the Creative Commons Attribution 4.0 License.
the Creative Commons Attribution 4.0 License.
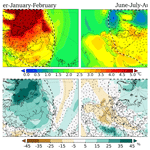
Overview: Recent advances in the understanding of the northern Eurasian environments and of the urban air quality in China – a Pan-Eurasian Experiment (PEEX) programme perspective
Hanna K. Lappalainen
Tuukka Petäjä
Timo Vihma
Jouni Räisänen
Alexander Baklanov
Sergey Chalov
Igor Esau
Ekaterina Ezhova
Matti Leppäranta
Dmitry Pozdnyakov
Jukka Pumpanen
Meinrat O. Andreae
Mikhail Arshinov
Eija Asmi
Jianhui Bai
Igor Bashmachnikov
Boris Belan
Federico Bianchi
Boris Biskaborn
Michael Boy
Jaana Bäck
Bin Cheng
Natalia Chubarova
Jonathan Duplissy
Egor Dyukarev
Konstantinos Eleftheriadis
Martin Forsius
Martin Heimann
Sirkku Juhola
Vladimir Konovalov
Igor Konovalov
Pavel Konstantinov
Kajar Köster
Elena Lapshina
Anna Lintunen
Alexander Mahura
Risto Makkonen
Svetlana Malkhazova
Ivan Mammarella
Stefano Mammola
Stephany Buenrostro Mazon
Outi Meinander
Eugene Mikhailov
Victoria Miles
Stanislav Myslenkov
Dmitry Orlov
Jean-Daniel Paris
Roberta Pirazzini
Olga Popovicheva
Jouni Pulliainen
Kimmo Rautiainen
Torsten Sachs
Vladimir Shevchenko
Andrey Skorokhod
Andreas Stohl
Elli Suhonen
Erik S. Thomson
Marina Tsidilina
Veli-Pekka Tynkkynen
Petteri Uotila
Aki Virkkula
Nadezhda Voropay
Tobias Wolf
Sayaka Yasunaka
Jiahua Zhang
Yubao Qiu
Aijun Ding
Huadong Guo
Valery Bondur
Nikolay Kasimov
Sergej Zilitinkevich
Veli-Matti Kerminen
Markku Kulmala
The Pan-Eurasian Experiment (PEEX) Science Plan, released in 2015, addressed a need for a holistic system understanding and outlined the most urgent research needs for the rapidly changing Arctic-boreal region. Air quality in China, together with the long-range transport of atmospheric pollutants, was also indicated as one of the most crucial topics of the research agenda. These two geographical regions, the northern Eurasian Arctic-boreal region and China, especially the megacities in China, were identified as a “PEEX region”. It is also important to recognize that the PEEX geographical region is an area where science-based policy actions would have significant impacts on the global climate. This paper summarizes results obtained during the last 5 years in the northern Eurasian region, together with recent observations of the air quality in the urban environments in China, in the context of the PEEX programme. The main regions of interest are the Russian Arctic, northern Eurasian boreal forests (Siberia) and peatlands, and the megacities in China. We frame our analysis against research themes introduced in the PEEX Science Plan in 2015. We summarize recent progress towards an enhanced holistic understanding of the land–atmosphere–ocean systems feedbacks. We conclude that although the scientific knowledge in these regions has increased, the new results are in many cases insufficient, and there are still gaps in our understanding of large-scale climate–Earth surface interactions and feedbacks. This arises from limitations in research infrastructures, especially the lack of coordinated, continuous and comprehensive in situ observations of the study region as well as integrative data analyses, hindering a comprehensive system analysis. The fast-changing environment and ecosystem changes driven by climate change, socio-economic activities like the China Silk Road Initiative, and the global trends like urbanization further complicate such analyses. We recognize new topics with an increasing importance in the near future, especially “the enhancing biological sequestration capacity of greenhouse gases into forests and soils to mitigate climate change” and the “socio-economic development to tackle air quality issues”.
- Article
(3622 KB) - Full-text XML
- BibTeX
- EndNote
The Earth system is facing major challenges, including climate change, biodiversity loss, ocean acidification, epidemics and energy demand on a global scale (Ripple et al., 2017). These “Grand Challenges” are highly connected and interlinked. This creates a need for an approach of a multi-disciplinary scientific programme, which could deliver science-based messages to fast-tracked policy making (Kulmala et al., 2015). The recent estimates based on observed atmospheric concentrations of CO2 (https://gml.noaa.gov/ccgg/trends/trends_log.html, last access: 17 January 2022) and business-as-usual scenarios show that humankind should find solutions to answer the Grand Challenges (IPCC, 2021). Deep understanding of the feedbacks and interactions between the land, atmosphere and ocean domains and accounting for social aspects in the regions of substantial changes are needed for effective technical solutions, mitigation and adaption policy actions. The northern regions (> 45∘ N), together with the Arctic coastal zone and Siberian region in Russia, are among the most critical areas for the global climate (Smith, 2011; Kulmala et al., 2015).
The idea of the Pan-Eurasian Experiment (PEEX) (https://www.atm.helsinki.fi/peex/, last access: 17 January 2022), originating from a group of Finnish and Russian scientists and research organizations in 2012, is a bottom-up research and capacity building programme concentrating on the sustainable development of the Arctic-boreal regions of northern Eurasia under changing climate and socio-economic megatrends (Kulmala et al., 2015; Lappalainen et al., 2016). The programme was based on four interconnected parts, namely research agenda, research infrastructures, capacity building, and societal impacts (Lappalainen et al., 2014; Kulmala et al., 2016a; Lappalainen et al., 2018a, b; Kulmala, 2018). In addition to a strong involvement of the Russian partners, the PEEX China collaboration was established in 2013. China has a strong economic interest in Arctic regions (Tillman et al., 2018). Furthermore, China is already facing extensive environmental and air pollution challenges and has major interests in finding technical solutions for environmental monitoring of the Silk Road Economic Belt (SREB) programme initiated by President Xi Jinping in 2013 (Kulmala, 2018; Lappalainen et al., 2018a; Dave and Kobayashi, 2018). The PEEX programme is an umbrella for several bilateral scientific projects and activities in Russia (e.g. Chalov et al., 2015, 2018; Esau et al., 2016; Alekseychik et al., 2017; Bobylev et al., 2018; Malkhazova et al., 2018; Kukkonen et al., 2020; Ezhova et al., 2018b; Zilitinkevich et al., 2019; Petäjä et al., 2020a, 2021; Bondur et al., 2019a, b, c, d, e, f; He et al., 2020), whereas in China the primary focus is on the development of atmospheric in situ stations and advanced air quality monitoring in megacity environments (e.g. Ding et al., 2016b; Yao et al., 2018; Wang et al., 2020). Furthermore, PEEX is closely collaborating with the Digital Belt and Road (DBAR) programme coordinated by the Institute for Digital Earth and Remote Sensing (RADI). The PEEX collaboration with DBAR is driven by a need for a novel in situ station network and ground-based data as complementary information for the remote sensing in the Silk Road economic region. Long-term development of a Station Measuring the Earth Surface and Atmosphere Relations (SMEAR) concept could provide baselines for this (Hari et al., 2016; Kulmala, 2015, 2018; Lappalainen et al., 2018a).
The PEEX programme is motivated by the need to obtain scientific information that combines research in the Arctic and boreal environments and to understand large-scale feedbacks and interactions operating in land–atmosphere–ocean systems (Kulmala et al., 2004, 2016a; Vihma et al., 2019) and large-scale weather impacts related to the Arctic amplification (e.g. Coumou et al., 2014; Vihma et al., 2020). Some of the scientific backbones of PEEX are the previously coordinated research frameworks and their synthesis. For example, the latest comprehensive overview of the interactions between the atmosphere, cryosphere and ecosystems at northern high latitudes was performed by the Nordic Center of Excellence in the Cryosphere-Atmosphere Interactions in a Changing Arctic Climate (CRAICC) community (Boy et al., 2019). The PEEX programme can upscale the CRAICC results into a wider geographical context.
Climate change, as a main driver of environmental changes in the northern Eurasian Arctic-boreal region and China, sets environmental boundaries for the future socio-economic activities of these regions in general. The harsh climate of this region puts pressure on the ecosystems and living conditions of the local people (e.g. IPCC, 2019). PEEX introduced 15 large-scale research questions, which would help us to fill the key gaps in our holistic understanding of land–atmosphere interactions and their connections to societies living in the northern Eurasian region (Kulmala et al., 2015; Lappalainen et al., 2018a). This approach also sets the framework of the current paper. Here we introduce the recent research progress in the large-scale scientific themes relevant to the PEEX region. The PEEX study region consists of the northern Eurasian Arctic and boreal (taiga) environments, and thus the major geographical part of the environments is located in the Russian territory. China was added to the study area in 2013 as it was seen as a locally and globally consequential region for climate change, air quality and long-term transport of atmospheric pollutants (Kulmala et al., 2015a, 2016a; Lappalainen et al., 2016, 2018a).
Here we introduce the scientific results from PEEX scientific output, review the main results from PEEX scientific papers and present an analysis of the key gaps in the current scientific understanding. We use the PEEX research agenda structure (Kulmala et al., 2015a; Lappalainen et al., 2018a) as a reference and mirror new rising themes and results against this plan. For the literature material, we used the following sources to demonstrate the results: (i) individual inputs sent by the PEEX research community, (ii) contents of the scientific papers published in the Atmospheric Chemistry and Physics (ACP) PEEX special issue in 2016–2019 (https://acp.copernicus.org/articles/special_issue395.html, last access: 17 January 2022) and (iii) scientific outputs from PEEX-labelled projects (https://www.atm.helsinki.fi/peex/index.php/portfolio-items/projects-subprograms/, last access: 17 January 2022) and other relevant results reported by the PEEX partners. For the individual input, we asked the PEEX research community to identify the main published papers in peer-reviewed journals for each question out of their own work and to connect the work with 1 of the 15 science questions introduced in the PEEX Science Plan. Based on the abstracts, we listed “addressed research themes” over the last 5 years per PEEX key topical area (Table 1), which we review in more detail in Sect. 2. The results are first discussed with a holistic approach, and then we categorize the advances through a set of identified feedbacks and interactions within the Arctic-boreal environment. We follow up with a discussion on the need for a future research infrastructure to be able to provide relevant data and underline the future socio-economic development of the region by setting the boundaries for proposed new science-based concepts and technical solutions.
Table 1Systems, key topical areas, and research question introduced in the PEEX Science Plan (SP) (Kulmala et al., 2015, 2016a; Lappalainen et al., 2018a) connected to the addressed research themes over the last 5 years by the PEEX questionnaire. The addressed research themes and the results are summarized in Sect. 3.
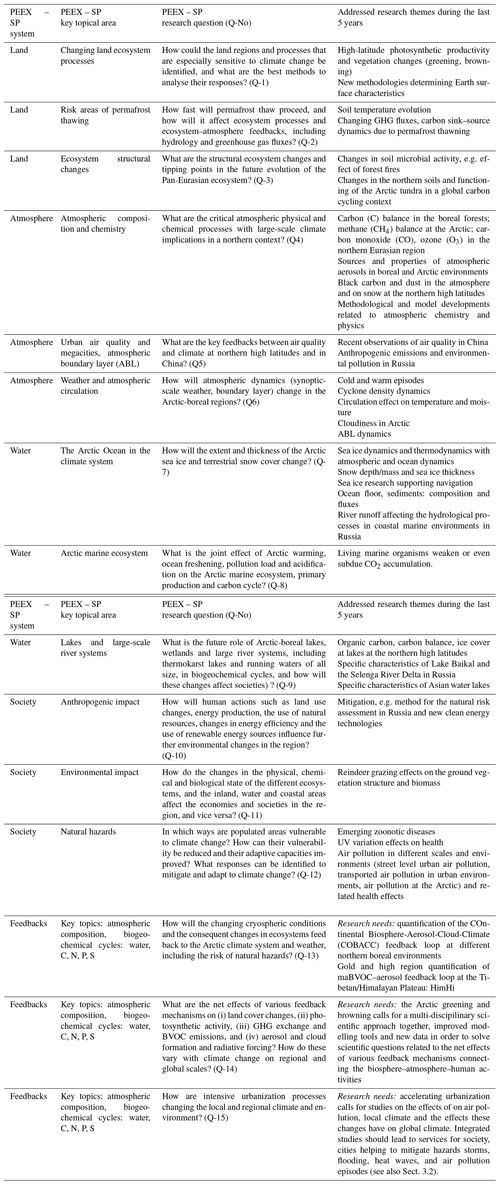
In the PEEX Science Plan, we indicated four main thematic research domains: the land system, the atmospheric system, the water system and the society with 15 thematic research areas and related large-scale research questions (Q) presented in Table 1 (Lappalainen et al., 2015). This is the framework we re-visited and utilized in the synthesis of new results of the PEEX community brought together in this paper. Furthermore, we synthesized the results and discuss their contribution to the large-scale feedbacks and interactions in the Arctic context in the sections below.
2.1 Land ecosystems
2.1.1 Changing land ecosystem processes (Q1)
High-latitude photosynthetic productivity
High-latitude terrestrial ecosystems are crucial to the global climate system and its regulation by vegetation. These ecosystems are typically temperature limited and thus also considered especially sensitive to climate warming. Better understanding of inter-annual and seasonal dynamics and resilience of the photosynthetic activity of forest vegetation as a whole is needed for the quantification of photosynthesis, or gross primary production (GPP), and for analysing the carbon balance of the boreal forests. The carbon sink and source dynamics of the boreal forests have been intensively studied during the last 5 years at the SMEAR II station in Finland (Hari and Kulmala, 2005; Hari et al., 2009). Recent results show that the Norway spruce and Scots pine ecosystems are rather resilient to a short-term weather variability (Matkala et al., 2020). Overall, the analyses by Kulmala et al. (2019) and Matkala et al. (2020) on subarctic Scots pine and Norway spruce stands at the northern timberline in Finland serve as examples of the canopy-scale dynamics, showing that these ecosystems are generally weak carbon sinks but have a clear annual variation. Kulmala et al. (2019) observed that there is a difference between tree canopy photosynthesis compared to forest floor photosynthesis, which starts to increase after the snowmelt. Thus, the models for photosynthesis should also address the snow cover period in order to better capture the seasonal dynamics of photosynthesis of the northern forests (Kulmala et al., 2019).
The abundance of tree species, stand biomass, increasing tree growth and coverage of broadleaf species may also affect biogenic volatile organic compound (BVOC) emissions from the forest floor and impact the total BVOC emissions from northern soils. At least the stand type has been shown to affect BVOC fluxes from the forest floor in a hemiboreal–boreal region (Mäki et al., 2019). As a whole, BVOCs emitted by boreal evergreen trees are connected to the photosynthetic activity with a strong seasonality and have a crucial role in atmospheric aerosol formation processes over the boreal forest zone. BVOC emissions have low rates during photosynthetically inactive winter and increasing rates towards summer (Aalto et al., 2015). High emission peaks caused by enhanced monoterpene synthesis were found in spring periods simultaneously with the photosynthetic spring recovery (Aalto et al., 2015). This suggests that monoterpene emissions may have a protective functional role for the foliage during the spring recovery state and that these emission peaks may contribute to atmospheric chemistry in the boreal forest in springtime. Vanhatalo et al. (2018) studied the interplay between needle monoterpene synthase activities, their endogenous storage pools and needle emissions in 2 consecutive years at a boreal forest in Finland. They found no direct correlation between monoterpene emissions and enzyme activity or the storage pool size. Monoterpene synthase activity of needles was different depending on seasonality and needle ontogenesis. However, the pool of stored monoterpenes did not change with the needle age (Vanhatalo et al., 2018). Also, clear annual patterns of primary biological aerosol particles have been measured from a boreal forest, with late spring and autumn being the seasons of a dominant occurrence. Increased levels of free amino acids and bacteria were observed during the pollen season in the SMEAR II station in Finland, whereas the highest levels for fungi were observed in autumn (Helin et al., 2017).
Extensive measurements of Scots pine photosynthesis and modelling resulted in optimized predictions of the daily behaviour and annual patterns of photosynthesis in a subarctic forest (Hari et al., 2017). The study connected theoretically the fundamental concepts affecting photosynthesis with the main environmental drivers (air temperature and light), and the theory gained strong support through empirical testing. Understanding stomatal regulation is fundamental in predicting the impact of changing environmental conditions on photosynthetic productivity. Lintunen et al. (2020) showed that the canopy conductance and soil-to-leaf hydraulic conductance are strongly coupled and that both soil temperature and soil water content influence the canopy conductance through changes in the below-ground hydraulic conductance. In particular, the finding that the soil temperature strongly influences the below-ground hydraulic conductance in mature, boreal trees may help to better understand tree behaviour and photosynthetic productivity in cold environments. The plant photosynthetic rate is concurrently limited by stomatal and non-stomatal limitations, and recent modelling (Hölttä et al., 2017) and empirical (Salmon et al., 2020) studies suggest that stomatal and non-stomatal controls are coordinated to maximize leaf photosynthesis; i.e. non-stomatal limitations to photosynthesis increase with a decreasing leaf water potential and/or increasing leaf sugar concentration. This new approach allows inclusion of the effects of non-stomatal limitations in models of tree–gas exchange (Fig. 1).
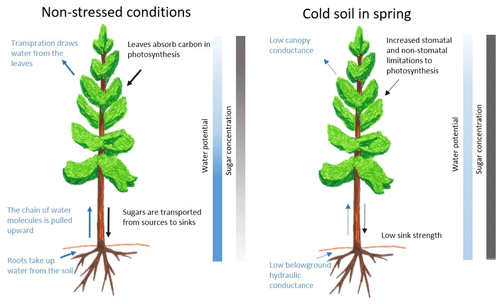
Figure 1A recent field and modelling study shows that cold soil decreases below-ground hydraulic conductance (i.e. less root water uptake) and further canopy conductance in mature, boreal trees in spring (Lintunen et al., 2020). Cold temperature also decreases sink strength (i.e. fewer sugars are needed for metabolism and growth). Low sink strength increases sugar concentration in leaves, which decreases photosynthesis due to increased stomatal and non-stomatal limitations for photosynthesis (Hölttä et al., 2017; Salmon et al., 2020).
Due to climate warming, it seems that trees at high latitudes have been progressively decreasing their regional growth coherence in the last decades (Shestakova et al., 2019). Shestakova et al. (2019) showed results that unequivocally linked a substantial decrease in the temporal coherence of forest productivity in boreal ecosystems to a less temperature-limited growth that is concurrent with regional warming trends. This emerging pattern points, for example, to an increasing dependence of the carbon balance on local drivers and the role of forests as carbon sinks in the northern Ural region.
Vegetation GPP is the largest CO2 flux of the carbon cycle in terrestrial ecosystems and impacts all of the carbon cycle variables (Beer et al., 2010). Ecosystem models usually overestimate GPP under drought and during spring, late autumn and winter (Ma et al., 2015). Several new methodological improvements for a better quantification and scaling of GPP have been reported (S. Zhang et al., 2018; Pulliainen et al., 2017; Kooijmans et al., 2019). The GPP, measuring photosynthesis, is crucially important for the global carbon cycle, and its accurate estimation is essential for ecosystem monitoring and simulation. Pulliainen et al. (2017) introduced a new proxy indicator for spring recovery from in situ flux data on CO2 exchange. This made it possible to quantify the relation between spring recovery and carbon uptake and to assess changes in the springtime carbon exchange, demonstrating a major increase in the CO2 sink. S. Zhang et al. (2018) introduced a new water-stress factor that effectively mitigates the overestimation of GPP under drought conditions, while J. H. Bai et al. (2018) and Y. Bai et al. (2018) developed a method for quantifying the evapotranspiration of crops by using a remote-sensing-based two-leaf canopy conductance model. These methods can provide novel insights into the quantification of GPP under different conditions and, in general, into the impacts of biosphere–atmosphere relations on a larger scale.
Methods for satellite-based remote sensing of photosynthesis have been developed recently based on a solar-induced chlorophyll fluorescence signal, such as the OCO-2 product that has an improved spatial resolution, data acquisition and retrieval precision as compared with earlier satellite missions with solar-induced chlorophyll fluorescence (SIF) capability, which allows for validation of the data directly against ground and airborne measurements (Sun et al., 2017). Interpretation of the solar-induced chlorophyll fluorescence signal has also been improved by many in situ studies. For example, J. Liu et al. (2019) and W. Liu et al. (2019) simulated SIF in a realistic three-dimensional birch stand reconstructed from terrestrial laser scanning data and found a large contribution of the understory layer to the remote-sensing signal.
Vegetation changes
The normalized difference vegetation index (NDVI) is used for detecting large-scale changes in vegetation productivity. In the past decades, these changes include an increasing NDVI, called “greening”, taking place in the tundra regions, and a decreasing NDVI, called “browning”, in the northern forest regions (Miles and Esau, 2016). A deeper analysis behind these changes is needed. For example, in northern West Siberia only 18 % of the total area had statistically significant changes in productivity either towards greening or browning and having these opposite trends for different species within the same bioclimatic zone. The observed complexity of the patterns and trends in the vegetation productivity underlines the need for new studies on how forest types and different species are responding to climate and environmental changes in the northern environments (Miles and Esau, 2016). Also, understanding the variations in small-scale plant communities, seasonality and biogeochemical properties is needed for modelling the functioning of the Arctic tundra in global carbon cycling. Also, the rapid development of the leaf area index (LAI, leaf area per ground area, m2 m2) during the short growing season and the yearly climatic variation address the importance of optimal timing of the satellite data images when they are compared with the field verification data in the Arctic region (Juutinen et al., 2017).
Bondur and Vorobyev (2015) analysed the vegetation indices and complex spectral transformations derived from processed long-term satellite data (1973–2013) for areas around the cities of Arkhangelsk and Zapolyarny (Murmansk oblast). They demonstrated that these areas are subject to a peak anthropogenic impact associated with specific industrial facilities, leading to changes in landscapes and depletion of natural ecosystems, consequently leading to the decline in the quality of life and health conditions (Bondur and Vorobyev, 2015). Region-wide changes in the vegetation cover and changes in and around several urbanized areas in Siberia reveal robust indications of an accelerated greening near the older urban areas. Many Siberian cities have turned greener, while their surroundings have been dominated by wider browning. The observed urban greening could be associated not only with a special tending of within-city green areas, but also with urban heat islands and succession of more productive shrub and tree species growing on warmer sandy soils (Koronatova and Milyaeva, 2011; Sizov and Lobotrosova, 2016). Tundra and forest–tundra biomes are sensitive to mean summer temperatures, which increases production and greening. Taiga biomes are sensitive to precipitation and soil moisture, with increased production in wet summers (Miles et al., 2019).
New methodologies determining Earth surface characteristics
Earth surface characteristics are fundamental knowledge for the understanding and quantification of land–atmosphere processes. The methods for determining Earth surface characteristics from satellites are improving. As an example, a method for recognition of the Earth surface types according to space images using an object-oriented classification was developed. The classification relies on Markov stochastic segmentation for object extraction and supervised classification of the objects (Gurchenkov et al., 2017). Furthermore, a prototype algorithm for hemispheric-scale detection of autumn soil freezing using spaceborne L-band passive microwave observations was developed (Rautiainen et al., 2016) and is currently an operative soil freeze and thaw product that delivers freely available data (ftp://litdb.fmi.fi/outgoing/SMOS-FTService/, last access: 17 January 2022). The CryoGrid 3 land surface model provides improved descriptions of possible pathways of ice-wedge polygon evolution and describes better the complex processes affecting ice-rich permafrost landscapes (Nitzbon et al., 2019). In addition, there are new observations for validating satellite observations and permafrost models. Boike et al. (2019) introduced a new, 16-year permafrost and meteorology data set from the Samoylov Island Arctic research site, north-eastern Siberia. Terentieva et al. (2016) introduced maps used as a baseline for validation of coarse-resolution land cover products and wetland data sets at high latitudes. Other examples of available data for the model validation are “BC emissions from agricultural burns and grass fires in Siberia” by Konovalov et al. (2018) and “permafrost records at the Lena River delta” by Boike et al. (2019).
Tundra ecosystems are under pressure and intensifying permafrost thawing, plant growth and ecosystem carbon exchange under the changing climate. The heterogeneity of Arctic landscapes is an extra challenge for environmental monitoring. For example, remote-sensing methods are not able to capture variations in moss biomass, which dominates the plant biomass and controls soil properties in the Arctic. The general accuracy of landscape level predictions in the land cover type (LCT) is good, but the spatial extrapolation of the vegetation and soil properties relevant for the regional ecosystem and global climate models still needs to be improved (Mikola et al., 2018). Furthermore, for the future, we need to have a land characterization in order to perform quantification and assessment of the ecosystem services at different scales using integrative techniques and integrated field observations together with remote sensing and modelling at the landscape scale (Burkhard et al., 2009; Fu and Forsius, 2015). At smaller scales, the isotopic composition of carbon and oxygen in peat can be used for the climate reconstruction (Granath et al., 2018).
2.1.2 Thawing permafrost (Q2)
Observations of ground temperature evolution
Permafrost regions of northern Eurasia are warming along with the climate (IPCC, 2019). During the Global Terrestrial Network for Permafrost reference decade, 2007–2016, the temperature at the depth of zero annual amplitude increased by 0.39 ± 0.15 ∘C in the continuous permafrost zone and by 0.20 ± 0.10 ∘C in the discontinuous permafrost zone. At the same time, the mountain permafrost warmed by 0.19 ± 0.05 ∘C. The global average of the permafrost temperature increased by 0.29 ± 0.12 ∘C (Biskaborn et al., 2019). The observed trend in the continuous permafrost zone follows the air temperature trend in the Northern Hemisphere.
The changes in the permafrost region affect climate, hydrology and ecology from local to global scales (Arneth et al., 2010; Hinzman et al., 2005). Several local studies focused on differences introduced by vegetation, soil and hydrological characteristics at the same site (Göckede et al., 2017, 2019). Göckede et al. (2017, 2019) presented findings on shifts in energy fluxes from paired ecosystem observations in north-eastern Siberia comprising a drained and corresponding control site. Drainage disturbance triggered a suite of secondary shifts in ecosystem properties, including alterations in vegetation community structure, which in turn influenced changes in snow cover dynamics and surface energy budget. First, the drainage reduced heat transfer into deeper soil layers, which may have led to shallower thaw depths. Second, the vegetation change due to the drainage led to an albedo increase, which decreased the total energy income, or net radiation, into the system. Third, the drainage reduced water content available for evapotranspiration, which resulted in a reduced latent heat flux and increased sensible heat flux, transferring more energy back into the atmosphere. The reported effects led to surface and permafrost cooling (Göckede et al., 2019).
Kukkonen et al. (2020) compared temperature data from several shallow boreholes in the Nadym region, Siberia, and predicted permafrost evolution for different climate scenarios. The Nadym area represents a typical site located in the discontinuous permafrost zone. Kukkonen et al. (2020) found that the permafrost thawed most rapidly in low-porosity soils, whereas high-porosity soils in the top layer (e.g. peatland) retarded thawing considerably. Similarly, the depth of a seasonally frozen layer and the temperature regime of peat soils in the oligotrophic bog in the southern taiga zone of West Siberia showed significant differences between the sites with high and low levels of bog waters (Kiselev et al., 2019). Both Kukkonen et al. (2020) and Kiselev et al. (2019) results are in line with previous conclusions on the importance of volumetric water content and unfrozen water content for soil thermal properties governing heat transfer and phase change processes (Romanovsky and Osterkamp, 2000). Locally, the sites with a thin snow cover (e.g. hilltops) demonstrated a higher resistance to the thawing (Williams and Smith, 1989; Kukkonen et al., 2020). To follow up on the development of permafrost thaw in different soil types requires continuous and comprehensive observations during the coming decades.
Changing greenhouse gas (GHG) fluxes and VOCs due to permafrost thaw
Biogenic GHG emissions are strongly connected to permafrost conditions and changes in other related environmental conditions, such as soil temperature and moisture conditions. Here we discuss recent results on the observed emissions from these permafrost perspectives and, later, in Sect. 2.2.1, address the connections between GHG fluxes and the other environmental factors, like deforestation and forest fires.
During the permafrost thaw, even small changes in the soil carbon cycle can turn a terrestrial ecosystem from a sink into a source (Schuur et al., 2008). Based on regional in situ observations of CO2 fluxes, Natali et al. (2019) estimated a wintertime carbon loss of 1662 Tg C yr−1, which is more than predicted by the process model estimates. Furthermore, Natalie et al. (2019) found that even if the soil CO2 loss were enhanced due to winter warming, the growing season might start earlier and onset the carbon uptake under warming climate conditions. For a better understanding of these connections and both spatial and temporal dynamics of the Arctic carbon cycle, we need more observations from permafrost ecosystems. Additional flux measurements are urgently needed to understand the variation between the current measurements and, especially, extended measurements on the CH4 emissions to better quantify their role in the carbon balance. For example, a new data assimilation system estimates an Arctic carbon sink of −67 g C m−2 yr−1, but this value is associated with very high uncertainties. Furthermore, these estimates do not include methane, which is even more difficult to evaluate (López-Blanco et al., 2019). Based on the field flux measurements, the Carbon Cycle Report 2018 (Schuur et al., 2018) estimated that the Eurasian boreal wetland is a source of 14 Tg CH4 yr−1. On the other hand, Kirschke et al. (2013) estimations, based on atmospheric measurements, end up at a value of 9 Tg CH4 yr−1. Locally, methane fluxes measured in 2005–2013 showed significant year-to-year variations. Interestingly, the observed variability in North America, specifically in the Hudson Bay lowlands, appears to have been driven partly by the soil temperature, while in the West Siberian lowlands the variability was dependent on the soil moisture (Thompson et al., 2017). The comparison of high-resolution modelling of atmospheric CH4 to CH4 observations already in 2012 from the East Siberian Arctic Shelf (ESAS), a potentially large CH4 source, confirms that methane releases are highly variable and inhomogeneous (Berchet et al., 2016).
Long-term flux measurements provide insight into the carbon sink–source dynamics. The flux measurements from the moist tussock tundra in north-eastern Siberia indicated that drainage influences the carbon cycle and that the tundra is changing to a weaker CO2 sink and CH4 source. Another relevant observation was that the time outside the growing season influences the carbon balance of ecosystem processes, especially during the zero-curtain period (Kittler et al., 2017). This is in line with similar studies in Alaska (Commane et al., 2017; Euskirchen et al., 2017). Therefore, the autumn temperature was identified as a major driving factor describing the differences between the annual GHG fluxes. Notably, however, the seasonal amplitudes of CO2 concentrations in Siberia were found to be significantly higher than those in the North American continent, likely due to the more intense biological activity here (Timokhina et al., 2015a). A recent study showed that the Siberian carbon cycle is a major contributor to the Northern Hemisphere amplitude of CO2 variation (Lin et al., 2020).
Observations from non-permafrost sites may give us a clue to the future dynamics of fluxes, as the permafrost is thawing. The chamber measurements of CH4 and CO2 fluxes from a non-permafrost site in the Siberian peatland in August 2015 showed that the highest values of methane fluxes were obtained in burned wet birch forest and the lowest ones in seasonally waterlogged forests (Glagolev et al., 2018). The fluxes can vary even between different sites of a bog, as measured by Dyukarev et al. (2019). The net ecosystem exchange (NEE), ecosystem respiration (ER) and GPP, based on the measured CO2 fluxes at a ridge–hollow complex bog and a model for ridge and hollow sites at oligotrophic bogs in the middle taiga zone of West Siberia, showed that a 2-year-average NEE at the hollow site was 1.7 times higher than at the ridge site (Dyukarev et al., 2019). The ecosystem processes are influenced by drying in tundra ecosystems. Kwon et al. (2019) reported from Alaska that drying in the tundra ecosystems increased contributions of modern soil carbon to the ecosystem respiration but, at the same time, decreased contributions of old soil carbon. These changes were attributed mainly to modified soil temperatures at different soil layers due to the altered thermal properties of organic soils following drainage. Furthermore, the drainage lowered CH4 fluxes by a factor of 20 during the growing season, with post-drainage changes in microbial communities, soil temperatures, and plant communities also affecting the flux reduction in an Arctic wetland ecosystem (Kwon et al., 2017).
Voigt et al. (2016) reported that, under warming conditions, the vegetated tundra in north-eastern European Russia shifted from a GHG sink to a source. The positive warming response was dominated by CO2; however, N2O emissions were also significant. N2O was emitted not only from bare peat, already identified as a strong source, but also from vast vegetated peat areas not emitting N2O under current climate conditions. These results can be explained by the dynamics between the temperature, nitrogen assimilation by plants and soil microbial activity, with a strong impact on the future GHG balance in the Arctic (Voigt et al., 2016; Gil et al., 2017). Studying N2O emissions from a typical permafrost peatland in Finnish Lapland, it was concluded that about 25 % of the Arctic territories are areas that potentially emit nitrous oxide (Voigt et al., 2017). It seems that there is a positive feedback mechanism between the permafrost thawing and moisture regime. Predicting the response of soils to climate change or land use is central to understanding and managing N2O emissions. According to recent results, the N2O flux can be predicted by models that incorporate soil nitrate concentration (NO), water content and temperature (Pärn et al., 2018).
In addition to CO2 and CH4, thawing or collapsing of Arctic permafrost can release volatile organic compounds (VOCs). H. Li et al. (2020) examined the release of VOCs from thawing permafrost peatland soils sampled from Finnish Lapland in the laboratory. The average VOC fluxes were 4 times as high as those from the active layer and mainly attributed to direct release of old, trapped gases from the permafrost. These results demonstrate a potential for substantive VOC releases from thawing permafrost and suggest that future global warming could stimulate VOC emissions from the Arctic permafrost.
2.1.3 Ecosystem structural change (Q3)
Changes in microbial activity
Climate change is likely to cause an increased appearance of trees on open peatlands, but we do not know how this vegetation change will influence the below-ground microbiology and composition. Changes along bog ecotones at three Russian peatland complexes suggest that tree encroachment may reduce the trophic level of testate amoeba communities and reduce the contribution of mixotrophic testate amoebae to primary production. Thus, it seems that increased tree recruitment on open peatlands will have important consequences for both microbial biodiversity and microbially mediated ecosystem processes (Payne et al., 2016). We also need to understand better the dynamics affecting the bacteria, fungi and other related species in the ground air layer. Recent studies by Korneykova and Evdokimova (2018) and Korneikova et al. (2018) showed the influences of anthropogenic sources (copper–nickel plant) and acidic soils in Russian northern taiga and tundra on the portion of the airborne fungi and on the structure of algological and mycological complexes (Korneikova et al., 2018). New methods were reported on how to improve soil conditions, developed on all kinds of materials made or exposed by human activity that otherwise would not occur at the Earth's surface, referred to as “Techno sol engineering” (Slukovskaya et al., 2019), and how to monitor climate change impacts on the functional state of bogs by using Sphagnum mosses (Preis et al., 2018).
Effects of forest fires on soils
Forest fires, a significant environmental factor in the northern Eurasian region, change soil chemical and physical properties and may influence greenhouse gas fluxes and emissions of BVOCs. Recent results indicate that a slower post-fire litter decomposition has a clear impact on the recovery of soil organic matter following forest fires in northern boreal coniferous forests due to accumulated soil organic matter. The soil recovery is related to slow litter composition and reduced enzymatic and microbial activity (E. Köster et al., 2016).
Post-fire studies on the long-term evolution of the structure and functioning of bacterial communities are sparse. Sun et al. (2016) showed that the major drivers influencing bacterial communities are the soil temperature, pH and moisture. Furthermore, E. Köster et al. (2015) analysed long-term effects of fire on soil CO2, CH4 and N2O fluxes in pine forest stands in Finnish Lapland and discussed the role of microbial biomass in this context. They did not detect significant effects of fires on CO2 emissions or N2O fluxes, but there was long-lasting strengthening of the CH4 sink by the soil. Interestingly, E. Köster et al. (2018) did not find a similar kind of long-term effect on the CH4 sink dynamics in studies carried out in Siberia.
Forest wildfires also regulate the BVOC emissions from boreal forest floors by changing the ground vegetation. Total BVOC emissions from a forest floor were found to decrease after a forest fire and then to increase again along with the succession of forests (Zhang-Turpeinen et al., 2020). For a comparison, Bai et al. (2017) showed that biomass burning resulted in increased BVOC emission fluxes and ozone concentration above canopy in a subtropical forest in China.
2.2 Atmospheric system
Concerning critical atmospheric processes and large-scale climate implications, we concentrate here on greenhouse gases and aerosol particles over northern Eurasia and the Arctic, urban air quality, and issues related to the weather and atmospheric circulation. We summarize the recent measurements on the atmospheric composition relevant to sink and source dynamics in Siberia, on the sources and properties of atmospheric aerosols in Arctic-boreal environments, including black carbon and dust in the atmosphere and snow, and on the methodological and model developments related to atmospheric chemistry and physics (Q4, Sect. 2.2.1). Furthermore, we introduce new results and observations of atmospheric pollution in rural, suburban and megacity environments in China and Russia (Q5, Sect. 2.2.2). We briefly show some recent results related to synoptic-scale weather in Arctic-boreal regions, focusing on cold and warm episodes, cyclone density and atmosphere–ocean interaction, effects of circulation on temperature and moisture, cloudiness in the Arctic, and boundary-layer dynamics (Q6, Sect. 2.2.3).
2.2.1 Atmospheric composition and chemistry (Q4)
Boreal forest carbon balance
As already discussed in Sect. 2.1.1, boreal forests as a carbon sink and the related role of forestation have been under international debate. It seems that early snowmelt increases springtime carbon uptake of the boreal forests of Eurasia and North America and shows a major advance in the CO2 sink (Pulliainen et al., 2017). A scenario of a complete global deforestation by Scott et al. (2018), combining radiative forcing with CO2, surface albedo and short-lived climate forcers (SLCFs), suggests that global deforestation could cause a 0.8 K warming after 100 years, with SLCFs contributing 8 % of the effect. However, deforestation as projected by the RCP8.5 scenario leads to zero net radiative forcing from SLCFs, primarily due to non-linearities in the aerosol indirect effect. Tuovinen et al. (2019) showed that methane fluxes vary strongly with a wind direction in a tundra ecosystem with heterogeneous vegetation. By combining very high-spatial-resolution satellite imagery and footprint modelling, they were able to estimate the relation between the main land cover types and ecosystem level measurements. CH4 emissions originated mainly from wet fen and graminoid tundra patches, whereas the areas of bare soil and lichen acted as strong CH4 sinks (Tuovinen et al., 2019; Tsuruta et al., 2017). Tsuruta et al. (2017) reported posterior mean global total emissions of 516±51 Tg CH4 yr−1 during 2000–2012, which indicates that these emissions had increased by 18 Tg CH4 yr−1 from the period 2001–2006 to 40 Tg CH4 yr−1 from the period 2007–2012. This increase can be explained by increased emissions from the temperate region in South America and from the temperate region and tropics in Asia.
Analysis of the trends and the diurnal, weekly and seasonal cycles of CO2 and CH4 mixing ratios derived from the long-term data of the Japan–Russia Siberian Tall Tower Inland Observation Network showed that the frequency of identified events of elevated concentration differs for CO2 and CH4 and may reach up to 20 % of days in some months (Belikov et al., 2019). These observations made it possible to reduce uncertainties in the biosphere surface CO2 uptake (Kim et al., 2017). Although the CO2 uptake in boreal Eurasia estimated by Kim et al. (2017) was about 30 % lower than that obtained without the assimilation of Siberian observation data, Siberia still remains a key contributor to the terrestrial CO2 sink in the Northern Hemisphere.
There are tendencies of a significant growth or suppression of soil CO2 fluxes across different types of human impacts, such as forest fires, trampling, settlements, reindeer grazing and clear cuts on cryogenic ecosystems in Russia (Karelin et al., 2017). For example, Ivakhov et al. (2019) analysed CO2 measurements during 2010–2017 and reported CO2 concentration increases of 20 ppm in Tiksi at a coast of the Laptev Sea and of 15 ppm at the Cape Baranov station. They also detected that wildfires in Siberia can lead to a parallel increase in the CO2 concentration in the Russian Arctic. Furthermore, the measurements showed that the atmospheric CO2 concentration increased on average by 2.0 ppm yr−1 during 2006–2013 in central Siberia, with a large inter-annual variation. The highest increases were found in 2010 and 2012 (3.6 and 4.3 ppm yr−1, respectively), when large wildfires released huge amounts of CO2 in Siberia (Timokhina et al., 2015b). Repeated wildfires in boreal forests can combust a portion of the thick organic soil layer characteristic of this ecosystem and change the forests from a carbon sink to a carbon source (Walker et al., 2019). A study on a fire chronosequence of the central Siberian permafrost soil showed that soils affected by fires over decades act as CO2 sources and that the CO2 emissions from these soils increased with an increasing time since the last fire (E. Köster et al., 2018). However, there were no similar effects on CH4 emissions, with soils acting as a CH4 sink without any connection to forest fires. In addition to CO2, wildfires also release large amounts of other trace gases and aerosols. Emission factors of several trace gases and aerosols from Siberian fires measured from the Trans-Siberian Railway were reported by Vasileva et al. (2017). The impact of Siberian fires as elevated aerosol concentrations was at times observed to extend up to the Arctic coast (Asmi et al., 2016).
