the Creative Commons Attribution 4.0 License.
the Creative Commons Attribution 4.0 License.
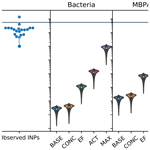
A numerical framework for simulating the atmospheric variability of supermicron marine biogenic ice nucleating particles
Isabelle Steinke
Paul J. DeMott
Grant B. Deane
Thomas C. J. Hill
Mathew Maltrud
Aishwarya Raman
Susannah M. Burrows
We present a framework for estimating concentrations of episodically elevated high-temperature marine ice nucleating particles (INPs) in the sea surface microlayer and their subsequent emission into the atmospheric boundary layer. These episodic INPs have been observed in multiple ship-based and coastal field campaigns, but the processes controlling their ocean concentrations and transfer to the atmosphere are not yet fully understood. We use a combination of empirical constraints and simulation outputs from an Earth system model to explore different hypotheses for explaining the variability of INP concentrations, and the occurrence of episodic INPs, in the marine atmosphere. In our calculations, we examine the following two proposed oceanic sources of high-temperature INPs: heterotrophic bacteria and marine biopolymer aggregates (MBPAs). Furthermore, we assume that the emission of these INPs is determined by the production of supermicron sea spray aerosol formed from jet drops, with an entrainment probability that is described by Poisson statistics. The concentration of jet drops is derived from the number concentration of supermicron sea spray aerosol calculated from model runs. We then derive the resulting number concentrations of marine high-temperature INPs (at 253 K) in the atmospheric boundary layer and compare their variability to atmospheric observations of INP variability. Specifically, we compare against concentrations of episodically occurring high-temperature INPs observed during field campaigns in the Southern Ocean, the Equatorial Pacific, and the North Atlantic. In this case study, we evaluate our framework at 253 K because reliable observational data at this temperature are available across three different ocean regions, but suitable data are sparse at higher temperatures.
We find that heterotrophic bacteria and MBPAs acting as INPs provide only a partial explanation for the observed high INP concentrations. We note, however, that there are still substantial knowledge gaps, particularly concerning the identity of the oceanic INPs contributing most frequently to episodic high-temperature INPs, their specific ice nucleation activity, and the enrichment of their concentrations during the sea–air transfer process. Therefore, targeted measurements investigating the composition of these marine INPs and drivers for their emissions are needed, ideally in combination with modeling studies focused on the potential cloud impacts of these high-temperature INPs.
- Article
(5482 KB) - Full-text XML
- BibTeX
- EndNote
Clouds are important components and drivers of the climate system (Boucher et al., 2013), with mixed-phase cloud processes being a major factor in determining cloud radiative properties (Cesana and Storelvmo, 2017; Vergara-Temprado et al., 2018), the strength of the cloud–climate feedback (McCoy et al., 2016), and the equilibrium climate sensitivity (Tan et al., 2016). The formation of ice crystals in mixed-phase clouds is typically initiated by a small fraction of atmospheric aerosol particles, i.e., ice nucleating particles (Hoose and Möhler, 2012; Murray et al., 2012; Vali et al., 2015; Kanji et al., 2017). While deserts are globally the largest source of ice nucleating particles (INPs) of mineral dust origin, there are areas such as the remote ocean regions where local marine sources dominate the emission of INPs (Burrows et al., 2013). It has been observed that the presence of marine INPs is associated with phytoplankton blooms and microbial degradation of organic material during the decay phase of those blooms (Wang et al., 2015; DeMott et al., 2016; McCluskey et al., 2017a) but the detailed fundamental physical, chemical, and biological processes controlling their production and emission to the atmosphere remain unclear (Schiffer et al., 2018).
One group of marine INPs in seawater originates from various types of organic matter in the ocean, with particles often being smaller than 200 nm in diameter (Wilson et al., 2015; Irish et al., 2017). In addition to these small ubiquitous INPs, recent studies have identified another contribution from INPs active at temperatures above 253 K (McCluskey et al., 2018a; Ickes et al., 2020; van Pinxteren et al., 2020), which can be larger and also heat labile (McCluskey et al., 2018a; Hartmann et al., 2020). The presence of heat-sensitive INPs suggests a contribution from complex organic (e.g., proteinaceous) macromolecules (Christner et al., 2008; Pummer et al., 2015). These high-temperature INPs occur episodically, e.g., after phytoplankton blooms following storm-induced mixing events (Wilbourn et al., 2020), and their identity remains elusive (Ickes et al., 2020).
Aerosolized organic matter in sea spray particles originates from the sea surface microlayer (and possibly the underlying surface layer) which is enriched in surfactants such as carbohydrates, lipids, proteins, and marine biogenic particles such as polymeric aggregates, cells (diatoms, bacteria, and viruses), and cell fragments (Garrett, 1967; Patterson et al., 2016; Bertram et al., 2018), with the latter group being the focus of this study. Sea–air transport of organic macromolecules and marine biogenic particles is driven by the bursting of bubbles formed primarily as the result of wave-driven entrainment of air into surface waters. Surface-active organic molecules accumulate in the sea surface microlayer and form films on the surfaces of rising bubbles (Burrows et al., 2014), become aerosolized once the bubbles burst at the air–sea interface, and are subsequently emitted within small film drops (O'Dowd and de Leeuw, 2007). Marine biogenic particles, in contrast, are preferentially emitted through jet drops forming when bubbles collapse and the ejected water jets break up into fragments (Blanchard and Syzdek, 1972; Wu, 2002; Pósfai et al., 2003; Wang et al., 2017). Jet drops are typically the dominant production mechanism for the supermicron sea spray particle population (Wang et al., 2017; Bertram et al., 2018).
Significant advances have been made in developing representations of marine organic INPs appropriate for global climate models (Burrows et al., 2013; Wilson et al., 2015; Huang et al., 2018; McCluskey et al., 2019). However, while modeling studies have demonstrated that INPs from surface-active macromolecules contained in submicron sea spray aerosol (SSA) can be predicted skillfully (Huang et al., 2018; McCluskey et al., 2019; Zhao et al., 2021), there are still major challenges in representing episodic contributions from marine, biogenic particulate INPs larger than 200 nm (Creamean et al., 2019; Trueblood et al., 2021). These INPs are not explicitly described by currently used parameterizations which depend solely on the observed aerosol surface area or the organic carbon content (Wilson et al., 2015; DeMott et al., 2016; McCluskey et al., 2018a). Recent studies have highlighted that supermicron sea spray particles may contribute significantly to observed marine INP concentrations (Creamean et al., 2019) by acting as carriers for these marine biogenic particles. So far, the sparsity and incompleteness of observational data have posed challenges to developing a process-based source function for these episodically occurring INPs.
There are numerous types of marine particles larger than 200 nm which may accumulate within the sea surface microlayer and act as INPs (McCluskey et al., 2018c), and their identity remains elusive, possibly because most studies have focused on phytoplankton species and their exudates (Alpert et al., 2011; Ladino et al., 2016; Ickes et al., 2020). The inconclusive findings of these studies point towards the presence of other non-plankton particles which may be only emitted episodically and in connection with individual events, e.g., related to storm-induced mixing (Wilbourn et al., 2020). These episodic INP emission events are clearly distinct from the background concentration of INPs over the remote oceans contributed by marine organics (McCluskey et al., 2018c). For example, a link between marine ecosystem processes has been hypothesized, as elevated enzymatic activity, higher bacteria concentrations, and increased INP emissions have been concurrently observed during simulated blooms (Wang et al., 2015). Marine gels form from marine biopolymers, which are excreted by microorganisms and phytoplankton (Verdugo et al., 2008). They are very complex, heterogeneous entities (i.e., biopolymer aggregates) with a potentially variable ice nucleation activity.
