the Creative Commons Attribution 4.0 License.
the Creative Commons Attribution 4.0 License.
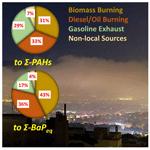
Annual exposure to polycyclic aromatic hydrocarbons in urban environments linked to wintertime wood-burning episodes
Irini Tsiodra
Georgios Grivas
Kalliopi Tavernaraki
Aikaterini Bougiatioti
Maria Apostolaki
Despina Paraskevopoulou
Alexandra Gogou
Constantine Parinos
Konstantina Oikonomou
Maria Tsagkaraki
Pavlos Zarmpas
Athanasios Nenes
Nikolaos Mihalopoulos
Polycyclic aromatic hydrocarbons (PAHs) are organic pollutants in fine particulate matter (PM) long known to have mutagenic and carcinogenic effects, but much is unknown about the importance of local and remote sources for PAH levels observed in population-dense urban environments. A year-long sampling campaign in Athens, Greece, where more than 150 samples were analyzed for 31 PAHs and a wide range of chemical markers, was combined with positive matrix factorization (PMF) to constrain the temporal variability, sources, and carcinogenic risk associated with PAHs. It was found that biomass burning (BB), a source mostly present during wintertime intense pollution events (observed for 18 % of measurement days in 2017), led to wintertime PAH levels that were 7 times higher than in other seasons and was as important for annual mean PAH concentrations (31 %) as diesel and oil (33 %) and gasoline (29 %) sources. The contribution of non-local sources, although limited on an annual basis (7 %), increased during summer, becoming comparable to that of local sources combined. The fraction of PAHs (12 members that were included in the PMF analysis) that was associated with BB was also linked to increased health risk compared to the other sources, accounting for almost half the annual PAH carcinogenic potential (43 %). This can result in a large number of excess cancer cases due to BB-related high PM levels and urges immediate action to reduce residential BB emissions in urban areas facing similar issues.
- Article
(2306 KB) - Full-text XML
-
Supplement
(1304 KB) - BibTeX
- EndNote
Polycyclic aromatic hydrocarbons (PAHs) are abundant in the atmosphere and can be present in the gas or particle phase (Finlayson-Pitts, 1997; Lin et al., 2015). Ambient PAHs originate mainly from internal combustion engines, stationary sources such as power and industrial plants, residential heating (including biomass burning), cooking, and wildfires (Finlayson-Pitts, 1997; Simoneit, 2002; Bond et al., 2013). Several PAHs are characterized as potential carcinogens and/or mutagens (IARC, 2010; Nowakowski et al., 2017). The IARC Group 1 carcinogen benzo[a]pyrene (BaP) is extensively studied among PAHs and is used as a marker for PAH toxicity. The European Union has set an air quality standard for the carcinogenic risk of PAHs with the directive 2004/107/EC. While the directive highlights the necessity of measurement for several PAHs (at least six other members), it lays down an annual target value only for BaP (1 ng m−3), which is selected as a marker for the carcinogenic risk of polycyclic aromatic hydrocarbons in ambient air.
The presence of PAHs tends to be highly enhanced in urban environments (Jiang et al., 2014; Mo et al., 2019; Ringuet et al., 2012), such as Athens, Greece. Studies to date in Athens show that PAH concentrations are significantly enhanced during the cold period of the year (Mantis et al., 2005) and linked to a multitude of incomplete combustion sources. Fireplace and woodstove usage for domestic heating in Greece has increased dramatically since the 2010 financial crisis, having considerable impacts on air quality in major urban centers such as Athens (Paraskevopoulou et al., 2014; Grivas et al., 2018; Theodosi et al., 2018) and Thessaloniki (Saffari et al., 2013), which host half the population of Greece.
It is worth noting that since 2013 several violations of the EU limit value for BaP have been recorded in Greek cities, with concentrations showing a clear wintertime enhancement (NAPMN, 2020). Greek urban centers are not unique in this regard, as residential biomass burning (BB) is a major issue for urban air quality throughout Europe. Studies in central European cities have reported BB contributions to cumulative particle PAH concentrations that even exceed 50 % (Li et al., 2018; Masiol et al., 2020; Schnelle-Kreis et al., 2007), while in other Mediterranean urban areas its impact appears less profound (Callén et al., 2014).
Uncontrolled residential BB emissions can lead to the appearance of intense pollution events (IPEs), during which very high urban levels of organic aerosols are observed in urban centers worldwide (Florou et al., 2017; Saffari et al., 2013; Fountoukis et al., 2016). Excessive exposure of urban populations to ambient woodsmoke pollution can cause severe health effects (Kocbach Bølling et al., 2009; Naeher et al., 2007; Wong et al., 2019), especially in view of the very high concentrations of highly oxidized organic species from nighttime chemistry and their potential to enhance toxicity (Kodros et al., 2020).
Moreover, the contribution of wildfires to regional background PAHs can be significant, given their increased incidence as a result of climate change (McCarty et al., 2020; O'Dell et al., 2020). Wildfires near urban centers can have especially direct and pronounced effects on population exposure. Every summer since 2017, for example, intense episodes of air quality degradation from wildfire smoke near major Greek urban regions (such as Athens, Patras, Heraklion, Kalamata, and Pyrgos) have been recorded. During these events, heavily increased PM2.5 and PM10 levels (reaching a few hundreds micrograms per cubic meter) that persist for several days (https://panacea-ri.gr, last access: 3 November 2021; Amiridis et al., 2012; Stavroulas et al., 2019) are observed.
Although much work has been done on PAH sources, their attribution using atmospheric samples is often pursued through highly uncertain diagnostic ratios for measured concentrations (Katsoyiannis et al., 2011). Relatively few studies exist in Europe and North America that utilize receptor modeling (e.g., Schnelle-Kreis et al., 2007; Sofowote et al., 2008; Iakovides et al., 2021a), an approach that can quantify the relative contributions of distinct local and regional sources to PAH levels and associated carcinogenic risks that urban populations are exposed to.
The present study aims to characterize the variability and sources of PAHs in Athens, Greece, one of the largest urban centers in the eastern Mediterranean. This is one of the first studies in Greece and southeastern Europe to utilize positive matrix factorization (PMF) receptor modeling for source apportionment of PAHs. For this, filter-based analysis is combined with online chemical speciation, allowing for the association of PAH groups with specific organic aerosol (OA) components and the verification of PMF-resolved sources. At present, there are only a few studies involving PAHs that comparatively assess offline and online organic aerosol source apportionment (e.g., Bozzetti et al., 2017; Srivastava et al., 2018). To specifically characterize the diurnal variability of PAHs and the BB source, separate daytime and nighttime filter samples were analyzed here, as done in a some previous studies in Greece (Saffari et al., 2013; Tsapakis and Stephanou, 2007). The separation of diesel and gasoline aerosol sources that is attempted in this work is also highly important for Athens, which stands out as a particularity among European cities, given the very small proportion of diesel cars in the passenger fleet due to the ban that existed up to 2011. The resulting identification of carbonaceous aerosol source profiles can provide useful reference for source apportionment studies that use long-term chemical speciation data in urban areas, thus enabling a source-specific toxicity assessment that considers local against regional contributions and is highly important for policies on PAH exposure reduction.
2.1 Study area
The Greater Athens Area (GAA, Fig. S1 in the Supplement), with 3.8 million inhabitants, is one of the largest metropolitan regions in southern Europe and a major commercial and transportation hub. Nearly 3 million private cars are registered for circulation in the GAA, along with 0.3 million trucks and buses (Hellenic Statistical Authority, 2020). Up to 2011, diesel-powered private cars were banned in Athens and, even though nowadays they comprise the majority of new sales, they only account for about 10 % of the fleet. Up to 2011, heating oil was the predominantly used fuel for domestic heating in Athens (in 76 % of residences). However, its consumption in the GAA decreased by 61 % in 2011–2018 (Hellenic Statistical Authority, 2019), with people resorting to alternative heating sources, including biomass burning in fireplaces and stoves. Industrial activity in the GAA is minor in the central basin as it is mostly concentrated in the Thriassion plain, 10–20 km to the northwest and separated by a low-altitude mountain range. The port of Piraeus to the southwest is the largest passenger port in Europe and also one of the busiest container ports in the Mediterranean (Fig. S1). The complex topography of the basin favors the appearance of mesoscale flows throughout the year and the frequent stagnation of air masses (Kassomenos et al., 1998).
2.2 Sampling and analyses
Ambient PM2.5 samples were collected between 4 December 2016–31 January 2018, at the urban background site of the National Observatory of Athens (NOA) at Thissio (37.97326∘ N, 23.71836∘ E), which is located 146 and is representative of the urban background conditions in central Athens (Stavroulas et al., 2019; Theodosi et al., 2018).
During the non-winter months, two samples per week were analyzed on alternating days between weeks. As such, a representative distribution between weekday and weekend samples (69 %–31 %) was achieved, since it is known that this is an important temporal scale inducing variability in urban PAH levels (Dutton et al., 2010; Lough et al., 2006). The same approach was followed for the winter period, focusing at the same time on as many daytime–nighttime pairs as possible to characterize the diurnal patterns. From December to February, separate daytime and nighttime filters were collected every 12 h (06:00–18:00 LST and 18:00–06:00 LST, respectively) for a total of 80 samples, while 76 total 24 h filters were sampled from March to November. The sampling schedule also satisfies the 2004/107/EC directive requirements for PAHs (roughly one measurement per week, equally distributed around the year), allowing the comparison with the BaP target value.
A low-volume sampler (3.1 PNS 15, Comde Derenda GmbH, Stahnsdorf, DE) with a flow rate of 2.3 m3 h−1 was used to collect particles onto quartz-fiber filters of 47 mm diameter (Flex Tissuquartz, Pall Corporation, Port Washington, NY, USA). The inlet height was 2 m above ground. Field and laboratory blanks were routinely collected. PAHs in the filter samples were quantified by gas chromatography–mass spectrometry (GC-MS, Agilent 6890N, Agilent Technologies Inc., Santa Clara, CA, USA). Prior to the analysis, samples were spiked with a mixture of deuterated internal standards for identification of PAHs and calculation of recovery efficiencies (16 members, LGC Standards, Middlesex, UK). Extraction was performed following the pre-established procedure of Gogou et al. (1998) with modifications (Parinos et al., 2019) (Sect. S1, Table S1 in the Supplement). Briefly, extracts were obtained using a 50:50 n-hexane/dichloromethane mixture and were purified on a silica column. PAHs were eluted with a 10 mL n-hexane/ethyl acetate (9:1 ) mixture and placed into a glass vial for further concentration under a gentle nitrogen stream. [2H12]perylene, used as an internal standard, was spiked into the vial before sealing and storage. Limits of detection (LODs) were calculated as 3 times the standard deviation of blanks. On the day of the analysis, injections with internal standards were also run to calculate relative response factors (RRFs). The identification of compounds was based on retention time, mass fractionation, and co-injection of standard mixtures.
Organic and elemental carbon (OC, EC) were determined by the thermal-optical transmission (TOT) method, using a Sunset carbon analyzer (Sunset Laboratory Inc., Portland, OR, USA) (Cavalli et al., 2010; Paraskevopoulou et al., 2014). Water-soluble ions were detected by ion chromatography (IC) (Paraskevopoulou et al., 2014) and monosaccharide anhydrides (levoglucosan, mannosan, and galactosan) by high-performance anion exchange chromatography with pulsed amperometric detection (HPAEC-PAD) (Iinuma et al., 2009).
The submicrometer organic aerosol (OA) fraction was monitored at the same site by an Aerosol Chemical Speciation Monitor (ACSM, Aerodyne Inc., Billerica, MA, USA) (Ng et al., 2011). The ACSM samples ambient air, through a critical orifice to a particle-focusing aerodynamic lens. The particle beam is driven in the detection chamber where non-refractory particles are flash-vaporized at 600 ∘C and ionized by electron impact. Finally, the resulting fragment ions are characterized by a quadrupole mass spectrometer. The obtained organic mass spectra are decomposed into OA components representative of specific sources and processes (namely hydrocarbon-like OA, biomass burning OA, cooking OA, and semi-volatile and low-volatility oxygenated OA), through PMF, based on the multilinear engine ME-2 algorithm, and using the Source Finder toolkit (SoFi) (Canonaco et al., 2013; Bougiatioti et al., 2014). The standard sampling duration of the Q-ACSM that was used is 30 min. ACSM monitoring was carried out for the majority of the filter-sampling period (December 2016 to January 2018). Data on OA, ionic composition, and organic spectra were available with the exception of September and October 2017. Validated, PMF-resolved OA source contributions were available during December 2016–July 2017. Additional details on the OA measurement and analysis can be found in Stavroulas et al., (2019).
Equivalent black carbon (BC) levels were monitored by a seven-wavelength aethalometer (AE33, Magee Scientific, Berkeley, CA, USA), with compensation for loading (DualSpot) and multiple scattering effects (Liakakou et al., 2020). The instrument internally applies the two-component aethalometer model (Drinovec et al., 2015; Sandradewi et al., 2008) and provides online estimates of the BB (%) contribution to BC at 880 nm, using the assumption that absorbing aerosols from fossil fuel (FF) combustion and BB have distinct spectral properties.