Arctic methane (CH4) balance
Deep understanding of the dynamics of methane emissions in the Arctic is needed for identifying and quantifying GHG-related feedbacks and the global methane cycle (Dean et al., 2018). During the winter, methane originates mostly from anthropogenic sources, while on smaller scales emissions from the oceans, including the ESAS, can also play an important role. During the warm season, the balance is dominated by emissions from wetlands and freshwater bodies. Thonat et al. (2017) employed the CHIMERE model for an assessment of the methane cycle in the Arctic. They reported that all methane sources, except biomass burning, contributed to measurements at six study sites. That study emphasizes the importance of a joint model–measurement approach for studies of complex phenomena at large spatial scales. Accounting for OH oxidation and soil uptake, two important sinks of methane, improved the agreement between observed and modelled methane concentrations (Thonat et al., 2017). Peltola et al. (2019) upscaled CH4 fluxes measured at 25 northern wetland sites and showed three different maps of wetland distribution, with the annual methane emissions varying from 31 to 38 Tg CH4 yr−1. (For the monthly upscaled CH4 flux data products, see https://doi.org/10.5281/zenodo.2560163.) Multiple sources, together with different spatiotemporal dynamics and magnitudes, are influencing the total Arctic CH4 budget and address the need for further improved assessments (Peltola et al., 2019; Thonat et al., 2017).
Northern Eurasian carbon monoxide (CO)
Analysis of long-term trends in the atmospheric composition in remote northern Eurasia (1998–2016) showed that the total column carbon monoxide (CO) amount has been stabilized or increased in summer and autumn months (Rakitin et al., 2018). The changes in the global photochemical system, especially changes in the ratio between the sources and sinks of minor atmospheric chemical species, could explain these trends (Skorokhod et al., 2017). A comparative study (1998–2014) on the atmospheric total column CO amount in background and polluted regions of Eurasia indicated that this amount has decreased remarkably in the Moscow urban environments (3.73 % yr−1 ± 0.39 % yr−1) compared to the background regions (0.9 % yr −1–1.7 % yr−1) (P. C. Wang et al., 2018).
Northern Eurasian ozone (O3)
Atmospheric measurements of ozone, its precursors and other pollutants over Siberia are important for the atmospheric chemistry modelling, satellite product validation and comparisons between Siberia and other regions of the Northern Hemisphere. Isoprene and monoterpenes together with nitrogen oxides impact tropospheric O3 formation and lead to an increase in the daytime ozone-forming potential (OFP) in urban environments. Bai et al. (2021) showed that O3 may respond either positively or negatively to isoprene and monoterpene emissions depending on the level of solar radiation and atmospheric loadings of trace gases and aerosol particles. It was demonstrated that monoterpenes have a major contribution to tropospheric O3 formation, especially in cities in Siberia with high atmospheric NOx concentrations (10–20 ppb) and daytime temperatures (> 25 ∘C) (Berezina et al., 2019). In contrast, isoprene is dominating the O3 formation and the increasing OFP in the cities in the Far East. The isoprene-derived OFP can originate from deciduous vegetation growing in city environments or nearby regions or from an anthropogenic isoprene source. The monoterpene-derived OFP was found to be lowest in medium-sized cities and highest in small cities (Berezina et al., 2019). The total contribution of benzene and toluene to photochemical O3 production was found to be up to 60 %–70 % in urbanized environments, indicating anthropogenic pollutant sources (Skorokhod et al., 2017). In addition to atmospheric chemistry, the connection between stratospheric O3, UV radiation and health effects needs to be addressed in the populated urban environments of Siberia. Chubarova et al. (2019b) estimated that the wintertime O3 depletion in northern regions of Siberia is not critical, but the much larger O3 reductions observed in the early spring can lead to dangerous levels of erythema UV radiation (see also Sect. 2.4.3, Natural hazards and UV variation).
Sources and properties of atmospheric aerosols in boreal and Arctic environments
Atmospheric new particle formation (NPF) is the largest contributor to the number concentration of aerosol particles in the global troposphere. During the past couple of decades, NPF has been measured in more than 10 boreal forest sites (Kerminen et al., 2018). The annual frequency of NPF event days varies between about 10 % and 30 % in the boreal forest zone, being highest in the western part of this region and lowest on the northern edge and in the Siberian part of it. Similar high nucleation frequencies were found at two very remote sites in boreal North America (Andreae et al., 2019). In contrast, annual NPF event frequencies below 2 % were reported from central Siberia (Wiedensohler et al., 2019). Similarly, in northern Siberia on the Arctic coast, NPF events were mainly connected to marine and coastal air masses and rarely observed in continental air masses (Asmi et al., 2016). Seasonally, NPF tends to be most frequent during spring, even though high NPF event frequencies can also be observed during summer or autumn. Wintertime NPF is rare throughout the boreal forest region. In the Arctic, NPF appears to be a major aerosol particle source from spring to summer, after which this source collapses during autumn and is practically absent over the whole winter (Freud et al., 2017). Twenty years of NPF observations in a boreal forest at the SMEAR II station in Finland show a higher frequency of NPF events under clear-sky conditions in comparison to cloudy conditions (Dada et al., 2017). Also, oxidized organic vapours showed a higher concentration during the clear-sky NPF event days, whereas the condensation sinks and some trace gases had higher concentrations during the non-event days.
The overall importance of atmospheric NPF in boreal and Arctic areas depends on the growth of freshly formed particles to cloud condensation nuclei. In the boreal forest environment, both observations and model simulations indicate that the particle growth is tied strongly to the oxidation products of biogenic volatile organic compounds originating from forest ecosystems (Paasonen et al., 2018; Östrom et al., 2017). The important compound group in this respect is monoterpenes, even though sesquiterpenes were also found to have high secondary organic aerosol yields in boreal forest environments (Hellén et al., 2018). The observed particle growth rates were found to increase with an increasing particle size and to be highest in summer (Paasonen et al., 2018). The chemistry of new particle growth in the Arctic atmosphere is not well characterized, even though available observations suggest that this growth is associated mainly with biogenic emissions from high-latitude marine areas (Giamarelou et al., 2016; Heintzenberg et al., 2017; Kecorius et al., 2019).
Wildfires are an important source of particulate pollutants on a global scale and are affecting both air quality and climate (Andreae, 2019; Bondur et al., 2020). Satellite observations indicate that the annual burned area by wildfires in Russia decreased by a factor of 2.6 during 2005–2016 owing to early detection and suppression of fire sources, whereas in Ukraine the relative size of burned-out areas increased by a factor of 6–9 from 2010–2013 to 2014–2016 (Bondur et al., 2017, 2019d). For Siberia, Ponomarev et al. (2016) reported strong increases in both number and burned areas based on satellite data, with burned areas doubling from 2005 to 2016. While biomass burning emissions have been measured widely over Russia (Bondur, 2016; Bondur and Ginzburg, 2016; Bondur et al., 2019d), there is still a need for further information on the atmospheric composition of wildfire emissions and related emission ratios from the Siberian region. Fire experiments provide important information on the emission and aging characteristics of smoke aerosols (e.g. Kalogridis et al., 2018).
Luoma et al. (2019) presented a detailed trend analysis for aerosol optical properties at the SMEAR II station in Finland. They found a statistically significantly decreasing trend for the scattering coefficient and even a stronger decreasing trend for the absorption coefficient during 2006–2017. These trends are very likely indicative of decreasing influence of anthropogenic emissions, with the contribution from emissions containing black carbon decreasing even more quickly.
Measurements of carbonaceous aerosols over central Siberia during 2010–2012 showed that, in autumn and winter, high concentrations of such aerosols were caused by long-range transport from the cities located in southern and south-western regions of Siberia (Mikhailov et al., 2015a). In spring and summer, pollution levels were high due to regional forest fires and agricultural burning in the Russian–Kazakh region. The variability of the background concentration of organic aerosols correlated with the air temperature in summer, implying that biogenic sources dominated the formation of organic particles at that time of the year (Mikhailov et al., 2015b). Based on a 5-year study by Mikhailov et al. (2017), it seems that the atmospheric pollution originating from the biomass burning and anthropogenic emissions is significantly affecting the Siberian region. However, in summer precipitation is removing the pollutants from the air and leading to relatively clean atmospheric conditions in this region.
At circumpolar sites over the Arctic, aerosol optical properties were found to vary both seasonally and spatially (Schmeisser et al., 2018). Arctic haze aerosols in late winter and spring are characterized by increased concentrations of sulfate, whereas in summer rich organic chemistry seems to be associated with vegetation, local urban and shipping sources as well as secondary aerosol formation influenced by emissions from low-latitude Siberia (O. Popovicheva et al., 2019). From a longer perspective, Arctic observations show large decreases in both sulfate and black carbon concentrations since the early 1980s (Breider et al., 2017).
Observations of the elemental composition of surface aerosols on the coastal Kandalaksha Bay of the White Sea were indicative of the dominance of biogenic aerosol particles during summertime, with heavy metal concentrations in aerosols being at Arctic background levels (Starodymova et al., 2016). Increases in Ni and Cu concentrations were observed in air masses arriving from the western part of the Kola Peninsula indicative of emissions from the smelters in that region.
Black carbon and dust in the atmosphere and snow
Black carbon (BC) is a potentially large contributor to climate forcing in the Arctic region; however, the assessment of its pollution is hampered by the lack of aerosol studies in northern Siberia (O. Popovicheva et al., 2019; O. B. Popovicheva et al., 2019). The spatial variability of Arctic BC was studied using a harmonized data set from six circumpolar Arctic observatories (Backman et al., 2017). These data suggested a significant spatial and seasonal variability (Schmeisser et al., 2018), addressing a need for more year-round data. BC observations (September 2014–September 2016) at the Hydrometeorological Observatory Tiksi at a coast of the Laptev Sea showed a seasonal variation, with the highest concentrations (up to 450 ng m−3) from January to March and the lowest ones (about 20 ng m−3) in June and September. During winter, stagnant weather and stable atmospheric stratification resulted in the accumulation of pollution, depending also on the wind direction and air mass transport (O. Popovicheva et al., 2019).
For the Arctic, important sources of BC include industrial regions of northern Europe, gas flares of the oil fields in the North Sea and Siberia, and Siberian biomass burning (Shevchenko et al., 2015; Konovalov et al., 2018). In 2012, for example, approximately a quarter of the biogenic BC emissions from Siberia after the fire season were transported into the Arctic (Konovalov et al., 2018). Popovicheva et al. (2017) analysed the BC origins over the Russian Arctic seas together with simulated BC concentrations. Concentrations were observed to be high (100–400 ng m−3) over the Kara Strait, Kara Sea and Kola Peninsula and extremely high (about 1000 ng m−3) over the White Sea. It seems that the gas-flaring emissions from the Yamal–Khanty–Mansiysk and Nenets–Komi regions affected the measurements made over the Kara Strait (north of 70∘ N) region, while the near-Arkhangelsk (White Sea) region was connected to the biomass burning at mid-latitudes. Combustion in central and eastern Europe was also identified as an important BC source.
Atmospheric aging promotes an internal mixing of BC with other aerosol constituents, leading to enhanced light absorption and radiative forcing. In situ observations at Arctic stations demonstrated an absorption enhancement due to the internal mixing of BC, which is a systematic effect and should be considered for quantifying the aerosol radiative forcing in this region (Zanatta et al., 2018).
Regional modelling of the Arctic aerosol pollution showed that long-range transported anthropogenic emissions and biomass burning are the main contributors to direct aerosol radiative effects in the region (Marelle et al., 2018). However, a scenario for 2050 indicates that shipping emissions in the Arctic Ocean could become the main source of surface aerosol and local flaring as a major source of BC, as the flaring is already a major source of BC in north-western Russia. Kühn et al. (2020) assessed the effects of different BC mitigation measures on Arctic climate and showed that reducing BC emissions by the Arctic Council member states can reduce BC deposition by about 30 % compared to the current situation. A full execution of recommendations by the Arctic Council member and observer countries could reduce the annual global premature deaths due to PM (particulate matter) by ∼9 % by 2030 (Kühn et al., 2020). Evangeliou et al. (2018) estimated the origin of elemental carbon (EC) in snow and showed that, for West Siberia, where gas flaring emissions are a major contributor, the model underestimation was significant. Furthermore, the model was evaluated by independent BC measurements in snow over the Arctic and, again, the model underestimated BC concentrations, especially in spring.
Climatically significant cryosphere effects of light-absorbing, high-latitude dust can be similar to the albedo and melt effects of BC (Peltoniemi et al., 2015; Svensson et al., 2016, 2018; Meinander et al., 2020a, b). Iceland is the most significant source of European Arctic dust and plays a role in the cryosphere–atmosphere–biosphere interactions and feedbacks (Boy et al., 2019; Dragosics et al., 2016; Dagsson-Waldhauserova and Meinander, 2019). Dust storms from technogenic mining industry tailing dumps on the Kola Peninsula are also an important source of local atmospheric pollution for neighbouring cities, e.g. Apatity and Kirovsk (Amosov et al., 2020). Besides regional-scale dust storms from deserts in Kazakhstan, Mongolia and China are also significant sources of aerosol pollution for these regions and for northern Asia.
Methodological and model developments related to atmospheric chemistry and physics
Several methods to characterize the atmospheric chemistry were introduced or improved. Motivated by the ability of atmospheric ion measurements to identify NPF events in the atmosphere (Leino et al., 2016), a new classification method for atmospheric NPF was developed (Dada et al., 2018). The new method uses both ion and aerosol particle number concentration measurements in the size ranges of 2–4 and 7–25 nm, respectively, is complementary to the traditional event analysis, and can also be used as an automatic way of determining NPF events from large data sets. Zaidan et al. (2018b) used a mutual information approach for a variety of simultaneously monitored ambient variables, including trace gas and aerosol particle concentrations and several meteorological variables, in order to identify key factors contributing to atmospheric NPF. This method can also be used in the atmospheric studies to discover other interesting phenomena and relevant variables. The NPF is directly observed by monitoring the time evolution of ambient aerosol particle size distributions. A new machine-learning-based approach, a Bayesian neural network (BNN) classifier, points out the potential of these methods and suggests further exploration in this direction (Zaidan et al., 2018a).
The condensation sink, being proportional to the surface area of an aerosol population, is one of the major parameters controlling NPF. A simple model for the time evolution of the condensation sink in the atmosphere for intermediate Knudsen numbers was developed to describe the coupled dynamics of the condensing vapour and the condensation sink (Ezhova et al., 2018a). The model gives reasonable predictions of condensation sink dynamics during periods of particle growth by condensation in the atmosphere. A new empirical relation between the atmospheric cloud condensation nuclei (CCN) concentration and aerosol optical properties was derived (Shen et al., 2019), making it possible to estimate CCN concentrations at sites with continuous observations of aerosol optical properties.
Empirical models of solar radiation were developed and used for calibrations of solar radiometers (Bai, 2019). This method can be used to calibrate all kinds of solar radiometers. A solar radiation model combined with ceilometer and pyranometer measurements was used to classify clouds at SMEAR II (Ylivinkka et al., 2020). It opens new possibilities for studies of aerosol–cloud interactions.
Che et al. (2016) made an inter-comparison of three satellite (AATSR Level-2) aerosol optical depth (AOD) products (SU, ADV and ORAC) over China. The SU algorithm performs very well over sites with different surface conditions in mainland China from March to October but slightly underestimates AOD over barren or sparsely vegetated surfaces in western China. The ADV product has the same precision and error distribution as the SU product. The main limits of the ADV algorithm are underestimation and applicability. The ORAC algorithm has the ability to retrieve AOD at different ranges, including high values of AOD, but its stability deceases significantly with an increasing AOD, especially when AOD > 1.0 (see also Sect. 2.2.2, Urban air quality and megacities).
One of the major problems for both interpretation of satellite data and applications of empirical models of solar radiation is related to elevated aerosol layers in the atmosphere. It was demonstrated that their origin can be attributed to a higher confidence when back trajectories are combined with lidar and radiosonde profiles (Nikandrova et al., 2018).
Black carbon measurement methods have progressed. A representative value for the multiple scattering enhancement factor, a fundamental quantity correcting atmospheric black carbon measurement using an aethalometer, was derived for the first time in the Arctic environment (Backman et al., 2017). By analysing BC measurements made with an aethalometer in Nanjing, Virkkula et al. (2015) showed that the compensation parameter of a widely used data processing method depends both on single-scattering albedo and the backscatter fraction of the aerosol (see also Sect. 2.2.2, Urban air quality and megacities). The multiple-scattering correction factor of quartz filters and the effect of filtering particles mixed in snow were estimated by Svensson et al. (2019), who applied the method for analysing light absorption and BC in snow samples taken from Finnish Lapland and the Indian Himalayas.
Measurement of atmospheric sub-10 nm particle number concentrations has been of substantial interest recently. A new high-flow differential mobility particle sizer (HF-DMPS) was built, calibrated and operated under field conditions for 1 month (Kangasluoma et al., 2018). The counting uncertainties of the HF-DMPS were reduced by about 50 % as compared to the traditional DMPS. The HF-DMPS detected about 2 times more particles than the DMPS in the size range of 3–10 nm. Below 3 nm, the HF-DMPS is currently limited by the inability of diethylene glycol to condense on biogenic particles. To collect BVOC samples, a novel collection method offering portability and improved selectivity and capacity was developed. Solid-phase micro-extraction (SPME) arrow sampling (Feijó Barreira et al., 2018) can be used for static and dynamic collection of BVOCs under the field conditions. A significant improvement in sampling capacity was observed with the new SPME arrow system over SPME fibres. A fully automated online dynamic in-tube extraction (ITEX)–gas chromatography/mass spectrometry (GC/MS) method was introduced for continuous and quantitative monitoring of volatile organic compounds in air (Lan et al., 2019). The stability and suitability of the developed system were validated with a measurement campaign, and the ITEX method provided 2–3 magnitude lower quantitation limits than established methods. Parshintsev et al. (2015) introduced a new, fast analysis method for the desorption atmospheric pressure photoionization high-resolution (Orbitrap) mass spectrometry (DAPPI-HRMS). The DAPPI results agreed with the aerosol particle number measured with an established method and were found to detect different compounds and give complementary information about the aerosol samples.
Fragmentation of molecular clusters inside mass spectrometers is a significant uncertainty source in many chemical applications. A novel model capable of quantitatively predicting the extent of fragmentation of sulfuric acid clusters was developed (Passananti et al., 2019). The fragmentation cannot be described in terms of rate constants under equilibrium conditions, because clusters accelerate under electric fields (Zapadinsky et al., 2019). A model describing an energy transfer to the cluster-internal modes caused by collisions with residual carrier gas molecules was developed. The model can be used to interpret experimental measurements done with atmospheric pressure interface mass spectrometers.
Recently, a new atmospheric observation site equipped with state-of-the-art atmospheric aerosol instrumentation was deployed in Beijing, China (Liu et al., 2020). At the Beijing University of Chemical Technology (BUCT), the Aerosol and Haze Laboratory (AHL) was established in 2018–2019, providing novel insights into air pollution in a comprehensive manner. The station hosts comprehensive instrumentation to concentrations of atmospheric trace gases, aerosol particle size distributions and mass concentrations, and particle chemical composition on the levels from molecules, clusters and nanometre- to micrometre-sized aerosol particles. For example, the first results showed increased cluster-mode particle number concentrations during NPF events, whereas during haze days accumulation-mode particle number concentrations were high (Zhou et al., 2020). The observations have enabled us to quantify number emission factors and underlined the importance of traffic (Kontkanen et al., 2020). Daytime sulfuric acid concentrations in Beijing were typically around 4.9 × 106 cm−3 (Lu et al., 2019). During these measurements, evidence was found of significant nighttime sulfuric acid production, yielding gaseous sulfuric acid concentrations of 1.0 to 3.0 × 106 cm−3 (Guo et al., 2021). For further results, see also Sect. 2.2.2, Urban air quality and megacities.
Besides Beijing, measurements have been performed in several other locations inside the PEEX area. We used novel instrumentation to measure new particle formation and its precursors at the background Fonovaya station in the Tomsk region (Russia, Siberia), at the Värriö subarctic research station (Finland), in Ny-Ålesund (Svalbard, Norway) and on the German icebreaker, the Polarstern, during the MOSAIC project. As an example, the first results from Fonovaya station are shown in Fig. 2. Thanks to these deployments, in the next years we will be able to understand the identity of NPF precursors in those remote places. This will help us to elucidate the human impact on aerosol formation and thereby on aerosol–cloud interactions at high latitudes. In Siberia, we will finally understand why new particle formation occurs infrequently and hopefully also identify the human role in this phenomenon. In the Arctic, we will understand the marine influence on NPF and will find out the detailed mechanism that leads to the formation of small clusters that initiate NPF.
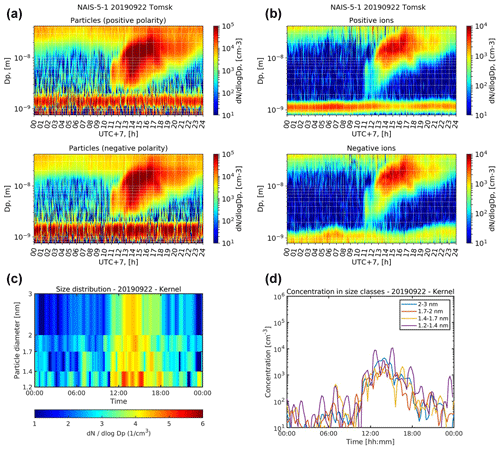
Figure 2Example of results from the state-of-the-art aerosol instruments NAIS and PSM displaying an NPF event at Fonovaya station, Siberia, on 22 September 2019. Particles of different polarity, NAIS (a), ions of different polarity, NAIS (b), particle number distribution at the smallest sizes, PSM (c), and number concentration of the smallest particles in different size bins, PSM (d).
Model developments were made at several scales. Aerosol–radiation and aerosol–cloud interactions are among the main sources of uncertainties in climate models, and detailed information on anthropogenic aerosol number emissions is needed to improve this situation. Anthropogenic aerosol number emissions in current large-scale models are usually converted from corresponding mass emissions in pre-compiled emission inventories using very simplistic methods. In the global aerosol–climate model ECHAM-HAM, the anthropogenic particle number emissions, converted originally from the AeroCom mass emissions, were replaced with recently formulated number emissions from the Greenhouse Gas and Air Pollution Interactions and Synergies (GAINS) model (Xausa et al., 2018). However, revisions are still needed in the new particle formation and growth schemes currently applied in global modelling frameworks.
For regional and urban scales, a fully integrated/online coupled meteorology–chemistry–aerosol model, Enviro-HIRLAM, was developed (Baklanov et al., 2017) and tested for several applications in Europe, the Russian Arctic and China (Shanghai) (Mahura et al., 2018, 2020). Key issues for seamless integrated chemistry–meteorology modelling for Earth system prediction were analysed and formulated (Baklanov et al., 2018), highlighting the scientific issues and emerging challenges that require proper consideration to improve the reliability and usability of these models for three main application areas: air quality, meteorology, and climate modelling. Baklanov et al. (2018) also present a synthesis of scientific progress in the form of answers to nine key questions and provide recommendations for future research directions and priorities in the development, application, and evaluation of online coupled models.
2.2.2 Urban air quality and megacities (Q5)
The rapid urbanization and growing number of megacities and urban agglomerations require new types of research and services that make the best use of science and available technology. There are urgent needs for examining what the rising number of megacities means for air pollution and local climate and what effects these changes have on global climate (Baklanov et al., 2016). Such integrated studies and services should assist cities in facing hazards, such as storm surge, flooding, heat waves and air pollution episodes, especially in changing climates (WMO, 2019). We discuss here the recent observation of the atmospheric pollution in China and Russia.
Air quality in China – recent observations
China is one of the regions with the highest concentrations of fine PM2.5 in the world (J. Wang et al., 2017). This has serious consequences for air pollution and the associated visibility reduction (haze) and adverse health effects (Zhao et al., 2017). The number of haze days in China has been growing during recent decades, but detailed understanding of the factors governing the occurrence of haze is still not clear (Wang et al., 2019). Both NO2 and SO2 concentrations showed increasing trends during the 2004–2012 period, and these trends could be linked to increased power plant and traffic missions (Wang et al., 2019). A key feature of haze formation seems to be an increased inorganic fraction of the aerosol, suggesting that the reduction of nitrate, sulfate and their precursor gases would improve air quality and visibility in China (Wang et al., 2019). In northern China, PM2.5 concentrations declined over the period 2013–2017, and approximately half of the inter-annual variability in this region was attributed to atmospheric circulation changes (M. Li et al., 2020). The maximum daily 8 h average O3 concentrations increased over most of northern China during the same time period, with large influences again due to atmospheric circulation on a daily basis (J. Liu et al., 2019).
Compared with most other urban environments investigated so far, measurements in urban China demonstrated a relatively frequent occurrence of atmospheric NPF, and the observed NPF events were typically characterized by high particle formation rates and strongly size-dependent growth of newly formed particles (Kulmala et al., 2016b; Z. B. Wang et al., 2017; Chu et al., 2019). Since the first reported sub-3 nm particle measurements in China a few years ago (Xiao et al., 2015), new insights into the formation pathways of molecular clusters and their growth have been obtained, including the relative roles of gaseous sulfuric acid, amines, ammonia and organic vapours in these processes (Yao et al., 2018; Yan et al., 2021). While high pre-existing particle loadings appear to suppress NPF during severe haze periods in Chinese megacities, it is unclear how NPF is possible at all under less but still quite polluted conditions typical of these environments (Kulmala et al., 2017). Overall, the available observations suggest NPF to be a major source of aerosol particles in urban China, with potentially large effects on haze formation (Kulmala et al., 2021) and cloud properties (Chu et al., 2019).
Urban measurements of particle number size distributions give deep understanding of the sources and atmospheric processing of fine particles. The longest urban continuous record is from the SORPES station in the Yangtze River Delta (Qi et al., 2015), covering almost a decade of measurements, whereas the broadest size range (1.5 nm–1 µm) was measured in the winter Beijing atmosphere (Zhou et al., 2020). The latter study found clear differences in particle sources in different size ranges: NPF was in general the largest source of clusters and nucleation-mode (< 25 nm) particles, while traffic contributed to all the size ranges and dominated both cluster and nucleation modes on haze days. Aitken-mode (25–100 nm) particles originated mainly from local emissions, with additional contributions from regional and transported pollution as well as from the growth of nucleation-mode particles. Regional and transported pollution was identified as the main source of accumulation-mode (> 100 nm) particles.
Air pollution and chemical transformation, including annual and seasonal variations of the concentrations of atmospheric constituents, were analysed for North China for the period 2005–2015 (Y. Bai et al., 2018). A photochemical link that related the production of fine PM and O3 to VOCs was detected, and this mechanism was found to be prominent in summer. An intensive measurement campaign (SORPES station, Yangtze River Delta) was carried out to investigate sulfate formation and the associated nitrogen chemistry (Xie et al., 2015). That study highlighted the effect of NOx in enhancing the atmospheric oxidizing capacity and indicated a potentially very important impact of increasing NO concentrations on particulate pollution formation and regional climate change in East Asia. In Changzhou, a highly populated city in the Yangtze River Delta, primary organic aerosol concentrations outweighed secondary ones, indicating an important role of local anthropogenic emissions in aerosol pollution (Ye et al., 2017). The measurement also showed the abundance of organic nitrogen compounds in water-soluble organic aerosol, suggesting that these compounds are likely associated with traffic emissions.
Aerosol impacts on warm cloud properties were investigated over three major urban clusters in East China and the East China Sea using multi-sensor satellite observations (Liu et al., 2017, 2018). In addition to the amount of aerosol, evidence was provided that aerosol types and environmental conditions need to be considered to understand the relationship between cloud properties and aerosols. Aerosol–cloud interactions were found to be more complex and of greater uncertainty over land than over the ocean.
The atmospheric boundary layer (ABL) and especially its dynamic behaviour are central to the evolution of near-surface air pollution. Using atmospheric observations combined with theoretical arguments, Petäjä et al. (2016) proposed a feedback mechanism connecting ABL properties with PM. According to such a mechanism, high concentrations of PM enhance the stability of an urban boundary layer (BL), decreasing its height and thus causing further accumulation of pollution inside the BL. Ding et al. (2016a) and Z. Wang et al. (2018) demonstrated an important role of BC aerosols in this feedback using model simulations combined with observations. A tight connection between the BL height and pollutant concentration and indications of the presence of the above feedback mechanism were also found based on comprehensive observations made on a 325 m tower in Beijing (Wang et al., 2020). In order to understand these feedbacks, Kulmala (2018) and Hari et al. (2016) emphasized the crucial role of continuous, comprehensive measurements in a network of flagship stations in tackling the air pollution problem in urban China and megacities elsewhere in the world. They also introduced the so-called SMEAR concept, which consists of integrated atmospheric and ecosystem observations allowing the analysis of Earth surface–atmosphere feedbacks and interactions. The first SMEAR-type station in China, the SORPES station located in the Yangtze River Delta, has been operating since 2011 (Ding et al., 2016b).
Anthropogenic emissions and environmental pollution in Russia
In the complex situation of the plurality of emissions, an important research task remains in the Moscow megacity environment for the assessment of the air quality and potential sources through aerosol composition analyses. Moscow aerosol pollution has been studied using a special AeroRadCity-2018 experiment (Chubarova et al., 2019a) and satellite data with the application of the new MAIAC/MODIS aerosol algorithm with a 1 km resolution (Zhdanova et al., 2020). An advanced source apportionment for this environment was performed using combined Fourier-transform infrared spectroscopy data and statistical principal component analysis (O. Popovicheva et al., 2020a). The main principal component loadings revealed the source impacts of transport and biomass burning, biogenic, dust and secondary aerosol in spring. Identification of biomass burning-affected periods discriminated between the daily aerosol composition change with respect to air mass transport and the number of fires detected in the surrounding areas. Measurements of particulate BC were conducted at an urban background site (Meteorological Observatory of MSU) during the spring period of 2017–2018 (O. Popovicheva et al., 2020b). The mean BC concentrations displayed significant diurnal variations, with a poorly prominent morning peak and minimum at daytime. BC mass concentrations were higher at nighttime due to the shallow boundary layer and intensive diesel traffic. The aerosol optical thickness (AOT) over Moscow showed a pronounced seasonal cycle, with a summer maximum and winter minimum (N. Y. Chubarova et al., 2016). It was found that during 2001–2014, the monthly-mean values of AOT declined by 1 % yr−1–5 % yr−1, and this decline was attributed to decreased emissions of aerosols and their precursors.
In general, the atmospheric environment over remote areas of Siberia and northern Asia is relatively clean compared with other surrounding regions of Asia and eastern Europe (Baklanov et al., 2013). However, air pollution from Siberian industrial centres poses significant environmental threats. For Siberian cities (e.g. Norilsk, Barnaul, Novokuznetsk), the air quality is among the worst in Russian and European cities. Similar to Arctic cities, stable atmospheric stratification and temperature inversions dominate for more than half a year. This leads to pollution accumulation near the surface, which influences ecosystems and people. Moreover, not only severe climatic conditions, but also man-made impacts on the environment in industrial areas and large cities have intensified. The impacts manifest themselves as the pollution of the environment, land use changes, hydrodynamic regimes and the local climate. Ultimately, these impacts feed back to people, affecting their health and well-being.
The Russian part of the Barents Euro-Arctic region includes severe emission “hotspots” for air pollutants. The Kola Peninsula, despite the presence of areas with undisturbed nature in the eastern part, is the most industrially developed and urbanized region in the Russian Arctic. The main polluters are the smelters of the Severonickel (Monchegorsk, central part of the peninsula) and Pechenganickel (Nickel and Zapolyarnyi near the Russian–Norwegian border) enterprises. For comparison, emissions of SO2 from the nickel smelter alone are 5–6 times larger than the total Norwegian emissions (Sandanger et al., 2013). In 2015, Norilsk Nickel in Siberia – the biggest mining and metallurgical complex – emitted about 1.9 million tonnes of SO2 (GGO, 2016). With the nickel factory (located in the southern part of the city), copper factory (just to its north) and metallurgical plant (12 km to the east), the city of Norilsk is influenced by heavy industry no matter which way the wind blows. The Blacksmith Institute declared in 2007 that Norilsk is one of the top 10 worst-polluted places in the world. The impacts of emissions are manifested as deterioration of forest ecosystems and acidification of soils and surface waters (Derome and Lukina, 2011), even at considerable distances from the smelters. Heavy metals and alkaline pollutants contaminate areas around the sources of pollution within a few hundred kilometres, while acid sulfates can be transported over long distances (Mahura et al., 2018).
A recent analysis of the total deposition and loading on the population in north-western Russia and Scandinavian countries caused by the continuous sulfur emissions from the Cu–Ni smelters in Murmansk indicates the dominance of wet deposition, especially in wintertime (Mahura et al., 2018). North-western Russia is influenced more by the Severonikel emissions compared with countries in the Scandinavian Peninsula. The cities of the Murmansk region (Kola Peninsula) are under the highest impacts. On a yearly scale, the individual loadings on the population are at the highest level (up to 120 kg/person) in the Murmansk region, much lower (15 kg/person) in northern Norway, and lowest (< 5 kg/person) in eastern Finland, the Republic of Karelia and the Arkhangelsk region. Distinct seasonal variability was identified, with the lowest contribution during summer and the highest contribution during winter–spring in Russia, during spring in Norway, and during autumn in Finland and Sweden.
The annual yearbook, the State of Atmospheric Pollution in cities in the Territory of Russia for 2018 (Roshydromet and GGO, 2019), states that the highest atmospheric emissions of PM were observed in Siberian and Ural cities. In Novokuznetsk and Omsk, the observed PM was highest (> 30 000 t yr−1), while emissions from other cities such as Angarsk and Chelyabinsk were lower (< 20 000 t yr−1). Note that in the 2015–2019 yearbooks, emissions from only stationary sources were provided due to revisions (approved and implemented in November 2019 by the Russian Ministry of Natural Resources and Ecology, MNRE) of methods applied for estimation of emissions into the atmosphere from mobile sources. Depending on source type, different methods to calculate emissions are applied (MNRE, 2019). For the gaseous compounds, such as SO2, the maximum emissions included were very high from Siberian cities (e.g. Norilsk, Novosibirsk, Novokuznetsk, Omsk, Ufa, Irkutsk, Angarsk) and from north-western Russian cities (Zapolyarny, Nickel, Monchegorsk). High NO2 emissions were observed in Novosibirsk, Omsk, Angarsk and Chelyabinsk. The CO integral urban emissions depend on a city's size. These varied from less than 10 Gg yr−1 (for small regional centres like Vladimir, Kursk, or Samara) to 406 and 804 Gg yr−1 for large metropolitan areas such as St. Petersburg and Moscow. As a whole, an analysis of spatiotemporal variation of trace gases in the boundary layer over Russian cities indicated significant emission variations between the urban environments and remote sites (Elansky et al., 2016).
Cities, not being isolated systems, may distribute as much pollution to the surrounding areas as they receive from outside them or from remote regions. The analysis of the transboundary atmospheric transport between Russian Siberia and bordering countries (e.g. China, Kazakhstan, and Mongolia) is part of a mutual risk assessment for urban areas/cities and their surroundings. For example, the city of Ulaanbaatar (Mongolia) suffers from high levels of pollution due to excessive airborne particulate matter emanating from coal combustion mixed with traffic emissions and resuspended soil dust, resulting in variable chemical source profiles (Gunchin et al., 2019). Long-range transport from remote sources might be an additional contributor. Moreover, there are indications that such transport of biomass burning emissions from Siberia could lead to pollution episodes and impact surface ozone as far as in western North America (Jaffe et al., 2004).
2.2.3 Weather and atmospheric circulation (Q6)
The observed evolution of weather and climate represents the combined effects of external forcing (changes in the concentrations of greenhouse gases and aerosols, etc.) and internal variability, related to a large extent to the atmospheric circulation. It is also affected by local factors, particularly urban heat islands in cities. Here we discuss these interconnected processes, focusing on cold and warm episodes, cyclone density and atmosphere–ocean interactions, effects of circulation on temperature and moisture, cloudiness in the Arctic, and boundary-layer dynamics relevant to the Arctic-boreal region.
Cold and warm episodes
The Arctic warming as well as the Arctic amplification have been associated with changes in atmospheric large-scale circulation together affecting the European winter temperatures. In large parts of Europe, severe cold (warm) winter events are significantly correlated with warm (cold) Arctic episodes (Vihma et al., 2020). Air mass trajectory analysis revealed that air masses associated with extreme cold (warm) events typically originate from over continents (sea areas). Despite Arctic and European-wide warming, winter cooling has occurred in north-eastern Europe in cases of air masses arriving from the south-east (Vihma et al., 2020).
Cyclone density dynamics and atmosphere–ocean interaction
Transporting large amounts of heat and moisture from mid-latitudes to the central Arctic, synoptic-scale cyclones are vital for the Arctic climate system. Recent findings, based on atmospheric reanalysis, above all the global ERA-Interim reanalysis available from 1 January 1979 to 31 August 2019, are summarized below. During 1979–2016 in winter (December, January, February), the cyclone density increased in the areas around Svalbard and in the north-western Barents Sea but decreased in the south-eastern Barents Sea (Wickström et al., 2020). This is related to a shift to more meridional winter storm tracks in the Norwegian, Barents, and Greenland seas. The shift is favoured by a positive trend in the Scandinavian pattern and, in the areas north of Svalbard, by a significant increase in the eddy growth rate (Wickström et al., 2020).
Numerical model simulations of the storm activity in the White, Baltic and Barents seas were analysed for the period 1979–2015 (Myslenkov et al., 2018). A high inter-annual variability in the storm number was observed for all studied seas. No significant trends in the storm number during the period 1979–2015 were found in the studied sea areas. On average, the connection with global atmospheric circulation is stronger for the Baltic Sea than for the other two seas. Also, the future changes in wind wave climate were analysed. According to the RCP8.5 scenario, in the second part of the 21st century the number of storm events will rise in the Baltic and Barents seas.
In the Bjerknes compensation, changes in atmospheric heat transport co-occur with opposing changes in ocean heat transport. Observations and model simulations indicate a central role for ocean–atmosphere heat exchange in the Barents Sea area in maintaining this compensation in the Arctic (Bashmachnikov et al., 2018a, b).
Circulation effect on temperature
The effect of atmospheric circulation on temperature trends in the years 1979–2018 was studied by Räisänen (2019, 2021) using a trajectory-based method. He found that the circulation trends had reduced the annual mean warming during this period in West and central Siberia locally by over 1 ∘C, with a much larger cooling effect in autumn and winter (Fig. 3). His findings also confirmed a circulation-induced amplification of warming over the Barents and Kara seas, particularly in winter. However, in most areas the circulation-related temperature trends have varied strongly from month to month, leaving only a relatively small effect on the annual mean temperature trends. The residual warming obtained after subtracting the circulation effect therefore tends to have a smoother seasonal cycle than the observed temperature trends, in better agreement with the multi-model mean trends in the CMIP5 simulations (Taylor et al., 2012).
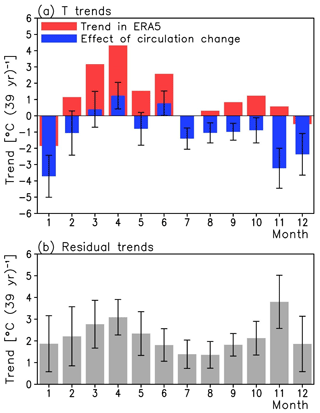
Figure 3Linear trends of monthly mean temperature in West Siberia (55–65∘ N, 65–90∘ E) in the years 1979–2018. In (a), the red bars show the trend in the ERA5 reanalysis and the blue bars the circulation-related trend. In (b), the residual trends are shown. The error bars indicate the 5 %–95 % uncertainty range in the circulation-related trend and the residual trend based on inter-annual variability. Redrawn from Räisänen (2021).