In this study, we focus on marine bacteria and marine biopolymer aggregates (MBPAs) as potential sources of episodically emitted marine biogenic INPs. Bacteria and MBPAs accumulate in the sea surface microlayer (Orellana and Leck, 2015; Engel et al., 2017; Rahlff et al., 2017) and have been found in aerosol particles emitted from the oceans (Aller et al., 2005; Leck and Bigg, 2008; Orellana et al., 2011). Note that, in this study, we assign a size of d=100 nm to MBPAs, with most nanogel particles found in clouds smaller than 200 nm, but with substantial uncertainties regarding their size due to annealing processes, leading to the formation of microgels that are up to several micrometers in size (Orellana et al., 2011). Therefore, we have chosen d=100 nm to represent the order-of-magnitude size of these gel particles. It should also be noted that it is not entirely clear how concentrations of these likely ice-nucleating entities may relate to concentrations of observed INPs.
Even though few studies have investigated the ice nucleation properties of marine bacteria (Fall and Schnell, 1985; Ladino et al., 2016), some bacteria from terrestrial sources are known to be very ice active (Huang et al., 2021) and ice-nucleating bacteria have been found in isolates derived from coastal air samples (Beall et al., 2021). Note, however, that a quantitative comparison between the ice nucleation efficiencies of marine and terrestrial bacteria remains challenging because only few studies have investigated specifically the immersion freezing properties of marine bacteria. Likewise, ice nucleation efficiencies (e.g., temperature-dependent ice-active surface site density values) of MBPAs have not been measured directly. They could also act as carriers for other potentially ice-active particles (Leck and Bigg, 2008), such as dust resuspended from the ocean surface (Cornwell et al., 2020).
Additionally, there is high uncertainty regarding a potentially selective transfer of marine organic particles across the sea–air interface, which may lead to an enrichment of these particles in marine aerosol droplets relative to the bulk seawater, with enrichment factors (EF) of up to 45 for marine particulate matter (Aller et al., 2005; Rastelli et al., 2017; Gong et al., 2020). EF represents the enhanced concentration of a trace species in the emitted aerosol, relative to its concentration in the seawater. It is typically calculated either relative to the sea salt or sodium mass. EF also depends on the organic/biological species in question and the SSA particle size (Quinn et al., 2015). Additionally, EF may also vary between different sampling techniques (Aller et al., 2017).
Here we use a combination of Earth system model simulation outputs and literature data to explore different hypotheses for explaining the variability of INP concentrations, and their episodic occurrence, in the marine atmosphere as part of a novel approach towards quantifying the contribution from episodically elevated high-temperature marine INPs in remote ocean regions. We evaluate to what extent the observed number concentrations and variability of high-temperature INPs can potentially be explained by emissions of sea spray containing either bacteria or marine gel particles and highlight gaps in our current understanding.
In this study, we focus on observations of marine INP concentrations from the literature data, with the aim to better quantify rare, episodic INP emissions events. INP data were analyzed for three ocean regions where ship-based or coastal campaigns took place, i.e., MAGIC, MARCUS, and MHD (see Table 1 for acronym definitions and details). All marine field campaign data analyzed in this study are derived from droplet freezing experiments conducted with the Colorado State University (CSU) ice spectrometer (McCluskey et al., 2018b). For these freezing experiments, aerosol particles were collected on polycarbonate membrane filters, frozen for transport, suspended in water and then analyzed with the CSU ice spectrometer. Number concentrations of INPs per liquid volume are evaluated following Vali (1971) and then converted into atmospheric INP concentrations using the known volumes of sampled air and water used to create suspensions from the filter samples (McCluskey et al., 2017b).
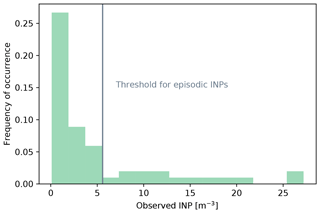
Figure 1Combined data set for three campaigns (Table 1) showing INP concentrations at 253 K. The threshold is defined as the 75th percentile for the combined INP data set (N=57).
Figure 1 shows combined observations of marine INPs at 253 K from the three campaigns. While the majority of observed INP concentrations are well below 5 m−3, there are a small number of samples with considerably higher INP concentrations. We define a concentration cutoff for episodic INP events at around 6 m−3, which corresponds to the 75th percentile in the distribution of INP concentrations. Note that while the impact from long-range transported and potentially ice-active dust cannot be excluded, it can be assumed that, for very remote regions such as the Southern Ocean, the contribution from dust INPs is most likely limited (Burrows et al., 2013). For the MHD measurements, high INP concentrations were observed for marine air masses, with data from air masses with terrestrial influence being excluded from our analysis (McCluskey et al., 2018b). The potential influence of dust INPs is also assumed to be limited for the MAGIC measurements (DeMott et al., 2016). For our analysis, we assume that all episodic INPs derived from the combined data set can be attributed to marine INP emissions.
Figure 2 shows a schematic overview of the numerical framework that we use to estimate concentrations of episodically emitted high-temperature INPs in remote ocean regions. Our framework accounts for bacteria and MBPA concentrations in the sea surface microlayer and their sea–air transfer through jet drop emission. Based on the abundance of these potentially ice-nucleating particles in the atmospheric boundary layer, we then estimate the range of INP concentrations in the atmospheric boundary, assuming that these marine biogenic particles are mostly transported across the sea–air interface as inclusions in jet drops.
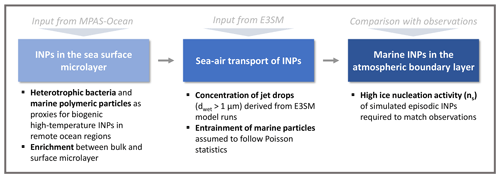
Figure 2Schematic overview of the numerical framework for estimating concentrations of marine high-temperature INPs.
3.1 Estimating the abundance of highly ice-active particles in the sea surface microlayer
We use ocean biogeochemistry output from the E3SMv1 MPAS-Ocean component, which relies on the Model for Prediction Across Scales (MPAS) framework (Petersen et al., 2019), to calculate concentrations of bacteria and MBPAs in the sea surface microlayer. Particle concentrations are calculated from monthly mean ocean biogeochemistry output fields for a Rossby radius of deformation scaling (RRS) mesh, with a grid size of RRS30to10, and then averaged over 10 consecutive years. Further details are described in Brady et al. (2019).
The abundance of heterotrophic bacteria is parameterized as a function of chlorophyll-a (chl-a) and sea surface temperature (SST). An empirical relationship between these variables was derived by Li et al. (2004), based on 15 years of observations across biogeochemical regimes in all major ocean regions (Li et al., 2004). Based on the chl-a concentrations cchl, heterotrophic bacteria concentrations cbac (cells per cubic meter) are given by the following:
For the MBPAs, we assume that polymers account for at least 10 % of dissolved organic carbon (DOC) in surface waters (Chin et al., 1998) and that 20 % of these polymers aggregate into MBPAs (Orellana and Leck, 2015). Additionally, we assume a density of 10 kg m−3 (Verdugo et al., 2008) and assume that MBPAs contribute 20 % of the total marine gel volume. Note that marine gel particles typically transition between different states of hydration, and therefore, their density changes over time.
Figures 3 and 4 show the seasonally averaged seawater concentrations of heterotrophic bacteria and MBPAs, respectively, that result from these assumptions. The reported concentrations refer to the top vertical layer of the ocean model, which is roughly 1.5 m deep in the MPAS-Ocean simulations. Inferred seawater bacteria concentrations agree with the range of observed values, e.g., Trueblood et al. (2021). Similarly, for MBPAs, the inferred concentrations agree with observations and with concentrations of marine polymer gel particles (polymers and colloidal gels smaller than 300 nm) up to 1022 L−1 (Orellana et al., 2011).
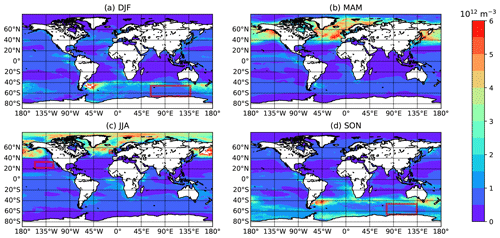
Figure 3Global maps of seasonally averaged concentrations of heterotrophic bacteria in bulk seawater as calculated from MPAS-Ocean output (averaged over 10 years), with (a) December–February, (b) March–May, (c) June–August, and (d) September–November. Red rectangles indicate areas/seasons for which observations are available (see Table 1).
