the Creative Commons Attribution 4.0 License.
the Creative Commons Attribution 4.0 License.
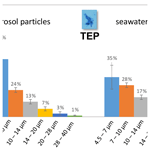
High number concentrations of transparent exopolymer particles in ambient aerosol particles and cloud water – a case study at the tropical Atlantic Ocean
Manuela van Pinxteren
Tiera-Brandy Robinson
Sebastian Zeppenfeld
Xianda Gong
Enno Bahlmann
Khanneh Wadinga Fomba
Nadja Triesch
Frank Stratmann
Oliver Wurl
Anja Engel
Heike Wex
Transparent exopolymer particles (TEPs) exhibit the properties of gels and are ubiquitously found in the world oceans. TEPs may enter the atmosphere as part of sea-spray aerosol. Here, we report number concentrations of TEPs with a diameter > 4.5 µm, hence covering a part of the supermicron particle range, in ambient aerosol and cloud water samples from the tropical Atlantic Ocean as well as in generated aerosol particles using a plunging waterfall tank that was filled with the ambient seawater. The ambient TEP concentrations ranged between 7×102 and 3×104 #TEP m−3 in the aerosol particles and correlations with sodium (Na+) and calcium (Ca2+) (R2=0.5) suggested some contribution via bubble bursting. Cloud water TEP concentrations were between 4×106 and 9×106 #TEP L−1 and, according to the measured cloud liquid water content, corresponding to equivalent air concentrations of 2–4×103 #TEP m−3.
Based on Na+ concentrations in seawater and in the atmosphere, the enrichment factors for TEPs in the atmosphere were calculated. The tank-generated TEPs were enriched by a factor of 50 compared with seawater and, therefore, in-line with published enrichment factors for supermicron organic matter in general and TEPs specifically. TEP enrichment in the ambient atmosphere was on average 1×103 in cloud water and 9×103 in ambient aerosol particles and therefore about two orders of magnitude higher than the corresponding enrichment from the tank study. Such high enrichment of supermicron particulate organic constituents in the atmosphere is uncommon and we propose that atmospheric TEP concentrations resulted from a combination of enrichment during bubble bursting transfer from the ocean and a secondary TEP in-situ formation in atmospheric phases. Abiotic in-situ formation might have occurred from aqueous reactions of dissolved organic precursors that were present in particle and cloud water samples, whereas biotic formation involves bacteria, which were abundant in the cloud water samples.
The ambient TEP number concentrations were two orders of magnitude higher than recently reported ice nucleating particle (INP) concentrations measured at the same location. As TEPs likely possess good properties to act as INPs, in future experiments it is worth studying if a certain part of TEPs contributes a fraction of the biogenic INP population.
- Article
(2537 KB) - Full-text XML
-
Supplement
(1040 KB) - BibTeX
- EndNote
In marine ecosystems, polymer gels and gel-like material play an important role in the biochemical cycling of organic matter (OM) (Passow, 2000, 2002b). One type of gel-like particles, transparent exopolymer particles (TEPs), have increasingly received attention. TEPs exist as individual particles rather than diffuse exopolymeric organic material and are operationally defined as particles that are stained on 0.2- or 0.4- µm pore-sized polycarbonate filters with the dye Alcian Blue (Passow, 2002b). TEPs have shown surface-active properties and are highly hydrated molecules (Passow, 2002a). Chemically, they consist of polysaccharide chains including uronic acids or sulphated monosaccharides that are bridged with divalent cations (mostly calcium) (Alldredge et al., 1993; Bittar et al., 2018).
In contrast to solid particles, TEPs have properties of gels; with similar constituents (carrageenans, alginic acid, and xanthan) to those that form gels, spontaneously forming from dissolved fibrillar colloids, and they can be broken up by calcium chelators such as EDTA. However, because TEPs have not yet been seen to undergo phase transition they can officially only be classified as gel-like particles (Verdugo et al., 2004). Regardless though, TEPs have been shown to be highly important in sedimentation processes and carbon cycling in the sea (Mari et al., 2017), as well as highly prevalent in the sea surface microlayer (SML) (Robinson et al., 2019a) with a potentially significant effect on air-sea release of marine aerosols.
Generally, TEPs can be formed via two pathways. First, the biotic pathway happens via a breakdown and secretion of precursor material or via a direct release as particles from aquatic organisms, e.g. as metabolic-excess waste products when nutrients are limited (Decho and Gutierrez, 2017; Engel et al., 2002, 2004). High TEP concentrations are usually associated with phytoplankton blooms, with the majority of precursor material being released by diatoms and to a lesser extent other plankton species. However, bacteria are also associated with TEP production, although their exact role is still not resolved (Passow, 2002a). Second, TEPs form through abiotic pathways. These could be formed spontaneously from dissolved organic precursors (e.g. dissolved polysaccharides) that are released by aquatic organisms. The abiotic formation is enhanced by turbulent or laminar shear (Engel et al., 2002; Passow, 2000). Recent studies confirmed that higher wind speeds, forming breaking waves, could be an effective transport and formation mechanism for TEPs to the ocean surface (Robinson et al., 2019b).
Transparent exopolymer particles are highly sticky and provide surfaces for other molecules and bacterial colonisation (Passow, 2002b), with between 0.5 % and 25 % (on average 3 %) of marine bacteria being attached to TEPs (Busch et al., 2017). TEPs naturally aggregate to other particles or highly dense matter and can sink in the ocean to contribute to downward carbon fluxes (Logan et al., 1995; Mari et al., 2017). However, TEPs that are not attached to sufficiently dense material will have a resulting low density and rise to the surface to form or stabilise the SML, which links the oceans to the atmosphere (Wurl and Holmes, 2008; Wurl et al., 2011).
From the ocean surface, TEPs have the potential to be transferred to the air. Owing to wind and breaking waves, sea-spray aerosol particles are formed (de Leeuw et al., 2011; Lewandowska and Falkowska, 2013; Liss and Johnson, 2014) that could be a transfer mechanism for TEPs from the ocean to the atmosphere. Recently, high TEP mass concentrations of 1.4 µg m−3 were reported in ambient marine aerosol particles measured in a size range between 0.1 and 1 µm, suggesting that gel-like particles can constitute more than half of the particulate OM mass (Aller et al., 2017).
Ocean-derived OM, of which TEPs are a part, has been reported to be enriched and selectively transferred (compared with sea salt) to the atmosphere (Facchini et al., 2008; Keene et al., 2007; van Pinxteren et al., 2017). Compared with seawater concentrations, OM in submicron aerosol particles is strongly enriched by factors of 103 and 104 (partly up to 105) (Quinn et al., 2015 and references therein) owing to processes (not yet resolved in detail) during the rise and burst of bubbles at the ocean surface (Blanchard, 1975). The enrichment of OM in supermicron aerosol particles is significantly lower, with average aerosol enrichment factors of 102 (Hoffman and Duce, 1976; Keene et al., 2007; Quinn et al., 2015). Aerosol enrichments have been studied for several organic compound groups such as lipids, carbohydrates, and proteins (e.g. Gao et al., 2012; Rastelli et al., 2017; Schmitt-Kopplin et al., 2012; Triesch et al., 2021a, b; Zeppenfeld et al., 2021). However, currently, data for TEP enrichment in the atmosphere are scarce. Aller et al. (2017) presented TEP mass concentrations in size-resolved aerosol particles and found them to contain more TEPs for submicron sizes than for larger sizes. Kuznetsova et al. (2005) reported TEP enrichment of a factor of 40 in freshly produced sea spray. Besides TEPs, other types of gel-like airborne particles in the size range of 100–300 nm (and even smaller) have been observed, e.g. in the Arctic atmosphere likely originating from the ocean surface (Bigg and Leck, 2008; Leck and Bigg, 2005a, b).
In addition to an oceanic transfer, atmospheric in-situ formation might contribute to OM abundance in the atmosphere. Ervens and Amato (2020) provided a framework to estimate the production of secondary biological aerosol mass in clouds by microbial cell growth and multiplication. It was recently shown that this pathway might represent a significant source of biological aerosol material (Ervens and Amato, 2020; Khaled et al., 2021; Zhang et al., 2021). In another recent study, cloud water in-situ formation of amino acids resulting from biotic and abiotic processes has been measured and modelled (Jaber et al., 2021). Moreover, a higher microbial enzymatic activity on the aerosol particles compared with seawater was observed and it was hypothesised that after ejection from the ocean, active enzymes can dynamically influence the OM concentration and composition of marine aerosol particles (Malfatti et al., 2019). Still, the atmospheric in-situ formation of important OM compounds and its importance has not been well investigated to date and no studies exist on atmospheric in-situ TEP formation.
Regarding the properties of ocean-derived OM in the atmosphere, its ability to act as cloud condensation nuclei (CCNs) (Orellana et al., 2011; Sellegri et al., 2021) or ice nucleating particles (INPs) (Burrows et al., 2013; Gong et al., 2020a; McCluskey et al., 2018a, b) is not well understood at present. Bigg and Leck (2008) and Leck and Bigg (2005b) demonstrated, based on morphology and chemical properties, that the biogenic particles collected in air and in the SML could be consistent with polymer gels. For regions that generally show a low total particle number concentration and low CCNs (such as the high Arctic), it was suggested that microgels are CCNs (Leck and Bigg, 2005a, b; Orellana et al., 2011), owing to their hydrated and hygroscopic nature and because of the absence of other significant aerosol particle sources.
In addition, oceanic biogenic INP sources have been discussed (Creamean et al., 2019; Hartmann et al., 2020; Wilson et al., 2015; Zeppenfeld et al., 2019). In regions, however, where other sources dominate, oceanic sources might not suffice to explain the INP population, and non-marine sources most likely contribute significantly to the local INP concentration (Gong et al., 2020a). According to their structure, biopolymers consisting of proteins, lipids, and higher saccharides have been shown to play a role in the ice-nucleating activity (Pummer et al., 2015). In this context, TEPs may provide excellent functionalities to act as INPs, as they form a 3D network where water molecules can attach, providing a structured surface for ice formation. A direct link between TEPs and INPs, however, has not yet been experimentally shown in field studies.
Within the present study, the number concentrations and size distributions of TEPs in the ambient atmosphere in the tropical Atlantic Ocean were elucidated. We aimed to investigate the TEP number concentrations in the ambient aerosol particles and cloud water and to derive connections to oceanic transfer and potential in-situ formation mechanisms. Finally, we compared the TEP number concentrations with recently published atmospheric INP number concentrations at the same location (Gong et al., 2020a) and analyse possible interconnections. To our knowledge, this is the first study with detailed measurements of TEP number size distribution in different atmospheric marine compartments within the tropical Atlantic environment.
2.1 Measurement site and ambient sampling
Samples were taken during the MarParCloud (“Marine biological production, organic aerosol particles and marine clouds: a process chain”) campaign that took place from 13 September to 13 October 2017 at the Cabo Verde archipelago island Sao Vicente located in the Eastern Tropical North Atlantic (ETNA). A detailed overview of the campaign, background, goals, and first results are available in van Pinxteren et al. (2020). Measurements were performed at the Cape Verde Atmospheric Observatory (CVAO) as described in more detail elsewhere (Triesch et al., 2021a, b; van Pinxteren et al., 2020). The CVAO is located directly at the shoreline at the northeastern tip of the São Vicente island at 10 m above seal level (a.s.l.) (Carpenter et al., 2010; Fomba et al., 2014). Owing to the trade winds, this site is free from local island pollution and provides reference conditions for studies of ocean–atmosphere interactions as there is a constant north-westerly wind from the open ocean towards the observatory. However, it also lies within the Saharan dust outflow corridor, and mainly in the winter months (January and February), dust outbreaks frequently occur.
