the Creative Commons Attribution 4.0 License.
the Creative Commons Attribution 4.0 License.
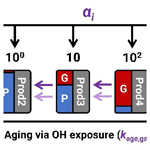
Volatile oxidation products and secondary organosiloxane aerosol from D5 + OH at varying OH exposures
Hyun Gu Kang
Yanfang Chen
Yoojin Park
Siloxanes are composed of silicon, oxygen, and alkyl groups and are emitted from consumer chemicals. Despite being entirely anthropogenic, siloxanes are being detected in remote regions and are ubiquitous in indoor and urban environments. Decamethylcyclopentasiloxane (D5) is one of the most common cyclic congeners, and smog chamber and oxidation flow reactor (OFR) experiments have found D5 + OH to form secondary organosiloxane aerosol (SOSiA). However, there is uncertainty about the reaction products and the reported SOSiA mass yields (YSOSiA) appear inconsistent. To quantify small volatile oxidation products (VOPs) and to consolidate the YSOSiA in the literature, we performed experiments using a potential aerosol mass OFR while varying D5 concentration, humidity, and OH exposure (OHexp). We use a proton transfer reaction time-of-flight mass spectrometer to quantify D5, HCHO, and HCOOH and to detect other VOPs, which we tentatively identify as siloxanols and siloxanyl formates. We determine molar yields of HCHO and HCOOH between 52 %–211 % and 45 %–127 %, respectively. With particle size distributions measured with a scanning mobility particle sizer, we find YSOSiA to be < 10 % at OHexp < 1.3 × 1011 s cm−3 and ∼ 20 % at OHexp, corresponding to that of the lifetime of D5 at atmospheric OH concentrations. We also find that YSOSiA is dependent on both organic aerosol mass loading and OHexp. We use a kinetic box model of SOSiA formation and oxidative aging to explain the YSOSiA values found in this study and the literature. The model uses a volatility basis set (VBS) of the primary oxidation products as well as an aging rate coefficient in the gas phase, kage,gas, of cm3 s−1 and an effective aging rate coefficient in the particle phase, kage,particle, of 2.0 × 10−12 cm3 s−1. The combination of a primary VBS and OH-dependent oxidative aging predicts SOSiA formation much better than a standard-VBS parameterization that does not consider aging (root mean square error = 42.6 vs. 96.5). In the model, multi-generational aging of SOSiA products occurred predominantly in the particle phase. The need for an aging-dependent parameterization to accurately model SOSiA formation shows that concepts developed for secondary organic aerosol precursors, which can form low-volatile products at low OHexp, do not necessarily apply to D5 + OH. The resulting yields of HCHO and HCOOH and the parameterization of YSOSiA may be used in larger-scale models to assess the implications of siloxanes for air quality.
- Article
(3263 KB) - Full-text XML
-
Supplement
(2504 KB) - BibTeX
- EndNote
Organosiloxanes are molecules composed of silicon–oxygen bonds with alkyl groups on the silicons and encompass linear and cyclic species, some of which have vapor pressures on par with volatile organic compounds (VOCs). Siloxanes are entirely anthropogenic pollutants (Rücker and Kümmerer, 2015) commonly used in consumer and industrial chemical products (Seltzer et al., 2021a; Gkatzelis et al., 2021) and their emissions are projected to increase in the coming decades (Tansel and Surita, 2017). Decamethylcyclopentasiloxane (D5, C10H30O5Si5), where “D” refers to silicon center atoms bonded to two oxygens, is a ubiquitous cyclosiloxane in the ambient environment.
Siloxanes have been detected in the indoor environment (Tang et al., 2015; Tran and Kannan, 2015; Arata et al., 2021; Katz et al., 2021; Kaikiti et al., 2022; Wang et al., 2022), near landfills (Schweigkofler and Niessner, 1999), and near sewage treatment sites (Lee et al., 2014; Horii et al., 2019). Siloxanes are also found in outdoor urban air (Xiang et al., 2021), and organosilicon compounds have been found in varying amounts in ambient particulates in China (Lu et al., 2019; Cheng et al., 2021; Meng et al., 2021; Song et al., 2022; Xu et al., 2022) and the United States (Milani et al., 2021).
Siloxanes are suspected to be environmentally persistent or emitted continuously to appear as such (Howard and Muir, 2010; Xiang et al., 2021), while other studies have found that methyl siloxanes would be removed on timescales of days to weeks (Graiver et al., 2003; Whelan and Kim, 2021). Reaction rate coefficients of D5 with atmospheric oxidants have been reported, and Atkinson (1991) found D5 to be effectively unreactive with atmospheric concentrations of O3 ( < cm3 s−1) and NO3 radicals ( < 3 × 10−16 cm3 s−1) at ∼ 298 K. While D5 is reactive with OH and Cl, Alton and Browne (2020) calculated that the removal of D5 by Cl radicals would only be a few percent of that by OH radicals at typical ambient oxidant concentrations.
Atkinson (1991), Safron et al. (2015), Xiao et al. (2015), Kim and Xu (2017), and Alton and Browne (2020) have measured at ∼ 298 K to be 1.55 × 10−12, 2.6 × 10−12, 2.46 × 10−12, 1.46 × 10−12, and cm3 s−1, respectively. These measurements are summarized in Table S1 in the Supplement. Xiao et al. (2015) derived computationally as 2.90 × 10−12 cm3 s−1. In this paper, we use 2.0 × 10−12 cm3 s−1, which is a rounded average of the empirically determined rate coefficients. This corresponds to a D5 atmospheric lifetime of about 4 d via removal by OH, assuming a daily average OH concentration ([OH]avg) of 1.5 × 106 cm−3.
D5 is expected to suppress O3 formation in urban environments. Carter et al. (1993) performed a series of chamber experiments mimicking urban air conditions and found that D5 siloxane would inhibit ozone formation by suppressing the OH radical. In contrast, formaldehyde (HCHO) is known to contribute to O3 formation (Derwent et al., 1996). Fu et al. (2020) predicted the formation of HCHO as a product of D5+ OH in low-NO / HO2 conditions using quantum chemical calculations and kinetics modeling, but an experimental yield of HCHO from D5+ OH has not been reported. Atkinson (1991) proposed HCHO as a product of the siloxane alkoxyl radical (RO) pathway, assuming an analogous mechanism to that of VOCs. Sommerlade et al. (1993) suggested that HCHO may arise from siloxane RO decomposition and from ROOH rearrangement in the presence of acids and H2O. Alton and Browne (2022) predicted HCHO as a product of RO2 rearrangement in the case of D3 siloxane. Because HCHO is a secondary product, the O3 formation potential of D5 may differ between at a source and at downwind locations.
Formic acid (HCOOH) is a common acid catalyst in the atmosphere (Hazra et al., 2014) and a particle-nucleating species (Yu, 2000). Studies have identified some HCOOH sources in the atmosphere (Millet et al., 2015; Franco et al., 2021); however, HCOOH is suspected to have unidentified anthropogenic sources in the troposphere (Millet et al., 2015; Chen et al., 2021) as some urban sources remain unaccounted for (Le Breton et al., 2012; Yuan et al., 2015). Chandramouli and Kamens (2001) proposed that the RO2 initially formed from D5+ OH makes a siloxanyl formate (D4T(OCHO), where “T” refers to a silicon center bonded to three oxygens) that reacts with H2O to form a siloxanol (D4T(OH)) and HCOOH. However, we are unaware of experimental HCOOH yields reported for D5 + OH.
Whelan et al. (2004) used known siloxane chemistry in a partitioning model to assess the atmospheric fate of siloxanes and found that silanols are the predominant oxidation products. These silanols are generally water soluble and either removed from the atmosphere via wet deposition or undergo a pH-dependent process of hydrolysis, forming smaller and smaller silanols (Whelan et al., 2004). Eventually, the small silanols are converted to SiO2, H2O, and CO2 through photolytic reactions in water or biological processes in soil (Spivack et al., 1997; Stevens, 1998; Graiver et al., 2003).
The intermediate products between D5 and those small silanols are less studied, and the OH-oxidation rate coefficients of these intermediates have not been reported. Sommerlade et al. (1993) and Alton and Browne (2022) used mass spectrometry to study the gaseous products of D5 oxidation in chambers, while Fu et al. (2020) used quantum chemistry modeling. These studies found that gaseous intermediates are composed of a variety of alcohols, aldehydes, esters, and hydroperoxides. Given that such volatile oxidation products (VOPs) in experiments with higher OHexp are likely to undergo multiple oxidation steps, there is a need to address their subsequent oxidation rate coefficients. Moreover, while the formation of HCHO and HCOOH has been predicted in mechanisms, it has not been quantified.
Secondary aerosol mass yield (Y, Eq. 1) is defined as the ratio of produced aerosol mass (Δm(SOSiA)) to reacted precursor mass (Δm(D5)), which we adopt here for secondary organosiloxane aerosol (SOSiA). Reports about secondary aerosol formation from D5 siloxane seem conflicting, with some experiments reporting much higher YSOSiA than others. For instance, Wu and Johnston (2017) and Janechek et al. (2019) saw maximum YSOSiA of 23 % and 50 %, respectively, in their photo-oxidation chamber and oxidation flow reactor (OFR) experiments, albeit at different OH exposures (OHexp). Charan et al. (2022) found a YSOSiA of 158 % with their OFR at an OHexp of 3.2 × 1012 s cm−3. Avery et al. (2023) reported a wide range of YSOSiA values (2 %–146 %) from their OFR experiments.
In contrast, Charan et al. (2022) reported almost negligible YSOSiA (< 5 %) from their chamber studies where [OH] was on the order of ∼ 106 cm−3, which is closer to [OH] found in ambient conditions (Peng and Jimenez, 2020). Han et al. (2022) conducted OFR experiments and found that YSOSiA would be 2 % at [OH] of 4.6×108 cm−3 or OHexp of 5.5 × 1010 s cm−3. The variation of YSOSiA reported in the literature suggests that oxidation conditions need to be considered to accurately parameterize YSOSiA, especially given that D5 is being considered in air-quality models as a part of volatile chemical product inventories (Pennington et al., 2021; Seltzer et al., 2021a, b).
In this study, we aim to assess the OH oxidation of D5 by determining the rate coefficients of secondary reactions of VOPs with OH (i.e., chemical aging) and providing a first quantification of HCHO and HCOOH yields. We also measure YSOSiA under diverse OHexp and [D5]0 conditions. Lastly, we develop parameterizations of SOSiA yield using a kinetic model with a chemical aging reaction scheme to reconcile the reported YSOSiA from D5+ OH in the literature and for use in air-quality models.