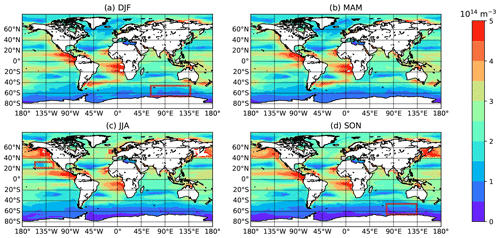
Figure 4Global maps of seasonally averaged concentrations of MBPA particles in bulk seawater as calculated from MPAS-Ocean output (averaged over 10 years), with (a) December–February, (b) March–May, (c) June–August, and (d) September–November. Red rectangles indicate areas/seasons for which observations are available (see Table 1).
3.2 Simulating jet drop concentrations in the marine boundary layer and the entrainment of marine particles into sea spray aerosol
In a second step, we simulate the entrainment (i.e., transfer) of heterotrophic bacteria and MBPAs into supermicron SSA, in order to estimate the number of marine particles (and, hence, potential INPs) transported to the atmospheric boundary layer (Fig. 2).
Supermicron sea spray aerosol (SSA) particles are a potential carrier of marine biogenic particles larger than 200 nm, e.g., bacteria, intact diatoms, and viruses (Blanchard and Syzdek, 1972; Prather et al., 2013; Patterson et al., 2016). Therefore, we focus on nascent sea spray droplets larger than 1 µm, which are predominantly emitted as jet drops (Blanchard and Syzdek, 1972). Laboratory studies such as Wang et al. (2017) have shown that film drop emission did not significantly contribute to the population of fresh sea spray particles larger than 1 µm. While it is possible for film drop particles to be larger than 1 µm, they are typically smaller than this, and most of the larger sea spray particles are produced through jet drop emissions (de Leeuw et al., 2011). Note, however, that the emission mechanisms of jet and film drops depend strongly on ocean surface processes, which may introduce uncertainties with regard to the size distributions of jet and film drops under atmospheric conditions.
We equate SSA concentrations (ddry>0.5 µm) from monthly averaged simulations with the E3SM Atmosphere Model to concentrations of large jet drops in the atmospheric boundary layer, without parameterizing the jet drop emission process explicitly. Aerosol concentrations in E3SM are simulated using the Modal Aerosol Module (MAM4) and the chemical species included in each mode are illustrated in Liu et al. (2016). The emission of SSA particles is based upon the whitecap parameterization which uses the wind speed at 10 m above the sea surface (Monahan, 1986). SSA particles are produced either directly or indirectly by raindrop evaporation and resuspension, and the major sinks include scavenging by precipitation (interstitial and cloud borne), wet removal, and dry deposition. Lifetimes of coarse SSA particles (1–10 µm) in E3SM are roughly 0.6 d (Wang et al., 2020). In this study, SSA mixing ratios were simulated by E3SM at 1∘ × 1∘ resolution with 72 vertical levels. Monthly averaged outputs of surface level SSA number concentrations (ddry>0.5 µm) were used to create maps of jet drop concentrations. Surface maps showing the seasonal variation of jet drop concentrations cjet (per cubic meter, hereafter m−3) are represented in Fig. 5.
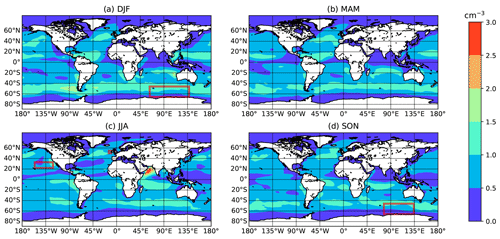
Figure 5Global maps of seasonally averaged concentrations of jet drop particles as calculated from E3SM SSA number concentration outputs, with (a) December–February, (b) March–May, (c) June–August, and (d) September–November. Red rectangles indicate areas/seasons for which observations are available (see Table 1).
The entrainment of marine particles in supermicron sea spray droplets follows a statistical distribution because of the particles' discrete nature. The probability of finding k marine particles in a drop can be modeled as a Poisson process with an entrainment rate λ dependent on the wet drop radius rj (meters; j is the number of bins) and the concentration of marine particles (cmar; m−3) in seawater as follows:
EF represents the enrichment of INPs between sea water and sea spray aerosol particles. Note that, for our base case (see Table 2), we assume that the processes controlling the transfer of particles from the seawater bulk into a drop are neither selective nor enriching (EF = 1), that each marine particle has the same probability of being entrained in a drop, and that the particles are distributed homogeneously in the seawater.
3.3 Estimating INP concentrations from episodic emissions of marine particles
To compare simulated INP concentrations and observations, we derive estimates for the INP concentrations, based on the number of entrained particles (MBPAs and bacteria), which are then aerosolized as inclusions in SSA particles. These entrained particles are then assigned a specific ice nucleation activity (quantified as the ice nucleation active surface site density, ns). INP concentrations cINP (m−3) are estimated from the average entrainment rate λ as follows:
with Amar being the surface of bacteria and MBPAs, respectively.
We base our analysis on a comparison between five different scenarios as outlined in Table 2. Note that, for our analysis, we assign particle sizes of dMBPA=100 nm and dbac=500 nm (Andersen et al., 2016; Orellana et al., 2011). Assuming a monodisperse size distribution allows us to better demonstrate the impact of different factors investigated in our scenario-based approach which will be described in the following paragraphs. Also, to our knowledge, there are currently no observations for size distributions of individual marine species acting as INPs.
For our base case (INP_BASE), we assume that marine particles (i.e., bacteria and MBPAs) are neither enriched nor depleted in the sea surface microlayer or in the freshly emitted SSA (EF = 1). For INP_BASE, we also assume an average ice nucleation active surface site density at 253 K (ns=105 m−2). Experimentally measured values of ns at 253 K have been reported to range from 104 to 106 m−2 (DeMott et al., 2016; McCluskey et al., 2018a; Gong et al., 2020), with values for individual marine species expected to be substantially higher, e.g., ns=109 m−2 at 248 K for marine algae (Ickes et al., 2020). Note that the range of observed values is substantially larger than the measurement uncertainties associated with individual values, and therefore, we do not consider the impact from measurement uncertainties related to ns in our study.
For INP_BASE, we prescribe the particle concentration in seawater as given by the median of cmar for each campaign and the corresponding season. We compare our baseline against four cases which are described in the following:
-
INP_CONC investigates the potential impact of high bacteria and MBPA concentrations in seawater, subsequently leading to a higher number of particles being included in supermicron SSA. In our analysis, we define high concentrations as the 90th percentile of the MBPA and bacteria concentrations across each campaign domain, respectively.
-
Values for EF are highly variable, depending on the species in question, the environmental conditions, and sampling techniques. For INP_EF, we use EF = 40, which is an upper limit based on the range of EFs found for different marine biogenic particles, e.g., bacteria, viruses, and exopolymers (Aller et al., 2005; Rahlff et al., 2017; Rastelli et al., 2017).
-
There is substantial uncertainty regarding the ice nucleation activity of individual marine species because most studies have only looked at the average ice nucleation activity of ambient or simulated SSA particles, which are complex mixtures of sea salt, organic surfactants, and marine biogenic particles. Recent studies, however, have found indications that individual species included in supermicron SSA might be characterized by ns values that are substantially higher (Ickes et al., 2020; Mitts et al., 2021) than previously observed for SSA. Therefore, for INP_ACT, we prescribe a high ns value with m−2, which roughly corresponds to the ice nucleation activity of two phytoplankton species characterized by Ickes et al. (2020). Note that both ns values are assumed to be representative of the observed ice nucleation efficiencies at 253 K.
-
INP_MAX is the upper limit of simulated INP concentrations, based on high estimates of EF, ns, and the concentration of bacteria and MBPAs in seawater.
