the Creative Commons Attribution 4.0 License.
the Creative Commons Attribution 4.0 License.
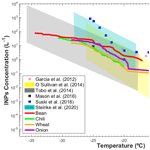
Mexican agricultural soil dust as a source of ice nucleating particles
Diana L. Pereira
Irma Gavilán
Consuelo Letechipía
Graciela B. Raga
Teresa Pi Puig
Violeta Mugica-Álvarez
Harry Alvarez-Ospina
Irma Rosas
Leticia Martinez
Eva Salinas
Erika T. Quintana
Daniel Rosas
Luis A. Ladino
Agricultural soil erosion, both mechanical and eolic, may impact cloud processes, as some aerosol particles are able to facilitate ice crystal formation. Given the large agricultural sector in Mexico, this study investigates the ice nucleating abilities of agricultural dust collected at different sites and generated in the laboratory. The immersion freezing mechanism of ice nucleation was simulated in the laboratory via the Universidad Nacional Autónoma de México (UNAM) microorifice uniform deposit impactor (MOUDI) droplet freezing technique (DFT), i.e., UNAM-MOUDI-DFT. The results show that agricultural dust from the Mexican territory promote ice formation in the temperature range from −11.8 to −34.5 ∘C, with ice nucleating particle (INP) concentrations between 0.11 and 41.8 L−1. Furthermore, aerosol samples generated in the laboratory are more efficient than those collected in the field, with T50 values (i.e., the temperature at which 50 % of the droplets freeze) higher by more than 2.9 ∘C. Mineralogical analysis indicated a high concentration of feldspars, i.e., K-feldspar and plagioclase (>40 %), in most of the aerosol and soil samples, with K-feldspar significantly correlated with the T50 of particles with aerodynamic diameters between 1.8 and 3.2 µm. Similarly, the organic carbon (OC) was correlated with the ice nucleation efficiency of aerosol samples from 3.2 to 5.6 and from 1.0 to 1.8 µm. Finally, a decrease in INP efficiency after heating the samples at 300 ∘C for 2 h indicates that the organic matter from agricultural soils plays a predominant role in the ice nucleating abilities of this type of aerosol sample.
- Article
(1275 KB) - Full-text XML
-
Supplement
(746 KB) - BibTeX
- EndNote
Agricultural activities may influence our environment and human health through the emission of aerosol particles (Telloli et al., 2014; Chen et al., 2017; Tomlin et al., 2020). It has been estimated that agricultural dust particles may represent between 25 % (Ginoux et al., 2012) and 50 % (Mahowald et al., 2004) of the global airborne dust. Dust particles impact visibility and water quality (Presley and Tatarko, 2009). Moreover, dust particles influence the global climate by affecting the Earth's radiative balance and cloud microphysical properties (Haywood and Boucher, 2000; Lagzi et al., 2013).
Aerosol particles influence cloud properties due to their ability to act as ice nucleating particles (INPs) or cloud condensation nuclei (CCN) (Cotton and Yuter, 2009). INPs promote ice formation in clouds, influencing precipitation development and the hydrological cycle (DeMott et al., 2010). The predominance of precipitation formation via the ice phase over the continents makes the presence of ice particles fundamental (Mülmenstädt et al., 2015), especially in mixed-phase clouds, where immersion freezing has been reported as the main ice formation pathway (Murray et al., 2012; Hande and Hoose, 2017). Immersion freezing allows ice activation from INPs embedded in liquid droplets when the temperature decreases below 0 ∘C (Murray et al., 2012).
Given that soils are complex mixtures of mineral and organic components, living organisms, air, and water (Kalev and Toor, 2018), a wide variety of aerosol particles with potential INP abilities can be emitted during agricultural activities, such as tilling (Steinke et al., 2016; Chen et al., 2017). Numerous studies have analyzed the ice nucleating abilities of the mineral components of dust particles (e.g., Eastwood et al., 2008; Zimmermann et al., 2008; Welti et al., 2009; Yakobi-Hancock et al., 2013; Broadley et al., 2012; Hiranuma et al., 2015; Boose et al., 2016). Their efficiency as INPs was found to be usually associated with the K-feldspar content (e.g., Yakobi-Hancock et al., 2013; Atkinson et al., 2013; Kiselev et al., 2016). On the other hand, Lee et al. (2006) and Tomlin et al. (2020) observed that the concentration of microorganisms is enhanced in agricultural soil samples. Thus, a variety of microorganisms (e.g., certain bacteria, fungi) or their residues are likely attached to mineral dust surfaces, enhancing their ice nucleating abilities, or they can also act as INPs by themselves (Conen et al., 2011; Després et al., 2012).
Biological particles have been reported to be one of the most efficient INPs (Schnell and Vali, 1972; Hoose and Möhler, 2012; Hader et al., 2014; Kanji et al., 2017), with activation temperatures as high as −3 ∘C (Christner et al., 2008; Huang et al., 2021). For example, Conen et al. (2011) observed that biological particles influence ice formation in aerosol samples at temperatures ∘C. Garcia et al. (2012) reported that soil samples contained ice nucleation active (INA) bacteria that were responsible for the nucleation events observed at −12 ∘C. Similarly, soils can serve as an important source of organic particles (Montgomery et al., 2000; Kelleher and Simpson, 2006; Hill et al., 2016), which may be able to catalyze ice formation (Hoose and Möhler, 2012; Knopf et al., 2018; Chen et al., 2018, 2021). Knopf et al. (2018) have shown that humic acids (HA) and humic-like substances (HULIS) influence ice nucleation in the immersion freezing and deposition nucleation modes. Chen et al. (2018) reported that droplets containing HULIS with concentrations from 15.8 to 96.7 mg L−1 freeze at temperatures between −9 and −22 ∘C. The ice nucleating abilities of HULIS may be a consequence of particle aggregation, which provides suitable surfaces for ice activation (Chen et al., 2021).
The ice nucleating abilities of agricultural soils from Argentina, England, Germany, Hungary, Mongolia, USA, and Russia have been evaluated in the last few decades (Conen et al., 2011; Garcia et al., 2012; Tobo et al., 2014; Steinke et al., 2016; Suski et al., 2018). Those studies suggest that the organic compounds in agricultural soils are more efficient at facilitating ice formation than the mineral components; however, Conen et al. (2011) highlighted the fact that the influence of organic components on ice formation is not considered in climate models. Also, it is important to note that although agriculture is a ubiquitous activity in tropical countries such as Mexico, the ice nucleating abilities of agricultural dust particles from the Mexican territory have not been reported to date.
Therefore, in the present study, the ice nucleating abilities of Mexican agricultural soils are evaluated for the first time using the Universidad Nacional Autónoma de México (UNAM) microorifice uniform deposit impactor (MOUDI) droplet freezing technique (DFT), i.e., UNAM-MOUDI-DFT, focusing on the influence of the mineralogical composition versus the organic content and the size of the INPs.
2.1 Sampling sites
Airborne and soil samples were collected in four different Mexican states characterized by important agricultural activities: Mexico City (CDMX), Morelos (MOR), Zacatecas (ZAC), and Yucatán (YUC) (Fig. 1). Soil samples and aerosol particles were collected at the ZAC site during a short-term field campaign between 24 and 27 February 2020. Soil samples from the CDMX, MOR, and YUC sites were collected in agricultural areas and provided through collaborations. The locations of the sampling sites and the number of samples collected are summarized in Table 1, where samples are labeled based on the crop previously present at each site.
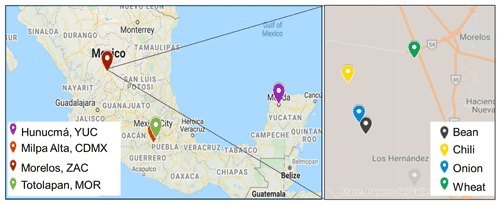
Figure 1Map showing the sampling sites in four different states in Mexico: Mexico City (CDMX), Morelos (MOR), Zacatecas (ZAC), and Yucatán (YUC). The zoom shows the sampling spots in ZAC, along with the corresponding crops (© Google Maps 2021).
