the Creative Commons Attribution 4.0 License.
the Creative Commons Attribution 4.0 License.
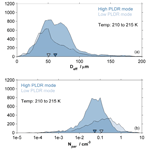
Investigating an indirect aviation effect on mid-latitude cirrus clouds – linking lidar-derived optical properties to in situ measurements
Tina Jurkat-Witschas
Martin Wirth
Benedikt Urbanek
Martina Krämer
Ralf Weigel
Christiane Voigt
Aviation has a large impact on the Earth's atmosphere and climate by various processes. Line-shaped contrails and contrail cirrus clouds lead to changes in the natural cirrus cloud cover and have a major contribution to the effective radiative forcing from aviation. In addition, aviation-induced aerosols might also change the microphysical properties and optical properties of naturally formed cirrus clouds. Latter aerosol–cloud interactions show large differences in the resulting effective radiative forcing, and our understanding on how aviation-induced aerosols affect cirrus cloud properties is still poor. Up to now, observations of this aviation-induced aerosol effect have been rare. In this study, we use combined airborne lidar and in situ ice cloud measurements to investigate differences in the microphysical and optical properties of naturally formed cirrus clouds, which formed in regions that are highly affected by aviation-induced aerosol emissions and, of those, which formed in regions rather unaffected by aviation. Urbanek et al. (2018) showed that those cirrus clouds, which are more affected by aviation-induced soot emission, are characterized by larger values of the particle linear depolarization ratio (PLDR). In this follow-on study we relate collocated lidar measurements performed aboard HALO during the ML-CIRRUS mission of the particle linear depolarization ratio with in situ cloud probe measurements of the number concentration and effective diameter of the ice particles. In situ measurements for both cloud types (high-PLDR-mode – aviation-affected – and low-PLDR-mode – pristine – cirrus) can be reliably compared in a temperature range between 210 and 215 K. Within this temperature range we find that high-PLDR-mode cirrus clouds tend to show larger effective ice particle diameters with a median value of 61.4 compared to 50.7 µm for low-PLDR-mode pristine cirrus clouds. Larger effective ice particles in aviation-influenced (high-PLDR-mode) cirrus are connected to lower ice particle number concentration with a median value of 0.05 compared to 0.11 cm−3 (low-PLDR-mode), which evolved in more pristine regions with only little impact from aviation. We suspect that a suppression of homogeneous ice formation by the heterogeneously freezing soot aerosol particles included in the areas affected by air traffic is the cause of the reduced ice crystal concentrations.
- Article
(2277 KB) - Full-text XML
-
Supplement
(382 KB) - BibTeX
- EndNote
Aviation has a large impact on the Earth's radiation budget and atmosphere (Lee et al., 2021) by various interactions; e.g., aerosols and trace gases are emitted, which directly interact with incoming and outgoing radiation (Lund et al., 2017). Line-shaped contrails can form in the exhaust plume of an aircraft (Voigt et al., 2010; Burkhardt et al., 2010) and might evolve into contrail-induced cirrus clouds in the aftermath (e.g., Haywood et al., 2009). A lot of research activities have been performed over the last few years to measure (Heymsfield et al., 2010a; Voigt et al., 2011, 2017) and understand contrails and contrail cirrus (e.g., Kärcher et al., 2015; Schuman et al., 2017), as well as to investigate their climate effect (Burkhardt and Kärcher, 2011; Kärcher, 2018; Bock and Burkhardt, 2019; Quaas et al., 2021). Contrails and contrail-induced cirrus clouds are supposed to have the largest aviation-induced impact on the Earth's radiation budget with a clearly warming effect (Lee et al., 2021). Recent studies show that the climate impact from contrails can be reduced by burning sustainable aviation fuels with a low aromatic content (Moore et al., 2017; Burkhardt et al., 2018; Voigt et al., 2021; Bräuer et al., 2021) or by climate friendly flight routing (Grewe et al., 2017). Contrails can further lead to an increase in the cirrus cloud optical properties (Tesche et al., 2016) and to changes in their ice crystal effective diameter (Haywood et al., 2009) and ice crystal number concentration (Marjani et al., 2022).
Besides contrail formation and its effect on already existing clouds, aviation-induced aerosols might also act as ice nuclei (ice nucleating particles, INPs) for naturally formed clouds. The aerosols change the microphysical properties – i.e., the number concentration and size of ice crystals of naturally formed cirrus clouds (Kärcher, 2017) and thus their optical and radiative effect. Model studies investigating the impact of this aviation-induced aerosol–cloud interaction show large differences in the resulting effective radiative forcing. In particular, the estimates of the impact of emitted soot particles on cirrus clouds are connected with large uncertainties. Several studies focused on the impact of aviation soot on cirrus clouds and thus on the resulting climate effect (e.g., Hendricks et al., 2005, 2011; Liu et al., 2009; Gettelman and Chen, 2013). Large differences in the magnitude and even in the sign of the effect (Penner et al., 2009, 2018; Zhou and Penner, 2014) were reported. The uncertainties in the estimate of the climate effect of aviation soot are mainly driven by the assumed efficiencies of soot particles to act as INPs (Righi et al., 2021). While some laboratory studies found soot particles to be efficient INPs (Möhler et al., 2005; Hoose and Möhler, 2012), others indicate soot particles are not efficient INPs (DeMott et al., 1999). In a recent laboratory study, Mahrt et al. (2020) found that soot particles would increase their efficiency to act as INPs after being pre-processed within contrails. This indicates an overestimation of the soot effect in some of the model studies.
Although the understanding of aviation's impact on the climate system has improved over the last few years, many uncertainties remain. However, observations of an aviation-induced indirect aerosol effect on cirrus clouds are rare. Urbanek et al. (2018) analyzed airborne lidar measurements over Europe, performed during the ML-CIRRUS mission (Voigt et al., 2017), and found larger median values of the particle linear depolarization ratio (PLDR) of cirrus clouds formed in air traffic regions compared to those evolved in regions with only little impact from aviation. Their analysis further showed lower supersaturation for those clouds with high PLDR formed in air traffic regions, which they interpreted as a signature of more heterogeneous freezing. The measurement study by Urbanek et al. (2018) is one of the first that could show traces of an indirect aerosol effect from aviation. During the first COVID-19 curfew in spring 2020, civil aviation over Europe was reduced by up to almost 90 % (https://www.eurocontrol.int/sites/default/files/2021-12/covid19-eurocontrol-comprehensive-air-traffic-assessment-16122021.pdf, last access: 5 July 2023). This reduction caused a unique opportunity to study the effect of aviation on cirrus clouds. Li and Groß (2021) used spaceborne lidar measurements aboard the CALIPSO satellite (Winker et al., 2010) in March and April 2020 to investigate differences in cloud occurrence and optical properties compared to previous years in the same time period. They found less cirrus occurrence mainly for colder height levels and for thinner cirrus clouds. Those findings were interpreted as a reduction of contrails and contrail-induced cloudiness due to reduced aviation. Schumann et al. (2021a, b) investigated the changes in contrail occurrence and the formation of persistent contrails by performing contrail simulations with the contrail cirrus prediction model, CoCiP (Schumann et al., 2012). They found that changes in the cirrus cloud occurrence from March to August 2020, compared to the same period in 2019, were partly caused by the air traffic reduction. Duda et al. (2023) used satellite measurements to investigate changes in contrail cover, optical properties and radiative forcing during April and May 2020. They could show a decrease in contrail coverage and shortwave contrail radiative forcing over the United States due to decreased air traffic. Teoh et al. (2022) found a significant decrease in contrail cirrus cover and energy forcing in 2020, when comparing to modeled contrail cirrus effects in the northern Atlantic flight corridor regions from 2016 to 2019.
