the Creative Commons Attribution 4.0 License.
the Creative Commons Attribution 4.0 License.
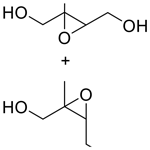
Technical note: Improved synthetic routes to cis- and trans-(2-methyloxirane-2,3-diyl)dimethanol (cis- and trans-β-isoprene epoxydiol)
Molly Frauenheim
Jason D. Surratt
Zhenfa Zhang
Avram Gold
We report improved synthetic routes to the isomeric isoprene-derived β-epoxydiols (β-IEPOX) in high yield (57 %–69 %) from inexpensive, readily available starting compounds. The syntheses do not require the protection/deprotection steps or time-consuming purification of intermediates and can readily be scaled up to yield the target IEPOX isomers in gram quantities. Emissions of isoprene (2-methyl-1,3-butadiene, C5H8), primarily from deciduous vegetation, constitute the largest source of nonmethane atmospheric hydrocarbons. In the gas phase under low-nitric-oxide (NO) conditions, addition of the atmospheric hydroxyl radical (OH) followed by rapid addition of O2 yields isoprene-derived hydroxyperoxyl radicals. The major sink (>90 %) for the peroxyl radicals is a sequential reaction with the hydroperoxyl radical (HO2), OH, and O2, which is then followed by the elimination of OH to yield a mixture of cis- and trans-(2-methyloxirane-2,3-diyl)dimethanol (cis- and trans-β-IEPOX). The IEPOX isomers account for about 80 % of closed-shell hydroxyperoxyl products and are rapidly taken up into acidic aerosols to form secondary organic aerosol (SOA). IEPOX-derived SOA makes a significant mass contribution to fine particulate matter (PM2.5), which is known to be a major factor in climate forcing as well as adversely affecting respiratory and cardiovascular systems of exposed populations. Prediction of ambient PM2.5 composition and distribution, both in regional- and global-scale atmospheric chemistry models, crucially depends on the accuracy of identification and quantitation of uptake product formation. Accessibility of authentic cis- and trans-β-IEPOX in high purity and in large quantity for laboratory studies underpins progress in developing models as well as identification and quantitation of PM2.5 components.
- Article
(832 KB) - Full-text XML
-
Supplement
(1439 KB) - BibTeX
- EndNote
We report here straightforward procedures for the synthesis of isomeric isoprene β-epoxydiols (β-IEPOX) in high yield from inexpensive, readily available starting compounds. The syntheses do not require the protection/deprotection steps or time-consuming purification of intermediates as used in past studies (Cole-Filipiak et al., 2010; Zhang et al., 2012; Bates et al., 2014, 2016; Chase et al., 2015) and can readily be scaled up to yield the target IEPOX isomers in gram quantities.
Yearly global emissions of isoprene (2-methyl-1,3-butadiene, C5H8), primarily from deciduous vegetation, are estimated to be between 500 and 600 Tg and constitute the largest source of nonmethane atmospheric hydrocarbons (Kanakidou et al., 2005; Guenther et al., 2006; Hallquist et al., 2009; St. Clair et al., 2016). In the gas phase under low-nitric-oxide (NO) conditions, the atmospheric hydroxyl radical (OH) adds rapidly to isoprene almost exclusively at C1 and C4, followed by addition of O2 to yield β- or δ-hydroperoxyl radicals (Hallquist et al., 2009). The major sink (>90 %) for the peroxyl radicals is reaction with the hydroperoxyl radical (HO2) to give closed-shell isoprene hydroxyhydroperoxides (ISOPOOHs). ISOPOOHs then undergo sequential addition with OH and O2, followed by the elimination of OH to yield a mixture of cis- and trans-(2-methyloxirane-2,3-diyl)dimethanol (cis- and trans-β-IEPOX). The IEPOX isomers account for about 80 % of the closed-shell hydroxyperoxy products (St. Clair et al., 2016; Wennberg et al., 2018; Paulot et al., 2009) and are rapidly taken up onto acidic aerosols (Lin et al., 2012; Gaston et al., 2014; Riedel et al., 2015). IEPOX isomers thus make a significant mass contribution to secondary organic aerosol (SOA) (Surratt et al., 2010; Riva et al., 2019) and the resulting atmospheric fine particulate matter (PM2.5) (Lin et al., 2013; Budisulistiorini et al., 2015, 2016; Rattanavaraha et al., 2016). PM2.5 is known to be a major factor in climate forcing (Hallquist et al., 2009) and adversely affects respiratory and cardiovascular systems of exposed populations (Pope and Dockery, 2006; Pye et al., 2021). Advancing the understanding of the impacts of PM2.5 requires the ability to predict PM2.5 composition and distribution, both in regional- and global-scale atmospheric chemistry and climate models (Pye et al., 2013; McNeill et al., 2015; Marais et al., 2016; Jo et al., 2019), which in turn depends crucially on the accuracy of identification and quantitation of uptake product formation. As major precursors of PM2.5, cis- and trans-β-IEPOX have been the focus of considerable effort to elucidate mechanisms underlying PM2.5 formation and aging (Lin et al., 2012, 2014; Gaston et al., 2014; Nguyen et al., 2014; Zhang et al., 2018; Riva et al., 2019; Armstrong et al., 2022; Cooke et al., 2022). Underpinning such efforts is the availability of authentic cis- and trans-β-IEPOX in high purity and in quantity.
