J. Geophys. Res., 103, 25905–25914, https://doi.org/10.1029/98JD02282, 1998.
Gettelman, A. and Chen, C.: The climate impact of aviation aerosols, Geophys. Res. Lett., 40, 2785–2789, https://doi.org/10.1002/grl.50539, 2013.
Gettelman, A. and Morrison, H.: Advanced Two-Moment Bulk Microphysics for Global Models. Part I: Off-Line Tests and Comparison with Other Schemes, J. Climate, 28, 1268–1287, https://doi.org/10.1175/JCLI-D-14-00102.1, 2015.
Gettelman, A., Fetzer, E. J., Irion, F. W., and Eldering, A.: The global distribution of supersaturation in the upper troposphere, J. Climate, 19, 6089–6103, 2006.
Gettelman, A., Hannay, C., Bacmeister, J. T., Neale, R. B., Pendergrass, A. G., Danabasoglu, G., Lamarque, J.-F., Fasullo, J. T., Bailey, D. A., Lawrence, D. M., and Mills, M. J.: High Climate Sensitivity in the Community Earth System Model Version 2 (CESM2), Geophys. Res. Lett., 46, 8329–8337, https://doi.org/10.1029/2019GL083978, 2019.
Gettelman, A., Bardeen, C. G., McCluskey, C. S., Järvinen, E., Stith, J., Bretherton, C., McFarquhar, G., Twohy, C., D'Alessandro, J., and Wu, W.: Simulating Observations of Southern Ocean Clouds and Implications for Climate, J. Geophys. Res., 125, https://doi.org/10.1029/2020JD032619, 2020.
Gettelman, A., Chen, C.-C., and Bardeen, C. G.: The climate impact of COVID-19-induced contrail changes, Atmos. Chem. Phys., 21, 9405–9416, https://doi.org/10.5194/acp-21-9405-2021, 2021.
Gierens, K.: Selected topics on the interaction between cirrus clouds and embedded contrails, Atmos. Chem. Phys., 12, 11943–11949, https://doi.org/10.5194/acp-12-11943-2012, 2012.
Gierens, K. and Vázquez-Navarro, M.: Statistical analysis of contrail lifetimes from a satellite perspective, Meteorol. Z., 27, 183–193, https://doi.org/10.1127/metz/2018/0888, 2018.
Gierens, K., Matthes, S., and Rohs, S.: How Well Can Persistent Contrails Be Predicted?, Aerospace, 7, 169, https://doi.org/10.3390/AEROSPACE7120169, 2020.
Gierens, K. M., Lim, L., and Eleftheratos, K.: A review of various strategies for contrail avoidance, Open Atmos. Sci. J., 2, 1–7, https://doi.org/10.2174/1874282300802010001, 2008.
Gounou, A. and Hogan, R. J.: A sensitivity study of the effect of horizontal photon transport on the radiative forcing of contrails, J. Atmos. Sci., 64, 1706–1716, https://doi.org/10.1175/JAS3943.1, 2007.
Graf, K., Schumann, U., Mannstein, H., and Mayer, B.: Aviation induced diurnal North Atlantic cirrus cover cycle, Geophys. Res. Lett., 39, https://doi.org/10.1029/2012GL052590, 2012.
Grewe, V., Gangoli Rao, A., Grönstedt, T., Xisto, C., Linke, F., Melkert, J., Middel, J., Ohlenforst, B., Blakey, S., Christie, S., Matthes, S., and Dahlmann, K.: Evaluating the climate impact of aviation emission scenarios towards the Paris agreement including COVID-19 effects, Nat. Commun., 12, 3841, https://doi.org/10.1038/s41467-021-24091-y, 2021.
Haywood, J. M., Allan, R. P., Bornemann, J., Forster, P. M., Francis, P. N., Milton, S., Rädel, G., Rap, A., Shine, K. P., and Thorpe, R.: A case study of the radiative forcing of persistent contrails evolving into contrail-induced cirrus, J. Geophys. Res.-Atmos., 114, https://doi.org/10.1029/2009JD012650, 2009.
Heymsfield, A. J., Krämer, M., Luebke, A., Brown, P., Cziczo, D. J., Franklin, C., Lawson, P., Lohmann, U., McFarquhar, G., Ulanowski, Z., and Van Tricht, K.: Cirrus clouds, Meteor. Mon., 58, 2.1–2.26, 2017.
Huszar, P., Teyssèdre, H., Michou, M., Voldoire, A., Olivié, D. J. L., Saint-Martin, D., Cariolle, D., Senesi, S., Salas Y Melia, D., Alias, A., Karcher, F., Ricaud, P., and Halenka, T.: Modeling the present and future impact of aviation on climate: an AOGCM approach with online coupled chemistry, Atmos. Chem. Phys., 13, 10027–10048, https://doi.org/10.5194/acp-13-10027-2013, 2013.
ICAO: Future Of Aviation 2012, https://www.icao.int/Meetings/FutureOfAviation/Pages/default.aspx (last access: 16 August 2024), 2012.
Immler, F., Treffeisen, R., Engelbart, D., Krüger, K., and Schrems, O.: Cirrus, contrails, and ice supersaturated regions in high pressure systems at northern mid latitudes, Atmos. Chem. Phys., 8, 1689–1699, https://doi.org/10.5194/acp-8-1689-2008, 2008.
IPCC: Aviation and the global atmosphere 1999, in: Intergovernmental Panel on Climate Change Special Report, edited by: Penner, J. E., Lister, D. H., Griggs, D. J., Dokken, D. J., and McFarland, M., Cambridge University Press, Cambridge, UK, 1999.
IPCC: Climate Change 2021: The Physical Science Basis. Contribution of Working Group I to the Sixth Assessment Report of the Intergovernmental Panel on Climate Change, edited by: Masson-Delmotte, V., Zhai, P., Pirani, A., Connors, S. L., Péan, C., Berger, S., Caud, N., Chen, Y., Goldfarb, L., Gomis, M. I., Huang, M., Leitzell, K., Lonnoy, E., Matthews, J. B. R., Maycock, T. K., Waterfield, T., Yelekçi, O., Yu, R., and Zhou, B., Cambridge University Press, Cambridge, United Kingdom and New York, NY, USA, 2391 pp., https://doi.org/10.1017/9781009157896, 2021.