Circulation effect on moisture
The effects of large-scale circulation on moisture, cloud and longwave radiation occur mostly via the impact of horizontal moisture transport (Nygård et al., 2019). Evaporation is typically not efficient enough to shape those distributions, and much of the moisture evaporated in the Arctic is transported southward (Nygård et al., 2019). Strong moisture transport events avail a large part of the northwards moisture transport. The meridional net transport is only a small part of the water vapour exchange between the Arctic and mid-latitudes (Naakka et al., 2019). When a high-pressure pattern across the Arctic Ocean from Siberia to North America is lacking, the amounts of moisture, clouds and downward longwave radiation are anomalously high near the North Pole (Nygård et al., 2019). Using vertically integrated water vapour as a metric, the Arctic (north of 70∘ N) has experienced a robust moistening trend since 1979, and in absolute numbers this trend is smallest in March and largest in August (Rinke et al., 2019). However, the relative trends are largest in winter. Although different atmospheric reanalyses are consistent in spatiotemporal trend patterns, they scatter in the trend magnitudes.
Analysis of moisture and aridity estimated using the web-GIS “CLIMATE” and the ECMWF ERA-Interim reanalysis data for southern Siberia (50–65∘ N, 60–120∘ E) from 1979 to 2010 with a 0.75∘ × 0.75∘ grid resolution showed that the mountain regions of eastern Siberia have become more arid each month during the last 30 years (Ryazanova and Voropay, 2017). In West Siberia, aridity increased in May and decreased in June, while in the other months positive and negative trends were found. The greatest differences in the trends of the aridity index, air temperature and precipitation were observed in July.
Cloudiness in the Arctic
The climatology and inter-annual variability of Arctic cloudiness remain a wildcard in regional climate change projections. Both climate models and satellite data products need in situ observations for calibration and validation. Chernokulsky et al. (2017) and Chernokulsky and Esau (2019) collected and processed manual cloud observations from meteorological stations in the PEEX area. The cloud records in the Arctic have been available since the end of the 19th century. Since 1936, cloud observations have representatively covered the Eurasian Arctic. This permits reconstructions of cloud type and cloud cover climatologies as well as studies of inter-decadal variability of cloudiness. A problem of special interest is related to the co-variability of the total cloud cover and sea ice concentration or extent. Both clouds and sea ice affect the surface heat balance through surface albedo, but their feedback mechanisms, dynamical impacts and climate sensitivities are different. Chernokulsky et al. (2017) found that the annual-mean total cloud cover (TCO) decreases during warmer climate periods with a lower sea ice concentration but increases over sea ice in the Barents Sea as more moisture is transported into the Arctic at higher temperatures. Furthermore, the increasing TCO reduces the deficit of the surface heat, and the intra- and inter-annual variability of TCO over solid ice is higher than that over open water (Chernokulsky et al., 2017). Long-term cloud climatological analysis based on meteorological observations of the total and low cloud cover and cloud types from the Barents Sea to the Chukchi Sea showed that significant transitions between cloud types have taken place, and especially the low-level stratus and stratocumulus types have been transformed to convective cloud types (Chernokulsky and Esau, 2019). Chernokulsky and Esau (2019) addressed how their results are relevant for understanding Arctic cloud processes and feedbacks and how new knowledge is needed to connect the changes in the Arctic radiation balance with the Arctic cloud cover–cloud type climatology.
Boundary-layer dynamics and urban heat islands
Against the background of accelerated and amplified Arctic warming, anthropogenic heat release and metabolism of cities add up to persistent warm temperature anomalies in urbanized areas (Fig. 4). Indeed, if the climate change forcing approaches 2 W m−2, the urban heat forcing could be 10–100 W m−2 (Konstantinov et al., 2018). The urban heating trapped in shallow planetary boundary layers is potent to raise the local temperatures by 1 to 10 ∘C or even more. This local climate phenomenon is known as an urban heat island (UHI) (Esau et al., 2020). A series of in situ and satellite UHI studies in the northern cities revealed strong and persistent warm temperature anomalies in almost all of the 28 northern West Siberian cities (Miles and Esau, 2017), in 5 cities covered by the UHIARC network (Konstantinov et al., 2018; Varentsov et al., 2018a) and in 57 Scandinavian cities (Miles and Esau, 2020). The mean wintertime temperature anomalies and the UHI intensity varied from 0.8 to 1.4 K and had extreme intensities of up to 7 K during cold anticyclone weather conditions. The complete data set of surface UHI intensity derived from MODIS land surface temperature (LST) data products is freely available and published in Miles (2020). Such a UHI-induced strong mediation of cold temperature spells might cause significant socio-economic and environmental impacts in the cities (Konstantinov et al., 2018, Fig. 4). A survey of other UHI studies in 11 Arctic cities and towns confirmed that even relatively small cities at high latitudes may exhibit intensive UHIs. A recent analysis confirms the important role of the surrounding temperature in explaining spatial–temporal variation of the UHI intensity (Miles and Esau, 2017). The major contribution to the UHI was revealed for water, sparse vegetation, grassland and scrubland. The mechanisms and pathways of the UHI maintenance require an involvement of numerical experiments with turbulence-resolving models to advance the understanding of the local climate features (urban heat islands – UHIARC data set; see http://urbanreanalysis.ru/uhi arc.html, last access: 18 January 2022). We would need a denser meteorological network, especially high-quality temperature data, to better understand the urban climatology and the thawing processes in urban soils and to better assess climatic trends relevant to Arctic societies and welfare (Konstantinov et al., 2018).
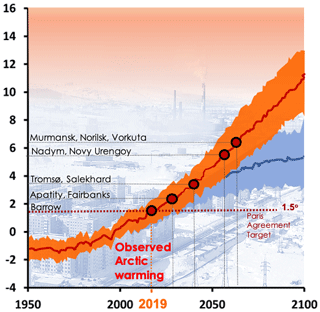
Figure 4The northern urban heat islands are forerunners of the global warming. Winter season future temperatures for the Arctic (60–90∘ N) averaged over 36 CMIP5 global climate models and expressed as departures from the means for the 1981–2005 period. The red line is the ensemble mean for RCP8.5, and the blue line is for RCP4.5. Shaded areas denote ±1 standard deviation from the ensemble mean (Overland et al., 2014 and Fig. 2.15 of AMAP, 2017). The observed surface UHIs are shown as red dots collocated with the expected future Arctic temperature anomalies; e.g. the observed wintertime urban temperature anomaly in Nadym corresponds to the regional warming as expected to be reached by 2060. Observe that the present Arctic climate is already 1.5 ∘C warmer than the historical normals 1960–1990.
Urban climate anomalies may cause more extreme weather and climate phenomena in densely populated megacities. The Moscow agglomeration – the largest megacity in the boreal continental climate within the PEEX domain – demonstrates a profound effect of interactions between the UHI and urban winds, known as a cross-over effect (Varentsov et al., 2018b). The UHI creates an urban heat “dome” with near-surface air inflow into the urban central districts and air outflow at higher levels in the atmosphere. The air uplift in the urban dome is connected to the increase in summer rainfall on the lee side and over the central urban districts. Stable atmospheric stratification over rural areas is strengthened by the downwind air motions coming from the urban region.
The atmospheric boundary layer over the Arctic Ocean has been studied on the basis of tethersonde sounding observations over sea ice (Palo et al., 2017) and research aircraft observations over the open ocean and sea ice (Suomi et al., 2016). Palo et al. (2017) found that in spring and summer, the occurrence and properties of temperature inversions were controlled by the surface melt and warm air advection rather than surface net radiation. During snowmelt/ice melt, temperature inversions were frequently surface-based and equally as strong as winter inversions over the Arctic Ocean. To better understand atmospheric boundary-layer processes in the Arctic, Suomi et al. (2016) developed a method to measure wind gusts from a research aircraft. It allows wind gust observations at altitudes not reached by traditional weather mast observations. The observed gust factors strongly depended on the surface roughness, which differed for sea ice and the open ocean.
2.3 Arctic-boreal aquatic system
We discuss the recent results on Arctic sea ice dynamics and thermodynamics, snow depth and sea ice thickness, sea ice research supporting navigation, and rare elements in snow and the ocean sediments, especially from the perspective of improvements in the observation and modelling methods (Q7, Sect. 3.3.1). We introduce new results on the Arctic marine ecosystem and focus on the primary production and carbon cycle (Q8, Sect. 3.3.2). In Sect. 3.3.3 for the Arctic-boreal lakes and rivers, we discuss the browning of lakes and lake sediment with special attention to the Selenga River system of Lake Baikal (Q9).
2.3.1 Changing water systems, snow, sea ice and ocean sediments (Q7)
Sea ice and thermodynamics with atmospheric and ocean dynamics
Referring to the earlier discussion in Sect. 2.2.3 on atmospheric circulation, we address here how the sea ice dynamics closely interacts with the atmospheric and ocean dynamics. A rapid decrease in the Arctic Ocean ice cover, particularly in the Barents and Kara seas, has been taking place since the late 1970s simultaneously with the cooling of winters in central Eurasia (McCusker et al., 2016). This unexpected winter cooling is related to increasing north-easterly winds over the south-eastern flank of an anomalous high that has developed over the north-western coast of Russia (McCusker et al., 2016; Mori et al., 2019, Räisänen, 2021). However, the causality between the atmospheric circulation changes and the Arctic sea ice decrease is debated. Observations suggest a strong correlation between these two, but climate model simulations forced by reduced ice cover produce much weaker circulation changes than observed, resulting in only weak cooling in central Eurasia (Mori et al., 2014, 2019; McCusker et al., 2016). This suggests either that most models are underestimating the sensitivity of the atmospheric circulation to sea ice decrease, supported by Romanowsky et al. (2019), or that the circulation change has not been primarily caused by the decreasing sea ice. In the latter case, the correlation between the reduced ice cover and atmospheric circulation would mainly reflect the effect of circulation on sea ice. In support of this, Blackport et al. (2019) showed that reduced sea ice coincides with an anomalous heat flux from the atmosphere to the ocean and that, on the sub-seasonal timescale, anomalies in atmospheric circulation tend to precede rather than follow those in sea ice. Thus, while the reduced sea ice might partly explain the observed changes in atmospheric circulation (Mori et al., 2019), the effect of circulation on sea ice appears to be stronger than the effect of sea ice on circulation.
Considering atmosphere–ice interactions, Jakobson et al. (2019) studied the linkages between sea ice concentration (SIC), atmospheric stratification, surface roughness and wind speed at the 10 m height (W10) and 850 hPa level (W850). In all the seasons except summer, a reduction in SIC favoured reduced atmospheric stratification and aerodynamic surface roughness, which resulted in a stronger W10. The effect was strongest in autumn, and positive trends in W10 and its ratio to W850 typically occurred in regions with the strongest negative trends in SIC. The relationships were stronger on inter-annual than sub-seasonal timescales. Large-scale atmospheric circulation, characterized e.g. by the dipole anomaly (DA), has also contributed to sea ice dynamics. A positive polarity in the DA has contributed to the recent rapid loss of summer sea ice in the Pacific part of the Arctic Ocean by bringing warmer air masses from the south and transporting more ice towards the north, enhancing the ice-albedo feedback (Lei et al., 2016). Another example of ice dynamics affecting the ice-albedo feedback was the weakened Transpolar Drift Stream in summer 2013. It reduced sea ice transport out of the Arctic Ocean and restrained ice melt because of the low air temperatures, weakened albedo feedback, and a relatively small oceanic heat flux in the central Arctic (Lei et al., 2018).
Solar radiation, being the main forcing factor for a sea ice melt in summer, is difficult to parameterize in thermodynamic models. This is due to the large variability in the optical properties of sea ice in space and time. A two-stream model provides a time-efficient parameterization of the apparent optical properties (AOPs) for ponded sea ice, accounting for both absorption and scattering, and has a potential to be implemented in sea ice thermodynamic models to explain the role of melt ponds in the summer decay of Arctic sea ice (Lu et al., 2016). This model was used to investigate the role of solar radiation in the Arctic sea ice during the melting season considering layers of melt ponds, underlying sea ice, and the ocean beneath the ice. It was found that the energy absorption profiles depend strongly on the incident irradiance and ice scattering but only weakly on the pond depth. It seems that the incident solar energy is largely absorbed by the melt pond rather than by the underlying sea ice (Lu et al., 2018a). The model was further applied to investigate the influence of a surface ice lid on the optical properties of a melt pond. The thickness of the ice lid determines the amount of solar energy absorbed. Visual inspections of the colour of refreezing melt ponds also help to judge the significance of the influence of the ice lid. This will allow for an accurate estimation of the role of surface ice lids during field investigations of the optical properties of melt ponds (Lu et al., 2018a). The modelled pond colour agrees with field observations from the Arctic sea ice in summer. The analysis of pond colour is a new potential method to obtain ice thickness in summer; however, more validation data and improvements to the radiative transfer model would be needed (Lu et al., 2018b).
Snow depth/mass and sea ice thickness
Snowpack on sea ice has a crucial role in insulating the sea ice from the colder atmosphere, accordingly reducing sea ice growth in winter, effectively reflecting the incoming solar radiation, reducing sea ice melt in spring and summer and contributing to its formation. The replacement of snowfall by rain strongly enhances the ice-albedo feedback in the Arctic Ocean (Dou et al., 2019). Shalina and Sandven (2018) refined the description of snow depth on sea ice in the central Arctic, providing new snow depth data for the Arctic marginal seas. High autumn and winter precipitation and thinning Arctic sea ice make snow–ice formation prevalent in the Atlantic sector of the Arctic (Merkouriadi et al., 2017).
Advances have been made in applying thermistor string-based autonomous high-resolution Snow and Ice Mass Balance Array (SIMBA) buoys to measure snow depth and ice thickness (Figs. 5 and 6). SIMBA has a lower cost, allowing deployment in large numbers (Lei et al., 2015). The determination of snow depth and ice thickness from SIMBA temperature profiles has so far been largely a manual process. A SIMBA algorithm was developed to process SIMBA data automatically (Liao et al., 2018), assuming a fixed snow–ice interface. Snow–ice formation results in the snow–ice interface moving upward. The SIMBA algorithm was further developed to tackle the moving interfaces (Cheng et al., 2020). The developed SIMBA algorithm works well under cold conditions for lakes and polar oceans. For polar oceans, the snow and ice are close to isothermal during summer, which prevents the identification of interfaces on the basis of the temperature gradient. Under such conditions, thermodynamic modelling yields valuable information on snow depth and ice thickness (Tian et al., 2017).
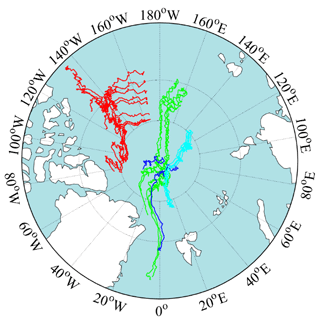
Figure 5Trajectories of SIMBA buoys deployed in the Arctic in the period 2018–2019. Red: Chinese National Arctic Research Expedition (CHINARE) (10 buoys), green: Nansen and Amundsen Basins Observational System (NABOS) (5 buoys), dark blue: CAATEX (2 buoys), and light blue: MOSAIC (15 buoys). SIMBA is a thermistor string-based ice mass balance (IMB) buoy. It measures high-resolution (2 cm) vertical environment temperature (ET) profiles (four times a day) through the air–snow–sea ice–ocean column. The heating temperature (HT) measured by the thermistor string once per day is based on the use of a small identical heater on each sensor. The ET and HT data are used to derive snow depth and ice thickness. SIMBA uses the GPS module to track the buoy location. The Iridium satellite is used for data transmission. A total 15 SIMBA buoys were deployed in the Arctic Ocean during the CHINARE 2018 and NABOS 2018 field expeditions in late autumn. In 2019 17 SIMBA buoys were deployed during the CAATEX (2) and MOSAIC expeditions (15, leg 1).
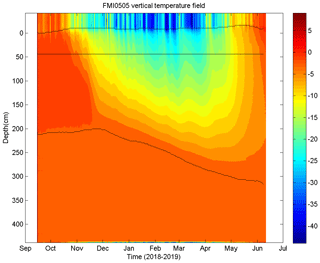
Figure 6SIMBA observations of the temporal evolution of the snow depth, ice thickness, and temperature profile from the ocean through snow and sea ice to air. The results were obtained by applying the algorithm by Liao et al. (2018). The black lines are snow surface (top), initial freeboard (middle) and ice base (bottom); 0 level refers to the snow–ice interface. The colours indicate the temperature in degrees Celsius.
A challenge in sea ice thermodynamic modelling is the uncertainty in the magnitude of the oceanic heat flux at the ice base, especially for land-fast sea ice. Yang et al. (2015) applied a one-dimensional thermodynamic model to investigate impact factors in land-fast sea ice in the East Siberian Sea. The modelled snow cover was less than 10 cm, having a small influence on the ice thickness, but surface albedo and oceanic heat fluxes were critical.
Also in the terrestrial Arctic and boreal zone, there is a need for better efficiency and coverage of an in situ snow observation network. Snow cover and snow mass are fundamental parameters for global energy and water cycles, and the changes in the regional snowpack have societal impacts, like on the amount of drinking water or the capacity for hydropower generation (Bormann et al., 2018). Snow depth data in the Arctic region are available from the synoptic weather stations and snow mass data are systematically collected from the snow courses, as demonstrated in extended data (Fig. 2) by Pulliainen et al. (2020). The use of automatic and cost-effective measurements together with harmonized snow measurement practices is the way forward. A survey on harmonized snow monitoring in Europe demonstrated that crucial parameters for operational services, such as parameters characterizing precipitating and suspended snow, are measured by 74 % of the European snow network contributors (COST Action ES1404), but the parameters characterizing the snow microstructural properties, electromagnetic properties and composition are currently measured by only 41 %, 26 % and 13 %, respectively, of the network contributors (Pirazzini et al., 2018). The observations at the continental scale, so far, demonstrate a widespread snow cover retreat since the 1970s across the Northern Hemisphere, particularly in the Arctic (Derksen and Brown, 2012; Bormann et al., 2018). By contrast, the results from the mountains are mixed, and there is no consistent picture of what is happening at the regional scale (Bormann et al., 2018). Pulliainen et al. (2020) provided new insight into the seasonal snow mass and its trend by using a bias-corrected GlobSnow 3.0 estimate. Pulliainen et al. (2020) were now able to demonstrate different continental trends based on the 39-year satellite record: a decrease in North America, a negligible trend in Eurasia, and a high regional variability in both areas.
Sea ice research supporting navigation
Recent research has addressed emerging opportunities for Arctic navigation and the importance of operational sea ice analysis. Lei et al. (2015) showed trends along the Arctic Northeast Passage (NEP) and demonstrated an increase in the spatially averaged length of the open period (the ice concentration less than 50 %) from 84 d in the 1980s to 114 d in the 2000s. The summer sea ice along the high-latitude sea route (HSR) north of the eastern Arctic islands has decreased during the last decade, with the ice-free period reaching 42 d in 2012. The HSR avoids shallow waters along the coast, which eases access for deeper-draft vessels (Lei et al., 2015). Considering operational sea ice analyses for the Bohai Sea, work has been done to combine thermodynamic modelling and Earth observation (EO) data from synthetic aperture radar (SAR) and microwave radiometers (Karvonen et al., 2017). The SAR-based discrimination between sea ice and open water works well, and areas of thinner and thicker ice can be distinguished. However, a larger comprehensive training data set is needed to set up an operational algorithm for the estimation of sea ice concentration and for the weighting scheme for sea ice thickness (Karvonen et al., 2017).
Multi-decadal Arctic sea ice state estimates are important for the strategic planning of Arctic navigation. These estimates are usually based on climate models with a thermodynamic–dynamic sea ice model. An up-to-date assessment of large-scale sea ice models was with the aid of sea ice models as a climate model component, and a comprehensive review was carried out by Leppäranta et al. (2020). Specifically, Uotila et al. (2015) found that a model with the subgrid-scale sea ice thickness distribution reproduces more realistic sea ice and upper ocean, due to better-captured spring evolution, than a model with just a single sea ice thickness category. In terms of the validity of initial conditions for multi-decadal predictions, Uotila et al. (2019) analysed a set of ocean reanalysis products, including Arctic sea ice, and found that the multi-model set mean is a useful product as a state estimate. This finding increases confidence in the use of the combination of ocean reanalysis for both initialization of multi-decadal predictions and analysis of multi-decadal variability.
Ocean floor and sediments: composition and fluxes
A significant content of illite and muscovite among layer silicates in most of the ice-rafted sediment samples taken from selected Arctic regions suggests that sources of the sedimentary material are mainly mineralogically similar to modern bottom sediments of the East Siberian and Chukchi seas as well as presumably sediments of the eastern Laptev Sea. A significant kaolinite fraction in the samples from the North Pole area can be caused by the influx of ice-rafted fine-grained sedimentary material from the Beaufort or Chukchi seas, where kaolinite is supplied from the Bering Sea. The samples contained variable proportions of erosion products of both mafic and felsic magmatic rocks and/or sufficiently mature sedimentary rocks (Maslov et al., 2018a).
Quantification of CH4 sources is fundamental information for the climate change mitigation (Fletcher and Schaefer, 2019). Methane stored in ocean floor reservoirs can reach the atmosphere in the form of bubbles or dissolved in water. Methane hydrates could destabilize with rising temperatures, further increasing greenhouse gas emissions in a warming climate. Subsea permafrost and hydrates in the ESAS act as a substantial carbon pool and source of methane to the atmosphere. Annual methane emissions of the region vary from 0.0 to 4.5 Tg CH4 yr−1 estimated by Berchet et al. (2016). Yasunaka et al. (2018) estimated the monthly air–sea CO2 fluxes in the Arctic Ocean and adjacent seas located north of 60∘ N for the period 1997–2014 and ended up at a net annual Arctic Ocean CO2 uptake of 180 ± 130 Tg C yr−1.
The Zeppelin Observatory data for 2014 suggest that the CH4 fluxes from the Svalbard continental platform are smaller than 0.2 Tg yr−1. All estimates are in the lower range of values reported earlier (Pisso et al., 2016). Platt et al. (2018) reported a potential region with high ocean–atmosphere CH4 fluxes located north of Svalbard but addressed how at the time of the measurements the meteorological conditions were unique, including a short episode of the highly sensitive emissions over an active seep site without a sensitivity to land-based emissions.
River runoff affecting the hydrological processes in coastal marine environments
The Arctic Ocean, including the Hudson Bay, receives 55.6 % of its river inflow from Russia, mostly via 19 large rivers (Shiklomanov and Shiklomanov, 2003). This freshwater inflow of approximately 2920 km3 yr−1 (Shiklomanov et al., 2008) is associated with large sediment and heat transports, which together affect the hydrography, marine climate and ecosystems across the Siberian shelf seas (Magritsky et al., 2018). A major part of seasonal and inter-annual variations in the river runoff is anthropogenic due to regulation in large reservoirs (Georgiadi et al., 2016). In addition, Magritsky et al. (2018) detected an increased runoff trend of 5 %–10 %, compared to a reference period of 1936 to 1975, in most of the major Russian rivers discharging into the Arctic Ocean. This trend is mostly due to a climate-induced increase since the second half of the 1980s (Magritsky et al., 2018). However, due to gaps in the monitoring programmes, these estimates have a large uncertainty: focusing on river discharges from the six largest Eurasian rivers to the Arctic Ocean, estimates of the increase range from 7 % (Peterson et al., 2002) to 1.5 % (Shiklomanov and Lammers, 2009).
Permafrost thawing has resulted in releases of old carbon storages, but so far there is no clear evidence of the impact of permafrost thawing on the net emissions of CO2 and CH4 into the atmosphere (IPCC, 2019). A potential explanation of no or weak net increase is that a fraction of the released methane has been taken by rivers instead of being emitted into the atmosphere. Increased amounts of organic carbon in rivers impact the regional and global biochemical and methane cycles (Shakhova et al., 2007; Wild et al., 2019). With the accelerating permafrost thaw, the atmospheric emissions are also expected to increase, in particular for CO2, but also for CH4. Expected future changes in river ice regime are consistent with the expected changes in the duration of the cold season and accumulated negative air temperatures. Significant changes are expected for the rivers in the Kola Peninsula and the lower reaches of the Northern Dvina and Pechora rivers, whereas the lowest changes are expected for the central parts of eastern Siberia (Agafonova et al., 2017). Due to anthropogenic activities (above all industry, municipal services, and filling of reservoirs), water withdrawal from Russian Arctic rivers and related groundwater systems is approximately 20.6 km3 yr−1, and it is expected to increase to 37 km3 yr−1 by 2025 to 2030 (Magritsky et al., 2018). Features of these changes at the marine margin of the Lena River Delta are different compared to changes in the delta head area.
The hydrological representativeness of a glacier is a new characteristic and of practical importance for basin-wide tasks of hydrology and glaciology. For its evaluation, it is proposed to replace the seasonal air temperatures with the glacier summer mass balance (BS) or to include BS in the multiple regression equations for calculating the runoff of rivers fed by melting of snow and ice. This method can be recommended for at least some glaciers in the existing network of the World Glacier Monitoring Service (WGMS) (Konovalov et al., 2019).
2.3.2 Marine ecology (Q8)
Living marine organisms weaken or even subdue CO2 accumulation
The important climatological role of the world's oceans is to reduce the CO2 accumulation into the atmosphere through its absorption. This mechanism is ordinarily viable as the partial pressure of dissolved CO2 in marine surface waters is less than the content of CO2 in the overlying atmosphere. Due to the organic pump, a net drawdown of atmospheric CO2 into the ocean is put into effect. It proceeds in the process of sinking of particulate organic carbon of algal origin: organically bound CO2 is released through remineralization and further accumulated in the deep ocean. In contrast, owing to the processes of a carbonate counter pump, CaCO3 is exported downward and, at depth, dissolves, causing a net release of CO2 into the atmosphere (Balch et al., 2016). However, there are living marine organisms that are able to weaken or even subdue CO2 accumulation, at least within their habitat. Among this group of marine organisms, the leading role belongs to coccolithophores. Among marine biosystems, coccolithophores (class Prymnesiophyceae) are the most productive calcifying algae (Taylor et al., 2017). They both produce particulate inorganic carbon (in the form of calcite) and promote the increase in CO2 partial pressure (pCO2) in the ambient marine surface waters. Thus, the biological activity of coccolithophores can exercise a direct influence on both the CO2 flux exchange at the atmosphere–ocean interface and the marine carbonate chemistry system (CCS). The rain ratio, i.e. the ratio of particulate inorganic carbon to organic carbon, determines the intensity and direction of CO2 flux at the atmosphere–ocean interface. In the case of coccolithophores, the rain ratio is above unity within their habitat area, which potentially can have climatic consequences but also drive alterations in marine CCSs (Balch, 2018).
Emiliania huxleyi is the most widespread coccolithophorid algal species in Earth's oceans, which, in light of the above, naturally explains why this is one of the best-studied marine algae. Of all other coccolithophores, E. huxleyi is probably the most successful in forming extensive blooms in world-wide marine waters ranging from oligotrophic to eutrophic. Unlike diatoms and dinoflagellates, this alga is phenomenally immune to both light limitation and very high light intensities. As high levels of incident light/irradiances enhance calcification (which is predominantly a light-dependent reaction), it is supposed that the calcification machinery enables E. huxleyi cells to resist photodamage by dissipating excess energy. This specialty is important in the case of nutrient-depleted waters, especially in combination with the high affinity of E. huxleyi for nutrients including nitrogen, but especially phosphorous. The properties of both mixotrophic nutrition and resistance, at least partially, to zooplankton grazing and virus attacks (due to a cell's coverage by calcite scales/coccoliths) contribute to this alga's ability to sustain a variety of unfavourable conditions and retain steadfastly its ecological niche (Godrijan et al., 2020). Thus, the elaborate biology of E. huxleyi cells imparts to them the intrinsic and rather rare property of pursuing growth-maximizing and loss-minimizing life strategies. This property reveals itself through multiple manifestations, two of which are vastness and sustainability of E. huxleyi bloom areas. A typical bloom surface is not less than thousands of square kilometres, but in many marine environments it is far larger (Kondrik et al., 2018b). For example, in some years, the value of S in the North and Norwegian seas can be well above 100 000 km2, in the Bering Sea maximum bloom area (S) values were registered at 250 000 km2, and particularly large E. huxleyi bloom areas (up to 380 000 km2) were observed in the Barents Sea (Kondrik et al., 2017). Within the subpolar and polar zones of the Northern Hemisphere, in the waters around Great Britain, in the North, Norwegian, Labrador, Greenland, Barents, and Bering seas, E. huxleyi blooms occur annually, although with largely varying intensities (Pozdnyakov et al., 2017). The duration of blooms in the North Atlantic and the Barents Sea is on average about 3–4 weeks. The moment of onset of the E. huxleyi bloom area maximum shifts from June–July to September–October for the seas located at the temperate, subpolar and polar latitudes of the Northern Hemisphere, respectively. This sequence mimics the flow pattern of the Gulf Stream. In the Bering Sea, the temporal pattern of S variations reveals two periods (1998–2001 and 2018–2020) of extraordinarily intense E. huxleyi outbursts. It is hypothesized that this phenomenon was driven by massive advection of Fe-depleted North Pacific waters due to a significant weakening of the Alaska Current. The latter is supposed to be a teleconnected aftermath of exceptionally strong El Niño events in 1996–1997 and 2017, respectively (Pozdnyakov et al., 2020).
Satellite-borne estimations made during 1998–2018 showed that E. huxleyi outbursts resulted in a release of particulate inorganic carbon (PIC) in the form of CaCO3 in surface waters in amounts ranging from ∼10 to several hundreds of kilotonnes. In the Barents Sea, the released PIC content varied between ∼ 100 and 250–300 kt, whereas in the Bering Sea, during the two periods of exceptional activity, the PIC content was as high as 500 kt (Kondrik et al., 2017). There is ample evidence that the release of PIC was accompanied by a significant increase in CO2 partial pressure (ΔpCO2) within the bloom area: between 1998 and 2016, the mean and maximum values of the ratio ΔpCO2 (ΔpCO2)background varied in the ranges of ∼ (20–40) % and ∼ (30–60) %, respectively. The highest numbers were registered in the Bering and Barents seas (Kondrik et al., 2018a; D. Kondrik et al., 2019). Also, there is spaceborne evidence of the atmospheric columnar ΔCO2 enhancement (ΔCO2)atm over E. huxleyi blooms: numerous case studies in the aforementioned North Atlantic seas as well as in the Barents and Black seas proved that (ΔCO2)atm could reach 2–3 ppm (D. V. Kondrik et al., 2019; Morozov et al., 2019).
Notwithstanding the remarkable ability of E. huxleyi to grow under conditions unfavourable for algae of other functional groups (e.g. diatoms, flagellates, cyanobacteria), a highly irregular pattern of the registered 2-decadal (1998–present) time series of S, PIC, and ΔpCO2 is indicative of susceptibility of this alga's outbursts to environmental conditions (Nissen et al., 2018; Kazakov et al., 2019; Silkin et al., 2018). Statistical prioritization of non-biogenic forcing factors (FFs) shows that the latter are sea- and time-period-specific (Pozdnyakov et al., 2019). Thus, in the Barents Sea, sea water temperature (SWT) is the highest-ranked FF, followed by PAR (photosynthetic active radiation). In the Bering Sea, beyond the aforementioned periods (1998–2001 and 2018–present), sea surface salinity (SSS) is the FF leader, with PAR as a runner-up, whereas SWT is only third in the series. Although these assessments are done without explicitly considered nutrient concentrations (NCs), implicitly NCs were among the FFs. Indeed, arguably, variations in SWT, SSS, CHL, MLD, and surface current speed/advection (tested as FFs) indirectly account for the variations in NCs as well in such CCS parameters as alkalinity and basicity (Durairaj et al., 2015; Pozdnyakov et al., 2019, and references therein).
In the long run, the steady accumulation of CO2 into the atmosphere should closely be considered (Rivero-Calle et al., 2015). The action of a rising atmospheric CO2 concentration is expected to proceed through a number of direct and indirect interactions (Fig. 7), both of which should ultimately cause alterations in the rain ratio. An increase in the atmospheric CO2 concentration leads to the rising of the global temperature and further to the strengthening of stratification, intensification of irradiance within the euphotic zone and cutting of nutrient fluxes from below. Although increases in CO2 fluxes to the surface ocean cause a reduction of pH and CO levels in water, the large pool of HCO remains to support the calcification machinery. Thus, it will lead to the establishment of environmental conditions unfavourable for non-calcifying phytoplankton (NCP) but beneficial (or at least endurable) for coccolithophores in general and E. huxleyi specifically. The reduction of NCP and uncontested growth of E. huxleyi drives a further reduction of dissolved CO2 consumption by other groups of phytoplankton, increase in pCO2 in the surface ocean and intensification of CO2 fluxes into the atmosphere. Concurrently, through a system of feedback interactions, alterations in the rain ratio are bound to affect the carbon fluxes at the water–atmosphere interface. Therefore, the scenario of further increases in atmospheric CO2 concentrations in the future, in all probability, implies a vaster proliferation of E. huxleyi in the world's oceans.
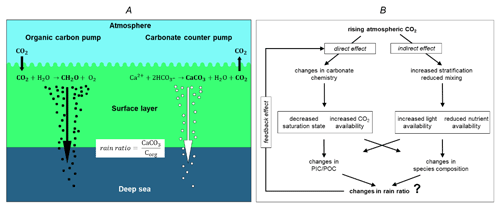
Figure 7(a) Biological pumps resulting in (i) an atmospheric CO2 sink and (ii) calcium carbonate transport from surface to deep ocean; (b) anticipated forward and feedback alterations in ocean ecology driven by atmospheric CO2 increase. PIC: particulate inorganic carbon; POC: particulate organic carbon (modified after Rost and Riebesell, 2004).
In combination with statistic-based mathematical models of E. huxleyi blooms (Pozdnyakov et al., 2019), the available IPCC climate models permit mid-term projections of the forthcoming changes (Gnatiuk et al., 2020). However, our knowledge of the reciprocal influence of climate change and both the structure and functioning of marine ecosystems (even at the level of primary producers) is still insufficient to confidently prognose the future dynamics of the E. huxleyi phenomenon. More studies are required even to fully understand the mechanism of intracellular light-dependent reaction of calcification and its dependency on both seawater carbonate chemistry and environmental FFs (Vihma et al., 2019). Creation of respective multi-decadal databases (as in Kazakov et al., 2019) as well as further delivery of satellite and in situ/shipborne/laboratory data are necessary to improve our capacity to assess with certainty the climatological and ecological role of E. huxleyi blooms on regional and global scales (Fig. 7).
2.3.3 Lakes and rivers (Q9)
Organic carbon in lakes
Spatial variability, an essential characteristic of lake ecosystems, has often been neglected in field research and monitoring. The detected spatial “noise” strongly suggests that, besides vertical variation, the horizontal variation should also be considered in the ecosystem monitoring and, most importantly, when the role of dissolved organic carbon (DOC) in the CO2 flux is estimated (Manasypov et al., 2015; Leppäranta et al., 2018). In natural waters with an increasing level of coloured dissolved organic matter (CDOM) concentration, the water colour is shifted towards brown. The key “permanent” landscape variables, the coverage by lakes and peatland in the catchment area, can be strongly correlated with lake elevation above the sea level. A high lake coverage indicates a low CDOM concentration, while a high peat coverage indicates the opposite (Arvola et al., 2016). For example, in Finland, recent results from inland water studies have not shown any overall, consistent large-scale changes in CDOM concentrations over the last 101-year period (Arvola et al., 2017). Rather, CDOM changes in individual lakes have been related to changes in land use in the drainage basin. Manasypov et al. (2015) reported results from Siberian lakes, representing a discontinuous permafrost zone, and addressed how although the concentrations of most elements in the lakes are lowest in spring, the maximal water coverage of land made it a significant reservoir of DOC. The soluble metals in the water column can be easily mobilized to the hydrological network.
In very shallow freezing lakes, the volume of liquid water is much reduced due to ice growth, and rejection of nutrients and pollutants in the ice growth causes major enrichment of the water body. This has major implications for the ecosystem of these lakes (Yang et al., 2016; Song et al., 2019). Freezing rejects some 80 %–90 % of the impurities in freshwater lakes. On the other hand, ice cover accumulates atmospheric deposition over several months but releases them into the water body within 1 month's melting phase. Rejection of nutrients and pollutants in lake ice growth causes major enrichment of the water body in shallow lakes and notable increases in nutrient concentrations in a shallow lake during seasonal ice growth (Fang et al., 2015).
Lake carbon balance
Arctic and boreal lakes are an important natural source of CH4 into the atmosphere (Bastviken et al., 2011). Methane is produced mainly in the bottom sediments and/or hypolimnion, where most of the anaerobic decomposition of organic matter takes place and then is either oxidized to CO2 in the water column or emitted into the atmosphere. At Kuivajärvi, a typical meso-humid lake located in southern Finland, it was found that 91 % of available CH4 was oxidized in the active CH4 oxidation zone during hypolimnetic hypoxia (Saarela et al., 2020). In warm springs, the early onset of thermal stratification with the cold and well-oxygenated hypolimnion delays the period of hypolimnetic hypoxia and thus limits the production of methane. At Kuivajärvi measured CO2 fluxes (F-CO2) showed that the lake acted as a net source of carbon during two open-water periods (Mammarella et al., 2015). During daytime, with typically high wind speeds, shear-induced water turbulence controls the water–air gas transfer efficiency, thus enhancing the vertical diffusive fluxes across the water–air interface. However, during calm nighttime conditions, buoyancy-driven turbulent mixing, associated with penetrative cooling of surface water, controls the gas exchange, and simple wind-speed-based transfer velocity models strongly underestimate F-CO2 (Mammarella et al., 2015). Kiuru et al. (2018) developed a model simulating CO2 dynamics of a boreal lake in a warming climate. The simulations for 2070–2099 showed a 20 %–35 % increase in the CO2 flux from the lake compared to the reference period of 1980–2009.
Lake ice cover
Wei et al. (2016) studied Lake Unari (67.14∘ N, 25.73∘ E), Finnish Lapland, in the winters 1980/1981–2012/2013, and observed an increasing trend in the air temperature during the freezing season associated with an increasing trend in the water precipitation during winter. Low temperatures with less precipitation led to the formation of columnar ice, while strong winds together with heavy snowfall favoured granular ice formation. Karetnikov et al. (2017) analysed long-term ice conditions in Lake Ladoga, Russia, for the period of 1913–2015 and showed that the mean freezing and breakup dates were 26 November and 15 May, respectively, and that the annual frequency of complete freeze-over of the lake was 0.83. The period from 1990 to present was much milder than the preceding years. The annual increase in the ice concentration depended on the accumulated freezing degree days (AFDDs) and the hypsographic curve, while the ice thickness increased with the square root of an AFDD.
An analysis of a Siberian thermokarst lake located in the Lena River Delta, characterized as a floating ice lake, showed that the temporal dynamics and magnitude of heat fluxes and surface energy balance closures are substantially different depending on lake surface conditions (Franz et al., 2018). Sensible heat and latent heat fluxes, modelled using available heat bulk transfer models (Woolway et al., 2015; Verburg and Antenucci, 2010; Andreas et al., 2002), tend to underestimate the measured fluxes and show less variability over freezing ice cover, melting ice in spring, as well as open water in summer. However, the performance of these models also depends on the accuracy of meteorological and hydrological input parameters, which should be carefully measured, especially during challenging winter conditions.
The seasonal lake ice cover is a sensitive indicator of climate variations in the Arctic (Kirillin et al., 2012; Leppäranta, 2015). To work more on this question, Lake Kilpisjärvi (surface 37.1 km2, maximum depth 57 m), a tundra lake in northern Finland, has been under intensive ice-related field programmes in recent years. The research covered the whole year but was focused on the melting period in May–June. The heat budget over the ice season was dominated by the radiation balance. Turbulent fluxes were significant before the freeze-up in autumn, but in the ice season they were small. The evolution of ice thickness served as a very good approximation to the total surface heat flux (Leppäranta et al., 2017) (Fig. 8). In the melting stage, solar radiation, the strongest forcing of the water body beneath ice cover, breaks the stability and initiates convective turbulent mixing. This brings heat from the deeper water to ice, enhancing melting at the ice bottom (Kirillin et al., 2018). Thus, the common assumption of the heat flux from the water to ice being due to molecular conduction does not hold in the melting stage, but it is much higher. The ice–water interaction under lake ice has not been well covered in earlier studies of ice growth and melting.
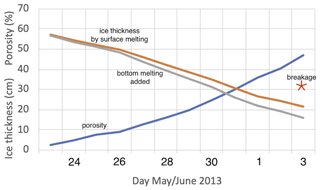
Figure 8Field data for ice decay in Lake Kilpisjärvi in 2013 showing decrease in ice thickness by surface melting and bottom melting and increase in porosity until breakage of ice cover.