The reactions described below should be performed under a fume hood.
The esterification and diisobutylaluminum hydride (DIBAL-H) reduction of mesaconic and citraconic acids generally followed the procedure reported by Klimovica et al. (2011). Epoxidation of the E- and Z-2-methylbut-2-ene-1,4-diols followed the procedure reported by Zhang et al. (2012). 1H nuclear magnetic resonance (NMR) spectra of all isolated products, as well as 13C NMR for target cis- and trans-β-IEPOX isomers, are provided in the Supplement. Mass spectra of cis- and trans-β-IEPOX, also provided in the Supplement, were obtained using the Agilent 1200 Series HPLC (high-performance liquid chromatography) system equipped with an ESI (electrospray ionization) source interfaced to an Agilent 6250 Series Accurate-Mass Q-TOF MS (quadrupole time-of-flight mass spectrometer) operated in negative ion (–) mode using instrumental conditions described elsewhere (Cui et al., 2018).
2.1 trans-β-IEPOX (trans-(2-methyloxirane-2,3-diyl)dimethanol) from mesaconic acid
2.1.1 Mesaconic acid, dimethyl ester
To a solution of mesaconic acid (11.00 g, 85 mmol, Sigma Aldrich, USD 80.20/10 g) in methanol (100 mL), concentrated H2SO4 (3 mL) was added. The reaction mixture was refluxed for 8 h until a complete conversion was observed by thin-layer chromatography (TLC; SiO2, 1:1 hexane : ethyl acetate), and the reaction was neutralized by addition of triethylamine (1.5 mL). The resulting mixture was dried on a rotary evaporator under house vacuum to afford mesaconic acid dimethyl ester as a colorless oil (13.4 g, 97 %), purity >98 %, by NMR. NMR (400 MHz, chloroform-d): δ6.71 (d, J=1.6 Hz, 1H), 3.74 (s, 3H), 3.71 (s, 3H), and 2.23 (d, J=1.6 Hz, 3H); see Fig. S1 in the Supplement.
2.1.2 E-2-Methylbut-2-ene-1,4-diol
A solution of mesaconic acid dimethyl ester (13.4 g, 85 mmol) in methylene chloride (120 mL) under argon was cooled to 0 ∘C, and diisobutylaluminum hydride (DIBAL-H; 400 mL 1.0 M solution in toluene, 400 mmol) was added dropwise over 2 h. The reaction mixture was stirred at 0 ∘C for 1 h. The reaction was diluted with ether (100 mL) and quenched with 16 mL water (0.04 volume equivalents of DIBAL-H), followed by 16 mL 15 % sodium hydroxide solution (0.04 volume equivalents) and 40 mL water (0.1 volume equivalents). After quenching, the mixture was allowed to warm to room temperature over 2 h and dried over magnesium sulfate. The aluminum salt was filtered under vacuum through a pad of Celite, and the collected solid was further washed with ethyl acetate (100 mL). The solvent was removed from the filtrate on a rotary evaporator under house vacuum to yield E-2-methylbut-2-ene-1,4-diol as a colorless oil (6.1 g, 71 %), purity >98 %, by NMR. NMR (400 MHz, D2O): δ5.54–5.43 (m, 1H), 4.06 (d, J=7.0 Hz, 2H), 3.90 (s, 2H), and 1.57 (s, 3H); see Fig. S2.
2.1.3 trans-(2-Methyloxirane-2,3-diyl)dimethanol (trans-β-IEPOX)
Epoxidation of E-2-methylbut-2-ene-1,4-diol followed a published procedure (Zhang et al., 2012). The butene diol (6.0 g, 59 mmol) was dissolved in acetonitrile (80 mL) and cooled in an ice-water bath. m-Chloroperoxybenzoic acid (m-CPBA; 15.5 g, 90 mmol) was added, and the clear solution was stirred in the ice-water bath for 2 h and then at room temperature for 1 h until complete transformation of the starting material as monitored by TLC (SiO2, 1:1 hexane : ethyl acetate). The reaction mixture was cooled at 4 ∘C, and the resulting precipitate was separated by filtration to remove the bulk of the 3-chlorobenzoic acid. The filtrate was concentrated under vacuum, and the residue was dissolved in water (30 mL) and washed repeatedly with chloroform. The aqueous solution was lyophilized to yield trans-(2-methyloxirane-2,3-diyl)dimethanol as a colorless oil (6.2 g, 89 %), purity >98 %, by NMR. The 1H NMR spectrum was identical to that reported in previous syntheses (Zhang et al., 2012). NMR (400 MHz, D2O): δ3.78 (dd, J=12.49, 4.29 Hz, 1H), 3.59 (d, J=12.56 Hz, 1H), 3.58 (dd, J=12.49, 7.08 Hz, 1H), 3.44 (d, J=12.56 Hz, 1H), 3.15 (dd, J=7.08, 4.29, 1H), and 1.23 (s, 3H); see Fig. S3. 13C NMR (400 MHz, D2O): δ65.06, 62.35, 60.89, 59.78, and 13.06; see Fig. S4. The (–)ESI-Q-TOF mass spectrum is provided in Fig. S5.