Furthermore, a significant decrease in the mean PLDR of cirrus clouds was found in spring 2020 compared to previous years (Li and Groß, 2021), which can be interpreted as a reduced, aviation-induced and indirect effect on naturally formed cirrus clouds. An integrated study, using aircraft, satellite and modeling data, showed a reduction of the aerosol optical depth (AOD) over Europe in May 2020 (Voigt et al., 2022). Although it is not clear whether the decrease in AOD was caused mainly by anthropogenic or meteorological influences, the authors suggest that it was partly caused by the 80 % decline in air traffic, as the aerosol number concentration decreased at flight altitudes compared to the reference years. In addition, comparisons of the measured black carbon mass concentration with model results from the ECHAM/MESSy Atmospheric Chemistry (EMAC) model indicated a 40 % reduction related to lockdown effects (Krüger et al., 2022). The reduction in air traffic over Europe furthermore led to a reduction in contrail cover and as a consequence in radiative forcing from contrails. Contrail radiative forcing was calculated with the contrail cirrus prediction model (CoCiP; Schumann, 2012) for 16 April 2020 for two scenarios: using air traffic data from 2019 and for 2020. For the same meteorology, the simulated contrail radiative forcing decreases by about 80 % for the reduced air traffic in 2020 compared to 2019. Voigt et al. (2022) also showed reduced effective optical depth of the cirrus clouds compared to previous years connected with reduced PLDR. Looking at long-term cirrus observations using CALIPSO measurements, Li and Groß (2022) found a significant increase in the PLDR over the last few years, which is clearly correlated with the increase in the number of flights over Europe. However, besides these advances in observing the change in optical properties due to the impact of aviation-induced soot, the link to the microphysical properties of the cirrus clouds is still missing. In a recent study, Zhu et al. (2022) examined CALIPSO satellite observations during the COVID-19 lockdowns and found a significant increase in ice crystal number concentration (Npart), which they linked to an increase in homogeneous freezing due to reduced aviation.
In this study we extend the work by Urbanek et al. (2018) using combined lidar and in situ measurements aboard HALO performed during the ML-CIRRUS mission (Voigt et al., 2017) to determine differences in the microphysical properties of natural cirrus clouds formed in air traffic regions and of those formed in regions less affected by aviation, and thus we expand our investigation on the non-CO2 effect of aviation. For this, we use the same clouds that were investigated within our previous study (Urbanek et al., 2018). In Sect. 2, we will present the campaign and the measurements. In Sect. 3, we will show the results focusing first on two case studies of different cirrus cloud types and afterwards on all the cloud measurements with collocated lidar and in situ measurements. Section 4 will discuss the results and conclude this study.
2.1 ML-CIRRUS campaign
The ML-CIRRUS campaign was conducted in March/April 2014 to study cirrus clouds in meteorological regimes typical for mid-latitudes. ML-CIRRUS aimed to investigate contrail cirrus, as well as to observe differences between anthropogenic and natural cirrus clouds. To achieve this goal, measurement flights with the German High Altitude and Long Range research aircraft (HALO), equipped with a combined remote sensing (including airborne lidar) and in situ (including cloud probes) payload, were performed out of Oberpfaffenhofen. An overview of the mission, the performed research flights and their main focus can be found in Voigt et al. (2017). Overall, 16 flights were performed covering the whole range of the mid-latitudes: from 36 to 58∘ N and from the Atlantic Ocean (∼ 15∘ W) to central Europe (∼ 15∘ E). However, only 8 of the 16 flights were designed in such a way that they provide coordinated lidar and in situ measurements. The sampling strategy during these flights were as follows. First, the HALO aircraft flew at higher altitudes above the cloud for cirrus cloud sounding with the lidar (lidar leg). Subsequently, the same cirrus clouds were probed by in situ measurements at several flight altitudes within the cirrus clouds (in situ leg). Typical lidar legs took about 30 to 50 min, with a typical aircraft speed of 200 m s−1 that results in an observed cloud dimension of about 360 to 600 km. The in situ legs took a minimum of 10 min per constant flight altitude. For our study only these eight flights with coordinated lidar and in situ measurements are relevant. Urbanek et al. (2018) grouped these flights into cirrus clouds developed in regions with enhanced background aerosols from aviation or in regions rather unaffected by aviation. By means of 24 h backward trajectories calculated with the trajectory module of CLaMS (McKenna et al., 2002), they investigated the maximum cloud ice water content to determine the most probable location of the cirrus formation and compared that to maps of enhanced background aerosols due to aircraft emissions (Stettler et al., 2013). Information on the flights (including their mission ID to make it comparable to Urbanek et al., 2018) is given in Table 1.
Table 1Overview of the combined in situ and lidar research missions during ML-CIRRUS showing the mission ID, date, measurement region and scope of the mission, median PLDR, temperatures of in situ measurements, and altitude range. Listed are only those missions with combined lidar and in situ measurements. Entries marked with an asterisk (*) after the mission ID show flight segments with cirrus clouds that were affected by aviation-induced aerosols (according to Urbanek et al., 2018), and the others indicate flight segments with cirrus clouds developed in regions with no or less aviation. The flight missions indicated in bold letters are shown in detail in the case studies.
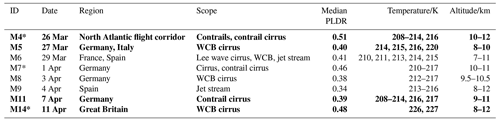
2.2 WALES lidar system
The WALES (WAter vapour Lidar Experiment in Space; Wirth et al., 2009) lidar is a combined high-spectral-resolution lidar (HSRL) and differential absorption lidar (DIAL) system, which was developed and built at the Institute of Atmospheric Physics of the German Aerospace Center. It measures directly the extinction coefficient at 532 nm, using the HSRL technique with a high vertical resolution of 15 m and of typically 0.2 s resolution in time (Esselborn et al., 2008). Additionally, the system is equipped with polarization sensitive channels at 532 and 1064 nm. For determining the particle linear polarization ratio (PLDR), which is the ratio of the measured perpendicular and the parallel component of polarization of the backscattered light, we apply the ±45∘ calibration method (Freudenthaler et al., 2009) and thus achieve an absolute accuracy of 5 % at typical cirrus PLDR values of about 0.35 to 0.55.
To assure that high-altitude aerosol residuals and liquid or mixed phase clouds are excluded from our study, we restrict the considered data to measurements of temperature ranges below 235 K and of a backscatter ratio (R) above a threshold of R=3. The backscatter ratio is defined as the ratio of the total backscatter coefficient (molecules and aerosol/cloud particles) to the molecular backscatter coefficient. This threshold was determined by carefully investigating all flights with lofted aerosol layers (Urbanek et al., 2018). Sensitivity studies showed that the further analyses only weakly depend on the chosen R value within a range from R=2 to R=25.
2.3 In situ instrumentation
Particle number concentrations (Npar) and effective diameter (Deff) are calculated using a composite of particle size distributions from three cloud probes, applying scattering detectors and light attenuation on optical arrays. Small particles in the size range from 3 to 50 µm were detected by the CAS (Voigt et al. 2017; Kleine et al., 2018). The data were grouped into 16 size bins, assuming rotationally symmetrical ellipsoids of random orientation with aspect ratios of 0.75, to avoid Mie ambiguities in the scattering signals. Larger particles were detected by a CIP (Cloud Imaging Probe; CIP) as part of the CCP (Cloud Combination Probe) and a PIP (Precipitation Imaging Probe) instrument (Weigel et al., 2016). Maximum dimension diameters were derived from 2-D images, and number concentrations were corrected for compression effects according to Weigel et al. (2016). The CIP was operated in the size range from 15 and 960 µm, and the PIP was operated in the size range from 100 to 6400 µm. The effective diameter is calculated from the effective radius (2⋅reff) according to Schumann et al. (2011). The data were averaged over 5 s intervals. For the majority of flights, the combined particle size distribution of the CAS, the CIP and the PIP was used, which is the same data set as used in the work of Righi et al. (2020) and Wang et al. (2023).
For the flight of 7 April 2014, only data from the NIXE-CAPS (Novel Ice Experiment–Cloud Aerosol and Precipitation Spectrometer; Krämer et al., 2016; Costa et al., 2017) instrument are available due to a failure of the CIP instrument. NIXE-CAPS is a combination probe that integrates two techniques for measuring the particle size distribution (PSD): the PSD of particles 0.6 to 50 µm in diameter is measured with NIXE's Cloud and Aerosol Spectrometer (NIXE-CAS) using light scattered from individual particles that pass through a focused laser beam. For measurements of particles 15–937 µm in diameter, NIXE's Cloud Imaging Probe (NIXE-CIP), which utilizes the optical array probe (OAP) technique, is used. Using the data analysis routines collected in the data processing library NIXE-Lib (Luebke et al., 2016), the PSDs of both instruments are analyzed simultaneously, whereby various error analyses and corrections are applied, including a correction of possible shattering of large ice crystals at the inlet tips. The data of NIXE-CAPS and the combined cloud probes are redundant, which was helpful in the event of a failure but also to assure good quality and consistency in the obtained data set. Comparison of the data sets for days when the two combined PSD were available showed in general a good agreement. As we only discuss relative changes in Npar and Deff, small differences arising by the use of different probes should not affect the results.
