the Creative Commons Attribution 4.0 License.
the Creative Commons Attribution 4.0 License.
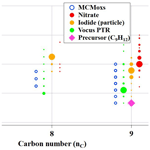
Oxygenated products formed from OH-initiated reactions of trimethylbenzene: autoxidation and accretion
Yuwei Wang
Archit Mehra
Jordan E. Krechmer
Gan Yang
Xiaoyu Hu
Andrew Lambe
Manjula Canagaratna
Jianmin Chen
Douglas Worsnop
Gas-phase oxidation pathways and products of anthropogenic volatile organic compounds (VOCs), mainly aromatics, are the subject of intensive research, with attention paid to their contributions to secondary organic aerosol (SOA) formation and potentially new particle formation (NPF) in the urban atmosphere. In this study, a series of OH-initiated oxidation experiments of trimethylbenzene (TMB, C9H12) including 1,2,4-TMB, 1,3,5-TMB, 1,2,3-TMB, and 1,2,4-(methyl-D3)-TMBs (C9H9D3) were investigated in an oxidation flow reactor (OFR) in the absence and presence of NOx. Products were measured using a suite of state-of-the-art instruments, i.e. a nitrate-based chemical ionization–atmospheric pressure interface time-of-flight mass spectrometer (nitrate CI-APi-TOF), an iodide-adduct chemical ionization time-of-flight mass spectrometer (iodide CI-TOF) equipped with a Filter Inlet for Gases and AEROsols (FIGAERO), and a Vocus proton-transfer-reaction mass spectrometer (Vocus PTR). A large number of C9 products with 1–11 oxygen atoms and C18 products presumably formed from dimerization of C9 peroxy radicals were observed, hinting at the extensive existence of autoxidation and accretion reaction pathways in the OH-initiated oxidation reactions of TMBs. Oxidation products of 1,2,4-(methyl-D3)-TMBs with deuterium atoms in different methyl substituents were then used as a molecular basis to propose potential autoxidation reaction pathways. Accretion of C9 peroxy radicals is the most significant for aromatics with meta-substituents and the least for aromatics with ortho-substituents if the number and size of substituted groups are identical. The presence of NOx would suppress the formation of highly oxygenated molecules (HOMs) of C18 and enhance the formation of organonitrates and even dinitrate organic compounds. Our results show that the oxidation products of TMB are much more diverse and could be more oxygenated than the current mechanisms predict.
- Article
(2544 KB) - Full-text XML
-
Supplement
(654 KB) - BibTeX
- EndNote
Oxidation products of volatile organic compounds (VOCs) contribute significantly to the formation of secondary organic aerosols (SOAs; Ng et al., 2010; Zhang et al., 2007), which raises a globally ubiquitous health and environmental concern (Hallquist et al., 2009). There have been numerous studies that aim to construct detailed VOC oxidation mechanisms to advance our understanding of VOC degradation, SOA formation, and ozone formation (Atkinson, 1986; Atkinson and Arey, 2003; Atkinson and Carter, 1984; Kroll and Seinfeld, 2008; Ziemann and Atkinson, 2012). Based on the hypothesis that the products and kinetics of many unstudied chemical reactions can be proposed by analogy to known reactions of similar chemical species (Ziemann and Atkinson, 2012) and/or predicted by the structure–activity relationships (Kwok and Atkinson, 1995), the Master Chemical Mechanism (MCM) is developed as a nearly explicit chemical mechanism, describing the degradation of numerous VOCs (Bloss et al., 2005; Jenkin et al., 2003; Saunders et al., 2003). Due to the high complexity of VOC oxidation processes, it is not surprising that mechanisms leading to the formation of previously unidentified species are still missing.
The formation of highly oxygenated organic molecules (HOMs) through the autoxidation pathway during VOC oxidation is such an example. HOMs refer to organic compounds typically containing six or more oxygen atoms that are formed in the gas phase (Bianchi et al., 2019). Autoxidation is a chemical process where an alkyl peroxy radical (RO2) undergoes an intramolecular hydrogen shift followed by the addition of a molecular oxygen, resulting in a more oxygenated RO2 radical (Crounse et al., 2013; Ehn et al., 2014). It is an effectively repetitive unimolecular reaction as the more oxidized RO2 will serve as a parent RO2 in the next autoxidation reaction, leading to the rapid formation of HOMs on very short timescales (Bianchi et al., 2019; Jørgensen et al., 2016).
Owing to recent developments in the analytical techniques such as nitrate-anion chemical ionization mass spectrometry (nitrate CIMS), our knowledge of the autoxidation pathway during the oxidation of biogenic volatile organic compounds (BVOCs) has been significantly improved. Certain systems, such as the oxidation of monoterpenes, have been studied extensively, of which ozonolysis has been confirmed as an important source for HOMs (Ehn et al., 2014; Jokinen et al., 2014). OH-initiated oxidation is also a considerable HOM formation source for monoterpenes and isoprene (Krechmer et al., 2015), albeit at lower yields for monoterpenes containing an endocyclic double bond (Jokinen et al., 2014, 2015; Rissanen et al., 2015). Detailed mechanisms of monoterpene-derived HOM formation reactions, initiated by ozone or OH, were investigated through theoretical calculations (Berndt et al., 2016) or by analogy to reactions of similar chemical species, i.e. cyclohexene (Rissanen et al., 2014). A couple of studies performed hydrogen and deuterium isotope exchange experiments, which can probe the number of hydrogen atoms other than that in C–H, strongly supporting the proposal of autoxidation mechanisms (Ehn et al., 2014; Rissanen et al., 2014). Research on other BVOCs, i.e. isoprene and sesquiterpenes (Crounse et al., 2013; Richters et al., 2016; Teng et al., 2017), and on other oxidants, i.e. NO3 and chlorine (Nah et al., 2016; Wang et al., 2020), indicate the widespread existence of autoxidation pathways in the oxidation of BVOCs. The products formed from autoxidation of biogenic precursors have been proven to play a vital role in atmospheric new particle formation (NPF) because of their low volatility (Ehn et al., 2014; Stolzenburg et al., 2018; Tröstl et al., 2016).
On the other hand, studies on autoxidation of anthropogenic VOCs are rather sparse. Wang et al. (2017) theoretically and experimentally showed the autoxidation route of alkylbenzenes to form HOMs in the gas phase. Identities and yields of HOM products from different aromatics were systematically measured, and the determined molar HOM yields were in the range of 0.1 % to 2.5 %, which are similar to the molar HOM yields of OH-initiated reactions of BVOCs (Jokinen et al., 2015; Molteni et al., 2018). Currently, aromatic-derived HOMs are believed to be formed via many reaction pathways, including accretion, bicyclic intermediate reactions, and multigeneration OH reactions (Berndt et al., 2018b; Garmash et al., 2020; Zaytsev et al., 2019). The unimolecular isomerization and autoxidation reactions of aromatic peroxy radicals have been shown to be fast enough to compete with other bimolecular reactions even under NO concentrations as high as in urban environments (Tsiligiannis et al., 2019).
Trimethylbenzene (TMB) including isomers of 1,3,5-TMB, 1,2,3-TMB, and 1,2,4-TMB is one of the most common anthropogenic VOCs in urban areas. OH-initiated oxidation of TMB is its dominant chemical loss in the atmosphere (Atkinson and Arey, 2003), which proceeds either via H atom abstraction from the methyl substituents or via addition of OH radicals onto the aromatic ring (Ziemann and Atkinson, 2012). The H atom abstraction channel is minor in the OH-induced oxidation reactions of TMB, forming dimethylbenzaldehyde. The major channels of OH addition consist of a peroxide-bicyclic pathway, phenolic pathway, and epoxy-oxy pathway (Bloss et al., 2005; Calvert et al., 2002; Jenkin et al., 2003). The three TMB isomers have different branching ratios for these pathways, resulting from the substitution, site, and stereospecificity; however, specific branching ratios are still in debate. Among these pathways, the peroxide-bicyclic pathway has the highest branching ratio and can form bicyclic peroxy radicals (BPRs), which are important intermediates that contribute significantly to the formation of HOMs (Wang et al., 2017). Subsequent reactions of the intermediates will lead to the formation of stabilized products (or non-radical products). On the other hand, the details of the autoxidation mechanisms for anthropogenic precursors remain elusive. Direct measurements of individual H-shift rates, the detailed structure of HOMs, and a robust quantification of HOM yields are still lacking. The detailed kinetics for termination reactions of different RO2 are also ambiguous. Consequently, it is hard to comprehensively judge the TMB oxidation reaction pathways and products under different atmospheric conditions and to evaluate the contribution of TMB oxidation to atmospheric NPF and SOA formation.
In this study, we study the OH-initiated oxidation of 1,3,5-TMB, 1,2,3-TMB, and 1,2,4-TMB with a focus on autoxidation and accretion products via the concurrent usage of a Vocus proton-transfer-reaction time-of-flight mass spectrometry (Vocus PTR), an iodide-adduct chemical ionization time-of-flight mass spectrometer equipped with a Filter Inlet for Gases and AEROsols (FIGAERO–iodide CI-TOF), and a nitrate-based chemical ionization–atmospheric pressure interface time-of-flight mass spectrometer (nitrate CI-APi-TOF). Oxidation of 1,2,4-(methyl-D3)-TMBs was investigated to elucidate the detailed autoxidation reaction pathway. The influence of NOx concentration on product distribution was also investigated.
As shown in Fig. 1, oxidation experiments of TMB were conducted in a potential aerosol mass (PAM) oxidation flow reactor (OFR; Aerodyne Research, Inc.). A self-prepared VOC cylinder was used to provide a constant source of gaseous TMB as a reactant. O3 and OH were produced in situ in the PAM, and the relative humidity (RH) was regulated by the PAM setup, which is introduced in detail later. A Vocus PTR (Krechmer et al., 2018), a FIGEARO–iodide CI-TOF (Lee et al., 2014; Lopez-Hilfiker et al., 2014), and a nitrate CI-APi-TOF (Ehn et al., 2014; Eisele and Tanner, 1993) were deployed to detect gaseous products as well as particulate ones. In addition, an ozone monitor (Model 106-M, 2B technologies) was utilized to measure ozone concentration, whereas a set of one scanning mobility particle sizer (SMPS; consisting of one TSI Model 3080 Long DMA and one TSI Model 3776 Condensation Particle Counter) was employed to measure the number size distribution of submicron aerosol particles.