Irvine, E. A., Hoskins, B. J., and Shine, K. P.: A Lagrangian analysis of ice-supersaturated air over the North Atlantic, J. Geophys. Res., 119, 90–100, https://doi.org/10.1002/2013jd020251, 2014.
Iwabuchi, H., Yang, P., Liou, K. N., and Minnis, P.: Physical and optical properties of persistent contrails: Climatology and interpretation, J. Geophys. Res.-Atmos., 117, D06215, https://doi.org/10.1029/2011JD017020, 2012.
Jacobson, M. Z., Wilkerson, J. T., Naiman, A. D., and Lele, S. K.: The effects of aircraft on climate and pollution. Part I: Numerical methods for treating the subgrid evolution of discrete size-and composition-resolved contrails from all commercial flights worldwide, J. Comput. Phys., 230, 5115–5132, https://doi.org/10.1016/j.jcp.2011.03.021, 2011.
Jensen, E., Ackermann, A. S., Stevens, D. E., Toon, O. B., and Minnis, P.: Spreading and growth of contrails in a sheared environment, J. Geophys. Res., 103, 13557–13567, https://doi.org/10.1029/98JD02594, 1998.
Jensen, E., Toon, O., Vay, S., Ovarlez, J., May, R., Bui, T., Twohy, C., Gandrud, B., Pueschel, R., and Schumann, U.: Prevalence of ice-supersaturated regions in the upper troposphere: Implications for optically thin ice cloud formation, J. Geophys. Res., 106, 17253–17266, https://doi.org/10.1029/2000JD900774, 2001.
Jones, H. M., Haywood, J., Marenco, F., O’Sullivan, D., Meyer, J., Thorpe, R., Gallagher, M. W., Krämer, M., Bower, K. N., Rädel, G., Rap, A., Woolley, A., Forster, P., and Coe, H.: A methodology for in-situ and remote sensing of microphysical and radiative properties of contrails as they evolve into cirrus, Atmos. Chem. Phys., 12, 8157–8175, https://doi.org/10.5194/acp-12-8157-2012, 2012.
Kärcher, B.: Formation and radiative forcing of contrail cirrus, Nat. Commun., 9, 1824, https://doi.org/10.1038/s41467-018-04068-0, 2018.
Kärcher, B. and Yu, F.: Role of aircraft soot emissions in contrail formation, Geophys. Res. Lett., 36, L01804, https://doi.org/10.1029/2008GL036649, 2009.
Kärcher, B., Peter, T., Biermann, U. M., and Schumann, U.: The initial composition of jet condensation trails, J. Atmos. Sci., 53, 3066–3083, 1996.
Kärcher, B., Burkhardt, U., Unterstrasser, S., and Minnis, P.: Factors controlling contrail cirrus optical depth, Atmos. Chem. Phys., 9, 6229–6254, https://doi.org/10.5194/acp-9-6229-2009, 2009.
Kärcher, B., Burkhardt, U., Bier, A., Bock, L., and Ford, I. J.: The microphysical pathway to contrail formation, J. Geophys. Res.-Atmos., 120, 7893–7927, https://doi.org/10.1002/2015JD023293, 2015.
Kärcher, B., Mahrt, F., and Marcolli, C.: Process-oriented analysis of aircraft soot-cirrus interactions constrains the climate impact of aviation, Commun. Earth Environ., 2, 113, https://doi.org/10.1038/s43247-021-00175-x, 2021.
Kaufmann, S., Voigt, C., Jeßberger, P., Jurkat, T., Schlager, H., Schwarzenboeck, A., Klingebiel, M., and Thornberry, T.: In-situ measurements of ice saturation in young contrails, Geophys. Res. Lett., 41, 702–709, https://doi.org/10.1002/2013GL058276, 2014.
Klöwer, M., Allen, M. R., Lee, D. S., Proud, S. R., Gallagher, L., and Skowron, A.: Quantifying aviation's contribution to global warming, Environ. Res. Lett., 16, 104027, https://doi.org/10.1088/1748-9326/ac286e, 2021.
Krämer, M., Schiller, C., Afchine, A., Bauer, R., Gensch, I., Mangold, A., Schlicht, S., Spelten, N., Sitnikov, N., Borrmann, S., de Reus, M., and Spichtinger, P.: Ice supersaturations and cirrus cloud crystal numbers, Atmos. Chem. Phys., 9, 3505–3522, https://doi.org/10.5194/acp-9-3505-2009, 2009.
Krämer, M., Rolf, C., Luebke, A., Afchine, A., Spelten, N., Costa, A., Meyer, J., Zöger, M., Smith, J., Herman, R. L., Buchholz, B., Ebert, V., Baumgardner, D., Borrmann, S., Klingebiel, M., and Avallone, L.: A microphysics guide to cirrus clouds – Part 1: Cirrus types, Atmos. Chem. Phys., 16, 3463–3483, https://doi.org/10.5194/acp-16-3463-2016, 2016.
Krämer, M., Rolf, C., Spelten, N., Afchine, A., Fahey, D., Jensen, E., Khaykin, S., Kuhn, T., Lawson, P., Lykov, A., Pan, L. L., Riese, M., Rollins, A., Stroh, F., Thornberry, T., Wolf, V., Woods, S., Spichtinger, P., Quaas, J., and Sourdeval, O.: A microphysics guide to cirrus – Part 2: Climatologies of clouds and humidity from observations, Atmos. Chem. Phys., 20, 12569–12608, https://doi.org/10.5194/acp-20-12569-2020, 2020.
Kristensson, A., Gayet, J.-F., Ström, J., and Auriol, F.: In situ observations of a reduction in effective crystal diameter in cirrus clouds near flight corridors, Geophys. Res. Lett., 27, 681–684, https://doi.org/10.1029/1999GL010934, 2000.
