the Creative Commons Attribution 4.0 License.
the Creative Commons Attribution 4.0 License.
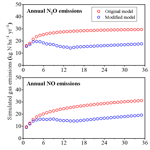
Effects of fertilization and stand age on N2O and NO emissions from tea plantations: a site-scale study in a subtropical region using a modified biogeochemical model
Wei Zhang
Zhisheng Yao
Xunhua Zheng
Chunyan Liu
Rui Wang
Kai Wang
Siqi Li
Shenghui Han
Qiang Zuo
Jianchu Shi
To meet increasing demands, tea plantations are rapidly expanding in China. Although the emissions of nitrous oxide (N2O) and nitric oxide (NO) from tea plantations may be substantially influenced by soil pH reduction and intensive nitrogen fertilization, process model-based studies on this issue are still rare. In this study, the process-oriented biogeochemical model, Catchment Nutrient Management Model – DeNitrification-DeComposition (CNMM-DNDC), was modified by adding tea-growth-related processes that may induce a soil pH reduction. Using a dataset for intensively managed tea plantations at a subtropical site, the performances of the original and modified models for simulating the emissions of both gases subject to different fertilization alternatives and stand ages were evaluated. Compared with the observations in the early stage of a tea plantation, the original and modified models showed comparable performances for simulating the daily gas fluxes (with a Nash–Sutcliffe index (NSI) of 0.10 versus 0.18 for N2O and 0.32 versus 0.33 for NO), annual emissions (with an NSI of 0.81 versus 0.94 for N2O and 0.92 versus 0.94 for NO) and annual direct emission factors (EFds). For the modified model, the observations and simulations demonstrated that the short-term replacement of urea with oil cake stimulated N2O emissions by ∼62 % and ∼36 % and mitigated NO emissions by ∼25 % and ∼14 %, respectively. The model simulations resulted in a positive dependence of EFds of either gas on nitrogen doses, implicating the importance of model-based quantification of this key parameter for inventory purposes. In addition, the modified model with pH-related scientific processes showed overall inhibitory effects on the gases' emissions in the middle to late stages during a full tea plant lifetime. In conclusion, the modified CNMM-DNDC exhibits the potential for quantifying N2O and NO emissions from tea plantations under various conditions. Nevertheless, wider validation is still required for simulation of long-term soil pH variations and emissions of both gases from tea plantations.
- Article
(2767 KB) - Full-text XML
-
Supplement
(838 KB) - BibTeX
- EndNote
Tea (Camellia sinensis (L.) Kuntze), as a perennial cash crop, has been widely cultivated long-term in the tropical and subtropical regions of the world, with nearly 90 % of the global tea harvest area currently located in Asia and over 50 % of that located within China (http://www.fao.org/faostat/, last access: 9 May 2019). To maximize the economic benefits, especially in China, tea production has expanded intensively, mostly through the conversion of arable uplands, rice paddies and forests into tea plantations (e.g., Xue et al., 2013; Yao et al., 2015). For instance, both the total harvest area and production dramatically increased by 166 % (from 1.09×106 to 2.90×106 ha) and 253 % (from 6.8×105 to 2.40×106 Mg), respectively, from 2000 to 2016 (http://www.fao.org/faostat/).
As a leaf- or bud-harvested crop, nitrogen is the key nutrient for yield. Thus, high tea yields are largely supported by the intensive application of nitrogen fertilizers. The nitrogen inputs amount to 450–1200 (mean: 553) kg N ha−1 yr−1 in the primary areas of tea cultivation in China (Han et al., 2013), which is much higher than the recommended doses of 250–375 kg N ha−1 yr−1 (Fu et al., 2012; Hirono and Nonaka, 2012, 2014; Hou et al., 2015; Li et al., 2016; Tokuda and Hayatsu, 2004; Yamamoto et al., 2014; Yao et al., 2015, 2018). This intensive nitrogen application results in superfluous reactive nitrogen remaining in the soil. The excessive reactive nitrogen induces the high potential for nitrous oxide (N2O) and nitric oxide (NO) emissions, thus leading to the detrimental consequences of global warming and air pollution.
The tea plant has been well known as one of the very few families tolerant to high levels of aluminum ion (Al3+) and thus can grow well in acidic soil (Taylor, 1991). Mature leaves of the tea plant may contain up to 30 g Al kg−1 on a dry-weight basis (Matsumoto et al., 1976) without experiencing Al toxicity (Morita et al., 2008). Part of the tissue Al returns to the soil through plant trimming, thus leading to Al accumulation in the surface soil of a tea plantation. In addition, the Al under an acidic condition can be recombined with the organic matter derived from root exudation. This process further facilitates the accumulation of Al in the upper soil layer of a tea plantation (Lin and Yang, 2014). The former mechanism of Al accumulation in surface soil almost does not occur for the absolute majority of plant families that much more weakly absorb of Al than tea plants do (Taylor, 1991; Matsumoto et al., 1976). Hence, the soil pH of tea plantations decreases with the increased stand age jointly due to the processes of (i) acid release by root exudation and (ii) hydrogen ion (H+) production in the hydrolysis of the accumulated Al3+ from residue decomposition in surface soil. The high nitrogen doses combined with the decreased soil pH may further promote the production of the harmful nitrogenous gases through both microbial processes (e.g., nitrification and denitrification) and nonbiological mechanisms (e.g., chemodenitrification; see, e.g., Chen et al., 2017; Fu et al., 2012; Yao et al., 2018), especially for low pH. A number of field studies have demonstrated that much more N2O and NO were emitted from tea plantations than from sites in other upland fields (e.g., Akiyama et al., 2006). In China, the N2O and NO emissions from tea plantations are 16.6 and 14.9 kg N ha−1 yr−1 on average, respectively (Fu et al., 2012; Han et al., 2013; Yao et al., 2015, 2018). In 2013, for instance, the N2O emissions from tea plantations in China accounted for more than one-tenth of the national total emissions of this gas from croplands and contributed to 85 % of the total N2O emissions from global tea plantations (Li et al., 2016). To alleviate the negative impacts on environmental quality and human health, organic fertilization has been strongly recommended in China and was adopted in nearly 4.5×104 ha of tea fields by 2011 (Han et al., 2013). Application of organic fertilizers in tea fields can improve soil fertility (e.g., Han et al., 2013), while stimulating N2O emissions but mitigating NO release (Yao et al., 2015). Therefore, investigating the impacts of replacing synthetic nitrogen fertilizer with organic manure and the effect of stand ages on the emissions of N2O and NO from tea plantations are necessary for understanding the mechanisms of nitrogen cycling and effectively mitigating the emissions of both of the nitrogenous gases from tea fields.
First-hand information of N2O and NO emissions can be obtained from field experiments, but such experiments can be time and labor intensive. Modeling approaches based on sufficient validation have been proposed to overcome the limits of field measurements (e.g., Chen et al., 2017). Because process-oriented biogeochemical models such as DNDC (e.g., Li, 2000), LandscapeDNDC (e.g., Haas et al., 2012), WNMM (e.g., Li et al., 2007) and CNMM-DNDC (Zhang et al., 2018) are generally designed following the basic theories of physics, chemistry, physiology and biology, they are expected to be widely applicable under various climates, soils, land uses and field management practices. These models, in principle, can facilitate the understanding of the interactions among various processes, identify gaps in current knowledge, and temporally and spatially extrapolate the results from experiments (Chen et al., 2008). Among these models, the Catchment Nutrient Management Model – DeNitrification-DeComposition (CNMM-DNDC) model is one of the latest versions of the DNDC. CNMM-DNDC was established by incorporating the core carbon and nitrogen biogeochemical processes of DNDC (including the processes of decomposition, nitrification, denitrification and fermentation) into the hydrological framework of the CNMM, and it therefore inherited the features from both CNMM and DNDC (Zhang et al., 2018). CNMM-DNDC was established to solve a common bottleneck problem of most biogeochemical models, i.e., the inability to simulate the lateral flows of water and nutrients. This solution potentially enables the model to identify the best management practices of intensive cropping systems. In its initial validation in a catchment with calcareous soils and complex landscapes, the CNMM-DNDC performed fairly well in simulating ecosystem productivity (represented by crop yields in croplands), hydrological nitrogen losses by soil leaching and nitrate discharge in streams, and emissions of gaseous carbon (carbon dioxide and methane) and nitrogenous gases (N2O, NO and ammonia) from different lands (forests and arable lands cultivated with maize, wheat, rapeseed or paddy rice; Zhang et al., 2018). However, the scientific processes of soil pH reduction due to tea growth are still lacking representation in the CNMM-DNDC. This gap may induce significant biases in simulating the fluxes in both nitrogenous gases from tea fields, especially for long-term prediction, because soil pH is the key factor regulating N2O and NO emissions from the soil (e.g., Chen et al., 2017; Yao et al., 2018). Therefore, the authors hypothesize that adding the missing scientific processes which lead to soil pH reduction into the internal model program codes can improve the performance of the CNMM-DNDC in simulating the N2O and NO emissions from tea plantations with different stand ages. Filling the gap in the model is especially necessary for predicting the long-term emissions of both gases from tea plantations.
To test the above hypothesis, the authors conducted a case study using a unique experimental dataset, which was obtained by Yao et al. (2015, 2018) in a tea plantation with field treatments of fertilization alternatives and stand ages. The aims of this case study were to (i) attempt to fill the gap in the CNMM-DNDC through the addition of the processes that may induce soil pH reduction due to tea growth; (ii) compare the performances of original and modified models in simulating N2O and NO emissions; and (iii) evaluate the modified model performance in simulating the direct emission factors (EFds) of different annual nitrogen doses and the N2O and NO emissions affected by the short-term replacement of a widely applied synthetic nitrogen fertilizer (urea) with a typical organic manure (oil cake), taking into account the stand ages within the early stage (1–6 years) of a new tea plantation.
