the Creative Commons Attribution 4.0 License.
the Creative Commons Attribution 4.0 License.
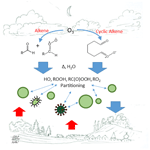
Opinion: The germicidal effect of ambient air (open-air factor) revisited
R. Anthony Cox
Paul T. Griffiths
Hartmut Herrmann
Erik H. Hoffmann
Michael E. Jenkin
V. Faye McNeill
Abdelwahid Mellouki
Christopher J. Penkett
Andreas Tilgner
Timothy J. Wallington
The term open-air factor (OAF) was coined following microbiological research in the 1960s and 1970s which established that rural air had powerful germicidal properties and attributed this to Criegee intermediates formed in the reaction of ozone with alkenes. We have re-evaluated those early experiments applying the current state of knowledge of ozone–alkene reactions. Contrary to previous speculation, neither Criegee intermediates nor the HO radicals formed in their decomposition are directly responsible for the germicidal activity attributed to the OAF. We identify other potential candidates, which are formed in ozone–alkene reactions and have known (and likely) germicidal properties, but the compounds responsible for the OAF remain a mystery. There has been very little research into the OAF since the 1970s, and this effect seems to have been largely forgotten. In this opinion piece we remind the community of the germicidal open-air factor. Given the current global pandemic spread by an airborne pathogen, understanding the natural germicidal effects of ambient air, solving the mystery of the open-air factor and determining how this effect can be used to improve human welfare should be a high priority for the atmospheric science community.
- Article
(780 KB) - Full-text XML
-
Supplement
(1872 KB) - BibTeX
- EndNote
The public health benefits of fresh air have been recognised since ancient times (Brimblecombe, 1995; Hobday, 2019). Over more than a century, knowledge has especially evolved during major medical emergencies, such as in wartime (Souttar, 1915; Nelson, 1915; Hobday, 1997), during the 1918 influenza pandemic (Hobday and Cason, 2009) or in the treatment of tuberculosis (Ransome, 1898; Ransome and Delphine, 1894). Fresh air was considered as a potential mass treatment for burns during a nuclear war (Blocker et al., 1951; Artz et al., 1953). Germicidal effects of fresh air were investigated as part of biodefence research in the 1970s (Hood, 2009). Fresh air has been considered an option in dealing with antimicrobial resistance (Wang et al., 2020). Phytotoxic and germicidal effects were related to the presence of pollutants produced during photochemical smog episodes in Los Angeles (Arnold, 1959; Druett and Packman, 1968). In experiments carried out at the Microbiological Research Establishment at Porton Down (MREPD) in southern England, Druett and May (1968) reported detection of a reactive airborne species in rural air which possessed powerful germicidal properties and called this the open-air factor (OAF). Flowing ambient air over microorganisms supported on spider-web filaments resulted in a loss of viability, which was reduced to zero after ∼3 h of exposure unless the air was first passed through a brass tube, which removed the germicidal agent (hence the term open air). Laboratory experiments exposing Escherichia coli to ozone, sulfur dioxide (SO2), nitrogen oxides (NOX), formaldehyde (HCHO) and ionised air at ambient levels showed that none of these were the responsible agent, and fluctuations of temperature and RH were also ruled out. Exposure to direct sunlight did, however, kill the microorganisms, so the experiments were carried out at night-time.
Druett and May (1968) postulated that the OAF was identical, or closely related, to the “ozone–olefin complex” identified earlier in phytotoxic air pollution. This postulate was supported by additional laboratory work at MREPD by Dark and Nash (1970), who showed that the ozonolysis of a series of alkenes resulted in significant, but variable, inactivation of E. coli and Micrococcus albus. It was not the first time that alkene ozonolysis was related to germicidal effects, as ozonated unsaturated oils have long been known as simple disinfectants (Harada, 1934; Travagli et al., 2010; Ugazio et al., 2020). Dark and Nash (1970) suggested that peroxide zwitterions (now known as Criegee intermediates) formed in the reaction of ozone with alkenes were the active agent of the OAF. Later work at MREPD by Hood (1974) further examined the effect of containment and found that the minimum rate of ventilation required to preserve OAF was correlated with the surface-to-volume area of the vessels used, consistent with Druett and May's idea that the OAF was removed at surfaces. Treating the OAF as a single or a small set of molecules in the gas phase enabled estimation of the diffusion coefficient of the elusive species to be made and thus the constraint of its molecular weight to the range 50–150. De Mik and De Groot (1978) at the Medical Biological Laboratory (TNO, Rijkswike, the Netherlands) found substantial damage to the DNA in aerosolised E. coli after exposure to air containing ozonised cyclohexene, indicating that OAF(s) could enter the outer lipid or fatty-acid protective layer(s) of the microorganisms and that they or free radicals deriving from them are accessing and interacting chemically with DNA within the cell. Note that DNA may additionally be protected by histone proteins (Willenbrock and Ussery, 2004). Very little additional work related to the OAF has been performed since the 1970s (Hobday, 2019). A few recent studies have attempted to generate the OAF to act against aerosolised microbes in the context of food preservation (Bailey et al., 2007; Nicholas et al., 2013) without providing new insight into the identity of the active agent.