Regulatory pollutants and meteorological parameters (temperature, wind speed and direction) were routinely measured at Thissio. Reference-grade instrumentation was used for measurement of CO (NDIR, APMA 360, Horiba Inc.), NOx (chemiluminescence, APNA 360, Horiba Inc.), and O3 (UV-Vis, 49-i, Thermo Fisher Scientific Inc.). NOx concentration data were also obtained from a nearby regulatory (Greek Ministry of Environment and Energy) traffic site (Athinas Str., 0.9 km NE of Thissio). ACSM, aethalometer, gaseous pollutant, temperature, and wind speed data were averaged on an hourly basis.
The 4 d air mass back trajectories, arriving at Thissio at 1000 m (Grivas et al., 2018; Kalkavouras et al., 2020; Stavroulas et al., 2019), were calculated using the Hybrid Single Particle Lagrangian Integrated Trajectory (HYSPLIT) model (Draxler and Rolph, 2016) and grouped daily using cluster analysis (Kalkavouras et al., 2020). The altitude of 1000 m was chosen to capture the regional transport with a high probability of affecting pollution within the boundary layer (hence, air quality and population exposure) and is consistent with typical planetary boundary layer estimates over Athens (Kokkalis et al., 2020).
2.3 Source apportionment
PMF analysis was performed using the EPA PMF 5.0 model (Norris et al., 2014). The 12 h samples collected during the winter intensive campaigns were appropriately combined to 24 h periods, between 06:00 LST of each day and 06:00 LST of the following day. This gives 104 total 24 h samples considered for the analysis. The carbonaceous aerosol speciation dataset that was used consisted of OC, EC, PAHs, oxalate, and levoglucosan. Total carbon (TC, i.e., the sum of OC and EC) was included as a total variable in the PMF model, for a meaningful mass balance approach (Piletic et al., 2013; Valotto et al., 2017). In total, 16 species were entered in the analysis, including 12 PAH members. Rotational and random errors were assessed using the bootstrap (BS) and displacement (DISP) error estimation methods included in the EPA PMF 5.0 software (Paatero et al., 2014). Details on PMF model design parameters, solution metrics, uncertainties, and error indices are provided in the Supplement (Sect. S2, Table S2).
2.4 PAH contribution to carcinogenic risk
The “toxic equivalence factor” (TEF) approach was used to estimate the carcinogenic potency of measured PAHs, in which the toxicity of each member is expressed using BaP as reference (Taghvaee et al., 2018a):
where Ci is the concentration (ng m−3) and TEFi is the toxicity equivalent factor of each member (Bari et al., 2010; Nisbet and LaGoy, 1992). The lifetime excess cancer risk (ECR) from inhalation was estimated as follows:
where URBaP (unit risk) refers to the number of excess cancer cases in the population with chronic inhalation exposure to 1 ng m−3 of BaP over a lifetime of 70 years. Estimations were made using reference URBaP values of (0.11 cases per 100 000 people) – according to the Office of Environmental Health Hazards Assessment (OEHHA) of the California Environmental Protection Agency (CalEPA) – and (8.7 cases per 100 000 people) – according to the World Health Organization (WHO) (Alves et al., 2017; Elzein et al., 2019).
3.1 Temporal variability
Figure 1 presents the monthly variation of total PAH concentrations, displaying a notable wintertime enhancement as compared to the summer period. Mean ΣPAH concentrations in winter 2016–2017, spring 2017, summer 2017, autumn 2017 and winter 2017–2018 were 7.0±10.1, 2.5±4.1, 0.9±0.9, 2.2±3.2 and 22.3±26.8 ng m−3, respectively, indicating a clear seasonal cycle. The mean annual (2017) BaP concentration in this study (0.26 ng m−3) did not exceed the EU target value, but nevertheless was higher than the WHO reference level (0.12 ng m−3). Largely increased BaP levels were observed during wintertime episodic events. These results can be placed in context by comparison against past studies for PAHs in the GAA (Table S3 in the Supplement) that identified similar seasonal profiles, although not with such pronounced winter-summer differences. Moreover, this study reports the highest mean annual BaP concentrations at a background location in the GAA in over two decades (Marino et al., 2000). In the few studies in the area that have compared traffic with background sites, there appears to be an important roadside enhancement of PAH levels (Andreou and Rapsomanikis, 2009; Mantis et al., 2005; Pateraki et al., 2019). Therefore, it is noteworthy that the present, urban background, BaP annual mean concentration is comparable to the mean BaP concentrations reported at 21 sites in the GAA by a study of annual duration in 2010–2011, 7 of which were high-traffic locations. Specifically, the street-site concentrations were reportedly higher by 44 % and 55 % for ΣPAHs and BaP, respectively, compared to urban background sites (Jedynska et al., 2014). This comparison likely indicates an increase of urban background levels in Athens, with implications for the population's exposure.
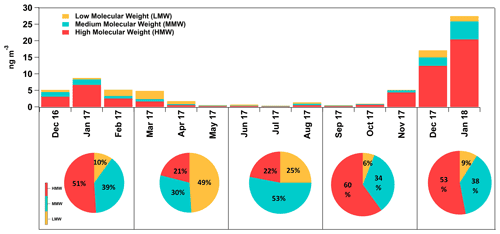
Figure 1The color bars represent the mean monthly concentrations of high (HMW), medium (MHW), and low (LMW) molecular weight PAHs, between December 2016 and January 2018. The pie charts in the lower row show the concentration fractions of LMW, MMW, and HMW PAHs in each seasonin each season, starting with winter on the far left, moving to spring, then to summer in the middle, autumn, and lastly winter again on the far right.
The classification of PAHs by molecular weight is thought to provide information about their sources. For example, low molecular weight PAHs (LMW; 128–178 g mol−1) and medium molecular weight PAHs (MMW; 202–228 g mol−1) have been linked to diesel engine emissions (Zheng et al., 2017), while MMW and high molecular weight (HMW; 252–300 g mol−1) PAH concentrations can be influenced by BB emissions (Han et al., 2019; Masiol et al., 2020). Figure 1 also displays the ΣPAH fractions of LMW, MMW, and HMW PAHs by season. During winter and autumn, HMW PAHs comprised the most abundant fraction (60 % and 51 %, respectively), implying a possible impact from BB. In spring there was an increase of the LMW fraction (49 %), while during summer, when regional fine aerosol sources maximize their influence (Grivas et al., 2018), the higher percentage (53 %) came from MMW species.
A prior study carried out during 2001–2002 (Table S3), well before the economic recession in Greece, at a GAA site not adjacent to a major road (Mantis et al., 2005), reported an LMW–MMW–HMW PAH fractionation similar to the present study (Fig. S2 in the Supplement), despite the considerably higher residential BB emissions here. However, the present concentrations of ΣPAHs and BaP were higher by a factor of 1.4 and 1.8, respectively. This most likely suggests a significant effect of the new BB source on an annual level, since PAH emissions from the road transport sector in Greece remained relatively comparable between the two periods (EIONET, 2021). These results indicate that approaches based on molecular weight (MW) alone have limitations, since many PAH members are emitted by both fossil fuel and biomass burning, and the attribution of PAH sources should be addressed through receptor modeling.
The seasonal variability seen in Fig. 1 is assessed statistically (t tests) to identify significant seasonal differences (winter against non-winter measurements), with results presented in Table S4 in the Supplement. Higher mean PAH concentrations were observed during wintertime, when emissions from processes like biomass burning are more intense. Lower PAH levels were recorded during the non-winter period due to the additional effects of volatilization, photochemical degradation, and enhanced atmospheric dispersion. For all PAH members, concentrations were significantly higher during the winter season at the 0.95 confidence level with the exception of Σ C1-202 (which instead registered a large number of below detection limit (BDL) values).
Temperature-dependent processes like gas and particle partitioning can affect the observed seasonal variability of semi-volatile PAH compounds. Previous studies in Greece (Iakovides et al., 2021b; Mandalakis et al., 2002; Sitaras and Siskos, 2001, 2008; Terzi and Samara, 2004; Vasilakos et al., 2007) that performed both gas- and particle-phase measurements reported that LMW PAHs were found predominantly in the gas phase (gas-phase fractions: 88 %–97 %), MMW PAHs in both gas and particle phase (gas phase: 46 %–90 %), and HMW PAHs mainly in the particle phase (particle phase: 71 %–100 %). In the present study, only the particle phase of PAHs was measured. In an effort to assess the fractionation of PAH members between the particle phase (measured) and the gas phase in the dataset, an approximation was performed, based on the partitioning theory of semi-volatile organics due to absorption by organic material in the particle phase (Pankow, 1994). This approach and the partitioning–absorption equations used to calculate the partitioning coefficient Kp, and therefore the gasparticle concentration ratios, have been used by numerous PAH studies (e.g., Andreou and Rapsomanikis, 2009; Xie et al., 2013). The estimations here were found to be in agreement with the above-mentioned studies in Athens and indicate that volatilization leads to minimal fractions of particle LMW PAHs (found in the gas phase in mean fractions of 71 %–100 %). Regarding MMW, fluoranthene (Flt) and pyrene (Pyr) are the main compounds affected by volatilization (15 %–25 %). HMW members remain relatively unaffected, being partitioned in the particle phase in fractions of 91 %–100 %, even though during regional transport and through successive dilutions a substantial part of HMW could end up being lost from the particle phase due to physical volatilization. The partitioning characterization methodology and results are presented in Sect. S3 of the Supplement (Fig. S3). Finally, as it has been proposed, some organic components can adopt an amorphous solid or semisolid state that can further impact the rate of heterogeneous reactions and multiphase processes (Shiraiwa et al., 2011). In our study for PAHs this does not seem to be the case, as PAHs from outside the region of Athens will be shown later in the paper to be present at low levels.
Apart from volatilization processes, the temporal variability of emission sources can account for a major part of observed seasonal differences. Correlations of PAHs with primary (NOx, CO) and secondary (O3) pollutants can provide insights into the related sources and atmospheric processes. The correlation analysis was performed separately for winter and non-winter months, and results are presented in Sect. S3 of the Supplement (Table S5). LMW members, Flt, and Pyr showed weak correlations with primary pollutants (NOx, CO) that were slightly higher during the cold period. MMW and HMW members were highly correlated with CO at Thissio and NOx at the traffic site during both the winter and non-winter periods. For those members, the highest wintertime correlations (r: 0.62–0.88, p<0.01) signify the additional contribution of biomass burning and heating oil combustion emissions that co-emit heavier PAHs, CO, and NOx. In the non-winter period there were relatively high correlations of coronene (Cor), indeno[1,2,3-cd]pyrene (IP), and benzo[ghi]perylene (BghiP) with CO and NOx as measured at Thissio (r: 0.55–0.81, p<0.01) that probably indicate an impact from light-duty vehicles (Weiss et al., 2011) in the absence of domestic burning.
3.2 Investigating intense pollution events: nighttime vs. daytime
Summary statistics for 12 and 24 h ΣPAH averages are provided in Table 1. The highest PAH concentrations were observed during intense pollution events (IPEs), which in this work are defined as periods with mean BC concentrations exceeding 2 µg m−3, and stagnant conditions with wind speeds below 3 ms−1 and a lack of precipitation (Fourtziou et al., 2017). Peak wintertime levels of BC (Fig. S4 in the Supplement) coincided with BCbb maxima, indicating a predominance of biomass burning over other local combustion sources for carbonaceous aerosols during these events (Liakakou et al., 2020). These high levels are considered to be mainly driven by emissions and not changes in mixing-layer height (Liakakou et al., 2020).
Table 1Average values ± standard deviation of PAH concentrations categorized according to their molecular weight during wintertime intense pollution events (IPEs).
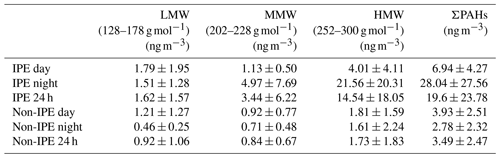
Among the 80 winter samples, 50 met the above criteria for IPE (20 daytime and 30 nighttime samples). IPEs occurred with higher frequency during the second winter (24 vs. 17 in the common months: December–January), due to more intense emissions (e.g., the BC mean concentration was 2.6 times higher during the second winter) and reduced atmospheric dispersion (average wind speeds of 3.0 and 1.9 m s−1 in the first and second winter periods, respectively).
During IPEs, ΣPAH concentrations displayed a strong night–day gradient, owing to the effect of nighttime residential BB emissions. Several studies have examined the diurnal variability of PAH levels worldwide (e.g., Yan et al., 2009). Similarly pronounced nighttime enhancements have been mostly reported for megacities in East Asia, such as Beijing and Nanjing (Elzein et al., 2019; Haque et al., 2019), where PAH levels are considerably higher than in Athens, due to the intensity and diversity of local sources that include burning of biomass, coal, and plastics. During non-IPE periods, ΣPAH levels were lower by 43 % in daytime and 90 % in nighttime, resulting in daytime levels being higher than nighttime and indicative of substantial contributions from other combustion sources like vehicular traffic.