2.1 Experiments
The Aerodyne Research (Billerica, MA, USA) potential aerosol mass OFR (PAM-OFR) (Kang et al., 2007) has a volume of 13.3 L and is made of chromated aluminum (Xu and Collins, 2021). We operated the PAM-OFR in OFR185 mode (Peng and Jimenez, 2020), where 185 nm lamps that also emit 254 nm light (GPH436T5VH, LightSources, Orange, CT, USA) generate OH and O3 with injected H2O vapor from a Nafion humidifier (FC-100-80-6MKK, Perma Pure, Lakewood, NJ, USA). There were two of these 185 nm lamps placed across from each other in clear fused quartz sleeves. The 185 nm lamps were wrapped with covers at even intervals to reduce the UV intensity so that 90 % of the lamp surface was covered. We operated the PAM-OFR at residence times (τres) of 120 and 180 s with flow rates of 6.65 and 4.43 L min−1, respectively. Additional details about the experiment setup are summarized in Fig. S1 and Sect. S1.
We use the D5 siloxane trace measured from the proton transfer reaction mass spectrometer (PTR-MS) to calculate OHexp with Eq. (2), where 2.0 × 10−12 cm3 s−1. [D5]0 and [D5]final are the D5 concentrations before and after the exposure to OH.
Prior to experiments, we checked the background particle and D5 concentrations with the scanning mobility particle sizer (SMPS) and PTR-MS. In all experiments, the background particle number concentrations were < 10 cm−3, and the background [D5] values were below the limit of detection (3σ=80 ppt). Then, we injected D5 with a syringe pump while monitoring the PTR-MS, with major ions at 371 and 355. We performed the experiments with a target [D5]0 of 50, 100, or 200 ppb. With these target [D5]0 values, we get external OH reactivities (OHRext) of 2.5–9.8 s−1 at 298.15 K and 1 atm, where OHRext is the reactivity caused by the injection of D5 into the PAM-OFR (Peng and Jimenez, 2020). With these OHRext values, we reduce the risk of OH suppression and VOCs photolysis (Peng and Jimenez, 2020).
When the D5 trace stabilized near the target [D5]0, we began the experiment by turning the UV lamps in the PAM-OFR on at either 2.4 or 8.0 V. We waited 30 min for the UV lamps to stabilize and for the PAM-OFR walls to equilibrate with gaseous species. The YSOSiA values (Eq. 1) were calculated using the average SOSiA mass concentration from four SMPS cycles following that 30 min. We obtained Δm(D5) as the difference between [D5]0 and [D5]final. At the end of an experiment, we turned off the UV lamps to check the D5 trace return.
To clean the PAM-OFR between experiments, we stopped the syringe pump and removed the syringe from the glass bulb while keeping the humid airflow in the PAM-OFR. We turned on the PAM-OFR UV lamps and connected the outlet directly to the exhaust, until D5 and particle number concentrations were below the limit of detection. We used Igor Pro 9 (WaveMetrics, Portland, OR, USA) for data post-processing and visualization.
2.2 Instrumentation
2.2.1 PTR-MS
To measure D5 and VOPs, we used a PTR-MS (PTR-TOF 1000, IONICON Analytik, Innsbruck, Austria) equipped with the extended volatility range (EVR) option (Piel et al., 2021), where the wetted inlet components and the drift tube are passivated with a silicon coating. The PTR-MS also had an ion transfer lens between the drift tube and time-of-flight mass spectrometer (Jordan et al., 2009). An internal permeation source (PerMaSCal) emitted a steady stream of 1,3-diiodobenzene into the mass spectrometer for mass calibration scale adjustments. Additional PTR-MS details are in Sect. S1.
To reduce H2O clusters at high humidities, we operated the PTR-MS at 137 Td (Udrift=600 V; Td is the Townsend unit where 1 Td = 10−17 V cm2) for quantification. The drift tube pressure and temperature were set to 2.30 mbar and 80 ∘C. For the reagent ion source, we set the Us, Uso, and H2O flow rate to 150 V, 80 V, and 6.00 sccm respectively. The ion source hollow-cathode discharge current was set to 5.0 mA. The PTR-MS drift tube was 9.6 cm long, and at 137 Td the [H2O]H+ reaction time (Δt) was 94 µs (de Gouw et al., 2003). We calculate the primary reagent ion signal, [H2O]H+, by multiplying the signal of its isotope, [HO]H+, by 500.
We use the PTR-MS data for the quantification of D5 ( 371), HCHO ( 31), and HCOOH ( 47), where the primary reagent ion counts were normalized to 106 counts per second (ncps). For D5, we used a calibration gas cylinder (Apel-Riemer Environmental, Miami, FL, USA) containing D5 to calibrate the PTR-MS. We also calculate the normalized measurement sensitivity (ncps ppb−1) of D5, HCHO, and HCOOH using Eq. (3) adapted from de Gouw and Warneke (2007). I(VOC)H+ and are the ion counts of the protonated VOCs and the reagent ion, respectively. Additional details on the mass spectra interpretation and quantification are in Sects. S1.5 and S3.
We tested the instrument sensitivity response with humidity by keeping the species concentrations constant while changing the sample air humidity. The sensitivity of D5 at 371 was not heavily affected by humidity at 137 Td, and we did not correct for humidity in the D5 quantification (Fig. S5). On the other hand, HCHO and HCOOH sensitivities varied with humidity, and we corrected their sensitivities as detailed in Sect. S3. Prior to experiments, we tuned the micro-channel plate (MCP) to prevent signal bias against higher-mass ions (Müller et al., 2014). We adjusted the MCP voltage in steps to increase the signal strength at 331, a diiodobenzene ion, until the relative signal increase was < 20 %.
2.2.2 Scanning mobility particle sizer
An SMPS 3938 (TSI, Shoreview, MN, USA) equipped with an impactor (0.0508 cm) measured the particle mobility diameter size distribution between diameters of 14.3 and 723.4 nm. The SMPS consisted of a model 3082 electrostatic classifier, a model 3081A differential mobility analyzer (DMA), a model 3088 soft X-ray neutralizer, and a model 3756 ultrafine condensation particle counter. We set the SMPS sheath flow at 3.0 L min−1 and the aerosol flow rate at 0.3 L min−1, and the DMA voltage ranged from 10.6 to 9921.4 V. The SMPS scanned for 150 s, followed by a 5 s retrace and 10 s purge while recording on a 3 min cycle. We referred to the manufacturer's recommendations when deciding these SMPS settings (TSI Inc., 2012), and a sample particle size distribution from experiment 12 (Table 1) is shown in Fig. S4.
Table 1Summary of SOSiA mass yields (YSOSiA) with aerosol sampling line corrections assuming ρSOSiA= 1.07 g cm−3 for all experiments. [H2O] is the molar mixing ratio of H2O in air. For COA and [D5] the errors are the standard deviation of the data points averaged, while for YSOSiA they are calculated with error propagation. For reference, at 25 ∘C and 1 atm, 1 ppb of D5 is ∼ 15 µ g m−3 and 1 d equivalent of OHexp is ∼ 1.3 × 1011 s cm−3 at a daily [OH]avg of 1.5 × 106 cm−3.
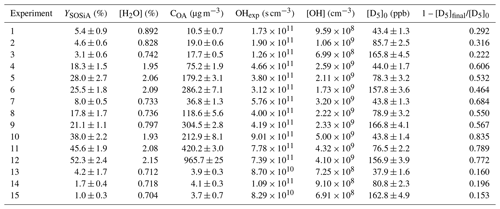
For the YSOSiA calculations, we convert the SMPS integrated particle volumes into mass using a SOSiA mass density (ρSOSiA) of 1.07 g cm−3 for all experiments. We obtained this ρSOSiA from PAM-OFR experiments separate from the ones described here, where we weighed the masses of SOSiA collected on filters and obtained particle volumes with the SMPS. Additional details on ρSOSiA are available in Sect. S2.
2.3 Volatility distribution parameterization
Janechek et al. (2019) and Charan et al. (2022) fitted their YSOSiA data to the Odum two-product model (Odum et al., 1996), and we follow the same methodology for comparison with the literature (Sect. S4). Similarly, we fit the standard volatility basis set (VBS) parameters α (Donahue et al., 2006) in Eq. (4) to the measured Δm(SOSiA) using the measured Δm(D5), where αi is the product mass yield for volatility bin i.
In the experiments, the organosiloxane aerosol mass loading (COA) was equivalent to the SOSiA mass concentrations. As the produced aerosol mass in the experiments ranged from 3.7 to 965.7 µg m−3, we use six logarithmically spaced effective saturation mass concentration (C*) bins ranging from 0.1 to 10 000 µg m−3 at 298.15 K to cover the low- and high-volatility products. For reference, D5 liquid has a vapor pressure of 20.4 Pa at 298.15 K or 106 µg m−3 (Lei et al., 2010). Since the experiments had slight variations in temperature, we correct for temperature impacts on C* between experiments using the Clausius–Clapeyron equation and an enthalpy of vaporization of 60 kJ mol−1, which is that of D5 siloxane (Lei et al., 2010).
As the experiments were performed for a range of OHexp, the products between experiments may have varied due to multi-generational aging (Zhao et al., 2015). To account for aging and parameterize YSOSiA as a function of OHexp, we also analyze the yield data using a kinetic box model with four chemical reactions (Reactions R1–R3) written in MATLAB (MathWorks, Natick, MA, USA).
Reaction (R1) describes the initial oxidation of D5 and immediate formation of products of varying volatility. Here, prod(i) refers to the sum of products (gas + particle) in volatility bin i, which are formed with a molar branching ratio αi. We assume that prod(i) values have the same molecular weights (g mol−1) as D5, and so the αi values are equivalent to the product mass yields at OHexp→0. In the model, a fraction fi of each oxidation product partitions instantaneously from the gas phase to the particle phase according to absorptive partitioning theory (Donahue et al., 2006) (Eq. 5).
Reactions (R2) and (R3) describe how OHexp causes volatility to decrease (Robinson et al., 2007). This decrease in volatility via “bin hopping” (Sommers et al., 2022) occurs at a rate proportional to the chemical aging rate coefficient for the gas-phase (kage,gas, cm3 s−1) and an effective aging rate coefficient for the particle-phase species (kage,particle, cm3 s−1). Here we assume that products in the lowest-volatility bin (i=1) cannot be removed from that bin and that the highest-volatility bin (i=6) does not receive product with aging. The [OH] values are set by dividing the experimental OHexp from Eq. (2) by the PAM-OFR residence times.