Based on recent advances in our understanding of the formation and fate of Criegee intermediates in alkene–O3 reactions (Cox et al., 2020) we show here that, contrary to previous speculation, neither Criegee intermediates nor the HO radicals formed in their decomposition are directly responsible for the germicidal activity attributed to the OAF but identify other potential candidates, which are formed from alkene–O3 reactions and which have known germicidal properties. The overall sequence of processes involved in the germicidal action of OAF is expected to be initiated by the reaction of ozone with alkenes producing oxygenated products in the gas phase that undergo transfer to the lipid membrane of the microorganism, where they may produce free radicals that initiate and propagate lipid phase oxidation processes. While the formation of the key species from alkene–O3 reactions has been demonstrated experimentally, it is likely that the same species (and/or similar species) are also formed from additional pathways in ambient air (e.g. HO- or NO3-initiated oxidation of volatile organic compounds) with a number of sources therefore contributing to OAF.
The experiments of Dark and Nash (1970) provide a basis for examining germicidal agent formation in relation to what is currently known about the mechanism of alkene ozonolysis. Dark and Nash (1970) investigated the effect of a series of reactive ozone–alkene mixtures on both E. coli and Micrococcus albus suspended on micro-threads produced by spiders, to mimic airborne particles, in a 670 L aluminium box. They selected a series of terminal alkenes, internal alkenes and cycloalkenes (and some unsaturated oxygenates). Experiments were carried out with 4, 11 and 33 ppb ozone, with alkene concentrations chosen such that 75 % ozone decay occurred over a period of 10 min. As shown in the Supplement (Sect. S1), the rate coefficients for reactions of ozone with alkenes inferred from the data reported by Dark and Nash (1970) are generally consistent with current recommended kinetic data (Cox et al., 2020) so that the relationship between germicidal effects and the structure of the alkene precursor reflected the differences in oxidation pathways and in OAF yields. A key result from Dark and Nash (1970) was the observation that (through their ozonolysis) cycloalkenes appeared to be more lethal to the microorganisms than linear acyclic alkenes, which, in turn, had a greater impact than branched acyclic alkenes. It is noted that the cycloalkenes are structurally similar to some common environmental terpenoid VOCs (e.g. α-pinene, limonene). The relative efficiency (expressed as an effective loss rate coefficient, kloss for the E. coli) of selected alkene–O3 mixtures is summarised in Fig. 1, illustrating the observed range of impacts. The derivation of kloss from the original data is described in the Supplement (Sect. S2). These may be compared with a value of kloss≈0.03 min−1 that can be inferred for open air for the typical conditions of the Druett and May (1968) study, based on information presented in Fig. 1 of their paper. The average values of kloss for the three ozone regimes in the Dark and Nash (1970) experiments, based on the data for the alkenes shown in Fig. 1, are about 0.04, 0.09 and 0.2 min−1 for the 4, 11 and 33 ppb ozone experiments respectively. In approximate terms, this suggests that the germicidal agent is formed, on average, at comparable levels to the ambient level in the 4 ppb experiments, and approaching an order of magnitude higher in the 33 ppb experiments – although with substantial variation between the different alkene–O3 systems.
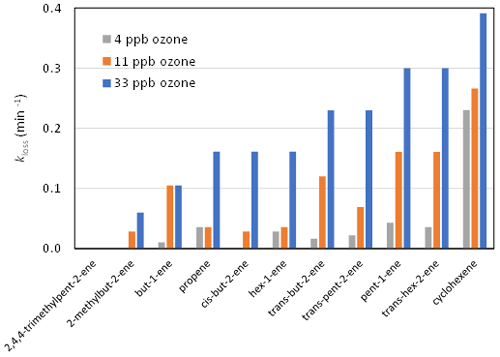
Figure 1Average E. coli loss rate (kloss) during the ozonolysis of selected alkenes in the experiments of Dark and Nash (1970), illustrating the variation of loss efficiency with alkene structure and ozone mixing ratio.
It is now well understood that the highly exothermic reaction of ozone with alkenes produces nascent Criegee intermediates (R1CR2OO∗) with substantial internal excitation. These can either be stabilised by transferring energy in collisions with other gas-phase atmospheric species (mainly N2 and O2) or undergo rapid unimolecular decomposition or internal rearrangement (Donahue et al., 2011). The yield of stabilised Criegee intermediates varies greatly (Cox et al., 2020). In the case of the reactions of O3 with cyclopentene, cyclohexene and cycloheptene, the yield is reported to be close to zero (Hatakeyama et al., 1984; Drozd and Donahue, 2011) and systematically lower than those for similarly sized acyclic alkenes. The observation by Dark and Nash (1970) that cycloalkenes are the most potent source of OAF, combined with our current understanding that there is a limited yield of stabilised Criegee intermediates formed in these systems, therefore rules out gas-phase Criegee intermediates as being the germicidal agent(s) in the OAF.