HMW PAHs had the highest contribution to ΣPAHs during IPEs and presented the most pronounced day–night differences. MMW PAHs were more abundant during nighttime, with 24 h levels more than double during IPEs. Levels of LMW PAHs were higher during daytime in both IPE and non-IPE periods, suggesting that biomass burning had a more limited effect in their particle-phase concentrations compared to the higher MW members.
To explore the various sources that drive the variability of PAHs, the correlations of ΣPAHs with source-specific tracers were examined (Table S6 in the Supplement). Given the very high correlations of ΣPAHs with BaP (Fig. S5 in the Supplement), especially during nighttime IPEs (r=0.95, p<0.01), similar associations with examined tracers can be expected for the corresponding PAH carcinogenicity. Strong correlations with biomass burning tracers such as non-sea salt K+ and BCbb were observed, particularly during IPE nights (r: 0.81 and 0.95, respectively, p<0.01). Similarly, levoglucosan, mannosan, and galactosan displayed very high correlations with ΣPAHs during nighttime IPEs (r: 0.90–0.93, p<0.01). Comparisons were also made with the “fingerprint” ACSM 60 and 73 that are linked to dissociation of levoglucosan produced as a pyrolytic product of biomass (Alfarra et al., 2007; Heringa et al., 2011; Weimer et al., 2008). In fact, filter-based levoglucosan measurements correlated excellently with 60 and 73 ACSM fragments (r=0.98 and 0.97, respectively, p<0.01). ΣPAHs exhibited strong correlations with 60 and 73 during nighttime IPEs, with r values of 0.88 and 0.86 (p<0.01), respectively, indicating the impact of BB emissions. A close association was also observed between ΣPAHs and submicrometer organic aerosol (OA). During the period when PMF-resolved ACSM OA components were available, the strongest correlations were observed with biomass burning organic aerosol (BBOA) and semi-volatile oxygenated organic aerosol (SV-OOA), the components that for the cold period in Athens correspond to fresh and processed BB organic aerosol, respectively (Stavroulas et al., 2019). On the contrary, ΣPAHs were uncorrelated with low-volatility oxygenated organic aerosol (LV-OOA), which mostly represents OA of regional origin. An absence of correlation between ΣPAHs and highly oxidized OA from ACSM data has been also reported by field and experimental studies (Cui et al., 2020; Zheng et al., 2020).
The results indicate a significant impact of BB emissions on PAH levels during nighttime IPEs. The ratio of ΣPAHs to levoglucosan during these events was 53 % lower than in daytime, when biomass burning and therefore levoglucosan concentrations decrease. This indicates the important effects of additional PAH sources such as vehicular traffic, a finding consistent with past studies in Greece (Saffari et al., 2013). The levoglucosan/(mannosan + galactosan) ratio can also indicate whether solid fuels used for heating are either “aged” (e.g., aged wood or lignite) producing more levoglucosan or more “fresh” (Saffari et al., 2013). The calculated value close to 6 for both daytime and nighttime samples suggests that solid fuels used for residential heating in Athens are mainly associated with fresh firewood and that their type has not changed since 2013, when a similar wintertime ratio was reported in Athens (Fourtziou et al., 2017). Finally, the ratio of levoglucosan to mannosan can be indicative of wood type, with hardwood use (e.g., olive, oak, beech) producing ratios around 14–15, while softwood (e.g., pine) burning leads to lower ratios, around 4 (Schmidl et al., 2008). In the present study, the levoglucosanmannosan ratio ranged from 7.3 to 9.7 (Table S7 in the Supplement), indicating a balanced use of both wood types, in agreement with observations at the same site during the 2013–2014 winter (Fourtziou et al., 2017), once again supporting that a mixture of fresh softwood and hardwood is consistently used for residential heating over the recent years.
3.3 PMF modeling and source characterization
Solutions with three to eight factors were examined, with the four-factor solution deemed as the most physically meaningful (a detailed presentation of the selected solution can be found in Sect. S5 of the Supplement). The four identified factors are presented below; a more extensive discussion on their validation is provided in Sect. S5 of the Supplement.
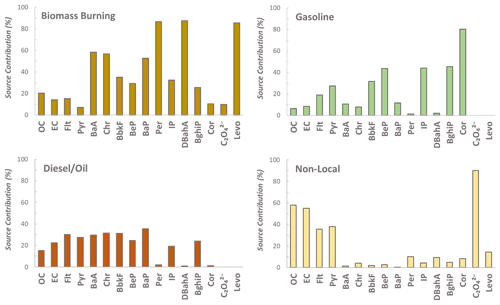
Figure 2Percentage contributions of individual sources to mean modeled concentrations of PAHs and other carbonaceous species obtained from PMF analysis for 24 h samples collected at Thissio, Athens, during December 2016–January 2018.
The first factor was attributed to biomass burning (BB). Levoglucosan, a well-established BB signature marker, is almost exclusively associated with this factor (Fig. 2). The factor is also characterized by important loadings in 5–6-ring PAHs, a feature that has been reported in BB source profiles of PMF studies in urban areas worldwide (Han et al., 2019; Masiol et al., 2020; Taghvaee et al., 2018b). The strong presence of BaP in the factor is consistent with results from studies in European cities (Li et al., 2018; Srivastava et al., 2018) and also with a recent study at Thissio (Fourtziou et al., 2017) that reported direct associations between wintertime BaP levels and several BB tracers. The OCEC ratio in the profile was 4.2, higher than the other local sources and characteristic of fresh BB emissions. It was also comparable to the value (3.7) calculated for the BB source at the same site by a long-term PM2.5 source apportionment study (Theodosi et al., 2018). Moreover, the source contributions registered the highest correlations, among the four factors, with BB external tracers and specifically with BCbb (r=0.93, p<0.01) and water-soluble K+ (r=0.61, p<0.01). Similarly, the strongest correlations were observed between the BB source and the 60 and 73 ACSM fragments (r=0.88 and 0.86, respectively, p<0.01), further validating its identification. The biomass burning source was closely related to the BBOA component (r=0.85, p<0.01) with a slope of 0.003 indicating that the 12 members included in the PMF analysis comprise about 0.3 % of BBOA (Poulain et al., 2011). Similar associations have been reported by studies comparing OA components from online aerosol mass spectrometry and source contributions from offline filter-based source apportionment (Bozzetti et al., 2017; Srivastava et al., 2021). The bivariate polar wind plot (calculated according to the methodology of Uria-Tellaetxe and Carslaw, 2014) for contributions of the BB source (Fig. S6a in the Supplement) indicates the local character of the source, with concentrations being enhanced during low-wind conditions, as it has been observed at the same site for fresh BB aerosols emitted in central Athens (Kaskaoutis et al., 2021; Stavroulas et al., 2019). The factor was present almost exclusively during the winter months (Fig. S7 in the Supplement), when local wood-burning emissions for residential heating intensify, leading to frequent IPE.
The second factor was linked to gasoline-powered vehicles, which constitute the major fraction (over 90 %) of the vehicular fleet in the GAA. The factor was characterized by stronger contributions to HMW PAHs (e.g., IP, BghiP, and Cor) over LMW members (Fig. 2). This feature has been used to differentiate the gasoline source from general traffic sources in several PAH source apportionment studies (Callén et al., 2013; Javed et al., 2019; Sofowote et al., 2008; Wu et al., 2014). The OCEC ratio in the source profile was 1.9, within the range (1.7–2.3) typically reported for fresh emissions from gasoline vehicles (Grivas et al., 2012). The source contribution time series presented the highest correlations with BCff (r=0.79, p<0.01), which is mostly a proxy of traffic emissions impacting Thissio (Liakakou et al., 2020). It was the only factor that correlated significantly during both non-winter (r=0.80, p<0.01) and winter (r=0.66, p<0.01) periods with CO, which is emitted mainly by gasoline vehicles in the GAA (Fameli and Assimakopoulos, 2016). In contrast, the BB source correlated with CO only in winter (r=0.83, p<0.01) due to strong CO emissions from wood burning during IPEs (Gratsea et al., 2017). Moreover, this was also the only local source that was correlated (r=0.57, p<0.01) with ACSM hydrocarbon-like organic aerosol (HOA) during the non-winter months, when HOA is exclusively associated with vehicular traffic (Stavroulas et al., 2019). However, unraveling the HOA contributions from gasoline and diesel vehicles is challenging (Shah et al., 2018), and while some experimental results support a close connection of ambient HOA profiles with gasoline vehicle emissions (Collier et al., 2015), there are field source apportionment studies that report high correlations of HOA with both fuel-type combustion sources (Al-Naiema et al., 2018). The wind plot for the factor contributions (Fig. S6b), given the absence of a strong directional pattern, is again suggestive of aerosols produced in the vicinity of the site (central Athens). Higher contributions were observed during the winter months (Fig. S7), consistent with the increased traffic in the center of Athens (especially during the December holiday period) relative to the vacation months of July–August.
The third factor was associated with emissions from diesel and oil combustion and is characterized by an increased abundance of lower MW members (Shirmohammadi et al., 2016; Zheng et al., 2017). It presented the highest contributions to Flt and Pyr among local sources and also substantial loadings of Benzo[a]anthracene (BaA), Chrysene (Chr), Benzo[b&k] fluoranthene (BbkF), and BaP, along with smaller – compared to the gasoline factor – loadings of IP and BghiP (Fig. 2). Comparable patterns can be observed in source profiles of other PMF studies that distinguish diesel and gasoline sources (e.g., Agudelo-Castañeda and Teixeira, 2014; Liu et al., 2019), where contributions in the diesel factor were found relatively increased for 4–5-ring PAHs and decreased for HMW members. The OCEC ratio was 1.6, higher than typically reported values for diesel exhaust, which could indicate moderate aging. Higher OCEC ratios can also be expected in the cases of heavy-duty diesel vehicles in creeping mode (Pio et al., 2011) and non-traffic oil combustion emissions (e.g., ships in the port). Based on its wind plot, the factor, while still local, presents a relative enhancement for moderate-speed winds transporting aerosols from the S–W sectors of the GAA (Fig. S6c). Primary pollution hot spots are found in this direction, such as the port of Piraeus (at a distance of 7–11 km) and the industrial and commercial hub of the Athens basin (2–4 km) that is traversed by the E75 international route, the most heavily trafficked – and frequently congested – highway in the GAA (Grivas et al., 2019). Therefore, the area to the SW of the site is characterized by increased circulation of light- and heavy-duty diesel vehicles. As with the gasoline factor, the contribution time series recorded statistically significant (p<0.01) correlations with external combustion indicators, albeit ones that are weaker (r=0.66, p<0.01 with CO; r=0.39, p<0.01 with BCff). Although the factor was uncorrelated with the HOA during the non-winter months, it recorded a large correlation during the first winter period (r=0.88, p<0.01), potentially signifying a measurable contribution of stationary heating fuel combustion – recognized as an additional contributing factor to HOA during winter in Athens (Stavroulas et al., 2019) – to the diesel factor during stagnation events. It is recognized, however, that this correlation could be inflated by a common boundary layer or a stagnation effect of traffic and residential emissions, and therefore more research is need to confirm the finding. When examining the entire period with availability of OA component data (December 2016–July 2017), it was observed that the source had a consistent association (r=0.74, p<0.01) with the HOACO ratio, which is expected to be higher for aerosols from diesel combustions (Reyes-Villegas et al., 2016).
The fourth factor was characteristic of non-local contributions to carbonaceous aerosol. It was mainly associated with high contributions to OC and EC, which at urban background locations are moderately impacted from local primary sources and mostly driven by aerosols regionally transported to the receptor site (Buzcu-Guven et al., 2007; Hasheminassab et al., 2014). The dominance of regional secondary sources at urban and suburban background sites has been identified by the majority of aerosol source apportionment studies in the GAA (Diapouli et al., 2017; Grivas et al., 2018; Paraskevopoulou et al., 2015; Theodosi et al., 2018) and other urban areas worldwide, such as Mexico City (Aiken et al., 2009) and Paris (Skyllakou et al., 2014). The factor was also characterized by a high contribution of oxalate, an important secondary constituent of water-soluble organic carbon (Myriokefalitakis et al., 2011). The contributions of non-local sources were positively correlated with sulfate and ammonium (r: 0.57 and 0.51, respectively, p<0.01), indicators of regionally transported secondary aerosol. The non-local PMF factor showed the strongest associations with SV-OOA and LV-OOA, the secondary, oxidized OA components (Srivastava et al., 2021; Xu et al., 2021). This was mainly observed during the non-winter months (r=0.77 and 0.55, p<0.01, respectively), while in the winter period the factor was again correlated with LV-OOA of regional origin (r=0.48, p<0.05) but not with SV-OOA, which in winter is instead associated with the fast processing of heating emissions (Stavroulas et al., 2019). This is the only factor that showed a statistically significant (p<0.01) enhancement (Fig. S7) during the non-winter months, due to increased photo-oxidation for production of secondary organics under stronger insolation. The polar plot of Fig. S6d displays the typically observed (Stavroulas et al., 2019) large dispersion of concentration enhancements along the SW–NE axis of the Athens basin, indicating the association of the factor with transport on a larger-than-urban spatial level. A considerable fraction of Flt and Pyr was attributed to this factor. Studies performed at regional background sites that are mainly impacted by long-range transport have attributed increased contributions of Flt and Pyr to aerosols deriving from distant combustion of coal and heavy oil (Lhotka et al., 2019; Mao et al., 2018; Miura et al., 2019; Wang et al., 2014). The OCEC ratio in the source profile (2.9) was increased compared to the petroleum-related sources (1.6–1.9), but not as much as usually reported for secondary aerosol factors. Given this, and the fact that PAHs are subject to oxidative aging and removal during atmospheric transport (Galarneau, 2008; Ravindra et al., 2008), it is likely that the non-local factor not only includes transboundary aerosols but also partially aged aerosol from a less extended spatial scale (e.g., from energy production using fossil fuels in continental Greece or from emissions from marine oil combustion in the Aegean Sea). In support of this, it is noted that the centroids of all identified air mass trajectory clusters during the study period converge to the north of the GAA before arriving in the Athens basin (see also the discussion in Sect. 3.4).
