the Creative Commons Attribution 4.0 License.
the Creative Commons Attribution 4.0 License.
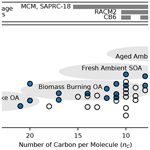
Linking gas, particulate, and toxic endpoints to air emissions in the Community Regional Atmospheric Chemistry Multiphase Mechanism (CRACMM)
Bryan K. Place
Benjamin N. Murphy
Karl M. Seltzer
Emma L. D'Ambro
Christine Allen
Ivan R. Piletic
Sara Farrell
Rebecca H. Schwantes
Matthew M. Coggon
Emily Saunders
Golam Sarwar
William T. Hutzell
Kristen M. Foley
George Pouliot
Jesse Bash
William R. Stockwell
Chemical mechanisms describe the atmospheric transformations of organic and inorganic species and connect air emissions to secondary species such as ozone, fine particles, and hazardous air pollutants (HAPs) like formaldehyde. Recent advances in our understanding of several chemical systems and shifts in the drivers of atmospheric chemistry warrant updates to mechanisms used in chemical transport models such as the Community Multiscale Air Quality (CMAQ) modeling system. This work builds on the Regional Atmospheric Chemistry Mechanism version 2 (RACM2) and develops the Community Regional Atmospheric Chemistry Multiphase Mechanism (CRACMM) version 1.0, which demonstrates a fully coupled representation of chemistry leading to ozone and secondary organic aerosol (SOA) with consideration of HAPs. CRACMMv1.0 includes 178 gas-phase species, 51 particulate species, and 508 reactions spanning gas-phase and heterogeneous pathways. To support estimation of health risks associated with HAPs, nine species in CRACMM cover 50 % of the total cancer and 60 % of the total non-cancer emission-weighted toxicity estimated for primary HAPs from anthropogenic and biomass burning sources in the US, with the coverage of toxicity higher (> 80 %) when secondary formaldehyde and acrolein are considered. In addition, new mechanism species were added based on the importance of their emissions for the ozone, organic aerosol, or atmospheric burden of total reactive organic carbon (ROC): sesquiterpenes, furans, propylene glycol, alkane-like low- to intermediate-volatility organic compounds (9 species), low- to intermediate-volatility oxygenated species (16 species), intermediate-volatility aromatic hydrocarbons (2 species), and slowly reacting organic carbon. Intermediate- and lower-volatility organic compounds were estimated to increase the coverage of anthropogenic and biomass burning ROC emissions by 40 % compared to current operational mechanisms. Autoxidation, a gas-phase reaction particularly effective in producing SOA, was added for C10 and larger alkanes, aromatic hydrocarbons, sesquiterpenes, and monoterpene systems including second-generation aldehydes. Integrating the radical and SOA chemistry put additional constraints on both systems and enabled the implementation of previously unconsidered SOA pathways from phenolic and furanone compounds, which were predicted to account for ∼ 30 % of total aromatic hydrocarbon SOA under typical atmospheric conditions. CRACMM organic aerosol species were found to span the atmospherically relevant range of species carbon number, number of oxygens per carbon, and oxidation state with a slight high bias in the number of hydrogens per carbon. In total, 11 new emitted species were implemented as precursors to SOA compared to current CMAQv5.3.3 representations, resulting in a bottom-up prediction of SOA, which is required for accurate source attribution and the design of control strategies. CRACMMv1.0 is available in CMAQv5.4.
- Article
(4980 KB) - Full-text XML
- Companion paper
-
Supplement
(1497 KB) - BibTeX
- EndNote
Reactive organic carbon (ROC) (Safieddine et al., 2017) includes all atmospheric organic species excluding methane and is abundant throughout the troposphere. Particulate forms of ROC are found in fine particles (PM2.5), and gaseous ROC is a major precursor to ozone (O3) and secondary organic aerosol (SOA) (Heald and Kroll, 2020). Recent work indicates that preferentially controlling emissions of ROC could yield significant health benefits by mitigating the mortality associated with ambient air pollution in the US (Pye et al., 2022). These predicted benefits come primarily from reductions in SOA, which is strongly associated with cardiorespiratory mortality (Pye et al., 2021; Pond et al., 2022). ROC also includes hazardous air pollutants (HAPs) such as benzene and formaldehyde that result in cancer and non-cancer risks to health (Scheffe et al., 2016).
Atmospheric chemical mechanisms connect ROC emissions to endpoints like SOA, O3, and secondary HAPs and are used to inform air quality management strategies to mitigate the impacts of air pollution. Chemical mechanisms were traditionally designed for estimating ambient O3 although not necessarily the lower levels of O3 observed today (Kaduwela et al., 2015) or sources of growing importance around the globe such as volatile chemical products (VCPs, also referred to as solvents) (Coggon et al., 2021; Karl et al., 2018; McDonald et al., 2018; Zheng et al., 2018) and biomass burning (Jaffe and Wigder, 2012) that are changing the composition of emissions towards increasingly oxygenated ROC (Venecek et al., 2018). While mechanisms may predict O3 reasonably well on broad spatial and temporal scales (Simon et al., 2012; Xing et al., 2015; Young et al., 2018), regional biases in predicted O3 can exceed 10 ppb (Young et al., 2018; Solazzo et al., 2017) or 20 % (Appel et al., 2012, 2021). Global model estimates of chemical production and loss of ozone also vary by a factor of ∼ 2 (Young et al., 2018), and emerging chemical pathways missing from standard models, such as particulate nitrate photolysis, can increase free-tropospheric ozone by 5 ppb (Shah et al., 2023), indicating a continued need for model development for ozone prediction. Furthermore, even when mechanisms are relatively similar in their O3 predictions, they can differ substantially in terms of predicted intermediates like the hydroxyl radical (HO) and nitrate radical (NO3) as well as products like formaldehyde and SOA (Knote et al., 2015). Model representations of organic aerosol are particularly diverse and span a factor of 10 in their estimates of global SOA source strength (Tsigaridis et al., 2014). Given parts of 22 different states are in marginal attainment to extreme non-attainment for the current US 8 h (2012) O3 standard (as of August 2022) (U.S. Environmental Protection Agency, 2022d) as well as recent work demonstrating health effects below the current fine-particle standards (Makar et al., 2017), increasingly accurate representations of emissions and how they connect to chemistry will be needed to inform air quality management strategies going forward. In addition, future implementation of global air quality guidelines, such as those from the World Health Organization, may need to account for the speciation of ambient aerosol since different species have different anthropogenic contributions (Pai et al., 2022).
In most chemical transport models used for air quality prediction, SOA algorithms are disconnected from the gas-phase radical chemistry leading to O3 formation (Pye et al., 2010; Ahmadov et al., 2012; Koo et al., 2014; Tilmes et al., 2015), leading to duplication of mass in the O3 and SOA representations. Gas-phase chemical mechanisms also typically exclude non-traditional species with saturation concentrations () in the low-volatility organic compound (LVOC; µg m−3) and semivolatile organic compound (SVOC; µg m−3) range. In addition, some gas-phase mechanisms also exclude intermediate-volatility organic compounds (IVOCs; 102.5 µg m−3) (Shah et al., 2020), which are potent SOA precursors but are somewhat less important for O3 formation than volatile organic compounds (VOCs; µg m−3). Recent studies have noted that the magnitude of VCP emissions exerts significant impact on model-predicted O3 but predicted SOA mass is relatively insensitive to VCP emissions due to a lack of suitable SOA precursors in standard mechanisms (Qin et al., 2021; Pennington et al., 2021; Zhu et al., 2019). This conclusion is consistent with the ROC budget analysis for Pasadena, California, by Heald et al. (2020) that suggests SOA formation requires consideration of precursors beyond traditional, non-oxygenated volatile hydrocarbons represented in most current SOA treatments.
Due to the challenges in representing SOA chemistry in mechanisms, some chemical transport models have opted to use empirical representations of anthropogenic SOA. These parameterizations are not tied to the behavior of specific parent hydrocarbon compounds or emission sources and fall into two classes: multigenerational and simplified. Multigenerational anthropogenic SOA treatments (Robinson et al., 2007) generally leverage the volatility basis set (VBS) framework and add IVOC and SVOC emissions thought to be missed by current measurement techniques (Koo et al., 2014; Ahmadov et al., 2012). Species throughout the µg m−3 volatility range are chemically processed over multiple HO reactions, leading to the production of lower-volatility species and SOA mass. Simplified representations use CO (Hodzic and Jimenez, 2011; Kim et al., 2015), primary organic aerosol (Murphy et al., 2017), or C4H10 (Dunne et al., 2020) as a surrogate for anthropogenic activity and precursor emissions that oxidize in one step to SOA. Since the SOA predicted from traditional anthropogenic hydrocarbon precursors has typically been small compared to observed SOA in urban locations (Woody et al., 2016), these schemes can be implemented in parallel to, or as a replacement for, explicit SOA precursor schemes based on traditional VOC precursors. The simplified surrogate approaches are fit to ambient data and thus have the advantage of reproducing observed levels of SOA (Qin et al., 2021; Nault et al., 2018; Murphy et al., 2017). For applications like the calculation of present-day aerosol optical depth or PM2.5 mass (e.g., Pye et al., 2021), empirical representations of anthropogenic SOA may be sufficient. However, the policy applications of empirical approaches are limited because they add emissions external to the regulatory reporting and model platform framework, do not allow for the separation of individual anthropogenic source contributions, and do not consider the representativeness of the emitted proxy in the context of a changing emission or chemical regime, all of which are needed for the design of regulatory control strategies.
In this work, the first version of the Community Regional Atmospheric Chemistry Multiphase Mechanism (CRACMM) is developed and presented. CRACMMv1.0 builds off the history of the Regional Atmospheric Chemistry Mechanism (RACM) development (Stockwell et al., 1997). RACM version 2 (Goliff et al., 2013) was chosen as a framework since it is implemented in regional models such as the Community Multiscale Air Quality (CMAQ) modeling system (Sarwar et al., 2013), provides a competitive computational speed with mechanisms used in regulatory applications (Sarwar et al., 2013), retains the carbon backbone of emitted species, represents individual peroxy radicals, and relies minimally on aggregated species for radical cycling (operators). Because of these features, RACM2 facilitates comparison with observations, provides transparency in emission mapping, and is relatively easy to modify and expand.
The purpose of the CRACMM version 1.0 effort described here is to demonstrate a coupled representation of NOx–ROC–O3 chemistry including SOA and the consideration of HAPs. In addition, this work includes the development of rules for mapping emitted ROC to mechanism species and updates to rate constants leading to a publicly available mechanism upon which further developments can be built. CRACMM is expected to become the default option in CMAQ in the future (U.S. Environmental Protection Agency, 2021c). While the mechanism is presented in the context of US conditions, it is informed by conditions outside the US (e.g., the work of Zhao et al., 2016, for China) and is meant to be generally relevant for tropospheric chemistry. CRACMM is available in the public release of CMAQv5.4 (U.S. EPA Office of Research and Development, 2022) and is distributed as a stand-alone mechanism (U.S. Environmental Protection Agency, 2022b). In this work, the aggregation of individual organic species to mechanism species (Sect. 2) and the chemistry (Sect. 3) and representation of HAPs (Sect. 4) are described for atmospheric ROC. The paper continues with a characterization of ROC in terms of oxidation state and van Krevelen space as well as estimated implications for O3 and fine-particle mass (Sect. 5). The paper concludes with a discussion on the importance of mechanism development with recommendations for future work (Sect. 6).
Various aspects of the development of CRACMM are related to the identity of ROC emissions. The methods behind characterizing emitted ROC and how it maps to mechanism species are described in the following section.
2.1 Individual emitted species
To inform the aggregation of individual species to mechanism species as well as estimate the contributions of mechanism species to endpoints like O3 and SOA, an emission inventory of individual ROC species was created for 2017 US conditions. Total ROC emissions from wildland fires, oil and gas extraction, vehicles, volatile chemical products, residential wood combustion, and other non-biogenic sectors were obtained following the Environmental Protection Agency's (EPA) Air QUAlity TimE Series (EQUATES) methods (Foley et al., 2023) based on the US National Emissions Inventory (NEI). The HAPs naphthalene, benzene, acetaldehyde, formaldehyde, and methanol (NBAFM) were included as specific species when available in the NEI. In the case of mobile emissions estimated with the MOVES model (MOtor Vehicle Emission Simulator; U.S. Environmental Protection Agency, 2020) and solvents estimated with the volatile chemical products in Python (VCPy) model (Seltzer et al., 2021), total ROC and individual HAPs (e.g., ethyl benzene, acrolein, styrene, and others in addition to NBAFM) were estimated consistently. For the remaining sectors, HAP species were estimated as a fraction of total ROC based on speciation profiles for different sources. In addition to the base EQUATES emissions, L/S/IVOC (LVOC, SVOC, and/or IVOC) emissions missing from the mobile-sector inventoried ROC mass, estimated at 4.6 % of non-methane organic gas (NMOG) for gasoline vehicles and 55 % of NMOG from diesel vehicles, were added using the volatility distribution from the work of Lu et al. (2020). An additional 20 % of NMOG from wood-burning sources (wildland, prescribed, and residential) was estimated to be an IVOC (assigned a of 104 µg m−3) following the estimates of Jathar et al. (2014). L/S/IVOC emissions inventoried as part of primary PM2.5 were estimated using published volatility profiles for vehicles (Lu et al., 2020) and wood burning (May et al., 2013; Woody et al., 2016). Other sources of primary organic aerosol (POA) were assumed to behave as a species with a of 10−2 µg m−3.
The identity of the individual species within inventoried ROC as well as the L/S/IVOCs (Jathar et al., 2014; Lu et al., 2020) were characterized using the EPA SPECIATE database version 5.2 (Simon et al., 2010) (pre-release version; see “Code and data availability”). To provide chemical structure information and facilitate automated property estimation, compounds in the SPECIATE database were assigned a unique Distributed Structure-Searchable Toxicity Database Substance Identifier (DTXSID) (Grulke et al., 2019) using the U.S. EPA's Chemicals Dashboard (referred to as the Dashboard; U.S. Environmental Protection Agency, 2021d; Williams et al., 2017). DTXSIDs allowed for each emitted species to be associated with structural identifiers like Simplified Molecular Input Line Entry System (SMILES) and IUPAC (International Union of Pure and Applied Chemistry) International Chemical Identifier (InChI) representations. In about two-thirds of cases, the emitted SPECIATE species could be exactly matched to a representative compound with a DTXSID in the Dashboard. In the other cases, an isomer or generally representative compound with similar functionality (e.g., presence of aromaticity or other functional groups) and carbon number (e.g., undecane for “isomers of undecane”) was manually selected. For the small number of cases in which the SPECIATE species was indicated as “unknown,” “unidentified”, or similarly undefined, n-decane was assigned as the representative compound. If the unidentified compound was also indicated as exempt from the regulatory definition of VOC (Code of Federal Regulations, 1986) (e.g., “aggregated exempt compounds”, “other, lumped, exempts, individually < 2 % of category”), acetone was used as the representative compound. The representative compound's preferred name from the Dashboard, DTXSID identifier, and a degree of assignment confidence score (1: species not well defined, 2: species manually mapped, 3: species automatically matched in the Dashboard but some properties inconsistent, 4: exact match in the Dashboard) were added to SPECIATEv5.2 (U.S. Environmental Protection Agency, 2022e). A logical (true/false) field in the SPECIATE database was also used to identify individual compounds classified as HAPs (see Sect. 4).
By mapping each emitted species (i) to a unique structural identifier, properties of the emissions could be estimated in a traceable manner. The batch feature of the Dashboard (Lowe and Williams, 2021) was used to obtain molecular weights, SMILES strings, and molecular formulas as well as perform OPEn structure–activity/property Relationship App (OPERA) (Mansouri et al., 2018) calculations for the Henry's Law coefficient, rate constant for atmospheric reaction with HO (kOH), and vapor pressure of each ROC species. Vapor pressures () and molecular weights (Mi) were used to calculate pure-species saturation concentrations (Donahue et al., 2006) at a temperature (T) of 298 K (, where R is the gas constant and is reported in µg m−3).
While actual mechanism calculations are required to estimate the contribution of any species to O3 and SOA in a specific location, two simple structure–activity relationships (SARs) were created for screening-level analysis of organic aerosol (OA) and O3 formation potentials of individual ROC species. In the case of OA potential, several sources, largely following high-NOx conditions outlined in the work of Seltzer et al. (2021), were aggregated to estimate the SOA yield of individual species. In this work, exponential or quadratic polynomial fits depending on what was most applicable were applied to data on the yield of SOA vs. by chemical class for oxygenated hydrocarbons, polycyclic aromatic hydrocarbons (PAHs), substituted aromatics, and alkenes and to the yield of SOA vs. the number of carbons for normal, branched, and cyclic alkanes. Most systems showed a good correlation between predicted and expected SOA yield with a coefficient of determination (r2) of 0.67 in the case of oxygenated hydrocarbons and greater for the other species types. Explicit yield assignments were made based on published data in the case of sesquiterpenes, monoterpenes, benzene, toluene, and xylene (Pye et al., 2010; Ng et al., 2007). Published single-ring aromatic yields were scaled up by the vapor wall loss factor (Zhang et al., 2014). An OA concentration of 10 µg m−3 and equal low-NOx vs. high-NOx behavior, typical of Northern Hemisphere July conditions (Porter et al., 2021), were assumed for these explicit yield assignments. While this OA concentration is on the high end of the atmospherically relevant range, it is on the low end of concentrations probed in laboratory studies (Porter et al., 2021), thus providing a bridge between observations and ambient conditions.
A second simple SAR was created to estimate the role of individual ROC species in O3 formation as indicated by maximum incremental reactivity (MIR). Input data for regression fits were obtained from the SAPRC database (Carter, 2019), which contains MIR data for over 1000 compounds. In the case of ill-defined compounds in the SAPRC database, representative compound structures with DTXSIDs were assigned. Compounds were filtered into various chemical classes (halocarbons, oxygenated, aromatic, alkenes, etc.). Within a given class, the MIR was fit as a function of the number of carbons per molecule, HO rate constant (from OPERA), number of oxygens, number of double bonds, number of ring structures, number of double bonded oxygen, and/or number of branches depending on the chemical class. The overall r2 between SAPRC-estimated and simple-SAR-predicted MIRs (Fig. S8) was 0.72. The MIRs are most appropriate for comparing species under a given set of conditions as changes in chemical (or meteorological) regime, such as those in the US between 1988 and 2010, have been found to decrease species MIRs by about 20 % on average (Venecek et al., 2018). The SARs were used to estimate average SOA yields and MIR for all ROC species in the SPECIATE database.
2.2 Mechanism species
CRACMM species were designed to leverage the original RACM2 chemistry while also considering the properties of present-day emitted species, including properties indicative of SOA formation potential, with a goal of maintaining a reasonable mechanism size (by species count) for computational efficiency. New explicit species were added for multiple reasons. First, certain species are known to contribute significantly to cancer and non-cancer health risk (Scheffe et al., 2016). Second, recent advances in measurement techniques, particularly for VOCs, have increased the number of measured species available, which motivates adding these newly measured species explicitly into models for direct comparison. Third, some individual species are emitted in significant quantities, and explicit representation facilitates better conservation of mass and the representation of product distributions. New lumped species were also added when existing RACM2 species did not provide a good fit in terms of molecular properties, SOA yields, or O3 formation potential for emissions.
A Python mapper (see “Code and data availability”) was developed to automate mapping of individual, emitted ROC species to mechanism species. Once initial rules were created with the intent of following RACM2, properties of the mechanism species were visualized and mapping rules were manually adjusted to better preserve mass (minimize the spread in the number of carbons per molecule, molecular weight, and molar oxygen–carbon ratio within the model species), estimate SOA (minimize spread in the saturation concentration, SOA yield, and Henry's law coefficient within the model species), and predict O3 (minimize spread in the HO rate constant and O3 formation potential within each model species). A decision tree summarizing the final mapper is provided schematically in Supplement Figs. S1–S4. The mapper uses as input the SMILES string for the ROC species, HO rate constant, and pure component . Both kOH and can be estimated from a SMILES string prior to mapper input using OPERA algorithms (Mansouri et al., 2018) available for any organic species through the EPA Chemical Transformation Simulator (U.S. Environmental Protection Agency, 2022f). This emission mapping follows a hierarchy of rules in which explicit species are mapped first followed by lumped biogenic VOCs (α-pinene and other monoterpenes with one double bond, API; limonene and other monoterpenes with two or more double bonds, LIM; and sesquiterpenes, SESQ). Other lumped species and mapping rules were created to consider volatility, functional groups (parsed in Python using the work of RDKit, 2022), and kOH. For L/SVOCs, mechanism assignment was based purely on volatility except in the case of PAHs (more than one aromatic ring), which were grouped with naphthalene into a NAPH species (Sect. 3.5). For IVOCs, assignments considered volatility and the presence of specific functional groups (aromatic, oxygenated, alkane). For VOCs, mapping considered only functional groups and kOH.
Figures 1–3 (and Supplement Figs. S5–S6) show the final 2017 US emission-weighted distributions of compound properties for all emitted ROC species in CRACMMv1.0. Looking across multiple properties illustrates the hierarchy of emission-mapping rules. For example, three classes of alkane-like species (discussed in Sect. 3.1) were inherited from RACM2: HC3, HC5, and HC10 (formerly HC8). In carbon number space (Fig. 1), these species overlap in their coverage of individual compounds with all three classes including species with two to eight carbons per molecule. Their saturation concentration distributions (Fig. 2) also show overlap. The log 10(kOH) (Fig. 3) highlights that HC3, HC5, and HC10 are defined by distinct and mutually exclusive ranges of the HO rate constant. Indeed, the HO rate constant is the classifying property for the HC3, HC5, and HC10 species and is implemented after volatility, functional-group identity, and other features of the species have been considered. As another example, SLOWROC is multimodal in the number of carbons per molecule (nC) and (Figs. 1–2), which could necessitate separation into more species. However, SLOWROC reacts so slowly (Fig. 3) that additional speciation is not warranted. The systems in Figs. 1–3 indicated by color coding will be further discussed in the next section.
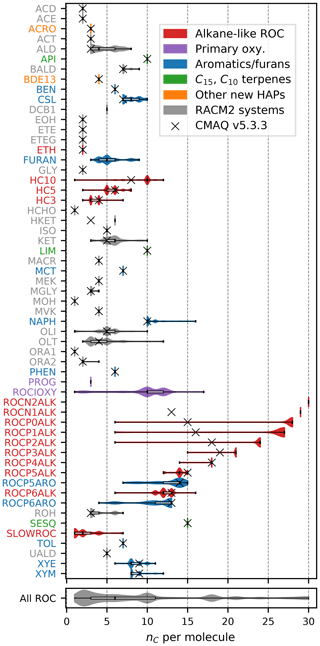
Figure 1Emission-weighted number of carbon atoms per molecule of individual ROC species grouped by CRACMM species. Violin plots (with shaded colors for families of species in Sect. 3 that are either new or substantially updated compared to RACM2) are weighted by the magnitude of US anthropogenic and biomass burning emissions in 2017. Overlaid boxplots indicate the 25th percentile, median, and 75th percentile values. Whiskers extend from the minimum to the maximum properties for species with emissions > 100 Mg yr−1. CMAQv5.3.3 values are for RACM2 with the aerosol module AERO6 or represent an individual HAP from CMAQ. In some cases, the CMAQv5.3.3 values represent similar species from RACM2 (e.g., HC8 values at CRACMM HC10). Emission magnitudes by species are available in Table D2 (Pye, 2022) in the Supplement. Species names and abbreviations can be found in Appendices A and B.
Multiple data sources were used to build the chemistry of CRACMM. As CRACMM will be a community mechanism in which different chemical systems are developed by different investigators, individual systems are expected to evolve at different rates and will be informed by different sources of data. Development of CRACMMv1.0 leveraged existing chemical mechanisms including the Generator for Explicit Chemistry and Kinetics of Organics in the Atmosphere (GECKO-A; Aumont et al., 2005), the Master Chemical Mechanism (MCM; Jenkin et al., 1997), the SAPRC-18 MechGen system (mechanism generation; Carter, 2020b), and RACM2, as well as literature. ROC systems not previously represented in RACM2 (such as furans and L/S/IVOCs), precursors to SOA, and systems with new kinetic data (Sect. 3.10) were targeted for development in this initial CRACMM version. Future work will continue to expand this initial representation by extending it to new chemical systems and/or updating these parameterizations with new data.
CRACMMv1.0 includes 178 gas-phase species (ROC species in Appendix A) and 508 reactions spanning gas-phase and heterogeneous pathways (Appendix B). In the CMAQv5.4 modal aerosol implementation, CRACMM includes 51 different chemical species in the particulate phase (81 model species across Aitken, accumulation, and coarse modes). These 51 particulate species in CRACMM include inorganic aerosol species such as sulfate, nitrate, ammonium, calcium, and other trace metals as in previous versions of CMAQ. To fully describe the state of atmospheric aerosol in CMAQ, CRACMM interacts with ISORROPIA II (Fountoukis and Nenes, 2007) and other algorithms describing nucleation and condensation. CRACMM specifically builds on the implementation of RACM2 chemistry coupled with aerosol chemistry of aerosol module 6 (AERO6) (411 reactions) in the CMAQv5.3.3 model, which differs slightly from the original RACM2 implementation (Goliff et al., 2013) (363 reactions) due to SOA pathways, parameterized effects of halogens on ozone (Sarwar et al., 2015), and other minor updates (see the work of Sarwar et al., 2013, and the “Code and data availability” section for the CMAQ implementation of RACM2).