2.1 Introduction to the field site and experimental treatments
The field site ( N, E; 441 m above sea level) selected for this modeling case study is located in Fangxian, Hubei province, China. The field site is subject to a northern subtropical monsoon climate, with annual precipitation of 914 mm and a mean air temperature of 14.2 ∘C in 2003–2011 (Yao et al., 2015). Two plots at the field site were involved in this study, encoded as T08 and T12. Both plots had been consecutively cultivated in the long term with paddy rice in summer and upland crops (or drained but fallowed) in winter until tea seedlings were transplanted in March 2008 for T08 and March 2012 for T12. Conventional fertilization practices had been adopted in both plots. A typical synthetic fertilizer (urea) was regularly applied at 450 (150 in autumn and 300 in spring) kg N ha−1 yr−1 (encoded as T08-UN and T12-UN). To determine the annual EFd (the fraction of the applied fertilizer nitrogen released in the form of N2O or NO within the 1-year period after fertilization) of either gas and to investigate the effects of short-term synthetic fertilizer replacement by organic manure on N2O and NO emissions, eight spatially replicated subplots were randomly set in either T08 or T12: four for the control without nitrogen fertilizer applied (NN) and the others for the exclusive application of organic manure (OM) in 2013 (only T08) and 2014 (both T08 and T12). Each daily flux was inferred from the single measurement based on five gas samples from a 30 min enclosure of a static opaque chamber between 09:00 and 11:00 (China standard time). Oil cake, one of the most widely applied organic manures in the subtropical regions of China, was exclusively used to amend the OM subplots to fully replace the urea. Nitrogen doses with the urea application were adopted for the subplots of UN. The NN and OM treatments were encoded as T08-NN, T08-OM, T12-NN and T12-OM. T08-NN and T08-OM were adopted consecutively in 2 full years (from October 2012 to March 2014) and T12-NN and T12-OM in 1 full year (from October 2013 to March 2014). The organic manure in dry weight contained 7.1 % nitrogen and 43.3 % carbon. The topsoil (0–15 cm depth) of the T08 and T12 plots had a loamy texture measured in 2013, and detailed information is provided in the Supplement (Table S1). The soil pH at the time of tea seedling transplanting was 6.0 (Yao et al., 2018). Irrigation was adopted following the typically regional management practice. Daily fluxes of N2O and NO, topsoil (5 cm) temperature, and surface soil (0–6 cm) moisture in water-filled pore space (WFPS) for each field treatment were observed over 2 full years for T08-NN, T08-UN and T08-OM (from mid-September 2012 to mid-October 2014) and 1 full year for T12-NN, T12-UN and T12-OM (from mid-September 2013 to mid-October 2014). For more detailed information on the field experiments and observed data, refer to Yao et al. (2015, 2018) and Table S2.
2.2 Model modifications
In this study, the CNMM-DNDC was modified through (i) defining and applying a soil pH regulating factor (fsph) on plant growth and (ii) adding two processes that produce H+ and thus acidify soils (Miao, 2015; Pang, 2014). These modifications were made to enable the model to simulate the responses and feedbacks between tea growth and soil pH changes.
Considering that the soil pH for tea growth is optimal within 5.0–5.4 and suitable within 4.0–6.5 (Cao et al., 2009), fsph, a dimensionless factor (0–1), is newly parameterized as a quadratic polynomial function utilizing an average soil pH of 0–20 cm (spha) as its single independent variable (Eq. 1). Based on Eq. (1), the value of fsph is around 1.0 when soil pH is within 5.0–5.4 and is above 0.85 when soil pH is within 4.0–6.5. However, the transient soil pH increase induced by urea hydrolysis is not considered for affecting plant growth, because it can be offset due to the soil-buffering effect within a few days. At each time step of the simulation, the value of spha is updated. This parameterized factor is introduced into the model to regulate photosynthesis and thus plant growth, even though the modification to the model has not yet been calibrated or validated due to a lack of sufficient field observations at the selected tea fields.
The two processes newly introduced into the model to simulate additional changes in the H+ concentration (Δ[H+]), thus modifying soil pH, are (i) ionization of the amino acids and other organic acids (HR) in root exudates (Reaction 1) and (ii) hydrolysis of the Al3+ from the decomposition of tea residues due to the trimming (tea leaves and young branches) or falling of old leaves (Reaction 2).
The ionization equilibrium of organic acids is formulated in Reaction (R1), wherein HR represents the category of amino acids or other organic acids in root exudates. Following Eqs. (2)–(4), the H+ concentration changes due to the ionization of these exudate-contained acids (; mol L−1) are calculated by solving Eqs. (3)–(4) (analytical method), which include the parameters of average ionization equilibrium constants for amino acids (Kami; mol L−1) and the other organic acids (Korg; mol L−1) in root exudates and the molar concentrations of amino acids and organic acids in the soil water (cami and corg, respectively; mol L−1). As the acid ionizations are thermodynamic processes, both Kaim and Korg vary with soil temperature (T; ∘C). Their values under various temperature conditions are given via the correction of their constant values at 25 ∘C for both acids, i.e., mol L−1 (Fu, 1999), using a temperature regulating factor, facid (Eqs. 5–6). The function form for parameterizing facid (Eq. 7) was adapted from Li (2016). The molar concentrations of the acids and H+ in the soil water are calculated using Eqs. (8)–(10). In these equations, 10−4 is a dimension adaptor (for each 3 h time step), h denotes the thickness of each soil layer (m), SM stands for the soil moisture in volumetric water content (m3 m−3), Mami and Morg represent the average molar mass of amino acids (128 g mol−1) and the other organic acids (119 g mol−1), respectively, in root exudates (Fu, 1999), aami and aorg are the mass fractions of the two categories of acids in root exudates (dimensionless), Ex is the root exudates in the soil layer (kg ha−1), sph′ denotes the soil pH, and cH(soil) is the H+ concentration corresponding to the most recently updated pH. At each time step (3 h) of the model simulation, 6 % of the net primary productivity is assumed to be released into the soil profile via root exudation. This assumption was made by referring to the experimental data of some other tree species (Miao, 2015). The Ex in the soil layer is determined by portioning the exudate quantity according to the vertical distribution of the root biomass in the soil profile of root depth.
According to Reaction (2), the H+ concentration changes due to the hydrolysis of Al3+ derived from decomposition of tea plant residues () are calculated by solving Eq. (11) (numerical method by Newton iteration). In this equation, Kw ((mol L−1)2) and Kb ((mol L−1)3) denote the water dissociation constant and ionization equilibrium constant of aluminum hydroxide (Al(OH)3), respectively, and cAl(III) is the molar concentration of Al3+ in the soil water (mol L−1). As both water dissociation and Al(OH)3 ionization are also thermodynamic processes, their equilibrium constants (dimensionless) vary with soil temperature and are thus determined following Eqs. (12)–(13), wherein the values at 25 ∘C, i.e., (mol L−1)2 and (mol L−1)3 for water and Al(OH)3, respectively (Fu, 1999), are corrected by the factors fw and fb, respectively. The parameterization for fw (Eq. 14) was cited from Li (2016), and fb was parameterized by Eq. (15). For the calculation of cAl(III) in Eq. (16), MAl denotes the molar mass of Al3+ (27 g mol−1), b the fraction of hydrolyzed Al(OH)3 (dimensionless), c the Al content in tea residues (kg per kg dry matter) and Res the quantity of tea residues in dry matter (kg ha−1). As the Al concentration in tea leaves varied from 1.2 to 2.7 mg per g dry matter, the c value was set as kg per kg dry matter (Hajiboland and Poschenrieder, 2015; Xu et al., 2006).
Using the H+ concentration changes calculated above, the soil pH most recently modified by the originally existing processes, or at the last time step of the simulation, is further updated by Eq. (17). The soil pH updated by Eq. (17) is used to update the independent variable of Eq. (1) so as to provide an update of fsph.
For the processes newly added above, the unknown parameters, aami, aorg and b, were calibrated in this study using the observed soil pH in the T08 and T12 plots. The independent variables of T, h, SM and Res, as well as the net primary production and the root biomass distribution in the soil profile required to calculate Ex, are provided by the model simulations at each time step.
The soil pH dynamics affected by the urea hydrolysis, soil buffering and manure application have already been considered in the original CNMM-DNDC (Table S3). The CNMM-DNDC with and without the above modifications is hereafter referred to as the original and modified model, respectively.