The overall yield for the synthesis of trans-β-IEPOX from mesaconic acid was 62. %.
2.2 cis-β-IEPOX (cis-(2-methyloxirane-2,3-diyl)dimethanol) from citraconic acid
2.2.1 Citraconic acid, dimethyl ester
To a solution of citraconic acid (2.0 g, 15 mmol, Sigma Aldrich, USD 46.10/5 g) in methanol (50 mL), concentrated H2SO4 (0.8 mL) was added. The reaction mixture was refluxed for 8 h until complete conversion as determined by TLC (SiO2, 1:1 hexane : ethyl acetate) and then neutralized by addition of triethylamine (0.5 mL). The resulting mixture was concentrated in vacuo to afford the desired citraconic acid dimethyl ester (2.2 g, 92 %) as a colorless oil, purity >98 %, by NMR. NMR (400 MHz, D2O): δ6.08 (d, J=1.6 Hz, 1H), 3.85 (s, 3H), 3.75 (s, 3H), and 2.07 (d, J=1.6 Hz, 3H); see Fig. S6.
2.2.2 Z-2-Methylbut-2-ene-1,4-diol
A solution of citraconic acid dimethyl ester (2.2 g, 14 mmol) in methylene chloride (25 mL) under argon was cooled to 0 ∘C, and DIBAL-H (70 mL 1.0 M solution in toluene, 70 mmol) was added dropwise. The reaction mixture was stirred at 0 ∘C for 1 h, diluted with ether (60 mL), and quenched with 2.8 mL water (0.04 of DIBAL-H volume equivalents), followed by 2.8 mL 15 % sodium hydroxide solution (0.04 volume equivalents) and 7.0 mL water (0.1 volume equivalents). After quenching, the mixture was allowed to warm to room temperature over 2 h and dried over magnesium sulfate. The aluminum salt was removed by filtration through a pad of Celite, and the collected solid was further washed with ethyl acetate (100 mL). The solvent was removed from filtrate, and the desired Z-2-methylbut-2-ene-1,4-diol (1.1 g, 75 % yield) was obtained as a colorless oil, purity >98 %, by NMR (Klimovica, et al., 2011). NMR (400 MHz, D2O): δ5.57 (t, J=7.2 Hz, 1H), 4.16 (d, J=7.2 Hz, 2H), 4.14 (s, 2H), and 1.82 (s, 3H); see Fig. S7.
2.2.3 cis-(2-Methyloxirane-2,3-diyl)dimethanol (cis-β-IEPOX)
The epoxidation of the butene diol was performed according to a published method (Zhang et al., 2012). The butene diol (1.1 g, 10 mmol) was dissolved in acetonitrile (25 mL) and cooled in an ice-water bath. m-CPBA (2.7 g, 16 mmol) was added, and the clear solution was stirred in the ice-water bath for 1 h and then at room temperature until complete transformation of the starting material, as monitored by TLC (SiO2, 1:1 hexane : ethyl acetate). The mixture was cooled at 0 ∘C, and the resulting precipitate was separated by filtration to remove the bulk of the 3-chlorobenzoic acid. The filtrate was concentrated under reduced pressure, and the residue was dissolved in water (15 mL) and washed repeatedly with chloroform. The aqueous solution was lyophilized to give Z-(2-methyloxirane-2,3-diyl)dimethanol as a colorless oil isolated as a crude product (1.0 g, 83 %), purity 98 %, by NMR. NMR (400 MHz, D2O): δ3.78 (dd, J=12.5, 3.9 Hz, 1H), 3.61 (d, J=12.3 Hz, 1H),3.54 (dd, J=12.6, 7.35 Hz, 1H), 3.52 (d, J=12.3 Hz, 1H), 3.10 (dd, J=7.35, 3.93 Hz, 1H), and 1.43 (s, 3H); see Fig. S8. 13C NMR (500 MHz, D2O): δ64.68, 62.61, 62.48, 59.39, and 18.82; see Fig. S9. The (–)ESI-Q-TOF mass spectrum is provided in Fig. S10.
The overall yield for the synthesis of cis-β-IEPOX from citraconic acid was 57 %.