2.4 Cirrus evolution classification scheme
To determine the evolution of the cirrus clouds, we use the cirrus lifetime classification method presented by Urbanek et al. (2017). The classification scheme is based on previous studies using differences of the RHi distribution in clouds at different stages of evolution (Groß et al., 2014). Based on combined temperature information and water vapor lidar measurements, it identifies regions outside and inside the cloud of supersaturation with respect to ice, heterogeneous and homogeneous nucleation, depositional growth, and ice sublimation in the 2-D field along the flight track.
In addition, measurements of the meteorological state parameters (e.g., T, RHi) at flight altitude were performed with the Basic HALO Measurement and Sensor System (BAHAMAS; Giez et al., 2023).
In a previous study (Urbanek et al., 2018) we found that cirrus clouds evolved in regions with enhanced air traffic show larger mean values of the PLDR than cirrus clouds forming in regions rather unaffected by aviation. In this study we extend the investigation of an impact of aviation on the microphysical properties of the cirrus clouds by comparing in situ measurements performed within these two cloud classes (of high and low PLDR). During the ML-CIRRUS campaign, eight missions were performed which provide coordinated lidar and in situ measurements (Table 1). The results of those coordinated measurements will be presented in the following. Two case studies show the differences of optical and microphysical properties of similar cirrus cloud types; one formed in a region of enhanced background aerosols due to aircraft emission and one evolved from aviation-unaffected air masses. One of the two case studies show measurements of cirrus clouds strongly affected by embedded contrails and the other of a warm conveyor belt cirrus. Those cases represent two of the main cirrus types in the European mid-latitudes. In a next step, we investigate the overall distribution of optical and microphysical properties of the observed cirrus clouds. The results of a significance test of the comparisons are given in the Supplement.
3.1 Case study – contrail cirrus
The first case study we choose for the comparison is a cirrus case with embedded fresh contrails (Wang et al., 2023). For the clouds observed on 26 March 2014 and 7 April 2014, the contrail cirrus prediction model (CoCiP; Schumann, 2012) indicated a large amount of embedded fresh contrails within the cirrus cloud (Urbanek, 2019). Back-trajectory analysis (Urbanek et al., 2018) indicates the origin of the cloud on 26 March over the North Atlantic with enhanced background aerosol due to aviation emission. The cirrus cloud on 7 April evolved further south over the Atlantic Ocean, in an area that is much less affected by aviation exhaust (Stettler et al., 2013).
The time–height cross sections of the PLDR of the two cirrus clouds with embedded fresh contrails is shown in Fig. 1 (a and b) along with the density distributions (c and d) of the particle linear depolarization ratio (PLDR). Both cirrus clouds are in approximately the same temperature and height range, so they are comparable. The values of the PLDR of the two cirrus clouds is quite different. The cirrus on 26 March 2014 shows larger values of the PLDR than the cirrus cloud on 7 April 2014. For the first one, we find values up to 0.6, while the PLDR of the cloud on 7 April barely exceeds 0.45. The mode of the PLDR distribution within the cirrus cloud on 26 March is 0.54, and its median is 0.52. In contrast, the mode and median of the PLDR distribution of the cirrus cloud on 7 April 2014 is much lower at values of 0.44 and 0.39, respectively. Both cirrus clouds show a large number of embedded contrails and are still different with respect to their PLDR. Thus, the freshly embedded contrails cannot be interpreted as a cause for the significant differences in the PLDR.
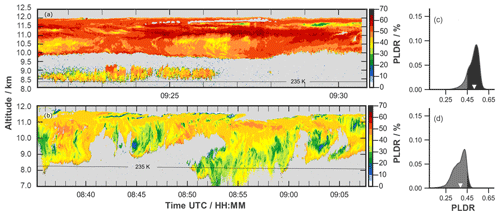
Figure 1Time–height cross section of the particle linear depolarization ratio (PLDR) for the contrail-affected cirrus cloud cases on 26 March (a) and 7 April 2014 (b), and the frequency distribution of the measured PLDR (c, d) of the cloud parts at temperature regions below 235 K. The color coding of the PLDR distribution (c, d) marks the threshold defined by Urbanek et al. (2018) for high (dark grey) and low (light grey) PLDR values.
In situ measurements were performed within the cirrus cloud at an altitude of approximately 11 km and show mean relative humidity with respect to ice (RHi) and temperature values of about 100 % and about 208 to 217 K, respectively, for both clouds. So, any differences due to different meteorological conditions within the cloud are not expected. The distributions of the derived Deff are narrow for both cirrus cases, with the main values below 100 µm (Fig. 2) potentially due to the high number of embedded contrails. The median Deff value for the cirrus cloud measured on 26 March of 58.5 µm is slightly larger than the median value of 53.2 µm measured on 7 April 2014. Differences are also found for the measured Npar for the two cloud cases. The distribution for the cirrus cloud evolving in regions with a large amount of aviation exhaust (26 March) shows a median value of 0.04 cm−3. In contrast, the distribution of Npar on 7 April is broader and shows a larger median value of 0.15 cm−3. In summary, the case study shows a larger median effective diameter and lower number concentration for the aviation-impacted cloud with the high particle linear depolarization ratio mode. This result will be discussed more in the conclusion.
3.2 Case study – warm conveyor belt cirrus
In a second case study, we compare the optical and microphysical properties of warm conveyor belt (WCB) cirrus. WCBs are a typical flow structure of the mid-latitudes leading to increased precipitation (Eckhardt et al., 2004). A warm conveyor belt (WCB) is characterized by warm humid air that is lifted fast (∼ 10 cm s−1; Browning, 1971) from the boundary layer to the upper troposphere on the timescale of 2 d. During the lifting process, liquid clouds form and freeze. This leads eventually to pure ice clouds. During ML-CIRRUS, we were able to observe and probe four cases of WCB cirrus (see Table 1). For this study, we choose the cloud observed on 27 March and on 11 April 2014. We determined the stage of evolution (Urbanek et al., 2017) to the clouds to ensure that we are comparing clouds in approximately the same stage of lifetime. Only about 0.4 %–0.5 % of the clouds are in the nucleation mode, and about 30 % are in the deposition mode. The majority of both clouds (∼ 70 %), however, is in the sublimation mode. This analysis proves that both clouds are comparable and that effects that might be caused by different evolution stages of the cirrus clouds can be excluded. Trajectory analysis for the two clouds shows that the first one evolved in rather clean conditions from aviation-exhaust-unaffected situations over northern Africa/the Mediterranean, while the nucleation process for the second one took place over the north Atlantic region, highly affected by air traffic exhaust (Urbanek et al., 2018).
Figure 3 shows the time–height cross sections (a and b) and density distributions (c and d) of the particle linear depolarization ratio (PLDR). While the PLDR of the WCB cirrus on 27 March 2014 does not exceed values of 0.55, the PLDR of the WCB cirrus on 11 April 2014 shows values as high as 0.7 at the top and in the lower part of the cloud. Considering all the measurement points within the observed WCB cirrus on 27 March, the overall distribution of PLDR has its maximum at a value of 0.41; the median of the distribution is at 0.4. In contrast, the distribution of the PLDR of the WCB cirrus on 11 April has its maximum at 0.5 and its median at a value of 0.48. As we find the differences in the measured PLDR for both WCB cases and as their stage of evolution is approximately the same, we suggest that neither the cirrus cloud type nor its lifetime has an effect on the differences in the PLDR.
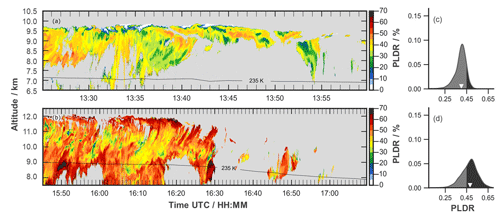
Figure 3Time–height cross section of particle linear depolarization ratio (PLDR) for the WCB cirrus observed on 27 March (a) and 11 April 2014 (b), and the frequency distribution of the measured PLDR (c, d) of the cloud parts at height regions below 235 K. The color coding of the PLDR distribution marks the threshold defined by Urbanek et al. (2018) for clouds characterized by high (dark grey) and low (light grey) PLDR values.