This conclusion can also be drawn from a kinetic perspective when examining the experiment by Druett and May (1968). If the germicidal agent were tied to the concentration of Criegee intermediates entering the tube from the atmosphere, it would be in steady state given by a balance between production from ozonolysis and removal via loss by unimolecular decomposition, reaction with trace gases and particles present in the air, and loss at the tube walls. As shown in the Supplement (Sect. S3), the lifetimes of common Criegee intermediates with respect to gas-phase sinks are <60 ms. The same kinetic argument also holds for HO radicals, generated from decomposition of (excited or stabilised) Criegee intermediates, which are removed by gas-phase reaction with most atmospheric trace species. The typical lifetime of HO radicals in clean background UK air is about 200 ms (Lee et al., 2009; Ingham et al., 2009). Thus, the species controlling the lifetime of the bacteria in the Druett and May (1968) experiment must be related to the precursors (O3) or the oxidation products that are lost to the walls. The kinetics of loss of viability observed in the brass-tube experiments of Druett and May (1968) (Fig. S2) may provide indications for the chemical properties of the OAF, when assuming that these are volatile gas-phase molecules taken up to the bacteria in a reactive process, and that the loss of these species at the wall controls the observed decay of germicidal activity. As shown in the Supplement (Sect. S4), based on the experimental gas flow rates and making reasonable assumptions about the properties of the brass tube, the uptake coefficient (γ, the probability per collision that the OAF is irreversibly lost at the cylindrical wall) falls in the range of 10−4 to 10−2. One possible explanation for the observed loss of bactericidal activity along the flow tube is loss of the precursor O3 to the brass walls. However, γ values for O3 are much lower than 10−4 (Crowley et al., 2010), especially when considering passivation effects over the several hours of the experiment. Peroxides, which are important products formed in alkene ozonolysis (and present in outside air from a variety of sources: see Sect. S3 of the Supplement), are well established disinfectants (Kampf et al., 2020) and hence are potential contributors to the OAF. Oxygenated VOCs (such as peroxides) in general strongly partition (reversibly and irreversibly) to tube walls (Deming et al., 2019). As discussed in the Supplement (Sect. S4), γ values for peroxides indeed fall into the range derived above to explain the wall-loss rates of the Druett and May (1968) experiment. The same is true for HO2 and possibly other peroxy radicals that could be precursors for further radicals.
To gain insight into the potential contribution of for example peroxy radicals and peroxides to the germicidal activity of OAF, we simulated the Dark and Nash (1970) experiments. Initial scoping simulations used the detailed alkene chemistry in the “Master Chemical Mechanism” (MCM v3.2: http://mcm.york.ac.uk, last access: 5 October 2015) for six of the linear acyclic alkene systems studied. The analysis not only generated gas-phase concentrations of product species, but also calculated respective vapour pressures and octanol–water partition coefficients (see Supplement, Sect. S5). These parameters are important as they impact partitioning to the condensed phase and the ability to penetrate cell membranes (see discussion below in Sect. 3). The simulations revealed that the concentrations of HO were suppressed by the high concentrations of alkenes. HO concentrations in the 33 ppb O3 experiments were (0.28–8.5)×105 molecule cm−3, with proportionately lower values in the 11 and 4 ppb O3 experiments. These were, therefore, generally lower than those typically present in air during daytime, but comparable to those reported at night in UK rural air (Emmerson and Carslaw, 2009). Simulated respective concentrations of HO2 and RO2, (1.3–1.8)×109 and (6.7–21)×109 molecule cm−3 in the 33 ppb O3 experiments, were however significantly higher than reported at night in UK rural air (Emmerson and Carslaw, 2009). As expected, some classes of non-radical products were found to be present in much higher concentrations with aldehydes, peroxides and organic acids dominating the product distribution (see Fig. S3). Important, and sometimes major, contributions to these product classes resulted from the secondary chemistry initiated by the reactions of HO with the parent alkenes. The simulations revealed no clear trend when considering the product concentrations (or the concentration scaled by vapour pressures or octanol–water distribution coefficients) with the germicidal activity for each alkene reported by Dark and Nash (1970). These results are summarised in detail in Sect. S5 of the Supplement.
As peroxides and peroxyacids (containing the −OOH group) such as H2O2 and peracetic acid are known germicides (Kampf et al., 2020; Kitis, 2004; Becker et al., 2017; Goyal et al., 2014) we examined their potential contribution to OAF in more detail. For this, bespoke mechanisms were written for the extended series of 11 alkenes shown in Fig. 1, which incorporated recent improvements in the current understanding of the ozonolysis chemistry (see Supplement, Sect. S6). The results show that (unlike the OAF) the collective formation of peroxides does not vary greatly from one alkene to the next, and total levels are similar in systems with low germicidal activity (e.g. 2,4,4-trimethylpent-2-ene) and in systems with high germicidal activity (e.g. cyclohexene) (Fig. 2a). The peroxide concentrations generated from the gas-phase chemistry cannot thus collectively explain the trend in bacterial destruction across the series of alkene–O3 systems. However, the peroxides formed in the various systems include a structurally diverse set of mono-, bi- and multifunctional peroxide species (see Supplement, Sect. S6), which will likely possess different propensities to penetrate the protective membrane of the microorganisms and initiate oxidation (see Sect. 3). These tend to be dominated by simple β-hydroxy peroxides (formed from secondary HO chemistry) in the acyclic systems (Fig. 2c and d). In the case of cyclohexene, however, about 30 % of the simulated peroxide burden is due to a series of highly oxygenated organic molecules (HOMs) containing hydroperoxide and/or peroxy acid groups, along with aldehyde and ketone groups (Fig. 2b). The formation of HOMs occurs via an autooxidation process (Ehn et al., 2014; Hansel et al., 2018), in which peroxy radicals generated sequentially in some alkene systems can isomerise via internal H-shift reactions resulting in rapid formation of a series of products containing increasing numbers of hydroperoxide or peroxy acid groups (Crounse et al., 2011, 2013; Mutzel et al., 2015; Kirkby et al., 2016). Cyclic alkenes, such as cyclohexene, tend to have higher HOM yields than most acyclic alkenes (Bianchi et al., 2019), with HOM formation mechanisms generally not being operative for small acyclic alkenes. While this idea is consistent with the observation by Dark and Nash (1970) that the most effective (or highest concentrations of) germicidal agents were produced in the ozonolysis of endocyclic alkenes, we note that several small acyclic alkenes (no HOMs formation) studied still exhibited notable germicidal properties. Nonetheless, given the plethora of (germicidal) peroxide, hydroperoxide and peracid groups in HOMs, and the fact that they partition readily to the particle phase (Riva et al., 2016; Bianchi et al., 2019), we hypothesise that HOMs may contribute to the OAF.