In this study, the sum of all the flows in the PAM, including a zero air flow, an ozone (O3) flow, a TMB∕N2 flow, and a N2O∕N2 flow depending upon experimental conditions, was kept at either 10 or 10.4 slpm (standard litres per minute, standard to 0 ∘C, 1 atm), resulting in calculated mean residence times of approximately 80 s (77.3 s at 10.4 slpm). Zero air was generated by a zero gas generator (Sabio Model 1001 Zero Gas Source). A fraction of the zero air was passed through a Nafion humidifier (Perma Pure Model FC100-80-6MSS) filled with ultra-pure water to achieve the desired RH in the OFR. Ozone was generated by passing 800 sccm (standard cubic centimetre, standard to 0 ∘C, 1 atm) of zero air through a separate ozone chamber and input into the OFR. In order to create a low-HO2∕RO2-ratio environment to promote the carbonyl and hydroxyl channels to terminate RO2 radicals, the OFR was operated with only the 254 nm lights on (Lambe et al., 2019), which is referred to as OFR254 mode in previous studies (Peng et al., 2015). In OFR254 mode, the primary oxidant production reactions in the OFR are
In some experiments, N2O (99.999 %, Air Liquide) was added at the OFR inlet, corresponding to mixing ratios of 3.4 % of the total gas flow rates, which produced NOx via the following reactions (Lambe et al., 2017):
A photochemical model (PAM_chem_v8; Lambe et al., 2017; Li et al., 2015; Peng et al., 2015) was implemented to constrain the NO∕NO2 profiles in the experiments, whose details are presented in Sect. S1.
Before each experiment, the PAM OFR was purged with zero air under the OFR254 operation mode until the signals of acetic acid and other common VOC oxidation products decreased to background levels of the Vocus PTR and CI-TOF that are described below.
The newly developed Vocus PTR has a high sensitivity to a wide range of VOCs and oxygenated volatile organic compounds (OVOCs; Krechmer et al., 2018; Li et al., 2019; Riva et al., 2019). Its mass-resolving power ( 000 at 200 Th, 1 Th , where e is the elementary charge, and u is the atomic mass unit) allows us to simultaneously monitor many isobaric species and even to distinguish the very minor mass discrepancy (0.001548 u) between one deuterium atom and two hydrogen atoms. The instrument background together with a quantitative calibration by injection of standards was measured between every two experiments to minimize potential inaccuracies. In our study, the pressure of the focusing ion-molecule reactor (FIMR) was actively maintained at 1.5 mbar, resulting in an E∕N of the FIMR at 110 Td (1 Td V cm2), which was generally a moderate operating condition, leading to relatively little fragmentation of compounds of interest (Gueneron et al., 2015; Yuan et al., 2017).
The iodide-adduct CI-TOF is able to determine elemental compositions of a suite of atmospheric oxygenated organic species (D'Ambro et al., 2017; Lee et al., 2014; Lopez-Hilfiker et al., 2016). It has increasing sensitivities toward more polar and acidic VOCs (Lee et al., 2014). The mass resolution of the iodide CI-TOF was tuned to be around 3000. The reagent ion (I−) was produced from permeated CH3I vapour in N2 by a radioactive source of Am-241 (0.1 mCi). The pressure in the ion-molecule reactor (IMR) was regulated at 100 mbar, whereas the small segmented quadrupole (SSQ) pressure was set to be around 2 mbar. The FIGAERO inlet manifold enables the iodide CI-TOF to measure both gas and particle compositions at a molecular level (Lopez-Hilfiker et al., 2014). In our study, aerosols were collected onto a PTFE filter (5 µm, Millipore) at 0.96 slpm for 20 min while the gases were measured simultaneously via a separate dedicated port. Then, a thermal desorption cycle was started 2 min after the FIGAERO filter was aligned to a heating tube, through which a heated ultra-high-purity nitrogen flow was passed and heated according to a pre-programmed temperature ramp. The ultra-high-purity nitrogen was initially held at 25 ∘C for 2 min and then heated at a rate of 10 ∘C min−1 to 200 ∘C, which was maintained for the remainder of the temperature ramp (50 min in total).
The nitrate CI-APi-TOF has been increasingly used for the measurement of low-volatility organic compounds (LVOCs) and extremely low-volatility organic compounds (ELVOCs; Ehn et al., 2014; Hyttinen et al., 2015; Jokinen et al., 2014), which mostly have a high O∕C ratio. The resolving power of the nitrate CI-APi-TOF was up to around 8000 in our study. The selectivity of nitrate ions keeps the spectrum clean from the more abundant, less oxidized compounds in our experiments. Most of the detected species were observed exclusively as adducts with , a very minor fraction of which contain odd hydrogen numbers and are hence postulated to be radicals but not presented in this paper.
The concurrent use of three mass spectrometers (MSs) with different reagent ions allows us to obtain a comprehensive picture of the oxidation products of TMB with OH radicals. The detection suitability of these three instruments for oxidation products with various levels of oxidation has been discussed a lot in previous studies (Isaacman-VanWertz et al., 2017; Krechmer et al., 2018; Riva et al., 2019). Generally, the Vocus PTR displays selectivity for less oxidized compounds, the iodide CI-TOF favours more oxygenated species, and the nitrate CI-APi-TOF shows the highest efficiency for the most oxidized compounds. Dimer products of TMB oxidation are expected to be detected by the nitrate CI-APi-TOF as clusters with , which is due to the potential hydrogen bond donor functional groups in these molecules, inferred from the abundant oxygen and hydrogen atoms in the formulas. These products should not be detected by the Vocus PTR. One explanation is that these molecules are likely to be fragile and therefore have fragmented owing to the protonation or the strong electric field in the FIMR of the Vocus PTR. Alternatively, these products might not go through the polyether ether ketone (PEEK) tube inlet of the Vocus PTR. At the same time, the sample inlet for the iodide CI-TOF in our experiments is not desirable for the detection of dimer products.
To ensure that the reported signal is truly from the sample flow instead of internal background or contamination, subtraction of the mass spectra for the OFR background from the samples has been performed for each instrument. In addition, since this study is mostly concerned with identification of oxidation products from OH-initiated reactions of TMBs and elucidation of the potential autoxidation pathway, the nitrate CI-APi-TOF and iodide CI-TOF were not calibrated, and only the arbitrary signals with MS transmission correction (Heinritzi et al., 2016; Krechmer et al., 2018) were compared within the same instrument. It should then be noted that the relative signal intensities are biased among the MSs because of their ionization methods and transmission efficiency.
In each experiment, the Vocus PTR was used to confirm the establishment of stable precursor gas concentrations, and then the pair of 254 nm Hg lamps were turned on to generate the OH radicals, and reaction products were analysed by the MSs. The input RH in the OFR was kept at a low level, and the voltage of the Hg lamps was slightly tuned in every experiment so that the OH exposure in the OFR was close to one oxidation lifetime of TMB, i.e. consumption of () of the initial TMB. Under this condition, the production of the first-generation products is generally favoured, and the multigeneration products are also present if the subsequent loss reactions for these products are assumed to proceed at a similar rate.
Table 1Summary of experimental conditions.
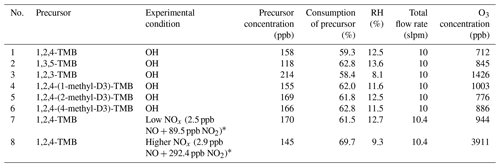
* Modelled mathematically averaged NO∕NO2 concentrations in the OFR are shown here because of the malfunction of a NOx monitor. The model underestimates [NO] and [NO2] by up to a factor of 2, according to separate experiments that are not presented.
Table 1 summarizes all the experiments that were performed. Studied were 1,3,5-TMB (≥99.0 %, Aladdin), 1,2,3-TMB (analytical standard, Aladdin), 1,2,4-TMB (≥99.5 %, Aladdin), 1,2,4-(1-methyl-D3)-TMB (≥95 %, Qingdao Tenglong Weibo Technology Co., Ltd., China), 1,2,4-(2-methyl-D3)-TMB (≥95 %, Qingdao Tenglong Weibo Technology Co., Ltd., China), and 1,2,4-(4-methyl-D3)-TMB (≥95 %, Qingdao Tenglong Weibo Technology Co., Ltd., China). The structure of these partially deuterated TMBs can be found in Fig. S1 in the Supplement. Note that ozone reactions were not taken into account in this study because ozone reacts with aromatics at negligible rates, and its reaction rate with oxidation products containing C=C double bonds is much slower compared with that of OH (Jenkin et al., 1997, 2003; Molteni et al., 2018; Saunders et al., 2003). Also note that the concentrations of precursors in our experiments were much higher than the atmospheric ones. These concentrations were deliberately chosen to help identify the highly oxygenated products that are of low volatility and easy to lose in the sampling but subject to the side effect that the relative significance of different pathways could be altered.
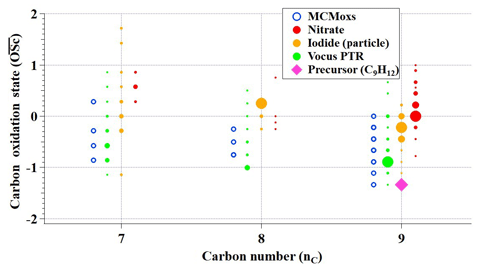
Figure 2Comparison of C7–C9 products observed in the OH-initiated oxidation of 1,2,4-TMB (Exp. 1 in Table 1) with those listed in the Master Chemical Mechanism (MCM; Bloss et al., 2005). Filled red, orange, and green circles denote observation by the nitrate CI-APi-TOF, iodide CI-TOF, and Vocus PTR, respectively, whereas open blue circles represent MCM species. The radii of filled circles are proportional to the signals of the compounds in each instrument. The signal of the most abundant product for each instrument is arbitrarily set to be 100 %, but note that the arbitrary signals are not comparable among instruments. Symbols have been offset horizontally to avoid overlap.
