the Creative Commons Attribution 4.0 License.
the Creative Commons Attribution 4.0 License.
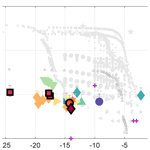
Cultivable halotolerant ice-nucleating bacteria and fungi in coastal precipitation
Charlotte M. Beall
Jennifer M. Michaud
Meredith A. Fish
Julie Dinasquet
Gavin C. Cornwell
M. Dale Stokes
Michael D. Burkart
Thomas C. Hill
Paul J. DeMott
Ice-nucleating particles (INPs) represent a rare subset of aerosol particles that initiate cloud droplet freezing at temperatures above the homogenous freezing point of water (−38 ∘C). Considering that the ocean covers 71 % of the Earth's surface and represents a large potential source of INPs, it is imperative that the identities, properties and relative emissions of ocean INPs become better understood. However, the specific underlying drivers of marine INP emissions remain largely unknown due to limited observations and the challenges associated with isolating rare INPs. By generating isolated nascent sea spray aerosol (SSA) over a range of biological conditions, mesocosm studies have shown that marine microbes can contribute to INPs. Here, we identify 14 (30 %) cultivable halotolerant ice-nucleating microbes and fungi among 47 total isolates recovered from precipitation and aerosol samples collected in coastal air in southern California. Ice-nucleating (IN) isolates collected in coastal air were nucleated ice from extremely warm to moderate freezing temperatures (−2.3 to −18 ∘C). While some Gammaproteobacteria and fungi are known to nucleate ice at temperatures as high as −2 ∘C, Brevibacterium sp. is the first Actinobacteria found to be capable of ice nucleation at a relatively high freezing temperature (−2.3 ∘C). Air mass trajectory analysis demonstrates that marine aerosol sources were dominant during all sampling periods, and phylogenetic analysis indicates that at least 2 of the 14 IN isolates are closely related to marine taxa. Moreover, results from cell-washing experiments demonstrate that most IN isolates maintained freezing activity in the absence of nutrients and cell growth media. This study supports previous studies that implicated microbes as a potential source of marine INPs, and it additionally demonstrates links between precipitation, marine aerosol and IN microbes.
- Article
(2196 KB) - Full-text XML
-
Supplement
(3055 KB) - BibTeX
- EndNote
Ice-nucleating particles (INPs) are rare aerosols, representing ∼1 in 105 or less of total particles in the free troposphere (Rogers et al., 1998) that induce freezing of cloud droplets at temperatures above the homogenous freezing point of water (−38 ∘C) and at relative humidities (RH) well below the homogenous freezing RH of aqueous solution droplets. They affect multiple climate-relevant properties of mixed-phase and cold clouds. For example, in-cloud INP distributions can influence the ice-phase partitioning processes that determine clouds' reflectivity, lifetime and precipitation efficiency (Creamean et al., 2013; DeLeon-Rodriguez et al., 2013; Fröhlich-Nowoisky et al., 2016; Ladino et al., 2016). However, numerical representations of cloud ice processes challenge climate models across all scales (Curry et al., 2000; Furtado and Field, 2017; Kay et al., 2016; Klein et al., 2009; Prenni et al., 2007). It has been hypothesized that an enhanced understanding of marine and terrestrial INP populations could contribute to improved representation of ice processes in models (Seinfeld et al., 2016; Storelvmo, 2017; Kanji et al., 2017).
Despite recent evidence showing that sea spray aerosol (SSA) represents a source of INPs (DeMott et al., 2016; McCluskey et al., 2016, 2018a, b), that these INPs can contribute significantly to total INP populations (particularly in remote marine regions where terrestrial aerosols are less abundant) (Burrows et al., 2013; Vergara-Temprado et al., 2017, 2018), and that specific parameterization of marine INPs can influence modeled radiative budgets (Wilson et al., 2015), little is known about the actual entities involved in forming marine INPs. Schnell and Vali (1975) were the first to associate phytoplankton blooms with increases in ice nucleation activity in seawater sampled shortly after a bloom in Bedford Basin, Nova Scotia. Recent mesocosm studies have linked SSA ice-nucleating (IN) activity specifically to the death phase of phytoplankton blooms. McCluskey et al. (2017) showed that increases in INP emissions corresponded to increased emissions of heterotrophic bacteria and the transfer of organic species in SSA, implicating microbes and biomolecules as contributors to marine INP populations. Marine microbes were further linked to INPs in McCluskey et al. (2018a): subsets of INPs in nascent SSA were found to be heat labile, with sizes greater than 0.2 µm, and INP emissions were found to be correlated with the increased emissions of cells or cellular material. An IN halotolerant strain of Pseudomonas fluorescens was detected in phytoplankton cultures derived from seawater (Fall and Schnell, 1985), and INPs have also been detected in seawater containing marine diatoms, green algae (Alpert et al., 2011; Junge and Swanson, 2008; Ladino et al., 2016; Parker et al., 1985) and sea-ice samples containing marine Antarctic bacteria (Junge and Swanson, 2008; Parker et al., 1985).
While indirect evidence indicates marine microbes and other biogenic entities as potential marine INPs, microbial contribution to marine INP populations has not yet been confirmed through direct observations (i.e., through isolation and identification in an atmospheric sample). Multiple factors make it difficult to determine INP origin, whether terrestrial or marine, including the low abundance of INPs and the diversity of aerosols that can ice nucleate (e.g., Kanji et al., 2017). However, cultivable IN microbes have been isolated from clouds and precipitation for decades (e.g., Sands et al., 1982; Failor et al., 2017; Morris et al., 2008), and the origins of IN isolates can be determined by comparing sequences with reference isolates of known origin. There are several caveats to consider when inferring in-cloud INP concentrations or properties from precipitation samples (Petters and Wright, 2015), including “sweep-out” of additional INPs as the hydrometeor traverses the atmosphere below the cloud (Vali, 1974). However, previous studies have derived estimates of in-cloud INP concentrations and origins from the concentrations and identities of IN microbes from ground-level collections (Christner et al., 2008; Failor et al., 2017a; Joyce et al., 2019; Monteil et al., 2014) by assuming that particles in precipitation originate from the cloud rather than the atmospheric column through which the hydrometeor descended. This assumption is supported by Vali (1971), who found that sub-cloud scavenging of aerosol did not affect INPs observed in precipitation collected at the surface in comparisons of INP spectra from surface samples with samples collected at cloud base. Furthermore, Wright et al. (2014) estimated that sweep-out contributed between 1.2 % and 14 % of INPs suspended in a precipitation sample collected at the surface.
While evidence exists for relationships between IN microbes and precipitation in terrestrial systems, studies on the relationship between marine INPs, marine microbes and precipitation remain quite limited. Here, we report the identities and freezing temperatures of 14 cultivable halotolerant IN species derived from marine and coastal precipitation and aerosol samples. Over the course of 11 precipitation events during an El Niño season, 47 cultivable halotolerant bacteria and fungi were recovered from aerosol and precipitation samples collected in a coastal subtropical climate in southern California. Bacterial and fungal species were isolated, identified and tested for ice nucleation behavior from 0 to −25 ∘C using an immersion-mode droplet freezing assay technique. Precipitating cloud altitudes and isolate source regions were estimated using the High-Resolution Rapid Refresh (HRRR) atmospheric model and the FLEXible PARTicle (FLEXPART) dispersion model (Stohl et al., 1998), respectively. Finally, the effect of media on the observed IN behavior of isolates was investigated through cell-washing experiments.
2.1 Precipitation and aerosol sample collection methods
Precipitation and ambient aerosol samples were collected on the Ellen Browning Scripps Memorial Pier at Scripps Institution of Oceanography (SIO; 32.8662∘ N, 117.2544∘ W) from 6 March to 6 May 2016. Sampling took place in the surf 8 m above Mean Lower Low Water (MLLW), and samples were only collected during westerly winds. Aerosol samples were collected over 1.5–5 h periods on polycarbonate filters (45 mm diameter, 0.2 µm pore size; Whatman® Nuclepore, Chicago, Illinois, USA) placed in open-face Nalgene® analytical filter units (Waltham, Massachusetts, USA). Prior to sampling, filters were pretreated for decontamination by soaking in 10 % H2O2 for 10 min and rinsing them three times with ultrapure water. Background levels of INPs from the sample handling processes were estimated using INP concentrations in aerosol sample field blanks assuming the average sampling volume (2270 L). Estimated INP concentrations across the three field blanks ranged between 0 and 0.1 L−1 at −20 ∘C (see Fig. S1). After collection, aerosol filters were placed in 50 mL sterile plastic Falcon® tubes (Corning Life Sciences, Corning, NY, USA) and immersed in 12 mL of ultrapure water using sterile polypropylene forceps that were pretreated using the 10 % H2O2 process described above. The samples were then hand shaken for 20 min to resuspend particles from the filter. The precipitation samples were collected using a modified Teledyne ISCO full-size portable sampler (Lincoln, Nebraska, USA), fitted with twenty-four 1 L polypropylene bottles. Prior to sampling, the bottles were immersed in 10 % hydrogen peroxide for 10 min and then rinsed three times with ultrapure water. The automated sampler would engage when triggered by precipitation of at least 0.13 cm h−1 and would sample using the first of 24 bottles for 30 min; thereafter, it would switch bottles at hourly intervals. Within 1–2 h of sample collection, INP concentrations were measured using the Scripps Institution of Oceanography Automated Ice Spectrometer (SIO-AIS) (Beall et al., 2017), an automated offline freezing assay technique for measurement of immersion-mode INPs. To decrease the effect of interstitial particle sweep-out by falling raindrops on the measured INP concentration, precipitation from the first 30 min was discarded. Sweep-out effects have been estimated to contribute between 1.2 % and 14 % to measured concentrations of INP in a precipitation sample (Wright et al., 2014).