3.4 Source contributions
Average source contributions to PAHs and TC are presented in Fig. 3 for the calendar year 2017 (January to December; 93 24 h values) to achieve consistent statistical sampling of each season. The following discussion is focused on the contributions to PAHs, while the contributions to TC are discussed in Sect. S5 of the Supplement. The mean annual contributions of sources to mean modeled OC and EC are displayed in Fig. S8 in the Supplement.
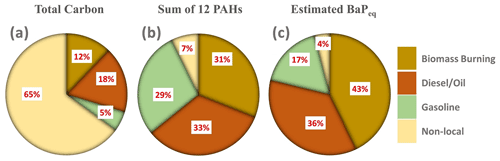
Figure 3Fractional contributions of PMF-resolved sources to mean modeled concentrations of (a) TC, (b) the sum of 12 PAHs included in the PMF analysis, and (c) estimated BaPeq.
Regarding local sources, the annual contribution of biomass burning to ΣPAHs is amplified compared to TC (31 % vs. 12 %, Fig. 2a and b) and even more so when assessing only the contributions to carcinogenic PAHs (36 %, Fig. 3c). The large impact of biomass burning on long- and short-term PAH exposure becomes more evident considering that it is essentially a source active mainly in wintertime and manifests mostly during IPEs (18 % of measurement days in 2017).
The other two local sources (diesel and/or oil and gasoline) accounted for a combined 62 % of ΣPAHs (Fig. 3b), highlighting the importance of urban vehicular emissions on a long-term basis but not during IPEs. Even though the participation of diesel cars is minimal in the passenger fleet in Athens (<10 %, the vast majority being Euro 5 and 6 vehicles), the contribution of the diesel and oil factor is at least comparable to the gasoline factor for ΣPAHs (and much larger for TC), indicating that it is probably emissions from older light- and heavy-duty vehicles (and to an extent stationary emissions) that should be associated with the significant contributions of this source. Manoli et al. (2016), reported an even larger gap between diesel and gasoline contributions to ΣPAHs (51 % vs. 30 % approximately) at an urban background location in Thessaloniki, Greece, during 2011–2012. Even before the lifting of the ban on private diesel cars in Athens and Thessaloniki (2011), diesel emissions were considered to be more important regarding the impact of road transport on PAH concentrations (Andreou and Rapsomanikis, 2009; Viras and Siskos, 1993). Comparing with these studies, a declining contribution to PAHs of diesel vehicles in Greek cities is implied.
Non-local sources were the major contributors to total carbon concentrations (65 %) at the Thissio site (Fig. 3a) – consistent with findings from previous studies at urban background sites in the GAA and elsewhere. The effect of non-local sources is greatly reduced (Fig. 3b) when examining contributions to ΣPAHs (7 %) against those of the three local sources (29 %–33 %). Figure 4a shows that non-local daily contributions remain lower than 20 % during winter but regularly exceed 50 % during summer.
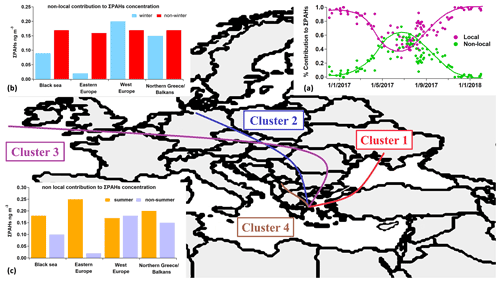
Figure 4The four dominant clusters of backward trajectories, Black Sea (cluster 1: red), eastern Europe (cluster 2: blue), western Europe (cluster 3: purple), and northern Greece/Balkans (cluster 4: brown). Panel (a) shows the local and non-local relative source contributions during the study period. Panels (b, c) show the non-local contribution to ΣPAHs concentration according to the respective air mass origin.
Non-local contributions are intimately linked with regional transport; therefore, an air mass trajectory cluster analysis can help understand their variability and origins. Four major air mass source regions are identified for the GAA during the study period using 96 h back trajectories: the Black Sea area (with a frequency of 43 %), northern Greece/Balkans (32 %), western Europe (20 %), and eastern Europe (5 %).
When examining total PAH levels per trajectory cluster, it should be considered that relevant synoptic circulation patterns can affect the intensity of local emission sources (e.g., during wintertime cold fronts may lead to increased BB), and thus this impedes a fully unbiased assessment. The source apportionment results were utilized here instead to remove the effect of local sources and to associate trajectory clusters more directly with non-local sources.
Figure 4b shows that the non-local contribution of the four clusters to ΣPAH concentrations during the non-winter period was practically the same (differences from the mean within 10 %). For the winter period it can be observed that the contribution from eastern Europe was very small compared to the other clusters. During summer (Fig. 4c), the highest contributions were related to trajectories from eastern Europe, an area identified as a hotspot of PAH production in Europe (EEA, 2018; Guerreiro et al., 2016; Rogula-Kozłowska et al., 2013). However, the small frequency (5 %) of the cluster in summer should be noted. An important summertime enhancement can be also observed for non-local contributions associated with trajectories from the Black Sea area, where extensive summer agricultural burning has been identified as a significant source of carbonaceous aerosol in the eastern Mediterranean (Amiridis et al., 2009; Sciare et al., 2005). This occurrence is mainly for the period from July to September (Sciare et al., 2008) and can be better observed using satellite imagery and fire maps (MODIS, VIIRS-NOAA). Moreover, emission inventories from GWIS (Global Wildfire Information System) verify the identified source area as a major hotspot of fire-related emissions. These fires derive predominantly from agricultural waste burning. For example, in 2017, the fractions of total burned area that were associated with croplands were 96.5 % and 96.1 % in Ukraine and Turkey, respectively. Relevant information is provided in Sect. S5, Fig. S9 in the Supplement. For the western Europe and northern Greece/Balkan clusters, the mean contributions remained effectively constant on a seasonal basis. In non-winter months, when non-local sources become much more prominent, contributions do not depend on air mass sector, possibly indicating that anthropogenic production of harmful aerosols is a continental-scale problem.
3.5 Contributions to carcinogenic potency and risk assessment
The calculated annual BaPeq value at the urban background site of Thissio for 2017 was 0.53 ng m−3, higher than the one reported during 2013 for a suburban background site in the GAA that is less affected by local emissions (0.3 ng m−3) (Alves et al., 2017). With the exception of sites in heavily industrialized areas (Kozielska et al., 2014), studies in other European and North American cities generally calculate BaPeq values in the same order of magnitude as in Athens. However, much higher values on the order of several ng m−3 BaPeq are usually reported for cities in China. (Table S8 in the Supplement). Figure S10 in the Supplement shows the members that contribute the most to BaPeq during the full measurement period; 50 % of the annual BaPeq is attributed to BaP, consistent with studies worldwide (Amador-Muñoz et al., 2010) and confirming its importance as an indicator of PAH carcinogenic risk. The BaPeq value displayed a clear seasonal variability, with the highest levels during winter and the lowest in summer (Table S9 in the Supplement). Especially during nighttime IPE in the winter of 2017–2018, the mean BaP concentration and the BaPeq estimate reached peak values of 2.75 and 5.18 ng m−3, respectively. Comparing to the mean BaPeq from all non-IPE samples (0.21 ng m−3), it appears that these short-duration wintertime events disproportionately influence the mean annual BaPeq value (0.53 ng m−3).
The PMF-resolved concentration profiles were used to obtain BaPeq contributions of each source. The annual contributions of biomass burning, diesel and oil, and gasoline to BaPeq were 43 %, 36 %, and 17 %, respectively, with the remaining 4 % attributed to non-local sources (Fig. 3d). These results clearly highlight BB, in spite of its seasonal and highly episodic character, as a principal driver of long-term carcinogenic risk. Moreover, the carcinogenic risk of PAHs should come under appraisal in the setting of wildfires impacting large urban agglomerations in vulnerable areas with increasing frequency, such as California, Australia, and southern Europe. Such events, especially in the summer months when ambient exposure duration is increased, might become an important factor for both short- and long-term health effects in the years ahead. In the few European studies, where BB factors were apportioned and their contributions to BaPeq were reported (mainly in southern European cities), these contributions were not that pronounced, even during the winter season (Callén et al., 2014; Masiol et al., 2012; Iakovides et al., 2021a).
Inhalation ECR values (Table S9), estimated for the annual period, were equal to (OEHHA method) and (WHO method). These estimates approached or exceeded 10−6, thought to be a threshold above which carcinogenic risks become not acceptable (U.S. EPA, 2011). At current levels, 76 % of the excess risk would be attributed to wintertime exposure, mostly during IPEs, a percentage largely superior to those corresponding to the other three seasons (4 %–14 %). Based on the stricter WHO unit risk, an excess of 4.6 cancer cases per 100 000 people can be linked to inhalation exposure to PAHs in urban background conditions in Athens. For the 3 million living in the central Athens basin, it can be projected that the number of excess cancer cases could be well over 100.
Domestic biomass burning is identified as a considerable source of carcinogenic PAHs in one of the most populated regions of the Mediterranean. Overutilization of wood burning for domestic heating during the economic recession in Greece persists even today despite the improved economy, leading to a significant increase in annual urban background levels of ΣPAHs and BaP with respect to the period preceding the recession. The local biomass burning source, which is present almost exclusively during the winter period, emerges as the most important contributor to carcinogenic toxicity of ΣPAHs (43 % on an annual basis). Therefore, wintertime exposure is seen as responsible for the largest part (76 %) of the estimated excess lifetime cancer risk. This large wintertime enhancement (estimated in 2017) can be mostly attributed to a few nighttime episodes (19 events in 105 d of measurement), revealing a disproportional impact of residential BB emissions but also an opportunity for targeted intervention measures. Given this and the extended usage of biomass burning throughout Europe (e.g., France, Germany, Ireland, and the UK), European action and policies aimed at the regulation of biomass burning emissions are immediately required as they can lead considerable benefits for public health.
Sources related to local road transport were found to be responsible for the major part of ΣPAH concentrations (62 %), indicating that current EU planning to further curb vehicular emissions and promote electromobility can lead to tangible results in reducing urban aerosol toxicity. However, caution is still needed for non-exhaust emissions that are also linked to enhanced particle toxicity and are expected to emerge as the principal vehicle-related particle source in the years to come (Alves et al., 2018; Daellenbach et al., 2020). In this work, there are some indications that the effects of diesel vehicles are weakening in Greek cities, which is consistent with the modernization of the commercial vehicle fleet over the last 2 decades. More research in other European cities, where diesel penetration in the passenger fleets is much higher, could be rather useful to study the relative contributions and trends of diesel and gasoline vehicle contributions.
Non-local sources had a relatively small contribution to ΣPAH level and toxicity, but their relative contribution during the warm period of the year becomes comparable with that of local sources. It should be noted that photochemistry of PAHs could be an important degradation pathway (mainly in summer) leading to formation of oxidized PAHs products, which can be considerably more toxic than parent PAHs. As this may revise the relative importance of non-local compared to local sources, oxy-PAHs are a subject of ongoing research with measurements at Thissio.
It was shown that a comprehensive observation dataset, combined with receptor modeling and back-trajectory analysis provides powerful insights into the source apportionment and contributions to the health risks from PAH exposure. Despite the large body of work to date on PAHs, similar studies are surprisingly scarce in Europe and the US, and thus it is intended that the present study will motivate urgently needed follow-ups in other urban environments.
Data are available upon request to the corresponding authors.
The supplement related to this article is available online at: https://doi.org/10.5194/acp-21-17865-2021-supplement.
Conceptualization was done by AN and NM. The methodology was developed by NM, AN, IT, and GG. The formal analysis was performed by IT and GG. The investigation was undertaken by IT, GG, AB, DP, MA, AG, CP, KT, PZ, MT, and KO. IT and GG prepared the original draft, which was reviewed and edited by IT, GG, AB, DP, NM, and AN. The study was supervised by NM and AN. Funding acquisition and project administration were performed by AN and NM.
The contact author has declared that neither they nor their co-authors have any competing interests.
Publisher's note: Copernicus Publications remains neutral with regard to jurisdictional claims in published maps and institutional affiliations.