The ice melting process was studied in detail in Lake Kilpisjärvi. The melting progressed in the upper and lower surfaces and in the interior, with proportions depending on the solar flux and optical properties of the ice and therefore being case-dependent. About one-third of the solar flux that penetrated the ice returned to the ice bottom, providing heat for melting. This was consistent with the under-ice results by Kirillin et al. (2018). In 2013 a rapid ice breakage event completed the ice breakup in a short time interval, with final breakage at the ice porosity 40 %–50 %. A lake ice melting model should include the thickness and porosity of ice, with porosity connected to an ice strength criterion (Leppäranta et al., 2019).
Lake Baikal and Selenga River Delta
The Selenga River, the main tributary of Lake Baikal, has a catchment area of 450 000 km2 in the boundary region between northern Mongolia and southern Siberia. This area is well known by its climate, land use and dynamic socio-economic changes which might have negative impacts on the ecosystems of Lake Baikal and thus was selected as a PEEX field laboratory within the PEEX sub-programme Selenga-Baikal Network (https://www.atm.helsinki.fi/peex/index.php/basenet/, last access: 18 January 2022). In the recent past, hydroclimatic development together with land use changes led to a contaminant influx from mining areas, and urban settlements increased. Additional hydrological modifications due to the construction of dams and abstractions/water diversions from the Selenga's Mongolian tributaries could lead to additional alterations (Karthe et al., 2017b). In addition to the Selenga River, a key issue for an improved understanding of regional impacts of the environmental change is to disentangle the influence of climate change from that of other pressures within the catchment (Lychagin et al., 2017). The PEEX sub-programme Selenga-Baikal Network aims at integrated field-based and modelling knowledge to develop a basin-wide conceptual framework of riverine fluxes (Kasimov et al., 2017; Karthe et al., 2019).
As a PEEX field laboratory, regional large-scale assessments made it possible to predict the comprehensive nature of hydrological and geochemical changes driven by climatic processes and human impacts. Heavy metals in water and sediments (Kasimov et al., 2020a, b) and fish communities (Kaus et al., 2017) were measured since 2011 in over 50 locations around the catchment. The mining zones are potential hotspots for increasing metal loads to downstream river systems. Several metals (Al, Cd, Fe, Mn, Pb and V) are exported from mining sites to the downstream river system, as shown by net increasing mass flows. Based on a novel partitioning coefficient approach (Table 2), contrasting patterns with domination of both particulate and dissolved phases in different parts of the basin were found. Such heterogeneity in the metal partitioning is likely to be found in many large river systems.
Table 2Hydrogeochemical signature of a large river system – Selenga River case study. The figure represents metal(loid) partitioning (median values) in the Selenga River basin in the upper (Mongolian) and downstream (Russian) parts between 20 July and 10 August 2011 under dominant high-water (a) and between 7 June and 10 July 2012 under dominant low-water conditions. Dark orange fill corresponds to the share of suspended forms of elements > 75 % (green), light orange to 75 %–50 %, light blue to 50 %–25 %, and dark blue to < 25 %. The figure indicates that in the large river system some metals are mostly found in dissolved form (84 %–96 % of Mo, U, B, and Sb on average), whereas many others predominantly existed in suspension (66 %–87 % of Al, Fe, Mn, Pb, Co, and Bi). A consistently increasing share of metals in suspended particulate modes (about 2–6 times) is observed under high-discharge conditions. For details and other hydrological seasons, see Kasimov et al. (2020b).
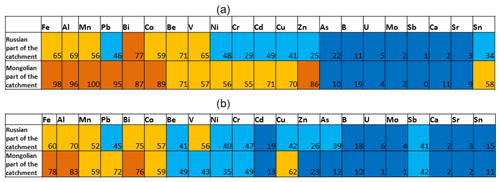
Multi-scale modelling ranged from the basin-wide (Malsy et al., 2017; Frolova et al., 2017) to specific sub-regions, such as particular segments of the river system (Kaus et al., 2017; Thorslund et al., 2017; Garmaev et al., 2019) or its delta (S. R. Chalov et al., 2017; S. Chalov et al., 2017; Shinkareva et al., 2019), and identified reactions of hydrogeochemical pathways to climate change. The mean flow reduction in the Selenga River was 3 %–5 % during the 2020s to 2030s and 4 %–25 % during the 2080s to 2090s, being a crucial driver of ongoing and future hydrogeochemical changes. Increases in temperatures with permafrost thaw and the expansion of agricultural, mining and urbanization processes may induce up to a 6 % increase in the particulate modes and 3 % in the dissolved modes of some metals in the river system (Chalov et al., 2018). Possible changes in the number or magnitudes of high-flow events, caused by climatic or other anthropogenic factors, could influence the total sediment deposition, which was primarily found to occur during relatively short high-flow events. Such potential changes have important implications for the possible spreading of polluted sediments (Pietroń et al., 2015) and their storage in the Selenga River Delta, which is an important wetland region forming the geochemical barrier which mitigates pollution of Lake Baikal by riverine fluxes (Voropay and Kichigina, 2018; Chalov et al., 2015). The Selenga River Delta region sequesters various metals bound to Selenga River sediments (Chalov et al., 2015, Pietroń et al., 2018). The water shortage decreases the processes of suspended sediment retention in the delta. The seasonal hydrogeochemical patterns are explained by wetland inundation during floods and channel erosion or Baikal wind surge during low-flow periods (S. R. Chalov et al., 2017; S. Chalov et al., 2017).
Asian water lakes
The largest internal drainage basins in the world are located in central Asia, with a limited availability of both surface water and groundwater (Karthe et al., 2017a). Since the 20th century, water resources of this region have been overexploited and, for example, from small Mongolian headwater streams to the mighty Aral Sea, surface waters have been partially desiccated. It seems that the implementation of the Integrated Water Resources Management and water–food–energy nexus approaches would lead to a more environmentally friendly future (Karthe et al., 2017a, b). The lake-rich Qinghai–Tibet Plateau (QTP) has recently been identified as the “Third Pole” of the Earth. Due to its high elevation and unique climate, the QTP affects the global and local climate and played an important role in the central and southern Asian water cycle (J. Zhang et al., 2018). Lake–atmosphere interactions have been quantified over open-water periods, yet little is known about the lake ice thermodynamics and heat and mass balance during the ice-covered season. A modelling study for a thermokarst lake in the QTP was performed (Huang et al., 2019a). Strong diurnal cycles were seen for all surface heat fluxes. The ice mass balance was dominated by the growth and melt at the base, but the surface sublimation was also crucial for the ice loss, accounting for up to 40 % of the maximum ice thickness and 41 % of the lake water loss during the ice-covered period. The strong penetration of solar radiative flux is the dominant contributor to the high value of upward sensible heat flux at the ice bottom, resulting in a relatively thin ice cover compared with the equivalent high-latitude climate.
2.4 Society
The anthropogenic impact has been addressed as one of the PEEX themes for the society system. The discussion on the mitigation and adaptation, including urban infrastructure design and risk assessment, is addressed in this context (Q10, Sect. 2.4.1). The social transformations are discussed in terms of how local reindeer grazing interacts with the environment (Q11, Sect. 2.4.2). The adaptive capacity of the northern societies depends on their environment, demographic structure and economic capacity, and the environmental hazards and environmental health under a changing climate are the key research areas in this context (Q12, Sect. 2.4.3).
2.4.1 Anthropogenic impact (Q10)
Mitigation
Arctic climate change generates a need for long-term planning and development of new socio-economic infrastructures, such as dams, bridges, roads and transnational and regional energy networks. For this task, new climate-based forecasting tools, cost and operational risk estimates as well as other methods and tools for an infrastructure and urban design are needed. As an example, engineering calculations for maximal discharges were provided for the Nadym River in Russia (Shevnina et al., 2017). Badina (2018) introduced a method for the natural risk assessment by using indices based on socio-economic potential data and spatial distribution of natural hazards. This method has been tested and used to identify the most vulnerable municipalities in southern Siberia. Another example of new methods is a “green factor tool” to increase the share and effectiveness of green areas in urban environments and cities. An ambitious target set in this tool could encourage or force urban developers to aim higher with the planning of green areas and construction; however, the existing regulations challenge the use of this approach (Juhola, 2018).
The energy production is of fundamental importance for the society's functions, and new clean energy technologies are needed to hinder the climate change. The potential of hydropower production under probabilistic projections of annual runoff rate and future changes in the potential hydropower production need to be evaluated (Shevnina et al., 2019). All the Nordic countries are vulnerable to various degrees to potential cross-border impacts due to their energy sectors being highly globalized and interconnected. However, cross-border impacts are not yet properly included in Nordic climate assessments or energy strategies. The EU's new Green Deal is pivotal in this respect, as for the first time emissions along the whole supply chain (oil, gas, coal, renewables) come under scrutiny as part of a normative governance. Therefore, policy makers and energy planners should be assisted in making comprehensive vulnerability assessments that address both domestic and international climate risks (Groundstroem and Juhola, 2019).
2.4.2 Environmental impact (Q11)
Reindeer (Rangifer tarandus L.) grazing and ground vegetation structure and biomass
Reindeer (Rangifer tarandus L.) grazing in the north affect the ground vegetation structure and biomass and cover of lichens. It seems that reindeer affect GHG fluxes from the forest field layer. Grazing changes affect the vegetation composition and thereby emissions (K. Köster et al., 2018). K. Köster et al. (2017) provided detailed information on soil CO2 effluxes, which were mostly affected by the year of measurement, time of measurement, soil temperature and the management, resulting in higher CO2 emissions in the grazed areas. Soil moisture content did not affect the soil CO2 efflux. For example, in Finnish Lapland the average soil CO2 efflux values were significantly higher in 2014 compared with 2013, mainly due to differences in the soil temperature at the beginning of the season (K. Köster et al., 2017). Furthermore, grazing significantly decreased the biomass and cover of lichens and also the amount of tree regeneration. In a subarctic mature pine forest, grazing did not affect the soil temperature or soil moisture. No statistically significant effect of grazing on the soil CO2 efflux, soil C stock or soil microbial C biomass was found. The soil microbial N biomass was significantly lower in the grazed areas compared with the non-grazed areas. It seems that in the boreal subarctic coniferous forests, grazing by reindeer can be considered “C neutral” (K. Köster et al., 2015). There is also an indication that reindeer grazing affects the boreal forest soils, e.g. their fungal community structure and litter degradation (Santalahti et al., 2018).
2.4.3 Natural hazards (Q12)
Under this theme, PEEX research has so far focused on environmental health issues. These include diseases, impact of UV radiation, and air pollution in urban environments. The spread of diseases caused by living pathogens is basically determined by environmental conditions. Medico-geographical assessments are usually based on identification of the links between the spread of diseases and factors of the geographical environment.
Naturally determined diseases
Climatic factors are deemed among the main determinants for the spread of naturally determined diseases (Malkhazova et al., 2018). Emerging zoonotic diseases are expected to be particularly vulnerable to climate and biodiversity disturbances. Anthrax is an archetypal zoonosis that manifests its most significant burden on vulnerable pastoralist communities. Ezhova et al. (2021) investigated the dynamics of environmental factors that led to an anthrax outbreak in the Yamal Peninsula, Siberia, during 2016. They found that the local permafrost was thawing rapidly for the last 6 years before the outbreak, supporting the hypothesized role of permafrost thaw in triggering this outbreak, and concluded further that the spread of anthrax was likely intensified by the extremely dry summer of 2016 in the region. Overall, the recent findings highlight the significance of warming temperatures for anthrax ecology at northern latitudes and suggest potential mitigating effects of interventions targeting megafauna biodiversity conservation in grassland ecosystems and animal health promotion among small to midsize livestock herds (Walsh et al., 2018). Equally important is the monitoring of climatic factors, such as warming and precipitation extremes, in Arctic regions previously contaminated by anthrax (Ezhova et al., 2021).
UV variations
Different geophysical parameters affecting the UV molecular number density show that, especially at high altitudes, the increased surface albedo has a significant effect on the UV growth. The new parameterization of the online UV tool (http://www.momsu.ru/uv/, last access: 18 January 2022) for northern Eurasia allows us to determine the altitude dependence of UV and to estimate the possible effects of UV on human health considering different skin types and various open body fractions for January and April conditions in the Alpine region (N. Chubarova et al., 2016). Using UV satellite retrievals, ERA-Interim data and the INM-RSHU chemistry–climate model, the changes in the UV irradiance and UV resources were estimated over northern Eurasia for the 1979–2015 period, demonstrating significant UV increases over vast areas (Chubarova et al., 2020). Referring to long-term UV measurements and model simulations in Moscow, a statistically significant positive trend of more than 5 % per decade since 1979 was evaluated (Chubarova et al., 2018). Related to the connection between UV variation and stratospheric O3, see also Sect. 2.2.1, Atmospheric composition and chemistry.
Examples of air pollution episodes
Street level urban air pollution is one of the key topics in urban environments. For example, in Bergen, Norway, the most extreme cases of repetitive wintertime air pollution episodes, followed by increased large-scale wind speeds above the valley, were transported by the local re-circulations to other less polluted areas with only slow dilution. This result underlines the need for better-described assumptions about transport paths and weak dispersion in classical air pollution models in order to improve the current air quality forecasts in urban areas (Wolf-Grosse et al., 2017b). A link between the persistence of the flow above the Bergen valley and the occurrence and severity of the local air pollution episodes was found. Analysis of the large-scale circulation over the North Atlantic–European region, with respect to air pollution in Bergen, revealed that the persistence in meteorological conditions connected to air pollution episodes is not necessarily caused by large-scale anomalies of the atmospheric circulation over the Norwegian western coast but is rather connected to anomalies as far away as Greenland (Wolf-Grosse et al., 2017a).
In Russia, especially intensive atmospheric pollution episodes have severe impacts on the environment and human health. O. B. Popovicheva et al. (2019) analysed the Tver region, north of Moscow, which was considerably affected by the secondary organic aerosol (SOA) formation originating from long-lasting peat bog fires. Spectral absorbance characteristics were similar to peat burning and traffic source emissions during fire- and non-fire-related days and confirmed the effect of transported peat smoke on air quality in a megacity environment (O. B. Popovicheva et al., 2019). O. B. Popovicheva et al. (2019) also showed that long-term transport from north-western Russia and Scandinavia influences the local population.
Local Arctic air pollution alone can seriously affect public health and ecosystems locally, especially in wintertime, when the pollution can accumulate under inversion layers (Schmale et al., 2018a). We need more research on the contributing emission sources and the relevant atmospheric pollution mechanisms and more detailed epidemiological or toxicological health impact studies in the Arctic. Socio-economic changes (shipping, tourism, natural resource extraction, increasing number of population) are already taking place in the Arctic, and they will increase in the future. It is also expected that the emission types and magnitudes will increase the number of exposed individuals (Arnold et al., 2016). There is still a large variation in the number of locations as a source of emissions. Future predictions are even more difficult due to the as yet unknown development of the Arctic economic activities and their emissions (Arnold et al., 2016; Schmale et al., 2018a, b).
3.1 Future research needs from the system perspectives
For the land ecosystem, the recent progress towards understanding of the northern Eurasian Arctic-boreal land ecosystems (Sect. 3.1) deals with improved methodologies relevant to land processes (Q1), observations of permafrost thawing (Q2), and observed changes in the northern ecosystems, especially soil conditions (Q3).
Improved satellite-based methods and (validation) data together with better quantification and, especially, the scaling of the GPP are enabling better identification and quantification of Earth surface characteristics and ecosystem carbon balance compared with the earlier capacity (Gurchenkov et al., 2017; Rautiainen et al., 2016; Nitzbon et al., 2019; Boike et al., 2019; Terentieva et al., 2016; S. Zhang et al., 2018; Pulliainen et al., 2017; Matkala et al., 2020; Bondur et al., 2008a, b). Intensive research has been carried out on the quantification of the GPP, a key variable for biological activity, in different conditions and at different scales (Pulliainen et al., 2017; Kulmala et al., 2019; Matkala et al., 2020). Further investigations are called for, for a more detailed understanding of the seasonal dynamics of the biological activity.
The northern Eurasian ecosystems' tipping points are related to multiple simultaneous stress factors. The key stress factors here are the permafrost thawing and factors important for ecosystems, such as the prolongation of the growing season, increase in the mean temperature of the growing season and forest fires (Kukkonen et al., 2020; Biskaborn et al., 2019; Payne et al., 2016; K. Köster et al., 2016; Miles and Esau, 2016; Miles et al., 2019). New evidence of the progress of permafrost thawing in Siberia has been introduced by Kukkonen et al. (2020) and Biskaborn et al. (2019). The permafrost thawing is also triggering as yet not clearly known processes related to changing fluxes, ecosystem processes and dynamics of greenhouse gas sinks and sources (Schuur et al., 2008; Thompson et al., 2017; Commane et al., 2017; Euskirchen et al., 2017; Dean et al., 2018; Thonat et al., 2017). The progress affecting permafrost thawing has not yet been analysed in detail. For example, we need more information on the dynamics of how the thawing processes vary between soil types due to differences in water movement and, in the wintertime, how the snow cover affects ground surface temperatures (Bartsch et al., 2010). In addition to permafrost processes, the recent advances in observed changes in the northern ecosystem reveal a significant role of soil processes in biogeochemical cycles, especially the nitrogen cycle (Voigt et al., 2016; Pärn et al., 2018). Knowledge of the soil microbiological composition and the effect of forest fires has been improved (K. Köster et al., 2015, 2016; Zhang-Turpeinen et al., 2020), but further research is needed for vegetation changes influencing the below-ground microbiology, its composition and enzymatic activity (Payne et al., 2016; K. Köster et al., 2016). The NDVI methods have made it possible to detect vegetation changes (Miles and Esau, 2016). A range of vegetation cover changes in Siberia have been reported, such as the Arctic greening and browning processes, but e.g. the greening of Siberian cities will also remain an issue of intensive research in the future (Miles and Esau, 2016; Miles et al., 2019).
For the atmospheric system, the recent progress in understanding the northern Eurasian Arctic-boreal land–atmosphere system and the aspects of the megacity air quality (Sect. 3.2) are dealing with atmospheric composition changes (Q4), key feedbacks between climate and air quality (Q5), and synoptic-scale weather (Q6). Recent results demonstrate improved quantification of the carbon balance and CO2 fluxes and concentrations due to land use change, forest fires in Siberia, and new understanding of aerosol sources and properties in the Arctic environment and across northern Eurasia (Pulliainen et al., 2017; Karelin et al., 2017; Rakitin et al., 2018; Skorokhod et al., 2017; Alekseychik et al., 2017). However, most of the results deal with atmospheric aerosol chemistry and physics in boreal and Arctic environments originating from measurements in the few flagship stations in Finland and Russia (Kerminen et al., 2018; Wiedensohler et al., 2019; Freud et al., 2017; Paasonen et al., 2018; Östrom et al., 2017; Kalogridis et al., 2018; Bondur et al., 2016; Bondur and Ginzburg, 2016; Bondur et al., 2019c, d; Bondur and Gordo, 2018; Mikhailov et al., 2017; Breider et al., 2017), indicating the need for a comprehensive station network in the PEEX region. Black carbon emitted by the Siberian forest fires and some other sources and its long-range transport to the Arctic are also widely discussed (Kalogridis et al., 2018; Bondur et al., 2016; Bondur and Ginzburg, 2016; Mikhailov et al., 2017; Breider et al., 2017; Shevchenko et al., 2015; Konovalov et al., 2018; Marelle et al., 2018). In addition, measurements of ozone in the troposphere and stratosphere provide insight into atmospheric chemistry in urban environments (Skorokhod et al., 2017), UV radiation and human health (Chubarova et al., 2019b). Environmental health, including the impacts of air quality and UV radiation, is foreseen as a high-momentum research topic in the PEEX domain, and further research is called for in this area.
Related to air pollution, we reported several new results on the dynamics between the haze pollution and boundary-layer meteorology in enhancing air pollution in megacity environments (Zhao et al., 2017; Ding et al., 2016a; Wang et al., 2018b; Y. Bai et al., 2018; Ye et al., 2017). The long-term and comprehensive measurements carried out especially at the SORPES station in Nanjing provide valuable data pools for such studies (Ding et al., 2016a). However, the backbone of the recent progress has been the improved online atmospheric measurements and the use of machine learning methods combined with different methodologies, such as back trajectories together with the lidar and radiosonde data. In addition, improved models of emission inventories together with the ECHAM-HAM and GAINS models have led to a better quantification of aerosol number emissions. New knowledge has enabled the introduction of new theoretical arguments on the feedbacks between high aerosol concentrations and the urban boundary layer (Petäjä et al., 2016). New measurements have also been obtained from Siberian cities (Elansky et al., 2016; N. Y. Chubarova et al., 2016; Mahura et al., 2018). However, we are still in the early phase of having a holistic picture of large-scale feedbacks due to the lack of long-term, comprehensive measurements in these regions.
Changes in the atmospheric dynamics in the north have potential impacts on short-term local/regional and sub-seasonal to seasonal large-scale weather predictions and on long-term projections on biogeochemical systems. It is therefore crucial to understand changes in boundary-layer processes as well as synoptic- and large-scale circulation in the Arctic and northern Eurasia. Recent results show potential, but causally arguable, connections between the alarming sea ice decline, evaporation, cloudiness, atmospheric circulation and moisture transport as well as Arctic and European winter temperatures (Nygård et al., 2019; Rinke et al., 2019; McCusker et al., 2016; Mori et al., 2014; Blackport et al., 2019; Cohen et al., 2020). Further investigations are needed for atmosphere–ice–ocean interactions, coupling between small-scale processes (such as clouds and turbulence) and synoptic-scale weather, as well as polar prediction and extreme events. Furthermore, more quantitative knowledge is needed on pan-Arctic energy budgets (Spengler et al., 2016). The UHI phenomena taking place in Arctic cities have received increasing attention, and there is a special need for improved forecasting services for Arctic cities (Miles and Esau, 2017; Konstantinov et al., 2018; Varentsov et al., 2018b).
For the water system, we discussed the Arctic sea ice dynamics and thermodynamics, snow depth and sea ice thickness, sea ice research supporting navigation, and rare elements in the snow and ocean sediments, especially from the perspective of improvements in the observation and modelling methods (Q7, Sect. 3.3.1). New evidence of atmosphere–Arctic sea ice interactions has been provided by Lei et al. (2018) and Jakobson et al. (2019). Lei at al. (2018) analysed how the climate warming would affect the winter growth rate of thin and thick ice, and Jakobson et al. (2019) gave new insight into the relation between sea ice concentration and wind speed. Furthermore, advances have been made in understanding the thermodynamics and metamorphosis of the snowpack on sea ice and their interactions with surface albedo changes (Dou et al., 2019). Operational sea ice analysis is increasingly important for Arctic shipping and navigation (Lei et al., 2015; Karvonen et al., 2017). New results on rare elements, mineral composition and CO2 and methane fluxes associated with ocean sediments have been attained (Maslov et al., 2018b; Yasunaka et al., 2018). This serves as important information for mitigation plans as well as for new estimates of the river runoff and discharge in Russian rivers into the Arctic seas (Grigoriev and Frolova, 2018; Agafonova et al., 2017).
The marine Arctic ecosystems are under a progressive increase in anthropogenic impacts, the main issues calling for better understanding being the integrated effect of Arctic warming, ice melt and snowmelt, ocean freshening, air quality and acidification of the Arctic marine ecosystems, primary production and the carbon cycle (Q8, Sect. 3.3.2). Quantitative information about the CO2 accumulation into the ocean has high momentum. Marine organisms, such as coccolithophorid algae, are influencing the CO2 flux exchange (Kondrik et al., 2018b; Pozdnyakov et al., 2017). In addition to changing marine environments, the Arctic-boreal lakes and rivers may undergo changes in flooding, increasing the amount of freshwater and allochthonous materials (Q9, Sect. 3.3.3). In addition to the Arctic Ocean, the ice and snow conditions of northern lakes are under pressure. Lake Kilpisjärvi (Finland) (Arvola et al., 2017; Leppäranta et al., 2017) and Lake Ladoga (Russia) (Karetnikov et al., 2017) have been subject to intensive research, and the recent results demonstrate changes in heat fluxes, ice cover periods and stratification. The browning of lakes and lake sediments was discussed, and new results were attained from the Selenga River of Lake Baikal. Dramatic changes will be expected in the water runoff and in the number of dissolved modes of metals, also having a serious impact on environmental health (S. R. Chalov et al., 2015, 2016, 2017; S. Chalov et al., 2017; Karthe et al., 2017a, b). As a comparison with the northern high latitudes, we also discussed freezing lakes in central Asia, where the climate is cold and arid. There the ice is typically snow-free or possesses only a thin snow cover, allowing penetration of sunlight into the water body (Huang et al., 2019b).
For the societal system, the anthropogenic impact has been addressed as one of the main themes (Q10). The discussion on the mitigation and adaptation, including the urban infrastructure design (Juhola, 2018) and risk assessment, was addressed in this context (Sect. 3.4.1). In social transformations, special attention was given to one of the most important local livelihoods in Lapland: reindeer grazing and how it interacts with the environment (Q11 Sect. 3.4.2). The adaptive capacity of the northern societies rests on their environment, demographic structure and economic activities (Q12). Referring to the earlier statement about future research needs for the atmospheric system with respect to environmental health, here again we would like to pay increasing attention to environmental health under a changing climate, including the spread of diseases and air pollution and their combined effects (Sect. 3.4.3).
3.2 Feedback mechanisms under changing climate, cryosphere conditions and urbanization
During recent years, Kulmala et al. (2004, 2021) focused on the quantification of the COntinental Biosphere-Aerosol-Cloud-Climate (COBACC) feedback loop relevant to the boreal region in northern Eurasia. Previous results on the COBACC feedback loop addressed the role of BVOC emission dynamics (Arneth et al., 2016). Both higher temperatures and increased CO2 concentrations are (separately) expected to increase emissions of BVOCs into the atmosphere. It also seems that the GPP is controlled by the BVOC effects on the clouds. Sporre et al. (2019) used an Earth system model to estimate aerosol scattering due to enhanced BVOC emissions and estimated the associated negative direct radiative effect (−0.06 W m−2). The total global radiative effect associated with this feedback was estimated to be −0.49 W m−2 (Sporre et al., 2019), indicating that it has the potential to offset about 13 % of the forcing associated with a doubling of CO2. The direct effect of aerosol on GPP due to an increase in the fraction of diffuse radiation was estimated at between 6 % and 14 % increase in GPP at maximum observed aerosol loading compared to low aerosol loading in northern Eurasian forests (Ezhova et al., 2018b).
The results from the Tibetan Plateau demonstrate notable feedbacks between vegetation, BVOC emissions and aerosol particles. The historical wetting of the TP region has increased the vegetation cover, allowing for feedback processes via biogenic aerosol formation and aerosol–cloud–precipitation interactions. A significant wetting trend since the early 1980s in the Tibetan Plateau is most conspicuous in central and eastern Asia. Fang et al. (2015) hypothesized that the current warming may enhance emissions of BVOCs, which can increase secondary organic aerosol concentrations, contributing to the precipitation increase. The wetting trend can increase the vegetation cover and has a positive feedback on the BVOC emissions. The simulations suggest a significant contribution of increased BVOC emissions to the regional organic aerosol mass, and the simulated increase in BVOC emissions is significantly correlated with the wetting trend in the Tibetan Plateau.
To estimate the net effects of various feedback mechanisms on land cover changes, photosynthetic activity, GHG exchange, BVOC emissions, formation of aerosols and clouds, and radiative forcing (Q14) call for intensive collaboration and integration between the Arctic Ocean sciences and terrestrial sciences across the pan-Arctic domain and across the Arctic and high-latitude domain. The Arctic greening and browning (Sect. 3.1.3) call for a multi-disciplinary scientific approach, improved modelling tools and new data to deeply understand the biosphere–atmosphere–anthroposphere interactions and feedbacks. Petäjä et al. (2020a, b) discussed the complexity of feedbacks, especially in the Arctic context, and the interplay between the temperature, GHG, permafrost, land cover and water bodies and between photosynthetic activity, aerosols, clouds and radiation budget. The current downturn of the Arctic cryosphere (Sect. 3.1.2) together with the changes in sea ice dynamics and glaciers and the permafrost thawing affect both marine and terrestrial carbon cycles in interconnected ways (Sect. 3.3.1). Parmentier et al. (2017) discussed the changing Arctic cryosphere and how the processes in the ocean and on land are too often studied as separate systems, although the sea ice decline connects the rapid warming of the Arctic, Arctic Ocean marine processes and air–sea exchange of CO2. Thus, future priorities would be on the development of our modelling tools towards an all-scale modelling approach to cover the feedbacks, processes and interactions at the land–ocean interface and also in urban environments in the Arctic region. We also need to support the further development of ground-based observation networks.
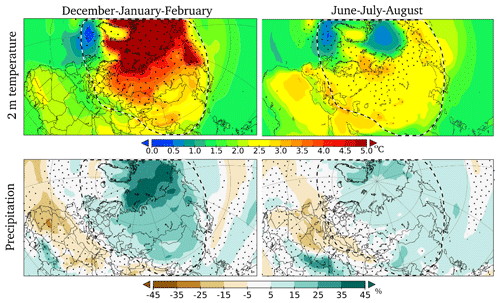
Figure 9Changes in 2 m temperature (∘C, upper panels) and precipitation (%, lower panels) during the 21st century. Present-day climatology is averaged over the years 1981–2010 and end-of-century climatology over 2070–2099. Winter (left) and summer (right) are shown separately. Dotted areas indicate high variability in the model ensemble (for temperature: standard deviation of 21st century change exceeds 1 ∘C; for precipitation: standard deviation of 21st century change exceeds 100 % or present-day precipitation). The model results are from IPCC AR5, based on 42 individual models in CMIP5 experiments under the RCP4.5 scenario.
3.3 Climate scenarios for the Arctic-boreal region
Climate scenarios set the urgency for the mitigation and adaptation actions for the northern Eurasian region. The Arctic-boreal region combines an area of both amplified climate change (Arctic amplification) and large diversity in the model predictions (Collins et al., 2013; Hoegh-Guldberg et al., 2018). Under the “low-to-medium” RCP4.5 forcing scenario (van Vuuren et al., 2011), the CMIP5 multi-model mean temperature changes during the 21st century indicate the strongest wintertime warming of > 5 ∘C in the Arctic Ocean, whereas the majority of the terrestrial region will warm by 2–4 ∘C (Fig. 9). Even during summertime, the continental warming over the region will generally exceed 2 ∘C. It is important to note that the diversity of model projections is accentuated over the Arctic and northern Eurasian domain: the mechanisms behind the Arctic amplification are implemented in varying details in the distinct models, and the associated interactions and feedback processes provide a diverse picture of the future in the Arctic-boreal regions.
In addition to considerable trends in atmospheric temperatures, the models further indicate prominent changes in precipitation (Collins et al., 2013; Hoegh-Guldberg et al., 2018). For the Arctic-boreal region, this is largely depicted as increasing rainfall during both winter and summer, extending to 15 %–25 % over most of the terrestrial domain over the winter and somewhat less during summer (Fig. 9). Contemporary warm Arctic temperatures and large sea ice deficits (75 % volume loss) demonstrate climate states outside our previous experience. The modelled changes in the Arctic cryosphere demonstrate that even limiting the global temperature increase to 2 ∘C will leave the Arctic a much different environment by mid-century, with less snow and sea ice, melted permafrost, altered ecosystems, and a projected annual mean Arctic temperature increase of +4 ∘C. Even under ambitious emission reduction scenarios, high-latitude land ice melt, including Greenland, is foreseen to continue due to internal lags, leading to accelerating global sea level rise throughout the century (Overland et al., 2019).
Only the integration of different observing networks and programmes into an inter-operable and integrated observation system can provide data needed for understanding the mechanisms of the Arctic-boreal system. There is a fundamental need for an integrated, comprehensive network of state-of-the-art in situ stations measuring Earth surface–atmosphere interactions (Kulmala et al., 2016a, 2018; Uttal et al., 2016; Hari et al., 2016; Alekseychik et al., 2016; Vihma et al., 2019). The results obtained in the Pan-Eurasian Experiment (PEEX) programme in Russian and China introduced in this paper are based on a combination of long-term observations and campaign data. In addition, the Arctic marine regions require comprehensive observations and subsequent synthesis, as these regions are under a lot of environmental stresses. Therefore, we need more in situ observations of the Arctic system covering the marine atmosphere, sea ice and ocean. However, there are pronounced technological and logistical challenges to set up such continuous, marine in situ observations (e.g. Vihma et al., 2019). Furthermore, improved monitoring is needed for river discharge and associated fluxes of greenhouse gases and other key compounds and more research on the understanding of coastal processes and atmospheric transport and specific regional socio-economic issues and their interactions with changing environments (Vihma et al., 2019; Petäjä et al., 2020a).
The international organizations and bodies like the Arctic Council (SAON's Roadmap for Arctic Observing and Data Systems, ROADS), EU Horizon 2020 (Blue Growth INTAROS and APPLICATE projects), GEO-CRI (high mountains and cold regions), the Belmont Forum COPERNICUS and the WMO are coordinating development of the Arctic data and services. New data products are expected from the large-scale MOSAIC campaign and projects like ERA-PLANET iCUPE (Petäjä et al., 2020b) or ArcticFLUX to monitor the interface between the marine Arctic and Eurasian continent. Also, national-based Arctic observations and research programmes like AC3 by German institutes are playing a significant role. Russia conducts extensive research in the Arctic region, notably on the manned drifting ice stations. These Arctic observation activities are coordinated and carried out by Roshydromet, universities and Russian Academy of Sciences institutes.
Concerning global energy markets, the Arctic region holds 25 % or more of the world's undiscovered oil and gas reservoirs (Bird et al., 2008). The plans of China and Russia to build an “Ice Silk Road” along the Northern Sea Route linking China and Russia to Europe highlights the growing economic and strategic importance of the polar regions and the increasing pressure on the Arctic environment and local communities. In addition, wide areas of the high latitudes and Arctic regions are under the pressure of the changing economic activities of the Arctic and also under high pressures of the changing environment and climate. A comprehensive observation network providing in situ data in close coordination with satellite observations and ground-based remote sensing is required to monitor the environmental impacts of the envisioned operations.
Over the last few years, Earth system science has been driven by the need to understand the scientific processes of climate change and air quality, their interrelations with the Earth system and their societal impacts. The interplay between science, politics and business and the analysis of the existing policies and strategies help us to recognize and analyse new and emerging trends of Arctic governance (e.g. protection and resilience vis-à-vis economic activities), geopolitics (e.g. state sovereignty vis-à-vis internationalization), geo-economics (e.g. tourism vis-à-vis reindeer herding), and science (e.g. climate change). The intensive work towards the new Arctic observations and data systems together with the intensive observations of the land–atmosphere interactions taking place at the high latitudes will provide the baseline for a cross-disciplinary research era. PEEX aims for these directions.
This is a review paper and the data availability is introduced in the original articles.
HKL had the initial idea. HKL, VMK, TP, SBM, and ES consolidated the overall text. Major contributions for the specific chapters were made by AL, EE for Sect. 2.1 Land, AB, FB, EE, IE, OP, AM, RM, and JR for Sect. 2.2 Atmospheric system, TV, SC, ML, and DP for Sect. 2.3 Arctic-boreal aquatic system, and V-P T and SJ for Sect. 2.4 Society. All the co-authors contributed to specific sections of the paper and commented on the paper and/or provided text and/or relevant references.
Some of the authors are members of the editorial board of Atmospheric Chemistry and Physics. The peer-review process was guided by an independent editor, and the authors also have no other competing interests to declare.
Publisher's note: Copernicus Publications remains neutral with regard to jurisdictional claims in published maps and institutional affiliations.
This article is part of the special issue “Pan-Eurasian Experiment (PEEX) – Part II”. It is not associated with a conference.
We thank the following funding agencies and projects: Academy of Finland contract nos. 280700, 294600, 296302 (Novel Assessment of Black Carbon in the Eurasian Arctic: From Historical Concentrations and Sources to Future Climate Impacts, NABCEA), 307331 (FCoE Atmospheric Sciences), 311932, 314798/99, 315203, 317999, and 337549 (Atmosphere and Climate Competence Center, ACCC), the Jane and Aatos Erkko Foundation, Ministry for Foreign Affairs of Finland (IBA-project no. PC0TQ4BT-20), PECC (Transferable Knowledge and Technologies for High-Resolution Environmental Impact Assessment and Management; TRAKT-2018) project, Russian Government megagrant project 1 075-15-2021-574 “Megapolis – heat and pollution island: interdisciplinary hydroclimatic, geochemical and ecological analysis”, Russian Foundation for Basic Research (RFBR) project nos. 17-29-05027 (Selenga–Baikal river system), 17-29-05102, 18-05-00306, 18-05-60037, 18-05-60219 (Arctic river), 18-35-20031, 18-44-860017, 18-45-700015, 18-05-60083 (Storm activity in the Barents Sea), 20-5512001 (An interdisciplinary study on the impact of aerosolized particulate matter from aged wildfire plumes on environment and human health), 19-05-50088, 19-05-00352, and 19-55-80021 (MOST and DST studies), Scientific and Educational School of Moscow State University “The Future of the Planet and Global Environmental Changes”, the IAO SB RAS supported by RFBR project no. 19-05-50024, Russian Science Foundation (RSF) projects 21-17-00181 (Monitoring at Lena River catchment), 17-17-01117, 18-17-00076 (Long-term measurement of aerosol chemical composition in central Siberia, 19-77-20109 (black carbon emissions from Siberian fires), 19-77-30004 (Moscow environment), and 19-77-300-12 (Measurement networks and field sites in the Kola Peninsula), Yugra State University grant no. 13-01-20/39, Ministry of Science and Higher Education of Russia agreement no. 13.1902.21.0003, State assignment no. 0148-2019-0006, project N75295423 launched by St. Petersburg State University, European Research Council (ERC) project nos. 742206 (ATM-GTP) and 850614 (CHAPAs), EU Horizon 2020 project nos. 727890 (Integrated Arctic Observing System, INTAROS), 689443 (Integrative and Comprehensive Understanding on Polar Environments, iCUPE), and 654109 (Aerosol Clouds and Trace Gases Research Infrastructure, ACTRIS-2), EU7 FP MarcoPolo grant no. 606953, Erasmus+ 561975-EPP-1-2015-1-FI-EPPKA2-CBHE-JP, Norwegian Research Council and Belmont Forum project SERUS no. 311986, National Natural Science Foundation of China grant no. 41275137, and ESA-MOST China Dragon Cooperation project nos. 10663 and 32771 (Dragons 3 and 4). We also thank the AASCO – Arena gap analysis of the existing Arctic science co-operations (contract 2858) funded by the Prince Albert Foundation of Monaco (contract no. 2858).
We especially thank Alla Borisova, INAR, University of Helsinki, for her help with the technical editing of the manuscript.
The work of Stanislav Myslenkov was supported by the Interdisciplinary Scientific and Educational School of Moscow State University “The Future of the Planet and Global Environmental Changes”.
Open-access funding was provided by the Helsinki University Library.
This paper was edited by Leiming Zhang and reviewed by three anonymous referees.
Aalto, J., Porcar-Castell, A., Atherton, J., Kolari, P., Pohja, T., Hari, P., Nikinmaa, E., Petäjä, T., and Bäck, J.: Onset of photosynthesis in spring speeds up monoterpene synthesis and leads to emission bursts, Plant Cell Environ., 38, 2299–2312, https://doi.org/10.1111/pce.12550, 2015.
Agafonova, S. A., Frolova, N. L., Surkova, G. V., and Koltermann, K. P.: Modern characteristics of the ice regime of Russian Arctic rivers and their possible changes in the 21st century, Geogr. Environ. Sustain., 10(, 4–15, https://doi.org/10.24057/2071-9388-2017-10-4-4-15, 2017.
Alekseychik, P., Lappalainen, H. K., Petäjä, T., Zaitseva, N., Heimann, M., Laurila, T., Lihavainen, H., Asmi, E., Arshinov, M., Shevchenko, V., Makshtas, A., Dubtsov, S., Mikhailov, E., Lapshina, E., Kirpotin, S., Kurbatova, Y., Ding, A., Guo, H., Park, S., Lavric, J. V., Reum, F., Panov, A., Prokushkin, A., and Kulmala, M.: Ground-based station network in Arctic and Subarctic Eurasia: An overview, Geogr. Environ. Sustain., 9, 75–88, 2016.
Alekseychik, P., Mammarella, I., Karpov, D., Dengel, S., Terentieva, I., Sabrekov, A., Glagolev, M., and Lapshina, E.: Net ecosystem exchange and energy fluxes measured with the eddy covariance technique in a western Siberian bog, Atmos. Chem. Phys., 17, 9333–9345, https://doi.org/10.5194/acp-17-9333-2017, 2017.
