the Creative Commons Attribution 4.0 License.
the Creative Commons Attribution 4.0 License.
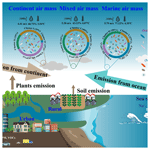
Influence of terrestrial and marine air mass on the constituents and intermixing of bioaerosols over a coastal atmosphere
Qun He
Zhaowen Wang
Houfeng Liu
Pengju Xu
Rongbao Duan
Caihong Xu
Jianmin Chen
Min Wei
Coastal environments provide an ideal setting for investigating the intermixing processes between terrestrial and marine aerosols. In this study, fine particulate matter (PM2.5) samples categorized into terrestrial, marine, and mixed air masses were collected from a coastal location in northern China. The chemical and biological constituents, including water-soluble ions (WSIs), metallic elements, and bacterial and fungal aerosols, were investigated from January to March 2018, encompassing both the winter heating and spring dust seasons. Terrestrial air masses constituted 59.94 % of the total air masses throughout the sampling period, with a significant increase during severe haze pollution (up to 90 %). These air masses exhibited a higher concentration of PM2.5 (240 µg m−3) and carried more water-soluble ions and metal elements. The terrestrial air mass also contained a larger number of animal parasites or symbionts, as well as human pathogens from anthropogenic emissions, such as Staphylococcus, Deinococcus, Sphingomonas, Lactobacillus, Cladosporium, and Malassezia. Conversely, a significant quantity of saprophytic bacteria such as hydrocarbon-degrading and gut bacteria from the genera Comamonas, Streptococcus, Novosphingobium, and Aerococcus and the saprophytic fungus Aspergillus were the most abundant species in the marine air mass samples. The mixed air mass elucidates the intermixing process of terrestrial and marine sources, a result of microorganisms originating from both anthropogenic and terrestrial emissions, which includes pathogenic microorganisms from hospitals and sewage treatment plants, and a multitude of soil bacteria. A stronger correlation was noted between microorganisms and continental elements in both terrestrial and mixed air mass samples, specifically K+, Mg2+, and Ca2+ derived from soil dust. Marine air masses exhibited a significant correlation with sea salt ions, specifically Na+. In the mixed air mass sample, a fusion of marine and terrestrial microorganisms is characterized by alterations in the ratio of pathogenic to saprophytic microorganisms when compared to samples derived from either terrestrial or marine sources. This study on the constituents and amalgamation of bioaerosols over the coastal atmosphere encompassing distinct air masses is crucial to understand the transport, intermixing processes, and health implications of terrestrial and marine air masses.
- Article
(5199 KB) - Full-text XML
-
Supplement
(1196 KB) - BibTeX
- EndNote
Bioaerosols, encompassing bacteria, fungi, viruses, pollen, and cellular debris, etc., are vital aerosol particles in the atmosphere. Notably, bacterial aerosols, which can be either free-floating or attached to airborne particles, typically measure between 0.3 and 10 µm (Shen and Yao, 2023; Zhao et al., 2022). Such characteristics allow for extended residence times and a heightened likelihood of long-distance transport from local to distant regions within the atmospheric circulation (Galbán et al., 2021; Smets et al., 2016). Fungal aerosols are prevalent in Earth's near-surface atmosphere, with their origins being diversely found in water, soil, and plants (Fröhlich-Nowoisky et al., 2009). Furthermore, the presence of pathogenic bacteria and fungi in the atmosphere may lead to significant penetration efficiency within the human respiratory system (Fakunle et al., 2021; Jiang et al., 2022).
Relevant studies have demonstrated that the bacterial and fungal concentration and community structure are significantly influenced by emission sources, atmospheric circulation, meteorological conditions, and geographical and topographical factors (Liu et al., 2021; Andrés et al., 2021; Li et al., 2019). The geographical and topographical factors, such as terrestrial and marine environments, exhibit significant differences in bioaerosol sources and pollution characteristics. Studies focusing on continental environments, particularly in large inland cities like Beijing (Zhang et al., 2019, 2022), Xian (Wang et al., 2020), Shanghai (Yao et al., 2019), Jinan (Wei et al., 2020), and Nanjing (Hu et al., 2020) during severe air pollution, have revealed that continental pollutant emissions significantly contribute to air pollution. Furthermore, transboundary transportation of terrestrial air masses on hazy days plays a crucial role in the progression of regional heavy pollution (Xie et al., 2021). The ocean serves as both a potential source and sink for airborne microorganisms (Archer et al., 2020; Mayol et al., 2017), exhibiting the intricate interplay between continental communities and their transmission across oceanic regions (Cho and Hwang, 2011; DeLeon-Rodriguez et al., 2013). Bioaerosols from the oceans may be influenced by long-distance transport from continental sources, such as plants and human pathogens (Elbert et al., 2007; Sharoni et al., 2015). Studies have shown that the concentration and diversity of bacterial and fungal aerosols from marine environment are typically lower than those derived from continental sources (Cao et al., 2024; Xue et al., 2022; Shi et al., 2022). Xu et al. (2019) undertook a thorough investigation of bacterial abundance on Mount Tai, China. Their findings indicate that variations in air mass from diverse sources could potentially influence the chemical composition of PM2.5, which in turn prompts shifts in bacterial groups. Several studies have examined the impacts of terrestrial and marine air masses on chemical constituents and microbial aerosols (Shi et al., 2022; Aswini and Hegde, 2021; Lang-Yona et al., 2022). Generally, the chemical aerosols can be affected by various sources of air masses, which may include local aerosols or remotely transported aerosols. However, it remains unclear whether different air trajectories contribute to the formation of bacterial communities within these particles. There is a dearth of research focused on the influence of different air masses on the chemical and biological composition in coastal cities.
Marine microbial aerosols can be released from the ocean micro-surface or transported from land (Prospero et al., 2005) and can settle thousands of kilometers away from their source of release (Mayol et al., 2014), exerting significant impacts on ecological and climate systems. Gong et al. (2020) conducted an examination of the microbial composition along Qingdao's coastlines, revealing a higher proportion of bacteria to total microorganisms from continental sources compared to marine sources. Some marine bacteria persist in aerosols after land transportation; Xu et al. (2021) studied the diversity of bacterial populations in PM2.5 across urban and rural areas of Shanghai, finding that airborne microbial communities over coastal cities are more influenced by long-distance transport than those inland. Air samples taken at high-altitude zones in coastal Europe and Japan demonstrated the continental transportation of marine microorganisms (Maki et al., 2014). The introduction of marine bacteria into the tropospheric free space may modify the airborne microbial composition in continental regions (Cho and Hwang, 2011; Amato et al., 2007; Maki et al., 2014; Polymenakou et al., 2008; Cáliz et al., 2018). Consequently, it is imperative to ascertain the impact of terrestrial and marine air masses on the composition of atmospheric microorganisms, with a particular focus on the influence of the mixing process between terrestrial and marine air masses on bioaerosols. Coastal environments offer optimal conditions for studying the mixing processes that occur between natural and anthropogenic air masses originating from terrestrial or marine sources.
Weihai, a coastal city situated at the confluence of the eastern Asia continent and the northwestern Pacific Ocean, is prone to the impact of marine and terrestrial air masses. Typically, Weihai maintains low pollutant emissions with an annual average PM2.5 concentration below 35 µg m−3. However, in winter and spring, regional air pollution intensifies, leading to severe air quality issues. This is due to increased inter-regional transportation, resulting in a daily average PM2.5 concentration greater than 150 µg m−3 (Wei et al., 2020). In this study, we conducted an integrated atmospheric observation experiment to examine the potential impact of terrestrial and marine air mass on the constituents and amalgamation of bioaerosols over the coastal atmosphere. This approach allows for a comprehensive exploration of the effects of sea–land air mass exchange on the spatial and temporal distribution of aerosols, as well as potential intermixing processes in coastal regions.
2.1 Sample collection
The sampling site was situated at the national air sampling station (37.53° N, 122.06° E; 41 m a.s.l.) approximately 1–2 km from the coast (Fig. S1 in the Supplement). The sampling platform was about 15 m a.g.l. (above ground level) and devoid of any significant obstructions. PM2.5 samples were gathered between January and March 2018, during the winter heating and spring dust seasons in northern China. Two parallel PM2.5 samplers (TH-150C-III, Wuhan Tianhong Instrument Co., Ltd., China) with a particle size of 2.5±0.2 µm were utilized, equipped with quartz membrane for the collection of PM2.5, inorganic ions, metal elements, and microorganisms at a flow rate of 100 L min−1. The quartz membranes were cauterized in a muffle furnace at 450 °C for 6 h to remove carbonaceous and contaminant materials. Intermittent sampling was used, and samples were collected twice a day (07:00 to 18:30 LT and 19:00 to 06:30 LT the following day). During the sampling process, the pre-weighed filter membrane was carefully positioned onto the filter mesh within a clean sampling folder using tweezers. The membrane's rough side should be oriented towards the direction of air intake, and it should be firmly pressed until there is no detectable air leakage. Prior to membrane replacement, the membrane tray is wiped with alcohol-coated cotton and dried. After sampling, the membrane is removed using tweezers. Blank samples were procured by putting the membrane into the sampler head without initiating the sampler. After sampling, the blank samples were extracted simultaneously with the collected sample membranes. All samples were then placed in a sterile filter cassette, encased in clean aluminum foil, and stored at −80 °C until further analysis. During the whole sampling period from 1 January to 31 March, a total of 102 PM2.5 samples over 51 d were obtained, out of which 24 samples were chosen for subsequent analysis based on air mass categories. Meteorological parameters, such as air temperature, relative humidity, wind direction, and wind speed, were monitored in situ utilizing a PC-4 automatic weather station (PC-4, JZYG, China). The hourly concentrations of PM2.5, PM10, CO, SO2, NO2, and O3 were systematically retrieved from the National Ambient Air Quality Monitoring System (http://www.cnemc.cn/, last access: 19 September 2024).