The in situ flight track for both clouds were performed approximately in the middle of the clouds' vertical extension: at about 9 km altitude for the cloud on 27 March and at about 9.5 km altitude for the cloud on 11 April. The relative humidity with respect to ice (RHi) along both flight tracks (Kaufmann et al., 2018) agrees well for both clouds with mean values of 105 % and 104 %. However, although the flights took place in approximately the same height range, the temperatures are quite different, with a mean value of 216 K for the measurements on 27 March and temperatures of 226–227 K for the flight track of 11 April. Thus, the comparison of the cloud microphysical properties has to be treated with care. The distribution of Deff (Fig. 4; left) for the WCB cirrus on 27 March 2014 has a narrow mono-modal structure with its maximum between about 80 to 110 µm. In contrast, Deff within the WCB cirrus on 11 April shows a broader distribution with its maximum at about 200 µm and a second smaller mode at about 100–120 µm. The overall mean (median) values of Deff for the WCB cirrus on 27 March and 11 April are 93.25 µm (95.12 µm) and 198.21 µm (193.78 µm), respectively. The distributions of Npar show only small differences for the two WCB cirrus cases. Both distributions show a skewness towards smaller values and a median of about 0.02 cm−3 for 27 March and of 0.04 cm−3 for 11 April. However, the temperatures in which the in situ data were sampled are quite different and thus might have a quite significant impact on the retrieved results, which will be discussed in Sect. 3.3. Earlier in the day on 11 April (∼ 07:50–08:25 UTC) we performed in situ measurements within the same cloud system at a height of about 10.5 km and a temperature range of 218–219 K. The mean (median) values of Deff and Npar are 132.09 µm (131.87 µm) and 0.07 cm−3 (0.02 cm−3). Unfortunately, no lidar measurements are available at the same time to make full use of this time range.
3.3 In situ data of all flight missions
Similar to its impact on the PLDR (Urbanek et al., 2018) the temperature is also correlated with the mean/median Deff and Npar showing lower values for Deff and higher values for Npar at the coldest temperature. Looking at Table 1, one can see that coordinated in situ and lidar measurements were mainly performed in cirrus clouds formed from aviation-unaffected air masses and only within a temperature range of 208 and 217 K and that only in the temperature range between 210 and 215 K are a sufficient number of in situ measurements available for a reliable comparison. Thus, we use only this temperature range for a first comparison of Deff and Npar for the two cloud classes (Fig. 5). For the temperature range colder than 209 K, in situ measurements of the high-depolarization-mode cirrus clouds dominate the available data. In contrast, in the temperature range warmer than 215 K, in situ measurements in cirrus clouds in the low depolarization mode dominate the data availability. Furthermore, the available number of data points for temperatures colder than 210 K and warmer than 215 K is small. This might affect the overall results, as no significant comparison is possible due to the small number of data points. Thus, we compare the derived Deff and Npar in a temperature range between 210 and 215 K (Fig. 5), where in situ measurements of both cirrus cloud types are available in about equal amount. Looking at this temperature regime (210–215 K) one can see slight differences between the two cirrus cloud classes. Although the main values of Deff for both cloud types are between about 25 and 100 µm, the median value of the Deff distribution (50.7 µm) within low-PLDR-mode cirrus clouds is slightly smaller than the median Deff value for the in situ measurements within high-PLDR-mode cirrus clouds (61.4 µm). The corresponding distributions of Npar show median values of about 0.05 for the high-PLDR-mode clouds and of 0.11 for the low-PLDR-mode clouds.
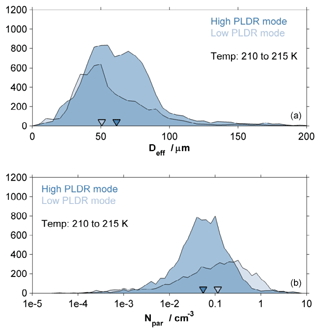
Figure 5Probability density function of the measured ice particle effective diameter (a) and ice particle number concentration (b) derived from CAS/CIP and NIXE-CAPS data (where available) for all cirrus clouds during ML-CIRRUS, where coordinated lidar and in situ measurements were available (Table 1) for both cirrus cloud types (high PLDR mode and low PLDR mode) with a significant amount of in situ samples. The light blue color indicates measurements in low-PLDR-mode clouds, and the dark blue color shows measurements for high-PLDR-mode clouds.
In a next step, we analyze the temperature dependence of the measured effective diameter and ice particle number concentration. For this evaluation, we again use only the temperature range from 210 to 215 K, as only in this temperature range can we perform a reliable comparison. We derive the distributions of Deff and Npar in 1 K steps (Fig. 6). One can see a tendency towards larger Deff with warmer temperatures, which is already known from previous studies (e.g., Heymsfield et al., 2010b; Bailey and Hallett, 2009). For the high-PLDR-mode clouds the median Deff is 40.6 µm at a temperature of 210 K and of 71.2 µm for a temperature of 215 K. The corresponding values for the low-PLDR-mode clouds are 37.7 and 75.9 µm, respectively.
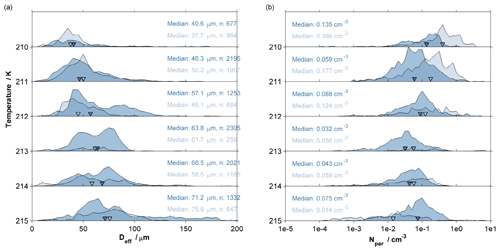
Figure 6Temperature-dependent relative distribution of the measured ice particle effective diameter (a) and ice particle number concentration (b) derived from CAS/CIP and NIXE-CAPS for all cirrus clouds during ML-CIRRUS, where coordinated lidar and in situ measurements were available (Table 1). The light blue color indicates measurements in low-PLDR-mode clouds, and the dark blue color shows measurements for high-PLDR-mode clouds. The triangles indicate the median values, and n gives the number of the available data points for each comparison.
The corresponding distributions of the ice particle number concentration show that Npar is larger for the low-PLDR-mode cirrus clouds than for the high-PLDR-mode cirrus clouds almost through the whole considered temperature ranges with median values of 0.39 cm−1 for the low-PLDR-mode cloud and 0.15 cm−1 for the high-PLDR-mode cloud at a temperature of 210 K, as well as about 0.01 cm−1 for the low-PLDR-mode cirrus and about 0.07 cm−1 for the high-PLDR-mode cirrus at a temperature of 215 K. However, as can be seen from the number of data points for each comparison given in Fig. 6, these last results have to be treated with care, as we tend to have a larger number of high-PLDR-mode cirrus cloud cases in the warm temperature range and a large spread of the results in the low-PLDR-mode cirrus cloud cases.
In our study, we used the same method and measurements as Urbanek et al. (2018), who showed for the first time a difference in the optical properties (i.e., the particle linear depolarization ratio) for cirrus clouds that formed in regions with large aviation-induced emissions (having higher values of the PLDR) and those that formed in less affected regions (lower values of the PLDR). We connected the lidar measurements with collocated in situ measurements of ice particle size and ice particle number concentration from cloud combination probes on HALO, where available. A reliable comparison of the in situ measurements of both types of clouds (high-PLDR-mode – aviation-affected – and low-PLDR-mode – pristine – cirrus) can be done in a temperature range between 210 and 215 K.