We use kage,gas and kage,particle as aggregate chemical aging rate coefficients, not specific to any species or volatility bin. Studies on chamber experiments (Robinson et al., 2007) and ambient measurements (Sommers et al., 2022) have applied chemical aging only to the gas phase as heterogeneous aging is slower. However, studies have found that the high oxidant concentrations in OFRs would appreciably oxidize organic aerosol (OA) within experiment timescales (Kessler et al., 2012; Kroll et al., 2015). To accommodate OH uptake to the bulk phase, we include particle-phase aging that also decreases the volatility of particle-phase products. The timescales and atmospheric relevance of heterogeneous oxidation in OFRs are areas of ongoing research (Zhao et al., 2019; Peng and Jimenez, 2020), but for now we opt to fit chemical aging rate coefficients for each phase. Note that surface and bulk accommodation processes are not explicitly resolved in the kinetic model and kage,particle acts on the concentrations of particle-phase products per unit gas volume (Sect. S1.6).
We fit kage,gas, kage,particle, and αiin the aging-VBS model to the experimental SOSiA mass using the Monte Carlo genetic algorithm (MCGA) (Berkemeier et al., 2017). We obtain a best model fit and a fit ensemble consisting of 1059 parameter sets for which the model's root mean square error (RMSE) is below the threshold of 50. We find this ensemble to estimate the parametric uncertainty associated with the model fit (Berkemeier et al., 2021).
We use the OFR chemistry template with KinSim (Peng and Jimenez, 2020) to estimate the RO2 fates and expect the fates to have been uniform across the experiments (Sect. S5). Although there are uncertainties in the RO2 reaction rate coefficients for siloxanes, we expect that the variation in YSOSiA is not driven by RO2 fate in these experiments. We also report the condensational sink and condensation lifetimes (Palm et al., 2016) calculated using the particle size distributions in Sect. S1.3.
3.1 Volatile organic products (VOPs)
3.1.1 Siloxanol and formate ester trends
In Fig. 1, the PTR-MS signals before and after D5 is oxidized are displayed relative to the protonated D5 ion at 371 on the y axis. We perform this scaling because the isotopologues of the product fragment ions overlap with the isotopologues of D5. Thus, changes in signal intensity are caused by both product formation and D5 oxidation. We choose to normalize the spectra at 371 because we assume that no product ion peaks overlap with the [D5]H+ signal at 371. While this scaling makes the product peaks appear larger, the changes in the mass spectrum are also qualitatively highlighted. For example, D5 loses a methyl group during the PTR, which forms a large signal at 355. The isotopologues of the -CH4 fragment of [D5]H+ overlap with fragments of VOPs. By scaling the mass spectrum with the ratio of [D5]H+ signal before and after oxidation, the signal of the VOPs is separated from that of remaining D5.
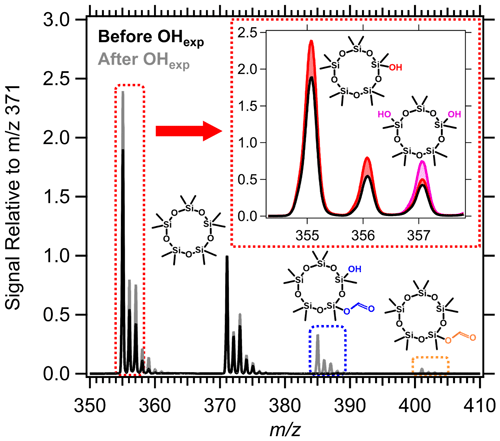
Figure 1Example PTR-MS mass spectra from experiment 12 and proposed VOP ions. For visualization, the signal intensities before (black) and after (gray) oxidation are shown relative to the maximum signal intensity of the [D5]H+ ion at 371, which is set to 1. The multifunctional species (blue, pink) are expected to be formed through multiple steps of OH oxidation. The red and pink areas in the inset each refer to the enhancement in signal attributed to D4T(OH) and D3T2(OH)2 over that of the -CH4 fragment of [D5]H+ and isotope signals, respectively.
Using the mass spectra and species reported by Alton and Browne (2022), we attribute the indicated ions in Fig. 1 to siloxanol (D4T(OH)), siloxanediol (D3T2(OH)2), siloxanyl formate (D4T(OCHO)), and siloxanolyl formate (D3T2(OH)(OCHO)). Here, D and T refer to silicon centers bonded to two and three oxygen atoms, respectively. The multifunctional VOPs are reported to arise from multiple steps of oxidation (Alton and Browne, 2022). The red and pink shaded areas in the inset of Fig. 1 refer to the enhancement in signal over that of the -CH4 fragment of [D5]H+, which we attribute to the -H2O fragments of [D4T(OH)]H+ and [D3T2(OH)2]H+, respectively. We use the masses of the -H2O fragments of the protonated siloxanols as large alcohols dissociate during the PTR (Brown et al., 2010). We also attribute the ions in the blue- and yellow-dotted boxes to the -H2O fragments of [D3T2(OH)(OCHO)]H+ and [D4T(OCHO)]H+.
As we did not have calibration standards to quantify these VOPs, we calculate the molar yields of the VOPs relative to that of protonated D5 siloxane at 371 to study the trends of siloxane VOPs (Fig. 2). For each VOP, we choose a characteristic peak in the mass spectrum and calculate the change in signal due to VOP formation (Sect. S1.5). Then, we calculate the ratios of the changes in the VOP and D5 signals to get the relative molar yields (Yrel,VOPi). In the right-side panels for each VOP in Fig. 2, the Yrel,VOPi decreases with increasing OHexp (x axes). This decrease in VOP signal is consistent with these gaseous products undergoing further oxidation or increased gas–particle partitioning due to higher COA at higher OHexp. We use the colors to highlight the functional groups on the D5 backbone.
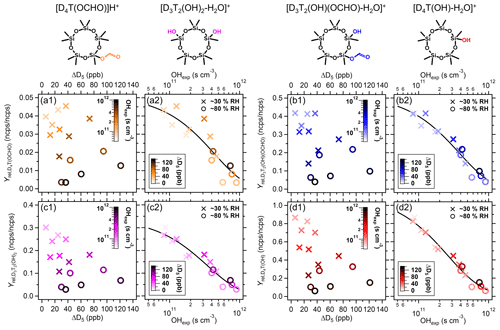
Figure 2Relative molar yields of VOPs as a function of OHexp and D5 consumed: (a1, a2) D4T(OCHO), (b1, b2) D3T2(OH)(OCHO), (c1, c2) D3T2(OH)2, and (d1, d2) D4T(OH). The colors correspond to the attributed mass ions and molecular structures shown at the top. We did not have a calibration for the suspected VOPs, so the y axes are relative molar yields (ncps ncps) calculated with the change in signal attributed to each VOP and that of D5 at 371. The relative molar yields decrease with OHexp, which is used to fit their OH-oxidation rate coefficients and γi (black lines).
In the left-side panels for each VOP in Fig. 2, the relative signals of the VOPs (y axes) decrease with increasing OHexp (color scale). Then, assuming that [OH] is constant throughout the PAM-OFR, that D5+ OH is the rate-limiting step in VOP formation, and that removal via gas–particle partitioning is negligible (Alton and Browne, 2022), we can consider a simplified D5+ OH chemical mechanism, Reactions (R4) and (R5).
In Reaction (R4), γi is the relative molar yield of a given VOPi found by extrapolating (y axes in Fig. 2) to OHexp→0. With ordinary differential equations (Eqs. 6 and 7) from these reactions and experimental inputs, we fit γi and the VOPi+ OH rate coefficient (kVOPi+OH, cm3 s−1). The fits are shown as black lines in the right-side panels of each VOP in Fig. 2.
The fitted kVOPi+OH values for each VOP are on the order of ∼ 10−12 cm3 s−1 (Table S7) but faster than , which suggests that these VOPs have atmospheric lifetimes shorter than that of D5. Alton and Browne (2022) have estimated these VOPs to be volatile with quantitative structure–activity relationship models. However, there are uncertainties in those models, and the VOPs may have lower-saturation mass concentrations than expected. Moreover, the chemical mechanism might be more complex than the one outlined with simple Reactions (R4) and (R5). Consequently, we present these kVOPi+OH values as estimates for secondary chemistry in this simplified reaction scheme, and future work using quantitative measurements should improve the calculated lifetimes of these intermediate D5+ OH products in the atmosphere.
3.1.2 Formaldehyde (HCHO) yields
As shown in Table S8 and Fig. 3, the experimental molar yields of HCHO (YHCHO, ΔHCHO/ΔD5 in ppb ppb−1) exceed 100 % at low OHexp and decrease with higher OHexp. We attribute the decreasing YHCHO with increasing OHexp to HCHO removal by OH in the PAM-OFR. HCHO has a lifetime of 0.91 d at [OH]avg= 1.5×106 cm−3 (Atkinson et al., 2006) or 78 s at [OH] = 1.5×109 cm−3. In such high-[OH] conditions, some HCHO is oxidized while being produced, which is consistent with the decreasing YHCHO with increasing OHexp (Fig. 3a). However, HCHO formation likely occurs over multiple oxidation steps (Fu et al., 2020), and how VOP + OH branches to produce HCHO and the rate coefficients for those reactions are not experimentally constrained.
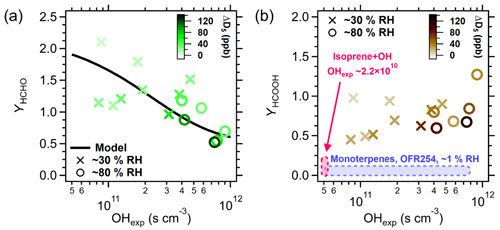
Figure 3Experimental molar yields of selected VOPs: (a) HCHO and (b) HCOOH as functions of OHexp. The blue shaded area in (b) is the range of YHCOOH (< 10 %) measured by Friedman and Farmer (2018) with monoterpenes under low-RH and low-NOx conditions. The pink shaded area refers to YHCOOH from isoprene + OH chamber experiments (Link et al., 2020) at lower OHexp.
Consequently, we implement a simplified mechanism (Reactions R6–R8) where D5+ OH produces a representative VOP (VOPrep) and yields HCHO at each oxidation step. The subsequent VOPrep+ OH reactions share the same rate coefficient as D5+ OH and produce HCHO with the same yield (γHCHO). This γHCHO is the cumulative molar yield of HCHO or the molar yield of HCHO as OHexp→0. This γHCHO is also used to correlate satellite column retrievals of HCHO with VOC emissions (Millet et al., 2006) where an empirical value can be used to constrain uncertainty.
We fit γHCHO to be 223 % (black line in Fig. 3a), assuming a constant [OH] in the PAM-OFR and that HCHO removal via partitioning or reactive uptake is negligible. This γHCHO is consistent with the modeled yields of those for VOCs used by Millet et al. (2006), who used γHCHO from chemical models ranging from 60 %–230 % for a variety of VOCs. Thus, D5 has a comparable γHCHO to that of isoprene or aromatic VOCs. An improved mechanism and additional rate coefficients are needed to accurately model HCHO formation.