The mass concentrations of PM2.5, water-soluble ions, and metal elements were quantified after sampling. The membranes were meticulously weighed utilizing a Mettler XP-6 balance with an accuracy of 10−6 g. Prior to the weighing, the membranes were maintained in a controlled environment with consistent temperature and humidity for a duration of 24 h. Ion chromatography (ICS-2100, Chameleon 6.8, AS-DV autosampler Thermo Fisher) was employed to determine the concentration of water-soluble ions such as Na+, K+, Ca2+, Mg2+, Cl−, NO, SO, and NH. These ions were extracted by sonication with ionized water for 45 min and then separated by anion (IonPacAS23) or cation column exchange (IonPacCS12A). Then, these ions were detected using a conductivity detector with an anion separation column of IonPacAS23 with a flow rate of 1.0 mL min−1, an anion suppressor of AERS500, and a conductivity detector. The injection volume was 25 µL, and the cation separation column was IonPacCS12A with a flow rate of 1.0 mL min−1; the cation suppressor was CERS500 (Zhang et al., 2022). Metallic elements, including Al, Fe, Ti, Mn, Co, Ni, Cu, Zn, Ga, Sr, Cd, Sn, Sb, Pb, V, Cr, and As, were extracted using microwave digestion extraction (ETHOS ONE, Milestone), with the concentrations determined by ICP-MS or ICP-OES (Thermo Fisher).
2.2 Air mass clustering and classification
The potential sources and transportation of air mass were examined using the MeteoInfo backward trajectory model (MeteoInfo 3.7.4 – Java, http://www.meteothink.org/downloads/index.html, last access: 19 September 2024) developed by the Chinese Academy of Meteorological Sciences. Backward trajectories were simulated at 1 h intervals and estimated over a 24 h period. The meteorological data were sourced from GDAS1 (ftp://arlftp.arlhq.noaa.gov/pub/archives/gdas1/, last access: 19 September 2024). Backward trajectories of air masses at an altitude of 500 m were categorized and clustered, with daily plots illustrating these trajectories. In this study, air mass categories were defined as follows:
-
If more than 90 % of the masses originated from the ocean, it was considered a typical sample affected by marine air mass.
-
If more than 90 % originated from the continent, it was considered a typical terrestrial air mass sample.
-
If the proportions of terrestrial and marine air masses were similar or accounted for more than 40 % of the total air masses in 1 d, it was considered a mixed air mass sample.
2.3 DNA extraction and qPCR amplification
Microbial genomic DNA was procured from filters utilizing the Fast-DNATM SPIN kit for soil (MO BIO Laboratories, Carlsbad, CA, USA). The concentration and quality of the extracted DNA was measured via a NanoDrop spectrometer (NanoDrop 2000, Thermo Scientific USA). The DNA concentration in the collected samples ranged from 10 to 20 ng µL−1, satisfying the prerequisites for subsequent PCR amplification. The quantitative polymerase chain reaction (qPCR) was employed to identify bacterial 16S rRNA and fungal ITS gene copy numbers per cubic meter of air. The bacterial 16S V3–V4 variable region was selected for PCR amplification using primer 338F (5′-ATCTACGGGGGGCAGCAG-3′) and 806R (5′GGACTACHVGGGTWTCTAAT-3′) (Masoud et al., 2011). The fungal ITS regions were amplified using the primers ITS1 (5′-CTTGGTCATTTAGAGGAAGTAA-3′) and ITS2 (5′-GCTGCGTTCTTCATCGATGC-3′) (Liu et al., 2021).
The PCR amplification conditions comprised an initial denaturation at 95 °C for 5 min, succeeded by 30 s at 95 °C, 30 s at 50 °C, and then 35 cycles at 72 °C for 40 s, followed by a final extension at 72 °C for 7 min to ensure comprehensive amplification. Fluorescent signals were gathered during the extension phase. For each sample, qPCR was conducted in triplicate, with ultrapure water serving as a negative control to identify potential PCR contamination. Standard curves were constructed using E. coli harboring the 16S rRNA gene and Streptomyces plasmids containing the ITS gene. Additionally, gradient dilutions of these plasmids were performed, ranging from 1×102–1×107 copies per microliter. The copy number of 16S rRNA gene in bacterial genome ranges from 1 to 21 with an average value of 5.5 according to the rrnDB database (https://rrndb.umms.med.umich.edu/, last access: 19 September 2024) (Stoddard et al., 2015). The number of rRNA copies per genome ranges from 50 to 100 in filamentous fungi (Rooney and Ward, 2005), and ascomycetes typically have relatively smaller genome sizes (Kullman et al., 2005). Consequently, the copy number of the fungal ITS gene in their genome was set at 50 in this study. The FTC-3000 real-time quantitative PCR system (Funglyn Biotech Inc., Scarborough, ON, Canada) was employed for standard curve construction and data processing.
2.4 16S rRNA and ITS gene sequencing and data processing
The V3–V4 regions of the bacterial 16S rRNA and the fungal ITS1 gene were targeted for PCR amplification utilizing barcode-specific primers 338F–806R and ITS1F–ITS2, respectively. To ensure optimal amplification efficiency and precision, a high-fidelity enzyme (Phusion® High Fidelity PCR Master Mix from New England Biolabs) in conjunction with a GC buffer was employed during PCR amplification. This procedure entailed a pre-denaturation step at 98 °C for 1 min, followed by 30 cycles of 98 °C for 10 s; 58 °C for bacteria (56 °C for fungi) for 30 s; and 72 °C for an additional 30 s, with a final extension at 72 °C for 5 min. Following amplification, samples were purified using the Agencourt AMPure XP kit (Beckman Coulter, Brea, CA, USA). Subsequently, the purified samples were combined to achieve equimolar concentrations and analyzed on the Illumina MiSeq PE300 platform (Illumina, Inc. in San Diego, CA).
Following sequencing, the barcode sequences were extracted and subsequently stored in FASTQ format utilizing the QIIME toolkit (Caporaso et al., 2010). Sequences with a length of shorter than 200 base pairs, a base quality value below 25, and those containing ambiguous bases were subjected to quality control screening using Trimmomatic (Bolger et al., 2014) and mothur (Schloss et al., 2009). The assembled sequences were then de-duplicated and trimmed to equal length. De-chimerized sequences were eliminated (Edgar, 2013), and sequences were clustered into operational taxonomic units (OTUs) using UPARSE at a 97 % identity threshold. Subsequently, individual OTUs were removed. Taxonomic assignments were determined using a Basic Local Comparison Search Tool (BLAST) search against the bacterial SILVA taxonomy release 138.2 dataset and fungal UNITE ITS reference v8.0 dataset. The original raw sequences were deposited in the Sequence Read Archive under the accession numbers PRJNA1101427 and PRJNA1101176.
2.5 Statistical analysis
Bacterial community functional analysis was conducted using FAPROTAX, a manually constructed database that maps prokaryotic taxa to metabolic or other ecological functions, such as sulfur, nitrogen, hydrogen, and carbon cycling (Chen et al., 2022). FUNGuild (Fungi Functional Guild) was used to predict the fungal ecological function. This tool could classify and analyze fungal communities by the microecological guild based on current published literature or data from authoritative websites to classify fungi functionally (Nguyen et al., 2016). Three primary groups are obtained based on the nutritional mode: pathotroph, symbiotroph, and saprotroph. Samples affected by different air masses were examined or intergroup species variability, based on community abundance data. This was achieved using rigorous statistical methods to identify species demonstrating differences in abundance within the microbial communities of different groups and hypothesis testing to evaluate the significance of these observed differences. Statistical analyses, including an analysis of variance (ANOVA) and Kruskal–Wallis tests, were employed to discern bacteria and fungi with varying abundances between samples and groups. A p value less than 0.05 or 0.01 was considered significant. ANOVA was used to analyze variation in a response variable measured under conditions defined by discrete factors (Larson, 2008). The Kruskal–Wallis test determines whether there is a statistically significant difference between the medians of three or more independent groups (Kassambara, 2019). The Mantel analysis was utilized to reveal the correlation between microbial community composition and various environmental factors. Pearson's r coefficient at p<0.05 and p<0.01 indicates the significant correlation.
3.1 Air mass categorization and typical pollution processes
The MeteoInfo backward trajectory model was employed to simulate the trajectories of air masses at an altitude of 500 m in Weihai over a 24 h period, identifying and classifying potential sources of air mass transport (Fig. S1). The terrestrial air mass accounted for 59.94 % of the total air masses at the sampling site throughout the period, exhibiting an average PM2.5 concentration of 36.15±26.52 µg m−3. Severe air pollution episodes occurred on 20 January, when PM2.5 was the primary pollutant and the concentration reached 240 µg m−3. During regional haze pollution, the terrestrial air masses that primarily influenced Weihai typically originated from the North China Plain and the surrounding areas. The primary contributors to emissions in the terrestrial air mass of this region are the dense population and industrial and agricultural activities. The impact of the spring dust is mainly reflected in the primary pollutant PM10 from 30 to 31 March. A significant increase in PM10 concentration was observed on 31 March, with an hourly maximum value of 167 µg m−3. A relatively low PM2.5 PM10 ratio of 0.25 indicated pronounced dust pollution. The typical marine air masses mainly from the eastern and southern areas are from the Yellow Sea and the western Pacific edge. Marine air masses from northern areas partly come from the Bohai Sea, crossing inland areas and possibly making contact with the land prior to reaching the study area (Fig. S1). A total of 14 % of the sampling days were influenced by marine air masses, resulting in an average PM2.5 concentration of 23.99±11.00 µg m−3. Mixed air masses, characterized by simultaneous influence from the northwestern winds of Inner Mongolia and the offshore air masses of the Bohai Sea or the Yellow Sea, accounted for 27 % of all affected sampling days. These mixed air masses yielded an average PM2.5 concentration of 45.11±12.69 µg m−3. In comparison, under the influence of mixed air masses, pollutant concentrations were notably high. During the spring dust season, there is a notable increase in the proportion of mixed air mass. This elevated concentration of particulate matter correlates with the transmission of sand dust (Xie et al., 2021).