Kübbeler, M., Hildebrandt, M., Meyer, J., Schiller, C., Hamburger, Th., Jurkat, T., Minikin, A., Petzold, A., Rautenhaus, M., Schlager, H., Schumann, U., Voigt, C., Spichtinger, P., Gayet, J.-F., Gourbeyre, C., and Krämer, M.: Thin and subvisible cirrus and contrails in a subsaturated environment, Atmos. Chem. Phys., 11, 5853–5865, https://doi.org/10.5194/acp-11-5853-2011, 2011.
Kulik, L.: Satellite-based detection of contrails using deep learning, Master's thesis, Massachusetts Institute of Technology, https://hdl.handle.net/1721.1/124179, 2019.
Lamquin, N., Stubenrauch, C. J., Gierens, K., Burkhardt, U., and Smit, H.: A global climatology of upper-tropospheric ice supersaturation occurrence inferred from the Atmospheric Infrared Sounder calibrated by MOZAIC, Atmos. Chem. Phys., 12, 381–405, https://doi.org/10.5194/acp-12-381-2012, 2012.
Lee, D. S., Fahey, D. W., Forster, P. M., Newton, P. J., Wit, R. C. N., Lim, L. L., Owen, B., and Sausen, R.: Aviation and global climate change in the 21st century, Atmos. Environ., 43, 3520–3537, https://doi.org/10.1016/j.atmosenv.2009.04.024, 2009.
Lee, D. S., Pitari, G., Grewe, V., Gierens, K., Penner, J. E., Petzold, A., Prather, M. J., Schumann, U., Bais, A., Berntsen, T., and Iachetti, D.: Transport impacts on atmosphere and climate: Aviation, Atmos. Environ., 44, 4678–4734, https://doi.org/10.1016/j.atmosenv.2010.06.045, 2010.
Lee, D. S., Fahey, D. W., Skowron, A., Allen, M. R., Burkhardt, U., Chen, Q., Doherty, S. J., Freeman, S., Forster, P. M., Fuglestvedt, J., Gettelman, A., De León, R. R., Lim, L. L., Lund, M. T., Millar, R. J., Owen, B., Penner, J. E., Pitari, G., Prather, M. J., Sausen, R., and Wilcox, L. J.: The contribution of global aviation to anthropogenic climate forcing for 2000 to 2018, Atmos. Environ., 244, 117834, https://doi.org/10.1016/j.atmosenv.2020.117834, 2021.
Lewellen, D. C.: Persistent contrails and contrail cirrus. Part II: Full lifetime behavior, J. Atmos. Sci., 71, 4420–4438, https://doi.org/10.1175/JAS-D-14-0004.1, 2014.
Lewellen, D. C., Meza, O., and Huebsch, W.: Persistent contrails and contrail cirrus. Part 1: Large-eddy simulations from inception to demise, J. Atmos. Sci., 71, 4399–4419, https://doi.org/10.1175/JAS-D-13-0316.1, 2014.
Li, J., Caiazzo, F., Chen, N. Y., Sridhar, B., Ng, H., and Barrett, S.: Evaluation of aircraft contrails using dynamic dispersion model, AIAA Guidance Navigation, and Control (GNC) Conf., 2013–5178, https://doi.org/10.2514/6.2013-5178, 2013.
Li, J., Kim, J. H., Sridhar, B., and Ng, H. K.: Ames Contrail Simulation Model: Modeling Aviation Induced Contrails and the Computation of Contrail Radiative Forcing Using Air Traffic Data, NASA Tech. Memo., NASA/TM-20230014633, 2023a.
Li, Y., Mahnke, C., Rohs, S., Bundke, U., Spelten, N., Dekoutsidis, G., Groß, S., Voigt, C., Schumann, U., Petzold, A., and Krämer, M.: Upper-tropospheric slightly ice-subsaturated regions: frequency of occurrence and statistical evidence for the appearance of contrail cirrus, Atmos. Chem. Phys., 23, 2251–2271, https://doi.org/10.5194/acp-23-2251-2023, 2023b.
Liou, K. N., Takano, Y., Yue, Q., and Yang, P.: On the radiative forcing of contrail cirrus contaminated by black carbon, Geophys. Res. Lett., 40, 778–784, https://doi.org/10.1002/grl.50214, 2013.
Liu, X., Ma, P.-L., Wang, H., Tilmes, S., Singh, B., Easter, R. C., Ghan, S. J., and Rasch, P. J.: Description and evaluation of a new four-mode version of the Modal Aerosol Module (MAM4) within version 5.3 of the Community Atmosphere Model, Geosci. Model Dev., 9, 505–522, https://doi.org/10.5194/gmd-9-505-2016, 2016.
Lohmann, U., Spichtinger, P., Heidt, S., Peter, T., and Smit, H.: Cirrus clouds and ice supersaturation regions in a global climate model, Environ. Res. Lett., 3, 045022, https://doi.org/10.1088/1748-9326/3/4/045022, 2008.
Luebke, A. E., Afchine, A., Costa, A., Grooß, J.-U., Meyer, J., Rolf, C., Spelten, N., Avallone, L. M., Baumgardner, D., and Krämer, M.: The origin of midlatitude ice clouds and the resulting influence on their microphysical properties, Atmos. Chem. Phys., 16, 5793–5809, https://doi.org/10.5194/acp-16-5793-2016, 2016.
Mahnke, C., Gomes, R., Bundke, U., Berg, M., Ziereis, H., Sharma, M., Righi, M., Hendricks, J., Zahn, A., Wahner, A., and Petzold, A.: Properties and Processing of Aviation Exhaust Aerosol at Cruise Altitude Observed from the IAGOS-CARIBIC Flying Laboratory, Environ. Sci. Technol., 58, 6945–6953, 2024.
Mannstein, H., Meyer, R., and Wendling, P.: Operational detection of contrails from NOAA-AVHRR data, Int. J. Remote Sens., 20, 1641–1660, https://doi.org/10.1080/014311699212470, 1999.
Mannstein, H., Brömser, A., and Bugliaro, L.: Ground-based observations for the validation of contrails and cirrus detection in satellite imagery, Atmos. Meas. Tech., 3, 655–669, https://doi.org/10.5194/amt-3-655-2010, 2010.