Fu et al. (2020) proposed a mechanism for D3 siloxane where high YHCHO is produced under low-NO / HO2 conditions. In that mechanism, RO2 rearrangement and RO H shift is fast, and HCHO is produced at each rearrangement step. The γHCHO exceeding 100 % in these D5 experiments is consistent with HCHO production over multiple rapid oxidation steps. The results we report suggest that a similar HCHO production mechanism exists for D5.
Mao et al. (2009) found that models under-predicted tropospheric HCHO during their aircraft campaign studying Asian pollution outflows into the Pacific Ocean. This discrepancy between the measurements and calculations was pronounced near the surface and up to 2 km. They proposed that there is some missing OH reactivity and that the unaccounted species would be reactive with OH and yield HCHO when oxidized. Based on the D5 experiments present here, the inclusion of siloxane species may reduce the HCHO formation gap; Coggon et al. (2021) already noted that including volatile chemical products in their model would increase HCHO production.
The large formation of HCHO may entail D5 siloxane contributing to O3 formation, albeit indirectly. We were unable to observe O3 enhancement due to the high concentrations of O3 produced from the PAM-OFR internal chemistry itself and the lack of NOx. Given that is relatively slow compared to that of other common anthropogenic VOCs, we suspect that the oxidation of D5 will occur downwind of urban sources in low-NOx conditions or in cases of air stagnation. Whether D5 has a net positive or negative effect on O3 formation in these VOC / NOx scenarios needs to be assessed with models. To get a rough estimate of O3 production, we consider a case where 20 ppt of D5 reacts with OH to form 40 ppt of HCHO, which also fully reacts. This D5 concentration is within the range reported by Coggon et al. (2018) in ambient urban air. The molar maximum incremental reactivity of HCHO under high-NOx conditions is ∼ 20 % (Carter et al., 1995), which makes HCHO a prominent precursor for tropospheric O3. By multiplying the maximum incremental reactivity with the HCHO reacted with OH, we can estimate an O3 formation potential of 8 ppt from D5 in urban air.
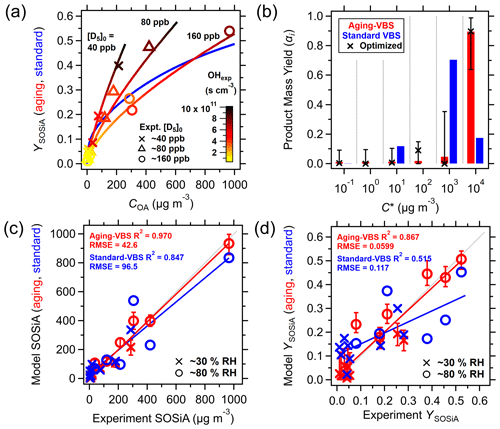
Figure 4Application of standard-VBS and aging-VBS models to experimental data. (a) YSOSiA as a function of COA, where the YSOSiA appears to be correlated with OHexp. The standard-VBS model is shown in blue, and the aging-VBS model is shown with OHexp (color scale) as it is a kinetic model. (b) VBS product mass yields for each volatility bin. For the aging-VBS model, the values are those of the first-generation products. (c) Comparison of SOSiA mass concentrations and (d) comparison of YSOSiA between the aging-VBS and standard-VBS models against measurements. The error bars indicate the minimum and maximum values from the parameter fit ensemble. The aging-VBS model shows a lower RMSE and higher R2.
3.1.3 Formic acid (HCOOH) yields
We find molar yields of HCOOH (YHCOOH, ΔHCOOH/ΔD5 ppb ppb−1) between 45 %–127 %, as shown in Fig. 3b, although a trend with OHexp is not obvious (Fig. 3b). We assume HCOOH loss via OH oxidation to be minor given the rate coefficient of 4.5 × 10−13 cm3 s−1 at 298.15 K (Atkinson et al., 2006), which corresponds to 17 d of OHexp at [OH]avg= 1.5 × 106 cm−3 or an OH-oxidation lifetime of 440 s in our highest-OHexp experiment. In addition to D4T(OCHO) hydrolysis, HCOOH may have been produced by heterogeneous reactions of HCHO at the surface of the SOSiA or the OFR walls in these humid experiments. In the atmosphere, HCOOH is presumed to form heterogeneously from HCHO and methanediol (HOCH2OH) in the presence of wet particles (Franco et al., 2021).
The YHCOOH values from D5+ OH we report are higher than the values from isoprene + OH (Link et al., 2020) or monoterpene + OH reported by Friedman and Farmer (2018), who quantified the YHCOOH of seven monoterpenes at varying OHexp without NOx. The range of YHCOOH values from these references is shown as shaded areas in Fig. 3b. The YHCOOH from D5 is on par with the humid isoprene ozonolysis cases reported by Link et al. (2020). Friedman and Farmer (2018) also used a PAM-OFR, but with 254 nm UV lamps in dry conditions (∼ 1 % RH), and Link et al. (2020) used a reaction chamber, which limits a direct comparison with our results. Nevertheless, Friedman and Farmer (2018) found YHCOOH of 0.64 %–8.5 % at OHexp= 2.0 × 1011 s cm−3. Aside from the different precursor VOCs and mechanism, Friedman and Farmer (2018) may have encountered less heterogenous production of HCOOH due to the dry OFR conditions. While D5+ OH may produce more HCOOH than isoprene + OH, the global emissions of D5 (McLachlan et al., 2010) are about 4 orders of magnitude smaller than those of isoprene (Guenther et al., 2012). Nevertheless, the product class of siloxanes may constitute a minor atmospheric HCOOH source in urban locations, especially if emissions were to increase.
3.2 SOSiA mass yields
3.2.1 Volatility basis set parameterization
The Odum two-product model does not accurately capture the YSOSiA in the literature in the high COA range (Sect. S4), so we apply a VBS model. Figure 4a shows the fitted aerosol mass yield curve (blue line) using a standard-VBS model (Eq. 4), but the experimental YSOSiA (y axis) appears to depend on both COA (x axis) and OHexp (color scale). To address whether accounting for the varying OHexp in these experiments would improve the VBS model outputs, we fit the produced SOSiA mass using a standard-VBS model (Eq. 4) and a kinetic model with VBS and chemical aging rate coefficients (“aging-VBS model”, Reactions R1–R3) based on OHexp and [D5]0 (Table 1).
We fit kage,gas and kage,particle in the aging-VBS model to be cm3 s−1 and 2.0 × 10−12 cm3 s−1, respectively. The fitted aging-VBS model parameters are summarized in Table S11. Figure 4a also shows the aerosol mass yield curves calculated with the aging-VBS model over varying OHexp. Since the aging-VBS model is kinetic, the YSOSiA values are dependent on both [D5]0 and OHexp, and we calculate three yield curves using the approximate experimental [D5]0. The yield curves generated with the aging-VBS model are more consistent with the experiments and show how YSOSiA, [D5]0, and OHexp are intertwined in the proposed aging mechanism.
Secondary organic aerosol (SOA) mass yield often exhibits a maximum as a function of OHexp, after which the yield decreases due to fragmentation becoming dominant at high OHexp (Isaacman-VanWertz et al., 2018). We do not find such a maximum in the range of OHexp studied, which suggests that an even higher YSOSiA could have been found at higher OHexp. Moreover, SOSiA is reported to be non-hygroscopic compared to SOA (Janechek et al., 2019), and we do not see an obvious relationship between the experiment humidity conditions and aerosol formation.
In both the standard and aging-VBS model fits (blue and red, respectively, in Fig. 4b), ∼ 95 % of the D5+ OH product mass is in the gas phase at a COA of 10 µ g m−3. The high fraction of gaseous products is consistent with low YSOSiA in the lower-OHexp experiments, whereas additional oxidation in the higher-OHexp experiments leads to a shift towards products that partition into the particle phase, thus increasing YSOSiA. The optimized αi values for the aging-VBS model are shown as markers in Fig. 4b. The error bars indicate the minimum and maximum values of the fitted αi in the ensemble parameter sets, which are further expanded in Fig. S12a. The fit ensemble suggests that products from D5+ OH must be largely volatile ( µ g m−3) in order to reproduce the experimental SOSiA yields.
Figure 4c and d show comparisons of the standard and aging-VBS models with experimental SOSiA mass concentrations and YSOSiA. The error bars indicate the range of model outcomes within the fit ensemble. We see an improvement in the RMSE and R2 with the aging-VBS model over the standard-VBS model, suggesting that incorporating OHexp into the yield parameterization improves model performance. Figure 5 compares the standard-VBS model with the aging-VBS model for a range of OHexp, showing that product volatility gradually decreases with increasing OHexp in the aging-VBS model. The high volatility of the initial products is consistent with the lack of the rapid formation of low-volatile species, such as highly oxygenated molecules known to form SOA (Isaacman-VanWertz et al., 2018).
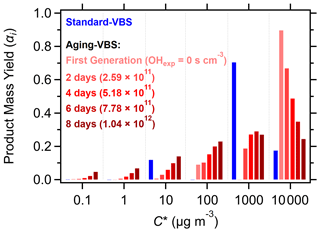
Figure 5Evolution of the volatility distribution with OHexp. The standard-VBS model parameterization (blue bars) is dominated by the µg m−3 volatility bin. In the aging-VBS model, the first-generation volatility distribution is dominated by the highest-volatility bin ( 000 µg m−3) but decreases with increasing OHexp (red bars).
We find that the model is sensitive to kage,particle, and a larger rate coefficient results in higher SOSiA formation (Fig. S11a). When fitting the model with deactivated particle-phase aging (), model-experiment RMSE is slightly increased (Fig. S11b). Thus, the fitting process provides a weak constraint on the parameter value (Fig. S12c). The numerical value of the fitted kage,particle is physically reasonable as it corresponds to an effective uptake coefficient of OH molecules colliding with the particle surface in the range of 0.1–1 (Sect. S1.6). On the other hand, kage,gas is very influential on the model output and tightly constraint in the ensemble of model fits around a value of cm3 s−1 (Fig. S12b). We hence postulate that multi-generational aging of in the gas phase is likely an important process for SOSiA formation, while particle-phase aging may only play a minor role under the investigated conditions.
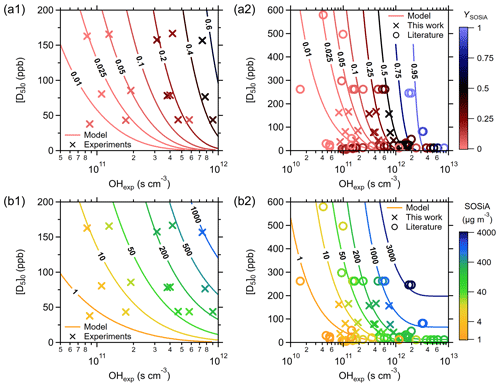
Figure 6Comparison of experiments, model results, and literature values: (a) YSOSiA and (b) SOSiA mass concentrations as a function of [D5]0 and OHexp. The aging-VBS model is fit using experimental data from (1) this study and (2) those in the literature. SOSiA formation generally increases with [D5]0 and OHexp. The aging-VBS model can capture the broad range of YSOSiA reported in the literature.