Total suspended aerosol particles (TSPs) for TEP analysis and PM10 sampling for analysis of further aerosol constituents (inorganic ions, INPs, dust) was performed on top of a 30-m sampling tower of the CVAO. Tower measurements there mainly represent the conditions above the ocean because the internal boundary layer (IBL), which can form when air passes a surface with changing roughness (i.e. the transfer from open water to island), is mainly below 30 m (Niedermeier et al., 2014). During the MarParCloud campaign, the marine boundary layer (MBL) was well mixed as indicated by an almost uniform particle number size distribution within the MBL (Gong et al., 2020b; van Pinxteren et al., 2020). Information on the meteorological conditions during the sampling period is given in Table S1 in the Supplement.
The TSPs were sampled with a filter sampler consisting of a filter holder equipped with a 0.2-µm pore-sized polycarbonate (PC) filter mounted to a pump. The PC filters had been cleaned with 10 % HCl and rinsed with ultrapure water (resistivity = 18.2 MΩ cm) before application. Sampling usually took place for 24 h and the flow of the pump was between 5 and 10 L min−1 and frequently measured with a flowmeter. Total air volumes between 10 and 15 m3 were sampled. In seawater TEP analysis, filtration is usually performed at a gentle pressure of 0.2 bar (Engel, 2009) which corresponds to a max flow rate of 21 or 38 L min−1. The flow rate of aerosol sampling was max. 10 L min−1 and therefore TEP losses during aerosol particle sampling were not expected.
PM10 particles were sampled with a high-volume sampler (Digitel, Riemer, Germany) equipped with preheated (105 ∘C for 24 h) 150-mm quartz fibre filters (Munktell, MK 360) at a flow rate of 700 L min−1, described in detail elsewhere (van Pinxteren et al., 2020). The sampling times for TSPs as well as PM10 were usually set to 24 h.
Cloud water was sampled on Mt. Verde, which is the highest point of the São Vicente island (744 m), situated in the northeast of the island (16∘52.11′ N, 24∘56.02′ W) and northwest to the CVAO (van Pinxteren et al., 2020). Again, Mt. Verde experiences direct trade winds from the ocean with no significant influence of anthropogenic activities from the island (Carpenter et al., 2010). Bulk cloud water was collected using a compact Caltech Active Strand Cloudwater Collectors (CASCC2) equipped with acid-cleaned Teflon®strands (508 µm in diameter). Cloud droplets were caught on the strands and gravitationally channelled into an Nalgene bottle. The 50 % lower size cut for the CASCC2 is approximately 3.5 µm in diameter. Much of the liquid water content (LWC) in clouds is contained of drops between 10 and 30 µm in diameter and the CASCC2 is predicted to collect drops in this size range with an efficiency greater than 80 % (Demoz et al., 1996).
Three cloud water samples collected on 20 September 2017, 28 September 2017, and 4 October 2017 were analysed for the TEP number concentrations. They were filtered (150–200 mL) through 0.2-µm pore-sized filters for TEP analysis using the same filter type and conditions as applied for the aerosol particle staining. All equipment that was in contact with the cloud water samples (Teflon® strands, sampling bottles, filters) had been cleaned with 10 % HCl and rinsed with ultrapure water (resistivity = 18.2 MΩ cm) before each application, as recommended in Engel (2009).
2.2 Particle sampling from the plunging waterfall tank
To investigate a direct oceanic transfer of TEPs via bubble bursting, TSPs were sampled from a plunging waterfall tank experiment that is described in detail in the MarParCloud overview paper (van Pinxteren et al., 2020, Supplement section). The tank was designed to study the bubble-driven transfer of organic matter from the bulk water into the aerosol phase. It consists of a 1400 L basin with a 500 L aerosol chamber on top. The bubble-driven transport of organic matter was induced using a skimmer on a plunging waterfall. A stainless steel inlet was inserted into the headspace of the tank and connected with three filter holders for offline aerosol particle sampling without size segregation (TSPs). The filter system for TEP analysis was equipped with a 0.2-µm pore-sized, acid-cleaned polycarbonate (PC) filter mounted to a pump. Sampling usually took place for ∼24 h, the flow of the pump was between 5 and 10 L min−1 and frequently measured with a flowmeter. Total volumes between 9 and 10 m3 were sampled. The sampling procedure was therefore identical to the ambient TEP filter sampling. Another filter holder was equipped with a preheated 47-mm quartz fibre filter (Munktell, MK 360) for sodium analysis. The stainless steel inlet was additionally connected to a TROPOS-type Scanning Mobility Particle Sizer (Wiedensohler et al., 2012) for online aerosol measurements. This method of aerosol generation resulted in an efficient generation of nascent sea-spray aerosol particles with an aerosol particle size distribution centred around 100 nm (van Pinxteren et al., 2020).
2.3 Analysis
The filters obtained from ambient and tank-generated TSP aerosol particle sampling and cloud water filtrations were stained with 3 mL of an Alcian blue stock solution (0.02 g Alcian blue in 100 mL of acetic acid solution, pH 2.5) for 5 s yielding an insoluble non-ionic pigment and afterward rinsed with milliQ water. The dye Alcian blue consists of a macromolecule with a central copper phthalocyanine ring linked to four isothiouronium groups via thioether bonds (Passow and Alldredge, 1995). The isothiouronium groups are strong bases and account for the cationic nature. The exact staining mechanism is not resolved but it is believed that the cationic isothiouronium groups bond via electrostatic linkages (ionic bonds) with the polyanionic molecules of the TEP molecule; hence, the carboxylic and sulfonic side groups are stained. Alcian Blue can also react with carbohydrate-conjuncted proteins at proteoglycans, but not with nucleic acids and neutral biopolymers (Villacorte et al., 2015). After staining, the filters were kept at −20 ∘C and transported to the laboratories of TROPOS.
For microscopic analysis, the protocol following Engel (2009) was applied. In short, abundance, area, and size-frequency distribution of TEP were determined using a light microscope (Zeiss Axio Scope A.1) connected to a camera (ColorView III). Filters were screened at 200× magnification. About 10 pictures were taken randomly from each filter in two perpendicular cross-sections (five pictures each cross-section; dimension 2576×1932 pixels, 8-bit colour depth) and microscopic pictures of TEPs in cloud water are shown in Fig. 1. Images were then semi-automatically analysed using ImageJ (Version 1.44). A minimum threshold value of 16 µm2 was set for particle size during particle analysis to remove the detection of non-aggregate material by the programme. This resulted in a minimum particle size of 4.5 µm (assuming spherical particles).
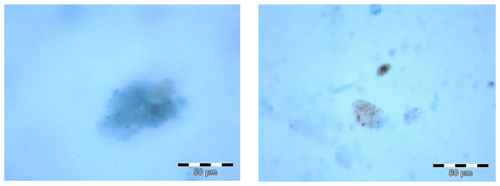
Figure 1Microscopic analysis of transparent exopolymer particles (TEPs) from the cloud water sample “WW5” (sampling interval: 28 September 19:30 LT–29 September 07:30 LT). Blue particles are TEPs, stained with Alcian Blue solution; brownish particles in the right picture are assumed to be dust particles. The scale refers to 50 µm.
Blank filters were taken for aerosol sampling (inserting filters in the aerosol sampler without probing them) and cloud water (filtering reagent water over a pre-cleaned filter), stained and treated the same way as the microscopic analysis. Blank number concentrations were on average 6 % of the cloud water results and between 5 % and 20 % for aerosol results and the blank values were subtracted from the samples.
The analysis of inorganic ions from PM10 samples was performed with ion chromatography and conductivity detection. Aqueous extracts of the aerosol samples were made by ca. 25 % of the PM10 filter in 1.5 mL ultra-pure water (resistivity = 18.2 MΩ cm) for 1 h. After the filtration (0.45-µm syringe filter) of the extracts sodium (Na+), calcium (Ca2+), magnesium (Mg2+), were analysed by using ion chromatography (Dionex ICS-6000, Thermo Scientific). The cations were separated in an isocratic mode (eluent: 36 mM methanesulfonic acid) on a Dionex IonPac CS16-4 µm column (2×250 mm) that was combined with a Dionex IonPac CG16-4 µm guard column (2×50 mm). The detection limits for the determined ions were between 5 and 20 µg L−1 (Zeppenfeld et al., 2021).
Non-sea-salt calcium was calculated from the ion ratio of in seawater of 0.038 (Turekian, 1968). Dust concentrations were estimated from the aerosol particle mass concentrations as the residual mass after the subtraction of all analytical concentrations from the PM10 mass, as described elsewhere (Fomba et al., 2014). Trace metal content was determined using a total reflection X-ray fluorescence S2 PICOFOX (Bruker AXS, Berlin, Germany) spectrometer equipped with a Molybdenum X-ray source (Fomba et al., 2013). The cloud LWC was measured using a particle volume monitor (PVM-100, Gerber Scientific, USA), which was mounted at the same height as the cloud water samplers.
Ice nucleating particle number concentrations (NINP) were measured using two droplet freezing techniques (LINA: Leipzig Ice Nucleation Array and INDA: Ice Nucleation Droplet Array) in different marine compartments. The uncertainties of NINP are given by the 5 % to 95 % confidence interval and the results are presented in Gong et al. (2020a).
All the samples of this study are summarised in Table 1. In addition to samples from the MarParCloud campaign, surface seawater samples obtained from the ETNA (Engel et al., 2020) were considered.
2.4 Enrichment factor
To determine enrichment or depletion of TEPs in the atmosphere (i.e. on the aerosol particles and in the cloud water) in relation to the TEP concentration in the ocean water, the concept of the aerosol enrichment factor can be applied. To this end, the concentration of the compound of interest in each compartment is related to the respective sodium mass concentration, as sodium is regarded as a conservative sea salt tracer transferred to the atmosphere in the process of bubble bursting (Sander et al., 2003). This concept is usually applied to calculate the enrichment of a compound in the aerosol particles (EFaer.) in relation to seawater (Quinn et al., 2015), but was recently extended to calculate the enrichment of organic compounds in cloud water (EFcloud) in relation to seawater (Triesch et al., 2021a). Therefore, the enrichment factor is defined as EFatm. (atmosphere enrichments factor) in Eq. (1).
For Eq. (1), TEP number concentrations were converted to TEP volume concentrations. To this end, for atmospheric and for oceanic samples, particle number concentrations of TEPs were extracted from the size distribution spectra and volume concentrations were calculated (assuming spherical particles). More detail on the conversion can be found in the Supplement (Tables S2–S5).