In contrast to almost all SOA representations in current chemical transport models, SOA systems in CRACMM are integrated with the gas-phase radical chemistry. Specifically, all condensible or soluble precursors to SOA are formed directly as gas-phase products with the ability to condense (systems in Sect. 3.1–3.7) or react heterogeneously (Sect. 3.8) and form SOA. Formation of SOA thus removes mass from the gas phase, sequestering RO2, NO, and/or hydrogen oxide (HOx) radicals with implications for ozone and species modulated by oxidant abundance such as sulfate.
All CRACMM species (both primary and secondary) have a representative structure (ROC species in Appendix A) based on the most abundantly emitted species or likely oxidation product. Representative structures were used to obtain properties such as the molecular weight, rate coefficient, solubility, and/or volatility of species except in two cases (SLOWROC in Sect. 3.1, VROCIOXY in Sect. 3.3). These representative structures can enable future prediction of other properties such as aerosol viscosity and the propensity to phase separate as well as deviations from ideal partitioning. They can also be used to synthesize CRACMM chemistry as demonstrated in Sect. 5. The species and chemistry of the major ROC systems updated compared to RACM2, reactions for two additional new HAPs, and rate constant updates (including many for inorganic reactions) are described in this section. Table 1 summarizes the SOA pathways.
Table 1Pathways to SOA in CRACMM by system. Some systems include a representation of autoxidation (Auto?: Yes). Actual SOA formation in CRACMM is modulated by the oxidant concentration (HO, NO3, O3), RO2 bimolecular fate (NO HO2), bimolecular RO2 lifetime (), abundance of the partitioning medium (OA), photolysis (hν), and/or aqueous environment (see heterogeneous reactions in Appendix B). When autoxidation is represented but is not listed here, autoxidation is assumed to be sufficiently fast so that it is not modulated by ambient conditions. SOA is modulated by temperature through gas-phase reaction rates and the effect of temperature on volatility (not explicitly listed). For estimated yield calculations, typical population-weighted values (Porter et al., 2021) of the bimolecular RO2 fate (rates of RO2 + HO2 and RO2 + NO), the bimolecular lifetime (10 s), and the amount of organic partitioning medium (10 µg m−3) are assumed (if applicable). Estimated yields exclude multigenerational oxidation of secondary oxygenated ROC species unless explicitly mentioned. Species names and abbreviations can be found in Appendices A and B. L/S/IVOC: LVOC, SVOC, and/or IVOC.
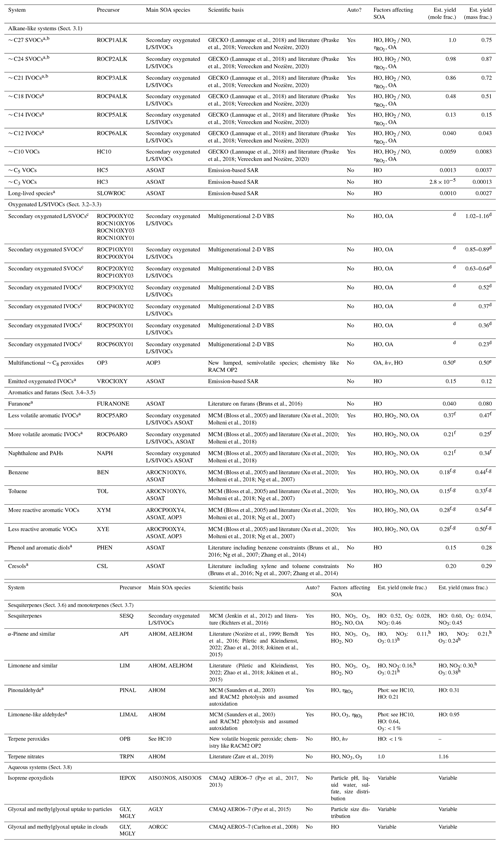
a New SOA precursor system compared to CMAQ AERO6–7 (Appel et al., 2021). b ROCN2ALK, ROCN1ALK, ROCP0ALK, ROCP1ALK, ROCP2ALK, and ROCP3ALK can partition directly to particles and form POA (see Sect. 3.1). Yields here are for chemical reaction. c While these species are envisioned as secondary oxygenated semivolatile emissions, those from sources such as biomass burning could be mapped to this system based on volatility. d Calculated for 12 h of reaction time across multiple generations. Only mass-based yields are provided. See Fig. 4. e Based on semivolatile partitioning of OP3. Further reaction of OP3 with HO produces < 1 % molar yield of SOA. f SOA yield includes furanone route contributions. g SOA yield includes phenolic (PHEN or CSL) route contributions. h SOA yield includes complete further reaction of TRPN but not aldehydes (PINAL or LIMAL).
3.1 Alkane-like ROC
CRACMM includes 14 classes of alkane-like species ranging from low-volatility compounds to ethane (Figs. 1–3 red series). Methane reaction with HO is from RACM2 and assumes a fixed background concentration (1.85 ppm for the late 2010s, Dlugokencky, 2022). After remapping all ROC species, the RACM2 alkane class HC8 (alkanes and other species with kOH > 6.8 × 10−12 cm3 molec.−1 s−1) was renamed to HC10 based on the nC (Fig. 1) and is consistent with a µg m−3 (Fig. 2). Nine new alkane-like mechanism species with high OA formation potential span the L/S/IVOC range and are grouped by into ROCN2ALK, ROCN1ALK, ROCP0ALK, ROCP1ALK, ROCP2ALK, ROCP3ALK, ROCP4ALK, ROCP5ALK, and ROCP6ALK, where the numbers indicate the negative (N) or positive (P) log 10([µg m−3]) value (Fig. 2). When the species reside in the gas phase as a vapor, it is prepended with a “V” (as in Appendix B), and when in the particulate aerosol phase, it is prepended an “A.” For example, VROCN2ALK is an alkane-like vapor species with a of 10−2 µg m−3, and AROCN2ALK is a particulate species of the same volatility.
The nine new alkane-like model species roughly correspond to carbon numbers of 30, 29, 28, 27, 24, 21, 18, 14, and 12 (Fig. 1) and are not represented in traditional atmospheric chemical mechanisms due to low ozone formation potential per unit mass (Fig. S5). For example, ∼ C8 is the largest alkane category in RACM2 and SAPRC-18, and n-dodecane (C12) is the largest alkane in MCM (Jenkin et al., 1997). Conceptually, for deposition and other processes, the gas-phase paraffinic species in the Carbon Bond version 6 (CB6) revision 3 is equivalent to a C4 species. Regardless of the chemical mechanism, regional modeling emission infrastructure previously used by CMAQ did not classify species with ∼ 20 or more carbons (Pye and Pouliot, 2012), and S/IVOC emissions were not propagated to model-ready species for CMAQ mechanisms (Shah et al., 2020). The CRACMM species with can exist in the gas or particle phase based on the local organic aerosol loading and absorptive partitioning theory (Pankow, 1994), while ROCP4ALK–ROCP6ALK exist meaningfully in the gas phase only (Appendix A). The low-volatility alkanes, µg m−3, are assumed to be primarily in the particulate phase and have a minor potential to react and contribute to O3 formation (Fig. S5) and so do not participate in gas-phase radical chemistry (Appendix B). Most of the L/S/IVOC emissions are expected to be unresolved at the individual-species level (Robinson et al., 2007) and are characterized through other means such as volatility analysis (e.g., Lu et al., 2018).
Gas-phase chemistry for the alkane species with 10 µg m−3 ≤ µg m−3 (ROCP1ALK–ROCP6ALK and HC10) is based on GECKO-A predictions for C10–C26 n-alkanes (Lannuque et al., 2018) and known H-shift pathways (Praske et al., 2018). The chemical reactions representing the major product channels and types of functionalities added to the parent hydrocarbon (RH) are the following:
where stable products are subscripted with their saturation concentration in (relative to a parent hydrocarbon with M) and the number of oxygens per molecule (nO). For chemical reactions such as Reactions (R1)–(R9), RNIT, ROOH, ROH, and RKET indicate a compound with specific functionality rather than a mechanism species. The products in Reactions (R1)–(R9) are mapped to mechanism species based on their properties. The initial product, RO2, is the prompt peroxy radical resulting from hydrogen abstraction followed by an O2 addition (Reaction R1). RO2 reactions lead to stable products like organic nitrates (nitrate functionality generally indicated as RNIT in the above reactions) and peroxides (peroxide functionality generally indicated as ROOH in the above reactions) (Reactions R2, R4) that can further react (following Sect. 3.2 for S/IVOCs and RACM2 for VOCs). The alkoxy radical generated from the prompt RO2 can also undergo a 1,5 H shift followed by addition of O2 leading to a new hydroxy peroxy radical, R(OH)O2 (Reactions R2, R3). The R(OH)O2 can undergo standard bimolecular peroxy radical fates leading to multifunctional nitrates (R(OH)NIT), ketones (R(OH)KET), and peroxides (R(OH)OOH) or a 1,6 H shift at a rate of 0.188 s−1 (Vereecken and Nozière, 2020) producing a ketohydroperoxide (R(O)OOH) and HO2 (Reaction R5) as described by Praske et al. (2018). Following GECKO-A (Lannuque et al., 2018), the yield of organic nitrates in Reaction (R2), β1, is 0.28 for S/IVOC alkanes and 0.26 for HC10, consistent with the plateau at ∼ 0.3 observed for C13 and larger alkanes (Yeh and Ziemann, 2014). The yield of organic nitrates for the hydroxy peroxy radical, β2, is 0.14 for S/IVOC alkanes and 0.12 for HC10 (Lannuque et al., 2018). Rate constants are provided in Appendix B.
Products are often 2–3 orders of magnitude lower in than their parent and can be 4–5 orders of magnitude lower in the case of the multifunctional nitrates and peroxides. For the alkane systems, product is based on vapor pressures obtained from GECKO-A output using the Nannoolal method (Nannoolal et al., 2008, 2004). With one exception, all stable products from the VOC, HC10 (M = 7), are expected to remain in the gas phase and thus map to the standard gas-phase species ONIT (organic nitrate), OP2 (organic peroxide), and KET (ketone) inherited from RACM2. The hydroxyhydroperoxide from HC10 oxidation is predicted to be sufficiently functionalized to be semivolatile. That C10 multifunctional peroxide along with all the stable products from alkane-like S/IVOCs are mapped to new CRACMM species of a matching and ratio of molar oxygen to carbon (nO:nC) (secondary oxygenated L/S/IVOC species, Sect. 3.2).
According to the SOA SAR (Fig. S5), as well as the prompt (one HO reaction) mechanism predictions (Table 1), SVOCs of µg m−3 and lower volatility have SOA yields that are near 100 % by mole (up to 150 % by mass), and the atmospherically relevant SOA yields will depend on competition between phase partitioning, reaction, and deposition. Much of the alkane-like L/SVOC contribution to ambient OA will be in the form of direct emission of the lower-volatility species as primary organic aerosol (POA). The mechanism-predicted prompt SOA yields for ROC3PALK and ROCP4ALK by mass (Table 1) are very similar to the emission-weighted SAR-based prediction of 0.83 and 0.55 by mass (Fig. S5). The mechanism-based prompt SOA yields for the more volatile alkane-like ROC species (ROCP5ALK, ROCP6ALK, and HC10) are lower than those predicted by the SOA SAR (28 %, 18 %, and 6 % by mass). Note that the HC10 class is estimated to contain substantial emissions (shown in Sect. 4 and accompanying Fig. 6b), some of which are poorly identified in SPECIATE (representative compound score of 1, Sect. 2.1).
The alkane-like ROC species differ from the previous CMAQ S/IVOC species implemented in AERO6–7 (× symbols in Figs. 1, 3) in terms of the trend in nC with volatility as they are all conceptualized as alkane-like structures because those are the representative structures currently populated with emissions in the S/IVOC range. SVOCs with log 10([µg m−3]) < 2.5 are lumped into ROCN2ALK–ROCP2ALK species based on volatility regardless of their functionality resulting in some higher nO:nC species being included (Fig. S6). CMAQ AERO6–7 previously assumed a slight increase in nO:nC and corresponding decrease in nC as volatility decreased (Figs. 1, S6). CRACMM alkane-like SVOCs with kOH from OPERA are also less reactive than AERO6–7 SVOCs (Fig. 3).
The reaction products of ethane (ETH), C3 alkanes and other slowly reacting species (3.5 × cm3 molec.−1 s−1, HC3), and C5 alkanes and other moderately reacting species (3.4 × cm3 molec.−1 s−1, HC5) (Fig. 3) are obtained directly from RACM2 with the addition of a very small yield of SOA from HC3 (2.8 × 10−5 by mole) and HC5 (1.3 × 10−3 by mole) (Table 1). Ethane is the only explicit alkane in CRACMM; its rate constant with the hydroxyl radical is updated to follow recent recommendations (Burkholder et al., 2019). In addition, CRACMM includes a new species called SLOWROC with a lifetime of about 1 month ( cm3 molec.−1 s−1) to prevent loss of emitted carbon that may contribute to the ambient atmospheric ROC burden (effective carbons per molecule of 2.1). SLOWROC also contains many HAPs (Sect. 4). Due to the highly empirical nature of SLOWROC, the molecular weight is based on an emission-weighted value rather than a representative compound. Oxidation of SLOWROC produces the ethylperoxy radical (ETHP) and a small yield of SOA (0.10 % by mole).
Effective SOA yields for the alkane-like VOC (log 10([µg m−3]) ≥6.5) systems except HC10 use the simple SAR for SOA and are driven by isopropyl acetate and methyl butanoate (estimated SOA yields of 2.8 % and 2.2 % by mass) in the case of HC3, by isopentane (estimated SOA yield of 1.9 % by mass) in the case of HC5, and by two long-lived aromatic species in the case of SLOWROC. The SOA from HC3, HC5, and SLOWROC is mapped to the species ASOAT, a general, non-volatile SOA species with a molecular weight of 200 g mol−1 (Table 1). HC3, HC5, and SLOWROC are estimated to contribute 0.003 %, 0.062 %, and 0.0002 % by mass, respectively, of the total OA potential for anthropogenic and biomass burning emissions in the US for 2017 conditions.
3.2 Secondary oxygenated L/S/IVOCs
Gas-phase oxidation of S/IVOC alkanes readily leads to oxygenated L/S/IVOC products with nO:nC ratios up to 0.3 (Reactions R1–R8). The products of these prompt reactions continue to be processed in the atmosphere, resulting in further functionalization as well as fragmentation (cleaving of the carbon backbone) with implications for increasing or decreasing SOA, respectively. Functionalization products of the secondary oxygenated L/S/IVOC chemistry can sequester radicals, but fragmentation products, like formaldehyde, can eventually release radicals via photolysis (Edwards et al., 2014).
The chemistry of secondary oxygenated L/S/IVOCs is parameterized using the 2-D VBS framework (Donahue et al., 2012) with some modifications. The decrease in per oxygen in the 2-D VBS box model was calculated using the parameterization from Donahue et al. (2011) with the oxygen–oxygen interaction term set to 2.3, the carbon–oxygen interaction parameter set to −0.3 to correct for the behavior of diacids, and the carbon–carbon interaction term set to 0.475. As identified in Donahue et al. (2011), the resulting decrease in log 10C* per oxygen is 1.7 as nO:nC approaches 0 and is 1.93 as nO:nC approaches 0.6. These values are consistent with the effect of adding carboxylic acids to an alkane-like molecule (Pankow and Asher, 2008). Homogeneous, gas-phase HO reaction rate constants were specified based on the parameterization proposed by Donahue et al. (2013): . Following the reaction with HO, the probability of functionalization was parameterized as , with subsequent probabilities of adding one, two, or three oxygens set at 30 %, 50 %, and 20 %, respectively, following the 2-D VBS functionalization kernel derived for photo-oxidation of POA and IVOCs (Zhao et al., 2016). The sensitivity of yields to NOx and formation of organic nitrates were not explicitly addressed in the 2-D-VBS-based aging mechanism, although both are addressed by CRACMM more broadly and some products mapped to secondary L/S/IVOCs contain nitrate functionality. Rather than recycling hydroxyl radicals as is standard practice for VBS-style reactions that are only meant to capture SOA, CRACMM sequesters HOx in oxygenated L/S/IVOC products as might be expected when peroxides form. For example, Reaction R1 followed by Reaction R4 sequester two HOx molecules for each initiating reaction.
L/S/IVOC products predicted by the 2-D VBS were lumped into a reduced series of 15 mechanism species spanning a of 10−2 through 106 µg m−3 and nO:nC of 0.1 through 0.8 for use in CRACMM: ROCN2OXY2, ROCN2OXY4, ROCN2OXY8, ROCN1OXY1, ROCN1OXY3, ROCN1OXY6, ROCP0OXY2, ROCP0OXY4, ROCP1OXY1, ROCP1OXY3, ROCP2OXY2, ROCP3OXY2, ROCP4OXY2, ROCP5OXY1, and ROCP6XY1. These species follow a naming convention similar to the S/IVOC alkanes, where numbers after “N” and “P” indicate the negative or positive value and the name ends in 10 × nO:nC (e.g., ROCN2OXY2 is µg m−3 with 0.2). VBS products of a known nC and nO were mapped to the available CRACMM model species, first by interpolating to the two nearest points and then to the two nearest species in nO:nC space. The number of nO:nC levels represented at a given volatility in CRACMM increases with decreasing to reflect increasing diversity in the chemical functionality and size of products with lower saturation concentrations.
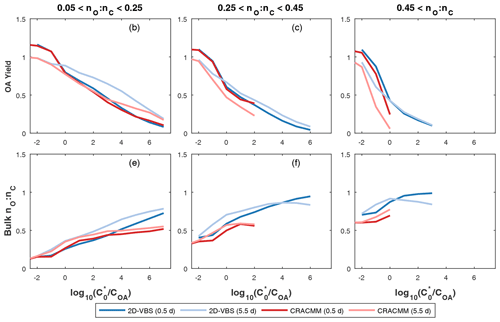
Figure 4Organic aerosol yield and bulk nO:nC predicted by the CRACMM oxygenated ROC aging mechanism (Sect. 3.2) and the 2-D VBS configuration reported by Zhao et al. (2016). The x axis is defined as , where COA is the background OA concentration and is the saturation concentration of the precursor. The aging of each species is simulated at a constant HO concentration of 106 molec. cm−3 for 12 h (darker colors) and 2.5 d (lighter colors) at four different COA conditions (0.1, 1, 10, and 100 µg m−3). In cases where multiple predictions are present for the same saturation ratio, values are averaged.
The portion of reacted mass following the fragmentation pathway, , was assumed to form fragments of sizes varying from one up to nC carbons. The distribution of fragments was estimated assuming the probability of attack on any carbon as . Fragments with greater than seven carbons were functionalized using the same oxygen addition probabilities and remapping to lumped model species as above. Stable fragmentation products with six or fewer carbons were mapped back to existing gas-phase species from RACM2 based on their carbon number as follows: C1 to formaldehyde (HCHO), C2 to acetaldehyde (ACD), C3 to higher aldehyde species (ALD), C4 to methyl ethyl ketone (MEK), C5 to a dicarbonyl (DCB1), C6 from low-nO:nC reactants to a hydroxy ketone (HKET), and C6 from high-nO:nC reactants to a higher-carbon-number ketone (KET) species. The choice of functionality of the product species (e.g., aldehydes vs. ketones) is entirely determined by the RACM2 species that were already available at each carbon number. Future measurements of the low-molecular-weight species produced by the oxidation of larger compounds would help constrain this choice and motivate the addition of new CRACMM species. A new semivolatile peroxide (OP3), equivalent to a C8H16O4 species with a of ∼ 10 µg m−3, in CRACMM provides an oxygenated peroxide species between the L/S/IVOC oxygenated series and RACM2's higher organic peroxide species (OP2). In addition, radical products are mapped to RACM2 peroxy radical species as follows: C1 to methylperoxy radical (MO2), C2 to ethylperoxy radicals (ETHP), C3 to isopropylperoxy radicals (HC3P), C4 to peroxy radicals from methyl ethyl ketone (MEKP), C5 to pentan-3-ylperoxy radicals (HC5P), and C6 to ketone-derived peroxy radicals (KETP). OP3 can photolyze or react with HO.
Overall, the CRACMM scheme performs similarly to the medium-yield 2-D VBS scheme optimized for S/IVOCs by Zhao et al. (2016) (Fig. 4). For precursors with nO:nC > 0.05 and 12 h of chemical processing, the 2-D VBS and CRACMM aging schemes are almost the same in terms of OA yield (Fig. 4a–c) with values ranging from near 0.1 to above 1 as a function of volatility (Table 1). Some deviations occur between the schemes for the most oxygenated and volatile precursors (nO:nC > 0.45 and , where COA is the mass-based concentration of the condensed-phase partitioning medium), for which CRACMM predicts a stronger dependence of yield on precursor volatility and also predicts less OA formation. Both CRACMM and the 2-D VBS predict consistent trends in OA yield as a function of precursor properties with more oxygenated and volatile precursors having lower yields due to an increased likelihood of fragmentation. At very long processing times CRACMM predicts OA yields will decrease (which has been observed in experimental systems in the work by He et al., 2022), while the 2-D VBS indicates yields continue to increase from 2.5 d (Fig. 4) to 5.5 d (Fig. S7). In CRACMM nO:nC ratios are predicted to increase with time, which can be due to both functionalization (Heald et al., 2010) and fragmentation (Kroll et al., 2009) reactions. CRACMM generally predicts lower nO:nC ratios in OA products from oxygenated ROC (0.1 to 0.5 for the least oxygenated and 0.6 to 0.7 for the most oxygenated precursors) than the 2-D VBS (Fig. 4d–f).
3.3 Primary oxygenated IVOCs
Volatile chemical products emit significant amounts of oxygenated IVOCs (Seltzer et al., 2021; McDonald et al., 2018). Many of these oxygenated species are structurally different than what is conceptualized in the secondary oxygenated L/S/IVOCs (Sect. 3.2) since they include siloxanes and ethers, while secondary oxygenated species are primarily alcohols, peroxides, nitrates, and ketones. Emitted oxygenated IVOCs have a significantly lower potential to form SOA than hydrocarbon IVOCs of a similar volatility (Pennington et al., 2021). In addition, oxygenated species generally differ from hydrocarbon-like emissions in their ability to form O3, peroxyacetyl nitrate (PAN), and formaldehyde (Coggon et al., 2021) and should be represented separately from hydrocarbon-like species.
Two new types of oxygenated IVOCs with direct emissions are included as distinct species in CRACMM (Figs. 1–3, purple): propylene glycol (PROG) and oxygenated IVOC species (VROCIOXY). 1,2-Propylene glycol is one of the most prevalent species in consumer product purchases (Stanfield et al., 2021) and is associated with increased allergic symptoms when inhaled (Choi et al., 2010). Propylene glycol is represented in CRACMM with chemistry based on MCM following the work of Coggon et al. (2021). The VROCIOXY class includes non-aromatic, saturated IVOCs with nO:nC > 0.1 and all species containing silicon. Decamethylcyclopentasiloxane is the most abundant individual species in VROCIOXY, and VROCIOXY has an emission-weighted effective carbon number of 9.5. Due to the highly aggregated nature of VROCIOXY, the kOH and molecular weight are emission-weighted properties rather than based on a representative compound. VROCIOXY produces the ethylperoxy radical with an 85.2 % molar yield and SOA with a 14.9 % molar yield (Table 1) upon reaction with HO in CRACMM. While the SOA yield may appear high, the lifetime of VROCIOXY is 40 h at typical daytime HO concentrations, which should limit the amount of SOA in urban source regions, similar to siloxane behavior in the work of Pennington et al. (2021). Future versions of CRACMM emission processing could redirect alcohols, carbonyls, and other oxygenated S/IVOCs from VROCIOXY to the secondary oxygenated L/S/IVOC series (Sect. 3.2) and readjust the effective VROCIOXY SOA yield.
3.4 Furans
FURAN is a new lumped ROC species introduced in CRACMM with the most abundant individual species in the category being furfural followed by furan. Furans were not previously an independent category in RACM2, and Carter (2020a) recommended mapping 2-furfural to ∼ C8 hydrocarbons (now HC10) and furan to the lumped o-xylene (XYO in RACM2). Given the abundance of furans (140 Gg yr−1 of emission, primarily from wood burning for 2017 US conditions), unique functional-group structure, HO reactivity (Koss et al., 2018), and O3 formation potential (Coggon et al., 2019), FURAN was implemented in CRACMM as a new species (Figs. 1–3, blue). Furans have been shown to form SOA with yields between 1.85 % and 8.5 % by mass depending on the structure (Gómez Alvarez et al., 2009), and the simple SAR predicts a yield of 2.6 % by mass (Fig. S5). The furan SOA yield is about a factor of 4 lower than that of xylenes, but products such as furanone (FURANONE, a new species in CRACMM) are also formed in aromatic systems like benzene (Sect. 3.5). The CRACMM species, FURAN, includes small amounts of other species with two double bonds (Fig. S3) including 2.4 Gg yr−1 of anthropogenic dienes.
The FURAN chemistry in CRACMM is based on a five-species weighted average using furan emission factors reported by Koss et al. (2018) and the furan chemistry outlined by Wang et al. (2021) and Coggon et al. (2019). FURAN will predominantly react with hydroxyl radicals, leading to gas-phase products including dicarbonyls (DCB1, DCB3), organic nitrates (ONIT), peroxides (OP2), furanones (FURANONE), and aldehydes (ALD) in addition to radicals (Appendix B). CRACMM assigns SOA from FURAN to further reactions in the ring-retaining product channel, FURANONE, consistent with products detected by Jiang et al. (2019). The effective SOA yield from FURAN is approximately 5 % by mass (Bruns et al., 2016) when branching between high- and low-NOx reactions is equal. The yield of SOA from FURANONE in CRACMM is set to 4 % by mole or 8 % by mass (Table 1).