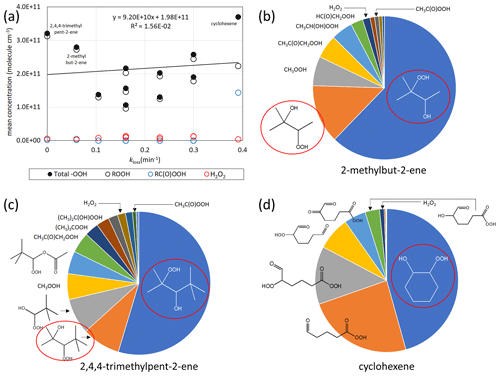
Figure 2(a) Simulated concentrations of hydroperoxide products vs. kloss for E. coli in 33 ppb O3 experiments for the series of 11 alkenes shown in Fig. 1, showing the concentrations for H2O2, ROOH species and RC(O)OOH species and their totals (total −OOH), as well as linear regression of the total −OOH data. (b–d) Simulated speciation of peroxidic compounds generated from 2-methylbut-2-ene, 2,4,4-trimethylpent-2-ene and cyclohexene in the 33 ppb O3 experiments. The total −OOH concentrations in the three cases were 2.8×1011 3.2×1011 and 3.7×1011 molecule cm−3, respectively. Species in red ellipses are those formed from the HO + alkene reactions.
In spite of the high degree of functionalisation of typical HOMs, the remaining vapour pressure could be sufficient that partitioning to the bacteria on the spider webs of the experiments and to the wall of vessels that was deduced by Hood (1974) becomes efficient. The air sampled in the Druett and May (1968) experiments certainly contained deliquesced, background aerosol on which condensation or dissolution of HOMs and other lower-volatility peroxides would have occurred. At relatively high humidity, the particle–gas equilibration timescale for an internally mixed aerosol was likely fast enough to enable the brass tube of the Druett and May (1968) experiment to act as a sink for the HOMs and other peroxidic or oxygenated species (Deming et al., 2019), meaning that the apparent OAF wall-loss kinetics could possibly have been driven by evaporation from the particles.
As mentioned above, the role of hydrogen peroxide (Kampf et al., 2020; Goyal et al., 2014), organic peroxides or peracids (Becker et al., 2017; Kitis, 2004) as disinfectants for a wide range of pathogens is well established. The work of De Mik and De Groot (1978) demonstrated that DNA damage resulted from primary or secondary attacks on the organisms. The organisms are protected from external damage by oxidising agents present in the air by external membranes surrounding the internal aqueous medium containing the key chemical entities controlling the functions in the cell. The chemical nature of the membrane layer is different in individual microorganisms. E. coli bacteria are surrounded by a lipid membrane, as are many viruses. Other micro-organisms have chemically different protective layers. The mechanism of action of peroxides and HOMs containing peroxidic and peroxyacidic groups is likely through their oxidation potential, probably initiated by their thermal decomposition. This yields smaller radicals as a source of reactive oxygen species that also drive their health impact when inhaled (Tong et al., 2019). The germicidal effects of ozonated unsaturated oils has been attributed to secondary ozonides and peroxides (Travagli et al., 2010). These result from the reaction of the stabilised Criegee intermediate (produced by ozonolysis in the condensed phase) with carbonyl compounds (Bailey, 1958; Zahardis and Petrucci, 2007; Pleik et al., 2018). In a recent experimental investigation of autoxidation of unsaturated lipids initiated by gas-phase HO, Zeng et al. (2020) observed substantial amplification of oxidation via lipid autoxidation propagated by Criegee intermediates and free radicals. These two examples emphasise that pathways specific for the condensed phase provide a route for radical precursors, such as HOMs or peroxides in general, to initiate a wealth of oxidative processes in the condensed phase. The finding of lipid membrane degradation initiated by reactive species is consistent with the fact that microbes with lipid membranes, including enveloped viruses and vegetative bacteria, are more susceptible to degradation by disinfectant agents than other microbes such as capsid viruses or prion proteins (Russell, 1999). The amplifying effect of radicals in lipid peroxidation also highlights that even very small amounts of OAF may have a substantial germicidal effect.