3.1 Characteristics of C9 products
Figure 2 presents an overview of C7, C8, and C9 products in a carbon oxidation state () carbon number (nC) space as observed by three MSs and also those predicted by MCM v3.3.1. Carbon oxidation state is a quantity that increases with the level of oxidation, which reveals the chemical ageing of atmospheric organics (Kroll et al., 2011). It is evident that more species were detected by the three MSs, and although there were clear differences between products detected from different MSs, results indicate missing oxidation pathways in the current versions of the MCM (MCM v3.3.1, available at: http://mcm.leeds.ac.uk/MCM, last access: 11 February 2020). Oxygen-containing C9 products were formed by adding functional groups to the carbon skeleton, whereas C7 and C8 products resulted from carbon–carbon scission of the original carbon skeleton together with functionalization. A large proportion of C7–C9 products were more oxidized than those predicted by MCM, hinting at the existence of highly efficient oxidation pathways.
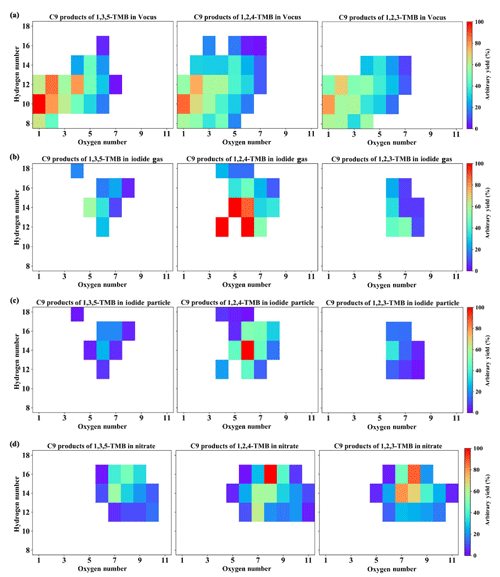
Figure 3Distribution of C9 products formed from OH-initiated reactions of TMBs (Exp. 1–3 in Table 1) by (a) the Vocus PTR, (b) the iodide CI-TOF for the gas phase, (c) the iodide CI-TOF for the particle phase, and (d) the nitrate CI-APi-TOF. The yield of the most abundant product for each instrument is arbitrarily set to be 100 %, but note that the arbitrary yields are not comparable among instruments. Also note that the signal of the Vocus PTR was processed in a logarithmic way before calculating the arbitrary yield.
Recent studies have emphasized the importance of the peroxide-bicyclic pathway in producing highly oxygenated compounds in the oxidation of alkylbenzenes (Wang et al., 2017; Zaytsev et al., 2019), which leads to the formation of ring-retaining products. Therefore, we further investigated C9 products of TMB oxidation detected by the three MSs (Fig. 3) here. C9H10O1−6, C9H12O1−7, and C9H14O4−6 contributed to most of the signal intensities in the Vocus PTR (Fig. 3a). Compounds with fewer hydrogen atoms than TMB in the Vocus PTR might be formed from hydrogen abstraction reactions. The iodide CI-TOF detected products with five to seven oxygen atoms (Fig. 3b and c), which is narrower compared with the Vocus PTR and nitrate CI-APi-TOF. Molecules with 18 hydrogen atoms were detected only in the iodide CI-TOF, which is an unexpectedly high number. These molecules, low in signal intensity in both gas and particle phases, might be formed from multiple OH attacks since each OH attack can only add a maximum of two hydrogens onto the parent molecule. The species with the highest signal intensities measured in the gas phase appeared to be C9H12O4, C9H12O6, C9H14O5, and C9H14O6 in the 1,2,4-TMB + OH experiment, C9H14O5 and C9H14O6 in the 1,3,5-TMB + OH experiment, and C9H12O6 and C9H12O7 in the 1,2,3-TMB + OH experiment (Fig. 3b). Compared with the gas phase, more oxidized particulate products tended to contribute a larger proportion of the signal in FIGAERO-Iodide-CI-APi-TOF (Fig. 3c). Nevertheless, the gas-phase products are emphasized in the current study, which can be detected by and compared among the three instruments. The nitrate CI-APi-TOF detected C9 products containing 12–16 hydrogen atoms and 5–11 oxygen atoms (Fig. 3d).
RO2 radicals can react in the absence of NO to form termination products including carbonyls, alcohols, and hydroperoxides via the following reactions (Mentel et al., 2015):
Here we present a criteria method based on the work of Mentel et al. (2015). For a parent peroxy radical with a molecular mass of m, its termination ought to lead to the formation of a carbonyl, an alcohol, and a hydroperoxyl, which have a molecular mass of m−17, m−15, and m+1, respectively. Since elemental formulas as determined by the high-resolution MS do not contain information regarding functional groups or the structure of a molecule, the identified mass spectral signals could be counted as either one of the three categories. Listed in Table 2 are detected stabilized oxidation products in categories of carbonyl, alcohol, and hydroperoxyl, which hint at the potential existence of the corresponding peroxy radicals. These stabilized products all contain six or more oxygen atoms, which meet the definition of HOMs (Bianchi et al., 2019). C9H12O6 is one of the only two signals that have been predicted by MCM, assumed to be a hydroperoxyl product from a ring-opening peroxy radical that goes through multiple OH attack reactions (MCM name: C7MOCOCO3H), which is unlikely to contribute a lot to the observed signal of C9H12O6 since the concentration of a multigeneration product is not expected to be high at OH exposure as short as one lifetime of TMB. C9H14O6 is the other one, presumed to be a hydroperoxyl product of a second-generation peroxy radical formed via epoxy-oxy pathway (MCM name: TM124MUOOH), which is unlikely to be formed through the MCM route with a considerable yield, either. Four pairs of peroxy radicals, i.e. C9H13O7• and C9H13O9•, C9H13O8• and C9H13O10•, C9H15O7• and C9H15O9•, and C9H15O8• and C9H15O10•, can be selected from the eight potential peroxy radicals in Table 2. The molecular formulas for the peroxy radicals within each pair differ by 2× O, which is initial evidence for the autoxidation pathway.
Table 2Oxidation products of 1,2,4-TMB in categories of carbonyl, hydroxyl, and hydroperoxyl according to their molecular mass as well as the potential peroxy radicals. Numbers in the parentheses denote the relative intensity detected by the nitrate CI-APi-TOF in the OH-initiated oxidation of 1,2,4-TMB when that of the largest HOM signal (C9H16O8) is arbitrarily set to be 100 %. The relative intensity has been corrected with the relative transmission efficiency of the nitrate CI-APi-TOF.
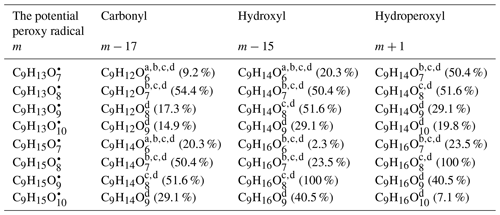
a These compounds are listed in the MCM mechanism of 1,2,4-TMB where they are formed by multiple OH oxidation steps. b These compounds were detected by the Vocus PTR. c These compounds were detected by the iodide CI-TOF in both gas and particle phases. d These compounds were detected by the nitrate CI-APi-TOF.
3.2 Autoxidation mechanisms of 1,2,4-TMB
The autoxidation pathways were then further elucidated by experiments with isotopically labelled precursors, 1,2,4-(1-methyl-D3)-TMB, 1,2,4-(2-methyl-D3)-TMB, and 1,2,4-(4-methyl-D3)-TMB, whose structure is shown in Fig. S1 in the Supplement.
If an intramolecular hydrogen shift happens during autoxidation with the abstracted hydrogen coming from a methyl group, molecular oxygen will rapidly attach to this carbon-centred radical to form a new alkyl peroxy radical (Bianchi et al., 2019, and references therein). One potential fate of this R−CH2OO• radical is to lose one of the two remaining hydrogen atoms, forming a carbonyl according to Reaction (R5). Thus, one of the three original hydrogen atoms in the methyl group will leave this molecule after an autoxidation step (Ehn et al., 2014; Mentel et al., 2015; Molteni et al., 2018; Otkjær et al., 2018; Rissanen et al., 2014; Wang et al., 2017). In the case of a deuterium abstraction from a methyl-D3 group during the autoxidation, an oxidation product with two deuterium atoms (CxHyD2Oz) will then be formed, which is presumably a carbonyl. Although an alcohol or a hydroperoxyl could also be formed from a peroxy radical, it is not suitable to utilize the presence of alcohol and hydroperoxyl products as a criterion to judge the existence of autoxidation. The hydroxyl channel of deuterated peroxy radicals can lead to the formation of alcohol products with either three or four deuterium atoms, depending on the nature of the other reacting RO2. The slow unimolecular reaction rate of the deuterated methyl group corresponds to little formation of the products with four deuterium atoms, whereas our MSs cannot differentiate three deuterium atoms either from a molecule with autoxidation and hydroxyl termination or from an untouched methyl-D3 group. On the other hand, the hydroperoxyl channel would lead to the formation of hydroperoxyl products with three deuterium atoms, too. Therefore, only the carbonyl channel products of a peroxy radical was used to suggest the potential autoxidation that has occurred.
Table 3Partially deuterated C9 products observed by the Vocus PTR and/or the nitrate CI-APi-TOF. “V” and “N” denote observation by the Vocus PTR and nitrate CI-APi-TOF, respectively, whereas “–” means that the product was not observed by any instrument.

Table 3 summarizes two-deuterium-containing C9 (C9HyD2Oz) products that were detected by the Vocus PTR and nitrate CI-APi-TOF in different isotope labelling experiments: C9H10D2O6 in the 1,2,4-(1-methyl-D3)-TMB + OH experiment by the Vocus PTR and nitrate CI-APi-TOF, C9H10D2O7 in the 1,2,4-(1-methyl-D3)-TMB + OH experiment by the Vocus PTR, and C9H12D2O8 in the 1,2,4-(4-methyl-D3)-TMB + OH experiment by the nitrate CI-APi-TOF. C9H10D2O7 (234.0703 Th) was expected to be detected by the nitrate CI-APi-TOF, but unfortunately an undefined peak (located at 295.9827 Th) covered the position where (296.0592 Th) was supposed to have been identified. C9H12D2O8 (252.0814 Th) was not detected by the Vocus PTR, likely owing to either its low proton affinity or its partitioning onto the inlet of the Vocus PTR given its high O∕C ratio and hence low volatility. However, the nitrate CI-APi-TOF was able to detect this very sticky compound because the nitrate source is constructed with concentric sample and sheath flows that minimize the diffusive losses of samples to the source wall. These results indicate that an intramolecular deuterium migration happened on the 1-methyl-D3 substituent of the C9H10D3O5• and C9H10D3O6• radicals and the 4-methyl-D3 substituent of the C9H12D3O7• radical, respectively. Then one oxygen was added to the resulting alkyl radicals, and the new peroxy radical reacted to form C9H10D2O6, C9H10D2O7, and C9H12D2O8, respectively.