3.2.2 Consolidating literature YSOSiA
To address the variation in the literature YSOSiA and to generate parameters for air-quality models, we fit the parameters in the aging-VBS model with all available data in the literature and those from our experiments. Given that the literature used differing ρSOSiA values to calculate YSOSiA from SMPS data, we adjust the YSOSiA and COA reported in the literature to that of the ρSOSiA used here (ρSOSiA=1.07 g cm−3). Similarly, we re-calculate the OHexp in the literature using Eq. (2) and the [D5]0 and [D5]final values.
Figure 6 shows experimental values (markers) and model outputs (contours) of YSOSiA (panels a1 and a2) and SOSiA mass concentrations (panels b1 and b2) as a function of [D5]0 and OHexp. Figure 6a1 and b1 are generated using the aging-VBS model fit using only data from experiments presented in this study, while Fig. 6a2 and b2 show a fit including data from the literature. The aging-VBS model captures the increasing YSOSiA with increasing [D5]0 and OHexp. At a given [D5]0, YSOSiA and the SOSiA mass concentrations increase with higher OHexp. Figure 6a2 shows that the relatively high YSOSiA (>50 %) is feasible at OHexp>1012 s cm3. Moreover, the aging-VBS model predicts that YSOSiA is almost negligible (< 5 %) under atmospheric concentrations of D5 and OHexp.
Figure S8 shows that the aging-VBS model used here leads to a much higher correlation between modeled and experimental values for SOSiA mass concentration compared to the same analysis with a standard-VBS model (RMSE = 189 vs. 378). The better correlation suggests that the volatility distribution evolves with OHexp and that chemical aging should be considered when evaluating the volatility distribution of SOSiA from D5 + OH.
We note that bulk-phase chemistry is more complex than logarithmic shifts in volatility with OHexp and is not fully captured in the proposed aging-VBS parameterization. For example, Wu and Johnston (2017), Avery et al. (2023), and Chen et al. (2023) characterized D5+ OH SOSiA with mass spectrometry and found spectra indicative of oligomers. The formation of oligomers may reduce the bulk volatility by more than one bin and change the gas–particle equilibrium timescales (Berkemeier et al., 2020). Here, we incorporate kage,gas and a simple bin hopping approach to illustrate that a change in the volatility distribution with OHexp can adequately capture the YSOSiA variation in the literature. Future work with more sophisticated chemical models should close that gap further.
With a PAM-OFR, PTR-MS, and SMPS, we studied the formation of VOPs and SOSiA under various OHexp conditions. Using a simplified VOP oxidation scheme (Reactions R4 and R5), we find that the VOPs of tentatively identified siloxanols and formate esters have shorter OH-oxidation lifetimes than their precursor D5 (Table S7). In addition, we find the mass yield of HCHO of D5 comparable to that of isoprene or aromatics (Millet et al., 2006), suggesting that D5 siloxane is a potential O3-contributing species in downwind scenarios. We find YHCOOH ranging from 45 %–127 %, which suggests that D5+ OH is a source of atmospheric HCOOH, albeit smaller than isoprene, which is emitted in higher amounts by orders of magnitude.
An aging-VBS model incorporating OHexp and chemical aging adequately describes gas–particle partitioning at atmospheric OHexp and COA. Based on these experiments, low-NOxYSOSiA should be < 5 % under commonly observed atmospheric OH s cm−3 (Fig. 6a1). The first-generation products of D5+ OH are likely volatile, but their volatility decreases with increasing OHexp (Fig. 5). This evolution in volatility suggests that further oxidation of secondary products would reduce the volatility enough to form SOSiA. Unlike α-pinene (Isaacman-VanWertz et al., 2018) or other precursors for SOA, D5+ OH does not appear to produce low-volatile species within a single oxidation step. Instead, additional OHexp is needed to form aerosol, which suggests that multiple oxidation steps lead to a gradual decrease of product volatility. Hence, concepts that can be successfully applied to SOA formation may not accurately capture SOSiA formation, for which models must consider chemical aging. In the atmosphere, SOSiA from D5+ OH may be easier to detect downwind of urban sources due to the higher OHexp and dilution and/or removal of competing OH-reactive species.
Based on KinSim calculations (Sect. S5), we expect that the RO2 fate is dominated by RO2+ HO2 and RO2+ OH, which is consistent with the calculations performed by Avery et al. (2023). However, we note that the reaction rate coefficients of RO2 and its subsequent products are uncertain for D5, and we cannot directly address the atmospheric relevance of these calculated RO2 fates at this time. To improve YSOSiA parameterizations for the atmosphere, there is a need to study the impact NOx has on siloxane RO2 chemistry, given that siloxanes are likely emitted from urban sources where [NOx] is high. In such scenarios, RO2+ NOx is likely an important fate (Peng et al., 2019; Newland et al., 2021). Han et al. (2022) found that the addition of N2O into their OFR would reduce YSOSiA, although the cause is unclear. However, Charan et al. (2022) did not find YSOSiA to change with NOx in their chamber experiments, which is consistent with rapid RO formation across RO2 fates. Quantifying secondary species across RO2 fates and identifying their subsequent oxidation reactions may also be useful to adapt the D5 oxidation mechanism into more sophisticated chemical kinetics models.
We find that the aging-VBS model is very sensitive to kage,gas (Fig. S12), suggesting that photochemical aging in the gas phase should be considered in these models. The condensation timescale calculations suggest that the loss of low-volatile species to the wall is small (Sect. S1.3); however, these calculations assume a high mass accommodation coefficient for SOSiA and do not account for particle nucleation. Should particle nucleation be delayed or happen slowly, the gas wall loss may be higher than expected, leading to under-quantification of SOSiA. Furthermore, the aging-VBS model assumes that kage,gas is uniform across products or that chemical aging results in a 10-fold decrease in volatility.
While the proposed model assumes that the particles are internally well mixed, the high [OH] used in OFRs may induce faster radical reactions and dimerization near the particle surface (Zhao et al., 2019), which affects particle composition and equilibrium timescales. While dimers and oligomers have been found in SOSiA (Wu and Johnston, 2017; Avery et al., 2023; Chen et al., 2023), the model currently does not account for particle-phase oligomer formation. How oligomerization in the D5+ OH SOSiA system evolves the volatility distribution and particle properties is currently not considered in the aging-VBS model. Moreover, high degrees of oxidation should lead to fragmentation and increasing volatility (Isaacman-VanWertz et al., 2018), which is also not considered in the aging-VBS model. Hence, multiphase modeling to evaluate SOSiA chemistry and translate experimental findings to atmospheric conditions remains a direction for future research.
COA: | organic aerosol mass loading |
C*: | effective saturation mass concentration |
D5: | decamethylcyclopentasiloxane |
EVR: | extended volatility range |
I254, I185: | flux of 254 and 185 nm photons |
ncps: | normalized counts per second |
NOx: | nitric oxide and nitrogen dioxide |
OA: | organic aerosol |
OFR: | oxidation flow reactor |
OH: | hydroxyl radical |
[OH]avg: | 24 h average daily hydroxyl |
radical concentration | |
OHexp: | hydroxyl radical exposure |
OHRext: | external hydroxyl radical reactivity |
O3: | ozone |
PAM: | potential aerosol mass |
PTR: | proton transfer reaction |
PTR-MS: | proton transfer reaction mass spectrometer |
RH: | relative humidity |
RMSE: | root mean square error |
RO: | alkoxyl radical |
RO2: | peroxyl radical |
SMPS: | scanning mobility particle sizer |
SOA: | secondary organic aerosol |
SOSiA: | secondary organosiloxane aerosol |
UV: | ultraviolet radiation |
VBS: | volatility basis set |
VOPs: | volatile oxidation products |
YHCHO: | formaldehyde molar yield from D5 |
YHCOOH: | formic acid molar yield from D5 |
Yrel,VOP: | relative molar yield of VOP from D5 |
YSOSiA: | SOSiA mass yield from D5 |
γ: | molar yields extrapolated to when OHexp→0 |
ρSOSiA: | SOSiA aerosol mass density |
τres: | residence time |
Summary data are available in the Supplement. Additional data will be provided upon reasonable request.
The supplement related to this article is available online at: https://doi.org/10.5194/acp-23-14307-2023-supplement.
HGK, YC, and YP conducted the experiments. YP performed the offline calibrations of OH exposure on the PAM-OFR. HGK analyzed the data. HGK and TB developed the kinetic models. HGK, TB, and HK wrote the manuscript with contributions from all co-authors. HK supervised the experiments, and TB supervised the model analyses.
At least one of the (co-)authors is a member of the editorial board of Atmospheric Chemistry and Physics. The peer-review process was guided by an independent editor, and the authors also have no other competing interests to declare.
Instruments and products used in the research are listed for reference and not as endorsements.
Publisher’s note: Copernicus Publications remains neutral with regard to jurisdictional claims made in the text, published maps, institutional affiliations, or any other geographical representation in this paper. While Copernicus Publications makes every effort to include appropriate place names, the final responsibility lies with the authors.
This work was supported by the FRIEND Project (Fine Particle Research Initiative in East Asia Considering National Differences), which is funded by the National Research Foundation (NRF) of Korea and the Ministry of Science and ICT of South Korea (2022M3G1A1020858). This work was also funded by the NRF under NRF-2021R1A2C2004365. Hyun Gu Kang is supported by the Max Planck Graduate Center with Johannes Gutenberg University Mainz. The authors thank APM Engineering (Gyeonggi-do, South Korea) for PTR-MS renting and for maintaining it. The authors acknowledge the two anonymous reviewers for their comments, which can be found on the EGUsphere website.
This research has been supported by the National Research Foundation of Korea (grant nos. NRF-2021R1A2C2004365 and 2022M3G1A1020858).
The article processing charges for this open-access publication were covered by the Max Planck Society.
This paper was edited by Allan Bertram and reviewed by two anonymous referees.
Alton, M. W. and Browne, E. C.: Atmospheric Chemistry of Volatile Methyl Siloxanes: Kinetics and Products of Oxidation by OH Radicals and Cl Atoms, Environ. Sci. Technol., 54, 5992–5999, https://doi.org/10.1021/acs.est.0c01368, 2020.
Alton, M. W. and Browne, E. C.: Atmospheric Degradation of Cyclic Volatile Methyl Siloxanes: Radical Chemistry and Oxidation Products, ACS Environmental Au, https://doi.org/10.1021/acsenvironau.1c00043, 2022.