The INP measurement technique is described in detail in Beall et al. (2017). Briefly, the precipitation samples and aerosol sample suspensions were distributed in twenty-four to thirty 50µL aliquots into a clean 96-well disposable polypropylene sample tray. An equal number and volume of aliquots of ultrapure water accompany each sample in the disposable tray as control for contamination from the loading and/or ultrapure water. The sample trays were then inserted into an aluminum block that is cooled until the samples are frozen. Cumulative INP number concentrations per temperature per volume are calculated using the fraction (f) of unfrozen wells per given temperature interval:
where Vd is the volume of the sample in each well. For aerosol filter samples, cumulative INP number concentrations are calculated using the ratio of the volume used for resuspension of the particles (Vre) to the volume of aerosol sampled (VA):
The fraction of unfrozen wells (f) is adjusted for contamination by subtracting the number of frozen ultrapure water wells per temperature interval from both the total number of unfrozen wells and total wells of the sample. For this study, thirty 50 µL droplets were deposited into the droplet assay, yielding a detection limit of 0.675 INPs per milliliter of liquid.
Within 1–2 h of collection, precipitation and aerosol samples were also inoculated in 5 mL ZoBell growth media (ZoBell, 1947; 5 g peptone, 1 g yeast extract per 1 L of filtered (0.22 µm) autoclaved seawater) and grown under ambient conditions (21–24 ∘C). Seawater was collected at the Ellen Browning Scripps Memorial Pier and was filtered prior to autoclaving. INP concentrations in ZoBell enrichments were measured 1 d post inoculation and for several days thereafter to monitor for sustained IN activity.
2.2 Bacterial and fungal isolation and characterization
Precipitation and SSA microorganisms were cultivated using the ZoBell enrichment described above (ZoBell, 1947) (Fisher Scientific, Houston, Texas, USA). Isolation was performed by successive plating on ZoBell agar (BD Bacto™ Agar, Sparks, MD, USA). Liquid cultures were inoculated from single colonies and grown to the late exponential phase. DNA was extracted from liquid cultures of isolates after an overnight lysis with proteinase K (100 µg mL−1) and lysozyme (5 mg mL−1) (MilliporeSigma, Burlington, Massachusetts, USA) (Boström et al., 2004) using a QIAamp® kit (QIAGEN, Hilden, Germany). The V4 region of the 16S rRNA gene was amplified using 515F (5' GTGYCAGCMGCCGCGGTAA 3') and 926R (5' CCGYCAATTCMTTTRAGT 3') primers (Walters et al., 2015). The PCR reaction contained 0.5 ng µL−1 genomic DNA, 0.2 µM of each primer, and one KAPA HiFi HotStart ReadyMix (KAPA Biosystems, KK2601), and the thermocycler was set to the following program: 95 ∘C for 30 s; 25 cycles of 95 ∘C for 30 s, 55 ∘C for 30 s and 72 ∘C for 30 s; 72 ∘C for 5 min. PCR products were purified using a GenElute™ PCR clean-up kit (MilliporeSigma). The sequences of the amplified 16S rRNA gene fragments were determined by Sanger sequencing (Retrogen Inc., San Diego, CA). Taxonomic assignments were determined from 16S rRNA gene sequences using the SILVA Incremental Aligner (SINA) (Pruesse et al., 2012) and the Basic Local Alignment Search Tool (BLAST) (https://www.ncbi.nlm.nih.gov/, last access: 1 May 2019). SINA aligns sequences to the SILVA database of rRNA genes using a combination of k-mer searching and partial order matching. Additionally, individual sequences were inspected using BLAST, and species identities were determined by >97 % sequence identity to reference rRNA sequences. The primers were specific to bacterial 16S rRNA gene sequences and were additionally able to capture some 18S fungal sequences. Primers specific to 18S rRNA were not used.
To assess for duplicate isolates within the sampling period, 16S rDNA sequences were compared. Sequences within the same genus were adjusted and aligned in DECIPHER (Alignseqs(), AdjustAlignment() with default settings) (Wright, 2015). DECIPHER uses an iterative process for multiple sequence alignment where two sequences are aligned and merged, and each successive sequence is added until all sequences are aligned. These sequence alignments were used to generate phylogenetic trees using ClustalW2 (the UPGMA method) (McWilliam et al., 2013) and visualized with the Interactive Tree Of Life (iTOL; Letunic and Bork, 2011). Alignments were not manually trimmed or adjusted prior to tree construction. Branch distances were used to evaluate sequence similarity. As the sequences resulting from rRNA amplification often covered unequal spans and had unequal lengths, their alignments often resulted in overestimates of tree distances (average mutations per base pair). This error was not seen in original taxonomic assignment where alignment of one sequence to a reference sequence in the database was more successful. To facilitate comparisons between organisms assigned to the same genus, identity assignments including divisions at distances >0.1 (e.g., 1, 2, 3 … ) were further subdivided by distances >0.01 (e.g., 1a, 1b, 1c … ). Nonzero distances <0.01 were given sub-labels (e.g., 1a1, 1a2 … ). Zero distances were given identical labels. In consideration of overestimations of tree distances and the risk of overreporting the numbers of isolates found, we applied more conservative criteria for the removal of potential duplication of the same isolate instead of only considering 100 % identical sequences. Distances <0.01 were determined to be possible duplicates if they were collected during the same sampling period unless the organisms had a different phenotype generally indicated by different pigmentation. Each duplicate was tested for its IN ability, and the results are reported in Table S1. If the duplicates had the same IN properties, only one representative isolate was retained, and the rest were discarded. Maximum likelihood phylogenetic trees were computed in MEGA7 (Tamura et al., 2013) after ClustalW alignment with reference sequences (https://www.ncbi.nlm.nih.gov/, last access: 18 May 2020).
2.3 Storm and aerosol source characterization methods
Cloud altitudes at the time of precipitation sample collection were estimated using the High-Resolution Rapid Refresh (HRRR) model. The altitudes and pressure levels of clouds were assumed to be located where the RH was >95 %–100 % in the model. The specific RH criteria applied to each sampling period are provided in Table S2. HRRR model output was compared with surface RH measurements from the SIO pier weather station during sampling periods, and the predicted RH was found to agree with observations with a root mean square error (RMSE) of <10 %–15 %, which aligns closely with previously reported RH accuracies over the continental USA (Benjamin et al., 2016). Three altitudes of the estimated cloud top, middle and bottom were used as release points for FLEXPART 10 d Lagrangian backward trajectories. Back trajectories were used to identify potential sources of INPs in the precipitation samples as well as to indicate potential sources of land-based contamination in aerosol and precipitation samples due to local wind patterns or land–sea breezes. Satellite composites from the National Weather Service Weather Prediction Center's North American Surface Analysis products were used for synoptic weather analysis to generally characterize meteorology during each rain event (see Table S3).
2.4 Isolate IN activity measurement and controls
To measure the IN activity of each isolate, liquid cultures were grown to the late exponential phase. Growth was monitored by optical density (OD) (590 nm). INP concentrations were measured as described in Sect. 2.1 in liquid cultures and compared to a ZoBell blank as a control. Isolate biomass was estimated from OD measurements using the distribution of OD to biomass conversion factors from Myers et al. (2013). As Myers et al. (2013) found, in a study of 17 diverse organisms, OD to biomass conversion factors ranged between 0.35 and 0.65 g DW OD−1 L−1 (where DW refers to dry weight); we assume that the INP per gram of biomass may be estimated from OD within a factor of 2. Thus, isolate INP concentrations as well as the upper and lower limits of the 95 % confidence intervals were scaled by , where m is the mean, minimum or maximum value of the biomass conversion factor distribution from Myers et al. (2013), respectively (i.e., 0.5, 0.65 and 0.35 g DW OD−1 L−1).
To investigate the effect of growth media on IN isolates, a subset of late exponential cultures were washed three times with filtered (0.22 µm) autoclaved seawater (FASW) by successive centrifugation and resuspension. The washing procedure removes everything that is water soluble as well as whole cells and insoluble molecules pellet upon centrifugation. INP measurements were taken as described within 2–4 h after the washing procedure and compared to sterile seawater controls (see Fig. S2b, c).
As ZoBell growth media contained INPs at moderate to cold freezing temperatures (−13 to −25 ∘C; see Fig. S2a), only isolates exhibiting INPs at significantly higher freezing temperatures (−2.3 to −15 ∘C) or at significantly higher concentrations than their respective ZoBell growth media sample were considered to be IN. The criterion for significance was chosen to be conservative: a data point along an isolate's measured IN spectrum was considered significant if there was no overlap between the 95 % binomial sampling confidence interval of the given data point (Agresti and Coull, 1998) and any ZoBell confidence interval within ±2.2 ∘C, the maximum uncertainty in freezing temperature measurement due to heterogeneity in heat transfer rates across the instrument's droplet assay (Beall et al., 2017). This equates to a significance threshold of p<0.005 (Krzywinski and Altman, 2013). The choice of ±2.2 ∘C is likely conservative given that the average and maximum ΔT observed across the droplet assay when cooling from 0 to −25 ∘C in a study of 11 cooling cycles was 0.38 and 0.98 ∘C, respectively (and following this study, the addition of a second thermistor under the second sample tray decreased the observed ΔT to within thermistor uncertainty, ±0.2 ∘C). The same criterion was applied to isolates washed and suspended in FASW as described above (Fig. S2b, c). Many isolates were diluted with their respective media (ZoBell or FASW) to decrease opacity such that freezing events could successfully be detected by the camera; thus, their respective dilution factors were applied to both the INP concentrations measured in the isolate suspension and the INP concentrations measured in the FASW or ZoBell samples for the significance analysis (see Figs. S2b, c and S3).