These three compounds (C9H10D2O6, C9H10D2O7, and C9H12D2O8) did not possess high signal intensities because the deuterium transfer reactions are typically significantly slower for D (2H) nuclei than hydrogen transfer reactions for H (1H) (Bianchi et al., 2019; Wang et al., 2017). There might be other two-deuterium-containing C9 products in these experiments. However, since many of these signals were at the instrument detection limits or even lower, the nonideal experimental conditions prevent us from confirming more such compounds.
Based on the observed signals of two-deuterium-containing C9 products and structures that have been previously proven to favour H-shift reactions (Otkjær et al., 2018), two plausible formation pathways for the observed products are proposed.
The first one starts with a BPR of C9H13O5• as shown in Scheme 1, which is the first BPR formed from C9H12 via the peroxide-bicyclic pathway. The structure of this particular C9H13O5• is different from what is proposed in MCM v3.3.1, but the position for the initial OH attack, i.e. the fourth carbon on the ring, is feasible owing to the attraction of a substituted group on its para-position (Li and Wang, 2014), and the subsequent addition of O2 after the initial OH attack along with bicyclization occurs in the same relative position as previous studies have suggested (Bloss et al., 2005; Jenkin et al., 2003). The resulting BPR of C9H13O5• undergoes a hydrogen shift, during which the abstracted hydrogen comes from the methyl terminal of an allylic group. This hydrogen is much easier to be abstracted compared to those in a normal methyl group that are unlikely to go through a hydrogen shift with a peroxy radical (Otkjær et al., 2018). The new BPR of C9H13O7• then reacts via (R5), (R6), and (R7) to form C9H12O6, C9H14O6, and C9H14O7, respectively. This pathway is suggested by the observation of C9H10D2O6 in the 1,2,4-(1-methyl-D3)-TMB + OH experiment.
It is noted that in all the three isotope experiments, we also detected products of C9H9D3O6 and C9H9D3O7 with much higher signal intensities, indicating the existence of other autoxidation pathways. Thus, it deserves a repeated emphasis here that we only point out feasible pathways that are supported by our isotope experiments in this work but do not rule out other possibilities.
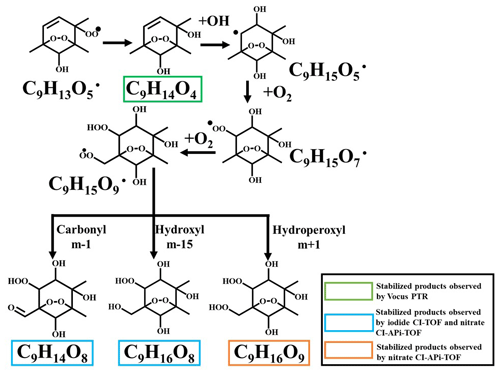
Scheme 2A proposed autoxidation reaction scheme involving a bicyclic peroxy radical of C9H13O5•. Note that the reaction has been terminated with the formation of C9H14O4 and reinitiated by a second OH attack.
The second pathway is described in Scheme 2. This pathway starts from a BPR of C9H13O5• that is formed by the initial OH attack and subsequent reactions. MCM v3.3.1 includes a BPR with the same structure but does not contain the subsequent reactions. The BPR of C9H13O5• can be terminated via (R5), forming a stabilized hydroxyl product of C9H14O4, which is subject to a second OH attack and a following addition of O2, resulting in a new peroxy radical of C9H15O7•. There are no systematic investigations on the effect of a peroxide-bicyclic substitution on the 1,5 H-shift rate constant. However, our data indicate that a hydrogen shift can occur on the 4-methyl group, based on which the structure of C9H15O9• is proposed. The new BPR of C9H15O9• is then terminated via (R5), (R6), and (R7), forming the stabilized products C9H14O8, C9H16O8, and C9H16O9, respectively. This pathway is suggested by the observation of C9H12D2O8 in the 1,2,4-(4-methyl-D3)-TMB + OH experiment, though other pathways could result in products with the same formula.
An autoxidation reaction pathway that can explain the observation of C9H10D2O7 in the 1,2,4-(1-methyl-D3)-TMB + OH experiment is currently unavailable, although we speculate that a “peroxy–alkoxy–peroxy” conversion is likely involved during the formation of C9H12O7 according to the number of oxygen atoms.
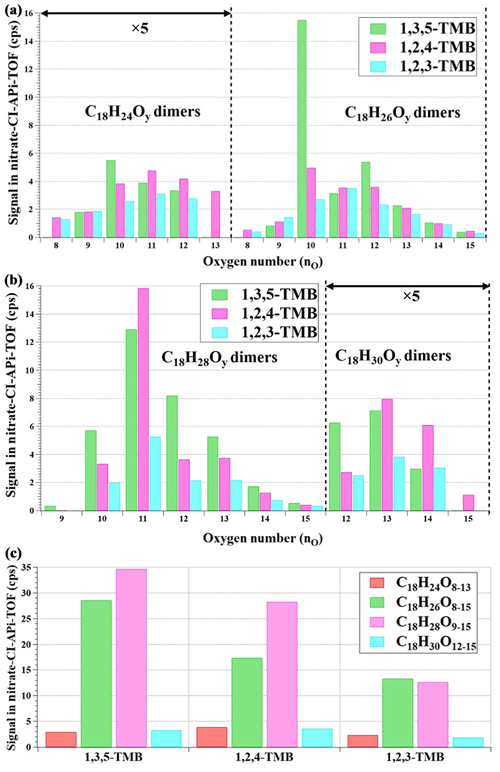
Figure 4(a) Distribution of C18H24O8−13 and C18H26O8−15 products formed from TMB oxidation experiments (Exp. 1–3 in Table 1) as measured by the nitrate CI-APi-TOF, (b) distribution of C18H28O9−15 and C18H30O12−15 formed from TMB oxidation experiments (Exp. 1–3 in Table 1) as measured by the nitrate CI-APi-TOF, and (c) the total signal of C18 products formed from TMB oxidation experiments (Exp. 1–3 in Table 1) as measured by the nitrate CI-APi-TOF.
3.3 Characteristics of C18 HOMs
Products with 18 carbon atoms were observed in our experiments by the nitrate CI-APi-TOF, all containing 24–30 hydrogen atoms and 8 or more oxygen atoms (O>8; Fig. 4). C18 products with 26 or 28 hydrogen atoms contributed most of the signal intensities, while those generated by 1,3,5-TMB were the most abundant. Recent studies revealed that long-neglected organic peroxide dimer (ROOR′) formation reactions might be an important source of gas-phase dimer compounds, through which two peroxy radicals form accretion products consisting of the carbon backbone of both reactants (Berndt et al., 2018a, b; Zhao et al., 2018).
This reaction has been proved to be another important loss process for RO2 radicals formed via autoxidation. On account of their extraordinarily low vapour pressure, HOM dimers contribute more significantly to the formation and growth of atmospheric new particles than HOM monomers.
Our C18 oxidation products have similar ion formulas to the dimer products in recent 1,3,5-TMB oxidation experiments (Molteni et al, 2018; Tsiligiannis et al., 2019). In our experiments, the formation of C18H26O8−15, C18H28O9−15, and C18H30O12−15 can be explained by reactions of two C9H13Ox•, one C9H13Ox• and one C9H15Ox•, and two C9H15Ox•, respectively. C18H24O8−13 with low signal intensities were detected by the nitrate CI-APi-TOF, hinting that H-abstraction reactions have occurred, leading to a lower hydrogen atom in the product than in the precursor.
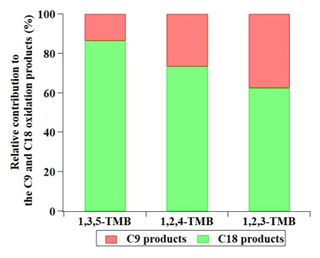
Figure 5Relative contribution of C9 and C18 products formed from TMB oxidation experiments, as measured by the nitrate CI-APi-TOF. The relative intensity has been corrected with the relative transmission efficiency.
Figure 5 summarizes the relative contribution of C9 and C18 products formed from TMB oxidation as detected by the nitrate CI-APi-TOF. The charging efficiency for C9 and C18 products is assumed to be identical in the nitrate CI-APi-TOF (Ehn et al., 2014; Hyttinen et al., 2015). Hence, the measured relative abundances of the oxidation products, with corrections of the transmission function in the MS, can faithfully represent the product distribution in the experiments. In Exp. 1–3, the dimers (C18H26O8−15) formed from two C9H13Ox• along those (C18H28O9−15) from one C9H13Ox• and one C9H15Ox• contributed the most intensity, whereas the most intense C9 products (C9H14O5−11) could be the alcohol or hydroperoxyl products of C9H13Ox• or the carbonyl products of C9H15Ox• (Table S1). 1,2,3-TMB produced the most C9 products, 1,2,4-TMB the second-most, and 1,3,5-TMB the least. An opposite trend was observed for C18 products. Therefore, the reduction in C9 products was likely due to the dimer formation. Here, we define the C18 fraction as the ratio of the signal intensities of C18 products to the sum of those of C9 and C18 products in the nitrate CI-APi-TOF and the C9 fraction in a similar way. According to our results, the dimer fraction was the highest for aromatics with meta-substituents and the least for aromatics with ortho-substituents if the number and size of substituted groups are identical, while the monomer fraction had an opposite tendency. This can be explained by the stereoselectivity of accretion formation reactions. In the 1,3,5-TMB oxidation experiments (Exp. 2), where the highest C18 dimer fraction was observed, the mole fraction of the C18 dimers is likely determined by the competition of Reactions (R5), (R6), (R7), and (R8), which can be mathematically expressed as
where kR5,R6 stands for the reaction rates for (R5) and (R6), assumed to be around cm3 molecule−1 s−1 by MCM; kR7 is the reaction rate for (R7), set at a typical value of cm3 molecule−1 s−1 (Berndt et al., 2018b; Bianchi et al., 2019); and kR8 is the reaction rate of (R8) for BPRs generated by 1,3,5-TMB, which has recently been measured to be as fast as 10−10 cm3 molecule−1 s−1 (Berndt et al., 2018b).