We found that for those temperature regimes, where we have a sufficient contribution of both cloud types, high-PLDR-mode clouds tend to show lower ice particle number concentrations with larger effective diameters compared to low-PLDR-mode clouds. That is an indication for more heterogeneous freezing due to aviation-induced emissions, as homogeneous nucleation is expected to be suppressed by heterogeneous nucleation (DeMott et al., 1997; Gierens, 2003). Homogeneous freezing might still occur sometime after the heterogeneous process according to Spichtinger and Cziczo (2010). They further showed that heterogeneous freezing takes place at lower RHi. In their study, Urbanek et al. (2018) investigated the distribution of RHi inside high-PLDR-mode clouds and low-PLDR-mode clouds and found differences in the supersaturation with larger values for the low-PLDR-mode clouds. These higher values can be thus interpreted such that homogeneous freezing plays a larger role in the low-PLDR-mode clouds. Homogeneous freezing is expected to produce high ice crystal number concentration and small crystal sizes (Kärcher et al., 2006; Spichtinger and Cziczo, 2010; Krämer et al., 2016). This was found during the COVID-19 curfew with strongly reduced aviation (Zhu et al., 2022) and thus decreased number of ice nucleating particles (INPs). In the presence of solid aerosol particles that act as INPs, ice crystals form at lower supersaturation. The INPs are comparably less numerous than the small aerosol solution droplets causing homogeneous freezing. Thus, the available water vapor deposits on a smaller number of ice crystals, but they grow to larger sizes and potentially also more complex ice crystals (Schnaiter et al., 2016). The availability of INPs and thus heterogeneous freezing processes lead furthermore to lower number ice particle number concentration in a subsequent homogeneous freezing process compared to pure homogenous freezing (Spichtinger and Cziczo, 2010; Krämer et al., 2016). This effect is stronger the more INPs are available.
The differences of Deff and Npar for the high-PLDR-mode and low-PLDR-mode clouds, with larger Npar but slightly smaller Deff for the low-PLDR-mode clouds, can thus also be interpreted as traces of more frequent heterogeneous freezing in the high-PLDR-mode clouds. Similar results were also found in a recent study investigating the changes in ice crystal number concentration during the COVID-19-caused air traffic closure (Zhu et al., 2022). They found a reduction in the ice crystal number concentration during that period and interpreted it as an increase in homogeneous freezing as soot from aircraft emissions was reduced. Li and Groß (2021) investigated the optical properties of cirrus clouds over the European mid-latitudes and found a reduction in the PLDR of cirrus clouds during the COVID-19 lockdown in spring 2020. However, number concentration and crystal size are not expected to be the only microphysical properties to affect the measured particle linear depolarization ratio, directly. It also depends on the crystal habit or surface roughness and thus on the complexity of the particles. Conditions during the nucleation process (e.g., temperature, relative humidity) impact the ice crystal shape (Bailey and Hallett, 2009). More heterogeneous freezing at lower supersaturation (Urbanek et al., 2018) and warmer temperature regimes (Kanitz et al., 2011) as expected for the high-PLDR-mode might lead to changes in the ice crystal complexity. Larger particles with more complex structure were found, e.g., from balloon-borne measurements of cirrus clouds (Heymsfield, 2003) under such conditions. In this study, we also find a temperature dependence of Deff and Npar with larger Deff and lower Npar for the warmer cloud temperature range.
Although the ML-CIRRUS campaign was not designed to investigate an indirect aviation effect of cirrus clouds and aerosols in the regions of cirrus cloud formation were not explored in detail, it was possible to derive important information of the data. Furthermore, the original focus of ML-CIRRUS was not on a sufficient collocation of lidar and in situ measurements for both cloud types. Therefore, additional measurements were performed during the CIRRUS-HL mission in 2021, and the flight planning for the CIRRUS-HL campaign learned from our experiences and the collocation of lidar and in situ measurements was particularly improved. Also, the sampling of aerosol properties in the region of the cloud formation and evolution was a focus of CIRRUS-HL. In the following, we expect that data from the CIRRUS-HL mission will address more of the open questions related to the impact of aviation on ice cloud properties, e.g., how the PLDR and microphysical properties depend on the aerosol concentration and size distribution in the region of cloud evolution.
The data used in this study are available at the HALO database (https://doi.org/10.17616/R39Q0T, Re3data.org, 2023).
The supplement related to this article is available online at: https://doi.org/10.5194/acp-23-8369-2023-supplement.
SG performed the lidar measurements. CV, TJW, MK and RW performed the in situ measurements during ML-CIRRUS. MW provided the basic analysis of the lidar data. MK and TJW provided the basic analysis of the in situ data. BU, QL and SG preformed the analyses in this study. SG wrote the manuscript. All authors discussed the data and findings.
At least one of the (co-)authors is a member of the editorial board of Atmospheric Chemistry and Physics. The peer-review process was guided by an independent editor, and the authors also have no other competing interests to declare.
Publisher’s note: Copernicus Publications remains neutral with regard to jurisdictional claims in published maps and institutional affiliations.
ML-CIRRUS was mainly funded by the Deutsches Zentrum für Luft- und Raumfahrt (DLR), the Deutsche Forschungsgemeinschaft (DFG) within the SPP1294-HALO project under contract VO1504/7-1, and the Helmholtz Association under contract W2/W3-60. This study was funded by CRC project TRR 301 under project ID 428312742 and DLR internal funding within the MABAK project.
This research has been supported by the
Deutsche Forschungsgemeinschaft (grant no. VO1504/7-1), by CRC project TRR 301 under project ID 428312742 and by DLR internal funding within the MABAK project.
The article processing charges for this open-access publication were covered by the German Aerospace Center (DLR).
This paper was edited by Johannes Quaas and reviewed by two anonymous referees.
Bailey, M. P. and Hallett, J.: A comprehensive habit diagram for atmospheric ice crystals: Confirmation from the laboratory, AIRS II, and other field studies, J. Atmos. Sci., 66, 2888–2899, 2009.
Bock, L. and Burkhardt, U.: Contrail cirrus radiative forcing for future air traffic, Atmos. Chem. Phys., 19, 8163–8174, https://doi.org/10.5194/acp-19-8163-2019, 2019.
Bräuer, T., Voigt, C., Sauer, D., Kaufmann, S., Hahn, V., Scheibe, M., Schlager, H., Huber, F., Le Clercq, P., Moore, R. H., and Anderson, B. E.: Reduced ice number concentrations in contrails from low-aromatic biofuel blends, Atmos. Chem. Phys., 21, 16817–16826, https://doi.org/10.5194/acp-21-16817-2021, 2021.
Browning, K. A.: Radar measurements of air motion near fronts, Weather, 26, 320–340, 1971.
Burkhardt, U. and Kärcher, B.: Global radiative forcing from contrail cirrus, Nat. Clim. Change, 1, 54–58, https://doi.org/10.1038/nclimate1068, 2011.
Burkhardt, U., Kärcher, B., and Schumann U.: Global modeling of the contrail and contrail cirrus climate impact, B. Am. Meteorol. Soc., 91, 479–484, https://doi.org/10.1175/2009bams2656.1, 2010.
Burkhardt, U., Bock, L., and Bier, A.: Mitigating the contrail cirrus climate impact by reducing aircraft soot number emissions, npj Clim. Atmos. Sci., 1, 37, https://doi.org/10.1038/s41612-018-0046-4, 2018.
Costa, A., Meyer, J., Afchine, A., Luebke, A., Günther, G., Dorsey, J. R., Gallagher, M. W., Ehrlich, A., Wendisch, M., Baumgardner, D., Wex, H., and Krämer, M.: Classification of Arctic, midlatitude and tropical clouds in the mixed-phase temperature regime, Atmos. Chem. Phys., 17, 12219–12238, https://doi.org/10.5194/acp-17-12219-2017, 2017.
DeMott, P. J., Rogers, D. C., and Kreideweis, S. M.: The susceptibility of ice formation in upper tropospheric clouds to insoluble aerosol components, J. Geophys. Res., 102, 19575–19584, https://doi.org/10.1029/97JD01138, 1997.
DeMott, P. J., Chen, Y., Kreideweis, S. M., Rogers, D. C., and Sherman, D. E.: Ice formation by black carbon particles, Geophys. Res. Lett., 26, 2429–2432, https://doi.org/10.1029/1999GL900580, 1999.
Duda, D. P., Smith, W. L., Bedka, S., Spangenberg, D., Chee, T., and Minnis, P.: Impact of COVID-19-related air traffic reductions on the coverage and radiative effects of linear persistent contrails over conterminous United States and surrounding oceanic routes, J. Geophys. Res.-Atmos., 128, e2022JD037554, https://doi.org/10.1029/2022JD037554, 2023.
Eckhardt, S., Stohl, A., Wernli, H., James, P., Forster, C., and Spichtinger, N.: A 15-year climatology of warm conveyor belt, J. Climate, 17, 218–237, 2004.