2.3 Evaluation of model simulations for emissions of both gases
The model performances in simulating N2O and NO emissions from the tea plantations were evaluated by comparing the simulations of the original and modified models with the field observations. The required input of hourly meteorological data (air temperature, precipitation, wind speed, solar radiation, humidity) for years with gas flux measurements (2012–2014) were obtained from the meteorological station at the field site, while those in 2008–2011 were adapted from the daily data at the nearby government meteorological station (provided by the National Meteorological Information Center, http://data.cma.cn/, last access: 5 October 2018) by referring to the diurnal patterns of the hourly data observed and provided by the Shennongjia Station (∼40 km south of the tea fields) of the Chinese Ecosystem Research Network. The aforementioned observations were used for the required inputs of soil properties (soil organic carbon (SOC), total nitrogen, mass fraction of clay, pH and bulk density). The required inputs of field capacity and wilting point (0.38 and 0.16, respectively, in volumetric water content) were calculated by the pedotransfer functions used by Li et al. (2019). According to the local survey, the initial biomass of tea seedling transplanting was set as 1500 kg dry matter (DM) ha−1. The harvesting of buds and the trimming of the canopy were started in the fourth year after transplanting (YAT), following the local conventional practices. The bud tea was harvested in T08 from April to May and August to October in the fourth, fifth and sixth YAT, with annual yields of 37.5–150 kg DM ha−1. The tea plants were trimmed twice per year in June and November, and nearly 40 % of the aboveground biomass was cut and left on the ground. The detailed management practices during the gas measurement period were obtained from Yao et al. (2015, 2018), which were also adopted during the remaining period of simulation. The simulated soil profile (0–100 cm depth) was divided into 20 layers. The thickness of each layer was 1, 5 and 10 cm for the top 10, middle 2 and other 8 layers, respectively. The time step of the simulation was set at 3 h. The measured data (Yao et al., 2015, 2018) used for evaluating the model performance included the topsoil temperature and moisture and the daily fluxes of N2O and NO emissions from T08-NN, T08-UN and T08-OM in 2012–2014 and T12-NN, T12-UN and T12-OM in 2013–2014 (Fig. 1).
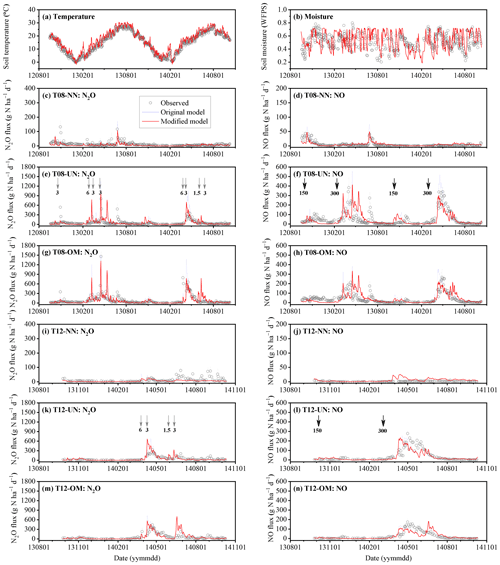
Figure 1Observed and simulated daily mean soil (5 cm) temperature, soil (0–6 cm) moisture, nitrous oxide (N2O) and nitric oxide (NO) fluxes from tea fields of different treatments by the original and modified models. T08 and T12 represent the fields with tea seedling transplanting in 2008 and 2012, respectively. NN, UN and OM encode the no nitrogen applied and fertilization with urea and oil cake, respectively. The gray and black arrows indicate the dates of irrigation and fertilization, respectively. The number under the arrow stands for the applied water amount in centimeters or fertilizer dose in kilograms of nitrogen per hectare. The vertical bar for each observation in panels (c)–(n) indicates the standard error in four spatial replicates. The legend in panel (c) applies to all panels.
2.4 Investigation of fertilization and stand age effects on emissions of both gases
In the field cases involved in this study, the short-term replacement of urea with oil cake was implemented in the second (T12) or fifth to sixth (T08) YAT following the land use change from long-term paddy rice cultivation to perennial tea plantation. Based on the field observations of N2O and NO emissions reported by Yao et al. (2015, 2018), the performance of the original and modified models in simulating the effects of the urea replacement by oil cake was examined through the comparison between the model relative bias (MRB) magnitudes and the observational error indicated by the coefficient of variation (CV). An absolute MRB () smaller than the 2 times the CV of the spatially replicated observations, which represented the observational uncertainty at the 95 % confidence interval (CI), was considered to indicate a statistically satisfactory performance (Dubache et al., 2019). For this examination, the urea replacement effects (Eur; %) on the N2O and NO emissions and their relative observational errors (εur; %) at the 95 % CI were calculated using Eqs. (18)–(19). In both equations, and (in kg N ha−1 yr−1) denote the mean annual emissions of N2O or NO from the OM and UN treatments, respectively, and δo and δu (in kg N ha−1 yr−1) signify the corresponding observational errors in 2 times the standard deviation (SD). Equation (19) is analytically established according to Eq. (18) and following the general error propagation theory. The observed data were directly cited or adapted from Yao et al. (2015, 2018).
The virtual experiments were designed to evaluate the performance of the original and modified models in simulating the annual EFds and to investigate the effects of fertilizer nitrogen doses on EFds. For each field treatment exclusively applied with urea or oil cake in 2013 or 2014, virtual experiments with nitrogen addition rates varying from zero to 600 (with an interval of 50) kg N ha−1 yr−1 were carried out. For each treatment, the gradient nitrogen doses were set only in the experimental year but remained at 450 kg N ha−1 yr−1 in the other year(s). The annual EFds (the fraction of the increased fertilizer nitrogen input released in the form of N2O or NO within the 1-year period after fertilization) as a percentage for the nitrogen dose gradients were simulated at each gradient with an interval (N50) of 50 kg N ha−1 yr−1, following Eq. (20), wherein E50+ and E50− denote the simulated annual emissions of N2O or NO at the higher and lower fertilizer nitrogen dose of the gradient, respectively.
The stand age effects on annual N2O and NO emissions in the early stage (1–6 years) or over a full tea plant lifetime (35 years) of a plantation can be investigated if the applicability of the model has been proven using available observations at the field site. Acceptable model applicability can be indicated by a smaller average than 2 times the CV of the spatially replicated observations. The effects of the stand ages during the early stage (first to sixth YAT) or the full tea lifetime (usually until approximately the 35th YAT in the region) can be investigated using a virtual experiment. The tea plantation in this virtual experiment was purely fertilized with urea at the conventional timings and with the conventional doses. Any influencing factor other than stand age should be excluded from this virtual experiment. To ensure the simulations of all the stand ages could be driven by the same meteorological conditions as those under which the measured data during the year-round period from 17 September 2013 to 9 October 2014 were collected, 35 independent scenarios were designed. Thus, the seedling transplanting for the stand ages of 35, 34, …, 1 year was set to occur in March of 1979, 1980, …, and 2013, respectively. The field management practices for T08-UN would be set for each stand age scenario.
2.5 Statistics and method to quantify uncertainties
The statistical criteria used in this study to evaluate the model performance include (i) the index of agreement (IA), (ii) the Nash–Sutcliffe index (NSI), (iii) the determination coefficient (R2) and slope of the zero-intercept univariate linear regression (ZIR) of the observations against the simulations, and (iv) the MRB. The IA falls between 0 and 1, with a value closer to 1 indicating a better simulation. An NSI value (ranged from minus infinity to 1) between 0 and 1 shows acceptable model performance, while closer to 1 is better. Better model performance is indicated by a slope and an R2 value that are both closer to 1 in a significant ZIR. The performance is regarded as acceptable if a significant ZIR with its slope closer to 1 can be obtained or the on average is smaller than the 2 times the CV of replicated observations. The Akaike information criterion (AIC) is applied to evaluate the significance of the multivariate linear regression. The additional independent variable is significant when the value of the AIC decreases. For more details on these criteria, refer to Eqs. (S1)–(S5) in Table S4 in the Supplement.
The model simulation error (εs) indicated the simulated bias diverging from the observation. It represented the total simulation uncertainty and was made of the uncertainty due to the model insufficiencies in scientific structure or process parameters (εmodel) and that due to the uncertainties in input items (εinput; Zhang et al., 2019). For the investigation of stand age effects, the mean relative εs and its random uncertainty (95 % CI) for either gas were estimated as the mean and the 2 times the SD of the MRBs relative to the observations for three stand ages (i.e., those in the T12-UN and T08-UN fields in the second and fifth to sixth YAT). The relative εs values for a gas were regarded to be equal among the different stand age scenarios. The mean or the 2 times the SD of the relative εs was converted to its absolute magnitude through multiplying it with the product of an adjustment factor and the simulated gas emission quantity. The adjustment factor was obtained from the model validation of the three stand ages, which was estimated as the mean of the ratios of individual observations to simulations. Since the uncertainties in the model input items were known as random errors, the εinput was a random error. It was estimated using the Monte Carlo test with Latin hypercube sampling (Helton and Davis, 2003) within the uncertain ranges (95 % CI) of sensitive input items, which included the soil properties (bulk density, pH, clay fraction, SOC and soil total nitrogen content; e.g., Li, 2016), thermal degree days (TDD) for maturity and nitrogen content in the different plant stages (seedling, early and harvest stages). According to the measurement errors, the uncertain ranges of the input items were 1.11–1.35 g cm−3 for bulk density, 5.6–6.4 for pH, 0.120–0.128 for clay fraction, 9.6–13.6 g kg−1 for SOC content and 1.00–1.48 g kg−1 for total nitrogen content. The uncertainties in the TDD and plant nitrogen content in the three stages were assumed to be ±5 % of the default values, which were 2500 ∘C and 7.8, 6.8 and 6.0 g N kg−1 DM, respectively. A uniform distribution for sampling was assumed in the Monte Carlo test, in which the simulations were iterated until the mean of the simulated gas emission quantities for all iterations converged to a certain level within the tolerance of 1 %. The εinput at the 95 % CI was presented as the double SDs of these iterated simulations.
If not specified, errors are presented hereafter at the 95 % CI.
In this study, the statistical analyses and graphical comparisons were performed with the SPSS Statistics 19.0 (SPSS Inc., Chicago, IL, USA) and Origin 8.0 (OriginLab, Northampton, MA, USA) software packages.