Three heavy pollution events were investigated to explore the alterations in air mass during these episodes (Figs. 1 and S2). Generally, the initiation and development stages of pollution events were predominantly characterized by terrestrial and mixed air masses. Cold northwestern air mass from the continent and marine air masses from east or south were the primary contributors during pollution mitigation. Pollution episode I occurred from 19 to 22 January, and the western terrestrial air mass was predominantly responsible for initiating the pollution, evidenced by a PM2.5 concentration of 51.35 µg m−3. As the pollution progressed, it transitioned into a mixed air mass with a PM2.5 concentration peaking at 240 µg m−3. This change was accompanied by significant increases in water-soluble ions (WSIs) and elemental concentrations. Pollution elimination was initiated with high winds and snowfall influenced by the cold northwestern air mass from the continent and marine air masses from the northeast sea. The average PM2.5 concentration was reduced to 7.92 µg m−3, accompanied by a decrease in both WSIs and elemental concentrations.
3.2 Water-soluble ions and metal elements over coastal atmosphere
During the sampling period, the concentration of water-soluble ions in PM2.5 was 22.92±12.19 µg m−3, ranging from 4.58 to 78.14 µg m−3. In marine air mass samples, a lower concentration of water-soluble ions was observed, with concentrations of 13.01±7.43, 27.94±13.61, and 30.38±11.38 µg m−3 in marine, terrestrial, and mixed air masses, respectively. Notably, NO had the highest proportion (26.94 %, 6.4 %–52.6 %), followed by SO (21.94 %, 9.4 %–33.4 %) and NH (20.26 %, 5.8 %–35.6 %). SO, NO, and NH constituted the majority of total ions in other studies conducted in Beijing, Shanghai, and Guangzhou (Pathak et al., 2009; Hu et al., 2014; Zhou et al., 2012). These inorganic secondary ions were significantly influenced by both terrestrial and mixed air masses, with the latter exhibiting a more pronounced effect (NO, 10.65±3.26 µg m−3; NH, 7.39±3.30 µg m−3; SO 6.76±1.77 µg m−3) (Fig. S3).
A high concentration of Na+ was observed, with a range of 3.15±1.69 µg m−3, and accounted for 14.47 % of the total water-soluble ions. The concentration of Na+ and Mg2+ exhibited analogous trends across terrestrial, marine, and mixed air mass samples. The ratio of Mg2+ Na+, typical components of sea salt, was determined to be 0.11. This value closely corresponded to the 0.12 found in seawater, indicating a potential origin from marine environments (Sun et al., 2022). The concentration of K+ was 0.24±0.20 and 0.26±0.10 µg m−3 in the terrestrial and mixed air mass samples and was twice as high as those in the marine air mass samples (0.11±0.05 µg m−3), which suggested an important contribution from anthropogenic emissions. The concentrations of Cl− and Ca2+ were high in terrestrial and mixed air mass samples. Generally, these two ions mostly come from anthropogenic or terrestrial environments. Cl− mainly comes from sea salt, coal, and biomass combustion, and Ca2+ is a crustal element associated with soil dust and sandstorms (Liang et al., 2021). Overall, from the composition and concentration of water-soluble ions in PM2.5, Weihai was more affected by sea salt, coal combustion, and dust events in early spring.
The element concentrations in different air mass samples are depicted in Fig. S3. The top 10 elements were found to be Fe, Al, Zn, Cu, Sn, Pb, Mn, Ti, Ni, and V. These metal elements were categorized into macro and trace elements. The macro metals, specifically iron (Fe), aluminum (Al), and zinc (Zn), constituted a significant proportion of the total heavy metal elements, accounting for 34 %, 25 %, and 23 %, respectively. In general, the concentration of both macro and trace metal elements in marine air masses was found to be lower than that in terrestrial and mixed air masses. The V Ni ratio in marine air mass samples was found to be 0.78, a value significantly higher than that of both terrestrial and mixed air masses. The V Ni ratio serves as an indicator to assess the influence of ship emissions. A ratio exceeding 0.7 typically indicates a significant impact from ship emission sources and is commonly used as an indicator in coastal cities (Zhang et al., 2014).
3.3 Microbial community over coastal atmosphere
Airborne bacterial and fungal concentrations in PM2.5 were copies per cubic meter ( bacteria per cubic meter, P=0.06) and copies per cubic meter ( fungal spores per cubic meter, P=0.04). This value was comparable to the average abundance of bacteria and fungi in the atmospheric boundary layer over land, which is approximately 1.9×104 bacteria per cubic meter and 2.4×104 fungal spores per cubic meter (Mayol et al., 2014). A high concentration was observed in terrestrial air mass samples, with the average value of copies per cubic meter (P=0.12) and copies per cubic meter (P=0.007) for bacteria and fungi, respectively. Microbial concentration in marine air mass samples was significantly lower, with the average concentrations of bacteria and fungi being copies per cubic meter (P=0.04) and copies per cubic meter (P=0.07).
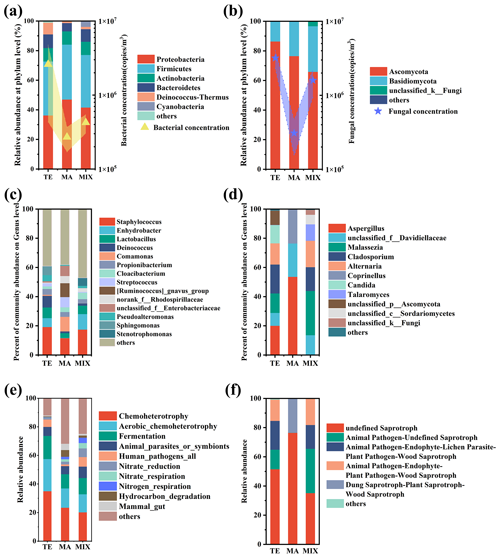
Figure 2Bacterial and fungal community composition and function influenced by different air masses. The community concentration of main the phylum (a, b), genus (c, d), and community function (e, f) are indicated.
Airborne microbial community structure exhibited significant variation influenced by marine and terrestrial air masses (Fig. 2). Predominantly, Proteobacteria (40.06 %), Firmicutes (36.30 %), Actinobacteria (8.97 %), Bacteroidetes (8.29 %), and Deinococcus-Thermus (4.59 %) were identified as the most abundant bacteria. Notably, Actinobacteria and Deinococcus-Thermus were found in high concentration in samples from terrestrial air masses. In particular, Deinococcus-Thermus demonstrated a relative abundance of 7.9 % in terrestrial air mass samples, significantly surpassing that of marine (1.2 %) and mixed air mass samples (1.5 %). Cyanobacteria exhibit a higher concentration in both terrestrial and mixed air mass samples. Cyanobacteria are prevalent in freshwater, soil, and surface crusts in deserts (Cordeiro et al., 2020; Temraleeva et al., 2016; Curren and Leong, 2020). In comparison to terrestrial environments, the prevalence of Cyanobacteria is notably diminished in colder marine environments (Koh et al., 2012). Atmospheric Cyanobacteria in Weihai may originate from the terrestrial environment, subsequently transported via terrestrial and mixed air masses. Proteobacteria were the most prevalent taxon in marine air mass samples. The predominant metabolic activity in deep-sea sediments is attributed to this group, with major taxa being found in marine sediments (Huang et al., 2021). The predominant bacterial genera included Staphylococcus (21.02 %), Enhydrobacter (6.43 %), Lactobacillus (6.03 %), and Deinococcus (4.56 %). These bacteria included a series of opportunistic pathogens and were found to be abundant in terrestrial and mixed air mass samples. Relative abundance of bacteria such as Comamonas, Streptococcus, Ruminococcus, and Enterobacteriaceae was higher in marine air mass samples. These predominantly consisted of saprophytic bacteria and intestinal microorganisms.
The dominant fungal phyla were Ascomycota (77.29 %) and Basidiomycota (21.58 %), which were similar to the previous studies (Du et al., 2018; Liu et al., 2019; Zeng et al., 2019). Fungal communities influenced by terrestrial and mixed air masses were quite similar, with relatively higher abundances of opportunistic pathogens such as Malassezia, Alternaria, and Cladosporium. In contrast the saprophytic Aspergillus, Davidiellaceae, and Coprinellus were abundant in marine air mass samples.
The FAPROTAX analysis revealed that the primary bacterial ecological functions were chemoheterotrophy, aerobic chemoheterotrophy, fermentation, and human pathogens. These accounted for 74 %, 47 %, and 44 %, respectively, in terrestrial, marine, and mixed air mass samples. Notably, human pathogens and animal parasites or symbionts were more prevalent in terrestrial and mixed air mass samples than in marine air mass samples. Marine air mass samples were enriched with mammal gut bacteria, as well as hydrocarbon and aromatic compound degradation bacteria. The fungal community functions in terrestrial and mixed air mass samples were similar, with undefined saprotroph, animal pathogen–undefined saprotroph, animal pathogen–endophyte–lichen parasite, and animal pathogen–endophyte–plant pathogen being the main functions, which totaled 99.27 %, 99.98 %, and 99.27 % in terrestrial, marine, and mixed air mass samples, respectively. In particular, the observed prevalence of saprotroph fungi was higher in samples from marine air masses, such as those containing Aspergillus. Notably, the fungi associated with terrestrial air masses predominantly carried animal pathogens and exhibited greater pathogenicity, including species such as Malassezia and Alternaria.