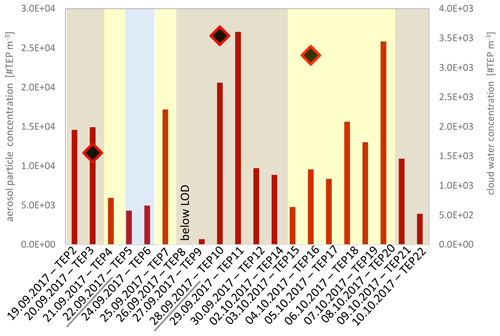
Figure 2Transparent exopolymer particle (TEP) number concentrations in the aerosol particles (red bars) and in the three cloud water samples (black-red squares). TEP concentrations were below the limit of detection (LOD) on 26 September 2017. The backgrounds represent the dust classification according to the ambient dust concentrations (blue: dust <5 µg m−3 marine conditions; yellow: dust < 20 µg m−3 low dust; brown: dust < 60 µg m−3 moderate dust). From underlined dates (22 September – > “TEP5” and 28 September 2017 – > “TEP10”) TEP number size distributions were measured.
3.1 Concentration and size distribution of TEPs
Within the 3-week sampling period, TEPs varied within one order of magnitude between 7×102 and 3×104 #TEP m−3 in the aerosol particles and between 4×106 and 9×106 #TEP L−1 in the cloud water (analysed diameter size range: ∼4.5 to ∼30 µm) as shown in Fig. 2. The cloud water concentrations were converted to atmospheric concentrations using the measured LWC of the cloud water (0.39 g m−3) and resulted in concentrations of 2–4×103 #TEP m−3 (Table S4). Comparing the #TEP concentrations in cloud water with those in the ambient aerosol particles suggested that about 20 % of the ambient TEP particles might be activated to cloud droplets when a cloud forms.
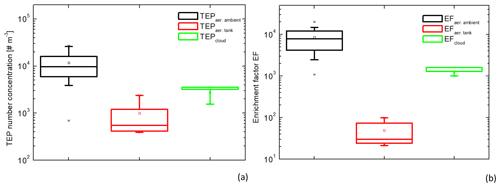
Figure 3Box and whisker plot of the transparent exopolymer particle (TEP) number concentrations (a) and the enrichment factors (b) in the ambient (n=18) and tank-generated (n=4) aerosol particles and in the cloud water samples (n=3). Each box encloses 50 % of the data with the mean value represented as an open square and the median value represented as a line. The bottom of the box marks the 25 % limit of the data, whereas the top marks the 75 % limit. The lines extending from the top and bottom of each box are the 5 % and 95 % percentiles within the data set, whereas the asterisks indicate the minimum and maximum values.
In addition, TEPs were measured in four aerosol particle samples from the plunging waterfall tank and the concentrations varied between 4×102 and 3×103 #TEP m−3 (Table S3). Although the TEP concentrations in ambient aerosol particles and cloud water were not significantly different (ANOVA, one-way, p=0.054 at a 0.05 level), the tank-generated TEP concentrations were significantly lower than the ambient aerosol TEP concentrations (ANOVA, one-way, p=0.004 at a 0.05 level). The TEP number concentrations measured in the different atmospheric compartments, the ambient aerosol particles, the tank-generated aerosol particles, and the cloud water are summarised in Fig. 3a and the individual values are presented in the Tables S2–S4.
In addition to the total number concentrations, TEP number size distribution was derived from all ambient aerosol particle samples and are shown in Fig. 4a–d in both, linear and logarithmic form. In addition, the TEP number size distribution of one cloud water sample is presented in Fig. 4e and f. All samples exhibited very similar trends in their size distribution, with higher number concentrations for smaller sizes.
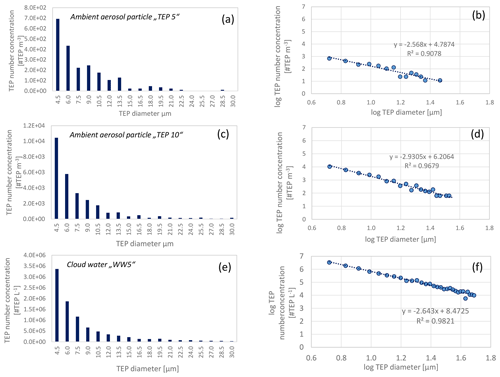
Figure 4Transparent exopolymer particle (TEP) number size distribution in the aerosol particles and cloud water in linear and logarithmic form; panels (a) and (b) show aerosol particle sample “TEP 5” (sampling start: 22 September 2017), panels (c) and (d) show aerosol sample “TEP 10” (sampling start: 28 September 2017), panels (e) and (f) show cloud water sample “WW5” (sampling interval: 28 September 19:30 LT–29 September 07:30 LT). The lower limit of the resolution of the microscope was 16 µm2 resulting in a particle diameter of 4.5 µm (assuming spherical particles). Each bar in (a), (c), and (e) represents the summed particle number concentrations (within 1.5 µm bins), e.g. the first column represents the summed concentrations between 4.5 and 6 µm.
From the observed size distributions, it can be assumed that the number concentrations will continue to increase towards smaller sizes. A comparison of TEP number concentrations in the ambient aerosol particles or cloud water with literature values is challenging owing to the availability of very few studies and different sample types and size ranges regarded in different studies. However, the trend in the TEP number size distributions observed here is consistent with studies by Kuznetsova et al. (2005) showing increased TEP concentrations in simulated sea spray regarding particle sizes from 50 µm to 10 µm in diameter. In addition, TEP mass concentrations showed a similar trend, with higher concentrations towards smaller particle sizes (size range 0.1–1 µm, Aller et al., 2017), that was, however not as pronounced as for the TEP number concentrations observed here.
Regarding polymer gels in general, a strong increase with decreasing sizes was observed in cloud water in the high Arctic (north of 80∘ N) in late summer using a very sensitive microscopic technique with epifluorescence (Orellana et al., 2011). 2×109 micrometre-sized polymer gels per mL−1 and 2–6×1011 nanometre-sized polymer gels per mL−1 were observed and the majority of the particles were smaller than 100 nm (Orellana et al., 2011). The measurements from Orellana et al. (2011) regarded a much smaller particle diameter range (down to the nanometre scale) than the present work and are therefore not directly comparable. However, from the logarithmic TEP number concentration vs. diameter relationship (Fig. 4) we calculated TEP number concentrations for smaller particle ranges (sub-micrometre size range). TEP number concentrations between 4.2×104 #TEP m−3 (low “TEP5” case, equation from Fig. 4b) and 1.6×106 #TEP m−3 (high “TEP10” case, equation from Fig. 4d) are calculated for 1-µm large particles. The high but varying concentrations for the two cases underlines the need for more measurements in the submicron range to derive robust numbers. Similarly, a concentration of 3.0×108 #TEP L−1 for 1-µm large particles in cloud water were calculated and 2.1×1010 #TEP L−1 for 200-nm large particles might exist in the submicron-size range (following the equation from Fig. 4f).
These calculations show that the number of gel-like particles in the high Arctic was still several orders of magnitude higher than TEP particles in the tropical Atlantic, e.g. 1010 #TEP L−1 (200-nm particles) in tropical cloud water observed here vs. 1011 #polymer gels per mL−1 (=1014 #polymer gels per L−1) from Orellana et al. (2011). If the TEPs in the tropical atmosphere comprise only a small subgroup of the total polymer gel number, or if the total amount of gel-like particles is generally higher in polar regions remains to be investigated.
3.2 Relating atmospheric TEPs to the ocean
From a recent study of TEP number concentrations in different oceanic regions, TEP number concentrations in surface waters (10-m depth) of the East Tropical North Atlantic (ETNA) were obtained (Engel et al., 2020). ETNA is the region that geographically includes the Cabo Verde islands. The oceanic TEP number concentrations are shown in Fig. 5. The TEPs in the ocean showed a similar size distribution to the TEPs in the atmosphere (i.e. aerosol particles and cloud water, Fig. 4) with increasing TEP number concentrations towards smaller particle sizes (Table S5 and more details in Engel et al., 2020).
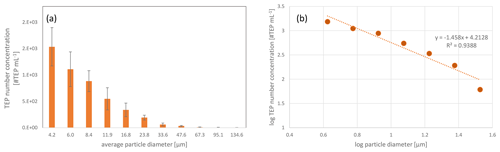
Figure 5Transparent exopolymer particle (TEP) number size distributions in the ocean surface water (sampling depth: 10 m) from the East Tropical North Atlantic (ETNA), averaged three stations from Engel et al. (2020). The data in this figure show the size distribution between ∼5 and ∼30 µm, matching the investigated aerosol size range (Fig. 4). The whole size spectrum is shown in Table S5.
A detailed comparison of #TEPs in the ocean and in the atmosphere regarding the identical size bins showed that the #TEP distribution among the different size bins was much more balanced for seawater than for aerosol particles. In aerosol particles, on average 52 % of the #TEPs were located in the smallest size bin analysed (4.5–7 µm) and show a sharp decrease towards the second size bin (which contained 24 % of the TEPs) (Fig. 6). For the seawater TEPs, however, around 35 % of the #TEPs were found in the first size bin and the relative contribution decreased uniformly towards the larger size bins (Fig. 6). This distribution is also visible in the correlation curves of Figs. 4b, d, f, and 5b. The correlation curves for the aerosol particles (and cloud water) have a steeper slope than the curve obtained for seawater TEPs. This could imply that (i) the transfer of TEPs from the ocean to the atmosphere is most efficient for small size ranges, (ii) larger TEPs are converted to smaller TEPs in the atmosphere (e.g. break down), and/or (iii) atmospheric in-situ formation mechanism of TEPs preferably occur in smaller particle size ranges. These considerations will be further evaluated in Sect. 3.3.
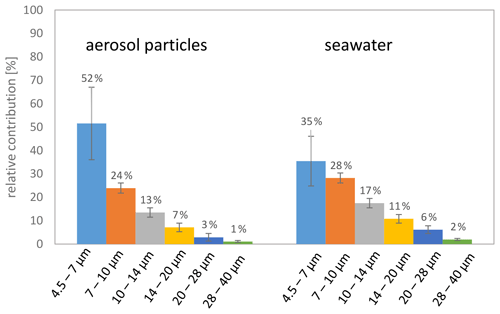
Figure 6Relative contribution of the transparent exopolymer particle number concentrations in the aerosol particles (left) and in the ocean surface water (right) regarding the identical size bins.
To compare seawater and atmospheric TEP concentrations in terms of enrichment or depletion, the atmospheric enrichment factor EFatm. (Eq. 1) was calculated. However, the TEP number concentrations in the ocean surface water were obtained from an additional measurement campaign, taking place in the biologically productive Mauritanian Upwelling region in the year 2012, hence, at another time and season (Table 1). Compared with other oceanic regions, the TEP values from the Mauritanian Upwelling region were at the higher end (Engel et al., 2020). The region around the CVAO is rather oligotrophic and chlorophyll-a values during the MarParCloud campaign were relatively low with 0.1 up to 0.6 µg L−1 (van Pinxteren et al., 2020). As TEP production is often connected to phytoplankton activity, the TEP concentration at the CVAO might be lower than that of more productive regions (Robinson et al., 2019a). A previous study showed that the total TEP number concentrations (covering TEP sizes between 1 and 200 µm) at the Cabo Verde islands (south of São Vicente at 16∘44.4′ N, 25∘09.4′ W) were by a factor of 2 lower than the data reported here, in detail 0.9×107 L−1 (Engel et al., 2015) vs. 2×107 L−1 (Table S5). Lower TEP concentrations would result in higher EFatm. (Eq. 1) regarding the ambient as well as the tank measurements as the same type of seawater was used for the calculations. Hence, the EFatm. reported here represent lower limits.