3.1 Calibration of modified model for soil pH simulation
Using the topsoil (0–15 cm) pH (6.0) prior to tea seedling transplanting and the values of 5.4 and 5.0 measured in T12-UN and T08-UN, respectively, in September 2013, each of the three parameters involved in the modified model (Eqs. 8–9 and 16) was calibrated to for aami and aorg and for b. The simulations of the modified CNMM-DNDC with these calibrated parameters resulted in topsoil (0–15 cm) pH values of 5.42 and 5.01 in the T12-UN and T08-UN fields, respectively, in September 2013, which were consistent with the observations. In contrast, the soil pH simulated by the original model remained nearly constant (approximately 6.0) during the 6-year period, despite the transient increases due to urea hydrolysis. Nevertheless, it is still required to validate the simulations of the modified model in terms of the soil pH changes due to tea growth using more field observations under different conditions.
3.2 Model validation for soil environment and emissions of both gases
Both the original and modified models accurately predicted the seasonal dynamics and magnitudes of topsoil temperature and moisture (Fig. 1a–b). The satisfactory model performance is indicated by the statistics in Table 1.
Table 1Statistical evaluation of the original (Ori) and modified (Mod) simulations on the soil temperature (ST), soil moisture (SM), nitrous oxide (N2O) and nitric oxide (NO) fluxes as daily means, annual emissions and annual direct emission factors (EFds).
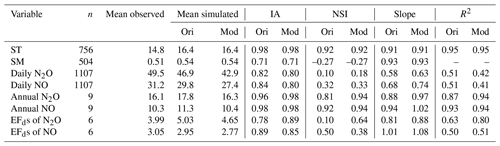
Note that n is number of data pairs. For definitions of IA, NSI, slope and R2, refer to Sect. 2.5 in the text.
The measured daily N2O and NO fluxes were highly variable across the entire observation period (Fig. 1c–n). The original and modified models generally captured the seasonal patterns of both gases for different field treatments, even though the magnitudes of some peak fluxes were inconsistent with the observations. In comparison, the original model generally overestimated the peak emissions of both gases. The performances of both models were similar and satisfying for the daily fluxes as indicated by the comparable IA, NSI, and ZIR slope and R2 values (Table 1). For the original model, three (NO) and five (N2O) of the nine individual simulations for each gas showed larger than the corresponding observed 2 times the CV, while the number of simulations with larger than the observed 2 times the CV were four (NO and N2O) for the modified model (Table S5). However, the statistics of both models still indicated agreements for annual emissions, with the IA and NSI values of 0.96–0.98 and 0.81–0.94, respectively, for N2O and NO (Table 1). In addition, the modified model improved the simulation of annual N2O emissions, with higher IA, NSI, ZIR slope and R2 values of 0.98, 0.94, 0.97 and 0.94 (p<0.001), respectively (Table 1, Fig. 2). These results indicate that the modified CNMM-DNDC can effectively simulate the daily and annual emissions of both gases from the tested tea plantations. Additionally, the modified model resulted in adjustment factors of 0.86 and 1.09 and relative εs values of 17±20 % and % for the annual N2O and NO emissions, respectively, from the UN treatments and adjustment factors of 1.00 and 0.97 and relative εs values of 0.2±24 % and 6±38 % for the N2O and NO emissions, respectively, from the OM plots. These adjustment factors and relative εs were used to estimate the absolute total errors in the simulated emissions.
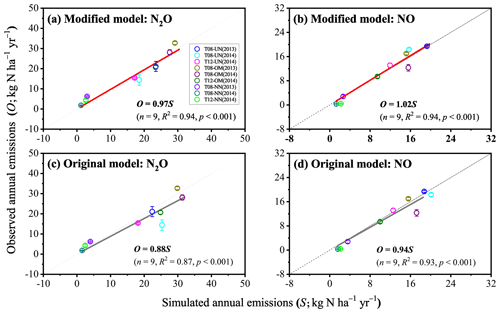
Figure 2Comparison between the observations and simulations of annual nitrous oxide (N2O) and nitric oxide (NO) emissions. The simulations were provided by the original and modified models. The red or gray solid lines illustrate the zero-intercept univariate linear regressions. The vertical bars indicate the standard error in four spatial replicates. The legend in panel (a) applies to all panels.
3.3 Effects of organic fertilization on emissions of both gases
According to the field observations, the short-term replacement of urea by oil cake stimulated the annual N2O emissions by ∼62 % (ranging between 35 % and 95 % or 5.3 and 13.7 kg N ha−1 yr−1) but simultaneously mitigated the annual NO emissions by ∼25 % (ranging between 12 % and 33 % or 2.4 and 6.0 kg N ha−1 yr−1). Based on the statistical analysis using linear mixed models, both the stimulation and mitigation effects were significant (p<0.05; Yao et al., 2015). The average relative observational errors in these effects were ∼97 % (ranging between 92 % and 106 %) for N2O and ∼73 % (ranging between 60 % and 83 %) for NO (adapted from Yao et al., 2015, 2018; Table S6). The simulated effects of the fertilizer replacement on annual N2O emissions by the modified model showed stimulations by ∼36 % (ranging between 24 % and 49 % or 5.7 and 9.1 kg N ha−1 yr−1), with an of ∼36 % (ranging between 4 % and 56 %; Table S6). The magnitudes were significantly lower than the relative observational errors (p=0.02), indicating consistency between the simulated and observed effects. The inhibition effects of the fertilizer replacement on annual NO emissions were about 14 % (varying between 1 % and 21 % or 0.1 and 4.1 kg N ha−1 yr−1) by the modified model except for some underestimation, which indicated the consistency of effects between the simulations and observations (Table S6). As these results suggest, the model with improvements in scientific processes could simulate the effects of short-term replacement of urea by oil cake on N2O and NO emissions in the early stage of the new tea plantations.
3.4 Nitrogen dose effects on annual direct emission factors of both gases
As Figs. 3a–b and S1a–b show, the simulated annual emissions of either gas varied nonlinearly with the nitrogen addition rate in the form of urea or oil cake. Accordingly, for the modified model, the simulated annual EFds of either gas at different levels of fertilizer doses increased linearly with the urea addition rates (Fig. 3c–d) but nonlinearly with the organic manure addition rates (Fig. 3e–f). In comparison with the linear fittings for the manure treatment, the relationships were better fitted the nonlinear curves, as indicated by the decreased AIC values (1.74 versus 1.72 for N2O and 0.53 versus 0.31 for NO). The simulations by the original model showed similar results to those of the modified model (Figs. 3c–f and S1c–f). The original and modified model simulations of annual gas emissions for the two experimental nitrogen doses (zero and 450 kg N ha−1 yr−1) resulted in EFds significantly consistent with the field observations for N2O (Fig. 4a). In comparison with the original model, the modified model performed better in simulating the EFds of N2O, increasing the IA from 0.78 to 0.89 and the NSI from 0.10 to 0.64 (Table 1). For NO, the simulated annual EFds by both models tended to be positively related with the field observations (Fig. 4b), with an acceptable IA of 0.85–0.89 and NSI of 0.38–0.50 (Table 1). These results imply that, compared with the original model, the modified version with the pH reduction processes added in this study could be applied to simulate the EFds of either gas from tea plantations under different field conditions.
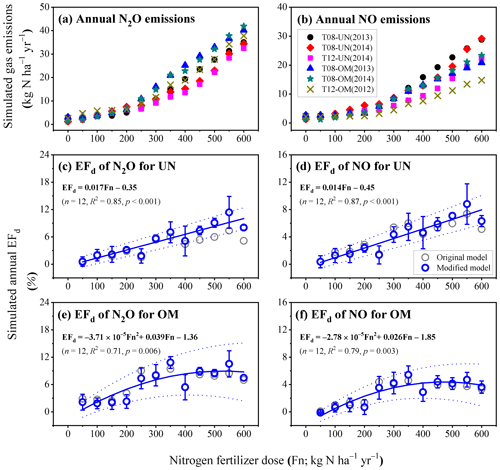
Figure 3Simulated annual emissions and direct emission factor (EFd) of nitrous oxide (N2O) and nitric oxide (NO) from tea plantations with early stand ages against nitrogen fertilizer doses. Data displayed in panels (a)–(b) were simulated by the modified model and those in panels (c)–(f) by the original (gray circle) and modified (blue circle) models. The legend in panel (b) also applies to panel (a), wherein T08 and T12 represent the plantations transplanted with seedlings in 2008 and 2012, respectively; UN and OM indicate the fields consecutively applied with urea since tea planting and short-term replacement of urea with oil cake, respectively; and 2013 and 2014 are the years with field observations of gas emissions. Each vertical bar in panel (c)–(f) is the standard deviation of the EFds for T08 in 2013 and 2014 and for T12 in 2013. Dashed lines are the lower and upper uncertain bounds at the 95 % confidence interval for regression curves. The legend in panel (d) also applies to panels (c), (e) and (f).
3.5 Effects of stand ages on emissions of both gases
The measured annual N2O and NO emissions from the T12-UN and T08-UN fields in the second and fifth to sixth year ranged from 14.4 to 21.1 and 13.1 to 19.4 kg N ha−1 yr−1, with double CVs of ∼43 % (ranging from 9 % to 72 %) and ∼13 % (ranging from 6 % to 21 %), respectively (Yao et al., 2015, 2018). The original model simulations of annual N2O and NO emissions showed an of ∼33 % (ranging from 6 % to 76 %) and ∼6 % (ranging from 3 % to 10 %), respectively, while of the annual N2O and NO emissions were ∼17 % (ranging from 11 % to 28 %) and ∼8 % (ranging from 1 % to 14 %) for the modified model. The on average for either gas (by both models) was smaller than the 2 times the CV on average in the observations. This evaluation indicates that the modified model with the new processes could also reliably simulate the emissions of both gases under different stand age conditions and therefore be applicable for investigating stand age effects in the long term using a virtual experiment.