AMAP: Arctic Pollution 2017. Arctic Monitoring and Assessment Programme (AMAP), Oslo, Norway, available at: https://www.amap.no/work-area/document/3283 (last access: 20 January 2020), 2017.
Amosov, P. V., Baklanov, A. A., Makarov, D. V., and Masloboev, V. A.: Estimating air pollution levels by numerical simulation depending on wind flow speed and dust source area, No. 5, https://doi.org/10.21440/0536-1028-2020-5-80-89, 2020.
Andreae, M. O.: Emission of trace gases and aerosols from biomass burning – an updated assessment, Atmos. Chem. Phys., 19, 8523–8546, https://doi.org/10.5194/acp-19-8523-2019, 2019.
Andreae, M. O., Andreae, T. W., and Ditas, F.: How frequent is new particle formation in the boundary layer over the remote temperate/boreal forest?, AGU Fall Meeting, San Francisco, USA, 9–13 December 2019, https://doi.org/10.1002/essoar.10501148.1, 2019.
Andreas, E. L., Guest, P. S., Persson, P. O. G., Fairall, C. W., Horst, T. W., Moritz, R. E., and Semmer, S. R.: Near-surface water vapor over polar sea ice is always near ice saturation, J. Geophys. Res.-Oceans, 107, 8033, https://doi.org/10.1029/2000JC000411, 2002.
Arneth, A., Harrison, S., Zaehle, S., Tsigaridis, K., Menon, S., Bartlein, P. J., Feichter, J., Korhola, A., Kulmala, M., O'Donnell, D., Schurgers, G., Sorvari, S., and Vesala, T.: Terrestrial biogeochemical feedbacks in the climate system. Nat. Geosci., 3, 525–532, https://doi.org/10.1038/ngeo905, 2010.
Arneth, A., Makkonen, R., Olin, S., Paasonen, P., Holst, T., Kajos, M. K., Kulmala, M., Maximov, T., Miller, P. A., and Schurgers, G.: Future vegetation–climate interactions in Eastern Siberia: an assessment of the competing effects of CO2 and secondary organic aerosols, Atmos. Chem. Phys., 16, 5243–5262, https://doi.org/10.5194/acp-16-5243-2016, 2016.
Arnold, S. R., Law, K. S., Brock, C. A., Thomas, J. L., Starkweather, S. M., von Salzen, K., Stohl, A., Sharma S., Lund, M. T., Flanner, M. G., Petaja, T., Tanimoto, H., Gamble, J., Dibb J. E., Melamed M., Johnson, N., Fidel, M., Tynkkynen, V.-P., Baklanov, A., Eckhardt, S., Monks, S. A., Browse, J., and Bozem, H.: Arctic air pollution: Challenges and opportunities for the next decade, Elementa, 4, 000104, https://doi.org/10.12952/journal.elementa.000104, 2016.
Arvola, L., Äijälä, C., and Leppäranta, M.: CDOM concentrations of large Finnish lakes relative to their landscape properties, Hydrobiologia, 780, 37–46, https://doi.org/10.1007/s10750-016-2906-4, 2016.
Arvola, L., Leppäranta, M., and Äijälä, C.: CDOM variations in Finnish lakes and rivers between 1913 and 2014, Sci. Total Environ., 601, 1638–1648, https://doi.org/10.1016/j.scitotenv.2017.06.034, 2017.
Asmi, E., Kondratyev, V., Brus, D., Laurila, T., Lihavainen, H., Backman, J., Vakkari, V., Aurela, M., Hatakka, J., Viisanen, Y., Uttal, T., Ivakhov, V., and Makshtas, A.: Aerosol size distribution seasonal characteristics measured in Tiksi, Russian Arctic, Atmos. Chem. Phys., 16, 1271–1287, https://doi.org/10.5194/acp-16-1271-2016, 2016.
Backman, J., Schmeisser, L., Virkkula, A., Ogren, J. A., Asmi, E., Starkweather, S., Sharma, S., Eleftheriadis, K., Uttal, T., Jefferson, A., Bergin, M., Makshtas, A., Tunved, P., and Fiebig, M.: On Aethalometer measurement uncertainties and an instrument correction factor for the Arctic, Atmos. Meas. Tech., 10, 5039–5062, https://doi.org/10.5194/amt-10-5039-2017, 2017.
Badina, S. V.: Socio-economic potential of municipalities in the context of natural risk (case study – Southern Siberian regions), IOP Conf. Ser.-Earth Env., 190, 012001, https://doi.org/10.1088/1755-1315/190/1/012001, 2018.
Bai, J. H.: UV extinction in the atmosphere and its spatial variation in North China, Atmos. Environ., 154, 318–330, https://doi.org/10.1016/j.atmosenv.2017.02.002, 2017.
Bai, J. H.: A calibration method of solar radiometers, Atmos. Pollut. Res., 10, 1365–1373, https://doi.org/10.1016/j.apr.2019.03.011, 2019.
Bai, J. H.: O3 Concentration and its relation with BVOC emissions in a subtropical plantation, Atmosphere, 12, 711, https://doi.org/10.3390/atmos12060711, 2021.
Bai, J. H., Guenther, A., Turnipseed, A., Duhl, T., and Greenberg, J.: Seasonal and interannual variations in whole-ecosystem BVOC emissions from a subtropical plantation in China, Atmos. Environ., 161, 176–190, https://doi.org/10.1016/j.atmosenv.2017.05.002, 2017.
Bai, J. H., de Leeuw, G., van der Ronald, A., De Smedt, I., Theys, N., Van Roozendael, M., Sogacheva, L., and Chai, W. H.: Variations and photochemical transformations of atmospheric constituents in North China, Atmos. Environ., 189, 213–226, https://doi.org/10.1016/j.atmosenv.2018.07.004, 2018.
Bai, Y., Zhang, J., Zhang, S., Yao, F., and Magliulo, V.: A remote sensing-based two-leaf canopy conductance model: Global optimization and applications in modeling gross primary productivity and evapotranspiration of crops, Remote Sens. Environ., 215, 411–437, https://doi.org/10.1016/j.rse.2018.06.005, 2018.
Baklanov, A., Molina, L. T., and Gauss, M.: Megacities, air quality and climate, Atmos. Environ., 126, 235–249, https://doi.org/10.1016/j.atmosenv.2015.11.059, 2016.
Baklanov, A., Smith Korsholm, U., Nuterman, R., Mahura, A., Nielsen, K. P., Sass, B. H., Rasmussen, A., Zakey, A., Kaas, E., Kurganskiy, A., Sørensen, B., and González-Aparicio, I.: Enviro-HIRLAM online integrated meteorology–chemistry modelling system: strategy, methodology, developments and applications (v7.2), Geosci. Model Dev., 10, 2971–2999, https://doi.org/10.5194/gmd-10-2971-2017, 2017.
Baklanov, A., Brunner,, D., Carmichael, G., Flemming, J., Freitas, S., Gauss, M., Hov, O., Mathur, R., Schlünzen, K., Seigneur, C., and Vogel, B.: Key issues for seamless integrated chemistry-meteorology modeling, B. Am. Meteorol. Soc., 98, 2285–2292, https://doi.org/10.1175/BAMS-D-15-00166.1, 2018.
Baklanov, A. A., Penenko, V. V., Mahura, A. G., Vinogradova, A. A., Elansky, N. F., Tsvetova, E. A., Rigina, O. Y., Maksimenkov, L. O., Nuterman, R. B., Pogarskii, F. A., and Zakey, A.: Aspects of Atmospheric Pollution in Siberia, in Regional Environmental Changes in Siberia and Their Global Consequences, edited by: Groismanm P. Y., and Gutman, G., 303–346, Springer, Dordrecht, Netherlands, https://doi.org/10.1007/978-94-007-4569-8, 2013.
Balch, W. M.: The ecology, biogeochemistry, and optical properties of coccolithophores, Ann. Rev. Mar. Sci., 109, 71–98, https://doi.org/10.1146/annurev-marine-121916-063319, 2018.
Balch, W. M., Bates, N. R., Lam, P. J., Twining, B. S., Rosengard, S. Z., Bowler, B. C., and Rauschenberg, S.: Factors regulating the Great Calcite Belt in the Southern Ocean, and its biogeochemical significance, Global Biogeochem. Cy., 30, 1124–1144, https://doi.org/10.1002/2016GB005414, 2016.
Bartsch, A., Kumpula, T., Forbes, B. C., and Stammler, F.: Detection of snow surface thawing and refreezing in the Eurasian Arctic with QuikSCAT: implications for reindeer herding, Ecol. Appl., 20, 2346–2358, https://doi.org/10.1890/09-1927.1, 2010.
Bashmachnikov, I. L., Yurova, A. Y., Bobylev, L. P., and Vesman, A. V.: Seasonal and Interannual Variations of Heat Fluxes in the Barents Sea Region, Izv. Atmos. Ocean. Phys., 54, 213–222, https://doi.org/10.1134/S0001433818020032, 2018a.
Bashmachnikov, I. L., Yurova, A. Y., Bobylev, L. P., and Vesman, A. V.: Seasonal and interannual variations of the heat fluxes in the Barents Sea region, Izv. Atmos. Ocean. Phys., 54, 239–249, https://doi.org/10.7868/S0003351518020149, 2018b.
Bastviken, D., Tranvik, L. J., Downing, J. A., Crilland, P. M., and Enrich-Prast, A.: Freshwater Methane Emissions Offset the Continental Carbon Sink, Science, 331, 50, https://doi.org/10.1126/science.1196808, 2011.
Beer, C., Reichstein, M., Tomelleri, E., Ciais, P., Jung, M., Carvalhais, N., Rödenbeck, C., Altaf Arain,M.., Baldocchi, D., Bonan, G. B., Bondeau, A.., Cescatti, A., Lasslop, G., Lindroth, A., Lomas, M., Luyssaert, S., Margolis, H., Oleson, K. W., Roupsard, O., Veenendaal, E., Viovy, N., Williams, C., Woodward, F., and Papale, D.: Terrestrial gross carbon dioxide uptake: global distribution and covariation with climate, Science, 329, 834–838, https://doi.org/10.1126/science.1184984, 2010.
Belikov, D., Arshinov, M., Belan, B., Davydov, D., Fofonov, A., Sasakawa, M., and Machida, T.: Analysis of the Diurnal, Weekly, and Seasonal Cycles and Annual Trends in Atmospheric CO2 and CH4 at Tower Network in Siberia from 2005 to 2016, Atmosphere, 10, 689, https://doi.org/10.3390/atmos10110689, 2019.
Berchet, A., Bousquet, P., Pison, I., Locatelli, R., Chevallier, F., Paris, J.-D., Dlugokencky, E. J., Laurila, T., Hatakka, J., Viisanen, Y., Worthy, D. E. J., Nisbet, E., Fisher, R., France, J., Lowry, D., Ivakhov, V., and Hermansen, O.: Atmospheric constraints on the methane emissions from the East Siberian Shelf, Atmos. Chem. Phys., 16, 4147–4157, https://doi.org/10.5194/acp-16-4147-2016, 2016.
Berezina, E., Moiseenko, K., Skorokhod, A., Elansky, N., Belikov, I., and Pankratova, N.: Isoprene and monoterpenes over Russia and their impacts in tropospheric ozone formation, Geogr. Environ. Sustain., 12, 63–74, https://doi.org/10.24057/2071-9388-2017-24, 2019.
Bird, K. J., Charpentier, R. R., Gautier, D. L., Houseknecht, D. W., Klett, T. R., Pitman, J. K., Moore, T. E., Schenk, C. J., Tennyson, M. E., and Wandrey, C. J.: Circum-Arctic resource appraisal; estimates of undiscovered oil and gas north of the Arctic Circle, U.S. Geological Survey Fact Sheet 2008–3049, 4, available at: http://pubs.usgs.gov/fs/2008/3049 (last access: 2 February 2022), 2008.
Biskaborn, B. K., Smith, S. L., Noetzli, J., Matthes, H., Vieira, G., Streletskiy, D. A., Schoeneich, P., Romanovsky, V. E., Lewkowicz, A. G., Abramov, A., Allard, M., Boike, J., Cable, W.L., Christiansen, H. H., Delaloye, R., Diekmann, B., Drozdov, D., Etzelmüller, B., Grosse, G., Guglielmin, M., Ingeman-Nielsen, T., Isaksen, K., Ishikawa, M., Johansson, M., Johannsson, H., Joo, A., Kaverin, D., Kholodov, A., Konstantinov, P., Kröger, T., Lambiel, C., Lanckman, J-P., Luo, D., Malkova, G., Meiklejohn, I., Moskalenko, N., Oliva, M., Phillips, M., Ramos, M., Sannel, A. B. K., Sergeev, D., Seybold, C., Skryabin, P., Vasiliev, A., Wu, Q., Yoshikawa, K., Zheleznyak, M., and Lantuit, H.: Permafrost is warming at a global scale, Nat. Commun., 10, 264, https://doi.org/10.1038/s41467-018-08240-4, 2019.
Blackport, R., Screen, J. A., van der Wiel, K., and Bintanja, R.: Minimal influence of reduced Arctic sea ice on coincident cold winters in mid-latitudes, Nat. Clim. Change, 9, 697–704, https://doi.org/10.1038/s41558-019-0551-4, 2019.
Bobylev, S. N., Chereshnya, O. Yu., Kulmala, M., Lappalainen, H. K., Petäjä, T., Solov’eva, S. V., Tikunov, V. S., and Tynkkynen, V.: Indicators for digitalization of sustainable development goals in PEEX program, Geography, Environment, Sustainability, 11, 145–156, https://doi.org/10.24057/2071-9388-2018-11-1-145-156, 2018.
Boike, J., Nitzbon, J., Anders, K., Grigoriev, M., Bolshiyanov, D., Langer, M., Lange, S., Bornemann, N., Morgenstern, A., Schreiber, P., Wille, C., Chadburn, S., Gouttevin, I., Burke, E., and Kutzbach, L.: A 16-year record (2002–2017) of permafrost, active-layer, and meteorological conditions at the Samoylov Island Arctic permafrost research site, Lena River delta, northern Siberia: an opportunity to validate remote-sensing data and land surface, snow, and permafrost models, Earth Syst. Sci. Data, 11, 261–299, https://doi.org/10.5194/essd-11-261-2019, 2019.
Bondur, V. G.: Satellite monitoring of trace gas and aerosol emissions during wildfires in Russia. Izv. Atmos. Ocean. Phys., 52, 1078–1091, https://doi.org/10.1134/s0001433816090103, 2016.
Bondur, V. G. and Ginzburg, A. S.: Emission of carbon-bearing gases and aerosols from natural fires on the territory of Russia based on space monitoring, Dokl. Earth Sci., 466, 148–152, https://doi.org/10.1134/s1028334x16020045, 2016.
Bondur, V. G. and Gordo, K. A.: Satellite Monitoring of Burned out Areas and Emissions of Harmful Contaminants due to Forest and other Wildfires in Russia, Izv. Atmos. Ocean. Phys., 54,955–965, https://doi.org/10.1134/S0001433818090104, 2018.
Bondur, V. G. and Vorobev, V. E.: Satellite monitoring of impact Arctic regions, Izv. Atmos. Ocean. Phys., 51, 949–968, https://doi.org/10.1134/S0001433815090054, 2015.
Bondur, V. G., Gordo, K. A., and Kladov, V. L.: Spacetime distributions of wildfire areas and emissions of carbon-containing gases and aerosols in northern eurasia according to satellite-Monitoring Data, Izv. Atmos. Ocean. Phys., 53, 859–874, https://doi.org/10.1134/s0001433817090055, 2017.
Bondur, V. G., Chimitdorzhiev, T. N., Dmitriev, A. V., and Dagurov, P. N.: Otsenka prostranstvennoy anizotropii neodnorodnostey lesnoy rastitelnosti pri razlichnykh azimutalnykh uglakh radarnogo polyarimetricheskogo zondirovaniya [Spatial anisotropy assessment of the forest vegetation heterogeneity at various azimuth angles of the radar polarimetric sensing], Issledovanie Zemli iz kosmosa, Russia, 3, 92–103, https://doi.org/10.31857/S0205-96142019392-103, 2019a.
Bondur, V. G., Chimitdorzhiev, T. N., Dmitriev, A. V., Dagurov, P. N., Zakharov, A. I., and Zakharova, L. N.: Metody radarnoj polyarimetrii dlya issledovaniya izmenenij mekhanizmov obratnogo rasseyaniya v zonah opolznej na primere obrusheniya sklona berega reki Bureya [Using radar polarimetry to monitor changes in backscattering mechanisms in landslide zones for the case study of the Bureya river bank collapse], Issledovanie Zemli iz kosmosa, Russia, 4, 3–17, https://doi.org/10.31857/S0205-9614201943-17, 2019b.
Bondur, V. G., Tsidilina, M. N., and Cherepanova, E. V.: Satellite Monitoring of Wildfire Impacts on The Conditions of Various Types of Vegetation Cover in The Federal Districts of the Russian Federation, Izv. Atmos. Ocean. Phys., 55, 1238–1253, https://doi.org/10.1134/s000143381909010X, 2019c.
Bondur, V. G., Tsidilina, M. N., Kladov, V. L., and Gordo, K. A.: Irregular Variability of Spatiotemporal Distributions of Wildfires and Emissions of Harmful Trace Gases in Europe Based on Satellite Monitoring Data, Dokl. Earth Sci., 485, 461–464, https://doi.org/10.1134/S1028334X19040202, 2019d.
Bondur, V. G., Zakharova, L. N., and Zakharov, A. I.: Monitoring of the landslide area state on Bureya river in 2018–2019 according to radar and optical satellite images, Issledovanie Zemli iz kosmosa, Russia, 6, 26–35, https://doi.org/10.31857/S0205-96142019626-35, 2019e.
Bondur, V. G., Zakharova, L. N., Zakharov, A. I., Chimitdorzhiev, T. N., Dmitriev, A. V., and Dagurov, P. N.: Monitoring of landslide processes by means of L-band radar interferometric observations: Bureya river bank caving case, Issledovanie Zemli iz kosmosa, Russia, 5, 3–14, https://doi.org/10.31857/S0205-9614201953-14, 2019f.
Bondur, V. G., Mokhov, I. I., Voronova, O. S., and Sitnov, S. A.: Satellite Monitoring of Siberian Wildfires and Their Effects: Features of 2019 Anomalies and Trends of 20-Year Changes, Dokl. Earth Sci., 492 370–375, https://doi.org/10.1134/S1028334X20050049, 2020.
Bormann, K. J., Brown, R. D., Derksen, C., and Painter, T. H.: Estimating snow-cover trends from space, Nat. Clim. Chang., 8, 924–928, 2018.
Boy, M., Thomson, E. S., Acosta Navarro, J.-C., Arnalds, O., Batchvarova, E., Bäck, J., Berninger, F., Bilde, M., Brasseur, Z., Dagsson-Waldhauserova, P., Castarède, D., Dalirian, M., de Leeuw, G., Dragosics, M., Duplissy, E.-M., Duplissy, J., Ekman, A. M. L., Fang, K., Gallet, J.-C., Glasius, M., Gryning, S.-E., Grythe, H., Hansson, H.-C., Hansson, M., Isaksson, E., Iversen, T., Jonsdottir, I., Kasurinen, V., Kirkevåg, A., Korhola, A., Krejci, R., Kristjansson, J. E., Lappalainen, H. K., Lauri, A., Leppäranta, M., Lihavainen, H., Makkonen, R., Massling, A., Meinander, O., Nilsson, E. D., Olafsson, H., Pettersson, J. B. C., Prisle, N. L., Riipinen, I., Roldin, P., Ruppel, M., Salter, M., Sand, M., Seland, Ø., Seppä, H., Skov, H., Soares, J., Stohl, A., Ström, J., Svensson, J., Swietlicki, E., Tabakova, K., Thorsteinsson, T., Virkkula, A., Weyhenmeyer, G. A., Wu, Y., Zieger, P., and Kulmala, M.: Interactions between the atmosphere, cryosphere, and ecosystems at northern high latitudes, Atmos. Chem. Phys., 19, 2015–2061, https://doi.org/10.5194/acp-19-2015-2019, 2019.
Breider, T. J., Mickley, L. J., Jacob, D. J., Ge, C., Wang, J., Sulprizio, M. P., Croft, B., Ridley, D. A., McConnell, J. R., Sharma, S., Husain, L., Dutkiewicz, V. A., Eleftheriadis, K., Skov, H., and Hopke, P. K.: Multidecadal trends in aerosol radiative forcing over the Arctic: Contribution of changes in anthropogenic aerosol to Arctic warming since 1980, J. Geophys. Res.-Atmos., 122, 3573–3594, https://doi.org/10.1002/2016JD025321, 2017.
Burkhard, B., Kroll, F., Müller, F., and Windhorst, W. F.: Landscapes Capacities to Provide Ecosystem Services – a Concept for Land-Cover Based Assessments, Landscape Online, 15, 1–12, https://doi.org/10.3097/LO.200915, 2009.
Chalov, S. R., Jarsjo, J., Kasimov, N. S., Romanchenko, A. O., Pietron, J., Thorslund, J., and Promakhova, E. V.: Spatio-temporal variation of sediment transport in the Selenga River Basin, Mongolia and Russia, Environ. Earth Sci., 73, 663–680, https://doi.org/10.1007/s12665-014-3106-z, 2015.
Chalov, S. R., Bazilova, V. O., and Tarasov, M. K.: Modelling suspended sediment distribution in the Selenga River Delta using LandSat data, Proc. IAHS, 375, 19–22, https://doi.org/10.5194/piahs-375-19-2017, 2017.
Chalov, S., Thorslund, J., Kasimov, N., Aybullatov, D., Ilyicheva, E., Karthe, D., Kositsky, A., Lychagin, M., Nittrouer, J., Pavlov, M., Pietron, J., Shinkareva, G., Tarasov, M., Garmaev, E., Akhtman, Y., and Jarsjö, J.: The Selenga River delta: a geochemical barrier protecting Lake Baikal waters, Reg. Environ. Change, 17, 2039–2053, https://doi.org/10.1007/s10113-016-0996-1, 2017.
Chalov, S. R., Millionshchikova, T. D., and Moreido, V. M.: Multi-Model Approach to Quantify Future Sediment and Pollutant Loads and Ecosystem Change in Selenga River System, Water Resour., 45, S22–S34, https://doi.org/10.1134/s0097807818060210, 2018.
Che, Y., Xue, Y., Mei, L., Guang, J., She, L., Guo, J., Hu, Y., Xu, H., He, X., Di, A., and Fan, C.: Technical note: Intercomparison of three AATSR Level 2 (L2) AOD products over China, Atmos. Chem. Phys., 16, 9655–9674, https://doi.org/10.5194/acp-16-9655-2016, 2016.
Cheng, Y., Cheng, B., Zheng, F., Vihma, T., Kontu, A., Yang, Q., and Liao, Z.: Air/snow, snow/ice and ice/water interfaces detection from high-resolution vertical temperature profiles measured by ice mass-balance buoys on an Arctic lake, Ann. Glaciol., 61, 309–319, https://doi.org/10.1017/aog.2020.51, 2020.
Chernokulsky, A. and Esau, I.: Cloud cover and cloud types in the Eurasian Arctic in 1936–2012, Int. J. Climatol., 39, 5771–5790, https://doi.org/10.1002/joc.6187, 2019.
Chernokulsky, A. V., Esau, I., Bulygina, O. N., Davy, R., Mokhov, I.I., Outten, S., and Semenov, V. A.: Climatology and interannual variability of cloudiness in the Atlantic Arctic from surface observations since the late nineteenth century, J. Climate, 30, 2103–2120, https://doi.org/10.1175/jcli-d-16-0329.1, 2017.
Chu, B., Kerminen, V.-M., Bianchi, F., Yan, C., Petäjä, T., and Kulmala, M.: Atmospheric new particle formation in China, Atmos. Chem. Phys., 19, 115–138, https://doi.org/10.5194/acp-19-115-2019, 2019.
Chubarova, N., Zhdanova, Y., and Nezval, Y.: A new parameterization of the UV irradiance altitude dependence for clear-sky conditions and its application in the on-line UV tool over Northern Eurasia, Atmos. Chem. Phys., 16, 11867–11881, https://doi.org/10.5194/acp-16-11867-2016, 2016.
Chubarova, N. Y., Poliukhov, A. A., and Gorlova, I. D.: Long-term variability of aerosol optical thickness in Eastern Europe over 2001–2014 according to the measurements at the Moscow MSU MO AERONET site with additional cloud and NO2 correction, Atmos. Meas. Tech., 9, 313–334, https://doi.org/10.5194/amt-9-313-2016, 2016.
Chubarova, N. E., Pastukhova, A. S., and Galin, V. Y.: Long-Term Variability of UV Irradiance in the Moscow Region according to Measurement and Modeling Data, Izv. Atmos. Ocean. Phys., 54, 139–146, https://doi.org/10.1134/S0001433818020056, 2018.
Chubarova, N. E., Androsova, E. E., Kirsanov, A. A., Vogel, B., Vogel, H., Popovicheva, O. B., and Rivin, G. S.: Aerosol and Its Radiative Effects during the Aeroradcity 2018 Moscow Experiment, Geography, Environment, Sustainability, 12, 114–131, https://doi.org/10.24057/2071-9388-2019-72 2019a.
Chubarova, N. E., Timofeev, Y. M., Virolainen, Y. A., and Polyakov, A. V.: Estimates of UV indices during the periods of reduced ozone content over Siberia in Winter-Spring 2016, Atmos. Oceanic Opt., 32, 177–179, https://doi.org/10.1134/s1024856019020040, 2019b.
Chubarova, N. E., Pastukhova, A. S., Zhdanova, E. Y., Volpert, E. V., Smyshlyaev, S. P., and Galin, V. Y.: Effects of Ozone and Clouds on Temporal Variability of Surface UV Radiation and UV Resources over Northern Eurasia Derived from Measurements and Modeling, Atmosphere, 11, 59, https://doi.org/10.3390/atmos11010059, 2020.
Cohen, J., Zhang, X., Francis, J., Jung, T., Kwok, R., Overland, J., Ballinger, T. J., Bhatt, U. S., Chen, H. W., Coumou, D., Feldstein, S., Gu, H., Handorf, D., Henderson, G., Ionita, M., Kretschmer, M., Laliberte, F., Lee, S., Linderholm, H. W., Maslowski, W., Peings, Y., Pfeiffer, K., Rigor, I., Semmler, T., Stroeve, J., Taylor, P. C., Vavrus, S., Vihma, T., Wang, S., Wendisch, M., Wu, Y., and Yoon, J.: Divergent consensuses on Arctic amplification influence on mid latitude severe winter weather, Nat. Clim. Change, 10, 20–29, https://doi.org/10.1038/s41558-019-0662-y, 2020.
Collins, M., Knutti, R., Arblaster, J., Dufresne, J.-L., Fichefet, T., Friedlingstein, P., Gao, X., Gutowski, W. J., Johns, T., Krinner, G., Shongwe, M., Tebaldi, C., Weaver, A. J., and Wehner, M.: Long-term climate change: Projections, commitments and irreversibility, in Climate Change 2013: The Physical Science Basis, Contribution of Working Group I to the Fifth Assessment Report of the Intergovernmental Panel on Climate Change, edited by: Stocker, T. F., Quin, D., Plattner, G.-K., Tignor, M., Allen, S. K., Doschung, J., Nauels, A., Xia, Y., Bex, V., and Midgley, P. M., Cambridge University Press, Cambridge, United-Kingdom, 1029–1136, https://doi.org/10.1017/CBO9781107415324.024, 2013.
Commane, R., Lindaas, J., Benmergui, J., Luus K. A., Chang, R. Y.-W., Daube, B. C., Euskirchen, E. S., Henderson, J. M., Karion, A., Miller, J. B., Miller, S. M., Parazoo, N. C., Randerson, J. T., Sweeney, C., Tans, P., Thoning, K., Veraverbeke, S., Miller, C. E., and Wofsy, S. C.: Carbon dioxide sources from Alaska driven by increasing early winter respiration from Arctic tundra, P. Natl. Acad. Sci. USA, 114, 5361–5366, 2017.
Coumou, C., Petoukhov, V., Rahmstorf, S., Petri, S., and Schellnhuber, H.-J.: Quasi-resonant circulation regimes and hemispheric synchronization of extreme weather in boreal summer, P. Natl. Acad. Sci. USA, 111, 331–12336, https://doi.org/10.1073/pnas.1412797111, 2014.
Dada, L., Paasonen, P., Nieminen, T., Buenrostro Mazon, S., Kontkanen, J., Peräkylä, O., Lehtipalo, K., Hussein, T., Petäjä, T., Kerminen, V.-M., Bäck, J., and Kulmala, M.: Long-term analysis of clear-sky new particle formation events and nonevents in Hyytiälä, Atmos. Chem. Phys., 17, 6227–6241, https://doi.org/10.5194/acp-17-6227-2017, 2017.
Dada, L., Chellapermal, R., Buenrostro Mazon, S., Paasonen, P., Lampilahti, J., Manninen, H. E., Junninen, H., Petäjä, T., Kerminen, V.-M., and Kulmala, M.: Refined classification and characterization of atmospheric new-particle formation events using air ions, Atmos. Chem. Phys., 18, 17883–17893, https://doi.org/10.5194/acp-18-17883-2018, 2018.
Dagsson-Waldhauserova, P. and Meinander, O.: Editorial: Atmosphere-cryosphere interaction in the Arctic, at high latitudes and mountains with focus on transport, deposition and effects of dust, black carbon, and other aerosols, at High Latitudes and Mountains With Focus on Transport, Deposition, and Effects of Dust, Black Carbon, and Other Aerosols, Front. Earth Sci., 7, 337, https://doi.org/10.3389/feart.2019.00337, 2019.
Dave, B. and Kobayashi, Y.: China's silk road economic belt initiative in Central Asia: economic and security implications, Asia Eur. J., 16, 1–15, https://doi.org/10.1007/s10308-018-0513-x, 2018.
Dean, J. F., Middelburg, J. J., Röckmann, T., Aerts, R., Blauw, L. G., Egger, M., Jetten, M. S. M., de Jong, A. E. E., Meisel, O. H., Rasigraf, O., Slomp, C. P., in't Zandt, M. H., and Dolman, A. J.: Methane feedbacks to the global climate system in a warmer world, Rev. Geophys., 56, 207–250, https://doi.org/10.1002/2017RG000559, 2018.
Derksen, C. and Brown, R.: Spring snow cover extent reductions in the 2008–2012 period exceeding climate model predictions, Geophys. Res. Lett., 39, L19504, https://doi.org/10.1029/2012GL053387, 2012.
Derome, J. and Lukina, N.: Interaction between environmental pollution and land-cover/land-use change in Arctic areas, in: Eurasian Arctic Land Cover and Land Use in a Changing Climate, edited by: Gutman, G. and Reissell, A., Springer, Amsterdam, Netherlands, 6, 269–290, https://doi.org/10.1007/978-90-481-9118-5_11, 2011.
Ding, A. J., Huang, X., Nie, W., Sun, J. N., Kerminen, V.-M., Petäjä, T., Su, H., Chen, Y. F., Yang, X.-Q., Wang, M. H., Chi, X. G., Wang, J. P., Virkkula, A., Guo, W. D., Yuan, J., Wang, S. Y., Zhang, R. J., Wu, Y. F., Song, Y. Zhu, T., Zilitinkevich, S., Kulmala, M., and Fu, C. B.: Enhanced haze pollution by black carbon in megacities in China, Geophys. Res. Lett., 43, 2873–2879, https://doi.org/10.1002/2016GL067745, 2016a.
Ding, A. J., Nie, W., Huang, X., Chi, X., Sun, J., Kerminen, V.-M., Xu, Z., Guo, W., Petäjä, T., Yang, X., Kulmala, M., and Fu, G.: Long-term observation of pollution-weather/climate interactions at the SORPES station: a review and outlook, Front. Environ. Sci. En., 10, 15, https://doi.org/10.1007/s11783-016-0877-3, 2016b.
Dou, T., Xiao, C., Liu, J., Han, W., Du, Z., Mahoney, A. R., Jones, J., and Eicken, H.: A key factor initiating surface ablation of Arctic sea ice: earlier and increasing liquid precipitation, The Cryosphere, 13, 1233–1246, https://doi.org/10.5194/tc-13-1233-2019, 2019.
Dragosics, M., Meinander, O., Jonsdottir, T., Durig, T., De Leeuw, G., Palsson, F., Dagsson-Waldhauserová, P., and Thorsteinsson, T.: Insulation effects of Icelandic dust and volcanic ash on snow and ice, Arab. J. Geosci., 9, 126, https://doi.org/10.1007/s12517-015-2224-6, 2016.
Durairaj, P., Sarangi., R., Ramalingam, S., Thirunavukarassu, T., and Chauhan, P.: Seasonal nitrate algorithms for nitrate retrieval using OCEANSAT-2 and MODIS-AQUA satellite data, Environ. Monit. Assess., 187, 176–189, https://doi.org/10.1007/s10661-015-4340-x, 2015.
Dyukarev, E. A., Godovnikov, E. A., Karpov, D. V., Kurakov, S. A., Lapshina, E. D., Filippov, I. V., Filippova, N. V., and Zarov, E. A.: Net ecosystem exchange, gross primary production and ecosystem respiration in ridge-hollow complex at Mukhrino Bog, Geography, Environment, Sustainability, 12, 227–244, doi.10.24057/2071-9388-2018-77, 2019.
Elansky, N. F., Lavrova, O. V., Skorokhod, A. I., and Belikov, I. B.: Trace gases in the atmophere over Russian cities, Atmos. Environ., 143, 108–119, https://doi.org/10.1016/j.atmosenv.2016.08.046, 2016.
Esau, I., Miles, V. V., Davy, R., Miles, M. W., and Kurchatova, A.: Trends in normalized difference vegetation index (NDVI) associated with urban development in northern West Siberia, Atmos. Chem. Phys., 16, 9563–9577, https://doi.org/10.5194/acp-16-9563-2016, 2016.
Esau, I., Varentsov, M., Laruelle, M., Miles, M. W., Konstantinov, P., Soromotin, A., Baklanov, A. A., and Miles, V. V.: Warmer Climate of Arctic Cities, chap. 3, in the monography “The Arctic: Current Issues and Challenges”, edited by: Pokrovsky, O., NOVA Publishers, ISBN 978-1-53617-306-2, available at: https://novapublishers.com/shop/the-arctic-current-issues-and-challenges/ (last access: 18 January 2022), 2020.
Euskirchen, E. S., Bret-Harte, M. S., Shaver, G. R., Edgar, C. W., and Romanovsky, V. E.: Long-term release of carbon dioxide from arctic tundra ecosystems in northern Alaska, Ecosystems, 20, 960–974, 2017.
Evangeliou, N., Shevchenko, V. P., Yttri, K. E., Eckhardt, S., Sollum, E., Pokrovsky, O. S., Kobelev, V. O., Korobov, V. B., Lobanov, A. A., Starodymova, D. P., Vorobiev, S. N., Thompson, R. L., and Stohl, A.: Origin of elemental carbon in snow from western Siberia and northwestern European Russia during winter–spring 2014, 2015 and 2016, Atmos. Chem. Phys., 18, 963–977, https://doi.org/10.5194/acp-18-963-2018, 2018.
Ezhova, E., Kerminen, V.-M., Lehtinen, K. E. J., and Kulmala, M.: A simple model for the time evolution of the condensation sink in the atmosphere for intermediate Knudsen numbers, Atmos. Chem. Phys., 18, 2431–2442, https://doi.org/10.5194/acp-18-2431-2018, 2018a.
Ezhova, E., Ylivinkka, I., Kuusk, J., Komsaare, K., Vana, M., Krasnova, A., Noe, S., Arshinov, M., Belan, B., Park, S.-B., Lavrič, J. V., Heimann, M., Petäjä, T., Vesala, T., Mammarella, I., Kolari, P., Bäck, J., Rannik, Ü., Kerminen, V.-M., and Kulmala, M.: Direct effect of aerosols on solar radiation and gross primary production in boreal and hemiboreal forests, Atmos. Chem. Phys., 18, 17863–17881, https://doi.org/10.5194/acp-18-17863-2018, 2018b.
Ezhova, E., Orlov, D., Suhonen, E., Kaverin, D., Mahura, A., Gennadinik, V., Kukkonen, I., Drozdov, D., Lappalainen, H. K., Melnikov, V., Petäjä, T., Kerminen, V.-M., Zilitinkevich, S., Malkhazova, S. M, Christensen, T. R., and Kulmala, M.: Climatic Factors Influencing the Anthrax Outbreak of 2016 in Siberia, Russia, EcoHealth, 18, 217–228, https://doi.org/10.1007/s10393-021-01549-5, 2021.
Fang, K., Makkonen, R., Guo, Z., and Seppa, H.: An increase in the biogenic aerosol concentration as a contributing factor to the recent wetting trend in Tibetan Plateau, Sci. Rep.-UK, 5, 14628, https://doi.org/10.1038/srep14628, 2015.
Feijó Barreira, L. M., Duporté, G., Rönkkö, T., Parshintsev, J., Hartonen, K., Hyrsky, L., Heikkinen, E., Jussila, M., Kulmala, M., and Riekkola, M.-L.: Field measurements of biogenic volatile organic compounds in the atmosphere using solid-phase microextraction Arrow, Atmos. Meas. Tech., 11, 881–893, https://doi.org/10.5194/amt-11-881-2018, 2018.
Fletcher, S. E. M. and Schaefer, H.: Rising methane: a new climate challenge, Science, 364, 932–933, 2019.
Franz, D., Mammarella, I., Boike, J., Kirillin, G., Vesala, T., Bornemann, N., Larmanou, E., Langer, M., and Sachs, T.: Lake-Atmosphere Heat Flux Dynamics of a Thermokarst Lake in Arctic Siberia, J. Geophys. Res.-Atmos., 123, 5222–5239, https://doi.org/10.1029/2017jd027751, 2018.
Freud, E., Krejci, R., Tunved, P., Leaitch, R., Nguyen, Q. T., Massling, A., Skov, H., and Barrie, L.: Pan-Arctic aerosol number size distributions: seasonality and transport patterns, Atmos. Chem. Phys., 17, 8101–8128, https://doi.org/10.5194/acp-17-8101-2017, 2017.
Frolova, N. L., Belyakova, P. A., Grigoriev, V. Y., Sazonov, A. A., Zotov, L. V., and Jarsjö, J.: Runoff fluctuations in the Selenga River Basin, Reg. Environ. Change, 17, 1965–1976, doi.org/10.1007/s10113-017-1199-0, 2017.
Fu, B. J. and Forsius, M.: Ecosystem services modeling in contrasting landscapes, Landscape Ecol., 30, 375–379, https://doi.org/10.1007/s10980-015-0176-6, 2015.
Garmaev, E. Z., Kulikov, A. I., Tsydypov, B. Z., Sodnomov, B. V., and Ayurzhanaev, A. A.: Environmental Conditions Of Zakamensk Town (Dzhida River Basin Hotspot), Geography, Environment, Sustainability, 12, 224–239, https://doi.org/10.24057/2071-9388-2019-32, 2019.
Georgiadi, A. G., Koronkevich, N. I., Milyukova, I. P., and Barabanova, E. A.: Integrated projection for runoff changes in large Russian river basins in the XXI Century, Geography, Environment, Sustainability, 9, 38–46, 2016.
Giamarelou, M., Eleftheriadis, K., Nyeki, S., Tunved, P., Torseth, K., and Biskos, G.: Indirect evidence of the composition of nucleation mode atmospheric particles in the high Arctic, J. Geophys. Res.-Atmos., 121, 965–975, https://doi.org/10.1002/2015jd023646, 2016.
Gil, J., Pérez, T., Boering, K., Martikainen, P. J., and Biasi, C.: Mechanisms responsible for high N2O emissions from subarctic permafrost peatlands studied via stable isotope techniques, Global Biogeochem. Cy., 31, 172, https://doi.org/10.1002/2015GB005370, 2017.
Glagolev, M. V., Ilyasov, D. V. I., Terentieva, E., Sabrekov, A. F., Yu Mochenov, S., and Maksutov, S. S.: Methane and carbon dioxide fluxes in the waterlogged forests of south and middle taiga of Western Siberia, IOP Conf. Ser.-Earth Environ. Sci., 138, 012005, https://doi.org/10.1088/1755-1315/138/1/012005, 2018.
Gnatiuk, N., Radchenko, I., Davy, R., Morozov, E., and Bobylev, L.: Simulation of factors affecting Emiliania huxleyi blooms in Arctic and sub-Arctic seas by CMIP5 climate models: model validation and selection, Biogeosciences, 17, 1199–1212, https://doi.org/10.5194/bg-17-1199-2020, 2020.