2.3 cis-β-IEPOX (cis-(2-methyloxirane-2,3-diyl)dimethanol) from 3-methyl-2(5H)-furanone
2.3.1 Z-2-Methylbut-2-ene-1,4-diol
A solution of 3-methyl-2(5H)-furanone (1.1 g, 11 mmol) in methylene chloride (30 mL) under argon was cooled to 0 ∘C, and DIBAL-H (21 mL 1.0 M solution in toluene, 21 mmol) was added dropwise. The reaction mixture was stirred at 0 ∘C for 1 h, diluted with ether (60 mL), and quenched with 0.85 mL water (0.04 of DIBAL-H volume equivalents), followed by 0.85 mL 15 % sodium hydroxide solution (0.04 DIBAL-H volume equivalents) and 2.1 mL water (0.1 volume equivalents). After quenching, the mixture was allowed to warm to room temperature over 2 h and dried over magnesium sulfate. The aluminum salt was filtered out through a pad of Celite, and the collected solid was further washed with ethyl acetate (100 mL). The solvent was removed on a rotary evaporator under house vacuum to yield Z-2-methylbut-2-ene-1,4-diol (1.0 g, 94 % yield) as a colorless oil, purity >98 %, by NMR; see Fig. S7.
2.3.2 cis-(2-Methyloxirane-2,3-diyl)dimethanol (cis-β-IEPOX)
Butene diol (0.92 g, 9.0 mmol) was dissolved in acetonitrile (50 mL) and cooled in an ice-water bath. m-CPBA (1.9 g, 14 mmol) was added, and the clear solution was stirred in the ice-water bath for 1 h and then at room temperature until complete transformation of the starting material, as monitored by TLC (SiO2, 1:1 hexane : ethyl acetate). The mixture was cooled at 0 ∘C, and the resulting precipitate was separated by filtration to remove the bulk of the 3-chlorobenzoic acid. The filtrate was dried on a rotary evaporator under house vacuum, and the residue was dissolved in water (30 mL). The aqueous solution was washed repeatedly with chloroform and lyophilized and isolated as a crude product to give cis-(2-methyloxirane-2,3-diyl)dimethanol as a colorless oil (0.88 g, 83 %), purity >98 %, by NMR; see Figs. S8 and S9.
The overall yield for the synthesis cis-β-IEPOX from 3-methyl-2(5H)-furanone was 69 %.
Several synthetic routes to the β-IEPOX isomers have been published to date. Procedures for the synthesis of trans-β-IEPOX followed the three strategies given in Figs. 1–3.
Figure 1, the first published route to trans-β-IEPOX (Cole-Filipiak et al., 2010), yielded a mixture of cis and trans products in four steps. The procedure is lengthy, and consecutive vacuum distillations for isolation of 1,4-dibromoisoprene from the 1,4-diol were required. The mixture was not separated, and the combined overall yield was 11 %.
The approach in Fig. 2 (Zhang et al., 2012) has been used in most syntheses reported subsequent to publication in 2012. Figure 2 targets synthesis of the trans isomer starting with prenol (3-methyl-2-buten-1-ol). SeO2 oxidation of the trisubstituted olefin yielded E-2-methylbut-2-ene-1,4-diol. Deprotection of the diol, followed by epoxidation with m-CPBA gave the target trans-β-IEPOX in an overall yield of 43 %. The expected trans geometry of the ultimate IEPOX isomer (Trachtenberg, et al., 1970; Sharpless and Lauer, 1972) was confirmed by the absence of a nuclear Overhauser effect correlation between the methyl group and the oxirane proton in the 1D NOESY (nuclear Overhauser effect spectroscopy) spectrum. An overall yield of ∼11 % can be calculated for synthesis by Fig. 2 in the only other report citing yields (Bates et al., 2014). The SeO2 oxidation–NaBH4 reduction sequence to generate 2-methylbut-2-ene-1,4-diol appears largely responsible for the discrepancy in yields. Isolation of the diol from the NaBH4 reduction step yields a mixture from which the isolation of a product is challenging and is most likely the source of the difference.
More recently, Fig. 3, a route to trans-β-IEPOX-d2, has been reported that could also serve as a route to the protio compound by substituting LAH4 for LAD4 as the reducing agent (Chase et al., 2015).
This route also involves a problematic metal hydride reduction step, and an overall yield of 31 % was reported. Figures 1–3 have steps that are difficult to accomplish in common, such as vacuum distillation, or they require carefully controlled conditions for the protection/deprotection of labile substituents, with the best reported yield being 43 % for Fig. 2 (Zhang et al., 2012).
Here we report a procedure for the synthesis of pure racemic trans-β-IEPOX that is efficient and simple and provides the target IEPOX with an overall yield of 62 %. No protection/deprotection steps, which add steps and can decrease yields, are involved, and no specialized glassware or instrumentation is required. The strategy for synthesis follows Fig. 4, which is based on inexpensive, readily available mesaconic acid as the starting material.
Mesaconic acid can be esterified to the dimethyl ester in high yield by refluxing in methanol containing 2 % concentrated sulfuric acid. The diester is reduced by diisobutylaluminum hydride (DIBAL-H) in methylene chloride to E-2-methylbut-2-en-1,4-diol, which is epoxidized by m-CPBA in acetonitrile. Key to the procedure is the efficient extraction of E-2-methylbut-2-ene-1,4-diol from the DIBAL-H reduction reaction with ethyl acetate, which allows for recovery of the diol in 70 % yield. The route in Fig. 4 will make trans-β-IEPOX readily available to laboratories without sophisticated synthesis capabilities. The procedure is particularly attractive because it can readily be scaled up to produce trans-β-IEPOX in gram quantities.