3.1 Subtropical coastal storm properties and origins
Aerosol and rain samples were collected from a pier on the coast of La Jolla, California (32∘52′01.4" N, 117∘15′26.5" W) during an El Niño event spanning 11 precipitation sampling periods from 6 March to 7 May 2016 (Table S3). Observations of INPs in precipitation generally fall within the bounds of previously reported INP concentrations from precipitation and cloud water samples (Fig. 1, gray shaded region, adapted from Petters and Wright, 2015). AIS measurement uncertainties are represented with 95 % binomial sampling intervals (Agresti and Coull, 1998). Observed freezing temperatures ranged from −6.5 to −22.0 ∘C, with concentrations up to the limit of testing at 105 INPs per liter of precipitation. Following the assumptions in Petters and Wright (2015) to estimate in-cloud INP concentrations from precipitation samples (i.e., condensed water content of 0.4 g m−3 air), observations of INP concentrations in fresh precipitation samples are additionally compared to studies on field measurements conducted in marine and coastal environments.
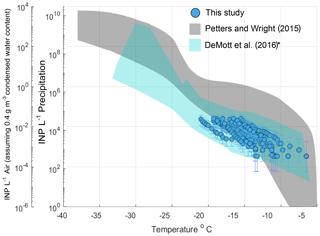
Figure 1INP concentrations per liter precipitation and estimated in-cloud INP concentrations per volume of air in 11 precipitation samples collected at Scripps Institution of Oceanography Ellen Browning Scripps Memorial Pier (32.8662∘ N, 117.2544∘ W; La Jolla, California, USA) between March and May 2016. The gray shaded region indicates the spectrum of INP concentrations reported in nine previous studies on precipitation and cloud water samples collected from various seasons and locations worldwide, adapted from Fig. 1 in Petters and Wright (2015). The blue shaded region represents the composite spectrum of INP concentrations observed in a range of marine and coastal environments including the Caribbean, the eastern Pacific and the Bering Sea as well as laboratory-generated nascent sea spray (DeMott et al., 2016). *DeMott et al. (2016) data have been updated with a complete dataset from the Ice in Clouds – Tropical (ICE-T) study, as shown in Yang et al. (2020).
Figure 1 shows that atmospheric INP concentration estimates compare well with INP concentrations observed in a range of marine and coastal environments, including the Caribbean, the eastern Pacific and the Bering Sea, as well as laboratory-generated nascent sea spray aerosol (DeMott et al., 2016). Observations of INPs in aerosol samples are shown in Fig. S4 and are also comparable with those of DeMott et al. (2016).
The source regions of aerosols present in precipitating clouds were estimated using 10 d FLEXPART back trajectories (Fig. 2). For each of the 11 sampling periods, back trajectories show that the mid- to high-latitude Pacific Ocean was the primary source region for precipitating cloud layers. Periods 5 through 11 were additionally influenced by west coast continental sources (particularly periods 6 and 7). The 10 d back-trajectory simulations for aerosol samples similarly indicated that marine sources dominated (see Fig. S5). Marine aerosols likely originated from regions near the coast (periods 2, 4–11, A1, A2 and A5) or in the mid-Pacific Ocean (periods 1 and 3), where trajectories descended below the marine boundary layer.
Cloud-bottom and cloud-top altitudes were estimated using the High-Resolution Rapid Refresh (HRRR) model, defined by the RH criteria in Table S2. Over the 11 precipitation sampling periods, cloud altitude ranged from 950 to 600 mbar, bottom to top, or from 500 to 4000 m, with temperatures ranging from 265 to 288 K.
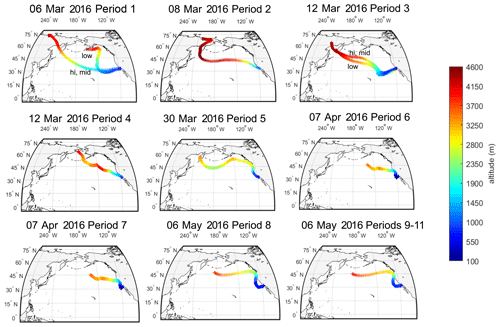
Figure 2The 10 d back trajectories from cloud base, mid-cloud and cloud top during 11 precipitation sampling periods at the SIO pier (32.8662∘ N, 117.2544∘ W). FLEXPART back trajectories were used to estimate potential source regions of INPs to the clouds during precipitation events. Shown are the particle centroids of back trajectories from three release altitudes within each cloud (see Table S2 for details on altitude selection criteria). If trajectories across the three selected release altitudes differed, they are labeled “hi” for cloud top, “mid” for halfway between base and top, and “low” for cloud bottom. Origins of particles in the 10 d simulation are shown to range from 4000 m over Russia to 2500–3500 m over the Sea of Okhostk, the Bering Sea and the North Pacific. FLEXPART results suggest a dominance of marine particle sources to clouds for sampling periods 1–11.
3.2 Bacterial and fungal taxonomy
Cultivable bacteria and fungi were enriched from rain and aerosol samples in marine bacterial growth media, and strains were further isolated on marine agar. This resulted in a total of 47 isolates, 34 from rain samples and 13 from aerosol samples, with 29 unique genera as determined by >97 % sequence identity of 16S rDNA sequences to reference sequences using BLAST (Table S1). The assignments by SINA agreed with the assignments by BLAST, although their sequence identities were lower in some cases (Table S4). Many of the isolates derived from rain and aerosol were highly pigmented, as observed in other studies (Delort et al., 2017; Fahlgren et al., 2010, 2015; Hwang and Cho, 2011; Tong and Lighthart, 1997), presumably aiding their survival under high UV exposure (Fig. S6). This pigmentation was especially prevalent in rain samples.
The taxonomy of the aerosol and rain isolates shows higher diversity in the precipitation samples (Fig. S7 and Table S1), which may be due to artificial biases from low aerosol isolate recovery or sweep-out of interstitial particles during raindrop descent. For example, sample handling may have decreased the isolate recovery rate from aerosol samples as cells were osmotically shocked during resuspension in ultrapure water (see Sect. 2.1). Decreased aerosol bacterial and fungal loads during rain events may have also contributed to lower isolate yield. INP concentration decreases in aerosol during precipitation events support this conclusion. For 3 of the 11 precipitation events featured in this study (see Fig. S1), INP concentrations in aerosol were measured immediately before, during and after precipitation events. In each of the three events, INP concentrations in aerosol decreased below detection levels during precipitation and increased again soon after the end of the precipitation event (in under 24 h), although not beyond the concentrations observed prior to the precipitation event. Interestingly, these features (i.e., the observed decreased INP concentrations during precipitation events and the absence of increased INP concentrations within 24 h of precipitation events) are in opposition to multiple studies on INP concentrations during and after rainfall events in terrestrial systems (Bigg, 1958; Conen et al., 2017; Huffman et al., 2013; Prenni et al., 2013). Additionally, Levin et al. (2019) observed an increase in INP concentrations after precipitation events in a coastal environment, although this increase may have been related to a shift from marine to terrestrial aerosol sources as indicated by the back trajectories. Thus, results in this study indicate that the positive feedbacks between rainfall and surface INP emissions observed in terrestrial systems (Bigg et al., 2015; Morris et al., 2017) may not always apply to marine environments.
The rain samples had a high proportion of Actinobacteria, whereas Firmicutes and Proteobacteria were more dominant in aerosol. As Michaud et al. (2018) showed, Actinobacteria, as well as select Proteobacteria and Firmicutes, have an increased ability to be aerosolized from seawater in SSA emissions. Two isolates (one from rain and one from aerosol, 3.5 % of total isolates) are related to Pantoea sp., strains of which are known to possess IN proteins (e.g., Hill et al., 2014). Pantoea sp. and Psychrobacter sp. were the only bacterial taxa identified previously known to possess ice nucleation activity (Hill et al., 2014; Ponder et al., 2005). However, both Psychrobacter sp. and Idiomarina sp. have been shown to be capable of inhibiting ice recrystallization, possibly through the production of antifreeze proteins (AFPs) which can both inhibit freezing at moderate temperatures and serve as INPs at colder temperatures (Wilson and Walker, 2010).
The phylogenetic relationships between isolates and reference sequences (Fig. 3) show that at least 2 of the 14 IN isolates are closely related to marine taxa, Idiomarina sp. and Psychrobacter sp. 1c2, both of which were derived from coastal aerosol. Additionally, considering the aerosol transport simulation data (Fig. 2), the evidence of marine influence in precipitation INP spectra (Fig. 1) and the use of marine growth media, multiple other IN isolates derived from the precipitation samples are also possibly marine. Furthermore, other IN isolates from precipitation samples cluster closely with marine reference sequences. For example, Pantoea sp. 1a and Brevibacterium sp. show high similarity to reference sequences derived from marine environments (Figs. 3, S8). However, several of the species identified in this study are likely more ubiquitous and closely related to reference isolates found in terrestrial and freshwater systems (Bowers et al., 2009; Fröhlich-Nowoisky et al., 2016; Santl-Temkiv et al., 2015; Vaïtilingom et al., 2012), including two of the IN isolates, Psychrobacter sp. 1b2 and Paenibacillus sp. 1.
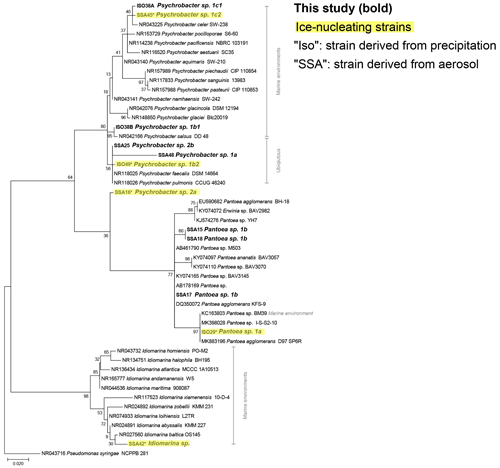
Figure 3Maximum likelihood phylogenetic tree based on 420 nucleotides of the 16S rRNA gene sequences showing the phylogenetic relationships of isolates (in bold) related to Gammaproteobacteria reference sequences. The environmental source of the reference sequences (based on National Center for Biotechnology Information, NCBI, metadata) is indicated in gray. Isolates with ice-nucleating properties are shaded in yellow; bootstrap values (n=500) are indicated at nodes; the scale bar represents changes per position.