In order to compare the same TEP diameters in all compartments, the size range between 5 µm (lower limit for atmospheric measurements) and 10 µm (typical upper limit for ambient aerosol particles) was regarded and converted from number to volume concentration (more details in Tables S2–S4 and Fig. S1 in the Supplement). For ocean water, TEP number concentrations of 3.5×103 #TEP mL−1 (= 3.5×106 #TEP L−1) and a TEP volume concentration of 4.6×105 µm3 TEP mL−1 (= 3.5×108 µm3 TEP L−1) were obtained. The respective values for the TEP volume concentration of ambient and tank-generated aerosol particles, as well as for the cloud water are listed in Tables S2–S4 and illustrated in Fig. 3b. As mentioned above, the factors given here are subject to some uncertainties and represent lower limits. An error discussion is introduced in the Supplement as an appendix to Table S2. It is clearly visible that the EFaer.ambient are significantly higher than the EFaer.tank (ANOVA, one-way, p=0.0017 at a 0.05 level) with average values of 9×103 and 50, respectively. The average EFcloud was 1×103. This means that the enrichment of TEPs derived from the plunging waterfall tank, representing the bubble-bursting transfer, is about two orders of magnitude lower than the enrichment of TEPs in the ambient aerosol particles.
It should be noted that the lower enrichment in the tank resulted from the lower TEP number concentrations in the generated aerosol particles, as the sodium concentrations in the tank aerosol were even higher than in the ambient particles (Table S3). This suggests that, although an artificial tank study cannot represent the ambient environment, the generation of sea-spray aerosol was in progress; however, TEP transfer seemed not to be pronounced.
In the following, the enrichment factors obtained here will be discussed in more detail considering studies available from the literature.
Atmospheric enrichment of ocean-derived OM has often been reported (e.g. Facchini et al., 2008; Keene et al., 2007; O'Dowd et al., 2004; Schmitt-Kopplin et al., 2012; Triesch et al., 2021a, b; van Pinxteren et al., 2017). Submicron particles are usually strongly enriched with OM with aerosol enrichment factors EFaer. of 103 up to 105 (Quinn et al., 2015 and references therein). The enrichment in supermicron aerosol particles is, however, significantly lower. Laboratory studies showed enrichment of OM in the order of 102 (Hoffman and Duce, 1976; Keene et al., 2007; Quinn et al., 2015). From the MarParCloud campaign, enrichment factors of free amino acids were between 10 and 30 in ambient supermicron particles (Triesch et al., 2021a). Kuznetsova et al. (2005) reported TEP enrichments in freshly produced sea spray with EF based on TEP number concentration. Consequently, the EFaer. tank (50±35) reported here are in line with published enrichment factors for OM in general and TEPs specifically. However, the EFaer.ambient (9×103) were orders of magnitude higher than reported enrichment factors for supermicron aerosol particles. Enrichment factors of OM in cloud water are scarce; we recently reported an enrichment of 103–104 for free amino acids in cloud water from the MarParCloud campaign (Triesch et al., 2021a) that was higher than the EFcloud observed here.
The concept of the aerosol enrichment factor originates from controlled tank experiments where a direct transfer of compounds from the ocean via sea-spray aerosol formation occurs. Obviously, this does not automatically correspond to the ambient environment as mixing processes, ageing, and further transformation reactions are not accounted for. However, the EFaer.ambient, which is much bigger than the EFaer.tank, and the comparison of EFcloud towards former literature data, clearly show the presence of significantly more TEPs in ambient aerosol and cloud water than in oceanic seawater, which will be discussed in detail in the following section.
3.3 Possible sources and atmospheric formation pathways of TEPs
3.3.1 Primary TEP sources
The high abundance of TEPs in the aerosol particles and cloud water may correspond to an oceanic transfer within the process of bubble bursting. To investigate linkage to the bubble-bursting transfer, TEP concentrations were correlated with the wind speed, as well as with the sea-spray tracers sodium and magnesium. To account for biases due to a number-based (TEPs) and mass-based (sodium, magnesium) comparison, the particle volume of TEPs was calculated from the particle number concentrations (regarding the size range: 5–10 µm). To this end, from each particle diameter within a size range of 5–10 µm, the respective volume was determined, assuming spherical particles, and summed up (data in Table S2). This transformation accounts for the fact that large TEPs likely possess a large mass but a low number concentration and vice versa.
Reasonably good correlations of TEPs with sodium, sea-salt calcium (Cass), and magnesium, (R2=0.5, Fig. 7a–c) were found, suggesting some connection to a bubble-bursting transfer. However, no correlation of TEPs with wind speed was found. It may be that as wind speed data represented an average value of 24 h, short but pronounced changes in the wind speed were not visible in the average wind speed value. No correlation was found between TEPs and non-sea-salt calcium or total calcium (Fig. 7d).
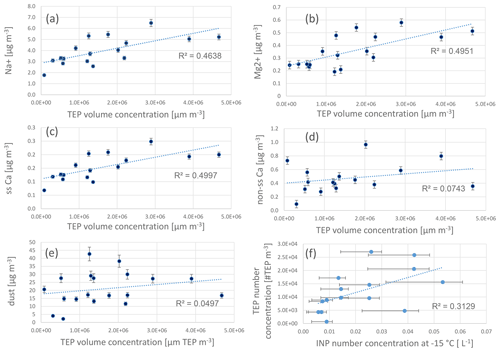
Figure 7Correlations of transparent exopolymer particle (TEP) volume concentrations (size range: 5–10 µm) with chemical parameters (inorganic constituents PM10) and dust (PM10), as well as correlation of TEP number concentration and ice nucleating particle (INP) number concentrations. Inorganic constituents were measured with ion chromatography and dust concentrations were derived from PM10 concentrations as reported elsewhere (Fomba et al., 2013; van Pinxteren et al., 2020). Measurements of INP number concentrations and error bars are explained in Gong et al. (2020a).
Despite the correlation of TEPs with sea-spray tracers, the high abundance and enrichment of #TEPs in the ambient aerosol particles compared with literature data and compared with the concentration and enrichment of the #TEPs from the plunging waterfall tank performed here, suggests that additional (secondary) TEP sources in the ambient atmosphere might exist from which TEPs are added to their primary transfer by bubble bursting from the oceans. At the Cabo Verde islands, besides the ocean, mineral dust is an important aerosol particle source (Fomba et al., 2014). TEPs are generally attributed to be ocean-derived compounds; however, dust has often been reported to transport attached biological particles (Maki et al., 2019; Marone et al., 2020). During the MarParCloud campaign, dust influences were low to moderate and the aerosol particle mass was found to be predominantly of marine origin (Fomba et al., 2014; van Pinxteren et al., 2020). Some dust influences were visible though, e.g. variations in the particle number concentrations, with elevated concentrations on (even low) dust-influenced air masses (Gong et al., 2020b). TEP number concentrations showed no clear connection to the ambient dust concentrations (Fig. 2). Within periods of moderate dust, TEP were partly below the detection limits (on 26 September 2017) and partly exhibited high concentrations (e.g. on 28 and 29 September 2017). No correlation between TEP and dust was found (R2=0.05, Fig. 7e); therefore, we do not consider dust to be a transport medium for TEPs to the particles or cloud water. However, dust may play a role in abiotic in situ TEP formation, as discussed in the section “Abiotic formation”.
3.3.2 In-situ formation
Abiotic formation
In aquatic environments, abiotic TEP formation has been reported to happen via several pathways, including spontaneous assembly from TEP precursors (Passow, 2002b). The aerosol particle and cloud water samples from the MarParCloud campaign investigated here showed high mass concentrations of amino acids (up to 6.3 ng m−3 in the submicron aerosol particles and up to 490 ng m−3 in the cloud water, published in Triesch et al., 2021a) as well as dissolved polysaccharides (up to 2 ng m−3 in the submicron aerosol particles and up to 2400 ng m−3 in the cloud water, results in preparation for publication). In the ocean, the dissolved polysaccharides are known TEP precursors (Passow, 2002b) and Wurl et al. (2011) determined abiotic TEP formation rates from dissolved polysaccharide concentration in various oceans. The rates were on average 7.9±5.0 µmol C L−1 d−1 and therefore significant considering that the average TEP concentration was 18.1±15.9 µmol C L−1 and the average dissolved polysaccharide concentration was 12.2±3.8 µmol C L−1 in the surface seawater (Wurl et al., 2011). Robinson et al. (2019b) showed that rising bubbles can lead to enhanced TEP formation after only a few minutes. The lifetime of supermicron aerosol particles, to which the TEPs studied here belong, is between hours and days, for example, Madry et al. (2011) calculated an average lifetime of supermicron sea salt particles of 50 h. Hence, abiotic TEP formation processes lie within the lifetime of supermicron aerosol particles and we suggest that spontaneous TEP formation from the (highly) abundant dissolved polysaccharides likely contributed to the high TEP concentrations observed in the ambient atmosphere in the present study. However, it needs to be considered that the abiotic TEP formation processes described by Wurl et al. (2011) and Robinson et al. (2019b) were relevant for the oceanic environment and might not be directly translated to atmospheric processes. Further studies are required on this topic.
Another important parameter likely impacting TEP formation is the presence of mineral dust. As already discussed above, dust mass concentrations were low to moderate, though not negligible, during the MarParCloud campaign. In laboratory minicosm studies, the addition of dust to oceanic water resulted in an acceleration of the kinetics of TEP formation leading to the formation of fast sinking particles (Louis et al., 2017). This process likely happens because of particle aggregation, meaning that dissolved OM and dust aggregate to form TEPs (Louis et al., 2017). In addition, dust particles in cloud water may promote turbulence, which, in aquatic media, has been suggested to enhance abiotic TEP formation (Passow, 2002b). The dust deposition at Cabo Verde has been recognised as a potentially large contributing factor to the TEP enrichment in the SML (Robinson et al., 2019a). Here, we speculate that even low concentrations of mineral dust can influence TEP formation on the aerosol particles and in the cloud water. This is further supported by the microscopic detection of dust in the cloud water (Fig. 1), that likely enhance the possibility that particles in the cloud water collide and stick. Consequently, although dust did not seem to serve as a transport medium for TEPs (see Sect. 3.3.1), dust may contribute to in-situ TEP formation in cloud water due to abiotic particle aggregation.
From atmospheric studies, marine gel particles have been reported to undergo a volume phase transition in response to environmental stimuli, such as pH and temperature, as well as cleavage of their polymers owing to ultraviolet (UV) radiation (Orellana et al., 2011). UV radiation can break down microgels in the ocean into a high number of smaller (nano-sized) particles (Orellana and Verdugo, 2003) – a mechanism that is expected to be highly relevant in the atmosphere where UV radiation is higher than in seawater. Furthermore, it has been shown that a lowering of the pH from neutral conditions (7 or 8) to 4.5 causes a sudden transition of gel particles in which the polymer network collapsed to a dense, non-porous array (Chin et al., 1998). As TEPs are reported to exhibit a gel-like character (Passow, 2002b), volume and number concentrations might be affected by the different factors such as pH, ion density, temperature, and pressure in the atmosphere. The measured cloud water pH value of the samples analysed here was between 6.3 and 6.6, at which marine gels could split into smaller units (Chin et al., 1998). Hence, a part of the cloud water TEPs might be below the minimum detectable particle size of 4.5 µm owing to the slightly acidic conditions. This could explain the lower concentrations in cloud water (2–4×103 #TEP m−3) compared with ambient aerosol particles (7×102–3×104 #TEP m−3). Hence, the different environmental stimuli likely impact atmospheric TEP formation and may lead to the formation of smaller particles. However, from our data we cannot fully explain the role of each of these effects and such investigations warrant further studies.