This work has been funded by the European Research Council, CoG-2016 project PyroTRACH (726165) H2020-EU.1.1. – Excellent Science. We also acknowledge support by the “PANhellenic infrastructure for Atmospheric Composition and climatE change” (MIS 5021516), which is implemented under the Action “Reinforcement of the Research and Innovation Infrastructure”, funded by the Operational Programme “Competitiveness, Entrepreneurship and Innovation” (NSRF 2014–2020) and co-financed by Greece and the European Union (European Regional Development Fund). The authors thank Eleni Liakakou for providing BC and BC speciation data (BCff and BCwb) for Thissio, Ioannis Hatzianestis of HCMR for providing guidance in the PAH analysis method, Iasonas Stavroulas for the contribution in the ACSM PMF analysis, Panayiotis Kalkavouras for the trajectory cluster analysis, and Maria Lianou for filter collection.
This research has been supported by the H2020 European Research Council (grant no. 726165) and the Hellenic Ministry of Environment and Energy (grant no. MIS 5021516).
This paper was edited by Lynn M. Russell and reviewed by two anonymous referees.
Agudelo-Castañeda, D. M. and Teixeira, E. C.: Seasonal changes, identification and source apportionment of PAH in PM1.0, Atmos. Environ., 96, 186–200, https://doi.org/10.1016/j.atmosenv.2014.07.030, 2014.
Aiken, A. C., Salcedo, D., Cubison, M. J., Huffman, J. A., DeCarlo, P. F., Ulbrich, I. M., Docherty, K. S., Sueper, D., Kimmel, J. R., Worsnop, D. R., Trimborn, A., Northway, M., Stone, E. A., Schauer, J. J., Volkamer, R. M., Fortner, E., de Foy, B., Wang, J., Laskin, A., Shutthanandan, V., Zheng, J., Zhang, R., Gaffney, J., Marley, N. A., Paredes-Miranda, G., Arnott, W. P., Molina, L. T., Sosa, G., and Jimenez, J. L.: Mexico City aerosol analysis during MILAGRO using high resolution aerosol mass spectrometry at the urban supersite (T0) – Part 1: Fine particle composition and organic source apportionment, Atmos. Chem. Phys., 9, 6633–6653, https://doi.org/10.5194/acp-9-6633-2009, 2009.
Al-Naiema, I. M., Hettiyadura, A. P. S., Wallace, H. W., Sanchez, N. P., Madler, C. J., Cevik, B. K., Bui, A. A. T., Kettler, J., Griffin, R. J., and Stone, E. A.: Source apportionment of fine particulate matter in Houston, Texas: insights to secondary organic aerosols, Atmos. Chem. Phys., 18, 15601–15622, https://doi.org/10.5194/acp-18-15601-2018, 2018.
Alfarra, M. R., Prevot, A. S. H., Szidat, S., Sandradewi, J., Weimer, S., Lanz, V. A., Schreiber, D., Mohr, M., and Baltensperger, U.: Identification of the Mass Spectral Signature of Organic Aerosols from Wood Burning Emissions, Environ. Sci. Technol., 41, 5770–5777, https://doi.org/10.1021/es062289b, 2007.
Alves, C. A., Vicente, A. M., Custódio, D., Cerqueira, M., Nunes, T., Pio, C., Lucarelli, F., Calzolai, G., Nava, S., Diapouli, E., Eleftheriadis, K., Querol, X., and Musa Bandowe, B. A.: Polycyclic aromatic hydrocarbons and their derivatives (nitro-PAHs, oxygenated PAHs, and azaarenes) in PM2.5 from Southern European cities, Sci. Total Environ., 595, 494–504, https://doi.org/10.1016/j.scitotenv.2017.03.256, 2017.
Alves, C. A., Evtyugina, M., Vicente, A. M. P., Vicente, E. D., Nunes, T. V., Silva, P. M. A., Duarte, M. A. C., Pio, C. A., Amato, F., and Querol, X.: Chemical profiling of PM10 from urban road dust, Sci. Total Environ., 634, 41–51, https://doi.org/10.1016/j.scitotenv.2018.03.338, 2018.
Amador-Muñoz, O., Villalobos-Pietrini, R., Agapito-Nadales, M. C., Munive-Colín, Z., Hernández-Mena, L., Sánchez-Sandoval, M., Gómez-Arroyo, S., Bravo-Cabrera, J. L., and Guzmán-Rincón, J.: Solvent extracted organic matter and polycyclic aromatic hydrocarbons distributed in size-segregated airborne particles in a zone of México City: Seasonal behavior and human exposure, Atmos. Environ., 44, 122–130, https://doi.org/10.1016/j.atmosenv.2009.07.012, 2010.
Amiridis, V., Balis, D. S., Giannakaki, E., Stohl, A., Kazadzis, S., Koukouli, M. E., and Zanis, P.: Optical characteristics of biomass burning aerosols over Southeastern Europe determined from UV-Raman lidar measurements, Atmos. Chem. Phys., 9, 2431–2440, https://doi.org/10.5194/acp-9-2431-2009, 2009.
Amiridis, V., Zerefos, C., Kazadzis, S., Gerasopoulos, E., Eleftheratos, K., Vrekoussis, M., Stohl, A., Mamouri, R. E., Kokkalis, P., Papayannis, A., Eleftheriadis, K., Diapouli, E., Keramitsoglou, I., Kontoes, C., Kotroni, V., Lagouvardos, K., Marinou, E., Giannakaki, E., Kostopoulou, E., Giannakopoulos, C., Richter, A., Burrows, J. P., and Mihalopoulos, N.: Impact of the 2009 Attica wild fires on the air quality in urban Athens, Atmos. Environ., 46, 536–544, https://doi.org/10.1016/j.atmosenv.2011.07.056, 2012.
Andreou, G. and Rapsomanikis, S.: Polycyclic aromatic hydrocarbons and their oxygenated derivatives in the urban atmosphere of Athens, J. Hazard. Mater., 172, 363–373, https://doi.org/10.1016/j.jhazmat.2009.07.023, 2009.
Bari, M. A., Baumbach, G., Kuch, B., and Scheffknecht, G.: Particle-phase concentrations of polycyclic aromatic hydrocarbons in ambient air of rural residential areas in southern Germany, Air Qual. Atmos. Hlth., 3, 103–116, https://doi.org/10.1007/s11869-009-0057-8, 2010.
Bond, T. C., Doherty, S. J., Fahey, D. W., Forster, P. M., Berntsen, T., DeAngelo, B. J., Flanner, M. G., Ghan, S., Kärcher, B., Koch, D., Kinne, S., Kondo, Y., Quinn, P. K., Sarofim, M. C., Schultz, M. G., Schulz, M., Venkataraman, C., Zhang, H., Zhang, S., Bellouin, N., Guttikunda, S. K., Hopke, P. K., Jacobson, M. Z., Kaiser, J. W., Klimont, Z., Lohmann, U., Schwarz, J. P., Shindell, D., Storelvmo, T., Warren, S. G., and Zender, C. S.: Bounding the role of black carbon in the climate system: A scientific assessment, J. Geophys. Res.-Atmos., 118, 5380–5552, https://doi.org/10.1002/jgrd.50171, 2013.
Bougiatioti, A., Stavroulas, I., Kostenidou, E., Zarmpas, P., Theodosi, C., Kouvarakis, G., Canonaco, F., Prévôt, A. S. H., Nenes, A., Pandis, S. N., and Mihalopoulos, N.: Processing of biomass-burning aerosol in the eastern Mediterranean during summertime, Atmos. Chem. Phys., 14, 4793–4807, https://doi.org/10.5194/acp-14-4793-2014, 2014.
Bozzetti, C., Sosedova, Y., Xiao, M., Daellenbach, K. R., Ulevicius, V., Dudoitis, V., Mordas, G., Byčenkienė, S., Plauškaitė, K., Vlachou, A., Golly, B., Chazeau, B., Besombes, J.-L., Baltensperger, U., Jaffrezo, J.-L., Slowik, J. G., El Haddad, I., and Prévôt, A. S. H.: Argon offline-AMS source apportionment of organic aerosol over yearly cycles for an urban, rural, and marine site in northern Europe, Atmos. Chem. Phys., 17, 117–141, https://doi.org/10.5194/acp-17-117-2017, 2017.
Buzcu-Guven, B., Brown, S. G., Frankel, A., Hafner, H. R., and Roberts, P. T.: Analysis and Apportionment of Organic Carbon and Fine Particulate Matter Sources at Multiple Sites in the Midwestern United States, J. Air Waste Manage., 57, 606–619, https://doi.org/10.3155/1047-3289.57.5.606, 2007.
Callén, M. S., López, J. M., Iturmendi, A., and Mastral, A. M.: Nature and sources of particle associated polycyclic aromatic hydrocarbons (PAH) in the atmospheric environment of an urban area, Environ. Pollut., 183, 166–174, https://doi.org/10.1016/j.envpol.2012.11.009, 2013.
Callén, M. S., Iturmendi, A., and López, J. M.: Source apportionment of atmospheric PM2.5-bound polycyclic aromatic hydrocarbons by a PMF receptor model. Assessment of potential risk for human health, Environ. Pollut., 195, 167–177, https://doi.org/10.1016/j.envpol.2014.08.025, 2014.
Canonaco, F., Crippa, M., Slowik, J. G., Baltensperger, U., and Prévôt, A. S. H.: SoFi, an IGOR-based interface for the efficient use of the generalized multilinear engine (ME-2) for the source apportionment: ME-2 application to aerosol mass spectrometer data, Atmos. Meas. Tech., 6, 3649–3661, https://doi.org/10.5194/amt-6-3649-2013, 2013.
Cavalli, F., Viana, M., Yttri, K. E., Genberg, J., and Putaud, J.-P.: Toward a standardised thermal-optical protocol for measuring atmospheric organic and elemental carbon: the EUSAAR protocol, Atmos. Meas. Tech., 3, 79–89, https://doi.org/10.5194/amt-3-79-2010, 2010.
Collier, S., Zhou, S., Kuwayama, T., Forestieri, S., Brady, J., Zhang, M., Kleeman, M., Cappa, C., Bertram, T., and Zhang, Q.: Organic PM Emissions from Vehicles: Composition, OC ratio, and Dependence on PM Concentration, Aerosol Sci. Tech., 49, 86–97, https://doi.org/10.1080/02786826.2014.1003364, 2015.
Cui, S., Lei, R., Wu, Y., Huang, D., Shen, F., Wang, J., Qiao, L., Zhou, M., Zhu, S., Ma, Y., and Ge, X.: Characteristics of Black Carbon Particle-Bound Polycyclic Aromatic Hydrocarbons in Two Sites of Nanjing and Shanghai, China, Atmosphere-Basel, 11, 202, https://doi.org/10.3390/atmos11020202, 2020.
Daellenbach, K. R., Uzu, G., Jiang, J., Cassagnes, L.-E., Leni, Z., Vlachou, A., Stefenelli, G., Canonaco, F., Weber, S., Segers, A., Kuenen, J. J. P., Schaap, M., Favez, O., Albinet, A., Aksoyoglu, S., Dommen, J., Baltensperger, U., Geiser, M., El Haddad, I., Jaffrezo, J.-L., and Prévôt, A. S. H.: Sources of particulate-matter air pollution and its oxidative potential in Europe, Nature, 587, 414–419, https://doi.org/10.1038/s41586-020-2902-8, 2020.
Diapouli, E., Manousakas, M., Vratolis, S., Vasilatou, V., Maggos, T., Saraga, D., Grigoratos, T., Argyropoulos, G., Voutsa, D., Samara, C., and Eleftheriadis, K.: Evolution of air pollution source contributions over one decade, derived by PM10 and PM2.5 source apportionment in two metropolitan urban areas in Greece, Atmos. Environ., 164, 416–430, https://doi.org/10.1016/j.atmosenv.2017.06.016, 2017.
Draxler, R. R. and Rolph, G. D.: HYSPLIT (HYbrid Single-particle Lagrangian Integrated Trajectory). Model Access via NOAA ARL READY NOAA Air Resources Laboratory, Silver Spring, 2016, available at: http://ready.arl.noaa.gov/HYSPLIT.php (last access: 21 September 2021), 2016.
Drinovec, L., Močnik, G., Zotter, P., Prévôt, A. S. H., Ruckstuhl, C., Coz, E., Rupakheti, M., Sciare, J., Müller, T., Wiedensohler, A., and Hansen, A. D. A.: The “dual-spot” Aethalometer: an improved measurement of aerosol black carbon with real-time loading compensation, Atmos. Meas. Tech., 8, 1965–1979, https://doi.org/10.5194/amt-8-1965-2015, 2015.
Dutton, S. J., Rajagopalan, B., Vedal, S., and Hannigan, M. P.: Temporal patterns in daily measurements of inorganic and organic speciated PM2.5 in Denver, Atmos. Environ., 44, 987–998, https://doi.org/10.1016/j.atmosenv.2009.06.006, 2010.
EIONET: National Emission Inventory for Greece, available at: https://cdr.eionet.europa.eu/gr/eu/nec_revised/inventories/envycotfw/, last access: 3 November 2021.