3.5 Aromatics
Aromatic hydrocarbons (Figs. 1–3, blue) were reorganized to reduce the number of aromatic VOC model species and increase the number of aromatic IVOC species in CRACMM. Instead of four aromatic VOC categories based on reactivity (kOH), CRACMM uses two categories of xylene-like hydrocarbon species based on reactivity: m-xylene and more reactive aromatics (XYM) and aromatics less reactive than m-xylene (XYE). Toluene (TOL), a HAP (Sect. 4), is now explicit in CRACMM, and benzene (BEN) was already explicit in RACM2. The three new IVOC aromatic hydrocarbons () are naphthalene and other polycyclic aromatic hydrocarbons (NAPH), single-ring aromatics of (ROCP5ARO), and single-ring aromatics of (ROCP6ARO). The ROCP5ARO and ROCP6ARO categories were previously found to be important for representing SOA from vehicle combustion sources (Lu et al., 2020), and the emissions for 2017 indicated insufficient mass and SOA formation potential to warrant another aromatic species at .
MCMv3.3.1 chemistry (Bloss et al., 2005; Jenkin et al., 2003) was used to obtain a basic mechanism for aromatic reaction for seven hydrocarbon-like aromatics in CRACMM (BEN, TOL, XYE, XYM, NAPH, ROCP6ARO, and ROCP5ARO). The MCM epoxide yield (which includes unidentified species mass, Birdsall and Elrod, 2011) was set to 0, and product mass was redirected to the bicyclic peroxy channel following Xu et al. (2020). In addition, the organic nitrate yield (β, Reaction R11) from RO2 + NO is 0.2 % in CRACMM (Xu et al., 2020). A fraction of the bicyclic peroxy radical channel is assumed to undergo autoxidation (Wang et al., 2017; Molteni et al., 2018; Xu et al., 2020). The following reactions describe this chemistry for a parent aromatic species (BEN, TOL, etc.), generally indicated as AROM:
Stable, individual species are subscripted with their relative to the parent volatility of M (estimated with SIMPOL (simple prediction method; Pankow and Asher, 2008) based on expected functionality) and number of oxygens per molecule. The phenolic product (PL) yield (αPL; 53 % for benzene and 16 %–18 % otherwise) is from MCM (o-xylene if a species was not available) and independent of NO level, in good agreement with experimental data for conditions below a few hundred parts per billion of NO (Bates et al., 2021). The PL product is mapped to phenol (for benzene), cresols (for toluene and xylenes), or a lumped secondary oxygenated product (described in Sect. 3.2) based on volatility and nO:nC (for all other aromatics). Aromatic peroxy radical (ARO2) products included peroxides, organic nitrates, and alkoxy radical decomposition products (ROPs). ROPs are produced by H abstraction (H), traditional HO addition resulting in bicylic peroxy radicals (B), and/or autoxidation (A). The fraction of all AROM + HO through the H-abstraction route (αH) is from MCM with the product mapped to benzaldehyde in the case of toluene and xylenes or a product based on expected volatility and nO:nC (H abstraction is not applicable for benzene). The ROPB from the bicylic peroxy radical alkoxy radical decomposition channel follows MCM and includes glyoxal and/or methylglyoxal, furanones, dicarbonyl(s), and HO2. αA is the fraction of products undergoing autoxidation and is a subset of the bicyclic RO2 products. Coefficients in Reactions (R9)–(R13) (αH, αPL, αA) are relative to total AROM + HO except for the fraction of RO2 + NO branching to organic nitrates (β) in Reaction (R11).
Aromatic peroxy radicals can react with other organic peroxy radicals (RRO2), with methylperoxy radicals and acetylperoxy radicals being the most abundant and always represented in RACM2 (Stockwell et al., 1990). The RRO2 product (RRO2P) is based on MCM at yields specified independently of the ARO2 product channels. Specifically, methylperoxy radicals (RRO2 as RACM2 species MO2) result in 0.68 formaldehyde, 0.37 HO2, and 0.32 higher alcohols (RRO2P = 0.68 HCHO + 0.37 HO2 + 0.32 MOH). Acetylperoxy radicals (RRO2 as RACM2 species ACO3) result in 0.7 methylperoxy radicals and 0.3 acetic acid (RRO2P = 0.7 MO2 + 0.3 ORA2).
Reactions (R9)–(R13) produce condensible gases and SOA precursors. In the case of volatile aromatics like benzene, toluene, and xylenes, further reaction of the phenolic product along with autoxidation is proposed as the major SOA channels in CRACMM since traditional bimolecular RO2 products are generally not of sufficiently low volatility. For aromatic IVOCs, peroxides, nitrates, and aldehydes from bimolecular RO2 reactions can be semivolatile and partition based on their saturation concentration. Further oxidation of furanone produced from aromatic oxidation (e.g., Reaction 477, Appendix B) also results in small amounts of SOA (Sect. 3.4). For products in Reactions (R9)–(R13) that are mapped to a corresponding surrogate of matching volatility and nO:nC, further chemical processing follows the secondary oxygenated S/IVOC chemistry in Sect. 3.2.
CRACMM retains the three phenolic species of RACM2 (hydroxy-substituted benzene like phenol and benzene diols, PHEN; cresol-like species, CSL; and methylcatechols and similar species, MCT) with the same gas-phase chemistry as RACM2 except for the addition of one non-volatile SOA product for PHEN and CSL. The yield of SOA from phenols and cresols is set to reproduce the high-NOx SOA yields from benzene and toluene oxidation observed in chamber experiments by Ng et al. (2007) with wall loss corrections based on Zhang et al. (2014) (see the Supplement for a detailed derivation). The molar SOA yield using this method is estimated as 15 % by mole for phenols and 20 % by mole for cresols (Table 1), within the range of 24 %–52 % by mass for phenols and 27 %–49 % by mass for cresols as summarized by Bruns et al. (2016). Future work should expand upon this phenolic SOA treatment as improvements in the phenoxy–phenylperoxy radical chemistry have been shown to modulate O3 formation and could improve predictions for laboratory conditions over MCM, RACM2, and SAPRC by breaking the catalytic radical cycles (Bates et al., 2021). Products like methylcatechols could also lead to SOA with implications for O3 and HO production in aromatic systems.
The bicyclic peroxy radical fate in aromatic hydrocarbon systems is not well characterized but includes autoxidation. Molteni et al. (2018) estimate molar yields of autoxidation products from aromatic oxidation of just under 3 % by mole, and that value is used for the aromatic IVOC systems in CRACMM (αA= 0.03). Higher values are not needed to produce significant SOA in IVOCs systems since traditional bimolecular RO2 fates result in sufficiently functionalized products to contribute to SOA. Specifically, with αA= 0.03, CRACMM predicts SOA yields for ROCP5ARO, ROCP6ARO, and NAPH of 37 %, 21 %, and 21 % by mole, respectively (Table 1). However, such low levels of autoxidation, even when combined with phenolic (PHEN and CSL) SOA, are insufficient to explain observed SOA production for the more volatile aromatics, particularly in RO2 + HO2-dominant conditions, where SOA yields are around 27 % by mole based on chamber experiments. Xu et al. (2020) indicate bicyclic peroxy radicals in the benzene system may predominantly form alkoxy radicals (even in RO2+ HO2 conditions) that continue to highly oxygenated organic molecules in addition to other products. Given the current lack of carbon closure for gas-phase aromatic chemistry (Xu et al., 2020) and low volatility of laboratory-generated RO2+ HO2 aromatic SOA (Ng et al., 2007), the amount of autoxidation in the benzene, toluene, and xylene aromatic systems is set in CRACMM to reproduce observed RO2+ HO2 chamber SOA yields when combined with the phenolic channel (see the Supplement for molar yield derivation). The resulting estimates for the fraction of AROM + HO reaction leading to autoxidation (αA) are 19 % by mole for benzene and 23 % by mole for toluene and xylenes. This results in the phenolic channel contributing 30 % of the SOA in the benzene system and 13 % in the toluene systems for RO2+ HO2 conditions, similar to the previously published estimate of 20 % for low-NOx conditions for benzene, toluene, and m-xylene (Nakao et al., 2011) and 20 %–40 % for toluene (Schwantes et al., 2017) as well as the relative abundance of phenolic products in benzene vs. toluene systems.
In general, autoxidation of the bicyclic RO2 in the aromatic systems is assumed to involve one H shift followed by O2 addition and result in peroxides and nitrates about seven values lower in volatility than the parent aromatic (products in Reactions R10–R11). The autoxidation product in benzene and toluene systems with only one H shift would have a of 10 µg m−3, making it semivolatile according to SIMPOL (Pankow and Asher, 2008). To improve consistency with Ng et al. (2007) yields and non-volatile partitioning behaviors under low-NOx conditions at low organic aerosol concentrations (< 10 µg m−3), the products from autoxidation in the toluene and benzene systems are assumed to result from two H shifts followed by O2 addition leading to two additional hydroperoxide functional groups and autoxidation products with 0.01 µg m−3. Xylene-like (XYM and XYE) autoxidation products assume one H shift with O2 addition resulting in autoxidation products with 1 µg m−3. ROOHB products from XYM and XYE are slightly lower in volatility than those from benzene and toluene and mapped to the new multifunctional C8 peroxide (OP3; see Sect. 3.2 and Table 1), resulting in SOA from channels other than autoxidation and phenolic routes for xylenes. SOA yields for benzene, toluene, and xylenes summarized in Table 1 generally reproduce wall-loss-corrected laboratory values (Ng et al., 2007; Zhang et al., 2014) due to the imposed autoxidation channel. Benzene and toluene are predicted to have lower SOA yields than the IVOC aromatics NAPH, ROCP5ARO, and ROCP6ARO. However, the amount of autoxidation for aromatic IVOCs was not adjusted to match literature SOA yields, since many traditional bimolecular products were already in the S/IVOC range and thus SOA for aromatic IVOCs could be underestimated compared to laboratory work (Srivastava et al., 2022).
Figure 5 shows the molar flows to organic aerosol in the combined aromatic, phenolic, and furan systems based on anthropogenic and biomass burning emissions in the US for 2017 and equal RO2 + HO2 vs. RO2 + NO branching. Most (69 %) phenol mass is directly emitted with the balance from benzene oxidation. In contrast, cresols are predominantly chemically produced (80 % of the source) rather than directly emitted. Approximately 22 % of furanone is produced directly from furan oxidation, but most furanone is predicted to be from oxidation of aromatic hydrocarbons like toluene and xylenes with smaller contributions from IVOC aromatics. About 32 % of the aromatic system SOA is predicted to come from phenols, cresols, and furanone through fixed yields and the formation of an empirical SOA species (ASOATJ). Peroxide species (specifically OP3) may be a substantial contributor to SOA mass. Autoxidation, leading to species such as ROCN1OXY6, also make meaningful contributions to the predicted SOA mass. By acknowledging further oxidation of phenolic species as contributors to overall aromatic hydrocarbon SOA, all phenolic emissions can now be considered SOA precursors. In addition, adding phenolic sources of SOA increases the overall amount of SOA from ROC emissions compared to previous CMAQ aerosol representations that did not include phenols or cresols as SOA precursors.
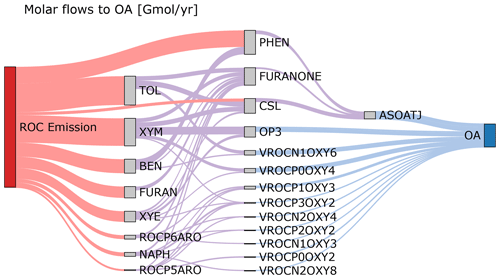
Figure 5Molar flows to organic aerosol in the aromatic–phenolic–furan systems for 2017 US emissions. Bimolecular RO2 reactions are split equally between RO2 + NO and RO2 + HO2 with the fraction of products undergoing autoxidation as specified in CRACMM. Partitioning of semivolatile species is calculated for 10 µg m−3 of organic aerosol. Precursor species include the following: toluene (TOL), m-xylene and more reactive aromatic VOCs (XYM), benzene (BEN), ethylbenzene and less reactive aromatic VOCs (XYE), phenolic species (PHEN), cresols (CSL), naphthalene and PAHs (NAPH), and other IVOC aromatics of higher (ROCP6ARO) and lower (ROCP5ARO) volatility. Aqueous pathways to SOA from glyoxal and methylglyoxal are not shown. Products that do not lead to OA are not shown but are indicated by the outflow from a species being smaller than the inflow. Red flows indicate emissions. Purple flows indicate hydroxyl radical oxidation chemistry. Blue flows indicate partitioning to the condensed phase.
3.6 Sesquiterpenes
Sesquiterpenes (C15H24) are a new radical system in CRACMM (previously only considered for SOA formation in CMAQ; Figs. 1–3, green) with chemistry built using β-caryophyllene from MCM (Jenkin et al., 2012) and autoxidation based on literature. β-Caryophyllene is an IVOC ( of 5.05 µg m−3), and MCM chemistry readily predicts sesquiterpene products that are S/IVOCs, consistent with the semivolatile nature of observed SOA (Griffin et al., 1999). Sesquiterpene species (SESQ) react with NO3, O3, and HO:
where αA is the fraction of ozonolysis products undergoing autoxidation and β is the fraction of RO2 + NO products resulting in organic nitrates (β= 0.25). The ozonolysis Reaction (R18) is highly simplified and predicted to result in a ketone (ketone functionality indicated by RKET) and autoxidation product (PA) of specified volatility and degree of oxygenation. Autoxidation is based on Richters et al. (2016) and αA is set to 1.8 % by mole. Observations indicate sesquiterpenes are not major contributors by mass to ambient SOA in the Amazon (Yee et al., 2018), southeastern US, or boreal forest (Lee et al., 2020). As a result, CRACMM does not retain the unique identity of sesquiterpene products, and all stable products in Reactions (R14)–(R22) are mapped to the corresponding secondary oxygenated S/IVOC of corresponding volatility and degree of oxygenation with further chemistry specified in Sect. 3.2.
CRACMM predicts prompt (first-generation) sesquiterpene SOA that is less volatile than previous CMAQ work (Carlton et al., 2010; Griffin et al., 1999), is NOx and oxidant dependent, and has the potential for higher yields through multigenerational chemistry. The yield of prompt SOA under RO2 + HO2-dominant conditions is predicted to be 50 % (OA = 1 µg m−3) to 91 % (OA = 10 µg m−3) by mole for HO and NO3 oxidation. These low-NO NO3 yields are within the range of those observed in NO3 oxidation experiments (SOA yields of 56 %–109 % by mole of C, Jaoui et al., 2013), although laboratory values corresponded to a higher concentration of organic aerosol (60–110 µg m−3) and the RO2 fate was not characterized. Under higher-NOx conditions (RO2 + NO dominant) and moderate organic aerosol loading (OA = 10 µg m−3), prompt SOA yields are expected to be ∼ 12 % by mole from HO oxidation, similar to the carbon-based yields of aerosol from laboratory work (19 % by mole for β-caryophyllene, Jaoui et al., 2013). Nitrate oxidation is not expected to produce significant SOA when RO2 reacts with NO or NO3 (Reactions R16–R17), and prompt SOA yields from ozonolysis are 2.7 % by mole, lower than the observed yield of 28 % by mole C for ozonolysis (Jaoui et al., 2013). Thus, further chemical processing of first-generation sesquiterpene-derived ketones (mapped to CRACMM species ROCP3OXY2; chemistry in Sect. 3.2) likely results in lower-volatility species that increase SOA yields beyond the prompt values, especially under high-NOx and ozonolysis conditions.
3.7 Monoterpenes
CRACMM retains the two monoterpene categories of RACM2 with α-pinene and Δ-limonene as the major representative compounds in each class (API and LIM, respectively; Figs. 1–3, green). The two classes differ in the number of double bonds per species, which is expected to influence reactivity and SOA formation potential (Hoffmann et al., 1997). In addition, species with two double bonds in their initial structure likely experience faster autoxidation (Møller et al., 2020). The two classes of monoterpenes (API vs. LIM) have different sources of emissions, with α-pinene being predominantly from vegetation but limonene having the potential for significant anthropogenic emissions from volatile chemical products (Coggon et al., 2021) in addition to biogenic sources. A new representation of API and LIM reaction with HO, NO3, and O3 was created to account for autoxidation leading to highly oxygenated molecules and SOA. In addition, bimolecular peroxy radical reactions leading to dimers of extremely low volatility (CRACMM species ELHOM) with the potential to contribute to new particle formation via nucleation (Bianchi et al., 2019) were added.
When a monoterpene (MT) species reacts with an oxidant like HO (or NO3), it directly forms a collection of peroxy radicals (generally indicated as MRO2 and ; see Appendices A and B for specific model species), a fraction of which (αA) can undergo autoxidation and form highly oxygenated molecules:
Autoxidation is implemented as a fixed yield rather than competitive fate since autoxidation in monoterpene + HO systems proceeds rapidly (rates of 3 to > 10 s−1) and only via specific peroxy radical isomers (Piletic and Kleindienst, 2022; Zhao et al., 2018; Berndt et al., 2016; Xu et al., 2019). This assumption of a fixed yield is valid for bimolecular RO2 lifetimes (timescale for RO2 reaction with NO or HO2) greater than ∼ 1 s (NO < ∼ 1 ppb), which is consistent with most current conditions near earth's surface except for select urban locations, more often in winter (Porter et al., 2021), and episodically near sources. The fraction of prompt API + HO peroxy radicals undergoing autoxidation and forming monoterpene-derived highly oxygenated molecules (tracked as CRACMM species HOM) (αA) is set to 2.5 % by mole (Berndt et al., 2016; Piletic and Kleindienst, 2022) with the uncertainty in the yield around a factor of 2. Limonene is expected to have rapid H-shift reactions (Møller et al., 2020) and higher amounts of autoxidation products than α-pinene (Jokinen et al., 2015), and αA is 5.5 % for LIM + HO (Piletic and Kleindienst, 2022) (Table S7).
The peroxy radicals from monoterpene (API and LIM) reactions with HO undergo traditional bimolecular RO2 fates leading to peroxides, alkoxy radical products, and nitrates:
MRO2 also reacts with MO2 and ACO3 (see Sect. 3.5) (Appendix B). Peroxides from an MRO2 reaction with HO2 (Reaction R24) map to a new organic peroxide, OPB, added specifically to represent the C10 hydroperoxides from monoterpene oxidation. Further reaction or photolysis of OPB is assumed to produce products like existing organic peroxide reactions in RACM2 with products fed back to the lumped aldehyde (ALD), ketone (KET), and saturated C10 RO2 (HC10P). To better conserve carbon and track the identity of monoterpene-derived nitrates, CRACMM includes a new C10 organic nitrate, TRPN (Reaction R25, RNIT product). The OPB peroxides and TRPN nitrates are assumed to remain in the gas phase (see representative structures in Appendix A).
The yield of organic nitrates (β, Reaction R25) is 18 % for API (Nozière et al., 1999) and 23 % for LIM based on MCMv3.3.1 (Saunders et al., 2003). Further reaction of the terpene nitrates produces LVOCs with a 100 % molar yield (Zare et al., 2019; Browne et al., 2014), with products mapped to then new lumped CRACMM species, HOM. While the yield of SOA from the TRPN reaction is 100 % by mole, chemical sinks will compete with deposition, resulting in less than 100 % of TRPN converted to SOA in chemical transport models.
In addition to terpene nitrates, major organic products from RO2 + NO (Reaction R25) are alkoxy radicals which decompose to either aldehydes and HO2 (ROPALD) with a yield of αALD or other smaller-carbon-number fragmentation products and HO2 (ROPFRAG). In the case of LIM (αALD= 64 %), the alkoxy radical decomposition products are assumed to be smaller fragments (HCHO and UALD), but αALD= 1 for α-pinene according to MCM. Since the aldehydes from API and LIM could undergo autoxidation as hinted by Rolletter et al. (2020), new aldehydes, PINAL and LIMAL, were added for the monoterpene systems. Autoxidation for PINAL and LIMAL is added as competitive fate with plausible autoxidation rate constant for terpene systems (k= 1 s−1) for HO-initiated peroxy radicals formed at a yield of 23 % (PINALP) or 70 % (LIMALP) based on MCMv3.3.1. LIMAL and PINAL can also be lost via photolysis, and LIMAL can react with O3. In general, rate constants in monoterpene systems (Appendix B) are from RACM2.
In the case of an API and LIM reaction with nitrate radicals, reactions analogous to Reactions (R23)–(R25) generally apply, but products are multifunctional and can release NO2. Nitrate radical reactions are assumed to behave similarly in terms of autoxidation and use the same αA as HO reactions, which is likely in the case of limonene (J. Chen et al., 2021) but an overestimate in the case of α-pinene (Kurtén et al., 2017). For reactions where multifunctional peroxy nitrates (or other multifunctional nitrates) are expected, the nitrate identity is prioritized for tracking and the product is mapped to TRPN. Reaction of nitrate-derived MRO2 with NO is expected to predominantly release all the nitrate as NO2 (β= 0) and convert NO to NO2 (additional NO2 product alongside aldehyde production) while yielding a terpene aldehyde (PINAL or LIMAL) (αALD= 1).
from autoxidation in monoterpene + HO systems is implemented using two new peroxy radicals (labeled APIP2 and LIMP2) that are assumed to result in C10O7 radicals (Berndt et al., 2016) that can undergo traditional bimolecular fates. For all API and LIM reactions with HO and NO3, the HO2 product is mapped to HOM. In the case of NO, all products that release NO2 (1−β) are also assumed to re-release HO via different fragmentation routes and the highly oxidized terpene nitrate as well as other carbon-containing products were mapped to HOM. MO2 and ACO3 aldehydes, ketones, and alcohols are also mapped to HOM. As a result, under all conditions, the yield of HOM from the initial API or LIM reaction with HO or NO3 is αA.
The speciation of HOM changes slightly when cross-react with other monoterpene or isoprene RO2. In addition to the traditional peroxy radical cross-reactions with other organic peroxy radicals (MO2 and ACO3), the monoterpene-derived peroxy radicals undergoing autoxidation, , react with the most abundant MRO2 from α-pinene and limonene + HO to produce C20 dimers. These reactions followed the basic form of
where αdim is the fraction of incorporated in dimers and set to 4 % based on the work of Zhao et al. (2018). Other products include highly oxygenated monomers (mapped to HOM), aldehydes (mapped to PINAL or LIMAL), and alcohols with branching between those products also as specified by Zhao et al. (2018). In the case of nitrate-initiated , NO2 rather than HO is released. The same approach is used for monoterpene + isoprene RO2 with HCHO and MVK produced rather than PINAL or LIMAL. Dimer reactions are assumed to proceed quickly, and the rate constant was set to 1 × 10−10 cm3 molec.−1 s−1 based on the work of Molteni et al. (2019). In both the monoterpene and isoprene cross-reactions, the dimer products are predicted to have a < −3 and are mapped to ELHOM.
The ozonolysis of monoterpenes in CRACMM also mimics Reaction (R23), where the oxidant in these reactions is O3. Initially, the ozonolysis reaction will break a monoterpene double bond and yield Criegee intermediates that self-react to release hydroxyl radicals and produce peroxy radicals which were classified into the same two types of peroxy radical categories as with HO reactions: either autoxidizable or non-autoxidizable. The yield of peroxy radicals able to undergo autoxidation () for ozonolysis is set to 5 % and 11 %, respectively, in the API and LIM systems. These yields are doubled compared to HO to fall within the uncertainty in laboratory and computational studies that indicated autoxidation yields from O3-initiated reactions are universally higher than autoxidation from HO-initiated chemistry (Jokinen et al., 2015; Ehn et al., 2014; J. Chen et al., 2021). The formation of HO, H2O2, CO, and aldehyde products from the ozonolysis reactions alongside were prescribed following MCM and RACM2, and further reaction of the MRO2 and peroxy radicals is the same as in the HO system.
Predicted SOA in the monoterpene systems comes from HOM and ELHOM products that are either promptly produced or from a further reaction of terpene nitrates or terpene aldehydes. The yield of SOA from an API reaction with HO or NO3 is expected to be 2.5 % by mole (4.6 % by mass) from the initial autoxidation HOM but is further increased to 11 % by mole (21 % by mass) when the terpene nitrates further react under typical ambient conditions (Table 1). Under high-NOx conditions (RO2 + NO as the dominant bimolecular fate), the yield of SOA from API + HO approaches 37 % by mass with most of the mass from terpene nitrate products, highlighting the importance of the terpene nitrate fate which is currently assumed to be a reaction with HO and functionalization. LIM SOA yields from HO and NO3 are similar with values of 16 % by mole or 30 % by mass for typical conditions but as much as 50 % by mass if RO2 + NO dominates and terpene nitrates react further. Yields also increase compared to the typical values if the terpene aldehydes react with HO, which is estimated to yield SOA of 21 % by mole (31 % by mass) or 64 % by mole (95 % by mass) for PINAL and LIMAL, respectively. Terpene aldehyde photolysis, OPB (and OP3) reaction with HO, or LIMAL reaction with O3 can also lead to trace amounts of SOA via a C10 RO2 product (< 1 % molar yield; chemistry in Sect. 3.1 for the HC10 peroxy radical).
The autoxidation-derived HOM yield for α-pinene from CRACMM is similar to the computed yield predicted by Weber et al. (2020) using a more detailed CRI-HOM (Common Representative Intermediates approach for highly oxygenated organic molecules) mechanism that invoked multigenerational peroxy radical chemistry in a global atmospheric chemistry model. Other models have applied numerous autoxidation mechanisms of varying complexity including a steady-state HOM yield assumption similar to CRACMM (Gordon et al., 2016), a volatility basis set model (Schervish and Donahue, 2020), and a near-explicit autoxidation mechanism involving 1773 reactions (Roldin et al., 2019). While the fixed HOM yields implemented in CRACMM consolidate the mechanism, additional species and reactions are considered here including NO3 oxidation chemistry, the chemistry of reactive monoterpenes like limonene, and many accretion reactions that may produce ELHOM. Further refinements to the autoxidation mechanism will be considered in future CRACMM versions including an implementation of the temperature dependence of H-shift reactions, potentially revised volatilities for HOM and ELHOM, and fragmentation reactions of highly oxidized peroxy radicals that may limit HOM production.