Biotic formation
Besides abiotic pathways, in aqueous media, TEPs can be directly released as particulates from aquatic organisms involving phytoplankton and bacteria (Passow, 2002a). Biotic TEP formation has by now been studied for seawater and lakes (Passow, 2002a); however, bacteria are also present in the atmosphere, are likely transferred from the ocean via sea spray (Rastelli et al., 2017), and can survive in cloud droplets (Deguillaume et al., 2020). The bacterial abundance in cloud water samples taken at Mt. Verde during the MarParCloud campaign ranged between 0.4 and 1.5×105 cells mL−1 (van Pinxteren et al., 2020). This concentration is one to two orders of magnitude higher than the TEP concentrations. The bacterial tracer muramic acid (Mimura and Romano, 1985) was detected in the aerosol particles and cloud water samples here in considerable concentrations (∼25 nM, data not shown), strongly suggesting bacterial activity in cloud water. We cannot derive conclusions on the origin of the bacteria measured in cloud water reported here, however the transfer of bacteria from the ocean to the atmosphere has been shown before (Rastelli et al., 2017; Uetake et al., 2020). TEPs are known to be closely connected to bacteria in different ways (Passow, 2002a, b); therefore, the presence of bacteria in the atmosphere exhibits a potential source of cloud water TEPs observed here. Furthermore, TEPs are strongly colonised by bacteria (Busch et al., 2017; Zäncker et al., 2019). Hence, TEPs can be a transfer vector for bacteria from the ocean to the atmosphere and/or act as a medium for bacterial colonisation in marine clouds.
The presence of active enzymes on ambient aerosol particles (enriched compared with seawater), and therefore biogenic in-situ cycling of OM through enzymatic reactions in atmospheric particles, was recently suggested (Malfatti et al., 2019). This is in line with the findings that the aerosol particles and cloud water from the MarParCloud campaign contained high concentrations of OM (amino acids, lipids), assumed to be connected to a biogenic formation (Triesch et al., 2021a, b). A combined approach of laboratory experiments and modelling recently underlined the importance of biotic (and abiotic) formation processes of OM in clouds (Jaber et al., 2021).
Regarding time scales of biotic processing, Matulova et al. (2014) showed that the Bacillus sp. 3B6 isolated from cloud water was able to bio-transform saccharides that are present in the atmosphere. The saccharides formed exopolymer substances (EPS), of which TEPs are a subgroup. The formation of EPS was revealed after 48 h of incubation and therefore within the lifetime of supermicron aerosol particles (Madry et al., 2011).
Considering recent literature and the data reported here, we suggest that in-situ TEP formation related to biogenic processes and likely connected to bacteria, as reported for seawater, might be important in the marine atmosphere as well. Besides, although not measured here, microalgae and cyanobacteria, which are relevant for direct TEP formation in seawater, have been reported to occur in the atmosphere (e.g. Lewandowska et al., 2017; Sharma et al., 2007; Wiśniewska et al., 2019, 2022). It is worth studying whether these species and their metabolic degradation products contribute to atmospheric TEP processing.
3.4 Connecting TEPs and INPs
Different kinds of ice-nucleating macromolecules have been found in a certain range of biological species and consist of a variety of chemical structures including proteins, polysaccharides (Pummer et al., 2015), and lipids (DeMott et al., 2018). TEPs, consisting of polysaccharidic chains bridged with divalent cations, may therefore possess good properties to act as INPs; however, such a link has not yet been shown in field experiments.
During the MarParCloud campaign INP number concentration (NINP) was measured in different marine compartments and the results are presented in Gong et al. (2020a). By combining INP concentration in the seawater, aerosol particles, and cloud water, it was found that NINP in the atmosphere was at least four orders of magnitude higher than what would be expected if all airborne INPs were to originate from sea spray. The measurements indicated that other sources besides the ocean, such as mineral dust or other long-ranged transported particles, contributed to the local INP concentration. However, some indications for contributions of biological particles to the INP population were obtained (details in Gong et al., 2020a). Nevertheless, the sources of INPs could not be revealed in detail.
In the present study, quantitative INP data (presented in Gong et al., 2020a) and TEP data measured from the same campaign were compared. To this end, INP concentrations achieved from PM10 quartz-fibre filters taken at the CVAO during the same period as the TSP filters were compared with the TEP measurements. In addition, cloud water INP and TEP data obtained from the same samples were combined.
Transparent exopolymer particle number concentrations were on average between 103 and 104 m−3 in the ambient aerosol particles, whereas INP number concentrations at −15 ∘C were between 10 and 102 m−3 (Gong et al., 2020a). It is interesting to note that the TEP concentrations in the ambient aerosol particles were about two orders of magnitude higher than the INP concentrations. Similar findings were obtained for the cloud water comparisons; TEP concentrations (∼106 L−1) were on average two orders of magnitude higher than INP number concentrations at −15 ∘C in cloud water (∼104 L−1) (Gong et al., 2020a).
The correlation between INP (active at −15 ∘C) and TEP concentrations was weak, with R2=0.3 (Fig. 7f), showing that a direct link between INP and the entire TEP number concentrations was not very pronounced. It needs to be underlined that TEP concentrations below a particle size of 4.5 µm are not included here and according to the size distribution, the TEP concentrations are increasing towards smaller sizes. Most of the TEPs reported here were in the supermicron size range between ∼4.5 and 14 µm (Fig. 4). However, the biologically active NINP at Cabo Verde was mainly present in the supermicron mode (>1 µm) (Gong et al., 2020a); hence, a comparison with the TEP particle concentrations above 5 µm seems justified. Nevertheless, future studies should concentrate on the exact same size ranges for TEPs and INPs.
The INP functionalities of biomolecules are not straightforward and whether a macromolecule acts as an INP is dependent on many factors, such as its size, the proper position of functional groups, and their allocation (Pummer et al., 2015). Typically, not the entire surface of an INP but rather specific areas (active sites) participate in ice nucleation. This means that despite TEPs likely providing INP properties, only a fraction of TEPs, if any, may be able to act as INPs. This hypothesis is supported by the findings that marine gels exhibit hydrophobic and hydrophilic surface-active segments, strongly suggesting a dichotomous, non-uniform behaviour of polymer gels (Leck et al., 2013; Orellana et al., 2011; Ovadnevaite et al., 2011). As mentioned in the sections “Abiotic formation” and “Biotic formation”, TEPs are often attached to, or colonised with bacteria. Bacteria themselves have been shown to provide excellent INP functionalities (Pandey et al., 2016) and TEPs may act as a carrying medium for INPs, such as bacteria. Bacteria concentrations were higher than TEP concentrations and also higher than INP concentrations. However, only a fraction of all bacteria (0.5 %–25 %) is associated with TEPs and, vice versa, not all TEPs are colonised by bacteria (Passow, 2002b). There is an indication that especially in oligotrophic waters, as the Cabo Verde islands are, the fraction of bacteria attached to TEPs is comparably low (Schuster and Herndl, 1995). Hence, the concentration range of bacteria-colonised TEPs in relation to INPs is worth further consideration. This may help to unravel whether a functional relationship between bacteria-colonised TEPs and INPs exists and whether a certain number of TEPs contain fragments in the biological INP population that, beyond dust, play a role in the Cabo Verde atmosphere.
This study presented TEP number concentrations > 4.5 µm in ambient atmospheric samples from the tropical Atlantic Ocean during the MarParCloud campaign, as well as in generated atmospheric particles using a plunging waterfall tank. The atmospheric TEPs showed a similar size distribution compared with the TEPs in the ocean, with increasing TEP number concentrations toward smaller particle sizes; however, the #TEP distribution among the different size bins was much more balanced for seawater than for aerosol particles, where half of the #TEPs were located in the smallest analysed size bin (4.5–7 µm). Based on Na+ concentrations in seawater and the atmosphere, the enrichment of TEPs in the tank-generated aerosol particles was in line with another study. The TEP enrichments in the ambient atmosphere were, however, up to two orders of magnitude higher than those in the tank study and such high values are thus far not reported for supermicron aerosol particles. We speculate that the high enrichment of TEPs in the particles and in cloud water result from a combination of enrichment during bubble-bursting transfer from the ocean and secondary in-situ atmospheric formation. We propose that similar (biotic and abiotic) formation mechanisms reported for TEP formation in (sea)water might take place in the atmosphere as well, as the required conditions (e.g. high concentrations of dissolved TEP precursors such as polysaccharides, presence of bacteria in the cloud water) were given. An assessment of the importance of the biotic versus the abiotic TEP formation pathways in the atmosphere, however, needs further investigations. TEP concentrations in the atmosphere were two orders of magnitude higher than INP concentrations in the aerosol particles and cloud water respectively. However, only a part of the TEP population, assumingly that colonised by bacteria, might contribute to INP population, and are worth further study. Finally, although dust may be a dominant INP source in the tropical Atlantic region close to the Saharan desert investigated here, in other remote oceanic locations, marine gel particles, their in-cloud formation and connection to bacteria and phytoplankton in the atmosphere could be highly relevant for a better understanding of marine cloud properties.
The TEP data are accessible under the following link https://doi.org/10.1594/PANGAEA.938169 (van Pinxteren, 2021). INP concentrations are accessible under the following link: https://doi.org/10.1594/PANGAEA.906946 (Gong et al., 2019).
The supplement related to this article is available online at: https://doi.org/10.5194/acp-22-5725-2022-supplement.
MvP led the MarParCloud campaign and, together with the campaign participants KWF, XG, EB, NT, BR, FS and HW performed the aerosol particle and could water sampling at the Cabo Verde islands. HH contributed to the campaign conceptualisation, coordination towards the CVAO and ACD, and manuscript development and revision. EB designed and operated the plunging waterfall tank. BR performed the microscopic TEP measurements and XG performed the INP analysis. AE contributed the seawater TEP data. MvP performed the data interpretation with help from SZ and BR. MvP wrote the paper with contributions from all authors.
The contact author has declared that neither they nor their co-authors has any competing interests.
Publisher's note: Copernicus Publications remains neutral with regard to jurisdictional claims in published maps and institutional affiliations.
This article is part of the special issue “Marine organic matter: from biological production in the ocean to organic aerosol particles and marine clouds (ACP/OS inter-journal SI)”. It is not associated with a conference.