3.3 Ice-nucleating properties of rain and SSA isolates
Of the 47 total isolates derived from precipitation and aerosol samples, 14 were found to be significantly ice nucleating according to the selection criterion described in Sect. 2.4. Within the technique's temperature and detection limit of 0.675 INPs per milliliter of liquid between 0 and −25 ∘C, 11 precipitation isolates exhibited freezing temperatures between −2.3 and −24.3 ∘C, and 3 aerosol isolates exhibited freezing temperatures between −14.0 and −24.5 ∘C (Table 1). Prior to this study, Lysinibacillus sp. was the only known gram-positive species found to be capable of ice nucleation (Failor et al., 2017a). However, several IN isolates identified in this study are also gram-positive, including isolates of Brevibacterium sp., Paenibacillus sp., Planococcus sp., Bacillus sp., Arthrobacter sp. and Cellulosimicrobium sp.
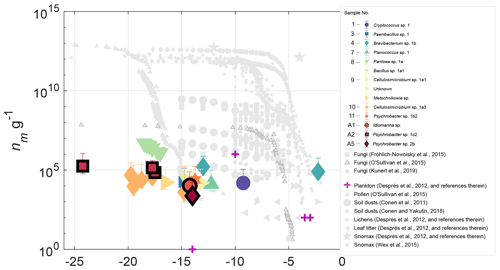
Figure 4INP concentrations (g−1 biomass) for 14 halotolerant isolates derived from precipitation and aerosol samples. Also shown are INP observations of various biological particles from published studies. The sample numbers in the legend indicate the precipitation or aerosol sample from which the isolate was derived (see Table S3). Data points corresponding to isolates from aerosol are outlined in black. Error bars indicate the 95 % confidence intervals and uncertainty associated with the biomass estimate (see Sect. 3.3 for details). Only freezing activity that was significantly enhanced (p<0.005) above ZoBell growth media is shown. Results show that with the exception of Brevibacterium sp., isolates are generally less efficient ice nucleators than most biological INPs of terrestrial origin.
Isolate INP spectra are shown in Fig. 4, normalized to biomass, (nm g−1; see Sect. 2.4 for details on biomass estimates). Also plotted in Fig. 4 are observations of a variety of marine and terrestrial bioaerosols from prior studies, including pollens, fungi, lichens, plankton, leaf litter and soil dusts (Conen et al., 2011; Conen and Yakutin, 2018; Després et al., 2012; Fröhlich-Nowoisky et al., 2015; Kunert et al., 2019; O'Sullivan et al., 2015; Wex et al., 2015). Results show that with the exception of Brevibacterium sp., isolates from this study are generally less efficient than most terrestrial IN biological particles, with lower concentrations and activation temperatures. Concentrations of INPs per milliliter in ZoBell suspension are additionally shown in Fig. S10.
Fungal isolates Cryptococcus sp. and Metschikowia sp. represent two new asomycotic and basidiomycotic IN fungal species, respectively, with INP concentrations 7–8 orders of magnitude lower than the highest reported values for fungal isolates F. armeniacum and F. acuminatum (Kunert et al., 2019). While multiple other IN species of the Ascomycota and Basidiomycota phyla have been previously reported (e.g., Jayaweera and Flanagan, 1982; Kieft et al., 1988; Pouleur et al., 1992), very little is known regarding the distribution and source potential of fungal INPs. Moreover, multiple issues pose challenges to the differentiation of marine vs. terrestrial fungal species (Amend et al., 2019). Many fungi found in the sea are also found in terrestrial environments, and strong correlations with abiotic environmental conditions (Orsi et al., 2013; Tisthammer et al., 2016) and gene expression data (Amend et al., 2012) suggest that some fungi are truly amphibious. Issues with amplicon sequencing pose additional challenges due to the co-amplification of other eukaryotes and large biases toward terrestrial species in internal transcribed spacer (ITS) rDNA primers, which were designed using sequence alignments from largely terrestrial representatives (Amend et al., 2019). However, future studies could take advantage of established marine fungi isolation and cultivation techniques to probe the INP source potential of various cultivable marine fungal species (e.g., Kjer et al., 2010; Overy et al., 2019).
To examine the IN properties of unique strains within samples, multiple sequence alignment of the 16S rDNA sequences was used to identity and remove duplicates. The relationship between 16S rDNA sequences of isolates within their genus is shown in Fig. S11. Ice-nucleating precipitation and aerosol isolates exhibit moderate IN freezing temperatures (<−10 ∘C) (Fig. 4), with the exception of two warm freezing isolates: a fungal isolate from sampling period 1, Cryptococcus sp., which triggered freezing at −9.3 ∘C; and a bacterial isolate from sampling period 4, Brevibacterium sp., which triggered freezing at a relatively high freezing temperature of −2.3 ∘C. The freezing temperatures of all but Brevibacterium sp. 1b overlap with previously reported freezing temperatures of INPs produced in fresh SSA (−7 to −33 ∘C) and, in particular, with the freezing temperatures shown to be likely associated with microbes or cellular material in SSA (−8 to −22 ∘C). (DeMott et al., 2016; McCluskey et al., 2017). Isolate freezing temperatures also overlap with INP freezing temperatures in samples of the Arctic marine sea surface microlayer (Irish et al., 2017; Wilson et al., 2015). However, INP measurements were not performed repeatedly on isolate suspensions, so the extent to which the observed freezing behavior was affected by the isolate's growth phase remains unknown.
Of the known IN bacteria, only Gammaproteobacteria have been shown to nucleate ice at high temperatures (Morris et al., 2004). Brevibacterium sp. was the first Actinobacteria to be shown capable of IN near 2 ∘C. Considering that only IN microbes of continental origins, such as Pseudomonas syringae, have been reported to have freezing temperatures as high as −2 or −3 ∘C (e.g., Fröhlich-Nowoisky et al., 2016, and references therein) and that SSA is associated with 1000 times fewer ice-nucleating active sites per unit surface area compared with mineral dust (McCluskey et al., 2018b), one would not expect to find a marine IN isolate with an extremely warm freezing onset temperature. However, the presence of bacteria closely related to Brevibacterium sp. in marine environments suggests that a marine origin is possible (Fig. S8, see also discussion in Sect. 3.2). Furthermore, the back-trajectory analysis for the sample from which Brevibacterium sp. was isolated indicates that the North Pacific region dominated the sampling period. Actinobacteria are common in marine environments (e.g., Bull et al., 2005) and have been identified in nascent SSA (Michaud et al., 2018). To explore the role of the growth media on the IN properties of isolates, controls were run on nine washed isolates (Fig. S2 and Table S5, see Sect. 2.4). Five of the selected isolates were found to not be significantly IN above sterile ZoBell background, whereas four were chosen from the subset of significantly IN isolates. Interestingly, the observed INP concentrations of washed isolates above that of the FASW were inconsistently related to activity when grown in ZoBell media and were generally enhanced. Seven of the nine media-free isolates exhibited significant IN behavior, including four isolates that were not IN in ZoBell. Some of the observed differences in ice nucleation above background between isolates suspended in ZoBell and those suspended in FASW could be a result of the differences in the background INP concentrations present in the suspension media (i.e., concentrations of INPs in FASW are less than in ZoBell, thereby increasing the temperature range in which IN activity could be detected). Another possibility is that the isolates' IN behavior varied depending on multiple factors, including their viability, environment, stress and nutrient availability. As washing cells removes soluble molecules, the apparent IN activity of washed suspensions could indicate that the source of IN activity is membrane-associated or, alternatively, that expression of IN activity is sensitive to environmental factors. The difference in IN activity between ZoBell and FASW suspensions indicates that in situ measurements of IN bacteria will be necessary to determine the abundance of active IN microbes in the atmosphere.
Table 1Identities of 14 cultivable halotolerant IN bacteria and fungi derived from aerosol and precipitation samples (see Table S2 for precipitation and aerosol sample details).
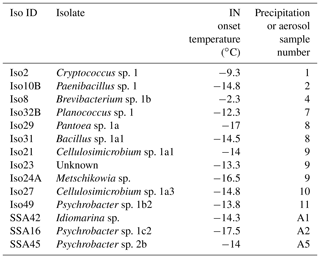
One study of note, Failor et al. (2017), used similar cultivation and INP measurement techniques on precipitation samples and additionally identified multiple halotolerant IN species using marine growth media. However, the IN species identified in Failor et al. (2017) were limited to Gammaproteobacteria, whereas we find greater diversity among the IN isolate taxonomies, including Actinobacteria, Bacilli, Saccharomycetes and Tremellomycetes. Two of the halotolerant IN Gammaproteobacteria identified in Failor et al. (2017) were also found here (see also Fall and Schnell, 1985). Additionally, whereas Failor et al. (2017) reports high freezing temperatures between −4 and −12 ∘C for multiple halotolerant Pseudomonas spp., none of the Pseudomonas spp. isolated in our study exhibited detectable IN activity. IN observations for Pantoea sp. also differ. The Pantoea sp. isolate in our study exhibited a moderate IN freezing temperature of −17 ∘C, but Failor et al. (2017) reported warm freezing activity between −4 and −10 ∘C. In addition to environment-dependent changes in isolate IN activity, the differences between the two studies could also be the result of inherent differences in IN activity between different strains of the same species (Morris et al., 2008).