Since the concentration of HO2 in the OFR was not measured, we utilized a kinetic reaction model (PAM_chem_v8) to characterize the concentration profiles of oxidants in the OFR, which include OH, O3, HO2, and H2O2. A detailed description of this model is given in Section S1 of the supplement, and the modelled profiles of oxidants and precursors are shown in Fig. S4. According to the model, the steady-state concentration of HO2 in Exp. 2 was around 18 ppt ( molecules cm−3). On the other hand, it is difficult to evaluate the effective concentration of the RO2 radicals in the system because RO2 with low oxidation states will not form HOMs via reactions (R5)–(R8). Therefore, we estimated the concentration of RO2 in Eq. (1) to be close to that of BPRs in the OFR. According to MCM v3.3.1, the branching ratio for the peroxide-bicyclic pathway in the OH oxidation of 1,3,5-TMB is 79 % so that the concentration of BPRs was roughly estimated to be 58.5 ppb ( molecules cm−3, 79 % of the reacted 1,3,5-TMB). Hence, the fraction of C18 dimer is estimated to be around 98 %. Clearly, this estimation itself comes with a large uncertainty, and the estimated fraction can only be regarded as an indication of explainable high yields of C18 dimers instead of a rigorous number.
In fact, under our experimental conditions, the C18 dimer fraction in the 1,3,5-TMB experiments was around 86.5 %, which is much higher than the dimer fraction of 42.6 %–56.5 % recalculated using the measured C9 and C18 signals by Tsiligiannis et al. (2019), 43.3 %–52.4 % modelled by Tsiligiannis et al. (2019), and 39 % reported by Molteni et al. (2018). The lack of a m∕z-transmission correction in the former two studies could partially explain the discrepancy (Molteni et al., 2018; Tsiligiannis et al., 2019). On the other hand, this observation could also be due to the much higher RO2 concentrations in our experiments. The amount of reacted 1,3,5-TMB in our experiment is around 74.1 ppb ( molecules cm−3), whereas in the experiments of Tsiligiannis et al. (2019) and Molteni et al. (2018), the numbers are 26 ppb ( molecules cm−3) and 22.3 ppb ( molecules cm−3), respectively. Again, it should be reminded that this result was obtained under the condition of very high concentrations of precursors, and thus the relative fractions of products could be different under the ambient conditions.
3.4 Influence of NOx
To constrain the NOx level in the OFR, the profiles of NO∕NO2 were modelled by PAM_chem_v8, as shown in Fig. S5. The mathematically averaged NOx levels in the low-NOx experiment (Exp. 7) and higher-NOx experiment (Exp. 8) were 92 ppb (2.5 ppb NO + 89.5 ppb NO2) and 295.3 ppb (2.9 ppb NO + 292.4 ppb NO2), respectively. The NOx∕VOC in our experiments is comparable to ambient values in polluted areas. The NOx∕(ΔVOC) was around 0.9 in the low-NOx experiment and 2.9 in the higher-NOx one.
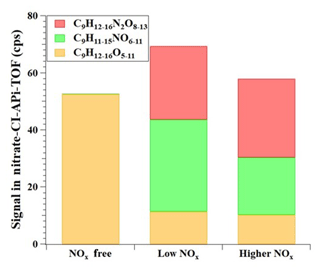
Figure 6Comparison of C9 products detected by the nitrate CI-APi-TOF with zero, one or two nitrogen atoms formed from 1,2,4-TMB oxidation with different NOx settings.
Figure 6 describes the distribution of C9 products detected by the nitrate CI-APi-TOF in the absence of NOx (Exp. 1), a low-NOx experiment (Exp. 7), and a higher-NOx experiment (Exp. 8), respectively. Once NOx was added, the formation of C9 non-nitrogen products declined down to around 20 % of those in Exp. 1. The production of C9 non-nitrogen products did not decrease much between low-NOx experiments and higher-NOx experiments, indicating a nonlinear effect of NOx on the production of C9 non-nitrogen products. Dinitrates (C9HxN2Oy) increased with the NOx concentration, but C9 organonitrates (ONs, C9HxNOy) slightly decreased in the higher-NOx experiment compared to that in the low one, which indicates a complex competition between RO2+RO2 and RO2+NOx.
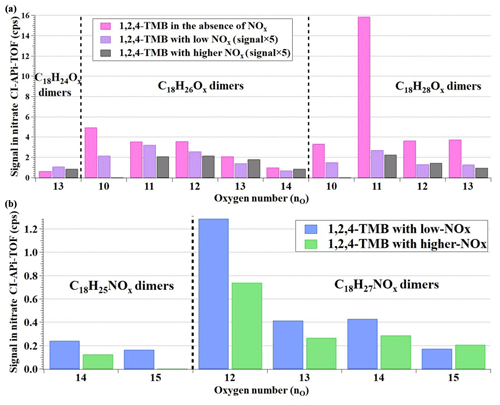
Figure 7(a) Comparison of C18 HOMs formed from 1,2,4-TMB oxidation with different NOx settings and (b) distribution of C18 organonitrates formed from 1,2,4-TMB oxidation.
The observation of C9 products containing one to two nitrogen atoms and C18 products with one nitrogen atom is similar to the results for 1,3,5-TMB oxidation experiments in the presence of NOx reported by Tsiligiannis et al. (2019). NOx can perturb the fate of peroxy radicals by the following reactions (Orlando and Tyndall, 2012; Rissanen, 2018):
Competing with the other RO2 reactions, NOx can dramatically reduce the formation of C9 non-nitrogen products.
Most organonitrates observed in our study were characterized with 13 hydrogen atoms, as detected by the nitrate CI-APi-TOF (Fig. S2). All of them contained more than six oxygen atoms, with molecular formulas corresponding to bicyclic organonitrates formed from termination reactions of C9H13Ox• with NO or NO2 (i.e. pathway R9 and R11, respectively). The dinitrates were dominated by species with 14 hydrogen atoms (Fig. S3). As suggested by Tsiligiannis et al. (2019), an OH radical could attack a nitrated compound that is formed from NOx termination of a peroxy radical, then an oxygen atom is added (similarly to the reactions from C9H14O4 to C9H15O7• in Scheme 2), and then the newly formed peroxy radical that already contained one nitrogen will be terminated by NO or NO2 again. Therefore, most of the detected dinitrates were also formed from C9H13Ox•.
Figure 7a describes the relative intensities of C18 HOMs in Exp. 7 and Exp. 8 as detected by the nitrate CI-APi-TOF in comparison with their relative intensities in Exp. 1. The relative intensities of most of the C18 HOMs decreased with the NOx∕(ΔVOC), while a few of the C18 HOMs including C18H24O13, C18H26O13, C18H26O14, and C18H28O12 increased slightly in the higher NOx experiment, potentially from a combined effect of NOx and OH. The injection of NOx can compete with the other RO2 reactions, and thus it consumes peroxy radicals that would otherwise go through accretion reactions, which explains the decrease in most C18 HOMs. On the other hand, the introduction of NOx can increase the oxidation capacity in the OFR, as it does in the ambient environment, leading to the slight enhancement of the few C18 HOMs. After the addition of NOx, all of the C18 HOMs decreased by a factor of more than 6 compared with those in no-NOx experiments, indicating that the dimers were more strongly influenced than monomers, which is in agreement with a previous study (Tsiligiannis et al., 2019).
The C18 ONs with 25 or 27 hydrogen atoms were detected in the NOx experiments (Fig. 7b). Other C18 products containing nitrogen atoms were not detected. The C18H25NOx might be formed from reactions between a C9H12NOx• radical and a C9H13Ox• radical or between a C9H14NOx• and a C9H11Ox• radical, all of which existed in the system. The C18H27NOx is most likely to be formed from reactions between a C9H14NOx• radical and a C9H13Ox• radical, which were the most abundant C9 radicals. All the C18 ONs decreased with the increase in NOx∕(ΔVOC), which is reasonable. Introduction of NOx into the system triggered reactions between C9 peroxy radicals and NOx, which consequently reduced the formation of accretion products like C18 ONs.
The identities and distribution of oxidation products formed from OH-initiated reactions of three TMBs were obtained with a suite of state-of-the-art chemical ionization mass spectrometers. Our recent study shows that the ring-retaining products are more oxygenated, and quite a lot of carbon–carbon scission products are missing in the current model, indicating that the degradation products of aromatics are much more diverse than what is available in MCM (Mehra et al., 2020). Because of its important contribution to the nucleation and SOA formation in urban areas, the ring-retaining products of TMB deserve a more detailed characterization. Here we have built on that work by showing the formation pathways of ring-retaining highly oxygenated products and through identification of accretion products.
With the assistance of three 1,2,4-(methyl-D3)-TMB experiments, we have demonstrated that the rapid formation of HOMs is attributable to the autoxidation pathway during the TMB oxidation. Several plausible autoxidation pathways for OH-initiated reactions of 1,2,4-TMB were proposed, emphasizing the ring-retaining pathways of aromatics, especially the bicyclic-peroxide channel, which is followed by autoxidation that is not shown in the current models, such as MCM. Oxidation of aromatic VOCs was shown in our study to produce HOM dimers, which might be underestimated or even completely ignored in previous studies which utilize techniques not capable of detecting dimers. The structural enhancement for accretion product formation via the reaction has been observed, of which the meta-substituents were shown to be strongest and ortho-substituents the weakest, though the detailed stereoselectivity for aromatics remains unclear now.