Figures 6–9 show results from simulated INP concentrations compared to observed episodic INP concentrations (see the cases described in Table 2). The violin plots represent the distribution of INP values for each season and across the geographic area in which each campaign has taken place. In each figure, we show INP concentrations observed at 253 K (left panel; with representative error bars for the lowest INP concentrations), with episodic events characterized by concentrations above the threshold derived from the combined INP data set (Fig. 1). Note that the number of available data points varies between the three campaigns, with the episodic nature of INP emissions most pronounced for the MARCUS data set (Figs. 6 and 7).
Observed INP concentrations are compared against simulated INP concentrations, using different scenarios (Table 2) to quantify the potential impact of varying ns, EF, and the concentration of marine biogenic particles in seawater.
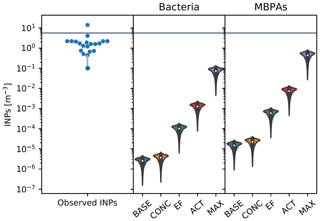
Figure 6In the left panel, each point corresponds to a single filter measurement, with the value of the INP concentration indicated by their location on the y axis. The points are arranged in the approximate shape of a violin as a visual cue to facilitate the comparison with the simulated values. The middle and right panels show simulated INP concentrations from bacteria (middle) and MBPAs (right) at 253 K, with violin plots representing the distribution of values across the relevant spatial domain and inner box plots indicating the median and interquartile values. The gray line indicates the threshold above which emissions are considered as episodic events. The parameters for the scenarios are listed in Table 2 (campaign: MARCUS, DJF).
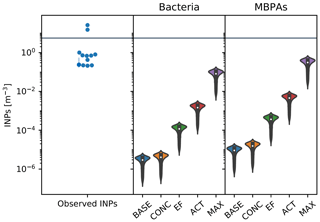
Figure 7In the left panel, each point corresponds to a single filter measurement, with the value of the INP concentration indicated by their location on the y axis. The points are arranged in the approximate shape of a violin as a visual cue to facilitate the comparison with the simulated values. The middle and right panels show simulated INP concentrations from bacteria (middle) and MBPAs (right) at 253 K, with violin plots representing the distribution of values across the relevant spatial domain and inner box plots indicating the median and interquartile values. The gray line indicates the threshold above which emissions are considered as episodic events. The parameters for the scenarios are listed in Table 2 (campaign: MARCUS, SON).
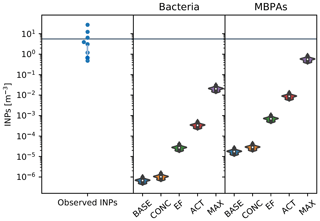
Figure 8In the left panel, each point corresponds to a single filter measurement, with the value of the INP concentration indicated by their location on the y axis. The points are arranged in the approximate shape of a violin as a visual cue to facilitate the comparison with the simulated values. The middle and right panels show simulated INP concentrations from bacteria (middle) and MBPAs (right) at 253 K, with violin plots representing the distribution of values across the relevant spatial domain and inner box plots indicating the median and interquartile values. The gray line indicates the threshold above which emissions are considered as episodic events. The parameters for the scenarios are listed in Table 2 (campaign: MAGIC, JJA).
Our results show that, compared to the variation in INP concentrations in sea water, the enrichment factor and the particle-type-dependent ice nucleation activity have a larger impact on the estimated jet drop INP concentrations. The relative variability in simulated INP concentrations – which is driven by the variability in jet drop concentrations across each domain – is largest for the two MARCUS cases. For all three campaigns, we observe a significant underprediction of INP concentrations by at least 1 order of magnitude. This finding indicates that contributions from larger, episodically occurring INPs might be limited to very specific conditions (e.g., ocean ecosystems, seasons, wind speed, and SST), or that there might be other processes leading to an enrichment of INPs associated with marine biogenic particles, as enrichment factors of ∼ 200 have been observed in recent laboratory experiments (Thomas C. J. Hill, personal communication, 2020). For the MBPAs, an enrichment factor of 200 would lead to a close agreement between observed and simulated INP concentrations (using the maximum assumptions). Also, the relative contribution of film and jet drops to the emission of SSA is virtually unknown under atmospheric conditions and, hence, also the number concentration of jet drop particles and their size distribution. Note that simulated film drop INP concentrations based on an average ice nucleation activity at 253 K of ns=105 m−2 (McCluskey et al., 2018c) generally range between 10−4 and 10−2 m−3 (data not shown), which is substantially lower than the observed INP concentrations. Therefore, model simulations may not fully capture the contribution of jet drop emission to the overall SSA population. Additionally, it should be noted that the factors that we varied for our sensitivity study are subject to substantial uncertainties, and therefore, additional studies are warranted.
In particular, we identify the following gaps based on our analysis:
-
To further improve our process-level understanding of the factors driving the occurrence of episodic INPs (e.g., marine biological activity), targeted observations of episodic INP emissions should be conducted, focusing on the frequency of these rare events, and a process-level understanding of the sea–air transport of relevant marine INPs (larger than 200 nm) should be gained. A more detailed understanding of the transport mechanisms is also a prerequisite for better quantifying enrichment factors under different conditions and for different particle species.
-
Another key uncertainty which could be addressed by laboratory studies is the temperature-dependent ice nucleation efficiency (e.g., as ns values) of individual marine species that contribute directly or indirectly to the observed INP population, particularly under mixed-phase conditions. Measurements of the ice nucleation efficiencies would also help to identify individual particle species which are most likely to contribute to elevated INP concentrations during episodic INP emission events.
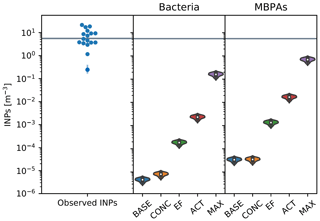
Figure 9In the left panel, each point corresponds to a single filter measurement, with the value of the INP concentration indicated by their location on the y axis. The points are arranged in the approximate shape of a violin as a visual cue to facilitate the comparison with the simulated values. The middle and right panels show simulated INP concentrations from bacteria (middle) and MBPAs (right) at 253 K, with violin plots representing the distribution of values across the relevant spatial domain and inner box plots indicating the median and interquartile values. The gray line indicates the threshold above which emissions are considered as episodic events. The parameters for the scenarios are listed in Table 2 (campaign: MHD, JJA).
In this study, we present a novel framework for exploring the hypotheses regarding the emissions of marine high-temperature INPs. This framework is used to simulate hypothetical sources of INPs in seawater (bacteria and MBPAs) and their transport to the marine atmospheric boundary layer via sea spray jet drop production. We also highlight the gaps that currently prevent the development of a source function for these INPs and which are mostly related to a lack of data to fully inform and constrain such a function.
Better quantifying the potential climate impacts from episodic INP emissions over the remote ocean requires a model-based assessment of their cloud impacts. More studies using cloud-resolving models (with appropriate cloud microphysics) are needed to evaluate the impacts of episodic increases in INPs on marine cloud processes. These studies can provide guidance on how accurately we need to be able to characterize the INP activities of marine biogenic particles in order to predict cloud impacts associated with these episodically emitted marine particles.
Targeted observations characterizing these episodic INPs would be needed to develop a more detailed process-level understanding of their occurrence. In particular, measurements are needed which comprise not only the quantification of the number concentration of INPs at a certain temperature but also measurements of the particle size distribution (including the range up to 5–10 µm) and single-particle analyses (e.g., using microscopy methods) that can provide insight into their chemical, physical, and biological identities. These measurements can serve as a starting point for identifying relevant INP types and for developing tailored parameterizations that can be tested in models, ranging from large-eddy simulations to large-scale climate models. Note, however, that the collection of a comprehensive data set allowing for the development of a globally representative source function for episodically occurring INPs in marine environments would be extremely challenging due to the complexity and variability of ocean ecosystems. Therefore, more modeling studies are needed to quantify the sensitivities of marine mixed-phase clouds to the presence of these episodic high-temperature INPs and to develop a targeted approach for future measurements.
All E3SM model codes (https://doi.org/10.11578/E3SM/dc.20180418.36, E3SM Project, 2018) may be accessed through GitHub (https://github.com/E3SM-Project/E3SM/releases/tag/v1.0.0, last access: 1 August 2021).