Finally, the results from Failor et al. (2017) show discrepancies between IN behavior of isolates directly plated from precipitation samples and those from suspensions of purified strains, and we also find that IN behavior can vary between different types of isolate suspensions (i.e., ZoBell vs. FASW). Failor et al. (2017) suggests that changes in an isolate's IN activity may be explained in part by growth conditions not conducive for the expression of ice nucleation activity (INA) and that INA molecules may generally be produced in higher amounts in oligotrophic conditions, such as those found in the atmosphere.
Through isolation and identification of multiple IN microbes in precipitation and aerosol, this study provides the identities of multiple halotolerant IN microbes that are likely of marine origin. Furthermore, we isolated six new IN gram-positive bacteria capable of ice nucleation. Prior to this study, Lysinibacillus sp. was thought to be the only gram-positive species capable of ice nucleation (Failor et al., 2017). Additionally, through cell-washing experiments in which soluble molecules and growth media are eliminated from isolate suspensions, we find that the IN activities of most isolates depend on growth conditions.
Due to the challenge of distinguishing between marine and terrestrial INPs in environmental samples, it is impossible to definitively claim marine or terrestrial origins for the 14 IN isolates measured in this study. In order to survive atmospheric transport and deposition in rainwater, cultivable isolates derived from precipitation must be tolerant of near-freshwater conditions. However, marine origin is highly likely for multiple isolates for the following reasons: aerosol back trajectories and INP observations during sampling events indicate that marine sources were dominant (Figs. 1, 2), multiple isolate sequences show similarity to marine isolation sources in reference sequences (Figs. 3, S8), and isolate freezing temperatures are generally in agreement with previously documented nascent SSA IN freezing temperatures (DeMott et al., 2016; McCluskey et al., 2017, 2018a).
While cultivation methods preclude the quantification of atmospheric abundance and exclude a large fraction of uncultivable microorganisms, we captured several possible contributors to precipitation IN populations and, through isolation, maintained the ability to assess their IN activity and other characteristics. Considering the general rarity of atmospheric INPs (1 in 105 at −20 ∘C) (Rogers et al., 1998), the relatively lower concentrations of INPs in marine air masses (DeMott et al., 2016; McCluskey et al., 2018c) and the rarity of cultivable microbes, it is quite surprising that a substantial fraction of the cultivable microbial isolates from precipitation samples were found to be IN at temperatures above −17 ∘C (11 out of 34 total, or 32 %), and this suggests that there is a significant fraction of IN species in aerosols among the substantially larger uncultivable community.
Finally, as cultivable populations represent a small fraction of the total microbial community, future studies should combine INP measurements with state-of-the-art sequencing approaches to identify relationships between specific microbial communities and INP freezing activity. Furthermore, a combination of advanced fractionation methods to identify the putative ice-nucleating metabolites associated with specific microbial communities and computational networking could illuminate molecular and microbial linkages to ice nucleation and the mechanisms by which the entities work individually or in concert. Further study is also needed to understand the factors, such as atmospheric processing or nutrient limitation, that inhibit or enhance microbe IN behavior, as well as the factors that modulate the emissions of IN bacteria from the ocean surface.
The 16S rRNA gene sequences from this study were deposited to GenBank under the accession numbers MW704027–MW704080. The dataset supporting this paper is hosted by the UC San Diego Library Digital Collections (https://doi.org/10.6075/J0GQ6W2Z) (Beall et al., 2020).
The supplement related to this article is available online at: https://doi.org/10.5194/acp-21-9031-2021-supplement.
CMB and JMM wrote the paper, prepared figures, and conducted the field campaign and laboratory measurements. MAF was responsible for High-Resolution Rapid Refresh analysis and characterized meteorology during sampling periods. JD provided phylogenetic analyses and prepared figures. GCC supported FLEXPART simulations. MDS, MDB, TCH, PJD and KAP provided feedback on the study design, analyses and paper.
The authors declare that they have no conflict of interest.
This work was supported by National Science Foundation (NSF) through the NSF Center for Aerosol Impacts on Chemistry of the Environment (CAICE grant nos. CHE-1801971, AGS-1451347 and IOS-1516156). This work was additionally supported by the U.S. Army Corps of Engineers (grant no. W912HZ-15-2-0019). The authors gratefully acknowledge Kelsey Krug for her assistance with sample archiving; Jesse DeWald, John Beall and Matt Merrill for assistance with sample collection; and the NOAA Earth Systems Research Laboratory (ESRL) for providing the High-Resolution Rapid Refresh model that was used in this study.
This research has been supported by the National Science Foundation (grant nos. CHE-1801971, AGS-1451347 and IOS-1516156) and the U.S. Army Corps of Engineers (grant no. W912HZ-15-2-0019).
This paper was edited by Maria Cristina Facchini and reviewed by two anonymous referees.
Agresti, A. and Coull, B. A.: Approximate Is Better than “Exact” for Interval Estimation of Binomial Proportions, Am. Stat., 52, 119–126, https://doi.org/10.2307/2685469, 1998.
Alpert, P. A., Aller, J. Y., and Knopf, D. A.: Initiation of the ice phase by marine biogenic surfaces in supersaturated gas and supercooled aqueous phases, Phys. Chem. Chem. Phys., 13, 19882, https://doi.org/10.1039/c1cp21844a, 2011.
Amend, A. S., Barshis, D. J., and Oliver, T. A.: Coral-associated marine fungi form novel lineages and heterogeneous assemblages, ISME J., 6, 1291–1301, https://doi.org/10.1038/ismej.2011.193, 2012.
Amend, A., Burgaud, G., Cunliffe, M., Edgcomb, V. P., Ettinger, C. L., Gutiérrez, M. H., Heitman, J., Hom, E. F. Y., Ianiri, G., Jones, A. C., Kagami, M., Picard, K. T., Quandt, C. A., Raghukumar, S., Riquelme, M., Stajich, J., Vargas-Muñiz, J., Walker, A. K., Yarden, O., and Gladfelter, A. S.: Fungi in the Marine Environment: Open Questions and Unsolved Problems, edited by: Garsin, D. A., MBio, 10, e01189-18, https://doi.org/10.1128/mBio.01189-18, 2019.
Beall, C. M., Stokes, M. D., Hill, T. C., DeMott, P. J., DeWald, J. T., and Prather, K. A.: Automation and heat transfer characterization of immersion mode spectroscopy for analysis of ice nucleating particles, Atmos. Meas. Tech., 10, 2613–2626, https://doi.org/10.5194/amt-10-2613-2017, 2017.
Beall, C. M., Michaud, J. M., Fish, M. A., Dinasquet, J., Cornwell, G. C., Stokes, M., D., Burkart, M. D., Hill, T. C., DeMott, P. J., and Prather, K. A: Data from: Cultivable, halotolerant ice nucleating bacteria and fungi in coastal precipitation, in: Center for Aerosol Impacts on Chemistry of the Environment (CAICE), UC San Diego Library Digital Collections, https://doi.org/10.6075/J0GQ6W2Z, 2020.
Benjamin, S. G., Weygandt, S. S., Brown, J. M., Hu, M., Alexander, C. R., Smirnova, T. G., Olson, J. B., James, E. P., Dowell, D. C., Grell, G. A., Lin, H., Peckham, S. E., Smith, T. L., Moninger, W. R., Kenyon, J. S., and Manikin, G. S.: A North American Hourly Assimilation and Model Forecast Cycle: The Rapid Refresh, Mon. Weather Rev., 144, 1669–1694, https://doi.org/10.1175/MWR-D-15-0242.1, 2016.
Bigg, E. K.: A long period fluctuation in freezing nucleus concentrations, J. Meteorol., 15, 561–562, https://doi.org/10.1175/1520-0469(1958)015<0561:alpfif>2.0.co;2, 1958
Bigg, E. K., Soubeyrand, S., and Morris, C. E.: Persistent after-effects of heavy rain on concentrations of ice nuclei and rainfall suggest a biological cause, Atmos. Chem. Phys., 15, 2313–2326, https://doi.org/10.5194/acp-15-2313-2015, 2015.
Boström, K. H., Simu, K., Hagström, Å., and Riemann, L.: Optimization of DNA extraction for quantitative marine bacterioplankton community analysis, Limnol. Oceanogr. Methods, 2, 365–373, 2004.
Bowers, R. M., Lauber, C. L., Wiedinmyer, C., Hamady, M., Hallar, A. G., Fall, R., Knight, R., and Fierer, N.: Characterization of airborne microbial communities at a high-elevation site and their potential to act as atmospheric ice nuclei, Appl. Environ. Microbiol., 75, 5121–5130, https://doi.org/10.1128/AEM.00447-09, 2009.
Bull, A. T., Stach, J., Ward, A. C., et al. Marine actinobacteria: perspectives, challenges, future directions, Antonie Van Leeuwenhoek, 87, 65–79, https://doi.org/10.1007/s10482-004-6562-8, 2005.
Burrows, S. M., Hoose, C., Pöschl, U., and Lawrence, M. G.: Ice nuclei in marine air: biogenic particles or dust?, Atmos. Chem. Phys., 13, 245–267, https://doi.org/10.5194/acp-13-245-2013, 2013.
Carro-Calvo, L., Hoose, C., Stengel, M., and Salcedo-Sanz, S.: Cloud glaciation temperature estimation from passive remote sensing data with evolutionary computing, J. Geophys. Res., 121, 13591–13608, https://doi.org/10.1002/2016JD025552, 2016.
Christner, B. C., Cai, R., Morris, C. E., McCarter, K. S., Foreman, C. M., Skidmore, M. L., Montross, S. N., and Sands, D. C.: Geographic, seasonal, and precipitation chemistry influence on the abundance and activity of biological ice nucleators in rain and snow., P. Natl. Acad. Sci. USA, 105, 18854–18859, https://doi.org/10.1073/pnas.0809816105, 2008.