The CRACMM approach to monoterpene organic nitrates differs from previous CMAQ approaches where organic nitrates were incorporated into the particle via heterogenous uptake driven by hydrolysis reactions (Pye et al., 2015; Zare et al., 2019). CRACMM indicates a potentially significant role for TRPN in forming SOA but via a different mechanism than previous work which assumed a 3 h lifetime against condensed-phase hydrolysis (kHET (defined in a footnote in Appendix B) of s−1). TRPN could also release NOx upon chemical reaction (Saunders et al., 2003) and fragment into smaller molecules (Weber et al., 2020) which are not considered here. Future versions of CRACMM should incorporate monoterpene nitrate hydrolysis and release NOx upon reaction where appropriate.
Note that the identity of terpene nitrates when they are lumped into HOM or ELHOM is not retained. Lower-volatility nitrates, peroxides, ketones, and alcohols from terpene oxidation are lumped together based on volatility with HOM having an effective of 0 to −3 and a representative structure with of −2.2. ELHOM species are nominally highly oxygenated C20 dimers with an effective of −5, but species with C15 structures are also mapped to ELHOM based on their volatility (estimated as ). Given the importance of volatility as a driver of new particle formation events (McFiggans et al., 2019), the resolution in volatility for highly oxidized products should be investigated in future work in the context of predicting new particle formation events.
3.8 Isoprene and aqueous aerosol pathways
The treatment of isoprene chemistry in CRACMM version 1.0 is the same as in RACM2–AERO6 as implemented in CMAQv5.3.3. Notably, the CMAQ implementation includes formation of isoprene epoxydiols (IEPOX) as a tracer. An investigation of isoprene chemistry in CRACMM using the Automated MOdel REduction (AMORE) condensation of a detailed isoprene mechanism (Wennberg et al., 2018) with isoprene nitrate hydrolysis (Vasquez et al., 2020) is available in the work of Wiser et al. (2023) and as CRACMM1AMORE in CMAQv5.4.
Precursors to SOA from aqueous reactions include IEPOX, glyoxal (GLY), and methylglyoxal (MGLY) and follow CMAQ AERO7. GLY is a lumped species, and emissions include glycolaldehyde (total 2017 US GLY emissions: 418 Gg yr−1). MGLY is also lumped and includes 2-oxobutanal and other carbonyl aldehydes (total 2017 US MGLY emissions: 1129 Gg yr−1). SOA from IEPOX uptake follows the reactive uptake formulation of Pye et al. (2013) with the Henry's law coefficient for IEPOX (3.0 × 107 M atm−1) and an organosulfate condensed-phase formation rate constant (8.83 × 10−3 M−2 s−1) from the work of Pye et al. (2017). New in CRACMM compared to the standard AERO7 in CMAQ are separate species for the organosulfate (AISO3OS) vs. non-sulfated (2-methyltetrol, AISO3NOS) IEPOX-derived SOA to facilitate tracking of sulfur. Reactive uptake of GLY and MGLY on aqueous particles uses a fixed uptake coefficient (2.9 × 10−3) (Liggio et al., 2005) as in CMAQ version 5.2–5.3.3 (Pye et al., 2015). Cloud-processed SOA from GLY and MGLY is based on the reaction with aqueous HO and the work of Carlton et al. (2008). Glyoxal SOA may include formation of salt-like structures in the aerosol phase (Paciga et al., 2014), but, for simplicity, the oligomeric structure of Loeffler et al. (2006) is used as the representative structure of all glyoxal and methylglyoxal SOA. Note that the molecular weight of GLY and MGLY SOA specified in CRACMM differs from the representative structure. Aqueous reaction products leading to SOA in CRACMM, as implemented in CMAQ, are not currently allowed to volatilize to the gas phase, which likely occurs for a subset of IEPOX products (Riedel et al., 2015; D'Ambro et al., 2019).
3.9 Acrolein and 1,3-butadiene
Acrolein (ACRO) is a major oxidation product of 1,3-butadiene (BDE13), and both species were added explicitly in CRACMM due to their importance for health (Scheffe et al., 2016) (see Sect. 4). For a BDE13 reaction with HO, which is likely its dominant removal pathway (Agency for Toxic Substances and Disease Registry, 2012; Tuazon et al., 1999), the SAPRC-18 MechGen utility (Carter, 2020b) was used to generate products that are mapped to the analogous CRACMM species. SAPRC-18 MechGen is convenient since the products are already aggregated to a similar degree as RACM2 and CRACMM. A peroxy radical specific to the BDE13 reaction with HO (BDE13P) is used so that formation of acrolein (from all channels except BDE13P + HO2) could be explicitly predicted. For BDE13 + O3, a Criegee biradical is predicted to be a significant product in SAPRC-18 and MCMv3.3.1. Criegee biradicals are not implemented in CRACMM due to their short lifetime, so MCMv3.3.1 was used to determine the likely products from Criegee decomposition. For simplicity, the BDE13 reaction with nitrate follows the diene + NO3 products from RACM2 with acrolein instead of MACR specified as the product. Products from a reaction of ACRO with HO and NO3 are taken from RACM2's lumped MACR species. In the case of ACRO ozonolysis, prompt products as well as the expected Criegee biradical products are from MCM. ACRO photolysis products are from SAPRC-18 MechGen.
3.10 Additional rate constant updates
The inorganic chemistry of RACM2 is retained in CRACMM with updated rate constants for some reactions. In CRACMM, rate expressions for 26 inorganic reactions and 2 organic reactions (carbon monoxide and methane with HO; ethane as mentioned in Sect. 3.1) were updated compared to RACM2 values (IUPAC, 2010; Sander et al., 2011; Goliff et al., 2013) to follow the NASA JPL (Jet Propulsion Laboratory) evaluation number 19 (Burkholder et al., 2019) and IUPAC recommendations (Atkinson et al., 2004). Photolysis rate coefficients were updated for five chemical species: C3 and higher aldehydes (ALD), acetone (ACT), methyl ethyl ketone (MEK), higher ketones (KET), and formaldehyde (HCHO). The photolysis rate coefficient for ALD is set to that of propionaldehyde from the NASA JPL evaluation number 19 recommendation (Burkholder et al., 2019). CRACMM adds the acetone photolysis pathway producing a methylperoxy radical and carbon monoxide in addition to the existing RACM2 pathway that produces methyl peroxy and acetyl peroxy radicals. Quantum yields of ACT are updated following the NASA JPL evaluation number 19 recommendation (Burkholder et al., 2019). In addition, the temperature and pressure effects on ACT photolysis rate coefficients now follow Blitz et al. (2004). Photolysis rate coefficients and products of MEK and KET use quantum yield from Raber and Moortgat (1996) and absorption cross-sections from Brewer et al. (2019). The photolysis pathway for formaldehyde in RACM2 contained an error in quantum yield data resulting in overestimated photolysis rate coefficients, which are now corrected in CRACMM using data from the NASA JPL evaluation number 19 recommendation. These general kinetic updates are expected to lead to minor decreases in O3 formation compared to RACM2–AERO6.
Hazardous air pollutants are known or suspected to cause serious adverse health or environmental effects and are therefore a priority to represent in chemical mechanisms. However, the number of HAPs routinely considered should be moderated for computational efficiency. While 189 substances are designated as HAPs by the U.S. EPA, HAP species such as polycyclic organic matter (POM) and glycol ethers contain many individual compounds such that the actual number of individual species meeting the definition of a HAP is well over 3000 (U.S. Environmental Protection Agency, 2022c). The SPECIATE database, which includes a HAP identifier, was used as the initial source of identification for the species-level emission inventory and supplemented with additional data sources. POM was identified based on species with more than one benzene ring and 0 in their representative structure (an additional 56 species on top of the HAP category in SPECIATE). The POM requirement of a boiling point above 100 ∘C was found to be duplicative with the aromaticity criteria based on the work of Achten and Andersson (2015). The identifier of 1-bromopropane, a newly designated HAP (U.S. Environmental Protection Agency, 2022a), was updated. SPECIATE was also cross-referenced with individual glycol ethers (U.S. Environmental Protection Agency, 2022c) (four additional HAPs). CAS (Chemical Abstracts Service) numbers of individual species and their representative structures were cross-referenced with the toxicity value file input to the Human Exposure Model (U.S. Environmental Protection Agency, 2021a) identifying an additional 39 HAPs. Overall, 491 HAPs were identified in SPECIATE, of which 188 had non-zero ROC emissions in the 2017 inventory used here.
To assess the coverage of HAPs and their toxicity in CRACMM, toxicity potentials were estimated using chronic inhalation metrics from the U.S. Environmental Protection Agency (2021b). The EPA's process for estimating a cancer risk is based on the unit risk estimate (URE), which is the estimated number of excess tumors per person due to inhalation of 1 µg m−3 of the pollutant over a lifetime. Non-cancer (mutagenicity, developmental toxicity, neurotoxicity, and/or reproductive toxicity) risk uses a reference concentration (RfC), which is an estimate of the concentration that could be inhaled over a lifetime without an appreciable risk. Species in SPECIATE were matched to the inhalation RfC and URE values (U.S. Environmental Protection Agency, 2021a) by the CAS number. A few SPECIATE species (2,4-toluene diisocyanate, an m- and p-xylene mixture, an m- and p-cresol mixture, and a chrysene mixture) were manually mapped to relevant exposure risk values. In cases where a species in SPECIATE did not have a CAS or unique structure, a representative structure was used for mapping. A relative non-cancer toxicity potential was estimated based on the emitted mass of a species divided by the RfC, and a relative cancer toxicity potential was estimated as the product of the emissions and URE (Simon et al., 2010). For species designated as HAPs but not included in the toxicity value table (U.S. Environmental Protection Agency, 2021a), an RfC of 20 mg m−3 and URE of µg−1 m3, corresponding to the maximum RfC and minimum URE values for known HAPs, were used to provide what is potentially a conservative underestimate of risk potential.
Nine species in CRACMM cover 50 % of the total cancer and 60 % of the total non-cancer emission-weighted toxicity estimated for the anthropogenic and biomass burning emissions for 2017 US conditions (Fig. 6a: ACD, ETEG, ACRO, TOL, NAPH, MOH, HCHO, BDE13, and BEN). Toluene (chemistry in Sect. 3.5) is now separated from other aromatics and explicit due to its role as a HAP and significant emissions on an individual basis (430 Gg yr−1 in 2017, Fig. 6b) as well as to facilitate comparison with routine measurements. Ethylene glycol, toluene, and methanol are, however, not particularly strong drivers of cancer and non-cancer inhalation toxicity risk potential (Fig. 6b). NAPH (chemistry in Sect. 3.5), ACRO (chemistry in Sect. 3.9), and BDE13 (chemistry in Sect.3.9) are new mechanism species and are estimated to carry significant emission-weighted toxicity (Scheffe et al., 2016) (Fig. 6b). NAPH emissions are dominated by naphthalene (74 %) but include POM as well, making it an aggregate of HAPs. Naphthalene alone accounts for 70 % of the cancer and 98 % of the non-cancer emission-weighted toxicity of NAPH. In the case of ACRO, significant secondary production (not shown in Fig. 6b) is expected, and acrolein has been previously shown to be the largest contributor to non-cancer inhalation risk in the US (Scheffe et al., 2016). Given acetaldehyde and formaldehyde are also produced by oxidation of biogenic and anthropogenic emissions, the actual coverage of toxicity by the nine major HAP species is likely much higher than estimated based on the emissions alone. Previous work including secondary production estimated that acetaldehyde, benzene, formaldehyde, methanol, acrolein, 1,3-butadiene, and naphthalene represented over 84 % of the cancer risk and 93 % of the non-cancer respiratory risk effects in the US in 2011 (Scheffe et al., 2016).
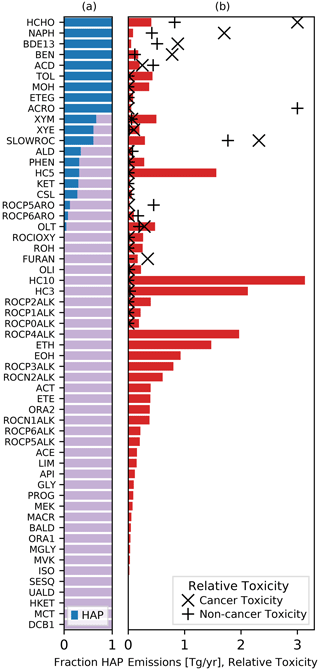
Figure 6Distribution of hazardous air pollutants (HAPs) across CRACMM emitted species. Panel (a) indicates the mass fraction of 2017 US anthropogenic and biomass burning ROC emissions by CRACMM species that are HAPs (blue). Panel (b) indicates the magnitude of emissions in teragrams per year by CRACMM species (bars) and the emission-weighted toxicity for cancer (×) or non-cancer (+) health effects. Cancer and non-cancer toxicity are normalized for purposes of display such that the species with the maximum value in each category is 3. Health risks are only shown for CRACMM species that contain non-zero emissions of HAPs. These data are available in the Supplement as Table D3 (Pye, 2022).
The lumped, slowly reacting ROC (SLOWROC, Sect. 3.1) is 61 % HAP by mass with enough emission-weighted toxicity to make it the second leading contributor to cancer and non-cancer health risk potential out of all CRACMM species (Fig. 6b). Species within SLOWROC have a lifetime against chemical reaction of about 1 month and are typically discarded from chemical transport model calculations for that reason. SLOWROC includes ethylene oxide and 1,2-dibromoethane, among many other species, that individually contribute to high levels of potential cancer risk (2nd and 10th highest emission-weighted toxicity out of all 188 individual HAPs in this work). Hydrogen cyanide is the most abundant individual species in SLOWROC and is the second largest contributor to non-cancer health risk potential for all HAPs considered. In standard CRACMM applications, SLOWROC concentrations could be used to indicate areas warranting additional investigation, but individual compound tracers would be required for studies specifically addressing the health impacts of these longer-lived pollutants. In CMAQv5.4, additional individual HAPs needed for air toxic assessments (e.g., Scheffe et al., 2016) can be added to a chemical mechanism as tracers with reactive decay.
In total, 29 ROC species in CRACMM contain some amount of HAP emissions (Fig. 6a). In terms of species with significant HAP emissions by mass, the two lumped, single-ring aromatic hydrocarbon categories (XYE and XYM) are 61 % and 67 % HAP by mass, with ethylbenzene (in XYE) and indene (in XYM) being the largest contributors to cancer toxicity and m-xylene (in XYM) and o-xylene (in XYE) being the largest contributors to non-cancer toxicity potential. The gas-phase chemistry of XYE is based on ethylbenzene (Sect. 3.5), so XYE could become an explicit HAP in CRACMM with changes only to emission mapping (redirecting single-ring species in XYE other than ethylbenzene to XYM). The two aromatic IVOCs are about 10 % HAP by emitted mass, with 2,4-toluene diisocyanate (ROCP5ARO) and aniline (ROCP6ARO) being the largest HAP contributors by mass as well as in terms of non-cancer health risk potential (5th and 10th out of 188 species). ALD (35 % HAP) includes the HAP propionaldehyde. OLT (5 % HAP by mass) includes acrylonitrile resulting in moderate cancer and non-cancer toxicity potential. Despite the low contributions by mass of HAPs to FURAN, FURAN shows moderate contributions to cancer potential due to the inclusion of chloroprene.
HAPs added in CRACMM provide greater explicit coverage of species contributing to chronic inhalation health risks, and many of the species classified as HAPs also contribute substantially to criteria pollutant formation. In total, HAPs are estimated to account for about 8 % of the total OA formation potential for 2017 US anthropogenic and biomass burning emissions (using SAR methods from Sect. 2.1). HAPs, with major contributors being formaldehyde, toluene, acetaldehyde, m-xylene, 1,3-butadiene, ethylbenzene, o-xylene, acrolein, ethylene glycol, and phenol, are predicted to contribute 31 % of the O3 formation potential for 2017 US anthropogenic and biomass burning emissions. Based on their potential for emission-weighted cancer toxicity (C), non-cancer toxicity (N), and O3 formation potential (O), priority HAPs to consider for purposes of protecting public health are the following: formaldehyde (CNO), ethylene oxide (C), naphthalene (C), 1,3-butadiene (CN), benzene (C), acrolein (N), hydrogen cyanide (N), toluene 2,4-diisocyanate (N), acetaldehyde (O), toluene (O), m-xylene (O), and methanol (O).
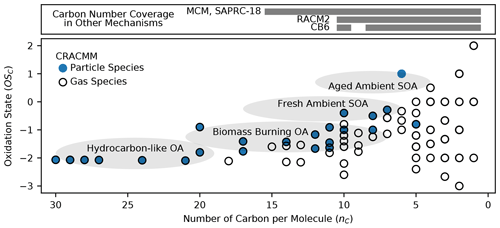
Figure 7Mean carbon oxidation state (OSC) and number of carbon atoms per molecule (nC) for all stable ROC species. Filled circles indicate at least one particulate species present in CRACMM. Black circles indicate the presence of at least one gas species in CRACMM. Grey ellipses indicate approximate ranges of observation-based bulk OSC and nC from the work by Kroll et al. (2011) for hydrocarbon-like OA (vehicle emissions and ambient hydrocarbon-like organic aerosol), biomass burning OA, fresh ambient SOA, and aged ambient SOA. Grey bars indicate nC coverage in mechanisms other than CRACMM.
In this section, CRACMM ROC species are visualized in terms of the carbon oxidation state and degree of oxygenation to understand if there are critical gaps in the atmospheric representation of ROC. The mean carbon oxidation state (OSC) of a species increases upon oxidation, and compounds generally move towards lower nC and higher OSC as they are chemically processed in the atmosphere (Kroll et al., 2011). This view emphasizes SOA as a chemical intermediate on the path toward smaller and more functionalized compounds with carbon dioxide (OSC= 4) as the ultimate endpoint. Using the CRACMM representative structures (Appendix A), each stable ROC species was plotted in the OSC vs. nC space (Fig. 7) using the OSC definition of Kroll et al. (2011) considering the number of carbons, hydrogens (nH), and oxygens (nO) per molecule and expanded to include nitrogen (nN) and sulfur (nS) (assuming sulfate and nitrate functionality) as follows:
CRACMM species cover the atmospherically relevant range of ROC oxidation state and nC (Fig. 7). The largest nC species in CRACMM are alkane like with 20 to 30 carbons and a low-oxidation state consistent with observations of particulate vehicle exhaust and ambient hydrocarbon-like organic aerosol (Kroll et al., 2011). Other OA species in CRACMM generally fall in the range of nC and OSC reported for ambient observations of biomass burning organic aerosol, fresh ambient (less oxygenated) SOA, and aged (more oxygenated) ambient SOA. These ambient observations are based on bulk analysis (Kroll et al., 2011), and thus the observed ranges shown do not identify each possible SOA contributor at the molecular level. Monoterpene SOA monomers (AHOM) and dimers (AELHOM) have an oxidation state of −0.4 and −0.9, respectively, similar to laboratory data (Kroll et al., 2011). Monoterpene SOA has also been linked with the less oxidized (fresh ambient SOA) aerosol mass spectrometer (AMS) surrogate (Xu et al., 2018).
Two species in CRACMM, the glyoxal and methylglyoxal SOA from uptake in aqueous particles (AGLY) and clouds (AORGC), have overlap with the observed ambient aged SOA, which is often identified via positive matrix factorization analysis as a more oxidized oxygenated organic aerosol (MO-OOA) (Zhang et al., 2011). The MO-OOA factor has been linked to SOA from aqueous processing (Xu et al., 2017), and 10 % by mass of the MO-OOA in the southeastern US has been attributed to low-molecular-weight carboxylic acids, of which dicarboxylic acids are primarily from aqueous processing (Y. Chen et al., 2021). Aqueous isoprene SOA species such as isoprene-derived organosulfates and 2-methyltetrols (nC= 5) match properties of known major isoprene SOA constituents (Kroll et al., 2011; Surratt et al., 2010), and aqueous isoprene SOA (not shown in Fig. 7) is often resolved separately from MO-OOA. If the aged SOA region described by MO-OOA does represent an intermediate through which significant amounts of carbon should pass, additional chemical pathways beyond those from glyoxal and methylglyoxal may be needed in CRACMM.
Other mechanisms besides CRACMM (top of Fig. 7) focus on the more volatile range of ROC. MCM and SAPRC-18 include a sesquiterpene species with 15 carbons but otherwise focus on smaller-carbon-number species. The range in nC for alkane-like species in current mechanisms was highlighted in Sect. 3.1 and never exceeds 12. In terms of aromatics, the largest aromatic in MCM is a C11 diethyltoluene. SAPRC-18 includes some naphthalene-like species with 12 carbons, and RACM2 represents single-ring aromatics with ∼ 9 carbons (Fig. 1, XYM). CB6 has a xylene species with 8 carbons, and RACM2 and CB6 both include monoterpenes as their largest species by nC. CRACMM S/IVOCs with alkane, aromatic, and oxygenated structures populate the higher-carbon-number (nC > 10) space that includes known organic aerosol species as well as precursors with high SOA yields and is not covered by current mechanisms due to their focus on gas-phase endpoints.
As a complement to OSC, van Krevelen diagrams of nH:nC vs. nO:nC for individual and bulk species have been used to provide insight into the evolution of ambient organic aerosol (Heald et al., 2010). Since hydrogen and oxygen are generally the most abundant non-carbon elements in organic aerosol, these diagrams can help identify types of chemical functionalization. Primary emissions, particularly for alkane-like sources like vehicles tend to reside near an nH:nC of 2 and nO:nC of 0. Atmospheric processing generally moves OA towards higher nO:nC and lower nH:nC with the trajectory determined by the abundance of alcohol and peroxide (slope of 0) vs. ketone and aldehyde (slope of −2) groups (Heald et al., 2010). Mean atmospheric transformation of OA has been observed to occur along a slope of −0.5 (Ng et al., 2011) to −0.6 (Chen et al., 2015), which reflects either carboxylic acids or a combination of alcohols, peroxides, ketones, and aldehydes. Figure 8 (black line) shows the observed trend and range in nO:nC from the ambient atmosphere from multiple field campaigns extended to an nO:nC of 0 for primary source measurements.
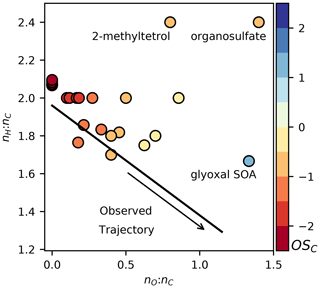
Figure 8Molar ratios of hydrogen to carbon (nH:nC) and oxygen to carbon (nO:nC) of CRACMM particulate ROC species. Color indicates the mean carbon oxidation state (OSC). The observed trajectory trend line with a slope of −0.6 is based on ambient measurements assembled by Chen et al. (2015) and extended to laboratory systems with nO:nC near 0. Three CRACMM species are labeled glyoxal SOA (AGLY), isoprene-derived organosulfates (AISO3OS), and non-sulfated isoprene SOA represented as 2-methyltetrols (AISO3NOS).
The 26 individual particulate organic species in CRACMM span the full range of observed nO:nC in bulk OA with excellent coverage for nO:nC < 0.5 (Fig. 8). The highest observed nO:nC conditions (∼ 1.2) were only present in remote regions sampled by aircraft as described in the work by Chen et al. (2015). While CRACMM includes species with high nO:nC, those species (glyoxal SOA, isoprene organosulfate SOA, and non-sulfated isoprene SOA) tend to have much higher nH:nC than the ambient trend suggests. Note that nO:nC based on measurement techniques may not include all the oxygen in organosulfate compounds and oxidation state is likely a more robust way to measure degree of oxidation than nO:nC based on techniques like use of an AMS (Canagaratna et al., 2015). Particularly for the nO:nC > 0.5 OA species, CRACMM indicates more hydrogen than ambient observations suggest. If the ambient observations are correct, future versions of CRACMM could resolve the overestimate in nH:nC by the following: (1) shifting the representative compound structures (for species like ROCN2OXY8) to reflect more ketones; (2) adjusting the assumed change in volatility per oxygen in the secondary oxygenated chemistry (Sect. 3.2); and/or (3) adding more chemical channels resulting in condensible ketones; carboxylic acids; or other high-nO:nC, low-nH:nC products (e.g., photolysis of SOA, Baboomian et al., 2020). Combined with the information from the oxidation state plot (Fig. 7), CRACMM may need SOA species that are both lower in H and higher in O and at smaller carbon numbers with implications for aerosol hygroscopicity and mass (Pye et al., 2017).
Chen et al. (2015) noted that SOA produced in laboratory experiments was generally too low in nH:nC at a given nO:nC and tended to reside below the black ambient line in Fig. 8. CRACMM species are above the ambient trend line, suggesting that our conceptual picture of atmospheric processing to SOA, informed by known gas-phase chemistry and 2-D VBS approaches, does not match what is observed in laboratory experiments. One possible reason is the preferential sampling of certain chemical space in laboratory experiments (Porter et al., 2021).