Observational data used in this publication are available through the data archive of the Atmospheric Radiation Measurement (ARM) User Facility, a U.S. Department of Energy (DOE) Office of Science User Facility managed by the Office of Biological and Environmental Research. (https://adc.arm.gov/discovery/#/results/primary_meas_type_code::icenuclei, last access: 6 June 2020).
IS and SMB designed the study. AR and MM provided output from the E3SM and MPAS-Ocean simulations. IS conceptualized the numerical framework presented in this study, with contributions from SMB, PJD, GD and TCJH. IS conducted all analyses and prepared the paper, with all co-authors contributing to reviewing and editing the draft.
The contact author has declared that neither they nor their co-authors have any competing interests.
Publisher’s note: Copernicus Publications remains neutral with regard to jurisdictional claims in published maps and institutional affiliations.
This research has been supported by the U.S. Department of Energy (DOE), Office of Science and Office of Biological and Environmental Research, through the Early Career Research Program, and used data from the Atmospheric Radiation Measurement Climate Research Facility, a DOE Office of Science User Facility. A portion of the research for this study was performed using resources available through Research Computing at the Pacific Northwest National Laboratory (PNNL). The E3SM simulation data were obtained from the Energy Exascale Earth System Model project, sponsored by the U.S. Department of Energy, Office of Science and Office of Biological and Environmental Research. Paul J. DeMott and Thomas C. J. Hill acknowledge support from the U.S. Department of Energy's Atmospheric System Research (ASR) program (grant nos. DE-SC0018929 and DE-SC0021116). Grant B. Deane acknowledges funding by the National Science Foundation through the NSF Center for Aerosol Impacts on Chemistry of the Environment (CAICE), a Center for Chemical Innovation (grant no. CHE-1801971).
The PNNL is operated for the DOE by the Battelle Memorial Institute (contract no. DE-AC05-76RL01830).
This research has been supported by the U.S. Department of Energy (Early Career Research Program and Atmospheric System Research Program; grant nos. DE-SC0018929 and DE-SC0021116) and the National Science Foundation (grant no. CHE-1801971).
This paper was edited by Hinrich Grothe and reviewed by two anonymous referees.
Aller, J. Y., Kuznetsova, M. R., Jahns, C. J., and Kemp, P. F.: The sea surface microlayer as a source of viral and bacterial enrichment in marine aerosols, J. Aerosol Sci., 36, 801–812, https://doi.org/10.1016/j.jaerosci.2004.10.012, 2005.
Aller, J. Y., Radway, J. C., Kilthau, W. P., Bothe, D. W., Wilson, T. W., Vaillancourt, R. D., Quinn, P. K., Coffman, D. J., Murray, B. J., and Knopf, D. A.: Size-resolved characterization of the polysaccharidic and proteinaceous components of sea spray aerosol, Atmos. Environ., 154, 331–347, https://doi.org/10.1016/j.atmosenv.2017.01.053, 2017.
Alpert, P. A., Aller, J. Y., and Knopf, D. A.: Ice nucleation from aqueous NaCl droplets with and without marine diatoms, Atmos. Chem. Phys., 11, 5539–5555, https://doi.org/10.5194/acp-11-5539-2011, 2011.
Andersen, K. H., Berge, T., Gonçalves, R. J., Hartvig, M., Heuschele, J., Hylander, S., Jacobsen, N. S., Lindemann, C., Martens, E. A., Neuheimer, A. B., Olsson, K., Palacz, A., Prowe, A. E. F., Sainmont, J., Traving, S. J., Visser, A. W., Wadhwa, N., and Kiørboe, T.: Characteristic Sizes of Life in the Oceans, from Bacteria to Whales, Annu. Rev. Mar. Sci., 8, 217–241, 2016.
Beall, C. M., Michaud, J. M., Fish, M. A., Dinasquet, J., Cornwell, G. C., Stokes, M. D., Burkart, M. D., Hill, T. C., DeMott, P. J., and Prather, K. A.: Cultivable halotolerant ice-nucleating bacteria and fungi in coastal precipitation, Atmos. Chem. Phys., 21, 9031–9045, https://doi.org/10.5194/acp-21-9031-2021, 2021.
Bertram, T. H., Cochran, R. E., Grassian, V. H., and Stone, E. A.: Sea spray aerosol chemical composition: elemental and molecular mimics for laboratory studies of heterogeneous and multiphase reactions, Chem. Soc. Rev., 47, 2374–2400, https://doi.org/10.1039/c7cs00008a, 2018.
Blanchard, D. C. and Syzdek, L. D.: Concentration of bacteria in jet drops from bursting bubbles, J. Geophys. Res., 77, 5087–5099, https://doi.org/10.1029/JC077i027p05087, 1972.
Boucher, O., Randall, D., Artaxo, P., Bretherton, C., Feingold, G., Forster, P., Kerminen, V.-M., Kondo, Y., Liao, H., Lohmann, U., Rasch, P., Satheesh, S.K., Sherwood, S., Stevens, B., and Zhang, X. Y.: Clouds and aerosols, Climate change 2013: the physical science basis. Contribution of Working Group I to the Fifth Assessment Report of the Intergovernmental Panel on Climate Change, edited by: Stocker, T. F., Qin, D., Plattner, G.-K., Tignor, M., Allen, S. K., Boschung, J., Nauels, A., Xia, Y., Bex, V., and Midgley, P. M., Cambridge University Press, Cambridge, 571–657, 2013.
Brady, S., Maltrud, R. X., Wolfram, M. E., Bujack, P. J. J., and Barbara, R.: Leveraging Lagrangian analysis for discriminating nutrient origins, in: Workshop on Visualisation in Environmental Sciences (EnvirVis), The Eurographics Association, Porto, Portugal, https://doi.org/10.2312/envirvis.20191100, 2019.
Burrows, S. M., Hoose, C., Pöschl, U., and Lawrence, M. G.: Ice nuclei in marine air: biogenic particles or dust?, Atmos. Chem. Phys., 13, 245–267, https://doi.org/10.5194/acp-13-245-2013, 2013.
Burrows, S. M., Ogunro, O., Frossard, A. A., Russell, L. M., Rasch, P. J., and Elliott, S. M.: A physically based framework for modeling the organic fractionation of sea spray aerosol from bubble film Langmuir equilibria, Atmos. Chem. Phys., 14, 13601–13629, https://doi.org/10.5194/acp-14-13601-2014, 2014.
Cesana, G. and Storelvmo, T.: Improving climate projections by understanding how cloud phase affects radiation: Radiative Effects of Cloud Phase, J. Geophys. Res.-Atmos., 122, 4594–4599, https://doi.org/10.1002/2017JD026927, 2017.
Chin, W.-C., Orellana, M. V., and Verdugo, P.: Spontaneous assembly of marine dissolved organic matter into polymer gels, Nature, 391, 568–572, https://doi.org/10.1038/35345, 1998.
Christner, B. C., Cai, R., Morris, C. E., McCarter, K. S., Foreman, C. M., Skidmore, M. L., Montross, S. N., and Sands, D. C.: Geographic, seasonal, and precipitation chemistry influence on the abundance and activity of biological ice nucleators in rain and snow, P. Natl. Acad. Sci. USA, 105, 18854–18859, https://doi.org/10.1073/pnas.0809816105, 2008.
Cornwell, G. C., Sultana, C. M., Prank, M., Cochran, R. E., Hill, T. C. J., Schill, G. P., DeMott, P. J., Mahowald, N., and Prather, K. A.: Ejection of dust from the ocean as a potential source of marine ice nucleating particles, J. Geophys. Res.-Atmos., 125, e2020JD033073, https://doi.org/10.1029/2020JD033073, 2020.