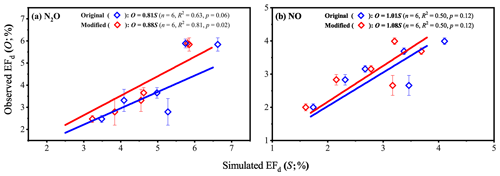
Figure 4Comparison between observed and simulated annual direct emission factor (EFd) of nitrous oxide (N2O) and nitric oxide (NO) by the original and modified models from tea plantations. The vertical bar indicates the standard error in four spatial replicates. The blue and red lines illustrate the zero-intercept univariate linear regressions by the original and modified models. Each simulated EFd is calculated from the simulated emissions of two nitrogen addition levels (zero and 450 kg N ha−1 yr−1).
For the modified model, the simulated daily topsoil (0–15 cm) pH during the early 6-year period basally declined gradually, with a temporary sudden pulse immediately following the urea application events in either spring or autumn (Fig. 5a). Although the simulated pH declined from the initial value of 6.0 to less than 5.0, it was still higher than 4.5 which was the threshold set in the model to trigger the chemodenitrification process. Different from the slightly nonlinear changes in the simulated basal pH, the simulated annual emissions of N2O and NO gradually increased with the stand ages in the first 4 or 5 years but then decreased gradually. The variation trend for the simulated annual emissions of either gas against the early stand ages (1–6 years) could be fitted by a quadratic polynomial equation instead of by a linear relationship as indicated by the decreased AIC values for the nonlinear fitting as compared with that for linear regression (−1.75 versus 0.66 for N2O and −3.67 versus 0.55 for NO). Similar nonlinear relationships were also obtained for the simulations by the original model (Fig. S2). As Fig. 5 indicates, almost all the field observations in the fertilized fields fell not only generally within the range of the uncertainty induced by the input items but also within the upper and lower bounds of uncertainty (95 % CI) of the regressions. Compared with the uncertainty induced by the inputs (εinput), the absolute values of the total model uncertainty (εs) were much smaller, only accounting for 32 % and 35 % of the εinput for N2O and NO, respectively.
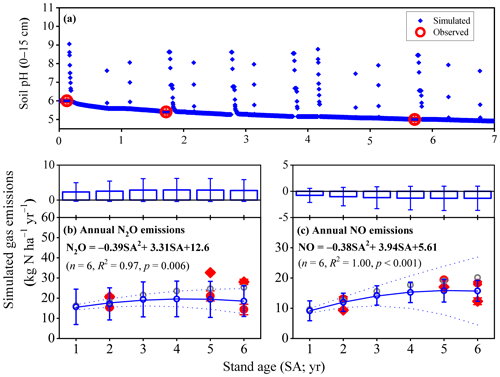
Figure 5Simulated topsoil (0–15 cm depth) pH and annual emissions of nitrous oxide (N2O) and nitric oxide (NO) against early tea stand ages by the modified model. The solid lines are the polynomial regression curves. Dashed lines are the lower and upper uncertain bounds at the 95 % confidence interval (CI) for regression curves. Each pH datum is given as the daily mean of eight diurnal simulations (3 h for each). The vertical bar crossing each datum point in panel (b) or (c) represents the uncertainty (95 % CI) induced by those of model inputs. Each box above panels (b)–(c) represents total model error that was estimated by referring to the mean of model relative biases (MRBs), with vertical bars representing the uncertainties (95 % CI) estimated by referring to the double standard deviations of . The red circles and diamonds in panel (b) and (c) represent the observed emissions of N2O (b) and NO (c) from urea and organic manure treatments. The gray circles in panel (b) and (c) represent the simulation by the original model.
Although the performances of both models in simulating the emissions of both gases were comparable in early stand ages, the original and modified model thereafter performed quite differently. The 35-year simulations demonstrated that the above polynomial functions derived from the original model simulation applied for both gases during the full tea lifetime; but those functions derived from the modified model did not apply for the middle to late stand stages (Fig. 6a). After the annual emissions of both gases simulated by the modified model reached peak values, they decreased near linearly until around the 15th YAT, when the chemodenitrification process was triggered by the pH threshold (4.5) set in the model. Thereafter, the emissions of either gas gradually increased by a very small annual increment (Fig. 6a). Thus, the emissions of both gases simulated by the original model were about 2 times those simulated by the modified model during the middle to late tea stand ages. The εs of the simulation by the modified model ranged from 2.11 to 2.89 and −1.63 to −0.78 for N2O and NO, respectively (Fig. 6a), indicating the potential overestimation or underestimation of either gas for 35-year simulations. Meanwhile, different from the stable topsoil pH (except for the sudden pulse due to urea hydrolysis) by the original model, the simulated basal pH of 0–15 cm by the modified model continued to decrease, finally reaching 3.74 (Fig. 6b–c). In addition, the 35-year simulation showed that the negative effects of soil pH on tea yield increased with the stand ages, resulting in a reduction by 0.3 %–3.4 % (Fig. 6d). These results suggest that the modifications by adding the processes regulating soil pH dynamics are necessary for accurately quantifying the long-term emissions of N2O and NO from tea plantations.
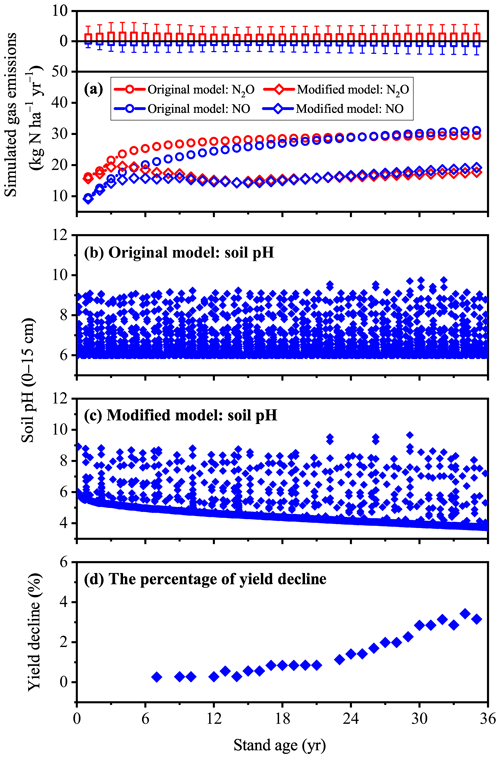
Figure 6Simulated emissions of nitrous oxide (N2O) and nitric oxide (NO) and topsoil (0–15 cm) pH of a urea-fertilized tea plantation against stand ages over the full lifetime of a tea plant (35 years). Each box above panel (a) represents total model error in the simulated emissions by the modified model that was estimated by referring to the mean of model relative biases (MRBs), with vertical bars representing the uncertainties at the 95 % confidence interval estimated by referring to the double standard deviations of . The given percentage of yield decline, simulated by the modified model, was due to the effect of soil pH on tea growth.
4.1 Model modifications
The modified CNMM-DNDC was hypothesized to reflect the general knowledge that tea can grow in soils with a suitable pH within 4.0–6.5 (Cao et al., 2009). But the transient increase in soil pH due to urea hydrolysis has no impact on plant growth, as the soil pH could be recovered within a few days due to the soil-buffering effect. Due to the lack of observed tea yields, the parameterized impact of soil pH on tea growth could not be calibrated or validated in this study, but virtual experiments showed increased yield reduction with increasing stand age, implicating the intensified negative effects on plant growth for older tea plantations. The newly added scientific processes relating to pH reduction were calibrated using the observed soil pH for different stand ages during the early stage of a tea plantation. Although the simulations showed that the modified CNMM-DNDC with the calibrated parameters could accurately reflect the basal soil pH decline during the early years, validation was still missing due to a lack of available independent observations of pH. However, the studies of the tea plantations in Jiangsu and Anhui provinces showed that the average soil pH (0–20 cm) decline rate was 0.06 pH yr−1 (Luo, 2006; Su, 2018). For the simulation of 35-year tea plantation in this study, the calculated average annual soil (0–20 cm) pH decline rate was close to the reports with the value of 0.064 pH yr−1. Therefore, the consistent decline rate indicates the modifications improve the scientific mechanisms of the biogeochemical model which could be applied for long-term simulation. As the actual soil pH would not decline constantly (Yao et al., 2018), the validation of soil pH dynamics over a long time is still necessary. The simulated annual emissions by both models were comparable in the early tea stand ages, but those by the modified model were much lower in the middle to late stages of tea lifetime. According to the modifications, the different annual emissions of both gases should be primarily attributed to the soil pH differences. Therefore, the proper simulation of soil pH decline for long time increased the reliability of the simulated variation in annual emissions even though validation of the differences was still missing due to lack of field observations. Thus, further study is still needed to confirm the general model applicability, especially for the simulations of long-term yields, soil pH dynamics, and N2O and NO emissions from tea plantations subject to different conditions.