Märkl, R. S., Voigt, C., Sauer, D., Dischl, R. K., Kaufmann, S., Harlaß, T., Hahn, V., Roiger, A., Weiß-Rehm, C., Burkhardt, U., Schumann, U., Marsing, A., Scheibe, M., Dörnbrack, A., Renard, C., Gauthier, M., Swann, P., Madden, P., Luff, D., Sallinen, R., Schripp, T., and Le Clercq, P.: Powering aircraft with 100 % sustainable aviation fuel reduces ice crystals in contrails, Atmos. Chem. Phys., 24, 3813–3837, https://doi.org/10.5194/acp-24-3813-2024, 2024.
Markowicz, K. M. and Witek, M. L.: Simulations of contrail optical properties and radiative forcing for various crystal shapes, J. Appl. Meteorol. Clim., 50, 1740–1755, https://doi.org/10.1175/2011JAMC2618.1, 2011.
Marquart, S., Ponater, M., Mager, F., and Sausen, R.: Future Development of Contrail Cover, Optical Depth, and Radiative Forcing: Impacts of Increasing Air Traffic and Climate Change, J. Climate, 16, 2890–2904, https://doi.org/10.1175/1520-0442(2003)016<2890:FDOCCO>2.0.CO;2, 2003.
Matthes, S., Lee, D. S., De Leon, R. R., Lim, L., Owen, B., Skowron, A., Thor, R. N., and Terrenoire, E.: The effects of supersonic aviation on ozone and climate, Aerospace, 9, 41, https://doi.org/10.3390/aerospace9010041, 2022.
McCloskey, K., Geraedts, S., Van Arsdale, C., and Brand, E.: A human-labeled landsat-8 contrails dataset, in: ICML 2021 Workshop on Tackling Climate Change with Machine Learning, https://www.climatechange.ai/events/iclr2023#about (last access: 16 August 2024), 2021.
Meerkötter, R., Schumann, U., Doelling, D. R., Minnis, P., Nakajima, T., and Tsushima, Y.: Radiative forcing by contrails, Ann. Geophys., 17, 1080–1094, https://doi.org/10.1007/s00585-999-1080-7, 1999.
Meijer, V. R., Kulik, L., Eastham, S. D., Allroggen, F., Speth, R. L., Karaman, S., and Barrett, S. R.: Contrail coverage over the United States before and during the COVID-19 pandemic, Environ. Res. Lett., 17, 034039, https://doi.org/10.1088/1748-9326/ac26f0, 2021.
Minnis, P., Young, D. F., Garber, D. P., Nguyen, L., Smith Jr., W. L., and Palikonda, R.: Transformation of contrails into cirrus during SUCCESS, Geophys. Res. Lett., 25, 1157–1160, https://doi.org/10.1029/97GL03314, 1998.
Minnis, P., Schumann, U., Doelling, D. R., Gierens, K. M., and Fahey, D. W.: Global distribution of contrail radiative forcing, Geophys. Res. Lett., 26, 1853–1856, https://doi.org/10.1029/1999GL900391, 1999.
Minnis, P., Bedka, S. T., Duda, D. P., Bedka, K. M., Chee, T., Ayers, J. K., Palikonda, R., Spangenberg, D. A., Khlopenkov, K. V., and Boeke, R.: Linear contrail and contrail cirrus properties determined from satellite data, Geophys. Res. Lett., 40, 3220–3226, https://doi.org/10.1002/grl.50557, 2013.
Molloy, J., Teoh, R., Harty, S., Koudis, G., Schumann, U., Poll, I., and Stettler, M. E.: Design principles for a contrail-minimizing trial in the north atlantic, Aerospace, 9, 37, https://doi.org/10.3390/aerospace9070375, 2022.
Moore, R. H., Thornhill, K. L., Weinzierl, B., Sauer, D., D'Ascoli, E., Kim, J., Lichtenstern, M., Scheibe, M., Beaton, B., Beyersdorf, A. J. and Barrick, J.: Biofuel blending reduces particle emissions from aircraft engines at cruise conditions, Nature, 543, 411–415, https://doi.org/10.1038/nature21420, 2017.
Myhre, G., Kvalevåg, M., Rädel, G., Cook, J., Shine, K. P., Clark, H., Kärcher, F., Markowicz, K., Kardas, A., Wolkenberg, P., Balkanski, Y., Ponater, M., Forster, P., Rap, A., and De Leon, R. R.: Intercomparison of radiative forcing calculations of stratospheric water vapour and contrails, Meteorol. Z., 18, 585–596, https://doi.org/10.1127/0941-2948/2009/0405, 2009.
Naiman, A. D., Lele, S. K., Wilkerson, J. T., and Jacobson, M. Z.: Parameterization of subgrid plume dilution for use in large-scale atmospheric simulations, Atmos. Chem. Phys., 10, 2551–2560, https://doi.org/10.5194/acp-10-2551-2010, 2010.
Naiman, A. D., Lele, S. K., and Jacobson, M. Z.: Large Eddy simulations of persistent aircraft contrails, 49th AIAA Aerospace Science Meeting, Orlando, https://doi.org/10.2514/6.2011-993, 2011a.
Naiman, A. D., Lele, S. K., Wilkerson, J. T., and Jacobson, M. Z.: A low order contrail model for use with global-scale climate models, 47th AIAA Aerospace Science Meeting, Orlando, FL, https://doi.org/10.2514/6.2009-557, 2011b.
Newinger, C. and Burkhardt, U.: Sensitivity of contrail cirrus radiative forcing to air traffic scheduling, J. Geophys. Res.-Atmos., 117, D1020, https://doi.org/10.1029/2011JD016815, 2012.
Ng, J. Y., McCloskey, K., Cui, J., Meijer, V. R., Brand, E., Sarna, A., Goyal, N., Van Arsdale, C., and Geraedts, S.: OpenContrails: Benchmarking contrail detection on GOES-16 ABI, arXiv [preprint], https://doi.org/10.48550/arXiv.2304.02122, 2023.
Ovarlez, J., van Velthoven, P., Sachse, G., Vay, S., Schlager, H., and Ovarlez, H.: Comparison of water vapor measurements from POLINAT 2 with ECMWF analyses in high-humidity conditions, J. Geophys. Res., 105, 3737–3744, https://doi.org/10.1029/1999JD900954, 2000.