Creamean, J. M., Cross, J. N., Pickart, R., McRaven, L., Lin, P., Pacini, A., Hanlon, R., Schmale, D. G., Ceniceros, J., Aydell, T., Colombi, N., Bolger, E., and DeMott, P. J.: Ice Nucleating Particles Carried From Below a Phytoplankton Bloom to the Arctic Atmosphere, Geophys. Res. Lett., 46, 8572–8581, https://doi.org/10.1029/2019GL083039, 2019.
de Leeuw, G., Andreas, E. L., Anguelova, M. D., Fairall, C. W., Lewis, E. R., O’Dowd, C., Schulz, M., and Schwartz, S. E.: Production Flux of Sea Spray Aerosol, Rev. Geophys., 49, https://doi.org/10.1029/2010rg000349, 2011.
DeMott, P. J., Hill, T. C. J., McCluskey, C. S., Prather, K. A., Collins, D. B., Sullivan, R. C., Ruppel, M. J., Mason, R. H., Irish, V. E., Lee, T., Hwang, C. Y., Rhee, T. S., Snider, J. R., McMeeking, G. R., Dhaniyala, S., Lewis, E. R., Wentzell, J. J. B., Abbatt, J., Lee, C., Sultana, C. M., Ault, A. P., Axson, J. L., Diaz Martinez, M., Venero, I., Santos-Figueroa, G., Stokes, M. D., Deane, G. B., Mayol-Bracero, O. L., Grassian, V. H., Bertram, T. H., Bertram, A. K., Moffett, B. F., and Franc, G. D.: Sea spray aerosol as a unique source of ice nucleating particles, P. Natl. Acad. Sci. USA, 113, 5797–5803, https://doi.org/10.1073/pnas.1514034112, 2016.
E3SM Project: Energy Exascale Earth System Model v1.0, DOE [code], https://doi.org/10.11578/E3SM/dc.20180418.36, 2018.
Engel, A., Piontek, J., Metfies, K., Endres, S., Sprong, P., Peeken, I., Gäbler-Schwarz, S., and Nöthig, E.-M.: Inter-annual variability of transparent exopolymer particles in the Arctic Ocean reveals high sensitivity to ecosystem changes, Sci. Rep.-UK, 7, 4129, https://doi.org/10.1038/s41598-017-04106-9, 2017.
Fall, R. and Schnell, R. C.: Association of an ice-nucleating pseudomonad with cultures of the marine dinoflagellate, Heterocapsa niei, J. Mar. Res., 43, 257–265, https://doi.org/10.1357/002224085788437370, 1985.
Garrett, W. D.: The organic chemical composition of the ocean surface, Deep-Sea Res. Abstracts, 14, 221–227, https://doi.org/10.1016/0011-7471(67)90007-1, 1967.
Gong, X., Wex, H., van Pinxteren, M., Triesch, N., Fomba, K. W., Lubitz, J., Stolle, C., Robinson, T.-B., Müller, T., Herrmann, H., and Stratmann, F.: Characterization of aerosol particles at Cabo Verde close to sea level and at the cloud level – Part 2: Ice-nucleating particles in air, cloud and seawater, Atmos. Chem. Phys., 20, 1451–1468, https://doi.org/10.5194/acp-20-1451-2020, 2020.
Hartmann, M., Adachi, K., Eppers, O., Haas, C., Herber, A., Holzinger, R., Hünerbein, A., Jäkel, E., Jentzsch, C., Pinxteren, M., Wex, H., Willmes, S., and Stratmann, F.: Wintertime Airborne Measurements of Ice Nucleating Particles in the High Arctic: A Hint to a Marine, Biogenic Source for Ice Nucleating Particles, Geophys. Res. Lett., 47, e2020GL087770, https://doi.org/10.1029/2020GL087770, 2020.
Hoose, C. and Möhler, O.: Heterogeneous ice nucleation on atmospheric aerosols: a review of results from laboratory experiments, Atmos. Chem. Phys., 12, 9817–9854, https://doi.org/10.5194/acp-12-9817-2012, 2012.
Huang, S., Hu, W., Chen, J., Wu, Z., Zhang, D., and Fu, P.: Overview of biological ice nucleating particles in the atmosphere, Environ. Int., 146, 106197, https://doi.org/10.1016/j.envint.2020.106197, 2021.
Huang, W. T. K., Ickes, L., Tegen, I., Rinaldi, M., Ceburnis, D., and Lohmann, U.: Global relevance of marine organic aerosol as ice nucleating particles, Atmos. Chem. Phys., 18, 11423–11445, https://doi.org/10.5194/acp-18-11423-2018, 2018.
Ickes, L., Porter, G. C. E., Wagner, R., Adams, M. P., Bierbauer, S., Bertram, A. K., Bilde, M., Christiansen, S., Ekman, A. M. L., Gorokhova, E., Höhler, K., Kiselev, A. A., Leck, C., Möhler, O., Murray, B. J., Schiebel, T., Ullrich, R., and Salter, M. E.: The ice-nucleating activity of Arctic sea surface microlayer samples and marine algal cultures, Atmos. Chem. Phys., 20, 11089–11117, https://doi.org/10.5194/acp-20-11089-2020, 2020.
Irish, V. E., Elizondo, P., Chen, J., Chou, C., Charette, J., Lizotte, M., Ladino, L. A., Wilson, T. W., Gosselin, M., Murray, B. J., Polishchuk, E., Abbatt, J. P. D., Miller, L. A., and Bertram, A. K.: Ice-nucleating particles in Canadian Arctic sea-surface microlayer and bulk seawater, Atmos. Chem. Phys., 17, 10583–10595, https://doi.org/10.5194/acp-17-10583-2017, 2017.
Kanji, Z. A., Ladino, L. A., Wex, H., Boose, Y., Burkert-Kohn, M., Cziczo, D. J., and Krämer, M.: Overview of Ice Nucleating Particles, Meteorol. Monogr., 58, 1.1–1.33, https://doi.org/10.1175/AMSMONOGRAPHS-D-16-0006.1, 2017.
Ladino, L. A., Yakobi-Hancock, J. D., Kilthau, W. P., Mason, R. H., Si, M., Li, J., Miller, L. A., Schiller, C. L., Huffman, J. A., Aller, J. Y., Knopf, D. A., Bertram, A. K., and Abbatt, J. P. D.: Addressing the ice nucleating abilities of marine aerosol: A combination of deposition mode laboratory and field measurements, Atmos. Environ., 132, 1–10, https://doi.org/10.1016/j.atmosenv.2016.02.028, 2016.
Leck, C. and Bigg, K. E.: Comparison of sources and nature of the tropical aerosol with the summer high Arctic aerosol, Tellus B, 60, 118–126, https://doi.org/10.1111/j.1600-0889.2007.00315.x, 2008.
Li, W. K. W., Head, E. J. H., and Glen Harrison, W.: Macroecological limits of heterotrophic bacterial abundance in the ocean, Deep-Sea Res. Pt. I, 51, 1529–1540, https://doi.org/10.1016/j.dsr.2004.06.012, 2004.
Liu, X., Ma, P.-L., Wang, H., Tilmes, S., Singh, B., Easter, R. C., Ghan, S. J., and Rasch, P. J.: Description and evaluation of a new four-mode version of the Modal Aerosol Module (MAM4) within version 5.3 of the Community Atmosphere Model, Geosci. Model Dev., 9, 505–522, https://doi.org/10.5194/gmd-9-505-2016, 2016.
McCluskey, C. S., Hill, T. C. J., Malfatti, F., Sultana, C. M., Lee, C., Santander, M. V., Beall, C. M., Moore, K. A., Cornwell, G. C., Collins, D. B., Prather, K. A., Jayarathne, T., Stone, E. A., Azam, F., Kreidenweis, S. M., and DeMott, P. J.: A Dynamic Link between Ice Nucleating Particles Released in Nascent Sea Spray Aerosol and Oceanic Biological Activity during Two Mesocosm Experiments, J. Atmos. Sci., 74, 151–166, https://doi.org/10.1175/JAS-D-16-0087.1, 2017a.
McCluskey, C. S., Hill, T. C. J., Malfatti, F., Sultana, C. M., Lee, C., Santander, M. V., Beall, C. M., Moore, K. A., Cornwell, G. C., Collins, D. B., Prather, K. A., Jayarathne, T., Stone, E. A., Azam, F., Kreidenweis, S. M., and DeMott, P. J.: A Dynamic Link between Ice Nucleating Particles Released in Nascent Sea Spray Aerosol and Oceanic Biological Activity during Two Mesocosm Experiments, J. Atmos. Sci., 74, 151–166, 2017b.