In the presence of NOx, whose reaction with RO2 can compete with RO2 + RO2 or RO2 + HO2 reactions, ONs and dinitrates will be generated via reactions of NOx with BPRs in the 1,2,4-TMB oxidation system, and dimer products with one nitrogen will be formed via the subsequent reactions. This is consistent with recent ambient observations in the polluted environment, where ONs, dinitrates, and nitrogen-containing dimers presumably formed from BVOCs and alkylbenzenes were detected (Brean et al., 2019). The formation of ONs and dinitrates from TMB is not linearly dependent on the NOx concentration, which excludes the possibility of extrapolating our laboratory results to ambient conditions. Nevertheless, the changes in HOM compositions in the presence of NOx, especially the accretion products, could have an effect on NPF and SOA formation. Previous work has shown that the ring-retaining product formation in the NOx environment tends to be more important for TMB than other single substituted C9 aromatics, i.e. isopropylbenzene and propylbenzene, which emphasized the significance of TMB ring-retaining oxidation in the urban environment (Mehra et al., 2020). Further research is needed to acquire a quantitative understanding of the role of NOx in HOM formation.
Clearly, these multifunctional gas-phase products appear at different stages of the oxidation chain. These mass spectra can be used as ideal “fingerprints” of TMB oxidation in the ambient gas-phase measurement to elucidate atmospheric oxidation conditions.
Data related to this article will be available from a persistent repository and upon request from the corresponding authors.
The supplement related to this article is available online at: https://doi.org/10.5194/acp-20-9563-2020-supplement.
LW and YW designed the experiments. YW, GY, XH, and YL carried out the instrument deployment and operation. AM, JK, and AL provided technical support. YW analysed the data. YW, LW, and JK wrote the paper. All co-authors discussed the results and commented on the manuscript.
The authors declare that they have no conflict of interest.
Lin Wang acknowledges the Newton Advanced Fellowship (grant no. NA140106).
This research has been supported by the National Natural Science Foundation of China (grant nos. 91644213 and 21925601) and the National Key R&D Program of China (grant no. 2017YFC0209505).
This paper was edited by Markus Ammann and reviewed by two anonymous referees.
Atkinson, R.: Kinetics and Mechanisms of the Gas-Phase Reactions of the Hydroxyl Radical with Organic Compounds under Atmospheric Conditions, Chem. Rev., 86, 69–201, https://doi.org/10.1021/cr00071a004, 1986.
Atkinson, R. and Arey, J.: Atmospheric Degradation of Volatile Organic Compounds, Chem. Rev., 103, 4605–4638, https://doi.org/10.1021/cr0206420, 2003.
Atkinson, R. and Carter, W. P. L.: Kinetics and Mechanisms of the Gas-Phase Reactions of Ozone with Organic Compounds under Atmospheric Conditions, Chem. Rev., 84, 437–470, https://doi.org/10.1021/cr00063a002, 1984.
Berndt, T., Richters, S., Jokinen, T., Hyttinen, N., Kurtén, T., Otkjær, R. V., Kjaergaard, H. G., Stratmann, F., Herrmann, H., Sipilä, M., Kulmala, M., and Ehn, M.: Hydroxyl radical-induced formation of highly oxidized organic compounds, Nat. Commun., 7, https://doi.org/10.1038/ncomms13677, 2016.
Berndt, T., Mentler, B., Scholz, W., Fischer, L., Herrmann, H., Kulmala, M., and Hansel, A.: Accretion Product Formation from Ozonolysis and OH Radical Reaction of á-Pinene: Mechanistic Insight and the Influence of Isoprene and Ethylene, Environ. Sci. Technol., 52, 11069–11077, https://doi.org/10.1021/acs.est.8b02210, 2018a.
Berndt, T., Scholz, W., Mentler, B., Fischer, L., Herrmann, H., Kulmala, M., and Hansel, A.: Accretion Product Formation from Self- and Cross-Reactions of RO2 Radicals in the Atmosphere, Angew. Chemie Int. Ed., 57, 3820–3824, https://doi.org/10.1002/anie.201710989, 2018b.
Bianchi, F., Kurtén, T., Riva, M., Mohr, C., Rissanen, M. P., Roldin, P., Berndt, T., Crounse, J. D., Wennberg, P. O., Mentel, T. F., Wildt, J., Junninen, H., Jokinen, T., Kulmala, M., Worsnop, D. R., Thornton, J. A., Donahue, N., Kjaergaard, H. G., and Ehn, M.: Highly Oxygenated Organic Molecules (HOM) from Gas-Phase Autoxidation Involving Peroxy Radicals: A Key Contributor to Atmospheric Aerosol, Chem. Rev., 119, 3472–3509, https://doi.org/10.1021/acs.chemrev.8b00395, 2019.
Bloss, C., Wagner, V., Jenkin, M. E., Volkamer, R., Bloss, W. J., Lee, J. D., Heard, D. E., Wirtz, K., Martin-Reviejo, M., Rea, G., Wenger, J. C., and Pilling, M. J.: Development of a detailed chemical mechanism (MCMv3.1) for the atmospheric oxidation of aromatic hydrocarbons, Atmos. Chem. Phys., 5, 641–664, https://doi.org/10.5194/acp-5-641-2005, 2005.
Brean, J., Harrison, R. M., Shi, Z., Beddows, D. C. S., Acton, W. J. F., Hewitt, C. N., Squires, F. A., and Lee, J.: Observations of highly oxidized molecules and particle nucleation in the atmosphere of Beijing, Atmos. Chem. Phys., 19, 14933–14947, https://doi.org/10.5194/acp-19-14933-2019, 2019.
Calvert, J., Atkinson, R., Becker, K. H., Kamens, R., Seinfeld, J., Wallington, T., and Yarwood, G.: The mechanisms of atmospheric oxidation of aromatic hydrocarbons, Oxford University Press, Inc., New York, 2002.
Crounse, J. D., Nielsen, L. B., Jørgensen, S., Kjaergaard, H. G., and Wennberg, P. O.: Autoxidation of organic compounds in the atmosphere, J. Phys. Chem. Lett., 4, 3513–3520, https://doi.org/10.1021/jz4019207, 2013.
D'Ambro, E. L., Lee, B. H., Liu, J., Shilling, J. E., Gaston, C. J., Lopez-Hilfiker, F. D., Schobesberger, S., Zaveri, R. A., Mohr, C., Lutz, A., Zhang, Z., Gold, A., Surratt, J. D., Rivera-Rios, J. C., Keutsch, F. N., and Thornton, J. A.: Molecular composition and volatility of isoprene photochemical oxidation secondary organic aerosol under low- and high-NOx conditions, Atmos. Chem. Phys., 17, 159–174, https://doi.org/10.5194/acp-17-159-2017, 2017.
Ehn, M., Thornton, J. A., Kleist, E., Sipilä, M., Junninen, H., Pullinen, I., Springer, M., Rubach, F., Tillmann, R., Lee, B., Lopez-Hilfiker, F., Andres, S., Acir, I. H., Rissanen, M., Jokinen, T., Schobesberger, S., Kangasluoma, J., Kontkanen, J., Nieminen, T., Kurtén, T., Nielsen, L. B., Jørgensen, S., Kjaergaard, H. G., Canagaratna, M., Maso, M. D., Berndt, T., Petäjä, T., Wahner, A., Kerminen, V. M., Kulmala, M., Worsnop, D. R., Wildt, J., and Mentel, T. F.: A large source of low-volatility secondary organic aerosol, Nature, 506, 476–479, https://doi.org/10.1038/nature13032, 2014.
Eisele, F. L. and Tanner, D. J.: Measurement of the gas phase concentration of H2SO4 and methane sulfonic acid and estimates of H2SO4 production and loss in the atmosphere, J. Geophys. Res., 98, 9001–9010, https://doi.org/10.1029/93JD00031, 1993.
Garmash, O., Rissanen, M. P., Pullinen, I., Schmitt, S., Kausiala, O., Tillmann, R., Zhao, D., Percival, C., Bannan, T. J., Priestley, M., Hallquist, Å. M., Kleist, E., Kiendler-Scharr, A., Hallquist, M., Berndt, T., McFiggans, G., Wildt, J., Mentel, T. F., and Ehn, M.: Multi-generation OH oxidation as a source for highly oxygenated organic molecules from aromatics, Atmos. Chem. Phys., 20, 515–537, https://doi.org/10.5194/acp-20-515-2020, 2020.
Gueneron, M., Erickson, M. H., Vanderschelden, G. S., and Jobson, B. T.: PTR-MS fragmentation patterns of gasoline hydrocarbons, Int. J. Mass Spectrom., 379, 97–109, https://doi.org/10.1016/j.ijms.2015.01.001, 2015.
Hallquist, M., Wenger, J. C., Baltensperger, U., Rudich, Y., Simpson, D., Claeys, M., Dommen, J., Donahue, N. M., George, C., Goldstein, A. H., Hamilton, J. F., Herrmann, H., Hoffmann, T., Iinuma, Y., Jang, M., Jenkin, M. E., Jimenez, J. L., Kiendler-Scharr, A., Maenhaut, W., McFiggans, G., Mentel, T. F., Monod, A., Prévôt, A. S. H., Seinfeld, J. H., Surratt, J. D., Szmigielski, R., and Wildt, J.: The formation, properties and impact of secondary organic aerosol: Current and emerging issues, Atmos. Chem. Phys., 9, 5155–5236, https://doi.org/10.5194/acp-9-5155-2009, 2009.
Heinritzi, M., Simon, M., Steiner, G., Wagner, A. C., Kürten, A., Hansel, A., and Curtius, J.: Characterization of the mass-dependent transmission efficiency of a CIMS, Atmos. Meas. Tech., 9, 1449–1460, https://doi.org/10.5194/amt-9-1449-2016, 2016.
Hyttinen, N., Kupiainen-Määttä, O., Rissanen, M. P., Muuronen, M., Ehn, M., and Kurtén, T.: Modeling the Charging of Highly Oxidized Cyclohexene Ozonolysis Products Using Nitrate-Based Chemical Ionization, J. Phys. Chem. A, 119, 6339–6345, https://doi.org/10.1021/acs.jpca.5b01818, 2015.