Table 1Summary of the details for the aerosol and soil samples collected in four different Mexican states: Mexico City (CDMX), Morelos (MOR), Zacatecas (ZAC), and Yucatán (YUC). The samples were labeled based on the crop previously present at the sampling location.
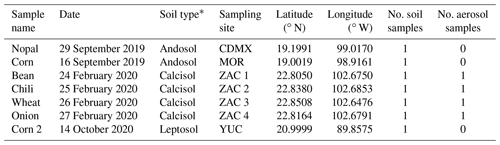
* The soil type was derived from SAGARPA (2017).
In the mentioned states (CDMX, MOR, ZAC, YUC), specific municipalities (Table 1) that grow different crops were analyzed. Milpa Alta is located in the southeast of CDMX and is well known as the first nopal producer in the state (Alcaldía Milpa Alta, 2019). Totolapan (MOR) is a traditional maize (corn) producer, and ∼50 % of its area is devoted to this crop (Ayuntamiento de Totolapan, 2018). Morelos (ZAC) is one of the main producers of chilies (its agricultural area represents 25 % of the territory), and some mining activities also reported for Morelos (Covarrubias and Peña Cabriales, 2017). Hunucmá is located in YUC, where 22 % of the territory is used for agricultural activities (INEGI, 2015).
2.2 Field samples
Soil samples from the top 10 cm were collected in CDMX, MOR, ZAC, and YUC, as shown in Table 1. In ZAC, soil samples were collected during soil tillage and aerosol samples were collected at ground level (at approximately 1.5 m a.g.l.). Aerosol particles were collected on siliconized glass substrates (HR3-215; Hampton Research) using a MOUDI cascade impactor (100R; MSP Corporation), which collects and classifies the particles according to their aerodynamic diameters (dp) onto eight stages (cut sizes of 0.18, 0.32, 0.56, 1.0, 1.8, 3.2, 5.6, and 10.0 µm) at a flow rate of 30 L min−1. Soil and aerosol samples were sealed and transported to the laboratory. Aerosol samples were stored at 3 ∘C and the soil samples at room temperature. Further information about the meteorological conditions observed during the sampling campaign are summarized in Table S1 in the Supplement.
2.3 Laboratory-generated samples
All soil samples were air dried, crushed, and sieved to a pore particle size of 425 µm. Aerosol particles were then generated using a dry system (Fig. 2) based on the Ladino and Abbatt (2013) design. Briefly, the experimental setup contained (a) an aerosol disperser consisting of a stirring plate and a metallic flask, in which the particles are generated by turbulence, (b) a mixing flask to homogenize the samples, and (c) an aerosol collector. A MOUDI 100R was used to collect aerosol particles for the analysis of INPs and the analysis of the mineralogical composition, while a MiniVol TAS (Airmetrics) was used to collect aerosol particles for the organic carbon (OC) analysis.
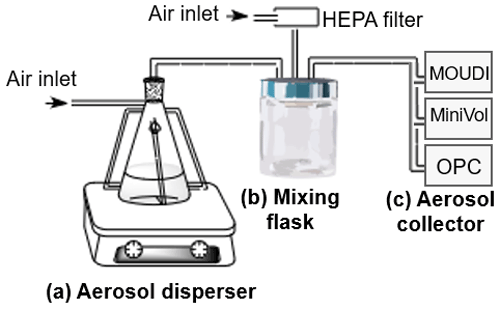
Figure 2Dry aerosol generation system setup. (a) Aerosol disperser, (b) mixing volume, and (c) aerosol collector (MOUDI 100R, MiniVol TAS, or OPC).
Siliconized glass substrates (HR3-215; Hampton Research) and 47 mm aluminum foil filters (0100-47-AF, TSI) were used in the MOUDI to sample the INPs and for mineral analysis, respectively. Aerosol particles collected over aluminum filters on the eight stages of the MOUDI (0.18 to 10 µm) were grouped into a single sample for the mineral analysis. Particulate matter with diameters of less than 10 µm (PM10) was collected over 47 mm quartz filters (2500QAO-UP, Pall Life Science) using the MiniVol at a flow of 5 L min−1 for the OC analysis. The quartz filters were previously conditioned at 500 ∘C for 4 h to remove trace pollutants, especially volatile organic compounds.
Additionally, a LasAir III optical particle counter (OPC; 310 B, Particle Measuring Systems) was used to obtain the particle size distributions (PSDs) of both the aerosol samples generated in the laboratory and those measured in situ during the field campaign. The OPC was operated at a flow rate of 28.3 L min−1, and the aerosol concentrations corresponded to particle sizes ranging between 0.3 and 10 µm.
2.4 Analysis of INPs
The ice nucleating abilities of the agricultural dust particles collected in the field and generated in the laboratory were analyzed through the immersion freezing mode using the UNAM-MOUDI-DFT (Córdoba et al., 2021). The equipment consisted of (a) a cold stage, (b) a humid/dry air system, (c) a Zeiss Axio Scope A1 optical microscope (Axiolab Zeiss) with a recording system, and (d) a data acquisition system (Córdoba et al., 2021).
Briefly, the glass substrates containing the aerosol particles that had impacted on them were introduced and fixed in the cold stage, where the temperature was controlled by a thermostat (LAUDA PRO-RP 1090) filled with polydimethylsiloxane. Afterwards, humid air was directed towards the sample to allow liquid droplet formation over the aerosol particles until the droplets reached a diameter of 170 µm (on average). Once most of the droplets had reached this size, dry nitrogen was used to shrink the size of the droplets to avoid contact between them. Finally, the system was isolated and the temperature was decreased from 0 to −40 ∘C at a cooling rate of 10 ∘C min−1 to freeze the droplets. The experiments were recorded, and the temperature was obtained with a resistance temperature detector (RTD) that was connected to a Fieldlogger device (RS485, NOVUS) and placed at the center of the cold stage. The recorded videos and the RTD temperatures allowed the determination of the freezing temperature for each droplet. The number of droplets formed during the experiments varied between 20 and 30.
The INP concentrations (L−1) were calculated using Eq. (1) (Mason et al., 2015a):
where Nu(T) is the number of unfrozen droplets (dimensionless) at temperature T (∘C), No is the total number of droplets (dimensionless), fne is a correction factor that accounts for the uncertainty associated with the number of nucleation events (dimensionless), Adeposit is the total area of the aerosol deposit on the glass substrates (mm2), ADFT is the area of the glass substrates analyzed by the DFT (mm2), V is the volume of air sampled with the MOUDI (L), and the fnu terms are correction factors to account for the aerosol deposit inhomogeneity (dimensionless).
2.5 Analytical techniques
X-ray diffraction (XRD) has been widely used for the characterization of crystalline materials (Kohli and Mittal, 2019). Therefore, the mineralogical compositions of the aerosol and soil samples were determined using an Empyrean X-ray diffractometer (Malvern Panalytical, with CuKα radiation) operated with a PIXcel 3D detector. The mineral phases were identified and quantified by the Rietveld method (Rietveld, 1969) using the HIGHScore v4.5 software and the ICDD (International Center for Diffraction Data) and ICSD (Inorganic Crystal Structure Database) databases.
The OC content was derived using a thermal-optical technique (Sunset Lab) based on the procedure from Birch and Cary (1996). Briefly, the quartz filters were introduced into an oven, where the samples were volatilized and oxidized to CO2. Finally, the CO2 was reduced and quantified by a flame ionization detector. To verify the influence of the organic matter on the ice nucleating abilities of the agricultural dust, the soil samples were heated at 300 ∘C for 2 h to remove the organic components, following Tobo et al. (2014).
2.6 Microbiological analysis
To determine the culturable microorganisms present on the soil samples collected in ZAC, 500 mg of each sample were added to 10 mL of sterile solution at 0.85 % (w/v of NaCl). After 1:100 dilution and vortex agitation, 0.1 mL of each solution were cultured on three growing media: trypticase soy agar (TSA) and MacConkey agar (MCA) for between 24 and 48 h at 35 ∘C, and malt extract agar (MEA) for 3 d at 25 ∘C. The TSA and MCA growing media were used to cultivate bacteria and the MEA for fungal propagules. Then, the colony forming units (CFU) were obtained for 1.0 g of soil.