Ovarlez, J., Gayet, J.-F., Gierens, K., Strom, J., Ovarlez, H., Auriol, F., Busen, R., and Schumann, U.: Water vapour measurements inside cirrus clouds in Northern and Southern hemispheres during INCA, Geophys. Res. Lett., 29, 1813–1817, https://doi.org/10.1029/2001GL014440, 2002.
Palikonda, R., Minnis, P., Duda, D. P., and Mannstein, H.: Contrail coverage derived from 2001 AVHRR data over the continental United States of America and surrounding areas, Meteorol. Z., 14, 525–536, https://doi.org/10.1127/0941-2948/2005/0055, 2005.
Paoli, R. and Shariff, K.: Contrail Modeling and Simulation, Annu. Rev. Fluid Mech., 48, 393–427, https://doi.org/10.1146/annurev-fluid-010814-013619, 2016.
Petzold, A., Busen, R., Schröder, F. P., Baumann, R., Kuhn, M., Ström, J., Hagen, D. E., Whitefield, P. D., Baumgardner, D., Arnold, F., Borrmann, S., and Schumann, U.: Near-field measurements on contrail properties from fuels with different sulfur content, J. Geophys. Res.-Atmos., 102, 29867–29880, https://doi.org/10.1029/97JD02209, 1997.
Petzold, A., Krämer, M., Neis, P., Rolf, C., Rohs, S., Berkes, F., Smit, H. G. J., Gallagher, M., Beswick, K., Lloyd, G., Baumgardner, D., Spichtinger, P., Nédélec, P., Ebert, V., Buchholz, B., Riese, M., and Wahner, A.: Upper tropospheric water vapor and its interaction with cirrus clouds as seen from IAGOS long-term routine in situ observations, Faraday Discuss., 200, 229–249, https://doi.org/10.1039/C7FD00006E, 2017.
Petzold, A., Neis, P., Rütimann, M., Rohs, S., Berkes, F., Smit, H. G. J., Krämer, M., Spelten, N., Spichtinger, P., Nédélec, P., and Wahner, A.: Ice-supersaturated air masses in the northern mid-latitudes from regular in situ observations by passenger aircraft: vertical distribution, seasonality and tropospheric fingerprint, Atmos. Chem. Phys., 20, 8157–8179, https://doi.org/10.5194/acp-20-8157-2020, 2020.
Poellot, M. R., Arnott, W. P., and Hallett, J.: In situ observations of contrail microphysics and implications for their radiative impact, J. Geophys. Res., 104, 12077–12084, https://doi.org/10.1029/1999JD900006, 1999.
Poll, D. I.: On the relationship between non-optimum operations and fuel requirement for large civil transport aircraft, with reference to environmental impact and contrail avoidance strategy, Aeronaut. J., 122, 1827–1870, 2018.
Pomroy, H. R. and Illingworth, J. A.: Ice cloud inhomogeneity: quantifying bias in emissivity from radar observations, Geophys. Res. Lett., 27, 2101–2104, https://doi.org/10.1029/2000GL011429, 2000.
Ponater, M., Marquart, S., and Sausen, R.: Contrails in a comprehensive global climate model: Parameterization and radiative forcing results, J. Geophys. Res.-Atmos., 107, ACL 2-1–ACL 2-15, https://doi.org/10.1029/2001JD001227, 2002.
Ponater, M., Bickel, M., Bock, L. and Burkhardt, U.: Towards determining the contrail cirrus efficacy, Aerospace, 8, 42, https://doi.org/10.3390/aerospace8020042, 2021.
Pruppacher, H. R. and Klett, J. D.: Microphysics of clouds and precipitation, Kluwer Academic, Norwell, Mass., https://doi.org/10.1080/02786829808965531, 2000.
Rädel, G. and Shine, K. P.: Radiative forcing by persistent contrails and its dependence on cruise altitudes, J. Geophys. Res.-Atmos., 113, D07105, https://doi.org/10.1029/2007JD009117, 2008.
Rap, A., Forster, P. M., Jones, A., Boucher, O., Haywood, J. M., Bellouin, N., and De Leon, R. R.: Parameterization of contrails in the UK Met Office Climate Model, J. Geophys. Res., 115, D10205, https://doi.org/10.1029/2009JD012152, 2010.
Reutter, P., Neis, P., Rohs, S., and Sauvage, B.: Ice supersaturated regions: properties and validation of ERA-Interim reanalysis with IAGOS in situ water vapour measurements, Atmos. Chem. Phys., 20, 787–804, https://doi.org/10.5194/acp-20-787-2020, 2020.
Righi, M., Hendricks, J., and Sausen, R.: The global impact of the transport sectors on atmospheric aerosol: simulations for year 2000 emissions, Atmos. Chem. Phys., 13, 9939–9970, https://doi.org/10.5194/acp-13-9939-2013, 2013.
Righi, M., Hendricks, J., and Beer, C. G.: Exploring the uncertainties in the aviation soot–cirrus effect, Atmos. Chem. Phys., 21, 17267–17289, https://doi.org/10.5194/acp-21-17267-2021, 2021.
Roeckner, E., Baeuml, G., Bonventura, L., Brokopf, R., Esch, M., Giorgetta, M., Hagemann, S., Kirchner, I., Kornblueh, L., Manzini, E., Rhodin, A., Schlese, U., Schulzweida, U., and Tompkins, A.: The atmospheric general circulation model ECHAM5. PART I Model description, Report 349, Max Planck Institute for Meteorology, Hamburg, Germany, ISSN 0937 – 1060, 2003.
Roosenbrand, E., Sun, J., and Hoekstra, J.: Optimizing Global Flight Altitudes for Contrail Reduction. Insights from Open Flight and Weather Balloon Data, in: Fifteenth USA/Europe Air Traffic Management Research and Development Seminar (ATM2023), 2023.
Sanz-Morère, I., Eastham, S. D., Allroggen, F., Speth, R. L., and Barrett, S. R. H.: Impacts of multi-layer overlap on contrail radiative forcing, Atmos. Chem. Phys., 21, 1649–1681, https://doi.org/10.5194/acp-21-1649-2021, 2021.