Elzein, A., Dunmore, R. E., Ward, M. W., Hamilton, J. F., and Lewis, A. C.: Variability of polycyclic aromatic hydrocarbons and their oxidative derivatives in wintertime Beijing, China, Atmos. Chem. Phys., 19, 8741–8758, https://doi.org/10.5194/acp-19-8741-2019, 2019.
European Environmental Agency: Air quality in Europe – 2018 report No 12/2018, available at: https://www.eea.europa.eu/publications/air-quality-in-europe-2018 (last access: 3 November 2021), 2018.
Fameli, K.-M. and Assimakopoulos, V. D.: The new open Flexible Emission Inventory for Greece and the Greater Athens Area (FEI-GREGAA): Account of pollutant sources and their importance from 2006 to 2012, Atmos. Environ., 137, 17–37, https://doi.org/10.1016/j.atmosenv.2016.04.004, 2016.
Finlayson-Pitts, B. J.: Tropospheric Air Pollution: Ozone, Airborne Toxics, Polycyclic Aromatic Hydrocarbons, and Particles, Science, 276, 1045–1051, https://doi.org/10.1126/science.276.5315.1045, 1997.
Florou, K., Papanastasiou, D. K., Pikridas, M., Kaltsonoudis, C., Louvaris, E., Gkatzelis, G. I., Patoulias, D., Mihalopoulos, N., and Pandis, S. N.: The contribution of wood burning and other pollution sources to wintertime organic aerosol levels in two Greek cities, Atmos. Chem. Phys., 17, 3145–3163, https://doi.org/10.5194/acp-17-3145-2017, 2017.
Fountoukis, C., Megaritis, A. G., Skyllakou, K., Charalampidis, P. E., Denier van der Gon, H. A. C., Crippa, M., Prévôt, A. S. H., Fachinger, F., Wiedensohler, A., Pilinis, C., and Pandis, S. N.: Simulating the formation of carbonaceous aerosol in a European Megacity (Paris) during the MEGAPOLI summer and winter campaigns, Atmos. Chem. Phys., 16, 3727–3741, https://doi.org/10.5194/acp-16-3727-2016, 2016.
Fourtziou, L., Liakakou, E., Stavroulas, I., Theodosi, C., Zarmpas, P., Psiloglou, B., Sciare, J., Maggos, T., Bairachtari, K., Bougiatioti, A., Gerasopoulos, E., Sarda-Estève, R., Bonnaire, N., and Mihalopoulos, N.: Multi-tracer approach to characterize domestic wood burning in Athens (Greece) during wintertime, Atmos. Environ., 148, 89–101, https://doi.org/10.1016/j.atmosenv.2016.10.011, 2017.
Galarneau, E.: Source specificity and atmospheric processing of airborne PAHs: Implications for source apportionment, Atmos. Environ., 42, 8139–8149, https://doi.org/10.1016/j.atmosenv.2008.07.025, 2008.
Gogou, A. I., Apostolaki, M., and Stephanou, E. G.: Determination of organic molecular markers in marine aerosols and sediments: one-step flash chromatography compound class fractionation and capillary gas chromatographic analysis, J. Chromatogr. A, 799, 215–231, https://doi.org/10.1016/S0021-9673(97)01106-0, 1998.
Gratsea, M., Liakakou, E., Mihalopoulos, N., Adamopoulos, A., Tsilibari, E., and Gerasopoulos, E.: The combined effect of reduced fossil fuel consumption and increasing biomass combustion on Athens' air quality, as inferred from long term CO measurements, Sci. Total Environ., 592, 115–123, https://doi.org/10.1016/j.scitotenv.2017.03.045, 2017.
Grivas, G., Cheristanidis, S., and Chaloulakou, A.: Elemental and organic carbon in the urban environment of Athens. Seasonal and diurnal variations and estimates of secondary organic carbon, Sci. Total Environ., 414, 535–545, https://doi.org/10.1016/j.scitotenv.2011.10.058, 2012.
Grivas, G., Cheristanidis, S., Chaloulakou, A., Koutrakis, P., and Mihalopoulos, N.: Elemental Composition and Source Apportionment of Fine and Coarse Particles at Traffic and Urban Background Locations in Athens, Greece, Aerosol Air Qual. Res., 18, 1642–1659, https://doi.org/10.4209/aaqr.2017.12.0567, 2018.
Grivas, G., Stavroulas, I., Liakakou, E., Kaskaoutis, D. G., Bougiatioti, A., Paraskevopoulou, D., Gerasopoulos, E., and Mihalopoulos, N.: Measuring the spatial variability of black carbon in Athens during wintertime, Air Qual. Atmos. Hlth., 12, 1405–1417, https://doi.org/10.1007/s11869-019-00756-y, 2019.
Guerreiro, C. B. B., Horálek, J., de Leeuw, F., and Couvidat, F.: Benzo(a)pyrene in Europe: Ambient air concentrations, population exposure and health effects, Environ. Pollut., 214, 657–667, https://doi.org/10.1016/j.envpol.2016.04.081, 2016.
Han, B., You, Y., Liu, Y., Xu, J., Zhou, J., Zhang, J., Niu, C., Zhang, N., He, F., Ding, X., and Bai, Z.: Inhalation cancer risk estimation of source-specific personal exposure for particulate matter–bound polycyclic aromatic hydrocarbons based on positive matrix factorization, Environ. Sci. Pollut. R., 26, 10230–10239, https://doi.org/10.1007/s11356-019-04198-y, 2019.
Haque, Md. M., Kawamura, K., Deshmukh, D. K., Fang, C., Song, W., Mengying, B., and Zhang, Y.-L.: Characterization of organic aerosols from a Chinese megacity during winter: predominance of fossil fuel combustion, Atmos. Chem. Phys., 19, 5147–5164, https://doi.org/10.5194/acp-19-5147-2019, 2019.
Hasheminassab, S., Daher, N., Saffari, A., Wang, D., Ostro, B. D., and Sioutas, C.: Spatial and temporal variability of sources of ambient fine particulate matter (PM2.5) in California, Atmos. Chem. Phys., 14, 12085–12097, https://doi.org/10.5194/acp-14-12085-2014, 2014.
Hellenic Statistical Authority: Consumption of petroleum products/2019, https://www.statistics.gr/en/statistics/-/publication/SDE15/2019 (last access: 3 November 2021), 2019.
Hellenic Statistical Authority: Vehicle fleet/January 2020, https://www.statistics.gr/en/statistics/-/publication/SME18/2020 (last access: 3 November 2021), 2020.
Heringa, M. F., DeCarlo, P. F., Chirico, R., Tritscher, T., Dommen, J., Weingartner, E., Richter, R., Wehrle, G., Prévôt, A. S. H., and Baltensperger, U.: Investigations of primary and secondary particulate matter of different wood combustion appliances with a high-resolution time-of-flight aerosol mass spectrometer, Atmos. Chem. Phys., 11, 5945–5957, https://doi.org/10.5194/acp-11-5945-2011, 2011.
Iakovides, M., Iakovides, G., and Stephanou, E. G.: Atmospheric particle-bound polycyclic aromatic hydrocarbons, n-alkanes, hopanes, steranes and trace metals: PM2.5 source identification, individual and cumulative multi-pathway lifetime cancer risk assessment in the urban environment, Sci. Total Environ., 752, 141834, https://doi.org/10.1016/j.scitotenv.2020.141834, 2021a.
Iakovides, M., Apostolaki, M., and Stephanou, E. G.: PAHs, PCBs and organochlorine pesticides in the atmosphere of Eastern Mediterranean: Investigation of their occurrence, sources and gas-particle partitioning in relation to air mass transport pathways, Atmos. Environ., 244, 117931, https://doi.org/10.1016/j.atmosenv.2020.117931, 2021b.
Iinuma, Y., Engling, G., Puxbaum, H., and Herrmann, H.: A highly resolved anion-exchange chromatographic method for determination of saccharidic tracers for biomass combustion and primary bio-particles in atmospheric aerosol, Atmos. Environ., 43, 1367–1371, https://doi.org/10.1016/j.atmosenv.2008.11.020, 2009.
IARC: IARC Monographs on the Evaluation of Carcinogenic Risks to Humans, Vol. 92, International Agency for Research on Cancer, Lyon, France, 2010.
Javed, W., Iakovides, M., Garaga, R., Stephanou, E. G., Kota, S. H., Ying, Q., Wolfson, J. M., Koutrakis, P., and Guo, B.: Source apportionment of organic pollutants in fine and coarse atmospheric particles in Doha, Qatar, J. Air Waste Manage., 69, 1277–1292, https://doi.org/10.1080/10962247.2019.1640803, 2019.
Jedynska, A., Hoek, G., Eeftens, M., Cyrys, J., Keuken, M., Ampe, C., Beelen, R., Cesaroni, G., Forastiere, F., Cirach, M., de Hoogh, K., De Nazelle, A., Madsen, C., Declercq, C., Eriksen, K. T., Katsouyanni, K., Akhlaghi, H. M., Lanki, T., Meliefste, K., Nieuwenhuijsen, M., Oldenwening, M., Pennanen, A., Raaschou-Nielsen, O., Brunekreef, B., and Kooter, I. M.: Spatial variations of PAH, hopanes/steranes and EC/OC concentrations within and between European study areas, Atmos. Environ., 87, 239–248, https://doi.org/10.1016/j.atmosenv.2014.01.026, 2014.
Jiang, B., Liang, Y., Xu, C., Zhang, J., Hu, M., and Shi, Q.: Polycyclic Aromatic Hydrocarbons (PAHs) in Ambient Aerosols from Beijing: Characterization of Low Volatile PAHs by Positive-Ion Atmospheric Pressure Photoionization (APPI) Coupled with Fourier Transform Ion Cyclotron Resonance, Environ. Sci. Technol., 48, 4716–4723, https://doi.org/10.1021/es405295p, 2014.
Kalkavouras, P., Bougiatioti, A., Grivas, G., Stavroulas, I., Kalivitis, N., Liakakou, E., Gerasopoulos, E., Pilinis, C., and Mihalopoulos, N.: On the regional aspects of new particle formation in the Eastern Mediterranean: A comparative study between a background and an urban site based on long term observations, Atmos. Res., 239, 104911, https://doi.org/10.1016/j.atmosres.2020.104911, 2020.
Kaskaoutis, D. G., Grivas, G., Stavroulas, I., Liakakou, E., Dumka, U. C., Dimitriou, K., Gerasopoulos, E., and Mihalopoulos, N.: In situ identification of aerosol types in Athens, Greece, based on long-term optical and on online chemical characterization, Atmos. Environ., 246, 118070, https://doi.org/10.1016/j.atmosenv.2020.118070, 2021.
Kassomenos, P., Flocas, H. A., Lykoudis, S., and Petrakis, M.: Analysis of Mesoscale Patterns in Relation to Synoptic Conditionsover an Urban Mediterranean Basin, Theor. Appl. Climatol., 59, 215–229, https://doi.org/10.1007/s007040050025, 1998.
Katsoyiannis, A., Sweetman, A. J., and Jones, K. C.: PAH Molecular Diagnostic Ratios Applied to Atmospheric Sources: A Critical Evaluation Using Two Decades of Source Inventory and Air Concentration Data from the UK, Environ. Sci. Technol., 45, 8897–8906, https://doi.org/10.1021/es202277u, 2011.
Kocbach Bølling, A., Pagels, J., Yttri, K. E., Barregard, L., Sallsten, G., Schwarze, P. E., and Boman, C.: Health effects of residential wood smoke particles: the importance of combustion conditions and physicochemical particle properties, Part. Fibre Toxicol., 6, 29, https://doi.org/10.1186/1743-8977-6-29, 2009.
Kodros, J. K., Papanastasiou, D. K., Paglione, M., Masiol, M., Squizzato, S., Florou, K., Skyllakou, K., Kaltsonoudis, C., Nenes, A., and Pandis, S. N.: Rapid dark aging of biomass burning as an overlooked source of oxidized organic aerosol, P. Natl. Acad. Sci. USA, 117, 33028–33033, https://doi.org/10.1073/pnas.2010365117, 2020.
Kokkalis, P., Alexiou, D., Papayannis, A., Rocadenbosch, F., Soupiona, O., Raptis, P.-I., Mylonaki, M., Tzanis, C. G., and Christodoulakis, J.: Application and Testing of the Extended-Kalman-Filtering Technique for Determining the Planetary Boundary-Layer Height over Athens, Greece, Bound.-Lay. Meteorol., 176, 125–147, https://doi.org/10.1007/s10546-020-00514-z, 2020.
Kozielska, B., Rogula-Kozłowska, W., and Klejnowski, K.: Seasonal Variations in Health Hazards from Polycyclic Aromatic Hydrocarbons Bound to Submicrometer Particles at Three Characteristic Sites in the Heavily Polluted Polish Region, Atmosphere-Basel, 6, 1–20, https://doi.org/10.3390/atmos6010001, 2014.
Lhotka, R., Pokorná, P., and Zíková, N.: Long-Term Trends in PAH Concentrations and Sources at Rural Background Site in Central Europe, Atmosphere-Basel, 10, 687, https://doi.org/10.3390/atmos10110687, 2019.