3.1 Ice nucleating abilities
Figure 3 summarizes the ice nucleating abilities of the different agricultural dust particles with sizes between 0.56 and 5.6 µm, corresponding to MOUDI stages 3 to 6. The present results focus on particles >0.56 µm, as it has been shown that particles >0.5 µm have a higher potential to act as INPs (e.g., DeMott et al., 2010). Two sets of samples are presented in Fig. 3: aerosol samples collected directly in the field (F) and those generated in the laboratory (L) from topsoil collected in the fields. The L samples (solid curves) acted as INPs between −11.0 and −26.0 ∘C, while the F samples (dashed curves) show a much wider temperature range (i.e., between −11.8 and −34.5 ∘C). In terms of T50 (i.e., the temperature at which 50 % of the droplets freeze), the highest and lowest mean values for the L samples were −19.7 ∘C (for dp = 1.8–3.2 µm) and −20.7 ∘C (for dp = 3.2–5.6 µm). Similarly, for the F samples, the highest mean T50 was −23.4 ∘C (for dp = 1.8–3.2 µm) and the lowest mean T50 was −26.1 ∘C (for dp = 0.56–1.0 µm). Therefore, the L samples were found to nucleate ice at higher temperatures compared to the F samples.
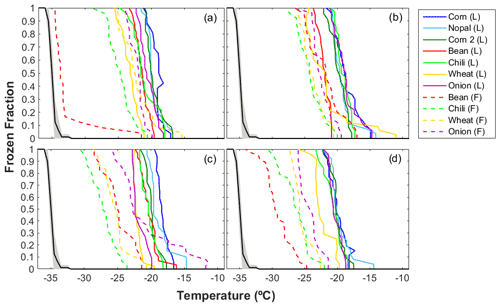
Figure 3Ice nucleating abilities of the agricultural soil particles as a function of temperature for particle aerodynamic diameters of (a) 3.2–5.6 µm, (b) 1.8–3.2 µm, (c) 1.0–1.8 µm, and (d) 0.56–1.0 µm. The black line depicts the average homogeneous freezing curve. The dashed and solid lines show the results for the samples collected in the field (F) and the samples generated in the laboratory (L), respectively.
The warmest freezing temperatures shown by L samples suggest that the aerosol particle generation during soil tillage is not fully simulated by the process used in the laboratory. The discrepancies in the INP abilities can be attributed to different environmental conditions, as the F samples are exposed to a variety of physicochemical processes while in the atmosphere (Boose et al., 2016; Cziczo et al., 2013), which is unlikely to be the case for the L samples. The differences between the laboratory and field environments are also reflected in different PSDs observed during the aerosolization process; see Fig. S1 in the Supplement. As this figure shows, mean particle concentrations of between and 1.2 particles cm−3 characterized the L samples, while lower values were observed for the F samples. Furthermore, the highest particle concentration for the L samples was found for particles between 1.0 and 5.0 µm (Fig. S1a), while the F samples were enriched in smaller particles, i.e., 0.3 µm (Fig. S1b). The difference in PSD could affect the ice nucleating abilities of the two types of samples; however, more experiments are needed to evaluate the contributions of different particle sizes to the total INP concentrations.
It was also found that the ice nucleating abilities of the different aerosol samples varied, with particles from the nopal, corn 1, and corn 2 samples showing the warmest freezing temperatures and the bean and wheat samples showing the coldest freezing temperatures (Fig. 3). The presence of additives on soils has been proposed to influence the organic content or soil properties (Peña-Méndez et al., 2005; Suski et al., 2018); therefore, it could influence the emission of aerosol particles from soils. However, more information is required to understand the nature of the soil components responsible for ice nucleating activity. As Table 1 shows, the ZAC samples were collected in calcisols; however, the concentrations of the mineral phases identified on the bean, chili, wheat, and onion samples differed, suggesting that additional parameters aside from the soil type may also influence the properties of the samples and their abilities to act as INPs. Kalev and Toor (2018) found that the composition of the soil determines its properties. This fact may influence the ice nucleating abilities of the soils, as shown in Fig. 3. Further details of the mineral composition and the organic content of each sample are discussed below.
Tegen and Fung (1995) and Tegen et al. (2004) also proposed that the PSD of the aerosol particles can vary according to soil type. The size of the aerosol particles is well known to influence their behavior as INPs (Diehl and Wurzler, 2004; DeMott et al., 2010; Mason et al., 2015b; Córdoba et al., 2021). This fact is evidenced in the different PSD distributions observed for each sample in Fig. S1. Figure S2 in the Supplement shows a clear trend for the F samples: the larger the particle size, the higher the T50. However, this behavior was not observed for the L samples, corroborating differences in the PSD between L and F samples.
Although the laboratory-generated aerosol particles do not fully reproduce the characteristics of the ambient agricultural particles, the ice nucleation experiments of the L samples highlight the importance of agricultural soils in ice formation in Mexico. As shown in Fig. S3 in the Supplement, the freezing temperatures and the ice nucleation active surface size (INAS) densities (nS) (Hoose and Möhler, 2012; Steinke et al., 2016) observed in the present study for aerosol particles collected in the field are on the same order as those reported for agricultural dust in Wyoming (USA), from 5.2×104 (−17 ∘C) to 3.5×107 (−35 ∘C) (Tobo et al., 2014). However, the nS values observed here are lower (by more than 2 orders of magnitude) than those reported for samples collected in Argentina, China, and Germany at temperatures ranging between −11 to −26 ∘C for dp <5 µm (Steinke et al., 2016). The similarities between the study of Tobo et al. (2014) and the present study suggest that aerosol particles emitted from agricultural soils are able to influence ice formation regardless of the origin or location of the agricultural soils. Further comparison of the present results with literature data is provided in Sect. 3.4.
3.2 The influence of organic matter
Figure 4 shows that the OC concentration represents a small fraction of the agricultural dust samples (dp <10 µm), with values between 5 % and 17 %, while the mineral components predominate (i.e., from 33 % to 95 %). Conen et al. (2011) found that the OC fraction for non-agricultural soils with dp <15 µm collected in Mongolia, Germany, Hungary, and Russia varied between 0.7 % and 12 %. O'Sullivan et al. (2014) also reported small values of OC, from 2 % to 13 %, for agricultural dust (bulk) in England. In contrast, Tobo et al. (2014) measured higher concentrations of organic compounds (37 %) for agricultural dust with dp = 0.6 µm in the US. The aforementioned studies reported that the organic components of soil dust (with different particle sizes and concentrations) can enhance the ice nucleating abilities of dust particles. This behavior is also observed for the agricultural dust analyzed here, as summarized in Fig. 5.
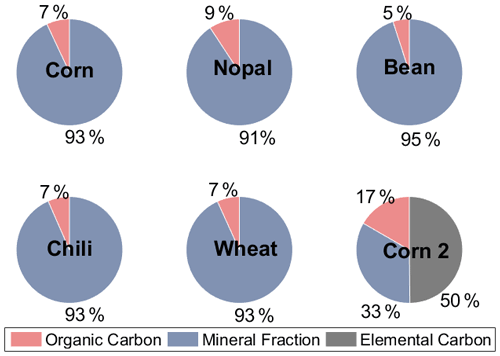
Figure 4Organic carbon, elemental carbon, and mineral contributions to the aerosol samples generated in the laboratory.