We acknowledge the funding by the Leibniz Association SAW in the project “Marine biological production, organic aerosol particles and marine clouds: a Process Chain (MarParCloud)” (SAW-2016-TROPOS-2), the Research and Innovation Staff Exchange EU project MARSU (69089) and the Deutsche Forschungsgemeinschaft (DFG, German Research Foundation) – Projektnummer 268020496 – TRR 172, within the Transregional Collaborative Research Center “ArctiC Amplification: Climate Relevant Atmospheric and SurfaCe Processes, and Feedback Mechanisms (AC)3” in the sub-project B04. We thank the CVAO site manager Luis Neves as well as René Rabe and Susanne Fuchs for technical and laboratory assistance. We further acknowledge the professional support provided by the Ocean Science Centre Mindelo (OSCM) and the Instituto do Mar (IMar).
This research has been supported by a Leibniz-Society SAW grant (MarParCloud) under SAW-2016-TROPOS-2.
The publication of this article was funded by the Open Access Fund of the Leibniz Association.
This paper was edited by Kimitaka Kawamura and reviewed by three anonymous referees.
Alldredge, A. L., Passow, U., and Logan, B. E.: The abundance and significance of a class of large, transparent organic particles in the ocean, Deep-Sea Res. Pt. I, 40, 1131–1140, https://doi.org/10.1016/0967-0637(93)90129-q, 1993.
Aller, J. Y., Radway, J. C., Kilthau, W. P., Bothe, D. W., Wilson, T. W., Vaillancourt, R. D., Quinn, P. K., Coffman, D. J., Murray, B. J., and Knopf, D. A.: Size-resolved characterization of the polysaccharidic and proteinaceous components of sea spray aerosol, Atmos. Environ., 154, 331–347, https://doi.org/10.1016/j.atmosenv.2017.01.053, 2017.
Bigg, E. K. and Leck, C.: The composition of fragments of bubbles bursting at the ocean surface, J. Geophys. Res.-Atmos., 113, D11209, https://doi.org/10.1029/2007jd009078, 2008.
Bittar, T. B., Passow, U., Hamaraty, L., Bidle, K. D., and Harvey, E. L.: An updated method for the calibration of transparent exopolymer particle measurements, Limnol. Oceanogr. Meth., 16, 621–628, https://doi.org/10.1002/lom3.10268, 2018.
Blanchard, D. C.: Bubble Scavenging and the Water-to-Air Transfer of Organic Material in the Sea, in: Applied Chemistry at Protein Interfaces, Advances in Chemistry, 145, American Chemical Society, 360–387, https://doi.org/10.1021/ba-1975-0145.ch018, 1975.
Burrows, S. M., Hoose, C., Poschl, U., and Lawrence, M. G.: Ice nuclei in marine air: biogenic particles or dust?, Atmos. Chem. Phys., 13, 245–267, https://doi.org/10.5194/acp-13-245-2013, 2013.
Busch, K., Endres, S., Iversen, M. H., Michels, J., Nothig, E. M., and Engel, A.: Bacterial Colonization and Vertical Distribution of Marine Gel Particles (TEP and CSP) in the Arctic Fram Strait, Front. Mar. Sci., 4, 166, https://doi.org/10.3389/fmars.2017.00166, 2017.
Carpenter, L. J., Fleming, Z. L., Read, K. A., Lee, J. D., Moller, S. J., Hopkins, J. R., Purvis, R. M., Lewis, A. C., Müller, K., Heinold, B., Herrmann, H., Fomba, K. W., van Pinxteren, D., Müller, C., Tegen, I., Wiedensohler, A., Müller, T., Niedermeier, N., Achterberg, E. P., Patey, M. D., Kozlova, E. A., Heimann, M., Heard, D. E., Plane, J. M. C., Mahajan, A., Oetjen, H., Ingham, T., Stone, D., Whalley, L. K., Evans, M. J., Pilling, M. J., Leigh, R. J., Monks, P. S., Karunaharan, A., Vaughan, S., Arnold, S. R., Tschritter, J., Pohler, D., Friess, U., Holla, R., Mendes, L. M., Lopez, H., Faria, B., Manning, A. J., and Wallace, D. W. R.: Seasonal characteristics of tropical marine boundary layer air measured at the Cape Verde Atmospheric Observatory, J. Atmos. Chem., 67, 87–140, https://doi.org/10.1007/s10874-011-9206-1, 2010.
Chin, W. C., Orellana, M. V., and Verdugo, P.: Spontaneous assembly of marine dissolved organic matter into polymer gels, Nature, 391, 568–572, https://doi.org/10.1038/35345, 1998.
Creamean, J. M., Cross, J. N., Pickart, R., McRaven, L., Lin, P., Pacini, A., Hanlon, R., Schmale, D. G., Ceniceros, J., Aydell, T., Colombi, N., Bolger, E., and DeMott, P. J.: Ice Nucleating Particles Carried From Below a Phytoplankton Bloom to the Arctic Atmosphere, Geophys. Res. Lett., 46, 8572–8581, https://doi.org/10.1029/2019gl083039, 2019.
Decho, A. W. and Gutierrez, T.: Microbial Extracellular Polymeric Substances (EPSs) in Ocean Systems, Front. Microbiol., 8, 922, https://doi.org/10.3389/fmicb.2017.00922, 2017.
Deguillaume, L., Perroux, H., Wirgot, N., Mouchel-Vallon, C. Chaumerliac, N., Joly, M., Vinatier, V., and Delort, A. M.: Biological Activity in Clouds: From the Laboratory to the Model, in: Air Pollution Modeling and its Application XXVI, ITM 2018, Proceedings in Complexity, edited by: Mensink, C. G. W. and Hakami, A., Springer, Cham, https://doi.org/10.1007/978-3-030-22055-6_64, 2020.
de Leeuw, G., Andreas, E. L., Anguelova, M. D., Fairall, C. W., Lewis, E. R., O'Dowd, C., Schulz, M., and Schwartz, S. E.: Production Flux of Sea Spray Aerosol, Rev. Geophys., 49, RG2001, https://doi.org/10.1029/2010rg000349, 2011.
DeMott, P. J., Mason, R. H., McCluskey, C. S., Hill, T. C. J., Perkins, R. J., Desyaterik, Y., Bertram, A. K., Trueblood, J. V., Grassian, V. H., Qiu, Y. Q., Molinero, V., Tobo, Y., Sultana, C. M., Lee, C., and Prather, K. A.: Ice nucleation by particles containing long-chain fatty acids of relevance to freezing by sea spray aerosols, Environ. Sci. Process. Imp., 20, 1559–1569, https://doi.org/10.1039/c8em00386f, 2018.
Demoz, B. B., Collett, J. L., and Daube, B. C.: On the Caltech Active Strand Cloudwater Collectors, Atmos. Res., 41, 47–62, https://doi.org/10.1016/0169-8095(95)00044-5, 1996.
Engel, A.: Determination of Marine Gel Particles, in: Practical guidelines for the analysis of seawater, edited by: Wurl, O., CRC Press, ISBN 9780429092763, 2009.
Engel, A., Goldthwait, S., Passow, U., and Alldredge, A.: Temporal decoupling of carbon and nitrogen dynamics in a mesocosm diatom bloom, Limnol. Oceanogr., 47, 753–761, https://doi.org/10.4319/lo.2002.47.3.0753, 2002.
Engel, A., Delille, B., Jacquet, S., Riebesell, U., Rochelle-Newall, E., Terbruggen, A., and Zondervan, I.: Transparent exopolymer particles and dissolved organic carbon production by Emiliania huxleyi exposed to different CO2 concentrations: a mesocosm experiment, Aquat. Microb. Ecol., 34, 93–104, https://doi.org/10.3354/ame034093, 2004.
Engel, A., Borchard, C., Loginova, A., Meyer, J., Hauss, H., and Kiko, R.: Effects of varied nitrate and phosphate supply on polysaccharidic and proteinaceous gel particle production during tropical phytoplankton bloom experiments, Biogeosciences, 12, 5647–5665, https://doi.org/10.5194/bg-12-5647-2015, 2015.
Engel, A., Endres, S., GaIgani, L., and Schartau, M.: Marvelous Marine Microgels: On the Distribution and Impact of Gel-Like Particles in the Oceanic Water-Column, Front. Mar. Sci., 7, 405, https://doi.org/10.3389/fmars.2020.00405, 2020.
Ervens, B. and Amato, P.: The global impact of bacterial processes on carbon mass, Atmos. Chem. Phys., 20, 1777–1794, https://doi.org/10.5194/acp-20-1777-2020, 2020.
Facchini, M. C., Rinaldi, M., Decesari, S., Carbone, C., Finessi, E., Mircea, M., Fuzzi, S., Ceburnis, D., Flanagan, R., Nilsson, E. D., de Leeuw, G., Martino, M., Woeltjen, J., and O'Dowd, C. D.: Primary submicron marine aerosol dominated by insoluble organic colloids and aggregates, Geophys. Res. Lett., 35, L17814, https://doi.org/10.1029/2008gl034210, 2008.
Fomba, K. W., Müller, K., van Pinxteren, D., and Herrmann, H.: Aerosol size-resolved trace metal composition in remote northern tropical Atlantic marine environment: case study Cape Verde islands, Atmos. Chem. Phys., 13, 4801–4814, https://doi.org/10.5194/acp-13-4801-2013, 2013.
Fomba, K. W., Mueller, K., van Pinxteren, D., Poulain, L., van Pinxteren, M., and Herrmann, H.: Long-term chemical characterization of tropical and marine aerosols at the Cape Verde Atmospheric Observatory (CVAO) from 2007 to 2011, Atmos. Chem. Phys., 14, 8883–8904, https://doi.org/10.5194/acp-14-8883-2014, 2014.
Gao, Q., Leck, C., Rauschenberg, C., and Matrai, P. A.: On the chemical dynamics of extracellular polysaccharides in the high Arctic surface microlayer, Ocean Sci., 8, 401–418, https://doi.org/10.5194/os-8-401-2012, 2012.
Gong, X., Wex, H., van Pinxteren, M., Triesch, N. Fomba, K. W., Lubitz, J., Stolle, C., Robinson, T.-B., Müller, T., Herrmann, H., and Stratmann, F.: Ice nucleating particles measured in air, cloud and seawater at the Cape Verde Atmospheric Observatory (CVAO), PANGAEA [data set], https://doi.org/10.1594/PANGAEA.906946, 2019.
Gong, X. D., Wex, H., van Pinxteren, M., Triesch, N., Fomba, K. W., Lubitz, J., Stolle, C., Robinson, T. B., Müller, T., Herrmann, H., and Stratmann, F.: Characterization of aerosol particles at Cabo Verde close to sea level and at the cloud level – Part 2: Ice-nucleating particles in air, cloud and seawater, Atmos. Chem. Phys., 20, 1451–1468, https://doi.org/10.5194/acp-20-1451-2020, 2020a.
Gong, X. D., Wex, H., Voigtlander, J., Fomba, K. W., Weinhold, K., van Pinxteren, M., Henning, S., Müller, T., Herrmann, H., and Stratmann, F.: Characterization of aerosol particles at Cabo Verde close to sea level and at the cloud level – Part 1: Particle number size distribution, cloud condensation nuclei and their origins, Atmos. Chem. Phys., 20, 1431–1449, https://doi.org/10.5194/acp-20-1431-2020, 2020b.