Conen, F. and Yakutin, M. V.: Soils rich in biological ice-nucleating particles abound in ice-nucleating macromolecules likely produced by fungi, Biogeosciences, 15, 4381–4385, https://doi.org/10.5194/bg-15-4381-2018, 2018.
Conen, F., Morris, C. E., Leifeld, J., Yakutin, M. V., and Alewell, C.: Biological residues define the ice nucleation properties of soil dust, Atmos. Chem. Phys., 11, 9643–9648, https://doi.org/10.5194/acp-11-9643-2011, 2011.
Conen, F., Eckhardt, S., Gundersen, H., Stohl, A., and Yttri, K. E.: Rainfall drives atmospheric ice-nucleating particles in the coastal climate of southern Norway, Atmos. Chem. Phys., 17, 11065–11073, https://doi.org/10.5194/acp-17-11065-2017, 2017.
Creamean, J. M., Suski, K. J., Rosenfeld, D., Cazorla, A., DeMott, P. J., Sullivan, R. C., White, A. B., Ralph, F. M., Minnis, P., Comstock, J. M., Tomlinson, J. M., and Prather, K. A.: Dust and biological aerosols from the Sahara and Asia influence precipitation in the Western U.S, Science, 340, 1572–1578, https://doi.org/10.1126/science.1227279, 2013.
Curry, J. A., Hobbs, P. V., King, M. D., Randall, D. A., Minnis, P., Isaac, G. A., Pinto, J. O., Uttal, T., Bucholtz, A., Cripe, D. G., Gerber, H., Fairall, C. W., Garrett, T. J., Hudson, J., Intrieri, J. M., Jakob, C., Jensen, T., Lawson, P., Marcotte, D., Nguyen, L., Pilewskie, P., Rangno, A., Rogers, D. C., Strawbridge, K. B., Valero, F. P. J., Williams, A. G., and Wylie, D.: FIRE arctic clouds experiment, B. Am. Meteorol. Soc., 81, 5–29, https://doi.org/10.1175/1520-0477(2000)081<0005:FACE>2.3.CO;2, 2000.
DeLeon-Rodriguez, N., Lathem, T. L., Rodriguez-R, L. M., Barazesh, J. M., Anderson, B. E., Beyersdorf, A. J., Ziemba, L. D., Bergin, M., Nenes, A., and Konstantinidis, K. T.: Microbiome of the upper troposphere: species composition and prevalence, effects of tropical storms, and atmospheric implications, P. Natl. Acad. Sci. USA, 110, 2575–80, https://doi.org/10.1073/pnas.1212089110, 2013.
Delort, A. M., Vaïtilingom, M., Joly, M., Amato, P., Wirgot, N., Lallement, A., Sancelme, M., Matulova, M., and Deguillaume, L.: Clouds: A Transient and Stressing Habitat for Microorganisms BT - Microbial Ecology of Extreme Environments, edited by: Chénard, C. and Lauro, F. M., 215–245, Springer International Publishing, Cham, 2017.
DeMott, P. J., Hill, T. C. J., McCluskey, C. S., Prather, K. A., Collins, D. B., Sullivan, R. C., Ruppel, M. J., Mason, R. H., Irish, V. E., Lee, T., Hwang, C. Y., Rhee, T. S., Snider, J. R., McMeeking, G. R., Dhaniyala, S., Lewis, E. R., Wentzell, J. J. B., Abbatt, J., Lee, C., Sultana, C. M., Ault, A. P., Axson, J. L., Diaz Martinez, M., Venero, I., Santos-Figueroa, G., Stokes, M. D., Deane, G. B., Mayol-Bracero, O. L., Grassian, V. H., Bertram, T. H., Bertram, A. K., Moffett, B. F., and Franc, G. D.: Sea spray aerosol as a unique source of ice nucleating particles, P. Natl. Acad. Sci., 113, 5797–5803, https://doi.org/10.1073/pnas.1514034112, 2016.
Després, V., Huffman, J. A., Burrows, S. M., Hoose, C., Safatov, A., Buryak, G., Fröhlich-Nowoisky, J., Elbert, W., Andreae, M., Pöschl, U., and Jaenicke, R.: Primary biological aerosol particles in the atmosphere: a review, Tellus B Chem. Phys. Meteorol., 64, 15598, https://doi.org/10.3402/tellusb.v64i0.15598, 2012.
Fahlgren, C., Hagström, Å., Nilsson, D., and Zweifel, U. L.: Annual Variations in the Diversity, Viability, and Origin of Airborne Bacteria, Appl. Environ. Microbiol., 76, 3015–3025, https://doi.org/10.1128/AEM.02092-09, 2010.
Fahlgren, C., Gómez-Consarnau, L., Zábori, J., Lindh, M. V, Krejci, R., Mårtensson, E. M., Nilsson, D., and Pinhassi, J.: Seawater mesocosm experiments in the Arctic uncover differential transfer of marine bacteria to aerosols, Environ. Microbiol. Rep., 7, 460–470, https://doi.org/10.1111/1758-2229.12273, 2015.
Failor, K. C., Schmale, D. G., Vinatzer, B. A., and Monteil, C. L.: Ice nucleation active bacteria in precipitation are genetically diverse and nucleate ice by employing different mechanisms, ISME J., 11, 2740–2753, https://doi.org/10.1038/ismej.2017.124, 2017.
Fall, R. and Schnell, R. C.: Association of an ice-nucleating pseudomonad with cultures of the marine dinoflagellate, Heterocapsa niei, J. Mar. Res., 43, 257–265, https://doi.org/10.1357/002224085788437370, 1985.
Fröhlich-Nowoisky, J., Hill, T. C. J., Pummer, B. G., Yordanova, P., Franc, G. D., and Pöschl, U.: Ice nucleation activity in the widespread soil fungus Mortierella alpina, Biogeosciences, 12, 1057–1071, https://doi.org/10.5194/bg-12-1057-2015, 2015.
Fröhlich-Nowoisky, J., Kampf, C. J., Weber, B., Huffman, J. A., Pöhlker, C., Andreae, M. O., Lang-Yona, N., Burrows, S. M., Gunthe, S. S., Elbert, W., Su, H., Hoor, P., Thines, E., Hoffmann, T., Després, V. R., and Pöschl, U.: Bioaerosols in the Earth system: Climate, health, and ecosystem interactions, Atmos. Res., 182, 346–376, https://doi.org/10.1016/j.atmosres.2016.07.018, 2016.
Furtado, K. and Field, P.: The Role of Ice Microphysics Parametrizations in Determining the Prevalence of Supercooled Liquid Water in High-Resolution Simulations of a Southern Ocean Midlatitude Cyclone, J. Atmos. Sci., 74, 2001–2021, https://doi.org/10.1175/JAS-D-16-0165.1, 2017.
Hill, T. C. J., Moffett, B. F., DeMott, P. J., Georgakopoulos, D. G., Stump, W. L., and Franc, G. D.: Measurement of ice nucleation-active bacteria on plants and in precipitation by quantitative PCR, Appl. Environ. Microbiol., 80, 1256–1267, https://doi.org/10.1128/AEM.02967-13, 2014.
Huffman, J. A., Prenni, A. J., DeMott, P. J., Pöhlker, C., Mason, R. H., Robinson, N. H., Fröhlich-Nowoisky, J., Tobo, Y., Després, V. R., Garcia, E., Gochis, D. J., Harris, E., Müller-Germann, I., Ruzene, C., Schmer, B., Sinha, B., Day, D. A., Andreae, M. O., Jimenez, J. L., Gallagher, M., Kreidenweis, S. M., Bertram, A. K., and Pöschl, U.: High concentrations of biological aerosol particles and ice nuclei during and after rain, Atmos. Chem. Phys., 13, 6151–6164, https://doi.org/10.5194/acp-13-6151-2013, 2013.
Hwang, C. Y. and Cho, B. C.: Prokaryotic abundance and 16S rRNA gene sequences detected in marine aerosols on the East Sea (Korea), FEMS Microbiol. Ecol., 76, 327–341, https://doi.org/10.1111/j.1574-6941.2011.01053.x, 2011.
Irish, V. E., Elizondo, P., Chen, J., Chou, C., Charette, J., Lizotte, M., Ladino, L. A., Wilson, T. W., Gosselin, M., Murray, B. J., Polishchuk, E., Abbatt, J. P. D., Miller, L. A., and Bertram, A. K.: Ice-nucleating particles in Canadian Arctic sea-surface microlayer and bulk seawater, Atmos. Chem. Phys., 17, 10583–10595, https://doi.org/10.5194/acp-17-10583-2017, 2017.
Jayaweera, K. and Flanagan, P.: Investigations on biogenic ice nuclei in the Arctic atmosphere, Geophys. Res. Lett., 9, 94–97, https://doi.org/10.1029/GL009i001p00094, 1982.
Joyce, R. E., Lavender, H., Farrar, J., Werth, J. T., Weber, C. F., D'Andrilli, J., Vaitilingom, M., and Christner, B. C.: Biological Ice-Nucleating Particles Deposited Year-Round in Subtropical Precipitation, edited by: Stams, A. J. M., Appl. Environ. Microbiol., 85, e01567-19, https://doi.org/10.1128/AEM.01567-19, 2019.
Junge, K. and Swanson, B. D.: High-resolution ice nucleation spectra of sea-ice bacteria: implications for cloud formation and life in frozen environments, Biogeosciences, 5, 865–873, https://doi.org/10.5194/bg-5-865-2008, 2008.
Kanji, Z. A., Ladino, L. A., Wex, H., Boose, Y., Burkert-Kohn, M., Cziczo, D. J., and Krämer, M.: Overview of Ice Nucleating Particles, Meteorol. Monogr., 58, 1.1–1.33, https://doi.org/10.1175/AMSMONOGRAPHS-D-16-0006.1, 2017.