In summary, the discussion above indicates that a range of products formed in the ozonolysis of alkenes could contribute to the OAF as distinct germicides. Chemical simulations of these chemical systems do not identify a clear individual gas-phase molecule or class of molecules that matches the ranking of bactericidal activity reported in the experiments of Dark and Nash (1970). However, we need to keep in mind that the gas-phase yields of the species in question may not correlate directly with their germicidal effects as the response of germs is related to the partitioning of these species to the condensed phase in a highly non-linear manner and may also depend on their ability to initiate condensed-phase free-radical chemistry that ultimately kills pathogens.
Although the airborne pathway, i.e. aerosol particles carrying pathogens and infecting a target, is recognised as a major route of transmission of many infectious diseases, including transmission of SARS-CoV-2 (Prather et al., 2020; Greenhalgh et al., 2021; Wang et al., 2021), the current understanding of the processes involved in the transmission of infection (e.g. during the current pandemic) is incomplete. An improved understanding of the OAF will help in the assessment of the importance of outdoor air on the transmission of COVID-19, as well as the importance of ventilation in indoor environments (Morawska and Milton, 2020; Morawska et al., 2020). It is therefore of substantial interest to further investigate the role of ambient air in pathogen viability and the mechanisms behind the OAF.
Numbers backing up the arguments of the main text are provided as tables in the Supplement. MCM simulations were performed with publicly available code as described in the Supplement. The mechanism extension in terms of reactions and species is provided in Tables S4 and S5.
The supplement related to this article is available online at: https://doi.org/10.5194/acp-21-13011-2021-supplement.
RAC initiated this study and provided the historic perspective. PTG and RAC performed preliminary analysis. CJP provided the biochemistry perspective. MA and JNC reanalysed the Druett and May brass-tube experiment. HH, AT and EHH performed the MCM model calculations and the species property estimation work. MEJ contributed the new and updated mechanisms and performed the corresponding model simulations. TJW, MA, MEJ and JNC prepared the paper, which was reviewed and refined by all co-authors.
Markus Ammann is a member of the editorial board of Atmospheric Chemistry and Physics.
Publisher's note: Copernicus Publications remains neutral with regard to jurisdictional claims in published maps and institutional affiliations.
We thank Richard Hobday for helpful discussions on the historical context of the open-air factor.
This research has been supported by the Swiss National Science Foundation (grant no. 188 662); the UKRI Natural Environment Research Council via the National Centre for Atmospheric Science (NCAS); and the International Union of Pure and Applied Chemistry, IUPAC (project no. 2017-024-1-100).
The article processing charges for this open-access publication were covered by the Max Planck Society.
This paper was edited by Ronald Cohen and reviewed by two anonymous referees.
Arnold, W. N.: The longevity of the phytotoxicant produced from gaseous ozone-olefin reactions, Int. J. Air Poll., 2, 167–174, 1959.
Artz, C. P., Reiss, E., Davis, J. H. J., and Amspacher, W. H.: The exposure treatment of burns, Ann. Surg., 137, 456–464, 1953.
Bailey, P. S.: The reactions of ozone with organic compounds, Chem. Rev., 58, 925–1010, 1958.
Bailey, R., Fielding, L., Young, A., and Griffith, C.: Effect of Ozone and Open Air Factor against Aerosolized Micrococcus luteus, J. Food Protect., 70, 2769–2773, https://doi.org/10.4315/0362-028X-70.12.2769, 2007.
Becker, B., Brill, F. H. H., Todt, D., Steinmann, E., Lenz, J., Paulmann, D., Bischoff, B., and Steinmann, J.: Virucidal efficacy of peracetic acid for instrument disinfection, Antimicrob. Resist. In., 6, 114, https://doi.org/10.1186/s13756-017-0271-3, 2017.
Bianchi, F., Kurtén, T., Riva, M., Mohr, C., Rissanen, M. P., Roldin, P., Berndt, T., Crounse, J. D., Wennberg, P. O., Mentel, T. F., Wildt, J., Junninen, H., Jokinen, T., Kulmala, M., Worsnop, D. R., Thornton, J. A., Donahue, N., Kjaergaard, H. G., and Ehn, M.: Highly Oxygenated Organic Molecules (HOM) from Gas-Phase Autoxidation Involving Peroxy Radicals: A Key Contributor to Atmospheric Aerosol, Chem. Rev., 119, 3472–3509, https://doi.org/10.1021/acs.chemrev.8b00395, 2019.
Blocker, T. G. J., Blocker, V., Lewis, S. R., and Snyder, C. C.: An approach to the problem of burn sepsis with the use of open-air therapy, Ann. Surg., 134, 574–580, 1951.
Brimblecombe, P.: History of air pollution, in: Composition, Chemistry and Climate of the Atmosphere, edited by: Singh, H. B., Van Nostrand, New York, 1–18, 1995.
Cox, R. A., Ammann, M., Crowley, J. N., Herrmann, H., Jenkin, M. E., McNeill, V. F., Mellouki, A., Troe, J., and Wallington, T. J.: Evaluated kinetic and photochemical data for atmospheric chemistry: Volume VII – Criegee intermediates, Atmos. Chem. Phys., 20, 13497–13519, https://doi.org/10.5194/acp-20-13497-2020, 2020.