Figure 5 shows that for the four particle size ranges analyzed here (i.e., 0.56–1.0, 1.0–1.8, 1.8–3.2, and 3.5–5.6 µm), there was a significant reduction in the freezing temperature, referred to as ΔT50, after the organic matter was degraded through a heat treatment. The T50 of the heated samples was reduced by between 0.7 and 14.0 ∘C, with the largest mean ΔT50 reported for particles in the size range between 1.8 and 3.2 µm, as shown in Fig. S4 in the Supplement. The highest reduction in ice nucleating ability as a consequence of the heat treatment was observed for the corn 2 sample ( ∘C, Table S1) with 17 % OC, while the other samples had OC values of <9 % (Fig. 4). The highest reduction was observed for particles with sizes ranging between 1.8 and 3.2 µm, which were also reported to be the most efficient INPs (Fig. 3). Even though the OC fraction is small compared to the mineral components, the reduction in the freezing temperatures of the soil samples analyzed here suggests that the organic components present in the samples increase their ice nucleating abilities. This behavior has been widely observed after the removal of the organic matter (O'Sullivan et al., 2014; Tobo et al., 2014; Suski et al., 2018) or the destruction of proteinaceous compounds (Conen et al., 2011; Garcia et al., 2012; Steinke et al., 2016) in agricultural soils. Organic matter in soils may come from microorganisms and/or their residues, humic (e.g., humic acids) and nonhumic (e.g., proteins, polysaccharides) substances, and refractory compounds (Hill et al., 2016).
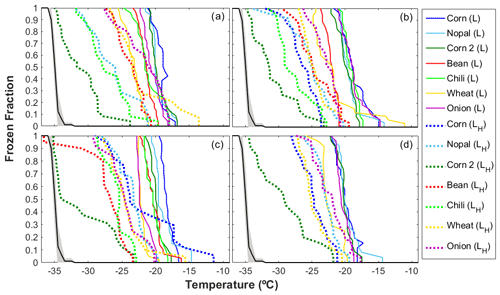
Figure 5Ice nucleating abilities of agricultural dust particles generated in the laboratory (L) before and after the heat treatment as a function of temperature for particle sizes of (a) 3.2–5.6 µm, (b) 1.8–3.2 µm, (c) 1.0–1.8 µm, and (d) 0.56–1.0 µm. The black line depicts the average homogeneous freezing curve. The dashed and solid lines show the results for the heated samples (H) and nonheated samples, respectively. For the heat treatment, the samples were heated at 300 ∘C for 2 h.
Different types of organic matter were not analyzed here; however, the presence of microorganisms as a possible source of organics in the ZAC soil samples was confirmed, as shown in Fig. S5 in the Supplement. Microorganism concentrations were observed to be as high as 5.6×106, 1.98×105, and 2.4×104 CFU g−1 for mesophilic bacteria, fungal propagules, and gram-negative bacteria, respectively. The concentrations of airborne microorganisms during harvesting activities in the US have been reported (Lighthart, 1984; Lee et al., 2006). Lighthart et al. (1984) observed that airborne concentrations of fungi and bacteria can reach values as high as 109 CFU m−3. During corn harvesting, concentrations of between 3.5×105 and 1.4×106 CFU m−3 were observed for culturable bacteria and between 1.6×106 and 7.4×106 CFU m−3 for culturable fungal spores (Lee et al., 2006). Although a direct intercomparison cannot be performed between these studies, the observed airborne concentrations of microorganisms suggest that an important fraction of the microorganisms in soils can be aerosolized during soil manipulation. Furthermore, it is important to highlight that gram-negative bacteria were found in the ZAC agricultural soils, as those are known to be active as INPs (Šantl-Temkiv et al., 2015)
As soils contain more than organic compounds, the effects of heat treatments on minerals have been questioned. Tobo et al. (2014) showed that the ice nucleating abilities of mineral samples are not affected by heat treatments for 2 h at 300 ∘C, and Perkins et al. (2020) observed similar behavior for the mineral dust proxy Arizona test dust (ATD) at 500 ∘C. Although those studies suggested that minerals are not strongly affected by dry heat treatments, studies performed by Zolles et al. (2015) and Daily et al. (2021) show that shifts in the efficiency of minerals as INPs cannot be neglected, as heat treatments at 250 ∘C might influence the ice nucleating abilities of feldspar compounds. This is not the case for the corn 2 sample (Table S2 in the Supplement), which lacks feldspars, highlighting the importance of organic compounds at temperatures ∘C.
Note that the observed decrease in freezing temperature varies as a function of particle size (Figs. 5 and S4), suggesting a relationship between the organic content and the size of the particles. These observations agree with those of Chen and Chiu (2003) and Lin et al. (2010), who reported that the composition of the organic matter in soils from Taiwan and the HULIS fraction in Chinese soils vary with particle size. In addition, significant positive correlations found between the T50 and the OC concentration for particles ranging between 1.0 and 1.8 µm (r=0.79, p value <0.05) and between 3.5 and 5.6 µm (r=0.86, p value <0.05), as shown in Fig. S6 in the Supplement, support the importance of particle size and chemical composition in the ice nucleating abilities of agricultural dust particles.
3.3 Mineralogical analysis
The presence of plagioclase, K-feldspar, quartz, kaolinite, smectite, mica-illite, and minor constituents was identified through XRD analysis. Figure 6 shows a high content of feldspars (i.e., K-feldspar, plagioclase) in both soil and aerosol samples, except in the corn 2 sample collected in Hunucmá, where the presence of feldspars was not identified. In particular, the plagioclase fraction (i.e., feldspar) and the K-feldspar together comprise more than 50 % of the total concentration in the corn, nopal, and bean samples. The absence of feldspar compounds in the corn 2 sample could be a consequence of the soil type, as leptosols are enriched in calcareous materials (SEMARNAT, 2002).
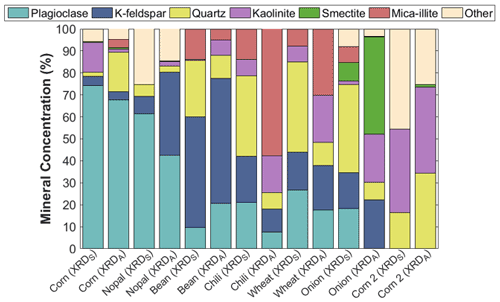
Figure 6Summary of the mineralogical compositions of the aerosol particles generated in the laboratory for dp = 0.18–10 µm (XRDA) and soil samples collected in the field for particle sizes of <425 µm (XRDS). The mineral phases were identified using X-ray diffraction (XRD).
Feldspars are well known for their efficiency as INPs (Atkinson et al., 2013; Peckhaus et al., 2016; Kiselev et al., 2016), which is mostly associated with the presence of active sites (i.e., cracks, defects, cavities) on their surfaces (Kiselev et al., 2016). Therefore, the high concentrations of feldspars in Mexican agricultural soils can also influence their ice nucleating abilities, as was observed in the high and statistically significant correlation between the K-feldspar concentration and the T50 of aerosol particles with sizes ranging between 1.8 and 3.2 µm (r=0.85, p value <0.05), as shown in Fig. S6. Boose et al. (2016) observed similar behavior for desert samples and the presence of feldspars, as they found good correlations between compounds containing feldspars and INP efficiency.
Differences in the mineral phases of the samples can be the source of different ice nucleating abilities, as shown in Fig. 3. The relationship between aerosol particle composition and their ice nucleating abilities has been previously reported (Baustian et al., 2012; O'Sullivan et al., 2014; Paramonov et al., 2018; Steinke et al., 2020; Hiranuma et al., 2021). Furthermore, the differences in mineralogical composition between the soil and aerosol samples indicate that not all the soil particles are aerosolized. These differences could also be a consequence of differences in the particle sizes analyzed, as the aerosol samples varied between 0.18 and 10 µm while the soil particles were smaller than 425 µm.
Feldspars, quartz, and clays (e.g., illite, kaolinite) have been commonly identified in mineral dust transported from Africa (Broadley et al., 2012) and other deserts around the world (e.g., Australia, Atacama; Boose et al., 2016) by XRD analysis. Similar mineral phases such as kaolinite, illite, quartz, and minor concentrations of feldspars were also observed in agricultural soils from England (O'Sullivan et al., 2014). Therefore, the present results and those from previous reports evidence that, although there are differences in the type and origin of the soils examined in these studies, the soils can have similar mineral components that determine the ice nucleation behavior of their aerosol particles.