The isolation of E-2-methylbut-2-ene-1,4-diol from the DIBAL-H reduction reaction in high yield by extraction with ethyl acetate led us to revisit published syntheses of cis-β-IEPOX in which metal hydrides were used as reduction reagents (Bates et al., 2014, 2016; Zhang et al., 2012). In Figs. 5 and 6, citraconic anhydride was the starting material, and the reducing agent was DIBAL-H.
The reported overall yield of cis-β-IEPOX from Fig. 5 was 12 % (Bates et al., 2014). Figure 6 streamlined the synthesis through bypassing steps 2 and 3 of Fig. 5 with direct reduction of citraconic anhydride to Z-2-methylbut-2-en-1,4-diol (Bates et al., 2016). Reduction of the anhydride required forcing conditions (five equivalents of DIBAL-H were used) to achieve reduction of citraconic anhydride, and possibly less efficient recovery of the diol resulted in the same overall yield reported for Fig. 5.
3-Methylfuran-2(5H)-one was the starting material for Fig. 7 and was reduced directly to Z-2-methylbut-2-en-1,4-diol by LAH (Zhang et al., 2012).
LAH is a powerful reducing agent leading to some unavoidable over-reduction of the furanone to the saturated diol, and the overall yield was 19 %. We repeated the synthesis of cis-β-IEPOX using either citraconic acid or 3-methylfuran-2(5H)-one as the starting point (Fig. 8). Because reduction of anhydrides to diols generally appears to be more difficult and requires forcing conditions (Bloomfield and Lee, 1967), we selected citraconic acid rather than the anhydride as the starting point for the synthesis. Citraconic acid requires esterification prior to reduction. Although the dimethyl ester is commercially available, the esterification reaction is very straightforward with a nearly quantitative yield, and the savings in cost are substantial.
Under the assumption that sterically hindered cis diester would nevertheless not proceed as readily as reduction of the trans diester, five equivalents of DIBAL-H were used for the reduction of citraconic diester. With a yield of 74.9 %, the reduction of the diester proved to be much more efficient than that of citraconic anhydride, for which the reported yield was 28 % (Bates et al., 2016). The overall yield for this route was 57 %, which represents a significant improvement over the yields reported by the routes in Figs. 5 and 6.
3-Methylfuran-2(5H)-one is a more expensive starting compound than citraconic acid or citraconic anhydride, but the procedure is streamlined to two steps, and the overall yield, at 69 %, is much higher than for any of the published routes. Cost of reagents would largely dictate the choice of citraconic acid or 3-methylfuran-2(5H)-one starting material. We note that trans- and cis-β-IEPOX were isolated directly following lyophilization. The purity of trans- and cis-β-IEPOX was >98 % by NMR (Figs. S3–S4 and S8–S9, respectively).
Unprocessed free induction decay signals (FIDs) and mass spectral data files are available on request from the corresponding author (golda@email.unc.edu).
Included in the Supplement are 1H NMR spectra of the target cis- and trans-β-IEPOX isomers and key intermediates in the synthetic routes. Additional 13C and (–)ESI-Q-TOF mass spectra are provided for the target cis- and trans-β-IEPOX isomers. The supplement related to this article is available online at: https://doi.org/10.5194/acp-23-7859-2023-supplement.
All authors contributed equally to the planning and performing of the experiments and the preparation of the manuscript. MF acquired spectroscopic data. JDS helped with editing and manuscript preparation.
At least one of the (co-)authors is a member of the editorial board of Atmospheric Chemistry and Physics. The peer-review process was guided by an independent editor, and the authors also have no other competing interests to declare.
Publisher’s note: Copernicus Publications remains neutral with regard to jurisdictional claims in published maps and institutional affiliations.
This research has been supported by the National Science Foundation (grant nos. AGS-2001027 and AGS-2039788).
This paper was edited by Ivan Kourtchev and reviewed by two anonymous referees.
Armstrong, N. C., Chen, Y., Cui, T., Zhang, Y., Christensen, C., Zhang, Z., Turpin, B. J., Chan, M.N., Gold, A., Ault, A. P., and Surratt, J. D.: Isoprene epoxydiol-derived sulfated and nonsulfated oligomers suppress particulate mass loss during oxidative aging of secondary organic aerosol, Environ. Sci. Technol., 56, 16611–16620, https://doi.org/10.1021/acs.est.2c03200, 2022.
Bates, K. H., Crounse, J. D., St Clair, J. M., Bennett, N. B., Nguyen, T. B., Seinfeld, J. H., Stoltz B. M., and Wennberg, P. O.: Gas phase production and loss of isoprene epoxydiols, J. Phys. Chem. A, 118, 1237–1246, https://doi.org/10.1021/jp4107958, 2014.
Bates, K. H., Nguyen, T. B., Teng, A. P., Crounse, J. D., Kjaergaard, H. G., Stoltz, B. M. Seinfeld, J. H., and Wennberg, P. O.: Production and fate of C4 dihydroxycarbonyl compounds from isoprene oxidation, J. Phys. Chem. A, 120, 106–117, https://doi.org/10.1021/acs.jpca.5b10335, 2016.