Esselborn, M., Wirth, M., Fix, A., Tesche, M., and Ehret, G.: Airborne high spectral resolution lidar for measuring aerosol extinction and backscatter coefficients, Appl. Opt., 47, 346, https://doi.org/10.1364/ao.47.000346, 2008.
Freudenthaler, V., Esselborn, M., Wiegner, M., Heese, B., Tesche, M., Ansmann, A., Müller, D., Althausen, D., Wirth, M., Fix, A., Ehret, G., Knippertz, P., Toledano, C., Gasteiger, J., Garhammer, M., and Seefeldner, M.: Depolarization ratio profiling at several wavelengths in pure saharan dust during SAMUM 2006, Tellus B, 61, 165–179, https://doi.org/10.1111/j.1600-0889.2008.00396.x, 2009.
Gettelman, A. and Chen, C.: The climate impact of aviation aerosols, Geophys. Res. Lett., 40, 2785–2789, https://doi.org/10.1002/grl.50520, 2013.
Gierens, K.: On the transition between heterogeneous and homogeneous freezing, Atmos. Chem. Phys., 3, 437–446, https://doi.org/10.5194/acp-3-437-2003, 2003.
Hendricks, J., Kärcher, B., Lohmann, U., and Ponater, M.: Do aircraft black carbon emissions affect cirrus clouds on the global scale?, Geophys. Res. Lett., 32, L12814, https://doi.org/10.1029/2005gl022740, 2005.
Hendricks, J., Kärcher, B., and Lohmann, U.: Effects of ice nuclei on cirrus clouds in a global climate model, J. Geophys. Res., 116, D18206, https://doi.org/10.1029/2010JD015302, 2011.
Giez, A., Zöger, M., Mallaun, C., Nenakhov, V., Schimpf, M., Grad, C., Numberger, A., and Raynor, K.: Determination of the measurement errors for the HSLO basic data System BAHAMAS by means of error propagation, DLR-FB 2022-27, 97, https://doi.org/10.57676/5rdc-q708, 2023.
Grewe, V., Dahlmann, K., Flink, J., Frömming, C., Ghosh, R., Gierens, K., Heller, R., Hendricks, J., Jöckel, P., Kaufmann, S., Kölker, K., Linke, F., Luchkova, T., Lührs, B., van Manen, J., Matthes, S., Minikin, A., Niklaß, M., Plohr, M., Righi, M., Rosanka, S., Schmitt, A., Schumann, U., Terekhov, I., Unterstrasser, S., Vázquez-Navarro, M., Voigt, C., Wicke, K., Yamashita, H., Zahn, A., and Ziereis, H.: Mitigating the Climate Impact from Aviation: Achievements and Results of the DLR WeCare Project Aerospace, 4, 34, https://doi.org/10.3390/aerospace4030034, 2017.
Groß, S., Wirth, M., Schäfler, A., Fix, A., Kaufmann, S., and Voigt, C.: Potential of airborne lidar measurements for cirrus cloud studies, Atmos. Meas. Tech., 7, 2745–2755, https://doi.org/10.5194/amt-7-2745-2014, 2014.
Haywood, J. M., Allan, R. P., Bornemann, J., Forster, P. M., Francis, P. N., Milton, S, Rädel, G., Rap, A., Shine, K. P., and Thorpe, R.: A case study of the radiative forcing of persistent contrails evolving into contrail-induced cirrus, J. Geophys. Res., 114, D24201, https://doi.org/10.1029/2009JD012650, 2009.
Hendricks, J., Kärcher, B., and Lohmann, U.: Effects of ice nuclei on cirrus clouds in a global climate model, J. Geophys. Res.-Atmos., 116, D18206, https://doi.org/10.1029/2010jd015302, 2011.
Heymsfield, A., Baumgardner, D., DeMott, P., Forster, P., Gierens, K., and Kärcher, B.: Contrail microphysics, B. Am. Meteorol. Soc., 91, 465–472, https://doi.org/10.1175/2009bams2839.1, 2010a.
Heymsfield, A., Schmitt, C., Bansemer, A., and Twohy, C. H.: Improved representation of ice particle masses based on observations in natural clouds, J. Atmos. Sci., 67, 3303–3318, 2010b.
Heymsfield, A. J.: Properties of tropical and midlatitude ice cloud particle ensembles. Part I: Median mass diameters and terminal velocities, J. Atmos. Sci., 60, 2573–2591, https://doi.org/10.1175/1520-0469(2003)060<2573:POTAMI>2.0.CO;2, 2003.
Hoose, C. and Möhler, O.: Heterogeneous ice nucleation on atmospheric aerosols: a review of results from laboratory experiments, Atmos. Chem. Phys., 12, 9817–9854, https://doi.org/10.5194/acp-12-9817-2012, 2012.
Kärcher, B., Hendricks, J., and Lohmann, U.: Physically based parameterization of cirrus cloud formation for use in global atmospheric models, J. Geopyhs. Res., 111, D01205, https://doi.org/10.1029/2005JD006219, 2006.
Kärcher, B., Burkhardt, U., Bier, A., Bock, L., and Ford, I. J.: The microphysical pathway to contrail formation, J. Geophys. Res.-Atmos., 120, 7893–7927, https://doi.org/10.1002/2015jd023491, 2015.
Kärcher, B.: Cirrus Clouds and Their Response to Anthropogenic Activities, Curr. Clim. Change Rep., 3, 45–57, https://doi.org/10.1007/s40641-017-0060-3, 2017.
Kärcher, B.: Formation and radiative forcing of contrail cirrus, Nat. Commun., 9, 1824, https://doi.org/10.1038/s41467-018-04068-0, 2018.
Kanitz, T., Seifert, P., Ansmann, A., Engelmann, R., Althausen, D., Casiccia, C., and Rohwer, E. G.: Contrasting the impact of aerosols at northern and southern midlatitudes on heterogeneous ice formation, Geophys. Res. Lett., 38, L17802, https://doi.org/10.1029/2011GL048532, 2011.
Kaufmann, S., Voigt, C., Heller, R., Jurkat-Witschas, T., Krämer, M., Rolf, C., Zöger, M., Giez, A., Buchholz, B., Ebert, V., Thornberry, T., and Schumann, U.: Intercomparison of midlatitude tropospheric and lower-stratospheric water vapor measurements and comparison to ECMWF humidity data, Atmos. Chem. Phys., 18, 16729–16745, https://doi.org/10.5194/acp-18-16729-2018, 2018.
Kleine, J., Voigt, C., Sauer, D., Schlager, H., Scheibe, M., Jurkat-Withschs, T., Kaufmann, S., Kärcher, B., and Anderson, B. E.: In Situ Observations of Ice Particle Losses in a Young Persistent Contrail, Geophys. Res. Lett., 45, 13553–13561, https://doi.org/10.1029/2018GL079390, 2018.
Krämer, M., Rolf, C., Luebke, A., Afchine, A., Spelten, N., Costa, A., Meyer, J., Zöger, M., Smith, J., Herman, R. L., Buchholz, B., Ebert, V., Baumgardner, D., Borrmann, S., Klingebiel, M., and Avallone, L.: A microphysics guide to cirrus clouds – Part 1: Cirrus types, Atmos. Chem. Phys., 16, 3463–3483, https://doi.org/10.5194/acp-16-3463-2016, 2016.
Krüger, O. O., Holanda, B. A., Chowdhury, S., Pozzer, A., Walter, D., Pöhlker, C., Andrés Hernández, M. D., Burrows, J. P., Voigt, C., Lelieveld, J., Quaas, J., Pöschl, U., and Pöhlker, M. L.: Black carbon aerosol reductions during COVID-19 confinement quantified by aircraft measurements over Europe, Atmos. Chem. Phys., 22, 8683–8699, https://doi.org/10.5194/acp-22-8683-2022, 2022.
Lee, D. S., Fahey, D.W., Skowron, A., Allen, M. R., Burkhardt, U., Chen, Q., Doherty, S. J., Freeman, S., Forster, P. M., Fuglestvedt, J., Gettelman, A., De León, R. R., Lim, L. L., Lund, M. T., Millar, R. J., Owen, B., Pennter, J. E., Pitari, G., Prather, M. J., Sausen, R., and Wilcox, L. J.: The contribution of global aviation to anthropogenic climate forcing for 2000 to 2018, Atmos. Enivron., 244, 117834, https://doi.org/10.1016/j.atmosenv.2020.117834, 2021.