3.4 INP concentrations and atmospheric implications
The total INP concentration emitted during tillage of the agricultural soils in ZAC was found to vary between 0.11 and 102 L−1 from −15.0 to −34.5 ∘C, as shown in Fig. 7. The INP concentrations measured in ZAC are on the same order as those reported for Colorado (USA) (Garcia et al., 2012), England (O'Sullivan et al., 2014), Kansas (USA) (Mason et al., 2016; Suski et al., 2018), and Wyoming (Tobo et al., 2014; Steinke et al., 2020). Garcia et al. (2012) reported INP concentrations between L−1 (−7.0 ∘C) and 5.5 L−1 (−20.2 ∘C) that were derived with a droplet freezing assay (DFA). Using the same method, O'Sullivan et al. (2014) observed INP concentrations that varied from L−1 (−6.2 ∘C) to 4.4×102 L−1 (−26.1 ∘C). Steinke et al. (2020) found INP concentrations between 0.15 L−1 (−18.2 ∘C) and 2.5×103 L−1 (−28.2 ∘C) using the Aerosol Interactions and Dynamics in the Atmosphere (AIDA) cloud chamber. Tobo et al. (2014) reported INP concentrations (analyzed in the Colorado State University Continuous Flow Diffusion Chamber, CSU-CFDC) between 10−2 L−1 (−18 ∘C) and 104 L−1 (−36 ∘C) for soils that were 25 % of agricultural origin and 75 % of desert origin. Suski et al. (2018) implemented an ice spectrometer and found INP concentrations from to 8.5×102 L−1 at temperatures between −5.5 and −25.5 ∘C. Using a similar setup to the present study, Mason et al. (2016) derived total INP concentrations at Colby (Kansas) of 0.5, 1.0, and 8.9 L−1 at −15, −20, and −25 ∘C, respectively.
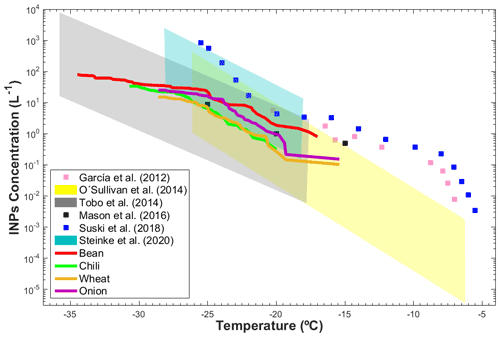
Figure 7Intercomparison of the INP concentrations from the present study (solid colored lines) and literature data (blue, gray, and yellow areas and filled symbols) as a function of temperature.
Although different devices, which present differences in their detection limits and sensitivities, have been used to determine the INP concentrations in agricultural dust samples, the INP concentrations reported here are within the same range, as shown in Fig. 7. The similarities between the present results and previous studies evidence the importance of the mineral and organic components of soil dust in ice formation, with organic components increasing the INP efficiency of soil particles, as observed in Sect. 3.2.
In agricultural areas, the organic components appear to be fundamental to the inherent capacity of soils to facilitate ice formation, even at low concentrations (Conen et al., 2011; O'Sullivan et al., 2014). This fact suggests that more attention must be paid to determining specific organic components in soils and to understanding their individual ice nucleating abilities. This efficiency can also be influenced by the size of the particles, as shown in Figs. 3 and S1. Furthermore, the present results show that emissions from tropical latitudes are consistent with those reported for mid- and high latitudes. Agricultural lands cover ca. 13 % of the total surface of Mexico (Torres and Martínez, 2019), and agriculture represents the economic activity with the highest income within the primary sector (CEDRSSA, 2021). Given that the economies of several tropical countries are based on agricultural activities, it is very important to determine the role that their associated emissions play in the local and regional hydrological cycle. Also, agricultural dust particles must be included in numerical studies aiming to predict the future climate, as the demand for food will increase, and hence the land used for food production will too.
This study reports, for the first time, the ice nucleating abilities of Mexican agricultural dust via immersion freezing. INP concentrations between 0.11 and 41.8 L−1 in the temperature range from −15 to −34.5 ∘C were observed. The measured concentrations are comparable to those reported for agricultural soils in the United States and England, confirming the contribution from tropical aerosol emissions and the potential they have to impact mixed-phase cloud formation if brought to altitudes higher than the cloud base. Furthermore, the comparison of the ice nucleating abilities of aerosol samples aerosolized in the laboratory and those collected at the field indicates a higher INP efficiency of the laboratory samples, with freezing temperature values varying between −11 and −26 ∘C and T50 values higher by more than 2.9 ∘C, as a consequence of the higher particle concentrations of larger particles.
The XRD analysis allowed the identification of the different mineral phases present in the aerosol and soil samples, where high concentrations of plagioclase, K-feldspar, quartz, kaolinite, smectite, and mica-illite were detected. These minerals have been previously identified in dust samples. In particular, feldspars were found in higher concentrations (>40 %) in most of the samples. Additionally, the significant correlation between the T50 and the K-feldspar concentration in particles with sizes of 1.8 to 3.2 µm shows the influence of K-feldspar in the INP efficiency of mineral particles. The concentrations of OC indicate that, despite the low percentage observed in most of the samples (<17 %) in comparison to the mineral concentration, the organic components increase the INP efficiency, promoting ice crystal formation. This is evidenced by the decrease in the efficiency of the ice nucleating ability after the removal of the organic matter, and the statistically significant correlations between the OC concentration and the T50 for particle sizes from 3.2 to 5.6 µm and from 1.0 to 1.8 µm. Therefore, the organic components seem to have a predominant role in the efficiency as INPs of the aerosol particles analyzed herein.
The present results improve the current gap in the knowledge of field measurements of aerosol particles at tropical latitudes, with this study focusing on agricultural emissions and highlighting the importance of both the chemical composition and the particle size to their efficiency as INPs. However, more analysis of and attention directed at the organic compounds in agricultural dust are needed to improve the current understanding of soil components and to develop new parametrizations.
Data are available upon the request to the corresponding author.
The supplement related to this article is available online at: https://doi.org/10.5194/acp-22-6435-2022-supplement.
LAL, GBR, and IG designed the field campaigns and the experimental process. DLP, LAL, CL, ETQ, and DR performed the field measurements. DLP, LAL, TPP, VMÁ, HAO, IR, LM, and ES carried out the experiments. DLP and LAL wrote the paper, with contributions from all coauthors.
The contact author has declared that neither they nor their co-authors have any competing interests.
Publisher’s note: Copernicus Publications remains neutral with regard to jurisdictional claims in published maps and institutional affiliations.
The authors thank Fernanda Córdoba, Luis Gonzales, Monserrat Adaya, Gabriel Cortes, and Dara Salcedo for their helpful support.
This research has been supported by the Consejo Nacional de Ciencia y Tecnología (grant nos. FC-2164 and CB-285023).
This paper was edited by Ottmar Möhler and reviewed by three anonymous referees.
Alcaldía Milpa Alta: Milpa Alta en la actualidad, https://www.milpa-alta.cdmx.gob.mx/ (last access: 26 August 2021), 2019 (in Spanish).
Atkinson, J. D., Murray, B. J., Woodhouse, M. T., Whale, T. F., Baustian, K. J., Carslaw, K. S., Dobbie, S., O'Sullivan, D., and Malkin, T. L.: The importance of feldspar for ice nucleation by mineral dust in mixed-phase clouds, Nature, 498, 355–358, https://doi.org/10.1038/nature12278, 2013.
Ayuntamiento de Totolapan: TOTOLAPAN, http://www.inafed.gob.mx/work/enciclopedia/EMM17morelos/municipios/17027a.html (last access: 26 August 2021), 2018 (in Spanish).
Baustian, K. J., Cziczo, D. J., Wise, M. E., Pratt, K. A., Kulkarni, G., Hallar, A. G., and Tolbert, M. A.: Importance of aerosol composition, mixing state, and morphology for heterogeneous ice nucleation: A combined field and laboratory approach, J. Geophys. Res., 117, D06217, https://doi.org/10.1029/2011JD016784, 2012.