Bloomfield, J. J. and Lee, S. L.: Control of lithium aluminum hydride reduction of cyclic dicarboxylic acid anhydrides to produce 7-lactones or diols, J. Org. Chem., 32, 3919–3924, 1967.
Budisulistiorini, S. H., Li, X., Bairai, S. T., Renfro, J., Liu, Y., Liu, Y. J., McKinney, K. A., Martin, S. T., McNeill, V. F., Pye, H. O. T., Nenes, A., Neff, M. E., Stone, E. A., Mueller, S., Knote, C., Shaw, S. L., Zhang, Z., Gold, A., and Surratt, J. D.: Examining the effects of anthropogenic emissions on isoprene-derived secondary organic aerosol formation during the 2013 Southern Oxidant and Aerosol Study (SOAS) at the Look Rock, Tennessee ground site, Atmos. Chem. Phys., 15, 8871–8888, https://doi.org/10.5194/acp-15-8871-2015, 2015.
Budisulistiorini, S. H., Baumann, K., Edgerton, E. S., Bairai, S. T., Mueller, S., Shaw, S. L., Knipping, E. M., Gold, A., and Surratt, J. D.: Seasonal characterization of submicron aerosol chemical composition and organic aerosol sources in the southeastern United States: Atlanta, Georgia,and Look Rock, Tennessee, Atmos. Chem. Phys., 16, 5171–5189, https://doi.org/10.5194/acp-16-5171-2016, 2016.
Chase, H. M., Psciuk, B. T., Strick, B. L., Thomson, R. J., Batista, V. S., and Geiger, F. M.: Beyond local group modes in vibrational sum frequency generation, J. Phys. Chem. A, 119, 3407–3414. https://doi.org/10.1021/jp511067d, 2015.
Cole-Filipiak, N. C., O'Connor, A. E., and Elrod, M. J.: Kinetics of the hydrolysis of atmospherically relevant isoprene-derived hydroxy epoxides, Environ. Sci. Technol., 44, 6718–6723, https://doi.org/10.1021/es102631b, 2010.
Cooke, M. E., Armstrong, N. C., Lei, Z., Chen, Y., Waters, C. M., Zhang, Y., Buchenau, N. A., Dibley, M. Q., Ledsky, I. R., Szalkowski, T., Lee, J. Y., Baumann, K., Zhang, Z., Vizuete, W., Gold, A., Surratt, J. D., and Ault, A. P.: Organosulfate formation in proxies for aged sea spray aerosol: reactive uptake of isoprene epoxydiols to acidic sodium sulfate, ACS Earth Space Chem., 6 , 2790–2800, https://doi.org/10.1021/acsearthspacechem.2c00156, 2022.
Cui, T., Zeng, Z., dos Santos, E. O., Zhang, Z., Chen, Y., Zhang, Y., Rose, C. A., Budisulistiorini, S. H., Collins, L. B., Bodnar, W. M., de Souza, R. A. F., Martin, S. T., Machado, C. M. D., Turpin, B. J., Gold, A., Ault, A. P., and Surratt, J. D.: Development of a Hydrophilic Interaction Liquid Chromatography (HILIC) method for the chemical characterization of water-soluble Isoprene Epoxydiol (IEPOX)-derived secondary organic aerosol, Environ. Sci. Process. Impacts, 20, 1524–1536, https://doi.org/10.1039/C8EM00308D, 2018.
Gaston, C. J., Riedel, T. P., Zhang, Z., Gold, A., Surratt, J. D., and Thornton, J. A.: Reactive uptake of an isoprene-derived epoxydiol to submicron aerosol particles, Environ. Sci. Technol., 48, 11178–11186, https://doi.org/10.1021/es5034266, 2014.
Guenther, A., Karl, T., Harley, P., Wiedinmyer, C., Palmer, P. I., and Geron, C.: Estimates of global terrestrial isoprene emissions using MEGAN (Model of Emissions of Gases and Aerosols from Nature), Atmos. Chem. Phys., 6, 3181–3210, https://doi.org/10.5194/acp-6-3181-2006, 2006.
Hallquist, M., Wenger, J. C., Baltensperger, U., Rudich, Y., Simpson, D., Claeys, M., Dommen, J., Donahue, N. M., George, C., Goldstein, A. H., Hamilton, J. F., Herrmann, H., Hoffmann, T., Iinuma, Y., Jang, M., Jenkin, M. E., Jimenez, J. L., Kiendler-Scharr, A., Maenhaut, W., McFiggans, G., Mentel, Th. F., Monod, A., Prévôt, A. S. H., Seinfeld, J. H., Surratt, J. D., Szmigielski, R., and Wildt, J.: The formation, properties and impact of secondary organic aerosol: current and emerging issues, Atmos. Chem. Phys., 9, 5155–5236, https://doi.org/10.5194/acp-9-5155-2009, 2009.