Godrijan, J., Drapeau, D., and Balch, W. M.: Mixotrophic uptake of organic compounds by coccolithphores, Limnol. Ocean., 65, 1410–1421, https://doi.org/10.1002/lno.11396, 2020.
Granath, G., Rydin, H., Baltzer, J. L., Bengtsson, F., Boncek, N., Bragazza, L., Bu, Z.-J., Caporn, S. J. M., Dorrepaal, E., Galanina, O., Gałka, M., Ganeva, A., Gillikin, D. P., Goia, I., Goncharova, N., Hájek, M., Haraguchi, A., Harris, L. I., Humphreys, E., Jiroušek, M., Kajukało, K., Karofeld, E., Koronatova, N. G., Kosykh, N. P., Lamentowicz, M., Lapshina, E., Limpens, J., Linkosalmi, M., Ma, J.-Z., Mauritz, M., Munir, T. M., Natali, S. M., Natcheva, R., Noskova, M., Payne, R. J., Pilkington, K., Robinson, S., Robroek, B. J. M., Rochefort, L., Singer, D., Stenøien, H. K., Tuittila, E.-S., Vellak, K., Verheyden, A., Waddington, J. M., and Rice, S. K.: Environmental and taxonomic controls of carbon and oxygen stable isotope composition in Sphagnum across broad climatic and geographic ranges, Biogeosciences, 15, 5189–5202, https://doi.org/10.5194/bg-15-5189-2018, 2018.
Grigoriev, V. Y. and Frolova, N. L.: Terrestrial water storage change of European Russia and its impact on water balance, Geography, Environment, Sustainability, 11, 38–50, https://doi.org/10.24057/2071-9388-2018-11-1-38-50, 2018.
Groundstroem, F. and Juhola, S.: A framework for identifying cross-border impacts of climate change on the energy sector, Environment Systems and Decisions, 39, 3–15, https://doi.org/10.1007/s10669-018-9697-2, 2019.
Gunchin, G., Manousakas, M., Osan, J., Karydas, A. G., Eleftheriadis, K., Lodoysamba, S., Shagjjamba, D., Migliori, A., Padilla-Alvarez, R., Streli, C., and Darby, I.: Three-year long source apportionment study of airborne particles in Ulaanbaatar using x-ray fluorescence and positive matrix factorization, Aerosol Air Qual. Res., 19, 1056–1067, https://doi.org/10.4209/aaqr.2018.09.0351, 2019.
Guo, Y., Yan, C., Li, C., Ma, W., Feng, Z., Zhou, Y., Lin, Z., Dada, L., Stolzenburg, D., Yin, R., Kontkanen, J., Daellenbach, K. R., Kangasluoma, J., Yao, L., Chu, B., Wang, Y., Cai, R., Bianchi, F., Liu, Y., and Kulmala, M.: Formation of nighttime sulfuric acid from the ozonolysis of alkenes in Beijing, Atmos. Chem. Phys., 21, 5499–5511, https://doi.org/10.5194/acp-21-5499-2021, 2021.
Gurchenkov, A. A., Murynin, A. B., Trekin, A. N., and Ignatyev, V. Y.: Object-oriented classification of substrate surface objects in Arctic impact regions aerospace monitoring, Herald of the Bauman Moscow State Technical University, 3, 135–146, https://doi.org/10.18698/1812-3368-2017-3-135-146, 2017.
Göckede, M., Kittler, F., Kwon, M. J., Burjack, I., Heimann, M., Kolle, O., Zimov, N., and Zimov, S.: Shifted energy fluxes, increased Bowen ratios, and reduced thaw depths linked with drainage-induced changes in permafrost ecosystem structure, The Cryosphere, 11, 2975–2996, https://doi.org/10.5194/tc-11-2975-2017, 2017.
Göckede, M., Kwon, M. J., Kittler, F., Heimann, M., Zimov, N., and Zimov, S.: Negative feedback processes following drainage slow down permafrost degradation, Glob. Change Biol., 25, 3254–3266, https://doi.org/10.1111/gcb.14744, 2019.
Hari, P. and Kulmala, M.: Station for Measuring Ecosystem–Atmosphere Relations (SMEAR II), Boreal Environ. Res., 10, 315–322, 2005.
Hari, P., Andreae, M., Kabat, P., and Kulmala, M.: A comprehensive network of measuring stations to monitor climate change, Boreal Environ. Res., 14, 442–446, 2009.
Hari, P., Petäjä, T., Bäck, J., Kerminen, V.-M., Lappalainen, H. K., Vihma, T., Laurila, T., Viisanen, Y., Vesala, T., and Kulmala, M.: Conceptual design of a measurement network of the global change, Atmos. Chem. Phys., 16, 1017–1028, https://doi.org/10.5194/acp-16-1017-2016, 2016.
Hari, P., Kerminen, V.-M., Kulmala, L., Kulmala, M., Noe, S., Petäjä, T., Vanhatalo, A., and Bäck, J.: Annual cycle of Scots pine photosynthesis, Atmos. Chem. Phys., 17, 15045–15053, https://doi.org/10.5194/acp-17-15045-2017, 2017.
He, Y., Chen, F., Jia, H., and Valery, G.: Bondur: Different Drought Legacies between Rain-fed and Irrigated Croplands in a Typical Russian Agricultural Region, Remote Sens., 12, 1700, https://doi.org/10.3390/rs12111700, 2020.
Heintzenberg, J., Tunved, P., Galí, M., and Leck, C.: New particle formation in the Svalbard region 2006–2015, Atmos. Chem. Phys., 17, 6153–6175, https://doi.org/10.5194/acp-17-6153-2017, 2017.
Helin, A., Sietiö, O.-M., Heinonsalo, J., Bäck, J., Riekkola, M.-L., and Parshintsev, J.: Characterization of free amino acids, bacteria and fungi in size-segregated atmospheric aerosols in boreal forest: seasonal patterns, abundances and size distributions, Atmos. Chem. Phys., 17, 13089–13101, https://doi.org/10.5194/acp-17-13089-2017, 2017.
Hellén, H., Praplan, A. P., Tykkä, T., Ylivinkka, I., Vakkari, V., Bäck, J., Petäjä, T., Kulmala, M., and Hakola, H.: Long-term measurements of volatile organic compounds highlight the importance of sesquiterpenes for the atmospheric chemistry of a boreal forest, Atmos. Chem. Phys., 18, 13839–13863, https://doi.org/10.5194/acp-18-13839-2018, 2018.
Hinzman, L. D., Bettez, N. D., Bolton, W. R., Chapin, F. S., Dyurgerov, M. B., Fastie, C. L., Griffith, B., Hollister, R. D., Hope, A., Huntington, H. P., Jensen, A. M., Jia, G.J., Jorgenson, T., Kane, D.L., Klein, D. R., Kofinas, G., Lynch, A. H., Lloyd, A. H., McGuire, A. D., Nelson, F. E., Oechel, W. C., Osterkamp, T. E., Racine, C. H., Romanovsky, V. E., Stone, R. S., Stow, D. A., Sturm, M., Tweedie, C. E., Vourlitis, G. L., Walker, M. D., Walker, D. A., Webber, P. J., Welker, J. M., Winker, K. S., and Yoshikawa, K.: Evidence and Implications of Recent Climate Change in Northern Alaska and Other Arctic Regions, Climatic Change, 72, 251–298, doi.org/10.1007/s10584-005-5352-2, 2005.
Hoegh-Guldberg, O., Jacob, D., Taylor, M., Bindi, M., Brown, S., Camilloni, I., Diedhiou, A., Djalante, R., Ebi, K. L., Engelbrecht, F., Guiot, J., Hijioka, Y., Mehrotra, S., Payne, A., Seneviratne, S. I., Thomas, A., Warren, R., and Zhou, G.: Impacts of 1.5 ∘C Global Warming on Natural and Human Systems, in: Global Warming of 1.5 ∘C: An IPCC Special Report on the impacts of global warming of 1.5 ∘C above pre-industrial levels and related global greenhouse gas emission pathways, in the context of strengthening the global response to the threat of climate change, sustainable development, and efforts to eradicate poverty, edited by: Masson-Delmotte, V., Zhai, P., Pörtner, H.-O., Roberts, D., Skea, J., Shukla, P. R., Pirani, A., Moufouma-Okia, W., Péan, C., Pidcock, R., Connors, S., Matthews, J. B. R., Chen, Y., Zhou, X., Gomis, M. I., Lonnoy, E., Maycock, T., Tignor, M., and Waterfield, T., WMO, Geneva, Switzerland, available at: https://www.ipcc.ch/sr15/ (last access: 1 February 2022), 2018.
Huang, W., Cheng, B., Zhang, J., Zhang, Z., Vihma, T., Li, Z., and Niu, F.: Modeling experiments on seasonal lake ice mass and energy balance in the Qinghai–Tibet Plateau: a case study, Hydrol. Earth Syst. Sci., 23, 2173–2186, https://doi.org/10.5194/hess-23-2173-2019, 2019a.
Huang, W., Zhang, J., Leppäranta, M., Li, Z., Cheng, B., and Lin, Z.: Thermal structure and water-ice heat transfer in a shallow ice–covered thermokarst lake in central Qinghai-Tibet Plateau, J. Hydrol., 578, 124122, https://doi.org/10.1016/j.jhydrol.2019.124122, 2019b.
Hölttä, T., Lintunen, A., Chan, T., Mäkelä, A., and Nikinmaa, E.: A steady state stomatal model of balanced leaf gas exchange, hydraulics and maximal source-sink flux, Tree Physiol., 37, 851–868, https://doi.org/10.1093/treephys/tpx011, 2017.
IPCC: Special Report on the Ocean and Cryosphere in a Changing Climate, edited by: Pörtner, H.-O., Roberts, D. C., Masson-Delmotte, V., Zhai, P., Tignor, M., Poloczanska, E., Mintenbeck, K., Alegriìa, A., Nicolai, M., Okem, A., Petzold, J., Rama, B., and Weyer, N. M., available at: https://www.ipcc.ch/srocc/ (last access: 1 February 2022), 2019.
IPCC: Climate Change 2021: The Physical Science Basis. Contribution of Working Group I to the Sixth Assessment Report of the Intergovernmental Panel on Climate Change, edited by: Masson-Delmotte, V., Zhai, P., Pirani, A., Connors, S. L., Péan, C., Berger, S., Caud, N., Chen, Y., Goldfarb, L., Gomis, M. I., Huang, M., Leitzell, K., Lonnoy, E., Matthews, J. B. R., Maycock, T. K., Waterfield, T., Yelekçi, O., Yu, R., and Zhou, B., Cambridge University Press, Summary for Policymakers IPCC, 2021: Summary for Policymakers, in: Climate Change 2021: The Physical Science Basis, Contribution of Working Group I to the Sixth Assessment Report of the Intergovernmental Panel on Climate Change, edited by: Masson-Delmotte, V., Zhai, P., Pirani, A., Connors, S. L., Péan, C., Berger, S., Caud, N., Chen, Y., Goldfarb, L., Gomis, M. I., Huang, M., Leitzell, K., Lonnoy, E., Matthews, J. B. R., Maycock, T. K., Waterfield, T., Yelekçi, O., Yu, R., and Zhou, B., Cambridge University Press, available at: https://www.ipcc.ch/report/sixth-assessment-report-working-group-i/ (last access: 1 February 2022), 2021.
Ivakhov, V. M., Paramonova, N. N., Privalov, V. I., Zinchenko, A. V., Loskutova, M. A., Makshtas, A. P., Kustov, V. Y., Laurila, T., Aurela, M., and Asmi, E.: Atmospheric Concentration of Carbon Dioxide at Tiksi and Cape Baranov Stations in 2010–2017, Russ. Meteorol. Hydrol., 44, 291–299, https://doi.org/10.3103/s1068373919040095, 2019.
Jaffe, D., Bertschi, I., Jaegle, L., Novelli, P., Reid, J. S., Tanimoto, H., Vingarzan, R., and Westphal, D. L.: Long-range transport of Siberian biomass burning emissions and impact on surface ozone in western North America, Geophys. Res. Lett., 31, L16106, https://doi.org/10.1029/2004GL020093, 2004.
Jakobson, L., Vihma, T., and Jakobson, E.: Relationships between Sea Ice Concentration and Wind Speed over the Arctic Ocean during 1979–2015, J. Climate, 32, 7783–7796, https://doi.org/10.1175/JCLI-D-19-0271.1, 2019.
Juhola, S.: Planning for a green city: The Green Factor tool, Urban For. Urban Gree., 34, 254–258, https://doi.org/10.1016/j.ufug.2018.07.019, 2018.
Juutinen, S., Virtanen, T., Kondratyev, V., Laurila, T., Linkosalmi, M., Mikola, J., Nyman, J., Räsänen, A., Tuovinen, J.-P., and Aurela, M.: Spatial variation and seasonal dynamics of leaf-area index in the arctic tundra-implications for linking ground observations and satellite images, Environ. Res. Lett., 12, 1748–9326, https://doi.org/10.1088/1748-9326/aa7f85, 2017.
Kalogridis, A. C., Popovicheva, O. B., Engling, G., Diapouli, E., Kawamura, K., Tachibana, E., Ono, K., Kozlov, V. S., and Eleftheriadis, K.: Smoke aerosol chemistry and aging of Siberian biomass burning emissions in a large aerosol chamber, Atmos. Environ., 185, 15–28, 2018.
Kangasluoma, J., Ahonen, L. R., Laurila, T., Cai, R., Enroth, J., Mazon, S., Korhonen, F., Aalto, P., Kulmala, M., Attoui, M., and Petäjä, T.: Laboratory verification of a new high flow differential mobility particle sizer, and field measurements in Hyytiälä, J. Aerosol Sci., 124, 1–9, 2018.
Karelin, D. V., Goryachkin, S. V., Zamolodchikov, D. G., Dolgikh, A. V., Zazovskaya, E. P., Shishkov, V. A., and Kraev, G. N.: Human footprints on greenhouse gas fluxes in cryogenic ecosystems, Dokl. Earth Sc., 477, 1467–1469, https://doi.org/10.1134/S1028334X17120133, 2017.
Karetnikov, S., Leppäranta, M., and Montonen, A.: A time series of over 100 years of ice seasons on Lake Ladoga, J. Great Lakes Res., 43, 979–988, https://doi.org/10.1016/j.jglr.2017.08.010, 2017.
Karthe, D., Abdullaev, I., Boldgiv, B., Borchardt, D., Chalov, S., Jarsjö, J., Li, L., and Nittrouer, J. A.: Water in Central Asia: an integrated assessment for science-based management, Environ. Earth Sci., 76, , 690, https://doi.org/10.1007/s12665-017-6994-x, 2017a.
Karthe, D., Chalov, S., Moreido, V., Pashkina, M., Romanchenko, A., Batbayar, G., Kalugin, A., Westphal, K., Malsy, M., and Flörke, M.: Assessment of runoff, water and sediment quality in the Selenga River basin aided by a web-based geoservice, Water Resour., 44, 399–416, 2017b.
Karthe, D., Chalov, S., Gradel, A., and Kusbach, A.: Special issue: Environment change on the Mongolian plateau: atmosphere, forests, soils and water, Geography, Environment, Sustainability, 12, 60–65, https://doi.org/10.24057/2071-9388-2019-1411, 2019.
Karvonen, J., Shi, L., Cheng, B., Similä, M., Mäkynen, M., and Vihma, T.: Bohai Sea ice parameter estimation based on thermodynamic ice model and Earth observation data, Remote Sensing, 9, 234, https://doi.org/10.3390/rs9030234, 2017.
Kasimov, N., Karthe, D., and Chalov, S.: Environmental change in the Selenga River–Lake Baikal Basin, Reg. Environ. Change, 17, 1945–1949, 2017.
Kasimov, N., Shinkareva, G., Lychagin, M., Kosheleva, N., Chalov, S., Pashkina, M., Thorslund, J., and Jarsjö, J.: River water quality of the selenga-baikal basin: Part i – spatio-temporal patterns of dissolved and suspended metals, Water, 12, 2137, https://doi.org/10.3390/w12082137, 2020a.
Kasimov, N., Shinkareva, G., Lychagin, M., Chalov, S., Pashkina, M., Thorslund, J., and Jarsjö, J.: River water quality of the selenga-baikal basin: part ii – metal partitioning under different hydroclimatic conditions, Water, 12, 2392, https://doi.org/10.3390/w12092392, 2020b.
Kaus, A., Schäffer, M., Karthe, D., Büttner, O., von Tümpling, W., and Borchardt, D.: Regional patterns of heavy metal exposure and contamination in the fish fauna of the Kharaa River basin, Mongolia, Reg. Environ. Change, 17, 2023–2037, https://doi.org/10.1007/s10113-016-0969-4, 2017.
Kondrik, D., Kazakov, E., and Pozdnyakov, D.: A synthetic satellite dataset of the spatio-temporal distributions of Emiliania huxleyi blooms and their impacts on Arctic and sub-Arctic marine environments (1998–2016), Earth Syst. Sci. Data, 11, 119–128, https://doi.org/10.5194/essd-11-119-2019, 2019.
Kecorius, S., Vogl, T., Paasonen, P., Lampilahti, J., Rothenberg, D., Wex, H., Zeppenfeld, S., van Pinxteren, M., Hartmann, M., Henning, S., Gong, X., Welti, A., Kulmala, M., Stratmann, F., Herrmann, H., and Wiedensohler, A.: New particle formation and its effect on cloud condensation nuclei abundance in the summer Arctic: a case study in the Fram Strait and Barents Sea, Atmos. Chem. Phys., 19, 14339–14364, https://doi.org/10.5194/acp-19-14339-2019, 2019.
Kerminen, V.-M., Chen, X., Vakkari, V., Petäjä, T., Kulmala, M., and Bianchi, F.: Atmospheric new particle formation and growth: review of field observations, Environ. Res. Lett., 13, 103003, https://doi.org/10.1088/1748-9326/aadf3c, 2018.
Kim, J., Kim, H. M., Cho, C.-H., Boo, K.-O., Jacobson, A. R., Sasakawa, M., Machida, T., Arshinov, M., and Fedoseev, N.: Impact of Siberian observations on the optimization of surface CO2 flux, Atmos. Chem. Phys., 17, 2881–2899, https://doi.org/10.5194/acp-17-2881-2017, 2017.
Kirillin, G., Leppäranta, M., Terzhevik, A., Bernhardt, J., Engelhardt, C., Granin, N., Golosov, S., Efremova, T., Palshin, N., Sherstyankin, P., Zdorovennova, G., and Zdorovennov, R.: Physics of seasonally ice-covered lakes: major drivers and temporal/spatial scales, Aquat. Ecol., 74, 659–682, 2012.
Kirillin, G., Aslamov, I., Leppäranta, M., and Lindgren, E.: Turbulent mixing and heat fluxes under lake ice: the role of seiche oscillations, Hydrol. Earth Syst. Sci., 22, 6493–6504, https://doi.org/10.5194/hess-22-6493-2018, 2018.
Kirschke, S., Bousquet, P., Ciais, P., Saunois, M., Canadell, J. G., Dlugokencky, E. J., Bergamaschi, P. Bergmann, D, Blake, D. R., Bruhwiler, L., Cameron-Smith, P., Castaldi, S., Chevallier, F., Feng, L., Fraser, A., Heimann, M., Hodson, E. L., Houweling, S., Josse, B., Fraser, P. J., Krummel, P. B., Lamarque, J.-F., Langenfelds, R. L., Le Quere, C., Naik, V., O'Doherty, S., Palmer, P. I., Pison, I., Plummer, D., Poulter, B., Prinn, R. G., Rigby, M., Ringeval, B., Santini, M., Schmidt, M., Shindell, D. T., Simpson, I. J., Spahni, R., Steele, L. P., Strode, S. A., Sudo, K., Szopa, S., van der Werf, G.R., Voulgarakis, A., van Weele, M., Weiss, R. F., Williams, J. E., and Zeng, G.: Three decades of global methane sources and sinks, Nat. Geosci., 6, 813–823, https://doi.org/10.1038/ngeo1955, 2013.
Kiselev, M. V., Voropay, N. N., and Cherkashina, A. A.: influence of anthropogenic activities on the temperature regime of soils of the South-Western Baikal region, IOP Conf. Ser.-Earth Environ. Sci., 381, 012043, https://doi.org/10.1088/1755-1315/381/1/012043, 2019.
Kittler, F., Heimann, M., Kolle, O., Zimov, N., Zimov, S., and Göckede, M.: Long-term drainage reduces CO2 uptake and CH4 emissions in a Siberian permafrost ecosystem, Global Biogeochem. Cy., 31, 1704–1717, https://doi.org/10.1002/2017GB005774, 2017.
Kiuru, P., Ojala, A., Mammarella, I., Heiskanen, J., Kämäräinen, M., Vesala, T., and Huttula, T.: Effects of Climate Change on CO2 Concentration and Efflux in a Humic Boreal Lake: A Modeling Study, J. Geophys. Res.-Biogeo., 123, 2212–2233, 2018.
Kondrik, D., Pozdnyakov, D., and Pettersson, L.: Particulate inorganic carbon production within E. huxleyi blooms in subpolar and polar seas: a satellite time series study (1998–2013), Int. J. Remote Sens., 38, 6179–6205, https://doi.org/10.1080/01431161.2017.1350304, 2017.
Kondrik, D. V., Pozdnyakov, D. V., and Johannessen, O. M.: Satellite evidence that E. huxleyi phytoplankton blooms weaken marine carbon sinks, Geophys. Res. Lett., 5, 846–854, https://doi.org/10.1002/2017GL076240, 2018a.
Kondrik, D. V., Pozdnyakov, D. V., and Pettersson, L. H.: Tendencies in Coccolithophorid Blooms in Some Marine Environments of the Northern Hemisphere according to the Data of Satellite Observations in 1998–2013, Izv. Atmos. Ocean. Phys., 53, 955–964, https://doi.org/10.1134/S000143381709016X, 2018b.
Kondrik, D., Kazakov, E., and Pozdnyakov, D.: A synthetic satellite dataset of the spatio-temporal distributions of Emiliania huxleyi blooms and their impacts on Arctic and sub-Arctic marine environments (1998–2016), Earth Syst. Sci. Data, 11, 119–128, https://doi.org/10.5194/essd-11-119-2019, 2019.
Kondrik, D. V., Kazakov, E. E., Pozdnyakov, D. V., and Johannessen, O. M.: Satellite evidence for enhancement of the column mixing ratio of atmospheric CO2 over E. huxleyi blooms, Transactions of the Karelian Research Centre of the Russian Academy of Sciences, Limnologoia i Oceanologia series, 9, 1–11, https://doi.org/10.17076/lim1107, 2019.
Konovalov, I. B., Lvova, D. A., Beekmann, M., Jethva, H., Mikhailov, E. F., Paris, J.-D., Belan, B. D., Kozlov, V. S., Ciais, P., and Andreae, M. O.: Estimation of black carbon emissions from Siberian fires using satellite observations of absorption and extinction optical depths, Atmos. Chem. Phys., 18, 14889–14924, https://doi.org/10.5194/acp-18-14889-2018, 2018.
Konovalov, V., Rets, E., and Pimankina, N.: Interrelation between glacier summer mass balance and runoff in mountain river basins, Geography, Environment, Sustainability, 12, 23–33, https://doi.org/10.24057/2071-9388-2018-26, 2019.
Konstantinov, P., Varentsov, M., and Esau, I.: A high density urban temperature network deployed in several cities of Eurasian Arctic, Environ. Res. Lett., 13, 075007, https://doi.org/10.1088/1748-9326/aacb84, 2018.
Kontkanen, J., Deng, C., Fu, Y., Dada, L., Zhou, Y., Cai, J., Daellenbach, K. R., Hakala, S., Kokkonen, T. V., Lin, Z., Liu, Y., Wang, Y., Yan, C., Petäjä, T., Jiang, J., Kulmala, M., and Paasonen, P.: Size-resolved particle number emissions in Beijing determined from measured particle size distributions, Atmos. Chem. Phys., 20, 11329–11348, https://doi.org/10.5194/acp-20-11329-2020, 2020.
Kooijmans, L. M. J., Sun, W., Aalto, J., Erkkilä, K.M., Maseyk, K., Seibt, U., Vesala, T., Mammarella, I., and Chen, H.: Influences of light and humidity on carbonyl sulfide-based estimates of photosynthesis, Proceedings of the National Academy of Sciences, 116 (7), 2470-2475, https://doi.org/10.1073/pnas.1807600116, 2019.
Korneykova, M. V. and Evdokimova, G. A.: Microbiota of the ground air layers in natural and industrial zones of the Kola Arctic, J. Environ. Sci. Health A, 53, 271–277, https://doi.org/10.1080/10934529.2017.1397444, 2018.
Korneikova, M. V., Redkina, V. V., and Shalygina, R. R.: Algological and mycological characterization of soils under pine and birch forests in the Pasvik Reserve, Eurasian Soil Sci., 51, 211–220, https://doi.org/10.1134/s1064229318020047, 2018.
Koronatova, N. G. and Milyaeva, E. V.: Plant community succession in post-mined quarries in the northern-taiga zone of West Siberia, Contemp. Probl. Ecol., 4, 513–518, 2011.
Köster, E., Köster, K., Berninger, F., and Pumpanen, J.: Carbon dioxide, methane and nitrous oxide fluxes from podzols of a fire chronosequence in the boreal forests in Värriö, Finnish Lapland, Geoderma Regional, 5, 181–187, https://doi.org/10.1016/j.geodrs.2015.07.001, 2015.
Köster, E., Köster, K., Berninger, F., Prokushkin, A., Aaltonen, H., Zhou, X., and Pumpanen, J.: Changes in fluxes of carbon dioxide and methane caused by fire in Siberian boreal forest with continuous permafrost, J. Environ. Manage., 228, 405–415, https://doi.org/10.1016/j.jenvman.2018.09.051, 2018.
Köster, K., Berninger, F., Koster, E., and Pumpanen, J.: Influences of reindeer grazing on above- and belowground biomass and soil carbon dynamics, Arct. Antarct. Alp. Res., 47, 495–503, https://doi.org/10.1657/aaar0014-062, 2015.
Köster, K., Berninger, F., Heinonsalo, J., Lindén, A., Köster, E., Ilvesniemi, H., and Pumpanen J.: The long-term impact of low-intensity surface fires on litter decomposition and enzyme activities in boreal coniferous forests, Int. J Wildland Fire, 25, 213–223, https://doi.org/10.1071/wf14217_co, 2016.
Köster, K., Koster, E., Kulmala, L., Berninger, F., and Pumpanen, J.: Are the climatic factors combined with reindeer grazing affecting the soil CO2 emissions in subarctic boreal pine forest?, Catena, 149, 616–622, https://doi.org/10.1016/j.catena.2016.06.011, 2017.
Köster, K., Koster, E., Berninger, F., Heinonsalo, J., and Pumpanen, J.: Contrasting effects of reindeer grazing on CO2, CH4, and N2O fluxes originating from the northern boreal forest floor, Land Degrad. Dev., 29, 374–381, https://doi.org/10.1002/ldr.2868, 2018.
Kukkonen, I., Ezhova, E., Suhonen, E. A. J., Lappalainen, H. K., Gennadinik, V., Ponomareva, O., Gravis, A., Miles, V., Kulmala, M., Melnikov, V., and Drozdov, D.: Observations and modelling of ground temperature evolution in the discontinuous permafrost zone in Nadym, North-West Siberia, Permafrost Periglac., 31, 264–280, https://doi.org/10.1002/ppp.2040, 2020.
Kulmala, M.: Atmospheric chemistry: China's choking cocktail, Nature, 526, 497–499, https://doi.org/10.1038/526497a, 2015.
Kulmala, M.: Build a global Earth observatory, Nature, 553, 21–23, https://doi.org/10.1038/d41586-017-08967-y, 2018.
Kulmala, M., Vehkamäki, H., Petäjä, T., Dal Maso, M., Lauri, A., Kerminen, V.-M., Birmili, W., and McMurry, P. H.: Formation and growth rates of ultrafine atmospheric particles: a review of observations, J. Aerosol Sci., 35, 143–176, 2004.
Kulmala, M., Lappalainen, H. K., Petäjä, T., Kurten, T., Kerminen, V.-M., Viisanen, Y., Hari, P., Sorvari, S., Bäck, J., Bondur, V., Kasimov, N., Kotlyakov, V., Matvienko, G., Baklanov, A., Guo, H. D., Ding, A., Hansson, H.-C., and Zilitinkevich, S.: Introduction: The Pan-Eurasian Experiment (PEEX) – multidisciplinary, multiscale and multicomponent research and capacity-building initiative, Atmos. Chem. Phys., 15, 13085–13096, https://doi.org/10.5194/acp-15-13085-2015, 2015.
Kulmala, M., Lappalainen, H. K., Petäjä, T., Kerminen, V.-M., Viisanen, Y., Matvienko, G., Melnikov, V., Baklanov, A., Bondur, V., Kasimov, N., and Zilitinkevich, S.: Pan-Eurasian Experiment (PEEX) Program: Grant Challenges in the Arctic-boreal context, J. Geography Environment Sustainability, 2, 5–18, 2016a.
Kulmala, M., Petäjä, T., Kerminen, V. M., Kujansuu, J., Ruuskanen, T., Ding, A., Nie, W., Hu, M., Wang, Z., Wu, Z., and Wang, L.: On secondary new particle formation in China, Front. Environ. Sci. En., 10, 8, https://doi.org/10.1007/s11783-016-0850-1, 2016b.
Kulmala, M., Kerminen, V.-M., Petäjä, T., Ding, A. J., and Wang L.: Atmospheric gas-to-particle conversion: why NPF events are observed in megacities?, Faraday Discuss., 200, 271–288, https://doi.org/10.1039/c6fd00257a, 2017.
Kulmala, L., Pumpanen, J., Kolari, P., Dengel, S., Berninger, F., Köster, K., Matkala, L., Vanhatalo, A., Vesala, T., and Bäck, J.: Inter- and intra-annual dynamics of photosynthesis differ between forest floor vegetation and tree canopy in a subarctic Scots pine stand, Agr. Forest Meteorol., 271, 1–11, https://doi.org/10.1016/j.agrformet.2019.02.029, 2019.
Kulmala, M., Dada, L., Daellenbach, K. R., Yan, C., Stolzenburg, D., Kontkanen, J., Ezhova, E., Hakala, S., Tuovinen, S., Kokkonen, T. V., Kurppa, M., Cai, R., Zhou, Y., Yin, R., Baalbaki, R., Chan, T., Chu, B., Deng, C., Fu, Y., Ge, M., He, H., Heikkinen, L., Junninen, H., Liu, Y., Lu, Y., Nie, W., Rusanen, A., Vakkari, V., Wang, V., Yang, G., Yao, L., Zheng, J., Kujansuu, J., Kangasluoma, J., Petäjä, T., Paasonen, P., Järvi, L., Worsnop, D., Ding, A., Liu, Y., Wang, L., Jiang, J., Bianchi, F., and Kerminen, V.-M.: Is reducing new particle formation a plausible solution to mitigate particulate air pollution in Beijing and other Chinese megacities?, Faraday Discuss., 226, 334–347, https://doi.org/10.1039/D0FD00078G, 2021.
Kwon, M. J., Beulig, F., Ilie, I., Wildner, M., Küsel, K., Merbold, L., Mahecha, M. D., Zimov, N., Zimov, S. A., Heimann, M., Schuur, E. A. G., Kostka, J. E., Kolle, O., Hilke, I., and Göckede, M.: Plants, microorganisms, and soil temperatures contribute to a decrease in methane fluxes on a drained Arctic floodplain, Glob. Change Biol., 23, 2396–2412, https://doi.org/10.1111/gcb.13558, 2017.
Kwon, M. J., Natali, S. M., Hicks Pries, C. E., Schuur, E. A., Steinhof, A., Crummer, K. G., Zimov, N., Zimov, S. A., Heimann, M., Kolle, O., and Göckede, M.: Drainage enhances modern soil carbon contribution but reduces old soil carbon contribution to ecosystem respiration in tundra ecosystems, Glob. Change Biol., 25, 1315–1325, https://doi.org/10.1111/gcb.14578, 2019.
Kühn, T., Kupiainen, K., Miinalainen, T., Kokkola, H., Paunu, V.-V., Laakso, A., Tonttila, J., Van Dingenen, R., Kulovesi, K., Karvosenoja, N., and Lehtinen, K. E. J.: Effects of black carbon mitigation on Arctic climate, Atmos. Chem. Phys., 20, 5527–5546, https://doi.org/10.5194/acp-20-5527-2020, 2020.
Lan, H., Holopainen, J., Hartonen, K., Jussila, M., Ritala, M., and Riekkola, M.-L.: Fully automated online dynamic in-tube extraction for continuous sampling of volatile organic compounds in air, Anal. Chem., 91, 8507–8515, https://doi.org/10.1021/acs.analchem.9b01668, 2019.
Lappalainen, H. K., Petäjä, T., Kujansuu, J., Kerminen, V., Shvidenko, A., Bäck, J., Vesala, T., Vihma, T., De Leeuw, G., Lauri, A., Ruuskanen, T., Lapshin, V. B., Zaitseva, N., Glezer, O., Arshinov, M., Spracklen, D. V., Arnold, S. R., Juhola, S., Lihavainen, H., Viisanen, Y., Chubarova, N., Chalov, S., Filatov, N., Skorokhod, A., Elansky, N., Dyukarev, E., Esau, I., Hari, P., Kotlyakov, V., Kasimov, N., Bondur, V., Matvienko, G., Baklanov, A., Mareev, E., Troitskaya, Y., Ding, A., Guo, H., Zilitinkevich, S., and Kulmala, M.: Pan-Eurasian Experiment (PEEX) –a research initiative meeting the grand challenges of the changing environment of the northern Pan-Eurasian arctic-boreal areas, J. Geography Environment Sustainability, 2, 13–48, https://doi.org/10.24057/2071-9388-2014-7-2-13-48, 2014.
Lappalainen, H. K., Kulmala, M., and Zilitinkevich, S. (Eds.): Pan Eurasian Experiment (PEEX) Science Plan, available at: https://www.atm.helsinki.fi/peex/ (last access: 26 January 2022), ISBN 978-951-51-0587-5 (printed), ISBN 978-951-51-0588-2 (online), 2015.
Lappalainen, H. K., Kerminen, V.-M., Petäjä, T., Kurten, T., Baklanov, A., Shvidenko, A., Bäck, J., Vihma, T., Alekseychik, P., Andreae, M. O., Arnold, S. R., Arshinov, M., Asmi, E., Belan, B., Bobylev, L., Chalov, S., Cheng, Y., Chubarova, N., de Leeuw, G., Ding, A., Dobrolyubov, S., Dubtsov, S., Dyukarev, E., Elansky, N., Eleftheriadis, K., Esau, I., Filatov, N., Flint, M., Fu, C., Glezer, O., Gliko, A., Heimann, M., Holtslag, A. A. M., Hõrrak, U., Janhunen, J., Juhola, S., Järvi, L., Järvinen, H., Kanukhina, A., Konstantinov, P., Kotlyakov, V., Kieloaho, A.-J., Komarov, A. S., Kujansuu, J., Kukkonen, I., Duplissy, E.-M., Laaksonen, A., Laurila, T., Lihavainen, H., Lisitzin, A., Mahura, A., Makshtas, A., Mareev, E., Mazon, S., Matishov, D., Melnikov, V., Mikhailov, E., Moisseev, D., Nigmatulin, R., Noe, S. M., Ojala, A., Pihlatie, M., Popovicheva, O., Pumpanen, J., Regerand, T., Repina, I., Shcherbinin, A., Shevchenko, V., Sipilä, M., Skorokhod, A., Spracklen, D. V., Su, H., Subetto, D. A., Sun, J., Terzhevik, A. Y., Timofeyev, Y., Troitskaya, Y., Tynkkynen, V.-P., Kharuk, V. I., Zaytseva, N., Zhang, J., Viisanen, Y., Vesala, T., Hari, P., Hansson, H. C., Matvienko, G. G., Kasimov, N. S., Guo, H., Bondur, V., Zilitinkevich, S., and Kulmala, M.: Pan-Eurasian Experiment (PEEX): towards a holistic understanding of the feedbacks and interactions in the land–atmosphere–ocean–society continuum in the northern Eurasian region, Atmos. Chem. Phys., 16, 14421–14461, https://doi.org/10.5194/acp-16-14421-2016, 2016.
Lappalainen, H. K., Kulmala, M., Kujansuu, J., Petäjä, T., Mahura, A., de Leeuw, G., Zilitinkevich, S., Juustila, M., Kerminen, V. M., Bornstein, B., and Jiahua, Z.: The Silk Road agenda of the Pan-Eurasian Experiment (PEEX) program, Big Earth Data, 2, 8–35, https://doi.org/10.1080/20964471.2018.1437704, 2018a.
Lappalainen, H. K., Altimir, N., Kerminen, V., Petäjä, T., Makkonen, R., Alekseychik, P., Zaitseva, N., Bashmakova, I., Kujansuu, J., Lauri, A., Haapanala, P., Mazon, S. B., Borisova, A., Konstantinov, P., Chalov, S., Laurila, T., Asmi, E., Lihavainen, H., Bäck, J., Arshinov, M., Mahura, A., Arnold, S., Vihma, T., Uotila, P., de Leeuw, G., Kukkonen, I., Malkhazova, S., Tynkkynen, V., Fedorova, I., Hansson, H. C., Dobrolyubov, S., Melnikov, V., Matvienko, G., Baklanov, A., Viisanen, Y., Kasimov, N., Guo, H., Bondur, V., Zilitinkevich, S., and Kulmala, M.: Pan-Eurasian Experiment (PEEX) Program: An overview of the first 5 years in operation and future prospects, Geography, Environment, Sustainability, 11, 6–19, https://doi.org/10.24057/2071-9388-2018-11-1-6-19, 2018b.
Lei, R., Tian-Kunze, X., Leppäranta, M., Wang, J., Kaleschke, L., and Zhang, Z.: Changes in summer sea ice, albedo, and portioning of surface solar radiation in the Pacific sector of Arctic Ocean during 1982–2009, J. Geophys. Res.-Oceans, 121, 5470–5486, https://doi.org/10.1002/2016jc011831, 2016.
Lei, R. B., Xie, H. J., Wang, J., Leppäranta, M., Jonsdottir, I., and Zhang, Z. H.: Changes in sea ice conditions along the Arctic Northeast Passage from 1979 to 2012, Cold Reg. Sci. Technol., 119, 132–144, https://doi.org/10.1016/j.coldregions.2015.08.004, 2015.
Lei, R. B., Cheng, B., Heil, P., Vihma, T., Wang, J., Ji, Q., and Zhang, Z. H.: Seasonal and interannual variations of sea ice mass balance from the Central Arctic to the Greenland Sea, J. Geophys. Res.-Oceans, 123, 2422–2439, https://doi.org/10.1002/2017jc013548, 2018.
Leino, K., Nieminen, T., Manninen, H. E., Petäjä, T., Kerminen, V.-M., and Kulmala, M.: Intermediate ions as a strong indicator of new particle formation bursts in a boreal forest, Boreal Environ. Res., 21, 274–286, 2016.
Leppäranta, M.: Structure and properties of lake ice. Freezing of Lakes and the Evolution of their Ice Cover, edited by: Leppäranta, M., Springer, Berlin/Heidelberg, Germany, 51–90, https://doi.org/10.1007/978-3-642-29081-7_3, 2015.
Leppäranta, M., Lindgren, E., and Shirasawa, K.: The heat budget of Lake Kilpisjarvi in the Arctic tundra, Hydrol. Res., 48, 969–980, https://doi.org/10.2166/nh.2016.171, 2017.
Leppäranta, M., Lewis, J. E., Heini, A., and Arvola, L.: Spatial statistics of hydrography and water chemistry in a eutrophic boreal lake based on sounding and water samples, Environ. Monit. Assess., 190, 378, https://doi.org/10.1007/s10661-018-6742-z, 2018.
Leppäranta, M., Lindgren, E., Wen, L., and Kirillin, G.: Ice cover decay and heat balance in Lake Kilpisjärvi in Arctic tundra, J. Limnol., 78, 163–175, https://doi.org/10.4081/jlimnol.2019.1879, 2019.
Leppäranta, M., Meleshko, V., Uotila, P., and Pavlova, T.: Sea ice modelling, Sea Ice in the Arctic, Editors Ola M. Johannessen, Leonid Bobylev, Elena V. Shalina, Stein Sandven, Springer, Cham, 315–388, ISBN (print) 978-3-030-21300-8, ISBN (electronic) 978-3-030-21301-5, 2020.