Birch, M. E. and Cary, R. A.: Elemental carbon-based method for monitoring occupational exposures to particulate diesel exhaust, Aerosol Sci. Tech., 25, 221–241, https://doi.org/10.1080/02786829608965393, 1996.
Boose, Y., Welti, A., Atkinson, J., Ramelli, F., Danielczok, A., Bingemer, H. G., Plötze, M., Sierau, B., Kanji, Z. A., and Lohmann, U.: Heterogeneous ice nucleation on dust particles sourced from nine deserts worldwide – Part 1: Immersion freezing, Atmos. Chem. Phys., 16, 15075–15095, https://doi.org/10.5194/acp-16-15075-2016, 2016.
Broadley, S. L., Murray, B. J., Herbert, R. J., Atkinson, J. D., Dobbie, S., Malkin, T. L., Condliffe, E., and Neve, L.: Immersion mode heterogeneous ice nucleation by an illite rich powder representative of atmospheric mineral dust, Atmos. Chem. Phys., 12, 287–307, https://doi.org/10.5194/acp-12-287-2012, 2012.
CEDRSSA: Situación del sector agropecuario en México, Mexico City, http://201.147.98.65/files/b/13/22Situacion_Sector_Agropecuario_Me%CC%81xico.pdf (last access 12 May 2022), 2021.
Chen, J., Wu, Z., Bai, Y., Hu, M., and Wex, H.: Freezing activity of droplets containing Humic-acid like substances (HULIS), EGU General Assembly, Vienna, Austria, 8–13 April 2018, EGU2018-18802, 2018.
Chen, J., Wu, Z. J., Zhao, X., Wang, Y. J., Chen, J. C., Qiu, Y. T., Zong, T. M., Chen, H. X., Wang, B. B., Lin, P., Liu, W., Guo, S., Yao, M. S., Zeng, L. M., Wex, H., Liu, X., Hu, M., and Li, S. M.: Atmospheric Humic-Like Substances (HULIS) Act as Ice Active Entities, Geophys. Res. Lett., 48, e2021GL092443, https://doi.org/10.1029/2021gl092443, 2021.
Chen, J. S. and Chiu, C. Y.: Characterization of soil organic matter in different particle-size fractions in humid subalpine soils by CP/MAS 13C NMR, Geoderma, 117, 129–141, https://doi.org/10.1016/S0016-7061(03)00160-5, 2003.
Chen, W., Tong, D. Q., Zhang, S., Zhang, X., and Zhao, H.: Local PM10 and PM2.5 emission inventories from agricultural tillage and harvest in northeastern China, J. Environm. Sci., 57, 15–23, https://doi.org/10.1016/j.jes.2016.02.024, 2017.
Christner, B. C., Cai, R., Morris, C. E., McCarter, K. S., Foreman, C. M., Skidmore, M. L., Montross, S. N., and Sands, D. C.: Geographic, seasonal, and precipitation chemistry influence on the abundance and activity of biological ice nucleators in rain and snow, P. Natl. Acad. Sci. USA., 105, 18854–18859, https://doi.org/10.1073/pnas.0809816105, 2008.
Conen, F., Morris, C. E., Leifeld, J., Yakutin, M. V., and Alewell, C.: Biological residues define the ice nucleation properties of soil dust, Atmos. Chem. Phys., 11, 9643–9648, https://doi.org/10.5194/acp-11-9643-2011, 2011.
Córdoba, F., Ramírez-Romero, C., Cabrera, D., Raga, G. B., Miranda, J., Alvarez-Ospina, H., Rosas, D., Figueroa, B., Kim, J. S., Yakobi-Hancock, J., Amador, T., Gutierrez, W., García, M., Bertram, A. K., Baumgardner, D., and Ladino, L. A.: Measurement report: Ice nucleating abilities of biomass burning, African dust, and sea spray aerosol particles over the Yucatán Peninsula, Atmos. Chem. Phys., 21, 4453–4470, https://doi.org/10.5194/acp-21-4453-2021, 2021.
Cotton, W. R. and Yuter, S.: Principles of cloud and precipitation formation, in: Aerosol pollution impact on precipitation, Springer, Dordrecht, 13–43, https://doi.org/10.1007/978-1-4020-8690-8_2, 2009.
Covarrubias, S. A. and Peña Cabriales, J. J.: Contaminación ambiental por metales pesados en México: Problemática y estrategias de fitorremediación, Rev. Int. Contam. Ambient., 33, 7–21, https://doi.org/10.20937/RICA.2017.33.esp01.01, 2017.
Cziczo, D. J., Froyd, K. D., Hoose, C., Jensen, E. J., Diao, M., Zondlo, M. A., Smith, J. B., Twohy, C. H., and Murphy, D. M.: Clarifying the dominant sources and mechanisms of cirrus cloud formation, Science, 340, 819–824, https://doi.org/10.1126/science.1234145, 2013.
Daily, M. I., Tarn, M. D., Whale, T. F., and Murray, B. J.: The sensitivity of the ice-nucleating ability of minerals to heat and the implications for the heat test for biological ice nucleators, Atmos. Meas. Tech. Discuss. [preprint], https://doi.org/10.5194/amt-2021-208, in review, 2021.
DeMott, P. J., Prenni, A. J., Liu, X., Kreidenweis, S. M., Petters, M. D., Twohy, C. H., Richardson, M. S., Eidhammer, T., and Rogers, D. C.: Predicting global atmospheric ice nuclei distributions and their impacts on climate, P. Natl. Acad. Sci. USA, 107, 11217–11222, https://doi.org/10.1073/pnas.0910818107, 2010.
Després, V. R., Alex Huffman, J., Burrows, S. M., Hoose, C., Safatov, A. S., Buryak, G., Fröhlich-Nowoisky, J., Elbert, W., Andreae, M. O., Pöschl, U., and Jaenicke, R.: Primary biological aerosol particles in the atmosphere: A review, Tellus B, 64, 15598, https://doi.org/10.3402/tellusb.v64i0.15598, 2012.
Diehl, K. and Wurzler, S.: Heterogeneous drop freezing in the immersion mode: Model calculations considering soluble and insoluble particles in the drops, J. Atmos. Sci., 61, 2063–2072, https://doi.org/10.1175/1520-0469(2004)061<2063:HDFITI>2.0.CO;2, 2004.
Eastwood, M. L., Cremel, S., Gehrke, C., Girard, E., and Bertram, A. K.: Ice nucleation on mineral dust particles: Onset conditions, nucleation rates and contact angles, J. Geophys. Res., 113, D22203, https://doi.org/10.1029/2008JD010639, 2008.
Garcia, E., Hill, T. C. J., Prenni, A. J., DeMott, P. J., Franc, G. D., and Kreidenweis, S. M.: Biogenic ice nuclei in boundary layer air over two U.S. high plains agricultural regions, J. Geophys. Res., 117, D18209, https://doi.org/10.1029/2012JD018343, 2012.
Ginoux, P., Prospero, J. M., Gill, T. E., Hsu, N. C., and Zhao, M.: Global-scale attribution of anthropogenic and natural dust sources and their emission rates based on MODIS Deep Blue aerosol products, Rev. Geophys., 50, RG3005, https://doi.org/10.1029/2012RG000388, 2012.
Hader, J. D., Wright, T. P., and Petters, M. D.: Contribution of pollen to atmospheric ice nuclei concentrations, Atmos. Chem. Phys., 14, 5433–5449, https://doi.org/10.5194/acp-14-5433-2014, 2014.
Hande, L. B. and Hoose, C.: Partitioning the primary ice formation modes in large eddy simulations of mixed-phase clouds, Atmos. Chem. Phys., 17, 14105–14118, https://doi.org/10.5194/acp-17-14105-2017, 2017.
Haywood, J. and Boucher, O.: Estimates of the direct and indirect radiative forcing due to tropospheric aerosols: A review, Rev. Geophys., 38, 513–543, https://doi.org/10.1029/1999RG000078, 2000.