Isaacman-VanWertz, G., Massoli, P., O'Brien, R. E., Nowak, J. B., Canagaratna, M. R., Jayne, J. T., Worsnop, D. R., Su, L., Knopf, D. A., Misztal, P. K., Arata, C., Goldstein, A. H., and Kroll, J. H.: Using advanced mass spectrometry techniques to fully characterize atmospheric organic carbon: current capabilities and remaining gaps, Faraday Discuss., 200, 579–598, 2017.
Jenkin, M. E., Saunders, S. M., and Pilling, M. J.: The tropospheric degradation of volatile organic compounds: A protocol for mechanism development, Atmos. Environ., 31, 81–104, https://doi.org/10.1016/S1352-2310(96)00105-7, 1997.
Jenkin, M. E., Saunders, S. M., Wagner, V., and Pilling, M. J.: Protocol for the development of the Master Chemical Mechanism, MCM v3 (Part B): tropospheric degradation of aromatic volatile organic compounds, Atmos. Chem. Phys., 3, 181–193, https://doi.org/10.5194/acp-3-181-2003, 2003.
Jokinen, T., Sipilä, M., Richters, S., Kerminen, V. M., Paasonen, P., Stratmann, F., Worsnop, D., Kulmala, M., Ehn, M., Herrmann, H., and Berndt, T.: Rapid autoxidation forms highly oxidized RO2 radicals in the atmosphere, Angew. Chemie – Int. Ed., 53, 14596–14600, https://doi.org/10.1002/anie.201408566, 2014.
Jokinen, T., Berndt, T., Makkonen, R., Kerminen, V. M., Junninen, H., Paasonen, P., Stratmann, F., Herrmann, H., Guenther, A. B., Worsnop, D. R., Kulmala, M., Ehn, M., and Sipilä, M.: Production of extremely low volatile organic compounds from biogenic emissions: Measured yields and atmospheric implications, P. Natl. Acad. Sci. USA, 112, 7123–7128, https://doi.org/10.1073/pnas.1423977112, 2015.
Jørgensen, S., Knap, H. C., Otkjær, R. V., Jensen, A. M., Kjeldsen, M. L. H., Wennberg, P. O., and Kjaergaard, H. G.: Rapid Hydrogen Shift Scrambling in Hydroperoxy-Substituted Organic Peroxy Radicals, J. Phys. Chem. A, 120, 266–275, https://doi.org/10.1021/acs.jpca.5b06768, 2016.
Krechmer, J., Lopez-Hilfiker, F., Koss, A., Hutterli, M., Stoermer, C., Deming, B., Kimmel, J., Warneke, C., Holzinger, R., Jayne, J., Worsnop, D., Fuhrer, K., Gonin, M., and De Gouw, J.: Evaluation of a New Reagent-Ion Source and Focusing Ion- Molecule Reactor for Use in Proton-Transfer-Reaction Mass Spectrometry, Anal. Chem., 90, 12011–12018, https://doi.org/10.1021/acs.analchem.8b02641, 2018.
Krechmer, J. E., Coggon, M. M., Massoli, P., Nguyen, T. B., Crounse, J. D., Hu, W., Day, D. A., Tyndall, G. S., Henze, D. K., Rivera-Rios, J. C., Nowak, J. B., Kimmel, J. R., Mauldin, R. L., Stark, H., Jayne, J. T., Sipilä, M., Junninen, H., St. Clair, J. M., Zhang, X., Feiner, P. A., Zhang, L., Miller, D. O., Brune, W. H., Keutsch, F. N., Wennberg, P. O., Seinfeld, J. H., Worsnop, D. R., Jimenez, J. L., and Canagaratna, M. R.: Formation of Low Volatility Organic Compounds and Secondary Organic Aerosol from Isoprene Hydroxyhydroperoxide Low-NO Oxidation, Environ. Sci. Technol., 49, 10330–10339, https://doi.org/10.1021/acs.est.5b02031, 2015.
Kroll, J. H. and Seinfeld, J. H.: Chemistry of secondary organic aerosol: Formation and evolution of low-volatility organics in the atmosphere, Atmos. Environ., 42, 3593–3624, https://doi.org/10.1016/j.atmosenv.2008.01.003, 2008.
Kroll, J. H., Donahue, N. M., Jimenez, J. L., Kessler, S. H., Canagaratna, M. R., Wilson, K. R., Altieri, K. E., Mazzoleni, L. R., Wozniak, A. S., Bluhm, H., Mysak, E. R., Smith, J. D., Kolb, C. E., and Worsnop, D. R.: Carbon oxidation state as a metric for describing the chemistry of atmospheric organic aerosol, Nat. Chem., 3, 133–139, https://doi.org/10.1038/nchem.948, 2011.
Kwok, E. S. C. and Atkinson, R.: Estimation of hydroxyl radical reaction rate constants for gas-phase organic compounds using a structure-reactivity relationship: An update, Atmos. Environ., 29, 1685–1695, https://doi.org/10.1016/1352-2310(95)00069-B, 1995.
Lambe, A., Massoli, P., Zhang, X., Canagaratna, M., Nowak, J., Daube, C., Yan, C., Nie, W., Onasch, T., Jayne, J., Kolb, C., Davidovits, P., Worsnop, D., and Brune, W.: Controlled nitric oxide production via O(1D) + N2O reactions for use in oxidation flow reactor studies, Atmos. Meas. Tech., 10, 2283–2298, https://doi.org/10.5194/amt-10-2283-2017, 2017.
Lambe, A. T., Krechmer, J. E., Peng, Z., Casar, J. R., Carrasquillo, A. J., Raff, J. D., Jimenez, J. L., and Worsnop, D. R.: HOx and NOx production in oxidation flow reactors via photolysis of isopropyl nitrite, isopropyl nitrite-d 7, and 1,3-propyl dinitrite at λ = 254, 350, and 369 nm, Atmos. Meas. Tech., 12, 299–311, https://doi.org/10.5194/amt-12-299-2019, 2019.
Lee, B. H., Lopez-Hilfiker, F. D., Mohr, C., Kurtén, T., Worsnop, D. R., and Thornton, J. A.: An iodide-adduct high-resolution time-of-flight chemical-ionization mass spectrometer: Application to atmospheric inorganic and organic compounds, Environ. Sci. Technol., 48, 6309–6317, https://doi.org/10.1021/es500362a, 2014.
Li, H., Riva, M., Rantala, P., Heikkinen, L., Daellenbach, K., Krechmer, J. E., Flaud, P.-M., Worsnop, D., Kulmala, M., Villenave, E., Perraudin, E., Ehn, M., and Bianchi, F.: Terpenes and their oxidation products in the French Landes forest: insights from Vocus PTR-TOF measurements, Atmos. Chem. Phys., 20, 1941–1959, https://doi.org/10.5194/acp-20-1941-2020, 2020.
Li, R., Palm, B. B., Ortega, A. M., Hlywiak, J., Hu, W., Peng, Z., Day, D. A., Knote, C., Brune, W. H., De Gouw, J. A., and Jimenez, J. L.: Modeling the radical chemistry in an oxidation flow reactor: Radical formation and recycling, sensitivities, and the OH exposure estimation equation, J. Phys. Chem. A, 119, 4418–4432, https://doi.org/10.1021/jp509534k, 2015.
Li, Y. and Wang, L.: The atmospheric oxidation mechanism of 1,2,4-trimethylbenzene initiated by OH radicals, Phys. Chem. Chem. Phys., 16, 17908, https://doi.org/10.1039/C4CP02027H, 2014.
Lopez-Hilfiker, F. D., Mohr, C., Ehn, M., Rubach, F., Kleist, E., Wildt, J., Mentel, T. F., Lutz, A., Hallquist, M., Worsnop, D., and Thornton, J. A.: A novel method for online analysis of gas and particle composition: Description and evaluation of a filter inlet for gases and AEROsols (FIGAERO), Atmos. Meas. Tech., 7, 983–1001, https://doi.org/10.5194/amt-7-983-2014, 2014.
Lopez-Hilfiker, F. D., Iyer, S., Mohr, C., Lee, B. H., D'ambro, E. L., Kurtén, T., and Thornton, J. A.: Constraining the sensitivity of iodide adduct chemical ionization mass spectrometry to multifunctional organic molecules using the collision limit and thermodynamic stability of iodide ion adducts, Atmos. Meas. Tech., 9, 1505–1512, https://doi.org/10.5194/amt-9-1505-2016, 2016.
Mentel, T. F., Springer, M., Ehn, M., Kleist, E., Pullinen, I., Kurtén, T., Rissanen, M., Wahner, A., and Wildt, J.: Formation of highly oxidized multifunctional compounds: Autoxidation of peroxy radicals formed in the ozonolysis of alkenes – Deduced from structure-product relationships, Atmos. Chem. Phys., 15, 6745–6765, https://doi.org/10.5194/acp-15-6745-2015, 2015.
Molteni, U., Bianchi, F., Klein, F., Haddad, I. El, Frege, C., Rossi, M. J., Dommen, J., and Baltensperger, U.: Formation of highly oxygenated organic molecules from aromatic compounds, Atmos. Chem. Phys, 18, 1909–1921, https://doi.org/10.5194/acp-18-1909-2018, 2018.
Nah, T., Sanchez, J., Boyd, C. M., and Ng, N. L.: Photochemical Aging of α-pinene and α-pinene Secondary Organic Aerosol formed from Nitrate Radical Oxidation, Environ. Sci. Technol., 50, 222–231, https://doi.org/10.1021/acs.est.5b04594, 2016.
Ng, N. L., Canagaratna, M. R., Zhang, Q., Jimenez, J. L., Tian, J., Ulbrich, I. M., Kroll, J. H., Docherty, K. S., Chhabra, P. S., Bahreini, R., Murphy, S. M., Seinfeld, J. H., Hildebrandt, L., Donahue, N. M., Decarlo, P. F., Lanz, V. A., Prévôt, A. S. H., Dinar, E., Rudich, Y., and Worsnop, D. R.: Organic aerosol components observed in Northern Hemispheric datasets from Aerosol Mass Spectrometry, Atmos. Chem. Phys., 10, 4625–4641, https://doi.org/10.5194/acp-10-4625-2010, 2010.
Orlando, J. J. and Tyndall, G. S.: Laboratory studies of organic peroxy radical chemistry: An overview with emphasis on recent issues of atmospheric significance, Chem. Soc. Rev., 41, 6294–6317, https://doi.org/10.1039/c2cs35166h, 2012.