4.1 Community disparities influenced by terrestrial and marine air masses
Overall, bacterial and fungal community concentrations influenced by terrestrial and mixed air masses were higher than those in marine air mass samples. Bioaerosols originating from anthropogenic environments, such as sewage treatment plants and hospitals, may harbor more resistant bacteria. Microorganisms from arid areas like deserts possess a higher number of species adapted to harsh conditions, including resistance to temperature fluctuations, dryness, and UV radiation. These include bacteria such as Bacillus, Streptococcus, and Deinococcus (Maki et al., 2010; Park et al., 2018; Qi et al., 2021). These microorganisms can adhere to particulate matter during long-distance transport of air masses after being released from their terrestrial habitats.
Community disparities influenced by terrestrial, marine, and mixed air masses are shown in Tables S2 and S3 in the Supplement and Figs. 3 and 4. The principal coordinates analysis (PCoA) revealed distinct clusters corresponding to terrestrial, marine, and mixed air mass samples (Fig. S4). In terms of bacterial community variations, numerous dominant microorganisms exhibited obvious differences in abundance between marine and terrestrial air mass samples, although these differences were not statistically significant (p>0.05). For instance, the bacterial enrichments in terrestrial and mixed air masses included Staphylococcus, Deinococcus, Lactobacillus, and Sphingomonas. Staphylococcus is a pathogenic bacterium widely found in human skin and intestines that can cause various infections (Cheung et al., 2021). As a radiation-resistant bacterium, Deinococcus-Thermus is capable of withstanding harsh environmental conditions (Callegan et al., 2008; Rainey et al., 2007). Lactobacillus, a genus of Gram-positive bacteria, has also been identified as abundant in atmospheric dust (Federici et al., 2018; Xu et al., 2017). Sphingomonas, typically found in water and soil, is widely known to have the metabolic mechanism ability to endure poor nutrients and degradation of refractory compounds, such as polycyclic aromatic hydrocarbons (PAHs) (Hu et al., 2007; Sun et al., 2018). The majority of these bacteria possess the ability to produce spores, which are capable of withstanding harsh conditions such as low temperatures, aridity, and radiation during long-distance transmission, thereby ensuring their survival throughout this process (Griffin et al., 2003). Comamonas was identified as the dominant bacterium in the coastal atmosphere of Weihai, particularly prevalent in marine air mass samples. This bacterium is commonly associated with environmental bioremediation and is predominant in oligotrophic environments (Yan et al., 2012; Zhang et al., 2024). Streptococcus is mostly found in the oral and gastrointestinal tracts and also play an important role in human infections (Brouwer et al., 2023). Ruminococcus and Enterobacteriaceae are typical gut microorganisms present in humans and animals. In the coastal atmosphere, the identified sequences of these gut bacteria might be associated with intestinal microorganisms in marine fish and mammal animals.
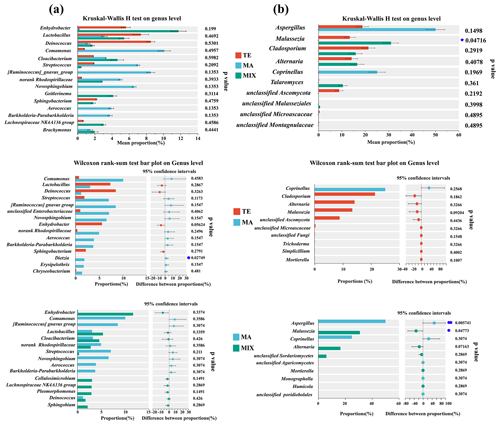
Figure 3Bacterial (a) and fungal (b) community disparities influenced by terrestrial (n=10), marine (n=6), and mixed air mass (n=8).
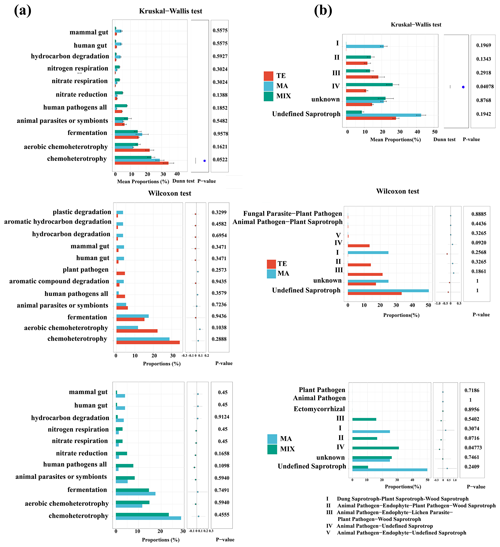
Figure 4Bacterial (a) and fungal (b) community function disparities influenced by terrestrial (n=10), marine (n=6), and mixed mass (n=8).
For fungal communities, Aspergillus demonstrated a significant differentiation between marine and mixed air mass samples (P=0.005). The highest proportion was noted in samples from marine air masses, at 53.7 %. In contrast, the values were 20.1 % and 0.3 % in terrestrial and mixed air masses, respectively. Previous studies have confirmed that Aspergillus is widely distributed in nature and unpolluted environments (Kendrick, 1995). This fungus is predominantly found in offshore regions, such as Qingdao, China (Li et al., 2011). Furthermore, the saprophytic Aspergillus was also prevalent in clean samples during periods of haze pollution and was frequently detected on non-haze days (Yan et al., 2016). Malassezia was higher in terrestrial and mixed air mass samples (P=0.047), which has been found to be widespread in a variety of animals. As a parasitic fungus, Malassezia causes the majority of skin diseases, such as dandruff and seborrheic dermatitis caused by Malassezia sphericalis (DeAngelis et al., 2007). Furthermore, Cladosporium exhibits higher abundance in terrestrial and mixed air mass samples compared to marine air masses, although this difference lacks statistical significance (P>0.05). This species is ubiquitous worldwide, commonly found in a wide variety of plants, known to be a common endophyte as well as a foliar fungus (El-Morsy, 2000; Islam and Hasin, 2000). It is frequently isolated from soil, foodstuff, and organic matter (Bensch et al., 2012). Moreover, Cladosporium have been commonly observed in terrestrial atmospheric environments, demonstrating the potential origins from continental environments (Frączek et al., 2017; Han et al., 2019).
The significant variance in the ecological function of microbial community was predominantly evident in the animal pathogen–undefined saprotrophs (P=0.04). These pathogenic fungi were more prevalent in terrestrial and mixed air mass samples than in marine air mass samples. Marine air mass samples were enriched with mammal gut bacteria, hydrocarbon and aromatic compound degradation bacteria, and undefined saprotroph fungi.
4.2 Implications of environmental factors on coastal airborne microbes
To elucidate the contributions of marine and terrestrial air masses to microbial aerosols in Weihai, a Mantel correlation analysis was conducted between microbial aerosols and various environmental factors (Figs. 5 and S4). The bacterial community exhibited a significant positive correlation with SO due to the influence of terrestrial and mixed air masses (P<0.05). Both bacterial and fungal communities demonstrated positive correlations with continental ions such as K+, Mg2+, and Ca2+ (Shi et al., 2022). Additionally, a positive correlation was observed between bacterial and fungal concentrations and NO2 (P<0.05), as well as a significant positive correlation between microbial concentration and PM10 (P<0.01). Air masses transported over long distances from the continent appear to harbor diverse and abundant microbial populations (Kakikawa et al., 2009; DeLeon-Rodriguez et al., 2013). For instance, the long-distance transportation of dust particles from northwestern winds in the Inner Mongolia region may have altered the community structure and abundance (Qi et al., 2021; Xia et al., 2024). Dust-borne bacteria, such as Staphylococcus, Delftia, Pseudoalteromonas, and Deinococcus, are likely introduced into the atmosphere during Asian dust events. Most of these bacteria accompany the transportation of dust particles to downwind coastal cities. Similarly, microbial communities showed a high positive correlation with ions from continental sources, such as K+, Mg2+, and Ca2+ in terrestrial and mixed air mass samples. K+ is the signature ion of biomass combustion (Mason et al., 2016; Yu et al., 2018). Mg2+ and Ca2+ are mostly derived from crustal elements (Zhang et al., 2012). The dominant bacteria within the microbial community exhibited a significant positive correlation with these three ions simultaneously, suggesting similar sources. They generally originate from terrestrial sources, including anthropogenic biomass combustion and soil dust.
The concentrations of particulate matter and water-soluble ions in samples affected by the marine air mass are notably low, indicating that the majority of chemical elements did not significantly influence the composition and abundance of the microbial community. However, a significant positive correlation was observed between Na+ and certain dominant species such as Talaromyces, Monographella, and Phoma (P<0.05). Similarly, Mg2+ was found to be significantly positively correlated with Malassezia in mixed air masses (P<0.01) (Fig. S5). Besides anthropogenic sources, such as industrial emissions, the origins of Mg2+ and Na+ in coastal regions should also consider the impact of sea salt (Sun et al., 2022). Samples collected under the influence of marine air masses had a positive correlation between Mg2+ and Na+ (r2=0.67), indicating that they have a similar origin of sea salt. Furthermore, the mean Mg2+ Na+ ratio was found to be 0.11, which is proximate to the seawater value of 0.12 (Seinfeld and Pandis, 1998).
Influenced by mixed air masses, temperature has a greater impact on fungal community, which was positively correlated with Malasseziales and Davidiellaceae. In marine air mass samples, a positive correlation between air temperature and certain microorganisms (Aerococcus, Cloacibacterium, Sphingobium, Enhydrobacterium, Davidiellaceae, and Malasseziales) also indicated that the increase in air temperature in spring favors the survival of airborne microbes (Jones and Harrison, 2004).