Hartmann, M., Adachi, K., Eppers, O., Haas, C., Herber, A., Holzinger, R., Hunerbein, A., Jakel, E., Jentzsch, C., van Pinxteren, M., Wex, H., Willmes, S., and Stratmann, F.: Wintertime Airborne Measurements of Ice Nucleating Particles in the High Arctic: A Hint to a Marine, Biogenic Source for Ice Nucleating Particles, Geophys. Res. Lett., 47, e2020GL087770, https://doi.org/10.1029/2020gl087770, 2020.
Hoffman, E. J. and Duce, R. A.: Factors influencing organic-carbon content of marine aerosols – Laboratory study, J. Geophys. Res., 81, 3667–3670, 1976.
Jaber, S., Joly, M., Brissy, M., Leremboure, M., Khaled, A., Ervens, B., and Delort, A. M.: Biotic and abiotic transformation of amino acids in cloud water: experimental studies and atmospheric implications, Biogeosciences, 18, 1067–1080, https://doi.org/10.5194/bg-18-1067-2021, 2021.
Keene, W. C., Maring, H., Maben, J. R., Kieber, D. J., Pszenny, A. A. P., Dahl, E. E., Izaguirre, M. A., Davis, A. J., Long, M. S., Zhou, X., Smoydzin, L., and Sander, R.: Chemical and physical characteristics of nascent aerosols produced by bursting bubbles at a model air-sea interface, J. Geophys. Res.-Atmos., 112, D21202, https://doi.org/10.1029/2007jd008464, 2007.
Khaled, A., Zhang, M. H., Amato, P., Delort, A. M., and Ervens, B.: Biodegradation by bacteria in clouds: an underestimated sink for some organics in the atmospheric multiphase system, Atmos. Chem. Phys., 21, 3123–3141, https://doi.org/10.5194/acp-21-3123-2021, 2021.
Kuznetsova, M., Lee, C., and Aller, J.: Characterization of the proteinaceous matter in marine aerosols, Mar. Chem., 96, 359–377, https://doi.org/10.1016/j.marchem.2005.03.007, 2005.
Leck, C. and Bigg, E. K.: Source and evolution of the marine aerosol – A new perspective, Geophys. Res. Lett., 32, L19803, https://doi.org/10.1029/2005gl023651, 2005a.
Leck, C. and Bigg, E. K.: Biogenic particles in the surface microlayer and overlaying atmosphere in the central Arctic Ocean during summer, Tellus B, 57, 305–316, https://doi.org/10.1111/j.1600-0889.2005.00148.x, 2005b.
Leck, C., Gao, Q., Rad, F. M., and Nilsson, U.: Size-resolved atmospheric particulate polysaccharides in the high summer Arctic, Atmos. Chem. Phys., 13, 12573–12588, https://doi.org/10.5194/acp-13-12573-2013, 2013.
Lewandowska, A. U. and Falkowska, L. M.: Sea salt in aerosols over the southern Baltic. Part 1. The generation and transportation of marine particles, Oceanologia, 55, 279–298, https://doi.org/10.5697/oc.55-2.279, 2013.
Lewandowska, A. U., Sliwinska-Wilczewska, S., and Wozniczka, D.: Identification of cyanobacteria and microalgae in aerosols of various sizes in the air over the Southern Baltic Sea, Mar. Pollut. Bull., 125, 30–38, https://doi.org/10.1016/j.marpolbul.2017.07.064, 2017.
Liss, P. S. and Johnson, M. T.: Ocean-Atmosphere Interactions of Gases and Particles, Springer, ISBN 978-3-662-52140-3, 2014.
Logan, B. E., Passow, U., Alldredge, A. L., Grossartt, H.-P., and Simont, M.: Rapid formation and sedimentation of large aggregates is predictable from coagulation rates (half-lives) of transparent exopolymer particles (TEP), Deep-Sea Res. Pt. II, 42, 203–214, https://doi.org/10.1016/0967-0645(95)00012-F, 1995.
Louis, J., Pedrotti, M. L., Gazeau, F., and Guieu, C.: Experimental evidence of formation of Transparent Exopolymer Particles (TEP) and POC export provoked by dust addition under current and high pCO2 conditions, Plos One, 12, e0171980, https://doi.org/10.1371/journal.pone.0171980, 2017.
Madry, W. L., Toon, O. B., and O'Dowd, C. D.: Modeled optical thickness of sea-salt aerosol, J. Geophys. Res.-Atmos., 116, https://doi.org/10.1029/2010jd014691, 2011.
Maki, T., Lee, K. C., Kawai, K., Onishi, K., Hong, C. S., Kurosaki, Y., Shinoda, M., Kai, K., Iwasaka, Y., Archer, S. D. J., Lacap-Bugler, D. C., Hasegawa, H., and Pointing, S. B.: Aeolian Dispersal of Bacteria Associated With Desert Dust and Anthropogenic Particles Over Continental and Oceanic Surfaces, J. Geophys. Res.-Atmos., 124, 5579–5588, https://doi.org/10.1029/2018jd029597, 2019.
Malfatti, F., Lee, C., Tinta, T., Pendergraft, M. A., Celussi, M., Zhou, Y. Y., Sultana, C. M., Rotter, A., Axson, J. L., Collins, D. B., Santander, M. V., Morales, A. L. A., Aluwihare, L. I., Riemer, N., Grassian, V. H., Azam, F., and Prather, K. A.: Detection of Active Microbial Enzymes in Nascent Sea Spray Aerosol: Implications for Atmospheric Chemistry and Climate, Environ. Sci. Technol. Lett., 6, 171–177, https://doi.org/10.1021/acs.estlett.8b00699, 2019.
Mari, X., Passow, U., Migon, C., Burd, A. B., and Legendre, L.: Transparent exopolymer particles: Effects on carbon cycling in the ocean, Prog. Oceanogr., 151, 13–37, https://doi.org/10.1016/j.pocean.2016.11.002, 2017.
Marone, A., Kane, C. T., Mbengue, M., Jenkins, G. S., Niang, D. N., Drame, M. S., and Gernand, J. M.: Characterization of Bacteria on Aerosols From Dust Events in Dakar, Senegal, West Africa, Geohealth, 4, e2019GH000216, https://doi.org/10.1029/2019gh000216, 2020.
Matulova, M., Husarova, S., Capek, P., Sancelme, M., and Delort, A. M.: Biotransformation of Various Saccharides and Production of Exopolymeric Substances by Cloud-Borne Bacillus sp 3B6, Environ. Sci. Technol., 48, 14238–14247, https://doi.org/10.1021/es501350s, 2014.
McCluskey, C. S., Hill, T. C. J., Humphries, R. S., Rauker, A. M., Moreau, S., Strutton, P. G., Chambers, S. D., Williams, A. G., McRobert, I., Ward, J., Keywood, M. D., Harnwell, J., Ponsonby, W., Loh, Z. M., Krummel, P. B., Protat, A., Kreidenweis, S. M., and DeMott, P. J.: Observations of Ice Nucleating Particles Over Southern Ocean Waters, Geophys. Res. Lett., 45, 11989–11997, https://doi.org/10.1029/2018gl079981, 2018a.
McCluskey, C. S., Ovadnevaite, J., Rinaldi, M., Atkinson, J., Belosi, F., Ceburnis, D., Marullo, S., Hill, T. C. J., Lohmann, U., Kanji, Z. A., O'Dowd, C., Kreidenweis, S. M., and DeMott, P. J.: Marine and Terrestrial Organic Ice-Nucleating Particles in Pristine Marine to Continentally Influenced Northeast Atlantic Air Masses, J. Geophys. Res.-Atmos., 123, 6196–6212, https://doi.org/10.1029/2017jd028033, 2018b.
Mimura, T. and Romano, J. C.: Muramin acid measurements for bacterial investigations in marine environments by high-pressure-liquid-chromatography, Appl. Environ. Microbiol., 50, 229–237, https://doi.org/10.1128/aem.50.2.229-237.1985, 1985.
Niedermeier, N., Held, A., Müller, T., Heinold, B., Schepanski, K., Tegen, I., Kandler, K., Ebert, M., Weinbruch, S., Read, K., Lee, J., Fomba, K. W., Müller, K., Herrmann, H., and Wiedensohler, A.: Mass deposition fluxes of Saharan mineral dust to the tropical northeast Atlantic Ocean: an intercomparison of methods, Atmos. Chem. Phys., 14, 2245–2266, https://doi.org/10.5194/acp-14-2245-2014, 2014.
O'Dowd, C. D., Facchini, M. C., Cavalli, F., Ceburnis, D., Mircea, M., Decesari, S., Fuzzi, S., Yoon, Y. J., and Putaud, J. P.: Biogenically driven organic contribution to marine aerosol, Nature, 431, 676–680, https://doi.org/10.1038/Nature02959, 2004.
Orellana, M. V. and Verdugo, P.: Ultraviolet radiation blocks the organic carbon exchange between the dissolved phase and the gel phase in the ocean, Limnol. Oceanogr., 48, 1618–1623, https://doi.org/10.4319/lo.2003.48.4.1618, 2003.
Orellana, M. V., Matrai, P. A., Leck, C., Rauschenberg, C. D., Lee, A. M., and Coz, E.: Marine microgels as a source of cloud condensation nuclei in the high Arctic, P. Natl. Acad. Sci. USA, 108, 13612–13617, https://doi.org/10.1073/pnas.1102457108, 2011.
Ovadnevaite, J., O'Dowd, C., Dall'Osto, M., Ceburnis, D., Worsnop, D. R., and Berresheim, H.: Detecting high contributions of primary organic matter to marine aerosol: A case study, Geophys. Res. Lett., 38, L02807, https://doi.org/10.1029/2010gl046083, 2011.
Pandey, R., Usui, K., Livingstone, R. A., Fischer, S. A., Pfaendtner, J., Backus, E. H. G., Nagata, Y., Frohlich-Nowoisky, J., Schmuser, L., Mauri, S., Scheel, J. F., Knopf, D. A., Poschl, U., Bonn, M., and Weidner, T.: Ice-nucleating bacteria control the order and dynamics of interfacial water, Sci. Adv., 2, e1501630, https://doi.org/10.1126/sciadv.1501630, 2016.
Passow, U.: Formation of transparent exopolymer particles, TEP, from dissolved precursor material, Mar. Ecol.-Prog. Ser., 192, 1–11, https://doi.org/10.3354/meps192001, 2000.
Passow, U.: Production of transparent exopolymer particles (TEP) by phyto- and bacterioplankton, Mar. Ecol.-Prog. Ser., 236, 1–12, https://doi.org/10.3354/meps236001, 2002a.
Passow, U.: Transparent exopolymer particles (TEP) in aquatic environments, Prog. Oceanogr., 55, 287–333, https://doi.org/10.1016/s0079-6611(02)00138-6, 2002b.
Passow, U. and Alldredge, A.: A dye-binding assay for the spectrophotometric measurement of transparent exopolymer particles (TEP), Limnol. Oceanogr., 40, 1326–1335, 1995.
Pummer, B. G., Budke, C., Augustin-Bauditz, S., Niedermeier, D., Felgitsch, L., Kampf, C. J., Huber, R. G., Liedl, K. R., Loerting, T., Moschen, T., Schauperl, M., Tollinger, M., Morris, C. E., Wex, H., Grothe, H., Pöschl, U., Koop, T., and Fröhlich-Nowoisky, J.: Ice nucleation by water-soluble macromolecules, Atmospheric Chemistry and Physics, 15, 4077–4091, https://doi.org/10.5194/acp-15-4077-2015, 2015.