Li, Q. and Groß, S.: Changes in cirrus cloud properties and occurrence over Europe during the COVID-19-caused air traffic reduction, Atmos. Chem. Phys., 21, 14573–14590, https://doi.org/10.5194/acp-21-14573-2021, 2021.
Li, Q. and Groß, S.: Satellite observations of seasonality and long-term trends in cirrus cloud properties over Europe: investigation of possible aviation impacts, Atmos. Chem. Phys., 22, 15963–15980, https://doi.org/10.5194/acp-22-15963-2022, 2022.
Liu, X., Penner, J. E., and Wang, M.: Influence of anthropogenic sulfate and black carbon on upper tropospheric clouds in the NCAR CAM3 model coupled to the IMPACT global aerosol model, J. Geophys. Res.-Atmos., 114, D03204, https://doi.org/10.1029/2008jd010492, 2009.
Luebke, A. E., Afchine, A., Costa, A., Grooß, J.-U., Meyer, J., Rolf, C., Spelten, N., Avallone, L. M., Baumgardner, D., and Krämer, M.: The origin of midlatitude ice clouds and the resulting influence on their microphysical properties, Atmos. Chem. Phys., 16, 5793–5809, https://doi.org/10.5194/acp-16-5793-2016, 2016.
Lund, M. T., Aamaas, B., Berntsen, T., Bock, L., Burkhardt, U., Fuglestvedt, J. S., and Shine, K. P.: Emission metrics for quantifying regional climate impacts of aviation, Earth Syst. Dynam., 8, 547–563, https://doi.org/10.5194/esd-8-547-2017, 2017.
Mahrt, F., Kilchhofer, K., Marcolli, C., Grönquist, P., David, R. O., Rösch, M., Lohmann, U., and Kanji, Z. A.: The Impact of Cloud Processing on the Ice Nucleation Abilities of Soot Particles at Cirrus Temperatures, J. Geophys. Res.-Atmos., 125, e2019JD030922, https://doi.org/10.1029/2019jd030922, 2020.
Marjani, S., Tesche, M., Bräuer, P., Sourdeval, O., and Quaas, J.: Satellite observations of the impact of individual aircraft on ice crystal number in thin cirrus clouds, Geophys. Res. Lett., 49, e2021GL096173, https://doi.org/10.1029/2021GL096173, 2022.
McKenna, D. S.: A new Chemical Lagrangian Model of the Stratosphere (CLaMS) 1. formulation of advection and mixing, J. Geophys. Res., 107, https://doi.org/10.1029/2000jd000114, 2002.
Möhler, O., Büttner, S., Linke, C., Schnaiter, M., Saathoff, H., Stetzer, O., Wagner, R., Krämer, M., Mangold, A., Ebert, V., and Schurath, U.: Effect of sulfuric acid coating on heterogeneous ice nucleation by soot aerosol particles, J. Geophys. Res.-Atmos., 110, 4309, https://doi.org/10.1029/2004jd005169, 2005.
Moore, R. H., Thornhill, K. L., Weinzierl, B., Sauer, D., D'Ascoli, E., Kim, J., Lichtenstern, M., Scheibe, M., Beaton, B., Beyersdorf, A. J., Barrick, J., Bulzan, D., Corr, C. A., Crosbie, E., Jurkat, T., Martin, R., Riddick, D., Shook, M., Slover, G., Voigt, C., White, R., Winstead, E., Yasky, R., Ziemba, L. D., Brown, A., Schlager, H., and Anderson, B. E.: Biofuel blending reduces particle emissions from aircraft engines at cruise conditions, Nature, 543, 411-415, https://doi.org/10.1038/nature21420, 2017.
Penner, J. E., Chen, Y., Wang, M., and Liu, X.: Possible influence of anthropogenic aerosols on cirrus clouds and anthropogenic forcing, Atmos. Chem. Phys., 9, 879–896, https://doi.org/10.5194/acp-9-879-2009, 2009.
Penner, J. E., Zhou, C., Garnier, A., and Mitchell, D. L.: Anthropogenic Aerosol Indirect Effects in Cirrus Clouds, J. Geophys. Res.-Atmos., 123, 11652–11677, https://doi.org/10.1029/2018jd029204, 2018.
Quaas, J., Gryspeerdt, E., Vautard, R., and Boucher, O.: Climate impact of aircraft-induced cirrus assessed from satellite observations before and during COVID-19, Environ. Res. Lett., 16, 064051, https://doi.org/10.1088/1748-9326/abf686, 2021.
Re3data.org: HALO database, re3data.org – Registry of Research Data Repositories [data set], https://doi.org/10.17616/R39Q0T, 2021.
Righi, M., Hendricks, J., Lohmann, U., Beer, C. G., Hahn, V., Heinold, B., Heller, R., Krämer, M., Ponater, M., Rolf, C., Tegen, I., and Voigt, C.: Coupling aerosols to (cirrus) clouds in the global EMAC-MADE3 aerosol–climate model, Geosci. Model Dev., 13, 1635–1661, https://doi.org/10.5194/gmd-13-1635-2020, 2020.
Righi, M., Hendricks, J., and Beer, C. G.: Exploring the uncertainties in the aviation soot–cirrus effect, Atmos. Chem. Phys., 21, 17267–17289, https://doi.org/10.5194/acp-21-17267-2021, 2021.
Schnaiter, M., Järvinen, E., Vochezer, P., Abdelmonem, A., Wagner, R., Jourdan, O., Mioche, G., Shcherbakov, V. N., Schmitt, C. G., Tricoli, U., Ulanowski, Z., and Heymsfield, A. J.: Cloud chamber experiments on the origin of ice crystal complexity in cirrus clouds, Atmos. Chem. Phys., 16, 5091–5110, https://doi.org/10.5194/acp-16-5091-2016, 2016.
Schumann, U.: A contrail cirrus prediction model, Geosci. Model Dev., 5, 543–580, https://doi.org/10.5194/gmd-5-543-2012, 2012.
Schumann, U., Mayer, B., Gierens, K., Unterstrasser, S., Jessberger, P., Petzold, A., Voigt, C., and Gayet, J.-F.: Effective Radius of Ice Particles in Cirrus and Contrails, Journal of the Atmospheric Sciences, American Meteorological Society, 68, 300–321, 2011.
Schumann, U., Baumann, R., Baumgardner, D., Bedka, S. T., Duda, D. P., Freudenthaler, V., Gayet, J.-F., Heymsfield, A. J., Minnis, P., Quante, M., Raschke, E., Schlager, H., Vázquez-Navarro, M., Voigt, C., and Wang, Z.: Properties of individual contrails: a compilation of observations and some comparisons, Atmos. Chem. Phys., 17, 403–438, https://doi.org/10.5194/acp-17-403-2017, 2017.
Schumann, U., Poll, I., Teoh, R., Koelle, R., Spinielli, E., Molloy, J., Koudis, G. S., Baumann, R., Bugliaro, L., Stettler, M., and Voigt, C.: Air traffic and contrail changes over Europe during COVID-19: a model study, Atmos. Chem. Phys., 21, 7429–7450, https://doi.org/10.5194/acp-21-7429-2021, 2021a.
Schumann, U., Bugliaro, L., Dörnbrack, A., Baumann, R., and Voigt, C.: Aviation contrail cirrus and radiative forcing over Europe during 6 months of COVID-19, Geophys. Res. Lett., 48, e2021GL092771, https://doi.org/10.1029/2021GL092771, 2021b.
Spichtinger, P. and Cziczo, D. J.: Impact of heterogeneous ice nuclei on homogeneous freezing events in cirrus clouds, J. Geophys. Res., 115, D14208, , https://doi.org/10.1029/2009JD012168, 2010.
Stettler, M. E. J., Boies, A., M., Petzold, A., and Barrett, S. R. H.: Global Civil Aviation Black Carbon Emissions, Environ. Sci. Technol. 47, 10397–10404, https://doi.org/10.1021/es401356v, 2013.
Teoh, R., Schumann, U., Gryspeerdt, E., Shapiro, M., Molloy, J., Koudis, G., Voigt, C., and Stettler, M. E. J.: Aviation contrail climate effects in the North Atlantic from 2016 to 2021, Atmos. Chem. Phys., 22, 10919–10935, https://doi.org/10.5194/acp-22-10919-2022, 2022.