Jo, D. S., Hodzic, A., Emmons, L. K., Marais, E. A., Peng, Z., Nault, B. A., Hu, W., Campuzano-Jost, P., and Jimenez, J. L.: A simplified parameterization of isoprene-epoxydiol-derived secondary organic aerosol (IEPOX-SOA) for global chemistry and climate models: a case study with GEOS-Chem v11-02-rc, Geosci. Model Dev., 12, 2983–3000, https://doi.org/10.5194/gmd-12-2983-2019, 2019.
Kanakidou, M., Seinfeld, J. H., Pandis, S. N., Barnes, I., Dentener, F. J., Facchini, M. C., Van Dingenen, R., Ervens, B., Nenes, A., Nielsen, C. J., Swietlicki, E., Putaud, J. P., Balkanski, Y., Fuzzi, S., Horth, J., Moortgat, G. K., Winterhalter, R., Myhre, C. E. L., Tsigaridis, K., Vignati, E., Stephanou, E. G., and Wilson, J.: Organic aerosol and global climate modelling: a review, Atmos. Chem. Phys., 5, 1053–1123, https://doi.org/10.5194/acp-5-1053-2005, 2005.
Klimovica, K., Grigorjeva, L., Maleckis, A., Popelis, J., and Jirgensons, A.: C-quaternary vinylglycinols by metal-catalyzed cyclization of allylic bistrichloroacetimidates, Synlett, 22, 2849–2851, https://doi.org/10.1055/s-00000083, 2011.
Lin, Y.-H., Zhang, Z., Docherty, K. S., Zhang, H., Budisulistiorini, S. H., Rubitschun, C. L., Shaw, S. L., Knipping, E. M., Edgerton, E. S., and Kleindienst, T. E.: Isoprene epoxydiols as precursors to secondary organic aerosol formation: acid-catalyzed reactive uptake studies with authentic compounds, Environ. Sci. Technol., 46, 250–258, 2012.
Lin, Y.-H., Knipping, E. M., Edgerton, E. S., Shaw, S. L., and Surratt, J. D.: Investigating the influences of SO2 and NH3 levels on isoprene-derived secondary organic aerosol formation using conditional sampling approaches, Atmos. Chem. Phys., 13, 8457–8470, https://doi.org/10.5194/acp-13-8457-2013, 2013.
Lin, Y.-H., Budisulistiorini, S. H., Chu, K., Siejack, R. A., Zhang, H., Riva, M., Zhang, Z., Gold, A., Kautzman, K. E., and Surratt, J. D.: Light-absorbing oligomer formation in secondary organic aerosol from reactive uptake of isoprene epoxydiols, Environ. Sci. Technol., 48, 12012–12021, 2014.
Marais, E. A., Jacob, D. J., Jimenez, J. L., Campuzano-Jost, P., Day, D. A., Hu, W., Krechmer, J., Zhu, L., Kim, P. S., Miller, C. C., Fisher, J. A., Travis, K., Yu, K., Hanisco, T. F., Wolfe, G. M., Arkinson, H. L., Pye, H. O. T., Froyd, K. D., Liao, J., and McNeill, V. F.: Aqueous-phase mechanism for secondary organic aerosol formation from isoprene: application to the southeast United States and co-benefit of SO2 emission controls, Atmos. Chem. Phys., 16, 1603–1618, https://doi.org/10.5194/acp-16-1603-2016, 2016.
McNeill, V. F.: Aqueous organic chemistry in the atmosphere: Sources and chemical processing of organic aerosols, Environ. Sci. Technol., 49, 1237–1244, 2015.
Nguyen, T. B., Coggon, M. M., Bates, K. H., Zhang, X., Schwantes, R. H., Schilling, K. A., Loza, C. L., Flagan, R. C., Wennberg, P. O., and Seinfeld, J. H.: Organic aerosol formation from the reactive uptake of isoprene epoxydiols (IEPOX) onto non-acidified inorganic seeds, Atmos. Chem. Phys., 14, 3497–3510, https://doi.org/10.5194/acp-14-3497-2014, 2014.
Paulot, F., Crounse, J. D., Kjaergaard, H. G., Kürten, A., St. Clair, J. M., Seinfeld, J. H., and Wennberg, P. O.: Unexpected epoxide formation in the gas-phase photooxidation of isoprene, Science, 325, 730–733, https://doi.org/10.1126/science.1172910, 2009.
Pope, C. A. and Dockery, D. W.: Health effects of fine particulate air pollution: lines that connect, J. Air Waste Manage., 56, 709–42, 1047–3289, 2006.
Pye, H. O., Pinder, R. W., Piletic, I. R., Xie, Y., Capps, S. L., Lin, Y.-H., Surratt, J. D., Zhang, Z., Gold, A., and Luecken, D. J.: Epoxide pathways improve model predictions of isoprene markers and reveal key role of acidity in aerosol formation, Environ. Sci. Technol., 47, 11056–11064, 2013.
Pye, H. O. T., Ward-Caviness, C. K., Murphy, B. N., Appel, K. W., and Seltzer, K. M.: Secondary organic aerosol association with cardiorespiratory disease mortality in the United States, Nat. Commun., 12, 7215, https://doi.org/10.1038/s41467-021-27484-1, 2021.