4.2 Model performance
This study was the first study testing the original or modified models against the measurements of N2O and NO emissions from a tea plantation. The results showed that both the original and modified models accurately captured the high temporal variations in daily N2O and NO emissions driven by the application of fertilizers, stand ages and weather conditions (Yao et al., 2015, 2018). Many previous studies did not report the R2 of regressions between the observed and simulated daily fluxes of either gas, usually due to poor model performance (Bell et al., 2012; Bouwman et al., 2010; Butterbach-Bahl et al., 2009). Considering the large uncertainties in field measurements as indicated by the SDs of the observations and the complexity of the management practices, the performance of the modified model for either gas was encouraging. Yao et al. (2015, 2018) obtained significant revised hole-in-the-pipe (HIP) regressions for the observed daily N2O plus NO fluxes as the dependent variable and the soil ammonium plus nitrate concentrations, temperature and moisture as the multiple independent variables. Compared with the R2 values of the original HIP regressions fitting the daily observations, those of the revised HIP model more than doubled and were up to 0.95–0.97 (Yao et al., 2015, 2018). Similarly, the daily simulations by the modified model also resulted in significant revised HIP regressions that showed more than doubled R2 (0.48–0.55) in comparison with the values (0.01–0.12) of the original HIP (Mei et al., 2011), despite the smaller determination coefficients than those for the field observations. The improvements of the revised HIP regressions by both observations and simulations were due to the consideration of the temperature- and moisture-regulated effects of nitrogen substrates for both nitrification and denitrification processes that produce N2O and NO.
For the annual N2O emissions, the statistics of the modified model were all better than the original model, indicating the modifications around soil pH reduction improve the model performance in tea plantations. Thus, the simulated corresponding effects of organic fertilization and EFds by the modified model were more consistent with the observations. However, the simulated annual NO emissions by the modified model were not much improved in comparison with those by the original model. The underestimation (2.56 kg N ha−1 yr−1) and overestimation (3.29 kg N ha−1 yr−1) of the NO emissions in 2014 for T08-UN and T08-OM, respectively, resulted in the significant underestimation of the inhibition effects and increased model relative bias for the modified model. The inhibited NO emissions were also partly attributed to the soil heterotrophic nitrification (Yao et al., 2015), which is the direct oxidation of organic nitrogen to nitrate without passing through mineralization. However, the heterotrophic nitrification was not considered in the model, which may result in the overestimated NO emissions in 2014 for the manure treatments by both models. In addition, compared with the original model, the underestimated NO emissions mentioned above were also the key reason for the unsatisfactory simulation of EFds, which led to the increment of the ZIR slope by 8 % (1.0 for the ZIR without T08-UN and 1.08 for the ZIR with T08-UN). Therefore, further study is still required for validating the model performance in simulating NO emissions under different fertilization conditions.
4.3 Contribution of the dominant process for emissions of both gases
The CNMM-DNDC model simulates the emissions of N2O and NO from nitrification and denitrification separately and then sums them up to give the overall emissions of either gas contributed by both processes (e.g., Li, 2016; Zhang et al., 2018). Some researchers have used the NO and N2O molar ratio levels higher or lower than 1 to indicate nitrification or denitrification as the dominant process for the emissions of either gas (e.g., Yamulki et al., 1995). However, Wang et al. (2013) have indicated that such criteria may not be applicable, as they commonly observed molar ratios greater than 1 under strict anaerobic conditions with low to moderate initial nitrate concentrations in a calcareous soil. This viewpoint could be supported by the simulated major contributions of the denitrification process by both models, accounting for 63 %–67 % and 59 %–62 % of the annual N2O and NO emissions, respectively, for all the fertilized fields. These larger contributions from the denitrification process could be at least partially attributed to the hot and humid climate from April to September, which resulted in favorable soil moisture and thus facilitated the N2O and NO emissions. This explanation could be supported by the simulated soil moisture and N2O emissions from the T08-UN treatment with observations in 2 consecutive full years. The simulated daily soil moisture falling in the range of 60 %–90 % WFPS appeared at a frequency of only 40 % during the 2-year period. However, the simulated cumulative N2O emissions (25.7 kg N ha−1) occurring on the days with such relatively high moisture content accounted for 61 % of the total modeled quantity of this gas (42.0 kg N ha−1). It is accepted that nitrification generally dominates N2O production in soils with less than 60 % WFPS (e.g., Chen et al., 2013). The dominant contributions of denitrification to N2O and NO emissions by the simulations could also be supported by previous experimental and modeling studies (Chen et al., 2017; Zhang et al., 2017). However, direct validation of the simulations by the original and modified model on the contributions of nitrification or denitrification is still lacking, due to no available direct measurement of N2O or NO emissions from either process. This challenge will need to be overcome in future studies.
4.4 Effects of organic fertilization on emissions of both gases
For the tea plantations, the applied fertilizers and the retained nitrogen in the soil are consumed by plant uptake, microbial processes and physical losses through ammonia volatilization and nitrate leaching (e.g., Zhang et al., 2015). Accordingly, changes in fertilizer types would affect the nitrogen transformation from the fertilizer to the available forms for the losses, thus altering the N2O and NO emissions (e.g., Deng et al., 2013; Goulding et al., 2008; Skinner et al., 2014). Organic fertilization has been widely encouraged in tea cultivation since it can reduce synthetic nitrogen inputs into the biosphere while improving both soil fertility and carbon sequestration (e.g., Skinner et al., 2014; Liang et al., 2011; Meng et al., 2005). Yao et al. (2015) observed that short-term replacement of urea with oil cake, which is characterized by a low carbon-to-nitrogen ratio, stimulated N2O emissions to a large extent while inhibiting NO releases to a relatively small extent. These observed effects were generally simulated by the original and modified CNMM-DNDC, especially the increased N2O emissions.
According to the model simulations, the stimulated N2O emissions were jointly attributed to (i) the enhanced production of this gas, as well as nitrate, in promoted nitrification and (ii) the enhanced production of this gas in promoted denitrification. The promoted nitrification was due to less ammonia volatilization derived from the organic nitrogen mineralization than from the urea hydrolysis (∼1.0 versus 13 kg N ha−1 yr−1). The oil cake mineralization slowly produced ammonium, while the deep placement of the fertilizer also inhibited ammonia volatilization. In comparison, the urea hydrolysis quickly transformed the fertilizer nitrogen form into ammonium within a few days following the fertilization event, when the hydrolysis-derived pulse increase in soil pH (Fig. 5a) stimulated ammonium loss by ammonia volatilization. The denitrification was promoted not only by the improved supply of nitrate (as the primary nitrogen substrate) from the promoted nitrification (Fig. S3) but also by the enhanced activity of denitrifiers that have a very high affinity for the carbon substrates provided by the organic manure decomposition (e.g., Li et al., 2005; Skinner et al., 2014; Snyder et al., 2009). For the annual NO emissions of the three paired OM-versus-UN cases, the modified model resulted in a consistent decrease (1 %–21 %) due to the full urea replacement by oil cake. The simulations showed that 0 %–44 % of the decreases were ascribed to the promoted nitrification (Table S5), whereby more nitrate was produced as the final product but less NO was produced as the by-product. The remaining 56 %–100 % of the decreases, however, were attributed to the promoted denitrification (Table S5), whereby more NO was reduced to N2O (e.g., Meijide et al., 2007; Snyder et al., 2009; Vallejo et al., 2006). Regarding the contributions of denitrification to the overall N2O or NO emissions, the simulations showed no significant effect of the full urea replacement by oil cake. However, validation of this simulated insignificance is still lacking, because no direct observations for the process contributions are currently available. Further study is still needed to validate the model's performance in simulating the contributions of nitrification or denitrification to the emissions of either gas from tea plantations.
4.5 Effects of nitrogen fertilizer doses on direct emission factors of both gases
Validation of the linear or nonlinear relationships for the urea or manure treatments from the virtual experiment was still lacking, since there were no available data from the experimental field site for the multiple fertilizer gradients. Nevertheless, the relationships of the simulated EFds against the nitrogen doses suggested that paired field observations of fertilized and unfertilized treatments, or those of two largely different nitrogen addition rates, as used in many field studies (e.g., Yao et al., 2015, 2018), would yield greatly biased EFds of either gas from the tea plantations, particularly creating a gross underestimation for moderate to high nitrogen addition rates. This conjecture from the virtual experiment was supported by two studies so far available for field observations of N2O emissions from tea plantations treated with nitrogen dose gradients (Han et al., 2013; Hou et al., 2015), even though similar literature support for NO was still lacking. These experimental studies showed that the EFd determined by the lowest nitrogen addition rates showed a 30 % underestimation on average as compared with the value by the highest nitrogen inputs (adapted from Han et al., 2013, and Hou et al., 2015). Obviously, this study implicates the potential capacity of the modified CNMM-DNDC as a robust tool to generate EFds of tea plantations subject to different conditions, although it is still necessary to widely validate the simulated EFds using field observations with multiple gradients of nitrogen fertilizer doses.