Arata, C., Misztal, P. K., Tian, Y., Lunderberg, D. M., Kristensen, K., Novoselac, A., Vance, M. E., Farmer, D. K., Nazaroff, W. W., and Goldstein, A. H.: Volatile organic compound emissions during HOMEChem, Indoor Air, 31, 2099–2117, https://doi.org/10.1111/ina.12906, 2021.
Atkinson, R.: Kinetics of the gas-phase reactions of a series of organosilicon compounds with hydroxyl and nitrate(NO3) radicals and ozone at 297 ± 2 K, Environ. Sci. Technol., 25, 863–866, https://doi.org/10.1021/es00017a005, 1991.
Atkinson, R., Baulch, D. L., Cox, R. A., Crowley, J. N., Hampson, R. F., Hynes, R. G., Jenkin, M. E., Rossi, M. J., Troe, J., and IUPAC Subcommittee: Evaluated kinetic and photochemical data for atmospheric chemistry: Volume II – gas phase reactions of organic species, Atmos. Chem. Phys., 6, 3625–4055, https://doi.org/10.5194/acp-6-3625-2006, 2006.
Avery, A. M., Alton, M. W., Canagaratna, M. R., Krechmer, J. E., Sueper, D. T., Bhattacharyya, N., Hildebrandt Ruiz, L., Brune, W. H., and Lambe, A. T.: Comparison of the Yield and Chemical Composition of Secondary Organic Aerosol Generated from the OH and Cl Oxidation of Decamethylcyclopentasiloxane, ACS Earth Space Chem., https://doi.org/10.1021/acsearthspacechem.2c00304, 2023.
Berkemeier, T., Ammann, M., Krieger, U. K., Peter, T., Spichtinger, P., Pöschl, U., Shiraiwa, M., and Huisman, A. J.: Technical note: Monte Carlo genetic algorithm (MCGA) for model analysis of multiphase chemical kinetics to determine transport and reaction rate coefficients using multiple experimental data sets, Atmos. Chem. Phys., 17, 8021–8029, https://doi.org/10.5194/acp-17-8021-2017, 2017.
Berkemeier, T., Takeuchi, M., Eris, G., and Ng, N. L.: Kinetic modeling of formation and evaporation of secondary organic aerosol from NO3 oxidation of pure and mixed monoterpenes, Atmos. Chem. Phys., 20, 15513–15535, https://doi.org/10.5194/acp-20-15513-2020, 2020.
Berkemeier, T., Mishra, A., Mattei, C., Huisman, A. J., Krieger, U. K., and Pöschl, U.: Ozonolysis of Oleic Acid Aerosol Revisited: Multiphase Chemical Kinetics and Reaction Mechanisms, ACS Earth Space Chem., 5, 3313–3323, https://doi.org/10.1021/acsearthspacechem.1c00232, 2021.
Brown, P., Watts, P., Märk, T. D., and Mayhew, C. A.: Proton transfer reaction mass spectrometry investigations on the effects of reduced electric field and reagent ion internal energy on product ion branching ratios for a series of saturated alcohols, Int. J. Mass Spectrom., 294, 103–111, https://doi.org/10.1016/j.ijms.2010.05.028, 2010.
Carter, W. P. L., Pierce, J. A., Malkina, I. L., Luo, D., and Long, W. D.: Environmental Chamber Studies of Maximum Incremental Reactivities of Volatile Organic Compounds, Statewide Air Pollution Research Center, University of California Riverside, 227 pp., 1993.
Carter, W. P. L., Pierce, J. A., Luo, D., and Malkina, I. L.: Environmental chamber study of maximum incremental reactivities of volatile organic compounds, Atmos. Environ., 29, 2499–2511, https://doi.org/10.1016/1352-2310(95)00149-S, 1995.
Chandramouli, B. and Kamens, R. M.: The photochemical formation and gas–particle partitioning of oxidation products of decamethyl cyclopentasiloxane and decamethyl tetrasiloxane in the atmosphere, Atmos. Environ., 35, 87–95, https://doi.org/10.1016/S1352-2310(00)00289-2, 2001.
Charan, S. M., Huang, Y., Buenconsejo, R. S., Li, Q., Cocker III, D. R., and Seinfeld, J. H.: Secondary organic aerosol formation from the oxidation of decamethylcyclopentasiloxane at atmospherically relevant OH concentrations, Atmos. Chem. Phys., 22, 917–928, https://doi.org/10.5194/acp-22-917-2022, 2022.
Chen, X., Millet, D. B., Neuman, J. A., Veres, P. R., Ray, E. A., Commane, R., Daube, B. C., McKain, K., Schwarz, J. P., Katich, J. M., Froyd, K. D., Schill, G. P., Kim, M. J., Crounse, J. D., Allen, H. M., Apel, E. C., Hornbrook, R. S., Blake, D. R., Nault, B. A., Campuzano-Jost, P., Jimenez, J. L., and Dibb, J. E.: HCOOH in the Remote Atmosphere: Constraints from Atmospheric Tomography (ATom) Airborne Observations, ACS Earth Space Chem., 5, 1436–1454, https://doi.org/10.1021/acsearthspacechem.1c00049, 2021.
Chen, Y., Park, Y., Kang, H. G., Jeong, J., and Kim, H.: Chemical characterization and formation of secondary organosiloxane aerosol (SOSiA) from OH oxidation of decamethylcyclopentasiloxane, Environ. Sci. Atmos., 3, 662–671, https://doi.org/10.1039/D2EA00161F, 2023.
Cheng, Z., Qiu, X., Shi, X., and Zhu, T.: Identification of organosiloxanes in ambient fine particulate matters using an untargeted strategy via gas chromatography and time-of-flight mass spectrometry, Environ. Pollut., 271, 116128, https://doi.org/10.1016/j.envpol.2020.116128, 2021.
Coggon, M. M., McDonald, B. C., Vlasenko, A., Veres, P. R., Bernard, F., Koss, A. R., Yuan, B., Gilman, J. B., Peischl, J., Aikin, K. C., DuRant, J., Warneke, C., Li, S.-M., and de Gouw, J. A.: Diurnal Variability and Emission Pattern of Decamethylcyclopentasiloxane (D5) from the Application of Personal Care Products in Two North American Cities, Environ. Sci. Technol., 52, 5610–5618, https://doi.org/10.1021/acs.est.8b00506, 2018.
Coggon, M. M., Gkatzelis, G. I., McDonald, B. C., Gilman, J. B., Schwantes, R. H., Abuhassan, N., Aikin, K. C., Arend, M. F., Berkoff, T. A., Brown, S. S., Campos, T. L., Dickerson, R. R., Gronoff, G., Hurley, J. F., Isaacman-VanWertz, G., Koss, A. R., Li, M., McKeen, S. A., Moshary, F., Peischl, J., Pospisilova, V., Ren, X., Wilson, A., Wu, Y., Trainer, M., and Warneke, C.: Volatile chemical product emissions enhance ozone and modulate urban chemistry, P. Natl. Acad. Sci. USA, 118, e2026653118, https://doi.org/10.1073/pnas.2026653118, 2021.
de Gouw, J. and Warneke, C.: Measurements of volatile organic compounds in the earth's atmosphere using proton-transfer-reaction mass spectrometry, Mass Spectrom. Rev., 26, 223–257, https://doi.org/10.1002/mas.20119, 2007.
de Gouw, J., Warneke, C., Karl, T., Eerdekens, G., van der Veen, C., and Fall, R.: Sensitivity and specificity of atmospheric trace gas detection by proton-transfer-reaction mass spectrometry, Int. J. Mass Spectrom., 223–224, 365–382, https://doi.org/10.1016/S1387-3806(02)00926-0, 2003.
Derwent, R. G., Jenkin, M. E., and Saunders, S. M.: Photochemical ozone creation potentials for a large number of reactive hydrocarbons under European conditions, Atmos. Environ., 30, 181–199, https://doi.org/10.1016/1352-2310(95)00303-G, 1996.
Donahue, N. M., Robinson, A. L., Stanier, C. O., and Pandis, S. N.: Coupled partitioning, dilution, and chemical aging of semivolatile organics, Environ. Sci. Technol., 40, 2635–2643, https://doi.org/10.1021/es052297c, 2006.
Franco, B., Blumenstock, T., Cho, C., Clarisse, L., Clerbaux, C., Coheur, P.-F., de Mazière, M., de Smedt, I., Dorn, H.-P., Emmerichs, T., Fuchs, H., Gkatzelis, G., Griffith, D. W. T., Gromov, S., Hannigan, J. W., Hase, F., Hohaus, T., Jones, N., Kerkweg, A., Kiendler-Scharr, A., Lutsch, E., Mahieu, E., Novelli, A., Ortega, I., Paton-Walsh, C., Pommier, M., Pozzer, A., Reimer, D., Rosanka, S., Sander, R., Schneider, M., Strong, K., Tillmann, R., van Roozendael, M., Vereecken, L., Vigouroux, C., Wahner, A., and Taraborrelli, D.: Ubiquitous atmospheric production of organic acids mediated by cloud droplets, Nature, 593, 233–237, https://doi.org/10.1038/s41586-021-03462-x, 2021.
Friedman, B. and Farmer, D. K.: SOA and gas phase organic acid yields from the sequential photooxidation of seven monoterpenes, Atmos. Environ., 187, 335–345, https://doi.org/10.1016/j.atmosenv.2018.06.003, 2018.
Fu, Z., Xie, H.-B., Elm, J., Guo, X., Fu, Z., and Chen, J.: Formation of Low-Volatile Products and Unexpected High Formaldehyde Yield from the Atmospheric Oxidation of Methylsiloxanes, Environ. Sci. Technol., 54, 7136–7145, https://doi.org/10.1021/acs.est.0c01090, 2020.
Gkatzelis, G. I., Coggon, M. M., McDonald, B. C., Peischl, J., Aikin, K. C., Gilman, J. B., Trainer, M., and Warneke, C.: Identifying Volatile Chemical Product Tracer Compounds in U.S. Cities, Environ. Sci. Technol., 55, 188–199, https://doi.org/10.1021/acs.est.0c05467, 2021.
Graiver, D., Farminer, K. W., and Narayan, R.: A Review of the Fate and Effects of Silicones in the Environment, J. Polym. Environ., 11, 129–136, https://doi.org/10.1023/A:1026056129717, 2003.
Guenther, A. B., Jiang, X., Heald, C. L., Sakulyanontvittaya, T., Duhl, T., Emmons, L. K., and Wang, X.: The Model of Emissions of Gases and Aerosols from Nature version 2.1 (MEGAN2.1): an extended and updated framework for modeling biogenic emissions, Geosci. Model Dev., 5, 1471–1492, https://doi.org/10.5194/gmd-5-1471-2012, 2012.