Li, H., Väliranta, M., Mäki, M., Kohl, L., Sannel, B., Pumpanen, J., Koskinen, M., Bäck, J., and Bianchi, F.: Overlooked organic vapor emissions from thawing Arctic permafrost, Environ. Res. Lett., 15, 104097, https://doi.org/10.1088/1748-9326/abb62d, 2020.
Li, M., Wang, L., Liu, J., Gao, W., Song, T., Sun, Y., Li, L., Li, X., Wang, Y., Liu, L., Daellenbach, K. R., Paasonen, P. J., Kerminen, V.-M., Kulmala, M., and Wang, Y.: Exploring the regional pollution characteristics and meteorological formation mechanism of PM2.5 in North China during 2013–2017, Env. Int., 134, 105283, https://doi.org/10.1016/j.envint.2019.105283, 2020.
Liao, Z., Cheng, B., Zhao, J., Vihma, T., Jackson, K., Yang, Q., Yang, Y., Zhang, L., Li, Z., Qiu, Y., and Cheng, X.: Snow depth and ice thickness derived from SIMBA ice mass balance buoy data using an automated algorithm, Int. J. Digit. Earth, 12, 962–979, https://doi.org/10.1080/17538947.2018.1545877, 2018.
Lin, X., Rogers, B. M., Sweeney, C., Chevallier, F., Arshinov, M., Dlugokencky, E., Machida, T., Sasakawa, M., Tans, P., and Keppel-Aleks, G.: Siberian and temperate ecosystems shape Northern Hemisphere atmospheric CO2 seasonal amplification, P. Natl. Acad. Sci. USA, 117, 21079–21087, https://doi.org/10.1073/pnas.1914135117, 2020.
Lintunen, A., Paljakka, T., Salmon, Y., Dewar, R., Riikonen, A., and Hölttä, T.: The influence of soil temperature and water content on belowground hydraulic conductance and leaf gas exchange in mature trees of three boreal species, Plant Cell Environ., 43, 532–547, https://doi.org/10.1111/pce.13709, 2020.
Liu, J., Wang, L., Li, M., Liao, Z., Sun, Y., Song, T., Gao, W., Wang, Y., Li, Y., Ji, D., Hu, B., Kerminen, V.-M., Wang, Y., and Kulmala, M.: Quantifying the impact of synoptic circulation patterns on ozone variability in northern China from April to October 2013–2017, Atmos. Chem. Phys., 19, 14477–14492, https://doi.org/10.5194/acp-19-14477-2019, 2019.
Liu, W., Atherton, J., Mõttus, M., Gastellu-Etchegorry, J. P., Malenovský, Z., Raumonen, P., Åkerblom, M., Mäkipää, R., and Porcar-Castell, A.: Simulating solar-induced chlorophyll fluorescence in a boreal forest stand reconstructed from terrestrial laser scanning measurements, Remote Sens. Environ., 232, 111274, https://doi.org/10.1016/j.rse.2019.111274, 2019.
Liu, Y., de Leeuw, G., Kerminen, V.-M., Zhang, J., Zhou, P., Nie, W., Qi, X., Hong, J., Wang, Y., Ding, A., Guo, H., Krüger, O., Kulmala, M., and Petäjä, T.: Analysis of aerosol effects on warm clouds over the Yangtze River Delta from multi-sensor satellite observations, Atmos. Chem. Phys., 17, 5623–5641, https://doi.org/10.5194/acp-17-5623-2017, 2017.
Liu, Y., Zhang, J., Zhou, P., Lin, T., Hong, J., Shi, L., Yao, F., Wu, J., Guo, H., and de Leeuw, G.: Satellite-based estimate of the variability of warm cloud properties associated with aerosol and meteorological conditions, Atmos. Chem. Phys., 18, 18187–18202, https://doi.org/10.5194/acp-18-18187-2018, 2018.
Liu, Y. C., Yan, C., Feng, Z., Zheng, F., Fan, X., Zhang, Y., Li, C., Zhou, Y., Lin, Z., Guo, Y., Zhang, Y., Ma, L., Zhou, W., Liu, Z., Dada, L., Dällenbach, K., Kontkanen, J., Cai, R., Chan, T., Chu, B., Du, W., Yao, L., Wang, Y., Cai, J., Kangasluoma, J., Kokkonen, T., Kujansuu, J., Rusanen, A., Deng, C., Fu, Y., Yin, R., Li, X., Lu, Y., Liu, Y., Lian, C., Yang, D., Wang, W., Ge, M., Wang, Y., Worsnop, D. R., Junninen, H., He, H., Kerminen, V.-M., Zheng, J., Wang, L., Jiang, J., Petäjä, T., Bianchi, F., and Kulmala, M.: Continuous and comprehensive atmospheric observation in Beijing: a station to understand the complex urban atmospheric environment, Big Earth Data, 4, 295–321, 2020.
López-Blanco, E., Exbrayat, J.-F., Lund, M., Christensen, T. R., Tamstorf, M. P., Slevin, D., Hugelius, G., Bloom, A. A., and Williams, M.: Evaluation of terrestrial pan-Arctic carbon cycling using a data-assimilation system, Earth Syst. Dynam., 10, 233–255, https://doi.org/10.5194/esd-10-233-2019, 2019.
Lu, P., Leppäranta, M., Cheng, B., and Li, Z.: Influence of melt-pond depth and ice thickness on Arctic sea-ice albedo and light transmittance, Cold Reg. Sci. Technol., 124, 1–10, https://doi.org/10.1016/j.coldregions.2015.12.010, 2016.
Lu, P., Cheng, B., Leppäranta, M., and Li, Z. J.: Partitioning of solar radiation in Arctic sea ice during melt season, Oceanologia, 60, 464–477, https://doi.org/10.1016/j.oceano.2018.03.002, 2018a.
Lu, P., Leppäranta, M., Cheng, B., Li, Z., Istomina, L., and Heygster, G.: The color of melt ponds on Arctic sea ice, The Cryosphere, 12, 1331–1345, https://doi.org/10.5194/tc-12-1331-2018, 2018b.
Lu, Y., Yan, C., Fu, Y., Chen, Y., Liu, Y., Yang, G., Wang, Y., Bianchi, F., Chu, B., Zhou, Y., Yin, R., Baalbaki, R., Garmash, O., Deng, C., Wang, W., Liu, Y., Petäjä, T., Kerminen, V.-M., Jiang, J., Kulmala, M., and Wang, L.: A proxy for atmospheric daytime gaseous sulfuric acid concentration in urban Beijing, Atmos. Chem. Phys., 19, 1971–1983, https://doi.org/10.5194/acp-19-1971-2019, 2019.
Luoma, K., Virkkula, A., Aalto, P., Petäjä, T., and Kulmala, M.: Over a 10-year record of aerosol optical properties at SMEAR II, Atmos. Chem. Phys., 19, 11363–11382, https://doi.org/10.5194/acp-19-11363-2019, 2019.
Lychagin, M., Chalov, S., Kasimov, N., Shinkareva, G., Jarsjo, J., and Thorslund, J.: Surface water pathways and fluxes of metals under changing environmental conditions and human interventions in the Selenga River system, Environ. Earth Sci., 76, 1, https://doi.org/10.1007/s12665-016-6304-z, 2017.
Ma, J., Yan, X., Dong, W., and Chou, J.: Gross primary production of global forest ecosystems has been overestimated, Sci. Rep.-UK, 5, 10820, https://doi.org/10.1038/srep10820, 2015.
Magritsky, D. V., Frolova, N. L., Evstigneev, V. M., Povalishnikova, E. S., Kireeva, M. B., and Pakhomova, O. M.: Long-term changes of river water inflow into the seas of the Russian Arctic sector, Polarforschung, 87, 177–194, https://doi.org/10.2312/polarforschung.87.2.177, 2018.
Mahura, A., Gonzalez-Aparacio, I., Nuterman, R., and Baklanov, A.: Seasonal Impact Analysis on Population due to Continuous Sulphur Emissions from Severonikel Smelters of the Kola Peninsula, Geography, Environment, Sustainability, 11, 130–144, https://doi.org/10.24057/2071-9388-2018-11-1-130-144, 2018.
Mahura, A., Baklanov, A., Petäjä, T., Nuterman, R., Ivanov, S., Michaelides, S., Ruban, I., Makkonen, R., Lappalainen, H. K., Zilitinkevich, S., and Kulmala, M.: PEEX Integrated Multi-scales and -Process Modelling for Environmental Applications, EGU General Assembly 2020, Online, 4–8 May 2020, EGU2020-11582, https://doi.org/10.5194/egusphere-egu2020-11582, 2020.
Mäki, M., Krasnov, D., Hellén, H., Noe, S. M., and Bäck, J.: Stand type affects fluxes of volatile organic compounds from the forest floor in hemiboreal and boreal climates, Plant Soil, 441, 363–381, https://doi.org/10.1007/s11104-019-04129-3, 2019.
Malkhazova, S. M., Mironova, V. A., Orlov, D. S., and Adishcheva, O. S.: Influence of climatic factor on naturally determined diseases in à regional context, Geography, Environment, Sustainability, 11, 157–170, https://doi.org/10.24057/2071-9388-2018-11-1-157-170, 2018.
Malsy, M., Flörke, M., and Borchardt, D.: What drives the water quality changes in the Selenga basin: climate change or socio-economic development?, Reg. Environ. Change, 17, 1977–1989, https://doi.org/10.1007/s10113-016-1005-4, 2017.
Mammarella, I., Nordbo, A., Rannik, Ü., Haapanala, S., Levula, J., Laakso, H., Ojala, A., Peltola, O., Heiskanen, J., Pumpanen, J., and Vesala, T.: Carbon dioxide and energy fluxes over a small boreal lake in Southern Finland, J. Geophys. Res.-Biogeo., 120, 1296–1314, https://doi.org/10.1002/2014jg002873, 2015.
Manasypov, R. M., Vorobyev, S. N., Loiko, S. V., Kritzkov, I. V., Shirokova, L. S., Shevchenko, V. P., Kirpotin, S. N., Kulizhsky, S. P., Kolesnichenko, L. G., Zemtzov, V. A., Sinkinov, V. V., and Pokrovsky, O. S.: Seasonal dynamics of organic carbon and metals in thermokarst lakes from the discontinuous permafrost zone of western Siberia, Biogeosciences, 12, 3009–3028, https://doi.org/10.5194/bg-12-3009-2015, 2015.
Marelle, L., Raut, J. C., Law, K. S., and Duclaux, O.: Current and future arctic aerosols and ozone from remote emissions and emerging local sources-modeled source contributions and radiative effects, J. Geophys. Res.-Atmos., 123, 12942–12963, https://doi.org/10.1029/2018jd028863, 2018.
Maslov, A. V., Shevchenko, V. P., Bobrov, V. A., Belogub, E. V., Ershova, V. B., Vereshchagin, O. S., and Khvorov, P. V.: Mineralogical-geochemical features of ice-rafted sediments in some arctic regions, Lithol. Miner. Resour., 53, 110–129, https://doi.org/10.1134/s0024490218020037, 2018a.
Maslov, A. V., Shevchenko, V. P., Kuznetsov, A. B., and Stein, R.: Geochemical and Sr-Nd-Pb-isotope characteristics of ice-rafted sediments of the Arctic Ocean, Geochem. Int., 56, 751–765, https://doi.org/10.1134/s0016702918080050, 2018b.
Matkala, L., Kulmala, L., Kolari, P., Aurela, M., and Bäck, J.: Resilience of carbon dioxide and water exchange to extreme weather events in subarctic Scots pine and Norway spruce stands, Agr. Forest Meteorol., 296, 108239, https://doi.org/10.1016/j.agrformet.2020.108239, 2020.
McCusker, K., Fyfe, J., and Sigmond, M.: Twenty-five winters of unexpected Eurasian cooling unlikely due to Arctic sea-ice loss, Nat. Geosci., 9, 838–842, https://doi.org/10.1038/ngeo2820, 2016.
Meinander, O., Heikkinen, E., Aurela, M., and Hyvärinen, A.: Sampling, Filtering, and Analysis Protocols to Detect Black Carbon, Organic Carbon, and Total Carbon in Seasonal Surface Snow in an Urban Background and Arctic Finland (> 60∘ N), Atmosphere, 11, 923, https://doi.org/10.3390/atmos11090923, 2020a.
Meinander, O., Kontu, A., Kouznetsov, R., and Sofiev, M.: Snow Samples Combined with Long-Range Transport Modeling to Reveal the Origin and Temporal Variability of Black Carbon in Seasonal Snow in Sodankylä (67∘ N), Front. Earth Sci., 8, 153, https://doi.org/10.3389/feart.2020.00153, 2020b.
Merkouriadi, I., Cheng, B., Graham, R. M., Rösel, A., and Granskog, M. A.: Critical role of snow on sea ice growth in the Atlantic sector of the Arctic Ocean, Geophys. Res. Lett., 44, 10479–10485, https://doi.org/10.1002/2017GL075494, 2017.
Mikhailov, E. F., Mironova, S. Y., Makarova, M. V., Vlasenko, S. S., Ryshkevich, T. I., Panov, A. V., and Andreae, M. O.: Studying seasonal variations in carbonaceous aerosol particles in the atmosphere over central Siberia, Izv. Atmos. Ocean. Phys., 51, 423–430, https://doi.org/10.1134/s000143381504009x, 2015a.
Mikhailov, E. F., Mironov, G. N., Pöhlker, C., Chi, X., Krüger, M. L., Shiraiwa, M., Förster, J.-D., Pöschl, U., Vlasenko, S. S., Ryshkevich, T. I., Weigand, M., Kilcoyne, A. L. D., and Andreae, M. O.: Chemical composition, microstructure, and hygroscopic properties of aerosol particles at the Zotino Tall Tower Observatory (ZOTTO), Siberia, during a summer campaign, Atmos. Chem. Phys., 15, 8847–8869, https://doi.org/10.5194/acp-15-8847-2015, 2015b.
Mikhailov, E. F., Mironova, S., Mironov, G., Vlasenko, S., Panov, A., Chi, X., Walter, D., Carbone, S., Artaxo, P., Heimann, M., Lavric, J., Pöschl, U., and Andreae, M. O.: Long-term measurements (2010–2014) of carbonaceous aerosol and carbon monoxide at the Zotino Tall Tower Observatory (ZOTTO) in central Siberia, Atmos. Chem. Phys., 17, 14365–14392, https://doi.org/10.5194/acp-17-14365-2017, 2017.
Mikola, J., Virtanen, T., Linkosalmi, M., Vähä, E., Nyman, J., Postanogova, O., Räsänen, A., Kotze, D. J., Laurila, T., Juutinen, S., Kondratyev, V., and Aurela, M.: Spatial variation and linkages of soil and vegetation in the Siberian Arctic tundra – coupling field observations with remote sensing data, Biogeosciences, 15, 2781–2801, https://doi.org/10.5194/bg-15-2781-2018, 2018.
Miles, M. W., Miles, V., and Esau, I.: Varying climate response across the tundra, forest–tundra and boreal forest biomes in northern West Siberia, Environ. Res. Lett., 14, 075008, https://doi.org/10.1088/1748-9326/ab2364, 2019.
Miles, V.: Arctic surface Urban Heat Island (UHI), MODIS Land Surface Temperature (LST) data, 2000–2016, Arctic Data Center, https://doi.org/10.18739/A2TB0XW4T, 2020.
Miles, V. and Esau, I.: Seasonal and Spatial Characteristics of Urban Heat Islands (UHIs) in Northern West Siberian Cities, Remote Sens., 9, 989, https://doi.org/10.3390/rs9100989, 2017.
Miles, V. and Esau, I.: Surface urban heat islands in 57 cities across different climates in northern Fennoscandia, Urban Climate, 31, 100575, https://doi.org/10.1016/j.uclim.2019.100575, 2020.
Miles, V. V. and Esau, I.: Spatial heterogeneity of greening and browning between and within bioclimatic zones in northern West Siberia, Environ. Res. Lett., 11, 115002, https://doi.org/10.1088/1748-9326/11/11/115002, 2016.
MNRE: Methods for calculating emissions of pollutants into the atmosphe from stationary sources. Ministry of Natural Resources and Ecology, available at: http://www.mnr.gov.ru/docs/metodiki_rascheta_vybrosov_vrednykh_zagryaznyayushchikh_veshchestv_v_atmosfernyy_vozdukh_statsionarn/perechen (last access: 18 January 2022), 2019 (in Russian).
Mori, M., Watanabe, M., Shiogama, H., Inoue, J., and Kimoton, M.: Robust Arctic sea-ice influence on the frequent Eurasian cold winters in past decades, Nat. Geosci., 7, 869–873, https://doi.org/10.1038/ngeo2277, 2014.
Mori, M., Kosaka, Y., Watanabe, M., Nakamura, H., and Kimoto, M.: A reconciled estimate of the influence of Arctic sea-ice loss on recent Eurasian cooling, Nat. Clim. Change, 9, 123–129, https://doi.org/10.1038/s41558-018-0379-3, 2019.
Morozov, E. A., Kondrik, D. V., Chepikova, S. S., and Pozdnyakov, D. V.: Atmospheric columnar CO2 enhancement over E. huxleyi blooms: case studies in the North Atlantic and Arctic waters. Transactions of the Karelian Research Centre of the Russian Academy of Sciences, Limnologoia i Oceanologia series, 3, 1–6, https://doi.org/10.17076/lim989, 2019.
Myslenkov, S., Medvedeva, A., Arkhipkin, V., Markina, M., Surkova, G., Krylov, A., Dobrolyubov, S., Zilitinkevich, S., and Koltermann, P.: Long-term statistics of storms in the Baltic, Barents and White Seas and their future climate projections, Geography, Environment, Sustainability, 11, 93–112, https://doi.org/10.24057/2071-9388-2018-11-1-93-112, 2018.
Naakka, T., Nygård, T., Vihma, T., Graversen, R., and Sedlar, J.: Atmospheric moisture transport between mid-latitudes and the Arctic: regional, seasonal and vertical distributions, Int. J. Climatol., 32, 1–18, https://doi.org/10.1002/joc.5988, 2019.
Natali, S. M., Watts, J. D., Rogers, B. M., Potter, S., Ludwig, S. M., Selbmann, A. K., Sullivan, P. F. Abbott, B. W., Arndt, K. A., Birch, L., Björkman, M. P., Bloom, A. A., Celis, G., Christensen, T. R., Christiansen, C. T., Commane, R., Cooper, E. J., Crill, P., Czimczik, C., Davydov, S., Du, J., Egan, J. E., Elberling, B., Euskirchen, E. S., Friborg, T., Genet, H., Göckede, M., Goodrich, J. P., Grogan, P., Helbig, M., Jafarov, E. E., Jastrow, J. D., Kalhori, A. A. M., Kim, Y., Kimball, J. S., Kutzbach, L., Lara, M.J., Larsen, K., S., Lee, B.-Y., Liu, Z., Loranty, M. M., Lund, M., Lupascu, M., Madani, N., Malhotra, A., Matamala, R., McFarland, J., McGuire, A. D., Michelsen, A., Minions, C., Oechel,W.C., Olefeldt, D., Parmentier, F.-J. W.,, Pirk, N., Poulter, B., Quinton, W., Rezanezhad, F., Risk, D., Sachs, T., Schaefer, K., Schmidt, N. M., Schuur, E.A.G., Semenchuk, P. R., Shaver, G., Sonnentag, O., Starr, G., Treat, C. C., Waldrop, M. P., Wang, Y., Welker, J., Wille, C., Xu, X., Zhang, Z., Zhuang, Q., and Zona, D.: Large loss of CO2 in winter observed across the northern permafrost region, Nat. Clim. Change, 9, 852–857, https://doi.org/10.1038/s41558-019-0592-8, 2019.
Nikandrova, A., Tabakova, K., Manninen, A., Väänänen, R., Petäjä, T., Kulmala, M., Kerminen, V.-M., and O'Connor, E.: Combining airborne in situ and ground-based lidar measurements for attribution of aerosol layers, Atmos. Chem. Phys., 18, 10575–10591, https://doi.org/10.5194/acp-18-10575-2018, 2018.
Nissen, C., Vogt, M., Münnich, M., Gruber, N., and Haumann, F. A.: Factors controlling coccolithophore biogeography in the Southern Ocean, Biogeosciences, 15, 6997–7024, https://doi.org/10.5194/bg-15-6997-2018, 2018.
Nitzbon, J., Langer, M., Westermann, S., Martin, L., Aas, K. S., and Boike, J.: Pathways of ice-wedge degradation in polygonal tundra under different hydrological conditions, The Cryosphere, 13, 1089–1123, https://doi.org/10.5194/tc-13-1089-2019, 2019.
Nygård, T., Graversen, R. G., Uotila, P., Naakka, T., and Vihma, T.: Strong dependence of wintertime Arctic moisture and cloud distributions on atmospheric large-scale circulation, J. Climate, 32, 8771–8790, https://doi.org/10.1175/JCLI-D-19-0242.1, 2019.
Öström, E., Putian, Z., Schurgers, G., Mishurov, M., Kivekäs, N., Lihavainen, H., Ehn, M., Rissanen, M. P., Kurtén, T., Boy, M., Swietlicki, E., and Roldin, P.: Modeling the role of highly oxidized multifunctional organic molecules for the growth of new particles over the boreal forest region, Atmos. Chem. Phys., 17, 8887–8901, https://doi.org/10.5194/acp-17-8887-2017, 2017.
Overland, J., Dunlea, E., Box, J. E., Corell, R., Forsius, M., Kattsov, V., Olsen, M. S., Pawlak, J., Reiersen, L. O., and Wang, M.: The urgency of Arctic change, Polar Sci., 21, 6-13, https://doi.org/10.1016/j.polar.2018.11.008, 2019.
Overland, J. E., Wang, M., Walsh, J. E., and Stroeve, J. C.: Future Arctic climate changes: Adaptation and mitigation time scales, Earth’s Future, 2, 68–74, https://doi.org/10.1002/2013EF000162, 2014.
Paasonen, P., Peltola, M., Kontkanen, J., Junninen, H., Kerminen, V.-M., and Kulmala, M.: Comprehensive analysis of particle growth rates from nucleation mode to cloud condensation nuclei in boreal forest, Atmos. Chem. Phys., 18, 12085–12103, https://doi.org/10.5194/acp-18-12085-2018, 2018.
Palo, T., Vihma, T., Jaagus, J., and Jakobson, E.: Observations on temperature inversion over central Arctic sea ice in summer, Q. J. Roy. Meteor. Soc., 143, 2741–2754, https://doi.org/10.1002/qj.3123, 2017.
Parmentier, F. W., Christensen, T. R., Rysgaard, S. Bendtsen, J., Glud, R. N., Else, B., van Huissteden, J., Sachs, T., Vonk, J. E., and Sejr, M. K.: A synthesis of the arctic terrestrial and marine carbon cycles under pressure from a dwindling cryosphere, Ambio, 46, 53–69, https://doi.org/10.1007/s13280-016-0872-8, 2017.
Pärn, J., Verhoeven, J. T., Butterbach-Bahl, K., Dise, N. B., Ullah, S., Aasa, A., Egorov, S., Espenberg, M., Järveoja, J., Jauhiainen, J., and Kasak, K.: Nitrogen-rich organic soils under warm well-drained conditions are global nitrous oxide emission hotspots, Nat. Commun., 9, 1135, https://doi.org/10.1038/s41467-018-03540-1, 2018.
Parshintsev, J., Vaikkinen, A., Lipponen, K., Vrkoslav, V., Cvačka, J., Kostiainen, R., Kotiaho, T., Hartonen, K., Riekkola, M.-L., and Kauppila, T. J.: Desorption atmospheric pressure photoionization high-resolution mass spectrometry: a complementary approach for the chemical analysis of atmospheric aerosols, Rapid Commun. Mass Sp., 29, 1233–1241, 2015.
Passananti, M., Zapadinsky, E., Zanca, T., Kangasluoma, J., Myllys, N., Rissanen, M. P., Kurtén, T., Ehn, M., Attoui, M., and Vehkamäki, H.: How well can we predict cluster fragmentation inside a mass spectrometer?, Chem. Commun., 55, 5946–5949, 2019.
Payne, R. J., Creevy, A., Malysheva, E., Ratcliffe, J., Andersen, R., Tsyganov, A. N., Rowson, J., Marcisz, K., Zielinska, M., Lamentowicz, M., Lapshina, E. D., and Mazei, Y.: Tree encroachment may lead to functionally-significant changes in peatland testate amoeba communities, Soil Biol, Biochem,, 98, 18–21, https://doi.org/10.1016/j.soilbio.2016.04.002, 2016.
Peltola, O., Vesala, T., Gao, Y., Räty, O., Alekseychik, P., Aurela, M., Chojnicki, B., Desai, A. R., Dolman, A. J., Euskirchen, E. S., Friborg, T., Göckede, M., Helbig, M., Humphreys, E., Jackson, R. B., Jocher, G., Joos, F., Klatt, J., Knox, S. H., Kowalska, N., Kutzbach, L., Lienert, S., Lohila, A., Mammarella, I., Nadeau, D. F., Nilsson, M. B., Oechel, W. C., Peichl, M., Pypker, T., Quinton, W., Rinne, J., Sachs, T., Samson, M., Schmid, H. P., Sonnentag, O., Wille, C., Zona, D., and Aalto, T.: Monthly gridded data product of northern wetland methane emissions based on upscaling eddy covariance observations, Earth Syst. Sci. Data, 11, 1263–1289, https://doi.org/10.5194/essd-11-1263-2019, 2019.
Peltoniemi, J. I., Gritsevich, M., Hakala, T., Dagsson-Waldhauserová, P., Arnalds, Ó., Anttila, K., Hannula, H.-R., Kivekäs, N., Lihavainen, H., Meinander, O., Svensson, J., Virkkula, A., and de Leeuw, G.: Soot on Snow experiment: bidirectional reflectance factor measurements of contaminated snow, The Cryosphere, 9, 2323–2337, https://doi.org/10.5194/tc-9-2323-2015, 2015.
Petäjä, T., Järvi, L., Kerminen, V.-M., Ding, A. J., Sun, J. N., Nie, W., Kujansuu, J., Virkkula, A., Yang, X.-Q., Fu, C. B., Zilitinkevich, S., and Kulmala M.: Enhanced air pollution via aerosol-boundary layer feedback in China, Sci. Rep.-UK, 6, 18998, https://doi.org/10.1038/srep18998, 2016.
Petäjä, T., Duplissy, E.-M., Tabakova, K., Schmale, J., Altstädter, B., Ancellet, G., Arshinov, M., Balin, Y., Baltensperger, U., Bange, J., Beamish, A., Belan, B., Berchet, A., Bossi, R., Cairns, W. R. L., Ebinghaus, R., El Haddad, I., Ferreira-Araujo, B., Franck, A., Huang, L., Hyvärinen, A., Humbert, A., Kalogridis, A.-C., Konstantinov, P., Lampert, A., MacLeod, M., Magand, O., Mahura, A., Marelle, L., Masloboev, V., Moisseev, D., Moschos, V., Neckel, N., Onishi, T., Osterwalder, S., Ovaska, A., Paasonen, P., Panchenko, M., Pankratov, F., Pernov, J. B., Platis, A., Popovicheva, O., Raut, J.-C., Riandet, A., Sachs, T., Salvatori, R., Salzano, R., Schröder, L., Schön, M., Shevchenko, V., Skov, H., Sonke, J. E., Spolaor, A., Stathopoulos, V. K., Strahlendorff, M., Thomas, J. L., Vitale, V., Vratolis, S., Barbante, C., Chabrillat, S., Dommergue, A., Eleftheriadis, K., Heilimo, J., Law, K. S., Massling, A., Noe, S. M., Paris, J.-D., Prévôt, A. S. H., Riipinen, I., Wehner, B., Xie, Z., and Lappalainen, H. K.: Overview: Integrative and Comprehensive Understanding on Polar Environments (iCUPE) – concept and initial results, Atmos. Chem. Phys., 20, 8551–8592, https://doi.org/10.5194/acp-20-8551-2020, 2020a.
Petäjä, T., Ganzei, K. S., Lappalainen, H. K., Tabakova, K., Makkonen, R., Räisänen, J., Chalov, S., Kulmala, M., Zilitinkevich, S. S., Baklanov, P., Shakirov, R. B., Mishina, N. V., Egidarev, E. G., and Kondrat'ev, I. I.: Research agenda for the Russian Far East and utilization of multi-platform compherensive environmental observations, J. Big Data, 5, 277–305, https://doi.org/10.1080/17538947.2020.1826589, 2020b.
Petäjä, T., Ganzei, K. S., Lappalainen, H. K., Tabakova, K., Makkonen, R., Räisänen, J., Chalov, S., Kulmala, M., Zilitinkevich, S., Baklanov, P. Y., Shakirov, R. B., Mishina, N. V., Egidarev, E. G., and Kondrat'ev, I. I.: Research agenda for the Russian Far East and utilization of multi-platform comprehensive environmental observations, Int. J. Digit. Earth, 14, 311–337, https://doi.org/10.1080/17538947.2020.1826589, 2021.
Peterson, B. J., Holmes, R. M., McClelland, J. W., Vörösmarty, C. J., Lammers, R. B., Shiklomanov, A. I., Shiklomanov, I. A., and Rahmstorf, S.: Increasing river discharge to the Arctic Ocean, Science, 80, 298, 2171–2173, https://doi.org/10.1126/science.1077445, 2002.
Pietroń, J., Jarsjo, J., Romanchenko, A. O., and Chalov, S. R.: Model analyses of the contribution of in-channel processes to sediment concentration hysteresis loops, J. Hydrol., 527, 576–589, https://doi.org/10.1016/j.jhydrol.2015.05.009, 2015.
Pietroń, J., Nittrouer, J. A., Chalov, S. R., Dong, T. Y., Kasimov, N., Shinkareva, G., and Jarsjö, J.: Sedimentation patterns in the Selenga River delta under changing hydroclimatic conditions, Hydrol. Process., 32, 278–292, https://doi.org/10.1002/hyp.11414, 2018.
Pirazzini, R., Leppanen, L., Picard, G., Lopez-Moreno, J. I., Marty, C., Macelloni, G., Kontu, A., Von Lerber, A., Tanis, C. M., Schneebeli, M., De Rosnay, P., and Arslan, A. N.: European in-situ snow measurements: practices and purposes, Sensors, 18, 2016, https://doi.org/10.3390/s18072016, 2018.
Pisso, I., Myhre, C. L., Platt, S. M., Eckhardt, S., Hermansen, O., Schmidbauer, N., Mienert, J., Vadakkepuliyambatta, S., Bauguitte, S., Pitt, J., Allen, G., Bower, K. N., O'Shea, S., Gallagher, M. W., Percival, C. J., Pyle, J., Cain, M., and Stohl, A.: Constraints on oceanic methane emissions west of Svalbard from atmospheric in situ measurements and Lagrangian transport modeling, J. Geophys. Res.-Atmos., 121, 14188–14200, https://doi.org/10.1002/2016jd025590, 2016.
Platt, S. M., Eckhardt, S., Ferré, B., Fisher, R. E., Hermansen, O., Jansson, P., Lowry, D., Nisbet, E. G., Pisso, I., Schmidbauer, N., Silyakova, A., Stohl, A., Svendby, T. M., Vadakkepuliyambatta, S., Mienert, J., and Lund Myhre, C.: Methane at Svalbard and over the European Arctic Ocean, Atmos. Chem. Phys., 18, 17207–17224, https://doi.org/10.5194/acp-18-17207-2018, 2018.
Ponomarev, E. I., Kharuk, V. I., and Ranson, K. J.: Wildfires Dynamics in Siberian Larch Forests, Forests, 7, 125, https://doi.org/10.3390/f7060125, 2016.
Popovicheva, O., Evangeliou, N., Eleftheriadis, K., Kalogridis, A. C., Sitnikov, V. N., Eckhardt, S., and Stohl, A.: Black carbon sources constrained by observations and modeling in the Russian high Arctic, Environ. Sci. Technol., 51, 3871–3879, 2017.
Popovicheva, O., Diapouli, E., Makshtas, A., Shonija, N., Manousakas, M., Saraga, D., Uttal, T., and Eleftheriadis, K.: East Siberian Arctic background and black carbon polluted aerosols at HMO Tiksi, Sci. Total Environ., 655, 924–938, https://doi.org/10.1016/j.scitotenv.2018.11.165, 2019.
Popovicheva, O., Ivanov, A., and Vojtisek, M.: Functional Factors of Biomass Burning Contribution to Spring Aerosol Composition in a Megacity: Combined FTIR-PCA Analyses, Atmosphere, 11, 319–339, 2020a.
Popovicheva, O., Volpert, E., Sitnikov, N., Chichaeva, M., and Padoan, S.: Black carbon in spring aerosols of Moscow urban background, Geography, Environment, Sustainability, 13, 233–243, 2020b.
Popovicheva, O. B., Engling, G., Ku, I. T., Timofeev, M. A., and Shonija, N. K.: Aerosol emissions from long-lasting smoldering of boreal peatlands: chemical composition, markers, and microstructure, Aerosol Air Qual. Res., 19, 484–503, https://doi.org/10.4209/aaqr.2018.08.0302, 2019.
Popovicheva, O. B., Padoan, S., Schnelle- Kreis, J., Nguyen, D. L., Adam, T. W., Kistler, M., Steinkogler, T., Kasper-Giebl, A., Zimmermann, R., and Chubarova, N. E.: Spring aerosol in urban atmosphere of megacity: analytical and statistical assessment for source impacts, Aerosol Air Qual. Res., 20, 702–719, 2020.
Pozdnyakov, D. V., Pettersson, L. H., and Korosov, A. A. (Eds.): Exploring the Marine Ecology from Space, Springer, Switzerland, ISBN 978-3-319-30074-0, ISBN 978-3-319-30075-5 (eBook), 2017.
Pozdnyakov, D. V., Kondrik, D. V., Kazakov, E. E., and Chepikova, S.: Environmental conditions favoring coccolithophore blooms in subarctic and arctic seas: a 20-year satellite and multi-dimensional statistical study, Proc. SPIE 11150, Remote Sensing of the Ocean, Sea Ice, Coastal Waters, and Large Water Regions, 11150, 11150W, https://doi.org/10.1117/12.2547868, 2019.
Pozdnyakov, D., Kondrik, D., Kazakov, E., and Chepikova, S.: Environmental conditions favoring coccolithophore blooms in subarctic and arctic seas: a 20-year satellite and multi-dimensional statistical study, in: SPIE Proceedings, 11150, SPIE: Remote Sensing of the Ocean, 9–12 September Strasbourg, France, https://doi.org/10.1117/12.2547868, 2020.
Preis, Y. I., Simonova, G. V., Voropay, N. N., and Dyukarev, E. A.: Estimation of the influence of hydrothermal conditions on the carbon isotope composition in Sphagnum mosses of bogs of Western Siberia, IOP Conf. Ser.-Earth Environ. Sci., 211, 012031, https://doi.org/10.1088/1755-1315/211/1/012031, 2018.
Pulliainen, J., Aurela, M., Laurila, T., Aalto, T., Takala, M., Salminen, M., Kulmala, M., Barr, A., Heimann, M., Lindroth, A., Laaksonen, A., Derksen, C., Mäkelä, A., Markkanen, T., Lemmetyinen, J., Susiluoto, J., Dengel, S., Mammarella, I., Tuovinen, J.-P., and Vesala, T.: Early snowmelt significantly enhances boreal springtime carbon uptake, P. Natl. Acad. Sci. USA, 114, 11081–11086, https://doi.org/10.1073/pnas.1707889114, 2017.
Pulliainen, J., Luojus, K., Derksen, C., Mudryk, L., Lemmetyinen, J., Salminen, M., Ikonen, J., Takala, M., Cohen, J., Smolander, T., and Norberg, J.: Patterns and trends of Northern Hemisphere snow mass from 1980 to 2018, Nature, 581, 294–298, https://doi.org/10.1038/s41586-020-2258-0, 2020.
Qi, X. M., Ding, A. J., Nie, W., Petäjä, T., Kerminen, V.-M., Herrmann, E., Xie, Y. N., Zheng, L. F., Manninen, H., Aalto, P., Sun, J. N., Xu, Z. N., Chi, X. G., Huang, X., Boy, M., Virkkula, A., Yang, X.-Q., Fu, C. B., and Kulmala, M.: Aerosol size distribution and new particle formation in the western Yangtze River Delta of China: 2 years of measurements at the SORPES station, Atmos. Chem. Phys., 15, 12445–12464, https://doi.org/10.5194/acp-15-12445-2015, 2015.
Räisänen, J.: Effect of atmospheric circulation on recent temperature changes in Finland, Clim. Dynam., 53, 5675–5687, https://doi.org/10.1007/s00382-019-04890-2, 2019.
Räisänen, J.: Effect of atmospheric circulation on surface air temperature trends in years 1979–2018, Clim. Dynam., 56, 2303–2320, https://doi.org/10.1007/s00382-020-05590-y, 2021.
Rakitin, V. S., Elansky, N. F., Wang, P., Wang, G., Pankratova, N. V., Shtabkin, Y. A., Skorokhod, A. I., Safronov, A. N., Makarova, M. V., and Grechko, E. I.: Changes in trends of atmospheric composition over urban and background regions of Eurasia: estimates based on spectroscopic observations, Geography, Environment, Sustainability, 11, 84–96, https://doi.org/10.24057/2071-9388-2018-11-2-84-96, 2018.
Rautiainen, K., Parkkinen, T., Lemmetyinen, J., Schwank, M., Wiesmann, A., Ikonen, J., Derksen, C., Davydov, S., Davydova, A., Boike, J., Langer, M., Druschg, M., and Pulliainen, J.: SMOS prototype algorithm for detecting autumn soil freezing, Remote Sens. Environ., 180, 346–360, https://doi.org/10.1016/j.rse.2016.01.012, 2016.
Rinke, A., Segger, B., Crewell, S., Maturilli, M., Naakka, T., Nygård, T., Vihma, T., Alshawaf, F., Dick, G., Wickert, J., and Keller, J.: Trends of vertically integrated water vapor over the Arctic during 1979–2016: Consistent moistening all over?, J. Climate, 32, 6097–6116, https://doi.org/10.1175/JCLI-D-19-0092.1, 2019.
Ripple, W. J., Wolf, C., Newsome, T. M., Galetti, M., Alamgir, M., Crist, E., Mahmoud, M. I., Laurance, W. F., and 15,364 scientist signatories from 184 countries: World scientists' warning to humanity: A second notice, BioScience, 67, 1026–1028, 2017.
Rivero-Calle, S., Gnanadesikan, A., Del Castilo, C., Balch, W., and Guikema, S.: Multidecadal increase in North Atlantic coccolithophores and the potential role of rising CO2, Science, 350, 1533–1537, https://doi.org/10.1126/science.aaa8026, 2015.
Romanovsky, V. E. and Osterkamp, T. E.: Effects of unfrozen water on heat and mass transport processes in the active layer and permafrost, Permafrost Periglac., 11, 219–39, 2000.
Romanowsky, E., Handorf, D., Jaiser, R., Wohltmann, I., Dorn, W., Ukita, J., Cohen, J., Dethloff, K., and Rex, M.: The role of stratospheric ozone for Arctic-midlatitude linkages, Sci. Rep.-UK, 9, 7962, https://doi.org/10.1038/s41598-019-43823-1, 2019.
Roshydromet and GGO: The State of Atmospheric Pollution in Cities on the Territory of Russia for 2018, Yearbook. Federal Service on Hydrometeorology and Monitoring Environment, Roshydromet & A.I. Voeikov Main Geophisical Observatory, St. Petersburg, 250 pp., ISBN 978-5-9500883-8-4, available at: http://voeikovmgo.ru/images/stories/publications/2019/ejegodnik_zagr_atm_2018+.pdf (last access: 18 January 2022), 2019 (in Russian).
Rost, B., and Riebesell, U.: Coccolithophores and the biological pump: responses to environmental changes, in: Coccolithophores, from molecular processes to global impact, edited by: Thierstein, H. R. and Young, J. R., Springer, Heidelberg, Germany, available at: https://doi.org/10.1007/978-3-662-06278-4_5, 99–125, 2004.
Ryazanova, A. and Voropay, N. N.: Droughts and Excessive Moisture Events in Southern Siberia in the Late XXth – Early XXIst Centuries, IOP Conf. Ser.-Earth Environ. Sci., 96, 012015, https://doi.org/10.1088/1755-1315/96/1/012015, 2017.