Crounse, J. D., Paulot, F., Kjaergaard, H. G., and Wennberg, P. O.: Peroxy radical isomerization in the oxidation of isoprene, Phys. Chem. Chem. Phys., 13, 13607–13613, https://doi.org/10.1039/C1CP21330J, 2011.
Crounse, J. D., Nielsen, L. B., Jørgensen, S., Kjaergaard, H. G., and Wennberg, P. O.: Autoxidation of Organic Compounds in the Atmosphere, J. Phys. Chem. Lett., 4, 3513–3520, https://doi.org/10.1021/jz4019207, 2013.
Crowley, J. N., Ammann, M., Cox, R. A., Hynes, R. G., Jenkin, M. E., Mellouki, A., Rossi, M. J., Troe, J., and Wallington, T. J.: Evaluated kinetic and photochemical data for atmospheric chemistry: Volume V – heterogeneous reactions on solid substrates, Atmos. Chem. Phys., 10, 9059–9223, https://doi.org/10.5194/acp-10-9059-2010, 2010.
Dark, F. A. and Nash, T.: Comparative toxicity of various ozonized olefins to bacteria suspended in air, J. Hyg.-Cambridge, 68, 245–252, https://doi.org/10.1017/S0022172400028710, 1970.
De Mik, G. and De Groot, I.: Breaks induced in the deoxyribonucleic acid of aerosolized Escherichia coli by ozonized cyclohexene, Appl. Environ. Microb., 35, 6–10, 1978.
Deming, B. L., Pagonis, D., Liu, X., Day, D. A., Talukdar, R., Krechmer, J. E., de Gouw, J. A., Jimenez, J. L., and Ziemann, P. J.: Measurements of delays of gas-phase compounds in a wide variety of tubing materials due to gas–wall interactions, Atmos. Meas. Tech., 12, 3453–3461, https://doi.org/10.5194/amt-12-3453-2019, 2019.
Donahue, N. M., Drozd, G. T., Epstein, S. A., Presto, A. A., and Kroll, J. H.: Adventures in ozoneland: down the rabbit-hole, Phys. Chem. Chem. Phys., 13, 10848–10857, https://doi.org/10.1039/C0CP02564J, 2011.
Drozd, G. T. and Donahue, N. M.: Pressure Dependence of Stabilized Criegee Intermediate Formation from a Sequence of Alkenes, J. Phys. Chem. A, 115, 4381–4387, https://doi.org/10.1021/jp2001089, 2011.
Druett, H. A. and May, K. R.: Unstable Germicidal Pollutant in Rural Air, Nature, 220, 395–396, https://doi.org/10.1038/220395a0, 1968.
Druett, H. A. and Packman, L. P.: Sensitive Microbiological Detector for Air Pollution, Nature, 218, 699–699, https://doi.org/10.1038/218699a0, 1968.
Ehn, M., Thornton, J. A., Kleist, E., Sipilä, M., Junninen, H., Pullinen, I., Springer, M., Rubach, F., Tillmann, R., Lee, B., Lopez-Hilfiker, F., Andres, S., Acir, I.-H., Rissanen, M., Jokinen, T., Schobesberger, S., Kangasluoma, J., Kontkanen, J., Nieminen, T., Kurtén, T., Nielsen, L. B., Jørgensen, S., Kjaergaard, H. G., Canagaratna, M., Maso, M. D., Berndt, T., Petäjä, T., Wahner, A., Kerminen, V.-M., Kulmala, M., Worsnop, D. R., Wildt, J., and Mentel, T. F.: A large source of low-volatility secondary organic aerosol, Nature, 506, 476, https://doi.org/10.1038/nature13032, 2014.
Emmerson, K. M. and Carslaw, N.: Night-time radical chemistry during the TORCH campaign, Atmos. Environ., 43, 3220–3226, https://doi.org/10.1016/j.atmosenv.2009.03.042, 2009.
Goyal, S. M., Chander, Y., Yezli, S., and Otter, J. A.: Evaluating the virucidal efficacy of hydrogen peroxide vapour, J. Hosp. Infect., 86, 255–259, https://doi.org/10.1016/j.jhin.2014.02.003, 2014.
Greenhalgh, T., Jimenez, J. L., Prather, K. A., Tufekci, Z., Fisman, D., and Schooley, R.: Ten scientific reasons in support of airborne transmission of SARS-CoV-2, The Lancet, 397, 1603–1605, https://doi.org/10.1016/S0140-6736(21)00869-2, 2021.
Hansel, A., Scholz, W., Mentler, B., Fischer, L., and Berndt, T.: Detection of RO2 radicals and other products from cyclohexene ozonolysis with and acetate chemical ionization mass spectrometry, Atmos. Environ., 186, 248–255, https://doi.org/10.1016/j.atmosenv.2018.04.023, 2018.
Harada, T.: Olive Oil Ozonide and its Fungicidal Quality, B. Chem. Soc. Jpn., 9, 192–197, https://doi.org/10.1246/bcsj.9.192, 1934.
Hatakeyama, S., Kobayashi, H., and Akimoto, H.: Gas-phase oxidation of sulfur dioxide in the ozone-olefin reactions, J. Phys. Chem., 88, 4736–4739, https://doi.org/10.1021/j150664a058, 1984.
Hobday, R. A.: Sunlight therapy and solar architecture, Med. Hist., 41, 455–472, https://doi.org/10.1017/S0025727300063043, 1997.