Han, C., Yang, H., Li, K., Lee, P., Liggio, J., Leithead, A., and Li, S.-M.: Secondary organic aerosols from OH oxidation of cyclic volatile methyl siloxanes as an important Si source in the atmosphere, Atmos. Chem. Phys., 22, 10827–10839, https://doi.org/10.5194/acp-22-10827-2022, 2022.
Hazra, M. K., Francisco, J. S., and Sinha, A.: Hydrolysis of Glyoxal in Water-Restricted Environments: Formation of Organic Aerosol Precursors through Formic Acid Catalysis, J. Phys. Chem. A, 118, 4095–4105, https://doi.org/10.1021/jp502126m, 2014.
Horii, Y., Nojiri, K., Minomo, K., Motegi, M., and Kannan, K.: Volatile methylsiloxanes in sewage treatment plants in Saitama, Japan: Mass distribution and emissions, Chemosphere, 233, 677–686, https://doi.org/10.1016/j.chemosphere.2019.05.247, 2019.
Howard, P. H. and Muir, D. C. G.: Identifying New Persistent and Bioaccumulative Organics Among Chemicals in Commerce, Environ. Sci. Technol., 44, 2277–2285, https://doi.org/10.1021/es903383a, 2010.
Isaacman-VanWertz, G., Massoli, P., O'Brien, R., Lim, C., Franklin, J. P., Moss, J. A., Hunter, J. F., Nowak, J. B., Canagaratna, M. R., Misztal, P. K., Arata, C., Roscioli, J. R., Herndon, S. T., Onasch, T. B., Lambe, A. T., Jayne, J. T., Su, L., Knopf, D. A., Goldstein, A. H., Worsnop, D. R., and Kroll, J. H.: Chemical evolution of atmospheric organic carbon over multiple generations of oxidation, Nat. Chem., 10, 462–468, https://doi.org/10.1038/s41557-018-0002-2, 2018.
Janechek, N. J., Marek, R. F., Bryngelson, N., Singh, A., Bullard, R. L., Brune, W. H., and Stanier, C. O.: Physical properties of secondary photochemical aerosol from OH oxidation of a cyclic siloxane, Atmos. Chem. Phys., 19, 1649–1664, https://doi.org/10.5194/acp-19-1649-2019, 2019.
Jordan, A., Haidacher, S., Hanel, G., Hartungen, E., Märk, L., Seehauser, H., Schottkowsky, R., Sulzer, P., and Märk, T. D.: A high resolution and high sensitivity proton-transfer-reaction time-of-flight mass spectrometer (PTR-TOF-MS), Int. J. Mass Spectrom., 286, 122–128, https://doi.org/10.1016/j.ijms.2009.07.005, 2009.
Kaikiti, C., Stylianou, M., and Agapiou, A.: TD-GC/MS analysis of indoor air pollutants (VOCs, PM) in hair salons, Chemosphere, 294, 133691, https://doi.org/10.1016/j.chemosphere.2022.133691, 2022.
Kang, E., Root, M. J., Toohey, D. W., and Brune, W. H.: Introducing the concept of Potential Aerosol Mass (PAM), Atmos. Chem. Phys., 7, 5727–5744, https://doi.org/10.5194/acp-7-5727-2007, 2007.
Katz, E. F., Lunderberg, D. M., Brown, W. L., Day, D. A., Jimenez, J. L., Nazaroff, W. W., Goldstein, A. H., and DeCarlo, P. F.: Large Emissions of Low-Volatility Siloxanes during Residential Oven Use, Environ. Sci. Technol. Lett., 8, 519–524, https://doi.org/10.1021/acs.estlett.1c00433, 2021.
Kessler, S. H., Nah, T., Daumit, K. E., Smith, J. D., Leone, S. R., Kolb, C. E., Worsnop, D. R., Wilson, K. R., and Kroll, J. H.: OH-Initiated Heterogeneous Aging of Highly Oxidized Organic Aerosol, J. Phys. Chem. A, 116, 6358–6365, https://doi.org/10.1021/jp212131m, 2012.
Kim, J. and Xu, S.: Quantitative structure-reactivity relationships of hydroxyl radical rate constants for linear and cyclic volatile methylsiloxanes, Environ. Toxicol. Chem., 36, 3240–3245, https://doi.org/10.1002/etc.3914, 2017.
Kroll, J. H., Lim, C. Y., Kessler, S. H., and Wilson, K. R.: Heterogeneous Oxidation of Atmospheric Organic Aerosol: Kinetics of Changes to the Amount and Oxidation State of Particle-Phase Organic Carbon, J. Phys. Chem. A, 119, 10767–10783, https://doi.org/10.1021/acs.jpca.5b06946, 2015.
Le Breton, M., McGillen, M. R., Muller, J. B. A., Bacak, A., Shallcross, D. E., Xiao, P., Huey, L. G., Tanner, D., Coe, H., and Percival, C. J.: Airborne observations of formic acid using a chemical ionization mass spectrometer, Atmos. Meas. Tech., 5, 3029–3039, https://doi.org/10.5194/amt-5-3029-2012, 2012.
Lee, S., Moon, H.-B., Song, G.-J., Ra, K., Lee, W.-C., and Kannan, K.: A nationwide survey and emission estimates of cyclic and linear siloxanes through sludge from wastewater treatment plants in Korea, Sci. Total Environ., 497–498, 106–112, https://doi.org/10.1016/j.scitotenv.2014.07.083, 2014.
Lei, Y. D., Wania, F., and Mathers, D.: Temperature-Dependent Vapor Pressure of Selected Cyclic and Linear Polydimethylsiloxane Oligomers, J. Chem. Eng. Data, 55, 5868–5873, https://doi.org/10.1021/je100835n, 2010.
Link, M. F., Nguyen, T. B., Bates, K., Müller, J.-F., and Farmer, D. K.: Can Isoprene Oxidation Explain High Concentrations of Atmospheric Formic and Acetic Acid over Forests?, ACS Earth Space Chem., 4, 730–740, https://doi.org/10.1021/acsearthspacechem.0c00010, 2020.
Lu, D., Tan, J., Yang, X., Sun, X., Liu, Q., and Jiang, G.: Unraveling the role of silicon in atmospheric aerosol secondary formation: a new conservative tracer for aerosol chemistry, Atmos. Chem. Phys., 19, 2861–2870, https://doi.org/10.5194/acp-19-2861-2019, 2019.
Mao, J., Ren, X., Brune, W. H., Olson, J. R., Crawford, J. H., Fried, A., Huey, L. G., Cohen, R. C., Heikes, B., Singh, H. B., Blake, D. R., Sachse, G. W., Diskin, G. S., Hall, S. R., and Shetter, R. E.: Airborne measurement of OH reactivity during INTEX-B, Atmos. Chem. Phys., 9, 163–173, https://doi.org/10.5194/acp-9-163-2009, 2009.
McLachlan, M. S., Kierkegaard, A., Hansen, K. M., van Egmond, R., Christensen, J. H., and Skjøth, C. A.: Concentrations and Fate of Decamethylcyclopentasiloxane (D5) in the Atmosphere, Environ. Sci. Technol., 44, 5365–5370, https://doi.org/10.1021/es100411w, 2010.
Meng, T., Su, S., Cheng, J., Zhong, F., and Tang, Z.: Methylsiloxanes in street dust from Hefei, China: Distribution, sources, and human exposure, Environ. Res., 201, 111513, https://doi.org/10.1016/j.envres.2021.111513, 2021.
Milani, A., Al-Naiema, I. M., and Stone, E. A.: Detection of a secondary organic aerosol tracer derived from personal care products, Atmos. Environ., 246, 118078, https://doi.org/10.1016/j.atmosenv.2020.118078, 2021.
Millet, D. B., Jacob, D. J., Turquety, S., Hudman, R. C., Wu, S., Fried, A., Walega, J., Heikes, B. G., Blake, D. R., Singh, H. B., Anderson, B. E., and Clarke, A. D.: Formaldehyde distribution over North America: Implications for satellite retrievals of formaldehyde columns and isoprene emission, J. Geophys. Res.-Atmos., 111, D24S02, https://doi.org/10.1029/2005JD006853, 2006.
Millet, D. B., Baasandorj, M., Farmer, D. K., Thornton, J. A., Baumann, K., Brophy, P., Chaliyakunnel, S., de Gouw, J. A., Graus, M., Hu, L., Koss, A., Lee, B. H., Lopez-Hilfiker, F. D., Neuman, J. A., Paulot, F., Peischl, J., Pollack, I. B., Ryerson, T. B., Warneke, C., Williams, B. J., and Xu, J.: A large and ubiquitous source of atmospheric formic acid, Atmos. Chem. Phys., 15, 6283–6304, https://doi.org/10.5194/acp-15-6283-2015, 2015.
Müller, M., Mikoviny, T., and Wisthaler, A.: Detector aging induced mass discrimination and non-linearity effects in PTR-ToF-MS, Int. J. Mass Spectrom., 365–366, 93–97, https://doi.org/10.1016/j.ijms.2013.12.008, 2014.
Newland, M. J., Bryant, D. J., Dunmore, R. E., Bannan, T. J., Acton, W. J. F., Langford, B., Hopkins, J. R., Squires, F. A., Dixon, W., Drysdale, W. S., Ivatt, P. D., Evans, M. J., Edwards, P. M., Whalley, L. K., Heard, D. E., Slater, E. J., Woodward-Massey, R., Ye, C., Mehra, A., Worrall, S. D., Bacak, A., Coe, H., Percival, C. J., Hewitt, C. N., Lee, J. D., Cui, T., Surratt, J. D., Wang, X., Lewis, A. C., Rickard, A. R., and Hamilton, J. F.: Low-NO atmospheric oxidation pathways in a polluted megacity, Atmos. Chem. Phys., 21, 1613–1625, https://doi.org/10.5194/acp-21-1613-2021, 2021.
Odum, J. R., Hoffmann, T., Bowman, F., Collins, D., Flagan, R. C., and Seinfeld, J. H.: Gas/Particle Partitioning and Secondary Organic Aerosol Yields, Environ. Sci. Technol., 30, 2580–2585, https://doi.org/10.1021/es950943+, 1996.
Palm, B. B., Campuzano-Jost, P., Ortega, A. M., Day, D. A., Kaser, L., Jud, W., Karl, T., Hansel, A., Hunter, J. F., Cross, E. S., Kroll, J. H., Peng, Z., Brune, W. H., and Jimenez, J. L.: In situ secondary organic aerosol formation from ambient pine forest air using an oxidation flow reactor, Atmos. Chem. Phys., 16, 2943–2970, https://doi.org/10.5194/acp-16-2943-2016, 2016.
Peng, Z. and Jimenez, J. L.: Radical chemistry in oxidation flow reactors for atmospheric chemistry research, Chem. Soc. Rev., 49, 2570–2616, https://doi.org/10.1039/C9CS00766K, 2020.