Li, F., Schnelle-Kreis, J., Cyrys, J., Karg, E., Gu, J., Abbaszade, G., Orasche, J., Peters, A., and Zimmermann, R.: Organic speciation of ambient quasi-ultrafine particulate matter (PM0.36) in Augsburg, Germany: Seasonal variability and source apportionment, Sci. Total Environ., 615, 828–837, https://doi.org/10.1016/j.scitotenv.2017.09.158, 2018.
Liakakou, E., Stavroulas, I., Kaskaoutis, D. G., Grivas, G., Paraskevopoulou, D., Dumka, U. C., Tsagkaraki, M., Bougiatioti, A., Oikonomou, K., Sciare, J., Gerasopoulos, E., and Mihalopoulos, N.: Long-term variability, source apportionment and spectral properties of black carbon at an urban background site in Athens, Greece, Atmos. Environ., 222, 117137, https://doi.org/10.1016/j.atmosenv.2019.117137, 2020.
Lin, Y., Ma, Y., Qiu, X., Li, R., Fang, Y., Wang, J., Zhu, Y., and Hu, D.: Sources, transformation, and health implications of PAHs and their nitrated, hydroxylated, and oxygenated derivatives in PM2.5 in Beijing, J. Geophys. Res.-Atmos., 120, 7219–7228, https://doi.org/10.1002/2015JD023628, 2015.
Liu, W., Xu, Y., Zhao, Y., Liu, Q., Yu, S., Liu, Y., Wang, X., Liu, Y., Tao, S., and Liu, W.: Occurrence, source, and risk assessment of atmospheric parent polycyclic aromatic hydrocarbons in the coastal cities of the Bohai and Yellow Seas, China, Environ. Pollut., 254, 113046, https://doi.org/10.1016/j.envpol.2019.113046, 2019.
Lough, G. C., Schauer, J. J., and Lawson, D. R.: Day-of-week trends in carbonaceous aerosol composition in the urban atmosphere, Atmos. Environ., 40, 4137–4149, https://doi.org/10.1016/j.atmosenv.2006.03.009, 2006.
Mandalakis, M., Tsapakis, M., Tsoga, A., and Stephanou, E. G.: Gas–particle concentrations and distribution of aliphatic hydrocarbons, PAHs, PCBs and PCDD/Fs in the atmosphere of Athens (Greece), Atmos. Environ., 36, 4023–4035, https://doi.org/10.1016/S1352-2310(02)00362-X, 2002.
Manoli, E., Kouras, A., Karagkiozidou, O., Argyropoulos, G., Voutsa, D., and Samara, C.: Polycyclic aromatic hydrocarbons (PAHs) at traffic and urban background sites of northern Greece: source apportionment of ambient PAH levels and PAH-induced lung cancer risk, Environ. Sci. Pollut. R., 23, 3556–3568, https://doi.org/10.1007/s11356-015-5573-5, 2016.
Mantis, J., Chaloulakou, A., and Samara, C.: PM10-bound polycyclic aromatic hydrocarbons (PAHs) in the Greater Area of Athens, Greece, Chemosphere, 59, 593–604, https://doi.org/10.1016/j.chemosphere.2004.10.019, 2005.
Mao, S., Li, J., Cheng, Z., Zhong, G., Li, K., Liu, X., and Zhang, G.: Contribution of Biomass Burning to Ambient Particulate Polycyclic Aromatic Hydrocarbons at a Regional Background Site in East China, Environ. Sci. Tech. Let., 5, 56–61, https://doi.org/10.1021/acs.estlett.8b00001, 2018.
Marino, F., Cecinato, A., and Siskos, P. A.: Nitro-PAH in ambient particulate matter in the atmosphere of Athens, Chemosphere, 40, 533–537, https://doi.org/10.1016/S0045-6535(99)00308-2, 2000.
Masiol, M., Hofer, A., Squizzato, S., Piazza, R., Rampazzo, G., and Pavoni, B.: Carcinogenic and mutagenic risk associated to airborne particle-phase polycyclic aromatic hydrocarbons: A source apportionment, Atmos. Environ., 60, 375–382, https://doi.org/10.1016/j.atmosenv.2012.06.073, 2012.
Masiol, M., Squizzato, S., Formenton, G., Khan, M. B., Hopke, P. K., Nenes, A., Pandis, S. N., Tositti, L., Benetello, F., Visin, F., and Pavoni, B.: Hybrid multiple-site mass closure and source apportionment of PM2.5 and aerosol acidity at major cities in the Po Valley, Sci. Total Environ., 704, 135287, https://doi.org/10.1016/j.scitotenv.2019.135287, 2020.
McCarty, J. L., Smith, T. E. L., and Turetsky, M. R.: Arctic fires re-emerging, Nat. Geosci., 13, 658–660, https://doi.org/10.1038/s41561-020-00645-5, 2020.
Miura, K., Shimada, K., Sugiyama, T., Sato, K., Takami, A., Chan, C. K., Kim, I. S., Kim, Y. P., Lin, N.-H., and Hatakeyama, S.: Seasonal and annual changes in PAH concentrations in a remote site in the Pacific Ocean, Sci. Rep.-UK, 9, 12591, https://doi.org/10.1038/s41598-019-47409-9, 2019.
Mo, Z., Wang, Z., Mao, G., Pan, X., Wu, L., Xu, P., Chen, S., Wang, A., Zhang, Y., Luo, J., Ye, X., Wang, X., Chen, Z., and Lou, X.: Characterization and health risk assessment of PM2.5-bound polycyclic aromatic hydrocarbons in 5 urban cities of Zhejiang Province, China, Sci. Rep.-UK, 9, 7296, https://doi.org/10.1038/s41598-019-43557-0, 2019.
Myriokefalitakis, S., Tsigaridis, K., Mihalopoulos, N., Sciare, J., Nenes, A., Kawamura, K., Segers, A., and Kanakidou, M.: In-cloud oxalate formation in the global troposphere: a 3-D modeling study, Atmos. Chem. Phys., 11, 5761–5782, https://doi.org/10.5194/acp-11-5761-2011, 2011.
Naeher, L. P., Brauer, M., Lipsett, M., Zelikoff, J. T., Simpson, C. D., Koenig, J. Q., and Smith, K. R.: Woodsmoke Health Effects: A Review, Inhal. Toxicol., 19, 67–106, https://doi.org/10.1080/08958370600985875, 2007.
NAPMN: Annual Air Quality Report 2019, Greek Ministry of Environment and Energy, Air Quality Department, Athens, 2020 (in Greek).
Ng, Y. L., Mann, V., and Gulabivala, K.: A prospective study of the factors affecting outcomes of nonsurgical root canal treatment: Part 1: Periapical health, Int. Endod. J., 44, 583–609, https://doi.org/10.1111/j.1365-2591.2011.01872.x, 2011.
Nisbet, I. C. T. and LaGoy, P. K.: Toxic equivalency factors (TEFs) for polycyclic aromatic hydrocarbons (PAHs), Regul. Toxicol. Pharm., 16, 290–300, https://doi.org/10.1016/0273-2300(92)90009-X, 1992.
Norris, G., Duvall, R., Brown, S., and Bai, S.: EPA Positive Matrix Factorization (PMF) 5.0 Fundamentals and User Guide, U.S. Environmental Protection Agency, Washington, DC, EPA/600/R-14/108 (NTIS PB2015-105147), 2014.
Nowakowski, T. J., Bhaduri, A., Pollen, A. A., Alvarado, B., Mostajo-radji, M. A., Lullo, E. Di, Haeussler, M., Sandoval-espinosa, C., Liu, S. J., Velmeshev, D., Ounadjela, J. R., Shuga, J., Wang, X., Lim, D. A., West, J. A., Leyrat, A. A., Kent, W. J., and Kriegstein, A. R.: Spatiotemporal gene expression trajectories reveal developmental hierarchies of the human cortex, Science, 1323, 1318–1323, https://doi.org/10.1126/science.aap8809, 2017.
O'Dell, K., Hornbrook, R. S., Permar, W., Levin, E. J. T., Garofalo, L. A., Apel, E. C., Blake, N. J., Jarnot, A., Pothier, M. A., Farmer, D. K., Hu, L., Campos, T., Ford, B., Pierce, J. R., and Fischer, E. V: Hazardous Air Pollutants in Fresh and Aged Western US Wildfire Smoke and Implications for Long-Term Exposure, Environ. Sci. Technol., 54, 11838–11847, https://doi.org/10.1021/acs.est.0c04497, 2020.
Paatero, P., Eberly, S., Brown, S. G., and Norris, G. A.: Methods for estimating uncertainty in factor analytic solutions, Atmos. Meas. Tech., 7, 781–797, https://doi.org/10.5194/amt-7-781-2014, 2014.
Pankow, J. F.: An absorption model of the gas/aerosol partitioning involved in the formation of secondary organic aerosol, Atmos. Environ., 28, 189–193, https://doi.org/10.1016/1352-2310(94)90094-9, 1994.
Paraskevopoulou, D., Liakakou, E., Gerasopoulos, E., Theodosi, C., and Mihalopoulos, N.: Long-term characterization of organic and elemental carbon in the PM2.5 fraction: the case of Athens, Greece, Atmos. Chem. Phys., 14, 13313–13325, https://doi.org/10.5194/acp-14-13313-2014, 2014.
Paraskevopoulou, D., Liakakou, E., Gerasopoulos, E., and Mihalopoulos, N.: Sources of atmospheric aerosol from long-term measurements (5 years) of chemical composition in Athens, Greece, Sci. Total Environ., 527–528, 165–178, https://doi.org/10.1016/j.scitotenv.2015.04.022, 2015.
Parinos, C., Hatzianestis, I., Chourdaki, S., Plakidi, E., and Gogou, A.: Imprint and short-term fate of the Agia Zoni II tanker oil spill on the marine ecosystem of Saronikos Gulf, Sci. Total Environ., 693, 133568, https://doi.org/10.1016/j.scitotenv.2019.07.374, 2019.
Pateraki, S., Manousakas, M., Bairachtari, K., Kantarelou, V., Eleftheriadis, K., Vasilakos, C., Assimakopoulos, V. D., and Maggos, T.: The traffic signature on the vertical PM profile: Environmental and health risks within an urban roadside environment, Sci. Total Environ., 646, 448–459, https://doi.org/10.1016/j.scitotenv.2018.07.289, 2019.
Piletic, I. R., Offenberg, J. H., Olson, D. A., Jaoui, M., Krug, J., Lewandowski, M., Turlington, J. M., and Kleindienst, T. E.: Constraining carbonaceous aerosol sources in a receptor model by including 14C data with redox species, organic tracers, and elemental/organic carbon measurements, Atmos. Environ., 80, 216–225, https://doi.org/10.1016/j.atmosenv.2013.07.062, 2013.
Pio, C., Cerqueira, M., Harrison, R. M., Nunes, T., Mirante, F., Alves, C., Oliveira, C., Sanchez de la Campa, A., Artíñano, B., and Matos, M.: OCEC ratio observations in Europe: Re-thinking the approach for apportionment between primary and secondary organic carbon, Atmos. Environ., 45, 6121–6132, https://doi.org/10.1016/j.atmosenv.2011.08.045, 2011.
Poulain, L., Iinuma, Y., Müller, K., Birmili, W., Weinhold, K., Brüggemann, E., Gnauk, T., Hausmann, A., Löschau, G., Wiedensohler, A., and Herrmann, H.: Diurnal variations of ambient particulate wood burning emissions and their contribution to the concentration of Polycyclic Aromatic Hydrocarbons (PAHs) in Seiffen, Germany, Atmos. Chem. Phys., 11, 12697–12713, https://doi.org/10.5194/acp-11-12697-2011, 2011.
Ravindra, K., Sokhi, R., and Van Grieken, R.: Atmospheric polycyclic aromatic hydrocarbons: Source attribution, emission factors and regulation, Atmos. Environ., 42, 2895–2921, https://doi.org/10.1016/j.atmosenv.2007.12.010, 2008.
Reyes-Villegas, E., Green, D. C., Priestman, M., Canonaco, F., Coe, H., Prévôt, A. S. H., and Allan, J. D.: Organic aerosol source apportionment in London 2013 with ME-2: exploring the solution space with annual and seasonal analysis, Atmos. Chem. Phys., 16, 15545–15559, https://doi.org/10.5194/acp-16-15545-2016, 2016.
Ringuet, J., Albinet, A., Leoz-Garziandia, E., Budzinski, H., and Villenave, E.: Diurnal/nocturnal concentrations and sources of particulate-bound PAHs, OPAHs and NPAHs at traffic and suburban sites in the region of Paris (France), Sci. Total Environ., 437, 297–305, https://doi.org/10.1016/j.scitotenv.2012.07.072, 2012.
Rogula-Kozłowska, W., Kozielska, B., and Klejnowski, K.: Concentration, Origin and Health Hazard from Fine Particle-Bound PAH at Three Characteristic Sites in Southern Poland, B. Environ. Contam. Tox., 91, 349–355, https://doi.org/10.1007/s00128-013-1060-1, 2013.