McCluskey, C. S., Hill, T. C. J., Sultana, C. M., Laskina, O., Trueblood, J., Santander, M. V., Beall, C. M., Michaud, J. M., Kreidenweis, S. M., Prather, K. A., Grassian, V., and DeMott, P. J.: A Mesocosm Double Feature: Insights into the Chemical Makeup of Marine Ice Nucleating Particles, J. Atmos. Sci., 75, 2405–2423, https://doi.org/10.1175/JAS-D-17-0155.1, 2018a.
McCluskey, C. S., Ovadnevaite, J., Rinaldi, M., Atkinson, J., Belosi, F., Ceburnis, D., Marullo, S., Hill, T. C. J., Lohmann, U., Kanji, Z. A., O'Dowd, C., Kreidenweis, S. M., and DeMott, P. J.: Marine and Terrestrial Organic Ice-Nucleating Particles in Pristine Marine to Continentally Influenced Northeast Atlantic Air Masses, J. Geophys. Res.-Atmos., 123, 6196–6212, https://doi.org/10.1029/2017JD028033, 2018b.
McCluskey, C. S., Hill, T. C. J., Humphries, R. S., Rauker, A. M., Moreau, S., Strutton, P. G., Chambers, S. D., Williams, A. G., McRobert, I., Ward, J., Keywood, M. D., Harnwell, J., Ponsonby, W., Loh, Z. M., Krummel, P. B., Protat, A., Kreidenweis, S. M., and DeMott, P. J.: Observations of Ice Nucleating Particles Over Southern Ocean Waters, Geophys. Res. Lett., 45, 11989–11997, https://doi.org/10.1029/2018GL079981, 2018c.
McCluskey, C. S., DeMott, P. J., Ma, P.-L., and Burrows, S. M.: Numerical Representations of Marine Ice-Nucleating Particles in Remote Marine Environments Evaluated Against Observations, Geophys. Res. Lett., 46, 7838–7847, https://doi.org/10.1029/2018GL081861, 2019.
McCoy, D. T., Tan, I., Hartmann, D. L., Zelinka, M. D., and Storelvmo, T.: On the relationships among cloud cover, mixed-phase partitioning, and planetary albedo in GCMs, J. Adv. Model. Earth Syst., 8, 650–668, https://doi.org/10.1002/2015MS000589, 2016.
Mitts, B. A., Wang, X., Lucero, D. D., Beall, C. M., Deane, G. B., DeMott, P. J., and Prather, K. A.: Importance of supermicron ice nucleating particles in nascent sea spray, Geophys. Res. Lett., 48, e2020GL089633, https://doi.org/10.1029/2020GL089633, 2021.
Monahan, E. C., Spiel, D. E., and Davidson, K. L.: A Model of Marine Aerosol Generation Via Whitecaps and Wave Disruption, in: Oceanic Whitecaps. Oceanographic Sciences Library, Vol. 2, edited by: Monahan, E. C. and Niocaill, G. M., Springer, Dordrecht, https://doi.org/10.1007/978-94-009-4668-2_16, 1986.
Murray, B. J., O'Sullivan, D., Atkinson, J. D., and Webb, M. E.: Ice nucleation by particles immersed in supercooled cloud droplets, Chem. Soc. Rev., 41, 6519–6554, https://doi.org/10.1039/c2cs35200a, 2012.
O'Dowd, C. D. and de Leeuw, G.: Marine aerosol production: a review of the current knowledge, Philos. T. R. Soc. A, 365, 1753–1774, https://doi.org/10.1098/rsta.2007.2043, 2007.
Orellana, M. V. and Leck, C.: Chapter 9 – Marine Microgels, in: Biogeochemistry of Marine Dissolved Organic Matter, 2nd Edn., edited by: Hansell, D. A. and Carlson, C. A., 451–480, Academic Press, Boston, https://doi.org/10.1016/B978-0-12-405940-5.00009-1, 2015.
Orellana, M. V., Matrai, P. A., Leck, C., Rauschenberg, C. D., Lee, A. M., and Coz, E.: Marine microgels as a source of cloud condensation nuclei in the high Arctic, P. Natl. Acad. Sci. USA, 108, 13612–13617, https://doi.org/10.1073/pnas.1102457108, 2011.
Patterson, J. P., Collins, D. B., Michaud, J. M., Axson, J. L., Sultana, C. M., Moser, T., Dommer, A. C., Conner, J., Grassian, V. H., Stokes, M. D., Deane, G. B., Evans, J. E., Burkart, M. D., Prather, K. A., and Gianneschi, N. C.: Sea Spray Aerosol Structure and Composition Using Cryogenic Transmission Electron Microscopy, ACS Cent. Sci., 2, 40–47, https://doi.org/10.1021/acscentsci.5b00344, 2016.
Petersen, M. R., Asay-Davis, X. S., Berres, A. S., Chen, Q., Feige, N., Hoffman, M. J., Jacobsen, D. W., Jones, P. W., Maltrud, M. E., Price, S. F., Ringler, T. D., Streletz, G. J., Turner, A. K., Van Roekel, L. P., Veneziani, M., Wolfe, J. D., Wolfram, P. J., and Woodring, J. L.: An Evaluation of the Ocean and Sea Ice Climate of E3SM Using MPAS and Interannual CORE-II Forcing, J. Adv. Model. Earth Syst., 11, 1438–1458, https://doi.org/10.1029/2018MS001373, 2019.
Pósfai, M., Li, J., Anderson, J. R., and Buseck, P. R.: Aerosol bacteria over the Southern Ocean during ACE-1, Atmos. Res., 66, 231–240, https://doi.org/10.1016/S0169-8095(03)00039-5, 2003.
Prather, K. A., Bertram, T. H., Grassian, V. H., Deane, G. B., Stokes, M. D., Demott, P. J., Aluwihare, L. I., Palenik, B. P., Azam, F., Seinfeld, J. H., Moffet, R. C., Molina, M. J., Cappa, C. D., Geiger, F. M., Roberts, G. C., Russell, L. M., Ault, A. P., Baltrusaitis, J., Collins, D. B., Corrigan, C. E., Cuadra-Rodriguez, L. A., Ebben, C. J., Forestieri, S. D., Guasco, T. L., Hersey, S. P., Kim, M. J., Lambert, W. F., Modini, R. L., Mui, W., Pedler, B. E., Ruppel, M. J., Ryder, O. S., Schoepp, N. G., Sullivan, R. C., and Zhao, D.: Bringing the ocean into the laboratory to probe the chemical complexity of sea spray aerosol, P. Natl. Acad. Sci. USA, 110, 7550–7555, https://doi.org/10.1073/pnas.1300262110, 2013.
Pummer, B. G., Budke, C., Augustin-Bauditz, S., Niedermeier, D., Felgitsch, L., Kampf, C. J., Huber, R. G., Liedl, K. R., Loerting, T., Moschen, T., Schauperl, M., Tollinger, M., Morris, C. E., Wex, H., Grothe, H., Pöschl, U., Koop, T., and Fröhlich-Nowoisky, J.: Ice nucleation by water-soluble macromolecules, Atmos. Chem. Phys., 15, 4077–4091, https://doi.org/10.5194/acp-15-4077-2015, 2015.
Quinn, P. K., Collins, D. B., Grassian, V. H., Prather, K. A., and Bates, T. S.: Chemistry and related properties of freshly emitted sea spray aerosol, Chem. Rev., 115, 4383–4399, 2015.
Rahlff, J., Stolle, C., Giebel, H.-A., Brinkhoff, T., Ribas-Ribas, M., Hodapp, D., and Wurl, O.: High wind speeds prevent formation of a distinct bacterioneuston community in the sea-surface microlayer, FEMS Microbiol. Ecol., 93, fix041, https://doi.org/10.1093/femsec/fix041, 2017.