Peng, Z., Lee-Taylor, J., Orlando, J. J., Tyndall, G. S., and Jimenez, J. L.: Organic peroxy radical chemistry in oxidation flow reactors and environmental chambers and their atmospheric relevance, Atmos. Chem. Phys., 19, 813–834, https://doi.org/10.5194/acp-19-813-2019, 2019.
Pennington, E. A., Seltzer, K. M., Murphy, B. N., Qin, M., Seinfeld, J. H., and Pye, H. O. T.: Modeling secondary organic aerosol formation from volatile chemical products, Atmos. Chem. Phys., 21, 18247–18261, https://doi.org/10.5194/acp-21-18247-2021, 2021.
Piel, F., Müller, M., Winkler, K., Skytte af Sätra, J., and Wisthaler, A.: Introducing the extended volatility range proton-transfer-reaction mass spectrometer (EVR PTR-MS), Atmos. Meas. Tech., 14, 1355–1363, https://doi.org/10.5194/amt-14-1355-2021, 2021.
Robinson, A. L., Donahue, N. M., Shrivastava, M. K., Weitkamp, E. A., Sage, A. M., Grieshop, A. P., Lane, T. E., Pierce, J. R., and Pandis, S. N.: Rethinking Organic Aerosols: Semivolatile Emissions and Photochemical Aging, Science (1979), 315, 1259–1262, https://doi.org/10.1126/science.1133061, 2007.
Rücker, C. and Kümmerer, K.: Environmental Chemistry of Organosiloxanes, Chem. Rev., 115, 466–524, https://doi.org/10.1021/cr500319v, 2015.
Safron, A., Strandell, M., Kierkegaard, A., and Macleod, M.: Rate Constants and Activation Energies for Gas-Phase Reactions of Three Cyclic Volatile Methyl Siloxanes with the Hydroxyl Radical, Int. J. Chem. Kinet., 47, 420–428, https://doi.org/10.1002/kin.20919, 2015.
Schweigkofler, M. and Niessner, R.: Determination of Siloxanes and VOC in Landfill Gas and Sewage Gas by Canister Sampling and GC-MS/AES Analysis, Environ. Sci. Technol., 33, 3680–3685, https://doi.org/10.1021/es9902569, 1999.
Seltzer, K. M., Pennington, E., Rao, V., Murphy, B. N., Strum, M., Isaacs, K. K., and Pye, H. O. T.: Reactive organic carbon emissions from volatile chemical products, Atmos. Chem. Phys., 21, 5079–5100, https://doi.org/10.5194/acp-21-5079-2021, 2021a.
Seltzer, K. M., Murphy, B. N., Pennington, E. A., Allen, C., Talgo, K., and Pye, H. O. T.: Volatile Chemical Product Enhancements to Criteria Pollutants in the United States, Environ. Sci. Technol., 56, 6905–6913, https://doi.org/10.1021/acs.est.1c04298, 2021b.
Sommerlade, R., Parlar, H., Wrobel, D., and Kochs, P.: Product analysis and kinetics of the gas-phase reactions of selected organosilicon compounds with OH radicals using a smog chamber-mass spectrometer system, Environ. Sci. Technol., 27, 2435–2440, https://doi.org/10.1021/es00048a019, 1993.
Sommers, J. M., Stroud, C. A., Adam, M. G., O'Brien, J., Brook, J. R., Hayden, K., Lee, A. K. Y., Li, K., Liggio, J., Mihele, C., Mittermeier, R. L., Stevens, R. G., Wolde, M., Zuend, A., and Hayes, P. L.: Evaluating SOA formation from different sources of semi- and intermediate-volatility organic compounds from the Athabasca oil sands, Environ. Sci.-Atmos., 2, 469–490, https://doi.org/10.1039/D1EA00053E, 2022.
Song, K., Gong, Y., Guo, S., Lv, D., Wang, H., Wan, Z., Yu, Y., Tang, R., Li, T., Tan, R., Zhu, W., Shen, R., and Lu, S.: Investigation of partition coefficients and fingerprints of atmospheric gas- and particle-phase intermediate volatility and semi-volatile organic compounds using pixel-based approaches, J. Chromatogr. A, 1665, 462808, https://doi.org/10.1016/j.chroma.2022.462808, 2022.
Spivack, J. L., Pohl, E. R., and Kochs, P.: Organoalkoxysilanes, Organosilanols, and Organosiloxanols, in: Organosilicon Materials, edited by: Chandra, G., Springer Berlin Heidelberg, Berlin, Heidelberg, 105–135, https://doi.org/10.1007/978-3-540-68331-5_5, 1997.
Stevens, C.: Environmental degradation pathways for the breakdown of polydimethylsiloxanes, J. Inorg. Biochem., 69, 203–207, https://doi.org/10.1016/S0162-0134(97)10019-8, 1998.
Tang, X., Misztal, P. K., Nazaroff, W. W., and Goldstein, A. H.: Siloxanes Are the Most Abundant Volatile Organic Compound Emitted from Engineering Students in a Classroom, Environ. Sci. Technol. Lett., 2, 303–307, https://doi.org/10.1021/acs.estlett.5b00256, 2015.
Tansel, B. and Surita, S. C.: Historical and projected trends of siloxane use in consumer products, associated impacts on municipal solid waste and landfill gas utilization, Int. J. Environ. Sci. Technol., 14, 795–802, https://doi.org/10.1007/s13762-016-1186-x, 2017.
Tran, T. M. and Kannan, K.: Occurrence of cyclic and linear siloxanes in indoor air from Albany, New York, USA, and its implications for inhalation exposure, Sci. Total Environ., 511, 138–144, https://doi.org/10.1016/j.scitotenv.2014.12.022, 2015.
TSI Inc.:Measuring Nanoparticle Size Distributions in Real-time: Key Factors for Accuracy, TSI Incorporated, Application Note SMPS-003, 7 pp., 2012.
Wang, N., Ernle, L., Bekö, G., Wargocki, P., and Williams, J.: Emission Rates of Volatile Organic Compounds from Humans, Environ. Sci. Technol., 56, 4838–4848, https://doi.org/10.1021/acs.est.1c08764, 2022.
Whelan, M. J. and Kim, J.: Application of multimedia models for understanding the environmental behavior of volatile methylsiloxanes: Fate, transport, and bioaccumulation, Integr. Environ. Assess. Manag., 18, 599–621, https://doi.org/10.1002/ieam.4507, 2021.
Whelan, M. J., Estrada, E., and van Egmond, R.: A modelling assessment of the atmospheric fate of volatile methyl siloxanes and their reaction products, Chemosphere, 57, 1427–1437, https://doi.org/10.1016/j.chemosphere.2004.08.100, 2004.
Wu, Y. and Johnston, M. V: Aerosol Formation from OH Oxidation of the Volatile Cyclic Methyl Siloxane (cVMS) Decamethylcyclopentasiloxane, Environ. Sci. Technol., 51, 4445–4451, https://doi.org/10.1021/acs.est.7b00655, 2017.
Xiang, X., Liu, N., Xu, L., and Cai, Y.: Review of recent findings on occurrence and fates of siloxanes in environmental compartments, Ecotoxicol Environ. Saf., 224, 112631, https://doi.org/10.1016/j.ecoenv.2021.112631, 2021.
Xiao, R., Zammit, I., Wei, Z., Hu, W.-P., MacLeod, M., and Spinney, R.: Kinetics and Mechanism of the Oxidation of Cyclic Methylsiloxanes by Hydroxyl Radical in the Gas Phase: An Experimental and Theoretical Study, Environ. Sci. Technol., 49, 13322–13330, https://doi.org/10.1021/acs.est.5b03744, 2015.
Xu, J., Harrison, R. M., Song, C., Hou, S., Wei, L., Fu, P., Li, H., Li, W., and Shi, Z.: PM2.5-bound silicon-containing secondary organic aerosols (Si-SOA) in Beijing ambient air, Chemosphere, 288, 132377, https://doi.org/10.1016/j.chemosphere.2021.132377, 2022.
Xu, N. and Collins, D. R.: Design and characterization of a new oxidation flow reactor for laboratory and long-term ambient studies, Atmos. Meas. Tech., 14, 2891–2906, https://doi.org/10.5194/amt-14-2891-2021, 2021.
Yu, S.: Role of organic acids (formic, acetic, pyruvic and oxalic) in the formation of cloud condensation nuclei (CCN): a review, Atmos. Res., 53, 185–217, https://doi.org/10.1016/S0169-8095(00)00037-5, 2000.
Yuan, B., Veres, P. R., Warneke, C., Roberts, J. M., Gilman, J. B., Koss, A., Edwards, P. M., Graus, M., Kuster, W. C., Li, S.-M., Wild, R. J., Brown, S. S., Dubé, W. P., Lerner, B. M., Williams, E. J., Johnson, J. E., Quinn, P. K., Bates, T. S., Lefer, B., Hayes, P. L., Jimenez, J. L., Weber, R. J., Zamora, R., Ervens, B., Millet, D. B., Rappenglück, B., and de Gouw, J. A.: Investigation of secondary formation of formic acid: urban environment vs. oil and gas producing region, Atmos. Chem. Phys., 15, 1975–1993, https://doi.org/10.5194/acp-15-1975-2015, 2015.
Zhao, B., Wang, S., Donahue, N. M., Chuang, W., Hildebrandt Ruiz, L., Ng, N. L., Wang, Y., and Hao, J.: Evaluation of One-Dimensional and Two-Dimensional Volatility Basis Sets in Simulating the Aging of Secondary Organic Aerosol with Smog-Chamber Experiments, Environ. Sci. Technol., 49, 2245–2254, https://doi.org/10.1021/es5048914, 2015.
Zhao, Z., Tolentino, R., Lee, J., Vuong, A., Yang, X., and Zhang, H.: Interfacial Dimerization by Organic Radical Reactions during Heterogeneous Oxidative Aging of Oxygenated Organic Aerosols, J. Phys. Chem. A, 123, 10782–10792, https://doi.org/10.1021/acs.jpca.9b10779, 2019.
- Abstract
- Graphical abstract: schematic of the kinetic box model
- Introduction
- Method and materials
- Results and discussion
- Conclusions and atmospheric implications
- Appendix A: Abbreviations
- Data availability
- Author contributions
- Competing interests
- Disclaimer
- Acknowledgements
- Financial support
- Review statement
- References
- Supplement
- Abstract
- Graphical abstract: schematic of the kinetic box model
- Introduction
- Method and materials
- Results and discussion
- Conclusions and atmospheric implications
- Appendix A: Abbreviations
- Data availability
- Author contributions
- Competing interests
- Disclaimer
- Acknowledgements
- Financial support
- Review statement
- References
- Supplement