Kay, J. E., Wall, C., Yettella, V., Medeiros, B., Hannay, C., Caldwell, P., and Bitz, C.: Global climate impacts of fixing the Southern Ocean shortwave radiation bias in the Community Earth System Model (CESM), J. Clim., 29, 4617–4636, https://doi.org/10.1175/JCLI-D-15-0358.1, 2016.
Kieft, T. L.: Ice Nucleation Activity in Lichens, Appl. Environ. Microbiol., 54, 1678–1681, 1988.
Kjer, J., Debbab, A., Aly, A. H., and Proksch, P.: Methods for isolation of marine-derived endophytic fungi and their bioactive secondary products, Nat. Protoc., 5, 479–490, https://doi.org/10.1038/nprot.2009.233, 2010.
Klein, S. A., McCoy, R. B., Morrison, H., Ackerman, A. S., Avramov, A., Boer, G. de, Chen, M., Cole, J. N. S., Del Genio, A. D., Falk, M., Foster, M. J., Fridlind, A., Golaz, J.-C., Hashino, T., Harrington, J. Y., Hoose, C., Khairoutdinov, M. F., Larson, V. E., Liu, X., Luo, Y., McFarquhar, G. M., Menon, S., Neggers, R. A. J., Park, S., Poellot, M. R., Schmidt, J. M., Sednev, I., Shipway, B. J., Shupe, M. D., Spangenberg, D. A., Sud, Y. C., Turner, D. D., Veron, D. E., Salzen, K. von, Walker, G. K., Wang, Z., Wolf, A. B., Xie, S., Xu, K.-M., Yang, F., and Zhang, G.: Intercomparison of model simulations of mixed-phase clouds observed during the ARM Mixed-Phase Arctic Cloud Experiment. I: single-layer cloud, Q. J. Roy. Meteorol. Soc., 135, 979–1002, https://doi.org/10.1002/qj.416, 2009.
Krzywinski, M. and Altman, N.: Error bars, Nat. Methods, 10, 921–922, https://doi.org/10.1038/nmeth.2659, 2013.
Kunert, A. T., Pöhlker, M. L., Tang, K., Krevert, C. S., Wieder, C., Speth, K. R., Hanson, L. E., Morris, C. E., Schmale III, D. G., Pöschl, U., and Fröhlich-Nowoisky, J.: Macromolecular fungal ice nuclei in Fusarium: effects of physical and chemical processing, Biogeosciences, 16, 4647–4659, https://doi.org/10.5194/bg-16-4647-2019, 2019.
Ladino, L. A., Yakobi-Hancock, J. D., Kilthau, W. P., Mason, R. H., Si, M., Li, J., Miller, L. A., Schiller, C. L., Huffman, J. A., Aller, J. Y., Knopf, D. A., Bertram, A. K., and Abbatt, J. P. D.: Addressing the ice nucleating abilities of marine aerosol: A combination of deposition mode laboratory and field measurements, Atmos. Environ., 132, 1–10, https://doi.org/10.1016/j.atmosenv.2016.02.028, 2016.
Letunic, I. and Bork, P.: Interactive Tree of Life v2: Online annotation and display of phylogenetic trees made easy, Nucleic Acids Res., 39, 475–478, https://doi.org/10.1093/nar/gkr201, 2011.
Levin, E. J. T., DeMott, P. J., Suski, K. J., Boose, Y., Hill, T. C. J., McCluskey, C. S., Schill, G. P., Rocci, K., Al-Mashat, H., Kristensen, L. J., Cornwell, G., Prather, K., Tomlinson, J., Mei, F., Hubbe, J., Pekour, M., Sullivan, R., Leung, L. R., and Kreidenweis, S. M.: Characteristics of Ice Nucleating Particles in and Around California Winter Storms, J. Geophys. Res.-Atmos., 124, 11530–11551, https://doi.org/10.1029/2019JD030831, 2019.
McCluskey, C. S., Hill, T. C. J., Malfatti, F., Sultana, C. M., Lee, C., Santander, M. V., Beall, C. M., Moore, K. A., Cornwell, G. C., Collins, D. B., Prather, K. A., Jayarathne, T., Stone, E. A., Azam, F., Kreidenweis, S. M., and DeMott, P. J.: A dynamic link between ice nucleating particles released in nascent sea spray aerosol and oceanic biological activity during two mesocosm experiments, J. Atmos. Sci., 74, 151–166, https://doi.org/10.1175/JAS-D-16-0087.1, 2017.
McCluskey, C. S., Hill, T. C. J., Sultana, C. M., Laskina, O., Trueblood, J., Santander, M. V, Beall, C. M., Michaud, J. M., Kreidenweis, S. M., Prather, K. A., Grassian, V., and DeMott, P. J.: A Mesocosm Double Feature: Insights into the Chemical Makeup of Marine Ice Nucleating Particles, J. Atmos. Sci., 75, 2405–2423, https://doi.org/10.1175/JAS-D-17-0155.1, 2018a.
McCluskey, C. S., Ovadnevaite, J., Rinaldi, M., Atkinson, J., Belosi, F., Ceburnis, D., Marullo, S., Hill, T. C. J., Lohmann, U., Kanji, Z. A., O'Dowd, C., Kreidenweis, S. M., and DeMott, P. J.: Marine and Terrestrial Organic Ice-Nucleating Particles in Pristine Marine to Continentally Influenced Northeast Atlantic Air Masses, J. Geophys. Res.-Atmos., 123, 6196–6212, https://doi.org/10.1029/2017JD028033, 2018b.
McCluskey, C. S., Hill, T. C. J., Humphries, R. S., Rauker, A. M., Moreau, S., Strutton, P. G., Chambers, S. D., Williams, A. G., McRobert, I., Ward, J., Keywood, M. D., Harnwell, J., Ponsonby, W., Loh, Z. M., Krummel, P. B., Protat, A., Kreidenweis, S. M., and DeMott, P. J.: Observations of Ice Nucleating Particles Over Southern Ocean Waters, Geophys. Res. Lett., 45, 11911–989997, https://doi.org/10.1029/2018GL079981, 2018c.
McWilliam, H., Li, W., Uludag, M., Squizzato, S., Park, Y. M., Buso, N., Cowley, A. P., and Lopez, R.: Analysis Tool Web Services from the EMBL-EBI., Nucleic Acids Res., 41, 597–600, https://doi.org/10.1093/nar/gkt376, 2013.
Michaud, J. M., Thompson, L. R., Kaul, D., Espinoza, J. L., Richter, R. A., Xu, Z. Z., Lee, C., Pham, K. M., Beall, C. M., Malfatti, F., Azam, F., Knight, R., Burkart, M. D., Dupont, C. L., and Prather, K. A.: Taxon-specific aerosolization of bacteria and viruses in an experimental ocean-atmosphere mesocosm, Nat. Commun., 9, https://doi.org/10.1038/s41467-018-04409-z, 2018.
Monteil, C. L., Bardin, M., and Morris, C. E.: Features of air masses associated with the deposition of Pseudomonas syringae and Botrytis cinerea by rain and snowfall, 8, 2290–2304, https://doi.org/10.1038/ismej.2014.55, 2014.
Morris, C. E., Georgakopoulos, D. G., and Sands, D. C.: Ice nucleation active bacteria and their potential role in precipitation, J. Phys., IV France, 121, 87–103, https://doi.org/10.1051/jp4:2004121004, 2004.
Morris, C. E., Sands, D. C., Vinatzer, B. A., Glaux, C., Guilbaud, C., Buffière, A., Yan, S., Dominguez, H., and Thompson, B. M.: The life history of the plant pathogen Pseudomonas syringae is linked to the water cycle, ISME J., 2, 321–334, https://doi.org/10.1038/ismej.2007.113, 2008.
Morris, C. E., Soubeyrand, S., Bigg, E. K., Creamean, J. M., and Sands, D. C.: Mapping rainfall feedback to reveal the potential sensitivity of precipitation to biological aerosols, B. Am. Meteorol. Soc., 98, 1109–1118, https://doi.org/10.1175/BAMS-D-15-00293.1, 2017.
Myers, J. A., Curtis, B. S., and Curtis, W. R.: Improving accuracy of cell and chromophore concentration measurements using optical density, BMC Biophys., 6, 4, https://doi.org/10.1186/2046-1682-6-4, 2013.
Nemecek-Marshall, M., LaDuca, R., and Fall, R.: High-level expression of ice nuclei in a Pseudomonas syringae strain is induced by nutrient limitation and low temperature, J. Bacteriol., 175, 4062–4070, 1993.
Orsi, W., Biddle, J. F., and Edgcomb, V.: Deep Sequencing of Subseafloor Eukaryotic rRNA Reveals Active Fungi across Marine Subsurface Provinces, PLoS One, 8, e56335, https://doi.org/10.1371/journal.pone.0056335, 2013.
O'Sullivan, D., Murray, B. J., Ross, J. F., Whale, T. F., Price, H. C., Atkinson, J. D., Umo, N. S., and Webb, M. E.: The relevance of nanoscale biological fragments for ice nucleation in clouds, Sci. Rep., 5, 8082, https://doi.org/10.1038/srep08082, 2015.
Overy, D. P., Rämä, T., Oosterhuis, R., Walker, A. K., and Pang, K.-L.: The Neglected Marine Fungi, Sensu stricto, and Their Isolation for Natural Products' Discovery, Mar. Drugs, 17, 42, https://doi.org/10.3390/md17010042, 2019.
Parker, L., Sullivan, C., Forest, T., and Ackley, S.: Ice nucleation activity of antarctic marine microorganisms, Antarct. J., 20, 126–127, 1985.
Petters, M. D. and Wright, T. P.: Revisiting ice nucleation from precipitation samples, Geophys. Res. Lett., 42, 8758–8766, https://doi.org/10.1002/2015GL065733, 2015.