Quinn, P. K., Collins, D. B., Grassian, V. H., Prather, K. A., and Bates, T. S.: Chemistry and Related Properties of Freshly Emitted Sea Spray Aerosol, Chem. Rev., 115, 4383–4399, https://doi.org/10.1021/cr500713g, 2015.
Rastelli, E., Corinaldesi, C., Dell'Anno, A., Lo Martire, M., Greco, S., Facchini, M. C., Rinaldi, M., O'Dowd, C., Ceburnis, D., and Danovaro, R.: Transfer of labile organic matter and microbes from the ocean surface to the marine aerosol: an experimental approach, Scient. Rep., 7, 11475, https://doi.org/10.1038/s41598-017-10563-z, 2017.
Robinson, T. B., Stolle, C., and Wurl, O.: Depth is relative: the importance of depth for transparent exopolymer particles in the near-surface environment, Ocean Sci., 15, 1653–1666, https://doi.org/10.5194/os-15-1653-2019, 2019a.
Robinson, T. B., Wurl, O., Bahlmann, E., Juergens, K., and Stolle, C.: Rising bubbles enhance the gelatinous nature of the air-sea interface, Limnol. Oceanogr., 64, 2358–2372, https://doi.org/10.1002/lno.11188, 2019b.
Sander, R., Keene, W. C., Pszenny, A. A. P., Arimoto, R., Ayers, G. P., Baboukas, E., Cainey, J. M., Crutzen, P. J., Duce, R. A., Hönninger, G., Huebert, B. J., Maenhaut, W., Mihalopoulos, N., Turekian, V. C., and Van Dingenen, R.: Inorganic bromine in the marine boundary layer: a critical review, Atmos. Chem. Phys., 3, 1301–1336, https://doi.org/10.5194/acp-3-1301-2003, 2003.
Schmitt-Kopplin, P., Liger-Belair, G., Koch, B. P., Flerus, R., Kattner, G., Harir, M., Kanawati, B., Lucio, M., Tziotis, D., Hertkorn, N., and Gebefügi, I.: Dissolved organic matter in sea spray: a transfer study from marine surface water to aerosols, Biogeosciences, 9, 1571–1582, https://doi.org/10.5194/bg-9-1571-2012, 2012.
Schuster, S. and Herndl, G. J.: Formation and significance of transparent exopolymeric particles in the northern adriatic sea, Mar. Ecol.-Prog. Ser., 124, 227–236, https://doi.org/10.3354/meps124227, 1995.
Sellegri, K., Nicosia, A., Freney, E., Uitz, J., Thyssen, M., Grégori, G., Engel, A., Zäncker, B., Haëntjens, N., Mas, S., Picard, D., Saint-Macary, A., Peltola, M., Rose, C., Trueblood, J., Lefevre, D., D'Anna, B., Desboeufs, K., Meskhidze, N., Guieu, C., and Law, C. S.: Surface ocean microbiota determine cloud precursors, Scient. Rep., 11, 281, https://doi.org/10.1038/s41598-020-78097-5, 2021.
Sharma, N. K., Rai, A. K., Singh, S., and Brown, R. M.: Airborne algae: Their present status and relevance, J. Phycol., 43, 615–627, https://doi.org/10.1111/j.1529-8817.2007.00373.x, 2007.
Triesch, N., van Pinxteren, M., Engel, A., and Herrmann, H.: Concerted measurements of free amino acids at the Cabo Verde islands: high enrichments in submicron sea spray aerosol particles and cloud droplets, Atmos. Chem. Phys., 21, 163–181, https://doi.org/10.5194/acp-21-163-2021, 2021a.
Triesch, N., van Pinxteren, M., Frka, S., Stolle, C., Spranger, T., Hoffmann, E. H., Gong, X., Wex, H., Schulz-Bull, D., Gašparović, B., and Herrmann, H.: Concerted measurements of lipids in seawater and on submicrometer aerosol particles at the Cabo Verde islands: biogenic sources, selective transfer and high enrichments, Atmos. Chem. Phys., 21, 4267–4283, https://doi.org/10.5194/acp-21-4267-2021, 2021b.
Turekian, K. K.: Oceans (Foundations of Earth Science), 1st Edn., Prentice Hall, ISBN 0780727819, 9780780727816, 1968.
Uetake, J., Hill, T. C. J., Moore, K. A., DeMott, P. J., Protat, A., and Kreidenweis, S. M.: Airborne bacteria confirm the pristine nature of the Southern Ocean boundary layer, P. Natl. Acad. Sci. USA, 117, 13275–13282, https://doi.org/10.1073/pnas.2000134117, 2020.
van Pinxteren, M.: Transparent exopolymer particles (TEP) and sodium in ambient aerosol particles and cloud water at Cape Verde islands, PANGAEA [data set], https://doi.org/10.1594/PANGAEA.938169, 2021
van Pinxteren, M., Barthel, S., Fomba, K., Müller, K., von Tümpling, W., and Herrmann, H.: The influence of environmental drivers on the enrichment of organic carbon in the sea surface microlayer and in submicron aerosol particles – measurements from the Atlantic Ocean, Elem. Sci. Anth., 5, 35, https://doi.org/10.1525/elementa.225, 2017.
van Pinxteren, M., Fomba, K. W., Triesch, N., Stolle, C., Wurl, O., Bahlmann, E., Gong, X. D., Voigtlander, J., Wex, H., Robinson, T. B., Barthel, S., Zeppenfeld, S., Hoffmann, E. H., Roveretto, M., Li, C. L., Grosselin, B., Daele, V., Senf, F., van Pinxteren, D., Manzi, M., Zabalegui, N., Frka, S., Gasparovic, B., Pereira, R., Li, T., Wen, L., Li, J. R., Zhu, C., Chen, H., Chen, J. M., Fiedler, B., Von Tumpling, W., Read, K. A., Punjabi, S., Lewis, A. C., Hopkins, J. R., Carpenter, L. J., Peeken, I., Rixen, T., Schulz-Bull, D., Monge, M. E., Mellouki, A., George, C., Stratmann, F., and Herrmann, H.: Marine organic matter in the remote environment of the Cape Verde islands - an introduction and overview to the MarParCloud campaign, Atmos. Chem. Phys., 20, 6921–6951, https://doi.org/10.5194/acp-20-6921-2020, 2020.
Verdugo, P., Alldredge, A. L., Azam, F., Kirchman, D. L., Passow, U., and Santschi, P. H.: The oceanic gel phase: a bridge in the DOM–POM continuum, Mar. Chem., 92, 67–85, 2004.
Villacorte, L. O., Ekowati, Y., Calix-Ponce, H. N., Schippers, J. C., Amy, G. L., and Kennedy, M. D.: Improved method for measuring transparent exopolymer particles (TEP) and their precursors in fresh and saline water, Water Res., 70, 300–312, https://doi.org/10.1016/j.watres.2014.12.012, 2015.
Wiedensohler, A., Birmili, W., Nowak, A., Sonntag, A., Weinhold, K., Merkel, M., Wehner, B., Tuch, T., Pfeifer, S., Fiebig, M., Fjaraa, A. M., Asmi, E., Sellegri, K., Depuy, R., Venzac, H., Villani, P., Laj, P., Aalto, P., Ogren, J. A., Swietlicki, E., Williams, P., Roldin, P., Quincey, P., Huglin, C., Fierz-Schmidhauser, R., Gysel, M., Weingartner, E., Riccobono, F., Santos, S., Gruning, C., Faloon, K., Beddows, D., Harrison, R. M., Monahan, C., Jennings, S. G., O'Dowd, C. D., Marinoni, A., Horn, H. G., Keck, L., Jiang, J., Scheckman, J., McMurry, P. H., Deng, Z., Zhao, C. S., Moerman, M., Henzing, B., de Leeuw, G., Loschau, G., and Bastian, S.: Mobility particle size spectrometers: harmonization of technical standards and data structure to facilitate high quality long-term observations of atmospheric particle number size distributions, Atmos. Meas. Tech., 5, 657–685, https://doi.org/10.5194/amt-5-657-2012, 2012.
Wilson, T. W., Ladino, L. A., Alpert, P. A., Breckels, M. N., Brooks, I. M., Browse, J., Burrows, S. M., Carslaw, K. S., Huffman, J. A., Judd, C., Kilthau, W. P., Mason, R. H., McFiggans, G., Miller, L. A., Najera, J. J., Polishchuk, E., Rae, S., Schiller, C. L., Si, M., Temprado, J. V., Whale, T. F., Wong, J. P. S., Wurl, O., Yakobi-Hancock, J. D., Abbatt, J. P. D., Aller, J. Y., Bertram, A. K., Knopf, D. A., and Murray, B. J.: A marine biogenic source of atmospheric ice-nucleating particles, Nature, 525, 234–238, https://doi.org/10.1038/nature14986, 2015.
Wiśniewska, K., Lewandowska, A. U., and Śliwińska-Wilczewska, S.: The importance of cyanobacteria and microalgae present in aerosols to human health and the environment – Review study, Environ. Int., 131, 104964, https://doi.org/10.1016/j.envint.2019.104964, 2019.
Wiśniewska, K. A., Śliwińska-Wilczewska, S., and Lewandowska, A. U.: Airborne microalgal and cyanobacterial diversity and composition during rain events in the southern Baltic Sea region, Scient. Rep., 12, 2029, https://doi.org/10.1038/s41598-022-06107-9, 2022.
Wurl, O. and Holmes, M.: The gelatinous nature of the sea-surface microlayer, Mar. Chem., 110, 89–97, https://doi.org/10.1016/j.marchem.2008.02.009, 2008.
Wurl, O., Miller, L., and Vagle, S.: Production and fate of transparent exopolymer particles in the ocean, J. Geophys. Res.-Oceans, 116, C00H13, https://doi.org/10.1029/2011jc007342, 2011.
Zäncker, B., Engel, A., and Cunliffe, M.: Bacterial communities associated with individual transparent exopolymer particles (TEP), J. Plankt. Res., 41, 561–565, https://doi.org/10.1093/plankt/fbz022, 2019.
Zeppenfeld, S., van Pinxteren, M., Hartmann, M., Bracher, A., Stratmann, F., and Herrmann, H.: Glucose as a Potential Chemical Marker for Ice Nucleating Activity in Arctic Seawater and Melt Pond Samples, Environ. Sci. Technol., 53, 8747–8756, https://doi.org/10.1021/acs.est.9b01469, 2019.
Zeppenfeld, S., van Pinxteren, M., van Pinxteren, D., Wex, H., Berdalet, E., Vaqué, D., Dall'Osto, M., and Herrmann, H.: Aerosol Marine Primary Carbohydrates and Atmospheric Transformation in the Western Antarctic Peninsula, ACS Earth Space Chem., 5, 1032–1047, https://doi.org/10.1021/acsearthspacechem.0c00351, 2021.
Zhang, M. H., Khaled, A., Amato, P., Delort, A. M., and Ervens, B.: Sensitivities to biological aerosol particle properties and ageing processes: potential implications for aerosol-cloud interactions and optical properties, Atmos. Chem. Phys., 21, 3699–3724, https://doi.org/10.5194/acp-21-3699-2021, 2021.