4.6 Effects of stand age on emissions of both gases
Relative to the N2O and NO emissions in the second or sixth YAT, more intensive emissions of both gases were observed in the fifth YAT (Yao et al., 2015, 2018). These relatively intensified emissions were thought to result from the comprehensive effects of increased soil nitrogen and carbon availability for nitrification and denitrification as well as reduced soil pH (Yao et al., 2018). For either gas, the observations in the tea fields purely applied with either urea or oil cake most likely implied a nonlinear trend in terms of stand ages, with the interannual maximum appearing between the second and fifth YAT. This implication was supported by the modified model simulations for a conventionally managed plantation over the full lifetime of tea plant, in which the interannual maximum of N2O emissions appeared in the fourth YAT when the initial harvest of tea bud and the first canopy trim occurred. The increases in the early years were mainly ascribed to the increasing root exudates and less-woody residues returning to soil promoted by the tea plant growth. The simulated interannual maximum emissions of N2O appeared in the year when basal soil pH reached the threshold of about 5.0. The inhibition effect of pH on microbial growth is intensified when soil pH is less than this threshold (Fig. S4). The adopted pH-influencing mechanisms in the model mainly induced the diminished annual emissions of N2O following the appearance of the peak, because the emissions of N2O were associated with the microbial production. In addition to the reduced microbial activity due to low pH inhibition, the postmaximum declines in the annual gas emissions against the stand ages were also attributed to the reduced availabilities of nitrogen substrates for the microbial processes, due to (i) the higher nitrogen demand for the tea growth stimulated by the multiple bud harvests and two trims per year, as well as (ii) the too slow decomposition of woody residues for old tea remaining on the ground surface. However, experimental support is far less sufficient for these explanations on the variations in gas emissions in relation to the stand ages in the early stage or during the full lifetime of tea growth, and thus further studies are still required. In addition, the smaller total model uncertainty, which only accounts for 33 % of the uncertainty induced by inputs, indicated that increasing the reliability of the inputs of soil properties and plant growth parameters can improve the model efficiency.
The decline of emissions following the peaks of both gases may not continue throughout the entire lifetime of tea growth, as the process of chemodenitrification would be triggered once the soil pH decreases to 4.5 or lower, thus promoting the emissions of either gas (e.g., Li, 2016; Pilegaard, 2013). Such a conjecture was well supported by the virtual experiment in this study, which demonstrated that the average soil pH (0–15 cm) decreased to the threshold in the 15th YAT and continued to decrease thereafter. In the model, the chemodenitrification process occurring under the low soil pH (≤4.5) is assumed to transform a portion of the NO produced in the microbial nitrification and denitrification processes into N2O. Before the chemodenitrification was triggered, the simulated microbial nitrification stably accounted for ∼36 % of the overall N2O and ∼41 % of the overall NO emissions. When the chemodenitrification occurred, its contributions to the overall simulated N2O emissions increased from ∼4 % to ∼8 % with increasing stand ages, while the microbial nitrification and denitrification accounted for ∼34 % and ∼59 %, respectively. However, these results of gas emissions from the virtual experiment still require validation with field experiments in future studies.
To fill a gap in the process-oriented biogeochemical model, Catchment Nutrient Management Model – DeNitrification-DeComposition (CNMM-DNDC), the effects of soil pH on tea growth and the processes that may induce soil pH reduction due to root exudation and residue decomposition during tea growth were added into the model in this study. Using the 2-year field measurements in tea plantations at a subtropical site in central China, the original and modified models were evaluated for simulating nitrous oxide (N2O) and nitric oxide (NO) emissions from this important type of agricultural ecosystem. Both the original and modified models showed comparable performance for simulating the daily and annual emissions of N2O and NO from the tested tea plantations at the early stage, especially before the initial tea harvest and the first trim. The modified model was further tested through simulating the emissions of both gases affected by the short-term replacement of synthetic fertilizer (urea) with organic manure (oil cake), gradient nitrogen doses of the two fertilizers and different stand ages of new tea plantations. Both observations and simulations demonstrated that short-term replacement of urea with oil cake can largely stimulate N2O emissions and mitigate NO emissions. The simulations by the modified model also showed linear relationships between the direct emission factors (EFds) of either gas and the nitrogen doses for tea plantations amended with synthetic fertilizer and nonlinear relationships for those plantations applied with organic manure. These relationships support the hypothesis that paired field observations of two largely different nitrogen addition rates, which have very often been implemented in field studies, lead to significant biases for the measured EFds of either gas from the tea plantations. These biases particularly induce significant underestimations for the moderate to high nitrogen doses that are typically applied by farms. The model simulations also showed that annual emissions of either gas increase with stand ages within the early stage of a new tea plantation and then gradually decrease until they slightly increase again due to chemodenitrification triggered by soil pH lower than 4.5. In conclusion, the modified CNMM-DNDC can reflect the comprehensive influences of weather, soil conditions, plant nitrogen demands and field management practices, thus showing potential to be a powerful tool for investigating long-term emissions of N2O and NO from tea plantations under specific field management alternatives at the site or regional scale. Nevertheless, experimental data are still too scarce to validate the model simulations of long-term soil pH changes and their effects on the emissions and EFds of both gases from tea plantations. To improve the robustness of the model for application in various tea plantations, comprehensive validations using simultaneous field observations are still necessary. The validations should include not only the variables involved in this study but also others, such as the emissions of other greenhouse gases (carbon dioxide and methane), volatilization of ammonia, hydrological nitrogen losses by leaching and surface runoff, and temporal changes in the soil organic carbon stock, which are urgently required.
The model and input and output datasets can be obtained from the first author, and all the observed datasets used in this study are available from the coauthors.
The supplement related to this article is available online at: https://doi.org/10.5194/acp-20-6903-2020-supplement.
XZ and WZ contributed to developing the idea and enhancing the science of this study. WZ improved the scientific processes of the model, designed and implemented the model simulations and virtual experiments, and prepared the manuscript with contributions from all coauthors. ZY, CL, RW and KW designed and carried out the field experiments. SL and SH collected and established the input database for modeling. QZ and JS provided the climate data observed in the field site.
The authors declare that they have no conflict of interest.
This article is part of the special issue “Regional assessment of air pollution and climate change over East and Southeast Asia: results from MICS-Asia Phase III”. It is not associated with a conference.
This research has been supported by the National Key R&D Program of China (grant no. 2016YFD0800103), the National Natural Science Foundation of China (grant nos. 41603075 and 41761144054) and the Chinese Academy of Sciences (grant no. ZDBS-LY-DQC007).
This paper was edited by Qiang Zhang and reviewed by two anonymous referees.
Akiyama, H., Yan, X., and Yagi, K.: Estimations of emissions factors for fertilizer-induced direct N2O emissions from agricultural soils in Japan: summary of available data, Soil Sci. Plant Nutr., 52, 774–787, 2006.
Bell, M. J., Jones, E., Smith, P., Yeluripati, J., Augustin, J., Juszczak, R., Olejnik, J., and Sommer, M.: Simulation of soil nitrogen, nitrous oxide emissions and mitigation scenarios at 3 European cropland sites using the ECOSSE model, Nutr. Cycl. Agroecosys., 92, 161–181, 2012.
Bouwman, A. F., Stehfest, E., and Vankessel, C.: Nitrous oxide emissions from the nitrogen cycle in arable agriculture: estimation and mitigation, in: Nitrous oxide and climate change, edited by: Smith, K. Earthscan, London, 2010.
Butterbach-Bahl, K., Kahl, M., Mykhayliv, L., Werner, C., Kiese, R., and Li, C.: A European-wide inventory of soil NO emissions using the biogeochemical models DNDC/Forest-DNDC, Atmos. Environ., 43, 1392–1402, 2009.
Cao, D., Zhang, Q., Xiao, J., and Zong, L.: Localized monitoring of soil acidification rate of tea garden in Jiangsu province, J. Tea Sci., 29, 443–448, 2009 (in Chinese).
Chen, D., Li, Y., Grace, P., and Mosier, A. R.: N2O emissions from agricultural lands: a synthesis of simulation approaches, Plant Soil, 309, 169–189, 2008.
Chen, D., Li, Y., Wang, C., Fu, X., Liu, X., Shen, J., Wang, Y., Xiao, R., Liu, D., and Wu, J.: Measurement and modeling of nitrous and nitric oxide emissions from a tea field in subtropical central China, Nutr. Cycl. Agroecosys., 107, 157–173, 2017.
Chen, H., Li, X., Hu, F., and Shi, W.: Soil nitrous oxide emissions following crop residue addition: a meta-analysis, Glob. Change Biol., 19, 2956–2964, 2013.
Deng, J., Zhou, Z., Zheng, X., and Li, C.: Modeling impacts of fertilization alternatives on nitrous oxide and nitric oxide emissions from conventional vegetable fields in southeastern China, Atmos. Environ., 81, 642–650, 2013.
Dubache, G., Li, S., Zheng, X., Zhang, W., and Deng, J.: Modeling ammonia volatilization following urea application to winter cereal fields in the United Kingdom by improving a biogeochemical model, Sci. Total Environ., 660, 1403–1418, 2019.
Fu, X. C.: University Chemistry, Higher Education Press, Beijing, 1999 (in Chinese).
Fu, X. Q., Li, Y., Su, W. J., Shen, J. L., Xiao, R. L., Tong, C. L., and Wu, J.: Annual dynamics of N2O emissions from a tea field in southern subtropical China, Plant Soil Environ., 58, 373–378, 2012.
Goulding, K., Jarvis, S., and Whitmore, A.: Optimizing nutrient management for farm systems, Philos. T. R. Soc. B, 363, 667–680, 2008.
Haas, E., Klatt, S., Fröhlich, A., Kraft, P., Werner, C., Kiese, R., Grote, R., Breuer, L., and Butterbach-Bahl, K.: LandscapeDNDC: a process model for simulation of biosphere–atmosphere–hydrosphere exchange processes at site and regional scale, Landscape Ecol., 28, 615–636, 2012.
Hajiboland, R. and Poschenrieder, C.: Localization and compartmentation of Al in the leaves and roots of tea plants, Phyton, 84, 86–100, 2015.
Han, W., Xu, J., Wei, K., Shi, Y., and Ma, L.: Estimation of N2O emission from tea garden soils, their adjacent vegetable garden and forest soils in eastern China, Environ. Earth Sci., 70, 2495–2500, 2013.