4.3 Intermixing of bioaerosols from terrestrial and marine air mass
Coastal atmospheres exhibit a complex amalgamation of terrestrial and marine aerosols, with the characteristics significantly influenced by the origins of the air masses. Consequently, these coastal aerosols offer ideal conditions for examining the mixing processes between natural and anthropogenic emission from terrestrial and marine sources, respectively. This work shows that enormous levels of haze aerosols from the terrestrial and mixed air mass can be rapidly transported into coastal cities during regional haze or dust pollution. During the long-distance transportation of air masses, a comprehensive mixture of soil-derived, biogenic, and anthropogenic microorganisms from terrestrial air masses and aquatic, saprophytic, and gut microorganisms from marine environments are fully mixed (Fig. 6). This amalgamation is particularly evident in the samples of mixed air masses. Within these, a significant quantity of chemical components, bacteria, and fungi, as well as opportunistic pathogens, were transported into coastal cities from the continent. Microbial communities were strongly correlated with haze aerosols, e.g., WSIs in PM2.5 from terrestrial and mixed air mass. The primary influence on terrestrial air mass was anthropogenic emissions, with coal combustion for winter heating and biomass burning being the predominant pollution sources. Moreover, dust events in spring carried a higher concentration of particulate matter. These air pollutants can act as an initial source of bioaerosols such as bacteria and fungi, thereby providing a site for attachment reproduction (Jiang et al., 2022). Additionally, water-soluble ions in PM, primarily secondary inorganic ions, such as sulfate, nitrate, and ammonium ions, can supply essential nutrients for microbial growth (Fan et al., 2019). This elucidates the elevated concentration of particulate matter and microorganisms in terrestrial air mass during heavy pollution. Simultaneously, it is pertinent to highlight that during such pollution events, the terrestrial air mass exacerbates the pollution process, leading to a marked increase in the proportion of pathogenic microorganisms.
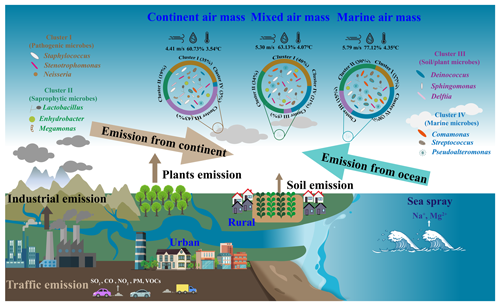
Figure 6Implications of intermixing of bioaerosols from terrestrial and marine air mass on the composition of airborne microbial communities.
In the coastal city of Weihai, marine air masses predominantly originate from the expansive western Pacific Ocean, located to the eastern and southern areas. These air masses are typically characterized by their cleanliness and contain a significant quantity of saprophytic and intestinal microorganisms that infiltrate the atmosphere via ocean droplets (Mayol et al., 2014). The presence of microorganisms in marine air masses exhibited a significant correlation with sea salt ions, specifically Na+ and Mg2+. These ions, when introduced into the atmosphere, form aerosolized particulate matter. The introduction of marine bacteria into tropospheric free space may alter the airborne microbial composition in continental regions (Cho and Hwang, 2011; Amato et al., 2007; Maki et al., 2014; Polymenakou et al., 2008; Cáliz et al., 2018), thereby significantly impacting bioaerosols over coastal atmospheres.
Notably, marine air masses from the northerly areas cross China's inland Bohai Sea and then pass through urban and rural areas with high human activity intensity. These areas have more anthropogenic microorganisms, including pathogenic microorganisms from hospitals and sewage treatment plants. In this study, samples within this category are defined as those influenced by mixed air mass. This also signifies the amalgamation of marine and terrestrial microorganisms. Consequently, within the mixed air mass samples, the prevalence of pathogenic and saprophytic microorganisms is comparable. This similarity underscores the complex impact of both terrestrial and marine sources. Populations in coastal cities may also be susceptible to exposure to these bioaerosols and pathogens, which are transported over long distances during regional air pollution.
This study evaluated the potential influence and the mixing effects between natural and anthropogenic aerosols, respectively, from terrestrial and marine air mass on biological constituents of PM2.5 over the coastal atmosphere. The concentrations of water-soluble ions, metal elements, and bioaerosols were higher in terrestrial and mixed air mass samples than in marine air mass samples. The V / Ni ratio greater than 0.7 in marine air mass samples indicated significant influence from marine ship emissions. Bacterial and fungal communities influenced by terrestrial and mixed air masses were enriched in pathogens, including Staphylococcus, Malassezia, and Alternaria. In contrast, marine air masses carried more gut and saprophytic microbes, such as Ruminococcus, Enterobacteriaceae, Aspergillus, and Davidiellaceae. The Mantel correlation analysis indicated a significant correlation between the bacterial community and SO, K+, Mg2+, Ca2+, and PM10, which was influenced by terrestrial and mixed air masses originating from continental sources. In contrast, influenced by marine air masses, bacterial and fungal communities were strongly correlated with sea salt ions, such as Na+. The predominant bacteria and fungi in marine and mixed air mass samples exhibited a positive correlation with air temperature in spring, suggesting elevated temperature is conducive to the survival and reproduction of bioaerosols. Our research offers a novel perspective on the amalgamation of terrestrial and marine aerosols in the coastal atmosphere. In the mixed air mass samples, a complex amalgamation of soil-derived and anthropogenic microorganisms from terrestrial air masses, along with aquatic, saprophytic, and gut microorganisms from marine environments, is fully intermixed during the long-distance transportation of air masses. This process significantly influences bioaerosols in coastal atmospheres. This understanding will enhance our comprehension of the environmental and climatic impacts on microbial aerosols within the marine boundary layer. Future research endeavors will concentrate on local emissions and long-distance transport, as well as the contributions of both terrestrial and marine air masses on bioaerosol components in coastal cities.
The hourly concentrations of PM2.5, PM10, CO, SO2, NO2, and O3 were systematically retrieved from the National Ambient Air Quality Monitoring System (http://www.cnemc.cn/, CNEMC, 2024). The historical air quality data were sourced from the nearest environmental monitoring center (https://www.aqistudy.cn/historydata/, Wang, 2024) and the MeteoInfo backward trajectory model (MeteoInfo 3.7.4 – Java, http://www.meteothink.org/downloads/index.html, MeteoInfo, 2024). Meteorological data were obtained from GDAS1 (ftp://arlftp.arlhq.noaa.gov/pub/archives/gdas1/, GDAS, 2024). Meteorological data and bacterial and fungal concentrations are available from the authors on request (minwei@sdnu.edu.cn). The raw sequences of the bacterial 16S rRNA and the fungal ITS gene are available in the Sequence Read Archive (SRA) under accession numbers PRJNA1101427 (https://www.ncbi.nlm.nih.gov/Traces/study/?acc=PRJNA1101427&o=acc_s:a, NIH, 2024a) and PRJNA1101176 (https://dataview.ncbi.nlm.nih.gov/object/PRJNA1101176?reviewer=mu6a68nm61vnh0g1lahff3qvma, NIH, 2024b).
The supplement related to this article is available online at: https://doi.org/10.5194/acp-24-12775-2024-supplement.
All authors contributed to the manuscript and have approved the final version. MW designed the study. QH performed the data analysis and wrote the original manuscript. MW assisted with the sampling. QH, ZW, and RD conducted the experiments and performed the statistical analyses. HL, MW, and PX contributed to the interpretation of results. MW, CX, and JC revised the manuscript.
The contact author has declared that none of the authors has any competing interests.
Publisher's note: Copernicus Publications remains neutral with regard to jurisdictional claims made in the text, published maps, institutional affiliations, or any other geographical representation in this paper. While Copernicus Publications makes every effort to include appropriate place names, the final responsibility lies with the authors.
The authors express their sincere gratitude to the Weihai Bureau of Environmental Protection for their assistance in sample collection and for providing reliable data during field observation. Further thanks go to the editors and reviewers for their insightful comments, patience, and support throughout the preparation of this paper.
This research has been supported by the National Key Research and Development Program of China (grant no. 2023YFC3710200), the National Natural Science Foundation of China (grant no. 42075183), and the China Postdoctoral Science Foundation (grant no. 2019T120606).
This paper was edited by Aurélien Dommergue and reviewed by three anonymous referees.
Amato, P., Parazols, M., Sancelme, M., Mailhot, G., Laj, P., and Delort, A.-M.: An important oceanic source of micro-organisms for cloud water at the Puy de Dôme (France), Atmos. Environ., 41, 8253–8263, https://doi.org/10.1016/j.atmosenv.2007.06.022, 2007.
Andrés, N., Ana, M. G., Diego, A. M., and Raúl, G.: Seasonal changes dominate long-term variability of the urban air microbiome across space and time, Environ. Int., 150, 106423, https://doi.org/10.1016/j.envint.2021.106423, 2021.
Archer, S. D. J., Lee, K. C., Caruso, T., King-Miaow, K., Harvey, M., Huang, D., Wainwright, B. J., and Pointing, S. B.: Air mass source determines airborne microbial diversity at the ocean–atmosphere interface of the Great Barrier Reef marine ecosystem, ISME J., 14, 871–876, https://doi.org/10.1038/s41396-019-0555-0, 2020.
Aswini, A. R. and Hegde, P.: Impact assessment of continental and marine air-mass on size-resolved aerosol chemical composition over coastal atmosphere: Significant organic contribution in coarse mode fraction, Atmos. Res., 248, 105216, https://doi.org/10.1016/j.atmosres.2020.105216, 2021.
Bensch, K., Braun, U., Groenewald, J. Z., and Crous, P. W.: The genus Cladosporium, Stud. Mycol., 72, 1–401, https://doi.org/10.3114/sim0003, 2012.
Bolger, A. M., Lohse, M., and Usadel, B.: Trimmomatic: a flexible trimmer for Illumina sequence data, Bioinformatics, 30, 2114–2120, https://doi.org/10.1093/bioinformatics/btu170, 2014.
Brouwer, S., Rivera-Hernandez, T., Curren, B. F., Harbison-Price, N., De Oliveira, D. M. P., Jespersen, M. G., Davies, M. R., and Walker, M. J.: Pathogenesis, epidemiology and control of Group A Streptococcus infection, Nat. Rev. Microbiol., 21, 431–447, https://doi.org/10.1038/s41579-023-00865-7, 2023.