Sausen, R., Gierens, K., Ponater, M., and Schumann, U.: A diagnostic study of the global distribution of contrails. Part I: Present-day climate, Theor. Appl. Climatol., 61, 127–141, https://doi.org/10.1007/s007040050076, 1998.
Sausen, R., Hofer, S., Gierens, K., Bugliaro, L., Ehrmanntraut, R., Sitova, I., Walczak, K., Burridge-Diesing, A., Bowman, M., and Miller, N.: Can we successfully avoid persistent contrails by small altitude adjustments of flights in the real world?, Meteorologische Zeitschrift, https://doi.org/10.1127/metz/2023/1157, 2023.
Schmidt, E.: Die Entstehung von Eisnebel aus den Auspuffgasen von Flugmotoren, Schr. Dtsch. Akad. Luftfahrtforsch., 44, 1–15, 1941.
Schröder, F., Kärcher, B., Duroure, C., Ström, J., Petzold, A., Gayet, J.-F., Strauss, B., Wendling, P., and Borrmann, S.: On the Transition of Contrails into Cirrus Clouds, J. Atmos. Sci., 57, 464–480, https://doi.org/10.1175/1520-0469(2000)057<0464:OTTOCI>2.0.CO;2, 2000.
Schumann, U.: On conditions for contrail formation from aircraft exhausts, Meteorol. Z., 5, 4–23, https://doi.org/10.1127/metz/5/1996/4, 1996.
Schumann, U.: Formation, properties and climatic effects of contrails, C. R. Phys., 6, 549–565, 2005.
Schumann, U.: A contrail cirrus prediction model, Geosci. Model Dev., 5, 543–580, https://doi.org/10.5194/gmd-5-543-2012, 2012.
Schumann, U. and Graf, K.: Aviation-induced cirrus and radiation changes at diurnal timescales, J. Geophys. Res., 118, 2404–2421, https://doi.org/10.1002/jgrd.50184, 2013.
Schumann, U. and Heymsfield, A.: On the lifecycle of individual contrails and contrail cirrus-Ice Formation and Evolution in Clouds and Precipitation: Measurement and Modeling Challenges, Chap. 3, Meteor. Mon, 58, 3.1–3.24, 2017.
Schumann, U. and Wendling, P.: Determination of contrails from satellite data and observational results, in: Air Traffic and the Environment-Background, Tendencies and Potential Global Atmospheric Effects, edited by: Schumann, U., Lecture Notes in Engineering, Springer-Verlag, 138–153, 1990.
Schumann, U., Konopka, P., Baumann, R., Busen, R., Gerz, T.,Schlager, H., Schulte, P., and Volkert, H.: Estimate of diffusion parameters of aircraft exhaust plumes near the tropopause from nitric oxide and turbulence measurements, J. Geophys. Res., 100, 14147–14162, https://doi.org/10.1029/95JD01277, 1995.
Schumann, U., Schlager, H., Arnold, F., Baumann, R., Haschberger, P., and Klemm, O.: Dilution of aircraft exhaust plumes at cruise altitudes, Atmos. Environ., 32, 3097–3103, 1998.
Schumann, U., Arnold, F., Busen, R., Curtius, J., Kärcher, B., Petzold, A., Schlager, H., Schröder, F., and Wohlfrom, K. H.: Influence of fuel sulfur on the composition of aircraft exhaust plumes: The experiments SULFUR 1-7, J. Geophys. Res., 107, 4247, https://doi.org/10.1029/2001JD000813, 2002.
Schumann, U., Mayer, B., Graf, K., and Mannstein, H.: A parametric radiative forcing model for contrail cirrus, J. Appl. Meteorol.Clim., 51, 1391–1406, https://doi.org/10.1175/JAMC-D-11-0242.1, 2012.
Schumann, U., Jeßberger, P., and Voigt, C.: Contrail ice particles in aircraft wakes and their climatic importance, Geophys. Res. Lett., 40, 2867–2872, 2013.
Schumann, U., Penner, J. E., Chen, Y., Zhou, C., and Graf, K.: Dehydration effects from contrails in a coupled contrail–climate model, Atmos. Chem. Phys., 15, 11179–11199, https://doi.org/10.5194/acp-15-11179-2015, 2015.
Schumann, U., Baumann, R., Baumgardner, D., Bedka, S. T., Duda, D. P., Freudenthaler, V., Gayet, J.-F., Heymsfield, A. J., Minnis, P., Quante, M., Raschke, E., Schlager, H., Vázquez-Navarro, M., Voigt, C., and Wang, Z.: Properties of individual contrails: a compilation of observations and some comparisons, Atmos. Chem. Phys., 17, 403–438, https://doi.org/10.5194/acp-17-403-2017, 2017.
Schumann, U., Bugliaro, L., Dörnbrack, A., Baumann, R., and Voigt, C.: Aviation Contrail Cirrus and Radiative Forcing Over Europe During 6 Months of COVID-19, Geophys. Res. Lett., 48, e2021GL092771, https://doi.org/10.1029/2021GL092771, 2021.
Siddiqui, N.: Atmospheric Contrail Detection with a Deep Learning Algorithm, Scholarly Horizons, Univ. Minnesota Morris Undergraduate Journal, 7, Article 5, https://doi.org/10.61366/2576-2176.1087, 2020.
Spichtinger, P. and Gierens, K. M.: Modelling of cirrus clouds – Part 1a: Model description and validation, Atmos. Chem. Phys., 9, 685–706, https://doi.org/10.5194/acp-9-685-2009, 2009.
Spinhirne, J. D., Hart, W. D., and Duda, D. P.: Evolution of the morphology and microphysics of contrail cirrus from airborne remote sensing, Geophys. Res. Lett., 25, 1153–1156, https://doi.org/10.1029/97GL03477, 1998.
Sridhar, B., Ng, H., and Chen, N.: Aircraft Trajectory Optimization and Contrails Avoidance in the Presence of Winds, J. Guid. Control Dynam., 34, 1577–1584, https://doi.org/10.2514/1.53378, 2011.