Figures 7 and 8 suggest that chemistry leading to OA needs to be considered in mechanism development to obtain an accurate representation of gas and particulate ROC including the correct properties of OA. Accurate properties of OA are critical for estimating hygroscopicity with implications for climate (Haywood and Boucher, 2000) as well as fine-particle mass (Pye et al., 2017). The linkages between gas and particulate endpoints are further emphasized by examining emissions from anthropogenic and biomass burning sources of ROC by volatility class and their propagation to endpoints (Fig. 9). Total emissions of ROC in 2017 (excluding biogenic VOCs) are estimated at 21 Tg yr−1, with VOCs as the most abundantly emitted volatility class of compounds. VOCs dominate ROC HO reactivity, accounting for 81 % of the total. In addition, the total US O3 formation potential is estimated as 47 Tg yr−1, with VOCs accounting for 90 % of it (based on the MIR SAR, Fig. 9). Thus, across all anthropogenic and biomass burning sources and locations for 2017, VOCs are the dominant contributors to gas-phase endpoints such as HO reactivity and O3; however, emitted IVOCs (generally excluded from mechanism development) make appreciable contributions to estimated gas-phase endpoints (18 % of HO reactivity and 10 % of the O3 formation potential). As a class, the O3 from IVOCs (about 4.5 Tg yr−1) exceeds the O3 estimated for any individual CRACMM species in Fig. 1. In terms of effective MIR, IVOCs (effective MIR of 1.1 g O3 g−1 ROC) are comparable to HC10 and exceed that of BEN, HC3, and ETH. L/SVOCs are not substantial contributors to HO reactivity or O3 formation (∼ 1 %) due to slower reaction rates (kOH, Fig. 3) and alkane-like structures with less potential for O3 formation (effective MIR of 0.14 to 0.27 g O3 g−1 ROC). The OA potential from ROC emissions in the US (excluding biogenic emissions) is estimated as 5 Tg yr−1 and emphasizes the need to consider L/S/IVOCs. Traditional VOCs (effective SOA yield of 5 %) are important (14 % of total) contributors to OA potential, but OA potential is dominated by IVOCs (38 %) and S/IVOCs (48 %) due to their initially lower volatility and ability to become condensible with only small additions in functionality.
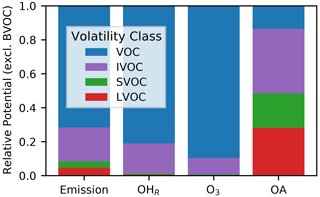
Figure 9Anthropogenic and wood-burning ROC emissions and their relative potential HO reactivity (OHR), ozone (O3) formation, and OA for 2017 US conditions by volatility class. Biogenic VOCs (BVOCs) are not considered here. Ozone and OA formation potentials are calculated using the MIR and OA simple-SAR approaches from Sect. 2.1. Metrics are aggregated from the individual-species level to the following volatility classes: low-volatility organic compounds (LVOCs), semivolatile organic compounds (SVOCs), intermediate-volatility organic compounds (IVOCs), and volatile organic compounds (VOCs).
CRACMM provides an integrated approach to the representation of O3, organic aerosol, and many HAPs in air. These endpoints are linked as O3, SOA, and secondary HAPs such as formaldehyde and acrolein are products of gas-phase precursor emissions including primary HAPs. This section highlights reasons why mechanism development remains important and provides specific recommendations for future work based on lessons from CRACMM development.
First, the magnitude and compound identity of ROC emissions is an active area of research, and mechanisms need to interface with this emerging information. Improving emission characterization without the accompanying mechanism linkages hinders accurate source apportionment and effective air quality management decisions. Much of the work on emission speciation is identifying new species in the IVOC range, which has been historically neglected by gas-phase mechanisms but is necessary for both O3 and SOA prediction. Emission speciation work should continue to characterize source profiles in databases and other forums at the highest level of individual compound detail available using representative structures when necessary so that compounds can be easily mapped to mechanisms. In addition, efforts to accurately determine the emissions of individual HAPs, especially formaldehyde, acetaldehyde, toluene, m-xylene, and methanol, which are important for O3, should be leveraged in the preparation of emission inputs for regional chemical transport models even when HAPs are not the primary objective. The development of emissions and mechanisms should continue to be an iterative process in which new measurement techniques better quantify and identify emissions resulting in new or refined mechanism species. Simultaneously, mechanisms can indicate which emitted species constitute a high priority to constrain due to their role in secondary pollutant formation or health impacts.
Second, current chemical transport model mechanisms do not characterize the full range of atmospheric ROC, and such analysis could help identify missing sources of SOA, HO reactivity, formaldehyde, and other secondary HAPs. The ability to account for all reactive tropospheric carbon and perform a ROC budget analysis in current mechanisms is limited due to the focus on the more volatile range of ROC, which excludes lower-volatility primary ROC. In addition, some carbon in secondary ROC, including species in the volatile range, is discarded in mechanisms like SAPRC-07 and RACM2 because of product lumping for computational efficiency. For example, the largest organic peroxide in RACM2 is OP2 with two carbons. So, peroxides formed from RO2 + HO2 reactions for xylene-like aromatics (nC= 9) result in a loss of seven carbons per reaction. In the RACM2 monoterpene system, eight carbons or 80 % of the parent carbon is lost each time a peroxide is formed, and SAPRC-07 loses four carbons for each monoterpene peroxide formed. While conservation of emitted mass is a priority in the design of CRACMM and more secondary mechanism species were added at the higher carbon numbers (e.g., a C8 and C10 peroxide), the chemical scheme in CRACMM is like RACM2 and SAPRC-07 in that it does not conserve mass upon reaction for all chemical systems. However, by curating structural identifiers (SMILES) for all species in CRACMM, conservation of carbon can now be calculated, and the importance of lost (or gained) carbon can be examined. The CMAQv5.4 implementation of CRACMM includes an updated chemical mechanism processor that creates an optional diagnostic file containing the elemental balance for each CRACMM reaction. Future work will aim to calculate mass balance across the mechanism and use it as a diagnostic tool to guide development.
Third, current gas-phase mechanisms do not couple radical chemistry with SOA formation, and linking the development provides additional constraints for ozone-forming reactions as well as secondary inorganic aerosol production. Particles and ozone are inherently linked systems (Ivatt et al., 2022; Womack et al., 2019). Molar yields for SOA are often comparable to molar yields of existing gas-phase product channels, and SOA mass should be removed from volatile gas-phase products. Properly sequestering products like peroxides in the particle will remove them as a potential photolytic source of radicals that releases HOx back to the atmosphere. Similarly, sequestering one organic nitrate in the particle phase could remove one HOx and one NO from the gas-phase system. Autoxidation, implemented in CRACMM primarily to produce SOA, effectively sequesters radicals since they are generally of sufficiently low volatility to condense. CRACMMv1.0 targeted SOA systems for development, but CRACMM updates impact O3 is demonstrated for the northeastern US in companion work (Place et al., 2023). Future versions of CRACMM should continue to consider chemical channels that lead to both gas-phase and particulate products to better constrain O3.
Fourth, linking gas-phase chemistry with SOA formation for the first time enabled the treatment of new SOA precursors with implications for the magnitude and source attribution of OA. Organic aerosol is dynamic with properties that evolve as a function of the precursor and chemical regime and need to be considered part of a holistic treatment of atmospheric chemistry. The interconnected nature of aromatic, phenolic, and furan systems highlights why mechanism development should consider SOA production alongside gas-phase chemistry. Developing phenolic and furanone gas-phase chemistry without consideration of SOA (as in CMAQv5.3.3) neglects a significant SOA source. Specifying SOA yields for phenolic and aromatic hydrocarbon precursors without recognizing they are also secondary would duplicate SOA mass. As a result, both phenolic and non-phenolic routes to SOA need to be specified consistently. The attribution of aromatic SOA to these two routes will affect how much SOA is predicted overall and how it is attributed to various sources. In the case of benzene SOA, the more SOA comes from phenol vs. non-phenol channels, the higher the total SOA potential of US emissions (as phenol > benzene emissions) and larger the attribution to sources with high ratios of phenol to benzene such as wildland fires and residential wood combustion. Previous work estimated oxidation of phenol, naphthalene, and benzene alone can account for 80 % of the SOA from residential wood combustion (Bruns et al., 2016). The importance of connecting SOA with multigenerational gas-phase chemistry also applies to the monoterpene system, where the fate of terpene nitrates and aldehydes will significantly modulate SOA formation. In the case of monoterpene SOA, the allocation of SOA between initial autoxidation, terpene nitrate, and aldehyde channels will affect the NOx dependence of total monoterpene SOA and therefore how much is considered controllable vs. non-controllable. The allocation of SOA among different later-generation species should continue to be evaluated and revised as new information becomes available which will improve source apportionment of fine-particle mass.
Fifth, new measurement techniques, observational studies, and computational methods are continually improving the characterization of many chemical systems, and their results need to be translated to model mechanisms. Autoxidation was determined to be an atmospherically relevant chemical pathway just under a decade ago (Crounse et al., 2013) and will be considered in CMAQ for the first time in CRACMMv1.0. Just this year, a new class of atmospherically relevant compounds, hydrotrioxides, were identified (Berndt et al., 2022). Even for traditional systems, information continues to emerge. For example, benzene mechanisms have been historically built on data that characterized about half of the product mass with recent work used to inform CRACMMv1.0 reaching ∼ 80 % carbon closure (Xu et al., 2020). Measurement techniques and the availability of observational data will only further improve, providing more complete data to design and evaluate mechanisms going forward.
Finally, the chemistry of the atmosphere in the US and elsewhere is changing, and previously acceptable representations of chemistry may need modification. Autoxidation is one example of a pathway likely to grow in importance, but indications of change can be seen in multiple systems. Deposition of nitrogen has shifted from primarily oxidized nitrogen (nitrate) to reduced nitrogen (ammonia) (Li et al., 2016). Fine-particle mass is no longer dominated by summertime sulfate (Chan et al., 2018), and the temperature dependence of summertime urban northeastern US PM2.5 is now being modulated by organic aerosol (Vannucci and Cohen, 2022). Particulate sulfur is also becoming increasingly recognized as organic (Riva et al., 2019; Moch et al., 2018). At the same time that sulfate and nitrate in cloud water have been decreasing at a mountaintop site in the northeastern US, total organic carbon in cloud water may be increasing (Lawrence et al., 2023). Organic compounds in air are changing with total US emissions of anthropogenic ROC going from ∼ 30 % lower than NOx in 2002 to exceeding NOx by ∼ 40 % in 2019 (Pye et al., 2022). The composition of ROC is also changing to more oxygenated forms, resulting in an average reduction in the O3 formation potential of an individual VOC of about 20 % due to mixture effects (Venecek et al., 2018). Questions chemical transport modeling and mechanisms are being asked to answer are also changing with increasing interest in wildland fires (McClure and Jaffe, 2018), volatile chemical products (Seltzer et al., 2022), and per- and polyfluoroalkyl substances (D'Ambro et al., 2021) among others. Changes in air pollution sources and questions of interest as well as chemical regimes over time require continued mechanism development, and CRACMM is now available as a community framework for further development.
Table A1ROC species in CRACMM and their description, phase (Phs) in which they can exist (G: gas, P: particle), and SMILES for representative compound structure. Appendix A along with additional ROC species information is also available in csv format in the data archive associated with this work (Table D1; Pye, 2022). Species properties such as molecular weights are determined from the representative structure except in the case of highly empirical species (SLOWROC, VROCIOXY, ASOAT). In CMAQ, aerosol species reside in Aitken, accumulation, and/or coarse modes and are appended with the letter to indicate the size mode. Five non-volatile, organic aerosol species start with the letter A (AISO3NOS, AISO3OS, AORGC, ASOAT, and AGLY). Some gas-phase species inherited from RACM2 (all indicated here) start with an A. In all other cases, an prepended A in CMAQ indicates a particulate form of the species below. A prepended V (if present) will indicate a gas-phase species.
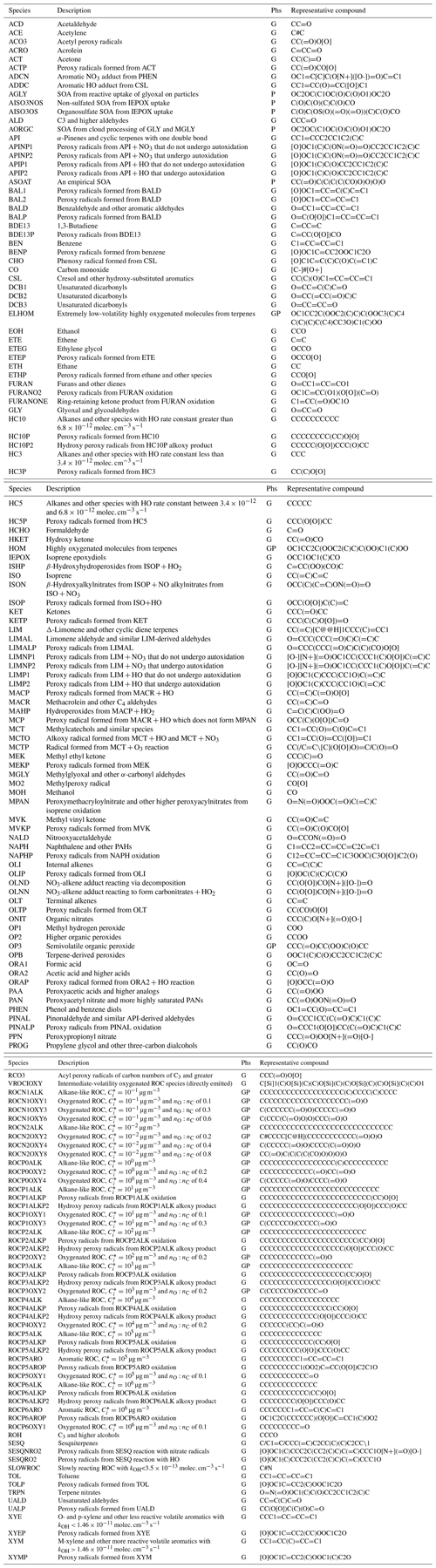
Table B1Chemistry of CRACMMv1.0. For photolysis and heterogenous reactions (rate constant values not provided), rates depend on radiation, predicted concentrations, and/or other conditions, so a reference to the underlying data and formulation is provided. Rate constant values (k), if provided, are specified at 298.15 K, M=2.4615 × 1019 molec. cm−3, and 1.00 atm. This information is also available in the supporting data archive and in CMAQv5.4. Partitioning of condensible organics is not listed here, and CMAQ assumes equilibrium partitioning calculated via operator splitting separate from the kinetic chemistry. Some coefficients have been rounded to the thousandths for brevity. Please see the “Code availability” section for where to find the mechanism files.

a Reaction rate constants following Arrhenius behavior are specified as k= Ae. Falloff or pressure-dependent reaction rate constants are specified as follows (M equals air number density): for rate constants with ko, ki, n, and F values, k= []FG, where G=(1+(log 10()2)−1; for rate constants with k1 and k2, k= k1+k2M; for rate constants with k0, k2, and k3, k= ; and for rate constants with k1, k2, and k3, k= . b Heterogeneous rates are specified as , where SA is the fine aerosol surface area, rp is the effective particle radius, Dg is the gas-phase diffusivity, ν is the mean molecular speed, and γ is the uptake coefficient. In the case of a heterogeneous NO2 reaction, the gas-phase diffusivity term in the denominator is neglected. c CMAQ calculates photolysis rate coefficients (J values) as follows: , where F(λ) is the actinic flux (photons cm−2 min−1 nm−1), σi(λ) is the absorption cross-section for the molecule undergoing a photolytic reaction (cm2 molec.−1), φi(λ) is the quantum yield of the photolysis reaction (molec. photon−1), and λ is the wavelength (nm). CMAQ uses seven-binned absorption cross-section and quantum yield data for calculating J values. Sources of absorption cross-section and quantum yield data are provided in the table. d The rate constant for R067 is scaled to the reverse equilibrium of R066. e The HAL_Ozone reaction represents a loss of ozone over ocean surfaces due to halogen chemistry. The rate is set to 0 if the sun is below the horizon and if the surface does not include sea or surf zones (P: air pressure in atmospheres) (Sarwar et al., 2015). f SULF represents sulfuric acid. In CMAQ, a tracking species, SULRXN, is used to implement sulfuric acid and subsequent condensation. g IEPOXP is an intermediate used for logistical reasons in CMAQ. It does not have a meaningful concentration.
The EPA's Chemicals Dashboard is available at https://comptox.epa.gov/dashboard (U.S. Environmental Protection Agency, 2021d). OPERA predictions of species properties can be obtained from the Chemicals Dashboard or for any species with a SMILES record using the EPA's Chemical Transformation Simulator at https://qed.epa.gov/cts/ (U.S. Environmental Protection Agency, 2022f). SPECIATE is distributed at https://www.epa.gov/air-emissions-modeling/speciate (U.S. Environmental Protection Agency, 2022g). RDKit version 2020.09.01 was used in Python (RDKit, 2020). The implementation of RACM2–AERO6 is available in CMAQv5.3.3 (https://doi.org/10.5281/zenodo.3585898, U.S. Environmental Protection Agency Office of Research and Development, 2019). RACM2 and CRACMMv1.0 in CMAQv5.4 (released October 2022) are available on GitHub (https://github.com/USEPA/CMAQ, last access: 21 April 2023) and Zenodo (https://doi.org/10.5281/zenodo.7218076, U.S. EPA Office of Research and Development, 2022). Supporting data for CRACMM, including the SPECIATE database mapped to CRACMM, input to the Speciation Tool, profile files output from the Speciation Tool for input to SMOKE, Python code for mapping species to CRACMM, chemical mechanism, and mechanism metadata, are available at https://github.com/USEPA/CRACMM (last access: 21 April 2023). Specific analyses and scripts used in this paper such as the 2017 US species-level inventory and code for figures are archived at https://doi.org/10.23719/1527956 (Pye, 2022).
The supplement related to this article is available online at: https://doi.org/10.5194/acp-23-5043-2023-supplement.
HOTP designed the overall scope and drafted the initial document with input from coauthors. The figures in the main text were prepared by BNM (Fig. 4) and HOTP (all others). HOTP, BNM, and KMS prepared the figures in the Supplement. The chemistry of various ROC systems was designed by HOTP (aromatics, sesquiterpenes, primary oxygenated IVOCs, and other miscellaneous SOA systems), BKP (monoterpenes), BNM (secondary oxygenated ROC), KMS (S/IVOC alkanes), ELD (1,3-butadiene and acrolein), IRP (monoterpenes), RHS (S/IVOC alkanes, furans), MMC (furans, propylene glycol), and LX (aromatics). HOTP, BKP, BNM, KMS, ELD, SF, GS, BH, and JB coded the CMAQ implementation of CRACMM. HOTP, KMS, ELD, IRP, and SF determined representative compound structures for SPECIATE. HOTP, KMS, CA, KMF, and GP developed the 2017 emission inventory and resulting SOA and ozone analysis. ES, GS, BH, and WRS updated rate constants and photolysis reactions in reactions ported from RACM2. HOTP performed the HAP analysis. All coauthors contributed to developing the mechanism and editing the paper.
The contact author has declared that none of the authors has any competing interests.
The views expressed in this article are those of the authors and do not
necessarily represent the views or policies of the U.S. Environmental
Protection Agency, Department of Energy (DOE), or Oak Ridge Institute of
Science and Education (ORISE).
Publisher’s note: Copernicus Publications remains neutral with regard to jurisdictional claims in published maps and institutional affiliations.
This work was supported by the U.S. Environmental Protection Agency Office of Research and Development. This research was supported in part by an appointment to the U.S. Environmental Protection Agency (EPA) Research Participation Program administered by the ORISE through an interagency agreement between the U.S. DOE and the U.S. Environmental Protection Agency. ORISE is managed by ORAU (Oak Ridge Associated Universities; DOE contract no. DE-SC0014664). We thank internal reviewers at EPA for providing comments on a draft of this paper. We thank Kelley Barsanti for useful discussion about emissions and mechanism development and Chris Nolte for perspectives on model development. We thank Rohit Mathur and Sergey Napelenok for comments on a draft version of the paper. Matthew M. Coggon, Rebecca H. Schwantes, and Lu Xu acknowledge support through the EPA STAR program (grant no. 84001001) and CIRES (cooperative agreement no. NA17OAR4320101). Lu Xu also acknowledges support from NASA (grant no. 80NSSC21K1704).
This research has been supported by the U.S. Environmental Protection Agency (grant no. 84001001), the National Oceanic and Atmospheric Administration (grant no. NA17OAR4320101), and the National Aeronautics and Space Administration (grant no. 80NSSC21K1704).
This paper was edited by Arthur Chan and reviewed by two anonymous referees.
Achten, C. and Andersson, J. T.: Overview of polycyclic aromatic compounds (PAC), Polycyclic Aromat. Compd., 35, 177–186, https://doi.org/10.1080/10406638.2014.994071, 2015.
Agency for Toxic Substances and Disease Registry: Toxicological profile for 1,3-butadiene, https://www.atsdr.cdc.gov/ToxProfiles/tp28.pdf (last access: 17 May 2022), 2012.
Ahmadov, R., McKeen, S. A., Robinson, A. L., Bahreini, R., Middlebrook, A. M., de Gouw, J. A., Meagher, J., Hsie, E.-Y., Edgerton, E., Shaw, S., and Trainer, M.: A volatility basis set model for summertime secondary organic aerosols over the eastern United States in 2006, J. Geophys. Res.-Atmos., 117, D06301, https://doi.org/10.1029/2011JD016831, 2012.
Appel, K. W., Chemel, C., Roselle, S. J., Francis, X. V., Hu, R.-M., Sokhi, R. S., Rao, S. T., and Galmarini, S.: Examination of the Community Multiscale Air Quality (CMAQ) model performance over the North American and European domains, Atmos. Environ., 53, 142–155, https://doi.org/10.1016/j.atmosenv.2011.11.016, 2012.
Appel, K. W., Bash, J. O., Fahey, K. M., Foley, K. M., Gilliam, R. C., Hogrefe, C., Hutzell, W. T., Kang, D., Mathur, R., Murphy, B. N., Napelenok, S. L., Nolte, C. G., Pleim, J. E., Pouliot, G. A., Pye, H. O. T., Ran, L., Roselle, S. J., Sarwar, G., Schwede, D. B., Sidi, F. I., Spero, T. L., and Wong, D. C.: The Community Multiscale Air Quality (CMAQ) model versions 5.3 and 5.3.1: system updates and evaluation, Geosci. Model Dev., 14, 2867–2897, https://doi.org/10.5194/gmd-14-2867-2021, 2021.
Atkinson, R., Baulch, D. L., Cox, R. A., Crowley, J. N., Hampson, R. F., Hynes, R. G., Jenkin, M. E., Rossi, M. J., and Troe, J.: Evaluated kinetic and photochemical data for atmospheric chemistry: Volume I – gas phase reactions of Ox, HOx, NOx and SOx species, Atmos. Chem. Phys., 4, 1461–1738, https://doi.org/10.5194/acp-4-1461-2004, 2004.
Atkinson, R., Baulch, D. L., Cox, R. A., Crowley, J. N., Hampson, R. F., Hynes, R. G., Jenkin, M. E., Rossi, M. J., Troe, J., and IUPAC Subcommittee: Evaluated kinetic and photochemical data for atmospheric chemistry: Volume II – gas phase reactions of organic species, Atmos. Chem. Phys., 6, 3625–4055, https://doi.org/10.5194/acp-6-3625-2006, 2006.
Aumont, B., Szopa, S., and Madronich, S.: Modelling the evolution of organic carbon during its gas-phase tropospheric oxidation: development of an explicit model based on a self generating approach, Atmos. Chem. Phys., 5, 2497–2517, https://doi.org/10.5194/acp-5-2497-2005, 2005.
Baboomian, V. J., Gu, Y., and Nizkorodov, S. A.: Photodegradation of Secondary Organic Aerosols by Long-Term Exposure to Solar Actinic Radiation, ACS Earth Space Chem., 4, 1078–1089, https://doi.org/10.1021/acsearthspacechem.0c00088, 2020.
Bates, K. H., Jacob, D. J., Li, K., Ivatt, P. D., Evans, M. J., Yan, Y., and Lin, J.: Development and evaluation of a new compact mechanism for aromatic oxidation in atmospheric models, Atmos. Chem. Phys., 21, 18351–18374, https://doi.org/10.5194/acp-21-18351-2021, 2021.
Berndt, T., Richters, S., Jokinen, T., Hyttinen, N., Kurtén, T., Otkjær, R. V., Kjaergaard, H. G., Stratmann, F., Herrmann, H., Sipilä, M., Kulmala, M., and Ehn, M.: Hydroxyl radical-induced formation of highly oxidized organic compounds, Nat. Commun., 7, 13677, https://doi.org/10.1038/ncomms13677, 2016.
Berndt, T., Chen, J., Kjærgaard, E. R., Møller, K. H., Tilgner, A., Hoffmann, E. H., Herrmann, H., Crounse, J. D., Wennberg, P. O., and Kjaergaard, H. G.: Hydrotrioxide (ROOOH) formation in the atmosphere, Science, 376, 979–982, https://doi.org/10.1126/science.abn6012, 2022.
Bianchi, F., Kurtén, T., Riva, M., Mohr, C., Rissanen, M. P., Roldin, P., Berndt, T., Crounse, J. D., Wennberg, P. O., Mentel, T. F., Wildt, J., Junninen, H., Jokinen, T., Kulmala, M., Worsnop, D. R., Thornton, J. A., Donahue, N., Kjaergaard, H. G., and Ehn, M.: Highly Oxygenated Organic Molecules (HOM) from gas-phase autoxidation involving peroxy radicals: A key contributor to atmospheric aerosol, Chem. Rev., 119, 3472–3509, https://doi.org/10.1021/acs.chemrev.8b00395, 2019.
Birdsall, A. W. and Elrod, M. J.: Comprehensive NO-dependent study of the products of the oxidation of atmospherically relevant aromatic compounds, J. Phys. Chem. A, 115, 5397–5407, https://doi.org/10.1021/jp2010327, 2011.