Hill, T. C. J., DeMott, P. J., Tobo, Y., Fröhlich-Nowoisky, J., Moffett, B. F., Franc, G. D., and Kreidenweis, S. M.: Sources of organic ice nucleating particles in soils, Atmos. Chem. Phys., 16, 7195–7211, https://doi.org/10.5194/acp-16-7195-2016, 2016.
Hiranuma, N., Augustin-Bauditz, S., Bingemer, H., Budke, C., Curtius, J., Danielczok, A., Diehl, K., Dreischmeier, K., Ebert, M., Frank, F., Hoffmann, N., Kandler, K., Kiselev, A., Koop, T., Leisner, T., Möhler, O., Nillius, B., Peckhaus, A., Rose, D., Weinbruch, S., Wex, H., Boose, Y., DeMott, P. J., Hader, J. D., Hill, T. C. J., Kanji, Z. A., Kulkarni, G., Levin, E. J. T., McCluskey, C. S., Murakami, M., Murray, B. J., Niedermeier, D., Petters, M. D., O'Sullivan, D., Saito, A., Schill, G. P., Tajiri, T., Tolbert, M. A., Welti, A., Whale, T. F., Wright, T. P., and Yamashita, K.: A comprehensive laboratory study on the immersion freezing behavior of illite NX particles: a comparison of 17 ice nucleation measurement techniques, Atmos. Chem. Phys., 15, 2489–2518, https://doi.org/10.5194/acp-15-2489-2015, 2015.
Hiranuma, N., Auvermann, B. W., Belosi, F., Bush, J., Cory, K. M., Georgakopoulos, D. G., Höhler, K., Hou, Y., Lacher, L., Saathoff, H., Santachiara, G., Shen, X., Steinke, I., Ullrich, R., Umo, N. S., Vepuri, H. S. K., Vogel, F., and Möhler, O.: Laboratory and field studies of ice-nucleating particles from open-lot livestock facilities in Texas, Atmos. Chem. Phys., 21, 14215–14234, https://doi.org/10.5194/acp-21-14215-2021, 2021.
Hoose, C. and Möhler, O.: Heterogeneous ice nucleation on atmospheric aerosols: a review of results from laboratory experiments, Atmos. Chem. Phys., 12, 9817–9854, https://doi.org/10.5194/acp-12-9817-2012, 2012.
Huang, S., Hu, W., Chen, J., Wu, Z., Zhang, D., and Fu, P.: Overview of biological ice nucleating particles in the atmosphere, Environ. Int., 146, 106197, https://doi.org/10.1016/j.envint.2020.106197, 2021.
INEGI: Información por entidad-Ciudad de México, http://cuentame.inegi.org.mx/default.aspx (last access: 26 August 2021), 2015 (in Spanish).
Kalev, S. D. and Toor, G. S.: Chapter 3.9 – The composition of soils and sediments, in: Green Chemistry: An Inclusive Approach, edited by: Török, B. and Dransfield, T., Elsevier, 339–357, https://doi.org/10.1016/B978-0-12-809270-5.00014-5, 2018.
Kanji, Z. A., Ladino, L. A., Wex, H., Boose, Y., Burkert-Kohn, M., Cziczo, D. J., and Krämer, M.: Overview of ice nucleating particles, Meteor. Mon., 58, 1.1–1.33, https://doi.org/10.1175/AMSMONOGRAPHS-D-16-0006.1, 2017.
Kelleher, B. P. and Simpson, A. J.: Humic substances in soils: Are they really chemically distinct?, Environ. Sci. Technol., 40, 4605–4611, https://doi.org/10.1021/es0608085, 2006.
Kiselev, A., Bachmann, F., Pedevilla, P., Cox, S. J., Michaelides, A., Gerthsen, D., and Leisner, T.: Active sites in heterogeneous ice nucleation-the example of K-rich feldspars, Science, 355, 367–371, https://doi.org/10.1126/science.aai8034, 2016.
Knopf, D. A., Alpert, P. A., and Wang, B.: The role of organic aerosol in atmospheric ice nucleation: A review, ACS Earth Space Chem., 2, 168–202, https://doi.org/10.1021/acsearthspacechem.7b00120, 2018.
Kohli, R. and Mittal, K. (Eds.): Chapter 3 – Methods for assessing surface cleanliness, in: Developments in Surface Contamination and Cleaning, Elsevier, 23–105, https://doi.org/10.1016/B978-0-12-816081-7.00003-6, 2019.
Ladino, L. A. and Abbatt, J. P. D.: Laboratory investigation of Martian water ice cloud formation using dust aerosol simulants, J. Geophys. Res.-Planet., 118, 14–25, https://doi.org/10.1029/2012JE004238, 2013.
Lagzi, I., Mészáros, R., Gelybó, G., and Leelossy, Á.: Atmospheric Chemistry, Etvs Lorand University, 2013.
Lee, S. A., Adhikari, A., Grinshpun, S. A., McKay, R., Shukla, R., and Reponen, T.: Personal exposure to airborne dust and microorganisms in agricultural environments, J. Occup. Environ. Hyg., 3, 118–130, https://doi.org/10.1080/15459620500524607, 2006.
Lighthart, B.: Microbial aerosols: estimated contribution of combine harvesting to an airshed, Appl. Environ. Microb., 47, 430–432, https://doi.org/10.1128/aem.47.2.430-432.1984, 1984.
Lin, P., Huang, X. F., He, L. Y., and Zhen Yu, J.: Abundance and size distribution of HULIS in ambient aerosols at a rural site in South China, J. Aerosol Sci., 41, 74–87, https://doi.org/10.1016/j.jaerosci.2009.09.001, 2010.
Mahowald, N. M., Rivera-Rivera, G. D., and Luo, C.: Comment on “Relative importance of climate and land use in determining present and future global soil dust emission” by I. Tegen et al., Geophys, Res. Lett., 31, L24105, https://doi.org/10.1029/2004GL021272, 2004.
Mason, R. H., Chou, C., McCluskey, C. S., Levin, E. J. T., Schiller, C. L., Hill, T. C. J., Huffman, J. A., DeMott, P. J., and Bertram, A. K.: The micro-orifice uniform deposit impactor–droplet freezing technique (MOUDI-DFT) for measuring concentrations of ice nucleating particles as a function of size: improvements and initial validation, Atmos. Meas. Tech., 8, 2449–2462, https://doi.org/10.5194/amt-8-2449-2015, 2015a.
Mason, R. H., Si, M., Li, J., Chou, C., Dickie, R., Toom-Sauntry, D., Pöhlker, C., Yakobi-Hancock, J. D., Ladino, L. A., Jones, K., Leaitch, W. R., Schiller, C. L., Abbatt, J. P. D., Huffman, J. A., and Bertram, A. K.: Ice nucleating particles at a coastal marine boundary layer site: correlations with aerosol type and meteorological conditions, Atmos. Chem. Phys., 15, 12547–12566, https://doi.org/10.5194/acp-15-12547-2015, 2015b.
Mason, R. H., Si, M., Chou, C., Irish, V. E., Dickie, R., Elizondo, P., Wong, R., Brintnell, M., Elsasser, M., Lassar, W. M., Pierce, K. M., Leaitch, W. R., MacDonald, A. M., Platt, A., Toom-Sauntry, D., Sarda-Estève, R., Schiller, C. L., Suski, K. J., Hill, T. C. J., Abbatt, J. P. D., Huffman, J. A., DeMott, P. J., and Bertram, A. K.: Size-resolved measurements of ice-nucleating particles at six locations in North America and one in Europe, Atmos. Chem. Phys., 16, 1637–1651, https://doi.org/10.5194/acp-16-1637-2016, 2016.
Montgomery, D. R., Zabowski, D. Ugolini, F. C., Hallberg R. O., and Spaltenstein, H.: Soils, watershed processes, and marine sediments, Int. Geophys., 72, 159–194, https://doi.org/10.1016/S0074-6142(00)80114-X, 2000.