Tesche, M., Achtert, P., Glantz, P., and Noone, K. J.: Aviation effects on already-existing cirrus clouds, Nat. Commun., 7, 12016, https://doi.org/10.1038/ncomms12016, 2016.
Urbanek, B.: Characterization of midlatitude cirrus clouds with airborne lidar – Investigating an indirect aviation effect, Diss. LMU, Ludwig-Maximilians-Universität München, https://doi.org/10.5282/edoc.25224, 2019.
Urbanek, B., Groß, S., Schäfler, A., and Wirth, M.: Determining stages of cirrus evolution: a cloud classification scheme, Atmos. Meas. Tech., 10, 1653–1664, https://doi.org/10.5194/amt-10-1653-2017, 2017.
Urbanek, B., Groß, S., Wirth, M., Rolf, C., Krämer, M., and Voigt, C.: High depolarization ratios of naturally occurring cirrus clouds near air traffic regions over Europe, Geophys. Res. Lett., 45, 13166–13172, https://doi.org/10.1029/2018GL079345, 2018.
Voigt, C., Schumann, U., Jurkat, T., Schäuble, D., Schlager, H., Petzold, A., Gayet, J.-F., Krämer, M., Schneider, J., Borrmann, S., Schmale, J., Jessberger, P., Hamburger, T., Lichtenstern, M., Scheibe, M., Gourbeyre, C., Meyer, J., Kübbeler, M., Frey, W., Kalesse, H., Butler, T., Lawrence, M. G., Holzäpfel, F., Arnold, F., Wendisch, M., Döpelheuer, A., Gottschaldt, K., Baumann, R., Zöger, M., Sölch, I., Rautenhaus, M., and Dörnbrack, A.: In-situ observations of young contrails – overview and selected results from the CONCERT campaign, Atmos. Chem. Phys., 10, 9039–9056, https://doi.org/10.5194/acp-10-9039-2010, 2010.
Voigt, C., Schumann, U., Jessberger, P., Jurkat, T., Petzold, A., Gayet, J.-F., Krämer, M., Thornberry, T., and Fahey, D. W.: Extinction and optical depth of contrails, Geophys. Res. Lett., 38, L11806, https://doi.org/10.1029/2011GL047189, 2011.
Voigt, C., Schumann, U., Minikin, A., Abdelmonem, A., Afchine, A., Borrmann, S., Boettcher, M., Buchholz, B., Bugliaro, L., Costa, A., Curtius, J., Dollner, M., Dörnbrack, A., Dreiling, V., Ebert, V., Ehrlich, A., Fix, A., Forster, L., Frank, F., Fütterer, D., Giez, A., Graf, K., Grooß, J.-U., Groß, S., Heimerl, K., Heinold, B., Hüneke, T., Järvinen, E., Jurkat, T., Kaufmann, S., Kenntner, M., Klingebiel, M., Klimach, T., Kohl, R., Krämer, M., Krisna, T. C., Luebke, A., Mayer, B., Mertes, S., Molleker, S., Petzold, A., Pfeilsticker, K., Port, M., Rapp, M., Reutter, P., Rolf, C., Rose, D., Sauer, D., Schäfler, A., Schlage, R., Schnaiter, M., Schneider, J., Spelten, N., Spichtinger, P., Stock, P., Walser, A., Weigel, R., Weinzierl, B., Wendisch, M., Werner, F., Wernli, H., Wirth, M., Zahn, A., Ziereis, H., and Zöger, M.: ML-CIRRUS: The airborne experiment on natural cirrus and contrail cirrus with the high-altitude long-range research aircraft HALO, B. Am. Meteorol. Soc., 98, 271–288, https://doi.org/10.1175/BAMS-D-15-00213.1, 2017.
Voigt, C., Kleine, J., Sauer, D., Moore, R. H.; Bräuer, T., Le Clercq, P., Kaufmann, S., Scheibe, M., Jurkat-Witschas, T., Aigner, M., Bauder, U., Boose, Y., Borrmann, S., Crosbie, E., Diskin, G. S., DiGangi, J., Han, V., Heckel, C., Huber, F., Nowak, J. B., Rapp, M., Rauch, B., Robinson, C., Schripp, T., Schook , M.,Winstead, W., Ziemba, L., Schlager, H., and Anderson, B. E.: Cleaner burning aviation fuels can reduce contrail cloudiness, Commun. Earth Environ., 2, 114, https://doi.org/10.1038/s43247-021-00174-y, 2021.
Voigt, C., Lelieveld, J., Schlager, H., Schneider, J., Curtius, J., Meerkötter, R., Sauer, D., Bugliaro, L., Bohn, B., Crowley, J. N., Erbertseder, T., Groβ, S., Hahn, V., Li, Q., Mertens, M., Pöhlker, M. L., Pozzer, A., Schumann, U., Tomsche, L., Williams, J., Zahn, A., Andreae, M., Borrmann, S., Bräuer, T., Dörich, R., Dörnbrack, A., Edtbauer, A., Ernle, L., Fischer, H., Giez, A., Granzin, M., Grewe, V., Harder, H., Heinritzi, M., Holanda, B. A., Jöckel, P., Kaiser, K., Krüger, O. O., Lucke, J., Marsing, A., Martin, A., Matthes, S., Pöhlker, C., Pöschl, U., Reifenberg, S., Ringsdorf, A., Scheibe, M., Tadic, I., Zauner-Wieczorek, M., Henke, R., and Rapp, M.: Cleaner skies during the COVID-19 lockdown, B. Am. Meteorol. Soc., 103, E1796–E1827, https://doi.org/10.1175/BAMS-D-21-0012.1, 2022.
Wang, Z., Bugliaro, L., Jurkat-Witschas, T., Heller, R., Burkhardt, U., Ziereis, H., Dekoutsidis, G., Wirth, M., Groß, S., Kirschler, S., Kaufmann, S., and Voigt, C.: Observations of microphysical properties and radiative effects of a contrail cirrus outbreak over the North Atlantic, Atmos. Chem. Phys., 23, 1941–1961, https://doi.org/10.5194/acp-23-1941-2023, 2023.
Weigel, R., Spichtinger, P., Mahnke, C., Klingebiel, M., Afchine, A., Petzold, A., Krämer, M., Costa, A., Molleker, S., Reutter, P., Szakáll, M., Port, M., Grulich, L., Jurkat, T., Minikin, A., and Borrmann, S.: Thermodynamic correction of particle concentrations measured by underwing probes on fast-flying aircraft, Atmos. Meas. Tech., 9, 5135–5162, https://doi.org/10.5194/amt-9-5135-2016, 2016.
Winker, D. M., Pelon, J., Coakley Jr., J. A., Ackerman, S. A., Charlson, R. J., Colarco, P. R., Flamant, P., Fu, Q., Hoff, R. M., Kittaka, C., Kubar, T. L., Le Treut, H., Mccormick, M. P., Mégie, G., Poole, L., Powell, K., Trepte, C., Vaughan, M. A., and Wielicki, B. A.: The CALIPSO Mission: A global 3D view of aerosols and clouds, B. Am. Meteorol. Soc., 91, 1211–1229, https://doi.org/10.1175/2010BAMS3009.1, 2010.
Wirth, M., Fix, A., Mahnke, P., Schwarzer, H., Schrandt, F., and Ehret, G.: The airborne multi-wavelength water vapor differential absorption lidar WALES: system design and performance, Appl. Phys. B, 96, 201–213, https://doi.org/10.1007/s00340-009-3365-7, 2009.
Zhou, C. and Penner, J. E.: Aircraft soot indirect effect on large-scale cirrus clouds: Is the indirect forcing by aircraft soot positive or negative?, J. Geophys. Res.-Atmos., 119, 11303–11320, https://doi.org/10.1002/2014jd021914, 2014.
Zhu, J., Penner, J. E., Garnier, A., Boucher, O., Gao, M., Song, L., Deng, J., Liu, C, and Fu, P.: Decreased aviation leads to increased ice crystal number and a positive radiative effect in cirrus clouds, AGU Advances, 3, e2021AV000546, https://doi.org/10.1029/2021AV000546, 2022.