Helton, J. C. and Davis, F. J.: Latin hypercube sampling and the propagation of uncertainty in analysis of complex systems, Reliab. Eng. Syst. Safe, 81, 23–69, 2003.
Hirono, Y. and Nonaka, K.: Nitrous oxide emissions from green tea fields in Japan: contribution of emissions from soil between rows and soil under the canopy of tea plants, J. Soil Sci. Plant Nut., 58, 384–392, 2012.
Hirono, Y. and Nonaka, K.: Effects of application of lime nitrogen and dicyandiamide on nitrous oxide emissions from green tea fields, J. Soil Sci. Plant Nut., 60, 276–285, 2014.
Hou, M., Ohkama-Ohtsu, N., Suzuki, S., Tanaka, H., Schmidhalter, U., and Bellingrath-Kimura, S. D.: Nitrous oxide emission from tea soil under different fertilizer managements in Japan, Catena, 135, 304–312, 2015.
Li, C.: Modeling trace gas emissions from agricultural ecosystems, Nutr. Cycl. Agroecosys., 58, 259–276, 2000.
Li, C.: Biogeochemistry: Scientific Fundamentals and Modelling Approach, Tsinghua University Press, Beijing, 2016 (in Chinese).
Li, C., Frolking, S., and Butterbach-Bahl, K.: Carbon sequestration in arable soils is likely to increase nitrous oxide emissions, offsetting reductions in climate aadiative forcing, Climatic Change, 72, 321–338, 2005.
Li, S., Zheng, X., Zhang, W., Han, S., Deng, J., Wang, K., Wang, R., Yao, Z., and Liu, C.: Modeling ammonia volatilization following the application of synthetic fertilizers to cultivated uplands with calcareous soils using an improved DNDC biogeochemistry model, Sci. Total Environ., 660, 931–946, 2019.
Li, Y., White, R., Chen, D., Zhang, J., Li, B., Zhang, Y., Huang, Y., and Edis, R.: A spatially referenced water and nitrogen management model (WNMM) for (irrigated) intensive cropping systems in the North China Plain, Ecol. Model., 203, 395–423, 2007.
Li, Y., Zheng, X., Fu, X., and Wu, Y.: Is green tea still “green”?, Geo: Geography and Environment, 3, e00021, https://doi.org/10.1002/geo2.21, 2016.
Liang, B., Yang, X., He, X., Murphy, D. V., and Zhou, J.: Long-term combined application of manure and NPK fertilizers influenced nitrogen retention and stabilization of organic C in Loess soil, Plant Soil, 353, 249–260, 2011.
Lin, T. and Yang, X.: The absorption and accumulation charactertics of aluminum in Camellia Sinensis and its safety evaluation of drinking tea, Tea Sci. Tech., 4, 1–4, 2014 (in Chinese).
Luo, M.: Study on soil acidification status and influence factors of tea plantations in Jiangsu province, Dissertation, Nanjing Agricultural University, 2006.
Matsumoto, H., Hirasawa, E., Morimura, S., and Takahashi, E.: Localization of aluminum in tea leaves, Plant Cell Physiol., 17, 627–631, 1976.
Mei, B., Zheng, X., Xie, B., Dong, H., Yao, Z., Liu, C., Zhou, Z., Wang, R., Deng, J., and Zhu, J.: Characteristics of multiple-year nitrous oxide emissions from conventional vegetable fields in southeastern China, J. Geophys. Res., 116, D12113, https://doi.org/10.1029/2010JD015059, 2011.
Meijide, A., Díez, J. A., Sánchez-Martín, L., López-Fernández, S., and Vallejo, A.: Nitrogen oxide emissions from an irrigated maize crop amended with treated pig slurries and composts in a Mediterranean climate, Agr. Ecosyst. Environ., 121, 383–394, 2007.
Meng, L., Ding, W., and Cai, Z.: Long-term application of organic manure and nitrogen fertilizer on N2O emissions, soil quality and crop production in a sandy loam soil, Soil Biol. Biochem., 37, 2037–2045, 2005.
Miao, X.: Effect of tea root secretion on the transformation mechnism of phophorus and aluminum forms in the soil (Dissertation), Huna Agricultural University, 2015 (in Chinese).
Morita, A., Yanagisawa, O., Takatsu, S., Maeda, S., and Hiradate, S.: Mechanism for the detoxification of aluminum in roots of tea plant (Camellia sinensis (L.) Kuntze), Phytochemistry, 69, 147–153, 2008.
Pang, X.: Effects of tea pruning and polyhoenols on organic acids secretion from roots and mineral contents in camellia sinensis (Dissertaiton), Nanjing Agricultural Univeristy, 2014 (in Chinese).
Pilegaard, K.: Processes regulating nitric oxide emissions from soils, Philos. T. R. Soc. B., 368, 20130126, https://doi.org/10.1098/rstb.2013.0126, 2013.
Skinner, C., Gattinger, A., Muller, A., Mader, P., Fliebetabach, A., Stolze, M., Ruser, R., and Niggli, U.: Greenhouse gas fluxes from agricultural soils under organic and non-organic management-a global meta-analysis, Sci. Total Environ., 468–469, 553–563, 2014.
Snyder, C. S., Bruulsema, T. W., Jensen, T. L., and Fixen, P. E.: Review of greenhouse gas emissions from crop production systems and fertilizer management effects, Agr. Ecosyst. Environ., 133, 247–266, 2009.
Su, Y.: Study on soil acidification characteristics and regulation mechanism of tea garderns in southern Anhui province, Dissertaion, Zhejiang University, 2018.
Taylor, G. J.: Current views of the aluminum stress response: the physiological basis of tolerance, Curr. T. Pl. B., 10, 57–93, 1991.
Tokuda, S. and Hayatsu, M.: Nitrous oxide flux from a tea field amended with a large amount of nitrogen fertilizer and soil environmental factors controlling the flux, J. Soil Sci. Plant Nut., 50, 365–374, 2004.
Vallejo, A., Skiba, U., Garciatorres, L., Arce, A., Lopezfernandez, S., and Sanchezmartin, L.: Nitrogen oxides emission from soils bearing a potato crop as influenced by fertilization with treated pig slurries and composts, Soil Biol. Biochem., 38, 2782–2793, 2006.
Wang, R., Feng, Q., Liao, T., Zheng, X., Butterbach-Bahl, K., Zhang, W., and Jin, C.: Effects of nitrate concentration on the denitrification potential of a calcic cambisol and its fractions of N2, N2O and NO, Plant Soil, 363, 175–189, 2013.
Xu, C., Yu, G., Sun, D., and Wan, G.: Aluminum content determination in brick-tea with chrome azurol S spectrophotometric method, Chin. J. Endemiol., 25, 703–704, 2006.
Xue, H., Ren, X., Li, S., Wu, X., Cheng, H., Xu, B., Gu, B., Yang, G., Peng, C., Ge, Y., and Chang, J.: Assessment of private economic benefits and positive environmental externalities of tea plantation in China, Environ. Monit. Assess., 185, 8501–8516, 2013.
Yamamoto, A., Akiyama, H., Naokawa, T., Miyakazi, Y., Honda, Y., Sano, Y., Nakajima, Y., and Yagi, K.: Lime-nitrogen application affects nitrification, denitrification, and N2O emission in an acidic tea soil, Biol. Fert. Soils, 50, 53–62, 2014.
Yamulki, S., Goulding, K. W. T., Webster, C. P., and Harrison, R. M.: Studies on NO and N2O fluxes from a wheat field, Atmos. Environ., 29, 1627–1635, 1995.
Yao, Z., Wei, Y., Liu, C., Zheng, X., and Xie, B.: Organically fertilized tea plantation stimulates N2O emissions and lowers NO fluxes in subtropical China, Biogeosciences, 12, 5915–5928, https://doi.org/10.5194/bg-12-5915-2015, 2015.
Yao, Z., Zheng, X., Liu, C., Wang, R., and Butterbach-Bahl, K.: Stand age amplifies greenhouse gas and NO releases following conversion of rice paddy to tea plantations in subtropical China, Agr. Forest. Meteorol., 248, 386–396, 2018.
Zhang, W., Liu, C., Zheng, X., Zhou, Z., Cui, F., Zhu, B., Haas, E., Klatt, S., Butterbach-Bahl, K., and Kiese, R.: Comparison of the DNDC, LandscapeDNDC and IAP-N-GAS models for simulating nitrous oxide and nitric oxide emissions from the winter wheat–summer maize rotation system, Agr. Syst., 140, 1–10, 2015.
Zhang, W., Li, Y., Zhu, B., Zheng, X., Liu, C., Tang, J., Su, F., Zhang, C., Ju, X., and Deng, J.: A process-oriented hydro-biogeochemical model enabling simulation of gaseous carbon and nitrogen emissions and hydrologic nitrogen losses from a subtropical catchment, Sci. Total Environ., 616–617, 305–317, 2018.
Zhang, W., Liu, C., Zheng, X., Wang, K., Cui, F., Wang, R., Li, S., Yao, Z., and Zhu, J.: Using a modified DNDC biogeochemical model to optimize field management of a multi-crop (cotton, wheat, and maize) system: a site-scale case study in northern China, Biogeosciences, 16, 2905–2922, https://doi.org/10.5194/bg-16-2905-2019, 2019.
Zhang, Y., Zhao, W., Zhang, J., and Cai, Z.: N2O production pathways relate to land use type in acidic soils in subtropical China, J. Soil Sediment, 17, 306–314, 2017.