Stenke, A., Grewe, V., and Pechtl, S.: Do supersonic aircraft avoid contrails?, Atmos. Chem. Phys., 8, 955–967, https://doi.org/10.5194/acp-8-955-2008, 2008.
Stier, P., Feichter, J., Kinne, S., Kloster, S., Vignati, E., Wilson, J., Ganzeveld, L., Tegen, I., Werner, M., Balkanski, Y., Schulz, M., Boucher, O., Minikin, A., and Petzold, A.: The aerosol-climate model ECHAM5-HAM, Atmos. Chem. Phys., 5, 1125–1156, https://doi.org/10.5194/acp-5-1125-2005, 2005.
Stuber, P., Radel, G., and Shine, K.: The importance of the diurnal and annual cycle of air traffic for contrail radiative forcing, Nature, 441, 864–867, https://doi.org/10.1038/nature04877, 2006.
Sun, J. and Roosenbrand, E.: Flight Contrail Segmentation via Augmented Transfer Learning with Novel SR Loss Function in Hough Space, arXiv [preprint], https://doi.org/10.48550/arXiv.2307.12032, 22 July 2023.
Teoh, R., Schumann, U., Majumdar, A., and Stettler, M. E.: Mitigating the climate forcing of aircraft contrails by small-scale diversions and technology adoption, Environ. Sci. Technol., 54, 2941–2950, https://doi.org/10.1021/acs.est.9b07102, 2020.
Teoh, R., Engberg, Z., Shapiro, M., Dray, L., and Stettler, M. E. J.: The high-resolution Global Aviation emissions Inventory based on ADS-B (GAIA) for 2019–2021, Atmos. Chem. Phys., 24, 725–744, https://doi.org/10.5194/acp-24-725-2024, 2024.
Tesche, M., Achtert, P., Glantz, P., and Noone, K. J.: Aviation effects on already-existing cirrus clouds, Nat. Commun., 7, 12016, https://doi.org/10.1038/ncomms12016, 2016.
Testa, B., Durdina, L., Edebeli, J., Spirig, C., and Kanji, Z. A.: Contrail processed aviation soot aerosol are poor ice nucleating particles at cirrus temperatures, EGUsphere [preprint], https://doi.org/10.5194/egusphere-2024-151, 2024.
Unterstrasser, S.: Properties of young contrails – a parametrisation based on large-eddy simulations, Atmos. Chem. Phys., 16, 2059–2082, https://doi.org/10.5194/acp-16-2059-2016, 2016.
Unterstrasser, S. and Görsch, N.: Aircraft-type dependency of contrail evolution, J. Geophys. Res.-Atmos., 119, 14015–14027, https://doi.org/10.1002/2014JD022083, 2014.
Unterstrasser, S., and Sölch, I.: Numerical modeling of contrail cluster formation, in: Proceedings of the 3rd International Conference on Transport, Atmosphere and Climate, 25–28 June 2012, Prien am Chiemsee, Germany, ISSN 1434-8454, 114–119, 2013.
Unterstrasser, S., Gierens, K., Sölch, I., and Wirth, M.: Numerical simulations of homogeneously nucleated natural cirrus and contrail-cirrus. Part 2: Interaction on a local scale, Meteorol. Z., 26, 643–661, https://doi.org/10.1127/metz/2017/0844, 2017.
Urbanek, B., Groß, S., Wirth, M., Rolf, C., Krämer, M., and Voigt, C.: High depolarization ratios of naturally occurring cirrus clouds near air traffic regions over Europe, Geophys. Res. Lett., 45, 13166–13173, 2018.
Vazquez-Navarro, M., Mannstein, H., and Mayer, B.: An automatic contrail tracking algorithm, Atmos. Meas. Tech., 3, 1089–1101, https://doi.org/10.5194/amt-3-1089-2010, 2010.
Vázquez-Navarro, M., Mayer, B., and Mannstein, H.: A fast method for the retrieval of integrated longwave and shortwave top-of-atmosphere upwelling irradiances from MSG/SEVIRI (RRUMS), Atmos. Meas. Tech., 6, 2627–2640, https://doi.org/10.5194/amt-6-2627-2013, 2013.
Vázquez-Navarro, M., Mannstein, H., and Kox, S.: Contrail life cycle and properties from 1 year of MSG/SEVIRI rapid-scan images, Atmos. Chem. Phys., 15, 8739–8749, https://doi.org/10.5194/acp-15-8739-2015, 2015.
Verma, P. and Burkhardt, U.: Contrail formation within cirrus: ICON-LEM simulations of the impact of cirrus cloud properties on contrail formation, Atmos. Chem. Phys., 22, 8819–8842, https://doi.org/10.5194/acp-22-8819-2022, 2022.
Voigt, C., Schumann, U., Jessberger, P., Jurkat, T., Petzold, A., Gayet, J.-F., Krämer, M., Thornberry, T., and Fahey, D. W.: Extinction and optical depth of contrails, Geophys. Res. Lett., 38, L11806, https://doi.org/10.1029/2011GL047189, 2011.
Voigt, C., Schumann, U., Jurkat, T., Schäuble, D., Schlager, H., Petzold, A., Gayet, J.-F., Krämer, M., Schneider, J., Borrmann, S., Schmale, J., Jessberger, P., Hamburger, T., Lichtenstern, M., Scheibe, M., Gourbeyre, C., Meyer, J., Kübbeler, M., Frey, W., Kalesse, H., Butler, T., Lawrence, M. G., Holzäpfel, F., Arnold, F., Wendisch, M., Döpelheuer, A., Gottschaldt, K., Baumann, R., Zöger, M., Sölch, I., Rautenhaus, M., and Dörnbrack, A.: In-situ observations of young contrails – overview and selected results from the CONCERT campaign, Atmos. Chem. Phys., 10, 9039–9056, https://doi.org/10.5194/acp-10-9039-2010, 2010.