Otkjær, R. V., Jakobsen, H. H., Tram, C. M., and Kjaergaard, H. G.: Calculated Hydrogen Shift Rate Constants in Substituted Alkyl Peroxy Radicals, J. Phys. Chem. A, 122, 8665–8673, https://doi.org/10.1021/acs.jpca.8b06223, 2018.
Peng, Z., Day, D. A., Stark, H., Li, R., Lee-Taylor, J., Palm, B. B., Brune, W. H., and Jimenez, J. L.: HOx radical chemistry in oxidation flow reactors with low-pressure mercury lamps systematically examined by modeling, Atmos. Meas. Tech, 8, 4863–4890, https://doi.org/10.5194/amt-8-4863-2015, 2015.
Richters, S., Herrmann, H., and Berndt, T.: Different pathways of the formation of highly oxidized multifunctional organic compounds (HOMs) from the gas-phase ozonolysis of α-caryophyllene, Atmos. Chem. Phys., 16, 9831–9845, https://doi.org/10.5194/acp-16-9831-2016, 2016.
Rissanen, M. P.: NO2 Suppression of Autoxidation-Inhibition of Gas-Phase Highly Oxidized Dimer Product Formation, ACS Earth Sp. Chem., 2, 1211–1219, https://doi.org/10.1021/acsearthspacechem.8b00123, 2018.
Rissanen, M. P., Kurtén, T., Sipilä, M., Thornton, J. A., Kangasluoma, J., Sarnela, N., Junninen, H., Jørgensen, S., Schallhart, S., Kajos, M. K., Taipale, R., Springer, M., Mentel, T. F., Ruuskanen, T., Petäjä, T., Worsnop, D. R., Kjaergaard, H. G., and Ehn, M.: The formation of highly oxidized multifunctional products in the ozonolysis of cyclohexene, J. Am. Chem. Soc., 136, 15596–15606, https://doi.org/10.1021/ja507146s, 2014.
Rissanen, M. P., Kurtén, T., Sipilä, M., Thornton, J. A., Kausiala, O., Garmash, O., Kjaergaard, H. G., Petäjä, T., Worsnop, D. R., Ehn, M., and Kulmala, M.: Effects of chemical complexity on the autoxidation mechanisms of endocyclic alkene ozonolysis products: From methylcyclohexenes toward understanding α-pinene, J. Phys. Chem. A, 119, 4633–4650, https://doi.org/10.1021/jp510966g, 2015.
Riva, M., Rantala, P., Krechmer, J. E., Peräkylä, O., Zhang, Y., Heikkinen, L., Garmash, O., Yan, C., Kulmala, M., Worsnop, D., and Ehn, M.: Evaluating the performance of five different chemical ionization techniques for detecting gaseous oxygenated organic species, Atmos. Meas. Tech, 12, 2403–2421, https://doi.org/10.5194/amt-12-2403-2019, 2019.
Saunders, S. M., Jenkin, M. E., Derwent, R. G., and Pilling, M. J.: Protocol for the development of the Master Chemical Mechanism, MCM v3 (Part A): tropospheric degradation of non-aromatic volatile organic compounds, Atmos. Chem. Phys., 3, 161–180, https://doi.org/10.5194/acp-3-161-2003, 2003.
Stolzenburg, D., Fischer, L., Vogel, A. L., Heinritzi, M., Schervish, M., Simon, M., Wagner, A. C., Dada, L., Ahonen, L. R., Amorim, A., Baccarini, A., Bauer, P. S., Baumgartner, B., Bergen, A., Bianchi, F., Breitenlechner, M., Brilke, S., Mazon, S. B., Chen, D., Dias, A., Draper, D. C., Duplissy, J., Haddad, I. El, Finkenzeller, H., Frege, C., Fuchs, C., Garmash, O., Gordon, H., He, X., Helm, J., Hofbauer, V., Hoyle, C. R., Kim, C., Kirkby, J., Kontkanen, J., Kürten, A., Lampilahti, J., Lawler, M., Lehtipalo, K., Leiminger, M., Mai, H., Mathot, S., Mentler, B., Molteni, U., Nie, W., Nieminen, T., Nowak, J. B., Ojdanic, A., Onnela, A., Passananti, M., Petäjä, T., Quéléver, L. L. J., Rissanen, M. P., Sarnela, N., Schallhart, S., Tauber, C., Tomé, A., Wagner, R., Wang, M., Weitz, L., Wimmer, D., Xiao, M., Yan, C., Ye, P., Zha, Q., Baltensperger, U., Curtius, J., Dommen, J., Flagan, R. C., Kulmala, M., Smith, J. N., Worsnop, D. R., Hansel, A., Donahue, N. M., and Winkler, P. M.: Rapid growth of organic aerosol nanoparticles over a wide tropospheric temperature range, P. Natl. Acad. Sci. USA, 115, 9122–9127, https://doi.org/10.1073/pnas.1807604115, 2018.
Teng, A. P., Crounse, J. D., and Wennberg, P. O.: Isoprene Peroxy Radical Dynamics, J. Am. Chem. Soc., 139, 5367–5377, https://doi.org/10.1021/jacs.6b12838, 2017.
Tröstl, J., Chuang, W. K., Gordon, H., Heinritzi, M., Yan, C., Molteni, U., Ahlm, L., Frege, C., Bianchi, F., Wagner, R., Simon, M., Lehtipalo, K., Williamson, C., Craven, J. S., Duplissy, J., Adamov, A., Almeida, J., Bernhammer, A. K., Breitenlechner, M., Brilke, S., Dias, A., Ehrhart, S., Flagan, R. C., Franchin, A., Fuchs, C., Guida, R., Gysel, M., Hansel, A., Hoyle, C. R., Jokinen, T., Junninen, H., Kangasluoma, J., Keskinen, H., Kim, J., Krapf, M., Kürten, A., Laaksonen, A., Lawler, M., Leiminger, M., Mathot, S., Möhler, O., Nieminen, T., Onnela, A., Petäjä, T., Piel, F. M., Miettinen, P., Rissanen, M. P., Rondo, L., Sarnela, N., Schobesberger, S., Sengupta, K., Sipilä, M., Smith, J. N., Steiner, G., Tomè, A., Virtanen, A., Wagner, A. C., Weingartner, E., Wimmer, D., Winkler, P. M., Ye, P., Carslaw, K. S., Curtius, J., Dommen, J., Kirkby, J., Kulmala, M., Riipinen, I., Worsnop, D. R., Donahue, N. M., and Baltensperger, U.: The role of low-volatility organic compounds in initial particle growth in the atmosphere, Nature, 533, 527–531, https://doi.org/10.1038/nature18271, 2016.
Tsiligiannis, E., Hammes, J., Salvador, C. M., Mentel, T. F., and Hallquist, M.: Effect of NOx on 1,3,5-trimethylbenzene (TMB) oxidation product distribution and particle formation, Atmos. Chem. Phys., 19, 15073–15086, https://doi.org/10.5194/acp-19-15073-2019, 2019.
Wang, S., Wu, R., Berndt, T., Ehn, M., and Wang, L.: Formation of Highly Oxidized Radicals and Multifunctional Products from the Atmospheric Oxidation of Alkylbenzenes, Environ. Sci. Technol., 51, 8442–8449, https://doi.org/10.1021/acs.est.7b02374, 2017.
Wang, Y., Riva, M., Xie, H., Heikkinen, L., Schallhart, S., Zha, Q., Yan, C., He, X.-C., Peräkylä, O., and Ehn, M.: Formation of highly oxygenated organic molecules from chlorine-atom-initiated oxidation of alpha-pinene, Atmos. Chem. Phys., 20, 5145–5155, https://doi.org/10.5194/acp-20-5145-2020, 2020.
Yuan, B., Koss, A. R., Warneke, C., Coggon, M., Sekimoto, K., and De Gouw, J. A.: Proton-Transfer-Reaction Mass Spectrometry: Applications in Atmospheric Sciences, Chem. Rev., 117, 13187–13229, https://doi.org/10.1021/acs.chemrev.7b00325, 2017.
Zaytsev, A., Koss, A. R., Breitenlechner, M., Krechmer, J. E., Nihill, K. J., Lim, C. Y., Rowe, J. C., Cox, J. L., Moss, J., Roscioli, J. R., Canagaratna, M. R., Worsnop, D. R., Kroll, J. H., and Keutsch, F. N.: Mechanistic study of the formation of ring-retaining and ring-opening products from the oxidation of aromatic compounds under urban atmospheric conditions, Atmos. Chem. Phys., 19, 15117–15129, https://doi.org/10.5194/acp-19-15117-2019, 2019.
Zhang, Q., Jimenez, J. L., Canagaratna, M. R., Allan, J. D., Coe, H., Ulbrich, I., Alfarra, M. R., Takami, A., Middlebrook, A. M., Sun, Y. L., Dzepina, K., Dunlea, E., Docherty, K., DeCarlo, P. F., Salcedo, D., Onasch, T., Jayne, J. T., Miyoshi, T., Shimono, A., Hatakeyama, S., Takegawa, N., Kondo, Y., Schneider, J., Drewnick, F., Borrmann, S., Weimer, S., Demerjian, K., Williams, P., Bower, K., Bahreini, R., Cottrell, L., Griffin, R. J., Rautiainen, J., Sun, J. Y., Zhang, Y. M., and Worsnop, D. R.: Ubiquity and dominance of oxygenated species in organic aerosols in anthropogenically-influenced Northern Hemisphere midlatitudes, Geophys. Res. Lett., 34, 1–6, https://doi.org/10.1029/2007GL029979, 2007.
Zhao, Y., Thornton, J. A., and Pye, H. O. T.: Quantitative constraints on autoxidation and dimer formation from direct probing of monoterpene-derived peroxy radical chemistry, P. Natl. Acad. Sci. USA, 115, 12142–12147, https://doi.org/10.1073/pnas.1812147115, 2018.
Ziemann, P. J. and Atkinson, R.: Kinetics, products, and mechanisms of secondary organic aerosol formation, Chem. Soc. Rev., 41, 6582–6605, https://doi.org/10.1039/c2cs35122f, 2012.