Rattanavaraha, W., Chu, K., Budisulistiorini, S. H., Riva, M., Lin, Y.-H., Edgerton, E. S., Baumann, K., Shaw, S. L., Guo, H., King, L., Weber, R. J., Neff, M. E., Stone, E. A., Offenberg, J. H., Zhang, Z., Gold, A., and Surratt, J. D.: Assessing the impact of anthropogenic pollution on isoprene-derived secondary organic aerosol formation in PM2.5 collected from the Birmingham, Alabama, ground site during the 2013 Southern Oxidant and Aerosol Study, Atmos. Chem. Phys., 16, 4897–4914, https://doi.org/10.5194/acp-16-4897-2016, 2016.
Riedel, T. P., Lin, Y.-H., Budisulistiorini, S. H., Gaston, C. J., Thornton, J. A., Zhang, Z., Vizuete, W., Gold, A., and Surratt, J. D.: Heterogeneous reactions of isoprene-derived epoxides: reaction probabilities and molar secondary organic aerosol yield estimates, Environ. Sci. Technol. Lett., 2, 38–42, 2015.
Riva, M., Chen, Y., Zhang, Y., Lei, Z., Olson, N. E., Boyer, H. C., Narayan, S., Yee, L D., Green, H. S., Cui, T., Zhang, Z., Baumann, K., Fort, M., Edgerton, E., Budisulistiorini, S. H., Rose, C. A., e Oliveira, I. O., Ribeiro, R. L., dos Santos, E. O., Machado, C. M. D., Szopa, S., Zhao, Y., Alves, E. G., de Sá, S. S., Hu, W., Knipping, E. M., Shaw, S. L., Junior, S. D., de Souza, R. A. F., Palm, B. B., Jimenez, J.-L., Glasius, M., Goldstein, A. H., Pye, H. O. T., Gold, A., Turpin, B. J., Vizuete, W., Martin, S. T., Thornton, J. A., Dutcher, C. S., Ault, A. P., Surratt, J. D.: Increasing isoprene epoxydiol-to-inorganic sulfate aerosol ratio results in extensive conversion of inorganic sulfate to organosulfur forms: Implications for aerosol physicochemical properties, Environ. Sci. Technol., 53, 8682–8694, https://doi.org/10.1021/acs.est.9b01019, 2019.
Sharpless, K. B. and Lauer, R. F.: Selenium dioxide oxidation of olefins. Evidence for the intermediacy of allylseleninic acids, J. Am. Chem. Soc., 94, 7154–7155, 1972.
St. Clair, J. M., Rivera-Rios, J. C., Crounse, J. D., Knap, H. C., Bates, K. H., Teng, A. P., Jorgensen, S., Kjaergaard, H. G., Keutsch, F. N., Wennberg, P. O.: Kinetics and products of the reaction of the first-generation isoprene hydroxy hydroperoxide (ISOPOOH) with OH, J. Phys. Chem. A, 120, 1441–1451, https://doi.org/10.1021/acs.jpca.5b06532, 2016.
Surratt, J. D., Chan, A. W., Eddingsaas, N. C., Chan, M., Loza, C. L., Kwan, A. J., Hersey, S. P., Flagan, R. C., Wennberg, P. O., and Seinfeld, J. H.: Reactive intermediates revealed in secondary organic aerosol formation from isoprene, P. Natl. Acad. Sci. USA, 107, 6640–6645, 2010.
Trachtenberg, E. N., Nelson, C. H., and Carver, J. R.: Mechanism of selenium dioxide oxidation of Olefins, J. Org. Chem., 35, 1653–1658, 1970.
Wennberg, P. O., Bates, K. H., Crounse, J. D., Dodson, L. G., McVay. R. C., Mertens, L. A., Nguyen, T. B., Praske, E., Schwantes, R. H., Smarte, M. D., St Clair, J. M., Teng, A. P., Zhang, X., and Seinfeld, J. H.: Gas-phase reactions of isoprene and its major oxidation products, Chem. Rev., 118, 3337–3390, https://doi.org/10.1021/acs.chemrev.7b00439, 2018.
Zhang, Y., Chen, Y., Lambe, A. T., Olson, N. E., Lei, Z., Craig, R. L., Zhang, Z., Gold, A., Onasch, T. B., Jayne, J. T., Worsnop, D. R., Gaston, C. J., Thornton, J. A., Vizuete, W., Ault, A. P., and Surratt, J. D.: Effect of the aerosol-phase state on secondary organic aerosol formation from the reactive uptake of isoprene-derived epoxydiols (IEPOX), Environ. Sci. Technol. Lett., 5, 167–174, https://doi.org/10.1021/acs.estlett.8b00044, 2018.
Zhang, Z., Lin, Y.-H., Zhang, H., Surratt, J. D., Ball, L. M., and Gold, A.: Technical Note: Synthesis of isoprene atmospheric oxidation products: isomeric epoxydiols and the rearrangement products cis- and trans-3-methyl-3,4-dihydroxytetrahydrofuran, Atmos. Chem. Phys., 12, 8529–8535, https://doi.org/10.5194/acp-12-8529-2012, 2012.