Blitz, M. A., Heard, D. E., Pilling, M. J., Arnold, S. R., and Chipperfield, M. P.: Pressure and temperature-dependent quantum yields for the photodissociation of acetone between 279 and 327.5 nm, Geophys. Res. Lett., 31, L06111, https://doi.org/10.1029/2003GL018793, 2004.
Bloss, C., Wagner, V., Jenkin, M. E., Volkamer, R., Bloss, W. J., Lee, J. D., Heard, D. E., Wirtz, K., Martin-Reviejo, M., Rea, G., Wenger, J. C., and Pilling, M. J.: Development of a detailed chemical mechanism (MCMv3.1) for the atmospheric oxidation of aromatic hydrocarbons, Atmos. Chem. Phys., 5, 641–664, https://doi.org/10.5194/acp-5-641-2005, 2005.
Brewer, J. F., Papanastasiou, D. K., Burkholder, J. B., Fischer, E. V., Ren, Y., Mellouki, A., and Ravishankara, A. R.: Atmospheric photolysis of methyl ethyl, diethyl, and propyl ethyl ketones: Temperature-dependent UV absorption cross sections, J. Geophys. Res.-Atmos., 124, 5906–5918, https://doi.org/10.1029/2019JD030391, 2019.
Browne, E. C., Wooldridge, P. J., Min, K.-E., and Cohen, R. C.: On the role of monoterpene chemistry in the remote continental boundary layer, Atmos. Chem. Phys., 14, 1225–1238, https://doi.org/10.5194/acp-14-1225-2014, 2014.
Bruns, E. A., El Haddad, I., Slowik, J. G., Kilic, D., Klein, F., Baltensperger, U., and Prévôt, A. S. H.: Identification of significant precursor gases of secondary organic aerosols from residential wood combustion, Sci. Rep.-UK, 6, 27881, https://doi.org/10.1038/srep27881, 2016.
Burkholder, J. B., Sander, S. P., Abbatt, J., Barker, J. R., Cappa, C., Crounse, J. D., Dibble, T. S., Huie, R. E., Kolb, C. E., Kurylo, M. J., Orkin, V. L., Percival, C. J., Wilmouth, D. M., and Wine, P. H.: Chemical Kinetics and Photochemical Data for Use in Atmospheric Studies, Evaluation No. 19 JPL Publication 19-5, https://jpldataeval.jpl.nasa.gov/pdf/NASA-JPL Evaluation 19-5.pdf (last access: 16 May 2022), 2019.
Canagaratna, M. R., Jimenez, J. L., Kroll, J. H., Chen, Q., Kessler, S. H., Massoli, P., Hildebrandt Ruiz, L., Fortner, E., Williams, L. R., Wilson, K. R., Surratt, J. D., Donahue, N. M., Jayne, J. T., and Worsnop, D. R.: Elemental ratio measurements of organic compounds using aerosol mass spectrometry: characterization, improved calibration, and implications, Atmos. Chem. Phys., 15, 253–272, https://doi.org/10.5194/acp-15-253-2015, 2015.
Carlton, A. G., Turpin, B. J., Altieri, K. E., Seitzinger, S. P., Mathur, R., Roselle, S. J., and Weber, R. J.: CMAQ Model Performance Enhanced When In-Cloud Secondary Organic Aerosol is Included: Comparisons of Organic Carbon Predictions with Measurements, Environ. Sci. Technol., 42, 8798–8802, https://doi.org/10.1021/es801192n, 2008.
Carlton, A. G., Bhave, P. V., Napelenok, S. L., Edney, E. O., Sarwar, G., Pinder, R. W., Pouliot, G. A., and Houyoux, M.: Model representation of secondary organic aerosol in CMAQv4.7, Environ. Sci. Technol., 44, 8553–8560, https://doi.org/10.1021/es100636q, 2010.
Carter, W. P. L.: Development of the SAPRC-07 chemical mechanism, Atmos. Environ., 44, 5324–5335, https://doi.org/10.1016/j.atmosenv.2010.01.026, 2010.
Carter, W. P. L.: Updated maximum incremental reactivity scale and hydrocarbon bin reactivities for regulatory applications and Reactivity values in an Excel File, https://intra.engr.ucr.edu/~carter/SAPRC/ (last access: 10 May 2022), 2019.
Carter, W. P. L.: Development of an Improved Chemical Speciation Database for Processing Emissions of Volatile Organic Compounds for Air Quality Models, https://intra.engr.ucr.edu/~carter/emitdb/ (last access: 11 March 2021), 2020a.
Carter, W. P. L.: Documentation of the SAPRC-18 mechanism, https://intra.engr.ucr.edu/~carter/SAPRC/18/S18doc.pdf (last access: 13 June 2022), 2020b.
Chan, E. A. W., Gantt, B., and McDow, S.: The reduction of summer sulfate and switch from summertime to wintertime PM2.5 concentration maxima in the United States, Atmos. Environ., 175, 25–32, https://doi.org/10.1016/j.atmosenv.2017.11.055, 2018.
Chen, J., Møller, K. H., Wennberg, P. O., and Kjaergaard, H. G.: Unimolecular reactions following indoor and outdoor limonene ozonolysis, J. Phys. Chem. A, 125, 669–680, https://doi.org/10.1021/acs.jpca.0c09882, 2021.
Chen, Q., Heald, C. L., Jimenez, J. L., Canagaratna, M. R., Zhang, Q., He, L.-Y., Huang, X.-F., Campuzano-Jost, P., Palm, B. B., Poulain, L., Kuwata, M., Martin, S. T., Abbatt, J. P. D., Lee, A. K. Y., and Liggio, J.: Elemental composition of organic aerosol: The gap between ambient and laboratory measurements, Geophys. Res. Lett., 42, 4182–4189, https://doi.org/10.1002/2015GL063693, 2015.
Chen, Y., Guo, H., Nah, T., Tanner, D. J., Sullivan, A. P., Takeuchi, M., Gao, Z., Vasilakos, P., Russell, A. G., Baumann, K., Huey, L. G., Weber, R. J., and Ng, N. L.: Low-molecular-weight carboxylic acids in the Southeastern U.S.: Formation, partitioning, and implications for organic aerosol aging, Environ. Sci. Technol., 55, 6688–6699, https://doi.org/10.1021/acs.est.1c01413, 2021.
Choi, H., Schmidbauer, N., Sundell, J., Hasselgren, M., Spengler, J., and Bornehag, C.-G.: Common household chemicals and the allergy risks in pre-school age children, PLoS One, 5, e13423, https://doi.org/10.1371/journal.pone.0013423, 2010.
Code of Federal Regulations: Volatile organic compounds (VOC), https://ecfr.federalregister.gov/current/title-40/chapter-I/subchapter-C/part-51 (last access: 17 June 2022), 1986.
Coggon, M. M., Lim, C. Y., Koss, A. R., Sekimoto, K., Yuan, B., Gilman, J. B., Hagan, D. H., Selimovic, V., Zarzana, K. J., Brown, S. S., Roberts, J. M., Müller, M., Yokelson, R., Wisthaler, A., Krechmer, J. E., Jimenez, J. L., Cappa, C., Kroll, J. H., de Gouw, J., and Warneke, C.: OH chemistry of non-methane organic gases (NMOGs) emitted from laboratory and ambient biomass burning smoke: evaluating the influence of furans and oxygenated aromatics on ozone and secondary NMOG formation, Atmos. Chem. Phys., 19, 14875–14899, https://doi.org/10.5194/acp-19-14875-2019, 2019.
Coggon, M. M., Gkatzelis, G. I., McDonald, B. C., Gilman, J. B., Schwantes, R. H., Abuhassan, N., Aikin, K. C., Arend, M. F., Berkoff, T. A., Brown, S. S., Campos, T. L., Dickerson, R. R., Gronoff, G., Hurley, J. F., Isaacman-VanWertz, G., Koss, A. R., Li, M., McKeen, S. A., Moshary, F., Peischl, J., Pospisilova, V., Ren, X., Wilson, A., Wu, Y., Trainer, M., and Warneke, C.: Volatile chemical product emissions enhance ozone and modulate urban chemistry, P. Natl. Acad. Sci. USA, 118, e2026653118, https://doi.org/10.1073/pnas.2026653118, 2021.
Crounse, J. D., Nielsen, L. B., Jørgensen, S., Kjaergaard, H. G., and Wennberg, P. O.: Autoxidation of Organic Compounds in the Atmosphere, J. Phys. Chem. Lett., 4, 3513–3520, https://doi.org/10.1021/jz4019207, 2013.
D'Ambro, E. L., Schobesberger, S., Gaston, C. J., Lopez-Hilfiker, F. D., Lee, B. H., Liu, J., Zelenyuk, A., Bell, D., Cappa, C. D., Helgestad, T., Li, Z., Guenther, A., Wang, J., Wise, M., Caylor, R., Surratt, J. D., Riedel, T., Hyttinen, N., Salo, V.-T., Hasan, G., Kurtén, T., Shilling, J. E., and Thornton, J. A.: Chamber-based insights into the factors controlling epoxydiol (IEPOX) secondary organic aerosol (SOA) yield, composition, and volatility, Atmos. Chem. Phys., 19, 11253–11265, https://doi.org/10.5194/acp-19-11253-2019, 2019.
D'Ambro, E. L., Pye, H. O. T., Bash, J. O., Bowyer, J., Allen, C., Efstathiou, C., Gilliam, R. C., Reynolds, L., Talgo, K., and Murphy, B. N.: Characterizing the air emissions, fransport, and deposition of per- and polyfluoroalkyl substances from a fluoropolymer manufacturing facility, Environ. Sci. Technol., 55, 862–870, https://doi.org/10.1021/acs.est.0c06580, 2021.
Davis, J. M., Bhave, P. V., and Foley, K. M.: Parameterization of N2O5 reaction probabilities on the surface of particles containing ammonium, sulfate, and nitrate, Atmos. Chem. Phys., 8, 5295–5311, https://doi.org/10.5194/acp-8-5295-2008, 2008.
Dlugokencky, E.: Trends in Atmospheric Methane, https://gml.noaa.gov/ccgg/trends_ch4/ (last access: 29 June 2022), 2022.
Donahue, N. M., Robinson, A. L., Stanier, C. O., and Pandis, S. N.: Coupled partitioning, dilution, and chemical aging of semivolatile organics, Environ. Sci. Technol., 40, 2635–2643, https:/doi.org/10.1021/es052297c, 2006.
Donahue, N. M., Epstein, S. A., Pandis, S. N., and Robinson, A. L.: A two-dimensional volatility basis set: 1. organic-aerosol mixing thermodynamics, Atmos. Chem. Phys., 11, 3303–3318, https://doi.org/10.5194/acp-11-3303-2011, 2011.
Donahue, N. M., Kroll, J. H., Pandis, S. N., and Robinson, A. L.: A two-dimensional volatility basis set – Part 2: Diagnostics of organic-aerosol evolution, Atmos. Chem. Phys., 12, 615–634, https://doi.org/10.5194/acp-12-615-2012, 2012.
Donahue, N. M., Chuang, W., Epstein, S. A., Kroll, J. H., Worsnop, D. R., Robinson, A. L., Adams, P. J., and Pandis, S. N.: Why do organic aerosols exist? Understanding aerosol lifetimes using the two-dimensional volatility basis set, Environ. Chem., 10, 151–157, https://doi.org/10.1071/EN13022, 2013.
Dunne, J. P., Horowitz, L. W., Adcroft, A. J., Ginoux, P., Held, I. M., John, J. G., Krasting, J. P., Malyshev, S., Naik, V., Paulot, F., Shevliakova, E., Stock, C. A., Zadeh, N., Balaji, V., Blanton, C., Dunne, K. A., Dupuis, C., Durachta, J., Dussin, R., Gauthier, P. P. G., Griffies, S. M., Guo, H., Hallberg, R. W., Harrison, M., He, J., Hurlin, W., McHugh, C., Menzel, R., Milly, P. C. D., Nikonov, S., Paynter, D. J., Ploshay, J., Radhakrishnan, A., Rand, K., Reichl, B. G., Robinson, T., Schwarzkopf, D. M., Sentman, L. T., Underwood, S., Vahlenkamp, H., Winton, M., Wittenberg, A. T., Wyman, B., Zeng, Y., and Zhao, M.: The GFDL Earth System Model Version 4.1 (GFDL-ESM 4.1): Overall coupled model description and simulation characteristics, J. Adv. Model. Earth Sy., 12, e2019MS002015, https://doi.org/10.1029/2019MS002015, 2020.
Edwards, P. M., Brown, S. S., Roberts, J. M., Ahmadov, R., Banta, R. M., deGouw, J. A., Dubé, W. P., Field, R. A., Flynn, J. H., Gilman, J. B., Graus, M., Helmig, D., Koss, A., Langford, A. O., Lefer, B. L., Lerner, B. M., Li, R., Li, S.-M., McKeen, S. A., Murphy, S. M., Parrish, D. D., Senff, C. J., Soltis, J., Stutz, J., Sweeney, C., Thompson, C. R., Trainer, M. K., Tsai, C., Veres, P. R., Washenfelder, R. A., Warneke, C., Wild, R. J., Young, C. J., Yuan, B., and Zamora, R.: High winter ozone pollution from carbonyl photolysis in an oil and gas basin, Nature, 514, 351–354, https://doi.org/10.1038/nature13767, 2014.
Ehn, M., Thornton, J. A., Kleist, E., Sipilä, M., Junninen, H., Pullinen, I., Springer, M., Rubach, F., Tillmann, R., Lee, B., Lopez-Hilfiker, F., Andres, S., Acir, I.-H., Rissanen, M., Jokinen, T., Schobesberger, S., Kangasluoma, J., Kontkanen, J., Nieminen, T., Kurtén, T., Nielsen, L. B., Jørgensen, S., Kjaergaard, H. G., Canagaratna, M., Maso, M. D., Berndt, T., Petäjä, T., Wahner, A., Kerminen, V.-M., Kulmala, M., Worsnop, D. R., Wildt, J., and Mentel, T. F.: A large source of low-volatility secondary organic aerosol, Nature, 506, 476–479, https://doi.org/10.1038/nature13032, 2014.
Foley, K. M., Pouliot, G. A., Eyth, A., Aldridge, M. F., Allen, C., Appel, K. W., Bash, J. O., Beardsley, M., Beidler, J., Choi, D., Farkas, C., Gilliam, R. C., Godfrey, J., Henderson, B. H., Hogrefe, C., Koplitz, S. N., Mason, R., Mathur, R., Misenis, C., Possiel, N., Pye, H. O. T., Reynolds, L., Roark, M., Roberts, S., Schwede, D. B., Seltzer, K. M., Sonntag, D., Talgo, K., Toro, C., Vukovich, J., Xing, J., and Adams, E.: 2002–2017 anthropogenic emissions data for air quality modeling over the United States, Data in Brief, 47, 109022, https://doi.org/10.1016/j.dib.2023.109022, 2023.
Fountoukis, C. and Nenes, A.: ISORROPIA II: a computationally efficient thermodynamic equilibrium model for K+–Ca2+–Mg2+–NH–Na+–SO−–NO–Cl−–H2O aerosols, Atmos. Chem. Phys., 7, 4639–4659, https://doi.org/10.5194/acp-7-4639-2007, 2007.
Gierczak, T., Burkholder, J. B., Talukdar, R. K., Mellouki, A., Barone, S. B., and Ravishankara, A. R.: Atmospheric fate of methyl vinyl ketone and methacrolein, J. Photch. Photobio. A, 110, 1–10, https://doi.org/10.1016/S1010-6030(97)00159-7, 1997.
Goliff, W. S., Stockwell, W. R., and Lawson, C. V.: The regional atmospheric chemistry mechanism, version 2, Atmos. Environ., 68, 174–185, https://doi.org/10.1016/j.atmosenv.2012.11.038, 2013.
Gómez Alvarez, E., Borrás, E., Viidanoja, J., and Hjorth, J.: Unsaturated dicarbonyl products from the OH-initiated photo-oxidation of furan, 2-methylfuran and 3-methylfuran, Atmos. Environ., 43, 1603–1612, https://doi.org/10.1016/j.atmosenv.2008.12.019, 2009.
Gordon, H., Sengupta, K., Rap, A., Duplissy, J., Frege, C., Williamson, C., Heinritzi, M., Simon, M., Yan, C., Almeida, J., Tröstl, J., Nieminen, T., Ortega, I. K., Wagner, R., Dunne, E. M., Adamov, A., Amorim, A., Bernhammer, A.-K., Bianchi, F., Breitenlechner, M., Brilke, S., Chen, X., Craven, J. S., Dias, A., Ehrhart, S., Fischer, L., Flagan, R. C., Franchin, A., Fuchs, C., Guida, R., Hakala, J., Hoyle, C. R., Jokinen, T., Junninen, H., Kangasluoma, J., Kim, J., Kirkby, J., Krapf, M., Kürten, A., Laaksonen, A., Lehtipalo, K., Makhmutov, V., Mathot, S., Molteni, U., Monks, S. A., Onnela, A., Peräkylä, O., Piel, F., Petäjä, T., Praplan, A. P., Pringle, K. J., Richards, N. A. D., Rissanen, M. P., Rondo, L., Sarnela, N., Schobesberger, S., Scott, C. E., Seinfeld, J. H., Sharma, S., Sipilä, M., Steiner, G., Stozhkov, Y., Stratmann, F., Tomé, A., Virtanen, A., Vogel, A. L., Wagner, A. C., Wagner, P. E., Weingartner, E., Wimmer, D., Winkler, P. M., Ye, P., Zhang, X., Hansel, A., Dommen, J., Donahue, N. M., Worsnop, D. R., Baltensperger, U., Kulmala, M., Curtius, J., and Carslaw, K. S.: Reduced anthropogenic aerosol radiative forcing caused by biogenic new particle formation, P. Natl. Acad. Sci. USA, 113, 12053–12058, https://doi.org/10.1073/pnas.1602360113, 2016.
Griffin, R. J., Cocker III, D. R., Flagan, R. C., and Seinfeld, J. H.: Organic aerosol formation from the oxidation of biogenic hydrocarbons, J. Geophys. Res.-Atmos., 104, 3555–3567, https://doi.org/10.1029/1998JD100049, 1999.
Grulke, C. M., Williams, A. J., Thillanadarajah, I., and Richard, A. M.: EPA's DSSTox database: History of development of a curated chemistry resource supporting computational toxicology research, Comput. Toxicol., 12, 100096, https://doi.org/10.1016/j.comtox.2019.100096, 2019.
Haywood, J. and Boucher, O.: Estimates of the direct and indirect radiative forcing due to tropospheric aerosols: A review, Rev. Geophys., 38, 513–543, https://doi.org/10.1029/1999RG000078, 2000.
He, Y., Lambe, A. T., Seinfeld, J. H., Cappa, C. D., Pierce, J. R., and Jathar, S. H.: Process-level modeling can simultaneously explain secondary organic aerosol evolution in chambers and flow reactors, Environ. Sci. Technol., 56, 6262–6273, https://doi.org/10.1021/acs.est.1c08520, 2022.
Heald, C. L. and Kroll, J. H.: The fuel of atmospheric chemistry: Toward a complete description of reactive organic carbon, Sci. Adv., 6, eaay8967, https://doi.org/10.1126/sciadv.aay8967, 2020.
Heald, C. L., Kroll, J. H., Jimenez, J. L., Docherty, K. S., DeCarlo, P. F., Aiken, A. C., Chen, Q., Martin, S. T., Farmer, D. K., and Artaxo, P.: A simplified description of the evolution of organic aerosol composition in the atmosphere, Geophys. Res. Lett., 37, L08803, https://doi.org/10.1029/2010GL042737, 2010.
Heald, C. L., de Gouw, J., Goldstein, A. H., Guenther, A. B., Hayes, P. L., Hu, W., Isaacman-VanWertz, G., Jimenez, J. L., Keutsch, F. N., Koss, A. R., Misztal, P. K., Rappenglück, B., Roberts, J. M., Stevens, P. S., Washenfelder, R. A., Warneke, C., and Young, C. J.: Contrasting reactive organic carbon observations in the Southeast United States (SOAS) and Southern California (CalNex), Environ. Sci. Technol., 54, 14923–14935, https://doi.org/10.1021/acs.est.0c05027, 2020.
Heicklen, J., Desai, J., Bahta, A., Harper, C., and Simonaitis, R.: The temperature and wavelength dependence of the photo-oxidation of propionaldehyde, J. Photochem., 34, 117–135, https://doi.org/10.1016/0047-2670(86)85014-6, 1986.
Hodzic, A. and Jimenez, J. L.: Modeling anthropogenically controlled secondary organic aerosols in a megacity: a simplified framework for global and climate models, Geosci. Model Dev., 4, 901–917, https://doi.org/10.5194/gmd-4-901-2011, 2011.
Hoffmann, T., Odum, J. R., Bowman, F., Collins, D., Klockow, D., Flagan, R. C., and Seinfeld, J. H.: Formation of organic aerosols from the oxidation of biogenic hydrocarbons, J. Atmos. Chem., 26, 189–222, https://doi.org/10.1023/A:1005734301837, 1997.
Hutzell, W. T., Luecken, D. J., Appel, K. W., and Carter, W. P. L.: Interpreting predictions from the SAPRC07 mechanism based on regional and continental simulations, Atmos. Environ., 46, 417–429, https://doi.org/10.1016/j.atmosenv.2011.09.030, 2012.
IUPAC: IUPAC subcommittee for gas kinetic data evaluation, http://www.iupac-kinetic.ch.cam.ac.uk/ (last access: 13 May 2022), 2010.
Ivatt, P. D., Evans, M. J., and Lewis, A. C.: Suppression of surface ozone by an aerosol-inhibited photochemical ozone regime, Nat. Geosci., 15, 536–540, https://doi.org/10.1038/s41561-022-00972-9, 2022.
Jaffe, D. A. and Wigder, N. L.: Ozone production from wildfires: A critical review, Atmos. Environ., 51, 1–10, https://doi.org/10.1016/j.atmosenv.2011.11.063, 2012.
Jaoui, M., Kleindienst, T. E., Docherty, K. S., Lewandowski, M., and Offenberg, J. H.: Secondary organic aerosol formation from the oxidation of a series of sesquiterpenes: α-cedrene, β-caryophyllene, α-humulene and α-farnesene with O3, OH and NO3 radicals, Environ. Chem., 10, 178–193, https://doi.org/10.1071/EN13025, 2013.
Jathar, S. H., Gordon, T. D., Hennigan, C. J., Pye, H. O. T., Pouliot, G., Adams, P. J., Donahue, N. M., and Robinson, A. L.: Unspeciated organic emissions from combustion sources and their influence on the secondary organic aerosol budget in the United States, P. Natl. Acad. Sci. USA, 111, 10473, https://doi.org/10.1073/pnas.1323740111, 2014.
Jenkin, M. E., Saunders, S. M., and Pilling, M. J.: The tropospheric degradation of volatile organic compounds: a protocol for mechanism development, Atmos. Environ., 31, 81–104, https://doi.org/10.1016/S1352-2310(96)00105-7, 1997.
Jenkin, M. E., Saunders, S. M., Wagner, V., and Pilling, M. J.: Protocol for the development of the Master Chemical Mechanism, MCM v3 (Part B): tropospheric degradation of aromatic volatile organic compounds, Atmos. Chem. Phys., 3, 181–193, https://doi.org/10.5194/acp-3-181-2003, 2003.
Jenkin, M. E., Wyche, K. P., Evans, C. J., Carr, T., Monks, P. S., Alfarra, M. R., Barley, M. H., McFiggans, G. B., Young, J. C., and Rickard, A. R.: Development and chamber evaluation of the MCM v3.2 degradation scheme for β-caryophyllene, Atmos. Chem. Phys., 12, 5275–5308, https://doi.org/10.5194/acp-12-5275-2012, 2012.
Jiang, X., Tsona, N. T., Jia, L., Liu, S., Zhang, H., Xu, Y., and Du, L.: Secondary organic aerosol formation from photooxidation of furan: effects of NOx and humidity, Atmos. Chem. Phys., 19, 13591–13609, https://doi.org/10.5194/acp-19-13591-2019, 2019.
Jokinen, T., Berndt, T., Makkonen, R., Kerminen, V.-M., Junninen, H., Paasonen, P., Stratmann, F., Herrmann, H., Guenther, A. B., Worsnop, D. R., Kulmala, M., Ehn, M., and Sipilä, M.: Production of extremely low volatile organic compounds from biogenic emissions: Measured yields and atmospheric implications, P. Natl. Acad. Sci. USA, 112, 7123–7128, https://doi.org/10.1073/pnas.1423977112, 2015.
Kaduwela, A., Luecken, D., Carter, W., and Derwent, R.: New directions: Atmospheric chemical mechanisms for the future, Atmos. Environ., 122, 609–610, https://doi.org/10.1016/j.atmosenv.2015.10.031, 2015.
Karl, T., Striednig, M., Graus, M., Hammerle, A., and Wohlfahrt, G.: Urban flux measurements reveal a large pool of oxygenated volatile organic compound emissions, P. Natl. Acad. Sci. USA, 115, 1186–1191, https://doi.org/10.1073/pnas.1714715115, 2018.
Kim, P. S., Jacob, D. J., Fisher, J. A., Travis, K., Yu, K., Zhu, L., Yantosca, R. M., Sulprizio, M. P., Jimenez, J. L., Campuzano-Jost, P., Froyd, K. D., Liao, J., Hair, J. W., Fenn, M. A., Butler, C. F., Wagner, N. L., Gordon, T. D., Welti, A., Wennberg, P. O., Crounse, J. D., St. Clair, J. M., Teng, A. P., Millet, D. B., Schwarz, J. P., Markovic, M. Z., and Perring, A. E.: Sources, seasonality, and trends of southeast US aerosol: an integrated analysis of surface, aircraft, and satellite observations with the GEOS-Chem chemical transport model, Atmos. Chem. Phys., 15, 10411–10433, https://doi.org/10.5194/acp-15-10411-2015, 2015.