Murray, B. J., O'Sullivan, D., Atkinson, J. D., and Webb, M. E.: Ice nucleation by particles immersed in supercooled cloud droplets, Chem. Soc. Rev., 41, 6519–6554, https://doi.org/10.1039/C2CS35200A, 2012.
Mülmenstädt, J., Sourdeval, O., Delanoë, J., and Quaas, J.: Frequency of occurrence of rain from liquid-, mixed-, and ice-phase clouds derived from A-Train satellite retrievals, Geophys. Res. Lett., 42, 6502–6509, https://doi.org/10.1002/2015GL064604, 2015.
O'Sullivan, D., Murray, B. J., Malkin, T. L., Whale, T. F., Umo, N. S., Atkinson, J. D., Price, H. C., Baustian, K. J., Browse, J., and Webb, M. E.: Ice nucleation by fertile soil dusts: relative importance of mineral and biogenic components, Atmos. Chem. Phys., 14, 1853–1867, https://doi.org/10.5194/acp-14-1853-2014, 2014.
Paramonov, M., David, R. O., Kretzschmar, R., and Kanji, Z. A.: A laboratory investigation of the ice nucleation efficiency of three types of mineral and soil dust, Atmos. Chem. Phys., 18, 16515–16536, https://doi.org/10.5194/acp-18-16515-2018, 2018.
Peckhaus, A., Kiselev, A., Hiron, T., Ebert, M., and Leisner, T.: A comparative study of K-rich and Na/Ca-rich feldspar ice-nucleating particles in a nanoliter droplet freezing assay, Atmos. Chem. Phys., 16, 11477–11496, https://doi.org/10.5194/acp-16-11477-2016, 2016.
Peña-Méndez, E. M., Havel, J., and Patočka, J.: Humic substance – Compounds of still unknown structure: Applications in agriculture, industry, environment, and biomedicine, J. Appl. Biomed., 3, 13–24, https://doi.org/10.32725/JAB.2005.002, 2005.
Perkins, R. J., Gillette, S. M., Hill, T. C. J., and DeMott, P. J.: The Labile Nature of Ice Nucleation by Arizona Test Dust, ACS Earth Space Chem., 4, 133–141, https://doi.org/10.1021/acsearthspacechem.9b00304, 2020.
Presley, D. and Tatarko, J.: Principles of Wind Erosion and its Control, Kans. State Univ. Res. Ext. MF-2860, 2009.
Rietveld, H. M.: A profile refinement method for nuclear and magnetic structures, J. Appl. Crystallogr., 2, 65–71, https://doi.org/10.1107/S0021889869006558, 1969.
SAGARPA: Caracterización por tipo de suelo de la República Mexicana, https://www.gob.mx/siap/prensa/mapa-de-caracterizacion-por-tipo-de-suelo?idiom=es (last access: 26 August 2021), 2017 (in Spanish).
Šantl-Temkiv, T., Sahyoun, M., Finster, K., Hartmann, S., Augustin-Bauditz, S., Stratmann, F., Wex, H., Clauss, T., Woetmann, N., Havskov Sorensen, J., Smith Korsholm, U., Wick L. Y., and Karlson, U. G.: Characterization of airborne ice-nucleation-active bacteria and bacterial fragments, Atmos. Environ., 109, 105–117, https://doi.org/10.1016/j.atmosenv.2015.02.060, 2015.
Schnell, R. C. and Vali, G.: Atmospheric ice nuclei from decomposing vegetation, Nature, 236, 163–165, https://doi.org/10.1038/236163a0, 1972.
SEMARNAT: Grupo principales de suelos en México, in: Suelos, 123–125, https://apps1.semarnat.gob.mx:8443/dgeia/informe_12/pdf/Cap3_suelos.pdf (last access: 12 May 2022), 2002.
Steinke, I., Funk, R., Busse, J., Iturri, A., Kirchen, S., Leue, M., Möhler, O., Schwartz, T., Schnaiter, M., Sierau, B., Toprak, E., Ullrich, R., Ulrich, A., Hoose, C., and Leisner, T.: Ice nucleation activity of agricultural soil dust aerosols from Mongolia, Argentina, and Germany, J. Geophys. Res.-Atmos., 121, 13559–13576, https://doi.org/10.1002/2016JD025160, 2016.
Steinke, I., Hiranuma, N., Funk, R., Höhler, K., Tüllmann, N., Umo, N. S., Weidler, P. G., Möhler, O., and Leisner, T.: Complex plant-derived organic aerosol as ice-nucleating particles – more than the sums of their parts?, Atmos. Chem. Phys., 20, 11387–11397, https://doi.org/10.5194/acp-20-11387-2020, 2020.
Suski, K. J., Hill, T. C. J., Levin, E. J. T., Miller, A., DeMott, P. J., and Kreidenweis, S. M.: Agricultural harvesting emissions of ice-nucleating particles, Atmos. Chem. Phys., 18, 13755–13771, https://doi.org/10.5194/acp-18-13755-2018, 2018.
Tegen, I. and Fung, I.: Contribution to the atmospheric mineral aerosol load from land surface modification, J. Geophys. Res., 100, 18707–18726, https://doi.org/10.1029/95JD02051, 1995.
Tegen, I., Werner, M., Harrison, S. P., and Kohfeld, K. E.: Relative importance of climate and land use in determining present and future global soil dust emission, Geophys. Res. Lett., 31, L05105, https://doi.org/10.1029/2003gl019216, 2004.
Telloli, C., Malaguti, A., Mircea, M., Tassinari, R., Vaccaro, C., and Berico, M.: Properties of agricultural aerosol released during wheat harvest threshing, plowing and sowing, J. Environ. Sci., 26, 1903–1912, https://doi.org/10.1016/j.jes.2014.07.004, 2014.
Tobo, Y., DeMott, P. J., Hill, T. C. J., Prenni, A. J., Swoboda-Colberg, N. G., Franc, G. D., and Kreidenweis, S. M.: Organic matter matters for ice nuclei of agricultural soil origin, Atmos. Chem. Phys., 14, 8521–8531, https://doi.org/10.5194/acp-14-8521-2014, 2014.
Tomlin, J. M., Jankowski, K. A., Rivera-Adorno, F. A., Fraund, M., China, S., Stirm, B. H., Kaeser, R., Eakins, G. S., Moffet, R. C., Shepson, P. B., and Laskin, A.: Chemical imaging of fine mode atmospheric particles collected from a research aircraft over agricultural fields, ACS Earth Space Chem., 4, 2171–2184, https://doi.org/10.1021/acsearthspacechem.0c00172, 2020.
Torres, T. F. and Martínez, A. R.: Suelo agrícola en México: retrospección y prospectiva para la seguridad alimentaria, https://rde.inegi.org.mx/index.php/2019/01/25/suelo-agricola- en-mexico-retrospeccion-prospectiva-la-seguridad-alimentaria/ (last access: 26 August 2021), 2019 (in Spanish).
Welti, A., Lüönd, F., Stetzer, O., and Lohmann, U.: Influence of particle size on the ice nucleating ability of mineral dusts, Atmos. Chem. Phys., 9, 6705–6715, https://doi.org/10.5194/acp-9-6705-2009, 2009.
Yakobi-Hancock, J. D., Ladino, L. A., and Abbatt, J. P.: Review of recent developments and shortcomings in the characterization of potential atmospheric ice nuclei: focus on the tropics, Revista de Ciencias, 17, 15–34, 2013.
Zimmermann, F., Weinbruch, S., Schütz, L., Hofmann, H., Ebert, M., Kandler, K., and Worringen, A.: Ice nucleation properties of the most abundant mineral dust phases, J. Geophys. Res., 113, D23204, https://doi.org/10.1029/2008JD010655, 2008.
Zolles, T., Burkart, J., Häusler, T., Pummer, B., Hitzenberger, R., and Grothe, H. Identification of ice nucleation active sites on feldspar dust particles, J. Phys. Chem. A, 119, 2692–2700, https://doi.org/10.1021/jp509839x, 2015.