Saffari, A., Daher, N., Samara, C., Voutsa, D., Kouras, A., Manoli, E., Karagkiozidou, O., Vlachokostas, C., Moussiopoulos, N., Shafer, M. M., Schauer, J. J., and Sioutas, C.: Increased Biomass Burning Due to the Economic Crisis in Greece and Its Adverse Impact on Wintertime Air Quality in Thessaloniki, Environ. Sci. Technol., 47, 13313–13320, https://doi.org/10.1021/es403847h, 2013.
Sandradewi, J., Prévôt, A. S. H., Szidat, S., Perron, N., Alfarra, M. R., Lanz, V. A., Weingartner, E., and Baltensperger, U.: Using Aerosol Light Absorption Measurements for the Quantitative Determination of Wood Burning and Traffic Emission Contributions to Particulate Matter, Environ. Sci. Technol., 42, 3316–3323, https://doi.org/10.1021/es702253m, 2008.
Schmidl, C., Marr, I. L., Caseiro, A., Kotianová, P., Berner, A., Bauer, H., Kasper-Giebl, A., and Puxbaum, H.: Chemical characterisation of fine particle emissions from wood stove combustion of common woods growing in mid-European Alpine regions, Atmos. Environ., 42, 126–141, https://doi.org/10.1016/j.atmosenv.2007.09.028, 2008.
Schnelle-Kreis, J., Sklorz, M., Orasche, J., Stölzel, M., Peters, A., and Zimmermann, R.: Semi Volatile Organic Compounds in Ambient PM2.5. Seasonal Trends and Daily Resolved Source Contributions, Environ. Sci. Technol., 41, 3821–3828, https://doi.org/10.1021/es060666e, 2007.
Sciare, J., Oikonomou, K., Cachier, H., Mihalopoulos, N., Andreae, M. O., Maenhaut, W., and Sarda-Estève, R.: Aerosol mass closure and reconstruction of the light scattering coefficient over the Eastern Mediterranean Sea during the MINOS campaign, Atmos. Chem. Phys., 5, 2253–2265, https://doi.org/10.5194/acp-5-2253-2005, 2005.
Sciare, J., Oikonomou, K., Favez, O., Liakakou, E., Markaki, Z., Cachier, H., and Mihalopoulos, N.: Long-term measurements of carbonaceous aerosols in the Eastern Mediterranean: evidence of long-range transport of biomass burning, Atmos. Chem. Phys., 8, 5551–5563, https://doi.org/10.5194/acp-8-5551-2008, 2008.
Shah, R. U., Robinson, E. S., Gu, P., Robinson, A. L., Apte, J. S., and Presto, A. A.: High-spatial-resolution mapping and source apportionment of aerosol composition in Oakland, California, using mobile aerosol mass spectrometry, Atmos. Chem. Phys., 18, 16325–16344, https://doi.org/10.5194/acp-18-16325-2018, 2018.
Shiraiwa, M., Ammann, M., Koop, T., and Pöschl, U.: Gas uptake and chemical aging of semisolid organic aerosol particles, P. Natl. Acad. Sci. USA, 108, 11003–11008, https://doi.org/10.1073/pnas.1103045108, 2011.
Shirmohammadi, F., Hasheminassab, S., Saffari, A., Schauer, J. J., Delfino, R. J., and Sioutas, C.: Fine and ultrafine particulate organic carbon in the Los Angeles basin: Trends in sources and composition, Sci. Total Environ., 541, 1083–1096, https://doi.org/10.1016/j.scitotenv.2015.09.133, 2016.
Simoneit, B. R.: Biomass burning – a review of organic tracers for smoke from incomplete combustion, Appl. Geochem., 17, 129–162, https://doi.org/10.1016/S0883-2927(01)00061-0, 2002.
Sitaras, I. E. and Siskos, P. A.: Levels of Volatile Polycyclic Aromatic Hydrocarbons in the Atmosphere of Athens, Greece, Polycycl. Aromat. Comp., 18, 451–467, https://doi.org/10.1080/10406630108233820, 2001.
Sitaras, I. E. and Siskos, P. A.: The role of primary and secondary air pollutants in atmospheric pollution: Athens urban area as a case study, Environ. Chem. Lett., 6, 59–69, https://doi.org/10.1007/s10311-007-0123-0, 2008.
Skyllakou, K., Murphy, B. N., Megaritis, A. G., Fountoukis, C., and Pandis, S. N.: Contributions of local and regional sources to fine PM in the megacity of Paris, Atmos. Chem. Phys., 14, 2343–2352, https://doi.org/10.5194/acp-14-2343-2014, 2014.
Sofowote, U. M., McCarry, B. E., and Marvin, C. H.: Source Apportionment of PAH in Hamilton Harbour Suspended Sediments: Comparison of Two Factor Analysis Methods, Environ. Sci. Technol., 42, 6007–6014, https://doi.org/10.1021/es800219z, 2008.
Srivastava, D., Tomaz, S., Favez, O., Lanzafame, G. M., Golly, B., Besombes, J.-L., Alleman, L. Y., Jaffrezo, J.-L., Jacob, V., Perraudin, E., Villenave, E., and Albinet, A.: Speciation of organic fraction does matter for source apportionment. Part 1: A one-year campaign in Grenoble (France), Sci. Total Environ., 624, 1598–1611, https://doi.org/10.1016/j.scitotenv.2017.12.135, 2018.
Srivastava, D., Daellenbach, K. R., Zhang, Y., Bonnaire, N., Chazeau, B., Perraudin, E., Gros, V., Lucarelli, F., Villenave, E., Prévôt, A. S. H., El Haddad, I., Favez, O., and Albinet, A.: Comparison of five methodologies to apportion organic aerosol sources during a PM pollution event, Sci. Total Environ., 757, 143168, https://doi.org/10.1016/j.scitotenv.2020.143168, 2021.
Stavroulas, I., Bougiatioti, A., Grivas, G., Paraskevopoulou, D., Tsagkaraki, M., Zarmpas, P., Liakakou, E., Gerasopoulos, E., and Mihalopoulos, N.: Sources and processes that control the submicron organic aerosol composition in an urban Mediterranean environment (Athens): a high temporal-resolution chemical composition measurement study, Atmos. Chem. Phys., 19, 901–919, https://doi.org/10.5194/acp-19-901-2019, 2019.
Taghvaee, S., Sowlat, M. H., Hassanvand, M. S., Yunesian, M., Naddafi, K., and Sioutas, C.: Source-specific lung cancer risk assessment of ambient PM2.5-bound polycyclic aromatic hydrocarbons (PAHs) in central Tehran, Environ. Int., 120, 321–332, https://doi.org/10.1016/j.envint.2018.08.003, 2018a.
Taghvaee, S., Sowlat, M. H., Mousavi, A., Hassanvand, M. S., Yunesian, M., Naddafi, K., and Sioutas, C.: Source apportionment of ambient PM2.5 in two locations in central Tehran using the Positive Matrix Factorization (PMF) model, Sci. Total Environ., 628–629, 672–686, https://doi.org/10.1016/j.scitotenv.2018.02.096, 2018b.
Terzi, E. and Samara, C.: Gas-Particle Partitioning of Polycyclic Aromatic Hydrocarbons in Urban, Adjacent Coastal, and Continental Background Sites of Western Greece, Environ. Sci. Technol., 38, 4973–4978, https://doi.org/10.1021/es040042d, 2004.
Theodosi, C., Tsagkaraki, M., Zarmpas, P., Grivas, G., Liakakou, E., Paraskevopoulou, D., Lianou, M., Gerasopoulos, E., and Mihalopoulos, N.: Multi-year chemical composition of the fine-aerosol fraction in Athens, Greece, with emphasis on the contribution of residential heating in wintertime, Atmos. Chem. Phys., 18, 14371–14391, https://doi.org/10.5194/acp-18-14371-2018, 2018.
Tsapakis, M. and Stephanou, E. G.: Diurnal Cycle of PAHs, Nitro-PAHs, and oxy-PAHs in a High Oxidation Capacity Marine Background Atmosphere, Environ. Sci. Technol., 41, 8011–8017, https://doi.org/10.1021/es071160e, 2007.
Uria-Tellaetxe, I. and Carslaw, D. C.: Conditional bivariate probability function for source identification, Environ. Modell. Softw., 59, 1–9, https://doi.org/10.1016/j.envsoft.2014.05.002, 2014.
U.S. EPA: Exposure Factors Handbook 2011 Edition (Final Report), U.S. Environmental Protection Agency, Washington, DC, EPA/600/R-09/052F, 2011.
Valotto, G., Rampazzo, G., Gonella, F., Formenton, G., Ficotto, S., and Giraldo, G.: Source apportionment of PAHs and n -alkanes bound to PM1 collected near the Venice highway, J. Environ. Sci., 54, 77–89, https://doi.org/10.1016/j.jes.2016.05.025, 2017.
Vasilakos, C., Levi, N., Maggos, T., Hatzianestis, J., Michopoulos, J., and Helmis, C.: Gas–particle concentration and characterization of sources of PAHs in the atmosphere of a suburban area in Athens, Greece, J. Hazard. Mater., 140, 45–51, https://doi.org/10.1016/j.jhazmat.2006.06.047, 2007.
Viras, L. G. and Siskos, P. A.: Spatial and Time Variation and Effect of Some Meteorological Parameters in Polycyclic Aromatic Hydrocarbons in Athens Greece, Polycycl. Aromat. Comp., 3, 89–100, https://doi.org/10.1080/10406639308047861, 1993.
Wang, F., Lin, T., Li, Y., Ji, T., Ma, C., and Guo, Z.: Sources of polycyclic aromatic hydrocarbons in PM2.5 over the East China Sea, a downwind domain of East Asian continental outflow, Atmos. Environ., 92, 484–492, https://doi.org/10.1016/j.atmosenv.2014.05.003, 2014.
Weimer, S., Alfarra, M. R., Schreiber, D., Mohr, M., Prévôt, A. S. H., and Baltensperger, U.: Organic aerosol mass spectral signatures from wood-burning emissions: Influence of burning conditions and wood type, J. Geophys. Res., 113, D10304, https://doi.org/10.1029/2007JD009309, 2008.
Weiss, M., Bonnel, P., Hummel, R., Provenza, A., and Manfredi, U.: On-Road Emissions of Light-Duty Vehicles in Europe, Environ. Sci. Technol., 45, 8575–8581, https://doi.org/10.1021/es2008424, 2011.
Wong, J. P. S., Tsagkaraki, M., Tsiodra, I., Mihalopoulos, N., Violaki, K., Kanakidou, M., Sciare, J., Nenes, A., and Weber, R. J.: Atmospheric evolution of molecular-weight-separated brown carbon from biomass burning, Atmos. Chem. Phys., 19, 7319–7334, https://doi.org/10.5194/acp-19-7319-2019, 2019.
Wu, Y., Yang, L., Zheng, X., Zhang, S., Song, S., Li, J., and Hao, J.: Characterization and source apportionment of particulate PAHs in the roadside environment in Beijing, Sci. Total Environ., 470–471, 76–83, https://doi.org/10.1016/j.scitotenv.2013.09.066, 2014.
Xie, M., Barsanti, K. C., Hannigan, M. P., Dutton, S. J., and Vedal, S.: Positive matrix factorization of PM2.5 – eliminating the effects of gas/particle partitioning of semivolatile organic compounds, Atmos. Chem. Phys., 13, 7381–7393, https://doi.org/10.5194/acp-13-7381-2013, 2013.
Xu, J., Liu, D., Wu, X., Vu, T. V., Zhang, Y., Fu, P., Sun, Y., Xu, W., Zheng, B., Harrison, R. M., and Shi, Z.: Source apportionment of fine organic carbon at an urban site of Beijing using a chemical mass balance model, Atmos. Chem. Phys., 21, 7321–7341, https://doi.org/10.5194/acp-21-7321-2021, 2021.
Yan, B., Zheng, M., Hu, Y., Ding, X., Sullivan, A. P., Weber, R. J., Baek, J., Edgerton, E. S., and Russell, A. G.: Roadside, Urban, and Rural Comparison of Primary and Secondary Organic Molecular Markers in Ambient PM2.5, Environ. Sci. Technol., 43, 4287–4293, https://doi.org/10.1021/es900316g, 2009.
Zheng, X., Wu, Y., Zhang, S., Hu, J., Zhang, K. M., Li, Z., He, L., and Hao, J.: Characterizing particulate polycyclic aromatic hydrocarbon emissions from diesel vehicles using a portable emissions measurement system, Sci. Rep.-UK, 7, 10058, https://doi.org/10.1038/s41598-017-09822-w, 2017.
Zheng, Y., Cheng, X., Liao, K., Li, Y., Li, Y. J., Huang, R.-J., Hu, W., Liu, Y., Zhu, T., Chen, S., Zeng, L., Worsnop, D. R., and Chen, Q.: Characterization of anthropogenic organic aerosols by TOF-ACSM with the new capture vaporizer, Atmos. Meas. Tech., 13, 2457–2472, https://doi.org/10.5194/amt-13-2457-2020, 2020.