Knote, C., Tuccella, P., Curci, G., Emmons, L., Orlando, J. J., Madronich, S., Baró, R., Jiménez-Guerrero, P., Luecken, D., Hogrefe, C., Forkel, R., Werhahn, J., Hirtl, M., Pérez, J. L., San José, R., Giordano, L., Brunner, D., Yahya, K., and Zhang, Y.: Influence of the choice of gas-phase mechanism on predictions of key gaseous pollutants during the AQMEII phase-2 intercomparison, Atmos. Environ., 115, 553–568, https://doi.org/10.1016/j.atmosenv.2014.11.066, 2015.
Koo, B., Knipping, E., and Yarwood, G.: 1.5-Dimensional volatility basis set approach for modeling organic aerosol in CAMx and CMAQ, Atmos. Environ., 95, 158–164, https://doi.org/10.1016/j.atmosenv.2014.06.031, 2014.
Koss, A. R., Sekimoto, K., Gilman, J. B., Selimovic, V., Coggon, M. M., Zarzana, K. J., Yuan, B., Lerner, B. M., Brown, S. S., Jimenez, J. L., Krechmer, J., Roberts, J. M., Warneke, C., Yokelson, R. J., and de Gouw, J.: Non-methane organic gas emissions from biomass burning: identification, quantification, and emission factors from PTR-ToF during the FIREX 2016 laboratory experiment, Atmos. Chem. Phys., 18, 3299–3319, https://doi.org/10.5194/acp-18-3299-2018, 2018.
Kroll, J. H., Smith, J. D., Che, D. L., Kessler, S. H., Worsnop, D. R., and Wilson, K. R.: Measurement of fragmentation and functionalization pathways in the heterogeneous oxidation of oxidized organic aerosol, Phys. Chem. Chem. Phys., 11, 8005–8014, https://doi.org/10.1039/B905289E, 2009.
Kroll, J. H., Donahue, N. M., Jimenez, J. L., Kessler, S. H., Canagaratna, M. R., Wilson, K. R., Altieri, K. E., Mazzoleni, L. R., Wozniak, A. S., Bluhm, H., Mysak, E. R., Smith, J. D., Kolb, C. E., and Worsnop, D. R.: Carbon oxidation state as a metric for describing the chemistry of atmospheric organic aerosol, Nat. Chem., 3, 133–139, https://doi.org/10.1038/nchem.948, 2011.
Kurtén, T., Møller, K. H., Nguyen, T. B., Schwantes, R. H., Misztal, P. K., Su, L., Wennberg, P. O., Fry, J. L., and Kjaergaard, H. G.: Alkoxy radical bond scissions explain the anomalously low secondary organic serosol and organonitrate yields from α-pinene + NO3, J. Phys. Chem. Lett., 8, 2826–2834, https://doi.org/10.1021/acs.jpclett.7b01038, 2017.
Lannuque, V., Camredon, M., Couvidat, F., Hodzic, A., Valorso, R., Madronich, S., Bessagnet, B., and Aumont, B.: Exploration of the influence of environmental conditions on secondary organic aerosol formation and organic species properties using explicit simulations: development of the VBS-GECKO parameterization, Atmos. Chem. Phys., 18, 13411–13428, https://doi.org/10.5194/acp-18-13411-2018, 2018.
Lawrence, C. E., Casson, P., Brandt, R., Schwab, J. J., Dukett, J. E., Snyder, P., Yerger, E., Kelting, D., VandenBoer, T. C., and Lance, S.: Long-term monitoring of cloud water chemistry at Whiteface Mountain: the emergence of a new chemical regime, Atmos. Chem. Phys., 23, 1619–1639, https://doi.org/10.5194/acp-23-1619-2023, 2023.
Lee, B. H., D'Ambro, E. L., Lopez-Hilfiker, F. D., Schobesberger, S., Mohr, C., Zawadowicz, M. A., Liu, J., Shilling, J. E., Hu, W., Palm, B. B., Jimenez, J. L., Hao, L., Virtanen, A., Zhang, H., Goldstein, A. H., Pye, H. O. T., and Thornton, J. A.: Resolving ambient organic aerosol formation and aging pathways with simultaneous molecular composition and volatility observations, ACS Earth Space Chem., 4, 391–402, https://doi.org/10.1021/acsearthspacechem.9b00302, 2020.
Li, Y., Schichtel Bret, A., Walker John, T., Schwede Donna, B., Chen, X., Lehmann Christopher, M. B., Puchalski Melissa, A., Gay David, A., and Collett Jeffrey, L.: Increasing importance of deposition of reduced nitrogen in the United States, P. Natl. Acad. Sci. USA, 113, 5874–5879, https://doi.org/10.1073/pnas.1525736113, 2016.
Liggio, J., Li, S.-M., and McLaren, R.: Reactive uptake of glyoxal by particulate matter, J. Geophys. Res.-Atmos., 110, D10304, https://doi.org/10.1029/2004JD005113, 2005.
Loeffler, K. W., Koehler, C. A., Paul, N. M., and De Haan, D. O.: Oligomer formation in evaporating aqueous glyoxal and methyl glyoxal solutions, Environ. Sci. Technol., 40, 6318–6323, https://doi.org/10.1021/es060810w, 2006.
Lowe, C. N. and Williams, A. J.: Enabling high-throughput searches for multiple chemical data using the U.S.-EPA CompTox Chemicals Dashboard, J. Chem. Inf. Model, 61, 565–570, https://doi.org/10.1021/acs.jcim.0c01273, 2021.
Lu, Q., Zhao, Y., and Robinson, A. L.: Comprehensive organic emission profiles for gasoline, diesel, and gas-turbine engines including intermediate and semi-volatile organic compound emissions, Atmos. Chem. Phys., 18, 17637–17654, https://doi.org/10.5194/acp-18-17637-2018, 2018.
Lu, Q., Murphy, B. N., Qin, M., Adams, P. J., Zhao, Y., Pye, H. O. T., Efstathiou, C., Allen, C., and Robinson, A. L.: Simulation of organic aerosol formation during the CalNex study: updated mobile emissions and secondary organic aerosol parameterization for intermediate-volatility organic compounds, Atmos. Chem. Phys., 20, 4313–4332, https://doi.org/10.5194/acp-20-4313-2020, 2020.
Magneron, I., Thévenet, R., Mellouki, A., Le Bras, G., Moortgat, G. K., and Wirtz, K.: A study of the photolysis and OH-initiated oxidation of acrolein and trans-crotonaldehyde, J. Phys. Chem. A, 106, 2526–2537, https://doi.org/10.1021/jp013413a, 2002.
Makar, M., Antonelli, J., Di, Q., Cutler, D., Schwartz, J., and Dominici, F.: Estimating the causal effect of low levels of fine particulate matter on hospitalization, Epidemiol., 28, 627–634, https://doi.org/10.1097/ede.0000000000000690, 2017.
May, A. A., Levin, E. J. T., Hennigan, C. J., Riipinen, I., Lee, T., Collett Jr., J. L., Jimenez, J. L., Kreidenweis, S. M., and Robinson, A. L.: Gas-particle partitioning of primary organic aerosol emissions: 3. Biomass burning, J. Geophys. Res.-Atmos., 118, 327–311, 338, https://doi.org/10.1002/jgrd.50828, 2013.
Mansouri, K., Grulke, C. M., Judson, R. S., and Williams, A. J.: OPERA models for predicting physicochemical properties and environmental fate endpoints, J. Cheminformatics, 10, 10, https://doi.org/10.1186/s13321-018-0263-1, 2018.
McClure, C. D. and Jaffe, D. A.: US particulate matter air quality improves except in wildfire-prone areas, P. Natl. Acad. Sci. USA, 115, 7901–7906, https://doi.org/10.1073/pnas.1804353115, 2018.
McDonald, B. C., de Gouw, J. A., Gilman, J. B., Jathar, S. H., Akherati, A., Cappa, C. D., Jimenez, J. L., Lee-Taylor, J., Hayes, P. L., McKeen, S. A., Cui, Y. Y., Kim, S.-W., Gentner, D. R., Isaacman-VanWertz, G., Goldstein, A. H., Harley, R. A., Frost, G. J., Roberts, J. M., Ryerson, T. B., and Trainer, M.: Volatile chemical products emerging as largest petrochemical source of urban organic emissions, Science, 359, 760, https://doi.org/10.1126/science.aaq0524, 2018.
McFiggans, G., Mentel, T. F., Wildt, J., Pullinen, I., Kang, S., Kleist, E., Schmitt, S., Springer, M., Tillmann, R., Wu, C., Zhao, D., Hallquist, M., Faxon, C., Le Breton, M., Hallquist, Å. M., Simpson, D., Bergström, R., Jenkin, M. E., Ehn, M., Thornton, J. A., Alfarra, M. R., Bannan, T. J., Percival, C. J., Priestley, M., Topping, D., and Kiendler-Scharr, A.: Secondary organic aerosol reduced by mixture of atmospheric vapours, Nature, 565, 587–593, https://doi.org/10.1038/s41586-018-0871-y, 2019.
Moch, J. M., Dovrou, E., Mickley, L. J., Keutsch, F. N., Cheng, Y., Jacob, D. J., Jiang, J., Li, M., Munger, J. W., Qiao, X., and Zhang, Q.: Contribution of hydroxymethane sulfonate to ambient particulate matter: A potential explanation for high particulate sulfur during severe winter haze in Beijing, Geophys. Res. Lett., 45, 11969–911979, https://doi.org/10.1029/2018GL079309, 2018.
Møller, K. H., Otkjær, R. V., Chen, J., and Kjaergaard, H. G.: Double bonds are key to fast unimolecular reactivity in first-generation monoterpene hydroxy peroxy radicals, J. Phys. Chem. A, 124, 2885–2896, https://doi.org/10.1021/acs.jpca.0c01079, 2020.
Molteni, U., Bianchi, F., Klein, F., El Haddad, I., Frege, C., Rossi, M. J., Dommen, J., and Baltensperger, U.: Formation of highly oxygenated organic molecules from aromatic compounds, Atmos. Chem. Phys., 18, 1909–1921, https://doi.org/10.5194/acp-18-1909-2018, 2018.
Molteni, U., Simon, M., Heinritzi, M., Hoyle, C. R., Bernhammer, A.-K., Bianchi, F., Breitenlechner, M., Brilke, S., Dias, A., Duplissy, J., Frege, C., Gordon, H., Heyn, C., Jokinen, T., Kürten, A., Lehtipalo, K., Makhmutov, V., Petäjä, T., Pieber, S. M., Praplan, A. P., Schobesberger, S., Steiner, G., Stozhkov, Y., Tomé, A., Tröstl, J., Wagner, A. C., Wagner, R., Williamson, C., Yan, C., Baltensperger, U., Curtius, J., Donahue, N. M., Hansel, A., Kirkby, J., Kulmala, M., Worsnop, D. R., and Dommen, J.: Formation of highly oxygenated organic molecules from α-pinene ozonolysis: Chemical characteristics, mechanism, and kinetic model development, ACS Earth Space Chem., 3, 873–883, https://doi.org/10.1021/acsearthspacechem.9b00035, 2019.
Murphy, B. N., Woody, M. C., Jimenez, J. L., Carlton, A. M. G., Hayes, P. L., Liu, S., Ng, N. L., Russell, L. M., Setyan, A., Xu, L., Young, J., Zaveri, R. A., Zhang, Q., and Pye, H. O. T.: Semivolatile POA and parameterized total combustion SOA in CMAQv5.2: impacts on source strength and partitioning, Atmos. Chem. Phys., 17, 11107–11133, https://doi.org/10.5194/acp-17-11107-2017, 2017.
Nakao, S., Clark, C., Tang, P., Sato, K., and Cocker III, D.: Secondary organic aerosol formation from phenolic compounds in the absence of NOx, Atmos. Chem. Phys., 11, 10649–10660, https://doi.org/10.5194/acp-11-10649-2011, 2011.
Nannoolal, Y., Rarey, J., Ramjugernath, D., and Cordes, W.: Estimation of pure component properties: Part 1. Estimation of the normal boiling point of non-electrolyte organic compounds via group contributions and group interactions, Fluid Phase Equilibr., 226, 45–63, https://doi.org/10.1016/j.fluid.2004.09.001, 2004.
Nannoolal, Y., Rarey, J., and Ramjugernath, D.: Estimation of pure component properties: Part 3. Estimation of the vapor pressure of non-electrolyte organic compounds via group contributions and group interactions, Fluid Phase Equilibr., 269, 117–133, https://doi.org/10.1016/j.fluid.2008.04.020, 2008.
Nault, B. A., Campuzano-Jost, P., Day, D. A., Schroder, J. C., Anderson, B., Beyersdorf, A. J., Blake, D. R., Brune, W. H., Choi, Y., Corr, C. A., de Gouw, J. A., Dibb, J., DiGangi, J. P., Diskin, G. S., Fried, A., Huey, L. G., Kim, M. J., Knote, C. J., Lamb, K. D., Lee, T., Park, T., Pusede, S. E., Scheuer, E., Thornhill, K. L., Woo, J.-H., and Jimenez, J. L.: Secondary organic aerosol production from local emissions dominates the organic aerosol budget over Seoul, South Korea, during KORUS-AQ, Atmos. Chem. Phys., 18, 17769–17800, https://doi.org/10.5194/acp-18-17769-2018, 2018.
Ng, N. L., Kroll, J. H., Chan, A. W. H., Chhabra, P. S., Flagan, R. C., and Seinfeld, J. H.: Secondary organic aerosol formation from m-xylene, toluene, and benzene, Atmos. Chem. Phys., 7, 3909–3922, https://doi.org/10.5194/acp-7-3909-2007, 2007.
Ng, N. L., Canagaratna, M. R., Jimenez, J. L., Chhabra, P. S., Seinfeld, J. H., and Worsnop, D. R.: Changes in organic aerosol composition with aging inferred from aerosol mass spectra, Atmos. Chem. Phys., 11, 6465–6474, https://doi.org/10.5194/acp-11-6465-2011, 2011.
Nozière, B., Barnes, I., and Becker, K.-H.: Product study and mechanisms of the reactions of α-pinene and of pinonaldehyde with OH radicals, J. Geophys. Res.-Atmos., 104, 23645–23656, https://doi.org/10.1029/1999JD900778, 1999.
Paciga, A. L., Riipinen, I., and Pandis, S. N.: Effect of Ammonia on the Volatility of Organic Diacids, Environ. Sci. Technol., 48, 13769–13775, 10.1021/es5037805, 2014.
Pai, S. J., Carter, T. S., Heald, C. L., and Kroll, J. H.: Updated World Health Organization Air Quality Guidelines highlight the importance of non-anthropogenic PM2.5, Environ. Sci. Tech. Lett., 9, 501-506, https://doi.org/10.1021/acs.estlett.2c00203, 2022.
Pankow, J. F.: An absorption model of the gas/aerosol partitioning involved in the formation of secondary organic aerosol, Atmos. Environ., 28, 189–193, https://doi.org/10.1016/1352-2310(94)90094-9, 1994.
Pankow, J. F. and Asher, W. E.: SIMPOL.1: a simple group contribution method for predicting vapor pressures and enthalpies of vaporization of multifunctional organic compounds, Atmos. Chem. Phys., 8, 2773–2796, https://doi.org/10.5194/acp-8-2773-2008, 2008.
Pennington, E. A., Seltzer, K. M., Murphy, B. N., Qin, M., Seinfeld, J. H., and Pye, H. O. T.: Modeling secondary organic aerosol formation from volatile chemical products, Atmos. Chem. Phys., 21, 18247–18261, https://doi.org/10.5194/acp-21-18247-2021, 2021.
Piletic, I. R., and Kleindienst, T. E.: Rates and yields of unimolecular reactions producing highly oxidized peroxy radicals in the OH-induced autoxidation of α-pinene, β-pinene, and limonene, J. Phys. Chem. A, 126, 88–100, https://doi.org/10.1021/acs.jpca.1c07961, 2022.
Place, B. K., Hutzell, W. T., Appel, K. W., Farrell, S., Valin, L., Murphy, B. N., Seltzer, K. M., Sarwar, G., Allen, C., Piletic, I. R., D'Ambro, E. L., Saunders, E., Simon, H., Torres-Vasquez, A., Pleim, J., Schwantes, R. H., Coggon, M. M., Xu, L., Stockwell, W. R., and Pye, H. O. T.: Sensitivity of Northeast U.S. surface ozone predictions to the representation of atmospheric chemistry in CRACMMv1.0, EGUsphere, 2023, 1–30, https://doi.org/10.5194/egusphere-2023-288, 2023.
Pond, Z. A., Hernandez, C. S., Adams, P. J., Pandis, S. N., Garcia, G. R., Robinson, A. L., Marshall, J. D., Burnett, R., Skyllakou, K., Garcia Rivera, P., Karnezi, E., Coleman, C. J., and Pope, C. A.: Cardiopulmonary mortality and fine particulate air pollution by species and source in a national U.S. cohort, Environ. Sci. Technol., 56, 7214–7223, https://doi.org/10.1021/acs.est.1c04176, 2022.
Porter, W. C., Jimenez, J. L., and Barsanti, K. C.: Quantifying atmospheric parameter ranges for ambient secondary organic aerosol formation, ACS Earth Space Chem., 5, 2380–2397, https://doi.org/10.1021/acsearthspacechem.1c00090, 2021.
Praske, E., Otkjær, R. V., Crounse, J. D., Hethcox, J. C., Stoltz, B. M., Kjaergaard, H. G., and Wennberg, P. O.: Atmospheric autoxidation is increasingly important in urban and suburban North America, P. Natl. Acad. Sci. USA, 115, 64–69, https://doi.org/10.1073/pnas.1715540115, 2018.
Pye, H. O. T.: Data for the Community Regional Atmospheric Chemistry Multiphase Mechanism (CRACMM) version 1.0, U.S. Environmental Protection Agency [data set], https://doi.org/10.23719/1527956, 2022.
Pye, H. O. T. and Pouliot, G. A.: Modeling the role of alkanes, polycyclic aromatic hydrocarbons, and their oligomers in secondary organic aerosol formation, Environ. Sci. Technol., 46, 6041–6047, https://doi.org/10.1021/es300409w, 2012.
Pye, H. O. T., Chan, A. W. H., Barkley, M. P., and Seinfeld, J. H.: Global modeling of organic aerosol: the importance of reactive nitrogen (NOx and NO3), Atmos. Chem. Phys., 10, 11261–11276, https://doi.org/10.5194/acp-10-11261-2010, 2010.
Pye, H. O. T., Pinder, R. W., Piletic, I. R., Xie, Y., Capps, S. L., Lin, Y.-H., Surratt, J. D., Zhang, Z., Gold, A., Luecken, D. J., Hutzell, W. T., Jaoui, M., Offenberg, J. H., Kleindienst, T. E., Lewandowski, M., and Edney, E. O.: Epoxide pathways improve model predictions of isoprene markers and reveal key role of acidity in aerosol formation, Environ. Sci. Technol., 47, 11056–11064, https://doi.org/10.1021/es402106h, 2013.
Pye, H. O. T., Luecken, D. J., Xu, L., Boyd, C. M., Ng, N. L., Baker, K. R., Ayres, B. R., Bash, J. O., Baumann, K., Carter, W. P. L., Edgerton, E., Fry, J. L., Hutzell, W. T., Schwede, D. B., and Shepson, P. B.: Modeling the current and future roles of particulate organic nitrates in the southeastern United States, Environ. Sci. Technol., 49, 14195–14203, https://doi.org/10.1021/acs.est.5b03738, 2015.
Pye, H. O. T., Murphy, B. N., Xu, L., Ng, N. L., Carlton, A. G., Guo, H., Weber, R., Vasilakos, P., Appel, K. W., Budisulistiorini, S. H., Surratt, J. D., Nenes, A., Hu, W., Jimenez, J. L., Isaacman-VanWertz, G., Misztal, P. K., and Goldstein, A. H.: On the implications of aerosol liquid water and phase separation for organic aerosol mass, Atmos. Chem. Phys., 17, 343–369, https://doi.org/10.5194/acp-17-343-2017, 2017.
Pye, H. O. T., Ward-Caviness, C. K., Murphy, B. N., Appel, K. W., and Seltzer, K. M.: Secondary organic aerosol association with cardiorespiratory disease mortality in the United States, Nat. Commun., 12, 7215, https://doi.org/10.1038/s41467-021-27484-1, 2021.
Pye, H. O. T., Appel, K. W., Seltzer, K. M., Ward-Caviness, C. K., and Murphy, B. N.: Human-health impacts of controlling secondary air pollution precursors, Environ. Sci. Tech. Lett., 9, 96–101, https://doi.org/10.1021/acs.estlett.1c00798, 2022.
Qin, M., Murphy, B. N., Isaacs, K. K., McDonald, B. C., Lu, Q., McKeen, S. A., Koval, L., Robinson, A. L., Efstathiou, C., Allen, C., and Pye, H. O. T.: Criteria pollutant impacts of volatile chemical products informed by near-field modelling, Nat. Sustain., 4, 129–137, https://doi.org/10.1038/s41893-020-00614-1, 2021.
Raber, W. H. and Moortgat, G. K.: Progress and Problems in Atmospheric Chemistry, World Scientific, edited by: Baker, J., Singapore, ISBN 9810218680, 1996.
RDKit: rdkit/rdkit: 2020_09_1 (Q3 2020) Release, Zenodo [code], https://doi.org/10.5281/zenodo.4107869, 2020.
RDKit: Open-source cheminformatics, https://www.rdkit.org, last access: 2 September 2022.
Richters, S., Herrmann, H., and Berndt, T.: Highly oxidized RO2 radicals and consecutive products from the ozonolysis of three sesquiterpenes, Environ. Sci. Technol., 50, 2354–2362, https://doi.org/10.1021/acs.est.5b05321, 2016.
Riedel, T. P., Lin, Y.-H., Budisulistiorini, S. H., Gaston, C. J., Thornton, J. A., Zhang, Z., Vizuete, W., Gold, A., and Surratt, J. D.: Heterogeneous reactions of isoprene-derived epoxides: Reaction probabilities and molar secondary organic aerosol yield estimates, Environ. Sci. Tech. Lett., 2, 38–42, https://doi.org/10.1021/ez500406f, 2015.
Riva, M., Chen, Y., Zhang, Y., Lei, Z., Olson, N. E., Boyer, H. C., Narayan, S., Yee, L. D., Green, H. S., Cui, T., Zhang, Z., Baumann, K., Fort, M., Edgerton, E., Budisulistiorini, S. H., Rose, C. A., Ribeiro, I. O., e Oliveira, R. L., dos Santos, E. O., Machado, C. M. D., Szopa, S., Zhao, Y., Alves, E. G., de Sá, S. S., Hu, W., Knipping, E. M., Shaw, S. L., Duvoisin Junior, S., de Souza, R. A. F., Palm, B. B., Jimenez, J.-L., Glasius, M., Goldstein, A. H., Pye, H. O. T., Gold, A., Turpin, B. J., Vizuete, W., Martin, S. T., Thornton, J. A., Dutcher, C. S., Ault, A. P., and Surratt, J. D.: Increasing isoprene epoxydiol-to-inorganic sulfate aerosol ratio results in extensive conversion of inorganic sulfate to organosulfur forms: Implications for aerosol physicochemical properties, Environ. Sci. Technol., 53, 8682–8694, https://doi.org/10.1021/acs.est.9b01019, 2019.
Robinson, A. L., Donahue, N. M., Shrivastava, M. K., Weitkamp, E. A., Sage, A. M., Grieshop, A. P., Lane, T. E., Pierce, J. R., and Pandis, S. N.: Rethinking organic aerosols: Semivolatile emissions and photochemical aging, Science, 315, 1259, https://doi.org/10.1126/science.1133061, 2007.
Roldin, P., Ehn, M., Kurtén, T., Olenius, T., Rissanen, M. P., Sarnela, N., Elm, J., Rantala, P., Hao, L., Hyttinen, N., Heikkinen, L., Worsnop, D. R., Pichelstorfer, L., Xavier, C., Clusius, P., Öström, E., Petäjä, T., Kulmala, M., Vehkamäki, H., Virtanen, A., Riipinen, I., and Boy, M.: The role of highly oxygenated organic molecules in the Boreal aerosol-cloud-climate system, Nat. Commun., 10, 4370, https://doi.org/10.1038/s41467-019-12338-8, 2019.
Rolletter, M., Blocquet, M., Kaminski, M., Bohn, B., Dorn, H.-P., Hofzumahaus, A., Holland, F., Li, X., Rohrer, F., Tillmann, R., Wegener, R., Kiendler-Scharr, A., Wahner, A., and Fuchs, H.: Photooxidation of pinonaldehyde at ambient conditions investigated in the atmospheric simulation chamber SAPHIR, Atmos. Chem. Phys., 20, 13701–13719, https://doi.org/10.5194/acp-20-13701-2020, 2020.
Safieddine, S. A., Heald, C. L., and Henderson, B. H.: The global nonmethane reactive organic carbon budget: A modeling perspective, Geophys. Res. Lett., 44, 3897–3906, https://doi.org/10.1002/2017GL072602, 2017.
Sander, S. P., Golden, D. M., Kurylo, M. J., Moortgat, G. K., Wine, P. H., Ravishankara, A. R., Kolb, C. E., Molina, M. J., Finlayson-Pitts, B. J., Huie, R. E., and Orkin, V. L.: Chemical kinetics and photochemical data for use in Atmospheric Studies Evaluation Number 15, JPL Publication 06-02, https://jpldataeval.jpl.nasa.gov/pdf/JPL_15_AllInOne.pdf (last access: 21 April 2023), 2006.