Saarela, T., Rissanen, A. J., Ojala, A., Pumpanen, J., Aalto, S. L., Tiirola, M., Vesala, T., and Jäntti, H.: CH4 oxidation in a boreal lake during the development of hypolimnetic hypoxia, Aquat. Sci., 82, 19, https://doi.org/10.1007/s00027-019-0690-8, 2020.
Salmon, Y., Lintunen, A., Dayet, A., Chan, T., Dewar, R., Vesala, T., and Hölttä, T.: Leaf carbon and water status control stomatal and non-stomatal limitations of photosynthesis in trees, New Phytol., 226, 690–703, https://doi.org/10.1111/nph.16436, 2020.
Sandanger, T. M., Anda, E., Berglen, T. F., Evenset, A., Christensen, G., and Heimstad, E. S.: Health and environmental impacts in the Norwegian border area related to local Russian industrial emissions, Knowledge status, Scientific Report, NILU OR No 40/2013, 88 pp., ISBN 978-82-425-2606-9, 2013.
Santalahti, M., Sun, H., Sietiö, O.M., Köster, K., Berninger, F., Laurila, T., Pumpanen, J., and Heinonsalo, J.: Reindeer grazing alter soil fungal community structure and litter decomposition related enzyme activities in boreal coniferous forests in Finnish Lapland, App. Soil Ecol., 132, 74–82, https://doi.org/10.1016/j.apsoil.2018.08.013, 2018.
Schmale, J., Arnold, S. R., Law, K. S., Thorp, T., Anenberg, S., Simpson, W. R., Mao, J., and Pratt, K. A.: Local Arctic air pollution: a neglected but serious problem, Earths Future, 6, 1385–1412, https://doi.org/10.1029/2018ef000952, 2018a.
Schmale, J., Henning, S., Decesari, S., Henzing, B., Keskinen, H., Sellegri, K., Ovadnevaite, J., Pöhlker, M. L., Brito, J., Bougiatioti, A., Kristensson, A., Kalivitis, N., Stavroulas, I., Carbone, S., Jefferson, A., Park, M., Schlag, P., Iwamoto, Y., Aalto, P., Äijälä, M., Bukowiecki, N., Ehn, M., Frank, G., Fröhlich, R., Frumau, A., Herrmann, E., Herrmann, H., Holzinger, R., Kos, G., Kulmala, M., Mihalopoulos, N., Nenes, A., O'Dowd, C., Petäjä, T., Picard, D., Pöhlker, C., Pöschl, U., Poulain, L., Prévôt, A. S. H., Swietlicki, E., Andreae, M. O., Artaxo, P., Wiedensohler, A., Ogren, J., Matsuki, A., Yum, S. S., Stratmann, F., Baltensperger, U., and Gysel, M.: Long-term cloud condensation nuclei number concentration, particle number size distribution and chemical composition measurements at regionally representative observatories, Atmos. Chem. Phys., 18, 2853–2881, https://doi.org/10.5194/acp-18-2853-2018, 2018b.
Schmeisser, L., Backman, J., Ogren, J. A., Andrews, E., Asmi, E., Starkweather, S., Uttal, T., Fiebig, M., Sharma, S., Eleftheriadis, K., Vratolis, S., Bergin, M., Tunved, P., and Jefferson, A.: Seasonality of aerosol optical properties in the Arctic, Atmos. Chem. Phys., 18, 11599–11622, https://doi.org/10.5194/acp-18-11599-2018, 2018.
Schuur, E. A. G., Bockheim, J., Canadell, J. G., Euskirchen, E., Field, C. B., Goryachkin, S. V., Hagemann, S., Kuhry, P., Lafleur, P.M., Lee, H., Mazhitova, G., Nelson, F. E., Rinke, A., Romanovsky, V. E., Shiklomanov, N., Tarnocai, C., Venevsky, S., Vogel, J. G., and Zimov, S. A.: Vulnerability of permafrost carbon to climate change: implications for the global carbon cycle, BioScience, 58, 701–714, https://doi.org/10.1641/B580807, 2008.
Schuur, E. A. G., McGuire, A. D., Romanovsky, V., Schädel, C., and Mack, M.: Arctic and boreal carbon, in: Second State of the Carbon Cycle Report (SOCCR2): A Sustained Assessment Report, chap. 11, edited by: Cavallaro, N., Shrestha, G., Birdsey, R., Mayes, M. A., Najjar, R. G., Reed, S. C., Romero-Lankao, P., and Zhu, Z., U.S. Global Change Research Program, Washington, DC, USA, 428–468, https://doi.org/10.7930/SOCCR2.2018.Ch11, 2018.
Scott, C. E., Monks, S. A., Spracklen, D. V., Arnold, S. R., Forster, P. M., Rap, A., Äijälä, M., Artaxo, P., Carslaw, K. S., Chipperfield, M. P., Ehn, M., Gilardoni, S., Heikkinen, L., Kulmala, M., Petäjä, T., Reddington, C. L. S., Rizzo, L. V., Swietlicki, E., Vignati, E., and Wilson, C.: Impact on short-lived climate forcers increases projected warming due to deforestation, Nat. Commun., 9, 157, https://doi.org/10.1038/s41467-017-02412-4, 2018.
Shakhova, N., Semiletov, I., and Belcheva, N.: The great Siberian rivers as a source of methane on the Russian Arctic shelf, Dokl. Earth Sci., 415, 734–736, https://doi.org/10.1134/S1028334X07050169, 2007.
Shalina, E. V. and Sandven, S.: Snow depth on Arctic sea ice from historical in situ data, The Cryosphere, 12, 1867–1886, https://doi.org/10.5194/tc-12-1867-2018, 2018.
Shen, Y., Virkkula, A., Ding, A., Wang, J., Chi, X., Nie, W., Qi, X., Huang, X., Liu, Q., Zheng, L., Xu, Z., Petäjä, T., Aalto, P. P., Fu, C., and Kulmala, M.: Aerosol optical properties at SORPES in Nanjing, east China, Atmos. Chem. Phys., 18, 5265–5292, https://doi.org/10.5194/acp-18-5265-2018, 2018.
Shen, Y., Virkkula, A., Ding, A., Luoma, K., Keskinen, H., Aalto, P. P., Chi, X., Qi, X., Nie, W., Huang, X., Petäjä, T., Kulmala, M., and Kerminen, V.-M.: Estimating cloud condensation nuclei number concentrations using aerosol optical properties: role of particle number size distribution and parameterization, Atmos. Chem. Phys., 19, 15483–15502, https://doi.org/10.5194/acp-19-15483-2019, 2019.
Shestakova, T. A., Gutierrez, E., Valeriano, C., Lapshina, E., and Voltas, J.: Recent loss of sensitivity to summer temperature constrains tree growth synchrony among boreal Eurasian forests, Agr. Forest Meteorol., 268, 318–330, https://doi.org/10.1016/j.agrformet.2019.01.039, 2019.
Shevchenko, V. P., Starodymova, D. P., Vinogradova, A. A., Lisitzin, A. P., Makarov, V. I., Popova, S. A., Sivonen, V. V., and Sivonen, V. P.: Elemental and organic carbon in atmospheric aerosols over the northwestern coast of Kandalaksha Bay of the White Sea, Dokl. Earth Sci., 461, 242–246, https://doi.org/10.1134/s1028334x1503006x, 2015.
Shevnina, E., Kourzeneva, E., Kovalenko, V., and Vihma, T.: Assessment of extreme flood events in a changing climate for a long-term planning of socio-economic infrastructure in the Russian Arctic, Hydrol. Earth Syst. Sci., 21, 2559–2578, https://doi.org/10.5194/hess-21-2559-2017, 2017.
Shevnina, E., Silaev, A., and Vihma, T.: Probabilistic projections of annual runoff and potential hydropower production in Finland, Universal Journal of Geoscience, 7, 43–55, https://doi.org/10.13189/ujg.2019.070201, 2019.
Shiklomanov, A. I. and Lammers, R. B.: Record Russian river discharge in 2007 and the limits of analysis, Environ. Res. Lett., 4, 045015, https://doi.org/10.1088/1748-9326/4/4/045015, 2009.
Shiklomanov, I. A. and Shiklomanov, A. I.: Climatic Change and the Dynamics of River Runoff into the Arctic Ocean, Water Resources, 30, 593–601, https://doi.org/10.1023/B:WARE.0000007584.73692.ca, 2003.
Shiklomanov, N. I., Nelson, F. E., Streletskiy, D. A., Hinkel, K. M., and Brown, J.: The Circumpolar Active Layer Monitoring (CALM) Program: Data collection, management, and dissemination strategies, In: Proceedings of the Ninth International Conference on Permafrost, Fairbanks, University of Alaska Press, 2, 1647–1652, 2008.
Shinkareva, G. L., Lychagin, M. Y., Tarasov, M. K., Pietroń, J., Chichaeva, M. A., and Chalov, S. R.: Biogeochemical specialization of macrophytes and their role as a biofilter in the Selenga delta, Geography, Environment, Sustainability, 12, 240–263, 2019.
Silkin, V. A., Pautova, L., Giordano, M., Chasovnikov, V., Vostokov, S., Podymov, O., Parkhomova, S., and Moskalenko, L.: Drivers of phytoplankton blooms in the northeastern Black Sea, Mar. Pollut. Bull., 138, 274–284, https://doi.org/10.1016/j.marpolbul.2018.11.042, 2018.
Sizov, O. S. and Lobotrosova, S. A.: Features of revegetation of drift sand sites in the northern taiga subzone of Western Siberia, Earth Cryosphere, 20, 3–13, https://doi.org/10.21782/kz1560-7496-2016-3(3-13), 2016.
Skorokhod, A. I., Berezina, E. V., Moiseenko, K. B., Elansky, N. F., and Belikov, I. B.: Benzene and toluene in the surface air of northern Eurasia from TROICA-12 campaign along the Trans-Siberian Railway, Atmos. Chem. Phys., 17, 5501–5514, https://doi.org/10.5194/acp-17-5501-2017, 2017.
Slukovskaya, M. V., Vasenev, V. I., Ivashchenko, K. V., Morev, D. V., Drogobuzhskaya, S. V., Ivanova, L. A., and Kremenetskaya, I. P.: Technosols on mining wastes in the subarctic: Efficiency of remediation under Cu-Ni atmospheric pollution, Int. Soil Water Conserv. Res., 7, 297–307, https://doi.org/10.1016/j.iswcr.2019.04.002, 2019.
Smith, L.: The new north: The world in 2050, Profile Books, London, UK, ISBN 978 1 84668 8768, eISBN 987 1 84765 312 3, 2011.
Song, S., Li, C., Shi, X., Zhao, S., Li, Z., Bai, Y., Cao, X., Wang, Q., Huotari, J., Tulonen, T., Uusheimo, S., Leppäranta, M., and Arvola, L.: Under-ice metabolism in a shallow lake (Wuliangsuhai) in Inner Mongolia, in cold and arid climate zone, Freshwater Biol., 64, 1710–1720, https://doi.org/10.1111/fwb.13363, 2019.
Spengler, T., Renfrew, I. A., Terpstra, A., Tjernström, M., Screen, J., Brooks, I. M., Andrew Carleton, A., Chechin, D., Chen, L., Doyle, J., Esau, I., Hezel, P. J., Jung, T., Kohyama, T., Lüpkes, C., McCusker, C. E., Nygård, T., Sergeev, D., Shupe, M. D., Sodemann, H., and Vihma, T.: High-latitude dynamics of atmosphere–ice–ocean interactions, B. Am. Meteorol. Soc., 97, ES179–ES182, https://doi.org/10.1175/bams-d-15-00302.1, 2016.
Sporre, M. K., Blichner, S. M., Karset, I. H. H., Makkonen, R., and Berntsen, T. K.: BVOC–aerosol–climate feedbacks investigated using NorESM, Atmos. Chem. Phys., 19, 4763–4782, https://doi.org/10.5194/acp-19-4763-2019, 2019.
Starodymova, D. P., Shevchenko, V. P., Sivonen, V. P., and Sivonen, V. V.: Material and elemental composition of surface aerosols on the north-western coast of the Kandalaksha Bay of the White Sea, Atmos. Oceanic Opt., 29, 507–511, https://doi.org/10.1134/s1024856016060154, 2016.
Sun, H., Santalahti, M., Pumpanen, J., Koster, K., Berninger, F., Raffaello, T., Asiegbu, F. O., and Heinonsalo, J.: Bacterial community structure and function shift across a northern boreal forest fire chronosequence, Sci. Rep.-UK, 6, 95, https://doi.org/10.1038/srep32411, 2016.
Sun, Y., Frankenberg, C., Wood, J. D., Schimel, D. S., Jung, M., Guanter, L., Drewry, D. T., Verma, M., Porcar-Castell, A., Griffis, T. J., Gu, L., Magney, S., Köhler, P., Evans, B., and Yuen, K.: OCO-2 advances photosynthesis observation from space via solar-induced chlorophyll fluorescence, Science, 358, 189, https://doi.org/10.1126/science.aam5747, 2017.
Suomi, I., Gryning, S.-E., O'Connor, E. J., and Vihma, T.: Methodology for obtaining wind gusts using Doppler lidar, Q. J. Roy. Meteor. Soc., 143, 2061–2072, doi.org/10.1002/qj.3059, 2016.
Svensson, J., Virkkula, A., Meinander, O., Kivekäs, N., Hannula, H.-R., Järvinen, O., Peltoniemi, J. I., Gritsevich, M., Heikkilä, A., Kontu, A., Neitola, K., Brus, D., DagssonWaldhauserova, P., Anttila, K., Vehkamäki, M., Hienola, A., de Leeuw, G., and Lihavainen, H.: Soot-doped natural snow and its albedo — results from field experiments, Boreal Environ. Res., 21, 481–503, 2016.
Svensson, J., Ström, J., Kivekäs, N., Dkhar, N. B., Tayal, S., Sharma, V. P., Jutila, A., Backman, J., Virkkula, A., Ruppel, M., Hyvärinen, A., Kontu, A., Hannula, H.-R., Leppäranta, M., Hooda, R. K., Korhola, A., Asmi, E., and Lihavainen, H.: Light-absorption of dust and elemental carbon in snow in the Indian Himalayas and the Finnish Arctic, Atmos. Meas. Tech., 11, 1403–1416, https://doi.org/10.5194/amt-11-1403-2018, 2018.
Svensson, J., Ström, J., and Virkkula, A.: Multiple-scattering correction factor of quartz filters and the effect of filtering particles mixed in water: implications for analyses of light absorption in snow samples, Atmos. Meas. Tech., 12, 5913–5925, https://doi.org/10.5194/amt-12-5913-2019, 2019.
Taylor, A., Brownlee., C., and Wheeler, G.: Coccolithophore cell biology: chalking up progress, Annu. Rev. Mar. Sci., 9, 283–310, https://doi.org/10.1146/annurev-marine-122414-034032, 2017.
Taylor, K. E., Stouffer, R. J., and Meehl, G. A.: An overview of CMIP5 and the experiment design, B. Am. Meteorol. Soc., 93, 485–498, https://doi.org/10.1175/BAMS-D-11-00094.1, 2012.
Terentieva, I. E., Glagolev, M. V., Lapshina, E. D., Sabrekov, A. F., and Maksyutov, S.: Mapping of West Siberian taiga wetland complexes using Landsat imagery: implications for methane emissions, Biogeosciences, 13, 4615–4626, https://doi.org/10.5194/bg-13-4615-2016, 2016.
Thompson, R. L., Sasakawa, M., Machida, T., Aalto, T., Worthy, D., Lavric, J. V., Lund Myhre, C., and Stohl, A.: Methane fluxes in the high northern latitudes for 2005–2013 estimated using a Bayesian atmospheric inversion, Atmos. Chem. Phys., 17, 3553–3572, https://doi.org/10.5194/acp-17-3553-2017, 2017.
Thonat, T., Saunois, M., Bousquet, P., Pison, I., Tan, Z., Zhuang, Q., Crill, P. M., Thornton, B. F., Bastviken, D., Dlugokencky, E. J., Zimov, N., Laurila, T., Hatakka, J., Hermansen, O., and Worthy, D. E. J.: Detectability of Arctic methane sources at six sites performing continuous atmospheric measurements, Atmos. Chem. Phys., 17, 8371–8394, https://doi.org/10.5194/acp-17-8371-2017, 2017.
Thorslund, J., Jarsjo, J., Wallstedt, T., Morth, C. M., Lychagin, M. Y., and Chalov, S. R.: Speciation and hydrological transport of metals in non-acidic river systems of the Lake Baikal basin: Field data and model predictions, Reg. Environ. Change, 17, 2007–2021, https://doi.org/10.1007/s10113-016-0982-7, 2017.
Tian, Z. X., Cheng, B., Zhao, J. C., Vihma, T., Zhang, W. L., Li, Z. J., and Zhang, Z. H.: Observed and modelled snow and ice thickness in the Arctic Ocean with CHINARE buoy data, Acta Oceanol. Sin., 36, 66–75, https://doi.org/10.1007/s13131-017-1020-4, 2017.
Tillman, H., Yang, J., and Nielsson, E.: The Polar Silk Road: China's New Frontier of International Cooperation, China Quarterly of International Strategic Studies, 4, 345–362, https://doi.org/10.1142/S2377740018500215, 2018.
Timokhina, A. V., Prokushkin, A. S., Onuchin, A. A., Panov, A. V., Kofman, G. B., and Heimann, M.: Variability of ground CO2 concentration in the middle taiga subzone of the Yenisei region of Siberia, Russ. J. Ecol., 46, 143–151, https://doi.org/10.1134/s1067413615020125, 2015a.
Timokhina, A. V., Prokushkin, A. S., Onuchin, A. A., Panov, A. V., Kofman, G. B., Verkhovets, S. V., and Heimann, M., Long-term trend in CO2 concentration in the surface atmosphere over Central Siberia, Russ. Meteorol. Hydrol., 40, 186–190, 2015b.
Tsuruta, A., Aalto, T., Backman, L., Hakkarainen, J., van der Laan-Luijkx, I. T., Krol, M. C., Spahni, R., Houweling, S., Laine, M., Dlugokencky, E., Gomez-Pelaez, A. J., van der Schoot, M., Langenfelds, R., Ellul, R., Arduini, J., Apadula, F., Gerbig, C., Feist, D. G., Kivi, R., Yoshida, Y., and Peters, W.: Global methane emission estimates for 2000–2012 from CarbonTracker Europe-CH4 v1.0, Geosci. Model Dev., 10, 1261–1289, https://doi.org/10.5194/gmd-10-1261-2017, 2017.
Tuovinen, J.-P., Aurela, M., Hatakka, J., Räsänen, A., Virtanen, T., Mikola, J., Ivakhov, V., Kondratyev, V., and Laurila, T.: Interpreting eddy covariance data from heterogeneous Siberian tundra: land-cover-specific methane fluxes and spatial representativeness, Biogeosciences, 16, 255–274, https://doi.org/10.5194/bg-16-255-2019, 2019.
Uotila, P., Vihma, T., and Haapala, J.: Atmospheric and oceanic conditions and the extremely low Bothnian Bay sea ice extent in 2014/2015, Geophys. Res. Lett., 42, 7740–7749, 2015.
Uotila, P., Goosse, H., Haines, K., Chevallier, M., Barthélemy, A., Bricaud, C., Carton, J., Fučkar, N., Garric, G., Iovino, D., Kauker, F., Korhonen, M., Lien, V. S., Marnela, M., Massonnet, F., Mignac, D., Peterson, K. A., Sadikni, R., Shi, L., Tietsche, S., Toyoda, T., Xie, J., and Zhang, A.: An assessment of ten ocean reanalyses in the polar regions, Clim. Dynam., 52, 1613–1650, https://doi.org/10.1007/s00382-018-4242-z, 2019.
Uttal, T., Starkweather, S., Drummond, J. R., Vihma, T., Makshtas, A. P., Darby, L. S., Burkhart, J. F., Cox, C. J., Schmeisser, L. N., Haiden, T., Maturilli, M., Shupe, M. D., De Boer, G., Saha, A., Grachev, A. A., Crepinsek, S. M., Bruhwiler, L., Goodison, B., McArthur, B., Walden, V. P., Dlugokencky, E. J., P. Persson, O., Lesins, G., Laurila, T., Ogren, J. A., Stone, R., Long, C. N., Sharma, S., Massling, A., Turner, D. D., Stanitski, D. M., Asmi, E., Aurela, M., Skov, H., Eleftheriadis, K., Virkkula, A., Platt, A., Førland, E. J., Iijima, Y., Nielsen, I. E., Bergin, M. H., Candlish, L., Zimov, N. S., Zimov, S. A., O'Neill, N. T., Fogal, P. F., Kivi, R., Konopleva-Akish, E. A., Verlinde, J., Kustov, V. Y., Vasel, B., Ivakhov, V. M., Viisanen Y., and Intrieri, J. M.: International Arctic Systems for Observing the Atmosphere: An International Polar Year Legacy Consortium, B. Am. Meteorol. Soc., 97, 1033–1056, https://doi.org/10.1175/BAMS-D-14-00145.1, 2016.
Vanhatalo, A., Ghirardo, A., Juurola, E., Schnitzler, J.-P., Zimmer, I., Hellén, H., Hakola, H., and Bäck, J.: Long-term dynamics of monoterpene synthase activities, monoterpene storage pools and emissions in boreal Scots pine, Biogeosciences, 15, 5047–5060, https://doi.org/10.5194/bg-15-5047-2018, 2018.
van Vuuren, D. P., Edmonds, J., Kainuma, M., Riahi, K., Thomson, A., Hibbard, K., Hurtt, G. C., Kram, T., Krey, V., Lamarque, J. F., Masui, T., Meinshausen, M., Nakicenovic, N., Smith, S. J., and Rose, S. K.: The representative concentration pathways: an overview, Clim. Chang., 109, 5–31, https://doi.org/10.1007/s10584-011-0148-z, 2011.
Varentsov, M., Konstantinov, P., Baklanov, A., Esau, I., Miles, V., and Davy, R.: Anthropogenic and natural drivers of a strong winter urban heat island in a typical Arctic city, Atmos. Chem. Phys., 18, 17573–17587, https://doi.org/10.5194/acp-18-17573-2018, 2018a.
Varentsov, M., Wouters, H., Platonov, V., and Konstantinov, P.: Megacity-induced mesoclimatic effects in the lower atmosphere: a modeling study for multiple summers over Moscow, Russia, Atmos., 9, 50, https://doi.org/10.3390/atmos9020050, 2018b.
Vasileva, A., Moiseenko, K., Skorokhod, A., Belikov, I., Kopeikin, V., and Lavrova, O.: Emission ratios of trace gases and particles for Siberian forest fires on the basis of mobile ground observations, Atmos. Chem. Phys., 17, 12303–12325, https://doi.org/10.5194/acp-17-12303-2017, 2017.
Verburg, P. and Antenucci, J. P.: Persistent unstable atmospheric boundary layer enhances sensible and latent heat loss in a tropical great lake: Lake Tanganyika, J. Geophys. Res.-Atmos., 115, D11109, https://doi.org/10.1029/2009JD012839, 2010.
Vihma, T., Uotila, P., Sandven, S., Pozdnyakov, D., Makshtas, A., Pelyasov, A., Pirazzini, R., Danielsen, F., Chalov, S., Lappalainen, H. K., Ivanov, V., Frolov, I., Albin, A., Cheng, B., Dobrolyubov, S., Arkhipkin, V., Myslenkov, S., Petäjä, T., and Kulmala, M.: Towards an advanced observation system for the marine Arctic in the framework of the Pan-Eurasian Experiment (PEEX), Atmos. Chem. Phys., 19, 1941–1970, https://doi.org/10.5194/acp-19-1941-2019, 2019.
Vihma, T., Graversen, R., Chen, L., Dörthe H., Skific, N., Francis, J.A., Tyrrell, N., Hall, R., Hanna, E., Uotila, P., Dethloff, K., Karpechko, A. Y., Björnsson, H., and Overland, J. E.: Effects of the tropospheric large-scale circulation on European winter temperatures during the period of amplified Arctic warming, Int. J. Climatol., 40, 509–529, https://doi.org/10.1002/joc.6225, 2020.
Virkkula, A., Chi, X., Ding, A., Shen, Y., Nie, W., Qi, X., Zheng, L., Huang, X., Xie, Y., Wang, J., Petäjä, T., and Kulmala, M.: On the interpretation of the loading correction of the aethalometer, Atmos. Meas. Tech., 8, 4415–4427, https://doi.org/10.5194/amt-8-4415-2015, 2015.
Voigt, C., Lamprecht, R. E., Marushchak, M. E., Lind, S. E., Novakovskiy, A., Aurela, M., Martikainen, P. J., and Biasi, C.: Warming of subarctic tundra increases emissions of all three important greenhouse gases – carbon dioxide, methane, and nitrous oxide, Glob. Change Biol., 23, 3121–3138, https://doi.org/10.1111/gcb.13563, 2016.
Voigt, C., Marushchak, M. E., Lamprecht, R. E., Jackowicz- Korczyński, M., Lindgren, A., Mastepanov, M., Granlund, L., Christensen, T. R., Tahvanainen, T., Martikainen, P. J., and Biasi, C.: Increased nitrous oxide emissions from Arctic peatlands after permafrost thaw, P. Natl. Acad. Sci. USA, 114, 6238–6243, https://doi.org/10.1073/pnas.1702902114, 2017.
Voropay, N. N. and Kichigina, N. V.: Long-term changes in the hydroclimatic characteristics in the Baikal region, IOP Conf. Ser.-Earth Environ. Sci., 107, 012042, https://doi.org/10.1088/1755-1315/107/1/012042, 2018.
Walker, X. J., Baltzer, J. L., Cumming, S. G., Day, N. J., Ebert, C., Goetz, S., Johnstone, J. F., Potter, S., Rogers, B. M., Schuur, E. A. G., Turetsky, M. R., and Mack, M. C., Increasing wildfires threaten historic carbon sink of boreal forest soils, Nature, 572, 520–523, https://doi.org/10.1038/s41586-019-1474-y, 2019.
Walsh, M. G., de Smalen, A. W., and Mor, S. M.: Climatic influence on anthrax suitability in warming northern latitudes, Sci. Rep.-UK, 8, 9269, https://doi.org/10.1038/s41598-018-27604-w, 2018.
Wang, J., Zhao, B., Wang, S., Yang, F., Xing, J., Morawska, L., Ding, A., Kulmala, M., Kerminen, V.-M., Kujansuu, J., Wang, Z., Ding, D., Zhang, X., Wang, H., Tian, M., Petäjä, T., Jiang, J., and Hao, J.: Particulate matter pollution over China and the effects of control policies, Sci. Total Environ., 584–585, 426–447, https://doi.org/10.1016/j.scitotenv.2017.01.027, 2017.
Wang, Z., Huang, X., and Ding, A.: Dome effect of black carbon and its key influencing factors: a one-dimensional modelling study, Atmos. Chem. Phys., 18, 2821–2834, https://doi.org/10.5194/acp-18-2821-2018, 2018.
Wang, Z. B., Wu, Z. J., Yue, D. L., Shang, D. J., Guo, S., Sun, J. Y., Ding, A. J., Wang, L., Jiang, J. K., Guo, H., Gao, J., Cheung, H. C., Morawska, L., Keywood, M., and Hu, M.: New particle formation in China: Current knowledge and further directions, Sci. Total Environ., 577, 258–266, https://doi.org/10.1016/j.scitotenv.2016.10.177, 2017.
Wang, P. C., Elansky, N. F., Timofeev, Y. M., Wang, G. C., Golitsyn, G. S., Makarova, M. V., Rakitin, V. S., Shtabkin, Yu., Skorokhod, A. I., Grechko, E. I., Fokeeva, E. V., Safronov, A. N., Ran, L., and Wang, T.: Long-term trends of carbon monoxide total columnar amount in urban areas and background regions: ground- and satellite-based spectroscopic measurements, Adv. Atmos. Sci., 35, 785–795, https://doi.org/10.1007/s00376-017-6327-8, 2018.
Wang, Y., Wang, Y., Wang, L., Petäjä, T., Zha, Q., Gong, C., Li, S., Pan, Y., Hu, B., Xin, J., and Kulmala, M.: Increased inorganic aerosol fraction contributes to air pollution and haze in China, Atmos. Chem. Phys., 19, 5881–5888, https://doi.org/10.5194/acp-19-5881-2019, 2019.
Wang, Y., Yu, M., Wang, Y., Tang, G., Song, T., Zhou, P., Liu, Z., Hu, B., Ji, D., Wang, L., Zhu, X., Yan, C., Ehn, M., Gao, W., Pan, Y., Xin, J., Sun, Y., Kerminen, V.-M., Kulmala, M., and Petäjä, T.: Rapid formation of intense haze episodes via aerosol–boundary layer feedback in Beijing, Atmos. Chem. Phys., 20, 45–53, https://doi.org/10.5194/acp-20-45-2020, 2020.
Wei, L. X., Deng, X. H., Cheng, B., Vihma, T., Hannula, H. R., Qin, T., and Pulliainen, J.: The impact of meteorological conditions on snow and ice thickness in an Arctic lake, Tellus A, 68, 31590, https://doi.org/10.3402/tellusa.v68.31590, 2016.
Wickström, S., Jonassen, M., Vihma, T., and Uotila, P.: Trends in cyclones in the high latitude North Atlantic during 1979–2016, Q. J. Roy. Meteor. Soc., 146, 762–779, https://doi.org/10.1002/qj.3707, 2020.
Wiedensohler, A., Ma, N., Birmili, W., Heintzenberg, J., Ditas, F., Andreae, M. O., and Panov, A.: Infrequent new particle formation over the remote boreal forest of Siberia, Atmos. Environ., 200, 167–169, https://doi.org/10.1016/j.atmosenv.2018.12.013, 2019.
Wild, B., Andersson, A., Bröder, L., Vonk, J., Hugelius, G., McClelland, J. W., Song, W., Raymond, P. A., and Gustafsson, Ö.: Rivers across the Siberian Arctic unearth the patterns of carbon release from thawing permafrost, P. Natl. Acad. Sci. USA, 116, 10280–10285, 2019.
Williams, P. J. and Smith, M. W.: The Frozen Earth: Fundamentals of Geocryology, Cambridge University Press, Cambridge, UK, ISBN 0-521-36534-1, 1989.
WMO: Guidance on Integrated Urban Hydrometeorological, Climate and Environmental Services, Volume 1, Concept and 115 Methodology, edited by: Grimmond, S., Bouchet, S., Molina, L., Baklanov, A., and Joe, P. (Lead authors), WMO-No. 1234, ISBN 978-92-63-11234-7, available at: https://library.wmo.int/doc_num.php?explnum_id=9903 (last access: 20 January 2022), 2019.
Wolf-Grosse, T., Esau, I., and Reuder, J.: The large-scale circulation during air quality hazards in Bergen, Norway, Tellus A, 69, 1406265, https://doi.org/10.1080/16000870.2017.1406265, 2017a.
Wolf-Grosse, T., Esau, I., and Reuder, J.: Sensitivity of local air quality to the interplay between small- and large-scale circulations: a large-eddy simulation study, Atmos. Chem. Phys., 17, 7261–7276, https://doi.org/10.5194/acp-17-7261-2017, 2017b.
Woolway, R. I., Jones, I. D., Hamilton, D. P., Maberly, S. C., Muraoka, K., Read, J. S., Smyth, R. L., and Winslow, L. A.: Automated calculation of surface energy fluxes with high-frequency lake buoy data, Environ. Modell. Softw., 70, 191–198, https://doi.org/10.1016/j.envsoft.2015.04.013, 2015.
Xausa, F., Paasonen, P., Makkonen, R., Arshinov, M., Ding, A., Denier Van Der Gon, H., Kerminen, V.-M., and Kulmala, M.: Advancing global aerosol simulations with size-segregated anthropogenic particle number emissions, Atmos. Chem. Phys., 18, 10039–10054, https://doi.org/10.5194/acp-18-10039-2018, 2018.
Xiao, S., Wang, M. Y., Yao, L., Kulmala, M., Zhou, B., Yang, X., Chen, J. M., Wang, D. F., Fu, Q. Y., Worsnop, D. R., and Wang, L.: Strong atmospheric new particle formation in winter in urban Shanghai, China, Atmos. Chem. Phys., 15, 1769–1781, https://doi.org/10.5194/acp-15-1769-2015, 2015.
Xie, Y. N., Ding, A. J., Nie, W., Mao, H. T., Qi, X. M., Huang, X., Xu, Z., Kerminen, V. K., Petäjä, T., Chi, X., Virkkula, A., Boy, M., Xue, L., Guo, J., Sun, J., Yang, X., Kulmala, M., and Fu, C. B.: Enhanced sulfate formation by nitrogen dioxide: Implications from in situ observations at the SORPES station, J. Geophys. Res.-Atmos., 120, 12679–12694, https://doi.org/10.1002/2015jd023607, 2015.
Yan, C., Yin, R., Lu, Y., Dada, L., Yang, D., Fu, Y., Kontkanen, J., Deng, C., Garmash, O., Ruan, J., Baalbaki, R., Schervish, M., Cai, R., Bloss, M., Chan, T., Chen, T., Chen, Q., Chen, X., Chen, Y., Chu, B., Dällenbach, K., Foreback, B., He, X., Heikkinen, L., Jokinen, T., Junninen, H., Kangasluoma, J., Kokkonen, T., Kurppa, M., Lehtipalo, K., Li, H., Li, H., Li, X., Liu, Y. Ma, Q., Paasonen, P., Rantala, P., Pileci, R. E., Rusanen, A., Sarnela, N., Simonen, P., Wang, S., Wang, W., Wang, Y. Xue, M., Yang, G., Yao, L., Zhou, Y., Kujansuu, J., Petäjä, T., Nie, W., Ma, N., Ge, M., He, H., Donahue, N. M., Worsnop, D. R., Kerminen, V.-M., Wang, L., Liu, Y., Zheng, J., Kulmala, M., Jiang, J., and Bianchi, F.: The synergistic role of sulfuric acid, bases, and oxidized or oganics governing new-particle formation in Beijing, Geophys. Res. Lett., 48, e2020GL091944, https://doi.org/10.1029/2020GL091944, 2021.
Yang, F., Li, C., Leppäranta, M., Shi, X., Zhao, S., and Zhang, C.: Notable increases in nutrient concentrations in a shallow lake during seasonal ice growth, Water Sci. Technol., 74, 2773–2883, 2016.
Yang, Y., Leppäranta, M. J., Ki, Z., Cheng, B., Zhai, M., and Demchev, D.: Model simulations of the annual cycle of the landfast ice thickness in the East Siberian Sea, Advances in Polar Sciences, 26, 168–178, https://doi.org/10.13679/j.advps.2015.2.00168, 2015.
Yao, L., Garmash, O., Bianchi, F., Zheng, J., Yan, C., Kontkanen, J., Junninen, H., Mazon, S. B., Ehn, M., Paasonen, P., Sipilä, M., Wang, M. Y., Wang, X. K., Xiao, S., Chen, H. F., Lu, Y. Q., Zhang, B. W., Wang, D. F., Fu, Q. Y., Geng, F. H., Li, L., Wang, H. L., Qiao, L. P., Yang, X., Chen, J. M., Kerminen, V.-M., Petäjä, T., Worsnop, D. R., Kulmala, M., and Wang, L.: Atmospheric new particle formation from sulfuric acid and amines in a Chinese megacity, Science, 361, 278–281, 2018.
Yasunaka, S., Siswanto, E., Olsen, A., Hoppema, M., Watanabe, E., Fransson, A., Chierici, M., Murata, A., Lauvset, S. K., Wanninkhof, R., Takahashi, T., Kosugi, N., Omar, A. M., van Heuven, S., and Mathis, J. T.: Arctic Ocean CO2 uptake: an improved multiyear estimate of the air–sea CO2 flux incorporating chlorophyll a concentrations, Biogeosciences, 15, 1643–1661, https://doi.org/10.5194/bg-15-1643-2018, 2018.
Ye, Z., Liu, J., Gu, A., Feng, F., Liu, Y., Bi, C., Xu, J., Li, L., Chen, H., Chen, Y., Dai, L., Zhou, Q., and Ge, X.: Chemical characterization of fine particulate matter in Changzhou, China, and source apportionment with offline aerosol mass spectrometry, Atmos. Chem. Phys., 17, 2573–2592, https://doi.org/10.5194/acp-17-2573-2017, 2017.
Ylivinkka, I., Kaupinmäki, S., Virman, M., Peltola, M., Taipale, D., Petäjä, T., Kerminen, V.-M., Kulmala, M., and Ezhova, E.: Clouds over Hyytiälä, Finland: an algorithm to classify clouds based on solar radiation and cloud base height measurements, Atmos. Meas. Tech., 13, 5595–5619, https://doi.org/10.5194/amt-13-5595-2020, 2020.
Zaidan, M. A., Haapasilta, V., Relan, R., Junninen, H., Aalto, P. P., Kulmala, M., Laurson, L., and Foster, A. S.: Predicting atmospheric particle formation days by Bayesian classification of the time series features, Tellus B, 70, 1–10, https://doi.org/10.1080/16000889.2018.1530031, 2018a.
Zaidan, M. A., Haapasilta, V., Relan, R., Paasonen, P., Kerminen, V.-M., Junninen, H., Kulmala, M., and Foster, A. S.: Exploring non-linear associations between atmospheric new-particle formation and ambient variables: a mutual information approach, Atmos. Chem. Phys., 18, 12699–12714, https://doi.org/10.5194/acp-18-12699-2018, 2018b.
Zanatta, M., Laj, P., Gysel, M., Baltensperger, U., Vratolis, S., Eleftheriadis, K., Kondo, Y., Dubuisson, P., Winiarek, V., Kazadzis, S., Tunved, P., and Jacobi, H.-W.: Effects of mixing state on optical and radiative properties of black carbon in the European Arctic, Atmos. Chem. Phys., 18, 14037–14057, https://doi.org/10.5194/acp-18-14037-2018, 2018.
Zapadinsky, E., Passananti, M., Myllys, N., Kurten, T., and Vehkamäki, H.: Modeling on Fragmentation of Clusters inside a Mass Spectrometer, J. Phys. Chem. A, 123, 611–624, https://doi.org/10.1021/acs.jpca.8b10744, 2019.
Zhang, J., Liu, C., and Chen, H.: The modulation of Tibetan Plateau heating on the multi-scale northernmost margin activity of East Asia summer monsoon in northern China, Global Planet. Change, 161, 149–161, 2018.
Zhang, S., Zhang, J. H., Bai, Y., Koju, U. A., Igbawua, T., Chang, Q., Zhang, D., and Yao, F. M.: Evaluation and improvement of the daily boreal ecosystem productivity simulator in simulating gross primary productivity at 41 flux sites across Europe, Ecol. Model., 368, 205–232, https://doi.org/10.1016/j.ecolmodel.2017.11.023, 2018.
Zhang-Turpeinen, H., Kivimäenpää, M., Aaltonen, H., Berninger, F., Köster, E., Köster, K., Menyailo, O., Prokushkin, A., and Pumpanen, J.: Wildfire effects on BVOC emissions from boreal forest floor on permafrost soil in Siberia, Sci. Total Environ., 134851, https://doi.org/10.1016/j.scitotenv.2019.134851, 2020.
Zhao, H., Li, X., Zhang, Q., Jiang, X., Lin, J., Peters, G. P., Li, M., Geng, G., Zheng, B., Huo, H., Zhang, L., Wang, H., Davis, S. J., and He, K.: Effects of atmospheric transport and trade on air pollution mortality in China, Atmos. Chem. Phys., 17, 10367–10381, https://doi.org/10.5194/acp-17-10367-2017, 2017.
Zhdanova, E. Y., Chubarova, N. Y., and Lyapustin, A. I.: Assessment of urban aerosol pollution over the Moscow megacity by the MAIAC aerosol product, Atmos. Meas. Tech., 13, 877–891, https://doi.org/10.5194/amt-13-877-2020, 2020.
Zhou, Y., Dada, L., Liu, Y., Fu, Y., Kangasluoma, J., Chan, T., Yan, C., Chu, B., Daellenbach, K. R., Bianchi, F., Kokkonen, T. V., Liu, Y., Kujansuu, J., Kerminen, V.-M., Petäjä, T., Wang, L., Jiang, J., and Kulmala, M.: Variation of size-segregated particle number concentrations in wintertime Beijing, Atmos. Chem. Phys., 20, 1201–1216, https://doi.org/10.5194/acp-20-1201-2020, 2020.
Zilitinkevich, S., Druzhinin, O., Glazunov, A., Kadantsev, E., Mortikov, E., Repina, I., and Troitskaya, Y.: Dissipation rate of turbulent kinetic energy in stably stratified sheared flows, Atmos. Chem. Phys., 19, 2489–2496, https://doi.org/10.5194/acp-19-2489-2019, 2019.