Cáliz, J., Triadó-Margarit, X., Camarero, L., and Casamayor, E. O.: A long-term survey unveils strong seasonal patterns in the airborne microbiome coupled to general and regional atmospheric circulations, P. Natl. Acad. Sci. USA, 115, 12229–12234, https://doi.org/10.1073/pnas.1812826115, 2018.
Callegan, R. P., Nobre, M. F., McTernan, P. M., Battista, J. R., Navarro-Gonzalez, R., McKay, C. P., da Costa, M. S., and Rainey, F. A.: Description of four novel psychrophilic, ionizing radiation-sensitive Deinococcus species from alpine environments, Int. J. Syst. Evol. Microbiol., 58, 1252–1258, https://doi.org/10.1099/ijs.0.65405-0, 2008.
Cao, F., Zhang, Y., Zhang, Y., Song, W., Zhang, Y., Lin, Y., Gul, C., and Haque, M. M.: Molecular compositions of marine organic aerosols over the Bohai and Yellow Seas: Influence of primary emission and secondary formation, Atmos. Res., 297, 107088, https://doi.org/10.1016/j.atmosres.2023.107088, 2024.
Caporaso, J. G., Kuczynski, J., Stombaugh, J., Bittinger, K., and Knight, R.: QIIME allows analysis of high-throughput community sequencing data, Nat. Meth., 7, 335–336, https://doi.org/10.1038/nmeth.f.303, 2010.
Chen, J., Zang, Y., Yang, Z., Qu, T., Sun, T., Liang, S., Zhu, M., Wang, Y., and Tang, X.: Composition and Functional Diversity of Epiphytic Bacterial and Fungal Communities on Marine Macrophytes in an Intertidal Zone, Front. Microbiol., 13, 839465, https://doi.org/10.3389/fmicb.2022.839465, 2022.
Cheung, G. Y. C., Bae, J. S., and Otto, M.: Pathogenicity and virulence of Staphylococcus aureus, Virulence, 12, 547–569, https://doi.org/10.1080/21505594.2021.1878688, 2021.
Cho, B. C. and Hwang, C. Y.: Prokaryotic abundance and 16S rRNA gene sequences detected in marine aerosols on the East Sea (Korea), FEMS Microbiol. Ecol., 76, 327–341, https://doi.org/10.1111/j.1574-6941.2011.01053.x, 2011.
CNEMC – China National Environmental Monitoring Centre: CNEMC Date, http://www.cnemc.cn/ (last access: 19 September 2024), 2024.
Cordeiro, R., Luz, R., Vasconcelos, V., Gonçalves, V., and Fonseca, A.: Cyanobacteria Phylogenetic Studies Reveal Evidence for Polyphyletic Genera from Thermal and Freshwater Habitats, Diversity, 12, 298–311, https://doi.org/10.3390/d12080298, 2020.
Curren, E. and Leong, S. C. Y.: Natural and anthropogenic dispersal of cyanobacteria: a review, Hydrobiologia, 847, 2801–2822, https://doi.org/10.1007/s10750-020-04286-y, 2020.
DeAngelis, Y. M., Saunders, C. W., Johnstone, K. R., Reeder, N. L., Coleman, C. G., Kaczvinsky, J. R., Gale, C., Walter, R., Mekel, M., Lacey, M. P., Keough, T. W., Fieno, A., Grant, R. A., Begley, B., Sun, Y., Fuentes, G., Scott Youngquist, R., Xu, J., and Dawson, T. L.: Isolation and Expression of a Malassezia globosa Lipase Gene, LIP1, J. Invest. Dermatol., 127, 2138–2146, https://doi.org/10.1038/sj.jid.5700844, 2007.
DeLeon-Rodriguez, N., Lathem, T. L., Rodriguez-R, L. M., Barazesh, J. M., Anderson, B. E., Beyersdorf, A. J., Ziemba, L. D., Bergin, M., Nenes, A., and Konstantinidis, K. T.: Microbiome of the upper troposphere: Species composition and prevalence, effects of tropical storms, and atmospheric implications, P. Natl. Acad. Sci. USA, 110, 2575–2580, https://doi.org/10.1073/pnas.1212089110, 2013.
Du, P., Du, R., Ren, W., Lu, Z., and Fu, P.: Seasonal variation characteristic of inhalable microbial communities in PM2.5 in Beijing city, China, Sci. Total Environ., 610–611, 308–315, https://doi.org/10.1016/j.scitotenv.2017.07.097, 2018.
Edgar, R. C.: UPARSE: highly accurate OTU sequences from microbial amplicon reads, Nat. Meth., 10, 996–998, https://doi.org/10.1038/nmeth.2604, 2013.
Elbert, W., Taylor, P. E., Andreae, M. O., and Pöschl, U.: Contribution of fungi to primary biogenic aerosols in the atmosphere: wet and dry discharged spores, carbohydrates, and inorganic ions, Atmos. Chem. Phys., 7, 4569–4588, https://doi.org/10.5194/acp-7-4569-2007, 2007.
El-Morsy, E. S. M.: Fungi isolated from the endorhizosphere of halophytic plants from the Red Sea Coast of Egypt, Fungal. Divers, 5, 43–54, 2000.
Fakunle, A. G., Jafta, N., Naidoo, R. N., and Smit, L. A. M.: Association of indoor microbial aerosols with respiratory symptoms among under-five children: a systematic review and meta-analysis, Environ. Health, 20, 77, https://doi.org/10.1186/s12940-021-00759-2, 2021.
Fan, X., Gao, J., Pan, K., Li, D., Dai, H., and Li, X.: More obvious air pollution impacts on variations in bacteria than fungi and their co-occurrences with ammonia-oxidizing microorganisms in PM2.5, Environ. Pollut., 251, 668–680, https://doi.org/10.1016/j.envpol.2019.05.004, 2019.
Federici, E., Petroselli, C., Montalbani, E., Casagrande, C., Ceci, E., Moroni, B., La Porta, G., Castellini, S., Selvaggi, R., Sebastiani, B., Crocchianti, S., Gandolfi, I., Franzetti, A., and Cappelletti, D.: Airborne bacteria and persistent organic pollutants associated with an intense Saharan dust event in the Central Mediterranean, Sci. Total Environ., 645, 401–410, https://doi.org/10.1016/j.scitotenv.2018.07.128, 2018.
Frączek, K., Kozdrój, J., Górny, R. L., Cyprowski, M., and Gołofit-Szymczak, M.: Fungal air contamination in distinct sites within a municipal landfill area, Int. J. Environ. Sci. Technol., 14, 2637–2648, https://doi.org/10.1007/s13762-017-1344-9, 2017.
Fröhlich-Nowoisky, J., Pickersgill, D. A., Després, V. R., and Pöschl, U.: High diversity of fungi in air particulate matter, P. Natl. Acad. Sci. USA, 106, 12814–12819, https://doi.org/10.1073/pnas.0811003106, 2009.
Galbán, S., Justel, A., González, S., and Quesada, A.: Local meteorological conditions, shape and desiccation influence dispersal capabilities for airborne microorganisms, Sci. Total Environ., 780, 146653, https://doi.org/10.1016/j.scitotenv.2021.146653, 2021.
GDAS – Global Data Assimilation System: GDAS Data, ftp://arlftp.arlhq.noaa.gov/pub/archives/gdas1/ (last access: 19 September 2024), 2024.
Gong, J., Qi, J., E, B., Yin, Y., and Gao, D.: Concentration, viability and size distribution of bacteria in atmospheric bioaerosols under different types of pollution, Environ. Pollut., 257, 113485, https://doi.org/10.1016/j.envpol.2019.113485, 2020.
Griffin, D. W., Kellogg, C. A., Garrison, V. H., Lisle, J. T., and Borden, T. C.: Atmospheric microbiology in the northern Caribbean during African dust events, Aerobiologia, 19, 143–157, https://doi.org/10.1023/B:AERO.0000006530.32845.8d, 2003.
Han, Y., Yang, K., Yang, T., Zhang, M., and Li, L.: Bioaerosols emission and exposure risk of a wastewater treatment plant with A2O treatment process, Ecotoxicol. Environ. Safe., 169, 161–168, https://doi.org/10.1016/j.ecoenv.2018.11.018, 2019.
Hu, G., Zhang, Y., Sun, J., Zhang, L., Shen, X., Lin, W., and Yang, Y.: Variability, formation and acidity of water-soluble ions in PM2.5 in Beijing based on the semi-continuous observations, Atmos. Res., 145–146, 1–11, https://doi.org/10.1016/j.atmosres.2014.03.014, 2014.
Hu, J., He, X., Li, D., and Liu, Q.: Progress in research of Sphingomonas, Chinese J. Appl. Environ. Biol., 13, 431–437, 2007.
Hu, W., Wang, Z., Huang, S., Ren, L., Yue, S., Li, P., Xie, Q., Zhao, W., Wei, L., Ren, H., Wu, L., Deng, J., and Fu, P.: Biological Aerosol Particles in Polluted Regions, Curr. Pollut. Rep., 6, 65–89, https://doi.org/10.1007/s40726-020-00138-4, 2020.
Huang, D., Zhang, Z., Sun, M., Feng, Z., and Ye, M.: Characterization and ecological function of bacterial communities in seabed sediments of the southwestern Yellow Sea and northwestern East China Sea, Western Pacific, Sci. Total Environ., 761, 143233, https://doi.org/10.1016/j.scitotenv.2020.143233, 2021.
Islam, M. and Hasin, F.: Studies on the phylloplane mycoflora of Amaranthus viridis. L., Natl. Acad. Sci. Lett., 23, 121–123, 2000.