Rastelli, E., Corinaldesi, C., Dell'Anno, A., Lo Martire, M., Greco, S., Cristina Facchini, M., Rinaldi, M., O'Dowd, C., Ceburnis, D., and Danovaro, R.: Transfer of labile organic matter and microbes from the ocean surface to the marine aerosol: an experimental approach, Sci. Rep.-UK, 7, 11475, https://doi.org/10.1038/s41598-017-10563-z, 2017.
Schiffer, J. M., Mael, L. E., Prather, K. A., Amaro, R. E., and Grassian, V. H.: Sea Spray Aerosol: Where Marine Biology Meets Atmospheric Chemistry, ACS Cent. Sci., 4, 1617–1623, https://doi.org/10.1021/acscentsci.8b00674, 2018.
Tan, I., Storelvmo, T., and Zelinka, M. D.: Observational constraints on mixed-phase clouds imply higher climate sensitivity, Science, 352, 224–227, https://doi.org/10.1126/science.aad5300, 2016.
Trueblood, J. V., Nicosia, A., Engel, A., Zäncker, B., Rinaldi, M., Freney, E., Thyssen, M., Obernosterer, I., Dinasquet, J., Belosi, F., Tovar-Sánchez, A., Rodriguez-Romero, A., Santachiara, G., Guieu, C., and Sellegri, K.: A two-component parameterization of marine ice-nucleating particles based on seawater biology and sea spray aerosol measurements in the Mediterranean Sea, Atmos. Chem. Phys., 21, 4659–4676, https://doi.org/10.5194/acp-21-4659-2021, 2021.
Vali, G.: Quantitative Evaluation of Experimental Results an the Heterogeneous Freezing Nucleation of Supercooled Liquids, J. Atmos. Sci., 28, 402–409, 1971.
Vali, G., DeMott, P. J., Möhler, O., and Whale, T. F.: Technical Note: A proposal for ice nucleation terminology, Atmos. Chem. Phys., 15, 10263–10270, https://doi.org/10.5194/acp-15-10263-2015, 2015.
van Pinxteren, M., Fomba, K. W., Triesch, N., Stolle, C., Wurl, O., Bahlmann, E., Gong, X., Voigtländer, J., Wex, H., Robinson, T.-B., Barthel, S., Zeppenfeld, S., Hoffmann, E. H., Roveretto, M., Li, C., Grosselin, B., Daële, V., Senf, F., van Pinxteren, D., Manzi, M., Zabalegui, N., Frka, S., Gašparović, B., Pereira, R., Li, T., Wen, L., Li, J., Zhu, C., Chen, H., Chen, J., Fiedler, B., von Tümpling, W., Read, K. A., Punjabi, S., Lewis, A. C., Hopkins, J. R., Carpenter, L. J., Peeken, I., Rixen, T., Schulz-Bull, D., Monge, M. E., Mellouki, A., George, C., Stratmann, F., and Herrmann, H.: Marine organic matter in the remote environment of the Cape Verde islands – an introduction and overview to the MarParCloud campaign, Atmos. Chem. Phys., 20, 6921–6951, https://doi.org/10.5194/acp-20-6921-2020, 2020.
Verdugo, P., Orellana, M. V., Chin, W.-C., Petersen, T. W., van den Eng, G., Benner, R., and Hedges, J. I.: Marine biopolymer self-assembly: implications for carbon cycling in the ocean, Faraday Discuss., 139, 393–398, https://doi.org/10.1039/b800149a, 2008.
Vergara-Temprado, J., Miltenberger, A. K., Furtado, K., Grosvenor, D. P., Shipway, B. J., Hill, A. A., Wilkinson, J. M., Field, P. R., Murray, B. J., and Carslaw, K. S.: Strong control of Southern Ocean cloud reflectivity by ice-nucleating particles, P. Natl. Acad. Sci. USA, 115, 2687–2692, https://doi.org/10.1073/pnas.1721627115, 2018.
Wang, H., Easter, R. C., Zhang, R., Ma, P.-L., Singh, B., Zhang, K., Ganguly, D., Rasch, P. J., Burrows, S. M., Ghan, S. J., Lou, S., Qian, Y., Yang, Y., Feng, Y., Flanner, M., Leung, L. R., Liu, X., Shrivastava, M., Sun, J., Tang, Q., Xie, S., and Yoon, J.-H.: Aerosols in the E3SM Version 1: New developments and their impacts on radiative forcing, J. Adv. Model. Earth Sy., 12, e2019MS001851, https://doi.org/10.1029/2019MS001851, 2020.
Wang, X., Sultana, C. M., Trueblood, J., Hill, T. C. J., Malfatti, F., Lee, C., Laskina, O., Moore, K. A., Beall, C. M., McCluskey, C. S., Cornwell, G. C., Zhou, Y., Cox, J. L., Pendergraft, M. A., Santander, M. V., Bertram, T. H., Cappa, C. D., Azam, F., DeMott, P. J., Grassian, V. H., and Prather, K. A.: Microbial Control of Sea Spray Aerosol Composition: A Tale of Two Blooms, ACS Cent. Sci., 1, 124–131, https://doi.org/10.1021/acscentsci.5b00148, 2015.
Wang, X., Deane, G. B., Moore, K. A., Ryder, O. S., Stokes, M. D., Beall, C. M., Collins, D. B., Santander, M. V., Burrows, S. M., Sultana, C. M., and Prather, K. A.: The role of jet and film drops in controlling the mixing state of submicron sea spray aerosol particles, P. Natl. Acad. Sci. USA, 114, 6978–6983, https://doi.org/10.1073/pnas.1702420114, 2017.
Wilbourn, E. K., Thornton, D. C. O., Ott, C., Graff, J., Quinn, P. K., Bates, T. S., Betha, R., Russell, L. M., Behrenfeld, M. J., and Brooks, S. D.: Ice Nucleation by Marine Aerosols Over the North Atlantic Ocean in Late Spring, J. Geophys. Res.-Atmos., 125, e2019JD030913, https://doi.org/10.1029/2019JD030913, 2020.
Wilson, T. W., Ladino, L. A., Alpert, P. A., Breckels, M. N., Brooks, I. M., Browse, J., Burrows, S. M., Carslaw, K. S., Huffman, J. A., Judd, C., Kilthau, W. P., Mason, R. H., McFiggans, G., Miller, L. A., Nájera, J. J., Polishchuk, E., Rae, S., Schiller, C. L., Si, M., Temprado, J. V., Whale, T. F., Wong, J. P. S., Wurl, O., Yakobi-Hancock, J. D., Abbatt, J. P. D., Aller, J. Y., Bertram, A. K., Knopf, D. A., and Murray, B. J.: A marine biogenic source of atmospheric ice-nucleating particles, Nature, 525, 234–238, https://doi.org/10.1038/nature14986, 2015.
Wu, J.: Jet Drops Produced by Bubbles Bursting at the Surface of Seawater, J. Phys. Oceanogr., 32, 3286–3290, https://doi.org/10.1175/1520-0485(2002)032<3286:JDPBBB>2.0.CO;2, 2002.
Zhao, X., Liu, X., Burrows, S. M., and Shi, Y.: Effects of marine organic aerosols as sources of immersion-mode ice-nucleating particles on high-latitude mixed-phase clouds, Atmos. Chem. Phys., 21, 2305–2327, https://doi.org/10.5194/acp-21-2305-2021, 2021.
- Abstract
- Introduction
- Analysis of episodic marine INP observations
- Numerical framework for estimating marine high-temperature INPs
- Comparison with observations of episodic marine INP emissions
- Conclusions and outlook
- Code availability
- Data availability
- Author contributions
- Competing interests
- Disclaimer
- Acknowledgements
- Financial support
- Review statement
- References
- Abstract
- Introduction
- Analysis of episodic marine INP observations
- Numerical framework for estimating marine high-temperature INPs
- Comparison with observations of episodic marine INP emissions
- Conclusions and outlook
- Code availability
- Data availability
- Author contributions
- Competing interests
- Disclaimer
- Acknowledgements
- Financial support
- Review statement
- References