Hobday, R. A.: The open-air factor and infection control, J. Hosp. Infect., 103, e23–e24, https://doi.org/10.1016/j.jhin.2019.04.003, 2019.
Hobday, R. A. and Cason, J. W.: The Open-Air treatment of pandemic influenza, Am. J. Public Health, 99, S236–S242, https://doi.org/10.2105/ajph.2008.134627, 2009.
Hood, A. M.: Open-Air Factors in Enclosed Systems, J. Hyg., 72, 53–60, https://doi.org/10.1017/s0022172400023202, 1974.
Hood, A. M.: The effect of open-air factors on the virulence and viability of airborne Francisella tularensis, Epidemiol. Infect., 137, 753–761, https://doi.org/10.1017/S0950268809002076, 2009.
Ingham, T., Goddard, A., Whalley, L. K., Furneaux, K. L., Edwards, P. M., Seal, C. P., Self, D. E., Johnson, G. P., Read, K. A., Lee, J. D., and Heard, D. E.: A flow-tube based laser-induced fluorescence instrument to measure OH reactivity in the troposphere, Atmos. Meas. Tech., 2, 465–477, https://doi.org/10.5194/amt-2-465-2009, 2009.
Kampf, G., Todt, D., Pfaender, S., and Steinmann, E.: Persistence of coronaviruses on inanimate surfaces and their inactivation with biocidal agents, J. Hosp. Infect., 104, 246–251, https://doi.org/10.1016/j.jhin.2020.01.022, 2020.
Kirkby, J., Duplissy, J., Sengupta, K., Frege, C., Gordon, H., Williamson, C., Heinritzi, M., Simon, M., Yan, C., Almeida, J., Tröstl, J., Nieminen, T., Ortega, I. K., Wagner, R., Adamov, A., Amorim, A., Bernhammer, A.-K., Bianchi, F., Breitenlechner, M., Brilke, S., Chen, X., Craven, J., Dias, A., Ehrhart, S., Flagan, R. C., Franchin, A., Fuchs, C., Guida, R., Hakala, J., Hoyle, C. R., Jokinen, T., Junninen, H., Kangasluoma, J., Kim, J., Krapf, M., Kürten, A., Laaksonen, A., Lehtipalo, K., Makhmutov, V., Mathot, S., Molteni, U., Onnela, A., Peräkylä, O., Piel, F., Petäjä, T., Praplan, A. P., Pringle, K., Rap, A., Richards, N. A. D., Riipinen, I., Rissanen, M. P., Rondo, L., Sarnela, N., Schobesberger, S., Scott, C. E., Seinfeld, J. H., Sipilä, M., Steiner, G., Stozhkov, Y., Stratmann, F., Tomé, A., Virtanen, A., Vogel, A. L., Wagner, A. C., Wagner, P. E., Weingartner, E., Wimmer, D., Winkler, P. M., Ye, P., Zhang, X., Hansel, A., Dommen, J., Donahue, N. M., Worsnop, D. R., Baltensperger, U., Kulmala, M., Carslaw, K. S., and Curtius, J.: Ion-induced nucleation of pure biogenic particles, Nature, 533, 521, https://doi.org/10.1038/nature17953, 2016.
Kitis, M.: Disinfection of wastewater with peracetic acid: a review, Environ. Int., 30, 47–55, https://doi.org/10.1016/S0160-4120(03)00147-8, 2004.
Lee, J. D., Young, J. C., Read, K. A., Hamilton, J. F., Hopkins, J. R., Lewis, A. C., Bandy, B. J., Davey, J., Edwards, P., Ingham, T., Self, D. E., Smith, S. C., Pilling, M. J., and Heard, D. E.: Measurement and calculation of OH reactivity at a United Kingdom coastal site, J. Atmos. Chem., 64, 53–76, https://doi.org/10.1007/s10874-010-9171-0, 2009.
Morawska, L. and Milton, D. K.: It Is Time to Address Airborne Transmission of Coronavirus Disease 2019 (COVID-19), Clin. Infect. Dis., 71, 2311–2313, https://doi.org/10.1093/cid/ciaa939, 2020.
Morawska, L., Tang, J. W., Bahnfleth, W., Bluyssen, P. M., Boerstra, A., Buonanno, G., Cao, J., Dancer, S., Floto, A., Franchimon, F., Haworth, C., Hogeling, J., Isaxon, C., Jimenez, J. L., Kurnitski, J., Li, Y., Loomans, M., Marks, G., Marr, L. C., Mazzarella, L., Melikov, A. K., Miller, S., Milton, D. K., Nazaroff, W., Nielsen, P. V., Noakes, C., Peccia, J., Querol, X., Sekhar, C., Seppänen, O., Tanabe, S.-i., Tellier, R., Tham, K. W., Wargocki, P., Wierzbicka, A., and Yao, M.: How can airborne transmission of COVID-19 indoors be minimised?, Environ. Int., 142, 105832, https://doi.org/10.1016/j.envint.2020.105832, 2020.
Mutzel, A., Poulain, L., Berndt, T., Iinuma, Y., Rodigast, M., Böge, O., Richters, S., Spindler, G., Sipilä, M., Jokinen, T., Kulmala, M., and Herrmann, H.: Highly Oxidized Multifunctional Organic Compounds Observed in Tropospheric Particles: A Field and Laboratory Study, Environ. Sci. Technol., 49, 7754–7761, https://doi.org/10.1021/acs.est.5b00885, 2015.