Jiang, X., Wang, C., Guo, J., Hou, J., Guo, X., Zhang, H., Tan, J., Li, M., Li, X., and Zhu, H.: Global Meta-analysis of Airborne Bacterial Communities and Associations with Anthropogenic Activities, Environ. Sci. Technol., 56, 9891–9902, https://doi.org/10.1021/acs.est.1c07923, 2022.
Jones, A. M. and Harrison, R. M.: The effects of meteorological factors on atmospheric bioaerosol concentrations – a review, Sci. Total Environ., 326, 151–180, https://doi.org/10.1016/j.scitotenv.2003.11.021, 2004.
Kakikawa, M., Kobayashi, F., Maki, T., Yamada, M., Higashi, T., Chen, B., Shi, G., Hong, C., Tobo, Y., and Iwasaka, Y.: Dustborne microorganisms in the atmosphere over an Asian dust source region, Dunhuang, Air Qual. Atmos. Health, 1, 195-202, https://doi.org/10.1007/s11869-008-0024-9, 2009.
Kassambara, A.: Comparing groups: Numerical variables, Datanovia, https://www.datanovia.com/en (last access: 19 September 2024), 2019.
Kendrick, D.-W. L. B.: A year-round study on functional relationships of airborne fungi with meteorological factors, Int. J. Biometeorol., 39, 74–80, https://doi.org/10.1007/BF01212584, 1995.
Koh, E. Y., Cowie, R. O. M., Simpson, A. M., O'Toole, R., and Ryan, K. G.: The origin of cyanobacteria in Antarctic sea ice: marine or freshwater?, Environ. Microbiol. Rep., 4, 479–483, https://doi.org/10.1111/j.1758-2229.2012.00346.x, 2012.
Kullman, B., Tamm, H., and Kullman, K.: Fungal genome size database, http://www.zbi.ee/fungal-genomesize (last access: 19 September 2024), 2005.
Lang-Yona, N., Flores, J. M., Haviv, R., Alberti, A., Poulain, J., Belser, C., Trainic, M., Gat, D., Ruscheweyh, H.-J., Wincker, P., Sunagawa, S., Rudich, Y., Koren, I., and Vardi, A.: Terrestrial and marine influence on atmospheric bacterial diversity over the north Atlantic and Pacific Oceans, Commun. Earth Environ., 3, 121–131, https://doi.org/10.1038/s43247-022-00441-6, 2022.
Larson, M. G.: Analysis of Variance, Circulation, 117, 115–121, https://doi.org/10.1161/circulationaha.107.654335, 2008.
Li, H., Zhou, X. Y., Yang, X. R., Zhu, Y. G., Hong, Y. W., and Su, J. Q.: Spatial and seasonal variation of the airborne microbiome in a rapidly developing city of China, Sci. Total Environ., 665, 61–68, https://doi.org/10.1016/j.scitotenv.2019.01.367, 2019.
Li, M., Qi, J., Zhang, H., Huang, S., Li, L., and Gao, D.: Concentration and size distribution of bioaerosols in an outdoor environment in the Qingdao coastal region, Sci. Total Environ., 409, 3812–3819, https://doi.org/10.1016/j.scitotenv.2011.06.001, 2011.
Liang, B., Cai, M., Sun, Q., Zhou, S., and Zhao, J.: Source apportionment of marine atmospheric aerosols in northern South China Sea during summertime 2018, Environ. Pollut., 289, 117948, https://doi.org/10.1016/j.envpol.2021.117948, 2021.
Liu, H., Hu, Z., Zhou, M., Hu, J., Yao, X., Zhang, H., Li, Z., Lou, L., Xi, C., Qian, H., Li, C., Xu, X., Zheng, P., and Hu, B.: The distribution variance of airborne microorganisms in urban and rural environments, Environ. Pollut., 247, 898–906, https://doi.org/10.1016/j.envpol.2019.01.090, 2019.
Liu, Y., Zhang, Y., Shi, Y., Shen, F., Yang, Y., Wang, M., Zhang, G., Deng, T., and Lai, S.: Characterization of fungal aerosol in a landfill and an incineration plants in Guangzhou, Southern China: The link to potential impacts, Sci. Total Environ., 764, 142908, https://doi.org/10.1016/j.scitotenv.2020.142908, 2021.
Maki, T., Susuki, S., Kobayashi, F., Kakikawa, M., Tobo, Y., Yamada, M., Higashi, T., Matsuki, A., Hong, C., Hasegawa, H., and Iwasaka, Y.: Phylogenetic analysis of atmospheric halotolerant bacterial communities at high altitude in an Asian dust (KOSA) arrival region, Suzu City, Sci. Total Environ., 408, 4556–4562, https://doi.org/10.1016/j.scitotenv.2010.04.002, 2010.
Maki, T., Puspitasari, F., Hara, K., Yamada, M., Kobayashi, F., Hasegawa, H., and Iwasaka, Y.: Variations in the structure of airborne bacterial communities in a downwind area during an Asian dust (Kosa) event, Sci. Total Environ., 488–489, 75–84, https://doi.org/10.1016/j.scitotenv.2014.04.044, 2014.
Mason, P. E., Darvell, L. I., Jones, J. M., and Williams, A.: Observations on the release of gas-phase potassium during the combustion of single particles of biomass, Fuel, 182, 110–117, https://doi.org/10.1016/j.fuel.2016.05.077, 2016.
Masoud, W., Takamiya, M., Vogensen, F. K., Lillevang, S., Al-Soud, W. A., Sørensen, S. J., and Jakobsen, M.: Characterization of bacterial populations in Danish raw milk cheeses made with different starter cultures by denaturating gradient gel electrophoresis and pyrosequencing, Int. Dairy J., 21, 142–148, https://doi.org/10.1016/j.idairyj.2010.10.007, 2011.
Mayol, E., Jiménez, M. A., Herndl, G. J., Duarte, C. M., and Arrieta, J. M.: Resolving the abundance and air-sea fluxes of airborne microorganisms in the North Atlantic Ocean, Front. Microbiol., 5, 557, https://doi.org/10.3389/fmicb.2014.00557, 2014.
Mayol, E., Arrieta, J. M., Jiménez, M. A., Martínez-Asensio, A., Garcias-Bonet, N., Dachs, J., González-Gaya, B., Royer, S.-J., Benítez-Barrios, V. M., Fraile-Nuez, E., and Duarte, C. M.: Long-range transport of airborne microbes over the global tropical and subtropical ocean, Nat. Commun., 8, 201–210, https://doi.org/10.1038/s41467-017-00110-9, 2017.
MeteoInfo: Downloads, http://www.meteothink.org/downloads/index.html (last access: 19 September 2024), 2024.
Nguyen, N. H., Song, Z., Bates, S. T., Branco, S., Tedersoo, L., Menke, J., Schilling, J. S., and Kennedy, P. G.: FUNGuild: An open annotation tool for parsing fungal community datasets by ecological guild, Fungal Ecol., 20, 241–248, https://doi.org/10.1016/j.funeco.2015.06.006, 2016.
NIH: PRJNA1101427, NIH [data set], https://www.ncbi.nlm.nih.gov/Traces/study/?acc=PRJNA1101427&o=acc_s:a (last access: 19 September 2024), 2024a.
NIH: BioProject: PRJNA1101176, NIH [data set], https://dataview.ncbi.nlm.nih.gov/object/PRJNA1101176?reviewer=mu6a68nm61vnh0g1lahff3qvma (last access: 19 September 2024), 2024b.
Park, J., Li, P.-F., Ichijo, T., Nasu, M., and Yamaguchi, N.: Effects of Asian dust events on atmospheric bacterial communities at different distances downwind of the source region, J. Environ. Sci., 72, 133–139, https://doi.org/10.1016/j.jes.2017.12.019, 2018.
Pathak, R. K., Wu, W. S., and Wang, T.: Summertime PM2.5 ionic species in four major cities of China: nitrate formation in an ammonia-deficient atmosphere, Atmos. Chem. Phys., 9, 1711–1722, https://doi.org/10.5194/acp-9-1711-2009, 2009.
Polymenakou, P. N., Mandalakis, M., Stephanou, E. G., and Tselepides, A.: Particle Size Distribution of Airborne Microorganisms and Pathogens during an Intense African Dust Event in the Eastern Mediterranean, Environ. Health Perspect., 116, 292–296, https://doi.org/10.1289/ehp.10684, 2008.
Prospero, J. M., Blades, E., Mathison, G., and Naidu, R.: Interhemispheric transport of viable fungi and bacteria from Africa to the Caribbean with soil dust, Aerobiologia, 21, 1–19, https://doi.org/10.1007/s10453-004-5872-7, 2005.
Qi, J., Huang, Z., Maki, T., Kang, S., Guo, J., Liu, K., and Liu, Y.: Airborne bacterial communities over the Tibetan and Mongolian Plateaus: variations and their possible sources, Atmos. Res., 247, 105215, https://doi.org/10.1016/j.atmosres.2020.105215, 2021.
Rainey, F. A., Ferreira, M., Nobre, M. F., Ray, K., Bagaley, D., Earl, A. M., Battista, J. R., Gómez-Silva, B., McKay, C. P., and da Costa, M. S.: Deinococcus peraridilitoris sp. nov., isolated from a coastal desert, Int. J. Syst. Evol. Microbiol., 57, 1408–1412, https://doi.org/10.1099/ijs.0.64956-0, 2007.
Rooney, A. P. and Ward, T. J.: Evolution of a large ribosomal RNA multigene family in filamentous fungi: birth and death of a concerted evolution paradigm, P. Natl. Acad. Sci. USA, 102, 5084–5089, https://doi.org/10.1073/pnas.0409689102, 2005.