Voigt, C., Schumann, U., Minikin, A., Abdelmonem, A., Afchine, A., Borrmann, S., Boettcher, M., Buchholz, B., Bugliaro, L., Costa, A., Curtius, J., Dollner, M., Dörnbrack, A., Dreiling, V., Ebert, V., Ehrlich, A., Fix, A., Forster, L., Frank, F., Fütterer, D., Giez, A., Graf, K., Grooß, J.-U., Groß, S., Heimerl, K., Heinold, B., Hüneke, T., Järvinen, E., Jurkat, T., Kaufmann, S., Kenntner, M., Klingebiel, M., Klimach, T., Kohl, R., Krämer, M., Krisna, T. C., Luebke, A., Mayer, B., Mertes, S., Molleker, S., Petzold, A., Pfeilsticker, K., Port, M., Rapp, M., Reutter, P., Rolf, C., Rose, D., Sauer, D., Schäfler, A., Schlage, R., Schnaiter, M., Schneider, J., Spelten, N., Spichtinger, P., Stock, P., Walser, Weigel, R., Weinzierl, B., Wendisch, M., Werner, F., Wernli, H., Wirth, M., Zahn, A., Ziereis, H., and Zöger, M.: ML-CIRRUS – The airborne experiment on natural cirrus and contrail cirrus with the high-altitude long-range research aircraft HALO, B. Am. Meteorol. Soc., online first, https://doi.org/10.1175/BAMS-D-15-00213.1, 2016.
Voigt, C., Schumann, U., Minikin, A., Abdelmonem, A., Afchine, A., Borrmann, S., Boettcher, M., Buchholz, B., Bugliaro, L., Costa, A., Curtius, J., Dollner, M., Dörnbrack, A., Dreiling, V., Ebert, V., Ehrlich, A., Fix, A., Forster, L., Frank, F., Fütterer, D., Giez, A., Graf, K., Grooß, J.-U., Groß, S., Heimerl, K., Heinold, B., Hüneke, T., Järvinen, E., Jurkat, T., Kaufmann, S., Kenntner, M., Klingebiel, M., Klimach, T., Kohl, R., Krämer, M., Krisna, T. C., Luebke, A., Mayer, B., Mertes, S., Molleker, S., Petzold, A., Pfeilsticker, K., Port, M., Rapp, M., Reutter, P., Rolf, C., Rose, D., Sauer, D., Schäfler, A., Schlage, R., Schnaiter, M., Schneider, J., Spelten, N., Spichtinger, P., Stock, P., Walser, A., Weigel, R., Weinzierl, B., Wendisch, M., Werner, F., Wernli, H., Wirth, M., Zahn, A., Ziereis, H., and Zöger, M.: ML-CIRRUS: The airborne experiment on natural cirrus and contrail cirrus with the high-altitude long-range research aircraft HALO, B. Am. Meteorol. Soc., 98, 271–288, https://doi.org/10.1175/BAMS-D-14-00193.1, 2017.
Wang, Z., Bugliaro, L., Jurkat-Witschas, T., Heller, R., Burkhardt, U., Ziereis, H., Dekoutsidis, G., Wirth, M., Groß, S., Kirschler, S., Kaufmann, S., and Voigt, C.: Observations of microphysical properties and radiative effects of a contrail cirrus outbreak over the North Atlantic, Atmos. Chem. Phys., 23, 1941–1961, https://doi.org/10.5194/acp-23-1941-2023, 2023.
Weickmann, H.: Formen und Bildung atmosphärischer Eiskristalle, Beitr. Phys. freien Atmos., 28, 33, 1945.
Wendisch, M. and Brenguier, J.-L.: Airborne Measurements for Environmental Research: Methods and Instruments, Wiley-VCH Verlag GmbH & Co. KGaA, Weinheim, Germany, 2013.
Wilkerson, J. T., Jacobson, M. Z., Malwitz, A., Balasubramanian, S., Wayson, R., Fleming, G., Naiman, A. D., and Lele, S. K.: Analysis of emission data from global commercial aviation: 2004 and 2006, Atmos. Chem. Phys., 10, 6391–6408, https://doi.org/10.5194/acp-10-6391-2010, 2010.
Wolf, K., Bellouin, N., and Boucher, O.: Sensitivity of cirrus and contrail radiative effect on cloud microphysical and environmental parameters, Atmos. Chem. Phys., 23, 14003–14037, https://doi.org/10.5194/acp-23-14003-2023, 2023.
Wuebbles, D., Gupta, M., and Ko, M.: Evaluating the impacts of aviation on climate change, Eos T. Am. Geophys. Un., 88, 157–160, https://doi.org/10.1029/2007EO140001, 2007.
Wuebbles, D., Forster, P., Rogers, H., and Herman, R.: Issues and uncertainties affecting metrics for aviation impacts on climate, B. Am. Meteorol. Soc., 91, 491–496, https://doi.org/10.1175/2009BAMS2840.1, 2010.
Zängl, G., Reinert, D., Rípodas, P., and Baldauf, M.: The ICON (ICOsahedral Non-hydrostatic) modelling framework of DWD and MPI-M: Description of the non-hydrostatic dynamical core, Q. J. Roy. Meteorol. Soc., 141, 563–579, https://doi.org/10.1002/qj.2378, 2014.
Zhou, C. and Penner, J. E.: Aircraft soot indirect effect on large-scale cirrus clouds: Is the indirect forcing by aircraft soot positive or negative?, J. Geophys. Res.-Atmos., 119, 11303–11320, https://doi.org/10.1002/2014JD021914, 2014.
- Abstract
- Introduction
- Microphysics of contrail formation, aging, and transition to contrail cirrus
- Modeling of contrails and their impacts
- Radiative forcing for contrail cirrus in global models
- Observation datasets for contrail studies
- Supersonic contrails
- Uncertainties and research gaps
- Conclusions
- Code and data availability
- Author contributions
- Competing interests
- Disclaimer
- Acknowledgement
- Financial support
- Review statement
- References
- Abstract
- Introduction
- Microphysics of contrail formation, aging, and transition to contrail cirrus
- Modeling of contrails and their impacts
- Radiative forcing for contrail cirrus in global models
- Observation datasets for contrail studies
- Supersonic contrails
- Uncertainties and research gaps
- Conclusions
- Code and data availability
- Author contributions
- Competing interests
- Disclaimer
- Acknowledgement
- Financial support
- Review statement
- References