Sander, S. P., Abbatt, J. P. D., Barker, J. R., Burkholder, J. B., Friedl, R. R., Golden, D. M., Huie, R. E., Kolb, C. E., Kurylo, M. J., Moortgat, G. K., Orkin, V. L., and Wine, P. H.: Chemical Kinetics and Photochemical Data for Use in Atmospheric Studies, Evaluation No. 17, JPL Publication 10-6, https://jpldataeval.jpl.nasa.gov/pdf/JPL 10-6 Final 15June2011.pdf (last access: 21 April 2023), 2011.
Sarwar, G., Godowitch, J., Henderson, B. H., Fahey, K., Pouliot, G., Hutzell, W. T., Mathur, R., Kang, D., Goliff, W. S., and Stockwell, W. R.: A comparison of atmospheric composition using the Carbon Bond and Regional Atmospheric Chemistry Mechanisms, Atmos. Chem. Phys., 13, 9695–9712, https://doi.org/10.5194/acp-13-9695-2013, 2013.
Sarwar, G., Gantt, B., Schwede, D., Foley, K., Mathur, R., and Saiz-Lopez, A.: Impact of enhanced ozone deposition and halogen chemistry on tropospheric ozone over the Northern Hemisphere, Environ. Sci. Technol., 49, 9203–9211, https://doi.org/10.1021/acs.est.5b01657, 2015.
Saunders, S. M., Jenkin, M. E., Derwent, R. G., and Pilling, M. J.: Protocol for the development of the Master Chemical Mechanism, MCM v3 (Part A): tropospheric degradation of non-aromatic volatile organic compounds, Atmos. Chem. Phys., 3, 161–180, https://doi.org/10.5194/acp-3-161-2003, 2003.
Scheffe, R. D., Strum, M., Phillips, S. B., Thurman, J., Eyth, A., Fudge, S., Morris, M., Palma, T., and Cook, R.: Hybrid modeling approach to estimate exposures of Hazardous Air Pollutants (HAPs) for the National Air Toxics Assessment (NATA), Environ. Sci. Technol., 50, 12356–12364, https://doi.org/10.1021/acs.est.6b04752, 2016.
Schervish, M. and Donahue, N. M.: Peroxy radical chemistry and the volatility basis set, Atmos. Chem. Phys., 20, 1183–1199, https://doi.org/10.5194/acp-20-1183-2020, 2020.
Schwantes, R. H., Schilling, K. A., McVay, R. C., Lignell, H., Coggon, M. M., Zhang, X., Wennberg, P. O., and Seinfeld, J. H.: Formation of highly oxygenated low-volatility products from cresol oxidation, Atmos. Chem. Phys., 17, 3453–3474, https://doi.org/10.5194/acp-17-3453-2017, 2017.
Seltzer, K. M., Pennington, E., Rao, V., Murphy, B. N., Strum, M., Isaacs, K. K., and Pye, H. O. T.: Reactive organic carbon emissions from volatile chemical products, Atmos. Chem. Phys., 21, 5079–5100, https://doi.org/10.5194/acp-21-5079-2021, 2021.
Seltzer, K. M., Murphy, B. N., Pennington, E. A., Allen, C., Talgo, K., and Pye, H. O. T.: Volatile Chemical Product Enhancements to Criteria Pollutants in the United States, Environ. Sci. Technol., 56, 6905–6913, https://doi.org/10.1021/acs.est.1c04298, 2022.
Shah, T., Shi, Y., Beardsley, R., and Yarwood, G.: Speciation Tool User's Guide Version 5.0, https://www.cmascenter.org/speciation_tool/documentation/5.0/Ramboll_sptool_users_guide_V5.pdf (last access: 2 August 2022), 2020.
Shah, V., Jacob, D. J., Dang, R., Lamsal, L. N., Strode, S. A., Steenrod, S. D., Boersma, K. F., Eastham, S. D., Fritz, T. M., Thompson, C., Peischl, J., Bourgeois, I., Pollack, I. B., Nault, B. A., Cohen, R. C., Campuzano-Jost, P., Jimenez, J. L., Andersen, S. T., Carpenter, L. J., Sherwen, T., and Evans, M. J.: Nitrogen oxides in the free troposphere: implications for tropospheric oxidants and the interpretation of satellite NO2 measurements, Atmos. Chem. Phys., 23, 1227–1257, https://doi.org/10.5194/acp-23-1227-2023, 2023.
Simon, H., Beck, L., Bhave, P. V., Divita, F., Hsu, Y., Luecken, D., Mobley, J. D., Pouliot, G. A., Reff, A., Sarwar, G., and Strum, M.: The development and uses of EPA's SPECIATE database, Atmos. Pollut. Res., 1, 196–206, https://doi.org/10.5094/APR.2010.026, 2010.
Simon, H., Baker, K. R., and Phillips, S.: Compilation and interpretation of photochemical model performance statistics published between 2006 and 2012, Atmos. Environ., 61, 124–139, https://doi.org/10.1016/j.atmosenv.2012.07.012, 2012.
Solazzo, E., Bianconi, R., Hogrefe, C., Curci, G., Tuccella, P., Alyuz, U., Balzarini, A., Baró, R., Bellasio, R., Bieser, J., Brandt, J., Christensen, J. H., Colette, A., Francis, X., Fraser, A., Vivanco, M. G., Jiménez-Guerrero, P., Im, U., Manders, A., Nopmongcol, U., Kitwiroon, N., Pirovano, G., Pozzoli, L., Prank, M., Sokhi, R. S., Unal, A., Yarwood, G., and Galmarini, S.: Evaluation and error apportionment of an ensemble of atmospheric chemistry transport modeling systems: multivariable temporal and spatial breakdown, Atmos. Chem. Phys., 17, 3001–3054, https://doi.org/10.5194/acp-17-3001-2017, 2017.
Srivastava, D., Vu, T. V., Tong, S., Shi, Z., and Harrison, R. M.: Formation of secondary organic aerosols from anthropogenic precursors in laboratory studies, NPJ Clim. Atmos., 5, 22, https://doi.org/10.1038/s41612-022-00238-6, 2022.
Stanfield, Z., Addington, C. K., Dionisio, K. L., Lyons, D., Tornero-Velez, R., Phillips, K. A., Buckley, T. J., and Isaacs, K. K.: Mining of Consumer Product Ingredient and Purchasing Data to Identify Potential Chemical Coexposures, Environ. Health Persp., 129, 067006, https://doi.org/10.1289/EHP8610, 2021.
Stockwell, W. R., Middleton, P., Chang, J. S., and Tang, X.: The second generation regional acid deposition model chemical mechanism for regional air quality modeling, J. Geophys. Res.-Atmos., 95, 16343–16367, https://doi.org/10.1029/JD095iD10p16343, 1990.
Stockwell, W. R., Kirchner, F., Kuhn, M., and Seefeld, S.: A new mechanism for regional atmospheric chemistry modeling, J. Geophys. Res.-Atmos., 102, 25847–25879, https://doi.org/10.1029/97JD00849, 1997.
Surratt, J., D., Chan, A., W. H., Eddingsaas, N., C., Chan, M., Loza, C., L., Kwan, A., J., Hersey, S., P., Flagan, R., C., Wennberg, P., O., and Seinfeld, J., H.: Reactive intermediates revealed in secondary organic aerosol formation from isoprene, P. Natl. Acad. Sci. USA, 107, 6640–6645, https://doi.org/10.1073/pnas.0911114107, 2010.
Talukdar, R. K., Burkholder, J. B., Hunter, M., Gilles, M. K., Roberts, J. M., and Ravishankara, A. R.: Atmospheric fate of several alkyl nitrates Part 2UV absorption cross-sections and photodissociation quantum yields, J. Chem. Soc., Faraday Trans., 93, 2797–2805, https://doi.org/10.1039/A701781B, 1997.
Tilmes, S., Lamarque, J.-F., Emmons, L. K., Kinnison, D. E., Ma, P.-L., Liu, X., Ghan, S., Bardeen, C., Arnold, S., Deeter, M., Vitt, F., Ryerson, T., Elkins, J. W., Moore, F., Spackman, J. R., and Val Martin, M.: Description and evaluation of tropospheric chemistry and aerosols in the Community Earth System Model (CESM1.2), Geosci. Model Dev., 8, 1395–1426, https://doi.org/10.5194/gmd-8-1395-2015, 2015.
Tsigaridis, K., Daskalakis, N., Kanakidou, M., Adams, P. J., Artaxo, P., Bahadur, R., Balkanski, Y., Bauer, S. E., Bellouin, N., Benedetti, A., Bergman, T., Berntsen, T. K., Beukes, J. P., Bian, H., Carslaw, K. S., Chin, M., Curci, G., Diehl, T., Easter, R. C., Ghan, S. J., Gong, S. L., Hodzic, A., Hoyle, C. R., Iversen, T., Jathar, S., Jimenez, J. L., Kaiser, J. W., Kirkevåg, A., Koch, D., Kokkola, H., Lee, Y. H., Lin, G., Liu, X., Luo, G., Ma, X., Mann, G. W., Mihalopoulos, N., Morcrette, J.-J., Müller, J.-F., Myhre, G., Myriokefalitakis, S., Ng, N. L., O'Donnell, D., Penner, J. E., Pozzoli, L., Pringle, K. J., Russell, L. M., Schulz, M., Sciare, J., Seland, Ø., Shindell, D. T., Sillman, S., Skeie, R. B., Spracklen, D., Stavrakou, T., Steenrod, S. D., Takemura, T., Tiitta, P., Tilmes, S., Tost, H., van Noije, T., van Zyl, P. G., von Salzen, K., Yu, F., Wang, Z., Wang, Z., Zaveri, R. A., Zhang, H., Zhang, K., Zhang, Q., and Zhang, X.: The AeroCom evaluation and intercomparison of organic aerosol in global models, Atmos. Chem. Phys., 14, 10845–10895, https://doi.org/10.5194/acp-14-10845-2014, 2014.
Tuazon, E. C., Alvarado, A., Aschmann, S. M., Atkinson, R., and Arey, J.: Products of the gas-phase reactions of 1,3-Butadiene with OH and NO3 Radicals, Environ. Sci. Technol., 33, 3586–3595, https://doi.org/10.1021/es990193u, 1999.
U.S. Environmental Protection Agency: Motor Vehicle EMission Simulator: MOVES3.0.0, https://www.epa.gov/moves (last access: 1 July 2022), 2020.
U.S. Environmental Protection Agency: Human Exposure Model, https://www.epa.gov/fera/download-human-exposure-model-hem (last access: 19 April 2022), 2021a.
U.S. Environmental Protection Agency: Dose-Response Assessment for Assessing Health Risks Associated With Exposure to Hazardous Air Pollutants, https://www.epa.gov/fera/dose-response-assessment-assessing-health-risks-associated-exposure-hazardous-air-pollutants, last access: 29 September 2021b.
U.S. Environmental Protection Agency: Community Regional Atmospheric Chemistry Multiphase Mechanism (CRACMM) for Improving Air Quality Modeling, https://www.epa.gov/system/files/documents/2021-11/cracmm-factsheet-october-2021-v2.pdf (last access: 21 October 2022), 2021c.
U.S. Environmental Protection Agency: CompTox Chemicals Dashboard, https://comptox.epa.gov/dashboard/, last access: 19 August 2021d.
U.S. Environmental Protection Agency: Federal Register, 40 CFR Part 63, [EPA–HQ–OAR–2014–0471; FRL–5562–08–OAR], RIN 2060–AS26, Clean Air Act Section 112 List of Hazardous Air Pollutant: Amendments to the List of Hazardous Air Pollutants (HAP), https://www.govinfo.gov/content/pkg/FR-2022-01-05/pdf/2021-28315.pdf, access: 13 July 2022a.
U.S. Environmental Protection Agency: CRACMM, GitHub [data set], https://github.com/USEPA/CRACMM, access: 21 November 2022b.
U.S. Environmental Protection Agency: List of chemicals within the certain glycol ethers category, https://ordspub.epa.gov/ords/guideme_ext/f?p=GUIDEME:GD::::RP:gd:glycol_ethers, last access: 19 April 2022c.
U.S. Environmental Protection Agency: Nonattainment Areas for Criteria Pollutants (Green Book), https://www.epa.gov/green-book, last access: 13 May 2022d.
U.S. Environmental Protection Agency: SPECIATE Version 5.2 Database Development Documentation, https://www.epa.gov/system/files/documents/2022-09/SPECIATE 5.2 Addendum.pdf (last access: 14 April 2023), 2022e.
U.S. Environmental Protection Agency: CTS: Chemical Transformation Simulator, https://qed.epa.gov/cts/, last access: 5 August 2022f.
U.S. Environmental Protection Agency: SPECIATE: https://www.epa.gov/air-emissions-modeling/speciate (last access: 14 April 2023), 2022g.
U.S. Environmental Protection Agency Office of Research and Development: CMAQ (Version 5.3.3), Zenodo [code], https://doi.org/10.5281/zenodo.3585898, 2019.
U.S. EPA Office of Research and Development: CMAQ Version 5.4, Zenodo [code], https://doi.org/10.5281/zenodo.7218076, 2022.
Vannucci, P. F. and Cohen, R. C.: Decadal trends in the temperature dependence of summertime urban PM2.5 in the Northeast United States, ACS Earth Space Chem., 1793–1798, https://doi.org/10.1021/acsearthspacechem.2c00077, 2022.
Vasquez, K. T., Crounse, J. D., Schulze, B. C., Bates, K. H., Teng, A. P., Xu, L., Allen, H. M., and Wennberg, P. O.: Rapid hydrolysis of tertiary isoprene nitrate efficiently removes NOX from the atmosphere, P. Natl. Acad. Sci. USA, 117, 33011–33016, https://doi.org/10.1073/pnas.2017442117, 2020.
Venecek, M. A., Carter, W. P. L., and Kleeman, M. J.: Updating the SAPRC Maximum Incremental Reactivity (MIR) scale for the United States from 1988 to 2010, J. Air Waste Manage., 68, 1301–1316, https://doi.org/10.1080/10962247.2018.1498410, 2018.
Vereecken, L. and Nozière, B.: H migration in peroxy radicals under atmospheric conditions, Atmos. Chem. Phys., 20, 7429–7458, https://doi.org/10.5194/acp-20-7429-2020, 2020.
Vogel, B., Vogel, H., Kleffmann, J., and Kurtenbach, R.: Measured and simulated vertical profiles of nitrous acid – Part II. Model simulations and indications for a photolytic source, Atmos. Environ., 37, 2957–2966, https://doi.org/10.1016/S1352-2310(03)00243-7, 2003.
Wang, S., Wu, R., Berndt, T., Ehn, M., and Wang, L.: Formation of highly oxidized radicals and multifunctional products from the atmospheric oxidation of alkylbenzenes, Environ. Sci. Technol., 51, 8442–8449, https://doi.org/10.1021/acs.est.7b02374, 2017.
Wang, S., Coggon, M. M., Gkatzelis, G. I., Warneke, C., Bourgeois, I., Ryerson, T., Peischl, J., Veres, P. R., Neuman, J. A., Hair, J., Shingler, T., Fenn, M., Diskin, G., Huey, L. G., Lee, Y. R., Apel, E. C., Hornbrook, R. S., Hills, A. J., Hall, S. R., Ullmann, K., Bela, M. M., Trainer, M. K., Kumar, R., Orlando, J. J., Flocke, F. M., and Emmons, L. K.: Chemical tomography in a fresh wildland fire plume: A Large Eddy Simulation (LES) Study, J. Geophys. Res.-Atmos., 126, e2021JD035203, https://doi.org/10.1029/2021JD035203, 2021.
Weber, J., Archer-Nicholls, S., Griffiths, P., Berndt, T., Jenkin, M., Gordon, H., Knote, C., and Archibald, A. T.: CRI-HOM: A novel chemical mechanism for simulating highly oxygenated organic molecules (HOMs) in global chemistry–aerosol–climate models, Atmos. Chem. Phys., 20, 10889–10910, https://doi.org/10.5194/acp-20-10889-2020, 2020.
Wennberg, P. O., Bates, K. H., Crounse, J. D., Dodson, L. G., McVay, R. C., Mertens, L. A., Nguyen, T. B., Praske, E., Schwantes, R. H., Smarte, M. D., St Clair, J. M., Teng, A. P., Zhang, X., and Seinfeld, J. H.: Gas-Phase reactions of isoprene and its major oxidation products, Chem. Rev., 118, 3337–3390, https://doi.org/10.1021/acs.chemrev.7b00439, 2018.
Williams, A. J., Grulke, C. M., Edwards, J., McEachran, A. D., Mansouri, K., Baker, N. C., Patlewicz, G., Shah, I., Wambaugh, J. F., Judson, R. S., and Richard, A. M.: The CompTox Chemistry Dashboard: a community data resource for environmental chemistry, J. Cheminformatics, 9, 61, https://doi.org/10.1186/s13321-017-0247-6, 2017.
Wiser, F., Place, B. K., Sen, S., Pye, H. O. T., Yang, B., Westervelt, D. M., Henze, D. K., Fiore, A. M., and McNeill, V. F.: AMORE-Isoprene v1.0: a new reduced mechanism for gas-phase isoprene oxidation, Geosci. Model Dev., 16, 1801–1821, https://doi.org/10.5194/gmd-16-1801-2023, 2023.
Womack, C. C., McDuffie, E. E., Edwards, P. M., Bares, R., de Gouw, J. A., Docherty, K. S., Dubé, W. P., Fibiger, D. L., Franchin, A., Gilman, J. B., Goldberger, L., Lee, B. H., Lin, J. C., Long, R., Middlebrook, A. M., Millet, D. B., Moravek, A., Murphy, J. G., Quinn, P. K., Riedel, T. P., Roberts, J. M., Thornton, J. A., Valin, L. C., Veres, P. R., Whitehill, A. R., Wild, R. J., Warneke, C., Yuan, B., Baasandorj, M., and Brown, S. S.: An Odd Oxygen Framework for Wintertime Ammonium Nitrate Aerosol Pollution in Urban Areas: NOx and VOC Control as Mitigation Strategies, Geophys. Res. Lett., 46, 4971–4979, https://doi.org/10.1029/2019GL082028, 2019.
Woody, M. C., Baker, K. R., Hayes, P. L., Jimenez, J. L., Koo, B., and Pye, H. O. T.: Understanding sources of organic aerosol during CalNex-2010 using the CMAQ-VBS, Atmos. Chem. Phys., 16, 4081–4100, https://doi.org/10.5194/acp-16-4081-2016, 2016.
Xing, J., Mathur, R., Pleim, J., Hogrefe, C., Gan, C.-M., Wong, D. C., Wei, C., Gilliam, R., and Pouliot, G.: Observations and modeling of air quality trends over 1990–2010 across the Northern Hemisphere: China, the United States and Europe, Atmos. Chem. Phys., 15, 2723–2747, https://doi.org/10.5194/acp-15-2723-2015, 2015.
Xu, L., Pye, H. O. T., He, J., Chen, Y., Murphy, B. N., and Ng, N. L.: Experimental and model estimates of the contributions from biogenic monoterpenes and sesquiterpenes to secondary organic aerosol in the southeastern United States, Atmos. Chem. Phys., 18, 12613–12637, https://doi.org/10.5194/acp-18-12613-2018, 2018.
Xu, L., Møller, K. H., Crounse, J. D., Otkjær, R. V., Kjaergaard, H. G., and Wennberg, P. O.: Unimolecular Reactions of Peroxy Radicals Formed in the Oxidation of α-Pinene and β-Pinene by Hydroxyl Radicals, J. Phys. Chem. A, 123, 1661–1674, https://doi.org/10.1021/acs.jpca.8b11726, 2019.
Xu, L., Møller, K. H., Crounse, J. D., Kjaergaard, H. G., and Wennberg, P. O.: New Insights into the Radical Chemistry and Product Distribution in the OH-Initiated Oxidation of Benzene, Environ. Sci. Technol., 54, 13467–13477, https://doi.org/10.1021/acs.est.0c04780, 2020.
Xu, W., Han, T., Du, W., Wang, Q., Chen, C., Zhao, J., Zhang, Y., Li, J., Fu, P., Wang, Z., Worsnop, D. R., and Sun, Y.: Effects of aqueous-phase and photochemical processing on secondary organic aerosol formation and evolution in Beijing, China, Environ. Sci. Technol., 51, 762–770, https://doi.org/10.1021/acs.est.6b04498, 2017.
Yee, L. D., Isaacman-VanWertz, G., Wernis, R. A., Meng, M., Rivera, V., Kreisberg, N. M., Hering, S. V., Bering, M. S., Glasius, M., Upshur, M. A., Gray Bé, A., Thomson, R. J., Geiger, F. M., Offenberg, J. H., Lewandowski, M., Kourtchev, I., Kalberer, M., de Sá, S., Martin, S. T., Alexander, M. L., Palm, B. B., Hu, W., Campuzano-Jost, P., Day, D. A., Jimenez, J. L., Liu, Y., McKinney, K. A., Artaxo, P., Viegas, J., Manzi, A., Oliveira, M. B., de Souza, R., Machado, L. A. T., Longo, K., and Goldstein, A. H.: Observations of sesquiterpenes and their oxidation products in central Amazonia during the wet and dry seasons, Atmos. Chem. Phys., 18, 10433–10457, https://doi.org/10.5194/acp-18-10433-2018, 2018.
Yeh, G. K., and Ziemann, P. J.: Alkyl nitrate formation from the reactions of C8–C14 n-alkanes with OH radicals in the presence of NOx: Measured yields with essential corrections for gas–wall partitioning, J. Phys. Chem. A, 118, 8147–8157, https://doi.org/10.1021/jp500631v, 2014.
Young, P. J., Naik, V., Fiore, A. M., Gaudel, A., Guo, J., Lin, M. Y., Neu, J. L., Parrish, D. D., Rieder, H. E., Schnell, J. L., Tilmes, S., Wild, O., Zhang, L., Ziemke, J., Brandt, J., Delcloo, A., Doherty, R. M., Geels, C., Hegglin, M. I., Hu, L., Im, U., Kumar, R., Luhar, A., Murray, L., Plummer, D., Rodriguez, J., Saiz-Lopez, A., Schultz, M. G., Woodhouse, M. T., and Zeng, G.: Tropospheric Ozone Assessment Report: Assessment of global-scale model performance for global and regional ozone distributions, variability, and trends, Elementa: Science of the Anthropocene, 6, 10, https://doi.org/10.1525/elementa.265, 2018.
Yujing, M. and Mellouki, A.: The near-UV absorption cross sections for several ketones, J. Photch. Photobio. A, 134, 31–36, https://doi.org/10.1016/S1010-6030(00)00243-4, 2000.
Zare, A., Fahey, K. M., Sarwar, G., Cohen, R. C., and Pye, H. O. T.: Vapor-Pressure pathways initiate but hydrolysis products dominate the aerosol estimated from organic nitrates, ACS Earth Space Chem., 3, 1426–1437, https://doi.org/10.1021/acsearthspacechem.9b00067, 2019.
Zhang, Q., Jimenez, J. L., Canagaratna, M. R., Ulbrich, I. M., Ng, N. L., Worsnop, D. R., and Sun, Y.: Understanding atmospheric organic aerosols via factor analysis of aerosol mass spectrometry: a review, Anal. Bioanal. Chem., 401, 3045–3067, https://doi.org/10.1007/s00216-011-5355-y, 2011.
Zhang, X., Cappa, C. D., Jathar, S. H., McVay, R. C., Ensberg, J. J., Kleeman, M. J., and Seinfeld, J. H.: Influence of vapor wall loss in laboratory chambers on yields of secondary organic aerosol, P. Natl. Acad. Sci. USA, 111, 5802, https://doi.org/10.1073/pnas.1404727111, 2014.
Zhao, B., Wang, S., Donahue, N. M., Jathar, S. H., Huang, X., Wu, W., Hao, J., and Robinson, A. L.: Quantifying the effect of organic aerosol aging and intermediate-volatility emissions on regional-scale aerosol pollution in China, Sci. Rep.-UK, 6, 28815–28815, https://doi.org/10.1038/srep28815, 2016.
Zhao, Y., Thornton Joel, A., and Pye Havala, O. T.: Quantitative constraints on autoxidation and dimer formation from direct probing of monoterpene-derived peroxy radical chemistry, P. Natl. Acad. Sci. USA, 115, 12142–12147, https://doi.org/10.1073/pnas.1812147115, 2018.
Zheng, B., Tong, D., Li, M., Liu, F., Hong, C., Geng, G., Li, H., Li, X., Peng, L., Qi, J., Yan, L., Zhang, Y., Zhao, H., Zheng, Y., He, K., and Zhang, Q.: Trends in China's anthropogenic emissions since 2010 as the consequence of clean air actions, Atmos. Chem. Phys., 18, 14095–14111, https://doi.org/10.5194/acp-18-14095-2018, 2018.
Zhu, S., Kinnon, M. M., Shaffer, B. P., Samuelsen, G. S., Brouwer, J., and Dabdub, D.: An uncertainty for clean air: Air quality modeling implications of underestimating VOC emissions in urban inventories, Atmos. Environ., 211, 256–267, https://doi.org/10.1016/j.atmosenv.2019.05.019, 2019.
- Abstract
- Introduction
- ROC emissions
- ROC chemistry
- ROC hazardous air pollutants
- Implications for the chemical evolution of ROC
- Discussion
- Appendix A
- Appendix B
- Code and data availability
- Author contributions
- Competing interests
- Disclaimer
- Acknowledgements
- Financial support
- Review statement
- References
- Supplement
- Abstract
- Introduction
- ROC emissions
- ROC chemistry
- ROC hazardous air pollutants
- Implications for the chemical evolution of ROC
- Discussion
- Appendix A
- Appendix B
- Code and data availability
- Author contributions
- Competing interests
- Disclaimer
- Acknowledgements
- Financial support
- Review statement
- References
- Supplement