Ponder, M. A., Gilmour, S. J., Bergholz, P. W., Mindock, C. A., Hollingsworth, R., Thomashow, M. F., and Tiedje, J. M.: Characterization of potential stress responses in ancient Siberian permafrost psychroactive bacteria, FEMS Microbiol. Ecol., 53, 103–115, https://doi.org/10.1016/j.femsec.2004.12.003, 2005.
Pouleur, S., Richard, C., Martin, J., and Antoun, H.: Ice nucleation activity in Fusarium acuminatum and Fusarium avenaceum, Appl. Environ. Microbiol., 58, 2960–2964, 1992.
Prenni, A. J., Harrington, J. Y., Tjernström, M., DeMott, P. J., Avramov, A., Long, C. N., Kreidenweis, S. M., Olsson, P. Q., and Verlinde, J.: Can Ice-Nucleating Aerosols Affect Arctic Seasonal Climate?, B. Am. Meteorol. Soc., 88, 541–550, https://doi.org/10.1175/BAMS-88-4-541, 2007.
Prenni, A. J., Tobo, Y., Garcia, E., DeMott, P. J., Huffman, J. A., McCluskey, C. S., Kreidenweis, S. M., Prenni, J. E., Pöhlker, C., and Pöschl, U.: The impact of rain on ice nuclei populations at a forested site in Colorado, Geophys. Res. Lett., 40, 227–231, https://doi.org/10.1029/2012GL053953, 2013.
Pruesse, E., Peplies, J., and Glöckner, F. O.: SINA: Accurate high-throughput multiple sequence alignment of ribosomal RNA genes, Bioinformatics, 28, 1823–1829, https://doi.org/10.1093/bioinformatics/bts252, 2012.
Rogers, D. C., DeMott, P. J., Kreidenweis, S. M., and Chen, Y.: Measurements of ice nucleating aerosols during SUCCESS, Geophys. Res. Lett., 25, 1383, https://doi.org/10.1029/97GL03478, 1998.
Sands, D., Langhans, V. E., Scharen, A. L., and Smet, G.: The association between bacteria and rain and possible resultant meteorological implications, J. Hungarian Meteorol. Serv., 86, 148–152, 1982.
Santl-Temkiv, T., Sahyoun, M., Finster, K., Hartmann, S., Augustin-Bauditz, S., Stratmann, F., Wex, H., Clauss, T., Nielsen, N. W., Sorensen, J. H., Korsholm, U. S., Wick, L. Y., and Karlson, U. G.: Characterization of airborne ice-nucleation-active bacteria and bacterial fragments, Atmos. Environ., 109, 105–117, https://doi.org/10.1016/j.atmosenv.2015.02.060, 2015.
Schnell, R. C. and Vali, G.: Freezing nuclei in marine waters, Tellus, 27, 321–323, https://doi.org/10.1111/j.2153-3490.1975.tb01682.x, 1975.
Seinfeld, J. H., Bretherton, C., Carslaw, K. S., Coe, H., DeMott, P. J., Dunlea, E. J., Feingold, G., Ghan, S., Guenther, A. B., Kahn, R., Kraucunas, I., Kreidenweis, S. M., Molina, M. J., Nenes, A., Penner, J. E., Prather, K. A., Ramanathan, V., Ramaswamy, V., Rasch, P. J., Ravishankara, A. R., Rosenfeld, D., Stephens, G., and Wood, R.: Improving our fundamental understanding of the role of aerosol−cloud interactions in the climate system, P. Natl. Acad. Sci. USA, 113, 5781–5790, https://doi.org/10.1073/pnas.1514043113, 2016.
Stohl, A., Hittenberger, M., and Wotawa, G.: Validation of the Lagrangian particle dispersion model FLEXPART against large scale tracer experiment data, Atmos. Environ., 32, 4245–4264, 1998.
Storelvmo, T.: Aerosol Effects on Climate via Mixed-Phase and Ice Clouds, Annu. Rev. Earth Planet. Sci., 45, 199–222, https://doi.org/10.1146/annurev-earth-060115-012240, 2017.
Tamura, K., Stecher, G., Peterson, D., Filipski, A., and Kumar, S.: MEGA6: Molecular Evolutionary Genetics Analysis version 6.0, Mol. Biol. Evol., 30, 2725–2729, https://doi.org/10.1093/molbev/mst197, 2013.
Tisthammer, K. H., Cobian, G. M., and Amend, A. S.: Global biogeography of marine fungi is shaped by the environment, Fungal Ecol., 19, 39–46, https://doi.org/10.1016/j.funeco.2015.09.003, 2016.
Tong, Y. and Lighthart, B.: Solar Radiation Is Shown to Select for Pigmented Bacteria in the Ambient Outdoor Atmosphere, Photochem. Photobiol., 65, 103–106, https://doi.org/10.1111/j.1751-1097.1997.tb01884.x, 1997.
Vaïtilingom, M., Attard, E., Gaiani, N., Sancelme, M., Deguillaume, L., Flossmann, A. I., Amato, P., and Delort, A. M.: Long-term features of cloud microbiology at the puy de Dôme (France), Atmos. Environ., 56, 88–100, https://doi.org/10.1016/j.atmosenv.2012.03.072, 2012.
Vali, G.: Freezing Nucleus Content of Hail and Rain in Alberta, J. Appl. Meteorol., 10, 73–78, 1971.
Vali, G.: Comments on “Freezing Nuclei Derived from Soil Particles,” J. Atmos. Sci., 31, 1457–1459, https://doi.org/10.1175/1520-0469(1974)031<1457:CONDFS>2.0.CO;2, 1974.
Vergara-Temprado, J., Murray, B. J., Wilson, T. W., O'Sullivan, D., Browse, J., Pringle, K. J., Ardon-Dryer, K., Bertram, A. K., Burrows, S. M., Ceburnis, D., DeMott, P. J., Mason, R. H., O'Dowd, C. D., Rinaldi, M., and Carslaw, K. S.: Contribution of feldspar and marine organic aerosols to global ice nucleating particle concentrations, Atmos. Chem. Phys., 17, 3637–3658, https://doi.org/10.5194/acp-17-3637-2017, 2017.
Vergara-Temprado, J., Miltenberger, A. K., Furtado, K., Grosvenor, D. P., Shipway, B. J., Hill, A. A., Wilkinson, J. M., Field, P. R., Murray, B. J., and Carslaw, K. S.: Strong control of Southern Ocean cloud reflectivity by ice-nucleating particles, P. Natl. Acad. Sci. USA, 115, 2687–2692, https://doi.org/10.1073/pnas.1721627115, 2018.
Walters, W., Hyde, E. R., Berg-lyons, D., Ackermann, G., Humphrey, G., Parada, A., Gilbert, J. A., and Jansson, J. K.: Improved bacterial 16S rRNA gene (V4 and V4-5) and fungal internal transcribed spacer marker gene primers for microbial community surveys, mSystems, 1, e0009-15, https://doi.org/10.1128/mSystems.00009-15, 2015.
Wex, H., Augustin-Bauditz, S., Boose, Y., Budke, C., Curtius, J., Diehl, K., Dreyer, A., Frank, F., Hartmann, S., Hiranuma, N., Jantsch, E., Kanji, Z. A., Kiselev, A., Koop, T., Möhler, O., Niedermeier, D., Nillius, B., Rösch, M., Rose, D., Schmidt, C., Steinke, I., and Stratmann, F.: Intercomparing different devices for the investigation of ice nucleating particles using Snomax® as test substance, Atmos. Chem. Phys., 15, 1463–1485, https://doi.org/10.5194/acp-15-1463-2015, 2015.
Wilson, S. L. and Walker, V. K.: Selection of low-temperature resistance in bacteria and potential applications, Environ. Technol., 31, 943–956, https://doi.org/10.1080/09593331003782417, 2010.
Wilson, T. W., Ladino, L. A., Alpert, P. A., Breckels, M. N., Brooks, I. M., Browse, J., Burrows, S. M., Carslaw, K. S., Huffman, J. A., Judd, C., Kilthau, W. P., Mason, R. H., McFiggans, G., Miller, L. A., Nájera, J. J., Polishchuk, E., Rae, S., Schiller, C. L., Si, M., Temprado, J. V., Whale, T. F., Wong, J. P. S., Wurl, O., Yakobi-Hancock, J. D., Abbatt, J. P. D., Aller, J. Y., Bertram, A. K., Knopf, D. A., and Murray, B. J.: A marine biogenic source of atmospheric ice-nucleating particles, Nature, 525, 234–238, https://doi.org/10.1038/nature14986, 2015.
Wright, E. S.: DECIPHER: harnessing local sequence context to improve protein multiple sequence alignment, BMC Bioinformatics 16, 322, https://doi.org/10.1186/s12859-015-0749-z, 2015.
Wright, T. P., Hader, J. D., McMeeking, G. R., and Petters, M. D.: High relative humidity as a trigger for widespread release of ice nuclei, Aerosol Sci. Technol., 48, i–v, https://doi.org/10.1080/02786826.2014.968244, 2014.
Wright E. S.: DECIPHER: harnessing local sequence context to improve protein multiple sequence alignment, BMC bioinformatics, 16, 322, https://doi.org/10.1186/s12859-015-0749-z, 2015.
Yang, J., Wang, Z., Heymsfield, A. J., DeMott, P. J., Twohy, C. H., Suski, K. J., and Toohey, D. W.: High ice concentration observed in tropical maritime stratiform mixed-phase clouds with top temperatures warmer than −8 ∘C, Atmos. Res., 233, 104719, https://doi.org/10.1016/j.atmosres.2019.104719, 2020.
ZoBell, C. E.: Marine Bacteriology, Annu. Rev. Biochem., 16, 565–586, 1947.