Schloss, P. D., Westcott, S. L., Ryabin, T., Hall, J. R., Hartmann, M., Hollister, E. B., Lesniewski, R. A., Oakley, B. B., Parks, D. H., Robinson, C. J., Sahl, J. W., Stres, B., Thallinger, G. G., Van Horn, D. J., and Weber, C. F.: Introducing mothur: Open-Source, Platform-Independent, Community-Supported Software for Describing and Comparing Microbial Communities, Appl. Environ. Microbiol., 75, 7537–7541, https://doi.org/10.1128/aem.01541-09, 2009.
Seinfeld, J. H. and Pandis, S. N.: Atmospheric Chemistry and Physics: From Air Pollution to Climate Change, Phys. Today, 51, 88–90, 1998.
Sharoni, S., Trainic, M., Schatz, D., Lehahn, Y., Flores, M. J., Bidle, K. D., Ben-Dor, S., Rudich, Y., Koren, I., and Vardi, A.: Infection of phytoplankton by aerosolized marine viruses, P. Natl. Acad. Sci. USA, 112, 6643–6647, https://doi.org/10.1073/pnas.1423667112, 2015.
Shen, F. and Yao, M.: Bioaerosol nexus of air quality, climate system and human health, Natl. Sci. Open, 2, 20220050, https://doi.org/10.1360/nso/20220050, 2023.
Shi, Y., Lai, S., Liu, Y., Gromov, S., and Zhang, Y.: Fungal Aerosol Diversity Over the Northern South China Sea: The Influence of Land and Ocean, J. Geophys. Res.-Atmos., 127, e2021JD035213, https://doi.org/10.1029/2021jd035213, 2022.
Smets, W., Moretti, S., Denys, S., and Lebeer, S.: Airborne bacteria in the atmosphere: Presence, purpose, and potential, Atmos. Environ., 139, 214–221, https://doi.org/10.1016/j.atmosenv.2016.05.038, 2016.
Stoddard, S. F., Smith, B. J., Hein, R., Roller, B. R. K., and Schmidt, T. M.: rrnDB: improved tools for interpreting rRNA gene abundance in bacteria and archaea and a new foundation for future development, Nucl. Acids Res., 43, D593–D598, https://doi.org/10.1093/nar/gku1201, 2015.
Sun, H., Sun, J., Zhu, C., Yu, L., Lou, Y., Li, R., and Lin, Z.: Chemical characterizations and sources of PM2.5 over the offshore Eastern China sea: Water soluble ions, stable isotopic compositions, and metal elements, Atmos. Pollut. Res., 13, 101410, https://doi.org/10.1016/j.apr.2022.101410, 2022.
Sun, Y., Xu, S., Zheng, D., Li, J., Tian, H., and Wang, Y.: Effects of haze pollution on microbial community changes and correlation with chemical components in atmospheric particulate matter, Sci. Total Environ., 637–638, 507–516, https://doi.org/10.1016/j.scitotenv.2018.04.203, 2018.
Temraleeva, A. D., Dronova, S. A., Moskalenko, S. V., and Didovich, S. V.: Modern methods for isolation, purification, and cultivation of soil cyanobacteria, Microbiology, 85, 389–399, https://doi.org/10.1134/s0026261716040159, 2016.
Wang, B., Li, Y., Xie, Z., Du, S., Zeng, X., Hou, J., and Ma, T.: Characteristics of microbial activity in atmospheric aerosols and its relationship to chemical composition of PM2.5 in Xi'an, China, J. Aerosol Sci., 146, 105572, https://doi.org/10.1016/j.jaerosci.2020.105572, 2020.
Wang, J.: Air quality historical data query, CNEMC [data set], https://www.aqistudy.cn/historydata/ (last access: 19 September 2024), 2024.
Wei, M., Li, M., Xu, C., Xu, P., and Liu, H.: Pollution characteristics of bioaerosols in PM2.5 during the winter heating season in a coastal city of northern China, Environ. Sci. Pollut. Res., 27, 27750–27761, https://doi.org/10.1007/s11356-020-09070-y, 2020.
Xia, F., Chen, Z., Tian, E., and Mo, J.: A super sandstorm altered the abundance and composition of airborne bacteria in Beijing, J. Environ. Sci., 144, 35–44, https://doi.org/10.1016/j.jes.2023.07.029, 2024.
Xie, W., Li, Y., Bai, W., Hou, J., Ma, T., Zeng, X., Zhang, L., and An, T.: The source and transport of bioaerosols in the air: A review, Front. Environ. Sci. Eng., 15, 44, https://doi.org/10.1007/s11783-020-1336-8, 2021.
Xu, C., Wei, M., Chen, J., Wang, X., Zhu, C., and Li, J.: Bacterial characterization in ambient submicron particles during severe haze episodes at Ji'nan, China, Sci. Total Environ., 580, 188–196, https://doi.org/10.1016/j.scitotenv.2016.11.145, 2017.
Xu, C., Wei, M., Chen, J., Zhu, C., Li, J., Xu, X., Wang, W., Zhang, Q., Ding, A., Kan, H., Zhao, Z., and Mellouki, A.: Profile of inhalable bacteria in PM2.5 at Mt. Tai, China: Abundance, community, and influence of air mass trajectories, Ecotoxicol. Environ. Safe., 168, 110–119, https://doi.org/10.1016/j.ecoenv.2018.10.071, 2019.
Xu, C., Chen, J., Wang, Z., Chen, H., Feng, H., Wang, L., Xie, Y., Wang, Z., Ye, X., Kan, H., Zhao, Z., and Mellouki, A.: Diverse bacterial populations of PM2.5 in urban and suburb Shanghai, China, Front. Environ. Sci. Eng., 15, 37, https://doi.org/10.1007/s11783-020-1329-7, 2021.
Xue, F., Yang, Y., Zou, S., Zhang, Y., Yue, D., Zhao, Y., and Lai, S.: Characterization of airborne bacteria and fungi at a land-sea transition site in Southern China, Sci. Total Environ., 849, 157786, https://doi.org/10.1016/j.scitotenv.2022.157786, 2022.
Yan, D., Zhang, T., Su, J., Zhao, L.-L., Wang, H., Fang, X.-M., Zhang, Y.-Q., Liu, H.-Y., and Yu, L.-Y.: Diversity and Composition of Airborne Fungal Community Associated with Particulate Matters in Beijing during Haze and Non-haze Days, Front. Microbiol., 7, 487, https://doi.org/10.3389/fmicb.2016.00487, 2016.
Yan, Z., Lingui, X., Lin, L. I., and Hongguang, Z.: Advance in environmental pollutants degradation of Comamonas, Microbiol. China, 39, 1471–1478, https://doi.org/10.13344/j.microbiol.china.2012.10.011, 2012.
Yao, L., Zhan, B., Xian, A., Sun, W., Li, Q., and Chen, J.: Contribution of transregional transport to particle pollution and health effects in Shanghai during 2013–2017, Sci. Total Environ., 677, 564–570, https://doi.org/10.1016/j.scitotenv.2019.03.488, 2019.
Yu, J., Yan, C., Liu, Y., Li, X., Zhou, T., and Zheng, M.: Potassium: A Tracer for Biomass Burning in Beijing?, Aerosol Air Qual. Res., 18, 2447–2459, https://doi.org/10.4209/aaqr.2017.11.0536, 2018.
Zeng, X., Kong, S., Zheng, S., Cheng, Y., Wu, F., Niu, Z., Yan, Q., Wu, J., Zheng, H., Zheng, M., Zeng, X., Chen, N., Xu, K., Zhu, B., Yan, Y., and Qi, S.: Variation of airborne DNA mass ratio and fungal diversity in fine particles with day-night difference during an entire winter haze evolution process of Central China, Sci. Total Environ., 694, 133802, https://doi.org/10.1016/j.scitotenv.2019.133802, 2019.
Zhang, F., Chen, Y., Tian, C., Wang, X., Huang, G., Fang, Y., and Zong, Z.: Identification and quantification of shipping emissions in Bohai Rim, China, Sci. Total Environ., 497–498, 570–577, https://doi.org/10.1016/j.scitotenv.2014.08.016, 2014.
Zhang, M., Zhao, B., Yan, Y., Cheng, Z., Li, Z., Han, L., Sun, Y., Zheng, Y., and Xia, Y.: Comamonas-dominant microbial community in carbon poor aquitard sediments revealed by metagenomic-based growth rate investigation, Sci. Total Environ., 912, 169203, https://doi.org/10.1016/j.scitotenv.2023.169203, 2024.
Zhang, N., Cao, J., Ho, K., and He, Y.: Chemical characterization of aerosol collected at Mt. Yulong in wintertime on the southeastern Tibetan Plateau, Atmos. Res., 107, 76–85, https://doi.org/10.1016/j.atmosres.2011.12.012, 2012.
Zhang, T., Li, X., Wang, M., Chen, H., and Yao, M.: Microbial aerosol chemistry characteristics in highly polluted air, Sci. China: Chem., 62, 1051–1063, https://doi.org/10.1007/s11426-019-9488-3, 2019.
Zhang, Y., Guo, C., Ma, K., Tang, A., Goulding, K., and Liu, X.: Characteristics of airborne bacterial communities across different PM2.5 levels in Beijing during winter and spring, Atmos. Res., 273, 106179, https://doi.org/10.1016/j.atmosres.2022.106179, 2022.
Zhao, J., Jin, L., Wu, D., Zhang, G., Xie, J., Li, J., and Fue, X.: Global airborne bacterial community – interactions with Earth's microbiomes and anthropogenic activities, P. Natl. Acad. Sci. USA, 119, e2204465119, https://doi.org/10.1073/pnas.2204465119, 2022.
Zhou, Y., Xue, L., Wang, T., Gao, X., Wang, Z., Wang, X., Zhang, J., Zhang, Q., and Wang, W.: Characterization of aerosol acidity at a high mountain site in central eastern China, Atmos. Environ., 51, 11–20, https://doi.org/10.1016/j.atmosenv.2012.01.061, 2012.