Nelson, W. E.: Open-Air treatment for wounds, a simple and inexpensive form of Open-Air ward, as used at the V. A. D. Hospital, Henley-in-Arden, Brit. Med. J., 2, 324–324, https://doi.org/10.1136/bmj.2.2852.324, 1915.
Nicholas, R., Dunton, P., Tatham, A., and Fielding, L.: The effect of ozone and open air factor on surface-attached and biofilm environmental Listeria monocytogenes, J. Appl. Microbiol., 115, 555–564, https://doi.org/10.1111/jam.12239, 2013.
Pleik, S., Spengler, B., Ram Bhandari, D., Luhn, S., Schäfer, T., Urbach, D., and Kirsch, D.: Ambient-air ozonolysis of triglycerides in aged fingerprint residues, Analyst, 143, 1197–1209, https://doi.org/10.1039/C7AN01506B, 2018.
Prather, K. A., Marr, L. C., Schooley, R. T., McDiarmid, M. A., Wilson, M. E., and Milton, D. K.: Airborne transmission of SARS-CoV-2, Science, 370, 303–304, https://doi.org/10.1126/science.abf0521, 2020.
Ransome, A.: Remarks on Sanatoria for the Open-Air Treatment of Consumption, Brit. Med. J., 2, 69–73, https://doi.org/10.1136/bmj.2.1958.69, 1898.
Ransome, A. and Delphine, S.: On the influence of certain natural agents on the virulence of the tubercle-bacillus, Proc. R. Soc. Lond., 56, 51–56, 1894.
Riva, M., Budisulistiorini, S. H., Chen, Y., Zhang, Z., D'Ambro, E. L., Zhang, X., Gold, A., Turpin, B. J., Thornton, J. A., Canagaratna, M. R., and Surratt, J. D.: Chemical Characterization of Secondary Organic Aerosol from Oxidation of Isoprene Hydroxyhydroperoxides, Environ. Sci. Technol., 50, 9889–9899, https://doi.org/10.1021/acs.est.6b02511, 2016.
Russell, A. D.: Bacterial resistance to disinfectants: present knowledge and future problems, J. Hosp. Infect., 43, S57–S68, https://doi.org/10.1016/S0195-6701(99)90066-X, 1999.
Souttar, H. S.: A Surgeon in Belgium, E. Arnold, London, 1915.
Tong, H., Zhang, Y., Filippi, A., Wang, T., Li, C., Liu, F., Leppla, D., Kourtchev, I., Wang, K., Keskinen, H.-M., Levula, J. T., Arangio, A. M., Shen, F., Ditas, F., Martin, S. T., Artaxo, P., Godoi, R. H. M., Yamamoto, C. I., de Souza, R. A. F., Huang, R.-J., Berkemeier, T., Wang, Y., Su, H., Cheng, Y., Pope, F. D., Fu, P., Yao, M., Pöhlker, C., Petäjä, T., Kulmala, M., Andreae, M. O., Shiraiwa, M., Pöschl, U., Hoffmann, T., and Kalberer, M.: Radical Formation by Fine Particulate Matter Associated with Highly Oxygenated Molecules, Environ. Sci. Technol., 53, 12506–12518, https://doi.org/10.1021/acs.est.9b05149, 2019.
Travagli, V., Zanardi, I., Valacchi, G., and Bocci, V.: Ozone and Ozonated Oils in Skin Diseases: A Review, Mediat. Inflamm., 2010, 610418, https://doi.org/10.1155/2010/610418, 2010.
Ugazio, E., Tullio, V., Binello, A., Tagliapietra, S., and Dosio, F.: Ozonated Oils as Antimicrobial Systems in Topical Applications. Their Characterization, Current Applications, and Advances in Improved Delivery Techniques, Molecules, 25, 334, 2020.
Wang, C. C., Prather, K. A., Sznitman, J., Jimenez, J. L., Lakdawala, S. S., Tufekci, Z., and Marr, L. C.: Airborne transmission of respiratory viruses, Science, 373, eabd9149, https://doi.org/10.1126/science.abd9149, 2021.
Wang, C.-H., Hsieh, Y.-H., Powers, Z. M., and Kao, C.-Y.: Defeating antibiotic-resistant bacteria: exploring alternative therapies for a post-antibiotic era, Int. J. Mol. Sci., 21, 1061, 2020.
Willenbrock, H. and Ussery, D. W.: Chromatin architecture and gene expression in Escherichia coli, Genome Biol., 5, 252–252, https://doi.org/10.1186/gb-2004-5-12-252, 2004.
Zahardis, J. and Petrucci, G. A.: The oleic acid-ozone heterogeneous reaction system: products, kinetics, secondary chemistry, and atmospheric implications of a model system – a review, Atmos. Chem. Phys., 7, 1237–1274, https://doi.org/10.5194/acp-7-1237-2007, 2007.
Zeng, M., Heine, N., and Wilson, K. R.: Evidence that Criegee intermediates drive autoxidation in unsaturated lipids, P. Natl. Acad. Sci. USA, 117, 4486–4490, https://doi.org/10.1073/pnas.1920765117, 2020.